- 1State Key Laboratory of Crop Stress Adaptation and Improvement, School of Life Sciences, Henan University, Kaifeng, China
- 2School of Food Science and Engineering, Wuhan Polytechnic University, Wuhan, China
- 3Academy for Advanced Interdisciplinary Studies, Henan University, Kaifeng, China
Algae play a crucial role in the earth’s primary productivity by producing not only oxygen but also a variety of high-value nutrients. One such nutrient is polyunsaturated fatty acids (PUFAs), which are accumulated in many algae and can be consumed by animals through the food chain and eventually by humans. Omega-3 and omega-6 PUFAs are essential nutrients for human and animal health. However, compared with plants and aquatic sourced PUFA, the production of PUFA-rich oil from microalgae is still in the early stages of exploration. This study has collected recent reports on algae-based PUFA production and analyzed related research hotspots and directions, including algae cultivation, lipids extraction, lipids purification, and PUFA enrichment processes. The entire technological process for the extraction, purification and enrichment of PUFA oils from algae is systemically summarized in this review, providing important guidance and technical reference for scientific research and industrialization of algae-based PUFA production.
Introduction
Algae are a group of photosynthetic organisms that evolved earlier than higher plants. Organisms that contain chlorophyll a in cells but lack differentiation of roots, stems, leaves and other tissues and organs are generally classified as algae (Lee, 2018). Humans have been utilizing algae as food for thousands of years, such as Nostoc, Aphanizomenon and Spirulina (Jensen et al., 2001). Macroalgae are typically consumed directly by humans, while microalgae are indirectly consumed through the consumption of fish and other animals. Algae are the primary contributors to water productivity on Earth, providing nutrients for many aquatic organisms (Chen H et al., 2021). Some algae are particularly suitable as feed (Becker, 2003a) for animals, and initial processing techniques have been established (Amorim et al., 2020).
Microalgae are a valuable source of biologically active substances and a treasure trove of resources. Recent articles have highlighted their ability to produce a variety of valuable products such as lipids, proteins, sugars, mannan (Capek et al., 2023), chitin (Chiriboga and Rorrer, 2019), terpenoids (Huang et al., 2021), vitamins (Ljubic et al., 2021). Some microalgae also produce essential fatty acids like alpha-linolenic acid (ALA) (Meng et al., 2018), eicosapentaenoic acid (EPA) (Peltomaa et al., 2018; Xia et al., 2020), docosahexaenoic acid (DHA) (Peltomaa et al., 2018), gamma-linolenic acid (GLA) (Ronda et al., 2012).
The analysis shows that lipids and fatty acids are the prominent research areas in algae research. Algal cells typically contain lipids ranging from 1% to 40% of their dry weight, while some species can accumulate up to 85% (Becker, 2003b; Chen and Wang, 2021). These lipids generally exist in cells in the form of glycerides, free fatty acids, glycolipids and phospholipids (Becker, 2003b). Certain algae species can produce both PUFA and pigment fucoxanthin simultaneously, such as diatom Nitzschia laevis (Lu et al., 2019), Tisochrysis lutea (Gao et al., 2022). Apart from nutrition and health applications, algae also have significant potential in environmental fields, such as biodiesel production (Zhu et al., 2016; Chen and Wang, 2022), hydrogen production (Chen et al., 2023), as well as the removal of nitrogen (Chen et al., 2016; Wang J et al., 2019; Chen and Wang, 2020), flame retardants (Zhang et al., 2021), and organics (Zheng et al., 2021) from wastewater. However, compared with fossil energy, due to the high cost of production, extraction, and conversion, algae biodiesel’s commercial advantages are limited (Chen et al., 2019; Chen H et al., 2020). Therefore, since 2014, the focus of algae research has gradually shifted towards high-value bioproducts and environmental applications (Rashid et al., 2018).
Algae are currently being developed as new sources of the polyunsaturated fatty acids (PUFAs), including linolenic acid (LA), ARA, EPA, docosapentaenoic acid (DPA), DHA (Chen W et al., 2021; Cui et al., 2021). Algal DHA has already been commercialized in the market. Although the deep-sea fish oil PUFAs currently available are derived from fish, they are essentially derived from the oil accumulated by algae ingested by fish (Ebm et al., 2021). To identify related research, we collected keywords related to algae and PUFA, and the 41 kinds of unsaturated fatty acids (UFAs) introduced on Wikipedia. Using these keywords, we searched the Web of Science and retrieved over 7,000 reports related to algae-PUFA research. From the perspective of statistics in Figure 1, algae-PUFA research is an important research hotspot and has shown continuous growth in recent years.
Analysis of research hotspots
The VOS viewer (version 1.6.18) was used to analyze the keywords found in the retrieved reports. Among the keywords, algae was found to be the central theme, and the keywords most closely related to algae were fatty-acid, composition, growth, and extraction (as shown in Figure 2).
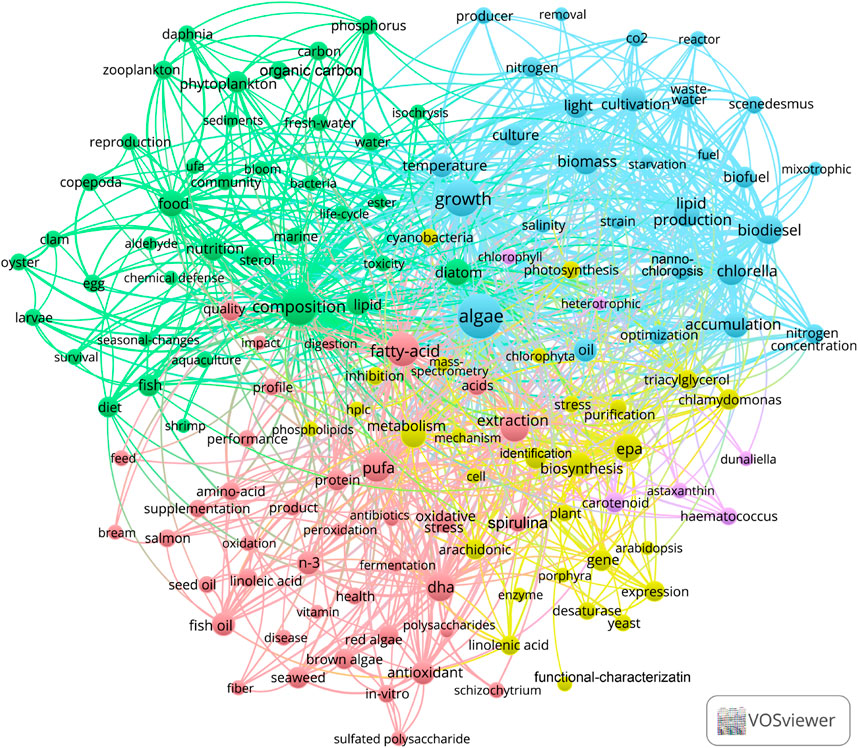
FIGURE 2. The most popular keywords in the articles of algae-PUFA in the Web of Science Core Collection.
In the blue cluster depicted in Figure 2, growth is found to be most closely related to light, biomass, cultivation, lipid production, biodiesel, accumulation. This indicates that there are some key factors involved in the accumulation of PUFA in the algae culture. Furthermore, algae oil has emerged as a critical area of research in the field of biofuel and biodiesel. However, the production of biodiesel from algae is considered to be expensive and less commercially viable (Kumar et al., 2013). With recent technological advancement, the production cost of microalgae biodiesel based on waste water and seawater has been reduced to US$2.2/kg, which is still much higher than that of fossil energy (Yu et al., 2023). Even after considering the revenues from wastewater treatment and carbon dioxide capture, the commercial competitiveness of microalgae biodiesel remains insufficient when compared with fossil energy. On the other hand, the production of PUFAs has proved to be commercially successful.
The microorganisms associated with PUFAs were ranked based on their occurrence, with green algae and diatoms being relatively more frequent (Figure 3A). Among green algae, Chlorella, Chlamydomonas, Nannochloropsis, Scenedesmus, Haematococcus, Dunaliella were reported more quantitatively. Among diatoms, there were more reports of Phaeodactylum tricornutum, Skeletononema costatum, and Thalassiosira rotula. It suggests that research on PUFAs production is mostly concentrated in these algae. In general, diatoms can accumulate a large amount of long-chain PUFA in cells, but their biomass production rate is generally relatively low even when supplemented with organic carbon sources (Marella et al., 2021; Cheah et al., 2022). On the other hand, Cryptheccdinium cohnii and Schizochytrium have a high content of long-chain PUFAs such as DHA and EPA, as well as high biomass production rates. Some green algae, such as Chromochloris zoffingiensis, can accumulate astaxanthin, but the accumulated PUFAs is mainly C18 PUFAs (Liu et al., 2016; Mao et al., 2018). Therefore, some research groups are also attempting to co-produce long-chain PUFAs and astaxanthin in algae cells, making it more cost-effective for commercial production (Pawar et al., 2021).
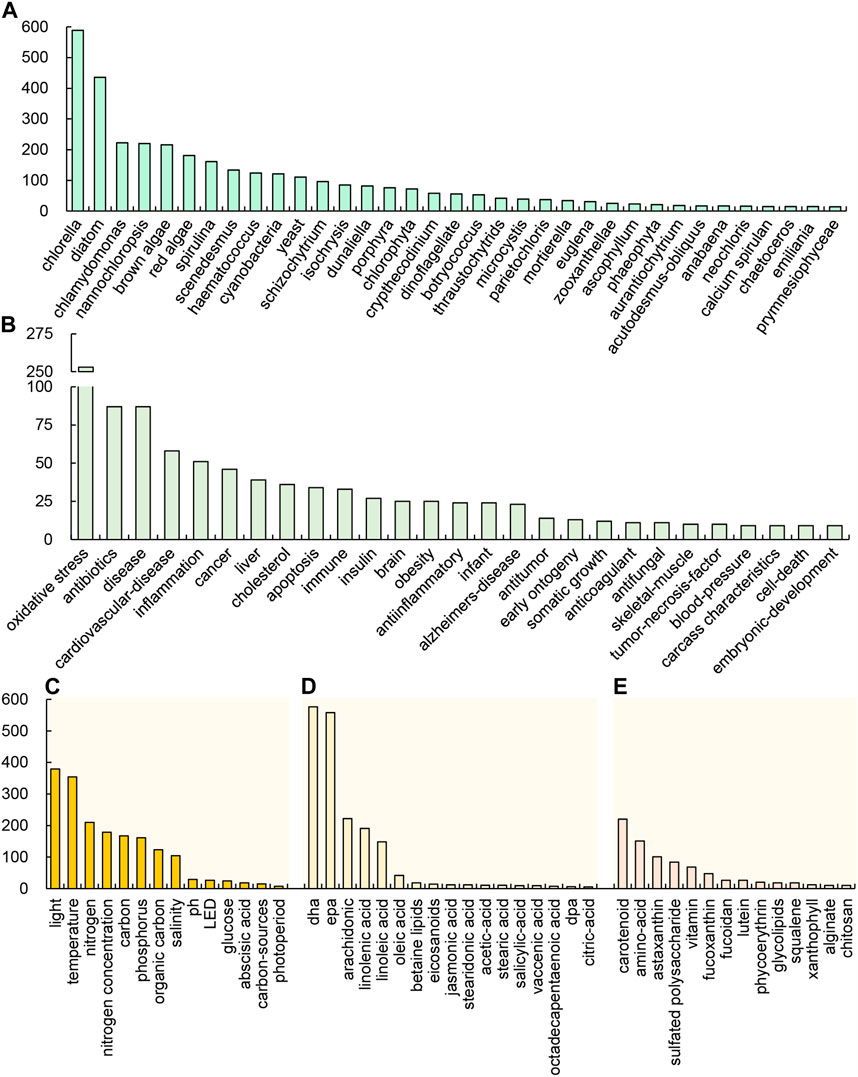
FIGURE 3. Occurrence of the main keywords in the reports of algae-PUFA according to the species (A), health functions (B), conditions (C), kinds of PUFAs (D), other high value products (E).
The keywords related to the cultivation conditions were ranked based on their occurrence, as shown in Figure 3C. Among these keywords, nitrogen (concentration), light, temperature, carbon, phosphorus, organic carbon, salinity, pH, LED, and glucose were found to be the key research factors. It is evident that the nitrogen source is the most relevant or critical for algal PUFA production. Other environmental or nutritional factors may also affect the accumulation of PUFAs. Therefore, to optimize culture conditions and increase the yield of algae PUFAs, the above environmental factors can be considered for trial testing.
In the green cluster composition shown in Figure 2, the focus is mainly on some keywords that PUFAs affect animal nutrition. This also indicates that PUFAs plays an important role in improving the nutritional value of aquatic products. Furthermore, the accumulation of PUFAs in many aquatic products is also due to the PUFAs accumulated by algae in the water (Brett and MÜller-Navarra, 1997; Nichols, 2003).
In the pink cluster in Figure 2, the keywords with higher occurrence are fatty-acid, PUFA, and extraction. These keywords are mainly related to the health benefits of PUFAs. Extraction is an important aspect of algae-PUFA production research, and closely related to growth, composition, PUFA, carotenoid. Additionally, in the exploration of algae biodiesel production, extraction is a significant cost factor (Kumar et al., 2013; Rashid et al., 2018).
In the yellow cluster in Figure 2, the keywords with higher occurrence are EPA and biosynthesis, which are related to the synthesis of PUFAs. The keywords of interest include desaturase and linolenic acid. Linolenic acid is not only an important PUFA, but also a precursor for the synthesis of other PUFAs, such as DHA and EPA. The human body has the ability to convert linolenic acid into other PUFAs. Ensuring the intake of linolenic acid may be beneficial for human health and meet the body’s demand for longer chain PUFAs such as DHA and EPA. In addition, the occurrence of various PUFA types is ranked by occurrence in Figure 3D, which shows that DHA and EPA have the highest occurrence. The occurrences of arachidonic acid (ARA), linolenic acid, and linoleic acid are also relatively high. The occurrence of oleic acid is very small, and the occurrence of other PUFAs is very low. This implies that the application value of these PUFAs is related to the current market demand.
In the purple cluster in Figure 2, carotenoid was the important keyword, closely related to astaxanthin, Haematococcus and Dunaliella, followed by heterotrophic and chlorophyll. Moreover, both carotenoid and PUFAs in the pink cluster are closely related to antioxidant. The keywords of other high-value products are ranked by their occurrences (Figure 3E). It can be seen that carotenoid, amino-acid, and astaxanthin are other high-value products with the highest correlation with algae-PUFA. This may be because the accumulation of these substances has a certain correlation with the accumulation of PUFAs. The simultaneous accumulation of PUFAs with other high-value products can greatly increase the potential for practical production applications of these algae.
Sources of PUFAs
Synthesis
Fatty acids are long-chain carboxylic acids that serve as crucial raw materials for oils and directly affect the properties and functions of oils. Fatty acids can be divided into saturated fatty acids and unsaturated fatty acids (UFAs). Fatty acids that lack carbon-carbon double bonds in the aliphatic chain are referred to as saturated fatty acids, which include palmitic acid and stearic acid, among others. PUFAs are straight-chain fatty acids with two or more double bonds (Metzner, 1984). Depending on the position of the first double bond from the methyl end of the fatty acid, PUFAs can be classified into ω-x fatty acids, including ω-9, ω-6, ω-3 (also referred to as n-9, n-6, n-3) (Djuricic and Calder, 2021).
The biosynthesis of ω-3, ω-6 and ω-9 PUFAs begins with acetyl-CoA and requires a series of carbon chain elongation and desaturation reactions, as illustrated in Figure 4 (Uemura, 2012; Scanferlato et al., 2019). The synthesis of ω-3 and ω-6 polyunsaturated fatty acids follows two distinct pathways, and intermediates from one pathway cannot be directly converted into intermediates of the other (Napier, 2002). The ω-3 PUFAs like DHA and EPA are derived from ALA, while the ω-6 PUFAs, such as GLA and ARA are derived from LA (9,12-linoleic acid) (Lehninger, 1982). The synthesis of PUFAs in microorganisms is similar to that in higher organisms. Acetyl-CoA and malonyl-CoA form LA under the action of fatty acid synthase complex, which is then further metabolized to PUFAs through the ω-6 and ω-3 pathways (Lehninger, 1982).
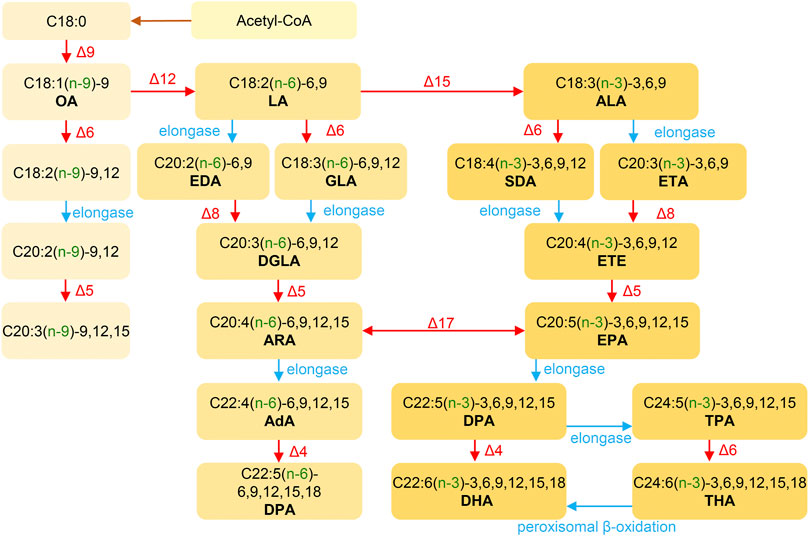
FIGURE 4. The general pathways of PUFAs synthesis. Desaturase: Δ4/Δ5/Δ6/Δ9/Δ15/Δ17; C18/20/22, carbon numbers; :1/:2/…/:6, number of olefinic bonds; (n-3/6/9), double bond between the (n–x) and (n-x+1) to last carbon atoms; −3/6/9/12/15/18, double bond between the x and x+1 to last carbon atoms. AdA, adrenic acid; ALA, α-linolenic acid; ARA, arachidonic acid; DGLA, dihomo-γ-linolenic acid; DHA, docosahexaenoic acid; DPA, osbond acid; DPA, Docosapentaenoic acid; EDA, eicosadienoic acid; EPA, eicosapentaenoic acid; ETA, eicosatrienoic acid; ETE, eicosatetraenoic acid; GLA, γ-linolenic acid; LA, linoleic acid; OA, oleic acid; SDA, stearidonic acid; THA, tetracosahexanoic acid; TPA, tetracosapentanoic acid.
Humans and other mammals are incapable of synthesizing ω-3 and ω-6 PUFAs de novo due to the absence of Δ12 and Δ15 dehydrogenases (Napier, 2002). Therefore, LA and ALA must be obtained from dietary sources (Djuricic and Calder, 2021). The synthetic pathways of ω-3 and ω-6 PUFAs share common desaturases and chain-elongation enzymes, resulting in a metabolic competition process (Smink et al., 2012). Therefore, a balanced intake of LA and ALA is crucial. The essential fatty acid ALA serves as a precursor to ω-3 PUFAs such as EPA and DHA (Brenna et al., 2009). Thus, supplementing these precursors can help to increase EPA or DHA levels, with a more comprehensive supplementing effect.
Main physiological and health functions
The health benefits of omega-3 PUFA was initially studied through the correlation between fish oil intake and health risks, mainly related to reducing triglycerides, lowering blood pressure, preventing thrombosis, and interfering with arachidonic acid cascade reaction, and thus playing an anti-atherosclerosis role (Simopoulos, 1991; Kris-Etherton et al., 2002). EPA and DHA have shown promising results in prevention, body weight management, and cognitive function in patients with mild Alzheimer’s disease (Swanson et al., 2012). Figure 3B displays the occurrence of keywords related to health and disease, where the antioxidant activity of PUFAs is the most prominent characteristic. PUFAs has anti-oxidation and anti-aging effects. Clinical studies have shown that after taking ALA, the activity of glutathione peroxidase and superoxide dismutase increases, the generation of malondialdehyde decreases, and it has an antioxidant effect (Mahsa et al., 2017; Boskabady et al., 2019; Korbecki et al., 2019). In addition, PUFAs have reported functions in antibiotics, cardiovascular-disease, anti-inflammation, cancer, liver health, blood lipids, diabetes, brain health, and ontogeny. In summary, PUFAs play an important role in human health, with their main physiological functions related to the circulatory system, immune system and nervous system. A summary is shown in Table 1. Omega-3 PUFAs have a strong market presence due to their crucial structural and physiological functions, as well as their beneficial health effects.
Food sources of PUFAs
According to Figure 4, ALA is the primary precursor of ω-3 PUFAs, and is mainly used in the pharmaceutical, food and cosmetic industries, with potential for even more new uses. Currently, the largest use of ALA is in dietary nutrition. The Center for Genetics, Nutrition and Health of the National Institutes of Health in the United States recommends a daily intake is 2.22 g/day of ALA (Simopoulos et al., 2000). However, ALA is found in low amounts in common foods, including various fresh vegetables. The content of ALA in plants is generally around 100 mg/100 g (fresh weight), and is mainly present in glycolipids in the leaves (Raper et al., 1992; Ayerza, 1995). Common sources and contents of ALA are shown in Table 2.
ALA-rich products available in the market are typically sourced from a few crops, such as flaxseed, as well as deep-sea fish oil. Marine fish are unable to synthesize DHA on their own. Instead, the DHA contained in their bodies originates from marine microorganisms, such as phytoplankton, and accumulates in marine fish, reptiles and mollusks through the marine food chain (Brett and MÜller-Navarra, 1997; Nichols, 2003). Research has shown that certain marine bacteria, algae, and fungi are capable of synthesizing PUFAs (Ratledge, 2001). Some algae, in particular, can accumulate ALA ranging from 10% to 40% of the total lipid fraction, as shown in Table 3.
Algae are the original source of ω-3 PUFAs in fish oil, making algae oil a crucial source of PUFAs. An increasing number of algae are now being cultivated on a large scale for the production of some bioactive substances, including PUFAs. Compared to planting terrestrial crops to produce oil to obtain ALA and other PUFAs, algae are easy to cultivate, have high photosynthesis efficiency, short reproduction cycle, and offer relatively more flexible production without the need for arable land. Furthermore, abundant microalgae germplasm resources contain numerous genetic resources, which can also be used as chassis organisms and living bioreactors to produce a variety of high value-added products that are beneficial to human and animal nutrition and health. There are several advantages to using microorganisms instead of fish oil as an alternative resource (Table 4).
Ways of producing PUFA
Currently, humans rely on fishery products as a source of omega-3 PUFA, which poses a risk of exposure to environmental pollutants such as methylmercury, polychlorinated biphenyls, algal toxins, and other contaminants (Ward and Singh, 2005; Hamilton et al., 2020; Zheng et al., 2023). The consumption of fish and fish oil is less safe due to these pollutants, and the limited fishery resources also make the sustainable supply of omega-3 PUFA challenging (Kris-Etherton et al., 2002; Hamilton et al., 2020). Furthermore, the efficiency of omega-3 PUFA digestion can be affected by the processing methods of aquatic products (Kris-Etherton et al., 2002; Adarme-Vega et al., 2012; Hamilton et al., 2020).
In contrast, microorganisms such as microalgae offer a flexible, efficient, and safe natural synthesis pathway for omega-3 PUFA (Hamilton et al., 2020; Gu et al., 2021). In terms of production costs, compared with fishing and aquaculture to obtain omega-3 PUFA, algae fermentation production still does not have a significant cost advantage. However, with the depletion of fishery resources and the advancement of microalgae processing technology, the cost and product quality of omega-3 PUFA from algae and other microorganisms can eventually capture the market.
For the industrialization of PUFA production from algae, DHA and EPA have been successfully commercialized on a large scale (Borowitzka, 2013; Xue et al., 2013). Currently, several species of microalgae and fungi, including Schizochytrium, Cryptocodinium cohnii, and diatoms, are being used for fermentation production of PUFA (Ward and Singh, 2005; Marella et al., 2021; Stramarkou et al., 2021; Chi et al., 2022). These studies primarily refer to the fermentation production mode of fungi such as yeast. Many algae can be photoautotrophically cultured in various reactors such as open ponds, and can also be heterotrophically cultured in fermentation tanks. However, commercial algae production mostly relies on autotrophic cultivation, including Spirulina sp. and Chlorella sp. (Mendes et al., 2022). Although the input cost of photoautotrophic culture is relatively low, the yield per unit cost is also low. Under mixotrophic and heterotrophic conditions, the biomass yield of many algae is even ten times higher than that under autotrophic conditions. If high-density fermentation can be achieved, the unit production cost of some microalgae is lower than that of photoautotrophic culture (Jin et al., 2020; Jin et al., 2021; Ruiz et al., 2022). It is estimated that at a production scale of 1,000 tons/year, the production cost per unit of microalgae biomass production by high-density fermentation method can be lower than that by open pond culture method (Jin et al., 2020). However, it should be noted that the oil content and composition of some algae in heterotrophic culture may not be as commercially valuable as in photoautotrophic culture (Jin et al., 2020). When choosing between photoautotrophic and heterotrophic cultivation, many manufacturers rely on the higher biomass yield per unit of input they can achieve (Ruiz et al., 2022). Therefore, achieving ultra-high-density fermentation is crucial for the commercialization of microalgae. It should be pointed out that, in theory, microalgae fermentation does not require the participation of photosynthesis. If the unnecessary physiological functions and structures of microalgae can be controlled during the proliferation process, or if the synthesis pathway of high-value products such as microalgae PUFA can be introduced into more robust and efficient chassis cells for expression, this could greatly enhance the economic viability of production.
Moreover, the development of sequencing, genetic engineering and bioinformatics technology has significantly contributed to the synthesis of omega-3 PUFA. It has provided essential information for optimizing the enzyme system for algae to synthesize high-value oil (Yang F et al., 2019; Degraeve-Guilbault et al., 2021). The synthetic pathways of PUFAs in algae are relatively well-understood, and many desaturases and elongases in algae or other species have been identified. Additionally, the enzymes present in algae have also provided crucial information for the synthesis pathways of omega-3 PUFA in other species, such as fungi and plants (Rezanka et al., 2017). Compared to the fermentation mode and genetic engineering of yeast and other microorganisms, the tools available for algae still need to be developed (Xue et al., 2013; Xie et al., 2015; Khera and Srivastava, 2022).
Advances in genetic engineering technology are essential for the synthetic biology of algae. However, many algae can only undergo genetic modification, such as RNAi, which cannot be stably inherited (Kugler et al., 2019). Alternatively, high-producing strains can be screened using blind mutagenesis. Nevertheless, if significant breakthroughs occur, many efficient photosynthetic chassis cells could provide a vital platform for the production of PUFA, carotenoids, and other substances. Algae, with their ability to use light energy and cheap carbon sources to produce PUFA, hold great potential for the future. With its high photosynthetic efficiency, algae can be used as chassis cells to transform into a cell factory that can synthesize omega-3 PUFA using solar energy and cheap carbon sources. Thus, genetic engineering technology to transform microbial fermentation for PUFA production is currently an important means to achieve commercialization.
Pretreatment of lipids extraction from algae
Typically, microalgal biomass obtained through fermentation or other cultures must be harvested prior to lipid extraction. Currently, algae biomass harvesting methods can be divided into four types, flocculation-sedimentation, centrifugation, filtration, and flotation, each with its own scope of application (Wang S et al., 2019; Li et al., 2020). Many materials have been developed to effectively flocculate algae, and various types of collection membranes have been developed to filter and collect algae. However, flocculation, sedimentation, filtration, and flotation are primarily used for the concentration and harvesting of algae with low biomass density, and only most of the medium residue can be removed by separation (Wang S et al., 2019; Li et al., 2020). On the other hand, centrifugation can achieve high-quality concentration and separation of algae, which has been utilized in commercial production and is more suitable for the collection of algae with high cell density (Wang S et al., 2019).
After being collected by centrifugation, the algae are still wet and need to be dehydrated in order to obtain dried biomass that is stable and easy to storage. While heat drying is a common method, it takes a long time and is not suitable for algae containing high-temperature-sensitive compositions (Hosseinizand et al., 2018). For algae rich in such compositions, like PUFAs, freeze-drying, vacuum drying, and spray drying are more appropriate as they do not involve long-term high-temperature heating. Spray drying involves dispersing wet algae into micro-droplets, which are then exposed to hot air for a very short time, greatly shortening the evaporation time (Zhang et al., 2022). Therefore, spray drying is suitable for large-scale production and is much more efficient than heat drying, freeze drying, and vacuum drying. After the purer algae biomass is collected, oil extraction, impurity removal, and PUFAs purification are required to obtain higher purity algae PUFAs (Ali et al., 2021; Chen W et al., 2021). The process of algae production, oil extraction and PUFAs enrichment is outlined in the Figure 5.
In addition to traditional processes, there is a process in which algae cells are directly converted into fatty acid methyl esters or fatty acid ethyl esters, bypassing the lipids extraction step. Dry or even wet microalgal cells can undergo in-situ transesterification to become fatty acid esters (Umamaheswari et al., 2020). Biodiesel production tests using wet Nannochloropsis gaditana and Schizochytium limacinum biomass have shown that the yield of fatty acid esters in total lipids can reach between 73% and 100% (Kim et al., 2015; Park et al., 2017). Generally, wet microalgae are not suitable for lipid extraction or transesterification reactions due to their moisture content, which prevents non-polar solvents from penetrating into the cells, thereby reducing lipid extraction efficiency (Nagappan et al., 2019). The in-situ transesterification reaction is a complex process that involves the reaction between an ionic liquid and the contents of a cell. This process requires breaking down the cell wall to react with the lipid present inside. To enhance the efficiency of this reaction and reduce associated costs, it is essential to conduct a meticulous study of the interaction between the appropriate ionic liquid and the cell components (Ding et al., 2022). However, these processes typically require high temperatures above 100°C, which may cause the degradation of PUFAs. If these limitations can be overcome, it would significantly reduce the production and enrichment process of microalgae PUFA and lower production costs, as illustrated in Figure 5.
Lipids extraction
Common oil crops such as rapeseed, peanut, soybean, corn, and sunflower can be extracted for lipids using physical pressing and solvent extraction methods. However, due to the powdered or block form of dried algae, physical pressing is not an effective method of extraction. Instead, solvent extraction is generally utilized using substances like n-hexane, chloroform, or supercritical CO2. Supplemental techniques such as microwave treatment, mechanical treatment, enzyme treatment, or ultrasonic cell wall breaking can also be applied to improve algae oil extraction (Teo and Idris, 2014).
Extraction methods
There are various methods available for extracting algae oil, but it is primarily utilized in biodiesel production to reduce costs (Liu et al., 2013). Due to the large number of other components that can be introduced, this method is typically used for extracting products not intended for human and animal consumption. Algae oil extraction methods include chemical solvent extraction, physical wall breaking treatment, supercritical fluid extraction (SFE), and biological extraction (Ali et al., 2021). These methods can be roughly divided into two categories: regular organic solvent extraction and SFE. The advantages, disadvantages, and scope of application of both extraction methods were summarized in Table 5.
Organic solvent extraction is widely used in the extraction of algae oil. That is to choose an organic solvent that can dissolve oil, infiltrate the algae and extract the intracellular oil (Ranjan et al., 2010). This method provides high oil yield, simple equipment, and convenient operation. However, the organic solvents used are often toxic and volatile, which can be harmful to human health. While static extraction can extract some substances from the material, its extraction efficiency is low and it takes a long time. To improve the extraction efficiency, dynamic extraction is generally employed, and the solvent is repeatedly flowed through the material (Grierson et al., 2012; Ramluckan et al., 2014; Aravind et al., 2021). For instance, Soxhlet extraction is commonly used to extract the total lipids. This method requires dry biomass with smaller particle size, continuous reflux of the solvent, continuous heating of the equipment, continuous reflux of the evaporated solvent, even continuous reflex with different solvent, making it a highly energy-intensive process that is unsuitable for components that are sensitive to high temperature (Chen W et al., 2020; Aravind et al., 2021).
In a fully enclosed device, SFE is a suitable method for temperature-sensitive products. Carbon dioxide, a non-polar molecule, can be transformed into a supercritical fluid at normal temperature and high pressure. By altering the temperature, pressure, and adding various entrainers, similar polarity molecules in the sample can be extracted (Cooney et al., 2009). Other substances, such as ammonia, ethylene, propane, and propylene, can also be utilized as supercritical fluids (Cooney et al., 2009). In some instances, lipid yields from SFE are comparable to those from Soxhlet extraction (Mercer and Armenta, 2011). Over the last 3 decades, SFE has been utilized to extract high-value compounds from algae, including astaxanthin, β-carotene, lutein, DHA, EPA, ALA, ARA (Solana et al., 2014; Molino et al., 2020). After 5% (w/v) ethanol was used as an entrainer to extract lipids from Chlorella vulgaris with supercritical CO2, the lipid extraction efficiency increased by 127% (Safi et al., 2014). Lipid extraction yields were also improved by using microwave radiation-assisted supercritical CO2 extraction strategies (Dejoye et al., 2011). However, the overall lipid extraction rates they reported, ranging from 47 to 127 mg/g cells, were not significantly higher than those of the physical and chemical methods mentioned above. Compared with Soxhlet extraction, SFE has several advantages in extracting PUFAs from Scenedesmus (Solana et al., 2014), Nannochloropsis sp. (Jiménez Callejón et al., 2022), Schizochytrium sp. (Rodríguez-España et al., 2022).
Extraction with wall breaking
The extraction of oils from certain algae can be a challenging task as they are protected by cell walls that need to be broken down. The cell wall of algae are complex structures made up of multiple layers, including cellulose and pectin, which are quite rigid and can impede the extraction of target compounds (Brennan and Owende, 2010). Currently, the main methods for breaking down algae cell walls are physical methods that mechanically destroy the cell wall, as well as biochemical methods that use enzymes or chemical agents to hydrolyze and degrade the cell wall. However, the appropriate cell wall breaking method largely depends on the unique biology and cell wall characteristics of the algae (Praveenkumar et al., 2015). Additionally, the efficiency of these methods is affected by operating conditions such as temperature, pressure, biomass (concentration, wet and dry state, growth stage), and scale (Rawat et al., 2011). If the synthesis of cell walls can be controlled through genetic engineering, the barrier to substance extraction can be eliminated, significantly reducing the cost of material processing, and also correspondingly increasing the utilization rate of nutrients in the culture medium. Therefore, creating excellent chassis cells and controlling the synthesis of cell walls is the most ideal solution.
Physical wall-breaking method
The physical wall-breaking method involves applying mechanical external force or non-mechanical physical methods to break up cells, which include homogenization, high-pressure homogenization, ultrasonic crushing, microwave crushing, electroporation, autoclave, repeated freezing and thawing (Madany et al., 2021).
The homogenization utilizes mechanical external force, such as grinding, to break the wall. When crushing Chlorococcum sp. by grinding, the average cell crushing rate reaches 17.5% (Halim et al., 2012). Homogenization is only appropriate when the product is easily separable. Although it is economical and environmentally friendly, and does not require mixing with other ingredients, it is not efficient for algae with stronger cell walls (Madany et al., 2021).
The high-pressure homogenization method uses high-speed impact and strong shearing under high pressure to break up cells. When treating Chlorococcum sp. with 85 MPa homogenization, the cell disruption rate reaches 73.8% (Halim et al., 2012). The crushing effect of the high-pressure homogenization method is closely related to the composition of the algae cell wall. This method usually has a high breaking rate and has little effect on fatty acid profile, but is energy intensive (Magpusao et al., 2022).
Ultrasonication is suitable for most algal cells and generates dense microbubbles in liquid media, resulting in strong cavitation effects when high-power ultrasound (typically exceeding 20 kHz) is used (Valizadeh Derakhshan et al., 2014). Proper ultrasonic treatment can even double the oil extraction rate from Chlorella (Ellison et al., 2019). Ultrasonic method is non-polluting and has a high wall-breaking rate, but the local temperature in the system may rise sharply, and its effect on the quality of the extracted oil is not yet clear.
The microwave crushing method employs microwave heating to rapidly increase the intracellular temperature, which results in the vaporization of liquid water and the generation of high pressure, ultimately breaking through the membrane-wall structure of the cell to create small holes (Lee et al., 2010). Although this method is fast and pollution-free, the sharp increase in temperature may cause denaturation of the active substance.
Electroporation generates minimal cell debris and can even promote cell growth and regeneration. It is also possible to extract intracellular lipids into the supernatant. However, the extraction rate is lower compared to chemical lysis or mechanical decomposition (Eleršek et al., 2020).
Autoclaving involves the use of high temperature and pressure to increase the diffusion of substances, allowing them to be released from cells (Zhou et al., 2020). However, it may cause side reactions in some substances and lead to changes in lipids. This method is typically used in bioenergy production (Sivaramakrishnan and Incharoensakdi, 2019).
In contrast, the repeated freezing and thawing method produces ice particles within the cells, causing the salt concentration of the remaining cytosol to increase, leading to cells swelling and breakage. This technique is simple to perform and does not damage heat-sensitive substances, making it suitable for the extraction of high-value substances, making it suitable for high-value product extraction using wall-broken extraction. However, it consumes more energy compared to other methods.
Biochemical wall-breaking method
The biochemical wall-breaking method involves adding biological enzymes or chemical reagents to assist in degrading cell wall. This approach is commonly used in conjunction with physical methods. The most prevalent methods are the osmotic shock method, solvent lysis method, ionic liquid lysis method, switchable polar solvent lysis method, and biological enzyme preparation digestion method.
The acid-heat method involves treating algae with acid to degrade the cell walls. Mixing Chlorococcum sp. with 8% sulfuric acid and heating the mixture at 160°C for 45 min achieved an algae cell wall breaking rate of 33.2% (Halim et al., 2012). Dilute H2SO4 can significantly affects the degradation of cell wall, even in the absence of any polar organic solvent such as methanol. However, it should also be noted that this sulfuric acid process can be efficiently applied to wet algal biomass, but appropriate material and facility costs should be addressed (Park et al., 2014).
Chemiosmosis involves using the difference in osmotic pressure between the inside and outside of algae cells in a hypertonic solution to break down the cells. Although this method is mild, it usually takes a long time, and the high osmotic pressure causes the cell contents to be squeezed out spontaneously. A low lipid extraction rate was obtained from wet algal biomass of C. vulgaris with 1 L of phosphorus hydrate P(CH2OH)4Cl (Olkiewicz et al., 2015).
Solvent lysis, ionic liquid lysis, and switchable polarity solvent lysis are based on the properties of the cell wall, where specific solvents or chemical substances are added to degrade the cell wall. The most common solvent lysis methods include the Bligh and Dyer method, Folch method, and Soxhlet extraction, which simultaneously achieve cell wall lysis and oil extraction. The ionic liquid lysis supplemented by physical treatment can significantly enhance the extraction of fatty acids. Switchable polarity solvents have unique chemical properties that can rearrange the lipid bilayer structure in the cell membrane, resulting in higher extraction efficiency than solvent lysis.
The enzymatic method utilizes hydrolytic enzymes to degrade algae cells. This method has a high rate of wall breaking, mild conditions, and does not cause denaturation of intracellular substances. However, the use of enzyme preparations also increases the difficulty of separating and purifying later products. Enzymatic hydrolysis of Chlorella vulgaris is superior to other physical breaking methods (Zheng et al., 2011). When comparing the effects of various cell disruption methods on lipid production in Schizochytrium sp., pretreatment with lysozyme and cellulase results in the highest extraction rate and highest DHA content (Hac İsa et al., 2022).
Decolorization and impurity removal
Crude or unrefined bio-oil often contains various other fat-soluble molecules, such as photosynthetic pigments, which are valuable nutritionally. However, as a commodity oil, these compositions not only impact the color and appearance of the oil but also reduce the stability of the oil quality, leading to oxidation or deterioration of the oil (Rodríguez-España et al., 2022). Therefore, it is crucial to remove unnecessary components such as pigments to improve the oil’s stability and quality.
Algae lipids generally contain a certain amount of carotenoids, which are fat-soluble. It has been found that carotenoids are typically synthesized in cells and stored in lipid droplets in a fat-soluble form to accumulate a substantial amount of astaxanthin, which requires synthesizing the necessary amount of lipid droplets (Collins et al., 2011; Han et al., 2013). Although there are several separation methods to separate carotenoids from lipids, such as silica gel column chromatography, central partition chromatography, and thin-layer chromatography (Sun et al., 2022), the separation cost is relatively high. Additionally, carotenoids and lipids can be separated by screening solvents and using the polarity difference (Lourenço-Lopes et al., 2020; Sun et al., 2022). Separation can improve the output value of production if the product value exceeds the increased process cost. Some algae can also synthesize carotenoids when accumulating large amounts of long chain PUFA under special conditions. For example, Chaetoceros gracilis (Tachihana et al., 2020), Phaeodactylum tricornutum (Derwenskus et al., 2020), Nitzschia sp (Ma et al., 2023) can simultaneously accumulate EPA and fucoxanthin, while Nannochloropsis gaditana (Menegol et al., 2019) can simultaneously accumulate EPA and carotenoids, while Aurantiochytrium sp (Yin et al., 2023) can simultaneously accumulate DHA, astaxanthin, and β-carotenoids. However, according to the reported content, in order to simultaneously accumulate both types of high-value products in large quantities, further in-depth research is still required.
Adsorbents commonly used for crude oil decolorization include various products such as activated clay and activated carbon. Among them, activated clay is widely used for decolorization in the oil industry. Activated clay is inexpensive, easy to obtain and prepare, and has a strong absorption capacity for chlorophyll, carotenoids and their derivatives, hydroxyl-containing polar molecules, free fatty acids, and colloidal substances (Kreps et al., 2014). For example, it effectively decolorizes crude oil from corn (Liu and Zheng, 2014) and Scenedesmus sp. (Chen W et al., 2021).
Activated clay is a commonly used adsorbent for crude oil decolorization, which can be obtained from various sources. However, activated clay from different production areas may have varying components, leading to differences in their decolorization performance. Therefore, modifications can be made to activated clay to enhance its adsorption and decolorization capabilities. Activated clay is preferred over other decolorization materials, such as activated carbon, activated fuller’s earth and attapulgite clay, making it a more suitable choice (Liu and Chen, 2012). Not only can activated clay effectively decolorize crudely extracted biodiesel, but it can also remove most peroxides (Su et al., 2014). For example, commercial montmorillonite clay from Leping, Jiangxi, China (Yang M et al., 2019), and upper Eocene sedimentary clay from Kairouan, Tunisia (Eloussaief et al., 2020) can be modified through proper treatment, greatly improving the adsorption capacity of pigment molecules in oil. Moreover, some activated clay used for oil decolorization can be regenerated by calcination and other treatments. Recycled activated clay can then be used again, reducing the cost of decolorization (Bachmann et al., 2020). To optimize the decolorization process, it is necessary to screen the activated clay with the best decolorization performance for the target oil. Appropriate improvement of the activated clay processing technology can also improve the decolorization performance. Finally, optimizing the decolorization conditions can lead to activated clay becoming an ideal tool for the decolorization of refined oil and algae oil (Chen et al., 2012).
Enrichment of PUFAs
Many algae have been approved as a new food resource and are already being incorporated into everyday meals, with related food products already on the market. Some people may need to supplement their omega-3 PUFA-rich intake through consuming foods and edible oils rich in these fatty acids, while others may need to turn to drugs, health products, or even milk powder. To manufacture these products, omega-3 PUFA raw materials with a single component and defined content are required. Algae oil, which is rich in omega-3 PUFAs, can be used as a raw material for these products. However, to meet the desired product characteristics when used in health products, pharmaceuticals, or auxiliary medicines, PUFAs in algae oil must be purified (Li X et al., 2019; Wei and Wang, 2020). For instance, several omega-3 PUFAs prescription drugs approved by the U.S. Food and Drug Administration for the prevention and treatment of high triglycerides require even higher purity (Hoy and Keating, 2009; Blair and Dhillon, 2014; Kim and McCormack, 2014).
Hydrolysis of lipids
Fatty acid hydrolysis can hydrolyze and separate ester-forming fatty acids, which can then be further separated and utilized based on their different properties.
Saponification-esterification method
PUFAs can be separated and concentrated by taking advantage of the difference in the solubility of fatty acid metal salts in certain organic solvents. To obtain refined oil with higher PUFAs content, lipids can be saponified under heating conditions using a specific ratio of ethanol and NaOH. The saponified mixture can then be filtered to obtain saponified liquid and solid particles, followed by esterification of the saponified compositions with dilute H2SO4.
Non-catalytic hydrolysis method
Transesterification of triglycerides to form fatty acid methyl esters or fatty acid ethyl esters can be achieved directly under high temperature and pressure (Felix et al., 2019), through the Thermal-Fenton mechanism (Yew et al., 2021), or in supercritical methanol conditions (Mohamadzadeh Shirazi et al., 2017). However, this process is mainly used in biodiesel production, which results in impurities and may easily damage PUFAs.
Chemical catalytic hydrolysis method
Acid catalysis, saponification-acidolysis, and high-pressure hydrolysis are common methods used in the oleochemical industry for lipid hydrolysis. The acid-catalyzed and saponification-acidification methods are cost-effective and have a hydrolysis rate of over 90%. However, they produce large amounts of waste acid or alkali (Zhuang et al., 2012; Asikainen et al., 2015). High-pressure steam hydrolysis is an effective alternative but requires higher equipment and operating costs. Generally, these methods are carried out at higher temperatures, particularly the high-pressure hydrolysis method (Zarli, 2020).
Chemical catalysts can be categorized into homogeneous and heterogeneous catalysts, which can be further subdivided into alkaline and acid catalysts (Neag et al., 2023). Commonly used homogeneous catalysts include NaOH, CH3ONa, KOH, concentrated HCl, or H2SO4 (Neag et al., 2023). Heterogeneous catalytic processes are suitable for raw materials containing more polar components, such as CaO/MgO, KF/CaO, magnetic nano-scale KOH/Fe2O3-Al2O3 (Kazemifard et al., 2019), CaMgO/Al2O3, zirconia (ZrO2), titanium oxide (TiO2), zeolites, ion exchange resins (Hidalgo et al., 2013), and algae carbon-based solid acid catalysts (Cao et al., 2021).
Lipase-catalyzed method
Enzymatic enrichment utilizes the selective specificity of lipase, an enzyme that catalyze various reactions such as hydrolysis, acidolysis, alcoholysis, transesterification and reverse synthesis of esters of triacylglycerides and other water-insoluble esters. The main reactions involved in enzymatic enrichment are hydrolysis, transesterification and esterification.
Enzymatic hydrolysis
The enzymatic hydrolysis method, which employs lipase for breaking down oil into fatty acids and glycerol, can be carried out at mild conditions without the need for steam boiling or pressurization. The reaction can be carried out at room temperature or slightly higher, and does not produce a large amount of waste acid or waste alkali. Lipase is a carboxyl ester hydrolase that can hydrolyze the α and β bonds of glycerides into fatty acids and glycerol and synthesize glycerol esters from fatty acids and glycerol. Depending on the characteristics of different lipases and the selected reaction conditions, both hydrolysis and transesterification reactions can be operated, as well as esterification of certain fatty acids. By undergoing hydrolysis, transesterification, and esterification reactions, lipases can produce high concentrations of specific fatty acids, which can be subsequently isolated and enriched by other methods (Akanbi et al., 2013). Lipase-catalyzed hydrolysis has proven successful in enriching EPA and DHA in oils from fish (Pan et al., 2012; Valverde et al., 2013; Valverde et al., 2014), Isochrysis galbana, Chaetocerous calcitran, Chlorella marina, and Tetraselmis gracillus (Jacob and Mathew, 2017), and ALA in flaxseed oil (Rupani et al., 2012; Zou et al., 2020). Hence, lipase can be an efficient and environmentally friendly tool to enrich fatty acids. The hydrolysis rate of some lipases for olive oil, soybean oil, rice bran oil, linseed oil, perilla seed oil, and rapeseed oil can reach 35%–100% (Zhao et al., 2022), and the hydrolysis rate of oil to Scenedesmus sp. can reach 66% (Chen W et al., 2021).
Enzymatic esterification method
Lipase selectively catalyzes the reaction of UFAs and alcohols to produce esters, or between saturated fatty acids and alcohols to generate esters, thereby enriching UFAs (Jithu Paul, 2019).
Enzymatic transesterification method
It involves the catalytic exchange of acyl groups between glycerides and free fatty acids, alcohols, or other esters under the catalysis of lipase. These reactions are referred to as acidolysis, alcoholysis and transesterification reactions, respectively. This method is particularly suitable for the preparation of high concentration PUFAs or PUFA methyl esters (ethyl esters) (Farmani et al., 2007; Castejón and Señoráns, 2020). During the transesterification process, lipase preferentially acts on saturated and monounsaturated chains, thereby substituting PUFAs into glycerides to achieve enrichment.
Chemical methods for enriching PUFAs usually require derivatization of raw oil to form PUFAs or their methyl and ethyl esters, which then need to be combined with other separation methods to achieve a good enrichment effect. Additionally, these methods often require further conversion into a glyceride form that can be easily absorbed by the human body. Physical and chemical methods also involve the use of a large amount of organic reagents, which can be harmful to health and must to be completely removed. In contrast, the lipase hydrolysis method only requires a single-step reaction under mild conditions and does not require a large amount of organic reagents or additional pretreatment. This method can enrich PUFAs on glycerides, and the hydrolysis rate of these fatty acid glycerides is faster than the methyl or ethyl ester enriched by physical and chemical methods (Yang et al., 1989). Therefore, the hydrolysis method is more suitable for human digestion and absorption. The immobilized lipase can still maintain 60% of its initial activity even after 10 reaction cycles (Liu et al., 2018). However, to separate the target components, it is often necessary to combine other methods. Lipase with high specificity must be extensively screened, which can increase the cost. To enhance the functional specificity of lipase, computer-aided design can be employed (Moharana and Rao, 2020).
Purification of PUFAs
Various methods are available for the separation and purification methods of PUFAs, such as low-temperature crystallization, urea inclusion, silver ion complexation, molecular distillation methods. The advantages and disadvantages of these methods are summarized in Table 6.
Ag+ complexation method
Silver ions have the ability to form polar complexes with carbon-carbon double bonds, and the more double bonds there are, the more Ag+ combined, the stronger the complexation, and the more stable the complex. The silver ion complexation method is typically categorized into the AgNO3 solution direct complexation method, AgNO3-silica gel column chromatography, AgNO3 membrane separation and adsorption method, etc. For instance, the Ag+ complexation method has been used to enrich omega-3 PUFA from Desmodesmus sp. oil, leading to an increase in the ALA content from 24% to 92% (Nagappan and Kumar Verma, 2018). These methods have high enrichment efficiency and product purity, but the cost of Ag+ is high and recovering it in large quantities is challenging. Moreover, after saponification, Ag+ needs to be eluted with organic reagents which may cause heavy metal pollution in the product and residues in organic eluents. These drawbacks have limited the widespread production and application of these methods.
Molecular distillation method
Molecular distillation is a method used to separate substances based on the difference in mean free path of the molecular thermal motion of each component in the mixture. The molecular mean free path is determined by the molecular diameter, system pressure, and temperature. Under specific temperature and pressure conditions, fatty acids with high degrees of unsaturation have a short molecular mean free path, resulting in a slower evaporation rate. Multistage distillation can efficiently separate different components (Zhang et al., 2013; He et al., 2020). Molecular distillation doesn’t require the introduction of foreign substances, does not need to be heated to the boiling point, has a short heating time, and can be produced continuously. However, it must be performed under high vacuum, has high energy consumption, and may yield products with low purity. Thus, when separating fatty acids with similar molecular mass, molecular distillation is often combined with other separation methods. For example, continuous four stages of molecular distillation can increase the mass fraction of ALA in silkworm chrysalis oil from 54.49% to 99.28% (Liu et al., 2018), and enrich ω-3 PUFAs in Schizochytrium limacinum oil to 92.98% (Zhang et al., 2013).
Low-temperature crystallization method
The low-temperature crystallization method is primarily based on the difference in freezing point and solubility at low temperature conditions for the purpose of separation and purification. The melting point of saturated fatty acids is usually higher than that of UFAs, meaning that the solidification temperature of fatty acids with more unsaturated bonds is lower. Additionally, temperature also affects the solubility of fatty acids in organic solvents. As a general rule, fatty acids with longer carbon chains exhibit lower solubility, while fatty acids with more double bonds have higher solubility in organic solvents, as shown in Figure 6 (Knothe and Dunn, 2009). Therefore, low-temperature crystallization permits saturated fatty acids in fatty acids to form a solid phase while UFAs can dissolve in organic solvents, thereby enriching UFAs in the remaining liquid phase. The separation technique involves two steps, namely oil crystallization and solid-liquid phase separation. It can be divided into dry fractionation, surfactant fractionation, and solvent fractionation.
Dry fractionation refers to the transesterification of crude oil under alkaline conditions to produce fatty acid methyl ester (ethyl ester), which is then cooled to a specific temperature without the addition of any organic solvent, allowing the solid components in the oil to crystallize first (Soleimanian et al., 2015). The solid and liquid phases are then separated by centrifugal filtration or other means. However, methanol-based alcoholysis is toxic and hazardous to human health, and thus many studies employ ethanol. Additionally, fatty acid ethyl ester is not easily hydrolyzed by pancreatic lipase, which is not beneficial for human absorption (Sigurgisladottir et al., 1992).
Surfactant fractionation entails freezing crude oil for natural crystallization, followed by the addition of a specific proportion of surfactant and electrolyte solution to emulsify the crystallized solid oil and suspend it in the water phase, thus increasing the separation efficiency of crystallized solid oil (Salminen et al., 2014; Islam et al., 2020). This method is more efficient than the dry method, but many surfactants cannot be utilized in food processing.
Solvent fractionation involves saponifying crude oil into free fatty acids, selecting an appropriate organic solvent to dissolve in a specific proportion, and refrigerating it at low temperature. Subsequently, large amounts of fatty acids with low degrees of unsaturation are crystallized and separated by filtration. Using the low-temperature crystallization method, the content of ALA in silkworm chrysalis oil increased from 15% to 47.6% (Wang et al., 2013), and the content of UFAs in the oil of Scenedesmus sp. can be increased to more than 90%, of which ALA can reach 63% (Chen W et al., 2021).
Urea inclusion
Urea can form clathrates with aliphatic compounds, specifically straight-chain compounds containing more than 4 carbon atoms (Domart et al., 1955; Hollingsworth et al., 1996). Urea inclusion has long been recognized as an effective method for enriching PUFA, as indicated in Table 7. The urea clathrate is based on the linear aliphatic compound as the axis, where there is a weaker van der Waals bond between urea and the linear aliphatic compound. Urea molecules spiral up around the axis in a counterclockwise direction through hydrogen bonding, tightly enclosing it (González-Fernández et al., 2020). During the crystallization process, urea forms relatively stable crystal inclusion complexes with saturated fatty acids or monounsaturated fatty acids. However, polyvalent unsaturated fatty acids are difficult to include due to their multiple double bonds and curved carbon chains, which have a specific spatial configuration (Shen et al., 2018). A variety of urea + X inclusion methods derived from the improvements can be used to design polymer-urea inclusion complexes more accurately. These methods make it easier to include straight chain molecules while making it more challenging to clathrate curved polymer molecules (Shen et al., 2018). The entire process of the urea inclusion method is simple to operate, and the necessary raw materials are readily available and inexpensive. This method is worth considering not only for large-scale application but also as it has a strong experimental foundation for reference.
Outlook
Essential PUFAs have numerous health and medical benefits. As people’s quality of life improves, their awareness of health protection increases, and they pay more attention to nutrition and health in their diet. Providing high-quality PUFAs for the population can help enhance their health and quality of life, and indirectly reduce medical expenses for society. Therefore, providing high-quality PUFAs for the general population has both significant social significance and good market prospects. However, there are few sources of PUFAs-rich oils on the market, and the production of PUFAs-rich algae oils is still at the laboratory-scale stage. Many algae that can produce high PUFAs are still in the verification stage and may be listed as new resource foods in the future. Thus, it is necessary to explore the technical model of large-scale production of these high-yield PUFAs algae, master practical industrial technology means, lay a technical foundation for the industrialization of algae edible oil, and promote algae resources to the market.
In the field of bioenergy research, the production of algae oil is currently facing a bottleneck due to high production costs. As a result, commercial promotion has become challenging, and progress in commercial applications have been slow. While the industrialization of high-value algal oils such as DHA and astaxanthin has already been successfully realized, producing high-value PUFAs from algae could potentially solve the cost problem of algae biodiesel by utilizing the separated by-product of saturated fatty acid as the raw material for algae biodiesel. Algae bioenergy currently faces a cost bottleneck that is difficult to overcome, but focusing on the development and utilization of high-value products could make it more economically feasible.
An analysis of the research hotspots and development direction of algae PUFAs production indicates that while there is considerable emphasis on the accumulation of algae PUFAs, downstream processes such as extraction, decolorization, impurity removal, enrichment, and purification require more attention. This work provides a systematic description of the necessary process methods for converting algae biomass to enriched PUFAs, and offers guidance for the production of omega fatty acids using algae.
Author contributions
QW conceptualized the idea for manuscript. WC, TL, SD, and HC drafted the manuscript. QW evaluated the manuscript and improved the content.
Funding
This work was supported jointly by the National Key R&D Program of China (2021YFA0909600), the National Natural Science Foundation of China (32170138 and 31870041), the Natural Science Foundation of Henan Province (212300410024), the Program for Innovative Research Team (in Science and Technology) in University of Henan Province (22IRTSTHN024), and the 111 Project (#D16014).
Conflict of interest
The authors declare that the research was conducted in the absence of any commercial or financial relationships that could be construed as a potential conflict of interest.
Publisher’s note
All claims expressed in this article are solely those of the authors and do not necessarily represent those of their affiliated organizations, or those of the publisher, the editors and the reviewers. Any product that may be evaluated in this article, or claim that may be made by its manufacturer, is not guaranteed or endorsed by the publisher.
Abbreviation
AdA, Adrenic acid; ARA, Arachidonic acid; ALA, Alpha-linolenic acid; DGLA, Dihomo-gamma-linolenic acid; DHA, Docosahexaenoic acid (Cervonic acid); DPA, Docosapentaenoic acid (Clupanodonic acid); EDA, Eicosadienoic acid; EPA, Eicosapentaenoic acid (Timnodonic acid); ETA, Eicosatetraenoic acid; ETE, Eicosatrienoic acid; GLA, Gamma-linolenic acid; HPA, Heneicosapentaenoic acid; HTA, Hexadecatrienoic acid; LA, Linoleic acid; OA, Oleic acid; PUFA(s), Polyunsaturated Fatty Acid(s); SDA, Stearidonic acid.
References
Abedin, L., Lien, E. L., Vingrys, A. J., and Sinclair, A. J. (1999). The effects of dietary alpha-linolenic acid compared with docosahexaenoic acid on brain, retina, liver, and heart in the Guinea pig. Lipids 34, 475–482. doi:10.1007/s11745-999-0387-3
Adarme-Vega, T. C., Lim, D. K. Y., Timmins, M., Vernen, F., Li, Y., and Schenk, P. M. (2012). Microalgal biofactories: A promising approach towards sustainable omega-3 fatty acid production. Microb. Cell Factories 11, 96. doi:10.1186/1475-2859-11-96
Aguillón-Páez, Y. J., Romero, L. A., and Diaz, G. J. (2020). Effect of full-fat sunflower or flaxseed seeds dietary inclusion on performance, egg yolk fatty acid profile and egg quality in laying hens. Anim. Nutr. 6, 179–184. doi:10.1016/j.aninu.2019.12.005
Akanbi, T. O., Adcock, J. L., and Barrow, C. J. (2013). Selective concentration of EPA and DHA using Thermomyces lanuginosus lipase is due to fatty acid selectivity and not regioselectivity. Food Chem. 138, 615–620. doi:10.1016/j.foodchem.2012.11.007
Ali, Z., Subeshan, B., Alam, M. A., Asmatulu, E., and Xu, J. (2021). Recent progress in extraction/transesterification techniques for the recovery of oil from algae biomass. Biomass Convers. Biorefinery 13, 2553–2569. doi:10.1007/s13399-021-01326-y
Amorim, M. L., Soares, J., Coimbra, J., Leite, M. O., Albino, L. F. T., and Martins, M. A. (2020). Microalgae proteins: Production, separation, isolation, quantification, and application in food and feed. Crit. Rev. food Sci. Nutr. 61, 1976–2002. doi:10.1080/10408398.2020.1768046
Anzhany, D., DespalToharmat, T., Nuraina, N., Hamidah, A. N., and Rofiah, N. (2021). Effect of different altitudes on milk fatty acid and conjugated linoleic acid (CLA) profiles. IOP Conf. Ser. Earth Environ. Sci. 667, 012102. doi:10.1088/1755-1315/667/1/012102
Aravind, S., Barik, D., Ragupathi, P., and Vignesh, G. (2021). Investigation on algae oil extraction from algae Spirogyra by Soxhlet extraction method. Mater. Today Proc. 43, 308–313. doi:10.1016/j.matpr.2020.11.668
Asikainen, M., Munter, T., and Linnekoski, J. (2015). Conversion of polar and non-polar algae oil lipids to fatty acid methyl esters with solid acid catalysts – a model compound study. Bioresour. Technol. 191, 300–305. doi:10.1016/j.biortech.2015.05.001
Aşkın, B., and Kaya, Y. (2020). Effect of deep frying process on the quality of the refined oleic/linoleic sunflower seed oil and olive oil. J. Food Sci. Technol. 57, 4716–4725. doi:10.1007/s13197-020-04655-4
Ayerza, R. (1995). Oil content and fatty acid composition of chia (Salvia hispanica L.) from five northwestern locations in Argentina. J. Am. Oil Chemists’ Soc. 72, 1079–1081. doi:10.1007/BF02660727
Bachmann, S. A. L., Valle, R. d. C. S. C., Vegini, A. A., and Tavares, L. B. B. (2020). Determination of optimum conditions for thermal regeneration and characterization of a spent bleaching Earth. J. Environ. Chem. Eng. 8, 103503. doi:10.1016/j.jece.2019.103503
Becker, W. (2003a). Microalgae for aquaculture: The nutritional value of microalgae for aquaculture. Handb. Microalgal Cult. 2003, 380–391. doi:10.1002/9780470995280.ch21
Becker, W. (2003b). Microalgae in human and animal nutrition. Handb. Microalgal Cult. 2003, 312–351. doi:10.1002/9780470995280.ch18
Bell, M. V., Batty, R. S., Dick, J. R., Fretwell, K., Navarro, J. C., and Sargent, J. R. (1995). Dietary deficiency of docosahexaenoic acid impairs vision at low light intensities in juvenile herring (Clupea harengus L.). Lipids 30, 443–449. doi:10.1007/Bf02536303
Blair, H. A., and Dhillon, S. (2014). Omega-3 carboxylic acids (Epanova®): A review of its use in patients with severe hypertriglyceridemia. Am. J. Cardiovasc. Drugs 14, 393–400. doi:10.1007/s40256-014-0090-3
Borowitzka, M. A. (2013). High-value products from microalgae—Their development and commercialisation. J. Appl. Phycol. 25, 743–756. doi:10.1007/s10811-013-9983-9
Boskabady, M. H., Kaveh, M., Shakeri, F., Mohammadian Roshan, N., and Rezaee, R. (2019). Alpha-linolenic acid ameliorates bronchial asthma features in ovalbumin-sensitized rats. J. Pharm. Pharmacol. 71, 1089–1099. doi:10.1111/jphp.13094
Brenna, J. T., Salem, N., Sinclair, A. J., and Cunnane, S. C. (2009). α-Linolenic acid supplementation and conversion to n-3 long-chain polyunsaturated fatty acids in humans. Prostagl. Leukot. Essent. Fat. Acids 80, 85–91. doi:10.1016/j.plefa.2009.01.004
Brennan, L., and Owende, P. (2010). Biofuels from microalgae—a review of technologies for production, processing, and extractions of biofuels and co-products. Renew. Sustain. Energy Rev. 14, 557–577. doi:10.1016/j.rser.2009.10.009
Brett, M., and Müller-Navarra, D. (1997). The role of highly unsaturated fatty acids in aquatic foodweb processes. Freshw. Biol. 38, 483–499. doi:10.1046/j.1365-2427.1997.00220.x
Calder, P. C., and Grimble, R. (2002). Polyunsaturated fatty acids, inflammation and immunity. Eur. J. Clin. Nutr. 56 (3), S14–S19. doi:10.1038/sj.ejcn.1601478
Cao, M., Peng, L., Xie, Q., Xing, K., Lu, M., and Ji, J. (2021). Sulfonated Sargassum horneri carbon as solid acid catalyst to produce biodiesel via esterification. Bioresour. Technol. 324, 124614. doi:10.1016/j.biortech.2020.124614
Capek, L., Uhliarikova, I., Kostalova, Z., Hindakova, A., and Capek, P. (2023). Structural properties of the extracellular biopolymer (beta-D-xylo-alpha-D-mannan) produced by the green microalga Gloeocystis vesiculosa Nageli. Carbohydr. Res. 525, 108766. doi:10.1016/j.carres.2023.108766
Castejón, N., and Señoráns, F. J. (2020). Enzymatic modification to produce health-promoting lipids from fish oil, algae and other new omega-3 sources: A review. New Biotechnol. 57, 45–54. doi:10.1016/j.nbt.2020.02.006
Chauhan, A. S., Patel, A. K., Chen, C.-W., Chang, J.-S., Michaud, P., Dong, C.-D., et al. (2023). Enhanced production of high-value polyunsaturated fatty acids (PUFAs) from potential thraustochytrid Aurantiochytrium sp. Bioresour. Technol. 370, 128536. doi:10.1016/j.biortech.2022.128536
Cheah, Y. T., Ng, B. W., Tan, T. L., Chia, Z. S., and Chan, D. J. C. (2022). Biomass and eicosapentaenoic acid production from Amphora sp. under different environmental and nutritional conditions. Biotechnol. Appl. Biochem. 2022, 2379. doi:10.1002/bab.2379
Chen, H., Li, K., Xue, C., and Wang, Q. (2021). A novel method for non-invasive estimation of primary productivity in aquatic ecosystems using a chlorophyll fluorescence-induced dynamic curve. Front. Microbiol. 12, 682250. doi:10.3389/fmicb.2021.682250
Chen, H., Li, T., and Wang, Q. (2019). Ten years of algal biofuel and bioproducts: Gains and pains. Planta 249, 195–219. doi:10.1007/s00425-018-3066-8
Chen, H., and Wang, Q. (2022). Microalgae-based green bio-manufacturing—how far from us. Front. Microbiol. 13, 832097. doi:10.3389/fmicb.2022.832097
Chen, H., and Wang, Q. (2020). Microalgae-based nitrogen bioremediation. Algal Res. 46, 101775. doi:10.1016/j.algal.2019.101775
Chen, H., and Wang, Q. (2021). Regulatory mechanisms of lipid biosynthesis in microalgae. Biol. Rev. 96, 2373–2391. doi:10.1111/brv.12759
Chen, H., Wang, X., and Wang, Q. (2020). Microalgal biofuels in China: The past, progress and prospects. GCB Bioenergy 00, 1044–1065. doi:10.1111/gcbb.12741
Chen, L., Liu, T., Zhang, W., Chen, X., and Wang, J. (2012). Biodiesel production from algae oil high in free fatty acids by two-step catalytic conversion. Bioresour. Technol. 111, 208–214. doi:10.1016/j.biortech.2012.02.033
Chen, W., Li, T., Ren, Y., Wang, J., Chen, H., and Wang, Q. (2023). Biological hydrogen with industrial potential: Improvement and prospection in biohydrogen production. J. Clean. Prod. 387, 135777. doi:10.1016/j.jclepro.2022.135777
Chen, W., Liu, Y., Song, L., Sommerfeld, M., and Hu, Q. (2020). Automated accelerated solvent extraction method for total lipid analysis of microalgae. Algal Res. 51, 102080. doi:10.1016/j.algal.2020.102080
Chen, W., Wang, J., Ren, Y., Chen, H., He, C., and Wang, Q. (2021). Optimized production and enrichment of α-linolenic acid by Scenedesmus sp. HSJ296. Algal Research-Biomass Biofuels Bioprod. 60, 102505. doi:10.1016/j.algal.2021.102505
Chen, W., Zhang, S., Rong, J., Li, X., Chen, H., He, C., et al. (2016). Effective biological DeNOx of industrial flue gas by the mixotrophic cultivation of an oil-producing green alga Chlorella sp. C2. Environ. Sci. Technol. 50, 1620–1627. doi:10.1021/acs.est.5b04696
Cheng, T., Yang, G., and Bi, Y. (2017). Enrichment of α-linoneic acid by tataric acid-assisted urea fractionation in methanol. J. Henan Univ. Technol. 38, 12–17.
Chi, G. X., Xu, Y. Y., Cao, X. Y., Li, Z. P., Cao, M. F., Chisti, Y., et al. (2022). Production of polyunsaturated fatty acids by Schizochytrium (Aurantiochytrium) spp. Biotechnol. Adv. 55, 107897. doi:10.1016/j.biotechadv.2021.107897
Chiriboga, O., and Rorrer, G. L. (2019). Phosphate addition strategies for enhancing the co-production of lipid and chitin nanofibers during fed-batch cultivation of the diatom Cyclotella sp. Algal Research-Biomass Biofuels Bioprod. 38, 101403. doi:10.1016/j.algal.2018.101403
Collins, A. M., Jones, H. D. T., Han, D., Hu, Q., Beechem, T. E., and Timlin, J. A. (2011). Carotenoid distribution in living cells of Haematococcus pluvialis (chlorophyceae). PLoS ONE 6, e24302. doi:10.1371/journal.pone.0024302
Cooney, M., Young, G., and Nagle, N. (2009). Extraction of bio-oils from microalgae. Sep. Purif. Rev. 38, 291–325. doi:10.1080/15422110903327919
Copolovici, D., Bungau, S., Boscencu, R., Tit, D. M., and Copolovici, L. (2017). The fatty acids composition and antioxidant activity of walnut cold press oil. Rev. Chim. 68, 507–509. doi:10.37358/rc.17.3.5489
Correia, N., Pereira, H., Silva, J. T., Santos, T., Soares, M., Sousa, C. B., et al. (2020). Isolation, identification and biotechnological applications of a novel, robust, free-living Chlorococcum (oophila) amblystomatis strain isolated from a local pond. Appl. Sci. 10, 3040. doi:10.3390/app10093040
Corsetto, P. A., Montorfano, G., Zava, S., Jovenitti, I. E., Cremona, A., Berra, B., et al. (2011). Effects of n-3 PUFAs on breast cancer cells through their incorporation in plasma membrane. Lipids Health Dis. 10, 73. doi:10.1186/1476-511X-10-73
Crexi, V. T., Monte, M. L., Monte, M. L., and Pinto, L. A. A. (2012). Polyunsaturated fatty acid concentrates of carp oil: Chemical hydrolysis and urea complexation. J. Am. Oil Chemists' Soc. 89, 329–334. doi:10.1007/s11746-011-1899-4
Cui, Y., Thomas-Hall, S. R., Chua, E. T., and Schenk, P. M. (2021). Development of high-level omega-3 eicosapentaenoic acid (EPA) production from Phaeodactylum tricornutum. J. Phycol. 57, 258–268. doi:10.1111/jpy.13082
de Souza, A. S., Fernandes, F. S., and Tavares do Carmo, M. d. G. (2011). Effects of maternal malnutrition and postnatal nutritional rehabilitation on brain fatty acids, learning, and memory. Nutr. Rev. 69, 132–144. doi:10.1111/j.1753-4887.2011.00374.x
Degraeve-Guilbault, C., Pankasem, N., Gueirrero, M., Lemoigne, C., Domergue, F., Kotajima, T., et al. (2021). Temperature acclimation of the picoalga Ostreococcus tauri triggers early fatty-acid variations and involves a plastidial omega 3-desaturase. Front. Plant Sci. 12, 639330. doi:10.3389/fpls.2021.639330
Dejoye, C., Vian, M. A., Lumia, G., Bouscarle, C., Charton, F., and Chemat, F. (2011). Combined extraction processes of lipid from Chlorella vulgaris microalgae: Microwave prior to supercritical carbon dioxide extraction. Int. J. Mol. Sci. 12, 9332–9341. doi:10.3390/ijms12129332
Derwenskus, F., Schäfer, B., Müller, J., Frick, K., Gille, A., Briviba, K., et al. (2020). Coproduction of EPA and fucoxanthin with P. Tricornutum – a promising approach for up- and downstream processing. Chem. Ing. Tech. 92, 1780–1789. doi:10.1002/cite.202000046
Devassy, J. G., Leng, S., Gabbs, M., Monirujjaman, M., and Aukema, H. M. (2016). Omega-3 polyunsaturated fatty acids and oxylipins in neuroinflammation and management of alzheimer disease. Adv. Nutr. 7, 905–916. doi:10.3945/an.116.012187
Ding, D., Shen, Z., Ma, T., Sun, Y., Shi, M., Lu, T., et al. (2022). Competition of dual roles of ionic liquids during in situ transesterification of wet algae. ACS Sustain. Chem. Eng. 10, 13692–13701. doi:10.1021/acssuschemeng.2c03641
Djuricic, I., and Calder, P. C. (2021). Beneficial outcomes of omega-6 and omega-3 polyunsaturated fatty acids on human health: An update for 2021. Nutrients 13, 2421. doi:10.3390/nu13072421
Domart, C., Miyauchi, D., and Sumerwell, W. (1955). The fractionation of marine-oil fatty acids with urea. J. Am. Oil Chem. Soc. 32, 481–483. doi:10.1007/bf02639943
Dovale-Rosabal, G., Rodríguez, A., Contreras, E., Ortiz-Viedma, J., Muñoz, M., Trigo, M., et al. (2019). Concentration of EPA and DHA from refined salmon oil by optimizing the urea–fatty acid adduction reaction conditions using response surface methodology. Molecules 24, 1642. doi:10.3390/molecules24091642
Ebm, N., Guo, F., Brett, M. T., Bunn, S. E., and Kainz, M. J. (2021). Polyunsaturated fatty acids in fish tissues more closely resemble algal than terrestrial diet sources. Hydrobiologia 848, 371–383. doi:10.1007/s10750-020-04445-1
Eleršek, T., Flisar, K., Likozar, B., Klemenčič, M., Golob, J., Kotnik, T., et al. (2020). Electroporation as a solvent-free green technique for non-destructive extraction of proteins and lipids from Chlorella vulgaris. Front. Bioeng. Biotechnol. 8, 443. doi:10.3389/fbioe.2020.00443
Ellison, C. R., Overa, S., and Boldor, D. (2019). Central composite design parameterization of microalgae/cyanobacteria co-culture pretreatment for enhanced lipid extraction using an external clamp-on ultrasonic transducer. Ultrason. Sonochemistry 51, 496–503. doi:10.1016/j.ultsonch.2018.05.006
Eloussaief, M., Chakroun, S., Kallel, N., and Benzina, M. (2020). Efficiency of clay materials collected from Ain Jeloula (Central Tunisia) in sunflower oil decolorization. Euro-Mediterranean J. Environ. Integration 5, 33. doi:10.1007/s41207-020-00171-1
Faraji Sarabmirza, R., Joolaei Ahranjani, P., Rashidi, L., Mousavi, M., Khodaiyan, F., and Rashidi Nodeh, H. (2023). An investigation on conjugated linoleic acid content, fatty acid composition, and physicochemical characteristics of Iranian Kurdish butter oil. Food Sci. Nutr. 11, 1051–1058. doi:10.1002/fsn3.3142
Farmani, J., Hamedi, M., Safari, M., and Madadlou, A. (2007). Trans-free Iranian vanaspati through enzymatic and chemical transesterification of triple blends of fully hydrogenated soybean, rapeseed and sunflower oils. Food Chem. 102, 827–833. doi:10.1016/j.foodchem.2006.06.015
Felix, C., Ubando, A., Madrazo, C., Gue, I. H., Sutanto, S., Tran-Nguyen, P. L., et al. (2019). Non-catalytic in-situ (trans) esterification of lipids in wet microalgae Chlorella vulgaris under subcritical conditions for the synthesis of fatty acid methyl esters. Appl. Energy 248, 526–537. doi:10.1016/j.apenergy.2019.04.149
Ferreira, M., Salgado, J. M., Fernandes, H., Peres, H., and Belo, I. (2022). Potential of red, green and Brown seaweeds as substrates for solid state fermentation to increase their nutritional value and to produce enzymes. Foods 11, 3864. doi:10.3390/foods11233864
Fonteh, A. N., Cipolla, M., Chiang, A. J., Edminster, S. P., Arakaki, X., and Harrington, M. G. (2020). Polyunsaturated fatty acid composition of cerebrospinal fluid fractions shows their contribution to cognitive resilience of a pre-symptomatic Alzheimer’s disease cohort. Front. Physiology 11, 83. doi:10.3389/fphys.2020.00083
Gao, F. Z., Cabanelas, I. T. D., Wijffels, R. H., and Barbosa, M. J. (2022). Fucoxanthin and docosahexaenoic acid production by cold-adapted Tisochrysis lutea. New Biotechnol. 66, 16–24. doi:10.1016/j.nbt.2021.08.005
Ge, F., Song, K., Yang, Z., Li, J., Yan, F., Zhang, M., et al. (2023). Enhancing docosahexaenoic acid production of Isochrysis galbana from starch-rich food processing byproducts. Fermentation 9, 158. doi:10.3390/fermentation9020158
Golanski, J., Szymanska, P., and Rozalski, M. (2021). Effects of omega-3 polyunsaturated fatty acids and their metabolites on haemostasis—current perspectives in cardiovascular disease. Int. J. Mol. Sci. 22, 2394. doi:10.3390/ijms22052394
González-Fernández, M. J., Fabrikov, D., Lyashenko, S., Ferrón-Carrillo, F., and Guil-Guerrero, J. L. (2020). Highly concentrated very long-chain PUFA obtainment by Urea complexation methodology. Environ. Technol. Innovation 18, 100736. doi:10.1016/j.eti.2020.100736
Grierson, S., Strezov, V., Bray, S., Mummacari, R., Danh, L. T., and Foster, N. (2012). Assessment of bio-oil extraction from Tetraselmis chui microalgae comparing supercritical CO2, solvent extraction, and thermal processing. Energy & Fuels 26, 248–255. doi:10.1021/ef2011222
Gu, W., Kavanagh, J. M., and McClure, D. D. (2021). Photoautotrophic production of eicosapentaenoic acid. Crit. Rev. Biotechnol. 41, 731–748. doi:10.1080/07388551.2021.1888065
Guesnet, P., and Alessandri, J.-M. (2011). Docosahexaenoic acid (DHA) and the developing central nervous system (CNS) – implications for dietary recommendations. Biochimie 93, 7–12. doi:10.1016/j.biochi.2010.05.005
Gunstone, F. (2011). Vegetable oils in food technology: Composition, properties and uses. New York, United States: John Wiley & Sons.
Hac İsa, M., Metin, C., Ercan, E., and Alparslan, Y. (2022). Effect of different cell disruption methods on lipid yield of Schizochytrium sp. J. Am. Oil Chemists' Soc. 99, 129–139. doi:10.1002/aocs.12551
Halim, R., Harun, R., Danquah, M. K., and Webley, P. A. (2012). Microalgal cell disruption for biofuel development. Appl. Energy 91, 116–121. doi:10.1016/j.apenergy.2011.08.048
Hamilton, H. A., Newton, R., Auchterlonie, N. A., and Müller, D. B. (2020). Systems approach to quantify the global omega-3 fatty acid cycle. Nat. Food 1, 59–62. doi:10.1038/s43016-019-0006-0
Han, D. X., Li, Y. T., and Hu, Q. (2013). Astaxanthin in microalgae: Pathways, functions and biotechnological implications. Algae 28, 131–147. doi:10.4490/algae.2013.28.2.131
Han, X., Xu, P., Meng, X., Liang, Z., Zhang, Z., and Yang, Z. (2004). Preparation of high-purity linolenic acid from oil of Lithospermum erythrorhizon by urea inclusion and column chromatography. J. Chin. Pharm. Sci. 13, 53–57.
Hashimoto, M., Tanabe, Y., Hossain, S., Matsuzaki, K., Ohno, M., Kato, S., et al. (2020). Intake of alpha-linolenic acid-rich perilla frutescens leaf powder decreases home blood pressure and serum oxidized low-density lipoprotein in Japanese adults. Molecules 25, 2099. doi:10.3390/molecules25092099
Hatlen, B., Larsson, T., Østbye, T.-K., Romarheim, O. H., Rubio, L. M., and Ruyter, B. (2022). Improved fillet quality in harvest-size Atlantic salmon fed high n-3 canola oil as a DHA-source. Aquaculture 560, 738555. doi:10.1016/j.aquaculture.2022.738555
He, J., Hong, B., Lu, R., Zhang, R., Fang, H., Huang, W., et al. (2020). Separation of saturated fatty acids from docosahexaenoic acid-rich algal oil by enzymatic ethanolysis in tandem with molecular distillation. Food Sci. Nutr. 8, 2234–2241. doi:10.1002/fsn3.1462
Hidalgo, P., Toro, C., Ciudad, G., and Navia, R. (2013). Advances in direct transesterification of microalgal biomass for biodiesel production. Rev. Environ. Sci. Bio/Technology 12, 179–199. doi:10.1007/s11157-013-9308-0
Hollingsworth, M. D., Brown, M. E., Hillier, A. C., Santarsiero, B. D., and Chaney, J. D. (1996). Superstructure control in the crystal growth and ordering of urea inclusion compounds. Science 273, 1355–1359. doi:10.1126/science.273.5280.1355
Hosseinizand, H., Sokhansanj, S., and Lim, C. J. (2018). Studying the drying mechanism of microalgae Chlorella vulgaris and the optimum drying temperature to preserve quality characteristics. Dry. Technol. 36, 1049–1060. doi:10.1080/07373937.2017.1369986
Hoy, S. M., and Keating, G. M. (2009). Omega-3 ethylester concentrate. Drugs 69, 1077–1105. doi:10.2165/00003495-200969080-00008
Huang, P. W., Wang, L. R., Geng, S. S., Ye, C., Sun, X. M., and Huang, H. (2021). Strategies for enhancing terpenoids accumulation in microalgae. Appl. Microbiol. Biotechnol. 105, 4919–4930. doi:10.1007/s00253-021-11368-x
Islam, M. S., Christopher, L. P., and Alam, M. N. (2020). Separation and purification of ω-6 linoleic acid from crude tall oil. Separations 7, 9. doi:10.3390/separations7010009
Jacob, J. P., and Mathew, S. (2017). Effect of lipases from Candida cylinderacea on enrichment of PUFA in marine microalgae. J. Food Process. Preserv. 41, e12928. doi:10.1111/jfpp.12928
James, M. J., Gibson, R. A., and Cleland, L. G. (2000). Dietary polyunsaturated fatty acids and inflammatory mediator production. Am. J. Clin. Nutr. 71, 343S–348S. doi:10.1093/ajcn/71.1.343s
Jeffrey, B. G., Mitchell, D. C., Gibson, R. A., and Neuringer, M. (2002a). n-3 fatty acid deficiency alters recovery of the rod photoresponse in rhesus monkeys. Investigative Ophthalmol. Vis. Sci. 43, 2806–2814. doi:10.1007/s00417-002-0511-X
Jeffrey, B. G., Mitchell, D. C., Hibbeln, J. R., Gibson, R. A., Lee Chedester, A., and Salem, N. (2002b). Visual acuity and retinal function in infant monkeys fed long-chain PUFA. Lipids 37, 839–848. doi:10.1007/s11745-002-0969-0
Jensen, G., Ginsberg, D. I., and Drapeau, C. (2001). Blue-green algae as an immuno-enhancer and biomodulator. J. Am. Nutraceutical Assoc. 3, 24–30.
Jiménez Callejón, M. J., Robles Medina, A., Macías Sánchez, M. D., González Moreno, P. A., Navarro López, E., Esteban Cerdán, L., et al. (2022). Supercritical fluid extraction and pressurized liquid extraction processes applied to eicosapentaenoic acid-rich polar lipid recovery from the microalga Nannochloropsis sp. Algal Res. 61, 102586. doi:10.1016/j.algal.2021.102586
Jin, H., Chuai, W., Li, K., Hou, G., Wu, M., Chen, J., et al. (2021). Ultrahigh-cell-density heterotrophic cultivation of the unicellular green alga Chlorella sorokiniana for biomass production. Biotechnol. Bioeng. 118, 4138–4151. doi:10.1002/bit.27890
Jin, H., Zhang, H., Zhou, Z., Li, K., Hou, G., Xu, Q., et al. (2020). Ultrahigh-cell-density heterotrophic cultivation of the unicellular green microalga Scenedesmus acuminatus and application of the cells to photoautotrophic culture enhance biomass and lipid production. Biotechnol. Bioeng. 117, 96–108. doi:10.1002/bit.27190
Jithu Paul, J. (2019). “Bioconcentration of marine algae using lipase enzyme,” in Microalgae. Editor V. Milada (Rijeka: IntechOpen). Ch. 8. doi:10.5772/intechopen.87026
Kadalag, N. L., Pawar, P. R., and Prakash, G. (2022). Co-cultivation of Phaeodactylum tricornutum and Aurantiochytrium limacinum for polyunsaturated omega-3 fatty acids production. Bioresour. Technol. 346, 126544. doi:10.1016/j.biortech.2021.126544
Kaveh, M., Eftekhar, N., and Boskabady, M. H. (2019). The effect of alpha linolenic acid on tracheal responsiveness, lung inflammation, and immune markers in sensitized rats. Iran. J. basic Med. Sci. 22, 255–261. doi:10.22038/ijbms.2019.27381.6684
Kazemifard, S., Nayebzadeh, H., Saghatoleslami, N., and Safakish, E. (2019). Application of magnetic alumina-ferric oxide nanocatalyst supported by KOH for in-situ transesterification of microalgae cultivated in wastewater medium. Biomass Bioenergy 129, 105338. doi:10.1016/j.biombioe.2019.105338
Khera, H. K., and Srivastava, A. K. (2022). “Chapter 22 - genetic engineering of algae,” in An integration of phycoremediation processes in wastewater treatment. Editors M. Shah, S. Rodriguez-Couto, C. B. V. De La Cruz, and J. Biswas (Amsterdam, Netherlands: Elsevier), 487–502. doi:10.1016/B978-0-12-823499-0.00018-3
Kim, B., Im, H., and Lee, J. W. (2015). In situ transesterification of highly wet microalgae using hydrochloric acid. Bioresour. Technol. 185, 421–425. doi:10.1016/j.biortech.2015.02.092
Kim, E. S., and McCormack, P. L. (2014). Icosapent ethyl: A review of its use in severe hypertriglyceridemia. Am. J. Cardiovasc. Drugs 14, 471–478. doi:10.1007/s40256-014-0099-7
Knothe, G., and Dunn, R. O. (2009). A comprehensive evaluation of the melting points of fatty acids and esters determined by differential scanning calorimetry. J. Am. Oil Chemists' Soc. 86, 843–856. doi:10.1007/s11746-009-1423-2
Korbecki, J., Bobiński, R., and Dutka, M. (2019). Self-regulation of the inflammatory response by peroxisome proliferator-activated receptors. Inflamm. Res. 68, 443–458. doi:10.1007/s00011-019-01231-1
Kreps, F., Vrbiková, L., and Schmidt, Š. (2014). Influence of industrial physical refining on tocopherol, chlorophyll and beta-carotene content in sunflower and rapeseed oil. Eur. J. Lipid Sci. Technol. 116, 1572–1582. doi:10.1002/ejlt.201300460
Kris-Etherton, P. M., Harris, W. S., and Appel, L. J. (2002). Fish consumption, fish oil, omega-3 fatty acids, and cardiovascular disease. Circulation 106, 2747–2757. doi:10.1161/01.CIR.0000038493.65177.94
Kugler, A., Zorin, B., Didi-Cohen, S., Sibiryak, M., Gorelova, O., Ismagulova, T., et al. (2019). Long-chain polyunsaturated fatty acids in the green microalga Lobosphaera incisa contribute to tolerance to abiotic stresses. Plant Cell Physiology 60, 1205–1223. doi:10.1093/pcp/pcz013
Kumar, N., Varun, , and Chauhan, S. R. (2013). Performance and emission characteristics of biodiesel from different origins: A review. Renew. Sustain. Energy Rev. 21, 633–658. doi:10.1016/j.rser.2013.01.006
Lee, J.-Y., Yoo, C., Jun, S.-Y., Ahn, C.-Y., and Oh, H.-M. (2010). Comparison of several methods for effective lipid extraction from microalgae. Bioresour. Technol. 101, S75–S77. doi:10.1016/j.biortech.2009.03.058
Li, S., Hu, T., Xu, Y., Wang, J., Chu, R., Yin, Z., et al. (2020). A review on flocculation as an efficient method to harvest energy microalgae: Mechanisms, performances, influencing factors and perspectives. Renew. Sustain. Energy Rev. 131, 110005. doi:10.1016/j.rser.2020.110005
Li, X., Liu, J., Chen, G., Zhang, J., Wang, C., and Liu, B. (2019). Extraction and purification of eicosapentaenoic acid and docosahexaenoic acid from microalgae: A critical review. Algal Res. 43, 101619. doi:10.1016/j.algal.2019.101619
Li Y, Y., Sun, H., Wu, T., Fu, Y., He, Y., Mao, X., et al. (2019). Storage carbon metabolism of Isochrysis zhangjiangensis under different light intensities and its application for co-production of fucoxanthin and stearidonic acid. Bioresour. Technol. 282, 94–102. doi:10.1016/j.biortech.2019.02.127
Liu, C.-Z., Zheng, S., Xu, L., Wang, F., and Guo, C. (2013). Algal oil extraction from wet biomass of Botryococcus braunii by 1,2-dimethoxyethane. Appl. Energy 102, 971–974. doi:10.1016/j.apenergy.2012.08.016
Liu, G. R., and Chen, G. Y. (2012). Study on the decolorization of biodiesel from waste cooking oil. Adv. Mater. Res. 347-353, 3781–3787. doi:10.4028/www.scientific.net/amr.347-353.3781.347-353.3781
Liu, J., Mao, X., Zhou, W., and Guarnieri, M. T. (2016). Simultaneous production of triacylglycerol and high-value carotenoids by the astaxanthin-producing oleaginous green microalga Chlorella zofingiensis. Bioresour. Technol. 214, 319–327. doi:10.1016/j.biortech.2016.04.112
Liu, N., Li, D., Wang, W., Hollmann, F., Xu, L., Ma, Y., et al. (2018). Production and immobilization of lipase PCL and its application in synthesis of α-linolenic acid-rich diacylglycerol. J. Food Biochem. 42, e12574. doi:10.1111/jfbc.12574
Liu, Y., and Zheng, L. (2014). The comparison of the quality and comprehensive decolorization effect of crude corn germ oil obtained respectively by pre-pressing and leaching process. Cereal & Food Industry 21, 6–13.
Ljubic, A., Thulesen, E. T., Jacobsen, C., and Jakobsen, J. (2021). UVB exposure stimulates production of vitamin D-3 in selected microalgae. Algal Research-Biomass Biofuels Bioprod. 59, 102472. doi:10.1016/j.algal.2021.102472
Lourenço-Lopes, C., Garcia-Oliveira, P., Carpena, M., Fraga-Corral, M., Jimenez-Lopez, C., Pereira, A. G., et al. (2020). Scientific approaches on extraction, purification and stability for the commercialization of fucoxanthin recovered from Brown algae. Foods 9, 1113. doi:10.3390/foods9081113
Lu, X., Liu, B., He, Y., Guo, B., Sun, H., and Chen, F. (2019). Novel insights into mixotrophic cultivation of Nitzschia laevis for co-production of fucoxanthin and eicosapentaenoic acid. Bioresour. Technol. 294, 122145. doi:10.1016/j.biortech.2019.122145
Ma, R., You, Y., Liu, X., Ho, S.-H., Xie, Y., and Chen, J. (2023). Highly efficient co-production of fucoxanthin and eicosapentaenoic acid by heterotrophic cultivation of a newly isolated microalga Nitzschia sp. FZU62. Algal Res. 71, 103046. doi:10.1016/j.algal.2023.103046
Ma, X.-N., Chen, T.-P., Yang, B., Liu, J., and Chen, F. (2016). Lipid production from Nannochloropsis. Mar. Drugs 14, 61. doi:10.3390/md14040061
Madany, M. A., Abdel-Kareem, M. S., Al-Oufy, A. K., Haroun, M., and Sheweita, S. A. (2021). The biopolymer ulvan from Ulva fasciata: Extraction towards nanofibers fabrication. Int. J. Biol. Macromol. 177, 401–412. doi:10.1016/j.ijbiomac.2021.02.047
Magpusao, J., Oey, I., and Kebede, B. (2022). Changes in volatile and fatty acid compositions of selected microalgal suspensions with high pressure homogenization. Algal Res. 67, 102867. doi:10.1016/j.algal.2022.102867
Mahsa, K., Akram, E., Ali, N., and Mohammad Hossein, B. (2017). The extract of Portulaca oleracea and its constituent, alpha linolenic acid affects serum oxidant levels and inflammatory cells in sensitized rats. Iran. J. Allergy, Asthma Immunol. 16, 256–270.
Mao, X., Wu, T., Sun, D., Zhang, Z., and Chen, F. (2018). Differential responses of the green microalga Chlorella zofingiensis to the starvation of various nutrients for oil and astaxanthin production. Bioresour. Technol. 249, 791–798. doi:10.1016/j.biortech.2017.10.090
Marella, T. K., Bhattacharjya, R., and Tiwari, A. (2021). Impact of organic carbon acquisition on growth and functional biomolecule production in diatoms. Microb. Cell Factories 20, 135. doi:10.1186/s12934-021-01627-x
Matsui, H., Sugihara, S., Wada, M., Ozaki, T., Saitoh, T., and Kotani, T. (2022). Application of genetic disruption of a Nannochloropsis oceanica cell wall synthesizing gene to n-3 HUFA enrichment of Brachionus plicatilis. Aquaculture 552, 738022. doi:10.1016/j.aquaculture.2022.738022
Mejía-Zepeda, R., and Pérez-Hernández, I. H. (2020). Effect of alpha linolenic acid on membrane fluidity and respiration of liver mitochondria in normoglycemic and diabetic Wistar rats. J. Bioenergetics Biomembr. 52, 421–430. doi:10.1007/s10863-020-09859-z
Mendes, M. C., Navalho, S., Ferreira, A., Paulino, C., Figueiredo, D., Silva, D., et al. (2022). Algae as food in europe: An overview of species diversity and their application. Foods 11, 1871. doi:10.3390/foods11131871
Menegol, T., Romero-Villegas, G. I., López-Rodríguez, M., Navarro-López, E., López-Rosales, L., Chisti, Y., et al. (2019). Mixotrophic production of polyunsaturated fatty acids and carotenoids by the microalga Nannochloropsis gaditana. J. Appl. Phycol. 31, 2823–2832. doi:10.1007/s10811-019-01828-3
Meng, X., Gao, H., Kong, R., and Xu, X. (2018). Optimization of heterotrophic growth conditions for a Scenedesmus strain with high content of α-linolenic acid. Acta Hydrobiol. Sin. 42, 819–823. doi:10.7541/2018.100
Mercer, P., and Armenta, R. E. (2011). Developments in oil extraction from microalgae. Eur. J. Lipid Sci. Technol. 113, 539–547. doi:10.1002/ejlt.201000455
Metzner, H. (1984). Geoffrey zubay (coordinating author). Reading, Menlo Park, London, Amsterdam, Don Mills, Sydney: Addison-Wesley Publishing Company. doi:10.1016/0302-4598(84)87027-0Biochemistry
Mizukami, T., Ikeda, K., Shimanaka, Y., Korogi, K., Zhou, C., Takase, H., et al. (2018). Reelin deficiency leads to aberrant lipid composition in mouse brain. Biochem. Biophysical Res. Commun. 505, 81–86. doi:10.1016/j.bbrc.2018.09.089
Mohamadzadeh Shirazi, H., Karimi-Sabet, J., and Ghotbi, C. (2017). Biodiesel production from Spirulina microalgae feedstock using direct transesterification near supercritical methanol condition. Bioresour. Technol. 239, 378–386. doi:10.1016/j.biortech.2017.04.073
Moharana, T. R., and Rao, N. M. (2020). Substrate structure and computation guided engineering of a lipase for omega-3 fatty acid selectivity. PLOS ONE 15, e0231177. doi:10.1371/journal.pone.0231177
Molino, A., Mehariya, S., Di Sanzo, G., Larocca, V., Martino, M., Leone, G. P., et al. (2020). Recent developments in supercritical fluid extraction of bioactive compounds from microalgae: Role of key parameters, technological achievements and challenges. J. CO2 Util. 36, 196–209. doi:10.1016/j.jcou.2019.11.014
Moniz, P., Silva, C., Oliveira, A. C., Reis, A., and Lopes da Silva, T. (2021). Raw glycerol based medium for DHA and lipids production, using the marine heterotrophic microalga Crypthecodinium cohnii. Processes 9, 2005. doi:10.3390/pr9112005
Muhlhausler, B. S., Collins, C. T., Gould, J. F., Best, K. P., and Leghi, G. E. (2018). “Chapter 7 - polyunsaturated fatty acids: Metabolism and nutritional requirements in pregnancy and infancy,” in Polyunsaturated fatty acid metabolism. Editor G. C. Burdge (Urbana: AOCS Press), 111–134. doi:10.1016/B978-0-12-811230-4.00007-7
Muramatsu, T., Yatsuya, H., Toyoshima, H., Sasaki, S., Li, Y., Otsuka, R., et al. (2010). Higher dietary intake of alpha-linolenic acid is associated with lower insulin resistance in middle-aged Japanese. Prev. Med. 50, 272–276. doi:10.1016/j.ypmed.2010.02.014
Mwakasege, E., Treydte, A., Hoeglinger, O., Kassim, N., and Makule, E. (2021). Fatty acid contents and stability of oyster nut oil (Telfairia pedata) compared to flaxseed and sunflower oil. Int. J. Food Sci. 2021, 1–8. doi:10.1155/2021/9985910
Nagappan, S., Devendran, S., Tsai, P.-C., Dinakaran, S., Dahms, H.-U., and Ponnusamy, V. K. (2019). Passive cell disruption lipid extraction methods of microalgae for biofuel production – a review. Fuel 252, 699–709. doi:10.1016/j.fuel.2019.04.092
Nagappan, S., and Kumar Verma, S. (2018). Co-production of biodiesel and alpha-linolenic acid (omega-3 fatty acid) from microalgae,Desmodesmus sp.MCC34. Desmodesmus sp. MCC34. Energy Sources, Part A Recovery, Util. Environ. Eff. 40, 2933–2940. doi:10.1080/15567036.2018.1514434
Najar-Villarreal, F., Boyle, E., Danler, R., O’quinn, T., Houser, T., and Gonzalez, J. (2019). Fatty acid composition, proximate analysis, and consumer sensory evaluation of United States retail grass-fed ground beef. Meat Muscle Biol. 3, 18. doi:10.22175/mmb2019.06.0018
Napier, J. A. (2002). Plumbing the depths of PUFA biosynthesis: A novel polyketide synthase-like pathway from marine organisms. Trends Plant Sci. 7, 51–54. doi:10.1016/S1360-1385(01)02191-4
Neag, E., Stupar, Z., Maicaneanu, S. A., and Roman, C. (2023). Advances in biodiesel production from microalgae. Energies 16, 1129. doi:10.3390/en16031129
Neuringer, M., Connor, W. E., Lin, D. S., Barstad, L., and Luck, S. (1986). Biochemical and functional effects of prenatal and postnatal omega 3 fatty acid deficiency on retina and brain in rhesus monkeys. Proc. Natl. Acad. Sci. 83, 4021–4025. doi:10.1073/pnas.83.11.4021
Nichols, D. S. (2003). Prokaryotes and the input of polyunsaturated fatty acids to the marine food web. FEMS Microbiol. Lett. 219, 1–7. doi:10.1016/s0378-1097(02)01200-4
Olkiewicz, M., Caporgno, M. P., Font, J., Legrand, J., Lepine, O., Plechkova, N. V., et al. (2015). A novel recovery process for lipids from microalgæ for biodiesel production using a hydrated phosphonium ionic liquid. Green Chem. 17, 2813–2824. doi:10.1039/C4GC02448F
Othman, F. S., Jamaluddin, H., Ibrahim, Z., Hara, H., Yahya, N. A., Mohamad, K. I. S. E., et al. (2019). Production of a-linolenic acid by an oleaginous green algae Acutodesmus obliquus isolated from Malaysia. J. Pure Appl. Microbiol. 13, 1297–1306. doi:10.22207/jpam.13.3.01
Özcan, M. M., Duman, E., and Duman, S. (2021). Influence of refining stages on the physicochemical properties and phytochemicals of canola oil. J. Food Process. Preserv. 45, e15164. doi:10.1111/jfpp.15164
Pan, X., Xu, L., Zhang, Y., Xiao, X., Wang, X., Liu, Y., et al. (2012). Efficient display of active Geotrichum sp. lipase on Pichia pastoris cell wall and its application as a whole-cell biocatalyst to enrich EPA and DHA in fish oil. J. Agric. Food Chem. 60, 9673–9679. doi:10.1021/jf301827y
Park, J.-Y., Oh, Y.-K., Lee, J.-S., Lee, K., Jeong, M.-J., and Choi, S.-A. (2014). Acid-catalyzed hot-water extraction of lipids from Chlorella vulgaris. Bioresour. Technol. 153, 408–412. doi:10.1016/j.biortech.2013.12.065
Park, J., Kim, B., Chang, Y. K., and Lee, J. W. (2017). Wet in situ transesterification of microalgae using ethyl acetate as a co-solvent and reactant. Bioresour. Technol. 230, 8–14. doi:10.1016/j.biortech.2017.01.027
Paschos, G. K., Magkos, F., Panagiotakos, D. B., Votteas, V., and Zampelas, A. (2007). Dietary supplementation with flaxseed oil lowers blood pressure in dyslipidaemic patients. Eur. J. Clin. Nutr. 61, 1201–1206. doi:10.1038/sj.ejcn.1602631
Pawar, P. R., Velani, S., Kumari, S., Lali, A. M., and Prakash, G. (2021). Isolation and optimization of a novel thraustochytrid strain for DHA rich and astaxanthin comprising biomass as aquafeed supplement. 3 Biotech. 11, 71. doi:10.1007/s13205-020-02616-4
Peltomaa, E., Johnson, M. D., and Taipale, S. J. (2018). Marine cryptophytes are great sources of EPA and DHA. Mar. Drugs 16, 3. doi:10.3390/md16010003
Petursdottir, A. L., Farr, S. A., Morley, J. E., Banks, W. A., and Skuladottir, G. V. (2008). Effect of dietary n-3 polyunsaturated fatty acids on brain lipid fatty acid composition, learning ability, and memory of senescence-accelerated mouse. Journals Gerontology Ser. A 63, 1153–1160. doi:10.1093/gerona/63.11.1153
Praveenkumar, R., Lee, K., Lee, J., and Oh, Y.-K. (2015). Breaking dormancy: An energy-efficient means of recovering astaxanthin from microalgae. Green Chem. 17, 1226–1234. doi:10.1039/C4GC01413H
Quilodrán, B., Hinzpeter, I., Hormazabal, E., Quiroz, A., and Shene, C. (2010). Docosahexaenoic acid (C22:6n−3, DHA) and astaxanthin production by Thraustochytriidae sp. AS4-A1 a native strain with high similitude to Ulkenia sp.: Evaluation of liquid residues from food industry as nutrient sources. Enzyme Microb. Technol. 47, 24–30. doi:10.1016/j.enzmictec.2010.04.002
Rajaram, S. (2014). Health benefits of plant-derived α-linolenic acid. Am. J. Clin. Nutr. 100, 443S–448S. doi:10.3945/ajcn.113.071514
Ramluckan, K., Moodley, K. G., and Bux, F. (2014). An evaluation of the efficacy of using selected solvents for the extraction of lipids from algal biomass by the soxhlet extraction method. Fuel 116, 103–108. doi:10.1016/j.fuel.2013.07.118
Ranjan, A., Patil, C., and Moholkar, V. S. (2010). Mechanistic assessment of microalgal lipid extraction. Industrial Eng. Chem. Res. 49, 2979–2985. doi:10.1021/ie9016557
Raper, N. R., Cronin, F. J., and Exler, J. (1992). Omega-3 fatty acid content of the US food supply. J. Am. Coll. Nutr. 11, 304–308. doi:10.1080/07315724.1992.10718231
Rashid, N., Park, W. K., and Selvaratnam, T. (2018). Binary culture of microalgae as an integrated approach for enhanced biomass and metabolites productivity, wastewater treatment, and bioflocculation. Chemosphere 194, 67–75. doi:10.1016/j.chemosphere.2017.11.108
Ratledge, C. (2001). Microbial lipids. Biotechnol. Set. 2001, 133–197. doi:10.1002/9783527620999.ch4g
Rawat, I., Ranjith Kumar, R., Mutanda, T., and Bux, F. (2011). Dual role of microalgae: Phycoremediation of domestic wastewater and biomass production for sustainable biofuels production. Appl. Energy 88, 3411–3424. doi:10.1016/j.apenergy.2010.11.025
Rezanka, T., Vitova, M., Kolouchova, I., Nedbalova, L., Dolezalova, J., Palyzova, A., et al. (2017). Polydatin and its derivatives inhibit fatty acid desaturases in microorganisms. Eur. J. Lipid Sci. Technol. 119, 1600369. doi:10.1002/ejlt.201600369
Rodríguez-España, M., Mendoza-Sánchez, L. G., Magallón-Servín, P., Salgado-Cervantes, M. A., Acosta-Osorio, A. A., and García, H. S. (2022). Supercritical fluid extraction of lipids rich in DHA from Schizochytrium sp. J. Supercrit. Fluids 179, 105391. doi:10.1016/j.supflu.2021.105391
Rodríguez-Miranda, J., Gallegos-Marín, I., Hernández-Santos, B., Herman-Lara, E., Medina-Juárez, L. A., Juárez-Barrientos, J. M., et al. (2019). Effect of frying and storage on oxidative quality of conjugated linoleic-acid-rich soybean oil produced by photoisomerization using plantain as a model system. J. Sci. Food Agric. 99, 3910–3916. doi:10.1002/jsfa.9614
Ronda, S. R., Bokka, C. S., Ketineni, C., Rijal, B., and Allu, P. R. (2012). Aeration effect on spirulina platensis growth and gamma-linolenic acid production. Braz. J. Microbiol. 43, 12–20. doi:10.1590/s1517-83822012000100002
Ruiz, J., Wijffels, R. H., Dominguez, M., and Barbosa, M. J. (2022). Heterotrophic vs autotrophic production of microalgae: Bringing some light into the everlasting cost controversy. Algal Res. 64, 102698. doi:10.1016/j.algal.2022.102698
Rupani, B., Kodam, K., Gadre, R., and Najafpour, G. D. (2012). Lipase-mediated hydrolysis of flax seed oil for selective enrichment of α-linolenic acid. Eur. J. Lipid Sci. 114, 1246–1253. doi:10.1002/ejlt.201100384
Safi, C., Camy, S., Frances, C., Varela, M. M., Badia, E. C., Pontalier, P.-Y., et al. (2014). Extraction of lipids and pigments of Chlorella vulgaris by supercritical carbon dioxide: Influence of bead milling on extraction performance. J. Appl. Phycol. 26, 1711–1718. doi:10.1007/s10811-013-0212-3
Salem, N., Kim, H.-Y., and Yergey, J. A. (1986). “Chapter 15 - docosahexaenoic acid: Membrane function and metabolism,” in Health effects of polyunsaturated fatty acids in seafoods. Editors R. R. Kifer, and R. E. Martin (Massachusetts, United Statespp: Academic Press), 263–317. doi:10.1016/B978-0-12-644360-8.50019-4
Salminen, H., Aulbach, S., Leuenberger, B. H., Tedeschi, C., and Weiss, J. (2014). Influence of surfactant composition on physical and oxidative stability of Quillaja saponin-stabilized lipid particles with encapsulated ω-3 fish oil. Colloids Surfaces B Biointerfaces 122, 46–55. doi:10.1016/j.colsurfb.2014.06.045
Scanferlato, R., Bortolotti, M., Sansone, A., Chatgilialoglu, C., Polito, L., De Spirito, M., et al. (2019). Hexadecenoic fatty acid positional isomers and de novo PUFA synthesis in colon cancer cells. Int. J. Mol. Sci. 20, 832. doi:10.3390/ijms20040832
Schmid, M., Guihéneuf, F., and Stengel, D. B. (2014). Fatty acid contents and profiles of 16 macroalgae collected from the Irish Coast at two seasons. J. Appl. Phycol. 26, 451–463. doi:10.1007/s10811-013-0132-2
Señoráns, M., Castejón, N., and Señoráns, F. J. (2020). Advanced extraction of lipids with DHA from Isochrysis galbana with enzymatic pre-treatment combined with pressurized liquids and ultrasound assisted extractions. Molecules 25, 3310. doi:10.3390/molecules25143310
Shen, J., Li, S., Caydamli, Y., Narayanan, G., Zhang, N., Harrison, O., et al. (2018). The role of polymer crystallizability on the formation of polymer-urea-inclusion compounds. Cryst. Growth & Des. 18, 3099–3106. doi:10.1021/acs.cgd.8b00240
Shim, S. J., Hong, M. E., Chang, W. S., and Sim, S. J. (2020). Repeated-batch production of omega-3 enriched biomass of Chlorella sorokiniana via calcium-induced homeoviscous adaptation. Bioresour. Technol. 303, 122944. doi:10.1016/j.biortech.2020.122944
Sigurgisladottir, S., Lall, S. P., Parrish, C. C., and Ackman, R. G. (1992). Cholestane as a digestibility marker in the absorption of polyunsaturated fatty acid ethyl esters in Atlantic salmon. Lipids 27, 418–424. doi:10.1007/BF02536382
Simopoulos, A. P., Leaf, A., and Salem, N. (2000). Workshop statement on the essentiality of and recommended dietary intakes for Omega-6 and Omega-3 fatty acids. Prostagl. Leukot. Essent. Fat. Acids 63, 119–121. doi:10.1054/plef.2000.0176
Simopoulos, A. P. (1991). Omega-3 fatty acids in health and disease and in growth and development. Am. J. Clin. Nutr. 54, 438–463. doi:10.1093/ajcn/54.3.438
Sivaramakrishnan, R., and Incharoensakdi, A. (2019). Enhancement of lipid extraction for efficient methyl ester production from Chlamydomonas sp. J. Appl. Phycol. 31, 2365–2377. doi:10.1007/s10811-019-1758-5
Smink, W., Gerrits, W. J. J., Gloaguen, M., Ruiter, A., and van Baal, J. (2012). Linoleic and α-linolenic acid as precursor and inhibitor for the synthesis of long-chain polyunsaturated fatty acids in liver and brain of growing pigs. Animal 6, 262–270. doi:10.1017/S1751731111001479
Solana, M., Rizza, C. S., and Bertucco, A. (2014). Exploiting microalgae as a source of essential fatty acids by supercritical fluid extraction of lipids: Comparison between Scenedesmus obliquus, Chlorella protothecoides and Nannochloropsis salina. J. Supercrit. Fluids 92, 311–318. doi:10.1016/j.supflu.2014.06.013
Soleimanian, Y., Sahari, M. A., and Barzegar, M. (2015). Influence of processing parameters on physicochemical properties of fractionated fish oil at low temperature crystallization. Nutr. Food Sci. 45, 2–19. doi:10.1108/NFS-05-2014-0038
Spurvey, S. A., and Shahidi, F. (2000). Concentration of gamma linolenic acid (gla) from borage oil by urea complexation: Optimization of reaction conditions. J. Food Lipids 7, 163–174. doi:10.1111/j.1745-4522.2000.tb00169.x
Stivala, S., Reiner, M. F., Lohmann, C., Lüscher, T. F., Matter, C. M., and Beer, J. H. (2013). Dietary α-linolenic acid increases the platelet count in ApoE−/− mice by reducing clearance. Blood 122, 1026–1033. doi:10.1182/blood-2013-02-484741
Stramarkou, M., Oikonomopoulou, V., Chalima, A., Boukouvalas, C., Topakas, E., and Krokida, M. (2021). Optimization of green extractions for the recovery of docosahexaenoic acid (DHA) from Crypthecodinium cohnii. Algal Research-Biomass Biofuels Bioprod. 58, 102374. doi:10.1016/j.algal.2021.102374
Su, H., Wang, X., Kim, Y. G., Kim, S. B., Seo, Y.-G., Kim, J. S., et al. (2014). Optimization of decoloring conditions of crude fatty acids recovered from crude glycerol by acid-activated clay using response surface method. Korean J. Chem. Eng. 31, 2070–2076. doi:10.1007/s11814-014-0158-4
Sun, J., Zhou, C., Cheng, P., Zhu, J., Hou, Y., Li, Y., et al. (2022). A simple and efficient strategy for fucoxanthin extraction from the microalga Phaeodactylum tricornutum. Algal Res. 61, 102610. doi:10.1016/j.algal.2021.102610
Swanson, D., Block, R., and Mousa, S. A. (2012). Omega-3 fatty acids EPA and DHA: Health benefits throughout life. Adv. Nutr. 3, 1–7. doi:10.3945/an.111.000893
Tachihana, S., Nagao, N., Katayama, T., Hirahara, M., Yusoff, F. M., Banerjee, S., et al. (2020). High productivity of eicosapentaenoic acid and fucoxanthin by a marine diatom Chaetoceros gracilis in a semi-continuous culture. Front. Bioeng. Biotechnol. 8, 602721. doi:10.3389/fbioe.2020.602721
Teo, C. L., and Idris, A. (2014). Enhancing the various solvent extraction method via microwave irradiation for extraction of lipids from marine microalgae in biodiesel production. Bioresour. Technol. 171, 477–481. doi:10.1016/j.biortech.2014.08.024
Uemura, H. (2012). Synthesis and production of unsaturated and polyunsaturated fatty acids in yeast: Current state and perspectives. Appl. Microbiol. Biotechnol. 95, 1–12. doi:10.1007/s00253-012-4105-1
Umamaheswari, J., Kavitha, M. S., and Shanthakumar, S. (2020). Outdoor cultivation of Chlorella pyrenoidosa in paddy-soaked wastewater and a feasibility study on biodiesel production from wet algal biomass through in-situ transesterification. Biomass Bioenergy 143, 105853. doi:10.1016/j.biombioe.2020.105853
Umezawa, M., Kogishi, K., Tojo, H., Yoshimura, S., Seriu, N., Ohta, A., et al. (1999). High-linoleate and high-α-linolenate diets affect learning ability and natural behavior in SAMR1 mice. J. Nutr. 129, 431–437. doi:10.1093/jn/129.2.431
Valizadeh Derakhshan, M., Nasernejad, B., Dadvar, M., and Hamidi, M. (2014). Pretreatment and kinetics of oil extraction from algae for biodiesel production. Asia-Pacific J. Chem. Eng. 9, 629–637. doi:10.1002/apj.1790
Valverde, L. M., Moreno, P. A. G., Callejón, M. J. J., Cerdán, L. E., and Medina, A. R. (2013). Concentration of eicosapentaenoic acid (EPA) by selective alcoholysis catalyzed by lipases. Eur. J. Lipid Sci. Technol. 115, 990–1004. doi:10.1002/ejlt.201300005
Valverde, L. M., Moreno, P. A. G., Cerdán, L. E., López, E. N., López, B. C., and Medina, A. R. (2014). Concentration of docosahexaenoic and eicosapentaenoic acids by enzymatic alcoholysis with different acyl-acceptors. Biochem. Eng. J. 91, 163–173. doi:10.1016/j.bej.2014.08.010
Varin, A., Thomas, C., Ishibashi, M., Ménégaut, L., Gautier, T., Trousson, A., et al. (2015). Liver X receptor activation promotes polyunsaturated fatty acid synthesis in macrophages. Arteriosclerosis, Thrombosis, Vasc. Biol. 35, 1357–1365. doi:10.1161/ATVBAHA.115.305539
Vidyashankar, S., VenuGopal, K. S., Swarnalatha, G. V., Kavitha, M. D., Chauhan, V. S., Ravi, R., et al. (2015). Characterization of fatty acids and hydrocarbons of chlorophycean microalgae towards their use as biofuel source. Biomass Bioenergy 77, 75–91. doi:10.1016/j.biombioe.2015.03.001
Wang, J., Zhang, J.-L., and Wu, F.-A. (2013). Enrichment process for α-linolenic acid from silkworm pupae oil. Eur. J. Lipid Sci. Technol. 115, 791–799. doi:10.1002/ejlt.201200324
Wang, J., Zhou, W., Chen, H., Zhan, J., He, C., and Wang, Q. (2019). Ammonium nitrogen tolerant Chlorella strain screening and its damaging effects on photosynthesis. Front. Microbiol. 9, 3250. doi:10.3389/fmicb.2018.03250
Wang, M., Ma, L., Yang, Y., Xiao, Z., and Wan, J. (2019). n-3 Polyunsaturated fatty acids for the management of alcoholic liver disease: A critical review. Crit. Rev. Food Sci. Nutr. 59, S116–S129. doi:10.1080/10408398.2018.1544542
Wang, S., Yerkebulan, M., Abomohra, A. E.-F., El-Khodary, S., and Wang, Q. (2019). Microalgae harvest influences the energy recovery: A case study on chemical flocculation of Scenedesmus obliquus for biodiesel and crude bio-oil production. Bioresour. Technol. 286, 121371. doi:10.1016/j.biortech.2019.121371
Wang X, X., Fosse, H. K., Li, K., Chauton, M. S., Vadstein, O., and Reitan, K. I. (2019). Influence of nitrogen limitation on lipid accumulation and EPA and DHA content in four marine microalgae for possible use in aquafeed. Front. Mar. Sci. 6. doi:10.3389/fmars.2019.00095
Ward, O. P., and Singh, A. (2005). Omega-3/6 fatty acids: Alternative sources of production. Process Biochem. 40, 3627–3652. doi:10.1016/j.procbio.2005.02.020
Wayan Suriani, N., and Komansilan, A. (2019). Enrichment of omega-3 fatty acids, waste oil by-products canning tuna (thunnus sp.) with urea crystallization. J. Phys. Conf. Ser. 1317, 012056. doi:10.1088/1742-6596/1317/1/012056
Wei, B., and Wang, S. (2020). Separation of eicosapentaenoic acid and docosahexaenoic acid by three-zone simulated moving bed chromatography. J. Chromatogr. A 1625, 461326. doi:10.1016/j.chroma.2020.461326
Winnik, S., Lohmann, C., Richter, E. K., Schäfer, N., Song, W.-L., Leiber, F., et al. (2011). Dietary α-linolenic acid diminishes experimental atherogenesis and restricts T cell-driven inflammation. Eur. Heart J. 32, 2573–2584. doi:10.1093/eurheartj/ehq501
Wu, C. e., Xu, K., Li, Y., Luo, G., and Li, T. (2005). Enrichment of α-linolenic acid from seed oil of kiwi fruit based on urea adduction fractionation method. Trans. Chin. Soc. Agric. Mach. 36, 57–61.
Xia, Y., Zhang, Y.-T., Sun, J.-Y., Huang, H., Zhao, Q., and Ren, L.-J. (2020). Strategies for enhancing eicosapentaenoic acid production: From fermentation to metabolic engineering. Algal Res. 51, 102038. doi:10.1016/j.algal.2020.102038
Xie, D., Jackson, E. N., and Zhu, Q. (2015). Sustainable source of omega-3 eicosapentaenoic acid from metabolically engineered yarrowia lipolytica: From fundamental research to commercial production. Appl. Microbiol. Biotechnol. 99, 1599–1610. doi:10.1007/s00253-014-6318-y
Xue, Z., Sharpe, P. L., Hong, S.-P., Yadav, N. S., Xie, D., Short, D. R., et al. (2013). Production of omega-3 eicosapentaenoic acid by metabolic engineering of Yarrowia lipolytica. Nat. Biotechnol. 31, 734–740. doi:10.1038/nbt.2622
Yamashita, T., Oda, E., Sano, T., Yamashita, T., Ijiru, Y., Giddings, J. C., et al. (2005). Varying the ratio of dietary n−6/n−3 polyunsaturated fatty acid alters the tendency to thrombosis and progress of atherosclerosis in apoE−/− LDLR−/− double knockout mouse. Thrombosis Res. 116, 393–401. doi:10.1016/j.thromres.2005.01.011
Yang, B., Ren, X.-L., Fu, Y.-Q., Gao, J.-L., and Li, D. (2014). Ratio of n-3/n-6 PUFAs and risk of breast cancer: A meta-analysis of 274135 adult females from 11 independent prospective studies. BMC Cancer 14, 105. doi:10.1186/1471-2407-14-105
Yang F, F., Yuan, W. S. Q., Ma, Y. H., Balamurugan, S., Li, H. Y., Fu, S. L., et al. (2019). Harnessing the lipogenic potential of delta 6-desaturase for simultaneous hyperaccumulation of lipids and polyunsaturated fatty acids in Nannochloropsis oceanica. Front. Mar. Sci. 6. doi:10.3389/fmars.2019.00682
Yang, J., Wen, C., Duan, Y., Deng, Q., Peng, D., Zhang, H., et al. (2021). The composition, extraction, analysis, bioactivities, bioavailability and applications in food system of flaxseed (Linum usitatissimum L.) oil: A review. Trends Food Sci. Technol. 118, 252–260. doi:10.1016/j.tifs.2021.09.025
Yang, L.-Y., Kuksis, A., and Myher, J. J. (1989). Lumenal hydrolysis of menhaden and rapeseed oils and their fatty acid methyl and ethyl esters in the rat. Biochem. Cell Biol. 67, 192–204. doi:10.1139/o89-030
Yang, M., Sun, H., Peng, T., Li, B., and Xie, Y. (2019). Structural alteration of montmorillonite by acid activation and its effect on the decolorization of rapeseed oil. J. Minerals 71, 3667–3672. doi:10.1007/s11837-019-03665-8
Yang, X., Li, Y., Li, Y., Ye, D., Yuan, L., Sun, Y., et al. (2019). Solid matrix-supported supercritical CO2 enhances extraction of γ-linolenic acid from the cyanobacterium arthrospira (spirulina) platensis and bioactivity evaluation of the molecule in zebrafish. Mar. Drugs 17, 203. doi:10.3390/md17040203
Yew, G. Y., Tan, X., Chew, K. W., Chang, J.-S., Tao, Y., Jiang, N., et al. (2021). Thermal-Fenton mechanism with sonoprocessing for rapid non-catalytic transesterification of microalgal to biofuel production. Chem. Eng. J. 408, 127264. doi:10.1016/j.cej.2020.127264
Yin, F.-W., Zhan, C.-T., Huang, J., Sun, X.-L., Yin, L.-F., Zheng, W.-L., et al. (2023). Efficient Co-production of docosahexaenoic acid oil and carotenoids in Aurantiochytrium sp. using a light intensity gradient strategy. Appl. Biochem. Biotechnol. 195, 623–638. doi:10.1007/s12010-022-04134-w
Youdim, K. A., Martin, A., and Joseph, J. A. (2000). Essential fatty acids and the brain: Possible health implications. Int. J. Dev. Neurosci. 18, 383–399. doi:10.1016/s0736-5748(00)00013-7
Yu, Z., Hou, Q., Liu, M., Xie, Z., Ma, M., Chen, H., et al. (2023). From lab to application: Cultivating limnetic microalgae in seawater coupled with wastewater for biodiesel production on a pilot scale. Water Res. 229, 119471. doi:10.1016/j.watres.2022.119471
Zainuddin, N. J., Babji, A. S., and Said, M. (2011). Extraction of lipids and purification of linoleic acid from Clarias macrocephalus oil. Aquac. Aquarium, Conservation Legislation 4, 423–429.
Zarli, A. (2020). “Chapter 6 - oleochemicals: All time players of green chemistry,” in Studies in surface science and catalysis. Editors A. Basile, G. Centi, M. D. Falco, and G. Iaquaniello (Amsterdam, Netherlands: Elsevier), 77–95. doi:10.1016/B978-0-444-64337-7.00006-9
Zhang, G., Liu, J., and Liu, Y. (2013). Concentration of omega-3 polyunsaturated fatty acids from oil of Schizochytrium limacinum by molecular distillation: Optimization of technological conditions. Industrial Eng. Chem. Res. 52, 3918–3925. doi:10.1021/ie3020044
Zhang, H., Fang, T., Pan, W., Liao, A., and Wei, Z. (2011). Optimization of the enrichment of alpha-linolenic acid in silkworm pupal oil by the method of urea adduction fractionation. Food Sci. 32, 74–77.
Zhang, H., Gong, T., Li, J., Pan, B., Hu, Q., Duan, M., et al. (2022). Study on the effect of spray drying process on the quality of microalgal biomass: A comprehensive biocomposition analysis of spray-dried S. acuminatus biomass. BioEnergy Res. 15, 320–333. doi:10.1007/s12155-021-10343-8
Zhang, X., Chen, H., Wang, H., and Wang, Q. (2021). Time-course effects of Tris(1,3-dichloro-2-propyl) phosphate (TDCPP) on Chlorella pyrenoidosa: Growth inhibition and adaptability mechanisms. J. Hazard. Mater. 402, 123784. doi:10.1016/j.jhazmat.2020.123784
Zhao, J., Ma, M., Yan, X., Zhang, G., Xia, J., Zeng, G., et al. (2022). Expression and characterization of a novel lipase from Bacillus licheniformis NCU CS-5 for application in enhancing fatty acids flavor release for low-fat cheeses. Food Chem. 368, 130868. doi:10.1016/j.foodchem.2021.130868
Zheng, H., Yin, J., Gao, Z., Huang, H., Ji, X., and Dou, C. (2011). Disruption of Chlorella vulgaris cells for the release of biodiesel-producing lipids: A comparison of grinding, ultrasonication, bead milling, enzymatic lysis, and microwaves. Appl. Biochem. Biotechnol. 164, 1215–1224. doi:10.1007/s12010-011-9207-1
Zheng, S., Chen, S., Zou, S., Yan, Y., Gao, G., He, M., et al. (2021). Bioremediation of Pyropia-processing wastewater coupled with lipid production using Chlorella sp. Bioresour. Technol. 321, 124428. doi:10.1016/j.biortech.2020.124428
Zheng, S., Zou, S., Feng, T., Sun, S., Guo, X., He, M., et al. (2022). Low temperature combined with high inoculum density improves alpha-linolenic acid production and biochemical characteristics of Chlamydomonas reinhardtii. Bioresour. Technol. 348, 126746. doi:10.1016/j.biortech.2022.126746
Zheng, Y., Xue, C., Chen, H., Jia, A., Zhao, L., Zhang, J., et al. (2023). Reconstitution and expression of mcy gene cluster in the model cyanobacterium Synechococcus 7942 reveals a role of MC-LR in cell division. New Phytol. 2023, 18766. doi:10.1111/nph.18766
Zheng, Z., Dai, Z., and Shen, Q. (2018). Enrichment of polyunsaturated fatty acids from seal oil through urea adduction and the fatty acids change rules during the process. J. Food Process. Preserv. 42, e13593. doi:10.1111/jfpp.13593
Zhou, Y., Chen, Y., Li, M., and Hu, C. (2020). Production of high-quality biofuel via ethanol liquefaction of pretreated natural microalgae. Renew. Energy 147, 293–301. doi:10.1016/j.renene.2019.08.136
Zhu, J., Chen, W., Chen, H., Zhang, X., He, C., Rong, J., et al. (2016). Improved productivity of neutral lipids in Chlorella sp. A2 by minimal nitrogen supply. Front. Microbiol. 7, 557. doi:10.3389/fmicb.2016.00557
Zhuang, Y., Guo, J., Chen, L., Li, D., Liu, J., and Ye, N. (2012). Microwave-assisted direct liquefaction of Ulva prolifera for bio-oil production by acid catalysis. Bioresour. Technol. 116, 133–139. doi:10.1016/j.biortech.2012.04.036
Zou, X., Ye, L., He, X., Wu, S., Zhang, H., and Jin, Q. (2020). Preparation of DHA-rich medium- and long-chain triacylglycerols by lipase-catalyzed acidolysis of microbial oil from Schizochytrium sp.with medium-chain fatty acids. Appl. Biochem. Biotechnol. 191, 1294–1314. doi:10.1007/s12010-020-03261-6
Keywords: algae, PUFA, research hotspots, extraction, enrichment, omega-fatty acids
Citation: Chen W, Li T, Du S, Chen H and Wang Q (2023) Microalgal polyunsaturated fatty acids: Hotspots and production techniques. Front. Bioeng. Biotechnol. 11:1146881. doi: 10.3389/fbioe.2023.1146881
Received: 18 January 2023; Accepted: 23 March 2023;
Published: 31 March 2023.
Edited by:
Jianhua Fan, East China University of Science and Technology, ChinaReviewed by:
Tonmoy Ghosh, Indian Institute of Technology Indore, IndiaDale McClure, Brunel University London, United Kingdom
Copyright © 2023 Chen, Li, Du, Chen and Wang. This is an open-access article distributed under the terms of the Creative Commons Attribution License (CC BY). The use, distribution or reproduction in other forums is permitted, provided the original author(s) and the copyright owner(s) are credited and that the original publication in this journal is cited, in accordance with accepted academic practice. No use, distribution or reproduction is permitted which does not comply with these terms.
*Correspondence: Qiang Wang, d2FuZ3FpYW5nQGhlbnUuZWR1LmNu