- 1State Key Laboratory of Southwestern Chinese Medicine Resources, School of Pharmacy, Chengdu University of Traditional Chinese Medicine, Chengdu, Sichuan, China
- 2Sichuan Purity Pharmaceutical Co. Ltd., Chengdu, Sichuan, China
Curcumin (CUR) is a natural polyphenol extract with significant antioxidant and anti-inflammatory effects, which indicates its great potential for neuroprotection. Lactoferrin (LF), a commonly used oral carrier and targeting ligand, has not been reported as a multifunctional nanocarrier for nose-to-brain delivery. This study aims to develop a nose-to-brain delivery system of curcumin-lactoferrin nanoparticles (CUR-LF NPs) and to further evaluate the neuroprotective effects in vitro and brain accumulation in vivo. Herein, CUR-LF NPs were prepared by the desolvation method with a particle size of 84.8 ± 6.5 nm and a zeta potential of +22.8 ± 4.3 mV. The permeability coefficient of CUR-LF NPs (4.36 ± 0.79 × 10−6 cm/s) was 50 times higher than that of CUR suspension (0.09 ± 0.04 × 10−6 cm/s) on MDCK monolayer, indicating that the nanoparticles could improve the absorption efficiency of CUR in the nasal cavity. Moreover, CUR-LF NPs showed excellent protection against Aβ25-35-induced nerve damage in PC12 cells. In vivo pharmacokinetic studies showed that the brain-targeting efficiency of CUR-LF NPs via IN administration was 248.1%, and the nose-to-brain direct transport percentage was 59.7%. Collectively, nose-to-brain delivery of CUR-LF NPs is capable of achieving superior brain enrichment and potential neuroprotective effects.
1 Introduction
As a class of typical central nervous system diseases, neurodegenerative diseases such as Alzheimer’s disease (AD), Parkinson’s disease (PD), and Huntington’s disease (HD) are characterized by high incidence and disability rates and are incurable. With the development of an aging population, such chronic diseases place a heavy burden on families and society (Miksys and Tyndale, 2010; Budd Haeberlein and Harris, 2015; Dugger and Dickson, 2017). Unfortunately, due to the complex pathogenesis of neurodegenerative diseases, current drug treatments have not shown satisfactory pharmacodynamic effects (Tofaris and Buckley, 2018). In addition, oral administration is a common route for most neurotherapeutic drugs, whereas the physiological protective effect of the blood-brain barrier (BBB) greatly limits drug exposure in the brain and further reduces the therapeutic effects (Patel et al., 2019; Dou et al., 2021). Meanwhile, excessive systemic exposure with oral administration may lead to intolerable toxicity and various side effects in the gastrointestinal tract and even in other organs (Lauzon et al., 2015). Intranasal (IN) administration has been considered an alternative route to oral administration for the delivery of neurotherapeutic drugs. Based on the better clarification of the physiological structure of the nasal cavity, it has been reported that active pharmaceutical ingredients (APIs) could be transported directly to the brain by bypassing the BBB via the trigeminal and olfactory regions (Agrawal et al., 2018; Crowe et al., 2018). Moreover, the respiratory region contains a large number of blood vessels, and APIs could be easily absorbed into the systemic blood circulation without enterohepatic circulation to avoid the first-pass effect (Erdo et al., 2018).
An increasing number of researchers believe that safe and mildly effective natural extracts, including but not limited to huperzine A, curcumin, hydroxysafflor yellow A, and resveratrol, are more beneficial for the long-term control and alleviation of chronic and persistent neurodegenerative diseases (Di Paolo et al., 2019). Among them, curcumin (CUR) is a natural polyphenolic compound that is extracted from the rhizomes of Zingiberaceae plants. It is widely used as a food additive and spice in India, where the incidence of AD is low (Reddy et al., 2018). It has been reported that CUR plays an important neuroprotective role in the early pathogenic stages of neurodegenerative diseases through antioxidant and anti-inflammatory effects (Vaz et al., 2017). However, similar to most natural compounds, the hydrophobic property, low permeability, short biological half-life, and difficulty in penetrating the BBB of CUR limit its further application in neurological diseases (Del Prado-Audelo et al., 2019; Zhang et al., 2020). Considering the properties of CUR, nanotechnology-based delivery systems have been recommended by numerous researchers for such complex natural compounds (Liu et al., 2013; Piazzini et al., 2019; Qizilbash et al., 2022). Among all biological nanomaterial candidates, lactoferrin (LF) is an 80 kDa monomeric glycoprotein belonging to the transferrin (TF) family (Wang et al., 2019). As a type of endogenous protein in the human body, LF is recognized to have not only high biological safety, but also to bind to TF and LF receptors that are highly expressed in brain endothelial cells (Sabra and Agwa, 2020). Therefore, it is commonly used as a ligand to modify nanoformulations to improve their efficiency in passing through the BBB (Nasr, 2016; Jiang et al., 2019; Agwa and Sabra, 2021; Kim et al., 2022). In addition, the excellent ability of LF as a drug carrier cannot be ignored. LF has been used as an oral carrier to cargo small molecule APIs (Li et al., 2018; Luo et al., 2021). As an amphipathic protein, the hydrophobic regions of LF provide suitable binding sites for insoluble APIs (Yan et al., 2017). Moreover, some studies have indicated that LF has a neuroprotective function (Zakharova et al., 2018; Elzoghby et al., 2020). It has been reported that LF could inhibit cognitive decline in AD mice by activating the expression of ADAM10 in the ERK1/2-CREB and HIF-1α signaling pathways via IN administration (Guo et al., 2017). As a consequence, LF could not only be used as a nose-to-brain nanocarrier and targeting ligand to promote efficient brain enrichment of CUR, but also play a synergistic neuroprotective effect with CUR. As shown in Figure 1, in this study, self-assembled CUR-LF NPs were prepared, and the neuroprotective mechanism of the nanoparticles on Aβ25-35-induced damage in PC12 cells was explored by antioxidant and anti-apoptosis analysis. We further studied the brain accumulation of CUR-LF NPs via IN administration to confirm their prospect and feasibility in neuroprotection.
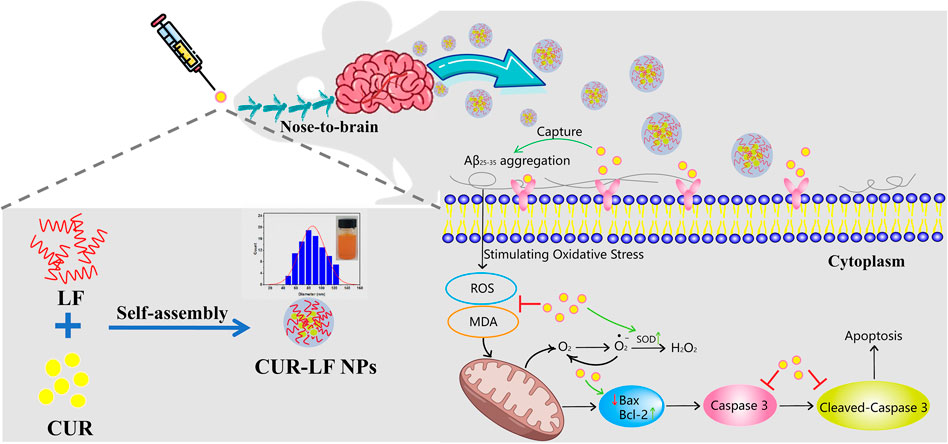
FIGURE 1. Schematic illustration of the preparation of curcumin-lactoferrin nanoparticles (CUR-LF NPs) and to evaluate the prospective and feasibility for neuroprotection via intranasal administration.
2 Materials and methods
2.1 Materials, cells, and animals
CUR was purchased from Chengdu McLean Biotechnology Co., Ltd (Analytical Reagent, Chengdu, China). LF was purchased from Shanghai Yuanye Biotechnology Co., Ltd (95%, Shanghai, China). CUR standard (98.5%) and magnolol standard (98.5%) were purchased from Chengdu Pusi Biotechnology Co., Ltd (Chengdu, China). Aβ25-35 and DMSO were obtained from Sigma-Aldrich (Saint Louis, MO, United States). The antibody against β-tubulin and 4,6-diamidino-2-phenylindole (DAPI) were obtained from Beyotime Biotechnology Co., Ltd (Shanghai, China). The antibodies against Bax (#ab32503) and Bcl-2 (#ab196495) were obtained from Abcam (Cambridge, United Kingdom). The antibody against Caspase 3/p17/p19 (66470-2-lg) was obtained from proteintech® (Chicago, United States). Goat Anti-Rabbit IgG H&L (HRP) and Goat Anti-Mouse IgG H&L (HRP) were obtained from Invitrogen (Carlsbad, CA, United States). Ultrapure water comes from the Milli-Q system (Millipore, Bedford, MA, United States). All solvents including glacial acetic acid, acetonitrile, formic acid, methanol, and absolute ethanol were HPLC grade and were obtained from Chengdu Cologne Chemical Co., Ltd. (Chengdu, China).
MDCK cells were purchased from Beina organisms (Beijing, China), and PC12 cells were kindly provided by Southwest Jiaotong University (Chengdu, China). The above cells were cultured in Dulbecco’s modified Eagle medium (DMEM), supplemented with 10% (v/v) fetal bovine serum (FBS), 1% (v/v) penicillin-streptomycin solution, and 1% (v/v) glutamine (Invitrogen). All reagents were freshly prepared, and free CUR was dissolved in DMSO and diluted more than 100 times with DMEM (containing 5% FBS) as the diluent.
Male Sprague-Dawley rats (SPF, 200–220 g) were purchased from Hunan Slake Jingda Laboratory Animal Co., Ltd (Hunan, China; permits SCXK 2019-0004). The protocol of animal experiments was approved by the Experimental Animal Ethics Committee of Chengdu University of Traditional Chinese Medicine. Rats were reared under standard conditions and had free access to food and distilled water.
2.2 Preparation of CUR-LF NPs
CUR-LF NPs were prepared by the desolvation method. Briefly, 1 mL of CUR ethanol solution (6 mg/mL) was added dropwise (1 mL/min) to 16 mL of LF solution (dispersed in ultrapure water, 3 mg/mL) under magnetic stirring (400 rpm). The mixture was homogenized using a probe sonicator at 100 W power, on for 2 s and off for 3 s, and cycled for 2 min. CUR has poor water solubility (11.33 ± 0.73 ng/mL) and the unencapsulated CUR exists in solution as microcrystalline material. Therefore, free CUR was separated by centrifugation precipitation (4,000 rpm, 10 min). The supernatant was filtered through a 0.22 μm filter to obtain CUR-LF NPs solution.
2.3 Characterization of CUR-LF NPs
2.3.1 Drug encapsulation capability
Encapsulation efficiency (EE, %) and drug loading (DL, %) of CUR-LF NPs were evaluated by HPLC (Agilent 1260 Infinity II, Agilent, Santa Clara, CA, United States) using an Agilent ZORBAX Eclipse Plus C18 (150 mm × 4.6 mm, 3.5 μm) chromatographic column at a detection wavelength of 430 nm. The mobile phase was 4% glacial acetic acid/acetonitrile (52/48, v/v) with a flow rate of 1 mL/min. The EE (%) and DL (%) were calculated by the following equations.
2.3.2 Particle size, zeta potential, and micromorphology
The mean particle size, polydispersity index (PDI), and zeta potential of CUR-LF NPs were conducted using a NanoBrook 90 Plus PALS instrument (Brookhaven Instruments Corporation, United States). The results were obtained from more than 3 parallel samples.
The micromorphology of CUR-LF NPs was observed by transmission electron microscopy (TEM) (HT7800, HITACHI, Japan). The observation was performed by applying a drop of CUR-LF NPs on a special copper mesh, followed by negative staining with 2.0% (w/v) sodium phosphotungstate.
2.3.3 Circular dichroism (CD)
UV circular dichroism (CD) spectra of the native LF and CUR-LF NPs were recorded in the range 190–250 nm with 0.25 mg/mL protein by a Chirascan qCD (Applied Photophysics Ltd., United Kingdom). The CD spectrum was the average of 3 measurements at a test speed of 100 nm/min, 0.2 nm resolution, 1.0 nm bandwidth, and nitrogen as shielding gas (Li et al., 2018). The collected data was analyzed using Dichroweb (Circular Dichroism Website http://dichroweb.cryst.bbk.ac.uk) to obtain the secondary structure of LF.
2.3.4 X-ray diffraction (XRD)
X-ray diffraction (XRD) measurements of CUR, LF, CUR/LF physical mixture, and CUR-LF NPs at 6°/min scanning from 5° to 40° (2θ) at 40 kV and 30 mA using the XRD instrument (DX-2700 BH, Dandong Haoyuan Instrument Co., Ltd. China).
2.3.5 Differential scanning calorimetry (DSC)
Calorimetric analyses were determined using differential scanning calorimetry (DSC) instrument (DSC 3+, Mettler Toledo Ltd. Switzerland). The aluminum pans containing approximately 3–5 mg of samples were heated from 30°C to 300°C at a rate of 10°C/min under a nitrogen environment.
2.3.6 Stability of CUR-LF NPs
To simulate the stability of CUR-LF NPs in vivo, we mixed CUR-LF NPs with PBS (pH 7.4), DMEM, simulated nasal fluid (SNF, pH 6.0), and artificial cerebrospinal fluid (ACSF, pH 7.4) at a volume ratio of 1:9 and incubated the mixture in a constant temperature shaker (100 rpm) at 37°C. CUR is extremely insoluble in water, thus, the leaked free CUR will be precipitated at the bottom of the test tube after the nanoparticles rupture. A total of 200 μL of supernatant containing CUR-LF NPs was collected, and the content of CUR-LF NPs was determined by HPLC at 0, 2, 4, 8, 12, and 24 h. Then, the nanoparticles’ stability rate was calculated (the stabilization ratio of CUR-LF NPs at 0 h was 100%).
2.4 In vitro delivery evaluation
2.4.1 In vitro release studies
A discriminatory and rugged dialysis method was developed for CUR-LF NPs to measure the drug release profiles (Li et al., 2022). Briefly, 1 mL of CUR solution (in 50% DMSO solution, v/v), CUR suspension (in 0.5% CMC-Na solution, w/v), and CUR-LF NPs with a concentration of 200 μg/mL were added into dialysis bags (MWCO 8000–14000 Da). They were immersed in 30 mL of release media composed of PBS buffer (pH 7.4) containing 30% ethanol (v/v) and placed in a constant-temperature shaker incubator (100 rpm) at 37°C. The release system was completely replaced with 30 mL of prewarmed, fresh media at 0.25, 0.5, 1, 2, 4, 6, 8, 12, and 24 h. Release tests for each sample were performed in triplicate. The concentration of CUR was determined by HPLC, and the cumulative release (Q, %) was obtained according to the following equation.
Where V is the volume of the release media; Ci is the curcumin concentration measured at each time point; M is the initial dosage.
2.4.2 MDCK monolayer penetration studies
First, the cytotoxicity of CUR formulations incubated with MDCK cells for 4 h was measured using Cell Counting Kit-8 (CCK-8, Dojindo Molecular Technologies, Inc. Rockville, MD, United States) (Supplementary Figure S1). MDCK cells (1.8×105/well) were seeded onto the apical chamber of cell culture inserts (1.12 cm2, 3 μm, polycarbonate membrane; Corning, New York, United States) in a 12-well plate and cultured for 3–5 days. The transepithelial electrical resistance (TEER) of each insert was monitored by an epithelial volt-ohm meter (MERS00002, Millipore) to reach higher than 0.9 kΩ cm2 prior to the penetration studies. Then, 0.5 mL of 100 μg/mL CUR solution (containing 1% DMSO), 0.5% carboxymethyl cellulose sodium (CMC-Na) CUR suspension, and CUR-LF NPs were added to the apical chamber, and 1.5 mL of HBSS solution (containing 0.05% Tween) was added to the basolateral chamber of the inserts. A 200 μL solution was collected from the basolateral chamber at 0.25, 0.5, 1, and 2 h, and the corresponding solution was replenished. The integrity of the MDCK monolayer cell membrane was determined by monitoring TEER changes throughout the process. The concentration of CUR in the basolateral chamber was determined by HPLC, and the apparent permeability coefficient (Papp, cm/s) was obtained according to the following equation.
Where dQ/dt is the permeability rate (μg/s); C0 is the initial curcumin concentration of the apical chamber (μg/mL); A is the polycarbonate membrane area (cm2).
2.5 In vitro efficacy evaluation of CUR-LF NPs in PC12 cells
2.5.1 Cellular uptake
Fluorescence microscopy (CKX53SF, Olympus Corporation, Japan) was used to qualitatively observe the cellular uptake of free CUR and CUR-LF NPs based on the spontaneous fluorescence of CUR (λEx/λEm was 425/530 nm). PC12 cells (4×105/well) were seeded into 6-well plates and cultured overnight. Then, free CUR and CUR-LF NPs (20 μM) with or without LF pretreatment were added and incubated for 60 min. After three washes with prechilled PBS, the cells were fixed in paraformaldehyde for 15 min. The nucleus was stained with DAPI for 5 min and then observed by fluorescence microscopy (Bonaccorso et al., 2020). Images were captured using the BioHD-C20 imaging system.
Quantitative analysis of cellular uptake was performed by HPLC. PC12 cells were treated with free CUR and CUR-LF NPs (20 μM) and incubated for 5, 30, 60, 120, and 240 min. The cells were collected by cell scraper and centrifugation (300 × g for 5 min at 4°C), cell lysis buffer containing the protein inhibitor cocktail (Merck) was added and lysed on ice for 30 min. Then, 4× volume of methanol was added to precipitate protein and extract CUR. HPLC analysis was performed on the supernatant. The protein concentration was determined by bicinchoninic acid assay (BCA assay, Beyotime). Quantitative cellular uptake was calculated by CUR concentration/protein content (μg/mg protein).
2.5.2 Cytotoxicity assays
CCK-8 was used to determine the safe dose range of CUR-LF NPs in PC12 cells. The cells were seeded into 96-well plates (1×105 cells/mL, 100 μL/well, 3 duplicate wells per group) and incubated overnight. Then, free CUR and CUR-LF NPs (0, 1, 10, 20, 30, and 50 μM) were added and incubated for 24 h. The negative control groups were cultured with the corresponding blank solvent. Each well was replaced with fresh DMEM and 10% CCK-8 in place of the previous medium, followed by incubation for 1–4 h. Pure CCK-8 medium was used as a blank well (Qian et al., 2018). The absorbance value of CCK-8 was detected at 450 nm with an automatic microplate reader (VICTOR NIVO, PerkinElmer, United States).
2.5.3 Protective effects of CUR-LF NPs on Aβ25-35-induced PC12 cells
Briefly, 1 mg of Aβ25-35 was evenly dissolved in 200 μL of DMSO, and then 743 μL of DPBS was added to obtain a 1 mM stock solution. The aliquoted stock solution was incubated at 37°C for 7 days to allow Aβ25-35 to aggregate and age prior to the experiment. The cytotoxic effect of Aβ25-35 (1, 10, 25, and 50 μM) in PC12 cells with 24 and 48 h induction times were investigated. The treatment groups (free CUR, LF NPs, LF NPs + free CUR, and CUR-LF NPs) were added to the medium after 24 h of Aβ25-35 induction, and then co-incubated with Aβ25-35 for another 24 h.
2.5.4 Oxidative stress assays
Oxidative stress was evaluated by detecting the levels of reactive oxygen species (ROS), superoxide dismutase (SOD), and malondialdehyde (MDA). Among them, ROS was detected using a DCFH-DA probe (Beyotime). The fluorescence of DCF was detected by an automatic microplate reader (λEx/λEm was 488/525 nm), and the results were expressed as relative fluorescence intensity (the control group was 100%). In addition, SOD and MDA were determined according to the protocol of the corresponding assay kits (Beyotime), and the protein concentration was determined as described above. The results of SOD and MDA were expressed as U/mg protein and μM/mg protein, respectively.
2.5.5 Cell apoptosis analysis
Cell apoptosis is commonly detected using an Annexin-V-FITC/PI apoptosis detection kit (Vazyme Biotechnology Co., Ltd. Nanjing, China). PC12 cells were treated according to the protocol of the apoptosis kit. The samples were analyzed by flow cytometry (EXFKOW-206, DAKEWE Biotech Co., Shenzhen, China) within 1 h.
2.5.6 Western blotting
Equal protein samples after heat denaturation were separated by SDS-PAGE (12%) at 80 V and transferred to 0.45 μm PVDF membranes. The membranes were blocked and incubated with antibodies. Visualization of the bands was performed using the ECL luminescence kit, and grayscale analysis was performed using ImageJ software (National Institute of Health, Bethesda, MD, United States). β-tubulin was used as an internal reference to facilitate data normalization.
2.6 In vivo studies of CUR-LF NPs
2.6.1 Grouping and administration of animals
Rats were randomized into 3 groups of 18 animals each (n = 3, at each time point). The experimental animals were anesthetized with 2% pentobarbital sodium intraperitoneally prior to administration. Rats in Groups 1 and 2 were administered 0.5% CMC-Na CUR suspension and CUR-LF NPs via the IN administration route (100 μL, 10 mg/kg), respectively. Group 3 was administered CUR-LF NPs via tail vein injection (0.5 mL, 10 mg/kg). The rats were sacrificed at predetermined time points at 0.25, 0.5, 1, 2, 4, and 8 h. Blood was taken from the abdominal aorta, and the whole brain was rapidly dissected and washed with saline. Subsequently, the plasma samples were collected by centrifugation (4,000 rpm, 10 min). Saline was added to the brain tissue (brain tissue:saline ratio 1:2 w/w) and homogenized using a SCIENTZ-24 High Throughput Tissue Grinder (Ningbo Scientz Biotechnology Co., Ltd. Ningbo, China) at 2000 rpm for 30 s. Samples were stored at −20°C, processed, and analyzed within 3 days.
2.6.2 Processing of samples and chromatographic conditions
Briefly, 20 μL of magnolol methanol solution was spiked into the samples as an internal standard (IS, 10 μg/mL). Then, 0.8 mL of acetonitrile was added to 200 μL of plasma, and 1 mL of ethyl acetate and n-hexane (1:1, v/v) was added to 200 μL of brain homogenate. Each sample was vortexed and centrifuged (10,000 rpm for 10 min at 4°C) to precipitate protein and extract CUR and magnolol. The supernatant was evaporated to dryness at 40°C using a dry nitrogen blower (Shanghai Bilang Instrument Manufacturing Co., Ltd. Shanghai, China). The residue was dissolved in 200 μL of methanol and filtered through a 0.22 μm filter.
The concentration of CUR was analyzed by UPLC-MS/MS using a Thermo Scientific Vanquish™ Flex coupled to TSQ Fortis™ MS (Thermo, Massachusetts, United States) on a ZORBAX SB-C18 column (2.1 × 150 mm, 3.5 µm, Agilent). The mobile phase consisted of acetonitrile (A) and 0.1% aqueous solution of formic acid (B) at a flow rate of 0.2 mL/min according to the following time gradient elution program: 0–6 min, 50%–85% (A), 6–8 min, 85%–95% (A), 8–10 min, 95% (A). Sample analysis was performed by multiple reaction monitoring (MRM) in negative ion mode using m/z 367.138/173.06 for CUR and m/z 265.088/224.071 for magnolol as monitor ions.
2.6.3 Pharmacokinetics parameters of CUR-LF NPs
The pharmacokinetic parameters were calculated by PK solver software (designed by China Pharmaceutical University, Nanjing, China) (Zhang et al., 2010) using the non-compartment model. Brain targeting efficiency (BTE, %) and nose-to-brain direct transport percentage (DTP, %) were calculated by the following equation (Hao et al., 2016):
2.7 Statistical analysis
The results were presented as mean ± standard deviation. All data were analyzed using SPSS Statistics 23 software (Chicago, IL). Statistical analysis was performed using independent samples t-test and one-way ANOVA. p* < 0.05 and p** < 0.01 were considered statistically significant.
3 Results and discussion
3.1 Preparation of CUR-LF NPs
The preparation process of CUR-LF NPs was optimized in our previous work (Li et al., 2022). We found that the volume ratio of ethanol to water was the key factor affecting the formation of the nanoparticles. It has been reported that CUR relies primarily on hydrophobic and hydrogen bonding forces to bind to the hydrophobic region of LF (Araujo et al., 2020). Adding an appropriate amount of ethanol could alter the permittivity of the protein mixed solvent (Hansen et al., 2021), promote the unfolding of protein molecules and thus expose the hydrophobic nucleus (Halder and Jana, 2021), which is conducive to reducing the hydration of protein and increasing the binding efficiency with the hydrophobic CUR molecule. However, when the volume ratio of ethanol to water was greater than 1:4, nanoparticles could not be formed. Similar results were also reported by Gong et al. (Gong et al., 2015). A possible explanation for this might be that hydrogen bonds were formed between excessive ethanol and protein. The protein was denatured and precipitated due to the destruction of the original hydrogen bond structure inside the protein. As a result, stable CUR-LF NPs could be formed well when the volume ratio of ethanol to water was between 1:8 and 1:16, where the ratio of 1:16 showed better EE, particle size, and PDI. Therefore, it was selected for preparation in this study.
3.2 Characterization of CUR-LF NPs
The HPLC method for the determination of CUR concentration showed reasonable linearity in the concentration range from 0.76 to 141.84 μg/mL (R2 = 0.9998) (Supplementary Figure S2). CUR-LF NPs showed an acceptable drug encapsulation capacity with EE and DL values of 91.2% ± 3.6% and 9.6% ± 0.8%, respectively.
As shown in Figure 2A, CUR-LF NPs appeared as a clear yellow solution. The particle size of CUR-LF NPs was 84.8 ± 6.5 nm, and the PDI was 0.078 ± 0.016. The particle size was within the most suitable range (<200 nm) for nose-to-brain delivery with a uniform particle size distribution (Samaridou and Alonso, 2018). In addition, the TEM image (Figure 2C) showed that CUR-LF NPs were in a regular uniform spherical shape. The zeta potential was +22.8 ± 4.3 mV, and the phase measured and phase fitted curves almost coincided, which indicated that the measured data were reliable (Figure 2B).
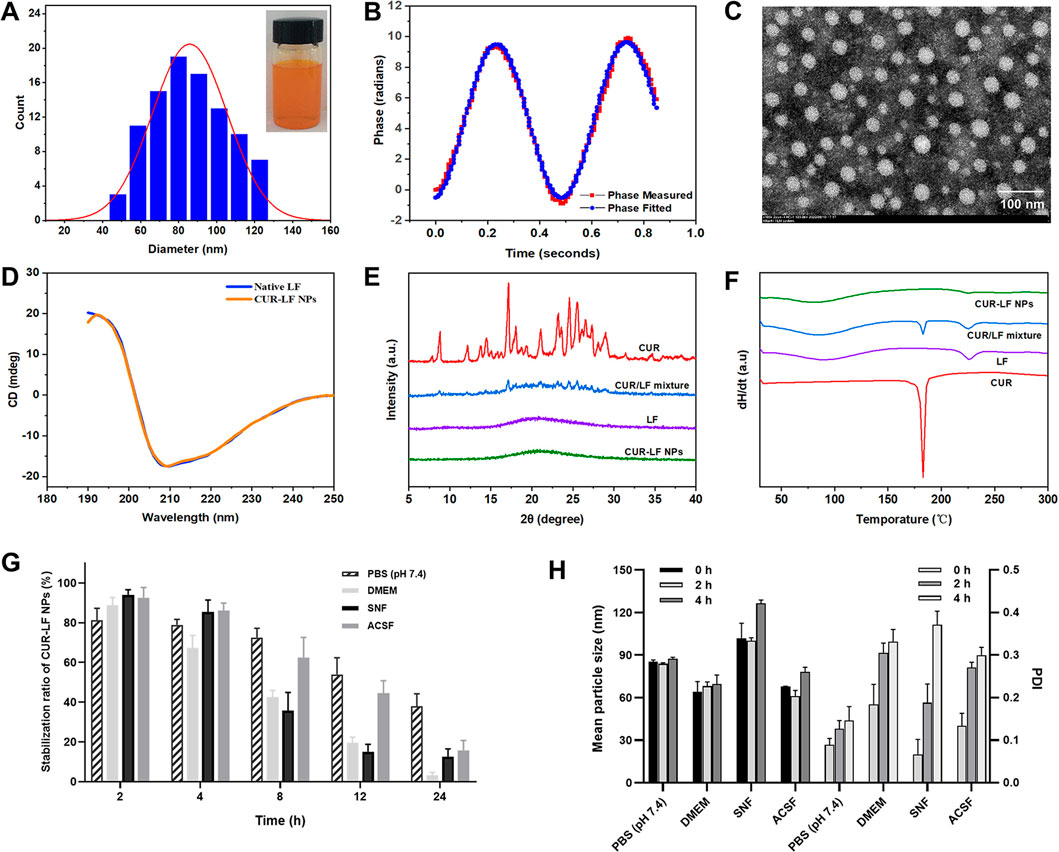
FIGURE 2. Characterization of CUR-LF NPs (A) Particle size distribution and appearance of CUR-LF NPs; (B) Zeta potential fitting diagram of CUR-LF NPs; (C) Transmission electron microscopy (× 100 000) of CUR-LF NPs; (D) Determination of secondary structure of native lactoferrin and CUR-LF NPs by circular dichroism; (E) X-ray diffractogram of curcumin, lactoferrin, curcumin/lactoferrin physical mixture, and CUR-LF NPs; (F) Differential scanning calorimetry of curcumin, lactoferrin, curcumin/lactoferrin physical mixture, and CUR-LF NPs; (G) Evaluation of the stabilization ratio of CUR-LF NPs incubated in PBS (pH 7.4), Dulbecco’s modified Eagle medium (DMEM), simulated nasal fluid (SNF), and artificial cerebrospinal fluid (ACSF) for 24 h; (H) Evaluation of the mean particle size and polydispersity index of CUR-LF NPs incubated in PBS (pH 7.4), DMEM, SNF, and ACSF for 4 h. Data were presented as mean ± SD (n = 3).
CD is a widely used method for the detection of the secondary structure of proteins in the far ultraviolet range (190–250 nm) (He et al., 2016). As shown in Figure 2D, native LF exhibited the largest positive peak and negative peak in the wavelength ranges of 190–195 nm and 205–210 nm, respectively, which is mainly characteristic of α-helix (Liu et al., 2015). The spectrum of β-folded CD has a negative band in the wavelength range of 215–220 nm. The secondary structure contents of native LF and CUR-LF NPs were calculated using the Dichroweb website. There was no significant difference between them (p > 0.05), as shown in Table 1, indicating that the binding of CUR to LF with non-covalent bonds did not significantly affect the backbone hydrogen bond structure in LF, which was similar to the study by Araujo et al. (2020).

TABLE 1. Secondary structural contents of native lactoferrin and CUR-LF NPs. Data were presented as mean ± SD (n = 3).
The XRD diffractograms of CUR, LF, CUR/LF physical mixture, and CUR-LF NPs were shown in Figure 2E. The strong signal characteristic diffraction peaks of crystalline CUR and the smooth XRD curve of amorphous LF could be observed. In addition, CUR and LF were physically mixed according to the preparation feeding ratio. The characteristic diffraction peaks of crystalline CUR were still observable, although the intensity was weakened. Finally, it was clear that the characteristic diffraction peaks of CUR in CUR-LF NPs disappeared, indicating that CUR was transformed into an amorphous state after being encapsulated with LF.
As shown in the DSC analysis (Figure 2F), the melting point of CUR was approximately 183°C, and the glass transition temperatures of LF were 89°C and 225°C. The specific endothermic peaks of CUR appeared in the physical mixture group at corresponding positions and disappeared in the CUR-LF NPs group. These phenomena could be explained by the fact that CUR was successfully encapsulated in the hydrophobic core of LF, rather than simply being physically mixed, which was consistent with the XRD analysis.
From Figures 2G, H, the stability magnitude of CUR-LF NPs in each culture system was PBS (pH 7.4) > ACSF > SNF > DMEM. Because nasal absorption is a rapid process, the stability of the nanoparticles in SNF ensures that CUR-LF NPs are absorbed by the nasal mucosa in a relatively complete nanostructure. Moreover, CUR-LF NPs showed more than 50% stability in ACSF within 12 h, which allows the nanoparticles to exert their effect in the brain to the greatest possible extent. Meanwhile, we found that the particle size of CUR-LF NPs did not significantly change within 4 h in each culture system, but the PDI showed an increasing trend.
3.3 In vitro release behaviors
Dialysis methods are frequently used for the simulation of the in vitro release kinetics of nanoparticle-based drug delivery systems (Yu et al., 2019). As shown in Figure 3A, free CUR was rapidly released within the first 2 h, and the equilibrium between the solutions inside and outside the dialysis bag was almost achieved at 8 h with a cumulative release rate of 82.0% ± 3.2%. Compared to free CUR, the equilibrium was not achieved during the release process for both the CUR-LF NPs and CUR suspension, with much lower cumulative release rates of 64.6% ± 1.6% and 52.3% ± 5.0%, respectively. In comparison, the release profile of CUR from the nanoparticles was significantly higher than that from the suspension. The obtained release curves were fitted with different mathematical models, and it was found that they could be described well by the first-order model (Supplementary Table S1). There is no doubt that first-order processes are only dependent on the concentration of the dissolving substance (May et al., 2012). Therefore, the release rate of CUR from the nanocarriers was faster than the dissolution rate of CUR particles during release.
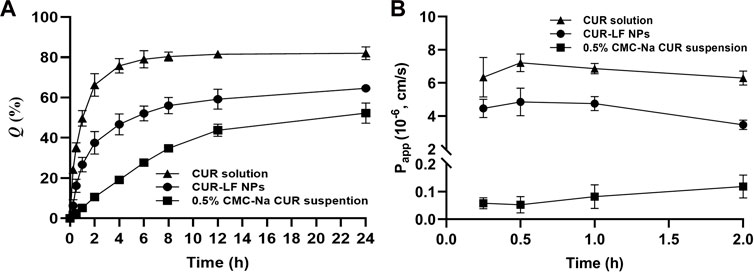
FIGURE 3. In vitro delivery evaluation (A) In vitro release of curcumin solution, 0.5% carboxymethyl cellulose sodium (CMC-Na) curcumin suspension, and CUR-LF NPs (B) The apparent permeability coefficient of curcumin solution, 0.5% CMC-Na curcumin suspension, and CUR-LF NPs on MDCK monolayer. Data were presented as mean ± SD (n = 3).
3.4 MDCK monolayer penetration studies
To evaluate the absorption and penetration of APIs in common in vitro cell models, some previous studies indicated that MDCK cells showed superior in vitro and in vivo correlation of in vitro permeability and in vivo nasal absorption in rats compared to Caco-2 and Calu-3 cells (Furubayashi et al., 2020). In addition, the MDCK cells formed tight junctions with a shorter molding time of 3 days and a high TEER value of over 0.9 kΩ.cm2, which is beneficial for the effective evaluation of nasal mucosal penetration efficiency of APIs in basic research. Therefore, the MDCK cell line was selected to establish a nasal mucosal model to investigate the in vitro penetration ability of different CUR formulations in this study. It is considered that APIs absorption through the nasal cavity requires rapid dissolution and passage through the mucus layer, which prevents the APIs from being removed from the absorption site by the nasal cilia, and then the APIs penetrate through the barrier of the epithelium, basement membrane, and lamina propria (He et al., 2020; Tai et al., 2022). As shown in Figure 3B, the insoluble CUR group was uniformly dispersed in a 0.5% CMC-Na suspension with a Papp of 0.09 ± 0.04×10−6 cm/s, which showed such a low permeability strength. CUR-LF NPs ameliorated the solubility of CUR, thus greatly improving the Papp (4.36 ± 0.79×10−6 cm/s) to a moderate permeability strength, which was very close to that of the CUR molecule in the solution state (6.68 ± 1.21×10−6 cm/s). APIs transport across the MDCK monolayer occurs mainly via paracellular and transcellular pathways. Due to the dense tight junctions between MDCK cells, the paracellular transport of CUR-LF NPs was greatly limited, and the CUR molecule was easier to transport via paracellular and transcellular pathways due to its low molecular weight and lipophilicity (Akel et al., 2020). As a result, the Papp of CUR solution was slightly higher than that of CUR-LF NPs.
3.5 Cellular uptake
PC12 cells were selected to analyze the cellular uptake of free CUR and CUR-LF NPs, as this cell line is widely used in neuroprotection mechanism research (Kwan et al., 2021). It is well known that increasing the cellular uptake efficiency is equivalent to effectively improving the therapeutic effect of APIs. Qualitative uptake was detected by fluorescence microscopy, as shown in Figure 4A. There was an interesting phenomenon that the fluorescence of free CUR was green, whereas that of CUR-LF NPs was yellow-green. We speculate that this occurred because CUR’s luminescent groups underwent subtle changes during binding to LF. This resulted in a shift in the emission wavelength and a further change in fluorescence. Similarly, a blue shift in the emission wavelength of CUR was found in Tween 80 micellar nanocurcumin prepared by Pinilla-Peñalver et al. (2020) and Curcumin-PLGA prepared by Zakaria et al. (2022). It was observed that the uptake of CUR-LF NPs was stronger than that of free CUR. Two types of explanations could be proposed for this result. The first explanation assumes that LF coating contributes to the uptake of CUR-LF NPs by additionally initiating the LF receptor-mediated endocytic pathway (Zhao et al., 2018a), which was demonstrated by performing experiments with or without pretreatment with LF. With the pretreatment of LF, LF receptors on the surface of PC12 cells were bound and blocked, and CUR-LF NPs were competitively inhibited via LF receptor-mediated endocytic channels, resulting in reduced uptake in PC12 cells. In contrast, pretreatment with LF did not make a significant difference in the uptake of free CUR by PC12 cells. Therefore, the uptake of free CUR does not depend on LF receptor-mediated cellular endocytosis. The second explanation assumes that the positively charged nature of CUR-LF NPs facilitates binding to negatively charged cell membranes, and thus enhances the internalization of PC12 cells via charge-based interactions (Agwa et al., 2020).
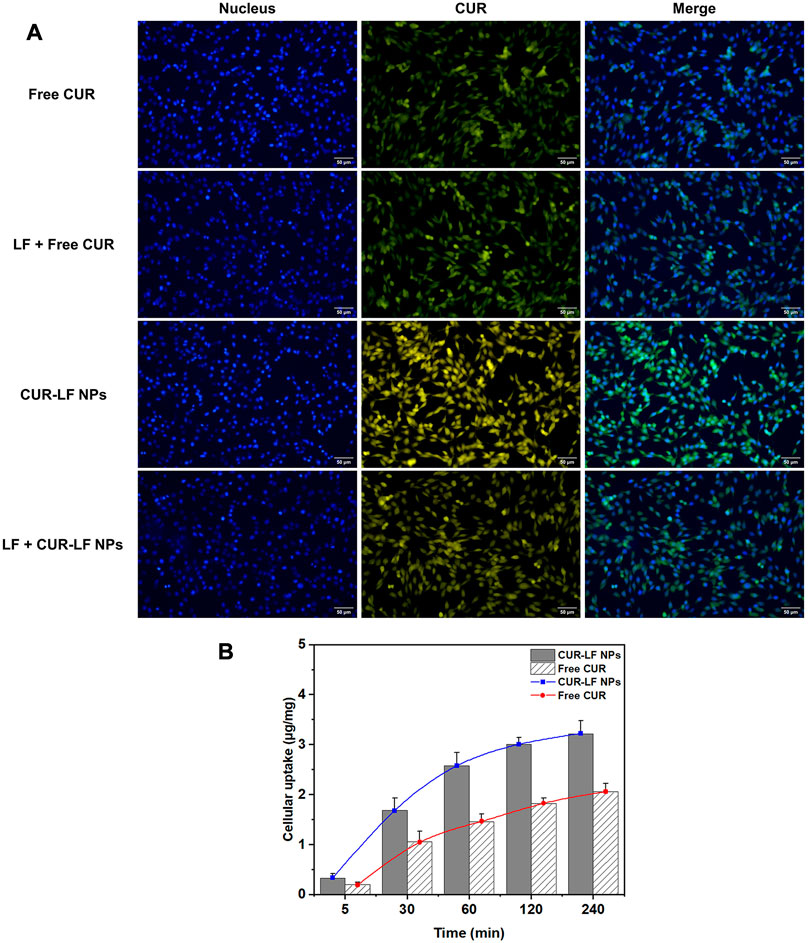
FIGURE 4. Cellular uptake of different curcumin formulations in PC12 cells (A) Fluorescence microscopy qualitative analysis of cellular uptake after incubation for 60 min with free curcumin and CUR-LF NPs with or without lactoferrin pretreatment at the concentration of 20 μM; (B) HPLC quantitative analysis of cellular uptake after incubation for 5, 30, 60, 120, and 240 min with the concentration of 20 μM. Data were presented as mean ± SD (n = 3).
Quantitative uptake was measured by HPLC (Figure 4B). We easily determined that the uptake of CUR-LF NPs by PC12 cells was higher than that of free CUR at each time point. The uptake rate of CUR-LF NPs was faster than that of free CUR in the first 60 min, as shown by the slope of the uptake curve. Overall, PC12 cells uptake in both groups was time-dependent. As the co-incubation time was extended, the uptake rate decreased, and the saturation trend gradually appeared.
3.6 Cytotoxicity assays
The potential cytotoxicity of CUR-LF NPs on PC12 cells was primarily evaluated using a CCK-8 assay and was utilized to ascertain the appropriate doses of CUR-LF NPs for further evaluation of their protective effect on Aβ25-35-induced PC12 cell damage. As shown in Figure 5A, free CUR did not exhibit cytotoxicity below 20 μM (cell viability >100%). Surprisingly, the safe dose of CUR was increased to 30 μM after encapsulation in LF nanoparticles. This could be attributable to the slow-release effect of CUR-LF NPs, which will not induce toxic reactions caused by excessive instantaneous drug concentrations. In addition, there were significant differences between free CUR and CUR-LF NPs at each concentration (1, 10, 20, 30, and 50 μM) (p < 0.05). It was clear that CUR-LF NPs displayed a better effect in promoting the proliferation of PC12 cells at concentrations of 1, 10, and 20 μM, and there was no significant difference in cell viability between 10 and 20 μM concentrations of CUR-LF NPs (p > 0.05). Therefore, in line with the principle of using as few drug doses as possible, 1 and 10 μM were selected as the low and high treatment doses of free CUR and CUR-LF NPs for the subsequent studies.
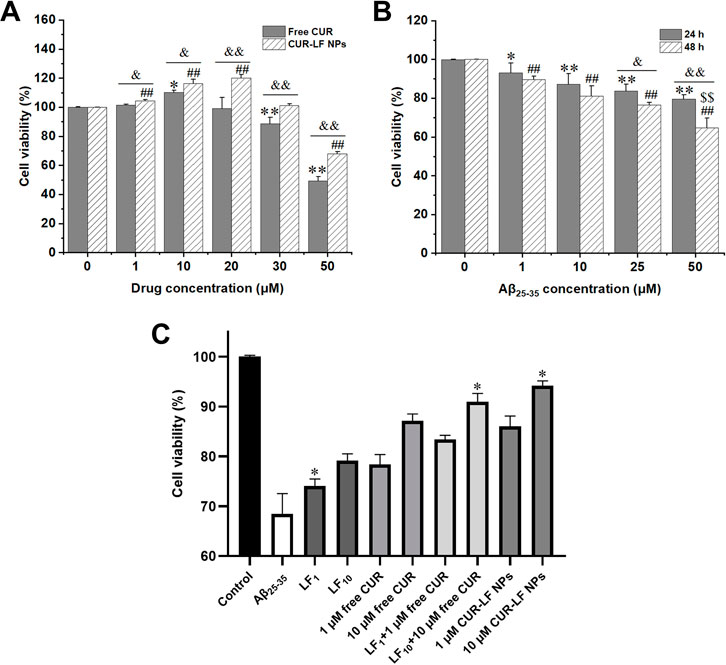
FIGURE 5. Cell viability assay (A) PC12 cells treated with free curcumin and CUR-LF NPs (1, 10, 20, 30, and 50 μM) for 24 h *p < 0.05, **p < 0.01 free curcumin group versus solvent control group; #p < 0.05, ##p < 0.01 CUR-LF NPs group versus solvent control group; &p < 0.05, &&p < 0.01 free curcumin group versus CUR-LF NPs group (B) Investigation of induction concentration (1, 10, 25, and 50 μM) and time (24 and 48 h) of Aβ25-35-induced PC12 cell damage. *p < 0.05, **p < 0.01 Aβ25-35 group versus control group at 24 h induction time; #p < 0.05, ##p < 0.01 Aβ25-35 group versus control group at 48 h induction time; &p < 0.05, &&p < 0.01 24 h versus 48 h induction time; $p < 0.01, $$p < 0.01 indicating the induction concentration and time was significantly different from other groups (C) Protective effect of lactoferrin nanoparticles, free curcumin, lactoferrin nanoparticles + free curcumin, and CUR-LF NPs on Aβ25-35-induced damage in PC12 cells. *p < 0.05, **p < 0.01 indicating the treatment group was significantly different from other treatment groups. Data were presented as mean ± SD (n = 3).
3.7 Protective effects of CUR-LF NPs on Aβ25-35-induced PC12 cells
The Aβ25-35-induced nerve damage model based on PC12 cells was constructed as previously described (Zhao et al., 2018b). From Figure 5B, it was found that the induction of 50 μM Aβ25-35 for 48 h could cause significant damage to PC12 cells to establish an appropriate in vitro AD model, and the cell viability under this condition was 68.4% ± 4.1%. Then, the protective effects of 1 and 10 μM free CUR and CUR-LF NPs against Aβ25-35-induced damage in PC12 cells were investigated. In addition, the protective effects of blank LF NPs (LF1, LF10) at different concentrations, whose LF content was equivalent to 1 and 10 μM CUR-LF NPs, were also evaluated in damaged PC12 cells.
As shown in Figure 5C, each formulation treatment group significantly improved the cell viability of Aβ25-35-induced damage in PC12 cells (p < 0.01). The protective capacity in order of magnitude was CUR-LF NPs > LF NPs + free CUR > free CUR > LF NPs and was dose-dependent (10 > 1 μM). Although LF NPs showed certain protective effects, the synergistic protective effect of simply mixing with free CUR was still lower than the equivalent dose of CUR-LF NPs in damaged PC12 cells. As mentioned in the cellular uptake studies, free CUR does not depend on the receptor-mediated endocytic pathway to access cells. Therefore, a simple mixture of free CUR and LF NPs does not promote the cellular uptake of free CUR, but merely superimposes their pharmacological effects. However, CUR-LF NPs not only had the synergistic pharmacological effects of CUR and LF, but also the LF coating increased the endocytosis of CUR, thus playing a stronger protective role. As a result, among all treatment groups, the 10 μM CUR-LF NPs group showed superior protective effects on Aβ25-35-induced damage in PC12 cells with cell viability of 91.0% ± 1.6%, and there was no significant difference between 1 μM CUR-LF NPs (86.1% ± 2.0%) and 10 μM free CUR (87.2% ± 1.3%) (p > 0.05). Therefore, 1 and 10 μM CUR-LF NPs were selected for further investigation.
3.8 Effect on antioxidant
The brain is highly sensitive to oxidative damage, and increased ROS in the brain could lead to excessive oxidation of proteins, lipids, and DNA, and reduce the activity of antioxidants such as glutathione, catalase, and SOD, thereby damaging neurons and even causing neuronal death (Ege, 2021). From Figures 6A–C, it was observed that the model group induced by Aβ25-35 significantly increased the generation of ROS from 100.4% ± 1.9% to 717.2% ± 60.2% and MDA from 3.3 ± 0.6 to 13.8 ± 1.8 μM/mg protein, and the content of antioxidant enzymes SOD significantly decreased from 39.5 ± 0.9 to 20.2 ± 3.0 U/mg protein compared to the control group. Adding low and high doses (1, 10 μM) of CUR-LF NPs to damaged PC12 cells greatly reduced the levels of ROS and MDA and improved the SOD activity, which was statistically significant compared to the model group (p < 0.05). There were significant differences between the 1 and 10 μM CUR-LF NPs treatment groups in all three indicators (p < 0.05), and the 10 μM CUR-LF NPs group showed better protective effects on Aβ25-35-induced oxidative stress in PC12 cells.
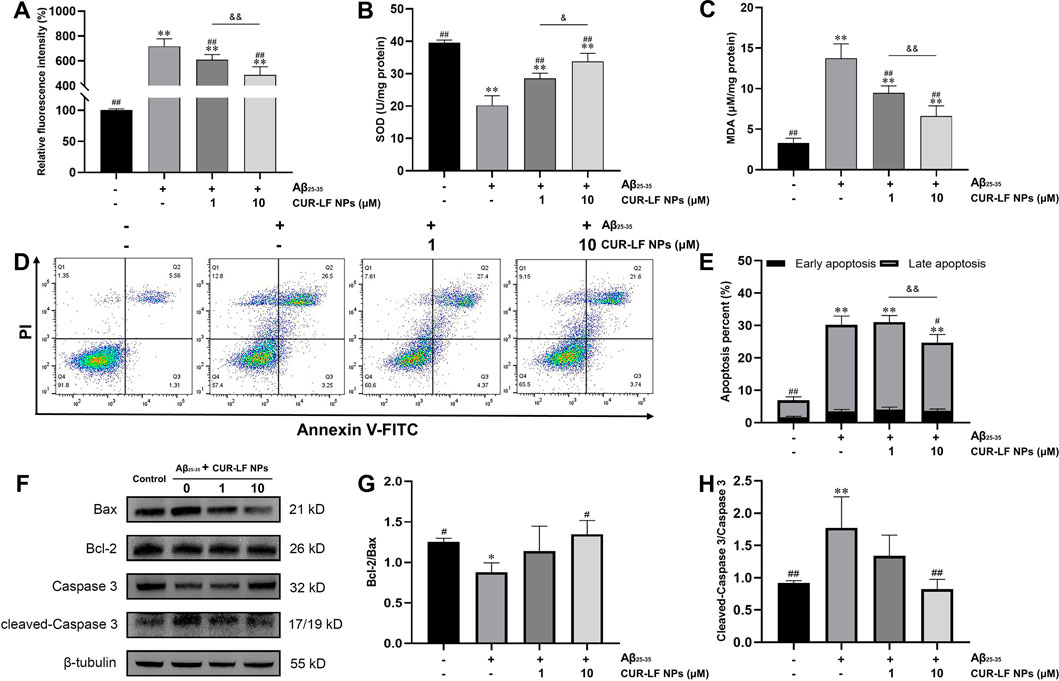
FIGURE 6. Protective effect of CUR-LF NPs on Aβ25-35-induced oxidative stress and apoptosis in PC12 cells (A) DCFH-DA fluorescence probe was used to detect intracellular ROS generation in PC12 cells (B) The intracellular SOD activity was measured using an SOD assay kit (C) The intracellular MDA level was evaluated with an MDA assay kit (D) Apoptosis of the treated PC12 cells was analyzed by flow cytometry using Annexin V-FITC/PI kit (E) Early and late apoptosis percent of PC12 cells (F) Representative western blotting images of Bax, Bcl-2, Caspase 3, cleaved-Caspase 3, and β-tubulin; Quantitation of Bcl-2/Bax ratio (G) and cleaved-Caspase 3/Caspase 3 ratio (H). *p < 0.05, **p < 0.01 versus control group; #p < 0.05, ##p < 0.01 versus Aβ25–35 group. &p < 0.05, &&p < 0.01 low dose treatment group (1 μM) versus high dose treatment group (10 μM). Data were presented as mean ± SD (n = 3).
3.9 Effect on cell apoptosis inhibition
Aβ25-35 could induce apoptosis and affect the morphology of PC12 cells with rounding and wrinkling of the cells (Supplementary Figure S3). Flow cytometry analysis showed that Aβ25-35 induced apoptosis of PC12 cells, with the proportion of living cells decreasing from 91.6% ± 1.2% to 55.9% ± 3.2%, and the proportion of apoptotic cells increasing from 6.9% ± 1.4% to 30.2% ± 2.1% compared to the control group (Figures 6D, E). CUR-LF NPs (1, 10 μM) treatment significantly improved the proportion of living cells (60.7% ± 2.2%, 65.9% ± 3.0%) compared to the model group (p < 0.05), where 10 μM CUR-LF NPs significantly inhibited Aβ25-35-induced apoptosis, with the proportion of apoptotic cells decreasing to 24.7% ± 2.0% (p < 0.05).
Western blotting analysis was performed to elucidate the efficacy mechanism of CUR-LF NPs to protect against Aβ25-35-induced apoptosis in PC12 cells. The apoptosis genes Bax and Bcl-2 play an essential role in regulating the apoptotic process. Caspase 3, as their downstream apoptotic executor protein, could be activated to cleaved-Caspase 3 at the initiation of the apoptotic process (Chen et al., 2020). As shown in Figures 6F–H, Aβ25-35 significantly reduced the Bcl-2/Bax ratio of PC12 cells, activated Caspase 3 to cleaved-Caspase 3, and induced apoptosis compared to the control group (p < 0.05). In this study, low and high doses (1, 10 μM) of CUR-LF NPs upregulated the Bcl-2/Bax ratio and downregulated the cleaved-Caspase 3/Caspase 3 ratio, where the 10 μM CUR-LF NPs group could better reverse Aβ25-35-induced apoptosis in PC12 cells.
3.10 In vivo pharmacokinetic studies
As shown in Figures 7A, B, we evaluated the pharmacokinetic profiles of CUR suspension, CUR-LF NPs via IN administration, and CUR-LF NPs via IV administration in plasma and brain at a dose of 10 mg/kg. The CUR concentrations were analyzed by UPLC-MS/MS, which was methodologically validated (Supplementary Figures S4, S5). The pharmacokinetic parameters, including AUC0–8h, t1/2, Tmax, and Cmax were shown in Table 2. We clearly observed that the encapsulation of CUR in LF nanoparticles could prolong the elimination half-life in the systemic blood circulation and brain. The AUCplasma of CUR-LF NPs was 2 times that of CUR suspension. In addition, the AUCbrain of CUR-LF NPs appeared to be 3 times that of CUR suspension. Overall, CUR-LF NPs could not only help CUR overcome defects, such as hydrophobic property and low permeability but also prolong the biological half-life and improve the bioavailability.
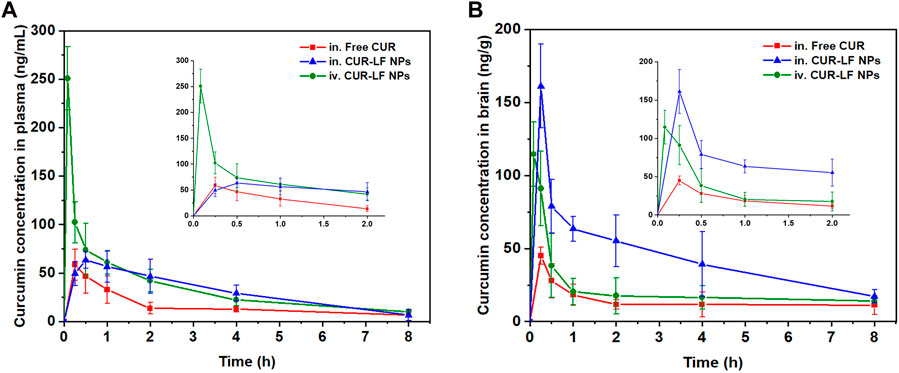
FIGURE 7. Concentration-time profiles of curcumin up to 8 h post-dosing in (A) plasma, (B) brain following intranasal administration of curcumin suspension and CUR-LF NPs, and intravenous injection administration of CUR-LF NPs at a dose of 10 mg/kg. Data were presented as mean ± SD (n = 3).
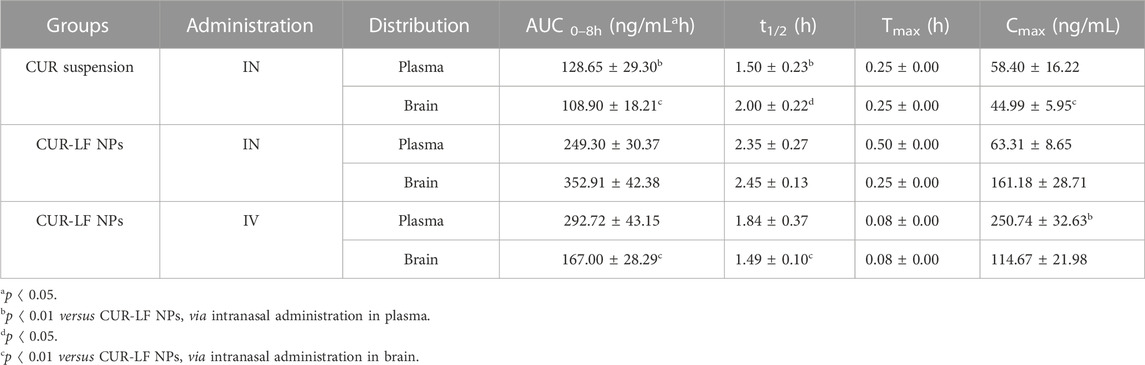
TABLE 2. The plasma and brain pharmacokinetic parameters after intranasal administration of curcumin suspension and CUR-LF NPs, and intravenous administration of CUR-LF NPs at a dose of 10 mg/kg. Data were presented as mean ± SD (n = 3).
Meanwhile, CUR-LF NPs via IV administration were also evaluated for comparison with IN administration. Although AUCplasma was considerably higher than AUCbrain of CUR-LF NPs via IV administration, it was reassuring to observe that a significant proportion of CUR-LF NPs could still cross the BBB into the brain because LF receptors were highly expressed in the BBB (Ye et al., 2013). The obtained results suggested that the brain accumulation of CUR via IN administration of CUR-LF NPs could be achieved mainly through two pathways: one was that CUR-LF NPs were absorbed into the peripheral circulation through the extensively vascularized respiratory area and then crossed the BBB via the LF receptor-mediated endocytic pathway, and the other was that CUR-LF NPs entered the brain via the direct nose-to-brain route (Tiozzo Fasiolo et al., 2021). For further studies, we calculated the BTE and DTP of CUR-LF NPs via IN administration by Equations 6, 7, which were 248.1% and 59.7%, respectively. The obtained results mean that the brain targeting of IN administration was higher than that of IV administration, and 59.7% of the CUR-LF NPs could be directly transported to the brain via the olfactory nerve pathway, olfactory mucosal epithelium, and trigeminal nerve.
4 Conclusion
Herein, we developed self-assembled CUR-LF NPs, which could be easily obtained by binding the hydrophobic region of LF to CUR without introducing cross-linking agents or toxic organic reagents. As a multifunctional glycoprotein, LF could be used as both a nanocarrier and targeting ligand in the nose-to-brain delivery of CUR-LF NPs. Moreover, it showed neuroprotective effects that could play a synergistic role with CUR. CUR-LF NPs could be favorably internalized by PC12 cells, thus playing an excellent role in protecting Aβ25-35-induced oxidative stress and apoptosis in PC12 cells. The in vivo pharmacokinetic results demonstrate that CUR-LF NPs could not only exhibit excellent brain accumulation via IN administration but also prolong the elimination half-life and improve the bioavailability of CUR. In conclusion, nose-to-brain delivery of CUR-LF NPs is a promising strategy for neuroprotection.
Data availability statement
The original contributions presented in the study are included in the article/Supplementary Material, further inquiries can be directed to the corresponding authors.
Ethics statement
The animal study was reviewed and approved by The Experimental Animal Ethics Committee of Chengdu University of Traditional Chinese Medicine.
Author contributions
Conceptualization, LL and LT; methodology, LL and QZ; validation, LT and QZ; formal analysis, LL and YC; data curation, LL and LT; writing-original draft preparation, LL; writing-review and editing, LT, YC, YL, and SH; funding acquisition, SH and RL. All authors have read and agreed to the published version of the manuscript.
Funding
This work was supported by the National Natural Science Foundation of China (Grant No. 82073994); the Outstanding Young Scientific and Technological Talents Project of Science and Technology Department of Sichuan Province (Grant No. 2020JDJQ0049); the Key Open Fund of Key Laboratory of Coarse Grain Processing, Ministry of Agriculture (Grant No. 2019CC02); the Xinglin Scholars Research Enhancement Program (QJJJ2021002).
Conflict of interest
LT, YC, and YL were employed by Sichuan Purity Pharmaceutical Co., Ltd.
The remaining authors declare that the research was conducted in the absence of any commercial or financial relationships that could be construed as a potential conflict of interest.
Publisher’s note
All claims expressed in this article are solely those of the authors and do not necessarily represent those of their affiliated organizations, or those of the publisher, the editors and the reviewers. Any product that may be evaluated in this article, or claim that may be made by its manufacturer, is not guaranteed or endorsed by the publisher.
Supplementary material
The Supplementary Material for this article can be found online at: https://www.frontiersin.org/articles/10.3389/fbioe.2023.1168408/full#supplementary-material
References
Agrawal, M., Saraf, S., Saraf, S., Antimisiaris, S. G., Chougule, M. B., Shoyele, S. A., et al. (2018). Nose-to-brain drug delivery: An update on clinical challenges and progress towards approval of anti-Alzheimer drugs. J. Control. Release 281, 139–177. doi:10.1016/j.jconrel.2018.05.011
Agwa, M. M., Abdelmonsif, D. A., Khattab, S. N., and Sabra, S. (2020). Self-assembled lactoferrin-conjugated linoleic acid micelles as an orally active targeted nanoplatform for Alzheimer's disease. Int. J. Biol. Macromol. 162, 246–261. doi:10.1016/j.ijbiomac.2020.06.058
Agwa, M. M., and Sabra, S. (2021). Lactoferrin coated or conjugated nanomaterials as an active targeting approach in nanomedicine. Int. J. Biol. Macromol. 167, 1527–1543. doi:10.1016/j.ijbiomac.2020.11.107
Akel, H., Ismail, R., and Csoka, I. (2020). Progress and perspectives of brain-targeting lipid-based nanosystems via the nasal route in Alzheimer's disease. Eur. J. Pharm. Biopharm. 148, 38–53. doi:10.1016/j.ejpb.2019.12.014
Araujo, J. F., Bourbon, A. I., Simoes, L. S., Vicente, A. A., Coutinho, P. J. G., and Ramos, O. L. (2020). Physicochemical characterisation and release behaviour of curcumin-loaded lactoferrin nanohydrogels into food simulants. Food Funct. 11 (1), 305–317. doi:10.1039/c9fo01963d
Bonaccorso, A., Gigliobianco, M. R., Pellitteri, R., Santonocito, D., Carbone, C., Di Martino, P., et al. (2020). Optimization of curcumin nanocrystals as promising strategy for nose-to-brain delivery application. Pharmaceutics 12 (5), 476. doi:10.3390/pharmaceutics12050476
Budd Haeberlein, S., and Harris, T. (2015). Promising targets for the treatment of neurodegenerative diseases. Clin. Pharmacol. Ther. 98 (5), 492–501. doi:10.1002/cpt.195
Chen, N., Wang, J., He, Y., Xu, Y., Zhang, Y., Gong, Q., et al. (2020). Trilobatin protects against Aβ25-35-induced hippocampal HT22 cells apoptosis through mediating ROS/p38/Caspase 3-dependent pathway. Front. Pharmacol. 11, 584. doi:10.3389/fphar.2020.00584
Crowe, T. P., Greenlee, M. H. W., Kanthasamy, A. G., and Hsu, W. H. (2018). Mechanism of intranasal drug delivery directly to the brain. Life Sci. 195, 44–52. doi:10.1016/j.lfs.2017.12.025
Del Prado-Audelo, M. L., Caballero-Floran, I. H., Meza-Toledo, J. A., Mendoza-Munoz, N., Gonzalez-Torres, M., Floran, B., et al. (2019). Formulations of curcumin nanoparticles for brain diseases. Biomolecules 9 (2), 56. doi:10.3390/biom9020056
Di Paolo, M., Papi, L., Gori, F., and Turillazzi, E. (2019). Natural products in neurodegenerative diseases: A great promise but an ethical challenge. Int. J. Mol. Sci. 20 (20), 5170. doi:10.3390/ijms20205170
Dou, Y., Zhao, D., Yang, F., Tang, Y., and Chang, J. (2021). Natural phyto-antioxidant albumin nanoagents to treat advanced Alzheimer's disease. ACS Appl. Mat. Interfaces 13 (26), 30373–30382. doi:10.1021/acsami.1c07281
Dugger, B., and Dickson, D. (2017). Pathology of neurodegenerative diseases. Cold Spring Harb. Symp. Quant. Biol. 9 (7), a028035. doi:10.1101/cshperspect.a028035
Ege, D. (2021). Action mechanisms of curcumin in Alzheimer's disease and its brain targeted delivery. Materials 14 (12), 3332. doi:10.3390/ma14123332
Elzoghby, A. O., Abdelmoneem, M. A., Hassanin, I. A., Abd Elwakil, M. M., Elnaggar, M. A., Mokhtar, S., et al. (2020). Lactoferrin, a multi-functional glycoprotein: Active therapeutic, drug nanocarrier & targeting ligand. Biomaterials 263, 120355. doi:10.1016/j.biomaterials.2020.120355
Erdo, F., Bors, L. A., Farkas, D., Bajza, A., and Gizurarson, S. (2018). Evaluation of intranasal delivery route of drug administration for brain targeting. Brain Res. Bull. 143, 155–170. doi:10.1016/j.brainresbull.2018.10.009
Furubayashi, T., Inoue, D., Nishiyama, N., Tanaka, A., Yutani, R., Kimura, S., et al. (2020). Comparison of various cell lines and three-dimensional mucociliary tissue model systems to estimate drug permeability using an in vitro transport study to predict nasal drug absorption in rats. Pharmaceutics 12 (1), 79. doi:10.3390/pharmaceutics12010079
Gong, G., Pan, Q., Wang, K., Wu, R., Sun, Y., and Lu, Y. (2015). Curcumin-incorporated albumin nanoparticles and its tumor image. Nanotechnology 26 (4), 045603. doi:10.1088/0957-4484/26/4/045603
Guo, C., Yang, Z. H., Zhang, S., Chai, R., Xue, H., Zhang, Y. H., et al. (2017). Intranasal lactoferrin enhances α-Secretase-dependent amyloid precursor protein processing via the ERK1/2-CREB and HIF-1α pathways in an Alzheimer's disease mouse model. Neuropsychopharmacology 42 (13), 2504–2515. doi:10.1038/npp.2017.8
Halder, R., and Jana, B. (2021). Exploring the role of hydrophilic amino acids in unfolding of protein in aqueous ethanol solution. Proteins 89 (1), 116–125. doi:10.1002/prot.25999
Hansen, J., Uthayakumar, R., Pedersen, J. S., Egelhaaf, S. U., and Platten, F. (2021). Interactions in protein solutions close to liquid-liquid phase separation: Ethanol reduces attractions via changes of the dielectric solution properties. Phys. Chem. Chem. Phys. 23, 22384–22394. doi:10.1039/d1cp03210k
Hao, J., Zhao, J., Zhang, S., Tong, T., Zhuang, Q., Jin, K., et al. (2016). Fabrication of an ionic-sensitive in situ gel loaded with resveratrol nanosuspensions intended for direct nose-to-brain delivery. Colloids Surf. B Biointerfaces 147, 376–386. doi:10.1016/j.colsurfb.2016.08.011
He, X., Mao, L., Gao, Y., and Yuan, F. (2016). Effects of high pressure processing on the structural and functional properties of bovine lactoferrin. Innov. Food Sci. Emerg. 38, 221–230. doi:10.1016/j.ifset.2016.10.014
He, Y., Liang, Y., Mak, J., Liao, Y., Li, T., Yan, R., et al. (2020). Size effect of curcumin nanocrystals on dissolution, airway mucosa penetration, lung tissue distribution and absorption by pulmonary delivery. Colloids Surf. B Biointerfaces 186, 110703. doi:10.1016/j.colsurfb.2019.110703
Jiang, Y., Liu, C., Zhai, W., Zhuang, N., Han, T., and Ding, Z. (2019). <p>The optimization design of lactoferrin loaded HupA nanoemulsion for targeted drug transport via intranasal route</p>. Int. J. Nanomedicine 14, 9217–9234. doi:10.2147/IJN.S214657
Kim, H. S., Lee, S. J., and Lee, D. Y. (2022). Milk protein-shelled gold nanoparticles with gastrointestinally active absorption for aurotherapy to brain tumor. Bioact. Mat. 8, 35–48. doi:10.1016/j.bioactmat.2021.06.026
Kwan, K., Yun, H., Dong, T., and Tsim, K. J. J. (2021). Ginsenosides attenuate bioenergetics and morphology of mitochondria in cultured PC12 cells under the insult of amyloid beta-peptide. J. Ginseng Res. 45 (4), 473–481. doi:10.1016/j.jgr.2020.09.005
Lauzon, M. A., Daviau, A., Marcos, B., and Faucheux, N. (2015). Nanoparticle-mediated growth factor delivery systems: A new way to treat Alzheimer's disease. J. Control. Release 206, 187–205. doi:10.1016/j.jconrel.2015.03.024
Li, J., Zhao, C., Wei, L., Li, X., Liu, F., Zhang, M., et al. (2018). Preservation of cichoric acid antioxidant properties loaded in heat treated lactoferrin nanoparticles. Molecules 23 (10), 2678. doi:10.3390/molecules23102678
Li, L., Liao, W., Zhang, Q., Yu, F., and Li, Z. (2022). Optimization of curcumin-lactoferrin nanoparticles prescription process based on Box-Behnken design-response surface method and in vitro evaluation. Chin. Traditional Herb. Drugs 53 (19), 5980–5990. (In Chinese). doi:10.7501/j.issn.0253-2670.2022.19.005
Liu, F., Sun, C., Yang, W., Yuan, F., and Gao, Y. (2015). Structural characterization and functional evaluation of lactoferrin-polyphenol conjugates formed by free-radical graft copolymerization. RSC Adv. 5 (20), 15641–15651. doi:10.1039/c4ra10802g
Liu, Z., Jiang, M., Kang, T., Miao, D., Gu, G., Song, Q., et al. (2013). Lactoferrin-modified PEG-co-PCL nanoparticles for enhanced brain delivery of NAP peptide following intranasal administration. Biomaterials 34 (15), 3870–3881. doi:10.1016/j.biomaterials.2013.02.003
Luo, R., Lin, M., Fu, C., Zhang, J., Chen, Q., Zhang, C., et al. (2021). Calcium pectinate and hyaluronic acid modified lactoferrin nanoparticles loaded rhein with dual-targeting for ulcerative colitis treatment. Carbohydr. Polym. 263, 117998. doi:10.1016/j.carbpol.2021.117998
May, S., Jensen, B., Wolkenhauer, M., Schneider, M., and Lehr, C. J. P. r. (2012). Dissolution techniques for in vitro testing of dry powders for inhalation. Pharm. Res. 29 (8), 2157–2166. doi:10.1007/s11095-012-0744-2
Miksys, S., and Tyndale, R. (2010). Neurodegenerative diseases: A growing challenge. Clin. Pharmacol. Ther. 88 (4), 427–430. doi:10.1038/clpt.2010.198
Nasr, M. (2016). Development of an optimized hyaluronic acid-based lipidic nanoemulsion co-encapsulating two polyphenols for nose to brain delivery. Drug Deliv. 23 (4), 1444–1452. doi:10.3109/10717544.2015.1092619
Patel, A., Surti, N., and Mahajan, A. (2019). Intranasal drug delivery: Novel delivery route for effective management of neurological disorders. J. Drug Deliv. Sci. Technol. 52, 130–137. doi:10.1016/j.jddst.2019.04.017
Piazzini, V., Landucci, E., D'Ambrosio, M., Tiozzo Fasiolo, L., Cinci, L., Colombo, G., et al. (2019). Chitosan coated human serum albumin nanoparticles: A promising strategy for nose-to-brain drug delivery. Int. J. Biol. Macromol. 129, 267–280. doi:10.1016/j.ijbiomac.2019.02.005
Pinilla-Peñalver, E., Soriano, M., Durán, G., Llorent-Martínez, E., Contento, A., and Ríos, Á. J. M. a. (2020). Discrimination between nanocurcumin and free curcumin using graphene quantum dots as a selective fluorescence probe. Microchim. Acta 187 (8), 446. doi:10.1007/s00604-020-04437-x
Qian, W., Li, H., Pan, N., and Zhang, C. (2018). Curcumin treatment is associated with increased expression of the N-methyl-D-aspartate receptor (NMDAR) subunit, NR2A, in a rat PC12 cell line model of Alzheimer's disease treated with the acetyl amyloid-β peptide, Aβ(25-35). Med. Sci. Monit. 24, 2693–2699. doi:10.12659/MSM.906933
Qizilbash, F. F., Ashhar, M. U., Zafar, A., Qamar, Z., Ali, J., Ali, A., et al. (2022). Thymoquinone-nnriched naringenin-loaded nanostructured lipid carrier for brain delivery via nasal route: In vitro prospect and in vivo therapeutic efficacy for the treatment of depression. Pharmaceutics 14 (3), 656. doi:10.3390/pharmaceutics14030656
Reddy, P. H., Manczak, M., Yin, X., Grady, M. C., Mitchell, A., Tonk, S., et al. (2018). Protective effects of Indian spice curcumin against amyloid-β in Alzheimer's disease. J. Alzheimers Dis. 61 (3), 843–866. doi:10.3233/jad-170512
Sabra, S., and Agwa, M. M. (2020). Lactoferrin, a unique molecule with diverse therapeutical and nanotechnological applications. Int. J. Biol. Macromol. 164, 1046–1060. doi:10.1016/j.ijbiomac.2020.07.167
Samaridou, E., and Alonso, M. J. (2018). Nose-to-brain peptide delivery - the potential of nanotechnology. Bioorg. Med. Chem. 26 (10), 2888–2905. doi:10.1016/j.bmc.2017.11.001
Tai, J., Han, M., Lee, D., Park, I.-H., Lee, S. H., and Kim, T. H. (2022). Different methods and formulations of drugs and vaccines for nasal administration. Pharmaceutics 14 (5), 1073. doi:10.3390/pharmaceutics14051073
Tiozzo Fasiolo, L., Manniello, M. D., Banella, S., Napoli, L., Bortolotti, F., Quarta, E., et al. (2021). Flurbiprofen sodium microparticles and soft pellets for nose-to-brain delivery: Serum and brain levels in rats after nasal insufflation. Int. J. Pharm. 605, 120827. doi:10.1016/j.ijpharm.2021.120827
Tofaris, G., and Buckley, N. (2018). Convergent molecular defects underpin diverse neurodegenerative diseases. J. Neurol. Neurosurg. Psychiatry 89 (9), 962–969. doi:10.1136/jnnp-2017-316988
Vaz, G. R., Hadrich, G., Bidone, J., Rodrigues, J. L., Falkembach, M. C., Putaux, J.-L., et al. (2017). Development of nasal lipid nanocarriers containing curcumin for brain targeting. J. Alzheimers Dis. 59 (3), 961–974. doi:10.3233/jad-160355
Wang, B., Timilsena, Y. P., Blanch, E., and Adhikari, B. (2019). Lactoferrin: Structure, function, denaturation and digestion. Crit. Rev. Food Sci. Nutr. 59 (4), 580–596. doi:10.1080/10408398.2017.1381583
Yan, J. K., Qiu, W. Y., Wang, Y. Y., and Wu, J. Y. (2017). Biocompatible polyelectrolyte complex nanoparticles from lactoferrin and pectin as potential vehicles for antioxidative curcumin. J. Agric. Food Chem. 65 (28), 5720–5730. doi:10.1021/acs.jafc.7b01848
Ye, Y., Sun, Y., Zhao, H., Lan, M., Gao, F., Song, C., et al. (2013). A novel lactoferrin-modified β-cyclodextrin nanocarrier for brain-targeting drug delivery. Int. J. Pharm. 458 (1), 110–117. doi:10.1016/j.ijpharm.2013.10.005
Yu, M., Yuan, W., Li, D., Schwendeman, A., and Schwendeman, S. (2019). Predicting drug release kinetics from nanocarriers inside dialysis bags. J. Control. Release 315, 23–30. doi:10.1016/j.jconrel.2019.09.016
Zakaria, H., El Kurdi, R., and Patra, D. J. R. a. (2022). Curcumin-PLGA based nanocapsule for the fluorescence spectroscopic detection of dopamine. RSC Adv. 12 (43), 28245–28253. doi:10.1039/d2ra01679f
Zakharova, E. T., Sokolov, A. V., Pavlichenko, N. N., Kostevich, V. A., Abdurasulova, I. N., Chechushkov, A. V., et al. (2018). Erythropoietin and Nrf2: Key factors in the neuroprotection provided by apo-lactoferrin. Biometals 31 (3), 425–443. doi:10.1007/s10534-018-0111-9
Zhang, L., Yang, S., Wong, L. R., Xie, H., and Ho, P. C.-L. (2020). In vitro and in vivo comparison of curcumin-encapsulated chitosan-coated poly(lactic-co-glycolic acid) nanoparticles and curcumin/hydroxypropyl-β-cyclodextrin inclusion complexes administered intranasally as therapeutic strategies for Alzheimer's disease. Mol. Pharm. 17 (11), 4256–4269. doi:10.1021/acs.molpharmaceut.0c00675
Zhang, Y., Huo, M., Zhou, J., and Xie, S. (2010). PKSolver: An add-in program for pharmacokinetic and pharmacodynamic data analysis in Microsoft Excel. Comput. Methods Programs Biomed. 99 (3), 306–314. doi:10.1016/j.cmpb.2010.01.007
Zhao, C., Zhang, J., Hu, H., Qiao, M., Chen, D., Zhao, X., et al. (2018a). Design of lactoferrin modified lipid nano-carriers for efficient brain-targeted delivery of nimodipine. Mat. Sci. Eng. 92, 1031–1040. doi:10.1016/j.msec.2018.02.004
Keywords: curcumin-lactoferrin nanoparticles, nose-to-brain, neuroprotective effect, Aβ25-35, pharmacokinetic
Citation: Li L, Tan L, Zhang Q, Cheng Y, Liu Y, Li R and Hou S (2023) Nose-to-brain delivery of self-assembled curcumin-lactoferrin nanoparticles: Characterization, neuroprotective effect and in vivo pharmacokinetic study. Front. Bioeng. Biotechnol. 11:1168408. doi: 10.3389/fbioe.2023.1168408
Received: 17 February 2023; Accepted: 15 March 2023;
Published: 27 March 2023.
Edited by:
Jinrong Peng, Sichuan University, ChinaReviewed by:
Wenliang Li, Jilin Medical University, ChinaCao Feng, China Pharmaceutical University, China
Copyright © 2023 Li, Tan, Zhang, Cheng, Liu, Li and Hou. This is an open-access article distributed under the terms of the Creative Commons Attribution License (CC BY). The use, distribution or reproduction in other forums is permitted, provided the original author(s) and the copyright owner(s) are credited and that the original publication in this journal is cited, in accordance with accepted academic practice. No use, distribution or reproduction is permitted which does not comply with these terms.
*Correspondence: Shuguang Hou, ZmFuc3oxOTMwQHlhaG9vLmNvbQ==; Rui Li, bGlydWlAY2R1dGNtLmVkdS5jbg==
†These authors have contributed equally to this work