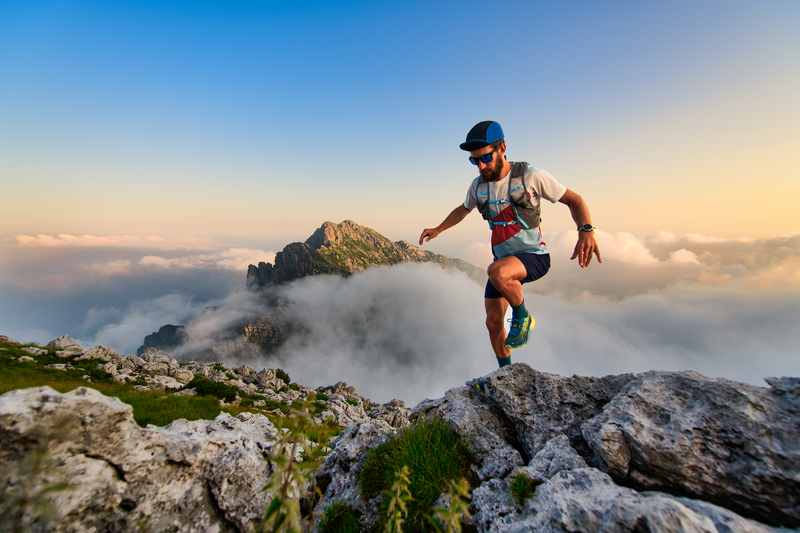
95% of researchers rate our articles as excellent or good
Learn more about the work of our research integrity team to safeguard the quality of each article we publish.
Find out more
REVIEW article
Front. Bioeng. Biotechnol. , 24 March 2023
Sec. Nanobiotechnology
Volume 11 - 2023 | https://doi.org/10.3389/fbioe.2023.1136583
This article is part of the Research Topic Advances in 3D Cell Culture for Drug Screening and Toxicology Evaluation View all 8 articles
Microgravity has been shown to induce many changes in cell growth and differentiation due to offloading the gravitational strain normally exerted on cells. Although many studies have used two-dimensional (2D) cell culture systems to investigate the effects of microgravity on cell growth, three-dimensional (3D) culture scaffolds can offer more direct indications of the modified cell response to microgravity-related dysregulations compared to 2D culture methods. Thus, knowledge of 3D cell culture is essential for better understanding the in vivo tissue function and physiological response under microgravity conditions. This review discusses the advances in 2D and 3D cell culture studies, particularly emphasizing the role of hydrogels, which can provide cells with a mimic in vivo environment to collect a more natural response. We also summarized recent studies about cell growth and differentiation under real microgravity or simulated microgravity conditions using ground-based equipment. Finally, we anticipate that hydrogel-based 3D culture models will play an essential role in constructing organoids, discovering the causes of microgravity-dependent molecular and cellular changes, improving space tissue regeneration, and developing innovative therapeutic strategies. Future research into the 3D culture in microgravity conditions could lead to valuable therapeutic applications in health and pharmaceuticals.
For a long time, two-dimensional (2D) cell culture has been used to study the physiological activities of cells in the complex human body using readily available flat plastic dishes. In a 2D culture system, the cells spread on flat and hard surfaces and proliferate unnaturally. As a result, their cellular morphology, functions, and overall behavior differ from those in the natural environment (Xu et al., 2000). Cells in the natural environment are embedded in the extracellular matrix (ECM), a fibrous three-dimensional (3D) structure, which ought to be viewed as a natural hydrogel (Schaefer and Schaefer, 2010). It is now possible to create these hydrogels that replicate ECM in vitro by using well-defined biopolymer models (collagen and fibrin) and other synthetic polymer models (Grinnell and Petroll, 2010; Liu et al., 2019). From an architectural perspective, the fibrous network of hydrogels with relatively large (on the order of millimeter size) holes makes it easy for cell growth, spreading, and metabolic chemicals to move between cells (Kikuchi et al., 2017). Because of these properties, hydrogels have been widely used in tissue engineering (Hou et al., 2022; Tarsitano et al., 2022).
Gravity impacts both physical and biological events on earth, influencing the development, equilibrium, and evolution of living systems. Reduced gravitational forces in space (microgravity: 10–3∼10–4 g) cause physiological changes in the human body, mainly in weight-bearing structures (White and Averner, 2001). The combined reactions to such changes and the re-adaptation of the human body during spaceflight and again upon re-entry pose substantial health dangers to space explorers. Space medicine research in the 21st century has tackled these health problems to better grasp the unknown mechanisms behind physiological alterations. Non-etheless, the medical curiosity about how these changes are organized extends beyond human space missions (Hides et al., 2017), calling for more extensive research in microgravity and countermeasure programs.
Cells were typically 2D exposed to real microgravity (RMG) in space or simulated microgravity (SMG) generated by a random positioning machine (RPM), which is a clinostat or a rotating wall vessel (RWV) bioreactor to investigate the effects of microgravity on cell growth (Briegleb, 1992; Goodwin et al., 1992). However, as previously stated, 2D-culture models are challenging to imitate real tissue. Thus, biotechnology and engineering advancements have permitted deploying more complex equipment and hydrogel-based 3D system for ground-based microgravity research. A fundamental understanding of biological alterations under microgravity conditions is critical not only for supporting human presence in space exploration but also for drug development and the potential development of novel tissue engineering and regenerative medicine.
This review focuses on the advances in cell growth and development achieved by culturing cells 2D and 3D under microgravity, particularly emphasizing the role of hydrogels-based 3D cell models. We anticipate that hydrogel-based 3D culture models will play an essential role in constructing organoids, discovering the causes of microgravity-dependent molecular and cellular changes, improving space medicine, and developing innovative therapeutic strategies.
The tissue’s function is determined by cellular and non-cellular components (Nerger and Nelson, 2020). The more accurately a cell culture system can replicate such settings, the better cells will mimic the behaviors and reactions of cells in vivo. This is why 3D cell cultures are intriguing. 3D cell culture takes another step toward keeping cells alive, growing, and behaving as they do in vivo by focusing on simulating natural cell-matrix and cell-cell interactions (Park et al., 2021). 3D cell culture, in conjunction with a biomaterials-based scaffold, provides researchers with an unequaled capacity to replicate physiological compositions and spatial arrangements of cells in vitro.
Various 3D culture methods use biomaterials to increase the efficiency of culture and cell activities in various forms, such as hydrogels, solid scaffolds, decellularized natural tissue, and ultra-low attachment (ULA) surfaces (Tibbitt and Anseth, 2009; Fan et al., 2020). Knowledge of 3D culture techniques has grown dramatically, resulting in the creation of several applications. For example, hydrogels have similar qualities to natural ECMs, such as biocompatibility, biodegradability, and adjustable properties (such as shape, gel state, and mechanical strength) (Hwang et al., 2009; Lei et al., 2011a; Lei et al., 2011b; Smeriglio et al., 2015; Zhou et al., 2019; Wang et al., 2021a; Fournier and Harrison, 2021; Ma et al., 2021; Okita et al., 2021). In recent years, hydrogels have gotten much interest in tissue engineering research, and they are the 3D culture materials we will primarily discuss.
Hydrogels comprise natural, synthetic, and semi-synthetic polymers. Natural hydrogels are composed of natural components such as collagen, alginate, hyaluronic acid (HA), and others that support numerous biological activities by including various endogenous elements that can improve the survival, proliferation, and differentiation of many cell types (Lou et al., 2018; Jose et al., 2020). Synthetic hydrogels are made up of artificial molecules such as polyvinyl alcohol (PVA), poly-2-hydroxyethyl methacrylate (pHEMA), polyethylene glycol (PEG), and polyisocyanopeptide (PIC), which can give mechanical support to various cell types. At the same time, they are physiologically inactive and lack endogenous components (Zhu, 2010; Wang et al., 2021b). Thus, synthetic hydrogels must be modified with appropriate biological components to increase cellular function signals. Recently, it was reported that the combination of arginine-glycine-aspartic acid (RGD) groups with PIC-supported human umbilical vein endothelial cells (HUVECs) spread and formed an endothelial cell network, which is similar to the tissue form in vivo for 3D cell culture (Ma et al., 2022).
A long-term 3D organoid culture system was established for mouse and human primary hepatocytes by Matrigel, which is a hydrogel based on solubilized basement membrane preparation extracted from Engelbreth-Holm-Swarm (EHS) mouse sarcoma, a tumor rich in ECM proteins (Kleinman et al., 1982). In this system, single hepatocytes can create organoids that can be cultivated for several months while keeping important morphological, functional, and gene expression characteristics (Hu et al., 2018). Furthermore, PEG hydrogels were employed to culture and expand a range of neuronal and glial cell types (Lampe et al., 2010) and hepatocytes (Christoffersson et al., 2019) by simply changing the material properties of the hydrogel. In addition, RGD-modified alginate hydrogels accelerated the development of retinal pigment epithelium and neuroretina in 3D-cultured human embryonic stem cells (hESCs)/human induced pluripotent stem cells (hiPSCs) (Hunt et al., 2017).
Since collagen is the most abundant natural type of fibrin hydrogel in vivo (Faraj et al., 2007), research has shown that collagen type II scaffolds may significantly improve the chondrogenic development of human mesenchymal stem cells (hMSCs) when compared to collagen type I hydrogel scaffolds (Tamaddon et al., 2017). Although type I and type II collagens support chondrogenic phenotypes in various ways, collagen hydrogel scaffolds can construct cartilage tissue (Tamaddon et al., 2017). For example, in a cartilage deficiency rat model, the collagen hydrogel scaffolds covered with rat mesenchymal stem cells (rMSCs) promoted rMSC chondrogenic development and had a statistically greater cartilage healing capability (Rouwkema and Khademhosseini, 2016). In addition, HA-based hydrogel scaffolds might stimulate the neural development of human-induced pluripotent stem cell-derived neural progenitors (hiPSC-NPCS) and the proliferation of neuroblastoma cells (Seidlits et al., 2010; Lei et al., 2011b; Yang et al., 2016). Another work encapsulated hiPSCs in HA-rich core-shell hydrogel microcapsules via microencapsulation to increase cell bulk and promote effective cardiac differentiation (Xu et al., 2021). In addition, growth factor-containing PVA hydrogels may accelerate the differentiation of mouse spermatogonial stem cells (mSCCs) into meiotic and postmeiotic cells (Kashani et al., 2020).
In a hydrogel-based 3D cell culture, cells can naturally form 3D structures rather than being restricted to a 2D surface. As shown in Figure 1, hepatocytes and hESCs form spheroids in PIC-based hydrogel (Bar = 200 μm). Similarly, the 3D culture experiments of chondrocytes showed that the collagen type I hydrogel scaffold could retain the chondrogenic phenotype of rat chondrocytes in a 3D growth pattern (Smeriglio et al., 2015; Jin and Kim, 2017; Kashani et al., 2020). Customizable HA hydrogels were also created with variable hardness for 3D rMSC growth (Wu et al., 2017). HA hydrogel scaffold can maintain the survival of bone marrow stromal cells (BMSCS) and promote direct tubular chondrogenic development (Ren et al., 2021). Furthermore, in 3D cultures of PVA hydrogel-coated cell plates, various human glioma cell lines (LN299, U87MG, and Gli36) may form tumor spheres like their morphology in vivo (Molyneaux et al., 2021). The alginate-collagen hydrogels improve cell adhesion of hiPSCs-derived neurons and stimulate the creation of complex neural networks in 3D culture models (Moxon et al., 2019).
The digested 2D cultured cells develop into a 3D cell mass after adding hydrogel into the cell suspension as a cell scaffold, as opposed to 2D culture with a flat morphology (Figure 1, left panel). Two types of cells, hepatocytes and hESCs, are depicted to show the difference between 2D patterned cells and 3D hydrogel-based cells (Figure 1, right panel). The hydrogel used here is polyisocyanopeptide (PIC) -based synthetic fiber hydrogels. hESCs, human embryonic stem cells; Bar = 200 μm.
It is known that a variety of growth factors may bind to ECM proteins. These secluded growth factors can produce gradients in concentration that direct the differentiation and morphogenesis of stem cells during in vivo development (Muncie and Weaver, 2018). Traditional 2D cell culture employs a liquid medium that does not allow for the storage and release of growth agents or the creation of concentration gradients. In contrast, 3D culture allows cells to construct 3D structures instead of being limited to a single layer in 2D (Jose et al., 2020). While more technically difficult than traditional 2D cell culture, hydrogel-based 3D cell culture facilitates normal cell-cell and cell-matrix interactions and tissue-specific activity. Materigel-based 3D cell culture, but not 2D models, increased tauopathy in human stem cell-derived neurons with familial Alzheimer’s disease by increasing the accumulation of β-amyloid aggregates in the ECM (Choi et al., 2014). PIC hydrogels enable the generation of mammary gland organoids from mammary fragments or pure single mammary epithelial cells by decorating with the adhesive peptide RGD for cell adhesion. Furthermore, the cell-gel interactions via the cell binding peptide density regulate the ratio of the major cell types in the mammary gland organoids (Zhang et al., 2020).
Given that alterations in cell-to-cell interactions and interactions with the ECM are the main effects of microgravity on cells and may alter cell fate through these effects (Andreeva et al., 2022), hydrogel-based 3D cell models should be employed for microgravity studies to study the response of human tissues and organs to microgravity.
The study of the bio-effects of SMG and RMG on cell growth and differentiation is a current subject in space medicine, contributing to the applications of biomedical sciences on earth by using technologies designed to simulate microgravity (Lei et al., 2011a; Herranz et al., 2013; Pietsch et al., 2013). The absence of gravity affects the creation of proteins, apoptosis, proliferation, differentiation, migration, adhesion, and other cellular changes (Pietsch et al., 2011a). In the following, we will discuss the effects of microgravity on cell proliferation, differentiation, morphology, and adhesion in general, and advanced researches about those topics are listed in Table 1. Firstly, Cell proliferation, cell cycle, and programmed cell death (apoptosis) are the three primary indicators of how microgravity affects cell growth. Different cell types differ in these aspects under microgravity. For example, the proliferation of murine osteoblasts was enhanced by 6–21 days of culture on RPM and the expression of osteogenic marker genes in osteogenic (Braveboy-Wagner et al., 2021). RMG resulted in enhanced proliferation and a shortened cell cycle of neural stem cells within 38 days of culture in space (Shaka et al., 2022). The amount of Ki67-positive cells and formation of canning epithelium could be observed after 10 days of culture in human epidermal stem cells (hEpSCs) on RCCS (Lei et al., 2011a). However, human promyelocytic leukemic HL-60 cells showed a significant decrease in cell proliferation and expression of proliferating cell nuclear antigen (PCNA) and phosphorylated ERK1/2 and AKT proteins under SMG. Moreover, SMG increased DNA damage, apoptosis, and ROS formation (Singh et al., 2021).
TABLE 1. Summary of advanced research on cell growth and differentiation under microgravity (Last 5 years).
Most studies show that microgravity affects cell differentiation by promoting reagent-induced differentiation of stem cells or specific cell types into specific tissue types. Short-term exposure (3 days) of cardiac progenitors to space microgravity upregulated genes involved in cardiac differentiation (Rampoldi et al., 2022). hBMSCs were affected by RMG and responded to RMG stresses, reverting to quiescence after a moderate osteogenic differentiation aboard ISS for 3 weeks (Bradamante et al., 2018). It was demonstrated that SMG facilitates hESCs to differentiate into hematopoietic stem cells (HSCs) and progenitor cells with more efficient induction of CD34+ CD31+ hemogenic endothelium progenitors (Ma et al., 2021). ADSCs displayed enhanced neural differentiation in neurons and increased neurotrophin expression and their specific Trk receptors, especially BDNF and TrkB, when cultured on fast rotating clinostat (Zarrinpour et al., 2017). When Postnatal stem cells from the apical papilla of teeth were cultured with NGF, EGF, and bFGF on high-aspect rotating vessels for 7 days, they formed nerve tissue (Kim et al., 2017). Limbal fibroblasts (LFs) cells could differentiate into adipocytes, osteocytes, and chondrocytes on RCCS/HARV for 3 days, compared to 1 g controls (Pao et al., 2017). Neonatal cardiac progenitor cells showed an increased expression of early developmental markers on ISS, 3 weeks (Baio et al., 2018). This less visible differentiation could be attributed to additional environmental factors or the poor cultural conditions of space travel experiments. However, some evidence suggests that microgravity can preserve pluripotency. Mouse ESCs can be maintained without leukemia inhibitory factor (LIF) and retain pluripotency under a simulated microgravity environment (Kawahara et al., 2009). Similar results were obtained for cancer stem cells (CSCs), which have similar differentiation potential to ESCs (Arun et al., 2017). SMG also increased stemness in human colorectal cancer cell HCT116 using RCCS, indicating CD133/CD44 dual-positive cells (Arun et al., 2019).
Another important aspect of the effect of microgravity on cells is the influence on cell adhesion, morphology, and cytoskeleton. When subjected to microgravity, some cells grew into a monolayer altering their growth behavior, while the remaining continued to develop naturally (Infanger et al., 2006; Ulbrich et al., 2010; Pietsch et al., 2011b). Although these cells detect the absence of gravity within seconds (Ulbrich et al., 2011; Grosse et al., 2012), it takes at least 12 h to see spheroids (Infanger et al., 2006; Pietsch et al., 2011b) and up to 7 days to see constructions like tubes resembling an intima floating in a culture flask (Grimm et al., 2009; Grimm et al., 2010). Our previous study found that SMG promotes hESCs to differentiate between HSCs and hematopoietic progenitor cells (HSPC). Interestingly, HSPC prefers floating growth, and transcriptome sequencing results showed that cell adhesion and ECM-related genes were downregulated (Ma et al., 2021). When retinal pigment epithelial cells, ARPE19 cells, were cultured on a 3D clinostat, actin cytoskeleton regulators were modulated, and the cells showed multilayered growth with increased expression of epithelial-mesenchymal transition (EMT) markers (Son et al., 2022).
Exposure of cells from epithelial tissue to microgravity creates 3D structures. For example, human microvascular endothelial cells (HMVEC) were exposed to RMG for 5 days and 12 days and SMG for 7 days, showing tubular structures (Pietsch et al., 2017). The yes-associated protein (YAP) and HIPPO signaling changes, known to be correlated with organ growth, cytoskeleton, and stress sensing, are also present in specific cells with enlarged morphology (Ma et al., 2019). For example, the upregulation of Hippo signaling with downstream genes, YAP1, was detected in cardiac progenitor cells (CPCs) when cultured on ISS and 2D clinostat (Camberos et al., 2019). Colorectal cancer cells, HCT116, are giant cancer cells formed with complete nuclear localization of YAP in the culture on RCCS (Arun et al., 2019). Changes in the cell adhesion, morphology, and cytoskeleton, which can impact various cellular functions, maybe a possible substrate for a cell’s response to microgravity. Since the 2D cell model differs from the in vivo stress environment, eliminating the effects of microgravity on cells from the experimental model should ensure that they are in a 3D development state akin to the in vivo environment.
In space circumstances, it has been discovered that there are some discrepancies in the biological effects between in vivo and in vitro, such as bone metabolism (Loomer, 2001), neuronal adaptation (Kohn and Ritzmann, 2018), skin health, and wound healing (Kasiviswanathan et al., 2020), which may be attributed to complex environments in vivo, as cell-cell contact. Given the obvious advantages of 3D culture methods, many studies have attempted to use 3D methods in microgravity research. Except for the scaffold-free clinostat for 3D culture (Aleshcheva et al., 2016), to better simulate the microenvironment of cell interaction in vivo, various biomaterials have been used as scaffolds for 3D culture, as a mixture of inorganic salt and collagen (Fournier and Harrison, 2021), matrix cell (Jackson et al., 2020) to study bio-effects of microgravity. As a result, these technologies constitute a novel paradigm for the organization of a wide range of tissues, including cartilage regeneration (Zhou et al., 2019), artificial vascular construction (Rouwkema and Khademhosseini, 2016), and generation of various organ tissues (Herranz et al., 2013; Luo et al., 2013; Zhang et al., 2014; Salerno-Goncalves et al., 2016) and cancer spheroids (Qian et al., 2008; Ulbrich et al., 2011; Ma et al., 2014). Furthermore, these aggregates are utilized to research the molecular pathways involved in angiogenesis (Rouwkema and Khademhosseini, 2016), osteogenesis (Braveboy-Wagner and Lelkes, 2022; Masini et al., 2022), cancer formation (Ma et al., 2014; Dietrichs et al., 2022), and pharmacological testing (Nishikawa et al., 2005) (Figure 2). The multiple advantages of the 3D culture of hydrogels are already discussed in Part 2. The specific applications of hydrogel-based 3D culture in microgravity are listed in Table 2.
For tissue engineering, various hydrogels were combined with a microgravity bioreactor. A multicellular 3D organotypic model used collagen I matrix of the human intestinal mucosa was composed of an intestinal epithelial cell line and primary human lymphocytes, endothelial cells, and fibroblasts cultured under microgravity provided by the RWV bioreactor (Salerno-Goncalves et al., 2016). When chondrocytes were seeded onto poly (DL-lactic-co-glycolic acid) (PLGA) sponges and cultured in a chondrogenic induction medium containing TGF-β 1 for 3 weeks, the engineered cartilage then emerged in a microgravity bioreactor for another 3 weeks. The results showed that it had a similar structure and composition to native rat cartilage (Emin et al., 2008). Human periodontal ligament fibroblasts (hPDLFs) were 3D cultivated on a mineralized PLGA scaffold to mimic microgravity in the NASA-approved bioreactor. The outcomes demonstrated a successful strategy for producing hPDLF-PLGA structures with improved osteogenic potential using a 3D system and microgravity settings (Inanc et al., 2006). hPDLFs encapsulated in C/HA microspheres exhibited significantly higher osteogenic differentiation potential when compared to those not encapsulated. The 3D-osteogenic culture environment can potentially improve the osteogenic differentiation of hPDLFs (Inanc et al., 2007). The collagen-HA is a type of implant material used to create a permanent implant. The collagen-HA material allowed the embedded MLO-Y4 cells to survive and grow for 6 months. This technology creates permanent implants for patients with spinal cord injuries (Fournier and Harrison, 2021). However, microgravity raises the likelihood of irreversible changes that weaken skeletal integrity and the gradual start of fracture injuries in space travelers (Nelson et al., 2009; Genah et al., 2021). According to the findings of the previous studies, the 3D -matrix bone differentiation model can be used to test potential drugs against bone loss or to promote bone regeneration.
Hydrogel 3D culture models were used to investigate the influence of microgravity on stem cell development, an important research area in regenerative medicine and tissue engineering. An efficient and integrated 3D bioprocess has developed based on the encapsulation of undifferentiated mouse embryonic stem cells (mESCs) within alginate hydrogels. The osteogenic lineage’s morphological, phenotypic, and molecular characteristics were represented in 3D mineralized constructions with mechanical strength and mineralized calcium/phosphate deposition. This bioprocess represents a significant advance in bone differentiation from mESCs (Hwang et al., 2009). Similar findings have been discovered in improved odontogenic differentiation abilities of Human dental pulp stem cells (hDPSCs) on PLGA scaffolds in the 3D SMG culture system (Li et al., 2017), indicating that these models have the potential to be used to explore tissue regeneration processes in regenerative medicine and microgravity conditions.
It may be preferable to create a physiological and pathological research model similar to that used in vivo. Human pancreatic cancer NOR-P1 cells, fibroblasts, or minced pancreatic carcinoma tissue were grown in solid collagen gels for seven days in a microgravity environment. Compared to NOR-P1 3D cultures treated to the static 1 g condition, cultures subjected to the SMG condition had more mitotic, cycling (Ki67-positive), nuclear factor-kappa B-activating cells and fewer apoptotic cells. Additionally, compared to static culture conditions, human pancreatic cancer specimens better preserved the original carcinoma tissue’s heterogeneous makeup and cellular activity (measured by the cycling cell ratio and mitotic index) (Nakamura et al., 2002). When fibroblast differentiation was studied in SMG using collagen-based 3D matrices to approximate interstitial tissue, SMG exposure decreased alpha-smooth muscle actin (SMA) expression and Smad2/3 translocation into the cell nucleus compared to the 1 g control (Sapudom et al., 2021). Compared to 2D cell culture, 3D cell culture attenuates the effects of SMG on the T cells transcriptome and nuclear abnormalities, which were closer to the in vivo findings (ElGindi et al., 2022).
Compared to 2D culture, 3D cell culture is more effective for enhancing cell differentiation and organ-like tissue formation from a variety of cells to study the bio-effects of microgravity (Figure 2, lower panel). Exposing 3D cells to SMG circumstances increased the knowledge of biological response mechanisms to SMG and RMG in several tissues, including the liver, bone, vasculature, skin, and capillary tissue. Microgravity affects physiology, pathology, and medical research (Figure 2, right panel). MSCs, mesenchymal stem cells; ESCs, embryonic stem cells (the Figure was created with BioRender.com).
As the studies above suggest, microgravity research would provide insight into the basic mechanisms of tissue dysfunction and regeneration under SMG or RMG, which could be applied to terrestrial settings. Additionally, combining tissue engineering approaches with ground-based platforms will open up new avenues for space physiology and aging research and accelerate the creation of novel tissue-engineered constructions. This can be done by using cells from the patient’s body or cell lines. These platforms can be made from various materials, including biomaterials (especially hydrogels), plastics, and composites. However, most of the 3D tissue and organ models cultured in microgravity environment are composed of single-cell types, which cannot simulate the complete function of organs that are comprise of complex multi-cell types. Moreover, the research on 3D cell matrix and cell interaction is not deep enough. Adjusting the physical and chemical properties of materials may give appropriate mechanical feedback to cells, that may be more conducive to the construction of some organizational models. In conclusion, 3D culture holds great promise for the future of biological research in microgravity. Tissue engineering and microgravity interfacial research will drive breakthroughs in both fields in the coming years.
CM: Writing original draft, revision and investigation. XD: Conceptualization, supervision and funding acquisition. XL: Conceptualization, supervision, manuscript revision and funding acquisition.
This work was supported by the National Key Research and Development Program of China (2021YFA0719303), the National Natural Science Foundation of China (32271284), the Key Research and Development Program of Shaanxi (Program No. 2020GXLH-Y-019, 2022KXJ-141), the China Manned Space Flight Technology Project Chinese Space Station (YYWT-0901-EXP-15) and the Innovation Capability Support Program of Shaanxi (Program No. 2019GHJD-14, 2021TD-40).
We thank Dr. Guanning Wei from the School of Life Sciences at Jilin University, for providing us with valuable suggestions in preparing the manuscript.
The authors declare that the research was conducted in the absence of any commercial or financial relationships that could be construed as a potential conflict of interest.
All claims expressed in this article are solely those of the authors and do not necessarily represent those of their affiliated organizations, or those of the publisher, the editors and the reviewers. Any product that may be evaluated in this article, or claim that may be made by its manufacturer, is not guaranteed or endorsed by the publisher.
Aleshcheva, G., Bauer, J., Hemmersbach, R., Slumstrup, L., Wehland, M., Infanger, M., et al. (2016). Scaffold-free tissue formation under real and simulated microgravity conditions. Basic Clin. Pharmacol. Toxicol. 119, 26–33. doi:10.1111/bcpt.12561
Alonzo, M., El Khoury, R., Nagiah, N., Thakur, V., Chattopadhyay, M., and Joddar, B. (2022). 3D biofabrication of a cardiac tissue construct for sustained longevity and function. ACS Appl. Mater Interfaces 14, 21800–21813. doi:10.1021/acsami.1c23883
Andreeva, E., Matveeva, D., Zhidkova, O., Zhivodernikov, I., Kotov, O., and Buravkova, L. (2022). Real and simulated microgravity: Focus on mammalian extracellular matrix. Life (Basel) 12, 1343. doi:10.3390/life12091343
Arun, R. P., Sivanesan, D., Patra, B., Varadaraj, S., and Verma, R. S. (2019). Simulated microgravity increases polyploid giant cancer cells and nuclear localization of YAP. Sci. Rep. 9, 10684. doi:10.1038/s41598-019-47116-5
Arun, R. P., Sivanesan, D., Vidyasekar, P., and Verma, R. S. (2017). PTEN/FOXO3/AKT pathway regulates cell death and mediates morphogenetic differentiation of Colorectal Cancer Cells under Simulated Microgravity. Sci. Rep. 7, 5952. doi:10.1038/s41598-017-06416-4
Baio, J., Martinez, A. F., Silva, I., Hoehn, C. V., Countryman, S., Bailey, L., et al. (2018). Cardiovascular progenitor cells cultured aboard the International Space Station exhibit altered developmental and functional properties. NPJ Microgravity 4, 13. doi:10.1038/s41526-018-0048-x
Bradamante, S., Rivero, D., Barenghi, L., Balsamo, M., Minardi, S. P., Vitali, F., et al. (2018). Scd – stem cell differentiation toward osteoblast onboard the international space station. Microgravity Sci. Technol. 30, 713–729. doi:10.1007/s12217-018-9653-2
Braveboy-Wagner, J., and Lelkes, P. I. (2022). Impairment of 7F2 osteoblast function by simulated partial gravity in a Random Positioning Machine. NPJ Microgravity 8, 20. doi:10.1038/s41526-022-00202-x
Braveboy-Wagner, J., Sharoni, Y., and Lelkes, P. I. (2021). Nutraceuticals synergistically promote osteogenesis in cultured 7F2 osteoblasts and mitigate inhibition of differentiation and maturation in simulated microgravity. Int. J. Mol. Sci. 23, 136. doi:10.3390/ijms23010136
Briegleb, W. (1992). Some qualitative and quantitative aspects of the fast-rotating clinostat as a research tool. ASGSB Bull. Publ. Am. Soc. Gravitational Space Biol. 5, 23–30.
Camberos, V., Baio, J., Bailey, L., Hasaniya, N., Lopez, L. V., and Kearns-Jonker, M. (2019). Effects of spaceflight and simulated microgravity on YAP1 expression in cardiovascular progenitors: Implications for cell-based repair. Int. J. Mol. Sci. 20, 2742. doi:10.3390/ijms20112742
Choi, D. H., Jeon, B., Lim, M. H., Lee, D. H., Ye, S. K., Jeong, S. Y., et al. (2021). 3D cell culture using a clinostat reproduces microgravity-induced skin changes. NPJ Microgravity 7, 20. doi:10.1038/s41526-021-00148-6
Choi, S. H., Kim, Y. H., Hebisch, M., Sliwinski, C., Lee, S., D'Avanzo, C., et al. (2014). A three-dimensional human neural cell culture model of Alzheimer's disease. Nature 515, 274–278. doi:10.1038/nature13800
Christoffersson, J., Aronsson, C., Jury, M., Selegard, R., Aili, D., and Mandenius, C.-F. (2019). Fabrication of modular hyaluronan-PEG hydrogels to support 3D cultures of hepatocytes in a perfused liver-on-a-chip device. Biofabrication 11, 015013. doi:10.1088/1758-5090/aaf657
Costantini, D., Overi, D., Casadei, L., Cardinale, V., Nevi, L., Carpino, G., et al. (2019). Simulated microgravity promotes the formation of tridimensional cultures and stimulates pluripotency and a glycolytic metabolism in human hepatic and biliary tree stem/progenitor cells. Sci. Rep. 9, 5559. doi:10.1038/s41598-019-41908-5
Devarasetty, M., Wang, E., Soker, S., and Skardal, A. (2017). Mesenchymal stem cells support growth and organization of host-liver colorectal-tumor organoids and possibly resistance to chemotherapy. Biofabrication 9, 021002. doi:10.1088/1758-5090/aa7484
Dietrichs, D., Grimm, D., Sahana, J., Melnik, D., Corydon, T. J., Wehland, M., et al. (2022). Three-dimensional growth of prostate cancer cells exposed to simulated microgravity. Front. Cell Dev. Biol. 10, 841017. doi:10.3389/fcell.2022.841017
Ebnerasuly, F., Hajebrahimi, Z., Tabaie, S. M., and Darbouy, M. (2017). Effect of simulated microgravity conditions on differentiation of adipose derived stem cells towards fibroblasts using connective tissue growth factor. Iran. J. Biotechnol. 15, 241–251. doi:10.15171/ijb.1747
ElGindi, M., Sapudom, J., Laws, P., Garcia-Sabate, A., Daqaq, M. F., and Teo, J. (2022). 3D microenvironment attenuates simulated microgravity-mediated changes in T cell transcriptome. Cell Mol. Life Sci. 79, 508. doi:10.1007/s00018-022-04531-8
Emin, N., Koc, A., Durkut, S., Elcin, A. E., and Elcin, Y. M. (2008). Engineering of rat articular cartilage on porous sponges: Effects of tgf-beta 1 and microgravity bioreactor culture. Artif. Cells Blood Substit. Immobil. Biotechnol. 36, 123–137. doi:10.1080/10731190801932116
Fan, Z., Cheng, P., Liu, M., Prakash, S., Han, J., Ding, Z., et al. (2020). Dynamic crosslinked and injectable biohydrogels as extracellular matrix mimics for the delivery of antibiotics and 3D cell culture. RSC Adv. 10, 19587–19599. doi:10.1039/d0ra02218g
Faraj, K. A., Van Kuppevelt, T. H., and Daamen, W. F. (2007). Construction of collagen scaffolds that mimic the three-dimensional architecture of specific tissues. Tissue Eng. 13, 2387–2394. doi:10.1089/ten.2006.0320
Fournier, R., and Harrison, R. E. (2021). Methods for studying MLO-Y4 osteocytes in collagen-hydroxyapatite scaffolds in the rotary cell culture system. Connect. Tissue Res. 62, 436–453. doi:10.1080/03008207.2020.1764548
Genah, S., Monici, M., and Morbidelli, L. (2021). The effect of space travel on bone metabolism: Considerations on today's major challenges and advances in pharmacology. Int. J. Mol. Sci. 22, 4585. doi:10.3390/ijms22094585
Goodwin, T. J., Jessup, J. M., and Wolf, D. A. (1992). Morphologic differentiation of colon carcinoma cell lines HT-29 and HT-29KM in rotating-wall vessels. Vitro Cell. Dev. Biology-Animal 28A, 47–60. doi:10.1007/BF02631079
Grimm, D., Bauer, J., Ulbrich, C., Westphal, K., Wehland, M., Infanger, M., et al. (2010). Different responsiveness of endothelial cells to vascular endothelial growth factor and basic fibroblast growth factor added to culture media under gravity and simulated microgravity. Tissue Eng. Part A 16, 1559–1573. doi:10.1089/ten.tea.2009.0524
Grimm, D., Infanger, M., Westphal, K., Ulbrich, C., Pietsch, J., Kossmehl, P., et al. (2009). A delayed type of three-dimensional growth of human endothelial cells under simulated weightlessness. Tissue Eng. Part A 15, 2267–2275. doi:10.1089/ten.tea.2008.0576
Grinnell, F., and Petroll, W. M. (2010). Cell motility and mechanics in three-dimensional collagen matrices. Annu. Rev. Cell Dev. Biol. 26, 335–361. doi:10.1146/annurev.cellbio.042308.113318
Grosse, J., Wehland, M., Pietsch, J., Ma, X., Ulbrich, C., Schulz, H., et al. (2012). Short-term weightlessness produced by parabolic flight maneuvers altered gene expression patterns in human endothelial cells. Faseb J. 26, 639–655. doi:10.1096/fj.11-194886
Herranz, R., Anken, R., Boonstra, J., Braun, M., Christianen, P. C. M., de Geest, M., et al. (2013). Ground-based facilities for simulation of microgravity: Organism-specific recommendations for their use, and recommended terminology. Astrobiology 13, 1–17. doi:10.1089/ast.2012.0876
Hides, J., Lambrecht, G., Ramdharry, G., Cusack, R., Bloomberg, J., and Stokes, M. (2017). Parallels between astronauts and terrestrial patients - taking physiotherapy rehabilitation "To infinity and beyond. Musculoskelet. Sci. Pract. 27, S32–S37. doi:10.1016/j.msksp.2016.12.008
Hou, Y., Ma, S., Hao, J., Lin, C., Zhao, J., and Sui, X. (2022). Construction and ion transport-related applications of the hydrogel-based membrane with 3D nanochannels. Polym. (Basel) 14, 4037. doi:10.3390/polym14194037
Hu, H., Gehart, H., Artegiani, B., Löpez-Iglesias, C., Dekkers, F., Basak, O., et al. (2018). Long-term expansion of functional mouse and human hepatocytes as 3D organoids. Cell 175, 1591–1606 e19. doi:10.1016/j.cell.2018.11.013
Hunt, N. C., Hallam, D., Karimi, A., Mellough, C. B., Chen, J., Steel, D. H. W., et al. (2017). 3D culture of human pluripotent stem cells in RGD-alginate hydrogel improves retinal tissue development. Acta Biomater. 49, 329–343. doi:10.1016/j.actbio.2016.11.016
Hwang, Y. S., Cho, J., Tay, F., Heng, J. Y., Ho, R., Kazarian, S. G., et al. (2009). The use of murine embryonic stem cells, alginate encapsulation, and rotary microgravity bioreactor in bone tissue engineering. Biomaterials 30, 499–507. doi:10.1016/j.biomaterials.2008.07.028
Inanc, B., Elcin, A. E., and Elcin, Y. M. (2006). Osteogenic induction of human periodontal ligament fibroblasts under two- and three-dimensional culture conditions. Tissue Eng. 12, 257–266. doi:10.1089/ten.2006.12.257
Inanc, B., Eser Elcin, A., Koc, A., Balos, K., Parlar, A., and Murat Elcin, Y. (2007). Encapsulation and osteoinduction of human periodontal ligament fibroblasts in chitosan-hydroxyapatite microspheres. J. Biomed. Mater Res. A 82, 917–926. doi:10.1002/jbm.a.31213
Infanger, M., Kossmehl, P., Shakibaei, M., Baatout, S., Witzing, A., Grosse, J., et al. (2006). Induction of three-dimensional assembly and increase in apoptosis of human endothelial cells by simulated microgravity: Impact of vascular endothelial growth factor. Apoptosis 11, 749–764. doi:10.1007/s10495-006-5697-7
Jackson, R., Maarsingh, J. D., Herbst-Kralovetz, M. M., and Van Doorslaer, K. (2020). 3D oral and cervical tissue models for studying papillomavirus host-pathogen interactions. Curr. Protoc. Microbiol. 59, e129. doi:10.1002/cpmc.129
Jin, G.-Z., and Kim, H.-W. (2017). Effects of type I collagen concentration in hydrogel on the growth and phenotypic expression of rat chondrocytes. Tissue Eng. Regen. Med. 14, 383–391. doi:10.1007/s13770-017-0060-3
Jose, G., Shalumon, K. T., and Chen, J.-P. (2020). Natural polymers based hydrogels for cell culture applications. Curr. Med. Chem. 27, 2734–2776. doi:10.2174/0929867326666190903113004
Kashani, M. Z., Bagher, Z., Asgari, H. R., Najafi, M., Koruji, M., and Mehraein, F. (2020). Differentiation of neonate mouse spermatogonial stem cells on three-dimensional agar/polyvinyl alcohol nanofiber scaffold. Syst. Biol. Reproductive Med. 66, 202–215. doi:10.1080/19396368.2020.1725927
Kasiviswanathan, D., Chinnasamy Perumal, R., Bhuvaneswari, S., Kumar, P., Sundaresan, L., Philip, M., et al. (2020). Interactome of miRNAs and transcriptome of human umbilical cord endothelial cells exposed to short-term simulated microgravity. NPJ Microgravity 6, 18. doi:10.1038/s41526-020-00108-6
Kawahara, Y., Manabe, T., Matsumoto, M., Kajiume, T., and Yuge, L. (2009). LIF-free embryonic stem cell culture in simulated microgravity. PLoS One 4, e6343. doi:10.1371/journal.pone.0006343
Kikuchi, I. S., Cardoso Galante, R. S., Dua, K., Malipeddi, V. R., Awasthi, R., Ghisleni, D. D. M., et al. (2017). Hydrogel based drug delivery systems: A review with special emphasis on challenges associated with decontamination of hydrogels and biomaterials. Curr. Drug Deliv. 14, 917–925. doi:10.2174/1567201813666161205130825
Kim, B. C., Jun, S. M., Kim, S. Y., Kwon, Y. D., Choe, S. C., Kim, E. C., et al. (2017). Engineering three dimensional micro nerve tissue using postnatal stem cells from human dental apical papilla. Biotechnol. Bioeng. 114, 903–914. doi:10.1002/bit.26205
Kleinman, H. K., McGarvey, M. L., Liotta, L. A., Robey, P. G., Tryggvason, K., and Martin, G. R. (1982). Isolation and characterization of type IV procollagen, laminin, and heparan sulfate proteoglycan from the EHS sarcoma. Biochemistry 21, 6188–6193. doi:10.1021/bi00267a025
Kohn, F. P. M., and Ritzmann, R. (2018). Gravity and neuronal adaptation, in vitro and in vivo-from neuronal cells up to neuromuscular responses: A first model. Eur. Biophys. J. 47, 97–107. doi:10.1007/s00249-017-1233-7
Lampe, K. J., Mooney, R. G., Bjugstad, K. B., and Mahoney, M. J. (2010). Effect of macromer weight percent on neural cell growth in 2D and 3D nondegradable PEG hydrogel culture. J. Biomed. Mater Res. A 94, 1162–1171. doi:10.1002/jbm.a.32787
Lei, X. H., Ning, L. N., Cao, Y. J., Liu, S., Zhang, S. B., Qiu, Z. F., et al. (2011). NASA-approved rotary bioreactor enhances proliferation of human epidermal stem cells and supports formation of 3D epidermis-like structure. PLoS One 6, e26603. doi:10.1371/journal.pone.0026603
Lei, Y., Gojgini, S., Lam, J., and Segura, T. (2011). The spreading, migration and proliferation of mouse mesenchymal stem cells cultured inside hyaluronic acid hydrogels. Biomaterials 32, 39–47. doi:10.1016/j.biomaterials.2010.08.103
Li, Y., He, L., Pan, S., Zhang, L., Zhang, W., Yi, H., et al. (2017). Three-dimensional simulated microgravity culture improves the proliferation and odontogenic differentiation of dental pulp stem cell in PLGA scaffolds implanted in mice. Mol. Med. Rep. 15, 873–878. doi:10.3892/mmr.2016.6042
Liu, K., Mihaila, S. M., Rowan, A., Oosterwijk, E., and Kouwer, P. H. J. (2019). Synthetic extracellular matrices with nonlinear elasticity regulate cellular organization. Biomacromolecules 20, 826–834. doi:10.1021/acs.biomac.8b01445
Long, R., Shi, L., He, P., Tian, J., Wang, S., and Zheng, J. (2022). 3D cell culture based on artificial cells and hydrogel under microgravity for bottom-up microtissue constructs. Front. Bioeng. Biotechnol. 10, 1056652. doi:10.3389/fbioe.2022.1056652
Loomer, P. M. (2001). The impact of microgravity on bone metabolism in vitro and in vivo. Crit. Rev. Oral Biol. Med. 12, 252–261. doi:10.1177/10454411010120030401
Lou, J., Stowers, R., Nam, S., Xia, Y., and Chaudhuri, O. (2018). Stress relaxing hyaluronic acid-collagen hydrogels promote cell spreading, fiber remodeling, and focal adhesion formation in 3D cell culture. Biomaterials 154, 213–222. doi:10.1016/j.biomaterials.2017.11.004
Luo, M. Z., Yang, Z. Q., Li, J. B., Xu, H. Y., Li, S. S., Zhang, W., et al. (2013). Calcium influx through stretch-activated channels mediates microfilament reorganization in osteoblasts under simulated weightlessness. Adv. Space Res. 51, 2058–2068. doi:10.1016/j.asr.2013.01.009
Ma, C., Liu, K., Li, Q., Xiong, Y., Xu, C., Zhang, W., et al. (2022). Synthetic extracellular matrices for 3D culture of schwann cells, hepatocytes, and HUVECs. Bioeng. (Basel) 9, 453. doi:10.3390/bioengineering9090453
Ma, C., Xiong, Y., Han, P., Zhang, X., Cao, Y., Wang, B., et al. (2021). Simulated microgravity potentiates hematopoietic differentiation of human pluripotent stem cells and supports formation of 3D hematopoietic cluster. Front. Cell Dev. Biol. 9, 797060. doi:10.3389/fcell.2021.797060
Ma, S., Meng, Z., Chen, R., and Guan, K. L. (2019). The Hippo pathway: Biology and pathophysiology. Annu. Rev. Biochem. 88, 577–604. doi:10.1146/annurev-biochem-013118-111829
Ma, X., Pietsch, J., Wehland, M., Schulz, H., Saar, K., Huebner, N., et al. (2014). Differential gene expression profile and altered cytokine secretion of thyroid cancer cells in space. Faseb J. 28, 813–835. doi:10.1096/fj.13-243287
Mashiko, T., Kanayama, K., Saito, N., Shirado, T., Asahi, R., Mori, M., et al. (2021). Selective proliferation of highly functional adipose-derived stem cells in microgravity culture with stirred microspheres. Cells 10, 560. doi:10.3390/cells10030560
Masini, M. A., Bonetto, V., Manfredi, M., Pasto, A., Barberis, E., Timo, S., et al. (2022). Prolonged exposure to simulated microgravity promotes stemness impairing morphological, metabolic and migratory profile of pancreatic cancer cells: A comprehensive proteomic, lipidomic and transcriptomic analysis. Cell Mol. Life Sci. 79, 226. doi:10.1007/s00018-022-04243-z
Molyneaux, K., Wnek, M. D., Craig, S. E. L., Vincent, J., Rucker, I., Wnek, G. E., et al. (2021). Physically-cross-linked poly(vinyl alcohol) cell culture plate coatings facilitate preservation of cell-cell interactions, spheroid formation, and stemness. J. Biomed. Mater. Res. Part B-Applied Biomaterials 109, 1744–1753. doi:10.1002/jbm.b.34832
Moxon, S. R., Corbett, N. J., Fisher, K., Potjewyd, G., Domingos, M., and Hooper, N. M. (2019). Blended alginate/collagen hydrogels promote neurogenesis and neuronal maturation. Mater. Sci. Eng. C-Materials Biol. Appl. 104, 109904. doi:10.1016/j.msec.2019.109904
Muncie, J. M., and Weaver, V. M. (2018). The physical and biochemical properties of the extracellular matrix regulate cell fate. Curr. Top. Dev. Biol. 130, 1–37. doi:10.1016/bs.ctdb.2018.02.002
Nakamura, K., Kuga, H., Morisaki, T., Baba, E., Sato, N., Mizumoto, K., et al. (2002). Simulated microgravity culture system for a 3-D carcinoma tissue model. Biotechniques 33, 1068-70–1072, 1074-1076. doi:10.2144/02335rr02
Nelson, E. S., Lewandowski, B., Licata, A., and Myers, J. G. (2009). Development and validation of a predictive bone fracture risk model for astronauts. Ann. Biomed. Eng. 37, 2337–2359. doi:10.1007/s10439-009-9779-x
Nerger, B. A., and Nelson, C. M. (2020). Engineered extracellular matrices: Emerging strategies for decoupling structural and molecular signals that regulate epithelial branching morphogenesis. Curr. Opin. Biomed. Eng. 13, 103–112. doi:10.1016/j.cobme.2019.12.013
Nishikawa, M., Ohgushi, H., Tamai, N., Osuga, K., Uemura, M., Yoshikawa, H., et al. (2005). The effect of simulated microgravity by three-dimensional clinostat on bone tissue engineering. Cell Transpl. 14, 829–835. doi:10.3727/000000005783982477
Okita, Y., Zheng, L., Kawanishi, K., Miyoshi, H., Yanagihara, K., and Kato, M. (2021). Polyvinyl alcohol scaffolds and supplementation support 3D and sphere culturing of human cancer cell lines by reducing apoptosis and promoting cellular proliferation. Genes Cells 26, 336–343. doi:10.1111/gtc.12843
Pao, S. I., Chien, K. H., Lin, H. T., Tai, M. C., Chen, J. T., and Liang, C. M. (2017). Effect of microgravity on the mesenchymal stem cell characteristics of limbal fibroblasts. J. Chin. Med. Assoc. 80, 595–607. doi:10.1016/j.jcma.2017.01.008
Park, Y., Huh, K. M., and Kang, S.-W. (2021). Applications of biomaterials in 3D cell culture and contributions of 3D cell culture to drug development and basic biomedical research. Int. J. Mol. Sci. 22, 2491. doi:10.3390/ijms22052491
Pietsch, J., Bauer, J., Egli, M., Infanger, M., Wise, P., Ulbrich, C., et al. (2011). The effects of weightlessness on the human organism and mammalian cells. Curr. Mol. Med. 11, 350–364. doi:10.2174/156652411795976600
Pietsch, J., Gass, S., Nebuloni, S., Echegoyen, D., Riwaldt, S., Baake, C., et al. (2017). Three-dimensional growth of human endothelial cells in an automated cell culture experiment container during the SpaceX CRS-8 ISS space mission - the SPHEROIDS project. Biomaterials 124, 126–156. doi:10.1016/j.biomaterials.2017.02.005
Pietsch, J., Ma, X., Wehland, M., Aleshcheva, G., Schwarzwaelder, A., Segerer, J., et al. (2013). Spheroid formation of human thyroid cancer cells in an automated culturing system during the Shenzhou-8 Space mission. Biomaterials 34, 7694–7705. doi:10.1016/j.biomaterials.2013.06.054
Pietsch, J., Sickmann, A., Weber, G., Bauer, J., Egli, M., Wildgruber, R., et al. (2011). A proteomic approach to analysing spheroid formation of two human thyroid cell lines cultured on a random positioning machine. Proteomics 11, 2095–2104. doi:10.1002/pmic.201000817
Qian, A. R., Zhang, W., Xie, L., Weng, Y. Y., Yang, P. F., Wang, Z., et al. (2008). Simulated weightlessness alters biological characteristics of human breast cancer cell line MCF-7. Acta Astronaut. 63, 947–958. doi:10.1016/j.actaastro.2008.01.024
Rampoldi, A., Forghani, P., Li, D., Hwang, H., Armand, L. C., Fite, J., et al. (2022). Space microgravity improves proliferation of human iPSC-derived cardiomyocytes. Stem Cell Rep. 17, 2272–2285. doi:10.1016/j.stemcr.2022.08.007
Ren, Y., Zhang, H., Wang, Y., Du, B., Yang, J., Liu, L., et al. (2021). Hyaluronic acid hydrogel with adjustable stiffness for mesenchymal stem cell 3D culture via related molecular mechanisms to maintain stemness and induce cartilage differentiation. ACS Appl. bio Mater. 4, 2601–2613. doi:10.1021/acsabm.0c01591
Rouwkema, J., and Khademhosseini, A. (2016). Vascularization and angiogenesis in tissue engineering: Beyond creating static networks. Trends Biotechnol. 34, 733–745. doi:10.1016/j.tibtech.2016.03.002
Salerno-Goncalves, R., Fasano, A., and Sztein, M. B. (2016). Development of a multicellular three-dimensional organotypic model of the human intestinal mucosa grown under microgravity. J. Vis. Exp. (113), 54148. doi:10.3791/54148
Sapudom, J., ElGindi, M., Arnoux, M., Drou, N., Garcia-Sabate, A., and Teo, J. C. M. (2021). Fibroblast differentiation and matrix remodeling impaired under simulated microgravity in 3D cell culture model. Int. J. Mol. Sci. 22, 11911. doi:10.3390/ijms222111911
Schaefer, L., and Schaefer, R. M. (2010). Proteoglycans: From structural compounds to signaling molecules. Cell Tissue Res. 339, 237–246. doi:10.1007/s00441-009-0821-y
Seidlits, S. K., Khaing, Z. Z., Petersen, R. R., Nickels, J. D., Vanscoy, J. E., Shear, J. B., et al. (2010). The effects of hyaluronic acid hydrogels with tunable mechanical properties on neural progenitor cell differentiation. Biomaterials 31, 3930–3940. doi:10.1016/j.biomaterials.2010.01.125
Shaka, S., Carpo, N., Tran, V., Cepeda, C., and Espinosa-Jeffrey, A. (2022). Space microgravity alters neural stem cell division: Implications for brain cancer research on earth and in space. Int. J. Mol. Sci. 23, 14320. doi:10.3390/ijms232214320
Singh, R., Rajput, M., and Singh, R. P. (2021). Simulated microgravity triggers DNA damage and mitochondria-mediated apoptosis through ROS generation in human promyelocytic leukemic cells. Mitochondrion 61, 114–124. doi:10.1016/j.mito.2021.09.006
Smeriglio, P., Dhulipala, L., Lai, J. H., Goodman, S. B., Dragoo, J. L., Smith, R. L., et al. (2015). Collagen VI enhances cartilage tissue generation by stimulating chondrocyte proliferation. Tissue Eng. Part A 21, 840–849. doi:10.1089/ten.tea.2014.0375
Son, M., Ryu, B., Je, J. G., Jeon, Y. J., and Kim, D. Y. (2022). Ishophloroglucin A ameliorates VEGF-induced epithelial-mesenchymal transition via VEGFR2 pathway inhibition in microgravity-stimulated human retinal pigment epithelial cells. Antioxidants (Basel) 11, 2212. doi:10.3390/antiox11112212
Tamaddon, M., Burrows, M., Ferreira, S. A., Dazzi, F., Apperley, J. F., Bradshaw, A., et al. (2017). Monomeric, porous type II collagen scaffolds promote chondrogenic differentiation of human bone marrow mesenchymal stem cells in vitro. Sci. Rep. 7, 43519. doi:10.1038/srep43519
Tarsitano, M., Cristiano, M. C., Fresta, M., Paolino, D., and Rafaniello, C. (2022). Alginate-based composites for corneal regeneration: The optimization of a biomaterial to overcome its limits. Gels 8, 431. doi:10.3390/gels8070431
Tibbitt, M. W., and Anseth, K. S. (2009). Hydrogels as extracellular matrix mimics for 3D cell culture. Biotechnol. Bioeng. 103, 655–663. doi:10.1002/bit.22361
Ulbrich, C., Pietsch, J., Grosse, J., Wehland, M., Schulz, H., Saar, K., et al. (2011). Differential gene regulation under altered gravity conditions in follicular thyroid cancer cells: Relationship between the extracellular matrix and the cytoskeleton. Cell. Physiology Biochem. 28, 185–198. doi:10.1159/000331730
Ulbrich, C., Westphal, K., Pietsch, J., Winkler, H. D. F., Leder, A., Bauer, J., et al. (2010). Characterization of human chondrocytes exposed to simulated microgravity. Cell. Physiology Biochem. 25, 551–560. doi:10.1159/000303059
Wang, G., An, Y., Zhang, X., Ding, P., Bi, H., and Zhao, Z. (2021). Chondrocyte spheroids laden in GelMA/HAMA hybrid hydrogel for tissue-engineered cartilage with enhanced proliferation, better phenotype maintenance, and natural morphological structure. Gels 7, 247. doi:10.3390/gels7040247
Wang, M., Bai, J., Shao, K., Tang, W., Zhao, X., Lin, D., et al. (2021). Poly(vinyl alcohol) hydrogels: The old and new functional materials. Int. J. Polym. Sci. 2021, 1–16. doi:10.1155/2021/2225426
Wang, P., Tian, H., Zhang, J., Qian, J., Li, L., Shi, L., et al. (2019). Spaceflight/microgravity inhibits the proliferation of hematopoietic stem cells by decreasing Kit-Ras/cAMP-CREB pathway networks as evidenced by RNA-Seq assays. FASEB J. 33, 5903–5913. doi:10.1096/fj.201802413r
Warnke, E., Pietsch, J., Kopp, S., Bauer, J., Sahana, J., Wehland, M., et al. (2017). Cytokine release and focal adhesion proteins in normal thyroid cells cultured on the random positioning machine. Cell Physiol. Biochem. 43, 257–270. doi:10.1159/000480368
Wnorowski, A., Sharma, A., Chen, H., Wu, H., Shao, N. Y., Sayed, N., et al. (2019). Effects of spaceflight on human induced pluripotent stem cell-derived cardiomyocyte structure and function. Stem Cell Rep. 13, 960–969. doi:10.1016/j.stemcr.2019.10.006
Wu, S., Xu, R., Duan, B., and Jiang, P. (2017). Three-dimensional hyaluronic acid hydrogel-based models for in vitro human iPSC-derived NPC culture and differentiation. J. Mater. Chem. B 5, 3870–3878. doi:10.1039/c7tb00721c
Xu, J., Shamul, J. G., Staten, N. A., White, A. M., Jiang, B., and He, X. (2021). Bioinspired 3D culture in nanoliter hyaluronic acid-rich core-shell hydrogel microcapsules isolates highly pluripotent human iPSCs. Small 17, 2102219. doi:10.1002/smll.202102219
Xu, K. P., Li, X. F., and Yu, F. S. (2000). Corneal organ culture model for assessing epithelial responses to surfactants. Toxicol. Sci. 58, 306–314. doi:10.1093/toxsci/58.2.306
Yang, M.-H., Chen, K.-C., Chiang, P.-W., Chung, T.-W., Chen, W.-J., Chu, P.-Y., et al. (2016). Proteomic profiling of neuroblastoma cells adhesion on hyaluronic acid-based surface for neural tissue engineering. Biomed Res. Int. 2016, 1–13. doi:10.1155/2016/1917394
Yin, H., Wang, Y., Sun, X., Cui, G., Sun, Z., Chen, P., et al. (2018). Functional tissue-engineered microtissue derived from cartilage extracellular matrix for articular cartilage regeneration. Acta Biomater. 77, 127–141. doi:10.1016/j.actbio.2018.07.031
Zarrinpour, V., Hajebrahimi, Z., and Jafarinia, M. (2017). Expression pattern of neurotrophins and their receptors during neuronal differentiation of adipose-derived stem cells in simulated microgravity condition. Iran. J. Basic Med. Sci. 20, 178–186. doi:10.22038/ijbms.2017.8244
Zhang, S., Zhang, B., Chen, X., Chen, L., Wang, Z., and Wang, Y. (2014). Three-dimensional culture in a microgravity bioreactor improves the engraftment efficiency of hepatic tissue constructs in mice. J. Mater Sci. Mater Med. 25, 2699–2709. doi:10.1007/s10856-014-5279-0
Zhang, Y., Tang, C., Span, P. N., Rowan, A. E., Aalders, T. W., Schalken, J. A., et al. (2020). Polyisocyanide hydrogels as a tunable platform for mammary gland organoid formation. Adv. Sci. (Weinh) 7, 2001797. doi:10.1002/advs.202001797
Zhou, X., Tang, X., Long, R., Wang, S., Wang, P., Cai, D., et al. (2019). The influence of bFGF on the fabrication of microencapsulated cartilage cells under different shaking modes. Polym. (Basel) 11, 471. doi:10.3390/polym11030471
Zhu, J. (2010). Bioactive modification of poly(ethylene glycol) hydrogels for tissue engineering. Biomaterials 31, 4639–4656. doi:10.1016/j.biomaterials.2010.02.044
Zhu, Y., Song, K., Jiang, S., Chen, J., Tang, L., Li, S., et al. (2017). Numerical simulation of mass transfer and three-dimensional fabrication of tissue-engineered cartilages based on chitosan/gelatin hybrid hydrogel scaffold in a rotating bioreactor. Appl. Biochem. Biotechnol. 181, 250–266. doi:10.1007/s12010-016-2210-9
Keywords: 3D culture, microgravity, hydrogel, tissue formation, dysfunction and regeneration
Citation: Ma C, Duan X and Lei X (2023) 3D cell culture model: From ground experiment to microgravity study. Front. Bioeng. Biotechnol. 11:1136583. doi: 10.3389/fbioe.2023.1136583
Received: 03 January 2023; Accepted: 13 March 2023;
Published: 24 March 2023.
Edited by:
Jinglong Tang, Qingdao University, ChinaCopyright © 2023 Ma, Duan and Lei. This is an open-access article distributed under the terms of the Creative Commons Attribution License (CC BY). The use, distribution or reproduction in other forums is permitted, provided the original author(s) and the copyright owner(s) are credited and that the original publication in this journal is cited, in accordance with accepted academic practice. No use, distribution or reproduction is permitted which does not comply with these terms.
*Correspondence: Xianglong Duan, ZHVhbnhpYW5nbG9uZ0Bud3B1LmVkdS5jbg==; Xiaohua Lei, eGgubGVpQHNpYXQuYWMuY24=
Disclaimer: All claims expressed in this article are solely those of the authors and do not necessarily represent those of their affiliated organizations, or those of the publisher, the editors and the reviewers. Any product that may be evaluated in this article or claim that may be made by its manufacturer is not guaranteed or endorsed by the publisher.
Research integrity at Frontiers
Learn more about the work of our research integrity team to safeguard the quality of each article we publish.