- 1Frontier Science Center for Synthetic Biology and Key Laboratory of Systems Bioengineering (Ministry of Education), School of Chemical Engineering and Technology, Tianjin University, Tianjin, China
- 2Georgia Tech Shenzhen Institute, Tianjin University, Shenzhen, China
Metabolic engineering strategies for terpenoid production have mainly focused on bottlenecks in the supply of precursor molecules and cytotoxicity to terpenoids. In recent years, the strategies involving compartmentalization in eukaryotic cells has rapidly developed and have provided several advantages in the supply of precursors, cofactors and a suitable physiochemical environment for product storage. In this review, we provide a comprehensive analysis of organelle compartmentalization for terpenoid production, which can guide the rewiring of subcellular metabolism to make full use of precursors, reduce metabolite toxicity, as well as provide suitable storage capacity and environment. Additionally, the strategies that can enhance the efficiency of a relocated pathway by increasing the number and size of organelles, expanding the cell membrane and targeting metabolic pathways in several organelles are also discussed. Finally, the challenges and future perspectives of this approach for the terpenoid biosynthesis are also discussed.
Introduction
Terpenoids are a large family of more than 8,000 natural compounds with a wide range of applications, such as pharmaceuticals, agrochemicals, food additives and biofuels. All terpenoids are derived from isopentenyl diphosphate (IPP) and dimethylallyl diphosphate (DMAPP), via the larger prenyl diphosphate compounds farnesyl diphosphate (FPP), geranyl diphosphate (GPP), or geranylgeranyl diphosphate (GGPP), which represent the universal pool of precursors for terpenoid biosynthesis. According to the number of isoprene units (Zhang and Hong, 2020), terpenoids are classified as monoterpenoids (C10), sesquiterpenoids (C15), diterpenoids (C20), triterpenoids (C30), and tetraterpenoids (carotenoids, C40). In recent years, the rapid development of metabolic engineering and synthetic biology has led to the development of alternative approaches meet the increasing demand for terpenoids (Chen et al., 2018). In eukaryotic cells, common strategies include increasing the supply of acetyl-CoA (Chen et al., 2013), upregulation of the mevalonate (MVA) pathway (Ro et al., 2006), and engineering that improves the supply of cofactors (Chen R. et al., 2022), some of which are common to all terpenoids (Zhang et al., 2022).
In spite of the common upstream pathway, the enormous structural diversity of terpenoids presents many unique challenges for their biosynthesis, necessitating specific optimization strategies for different types of terpenoids (Figure 1). The insufficient precursor supply is an important factor restricting the production of monoterpenes and sesquiterpenes (Moser and Pichler, 2019). GPP, the precursor of monoterpenes, is also an intermediate of the pathway leading to FPP. Once synthesized, GPP is rapidly converted by FPP synthase (ERG20) which limits the GPP pools and blocks the production of monoterpenoids (Ignea et al., 2014). Likewise, FPP is channeled toward sterol biosynthesis by ERG9, which is essential for cell survival and cannot be completely knocked out. In addition, the cytotoxicity of monoterpenes can have a negative effect on the producing hosts and thereby limits further improvement of monoterpenoid production (Zhu K. et al., 2021). Triterpenes and tetraterpenes are large molecules that accumulate intracellularly in specific subcellular compartments, while some terpenoids are not suitable for intracellular accumulation due to their cytotoxicity. The storge capacity of organelles with hydrophobic environments are essential for the terpenoid production. To overcome these challenges caused by terpenoids, strategies involving the optimization of the expression of key enzymes, tolerance engineering and transporter engineering have been proposed to reduce precursor limitations and cytotoxicity, have resulted in significant improvements in terpenoid production (Peng et al., 2017; Zhang et al., 2017).
Strategies to control the subcellular arrangement of metabolic enzymes are a promising approach for resolving the challenges of terpenoid production mentioned above. Eukaryotic cells contain several specific organelles, including mitochondria, peroxisomes, endoplasmic reticulum (ER), lipid bodies (LDs) and the cell membrane (Dyall et al., 2004). All these organelles possess a complex structure, whereby the specific cofactors, metabolites, and unique physicochemical environments of these organelles offers different conditions for different metabolic pathways (Cao et al., 2020). Some organelles, such as mitochondria, peroxisomes and LDs, have phospholipid membranes, which can increase the local concentration of substrates and enzymes inside the smaller organelle compartments. Compartmentalization also blocks competing reactions and reduces the toxicity of intermediates or substrates by organellar insulation (Gao and Zhou, 2019). The production of terpenoids involves complex metabolic compartmentalization, which partly explains the low yield in terpenoid production achieved to date (Basiony et al., 2022), especially using heterologous yeasts. In heterologous biosynthesis in yeasts, enzymes re-locate to specific organelles and some terpenoids accumulate in membrane-like structures such as LDs. The enzymes and its substates are distributed in different organelles in yeast, which result in the separation of enzyme and substate. (Zhu Z-T. et al., 2021). Targeting the biosynthetic pathway to the same organelle where the desired products are stored enhances the biosynthesis of terpenoids. Subcellular compartmentalization for rewiring of metabolic flux can overcome serveral tackles and enhances the productivity of terpenoids pathways.
Many studies have harnessed subcellular compartments to improve terpenoid biosynthesis in yeasts (Hammer and Avalos, 2017; Yocum et al., 2021; Jin et al., 2022), as shown in Table 1. The physiological properties of various organelles as well as their benefits and drawbacks for organelle compartmentalization in terpenoid production (Jin et al., 2022), which will not be discussed below. In this review, we summarize the characteristics of monoterpenoids, sesquiterpenoids and tetraterpenoids and focuse on their unique requirements for improving terpenoid production through organelle compartmentalization. In addition, we provide an overview of recent progress in the modification of organellar morphology for subcellular compartmentalization in terpenoid production.
Monoterpenoids and sesquiterpenoids
Enhancing the supply and utilization of GPP and FPP
The difficulty in creating a sufficient precursor pool of GPP and FPP limits the biosynthesis of all monoterpenoids and sesquiterpenoids (Lei et al., 2021). The traditional monoterpenoid and sesquiterpenoid production processes utilize cytoplasmic GPP and FPP (Figure 2A), which is generally limited. However, additional pools of GPP and FPP are distributed in different organelles. Inspired by the concept that organelles of eukaryotic cells can be harnessed to reconstruct metabolic pathways and thereby increase the availability of precursors (Hammer and Avalos, 2017). Targeting the enzymes or the whole biosynthesis pathway to the organelles can effectively optimize monoterpene and sesquiterpene production (Figure 2B). Optimal utilization of GPP and FPP pools distributed in yeast organelles provides a method for microbial overproduction of monoterpenes and sesquiterpenes (Jia et al., 2020). To harness the pool of FPP in mitochondria, Farhi et al. (2011) targeted FPP synthase and sesquiterpene synthase in mitochondria, achieving an eight- and 20-fold improvements in the production of valencene and amorphadiene, respectively. Upstream of FPP and GPP, acetyl-CoA is the central precursor of the MVA pathway for the biosynthesis of terpenoids, and an insufficient supply of acetyl-CoA limits the metabolic flux toward desired compounds (Krivoruchko et al., 2015). As sites of the β-oxidation of fatty acids, peroxisomes can supply ample acetyl-CoA (van der Klei and Veenhuis, 1997). Similarly, mitochondria have nearly 20–30 times higher acetyl-CoA content than the cytosol (Duran et al., 2020). As shown in Figure 2C, rational organelle compartmentalization can greatly improve the utilization efficiency of the acetyl-CoA pools in different organelles (Son et al., 2022a; Lu et al., 2022). Dong et al. (2021) compartmentalized the whole MVA pathway into mitochondria, leading to a 3.7-fold improvement in the production of α-santalene.
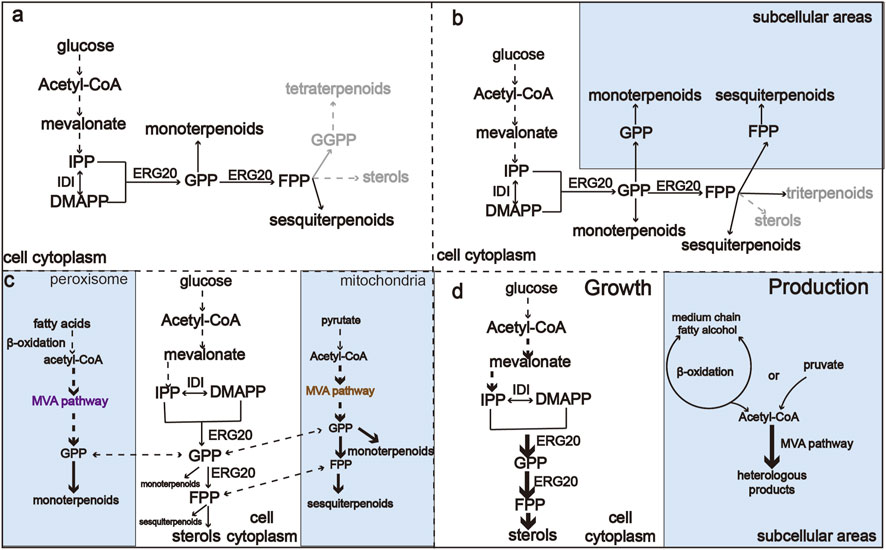
FIGURE 2. An overview of metabolic regulation of monoterpenoid and sesquiterpenoid biosynthesis pathways. (A) Monoterpenoid and sesquiterpenoid biosynthesis pathway in the cytoplasm. (B) Harnessing GPP and FPP in both cytoplasm and organelles to improve the production of monoterpenoids and sesquiterpenoids. (C) Harnessing acetyl-CoA in organelles to improve the production of monoterpenoids and sesquiterpenoids. (D) Engineering compartmentalization for orthogonal biosynthesis of monoterpenoids and sesquiterpenoids to reduce competing reactions.
In addition to increasing the supply of precursors to enhance the production of mono- and sesquiterpenes, inhibiting competing pathways is also a major strategy to increase the production of desired compounds (Mai et al., 2021). The FPP-derived squalene and native sterol biosynthesis pathways are the main competing pathways of monoterpenoid and sesquiterpene synthesis. However, these pathways are essential for the fluidity of the yeast cell membrane and host growth (Fischer et al., 2011), which limits heterologous monoterpene and sesquiterpene production (Peng et al., 2017). A commonly used method to reduce the competing reactions is to construct and screen mutants of ERG20 (Zhang et al., 2020), degrade the rate-limiting enzymes ERG20 and ERG9 (Peng et al., 2018), or establish an orthogonal pathway that uses neryl diphosphate (NPP) as an alternative substrate (Ignea et al., 2019). Due to the necessity of ergosterol for cells, the heterologous pathway affects cellular function due to ubiquitous metabolic interactions. The regulation of metabolic fluxes is crucial to balance growth and production of the desired molecule. However, metabolic rewiring faces many biological challenges that affect the growth and fitness of the hosts (Zhu Z-T. et al., 2021). A method to overcome this obstacle would be to minimize the interactions between heterologous pathway and native metabolism (Pandit et al., 2017; Ignea et al., 2019). This can be achieved by constructing orthogonal pathways through subcellular compartmentalization (Figure 2D). For example, targeting the MVA biosynthesis pathway and the product biosynthesis pathway toorganelles can separate chassis growth from metabolite production. In addition, organelles such as peroxisome and mitochondria can isolate the intermediates from competing reactions, thus increasing the GPP or FPP pool by avoiding consumption by cytoplasmic enzymes (Yuan and Ching, 2016; Yee et al., 2019; Yee et al., 2019; Zhang et al., 2020). By introducing the complete MVA pathway into the peroxisome, Dusséaux et al. (2020) achieve up to a 125-fold increases in the production of geranyl diphosphate-derived compounds compared to the cytosolic pathway. This strategy can be used to produce GPP- or FPP-derived compounds in eukaryotic cells without noticeable effects on strain fitness or viability. Metabolic rewiring has become a promising strategy for enhancing terpenoid production in yeasts.
Reducing the cytotoxicity of monoterpenoids and sesquiterpenoids
The high toxicity of monoterpenoids remains a challenging issue (Erdogan and Ozkan, 2017). Notably, monoterpenoids not only interfere with cell walls and organellar membranes by altering membrane fluidity, structural membrane integrity, and membrane composition, but also induce oxidative stress, and bring more toxic monoterpene hydroperoxides (Brennan et al., 2013; Moon et al., 2020). As shown in Table 2, traditional strategies such as transporter engineering (Hu et al., 2020), in situ product extraction (Rolf et al., 2020), and tolerance engineering can improve the tolerance of strains to monoterpenes and reducetoxicity (Brennan et al., 2015; Jezierska and Van Bogaert, 2017; Zhu K. et al., 2021). To address the metabolic toxicity of monoterpenoids, intracellular compartmentalization of their biosynthetic pathways is commonly used to insolate the monoterpenoids into specific organelles (Kulagina et al., 2021). The monoterpene indole alkaloids produced in Catharanthus roseus were distributed into several organelles to relieve cell toxicity, which provides new insight into how subcellular compartmentalization can enhance the metabolic flux towards the biosynthesis of target compounds (Courdavault et al., 2014; Guirimand et al., 2020). Peroxisomes are not essential for cell growth (Sibirny, 2016), and are also detoxifying organelles (Breitling et al., 2002). Accordingly, the localization of biosynthetic enzymes or whole biosynthetic pathways to peroxisomes may insulate the toxic components away from the host cell cytosol. The peroxisome can be used as a detoxifying organelle for the synthesis of monoterpenes, including limonene, geraniol, α-pinene, sabinene and camphene (Dusséaux et al., 2020). Dusséaux et al. (2020) constructed peroxisome microfactorties able to accelerate recovery growth by the reducing cytotoxicity of monoterpene products. Gerke et al. (2020) targeted the geraniol biosynthetic enzymes to peroxisomes and improved the geraniol tolerance of the yeast cells to reduce product toxicity, resulting in an 80% increase in the geraniol titer. A combination of traditional metabolic strategies and organelle compartmentalization to alleviate product cytotoxicity may provide new insights.
Triterpenoids and tetraterpenoids
Reducing cytotoxicity of triterpenoids and tetraterpenoids
The long-chain triterpenoids and tetraterpenoids are highly polyunsaturated lipophilic hydrocarbons. Similar to triacylglycerols (TAGs) and sterol esters (SEs), triterpenoids and tetraterpenoids are generally sequestered in specific subcellular areas because of their hydrophobicity (Spanova et al., 2010; Wei et al., 2018). As shown in Figure 3, different organelles such as LDs, peroxisomes, ER and plasma membrane can be used as terpenoid reservoirs due to their similar hydrophobicity (Cunningham and Gantt, 1998; Lee et al., 2009). Cytotoxicity is caused by the excessive accumulation of triterpenoids and tetraterpenoids in the host. The plasma membrane provides storage space for the accumulation of heterologous hydrophobic target compounds (Wu et al., 2017), such as carotenoids (Liu et al., 2016). Targeting the key enzymes to the plasma membrane can effectively alleviate the toxicity of hydrophobic compounds and increase production (Bian et al., 2021; Chen M. et al., 2022). Ye et al. (2018) fused a β-carotene ketolase (CrtW) and β-carotene hydroxylase (CrtZ) and targeted the fusion protein to the cell membrane, which resulted in a 215.4% increase in astaxanthin production. By regulating steady-state fluxes and the availability of intermediate metabolites, compartmentalization engineering in yeasts can significantly shorten the distance between the enzyme and the substrate and increase local concentration of the enzyme and the substrate, thereby improve the product yield (Valm et al., 2017).
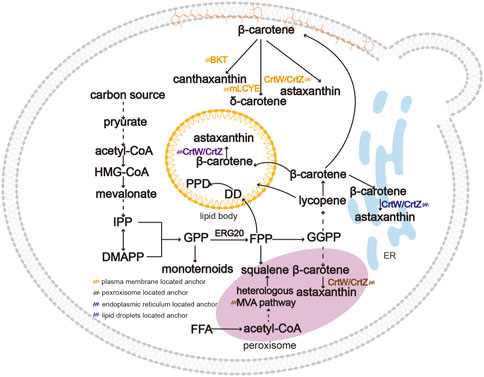
FIGURE 3. An overview of subcellular compartmentalization for the biosynthesis of triterpenoids and tetraterpenoids. Due to their hydrophobicity, triterpenoids and tetraterpenoids are generally sequestered in specific organelles such as LDs, peroxisomes, ER and plasma membrane. Product distribution in strains reduces cytotoxicity and causes spatial separation between substates and products. Moreover, targeting the pathway to more than one compartment can increase the product titer compared to single subcellular compartmentalization. Abbreviations: BKT, β-carotene ketolase; mLCYE, lycopene ε-cyclase.
Providing a suitable environment for the production of triterpenoids and tetraterpenoids
Organelles such as LDs and peroxisomes can provide a suitable storage environment to relive the cytotoxicity of triterpenoids and tetraterpenoids. LDs and peroxisomes are involved in cellular lipid metabolism, among which peroxisomes play a vital role in α- and β-oxidation of fatty acids. These compartments can provide different intracellular environments for enzyme catalysis, such as adequate substrate supply and cofactor balance (Gao and Zhou, 2019). Peroxisomes are enclosed by a single membrane and are speculated to be an additional cellular storage space for lipophilic products in eukaryotic microbial hosts, acting as an advantageous dynamic depot for storing hydrophobic compounds like squalene (Liu et al., 2020). Liu et al. (2020) compartmentalized the entire squalene synthesis pathway into peroxisomes, followed by dual modulation through cytoplasmic and peroxisomal engineering, which improved the squalene titer to 1.7 g/L. Squalene is an important precursor for triterpenoid biosynthesis, and re-locating triterpene synthases to peroxisomes would be a promising solution for triterpenoid production. Recently, the importance of LDs for the storage of hydrophobic carotenoids was highlighted (Larroude et al., 2018; Bu et al., 2022). LDs serve as specialized platforms for lipid metabolism and storage, with a phospholipid monolayer in which molecules are transported in and out of a LDs (Henne et al., 2020). Ma et al. (2019) observed lycopene accumulated in LDs of S. cerevisiae. The hydrophobic compounds are distributed in different parts of LDs due to their different structures (Son et al., 2022b). Adjusting organelle morphology can be a useful strategy for increasing terpenoid production, which will be introduced in detail below. Therefore, the selection of organelles that are naturally lipophilic or hydrophobic may be more conducive to the production of triterpenoids and tetraterpenoids.
Reducing spatial separation between enzyme and product
The triterpenoids and tetraterpenoids always accumulate intracellularly in specific organelles, while enzymes locate in other organelles such as the cytoplasm or ER; thus, the spatial distance between the enzyme and its product may influence the yield of the resulting products. Breaking the barrier between the enzyme and the substrate can reinforce metabolic flux and improve biosynthesis efficiency. The accumulation of sterol metabolites (e.g., zymosterol) in LDs prevents their conversion by enzymes located in ER. Guo et al. reconstituted the post-squalene pathway in LDs and revealed an effective method of pathway compartmentalization (Guo X-J. et al., 2021). The location of enzymes of biosynthesis pathways in membrane-enclosed LDs or peroxisomes can significantly enhance the metabolic flux and promote product titers. Shi et al. (2021) co-localized the pathway enzymes in LDs to eliminate the separation between the substrate dammarenediol-II (DD) and protopanaxadiol synthase (PPDS) of the PPD biosynthesis pathway, which increased the conversion rate of DD to PPD from 17.4% to 86.0%. Compartmentalized reconstitution is an effective way of providing adequate storage and improving the output of triterpenoids and tetraterpenoids.
Modification of organellar morphology
Lipid droplets
Subcellular compartmentalization leads to the co-accumulation and co-localization of enzymes and products in organelles. Son et al. reported that flexible lipids such as squalene with conjugated π bonds are readily dispersed among TAGs, and squalene production can be enhanced by expanding the volume of the LD. Similarly, rigid lipids such as zeaxanthin and β-carotene are retained between TAGs, and the production of zeaxanthin was enhanced by increasing the LD surface area within the cell (Son et al., 2022b). Thus, LDs are suitable organelles for storing triterpenoids and tetraterpenoids, while strategies that control the size and the number of organelles influence their storage capacity (Figure 4). To achieve a high production of lipophilic terpenoids, enhanced the TAG biosynthesis and reduced TAG degradation have been applied to increase LD volume, thereby promoting intracellular terpenoids accumulation (Wei et al., 2018; Ma et al., 2019). Diacylglycerol acyltransferases (DGATs) and phosphatidate phosphatase (PAP) are crucial enzymes that catalyze the ultimate step of TAG synthesis (Adeyo et al., 2011). Overexpression of DGA1 increase lipid content in yeasts, expanding the intracellular storage pool and significantly promoting the accumulation of lycopene (Ma et al., 2019), squalene (Wei et al., 2021) and other terpenoids (Yu et al., 2020). Shi et al. (2021) targeted the limiting enzymes PPDS to LDs and overexpressed DGA1 to increase LD volume, after which the CK titer of the resulting strain reached 5 g/L in 5 L of fed-batch fermentation. Relevant genes that encoding phosphatidic acid phosphatase (PAH1), acetyl-CoA carboxylase (ACC1), and fatty acid desaturase (OLE1) can also regulate TAG biosynthesis (Karanasios et al., 2013; Shi et al., 2014). In addition to LD volume, regulating the number of LDs to increases the monolayer surface is another important strategy affecting terpenoid production (Shi et al., 2021). Overexpressing the LOA1 gene and deleting ERD1 can induce the stress response of the ER and stimulate LD formation, which leads to large numbers of LDs (Ayciriex et al., 2012; Teixeira et al., 2018).
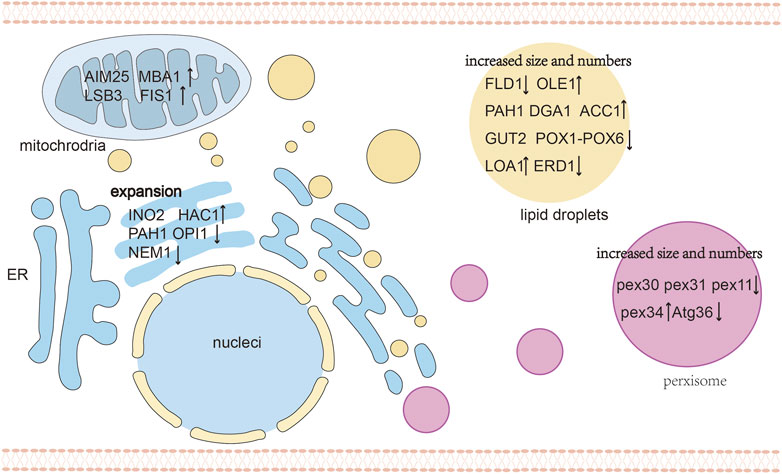
FIGURE 4. An overview of organelle morphology engineering. The target genes of different organelles are shown to regulate organelle morphology, including increased size and number of LDs and peroxisomes, expansion for ER and engineering mitochondrial physiology. Abbreviations: FLD1, seipin; OLE1, fatty acid desaturase; GUT2, G3P dehydrogenase; POX1-6, acyl-CoA oxidases 1 through 6; PEX11, PEX30, PEX32, PEX34, peroxisome-population-regulated proteins; ATG36, autophagy-related protein; OPI1, negative regulator of phospholipid biosynthesis; NEM1, nuclear envelope morphology protein 1; HAC1, Transcriptional activator; ↑ Gene overexpression; ↓ Gene knockdown.
Peroxisome
Similar to LDs, alternative methods that manipulate the number and volume of peroxisomes can effectively enhance terpenoid production. By regulating the specific surface area, quantity and size of the organelles, it is possible to enhance the content of its host localization proteins and pathway metabolites, which can further increase the flux of the localized pathway. The morphology of peroxisomes is regulated by peroxins (PEX) and dynamin-related proteins (DRPs), as well as the autophagy (ATG) protein family, which are responsible for peroxisome biogenesis, fission and pexophagy, respectively (Islinger et al., 2018). Most PEX genes are involved in the import of matrix proteins (Heiland and Erdmann, 2005), and the remaining PEX genes are involved in regulation of the abundance and size of peroxisomes (Vizeacoumar et al., 2003; Vizeacoumar et al., 2004; Tower et al., 2011; Yofe et al., 2017). To maximize the storage capacity of the peroxisome membrane of S. cerevisiae, Choi et al. increased the expression of PEX34, deleted the PEX11 and ATG36 gene, after which they introduced a heterologous protopanaxadiol pathway (Choi et al., 2022). Deletion of PEX11 generated enlarged and clustered peroxisomes, while PEX34, overexpression of PEX34 and deletion of ATG36 significantly increased the number of peroxisomes. The deletion of PEX30, PEX31, and ATG36 in a geraniol producing strains with biosynthetic enzymes targeted to the peroxisome resulted in a larger number of peroxisomes and increased the geraniol titer by 80% (Gerke et al., 2020).
ER and membrane engineering
The expanded membranes of the engineered strains can also be used for additional storage of hydrophobic compounds. Expansion of the ER can increase its capacity to promote the expression of ER-localized proteins and boost terpene accumulation (Kim et al., 2019). Furthermore, suppressing the dephosphorylation and activation of PAH1 can enhance membrane protein productivity and increase the surface area of the ER membrane (Ju et al., 2022). The disruption of PAH1 resulted in a decreased number of LDs, accompanied by the accumulation of neutral lipids in the ER (Adeyo et al., 2011). Similarly to PAH1, overexpression of INO2 in yeast increased its capacity to synthesize endogenous and heterologous ER-associated proteins, leading to a significantly increased squalene titer of 634 mg/L (Kim et al., 2019). Engineering the membrane morphology and improving the membrane synthesis pathway can also increase the stability of membrane-anchored biosynthetic pathways and enhance terpenoid production. A Tsr-augmented recombinant Escherichia coli was able to extend the membrane network, enhance the squalene storage and improved the squalene titer to 712 mg/L (Meng et al., 2020). The native frd operon and UncF protein also induce membrane invagination and increase the membrane lipid volume (Elmes et al., 1986; Arechaga et al., 2000). These findings suggest that membrane engineering has achieved good progress in terpenoid production in E. coli, and suggest a great potential for the utilization of similar mechanisms to modify the membrane area of yeasts. Membrane engineering is a rapidly developing field with great potential for organelle compartmentalization and metabolic engineering.
Mitochondria
Yeast mitochondria are highly dynamic in size, activity, number, and surface area depending on growth conditions, carbon source and metabolic state (Okamoto and Shaw, 2005). The engineering of mitochondria to increase their activity, morphology, number, and localization can increase the activity of mitochondrial biosynthetic pathways. Jia et al. (2020) overexpressed mitochondria-related proteins including FIS1 for mitochondrial division, LSB3 for mitochondrial motility, MBA1 and AIM25 encoding other functional proteins to enhance the compartmentalized pathways, and the AIM25 gene boosted the sabinene titer to 154.9 mg/L. The understanding of mitochondrial physiology and metabolism could be useful to expand the applicability of mitochondrial engineering and realizing its full potential (Ferramosca and Zara, 2021).
Anchoring enzymes simultaneously to several organelles
Organelles such as mitochondria, ER, LDs, and peroxisomes provide different advantages in regulating metabolic fluxes. To further make use of precursors and promote the dynamic balance between different organelles, dual or triple metabolic regulation of the terpenoid synthesis pathway in different subcellular areas could open new possibilities for the production of terpenoids (Lv et al., 2016; Zhu Z-T. et al., 2021; Guo et al., 2022). Comprehensive utilization of multiple organelles can not only enhance the utilization of the limited hydrophobic storage environment, but can also make full use of hydrophobic substrates such as carotene located in different organelles. Ma et al. (2021) expressed the astaxanthin pathway in LDs, endoplasmic reticulum and peroxisomes, and the best strain ultimately produced 858 mg/L of astaxanthin in fed-batch fermentation. Zhu et al. compartmentalized the MVA pathway to mitochondria to improve cell growth and synthesized squalene in the cytoplasm to improve production (Zhu Z-T. et al., 2021). The simultaneous localization of terpenes in different organelles can serve as a promising strategy for optimizing the biosynthetic pathway module and cell growth in parallel.
Conclusion and perspectives
Anchoring the key enzymes or the MVA pathway to specific organelles by localization tags can yield substantial improvements in terpenoid production. In this review, we have systematically analyzed successful approaches using organelle compartmentalization applied to overcome problems associated with the production of different terpenoids, including inadequate supply of GPP/FPP pools and other substrates, cytotoxicity of terpenoids, insufficient storge space, and special challenges of tetraterpenoids. However, more work to improve terpenoid production is still needed in the future. Diterpenoids are one of the most important families of bioactive compounds, and compartmentalization is still limited in diterpenoid production in yeasts. Li et al. (2019) compartmentalized taxadiene synthase, taxadiene-5ahydroxylase and cytochrome P450 reductase to the chloroplast of Nicotiana benthamiana to improve the supply of precursor for taxadiene synthesis. The accumulation of different diterpenoids has temporal and spatial specificity, and compartmentalization of key enzymes and biosynthetic pathway of diterpenoids is a promising alternative to current methods.
As the most extensively studied organelles in cellular physiology, the engineering of mitochondria, peroxisomes, ER, LDs, and plasma membranes has already led to great progress in terpenoid production. However, future studies should target new organelles like the Golgi apparatus and the vacuole whose potential to improve terpenoid production remains unexplored. For secologanin biosynthesis in C. roseus, geraniol from the MEP pathway is directed toward the vacuole following a hydroxylation reaction by G10H (Verma et al., 2012). Some terpenoids and relevant enzymes are naturally located in the vacuoles (Guirimand et al., 2011), which may provide an alternative option for terpenoid production as a detoxification compartment and proper site for enzyme catalysis. In eukaryotic cells, cofactors are distributed in different subcellular compartments, which necessitates systematically engineering the supply and recycling of cofactors to couple compartmentalized cellular metabolism (Chen R. et al., 2022). Engineering cofactors in organelle compartmentalization benefits the biosynthesis of terpenoids that require cofactors (Cataldo et al., 2020; Zhang et al., 2022). A large number of terpene synthases like P450s and key limiting enzymes such as HMG1 are NADH-dependent and FADH-dependent enzymes. For instance, CrtI which converts phytoene to lycopene is also a FAD-dependent enzyme (Shen et al., 2016). FAD (H2)-dependent catalytic reactions in the cytosol may be relatively inefficient due to the lower FAD(H) concentration (Pallotta et al., 1998), as the FAD (H2) concentration in mitochondria is 20 times higher than in the cytosol of S. cerevisiae. Different cofactors have their unique organelle localization and construction of a cofactor biosynthesis pathway in the cytosol or targeted organelles is an alternative method for increasing terpenoid production.
Author contributions
LC wrote the manuscript. MY, WX, and YY helped to draft the manuscript and revised the manuscript. YW revised and supervised the manuscript. All authors read and approved the final manuscript.
Funding
This work was supported by the National Natural Science Foundation of China (32071415).
Conflict of interest
The authors declare that the research was conducted in the absence of any commercial or financial relationships that could be construed as a potential conflict of interest.
Publisher’s note
All claims expressed in this article are solely those of the authors and do not necessarily represent those of their affiliated organizations, or those of the publisher, the editors and the reviewers. Any product that may be evaluated in this article, or claim that may be made by its manufacturer, is not guaranteed or endorsed by the publisher.
References
Adeyo, O., Horn, P. J., Lee, S., Binns, D. D., Chandrahas, A., Chapman, K. D., et al. (2011). The yeast lipin orthologue Pah1p is important for biogenesis of lipid droplets. J. Cell Biol. 192 (6), 1043–1055. doi:10.1083/jcb.201010111
Arechaga, I., Miroux, B., Karrasch, S., Huijbregts, R., de Kruijff, B., Runswick, M. J., et al. (2000). Characterisation of new intracellular membranes in Escherichia coli accompanying large scale over-production of the b subunit of F(1)F(o) ATP synthase. FEBS Lett. 482 (3), 215–219. doi:10.1016/s0014-5793(00)02054-8
Ayciriex, S., Le Guédard, M., Camougrand, N., Velours, G., Schoene, M., Leone, S., et al. (2012). YPR139c/LOA1 encodes a novel lysophosphatidic acid acyltransferase associated with lipid droplets and involved in TAG homeostasis. Mol. Biol. Cell 23 (2), 233–246. doi:10.1091/mbc.E11-07-0650
Basiony, M., Ouyang, L., Wang, D., Yu, J., Zhou, L., Zhu, M., et al. (2022). Optimization of microbial cell factories for astaxanthin production: Biosynthesis and regulations, engineering strategies and fermentation optimization strategies. Synthetic Syst. Biotechnol. 7 (2), 689–704. doi:10.1016/j.synbio.2022.01.002
Bhataya, A., Schmidt-Dannert, C., and Lee, P. C. (2009). Metabolic engineering of Pichia pastoris X-33 for lycopene production. Process Biochem. 44 (10), 1095–1102. doi:10.1016/j.procbio.2009.05.012
Bian, Q., Zhou, P., Yao, Z., Li, M., Yu, H., and Ye, L. (2021). Heterologous biosynthesis of lutein in S. cerevisiae enabled by temporospatial pathway control. Metab. Eng. 67, 19–28. doi:10.1016/j.ymben.2021.05.008
Breitling, R., Sharif, O., Hartman, M. L., and Krisans, S. K. (2002). Loss of compartmentalization causes misregulation of lysine biosynthesis in peroxisome-deficient yeast cells. Eukaryot. Cell 1 (6), 978–986. doi:10.1128/ec.1.6.978-986.2002
Brennan, T. C., Krömer, J. O., and Nielsen, L. K. (2013). Physiological and transcriptional responses of Saccharomyces cerevisiae to d-limonene show changes to the cell wall but not to the plasma membrane. Appl. Environ. Microbiol. 79 (12), 3590–3600. doi:10.1128/aem.00463-13
Brennan, T. C., Turner, C. D., Krömer, J. O., and Nielsen, L. K. (2012). Alleviating monoterpene toxicity using a two-phase extractive fermentation for the bioproduction of jet fuel mixtures in Saccharomyces cerevisiae. Biotechnol. Bioeng. 109 (10), 2513–2522. doi:10.1002/bit.24536
Brennan, T. C., Williams, T. C., Schulz, B. L., Palfreyman, R. W., Krömer, J. O., and Nielsen, L. K. (2015). Evolutionary engineering improves tolerance for replacement jet fuels in Saccharomyces cerevisiae. Appl. Environ. Microbiol. 81 (10), 3316–3325. doi:10.1128/aem.04144-14
Bu, X., Lin, J. Y., Duan, C. Q., Koffas, M. A. G., and Yan, G. L. (2022). Dual regulation of lipid droplet-triacylglycerol metabolism and ERG9 expression for improved β-carotene production in Saccharomyces cerevisiae. Microb. Cell Fact. 21 (1), 3. doi:10.1186/s12934-021-01723-y
Cao, X., Yang, S., Cao, C., and Zhou, Y. J. (2020). Harnessing sub-organelle metabolism for biosynthesis of isoprenoids in yeast. Synth. Syst. Biotechnol. 5 (3), 179–186. doi:10.1016/j.synbio.2020.06.005
Cataldo, V. F., Arenas, N., Salgado, V., Camilo, C., Ibáñez, F., and Agosin, E. (2020). Heterologous production of the epoxycarotenoid violaxanthin in Saccharomyces cerevisiae. Metab. Eng. 59, 53–63. doi:10.1016/j.ymben.2020.01.006
Chen, M., Li, M., Ye, L., and Yu, H. (2022). Construction of canthaxanthin-producing yeast by combining spatiotemporal regulation and pleiotropic drug resistance engineering. ACS Synth. Biol. 11 (1), 325–333. doi:10.1021/acssynbio.1c00437
Chen, R., Gao, J., Yu, W., Chen, X., Zhai, X., Chen, Y., et al. (2022). Engineering cofactor supply and recycling to drive phenolic acid biosynthesis in yeast. Nat. Chem. Biol. 18 (5), 520–529. doi:10.1038/s41589-022-01014-6
Chen, X., Gao, C., Guo, L., Hu, G., Luo, Q., Liu, J., et al. (2018). DCEO biotechnology: Tools to design, construct, evaluate, and optimize the metabolic pathway for biosynthesis of chemicals. Chem. Rev. 118 (1), 4–72. doi:10.1021/acs.chemrev.6b00804
Chen, Y., Daviet, L., Schalk, M., Siewers, V., and Nielsen, J. (2013). Establishing a platform cell factory through engineering of yeast acetyl-CoA metabolism. Metab. Eng. 15, 48–54. doi:10.1016/j.ymben.2012.11.002
Choi, B. H., Kang, H. J., Kim, S. C., and Lee, P. C. (2022). Organelle engineering in yeast: Enhanced production of protopanaxadiol through manipulation of peroxisome proliferation in Saccharomyces cerevisiae. Microorg. [Online] 10 (3), 650. doi:10.3390/microorganisms10030650
Courdavault, V., Papon, N., Clastre, M., Giglioli-Guivarc’h, N., St-Pierre, B., and Burlat, V. (2014). A look inside an alkaloid multisite plant: The Catharanthus logistics. Curr. Opin. Plant Biol. 19, 43–50. doi:10.1016/j.pbi.2014.03.010
Cunningham, F. X., and Gantt, E. (1998). Genes and enzymes of carotenoid biosynthesis in plants. Annu. Rev. PLANT PHYSIOLOGY PLANT Mol. Biol. 49, 557–583. doi:10.1146/annurev.arplant.49.1.557
Dong, C., Shi, Z., Huang, L., Zhao, H., Xu, Z., and Lian, J. (2021). Cloning and characterization of a panel of mitochondrial targeting sequences for compartmentalization engineering in Saccharomyces cerevisiae. Biotechnol. Bioeng. 118 (11), 4269–4277. doi:10.1002/bit.27896
Duran, L., López, J. M., and Avalos, J. L. (2020). ¡Viva la mitochondria!: Harnessing yeast mitochondria for chemical production. FEMS Yeast Res. 20 (6), foaa037. doi:10.1093/femsyr/foaa037
Dusséaux, S., Wajn, W. T., Liu, Y., Ignea, C., and Kampranis, S. C. (2020). Transforming yeast peroxisomes into microfactories for the efficient production of high-value isoprenoids. Proc. Natl. Acad. Sci. U. S. A. 117 (50), 31789–31799. doi:10.1073/pnas.2013968117
Dyall, S. D., Brown, M. T., and Johnson, P. J. (2004). Ancient invasions: From endosymbionts to organelles. Science 304 (5668), 253–257. doi:10.1126/science.1094884
Elmes, M. L., Scraba, D. G., and Weiner, J. H. (1986). Isolation and characterization of the tubular organelles induced by fumarate reductase overproduction in Escherichia coli. J. Gen. Microbiol. 132 (6), 1429–1439. doi:10.1099/00221287-132-6-1429
Erdogan, A., and Ozkan, A. (2017). Investigatıon of antioxıdative, cytotoxic, membrane-damaging and membrane-protective effects of the essentıal oil of Origanum majorana and its oxygenated monoterpene component linalool in human-derived hep G2 cell line. Iran. J. Pharm. Res. 16 (1), 24–34.
Farhi, M., Marhevka, E., Masci, T., Marcos, E., Eyal, Y., Ovadis, M., et al. (2011). Harnessing yeast subcellular compartments for the production of plant terpenoids. Metab. Eng. 13 (5), 474–481. doi:10.1016/j.ymben.2011.05.001
Ferramosca, A., and Zara, V. (2021). Mitochondrial carriers and substrates transport network: A lesson from Saccharomyces cerevisiae. Int. J. Mol. Sci. 22 (16), 8496. doi:10.3390/ijms22168496
Fischer, M. J., Meyer, S., Claudel, P., Bergdoll, M., and Karst, F. (2011). Metabolic engineering of monoterpene synthesis in yeast. Biotechnol. Bioeng. 108 (8), 1883–1892. doi:10.1002/bit.23129
Gao, J., and Zhou, Y. J. (2019). Repurposing peroxisomes for microbial synthesis for biomolecules. Methods Enzymol. 617, 83–111. doi:10.1016/bs.mie.2018.12.004
Gerke, J., Frauendorf, H., Schneider, D., Wintergoller, M., Hofmeister, T., Poehlein, A., et al. (2020). Production of the fragrance geraniol in peroxisomes of a product-tolerant baker's yeast. Front. Bioeng. Biotechnol. 8, 582052. doi:10.3389/fbioe.2020.582052
Grewal, P. S., Samson, J. A., Baker, J. J., Choi, B., and Dueber, J. E. (2021). Peroxisome compartmentalization of a toxic enzyme improves alkaloid production. Nat. Chem. Biol. 17 (1), 96–103. doi:10.1038/s41589-020-00668-4
Guirimand, G., Guihur, A., Ginis, O., Poutrain, P., Héricourt, F., Oudin, A., et al. (2011). The subcellular organization of strictosidine biosynthesis in Catharanthus roseus epidermis highlights several trans-tonoplast translocations of intermediate metabolites. Febs J. 278 (5), 749–763. doi:10.1111/j.1742-4658.2010.07994.x
Guirimand, G., Guihur, A., Perello, C., Phillips, M., Mahroug, S., Oudin, A., et al. (2020). Cellular and subcellular compartmentation of the 2C-Methyl-D-Erythritol 4-phosphate pathway in the Madagascar periwinkle. Plants [Online] 9 (4), 462. doi:10.3390/plants9040462
Guo, Q., Li, Y. W., Yan, F., Li, K., Wang, Y. T., Ye, C., et al. (2022). Dual cytoplasmic-peroxisomal engineering for high-yield production of sesquiterpene α-humulene in Yarrowia lipolytica. Biotechnol. Bioeng. 119 (10), 2819–2830. doi:10.1002/bit.28176
Guo, Q., Shi, T. Q., Peng, Q. Q., Sun, X. M., Ji, X. J., and Huang, H. (2021). Harnessing yarrowia lipolytica peroxisomes as a subcellular factory for α-humulene overproduction. J. Agric. Food Chem. 69 (46), 13831–13837. doi:10.1021/acs.jafc.1c05897
Guo, X.-J., Yao, M.-D., Xiao, W.-H., Wang, Y., Zhao, G.-R., and Yuan, Y.-J. (2021). Compartmentalized reconstitution of post-squalene pathway for 7-dehydrocholesterol overproduction in Saccharomyces cerevisiae. Front. Microbiol. 12, 663973. doi:10.3389/fmicb.2021.663973
Hammer, S. K., and Avalos, J. L. (2017). Harnessing yeast organelles for metabolic engineering. Nat. Chem. Biol. 13 (8), 823–832. doi:10.1038/nchembio.2429
Heiland, I., and Erdmann, R. (2005). Biogenesis of peroxisomes. Topogenesis of the peroxisomal membrane and matrix proteins. Febs J. 272 (10), 2362–2372. doi:10.1111/j.1742-4658.2005.04690.x
Henne, M., Goodman, J. M., and Hariri, H. (2020). Spatial compartmentalization of lipid droplet biogenesis. Biochimica Biophysica Acta (BBA) - Mol. Cell Biol. Lipids 1865 (1), 158499. doi:10.1016/j.bbalip.2019.07.008
Hu, Z., Li, H., Weng, Y., Li, P., Zhang, C., and Xiao, D. (2020). Improve the production of D-limonene by regulating the mevalonate pathway of Saccharomyces cerevisiae during alcoholic beverage fermentation. J. Ind. Microbiol. Biotechnol. 47 (12), 1083–1097. doi:10.1007/s10295-020-02329-w
Ignea, C., Pontini, M., Maffei, M. E., Makris, A. M., and Kampranis, S. C. (2014). Engineering monoterpene production in yeast using a synthetic dominant negative geranyl diphosphate synthase. ACS Synth. Biol. 3 (5), 298–306. doi:10.1021/sb400115e
Ignea, C., Raadam, M. H., Motawia, M. S., Makris, A. M., Vickers, C. E., and Kampranis, S. C. (2019). Orthogonal monoterpenoid biosynthesis in yeast constructed on an isomeric substrate. Nat. Commun. 10 (1), 3799. doi:10.1038/s41467-019-11290-x
Islinger, M., Voelkl, A., Fahimi, H. D., and Schrader, M. (2018). The peroxisome: An update on mysteries 2.0. Histochem Cell Biol. 150 (5), 443–471. doi:10.1007/s00418-018-1722-5
Jezierska, S., and Van Bogaert, I. N. A. (2017). Crossing boundaries: The importance of cellular membranes in industrial biotechnology. J. Ind. Microbiol. Biotechnol. 44 (4-5), 721–733. doi:10.1007/s10295-016-1858-z
Jia, H., Chen, T., Qu, J., Yao, M., Xiao, W., Wang, Y., et al. (2020). Collaborative subcellular compartmentalization to improve GPP utilization and boost sabinene accumulation in Saccharomyces cerevisiae. Biochem. Eng. J. 164, 107768. doi:10.1016/j.bej.2020.107768
Jin, K., Xia, H., Liu, Y., Li, J., Du, G., Lv, X., et al. (2022). Compartmentalization and transporter engineering strategies for terpenoid synthesis. Microb. Cell Fact. 21 (1), 92. doi:10.1186/s12934-022-01819-z
Ju, H., Zhang, C., He, S., Nan, W., and Lu, W. (2022). Construction and optimization of Saccharomyces cerevisiae for synthesizing forskolin. Appl. Microbiol. Biotechnol. 106 (5), 1933–1944. doi:10.1007/s00253-022-11819-z
Karanasios, E., Barbosa, A. D., Sembongi, H., Mari, M., Han, G. S., Reggiori, F., et al. (2013). Regulation of lipid droplet and membrane biogenesis by the acidic tail of the phosphatidate phosphatase Pah1p. Mol. Biol. Cell 24 (13), 2124–2133. doi:10.1091/mbc.E13-01-0021
Kim, J.-E., Jang, I.-S., Son, S.-H., Ko, Y.-J., Cho, B.-K., Kim, S. C., et al. (2019). Tailoring the Saccharomyces cerevisiae endoplasmic reticulum for functional assembly of terpene synthesis pathway. Metab. Eng. 56, 50–59. doi:10.1016/j.ymben.2019.08.013
Krivoruchko, A., Zhang, Y., Siewers, V., Chen, Y., and Nielsen, J. (2015). Microbial acetyl-CoA metabolism and metabolic engineering. Metab. Eng. 28, 28–42. doi:10.1016/j.ymben.2014.11.009
Kulagina, N., Besseau, S., Papon, N., and Courdavault, V. (2021). Peroxisomes: A new hub for metabolic engineering in yeast. Front. Bioeng. Biotechnol. 9, 659431. doi:10.3389/fbioe.2021.659431
Langevin, A. M., El Meouche, I., and Dunlop, M. J. (2020). Mapping the role of AcrAB-TolC efflux pumps in the evolution of antibiotic resistance reveals near-MIC treatments facilitate resistance acquisition. mSphere 5 (6), 010566–e1120. doi:10.1128/mSphere.01056-20
Larroude, M., Celinska, E., Back, A., Thomas, S., Nicaud, J. M., and Ledesma-Amaro, R. (2018). A synthetic biology approach to transform Yarrowia lipolytica into a competitive biotechnological producer of β-carotene. Biotechnol. Bioeng. 115 (2), 464–472. doi:10.1002/bit.26473
Lee, P. C., Yoon, Y., and Schmidt-Dannert, C. (2009). Investigation of cellular targeting of carotenoid pathway enzymes in Pichia pastoris. J. Biotechnol. 140 (3-4), 227–233. doi:10.1016/j.jbiotec.2009.01.019
Lei, D., Qiu, Z., Qiao, J., and Zhao, G.-R. (2021). Plasticity engineering of plant monoterpene synthases and application for microbial production of monoterpenoids. Biotechnol. Biofuels 14 (1), 147. doi:10.1186/s13068-021-01998-8
Li, J., Mutanda, I., Wang, K., Yang, L., Wang, J., and Wang, Y. (2019). Chloroplastic metabolic engineering coupled with isoprenoid pool enhancement for committed taxanes biosynthesis in Nicotiana benthamiana. Nat. Commun. 10 (1), 4850. doi:10.1038/s41467-019-12879-y
Li, J., Zhu, K., Miao, L., Rong, L., Zhao, Y., Li, S., et al. (2021). Simultaneous improvement of limonene production and tolerance in yarrowia lipolytica through tolerance engineering and evolutionary engineering. ACS Synth. Biol. 10 (4), 884–896. doi:10.1021/acssynbio.1c00052
Liu, G. S., Li, T., Zhou, W., Jiang, M., Tao, X. Y., Liu, M., et al. (2020). The yeast peroxisome: A dynamic storage depot and subcellular factory for squalene overproduction. Metab. Eng. 57, 151–161. doi:10.1016/j.ymben.2019.11.001
Liu, P., Sun, L., Sun, Y., Shang, F., and Yan, G. (2016). Decreased fluidity of cell membranes causes a metal ion deficiency in recombinant Saccharomyces cerevisiae producing carotenoids. J. Ind. Microbiol. Biotechnol. 43 (4), 525–535. doi:10.1007/s10295-015-1728-0
Lu, S., Zhou, C., Guo, X., Du, Z., Cheng, Y., Wang, Z., et al. (2022). Enhancing fluxes through the mevalonate pathway in Saccharomyces cerevisiae by engineering the HMGR and β-alanine metabolism. Microb. Biotechnol. 15 (8), 2292–2306. doi:10.1111/1751-7915.14072
Lv, X., Wang, F., Zhou, P., Ye, L., Xie, W., Xu, H., et al. (2016). Dual regulation of cytoplasmic and mitochondrial acetyl-CoA utilization for improved isoprene production in Saccharomyces cerevisiae. Nat. Commun. 7, 12851. doi:10.1038/ncomms12851
Ma, T., Shi, B., Ye, Z., Li, X., Liu, M., Chen, Y., et al. (2019). Lipid engineering combined with systematic metabolic engineering of Saccharomyces cerevisiae for high-yield production of lycopene. Metab. Eng. 52, 134–142. doi:10.1016/j.ymben.2018.11.009
Ma, Y., Li, J., Huang, S., and Stephanopoulos, G. (2021). Targeting pathway expression to subcellular organelles improves astaxanthin synthesis in Yarrowia lipolytica. Metab. Eng. 68, 152–161. doi:10.1016/j.ymben.2021.10.004
Mai, J., Li, W., Ledesma-Amaro, R., and Ji, X. J. (2021). Engineering plant sesquiterpene synthesis into yeasts: A review. J. Agric. Food Chem. 69 (33), 9498–9510. doi:10.1021/acs.jafc.1c03864
Meng, Y., Shao, X., Wang, Y., Li, Y., Zheng, X., Wei, G., et al. (2020). Extension of cell membrane boosting squalene production in the engineered Escherichia coli. Biotechnol. Bioeng. 117 (11), 3499–3507. doi:10.1002/bit.27511
Moon, J. E., Heo, W., Lee, S. H., Lee, S. H., Lee, H. G., Lee, J. H., et al. (2020). Trehalose protects the probiotic yeast Saccharomyces boulardii against oxidative stress-induced cell death. J. Microbiol. Biotechnol. 30 (1), 54–61. doi:10.4014/jmb.1906.06041
Moser, S., and Pichler, H. (2019). Identifying and engineering the ideal microbial terpenoid production host. Appl. Microbiol. Biotechnol. 103 (14), 5501–5516. doi:10.1007/s00253-019-09892-y
Okamoto, K., and Shaw, J. M. (2005). Mitochondrial morphology and dynamics in yeast and multicellular eukaryotes. Annu. Rev. Genet. 39, 503–536. doi:10.1146/annurev.genet.38.072902.093019
Pallotta, M. L., Brizio, C., Fratianni, A., De Virgilio, C., Barile, M., and Passarella, S. (1998). Saccharomyces cerevisiae mitochondria can synthesise FMN and FAD from externally added riboflavin and export them to the extramitochondrial phase. FEBS Lett. 428 (3), 245–249. doi:10.1016/s0014-5793(98)00544-4
Pandit, A. V., Srinivasan, S., and Mahadevan, R. (2017). Redesigning metabolism based on orthogonality principles. Nat. Commun. 8 (1), 15188. doi:10.1038/ncomms15188
Peng, B., Nielsen, L. K., Kampranis, S. C., and Vickers, C. E. (2018). Engineered protein degradation of farnesyl pyrophosphate synthase is an effective regulatory mechanism to increase monoterpene production in Saccharomyces cerevisiae. Metab. Eng. 47, 83–93. doi:10.1016/j.ymben.2018.02.005
Peng, B., Plan, M. R., Chrysanthopoulos, P., Hodson, M. P., Nielsen, L. K., and Vickers, C. E. (2017). A squalene synthase protein degradation method for improved sesquiterpene production in Saccharomyces cerevisiae. Metab. Eng. 39, 209–219. doi:10.1016/j.ymben.2016.12.003
Ro, D. K., Paradise, E. M., Ouellet, M., Fisher, K. J., Newman, K. L., Ndungu, J. M., et al. (2006). Production of the antimalarial drug precursor artemisinic acid in engineered yeast. Nature 440 (7086), 940–943. doi:10.1038/nature04640
Rolf, J., Julsing, M. K., Rosenthal, K., and Lütz, S. (2020). A gram-scale limonene production process with engineered Escherichia coli. Molecules 25 (8), 1881. doi:10.3390/molecules25081881
Shen, H. J., Cheng, B. Y., Zhang, Y. M., Tang, L., Li, Z., Bu, Y. F., et al. (2016). Dynamic control of the mevalonate pathway expression for improved zeaxanthin production in Escherichia coli and comparative proteome analysis. Metab. Eng. 38, 180–190. doi:10.1016/j.ymben.2016.07.012
Shi, S., Chen, Y., Siewers, V., and Nielsen, J. (2014). Improving production of malonyl coenzyme A-derived metabolites by abolishing Snf1-dependent regulation of Acc1. mBio 5 (3), e01130–e01114. doi:10.1128/mBio.01130-14
Shi, Y., Wang, D., Li, R., Huang, L., Dai, Z., and Zhang, X. (2021). Engineering yeast subcellular compartments for increased production of the lipophilic natural products ginsenosides. Metab. Eng. 67, 104–111. doi:10.1016/j.ymben.2021.06.002
Sibirny, A. A. (2016). Yeast peroxisomes: Structure, functions and biotechnological opportunities. FEMS Yeast Res. 16 (4), fow038. doi:10.1093/femsyr/fow038
Son, S. H., Kim, J. E., Moon, S. Y., Jang, I. S., Yu, B. J., and Lee, J. Y. (2022a). Metabolic recycling of storage lipids promotes squalene biosynthesis in yeast. Biotechnol. Biofuels Bioprod. 15 (1), 108. doi:10.1186/s13068-022-02208-9
Son, S. H., Park, G., Lim, J., Son, C. Y., Oh, S. S., and Lee, J. Y. (2022b). Chain flexibility of medicinal lipids determines their selective partitioning into lipid droplets. Nat. Commun. 13 (1), 3612. doi:10.1038/s41467-022-31400-6
Spanova, M., Czabany, T., Zellnig, G., Leitner, E., Hapala, I., and Daum, G. (2010). Effect of lipid particle biogenesis on the subcellular distribution of squalene in the yeast Saccharomyces cerevisiae. J. Biol. Chem. 285 (9), 6127–6133. doi:10.1074/jbc.M109.074229
Teixeira, P. G., David, F., Siewers, V., and Nielsen, J. (2018). Engineering lipid droplet assembly mechanisms for improved triacylglycerol accumulation in Saccharomyces cerevisiae. FEMS Yeast Res. 18 (6). doi:10.1093/femsyr/foy060
Tower, R. J., Fagarasanu, A., Aitchison, J. D., and Rachubinski, R. A. (2011). The peroxin Pex34p functions with the Pex11 family of peroxisomal divisional proteins to regulate the peroxisome population in yeast. Mol. Biol. Cell 22 (10), 1727–1738. doi:10.1091/mbc.E11-01-0084
Valm, A. M., Cohen, S., Legant, W. R., Melunis, J., Hershberg, U., Wait, E., et al. (2017). Applying systems-level spectral imaging and analysis to reveal the organelle interactome. Nature 546 (7656), 162–167. doi:10.1038/nature22369
van der Klei, I. J., and Veenhuis, M. (1997). Yeast peroxisomes: Function and biogenesis of a versatile cell organelle. Trends Microbiol. 5 (12), 502–509. doi:10.1016/s0966-842x(97)01156-6
Verma, P., Mathur, A. K., Srivastava, A., and Mathur, A. (2012). Emerging trends in research on spatial and temporal organization of terpenoid indole alkaloid pathway in Catharanthus roseus: A literature update. Protoplasma 249 (2), 255–268. doi:10.1007/s00709-011-0291-4
Vizeacoumar, F. J., Torres-Guzman, J. C., Bouard, D., Aitchison, J. D., and Rachubinski, R. A. (2004). Pex30p, Pex31p, and Pex32p form a family of peroxisomal integral membrane proteins regulating peroxisome size and number in Saccharomyces cerevisiae. Mol. Biol. Cell 15 (2), 665–677. doi:10.1091/mbc.e03-09-0681
Vizeacoumar, F. J., Torres-Guzman, J. C., Tam, Y. Y., Aitchison, J. D., and Rachubinski, R. A. (2003). YHR150w and YDR479c encode peroxisomal integral membrane proteins involved in the regulation of peroxisome number, size, and distribution in Saccharomyces cerevisiae. J. Cell Biol. 161 (2), 321–332. doi:10.1083/jcb.200210130
Wei, L.-J., Cao, X., Liu, J.-J., Kwak, S., Jin, Y.-S., Wang, W., et al. (2021). Increased accumulation of squalene in engineered yarrowia lipolytica through deletion of PEX10 and URE2. Appl. Environ. Microbiol. 87 (17), 00481211–e100421. doi:10.1128/AEM.00481-21
Wei, L. J., Kwak, S., Liu, J. J., Lane, S., Hua, Q., Kweon, D. H., et al. (2018). Improved squalene production through increasing lipid contents in Saccharomyces cerevisiae. Biotechnol. Bioeng. 115 (7), 1793–1800. doi:10.1002/bit.26595
Wu, T., Ye, L., Zhao, D., Li, S., Li, Q., Zhang, B., et al. (2017). Membrane engineering - a novel strategy to enhance the production and accumulation of β-carotene in Escherichia coli. Metab. Eng. 43, 85–91. doi:10.1016/j.ymben.2017.07.001
Ye, L., Zhu, X., Wu, T., Wang, W., Zhao, D., Bi, C., et al. (2018). Optimizing the localization of astaxanthin enzymes for improved productivity. Biotechnol. Biofuels 11, 278. doi:10.1186/s13068-018-1270-1
Yee, D. A., DeNicola, A. B., Billingsley, J. M., Creso, J. G., Subrahmanyam, V., and Tang, Y. (2019). Engineered mitochondrial production of monoterpenes in Saccharomyces cerevisiae. Metab. Eng. 55, 76–84. doi:10.1016/j.ymben.2019.06.004
Yocum, H. C., Pham, A., and Da Silva, N. A. (2021). Successful enzyme colocalization strategies in yeast for increased synthesis of non-native products. Front. Bioeng. Biotechnol. 9, 606795. doi:10.3389/fbioe.2021.606795
Yofe, I., Soliman, K., Chuartzman, S. G., Morgan, B., Weill, U., Yifrach, E., et al. (2017). Pex35 is a regulator of peroxisome abundance. J. Cell Sci. 130 (4), 791–804. doi:10.1242/jcs.187914
Yu, Y., Rasool, A., Liu, H., Lv, B., Chang, P., Song, H., et al. (2020). Engineering Saccharomyces cerevisiae for high yield production of α-amyrin via synergistic remodeling of α-amyrin synthase and expanding the storage pool. Metab. Eng. 62, 72–83. doi:10.1016/j.ymben.2020.08.010
Yuan, J., and Ching, C. B. (2016). Mitochondrial acetyl-CoA utilization pathway for terpenoid productions. Metab. Eng. 38, 303–309. doi:10.1016/j.ymben.2016.07.008
Zhang, C., and Hong, K. (2020). Production of terpenoids by synthetic biology approaches. Front. Bioeng. Biotechnol. 8, 347. doi:10.3389/fbioe.2020.00347
Zhang, G., Wang, H., Zhang, Z., Verstrepen, K. J., Wang, Q., and Dai, Z. (2022). Metabolic engineering of yarrowia lipolytica for terpenoids production: Advances and perspectives. Crit. Rev. Biotechnol. 42 (4), 618–633. doi:10.1080/07388551.2021.1947183
Zhang, L., Xiao, W.-H., Wang, Y., Yao, M.-D., Jiang, G.-Z., Zeng, B.-X., et al. (2017). Chassis and key enzymes engineering for monoterpenes production. Biotechnol. Adv. 35 (8), 1022–1031. doi:10.1016/j.biotechadv.2017.09.002
Zhang, Y., Wang, J., Cao, X., Liu, W., Yu, H., and Ye, L. (2020). High-level production of linalool by engineered Saccharomyces cerevisiae harboring dual mevalonate pathways in mitochondria and cytoplasm. Enzyme Microb. Technol. 134, 109462. doi:10.1016/j.enzmictec.2019.109462
Zhu, K., Kong, J., Zhao, B., Rong, L., Liu, S., Lu, Z., et al. (2021). Metabolic engineering of microbes for monoterpenoid production. Biotechnol. Adv. 53, 107837. doi:10.1016/j.biotechadv.2021.107837
Keywords: compartmentalization, terpenoids, precursor limitation, cell cytotoxicity, metabolic engineering
Citation: Chen L, Xiao W, Yao M, Wang Y and Yuan Y (2023) Compartmentalization engineering of yeasts to overcome precursor limitations and cytotoxicity in terpenoid production. Front. Bioeng. Biotechnol. 11:1132244. doi: 10.3389/fbioe.2023.1132244
Received: 27 December 2022; Accepted: 13 February 2023;
Published: 23 February 2023.
Edited by:
Wei Luo, Jiangnan University, ChinaReviewed by:
Jifeng Yuan, Xiamen University, ChinaZhigang Sui, Dalian Institute of Chemical Physics (CAS), China
Copyright © 2023 Chen, Xiao, Yao, Wang and Yuan. This is an open-access article distributed under the terms of the Creative Commons Attribution License (CC BY). The use, distribution or reproduction in other forums is permitted, provided the original author(s) and the copyright owner(s) are credited and that the original publication in this journal is cited, in accordance with accepted academic practice. No use, distribution or reproduction is permitted which does not comply with these terms.
*Correspondence: Ying Wang, ying.wang@tju.edu.cn