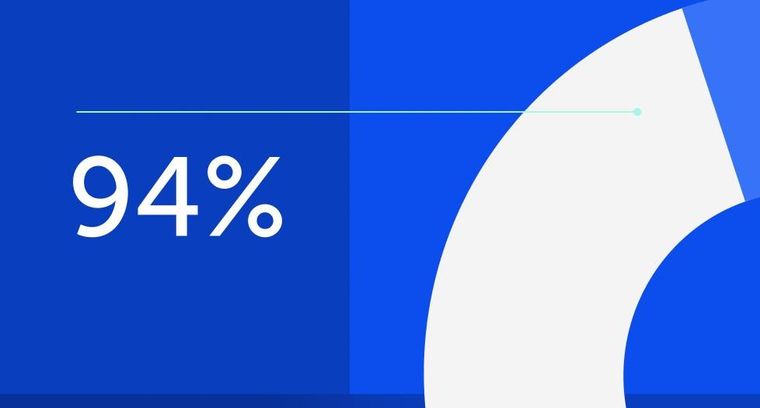
94% of researchers rate our articles as excellent or good
Learn more about the work of our research integrity team to safeguard the quality of each article we publish.
Find out more
REVIEW article
Front. Bioeng. Biotechnol., 27 March 2023
Sec. Biosensors and Biomolecular Electronics
Volume 11 - 2023 | https://doi.org/10.3389/fbioe.2023.1141523
This article is part of the Research TopicAdvanced Nanobiosensors for Non-invasive Disease DiagnosisView all 6 articles
Endocrine-disrupting chemicals (EDCs) are a class of man-made substances with potential to disrupt the standard function of the endocrine system. These EDCs include phthalates, perchlorates, phenols, some heavy metals, furans, dimethoate, aromatic hydrocarbons, some pesticides, and per- and polyfluoroalkyl substances (PFAS). EDCs are widespread in the environment given their frequent use in daily life. Their production, usage, and consumption have increased many-fold in recent years. Their ability to interact and mimic normal endocrine functions makes them a potential threat to human health, aquatics, and wild life. Detection of these toxins has predominantly been done by mass spectroscopy and/or chromatography-based methods and to a lesser extent by advanced sensing approaches such as electrochemical and/or colorimetric methods. Instrument-based analytical techniques are often not amenable for onsite detection due to the lab-based nature of these detecting systems. Alternatively, analytical approaches based on sensor/biosensor techniques are more attractive because they are rapid, portable, equally sensitive, and eco-friendly. Advanced sensing systems have been adopted to detect a range of EDCs in the environment and food production systems. This review will focus on advances and developments in portable sensing techniques for EDCs, encompassing electrochemical, colorimetric, optical, aptamer-based, and microbial sensing approaches. We have also delineated the advantages and limitations of some of these sensing techniques and discussed future developments in sensor technology for the environmental sensing of EDCs.
Due to rapid industrial advances, hundreds of new chemicals have been synthesized for domestic and industrial use without a clear understanding of the toxic effects on humans and the long-term deleterious effects on the ecosystem (Ren et al., 2015b; Esteso, 2022). EDCs are a group of structurally diverse, artificially synthesized exogenous chemicals with the ability to perturb the normal function, secretion, transport, and metabolism of natural hormones (Chen H. et al., 2019; Charitos et al., 2022). These hormones are produced by the endocrine glands (hypothalamus, pituitary, thyroid, parathyroid, thymus, and adrenal), have many regulatory effects on natural homeostasis, and affect the development and overall function of the endocrine system. EDCs can enter the body through several routes such as inhalation, skin contact, and ingestion to affect the natural functions of the endocrine hormones (Roy et al., 1997; Bergman et al., 2013; Preciados et al., 2016). Several EDC compounds are found contemporaneously in pesticides, insecticides, pharmaceutical agents, compounds of heavy metals, drugs, feed additives, industrial chemicals, and electronic waste (e-waste). Because of the impact of the endocrine system on healthy development and regulation of hormones, EDCs have a devastating potential to adversely affect individuals of every group from childhood to old age (Lee et al., 2015; Preciados et al., 2016).
EDCs are present in a wide range of synthetic plastic products such as toys, bottles, and PVC pipes. They are also commonly found in detergents, toothpaste, flame retardants, and in different cosmetic items (Flint et al., 2012). EDCs as pesticides are extensively applied in the agriculture industry for the improvements of crop yields. The residues of these toxins are detected in diverse food products and their related environments (Yang et al., 2020). For example, Atrazine is detected in surface and groundwater all over the world (Wan et al., 2021). EDCs enter the environment via wastewater discharge, hospital waste disposal, leaching of chemicals from industrial plants and households, and pesticide residues. They often enter landfills and reach aquatic bodies, such as surface water, groundwater (Gao et al., 2020), the marine environment (Muller et al., 2020), freshwater reservoirs (Rocha et al., 2019), and ultimately enter the food chain through sea food contamination (Muller et al., 2020; Chen et al., 2022; Shah et al., 2022).
Per- and poly-fluoroalkyl substances (PFAS) which have been brought to the limelight are ubiquitous and reported to be present in surfactants, lubricants, fire retardants, and polymer additives (Hoisaeter et al., 2019; Mumtaz et al., 2019; Xu et al., 2021). Dichlorodiphenyltrichloroethane (DDT) is an insecticide commonly used to combat malaria (Meeker, 2012). Polychlorinated biphenyls (PCBs) have been extensively used in electrical equipment, mainly in transformers and capacitors (Habibullah-Al-Mamun et al., 2019). Phthalates are extensively used in children’s products, medical devices, cosmetics goods, plastic items, packing, and building materials (Vieira et al., 2021). Bisphenol A, an estrogen mimic, is widely used in the plastics industry and in food packaging, thermal papers, DVDs, plastic pipes, vehicle panels, medical equipment, personal care products, and dental sealants (Fisher et al., 2017). It has also been used as a supplement in cattle and poultry production to increase growth rates (Pironti et al., 2021). Cadmium is used in the production of batteries, pigments, plastic, and alloys (Friberg et al., 2019). The use of Lead is reported in plastic, glass, batteries, and welding materials. Mercury is used in the production of pigments, caustic soda, thermometers, and in dental products. As a result of extensive uses and applications of these toxins, EDCs are widespread in our ecosystem contributing to conditions such as diabetes, obesity, nervous disorders, gland dysfunction, discomfort in respiration, abnormalities in muscular functions, seizures, cardiovascular diseases, fluctuation in blood pressure, early puberty, and infertility (Vieira et al., 2021).
Many EDCs have the ability to mimic the functions of natural hormones, impacting many physiological functions such as growth, development, and reproduction through the overstimulation of receptors (Lu et al., 2020). Other EDCs block the activity of natural hormones, disrupting normal signaling through the inhibition of binding. This leads to the disturbance in the function of glands, abnormal levels of hormone production, secretions, and metabolism, which ultimately lead to adverse health outcomes for the exposed individual (Vandenberg et al., 2012; Hu et al., 2015; Vandenberg, 2019). Exposure to EDCs leads to many diseases which are caused by a disturbance in the normal functionality of estrogen, androgen, and thyroid hormones. Structural similarities of EDCs provide the potential to interact with nuclear and hormonal receptors, resulting in the activation of hormone transporter proteins, dysregulation of healthy hormone metabolism, and affect the number of receptors on cell surfaces, which lead to the interruption in normal hormonal responses. EDCs affect the signal transduction to the immune as well as the nervous systems and often lead to alteration of the production and function of natural hormones (De Coster and Van Larebeke, 2012). The lipophilic nature of many of these chemicals causes them to be retained in adipose tissues, which leads to prolonged and elevated levels of these chemicals inside living beings (Heindel et al., 2015; Sargis, 2015). EDCs also have negative impacts on genome regulation. Epigenetic changes such as methylation and acetylation of DNA and histone modifications are also being reported (Zama and Uzumcu, 2010; Lauretta et al., 2019). Even a low dose of these chemicals have the potential to either directly or indirectly affect the exposed individuals. Experimental results suggest that the low concentration of these chemicals showing impairment during in vitro experiments can also be detrimental not only to wildlife but to human health as well (Lauretta et al., 2019).
Many in vitro and in vivo studies report the health damages due to exposure to EDCs, including short-term acute and long-term chronic effects, which may appear months or years after exposure (Hayes et al., 2011; Sabuz Vidal et al., 2021; Sakhteman et al., 2021). Acute effects which are observed in persons involved in the use of pesticides include painful eyes, rashes, wounds, blindness, nausea, respiratory issues, and diarrhea (De Coster and Van Larebeke, 2012; Ren et al., 2015a). The chronic effects which are reported include cancer, congenital disabilities, neural and developmental deformation as well as immunotoxicity (Nalbone et al., 2013; Scognamiglio et al., 2016). The brain and nerve cells are very prone to be affected by these EDCs, especially at the developmental stages (Marinello and Patisaul, 2021). Experimental results and clinical and epidemiological studies confirm that exposure to EDCs can result in harmful effects on brain health (Lopez-Rodriguez et al., 2021). The toxic nature of EDCs has the potential to alter normal functions of neural cells that result in neurogenesis and affect neural transmission. This can lead to neurological disorders that can disrupt learning and cause aggressive behavior (Kajta and Wojtowicz, 2013; Preciados et al., 2016; Streifer and Gore, 2021).
Conventional measurement of EDCs has been primarily performed using liquid or gas chromatography-mass spectrometry (LC- or GC-MS) in a variety of matrices such as water, breast milk, urine, serum, food, and soil (Metcalfe et al., 2022). PFAS and phthalates have most commonly been measured by LC-MS using tandem mass spectrometry (MS/MS) (Dima et al., 2020; Abafe et al., 2021) or high resolution mass spectrometry (HRMS) (Charbonnet et al., 2022) In contrast, aromatic hydrocarbons have been primarily measured using GC-MS or GC-HRMS (Wang et al., 2019; Zastrow et al., 2021) while BPA, insecticides, and pesticides have been analyzed using either LC-MS/MS or GC-MS (Lee et al., 2017; Dima et al., 2020). Metal EDCs such as arsenic and lead have most often been tested by inductively coupled plasma mass spectrometry (ICP-MS) (Luo et al., 2017). In general, while targeting specific EDCs using such techniques as MS/MS provides the optimal ability to detect low abundant compounds, HRMS enables the greater ability to discover unknown EDC metabolites through untargeted screening (Metcalfe et al., 2022). Due to the variety of sample matrices and extraction methods used prior to MS analysis, the use of internal standards is critical for ensuring precision and accuracy of quantitation (Cortese et al., 2020). Surrogate internal standards, consisting of stable isotope or deuterium labeled versions of target compounds, are added at the beginning of extraction to later adjust data for factors including extraction efficiency and matrix effects. In some instances, such as with PFAS, internal standard mixtures consisting of a large number of compounds within an EDC class can be purchased to facilitate inclusion in extraction protocols (Genualdi and deJager, 2021).
Monitoring and detection of EDCs compounds, as noted earlier, have been traditionally performed with analytical methods such as gas chromatography-mass spectrometry (GC-MS) (Azzouz et al., 2020), Liquid chromatography (Zatrochova et al., 2022), ultra-performance liquid chromatography-tandem mass spectrometry (Zhou et al., 2022), capillary electrophoresis (Chen et al., 2021) surface plasmon resonance (Bakhshpour and Denizli, 2020), and solid phase extraction (Zhang et al., 2020). Although conventional analytical methods are standardized, highly sensitive and selective, these approaches are time-consuming, have complex protocols, often require sample pre-treatment and sophisticated instrumentation, trained and skilled personnel. Novel sensing systems have been reported and well-established in the last decades to monitor several toxins. Recent advances in materials and detection systems provide rapid, amenable for on-site, real-time, and cost-effective monitoring at very high sensitivity of EDCs at trace levels. Table 1 provides a concise account of technologies used for detection various EDCs.
The impact of EDCs on health is amplified by both a dearth of knowledge of how these chemicals affect our wellbeing and by a lack of awareness of how pervasive these compounds are in our environment (Kabir et al., 2015). In this review, we will focus on new or emerging technologies for the detection of EDCs. We discuss biosensors based on electrochemical, colorimetric, optical, aptamer, and microbial sensing approaches for EDC detection. The advantages and limitations of different sensing systems are compared and evaluated in the context of more conventional, lab-based detection techniques. Future developments and applications of EDC sensors will be discussed in the last section.
Sensors are integrated devices that require small sample volumes and minimum sample preparation, are fast, cheap, portable, user-friendly, and capable of performing real-time monitoring without the production of toxic chemicals. While there are several success stories, summarized in Table 2, numerous systems are still in development. In the subsequent sections, we will discuss the different biosensing modalities for the detection of EDCs.
An electrochemical sensor is made of two electrodes, i.e., reference and sensing electrodes. A suitable electrolyte separates both electrodes. The presence of a target analyte generates electrochemical signals which are used to monitor the concentration of the analyte (Kaya et al., 2021). These types of sensing systems provide high selectivity, low power requirements, linear and stable output, excellent accuracy, repeatability, and economical sensing. With all these features, the easy integration of these electrochemical sensors into smart portable units makes them a useful option for in-field deployment (Hayat and Marty, 2014). Their compatibility with complicated sample matrices reduces the need for lengthy sample pretreatments and leads to the simplification of sensing protocols (Lee and Irudayaraj, 2013; Huang et al., 2017). All these are attractive features in the development of electrochemical sensors for the detection of EDCs.
EDCs such BPA and phthalates, due to their electroactive nature, can be detected directly by adopting suitable electrochemical sensing approaches as illustrated in Figure 1. Some common EDCs along with their chemical structures are depicted in Figure 1A. Figure 1B illustrates the direct detection of electroactive species. Figure 1C elaborates on the sensing of EDCs such as polychlorinated biphenyls (PCBs) and PFAS that need an adjunct electro-active species to be measured. These non-electrostatic targets can be identified after interaction with electro-active substances. Figure 1D, shows the detection of binding functionalities of microorganisms via electrochemical sandwich assay (Ranaweera et al., 2019; Sofen and Furst, 2019). An electrochemical assay was constructed by expressing estrogen receptors on the surface of Escherichia coli (E. Coli) for monitoring various EDCs by signal variants from impedance measurements (Furst et al., 2017).
FIGURE 1. Schematic diagram representing well-developed electrochemical approaches for EDCs monitoring (A) Common EDCs with chemical structures (B) BPA can be detected by the electro-chemical oxidation methods to find out the presence of its phenolic groups, (C) Competition-based immunoassay assay for PCB sensing, enzymes connect with circular dots of different colors which is pre link to the surface, as a result of this connection the electrochemical signals are being generated depending upon PCB amounts in the solution, (D) An electrochemical sandwich assay, functioning on the binding of E-coli to an electrode is being sketched to monitor EDCs.
Recent electrochemical sensing systems have also used nanomaterials to enhance the selectivity and sensitivity of the systems (Guo and Irudayaraj, 2011; Stefan-van Staden et al., 2019). The incorporation of nanomaterials such as carbon nanotubes, graphene, and other metal-based nanomaterials onto electrodes provides more selective and sensitive results by improving signal-to-noise ratios on the sensing electrode (Qu et al., 2019; Sofen and Furst, 2019; Ahmad et al., 2022).
BPA is an ideal candidate for direct detection due to its electroactive nature, undergoing two-electron, two-proton oxidation, and detection can be amplified with combinations of enzyme reactivity and signal enhancement. Liu et al. (2019) developed an electrochemical biosensor for the monitoring of BPA with the help of a tyrosinase enzyme. In this method, biochar nanoparticles (BCNPs) are synthesized from sugarcane sources by applying the Nafion embedding method, which is being used as a transducer and signal enhancer. The presence of highly conductive BCNPs not only increased the sensor signals but also decreased the impedance and reduction potential, which ultimately improved the output from amperometric sensing. By utilizing this sensor, as little as 3.18 nM of BPA can be detected with a linear range between 0.02 and 10 µM. One important aspect of implementing these technologies is the ability to commercialize testing platforms. These BNCP-enzyme platforms show 86.9% signal retention after 1 month of sensor storage, which confirms the stability of BCNPs/Tyr complex. BNCP-enzyme coupled biosensors provide easy electrode preparation, non-complicated operation, and better performance for the detection of BPA from water samples (Liu et al., 2019).
Atrazine is an herbicide commonly found in ground water and surface runoff (Bachetti et al., 2021; Urseler et al., 2022). Supraja and co-researchers created a real-time sensor for atrazine using an electrospun SnO2 nanofiber platform. Using label-free transductions on this platform, atrazine can be detected at 0.9 zM with a linear range of 1.0 zM to 1.0 μM. This system exhibits excellent interference resistance as spiked ground and mineral water samples were successfully tested for atrazine (Supraja et al., 2019). Zahran et al. (2020), used silver nanoparticles (AgNPs) synthesized by the direct reduction technique for the electrochemical sensing of atrazine. Signal enhancement was facilitated with dissolved organic matter (DOM) interacting with AgNPs on the surface of the glassy carbon electrode (GC), leading to the formation of a DOM/AgNP/GC composite. After optimizing the composite manufacture, atrazine was detected in the nM range in stream water samples with this method (Zahran et al., 2020).
Dibutyl phthalate (DBP) is used during the manufacture of flexible plastics, as an insect repellent, and as a solvent for oil and resins in several products (Batool et al., 2022; Sun et al., 2022). Bolat et al. (2019), developed a sensor using molecular imprinting for the targeted detection of DBP. An integrated approach using a conducting polymer, polypyrrole (PPY), molecularly imprinted by polymerization in the presence of DBP on a graphite electrode was used. Electrochemical impedance spectroscopy (EIS) is used to detect the interaction of solution DBP with the molecularly imprinted sensor. After sensor optimization, the device can detect 4.5 nM DBP with a linear response range of 0.01–1.0 μM. This sensor provides a rapid, sensitive, economical, disposable, and easy-to-use platform for the detection of DBP (Bolat et al., 2019). Also to detect DBP, a biosensing platform based on a competitive binding assay similar to enzyme-linked immunosorbent assay (ELISA) was developed using gold nanoparticles (AuNPs) for signal amplification. The amplification results from the enlargement of AuNPs via catalytic precipitation. Under optimized conditions, the LOD of this system at 7.0 nM is 10 times lower than conventional ELISA. Detection efficiency remains stable at a wide range of pH (6–9) and ionic content (Na+8%, Ca+2 4%; w/v) as well as in matrices of pond water and river water. The overall performance of this immunosensor indicates the potential to be developed into a rapid and low-cost sensor for monitoring DBP in water samples (Liang et al., 2017).
Perchlorate contamination is primarily associated with military installations due to its use as a strong oxidant in explosives but is also found in food packaging (Feng et al., 2022). It has been found in both drinking water and food (Tian et al., 2020). Hassan and his colleagues developed an electrochemical sensor for perchlorate ions using a solid-contact ion-selective electrode (SC-ISE). The use of single-walled carbon nanotubes as a solid-contact material provides a high double layer capacitance as a result of the high surface area of the nanomaterial. The sensor showed enhanced selectivity toward perchlorate ions with a broad linear range spanning 1 μM–10 mM concentrations and a detection limit under 200 nM. The system showed quick response time, high sensitivity, and accuracy. These types of sensors could be used for the perchlorate determination in a flow system for continuous monitoring (Hassan et al., 2019).
Perfluorooctane sulfonate (PFOS) was widely used in stain-resistant fabrics, fire-fighting foams, food packaging, and as a surfactant in industrial processes, and PFOS can also be generated in the environment after release and transformation of precursors (Kancharla et al., 2022; Nilsson et al., 2022). Although no longer produced in the United States, PFOS remains an environmental contaminant and is still being produced and used in products internationally (Pontius, 2019). Li et al., have developed a low-cost, disposable photoelectrochemical (PEC) strip to monitor the PFOS precursor perfluorooctane sulfonyl fluoride (PFOSF). PFOSF has been recalcitrant to measurement without chemical derivatization. The PEC strips are fabricated via a facile one-step electrodeposition method, coated with bismuth oxyiodide (BiOI) nanoparticles, and targeted to PFOSF detection with molecular imprinted polymers grafted to the electrode. The resulting sensors have a linear range of detection of 0.1–1 μM and are capable of detecting PFOSF in spiked river and lake water (Li et al., 2018c). An electrochemical biosensor for PFOS based on the inhibition of the biocatalytic process in enzymatic biofuel cells (BFC) was established. This single-compartment BFC was equipped with multi-walled carbon nanohorns (MWHNs). Glassy carbon electrodes (GCE) acts as a substrate for bio-anode as well as for bio-cathode and glutamic dehydrogenase (GLDH) behaved as biocatalyst for the bio-anode. It had previously been noted that PFOS altered the glutamate mediated current in rat hippocampal cells (Liao et al., 2009). In general, the detection levels from the biosensors were not currently on par with the conventional methods but future improvements are expected. Sensitivity targets to monitor the minimum reporting levels (usually in the 2–4 ppt range) and lifetime health advisory levels (0.004 ppt for PFOA, 0.02 ppt for PFOS, 10 ppt for GenX, and 2,000 ppt for PFOS) are mandated by the EPA in their 2022 reports (Hogue, 2022).
Colorimetric-based sensing systems depend on the color variations in the reaction mixture due to the presence of a specific analyte. The presence of an analyte produces a reaction with the sensing materials, which causes visual color variation (Rodrigues et al., 2021). These sensor platforms provide advantages over existing lab-based methods such as ease of use, quick response, and the ability to obtain test results without any accessory equipment or access to power sources. The quick and distinct color change of the reaction enables users to interpret the positive results qualitatively with the naked eye or quantitatively with optical sensors. The colorimetric assay has proven to be a powerful analytical approach for EDCs, owing to its convenience and simplicity (Shah et al., 2013; Che Sulaiman et al., 2020). An example schematic for novel colorimetric sensing comprising of synthesis steps, characterization, and application (AuNCs-MnO2) for monitoring target analytes is outlined in Figure 2. The fabrication of MnO2 (AuNCs-MnO2) NPs from bacteria/green source is depicted in Figure 2A, and their characterization with various techniques in Figure 2B, while Figure 2C illustrates sample analysis and signal detection by colorimetry.
FIGURE 2. Steps for the colorimetric monitoring of analytes (A) Synthesis of MnO2 (AuNCs-MnO2) nanoparticle from plant/bacteria source, (B) fabricated nanoparticles are characterized with different techniques, (C) colorimetric detection of an analyte by adapting fluorescence and colorimetric signal variation.
The physio-chemical nature of gold nanoparticles is being exploited for onsite visual detection of perchlorate. A reliable nanoparticle-based analytical method for perchlorate detection was proposed with the help of methylene blue (MB) and negatively charged gold nanoparticles. The presence of MB gives an overall positive charge to the AuNPs-MB nanocomposite, which enables the surface to electrostatically attract negatively charged perchlorate which leads to the ion-pair formation between the MB and perchlorate anion. This ion pair formation causes agglomeration in the AuNPs, which is confirmed by the localized SPR absorption band. The LOD of this system was found to be 240 mM and the limit of quantification (LOQ) was observed as 830 mM. The efficacy of this system for percolate ions is being investigated statistically for different interfering common ions (Keskin et al., 2020).
In a study by Yan et al. (2019), a nanocomposite based on gold nanoclusters and MnO2 (AuNCs-MnO2) was used to detect carbamate pesticides by adopting fluorescence and colorimetric signal variations. MnO2 quenched the fluorescence of AuNCs, which cause a decrease in fluorescence as well as a color change. The presence of acetylcholinesterase (AChE), its substrate, and choline oxidase lead to the generation of H2O2 due to enzymatic hydrolysis resulting in the decomposition of MnO2, affecting the fluorescence from AuNCs. But when carbamate is in the system, its blockage of AChE leads to the reduction of the enzymatic reaction, hence a drop in H2O2 generation, which eventually results in the blockage of MnO2 breakage and increase in quenching of AuNCs fluorescence (Yan et al., 2019).
A simple, easy-to-use dual-sensing system for organophosphate (OP) pesticides using adenosine triphosphate (ATP) and rhodamine B-modified gold nanoparticles (RB-AuNPs) has been successfully developed (Li et al., 2018b). This sensing system is based on the aggregation of AuNPs due to ligand replacement, which is caused by the presence of OP. Ethoprophos, an OP ester, can be detected by the variations in colorimetric and fluorescence signals grom the RB-AuNPs. The linear range for this detection system was 4.0–15.0 µM, and the LOD of this system was as low as 37.0 nM. This cost-effective, sensitive and reliable sensing platform can be applied to real-time sensing of ethoprophos in water samples (Li et al., 2018b).
A colorimetric method for the monitoring of OP is being developed by the aggregation of lipoic acid-capped AuNPs and subsequent color change due to AChE/ATCh (acetylthiocholine) reaction. In this scheme, the enzymatic product of AChE, cationic thiocholine (TCh), causes the aggregation of AuNPs which leads to a change in color from red to blue. The presence of OP in this system causes the blockage of AChE, which ultimately reduces TCh generation, preventing aggregation of AuNPs and the absence of a red-shift in the system. This detection system was applied to detect OP from fruit samples (Sun et al., 2011).
Optical sensors are highly attractive devices that use light to detect and measure changes in a physical entity, such as position, motion, light intensity, wavelength, and temperature. These sensors have been applied to detect very low concentrations of EDCs in various samples, such as food, beverages, blood, urine, soil, crops, industrial effluents, wastewater, and surface water (rivers, lakes, and oceans). Detection of EDCs in blood and urine helps in the diagnosis of EDC-related disorders and monitoring treatment progress. These chemicals were monitored in soil and crop samples by employing optical sensors to identify the sources of contamination and to undertake measures to reduce their impact on crop yields and soil fertility. Monitoring industrial effluents and wastewater can help in the identification of sources of contamination whereby measures can be undertaken to reduce their release into the environment. Optical sensors can be used to assess the extent of contamination and to take measures to mitigate its effects.
A fluorescent-based photo-stable detection system of atrazine was developed by Mohapatra et al. (2018), with the help of a nitrogen-doped carbon quantum dot. The presence of atrazine induces the fluorescence system to luminesce at increasing intensity depending on the concentration of atrazine. The hydrogen bonding interaction between the atrazine and amino groups on the surface of the quantum dots makes the sensing system selective and highly sensitive to atrazine. With this approach, as low as 3.0 pM of atrazine could be detected in the broad linear range of 5.0 pM–7.0 nM. This system is being applied to not only the real-time detection of atrazine in agricultural samples but also to detect atrazine in bacterial cell lines were the nitrogen-doped carbon quantum dots show good cell permeability and emission intensity in bacterial cell lines (Mohapatra et al., 2018).
The detection principle of a novel fluorescence immunoassay for atrazine is shown in Figure 3. Herein, the probe [comprising of the antigen-polystyrene magnetic microspheres (PMMs) complex] can be used to capture the target [coating antibody-upconversion nanoparticles (UCNPs) blend] to form an immune conjugate (antibody-UCNPs-atrazine-PMMs) and detected with a spectrometer. The use of an association between the atrazine and the fluorescence signal allows for quantitative sensing of atrazine in the sample (Sheng et al., 2019).
FIGURE 3. An illustration of probe fabrication and analysis based on immunofluorescence (competition assay for atrazine detection).
Li et al. (2021), adopted a fluorescence-based method for the ultrasensitive detection of BPA in water samples with the application of up-conversion nanoparticles (UCNPs) and tetramethylrhodamine. The use of UCNPs not only enhanced the sensitivity of the system, but the luminous efficiency also increased. A simple pretreatment for the water sample measured makes this method time-saving and easy to adopt (Li Q et al., 2019).
An assay for detecting BPA using a material created by compounding magnetic Fe3O4 onto the surface of oxidized graphene to make magnetic oxidation graphene (MGO) was developed. The magnetic material is a strong quenching agent. Fluorescently labeled BPA-targeted single-stranded DNA (ssDNA) aptamer sequences bind to the surface in a quenched state. The presence of free BPA releases the aptamer and restores the fluorescence. Magnetic separation of the MGO material after release of fluorescent aptamer provides a sensitive indicator of the presence of BPA. The LOD of this system was 0.071 ng/mL and a linear range of 0.2–10 ng/mL. The specificity of the aptamer for BPA and the use of fluorescence proves to be highly sensitive and selective (Hu et al., 2017). Using a similar targeted binding strategy, Inuyama and co-researchers capitalized targeted dioxin-binding peptide in a competitive binding assay with fluorescence readout. The fluorescent compound N-NBD-3-(3′, 4′-dichlorophenoxy)-1-propylamine binds to the dioxin-targeted peptide which is conjugated to the surface of a bead. The fluorescence of the beads decreases as increasing concentrations of dioxin displace the fluorescent molecule from the bead. The fluorescence intensity decreases with the increase in the concentration of dioxin. With optimized bead conjugations an LOD of 0.5 nM dioxin is achieved. This system is robust enough to identify soil samples that have a higher concentration of dioxin than standards (Inuyama et al., 2007).
A Surface Plasmon resonance (SPR) based sensing assay was developed by capitalizing on functionalized gold nanoparticles to enhance sensor response. By adopting this strategy BPA was successfully detected in the concentrations ranging from pM to mM with a detection limit of 22.7 pM (Xue et al., 2019). Another sensing system was designed for monitoring BPA based on molecular imprinted photonic crystals. The high specificity and selectivity of the developed sensing system were associated with the interactions of binding sites of nano-cavities with BPA (Griffete et al., 2011). An efficient, regenerative two dimensional, dually cross-linked photonic crystal for colorimetric and florescent-based sensing system for BPA monitoring was developed. The detection limit of this cost-effective and rapid system was 4.38 nM (Du et al., 2023).
Another SPR-based sensing system was developed for monitoring of triclosan in wastewater. The sensor was modified to enhance its functionality by the incorporation of allylmercaptane-modified gold SPR chip and imprinted poly(2-hydroxyethyl methacrylate–methacryloylamidoglutamic acid) nanofilm. The linearity range and detection limit of this sensor were 0.173–3.45 nM and 0.058 nM, respectively (Atar et al., 2015). A modified quartz crystal microbalance (QCM) was established to inspect four EDCs including BPA, oestrone, oestradiol, and sulfamethoxazole, by employing an adsorption mechanism via a zeolite filter in real-time in water samples. Observed results correlate with the models of pseudo-first-order kinetic and Sips isotherms. Adsorption efficiency was influenced by the molecular structure and polarity of these analytes, but the variations in pH and ionic strength of the sample did not impact adsorption efficiency (Li et al., 2021).
Chapartegui-Arias et al. (2019), established a zeolitic imidazole framework (ZIF) to measure phthalate esters (PAEs) in solution by an optical sensing protocol. In this method, the fluorescence emission intensity of aminopyrine conjugated to the ZIF changed due to the presence of Phthalate. Aminopyrene is covalently bound to the zeolitic imidazolate framework (ZIF-8) and the addition of a butylamine as a modulating agent during synthesis resulted in nanoparticles of ZIF-8. The quenching of the fluorescence is observed when PAEs are present and interact with the material by their aromatic structures. The ability to capture transient changes in fluorescence intensity without expensive and time-consuming regeneration steps show the possibility of a cheap, fast, and easy-to-use PAEs detection system. With some initial pretreatment of industrial wastewater, this detection system can be used for quick and reliable PAEs sensing with the LOD range from 0.064–0.19 mM (Chapartegui-Arias et al., 2019).
A novel fluorescence-based molecularly imprinted polymer (MIP) synthesized by precipitation polymerization for the monitoring of dibutyl phthalate (DBP) has been described. Mn-doped ZnS quantum dots (QDs), which anchor a MIPs layer on the SiO2 nanoparticles (SiO2@QDs@MIPs), were synthesized. Acrylamide was used as a functional monomer and ethyl glycol dimethacrylate as the cross-linker in the presence of DBP to create the MIP layer on the surface of the SiO2 nanoparticles. The sensor can selectively and sensitively recognize DBP resulting in a decreasing fluorescence intensity of the particles with increasing binding of DBP. In optimized conditions, the fluorescence signal changes linearly with concentration of DBP. The SiO2@QDs@MIPs was applied to tap water analysis, and on average, a 97.80% recovery rate of DBP was observed with a relative standard deviation (RSD) of less than 3.25%. This new method provides excellent, quick, responsive, stable, and applied alternatives to sophisticated analytical tools for the onsite detection of DBP in the linear range from 5 to 50 µM (Zhou et al., 2017).
An immunofluorescence plate assay was developed by (Zhang et al., 2006). A polyclonal antiserum was generated in rabbits targeting DBP. A very low cross-reactivity rate (10%) with similar phthalate compounds suggests polyclonal antiserum can increase sensitivity with an acceptable loss of specificity. The resulting antibody-coated plate format was used for the detection of dibutyl phthalate (DBP) via fluorescence immunoassay. With the help of this assay, water samples from different sources such as river water, tap water, and drinking water can be tested for the DBP with simplicity, sensitivity, and reliability (Zhang et al., 2006).
A novel, indirect strategy for detecting the insecticide parathion-methyl (PM) based on the measurement of its hydrolysate, p-nitrophenol, was established by Yan et al. (2015). The fluorescence of CdTe quantum dots (QDs) assembled with positively charged cetyltrimethylammonium bromide (CTAB) is quenched when the alkyl chain of CTAB interacts with the aromatic ring of p-nitrophenol. The compound p-nitrophenol is produced by the hydrolysis of PM by organophosphorus hydrolase (OPH) and is subsequently involved in the electron transfer from CdTe QDs/CTAB. The long alkyl chain of CTAB possesses a positive charge that is an absorbent for p-nitrophenol due to hydrophobic interactions. The presence of PM is indirectly assessed from the fluorescence variation of CdTe QDs/CTAB by this electron transfer approach at a detection limit of 0.062 µM (Yan et al., 2015). For real-time, precise, and accurate detection of a herbicide (2,4-dichlorophenoxyacetic acid), molecularly imprinted QCM and SPR systems were proposed. Polymeric surfaces were designed by exploiting the molecular imprinting procedure to develop sensors with high sensitivity. Observed LOD of the QCM and SPR sensors was 0.091 and 0.11 nM respectively (Cakir et al., 2019).
A spectrophotometric turn-on method was devised for the detection of PFOS/PFOA in environmental water samples using an erythrosine B (EB) and CTAB fluorescence system. The fluorescence of EB in the presence of a large excess of CTAB results in both a quenching and a fluorescence redshift of 11 nm. The addition of PFOS/PFOA induces enhanced fluorescence intensity at the new redshifted wavelength. It is found that the presence of PFOS/PFOA enhanced the fluorescence in proportion to the concentration of these toxins. The LOD for PFOS was 12.8 nM and for PFOA it was 11.8 nM. The established method is a natural, simple, quick, economical, and sensitive way to monitor the PFOS/PFOA in the water sample (Cheng et al., 2018). However, the detection limits achieved may not be sufficient to detect trace levels of PFAS. Using a similar strategy (Cheng et al., 2019), further discovered that in a sensing setup, PFOS can be visually detected from the aqueous samples with the help of carbon dots (CDs) and berberine chloride hydrate (BH). The presence of BH decreases the fluorescence of CDs in the medium of the Britton-Robinson (BR) buffer solution (pH 6.09). The addition of PFOS in the system recovers the fluorescence slightly at 488 nm but greatly enhances fluorescence at 533 nm. Interestingly this effect was not observed with other perfluorinated compounds of this family. Visual color variations from blue to light yellow were easily perceived in the presence of PFOS. The enhanced intensity responses at 533 nm are linear in the range of 0.22–50.0 µM PFOS concentrations with a LOD at 21.7 nM (Cheng et al., 2019).
Recognition and detection of EDCs with the help of antibodies, aptamers, and hormones integrated into a sensing setup provide sensitive and selective detection. This setup also provides and enhances the knowledge of the interaction of EDCs with living systems (Sofen and Furst, 2019). Aptamers are artificial manmade nucleic acid ligands that can be produced to bind to any target molecules including proteins, amino acids, and drugs (O'Sullivan, 2002).
An aptamer-based electrochemical sensor for atrazine (ATZ) was developed by Fan et al. (2019). Nickel hexacyanoferrate nanoparticles (NiHCF NPs) and electrochemically reduced graphene oxide (ERGO) were applied to modify a glassy carbon electrode (GCE) surface. AuNPs were deposited on the electrode to facilitate the anchoring of atrazine-targeted aptamer. When atrazine is introduced into the system, it forms a complex with the aptamer. This ATZ-aptamer complex leads to a decrease in the electrochemical signal due to the hindrance of electron transfer. The linear curve with this sensing system was in the range of 0.25–250 pM with the LOD 0.1 pM. This method could apply to the detection of ATZ in water and soil samples (Fan et al., 2019). In another study, Jia et al. (2020), took a previously developed 63-mer aptamer targeting BPA and rationally truncated the sequence into two shorter, higher affinity aptamers of 38-mer and 12-mer length. A comparison of the sensing system with original and truncated aptamers was performed. In comparison to the parent aptamer, the LOD of the 38-mer was 265-fold higher the original aptamter and the LOD of the 12-mer was 14-fold higher. In the same way, the selectivity of truncated aptamers was found to be much higher than the original aptamer. The sensor shows the potential for sequential development and optimization of aptamers for EDC targeted sensors. The developed sensor was used to detect BPA in milk, orange juice, and water showing potential for use in food and environmental samples (Jia et al., 2020).
A similar strategy of optimizing aptamer binding was used to develop a sensor for the detection of the plasticizer di-2-ethylhexyl phthalate (DEHP). A Quantum dot aptasensor (QD-aptasensor) with a portable analyzer was developed. Three truncated aptamers of 45-, 28-, and 22-mer were taken from a previously identified 60-mer aptamer targeting DEHP along with three unique DNA probes. Of all the different combinations, the 22-mer aptamer with 12mer DNA probe provides excellent sensitivity and selectivity even in the presence of phthalate analogs and a linear signal response to increasing concentrations of DEHP. The selected aptamer-probe combination is being tested further to make the sensing system more amenable to practical use (Lim et al., 2018). Using a 24-mer aptamer coupled to gold nanoparticles (AuNP-aptamer), Lee and co-researchers created an easy and quick colorimetric assay for the detection of BPA. In the addition of BPA, the AuNP-aptamer undergoes electrolytic induced aggregation, resulting in a visual color shift from red to blue in presence of part per billion concentrations of BPA. With the help of this method, BPA can be detected at concentrations as low as 4.0 pM. Additionally, the performance of this system is comparable to analytical methods such as chromatography and ELISA (Lee et al., 2019).
An enzyme-based detection system for the organophosphate pesticides dichlorvos and methyl-paraoxon was developed by (Zhang et al., 2016) by exploiting the toxic, acetylcholinesterase (AChE) inhibition caused by OPs. The system uses polyacrylic acid-coated cerium oxide nanoparticles (PAA-CeO2) to oxidize the chromogenic substrate tetramethylbenzidine (TMB) to create a blue colored solution. The enzymatic activity of AChE in the system reduces acetylthiocholine to thiocholine (TCh). The resulting TCh inhibits the oxidation of TMB, resulting in a clear color. The addition of OPs inhibits the creation of TCh, allowing more TMB to be oxidized and create a blue color. The system can detect dichlorvos as low as 8.62 ppb and methyl-paraoxon at 26.73 ppb. This colorimetric approach is a potential tool for rapid screening for the presence of OPs (Zhang et al., 2016).
A surface plasmon resonance (SPR) optical fiber biosensor was developed by Cennamo et al. (2018b), to detect perfluorooctanoate (PFOA) and Perfluorooctane sulfonate (PFOS) compounds. Polyclonal antibodies were produced in rabbits and the resulting serum was enriched for target specificity using PFOA-EAH sepharose columns. The resulting antibodies show similar affinity to both PFOA and PFOS, likely due to the method of binding PFOA to the sepharose column. This system has been applied for the sensing of PFOA/PFOS in seawater. This sensing platform can detect concentrations of PFOA/PFOS as low as 0.221 ppb, lower than the maximum residue limit for PFOA/PFOS set by European Union regulations (Cennamo et al., 2018b). A conceptually similar approach was adopted to create SPR optical fiber biosensors using Molecularly Imprinted Polymer (MIP) materials instead of antibodies. This system was selective toward a range of per-and polyfluoroalkyl substances (PFAS) with carbon backbones in the C4-C11 range. The sensor detected these compounds with LOD of 0.13–0.15 ppb. Its performance was comparable to the antibody-based SPR-POF system but possessed the advantages of cost-effectiveness, reproducibility, and increased stability with natural, environmental solutions (Cennamo et al., 2018a).
Various microbial sensors have been reported for the monitoring of EDCs. Generally, this class of sensors consists of a microbe as both a detector and a transducing agent as elaborated in Figure 4. These systems build on detection and signaling pathways that have previously evolved in organisms to improve survival potential in the environment. The specificity and sensitivity of these sensors to EDCs has been enhanced by the genetic modification and regulation of gene expressions of these microbes. As a result, the efficiency and sensitivity of these systems has increased to compete with standard immunosensor technology (Bilal and Iqbal, 2019). These sensors provide some advantages over standard sensors such as robust response in a range of measurement conditions, extended lifetime, and low cost. One disadvantage of these systems is the need for a prolonged response time compared to other sensing systems.
FIGURE 4. The illustration of cell free microbial sensors for monitoring EDCs. Cell free microbial sensors were used to detect EDCs. The downstream reporter gene is activated for transcription and then translated for reporter protein production in a cell free system.
An E. coli-based lux operon biosensor for the efficient testing of perchlorate was developed. The lux biosensor strains tested generate a bioluminescence in response to reactive oxygen species. The presence of ammonium perchlorate (AP) in the media causes the generation of hydrogen peroxide and superoxide anion, which causes oxidative stress in the sensor cells. During the design of lux biosensors, hybrid plasmids are fused with bacterial luciferase lux genes. The concentration of AP at 5.0 and 50 mM induces 5 and 10 times higher luminescence respectively in bacterial cells relative to untreated cells (Balabanov et al., 2017). An array of M13 bacteriophage is being exploited to develop a colorimetric sensor for the detection and categorization of EDCs, including benzene, phthalates, and polychlorinated biphenyls (PCBs). Structurally and genetically modified M13 bacteriophages are being used for the biomimetic sensor, which changes the color patterns depending on the compound. This promising, portable, and easy-to-use sensing system could be applied for the monitoring of food and pharmaceutical samples (Moon et al., 2016).
Various types of sensing techniques including electrochemical, colorimetric, and optical sensing technologies have been developed for monitoring EDCs in various environments, such as water, food, and consumer products. However, these technologies also face several challenges and limitations. As such, these sensors have varying levels of sensitivity to the different EDCs, and poses a challenge for detection with sufficient sensitivity. These sensors may also face challenges in distinguishing between different EDCs or between EDCs and other chemicals that may be present in the sample. The presence of other chemicals or compounds in the sample may interfere with the functioning of the sensor, leading to false positives or false negatives. In some cases, sample preparation may be required before analysis, which can add to the complexity of detection. In addition, the performance of these sensors may be affected by environmental factors such as temperature, humidity, and pH, which can lead to variations in their accuracy and reliability. The presence of different types of matrices (e.g., soil, water, food) can also affect the performance of these monitoring systems since the properties of the matrix may interfere with the functioning of the sensor or affect the detection signal. Overall, while sensing technologies offer promising solutions for the monitoring of EDCs, there are still several challenges and limitations that need to be addressed to improve their accuracy, sensitivity, and practicality for use in real-world settings.
Advanced sensing techniques hold significant promise in the monitoring of EDCs in the environment. New nanomaterial-based sensors can provide increased sensitivity to detect EDCs at very low concentrations. This can lead to more accurate and reliable monitoring even in complex matrices. Sensing techniques based on molecularly imprinted polymers (MIPs), aptamers, or other biomimetic materials have the potential to be highly specific to the target EDC. This can minimize interference from other compounds in the sample and reduce the occurrence of false positives and has the potential for rapid response. This can be especially important in industrial or agricultural settings where there is a higher risk of EDC contamination. Sensor technologies can also allow for remote monitoring of EDCs using wireless or internet of things (IoT) technologies. Advance techniques can provide timely data to environmental agencies or other stakeholders, enabling them to take appropriate actions to reduce health hazards. Sensor-based methods can render monitoring EDCs more accessible to a wider audience, including communities and citizen scientists. Platforms can be designed to detect multiple EDCs simultaneously, providing a more comprehensive understanding of environmental contamination. This can enable stakeholders to develop targeted mitigation strategies to reduce exposure to multiple EDCs.
There are several new trends in the development of sensors for the monitoring of EDCs, including integration with fluidics, miniaturization, and multiplexing. The integration of sensors with microfluidics can enable automated and high-throughput sample processing, reducing the time and cost of EDC detection. Microfluidic platforms can also enable precise control of sample flow and reaction conditions, leading to increased sensitivity and specificity of EDC detection. Miniaturization of sensors can enable portable and field-deployable devices. Miniaturized sensors can also reduce the amount of samples and reagents required for detection, making them cost-effective and environmentally friendly. These sensors can also enable in situ and real-time monitoring of EDCs in environmental and biological samples. Multiplexed sensors can detect multiple EDCs simultaneously in a single sample, reducing the time and cost of EDC detection. Multiplexed sensors can also enable the detection of complex mixtures of EDCs which often occur in environmental samples. Multi-target sensors can be achieved through the use of arrays of sensors with different sensing elements, or the use of microarrays on lab-on-a-chip platforms. Overall, these trends are enabling the development of advanced sensors for EDC detection that are more sensitive, specific, cost-effective, and portable than traditional methods. These sensors have the potential to revolutionize EDC monitoring and risk assessment, leading to improved public health and environmental protection.
EDCs are found in a broad spectrum of locations ranging from commercial to residential areas. Many are ubiquitous, posing serious threats to human health. Given the increase in EDCs in the domestic and industrial sectors, improved sensors for point of exposure detection are of the highest need. In the future, advanced EDC sensors for routine monitoring will become imperative to control the deleterious health effects of these compounds. Devices to detect these chemicals at home, in agricultural fields, wastewater treatment units and in manufacturing plants will become the norm. EDC detection is a potentially growing field; non-analytical advanced sensing systems have emerged as a promising means for the detection and monitoring of EDCs due to their high sensitivity, selectivity, cost-effective, portable, rapid response time as well as user-friendly approach. The development of sensors for EDCs has been driven by the need to monitor these chemicals in various settings, including water, air, and food and stringent limits by the regulatory agencies. Biosensors have gained significant attention in the medical industry and are already emerging as an alternative to traditional and conventional methods for the sensing of EDCs. In contrast to traditional analytical sensing techniques, modern sensing methods can provide for the possibility of detecting an array of pollutants and toxic chemicals on site, rapidly and with excellent sensitivity and selectivity.
Despite the significant progress made in the development of sensors for EDCs, challenges remain along with significant opportunities. One of the main challenges is the detection of EDCs in complex environmental sample matrices. Other limitations such as sustainability and utilization under harsh environmental factors such as temperature, alkalinity, pH, salinity, and other adverse conditions can lead to poor results. With advances in sample preparation methods, device durability, and cost effectiveness these limitations can potentially be overcome in the near future. Given the emphasis to reduce pollution in the coming decades, explosive growth in the development and deployment of these state-of-the-art technologies for environmental sensing and detection of EDCs is expected. Overall, the development of sensors for EDCs is an active and growing field. The continued development of sensors for EDCs has the potential to provide valuable insights into the exposure and health effects associated with these chemicals, as well as inform public health policies aimed at reducing health risk due to exposure in a timely manner.
MS contributed to the conceptualization, data collection, investigation and writing original draft; KA and SB on writing the original draft and figures, ML and TJ contributed to visualization, writing, reviewing and editing, JI contributed to conceptualization, investigation, funding acquisition, and overall supervision. All authors read and approved this version of the manuscript.
The authors declare that the research was conducted in the absence of any commercial or financial relationships that could be construed as a potential conflict of interest.
All claims expressed in this article are solely those of the authors and do not necessarily represent those of their affiliated organizations, or those of the publisher, the editors and the reviewers. Any product that may be evaluated in this article, or claim that may be made by its manufacturer, is not guaranteed or endorsed by the publisher.
Abafe, O. A., Macheka, L. R., and Olowoyo, J. O. (2021). Confirmatory analysis of per and polyfluoroalkyl substances in milk and infant formula using uhplc–ms/ms. Molecules 26, 3664. doi:10.3390/molecules26123664
Ahmad, K., Khan, S., Afridi, M., Hassan, A., Shah, M. M., Rasheed, H., et al. (2022). Marine macroalgae polysaccharides-based nanomaterials: An overview with respect to nanoscience applications. Beni-Suef Univ. J. Basic Appl. Sci. 11, 156–214. doi:10.1186/s43088-022-00335-8
Alberti, G., Zanoni, C., Spina, S., Magnaghi, L. R., and Biesuz, R. (2022). MIP-based screen-printed potentiometric cell for atrazine sensing. Chemosensors 10, 339. doi:10.3390/chemosensors10080339
Alimzhanova, M., Mamedova, M., Ashimuly, K., Alipuly, A., and Adilbekov, Y. (2022). Miniaturized solid-phase microextraction coupled with gas chromatography-mass spectrometry for determination of endocrine disruptors in drinking water. Food Chem. X, 100345. doi:10.1016/j.fochx.2022.100345
Atar, N., Eren, T., Yola, M. L., and Wang, S. (2015). A sensitive molecular imprinted surface plasmon resonance nanosensor for selective determination of trace triclosan in wastewater. Sensors Actuators B Chem. 216, 638–644. doi:10.1016/j.snb.2015.04.076
Azzouz, A., Colón, L. P., Hejji, L., and Ballesteros, E. (2020). Determination of alkylphenols, phenylphenols, bisphenol A, parabens, organophosphorus pesticides and triclosan in different cereal-based foodstuffs by gas chromatography–mass spectrometry. Anal. Bioanal. Chem. 412, 2621–2631. doi:10.1007/s00216-020-02491-1
Bachetti, R. A., Urseler, N., Morgante, V., Damilano, G., Porporatto, C., Agostini, E., et al. (2021). Monitoring of atrazine pollution and its spatial-seasonal variation on surface water sources of an agricultural river basin. Bull. Environ. Contam. 106, 929–935. doi:10.1007/s00128-021-03264-x
Bakhshpour, M., and Denizli, A. (2020). Highly sensitive detection of Cd (II) ions using ion-imprinted surface plasmon resonance sensors. Microchem. J. 159, 105572. doi:10.1016/j.microc.2020.105572
Balabanov, V., Khrulnova, S., Kotova, V. Y., and Zavilgelsky, G. (2017). Ammonium perchlorate detection in natural environments using specific lux biosensors. Russ. J. Phys. Chem. B 11, 663–665. doi:10.1134/s1990793117040133
Batool, S., Batool, S., Shameem, S., Batool, T., and Batool, S. (2022). Effects of dibutyl phthalate and di (2-ethylhexyl) phthalate on hepatic structure and function of adult male mice. Toxicol. industrial health 38, 470–480. doi:10.1177/07482337221108578
Belkhamssa, N., Justino, C. I., Santos, P. S., Cardoso, S., Lopes, I., Duarte, A. C., et al. (2016). Label-free disposable immunosensor for detection of atrazine. Talanta 146, 430–434. doi:10.1016/j.talanta.2015.09.015
Bergman, A., Heindel, J. J., Jobling, S., Kidd, K., Zoeller, T. R., and Organization, W. H. (2013). State of the science of endocrine disrupting chemicals 2012. World Health Organization.
Bilal, M., and Iqbal, H. M. (2019). Microbial-derived biosensors for monitoring environmental contaminants: Recent advances and future outlook. Process Saf. Environ. Prot. 124, 8–17. doi:10.1016/j.psep.2019.01.032
Bolat, G., Yaman, Y. T., and Abaci, S. (2019). Molecularly imprinted electrochemical impedance sensor for sensitive dibutyl phthalate (DBP) determination. Sensors Actuators B Chem. 299, 127000. doi:10.1016/j.snb.2019.127000
Cakir, O., Bakhshpour, M., Yilmaz, F., and Baysal, Z. (2019). Novel QCM and SPR sensors based on molecular imprinting for highly sensitive and selective detection of 2, 4-dichlorophenoxyacetic acid in apple samples. Mater. Sci. Eng. C 102, 483–491. doi:10.1016/j.msec.2019.04.056
Cakır, O., and Baysal, Z. (2019). Pesticide analysis with molecularly imprinted nanofilms using surface plasmon resonance sensor and LC-MS/MS: Comparative study for environmental water samples. Sensors Actuators B Chem. 297, 126764. doi:10.1016/j.snb.2019.126764
Cennamo, N., D Agostino, G., Porto, G., Biasiolo, A., Perri, C., Arcadio, F., et al. (2018a). A molecularly imprinted polymer on a plasmonic plastic optical fiber to detect perfluorinated compounds in water. Sensors 18, 1836. doi:10.3390/s18061836
Cennamo, N., Zeni, L., Tortora, P., Regonesi, M. E., Giusti, A., Staiano, M., et al. (2018b). A High Sensitivity Biosensor to detect the presence of perfluorinated compounds in environment. Talanta 178, 955–961. doi:10.1016/j.talanta.2017.10.034
Chapartegui-Arias, A., Villajos, J. A., Myxa, A., Beyer, S., Falkenhagen, J., Schneider, R. J., et al. (2019). Covalently fluorophore-functionalized ZIF-8 colloidal particles as a sensing platform for endocrine-disrupting chemicals such as phthalates plasticizers. ACS omega 4, 17090–17097. doi:10.1021/acsomega.9b01051
Charbonnet, J. A., Mcdonough, C. A., Xiao, F., Schwichtenberg, T., Cao, D., Kaserzon, S., et al. (2022). Communicating confidence of per-and polyfluoroalkyl substance identification via high-resolution mass spectrometry. Environ. Sci. Technol. Lett. 9, 473–481. doi:10.1021/acs.estlett.2c00206
Charitos, I. A., Topi, S., Gagliano-Candela, R., De Nitto, E., Polimeno, L., Montagnani, M., et al. (2022). The toxic effects of endocrine disrupting chemicals (EDCs) on gut microbiota: Bisphenol A (BPA) A review. Endocr. Metab. Immune Disorders-Drug Targets 22, 716–727. doi:10.2174/1871530322666220325114045
Che Lah, N. F., Ahmad, A. L., Low, S. C., and Zaulkiflee, N. D. (2021). Isotherm and electrochemical properties of atrazine sensing using PVC/MIP: Effect of porogenic solvent concentration ratio. Membranes 11, 657. doi:10.3390/membranes11090657
Che Sulaiman, I., Chieng, B., Osman, M., Ong, K., Rashid, J., Wan Yunus, W., et al. (2020). A review on colorimetric methods for determination of organophosphate pesticides using gold and silver nanoparticles. Microchim. Acta 187, 131–222. doi:10.1007/s00604-019-3893-8
Chen, G. Y., Zhang, H., Zhao, C.-P., Zhang, C.-Y., Wang, Y., Chen, H., et al. (2021). On-line immobilized acetylcholinesterase microreactor based on capillary electrophoresis for the determination of organophosphorus pesticide residues. Chin. J. Anal. Chem. 49, 11–17. doi:10.1016/j.cjac.2021.09.004
Chen, H., Wang, C., Li, H., Ma, R., Yu, Z., Li, L., et al. (2019a). A review of toxicity induced by persistent organic pollutants (POPs) and endocrine-disrupting chemicals (EDCs) in the nematode Caenorhabditis elegans. J. Environ. Manag. 237, 519–525. doi:10.1016/j.jenvman.2019.02.102
Chen, Q., Zhu, P., Xiong, J., Gao, L., and Tan, K. (2019b). A sensitive and selective triple-channel optical assay based on red-emissive carbon dots for the determination of PFOS. Microchem. J. 145, 388–396. doi:10.1016/j.microc.2018.11.003
Chen, Y., Yang, J., Yao, B., Zhi, D., Luo, L., and Zhou, Y. (2022). Endocrine disrupting chemicals in the environment: Environmental sources, biological effects, remediation techniques, and perspective. Environmental Pollution.119918.
Chen, Z., Han, K., and Zhang, Y.-N. (2019c). Reflective fiber surface plasmon resonance sensor for high-sensitive mercury ion detection. Appl. Sci. 9, 1480. doi:10.3390/app9071480
Cheng, Z., Dong, H., Liang, J., Zhang, F., Chen, X., Du, L., et al. (2019). Highly selective fluorescent visual detection of perfluorooctane sulfonate via blue fluorescent carbon dots and berberine chloride hydrate. Spectrochimica Acta Part A Mol. Biomol. Spectrosc. 207, 262–269. doi:10.1016/j.saa.2018.09.028
Cheng, Z., Du, L., Zhu, P., Chen, Q., and Tan, K. (2018). An erythrosin B-based “turn on” fluorescent sensor for detecting perfluorooctane sulfonate and perfluorooctanoic acid in environmental water samples. Spectrochimica Acta Part A Mol. Biomol. Spectrosc. 201, 281–287. doi:10.1016/j.saa.2018.05.013
Cortese, M., Gigliobianco, M. R., Magnoni, F., Censi, R., and Di Martino, P. (2020). Compensate for or minimize matrix effects? Strategies for overcoming matrix effects in liquid chromatography-mass spectrometry technique: A tutorial review. Molecules 25, 3047. doi:10.3390/molecules25133047
De Coster, S., and Van Larebeke, N. (2012). Endocrine-disrupting chemicals: Associated disorders and mechanisms of action. J. Environ. public health 2012, 713696. doi:10.1155/2012/713696
Dhara, P., Kumar, R., Binetti, L., Nguyen, H. T., Alwis, L. S., Sun, T., et al. (2019). Optical fiber-based heavy metal detection using the localized surface plasmon resonance technique. IEEE Sensors J. 19, 8720–8726. doi:10.1109/jsen.2019.2921701
Dima, A. P., De Santis, L., Verlengia, C., Lombardo, F., Lenzi, A., Mazzarino, M., et al. (2020). Development and validation of a liquid chromatography-tandem mass spectrometry method for the simultaneous determination of phthalates and bisphenol a in serum, urine and follicular fluid. Clin. Mass Spectrom. 18, 54–65. doi:10.1016/j.clinms.2020.10.002
Du, W., Liu, J., Li, H., Deng, C., Luo, J., Feng, Q., et al. (2023). Competition-based two-dimensional photonic crystal dually cross-linked supramolecular hydrogel for colorimetric and fluorescent dual-mode sensing of bisphenol A. Anal. Chem. 95, 4220–4226. doi:10.1021/acs.analchem.2c05662
Faghiri, F., Hajjami, M., and Ghorbani, F. (2021). Development of a sensing system based on coupling magnetic solid phase extraction and colorimetric detection for determination of organophosphorus pesticides in fruit extract and environmental sample. Sensors Actuators B Chem. 343, 130157. doi:10.1016/j.snb.2021.130157
Fan, L., Zhang, C., Yan, W., Guo, Y., Shuang, S., Dong, C., et al. (2019). Design of a facile and label-free electrochemical aptasensor for detection of atrazine. Talanta 201, 156–164. doi:10.1016/j.talanta.2019.03.114
Feng, X.-J., Zhang, K., Shang, Y., and Pan, W. (2022). Thermal decomposition study of perchlorate-based metal-free molecular perovskite DAP-4 mixed with ammonium perchlorate. Case Stud. Therm. Eng. 34, 102013. doi:10.1016/j.csite.2022.102013
Fernandez, M., Mustieles, V., Suarez, B., Reina-Perez, I., Olivas-Martinez, A., and Vela-Soria, F. (2021). Determination of bisphenols, parabens, and benzophenones in placenta by dispersive liquid-liquid microextraction and gas chromatography-tandem mass spectrometry. Chemosphere 274, 129707. doi:10.1016/j.chemosphere.2021.129707
Fisher, M., Macpherson, S., Braun, J. M., Hauser, R., Walker, M., Feeley, M., et al. (2017). Paraben concentrations in maternal urine and breast milk and its association with personal care product use. Environ. Sci. Technol. 51, 4009–4017. doi:10.1021/acs.est.6b04302
Flint, S., Markle, T., Thompson, S., and Wallace, E. (2012). Bisphenol A exposure, effects, and policy: A wildlife perspective. J. Environ. Manag. 104, 19–34. doi:10.1016/j.jenvman.2012.03.021
Friberg, L. T., Elinder, G.-G., Kjellstrom, T., and Nordberg, G. F. (2019). Cadmium and health: A toxicological and epidemiological appraisal: Volume 2: Effects and response. CRC Press.
Furst, A. L., Hoepker, A. C., and Francis, M. B. (2017). Quantifying hormone disruptors with an engineered bacterial biosensor. ACS central Sci. 3, 110–116. doi:10.1021/acscentsci.6b00322
Gao, X., Kang, S., Xiong, R., and Chen, M. (2020). Environment-friendly removal methods for endocrine disrupting chemicals. Sustainability 12, 7615. doi:10.3390/su12187615
Genualdi, S., and deJager, L. (2021). Determination of 16 per and polyfluoroalkyl substances (PFAS) in processed food using liquid chromatography-tandem mass spectrometry (LC-MS/MS). U. S. Food Drug Adm. (FDA). Appl. Noteb. 39.
Griffete, N., Frederich, H., Maitre, A., Schwob, C., Ravaine, S., Carbonnier, B., et al. (2011). Introduction of a planar defect in a molecularly imprinted photonic crystal sensor for the detection of bisphenol A. J. colloid interface Sci. 364, 18–23. doi:10.1016/j.jcis.2011.07.028
Gunupuru, R., Maity, D., Vyas, G., and Paul, P. (2022). Water dispersible glycylglycine functionalized gold nanoparticles: Application in colorimetric sensing of Hg (II), Pb (II) and Cr (III) in aqueous media. J. Chem. Sci. 134, 80–10. doi:10.1007/s12039-022-02078-6
Guo, C., and Irudayaraj, J. (2011). Fluorescent Ag clusters via a protein-directed approach as a Hg (II) ion sensor. Anal. Chem. 83, 2883–2889. doi:10.1021/ac1032403
Habibullah-Al-Mamun, M., Ahmed, M. K., Islam, M. S., Tokumura, M., and Masunaga, S. J. E. (2019). Occurrence, distribution and possible sources of polychlorinated biphenyls (PCBs) in the surface water from the Bay of Bengal coast of Bangladesh. Ecotoxicol. Environ. Saf. 167, 450–458. doi:10.1016/j.ecoenv.2018.10.052
Hasan, G. A., Shaikh, M. a. A., Satter, M. A., and Hossain, M. S. (2022). Detection of indicator polychlorinated biphenyls (I-PCBs) and polycyclic aromatic hydrocarbons (PAHs) in cow milk from selected areas of Dhaka, Bangladesh and potential human health risks assessment. Toxicol. Rep. 9, 1514–1522. doi:10.1016/j.toxrep.2022.07.008
Hassan, S. S., Galal Eldin, A., Amr, A. E.-G. E., Al-Omar, M. A., and Kamel, A. H. (2019). Single-walled carbon nanotubes (SWCNTs) as solid-contact in all-solid-state perchlorate ISEs: Applications to fireworks and propellants analysis. Sensors 19, 2697. doi:10.3390/s19122697
Hayat, A., and Marty, J. L. (2014). Disposable screen printed electrochemical sensors: Tools for environmental monitoring. Sensors 14, 10432–10453. doi:10.3390/s140610432
Hayes, T. B., Anderson, L. L., Beasley, V. R., De Solla, S. R., Iguchi, T., Ingraham, H., et al. (2011). Demasculinization and feminization of male gonads by atrazine: Consistent effects across vertebrate classes. J. steroid Biochem. Mol. Biol. 127, 64–73. doi:10.1016/j.jsbmb.2011.03.015
Heindel, J. J., Newbold, R., and Schug, T. T. (2015). Endocrine disruptors and obesity. Nat. Rev. Endocrinol. 11, 653–661. doi:10.1038/nrendo.2015.163
Hoisaeter, A., Pfaff, A., and Breedveld, G. D. (2019). Leaching and transport of PFAS from aqueous film-forming foam (AFFF) in the unsaturated soil at a firefighting training facility under cold climatic conditions. J. Contam. hydrology 222, 112–122. doi:10.1016/j.jconhyd.2019.02.010
Hu, L.-Y., Niu, C.-G., Wang, X.-Y., Huang, D.-W., Zhang, L., and Zeng, G.-M. (2017). Magnetic separate" turn-on" fluorescent biosensor for Bisphenol A based on magnetic oxidation graphene. Talanta 168, 196–202. doi:10.1016/j.talanta.2017.03.055
Hu, Y., Lee, S., Kumar, P., Nian, Q., Wang, W., Irudayaraj, J., et al. (2015). Water flattens graphene wrinkles: Laser shock wrapping of graphene onto substrate-supported crystalline plasmonic nanoparticle arrays. Nanoscale 7, 19885–19893. doi:10.1039/c5nr04810a
Huang, Y., Xu, J., Liu, J., Wang, X., and Chen, B. (2017). Disease-related detection with electrochemical biosensors: A review. Sensors 17, 2375. doi:10.3390/s17102375
Inuyama, Y., Nakamura, C., Oka, T., Yoneda, Y., Obataya, I., Santo, N., et al. (2007). Simple and high-sensitivity detection of dioxin using dioxin-binding pentapeptide. Biosens. Bioelectron. 22, 2093–2099. doi:10.1016/j.bios.2006.09.016
Jebril, S., Cubillana Aguilera, L., Palacios Santander, J. M., and Dridi, C. (2021). A novel electrochemical sensor modified with green gold sononanoparticles and carbon black nanocomposite for bisphenol A detection. Mater. Sci. Eng. B 264, 114951. doi:10.1016/j.mseb.2020.114951
Jia, M., Sha, J., Li, Z., Wang, W., and Zhang, H. (2020). High affinity truncated aptamers for ultra-sensitive colorimetric detection of bisphenol A with label-free aptasensor. Food Chem. 317, 126459. doi:10.1016/j.foodchem.2020.126459
Kabir, E. R., Rahman, M. S., and Rahman, I. (2015). A review on endocrine disruptors and their possible impacts on human health. Environ. Toxicol. Pharmacol. 40, 241–258. doi:10.1016/j.etap.2015.06.009
Kajta, M., and Wojtowicz, A. K. (2013). Impact of endocrine-disrupting chemicals on neural development and the onset of neurological disorders. Pharmacol. Rep. 65, 1632–1639. doi:10.1016/s1734-1140(13)71524-x
Kancharla, S., Alexandridis, P., and Tsianou, M. (2022). Sequestration of per- and polyfluoroalkyl substances (PFAS) by adsorption: Surfactant and surface aspects. Curr. Opin. Colloid Interface Sci. 58, 101571. doi:10.1016/j.cocis.2022.101571
Kaya, H. K., Cinar, S., Altundal, G., Bayramlı, Y., Unaleroglu, C., and Kuralay, F. J. S. (2021). A novel design thia-bilane structure-based molecular imprinted electrochemical sensor for sensitive and selective dopamine determination. Sensors Actuators B Chem. 346, 130425. doi:10.1016/j.snb.2021.130425
Keskin, B., Uzer, A., and Apak, R. (2020). Colorimetric sensing of ammonium perchlorate using methylene blue− modified gold nanoparticles. Talanta 206, 120240. doi:10.1016/j.talanta.2019.120240
Keskin, B., Uzer, A., and Apak, R. (2022). Ionic liquid-modified gold nanoparticle-based colorimetric sensor for perchlorate detection via Anion− π interaction. ACS omega.
Lauretta, R., Sansone, A., Sansone, M., Romanelli, F., and Appetecchia, M. (2019). Endocrine disrupting chemicals: Effects on endocrine glands. Front. Endocrinol. 10, 178. doi:10.3389/fendo.2019.00178
Lee, E.-H., Lee, S. K., Kim, M. J., and Lee, S.-W. (2019). Simple and rapid detection of bisphenol A using a gold nanoparticle-based colorimetric aptasensor. Food Chem. 287, 205–213. doi:10.1016/j.foodchem.2019.02.079
Lee, J., Kim, L., Shin, Y., Lee, J., Lee, J., Kim, E., et al. (2017). Rapid and simultaneous analysis of 360 pesticides in Brown rice, spinach, orange, and potato using microbore GC-MS/MS. J. Agric. Food Chem. 65, 3387–3395. doi:10.1021/acs.jafc.7b00576
Lee, K., and Irudayaraj, J. (2013). Correct spectral conversion between surface-enhanced Raman and plasmon resonance scattering from nanoparticle dimers for single-molecule detection. Small 9, 1106–1115. doi:10.1002/smll.201201985
Lee, S., Kumar, P., Hu, Y., Cheng, G. J., and Irudayaraj, J. (2015). Graphene laminated gold bipyramids as sensitive detection platforms for antibiotic molecules. Chem. Commun. 51, 15494–15497. doi:10.1039/c5cc04890g
Lei, S.-N., and Cong, H. (2022). Fluorescence detection of perfluorooctane sulfonate in water employing a tetraphenylethylene-derived dual macrocycle BowtieCyclophane. Chin. Chem. Lett. 33, 1493–1496. doi:10.1016/j.cclet.2021.08.068
Li, D., Qiao, X., Lu, J., and Xu, Z. (2018a). Synthesis and evaluation of a magnetic molecularly imprinted polymer sorbent for determination of trace trichlorfon residue in vegetables by capillary electrophoresis. Adv. Polym. Technol. 37, 968–976. doi:10.1002/adv.21745
Li, J., Hu, X., Zhou, Y., Zhang, L., Ge, Z., Wang, X., et al. (2019). β-Cyclodextrin-stabilized Au nanoparticles for the detection of butyl benzyl phthalate. ACS Appl. Nano Mater. 2, 2743–2751. doi:10.1021/acsanm.9b00258
Li, Q., Bai, J., Ren, S., Wang, J., Gao, Y., Li, S., et al. (2019). An ultrasensitive sensor based on quantitatively modified upconversion particles for trace bisphenol A detection. Anal. Bioanal. Chem. 411, 171–179. doi:10.1007/s00216-018-1425-8
Li, W.-M., Tang, Y.-J., Chen, L., Chen, Y.-P., and Yan, P. (2021). Quartz crystal microbalance-based method to study adsorption of endocrine disruptor compounds on zeolite. Environ. Technol. 42, 3025–3035. doi:10.1080/09593330.2020.1720308
Li, X., Cui, H., and Zeng, Z. (2018b). A simple colorimetric and fluorescent sensor to detect organophosphate pesticides based on adenosine triphosphate-modified gold nanoparticles. Sensors 18, 4302. doi:10.3390/s18124302
Li, X., Wang, X., Fang, T., Zhang, L., and Gong, J. (2018c). Disposable photoelectrochemical sensing strip for highly sensitive determination of perfluorooctane sulfonyl fluoride on functionalized screen-printed carbon electrode. Talanta 181, 147–153. doi:10.1016/j.talanta.2018.01.005
Liang, Y.-R., Zhang, Z.-M., Liu, Z.-J., Wang, K., Wu, X.-Y., Zeng, K., et al. (2017). A highly sensitive signal-amplified gold nanoparticle-based electrochemical immunosensor for dibutyl phthalate detection. Biosens. Bioelectron. 91, 199–202. doi:10.1016/j.bios.2016.12.007
Liao, C.-Y., Cui, L., Zhou, Q.-F., Duan, S.-M., and Jiang, G.-B. (2009). Effects of perfluorooctane sulfonate on ion channels and glutamate-activated current in cultured rat hippocampal neurons. Environ. Toxicol. Pharmacol. 27, 338–344. doi:10.1016/j.etap.2008.11.013
Lim, H. J., Kim, A.-R., Yoon, M.-Y., You, Y., Chua, B., and Son, A. (2018). Development of quantum dot aptasensor and its portable analyzer for the detection of di-2-ethylhexyl phthalate. Biosens. Bioelectron. 121, 1–9. doi:10.1016/j.bios.2018.08.065
Liu, Y., Lin, X., Ji, X., Hao, Z., and Tao, Z. (2020). Smartphone-based enzyme-free fluorescence sensing of organophosphate DDVP. Microchim. Acta 187, 419–428. doi:10.1007/s00604-020-04384-7
Liu, Y., Yao, L., He, L., Liu, N., and Piao, Y. (2019). Electrochemical enzyme biosensor bearing biochar nanoparticle as signal enhancer for bisphenol a detection in water. Sensors 19, 1619. doi:10.3390/s19071619
Lopez-Rodriguez, D., Franssen, D., Bakker, J., Lomniczi, A., and Parent, A.-S. (2021). Cellular and molecular features of EDC exposure: Consequences for the GnRH network. Nat. Rev. Endocrinol. 17, 83–96. doi:10.1038/s41574-020-00436-3
Lu, X., Sun, J., and Sun, X. (2020). Recent advances in biosensors for the detection of estrogens in the environment and food. TrAC Trends Anal. Chem. 127, 115882. doi:10.1016/j.trac.2020.115882
Luo, R., Su, X., Xu, W., Zhang, S., Zhuo, X., and Ma, D. (2017). Determination of arsenic and lead in single hair strands by laser ablation inductively coupled plasma mass spectrometry. Sci. Rep. 7, 3426–3427. doi:10.1038/s41598-017-03660-6
Ma, Y., Jiang, H., Shen, C., Hou, C., Huo, D., Wu, H., et al. (2017). Detection of carbendazim residues with a colorimetric sensor based on gold nanoparticles. J. Appl. Spectrosc. 84, 460–465. doi:10.1007/s10812-017-0492-5
Marinello, W. P., and Patisaul, H. B. (2021). Endocrine disrupting chemicals (EDCs) and placental function: Impact on fetal brain development. Adv. Pharmacol. 92, 347–400. doi:10.1016/bs.apha.2021.04.003
Meeker, J. D. (2012). Exposure to environmental endocrine disruptors and child development. Archives Pediatr. Adolesc. Med. 166, E1–E7. doi:10.1001/archpediatrics.2012.241
Mesa, R., Kabir, A., Samanidou, V., and Furton, K. G. (2019). Simultaneous determination of selected estrogenic endocrine disrupting chemicals and bisphenol A residues in whole milk using fabric phase sorptive extraction coupled to HPLC-UV detection and LC-MS/MS. J. Sep. Sci. 42, 598–608. doi:10.1002/jssc.201800901
Metcalfe, C., Bayen, S., Desrosiers, M., Munoz, G., Sauve, S., and Yargeau, V. (2022). Methods for the analysis of endocrine disrupting chemicals in selected environmental matrixes. Environ. Res. 206, 112616. doi:10.1016/j.envres.2021.112616
Mohapatra, S., Bera, M. K., and Das, R. K. (2018). Rapid “turn-on” detection of atrazine using highly luminescent N-doped carbon quantum dot. Sensors Actuators B Chem. 263, 459–468. doi:10.1016/j.snb.2018.02.155
Moon, J. S., Lee, Y., Shin, D. M., Kim, C., Kim, W. G., Park, M., et al. (2016). Identification of endocrine disrupting chemicals using a virus-based colorimetric sensor. Chemistry–An Asian J. 11, 3097–3101. doi:10.1002/asia.201601079
Muller, A.-K., Markert, N., Leser, K., Kampfer, D., Crawford, S. E., Schaffer, A., et al. (2020). Assessing endocrine disruption in freshwater fish species from a “hotspot” for estrogenic activity in sediment. Environ. Pollut. 257, 113636. doi:10.1016/j.envpol.2019.113636
Mumtaz, M., Bao, Y., Liu, L., Huang, J., Cagnetta, G., and Yu, G. J. E. S. (2019). Per-and polyfluoroalkyl substances in representative fluorocarbon surfactants used in Chinese film-forming foams: Levels, profile shift, and environmental implications. Environ. Sci. Technol. Lett. 6, 259–264. doi:10.1021/acs.estlett.9b00154
Naing, N. N., Goh, E. X. Y., and Lee, H. K. (2021). Enhanced microextraction of endocrine disrupting chemicals adsorbed on airborne fine particulate matter with gas chromatography–tandem mass spectrometric analysis. J. Chromatogr. A 1637, 461828. doi:10.1016/j.chroma.2020.461828
Nalbone, G., Cicolella, A., and Laot-Cabon, S. (2013). Perturbateurs endocriniens et maladies métaboliques: Un défi majeur en santé publique. Sante Publique 25, 45–49. doi:10.3917/spub.131.0045
Nilsson, S., Thompson, J., Mueller, J. F., and BraUnig, J. (2022). Apparent half-lives of chlorinated-perfluorooctane sulfonate and perfluorooctane sulfonate isomers in aviation firefighters. Environ. Sci. Technol. 56, 17052–17060. doi:10.1021/acs.est.2c04637
Nsibande, S. A., and Forbes, P. B. (2019). Development of a quantum dot molecularly imprinted polymer sensor for fluorescence detection of atrazine. Luminescence 34, 480–488. doi:10.1002/bio.3620
O'sullivan, C. K. (2002). Aptasensors–the future of biosensing? Anal. Bioanal. Chem. 372, 44–48. doi:10.1007/s00216-001-1189-3
Ouyang, Q., Wang, L., Ahmad, W., Rong, Y., Li, H., Hu, Y., et al. (2021). A highly sensitive detection of carbendazim pesticide in food based on the upconversion-MnO2 luminescent resonance energy transfer biosensor. Food Chem. 349, 129157. doi:10.1016/j.foodchem.2021.129157
Palacios Colon, L., Rascon, A. J., Hejji, L., Azzouz, A., and Ballesteros, E. (2021). Validation and use of an accurate, sensitive method for sample preparation and gas chromatography–mass spectrometry determination of different endocrine-disrupting chemicals in dairy products. Foods 10, 1040. doi:10.3390/foods10051040
Petrarca, M. H., Menezes-Sousa, D., Ferreira, R., Fernandes, J. O., Vieira, L. R., Guilhermino, L., et al. (2022). Occurrence and risk assessment of endocrine-disrupting compounds in fish muscle: The case study of the Douro River (North East Atlantic Ocean). Environmental Research, 114236.
Pironti, C., Ricciardi, M., Proto, A., Bianco, P. M., Montano, L., and Motta, O. (2021). Endocrine-disrupting compounds: An overview on their occurrence in the aquatic environment and human exposure. Water 13, 1347. doi:10.3390/w13101347
Pontius, F. (2019). Regulation of perfluorooctanoic acid (pfoa) and perfluorooctane sulfonic acid (pfos) in drinking water: A comprehensive review. Water 11, 2003. doi:10.3390/w11102003
Preciados, M., Yoo, C., and Roy, D. (2016). Estrogenic endocrine disrupting chemicals influencing NRF1 regulated gene networks in the development of complex human brain diseases. Int. J. Mol. Sci. 17, 2086. doi:10.3390/ijms17122086
Qu, K., Bai, Y., Liu, X., Deng, M., Wu, L., and Chen, Z. (2019). Electro-catalytic behavior of multiwall carbon nanotube-doped poly (3, 4-ethylenedioxythiophene) toward environmental pollutant bisphenol A. J. Electrochem. Soc. 166, H810–H818. doi:10.1149/2.0951915jes
Ranaweera, R., Ghafari, C., and Luo, L. (2019). Bubble-nucleation-based method for the selective and sensitive electrochemical detection of surfactants. Anal. Chem. 91, 7744–7748. doi:10.1021/acs.analchem.9b01060
Ren, W., Mura, S., and Irudayaraj, J. M. (2015a). Modified graphene oxide sensors for ultra-sensitive detection of nitrate ions in water. Talanta 143, 234–239. doi:10.1016/j.talanta.2015.05.073
Ren, W., Zhou, Z., and Irudayaraj, J. M. (2015b). Trichloroethylene sensing in water based on SERS with multifunctional Au/TiO 2 core–shell nanocomposites. Analyst 140, 6625–6630. doi:10.1039/c5an01294e
Rocha, M. J., Madureira, T. V., Venade, C. S., Martins, I., Campos, J., and Rocha, E. (2019). Presence of estrogenic endocrine disruptors in three European estuaries in Northwest Iberian Peninsula (Portugal). Toxicol. Environ. Chem. 101, 244–264. doi:10.1080/02772248.2019.1692019
Rodrigues, C., Souza, V. G. L., Coelhoso, I., and Fernando, A. L. (2021). Bio-based sensors for smart food packaging—current applications and future trends. Sensors 21, 2148. doi:10.3390/s21062148
Roy, D., Palangat, M., Chen, C.-W., Thomas, R. D., Colerangle, J., Atkinson, A., et al. (1997). Biochemical and molecular changes at the cellular level in response to exposure to environmental estrogen-like chemicals. J. Toxicol. Environ. Health 50, 1–30. doi:10.1080/009841097160573
Sabuz Vidal, O., Deepika, D., Schuhmacher, M., and Kumar, V. J. C. R. I. T. (2021). EDC-Induced mechanisms of immunotoxicity: A systematic review. Crit. Rev. Toxicol. 51, 634–652. doi:10.1080/10408444.2021.2009438
Sahin, S., Ustundag, Z., and Caglayan, M. O. (2022). Spectroscopic ellipsometry-based aptasensor platform for bisphenol a detection. Talanta, 123885.
Sakhteman, A., Failli, M., Kublbeck, J., Levonen, A., and Fortino, V. (2021). A toxicogenomic data space for system-level understanding and prediction of EDC-induced toxicity. Environ. Int. 156, 106751. doi:10.1016/j.envint.2021.106751
Sargis, R. M. (2015). Metabolic disruption in context: Clinical avenues for synergistic perturbations in energy homeostasis by endocrine disrupting chemicals. Endocr. Disruptors 3, e1080788. doi:10.1080/23273747.2015.1080788
Scognamiglio, V., Antonacci, A., Patrolecco, L., Lambreva, M. D., Litescu, S. C., Ghuge, S. A., et al. (2016). Analytical tools monitoring endocrine disrupting chemicals. TrAC Trends Anal. Chem. 80, 555–567. doi:10.1016/j.trac.2016.04.014
Shah, M. M., Ahmad, K., Ahmad, B., Shah, S. M., Masood, H., Siddique, M. a. R., et al. (2022). Recent trends in green synthesis of silver, gold, and zinc oxide nanoparticles and their application in nanosciences and toxicity: A review. Nanotechnol. Environ. Eng. 7, 907–922. doi:10.1007/s41204-022-00287-5
Shah, M. M., Ren, W., Irudayaraj, J., Sajini, A. A., Ali, M. I., and Ahmad, B. (2021). Colorimetric detection of organophosphate pesticides based on acetylcholinesterase and cysteamine capped gold nanoparticles as nanozyme. Sensors 21, 8050. doi:10.3390/s21238050
Shah, P., Zhu, X., and Li, C.-Z. (2013). Development of paper-based analytical kit for point-of-care testing. Expert Rev. Mol. diagnostics 13, 83–91. doi:10.1586/erm.12.130
Sheng, W., Shi, Y., Ma, J., Wang, L., Zhang, B., Chang, Q., et al. (2019). Highly sensitive atrazine fluorescence immunoassay by using magnetic separation and upconversion nanoparticles as labels. Microchim. Acta 186, 564–569. doi:10.1007/s00604-019-3667-3
Sofen, L. E., and Furst, A. L. (2019). Perspective—Electrochemical sensors to monitor endocrine disrupting pollutants. J. Electrochem. Soc. 167, 037524. doi:10.1149/2.0242003jes
Stefan-Van Staden, R.-I., Moscalu-Lungu, A., and Van Staden, J. F. (2019). Nanostructured materials used for pattern recognition of bisphenols in waste water samples. J. Electrochem. Soc. 166, B903–B907. doi:10.1149/2.0521912jes
St. John, P. M., Kienzle, S. D., Flood, B. M., Moody, T. V., Depaola, N. F., and Mass, S. (2021). Extracting and quantifying deuterated bisphenol A from planaria using gas chromatography/mass spectrometry: An integrative laboratory experiment. J. Chem. Educ. 98, 2090–2096. doi:10.1021/acs.jchemed.0c01088
Streifer, M., and Gore, A. C. (2021). Epigenetics, estrogenic endocrine-disrupting chemicals (EDCs), and the brain. Adv. Pharmacol. 92, 73–99. doi:10.1016/bs.apha.2021.03.006
Su, Z., Li, Y., Li, J., and Dou, X. (2021). Ultrasensitive luminescent turn-on detection of perchlorate particulates by triggering supramolecular self-assembly of platinum (II) complex in hydrogel matrix. Sensors Actuators B Chem. 336, 129728. doi:10.1016/j.snb.2021.129728
Su, Z., Li, Y., Li, J., Li, K., and Dou, X. (2022). Ultrasensitive dual-mode visualization of perchlorate in water, soil and air boosted by close and stable Pt–Pt packing endowed low-energy absorption and emission. J. Mater. Chem. A 10, 8195–8207. doi:10.1039/d2ta00843b
Sun, C., Li, K., Zhang, J., and Huang, C. (2022). Prediction of phthalates concentration in household dust based on back propagation neural network. Indoor Built Environ. 31, 230–244. doi:10.1177/1420326x211010212
Sun, J., Guo, L., Bao, Y., and Xie, J. (2011). A simple, label-free AuNPs-based colorimetric ultrasensitive detection of nerve agents and highly toxic organophosphate pesticide. Biosens. Bioelectron. 28, 152–157. doi:10.1016/j.bios.2011.07.012
Supraja, P., Tripathy, S., Vanjari, S. R. K., Singh, V., and Singh, S. G. (2019). Electrospun tin (IV) oxide nanofiber based electrochemical sensor for ultra-sensitive and selective detection of atrazine in water at trace levels. Biosens. Bioelectron. 141, 111441. doi:10.1016/j.bios.2019.111441
Tian, Y., Xu, H., Liu, S., Fang, M., Wu, Y., and Gong, Z. (2020). Study on the bioaccessibility and bioavailability of perchlorate in different food matrices in vitro. Food Chem. 333, 127470. doi:10.1016/j.foodchem.2020.127470
Urseler, N., Bachetti, R., Biolé, F., Morgante, V., and Morgante, C. (2022). Atrazine pollution in groundwater and raw bovine milk: Water quality, bioaccumulation and human risk assessment. Sci. Total Environ. 852, 158498. doi:10.1016/j.scitotenv.2022.158498
Vandenberg, L. N., Colborn, T., Hayes, T. B., Heindel, J. J., Jacobs, D. R., Lee, D.-H., et al. (2012). Hormones and endocrine-disrupting chemicals: Low-dose effects and nonmonotonic dose responses. Endocr. Rev. 33, 378–455. doi:10.1210/er.2011-1050
Vandenberg, L. N. (2019). Low dose effects challenge the evaluation of endocrine disrupting chemicals. Trends Food Sci. Technol. 84, 58–61. doi:10.1016/j.tifs.2018.11.029
Vieira, W. T., De Farias, M. B., Spaolonzi, M. P., Da Silva, M. G. C., and Vieira, M. G. A. (2021). Endocrine-disrupting compounds: Occurrence, detection methods, effects and promising treatment pathways—a critical review. J. Environ. Chem. Eng. 9, 104558. doi:10.1016/j.jece.2020.104558
Vu, T. H. V., Lim, H. H., and Shin, H. S. (2020). Determination of 15 biomarkers of endocrine disrupting chemicals in human saliva by gas chromatography–mass spectrometry. Bull. Korean Chem. Soc. 41, 424–432. doi:10.1002/bkcs.11986
Wan, Y., Tran, T. M., Nguyen, V. T., Wang, A., Wang, J., and Kannan, K. (2021). Neonicotinoids, fipronil, chlorpyrifos, carbendazim, chlorotriazines, chlorophenoxy herbicides, bentazon, and selected pesticide transformation products in surface water and drinking water from northern Vietnam. Sci. Total Environ. 750, 141507. doi:10.1016/j.scitotenv.2020.141507
Wang, S.-W., Hsu, K.-H., Huang, S.-C., Tseng, S.-H., Wang, D.-Y., and Cheng, H.-F. (2019). Determination of polycyclic aromatic hydrocarbons (PAHs) in cosmetic products by gas chromatography-tandem mass spectrometry. J. food drug analysis 27, 815–824. doi:10.1016/j.jfda.2019.01.003
Wang, S., Pan, M., Liu, K., Xie, X., Yang, J., Hong, L., et al. (2022). A SiO2@ MIP electrochemical sensor based on MWCNTs and AuNPs for highly sensitive and selective recognition and detection of dibutyl phthalate. Food Chem. 381, 132225. doi:10.1016/j.foodchem.2022.132225
Xu, B., Liu, S., Zhou, J. L., Zheng, C., Weifeng, J., Chen, B., et al. (2021). PFAS and their substitutes in groundwater: Occurrence, transformation and remediation. J. Hazard. Mater. 412, 125159. doi:10.1016/j.jhazmat.2021.125159
Xue, C. S., Erika, G., and Jiri, H. (2019). Surface plasmon resonance biosensor for the ultrasensitive detection of bisphenol A. Anal. Bioanal. Chem. 411, 5655–5658. doi:10.1007/s00216-019-01996-8
Yan, X., Kong, D., Jin, R., Zhao, X., Li, H., Liu, F., et al. (2019). Fluorometric and colorimetric analysis of carbamate pesticide via enzyme-triggered decomposition of Gold nanoclusters-anchored MnO2 nanocomposite. Sensors Actuators B Chem. 290, 640–647. doi:10.1016/j.snb.2019.04.045
Yan, X., Li, H., Wang, X., and Su, X. (2015). A novel fluorescence probing strategy for the determination of parathion-methyl. Talanta 131, 88–94. doi:10.1016/j.talanta.2014.07.032
Yang, F.-W., Zhao, G.-P., Ren, F.-Z., Pang, G.-F., and Li, Y.-X. (2020). Assessment of the endocrine-disrupting effects of diethyl phosphate, a nonspecific metabolite of organophosphorus pesticides, by in vivo and in silico approaches. Environ. Int. 135, 105383. doi:10.1016/j.envint.2019.105383
Yin, X. L., Liu, Y. Q., Gu, H.-W., Zhang, Q., Zhang, Z. W., Li, H., et al. (2021). Multicolor enzyme-linked immunosorbent sensor for sensitive detection of organophosphorus pesticides based on TMB2+-mediated etching of gold nanorods. Microchem. J. 168, 106411. doi:10.1016/j.microc.2021.106411
Zahran, M., Khalifa, Z., Zahran, M. a.-H., and Abdel Azzem, M. (2020). Dissolved organic matter-capped silver nanoparticles for electrochemical aggregation sensing of atrazine in aqueous systems. ACS Appl. Nano Mater. 3, 3868–3875. doi:10.1021/acsanm.0c00597
Zama, A. M., and Uzumcu, M. (2010). Epigenetic effects of endocrine-disrupting chemicals on female reproduction: An ovarian perspective. Front. Neuroendocrinol. 31, 420–439. doi:10.1016/j.yfrne.2010.06.003
Zastrow, L., Speer, K., Schwind, K.-H., and Jira, W. (2021). A sensitive GC–HRMS method for the simultaneous determination of parent and oxygenated polycyclic aromatic hydrocarbons in barbecued meat and meat substitutes. Food Chem. 365, 130625. doi:10.1016/j.foodchem.2021.130625
Zatrochova, S., Martinez-Perez-Cejuela, H., Catala-Icardo, M., Simo-Alfonso, E., Lhotska, I., Satinsky, D., et al. (2022). Development of hybrid monoliths incorporating metal–organic frameworks for stir bar sorptive extraction coupled with liquid chromatography for determination of estrogen endocrine disruptors in water and human urine samples. Microchim. Acta 189, 92–10. doi:10.1007/s00604-022-05208-6
Zhang, J., Zang, L., Wang, T., Wang, X., Jia, M., Zhang, D., et al. (2020). A solid-phase extraction method for estrogenic disrupting compounds based on the estrogen response element. Food Chem. 333, 127529. doi:10.1016/j.foodchem.2020.127529
Zhang, M.-C., Wang, Q.-E., and Zhuang, H.-S. (2006). A novel competitive fluorescence immunoassay for the determination of dibutyl phthalate. Anal. Bioanal. Chem. 386, 1401–1406. doi:10.1007/s00216-006-0703-z
Zhang, S. X., Xue, S.-F., Deng, J., Zhang, M., Shi, G., and Zhou, T. (2016). Polyacrylic acid-coated cerium oxide nanoparticles: An oxidase mimic applied for colorimetric assay to organophosphorus pesticides. Biosens. Bioelectron. 85, 457–463. doi:10.1016/j.bios.2016.05.040
Zhao, G., Zhou, B., Wang, X., Shen, J., and Zhao, B. (2021). Detection of organophosphorus pesticides by nanogold/mercaptomethamidophos multi-residue electrochemical biosensor. Food Chem. 354, 129511. doi:10.1016/j.foodchem.2021.129511
Zhao, S., Zhou, T., Khan, A., Chen, Z., Liu, P., and Li, X. (2022). A novel electrochemical biosensor for bisphenol A detection based on engineered Escherichia coli cells with a surface-display of tyrosinase. Sensors Actuators B Chem. 353, 131063. doi:10.1016/j.snb.2021.131063
Zhou, H. M., Bao, Y. F., Huang, W. P., Yin, D. Q., and Hu, X. L. (2022). Simultaneous determination of 31 endocrine disrupting chemicals in fish plasma by solid-phase extraction coupled with ultra-performance liquid chromatography-tandem mass spectrometry. J. Sep. Sci. 45, 4128–4140. doi:10.1002/jssc.202200455
Zhou, Z., Li, T., Xu, W., Huang, W., Wang, N., and Yang, W. (2017). Synthesis and characterization of fluorescence molecularly imprinted polymers as sensor for highly sensitive detection of dibutyl phthalate from tap water samples. Sensors Actuators B Chem. 240, 1114–1122. doi:10.1016/j.snb.2016.09.092
Keywords: endocrine-disrupting chemicals, environmental contaminants detection, analytical technics, biosensors, human health and disease
Citation: Shah MM, Ahmad K, Boota S, Jensen T, La Frano MR and Irudayaraj J (2023) Sensor technologies for the detection and monitoring of endocrine-disrupting chemicals. Front. Bioeng. Biotechnol. 11:1141523. doi: 10.3389/fbioe.2023.1141523
Received: 10 January 2023; Accepted: 14 March 2023;
Published: 27 March 2023.
Edited by:
Xiaodong Zhang, Nanyang Technological University, SingaporeReviewed by:
Forough Ghasemi, Agricultural Biotechnology Research Institute of Iran, IranCopyright © 2023 Shah, Ahmad, Boota, Jensen, La Frano and Irudayaraj. This is an open-access article distributed under the terms of the Creative Commons Attribution License (CC BY). The use, distribution or reproduction in other forums is permitted, provided the original author(s) and the copyright owner(s) are credited and that the original publication in this journal is cited, in accordance with accepted academic practice. No use, distribution or reproduction is permitted which does not comply with these terms.
*Correspondence: Joseph Irudayaraj, amlydWRheWFAaWxsaW5vaXMuZWR1
Disclaimer: All claims expressed in this article are solely those of the authors and do not necessarily represent those of their affiliated organizations, or those of the publisher, the editors and the reviewers. Any product that may be evaluated in this article or claim that may be made by its manufacturer is not guaranteed or endorsed by the publisher.
Research integrity at Frontiers
Learn more about the work of our research integrity team to safeguard the quality of each article we publish.