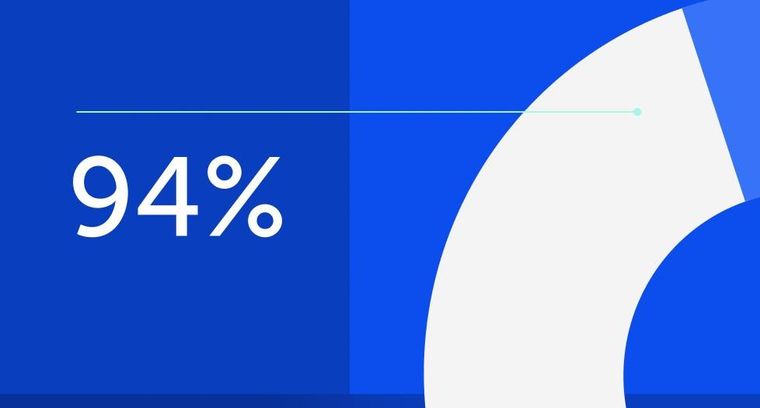
94% of researchers rate our articles as excellent or good
Learn more about the work of our research integrity team to safeguard the quality of each article we publish.
Find out more
ORIGINAL RESEARCH article
Front. Bioeng. Biotechnol., 06 June 2023
Sec. Biomaterials
Volume 11 - 2023 | https://doi.org/10.3389/fbioe.2023.1179332
Tissue Engineering of cartilage has been hampered by the inability of engineered tissue to express native levels of type II collagen in vitro. Inadequate levels of type II collagen are, in part, due to a failure to recapitulate the physiological environment in culture. In this study, we engineered primary rabbit chondrocytes to express a secreted reporter, Gaussia Luciferase, driven by the type II collagen promoter, and applied a Design of Experiments approach to assess chondrogenic differentiation in micronutrient-supplemented medium. Using a Response Surface Model, 240 combinations of micronutrients absent in standard chondrogenic differentiation medium, were screened and assessed for type II collagen promoter-driven Gaussia luciferase expression. While the target of this study was to establish a combination of all micronutrients, alpha-linolenic acid, copper, cobalt, chromium, manganese, molybdenum, vitamins A, E, D and B7 were all found to have a significant effect on type II collagen promoter activity. Five conditions containing all micronutrients predicted to produce the greatest luciferase expression were selected for further study. Validation of these conditions in 3D aggregates identified an optimal condition for type II collagen promoter activity. Engineered cartilage grown in this condition, showed a 170% increase in type II collagen expression (Day 22 Luminescence) and in Young’s tensile modulus compared to engineered cartilage in basal media alone.Collagen cross-linking analysis confirmed formation of type II-type II collagen and type II-type IX collagen cross-linked heteropolymeric fibrils, characteristic of mature native cartilage. Combining a Design of Experiments approach and secreted reporter cells in 3D aggregate culture enabled a high-throughput platform that can be used to identify more optimal physiological culture parameters for chondrogenesis.
Osteoarthritis (OA) is the most common degenerative musculoskeletal disease and is projected to increase in prevalence (Mueller and Tuan, 2011; Sacitharan, 2019). OA is characterized by progressive degeneration of articular cartilage in the joints of the hands, knees, and hip due to an imbalance of cartilage matrix anabolism and catabolism (Mueller and Tuan, 2011; Sacitharan, 2019). Articular cartilage is a form of specialized connective tissue, primarily composed of type II collagen, water, and proteoglycans with sparsely distributed chondrocytes (Sophia Fox et al., 2009). Cartilage has limited healing and regenerative abilities given that its avascular nature limits access to circulating progenitor cells following physical insult (Sophia Fox et al., 2009; Kean and Dennis, 2015). Currently, there are no disease-modifying treatments for OA (Kean and Dennis, 2015; Sacitharan, 2019). Available therapeutics offer short-lived relief of acute symptoms and do not prevent endpoint joint damage, therefore there is a strong need for the development of new disease-modifying therapeutics (Kean and Dennis, 2015; Sacitharan, 2019; Dennis et al., 2020a).
Tissue engineering of cartilage has the potential to revolutionize the field by providing improved in vitro models for drug discovery and/or a biological replacement (Dennis et al., 2020a). Tissue engineering incorporates the use of components such as cells, scaffolds, growth factors, and physical stimulation to generate biomimetic tissue (Francis et al., 2018). However, tissue engineering of cartilage has been hampered by an inability to recapitulate the properties of native cartilage tissue, which we hypothesize is primarily due to insufficient type II collagen production. Whereas 90%–95% of collagen in native tissue is type II collagen, several studies have reported much lower type II collagen levels in engineered tissue with values hovering around 20% of dry weight despite modifications to increase collagen deposition (Vunjak-Novakovic et al., 1999; Gemmiti and Guldberg, 2006; Bian et al., 2011; Sato et al., 2017; Whitney et al., 2018; Dennis et al., 2020a). We postulate that part of this deficiency in type II collagen is due to sub-optimal formulation of the culture medium used for cartilage engineering in vitro.
Differentiation media traditionally used for chondrocyte cell culture was noted to lack several micronutrients which are known to be physiologically essential for a host of biological processes (Dennis et al., 2020b). Although the specific roles that some of these micronutrients have in chondrogenesis remain undefined, there are several findings that point towards these biomolecules having significant effects on cartilage generation and maintenance (Koyano et al., 1996; Booth and Mayer, 1997; Litchfield et al., 1998; Connor, 1999; Finch, 2015; Chin and Ima-Nirwana, 2018; Dennis et al., 2020b). In our ATDC5 study, we found the combination of copper and vitamin K to have the most beneficial effect on type II collagen production (Dennis et al., 2020a). We hypothesize the addition of these vitamins and minerals to basal differentiation medium will promote type II collagen production in vitro and better mimic the physiological environment.
A Design of Experiments (DoE) approach was implemented in this study to screen different combinations of vitamins and minerals. DoE is a statistical technique that facilitates systematic optimization by producing experimental design models to study interactions of multiple factors on a desired outcome or response. DoE allows for a multi-factor, rather than a one-factor approach, that evaluates synergistic effects, and can predict optimal conditions while reducing the burden of conducting repetitive experiments. DoE has provided significant benefits to other fields of engineering and biotechnology but has rarely been used in cartilage tissue engineering and regenerative medicine (Enochson et al., 2012; Kuterbekov et al., 2019). A defined chondrogenic media containing all the missing vitamins and minerals, essential for human health, has not been established prior to this study.
In this study, we have identified, for the first time, an optimal supplementation of physiologically necessary micronutrients to chondrogenic media, using a streamlined platform that includes a type II collagen promoter-driven Gaussia luciferase construct in primary rabbit articular chondrocytes combined with a DoE approach. This optimized chondrogenic media significantly enhances type II collagen expression in primary rabbit chondrocytes cultured in 3D cell aggregates and engineered cartilage sheets.
To characterize the TGF-β1 response of engineered type II collagen promoter-driven Gaussia luciferase reporter (COL2A1-Gluc) in primary rabbit chondrocytes, cells were cultured in 3D aggregates in defined chondrogenic media supplemented with 0–10 ng/mL of TGF-β1, a known stimulator of type II collagen (Han et al., 2005; Jennifer et al., 2010; Dennis et al., 2020a). Conditioned media, containing the secreted Gaussia luciferase, was assayed for luminescence over 3 weeks. Dose response curves were generated from luminescence data at Day 7 (Figure 1A) and Day 21 (Figure 1B). As seen in Figure 1, there was a dose dependent increase in luminescence with a calculated 50% effective concentration (EC50) of 0.17 ng/ml and 0.10 ng/ml for Day 7 and Day 21 respectively. Curve fit was tested with an extra sum-of-squares F test (GraphPad Prism) and p < 0.0001 that the data could not be fit to a single curve.
FIGURE 1. Dose effect of TGF-β1 on COL2A1-GLuc reporter rabbit chondrocytes. (A, B) Primary COL2A1-GLuc rabbit chondrocytes were grown in aggregate culture in the presence of different concentrations of TGF-β1 (0–10 ng/mL). Dose response curves were generated from transformed luminescence data at day 7 (A) and day 21 (B) after seeding. EC50s for each day are shown within each curve. Values are the mean ± S.D. n = 4.
To identify potential interactions between factors and their effect on type II collagen expression, COL2-GLuc rabbit chondrocytes were seeded in 3D aggregate culture with DoE generated combinations of vitamins and minerals. Media was sampled and replaced every 2–3 days over 3 weeks. Combinations and concentrations are defined by the parameters set in the response surface model (Table 1) and are listed in Supplementary Table S2 in Supplementary Data Sheet S2.
TABLE 1. Concentrations of micronutrients absent in chondrogenic media with input parameters for DoE screen.
In the surface response model, each vitamin or mineral is introduced as an independent variable and is defined in Design-Expert (V.12, StatEase) as a model term. Luminescence signal over time, cumulative luminescence and resazurin data are defined as responses. The response surface study was designed as a quadratic model. Figures 2A, B shows the normal probability plot after the data was transformed to fit the quadratic model for week 2 and week 3. The residuals are the deviation of each sample compared to its predicted value. For the residuals to be normally distributed they must show a linear trend, indicated by the red line, with little variation outside of it. As seen in Figures 2A, B the residuals are normally distributed for all timepoints.
FIGURE 2. Dose effect of basal chondrogenic media supplemented with DoE micronutrient combinations on COL2A1-GLuc reporter Rabbit chondrocytes. Normal probability plots of the residuals for Gaussia Luciferase signal at weeks 2 (A) and 3 (B) after seeding. 3D surface response plots for interactive effects between vitamin A and linolenic acid at indicated weeks after seeding. End of week 2 (C), and week 3 (D).
ANOVA analysis of luminescence expression for week 2 and week 3 of chondrogenesis identified significant model terms, i.e., factors that have significant effects on the type II collagen responses (Table 2).
TABLE 2. ANOVA results of surface response model for micronutrients significantly regulating COL2A1-GLuc reporter activity in rabbit chondrocytes.
Results of this analysis include, F-values, p-values, and lack of fit test, which indicate how well the responses fit the model. As shown in Table 2 the F- and P- values of the model, as well as the lack of fit test, over the 3 weeks support that the model is significant and thus the analysis for the associated terms is valid. Table 2 displays model terms that were significant for at least one of the timepoints indicated shown by a p-value <0.05. Significant single terms for all timepoints were linolenic acid, copper, and vitamin A. Several interactions were defined as significant for at least one of the timepoints shown (orange highlighted), while others including: chromium and cobalt, manganese and molybdenum, and cobalt and vitamin D were significant for all timepoints.
To contrast these results, testing micronutrients in a one factor at a time approach previously identified basal media supplemented with cobalt, chromium, thyroxine, or Vitamin B7, as having higher luminescence at multiple concentrations as compared to basal media alone (Supplementary Figure S1A). Combinations of these factors, with factors previously shown to have an effect (Dennis et al., 2020a), are shown in Supplementary Figure S1B. Copper and Vitamin B7 alone or in combination with each other seemingly had no effect on luminescence, ∼40,000 RLU; however, in combination with thyroxine they significantly increased luminescence ∼70,000 RLU as compared to basal media alone, ∼40,000 RLU. This supports that there a synergistic effect between copper, biotin, and thyroxine. Furthermore, this supports the use of a Design of Experiments approach over a one factor a time approach as the DoE screen also identified factors that were significant in interactions but were not identified as individually significant.
Using a response surface model allows us to determine significant interactions between terms as well as determine and predict optimal concentrations of the terms within the parameters input into the initial model. 3D surface plots in Figures 2C, D show the dose effect of two terms (linolenic acid and vitamin A) in relation to each other and to the response (luminescence) at week 2 (Figure 2C) and week 3 (Figure 2D) of chondrogenesis. During week 2 (Figure 2C) there is a predicted optimal concentration for linolenic acid and vitamin A (approximately 3 × 10−6 fg/mL and 5 × 10−1 fg/mL respectively) that results in a maximal response that increases in week 3 (Figure 2D), from 101.8 RLU to 102.4 RLU, although the optimal concentrations of the terms remain the same. Interestingly, the DoE model predicts that there is an optimal concentration for these in the femtomolar range (Figures 2C, D) while each of the two factors, when analyzed as sole additives, were detrimental to chondrogenesis at higher concentrations (Supplementary Figure S1A).
Figures 2C, D is a representation of only two of the terms and a predicted optimal for each individual timepoint. Through Design-Expert, multiple terms and responses can be analyzed together to derive predicted optimal concentrations for all terms. These predicted optima account for individual responses as well interactions between terms. Out of the generated predicted optimal conditions, 5 were selected for validation, designated as conditions 12, 25, 52, 72, and 89 (Supplementary Table S2 in Supplementary Data Sheet S2).
To validate the Design-Expert generated predicted conditions, COL2A1-GLuc cells in aggregate culture were maintained in media supplemented with the predicted conditions (Supplementary Table S2 in Supplementary Data Sheet S2) for 3 weeks. As seen in Figure 3A, all conditions tested had increased luminescence over basal media control for all timepoints after day 8, which suggests that predicted conditions have an anabolic effect early in chondrogenesis. Cumulative luminescence seen in Figure 3B is the sum of luminescence signal over all days in culture and confirms that increased luminescence at each timepoint results in an overall significant increase in type II collagen promoter-driven activity for all predicted conditions, with condition 25 having a higher cumulative signal of ∼1 × 106 RLU as compared to basal media, ∼6 × 105 RLU. Single day luminescence shown for day 10 (Figure 3C) and day 22 (Figure 3D) supports an increase in luminescence that is statistically significant for all conditions tested as compared to basal media with condition 25 having an average luminescence signal that is twice of that in basal media, ∼2 × 105 RLU vs. 1 × 105 RLU respectively, for both timepoints.
FIGURE 3. Validation of DoE predicted optimal conditions. (A–D) Conditions predicted by DoE analysis were tested in aggregate culture of COL2A1-GLuc reporter rabbit chondrocytes over 22 days. Results are shown as luminescence over 22 days sampled (A), as well as cumulative luminescence signal (B). To explore temporal effects, data was also analyzed at single day luminescence shown here for Day 10 (C) and Day 22 (D). At day 22, aggregate cultures were assessed for total DNA (E), glycosaminoglycan (F) and collagen (G) content. Results are also shown as total glycosaminoglycan and collagen normalized to DNA content (H, I) and to each other (J). Alternatively, aggregates were fixed, embedded in paraffin and sectioned. (K) Sections were analyzed for type II collagen. Scale Bars, 200 um. (A–D) N = 6. (E–J) N = 5. Replicates or means ± S.D. and * p <0.05, ** p <0.01 and *** p <0.001 vs. Basal Media control.
To corroborate results seen by luminescence output, endpoint biochemical assays were performed at day 22 of the experiment to quantitate DNA, glycosaminoglycan (GAG) and total collagen content. Figure 3E shows an average of ∼0.4 μg of DNA per sample with no significant difference between conditions tested, which suggests that predicted conditions have no effect on cell proliferation or viability over 22 days. Total glycosaminoglycan content is shown in Figure 3F and as amount per microgram of DNA in Figure 3H. As expected, DoE predicted conditions did not significantly affect glycosaminoglycan production over 22 days, although condition 89 shows large variability between samples as compared to other conditions. Quantification of total collagen, as seen in Figure 3G shows an increase in aggregates cultured in predicted conditions to ∼10 µg over basal media (∼6 µg). However, when normalized to micrograms of DNA (Figure 3I) only conditions 12, 25 and 89, show increased collagen as compared to aggregates cultured in basal media with significant variability within each group leading to lack of statistical significance. Glycosaminoglycan to collagen ratio (Figure 3J) further support an increase in collagen with no change in GAG content (see Supplementary Data Sheet S2 for GAG histology). Immunohistochemistry for type II collagen (Figure 3K) confirms the presence of type II collagen for cell aggregates in all conditions at day 22 with similar staining pattern across all conditions.
When using a one factor at a time approach, thyroxine (T4) was required in the tested combinations for type II collagen stimulation over basal media (Supplementary Figure S1B). To test if one factor was solely responsible for the enhancement in luminescence seen over basal media, aggregates were cultured in the predicted DoE condition 25, or combinations where one factor was removed from condition 25 for 22 days Figure 4A represents luminescence data for all days tested with basal media shown by a thicker black line and complete condition 25 shown by the thicker red line. All conditions had increased luminescence as compared to basal media after day 8. For statistical analysis, day 10 luminescence (Figure 4B) is shown for all conditions tested.
FIGURE 4. Effect of a single micronutrient removal from DoE predicted condition 25 on type II collagen driven expression of Gaussia Luciferase. Aggregates of COL2A1-GLuc primary rabbit chondrocytes were cultured with condition 25 or condition 25 with a single micronutrient removed as indicated. Results are shown as luminescence over 22 days (A). Luminescence is shown for a single timepoint, Day 10 (B). φ indicates p < 0.05 vs Basal Media control. N = 7. Mean ± S.D.
Aggregates cultured in all conditions had increased luminescence. However, effects by condition 25 with Linolenic acid, vitamin A or with vitamin D removed, were not statistically significant as compared to basal media, suggestive of a major role for these biomolecules. Formulations where any of the other factors were removed from Condition 25 had significant increases in luminescence as compared to basal media alone. There was no statistical significance between any of the conditions with a single factor removed as compared to complete condition 25. These results are evidence that no single factor within DoE predicted condition 25 is solely responsible for the higher stimulation of type II collagen promoter activity observed.
To determine if ratios, regardless of the absolute concentration, within the DoE predicted conditions were sufficient for type II collagen stimulation, aggregate cultures were treated with the DoE predicted conditions at the concentrations given or at 1/15th of the predicted concentrations. Two-way ANOVA analysis of luminescence at Day 10 (Figure 5A) and Day 17 (Figure 5B) determined that media conditions at 1x or 1/15x of their DoE predicted concentrations had an overall p = 0.0149 for 6a and p = 0.0004 for 6b but that none of the individual comparisons had p < 0.05.
FIGURE 5. Effect of absolute versus relative concentration of micronutrients on Type II collagen driven expression of Gaussia Luciferase. Aggregates of COL2A1-GLuc primary rabbit chondrocytes were cultured with predicted DoE conditions (1x) or the same ratios of these conditions at 1/15th the optimal predicted concentration (1/15x) over 22 days. Luminescence results are shown for day 10 (A) and day 17 (B). φ indicates p < 0.05 vs Basal Media control. N = 5 for DoE at 1/15x and N = 6 for DoE at 1x. Individual replicates and the mean ± S.D. are shown.
Interestingly, while aggregates cultured at the 1x DoE predicted conditions had significantly higher luminescence as compared to those in basal media, aggregates cultured in most conditions at 1/15th did not. Only condition 25 at 1/15th the concentration showed a significantly higher level of luminescence at both days vs Basal Media, ∼1.5 to ∼1.7 × 105 RLU vs. ∼1 × 105 RLU. This suggests that the combinatorial effect of the vitamins and minerals plays a significant role in type II collagen promoter activity in primary rabbit chondrocytes, but concentrations as predicted by the DoE are optimal for type II collagen stimulation.
To determine if condition 25, the best performing DoE predicted condition, could have an effect in cartilage tissue engineering, we cultured COL2A1-GLuc or Non-transduced (NonTr) primary rabbit chondrocytes in custom, 3D printed bioreactors adapted from Whitney GA, et al. shown in Figures 6A–C over 22 days (Whitney et al., 2012; Dennis et al., 2020b).
FIGURE 6. Biochamber for the generation of tissue engineered cartilage sheets Custom 3D printed ABS biochambers were designed to generate tissue engineered articular cartilage sheets. (A) Model of the biochamber assembly. (B) Photo of printed biochamber and Nalgene container fitted with sterile filter top. (C) Diagram of cell seeding and media top up media levels.
At the end of this culture period, 1.2 cm2 cartilage sheets were collected as shown in Figure 7A. Over the 22 days bioreactors housing COL2A1-Gluc cells were regularly sampled for luminescence. Figure 7B represents luminescence data for all days tested.
FIGURE 7. Tissue engineered cartilage sheet response to supplementation with condition 25. COL2A1-GLuc primary rabbit chondrocytes were cultured in bioreactors with condition 25 (1x) or condition 25 at 1/15th the concentration (1/15x) over 22 days (A) On day 22, engineered sheets were collected. (B) Luminescence signal over 22 days in culture. Max tensile force (C) and tensile modulus (D) are shown. DNA (E), glycosaminoglycan (F) and collagen content (G) of the tissue were assessed. Data is also calculated as GAG/DNA (H), collagen/DNA (I) and GAG/collagen (J).
Similar to results seen when conditions were tested in aggregates (Figure 3), both condition 25 at 1x and at 1/15th concentration showed increased luminescence as compared to basal media after day 8. A different curve in luminescence is observed in Figure 7B as compared to Figure 3A because samples were collected from inside of the biochamber for day 1 and day 3. After day 3, medium was added to increase exchange between the inside and outside of the chamber, thus samples collected are from a larger volume resulting in a decrease in luminescence from day 3 to day 8. After sheets were collected, several biopsy punches of the sheets were obtained for endpoint analysis. To determine whether increased COL2A1 reporter activity translates to improved mechanical properties of engineered cartilage, we assessed tissue elasticity via tensile testing. As expected, we observed a marked increase in the Tensile modulus (Figure 7C) as well as maximal tensile force (Figure 7D), in sheets generated in condition 25 media at 1x and 1/15th of the concentration as compared to basal media, from ∼6 MPa in basal media to ∼8 MPa in condition 25 and ∼10 MPa in condition 25 at 1/15th concentration. In contrast, compression testing resulted in a marked decrease in stiffness for sheets generated in conditions 25 regardless of concentration (Supplementary Figure S2).
To evaluate whether the luminescence results reflected matrix accumulation, biochemical assays were performed at day 22 of the experiment to quantitate DNA, glycosaminoglycan (GAG) and total collagen content from bioreactors seeded with COL2A1-Gluc and non-transduced cells. Both COL2A1-gLuc and non-transduced cell generated sheets had similar trends in DNA (Figure 7E), GAG (Figure 7F), and total collagen (Figure 7G) except for the sheet generated with COL2A1-Gluc cells in condition 25 at (1x), which shows lower collagen as compared to its non-transduced counterpart (Figure 7G). Sheets in supplemented groups had overall higher collagen content per milligram of tissue, as well as per mi-crogram of DNA (Figures 7G,I). Total glycosaminoglycan content is relatively constant for all groups at ∼40 μg per mg of wet tissue weight (Figure 7F), was potentially stimulated in condition 25 when normalized to DNA (Figure 7H). When normalized to collagen content (Figure 7J) there is a noticeable decrease in both reporter cell and non-transduced sheets generated in condition 25 (both 1x and 1/15x) suggesting that condition 25 specifically affects collagen content and not glycosaminoglycan content.
Immunofluorescence of sections of the engineered cartilage confirmed the presence of type II collagen (Figure 8A). Interestingly, condition 25 (1/15th) had a more even distribution of type II collagen signal as compared to basal media alone which showed an uneven pattern of staining. Collagen heteropolymer analysis was also carried out on samples of the engineered cartilage sheets and compared to native rabbit articular cartilage to further analyze collagen content. Figure 8B shows that the major pepsin-resistant Coomassie blue-stained band in both transfected and non-transfected articular chondrocyte cultures migrated identically to the α1(II) chain of type II collagen in the adult rabbit articular cartilage. β1(II) chains (dimers of α1(II) chains) were also observed in all lanes (Figure 8B). Two faintly stained bands migrating slightly slower than that of the α1(II) chains, are the α1 (XI) and α2 (XI) chains of type XI collagen as previously identified by mass spectrometry and described by McAlinden et al. (Fernandes et al., 2007; McAlinden et al., 2014). The α1(I) and α2(I) chains of type I collagen were neither detected in engineered cartilages nor in the control articular cartilage (if present the α2(I) chain migrates slightly faster than the α1(II) chain), indicating that type II collagen and type XI collagen were the major collagens synthesized by the cultured chondrocytes and the cartilage collagen phenotype was maintained (Figure 8B). The Western blot shown in Figure 8C confirms that the Coomassie blue stained bands were indeed α1(II) chains of type II collagen.
FIGURE 8. Analysis of Type II collagen and heteropolymer formation with Type IX collagen in tissue engineered cartilage. (A) Engineered cartilage was analyzed for type II collagen. Scale Bars, 300 um. (B) Coomassie blue-stained SDS-PAGE gel of pepsin solubilized collagen showing β1(II), α1 (XI), α2 (XI) and α1(II) chains. Equivalent dry weight (25 µg) was loaded. (C) Western blot of samples equivalent to those in (B) and probed with anti-type II collagen antibody (1C10). (D) Western blot of samples equivalent to those electrophoresed in (B) (above) and probed with mAb 10F2. This antibody specifically recognizes the C- telopeptide domain of type II collagen when it is cross-linked to another α1(II) collagen chain. (E) Western blot of samples identical to those in (B) probed with antibody 5890. This antibody specifically recognizes N-telopeptide domain of α1 (XI) collagen when cross-linked to chains of α1(II) and β1(II). X denotes crosslinks.
Using mAb 1C10, which specifically recognizes type II collagen chains (Fernandes et al., 2001), intense staining of both the α1(II) and β1(II) chains were observed in all the cultures and the adult rabbit cartilage. Since equivalent engineered cartilage dry weights loads were electrophoresed in all the lanes, densitometry of the Western blot revealed increased levels of type II collagen (α1(II) + β1(II)) reactivity under condition 25 in both transfected and non-transfected cultures compared to basal conditions. Condition 25 (1/15x) however showed highest levels of reactivity indicating highest type II collagen retained in the Condition 25 (1/15x) sheet. This is consistent with the analytical results in Figure 7G showing highest collagen content under this condition on a per mass basis. Using a refined Western blot method (Dennis et al., 2020b) we were able to identify precise domains of collagen chains that were cross-linked in these cultures. As seen in Figure 8D, Western blotting using mAb 10F2 (Fernandes et al., 2003; McAlinden et al., 2014) recognized the α1(II) and β1(II) chains in all the cultures and the adult rabbit cartilage. This is evidence that the C-telopeptide of the α1(II) chain specifically recognized by this antibody was cross-linked to the helical lysine (K87) residues in a fraction of α1(II) collagen chains and, thus, type II to type II collagen cross-links had formed in these cultures (Dennis et al., 2020b). It must be reiterated that pepsin-extracted α1(II) collagen chains are devoid of telopeptides unless they are cross-linked to the lysine residues in the helical regions of α1(II) chains (McAlinden et al., 2014; Dennis et al., 2020b).
To examine if type II and type XI collagen molecules in these cultures were stabilized by these cross-links, we used the pAb 5890 (Fernandes et al., 2007; McAlinden et al., 2014). As seen in Figure 8E, this antibody also recognized the α1(II) chains and the β1(II) chains of type II collagen in the tissue engineered sheets and adult rabbit cartilage. As shown before (McAlinden et al., 2014), this means that the N-telopeptide of the α1 (XI) chain to which this antibody was raised was cross-linked to the helical lysine (K930) residue in a fraction of α1(II) chains of type II collagen molecules and thus a hetero-polymer of type II and type XI collagens had formed in all these cultures. A faint reactivity of the α1 (XI) chain was observed in some of the engineered cartilage cultures that probably indicates that N-telopeptides of α1 (XI) chain are cross-linked to helical lysine of another α1 (XI) chain and a homo-polymer in a fraction of type XI collagen had also formed in these cultures. We have published on the locations of the antibody epitopes in the α1(II) chain or in telopeptide stubs crosslinked to the collagen chains in type II-IX-XI heteropolymer (Dennis et al., 2020b).
The data confirms that a polymer of type II collagen had formed in tissue engineered cartilage sheets and a mature collagenous heteropolymer of cross-linked type II-XI collagen fibrils had formed as seen for control type II collagen extracted from rabbit articular cartilage (Figures 8C–E, last lane).
Our previous efforts to optimize defined chondrogenic media, tested the effect of 15 different micronutrients and thyroxine on murine chondrocytes using proposed concentrations based on physiologic levels in a one-factor at a time approach (Dennis et al., 2020a). In that work, we identified copper, vitamin A and linolenic acid as having a positive effect on chondrogenesis. Overall, we found that combinations of these micronutrients were able to increase the type II collagen promoter activity when tested temporally and in a dose-dependent manner (Dennis et al., 2020a). While our previous work showed that vitamins and minerals affect type II collagen production in murine chondrocytes in vitro, we noted several limitations of using a traditional one factor at a time approach. This approach consists of experimental runs that are executed to hold every factor constant except for the variable of interest. This approach poorly reflects the complexity of in vivo conditions by failing to account for important interactions and largely relies on iterative experiments and trial and error for optimization. In the current study, we combined a non-destructive reporter, primary rabbit chondrocytes, 3D culture in 96-well plates, automated pipetting, and Design of Experiments approach as an efficient high throughput platform. We were able to not only identify interactions of micronutrients that had an effect on type II collagen promoter activity, but to also derive an optimal combination containing all missing factors as a supplement to traditional basal media, that simultaneously increased type II collagen expression.
There are several advantages to the platform we implemented in this study. 1) we made use of primary rabbit articular chondrocytes as a model for healthy cartilage. Primary cells have the advantage of being more relevant in orthopedic research than cell lines and thus more likely to mimic responses in vivo (Horton et al., 1988; Thenet et al., 1992; Steimberg et al., 1999; Santoro et al., 2015). 2) using 3D cell aggregates adapted to 96-well plates cultured in physioxic conditions, which we adapted for use in an automated system, allows chondrocytes to maintain their phenotype as compared to 2D culture (Benya and Shaffer, 1982; Bonaventure et al., 1994; Yoo et al., 1998; Penick et al., 2005; Takahashi et al., 2007). 3) We made use of a secreted Gaussia Luciferase reporter (Tannous et al., 2005; Badr et al., 2007; Ruecker et al., 2008; Wurdinger et al., 2008; Tannous, 2009). Traditional biochemical assays to evaluate chondrogenesis typically rely on destructive endpoint analysis, and due to the length of culture of the samples, low cell number in aggregates, and long and laborious processes, can result in high variability as seen in Figures 3E–J. Using a secreted reporter allows us to sample the media without lysis of the aggregate and thus provides the ability to examine the temporal effects of the treatment conditions on chondrogenesis. Because media is replaced every 2–3 days Gaussia luciferase readings provide a readout of the activity of the type II collagen promoter at early, mid and late timepoints in chondrogenesis. This was seen and confirmed by the TGF-β1 dose response curve where we have shown, for the first time, that the effective dose of TGF-β1 on type II collagen stimulation differs throughout the process of chondrogenesis. Furthermore, type II collagen expression levels are 66% higher at early timepoints with a decrease in activity at later timepoints of chondrogenesis (Figures 1A, B). As maximal type II collagen production was achieved with 1 ng/ml TGFβ1 (Figure 1), this concentration was used to screen for micronutrient effects. The Gaussia luciferase assay is simple, sensitive and fast to perform and thus reduces variability between samples.
Using this platform, we successfully screened 240 combinations of vitamins and minerals for their ability to promote type II collagen. Previous studies have shown that several of the micronutrients we tested can play a role in chondrogenesis (Pacifici et al., 1980; Barone et al., 1991; Ren et al., 2007; Chin and Ima-Nirwana, 2018; Whitney et al., 2018; Dennis et al., 2020a; Sumitani et al., 2020). Previously, vitamins D and K were shown to play a role in the development and regulation of chondrogenesis, while vitamin A exhibited inhibitory action on in vitro chondrogenic differentiation (Barone et al., 1991; Connor, 1999). Additionally, vitamin E has exhibited oxidative stress inhibition during in vivo and clinical studies (Chin and Ima-Nirwana, 2018). Other trace minerals such as copper and zinc promote extracellular matrix formation and deficiencies in selenium and iodine have been shown to impair bone and growth formation (Ren et al., 2007). Molecules like linoleic acid are known to enhance the metabolic activity of differentiating cells, while thyroxine was shown to increase type II collagen expression and glycosaminoglycan (GAG) deposition in scaffold-free engineered cartilage tissue (Connor, 1999; Whitney et al., 2018). In this study we identified linolenic acid, copper, and vitamin A, as well as interactions between various vitamins and minerals (Table 2) as having significant effects on type II collagen stimulation promoter activity. With both vitamin A and linolenic acid there was an optimal concentration above which deleterious effects on type II collagen production were evident (Figure 2).
The role of each micronutrient in chondrogenesis, particularly in the production of type II collagen, is not clear but effects of the highlighted micronutrients on cell function are briefly described here. Linolenic acid, an essential omega-3 fatty acid, can be synthesized into eicosanoids potentially leading to an anti-inflammatory effect or, through cell membrane integration, alter lipid raft composition, membrane transport and signaling (Finch and Finch, 2007; Shaikh, 2012). Chromium affects metabolism through insulin activity and glucose oxidation, both components of defined chondrogenic media, and could therefore promote cartilage anabolism (Lukaski, 2000; Pechova and Pavlata, 2007). Copper is a co-factor in many enzymes, perhaps the most relevant in cartilage tissue engineering is lysyl oxidase, where supplementation has enhanced collagen crosslinking (Makris et al., 2013a; Dennis et al., 2020a). Interestingly, copper also regulates HIF-1α binding to hypoxic response elements, significant due to the chondrogenic activity of HIF-1α (Liu et al., 2018). Cobalt, while mainly known for its vitamin B12 functions, has several noncorrin-cobalt containing enzymes including prolidase which could increase collagen synthesis; cobalt is also able to stabilize HIF-1α (Kobayashi and Shimizu, 1999; Surazynski et al., 2008). Manganese is a cofactor for many enzymes including superoxidase dismutase, potentially protecting the cartilage against reactive oxygen (Crowley et al., 2000). Additionally, the level of manganese was higher in hip articular cartilage than in the bone (Brodziak-Dopierala et al., 2013). Molybdenum is a cofactor of more than 50 enzymes including sulfite oxidases which play a role in sulfated glycosaminoglycans, an essential component of cartilage (Pecora et al., 2006; Hille et al., 2014). Vitamin A, known for its anti-chondrogenic properties (Pacifici et al., 1980), was stimulatory to type II collagen production in the femtomolar range potentially through an FGF/Dlx mechanism (Frenz et al., 2010). Vitamin E has a complex and poorly understood role overall, with little data in cartilage where it seems to have an anti-inflammatory/anti-oxidant effect (Galli et al., 2017; Chin and Ima-Nirwana, 2018; Hassan et al., 2019). Vitamin D, well known for its promotion of bone homeostasis, also has a role in chondrogenesis—increasing cartilage thickness, proteoglycan content, type II collagen and lubricin expression (Li et al., 2016; Szychlinska et al., 2019). Vitamin B7’s role in chondrogenesis is unclear; however, its role in gluconeogenesis, amino acid metabolism and fatty acid synthesis would undoubtedly impact chondrogenesis (Saleem and Soos, 2023).
These findings relied on the use of Response Surface Methodology based on Design of Experiments which significantly reduces the number of trials, accounts for errors in the model, and for interactions between factors (Hanrahan and Lu, 2006; Myers et al., 2016; Yolmeh and Jafari, 2017; Aydar, 2018). Statistical analysis with this approach allowed us to predict an optimal combination of vitamins and minerals, condition 25, that when tested in vitro showed significant increases in type II collagen as compared to the basal media control. Furthermore, we removed one factor at a time from condition 25 and confirmed the importance of linolenic acid, vitamin A and vitamin D and their interactions in type II collagen stimulation, further confirming the validity of the DoE results.
While Response Surface Methodology has significant advantages, its effectiveness does rely on the data fitting a second order polynomial model, thus fit statistics are crucial to ensure that the data fits the model (Koç and Kaymak-Ertekin, 2017; Aydar, 2018). In addition, the validation of any findings is essential. Other aspects to consider include parameter selection for optimization of factors and response, as well as examining predicted values before validation. In our study, this is seen by our predicted condition 25, while having a predicted desirability of 0.595, it was selected for validation due to the high predicted individual responses during analysis. When tested in vitro, it showed similar if not better responses than other selected conditions. There are few studies that have used Design of Experiments to look at biological processes, typically investigating fewer factors (Enochson et al., 2012; Renner and Liu, 2013; Kuterbekov et al., 2019). To date, this study is the first to apply a response surface model to primary chondrocytes.
After validation of condition 25 in aggregates we explored this supplementation in tissue engineered cartilage. Effects generated in one tissue engineered system are often found to be context dependent. To broaden the impact of this study and provide proof of concept data for the screening strategy, we used custom 3D printed bioreactors adapted from Whitney, GA et al. to generate cartilage sheets in vitro (Figures 6, 7A) (Whitney et al., 2012; Kean, 2019; Dennis et al., 2020b). Similar to our findings in cell aggregates, type II collagen promoter-driven expression of Gaussia Luciferase was significantly increased as compared to cells in basal media in engineered cartilage. Biochemical studies supported an increase in total collagen content. Western blots of pepsin extracted samples confirm the increase is type II collagen, specifically, in sheets supplemented with condition 25 (Figure 8C). Collagen x-link analysis supports the formation of type II collagen to type II collagen and type II collagen to type IX collagen heteropolymers, as in native rabbit cartilage (Figures 8D, E). These crosslinks are characteristic of mature cartilage. This is significant as cell processes, particularly in tissue engineering, are often context dependent (Day et al., 2016; Vermeulen et al., 2020; Luo and Li, 2022). It is interesting to note that condition 25 at 1/15th was optimal for type II collagen expression as compared to condition 25 at 1x, as seen by luminescence, immunofluorescence and Western blot. It is possible that higher concentrations are not needed by chondrocytes and could even be detrimental for chondrogenesis resulting in greater type II collagen expression when the concentrations are decreased. Multiple cell types, like osteoblasts, endothelial cells and vascular smooth muscle cells, have specialized mechanisms to recycle and fully utilize vitamins and minerals, as these cannot be synthesized by humans (Kagan and Tyurina, 1998; May et al., 2003; Schurgers et al., 2013; Shearer and Newman, 2014). Investigation of micronutrient recycling in chondrocytes has not been well studied and was beyond the scope of this work.
Supplementation with condition 25 also altered the mechanical properties of the engineered cartilage. While it increased the tensile modulus of engineered cartilage, unexpectedly, we observed a decrease in Young’s modulus in compressive tests as compared to basal media (Supplementary Figure S2), suggestive of decreased stiffness. While it is thought that type II collagen generally increases the tensile properties of cartilage, there is no clear correlation between type II collagen and stiffness (Mansour, 2009). Furthermore, mechanical testing of live biological tissue is also confounded by the method of testing. Patel JM et al. (Patel et al., 2019), has explored the inconsistencies present with various modes of mechanical testing which make any comparison of our findings to previous literature extremely difficult. Despite a decrease in the compressive modulus the engineered cartilage generated with condition 25 shows mechanical and biochemical properties closer to that of native cartilage than engineered cartilage generated in basal media alone.
This study demonstrates that the physiologic environment of micronutrients to culture chondrocytes has a far greater impact on chondrogenesis than previously appreciated. Supplementation of culture medium with 15 micronutrients, that are physiologically present in the articular joint, can be tailored to improve in vitro chondrogenesis, and the biochemical and mechanical properties of tissue engineered cartilage. Our results show that the presence and concentrations of seemingly minor components of culture medium can have a major impact on chondrogenesis. Furthermore, we established a streamlined process using Design of Experiments and primary reporter chondrocytes as a way to identify optimal chondrogenic conditions in vitro.
Rabbits were euthanized under American Veterinary Medical Association guidelines and knees were isolated within 2 h of euthanasia. This study uses rabbits obtained from University of Texas Health Sciences (Dr. Steven Mills). Baylor College of Medicine did not require the study to be reviewed or approved by an ethics committee because we received dead animals. The articular knee joints were dissected under sterile conditions, and articular cartilage was isolated from both the femoral condyle and the tibial plateau. Isolated cartilage was diced into <1 mm (Mueller and Tuan, 2011) pieces before sequential digest, first in hyaluronidase for 30 min (660 Units/ml Sigma, H3506; in DMEM/F12 with pen/strep/amphotericin B, 30 mL), followed by collagenase type II for ∼16 h at 37°C (583 Units/ml Worthington Biochemical Corp.; in DMEM/F12 with 10% FBS, 1% pen/strep/fungizone, 30 mL). The digest was then filtered through a 70 μm cell strainer, washed with DMEM/F12, and resuspended in growth media (DMEM/F12 supplemented with 10% FBS, 1% pen/strep). Cells were subsequently infected as described below or cryopreserved (95% FBS, 5% DMSO).
Lentivirus was generated as previously described (Dennis et al., 2020a). Briefly, an HIV based lentiviral third generation system from GeneCopoeia was used to generate pseudolentiviral particles. Custom ordered COL2A1-Gaussia Luciferase plasmid (1.4 kb promoter, HPRM22364-LvPG02, GeneCopoeia, Inc., Supplementary Data Sheet S2), envelope (pMD2. G) and packaging (psPAX2) plasmids were amplified in Escherichia coli (GCI-L3, GeneCopoeia) and silica column purified (Qiagen Maxiprep) before being co-transfected into HEK293Ta (GeneCopoeia) cells via calcium chloride precipitation. Pseudolentiviral particles were harvested from conditioned media after 48 h and concentrated via ultracentrifugation (10,000 RCF, 4°C, overnight). Titers for COL2A1-Gluc lentivirus were estimated via real-time PCR and aliquots stored at −80°C.
Isolated rabbit chondrocytes were seeded at 6,200 cells/cm2 in growth media and allowed to adhere overnight (∼20% confluency). Cells were infected with lentivirus (COL2A1-GLuc; MOI 25 in growth media) in the presence of 4 μg/ml polybrene (Opti-mem, Gibco) for 12 h. Lentiviral medium was replaced with growth medium and cells expanded to ∼90% confluency. Cells were subsequently plated on flasks coated in porcine synoviocyte matrix (Kean and Dennis, 2015; Kean et al., 2019) and selected with puromycin (2 μg/mL) when 70% confluent for 48 h. Culturing of rabbit chondrocytes during infection was done in physioxic (37°C, 5% O2, 5% CO2) conditions. Newly generated COL2A1-GLuc cells were cryopreserved at the end of this first passage (95% FBS, 5% DMSO). These cells were used for all subsequent studies.
Rabbit COL2A1-GLuc were thawed and seeded in growth media at 6000 cells/cm2 and expanded to 90%–100% confluence in physioxia. Cells were trypsinized (0.25% Trypsin/EDTA; Corning), resuspended in basal chondrogenic media (93.24% High-Glucose DMEM (Gibco), 1% dexamethasone 10−5 M (Sigma), 1% ITS + premix (Becton-Dickinson), 1% Glutamax (Hyclone), 1% 100 mM Sodium Pyruvate (Hyclone), 1% MEM Non-Essential Amino Acids (Hyclone), 0.26% L-Ascorbic Acid Phosphate 50 mM (Wako), 0.5% Fungizone (Life Technologies) with TGF-β1 (Peprotech) and seeded as described below.
To generate 3D aggregates, cells were seeded at 50,000 cells per well (in 96-well cell repellent u-bottom plates, GreinerBio) and then centrifuged at 500 RCF, 5 min. For the TGF-β1 dose response studies, that serve as positive controls for the reporter cells, aggregates were cultured in basal chondrogenic media and different concentrations of TGF-β1 ranging from 0–10 ng/mL. For the DoE studies, aggregates were cultured in basal chondrogenic media (1 ng/ml TGF-β1) as a control or basal media supplemented with vitamins and minerals (Table 1; Supplementary Tables S1, S2). Plates were cultured for 3 weeks in physioxia, media was sampled and replaced three times a week with respective medium. An OT-2 (Opentrons) python coded robotic pipette, programmed at an aspiration height of 2 mm from the bottom of the wells and aspiration rate of 40 μL/s was utilized for media preparation, cell feeding, and media sampling for luciferase assay (Supplementary File S1). After 3 weeks, cell aggregates were either fixed in neutral buffered formalin for histology or medium removed and aggregates frozen dry (−20°C) for biochemical assays.
Custom 3D printed biochambers (Kean, 2019) that produce 1.2 cm2 cartilage sheets are shown in Figure 6. The chambers are made of an acrylonitrile butadiene styrene (ABS) seeding chamber and a 10 µm pore polyester membrane (Sterlitech). Screws, a silicone washer and ABS frits hold everything securely and prevent any leaking in between the different pieces. Furthermore, they keep the chamber elevated to allow medium to reach the membrane from the top and bottom for efficient media exchange. The chambers are contained within Nalgene containers modified to have a 0.2 µm sterile filter on the top to allow gas exchange.
The Nalgene containers along with screws, silicone washer, polyester membrane and nuts were autoclaved and sterile filters fitted to the containers in a biosafety cabinet. ABS pieces were placed in a sealable container for sterilization by immersion in a 10% bleach solution, water rinse, followed by a 10% sodium thiosulfate treatment to neutralize any remaining chlorine, sterile water and isopropanol wash before drying in the biosafety cabinet. Biochambers were assembled as shown in Figure 6A inside a biosafety hood using sterile surgical gloves and autoclaved surgical tools to handle biochamber parts. Once assembled, the polyester membrane was coated with fibronectin (50 μg/cm2, Corning, in PBS) and allowed to dry in a biosafety cabinet for 1h.
COL2A1-Gluc cells or uninfected primary rabbit chondrocytes were seeded at 5 × 106 cells/cm2 in ABS biochambers with a 1.2 cm2 seeding area in basal chondrogenic media alone or in basal media with condition 25 at 1x and 1/15x (Supplementary Table S2) (Kean, 2019). Media were added in the Nalgene container outside of the biochamber making sure it did not reach the top of the biochamber and combine with the cell suspension inside the biochamber in order to allow cells to adhere to the membrane. After 1 day, medium was added to the top of the biochamber so that media exchange occurs with the inside of the whole biochamber (Figure 6C). These were cultured in physioxia on a shaker (10 RPM) for 3-week with media changes three times a week. During media replacement, samples from COL2A1-Gluc biochambers were assessed for luciferase. After 3 weeks, cartilage sheets were collected, four (4 mm) biopsy punches were taken for mechanical assessment, and collagen cross-linking analysis, remaining pieces of the sheets were frozen (−80°C) for biochemical assessment or stored in formalin for histology.
Cell culture medium sampled from the seeded 96-wells (20 µL/well) was assessed using a stabilized Gaussia Luciferase buffer at a final concentration of 0.09 M MES, 0.15M Ascorbic Acid, and 4.2 µM Coelenterazine in white 96-well plates. Luminescence was measured in a plate reader (25°C, relative light units, EnVision plate reader). An OT-2 (Opentrons) python coded robotic pipette was utilized for luciferase buffer addition to white plates (GreinerBio).
At the end of 3-week culture, cell aggregates were fixed in 10% Neutral Buffered Formalin overnight, embedded in paraffin wax and sectioned (5 µm sections). Sections were deparaffinized and hydrated, followed by treatment with pronase (1 mg/ml, Sigma P5147, in PBS with 5 mM CaCl2) for epitope retrieval and incubation with primary anti-Collagen Type II (DSHB II-II6B3) followed by a biotinylated secondary and Streptavidin-HRP (BD Biosciences). II-II6B3 was deposited to the DSHB by Linsenmayer, T.F. (DSHB Hybridoma Product II-II6B3). Sections were stained with a chromogen-based substrate kit (Vector labs, VIP substrate vector kit). Engineered cartilage sheet sections were also treated with pronase and primary anti-Collagen Type II (DSHB II-II6B3) followed by VectaFluor R.T.U Antibody Kit DyLight® 488 (Vector Labs DI-2788) following manufacturer’s protocol. All sections were imaged at ×10 magnification.
Frozen cell aggregates, or pieces of engineered cartilage were thawed in PBS, and enzymatically digested with Papain (25 μg/mL, Sigma, P4762, in 2 mM cysteine; 50 mM sodium phosphate; 2 mM EDTA at a pH 6.5, 100 µL) at 65°C overnight. During digestion, plates were covered with a qPCR adhesive sealing film (USA Scientific), a silicone sheet, and steel plates clamped to the plate to prevent evaporation. After digestion half of the digest was transferred to another plate and frozen for hydroxyproline assessment. For the remaining half of the digest, papain was inactivated with 0.1M NaOH (50 µL) followed by neutralization (100 mM Na2HPO4, 0.1 N HCL, pH 1.82, 50 µL). To assess DNA, samples of the digests (20 µL) were combined with buffered Hoechst dye (#33258, 667 ng/ml, phosphate buffer pH 8, 100 µL) and fluorescence measured at an excitation of 365 nm and emission of 460 nm. For GAG assessment, samples of aggregate digest (5 µL) were combined with a 1,9-Dimethyl-methylene blue solution (195 µL) and absorbance was measured at 595 nm and 525 nm (Farndale et al., 1982). Absorbance readings were corrected by subtracting 595 nm reading from 525 nm. Total micrograms of DNA and GAG were calculated using a Calf thymus DNA standard (Sigma) and Chondroitin Sulfate standard (Seikagaku Corp.), respectively.
For hydroxyproline (HP), the frozen digest was thawed and incubated overnight at 105°C with 6M HCL (200 µL). Plates were covered as described above to prevent evaporation. Samples were subsequently dried at 70°C overnight with a hydroxyproline standard (Sigma). Copper sulfate (0.15M, 10 µL) and sodium hydroxide (2.5 M, 10 µL) were added to each well and incubated at 50°C for 5 min, followed by hydrogen peroxide (6%, 10 µL) for 10 min. Sulfuric acid (1.5 M, 40 μL) and Ehrlich’s reagent (Dennis et al., 2020b) (20 µL) were added and samples further incubated at 70°C for 15 min before reading absorbance at 505 nm. Total micrograms of hydroxyproline were calculated from the standard curve. Total collagen was calculated by the following formula (Dennis et al., 2020b) (µg of HP X 7.6 = µg Total Collagen). (Dennis et al., 2020b).
After harvest, samples were frozen at −80°C until use. Samples were lyophilized, and dry weights obtained. Proteoglycans were extracted using 4M guanidine hydrochloride (GuHCl) in 50 mM Tris buffer pH 7.4. The residue was exhaustively rinsed using MilliQ water to remove residual GuHCl, lyophilized and weighed. The cross-linked collagen network was depolymerized using equal volumes of pepsin (0.5 mg/mL in 0.5M acetic acid) (Fernandes et al., 2003). Equivalent aliquots of dry weights were loaded on 6% polyacrylamide gels. Pepsin-extracted type II collagen from adult rabbit articular cartilage was used as a control. After electrophoresis, collagen chains were stained using Coomassie Blue. For Western blots, following SDS-PAGE the separated collagen chains were transferred, by electrophoresis, onto a polyvinyl difluoride (PVDF) membrane and probed with monoclonal antibody (mAb) 10F2 raised against a synthetic peptide EKGPDPLQ (Fernandes et al., 2003) to identify the C-telopeptide of α1(II) collagen chains cross-linked to α1(II) chains. Another blot was probed with polyclonal antibody (pAb) 5890 to identify the N-telopeptide of α1 (XI) chains cross-linked to α1(II) chains (Dennis et al., 2020b). This blot was then stripped and probed with mAb 1C10 to identify α1(II) chains (Dennis et al., 2020b). As we have described before, this determines if a heteropolymer of type II and type XI collagen had formed (Fernandes et al., 2003). All three antibodies (10F2, 5890 and 1C10) were a gift from Dr. David R. Eyre, University of Washington, Seattle. Type II collagen from adult rabbit articular cartilage was extracted using pepsin as above and used as a control.
Biopsy punches (4 mm) were thawed in Tyrode’s solution (Sigma) with protease inhibitors (Sigma, P8465) and equilibrated to room temperature. Using a TA. XTPlus connect Texture analyzer a trigger force of 0.1 g determined the height of the tissue, then 5%–20% strain was applied in 5% increments at 0.1 mm/s with a 20-min hold to reach equilibrium. From these results, the equilibrium force was calculated, and a stress vs. strain curve generated (Dennis et al., 2020b). Young’s modulus in compression was determined from the slope of these curves.
Biopsy punches (4 mm) were thawed in Tyrode’s solution (Sigma) with protease inhibitors and equilibrated to room temperature. As previously described, a custom dog-bone punch was made from biopsy punches and punches taken from the 4 mm punch (Dennis et al., 2020b). Custom holders were made from laminate projector sheets and dog-bone punches attached using cyanoacrylate glue (Ultra Gel Control, Loctite), tissue was continuously bathed in PBS during this process (Dennis et al., 2020b). Using a TA. XTPlus connect Texture analyzer with a trigger force of 0.1 G, tissues were stretched to failure at 3 mm/s, the equilibrium tensile force was calculated and a stress vs. strain curve generated (Dennis et al., 2020b). Young’s modulus in tension was determined from the slope of these curves.
Design-Expert 12 (StatEase) was used to generate a surface response model to assess the effect of 15 factors: linoleic acid, cobalt, copper, chromium, iodine, manganese, molybdenum, thyroxine, vitamin A, vitamin B12, vitamin B7, vitamin D, vitamin E, vitamin K, and zinc. Table 1 shows minimum and maximum concentrations input into Design-Expert. The response surface I-optimal blocked design generated 240 total conditions to assess the response (Supplemental Table S1).
At the end of this experiment, responses (luminescence, metabolic activity and aggregate area) from the screen of 240 conditions as well as results from the one factor at a time approach, were analyzed (Design-Expert, StatEase). Analysis of the results suggested a quadratic model as the best fit. After transformation of the data to fit a quadratic model, Analysis of Variance (ANOVA) was used to identify the positive and negative effects on chondrogenesis as well as fit statistics for the model. The optimization module of the software was used to generate five predicted optimal combinations of factors (Supplementary Table S2, Supplementary Data Sheet S2). Two sets of parameters were used to generate predicted conditions. For condition 25 from Supplementary Table S2, all vitamins and minerals were targeted at 75% serum max except for linolenic acid, vitamin A, copper and vitamin D which are set at their predicted optima. For the other predicted conditions vitamins and minerals were set between 0.01% of serum max and serum max except for vitamin A, E and linolenic acid which were at their approximate optima. All conditions were selected to maximize luminescence for week two and week three as well as aggregate area for week three. Condition 25 also had a target of 0.2, for Resazurin (metabolic activity), the average measurement for chondrocyte aggregates, at week three.
Rabbits were euthanized under American Veterinary Medical Association guidelines for another study (Dr. Steven Mills (University of Texas Health Sciences)).
Statistical analysis for all experiments except for the Design of Experiments screen (analysis described above) were performed using GraphPad Prism 9 and One-way or two-way ANOVA. All data passed tests for normality. In all figures * indicates p-value <0.05, ** indicates p-value <0.01, and *** indicates p-value <0.001.
The original contributions presented in the study are included in the article/Supplementary Material, further inquiries can be directed to the corresponding author.
Rabbits were euthanized under American Veterinary Medical Association guidelines and knees were isolated within 2 hours of euthanasia. This study uses rabbits obtained from University of Texas Health Sciences (Dr. Steven Mills). Baylor College of Medicine did not require the study to be reviewed or approved by an ethics committee because we received dead animals.
Research design: MC, RF, JD, and TK. Conducted experiments: MC, YG, JV, MK, AR, LM, RM, TS, MA, and TK. Analyzed and interpreted data: MC, AR, RF, and TK. Prepared the manuscript: MC, YG, and TK. Contributed to the discussion, language and proofreading: MC, RF, JD, and TK. All authors contributed to the article and approved the submitted version.
Supplied by the University of Central Florida (TK), University of Central Florida College of Medicine (TK), the Rolanette and Berdon Lawrence Bone Disease Program (TK), NIH grant AR057025 (RF) and the Ernest M. Burgess Endowed Chair research program at the University of Washington (RF).
We would like to thank Dr. Steven Mills (University of Texas Health Sciences) for the donation of rabbit tissue. The plasmids, pMD2. G, and psPAX2, were a gift from Didier Trono (Addgene plasmid # 12259; http://n2t.net/addgene:12259; RRID:Addgene_12259) and (Addgene plasmid # 12260; http://n2t.net/addgene:12260; RRID:Addgene_12260) respectively. This article was published as a pre-print on BioRxiv (Cruz, 2022). Article processing charges were provided in part by the UCF College of Graduate Studies Open Access Publishing Fund.
TK and MC are inventors named on a patent application on “Anabolic Drugs Stimulating Type II Collagen Production from Chondrocytes or their Progenitors” is in process and is tangentially related.
The remaining authors declare that the research was conducted in the absence of any commercial or financial relationships that could be construed as a potential conflict of interest.
All claims expressed in this article are solely those of the authors and do not necessarily represent those of their affiliated organizations, or those of the publisher, the editors and the reviewers. Any product that may be evaluated in this article, or claim that may be made by its manufacturer, is not guaranteed or endorsed by the publisher.
The Supplementary Material for this article can be found online at: https://www.frontiersin.org/articles/10.3389/fbioe.2023.1179332/full#supplementary-material
Aydar, A. Y. (2018). Utilization of response surface methodology in optimization of extraction of plant materials. IntechOpen.
Badr, C. E., Hewett, J. W., Breakefield, X. O., and Tannous, B. A. (2007). A highly sensitive assay for monitoring the secretory pathway and ER stress. PLoS ONE 2, e571. doi:10.1371/journal.pone.0000571
Barone, L. M., Owen, T. A., Tassinari, M. S., Bortell, R., Stein, G. S., and Lian, J. B. (1991). Developmental expression and hormonal regulation of the rat matrix Gla protein (MGP) gene in chondrogenesis and osteogenesis. J. Cell Biochem. 46, 351–365. doi:10.1002/jcb.240460410
Benya, P. D., and Shaffer, J. D. (1982). Dedifferentiated chondrocytes reexpress the differentiated collagen phenotype when cultured in agarose gels. Cell 30, 215–224. doi:10.1016/0092-8674(82)90027-7
Bian, L., Zhai, D. Y., Mauck, R. L., and Burdick, J. A. (2011). Coculture of human mesenchymal stem cells and articular chondrocytes reduces hypertrophy and enhances functional properties of engineered cartilage. Tissue Eng. Part A 17, 1137–1145. doi:10.1089/ten.tea.2010.0531
Bonaventure, J., Kadhom, N., Cohen-Solal, L., Ng, K., Bourguignon, J., Lasselin, C., et al. (1994). Reexpression of cartilage-specific genes by dedifferentiated human articular chondrocytes cultured in alginate beads. Exp. Cell Res. 212, 97–104. doi:10.1006/excr.1994.1123
Booth, S. L., and Mayer, (1997). Skeletal functions of vitamin K-dependent proteins: Not just for clotting anymore. Nutr. Rev. 55, 282–284. doi:10.1111/j.1753-4887.1997.tb01619.x
Brodziak-Dopierala, B., Kwapulinski, J., Sobczyk, K., and Wiechula, D. (2013). The content of manganese and iron in hip joint tissue. J. Trace Elem. Med. Biol. 27, 208–212. doi:10.1016/j.jtemb.2012.12.005
Chin, K. Y., and Ima-Nirwana, S. (2018). The role of vitamin E in preventing and treating osteoarthritis - a review of the current evidence. Front. Pharmacol. 9, 946. doi:10.3389/fphar.2018.00946
Connor, W. E. (1999). α-Linolenic acid in health and disease. Am. J. Clin. Nutr. 69, 827–828. doi:10.1093/ajcn/69.5.827
Crowley, J., Traynor, D., and Weatherburn, D. (2000). Metal ions in biological systems, 37. New York: Marcel Dekker.
Cruz, M. A., Gonzalez, Y., Vélez Toro, J. A., Karimzadeh, M., Rubbo, A., Morris, L., et al. (2022). Micronutrient optimization using design of experiments approach in tissue engineered articular cartilage for production of type II collagen. BioRxiv 2022, 519522. doi:10.1101/2022.12.07.519522
Day, E. K., Sosale, N. G., and Lazzara, M. J. (2016). Cell signaling regulation by protein phosphorylation: A multivariate, heterogeneous, and context-dependent process. Curr. Opin. Biotechnol. 40, 185–192. doi:10.1016/j.copbio.2016.06.005
Dennis, J. E., Splawn, T., and Kean, T. J. (2020). High-throughput, temporal and dose dependent, effect of vitamins and minerals on chondrogenesis. Front. Cell Dev. Biol. 8, 92. doi:10.3389/fcell.2020.00092
Dennis, J. E., Whitney, G. A., Rai, J., Fernandes, R. J., and Kean, T. J. (2020). Physioxia stimulates extracellular matrix deposition and increases mechanical properties of human chondrocyte-derived tissue-engineered cartilage. Front. Bioeng. Biotechnol. 8, 590743. doi:10.3389/fbioe.2020.590743
Enochson, L., Brittberg, M., and Lindahl, A. (2012). Optimization of a chondrogenic medium through the use of factorial design of experiments. Biores Open Access 1, 306–313. doi:10.1089/biores.2012.0277
Farndale, R. W., Sayers, C. A., and Barrett, A. J. (1982). A direct spectrophotometric microassay for sulfated glycosaminoglycans in cartilage cultures. Connect. Tissue Res. 9, 247–248. doi:10.3109/03008208209160269
Fernandes, R. J., Hirohata, S., Engle, J. M., Colige, A., Cohn, D. H., Eyre, D. R., et al. (2001). Procollagen II amino propeptide processing by ADAMTS-3. J. Biol. Chem. 276, 31502–31509. doi:10.1074/jbc.m103466200
Fernandes, R. J., Schmid, T. M., and Eyre, D. R. (2003). Assembly of collagen types II, IX and XI into nascent hetero-fibrils by a rat chondrocyte cell line. Eur. J. Biochem. 270, 3243–3250. doi:10.1046/j.1432-1033.2003.03711.x
Fernandes, R. J., Weis, M., Scott, M. A., Seegmiller, R. E., and Eyre, D. R. (2007). Collagen XI chain misassembly in cartilage of the chondrodysplasia (cho) mouse. Matrix Biol. 26, 597–603. doi:10.1016/j.matbio.2007.06.007
Finch, C. E. (2007). “Chapter 2 - infections, inflammogens, and drugs,” in The biology of human longevity. Editor C. E. Finch (Burlington: Academic Press), 113–173.
Finch, C. W. (2015). Review of trace mineral requirements for preterm infants: What are the current recommendations for clinical practice? Nutr. Clin. Pract. 30, 44–58. doi:10.1177/0884533614563353
Francis, S. L., Di Bella, C., Wallace, G. G., and Choong, P. F. M. (2018). Cartilage tissue engineering using stem cells and bioprinting technology—barriers to clinical translation. Front. Surg. 5, 70. doi:10.3389/fsurg.2018.00070
Frenz, D. A., Liu, W., Cvekl, A., Xie, Q., Wassef, L., Quadro, L., et al. (2010). Retinoid signaling in inner ear development: A "goldilocks" phenomenon. Am. J. Med. Genet. A 152A, 2947–2961. doi:10.1002/ajmg.a.33670
Galli, F., Azzi, A., Birringer, M., Cook-Mills, J. M., Eggersdorfer, M., Frank, J., et al. (2017). Vitamin E: Emerging aspects and new directions. Free Radic. Biol. Med. 102, 16–36. doi:10.1016/j.freeradbiomed.2016.09.017
Gemmiti, C. V., and Guldberg, R. E. (2006). Fluid flow increases type II collagen deposition and tensile mechanical properties in bioreactor-grown tissue-engineered cartilage. Tissue Eng. 12, 060320122646001–060320122646479. doi:10.1089/ten.2006.12.ft-53
Han, F., Adams, C. S., Tao, Z., Williams, C. J., Zaka, R., Tuan, R. S., et al. (2005). Transforming growth factor-β1 (TGF-β1) regulates ATDC5 chondrogenic differentiation and fibronectin isoform expression. J. Cell. Biochem. 95, 750–762. doi:10.1002/jcb.20427
Hanrahan, G., and Lu, K. (2006). Application of factorial and response surface methodology in modern experimental design and optimization. Crit. Rev. Anal. Chem. 36, 141–151. doi:10.1080/10408340600969478
Hassan, W. N., Bin-Jaliah, I., Haidara, M. A., Eid, R. A., Heidar, E. H. A., Dallak, M., et al. (2019). Vitamin E ameliorates alterations to the articular cartilage of knee joints induced by monoiodoacetate and diabetes mellitus in rats. Ultrastruct. Pathol. 43, 126–134. doi:10.1080/01913123.2019.1627446
Hille, R., Hall, J., and Basu, P. (2014). The mononuclear molybdenum enzymes. Chem. Rev. 114, 3963–4038. doi:10.1021/cr400443z
Horton, W. E., Cleveland, J., Rapp, U., Nemuth, G., Bolander, M., Doege, K., et al. (1988). An established rat cell line expressing chondrocyte properties. Exp. Cell Res. 178, 457–468. doi:10.1016/0014-4827(88)90414-4
Jennifer, L., Puetzer, J. N. P., and Loboa, E. G. (2010). Comparative review of growth factors for induction of three-dimensional in vitro chondrogenesis in human mesenchymal stem cells isolated from bone marrow and adipose tissue. Tissue Eng. Part B Rev. 16, 435–444. doi:10.1089/ten.teb.2009.0705
Kagan, V. E., and Tyurina, Y. Y. (1998). Recycling and redox cycling of phenolic antioxidants. Ann. N. Y. Acad. Sci. 854, 425–434. doi:10.1111/j.1749-6632.1998.tb09921.x
Kean, T. J. (2019). 1 cm^2 biochamber. Available at: https://3dprint.nih.gov/discover/3dpx-010438.2022.
Kean, T. J., and Dennis, J. E. (2015). Synoviocyte derived-extracellular matrix enhances human articular chondrocyte proliferation and maintains Re-differentiation capacity at both low and atmospheric oxygen tensions. PLoS One 10, e0129961. doi:10.1371/journal.pone.0129961
Kean, T. J., Ge, Z., Li, Y., Chen, R., and Dennis, J. E. (2019). Transcriptome-wide analysis of human chondrocyte expansion on synoviocyte matrix. Cells 8, 85. doi:10.3390/cells8020085
Kobayashi, M., and Shimizu, S. (1999). Cobalt proteins. Eur. J. Biochem. 261, 1–9. doi:10.1046/j.1432-1327.1999.00186.x
Koç, B., and Kaymak-Ertekin, F. (2017). Response surface methodology and food processing applications. Gıda. (Turkish with English Abstract).
Koyano, Y., Hejna, M., Flechtenmacher, J., Schmid, T. M., Thonar, E. J. M. A., and Mollenhauer, J. (1996). Collagen and proteoglycan production by bovine fetal and adult chondrocytes under low levels of calcium and zinc ions. Connect. tissue Res. 34, 213–225. doi:10.3109/03008209609000700
Kuterbekov, M., Machillot, P., Baillet, F., Jonas, A. M., Glinel, K., and Picart, C. (2019). Design of experiments to assess the effect of culture parameters on the osteogenic differentiation of human adipose stromal cells. Stem Cell Res. Ther. 10, 256. doi:10.1186/s13287-019-1333-7
Li, S., Niu, G., Wu, Y., Du, G., Huang, C., Yin, X., et al. (2016). Vitamin D prevents articular cartilage erosion by regulating collagen II turnover through TGF-β1 in ovariectomized rats. Osteoarthr. Cartil. 24, 345–353. doi:10.1016/j.joca.2015.08.013
Lind, T., Sundqvist, A., Hu, L., Pejler, G., Andersson, G., Jacobson, A., et al. (2013). Vitamin a is a negative regulator of osteoblast mineralization. PloS one 8, e82388. doi:10.1371/journal.pone.0082388
Litchfield, T. M., Ishikawa, Y., Wu, L. N., Wuthier, R. E., and Sauer, G. R. (1998). Effect of metal ions on calcifying growth plate cartilage chondrocytes. Calcif. Tissue Int. 62, 341–349. doi:10.1007/s002239900442
Liu, X., Zhang, W., Wu, Z., Yang, Y., and Kang, Y. J. (2018). Copper levels affect targeting of hypoxia-inducible factor 1α to the promoters of hypoxia-regulated genes. J. Biol. Chem. 293, 14669–14677. doi:10.1074/jbc.ra118.001764
Lukaski, H. C. (2000). Magnesium, zinc, and chromium nutriture and physical activity. Am. J. Clin. Nutr. 72, 585S–593S. doi:10.1093/ajcn/72.2.585s
Luo, J., and Li, P. (2022). Context-dependent transcriptional regulations of YAP/TAZ in stem cell and differentiation. Stem Cell Res. Ther. 13, 10. doi:10.1186/s13287-021-02686-y
Makris, E. A., Hu, J. C., and Athanasiou, K. A. (2013). Hypoxia-induced collagen crosslinking as a mechanism for enhancing mechanical properties of engineered articular cartilage. Osteoarthr. Cartil. 21, 634–641. doi:10.1016/j.joca.2013.01.007
Makris, E. A., MacBarb, R. F., Responte, D. J., Hu, J. C., and Athanasiou, K. A. (2013). A copper sulfate and hydroxylysine treatment regimen for enhancing collagen cross-linking and biomechanical properties in engineered neocartilage. FASEB J. 27, 2421–2430. doi:10.1096/fj.12-224030
Mansour, J. M. (2009). “Biomechanics of cartilage,” in Kinesiology: The mechanics and pathomechanics of human movement (Lippincott Williams and Wilkins).
Masuda, E., Shirai, K., Maekubo, K., and Hirai, Y. (2015). A newly established culture method highlights regulatory roles of retinoic acid on morphogenesis and calcification of mammalian limb cartilage. BioTechniques 58, 318–324. doi:10.2144/000114300
May, J. M., Qu, Z.-c., Neel, D. R., and Li, X. (2003). Recycling of vitamin C from its oxidized forms by human endothelial cells. Biochimica Biophysica Acta (BBA) - Mol. Cell Res. 1640, 153–161. doi:10.1016/s0167-4889(03)00043-0
MayoClinic (2022). Mayo medical laboratories rochester test catalog. Available at: https://www.mayocliniclabs.com/test-catalog/print-catalog.html.
McAlinden, A., Traeger, G., Hansen, U., Weis, M. A., Ravindran, S., Wirthlin, L., et al. (2014). Molecular properties and fibril ultrastructure of types II and XI collagens in cartilage of mice expressing exclusively the α1(IIA) collagen isoform. Matrix Biol. 34, 105–113. doi:10.1016/j.matbio.2013.09.006
Mello, M. A., and Tuan, R. S. (2006). Effects of TGF-β1 and triiodothyronine on cartilage maturation: In vitro analysis using long-term high-density micromass cultures of chick embryonic limb mesenchymal cells. J. Orthop. Res. 24, 2095–2105. doi:10.1002/jor.20233
Mobasheri, A. L. I., Platt, N., Thorpe, C., and Shakibaei, M. (2006). Regulation of 2-Deoxy-d-Glucose transport, lactate metabolism, and MMP-2 secretion by the hypoxia mimetic cobalt chloride in articular chondrocytes. Ann. N. Y. Acad. Sci. 1091, 83–93. doi:10.1196/annals.1378.057
Mueller, M. B. M. D., and Tuan, R. S. P. (2011). Anabolic/catabolic balance in pathogenesis of osteoarthritis: Identifying molecular targets. PM R 3, S3–S11. doi:10.1016/j.pmrj.2011.05.009
Myers, R. H., Montgomery, D. C., and Anderson-Cook, C. M. (2016). Response surface methodology: Process and product optimization using designed experiments. Incorporated, New York: John Wiley and Sons.
Pacifici, M., Cossu, G., Molinaro, M., and Tato, F. (1980). Vitamin A inhibits chondrogenesis but not myogenesis. Exp. Cell Res. 129, 469–474. doi:10.1016/0014-4827(80)90517-0
Patel, J. M., Wise, B. C., Bonnevie, E. D., and Mauck, R. L. (2019). A systematic review and guide to mechanical testing for articular cartilage tissue engineering. Tissue Eng. Part C Methods 25, 593–608. doi:10.1089/ten.tec.2019.0116
Pechova, A., and Pavlata, L. (2007). Chromium as an essential nutrient: A review. Veterinární medicína 52, 1–18. doi:10.17221/2010-vetmed
Pecora, F., Gualeni, B., Forlino, A., Superti-Furga, A., Tenni, R., Cetta, G., et al. (2006). In vivo contribution of amino acid sulfur to cartilage proteoglycan sulfation. Biochem. J. 398, 509–514. doi:10.1042/bj20060566
Penick, K. J., Solchaga, L. A., and Welter, J. F. (2005). High-throughput aggregate culture system to assess the chondrogenic potential of mesenchymal stem cells. Biotechniques 39, 687–691. doi:10.2144/000112009
Ren, F. L., Guo, X., Zhang, R., Wang, S., Zuo, H., Zhang, Z., et al. (2007). Effects of selenium and iodine deficiency on bone, cartilage growth plate and chondrocyte differentiation in two generations of rats. Osteoarthr. Cartil. 15, 1171–1177. doi:10.1016/j.joca.2007.03.013
Renner, J. N., and Liu, J. C. (2013). Investigating the effect of peptide agonists on the chondrogenic differentiation of human mesenchymal stem cells using design of experiments. Biotechnol. Prog. 29, 1550–1557. doi:10.1002/btpr.1808
Ruecker, O., Zillner, K., Groebner-Ferreira, R., and Heitzer, M. (2008). Gaussia-luciferase as a sensitive reporter gene for monitoring promoter activity in the nucleus of the green alga Chlamydomonas reinhardtii. Mol. Genet. genomics MGG 280, 153–162. doi:10.1007/s00438-008-0352-3
Sacitharan, P. K. (2019). Ageing and osteoarthritis. Subcell. Biochem. 91, 123–159. doi:10.1007/978-981-13-3681-2_6
Santoro, A., Conde, J., Scotece, M., Abella, V., López, V., Pino, J., et al. (2015). Choosing the right chondrocyte cell line: Focus on nitric oxide: Choosing the right chondrocyte cell line: Focus. J. Orthop. Res. 33, 1784–1788. doi:10.1002/jor.22954
Sardesai, V. M. (1993). Molybdenum: An essential trace element. Nutr. Clin. Pract. 8, 277–281. doi:10.1177/0115426593008006277
Sato, K., Mera, H., Wakitani, S., and Takagi, M. (2017). Effect of epigallocatechin-3-gallate on the increase in type II collagen accumulation in cartilage-like MSC sheets. Biosci. Biotechnol. Biochem. 81, 1241–1245. doi:10.1080/09168451.2017.1282809
Schurgers, L. J., Uitto, J., and Reutelingsperger, C. P. (2013). Vitamin K-dependent carboxylation of matrix gla-protein: A crucial switch to control ectopic mineralization. Trends Mol. Med. 19, 217–226. doi:10.1016/j.molmed.2012.12.008
Shaikh, S. R. (2012). Biophysical and biochemical mechanisms by which dietary N-3 polyunsaturated fatty acids from fish oil disrupt membrane lipid rafts. J. Nutr. Biochem. 23, 101–105. doi:10.1016/j.jnutbio.2011.07.001
Shearer, M. J., and Newman, P. (2014). Recent trends in the metabolism and cell biology of vitamin K with special reference to vitamin K cycling and MK-4 biosynthesis. J. Lipid Res. 55, 345–362. doi:10.1194/jlr.r045559
Sophia Fox, A. J., Bedi, A., and Rodeo, S. A. (2009). The basic science of articular cartilage: Structure, composition, and function. Sports Health 1, 461–468. doi:10.1177/1941738109350438
Stabler, S. P. (2013). Vitamin B12 deficiency. N. Engl. J. Med. 368, 149–160. doi:10.1056/nejmcp1113996
Steimberg, N., Viengchareun, S., Biehlmann, F., Guénal, I., Mignotte, B., Adolphe, M., et al. (1999). SV40 large T antigen expression driven by col2a1 regulatory sequences immortalizes articular chondrocytes but does not allow stabilization of type II collagen expression. Exp. Cell Res. 249, 248–259. doi:10.1006/excr.1999.4478
Sumitani, Y., Uchibe, K., Yoshida, K., Weng, Y., Guo, J., Yuan, H., et al. (2020). Inhibitory effect of retinoic acid receptor agonists on in vitro chondrogenic differentiation. Anat. Sci. Int. 95, 202–208. doi:10.1007/s12565-019-00512-3
Surazynski, A., Miltyk, W., Palka, J., and Phang, J. M. (2008). Prolidase-dependent regulation of collagen biosynthesis. Amino Acids 35, 731–738. doi:10.1007/s00726-008-0051-8
Szychlinska, M. A., Imbesi, R., Castrogiovanni, P., Guglielmino, C., Ravalli, S., Di Rosa, M., et al. (2019). Assessment of vitamin D supplementation on articular cartilage morphology in a Young healthy sedentary rat model. Nutrients 11, 1260. doi:10.3390/nu11061260
Takahashi, T., Ogasawara, T., Asawa, Y., Mori, Y., Uchinuma, E., Takato, T., et al. (2007). Three-dimensional microenvironments retain chondrocyte phenotypes during proliferation culture. Tissue Eng. 13, 1583–1592. doi:10.1089/ten.2006.0322
Tannous, B. A. (2009). Gaussia luciferase reporter assay for monitoring biological processes in culture and in vivo. Nat. Protoc. 4, 582–591. doi:10.1038/nprot.2009.28
Tannous, B. A., Kim, D. E., Fernandez, J. L., Weissleder, R., and Breakefield, X. O. (2005). Codon-optimized Gaussia luciferase cDNA for mammalian gene expression in culture and in vivo. Mol. Ther. 11, 435–443. doi:10.1016/j.ymthe.2004.10.016
Thenet, S., Benya, P. D., Demignot, S., Feunteun, J., and Adolphe, M. (1992). SV40-immortalization of rabbit articular chondrocytes: Alteration of differentiated functions. J. Cell. physiology 150, 158–167. doi:10.1002/jcp.1041500121
Tsonis, P. A. (1991). 1,25-dihydroxyvitamin D3 stimulates chondrogenesis of the chick limb bud mesenchymal cells. Dev. Biol. 143, 130–134. doi:10.1016/0012-1606(91)90060-g
Vermeulen, S., Roumans, N., Honig, F., Carlier, A., Hebels, D. G., Eren, A. D., et al. (2020). Mechanotransduction is a context-dependent activator of TGF-beta signaling in mesenchymal stem cells. Biomaterials 259, 120331. doi:10.1016/j.biomaterials.2020.120331
Vunjak-Novakovic, G., Martin, I., Obradovic, B., Treppo, S., Grodzinsky, A. J., Langer, R., et al. (1999). Bioreactor cultivation conditions modulate the composition and mechanical properties of tissue-engineered cartilage. J. Orthop. Res. 17, 130–138. doi:10.1002/jor.1100170119
Whitney, G. A., Kean, T. J., Fernandes, R. J., Waldman, S., Tse, M. Y., Pang, S. C., et al. (2018). Thyroxine increases collagen type II expression and accumulation in scaffold-free tissue-engineered articular cartilage. Tissue Eng. Part A 24, 369–381. doi:10.1089/ten.tea.2016.0533
Whitney, G. A., Mera, H., Weidenbecher, M., Awadallah, A., Mansour, J. M., and Dennis, J. E. (2012). Methods for producing scaffold-free engineered cartilage sheets from auricular and articular chondrocyte cell sources and attachment to porous tantalum. Biores Open Access 1, 157–165. doi:10.1089/biores.2012.0231
Wluka, A. E., Stuckey, S., Brand, C., and Cicuttini, F. M. (2002). Supplementary vitamin E does not affect the loss of cartilage volume in knee osteoarthritis: A 2 year double blind randomized placebo controlled study. J. rheumatology 29, 2585–2591.
Wurdinger, T., Badr, C., Pike, L., de Kleine, R., Weissleder, R., Breakefield, X. O., et al. (2008). A secreted luciferase for ex vivo monitoring of in vivo processes. Nat. methods 5, 171–173. doi:10.1038/nmeth.1177
Yolmeh, M., and Jafari, S. M. (2017). Applications of response surface methodology in the food industry processes. Food bioprocess Technol. 10, 413–433. doi:10.1007/s11947-016-1855-2
Keywords: primary chondrocyte, media optimization, tissue engineered cartilage, design of experiments (DoE), scaffold-free tissue engineered cartilage, vitamins and minerals, extracellular matrix reporter, type II collagen heteropolymer
Citation: Cruz MA, Gonzalez Y, Vélez Toro JA, Karimzadeh M, Rubbo A, Morris L, Medam R, Splawn T, Archer M, Fernandes RJ, Dennis JE and Kean TJ (2023) Micronutrient optimization for tissue engineered articular cartilage production of type II collagen. Front. Bioeng. Biotechnol. 11:1179332. doi: 10.3389/fbioe.2023.1179332
Received: 06 March 2023; Accepted: 23 May 2023;
Published: 06 June 2023.
Edited by:
Oommen Varghese, Uppsala University, SwedenReviewed by:
Ron June, Montana State University, United StatesCopyright © 2023 Cruz, Gonzalez, Vélez Toro, Karimzadeh, Rubbo, Morris, Medam, Splawn, Archer, Fernandes, Dennis and Kean. This is an open-access article distributed under the terms of the Creative Commons Attribution License (CC BY). The use, distribution or reproduction in other forums is permitted, provided the original author(s) and the copyright owner(s) are credited and that the original publication in this journal is cited, in accordance with accepted academic practice. No use, distribution or reproduction is permitted which does not comply with these terms.
*Correspondence: Thomas J. Kean, dGhvbWFzLmtlYW5AdWNmLmVkdQ==
Disclaimer: All claims expressed in this article are solely those of the authors and do not necessarily represent those of their affiliated organizations, or those of the publisher, the editors and the reviewers. Any product that may be evaluated in this article or claim that may be made by its manufacturer is not guaranteed or endorsed by the publisher.
Research integrity at Frontiers
Learn more about the work of our research integrity team to safeguard the quality of each article we publish.