- 1Centre for Tissue Engineering and Regenerative Medicine, Faculty of Medicine, Universiti Kebangsaan Malaysia, Kuala Lumpur, Malaysia
- 2Department of Surgery, Hospital Canselor Tuanku Muhriz, Universiti Kebangsaan Malaysia, Kuala Lumpur, Malaysia
- 3My Cytohealth Sdn Bhd Kuala Lumpur, Kuala Lumpur, Malaysia
- 4Business Innovation and Incubation Centre, Mahatma Gandhi University, Kottayam, Kerala, India
- 5International and Inter University Centre for Nanosciences and Nanotechnology, Mahatma Gandhi University, Kottayam, Kerala, India
- 6Department of Industrial Engineering, University of Trento, Trento, Italy
Skin tissue engineering possesses great promise in providing successful wound injury and tissue loss treatments that current methods cannot treat or achieve a satisfactory clinical outcome. A major field direction is exploring bioscaffolds with multifunctional properties to enhance biological performance and expedite complex skin tissue regeneration. Multifunctional bioscaffolds are three-dimensional (3D) constructs manufactured from natural and synthetic biomaterials using cutting-edge tissue fabrication techniques incorporated with cells, growth factors, secretomes, antibacterial compounds, and bioactive molecules. It offers a physical, chemical, and biological environment with a biomimetic framework to direct cells toward higher-order tissue regeneration during wound healing. Multifunctional bioscaffolds are a promising possibility for skin regeneration because of the variety of structures they provide and the capacity to customise the chemistry of their surfaces, which allows for the regulated distribution of bioactive chemicals or cells. Meanwhile, the current gap is through advanced fabrication techniques such as computational designing, electrospinning, and 3D bioprinting to fabricate multifunctional scaffolds with long-term safety. This review stipulates the wound healing processes used by commercially available engineered skin replacements (ESS), highlighting the demand for a multifunctional, and next-generation ESS replacement as the goals and significance study in tissue engineering and regenerative medicine (TERM). This work also scrutinise the use of multifunctional bioscaffolds in wound healing applications, demonstrating successful biological performance in the in vitro and in vivo animal models. Further, we also provided a comprehensive review in requiring new viewpoints and technological innovations for the clinical application of multifunctional bioscaffolds for wound healing that have been found in the literature in the last 5 years.
1 Introduction
Skin, the “first line of defense” in the human body, acts as a shield against the external environment and assists in thermal regulation and hydration retention (Bacakova et al., 2016). In addition, it helps to prevent microbial attack via infiltration of immune cells, including neutrophils or macrophages, and restoring damaged tissue function through rapid regeneration (Chaudhari et al., 2016). However, any deep partial-thickness or full-thickness skin wounds >4 cm like diabetic ulcer and burn usually take a longer time to heal. This is due to a lack of epithelialisation foci from hair follicles, sweat glands, and other dermal appendages and requires additional surgery, necessitating the utmost requirements of skin substitute for tissue repair and regeneration (Bhardwaj et al., 2018). Any damage to this tissue represents a substantial imbalance of physiological processes that may lead to mortality, hospitalisation, or long-time morbidity (Korrapati et al., 2016). In response to the injury, most skin wounds like skin cuts heal naturally by stopping hemorrhage, and avoiding excessive blood loss leading to death. Additionally, skin and subcutaneous disorders were rated as the fourth most common cause of non-fatal disease burden globally, highlighting the importance of dermatology in the rapidly developing field of global health (Hay et al., 2014). According to Rachel et al. (Giesey et al., 2021), skin and subcutaneous disease grew from 46.8% between 1990 and 2017 and is ranked fourth by the incidence of all causes of disease. There is global variation in disease burden when stratified by age, sex, geographic regions, and sociodemographic index.
In the past, the split skin graft (SSG) method was used to correct skin defects by harvesting skin from parts of the body that had not been harmed. This skin had the entire epidermal layer as well as a small amount of the dermal layer. The role of SSG is to direct self-renewing keratinocyte stem cell proliferation to the injured location for skin regeneration. However, due to a lack of donor sites and keloid formation, the use of SSG in full-thickness skin wound treatment is currently restricted. In addition, collecting skin grafts from severe burns will result in fresh wounds and more physiological harm (Shimizu and Kishi, 2012). In order to cure skin defects such as diabetic ulcers and burn injuries, engineered skin substitute (ESS) developed as a promising therapeutic option to conventional dressings and autologous skin grafts (Kennedy et al., 2017). Despite showing promising results in wound healing applications, ESS bears the threat of causing further infection at the wound site that could elevate the severity of the patient’s health condition.
The main component of conventional tissue engineering approaches is defined as the use of a scaffold as a structural element with well-defined physical, chemical, mechanical, and biological properties, as well as the right structure and porosity to support the metabolism and healing mechanisms specific to the cell tissue (Kaliva et al., 2017). Decellularised allogeneic dermis, reconstituted collagen gels and sponge, natural and synthetic polymer films make up the components of commercially available skin substitutes. However, these foreign bodies necessitate several transplantation procedures. The lack of the structural and biological cues necessary to promote fast vascularisation and regenerative cell propensity after implantation into the patient’s body may cause prolonged inflammation leading to immunological rejection. In tissue engineering approaches, the construction of a multifunctional scaffold is focused on combining three key elements to address these limitations; biomaterials as a microenvironment to trigger and guide specific cells bioactivity necessary for tissue development eventually loaded with an appropriate cells type, active agents, nanoparticles (NPs) and/or biomolecules (Alaribe et al., 2016; Fadilah et al., 2022a) (Figure 1).
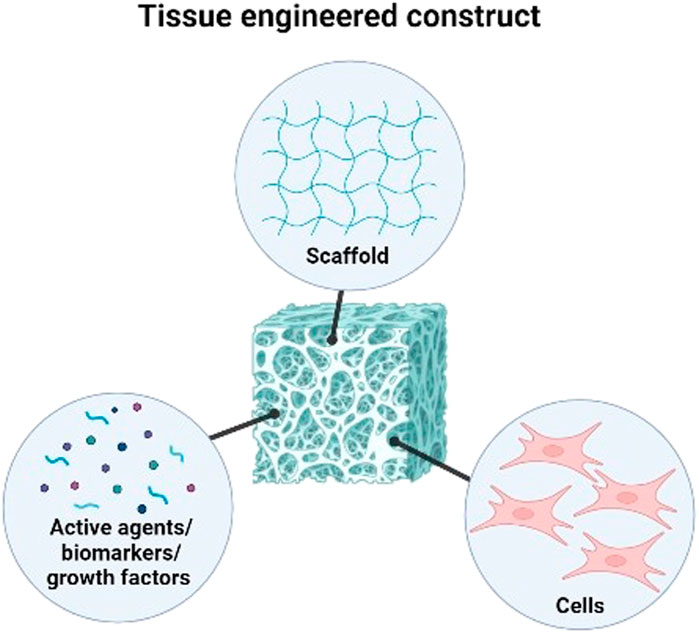
FIGURE 1. Three key elements in combining to form tissue engineered construct for tissue engineering and regenerative medicine.
The term multifunctional bioscaffolds refers to a three-dimensional (3D) structure that consists of various combinations of bioactive agents, bioinert components, and molecules to improve cells biomaterial contacts, prevent infections while biodegrading at a certain controlled-rate, overall contributing to skin regeneration (Swartjes, 2017). The properties and behavior of multifunctional bioscaffolds made from various natural and synthetic biomaterials can be tuned, and they can carry out multiple tasks at once, including delivering bioactive agents and pharmaceutical molecules, controlling stem cells behavior, and directing cells growth and differentiation (Qazi et al., 2015; Fadilah et al., 2021). The development of multifunctional bioscaffolds that can actively engage in the process of providing the biological signals that guide and drive cells activity (attachment, proliferation, migration, growth, and differentiation) is thoroughly researched using organic, inorganic, and hybrid (organic-inorganic) materials (Gupta et al., 2022; Selvakumar and Lonchin, 2022). Using multifunctional bioscaffolds, various degrees of success in skin regeneration has been demonstrated in vitro and in vivo models (Li et al., 2018a; Rahmanian et al., 2019; Ramadass et al., 2019; Abdel-Mohsen et al., 2020; Yang et al., 2020a; Sallehuddin et al., 2022). Though, just a few of these approaches outlined above have been applied to human clinical trials to be commercialised. This is because the fabrication of multifunctional bioscaffolds for clinical use requires controllable properties with uniform porous 3D structure, interconnected porosity, and proper mechanical properties to carry cells and bioactive molecules for wound healing and skin regeneration (Zhao et al., 2017). Additionally, to our knowledge several factors might contribute to the failure of clinical trials instead of the effect of implanted multifunctional bioscaffolds. Proper planning for execution is essential to ensure the integrity and related procedures can take place smoothly. To accomplish the whole activity, the sustainability of machinery and facilities for a planned clinical trial should be in place throughout the particular time frame. Any critical trial should dedicate a competent and permanent data collector to securely keep all the data information before further analysis. Besides, the difficulty in patient recruitment is because of the stringent inclusion criteria which could limit the success rate of any clinical trial. The major adverse event of the implanted prodder such as safety issue, may contribute the most to clinical trial failure. However, the successful implantation into the targeted area needs to strategies for effective post-implantation arrangement mainly for trial participants (Fadilah et al., 2022b). It includes knowledge about motivation, good dressing, and proper education that emphasises post-application care or post-operative care (Gizaw et al., 2022). For example, proper dressing and splinting are essential for skin wound care management to immobilize the specific area post-operative. The splinting can assist in managing better wound healing, including vacuum or back slab splint.
The range of biomaterials, either in natural or synthetic polymers and their composites incorporated with bioactive agents like growth factors have been considered in several reviews to promote skin regeneration (Follmann et al., 2017; Sharma et al., 2019; Mertgen et al., 2020). This review emphasises how cells including fibroblasts and stem cells, interact with traditional bioscaffolds to encourage their growth, migration, and differentiation toward skin regeneration. The geometric structure, mechanical, and biochemical characteristics of the matrix, in addition to the biomaterials and biomolecules utilised, also control how cells behave. However, in recent years, special interest has been developed in multifunctional bioscaffolds with multiple wound healing properties and to ensure their translational potential for human clinical application. This is because wound healing requirements differ for each clinical application, making multifunctionality a prerequisite in most applications. For instance, ESS used in burn treatment not only needs to integrate with the native tissue without causing the problem, but also needs to prevent infection and promote improved recovery. Hence, there is a need for literature focusing on the impact of multifunctional bioscaffolds on wound healing. Therefore, in this review, we evaluated the commercial skin substitute products and their limitations, identifying key factors to improve the translation of multifunctional bioscaffolds for clinical settings as summarised in the graphical abstract in the Supplementary Materials. In addition, we evaluate the therapeutic results of these in vitro and pre-clinical multifunctional bioscaffolds research, and we discuss potential future directions for the advancement of multifunctional bioscaffolds towards clinical applications.
2 Data extraction management
A literature search was conducted within 5 years of publications (2018–2022) through the platform of PubMed, EBSCO host, Web of Science (WoS), Scopus, and Google Scholar. The search strategy used the terms of: “bioscaffolds,” “skin substitutes,” “biomaterials,” “wound healing,” “skin tissue engineering.” The exclusion criteria for this review would be all secondary literature and any original articles that have been wrote and submitted in other languages other than English.
3 Skin substitutes for treating wounds
Several factors, including the type of wounds (epidermal, deep dermal, full-thickness), the origin of tissue damage (first-, second-, or third-degree burn) or trauma, the amount of moisture in the wound, inflammation, and secondary infection, all play a role in the coordinated process of wound healing followed by skin regeneration (Fadilah et al., 2022). Skin wound healing is comprised of four phases (hemostasis, inflammation, proliferation, and wound remodeling), including the regeneration of the new cells induced via extracellular matrix (ECM) secretion by fibroblasts, followed by keratinocytes replication as well as layered-proliferation and finally, the differentiation of keratinocytes to form the outermost epidermis layer (Fadilah et al., 2020; Tottoli et al., 2020). For minor skin injuries to heal, simple cell contraction and proliferation within the wound site are required. On the other hand, more extensive skin wounds take much longer to heal and are more likely to experience unanticipated dangers, including inflammation, infection, and scarring, which can lead to chronic wounds (Negut et al., 2020). Furthermore, additional elements like illness states (such as diabetes and kidney infections), the presence of foreign bodies, malnutrition, an immunocompromised body, and older age may affect the process of tissue regeneration and wound healing (Guo and Dipietro, 2010). Hence, it is essential to consider these multifactorials (wound type and stages of wound healing) while developing engineered grafts for skin tissue regeneration.
Wound healing and skin tissue restoration have shown promising results in the last few years with the manufacture of novel skin tissue-engineered products. Various grafts (allo-, auto-, xeno-) of dermal, epidermal, or dermo-epidermal origin, such as Alloderm, Epicel, and OrCel have been used commercially and reported to exhibit improved wound healing and tissue regeneration efficiency (Chaudhari et al., 2016). Such grafts contributed to the regeneration of skin tissue structure by repairing the wound effectively. ESS is currently designed to repair wounds and provide supplements like bioactive molecules, growth factors, secretomes, antibiotics, and anti-inflammatory drugs, which eventually accelerate the process of wound healing (Figure 2). Multifunctional bioscaffolds have been developed as a part of these bioengineered substitutes to promote cells growth in 3D structures; which exhibit high biocompatibility and biodegradation, acting as a suitable graft for wounded skin tissue (Negut et al., 2020).
3.1 In vitro effect on cell sub-organelles
Biomatrices are frequently utilised in tissue engineering and regenerative medicine applications as to provide a scaffold for cells to adhere to and grow on it (Dhandayuthapani et al., 2011). The in vitro effect of a functionalized biomatrix on cell sub-organelles would differ depending on the specific type of functionalisation and the particular sub-organelles being studied. The functionalisation of the biomatrices can be done through various ways and methods, such as the incorporation of specific proteins, peptides, or growth factors, or the modification of surface chemistry to encourage cell adhesion and provide specific signaling cues (Krishna and Kiick, 2010; Tallawi et al., 2015; Mitchell et al., 2016).
Researchers may be interested in evaluating the changes in organelle morphology, localisation, or function with regard to the impact of a functionalised biomatrix on cell sub-organelles. One of the main organelles involved in the wound healing is the mitochondrion, which plays a critical role in energy production and cells metabolism (Yan et al., 2021). Another important organelle in the wound healing is the endoplasmic reticulum (ER), which is responsible for protein synthesis, folding, and transport. Changes in ER morphology and function have been observed in response to biomatrix functionalisation. For example, functionalisation of a biomatrix with a protein can have a significant effect on wound healing. One example is the use of ECM proteins such as collagen and fibronectin. It was known to promote mitochondrial biogenesis may lead to an increase in mitochondrial mass and improved mitochondrial function in cultured cells. Alternatively, modification of the ECM to mimic the basement membrane may promote the formation of tight junctions and increase the stability of the Golgi apparatus in the cultured cells (Jain et al., 2022). Fauzi et al. have found that collagen scaffolds can promote cell adhesion, migration, and proliferation, which are essential for the formation of new tissue (Fauzi et al., 2016; Busra et al., 2017; Mh Busra et al., 2019). Additionally, collagen can activate various signaling pathways that regulate the expression of genes involved in cell proliferation, ECM remodeling, and angiogenesis (Mathew-Steiner et al., 2021).
On the other hand, fibronectin is another ECM protein that is often used for biomatrix functionalisation in wound healing applications. It has been shown to promote cell adhesion and migration, as well as the formation of new blood vessels. It promotes the spreading of platelets at the site of injury, the adhesion and migration of neutrophils, monocytes, fibroblasts, and endothelial cells into the wound region, and the migration of epidermal cells through the granulation tissue. During matrix synthesis, fibronectin appears to be involved both in the organization of the granulation tissue and basement membrane. In terms of tissue remodeling, fibronectin functions as a nonimmune opsonin for phagocytosis of debris by fibroblasts, keratinocytes, and under some circumstances, macrophages (Grinnell, 1984).
In summary, the functionalisation of a biomatrix can have a significant impact on the morphology, localisation, and function of organelles involved in wound healing, such as mitochondria, ER, and Golgi apparatus. These changes can promote cellular energy production, ECM remodeling, and growth factor secretion, ultimately enhancing the process of skin tissue repair. However, in vitro studies can provide important insights into these effects, but further investigation will be needed to determine how they translate to the in vivo systems.
4 Commercially available skin substitutes
Whether the injury is acute or chronic, the optimal wound therapy in a clinical setting relies on the depth of the wound. Several commercial wound dressings can guard the wound site while maintaining the proper moisture levels and avoiding bacterial infection since they are based on polymer films, hydrogel, foams, sponges, and beads (Alven et al., 2022). However, the ECM modeling matrix and cells proliferation are not sufficiently provided by the wound dressings (Mele, 2016). In such cases, commercially available ESS is required (Table 1) to ensure proper epidermal, dermal, or/and full-thickness wound healing through inflammation, proliferation, and remodeling stage.
Despite improving wound healing, commercially available skin graft shows several limitations, such as low blood vessel formation, reduced mechanical strength, poor integration, scarring, and immune rejection (Alrubaiy and Al-Rubaiy, 2009). For instance, autologous grafts are considered a gold standard for full-thickness wounds; nevertheless, their use is restricted by the patient’s availability of healthy skin and necessitates additional surgery to remove donor tissue. Moreover, operative assessment on a ten-year-old boy using an autologous skin graft reported the development of progressive chest scarring, contraction and keloid formation with chronic pain limiting range of motion for the patient (Patterson et al., 2019). On the other hand, allogeneic grafts can act as a replacement, but the patient may suffer from immunological rejection and the risk of infection in the long run. The OrCel® (New York, NY) ESS is constructed by seeding allogeneic fibroblasts and keratinocytes into bovine collagen sponge scaffolds; however, these cells can only survive for less than 2 months in vivo, making it a temporary solution for wound repair (Stojic et al., 2019). To overcome this challenge, Amarantus Bioscience (San Francisco, CA) came up with an alternative approach to use autologous cells in a collagen sponge (Boyce et al., 2017). The majority of epidermal substitutes are made from cultural epithelial autografts (CEA), in which the patient’s cells are extracted, grown in culture, and then reapplied to the injured spot (Barret et al., 2000; Brockmann et al., 2018). Despite its extensive use, there is still ongoing development of CEA for improved physiological integration with the underlying dermis to reduce the production time of grafts in vitro (Dreifke et al., 2015).
Other than epidermal grafts, dermal substitutes received industrial attention in skin regeneration treatment. Although dermal replacements frequently necessitate two-step operations (followed by grafting of a CEA or other epidermal product), regulatory approval is easier for dermal replacements than for full-thickness skin substitutes that necessitate cell seeding, which makes regulation more severe (Savoji et al., 2018). Furthermore, many existing skin substitutes are costly due to high production costs (particularly those needing human cell culture), and those utilising allogeneic cells or ECM pose a risk of infection (Przekora, 2020). A total of sixteen well-designed clinical studies and ongoing clinical trials were identified in 2019. Table 2 summarizes the previous randomised clinical trials (RCTS) and ongoing clinical trials on skin substitutes. From sixteen RCTs, thirteen RCTs compared the efficacy of acellular skin substitute with standard of care such as sharp debridement, pressure redistribution support, glucose control, compression bandages, infection control, offloading, and daily dressing changes, while three studies investigated the safety and efficacy of cellular dermal substitutes. Furthermore, the lack of rigorous, well-controlled clinical trials of these products in this category may add to the paucity of clinical data for cellular dermal substitutes.
None of the ESS shown in Tables 1, 2 appear to provide the optimal combination of biodegradable, biomimetic structure that promotes rapid wound healing and regeneration of native skin structure, including vascularisation, with little to no scarring. Furthermore, because they are made of fibroblasts and keratinocytes, the currently available skin substitutes lack the potential to form differentiated structures, such as hair and sweat glands; thus, it is critical to integrate additional cell types, such as endothelial cells, in ESS. As a result, the improvement of commercially accessible goods necessitates the development of multifunctional bioscaffolds with linked, porous, 3D geometry; mechanical strength; customised degradability; and biological signals for cells to repair and remodel tissue (Ghilardi et al., 2020).
5 Multifunctional bioscaffolds
A multifunctional bioscaffold is a porous, fibrous, or permeable 3D structure made of biomaterials infused with cells, bioactive molecules, secretomes, and antibacterial agents to facilitate the transport of body liquids and gases, promote cell interaction, sustain cell viability, and ECM deposition with minimal inflammation and toxicity while biodegrading at a controlled rate (Nikolova and Chavali, 2019). The 3D networks have unique properties that can imitate the skin ECM and have been shown to facilitate wound healing and skin regeneration (Zawani and Fauzi, 2021). These structures give mechanical stability and support to the tissue, as well as a vehicle for transferring bioactive compounds (drugs, antibiotics, growth factors, etc.) and templates for the attachment of genetically modified cells that generate new tissue regeneration centers (Chan and Leong, 2008; Hanczar et al., 2021). Moreover, the complex structure of the native tissue can be replicated using multifunctional bioscaffolds as potential tissue models, which makes biomaterials a component of prime importance for manufacturing multifunctional bioscaffolds. However, sometimes the multifunctional bioscaffolds present an associated risk of toxicity. Various scaffold materials showed different toxicity and pharmacology effects. Hence, the toxicity and biocompatibility tests are needed to evaluate scaffold material in mediating cell proliferation and differentiation, secreting ECM and carrying biomolecular signals for cell communication (Rahyussalim et al., 2017). Kamal et al. stated that a scaffold is considered toxic if it inhibits more than 50% of cell proliferation. The least inhibitory value means the scaffold is not toxic (Kamal et al., 2013).
5.1 Biomaterials used in developing multifunctional bioscaffolds
Biomaterials used for manufacturing multifunctional bioscaffolds in skin tissue engineering can be classified into natural and synthetic polymers, and composites (Figure 3). Currently, the construction of multifunctional bioscaffolds focuses on biodegradable biomaterials that do not require extraction from the organism (Litowczenko et al., 2021). Table 3 outlines the major kinds of biomaterials employed in the manufacturing of scaffolds with multifunctional properties, as well as their fabrication process and common use. Each biomaterial has unique physical, chemical, and mechanical properties, necessitating specific production procedures to manage 3D shapes and geometry. The manufacturing procedures for multifunctional bioscaffolds are selected based on the scaffold’s specific requirements, the material of interest, and machine limitations (Mazzoni et al., 2021). The emergence of computer-aided design (CAD) software and rapid prototyping have paved the way for multi-bio development with macro, micro, and nano-architecture (Nikolova and Chavali, 2019). Moreover, using acquired patient data and advanced fabrication technology, the manufacture of multifunctional bioscaffolds could be personalised by fabricating a unique 3D model with specific geometry containing functional molecules compatible with multiple biomaterials and cells (Litowczenko et al., 2021; Moysidou et al., 2021). Table 4 outlined the fabrication techniques for multifunctional bioscaffolds with their advantages and disadvantages.
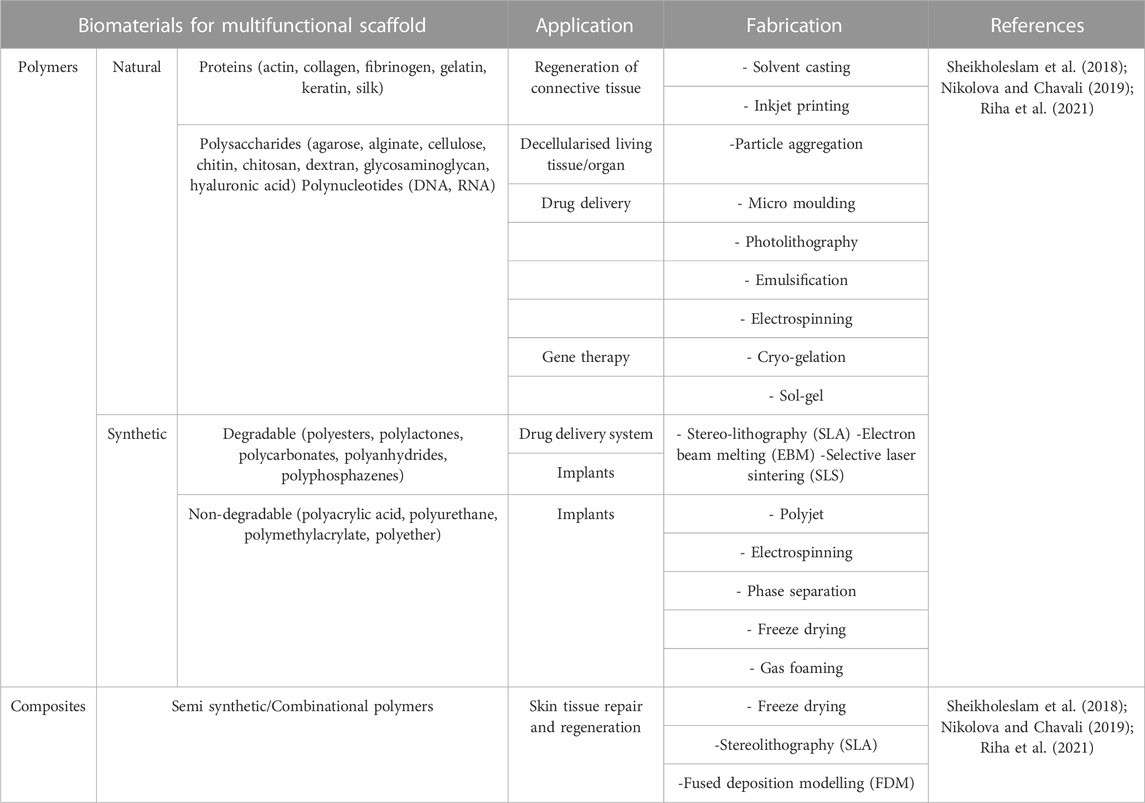
TABLE 3. Biomaterials are used in manufacturing multifunctional scaffold along with their application and fabrication technique.
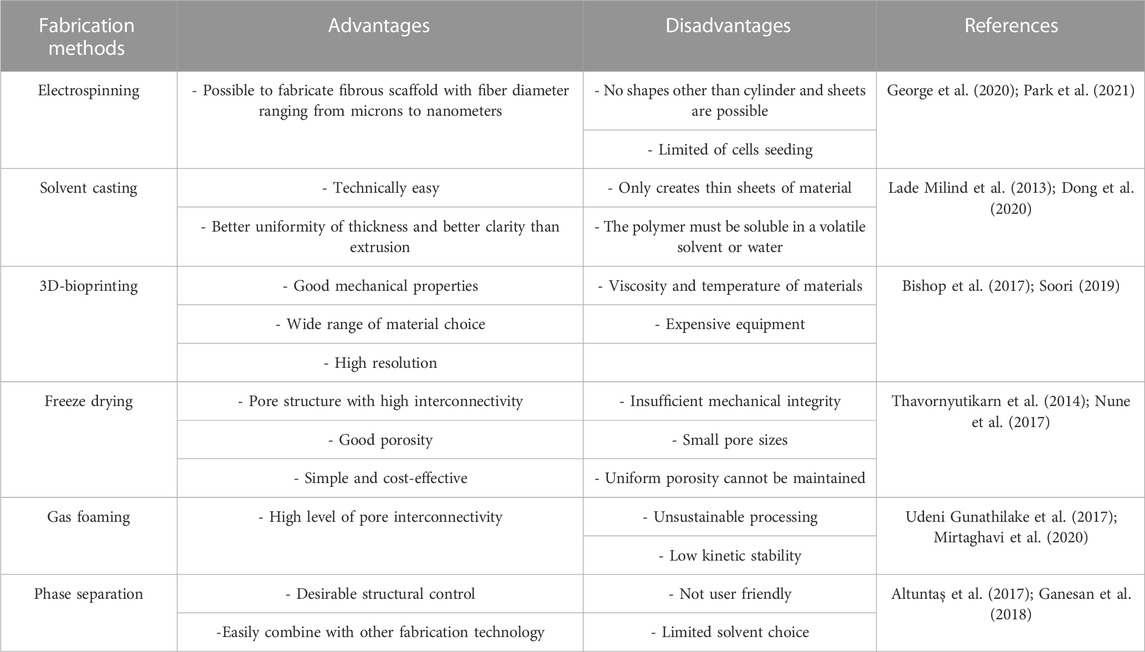
TABLE 4. Advantages and disadvantages of different fabrication methods for multifunctional bioscaffolds.
Besides, the advent of modern techniques such as electrospinning and 3D printing have revolutionised the development of multifunctional bioscaffolds at a minimal cost, making it affordable for users (Masri et al., 2022). The minimal cost of manufacturing refers to printing 5,000 pieces of a physical library of mix-and-match channel scaffolds (100 μm) for USD$ 0.50 and making it available for researchers who lack access to suitable technology (Felton et al., 2021). Such proof was obtained in a study conducted by Felton (Felton et al., 2021) and his team, which revealed that it is possible to produce a 5000-piece library of microchannel modules using the 0.4 mm nozzle for less than USD$ 1.50. Recently, the significant demand for biomaterials has shown a gradual increase in the clinical industry to support the current standard treatment regarding cutaneous wound healing. Contributing to the high demand for therapeutic implants biomaterial usage has reached an annual growth rate of 16%, creating an estimated global market for biomaterials worth USD$ 207 billion by 2024 (Naomi et al., 2020). In addition, Smandri et al. reported the importance of many natural materials that could be introduced as bioinks for a better therapeutic approach in wound healing (Smandri et al., 2020). Still, the printable quality of the currently available bioinks demonstrated shortcomings in the physicochemical and mechanical properties of the printed bioscaffolds. It is necessary to obtain optimum pore sizes and porosity that will allow the migration of cells (Masri and Fauzi, 2021).
5.2 Multifunctional bioscaffolds in wound healing treatment
In recent years, increased research efforts have been made to construct multifunctional bioscaffolds comprising signaling components and active compounds for tissue engineering applications. Figure 4 presented several types of bioscaffold for the purpose of wound healing. These bioscaffolds’ ability to contribute to wound healing applications by encouraging tissue development and regeneration has been investigated primarily in vitro and in vivo research. Table 5 summarises the use of multifunctional bioscaffolds in wound healing applications along with their treatment outcomes. Yang et al. created a lidocaine hydrochloride (LID) and mupirocin loaded (Yang et al., 2020b), chitosan/polycaprolactone (CSLD-PCLM) nanofiber with variable dual drug release and multifunctional properties such as hydrophilicity, absorbing capacity, cytocompatibility, and antibacterial functions. So, it can improve wound healing conditions in both in vitro and in vivo models. The MTT assay in the in vitro study showed improved proliferation of human dermal fibroblasts after 2 days of culturing compared to the control group. However, after 3 days of culture, no significant difference was seen between the control group and the CSLD-PCLM scaffold. The study also showed 94.4% contraction of full-thickness excisional wound created in rats on day 7, followed by complete wound closure on day 14. Also, on day 14, similar in vivo wounds treated with solely CSLD and PCLM had 2% and 4.1% required closure, indicating the importance of the CSLD-PCLM nanofiber scaffold in facilitating quicker wound healing. Besides, the flexibility of electrospun materials contributed to 73% LID (the drug that reduces pain at the wound site in the early stages) release in the first 30 min and 28.7% mupirocin (antibacterial agent) within 24 h from the CSLD-PCLM scaffold.
In addition to the mentioned works, one well-known biomaterial used for wound healing research is collagen, which can be classified into four main types: type-I, II, III, and IV. Fauzi et al. introduced collagen-type -I- based scaffolds for tissue engineering applications. The extraction of the collagen-type-I from the ovine tendon (OTC-1) demonstrated a high yield and comparable characteristics chemically with the commercial collagen-type-I (Amirrah et al., 2022; Salleh et al., 2022). In the previous study, the physicochemical properties of OTC-1 were evaluated with Fourier transform infrared spectrometry (FTIR), X-ray diffraction (XRD) and Energy dispersive X-ray spectroscopy (EDX) (Fauzi et al., 2016). Basically, the OTC-1 has the ability to be fabricated into 3D scaffolds and can be moulded into various types including sponge, film, and hydrogel that are biocompatible. Therefore, they are suitable to be used as scaffolds for developing tissue substitutes for in vitro tissue models, in vivo, and clinical applications. However, a study reported that the mechanical strength of the OTC-1 sponge crosslinked with genipin was significantly higher in terms of Young’s modulus (0.8290 ± 0.10 Gpa) and tensile strain (42.7% ± 1.59%) compared to the other crosslinked scaffolds (Busra et al., 2017). By in vitro studies, OTC-1 demonstrated no toxic effects on cells as it promoted higher cell attachment and proliferation towards both primary human epidermal keratinocytes and dermal fibroblasts. The efficacy of OTC-1 sponge crosslinked with genipin was evaluated in an in vivo full-thickness skin model. The results revealed no sign of immune response and the wound presented enhanced healing with superior skin maturity and microstructure features (Mh Busra et al., 2019).
Several studies have examined the effects of chitosan (CS) and polycaprolactone (PCL) on fibroblast cell proliferation (Shalumon et al., 2011; Mosallanezhad et al., 2022; Querido et al., 2022). Li et al. (Li et al., 2018b) investigated the properties of a double-layered fibrous PCL mat blended with CS, which demonstrated significant cell proliferation with spreading cellular morphology in vitro, whereas a PCL fibrous mat without CS showed minimal cell adherence. The double-layered multifunctional bioscaffolds were non-toxic to human dermal fibroblasts. Live/dead cell assay presented green fluorescence staining after indicating live cells without toxicity after 24 h of incubation. Moreover, MTT assay showed no inhibition of cell viability after 72 h of incubation which is attributed to the addition of CS.
Ozkan and Turkoglu Sasmazel (Ozkan and Turkoglu Sasmazel, 2016) demonstrated similar results, whereby the mouse fibroblast line (L929) showed improved cell adhesion and proliferation within a PCL/CS/PCL layer-by-layer hybrid scaffolds. The results aligned with Yang and his team (Yang et al., 2020b), where the bioscaffolds described the highest wound contraction area (94.4%) in rats on day 7 and was completely closed after 7 days. The improved efficiency of the scaffold in wound healing is due to the presence of CS and PCL. Positively charged CS acts as a hemostatic agent, promoting erythrocyte aggregation, improved platelet adhesion, full blood coagulation, homeostasis, and serving as a carrier for antibacterial drug dressings (Feng et al., 2016; Zhang et al., 2019a); whereas, PCL carried out the controlled release of mupirocin (antibacterial agent) preventing bacterial growth (Yang et al., 2020b). Furthermore, cell viability and density improved with time, and the bioscaffolds had no harmful effects on cells for 7 days, according to the absorbance value of the MTT cell viability assay (Podgórski et al., 2022). Besides, the nanofibrous nature of the scaffold constructed via electrospinning allowed drug loading and encapsulation of mupirocin, followed by its controlled release (Saghazadeh et al., 2018). Another study by Abdel-Mohsen and his co-workers (Abdel-Mohsen et al., 2020) stated that a novel multi-functional collagen (CO)/chitosan-glucan (CSGC) hollow fiber/(CO)/aloe-vera (AV) composite bioscaffolds demonstrated increased swelling, hydrolytic degradation, controlled porosity, and hemostatic properties. Within 8 days of administration to the full-thickness wound region of rats, there was increased fibroblasts migration during the creation of granulation tissue, collagen, and re-epithelialisation in proliferative phases. Furthermore, the composite multifunctional scaffold also maintains optimum hydration of the exposed tissue, reducing wound healing time. Besides, the scaffold further demonstrated improved viability and biocompatibility of normal human dermal fibroblast in vitro. The results are in coherence with Chowdhury and his team (Chowdhury et al., 2011) whereby a porous sheep collagen sponge successfully fabricated using freeze-drying method showed no cytotoxic and genotoxic effect towards human dermal fibroblasts, thus ensuring biocompatibility.
In addition, in vitro study of gallium-incorporated mesoporous bioactive glass/chitosan composite multifunctional bioscaffolds constructed via lyophilisation by Pourshahrestani and her team (Pourshahrestani et al., 2017), exhibited increased thrombus generation, blood clotting, and platelet adhesion and aggregation after 4 days of culture. Moreover, the highly ordered mesoporous channel structure of the composite bioscaffold provides high porosity, huge surface area, and pore volume, which allows easy incorporation and delivery of low concentration (1 mol%) of therapeutic gallium ion, improving the biodegradability and biocompatibility of the bioscaffold. Besides, in vitro cytotoxicity evaluation of the scaffold demonstrated non-cytotoxicity towards human dermal fibroblasts and exhibited a large number of viable cells on the surface of the bioscaffold after 4 days of culture, attributing its cytocompatibility towards human dermal fibroblasts (Wang et al., 2013).
Apart from fibrous and composite multifunctional bioscaffolds, Chen and his fellow members (Chen et al., 2018) reported that chitosan hydrogel inverse opal particles loaded with fibroblast growth factor demonstrated facilitated cell distribution and migration, followed by improved angiogenesis, collagen deposition, granulation-tissue formation, and reduced inflammation within 7 days in acute infectious rat wound. The study also suggests that the multifunctional bioscaffold supports the effective transfer of oxygen, nutrients, and metabolic wastes following the release of growth factors, which is crucial for maintaining high viability of the tissue during the wound healing process prior to angiogenesis. Moreover, another study by Hao and his team (Hao et al., 2020) used a multifunctional composite sponge made of alginate/chitosan/fucoidan (ACF) developed through electrostatic interaction, Ca2+ crosslinking, and freeze-drying process, which showed improved wound healing properties both in vitro and in vivo. The study reported that the bioscaffold possessed flexible mechanical properties as its pore size and porosity could be tailored using various fucoidan and alginate/chitosan concentrations. The study found that 10% fucoidan had better hemostatic and antibacterial activities in vitro than 30% fucoidan. Furthermore, in a full-thickness rat wound model, ACF bioscaffold with 10% fucoidan significantly promoted dermal re-epithelialisation and collagen formation, enhanced vascularisation by upregulating the specific protein expression of CD31, and hair follicle regeneration, as well as suppressed inflammation by downregulating TNF-specific protein expression.
Apart from the excisional wound model, bioscaffolds with multifunctional properties also show promising results in diabetic wound healing. Ramadass and his co-workers (Ramadass et al., 2019) developed a type I collagen peptide (CP) and S-Nitrosoglutathione (GSNO) incorporated with silk fibroin-based multifunctional scaffold to improve diabetic wound healing in clinical and experimental studies. The study demonstrated that NIH3T3 cells continued to proliferate on the surface of the scaffold, implying that the scaffold was non-toxic to fibroblast cells. In this scenario, the use of type I collagen in smaller peptide form may facilitate in the regeneration of lost ECM by providing better bioavailability to the wound bed compared to parent collagen as well as benefit the cell-matrix interaction via improved hydrophilicity, whereas, S-Nitrosoglutathione formulations could enhance the microvascular blood supply in both clinical and experimental studies (Ramadass et al., 2019). Moreover, the nanofibrous structure made of silk fibroin with regenerative and functional components serves as a potential delivery vehicle for transporting functional moieties to the wound site in treating non-healing diabetic ulcers by providing mechanical support, biocompatibility, and a moist healing environment (Chouhan et al., 2017). Similarly, Wang et al. with his team (Wang et al., 2020) fabricated injectable hydrogel-based multifunctional bioscaffolds with self-healing, antibacterial, hemostatic, and UV shielding properties which can facilitate the release of therapeutic exosome promoting diabetic wound healing. The bioscaffold aided in cell proliferation, migration, and angiogenesis in newly formed tissue, resulting in the production of granulation tissue, collagen deposition, and remodeling, expediting diabetic wound healing and even restoring skin appendages in healed wounds. Exosomes (produced from mesenchymal stem cells) were used in this investigation because they include mRNA, miRNA, growth factors, and protein molecules that can be transported to target cells to facilitate intercellular communication and the physiologic wound healing process (Fadilah et al., 2022). Besides, wound healing was attributed to the bioscaffold’s fabrication and multifunctional properties, as the injected hydrogel nature of the bioscaffolds can absorb wound secretion, maintain a moist environment to keep a relatively favorable local environment for healing, and result in better healing compared to control (Hu et al., 2023; Liu et al., 2023; Wang et al., 2023). Furthermore, the multi-functional bioscaffold’s antibacterial and UV-shielding properties can protect the wound bed from bacterial invasion and light-induced damage, as demonstrated in earlier research (Wang et al., 2019; Li et al., 2022). Also, the bioscaffolds showed good biocompatibility in vivo as no hemorrhage and inflammatory infiltration was observed in the mouse model’s heart, lung, liver, and kidney (Li et al., 2023).
Nanofiber materials have often been reported as transporters for clinical drugs but face the limitation of burst releasing the drugs (Fadilah et al., 2019). The 3D scaffolds such as nanofibers might offer promising results to facilitate the restoration of target tissues. Moreover, the advances in nanotechnology have offered groundbreaking progress in the field of tissue engineering by providing a microenvironment for the induction of cell expansion and differentiation (Ahmadian et al., 2023). In another study, Liao et al. (2021) created an aligned poly(lactide-co-glycolic acid) (PLGA)/curcumin nanofibrous multi-bio with a high-density heparin surface coating to attract endogenous growth factor and facilitate curcumin release, which could serve as an exogenous factor for wound healing. According to the study, the multifunctional scaffold displayed good tensile strength, minimal cytotoxicity, and a sufficient water vapor transmission rate for use as wound dressings. Furthermore, the bioscaffold’s aligned nanofiber orientation may facilitate migration of fibroblasts and keratinocytes from the peripheral wound area to the center, as well as promote re-epithelization and collagen deposition, hence shortening wound healing time (Ottosson et al., 2017). Despite this, the multifunctional bioscaffolds demonstrated a faster rate of curcumin release than the control due to increased hydrophilicity, which contributes to a higher cell migration rate and induced oxidative stress protection of HS68 fibroblast cells in vitro. Furthermore, an in vivo investigation revealed a rapid rate of wound closure, quicker re-epithelisation, increased angiogenesis, and increased collagen deposition at the wound site (Liao et al., 2021). Furthermore, the rapid skin regeneration may be related to the multifunctionality of nanofibers, where grafted heparin attracted and stabilized the growth factors required for wound healing in situ (Veith et al., 2019), as well as relieving the high oxidative stress and inflammatory cascade caused by released curcumin during diabetic wound healing (Ranjbar-Mohammadi et al., 2016; Mohanty and Sahoo, 2017).
Recently, the combining NPs into bioscaffolds represents the targeted approach of advance treatment that has gain significant attraction to the existing therapeutics (Kalantari et al., 2020; Pormohammad et al., 2021). In addition to the bioscaffolds indicated in Table 5, inserting metal-based NPs in scaffolds has sparked considerable interest in TERM. Depending on the application, many synthetic methods have been used to prepare NPs with low toxicity, contrasting agent properties, tailorable characteristics, targeted stimuli/response delivery potential, and precise control over behavior (via external stimuli such as magnetic field), which has found applications in various areas of TERM (Bahal et al., 2016; Mili et al., 2018). In animal models, for example, integration of chitosan (Kaparekar et al., 2020), gold (Nanda et al., 2022), and silver (You et al., 2017) based NPs in multifunctional bioscaffolds successfully increased re-epithelialisation, accelerated fibroblast cell migration, wound healing, and considerable wound contraction. Besides that, Sharma and Kaushal reviewed the formation of nanoparticles in green synthesised where they have played a significant role due to their higher reactivity and stability from different sources such as bacteria, fungus, algae, and plants (Sharma and NavneetKaushal, 2022). The nanoparticles can stimulate numerous cellular and molecular processes that aid in wound microenvironment via different mechanisms such as anti-inflammatory, antimicrobial, and angiogenic effects, possibly changing the milieu from non-healing to healing (Kushwaha et al., 2022). However, due to the prominent nature of the associated toxicity and environmental concerns contained in most of these conventional processes, the demand for more clean, trustworthy, eco-friendly, and biologically compatible procedures has limited their continuous usage.
Metal NPs can now be synthesised utilizing plant-mediated methods, which avoid many of the drawbacks of traditional synthetic methods. Bioresources are used as a scaffold to reduce and stabilise the materials, making them biocompatible with living cells (Jo et al., 2022). Many studies have been conducted in biochemical synthesis and analysis due to plants’ intrinsic ability to reorganise inorganic metal ions into NPs via organic processes. Several published research findings strongly suggest that natural-based products containing NPs are beneficial and safe for developing of scaffolds (Zhang et al., 2019b; Kaparekar et al., 2020; Konop et al., 2020; Fahimirad et al., 2021). The presence of beneficial phytochemicals inside the plants has been linked to these healing properties. However, there are still significant health concerns, particularly regarding toxicity due to the NPs’ uncontrollable use and release. As a result, the inclusion of NPs with natural-based biomaterials for scaffold construction should be addressed to make the use of slow-release NPs easier, safer, and more ecologically friendly to improve wound healing.
6 Challenges and improvement of multifunctional bioscaffolds towards future translational applications
Although a wide range of successes in wound healing and skin regeneration has been shown in vitro and in vivo in animal models using multifunctional bioscaffolds, almost no progress has been made in translating to human clinical trials towards commercialisation. This is due to the heterogenous nature of wounds among the patients depending on the considered pathology, which cannot be treated using a single dressing or scaffold that can meet the diverse needs of all wounds (Memic et al., 2019). Hence, treatment for skin regeneration should progress towards a personalised therapy-based multifunctional bioscaffolds production for tissue regeneration. Despite making considerable progress in multifunctional bioscaffolds research, multiple challenges must be resolved to make it available for clinical use. The significant hurdles include i) modulating the properties (structural and biomechanical), and degradation rate of multifunctional bioscaffolds depending on the intended use by optimising surface characteristics to improve cell adhesion and ECM deposition; ii) efforts in manufacturing multifunctional bioscaffolds for intended application, using various fabrication techniques by combining different biomaterials incorporated with bioactive molecules; iii) customising therapeutic approach via selection of biomolecules and optimising appropriate dose considering the level of skin damage and patient condition iv) increased vascularisation preventing localised necrosis, and implant failure minimising immune response; v) improving the clarity and accuracy of technology for producing a multifunctional bioscaffolds, and precisely replicating the composition of the ECM; vi) reducing the post implantation effects like immune rejection, and secondary damages; vii) minimising complexity in fabrication process; and (viii) industrial scale production and commercialisation outside the laboratory environment (Nikolova and Chavali, 2019). Hence, eliminating these challenges requires optimising the multi-bio architecture and providing a conducive biological environment for its effective outcome in skin regeneration treatment. In this scenario, using advanced technologies such as computational modelling, electrospinning, and 3D-printing for skin regeneration would optimise bioscaffolds depending on wound requirements.
Computational models can play an important role in designing multifunctional bioscaffolds since they can be used to determine the best mix of physical, chemical, and biological properties and give a precise simulation of wound healing and deformation (Chang et al., 2017). Prevailing computer-aided design (CAD) software is mainly used for manufacturing existing scaffolds, which emphasises geometric shapes; however, the CAD system cannot guarantee multifunctional capability as it is difficult to incorporate and optimise these attributes manually design (Leung et al., 2019). Researchers currently use CAD software coupled with finite element analysis (FEA) and a trail error approach to design multifunctional properties (Chang et al., 2017). Electrospinning has also received attention in the development of multifunctional bioscaffolds for wound healing applications and is already in use by companies to manufacture nanofiber-based wound dressings and skin substitutes for commercial purposes. This technology is used to fabricate biomimetic multifunctional bioscaffolds with growth factors, antimicrobial agents, anti-inflammatory drugs, and anesthetics which can be used for epidermal grafts (Memic et al., 2019). Recent studies by Li (Li et al., 2018c); Liao (Liao et al., 2021), Ramadass (Ramadass et al., 2019) and their co-workers have demonstrated satisfactory outcome in skin tissue regeneration when combined with stem cells, bioactive compounds, and nanomaterial. Nonetheless, the bulky and expensive setup of electrospinning devices limited its use in tissue engineering application. However, the recent advances in nanotechnology has allowed the manufacturing of light weight, battery operated and portable devices, which hold the potential of increased production and utilisation of electrospun nanofibers for clinical application (Memic et al., 2019).
Apart from electrospinning, the 3D bioprinting method has emerged as potential alternative technology which is used in fabricating more complex skin substitutes with precision by incorporating several cell types, bioactive molecules, and NPs in an automated way reducing cost and time in the manufacturing process (Jamróz et al., 2018). This new technology operates by precisely depositing biological agents in layers to form complex structures. The biological agents include using natural and synthetic biomaterials to form structural matrix, different types of cells, growth factors, antimicrobial or anti-inflammatory drugs, secretomes, and other bioactive materials (Leung et al., 2019). A patient with full-thickness burns or other deep skin damage may benefit from the implantation of a multifunctional scaffold made using 3D bioprinting because it allows for the precise placement of cells to repair damaged skin while minimising the number of surgeries and length of the patient’s stay. Additionally, it may be possible to manage the geographic integration of multifunctional scaffold and cells in 3D printed skin structures, which could result in a more effective system that speeds up regeneration while possibly requiring less intervention (Masri et al., 2022). Despite being a developed technology, 3D bioprinting suffers from multiple challenges which need to be resolved. Some of the hurdles include i) the capacity of 3D bioprinting to produce tissues at the human scale; ii) the reproducibility of the intricate structure resembling ECM; iii) the embedding of desirable cells capable of developing into a mature tissue; iv) the time spent to print out a clinically relevant skin construct; and v) the ethical, social, and regulatory issues associated with 3D printed skin (Kačarević et al., 2018). The urge to produce quick and instant bioengineered skin construct for immediate implantation gave birth to the idea of in situ bioprinting. In most cases, the application of 3D bioprinting under laboratory setting is carried out by printing the 3D skin under in vitro conditions, followed by post-processing and subsequent engrafting in animal models. However, in clinical setting this approach could give rise to potential complications like construct damage upon transportation or manipulation, and haphazard placement of a construct with a complicated 3D topology on the wound bed (Murphy and Atala, 2014; Singh and Jonnalagadda, 2020). Such complications can be avoided via in situ bioprinting whereby a printing device can directly graft 3D bio-printed skin on the patients’ wound site, regardless of the size using their body as a bioreactor to develop engrafted skin construct functionally (Manita et al., 2021).
Biological research may significantly influence the best multifunctional bioscaffolds design for skin regeneration to understand better the wound milieu (physical and biochemical) (Muzzio et al., 2021; Qin et al., 2022). Additionally, it is crucial to take caution when modifying the physicochemical characteristics of multifunctional bioscaffolds because their size, shape, composition, charge, and topography can affect the host’s inherent immunogenicity (Echeverria Molina et al., 2021). A chain of events known as the foreign body response (FBR) is triggered by the implantation of bioscaffolds in vivo and typically lasts for 1–2 weeks (Chen et al., 2019; Veiseh and Vegas, 2019; Capuani et al., 2022). For instance, the bioscaffold’s pore size can affect the development of fibrotic capsules and change the phenotype of macrophages, both of which are crucial to the healing of wounds. Increased pore size of electrospun bioscaffolds has been linked in studies to a change in the M2 macrophage phenotype (Garg et al., 2013; Sussman et al., 2014). Porosity may harm the mechanical strength of the construct, which may be inadequate for reproducing the strength of the native tissue, even though it can be used to foster a regenerative environment by modifying the macrophage phenotype. Additionally, via affecting FBR and fibrosis, scaffold size and shape have an impact on the phenotypes of responding immune cells. The host’s ability to recognise the implanted bioscaffolds and the propagation of foreign body reactions can both be impacted by changing its geometry. For example, it has been established that spherically shaped implants are necessary for resisting host fibrosis and that increasing implant size is insufficient to prevent FBR (Veiseh et al., 2015). Moreover, the chemical properties of multifunctional bioscaffolds can also have a significant impact on the host response. Carbodiimide or glutaraldehyde are crosslinking agents that strengthen ECM-based scaffolds and have been shown to encourage an early pro-inflammatory immune cell phenotype and inhibit macrophage ECM degradation (Brown et al., 2012; Sadtler et al., 2019). On the other hand, genipin is preferable to other plant-based crosslinkers due to its biological activities, such as antioxidant and anti-inflammatory which are key players in boosting skin wound healing (Utami Nike et al., 2022). The integration of natural-based antioxidant compounds in the implanted biomaterial has revealed an advancement in supporting and expediting tissue regeneration where the scaffolds should be able to maintain their antioxidant activity while facilitating skin tissue recovery. Fadilah et al. reviewed the antioxidant-based therapies by summarised the recent literature that reported the role of natural antioxidant-incorporated biomaterials in promoting skin wound healing and tissue regeneration (Fadilah et al., 2023). For future directions on antioxidant therapy, earlier intervention seems to have a higher chance of success. Antioxidants do not appear to be very effective when the disease is already well-established and compelling evidence shows that they are more effective in prevention rather than treatment. This leads to the assumption that the combination of different antioxidants compounds or materials might have a synergistic effect towards the treatment (Firuzi et al., 2011). Therefore, tissue repair and regeneration must build multifunctional bioscaffolds that actively modify the immune response rather than avoid or inhibit it. As such, researchers are working on developing new strategies at harnessing the immunomodulatory response with the help of advanced fabrication techniques via cautious morphology and architectural selection and design to promote multifunctional scaffold tolerance.
7 Conclusion and future perspective
In summary, the successful recovery of the complex wound healing process demands an orderly cascade of biological events that involves interaction of cells with their physical and biochemical environment to promote skin regeneration. It is a significant problem for clinicians to treat varied wound types; and therefore, an extensive understanding of wound types and appropriate skin grafts is required. Thus, this review has depicted an overview of the development of multifunctional bioscaffolds, which led to significant improvements in skin tissue engineering. Implantation of such scaffolds with multifunctional properties to the wounded area not only provide accurate moisture level and prevent bacterial infection but also contributes to the healing process, cell proliferation and ECM modelling due to the combined work of the polymeric constituents, architecture, and biological agents, as shown in several in vitro and in vivo studies summarised here. The review further emphasises that multifunctional bioscaffolds must not only be passive supports for stem cell activity after implantation to improve regeneration, but they can also be engineered in various ways to modify inflammatory response and influence stem cell activity. Though the wound healing efficacy of multifunctional scaffold has been tested primarily on excisional wounds created in animal models, its translational use for clinical application faces some challenges in terms of affordable cost, industrial-scale production, optimised fabrication technique, and off-the-shelf availability to meet high demands in hospitals. Hence, we suggest further investigation into advanced fabrication techniques such as computational designing, electrospinning, and 3D bioprinting to fabricate multifunctional scaffolds with long-term safety. Understanding immune cell scaffold cross-interactions and their implications for host response are equally critical. Finally, comparative studies on commercially available engineered skin substitute and multifunctional bioscaffolds used in various wound healing treatments and their long-term follow-up studies will help consolidate healing efficacy.
Author contributions
Conceptualization, NF and MF; Methodology, NF and SR; Writing and original draft preparation, NF, SR, and ZM; Writing, review and editing, NF, SR, ZW, AW, LH, BJ, MM, ST, AM, and MF; Supervision, MF; Project administration, MF; Funding acquisition, MF. All authors contributed to manuscript revision, read, and approved the submitted version.
Funding
This review study was funded by Universiti Kebangsaan Malaysia through the Fundamental Grant (Grant Code: FF-2021-234). Besides, this project has received funding from the European Union's Horizon 2020 research and innovation program through Shaping Innovative Products for Sustainable Tissue Engineering Strategies (SHIFT), under the Marie Sklodowska-Curie grant agreement numbers 101008041.
Acknowledgments
All authors would like to thank the Faculty of Medicine, UKM for the direction and resources used to complete this review.
Conflict of interest
The authors declare that the research was conducted in the absence of any commercial or financial relationships that could be construed as a potential conflict of interest.
Publisher’s note
All claims expressed in this article are solely those of the authors and do not necessarily represent those of their affiliated organizations, or those of the publisher, the editors and the reviewers. Any product that may be evaluated in this article, or claim that may be made by its manufacturer, is not guaranteed or endorsed by the publisher.
Supplementary material
The Supplementary Material for this article can be found online at: https://www.frontiersin.org/articles/10.3389/fbioe.2023.1160577/full#supplementary-material
References
Abdel-Mohsen, A., Frankova, J., Abdel-Rahman, R. M., Salem, A., Sahffie, N., Kubena, I., et al. (2020). Chitosan-glucan complex hollow fibers reinforced collagen wound dressing embedded with aloe vera. II. Multifunctional properties to promote cutaneous wound healing. Int. J. Pharm. 582, 119349. doi:10.1016/j.ijpharm.2020.119349
Ahmadian, E., Eftekhari, A., Janas, D., and Vahedi, P. (2023). Nanofiber scaffolds based on extracellular matrix for articular cartilage engineering: A perspective. Nanotheranostics 7, 61–69. doi:10.7150/ntno.78611
Alaribe, F. N., Manoto, S. L., and Motaung, S. C. (2016). Scaffolds from biomaterials: Advantages and limitations in bone and tissue engineering. Biologia 71, 353–366. doi:10.1515/biolog-2016-0056
Alrubaiy, L., and Al-Rubaiy, K. K. (2009). Skin substitutes: A brief review of types and clinical applications. Oman Med. J. 24, 4–6. doi:10.5001/omj.2009.2
Altuntaş, E., Özkan, B., and Yener, G. (2017). Nanobiomaterials science, development and evaluation. The Netherlands: Elsevier Amsterdam.
Alvarez, O. M., Makowitz, L., and Patel, M. (2017). Venous ulcers treated with a hyaluronic acid extracellular matrix and compression therapy: Interim analysis of a randomized controlled trial. Wounds 29, E51–E54.
Alvarez, O. M., Smith, T., Gilbert, T. W., Onumah, N. J., Wendelken, M. E., Parker, R., et al. (2017). Diabetic foot ulcers treated with porcine urinary bladder extracellular matrix and total contact cast: Interim analysis of a randomized, controlled trial. Wounds 29, 140–146.
Alven, S., Peter, S., Mbese, Z., and Aderibigbe, B. A. (2022). Polymer-based wound dressing materials loaded with bioactive agents: Potential materials for the treatment of diabetic wounds. Polym. (Basel) 14, 724. doi:10.3390/polym14040724
Amirrah, I. N., Lokanathan, Y., Zulkiflee, I., Wee, M. F. M. R., Motta, A., and Fauzi, M. B. (2022). A comprehensive review on collagen type I development of biomaterials for tissue engineering: From biosynthesis to bioscaffold. Biomedicines 10, 2307. doi:10.3390/biomedicines10092307
Bacakova, M., Musilkova, J., Riedel, T., Stranska, D., Brynda, E., Bacakova, L., et al. (2016). The potential applications of fibrin-coated electrospun polylactide nanofibers in skin tissue engineering. Int. J. Nanomedicine 11, 771–789. doi:10.2147/IJN.S99317
Bahal, R., Ali McNeer, N., Quijano, E., Liu, Y., Sulkowski, P., Turchick, A., et al. (2016). In vivo correction of anaemia in β-thalassemic mice by γPNA-mediated gene editing with nanoparticle delivery. Nat. Commun. 7, 13304–13314. doi:10.1038/ncomms13304
Barret, J. P., Wolf, S. E., Desai, M. H., and Herndon, D. N. (2000). Cost-efficacy of cultured epidermal autografts in massive pediatric burns. Ann. Surg. 231, 869–876. doi:10.1097/00000658-200006000-00011
Bello, Y. M., Falabella, A. F., and Eaglstein, W. H. (2001). Tissue-engineered skin. Current status in wound healing. Am. J. Clin. Dermatol 2, 305–313. doi:10.2165/00128071-200102050-00005
Bhardwaj, N., Chouhan, D., and Mandal, B. B. (2018). 3D functional scaffolds for skin tissue engineering. Funct. 3D Tissue Eng. Scaffolds 2018, 345–365. doi:10.1016/B978-0-08-100979-6.00014-8
Bianchi, C., Cazzell, S., Vayser, D., Reyzelman, A. M., Dosluoglu, H., and Tovmassian, G. (2018). A multicentre randomised controlled trial evaluating the efficacy of dehydrated human amnion/chorion membrane (EpiFix(®)) allograft for the treatment of venous leg ulcers. Int. Wound J. 15, 114–122. doi:10.1111/iwj.12843
Bishop, E. S., Mostafa, S., Pakvasa, M., Luu, H. H., Lee, M. J., Wolf, J. M., et al. (2017). 3-D bioprinting technologies in tissue engineering and regenerative medicine: Current and future trends. Genes & Dis. 4, 185–195. doi:10.1016/j.gendis.2017.10.002
Bock, A.-K., Ibarreta, D., and Rodríguez-Cerezo, E. (2003). Human tissue-engineered products-Today ’ s markets and future prospects. Technical report Series
Boyce, S. T., Simpson, P. S., Rieman, M. T., Warner, P. M., Yakuboff, K. P., Bailey, J. K., et al. (2017). Randomized, paired-site comparison of autologous engineered skin substitutes and split-thickness skin graft for closure of extensive, full-thickness burns. J. Burn Care Res. 38, 61–70. doi:10.1097/bcr.0000000000000401
Brockmann, I., Ehrenpfordt, J., Sturmheit, T., Brandenburger, M., Kruse, C., Zille, M., et al. (2018). Skin-Derived stem cells for wound treatment using cultured epidermal autografts: Clinical applications and challenges. Stem Cells Int. 2018, 1–9. doi:10.1155/2018/4623615
Brown, B. N., Londono, R., Tottey, S., Zhang, L., Kukla, K. A., Wolf, M. T., et al. (2012). Macrophage phenotype as a predictor of constructive remodeling following the implantation of biologically derived surgical mesh materials. Acta biomater. 8, 978–987. doi:10.1016/j.actbio.2011.11.031
Brown-Etris, M., Milne, C. T., and Hodde, J. P. (2019). An extracellular matrix graft (Oasis(®) wound matrix) for treating full-thickness pressure ulcers: A randomized clinical trial. J. Tissue Viability 28, 21–26. doi:10.1016/j.jtv.2018.11.001
Busra, F. M., Lokanathan, Y., Nadzir, M. M., Saim, A., Hj Idrus, R., and Chowdhury, S. R. (2017). Attachment, proliferation, and morphological properties of human dermal fibroblasts on ovine tendon collagen scaffolds: A comparative study. Malays. J. Med. Sci. MJMS 24, 33–43. doi:10.21315/mjms2016.24.2.5
Capuani, S., Malgir, G., Chua, C. Y. X., and Grattoni, A. (2022). Advanced strategies to thwart foreign body response to implantable devices. Bioeng. Transl. Med. 7, e10300. doi:10.1002/btm2.10300
Carsin, H., Ainaud, P., Le Bever, H., Rives, J. M., Lakhel, A., Stephanazzi, J., et al. (2000). Cultured epithelial autografts in extensive burn coverage of severely traumatized patients: A five year single-center experience with 30 patients. Burns 26, 379–387. doi:10.1016/S0305-4179(99)00143-6
Cazzell, S. (2019). A randomized controlled trial comparing a human acellular dermal matrix versus conventional care for the treatment of venous leg ulcers. Wounds 31, 68–74.
Chan, B. P., and Leong, K. W. (2008). Scaffolding in tissue engineering: General approaches and tissue-specific considerations. Eur. Spine J. 17 (4), 467–479. doi:10.1007/s00586-008-0745-3
Chang, C.-C., Chen, Y., Zhou, S., Mai, Y. W., and Li, Q. (2017). “Computational design for scaffold tissue engineering,” in Biomaterials for implants and scaffolds (Berlin, Germany: Springer), 349–369.
Chaudhari, A. A., Vig, K., Baganizi, D. R., Sahu, R., Dixit, S., Dennis, V., et al. (2016). Future prospects for scaffolding methods and biomaterials in skin tissue engineering: A review. Int. J. Mol. Sci. 17, 1974. doi:10.3390/ijms17121974
Cheah, A. K. W., Chong, S. J., and Tan, B. K. (2014). Early experience with Biobrane™ in Singapore in the management of partial thickness burns. Proc. Singap. Healthc. 23, 196–200. doi:10.1177/201010581402300304
Chen, C., Liu, Y., Wang, H., Chen, G., Wu, X., Ren, J., et al. (2018). Multifunctional chitosan inverse opal particles for wound healing. ACS Nano 12, 10493–10500. doi:10.1021/acsnano.8b06237
Chen, Y., Shu, Z., Qian, K., Wang, J., and Zhu, H. (2019). Harnessing the properties of biomaterial to enhance the immunomodulation of mesenchymal stem cells. Tissue Eng. Part B Rev. 25, 492–499. doi:10.1089/ten.teb.2019.0131
Chouhan, D., Chakraborty, B., Nandi, S. K., and Mandal, B. B. (2017). Role of non-mulberry silk fibroin in deposition and regulation of extracellular matrix towards accelerated wound healing. Acta Biomater. 48, 157–174. doi:10.1016/j.actbio.2016.10.019
Chowdhury, S. R., Busra, F., Saim, B. A., and Idrus, R. B. (2011). Genotoxicity and cytotoxicity of ovine collagen on human dermal fibroblasts. Saudi Med. J. 32, 1311–1312.
Curran, M. P., and Plosker, G. L. (2002). Bilayered bioengineered skin substitute (apligraf): A review of its use in the treatment of venous leg ulcers and diabetic foot ulcers. BioDrugs 16, 439–455. doi:10.2165/00063030-200216060-00005
Dhandayuthapani, B., Yoshida, Y., Maekawa, T., and Kumar, D. S. (2011). Polymeric scaffolds in tissue engineering application: A review. Int. J. Polym. Sci. 2011, 1–19. doi:10.1155/2011/290602
DiDomenico, L. A., Orgill, D. P., Galiano, R. D., Serena, T. E., Carter, M. J., Kaufman, J. P., et al. (2018). Use of an aseptically processed, dehydrated human amnion and chorion membrane improves likelihood and rate of healing in chronic diabetic foot ulcers: A prospective, randomised, multi-centre clinical trial in 80 patients. Int. Wound J. 15, 950–957. doi:10.1111/iwj.12954
Dodson, B. P., and Levine, A. D. (2015). Challenges in the translation and commercialization of cell therapies. BMC Biotechnol. 15, 70. doi:10.1186/s12896-015-0190-4
Dong, J., Li, Y., Lin, P., Leeflang, M., van Asperen, S., Yu, K., et al. (2020). Solvent-cast 3D printing of magnesium scaffolds. Acta Biomater. 114, 497–514. doi:10.1016/j.actbio.2020.08.002
Dreifke, M. B., Jayasuriya, A. A., and Jayasuriya, A. C. (2015). Current wound healing procedures and potential care. Mater Sci. Eng. C Mater Biol. Appl. 48, 651–662. doi:10.1016/j.msec.2014.12.068
Driver, V. R., Lavery, L. A., Reyzelman, A. M., Dutra, T. G., Dove, C. R., Kotsis, S. V., et al. (2015). A clinical trial of Integra Template for diabetic foot ulcer treatment. Wound Repair Regen. 23, 891–900. doi:10.1111/wrr.12357
Echeverria Molina, M. I., Malollari, K. G., and Komvopoulos, K. (2021). Design challenges in polymeric scaffolds for tissue engineering. Front. Bioeng. Biotechnol. 9, 617141–20210614. doi:10.3389/fbioe.2021.617141
Fadilah, N. I. M., Ahmad, H., and Abd Rahman, M. F. (2019). Electrospun poly (vinyl alcohol) nanofibers doped with mesoporous silica nanoparticles for controlled release of hydrophilic model drug. Malays J. Anal. Sci. 23, 212–218.
Fadilah, N. I. M., Ahmad, H., Rahman, M. B. A., Chia, S. L., Ng, S. F., and Leong, S. W. (2020). Synthesis and in vitro biological evaluations of novel tetrapeptide as therapeutic agent for wound treatment. J. Saudi Chem. Soc. 24, 606–619. doi:10.1016/j.jscs.2020.06.003
Fadilah, N. M, Badrul Hisham, M. A. I., Sunthar, N. R., Shamsuddin, S. A., Maarof, F., Ng, M. H., et al. (2022). Cell secretomes for wound healing and tissue regeneration: Next generation acellular based tissue engineered products. J. Tissue Eng. 13, 20417314221114273. doi:10.1177/20417314221114273
Fadilah, N. I. M., Isa, I. L. M., Zaman, W. S. W. K., Tabata, Y., and Fauzi, M. B. (2022). The effect of nanoparticle-incorporated natural-based biomaterials towards cells on activated pathways: A systematic review. Polymers 14, 476. doi:10.3390/polym14030476
Fadilah, N. I. M., Maarof, M., Motta, A., Tabata, Y., and Fauzi, M. B. (2022). The discovery and development of natural-based biomaterials with demonstrated wound healing properties: A reliable approach in clinical trials. Biomedicines 10, 2226. doi:10.3390/biomedicines10092226
Fadilah, N. I. M., Phang, S. J., Kamaruzaman, N., Salleh, A., Zawani, M., Sanyal, A., et al. (2023). Antioxidant biomaterials in cutaneous wound healing and tissue regeneration: A critical review. Antioxidants 12, 787. doi:10.3390/antiox12040787
Fadilah, N. I. M., Rahman, M. B. A., Yusof, L. M., Mustapha, N. M., and Ahmad, H. (2021). The therapeutic effect and in vivo assessment of Palmitoyl-GDPH on the wound healing process. Pharmaceutics 13, 193. doi:10.3390/pharmaceutics13020193
Fahimirad, S., Abtahi, H., Satei, P., Ghaznavi-Rad, E., Moslehi, M., and Ganji, A. (2021). Wound healing performance of PCL/chitosan based electrospun nanofiber electrosprayed with curcumin loaded chitosan nanoparticles. Carbohydr. Polym. 259, 117640. doi:10.1016/j.carbpol.2021.117640
Fauzi, M., Lokanathan, Y., Aminuddin, B., Ruszymah, B., and Chowdhury, S. (2016). Ovine tendon collagen: Extraction, characterisation and fabrication of thin films for tissue engineering applications. Mater. Sci. Eng. C 68, 163–171. doi:10.1016/j.msec.2016.05.109
Felton, H., Hughes, R., and Diaz-Gaxiola, A. (2021). Negligible-cost microfluidic device fabrication using 3D-printed interconnecting channel scaffolds. PloS one 16, e0245206. doi:10.1371/journal.pone.0245206
Feng, C., Li, J., Wu, G. S., Mu, Y. Z., Kong, M., Jiang, C. Q., et al. (2016). Chitosan-Coated diatom silica as hemostatic agent for hemorrhage control. ACS Appl. Mater. Interfaces 8, 34234–34243. doi:10.1021/acsami.6b12317
Firuzi, O., Miri, R., Tavakkoli, M., and Saso, L. (2011). Antioxidant therapy: Current status and future prospects. Curr. Med. Chem. 18, 3871–3888. doi:10.2174/092986711803414368
Follmann, H. D. M., Naves, A. F., Araujo, R. A., Dubovoy, V., Huang, X., Asefa, T., et al. (2017). Hybrid materials and nanocomposites as multifunctional biomaterials. Curr. Pharm. Des. 23, 3794–3813. doi:10.2174/1381612823666170710160615
Ganesan, K., Budtova, T., Ratke, L., Gurikov, P., Baudron, V., Preibisch, I., et al. (2018). Review on the production of polysaccharide aerogel particles. Materials 11, 2144. doi:10.3390/ma11112144
Garg, K., Pullen, N. A., Oskeritzian, C. A., Ryan, J. J., and Bowlin, G. L. (2013). Macrophage functional polarization (M1/M2) in response to varying fiber and pore dimensions of electrospun scaffolds. Biomaterials 34, 4439–4451. doi:10.1016/j.biomaterials.2013.02.065
George, A. M., Peddireddy, S. P. R., Thakur, G., and Rodrigues, F. C. (2020). Biopolymer-based scaffolds: Development and biomedical applications. Biopolymer-based Formul. 2020, 717–749. doi:10.1016/B978-0-12-816897-4.00029-1
Ghilardi, S. J., O'Reilly, B. M., and Sgro, A. E. (2020). Intracellular signaling dynamics and their role in coordinating tissue repair. WIREs Syst. Biol. Med. 12, e1479. doi:10.1002/wsbm.1479
Giesey, R. L., Mehrmal, S., Uppal, P., and Delost, G. (2021). Global burden of skin and subcutaneous disease: A longitudinal analysis from the global burden of disease study from 1990-2017. SKIN J. Cutan. Med. 5, 125–136. doi:10.25251/skin.5.2.7
Gizaw, M. A., Negawo, M. K., Bala, E. T., and Daba, D. B. (2022). Knowledge, practice, and associated factors towards postoperative wound care among nurses working in public hospitals in Ethiopia: A multicenter cross-sectional study in low resource setting area. Health Sci. Rep. 5, e677–e20220603. doi:10.1002/hsr2.677
Goedkoop, R., Juliet, R., You, P. H., Daroczy, J., de Roos, K. P., Lijnen, R., et al. (2010). Wound stimulation by growth-arrested human keratinocytes and fibroblasts: HP802-247, a new-generation allogeneic tissue engineering product. Dermatology 220, 114–120. doi:10.1159/000277380
Gonzalez, S. R., Wolter, K. G., and Yuen, J. C. (2020). Infectious complications associated with the use of integra: A systematic review of the literature. Plast. Reconstr. Surg. Glob. Open 8, e2869–e20200715. doi:10.1097/gox.0000000000002869
Gordley, K., Cole, P., Hicks, J., and Hollier, L. (2009). A comparative, long term assessment of soft tissue substitutes: AlloDerm, enduragen, and dermamatrix. J. Plast. Reconstr. Aesthet. Surg. 62, 849–850. doi:10.1016/j.bjps.2008.05.006
Grinnell, F. (1984). Fibronectin and wound healing. J. Cell Biochem. 26, 107–116. doi:10.1002/jcb.240260206
Groeber, F., Holeiter, M., Hampel, M., Hinderer, S., and Schenke-Layland, K. (2011). Skin tissue engineering-in vivo and in vitro applications. Adv. Drug Deliv. Rev. 63, 352–366. doi:10.1016/j.addr.2011.01.005
Guo, S., and Dipietro, L. A. (2010). Factors affecting wound healing. J. Dent. Res. 89, 219–229. doi:10.1177/0022034509359125
Gupta, M., Sharma, A., Beniwal, C. S., and Tyagi, P. (2022). Curcumin coated 3D biocomposite scaffolds based on chitosan and cellulose for diabetic wound healing. Heliyon 8, e11442. doi:10.1016/j.heliyon.2022.e11442
Haddow, D. B., Steele, D. A., Short, R. D., Dawson, R. A., and Macneil, S. (2003). Plasma-polymerized surfaces for culture of human keratinocytes and transfer of cells to an in vitro wound-bed model. J. Biomed. Mater Res. A 64, 80–87. doi:10.1002/jbm.a.10356
Hanczar, M., Moazen, M., and Day, R. (2021). The significance of biomechanics and scaffold structure for bladder tissue engineering. Int. J. Mol. Sci. 22, 12657. doi:10.3390/ijms222312657
Hao, Y., Zhao, W., Zhang, L., Zeng, X., Sun, Z., Zhang, D., et al. (2020). Bio-multifunctional alginate/chitosan/fucoidan sponges with enhanced angiogenesis and hair follicle regeneration for promoting full-thickness wound healing. Mater. Des. 193, 108863. doi:10.1016/j.matdes.2020.108863
Harding, K., Sumner, M., and Cardinal, M. (2013). A prospective, multicentre, randomised controlled study of human fibroblast-derived dermal substitute (Dermagraft) in patients with venous leg ulcers. Int. Wound J. 10, 132–137. doi:10.1111/iwj.12053
Harding, K. G., Krieg, T., Eming, S. A., Flour, M. L., Jawien, A., Cencora, A., et al. (2005). Efficacy and safety of the freeze-dried cultured human keratinocyte lysate, LyphoDermtm 0.9%, in the treatment of hard-to-heal venous leg ulcers. Wound Repair Regen. 13, 138–147. doi:10.1111/j.1067-1927.2005.130204.x
Hart, J., Silcock, D., Gunnigle, S., Cullen, B., Light, N. D., and Watt, P. W. (2002). The role of oxidised regenerated cellulose/collagen in wound repair: Effects in vitro on fibroblast biology and in vivo in a model of compromised healing. Int. J. Biochem. Cell Biol. 34, 1557–1570. doi:10.1016/s1357-2725(02)00062-6
Hay, R. J., Johns, N. E., Williams, H. C., Bolliger, I. W., Dellavalle, R. P., Margolis, D. J., et al. (2014). The global burden of skin disease in 2010: An analysis of the prevalence and impact of skin conditions. J. Investigative Dermatology 134, 1527–1534. doi:10.1038/jid.2013.446
Hsu, K. F., Chiu, Y. L., Chiao, H. Y., Chen, C. Y., Chang, C. K., Wu, C. J., et al. (2021). Negative-pressure wound therapy combined with artificial dermis (terudermis) followed by split-thickness skin graft might be an effective treatment option for wounds exposing tendon and bone: A retrospective observation study. Med. Baltim. 100, e25395. doi:10.1097/md.0000000000025395
Hu, K., Jia, E., Zhang, Q., Zheng, W., Sun, R., Qian, M., et al. (2023). Injectable carboxymethyl chitosan-genipin hydrogels encapsulating tea tree oil for wound healing. Carbohydr. Polym. 301, 120348. doi:10.1016/j.carbpol.2022.120348
Jain, P., Rauer, S. B., Möller, M., and Singh, S. (2022). Mimicking the natural basement membrane for advanced tissue engineering. Biomacromolecules 23, 3081–3103. doi:10.1021/acs.biomac.2c00402
Jamróz, W., Szafraniec, J., Kurek, M., and Jachowicz, R. (2018). 3D printing in pharmaceutical and medical applications – recent achievements and challenges. Pharm. Res. 35, 176. doi:10.1007/s11095-018-2454-x
Jo, A., Oriola, A. O., and Onwudiwe, D. C. (2022). Plant extracts mediated metal-based nanoparticles: Synthesis and biological applications. Biomolecules 12, 20220424. doi:10.3390/biom12050627
Kačarević, Ž. P., Rider, P. M., Alkildani, S., Retnasingh, S., Smeets, R., Jung, O., et al. (2018). An introduction to 3D bioprinting: Possibilities, challenges and future aspects. Materials 11, 2199. doi:10.3390/ma11112199
Kalantari, K., Mostafavi, E., Afifi, A. M., Izadiyan, Z., Jahangirian, H., Rafiee-Moghaddam, R., et al. (2020). Wound dressings functionalized with silver nanoparticles: Promises and pitfalls. Nanoscale 12, 2268–2291. doi:10.1039/c9nr08234d
Kaliva, M., Chatzinikolaidou, M., and Vamvakaki, M. (2017). “CHAPTER 1 applications of smart multifunctional tissue engineering scaffolds,” in Smart materials for tissue engineering: Applications (London, United Kingdom: The Royal Society of Chemistry), 1–38.
Kamal, A. F., Iskandriati, D., Dilogo, I. H., Siregar, N. C., Hutagalung, E. U., Susworo, R., et al. (2013). Biocompatibility of various hydoxyapatite scaffolds evaluated by proliferation of rat’s bone marrow mesenchymal stem cells: An <em>in vitro</em> study. Med. J. Indonesia 22, 202–208. doi:10.13181/mji.v22i4.600
Kaparekar, P. S., Pathmanapan, S., and Anandasadagopan, S. K. (2020). Polymeric scaffold of Gallic acid loaded chitosan nanoparticles infused with collagen-fibrin for wound dressing application. Int. J. Biol. Macromol. 165, 930–947. doi:10.1016/j.ijbiomac.2020.09.212
Kennedy, K. M., Bhaw-Luximon, A., and Jhurry, D. (2017). Skin tissue engineering: Biological performance of electrospun polymer scaffolds and translational challenges. Regen. Eng. Transl. Med. 3, 201–214. doi:10.1007/s40883-017-0035-x
Konop, M., Czuwara, J., Kłodzińska, E., Laskowska, A. K., Sulejczak, D., Damps, T., et al. (2020). Evaluation of keratin biomaterial containing silver nanoparticles as a potential wound dressing in full-thickness skin wound model in diabetic mice. J. tissue Eng. Regen. Med. 14, 334–346. doi:10.1002/term.2998
Korrapati, P. S., Karthikeyan, K., Satish, A., Krishnaswamy, V. R., Venugopal, J. R., and Ramakrishna, S. (2016). Recent advancements in nanotechnological strategies in selection, design and delivery of biomolecules for skin regeneration. Mater Sci. Eng. C Mater Biol. Appl. 67, 747–765. doi:10.1016/j.msec.2016.05.074
Krishna, O. D., and Kiick, K. L. (2010). Protein- and peptide-modified synthetic polymeric biomaterials. Biopolymers 94, 32–48. doi:10.1002/bip.21333
Kushwaha, A., Goswami, L., and Kim, B. S. (2022). Nanomaterial-based therapy for wound healing. Nanomater. (Basel) 12, 618. doi:10.3390/nano12040618
Lade Milind, S., Payghan Santosh, A., and Tamboli Zaki, J. (2013). Polymer based wafer technology: A review. Int. J. Pharm. Biol. Archives 4, 1060–1074.
Lavery, L. A., Fulmer, J., Shebetka, K. A., Regulski, M., Vayser, D., Fried, D., et al. (2014). The efficacy and safety of Grafix(®) for the treatment of chronic diabetic foot ulcers: Results of a multi-centre, controlled, randomised, blinded, clinical trial. Int. Wound J. 11, 554–560. doi:10.1111/iwj.12329
Leung, Y.-S., Kwok, T.-H., Li, X., Yang, Y., Wang, C. C. L., and Chen, Y. (2019). Challenges and status on design and computation for emerging additive manufacturing technologies. J. Comput. Inf. Sci. Eng. 19. doi:10.1115/1.4041913
Li, H., Xue, Y., Jia, B., Bai, Y., Zuo, Y., Wang, S., et al. (2018). The preparation of hyaluronic acid grafted pullulan polymers and their use in the formation of novel biocompatible wound healing film. Carbohydr. Polym. 188, 92–100. doi:10.1016/j.carbpol.2018.01.102
Li, W., Zheng, Y., Pang, W., and Lai, P. (2023). Bio-inspired adhesive hydrogel for wound healing. Biomed. Technol. 1, 65–72. doi:10.1016/j.bmt.2022.11.009
Li, X., Wang, C., Yang, S., Liu, P., and Zhang, B. (2018). Electrospun PCL/mupirocin and chitosan/lidocaine hydrochloride multifunctional double layer nanofibrous scaffolds for wound dressing applications. Int. J. nanomedicine 13, 5287–5299. doi:10.2147/ijn.s177256
Li, X., Wang, C., Yang, S., Liu, P., and Zhang, B. (2018). Electrospun PCL/mupirocin and chitosan/lidocaine hydrochloride multifunctional double layer nanofibrous scaffolds for wound dressing applications. Int. J. Nanomedicine 13, 5287–5299. doi:10.2147/ijn.S177256
Li, Y., Chen, Y., Wu, Q., Huang, J., Zhao, Y., Li, Q., et al. (2022). Improved hydrophobic, UV barrier and antibacterial properties of multifunctional PVA nanocomposite films reinforced with modified lignin contained cellulose nanofibers. Polymers 14, 1705. doi:10.3390/polym14091705
Liao, H. T., Lai, Y.-T., Kuo, C.-Y., and Chen, J. P. (2021). A bioactive multi-functional heparin-grafted aligned poly(lactide-co-glycolide)/curcumin nanofiber membrane to accelerate diabetic wound healing. Mater. Sci. Eng. C 120, 111689. doi:10.1016/j.msec.2020.111689
Límová, M. (2010). Active wound coverings: Bioengineered skin and dermal substitutes. Surg. Clin. North Am. 90, 1237–1255. doi:10.1016/j.suc.2010.08.004
Litowczenko, J., Woźniak-Budych, M. J., Staszak, K., Wieszczycka, K., Jurga, S., and Tylkowski, B. (2021). Milestones and current achievements in development of multifunctional bioscaffolds for medical application. Bioact. Mater. 6, 2412–2438. doi:10.1016/j.bioactmat.2021.01.007
Liu, N., Zhou, Z., Ning, X., Zhang, X., Guo, Q., Guo, M., et al. (2023). Enhancing the paracrine effects of adipose stem cells using nanofiber-based meshes prepared by light-welding for accelerating wound healing. Mater. Des. 2023, 111582. doi:10.1016/j.matdes.2022.111582
Magnusson, M., Papini, R. P., Rea, S. M., Reed, C. C., and Wood, F. M. (2007). Cultured autologous keratinocytes in suspension accelerate epithelial maturation in an in vivo wound model as measured by surface electrical capacitance. Plast. Reconstr. Surg. 119, 495–499. doi:10.1097/01.prs.0000246315.80133.8d
Manita, P., García Orue, I., Santos Vizcaíno, E., Hernandez, R. M., and Igartua, M. (2021). 3D bioprinting of functional skin substitutes: From current achievements to future goals. Pharmaceuticals 14, 362. doi:10.3390/ph14040362
Masri, S., and Fauzi, M. B. (2021). Current insight of printability quality improvement strategies in natural-based bioinks for skin regeneration and wound healing. Polymers 13, 1011. doi:10.3390/polym13071011
Masri, S., Zawani, M., Zulkiflee, I., Salleh, A., Fadilah, N. I. M., Maarof, M., et al. (2022). Cellular interaction of human skin cells towards natural bioink via 3D-bioprinting technologies for chronic wound: A comprehensive review. Int. J. Mol. Sci. 23, 476. doi:10.3390/ijms23010476
Mathew-Steiner, S. S., Roy, S., and Sen, C. K. (2021). Collagen in wound healing. Bioeng. (Basel) 8, 63. doi:10.3390/bioengineering8050063
Mazzoni, E., Iaquinta, M. R., Lanzillotti, C., Mazziotta, C., Maritati, M., Montesi, M., et al. (2021). Bioactive materials for soft tissue repair. Front. Bioeng. Biotechnol. 9, 613787. doi:10.3389/fbioe.2021.613787
Mele, E. (2016), Electrospinning of natural polymers for advanced wound care: Towards responsive and adaptive dressings. J. Mater. Chem. B 4, 4801–4812. doi:10.1039/C6TB00804F
Memic, A., Abudula, T., Mohammed, H. S., Joshi Navare, K., Colombani, T., and Bencherif, S. A. (2019). Latest progress in electrospun nanofibers for wound healing applications. ACS Appl. Bio Mater. 2, 952–969. doi:10.1021/acsabm.8b00637
Mertgen, A.-S., Trossmann, V. T., Guex, A. G., Maniura-Weber, K., Scheibel, T., and Rottmar, M. (2020). Multifunctional biomaterials: Combining material modification strategies for engineering of cell-contacting surfaces. ACS Appl. Mater. Interfaces 12, 21342–21367. doi:10.1021/acsami.0c01893
Mh Busra, F., Rajab, N. F., Tabata, Y., Saim, A. B., B.H. Idrus, R., and Chowdhury, S. R. (2019). Rapid treatment of full-thickness skin loss using ovine tendon collagen type I scaffold with skin cells. J. tissue Eng. Regen. Med. 13, 874–891. doi:10.1002/term.2842
Mili, B., Das, K., Kumar, A., Saxena, A. C., Singh, P., Ghosh, S., et al. (2018). Preparation of NGF encapsulated chitosan nanoparticles and its evaluation on neuronal differentiation potentiality of canine mesenchymal stem cells. J. Mater. Sci. Mater. Med. 29, 4–13. doi:10.1007/s10856-017-6008-2
Mirtaghavi, A., Luo, J., and Muthuraj, R. (2020). Recent advances in porous 3D cellulose aerogels for tissue engineering applications: A review. J. Compos. Sci. 4, 152. doi:10.3390/jcs4040152
Mitchell, A. C., Briquez, P. S., Hubbell, J. A., and Cochran, J. R. (2016). Engineering growth factors for regenerative medicine applications. Acta Biomater. 30, 1–12. doi:10.1016/j.actbio.2015.11.007
Mohanty, C., and Sahoo, S. K. (2017). Curcumin and its topical formulations for wound healing applications. Drug Discov. Today 22, 1582–1592. doi:10.1016/j.drudis.2017.07.001
Mosallanezhad, P., Nazockdast, H., Ahmadi, Z., et al. (2022). Fabrication and characterization of polycaprolactone/chitosan nanofibers containing antibacterial agents of curcumin and ZnO nanoparticles for use as wound dressing. Front. Bioeng. Biotechnol. 2022, 10. doi:10.3389/fbioe.2022.1027351
Moysidou, C.-M., Barberio, C., and Owens, R. M. (2021). Advances in engineering human tissue models. Front. Bioeng. Biotechnol. 8, 620962. Systematic Review. doi:10.3389/fbioe.2020.620962
Murphy, S. V., and Atala, A. (2014). 3D bioprinting of tissues and organs. Nat. Biotechnol. 32, 773–785. doi:10.1038/nbt.2958
Muzzio, N., Moya, S., and Romero, G. (2021). Multifunctional scaffolds and synergistic strategies in tissue engineering and regenerative medicine. Pharmaceutics 13, 792. doi:10.3390/pharmaceutics13060792
Nanda, S. S., Wang, T., Hossain, M. I., Yoon, H. Y., Selvan, S. T., Kim, K., et al. (2022). Gold-nanorod-based scaffolds for wound-healing applications. ACS Appl. Nano Mater. 5, 8640–8648. doi:10.1021/acsanm.2c02230
Naomi, R., Ratanavaraporn, J., and Fauzi, M. B. (2020). Comprehensive review of hybrid collagen and silk fibroin for cutaneous wound healing. Materials 13, 3097. doi:10.3390/ma13143097
Nathoo, R., Howe, N., and Cohen, G. (2014). Skin substitutes: An overview of the key players in wound management. J. Clin. Aesthet. Dermatol 7, 44–48.
Negut, I., Dorcioman, G., and Grumezescu, V. (2020). Scaffolds for wound healing applications. Polymers 12, 2010. doi:10.3390/polym12092010
Nikolova, M. P., and Chavali, M. S. (2019). Recent advances in biomaterials for 3D scaffolds: A review. Bioact. Mater 4, 271–292. doi:10.1016/j.bioactmat.2019.10.005
Nune, S. K., Rama, K. S., Dirisala, V. R., and Chavali, M. Y. (2017). Electrospinning of collagen nanofiber scaffolds for tissue repair and regeneration. Nanostructures Nov. Ther. 2017, 281–311. doi:10.1016/B978-0-323-46142-9.00011-6
Ottosson, M., Jakobsson, A., and Johansson, F. (2017). Accelerated wound closure - differently organized nanofibers affect cell migration and hence the closure of artificial wounds in a cell based in vitro model. PLoS One 12, e0169419. doi:10.1371/journal.pone.0169419
Ozkan, O., and Turkoglu Sasmazel, H. (2016). Effects of nozzle type atmospheric dry air plasma on L929 fibroblast cells hybrid poly (ε-caprolactone)/chitosan/poly (ε-caprolactone) scaffolds interactions. J. Biosci. Bioeng. 122, 232–239. doi:10.1016/j.jbiosc.2016.01.004
Park, Y., Huh, K. M., and Kang, S.-W. (2021). Applications of biomaterials in 3D cell culture and contributions of 3D cell culture to drug development and basic biomedical research. Int. J. Mol. Sci. 22, 2491. doi:10.3390/ijms22052491
Patterson, C. W., Stark, M., Sharma, S., and Mundinger, G. S. (2019). Regeneration and expansion of autologous full-thickness skin through a self-propagating autologous skin graft technology. Clin. Case Rep. 7, 2449–2455. doi:10.1002/ccr3.2533
Podgórski, R., Wojasiński, M., and Ciach, T. (2022). Nanofibrous materials affect the reaction of cytotoxicity assays. Sci. Rep. 12, 9047. doi:10.1038/s41598-022-13002-w
Pormohammad, A., Monych, N. K., Ghosh, S., Turner, D. L., and Turner, R. J. (2021). Nanomaterials in wound healing and infection control. Antibiot. (Basel) 10, 473. doi:10.3390/antibiotics10050473
Pourshahrestani, S., Zeimaran, E., Kadri, N. A., Gargiulo, N., Jindal, H. M., Naveen, S. V., et al. (2017). Potency and cytotoxicity of a novel gallium-containing mesoporous bioactive glass/chitosan composite scaffold as hemostatic agents. ACS Appl. Mater. interfaces 9, 31381–31392. doi:10.1021/acsami.7b07769
Przekora, A. (2020). A concise review on tissue engineered artificial skin grafts for chronic wound treatment: Can we reconstruct functional skin tissue in vitro? Cells 9, 1622. doi:10.3390/cells9071622
Qazi, T. H., Mooney, D. J., Pumberger, M., Geißler, S., and Duda, G. N. (2015). Biomaterials based strategies for skeletal muscle tissue engineering: Existing technologies and future trends. Biomaterials 53, 502–521. doi:10.1016/j.biomaterials.2015.02.110
Qin, J., Chen, F., Wu, P., and Sun, G. (2022). Recent advances in bioengineered scaffolds for cutaneous wound healing. Front. Bioeng. Biotechnol. 10, 841583–20220301. doi:10.3389/fbioe.2022.841583
Querido, D., Vieira, T., Ferreira, J. L., Henriques, C., Borges, J. P., and Silva, J. C. (2022). Study on the incorporation of chitosan flakes in electrospun polycaprolactone scaffolds. Polym. (Basel) 14, 1496. doi:10.3390/polym14081496
Rahmanian, M., Dehghan, M. M., Eini, L., Naghib, S. M., Gholami, H., et al. (2019). Multifunctional gelatin–tricalcium phosphate porous nanocomposite scaffolds for tissue engineering and local drug delivery: In vitro and in vivo studies. J. Taiwan Inst. Chem. Eng. 101, 214–220. doi:10.1016/j.jtice.2019.04.028
Rahyussalim, A., Kurniawati, T., Aprilya, D., Anggraini, R., Ramahdita, G., and Whulanza, Y. (2017). Toxicity and biocompatibility profile of 3D bone scaffold developed by universitas Indonesia: A preliminary study. Jakarta, Indonesia: AIP Conference Proceedings.
Ramadass, S. K., Nazir, L. S., Thangam, R., Perumal, R. K., Manjubala, I., Madhan, B., et al. (2019). Type I collagen peptides and nitric oxide releasing electrospun silk fibroin scaffold: A multifunctional approach for the treatment of ischemic chronic wounds. Colloids Surfaces B Biointerfaces 175, 636–643. doi:10.1016/j.colsurfb.2018.12.025
Ranjbar-Mohammadi, M., Rabbani, S., Bahrami, S. H., Joghataei, M., and Moayer, F. (2016). Antibacterial performance and in vivo diabetic wound healing of curcumin loaded gum tragacanth/poly(ε-caprolactone) electrospun nanofibers. Mater. Sci. Eng. C 69, 1183–1191. doi:10.1016/j.msec.2016.08.032
Riha, S. M., Maarof, M., and Fauzi, M. B. (2021). Synergistic effect of biomaterial and stem cell for skin tissue engineering in cutaneous wound healing: A concise review. Polymers 13, 1546. doi:10.3390/polym13101546
Sadtler, K., Wolf, M. T., Ganguly, S., Moad, C. A., Chung, L., Majumdar, S., et al. (2019). Divergent immune responses to synthetic and biological scaffolds. Biomaterials 192, 405–415. doi:10.1016/j.biomaterials.2018.11.002
Saghazadeh, S., Rinoldi, C., Schot, M., Kashaf, S. S., Sharifi, F., Jalilian, E., et al. (2018). Drug delivery systems and materials for wound healing applications. Adv. Drug Deliv. Rev. 127, 138–166. doi:10.1016/j.addr.2018.04.008
Salleh, A., Mustafa, N., Teow, Y. H., Fatimah, M. N., Khairudin, F. A., Ahmad, I., et al. (2022). Dual-layered approach of ovine collagen-gelatin/cellulose hybrid biomatrix containing graphene oxide-silver nanoparticles for cutaneous wound healing: Fabrication, physicochemical, cytotoxicity and antibacterial characterisation. Biomedicines 10, 816. doi:10.3390/biomedicines10040816
Sallehuddin, N., Md Fadilah, N. I., Hwei, N. M., Wen, A. P. Y., Yusop, S. M., Rajab, N. F., et al. (2022). Characterization and cytocompatibility of collagen–gelatin–elastin (CollaGee) acellular skin substitute towards human dermal fibroblasts: In vitro assessment. Biomedicines 10, 1327. doi:10.3390/biomedicines10061327
Savoji, H., Godau, B., Hassani, M. S., and Akbari, M. (2018). Skin tissue substitutes and biomaterial risk assessment and testing. Front. Bioeng. Biotechnol. 6, 86–20180726. doi:10.3389/fbioe.2018.00086
Selvakumar, G., and Lonchin, S. (2022). Bioactive functional collagen-oxidized pullulan scaffold loaded with polydatin for treating chronic wounds. Biomater. Adv. 140, 213078. doi:10.1016/j.bioadv.2022.213078
Serena, T. E., Carter, M. J., Le, L. T., Sabo, M. J., and DiMarco, D. T. (2014). A multicenter, randomized, controlled clinical trial evaluating the use of dehydrated human amnion/chorion membrane allografts and multilayer compression therapy vs. multilayer compression therapy alone in the treatment of venous leg ulcers. Wound Repair Regen. 22, 688–693. doi:10.1111/wrr.12227
Serena, T. E., Yaakov, R., Moore, S., Cole, W., Coe, S., Snyder, R., et al. (2020). A randomized controlled clinical trial of a hypothermically stored amniotic membrane for use in diabetic foot ulcers. J. Comp. Eff. Res. 9, 23–34. doi:10.2217/cer-2019-0142
Shalumon, K. T., Anulekha, K. H., Chennazhi, K. P., Tamura, H., Nair, S., and Jayakumar, R. (2011). Fabrication of chitosan/poly(caprolactone) nanofibrous scaffold for bone and skin tissue engineering. Int. J. Biol. Macromol. 48, 571–576. doi:10.1016/j.ijbiomac.2011.01.020
Sharma, K., Mujawar, M. A., and Kaushik, A. (2019) State-of-Art functional biomaterials for tissue engineering. Front. Mater. Mini Rev. 6. doi:10.3389/fmats.2019.00172
Sharma, P., Navneet, , and Kaushal, A. (2022). Green nanoparticle formation toward wound healing, and its application in drug delivery approaches. Eur. J. Med. Chem. Rep. 6, 100088. doi:10.1016/j.ejmcr.2022.100088
Sheikholeslam, M., Wright, M. E. E., Jeschke, M. G., and Amini-Nik, S. (2018). Biomaterials for skin substitutes. Adv. Healthc. Mater 7, 1700897–1700923. doi:10.1002/adhm.201700897
Shevchenko, R. V., James, S. L., and James, S. E. (2010). A review of tissue-engineered skin bioconstructs available for skin reconstruction. J. R. Soc. Interface 7, 229–258. doi:10.1098/rsif.2009.0403
Singh, M., and Jonnalagadda, S. (2020). Advances in bioprinting using additive manufacturing. Eur. J. Pharm. Sci. 143, 105167. doi:10.1016/j.ejps.2019.105167
Smandri, A., Nordin, A., Hwei, N. M., Chin, K. Y., Abd Aziz, I., and Fauzi, M. B. (2020). Natural 3D-printed bioinks for skin regeneration and wound healing: A systematic review. Polymers 12, 1782. doi:10.3390/polym12081782
Snyder, R. J., Shimozaki, K., Tallis, A., Kerzner, M., Reyzelman, A., Lintzeris, D., et al. (2016). A prospective, randomized, multicenter, controlled evaluation of the use of dehydrated amniotic membrane allograft compared to standard of care for the closure of chronic diabetic foot ulcer. Wounds 28, 70–77.
Stark, H. J., Boehnke, K., Mirancea, N., Willhauck, M. J., Pavesio, A., Fusenig, N. E., et al. (2006). Epidermal homeostasis in long-term scaffold-enforced skin equivalents. J. Investig. Dermatol Symp. Proc. 11, 93–105. doi:10.1038/sj.jidsymp.5650015
Still, J., Glat, P., Silverstein, P., Griswold, J., and Mozingo, D. (2003). The use of a collagen sponge/living cell composite material to treat donor sites in burn patients. Burns 29, 837–841. doi:10.1016/S0305-4179(03)00164-5
Stojic, M., López, V., and Montero, A. (2019). “3 - skin tissue engineering,” in Biomaterials for skin repair and regeneration Editor E García-Gareta (Sawston, United Kingdom: Woodhead Publishing), 59–99.
Supp, D. M. (2011). Skin substitutes for burn wound healing: Current and future approaches. Expert Rev. Dermatology 6, 217–227. doi:10.1586/edm.10.73
Sussman, E. M., Halpin, M. C., Muster, J., Moon, R. T., and Ratner, B. D. (2014). Porous implants modulate healing and induce shifts in local macrophage polarization in the foreign body reaction. Ann. Biomed. Eng. 42, 1508–1516. doi:10.1007/s10439-013-0933-0
Swartjes, J. (2017). “Chapter 5: Multifunctional biomaterials and their bioinspired systems for bioactive molecules delivery,” in Bioinspired materials for medical applications (Sawston, United Kingdom: Woodhead Publishing), 119–137.
Tallawi, M., Rosellini, E., Barbani, N., Cascone, M. G., Rai, R., Saint-Pierre, G., et al. (2015). Strategies for the chemical and biological functionalization of scaffolds for cardiac tissue engineering: A review. J. R. Soc. Interface 12, 20150254. doi:10.1098/rsif.2015.0254
Tan, H., Wasiak, J., Paul, E., and Cleland, H. (2015). Effective use of biobrane as a temporary wound dressing prior to definitive split-skin graft in the treatment of severe burn: A retrospective analysis. Burns 41, 969–976. doi:10.1016/j.burns.2014.07.015
Tavakoli, S., and Klar, A. S. (2021). Bioengineered skin substitutes: Advances and future trends. Appl. Sci. 11, 1493. doi:10.3390/app11041493
Tettelbach, W., Cazzell, S., Reyzelman, A. M., Sigal, F., Caporusso, J. M., and Agnew, P. S. (2019). A confirmatory study on the efficacy of dehydrated human amnion/chorion membrane dHACM allograft in the management of diabetic foot ulcers: A prospective, multicentre, randomised, controlled study of 110 patients from 14 wound clinics. Int. Wound J. 16, 19–29. doi:10.1111/iwj.12976
Tettelbach, W., Cazzell, S., Sigal, F., Caporusso, J. M., Agnew, P. S., Hanft, J., et al. (2019). A multicentre prospective randomised controlled comparative parallel study of dehydrated human umbilical cord (EpiCord) allograft for the treatment of diabetic foot ulcers. Int. Wound J. 16, 122–130. doi:10.1111/iwj.13001
Thavornyutikarn, B., Chantarapanich, N., Sitthiseripratip, K., Thouas, G. A., and Chen, Q. (2014). Bone tissue engineering scaffolding: Computer-aided scaffolding techniques. Prog. biomaterials 3, 61–102. doi:10.1007/s40204-014-0026-7
Tottoli, E. M., Dorati, R., Genta, I., Chiesa, E., Pisani, S., and Conti, B. (2020). Skin wound healing process and new emerging technologies for skin wound care and regeneration. Pharmaceutics 12, 735. doi:10.3390/pharmaceutics12080735
Udeni Gunathilake, T. M. S., Ching, Y. C., and Chuah, C. H. (2017). Enhancement of curcumin bioavailability using nanocellulose reinforced chitosan hydrogel. Polymers 9, 64. doi:10.3390/polym9020064
Urciuolo, F., Casale, C., Imparato, G., and Netti, P. A. (2019). Bioengineered skin substitutes: The role of extracellular matrix and vascularization in the healing of deep wounds. J. Clin. Med. 8, 2083. doi:10.3390/jcm8122083
Utami Nike, D., Md Fadilah, N. I., Sallehuddin, N., Nor Azlan, A. Y. H., Imran, F. H., Maarof, M., et al. (2022). Genipin-crosslinking effects on biomatrix development for cutaneous wound healing: A concise review. Front. Bioeng. Biotechnol. 10, 865014. doi:10.3389/fbioe.2022.865014
Varkey, M., Ding, J., and Tredget, E. E. (2015). Advances in skin substitutes-potential of tissue engineered skin for facilitating anti-fibrotic healing. J. Funct. Biomater. 6, 547–563. doi:10.3390/jfb6030547
Veiseh, O., Doloff, J. C., Ma, M., Vegas, A. J., Tam, H. H., Bader, A., et al. (2015). Size-and shape-dependent foreign body immune response to materials implanted in rodents and non-human primates. Nat. Mater. 14, 643–651. doi:10.1038/nmat4290
Veiseh, O., and Vegas, A. J. (2019). Domesticating the foreign body response: Recent advances and applications. Adv. Drug Deliv. Rev. 144, 148–161. doi:10.1016/j.addr.2019.08.010
Veith, A. P., Henderson, K., Spencer, A., Sligar, A. D., and Baker, A. B. (2019). Therapeutic strategies for enhancing angiogenesis in wound healing. Adv. Drug Deliv. Rev. 146, 97–125. doi:10.1016/j.addr.2018.09.010
Wang, M., Chen, M., Niu, W., Winston, D. D., Cheng, W., and Lei, B. (2020). Injectable biodegradation-visual self-healing citrate hydrogel with high tissue penetration for microenvironment-responsive degradation and local tumor therapy. Biomaterials 261, 120301. doi:10.1016/j.biomaterials.2020.120301
Wang, M., Wang, C., Chen, M., Xi, Y., Cheng, W., Mao, C., et al. (2019). Efficient angiogenesis-based diabetic wound healing/skin reconstruction through bioactive antibacterial adhesive ultraviolet shielding nanodressing with exosome release. ACS Nano 13, 10279–10293. doi:10.1021/acsnano.9b03656
Wang, M. O., Etheridge, J. M., Thompson, J. A., Vorwald, C. E., Dean, D., and Fisher, J. P. (2013). Evaluation of the in vitro cytotoxicity of cross-linked biomaterials. Biomacromolecules 14, 1321–1329. doi:10.1021/bm301962f
Wang, S., Zhang, Y., Sun, F., Xi, K., Sun, Z., Zheng, X., et al. (2023). Catalase-like nanozymes combined with hydrogel to facilitate wound healing by improving the microenvironment of diabetic ulcers. Mater. Des. 225, 111557. doi:10.1016/j.matdes.2022.111557
Yan, W., Diao, S., and Fan, Z. (2021). The role and mechanism of mitochondrial functions and energy metabolism in the function regulation of the mesenchymal stem cells. Stem Cell Res. Ther. 12, 140–20210217. doi:10.1186/s13287-021-02194-z
Yang, S., Li, X., Liu, P., Zhang, M., Wang, C., and Zhang, B. (2020). Multifunctional chitosan/polycaprolactone nanofiber scaffolds with varied dual-drug release for wound-healing applications. ACS Biomaterials Sci. Eng. 6, 4666–4676. doi:10.1021/acsbiomaterials.0c00674
Yang, S., Li, X., Liu, P., Zhang, M., Wang, C., and Zhang, B. (2020). Multifunctional chitosan/polycaprolactone nanofiber scaffolds with varied dual-drug release for wound-healing applications. ACS Biomater. Sci. Eng. 6, 4666–4676. doi:10.1021/acsbiomaterials.0c00674
You, C., Li, Q., Wang, X., Wu, P., Ho, J. K., Jin, R., et al. (2017). Silver nanoparticle loaded collagen/chitosan scaffolds promote wound healing via regulating fibroblast migration and macrophage activation. Sci. Rep. 7, 10489–10511. doi:10.1038/s41598-017-10481-0
Zawani, M., and Fauzi, M. B. (2021). Injectable hydrogels for chronic skin wound management: A concise review. Biomedicines 9, 527. doi:10.3390/biomedicines9050527
Zelen, C. M., Orgill, D. P., Serena, T. E., Galiano, R. E., Carter, M. J., DiDomenico, L. A., et al. (2018). An aseptically processed, acellular, reticular, allogenic human dermis improves healing in diabetic foot ulcers: A prospective, randomised, controlled, multicentre follow-up trial. Int. Wound J. 15, 731–739. doi:10.1111/iwj.12920
Zelen, C. M., Serena, T. E., Denoziere, G., and Fetterolf, D. E. (2013). A prospective randomised comparative parallel study of amniotic membrane wound graft in the management of diabetic foot ulcers. Int. Wound J. 10, 502–507. doi:10.1111/iwj.12097
Zhang, K., Bai, X., Yuan, Z., Cao, X., Jiao, X., Li, Y., et al. (2019). Layered nanofiber sponge with an improved capacity for promoting blood coagulation and wound healing. Biomaterials 204, 70–79. doi:10.1016/j.biomaterials.2019.03.008
Zhang, S., Ou, Q., Xin, P., Yuan, Q., Wang, Y., and Wu, J. (2019). Polydopamine/puerarin nanoparticle-incorporated hybrid hydrogels for enhanced wound healing. Biomaterials Sci. 7, 4230–4236. doi:10.1039/c9bm00991d
Keywords: multifunctional bioscaffolds, skin substitutes, biomaterials, wound healing, skin tissue engineering
Citation: Fadilah NIM, Riha SM, Mazlan Z, Wen APY, Hao LQ, Joseph B, Maarof M, Thomas S, Motta A and Fauzi MB (2023) Functionalised-biomatrix for wound healing and cutaneous regeneration: future impactful medical products in clinical translation and precision medicine. Front. Bioeng. Biotechnol. 11:1160577. doi: 10.3389/fbioe.2023.1160577
Received: 07 February 2023; Accepted: 08 May 2023;
Published: 24 May 2023.
Edited by:
Hilal Turkoglu Sasmazel, Atılım University, TürkiyeReviewed by:
Soodabeh Davaran, Tabriz University of Medical Sciences, IranPriyanka Tyagi, GD Goenka University, India
Copyright © 2023 Fadilah, Riha, Mazlan, Wen, Hao, Joseph, Maarof, Thomas, Motta and Fauzi. This is an open-access article distributed under the terms of the Creative Commons Attribution License (CC BY). The use, distribution or reproduction in other forums is permitted, provided the original author(s) and the copyright owner(s) are credited and that the original publication in this journal is cited, in accordance with accepted academic practice. No use, distribution or reproduction is permitted which does not comply with these terms.
*Correspondence: Mh Busra Fauzi, ZmF1emlidXNyYUB1a20uZWR1Lm15