- 1School of Medicine, Trinity College, Trinity Biomedical Sciences Institute, Dublin, Ireland
- 2Department of Cell and Molecular Sciences, Faculty of Biological Sciences, Kharazmi University, Tehran, Iran
- 3Department of Clinical Medicine, Trinity College Dublin, Tallaght University Hospital, Dublin, Ireland
Lung cancer is the major cause of cancer death worldwide. Cancer immunotherapy has been introduced as a promising and effective treatment that can improve the immune system’s ability to eliminate cancer cells and help establish immunological memory. Nanoparticles can contribute to the rapidly evolving field of immunotherapy by simultaneously delivering a variety of immunological agents to the target site and tumor microenvironment. Nano drug delivery systems can precisely target biological pathways and be implemented to reprogram or regulate immune responses. Numerous investigations have been conducted to employ different types of nanoparticles for immunotherapy of lung cancer. Nano-based immunotherapy adds a strong tool to the diverse collection of cancer therapies. This review briefly summarizes the remarkable potential opportunities for nanoparticles in lung cancer immunotherapy and its challenges.
1 Introduction
Humankind’s quest to defeat cancer continues by developing targeted treatments. Among the frequently used cancer treatments with significant improvements are chemotherapy, radiation therapy, surgery, and combinations of them. However, these strategies have various limitations; for instance, although surgery offers the best outcome for cancers detected at early stages, this approach often falls short for cancers detected at late stages which have already spread throughout the body. Furthermore, chemotherapy has low specificity, drug-induced side effects, and drug resistance, and has shown higher cancer relapse rates similar to radiation therapy (Velpurisiva et al., 2017; Doroudian et al., 2020; Niloy et al., 2021; Anconina et al., 2022; Hosseinkazemi et al., 2022). As a result, researchers were encouraged to make use of the human body’s own defense system as a tool to fight cancer. Cancer immunotherapy is a rapidly evolving treatment in clinical medicine that enhances the ability of the immune system to recognize and eliminate malignant tumors (Caster et al., 2019).
Cancer immunotherapy has already received FDA approval and can be effective even for late-stage diagnosis. Clinical research has shown that when cancer immunotherapies are effectively formulated into nanoparticles, they generate much stronger and longer-lasting anticancer activity than administered as free drugs, which is an adaptive and enduring treatment method (Chen et al., 2022). Nanoparticles have shown vast improvements in the delivery of immunostimulatory agents through physicochemical manipulation for cancer immunotherapy. In addition to playing a significant role in selective targeting and efficient delivery, material composition and surface modification are also important for stimulating the immune response. Thus, using nanomaterials to engineer cancer immunotherapies is a promising approach worthy of further investigation (Velpurisiva et al., 2017; Doroudian et al., 2019; Farjadian et al., 2022; Hejabi et al., 2022).
As the deadliest cancer, lung cancer has a poor prognosis (Hanjani et al., 2022). Several studies include a broad range of nanoparticles for lung cancer immunotherapy and provide convincing evidence that nanocarriers can stimulate effective immune responses to cancer. An overview of recent advances in lung cancer immune engineering using nanoparticles to improve the therapeutic efficacy of various immunotherapies is presented in this article.
2 Immunotherapy
Immunotherapy has brought meaningful improvements in the treatment of patients with cancer. Immunotherapies have limitations because of their immune-related adverse events (irAEs), an immune system, and inflammatory situations against the host’s healthy tissues (Das and Johnson, 2019). The immune system’s action against the host’s tumor is a suitable result, but prediction, diagnosis, and treatment of irAEs is challenging. Immunotherapy has now noticeably introduced itself as one of the most important treatments of cancer, from the metastatic level to the adjuvant and neoadjuvant settings in multiple cancers. Immunotherapy is still at the beginning of improvements, with many obstacles to overcome (Esfahani et al., 2020).
Immune checkpoint inhibitors (ICIs) have demonstrated a significant role in the treatment of non-small-cell lung cancer (NSCLC) patients and have appeared as an effective treatment selection. ICIs have the ability to prevent inhibitory pathways restraining immune response against cancer, restoring, along with sustaining anti-tumor immunity. The blocking agents of 4 Programmed Cell Death Ligand 1 (PD-L1) as well as Programmed Cell Death Protein 1 (PD-1) are adopted in clinics, and using immunotherapy alone or combined with chemotherapy is preferred (Lim et al., 2020). One of the most important members of anti-tumor immunity is T-cell, which plays a critical role in anti-tumor immunity. A great range of immune checkpoint molecules, such as PD-1 (Okazaki and Honjo, 2007), PD-L1 (Herbst et al., 2014), CD73 (Allard et al., 2017), Lag-3 (Andrews et al., 2017), B7-H3 (Suh et al., 2003), TIM-3 (Anderson et al., 2016), CTLA-4 (Krummel and Allison, 1995), TIGIT (Kurtulus et al., 2015), and VISTA (Wang et al., 2011) in the tumor microenvironment (TME) are involved in important mechanisms that prevent effector T cells’ anti-tumor activities. Immune checkpoint antibodies have established meaningful clinical advantages for mono- or combination cancer immunotherapies. The antibody Fc-hinge region binding to Fc gamma receptors is recognized to deeply affect antibody function as well as in vivo efficacy. The analysis of immune checkpoint antibodies depends primarily on their effector functions, and its impact on therapeutic efficiency has recently attracted attention (Chen et al., 2019).
Immunotherapies depending on T cells have achieved remarkable results in cancer treatment, however, assorted challenges exist, such as severe side effects, low response rate, and the acquired resistance, causing unsuitable outcomes. The immunosuppressive TME and the dysfunctional anti-tumor T cells characterize cancer immunity. TME involves tumor cells, vascular elements, stroma, tumor-draining lymph nodes, alongside the biomolecules surrounding them. In TME, numerous cellular and molecular reactions play a key role in shaping the anti-tumor immune mechanism and determining the conditional effectiveness of immunotherapy. In general, T-cell-mediated antitumor immunity involves the following steps in order: In the first step, dying tumor cells produce neoantigens that are caught by antigen-presenting cells (APCs); In the second, APCs show the captured antigens on MHC-I and MHC-II molecules to T cells; The third one is the particular activation of effector T cells against the cancer-specific antigens; In the next level the stimulated effector T cells circulate to tumor site; In the following step T cells enter the tumor; Finally, activated effector T cells bind specifically to cancer cells, and antigen-specific T cells destroy cancer cells (Figure 1) (Deng et al., 2020).
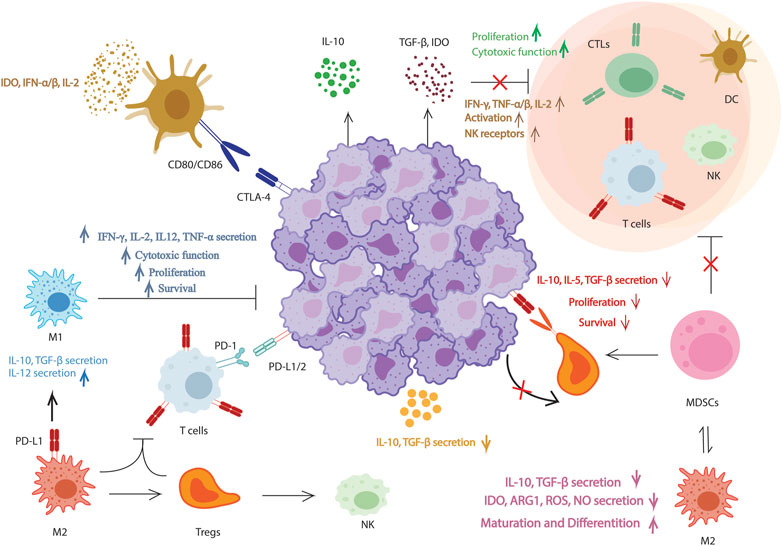
FIGURE 1. Proposed pathways for molecular procedures of natural immunomodulatory effects. Natural products have inhibitory effects on tumors by enhancing effector T-cells and natural killer cells, reducing CD4+ CD25+ Foxp3+ regulatory T-cells (Tregs), decreasing myeloid-derived suppressor cells, lowering TGF-, IL-5, IL-10, indoleamine 2,3-dioxygenase, and ARG1 levels, promoting IL-12, IFN-γ, and TNF-α/β production, and inhibiting PD-L1 and CTLA-4 in order to prevent Treg cell-cell interaction.
In most cases, immunotherapy does not work or unavoidably develops opposition to treatment after a period, manifested by primary resistance or acquired resistance after the initial response. At the moment, various immunotherapy resistance mechanisms have been recognized, and more keep on being exposed. It is through such efforts that we can improve cancer diagnosis and treatment, and ultimately deliver accurate individualized treatment for patients (Steven et al., 2016; Bai et al., 2020). Immunotherapy based on antibodies has some drawbacks including immunogenicity, membrane permeability, stability, along with production costs. As a result, replacing mechanisms involving small-molecule-regulated immune response are presented (Zhu and Li, 2018).
There are several ongoing immunotherapies for lung cancer including Programmed cell death protein 1 (PD-1) inhibitors, Programmed death-ligand 1 (PD-L1) inhibitors, Chimeric antigen receptor (CAR) T-cell therapy, Oncolytic viruses, and Cancer vaccines. The success rates, failures, and limitations of immunotherapies for lung cancer can vary depending on the specific type of immunotherapy and the individual patient’s circumstances. Pembrolizumab (Herbst et al., 2016) and Nivolumab (Borghaei et al., 2015) have both been shown to improve overall survival in patients with advanced NSCLC. In one study, patients treated with pembrolizumab had a median overall survival of 10.4 months, compared to 6.1 months for those treated with chemotherapy. However, not all patients respond to PD-1 inhibitors, and the response rates can vary widely depending on factors such as the level of PD-L1 expression in the tumor. In one study, the overall response rate to pembrolizumab was 44.8% in patients with PD-L1 expression of 50% or greater, compared to 16.7% in patients with PD-L1 expression of less than 1%. Side effects of PD-1 inhibitors can also occur, with fatigue, nausea, and diarrhea being some of the most common (Borghaei et al., 2015; Herbst et al., 2016). Furthermore, Atezolizumab (Rittmeyer et al., 2017), Durvalumab (Antonia et al., 2017), and Avelumab (Paz-Ares et al., 2018; Borghaei et al., 2020; Paz-Ares et al., 2020) have also been shown to improve overall survival in patients with advanced NSCLC. In one study, patients treated with atezolizumab had a median overall survival of 15.7 months, compared to 11.4 months for those treated with chemotherapy. As with PD-1 inhibitors, response rates to PD-L1 inhibitors can vary depending on factors such as PD-L1 expression levels. In one study, the overall response rate to atezolizumab was 38% in patients with high PD-L1 expression levels, compared to 16% in patients with low PD-L1 expression levels. Side effects of PD-L1 inhibitors can include fatigue, cough, and decreased appetite (Antonia et al., 2017; Rittmeyer et al., 2017; Paz-Ares et al., 2020). Moreover, CAR T-cell therapy is a newer type of immunotherapy that has shown promise in treating certain types of blood cancers. In one study, 7 out of 11 patients with NSCLC who received CAR T-cell therapy had a partial response or stable disease, with a median duration of response of 5.3 months. However, CAR T-cell therapy can also cause serious side effects, such as cytokine release syndrome and neurotoxicity, which occurred in 9 out of the 11 patients in the aforementioned study (Liu B. et al., 2017). There are limited statistics available on the success rates of oncolytic viruses and cancer vaccines in lung cancer, as these types of immunotherapy are still being studied in clinical trials. One phase two trial of a cancer vaccine in patients with NSCLC found that the vaccine improved progression-free survival compared to placebo, but did not improve overall survival (Butts et al., 2005; Butts et al., 2011).
Nanomedicine has shown potential in improving the delivery and efficacy of immunotherapeutic treatments in lung cancer. Nanoparticles can be designed to specifically target cancer cells, thereby reducing toxicity to healthy cells and improving drug delivery to the tumor site. Nanoparticles can be engineered to enhance the immune response against cancer cells and be used to deliver multiple immunotherapeutic agents simultaneously, allowing for combination therapy that can target different pathways involved in cancer progression. Nanomedicine-based immunotherapeutic treatments hold great promise for improving the effectiveness of lung cancer treatments. However, more research is needed to fully understand the potential of this approach and to develop safe and effective nanomedicine-based immunotherapies for clinical use (Chen et al., 2020; Chauhan et al., 2021; Jin et al., 2021; Wang et al., 2022).
3 Nanoparticle-based immunotherapy
Nanoparticles with immunomodulatory functions can trigger immune cells and adjust TME to improve antitumor immunity (Yang Z. et al., 2020). Cancer nanomedicine combined with immunotherapies promise an enhancement in the anti-tumor immune responses and sensitization of tumors to immunotherapies (He et al., 2021; Ghasempour et al., 2022). Cancer immune therapeutics limitation is the ability to deliver cancer antigens to immune cells. Nanoparticle systems have a variety of types, among which polymer-based nanoparticles are the most popular platform for cancer immunotherapy and are approved by FDA. A broad range of polymers such as poly (lactide-o-glycolic) acid, chitosan, and polyethylene glycol, have favorable characteristics like biodegradability, biocompatibility, as well as non-toxic features (Buabeid et al., 2020; Doroudian et al., 2021b).
Cancer immunotherapy is limited and cannot respond to treatments because of insufficient efficacy, toxicity concerns, and adverse side effects. Incorporating pH-responsive nanoparticles into immunotherapy brings great access to tackle these disputes since they can target the tumor tissues and organelles of APCs in acidic microenvironment (Yan and Ding, 2020).
The structure and function of TME impinge on the development and metastasis of tumors. TME modulation methods have recently become popular in cancer immunotherapy. Nanoparticles with special environmental characteristics and sophisticated arrangements can efficiently infiltrate TME and significantly deliver the specific components to TME (Yang et al., 2021).
Nowadays, nanoparticles are implemented in both recognition and treatment. They can be used as adjuvants, able to perform immune-modulating activities, or as a transporter for molecules to be passaged to a special target, after all charged with specific ligands favoring specific uptake (Figure 2) (Di Gioacchino et al., 2020). Cancer immunotherapy is being raised as a significant therapeutic method, and nanoparticles may contribute as an absolute blueprint for systemic delivery of the immune modulators as shown in Table 1 (Castro et al., 2020).
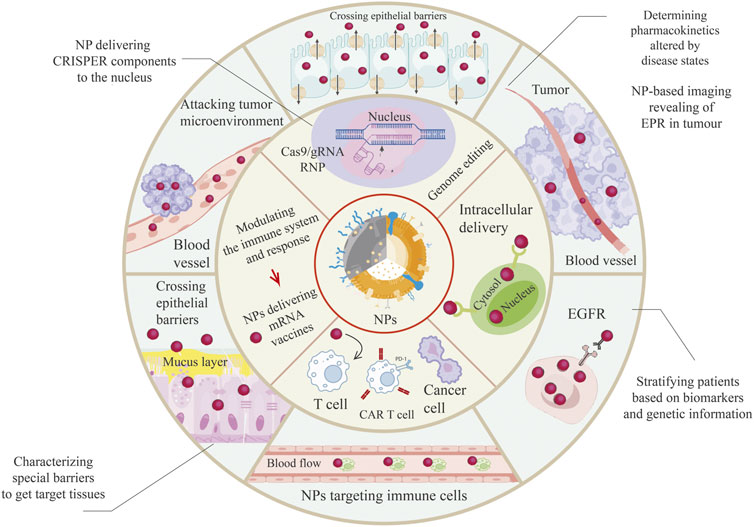
FIGURE 2. A broad range of applications of Nanoparticles (NPs) in the field of lung cancer treatment. NPs have been increasingly used to improve drug delivery and enhance the effectiveness of precision medicine. NPs can overcome biological barriers such as the blood-brain barrier, which can limit the effectiveness of certain drugs. By encapsulating drugs in NPs, it is possible to improve their solubility, bioavailability, and stability, and target them to specific cells or tissues. For instance, RNA nanoparticles (RNPs) have shown promise in delivering therapeutic RNAs such as siRNA and miRNA for the treatment of various diseases, including cancer and genetic disorders. Similarly, guide RNA (gRNA) delivered via NPs has been used in gene editing applications such as CRISPR-Cas9. Enhanced permeation and retention (EPR) is another mechanism that NPs can exploit to improve drug delivery. EPR takes advantage of the leaky blood vessels and poor lymphatic drainage of tumors to accumulate NPs at the tumor site, allowing for targeted drug delivery and minimizing systemic side effects. Epidermal growth factor receptor (EGFR)-targeted NPs have also been used for targeted drug delivery to cancer cells, while chimeric antigen receptor (CAR) T-cell therapy uses NPs to engineer T cells to target and destroy cancer cells. They can be used to deliver CARs directly to T cells, stimulate CAR T cells to proliferate and persist in the body, silence genes that inhibit CAR T-cell function or promote their exhaustion, and deliver CAR T cells directly to the tumor site with enhanced specificity, efficacy, and safety of this promising cancer treatment. Overall, the use of NPs in precision medicine applications holds great promise for improving drug delivery and enhancing the effectiveness of precision medicine therapies.
4 Nanoparticles in lung Cancer immunotherapy
A number of studies have been conducted on nanoparticles as passive delivery routes for cancer therapy, particularly for lung cancer. Cancer cells can be precisely identified using this targeting mechanism by interacting with specific ligands and receptors expressed abundantly in cancer cells (Wathoni et al., 2022). Ongoing and completed clinical trials have already reported positive results using nano-immunotherapy, and preclinical studies have also demonstrated significant improvements in therapeutic efficacy (Sun et al., 2020).
There is evidence that ICI treatment targeting PD-1 and PD-L1 can improve survival in patients with NSCLC. ICIs are only effective in a minority of NSCLC patients, and therefore, superior immunotherapy is promptly required. Reda et al. have designed a nanoparticle-based immunotherapy system called Antigen Release Agent and Checkpoint Inhibitor (ARAC) in order to improve the PD-L1 inhibitor’s efficiency. In the ARAC platform, a nanoparticle co-delivers both Volasertib, the inhibitor of Polo-like kinase 1 (PLK1), along with PD-L1 antibody. In NSCLC and multiple other cancers, PLK1 is overexpressed as a key mitotic kinase that stimulates cell proliferation. Through the inhibition of PLK1, cancer cells are selectively killed, and PD-L1 expression is upregulated in surviving cancer cells, resulting a feedforward targeted delivery of ARAC using PD-L1 antibody-conjugated nanoparticles. It reduces volasertib and PD-L1 antibody effective doses by five times in a metastatic lung tumor model (LLC-JSP) by primarily influencing CD8+ T cells. Furthermore, ARAC has been shown to be effective in KLN-205, a lung tumor model that is not responsive to the combinations of CTLA-4 and PD-1 inhibitors (Liu Z. et al., 2017; Zhang et al., 2020; Islam and Stanslas, 2021; Reda et al., 2022).
It has been proposed that blocking the PD-1/PD-L1 pathway would be a promising immunotherapeutic approach, but the response rate of their inhibition is low, resulting in minimal infiltration of cytotoxic T cells. Using an immunosuppressive microenvironment to alleviate intractable tumor microenvironments, Wang et al. developed a novel mesoporous nanocarrier with a core made of upconverting nanoparticles and a shell made of large pores of mesoporous silica (UCMS) simultaneously loaded with photosensitizer molecules, the AL-9 peptide vaccine from the IDO, and the inhibitors of PD-L1, and tested it in Lewis murine lung carcinoma cell line and mouse model. The components of the designed UCMS@Pep-aPDL1 were shown to be capable of potentiated local and systemic anti-tumor immunity through stimulating the killing of IDO-expressed tumor cells, inducing immunogenic cell death, and promoting the infiltration of effector T-cell (Wang et al., 2021; Chen et al., 2022).
The STK11 gene (LKB1), encoding serine/threonine kinase 11, is deleted or inactivated in nearly half of NSCLC patients with activated KRAS mutations. KRAS mutant NSCLC metastatic tumors are mostly resistant to anti-PD-L1 or PD-1 immunotherapy in nature. An animal model of humanized mice has been used by Meraz et al. to indicate that whereas carboplatin and pembrolizumab inhibit tumor growth moderately and transiently, TUSC2, a tumor suppressor gene delivered in nanovesicles, eradicates tumor growth in most animals. A pro-immune microenvironment in the tumor is modulated by TUSC2, which makes KRAS/LKB1 tumors more sensitive to carboplatin plus pembrolizumab. They also found that TUSC2 restoration reduced PD-L1 expression and synergized with anti-PD-1 treatment in KRAS mutant lung cancer models of mice (Meraz et al., 2018; Meraz et al., 2022).
Toll-like receptor (TLR) agonists are immunomodulators capable of inducing innate immune responses and controlling adaptive immunity which have attracted attention as anticancer molecules in the early stages of clinical development. CpG oligodeoxynucleotides bind to TLR9 as a TLR9 agonist and evoke a cascade of innate and adaptive immune responses. CpG stimulates dendritic cells and causes secondary effects such as pro-inflammatory cytokines secretion, natural killer cell activation, and T-cell expansion as well as superior antitumor effects while being locally delivered in tumors. Perry et al. (2020) have employed Particle Replication In Non-wetting Templates (PRINT) nanoparticles for the delivery of CpG into two murine orthotopic metastasis lung cancers (NSCLC-344SQ and KAL-LN2E1) via orotracheal instillations. The PRINT-CpG Nano-platform local delivery into lungs resulted in extensive tumor regression, resistance to tumor rechallenge, sustained boost of antitumor cytokine levels and extended CpG residence time in the lungs, as well as lower toxicity compared to soluble CpG .
MicroRNAs (miR) are small endogenous non-coding RNAs, which are actively involved in the adjustment of adaptive and innate immune responses. Some miRs including miR-155, miR-125, as well as miR-29 are able to stimulate repolarization of tumor-associated macrophages (TAMs) from pro-tumor M2 phenotype towards antitumor/pro-inflammatory M1 phenotype. Macrophage activation is regulated by miR-125b, which is expressed at a higher level in macrophages compared to other immune cells. By promoting the M1 macrophage phenotype through miR-125b overexpression by a virus-based vector, the anti-tumor efficacy is enhanced by improving the killing of cancer cells directly or inhibiting their proliferation in an indirect manner in a model of subcutaneous EL4 tumor. By using hyaluronic acid-poly (ethylenimine) (HA-PEI)-based nanoparticles with encapsulated miR-125b to target CD44, Parayath et al. (2018) demonstrated macrophage-specific delivery and transfection after intraperitoneal administration. The miR-125b transfectants increased the ratio of M1 to M2 macrophages by > 6 fold as well as iNOS (M1 marker) and Arg-1 (M2 marker) ratios in TAMs by 300 fold over the untreated controls, indicating the successful repolarization of TAMs in a KRAS/p53 double mutant genetically engineered NSCLC mouse model and naïve mice through the intraperitoneal administration of macrophage-specific HA-PEI nanoparticles in their lung tissues as a promising strategy in anticancer immunotherapy (Gharavi et al., 2022; Zanganeh et al., 2022).
Other viral vectors have also been used as a nanotechnology tool for immunotherapy against cancer. Inhaling self-assembled virus-like nanoparticles from Cowpea Mosaic Virus, as an immunostimulatory agent, triggers neutrophils in the microenvironment of tumors and interacts with the immune system, which has been studied by Lizotte et al. in lung cancer metastasis. Furthermore, Feldmann et al. (2018) designed an adenovirus-inspired polymer system for endosomal release and linked this nanoparticle to siRNA, delivered it to NCI-H1299 cells which is a NSCLC cell line in humans, and knocked down the genes involved in cancer development or progression (Lizotte et al., 2016; Carrasco-Esteban et al., 2021).
Combination therapy has also shown promising potential for cancer treatment. Yang et al. have developed hyaluronic acid-cisplatin/polystyrene-polymetformin dual-prodrug co-assembled nanoparticles as a combination therapy against lung cancer which can overcome the asynchronous pharmacokinetic behavior barriers of cisplatin and metformin occurring while utilized in the free drug form. The results of in vitro and in vivo tests of this nano-platform on Lewis lung cancer cells include higher tumor accumulation and proliferation inhibition, induction of apoptosis, increase in CD4+ and CD8+ T cells as well as the expression of TNF-α and IFN-γ cytokines, and prolonged overall survival of mouse models through this potent immunotherapeutic function (Yang T. et al., 2020).
Chiang et al. have designed a novel drug delivery platform including magnetic Iron Oxide nanoparticles along with Fucoidan accompanied by aldehyde-functionalized dextran (Dex) and combined it with T-cell activators and checkpoint inhibitors. As the ICI, they added anti-PD-L1 antibody and supplemented it with anti-CD3/CD28 antibody to act as the activator of the immune system. The application of IO-FuDex3-nano-biohybrid immunotherapy in a 4T1-metastatic lung carcinoma model resulted in low systemic toxicity, higher infiltration CD4+ and CD8+ T cells, lower number of metastatic nodules, improved immune responses, and an extended median survival rate of animals (Chiang et al., 2018; Rawal and Patel, 2021; Sanaei et al., 2021).
Su et al. demonstrated the potential of Au@PG nanoparticles consisting of Au and polyaniline-based glyco structures. Employing Au@PG nanoparticles in lung cancer immunotherapy resulted in the promising polarization activity of M1 macrophage and the promotion of the cytotoxic T cell response as well as tumor inhibition and the secretion of immunogenic cytokines when combined with PD-1 therapy (Su et al., 2022). Another active targeted nanoparticle is SGT-53 which is capable of restoring the function of the p53 protein and improving anti-PD-1 immunotherapy by delivering the TP53 tumor suppressor gene to tumor cells. SGT-53 has gone through several stages of clinical trials and is considered a potent strategy against lung cancer (Kim et al., 2018; Doroudian et al., 2021a).
There are some specific limitations in immunotherapy for lung cancer compared to other cancers where nanomedicine can aid in developing better treatments including limited response rates, toxicity, and resistance. Immunotherapy has shown limited response rates in lung cancer compared to other cancer types, such as melanoma and renal cell carcinoma. This may be due to the complex immune environment of lung cancer, which involves multiple immune suppressive pathways (Gettinger et al., 2018; Hellmann et al., 2018). Furthermore, immunotherapy can cause significant toxicity, particularly when used in combination with other treatments such as chemotherapy. This can limit the dose and duration of treatment, which can impact treatment efficacy (Gandhi et al., 2018; Postow et al., 2018). Some lung cancers may also develop resistance to immunotherapy, leading to treatment failure. This may be due to multiple factors, including the development of new mutations, alterations in the tumor microenvironment, and immune escape mechanisms (Pardoll, 2012; Dong et al., 2017; Skoulidis et al., 2018). Nanomedicine has the potential to address some of these limitations by improving the delivery and efficacy of immunotherapeutic treatments in lung cancer. For example, nanoparticles can be designed to specifically target cancer cells and reduce toxicity to healthy cells, potentially improving treatment response rates and reducing toxicity. Additionally, nanoparticles can be used to deliver multiple immunotherapeutic agents simultaneously, allowing for combination therapy that can target different pathways involved in cancer progression (Shi et al., 2017; Cheng et al., 2021; Jagdale Swati et al., 2023).
As advanced drug delivery vehicles, nanoparticles are expected to leverage existing platforms to enhance specific subpopulations’ targeting as well as co-stimulatory signals’ synchronous delivery. Nanomedicines with novel properties, including engineered immune mimics, may have the greatest potential to alter paradigms in immunotherapy.
5 Conclusion and perspectives
Nanoparticle immunotherapy is demonstrating tremendous potential at its early stages of development. Nanoparticles can deliver the optimal dose of immunotherapy to the target region without stimulating adverse side effects. These versatile nano-platforms can be engineered in a variety of characteristics such as shape, size, elasticity, kinetics, surface modifications, and specific targeting. Smart nanoparticles can be formulated with stimuli-sensitive materials to limit immunotherapeutic release to when the engineered nanoparticles arrive at their target tumor microenvironment and specific destination. Many factors must be considered and enhanced before nanoparticles can be clinically adopted for the immunotherapy of lung cancer. The design of nanoparticle immunotherapies should be improved for a better targeting specificity. The efficacy of nanoparticle immunotherapies which are under development stages should be boosted so that their application over conventional therapies would be justified. Mechanistic, in silico, and in vivo studies need to be conducted to determine the immune responses to this therapy and how it evolves over time, the homogenous size biodistribution of nanoparticles, the toxicity and safety concerns of such approach, and the immunogenicity of these nano-delivery vehicles as well as the therapeutics they carry. Additionally, a comprehensive grasp of the tumor biology, its microenvironment, and all the interactions between cancer cells and nanoparticles should be obtained for nanoparticles to be able to be developed for immunotherapy of lung cancer tumors and its metastases.
Author contributions
MD, SZ, and EA writing—original draft. EA generating figures. MD and SD reviewing and editing the final manuscript. MD and SD editing, conceptualization, preparation, reviewing, and editing the final manuscript.
Conflict of interest
The authors declare that the research was conducted in the absence of any commercial or financial relationships that could be construed as a potential conflict of interest.
Publisher’s note
All claims expressed in this article are solely those of the authors and do not necessarily represent those of their affiliated organizations, or those of the publisher, the editors and the reviewers. Any product that may be evaluated in this article, or claim that may be made by its manufacturer, is not guaranteed or endorsed by the publisher.
References
Allard, B., Longhi, M. S., Robson, S. C., and Stagg, J. (2017). The ectonucleotidases CD 39 and CD 73: Novel checkpoint inhibitor targets. Immunol. Rev. 276 (1), 121–144. doi:10.1111/imr.12528
Anconina, R., Ortega, C., Metser, U., Liu, Z. A., Suzuki, C., McInnis, M., et al. (2022). Influence of sarcopenia, clinical data, and 2-[18F] FDG PET/CT in outcome prediction of patients with early-stage adenocarcinoma esophageal cancer. Eur. J. Nucl. Med. Mol. Imaging 49 (3), 1012–1020. doi:10.1007/s00259-021-05514-w
Anderson, A. C., Joller, N., and Kuchroo, V. K. (2016). Lag-3, tim-3, and TIGIT: Co-inhibitory receptors with specialized functions in immune regulation. Immunity 44 (5), 989–1004. doi:10.1016/j.immuni.2016.05.001
Andrews, L. P., Marciscano, A. E., Drake, C. G., and Vignali, D. A. (2017). LAG 3 (CD 223) as a cancer immunotherapy target. Immunol. Rev. 276 (1), 80–96. doi:10.1111/imr.12519
Antonia, S. J., Villegas, A., Daniel, D., Vicente, D., Murakami, S., Hui, R., et al. (2017). Durvalumab after chemoradiotherapy in stage III non–small-cell lung cancer. N. Engl. J. Med. 377 (20), 1919–1929. doi:10.1056/nejmoa1709937
Bai, R., Chen, N., Li, L., Du, N., Bai, L., Lv, Z., et al. (2020). Mechanisms of cancer resistance to immunotherapy. Front. Oncol. 10, 1290. doi:10.3389/fonc.2020.01290
Borghaei, H., Langer, C. J., Paz-Ares, L., Rodríguez-Abreu, D., Halmos, B., Garassino, M. C., et al. (2020). Pembrolizumab plus chemotherapy versus chemotherapy alone in patients with advanced non–small cell lung cancer without tumor PD-L1 expression: A pooled analysis of 3 randomized controlled trials. Cancer 126 (22), 4867–4877. doi:10.1002/cncr.33142
Borghaei, H., Paz-Ares, L., Horn, L., Spigel, D. R., Steins, M., Ready, N. E., et al. (2015). Nivolumab versus docetaxel in advanced nonsquamous non–small-cell lung cancer. N. Engl. J. Med. 373 (17), 1627–1639. doi:10.1056/nejmoa1507643
Buabeid, M. A., Arafa, E.-S. A., and Murtaza, G. (2020). Emerging prospects for nanoparticle-enabled cancer immunotherapy. J. Immunol. Res., 2020, 1–11. doi:10.1155/2020/9624532
Butts, C., Maksymiuk, A., Goss, G., Soulieres, D., Marshall, E., Cormier, Y., et al. (2011). Updated survival analysis in patients with stage IIIB or IV non-small-cell lung cancer receiving BLP25 liposome vaccine (L-BLP25): Phase IIB randomized, multicenter, open-label trial. J. cancer Res. Clin. Oncol. 137, 1337–1342. doi:10.1007/s00432-011-1003-3
Butts, C., Murray, N., Maksymiuk, A., Goss, G., Marshall, E., Soulières, D., et al. (2005). Randomized phase IIB trial of BLP25 liposome vaccine in stage IIIB and IV non–small-cell lung cancer. J. Clin. Oncol. 23 (27), 6674–6681. doi:10.1200/jco.2005.13.011
Carrasco-Esteban, E., Domínguez-Rullán, J. A., Barrionuevo-Castillo, P., Pelari-Mici, L., Leaman, O., Sastre-Gallego, S., et al. (2021). Current role of nanoparticles in the treatment of lung cancer. J. Clin. Transl. Res. 7 (2), 140–155.
Caster, J. M., Callaghan, C., Seyedin, S. N., Henderson, K., Sun, B., and Wang, A. Z. (2019). Optimizing advances in nanoparticle delivery for cancer immunotherapy. Adv. Drug Deliv. Rev. 144, 3–15. doi:10.1016/j.addr.2019.07.009
Castro, F., Pinto, M. L., Pereira, C. L., Serre, K., Barbosa, M. A., Vermaelen, K., et al. (2020). Chitosan/γ-PGA nanoparticles-based immunotherapy as adjuvant to radiotherapy in breast cancer. Biomaterials 257, 120218. doi:10.1016/j.biomaterials.2020.120218
Chauhan, A., Khan, T., and Omri, A. (2021). Design and encapsulation of immunomodulators onto gold nanoparticles in cancer immunotherapy. Int. J. Mol. Sci. 22 (15), 8037. doi:10.3390/ijms22158037
Chen, X., Song, X., Li, K., and Zhang, T. (2019). FcγR-binding is an important functional attribute for immune checkpoint antibodies in cancer immunotherapy. Front. Immunol. 10, 292. doi:10.3389/fimmu.2019.00292
Chen, Z.-J., Yang, S.-C., Liu, X.-L., Gao, Y., Dong, X., Lai, X., et al. (2020). Nanobowl-supported liposomes improve drug loading and delivery. Nano Lett. 20 (6), 4177–4187. doi:10.1021/acs.nanolett.0c00495
Chen, Z., Yue, Z., Yang, K., and Li, S. (2022). Nanomaterials: Small particles show huge possibilities for cancer immunotherapy. J. Nanobiotechnology 20 (1), 484–538. doi:10.1186/s12951-022-01692-3
Cheng, Z., Li, M., Dey, R., and Chen, Y. (2021). Nanomaterials for cancer therapy: Current progress and perspectives. J. Hematol. Oncol. 14 (1), 85–27. doi:10.1186/s13045-021-01096-0
Chiang, C.-S., Lin, Y.-J., Lee, R., Lai, Y.-H., Cheng, H.-W., Hsieh, C.-H., et al. (2018). Combination of fucoidan-based magnetic nanoparticles and immunomodulators enhances tumour-localized immunotherapy. Nat. Nanotechnol. 13 (8), 746–754. doi:10.1038/s41565-018-0146-7
Dai, Z., Song, X.-Z., Cao, J., He, Y., Wen, W., Xu, X., et al. (2018). Dual-stimuli-responsive TiO x/DOX nanodrug system for lung cancer synergistic therapy. RSC Adv. 8 (39), 21975–21984. doi:10.1039/c8ra02899k
Das, S., and Johnson, D. B. (2019). Immune-related adverse events and anti-tumor efficacy of immune checkpoint inhibitors. J. Immunother. cancer 7 (1), 306–311. doi:10.1186/s40425-019-0805-8
Deng, L. J., Qi, M., Li, N., Lei, Y. H., Zhang, D. M., and Chen, J. X. (2020). Natural products and their derivatives: Promising modulators of tumor immunotherapy. J. Leukoc. Biol. 108 (2), 493–508. doi:10.1002/jlb.3mr0320-444r
Di Gioacchino, M., Petrarca, C., Gatta, A., Scarano, G., Farinelli, A., Della Valle, L., et al. (2020). Nanoparticle-based immunotherapy: State of the art and future perspectives. Expert Rev. Clin. Immunol. 16 (5), 513–525. doi:10.1080/1744666x.2020.1762572
Dong, Z.-Y., Zhang, J.-T., Liu, S.-Y., Su, J., Zhang, C., Xie, Z., et al. (2017). EGFR mutation correlates with uninflamed phenotype and weak immunogenicity, causing impaired response to PD-1 blockade in non-small cell lung cancer. Oncoimmunology 6 (11), e1356145. doi:10.1080/2162402x.2017.1356145
Doroudian, M., Azhdari, M. H., Goodarzi, N., O’Sullivan, D., and Donnelly, S. C. (2021a). Smart nanotherapeutics and lung cancer. Pharmaceutics 13 (11), 1972. doi:10.3390/pharmaceutics13111972
Doroudian, M., MacLoughlin, R., Poynton, F., Prina-Mello, A., and Donnelly, S. C. (2019). Nanotechnology based therapeutics for lung disease. Thorax 74 (10), 965–976. doi:10.1136/thoraxjnl-2019-213037
Doroudian, M., O'Neill, A., O'Reilly, C., Tynan, A., Mawhinney, L., McElroy, A., et al. (2020). Aerosolized drug-loaded nanoparticles targeting migration inhibitory factors inhibit Pseudomonas aeruginosa-induced inflammation and biofilm formation. Nanomedicine 15 (30), 2933–2953. doi:10.2217/nnm-2020-0344
Doroudian, M., O’Neill, A., Mac Loughlin, R., Prina-Mello, A., Volkov, Y., and Donnelly, S. C. (2021b). Nanotechnology in pulmonary medicine. Curr. Opin. Pharmacol. 56, 85–92. doi:10.1016/j.coph.2020.11.002
Esfahani, K., Roudaia, L., Buhlaiga, N. a., Del Rincon, S., Papneja, N., and Miller, W. (2020). A review of cancer immunotherapy: From the past, to the present, to the future. Curr. Oncol. 27 (2), 87–97. doi:10.3747/co.27.5223
Farjadian, F., Ghasemi, S., Akbarian, M., Hoseini-Ghahfarokhi, M., Moghoofei, M., and Doroudian, M. (2022). Physically stimulus-responsive nanoparticles for therapy and diagnosis. Front. Chem. 10, 952675. doi:10.3389/fchem.2022.952675
Feldmann, D. P., Cheng, Y., Kandil, R., Xie, Y., Mohammadi, M., Harz, H., et al. (2018). In vitro and in vivo delivery of siRNA via VIPER polymer system to lung cells. J. Control. Release 276, 50–58. doi:10.1016/j.jconrel.2018.02.017
Gandhi, L., Rodríguez-Abreu, D., Gadgeel, S., Esteban, E., Felip, E., De Angelis, F., et al. (2018). Pembrolizumab plus chemotherapy in metastatic non–small-cell lung cancer. N. Engl. J. Med. 378 (22), 2078–2092. doi:10.1056/nejmoa1801005
Gao, L., Wang, H., Nan, L., Peng, T., Sun, L., Zhou, J., et al. (2017). Erythrocyte membrane-wrapped pH sensitive polymeric nanoparticles for non-small cell lung cancer therapy. Bioconjugate Chem. 28 (10), 2591–2598. doi:10.1021/acs.bioconjchem.7b00428
Garg, S., Garg, A., Enaganti, S., and Yadav, A. K. (2019). Chondroitin sulphate decorated polymeric nanoparticles: An effective carrier for enhancement of lung cancer targeting capabilities of anticancer drug. Curr. Nanomedicine Former. Recent Pat. Nanomedicine) 9 (3), 243–261. doi:10.2174/2468187309666190126112933
Gettinger, S., Horn, L., Jackman, D., Spigel, D., Antonia, S., Hellmann, M., et al. (2018). Five-year follow-up of nivolumab in previously treated advanced non–small-cell lung cancer: Results from the ca209-003 study. J. Clin. Oncol. 36 (17), 1675–1684. doi:10.1200/jco.2017.77.0412
Gharavi, A. T., Hanjani, N. A., Movahed, E., and Doroudian, M. (2022). The role of macrophage subtypes and exosomes in immunomodulation. Cell. Mol. Biol. Lett. 27 (1), 83–18. doi:10.1186/s11658-022-00384-y
Ghasempour, E., Hesami, S., Movahed, E., and Doroudian, M. (2022). Mesenchymal stem cell-derived exosomes as a new therapeutic strategy in the brain tumors. Stem Cell Res. Ther. 13 (1), 527–612. doi:10.1186/s13287-022-03212-4
Hanjani, N. A., Esmaelizad, N., Zanganeh, S., Gharavi, A. T., Heidarizadeh, P., Radfar, M., et al. (2022). Emerging role of exosomes as biomarkers in cancer treatment and diagnosis. Crit. Rev. oncology/hematology 169, 103565. doi:10.1016/j.critrevonc.2021.103565
He, J. S., Liu, S. J., Zhang, Y. R., Chu, X. D., Lin, Z. B., Zhao, Z., et al. (2021). The application of and strategy for gold nanoparticles in cancer immunotherapy. Front. Pharmacol. 12, 687399. doi:10.3389/fphar.2021.687399
Hejabi, F., Abbaszadeh, M. S., Taji, S., O’Neill, A., Farjadian, F., and Doroudian, M. (2022). Nanocarriers: A novel strategy for the delivery of CRISPR/cas systems. Front. Chem. 10, 957572. doi:10.3389/fchem.2022.957572
Hellmann, M. D., Nathanson, T., Rizvi, H., Creelan, B. C., Sanchez-Vega, F., Ahuja, A., et al. (2018). Genomic features of response to combination immunotherapy in patients with advanced non-small-cell lung cancer. Cancer Cell 33 (5), 843–852.e4. doi:10.1016/j.ccell.2018.03.018
Herbst, R. S., Baas, P., Kim, D.-W., Felip, E., Pérez-Gracia, J. L., Han, J.-Y., et al. (2016). Pembrolizumab versus docetaxel for previously treated, PD-L1-positive, advanced non-small-cell lung cancer (KEYNOTE-010): A randomised controlled trial. Lancet 387 (10027), 1540–1550. doi:10.1016/s0140-6736(15)01281-7
Herbst, R. S., Soria, J.-C., Kowanetz, M., Fine, G. D., Hamid, O., Gordon, M. S., et al. (2014). Predictive correlates of response to the anti-PD-L1 antibody MPDL3280A in cancer patients. Nature 515 (7528), 563–567. doi:10.1038/nature14011
Hosseinkazemi, H., Samani, S., O’Neill, A., Soezi, M., Moghoofei, M., Azhdari, M. H., et al. (2022). Applications of Iron Oxide nanoparticles against breast cancer. J. Nanomater., 2022, 1–12. doi:10.1155/2022/6493458
Hu, J., Fu, S., Peng, Q., Han, Y., Xie, J., Zan, N., et al. (2017). Paclitaxel-loaded polymeric nanoparticles combined with chronomodulated chemotherapy on lung cancer: In vitro and in vivo evaluation. Int. J. Pharm. 516 (1-2), 313–322. doi:10.1016/j.ijpharm.2016.11.047
Islam, M. K., and Stanslas, J. (2021). Peptide-based and small molecule PD-1 and PD-L1 pharmacological modulators in the treatment of cancer. Pharmacol. Ther. 227, 107870. doi:10.1016/j.pharmthera.2021.107870
Jagdale Swati, C., HableAsawaree, A., and ChabukswarAnuruddha, R. (2023). Nanomedicine in lung cancer therapy. Adv. Nov. Formulations Drug Deliv. 25, 433–448. doi:10.1002/9781394167708.ch23
Jin, Q., Zhu, W., Zhu, J., Zhu, J., Shen, J., Liu, Z., et al. (2021). Nanoparticle-mediated delivery of inhaled immunotherapeutics for treating lung metastasis. Adv. Mater. 33 (7), 2007557. doi:10.1002/adma.202007557
Kim, S.-S., Harford, J. B., Moghe, M., Rait, A., and Chang, E. H. (2018). Combination with SGT-53 overcomes tumor resistance to a checkpoint inhibitor. Oncoimmunology 7 (10), e1484982. doi:10.1080/2162402x.2018.1484982
Krummel, M. F., and Allison, J. P. (1995). CD28 and CTLA-4 have opposing effects on the response of T cells to stimulation. J. Exp. Med. 182 (2), 459–465. doi:10.1084/jem.182.2.459
Kurtulus, S., Sakuishi, K., Ngiow, S.-F., Joller, N., Tan, D. J., Teng, M. W., et al. (2015). TIGIT predominantly regulates the immune response via regulatory T cells. J. Clin. investigation 125 (11), 4053–4062. doi:10.1172/jci81187
Lian, G.-Y., Wan, Y., Mak, T. S.-K., Wang, Q.-M., Zhang, J., Chen, J., et al. (2022). Self-carried nanodrug (SCND-SIS3): A targeted therapy for lung cancer with superior biocompatibility and immune boosting effects. Biomaterials 288, 121730. doi:10.1016/j.biomaterials.2022.121730
Lim, S. M., Hong, M. H., and Kim, H. R. (2020). Immunotherapy for non-small cell lung cancer: Current landscape and future perspectives. Immune Netw. 20 (1), e10. doi:10.4110/in.2020.20.e10
Liu, B., Song, Y., and Liu, D. (2017). Clinical trials of CAR-T cells in China. J. Hematol. Oncol. 10 (1), 166–210. doi:10.1186/s13045-017-0535-7
Liu, Z., Sun, Q., and Wang, X. (2017). PLK1, a potential target for cancer therapy. Transl. Oncol. 10 (1), 22–32. doi:10.1016/j.tranon.2016.10.003
Lizotte, P., Wen, A., Sheen, M., Fields, J., Rojanasopondist, P., Steinmetz, N., et al. (2016). In situ vaccination with cowpea mosaic virus nanoparticles suppresses metastatic cancer. Nat. Nanotechnol. 11 (3), 295–303. doi:10.1038/nnano.2015.292
Meraz, I. M., Majidi, M., Cao, X., Lin, H., Li, L., Wang, J., et al. (2018). TUSC2 immunogene therapy synergizes with anti–PD-1 through enhanced proliferation and infiltration of natural killer cells in syngeneic kras-mutant mouse lung cancer models. Cancer Immunol. Res. 6 (2), 163–177. doi:10.1158/2326-6066.cir-17-0273
Meraz, I. M., Majidi, M., Shao, R., Meng, F., Ha, M. J., Shpall, E., et al. (2022). TUSC2 immunogene enhances efficacy of chemo-immuno combination on KRAS/LKB1 mutant NSCLC in humanized mouse model. Commun. Biol. 5 (1), 167–213. doi:10.1038/s42003-022-03103-7
Niloy, M. S., Shakil, M., Hossen, M., Alam, M., and Rosengren, R. J. (2021). Promise of gold nanomaterials as a lung cancer theranostic agent: A systematic review. Int. Nano Lett. 11 (2), 93–111. doi:10.1007/s40089-021-00332-2
Okazaki, T., and Honjo, T. (2007). PD-1 and PD-1 ligands: From discovery to clinical application. Int. Immunol. 19 (7), 813–824. doi:10.1093/intimm/dxm057
Parayath, N. N., Parikh, A., and Amiji, M. M. (2018). Repolarization of tumor-associated macrophages in a genetically engineered nonsmall cell lung cancer model by intraperitoneal administration of hyaluronic acid-based nanoparticles encapsulating microRNA-125b. Nano Lett. 18 (6), 3571–3579. doi:10.1021/acs.nanolett.8b00689
Pardoll, D. M. (2012). The blockade of immune checkpoints in cancer immunotherapy. Nat. Rev. Cancer 12 (4), 252–264. doi:10.1038/nrc3239
Paz-Ares, L., Luft, A., Vicente, D., Tafreshi, A., Gümüş, M., Mazières, J., et al. (2018). Pembrolizumab plus chemotherapy for squamous non–small-cell lung cancer. N. Engl. J. Med. 379 (21), 2040–2051. doi:10.1056/nejmoa1810865
Paz-Ares, L., Vicente, D., Tafreshi, A., Robinson, A., Parra, H. S., Mazières, J., et al. (2020). A randomized, placebo-controlled trial of pembrolizumab plus chemotherapy in patients with metastatic squamous NSCLC: Protocol-specified final analysis of KEYNOTE-407. J. Thorac. Oncol. 15 (10), 1657–1669. doi:10.1016/j.jtho.2020.06.015
Perry, J. L., Tian, S., Sengottuvel, N., Harrison, E. B., Gorentla, B. K., Kapadia, C. H., et al. (2020). Pulmonary delivery of nanoparticle-bound toll-like receptor 9 agonist for the treatment of metastatic lung cancer. ACS nano 14 (6), 7200–7215. doi:10.1021/acsnano.0c02207
Postow, M. A., Sidlow, R., and Hellmann, M. D. (2018). Immune-related adverse events associated with immune checkpoint blockade. N. Engl. J. Med. 378 (2), 158–168. doi:10.1056/nejmra1703481
Rawal, S., and Patel, M. (2021). Bio-nanocarriers for lung cancer management: Befriending the barriers. Nano-Micro Lett. 13 (1), 142–154. doi:10.1007/s40820-021-00630-6
Reda, M., Ngamcherdtrakul, W., Nelson, M. A., Siriwon, N., Wang, R., Zaidan, H. Y., et al. (2022). Development of a nanoparticle-based immunotherapy targeting PD-L1 and PLK1 for lung cancer treatment. Nat. Commun. 13 (1), 4261–4311. doi:10.1038/s41467-022-31926-9
Rittmeyer, A., Barlesi, F., Waterkamp, D., Park, K., Ciardiello, F., Von Pawel, J., et al. (2017). Atezolizumab versus docetaxel in patients with previously treated non-small-cell lung cancer (OAK): A phase 3, open-label, multicentre randomised controlled trial. Lancet 389 (10066), 255–265. doi:10.1016/s0140-6736(16)32517-x
Sanaei, M.-J., Pourbagheri-Sigaroodi, A., Kaveh, V., Abolghasemi, H., Ghaffari, S. H., Momeny, M., et al. (2021). Recent advances in immune checkpoint therapy in non-small cell lung cancer and opportunities for nanoparticle-based therapy. Eur. J. Pharmacol. 909, 174404. doi:10.1016/j.ejphar.2021.174404
Shi, J., Kantoff, P. W., Wooster, R., and Farokhzad, O. C. (2017). Cancer nanomedicine: Progress, challenges and opportunities. Nat. Rev. Cancer 17 (1), 20–37. doi:10.1038/nrc.2016.108
Shi, Y. (2020). Clinical translation of nanomedicine and biomaterials for cancer immunotherapy: Progress and perspectives. Adv. Ther. 3 (9), 1900215. doi:10.1002/adtp.201900215
Skoulidis, F., Goldberg, M. E., Greenawalt, D. M., Hellmann, M. D., Awad, M. M., Gainor, J. F., et al. (2018). STK11/LKB1 mutations and PD-1 inhibitor resistance in KRAS-mutant lung adenocarcinoma. Cancer Discov. 8 (7), 822–835. doi:10.1158/2159-8290.cd-18-0099
Steven, A., Fisher, S. A., and Robinson, B. W. (2016). Immunotherapy for lung cancer. Respirology 21 (5), 821–833. doi:10.1111/resp.12789
Su, W.-P., Chang, L.-C., Song, W.-H., Yang, L.-X., Wang, L.-C., Chia, Z.-C., et al. (2022). Polyaniline-based glyco-condensation on Au nanoparticles enhances immunotherapy in lung cancer. ACS Appl. Mater. Interfaces 14, 24144–24159. doi:10.1021/acsami.2c03839
Suh, W.-K., Gajewska, B. U., Okada, H., Gronski, M. A., Bertram, E. M., Dawicki, W., et al. (2003). The B7 family member B7-H3 preferentially down-regulates T helper type 1–mediated immune responses. Nat. Immunol. 4 (9), 899–906. doi:10.1038/ni967
Sun, Q., Bai, X., Sofias, A. M., van der Meel, R., Ruiz-Hernandez, E., Storm, G., et al. (2020). Cancer nanomedicine meets immunotherapy: Opportunities and challenges. Acta Pharmacol. Sin. 41 (7), 954–958. doi:10.1038/s41401-020-0448-9
Velpurisiva, P., Gad, A., Piel, B., Jadia, R., and Rai, P. (2017). Nanoparticle design strategies for effective cancer immunotherapy. J. Biomed. Syd. NSW) 2 (2), 64–77. doi:10.7150/jbm.18877
Wang, L., Rubinstein, R., Lines, J. L., Wasiuk, A., Ahonen, C., Guo, Y., et al. (2011). VISTA, a novel mouse Ig superfamily ligand that negatively regulates T cell responses. J. Exp. Med. 208 (3), 577–592. doi:10.1084/jem.20100619
Wang, Q.-y., Hu, Q.-h., Huang, S.-h., Lin, J., and Zhou, Q.-h. (2022). Surface charge switchable nano-micelle for pH/redox-triggered and endosomal escape mediated co-delivery of doxorubicin and paclitaxel in treatment of lung adenocarcinoma. Colloids Surfaces B Biointerfaces 216, 112588. doi:10.1016/j.colsurfb.2022.112588
Wang, Z., Chen, L., Ma, Y., Li, X., Hu, A., Wang, H., et al. (2021). Peptide vaccine-conjugated mesoporous carriers synergize with immunogenic cell death and PD-L1 blockade for amplified immunotherapy of metastatic spinal. J. Nanobiotechnology 19 (1), 243–316. doi:10.1186/s12951-021-00975-5
Wathoni, N., Puluhulawa, L. E., Joni, I. M., Muchtaridi, M., Mohammed, A. F. A., Elamin, K. M., et al. (2022). Monoclonal antibody as a targeting mediator for nanoparticle targeted delivery system for lung cancer. Drug Deliv. 29 (1), 2959–2970. doi:10.1080/10717544.2022.2120566
Yan, Y., and Ding, H. (2020). pH-responsive nanoparticles for cancer immunotherapy: a brief review. Nanomaterials 10 (8), 1613. doi:10.3390/nano10081613
Yang, M., Li, J., Gu, P., and Fan, X. (2021). The application of nanoparticles in cancer immunotherapy: Targeting tumor microenvironment. Bioact. Mater. 6 (7), 1973–1987. doi:10.1016/j.bioactmat.2020.12.010
Yang, T., Yu, S., Liu, L., Sun, Y., Lan, Y., Ma, X., et al. (2020). Dual polymeric prodrug co-assembled nanoparticles with precise ratiometric co-delivery of cisplatin and metformin for lung cancer chemoimmunotherapy. Biomaterials Sci. 8 (20), 5698–5714. doi:10.1039/d0bm01191f
Yang, Z., Ma, Y., Zhao, H., Yuan, Y., and Kim, B. Y. (2020). Nanotechnology platforms for cancer immunotherapy. Wiley Interdiscip. Rev. Nanomedicine Nanobiotechnology 12 (2), e1590. doi:10.1002/wnan.1590
Zanganeh, S., Goodarzi, N., Doroudian, M., and Movahed, E. (2022). Potential COVID-19 therapeutic approaches targeting angiotensin-converting enzyme 2; an updated review. Rev. Med. Virology 32 (4), e2321. doi:10.1002/rmv.2321
Zhang, S., Bai, X., and Shan, F. (2020). The progress and confusion of anti-PD1/PD-L1 immunotherapy for patients with advanced non-small cell lung cancer. Int. Immunopharmacol. 80, 106247. doi:10.1016/j.intimp.2020.106247
Keywords: nanomedicine, immunotherapy, lung cancer, nanocarriers, drug delivery
Citation: Doroudian M, Zanganeh S, Abbasgholinejad E and Donnelly SC (2023) Nanomedicine in Lung Cancer Immunotherapy. Front. Bioeng. Biotechnol. 11:1144653. doi: 10.3389/fbioe.2023.1144653
Received: 14 January 2023; Accepted: 07 March 2023;
Published: 17 March 2023.
Edited by:
Md Nurunnabi, The University of Texas at El Paso, United StatesReviewed by:
Uday Chintapula, University of Pennsylvania, United StatesCopyright © 2023 Doroudian, Zanganeh, Abbasgholinejad and Donnelly. This is an open-access article distributed under the terms of the Creative Commons Attribution License (CC BY). The use, distribution or reproduction in other forums is permitted, provided the original author(s) and the copyright owner(s) are credited and that the original publication in this journal is cited, in accordance with accepted academic practice. No use, distribution or reproduction is permitted which does not comply with these terms.
*Correspondence: Seamas C. Donnelly, c2VhbWFzLmRvbm5lbGx5QHRjZC5pZQ==
†These authors have contributed equally to this work