- 1Department of Joint Surgery, Shanghai Sixth People’s Hospital Affiliated to Shanghai Jiao Tong University School of Medicine, Shanghai, China
- 2Department of Rehabilitation, The Second Affiliated Hospital of Nanjing Medical University, Nanjing, China
With the development of nanotechnology, nanomaterials are widely applied in different areas. Some nanomaterials are designed to be biocompatible and can be used in the medical field, playing an important role in disease treatment. Exosomes are nanoscale vesicles with a diameter of 30–200 nm. Studies have shown that exosomes have the effect of angiogenesis, tissue (skin, tendon, cartilage, et al.) repair and reconstruction. Nano-hydrogels are hydrogels with a diameter of 200 nm or less and can be used as the carrier to transport the exosomes into the body. Some orthopedic diseases, such as bone defects and bone infections, are difficult to handle. The emergence of nano-hydrogels coated exosomes may provide a new idea to solve these problems, improving the prognosis of patients. This review summarizes the function of nano-hydrogels coated exosomes in bone tissue repair, intending to illustrate the potential use and application of nano-hydrogels coated exosomes in bone disease.
Introduction
Bone tissue can regenerate as part of the repair process following bone disease, such as trauma, bone tumor, and bone defect. The commonest form of bone repair is bone fracture healing. Intramembranous and endochondral ossification are involved in the process (Dimitriou et al., 2011). However, the ability of bone tissue repair is limited. In severe bone diseases, the bone’s compensatory capacity is impaired, in which interventions are needed. Sometimes it is necessary to perform surgery because conservative therapies usually have little effect on bone tissue repair. Although surgery can achieve good results in most cases, it is, after all, an invasive procedure, and in patients who already had bone surgery before, a second revision surgery requires careful consideration. So, finding ways to reduce or even avoid surgery intervention and effectively promote bone repair is a new trend in the treatment of bone diseases.
Johnstone et al. discovered a vesicle-like structure during the period of sheep reticulocyte maturation and isolated these membrane-bound vesicles by ultracentrifugation. The vesicle-like structures were given the name “exosomes” for the first time (Johnstone et al., 1987; Johnstone, 2005). Exosomes are nanoscale extracellular vesicles with 30–200 nm in diameter that are produced through budding from the plasma membrane and endosome membrane. Exosome contain a variety of substances, such as nucleic acids, lipids, and proteins, expressing transmembrane proteins and receptors that facilitate intracellular communication and materials transportation. Donor cells can transfer exogenous substances such as proteins, mRNAs, microRNAs (miRNAs), and lipids to recipient cells via exosomes (Liang et al., 2021a). Exosomes carry mRNAs and miRNAs, playing a key role in the repair of various tissue injuries (Wang et al., 2020a; Liu et al., 2021). It has been shown that mesenchymal stem cells (MSCs) derived exosomes can protect cartilage, downregulate the expression of inflammatory factors, and inhibit the conversion of macrophages to the M1 type (M1 type promotes inflammatory reaction), which can be used as a novel treatment for osteoarthritis (Pandey et al., 2022). Bone morphogenetic protein 2 (BMP2)/macrophage-derived exosomes can regulate the osteoblast differentiation of MSCs, stimulating bone regeneration. Exosomes also play an important role in angiogenesis and in the repair and reconstruction process of many tissues (e.g., skin, tendon, cartilage) (Zhang et al., 2021a). The excellent properties of exosomes make them ideal materials for bone tissue repair. Many studies have focused on this hot spot.
On the other hand, cutting-edge research in nanomaterials also brings new opportunities for bone repair and reconstruction. Hydrogels are a class of polymeric materials with high water contents that have been widely used in cell culture, drug delivery, tissue engineering, and other biomedical fields. Hydrogels have a unique three-dimensional structure that provides sufficient space to accommodate a variety of substances, including small molecules, polymers, and particles. Due to their soft, moist, and biocompatible properties, hydrogels can be used as matrix components for engineering living cells, leading the nascent field of engineering material science (Liu et al., 2022a). Hydrogels can also be used as drug carriers. After relevant modifications, Hydrogels enable precise target positioning and controlled release of drugs, which is safe and effective. Some wearable tissue-adhesive electronic devices also form interfaces with tissues (e.g., skin) through the good adhesion and electrical conductivity of hydrogels, and are used for real-time in vitro sensing and organ repair (Li et al., 2021a). The use of biomaterials with antimicrobial hydrogel coatings is an effective way to combat colonizing bacteria. As for bone tissue repair, some studies demonstrated hydrogels can accelerate bone growth (Anada et al., 2019; Hasani-Sadrabadi et al., 2020; Datta et al., 2021), which can be used in the treatment of bone diseases.
According to the previous study, exosomes and hydrogels have positive functions in the process of bone tissue repair, bringing new hope for the treatment of orthopedic diseases. And there are studies focusing on the nano-hydrogels coated exosomes in bone tissue repair aspects.
This review intends to explore the role of nano-hydrogels coated exosomes in the bone tissue repair process and discover its functions in various bone diseases.
Exosomes
Exosomes are single-membrane vesicles with the same topology as cells, with diameters ranging from 30 to 200 nm (Pegtel and Gould, 2019). Exosomes are a subset of extracellular vesicles which are produced as a result of double invagination of the plasma membrane and the formation of intracellular multivesicular bodies (MVBs) containing intraluminal vesicles (ILVs). ILVs are eventually secreted as exosomes with diameters ranging from 40 to 160 nm via fusions of MVBs to the plasma membrane and exocytosis (Kalluri and LeBleu, 2020). MVBs and exosomes’ production and release are controlled by the endosomal sorting complexes needed for the transport (ESCRT) pathway (Wollert and Hurley, 2010). Exosomes are now known to be released into the extracellular environment by donor cells to perform a variety of biological functions, such as intracellular communication and the exchange of genetic material and proteins between a parent cell and surrounding cells. Their functions for drug delivery in cancer immunotherapy have been proven. Because of their microRNA and mRNA contents, they are also a promising biological gene delivery system (Hade et al., 2021). As a novel material, its application in the medical field remains to be explored further. Figure 1 shows the morphology of exosomes (Red arrow) under electron microscopy. Figure 2 is the diagram of exosomes secretion.
Exosomes components
Exosomes are made up of a wide range of materials, including lipids, proteins, DNA, and RNA. Some specific components, including lipids, proteins, DNA, mRNA, and noncoding RNAs, can function as autocrine and/or paracrine agents (Dai et al., 2020).
Lipid
Exosomes’ lipid composition includes sphingolipids, cholesterol, phosphatidylserine, saturated fatty acids, and ceramides, all of which can be discovered in plasma membranes (Skotland et al., 2020). Exosomes’ lipid composition is cell-specific or conserved. Lipids have a crucial role in exosomes shape preservation, exosomes synthesis, and exosomes’ homeostasis maintenance in the recipient cells (Mashouri et al., 2019).
Protein
Exosomes’ protein composition includes membrane trafficking-related proteins, such as the tetraspanins (CD63, CD81, CD82, and CD9) (Larios et al., 2020). Additionally, integrins (cell adhesion-related proteins), actin, myosin (participating in cytoskeletal construction), MHC class II proteins, and heat-shock proteins (Hsp60, Hsp70, and Hsp90) can be concentrated in exosomes (Clayton et al., 2005; Dai et al., 2020). Regardless of the type of cells that exosomes originate from, ESCRT proteins and their accessory proteins (Alix, TSG101, HSC70, and HSP90) are predicted to be present in exosomes as they govern exosomal production and MVBs transit (Tauro et al., 2012). This group of proteins are hence known as “exosomal marker proteins”. (Tauro et al., 2012).
DNA and RNA
Exosomal DNA (exoDNA) can be single-stranded or double-stranded DNA. It contains both nuclear and mitochondrial DNA. ExoDNA exists on the surface of the vesicle or inside the vesicle (Wortzel et al., 2019).
The most frequently researched exosomal RNA species are mRNAs and miRNAs (Carnino et al., 2020). Some exosomal mRNAs have been shown by Valadi et al. to be intact and capable of being translated into useful proteins in recipient cells (Valadi et al., 2007). MicroRNAs (miRNAs), which are significant members of the small non-coding RNA family and range in length from 20 to 22 nucleotides, have been extensively studied in a wide range of physiological and pathological processes. They mediate post-transcriptional gene silencing by binding to the 3′-untranslated region or open reading frames of the target mRNA (Treiber et al., 2019). MiRNAs can also be found in exosomes that have been extracted from bodily fluids (such as saliva, blood, or serum), suggesting the potential benefits of employing exosomal miRNAs as the novel, non-invasive biomarkers (Nedaeinia et al., 2017). RNA species are sorted into exosomes in a variety of ways.
Classifications
Depending on whether they have undergone artificial modification, exosomes are classified as natural exosomes or designed exosomes. Natural exosomes can be divided into animal-derived exosomes and plant-derived exosomes. Animal-derived exosomes are further separated into normal exosomes and tumor exosomes according to their environments (normal or malignant) (Zhang et al., 2020a).
Exosomes from different origins
Nearly all normal cell types, including human umbilical vein endothelial cells, mesenchymal stem cells (MSCs), T cells, B cells, macrophages, dendritic cells (DC), and natural killer (NK) cells, have the ability to create exosomes (Cheng et al., 2017; He et al., 2020; Li et al., 2020; Zhao et al., 2020).
Additionally, the previously described normal exosomes can be found in large quantities in biofluids like saliva, plasma, urine, ascites, milk, and bile.
Below we have listed several common sources of exosomes. (See Figure 3).
Mesenchymal stem cells exosomes
Mesenchymal stem cells (MSCs) are multipotent mesenchymal stromal cells that can differentiate into a variety of cell types, including osteocytes, chondrocytes, adipocytes, cardiomyocytes, and endothelial cells. They also have the self-renew ablity (generate more MSCs themselves) (Akbari et al., 2020). MSCs can be extracted from a variety of body fluids and tissues, including adipose tissue, bone marrow, tooth pulp, synovial fluid, amniotic fluid, placenta, umbilical cord, and Wharton’s jelly (Andrzejewska et al., 2019). MSCs exosomes are widely used in tissue repair aspects.
Adipocyte exosomes
Adipocytes can secret exosomes and act locally in paracrine ways or enter the bloodstream to exert systemic effects (Isaac et al., 2021). Using a fat-specific Dicer deletion, Thomou et al. demonstrated that adipocytes were a significant source of circulating exosomal miRNAs (Thomou et al., 2017). MiR-16, miR-27a, miR-146b, and miR-222 are found in exosomes generated by giant adipocytes, and they can be transferred to tiny adipocytes to promote lipogenesis and adipocyte hypertrophy (Müller et al., 2011). Adipocyte exosomes also contain non-miRNA that have distant biologic effects (Isaac et al., 2021).
Endothelial cell exosomes
The endothelial cell (EC) activation or apoptosis can trigger the release of generated exosomes. In peripheral circulation, EC exosomes account for 5%–15% of the total circulating vesicles (Arraud et al., 2014). Proteins detected in released EC exosomes match those in the generating EC, according to proteomic investigations; some of these proteins can be transported to recipient cells (Liu et al., 2013).
Skeletal muscle exosomes
Exosomes produced by skeletal muscle have been found to have both paracrine and endocrine impacts on the preservation of muscle homeostasis and communication with other tissues (Qin and Dallas, 2019). Myoblasts and myotubes, two types of muscle cells, are sources of exosomes that express the Tsg101 and Alix protein markers as well as other signals transduction related proteins (Rome et al., 2019). Exosomes may play a role in the differentiation and maturation of skeletal muscle as numerous proteins involved in the transition from myoblast to myotube are found in exosomes, (Le Bihan et al., 2012).
Tumor-cell derived exosomes
Exosomes can be produced in huge quantities by tumor cells. The unique antigens on their surface may reveal information about the origins of donor cells. Tumor exosomes contribute to the growth, metastasis, and immunological control of tumors. They also monitor the onset of diseases and act as diagnostic indicators for illnesses (Poggio et al., 2019; Sanderson et al., 2019).
Exosomes and bone tissue repair
Exosomes can be secreted by cells such as osteoblasts, osteoclasts, osteocytes, and MSCs, which are known to mediate cellular communication and participate in the regulation of the bone microenvironment. Exosomes play critical regulatory roles in bone remodeling. Exosomes derived from osteoblasts stimulate osteoclast differentiation in vivo, and thus exosomes treatment can be used to enhance the removal of damaged tissue (Hade et al., 2021). Exosomes from different origins can be made in different forms and injected into the bone defect area to facilitate bone regeneration. Stem cells derived exosomes can promote osteogenesis through four main mechanisms: reducing apoptosis, recruiting mesenchymal stem cells and promoting their proliferation, creating an osteogenic-inducing environment to promote osteogenic differentiation of stem cells, and accelerating angiogenesis and bone vascularization (Girón et al., 2022). Its applications for bone defect, bone and cartilage regeneration, osteoarthritis, osteoporosis, and osteonecrosis have been described (Bei et al., 2021). The most commonly used exosomes in medical fields are MSCs exosomes. MSCs can secret exosomes, which are the primary therapeutic agents for encouraging tissue regeneration (Yu et al., 2014). MSCs-exosomes have anti-inflammatory and immunomodulatory functions and can be a perfect alternative to MSCs therapy because they possess similar biological functions to their originating cells while they are stabler and have lower immunogenicity (Ha et al., 2020). MSCs-exosomes were proven to have a protective function against the cardiovascular system by Lai et al. in a cardiac ischemia-reperfusion damage mouse model (Lai et al., 2010). And later, exosomes’ therapeutic use was extended to models of additional diseases. Studies have shown exosomes from MSCs have a significant impact on bone regeneration and exhibit osteoinductive properties (Li et al., 2018).
Hydrogels
The chemical structure of hydrogels is a three-dimensional polymeric network connecting hydrophilic components (Gradinaru et al., 2018). Hydrogels are made up of hydrophilic polymers with polar functional groups and have a high water content (Zhang et al., 2020b). The network is composed of crosslinking polymers with covalent bonds or noncovalent interactions. Their structure can be changed for different applications. Due to their stable physicochemical properties, hydrogels have been widely studied. The biomedical applications of hydrogels include 3D cell culture, drug delivery, wound dressing, tissue engineering and so on (Ho et al., 2022).
Common materials of the hydrogels
Natural polymers
Natural hydrogels have good biocompatibility and bioactivity, which can promote cell adhesion, proliferation, and tissue repair and regeneration. Natural polymer molecules from living beings, such as proteins and polysaccharides (hyaluronic acid, sodium alginate, chitosan, and agarose), make up the raw ingredients [e.g., collagen, tropocollagen, gelatin, silk fibroin (Ju et al., 2023)]. But they are unstable, needing to be crosslinked with additional polymers due to their poor durability, mechanical characteristics, and tissue adhesion capabilities.
Polysaccharides
Polysaccharides that have been used in hydrogels making include hyaluronic acid (HA), chitosan, and alginate.
HA is a linear nonsulfated glycosaminoglycan, which is a significant element of the ECM and is present in practically all bodily fluids and tissues (Yasin et al., 2022). Since HA is an important part of cartilage tissue and has good biocompatibility, HA hydrogels are crucial in the cartilage tissue engineering (Ngadimin et al., 2021).
Chitosan is a frequently used ingredient in the process of creating natural hydrogels, which is hydrophilic, biocompatible, and biodegradable. It can be degraded by lysozyme, acid, and colonic bacteria in the human body (Ways et al., 2018). Chitosan has an amine group and can react with other aldehyde-containing polysaccharides through Schiff base reaction, making it a good material for the preparation of self-healing hydrogels (Mo et al., 2021).
Alginate is a natural marine polysaccharide, and sodium alginate is the most often used extract (Zhang et al., 2021b). Alginate is an abundant and easily accessible biopolymer with exceptional biocompatibility, good porosity, high capacity to retain water, and changeable viscosity (Liu et al., 2022b). Through ion-exchange interactions with cations, sodium alginate has exceptional pH sensitivity and can quickly form gels under incredibly mild conditions (Maity and Das, 2021).
Proteins
Proteins used for hydrogels synthesis include collagen, gelatin, silk fibroin (SF), and polydopamine (PDA).
As the most prevalent protein in the ECM, collagen has a triple helix shape that offers high tensile strength. Since natural ECM contains a lot of collagen, collagen-based hydrogels are becoming more and more common as scaffolds for tissue engineering. Type I collagen is by far the most common kind of collagen among the other types. Collagen’s structural flexibility enables cross-linking to create a three-dimensional porous fibrous meshwork that makes extracellular vesicles (Evs) loading easier (Antoine et al., 2014).
The processing, molecular weight, and isoelectric point of collagen have a significant impact on the characteristics of gelatin, a partially hydrolyzed derivative of collagen (Salahuddin et al., 2021). Gelatin, as opposed to collagen, has greater temperature stability and biocompatibility (Mahmood et al., 2022). Gelatin methacrylate (GelMA), a composite improved form of gelatin hydrogel, was created in 2000 by Bulcke et al. (Van Den Bulcke et al., 2000) and is a typical form of hydrogels. Methacrylic anhydride and gelatin make up the photosensitive biohydrogel material known as GelMA, which can be activated by ultraviolet (UV) or visible light to create three-dimensional structures strong enough to sustain cell growth and differentiation (Kurian et al., 2022).
Sericin protein (SF) is a natural polymeric protein polymer extracted from natural silk and widely used in biomanufacturing. SF is highly biocompatible, biodegradable, and has a high tensile biomechanical strength (Lujerdean et al., 2022). Because SF biopolymers naturally form regular-sheet stacks, they can be treated to create hydrogels that are only physically cross-linked without the use of chemical cross-linking agents (Ju et al., 2023).
Polydopamine (PDA) is a biopolymer created when dopamine undergoes oxidative polymerization (Li et al., 2021b). PDA is easily and cheaply made without the use of hazardous solvents, and as a result, it has low cytotoxicity and high biocompatibility (>80%). PDA has also been demonstrated to improve cell adhesion and proliferation (Li et al., 2021b). Additionally, PDA is especially suited for biological applications because of its hydrophilicity and capacity to functionalize a variety of substrates.
Synthetic polymers
By crosslinking synthetic hydrophilic polymers, such as polyethylene glycol (PEG), polyvinyl alcohol (PVA), polyacrylic acid and its derivatives, polylactic acid-hydroxy acetic acid copolymer (PLGA), and phenoxyethyl methacrylate (PHEMA), synthetic hydrogels are created (Ju et al., 2023).
Compared with natural polymers, synthetic polymers contain particular molecular weights and fundamental structural units, and can be pre-designed to get desired qualities, such as particular porosity, degradation times, and tensile properties (Sánchez-Cid et al., 2022).
Synthetic hydrogels have a reliable material source and a longer quality guarantee period, with wider varieties and stable properties. However, the synthesis process may introduce chemical cross-linking agents and other toxic components. Their degradability and biocompatibility are poor (El-Sherbiny and Yacoub, 2013; Naahidi et al., 2017; Catoira et al., 2019).
Hydrogels classification
There are different types of hydrogels according to different classification methods. Except for the natural hydrogels and synthetic hydrogels mentioned above, we classify the hydrogels by their size, crosslinking modes, and properties (See Figure 4).
Size
According to the different sizes and shapes of hydrogels, there are macroscopic gels and microscopic gels (microspheres). Macroscopic gels can be divided into columnar, porous sponge, fibrous, membrane, and spherical according to the different shapes. The microscopic gels include micron-sized microspheres and nano-sized microspheres.
Particulate hydrogels with particle sizes in the micron and nanometer ranges, respectively, are known as microgels (hydrogel microspheres) and nanogels. Microgels and nanogels can be directly injected since they are significantly smaller than the inner diameter of a syringe needle than macroscopic hydrogels. Additionally, their greater relative surface area improves the capacity to penetrate tissue barriers and aids natural clearance. Different hydrogel sizes are suited for various drug delivery methods. For instance, microgels with a diameter of fewer than 5 nm are typically employed for pulmonary or oral delivery but are frequently viewed as unsuitable for intravascular injection due to their quicker circulation clearance rates. Because they can exit tiny blood arteries through the windows opened by the endothelium lining, nanogels with diameters between 10 and 100 nm are appropriate for systemic delivery and allow extravasation into tissue (Li and Mooney, 2016).
Cross-linking methods
Hydrogels can be divided into physical crosslinking and chemical crosslinking according to the bonding mode of the network structure.
Physical crosslinking refers to the formation of a cross-linked network structure through polymer chain entangling, crystallization points, or other weak interactions (such as ionic bonds, van der Waals forces, hydrogen bonds, etc.) without the formation of new bonds. Due to the formation of cluster structures between molecules during physical crosslinking, the uniformity of crosslinking is poor. Moreover, the mechanical strength and gel time of hydrogel were affected and the degradation process was hindered due to the defects of the network structure.
Chemical crosslinking refers to the formation of new covalent bonds, which form a three-dimensional network structure between molecules through copolymerization or condensation reaction. This kind of hydrogel is permanent and irreversible, and cannot be dissolved or fused by heating, which is also known as true gel. The common bonds and modes of chemical crosslinking are Schiff base reaction, Michael addition reaction, and light/heat initiated crosslinking.
Self-healing hydrogels
The use of self-healing hydrogels in 3D printing, medication delivery, and tissue regeneration has shown considerable promise (Mondal and Chatterjee, 2022; Bertsch et al., 2023). Self-healing hydrogels are often created using dynamic covalent bonding or non-covalent interaction principles. These hydrogels’ capacity for self-healing enables them to conform to damaged tissues and organs, hence enabling their protection. Additionally, the self-healing hydrogels have injectable qualities: under high shear conditions, it briefly become fluid before returning to their gel state. Importantly, the self-healing hydrogel is physically stable in place, making it possible for the encapsulated drug to be protected for a longer period of time and, as a result, to release drugs slowly. Physical crosslinking causes the shear-thinning characteristic (Ju et al., 2023).
Conductive hydrogels
Cellular actions that can encourage cytokine release and enhance the microenvironment of damaged tissue are controlled by bioelectrical signals, which are essential. In order to create composite conductive hydrogels, conductive nanomaterials like graphene and carbon nanotubes as well as conductive polymers like polyaniline and polypyrrole are frequently integrated into hydrogel networks (Walker et al., 2019).
Stimulus sources
Stimuli-responsive hydrogels are aqueous-swollen polymer networks that have the ability to perform a volume phase transition on the basis of external stimuli (Jalili et al., 2017). They can respond to a variety of external stimuli, changing their structure, physical makeup, chemical composition, or mechanical properties (Mellati et al., 2021).
Hydrogels are primarily split into three categories based on the types of stimulus sources: physical-responsive hydrogels, chemical-responsive hydrogels, and biochemical-responsive hydrogels. By the design of polymer molecules, the character of the hydrogels can be changed, which makes them more “smart” (Chang et al., 2022).
Hydrogels and bone tissue repair
Bone tissue engineering, as a new technological innovation, helps to create three-dimensional substitutes similar to the human bone tissue thereby maintaining the bone’s structural as well as functional integrity. Scaffolds play a vital role in the bone tissue engineering aspect, which forms the bioengineered structure. Among the scaffold materials, hydrogels have porous structures which simulate the extracellular matrix and can serve as a carrier to facilitate growth factor promotion (Nallusamy and Das, 2021). With their hydrophilic nature, three-dimensional structure, and comparable ECM components, hydrogels are appropriate scaffolds for cellular infiltration, adhesion, growth, proliferation, migration, and differentiation. They are also easily chemically changeable and can be further modified to demonstrate a favorable degradation profile and mechanical integrity. It has been demonstrated that hydrogels derived from natural bone tissue, particularly those from periosteal and demineralized bone matrix sources, can promote osteoconduction and osteoinduction (Xia and Chen, 2022).
Hydrogels coated exosomes
Although exosomes have many benefits and their therapeutic effect are promising, they still have some drawbacks (Shiue et al., 2019). Exosomes must be absorbed by the targeted cell by endocytosis in order for their biological effects to be triggered; otherwise, they would be quickly eliminated from the blood circulation and might even build up in the liver, spleen, lungs, and digestive system. Direct injections of exosomes intravenously, intraperitoneally, or subcutaneously may cause macrophages in the reticuloendothelial system to respond, which may result in their rejection. After interacting with sweat, tears, and the epithelial barrier (tight junctions), bodily and topical treatments on the skin or ocular surfaces have demonstrated limited half-lives. What’s more, the costly manufacturing methods that demand consistency and purity of nanometer-sized biomaterials are the root of the challenges in exosome purification and mass production. Exosome delivery, therefore, requires a more effective means of avoiding clearance by the host organism (Khayambashi et al., 2021). The most appropriate way to use exosomes in regenerative medicine is to combine them with biomaterials (Shi et al., 2017). Hydrogels, as versatile nanomaterials which can mimic natural tissue, have been extensively utilized as a vehicle for the local medication delivery of treated exosomes. Hydrogels’ hydrophilic and cross-linking properties aid in their capacity for controlled medication release (Khayambashi et al., 2021). Exosomes from various cell origins have so far been enclosed in hydrogels made of HA, gelatin, chitosan, and polypeptides (Qin et al., 2016; Liu et al., 2017).
Preparation methods of hydrogels coated exosomes
Three strategies were mentioned in a review by Parisa Khayambashi et al. to make hydrogels coated exosomes: 1) Exosomes and hydrogels are combined, then crosslinkers are added to cause gelation. 2) Physical incorporation of hydrogels or “breathing” technique. (Two basic steps are involved: the already inflated hydrogels are submerged in a solvent in order to remove the water from the hydrogel; then the hydrogels are soaked in an aqueous solution containing the exosomes, making the breathing-in of the exosomes into the porous hydrogel. 3) Simultaneous blending of the exosomes with the crosslinkers and the exosomes in solution, causing an in situ gelation that enables the exosomes to be delivered specifically (Khayambashi et al., 2021). Figure 5 demonstrates the preparation methods of hydrogels coated exosomes.
Hydrogels coated exosomes and bone diseases
We have summarized the application of hydrogels coated exosomes in bone defect, osteoarthritis/cartilage defect, intervertebral disc degeneration, and rotator cuff tear/tendon repair (See Figure 6). Previous studies showed the potential therapeutic effect of hydrogels coated exosomes on these diseases.
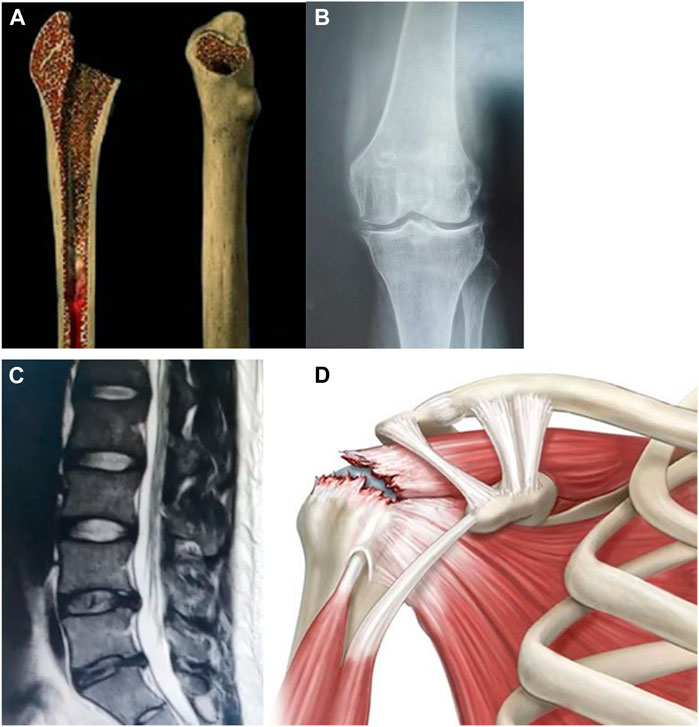
FIGURE 6. Hydrogels-coated exosomes have a potential therapeutic effect on bone diseases. (A): Bone defect (B): Knee osteoarthritis (C): Intervertebral disc degeneration (D): Rotator cuff tear.
Hydrogels coated exosomes in bone defect
A safe and effective treatment must be utilized to achieve bone tissue regeneration and repair since bone defects brought on by trauma, bone tumor removal, infection (such as osteomyelitis) and other diseases have emerged as major problems substantially impacting patients’ limb function (Chang et al., 2022). There are studies showing hydrogels coated exosomes have the potential therapeutic effect on the bone defect. Li Wang et al. studied a new type of self-healing hydrogels using coralline hydroxyapatite (CHA)/silk fibroin (SF)/glycol chitosan (GCS)/difunctionalized polyethylene glycol (DF-PEG) and made it a carrier of HucMSCs (human umbilical cord) derived exosomes. They injected the mixture of exosomes and hydrogels into the femoral condyle defect area in SD rats and found the bone defect area had effectively healed (Wang et al., 2020b). In another study, Zhang Yuntong et al. used hyaluronic acid hydrogel (HA-Gel)-encapsulated umbilical MSC-derived exosomes (uMSCEXOs) in conjunction with specialized nanohydroxyapatite/polycaprolactone (nHP) scaffold folds to heal cranial lesions in rats and demonstrated the uMSCEXOs combined with the novel composite can stimulate both angiogenesis and osteogenesis in a critical-size cranial defect model (Zhang et al., 2021a). Rui Li et al. injected the adipose-derived exosomes loaded by gelatine hydrogels into a rat skull defect model and found it can regulate bone immune metabolism and promote bone healing (Li et al., 2022).
Hydrogels coated exosomes in osteoarthritis/cartilage defect
Osteoarthritis (OA) is a common bone disease that can cause joint pain, stiffness, and limited activity, which is the main cause of disability in the elderly. OA is primarily characterized by degenerative cartilage lesions. Chondrocytes and extracellular matrix make up the majority of the cartilage in the joint while there are no blood vessels or nerves. Chondrocytes, which are highly differentiated cells, react primarily by synthesizing and secreting the cartilage matrix, which is crucial for preserving the metabolic equilibrium of cartilage tissue (Liang et al., 2021b). There is currently no medication that can stop the progression of OA. Surgical intervention may solve the problems, but any surgery has risks and the possibility of failure. To date, injecting therapeutic materials into the joint cavity has become a new treatment method for osteoarthritis. And hydrogels-coated exosomes have been reported to have the function of attenuating cartilage degradation and enhancing cartilage regeneration, which shows an attractive prospect in the treatment of osteoarthritis (Zhang et al., 2021c; Luo et al., 2022; Shen et al., 2022). Yu Zhang et al. studied the thermosensitive gel with poloxamers as a delivery platform for PRP (platelet-rich plasma) -Exosomes, finding the new therapy can induce cartilage proliferation and inhibit cartilage degradation in subtalar osteoarthritis rat model (Zhang et al., 2022). Xuehan Sang et al. used thermosensitive hydrogel loaded with chondrocyte-derived exosomes to treat damaged cartilage and proved its function to relieve OA through positively regulating chondrocytes on the proliferation, migration, and differentiation (Sang et al., 2022). Gelatin methacrylate loaded with MSCs exosomes was described to fabricate a 3D-printed decellularized extracellular matrix (ECM), according to Chen et al. (Mahmood et al., 2022). This technology made it possible to create radically directed channels, which improve cartilage regeneration by controlling chondrocyte migration and healing osteochondral defects. Fang-Xue Zhang et al. (Zhang et al., 2021c) discussed the effect of an injectable mussel-inspired highly adhesive hydrogel with exosomes to repair cartilage defects in rat patellar grooves, which can be a potential treatment for cartilage defects. Xiaolin Liu et al. reported photoinduced imine crosslinking (PIC) hydrogel coated MSCs exosomes and demonstrated its ability to facilitate articular cartilage regeneration (Liu et al., 2017).
Hydrogels coated exosomes in intervertebral disc degeneration
The intervertebral disc has the function of increasing the range of spinal motion, bearing pressure, cushioning vibration, and protecting the brain and spinal cord. Similar to osteoarthritis, degenerative intervertebral disc disease is also a common orthopedic condition, having a severe impact on people’s life quality. Intervertebral disc consists of fibrous ring, nucleus pulposus and hyaline cartilage plate. The ECM’s imbalance of catabolism and anabolism as well as changes in the intervertebral disc microenvironment are the primary causes of intervertebral disc degeneration (Binch et al., 2021). MSCs transplantation can be a treatment method for intervertebral disc degeneration (Sakai and Andersson, 2015). However, stem cell transplantation still faces potential risks of in vivo survival, immunogenicity, and tumorigenicity (Hao et al., 2017). MSCs-derived exosomes can inherit MSCs’ properties, restrain the aptosis of nucleus pulposus cells and promote ECM synthesis, thus mitigating inflammatory responses. What’s more, low immunogenicity makes exosomes an ideal therapeutic material for intervertebral disc degeneration (Xing et al., 2021). However, the interaction time of exosome injection alone is too short to achieve long-term effects. So, it’s proper to load the exosomes into a container. Hydrogels, as mentioned before, show stable property and biocompatibility and can serve as the carrier of exosomes. As for the applications of hydrogels coated exosomes on intervertebral disc degeneration, some studies have been done. Liwen Luo et al. tried to inhibit intervertebral disc degeneration by injecting transcostal cartilage ECM modified hydrogels coated exosomes from cartilage endplate stem cells into the impaired intervertebral disc. They collected intervertebral disc samples from rats and patients who underwent elective intervertebral removal surgery. The outcomes revealed that the compound material can release exosomes stably and prevent the degeneration of intervertebral disc (Luo et al., 2022). Ming Guan et al. developed an injectable, self-healing biocompatible hydrogel to load MSCs-Exosomes and drew the conclusion that the hydrogels coated exosomes can treat disc degeneration by attenuating nucleus pulposus cellular senescence (Guan et al., 2023).
Hydrogels coated exosomes in rotator cuff tear/tendon repair
The rotator cuff is composed of the tendons of the supraspinatus, infraspinatus, teres minor and subscapularis muscles. It’s an important structure to maintain shoulder stability. Rotator cuff tear is a common injury in sports. Tendon-bone interface recovery is the key factor in rotator cuff tear repair. The formation of fibrovascular scar tissue after rotator cuff injury can affect tendon-bone healing (Gulotta et al., 2009). Currently, biological intervention is applied to treat rotator cuff tear to promote tendon-bone healing, which may be crucial for preventing retear (Zhang et al., 2021d). With the discovery of exosomes and the emergence of various hydrogels, there are researches focusing on rotator cuff tear repair using the combination of the two materials. Jiangyu Cai et al. evaluated the effect of sodium alginate hydrogel coated Kartogenin-Preconditioned MSCs exosomes on the rotator cuff tear model of the rat. The outcomes showed that it can accelerate the rotator cuff tendon-bone repair, enhancing its biomechanical properties (Cai et al., 2023). Xiaopeng Tong et al. analyzed the function of hydrogels coated human urine-derived stem cells (USCs) exosomes on a rat rotator cuff tear model. The results proved its ability to heal tendon-bone interface (Tong et al., 2023). Jinwei Lu et al. developed GelMA hydrogels coated platelet-derived exosomes and verified their capacity to promote tendon stem/progenitor cell proliferation and regeneration, facilitating tendon repair (Lu et al., 2023).
Conclusion
Exosomes are a subset of extracellular vesicles with a diameter of 30–200 nm. Their applications in bone tissue repair have been studied. Hydrogels have 3D structure work and can be used in tissue repair as well as drug carriers. A combination of the exosomes and the hydrogels may utilize their advantages and make up for the disadvantages. We summarize the hydrogels coated exosomes in bone tissue repair and reviewed their therapeutic effects on the bone defect, osteoarthritis/cartilage defect, intervertebral disc degeneration, and rotator cuff tear/tendon repair. Hydrogels coated exosomes are promising, and their role in bone tissue repair needs to be further explored. In the future, through the improvement of exosomes extraction and hydrogels synthesis, hydrogels coated exosomes are expected to play a greater role in the diagnosis and treatment of orthopedic diseases, solving bone tissue repair problems.
Author contributions
All authors listed have made a substantial, direct, and intellectual contribution to the work and approved it for publication.
Conflict of interest
The authors declare that the research was conducted in the absence of any commercial or financial relationships that could be construed as a potential conflict of interest.
Publisher’s note
All claims expressed in this article are solely those of the authors and do not necessarily represent those of their affiliated organizations, or those of the publisher, the editors and the reviewers. Any product that may be evaluated in this article, or claim that may be made by its manufacturer, is not guaranteed or endorsed by the publisher.
References
Akbari, A., Jabbari, N., Sharifi, R., Ahmadi, M., Vahhabi, A., Seyedzadeh, S. J., et al. (2020). Free and hydrogel encapsulated exosome-based therapies in regenerative medicine. Life Sci. 249, 117447. doi:10.1016/j.lfs.2020.117447
Anada, T., Pan, C. C., Stahl, A. M., Mori, S., Fukuda, J., Suzuki, O., et al. (2019). Vascularized bone-mimetic hydrogel constructs by 3D bioprinting to promote osteogenesis and angiogenesis. Int. J. Mol. Sci. 20, 1096. doi:10.3390/ijms20051096
Andrzejewska, A., Lukomska, B., and Janowski, M. (2019). Concise review: Mesenchymal stem cells: From roots to boost. Stem Cells 37, 855–864. doi:10.1002/stem.3016
Antoine, E. E., Vlachos, P. P., and Rylander, M. N. (2014). Review of collagen I hydrogels for bioengineered tissue microenvironments: Characterization of mechanics, structure, and transport. Tissue Eng. Part B Rev. 20, 683–696. doi:10.1089/ten.teb.2014.0086
Arraud, N., Linares, R., Tan, S., Gounou, C., Pasquet, J. M., Mornet, S., et al. (2014). Extracellular vesicles from blood plasma: Determination of their morphology, size, phenotype and concentration. J. Thromb. Haemost. 12, 614–627. doi:10.1111/jth.12554
Bei, H. P., Hung, P. M., Yeung, H. L., Wang, S., and Zhao, X. (2021). Bone-a-Petite: Engineering exosomes towards bone, osteochondral, and cartilage repair. Small 17, e2101741. doi:10.1002/smll.202101741
Bertsch, P., Diba, M., Mooney, D. J., and Leeuwenburgh, S. C. G. (2023). Self-healing injectable hydrogels for tissue regeneration. Chem. Rev. 123, 834–873. doi:10.1021/acs.chemrev.2c00179
Binch, A. L. A., Fitzgerald, J. C., Growney, E. A., and Barry, F. (2021). Cell-based strategies for IVD repair: Clinical progress and translational obstacles. Nat. Rev. Rheumatol. 17, 158–175. doi:10.1038/s41584-020-00568-w
Cai, J., Xu, J., Ye, Z., Wang, L., Zheng, T., Zhang, T., et al. (2023). Exosomes derived from kartogenin-preconditioned mesenchymal stem cells promote cartilage formation and collagen maturation for enthesis regeneration in a rat model of chronic rotator cuff tear. Am. J. Sports Med. 51, 1267–1276. doi:10.1177/03635465231155927
Carnino, J. M., Ni, K., and Jin, Y. (2020). Post-translational modification regulates formation and cargo-loading of extracellular vesicles. Front. Immunol. 11, 948. doi:10.3389/fimmu.2020.00948
Catoira, M. C., Fusaro, L., Di Francesco, D., Ramella, M., and Boccafoschi, F. (2019). Overview of natural hydrogels for regenerative medicine applications. J. Mater Sci. Mater Med. 30, 115. doi:10.1007/s10856-019-6318-7
Chang, S., Wang, S., Liu, Z., and Wang, X. (2022). Advances of stimulus-responsive hydrogels for bone defects repair in tissue engineering. Gels 8, 389. doi:10.3390/gels8060389
Cheng, L., Zhang, K., Wu, S., Cui, M., and Xu, T. (2017). Focus on mesenchymal stem cell-derived exosomes: Opportunities and challenges in cell-free therapy. Stem Cells Int. 2017, 1–10. doi:10.1155/2017/6305295
Clayton, A., Turkes, A., Navabi, H., Mason, M. D., and Tabi, Z. (2005). Induction of heat shock proteins in B-cell exosomes. J. Cell Sci. 118, 3631–3638. doi:10.1242/jcs.02494
Dai, J., Su, Y., Zhong, S., Cong, L., Liu, B., Yang, J., et al. (2020). Exosomes: Key players in cancer and potential therapeutic strategy. Signal Transduct. Target Ther. 5, 145. doi:10.1038/s41392-020-00261-0
Datta, S., Rameshbabu, A. P., Bankoti, K., Roy, M., Gupta, C., Jana, S., et al. (2021). Decellularized bone matrix/oleoyl chitosan derived supramolecular injectable hydrogel promotes efficient bone integration. Mater Sci. Eng. C Mater Biol. Appl. 119, 111604. doi:10.1016/j.msec.2020.111604
Dimitriou, R., Jones, E., McGonagle, D., and Giannoudis, P. V. (2011). Bone regeneration: Current concepts and future directions. BMC Med. 9, 66. doi:10.1186/1741-7015-9-66
El-Sherbiny, I. M., and Yacoub, M. H. (2013). Hydrogel scaffolds for tissue engineering: Progress and challenges. Glob. Cardiol. Sci. Pract. 2013, 38–42. doi:10.5339/gcsp.2013.38
Girón, J., Maurmann, N., and Pranke, P. (2022). The role of stem cell-derived exosomes in the repair of cutaneous and bone tissue. J. Cell Biochem. 123, 183–201. doi:10.1002/jcb.30144
Gradinaru, V., Treweek, J., Overton, K., and Deisseroth, K. (2018). Hydrogel-tissue chemistry: Principles and applications. Annu. Rev. Biophys. 47, 355–376. doi:10.1146/annurev-biophys-070317-032905
Guan, M., Liu, C., Zheng, Q., Chu, G., Wang, H., Jin, J., et al. (2023). Exosome-laden injectable self-healing hydrogel based on quaternized chitosan and oxidized starch attenuates disc degeneration by suppressing nucleus pulposus senescence. Int. J. Biol. Macromol. 232, 123479. doi:10.1016/j.ijbiomac.2023.123479
Gulotta, L. V., Kovacevic, D., Ehteshami, J. R., Dagher, E., Packer, J. D., and Rodeo, S. A. (2009). Application of bone marrow-derived mesenchymal stem cells in a rotator cuff repair model. Am. J. Sports Med. 37, 2126–2133. doi:10.1177/0363546509339582
Ha, D. H., Kim, H. K., Lee, J., Kwon, H. H., Park, G. H., Yang, S. H., et al. (2020). Mesenchymal stem/stromal cell-derived exosomes for immunomodulatory therapeutics and skin regeneration. Cells 9, 1157. doi:10.3390/cells9051157
Hade, M. D., Suire, C. N., and Suo, Z. (2021). Mesenchymal stem cell-derived exosomes: Applications in regenerative medicine. Cells 10, 1959. doi:10.3390/cells10081959
Hao, Z. C., Lu, J., Wang, S. Z., Wu, H., Zhang, Y. T., and Xu, S. G. (2017). Stem cell-derived exosomes: A promising strategy for fracture healing. Cell Prolif. 50, e12359. doi:10.1111/cpr.12359
Hasani-Sadrabadi, M. M., Sarrion, P., Pouraghaei, S., Chau, Y., Ansari, S., Li, S., et al. (2020). An engineered cell-laden adhesive hydrogel promotes craniofacial bone tissue regeneration in rats. Sci. Transl. Med. 12, eaay6853. doi:10.1126/scitranslmed.aay6853
He, C., Hua, W., Liu, J., Fan, L., Wang, H., and Sun, G. (2020). Exosomes derived from endoplasmic reticulum-stressed liver cancer cells enhance the expression of cytokines in macrophages via the STAT3 signaling pathway. Oncol. Lett. 20, 589–600. doi:10.3892/ol.2020.11609
Ho, T. C., Chang, C. C., Chan, H. P., Chung, T. W., Shu, C. W., Chuang, K. P., et al. (2022). Hydrogels: Properties and applications in biomedicine. Molecules 27, 2902. doi:10.3390/molecules27092902
Isaac, R., Reis, F. C. G., Ying, W., and Olefsky, J. M. (2021). Exosomes as mediators of intercellular crosstalk in metabolism. Cell Metab. 33, 1744–1762. doi:10.1016/j.cmet.2021.08.006
Jalili, N. A., Jaiswal, M. K., Peak, C. W., Cross, L. M., and Gaharwar, A. K. (2017). Injectable nanoengineered stimuli-responsive hydrogels for on-demand and localized therapeutic delivery. Nanoscale 9, 15379–15389. doi:10.1039/c7nr02327h
Johnstone, R. M., Adam, M., Hammond, J. R., Orr, L., and Turbide, C. (1987). Vesicle formation during reticulocyte maturation. Association of plasma membrane activities with released vesicles (exosomes). J. Biol. Chem. 262, 9412–9420. doi:10.1016/s0021-9258(18)48095-7
Johnstone, R. M. (2005). Revisiting the road to the discovery of exosomes. Blood Cells Mol. Dis. 34, 214–219. doi:10.1016/j.bcmd.2005.03.002
Ju, Y., Hu, Y., Yang, P., Xie, X., and Fang, B. (2023). Extracellular vesicle-loaded hydrogels for tissue repair and regeneration. Mater Today Bio 18, 100522. doi:10.1016/j.mtbio.2022.100522
Kalluri, R., and LeBleu, V. S. (2020). The biology, function, and biomedical applications of exosomes. Science 367, eaau6977. doi:10.1126/science.aau6977
Khayambashi, P., Iyer, J., Pillai, S., Upadhyay, A., Zhang, Y., and Tran, S. D. (2021). Hydrogel encapsulation of mesenchymal stem cells and their derived exosomes for tissue engineering. Int. J. Mol. Sci. 22, 684. doi:10.3390/ijms22020684
Kurian, A. G., Singh, R. K., Patel, K. D., Lee, J. H., and Kim, H. W. (2022). Multifunctional GelMA platforms with nanomaterials for advanced tissue therapeutics. Bioact. Mater 8, 267–295. doi:10.1016/j.bioactmat.2021.06.027
Lai, R. C., Arslan, F., Lee, M. M., Sze, N. S., Choo, A., Chen, T. S., et al. (2010). Exosome secreted by MSC reduces myocardial ischemia/reperfusion injury. Stem Cell Res. 4, 214–222. doi:10.1016/j.scr.2009.12.003
Larios, J., Mercier, V., Roux, A., and Gruenberg, J. (2020). ALIX- and ESCRT-III-dependent sorting of tetraspanins to exosomes. J. Cell Biol. 219, e201904113. doi:10.1083/jcb.201904113
Le Bihan, M. C., Bigot, A., Jensen, S. S., Dennis, J. L., Rogowska-Wrzesinska, A., Lainé, J., et al. (2012). In-depth analysis of the secretome identifies three major independent secretory pathways in differentiating human myoblasts. J. Proteomics 77, 344–356. doi:10.1016/j.jprot.2012.09.008
Li, D., Wang, Y., Jin, X., Hu, D., Xia, C., Xu, H., et al. (2020). NK cell-derived exosomes carry miR-207 and alleviate depression-like symptoms in mice. J. Neuroinflammation 17, 126. doi:10.1186/s12974-020-01787-4
Li, H., Yin, D., Li, W., Tang, Q., Zou, L., and Peng, Q. (2021). Polydopamine-based nanomaterials and their potentials in advanced drug delivery and therapy. Colloids Surf. B Biointerfaces 199, 111502. doi:10.1016/j.colsurfb.2020.111502
Li, J., and Mooney, D. J. (2016). Designing hydrogels for controlled drug delivery. Nat. Rev. Mater 1, 16071. doi:10.1038/natrevmats.2016.71
Li, R., Li, D., Wang, H., Chen, K., Wang, S., Xu, J., et al. (2022). Exosomes from adipose-derived stem cells regulate M1/M2 macrophage phenotypic polarization to promote bone healing via miR-451a/MIF. Stem Cell Res. Ther. 13, 149. doi:10.1186/s13287-022-02823-1
Li, S., Cong, Y., and Fu, J. (2021). Tissue adhesive hydrogel bioelectronics. J. Mater. Chem. B 9, 4423–4443. doi:10.1039/d1tb00523e
Li, W., Liu, Y., Zhang, P., Tang, Y., Zhou, M., Jiang, W., et al. (2018). Tissue-Engineered bone immobilized with human adipose stem cells-derived exosomes promotes bone regeneration. ACS Appl. Mater Interfaces 10, 5240–5254. doi:10.1021/acsami.7b17620
Liang, Y., Duan, L., Lu, J., and Xia, J. (2021). Engineering exosomes for targeted drug delivery. Theranostics 11, 3183–3195. doi:10.7150/thno.52570
Liang, Y., Xu, X., Xu, L., Prasadam, I., Duan, L., Xiao, Y., et al. (2021). Non-surgical osteoarthritis therapy, intra-articular drug delivery towards clinical applications. J. Drug Target 29, 609–616. doi:10.1080/1061186x.2020.1870231
Liu, A., Lin, D., Zhao, H., Chen, L., Cai, B., Lin, K., et al. (2021). Optimized BMSC-derived osteoinductive exosomes immobilized in hierarchical scaffold via lyophilization for bone repair through Bmpr2/Acvr2b competitive receptor-activated Smad pathway. Biomaterials 272, 120718. doi:10.1016/j.biomaterials.2021.120718
Liu, W., Madry, H., and Cucchiarini, M. (2022). Application of alginate hydrogels for next-generation articular cartilage regeneration. Int. J. Mol. Sci. 23, 1147. doi:10.3390/ijms23031147
Liu, X., Inda, M. E., Lai, Y., Lu, T. K., and Zhao, X. (2022). Engineered living hydrogels. Adv. Mater 34, e2201326. doi:10.1002/adma.202201326
Liu, X., Yang, Y., Li, Y., Niu, X., Zhao, B., Wang, Y., et al. (2017). Integration of stem cell-derived exosomes with in situ hydrogel glue as a promising tissue patch for articular cartilage regeneration. Nanoscale 9, 4430–4438. doi:10.1039/c7nr00352h
Liu, Y., Huang, W., Zhang, R., Wu, J., Li, L., and Tang, Y. (2013). Proteomic analysis of TNF-α-activated endothelial cells and endothelial microparticles. Mol. Med. Rep. 7, 318–326. doi:10.3892/mmr.2012.1139
Lu, J., Yang, X., He, C., Chen, Y., Li, C., Li, S., et al. (2023). Rejuvenation of tendon stem/progenitor cells for functional tendon regeneration through platelet-derived exosomes loaded with recombinant Yap1. Acta Biomater. 161, 80–99. doi:10.1016/j.actbio.2023.02.018
Lujerdean, C., Baci, G. M., Cucu, A. A., and Dezmirean, D. S. (2022). The contribution of silk fibroin in biomedical engineering. Insects 13, 286. doi:10.3390/insects13030286
Luo, L., Gong, J., Wang, Z., Liu, Y., Cao, J., Qin, J., et al. (2022). Injectable cartilage matrix hydrogel loaded with cartilage endplate stem cells engineered to release exosomes for non-invasive treatment of intervertebral disc degeneration. Bioact. Mater 15, 29–43. doi:10.1016/j.bioactmat.2021.12.007
Mahmood, A., Patel, D., Hickson, B., DesRochers, J., and Hu, X. (2022). Recent progress in biopolymer-based hydrogel materials for biomedical applications. Int. J. Mol. Sci. 23, 1415. doi:10.3390/ijms23031415
Maity, C., and Das, N. (2021). Alginate-based smart materials and their application: Recent advances and perspectives. Top. Curr. Chem. (Cham) 380, 3. doi:10.1007/s41061-021-00360-8
Mashouri, L., Yousefi, H., Aref, A. R., Ahadi, A. M., Molaei, F., and Alahari, S. K. (2019). Exosomes: Composition, biogenesis, and mechanisms in cancer metastasis and drug resistance. Mol. Cancer 18, 75. doi:10.1186/s12943-019-0991-5
Mellati, A., Hasanzadeh, E., Gholipourmalekabadi, M., and Enderami, S. E. (2021). Injectable nanocomposite hydrogels as an emerging platform for biomedical applications: A review. Mater Sci. Eng. C Mater Biol. Appl. 131, 112489. doi:10.1016/j.msec.2021.112489
Mo, C., Xiang, L., and Chen, Y. (2021). Advances in injectable and self-healing polysaccharide hydrogel based on the Schiff base reaction. Macromol. Rapid Commun. 42, e2100025. doi:10.1002/marc.202100025
Mondal, P., and Chatterjee, K. (2022). Injectable and self-healing double network polysaccharide hydrogel as a minimally-invasive delivery platform. Carbohydr. Polym. 291, 119585. doi:10.1016/j.carbpol.2022.119585
Müller, G., Schneider, M., Biemer-Daub, G., and Wied, S. (2011). Microvesicles released from rat adipocytes and harboring glycosylphosphatidylinositol-anchored proteins transfer RNA stimulating lipid synthesis. Cell Signal 23, 1207–1223. doi:10.1016/j.cellsig.2011.03.013
Naahidi, S., Jafari, M., Logan, M., Wang, Y., Yuan, Y., Bae, H., et al. (2017). Biocompatibility of hydrogel-based scaffolds for tissue engineering applications. Biotechnol. Adv. 35, 530–544. doi:10.1016/j.biotechadv.2017.05.006
Nallusamy, J., and Das, R. K. (2021). Hydrogels and their role in bone tissue engineering: An overview. J. Pharm. Bioallied Sci. 13, S908–s12. doi:10.4103/jpbs.jpbs_237_21
Nedaeinia, R., Manian, M., Jazayeri, M. H., Ranjbar, M., Salehi, R., Sharifi, M., et al. (2017). Circulating exosomes and exosomal microRNAs as biomarkers in gastrointestinal cancer. Cancer Gene Ther. 24, 48–56. doi:10.1038/cgt.2016.77
Ngadimin, K. D., Stokes, A., Gentile, P., and Ferreira, A. M. (2021). Biomimetic hydrogels designed for cartilage tissue engineering. Biomater. Sci. 9, 4246–4259. doi:10.1039/d0bm01852j
Pandey, V., Madi, S., and Gupta, P. (2022). The promising role of autologous and allogeneic mesenchymal stromal cells in managing knee osteoarthritis. What is beyond Mesenchymal stromal cells? J. Clin. Orthop. Trauma 26, 101804. doi:10.1016/j.jcot.2022.101804
Pegtel, D. M., and Gould, S. J. (2019). Exosomes. Annu. Rev. Biochem. 88, 487–514. doi:10.1146/annurev-biochem-013118-111902
Poggio, M., Hu, T., Pai, C. C., Chu, B., Belair, C. D., Chang, A., et al. (2019). Suppression of exosomal PD-L1 induces systemic anti-tumor immunity and memory. Cell 177, 414–427.e13. doi:10.1016/j.cell.2019.02.016
Qin, W., and Dallas, S. L. (2019). Exosomes and extracellular RNA in muscle and bone aging and crosstalk. Curr. Osteoporos. Rep. 17, 548–559. doi:10.1007/s11914-019-00537-7
Qin, Y., Wang, L., Gao, Z., Chen, G., and Zhang, C. (2016). Bone marrow stromal/stem cell-derived extracellular vesicles regulate osteoblast activity and differentiation in vitro and promote bone regeneration in vivo. Sci. Rep. 6, 21961. doi:10.1038/srep21961
Rome, S., Forterre, A., Mizgier, M. L., and Bouzakri, K. (2019). Skeletal muscle-released extracellular vesicles: State of the art. Front. Physiol. 10, 929. doi:10.3389/fphys.2019.00929
Sakai, D., and Andersson, G. B. (2015). Stem cell therapy for intervertebral disc regeneration: Obstacles and solutions. Nat. Rev. Rheumatol. 11, 243–256. doi:10.1038/nrrheum.2015.13
Salahuddin, B., Wang, S., Sangian, D., Aziz, S., and Gu, Q. (2021). Hybrid gelatin hydrogels in nanomedicine applications. ACS Appl. Bio Mater 4, 2886–2906. doi:10.1021/acsabm.0c01630
Sánchez-Cid, P., Jiménez-Rosado, M., Romero, A., and Pérez-Puyana, V. (2022). Novel trends in hydrogel development for biomedical applications: A review. Polym. (Basel) 14, 3023. doi:10.3390/polym14153023
Sanderson, R. D., Bandari, S. K., and Vlodavsky, I. (2019). Proteases and glycosidases on the surface of exosomes: Newly discovered mechanisms for extracellular remodeling. Matrix Biol. 75-76, 160–169. doi:10.1016/j.matbio.2017.10.007
Sang, X., Zhao, X., Yan, L., Jin, X., Wang, X., Wang, J., et al. (2022). Thermosensitive hydrogel loaded with primary chondrocyte-derived exosomes promotes cartilage repair by regulating macrophage polarization in osteoarthritis. Tissue Eng. Regen. Med. 19, 629–642. doi:10.1007/s13770-022-00437-5
Shen, K., Duan, A., Cheng, J., Yuan, T., Zhou, J., Song, H., et al. (2022). Exosomes derived from hypoxia preconditioned mesenchymal stem cells laden in a silk hydrogel promote cartilage regeneration via the miR-205-5p/PTEN/AKT pathway. Acta Biomater. 143, 173–188. doi:10.1016/j.actbio.2022.02.026
Shi, Q., Qian, Z., Liu, D., Sun, J., Wang, X., Liu, H., et al. (2017). GMSC-derived exosomes combined with a chitosan/silk hydrogel sponge accelerates wound healing in a diabetic rat skin defect model. Front. Physiol. 8, 904. doi:10.3389/fphys.2017.00904
Shiue, S. J., Rau, R. H., Shiue, H. S., Hung, Y. W., Li, Z. X., Yang, K. D., et al. (2019). Mesenchymal stem cell exosomes as a cell-free therapy for nerve injury-induced pain in rats. Pain 160, 210–223. doi:10.1097/j.pain.0000000000001395
Skotland, T., Sagini, K., Sandvig, K., and Llorente, A. (2020). An emerging focus on lipids in extracellular vesicles. Adv. Drug Deliv. Rev. 159, 308–321. doi:10.1016/j.addr.2020.03.002
Tauro, B. J., Greening, D. W., Mathias, R. A., Ji, H., Mathivanan, S., Scott, A. M., et al. (2012). Comparison of ultracentrifugation, density gradient separation, and immunoaffinity capture methods for isolating human colon cancer cell line LIM1863-derived exosomes. Methods 56, 293–304. doi:10.1016/j.ymeth.2012.01.002
Thomou, T., Mori, M. A., Dreyfuss, J. M., Konishi, M., Sakaguchi, M., Wolfrum, C., et al. (2017). Adipose-derived circulating miRNAs regulate gene expression in other tissues. Nature 542, 450–455. doi:10.1038/nature21365
Tong, X., Xu, Y., Zhang, T., Deng, C., Xun, J., Sun, D., et al. (2023). Exosomes from CD133(+) human urine-derived stem cells combined adhesive hydrogel facilitate rotator cuff healing by mediating bone marrow mesenchymal stem cells. J. Orthop. Transl. 39, 100–112. doi:10.1016/j.jot.2023.02.002
Treiber, T., Treiber, N., and Meister, G. (2019). Regulation of microRNA biogenesis and its crosstalk with other cellular pathways. Nat. Rev. Mol. Cell Biol. 20, 5–20. doi:10.1038/s41580-018-0059-1
Valadi, H., Ekström, K., Bossios, A., Sjöstrand, M., Lee, J. J., and Lötvall, J. O. (2007). Exosome-mediated transfer of mRNAs and microRNAs is a novel mechanism of genetic exchange between cells. Nat. Cell Biol. 9, 654–659. doi:10.1038/ncb1596
Van Den Bulcke, A. I., Bogdanov, B., De Rooze, N., Schacht, E. H., Cornelissen, M., and Berghmans, H. (2000). Structural and rheological properties of methacrylamide modified gelatin hydrogels. Biomacromolecules 1, 31–38. doi:10.1021/bm990017d
Ways, T. M. M., Lau, W. M., and Khutoryanskiy, V. V. (2018). Chitosan and its derivatives for application in mucoadhesive drug delivery systems. Polym. (Basel), 10
Walker, B. W., Lara, R. P., Mogadam, E., Yu, C. H., Kimball, W., and Annabi, N. (2019). Rational design of microfabricated electroconductive hydrogels for biomedical applications. Prog. Polym. Sci. 92, 135–157. doi:10.1016/j.progpolymsci.2019.02.007
Wang, L., Wang, J., Zhou, X., Sun, J., Zhu, B., Duan, C., et al. (2020). A new self-healing hydrogel containing hucMSC-derived exosomes promotes bone regeneration. Front. Bioeng. Biotechnol. 8, 564731. doi:10.3389/fbioe.2020.564731
Wang, T., Jian, Z., Baskys, A., Yang, J., Li, J., Guo, H., et al. (2020). MSC-derived exosomes protect against oxidative stress-induced skin injury via adaptive regulation of the NRF2 defense system. Biomaterials 257, 120264. doi:10.1016/j.biomaterials.2020.120264
Wollert, T., and Hurley, J. H. (2010). Molecular mechanism of multivesicular body biogenesis by ESCRT complexes. Nature 464, 864–869. doi:10.1038/nature08849
Wortzel, I., Dror, S., Kenific, C. M., and Lyden, D. (2019). Exosome-Mediated metastasis: Communication from a distance. Dev. Cell 49, 347–360. doi:10.1016/j.devcel.2019.04.011
Xia, B., and Chen, G. (2022). Research progress of natural tissue-derived hydrogels for tissue repair and reconstruction. Int. J. Biol. Macromol. 214, 480–491. doi:10.1016/j.ijbiomac.2022.06.137
Xing, H., Zhang, Z., Mao, Q., Wang, C., Zhou, Y., Zhou, X., et al. (2021). Injectable exosome-functionalized extracellular matrix hydrogel for metabolism balance and pyroptosis regulation in intervertebral disc degeneration. J. Nanobiotechnology 19, 264. doi:10.1186/s12951-021-00991-5
Yasin, A., Ren, Y., Li, J., Sheng, Y., Cao, C., and Zhang, K. (2022). Advances in hyaluronic acid for biomedical applications. Front. Bioeng. Biotechnol. 10, 910290. doi:10.3389/fbioe.2022.910290
Yu, B., Zhang, X., and Li, X. (2014). Exosomes derived from mesenchymal stem cells. Int. J. Mol. Sci. 15, 4142–4157. doi:10.3390/ijms15034142
Zhang, A., Liu, Y., Qin, D., Sun, M., Wang, T., and Chen, X. (2020). Research status of self-healing hydrogel for wound management: A review. Int. J. Biol. Macromol. 164, 2108–2123. doi:10.1016/j.ijbiomac.2020.08.109
Zhang, C., Wu, J., Li, X., Wang, Z., Lu, W. W., and Wong, T. M. (2021). Current biological strategies to enhance surgical treatment for rotator cuff repair. Front. Bioeng. Biotechnol. 9, 657584. doi:10.3389/fbioe.2021.657584
Zhang, F. X., Liu, P., Ding, W., Meng, Q. B., Su, D. H., Zhang, Q. C., et al. (2021). Injectable Mussel-Inspired highly adhesive hydrogel with exosomes for endogenous cell recruitment and cartilage defect regeneration. Biomaterials 278, 121169. doi:10.1016/j.biomaterials.2021.121169
Zhang, H., Cheng, J., and Ao, Q. (2021). Preparation of alginate-based biomaterials and their applications in biomedicine. Mar. Drugs 19, 264. doi:10.3390/md19050264
Zhang, Y., Bi, J., Huang, J., Tang, Y., Du, S., and Li, P. (2020). <p>Exosome: A review of its classification, isolation techniques, storage, diagnostic and targeted therapy applications</p>. Int. J. Nanomedicine 15, 6917–6934. doi:10.2147/ijn.s264498
Zhang, Y., Wang, X., Chen, J., Qian, D., Gao, P., Qin, T., et al. (2022). Exosomes derived from platelet-rich plasma administration in site mediate cartilage protection in subtalar osteoarthritis. J. Nanobiotechnology 20, 56. doi:10.1186/s12951-022-01245-8
Zhang, Y., Xie, Y., Hao, Z., Zhou, P., Wang, P., Fang, S., et al. (2021). Umbilical mesenchymal stem cell-derived exosome-encapsulated hydrogels accelerate bone repair by enhancing angiogenesis. ACS Appl. Mater Interfaces 13, 18472–18487. doi:10.1021/acsami.0c22671
Keywords: hydrogels, exosomes, hydrogels coated exosomes, bone tissue, repair and regeneration
Citation: Pan Y, Li Y, Dong W, Jiang B, Yu Y and Chen Y (2023) Role of nano-hydrogels coated exosomes in bone tissue repair. Front. Bioeng. Biotechnol. 11:1167012. doi: 10.3389/fbioe.2023.1167012
Received: 15 February 2023; Accepted: 26 April 2023;
Published: 09 May 2023.
Edited by:
Shen Liu, Shanghai Jiao Tong University, ChinaReviewed by:
Yan Hu, Shanghai University, ChinaDeng-Guang Yu, University of Shanghai for Science and Technology, China
Copyright © 2023 Pan, Li, Dong, Jiang, Yu and Chen. This is an open-access article distributed under the terms of the Creative Commons Attribution License (CC BY). The use, distribution or reproduction in other forums is permitted, provided the original author(s) and the copyright owner(s) are credited and that the original publication in this journal is cited, in accordance with accepted academic practice. No use, distribution or reproduction is permitted which does not comply with these terms.
*Correspondence: Yunsu Chen, yschenb@163.com
†These authors have contributed equally to this work