- Department of Microsystems, Faculty of Technology, Natural Sciences and Maritime Sciences, University of South-Eastern Norway, Horten, Norway
Recent research aiming at the development of electroceuticals for the treatment of medical conditions such as degenerative diseases, cardiac arrhythmia and chronic pain, has given rise to microfabricated implanted bioelectronic devices capable of interacting with host biological tissues in synergistic modalities. Owing to their multimodal affinity to biological tissues, hydrogels have emerged as promising interface materials for bioelectronic devices. Here, we review the state-of-the-art and forefront in the techniques used by research groups for the integration of hydrogels into the microfabrication processes of bioelectronic devices, and present the manufacturability challenges to unlock their further clinical deployment.
1 Introduction
Since inception of the first-in-human implanted device in the form of an artificial pacemaker in 1932 (Aquilina, 2006), integrated electronics interfacing directly with biological tissue have widely grown in interest. Conditions such as bladder dysfunction (Clausen et al., 2018), chronic pain (Finch et al., 2019), spinal cord injury (Wagner et al., 2018), neurodegenerative diseases (Khedr et al., 2019) and heart failure (Nguyen et al., 2016; Jorbendaze et al., 2022) are increasingly treated with medical devices establishing electrical interactions with the targeted organs or the nervous structures governing their functions. Bioelectronic medical devices are implanted into the biological host to provide diagnostic and/or therapeutic capabilities, most commonly in the form of signal recording and electrical stimulation. Recent research bridging the fields of medicine and micro- and nanotechnology aims to unlock the fabrication of bioelectronic devices that leverage microfabrication techniques and their inherent advantages of miniaturization and design flexibility for personalization (Vomero and Schiavone, 2021). Historically, the first micrometre-sized bioelectronic devices, embodied as recording neural electrode arrays, spurred from the advances in semiconductor manufacturing of the 1970s (Scholten and Meng, 2015). Despite the maturity and reliability of silicon technology, however, the rigidity of the associated substrate, conductor and insulating materials, quantified by Young’s moduli E in the 100 GPa range, together with planar form factors, introduces a mechanical mismatch to soft biological tissues (E in the kPa range), hindering seamless device biointegration (Lacour et al., 2016).
2 Biointerface matching: Mechanical, chemical and electrical
Mechanical mismatch results from a combination of material properties and device geometry. In the case of surface-deployed devices (membrane-like), their stiffness can be estimated by the flexural rigidity, which is proportional to the materials’ Young’s moduli and the cubic power of the thickness. A possible solution to lower the mechanical mismatch is therefore to reduce the substrate thickness. This strategy has materialized in ultra-thin (<10 µm) bioelectronic interfaces using GPa-range polymers such as SU-8, Polyimide and Parylene-C as substrate material (Cho et al., 2008; Luan et al., 2017; Vomero et al., 2022). While effectively lowering the flexural rigidity, ultra-thin form factors introduce significant challenges in device handling, requiring complex ad hoc tools to enable surgical manipulation (Luan et al., 2017; Vomero et al., 2020), and possible limitations to area coverage (less than few cm2). An alternative strategy to lower the mechanical mismatch is to use softer materials (E < 1 MPa) for the structural elements of the devices. For a given flexural rigidity value, using low modulus materials enables larger thicknesses and therefore easier handling (Fallegger et al., 2021). While initial steps in this direction have been explored in recent years with elastomer-based bioelectronic interfaces (Lee K. Y. et al., 2020; Renz et al., 2020; Schiavone et al., 2020a), further advances may be achieved by using even softer materials. Hydrogels (E < 100 kPa) for instance, can be engineered to present mechanical, chemical and electrical properties enabling a more comprehensive matching at the device-tissue interface.
Hydrogels are defined as cross-linked polymeric networks capable of absorbing large quantity of water (up to 90% in weight) without dissolving (Li et al., 2015). Hydrophilic functional groups (e.g., COOH, NH2, OH) bonded to the polymer introduce affinity with water. The cross-linking between the polymer macromolecules provides the resistance to dissolution and the ability to maintain a 3D structure in the swollen state (Bashir et al., 2020). In addition to the mechanical advantages, the nature of such materials provides affinity towards the chemical properties of biological tissues (Yuk et al., 2020), characterized by high water-to-weight ratios of approximately 70% for muscles and skin, 75% for the heart, 80% for the lungs and 85% for the brain (Yuk et al., 2022). The immune system recognizes and targets hydrophobic regions of biomolecules as a universal molecular pattern associated with damage (Seong and Matzinger, 2004; Roh and Sohn, 2018). Although non-cytotoxic, the hydrophobic nature of most bioelectronic implants is thought to trigger proinflammatory protein responses (Scholten and Meng, 2015; Mariani et al., 2019), which in turn activate the immune cells responsible for the scar formation around the implanted device (Franz et al., 2011). Over long implantation periods, this phenomenon can lead to the formation of a fibrotic tissue encapsulation around the device (Prodanov and Delbeke, 2016), compromising its functionality (Williams, 2008) and potentially damaging both tissue (Biran et al., 2005) and device (Song et al., 2022). With their ability to absorb water, hydrogels are intrinsically hydrophilic. Used at the biological interface, they limit the adsorption of proinflammatory proteins by inhibiting their hydrophobic domain to attach to the surface of the device (Wellman and Kozai, 2017). At the current preclinical state of research, implants using hydrogels as interface have been shown to benefit from decreased foreign body reaction (Zhang et al., 2013; Yan et al., 2019; Gori et al., 2022).
For a bioelectronic interface to electrically interact and relay information to and from biological tissue, several components are required, as shown in Figure 1. Biological tissue generates biopotentials through ionic interactions in the vicinity of a working electrode, which can use different charge transduction mechanisms to convert them into a measurable signal (Schiavone et al., 2021). Electrodes transmit such signals through embedded conductors to the electronic circuitry (e.g., solid state amplifiers, logic, power supply) that processes and relays them to the user instrumentation via cables, connectors and/or telemetry modules. Similarly for electrical stimulation, pulses of current are relayed through electronic circuitry to the implanted working electrode, which injects the charge to the ionic carriers in the tissue. The performance of bioelectrodes is typically measured by their electrochemical impedance and charge transduction properties, that reflect how efficiently the interface mediates the transmission of signals of different waveform, frequency and amplitude between the electronic circuitry and the ionic medium (Schiavone et al., 2020b). Although metals offer high electrical conductivity, this property per se does not necessarily guarantee efficient charge transduction (Seyedkhani et al., 2022), and extensive research has focused on developing optimal interface materials mediating electronic-ionic signal transmission. For instance, it has been demonstrated that metal electrodes coated with organic conductors can outperform uncoated controls (Khodagholy et al., 2011; Venkatraman et al., 2011). Materials offering mixed electronic and ionic electrical conduction exhibit in particular high charge transduction properties (Cogan, 2008). Adding to the mechanical and chemical matching advantages described in the previous paragraphs, hydrogels can be engineered to embed mixed-conduction polymers (typified by PEDOT:PSS), and therefore offer efficient ionic-electronic signal transduction at the interface with biological tissue (Ido et al., 2012; Kleber et al., 2017).
3 Classification, engineering and functionalization of hydrogels
Numerous classifications have been proposed for hydrogels according to different categories of material properties. Table 1 provides a comprehensive list of the possible classification criteria found in the literature, accompanied by corresponding examples of published research.
One of the most desirable features of hydrogels is the ability to modify and fine-tune their properties to render them adapted to a broad range of applications. They can be customized to achieve better affinity with the cellular environment, tailored to mimic the water content of biological tissue, and synthesized with precise mechanical properties such as higher elasticity and conformability. In the following section, we present techniques that have been used to engineer hydrogel materials to optimize their function in bioelectronic devices.
3.1 Mechanical properties and porosity
The elastic modulus of hydrogels is proportional to the density of crosslinks between the polymer chains, the crosslink length and the molecular weight of the precursor (Li et al., 2018). One of the properties that limits hydrogel use, particularly in medical applications, is their brittle nature. Several strategies have been proposed to overcome this challenge, such as using IPN matrix gels (Dragan, 2014), loading the hydrogel with nanoparticles (Chen et al., 2021) or nanofibers such as silk fibroin (Cui et al., 2021), as well as chitin (Ge et al., 2018; Suo et al., 2018). Plasticizers such as lauric acid, glycerol, sorbitol and PEG constitute an alternative option to increase flexibility and tensile strength especially in natural hydrogels without compromising cell viability (Reis et al., 2006; Snejdrova and Dittrich, 2012; Sun et al., 2018; Tarique et al., 2021).
In biointerfaces, the pore size and distribution within the 3D hydrogel matrix play an important role in cell growth (Přádný et al., 2014), with large pores (20–80 µm) enabling cell proliferation and smaller pores (1 µm) allowing for nutrient and oxygen supply (Janoušková et al., 2019). For electrochemical electrode interfaces, porosity increases the electroactive surface area of a working electrode (Zhu and Zhao, 2017) allowing for more efficient charge injection or higher quality signal recording (Cogan, 2008). Porosity can be engineered by lyophilization, a process in which the hydrogel is quickly frozen to induce a phase separation between the polymeric network and the solvent. The latter is then removed by sublimation, leaving cavities in the parts it previously occupied. Another method is gas foaming, where a foaming agent chemically reacts with the precursor hydrogel solution to create bubbles (e.g., CO2 formed in acidic environment). Electrospinning can also be used to fabricate fibrous and porous hydrogel scaffolds. The fibres are created by applying an external voltage to the precursor polymeric solution, which is then ejected through a spinneret. Part of the solvent volatilizes and the filaments are collected on a collector plate (Annabi et al., 2010).
3.2 Electrical conductivity
Electrical conductivity can be mediated either ionically or electronically. Owing to their ability to absorb biological fluids where ionic interactions are predominant, hydrogels can indirectly act as ionic conductors. In this case, the electrical conductivity of ionic hydrogels (0.1–10 S/m) (Yang and Suo, 2018) remains comparable to that of biological tissue (0.03–1.6 S/m) (Martinsen et al., 2008). It is possible to further increase ionic conductivity by doping the hydrogel with salts such as NaCl, LiCl, FeCl3, KCl or CaCl2, but only to a certain extent, since excessive concentrations can cause tissue damage (Peng et al., 2020). Another option is to use conductive polymers such as PPy, PEDOT, PANi. The aromatic groups in the polymeric chains contain π-conjugations (alternating single and double covalent bonds) with free electrons, conferring electronic conductivity (10–3–105 S/cm) (Nezakati et al., 2018; Gao et al., 2022).
More recently, doping of hydrogels with conductive nanomaterials has gained interest as a method to achieve higher conductivity ranges. As notable examples, hydrogels doped with silver particles have displayed conductivities in the range of 1.36–374 S/cm (Devaki et al., 2014; Ohm et al., 2021), while doping with graphene or carbon nanotubes has enabled conductivities of 4 × 10−5–4.2 × 10−3 S/cm (Zhou et al., 2018; Park et al., 2019) and 0.01–10 S/cm (Cho and Borgens, 2010; Liu et al., 2014), respectively.
When discussing conductivity in biointerfaces, it is worth noting that bioelectronic devices require spatially confined electrically conductive regions in contact with tissue (i.e., electrode contacts), while the remaining device surface must be electrically insulated. This ensures that electrical interfacing is established at precisely defined locations only. As hydrogels absorb the surrounding ionically conductive fluids, they cannot be used as insulation for electrodes and conductors, and careful device design integrating suitable barrier layers is required to ensure correct functionality.
3.3 Adhesion
When hydrogels are used as interface material between a bioelectronic device and biological tissue, controlling the adhesion to both parts is crucial. The abundant functional groups present on biological tissues such as hydroxyl, carboxylic acid, thiol and amino groups can be leveraged to anchor the hydrogel via chemical or physical bonds (Yang et al., 2022). Physical binding results mainly from electrostatic, Van der Walls and hydrogen bonds, and is by nature weaker than covalent bonding. To promote adhesion, hydrogels can be functionalized with chemical moieties capable of binding to the functional groups naturally present in tissues. Common chemical reactions to form covalent bonds to tissue are Michaelson’s addition and Schiff’s base reaction (Cong and Fu, 2022). Additionally, several nature-inspired approaches have also been demonstrated. Mimicking mussels’ ability to adhere to surfaces in sea water, polydopamine has been introduced as a plaster to promote adhesion to wet surfaces (Chalmers et al., 2020). The catechol, imine and amine groups present in polydopamine allows adhesion on different surfaces (e.g., PDMS, paper, PI, glass, metals) through π-π stacking, hydrogen and covalent bonding (Ryu et al., 2018). A third option is mechanical intermeshing, achievable by surface roughness or microstructured patterns (Fan and Gong, 2021). Notable examples are reversible adhesion by octopus-inspired microstructures (Lee et al., 2016) and hexagonal mesh grids of clingfish (Rao et al., 2018).
3.4 Self-healing
In analogy to the resilience of biological tissues, self-healing hydrogels have been introduced with the ability of restoring their electrical and mechanical properties after rupture (Lei et al., 2017; Deng et al., 2018; Robby et al., 2019; Talebian et al., 2019; Ge et al., 2020; Su et al., 2021). This recovery property originates from reversible bonds present within the material architecture (Taylor and in het Panhuis, 2016), and more specifically owing to the intrinsic ability of chemical molecules to recreate a bond after rupture. Healing mechanisms have been reported involving both covalent and non-covalent, ionic or hydrogen, bonds. For example, hydrogen or ionic bonds can be broken and re-established between, respectively, two hydroxyl groups (OH-OH), or carboxyl groups (COOH) and Fe3+ (Devi V. K. et al., 2021). Reversible covalent bond break and formation has equally been reported between disulfide (S-S) and imine bonds (C=N) (Talebian et al., 2019).
3.5 Loading with pharmacological agents
In pharmacological applications, hydrogels have been extensively employed to facilitate the release of concentrated drugs or chemical molecules over a prolonged period of time through diffusion, swelling or environmental stimuli. The problems of systemic toxicity and repeated administration that conventional drug carriers might cause can be avoided by hydrogel-mediated release (Narayanaswamy and Torchilin, 2019). Specifically for implanted bioelectronic devices, hydrogel loading with drugs to inhibit inflammatory response in the surrounding tissue has been proposed (Nguyen et al., 2022). Growth factors are another type of bioactive molecules that can be incorporated into hydrogel matrices. They are crucial factors in tissue repair and regeneration, however biostability and yield challenges related to their rapid degradation before reaching the target are yet to be fully solved (Tayalia and Mooney, 2009). The use of electrical stimulation to facilitate the release of growth factor molecules (e.g., neurotrophins, myostatin, thrombopoietin) embedded in conductive hydrogel carriers in a controlled manner has shown great potential for cell adhesion, proliferation and differentiation (Liu et al., 2021; Cheah et al., 2023). The porous structure of conductive hydrogels offers an additional advantage compared to the polymer counterparts, as larger amounts of bioactive molecules can be stored within the matrix and released for a prolonged period of time (Caballero Aguilar et al., 2019).
3.6 Degradability
Degradability is an essential parameter for implantable devices. Depending on the therapeutic timeframes, strategies towards long- or short-term degradation can be employed. Degradability can be tailored by using intrinsically biodegradable material (e.g., HA, collagen) or by integrating molecules or degradable polymeric segments into the hydrogel matrix. Functional groups such as esters, anhydrides and thioesters are subject to hydrolysis. Incorporated to the polymeric chains, they can react with water and/or enzymes, leading to the dissolution of the hydrogel (Ozcelik, 2016). Copolymeric hydrogels containing alternatively synthetic polymeric sequences and peptide or protein units are commonly used to fabricate biodegradable composites (Kopeček and Yang, 2007; Patterson et al., 2010). The use of low crosslinking degree and low molecular weight crosslinkers is another way to promote degradation (Kong et al., 2004). In cases where degradation is unwanted, non-toxic synthetic hydrogels such as PEG and PHEMA are viable options. However, it has been demonstrated that PEG can trigger foreign body response and although non-biodegradable it can be damaged by acids, reactive oxygen intermediates, enzymes, etc., discharged by macrophages and foreign body giant cells (Browning et al., 2014). Zwitterionic hydrogels have recently been introduced as an excellent antifouling and non-degradable material. Owing to their superhydrophilicity, their stability in vivo has for instance been proven for up to 1 year in mice (Dong et al., 2021).
3.7 Other uses
Finally, beyond microfabricated bioelectronic interfaces per se, hydrogels have found employment as structural materials in related applications. Saeki et al. (2020) have reported on the use of alginate as sacrificial matrix to fabricate protein-based microfibers (Li S. et al., 2020). Another scope of particular interest is the fabrication of scaffolds for artificial organs, enabling biomimetic replicas of biological structures for in vitro testing prior to implantation, contributing to a reduction in the use of animals for experimentation (Tringides et al., 2021).
In sum, extensive research has been published to date on the synthesis, functionalization and characterization of hydrogels. However, the integration of this class of materials into manufacturing processes for complete microfabricated devices remains today at a seminal stage. The following section introduces the methods that have been used by researchers to integrate hydrogels into the process flow for microfabricated bioelectronic devices, and presents an understanding of the status of the technology as well as future challenges.
4 Integration of hydrogels in microfabricated bioelectronic devices
We classify microfabricated bioelectronic devices with integrated hydrogel layers into two different categories: devices using hydrogels as encapsulation only, to form the chemo-mechanical interface with biological tissue; and devices using hydrogels as elements performing engineered functions (electrical, drug release, etc.). This classification based on device architecture is matched by a corresponding classification of the associated manufacturing methods. Typically, encapsulating devices with a hydrogel layer or shell is achieved using non-selective coating processes such as dip coating, drop casting or spin coating. When, however, devices integrate functional hydrogel elements, further micropatterning processes are required in addition to the previous coating processes, introducing a higher level of manufacturing complexity. To this end, conventional silicon and MEMS foundry techniques are not suited for soft and wet materials, and manufacturing processes must therefore be adapted or developed anew by avoiding, for instance, high temperatures and incompatible chemicals. Ad-hoc microfabrication methods for the patterning of hydrogel structures include both subtractive techniques, such as photo- and soft lithography and laser patterning, and additive techniques such as inkjet, direct ink, and screen printing. These are illustrated in Figure 2 and discussed in the following sections.
4.1 Coating techniques
Bioelectronic devices that use hydrogels as substrate and/or encapsulation can be fabricated using drop casting and spin-coating methods to deposit the gel on a flat surface such as a glass or silicon carrier (Macron et al., 2019; Zhou et al., 2020), or dip coating of arbitrarily-shaped surfaces (Yin et al., 2018). An alternative technique to coat hydrogels on organic or inorganic surfaces is initiated chemical vapor deposition (iCVD), where monomer, initiator and crosslinker are introduced in vapour phase in a vacuum chamber. A heated filament activates the initiator and hydrogel synthesis occurs on the substrate surface kept at room temperature. High precision thickness and topography can be achieved without subjecting the substrate to high temperatures or solvents (Yagüe and Gleason, 2012).
4.2 Photolithography
Photolithography is one of the most common patterning techniques, where a hydrogel precursor solution (prehydrogel) previously coated on a carrier surface is illuminated through a photomask. The light patterns generated by the mask lead to selective crosslinking or polymerization of the illuminated areas, while leaving the rest of the material soluble to a developing agent. This well-established technique offers several advantages such as repeatability, infrastructure availability, the ability to form high resolution patterns (down to 100 nm, and 50 nm using deep UV) (Xu and Siedlecki, 2017), arbitrarily complex in-plane shapes, and multilayer structures by sequential lithographic steps (Tenje et al., 2020). However, the use of photolithography is limited to photosensitive crosslinkers or initiators that are usually cytotoxic due to the radical molecules needed to induce the chemical reactions (Fedorovich et al., 2009; Mironi-Harpaz et al., 2012). In some specific cases of prehydrogels loaded with cells or proteins, UV exposure is of concern for cell viability (Masuma et al., 2013). Lastly, it has been reported that the opacity of photolithographically synthesized hydrogel can cause uneven light exposure, leading to crosslinking gradients within the matrix (Tenje et al., 2020).
4.3 Soft lithography
Soft lithography refers to the fabrication or replication of patterns using elastomeric stamps or moulds, commonly PDMS, enabling resolutions down to 100 nm. Patterned stamps are placed in contact with the precursor solution, and capillary forces, heat, physical gelation, chemical or UV crosslinking/polymerization form hydrogel patterns matching the stamp. Contrary to photolithography, the resolution is not limited by optical diffraction, but wettability, Van Der Walls and capillary forces (Nur and Willander, 2020). The main benefits of soft lithography are high resolution and low cost for mass production, suitability to biological samples, compatibility with a wide variety of materials, regardless of their photosensitivity. However, moulds and stamps are manufactured using photo- or e-beam lithography (for nanostructures), which may reduce the cost benefits. For precursor hydrogel solutions requiring UV light to induce polymerization, photocrosslinkers can be detrimental to cells and proteins. Multilayer devices have not yet been demonstrated with soft lithography due to alignment challenges (Nur and Willander, 2020).
4.4 Laser patterning
Laser patterning refers to additive and subtractive methods of shaping hydrogels. Direct laser patterning exploits a highly focused light beam to induce localized polymerization or crosslinking to create complex 3D structures. Stereolithography and two-photon photopolymerization are the most reported techniques using lasers. In stereolithography, the precursor hydrogel solution is placed in a tank where the hydrogel is shaped layer by layer through light exposure according to a predefined pattern. The thickness of each layer of the final hydrogel is determined by the motion of a vertical stage. Two-photon polymerization uses femtosecond pulsed lasers to locally crosslink the precursor solution placed in a reservoir in a spatially controlled manner. Similarly, to photolithography, these methods are limited to photosensitive hydrogels precursors. However, it is worth mentioning that hydrogels loaded with cells or proteins would benefit from two-photon polymerization process, as this technique uses near IR which is less harmful for living organisms than UV light (Tenje et al., 2020).
Laser cutting is the subtractive alternative to laser polymerization, where the laser is used to locally break bonds and shape hydrogel structures. It is noteworthy that the opacity of the hydrogel limits the penetration depth of light. Both techniques can achieve µm resolution, but are time consuming (serial process) and require ad hoc tools (Verhulsel et al., 2014).
4.5 Inkjet and direct ink writing
Inkjet printing is technique in which hydrogel drops are dispensed at precise locations to form predefined patterns. A heater or piezoelectric actuator is used to eject droplets with resolution of 50–500 μm, at speeds up to 5000 drops/s. For hydrogels, the process requires rapid crosslinking and is constrained by the viscosity of the ink (1–15 mPa s in the case of thermal actuators and up to 100 mPa s for piezoelectric actuators), so as to avoid nozzle clogging (Yanagawa et al., 2016; Makrygianni et al., 2018).
Building on inkjet printing, direct ink writing is an additive method enabling the fabrication of complex three-dimensional structures. A viscous hydrogel precursor solution is extruded through a nozzle using pneumatic or screw actuation, and 3D structures are built layer-by-layer on a stage by temperature solidification, physical or chemical gelation. Viscosity, gelation kinetics, sheer-thinning and thixotropic properties are crucial parameters for process development (Liu S. et al., 2020). Direct ink writing has been demonstrated with collagen (Kim et al., 2016), gelatin (Billiet et al., 2014), chitosan (Wu et al., 2018) and alginate (Li et al., 2017). Resolutions down to 30 µm can be achieved (Yuk et al., 2020).
For both techniques, it is possible to integrate biological elements to the ink (e.g., proteins), notably to replicate extracellular matrix and favour cellular growth and differentiation (Nam and Park, 2018). In this case, this method is referred to as bioprinting.
4.6 Transfer printing
Transfer printing enables electrical circuits fabricated on a separate donor substrate using conventional methods to be transferred onto a hydrogel acceptor substrate. The electrical conductors are first patterned on the donor substrate. Next, a hydrogel layer is deposited on top of the patterned conductors and then lifted off. This method eliminates the need for the hydrogel to be suitable as substrate for subsequent conductor deposition and patterning. However, the technique relies on the careful interplay of adhesion forces between donor, transfer patterns, and the hydrogel substrate (Zhou et al., 2019).
4.7 Screen printing
Screen printing enables the formation of patterns on a surface by applying a viscous material through a screen and a stencil mask, which is machined to match the desired patterns and aligned to the underlying substrate. This technique offers the advantages of low cost and ease of manufacturing, a resolution down to 300 µm (He et al., 2019; Pandala et al., 2020) and sterilized stencils can be used to fabricate cell culture hydrogel scaffolds (Pandala et al., 2021).
Despite the wide range of possible techniques made available from the microelectronic manufacturing industry, their application to hydrogel materials as part of complete bioelectronic devices has not yet benefitted from collective efforts in standardization and adoption. The technological processes used today to fabricate the devices presented in the scientific literature are inherited from silicon or MEMS foundry, and adapted case-by-case to specific materials and designs. Wide applicability of these techniques has therefore not yet been achieved.
5 Applications in bioelectronic medicine and technology readiness levels
Due to their versatility in functionalisation, shape and stimuli-responsiveness, hydrogels have been extensively explored for medical applications: from contact lenses, dentistry (Han et al., 2017), surgical adhesives (Zhang et al., 2020), cartilage treatment (Chuang et al., 2018; Wei et al., 2021), bone regeneration (Kuroda et al., 2019; Lee C.-S. et al., 2020), to drug delivery (Raina et al., 2022). Several hydrogel materials developed for tissue engineering are currently being tested in clinical trials or have been granted authorization for commercialization (Mandal et al., 2020). However, microfabricated bioelectronic devices using hydrogels either as functional electrical elements or as passive interface layers are still in their infancy, with research still at an early stage, as shown by the range of publication dates. While many proofs of concept in the literature provide elements of feasibility and relevance, a clinic-ready, let alone commercially available, bioelectronic device, is yet to be found. Figure 3A displays a selection of hydrogel-based bioelectronic devices reported in the scientific literature, with illustrations of their intended deployment. Table 2 lists a selection of notable examples of complete bioelectronic devices, classified according to the microfabrication process employed, and scored on a qualitative scale indicating the level of maturity based on the preclinical validation data reported.
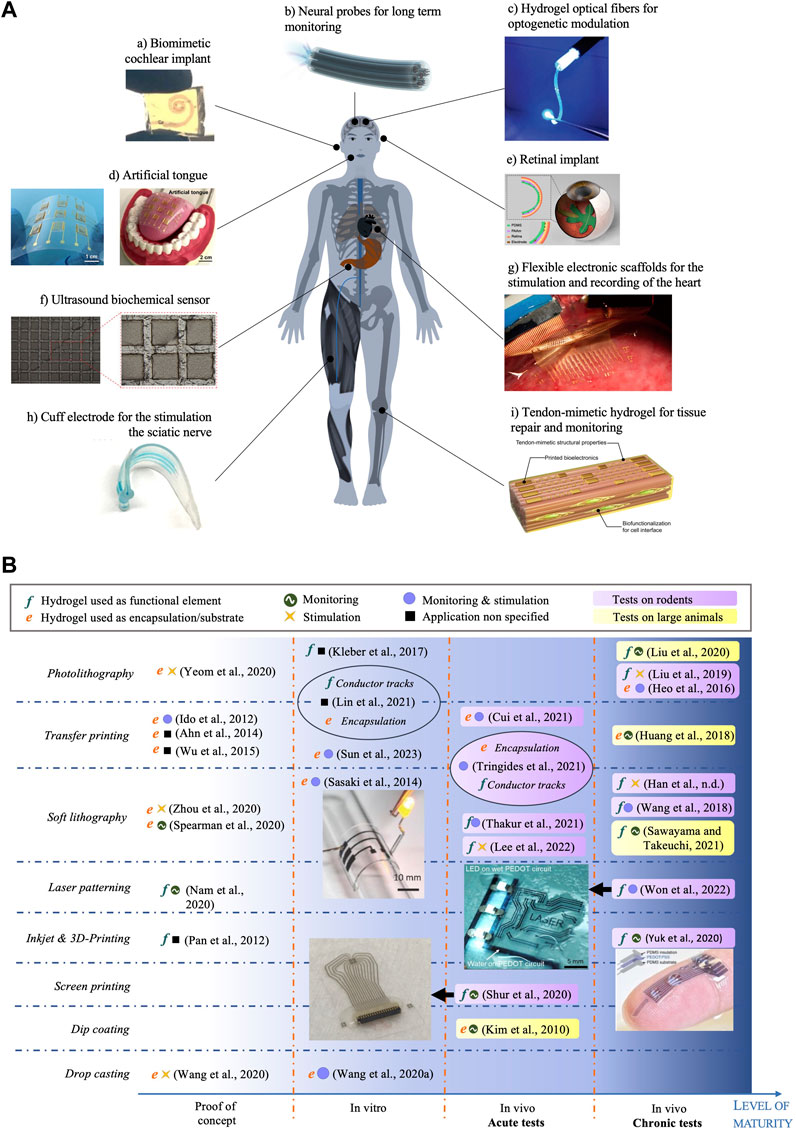
FIGURE 3. (A) Example applications of hydrogel-based bioelectronic devices. Reproduced with permission from reference: (a) (Lei et al., 2017), copyright 2021 Springer Nature. (b) (Park et al., 2019), copyright 2020 Springer Nature. (c) (Wang et al., 2017), copyright 2018 Wiley-VCH. (d) (Yeom et al., 2020), copyright 2020 American Academy for the Advancement of Science. (E) (Zhou et al., 2020). (f) (Nam and Park, 2018), copyright 2020 Frontiers. (g) (Li J. et al., 2020). (h) (Thakur et al., 2021). (i) (Sun et al., 2023), copyright 2023 American Academy for the Advancement of Science. (B) Map of hydrogel-based bioelectronic devices according to the microfabrication technique to process hydrogel layers (rows) and their level of maturity (x-axis). Reproduced with permission from reference (Sasaki et al., 2014), (Won et al., 2022), (Yuk et al., 2020) and (Shur et al., 2020). Copyright 2014 Wiley, 2022 American Academy for the Advancement of Science, 2020 Springer Nature and 2020 American Chemical Society respectively.
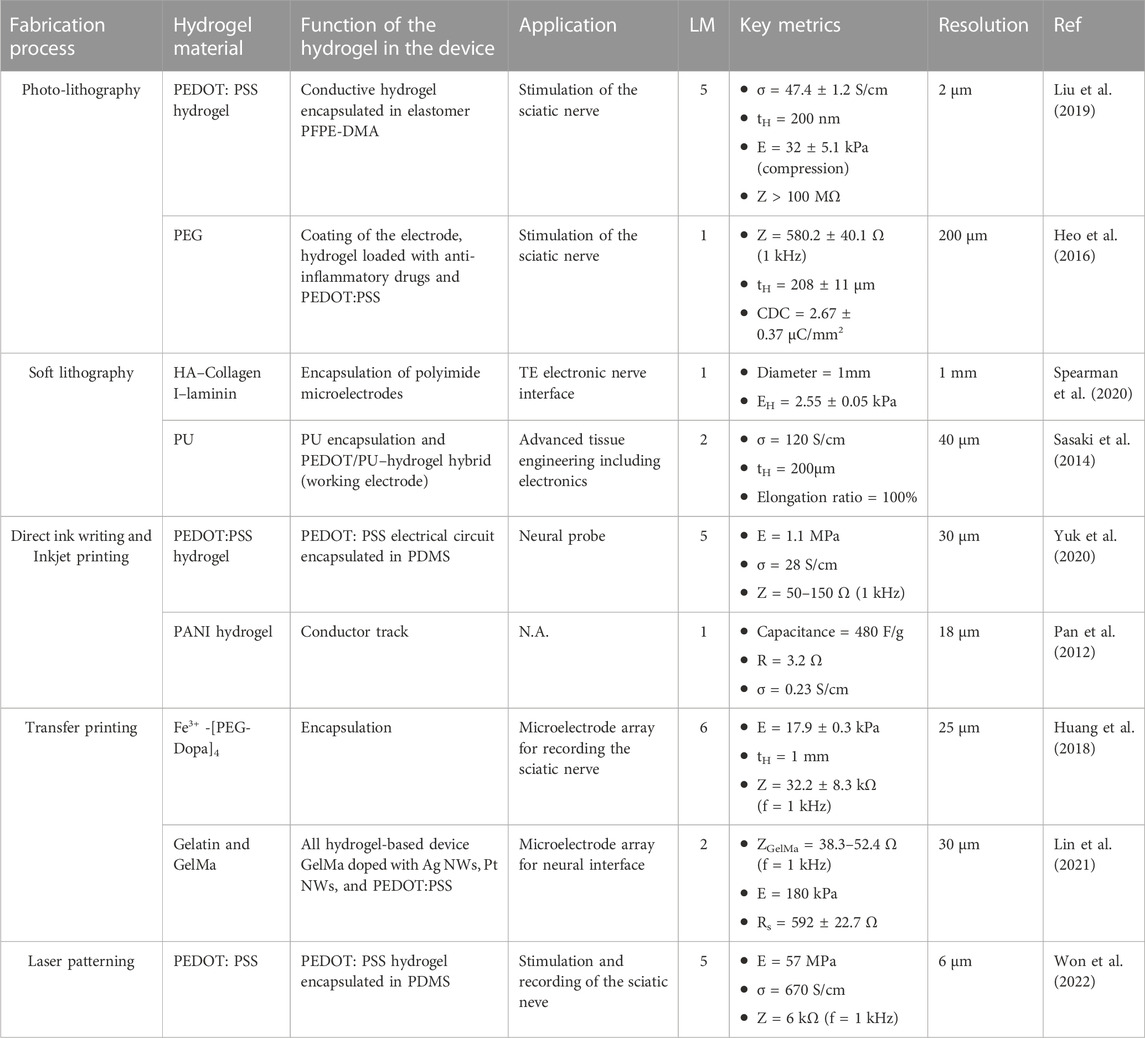
TABLE 2. Selection of hydrogel-based bioelectronic devices, classified according to the fabrication technique. Devices are qualitatively scored based on a level of maturity (LM) scale: 1- Proof of concept (mechanical, electrical and electrochemical testing); 2- In vitro testing (e.g., cytotoxicity); 3- In vivo acute testing on small animals (rodents); 4- In vivo acute testing on large animals (pigs, monkeys, cats, dogs); 5- In vivo chronic testing on rodents; 6- In vivo chronic testing on large animals; 7- Clinical trials.
Figure 3B provides an overview on the status of the technology, with a map of all complete hydrogel-based microfabricated bioelectronic devices published in the literature, to the best of our knowledge. We classify the devices according to the technique used to process the hydrogel material (rows) and the level of maturity based on the validation data presented (x-axis). This map reveals that most of the published work includes acute and chronic tests conducted with rodents to confirm the ability to deliver electrical stimulation or to record electrophysiological responses. In smaller proportion, device operation tests with pigs (Sawayama and Takeuchi, 2021), cats (Huang et al., 2018) and rabbits (Xue et al., 2021) have been reported. In terms of microfabrication approach, the majority of the reported devices are manufactured using photolithography, soft lithography and transfer printing techniques, owing to the wider availability of the associated equipment. Some of the devices integrate hydrogels both as a functional electrical element and as an encapsulation using different microfabrication methods (Lin et al., 2021; Tringides et al., 2021). Finally, we note that no device has been tested in clinical trials yet, testifying to the novelty of this material technology. Some devices were tested chronically in vivo on both rodents and large animal models, suggesting a translational pathway planning. The longest in vivo implantation period reported thus far is 6 weeks. For example, Liu et al. (2020a) successfully implanted a hydrogel based electrode for chronic epicardial and endocardial mapping of the heart in a pig for 6 weeks. Won et al. (2022) showed that electrodes made of PEDOT:PSS encapsulated in soft SBS produced minimal damage to the sciatic nerve tissue in comparison with a rigid Au cuff electrode control, after 4 weeks implantation in mice.
6 Discussion
Hydrogels have extensively gained research interest over the past two decades (from less than 500 publications per year in 2002 to over 10,000 in 2022) (Correa et al., 2021). Owing to their wide range of property tunability, they are promising interface materials for mimicking the mechanical, chemical and electrical properties of biological tissues. Most contributions to the scientific literature focus on the synthesis and structuring of hydrogels, both at the micro and macro scale. However, full integration of hydrogel materials into minimally invasive and biomimetic bioelectronic devices is yet to be achieved at large scale, as numerous important technological and manufacturability challenges remain unsolved.
Patterning small features on hydrogels for long term implantation is a challenging endeavour, as they tend to degrade much faster compared to inorganic materials. Biostability data varies widely: Wang et al. (2017) reported that 87% of a chitosan/alginate hydrogel degraded after 12 days in vivo, while Browning et al. (2014) showed PEGDA hydrogels, subcutaneously implanted, stabile for up to 12 weeks. Stability in biological media over time is an essential requirement that has yet to be widely demonstrated with hydrogel-based devices. A possible strategy to address this challenge is to use synthetic monomers to further increase the lifetime of the structure (Takeda et al., 2015; Loh et al., 2020). In general, however, beside isolated examples, developing robust and standardized manufacturing methods accessible to the wider bioelectronic microfabrication community has yet to be achieved to unlock reproducible manufacturability of hydrogels at the microscale.
Another consideration that is specific to bioelectronic interfaces, is the unsuitability of hydrogels as barrier layers encapsulating electrical conductors. While the advantages of soft materials in reducing the mechanical mismatch to biological tissue are well-established, complete and stable bioelectronic devices cannot be made entirely of hydrogels, as these cannot guarantee the necessary insulation when biological fluids are absorbed. Given enough diffusion time, their intrinsic permeability to ionic fluids renders the entire volume conductive, bridging conductors intended to carry separate electrical signals. To overcome this challenge, elastomers with a slightly higher modulus (MPa range) have been proposed to encapsulate and electrically insulate the conductors, while containing the consequent decrease in mechanical compliance (Liu et al., 2019). Hydrogels can also be coated on thin elastomer substrates to provide the interface with the biological tissue at specific locations (Tringides et al., 2021). This strategy trades off elasticity with acceptable barrier performance. In general, although considerable advances have been reported in building interfaces that mimic biological tissue, the path to a fully synergistic bioelectronic device remains yet unpaved.
The limitations above, coupled to other specific design challenges such as the management of swelling and the associated interfacial stresses, constitute a significant set of roadblocks that today hinders the surfacing of complete hydrogel-based bioelectronic devices ready for clinical use or long-term preclinical validation. The authors expect a near-future increase in the research momentum to address the challenges presented herein, with the aim of enabling microelectronic manufacturing of fully biomimetic bioelectronic interfaces.
Author contributions
Conceptualization: SS and GS. Resources: KI and GS. Formal analysis: SS and GS. Writing—original draft: SS and GS. Writing—review and editing: SS, KI, and GS. Visualization: SS. Supervision: KI and GS. Funding acquisition: GS.
Acknowledgments
The authors would like to acknowledge the University of South-Eastern Norway library for their administrative and financial support.
Conflict of interest
The authors declare that the research was conducted in the absence of any commercial or financial relationships that could be construed as a potential conflict of interest.
Publisher’s note
All claims expressed in this article are solely those of the authors and do not necessarily represent those of their affiliated organizations, or those of the publisher, the editors and the reviewers. Any product that may be evaluated in this article, or claim that may be made by its manufacturer, is not guaranteed or endorsed by the publisher.
References
Ahmadi, M., Panahi, F., Bahri-Laleh, N., Sabzi, M., Pareras, G., Falcone, B. N., et al. (2022). pH-Responsive gelation in metallo-supramolecular polymers based on the protic pyridinedicarboxamide ligand. Chem. Mat. 34, 6155–6169. doi:10.1021/acs.chemmater.2c01346
Ambrožič, R., and Plazl, I. (2021). Development of an electrically responsive hydrogel for programmable in situ immobilization within a microfluidic device. Soft Matter 17, 6751–6764. doi:10.1039/D1SM00510C
Annabi, N., Nichol, J. W., Zhong, X., Ji, C., Koshy, S., Khademhosseini, A., et al. (2010). Controlling the porosity and microarchitecture of hydrogels for tissue engineering. Tissue Eng. Part B Rev. 16, 371–383. doi:10.1089/ten.teb.2009.0639
Araújo-Custódio, S., Gomez-Florit, M., Tomás, A. R., Mendes, B. B., Babo, P. S., Mithieux, S. M., et al. (2019). Injectable and magnetic responsive hydrogels with bioinspired ordered structures. ACS Biomater. Sci. Eng. 5, 1392–1404. doi:10.1021/acsbiomaterials.8b01179
Bansal, M., Raos, B., Aqrawe, Z., Wu, Z., and Svirskis, D. (2022). An interpenetrating and patternable conducting polymer hydrogel for electrically stimulated release of glutamate. Acta Biomater. 137, 124–135. doi:10.1016/j.actbio.2021.10.010
Bashir, S., Hina, M., Iqbal, J., Rajpar, A. H., Mujtaba, M. A., Alghamdi, N. A., et al. (2020). Fundamental concepts of hydrogels: Synthesis, properties, and their applications. Polym. (Basel) 12, 2702. doi:10.3390/polym12112702
Bichara, D. A., Zhao, X., Hwang, N. S., Bodugoz-Senturk, H., Yaremchuk, M. J., Randolph, M. A., et al. (2010). Porous poly(vinyl alcohol)-alginate gel hybrid construct for neocartilage formation using human nasoseptal cells. J. Surg. Res. 163, 331–336. doi:10.1016/j.jss.2010.03.070
Billiet, T., Gevaert, E., De Schryver, T., Cornelissen, M., and Dubruel, P. (2014). The 3D printing of gelatin methacrylamide cell-laden tissue-engineered constructs with high cell viability. Biomaterials 35, 49–62. doi:10.1016/j.biomaterials.2013.09.078
Biran, R., Martin, D. C., and Tresco, P. A. (2005). Neuronal cell loss accompanies the brain tissue response to chronically implanted silicon microelectrode arrays. Exp. Neurol. 195, 115–126. doi:10.1016/j.expneurol.2005.04.020
Brown, G. L., and Brown, M. T. (2022). Transcranial electrical stimulation in neurological disease. Neural Regen. Res. 17, 2221–2222. doi:10.4103/1673-5374.335796
Browning, M. B., Cereceres, S. N., Luong, P. T., and Cosgriff-Hernandez, E. M. (2014). Determination of the in vivo degradation mechanism of PEGDA hydrogels. J. Biomed. Mater. Res. Part A 102, 4244–4251. doi:10.1002/jbm.a.35096
Buwalda, S. J., Bethry, A., Hunger, S., Kandoussi, S., Coudane, J., and Nottelet, B. (2019). Ultrafast in situ forming poly(ethylene glycol)-poly(amido amine) hydrogels with tunable drug release properties via controllable degradation rates. Eur. J. Pharm. Biopharm. 139, 232–239. doi:10.1016/j.ejpb.2019.04.006
Caballero Aguilar, L. M., Silva, S. M., and Moulton, S. E. (2019). Growth factor delivery: Defining the next generation platforms for tissue engineering. J. Control. Release 306, 40–58. doi:10.1016/j.jconrel.2019.05.028
Chalmers, E., Lee, H., Zhu, C., and Liu, X. (2020). Increasing the conductivity and adhesion of polypyrrole hydrogels with electropolymerized polydopamine. Chem. Mat. 32, 234–244. doi:10.1021/acs.chemmater.9b03655
Cheah, E., Bansal, M., Nguyen, L., Chalard, A., Malmström, J., O’Carroll, S. J., et al. (2023). Electrically responsive release of proteins from conducting polymer hydrogels. Acta Biomater. 158, 87–100. doi:10.1016/j.actbio.2023.01.013
Chen, Y., Li, C., Wang, Z., Long, J., Wang, R., Zhao, J., et al. (2021). Self-assembled nanocomposite hydrogels enhanced by nanoparticles phosphonate-magnesium coordination for bone regeneration. Appl. Mater. Today 25, 101182. doi:10.1016/j.apmt.2021.101182
Cho, S.-H., Lu, H. M., Cauller, L., Romero-Ortega, M. I., Lee, J.-B., and Hughes, G. A. (2008). Biocompatible SU-8-Based microprobes for recording neural spike signals from regenerated peripheral nerve fibers. IEEE Sensors J. 8, 1830–1836. doi:10.1109/JSEN.2008.2006261
Cho, Y., and Borgens, R. B. (2010). The effect of an electrically conductive carbon nanotube/collagen composite on neurite outgrowth of PC12 cells. J. Biomed. Mater Res. A 95, 510–517. doi:10.1002/jbm.a.32841
Chuang, E.-Y., Chiang, C.-W., Wong, P.-C., and Chen, C.-H. (2018). Hydrogels for the application of articular cartilage tissue engineering: A review of hydrogels. Adv. Mater. Sci. Eng. 2018, 1–13. doi:10.1155/2018/4368910
Clausen, I., Tvedt, W. L. G., and Glott, T. (2018). Measurement of urinary bladder pressure: A comparison of methods. Sensors 18, 2128. doi:10.3390/s18072128
Cogan, S. F. (2008). Neural stimulation and recording electrodes. Annu. Rev. Biomed. Eng. 10, 275–309. doi:10.1146/annurev.bioeng.10.061807.160518
Cong, Y., and Fu, J. (2022). Hydrogel–tissue interface interactions for implantable flexible bioelectronics. Langmuir 38, 11503–11513. doi:10.1021/acs.langmuir.2c01674
Correa, S., Grosskopf, A. K., Lopez Hernandez, H., Chan, D., Yu, A. C., Stapleton, L. M., et al. (2021). Translational applications of hydrogels. Chem. Rev. 121, 11385–11457. doi:10.1021/acs.chemrev.0c01177
Cui, Y., Zhang, F., Chen, G., Yao, L., Zhang, N., Liu, Z., et al. (2021). A stretchable and transparent electrode based on PEGylated silk fibroin for in vivo dual-modal neural-vascular activity probing. Adv. Mater. 33, 2100221. doi:10.1002/adma.202100221
Deng, Y., Hussain, I., Kang, M., Li, K., Yao, F., Liu, S., et al. (2018). Self-recoverable and mechanical-reinforced hydrogel based on hydrophobic interaction with self-healable and conductive properties. Chem. Eng. J. C 353, 900–910. doi:10.1016/j.cej.2018.07.187
Devaki, S. J., Narayanan, R. K., and Sarojam, S. (2014). Electrically conducting silver nanoparticle–polyacrylic acid hydrogel by in situ reduction and polymerization approach. Mater. Lett. 116, 135–138. doi:10.1016/j.matlet.2013.10.110
Devi, V. K. A., Shyam, R., Palaniappan, A., Jaiswal, A. K., Oh, T.-H., and Nathanael, A. J. (2021). Self-healing hydrogels: Preparation, mechanism and advancement in biomedical applications. Polym. (Basel) 13, 3782. doi:10.3390/polym13213782
Dong, D., Tsao, C., Hung, H.-C., Yao, F., Tang, C., Niu, L., et al. (2021). High-strength and fibrous capsule–resistant zwitterionic elastomers. Sci. Adv. 7, eabc5442. doi:10.1126/sciadv.abc5442
Dragan, E. S. (2014). Design and applications of interpenetrating polymer network hydrogels. A review. Chem. Eng. J. 243, 572–590. doi:10.1016/j.cej.2014.01.065
Fallegger, F., Schiavone, G., Pirondini, E., Wagner, F. B., Vachicouras, N., Serex, L., et al. (2021). MRI-compatible and conformal electrocorticography grids for translational research. Adv. Sci. 8, 2003761. doi:10.1002/advs.202003761
Fan, H., and Gong, J. P. (2021). Bioinspired underwater adhesives. Adv. Mater. 33, 2102983. doi:10.1002/adma.202102983
Fedorovich, N. E., Oudshoorn, M. H., van Geemen, D., Hennink, W. E., Alblas, J., and Dhert, W. J. A. (2009). The effect of photopolymerization on stem cells embedded in hydrogels. Biomaterials 30, 344–353. doi:10.1016/j.biomaterials.2008.09.037
Finch, P., Price, L., and Drummond, P. (2019). High-frequency (10 kHz) electrical stimulation of peripheral nerves for treating chronic pain: A double-blind trial of presence vs absence of stimulation. Neuromodulation Technol. A. T. Neural Interface 22, 529–536. doi:10.1111/ner.12877
Franz, S., Rammelt, S., Scharnweber, D., and Simon, J. C. (2011). Immune responses to implants – a review of the implications for the design of immunomodulatory biomaterials. Biomaterials 32, 6692–6709. doi:10.1016/j.biomaterials.2011.05.078
Fu, F., Wang, J., and Yu, J. (2021). Interpenetrating PAA-PEDOT conductive hydrogels for flexible skin sensors. J. Mat. Chem. C 9, 11794–11800. doi:10.1039/D1TC01578H
Gao, C., Song, S., Lv, Y., Huang, J., and Zhang, Z. (2022). Recent development of conductive hydrogels for tissue engineering: Review and perspective. Macromol. Biosci. 22, 2200051. doi:10.1002/mabi.202200051
Ge, G., Lu, Y., Qu, X., Zhao, W., Ren, Y., Wang, W., et al. (2020). Muscle-inspired self-healing hydrogels for strain and temperature sensor. ACS Nano 14, 218–228. doi:10.1021/acsnano.9b07874
Ge, S., Liu, Q., Li, M., Liu, J., Lu, H., Li, F., et al. (2018). Enhanced mechanical properties and gelling ability of gelatin hydrogels reinforced with chitin whiskers. Food Hydrocoll. 75, 1–12. doi:10.1016/j.foodhyd.2017.09.023
Gori, M., Giannitelli, S. M., Vadalà, G., Papalia, R., Zollo, L., Sanchez, M., et al. (2022). A soft zwitterionic hydrogel as potential coating on a polyimide surface to reduce foreign body reaction to intraneural electrodes. Molecules 27, 3126. doi:10.3390/molecules27103126
Han, M., Li, Q.-L., Cao, Y., Fang, H., Xia, R., and Zhang, Z.-H. (2017). In vivo remineralization of dentin using an agarose hydrogel biomimetic mineralization system. Sci. Rep. 7, 41955. doi:10.1038/srep41955
He, P., Cao, J., Ding, H., Liu, C., Neilson, J., Li, Z., et al. (2019). Screen-printing of a highly conductive graphene ink for flexible printed electronics. ACS Appl. Mat. Interfaces 11, 32225–32234. doi:10.1021/acsami.9b04589
Heo, D. N., Song, S.-J., Kim, H.-J., Lee, Y. J., Ko, W.-K., Lee, S. J., et al. (2016). Multifunctional hydrogel coatings on the surface of neural cuff electrode for improving electrode-nerve tissue interfaces. Acta Biomater. 39, 25–33. doi:10.1016/j.actbio.2016.05.009
Huang, W.-C., Ong, X. C., Kwon, I. S., Gopinath, C., Fisher, L. E., Wu, H., et al. (2018). Ultracompliant hydrogel-based neural interfaces fabricated by aqueous-phase microtransfer printing. Adv. Funct. Mater. 28, 1801059. doi:10.1002/adfm.201801059
Ido, Y., Takahashi, D., Sasaki, M., Nagamine, K., Miyake, T., Jasinski, P., et al. (2012). Conducting polymer microelectrodes anchored to hydrogel films. ACS Macro Lett. 1, 400–403. doi:10.1021/mz2002406
Janoušková, O., Přádný, M., Vetrík, M., Krumbholcová, E. C., Michálek, J., and Smrčková, M. D. (2019). Biomimetic modification of dual porosity poly(2-hydroxyethyl methacrylate) hydrogel scaffolds—Porosity and stem cell growth evaluation. Biomed. Mat. 14, 055004. doi:10.1088/1748-605X/ab2856
Jorbendaze, A., Young, R., Shaburishvili, T., Demyanchuk, V., Buriak, R., Todurov, B., et al. (2022). Synchronized diaphragmatic stimulation for heart failure using the VisONE system: A first-in-patient study. Esc. Heart Fail. 9, 2207–2214. doi:10.1002/ehf2.13984
Kaczmarek, B., Nadolna, K., and Owczarek, A. (2020). “Chapter 6 - the physical and chemical properties of hydrogels based on natural polymers,” in Hydrogels based on natural polymers. Editor Y. Chen (Netherlands: Elsevier).
Kanick, S. C., Schneider, P. A., Klitzman, B., Wisniewski, N. A., and Rebrin, K. (2019). Continuous monitoring of interstitial tissue oxygen using subcutaneous oxygen microsensors: In vivo characterization in healthy volunteers. Microvasc. Res. 124, 6–18. doi:10.1016/j.mvr.2019.02.002
Khedr, E. M., Salama, R. H., Abdel Hameed, M., Abo Elfetoh, N., and Seif, P. (2019). Therapeutic role of transcranial direct current stimulation in alzheimer disease patients: Double-blind, placebo-controlled clinical trial. Neurorehabil Neural Repair 33, 384–394. doi:10.1177/1545968319840285
Khodagholy, D., Doublet, T., Gurfinkel, M., Quilichini, P., Ismailova, E., Leleux, P., et al. (2011). Highly conformable conducting polymer electrodes for in vivo recordings. Adv. Mater. 23, H268–H272. doi:10.1002/adma.201102378
Kim, Y. B., Lee, H., and Kim, G. H. (2016). Strategy to achieve highly porous/biocompatible macroscale cell blocks, using a collagen/genipin-bioink and an optimal 3D printing process. ACS Appl. Mat. Interfaces 8, 32230–32240. doi:10.1021/acsami.6b11669
Kleber, C., Bruns, M., Lienkamp, K., Rühe, J., and Asplund, M. (2017). An interpenetrating, microstructurable and covalently attached conducting polymer hydrogel for neural interfaces. Acta Biomater. 58, 365–375. doi:10.1016/j.actbio.2017.05.056
Kong, H. J., Kaigler, D., Kim, K., and Mooney, D. J. (2004). Controlling rigidity and degradation of alginate hydrogels via molecular weight distribution. Biomacromolecules 5, 1720–1727. doi:10.1021/bm049879r
Konieczynska, M. D., and Grinstaff, M. W. (2017). On-demand dissolution of chemically cross-linked hydrogels. Acc. Chem. Res. 50, 151–160. doi:10.1021/acs.accounts.6b00547
Kopeček, J., and Yang, J. (2007). Hydrogels as smart biomaterials. Polym. Int. 56, 1078–1098. doi:10.1002/pi.2253
Kuroda, Y., Kawai, T., Goto, K., and Matsuda, S. (2019). Clinical application of injectable growth factor for bone regeneration: A systematic review. Inflamm. Regen. 39, 20. doi:10.1186/s41232-019-0109-x
Lacour, S. P., Courtine, G., and Guck, J. (2016). Materials and technologies for soft implantable neuroprostheses. Nat. Rev. Mater 1, 1–14. doi:10.1038/natrevmats.2016.63
Lee, C.-S., Hwang, H. S., Kim, S., Fan, J., Aghaloo, T., and Lee, M. (2020a). Inspired by nature: Facile design of nanoclay–organic hydrogel bone sealant with multifunctional properties for robust bone regeneration. Adv. Funct. Mater. 30, 2003717. doi:10.1002/adfm.202003717
Lee, H., Um, D.-S., Lee, Y., Lim, S., Kim, H., and Ko, H. (2016). Octopus-inspired smart adhesive pads for transfer printing of semiconducting nanomembranes. Adv. Mater. 28, 7457–7465. doi:10.1002/adma.201601407
Lee, K. Y., Moon, H., Kim, B., Kang, Y. N., Jang, J.-W., Choe, H. K., et al. (2020b). Development of a polydimethylsiloxane-based electrode array for electrocorticography. Adv. Mater. Interfaces 7, 2001152. doi:10.1002/admi.202001152
Lei, Z., Wang, Q., Sun, S., Zhu, W., and Wu, P. (2017). A bioinspired mineral hydrogel as a self-healable, mechanically adaptable ionic skin for highly sensitive pressure sensing. Adv. Mater. 29, 1700321. doi:10.1002/adma.201700321
Li, H., Tan, Y. J., Leong, K. F., and Li, L. (2017). 3D bioprinting of highly thixotropic alginate/methylcellulose hydrogel with strong interface bonding. ACS Appl. Mat. Interfaces 9, 20086–20097. doi:10.1021/acsami.7b04216
Li, J., Ma, L., Chen, G., Zhou, Z., and Li, Q. (2015). A high water-content and high elastic dual-responsive polyurethane hydrogel for drug delivery. J. Mat. Chem. B 3, 8401–8409. doi:10.1039/C5TB01702E
Li, J., Wu, C., Chu, P. K., and Gelinsky, M. (2020a). 3D printing of hydrogels: Rational design strategies and emerging biomedical applications. Mater. Sci. Eng. R Rep. 140, 100543. doi:10.1016/j.mser.2020.100543
Li, S., Wang, K., Jiang, X., Hu, Q., Zhang, C., and Wang, B. (2020b). Rapid fabrication of ready-to-use gelatin scaffolds with prevascular networks using alginate hollow fibers as sacrificial templates. ACS Biomater. Sci. Eng. 6, 2297–2311. doi:10.1021/acsbiomaterials.9b01834
Li, X., Sun, Q., Li, Q., Kawazoe, N., and Chen, G. (2018). Functional hydrogels with tunable structures and properties for tissue engineering applications. Front. Chem. 6, 499. doi:10.3389/fchem.2018.00499
Lim, S. L., Ooi, C.-W., Low, L. E., Tan, W. S., Chan, E.-S., Ho, K. L., et al. (2020). Synthesis of poly(acrylamide)-based hydrogel for bio-sensing of Hepatitis B core antigen. Mater. Chem. Phys. 243, 122578. doi:10.1016/j.matchemphys.2019.122578
Lin, Y.-X., Li, S.-H., and Huang, W.-C. (2021). Fabrication of soft tissue scaffold-mimicked microelectrode arrays using enzyme-mediated transfer printing. Micromachines 12, 1057. doi:10.3390/mi12091057
Liu, H., Rong, L., Wang, B., Xie, R., Sui, X., Xu, H., et al. (2017). Facile fabrication of redox/pH dual stimuli responsive cellulose hydrogel. Carbohydr. Polym. 176, 299–306. doi:10.1016/j.carbpol.2017.08.085
Liu, J., Zhang, X., Liu, Y., Rodrigo, M., Loftus, P. D., Aparicio-Valenzuela, J., et al. (2020a). Intrinsically stretchable electrode array enabled in vivo electrophysiological mapping of atrial fibrillation at cellular resolution. Proc. Natl. Acad. Sci. U. S. A. 117, 14769–14778. doi:10.1073/pnas.2000207117
Liu, S., Chen, X., and Zhang, Y. (2020b). “Chapter 14 - hydrogels and hydrogel composites for 3D and 4D printing applications,” in 3D and 4D printing of polymer nanocomposite materials. Editors K. K. Sadasivuni, K. Deshmukh, and M. A. Almaadeed (Netherlands: Elsevier).
Liu, W., Luo, Y., Ning, C., Zhang, W., Zhang, Q., Zou, H., et al. (2021). Thermo-sensitive electroactive hydrogel combined with electrical stimulation for repair of spinal cord injury. J. Nanobiotechnology 19, 286. doi:10.1186/s12951-021-01031-y
Liu, X.-W., Huang, Y.-X., Sun, X.-F., Sheng, G.-P., Zhao, F., Wang, S.-G., et al. (2014). Conductive carbon nanotube hydrogel as a bioanode for enhanced microbial electrocatalysis. ACS Appl. Mat. Interfaces 6, 8158–8164. doi:10.1021/am500624k
Liu, Y., Liu, J., Chen, S., Lei, T., Kim, Y., Niu, S., et al. (2019). Soft and elastic hydrogel-based microelectronics for localized low-voltage neuromodulation. Nat. Biomed. Eng. 3, 58–68. doi:10.1038/s41551-018-0335-6
Loh, E. Y. X., Fauzi, Mh. B., Ng, M. H., Ng, P. Y., Ng, S. F., and Mohd Amin, M. C. I. (2020). Insight into delivery of dermal fibroblast by non-biodegradable bacterial nanocellulose composite hydrogel on wound healing. Int. J. Biol. Macromol. 159, 497–509. doi:10.1016/j.ijbiomac.2020.05.011
Luan, L., Wei, X., Zhao, Z., Siegel, J. J., Potnis, O., Tuppen, C. A., et al. (2017). Ultraflexible nanoelectronic probes form reliable, glial scar–free neural integration. Sci. Adv. 3, e1601966. doi:10.1126/sciadv.1601966
Macron, J., Gerratt, A. P., and Lacour, S. P. (2019). Thin hydrogel–elastomer multilayer encapsulation for soft electronics. Adv. Mater. Technol. 4, 1900331. doi:10.1002/admt.201900331
Makrygianni, M., Milionis, A., Kryou, C., Trantakis, I., Poulikakos, D., and Zergioti, I. (2018). On-demand laser printing of picoliter-sized, highly viscous, adhesive fluids: Beyond inkjet limitations. Adv. Mater. Interfaces 5, 1800440. doi:10.1002/admi.201800440
Mandal, A., Clegg, J. R., Anselmo, A. C., and Mitragotri, S. (2020). Hydrogels in the clinic. Bioeng. Transl. Med. 5, e10158. doi:10.1002/btm2.10158
Mariani, E., Lisignoli, G., Borzì, R. M., and Pulsatelli, L. (2019). Biomaterials: Foreign bodies or tuners for the immune response? Int. J. Mol. Sci. 20, 636. doi:10.3390/ijms20030636
Martinsen, O. G., Grimnes, S., and Martinsen, O. G. (2008). Bioimpedance and bioelectricity basics. Oxford, UNITED KINGDOM: Elsevier Science and Technology.
Masuma, R., Kashima, S., Kurasaki, M., and Okuno, T. (2013). Effects of UV wavelength on cell damages caused by UV irradiation in PC12 cells. J. Photochem. Photobiol. B Biol. 125, 202–208. doi:10.1016/j.jphotobiol.2013.06.003
Mironi-Harpaz, I., Wang, D. Y., Venkatraman, S., and Seliktar, D. (2012). Photopolymerization of cell-encapsulating hydrogels: Crosslinking efficiency versus cytotoxicity. Acta Biomater. 8, 1838–1848. doi:10.1016/j.actbio.2011.12.034
Nam, S. Y., and Park, S.-H. (2018). ECM based bioink for tissue mimetic 3D bioprinting. Adv. Exp. Med. Biol. 1064, 335–353. doi:10.1007/978-981-13-0445-3_20
Narayanaswamy, R., and Torchilin, V. P. (2019). Hydrogels and their applications in targeted drug delivery. Molecules 24, 603. doi:10.3390/molecules24030603
Negro, A., Cherbuin, T., and Lutolf, M. P. (2018). 3D inkjet printing of complex, cell-laden hydrogel structures. Sci. Rep. 8, 17099. doi:10.1038/s41598-018-35504-2
Nezakati, T., Seifalian, A., Tan, A., and Seifalian, A. M. (2018). Conductive polymers: Opportunities and challenges in biomedical applications. Chem. Rev. 118, 6766–6843. doi:10.1021/acs.chemrev.6b00275
Nguyen, A.-T. T., Aasmundtveit, K. E., Hoff, L., Imenes, K., Tyssø, J., and Grymyr, O.-J. (2016). “Improved design of an implantable heart monitoring device,” in 2016 Symposium on Design, Test, Integration and Packaging of MEMS/MOEMS (DTIP), Hungary, 02 June 2016. doi:10.1109/DTIP.2016.7514849
Nguyen, H. T. T., Do, N. H. N., Lac, H. D., Nguyen, P. L. N., and Le, P. K. (2022). Synthesis, properties, and applications of chitosan hydrogels as anti-inflammatory drug delivery system. J. Porous Mater. doi:10.1007/s10934-022-01371-6
Nur, O., and Willander, M. (2020). “Chapter 3 - conventional nanofabrication methods,” in Low temperature chemical nanofabrication micro and nano technologies. Editors O. Nur, and M. Willander (England: William Andrew Publishing).
Ohm, Y., Pan, C., Ford, M. J., Huang, X., Liao, J., and Majidi, C. (2021). An electrically conductive silver–polyacrylamide–alginate hydrogel composite for soft electronics. Nat. Electron 4, 185–192. doi:10.1038/s41928-021-00545-5
Onaciu, A., Munteanu, R. A., Moldovan, A. I., Moldovan, C. S., and Berindan-Neagoe, I. (2019). Hydrogels based drug delivery synthesis, characterization and administration. Pharmaceutics 11, 432. doi:10.3390/pharmaceutics11090432
Ozcelik, B. (2016). “7 - degradable hydrogel systems for biomedical applications,” in Biosynthetic Polymers for medical applications woodhead publishing series in biomaterials. Editors L. Poole-Warren, P. Martens, and R. Green (United Kingdom: Woodhead Publishing).
Pan, L., Yu, G., Zhai, D., Lee, H. R., Zhao, W., Liu, N., et al. (2012). Hierarchical nanostructured conducting polymer hydrogel with high electrochemical activity. Proc. Natl. Acad. Sci. U. S. A. 109, 9287–9292. doi:10.1073/pnas.1202636109
Pandala, N., Haywood, S., LaScola, M. A., Day, A., Leckron, J., and Lavik, E. (2020). Screen printing to create 3D tissue models. ACS Appl. Bio Mat. 3, 8113–8120. doi:10.1021/acsabm.0c01256
Pandala, N., LaScola, M. A., Tang, Y., Bieberich, M., Korley, L. T. J., and Lavik, E. (2021). Screen printing tissue models using chemically cross-linked hydrogel systems: A simple approach to efficiently make highly tunable matrices. ACS Biomater. Sci. Eng. 7, 5007–5013. doi:10.1021/acsbiomaterials.1c00902
Park, J., Choi, J. H., Kim, S., Jang, I., Jeong, S., and Lee, J. Y. (2019). Micropatterned conductive hydrogels as multifunctional muscle-mimicking biomaterials: Graphene-incorporated hydrogels directly patterned with femtosecond laser ablation. Acta Biomater. 97, 141–153. doi:10.1016/j.actbio.2019.07.044
Patterson, J., Siew, R., Herring, S. W., Lin, A. S. P., Guldberg, R., and Stayton, P. S. (2010). Hyaluronic acid hydrogels with controlled degradation properties for oriented bone regeneration. Biomaterials 31, 6772–6781. doi:10.1016/j.biomaterials.2010.05.047
Peng, K., Tomatsu, I., and Kros, A. (2010). Light controlled protein release from a supramolecular hydrogel. Chem. Commun. 46, 4094–4096. doi:10.1039/C002565H
Peng, Q., Chen, J., Wang, T., Peng, X., Liu, J., Wang, X., et al. (2020). Recent advances in designing conductive hydrogels for flexible electronics. InfoMat 2, 843–865. doi:10.1002/inf2.12113
Přádný, M., Dušková-Smrčková, M., Dušek, K., Janoušková, O., Sadakbayeva, Z., Šlouf, M., et al. (2014). Macroporous 2-hydroxyethyl methacrylate hydrogels of dual porosity for cell cultivation: Morphology, swelling, permeability, and mechanical behavior. J. Polym. Res. 21, 579. doi:10.1007/s10965-014-0579-0
Prodanov, D., and Delbeke, J. (2016). Mechanical and biological interactions of implants with the brain and their impact on implant design. Front. Neurosci. 10, 11. doi:10.3389/fnins.2016.00011
Raina, N., Pahwa, R., Bhattacharya, J., Paul, A. K., Nissapatorn, V., de Lourdes Pereira, M., et al. (2022). Drug delivery strategies and biomedical significance of hydrogels: Translational considerations. Pharmaceutics 14, 574. doi:10.3390/pharmaceutics14030574
Raina, N., Rani, R., Khan, A., Nagpal, K., and Gupta, M. (2020). Interpenetrating polymer network as a pioneer drug delivery system: A review. Polym. Bull. 77, 5027–5050. doi:10.1007/s00289-019-02996-5
Rao, P., Sun, T. L., Chen, L., Takahashi, R., Shinohara, G., Guo, H., et al. (2018). Tough hydrogels with fast, strong, and reversible underwater adhesion based on a multiscale design. Adv. Mater. 30, 1801884. doi:10.1002/adma.201801884
Reis, A. V., Guilherme, M. R., Cavalcanti, O. A., Rubira, A. F., and Muniz, E. C. (2006). Synthesis and characterization of pH-responsive hydrogels based on chemically modified Arabic gum polysaccharide. Polymer 47, 2023–2029. doi:10.1016/j.polymer.2006.01.058
Renz, A. F., Lee, J., Tybrandt, K., Brzezinski, M., Lorenzo, D. A., Cerra Cheraka, M., et al. (2020). Opto-E-dura: A soft, stretchable ECoG array for multimodal, multiscale neuroscience. Adv. Healthc. Mater. 9, 2000814. doi:10.1002/adhm.202000814
Robby, A. I., Lee, G., and Park, S. Y. (2019). NIR-induced pH-reversible self-healing monitoring with smartphone by wireless hydrogel sensor. Sensors Actuators B Chem. 297, 126783. doi:10.1016/j.snb.2019.126783
Roh, J. S., and Sohn, D. H. (2018). Damage-associated molecular patterns in inflammatory diseases. Immune Netw. 18, e27. doi:10.4110/in.2018.18.e27
Ryu, J. H., Messersmith, P. B., and Lee, H. (2018). Polydopamine surface chemistry: A decade of discovery. ACS Appl. Mat. Interfaces 10, 7523–7540. doi:10.1021/acsami.7b19865
Saeki, K., Hiramatsu, H., Hori, A., Hirai, Y., Yamada, M., Utoh, R., et al. (2020). Sacrificial alginate-assisted microfluidic engineering of cell-supportive protein microfibers for hydrogel-based cell encapsulation. ACS Omega 5, 21641–21650. doi:10.1021/acsomega.0c02385
Sasaki, M., Karikkineth, B. C., Nagamine, K., Kaji, H., Torimitsu, K., and Nishizawa, M. (2014). Highly conductive stretchable and biocompatible electrode–hydrogel hybrids for advanced tissue engineering. Adv. Healthc. Mater. 3, 1919–1927. doi:10.1002/adhm.201400209
Sawayama, J., and Takeuchi, S. (2021). Long-term continuous glucose monitoring using a fluorescence-based biocompatible hydrogel glucose sensor. Adv. Healthc. Mater. 10, 2001286. doi:10.1002/adhm.202001286
Schiavone, G., Fallegger, F., Kang, X., Barra, B., Vachicouras, N., Roussinova, E., et al. (2020a). Soft, implantable bioelectronic interfaces for translational research. Adv. Mater. 32, 1906512. doi:10.1002/adma.201906512
Schiavone, G., Kang, X., Fallegger, F., Gandar, J., Courtine, G., and Lacour, S. P. (2020b). Guidelines to study and develop soft electrode systems for neural stimulation. Neuron 108, 238–258. doi:10.1016/j.neuron.2020.10.010
Schiavone, G., Vachicouras, N., Vyza, Y., and Lacour, S. P. (2021). Dimensional scaling of thin-film stimulation electrode systems in translational research. J. Neural Eng. 18, 046054. doi:10.1088/1741-2552/abf607
Scholten, K., and Meng, E. (2015). Materials for microfabricated implantable devices: A review. Lab. Chip 15, 4256–4272. doi:10.1039/C5LC00809C
Seong, S.-Y., and Matzinger, P. (2004). Hydrophobicity: An ancient damage-associated molecular pattern that initiates innate immune responses. Nat. Rev. Immunol. 4, 469–478. doi:10.1038/nri1372
Seyedkhani, S. A., Mohammadpour, R., Seyedkhani, S. A., and Mohammadpour, R. (2022). Tissue-electronics interfaces. IntechOpen, 6–8. doi:10.5772/intechopen.108129
Shur, M., Fallegger, F., Pirondini, E., Roux, A., Bichat, A., Barraud, Q., et al. (2020). Soft printable electrode coating for neural interfaces. ACS Appl. Bio Mater 3, 4388–4397. doi:10.1021/acsabm.0c00401
Snejdrova, E., and Dittrich, M. (2012). “Pharmaceutically used plasticizers,” in Recent advances in plasticizers (London: IntechOpen).
Song, S., Regan, B., Ereifej, E. S., Chan, E. R., and Capadona, J. R. (2022). Neuroinflammatory gene expression analysis reveals pathways of interest as potential targets to improve the recording performance of intracortical microelectrodes. Cells 11, 2348. doi:10.3390/cells11152348
Spearman, B. S., Kuliasha, C. A., Judy, J. W., and Schmidt, C. E. (2020). Integration of flexible polyimide arrays into soft extracellular matrix-based hydrogel materials for a tissue-engineered electronic nerve interface (TEENI). J. Neurosci. Methods 341, 108762. doi:10.1016/j.jneumeth.2020.108762
Sperling, L. H. (2012). Interpenetrating polymer networks and related materials. Germany: Springer Science and Business Media.
Su, G., Yin, S., Guo, Y., Zhao, F., Guo, Q., Zhang, X., et al. (2021). Balancing the mechanical, electronic, and self-healing properties in conductive self-healing hydrogel for wearable sensor applications. Mat. Horiz. 8, 1795–1804. doi:10.1039/D1MH00085C
Sun, G., Liang, T., Tan, W., and Wang, L. (2018). Rheological behaviors and physical properties of plasticized hydrogel films developed from κ-carrageenan incorporating hydroxypropyl methylcellulose. Food Hydrocoll. 85, 61–68. doi:10.1016/j.foodhyd.2018.07.002
Sun, M., Li, H., Hou, Y., Huang, N., Xia, X., Zhu, H., et al. (2023). Multifunctional tendon-mimetic hydrogels. Sci. Adv. 9, eade6973. doi:10.1126/sciadv.ade6973
Suo, H., Zhang, D., Yin, J., Qian, J., Wu, Z. L., and Fu, J. (2018). Interpenetrating polymer network hydrogels composed of chitosan and photocrosslinkable gelatin with enhanced mechanical properties for tissue engineering. Mater. Sci. Eng. C 92, 612–620. doi:10.1016/j.msec.2018.07.016
Takeda, K., Kitagawa, H., Tsuboi, R., Kiba, W., Sasaki, J.-I., Hayashi, M., et al. (2015). Effectiveness of non-biodegradable poly(2-hydroxyethyl methacrylate)-based hydrogel particles as a fibroblast growth factor-2 releasing carrier. Dent. Mater. 31, 1406–1414. doi:10.1016/j.dental.2015.09.007
Talebian, S., Mehrali, M., Taebnia, N., Pennisi, C. P., Kadumudi, F. B., Foroughi, J., et al. (2019). Self-healing hydrogels: The next paradigm shift in tissue engineering? Adv. Sci. 6, 1801664. doi:10.1002/advs.201801664
Tao, X.-Y., Wang, Y., Ma, W., Ye, S.-F., Zhu, K.-H., Guo, L.-T., et al. (2021). Copolymer hydrogel as self-standing electrode for high performance all-hydrogel-state supercapacitor. J. Mater Sci. 56, 16028–16043. doi:10.1007/s10853-021-06304-3
Tarique, J., Sapuan, S. M., and Khalina, A. (2021). Effect of glycerol plasticizer loading on the physical, mechanical, thermal, and barrier properties of arrowroot (Maranta arundinacea) starch biopolymers. Sci. Rep. 11, 13900. doi:10.1038/s41598-021-93094-y
Tayalia, P., and Mooney, D. J. (2009). Controlled growth factor delivery for tissue engineering. Adv. Mater. 21, 3269–3285. doi:10.1002/adma.200900241
Taylor, D. L., and in het Panhuis, M. (2016). Self-healing hydrogels. Adv. Mater. 28, 9060–9093. doi:10.1002/adma.201601613
Tee, H. T., Zipp, R., Koynov, K., Tremel, W., and Wurm, F. R. (2020). Poly(methyl ethylene phosphate) hydrogels: Degradable and cell-repellent alternatives to PEG-hydrogels. Eur. Polym. J. 141, 110075. doi:10.1016/j.eurpolymj.2020.110075
Tenje, M., Cantoni, F., Porras Hernández, A. M., Searle, S. S., Johansson, S., Barbe, L., et al. (2020). A practical guide to microfabrication and patterning of hydrogels for biomimetic cell culture scaffolds. Organs-on-a-Chip 2, 100003. doi:10.1016/j.ooc.2020.100003
Thakur, R., Aplin, F. P., and Fridman, G. Y. (2021). A hydrogel-based microfluidic nerve cuff for neuromodulation of peripheral nerves. Micromachines 12 (12), 1522. doi:10.3390/mi12121522
Tirella, A., Mattei, G., La Marca, M., Ahluwalia, A., and Tirelli, N. (2020). Functionalized enzyme-responsive biomaterials to model tissue stiffening in vitro. Front. Bioeng. Biotechnol. 8, 208. doi:10.3389/fbioe.2020.00208
Tringides, C. M., Vachicouras, N., de Lázaro, I., Wang, H., Trouillet, A., Seo, B. R., et al. (2021). Viscoelastic surface electrode arrays to interface with viscoelastic tissues. Nat. Nanotechnol. 16, 1019–1029. doi:10.1038/s41565-021-00926-z
Venkatraman, S., Hendricks, J., King, Z. A., Sereno, A. J., Richardson-Burns, S., Martin, D., et al. (2011). In vitro and in vivo evaluation of PEDOT microelectrodes for neural stimulation and recording. IEEE Trans. Neural Syst. Rehabil. Eng. 19, 307–316. doi:10.1109/TNSRE.2011.2109399
Verhulsel, M., Vignes, M., Descroix, S., Malaquin, L., Vignjevic, D. M., and Viovy, J.-L. (2014). A review of microfabrication and hydrogel engineering for micro-organs on chips. Biomaterials 35, 1816–1832. doi:10.1016/j.biomaterials.2013.11.021
Vomero, M., Ciarpella, F., Zucchini, E., Kirsch, M., Fadiga, L., Stieglitz, T., et al. (2022). On the longevity of flexible neural interfaces: Establishing biostability of polyimide-based intracortical implants. Biomaterials 281, 121372. doi:10.1016/j.biomaterials.2022.121372
Vomero, M., Porto Cruz, M. F., Zucchini, E., Ciarpella, F., Delfino, E., Carli, S., et al. (2020). Conformable polyimide-based μECoGs: Bringing the electrodes closer to the signal source. Biomaterials 255, 120178. doi:10.1016/j.biomaterials.2020.120178
Vomero, M., and Schiavone, G. (2021). Biomedical microtechnologies beyond scholarly impact. Micromachines 12, 1471. doi:10.3390/mi12121471
Wagner, F. B., Mignardot, J.-B., Le Goff-Mignardot, C. G., Demesmaeker, R., Komi, S., Capogrosso, M., et al. (2018). Targeted neurotechnology restores walking in humans with spinal cord injury. Nature 563, 65–71. doi:10.1038/s41586-018-0649-2
Wang, L., Li, B., Xu, F., Li, Y., Xu, Z., Wei, D., et al. (2017). Visual in vivo degradation of injectable hydrogel by real-time and non-invasive tracking using carbon nanodots as fluorescent indicator. Biomaterials 145, 192–206. doi:10.1016/j.biomaterials.2017.08.039
Wang, X., Li, M., Wang, D., Zhang, H., Duan, R., Zhang, D., et al. (2020). Low-cost, robust pressure-responsive smart windows with dynamic switchable transmittance. ACS Appl. Mat. Interfaces 12, 15695–15702. doi:10.1021/acsami.0c00300
Wei, W., Ma, Y., Yao, X., Zhou, W., Wang, X., Li, C., et al. (2021). Advanced hydrogels for the repair of cartilage defects and regeneration. Bioact. Mater. 6, 998–1011. doi:10.1016/j.bioactmat.2020.09.030
Wellman, S. M., and Kozai, T. D. Y. (2017). Understanding the inflammatory tissue reaction to brain implants to improve neurochemical sensing performance. ACS Chem. Neurosci. 8, 2578–2582. doi:10.1021/acschemneuro.7b00403
Williams, D. F. (2008). On the mechanisms of biocompatibility. Biomaterials 29, 2941–2953. doi:10.1016/j.biomaterials.2008.04.023
Won, D., Kim, J., Choi, J., Kim, H., Han, S., Ha, I., et al. (2022). Digital selective transformation and patterning of highly conductive hydrogel bioelectronics by laser-induced phase separation. Sci. Adv. 8, eabo3209. doi:10.1126/sciadv.abo3209
Wu, Q., Therriault, D., and Heuzey, M.-C. (2018). Processing and properties of chitosan inks for 3D printing of hydrogel microstructures. ACS Biomater. Sci. Eng. 4, 2643–2652. doi:10.1021/acsbiomaterials.8b00415
Xu, L.-C., and Siedlecki, C. A. (2017). “4.18 surface texturing and control of bacterial adhesion,” in Comprehensive biomaterials II. Editor P. Ducheyne (Oxford: Elsevier).
Xue, Y., Zhang, J., Chen, X., Zhang, J., Chen, G., Zhang, K., et al. (2021). Trigger-detachable hydrogel adhesives for bioelectronic interfaces. Adv. Funct. Mater. 31, 2106446. doi:10.1002/adfm.202106446
Yagüe, J. L., and Gleason, K. K. (2012). Systematic control of mesh size in hydrogels by initiated chemical vapor deposition. Soft Matter 8, 2890–2894. doi:10.1039/C2SM07137A
Yan, H., Seignez, C., Hjorth, M., Winkeljann, B., Blakeley, M., Lieleg, O., et al. (2019). Immune-informed mucin hydrogels evade fibrotic foreign body response in vivo. Adv. Funct. Mater. 29, 1902581. doi:10.1002/adfm.201902581
Yanagawa, F., Sugiura, S., and Kanamori, T. (2016). Hydrogel microfabrication technology toward three dimensional tissue engineering. Regen. Ther. 3, 45–57. doi:10.1016/j.reth.2016.02.007
Yang, C., and Suo, Z. (2018). Hydrogel ionotronics. Nat. Rev. Mater 3, 125–142. doi:10.1038/s41578-018-0018-7
Yang, Y., Ren, Y., Song, W., Yu, B., and Liu, H. (2022). Rational design in functional hydrogels towards biotherapeutics. Mater. Des. 223, 111086. doi:10.1016/j.matdes.2022.111086
Yeom, J., Choe, A., Lim, S., Lee, Y., Na, S., and Ko, H. (2020). Soft and ion-conducting hydrogel artificial tongue for astringency perception. Sci. Adv. 6, eaba5785. doi:10.1126/sciadv.aba5785
Yin, M., Zhang, Y., Yin, Z., Zheng, Q., and Zhang, A. P. (2018). Micropatterned elastic gold-nanowire/polyacrylamide composite hydrogels for wearable pressure sensors. Adv. Mater. Technol. 3, 1800051. doi:10.1002/admt.201800051
Yuk, H., Lu, B., Lin, S., Qu, K., Xu, J., Luo, J., et al. (2020). 3D printing of conducting polymers. Nat. Commun. 11, 1604. doi:10.1038/s41467-020-15316-7
Yuk, H., Wu, J., and Zhao, X. (2022). Hydrogel interfaces for merging humans and machines. Nat. Rev. Mater 7, 935–952. doi:10.1038/s41578-022-00483-4
Zhang, H. J., Luo, F., Ye, Y., Sun, T. L., Nonoyama, T., Kurokawa, T., et al. (2019). Tough triblock copolymer hydrogels with different micromorphologies for medical and sensory materials. ACS Appl. Polym. Mat. 1, 1948–1953. doi:10.1021/acsapm.9b00395
Zhang, L., Cao, Z., Bai, T., Carr, L., Ella-Menye, J.-R., Irvin, C., et al. (2013). Zwitterionic hydrogels implanted in mice resist the foreign-body reaction. Nat. Biotechnol. 31, 553–556. doi:10.1038/nbt.2580
Zhang, L., Liu, M., Zhang, Y., and Pei, R. (2020). Recent progress of highly adhesive hydrogels as wound dressings. Biomacromolecules 21, 3966–3983. doi:10.1021/acs.biomac.0c01069
Zhang, M., Song, C.-C., Du, F.-S., and Li, Z.-C. (2017). Supersensitive oxidation-responsive biodegradable PEG hydrogels for glucose-triggered insulin delivery. ACS Appl. Mat. Interfaces 9, 25905–25914. doi:10.1021/acsami.7b08372
Zhao, C., Zhuang, X., He, P., Xiao, C., He, C., Sun, J., et al. (2009). Synthesis of biodegradable thermo- and pH-responsive hydrogels for controlled drug release. Polymer 50, 4308–4316. doi:10.1016/j.polymer.2009.07.010
Zhou, H., Qin, W., Yu, Q., Cheng, H., Yu, X., and Wu, H. (2019). Transfer printing and its applications in flexible electronic devices. Nanomaterials 9, 283. doi:10.3390/nano9020283
Zhou, J., Yang, X., Liu, W., Wang, C., Shen, Y., Zhang, F., et al. (2018). Injectable OPF/graphene oxide hydrogels provide mechanical support and enhance cell electrical signaling after implantation into myocardial infarct. Theranostics 8, 3317–3330. doi:10.7150/thno.25504
Zhou, M., Kang, D. H., Kim, J., and Weiland, J. D. (2020). Shape morphable hydrogel/elastomer bilayer for implanted retinal electronics. Micromachines 11, 392. doi:10.3390/mi11040392
Zhu, P., and Zhao, Y. (2017). Effects of electrochemical reaction and surface morphology on electroactive surface area of porous copper manufactured by Lost Carbonate Sintering. RSC Adv. 7, 26392–26400. doi:10.1039/C7RA04204C
Zustiak, S. P., and Leach, J. B. (2010). Hydrolytically degradable poly(ethylene glycol) hydrogel scaffolds with tunable degradation and mechanical properties. Biomacromolecules 11, 1348–1357. doi:10.1021/bm100137q
Glossary
2PP Two-photon polymerization
AG Arabic gum
Anti-HBc Hepatitis B core antibody
CDC Charge delivery capacity (charge capacity per unit area)
CNC Cellulose nanocrystal
DA Dimethylacrylate
DE Diglycidyl ether
Dopa Dopamine
E Young’s modulus
GelMa Gelatin methacryloyl
GMA Glycidyl methacrylate
IPN Interpenetrating polymer network
LOx Lysyl oxidase
NWs Nanowires
PAA Polyacrylamide
PAAN Polysodium acrylate
PACP Poly(aniline-co-pyrrole)
PAMAM Poly(amido amine)
PANi Polyaniline
PBMA Poly(butyl methacrylate)
PCL Polycaprolactone
PDCA Pyridinedicarboxamide
PDMS Polydimethylsiloxane
PEDOT Poly(3,4-ethylenedioxythiophene)
PEG Polyethylene glycol
PEG-SVA Poly(ethylene glycol disuccinimidyl valerate)
PEG-VS Poly(ethylene glycol) vinyl sulfone
PFPE-DMA Dimethacrylate-functionalized perfluoropolyether
PGA Poly(glycolic acid)
PHEMA Poly(hydroxyethyl methacrylate)
PI Polyimide
PMEP-DMA Poly(methyl ethylene phosphate)-dimethacrylate
PMMA Poly(methacrylic acid)
PNH Poly(N-isopropylacrylamide-co-2-hydroxyethyl methacrylate)
PPy Polypyrrole
PU Polyurethane
PSS Polystyrene sulfonate
PVA Polyvinyl alcohol
R Resistance
Rs Sheet resistance
SBS Styrene-butadiene-styrene
SU-8 Structured by UV (refers to the epoxy-based negative photoresist)
tH Thickness of the hydrogel layer
Z Impedance
σ Electrical conductivity
Keywords: hydrogels, bioelectronic interfaces, microfabrication processes, implantable medical devices, biomaterials, soft bioelectronics, medical device encapsulation, biomimetic coatings
Citation: Saghir S, Imenes K and Schiavone G (2023) Integration of hydrogels in microfabrication processes for bioelectronic medicine: Progress and outlook. Front. Bioeng. Biotechnol. 11:1150147. doi: 10.3389/fbioe.2023.1150147
Received: 23 January 2023; Accepted: 10 March 2023;
Published: 24 March 2023.
Edited by:
Anna-Maria Pappa, Khalifa University, United Arab EmiratesReviewed by:
Nicholas Hallfors, Khalifa University, United Arab EmiratesMadhur Atreya, University of Colorado Boulder, United States
Copyright © 2023 Saghir, Imenes and Schiavone. This is an open-access article distributed under the terms of the Creative Commons Attribution License (CC BY). The use, distribution or reproduction in other forums is permitted, provided the original author(s) and the copyright owner(s) are credited and that the original publication in this journal is cited, in accordance with accepted academic practice. No use, distribution or reproduction is permitted which does not comply with these terms.
*Correspondence: Giuseppe Schiavone, Z2l1c2VwcGUuc2NoaWF2b25lQHVzbi5ubw==