- Department of Endodontics, Stomatological Hospital, School of Stomatology, Southern Medical University, Guangzhou, Guangdong, China
One of the difficulties of pulp regeneration is the rapid vascularization of transplanted engineered tissue, which is crucial for the initial survival of the graft and subsequent pulp regeneration. At present, prevascularization techniques, as emerging techniques in the field of pulp regeneration, has been proposed to solve this challenge and have broad application prospects. In these techniques, endothelial cells and pericytes are cocultured to induce intercellular communication, and the cell coculture is then introduced into the customized artificial vascular bed or induced to self-assembly to simulate the interaction between cells and extracellular matrix, which would result in construction of a prevascularization system, preformation of a functional capillary network, and rapid reconstruction of a sufficient blood supply in engineered tissue after transplantation. However, prevascularization techniques for pulp regeneration remain in their infancy, and there remain unresolved problems regarding cell sources, intercellular communication and the construction of prevascularization systems. This review focuses on the recent advances in the application of prevascularization techniques for pulp regeneration, considers dental stem cells as a potential cell source of endothelial cells and pericytes, discusses strategies for their directional differentiation, sketches the mechanism of intercellular communication and the potential application of communication mediators, and summarizes construction strategies for prevascularized systems. We also provide novel ideas for the extensive application and follow-up development of prevascularization techniques for dental pulp regeneration.
1 Introduction
The loss of tooth vitality and subsequent complications caused by traditional root canal therapy are contrary to the concept of regeneration in modern stomatology. Pulp regeneration tissue engineering has become a new approach to solving this dilemma. The basic metabolism of the engineered tissue transplanted into the pulp chamber requires microvasculature to exchange nutrients and gases, remove metabolic waste and maintain a series of physiological functions. However, the diffusion limit of oxygen in the vasculature is only 200 μm (Hoeben et al., 2004). Therefore, the rapid vascularization of engineered tissue with a diameter larger than the critical value is the key to its survival, and the formation of a functional vascular network is the decisive factor in pulp tissue regeneration (Xie et al., 2021). In recent years, a large number of studies have used cell homing or stem cell transplantation to construct engineered tissue for in situ angiogenesis in the pulp chamber. By inducing the invasion of the host vasculature, the vascular network of engineered tissue was formed by the sprouting, extension and fusion of blood vessels to promote tissue regeneration. However, three points must be considered: 1) the host blood vessel could enter the pulp chamber only through a narrow apical foramen and grew along the root canal to the crown at a slow extension rate of 5 μm/h (Utzinger et al., 2015); 2) the pulp chamber constituted a naturally ischemic and hypoxic environment, which was not conducive to the survival of engineered tissue or to pulp regeneration; and 3) it was difficult to monitor and control the survival and vascularization process after tissue implantation. Therefore, researchers proposed prevascularization techniques in which a functional vascular network is preformed in engineered tissue constructs of dental pulp to ensure the rapid delivery of a sufficient blood supply. Subsequently, the vascular network anastomoses with the host vasculature (Ben-Shaul et al., 2019) and finally induces the regeneration of vascularized dental pulp (Mazio et al., 2019; Rademakers et al., 2019). Compared with traditional methods, this method could accurately control the formation of the vascular network, accelerate the process of vascularization in vivo, and facilitate large-scale production and clinical transformation.
To achieve the prevascularization of dental pulp, researchers obtain endothelial cells (ECs) and pericytes, introduce the cell coculture into the customized artificial vascular bed or induce self-assembly of the cell coculture to construct a prevascularization system, and then induce formation of a functional capillary network through intercellular communication and the interaction between cells and the extracellular matrix (cell‒ECM interaction). However, the cell sources and their quality of prevascularized dental pulp-engineered tissue are limited, the utilization of intercellular communication mechanisms is inadequate, and the construction strategies of prevascularization systems are still in their infancy. Therefore, this review considers dental stem cells as a potential cell source of endothelial cells and pericytes, discusses strategies for their directional differentiation, sketches the mechanism of intercellular communication and the potential application of communication mediators, summarizes construction strategies for prevascularized systems, and focuses on the recent advances in the application of prevascularization techniques for pulp regeneration.
2 Principles of vasculogenesis and angiogenesis
Prevascularization techniques preconstruct a functional vascular network through angiogenesis and vasculogenesis of ECs and perivascular cells (pericytes and vascular smooth muscle cells) (Liu et al., 2019). Vasculogenesis begins with the differentiation of the mesoderm into vascular cells and the formation of blood islands during embryonic development. The outer cells of the blood island are induced by vascular endothelial growth factor (VEGF) and fibroblast growth factor (FGF) to differentiate into ECs, while the inner region forms a lacuna in which the free cells differentiate into hematopoietic stem cells. Later, primitive blood vessels are formed (Carmeliet, 2000a). The maturation of primitive blood vessels is completed by the basement membrane and perivascular cells. Angiogenesis begins with the secretion of proangiogenic factors such as VEGF and FGF at injured or hypoxic sites (Draoui et al., 2017), forming a concentration gradient, activating the formation of endothelial tip cells, and directionally invading and decomposing the extracellular matrix (ECM). Tip cells and adjacent perivascular cells migrate along the concentration gradient of proangiogenic factors, followed by endothelial stem cells. These ECs proliferate to form new endothelial cell strips, then become lumen, and finally mature into functional vessels under the action of perivascular cells (Armulik et al., 2011; Wang et al., 2020).
The development of prevascularization techniques in the field of dental pulp regeneration originated from an attempt to construct blood vessels with endothelial cells (Athirasala et al., 2017). At present, this has progressed to the successful integration of ECs and pericytes and the formation of capillary networks (Qi et al., 2021). Few studies have investigated vascular smooth muscle cells. Therefore, this article mainly refers to ECs and pericytes. An in-depth study of vascular smooth muscle cells in dental pulp is likely to be a future development direction that has the potential to achieve the regeneration of microvasculature including capillaries, arterioles, and venules.
3 Potential cell sources of prevascularization techniques
3.1 Potential cell sources of ECs
ECs line the inner surface of blood vessels to form an inner wall, which is an indispensable part of the process of vascularization. Autologous ECs, such as human umbilical vein endothelial cells (HUVECs), human umbilical artery endothelial cells, human microvascular endothelial cells and endothelial progenitor cells, are the most direct sources of cells that can minimize the risk of immune rejection. These ECs are widely used in tissue engineering and vascular engineering for pulp regeneration. However, problems such as difficulty in obtaining samples, inefficient proliferation and expansion, rapid loss of expression of phenotypic markers in vitro, and potential dysfunction of ECs in some patients with systemic diseases have limited the application of autologous ECs (Kim and von Recum, 2008; Afra and Matin, 2020). With the rapid development of cell engineering and genetic engineering, the differentiation of stem cells, such as embryonic stem cells, induced pluripotent stem cells (iPSCs) and adult stem cells (ASCs), has been a promising method to obtain ECs.
Embryonic stem cells are not included in this discussion due to the destructive acquisition process and ethical limitations.
Pluripotent cells transformed from terminally differentiated cells by introducing specific transcription factors (Takahashi et al., 2007; Yu et al., 2007) or using small-molecule compounds (Guan et al., 2022) are called iPSCs. iPSCs have the potential to differentiate into all three germ lineages and have greater inherited plasticity than autologous ECs (Sivarapatna et al., 2015; Xu M. et al., 2019). However, the unlimited proliferative potential of iPSCs creates a high risk of teratoma or tumor formation. The process of producing iPSC-ECs is complex and time-consuming, and the phenotypes of cells that are recoded into a pluripotent state and then redifferentiated are usually immature (Yamanaka, 2020).
ASCs are undifferentiated cells that exist in differentiated organs and tissues. Among them, mesenchymal stem cells (MSCs) are multipotent stem cells derived from the mesoderm that were first successfully isolated from bone marrow (Friedenstein et al., 1976) and later obtained from adipose tissue (Zuk et al., 2001), teeth (dental stem cells) and other tissues. Among them, dental stem cells (DSCs), including dental pulp stem cells (DPSCs) (Gronthos et al., 2000), stem cells of human deciduous exfoliated teeth (SHEDs) (Miura et al., 2003), stem cells from apical papilla (SCAPs) (Sonoyama et al., 2006) and dental papilla cells, which are separated from permanent dental pulp, deciduous dental pulp, apical papilla tissue of immature young permanent teeth and dental papilla in tooth germ respectively, have the advantages of easy access and culture, high proliferative activity and the potential to differentiate into three germ layers while avoiding ethical and tumorigenic risks. Previous studies have verified that endothelial differentiated DPSCs promote the formation of prevascularized structures and the regeneration of vascularized pulp-like tissue (Katata et al., 2021). Therefore, the following section considers DSCs as a source of endothelial cells and reviews strategies for the endothelial differentiation of DSCs.
3.1.1 Strategies for endothelial differentiation of DSCs
Existing studies mainly induce endothelial differentiation of DSCs through in vivo differentiation models or in vitro induction methods. The latter contains gene transduction, addition of differentiation inducers, cell coculture, and auxiliary strategies including mechanical stimulation and cell subgroup selection (Figure 1).
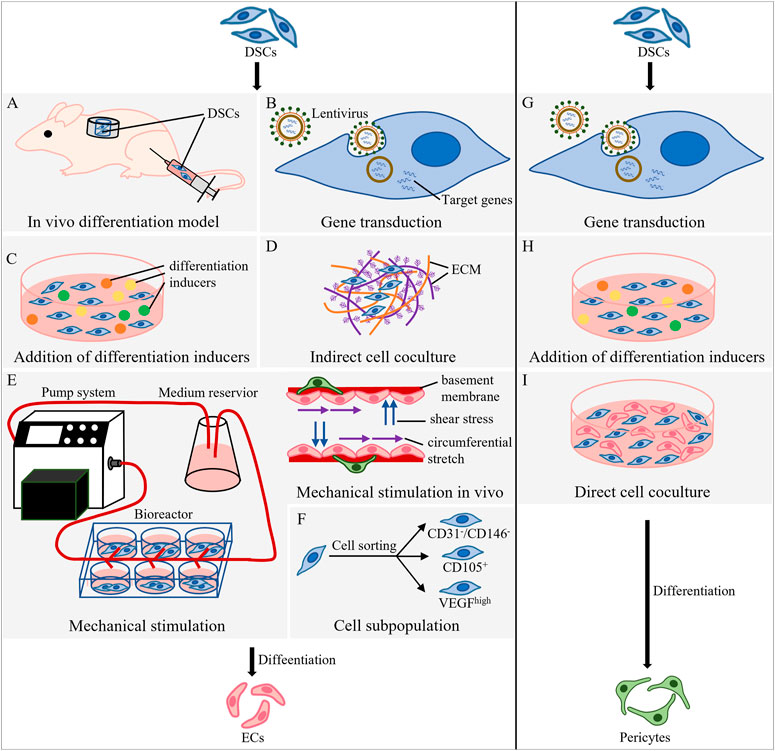
FIGURE 1. Methods of DSC differentiation into ECs and pericytes. (A–F) Strategies for endothelial differentiation of DSCs. (A) Root ectopia model and hindlimb ischemia model. In the root ectopia model, tooth slices loaded with DSCs are transplanted subcutaneously into nude mice; in the hindlimb ischemia model, DSCs are injected into the ischemic gastrocnemius muscle of the thigh. (B) Use lentivirus as a vector to integrate the target gene into DSCs and guide endothelial differentiation. (C) Addition of differentiation inducers to induce endothelial differentiation of DSCs. (D) Indirect contact coculture of DSCs and ECM of cells. (E) A pump system, medium reservoir and bioreactor were used to simulate mechanical stimulation in vivo. (F) Cell subpopulations are selected from DSCs. (G–I) Methods of DSC differentiation into pericytes. (G) Use lentivirus as a vector to integrate the target gene into the host and guide differentiation into pericytes. (H) Addition of differentiation inducers to induce DSCs to differentiate into pericytes. (I) Direct cell coculture of DSCs and ECs.
3.1.1.1 In vivo differentiation model
In vivo differentiation models (Figure 1A) include the root ectopia model and the hindlimb ischemia model (Xu M. et al., 2019). In the root ectopia model, tooth slices loaded with labeled human DPSCs (hDPSCs) (Zhang et al., 2016) or SHEDs (Cordeiro et al., 2008) were transplanted subcutaneously into nude mice. The microvascular structure was observed in the newly formed pulp tissue, and the vascular wall was found to be lined with human hDPSCs or SHEDs differentiated into ECs (Sakai et al., 2010). The hindlimb ischemia model was established by ligating or cutting off the blood supply artery of the rat or mouse hindlimb, and then DSCs were injected into the ischemic gastrocnemius muscle of the thigh to obtain endothelial differentiation. Injection of DPSCs mobilized by granulocyte-colony stimulating factor into this model, which compared with colony-derived DPSCs, significantly increased capillary density and blood flow perfusion, and the area of regenerated pulp and the vascularized area formed in the heterotopic root model were significantly increased (Murakami et al., 2013).
Although in vivo models are closer to the human microenvironment, such models involve complex cellular and molecular cascade reactions, which often lead to failure due to local chronic inflammation (Xu M. et al., 2019). Therefore, in vitro induction methods have become the mainstream of endothelial differentiation of DSCs.
3.1.1.2 In vitro induction method: gene transduction
Gene translation integrates target genes into the host to guide the expression of specific genes. Most studies apply lentivirus as a vector for the transfection target genes (Figure 1B). The ETS variant transcription factor 2 (ETV2) is the key gene for endothelial cell differentiation (Koyano-Nakagawa and Garry, 2017; Kim et al., 2023). Overexpression of ETV2 was found to significantly enhance the endothelial differentiation of DPSCs (Li et al., 2021). VEGF regulates ECM remodeling and angiogenesis (Carmeliet, 2000b), while C-X-C motif chemokine ligand 12 (CXCL12) matures and stabilizes VEGF-induced neovascularization (Grunewald et al., 2006). Overexpression of VEGF and CXCL12 in DPSCs resulted in a significant increase in the length and vascular area density of regenerated pulp-like tissue in the root canal (Zhu et al., 2019). Wnt ligand binds low-density lipoprotein receptor-associated protein 6 expressed by DPSCs to activate the Wnt/β-catenin pathway, inducing the endothelial differentiation of DPSCs. Therefore, forced activation of low-density lipoprotein receptor-associated protein 6 signaling may accelerate the generation of functional vessels (Silva et al., 2017). Hypoxia causes the stable expression of hypoxia inducible factor 1 subunit alpha (HIF-1α) and upregulation of VEGF (Pugh and Ratcliffe, 2003), promoting endothelial differentiation of SHEDs (Han Y. et al., 2022), while BCL2 apoptosis regulator (BCL2) is an anti-apoptotic gene that can inhibit cell apoptosis and prolong cell life (Vaux et al., 1988). Overexpression of BCL2 and hypoxic preconditioning could enhance cell survival and angiogenesis after DPSC implantation (Dissanayaka et al., 2020). In addition, some microRNAs are involved in regulating the angiogenesis of human dental pulp cells (hDPCs). Knocking down the expression of miR-424 in hDPCs could promote endothelial differentiation and tube formation (Liu et al., 2014).
3.1.1.3 In vitro induction method: addition of differentiation inducers
The addition of differentiation inducers, including growth factors and signaling pathway regulators (Le Bras et al., 2010), can induce endothelial differentiation of DSCs (Figure 1C), and this process which can be further enhanced by epigenetic regulators and epigenetic regulatory factors.
Efficient endothelial differentiation and angiogenesis of DPSCs has been achieved in serum-free medium supplemented with vascular growth factors VEGF-A165, B27 and heparin (Luzuriaga et al., 2020). Commercially available endothelial cell growth medium EGM-2™ similar to this formula achieve the same goal (Aksel and Huang, 2017). Endothelial differentiation medium supplemented with 50 ng/mL recombinant human VEGF165 induces three-dimensional (3D) DPSC constructs to form endothelial differentiation cell constructs. On the 10th day, the outer layer of endothelial differentiation cell constructs has formed a vascular network composed of VWF-positive cells. Pulp-like tissue with a high density of CD31+ blood vessels will ultimately form in the root ectopia model (Katata et al., 2021). Under hypoxic conditions, the kidney produces erythropoietin, whose angiogenic potential is close to that of some other known angiogenic factors, such as VEGF and basic fibroblast growth factor (FGF2). Therefore, short-term exposure to the combination of serum deprivation, glucose deprivation and hypoxia/oxygen deprivation promoted the differentiation of SCAPs into endothelial cells; the addition of recombinant human erythropoietin to complete culture medium-α, SCAPs formed a microvascular structure, and the upregulation of vascular endothelial growth factor receptor 2 (VEGFR2), von Willebrand factor (VWF), vascular endothelial cadherin (VE-cadherin) and other endothelial markers was observed (Koutsoumparis et al., 2018).
Semaphorin-4D is a transmembrane class IV semaphorin that participates in the regulation of nerve signal transduction, immunity, angiogenesis and other processes (Zhang L. et al., 2022). By binding with plexin B1 on the cell membrane of DPSCs, Semaphorin-4D activates the PI3K/AKT and MEK/ERK1/2 signaling pathways, leading to the upregulation of endothelial differentiation-related genes (VEGFR1, VEGFR2, CD31 and VWF) and angiogenesis-related genes (HIF, angiopoietin 1 and angiopoietin like 4) and thereby inducing the endothelial differentiation of DPSCs (Zou et al., 2019). Transforming growth factor beta (TGF-β) inhibitor enhances the differentiation of dental pulp-derived stem cells into endodermal cells (Xu et al., 2018). This inhibitor acts on the TGF signaling pathway (TGFβ/Smad2/3), inhibits the transformation of SHEDs into smooth muscle cells (SMCs), and promotes endothelial differentiation of SHEDs through the VEGF signaling pathway. VEGF triggers MEK1/ERK phosphorylation and downstream signaling pathway activation and participates in SHED endothelial differentiation (Bento et al., 2013) and the sprouting and anastomosis of vessels derived from DPSCs (Sasaki et al., 2020). Therefore, the MEK1/ERK activator may contribute to the endothelial differentiation of DPSCs. In addition, a glycogen synthase kinase 3 β (GSK-3b) inhibitor activated the canonical Wnt/β-catenin pathway to induce the endothelial differentiation of DPSCs (Zhang et al., 2016).
VPA is the epigenetic regulator most widely used in chemical reprogramming to overcome the epigenetic barriers between different types of cells. The combination of VPA with signal pathway regulators (VPA, GSK signal inhibitor, TGF-β signal inhibitor, cAMP signal activator and ROCK inhibitor) and three key growth factors (VEGF, BMP-4 and 8-Br-cAMP) effectively induced SCAPs into EC-like cells that formed vascular structures in vivo and in vitro (Yi et al., 2021). In addition, p53 is a tumor suppressor that not only induces cell apoptosis but also balances cell stemness and differentiation through p21. Bmi-1 is a polycomb protein that is involved in regulating stem cell differentiation as an epigenetic regulatory factor. p53 inhibitors can induce high expression of Bmi-1 and promote the endothelial differentiation of DPSCs by blocking the p53/p21 signaling pathway (Zhang Z. et al., 2021).
3.1.1.4 In vitro induction method: cell coculture
The main methods of cell coculture are direct contact and indirect contact coculture (Figure 1D). The study of endothelial differentiation of DSCs by direct contact culture is still lacking. In indirect coculture, the vascular tissue with multiple cells or ECM of ECs provides DSCs with a microenvironment containing endothelial differentiation guidance clues consisting of a complex combination of proteins, polysaccharides, growth factors and cytokines (Xu M. et al., 2019). Bovine acellular dental pulp matrix retains the natural vascular system and angiogenic factors. The inoculation of hDPSCs into this matrix could upregulate the expression of hDPSC angiogenesis markers and cause vascular system repopulation (Alghutaimel et al., 2021). Moreover, after 7 days of coculture of acellular ECM from SHEDs and HUVECs, significant upregulation of the specific endothelial markers CD31 and VEGFR2 and the formation of more capillary structures on the matrix gel were observed (Gong et al., 2017). Furthermore, heparan sulfate in the ECM plays a key role in the endothelial differentiation of DPSCs (Li et al., 2023).
Among the above methods, chemical stimulation is the most common induction method with the highest efficiency, convenient operation, fine adjustment and low cost. However, the chemical stimulation method based on two-dimensional monolayer cell culture has difficulty imitating intercellular communication and cell‒ECM interactions in the microenvironment. Therefore, some scholars have chosen to apply natural ECM after acellularization treatment. However, decellularization techniques cannot balance the complete removal of cells and the maximum retention of ECM components (Gong et al., 2017). Other researchers have chosen a 3D culture that forms a 3D structure through the self-assembly of SHEDs (Guo et al., 2020) and DPSCs (Katata et al., 2021) or have prepared 3D tooth germ organ conditioned medium (Zhou et al., 2021), which is conducive to the endothelial differentiation of SHEDs and DPSCs and the formation of vascularized pulp tissue. However, 3D cultivation faces the risks of complex operation, high cost and low output. These methods need to be further developed.
3.1.1.5 Auxiliary method for in vitro induction: mechanical stimulation
The life activities of cells occur in a dynamic, balanced microenvironment. Therefore, static culture conditions without mechanical stimulation, such as the hardness of the vascular intima or shear stress of blood flow, are not conducive to endothelial differentiation. ECs and pericytes jointly secrete ECM to form the basement membrane, which is composed of a protein fiber soft matrix with a low compression modulus (Peloquin et al., 2011). VEGF alone induced the expression of the early endothelial markers VEGFR1 and smooth muscle α-actin (SMA) in MSCs, which induced the MSCs to become vascular progenitor cells or enter a pathological vascular phenotype. The addition of soft matrix elasticity (∼2 kPa) could inhibit SMA and promote the expression of the mature endothelial cell markers VWF and VEGFR2 (Wingate et al., 2014). Therefore, the combination of mechanical and chemical stimulation accelerated the differentiation of MSCs into a mature vascular phenotype. In addition, SHEDs need physiological shear stress to activate angiogenic factors, initiate the VEGF-DLL4/Notch-ephrin B2 cascade, promote SHED differentiation into ECs (Wang et al., 2018) and maintain the function of vascular-related cells (Sharmin et al., 2021). Furthermore, the combination of fluid shear stress and cyclic stress upregulates the expression of EC markers in MSCs (Kim D. H. et al., 2016).
There are few literature reports relevant to dynamic cell culture conditions in dentistry, so we can learn from customized bioreactors in other fields. The cell culture medium inside this instrument was supplied by a fluid reservoir and perfused by a digital permanent pump to simulate an in vivo environment containing stimulatory effects such as fluid shear stress (Hann et al., 2021) (Figure 1E).
3.1.1.6 Auxiliary method for in vitro induction: cell subpopulation
Sorting dental stem cell subpopulations with high vascular potential contributed to improving the efficiency of endothelial differentiation (Figure 1F). CD31-/CD146- side population and CD105+ cells in the dental pulp group promoted an increase in capillary density and blood flow in a mouse hindlimb ischemia model (Nakashima et al., 2009). After 2 years, collagen scaffolds loaded with this subgroup of cells and CXCL12 were transplanted into the root canal, achieving the first complete in situ dental pulp regeneration full of blood vessels and nerves (Nakashima and Iohara, 2011). In cell subpopulation with VEGFR1high SHEDs or DPSCs, VEGF signaling activates MEK1/ERK signaling through VEGFR1, inhibits STAT3 transcriptional activity, and differentiates DPSCs into ECs (Bergamo et al., 2021). A group of CD24a+ multipotent cells isolated from dental papilla cells by 3D sphere culture had stronger stemness and differentiation potential than other DSCs, and can be used as a cell source for the efficient regeneration of vascularized dental pulp (Chen et al., 2020).
3.2 Potential cell sources of pericytes
Pericytes cover the outside of the microvascular walls and are widely distributed in all tissues and organs of the body, regulating the sprouting of ECs, enhancing the formation of the EC barrier, and promoting the stability and maturation of capillaries (Armulik et al., 2011). Primary pericytes can be obtained by microvascular isolation and culture (Crisan et al., 2008). However, similar defects to those of autologous ECs limit the mass production of pericytes. Therefore, the identification of alternative sources of pericytes is helpful for experimental research and clinical transformation.
Studies have found that pericytes express surface markers of MSCs, namely, CD146+, CD45−, CD34−, CD73+, CD44+ and CD105+ (Crisan et al., 2008; Wang et al., 2019; Manocha et al., 2022), and exhibit multidirectional differentiation ability similar to that of MSCs (Farrington-Rock et al., 2004; Tsang et al., 2013; Chou et al., 2020; Kesavan et al., 2023). The MSCs residing in tissue are located in the pericyte niche in the microvasculature (Murray et al., 2014) and express perivascular cell markers: CD146, desmin, NG2, and platelet-derived growth factor receptor (PDGFR) (Crisan et al., 2008). Therefore, MSCs could be considered a source of pericytes for prevascularized tissue. In addition, pericytes are tissue specific (Yianni and Sharpe, 2018), and MSCs derived from the same tissue are more conducive to revascularization (Meijer et al., 2022). Therefore, DSCs are preferred as the periodontal cell pool for dental pulp engineering tissue (Figure 1).
3.2.1 Methods for DSC differentiation into pericytes
The strategies for acquiring pericytes included gene transduction (Figure 1G), chemical stimulation (Figure 1H) and cell coculture (Figure 1I). DPSCs transfected with hypoxia-responsive elements and FGF2 differentiated into CD13+ pericytes and secreted FGF2 to improve the expression of N-cadherin and promote the adhesion of CD13+ pericytes to endothelial cells (Zhu S. et al., 2021). NG2+ hDPCs sorted from hDPCs were cultured in human pericyte medium and differentiated into NG2+ pericytes (Fu et al., 2023). In addition, FGF2 in endothelial cell growth medium induced DPSCs to differentiate into NG2+ pericytes and further supported the proliferation and migration of pericytes (Delle Monache et al., 2019).
Cell coculture is not only a strategy to obtain pericytes but also a method to identify the function of pericytes. Coculture with HUVECs verified the pericyte function of SHEDs and DPSCs (Zhu S. Y. et al., 2021). Among them, SHEDs and HUVECs separately expressed angiogenesis-related factors and their corresponding receptors: SHEDs expressed VEGF, CXCL12, and platelet-derived growth factor receptor (PDGFRB), while HUVECs expressed VEGFR1, VEGFR2, C-X-C motif chemokine receptor 4 (CXCR4), and platelet-derived growth factor BB (PDGF-BB), mediating the interaction between pericytes and ECs (Kim J. H. et al., 2016). DPSCs mainly play a VEGFR2-dependent pericyte function when cocultured with HUVECs or primary endothelial cells isolated from the hearts of mice (Janebodin et al., 2013). In addition, Aksel induced human DPSCs to differentiate into ECs in endothelial growth medium and cocultured them with noninduced DPSCs to form a vascular network. As a result, noninduced DPSCs play the role of pericytes and maintain the stability and integrity of blood vessels (Aksel and Huang, 2017). In fact, DSC-EC coculture is the mainstream technique for pulp prevascularization at present. Intercellular communication between DSCs and ECs could effectively induce ECs and DSCs to perform angiogenesis and act as pericytes, respectively, and further form vascularized engineered tissue.
4 Intercellular communication in prevascularization techniques
The processes of vasculogenesis and angiogenesis in prevascularization techniques are complex and precise, and are induced via intercellular communication between ECs and pericytes (Armulik et al., 2005). This intercellular communication involves paracrine and juxtacrine signal transduction (Table 1). Paracrine signaling mediators are mainly bioactive molecules, so artificial addition of angiogenic bioactive molecules can improve the efficiency of revascularization of engineered tissue. Ratajczak’s review has fully revealed the angiogenic factors in DSCs, which can be referred to in the table in this paper (Ratajczak et al., 2016). Furthermore, the natural bioactive molecular pool taken from organisms provides a large number of angiogenic factors on the basis of preserving the microenvironment in vivo. Rapid progress has been made in laboratory research and clinical transformation (Table 2).
In recent years, in-depth research on the paracrine substances of cells has revealed that in addition to common biologically active molecules, there are also extracellular vesicles (Table 2), which have been widely applied in regenerative medicine and vascular engineering (Hade et al., 2021; Huang et al., 2021). Although extracellular vesicles have not been used in the prevascularization of dental pulp engineered tissue, they have broad application prospects in the future.
4.1 Paracrine and juxtacrine pathways
The interaction between pericytes derived from DSCs and ECs involves paracrine and juxtacrine signal transduction. Paracrine signals are soluble molecules released to act on receptor cells in the microenvironment, and juxtacrine signals are transmitted through direct contact between adjacent cells. The following sections introduce VEGF/VEGFR, PDGF-BB/PDGFRB, TGFβ, angiopoietin-1/TEK receptor tyrosine kinase (ANGPT1/TIE2) and ephrin B2/EPH receptor B4 (EFNB2/EPHB4) (Table 1) (Gaengel et al., 2009).
VEGF is the most critical growth factor in vasculogenesis and angiogenesis and activates the PKC, MAPK, AKT and Ca2+-calcineurin signaling pathways. Endothelial differentiation of DPSCs is caused by the binding of VEGF and VEGFR1 (Bergamo et al., 2021), while the function of DPSCs as pericytes is VEGFR2 dependent, participating in tube formation of ECs in the early stage of EC angiogenesis (Yuan et al., 2015; Delle Monache et al., 2019). VEGF secreted by DPSCs acts on VEGFR2 on ECs via the paracrine pathway, activating the PLC/PIP2 (Wong and Jin, 2005), RAS/RAF/MAPK and PI3K/AKT signaling pathways and inducing the promotion, permeability, invasion, migration, survival and activation of ECs. At the same time, VEGF acts on VEGFR2 on DPSCs in an autocrine manner (Grando Mattuella et al., 2007), further enhancing the VEGF signal (Janebodin et al., 2013). In addition, few studies have investigated the VEGFR2/Calcineurin/NFAT signaling pathway in the field of pulp prevascularization and regeneration, although this pathway has been confirmed to be involved in tumor angiogenesis and vasculogenesis (Alghanem et al., 2017). The activity of NFAT target genes is closely related to the activation of ECs (Suehiro et al., 2014).
ECs release PDGF-BB, which combines with PDGFRB on the surface of pericytes. Then, PDGFR-positive pericytes are recruited to cover the vascular wall. When cocultured with human umbilical artery smooth muscle cells, human DPSCs differentiated into ECs and released PDGF-BB to recruit SMCs. Inhibition of PDGFRB signaling reduced the number of vascular buds and the recruitment of SMCs around the vessels (Zhang Z. et al., 2022). When cocultured with HUVECs, SHEDs were recruited by PDGF-BB released by HUVECs to the periphery of blood vessels to obtain a pericyte phenotype and deposit type IV collagen on the basement membrane. This coculture could effectively prevascularize constructs and mature microvessels, and improve blood perfusion and cell survival after transplantation (Atlas et al., 2021).
Both ECs and pericytes express TGF-β and TGF-β receptors; therefore, the TGF-β signaling pathway is related to the proliferation and differentiation of ECs and pericytes. TGF-β receptors, including type I TGF-β receptor activin receptor-like kinase-1 (ALK1) and activin receptor-like kinase-5 (ALK5), trigger different cellular effects (Goumans et al., 2002; Goumans et al., 2003). In most cell types, TGF-β activates ALK5, causes Smad2/3 phosphorylation (Van Geest et al., 2010), induces MSCs to differentiate into SMCs (Xu et al., 2017), inhibits the proliferation and migration of ECs, and promotes ECM production and vascular maturation. The TGF-β inhibitors used in a previous article inhibited this pathway and induced the endothelial differentiation of DPSCs (Xu et al., 2018). Only ECs expressed ALK1, and the activation of ALK1 caused Smad1/5 phosphorylation, promoted the proliferation and migration of ECs, and led to the formation of new blood vessels (Goumans et al., 2002; Kritharis et al., 2018).
Perivascular cells, including perivascular MSCs, express ANGPT1 to recruit and maintain supporting cells around endothelial cells (Kim et al., 2000) and promote vascular maturation by strengthening the interaction between perivascular cells and ECs (Davis et al., 1996). ANGPT1 binds with the receptor TIE2 on the surface of the EC membrane and participates in the regulation of vascular stability. In a previous study, DPSCs treated with TGF-β (T-DPSCs) triggered the phosphorylation of Smad2/3. Activated Smad2/3 further induced the upregulation of ANGPT1 and VEGF gene expression. First, the binding of VEGF secreted by T-DPSCs and VEGFR2 on ECs induces vascular germination. Subsequently, ANGPT1 secreted by T-DPSCs activated the receptor TIE2 on the surface of the HUVEC membrane. Activated ANGPT1/TIE2 increased the expression of VE-cadherin, enhanced intercellular adhesion, and inhibited the migration of ECs and endothelial sprouting, thus stabilizing blood vessels. Meanwhile, T-DPSCs differentiated into pericyte-like cells, so the VEGF secreted by DPSCs gradually decreased, which weakened the VEGF/VEGFR2 signal, inhibited HUVEC migration, and promoted vascular maturation in cooperation with ANGPT1/TIE2 (Zhang Y. et al., 2021).
In addition, the EFNB2/EPHB4 system is a contact-dependent bidirectional juxtacrine signaling pathway that regulates embryonic vascular development and postnatal angiogenesis (Salvucci and Tosato, 2012). Gong first revealed that the EFNB2/EPHB4 signaling pathway played a key role in the regulation of EC sprouting and lumen formation by DPSCs (Gong et al., 2019), while Yuan found that EPHB2 stabilized the vascular structure produced by HUVECs and SCAPs (Yuan et al., 2016). SCAPs overexpressing EPHB2 secreted more VEGF and accelerated angiogenesis by binding with VEGFR2 on HUVECs. Simultaneously, activation of the EPHB2 reversed signal in SCAPs might activate the ANGPT1/TIE2 axis in HUVECs and regulate vascular permeability and morphogenesis (Yuan et al., 2019).
Through an understanding of the mechanism of EC-pericyte intercellular communication, we can consider the incorporation of key signal molecules or signal pathway regulators to accelerate the process of prevascularization of dental pulp constructs, which requires further research.
4.2 Natural signal molecular storage
Adipose tissue-derived microvessel fragments are functional vascular fragments isolated from adipose tissue and contain angiogenic factors such as VEGF and FGF, as well as cell components such as ECs and pericytes, which are required for vascularization. Aggregates obtained by the coculture of these microvessel fragments and DPSCs have been found to promote the vascularization of regenerated pulp (Xu et al., 2021).
There are many kinds of platelet derivatives. The concentrated growth factor obtained by the differential centrifugation of human venous blood is a third-generation platelet concentrate containing a large number of angiogenesis-related factors, including PDGF-BB, TGF-β1, insulin like growth factor 1, VEGF and FGF2 in natural fibrin network structures were considered likely to serve as signal molecule reservoirs and scaffolds for pulp prevascularization. After implanting concentrated growth factor into the root canal of immature permanent teeth of beagle dogs, pulp-like tissues that resembled the natural tissues and exhibited vascularization and innervation were regenerated after 8 weeks (Xu F. et al., 2019). Platelet lysate (PL) is obtained by repeatedly freezing and thawing platelets or by ultrasound and contains a high concentration of bioactive molecules. Many studies have incorporated PL into bioactive scaffold materials to encapsulate HUVECs and DPSCs (detailed in the next chapter) to accelerate the process of vascularization in engineered tissues.
MSCs secrete a great quantity of paracrine factors into the medium during the culture process. Such conditioned medium has been proven to contribute to tissue regeneration. Conditioned medium from DPSCs promoted the adhesion, proliferation, migration and tubular network formation of ECs (Gharaei et al., 2018). Based on this study, conditioned medium from SHEDs induced the formation of vascularized pulp-like tissue in the root canal of rats (de Cara et al., 2019).
4.3 Potential applications of extracellular vesicles
Extracellular vesicles (EVs) are membranous vesicles released from cells (van Niel et al., 2018) with subgroups that include exosomes, apoptotic bodies, and microvesicles, all containing a large number of nucleic acids and proteins. Zhang fabricated an EV-fibrin gel composite in which EVs were isolated from DPSCs and then mixed with fibrin gel. Thereafter, ECs and DPSCs were cocultured in EV-fibrin gels. DPSC-EVs promoted the rapid formation of vascular structures by increasing the release of VEGF (Zhang et al., 2020). hDPSCs pretreated with lipopolysaccharide (in a mild inflammatory environment) could increase EV production and further enhance angiogenic potential, possibly through TGF-β signaling (Chen et al., 2021). Schwann cells are glial cells in dental pulp tissue, and their extracellular vesicles enhance the recruitment of ECs and MSCs and introduce the formation of dental pulp-like tissue in in vivo models (Wang et al., 2022).
Exosomes are secreted by the fusion of multivesicular bodies and the plasma membrane (Han Q. F. et al., 2022). MiR-26a secreted by exosomes derived from SHEDs (SHED-Exos) regulates the TGF-β/SMAD2/3 signaling pathway to improve angiogenesis in SHEDs and HUVECs, contributing to pulp tissue regeneration (Wu et al., 2021). Compared with SHED-Exos, SHED-Exos pretreated with hypoxia further promote the proliferation, migration and tubular formation of ECs and contribute to increasing the expression of VEGF and the number of CD31+ lumenal structures by transferring let-7f-5p and miR-210-3p (Liu et al., 2022). Exosomes isolated from DPSCs cultured under a growth or angiogenic differentiation condition contain key miRNAs for angiogenesis (miR-199a-3p, miR-221-3p, miR-24-3p, miR-21-5p). The miRNA sequences loaded in exosomes have significant therapeutic potential for pulp prevascularization (Ganesh et al., 2022).
Apoptosis bodies are produced during cell apoptosis, by being released from SHEDs. These bodies carried mitochondrial Tu translation elongation factor, regulate the angiogenic activation of ECs via the transcription factor EB-autophagy pathway, and promote the formation of vascularized dental pulp tissue (Li et al., 2022).
5 Construction strategies for prevascularization systems
Construction strategies for prevascularization systems are mainly divided into two types (Figure 2): 1) bottom-up strategies, in which the microenvironment in an organism is simulated to induce the formation of a self-assembled vascular structure (Figure 2A), and 2) top-down strategies, in which a vascular bed with specific geometry and structure is designed and prepared in advance before introducing cells (Figure 2D).
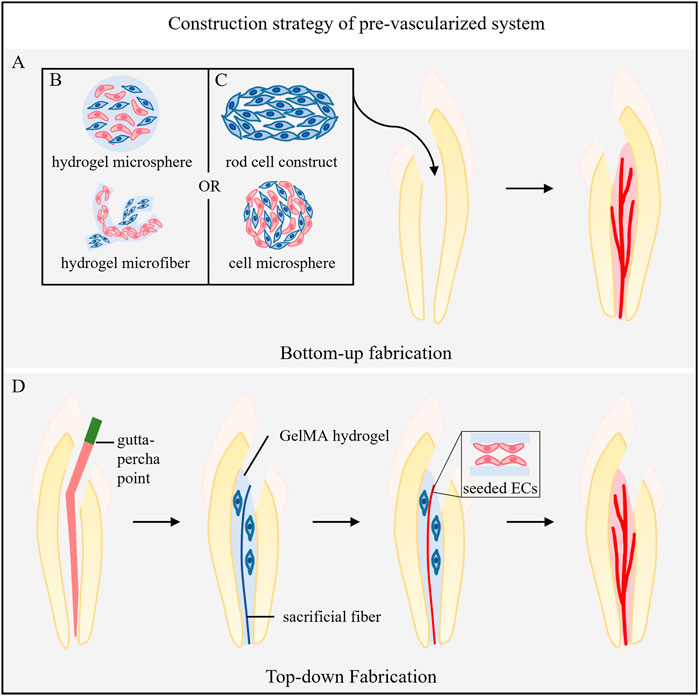
FIGURE 2. Construction strategy of the prevascularized system. (A) Bottom-up fabrication. (B) The incorporation of ECs and pericytes into the materials. (C) Cultivation of vascular-related cells into scaffold-free, self-assembled 3D microtissue structures. (D) Top-down Fabrication. The gutta-percha points into the root canal to form tapered templates, and the hydrogel loaded with cells around it is injected. Then, gutta-percha points were removed after hydrogel coagulation, and sacrificial agarose material was injected. Finally, the sacrificial material is removed, and ECs are seeded in the formed microchannel.
5.1 Bottom-up fabrication
Bottom-up fabrication includes two methods: 1) the incorporation of ECs and pericytes into the biological microenvironment simulated by the materials (Figure 2B) or 2) the cultivation of vascular-related cells into scaffold-free and self-assembled 3D microtissue structures (Figure 2C). Both methods aim to induce vasculogenesis and angiogenesis and eventually form microvascular networks.
5.1.1 Scaffold system made from natural materials or synthetic materials
Each step in the process of vascularization is regulated by microenvironmental clues provided by the surrounding ECM (cell‒ECM interaction). Therefore, the type of scaffold material and its physicochemical properties and biological characteristics affect the formation of the vascular system of the engineered tissue (Marchand et al., 2019). During angiogenesis, fibronectin, laminin, collagen, hyaluronic acid and other proteins or polysaccharides in the ECM participate in regulating the proliferation, differentiation and sprouting process of ECs (Shafiee et al., 2021) Natural hydrogel materials, such as collagen, gelatin, alginate and hyaluronic acid, are rich in the above ingredients and have a strong ability to promote angiogenesis (Shokrani et al., 2022). Their high water content and porosity can simulate the natural ECM (Klotz et al., 2019). In previous studies, 3D collagen hydrogel and 3D fibrin gel were separately used as scaffolds for coculturing ECs and pericytes to achieve prevascularization of the construct (Gong et al., 2019; Atlas et al., 2021). Synthetic materials could be produced by the artificial modification of natural materials or completely synthesized to obtain customized properties suitable for the formation of vascular networks. Therefore, synthetic materials can break through the limitations of natural materials and are more favored by researchers.
5.1.1.1 Natural scaffold based on chitosan
Chitosan hydrogel is a natural linear polysaccharide. In addition to its antibacterial properties, its application in hydrogel carriers can promote cell adhesion and migration. The author loaded the SHED secretome (conditioned medium from SHEDs) into chitosan hydrogel, which significantly increased the release of VEGF and CXCL12, and contributed to rapidly restoring the blood perfusion of dental pulp engineered tissues. Subsequently, the formation of controlled and sustained vascular structures was observed in the tube formation experiment when SCAPs were cocultured with HUVECs (Carvalho et al., 2022).
5.1.1.2 Natural scaffold based on self-assembled peptide
The peptide components of the self-assembled peptide hydrogel PuraMatrix ™ self-assembled into a nanofiber network, creating a real 3D environment for cells. Its injectability was conducive to accurately matching the 3D shape of the dental root canal. Prevascularized microtissues formed by encapsulated DPSCs/HUVECs were transplanted into the root heterotopic model. Then, the formation of vascular structure was observed after 24 hours, and the prevascularized microtissues were integrated with the host vascular system after 4 weeks (Dissanayaka et al., 2015a).
5.1.1.3 Synthetic scaffold based on gelatin methacrylate
Gelatin methacrylate (GelMA) is an inexpensive ultraviolet-cured synthetic material with excellent cell adhesion ability, physical and chemical adjustability and biodegradability and is beneficial to cell encapsulation, survival and proliferation. Khayat et al. encapsulated hDPSCs and HUVECs with GelMA and observed well-organized neovasculature formation (Khayat et al., 2017). However, the traditional ultraviolet irradiation crosslinking method produced oxygen free radicals, which destroyed endogenous DNA and led to tissue aging. Monteiro used an LED blue light source to irradiate GelMA containing encapsulated SCAPs/HUVECs, which not only avoided the harmful consequences of ultraviolet photopolymerization but also accelerated the formation of a wide range of vascular networks (Monteiro et al., 2018). Tian et al. incorporated human PL into a GelMA microsphere system to optimize the angiogenic activity and further modified it with laponite, which has a high drug delivery capacity, to improve the physical properties (Zhang Q. et al., 2021). One year later, this research team used GelMA sodium alginate core-shell microcapsules to solve the difficulty of collecting hydrogel microspheres and their easy aggregation (Liang X. et al., 2022). Both studies encapsulated hDPSCs and HUVECs to obtain highly prevascularized microtissues. In addition, the research team injected GelMA loaded with DPSCs or endothelial cells into transparent silicone tubes and crosslinked with ultraviolet to fabricate cell-laden microfibers. Two types of GelMA hydrogel microfibers loaded with HUVECs and DPSCs were respectively prepared and combined at a ratio of 2:1 to form microfiber aggregates (MAs). Compared with GelMA hydrogel blocks, the MAs have better mechanical properties, and their diameter of 400 μm was similar to that of microvessels, which enabled these materials to improve the survival rate of cells and induce the formation of more pulp-like tissue, blood vessels and odontoblast-like cells in an ectopic root model (Liang Q. et al., 2022).
5.1.1.4 Synthetic scaffold based on other materials
The modification of other materials also achieved excellent results. Silva modified an injectable HA hydrogel with cellulose nanocrystals and added PL. Cellulose nanocrystals improved the mechanical properties and biological activities of HA while optimizing the release curve of angiogenic factors in PL (Silva et al., 2018). Lai took advantage of the potential of Si ions to promote angiogenesis by preparing cell blocks with alginate/fish gelatin gel rich in human DPSCs in the center and fish gelatin methacrylate rich in silicon ion implanted HUVECs in the periphery. The combination of alginate and gelatin optimized the physical and biological properties, and the modification of gelatin by methacrylate caused the gelatin to photopolymerize, improving the mechanical and physical properties. Finally, the synergistic cell block induced the formation of vascular structure and odontogenesis (Lai et al., 2021). Li combined VEGF with heparin and encapsulated VEGF in heparin-conjugated gelatin nanospheres. The nanospheres were fixed in nanofibers of injectable poly (l-lactic acid) microspheres. In summary, the VEGF binding domain of heparin prevented the denaturation and degradation of VEGF and controlled its slow release; the double-layer microsphere system simulated the ECM structure, effectively accommodated DPSCs to promote their proliferation, migration and differentiation, and cooperatively induced the formation of vascularized dental pulp tissue (Li et al., 2016).
5.1.2 3D self-assembled microtissue constructs
The communication between cells and the cell‒ECM interaction in a 3D microtissue construct assembled by cells can mostly simulate the microenvironment in vivo and is not affected by materials. Dissanayaka constructed a scaffold-free microtissue sphere of hDPCs that was prevascularized by HUVECs in agarose 3D petri dishes and ultimately induced the regeneration of vascularized pulp-like tissue (Dissanayaka et al., 2014; 2015b). Itoh fabricated a 3D scaffold-free rod cell construct composed of DPSCs in a thermoresponsive hydrogel mold and formed dental pulp-like tissue with rich blood vessels in a model of tooth root ectopia. The part of the tissue in contact with the dentin wall differentiated into odontoblast-like cells, and human CD31+ ECs were found in the center of the tissue (Itoh et al., 2018). A previous study referred to Itoh’s method to fabricate 3D DPSCs constructs (cube structure), and then induced the formation of prevascularized endothelial differentiated cell constructs to achieve the regeneration of vascularized pulp tissue (Katata et al., 2021).
5.2 Top-down fabrication
Top-down fabrication uses different technologies, such as dissolution and sacrifice molding, 3D bioprinting, laser degradation, layer-by-layer fabrication and microfluidics (Miller et al., 2012; Song et al., 2018; Sharma et al., 2019), to prebuild vascular beds with specific geometric shapes. In the field of dental pulp regeneration, dissolution sacrificial molding is most commonly used. Sacrificial materials are added to the hydrogel to form hollow microchannels. ECs and pericytes or other related vascular cells are seeded into the inner wall of the channel to form microvessels. Other technologies in this field have yet to be developed.
Athirasala placed a prefabricated sacrificial agarose fiber in the root canal to create a microchannel through the full length of the root canal from the pulp chamber to the apical foramen. They placed GelMA hydrogel prepolymer loaded with odontocyte-like cells (OD21) in the root canal (around the microchannel) and aspirated the sacrificial fiber with a vacuum glass straw after photopolymerization. Then, endothelial colony forming cells were seeded into the microchannel, differentiated into monolayer ECs with vascular bud sprouting on the seventh day, and formed a full-length prevascularized pulp-like tissue structure (Athirasala et al., 2017).
Qi et al. utilized the template micromolding technique to create in vitro 3D engineered tissue constructs with inbuilt fluidic microchannels for dental pulp tissue regeneration. They first built PDMS molds that formed the fundamental part of the microchannel-on-a-chip and prepared agarose sacrificial template material. Then, parallel and different tapered templates were fabricated by injecting agarose solution into the PDMS molds using a glass capillary and gutta-percha points, respectively. The agarose template was assembled in the middle of the PDMS chip. Subsequently, the microchannel was formed by dispensing different concentrations of GelMA hydrogel precursor to cover the template, followed by photocrosslinking and removal of the template. Finally, HUVECs were seeded alone or with SCAPs in coculture into these microchannels. The coculture of HUVECs and SCAPs at a 1:1 ratio in 0.04 taper microchannels within 5% GelMA hydrogel scaffolds was the most conducive to stimulating sprouting angiogenesis. In addition, the preencapsulation of SCAPs in the GelMA hydrogel around the microchannel could further accelerate the process of sprouting (Qi et al., 2021).
5.3 Future directions
Regarding construction strategies for prevascularization systems, we can focus on the following points in future research: further development of materials, including reducing the complexity of their preparation and improving their yield and properties. In addition, natural and synthetic materials are not completely oppositing and could potentially be combined. Zheng combined GelMA hydrogel microspheres with dental pulp derived growth factors to achieve multifunctional dental pulp regeneration by taking advantage of the former’s plasticity, biocompatibility and easy functionalization, and the latter’s ability to reduce the dental pulp ECM to induce DPSCs to differentiate in multiple directions (Zheng et al., 2023). Therefore, the proper combination of the two types of materials will be helpful to further optimize their performance based on the maximum simulation of the ECM and surpass the functional limitations of natural tissue. Although 3D self-assembled constructs can avoid the influence of materials and rely on the ECM secreted by cells to restore the microenvironment in vivo, challenges such as low production, high technical difficulty and high cost still need to be solved (Liang X. et al., 2022). The unique advantage of top-down fabrication is that it can personalize the root canals of different patients. However, at present, this strategy is rarely used in the field of pulp regeneration. Therefore, the development of other technologies can be considered while further studying dissolution sacrificial molding. In a word, the enrichment and optimization of the methodology can help the rapid development of prevascularization technology that can be initially applied in pulp regeneration and identify the most suitable construction strategy in the future.
6 Conclusion
At present, prevascularization techniques for dental pulp tissue engineering remain in their infancy. The cell sources of ECs and pericytes are limited and of low quality; the application and development of intercellular communication mechanisms and mediators are insufficient; the construction of prevascularization systems is based mainly on dissolution and sacrifice molding technology, and research on other technologies remains insufficient. In fact, expanding the range of cell sources and enriching and optimizing the acquisition methods could significantly improve the output and quality of ECs and pericytes, providing strong support for laboratory research and subsequent clinical transformation; an improved understanding and application of intercellular communication, such as taking signaling pathways as therapeutic targets or developing potential signaling mediators, including natural signal molecular storage and EVs, could further accelerate the process of vasculogenesis and angiogenesis in the coculture of ECs and pericytes; cell‒ECM interaction mainly occurs in the prevascularized systems after the implantation of EC-pericyte coculture (dental pulp engineered tissue). Therefore, suitable construction strategies for prevascularization systems, including bottom-up and top-down fabrication, would be conducive to the efficient prevascularization of engineered tissue before implantation and to rapid anastomosis with the host vessel after implantation. The combination of these three factors would ultimately achieve prevascularization in pulp regeneration. This manuscript provides novel ideas for the extensive application and follow-up development of prevascularization techniques for dental pulp regeneration and could thus be used as a reference for future research.
Author contributions
QR, DM, and JW contributed to the conception and design of this manuscript. QR and LG collected data and evidence. QR wrote the manuscript. QR drew the tables and figures. QR, DM, ST, and JW revised the manuscript. All authors listed have made a substantial, direct, and intellectual contribution to the work and approved it for publication.
Conflict of interest
The authors declare that the research was conducted in the absence of any commercial or financial relationships that could be construed as a potential conflict of interest.
Publisher’s note
All claims expressed in this article are solely those of the authors and do not necessarily represent those of their affiliated organizations, or those of the publisher, the editors and the reviewers. Any product that may be evaluated in this article, or claim that may be made by its manufacturer, is not guaranteed or endorsed by the publisher.
References
Afra, S., and Matin, M. M. (2020). Potential of mesenchymal stem cells for bioengineered blood vessels in comparison with other eligible cell sources. Cell Tissue Res. 380 (1), 1–13. doi:10.1007/s00441-019-03161-0
Aksel, H., and Huang, G. T. (2017). Human and swine dental pulp stem cells form a vascularlike network after angiogenic differentiation in comparison with endothelial cells: A quantitative analysis. J. Endod. 43 (4), 588–595. doi:10.1016/j.joen.2016.11.015
Alghanem, A. F., Wilkinson, E. L., Emmett, M. S., Aljasir, M. A., Holmes, K., Rothermel, B. A., et al. (2017). RCAN1.4 regulates VEGFR-2 internalisation, cell polarity and migration in human microvascular endothelial cells. Angiogenesis 20 (3), 341–358. doi:10.1007/s10456-017-9542-0
Alghutaimel, H., Yang, X., Drummond, B., Nazzal, H., Duggal, M., and Raïf, E. (2021). Investigating the vascularization capacity of a decellularized dental pulp matrix seeded with human dental pulp stem cells: In vitro and preliminary in vivo evaluations. Int. Endod. J. 54 (8), 1300–1316. doi:10.1111/iej.13510
Armulik, A., Abramsson, A., and Betsholtz, C. (2005). Endothelial/pericyte interactions. Circ. Res. 97 (6), 512–523. doi:10.1161/01.RES.0000182903.16652.d7
Armulik, A., Genové, G., and Betsholtz, C. (2011). Pericytes: Developmental, physiological, and pathological perspectives, problems, and promises. Dev. Cell 21 (2), 193–215. doi:10.1016/j.devcel.2011.07.001
Athirasala, A., Lins, F., Tahayeri, A., Hinds, M., Smith, A. J., Sedgley, C., et al. (2017). A novel strategy to engineer pre-vascularized full-length dental pulp-like tissue constructs. Sci. Rep. 7 (1), 3323. doi:10.1038/s41598-017-02532-3
Atlas, Y., Gorin, C., Novais, A., Marchand, M. F., Chatzopoulou, E., Lesieur, J., et al. (2021). Microvascular maturation by mesenchymal stem cells in vitro improves blood perfusion in implanted tissue constructs. Biomaterials 268, 120594. doi:10.1016/j.biomaterials.2020.120594
Ben-Shaul, S., Landau, S., Merdler, U., and Levenberg, S. (2019). Mature vessel networks in engineered tissue promote graft-host anastomosis and prevent graft thrombosis. Proc. Natl. Acad. Sci. U. S. A. 116 (8), 2955–2960. doi:10.1073/pnas.1814238116
Bento, L. W., Zhang, Z., Imai, A., Nör, F., Dong, Z., Shi, S., et al. (2013). Endothelial differentiation of SHED requires MEK1/ERK signaling. J. Dent. Res. 92 (1), 51–57. doi:10.1177/0022034512466263
Bergamo, M. T., Zhang, Z., Oliveira, T. M., and Nör, J. E. (2021). VEGFR1 primes a unique cohort of dental pulp stem cells for vasculogenic differentiation. Eur. Cell Mater 41, 332–344. doi:10.22203/eCM.v041a21
Carmeliet, P. (2000a). Mechanisms of angiogenesis and arteriogenesis. Nat. Med. 6 (4), 389–395. doi:10.1038/74651
Carmeliet, P. (2000b). VEGF gene therapy: Stimulating angiogenesis or angioma-genesis? Nat. Med. 6 (10), 1102–1103. doi:10.1038/80430
Carvalho, G. L., Sarra, G., Schröter, G. T., Silva, L., Ariga, S. K. K., Gonçalves, F., et al. (2022). Pro-angiogenic potential of a functionalized hydrogel scaffold as a secretome delivery platform: An innovative strategy for cell homing-based dental pulp tissue engineering. J. Tissue Eng. Regen. Med. 16 (5), 472–483. doi:10.1002/term.3294
Chen, H., Fu, H., Wu, X., Duan, Y., Zhang, S., Hu, H., et al. (2020). Regeneration of pulpo-dentinal-like complex by a group of unique multipotent CD24a(+) stem cells. Sci. Adv. 6 (15), eaay1514. doi:10.1126/sciadv.aay1514
Chen, W. J., Xie, J., Lin, X., Ou, M. H., Zhou, J., Wei, X. L., et al. (2021). The role of small extracellular vesicles derived from lipopolysaccharide-preconditioned human dental pulp stem cells in dental pulp regeneration. J. Endod. 47 (6), 961–969. doi:10.1016/j.joen.2021.03.010
Chou, Y. H., Pan, S. Y., Shao, Y. H., Shih, H. M., Wei, S. Y., Lai, C. F., et al. (2020). Methylation in pericytes after acute injury promotes chronic kidney disease. J. Clin. Invest. 130 (9), 4845–4857. doi:10.1172/jci135773
Cordeiro, M. M., Dong, Z., Kaneko, T., Zhang, Z., Miyazawa, M., Shi, S., et al. (2008). Dental pulp tissue engineering with stem cells from exfoliated deciduous teeth. J. Endod. 34 (8), 962–969. doi:10.1016/j.joen.2008.04.009
Crisan, M., Yap, S., Casteilla, L., Chen, C. W., Corselli, M., Park, T. S., et al. (2008). A perivascular origin for mesenchymal stem cells in multiple human organs. Cell Stem Cell 3 (3), 301–313. doi:10.1016/j.stem.2008.07.003
Davis, S., Aldrich, T. H., Jones, P. F., Acheson, A., Compton, D. L., Jain, V., et al. (1996). Isolation of angiopoietin-1, a ligand for the TIE2 receptor, by secretion-trap expression cloning. Cell 87 (7), 1161–1169. doi:10.1016/s0092-8674(00)81812-7
de Cara, S., Origassa, C. S. T., de Sá Silva, F., Moreira, M., de Almeida, D. C., Pedroni, A. C. F., et al. (2019). Angiogenic properties of dental pulp stem cells conditioned medium on endothelial cells in vitro and in rodent orthotopic dental pulp regeneration. Heliyon 5 (4), e01560. doi:10.1016/j.heliyon.2019.e01560
Delle Monache, S., Martellucci, S., Clementi, L., Pulcini, F., Santilli, F., Mei, C., et al. (2019). In vitro conditioning determines the capacity of dental pulp stem cells to function as pericyte-like cells. Stem Cells Dev. 28 (10), 695–706. doi:10.1089/scd.2018.0192
Dissanayaka, W. L., Han, Y., Zhang, L., Zou, T., and Zhang, C. (2020). Bcl-2 overexpression and hypoxia synergistically enhance angiogenic properties of dental pulp stem cells. Int. J. Mol. Sci. 21 (17), 6159. doi:10.3390/ijms21176159
Dissanayaka, W. L., Hargreaves, K. M., Jin, L., Samaranayake, L. P., and Zhang, C. (2015a). The interplay of dental pulp stem cells and endothelial cells in an injectable peptide hydrogel on angiogenesis and pulp regeneration in vivo. Tissue Eng. Part A 21 (3-4), 550–563. doi:10.1089/ten.TEA.2014.0154
Dissanayaka, W. L., Zhu, L., Hargreaves, K. M., Jin, L., and Zhang, C. (2015b). In vitro analysis of scaffold-free prevascularized microtissue spheroids containing human dental pulp cells and endothelial cells. J. Endod. 41 (5), 663–670. doi:10.1016/j.joen.2014.12.017
Dissanayaka, W. L., Zhu, L., Hargreaves, K. M., Jin, L., and Zhang, C. (2014). Scaffold-free prevascularized microtissue spheroids for pulp regeneration. J. Dent. Res. 93 (12), 1296–1303. doi:10.1177/0022034514550040
Draoui, N., de Zeeuw, P., and Carmeliet, P. (2017). Angiogenesis revisited from a metabolic perspective: Role and therapeutic implications of endothelial cell metabolism. Open Biol. 7 (12), 170219. doi:10.1098/rsob.170219
Farrington-Rock, C., Crofts, N. J., Doherty, M. J., Ashton, B. A., Griffin-Jones, C., and Canfield, A. E. (2004). Chondrogenic and adipogenic potential of microvascular pericytes. Circulation 110 (15), 2226–2232. doi:10.1161/01.Cir.0000144457.55518.E5
Friedenstein, A. J., Gorskaja, J. F., and Kulagina, N. N. (1976). Fibroblast precursors in normal and irradiated mouse hematopoietic organs. Exp. Hematol. 4 (5), 267–274.
Fu, Y., Ju, Y., and Zhao, S. (2023). Ca(v)1.2 regulated odontogenic differentiation of NG2(+) pericytes during pulp injury. Odontology 111 (1), 57–67. doi:10.1007/s10266-022-00720-w
Gaengel, K., Genové, G., Armulik, A., and Betsholtz, C. (2009). Endothelial-mural cell signaling in vascular development and angiogenesis. Arterioscler. Thromb. Vasc. Biol. 29 (5), 630–638. doi:10.1161/atvbaha.107.161521
Ganesh, V., Seol, D., Gomez-Contreras, P. C., Keen, H. L., Shin, K., and Martin, J. A. (2022). Exosome-based cell homing and angiogenic differentiation for dental pulp regeneration. Int. J. Mol. Sci. 24 (1), 466. doi:10.3390/ijms24010466
Gharaei, M. A., Xue, Y., Mustafa, K., Lie, S. A., and Fristad, I. (2018). Human dental pulp stromal cell conditioned medium alters endothelial cell behavior. Stem Cell Res. Ther. 9 (1), 69. doi:10.1186/s13287-018-0815-3
Gong, T., Heng, B. C., Xu, J., Zhu, S., Yuan, C., Lo, E. C., et al. (2017). Decellularized extracellular matrix of human umbilical vein endothelial cells promotes endothelial differentiation of stem cells from exfoliated deciduous teeth. J. Biomed. Mater Res. A 105 (4), 1083–1093. doi:10.1002/jbm.a.36003
Gong, T., Xu, J., Heng, B., Qiu, S., Yi, B., Han, Y., et al. (2019). EphrinB2/EphB4 signaling regulates DPSCs to induce sprouting angiogenesis of endothelial cells. J. Dent. Res. 98 (7), 803–812. doi:10.1177/0022034519843886
Goumans, M. J., Valdimarsdottir, G., Itoh, S., Lebrin, F., Larsson, J., Mummery, C., et al. (2003). Activin receptor-like kinase (ALK)1 is an antagonistic mediator of lateral tgfβ/ALK5 signaling. Mol. Cell 12 (4), 817–828. doi:10.1016/s1097-2765(03)00386-1
Goumans, M. J., Valdimarsdottir, G., Itoh, S., Rosendahl, A., Sideras, P., and ten Dijke, P. (2002). Balancing the activation state of the endothelium via two distinct TGF-beta type I receptors. Embo J. 21 (7), 1743–1753. doi:10.1093/emboj/21.7.1743
Grando Mattuella, L., Westphalen Bento, L., de Figueiredo, J. A., Nör, J. E., de Araujo, F. B., and Fossati, A. C. (2007). Vascular endothelial growth factor and its relationship with the dental pulp. J. Endod. 33 (5), 524–530. doi:10.1016/j.joen.2007.01.003
Gronthos, S., Mankani, M., Brahim, J., Robey, P. G., and Shi, S. (2000). Postnatal human dental pulp stem cells (DPSCs) in vitro and in vivo. Proc. Natl. Acad. Sci. U. S. A. 97 (25), 13625–13630. doi:10.1073/pnas.240309797
Grunewald, M., Avraham, I., Dor, Y., Bachar-Lustig, E., Itin, A., Jung, S., et al. (2006). VEGF-Induced adult neovascularization: Recruitment, retention, and role of accessory cells. Cell 124 (1), 175–189. doi:10.1016/j.cell.2005.10.036
Guan, J., Wang, G., Wang, J., Zhang, Z., Fu, Y., Cheng, L., et al. (2022). Chemical reprogramming of human somatic cells to pluripotent stem cells. Nature 605 (7909), 325–331. doi:10.1038/s41586-022-04593-5
Guo, H., Zhao, W., Liu, A., Wu, M., Shuai, Y., Li, B., et al. (2020). SHED promote angiogenesis in stem cell-mediated dental pulp regeneration. Biochem. Biophys. Res. Commun. 529 (4), 1158–1164. doi:10.1016/j.bbrc.2020.06.151
Hade, M. D., Suire, C. N., and Suo, Z. (2021). Mesenchymal stem cell-derived exosomes: Applications in regenerative medicine. Cells 10 (8), 1959. doi:10.3390/cells10081959
Han, Q. F., Li, W. J., Hu, K. S., Gao, J., Zhai, W. L., Yang, J. H., et al. (2022a). Exosome biogenesis: Machinery, regulation, and therapeutic implications in cancer. Mol. Cancer 21 (1), 207. doi:10.1186/s12943-022-01671-0
Han, Y., Koohi-Moghadam, M., Chen, Q., Zhang, L., Chopra, H., Zhang, J., et al. (2022b). HIF-1α stabilization boosts pulp regeneration by modulating cell metabolism. J. Dent. Res. 101 (10), 1214–1226. doi:10.1177/00220345221091528
Hann, S. Y., Cui, H., Esworthy, T., Zhou, X., Lee, S. J., Plesniak, M. W., et al. (2021). Dual 3D printing for vascularized bone tissue regeneration. Acta Biomater. 123, 263–274. doi:10.1016/j.actbio.2021.01.012
Hoeben, A., Landuyt, B., Highley, M. S., Wildiers, H., Van Oosterom, A. T., and De Bruijn, E. A. (2004). Vascular endothelial growth factor and angiogenesis. Pharmacol. Rev. 56 (4), 549–580. doi:10.1124/pr.56.4.3
Huang, J., Xiong, J., Yang, L., Zhang, J., Sun, S., and Liang, Y. (2021). Cell-free exosome-laden scaffolds for tissue repair. Nanoscale 13 (19), 8740–8750. doi:10.1039/d1nr01314a
Itoh, Y., Sasaki, J. I., Hashimoto, M., Katata, C., Hayashi, M., and Imazato, S. (2018). Pulp regeneration by 3-dimensional dental pulp stem cell constructs. J. Dent. Res. 97 (10), 1137–1143. doi:10.1177/0022034518772260
Janebodin, K., Zeng, Y., Buranaphatthana, W., Ieronimakis, N., and Reyes, M. (2013). VEGFR2-dependent angiogenic capacity of pericyte-like dental pulp stem cells. J. Dent. Res. 92 (6), 524–531. doi:10.1177/0022034513485599
Katata, C., Sasaki, J. I., Li, A., Abe, G. L., Nör, J. E., Hayashi, M., et al. (2021). Fabrication of vascularized DPSC constructs for efficient pulp regeneration. J. Dent. Res. 100 (12), 1351–1358. doi:10.1177/00220345211007427
Kesavan, C., Gomez, G. A., Pourteymoor, S., and Mohan, S. (2023). Development of an animal model for traumatic brain injury augmentation of heterotopic ossification in response to local injury. Biomedicines 11 (3), 943. doi:10.3390/biomedicines11030943
Khayat, A., Monteiro, N., Smith, E. E., Pagni, S., Zhang, W., Khademhosseini, A., et al. (2017). GelMA-encapsulated hDPSCs and HUVECs for dental pulp regeneration. J. Dent. Res. 96 (2), 192–199. doi:10.1177/0022034516682005
Kim, D. H., Heo, S. J., Kang, Y. G., Shin, J. W., Park, S. H., and Shin, J. W. (2016a). Shear stress and circumferential stretch by pulsatile flow direct vascular endothelial lineage commitment of mesenchymal stem cells in engineered blood vessels. J. Mater Sci. Mater Med. 27 (3), 60. doi:10.1007/s10856-016-5670-0
Kim, I., Kim, H. G., So, J. N., Kim, J. H., Kwak, H. J., and Koh, G. Y. (2000). Angiopoietin-1 regulates endothelial cell survival through the phosphatidylinositol 3'-Kinase/Akt signal transduction pathway. Circ. Res. 86 (1), 24–29. doi:10.1161/01.res.86.1.24
Kim, J. H., Kim, G. H., Kim, J. W., Pyeon, H. J., Lee, J. C., Lee, G., et al. (2016b). In vivo angiogenic capacity of stem cells from human exfoliated deciduous teeth with human umbilical vein endothelial cells. Mol. Cells 39 (11), 790–796. doi:10.14348/molcells.2016.0131
Kim, S., and von Recum, H. (2008). Endothelial stem cells and precursors for tissue engineering: Cell source, differentiation, selection, and application. Tissue Eng. Part B Rev. 14 (1), 133–147. doi:10.1089/teb.2007.0304
Kim, T. M., Lee, R. H., Kim, M. S., Lewis, C. A., and Park, C. (2023). ETV2/ER71, the key factor leading the paths to vascular regeneration and angiogenic reprogramming. Stem Cell Res. Ther. 14 (1), 41. doi:10.1186/s13287-023-03267-x
Klotz, B. J., Oosterhoff, L. A., Utomo, L., Lim, K. S., Vallmajo-Martin, Q., Clevers, H., et al. (2019). A versatile biosynthetic hydrogel platform for engineering of tissue analogues. Adv. Healthc. Mater 8 (19), e1900979. doi:10.1002/adhm.201900979
Koutsoumparis, A., Vassili, A., Bakopoulou, A., Ziouta, A., and Tsiftsoglou, A. S. (2018). Erythropoietin (rhEPOa) promotes endothelial transdifferentiation of stem cells of the apical papilla (SCAP). Arch. Oral Biol. 96, 96–103. doi:10.1016/j.archoralbio.2018.09.001
Koyano-Nakagawa, N., and Garry, D. J. (2017). Etv2 as an essential regulator of mesodermal lineage development. Cardiovasc Res. 113 (11), 1294–1306. doi:10.1093/cvr/cvx133
Kritharis, A., Al-Samkari, H., and Kuter, D. J. (2018). Hereditary hemorrhagic telangiectasia: Diagnosis and management from the hematologist's perspective. Haematologica 103 (9), 1433–1443. doi:10.3324/haematol.2018.193003
Lai, W. Y., Lee, T. H., Chen, J. X., Ng, H. Y., Huang, T. H., and Shie, M. Y. (2021). Synergies of human umbilical vein endothelial cell-laden calcium silicate-activated gelatin methacrylate for accelerating 3D human dental pulp stem cell differentiation for endodontic regeneration. Polym. (Basel) 13 (19), 3301. doi:10.3390/polym13193301
Le Bras, A., Vijayaraj, P., and Oettgen, P. (2010). Molecular mechanisms of endothelial differentiation. Vasc. Med. 15 (4), 321–331. doi:10.1177/1358863x10371685
Li, A., Sasaki, J. I., Inubushi, T., Abe, G. L., Nör, J. E., Yamashiro, T., et al. (2023). Role of heparan sulfate in vasculogenesis of dental pulp stem cells. J. Dent. Res. 102 (2), 207–216. doi:10.1177/00220345221130682
Li, J., Zhu, Y., Li, N., Wu, T., Zheng, X., Heng, B. C., et al. (2021). Upregulation of ETV2 expression promotes endothelial differentiation of human dental pulp stem cells. Cell Transpl. 30, 096368972097873. doi:10.1177/0963689720978739
Li, X., Ma, C., Xie, X., Sun, H., and Liu, X. (2016). Pulp regeneration in a full-length human tooth root using a hierarchical nanofibrous microsphere system. Acta Biomater. 35, 57–67. doi:10.1016/j.actbio.2016.02.040
Li, Z., Wu, M., Liu, S., Liu, X., Huan, Y., Ye, Q., et al. (2022). Apoptotic vesicles activate autophagy in recipient cells to induce angiogenesis and dental pulp regeneration. Mol. Ther. 30 (10), 3193–3208. doi:10.1016/j.ymthe.2022.05.006
Liang, Q., Liang, C., Liu, X., Xing, X., Ma, S., Huang, H., et al. (2022a). Vascularized dental pulp regeneration using cell-laden microfiber aggregates. J. Mater Chem. B 10 (48), 10097–10111. doi:10.1039/d2tb01825j
Liang, X., Xie, L., Zhang, Q., Wang, G., Zhang, S., Jiang, M., et al. (2022b). Gelatin methacryloyl-alginate core-shell microcapsules as efficient delivery platforms for prevascularized microtissues in endodontic regeneration. Acta Biomater. 144, 242–257. doi:10.1016/j.actbio.2022.03.045
Liu, J., Chuah, Y. J., Fu, J., Zhu, W., and Wang, D. A. (2019). Co-culture of human umbilical vein endothelial cells and human bone marrow stromal cells into a micro-cavitary gelatin-methacrylate hydrogel system to enhance angiogenesis. Mater Sci. Eng. C Mater Biol. Appl. 102, 906–916. doi:10.1016/j.msec.2019.04.089
Liu, P., Qin, L., Liu, C., Mi, J., Zhang, Q., Wang, S., et al. (2022). Exosomes derived from hypoxia-conditioned stem cells of human deciduous exfoliated teeth enhance angiogenesis via the transfer of let-7f-5p and miR-210-3p. Front. Cell Dev. Biol. 10, 879877. doi:10.3389/fcell.2022.879877
Liu, W., Gong, Q., Ling, J., Zhang, W., Liu, Z., and Quan, J. (2014). Role of miR-424 on angiogenic potential in human dental pulp cells. J. Endod. 40 (1), 76–82. doi:10.1016/j.joen.2013.09.035
Luzuriaga, J., Irurzun, J., Irastorza, I., Unda, F., Ibarretxe, G., and Pineda, J. R. (2020). Vasculogenesis from human dental pulp stem cells grown in matrigel with fully defined serum-free culture media. Biomedicines 8 (11), 483. doi:10.3390/biomedicines8110483
Manocha, E., Consonni, A., Baggi, F., Ciusani, E., Cocce, V., Paino, F., et al. (2022). CD146(+) pericytes subset isolated from human micro-fragmented fat tissue display a strong interaction with endothelial cells: A potential cell target for therapeutic angiogenesis. Int. J. Mol. Sci. 23 (10), 5806. doi:10.3390/ijms23105806
Marchand, M., Monnot, C., Muller, L., and Germain, S. (2019). Extracellular matrix scaffolding in angiogenesis and capillary homeostasis. Semin. Cell Dev. Biol. 89, 147–156. doi:10.1016/j.semcdb.2018.08.007
Mazio, C., Casale, C., Imparato, G., Urciuolo, F., Attanasio, C., De Gregorio, M., et al. (2019). Pre-vascularized dermis model for fast and functional anastomosis with host vasculature. Biomaterials 192, 159–170. doi:10.1016/j.biomaterials.2018.11.018
Meijer, E. M., van Dijk, C. G. M., Kramann, R., Verhaar, M. C., and Cheng, C. (2022). Implementation of pericytes in vascular regeneration strategies. Tissue Eng. Part B Rev. 28 (1), 1–21. doi:10.1089/ten.TEB.2020.0229
Miller, J. S., Stevens, K. R., Yang, M. T., Baker, B. M., Nguyen, D. H., Cohen, D. M., et al. (2012). Rapid casting of patterned vascular networks for perfusable engineered three-dimensional tissues. Nat. Mater 11 (9), 768–774. doi:10.1038/nmat3357
Miura, M., Gronthos, S., Zhao, M., Lu, B., Fisher, L. W., Robey, P. G., et al. (2003). Shed: Stem cells from human exfoliated deciduous teeth. Proc. Natl. Acad. Sci. U. S. A. 100 (10), 5807–5812. doi:10.1073/pnas.0937635100
Monteiro, N., He, W., Franca, C. M., Athirasala, A., and Bertassoni, L. E. (2018). Engineering microvascular networks in LED light-cured cell-laden hydrogels. ACS Biomater. Sci. Eng. 4 (7), 2563–2570. doi:10.1021/acsbiomaterials.8b00502
Murakami, M., Horibe, H., Iohara, K., Hayashi, Y., Osako, Y., Takei, Y., et al. (2013). The use of granulocyte-colony stimulating factor induced mobilization for isolation of dental pulp stem cells with high regenerative potential. Biomaterials 34 (36), 9036–9047. doi:10.1016/j.biomaterials.2013.08.011
Murray, I. R., West, C. C., Hardy, W. R., James, A. W., Park, T. S., Nguyen, A., et al. (2014). Natural history of mesenchymal stem cells, from vessel walls to culture vessels. Cell Mol. Life Sci. 71 (8), 1353–1374. doi:10.1007/s00018-013-1462-6
Nakashima, M., and Iohara, K. J. A. i. D. R. (2011). Regeneration of dental pulp by stem cells. Adv. Dent. Res. 23 (3), 313–319. doi:10.1177/0022034511405323
Nakashima, M., Iohara, K., and Sugiyama, M. (2009). Human dental pulp stem cells with highly angiogenic and neurogenic potential for possible use in pulp regeneration. Cytokine Growth Factor Rev. 20 (5-6), 435–440. doi:10.1016/j.cytogfr.2009.10.012
Peloquin, J., Huynh, J., Williams, R. M., and Reinhart-King, C. A. (2011). Indentation measurements of the subendothelial matrix in bovine carotid arteries. J. Biomech. 44 (5), 815–821. doi:10.1016/j.jbiomech.2010.12.018
Pugh, C. W., and Ratcliffe, P. J. (2003). Regulation of angiogenesis by hypoxia: Role of the HIF system. Nat. Med. 9 (6), 677–684. doi:10.1038/nm0603-677
Qi, Y., Zou, T., Dissanayaka, W. L., Wong, H. M., Bertassoni, L. E., and Zhang, C. (2021). Fabrication of tapered fluidic microchannels conducive to angiogenic sprouting within gelatin methacryloyl hydrogels. J. Endod. 47 (1), 52–61. doi:10.1016/j.joen.2020.08.026
Rademakers, T., Horvath, J. M., van Blitterswijk, C. A., and LaPointe, V. L. S. (2019). Oxygen and nutrient delivery in tissue engineering: Approaches to graft vascularization. J. Tissue Eng. Regen. Med. 13 (10), 1815–1829. doi:10.1002/term.2932
Ratajczak, J., Bronckaers, A., Dillen, Y., Gervois, P., Vangansewinkel, T., Driesen, R. B., et al. (2016). The neurovascular properties of dental stem cells and their importance in dental tissue engineering. Stem Cells Int. 2016, 17. doi:10.1155/2016/9762871
Sakai, V. T., Zhang, Z., Dong, Z., Neiva, K. G., Machado, M. A., Shi, S., et al. (2010). SHED differentiate into functional odontoblasts and endothelium. J. Dent. Res. 89 (8), 791–796. doi:10.1177/0022034510368647
Salvucci, O., and Tosato, G. (2012). Essential roles of EphB receptors and EphrinB ligands in endothelial cell function and angiogenesis. Adv. Cancer Res. 114, 21–57. doi:10.1016/b978-0-12-386503-8.00002-8
Sasaki, J. I., Zhang, Z., Oh, M., Pobocik, A. M., Imazato, S., Shi, S., et al. (2020). VE-cadherin and anastomosis of blood vessels formed by dental stem cells. J. Dent. Res. 99 (4), 437–445. doi:10.1177/0022034520902458
Shafiee, S., Shariatzadeh, S., Zafari, A., Majd, A., and Niknejad, H. (2021). Recent advances on cell-based Co-culture strategies for prevascularization in tissue engineering. Front. Bioeng. Biotechnol. 9, 745314. doi:10.3389/fbioe.2021.745314
Sharma, D., Ross, D., Wang, G., Jia, W., Kirkpatrick, S. J., and Zhao, F. (2019). Upgrading prevascularization in tissue engineering: A review of strategies for promoting highly organized microvascular network formation. Acta Biomater. 95, 112–130. doi:10.1016/j.actbio.2019.03.016
Sharmin, R., Hamoh, T., Sigaeva, A., Mzyk, A., Damle, V. G., Morita, A., et al. (2021). Fluorescent nanodiamonds for detecting free-radical generation in real time during shear stress in human umbilical vein endothelial cells. ACS Sens. 6 (12), 4349–4359. doi:10.1021/acssensors.1c01582
Shokrani, H., Shokrani, A., Sajadi, S. M., Seidi, F., Mashhadzadeh, A. H., Rabiee, N., et al. (2022). Cell-seeded biomaterial scaffolds: The urgent need for unanswered accelerated angiogenesis. Int. J. Nanomedicine 17, 1035–1068. doi:10.2147/ijn.S353062
Silva, C. R., Babo, P. S., Gulino, M., Costa, L., Oliveira, J. M., Silva-Correia, J., et al. (2018). Injectable and tunable hyaluronic acid hydrogels releasing chemotactic and angiogenic growth factors for endodontic regeneration. Acta Biomater. 77, 155–171. doi:10.1016/j.actbio.2018.07.035
Silva, G. O., Zhang, Z., Cucco, C., Oh, M., Camargo, C. H. R., and Nör, J. E. (2017). Lipoprotein receptor-related protein 6 signaling is necessary for vasculogenic differentiation of human dental pulp stem cells. J. Endod. 43, S25–s30. doi:10.1016/j.joen.2017.06.006
Sivarapatna, A., Ghaedi, M., Le, A. V., Mendez, J. J., Qyang, Y., and Niklason, L. E. (2015). Arterial specification of endothelial cells derived from human induced pluripotent stem cells in a biomimetic flow bioreactor. Biomaterials 53, 621–633. doi:10.1016/j.biomaterials.2015.02.121
Song, H. G., Rumma, R. T., Ozaki, C. K., Edelman, E. R., and Chen, C. S. (2018). Vascular tissue engineering: Progress, challenges, and clinical promise. Cell Stem Cell 22 (3), 340–354. doi:10.1016/j.stem.2018.02.009
Sonoyama, W., Liu, Y., Fang, D., Yamaza, T., Seo, B. M., Zhang, C., et al. (2006). Mesenchymal stem cell-mediated functional tooth regeneration in swine. PLoS One 1 (1), e79. doi:10.1371/journal.pone.0000079
Suehiro, J., Kanki, Y., Makihara, C., Schadler, K., Miura, M., Manabe, Y., et al. (2014). Genome-wide approaches reveal functional vascular endothelial growth factor (VEGF)-inducible nuclear factor of activated T cells (NFAT) c1 binding to angiogenesis-related genes in the endothelium. J. Biol. Chem. 289 (42), 29044–29059. doi:10.1074/jbc.M114.555235
Takahashi, K., Tanabe, K., Ohnuki, M., Narita, M., Ichisaka, T., Tomoda, K., et al. (2007). Induction of pluripotent stem cells from adult human fibroblasts by defined factors. Cell 131 (5), 861–872. doi:10.1016/j.cell.2007.11.019
Tsang, W. P., Shu, Y., Kwok, P. L., Zhang, F., Lee, K. K., Tang, M. K., et al. (2013). CD146+ human umbilical cord perivascular cells maintain stemness under hypoxia and as a cell source for skeletal regeneration. PLoS One 8 (10), e76153. doi:10.1371/journal.pone.0076153
Utzinger, U., Baggett, B., Weiss, J. A., Hoying, J. B., and Edgar, L. T. (2015). Large-scale time series microscopy of neovessel growth during angiogenesis. Angiogenesis 18 (3), 219–232. doi:10.1007/s10456-015-9461-x
Van Geest, R. J., Klaassen, I., Vogels, I. M., Van Noorden, C. J., and Schlingemann, R. O. (2010). Differential TGF-{beta} signaling in retinal vascular cells: A role in diabetic retinopathy? Invest. Ophthalmol. Vis. Sci. 51 (4), 1857–1865. doi:10.1167/iovs.09-4181
van Niel, G., D'Angelo, G., and Raposo, G. (2018). Shedding light on the cell biology of extracellular vesicles. Nat. Rev. Mol. Cell Biol. 19 (4), 213–228. doi:10.1038/nrm.2017.125
Vaux, D. L., Cory, S., and Adams, J. M. (1988). Bcl-2 gene promotes haemopoietic cell survival and cooperates with c-myc to immortalize pre-B cells. Nature 335 (6189), 440–442. doi:10.1038/335440a0
Wang, D., Lyu, Y., Yang, Y., Zhang, S., Chen, G., Pan, J., et al. (2022). Schwann cell-derived EVs facilitate dental pulp regeneration through endogenous stem cell recruitment via SDF-1/CXCR4 axis. Acta Biomater. 140, 610–624. doi:10.1016/j.actbio.2021.11.039
Wang, P., Zhu, S., Yuan, C., Wang, L., Xu, J., and Liu, Z. (2018). Shear stress promotes differentiation of stem cells from human exfoliated deciduous teeth into endothelial cells via the downstream pathway of VEGF-Notch signaling. Int. J. Mol. Med. 42 (4), 1827–1836. doi:10.3892/ijmm.2018.3761
Wang, W. Y., Lin, D., Jarman, E. H., Polacheck, W. J., and Baker, B. M. (2020). Functional angiogenesis requires microenvironmental cues balancing endothelial cell migration and proliferation. Lab. Chip 20 (6), 1153–1166. doi:10.1039/c9lc01170f
Wang, Y., Xu, J., Chang, L., Meyers, C. A., Zhang, L., Broderick, K., et al. (2019). Relative contributions of adipose-resident CD146(+) pericytes and CD34(+) adventitial progenitor cells in bone tissue engineering. NPJ Regen. Med. 4, 1. doi:10.1038/s41536-018-0063-2
Wingate, K., Floren, M., Tan, Y., Tseng, P., and Tan, W. J. T. E. P. A. (2014). Synergism of matrix stiffness and vascular endothelial growth factor on mesenchymal stem cells for vascular endothelial regeneration. Tissue Eng. Part A 20 (17-18), 2503–2512. doi:10.1089/ten.tea.2013.0249
Wong, C., and Jin, Z. G. (2005). Protein kinase C-dependent protein kinase D activation modulates ERK signal pathway and endothelial cell proliferation by vascular endothelial growth factor. J. Biol. Chem. 280 (39), 33262–33269. doi:10.1074/jbc.M503198200
Wu, M., Liu, X., Li, Z., Huang, X., Guo, H., Guo, X., et al. (2021). SHED aggregate exosomes shuttled miR-26a promote angiogenesis in pulp regeneration via TGF-β/SMAD2/3 signalling. Cell Prolif. 54 (7), e13074. doi:10.1111/cpr.13074
Xie, Z., Shen, Z., Zhan, P., Yang, J., Huang, Q., Huang, S., et al. (2021). Functional dental pulp regeneration: Basic research and clinical translation. Int. J. Mol. Sci. 22 (16), 8991. doi:10.3390/ijms22168991
Xu, F., Qiao, L., Zhao, Y., Chen, W., Hong, S., Pan, J., et al. (2019a). The potential application of concentrated growth factor in pulp regeneration: An in vitro and in vivo study. Stem Cell Res. Ther. 10 (1), 134. doi:10.1186/s13287-019-1247-4
Xu, J. G., Gong, T., Wang, Y. Y., Zou, T., Heng, B. C., Yang, Y. Q., et al. (2018). Inhibition of TGF-β signaling in SHED enhances endothelial differentiation. J. Dent. Res. 97 (2), 218–225. doi:10.1177/0022034517733741
Xu, J. G., Zhu, S. Y., Heng, B. C., Dissanayaka, W. L., and Zhang, C. F. (2017). TGF-β1-induced differentiation of SHED into functional smooth muscle cells. Stem Cell Res. Ther. 8 (1), 10. doi:10.1186/s13287-016-0459-0
Xu, M., He, J., Zhang, C., Xu, J., and Wang, Y. (2019b). Strategies for derivation of endothelial lineages from human stem cells. Stem Cell Res. Ther. 10 (1), 200. doi:10.1186/s13287-019-1274-1
Xu, X., Liang, C., Gao, X., Huang, H., Xing, X., Tang, Q., et al. (2021). Adipose tissue-derived microvascular fragments as vascularization units for dental pulp regeneration. J. Endod. 47 (7), 1092–1100. doi:10.1016/j.joen.2021.04.012
Yamanaka, S. (2020). Pluripotent stem cell-based cell therapy-promise and challenges. Cell Stem Cell 27 (4), 523–531. doi:10.1016/j.stem.2020.09.014
Yi, B., Ding, T., Jiang, S., Gong, T., Chopra, H., Sha, O., et al. (2021). Conversion of stem cells from apical papilla into endothelial cells by small molecules and growth factors. Stem Cell Res. Ther. 12 (1), 266. doi:10.1186/s13287-021-02350-5
Yianni, V., and Sharpe, P. T. (2018). Molecular programming of perivascular stem cell precursors. Stem Cells 36 (12), 1890–1904. doi:10.1002/stem.2895
Yu, J., Vodyanik, M. A., Smuga-Otto, K., Antosiewicz-Bourget, J., Frane, J. L., Tian, S., et al. (2007). Induced pluripotent stem cell lines derived from human somatic cells. Science 318 (5858), 1917–1920. doi:10.1126/science.1151526
Yuan, C., Wang, P., Zhu, L., Dissanayaka, W. L., Green, D. W., Tong, E. H., et al. (2015). Coculture of stem cells from apical papilla and human umbilical vein endothelial cell under hypoxia increases the formation of three-dimensional vessel-like structures in vitro. Tissue Eng. Part A 21 (5-6), 1163–1172. doi:10.1089/ten.TEA.2014.0058
Yuan, C., Wang, P., Zhu, S., Liu, Z., Wang, W., Geng, T., et al. (2019). Overexpression of ephrinB2 in stem cells from apical papilla accelerates angiogenesis. Oral Dis. 25 (3), 848–859. doi:10.1111/odi.13042
Yuan, C., Wang, P., Zhu, S., Zou, T., Wang, S., Xu, J., et al. (2016). EphrinB2 stabilizes vascularlike structures generated by endothelial cells and stem cells from apical papilla. J. Endod. 42 (9), 1362–1370. doi:10.1016/j.joen.2016.05.012
Zhang, L., Han, Y., Chen, Q., and Dissanayaka, W. L. (2022a). Sema4D-plexin-B1 signaling in recruiting dental stem cells for vascular stabilization on a microfluidic platform. Lab. Chip 22 (23), 4632–4644. doi:10.1039/d2lc00632d
Zhang, Q., Yang, T., Zhang, R., Liang, X., Wang, G., Tian, Y., et al. (2021a). Platelet lysate functionalized gelatin methacrylate microspheres for improving angiogenesis in endodontic regeneration. Acta Biomater. 136, 441–455. doi:10.1016/j.actbio.2021.09.024
Zhang, S., Thiebes, A. L., Kreimendahl, F., Ruetten, S., Buhl, E. M., Wolf, M., et al. (2020). Extracellular vesicles-loaded fibrin gel supports rapid neovascularization for dental pulp regeneration. Int. J. Mol. Sci. 21 (12), 4226. doi:10.3390/ijms21124226
Zhang, Y., Liu, J., Zou, T., Qi, Y., Yi, B., Dissanayaka, W. L., et al. (2021b). DPSCs treated by TGF-β1 regulate angiogenic sprouting of three-dimensionally co-cultured HUVECs and DPSCs through VEGF-Ang-Tie2 signaling. Stem Cell Res. Ther. 12 (1), 281. doi:10.1186/s13287-021-02349-y
Zhang, Z., Nör, F., Oh, M., Cucco, C., Shi, S., and Nör, J. E. (2016). Wnt/β-Catenin signaling determines the vasculogenic fate of postnatal mesenchymal stem cells. Stem Cells 34 (6), 1576–1587. doi:10.1002/stem.2334
Zhang, Z., Oh, M., Sasaki, J. I., and Nör, J. E. (2021c). Inverse and reciprocal regulation of p53/p21 and Bmi-1 modulates vasculogenic differentiation of dental pulp stem cells. Cell Death Dis. 12 (7), 644. doi:10.1038/s41419-021-03925-z
Zhang, Z., Warner, K. A., Mantesso, A., and Nör, J. E. (2022b). PDGF-BB signaling via PDGFR-β regulates the maturation of blood vessels generated upon vasculogenic differentiation of dental pulp stem cells. Front. Cell Dev. Biol. 10, 977725. doi:10.3389/fcell.2022.977725
Zheng, L., Liu, Y., Jiang, L., Wang, X., Chen, Y., Li, L., et al. (2023). Injectable decellularized dental pulp matrix-functionalized hydrogel microspheres for endodontic regeneration. Acta Biomater. 156, 37–48. doi:10.1016/j.actbio.2022.11.047
Zhou, T., Rong, M., Wang, Z., Chu, H., Chen, C., Zhang, J., et al. (2021). Conditioned medium derived from 3D tooth germs: A novel cocktail for stem cell priming and early in vivo pulp regeneration. Cell Prolif. 54 (11), e13129. doi:10.1111/cpr.13129
Zhu, L., Dissanayaka, W. L., and Zhang, C. (2019). Dental pulp stem cells overexpressing stromal-derived factor-1α and vascular endothelial growth factor in dental pulp regeneration. Clin. Oral Investig. 23 (5), 2497–2509. doi:10.1007/s00784-018-2699-0
Zhu, S., Ying, Y., He, Y., Zhong, X., Ye, J., Huang, Z., et al. (2021a). Hypoxia response element-directed expression of bFGF in dental pulp stem cells improve the hypoxic environment by targeting pericytes in SCI rats. Bioact. Mater 6 (8), 2452–2466. doi:10.1016/j.bioactmat.2021.01.024
Zhu, S. Y., Yuan, C. Y., Lin, Y. F., Liu, H., Yang, Y. Q., Wong, H. M., et al. (2021b). Stem cells from human exfoliated deciduous teeth (SHEDs) and dental pulp stem cells (DPSCs) display a similar profile with pericytes. Stem Cells Int. 2021, 1–12. doi:10.1155/2021/8859902
Zou, T., Jiang, S., Dissanayaka, W. L., Heng, B. C., Xu, J., Gong, T., et al. (2019). Sema4D/PlexinB1 promotes endothelial differentiation of dental pulp stem cells via activation of AKT and ERK1/2 signaling. J. Cell Biochem. 120 (8), 13614–13624. doi:10.1002/jcb.28635
Zuk, P. A., Zhu, M., Mizuno, H., Huang, J., Futrell, J. W., Katz, A. J., et al. (2001). Multilineage cells from human adipose tissue: Implications for cell-based therapies. Tissue Eng. 7 (2), 211–228. doi:10.1089/107632701300062859
Glossary
Keywords: prevascularization, dental pulp regeneration, tissue engineering, dental stem cells, endothelial cells, pericytes
Citation: Ruan Q, Tan S, Guo L, Ma D and Wen J (2023) Prevascularization techniques for dental pulp regeneration: potential cell sources, intercellular communication and construction strategies. Front. Bioeng. Biotechnol. 11:1186030. doi: 10.3389/fbioe.2023.1186030
Received: 14 March 2023; Accepted: 10 May 2023;
Published: 18 May 2023.
Edited by:
Mona Kamal Marei, Alexandria University, EgyptReviewed by:
Lei Hu, Capital Medical University, ChinaJon Luzuriaga, University of the Basque Country, Spain
Copyright © 2023 Ruan, Tan, Guo, Ma and Wen. This is an open-access article distributed under the terms of the Creative Commons Attribution License (CC BY). The use, distribution or reproduction in other forums is permitted, provided the original author(s) and the copyright owner(s) are credited and that the original publication in this journal is cited, in accordance with accepted academic practice. No use, distribution or reproduction is permitted which does not comply with these terms.
*Correspondence: Dandan Ma, bWRkQHNtdS5lZHUuY24=; Jun Wen, ZHJ3ZW5qdW5Ac211LmVkdS5jbg==