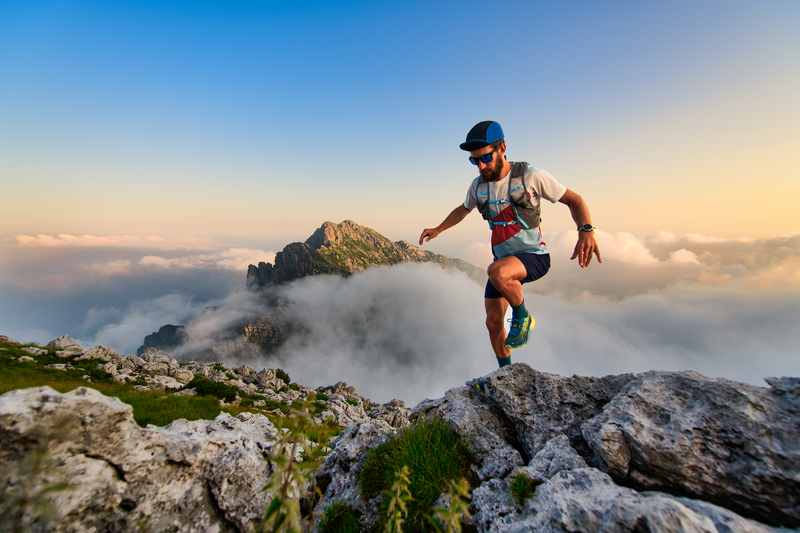
95% of researchers rate our articles as excellent or good
Learn more about the work of our research integrity team to safeguard the quality of each article we publish.
Find out more
REVIEW article
Front. Bioeng. Biotechnol. , 27 July 2023
Sec. Tissue Engineering and Regenerative Medicine
Volume 11 - 2023 | https://doi.org/10.3389/fbioe.2023.1136077
This article is part of the Research Topic Bioactive Peptides for Skin Regeneration View all 5 articles
Skin indicates a person’s state of health and is so important that it influences a person’s emotional and psychological behavior. In this context, the effective treatment of wounds is a major concern, since several conventional wound healing materials have not been able to provide adequate healing, often leading to scar formation. Hence, the development of innovative biomaterials for wound healing is essential. Natural and synthetic polymers are used extensively for wound dressings and scaffold production. Both natural and synthetic polymers have beneficial properties and limitations, so they are often used in combination to overcome overcome their individual limitations. The use of different polymers in the production of biomaterials has proven to be a promising alternative for the treatment of wounds, as their capacity to accelerate the healing process has been demonstrated in many studies. Thus, this work focuses on describing several currently commercially available solutions used for the management of skin wounds, such as polymeric biomaterials for skin substitutes. New directions, strategies, and innovative technologies for the design of polymeric biomaterials are also addressed, providing solutions for deep burns, personalized care and faster healing.
Skin is the first biological barrier against several invaders, such as external biological, physical, and chemical agents, and radiation. This organ has various important roles: body temperature regulation and vitamin D production. Moreover, the skin functions as a scaffold for other tissues and organs such as hair follicles (Hazrati et al., 2022). Given the clear importance of skin, not only in physical health, but in people’s mental health and social behaviour, it is crucial that this tissue is well cared for. Skin is often subject to injury, so effective wound healing is essential.
Wound healing is the biological process of tissue repair, which includes a sequence of cellular events that at a given time lead to new healthy tissue (Suamte et al., 2023), (Ghosh and Gaba, 2013) (Figure 1). This sequence of events comprises i) hemostasis, which consists of the restriction of the microvasculature system, platelets aggregation, degranulation, and fibrin formation, ii) inflammation, when neutrophil, monocyte, and lymphocyte infiltration and macrophage differentiation occur, iii) cellular proliferation, comprising mesenchymal cell differentiation/migration to the wound site, re-epithelialization, and angiogenesis, iv) collagen synthesis and extracellular matrix formation (ECM), and v) remodeling process, including the maturation of vascular functions and ECM remodeling (Hazrati et al., 2022), (Guo and Dipietro, 2010), (Thomas Hess, 2011). Hemostasis and chemotaxis seem to be the first evidence of the inflammatory phase. White blood cells and thrombocytes equally generate mediators and cytokines, contributing to the inflammatory response. In addition to the platelet growth factor, which is responsible for collagen destruction, fibroblasts attraction, new blood vessel formation, and re-epithelialization, mediators such as serotonin and histamine, are released from platelets to enhance the cell permeability. In turn, fibroblasts settle on fibrin and proliferate, beginning to synthesize collagen and some glycosaminoglycans. This step allows wound stabilization and the completion of the wound healing process through the migration of pre-existing cells or the circulation of stem/progenitor cells to the damaged site and their differentiation into epithelial cells. This step is followed by the maturation of the vascular function and ECM remodeling (Hazrati et al., 2022), (Eming et al., 2007).
Wounds can be classified as acute or chronic, depending on the type and magnitude of the skin injury. In this way, small wounds consisting of superficial-partial thickness skin defects are classified as acute wounds and are re-established by a simple contraction along with cell growth in the wound area. On the other hand, large skin wounds consisting of deep-partial and full-thickness defects require a longer time for healing. If the healing process is halted, due to an arrest at the inflammation stage and/or infection for example, the wound becomes chronic (Mogoşanu and Grumezescu, 2014), (Hosseini and Shafiee, 2021). The development of chronic wounds may be triggered by malnutrition, kidney infections, immunodeficiency, diabetes, and age, among other factors (Mogoşanu and Grumezescu, 2014), (Nyame et al., 2015).
Several biological phenomena can occur, such as fibroplasia, granulation, contraction, epithelialization, and scar maturation, leading to significant scaring and poor diagnosis for the patient (Hazrati et al., 2022). Therefore, the need to develop better systems to mimic wound healing physiology is of upmost importance. Polymer-based biomaterials can be used for wound treatment, as wound dressings and regenerative scaffolds. Indeed, polymers have an important feature, as they can form hydrogels, which can absorb and release water, being therefore effective for controlling excessive wound exudates, while keeping a moist environment at the wound site enabling healing (Kohli et al., 2019), (ter Horst et al., 2019).
This review paper aims to describe several solutions used for the management of skin wounds, such as polymeric biomaterials for skin substitutes. Moreover, this research focuses on comparing and discussing the advantages and disadvantages of currently commercially available distinct natural and synthetic polymers used in cutaneous tissue engineering. New directions, strategies and innovative technologies for the design of polymeric biomaterials are also addressed, providing solutions for deep burns, personalized care, and faster healing.
Materials to be used for wound treatment must have biocompatibility, like chitosan (Liu, 2018). Polymers have interesting properties. They are easily modified through chemical processes, with the ability to form 3D structures for scaffolds and tailor their surface functionality (Paul and Sharma, 2004). Polymers flexibility in terms of topology, dimensions, and chemistry make them suitable to act as a drug delivery system enhancing wound repair. Therefore, polymers are great candidates to be used as skin substitutes (Talikowska et al., 2019), (Negut et al., 2020). Table 1 describes the application of different polymers for wound healing and skin applications.
TABLE 1. Polymers commonly employed as scaffolds for wound healing and skin tissue engineering applications.
Natural polymers, as the name states, are obtained from natural sources such as microbial, vegetable, and animal biomass. The characteristics of these polymers, namely biodegradability, biocompatibility, and biological activity, make them ideal for health-related applications (Negut et al., 2020). Moreover, natural-derived polymers can be used to replace natural ECM structural components and skin cellular background (Negut et al., 2020), (Portela et al., 2019). Indeed, these biopolymers are an asset for the design of versatile materials, as they meet the requirements for tissue engineering applications. When subjected to enzymatic degradation, natural polymers form by-products with low toxicity, which in most cases are well accepted by living organisms. However, natural polymers have some limitations, namely the difficulty to control their degradation rates/processes (Negut et al., 2020), (Bao et al., 2009), (Sill and von Recum, 2008). Regarding wound healing applications, natural polymers can be used as bioactive materials enhancing regeneration, as vehicles for drug delivery, and as scaffold formations with 3D networks promoting local tissue regeneration. When selecting polymeric materials for biomedical applications, several characteristics must be considered, including architecture, solubility, pore size, degradability, water absorption capacity, and electrical charge (ter Horst et al., 2019).
In the following sub-sections, several types of natural biopolymers already used in regenerative medicine processes will be discussed.
Alginate has been used for skin regeneration for several years (Barbu, 2021). Alginate is a linear anionic polymer consisting of mannuronic acid (M blocks) and guluronic acid (G blocks) units. Alginate can be obtained from some bacteria, brown algae and kelp (ref). Alginate is a versatile polymer, that can form hydrogels and its mechanical properties can be easily adapted by the type and concentration of a cross-linker (ter Horst et al., 2019), (Augst et al., 2006). Alginate has several advantageous properties, such as biocompatibility, biodegradability, low-immunogenicity, simple gelation, ability to control its degradation, and relatively low cost (Suamte et al., 2023), (ter Horst et al., 2019), (Ceccaldi et al., 2017). When this polymer has a higher ratio of G blocks it is easier to process and it seems to have lower immunogenicity (ter Horst et al., 2019), (Aravamudhan et al., 2014). This polymer also has antiseptic properties, low toxicity, conformability, good water absorption and optimal water vapor transmission rate, which are some of the main characteristics required for wound dressings (Suamte et al., 2023), (Barnett and Varley, 1987). Given its beneficial properties, alginate can also be used in the creation of scaffolds for tissue engineering. Indeed, scaffolds consisting of alginate microspheres, alginate-chitosan, chito-oligosaccharides (COS) with collagen (alginate–chitosan–COS–collagen) and a combination of alginate, chitosan and COS (alginate–chitosan–COS) have been applied as a skin substitute, revealing improved biocompatibility and replicating the skin microenvironment (Suamte et al., 2023), (Chandika et al., 2015). Mobaraki et al. (2021) developed a drug delivery scaffold by conjugating alginate and collagen and incorporated curcumin nanoparticles to enhance wound healing properties. The results showed that wound healing increased by over 90% within 14 days. This study also demonstrated the ability of an alginate/collagen scaffold to incorporate curcumin nanoparticles and consequently improve skin healing. Gao et al. (2022) developed an innovative 3D culture system using an alginate microsphere-collagen hydrogel, which demonstrated the ability to enhance human umbilical cord mesenchymal stem cell survival, allowing their sustained release to boost wound healing.
Cellulose is an abundant polymer in nature and can be obtained from plants (vascular plants or algae) and bacteria (Acetobacter xylinum) (ter Horst et al., 2019). This polymer offers a stable matrix necessary for tissue engineering purposes and is available in diverse forms with exceptional biocompatibility, as is the case of viscose cellulose sponge (Suamte et al., 2023), (Märtson et al., 1998).
Bacterial cellulose has an interesting feature, a porous structure similar to skin. It is a highly hydrophilic material, which is a very important feature for a wound dressing, as a wound requires a moist environment (Kucińska-Lipka et al., 2015). Diaz-Gomez et al. (2022) developed a 3D printed carboxymethyl cellulose scaffold loaded with platelet rich plasma for the treatment of diabetic wounds. This scaffold revealed a sustained release of growth factors and boosted angiogenesis, granulation, and re-epithelialization in skin defects, highlighting its suitability to act as an active dressing for patients with diabetes (Diaz-Gomez et al., 2022). Cellulose can also be associated with different components to further enhance its effect, as demonstrated by Alavi and Nokhodchi (2020). The authors combined cellulose with zinc oxide nanoparticles and reported that this combination improves the mechanical and anti-bacterial properties of the wound dressing. Additionally, Madub et al. (2021) reported the possibility to prepare a nanofibrous scaffold using ulvan cellulose. Using an in vitro assay with fibroblast cells, the study demonstrated that the scaffold enhanced cell growth and accelerated angiogenesis without foreign body response in an in vitro biocompatibility test. Xu et al. (2022) prepared a chitosan-cellulose hydrogel dressing integrating microspheres for wound healing. This hydrogel exhibited good drug release performance, improved antibacterial activity, and had the ability to support the survival and proliferation of human adipose-derived stem cells. The main limitation of this natural polymer is the high cost associated with its purification process.
Chitosan is a polysaccharide obtained from the deacetylation of chitin. Chitin is the structural element crustaceans or fungi exoskeleton (ter Horst et al., 2019), (Ahmadi et al., 2015). The main limitation of chitosan/chitin is its poor stability. As it is a weak base, chitosan/chitin is soluble in an acidic solution, but is not soluble in a neutral solution (ter Horst et al., 2019). This polymer has several beneficial properties, such as homeostasis, mucoadhesion, hydrophilicity and non-toxicity. Its similarity to the ECM structure improves cell adhesion and proliferation, making chitosan/chitin an auspicious candidate for tissue engineering applications (Suamte et al., 2023), (Amado et al., 2008). Indeed, studies have shown that chitosan can improve the function of inflammatory cells during wound healing (Feng et al., 2021). Moreover, it has been reported that a chitosan coating enhances the biocompatibility and hydrophilicity of different biomaterials (Kweon et al., 2003), (Shalumon et al., 2011). Fujita et al. (2004) developed a chitosan hydrogel cross-linked by UV light irradiation that revealed resistance against Escherichia coli growth. Dacron grafts combined with this chitosan hydrogel demonstrated antibacterial activity with considerable local infection inhibition. A study with diabetic rats demonstrated that a chitosan/collagen scaffold fused with Thymosin beta-4 (TB4) presented a sustained release of TB4 leading to faster proliferation and migration of glucose-treated human umbilical vein endothelial cells with heightened angiogenesis (Ti et al., 2014). Rezaii et al. also prepared a chitosan-collagen scaffold with the incorporation of curcumin nanoparticles. This scaffold led to the successful healing of full-thickness punch wounds on a Wistar rat model (Rezaii et al., 2019). The developed scaffold revealed an excellent wound-healing potential since it allowed new blood vessel formation and increased collagen production. Additionally it led to changes at the wound site regarding the granulation tissue density and epidermis thickness (Rezaii et al., 2019). A porous 3D scaffold composed of chitosan hydrochloride, collagen, carboxymethyl cellulose and β-glycerophosphate presented water absorption capacity and sustained released of rosuvastatin. Maged et al. also reported that the loading of mesenchymal stem cells into the scaffold further enhanced fibroblast proliferation and skin regeneration in a wound-healing model of Albino rats (Maged et al., 2019). Vakilian et al. (2021) produced a three-layered scaffold based on chitosan and alginate, which demonstrated excellent biocompatibility and the capacity to promote tissue regeneration.
Dextran is a hydrophilic natural polysaccharide with linear chains containing α-1,6 linked D-glucopyranose residues produced by several strains of bacteria. This polymer contains a large number of hydroxyl groups that can be easily conjugated to drugs and proteins by either direct attachment or through a linker, and its degradation occurs via phagocytosis (Suamte et al., 2023), (Mehvar, 2000). These polymer properties are described in Figure 2. Dextran-derived hydrogels can be prepared by chemical or physical cross-linking or even by radical polymerization (ter Horst et al., 2019). These hydrogels have restricted cell adhesivity and do not appear to influence cell viability, so they are normally used for drug delivery. In addition, dextran has a slow degradation rate, which limits its application for wound healing (ter Horst et al., 2019). Researchers have tried to alter dextran hydrogels properties to enhance cell adhesion (Massia and Stark, 2001) and improve biocompatibility (Sun et al., 2010). For that Möller et al. (2007) modified a dextran degradation profile by treating dextran with glycidyl methacrylate to improve its functionality as a tissue engineered scaffold for burn wound healing (Sun et al., 2011). Sun et al. (2011) developed an acellular dextran hydrogel and studied its application in full thickness burns using a mice model. This study revealed that the prepared hydrogel stimulates angiogenesis and skin regeneration. Shen et al. (2015) reported similar results in a full thickness porcine model, demonstrating an additional improvement in dermal reconstruction and reinnervation. Furthermore, Park et al. (2014) evidenced the capacity of a hypoxia-inducible dextran hydrogel to promote neovascularization. Regarding cell delivery to wounds, Voigt et al. (1999) seeded keratinocytes into collagen-coated dextran microspheres. These microspheres were transplanted as micrograft’s to full thickness wounds in an athymic nude mouse model, revealing the reconstitution of the epithelium, which was multi-layered and keratinized within 14 days. Yang et al. (2022) fabricated a multifunctional hydrogel created through polylysine-graft-cysteine/oxidized dextran for supporting skin tissue regeneration. This hydrogel exhibited not only good antibacterial and free radical scavenging capacity, but also a better efficacy for promoting wound repair than commercial dressing.
Hyaluronic acid is a non-sulfated glycosaminoglycan consisting of repeating polymeric disaccharides of D-glucuronic acid and N-acetyl-D-glucosamine linked by a glucuronidic β (1→3) bond (Weissmann and Meyer, 1954). It is an anionic, linear polymer that exists in all living organisms and can be found in connective tissue particularly in the dermis of the skin (ter Horst et al., 2019). This polymer has several beneficial properties that are described in Figure 3. Hyaluronic acid plays important roles in cell growth and differentiation, and it facilitates early inflammation, which is essential for wound healing (Suamte et al., 2023), (Chen et al., 2002). However, its main limitation is due to its rapid enzymatic degradation in physiological media (Negut et al., 2020). Due to its properties, easy preparation and modification, hydrophilicity, biodegradability and non-adhesiveness, this polymer is normally used for the development of cosmetic products, as well as, tissue scaffolds (Suamte et al., 2023), (Gravante et al., 2007). In addition, hyaluronic acid has physical and biochemical features either in solution or hydrogel form that improves body repair (Collins and Birkinshaw, 2013). Su et al. developed a decellularized scaffold of hyaluronic acid loaded with epidermal growth factor, which exhibited good healing rates (over 87%) on wounds with thick epidermis and skin appendage formation (Su et al., 2014). A study conducted by Sanad and co-workers demonstrated the potential of a novel chitosan–hyaluronic acid composite sponge scaffold enriched with andrographolide lipid nanocarriers for use in wound treatment. Indeed, it has been demonstrated by in vivo evaluation using a rat model that the developed scaffold heightened wound healing with no scar formation and improved tissue quality (Sanad and Abdel-Bar, 2017). Chang et al. developed an enzyme-crosslinked hyaluronic acid-tyramine hydrogel loaded with antioxidant and photothermal silver nanoparticles to be used as a functional wound dressing. This nanocomposite hydrogel has good antibacterial and antioxidant properties, decreases inflammation, supports angiogenesis, and accelerates the healing process. This study demonstrates the great potential of the developed hydrogel for the treatment of infected skin wounds (Chang et al., 2022).
Starch is a storage polysaccharide that has interesting properties, such as biodegradability, non-cytotoxicity, and renewability (MORRISON et al., 1990). Starch is also easily modified by chemical procedures opening the door to its use for drug delivery and tissue engineering. Indeed, Ngoenkam et al. (2010) developed an injectable starch-chitosan hydrogel for the delivery of chondrocytes. Starch can be easily blended with other polymers due to its non-ionic nature. It can increase pore size and water uptake of the resulting polymer blend, as demonstrated in this study. Sundaram et al. also reported excellent mechanical properties with increased porosity (82.51%) of a gelatine-starch scaffold making it suitable for cell growth, vascularization and cellular interaction (Sundaram et al., 2008). Starch-based scaffolds have a good biodegradation rate and can act as a substrate for cell adhesion (Roslan et al., 2016). The potential for these scaffolds in wound healing applications has been highlighted by Waghmare et al. (2018) who showed an increase in mouse fibroblast cells (L929), growth and proliferation with low toxicity. Salehi et al. (2017) prepared starch-based nanocomposite hydrogel scaffolds with zeolite nanoparticles and chamomile extracts for the treatment of chronic ulcers. They demonstrated the successful healing of the refractory ulcers without any hypersensitivity reaction towards the scaffold. Chhabra et al. (2020) reported the development of starch-gelatine scaffolds for wound healing applications. This study revealed the ability of the scaffold to accelerate wound closure and promote tissue reorganization and remodelling.
Agar is a polymer with high biocompatibility and non-immunogenicity (Zarrintaj et al., 2018), which can form a gel that mimics ECM physical characteristics of the tissue with the ability to transport cell growth factors to injured tissues and organs (Lewitus et al., 2011). Nayak and Gupta (2015) demonstrated that a binary blend of agar with keratin is non-toxic and allows cell growth, which makes it appropriate to be used in wound healing and skin regeneration. Furthermore, it has been confirmed that a novel squalene loaded agar-based emulgel scaffold can accelerate the healing of burn wounds by significantly contracting the wound area with high re-vascularization and macrophage polarization (Shanmugarajan et al., 2020). Eivazzadeh-Keihan et al. (2021) developed a nanobiocomposite scaffold based on cross-linked lignin-agarose hydrogel, extracted silk fibroin solution, and zinc chromite nanoparticles for wound healing applications. This study revealed that mice wounds treated with this nanobiocomposite were almost completely healed in 5 days. Basha et al. (2020) synthesized fumaric acid and incorporated it into an agar-silver hydrogel to tackle wound healing. This hydrogel showed a synergistic antibacterial effect against microbes, a quicker rate of wound healing, and augmented collagen deposition and angiogenesis.
Silk is a natural fibrous protein acquired from the larvae of Lepidoptera, consisting of two key proteins: a fibrous protein and sericin which is soluble in water (Suamte et al., 2023), (Reddy et al., 2021). This biopolymer has several beneficial properties such as low weight, high strength, excellent elasticity and durability; and it can be used as a coating material or support for the development of biocompatible scaffolds (Choi et al., 2018). Wang et al. (2021) developed a bioactive scaffold composed of hyaluronic acid and natural silk fibroin nanofiber, which has a similar chemical and biophysical composition, as well as, a similar nanostructure to the ECM. This study revealed the cytocompatibility of the scaffold with cell proliferation and differentiation. The presence of this material increased wound healing with collagen assembly and inhibited scar formation. Babu et al. (2018) conducted a study involving the use of silk fibroin with silver oxide nanoparticles to evaluate their synergistic effect on wound healing. The developed biomaterial revealed a noteworthy antibacterial activity against pathogenic and non-pathogenic bacteria and demonstrated through an in vitro scratch assay that the T3T fibroblast cells quickly migrated to the scratch area with complete coverage of the area upon 24 h. This study also confirmed the biocompatibility of the scaffold through a cytotoxicity assay highlighting its potential for wound healing applications (Babu et al., 2018). Xie et al. (2022) developed an allantoin-functionalized silk fibroin-sodium alginate composite scaffold for the treatment of cutaneous wounds. The scaffolds revealed excellent biocompatibility and enabled the wound healing process by boosting collagen deposition, re-epithelialization, and vascularization at the wound site.
Fibrin is an insoluble protein, formed by the cleavage of soluble fibrinogen by the proteolytic thrombin, which is activated upon tissue injury. Given the ability of fibrin to act as a blood clotting agent, it is natural that it forms a fibrous mesh (ter Horst et al., 2019). This polymer has been widely studied for wound healing applications (ter Horst et al., 2019). Fibrin-based scaffolds have demonstrated that they let binding of a series of active biological molecules to themselves which in turn enables specified cell matrix relations and augments tissue regeneration (Suamte et al., 2023). These scaffolds offer the required time for the development of neo matrix with a gradual reassimilation through proteases (Bencherif et al., 2017). Fibrin can also be used in combination with distinct polymers, as a biological scaffold, with potential biomaterial for the regeneration of primary cells, skin, and bones (Ahmed et al., 2008). The use of this polymer in the design of scaffolds, may not be ideal, as fibrin can be rapidly degraded (SAKIYAMA et al., 1999). Bastidas et al. (2020) prepared a fibrin-based scaffold composed of poly (lactic-co-glycolic acid) (PLGA) by electrospinning. They evaluated its potential to be used as a skin substitute, and results demonstrated that this scaffold presents blood compatibility and incorporation of fibrin improved cell adhesion and viability. Furthermore, the effect of an injectable fibrin scaffold loaded with growth factors for the repair of full thickness skin defects was assessed (Shao et al., 2021). Shao et al. (2021) showed that this scaffold, which has a fine network of platelets and white blood cells, allowed a sustained release of growth hormones, facilitating the cellular proliferation, revascularization and deposition of collagen. These results highlighted the potential of the scaffold to promote skin repair. Mirhaj et al. (2022) fabricated a polycaprolactone/keratin/platelet-rich fibrin fibrous scaffold by electrospinning and demonstrated its angiogenic potential, as well as its ability to accelerate wound healing, collagen deposition and formation of skin appendages.
Collagen is the most abundant protein in the human body and the major component of the extracellular matrix (Sherman et al., 2015). It is the main structural protein in the dermis, having an important role in wound healing. Thereby, collagen has been employed in the development of several skin scaffolds (Chattopadhyay and Raines, 2014). Collagen type I is the most used in skin scaffolds, since it is the most abundant protein in native dermal tissue (ter Horst et al., 2019). This polymer can easily be blended with other polymers such as chitosan, hyaluronic acid, and chondroitin sulfate to obtain improved biological and mechanical features (Matsiko et al., 2012). The properties of the scaffolds can be modulated by varying the concentration of collagen (Wozney et al., 1988). Collagen presents excellent properties, such as biocompatibility, low antigenicity, and high mechanical strength, and it facilitates cell binding, proliferation, differentiation, and production of ECM (Figure 4), which make it suitable for wound healing applications (Reis et al., 2008). Natarajan and co-workers developed tannic acid cross-linked collagen scaffold using a casting technique and evaluated its effects on wound healing (Natarajan et al., 2013). The study included an in vivo test using excision wound model rats, demonstrating improved healing rates, and an efficient wound closure. This new biomaterial also revealed the potential for controlled drug release. Indeed, Tezgel et al. (2020) loaded nanostructured lipid carriers and siRNA complexes in collagen scaffolds and coated them with a 1,2-Dioleoyl-sn-glycero-3-phosphoethanolamine (DOPE) shell. This study showed a prolonged release of siRNA with good transfection efficiency and a prolonged downregulation of ERK-1 protein for 7 days. These results confirmed that the developed collagen scaffolds are promising materials to be applied into wounds to promote healing through controlling the expression of proteins involved in this complex process (Tezgel et al., 2020). Kumar Reddy Sanapalli et al. (2022) developed an L-Glutamic acid loaded collagen/chitosan composite scaffold for the faster healing of diabetic wounds. The scaffolds have not exhibited any toxicity and showed higher rates of wound contraction, having also a modulatory effect on the inflammatory biomarker MMP-9.
Fibronectin is a high molecular weight glycoprotein obtained from human and bovine plasma that can be used as a scaffold to assist cell adherence and migration, thus regulating cell proliferation and differentiation (Suamte et al., 2023), (Pankov and Yamada, 2002). Chantre et al. (2018) produced scaffolds based on fibronectin nanofibers for wound healing applications. The obtained results revealed that this scaffold has the ability to accelerate wound closure, as well as, to increase tissue restoration and recovery of dermal and epidermal constructs including adipose tissue and skin appendages. Jara et al. (2020) synthesized a novel fibrin matrix composed of fibronectin and plasma fibrinogen combined with fibrin nanofibers with fibronectin nanoclusters. These nanoclusters stimulated the pro-healing cells such as endothelial cells, fibroblasts and keratinocytes and promoted their migration, survival, proliferation and function, resulting in quicker and improved wound healing.
Elastin is another ECM protein that is hydrophobic and insoluble in nature. This polymer can be used in the development of biomaterials in its different forms: hydrolyzed soluble elastin, insoluble elastin fibres, recombinant tropoelastin, block copolymers of elastin, repeats of synthetic peptide sequences and in combination with other polymers (Suamte et al., 2023), (Daamen et al., 2007). Boekema et al. (2014) developed a novel collagen-elastin scaffold to be used as a dermal replacement for treating full-thickness skin defects such as burn wounds. Furthermore, a study reported that a triple-polymer scaffold made of a collagen-elastin-polycaprolactone composite was developed (Chong et al., 2019). The inclusion of elastin was revealed to decrease the stiffness of the scaffold, while also decreasing hysteresis and increasing elasticity. Additionally, the developed scaffold promoted keratinocyte and fibroblast proliferation, tissue integration and accelerated early-stage angiogenesis. Thus, this study revealed the potential use of the triple-polymer scaffold in supporting full growth with tissue regeneration in a severe burn injury (Chong et al., 2019).
Keratin is a fibrous protein present in skin, nails and hair. It has interesting properties, like good biocompatibility and the ability to form self-organized structures that synchronize cellular recognition and behavior (Bragulla and Homberger, 2009), (Rouse and Van Dyke, 2010). Moreover, wool keratin has shown its ability to promote cell adhesion, due to the presence of the cell adhesion sequences, arginine-glycine-aspartic acid and leucine-aspartic acid-valine (Tachibana et al., 2002). Xu et al. (2013) prepared a human hair keratin scaffold by freeze-dying and demonstrated its ability for subcutaneous implantation and for treating full-thickness skin defects in rats. Indeed, this study confirmed the potential of keratin for skin regeneration since the scaffold provided a more rapid vascularization, thicker epidermis, lower contractions and formation of new hair follicles. A study involving the preparation of a porous keratin/chitosan scaffold by lyophilization revealed enhanced antibacterial activity and cell proliferation rates, highlighting its promising use for wound dressings (TAN et al., 2015). The development of an in situ forming keratin hydrogel and its application as a drug depot for wound repair revealed that the deferoxamine-loaded scaffold accelerated healing in the full-thickness wounds of streptozotocin-induced diabetic rats by raising angiogenesis and neovascularization in wounds (Chen et al., 2021).
Gelatine is a natural polymer obtained from collagen and it has beneficial features such as low antigenicity, biocompatibility and biodegradability making it suitable for medicinal and pharmaceutical applications (Young et al., 2005). This polymer has acidic and basic groups giving it a high flexibility in terms of its structure in comparison to other polymers. Gelatine can be processed in several forms, for example as injectable hydrogels, sponges and microspheres, given its gelation abilities and ease of processibility (Malafaya et al., 2007). Moreover, other peptides and cell adhesion molecules can be incorporated into gelatine for its use in distinct tissue engineering approaches (Ito et al., 2003). Ninan and co-workers developed porous gelatine/zeolite scaffolds by freeze drying, revealing their cytocompatibility and antibacterial activity, as well as, their ability to improve wound healing in Sprague Dawley rats (Ninan et al., 2014). A study described the development of a gelatine-based scaffold combined with alginate hollow fibers, to attain a vascular network for nutrients and oxygen supply for embedded cells. In this study, human umbilical vein endothelial cells were embedded into the scaffolds and the network formed by the hollow fibers supplied the oxygen and required nutrients and aided the waste removal (Li et al., 2020). Sadeghi et al. (2023) developed a gelatine/alginate sulfate hybrid scaffold as a dermal substitute to hasten the healing of full-thickness diabetic ulcers in a diabetic mouse model. The scaffolds revealed no cytotoxicity, as well as, an increase in cell growth. This increase was related to the increase in the alginate sulfate content. Additionally, this study confirmed the potential of hybrid scaffolds to act as dermal substitutes, as they lead to the formation of an epidermal layer with a homogeneous distribution of collagenous tissue and low penetration of immune cells.
In contrast to natural polymers, synthetic polymers are chemically synthesized. Therefore, its properties can be adjusted in a controlled manner, having constant and homogeneous physico-chemical properties and stability. These polymers do not enclose impurities, being usually mechanically stable and with controlled degradation (Negut et al., 2020). They have several biological properties, namely, biocompatibility, chemical structure versatility, tuneable mechanical properties, and degradation rate. Moreover, some synthetic polymers (e.g., polyesters) can be biodegradable, and, in general, these materials are cost-effective compared to natural polymer preparation (Kohli et al., 2019). However, synthetic polymers also have limitations like an associated toxicity risk, and they do not offer a therapeutical advantage like natural polymers, as they are biologically inert (Negut et al., 2020), (Zhong et al., 2010). In addition, these materials have relatively poor cellular interaction, so they normally require surface treatment or are blended into a composite material (e.g., natural polymers) to enhance their cellular compatibility (Kohli et al., 2019). These surface treatments generally included chemical alterations to reduce hydrophobicity, like inserting polar groups on the polymeric surface increasing direct cell adhesion and/or the addition of a biological component like adhesion peptides (Kohli et al., 2019). The most commonly used strategy consists of the combination of synthetic and natural polymers, as natural polymers contribute with their natural biological activity and their proximity to the ECM tissues (Davison-Kotler et al., 2018; Sharma et al., 2017; V Shevchenko et al., 2010).
Polydimethylsiloxane (PDMS) is a part of the siloxane (silicone) family and is the most commonly silicone elastomer used (Kohli et al., 2019). This polymer has several properties, including its inertia, compatibility with blood, low toxicity, low glass transition temperature, among others (Figure 5) (Akhtar et al., 2016), (Polmanteer, 1988). Given its beneficial features, PDMS is used in several applications, like in cardiac pacemaker leads, artificial skin, contact lenses, medical adhesives, finger joints, catheters and drug delivery systems (Akhtar et al., 2016)– (Leeper and Wright, 1983). Regarding the tissue engineering field, Nikpour and co-workers developed a curcumin-loaded Fe(II) metal–organic framework/polydimethylsiloxane sponge that showed its ability to enhance healing and revascularization, highlighting its potential to be used as a 3D porous substrate for tissue engineering and regenerative medicine (Nikpour et al., 2022).
Polyvinyl Alcohol (PVA) is a water-soluble polymer with a repeating hydroxyl group in its backbone (Kohli et al., 2019). This polymer has desirable features such as biodegradability, biocompatibility, and non-toxicity which make them suitable to be used in several biomedical applications (Allen et al., 2004). PVA is frequently used in conjugation with other polymers, such as chitosan and polyhydroxy butyrate, for the production of nanofibers that can be used in tissue engineering applications (e.g., wound healing) (Rahmani Del Bakhshayesh et al., 2018). Ahlawat et al. (2019) developed Carica papaya-loaded PVA/Gelatine nanofibers for wound dressing application. This study revealed that the fabricated material provided a moist environment at the wound site, allowing re-epithelialization. In addition, the cytotoxicity assay performed on NIH 3T3 fibroblast cells demonstrated that the nanofibers have good compatibility with no cytotoxic effects. It is also worth noting the excellent antibacterial activity of the material against gram-positive and gram-negative bacteria, evidencing the promising use of these nanofibers in wound healing applications. Rajora and colleagues prepared a nanofiber mat of neem gum polysaccharide with PVA using an electrospinning technique (Rajora and Bal, 2022). This nanofiber showed hemocompatibility, as well as, biodegradability having antimicrobial activity against Escherichia coli and Staphylococcus aureus. Furthermore, this study revealed that the prepared device speed up wound healing in mice with dense collagen and fibroblasts highlighting.
Poly-N-vinylpyrrolidone (PVP) is a biocompatible, water-soluble, and biodegradable polymer extensively used as a hydrogel membrane for skin alternative products, since it is no irritating to the skin (Kamoun et al., 2017). PVP also has other beneficial features, such as its environmental stability, low cytotoxicity, high chemical and thermal resistance, affinity to complex hydrophilic and hydrophobic substances and very good solubility in organic solvents (Negut et al., 2020). Moreover, the treatment of PVP through coating methods and copolymers combination gives it a variety of features (e.g., prevention of microbial penetration) useful for wound healing applications (Kohli et al., 2019). A study reported the development of a phlorizin-loaded silk protein/PVP composite nanofibrous membrane to be used as a wound dressing. The study demonstrated its ability to promote collagen deposition and angiogenesis in the wound site, as well as, its capacity to increase autophagy, accelerating wound repair (Sun et al., 2022). Zhang and co-workers prepared a chitosan, PVP, and dihydroquercetin nanofiber film to be used as wound excipients. The fibre film showed antioxidant and antibacterial activity, acceleration of the healing process and promotion of wound healing by inducing the autophagy pathway. The cytotoxicity assay performed on keratinocytes (HaCat cells) proved that the film is non-toxic (Zhang et al., 2022).
Polyurethane (PU) has versatile features such as toughness, durability, biocompatibility, and degradation rates which can be customized depending on the application (Zhang et al., 2016). PU can be employed in several combinations such as with olive oil to obtain antioxidant properties and a photoprotective mechanism or combined with propolis to enhance antibacterial activity and mechanical strength (Amna et al., 2014), (Kim et al., 2014). The combination of PU with dextran fibres through electrospinning revealed good angiogenesis activity alongside high anti-inflammatory action leading to the acceleration of cutaneous wound healing (Unnithan et al., 2015). A study, in which a nanocomposite PU/polycaprolactone scaffold with graphene oxide was developed through electrospinning, revealed great biocompatibility in contact with skin fibroblast cells and improved hydrophilicity (Sadeghianmaryan et al., 2020). Jaganathan et al. fabricated an electrospun PU scaffold loaded with nanofibers of zinc nitrate and evaluated its use as a wound dressing material (Jaganathan and Mani, 2019). The cytocompatibility and hemolytic study demonstrated a lower hemolytic index with increased fibroblasts proliferation. The developed material also exhibited enhanced physiochemical properties. Therefore, this material seems to be a good alternative for wound dressing materials. A PU cellulose acetate electrospun fibbers loaded with rosemary essential oil and adsorbed silver nanoparticles exhibited antibacterial activity and improved hydrophilicity of the fibbers, leading to a better attachment of cells to the micro-nanofibers, similar to the natural ECM (Rather et al., 2023).
Polylactic acid (PLA) is a biodegradable polyester obtained from renewable sources, like corn, starch, or sugar cane (Kohli et al., 2019), (Avinc and Khoddami, 2009). This polymer has several characteristics: inexpensive, non-toxic, hydrophobic, structurally stable, and excellent biocompatibility and mechanical integrity making it suitable for medical applications. PLA is an aliphatic polyester of naturally occurring lactic acid, thus, its degradation by enzymes or hydrolysis under physiological conditions originate products that are easily absorbed by the body (Avinc and Khoddami, 2009; Ulery et al., 2011; Sin et al., 2013; Yoon et al., 2017). Regarding tissue engineering applications, a novel dressing scaffold composed of Polyvinylpyrrolidone/PLA-Polyethylene oxide fibers with encapsulated collagen and cefazolin was evaluated as a wound healing material. Different scaffolds were prepared by varying the collagen concentration (10%, 20%, and 40% w/w based on PVP). The study showed that the scaffold adhesion rises with collagen increase boosting the healing effect. Moreover, the fabricated material exhibited antimicrobial activity and in vivo histological tests revealed that 10% and 20% collagen doses had the highest healing rate (Hajikhani et al., 2021). Another study reported the encapsulation of hydroxyapatite, zirconia, and graphene oxide nanosheets in mono, di, or tri phases, into nanofibrous scaffolds of PLA. It has been demonstrated that the scaffolds exhibited enhanced antibacterial activity and promoted cell viability. In addition, human fibroblasts proliferate on its surface, as well as, in the pores of the nanofibrous scaffold (Al-Wafi et al., 2021).
Polyglycolic acid (PGA) is a biodegradable polymer that belongs to the family of linear aliphatic polyesters (Kohli et al., 2019). This polymer is biocompatible, more hydrophilic than PLA and has high tensile strength (Negut et al., 2020). However, the use of PGA in biomedical applications is limited by its rapid degradation through hydrolysis, which results in a pH decrease in its microenvironment due to carbon dioxide production, leading to local cell and tissue necrosis. Additionally, the excess glycolic acid production from PGA degradation can elicit an inflammatory response (Ceonzo et al., 2006), (Pihlajamäki et al., 2006). Sekiya and collaborators reported that fusing PGA with collagen decreased inflammation and induced neovascularization in a mouse skin defect model (Sekiya et al., 2013). The incorporation of sodium tripolyphosphate into PGA scaffolds fillers via CO2 foaming relieved inflammation and enhanced phagocytosis in a porcine model (Zhang et al., 2020).
Poly(lactic-co-glycolic) acid (PLGA) is a biodegradable polyester copolymer manufactured from random copolymerization of PLA and PGA (Kohli et al., 2019). The main properties of this polymer are described in Figure 6 (Negut et al., 2020). Given its beneficial features, it has been extensively used for sutures, drug delivery devices, and tissue engineering scaffolds (Maitz, 2015), (Pan and Ding, 2012). The preparation of a PLGA-liposome scaffold loaded with microRNA 145 and platelet-derived growth factor promoted angiogenesis in vitro and wound healing in vivo (Hu et al., 2021). Silk sericin-PLGA scaffolds obtained by electrospinning with ketoprofen for the treatment of periodontal diseases were developed by Chachlioutaki et al. (2022). The scaffold had enhanced hydrophilicity and mechanical strength, as well as, an anti-inflammatory effect on LPS-stimulated RAW-264.7 cells. Additionally, the developed material favours attachment and proliferation of human gingival fibroblasts (Chachlioutaki et al., 2022).
Poly-ε-caprolactone (PCL) is an extremely tough aliphatic polymer with good solubility, biocompatibility, exceptional elasticity and mechanical characteristics, low melting point and low decomposition rates along with non-toxicity and cost-effectiveness (Suamte et al., 2023), (Woodruff and Hutmacher, 2010). However, the main limitation of PCL is its low bioactivity and high hydrophobicity leading to the decrease of cell affinity with minimal tissue regeneration. Aiming to minimize these limitations PCL can be combined with other polymers like PLA to form a lesser hydrophobic construct with better degradability and mechanical properties (Patrício et al., 2013). For tissue engineering applications, a bilayer nanofibrous scaffold consisting of fish collagen and PCL produced using electrospinning, with the covalent attachment of chitooligosaccharides via carbodiimide chemistry demonstrated in vitro and in vivo assays and enhanced healing process (Chandika et al., 2021). Ekambaram et al. (2022) developed a novel wheatgrass extract infused PCL/chitosan nanofibrous scaffold through electrospinning for wound infections treatment and enhanced wound healing. The material revealed cytocompatibility against vero cells and exhibited higher anti-bacterial activity than commercial anti-biotic gentamicin. Additionally, the scaffold exhibited higher affinity with binding energy of−9.6 kcal/mol towards wound the healing receptor COX-2. This fact is very important as COX-2 induction enhances faster wound healing.
Polyethylene Glycol (PEG) is a biocompatible, nonbiodegradable, bioinert and hydrophilic polymer that has optimal biological and physicochemical properties, as well as, resistance to protein adsorption (Kohli et al., 2019), (Negut et al., 2020). Given the easiness to control its architecture along with its chemical composition, this polymer is an attractive scaffold material for tissue engineering applications (Suamte et al., 2023). A study in which an exosome secreted by adipose-derived stem cell was loaded into the matrix of a metalloproteinase degradable PEG smart hydrogel promoted diabetic wound healing by improving cellular functions (Jiang et al., 2022). Jin et al. (2021) developed a hybrid hydrogel composed of chitosan and poly (D,l-lactide)-PEG-poly (D,l-lactide) that tightly adhered to the skin with good antibacterial properties and accelerated the wound healing process.
Tissue engineered skin substitutes have emerged as excellent alternatives to traditional wound healing strategies and tissue regeneration. It should be noted that skin was the first engineered organ that went from the bench to patient care (Rheinwald, 1989). Over the last few years, several synthetic and bioengineered substitutes have been prepared. Usually, these substitutes are placed within the wound providing several benefits, like the provision of barrier function along with protection against microorganisms, pain reduction and wound healing promotion (Alonso and Fuchs, 2003)–(de Mel et al., 2012). The research from the past years led to the commercialization of several skin substitutes, which can be classified in different ways (V Shevchenko et al., 2010), (Clark et al., 2007), (Horch et al., 2005; Atiyeh and Costagliola, 2007; MacNeil, 2007). They can be classified according to their cover duration as permanent, semi-permanent, or temporary, according to their anatomical structure as epidermal, dermal, or dermo-epidermal (composite), based on their composition as cellular or acellular, and according to the type of biomaterial used as biological (autologous, allogeneic, xenogeneic) or synthetic (biodegradable, non-biodegradable). Synthetic skin substitutes are composed of acellular materials, usually used as a barrier to fluid loss and microbial contamination. These skin substitutes normally comprise a nylon mesh or collagen that acts as a “dermis” and a silicon membrane that acts as an “epidermis” (Vig et al., 2017). They are used principally as temporary skin substitutes for superficial or mid-dermal partial thickness wounds and burns (Shakespeare and Shakespeare, 2002), (Shakespeare, 2005). Biobrane®, Integra® and Alloderm® are some of the synthetic acellular skin substitutes frequently used. On the other hand, the natural skin substitutes are primarily cultured allogeneic or autologous cell suspensions or sheets, which can be used on their own or along with a dermal matrix (Vig et al., 2017). Cellular allogeneic skin substitutes are generally developed using living neonatal foreskin fibroblasts with a mesh or matrix. These biomaterials have been used for the treatment of venous and diabetic ulcers, as well as, for wound management in epidermolysis bullosa, skin cancer, and in burns (Horch et al., 2005), (Supp and Boyce, 2005). Dermagraft®, Apligraf®, TransCyte® and OrCel® are some examples of commonly used natural skin substitutes with allogeneic cells. In the case of cellular autologous skin substitutes, there are two types available - Cultured Epidermal Autograft (CEA) and Cultured Skin Substitutes (CSS) (Vig et al., 2017). CEA involves the culture of autologous keratinocytes, derived from the skin biopsy of the patient (Supp and Boyce, 2005), while CSS is an autologous graft with both epidermal and dermal components. Epicel® is an example of a skin substitute with autologous cells. Table 2 lists some of the commercially available skin substitutes, including those already mentioned.
Being one of the most complex processes in the human body, wound healing involves spatial and temporal synchronization of the inflammatory phase, with tissue regeneration and remodeling phases (Gethin, 2012), (Velnar et al., 2009).
Nowadays, skin wound treatment follows a combination between regeneration strategies and conventional treatments, like debridement (Tottoli et al., 2020). Wound treatment is still a challenging field, for example the difficulty to use skin grafts for the replacement of surface deficits, due to the impossibility to fixed them by simply approaching it to the injured skin margins. Also, skin grafts are major surgical procedures that are Invasive and can expose the patients to severe complications, since there will be an area of the skin that will know be exposed due to the skin that was removed to cover the wound (Dunkin et al., 2007). Regenerative skin wound healing is a novel and fast developing medical science in the biomedical field that seeks to restore skin to its original function, aiming to reestablish injured cells and skin. It focuses on the enhancement of the regeneration process using different strategies, based on a reparative approach to the physiological process of wound healing, minimizing the scarring (Boyce and Lalley, 2018), (Pang et al., 2017).
There are key components in regenerative medicine, particularly for wound healing, namely growth factors, involved in the stimulation of the wounds (WERNER and GROSE, 2003); cellular skin substitutes, that provides all the elements needed for skin regeneration (e.g., cells, mediators and materials that mimic the extracellular matrix (ECM)) (Kallis et al., 2018); gene therapy, for the prevention or treatment of skin diseases (for example, the use of holoclones in a patient with junctional epidermolysis, a disease that results in fatal skin adhesion disorder) (Mavilio et al., 2006; Aragona and Blanpain, 2017; High and Roncarolo, 2019); induced pluripotent stem cells (iPSCs), where the cell reprograming technology consists in reprogramming the adult stomatic cells into those iPSCs. iPSCs are expected to have a major role in regenerative medicine as an important source for transplant therapy, since in vitro and in vivo studies on mouse models have demonstrated the vast potential offered by them. iPSCs can generate a number of human autologous cells for degenerative skin disorders and advanced chronic wound treatment (Takahashi et al., 2007; Hu et al., 2010; Villa-Diaz et al., 2012; Zhang et al., 2015; Wang et al., 2019); skin tissue engineering, consisting in different methods to promote biological regeneration, repair the tissues, or replace tissues functions with systems that contain living tissue or cells. The variety of elements in the tissue engineering area comprises biomaterials, growth factors, cells, and engineering components such as scaffolds, tubes, bioreactors, oxygenators and pumps (Ehrenreich and Ruszczak, 2006).
Scaffolds are the best strategy to restore, maintain and improve tissue functionality in wound treatments according to the definition of tissue engineering as described by the National Science Foundation seminar. Scaffolds have a specific role in the reparation and regeneration of tissues, providing a foundation for cell survival, proliferation and differentiation (O’Brien, 2011), (Chaudhari et al., 2016). Scaffolds should be as similar as possible to the native ECM since all cells are constantly in contact with the ECM. Isolated cells are not capable of spontaneously organizing themselves into new tissues. Furthermore, the ECM is widely known to offer structural support to cells and tissues (Hynes, 2009). Scaffolds can be produced using natural and/or synthetic biomaterials. Materials which can remain stable in a biological environment and/or from materials that can be degraded in the human body (Chaudhari et al., 2016), (Chen and Wang, 2002). The use of synthetic materials has several advantages: production on large scales, the ability to control the material’s molecular weight and degradation time, adapting its properties to different applications; their tunable chemical properties, by cross-linking for example; and their biocompatibility and biodegradability properties (Eltom et al., 2019). Polymers are the most frequently used material in tissue engineering, particularly biodegradable polyesters, which have greater compatibility with body tissues. Synthetic polymers such as PLA, PGA, PLGA and PCL can form matrices individually or as composites. These polymers have been used due to their process easiness, adjustable mechanical and chemical properties, and their low cost. A work conducted by Ferri et al. (2018) analyzed cell growth and healing effectiveness in composite systems containing synthetic polymers and bioactive substances, where bioactive glasses were mixed with PLGA copolymer and the results showed encouraging evidence in vitro and in vivo scaffolds neovascularization. In order to overcome the previously described limitations of synthetic materials, natural materials have been studied since they have good biocompatibility and biodegradability when compared with synthetic materials. Some natural materials contain signal sequences that promote and maintain cell attachment, function, and structure. Natural polymers such as silk, fibrin, collagen, and gelatin are the most used natural biomaterials, particularly for protein derivation and are intended for tissue engineering, because these polymers are already present in the human body as ECM elements (Tottoli et al., 2020). Recently, a collagen-based scaffold developed in the form of a wound graft, marketed as Integra, was approved by the U.S. Food and Drug Administration for the treatment of severe burns. The new treatment decreased patients’ hospital stays (Iovene et al., 2021), (Ryan et al., 2002).
Recently natural polymers have been studied in combination with synthetic polymers, or even combined with drugs and bioactive molecules. The combination of these materials improves bioactivity, including mechanical and chemical properties, and the chemical release is more controlled for regenerative medicine. Singaravelu et al. conducted a study where a complex matrix made by a porous keratin-fibrin-gelatin 3D sponge scaffold was designed and the drug mupirocin was incorporated for tissue engineering applications (Frykberg and Banks, 2015). For tissue regeneration the combination of multi-polymer-type materials in their composition offers the capability to adjust mechanical strength, degradation rate, chemical properties, and cellular adhesion.
With all the research being made with the combination of both natural and synthetic materials, and since it has been proven to create porous and local bioactive environment to regenerate injured tissues. It is probably the route that will be followed in the skin regeneration field.
Skin appearance is of extreme importance physiologically and psychologically. Since skin tissue is often subject to injury and consequently scarring, it is necessary to develop new alternatives to enhance its healing process, minimize scar formation, and ensure rapid and efficient skin regeneration. Thus, the use of polymer-based biomaterials in wound treatment as wound dressings and regenerative scaffolds is noteworthy.
Natural polymers present excellent features such as biodegradability, biocompatibility, and biological activity. On the other hand, synthetic polymers are biocompatible, present a versatile chemical structure and are easily modified to obtain distinct mechanical properties and degradation rates. In general, these polymers are cost-effective compared to natural polymers. However, synthetic polymers also have disadvantages when compared to natural polymers, namely, higher toxicity and lack of therapeutic advantage as they are biologically inert. However, it is possible to overcome this by coating the polymer with bioactive molecules and or natural polymers, leading to the formation of a composite material with enhanced bioactivity.
This review presents a detailed description of the polymers from natural polymers, synthetic polymers, or a combination of both, and the newer strategies to improve wound healing treatment.
CO–Research and Writing JT–Revision PF-S–Revision CB–Writing, Supervision and Revision. All authors contributed to the article and approved the submitted version.
This research was supported by the Portuguese Foundation for Science and Technology (FCT) under the scope of the strategic funding of UIDB/04469/2020 unit, and by LABBELS—Associate Laboratory in Biotechnology, Bioengineering and Microelectromechanical Systems, LA/P/0029/2020. This study was also funded by program Marie Skłodowska-Curie grant (MSCA-RISE; FODIAC; 778388), and by the European Regional Development Fund (ERDF) through the Competitiveness factors Operational program—Norte 2020, COMPETE and by National Funds through the FCT—under the project AgriFood XXI (NORTE- 01-0145-FEDER-000041).
PF-S would like to express gratitude to the Spanish Ministry of Science, Innovation and Universities and the European Union–NextGenerationEU/PRTR–for financial support (FJC2021-046978-I).
The authors declare that the research was conducted in the absence of any commercial or financial relationships that could be construed as a potential conflict of interest.
All claims expressed in this article are solely those of the authors and do not necessarily represent those of their affiliated organizations, or those of the publisher, the editors and the reviewers. Any product that may be evaluated in this article, or claim that may be made by its manufacturer, is not guaranteed or endorsed by the publisher.
Ackermann, K., Borgia, S. L., Korting, H. C., Mewes, K. R., and Schäfer-Korting, M. (2010). The Phenion full-thickness skin model for percutaneous absorption testing. Skin. Pharmacol. Physiol. 23 (2), 105–112. doi:10.1159/000265681
Ahlawat, J., Kumar, V., and Gopinath, P. (2019). Carica papaya loaded poly (vinyl alcohol)-gelatin nanofibrous scaffold for potential application in wound dressing. Mat. Sci. Eng. C 103, 109834. doi:10.1016/j.msec.2019.109834
Ahmadi, F., Oveisi, Z., Samani, S. M., and Amoozgar, Z. (2015). Chitosan based hydrogels: Characteristics and pharmaceutical applications. Res. Pharm. Sci. 10 (1), 1–16.
Ahmed, T. A. E., V Dare, E., and Hincke, M. (2008). Fibrin: A versatile scaffold for tissue engineering applications. Tissue Eng. Part B Rev. 14 (2), 199–215. doi:10.1089/ten.teb.2007.0435
Akhtar, M. F., Hanif, M., and Ranjha, N. M. (2016). Methods of synthesis of hydrogels … A review, A review. Saudi Pharm. J. 24 (5), 554–559. doi:10.1016/j.jsps.2015.03.022
Al-Wafi, R., Mansour, S. F., AlHammad, M. S., and Ahmed, M. K. (2021). Biological response, antibacterial properties of ZrO2/hydroxyapatite/graphene oxide encapsulated into nanofibrous scaffolds of polylactic acid for wound healing applications. Int. J. Pharm. 601, 120517. doi:10.1016/j.ijpharm.2021.120517
Alavi, M., and Nokhodchi, A. (2020). An overview on antimicrobial and wound healing properties of ZnO nanobiofilms, hydrogels, and bionanocomposites based on cellulose, chitosan, and alginate polymers. Carbohydr. Polym. 227, 115349. doi:10.1016/j.carbpol.2019.115349
Allen, M. J., Schoonmaker, J. E., Bauer, T. W., Williams, P. F., Higham, P. A., and Yuan, H. A. (2004). Preclinical evaluation of a poly (vinyl alcohol) hydrogel implant as a replacement for the nucleus pulposus. Spine (Phila. pa. 1976) 29 (5), 515–523. doi:10.1097/01.brs.0000113871.67305.38
Alonso, L., and Fuchs, E. (2003). Stem cells of the skin epithelium. Proc. Natl. Acad. Sci. 100. 11830–11835. doi:10.1073/pnas.1734203100
Amado, S., Simões, M., Armada da Silva, P., Luís, A., Shirosaki, Y., Lopes, M., et al. (2008). Use of hybrid chitosan membranes and N1E-115 cells for promoting nerve regeneration in an axonotmesis rat model. Biomaterials 29 (33), 4409–4419. doi:10.1016/j.biomaterials.2008.07.043
Amna, T., Hassan, M., Yang, J., Khil, M. S., Song, K. D., Hwang, , et al. (2014). Virgin olive oil blended polyurethane micro/nanofibers ornamented with copper oxide nanocrystals for biomedical applications. Int. J. Nanomedicine 9, 891–898. doi:10.2147/IJN.S54113
Aragona, M., and Blanpain, C. (2017). Transgenic stem cells replace skin. Nature 551 (7680), 306–307. doi:10.1038/nature24753
Aravamudhan, A., Ramos, D. M., Nada, A. A., and Kumbar, S. G. (2014). “Chapter 4 - natural polymers: Polysaccharides and their derivatives for biomedical applications,” in Natural and synthetic biomedical polymers. Editors S. G. Kumbar, C. T. Laurencin, and S. B. P. Deng (Oxford: Elsevier), 67–89.
Atiyeh, B. S., and Costagliola, M. (2007). Cultured epithelial autograft (CEA) in burn treatment: Three decades later. Burns 33 (4), 405–413. doi:10.1016/j.burns.2006.11.002
Augst, A. D., Kong, H. J., and Mooney, D. J. (2006). Alginate hydrogels as biomaterials. Macromol. Biosci. 6 (8), 623–633. doi:10.1002/mabi.200600069
Avinc, O., and Khoddami, A. (2009). Overview of poly(lactic acid) (PLA) fibre. Fibre Chem. 41 (6), 391–401. doi:10.1007/s10692-010-9213-z
Babu, P. J., Doble, M., and Raichur, A. M. (2018). Silver oxide nanoparticles embedded silk fibroin spuns: Microwave mediated preparation, characterization and their synergistic wound healing and anti-bacterial activity. J. Colloid Interface Sci. 513, 62–71. doi:10.1016/j.jcis.2017.11.001
Bao, P., Kodra, A., Tomic-Canic, M., Golinko, M. S., Ehrlich, H. P., and Brem, H. (2009). The role of vascular endothelial growth factor in wound healing. J. Surg. Res. 153 (2), 347–358. doi:10.1016/j.jss.2008.04.023
Barbu, A. (2021). Personalized medicine review current trends in advanced alginate-based wound dressings for chronic wounds. doi:10.3390/jpm11090890
Barnett, S. E., and Varley, S. J. (1987). The effects of calcium alginate on wound healing. Ann. R. Coll. Surg. Engl. 69 (4), 153–155.
Barzegar, S., Zare, M. R., Shojaei, F., Zareshahrabadi, Z., Koohi-Hosseinabadi, O., Saharkhiz, M. J., et al. (2021). Core-shell chitosan/PVA-based nanofibrous scaffolds loaded with Satureja mutica or Oliveria decumbens essential oils as enhanced antimicrobial wound dressing. Int. J. Pharm. 597, 120288. doi:10.1016/j.ijpharm.2021.120288
Basha, S. I., Ghosh, S., Vinothkumar, K., Ramesh, B., kumari, P. H. p., Mohan, K. M., et al. (2020). Fumaric acid incorporated Ag/agar-agar hybrid hydrogel: A multifunctional avenue to tackle wound healing. Mat. Sci. Eng. C 111, 110743. doi:10.1016/j.msec.2020.110743
Bastidas, J. G., Maurmann, N., da Silveira, M. R., Ferreira, C. A., and Pranke, P. (2020). Development of fibrous PLGA/fibrin scaffolds as a potential skin substitute. Biomed. Mat. 15 (5), 055014. doi:10.1088/1748-605X/aba086
Bello, Y. M., Falabella, A. F., and Eaglstein, W. H. (2001). Tissue-engineered skin. Am. J. Clin. Dermatol. 2 (5), 305–313. doi:10.2165/00128071-200102050-00005
Bencherif, S. A., Gsib, O., and Egles, C. (2017). Fibrin: An underrated biopolymer for skin tissue engineering. J. Mol. Biol. Biotech. 2 (1).
Boekema, B. K. H. L., Vlig, M., Olde Damink, L., Middelkoop, E., Eummelen, L., Bühren, A. V., et al. (2014). Effect of pore size and cross-linking of a novel collagen-elastin dermal substitute on wound healing. J. Mat. Sci. Mat. Med. 25 (2), 423–433. doi:10.1007/s10856-013-5075-2
Boyce, S. T., and Lalley, A. L. (2018). Tissue engineering of skin and regenerative medicine for wound care. Burn. Trauma 6, 4. doi:10.1186/s41038-017-0103-y
Bragulla, H. H., and Homberger, D. G. (2009). Structure and functions of keratin proteins in simple, stratified, keratinized and cornified epithelia. J. Anat. 214 (4), 516–559. doi:10.1111/j.1469-7580.2009.01066.x
Caravaggi, C., De Giglio, R., Pritelli, C., Sommaria, M., Dalla Noce, S., Faglia, E., et al. (2003). HYAFF 11-based autologous dermal and epidermal grafts in the treatment of noninfected diabetic plantar and dorsal foot ulcers: A prospective, multicenter, controlled, randomized clinical trial. Diabetes Care 26 (10), 2853–2859. doi:10.2337/diacare.26.10.2853
Carsin, H., Ainaud, P., Le Bever, H., Rives, J. M., Lakhel, A., Stephanazzi, J., et al. (2000). Cultured epithelial autografts in extensive burn coverage of severely traumatized patients: A five year single-center experience with 30 patients. Burns 26 (4), 379–387. doi:10.1016/S0305-4179(99)00143-6
Ceccaldi, C., Bushkalova, R., Cussac, D., Duployer, B., Tenailleau, C., Bourin, P., et al. (2017). Elaboration and evaluation of alginate foam scaffolds for soft tissue engineering. Int. J. Pharm. 524 (1), 433–442. doi:10.1016/j.ijpharm.2017.02.060
Centanni, J. M., Straseski, J. A., Wicks, A., Hank, J. A., Rasmussen, C. A., Lokuta, M. A., et al. (2011). StrataGraft skin substitute is well-tolerated and is not acutely immunogenic in patients with traumatic wounds: Results from a prospective, randomized, controlled dose escalation trial. Ann. Surg. 253 (4), 672–683. doi:10.1097/SLA.0b013e318210f3bd
Ceonzo, K., Gaynor, A., Shaffer, L., Kojima, K., Vacanti, C. A., and Stahl, G. L. (2006). Polyglycolic acid-induced inflammation: Role of hydrolysis and resulting complement activation. Tissue Eng. 12 (2), 301–308. doi:10.1089/ten.2006.12.301
Chachlioutaki, K., Karavasili, C., Adamoudi, E., Bouropoulos, N., Tzetzis, D., Bakopoulou, A., et al. (2022). Silk sericin/PLGA electrospun scaffolds with anti-inflammatory drug-eluting properties for periodontal tissue engineering. Biomater. Adv. 133, 112723. doi:10.1016/j.msec.2022.112723
Chandika, P., Khan, F., Heo, S.-Y., Kim, Y.-M., Yi, M., and Jung, W.-K. (2022). Enhanced wound-healing capability with inherent antimicrobial activities of usnic acid incorporated poly(ε-caprolactone)/decellularized extracellular matrix nanofibrous scaffold. Biomater. Adv. 140, 213046. doi:10.1016/j.bioadv.2022.213046
Chandika, P., Ko, S. C., Oh, G. W., Heo, S. Y., Nguyen, V. T., Jeon, Y. J., et al. (2015). Fish collagen/alginate/chitooligosaccharides integrated scaffold for skin tissue regeneration application. Int. J. Biol. Macromol. 81, 504–513. doi:10.1016/j.ijbiomac.2015.08.038
Chandika, P., Oh, G. W., Heo, S. Y., Kim, S. C., Kim, T. H., Kim, M. S., et al. (2021). Electrospun porous bilayer nano-fibrous fish collagen/PCL bio-composite scaffolds with covalently cross-linked chitooligosaccharides for full-thickness wound-healing applications. Mat. Sci. Eng. C 121, 111871. doi:10.1016/j.msec.2021.111871
Chang, R., Zhao, D., Zhang, C., Liu, K., He, Y., Guan, F., et al. (2022). Nanocomposite multifunctional hyaluronic acid hydrogel with photothermal antibacterial and antioxidant properties for infected wound healing. Int. J. Biol. Macromol. 226, 870–884. doi:10.1016/j.ijbiomac.2022.12.116
Chantre, C. O., Campbell, P. H., Golecki, H. M., Buganza, A. T., Capulli, A. K., Deravi, L. F., et al. (2018). Production-scale fibronectin nanofibers promote wound closure and tissue repair in a dermal mouse model. Biomaterials 166, 96–108. doi:10.1016/j.biomaterials.2018.03.006
Chattopadhyay, S., and Raines, R. T. (2014). Collagen-based biomaterials for wound healing. Biopolymers 101 (8), 821–833. doi:10.1002/bip.22486
Chaudhari, A., Vig, K., Baganizi, D., Sahu, R., Dixit, S., Dennis, V., et al. (2016). Future prospects for scaffolding methods and biomaterials in skin tissue engineering: A review. Int. J. Mol. Sci. 17 (12), 1974. doi:10.3390/ijms17121974
Chen, L. J., and Wang, M. (2002). Production and evaluation of biodegradable composites based on PHB–PHV copolymer. Biomaterials 23 (13), 2631–2639. doi:10.1016/S0142-9612(01)00394-5
Chen, W. Y. (2002). “Functions of hyaluronan in wound repair,” in Hyaluronan. Editors J. F. KENNEDY, G. O. PHILLIPS, and P. A. B. T.-H. WILLIAMS (Woodhead Publishing), 147–156.
Chen, Y., Li, Y., Yang, X., Cao, Z., Nie, H., Bian, Y., et al. (2021). Glucose-triggered in situ forming keratin hydrogel for the treatment of diabetic wounds. Acta Biomater. 125, 208–218. doi:10.1016/j.actbio.2021.02.035
Chhabra, R., Peshattiwar, V., Pant, T., Deshpande, A., Modi, D., Sathaye, S., et al. (2020). In vivo studies of 3D starch–gelatin scaffolds for full-thickness wound healing. ACS Appl. Bio Mat. 3 (5), 2920–2929. doi:10.1021/acsabm.9b01139
Choi, J. H., Kim, D. K., Song, J. E., Oliveira, J. M., Reis, R. L., and Khang, G. (2018). in Silk fibroin-based scaffold for bone tissue engineering BT - novel biomaterials for regenerative medicine. Editors H. J. Chun, K. Park, C.-H. Kim, and G. Khang (Singapore: Springer Singapore), 371–387.
Chong, C., Wang, Y., Fathi, A., Parungao, R., Maitz, P. K., and Li, Z. (2019). Skin wound repair: Results of a pre-clinical study to evaluate electropsun collagen–elastin–PCL scaffolds as dermal substitutes. Burns 45 (7), 1639–1648. doi:10.1016/j.burns.2019.04.014
Clark, R. A. F., Ghosh, K., and Tonnesen, M. G. (2007). Tissue engineering for cutaneous wounds. J. Invest. Dermatol. 127 (5), 1018–1029. doi:10.1038/sj.jid.5700715
Collins, M. N., and Birkinshaw, C. (2013). Hyaluronic acid based scaffolds for tissue engineering—a review. Carbohydr. Polym. 92 (2), 1262–1279. doi:10.1016/j.carbpol.2012.10.028
Cullen, B., Watt, P. W., Lundqvist, C., Silcock, D., Schmidt, R. J., Bogan, D., et al. (2002). The role of oxidised regenerated cellulose/collagen in chronic wound repair and its potential mechanism of action. Int. J. Biochem. Cell Biol. 34 (12), 1544–1556. doi:10.1016/s1357-2725(02)00054-7
Daamen, W. F., Veerkamp, J. H., van Hest, J. C. M., and van Kuppevelt, T. H. (2007). Elastin as a biomaterial for tissue engineering. Biomaterials 28 (30), 4378–4398. doi:10.1016/j.biomaterials.2007.06.025
Davison-Kotler, E., Sharma, V., Kang, N. V., and García-Gareta, E. (2018). A universal classification system of skin substitutes inspired by factorial design. Tissue Eng. Part B Rev. 24 (4), 279–288. doi:10.1089/ten.teb.2017.0477
de Mel, A., Seifalian, A. M., and Birchall, M. A. (2012). Orchestrating cell/material interactions for tissue engineering of surgical implants. Macromol. Biosci. 12, 1010–1021. doi:10.1002/mabi.201200039
Diaz-Gomez, L., Gonzalez-Prada, I., Millan, R., Da Silva-Candal, A., Bugallo-Casal, A., Campos, F., et al. (2022). 3D printed carboxymethyl cellulose scaffolds for autologous growth factors delivery in wound healing. Carbohydr. Polym. 278, 118924. doi:10.1016/j.carbpol.2021.118924
Dodson, B. P., and Levine, A. D. (2015). Challenges in the translation and commercialization of cell therapies. BMC Biotechnol. 15, 70. doi:10.1186/s12896-015-0190-4
Dunkin, C. S. J., Pleat, J. M., Gillespie, P. H., Tyler, M. P. H., Roberts, A. H. N., and McGrouther, D. A. (2007). Scarring occurs at a critical depth of skin injury: Precise measurement in a graduated dermal scratch in human volunteers. Plast. Reconstr. Surg. 119 (6), 1722–1732. doi:10.1097/01.prs.0000258829.07399.f0
Ehrenreich, M., and Ruszczak, Z. (2006). Update on tissue-engineered biological dressings. Tissue Eng. 12 (9), 2407–2424. doi:10.1089/ten.2006.12.2407
Eivazzadeh-Keihan, R., Moghim Aliabadi, H. A., Radinekiyan, F., Sobhani, M., Farzane khalili, , Maleki, A., et al. (2021). Investigation of the biological activity, mechanical properties and wound healing application of a novel scaffold based on lignin–agarose hydrogel and silk fibroin embedded zinc chromite nanoparticles. RSC Adv. 11 (29), 17914–17923. doi:10.1039/D1RA01300A
Ekambaram, R., Sugumar, M., Karuppasamy, S., Prasad, P., and Dharmalingam, S. (2022). Fabrication of wheatgrass incorporated PCL/chitosan biomimetic nanoscaffold for skin wound healing: In vitro and in silico analysis. J. Drug Deliv. Sci. Technol. 71, 103286. doi:10.1016/j.jddst.2022.103286
Eltom, A., Zhong, G., and Muhammad, A. (2019). Scaffold techniques and designs in tissue engineering functions and purposes: A review. Adv. Mat. Sci. Eng. 2019, 1–13. doi:10.1155/2019/3429527
Eming, S. A., Krieg, T., and Davidson, J. M. (2007). Inflammation in wound repair: Molecular and cellular mechanisms. J. Invest. Dermatol. 127 (3), 514–525. doi:10.1038/sj.jid.5700701
Feng, P., Luo, Y., Ke, C., Qiu, H., Wang, W., Zhu, Y., et al. (2021). Chitosan-based functional materials for skin wound repair: Mechanisms and applications. Front. Bioeng. Biotechnol. 9, 650598. doi:10.3389/FBIOE.2021.650598
Ferreira, M. C., Paggiaro, A. O., Isaac, C., Teixeira Neto, N., and dos Santos, G. B. (2011). Substitutos cutâneos: Conceitos atuais e proposta de classificação. Rev. Bras. Cir. Plástica 26, 696–702. doi:10.1590/s1983-51752011000400028
Ferri, J., Jordá, J., Montanes, N., Fenollar, O., and Balart, R. (2018). Manufacturing and characterization of poly(lactic acid) composites with hydroxyapatite. J. Thermoplast. Compos. Mat. 31 (7), 865–881. doi:10.1177/0892705717729014
Frykberg, R. G., and Banks, J. (2015). Challenges in the treatment of chronic wounds. Adv. wound care 4 (9), 560–582. doi:10.1089/wound.2015.0635
Fujita, M., Kinoshita, M., Ishihara, M., Kanatani, Y., Morimoto, Y., Simizu, M., et al. (2004). Inhibition of vascular prosthetic graft infection using a photocrosslinkable chitosan hydrogel. J. Surg. Res. 121 (1), 135–140. doi:10.1016/j.jss.2004.04.010
Gao, Y., Kang, Y., Wang, T., Li, C., Shen, S., Qu, C., et al. (2022). Alginate microspheres-collagen hydrogel, as a novel 3D culture system, enhanced skin wound healing of hUCMSCs in rats model. Colloids Surfaces B Biointerfaces 219, 112799. doi:10.1016/j.colsurfb.2022.112799
Gethin, G. (2012). Understanding the inflammatory process in wound healing. Br. J. Community Nurs. 17, S17–S22. doi:10.12968/bjcn.2012.17.Sup3.S17
Ghorbani, M., Nezhad-Mokhtari, P., and Ramazani, S. (2020). Aloe vera-loaded nanofibrous scaffold based on Zein/Polycaprolactone/Collagen for wound healing. Int. J. Biol. Macromol. 153, 921–930. doi:10.1016/j.ijbiomac.2020.03.036
Ghosh, P. K., and Gaba, A. (2013). Phyto-extracts in wound healing. J. Pharm. Pharm. Sci. 16, 760–820. 5 SE-Review Articles. doi:10.18433/J3831V
Goedkoop, R., Juliet, R., You, P. H. K., Daroczy, J., de Roos, K. P., Lijnen, R., et al. (2010). Wound stimulation by growth-arrested human keratinocytes and fibroblasts: HP802-247, a new-generation allogeneic tissue engineering product. Dermatology 220 (2), 114–120. doi:10.1159/000277380
Gordley, K., Cole, P., Hicks, J., and Hollier, L. (2009). A comparative, long term assessment of soft tissue substitutes: AlloDerm, enduragen, and dermamatrix. J. plastic, Reconstr. aesthetic Surg. JPRAS 62, 849–850. doi:10.1016/j.bjps.2008.05.006
Gravante, G., Delogu, D., Giordan, N., Morano, G., Montone, A., and Esposito, G. (2007). The use of hyalomatrix PA in the treatment of deep partial-thickness burns. J. Burn Care Res. 28 (2), 269–274. doi:10.1097/BCR.0B013E318031A236
Groeber, F., Holeiter, M., Hampel, M., Hinderer, S., and Schenke-Layland, K. (2011). Skin tissue engineering-in vivo and in vitro applications. Adv. Drug Deliv. Rev. 63 (4–5), 352–366. doi:10.1016/j.addr.2011.01.005
Guo, S., and Dipietro, L. A. (2010). Factors affecting wound healing. J. Dent. Res. 89 (3), 219–229. doi:10.1177/0022034509359125
Haddow, D. B., Steele, D. A., Short, R. D., Dawson, R. A., and Macneil, S. (2003). Plasma-polymerized surfaces for culture of human keratinocytes and transfer of cells to an in vitro wound-bed model. J. Biomed. Mat. Res. A 64 (1), 80–87. doi:10.1002/jbm.a.10356
Hajikhani, M., Emam-Djomeh, Z., and Askari, G. (2021). Fabrication and characterization of mucoadhesive bioplastic patch via coaxial polylactic acid (PLA) based electrospun nanofibers with antimicrobial and wound healing application. Int. J. Biol. Macromol. 172, 143–153. doi:10.1016/j.ijbiomac.2021.01.051
Halim, A. S., Khoo, T. L., and Mohd Yussof, S. J. (2010). Biologic and synthetic skin substitutes: An overview. Indian J. Plast. Surg. Off. Publ. Assoc. Plast. Surg. India 43 (l), S23–S28. doi:10.4103/0970-0358.70712
Hazrati, R., Davaran, S., and Omidi, Y. (2022). Bioactive functional scaffolds for stem cells delivery in wound healing and skin regeneration. React. Funct. Polym. 174, 105233. doi:10.1016/j.reactfunctpolym.2022.105233
Hellman, K. B. (2006). Engineered tissues: The regulatory path from concept to market. Adv. Exp. Med. Biol. 585, 363–376. doi:10.1007/978-0-387-34133-0_23
High, K. A., and Roncarolo, M. G. (2019). Gene therapy. N. Engl. J. Med. 381 (5), 455–464. doi:10.1056/NEJMra1706910
Hodde, J. P., Ernst, D. M. J., and Hiles, M. C. (2005). An investigation of the long-term bioactivity of endogenous growth factor in OASIS Wound Matrix. J. Wound Care 14 (1), 23–25. doi:10.12968/jowc.2005.14.1.26721
Horch, R. E., Kopp, J., Kneser, U., Beier, J., and Bach, A. D. (2005). Tissue engineering of cultured skin substitutes. J. Cell. Mol. Med. 9 (3), 592–608. doi:10.1111/j.1582-4934.2005.tb00491.x
Hosseini, M., and Shafiee, A. (2021). Engineering bioactive scaffolds for skin regeneration. Small 17 (41), 2101384. doi:10.1002/smll.202101384
Hu, B.-Y., Weick, J. P., Yu, J., Ma, L. X., Zhang, X. Q., Thomson, J. A., et al. (2010). Neural differentiation of human induced pluripotent stem cells follows developmental principles but with variable potency. Proc. Natl. Acad. Sci. 107 (9), 4335–4340. doi:10.1073/pnas.0910012107
Hu, K., Xiang, L., Chen, J., Qu, H., Wan, Y., and Xiang, D. (2021). PLGA-liposome electrospun fiber delivery of miR-145 and PDGF-BB synergistically promoted wound healing. Chem. Eng. J. 422, 129951. doi:10.1016/j.cej.2021.129951
Huang, L., Li, W., Guo, M., Huang, Z., Chen, Y., Dong, X., et al. (2023). Silver doped-silica nanoparticles reinforced poly (ethylene glycol) diacrylate/hyaluronic acid hydrogel dressings for synergistically accelerating bacterial-infected wound healing. Carbohydr. Polym. 304, 2022, 120450. doi:10.1016/j.carbpol.2022.120450
Hynes, R. O. (2009). The extracellular matrix: Not just pretty fibrils. Science 326 (5957), 1216–1219, Nov. doi:10.1126/science.1176009
Iovene, A., Zhao, Y., Wang, S., and Amoako, K. (2021). Bioactive polymeric materials for the advancement of regenerative medicine. J. Funct. Biomater. 12 (1), 14. doi:10.3390/jfb12010014
Ito, A., Mase, A., Takizawa, Y., Shinkai, M., Honda, H., Hata, K. I., et al. (2003). Transglutaminase-mediated gelatin matrices incorporating cell adhesion factors as a biomaterial for tissue engineering. J. Biosci. Bioeng. 95 (2), 196–199. doi:10.1016/S1389-1723(03)80129-9
Jaganathan, S. K., and Mani, M. P. (2019). Single-stage synthesis of electrospun polyurethane scaffold impregnated with zinc nitrate nanofibers for wound healing applications. J. Appl. Polym. Sci. 136 (3), 46942. doi:10.1002/app.46942
Jara, C. P., Wang, O., Paulino do Prado, T., Ismail, A., Fabian, F. M., et al. (2020). Novel fibrin-fibronectin matrix accelerates mice skin wound healing. Bioact. Mat. 5 (4), 949–962. doi:10.1016/j.bioactmat.2020.06.015
Jiang, T., Liu, S., Wu, Z., Li, Q., Ren, S., Chen, J., et al. (2022). ADSC-exo@MMP-PEG smart hydrogel promotes diabetic wound healing by optimizing cellular functions and relieving oxidative stress. Mat. Today Bio 16, 100365. doi:10.1016/j.mtbio.2022.100365
Jin, X., Fu, Q., Gu, Z., Zhang, Z., and Lv, H. (2021). Chitosan/PDLLA-PEG-PDLLA solution preparation by simple stirring and formation into a hydrogel at body temperature for whole wound healing. Int. J. Biol. Macromol. 184, 787–796. doi:10.1016/j.ijbiomac.2021.06.087
Kallis, P. J., Friedman, A. J., and Lev-Tov, H. (2018). A guide to tissue-engineered skin substitutes. J. Drugs Dermatol. 17 (1), 57–64.
Kamoun, E. A., Kenawy, E.-R. S., and Chen, X. (2017). A review on polymeric hydrogel membranes for wound dressing applications: PVA-based hydrogel dressings. J. Adv. Res. 8 (3), 217–233. doi:10.1016/j.jare.2017.01.005
Kim, J. I., Pant, H. R., Sim, H.-J., Lee, K. M., and Kim, C. S. (2014). Electrospun propolis/polyurethane composite nanofibers for biomedical applications. Mat. Sci. Eng. C 44, 52–57. doi:10.1016/j.msec.2014.07.062
Kirsner, R. S., Marston, W. A., Snyder, R. J., Lee, T. D., Cargill, D. I., and Slade, H. B. (2012). Spray-applied cell therapy with human allogeneic fibroblasts and keratinocytes for the treatment of chronic venous leg ulcers: A phase 2, multicentre, double-blind, randomised, placebo-controlled trial. Lancet 380 (9846), 977–985. doi:10.1016/S0140-6736(12)60644-8
Kohli, N., Sharma, V., Brown, S. J., and García-Gareta, E. (2019). “5 - synthetic polymers for skin biomaterials,” in Biomaterials for skin repair and regeneration (Woodhead Publishing), 125–149.
Koyuncu, A., Koç, S., Akdere, Ö. E., Çakmak, A. S., and Gümüşderelioğlu, M. (2022). Investigation of the synergistic effect of platelet-rich plasma and polychromatic light on human dermal fibroblasts seeded chitosan/gelatin scaffolds for wound healing. J. Photochem. Photobiol. B Biol. 232, 112476. doi:10.1016/j.jphotobiol.2022.112476
Kucińska-Lipka, J., Gubanska, I., and Janik, H. (2015). Bacterial cellulose in the field of wound healing and regenerative medicine of skin: Recent trends and future prospectives. Polym. Bull. 72 (9), 2399–2419. doi:10.1007/s00289-015-1407-3
kumar Kesavan, S., Selvaraj, D., Perumal, S., Arunachalakasi, A., Ganesan, N., Chinnaiyan, S. K., et al. (2022). Fabrication of hybrid povidone-iodine impregnated collagen-hydroxypropyl methylcellulose composite scaffolds for wound-healing application. J. Drug Deliv. Sci. Technol. 70, 103247. doi:10.1016/j.jddst.2022.103247
Kumar Reddy Sanapalli, B., Tyagi, R., Shaik, A. B., Pelluri, R., Bhandare, R. R., Annadurai, S., et al. (2022). L-Glutamic acid loaded collagen chitosan composite scaffold as regenerative medicine for the accelerated healing of diabetic wounds. Arab. J. Chem. 15 (6), 103841. doi:10.1016/j.arabjc.2022.103841
Kweon, D.-K., Song, S.-B., and Park, Y.-Y. (2003). Preparation of water-soluble chitosan/heparin complex and its application as wound healing accelerator. Biomaterials 24 (9), 1595–1601. doi:10.1016/S0142-9612(02)00566-5
Lee, C.-H., Chang, S. H., Chen, W. J., Hung, K. C., Lin, Y. H., Liu, S. J., et al. (2015). Augmentation of diabetic wound healing and enhancement of collagen content using nanofibrous glucophage-loaded collagen/PLGA scaffold membranes. J. Colloid Interface Sci. 439, 88–97. doi:10.1016/j.jcis.2014.10.028
Leeper, H. M., and Wright, R. M. (1983). Elastomers in medicine. Rubber Chem. Technol. 56 (3), 523–556. doi:10.5254/1.3538140
Lewitus, D. Y., Landers, J., Branch, J. R., Smith, K. L., Callegari, G., Kohn, J., et al. (2011). Biohybrid carbon nanotube/agarose fibers for neural tissue engineering. Adv. Funct. Mat. 21 (14), 2624–2632. doi:10.1002/adfm.201002429
Li, S., Wang, K., Jiang, X., Hu, Q., Zhang, C., and Wang, B. (2020). Rapid fabrication of ready-to-use gelatin scaffolds with prevascular networks using alginate hollow fibers as sacrificial templates. ACS Biomater. Sci. Eng. 6 (4), 2297–2311. doi:10.1021/acsbiomaterials.9b01834
Límová, M. (2010). Active wound coverings: Bioengineered skin and dermal substitutes. Surg. Clin. North Am. 90 (6), 1237–1255. doi:10.1016/j.suc.2010.08.004
Lindberg, K., and Badylak, S. F. (2001). Porcine small intestinal submucosa (SIS): A bioscaffold supporting in vitro primary human epidermal cell differentiation and synthesis of basement membrane proteins. Burns 27 (3), 254–266. doi:10.1016/S0305-4179(00)00113-3
Liu, H. (2018). A functional chitosan-based hydrogel as a wound dressing and drug delivery system in the treatment of wound healing. doi:10.1039/c7ra13510f
MacNeil, S. (2007). Progress and opportunities for tissue-engineered skin. Nature 445 (7130), 874–880. doi:10.1038/nature05664
Madub, K., Goonoo, N., Gimié, F., Ait Arsa, I., Schönherr, H., and Bhaw-Luximon, A. (2021). Green seaweeds ulvan-cellulose scaffolds enhance in vitro cell growth and in vivo angiogenesis for skin tissue engineering. Carbohydr. Polym. 251, 117025. doi:10.1016/j.carbpol.2020.117025
Maged, A., Abdelkhalek, A. A., Mahmoud, A. A., Salah, S., Ammar, M. M., and Ghorab, M. M. (2019). Mesenchymal stem cells associated with chitosan scaffolds loaded with rosuvastatin to improve wound healing. Eur. J. Pharm. Sci. 127, 185–198. doi:10.1016/j.ejps.2018.11.002
Magnusson, M., Papini, R. P., Rea, S. M., Reed, C. C., and Wood, F. M. (2007). Cultured autologous keratinocytes in suspension accelerate epithelial maturation in an in vivo wound model as measured by surface electrical capacitance. Plast. Reconstr. Surg. 119 (2), 495–499. doi:10.1097/01.prs.0000246315.80133.8d
Maitz, M. F. (2015). Applications of synthetic polymers in clinical medicine. Biosurface Biotribology 1 (3), 161–176. doi:10.1016/j.bsbt.2015.08.002
Malafaya, P. B., Silva, G. A., and Reis, R. L. (2007). Natural–origin polymers as carriers and scaffolds for biomolecules and cell delivery in tissue engineering applications. Adv. Drug Deliv. Rev. 59 (4), 207–233. doi:10.1016/j.addr.2007.03.012
Märtson, M., Viljanto, J., Hurme, T., and Saukko, P. (1998). Biocompatibility of cellulose sponge with bone. Eur. Surg. Res. Eur. Chir. Forsch. Rech. Chir. Eur. 30 (6), 426–432. doi:10.1159/000008609
Massia, S. P., and Stark, J. (2001). Immobilized RGD peptides on surface-grafted dextran promote biospecific cell attachment. J. Biomed. Mat. Res. 56 (3), 390–399. doi:10.1002/1097-4636(20010905)56:3<390:aid-jbm1108>3.0.co;2-l
Matsiko, A., Levingstone, T. J., O’Brien, F. J., and Gleeson, J. P. (2012). Addition of hyaluronic acid improves cellular infiltration and promotes early-stage chondrogenesis in a collagen-based scaffold for cartilage tissue engineering. J. Mech. Behav. Biomed. Mat. 11, 41–52. doi:10.1016/j.jmbbm.2011.11.012
Mavilio, F., Pellegrini, G., Ferrari, S., Di Nunzio, F., Di Iorio, E., Recchia, A., et al. (2006). Correction of junctional epidermolysis bullosa by transplantation of genetically modified epidermal stem cells. Nat. Med. 12 (12), 1397–1402. doi:10.1038/nm1504
Mehvar, R. (2000). Dextrans for targeted and sustained delivery of therapeutic and imaging agents. J. Control. release Off. J. Control. Release Soc. 69 (1), 1–25. doi:10.1016/s0168-3659(00)00302-3
Mirhaj, M., Tavakoli, M., Varshosaz, J., Labbaf, S., Jafarpour, F., Ahmaditabar, P., et al. (2022). Platelet rich fibrin containing nanofibrous dressing for wound healing application: Fabrication, characterization and biological evaluations. Biomater. Adv. 134, 112541. doi:10.1016/j.msec.2021.112541
Mobaraki, M., Bizari, D., Soltani, M., Khshmohabat, H., Raahemifar, K., and Akbarzade Amirdehi, M. (2021). The effects of curcumin nanoparticles incorporated into collagen-alginate scaffold on wound healing of skin tissue in trauma patients. Polymers 13, 4291–4324. doi:10.3390/polym13244291
Mogoşanu, G. D., and Grumezescu, A. M. (2014). Natural and synthetic polymers for wounds and burns dressing. Int. J. Pharm. 463 (2), 127–136. doi:10.1016/j.ijpharm.2013.12.015
Mohamadi, P. S., Hivechi, A., Bahrami, S. H., Nezari, S., Milan, P. B., and Amoupour, M. (2022). Fabrication and investigating in vivo wound healing property of coconut oil loaded nanofiber/hydrogel hybrid scaffold. Biomater. Adv. 142, 213139. doi:10.1016/j.bioadv.2022.213139
Möller, S., Weisser, J., Bischoff, S., and Schnabelrauch, M. (2007). Dextran and hyaluronan methacrylate based hydrogels as matrices for soft tissue reconstruction. Biomol. Eng. 24 (5), 496–504. doi:10.1016/j.bioeng.2007.08.014
Morrison, W. R., and Karkalas, J. (1990). “9 - starch,” in Carbohydrates. Editor P. B. DEY (Academic Press), 2, 323–352.
Myers, S. R., Partha, V. N., Soranzo, C., Price, R. D., and Navsaria, H. A. (2007). Hyalomatrix: A temporary epidermal barrier, hyaluronan delivery, and neodermis induction system for keratinocyte stem cell therapy. Tissue Eng. 13 (11), 2733–2741, Nov. doi:10.1089/ten.2007.0109
Natarajan, V., Krithica, N., Madhan, B., and Sehgal, P. K. (2013). Preparation and properties of tannic acid cross-linked collagen scaffold and its application in wound healing. J. Biomed. Mat. Res. Part B Appl. Biomater. 101B (4), 560–567. doi:10.1002/jbm.b.32856
Nathoo, R., Howe, N., and Cohen, G. (2014). Skin substitutes: An overview of the key players in wound management. J. Clin. Aesthet. Dermatol. 7 (10), 44–48.
Nayak, K. K., and Gupta, P. (2015). In vitro biocompatibility study of keratin/agar scaffold for tissue engineering. Int. J. Biol. Macromol. 81, 1–10. doi:10.1016/j.ijbiomac.2015.07.025
Negut, I., Dorcioman, G., and Grumezescu, V. (2020). Scaffolds for wound healing applications. Polymers 12, 2010. doi:10.3390/polym12092010
Ng, K. W., and Hutmacher, D. W. (2006). Reduced contraction of skin equivalent engineered using cell sheets cultured in 3D matrices. Biomaterials 27 (26), 4591–4598. doi:10.1016/j.biomaterials.2006.04.020
Ngoenkam, J., Faikrua, A., Yasothornsrikul, S., and Viyoch, J. (2010). Potential of an injectable chitosan/starch/β-glycerol phosphate hydrogel for sustaining normal chondrocyte function. Int. J. Pharm. 391 (1), 115–124. doi:10.1016/j.ijpharm.2010.02.028
Nikpour, S., Ansari-Asl, Z., Sedaghat, T., and Hoveizi, E. (2022). Curcumin-loaded Fe-MOF/PDMS porous scaffold: Fabrication, characterization, and biocompatibility assessment. J. Ind. Eng. Chem. 110, 188–197. doi:10.1016/j.jiec.2022.02.052
Ninan, N., Muthiah, M., Bt.Yahaya, N. A., Park, I. K., Elain, A., Wong, T. W., et al. (2014). Antibacterial and wound healing analysis of gelatin/zeolite scaffolds. Colloids Surfaces B Biointerfaces 115, 244–252. doi:10.1016/j.colsurfb.2013.11.048
Noordenbos, J., Doré, C., and Hansbrough, J. F. (1999). Safety and efficacy of TransCyte for the treatment of partial-thickness burns. J. Burn Care Rehabil. 20 (4), 275–281. doi:10.1097/00004630-199907000-00002
Nyame, T. T., Chiang, H. A., Leavitt, T., Ozambela, M., and Orgill, D. P. (2015). Tissue-engineered skin substitutes. Plast. Reconstr. Surg. 136 (6), 1379–1388. doi:10.1097/PRS.0000000000001748
O’Brien, F. J. (2011). Biomaterials & scaffolds for tissue engineering. Mat. Today 14 (3), 88–95. doi:10.1016/S1369-7021(11)70058-X
Pan, Z., and Ding, J. (2012). Poly(lactide-co-glycolide) porous scaffolds for tissue engineering and regenerative medicine. Interface Focus 2 (3), 366–377. doi:10.1098/rsfs.2011.0123
Pang, C., Ibrahim, A., Bulstrode, N. W., and Ferretti, P. (2017). An overview of the therapeutic potential of regenerative medicine in cutaneous wound healing. Int. Wound J. 14 (3), 450–459. doi:10.1111/iwj.12735
Pankov, R., and Yamada, K. M. (2002). Fibronectin at a glance. J. Cell Sci. 115 (20), 3861–3863. doi:10.1242/jcs.00059
Park, K. M., Blatchley, M. R., and Gerecht, S. (2014). The design of dextran-based hypoxia-inducible hydrogels via in situ oxygen-consuming reaction. Macromol. Rapid Commun. 35 (22), 1968–1975. doi:10.1002/marc.201400369
Patrício, T., Domingos, M., Gloria, A., and Bártolo, P. (2013). Characterisation of PCL and PCL/PLA scaffolds for tissue engineering. Procedia CIRP 5, 110–114. doi:10.1016/j.procir.2013.01.022
Paul, W., and Sharma, C. P. (2004). Chitosan and alginate wound dressings: A short review. Trends Biomater. Artif. Organs 18.
Pihlajamäki, H., Salminen, S., Laitinen, O., Tynninen, O., and Böstman, O. (2006). Tissue response to polyglycolide, polydioxanone, polylevolactide, and metallic pins in cancellous bone: An experimental study on rabbits. J. Orthop. Res. 24 (8), 1597–1606. doi:10.1002/jor.20191
Polmanteer, K. E. (1988). Silicone rubber, its development and technological progress. Rubber Chem. Technol. 61 (3), 470–502. doi:10.5254/1.3536197
Portela, R., Leal, C. R., Almeida, P. L., and Sobral, R. G. (2019). Bacterial cellulose: A versatile biopolymer for wound dressing applications. Microb. Biotechnol. 12 (4), 586–610. doi:10.1111/1751-7915.13392
Rahmani Del Bakhshayesh, A., Annabi, N., Khalilov, R., Akbarzadeh, A., Samiei, M., Alizadeh, E., et al. (2018). Recent advances on biomedical applications of scaffolds in wound healing and dermal tissue engineering. Artif. Cells, Nanomedicine, Biotechnol. 46 (4), 691–705. doi:10.1080/21691401.2017.1349778
Rajora, A. D., and Bal, T. (2022). Evaluating neem gum-polyvinyl alcohol (NGP-PVA) blend nanofiber mat as a novel platform for wound healing in murine model. Int. J. Biol. Macromol. 226, 760–771. doi:10.1016/j.ijbiomac.2022.12.014
Rather, A. H., Khan, R. S., Wani, T. U., Rafiq, M., Jadhav, A. H., Srinivasappa, P. M., et al. (2023). Polyurethane and cellulose acetate micro-nanofibers containing rosemary essential oil, and decorated with silver nanoparticles for wound healing application. Int. J. Biol. Macromol. 226, 690–705. doi:10.1016/j.ijbiomac.2022.12.048
Reddy, M. S., Ponnamma, D., Choudhary, R., and Sadasivuni, K. K. (2021). A comparative review of natural and synthetic biopolymer composite scaffolds. Polymers 13, 1105–1107. doi:10.3390/polym13071105
Reis, R. L., Neves, N. M., Mano, J. F., Gomes, M. E., Marques, A. P., and Azevedo, H. S. (2008). Natural-based polymers for biomedical applications. Elsevier.
Rezaii, M., Oryan, S., and Javeri, A. (2019). Curcumin nanoparticles incorporated collagen-chitosan scaffold promotes cutaneous wound healing through regulation of TGF-β1/Smad7 gene expression. Mat. Sci. Eng. C 98, 347–357. doi:10.1016/j.msec.2018.12.143
Rheinwald, J. G. (1989). Human epidermal keratinocyte cell culture and xenograft systems: Applications in the detection of potential chemical carcinogens and the study of epidermal transformation. Prog. Clin. Biol. Res. 298, 113–125.
Rockwood, D. N., Woodhouse, K. A., Fromstein, J. D., Chase, D. B., and Rabolt, J. F. (2007). Characterization of biodegradable polyurethane microfibers for tissue engineering. J. Biomater. Sci. Polym. Ed. 18 (6), 743–758. doi:10.1163/156856207781034115
Roslan, M. R., Nasir, N. F. M., Cheng, E. M., and Amin, N. A. M. (2016). “Tissue engineering scaffold based on starch: A review,” in 2016 international conference on electrical, electronics, and optimization techniques (ICEEOT), 1857–1860. doi:10.1109/ICEEOT.2016.7755010
Rouse, J. G., and Van Dyke, M. E. (2010). A review of keratin-based biomaterials for biomedical applications. Materials 3 (2), 999–1014. doi:10.3390/ma3020999
Ryan, C. M., Schoenfeld, D. A., Malloy, M., Schulz, J. T., Sheridan, R. L., and Tompkins, R. G. (2002). Use of Integra® artificial skin is associated with decreased length of stay for severely injured adult burn survivors. J. Burn Care Rehabil. 23 (5), 311–317. doi:10.1097/00004630-200209000-00002
Sadeghi, A., Zare-Gachi, M., Najjar-Asl, M., Rajabi, S., Fatemi, M. J., Forghani, S. F., et al. (2023). Hybrid gelatin-sulfated alginate scaffolds as dermal substitutes can dramatically accelerate healing of full-thickness diabetic wounds. Carbohydr. Polym. 302, 120404. doi:10.1016/j.carbpol.2022.120404
Sadeghianmaryan, A., Karimi, Y., Naghieh, S., Alizadeh Sardroud, H., Gorji, M., and Chen, X. (2020). Electrospinning of scaffolds from the polycaprolactone/polyurethane composite with graphene oxide for skin tissue engineering. Appl. Biochem. Biotechnol. 191 (2), 567–578. doi:10.1007/s12010-019-03192-x
Sakiyama, S. E., Schense, J. C., and Hubbell, J. A. (1999). Incorporation of heparin-binding peptides into fibrin gels enhances neurite extension: An example of designer matrices in tissue engineering. FASEB J. 13 (15), 2214–2224. doi:10.1096/fasebj.13.15.2214
Salehi, H., Mehrasa, M., Nasri-Nasrabadi, B., Doostmohammadi, M., Seyedebrahimi, R., et al. (2017). Effects of nanozeolite/starch thermoplastic hydrogels on wound healing. J. Res. Med. Sci. Off. J. Isfahan Univ. Med. Sci. 22, 110. doi:10.4103/jrms.JRMS_1037_16
Sanad, R. A.-B., and Abdel-Bar, H. M. (2017). Chitosan–hyaluronic acid composite sponge scaffold enriched with Andrographolide-loaded lipid nanoparticles for enhanced wound healing. Carbohydr. Polym. 173, 441–450. doi:10.1016/j.carbpol.2017.05.098
Schurr, M. J., Foster, K. N., Centanni, J. M., Comer, A. R., Wicks, A., Gibson, A. L., et al. (2009). Phase I/II clinical evaluation of StrataGraft: A consistent, pathogen-free human skin substitute. J. Trauma 66 (3), 866–874. doi:10.1097/TA.0b013e31819849d6
Sekiya, N., Ichioka, S., Terada, D., Tsuchiya, S., and Kobayashi, H. (2013). Efficacy of a poly glycolic acid (PGA)/collagen composite nanofibre scaffold on cell migration and neovascularisation in vivo skin defect model. J. Plast. Surg. Hand Surg. 47 (6), 498–502. doi:10.3109/2000656X.2013.788507
Selvakumar, G., and Lonchin, S. (2022). Bioactive functional collagen-oxidized pullulan scaffold loaded with polydatin for treating chronic wounds. Biomater. Adv. 140, 213078. doi:10.1016/j.bioadv.2022.213078
Shakespeare, P. G. (2005). The role of skin substitutes in the treatment of burn injuries. Clin. Dermatol. 23 (4), 413–418. doi:10.1016/j.clindermatol.2004.07.015
Shakespeare, P., and Shakespeare, V. (2002). Survey: Use of skin substitute materials in UK burn treatment centres. Burns 28 (4), 295–297. doi:10.1016/S0305-4179(02)00062-1
Shalumon, K. T., Anulekha, K. H., Chennazhi, K. P., Tamura, H., V Nair, S., and Jayakumar, R. (2011). Fabrication of chitosan/poly(caprolactone) nanofibrous scaffold for bone and skin tissue engineering. Int. J. Biol. Macromol. 48 (4), 571–576. doi:10.1016/j.ijbiomac.2011.01.020
Shanmugarajan, T. S., Selvan, N. K., and Uppuluri, V. N. V. A. (2020). Development and characterization of squalene-loaded topical agar-based emulgel scaffold: Wound healing potential in full-thickness burn model. Int. J. Low. Extrem. Wounds 20 (4), 364–373. doi:10.1177/1534734620921629
Shao, Z., Lyu, C., Teng, L., Xie, X., Sun, J., Zou, D., et al. (2021). An injectable fibrin scaffold rich in growth factors for skin repair. Biomed. Res. Int. 2021, 1–13. doi:10.1155/2021/8094932
Sharma, V., Kohli, N., Moulding, D., Afolabi, H., Hook, L., Mason, C., et al. (2017). Design of a novel two-component hybrid dermal scaffold for the treatment of pressure sores. Macromol. Biosci. 17 (11), 1700185, Nov. doi:10.1002/mabi.201700185
Shen, Y.-I., Song, H.-H. G., Papa, A. E., Burke, J. A., Volk, S. W., and Gerecht, S. (2015). Acellular hydrogels for regenerative burn wound healing: Translation from a porcine model. J. Invest. Dermatol. 135 (10), 2519–2529. doi:10.1038/jid.2015.182
Sherman, V. R., Yang, W., and Meyers, M. A. (2015). The materials science of collagen. J. Mech. Behav. Biomed. Mat. 52, 22–50. doi:10.1016/j.jmbbm.2015.05.023
Sill, T. J., and von Recum, H. A. (2008). Electrospinning: Applications in drug delivery and tissue engineering. Biomaterials 29 (13), 1989–2006. doi:10.1016/j.biomaterials.2008.01.011
Sin, L. T., Rahmat, A. R., and Rahman, W. A. W. A. (2013). “3 - applications of poly(lactic acid),” in Plastics design library. Editor B. P. Ebnesajjad (Boston: William Andrew Publishing), 55–69.
Sivakumar, S., Murali, R., Arathanaikotti, D., Gopinath, A., Senthilkumar, C., Kesavan, S., et al. (2021). Ferulic acid loaded microspheres reinforced in 3D hybrid scaffold for antimicrobial wound dressing. Int. J. Biol. Macromol. 177, 463–473. doi:10.1016/j.ijbiomac.2021.02.124
Snyder, D., Sullivan, N., Margolis, D., and Schoelles, K. (2020). Skin substitutes for treating chronic wounds. Rockville (MD).
Stark, H.-J., Boehnke, K., Mirancea, N., Willhauck, M. J., Pavesio, A., Fusenig, N. E., et al. (2006). Epidermal homeostasis in long-term scaffold-enforced skin equivalents. J. Investig. Dermatol. Symp. Proc. 11 (1), 93–105. doi:10.1038/sj.jidsymp.5650015
Still, J., Glat, P., Silverstein, P., Griswold, J., and Mozingo, D. (2003). The use of a collagen sponge/living cell composite material to treat donor sites in burn patients. Burns 29 (8), 837–841. doi:10.1016/S0305-4179(03)00164-5
Su, Z., Ma, H., Wu, Z., Zeng, H., Li, Z., Wang, Y., et al. (2014). Enhancement of skin wound healing with decellularized scaffolds loaded with hyaluronic acid and epidermal growth factor. Mat. Sci. Eng. C 44, 440–448. doi:10.1016/j.msec.2014.07.039
Suamte, L., Tirkey, A., and Babu, P. J. (2023). Design of 3D smart scaffolds using natural, synthetic and hybrid derived polymers for skin regenerative applications. Smart Mat. Med. 4, 243–256. doi:10.1016/j.smaim.2022.09.005
Sun, G., Shen, Y.-I., Ho, C. C., Kusuma, S., and Gerecht, S. (2010). Functional groups affect physical and biological properties of dextran-based hydrogels. J. Biomed. Mat. Res. Part A 93A (3), 1080–1090. doi:10.1002/jbm.a.32604
Sun, G., Zhang, X., Shen, Y. I., Sebastian, R., Dickinson, L. E., Fox-Talbot, K., et al. (2011). Dextran hydrogel scaffolds enhance angiogenic responses and promote complete skin regeneration during burn wound healing. Proc. Natl. Acad. Sci. 108 (52), 20976–20981. doi:10.1073/pnas.1115973108
Sun, S., Hao, M., Ding, C., Zhang, J., Ding, Q., Zhang, Y., et al. (2022). SF/PVP nanofiber wound dressings loaded with phlorizin: Preparation, characterization, in vivo and in vitro evaluation. Colloids Surfaces B Biointerfaces 217, 112692. doi:10.1016/j.colsurfb.2022.112692
Sundaram, J., Durance, T. D., and Wang, R. (2008). Porous scaffold of gelatin–starch with nanohydroxyapatite composite processed via novel microwave vacuum drying. Acta Biomater. 4 (4), 932–942. doi:10.1016/j.actbio.2008.01.019
Supp, D. M., and Boyce, S. T. (2005). Engineered skin substitutes: Practices and potentials. Clin. Dermatol. 23 (4), 403–412. doi:10.1016/j.clindermatol.2004.07.023
Supp, D. M., Karpinski, A. C., and Boyce, S. T. (2004). Expression of human beta-defensins HBD-1, HBD-2, and HBD-3 in cultured keratinocytes and skin substitutes. Burns 30 (7), 643–648. doi:10.1016/j.burns.2004.03.012
Tachibana, A., Furuta, Y., Takeshima, H., Tanabe, T., and Yamauchi, K. (2002). Fabrication of wool keratin sponge scaffolds for long-term cell cultivation. J. Biotechnol. 93 (2), 165–170. doi:10.1016/S0168-1656(01)00395-9
Takahashi, K., Tanabe, K., Ohnuki, M., Narita, M., Ichisaka, T., Tomoda, K., et al. (2007). Induction of pluripotent stem cells from adult human fibroblasts by defined factors. Cell 131 (5), 861–872. doi:10.1016/j.cell.2007.11.019
Talikowska, M., Fu, X., and Lisak, G. (2019). Application of conducting polymers to wound care and skin tissue engineering: A review. Biosens. Bioelectron. 135, 50–63. doi:10.1016/j.bios.2019.04.001
Tan, H. B., Wang, F. Y., Ding, W., Zhang, Y., Ding, J., Cai, D. X., et al. (2015). Fabrication and evaluation of porous keratin/chitosan (KCS) scaffolds for effectively accelerating wound healing. Biomed. Environ. Sci. 28 (3), 178–189. doi:10.3967/bes2015.024
Tausche, A.-K., Skaria, M., Bohlen, L., Liebold, K., Hafner, J., Friedlein, H., et al. (2003). An autologous epidermal equivalent tissue-engineered from follicular outer root sheath keratinocytes is as effective as split-thickness skin autograft in recalcitrant vascular leg ulcers. Wound repair Regen. Off. Publ. Wound heal. Soc. And. Eur. Tissue Repair Soc. 11 (4), 248–252. doi:10.1046/j.1524-475x.2003.11403.x
Teaima, M. H., Elasaly, M. K., Omar, S. A., El-Nabarawi, M. A., and Shoueir, K. R. (2022). Wound healing activities of polyurethane modified chitosan nanofibers loaded with different concentrations of linezolid in an experimental model of diabetes. J. Drug Deliv. Sci. Technol. 67, 102982. doi:10.1016/j.jddst.2021.102982
ter Horst, B., Moiemen, N. S., and Grover, L. M. (2019). “6 - natural polymers: Biomaterials for skin scaffolds,” in Biomaterials for skin repair and regeneration (Woodhead Publishing), 151–192.
Tezgel, Ö., DiStasio, N., Laghezza-Masci, V., Taddei, A. R., Szarpak-Jankowska, A., Auzély-Velty, R., et al. (2020). Collagen scaffold-mediated delivery of NLC/siRNA as wound healing materials. J. Drug Deliv. Sci. Technol. 55, 101421. doi:10.1016/j.jddst.2019.101421
Thomas Hess, C. (2011). Checklist for factors affecting wound healing. Adv. Skin. Wound Care 24 (4), 192. doi:10.1097/01.ASW.0000396300.04173.ec
Ti, D., Hao, H., Xia, L., Tong, C., Liu, J., Dong, L., et al. (2014). Controlled release of Thymosin beta 4 using a collagen–chitosan sponge scaffold augments cutaneous wound healing and increases angiogenesis in diabetic rats with hindlimb ischemia. Tissue Eng. Part A 21, 541–549. doi:10.1089/ten.tea.2013.0750
Tottoli, E. M., Dorati, R., Genta, I., Chiesa, E., Pisani, S., and Conti, B. (2020). Skin wound healing process and new emerging technologies for skin wound care and regeneration. Pharmaceutics 12 (8), 735. doi:10.3390/pharmaceutics12080735
Uccioli, L. (2003). A clinical investigation on the characteristics and outcomes of treating chronic lower extremity wounds using the tissuetech autograft system. Int. J. Low. Extrem. Wounds 2 (3), 140–151. doi:10.1177/1534734603258480
Ulery, B. D., Nair, L. S., and Laurencin, C. T. (2011). Biomedical applications of biodegradable polymers. J. Polym. Sci. Part B Polym. Phys. 49 (12), 832–864. doi:10.1002/polb.22259
Unnithan, A. R., Sasikala, A. R. K., Murugesan, P., Gurusamy, M., Wu, D., Park, C. H., et al. (2015). Electrospun polyurethane-dextran nanofiber mats loaded with Estradiol for post-menopausal wound dressing. Int. J. Biol. Macromol. 77, 1–8. doi:10.1016/j.ijbiomac.2015.02.044
V Shevchenko, R., James, S. L., and James, S. E. (2010). A review of tissue-engineered skin bioconstructs available for skin reconstruction. J. R. Soc. Interface 7 (43), 229–258. doi:10.1098/rsif.2009.0403
Vakilian, S., Jamshidi-adegani, F., Al Yahmadi, A., Al-Broumi, M., Ur Rehman, N., Anwar, M. U., et al. (2021). A competitive nature-derived multilayered scaffold based on chitosan and alginate, for full-thickness wound healing. Carbohydr. Polym. 262, 117921. doi:10.1016/j.carbpol.2021.117921
van Dorp, A. G., Verhoeven, M. C., Koerten, H. K., van Blitterswijk, C. A., and Ponec, M. (1999). Bilayered biodegradable poly(ethylene glycol)/poly(butylene terephthalate) copolymer (Polyactive) as substrate for human fibroblasts and keratinocytes. J. Biomed. Mat. Res. 47 (3), 292–300. doi:10.1002/(sici)1097-4636(19991205)47:3<292:aid-jbm2>3.0.co;2-b
Varkey, M., Ding, J., and Tredget, E. E. (2015). Advances in skin substitutes-potential of tissue engineered skin for facilitating anti-fibrotic healing. J. Funct. Biomater. 6 (3), 547–563. doi:10.3390/jfb6030547
Velnar, T., Bailey, T., and Smrkolj, V. (2009). The wound healing process: An overview of the cellular and molecular mechanisms. J. Int. Med. Res. 37 (5), 1528–1542. doi:10.1177/147323000903700531
Vig, K., Chaudhari, A., Tripathi, S., Dixit, S., Sahu, R., Pillai, S., et al. (2017). Advances in skin regeneration using tissue engineering. Int. J. Mol. Sci. 18 (4), 789. doi:10.3390/ijms18040789
Villa-Diaz, L. G., Brown, S., Liu, Y., Ross, A., Lahann, J., Parent, J., et al. (2012). Derivation of mesenchymal stem cells from human induced pluripotent stem cells cultured on synthetic substrates. Stem Cells 30 (6), 1174–1181. doi:10.1002/stem.1084
Voigt, M., Schauer, M., Schaefer, D. J., Andree, C., Horch, R., and Stark, G. B. (1999). Cultured epidermal keratinocytes on a microspherical transport system are feasible to reconstitute the epidermis in full-thickness wounds. Tissue Eng. 5 (6), 563–572. doi:10.1089/ten.1999.5.563
Waghmare, V. S., Wadke, P. R., Dyawanapelly, S., Deshpande, A., Jain, R., and Dandekar, P. (2018). Starch based nanofibrous scaffolds for wound healing applications. Bioact. Mat. 3 (3), 255–266. doi:10.1016/j.bioactmat.2017.11.006
Wang, L., Su, Y., Huang, C., Yin, Y., Chu, A., Knupp, A., et al. (2019). NANOG and LIN28 dramatically improve human cell reprogramming by modulating LIN41 and canonical WNT activities. Biol. Open 8 (12), bio047225. doi:10.1242/bio.047225
Wang, Q., Zhou, S., Wang, L., You, R., Yan, S., Zhang, Q., et al. (2021). Bioactive silk fibroin scaffold with nanoarchitecture for wound healing. Compos. Part B Eng. 224, 109165. doi:10.1016/j.compositesb.2021.109165
Weissmann, B., and Meyer, K. (1954). The structure of hyalobiuronic acid and of hyaluronic acid from umbilical cord. J. Am. Chem. Soc. 76 (7), 1753–1757. doi:10.1021/ja01636a010
Werner, S., and Grose, R. (2003). Regulation of wound healing by growth factors and cytokines. Physiol. Rev. 83 (3), 835–870. doi:10.1152/physrev.2003.83.3.835
Woodruff, M. A., and Hutmacher, D. W. (2010). The return of a forgotten polymer—polycaprolactone in the 21st century. Prog. Polym. Sci. 35 (10), 1217–1256. doi:10.1016/j.progpolymsci.2010.04.002
Wozney, J. M., Rosen, V., Celeste, A. J., Mitsock, L. M., Whitters, M. J., Kriz, R. W., et al. (1988). Novel regulators of bone formation: Molecular clones and activities. Science 242 (4885), 1528–1534. doi:10.1126/science.3201241
Xie, H., Bai, Q., Kong, F., Li, Y., Zha, X., Zhang, L., et al. (2022). Allantoin-functionalized silk fibroin/sodium alginate transparent scaffold for cutaneous wound healing. Int. J. Biol. Macromol. 207, 859–872. doi:10.1016/j.ijbiomac.2022.03.147
Xu, S., Sang, L., Zhang, Y., Wang, X., and Li, X. (2013). Biological evaluation of human hair keratin scaffolds for skin wound repair and regeneration. Mat. Sci. Eng. C 33 (2), 648–655. doi:10.1016/j.msec.2012.10.011
Xu, W., Gao, X., Tan, H., Li, S., Zhou, T., Li, J., et al. (2022). Covalent and biodegradable chitosan-cellulose hydrogel dressing containing microspheres for drug delivery and wound healing. Mat. Today Commun. 33, 104163. doi:10.1016/j.mtcomm.2022.104163
Yang, R., Xue, W., Liao, H., Wu, F., Guo, H., Zhang, W., et al. (2022). Injectable polylysine and dextran hydrogels with robust antibacterial and ROS-scavenging activity for wound healing. Int. J. Biol. Macromol. 223, 950–960. doi:10.1016/j.ijbiomac.2022.11.065
Yoon, S.-D., Kwon, Y.-S., and Lee, K.-S. (2017). Biodegradation and biocompatibility of poly L-lactic acid implantable mesh. Int. Neurourol. J. 21 (1), S48–S54. doi:10.5213/inj.1734882.441
Young, S., Wong, M., Tabata, Y., and Mikos, A. G. (2005). Gelatin as a delivery vehicle for the controlled release of bioactive molecules. J. Control. Release 109 (1), 256–274. doi:10.1016/j.jconrel.2005.09.023
Yu, H., Gong, W., Mei, J., Qin, L., Piao, Z., You, D., et al. (2022a). The efficacy of a paeoniflorin-sodium alginate-gelatin skin scaffold for the treatment of diabetic wound: An in vivo study in a rat model. Biomed. Pharmacother. 151, 113165. doi:10.1016/j.biopha.2022.113165
Yu, Y., Yang, B., Tian, D., Liu, J., Yu, A., and Wan, Y. (2022b). Thiolated hyaluronic acid/silk fibroin dual-network hydrogel incorporated with bioglass nanoparticles for wound healing. Carbohydr. Polym. 288, 119334. doi:10.1016/j.carbpol.2022.119334
Zarrintaj, P., Manouchehri, S., Ahmadi, Z., Saeb, M. R., Urbanska, A. M., Kaplan, D. L., et al. (2018). Agarose-based biomaterials for tissue engineering. Carbohydr. Polym. 187, 66–84. doi:10.1016/j.carbpol.2018.01.060
Zhang, J., Chen, K., Ding, C., Sun, S., Zheng, Y., Ding, Q., et al. (2022). Fabrication of chitosan/PVP/dihydroquercetin nanocomposite film for in vitro and in vivo evaluation of wound healing. Int. J. Biol. Macromol. 206, 591–604. doi:10.1016/j.ijbiomac.2022.02.110
Zhang, J., Guan, J., Niu, X., Hu, G., Guo, S., Li, Q., et al. (2015). Exosomes released from human induced pluripotent stem cells-derived MSCs facilitate cutaneous wound healing by promoting collagen synthesis and angiogenesis. J. Transl. Med. 13 (1), 49. doi:10.1186/s12967-015-0417-0
Zhang, J., Song, C., Han, Y., Xi, Z., Zhao, L., Cen, L., et al. (2020). Regulation of inflammatory response to polyglycolic acid scaffolds through incorporation of sodium tripolyphosphate. Eur. Polym. J. 122, 109349. doi:10.1016/j.eurpolymj.2019.109349
Zhang, X., Battiston, K. G., McBane, J. E., Matheson, L. A., Labow, R. S., and Santerre, J. P. (2016). in 3 - design of biodegradable polyurethanes and the interactions of the polymers and their degradation by-products within in vitro and in vivo environments. Editors S. L. Cooper, and P. B. Guan (Woodhead Publishing), 75–114.
Zhong, S. P., Zhang, Y. Z., and Lim, C. T. (2010). Tissue scaffolds for skin wound healing and dermal reconstruction. WIREs Nanomedicine Nanobiotechnology 2 (5), 510–525. doi:10.1002/wnan.100
Zhou, K., Zhang, Z., Xue, J., Shang, J., Ding, D., Zhang, W., et al. (2022). Hybrid Ag nanoparticles/polyoxometalate-polydopamine nano-flowers loaded chitosan/gelatin hydrogel scaffolds with synergistic photothermal/chemodynamic/Ag+ anti-bacterial action for accelerated wound healing. Int. J. Biol. Macromol. 221, 135–148. doi:10.1016/j.ijbiomac.2022.08.151
Keywords: wound healing, polymeric biomaterials, skin regeneration, natural materials, health
Citation: Oliveira C, Sousa D, Teixeira JA, Ferreira-Santos P and Botelho CM (2023) Polymeric biomaterials for wound healing. Front. Bioeng. Biotechnol. 11:1136077. doi: 10.3389/fbioe.2023.1136077
Received: 02 January 2023; Accepted: 19 June 2023;
Published: 27 July 2023.
Edited by:
Antonella Motta, University of Trento, ItalyReviewed by:
Mh Busra Fauzi, National University of Malaysia, MalaysiaCopyright © 2023 Oliveira, Sousa, Teixeira, Ferreira-Santos and Botelho. This is an open-access article distributed under the terms of the Creative Commons Attribution License (CC BY). The use, distribution or reproduction in other forums is permitted, provided the original author(s) and the copyright owner(s) are credited and that the original publication in this journal is cited, in accordance with accepted academic practice. No use, distribution or reproduction is permitted which does not comply with these terms.
*Correspondence: Claudia M. Botelho, Y2xhdWRpYWJvdGVsaG9AZGViLnVtaW5oby5wdA==
Disclaimer: All claims expressed in this article are solely those of the authors and do not necessarily represent those of their affiliated organizations, or those of the publisher, the editors and the reviewers. Any product that may be evaluated in this article or claim that may be made by its manufacturer is not guaranteed or endorsed by the publisher.
Research integrity at Frontiers
Learn more about the work of our research integrity team to safeguard the quality of each article we publish.