- 1Department of Molecular Science and Technology, Ajou University, Suwon, Republic of Korea
- 2Department of Applied Chemistry and Biological Engineering, Ajou University, Suwon, Republic of Korea
Accurate and reliable detection of biological molecules such as nucleic acids, proteins, and small molecules is essential for the diagnosis and treatment of diseases. While simple homogeneous assays have been developed and are widely used for detecting nucleic acids, non-nucleic acid molecules such as proteins and small molecules are usually analyzed using methods that require time-consuming procedures and highly trained personnel. Recently, methods using proximity-enhanced reactions (PERs) have been developed for detecting non-nucleic acids. These reactions can be conducted in a homogeneous liquid phase via a single-step procedure. Herein, we review three assays based on PERs for the detection of non-nucleic acid molecules: proximity ligation assay, proximity extension assay, and proximity proteolysis assay.
1 Introduction
Accurately identifying and quantifying biological molecules such as proteins, small molecules, and nucleic acids plays a crucial role in the diagnosis and treatment of disease. Therefore, developing precise and reliable assays is essential for clinical medicine. Homogeneous assays have recently been developed for nucleic acids based on the specific hybridization between nucleic acid strands. This has enabled the development of simple methods for the early detection of pathogens and abnormal cells (Yan et al., 2014; Trotter et al., 2020). However, for non-nucleic molecules such as proteins and small molecules, detection methods that make use of heterogeneous assays involving solid surfaces have been the standard for several decades (Zhang et al., 2014; Cohen and Walt, 2019). Typical examples of these assays are the enzyme-linked immunosorbent assay and its modified versions, which satisfy key diagnostic features, including robustness, sensitivity, and specificity. However, the procedures involved in performing the assays involve multiple steps and require trained personnel or automated instruments. These assays can therefore be time-consuming and/or expensive. Consequently, there is an increasing demand for homogeneous assays that can detect non-nucleic acid molecules precisely without separating the target analyte from the detection molecule.
Strategies for inducing molecular assembly in the presence of target molecules have been proposed to develop homogeneous methods for detecting proteins and small molecules (Liu et al., 2016; Park and Yoo, 2018; Hwang et al., 2020). For instance, the colocalization of sensors generates a detectable signal, thereby enabling assay performance in the liquid phase with minimal background signal. Förster resonance energy transfer pairs (PJ Santangelo, 2004; Blackstock and Chen, 2014; Graham et al., 2022) and split proteins (Shekhawat and Ghosh, 2011) have both been used to monitor molecular interactions. Covalently or physically linking these molecules to two binders that target independent regions of a molecule can result in detection of the target in the homogeneous phase. When molecules linked to target binders participate in chemical or biological reactions, this reaction can be enhanced in the presence of the target via increased effective concentrations This system, called proximity-enhanced reactions (PERs), has been used to design chemical (Al Sulaiman et al., 2017; Velema and Kool, 2017; Rossetti et al., 2020) and biological reactions (Gullberg et al., 2003; Weibrecht et al., 2010; Blokzijl et al., 2014; Greenwood et al., 2015a; Greenwood et al., 2015b; Liu et al., 2016; Alam, 2018; Park and Yoo, 2018; Rossetti et al., 2020; Wang et al., 2021) for detecting various molecules, including proteins, antibodies, and nucleic acids as well as to characterize molecular interactions (Figure 1). In this review, we describe how PERs have been used to develop methods to detect non-nucleic acid molecules. We focus on three types of assays: proximity ligation assay (PLA; Figure 2A), proximity extension assay (PEA; Figure 2B), and proximity proteolysis assay (PPA; Figure 2C).
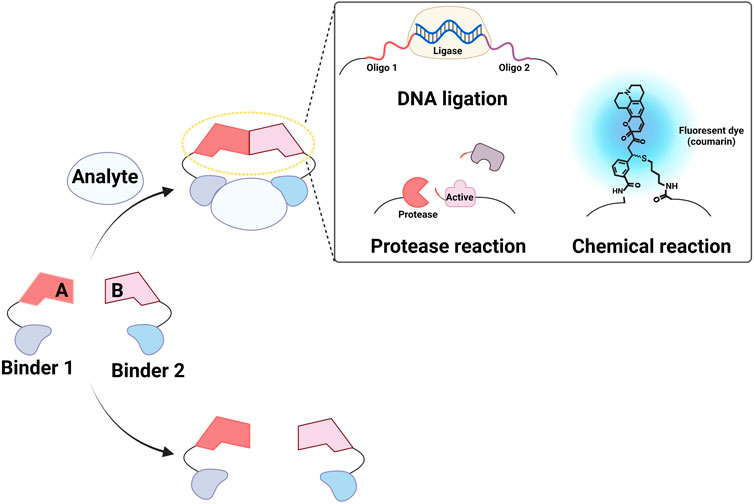
FIGURE 1. Conceptual diagram of proximity-enhanced reactions (PERs). Created with BioRender.com.
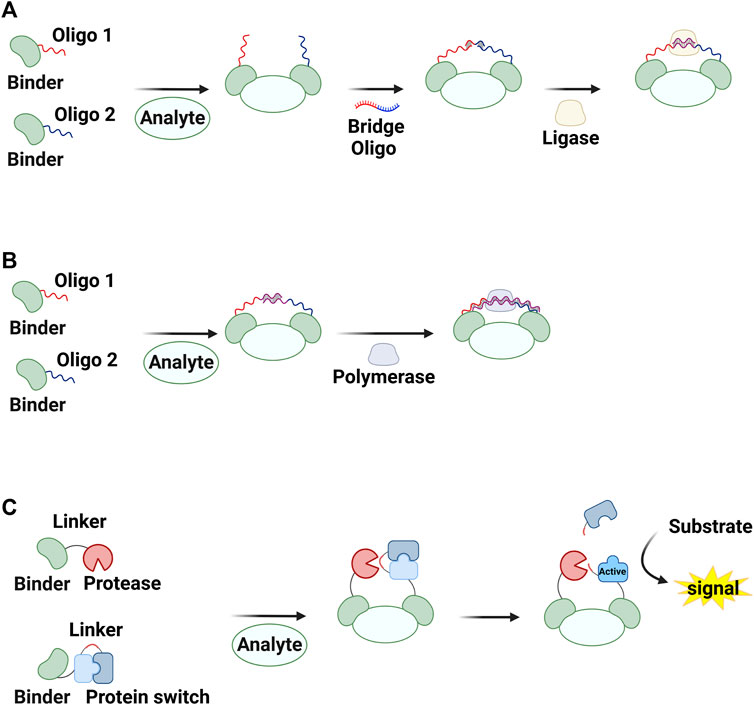
FIGURE 2. Assays based on proximity-enhanced reactions (PERs) described in this review. Shown are (A) a proximity ligation assay (PLA), (B) a proximity extension assay (PEA), and (C) a proximity proteolysis assay (PPA). Created with BioRender.com.
2 PLA
PLA, first reported 20 years ago (Fredriksson et al., 2002), involves a ligation reaction between single-stranded oligonucleotides conjugated to target-binding molecules (Figure 2A). For this reaction, a pair of binder–oligonucleotide conjugates is first placed in proximity in the presence of a target molecule. The ligation reaction, which is initiated via a bridge oligonucleotide and DNA ligase, can be enhanced in the presence of a target molecule via proximity effects. That is, the target concentration increases the yield of the ligated product. The product molecule can then be detected using various DNA amplification techniques. In this section, we first describe conjugates of target binders and oligonucleotides and then discuss methods for detecting ligated oligonucleotides.
2.1 Binder–oligonucleotide conjugates
Antibodies and their fragments, such as scFv and Fab, have been frequently used to prepare binder–oligonucleotide conjugates (Gullberg et al., 2004) (Table 1). Proteins based on alternative scaffolds—e.g., designed ankyrin repeat proteins (DARPins)—have also been developed as binders for targets and have been used to generate binder–oligonucleotide conjugates (Gu et al., 2013) (Table 1). Additionally, antigens have been used as binders for the detection of antibodies (Tsai et al., 2016; Tsai et al., 2018; Cortez et al., 2020; Karp et al., 2020; Cortez et al., 2022; Lind et al., 2022) (Table 1). Aptamers have also been used as binders (Fredriksson et al., 2002; Yang and Ellington, 2008; Joonyul Kim, 2010; Liu et al., 2020; Zhao et al., 2020; Marnissi et al., 2021) (Table 1); they possess a key advantage over protein binders in that binder (aptamer)–oligonucleotide molecules can be prepared as a single molecule via chemical or biological pathways (Fredriksson et al., 2002; Yang and Ellington, 2008; Joonyul Kim, 2010; Liu et al., 2020; Zhao et al., 2020; Marnissi et al., 2021).
Various methods have been used to link protein binders with oligonucleotides. Nucleophilic groups present in proteins, such as the primary amine of lysine and the thiol of cysteine, are frequently employed to conjugate protein binders with oligonucleotides. Oligonucleotides are synthesized chemically to be capable of reacting with the nucleophiles of proteins. For example, in one study, the lysine residues of protein binders were reacted with N-hydroxysuccinimide (NHS)-modified oligonucleotides to prepare protein–oligonucleotide conjugates (Li et al., 2019). The nearly irreversible binding between biotin and streptavidin has also been used to link binders and oligonucleotides. Protein binders can be modified with biotin via the amine–NHS coupling reaction. The resulting biotinylated binders and oligonucleotides can then be assembled via streptavidin, which has four binding sites for biotin (Gustafsdottir et al., 2006; Lundberg et al., 2011a; Dhillon et al., 2016; Chen and Liang, 2022) (Table 1). In another study, the authors made use of the fast and biorthogonal reaction between tetrazine and trans-cyclooctene (TCO). Protein binders were first reacted with NHS–tetrazine and then conjugated with TCO-modified oligonucleotides (van Buggenum et al., 2016). The protein has more than one lysine residue, and its N-terminus is the primary amine. That is, conjugation strategies using amine–NHS coupling inevitably yield heterogeneous products. To overcome this limitation, a method to site-specifically introduce unnatural amino acids into proteins was used to generate binder–oligonucleotide conjugates. Acetyl phenylalanine (AcF) was incorporated into protein binders using an engineered orthogonal amber suppressor aminoacyl-tRNA synthetase/tRNA pair derived from Methanococcus jannaschii (Kazane et al., 2012). The acetyl group was then reacted with aminooxy-modified oligonucleotides, which produced homogeneous products.
2.2 Methods to detect ligated oligonucleotides
2.2.1 Polymerase chain reaction (PCR)
A typical PCR produces millions to billions of copies of a specific segment of DNA. Methods of monitoring the amplification process in a real-time manner, usually via tracking fluorescent signals, are known as quantitative PCR (qPCR). They can quantify the abundance of template DNA with very high sensitivity and specificity (Fredriksson et al., 2002; Gullberg et al., 2003; Gullberg et al., 2004; Gustafsdottir et al., 2006; Fredriksson et al., 2007; Schallmeiner et al., 2007; Fredriksson et al., 2008; Yang and Ellington, 2008; Chang et al., 2009; Joonyul Kim, 2010; Masood Kamali-Moghaddam and Gholamreza Tavoosidana, 2010; Lundberg et al., 2011b; Tavoosidana et al., 2011; Cheng et al., 2012; Gu et al., 2013; Blokzijl et al., 2014; Dhillon et al., 2016; Robinson et al., 2016; Tsai et al., 2016; Jalili et al., 2018; Tsai et al., 2018; Li et al., 2019; Cortez et al., 2020; Karp et al., 2020; Liu et al., 2020; Marnissi et al., 2021; Song et al., 2021; Chen and Liang, 2022; Cortez et al., 2022; Lind et al., 2022) (Table 1). Recently, next-generation sequencing (NGS) following a short PCR has been used to detect PLA products (Darmanis et al., 2011; Nong et al., 2013).
2.2.2 Rolling circle amplification (RCA)
Relying on repeated heating and cooling steps limits the application of PCR-based techniques despite their advantages. Therefore, isothermal amplification methods, such as RCA, have been used to detect the ligated nucleotides produced by PLA (Soderberg et al., 2006; Jarvius et al., 2007; Nilsson et al., 2010; Andersen et al., 2013; Gu et al., 2013; Lioudmila Elfineh, 2014; Li et al., 2017; Poulard et al., 2020; Zhao et al., 2020; Fu et al., 2021) (Table 1). For this procedure, oligonucleotides conjugated to binders are designed to yield circular DNA PLA products; these can then be used as templates for RCA reactions. The amplified products are long single-stranded DNA molecules, and in combination with fluorescent probes, this method can be useful for imaging targets (Soderberg et al., 2006; Jarvius et al., 2007; Nilsson et al., 2010; Andersen et al., 2013; Gu et al., 2013; Lioudmila Elfineh, 2014; Li et al., 2017; Poulard et al., 2020; Zhao et al., 2020; Fu et al., 2021).
2.3 Non-nucleic acid targets detected by PLA
The first PLA method was developed to detect platelet-derived growth factor using two aptamer binders and qPCR (Fredriksson et al., 2002). Since the development of this method, PLA methods coupled with PCR have been developed to detect various non-nucleic acid target molecules, including cytokines (Gullberg et al., 2004), pathogens (Gustafsdottir et al., 2006; Wang et al., 2021), thrombin (Yang and Ellington, 2008; Joonyul Kim, 2010), clenbuterol (Cheng et al., 2012), ractopamine (Cheng et al., 2012), toxins (Dhillon et al., 2016), antibodies (Tsai et al., 2016; Tsai et al., 2018; Cortez et al., 2020; Karp et al., 2020; Cortez et al., 2022), viruses (Liu et al., 2020; Marnissi et al., 2021), and post-translationally modified proteins (Robinson et al., 2016; Oliveira et al., 2018; Song et al., 2021; Chen and Liang, 2022) (Table 1). Multiplexed protein marker detection using PLA methods has been reported using qPCR (Fredriksson et al., 2007; Fredriksson et al., 2008; Chang et al., 2009; Lundberg et al., 2011b; Blokzijl et al., 2014) (Table 1). Moreover, PLA methods for detecting protein–protein interactions (PPIs) (Jarvius et al., 2007; Nilsson et al., 2010; Andersen et al., 2013) and post-translational modifications (PTMs) (Lioudmila Elfineh, 2014; Li et al., 2017; Poulard et al., 2020; Fu et al., 2021) via RCA have also been reported (Table 1).
2.3.1 Antibodies
Antibodies are also widely used as disease biomarkers. In one case, protein antigens were conjugated with oligonucleotides to develop an antibody detection method based on PLA (Tsai et al., 2016). The presence of antibodies enhanced the ligation reaction between the oligonucleotides conjugated with antigens. The ligated products were then detected and quantified using qPCR. Because antibodies can induce the formation of aggregates in the presence of their antigen, this strategy was named “antibody detection by agglutination-PCR” (ADAP; Figure 3A). A method for detecting anti-thyroglobulin antibodies based on ADAP was found to be 1,000-fold more sensitive than an FDA-approved radioimmunoassay (Tsai et al., 2016). The ADAP method has also been used for the early diagnosis of HIV infection (Tsai et al., 2018) and for detecting multiple islet-specific autoantibodies associated with type 1 diabetes (Cortez et al., 2020). Recently, robotic systems have been developed to fully automate ADAP assays (Karp et al., 2020; Cortez et al., 2022).
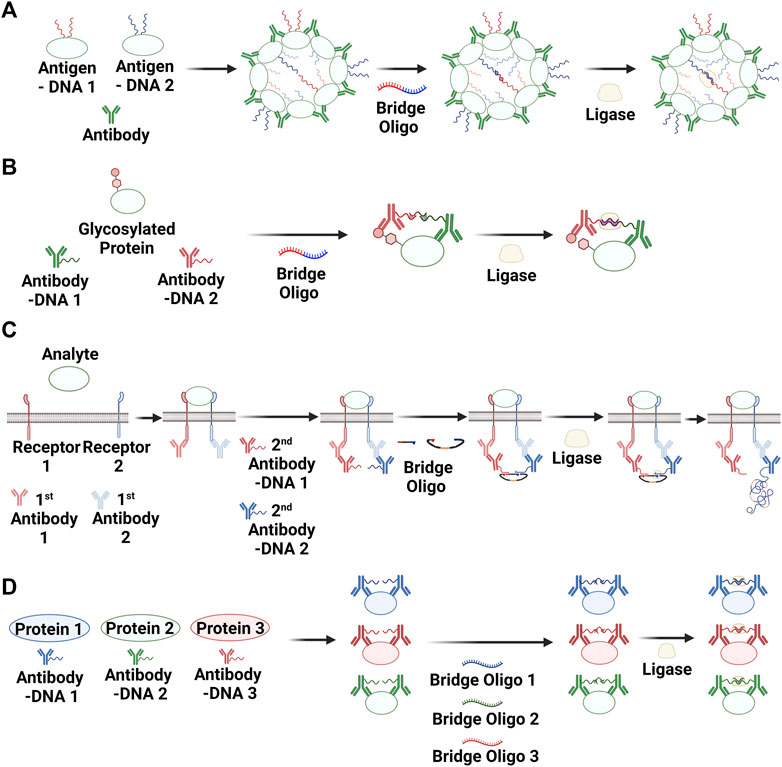
FIGURE 3. PLA methods for detecting antibodies (i.e., via agglutination-PCR) (A), post-translational modifications (B), protein–protein interactions (C), and simultaneous multiple targeting (D). Created with BioRender.com.
2.3.2 PTMs
PTMs are involved in various protein functions and are thus important for understanding diseases (Karve and Cheema, 2011; Darling and Uversky, 2018; Conibear, 2020). Immunoblotting and mass spectrometry have been widely used to identify and elucidate protein modifications (Seo and Lee, 2004; Hermann et al., 2022). Robinson et al. (2016) developed an ultrasensitive method for detecting protein glycosylation based on PLA (Figure 3B). An N-acetylgalactosamine derivative containing an azide group (N-azidoacetylgalatosaime; GalNAz) was transferred to O-N-acetylglucosamine residues on proteins via an enzymatic reaction involving glycosyl transferase. Once this step was complete, the azide group was reacted with biotin–alkyne via click chemistry. Two antibody–oligonucleotide conjugates, one for the protein of interest and the other for biotin, were used for PLA, and the ligated products were quantified using qPCR. PLA-based methods have also been reported to image glycosylated proteins in situ (Li et al., 2017; Fu et al., 2021). Cells were first incubated with tetra-acetylated N-azidoacetylmannosamine, and the monosaccharide as a surrogate of N-acetylmannosamine was incorporated via glycosylation into glycosylated proteins. As in the GalNAz case described above, the azide group was reacted with biotin–alkyne via the click chemistry. RCA instead of PCR was used to image the glycosylated proteins following the ligation reaction. Lectins (Oliveira et al., 2018) and an engineered β-D-N-acetylglucosaminidase mutant (Song et al., 2021) have also been used as binders for glycosylated proteins.
2.3.3 PPIs
The ability to observe transient PPIs is an important tool for understanding key cellular events and their consequences. In particular, the in situ visualization of PPIs can provide additional information for studying dynamic biological processes. Methods consisting of proximity ligation reaction and imaging of the product with RCA and fluorescent probes have been applied to detect numerous PPIs, including c-Myc and Max (Soderberg et al., 2006), VEGFR2 and VEFGR3 (Nilsson et al., 2010), and the IL-7 receptor hetero-complex (Andersen et al., 2013) (Figure 3C).
2.3.4 Multiple targets
The discovery of biomarkers and their applications for diagnosis or treatment have played a central role in clinical medicine (Blokzijl et al., 2014; Davis et al., 2020). Given the complexity of biological processes, detecting one biomarker within a sample does not usually provide enough information for understanding the disease state (Chang et al., 2009; Zemans et al., 2017). Thus, there is an ongoing need for methods capable of analyzing multiple biomarkers from a small amount of a single sample. A multiplexed protein detection method based on PLA was first reported in 2007 (Fredriksson et al., 2007), and this strategy has been used to identify biomarkers for various cancers (Figure 3D). Furthermore, NGS after a short PCR has been used to examine the composition of a mixture of ligated oligonucleotides (Darmanis et al., 2011; Nong et al., 2013), which enables the analysis of many biomarkers from one sample.
3 PEA
PEAs also rely on reaction enhancement based on a pair of binder–oligonucleotide conjugates. In this procedure, two single-stranded oligonucleotides conjugated to binders hybridize with each other in the presence of a target molecule via the proximity effect, and DNA polymerase then generates double-stranded DNAs (Figure 2B). The resulting amplicons can be analyzed using PCR or isothermal amplification methods (Di Giusto et al., 2005; Lundberg et al., 2011a; Lundberg et al., 2011b; Stine Buch Thorsen, 2013; Assarsson et al., 2014; Berggrund et al., 2019; Li et al., 2021b; Zhang et al., 2021; Hu et al., 2022) (Table 1). PEA has the same advantages as PLA, including reaction homogeneity, high sensitivity, high specificity, and low sample consumption. Moreover, PEA has been found to be less sensitive to reaction conditions. For example, T4 DNA polymerase used in a typical PEA protocol performed well in blood plasma, which is different from DNA ligases for PLA (Lundberg et al., 2011a; Lundberg et al., 2011b). Lundberg et al. (2011a) reported a PEA method for analyses of human blood. This method enabled the sensitive and specific detection of low-abundance proteins from human blood plasma. In combination with qPCR or NGS, this method has been used for multiplex analyses of human proteomes in high throughput ways (Lundberg et al., 2011a; Stine Buch Thorsen, 2013; Assarsson et al., 2014; Berggrund et al., 2019; Wik et al., 2021). The assay itself has been commercialized by Olink (Uppsala, Sweden). While RCA is typically used as an isothermal amplification method for PLA, another strategy, termed the exponential amplification reaction (EXPAR) has been used for PEA (Figure 4) (Li et al., 2021b; Zhang et al., 2021; Hu et al., 2022). Strand-displacing DNA polymerases such as phi29 DNA polymerase and Bst DNA polymerase (large fragment) are used instead of T4 DNA polymerase to generate double-stranded DNAs. One strand of the product is cleaved by nicking endonucleases, and then the strand-displacing DNA polymerases synthesize DNA strands starting from the nicked site, yielding ssDNA amplicons. These amplicons can be detected in various ways, including fluorescence signals coming from G-quadruplexes complexed with thioflavin T (Zhang et al., 2021), molecular beacons (Hu et al., 2022), and Cas12a activation (Li et al., 2021b).
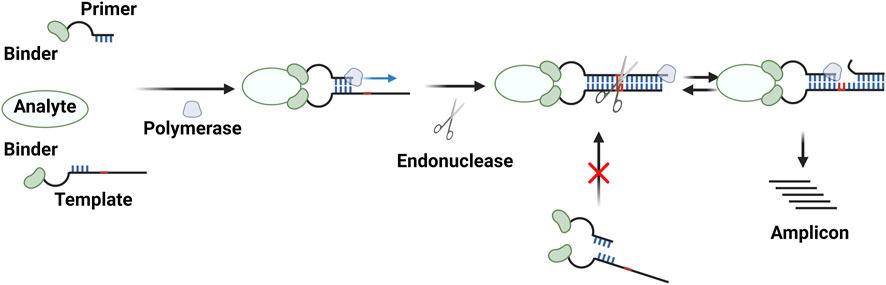
FIGURE 4. Diagram showing the PEA method with an exponential amplification reaction (EXPAR). Created with BioRender.com.
4 PPA
The concept of enhancing reaction rates via proximity can be generalized from ligase- and polymerase-mediated reactions to many other types of chemical and enzymatic reactions. Several research groups, including us, have recently reported PER-based assay methods. One of these, termed PPA, has been successfully developed into methods for detecting non-nucleic acid targets (Table 1). A protease and its substrate (i.e., zymogen) are linked to target binders. The proteolysis reaction is enhanced in the presence of the target, and the activated zymogen generates a detectable signal (Figure 2C). The first example of a PPA method used to detect a non-nucleic acid molecule was reported by Stein and Alexandrov (Stein and Alexandrov, 2014) (Figure 5A). Zymogen was designed as a hepatitis C virus NS3 serine protease (HCV) was connected to its inhibitory peptide via a flexible linker. The linker includes an amino acid sequence that is cleavable by a nuclear inclusion a (NIa) protease from tobacco vein mottling virus (TVMV). TVMV was also fused to its inhibitory peptide to decrease its activity, and this can reduce background signal in the absence of a target of interest. The authors developed a homogeneous method to detect rapamycin by fusing two engineered proteases to rapamycin-binding proteins (the FRB and FKBP12 domains). A proteolysis reaction by TVMV was found to be enhanced in the presence of rapamycin, and the activated HCV generated a fluorescence signal by hydrolyzing a quenched substrate.
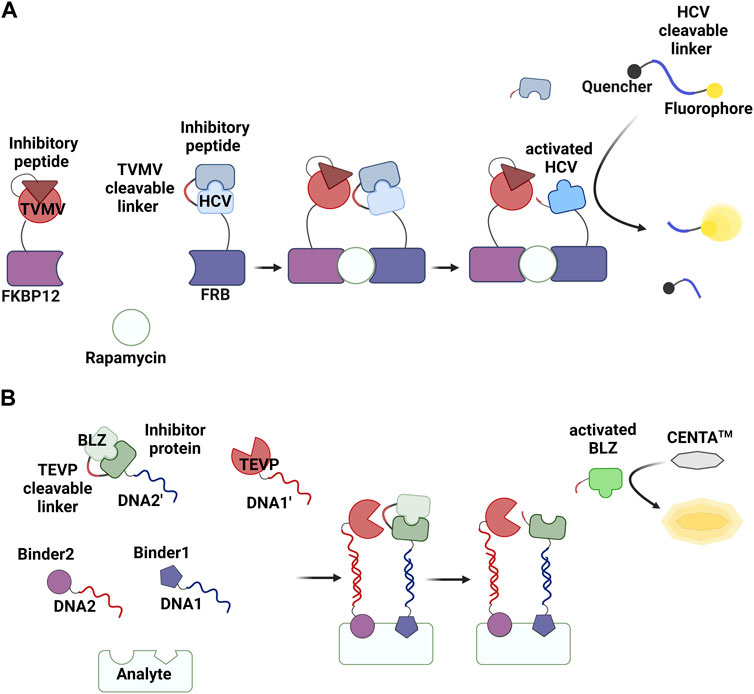
FIGURE 5. PPA methods reported by Stein and Alexandrov (Stein and Alexandrov, 2014) (A) and by Park et al. (Park et al., 2021) (B). Created with BioRender.com.
Park et al. (2021) developed a PPA system that can be used for detecting various molecules (Figure 5B). In this method, a protease (tobacco, etch virus protease; TEVP) and an engineered β-lactamase zymogen (BLZ) (Kim et al., 2014) that can be activated by TEVP are linked to target binders via specific hybridization between complementary DNAs. Conjugation between proteins and oligonucleotide was achieved via a strain-promoted click reaction between azide and cyclooctyne (Park and Yoo, 2018). An azide-containing unnatural amino acid (4-azido-phenylalanine; AzF) was site-specifically introduced into these proteins using an orthogonal pair of tRNA and an aminoacyl-tRNA synthetase engineered to specifically incorporate AzF into the amber codon (Park et al., 2021; Park et al., 2022). The single-stranded DNAs were modified with dibenzocycloocyne. Various molecules, including antibodies, proteins, aptamers, and small molecules, have been used as target binders, and different strategies were employed to conjugate target binders with oligonucleotides. This assay can be conducted in a one-pot format by incubating four conjugates (TEVP-DNA1’, BLZ-DNA2’, Binder1-DNA1, and Binder2-DNA2) and a chromogenic substrate for β-lactamase (CENTA™) with samples, after which a change in absorbance is measured. Homogeneous assay methods have successfully been developed for detecting various analytes, including the ectodomain of human epidermal growth factor receptor-2, cardiac troponin I, thrombin, digoxigenin (Dig), and anti-Dig antibody, at subnanomolar concentrations using a one-step procedure and color signal.
5 Conclusion
One strategy to develop methods for detecting biomarkers is via inducing molecular assembly in the presence of a target, which in turn generates a detectable signal. When molecules participating in chemical or biological reactions are parts of the molecular assembly, the reaction between the molecules can be enhanced by their increased effective concentrations. This concept, known as PER, has been used to develop methods for detecting and quantifying non-nucleic acid molecules in many studies. Herein, we described three types of assays based on PERs: PLA, PEA, and PPA. While assays for detecting nucleic acids are usually conducted in a homogenous liquid, the detection of non-nucleic acid molecules often depends on methods involving multiple steps (ELISA) or suffers from limited sensitivities (LFA) (Table 2). PER-based approaches enable the development of homogenous assays for detecting targets such as proteins, small molecules, molecular interactions, and nucleic acids. These simple and sensitive assays can identify and quantify the content of many biological molecules, which is crucial for the diagnosis and treatment of diseases.
The strategy used to design binder–oligonucleotide conjugates is modular, and various binders—including ones that were previously investigated for targets—can be used to develop assay methods based on PERs. At present, antibodies are the most used binders for PERs; which sometimes involve complicated processes to link antibodies and oligonucleotides (Tables 1, 2). On the contrary, aptamers have several advantages over antibodies, including their small size, high stability, and production via chemical synthesis (Thiviyanathan and Gorenstein, 2012; Li et al., 2021a). Moreover, binder (aptamer)–oligonucleotide molecules can be synthesized as one-strand oligonucleotides, and this process is much simpler and cheaper than many of the methods used to conjugate protein binders and chemically modified oligonucleotides. Currently, the number of available aptamers is much smaller than that of antibodies. However, technological improvement of instruments and advances in automation are expected to accelerate the discovery of target-specific aptamers (Michael Famulok and blind, 2000; Shaban and Kim, 2021).
Olink (Uppsala, Sweden) has successfully launched products based on its PEA technology, and one of them (Olink® Explore 3072) coupled with NGS readouts enables ∼3000 protein assays in a high-throughput way. However, point-of-care tests (POCTs) based on PERs have not yet been widely developed, probably because of the detection methods of PER-based assays. For example, fluorescent signals are most commonly used to measure the products of PLAs and PEAs, and the detection of these signals requires complex instruments for quantitative analysis. Several POCTs for detecting nucleic acids have been very recently developed, and these were mainly related to the SARS-CoV-2 pandemic (Islam and Iqbal, 2020; Kang et al., 2022; Ye et al., 2022). Incorporating the newly development methods would be one approach to developing POCTs based on PLAs and PEAs. The PPA (Figure 4B) used the β-lactamase zymogen as a reporter to produce an absorbance signal. This can be quantified by a relatively simple instrument, such as a smartphone (Bergua et al., 2022). Thus, this method has potential for the development of POCTs to detect non-nucleic acid targets. Moreover, the strategy used to design the β-lactamase zymogen can be applied to engineer other reporter enzymes (Inoue et al., 2010; Stein and Alexandrov, 2015) into zymogens, and various such PPAs are expected to be developed in the future.
Author contributions
All authors listed have made a substantial, direct, and intellectual contribution to the work and approved it for publication.
Funding
This research was supported by the Challengeable Future Defense Technology Research and Development Program through the Agency for Defence Development (ADD) funded by the Defense Acquisition Program Administration (No. UI220005TD).
Acknowledgments
All figures were created using BioRender.com.
Conflict of interest
The authors declare that the research was conducted in the absence of any commercial or financial relationships that could be construed as a potential conflict of interest.
Publisher’s note
All claims expressed in this article are solely those of the authors and do not necessarily represent those of their affiliated organizations, or those of the publisher, the editors and the reviewers. Any product that may be evaluated in this article, or claim that may be made by its manufacturer, is not guaranteed or endorsed by the publisher.
References
Al Sulaiman, D., Chang, J. Y. H., and Ladame, S. (2017). Subnanomolar detection of oligonucleotides through templated fluorogenic reaction in hydrogels: Controlling diffusion to improve sensitivity. Angew. Chem. Int. Ed. Engl. 56, 5247–5251. doi:10.1002/anie.201701356
Alam, M. S. (2018). Proximity ligation assay (PLA). Curr. Protoc. Immunol. 123, e58. doi:10.1002/cpim.58
Andersen, S. S., Hvid, M., Pedersen, F. S., and Deleuran, B. (2013). Proximity ligation assay combined with flow cytometry is a powerful tool for the detection of cytokine receptor dimerization. Cytokine 64, 54–57. doi:10.1016/j.cyto.2013.04.026
Assarsson, E., Lundberg, M., Holmquist, G., Bjorkesten, J., Thorsen, S. B., Ekman, D., et al. (2014). Homogenous 96-plex PEA immunoassay exhibiting high sensitivity, specificity, and excellent scalability. PLoS One 9, e95192. doi:10.1371/journal.pone.0095192
Berggrund, M., Enroth, S., Lundberg, M., Assarsson, E., Stalberg, K., Lindquist, D., et al. (2019). Identification of candidate plasma protein biomarkers for cervical cancer using the multiplex proximity extension assay. Mol. Cell. Proteomics 18, 735–743. doi:10.1074/mcp.ra118.001208
Bergua, J. F., Alvarez-Diduk, R., Idili, A., Parolo, C., Maymo, M., Hu, L., et al. (2022). Low-cost, user-friendly, all-integrated smartphone-based microplate reader for optical-based biological and chemical analyses. Anal. Chem. 94, 1271–1285. doi:10.1021/acs.analchem.1c04491
Blackstock, D., and Chen, W. (2014). Halo-tag mediated self-labeling of fluorescent proteins to molecular beacons for nucleic acid detection. Chem. Commun. (Camb) 50, 13735–13738. doi:10.1039/c4cc07118b
Blokzijl, A., Nong, R., Darmanis, S., Hertz, E., Landegren, U., and Kamali-Moghaddam, M. (2014). Protein biomarker validation via proximity ligation assays. Biochim. Biophys. Acta 1844, 933–939. doi:10.1016/j.bbapap.2013.07.016
Chang, S. T., Zahn, J. M., Horecka, J., Kunz, P. L., Ford, J. M., Fisher, G. A., et al. (2009). Identification of a biomarker panel using a multiplex proximity ligation assay improves accuracy of pancreatic cancer diagnosis. J. Transl. Med. 7, 105. doi:10.1186/1479-5876-7-105
Chen, L., and Liang, J. (2022). A proximity ligation assay (PLA) based sensing platform for the ultrasensitive detection of P53 protein-specific SUMOylation. Process Biochem. 112, 1–5. doi:10.1016/j.procbio.2021.11.003
Cheng, S., Shi, F., Jiang, X., Wang, L., Chen, W., and Zhu, C. (2012). Sensitive detection of small molecules by competitive immunomagnetic-proximity ligation assay. Anal. Chem. 84, 2129–2132. doi:10.1021/ac3001463
Cohen, L., and Walt, D. R. (2019). Highly sensitive and multiplexed protein measurements. Chem. Rev. 119, 293–321. doi:10.1021/acs.chemrev.8b00257
Conibear, A. C. (2020). Deciphering protein post-translational modifications using chemical biology tools. Nat. Rev. Chem. 4, 674–695. doi:10.1038/s41570-020-00223-8
Cortez, F. J., Gebhart, D., Robinson, P. V., Seftel, D., Pourmandi, N., Owyoung, J., et al. (2020). Sensitive detection of multiple islet autoantibodies in type 1 diabetes using small sample volumes by agglutination-PCR. PLoS One 15, e0242049. doi:10.1371/journal.pone.0242049
Cortez, F. J., Gebhart, D., Tandel, D., Robinson, P. V., Seftel, D., Wilson, D. M., et al. (2022). Automation of a multiplex agglutination-PCR (ADAP) type 1 diabetes (T1D) assay for the rapid analysis of islet autoantibodies. SLAS Technol. 27, 26–31. doi:10.1016/j.slast.2021.10.001
Darling, A. L., and Uversky, V. N. (2018). Intrinsic disorder and posttranslational modifications: The darker side of the biological dark matter. Front. Genet. 9, 158. doi:10.3389/fgene.2018.00158
Darmanis, S., Nong, R. Y., Vanelid, J., Siegbahn, A., Ericsson, O., Fredriksson, S., et al. (2011). ProteinSeq: High-performance proteomic analyses by proximity ligation and next generation sequencing. PLoS One 6, e25583. doi:10.1371/journal.pone.0025583
Davis, K. D., Aghaeepour, N., Ahn, A. H., Angst, M. S., Borsook, D., Brenton, A., et al. (2020). Discovery and validation of biomarkers to aid the development of safe and effective pain therapeutics: Challenges and opportunities. Nat. Rev. Neurol. 16, 381–400. doi:10.1038/s41582-020-0362-2
Dhillon, H. S., Johnson, G., Shannon, M., Greenwood, C., Roberts, D., and Bustin, S. (2016). Homogeneous and digital proximity ligation assays for the detection of Clostridium difficile toxins A and B. Biomol. Detect Quantif. 10, 2–8. doi:10.1016/j.bdq.2016.06.003
Di Giusto, D. A., Wlassoff, W. A., Gooding, J. J., Messerle, B. A., and King, G. C. (2005). Proximity extension of circular DNA aptamers with real-time protein detection. Nucleic Acids Res. 33, e64. doi:10.1093/nar/gni063
Fredriksson, S., Dixon, W., Ji, H., Koong, A. C., Mindrinos, M., and Davis, R. W. (2007). Multiplexed protein detection by proximity ligation for cancer biomarker validation. Nat. Methods 4, 327–329. doi:10.1038/nmeth1020
Fredriksson, S., Gullberg, M., Jarvius, J., Olsson, C., Pietras, K., G Stafsd Ttir, S. M., et al. (2002). Protein detection using proximity-dependent DNA ligation assays. Nat. Biotechnol. 20, 473–477. doi:10.1038/nbt0502-473
Fredriksson, S., Horecka, J., Brustugun, O. T., Schlingemann, J., Koong, A. C., Tibshirani, R., et al. (2008). Multiplexed proximity ligation assays to profile putative plasma biomarkers relevant to pancreatic and ovarian cancer. Clin. Chem. 54, 582–589. doi:10.1373/clinchem.2007.093195
Fu, Y., Qian, H., Zhou, X., Wu, Y., Song, L., Chen, K., et al. (2021). Proximity ligation assay mediated rolling circle amplification strategy for in situ amplified imaging of glycosylated PD-L1. Anal. Bioanal. Chem. 413, 6929–6939. doi:10.1007/s00216-021-03659-z
Graham, T. G. W., Ferrie, J. J., Dailey, G. M., Tjian, R., and Darzacq, X. (2022). Detecting molecular interactions in live-cell single-molecule imaging with proximity-assisted photoactivation (PAPA). Elife 11, e76870. doi:10.7554/elife.76870
Greenwood, C., Johnson, G., Dhillon, H. S., and Bustin, S. (2015a). Recent progress in developing proximity ligation assays for pathogen detection. Expert Rev. Mol. Diagn 15, 861–867. doi:10.1586/14737159.2015.1044440
Greenwood, C., Ruff, D., Kirvell, S., Johnson, G., Dhillon, H. S., and Bustin, S. A. (2015b). Proximity assays for sensitive quantification of proteins. Biomol. Detect Quantif. 4, 10–16. doi:10.1016/j.bdq.2015.04.002
Gu, G. J., Friedman, M., Jost, C., Johnsson, K., Kamali-Moghaddam, M., Pluckthun, A., et al. (2013). Protein tag-mediated conjugation of oligonucleotides to recombinant affinity binders for proximity ligation. N. Biotechnol. 30, 144–152. doi:10.1016/j.nbt.2012.05.005
Gullberg, M., Fredriksson, S., Taussig, M., Jarvius, J., Gustafsdottir, S., and Landegren, U. (2003). A sense of closeness protein detection by proximity ligation. Curr. Opin. Biotechnol. 14, 82–86. doi:10.1016/s0958-1669(02)00011-3
Gullberg, M., Gustafsdottir, S. M., Schallmeiner, E., Jarvius, J., Bjarnegard, M., Betsholtz, C., et al. (2004). Cytokine detection by antibody-based proximity ligation. Proc. Natl. Acad. Sci. U. S. A. 101, 8420–8424. doi:10.1073/pnas.0400552101
Gustafsdottir, S. M., Nordengrahn, A., Fredriksson, S., Wallgren, P., Rivera, E., Schallmeiner, E., et al. (2006). Detection of individual microbial pathogens by proximity ligation. Clin. Chem. 52, 1152–1160. doi:10.1373/clinchem.2005.065847
Hermann, J., Schurgers, L., and Jankowski, V. (2022). Identification and characterization of post-translational modifications: Clinical implications. Mol. Asp. Med. 86, 101066. doi:10.1016/j.mam.2022.101066
Hu, C., Zhang, J., Jin, Y., Ma, W., Zhou, R., Du, H., et al. (2022). Protein-Recognition-initiated exponential amplification reaction (PRIEAR) and its application in clinical diagnosis. Chembiochem 23, e202100548. doi:10.1002/cbic.202100548
Hwang, B. B., Engel, L., Goueli, S. A., and Zegzouti, H. (2020). A homogeneous bioluminescent immunoassay to probe cellular signaling pathway regulation. Commun. Biol. 3, 8. doi:10.1038/s42003-019-0723-9
Inoue, K. Y., Ino, K., Shiku, H., and Matsue, T. (2010). Electrochemical detection of endotoxin using recombinant factor C zymogen. Electrochem. Commun. 12, 1066–1069. doi:10.1016/j.elecom.2010.05.028
Islam, K. U., and Iqbal, J. (2020). An update on molecular diagnostics for COVID-19. Front. Cell. Infect. Microbiol. 10, 560616. doi:10.3389/fcimb.2020.560616
Jalili, R., Horecka, J., Swartz, J. R., Davis, R. W., and Persson, H. H. J. (2018). Streamlined circular proximity ligation assay provides high stringency and compatibility with low-affinity antibodies. Proc. Natl. Acad. Sci. U. S. A. 115, E925–E933. doi:10.1073/pnas.1718283115
Jarvius, M., Paulsson, J., Weibrecht, I., Leuchowius, K.-J., Andersson, A.-C., W Hlby, C., et al. (2007). In situ detection of phosphorylated platelet-derived growth factor receptor beta using a generalized proximity ligation method. Mol. Cell. Proteomics 6, 1500–1509. doi:10.1074/mcp.m700166-mcp200
Joonyul Kim, J. H., Rebecca, S., Easley, C. J., and Easley, C. J. (2010). Improvement of sensitivity and dynamic range in proximity ligation assays by asymmetric connector hybridization. Anal. Chem. 82, 6976–6982. doi:10.1021/ac101762m
Kang, T., Lu, J., Yu, T., Long, Y., and Liu, G. (2022). Advances in nucleic acid amplification techniques (NAATs): COVID-19 point-of-care diagnostics as an example. Biosens. Bioelectron. 206, 114109. doi:10.1016/j.bios.2022.114109
Karp, D. G., Cuda, D., Tandel, D., Danh, K., Robinson, P. V., Seftel, D., et al. (2020). Sensitive and specific detection of SARS-CoV-2 antibodies using a high-throughput, fully automated liquid-handling robotic system. SLAS Technol. 25, 545–552. doi:10.1177/2472630320950663
Karve, T. M., and Cheema, A. K. (2011). Small changes huge impact: The role of protein posttranslational modifications in cellular homeostasis and disease. J. Amino Acids 2011, 207691–207713. doi:10.4061/2011/207691
Kazane, S. A., Sok, D., Cho, E. H., Uson, M. L., Kuhn, P., Schultz, P. G., et al. (2012). Site-specific DNA-antibody conjugates for specific and sensitive immuno-PCR. Proc. Natl. Acad. Sci. U. S. A. 109, 3731–3736. doi:10.1073/pnas.1120682109
Kim, H., Yoon, H. K., and Yoo, T. H. (2014). Engineering beta-lactamase zymogens for use in protease activity assays. Chem. Commun. (Camb) 50, 10155–10157. doi:10.1039/c4cc04549a
Li, G., Eckert, M. A., Chang, J. W., Montgomery, J. E., Chryplewicz, A., Lengyel, E., et al. (2019). Ultrasensitive, multiplexed chemoproteomic profiling with soluble activity-dependent proximity ligation. Proc. Natl. Acad. Sci. U. S. A. 116, 21493–21500. doi:10.1073/pnas.1912934116
Li, L., Xu, S., Yan, H., Li, X., Yazd, H. S., Li, X., et al. (2021a). Nucleic acid aptamers for molecular diagnostics and therapeutics: Advances and perspectives. Angew. Chem. Int. Ed. Engl. 60, 2249–2259. doi:10.1002/ange.202003563
Li, X., Jiang, X., Xu, X., Zhu, C., and Yi, W. (2017). Imaging of protein-specific glycosylation by glycan metabolic tagging and in situ proximity ligation. Carbohydr. Res. 448, 148–154. doi:10.1016/j.carres.2017.06.015
Li, Y., Mansour, H., Watson, C. J. F., Tang, Y., Macneil, A. J., and Li, F. (2021b). Amplified detection of nucleic acids and proteins using an isothermal proximity CRISPR Cas12a assay. Chem. Sci. 12, 2133–2137. doi:10.1039/d0sc06113a
Lind, A., De Jesus Cortez, F., Ramelius, A., Bennet, R., Robinson, P. V., Seftel, D., et al. (2022). Multiplex agglutination-PCR (ADAP) autoantibody assays compared to radiobinding autoantibodies in type 1 diabetes and celiac disease. J. Immunol. Methods 506, 113265. doi:10.1016/j.jim.2022.113265
Lioudmila Elfineh, C. C., Anna, A. S. P. L. U. N. D., Pettersson, U. L. F., Masood, K. M., Sara Bergstr, M. L. I. N. D., and Lind, S. B. (2014). Tyrosine phosphorylation profiling via in situ proximity ligation assay. BMC Cancer 14, 435. doi:10.1186/1471-2407-14-435
Liu, H., Rong, P., Jia, H., Yang, J., Dong, B., Dong, Q., et al. (2016). A wash-free homogeneous colorimetric immunoassay method. Theranostics 6, 54–64. doi:10.7150/thno.13159
Liu, R., He, L., Hu, Y., Luo, Z., and Zhang, J. (2020). A serological aptamer-assisted proximity ligation assay for COVID-19 diagnosis and seeking neutralizing aptamers. Chem. Sci. 11, 12157–12164. doi:10.1039/d0sc03920a
Lundberg, M., Eriksson, A., Tran, B., Assarsson, E., and Fredriksson, S. (2011a). Homogeneous antibody-based proximity extension assays provide sensitive and specific detection of low-abundant proteins in human blood. Nucleic Acids Res. 39, e102. doi:10.1093/nar/gkr424
Lundberg, M., Stine Buch, T., Erika, A. S., Andrea, V. I., Bonnie, T. R., et al. (2011b). Multiplexed homogeneous proximity ligation assays for high-throughput protein biomarker research in serological material. Mol. Cell. Proteom. 10, M110.004978. doi:10.1074/mcp.m110.004978
Marnissi, B., Khalfaoui, K., Ebai, T., Marques Souza De Oliveira, F., Ghram, A., Kamali-Moghaddam, M., et al. (2021). Accurate detection of Newcastle disease virus using proximity-dependent DNA aptamer ligation assays. FEBS Open Bio 11, 1122–1131. doi:10.1002/2211-5463.13117
Masood Kamali-Moghaddam, F. E. P., Wu, D. I., Hillevi, E. N. G. L. U. N. D., Spyros, D., Anna, L. O. R. D., Gholamreza Tavoosidana, D. S., et al. (2010). Sensitive detection of Ab protofibrils by proximity ligation. relevance for alzheimer's disease. BMC Neurosci.
Michael Famulok, G. M., and Blind, M. (2000). Nucleic acid AptamersFrom selection in vitro to applications in vivo. Acc. Chem. Res. 33, 591–599. doi:10.1021/ar960167q
Nilsson, I., Bahram, F., Li, X., Gualandi, L., Koch, S., Jarvius, M., et al. (2010). VEGF receptor 2/-3 heterodimers detected in situ by proximity ligation on angiogenic sprouts. EMBO J. 29, 1377–1388. doi:10.1038/emboj.2010.30
Nong, R. Y., Wu, D., Yan, J., Hammond, M., Gu, G. J., Kamali-Moghaddam, M., et al. (2013). Solid-phase proximity ligation assays for individual or parallel protein analyses with readout via real-time PCR or sequencing. Nat. Protoc. 8, 1234–1248. doi:10.1038/nprot.2013.070
Oliveira, F. M. S., Mereiter, S., Lonn, P., Siart, B., Shen, Q., Heldin, J., et al. (2018). Detection of post-translational modifications using solid-phase proximity ligation assay. N. Biotechnol. 45, 51–59. doi:10.1016/j.nbt.2017.10.005
Park, H. J., Jung, C., and Yoo, T. H. (2022). Development of one-step isothermal methods to detect RNAs using hairpin-loop signal converters and proximity proteolysis reaction. Biosens. Bioelectron. 197, 113769. doi:10.1016/j.bios.2021.113769
Park, H. J., Kim, Y., and Yoo, T. H. (2021). One-pot colorimetric detection of molecules based on proximity proteolysis reaction. Biosens. Bioelectron. 188, 113349. doi:10.1016/j.bios.2021.113349
Park, H. J., and Yoo, T. H. (2018). Nucleic acid detection by a target-assisted proximity proteolysis reaction. ACS Sens. 3, 2066–2070. doi:10.1021/acssensors.8b00821
Pj Santangelo, B. N., Andrew, T. S. O. U. R. K. A. S., and Bao, G. A. N. G. (2004). Dual FRET molecular beacons for mRNA detection in living cells. Nucleic Acids Res. 32, e57. doi:10.1093/nar/gnh062
Poulard, C., Jacquemetton, J., Pham, T. H., and Le Romancer, M. (2020). Using proximity ligation assay to detect protein arginine methylation. Methods 175, 66–71. doi:10.1016/j.ymeth.2019.09.007
Robinson, P. V., Tsai, C. T., De Groot, A. E., Mckechnie, J. L., and Bertozzi, C. R. (2016). Glyco-seek: Ultrasensitive detection of protein-specific glycosylation by proximity ligation polymerase chain reaction. J. Am. Chem. Soc. 138, 10722–10725. doi:10.1021/jacs.6b03861
Rossetti, M., Bertucci, A., Patino, T., Baranda, L., and Porchetta, A. (2020). Programming DNA-based systems through effective molarity enforced by biomolecular confinement. Chemistry 26, 9826–9834. doi:10.1002/chem.202001660
Schallmeiner, E., Oksanen, E., Ericsson, O., Spangberg, L., Eriksson, S., Stenman, U. H., et al. (2007). Sensitive protein detection via triple-binder proximity ligation assays. Nat. Methods 4, 135–137. doi:10.1038/nmeth974
Seo, J., and Lee, K.-J. (2004). Post-translational modifications and their biological functions: Proteomic analysis and systematic approaches. J. Biochem. Mol. Biol. 37, 35–44. doi:10.5483/bmbrep.2004.37.1.035
Shaban, S. M., and Kim, D. H. (2021). Recent advances in aptamer sensors. Sensors (Basel) 21, 979. doi:10.3390/s21030979
Shekhawat, S. S., and Ghosh, I. (2011). Split-protein systems: Beyond binary protein-protein interactions. Curr. Opin. Chem. Biol. 15, 789–797. doi:10.1016/j.cbpa.2011.10.014
Soderberg, O., Gullberg, M., Jarvius, M., Ridderstrale, K., Leuchowius, K. J., Jarvius, J., et al. (2006). Direct observation of individual endogenous protein complexes in situ by proximity ligation. Nat. Methods 3, 995–1000. doi:10.1038/nmeth947
Song, J., Liu, C., Wang, X., Xu, B., Liu, X., Li, Y., et al. (2021). O-GlcNAcylation quantification of certain protein by the proximity ligation assay and Clostridium perfringen OGA(D298N)(CpOGA(D298N)). ACS Chem. Biol. 16, 1040–1049. doi:10.1021/acschembio.1c00185
Stein, V., and Alexandrov, K. (2014). Protease-based synthetic sensing and signal amplification. PNAS 111, 15934–15939. doi:10.1073/pnas.1405220111
Stein, V., and Alexandrov, K. (2015). Synthetic protein switches: Design principles and applications. Trends Biotechnol. 33, 101–110. doi:10.1016/j.tibtech.2014.11.010
Stine Buch Thorsen, M. L., Andrea, V. I. L. L. A. B. L. A. N. C. A., Louise, S. A. R. A. H., Christensen, T., Kirstine Christensen, B. E. L. L. I. N. G., Birgittenielsen, S. A. N. D. E. R., et al. (2013). Detection of serological biomarkers by proximity extension assay for detection of colorectal neoplasias in symptomatic individuals. J. Transl. Med. 11, 253. doi:10.1186/1479-5876-11-253
Tavoosidana, G., Ronquist, G., Darmanis, S., Yan, J., Carlsson, L., Wu, D., et al. (2011). Multiple recognition assay reveals prostasomes as promising plasma biomarkers for prostate cancer. Proc. Natl. Acad. Sci. U. S. A. 108, 8809–8814. doi:10.1073/pnas.1019330108
Thiviyanathan, V., and Gorenstein, D. G. (2012). Aptamers and the next generation of diagnostic reagents. Proteomics Clin. Appl. 6, 563–573. doi:10.1002/prca.201200042
Trotter, M., Borst, N., Thewes, R., and Von Stetten, F. (2020). Review: Electrochemical DNA sensing - principles, commercial systems, and applications. Biosens. Bioelectron. 154, 112069. doi:10.1016/j.bios.2020.112069
Tsai, C.-T., Robinson, P. V., Cortez, F. D. J., Elma, M. L. B., Seftel, D., Pourmandi, N., et al. (2018). Antibody detection by agglutination-PCR (ADAP) enables early diagnosis of HIV infection by oral fluid analysis. Proc. Natl. Acad. Sci. U. S. A. 115, 1250–1255. doi:10.1073/pnas.1711004115
Tsai, C. T., Robinson, P. V., Spencer, C. A., and Bertozzi, C. R. (2016). Ultrasensitive antibody detection by agglutination-PCR (ADAP). ACS Cent. Sci. 2, 139–147. doi:10.1021/acscentsci.5b00340
Van Buggenum, J. A., Gerlach, J. P., Eising, S., Schoonen, L., Van Eijl, R. A., Tanis, S. E., et al. (2016). A covalent and cleavable antibody-DNA conjugation strategy for sensitive protein detection via immuno-PCR. Sci. Rep. 6, 22675. doi:10.1038/srep22675
Velema, W. A., and Kool, E. T. (2017). Fluorogenic templated reaction cascades for RNA detection. J. Am. Chem. Soc. 139, 5405–5411. doi:10.1021/jacs.7b00466
Wang, P., Yang, Y., Hong, T., and Zhu, G. (2021). Proximity ligation assay: An ultrasensitive method for protein quantification and its applications in pathogen detection. Appl. Microbiol. Biotechnol. 105, 923–935. doi:10.1007/s00253-020-11049-1
Weibrecht, I., Leuchowius, K. J., Clausson, C. M., Conze, T., Jarvius, M., Howell, W. M., et al. (2010). Proximity ligation assays: A recent addition to the proteomics toolbox. Expert Rev. Proteomics 7, 401–409. doi:10.1586/epr.10.10
Wik, L., Nordberg, N., Broberg, J., Bjorkesten, J., Assarsson, E., Henriksson, S., et al. (2021). Proximity extension assay in combination with next-generation sequencing for high-throughput proteome-wide analysis. Mol. Cell. Proteomics 20, 100168. doi:10.1016/j.mcpro.2021.100168
Yan, L., Zhou, J., Zheng, Y., Gamson, A. S., Roembke, B. T., Nakayama, S., et al. (2014). Isothermal amplified detection of DNA and RNA. Mol. Biosyst. 10, 970–1003. doi:10.1039/c3mb70304e
Yang, L., and Ellington, A. D. (2008). Real-time PCR detection of protein analytes with conformation-switching aptamers. Anal. Biochem. 380, 164–173. doi:10.1016/j.ab.2008.05.018
Ye, Q., Lu, D., Zhang, T., Mao, J., and Shang, S. (2022). Application experience of a rapid nucleic acid detection system for COVID-19. Microbes Infect. 24, 104945. doi:10.1016/j.micinf.2022.104945
Zemans, R. L., Jacobson, S., Keene, J., Kechris, K., Miller, B. E., Tal-Singer, R., et al. (2017). Multiple biomarkers predict disease severity, progression and mortality in COPD. Respir. Res. 18, 117. doi:10.1186/s12931-017-0597-7
Zhang, S., Garcia-D'Angeli, A., Brennan, J. P., and Huo, Q. (2014). Predicting detection limits of enzyme-linked immunosorbent assay (ELISA) and bioanalytical techniques in general. Analyst 139, 439–445. doi:10.1039/c3an01835k
Zhang, Y. P., Wang, H. P., Dong, R. L., Li, S. Y., Wang, Z. G., Liu, S. L., et al. (2021). Proximity-induced exponential amplification reaction triggered by proteins and small molecules. Chem. Commun. (Camb) 57, 4714–4717. doi:10.1039/d1cc00583a
Keywords: biosensor, proximity-enhanced reaction, proximity ligation assay, proximity extension assay, proximity proteolysis assay
Citation: Park YS, Choi S, Jang HJ and Yoo TH (2023) Assay methods based on proximity-enhanced reactions for detecting non-nucleic acid molecules. Front. Bioeng. Biotechnol. 11:1188313. doi: 10.3389/fbioe.2023.1188313
Received: 17 March 2023; Accepted: 21 June 2023;
Published: 29 June 2023.
Edited by:
Eden Morales-Narváez, Universidad Nacional Autónoma de México, MexicoReviewed by:
Xiaobo Yu, Beijing Proteome Research Center, ChinaAndrea Idili, University of Rome Tor Vergata, Italy
Copyright © 2023 Park, Choi, Jang and Yoo. This is an open-access article distributed under the terms of the Creative Commons Attribution License (CC BY). The use, distribution or reproduction in other forums is permitted, provided the original author(s) and the copyright owner(s) are credited and that the original publication in this journal is cited, in accordance with accepted academic practice. No use, distribution or reproduction is permitted which does not comply with these terms.
*Correspondence: Tae Hyeon Yoo, dGFlaHllb255b29AYWpvdS5hYy5rcg==