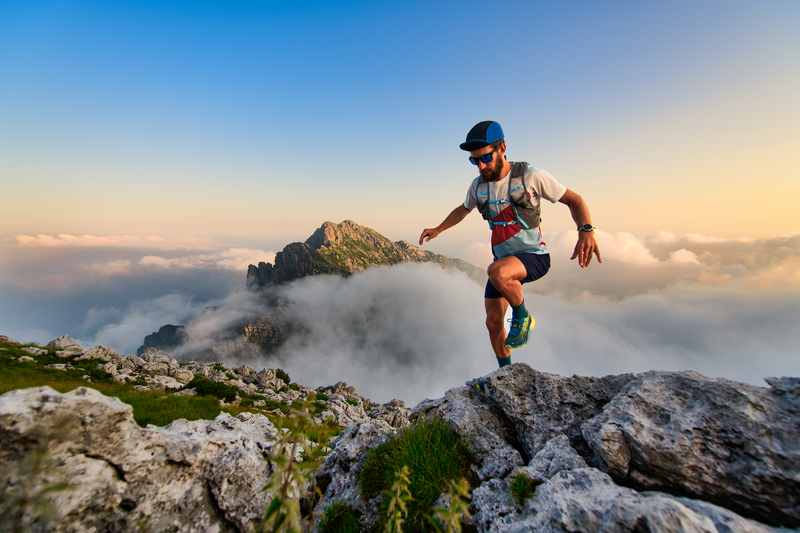
95% of researchers rate our articles as excellent or good
Learn more about the work of our research integrity team to safeguard the quality of each article we publish.
Find out more
MINI REVIEW article
Front. Bioeng. Biotechnol. , 02 March 2023
Sec. Biomaterials
Volume 11 - 2023 | https://doi.org/10.3389/fbioe.2023.1127757
Natural polymers have been widely used in scaffolds for tissue engineering due to their superior biocompatibility, biodegradability, and low cytotoxicity compared to synthetic polymers. Despite these advantages, there remain drawbacks such as unsatisfying mechanical properties or low processability, which hinder natural tissue substitution. Several non-covalent or covalent crosslinking methods induced by chemicals, temperatures, pH, or light sources have been suggested to overcome these limitations. Among them, light-assisted crosslinking has been considered as a promising strategy for fabricating microstructures of scaffolds. This is due to the merits of non-invasiveness, relatively high crosslinking efficiency via light penetration, and easily controllable parameters, including light intensity or exposure time. This review focuses on photo-reactive moieties and their reaction mechanisms, which are widely exploited along with natural polymer and its tissue engineering applications.
Artificial scaffold has been engineered to mimic the spatial dimension of the extracellular matrix (ECM). This is because the 3D network affects the diffusion and release kinetics of biomolecules as well as the mechanical modulus and rheological properties of the whole scaffold (Ko et al., 2010; Pei et al., 2019). The macro/microstructure of the scaffold can be tailored by chemical or physical crosslinking with a suitable choice of reactive moieties (Mihaila et al., 2012; Marizza et al., 2016).
While the physical intramolecular interactions involve weak non-covalent interactions, the chemical crosslinking is not transient due to covalent interactions (Marizza et al., 2016; Samani et al., 2020). In most cases, the stimulus of chemical crosslinking is a chemical agent or light source. Remarkably, light-initiated photocrosslinking has attracted attention due to its easily controllable parameters such as light intensity, exposure time, or irradiation distance (Chandrasekharan et al., 2019; Choi and Cha, 2019; Yano et al., 2020). Most of all, the non-invasiveness and capability of in situ photopolymerizations represent the unique and powerful merits of light-initiated photocrosslinking.
The polymerization between the functional groups of polymer chains activated by light exposure with or without photoinitiators is called photocrosslinking (Smeds et al., 2001). During this process, highly reactive free radicals are generated by photocleavage. The photoinitiators absorb light photons and convert light energy into chemical energy (Maiz-Fernández et al., 2022). The free radicals are covalently crosslinked with intra- or extra-molecular groups. Tyrosine/tyramine and methacryloyl are the most widely used photo-responsive moieties of biomaterials, followed by cinnamoyl, benzophenone, norbornene, aryl azide, and diazirine.
Photo-reactions triggered by functional groups activation facilitate bulk polymer crosslinking, photo-immobilization, surface modification, molecular labeling, or particle fabrication (Fernández and Orozco, 2021). These are applicable to both two-dimensional and three-dimensional structures (spatially addressable effects) with high selectivity and efficiency without producing toxic or reactive side products (Kim et al., 2017). It is important to select the appropriate photo-reactive moiety of natural polymer derivatives which meet the characteristics of the purposed applications in tissue engineering.
As well known, numerous reviews have covered the crosslinked natural polymers utilized in biomedical applications. In this review, photo-reactive or photo-responsive moieties and their reaction mechanisms were covered, which are widely exploited along with natural polymer and its tissue engineering applications. The characteristics of reactions, the chemical substitution of functional groups on target biopolymers, and types of photoinitiators depending on the light source for each moiety are discussed. Additionally, recent studies on each photopolymerization technique are introduced to enhance readers’ understanding.
Tyrosine is a reactive amino acid with a phenolic ring that can easily be transformed into hydrogels with or without chemical reagents via phenolic oxidation (Sogawa et al., 2020; Liu et al., 2021). The phenolic functional group of tyrosine contributes to the π-π interaction in terms of structural stability and the proton-coupled electron transfer reactions in energy transportation. The phenolic side chain allows crucial biosynthesis in nature systems by forming dityrosine crosslinks via electron transfer (Raven et al., 1971; Partlow et al., 2016; Lee et al., 2019). The tyrosine-tyrosine chemical bonds provide elasticity to biomaterials, as seen in diverse organisms. Examples of elastic biomaterials are the wing tendon of dragonflies (the protein named resilin), the cuticles of locus, and the silk fibroin of silkworms (Partlow et al., 2016). The covalent bonds within dityrosine can be emulated in vitro via enzymes or photo-based radical reactions to attain the physical and functional properties of polymeric biomaterials (Partlow et al., 2016).
These phenolic crosslinking systems are not only possible in tyrosine rich-natural proteins or peptides, but also in tyramine-modified materials including, polysaccharides (alginate, hyaluronic acid, dextran, etc.) or synthetic polymers (poly (ethylene glycol), poly (vinyl alcohol), etc.) (Roberts et al., 2016). As mentioned previously, the in vitro dityrosine crosslink has been employed to increase the mechanical properties of biomaterials containing silk fibroin (5.3% tyrosine) (Gong et al., 2020; Mu et al., 2020; Huang Y. et al., 2022), keratin (22% tyrosine) (Gillespie, 1972; Sando et al., 2010; Navarro et al., 2018), fibrinogen (5.6% tyrosine in γ-chain; 4.9% tyrosine in β-chain; .65% tyrosine in α-chain) (Elvin et al., 2009), marine-derived silk (aneroin, 5% tyrosine) (Park et al., 2019), and recombinant resilin (rec1-resilin, 6% tyrosine) (Truong et al., 2011). In order to increase the mechanical durability of polysaccharides (alginate, hyaluronic acid, etc.), the phenolic groups have been introduced through tyramine conjugation, synthesized by the EDC/NHS [N-(3-dimethylaminopropyl)-N′-ethylcarbodiimide hydrochloride/N-hydroxysuccinimide] chemistry (Schulz et al., 2019; Wang et al., 2020; Kim E. et al., 2021). The crosslinking density is also adjustable with diverse photoinitiator concentrations and photo-irradiation intensities (distance- and time-dependent).
Among the diverse dityrosine crosslinking triggers, photo-based crosslinking has significant advantages in terms of non-invasiveness, fast reaction rate, fine tunability, and crosslinking efficiency (Choi and Cha, 2019; Mu et al., 2020; Perin et al., 2022). The light source can be ultraviolet (UV) or visible light. UV irradiation generates radical cations (Tyr-OH·+) and solvated electrons to form tyrosyl radicals. These radicals induce continuous polymerization between tyrosine-tyrosine residues via deprotonation, radical isomerization, diradical production, and enolization (Figure 1A) (Houée-Lévin et al., 2015; Min et al., 2016). This one-step UV-mediated dityrosine crosslink is a covalent self-assembly system within short peptides and does not require additives (Min et al., 2016). Dityrosine units in tyrosine-rich peptides or other biomaterials function as not only stable assembly motifs but also multifunctional templates which allows self-assembled structure of organic/inorganic hybrid biomaterials to feature its chemical, electrochemical and structural properties triggered by the proton-coupled electron-transfer reactions (Lee et al., 2019). However, UV irradiation also regulates the antibody binding capability of peptide hormones, and in vitro hormonal function which could be exploited in pharmaceutical industry to estimate hormone’s structure and bioactivity (Correia et al., 2012). For example, the continuous UV excitation induced structural changes by forming tyrosine photo-product dityrosine, leading to covalent insulin dimerization and decreased antibody binding affinity up to 62.1% when irradiated 276 nm for 3.5 h (Correia et al., 2012).
FIGURE 1. Overview of tyrosyl residue crosslinking mechanisms initiated by (A) UV light (Correia et al., 2012), (B) Ru (Ⅱ) polypyridine (Fancy and Kodadek, 1999), and (C) riboflavin (Liu et al., 2021).
As for the visible light system, ruthenium (Ⅱ) polypyridine [Ru (Ⅱ) polypyridine] and persulfate are essentially needed. Ru (Ⅱ) polypyridine generates electrons under visible light. The generated electrons are transferred to the persulfate acceptor. At the same time, tyrosine radicals are oxidated and crosslinked into dityrosine (Figure 1B) (Fancy and Kodadek, 1999). Here, the ruthenium complexes have higher excitation selectivity, a relatively longer excited state period, and higher chemical stability than other photoinitiators (Kim H. et al., 2021). Thus, many previous works of literature on silk fibroin, collagen, gelatin, and recombinant resilin have exploited ruthenium-mediated photo-crosslinking (Liu et al., 2021). In one study, the tyramine-modified gelatin methacrylate (GelMA-Tyr) hydrogels were photo-irradiated by visible light with Ru/SPS initiators for cartilage-binding glue (Lim et al., 2020a). Its adhesion strength (13.25 kPa) was 15 times higher than that of UV(365 nm)-crosslinked GelMA/Irgacure 2959 hydrogels because of the number of residues involved in photo-crosslinks (Lim et al., 2020a).
Under UV and visible light, riboflavin (vitamin B2) and its derivatives activate photo-catalysis by changing its state into single- or triplet-excited riboflavin (Huang et al., 2004). The single riboflavin generates oxygen intermediates and oxidizes tyrosine (Daood et al., 2013). Equivalently, the triplet riboflavin produces tyrosyl radicals, which form dityrosine linkages (Figure 1C) (Liu et al., 2021). In the presence of riboflavin, the silk fibroin gel produced by the photolithography can achieve ∼50 μm resolution due to fast photo-reactivity. The sophisticated fabrication with non-toxic photoinitiators and its own transparency has allowed elastic fibroin gel to be applied to ocular prostheses (Applegate et al., 2016).
For synergetic enhancement in mechanical properties and complexity of scaffolds, dual crosslinking is another strategy. In one research, the photo-crosslinked alginate-tyramine microfibers were further crosslinked ionically (Lim et al., 2021). The photo-crosslinking resulted in fast gelation within a few seconds, allowing the polysaccharide inks to be stacked layer by layer. The following ionic crosslinking was also beneficial to structural integrity (Park et al., 2019). Thermal and light-induced crosslinking method was used to fabricate 3D cell-laden scaffolds with decellularized extracellular matrix (dECM)-based bioinks at the centimeter scale with high printability where the minimal strand width was 100 μm (Kim H. et al., 2021). This dual-crosslinked product not only achieved 3.79 and 20.04-folds increases in elastic and resilient modulus respectively compared to of which the only thermo-physically crosslinked dECM products, but also have the ability of working as a functional tissue (Kim H. et al., 2021).
Overall, the dityrosine photo-crosslinking allows fabricating tissue mimicking polymeric materials due to its rapid gelation with improved homogeneity of network and superior mechanical integrity which meets the sufficient range of targeted elastic moduli (Camp et al., 2020; Liu et al., 2021). Besides, distinctive properties of UV-excited dityrosine crosslinking facilitate to regulate of the peptide hormones’ bioactivity and act as a novel bioreactor or probe for fluorescence detection of functional nanomaterials by UV-excited inherent blue fluorescence which is exploited in medical and pharmaceutical engineering (Correia et al., 2012; Min et al., 2018; Mukherjee et al., 2019).
Methacrylates and their derivatives have been extensively manipulated for light-activated crosslinking (Reis et al., 2009; Mu et al., 2020). Under UV conditions, the methacryloyl side chain generates free radicals, which rapidly crosslink each other. Methacrylate networks are not sensitive to the surrounding environmental conditions such as pH and temperature allowing good stability (Liu et al., 2022b). The degree of crosslinking is dependent on the number of methacryloyl substitutions (Kim S. H. et al., 2021).
Unfortunately, methacryloyl is not an intrinsic moiety of natural polymers, however, diverse methacrylating agents allow modify the chemical structures of the wide range of biomolecules and biopolymers including proteins, polysaccharides, synthetic polymers, as well as nanoparticles with high substitution yields depend on their functional groups (Samani et al., 2020). Methacrylates substitute the specific reactive residues of amine (-NH2), carboxyl (-COOH), and hydroxyl (-OH) groups, which are adjusted based on the reaction temperature, pH, and agitation speed (Chou and Nicoll, 2009; Yue et al., 2017). Therefore, the final product methacryloyl-modified biomaterials should be characterized by production conditions to confirm reproducibility regarding purity, methacrylation degree, and physiochemical properties as commercial utilization (Hasany et al., 2021).
Methacrylic anhydride (MA) and glycidyl methacrylate (GMA) are commonly used reagents to introduce methacryloyl groups. Modification with MA is the most cost-effective and straightforward method for methacryloyl incorporation (Hasany et al., 2021). MA has a carbon-carbon double bond which readily undergoes free-radical polymerization and esterification with all three functional groups (-NH2, -COOH, and–OH) (Figure 2A). Therefore, it is widely utilized in various biopolymers, including silk, gelatin, hyaluronic acid, chitosan, alginate, starch, and pectin (Messager et al., 2013; Pereira et al., 2018b; Han et al., 2020; Noè et al., 2020; He et al., 2021). In some cases, GMA utilization is more plausible due to the problematic assessability of MA in the target material, such as silk, which has high crystallinity in acidic conditions (Mu et al., 2020). GMA is capable of epoxide ring-opening that facilitates methacrylate modification, even in acidic conditions. Additionally, the epoxide ring-opening reaction is processed predominantly over transesterification in acidic conditions (Li et al., 2003, 2017; Hasany et al., 2021). In addition to MA and GMA, AEMA (2-aminoethyl methacrylate) activates the carboxyl groups (-COOH) in hyaluronic acid or alginate under EDC/HNS reaction (Jeon et al., 2009).
FIGURE 2. (A) Diverse methacryloyl substitution in methacrylic alginate induced by methacrylic anhydride, glycidyl methacrylate, and 2-aminoethyl methacrylate (Hasany et al., 2021), and (B) light-activated chain polymerization that occurred between two methacryloyl moieties (red: photo-reactive residues) (Bupphathong et al., 2022).
The methacryloyl-initiated photocrosslinking is activated by diverse photoinitiators (Figure 3). Irgacure 2959 (2-hydroxy-1-[4-(2-hydroxyethoxy) phenyl]-2-methyl-1-propanone) and LAP (lithium acylphosphinate salt) generate free radicals under UV (365 and 405 nm, respectively) light, and simultaneously participate in chain polymerization, which occurs between two methacryloyl moieties (Figure 2B) (Basara et al., 2019). Tris (2,20-bipyridyl) dichlororuthenium (II) [Ru(II)] and eosin Y also induce photo-activated crosslinking under visible light, where their ranges are 420–450 and 450–550 nm, respectively (Noshadi et al., 2017; Lim et al., 2020a).
FIGURE 3. Common photoinitiators: (A) Irgacure 2959, (B) lithium phenyl-2,4,6-trimethylbenzoylphosphinate, (C) ruthenium (Ⅱ)/persulfate, (D) eosin Y, and (E) riboflavin (Mu et al., 2020).
Gelatin-methacrylate (GelMA) is the first methacryloyl-modified form developed using GMA in the 1990s; since then, there have been many different methacryloyl-modified biomaterials, such as silk fibroin-methacrylate (SilMA), methacrylate hyaluronic acid (HAMA), methacrylate pectin (PECMA), and methacrylate carboxymethyl cellulose (CMCMA) (Choi and Cha, 2019).
Photocrosslinkable sites of PECMA based bioink enabled to print chemically defined single-component 3D scaffolds with tunable mechanical strength (Pereira et al., 2018b). With increase in ink concentration from 1.5 wt% to 2.5 wt%, elastic modulus of hydrogel was adjusted from 79.6 Pa to 2,600 kPa under UV irradiation for 160 s (Pereira et al., 2018b). Moreover, extended UV exposure time from 160 s to 300 s, 3.2-fold increased stiffness modulus gels could be obtained in low polymer concentration (1.5 wt%) (Pereira et al., 2018b). In one study, methacryloyl-substituted tropoelastin (MeTro) and GelMA blend were photo-cured under UV (320–390 nm) light for 0.23 s per μm of thickness (Soucy et al., 2018). It showed 15 times higher adhesive strength than fibrin-based hydrogel with 85% encapsulated viable Schwann cells for 5 days (Soucy et al., 2018). It is expected to be used as a rapid tissue adhesive. In another research, HAMA/CMCMA hydrogel showed a much faster crosslink time of 0.018 s per μm thickness, under 400 nm visible light (Huang Y. C. et al., 2022). Based on its durable compressive elastic modulus (0.82 MPa), it is expected to be employed as an anti-adhesion barrier for post-operative measures (Huang Y. C. et al., 2022). By filling in the epidural defect space, the HAMA/CMCMA based scaffolds hinders the attachment and migration of 3T3 fibroblasts (Huang Y. C. et al., 2022). Thus, the fast polymerization time of methacrylate-based polymers has allowed them to be utilized as instantly and urgently needed biomaterials.
Especially, the intimate polymeric network between different types of biomaterials is the distinctive advantage of methacryloyl-mediated cross-linking. UV-induced dual crosslinked hydrogel consisting of methacryloyl-substituted Bletilla Striata polysaccharide and gelatin has suitable pore size (85.20 ± 4.99 µm), porosity (72.13% ± 2.15%) and significantly enhanced compression modulus (62.93 ± 8.24 kPa) compared to that of the single network which showed effective wound closure ability for diabetic wound treatment due to the tightly dual crosslinked network structure (Liu et al., 2022a).
Likewise, a wide range of spatially cross-linkable methacryloyl facilitates the fabrication of complex micro- or macro-structures as tissue engineering scaffolds using photo-patterning, bioprinting, and microfluidics (Hasany et al., 2021). Although both the biocompatibility and cytotoxicity of each methacryloyl-modified biomaterial should be identified for clinical applications, methacrylate crosslinked polymer is an ideal candidate for various tissue implants from the brain to bone now that it represents a wide range of elastic moduli (from mPa to GPa) (Chandrasekharan et al., 2019; Kono et al., 2020).
Diazirine and benzophenone are occurred photocrosslinking related with redox and generate intermediates during the reaction process (Wu and Kohler, 2019). The intermediates, which are comprised of covalent bond have greatly reactive crosslinking activity and can interaction with various ligand of proteins or residue of polymers (Deseke et al., 1998).
Specifically, the diazirine has aromatic azides structure (Kuboe et al., 2010). It comprises one carbon and two nitrogen atoms connected with a double bond, forming a cyclopropene-like ring (Zhou et al., 2020). The carbene in diazirine rapidly binds with other biomolecules through C-H, O-H, and N-H bonds under UV (350–380 nm) irradiation (Hill and Robertson, 2018; Musolino et al., 2021). Specifically, when the UV light is irradiated to diazirine, N2 gas is extruded and forms singlet carbene (Dey et al., 2021). The singlet carbene combines with nearby biomolecules through covalent, C-H, and heteroatom-H bonds, thus forming isomerized carbene (Figure 4A) (Murale et al., 2017; West et al., 2021; Yu and Baskin, 2022).
FIGURE 4. The protein polymerization mechanism through photocrosslinking of (A) diazirine and (B) benzophenone.
This reactivity is not constrained to light only, but it also occurs due to heat and electricity (Álvarez-Hernández et al., 2018; Lepage et al., 2019). Although the diazirine has high thermal and chemical stability, the process of photocrosslinking reaction is more complex than benzophenone (Ge et al., 2018; Jakubovska et al., 2018). However, the carbene, which is intermediate material during reaction, is not uneconomical because carbene can be utilized for crosslinking (Temel et al., 2011). The carbene and diazirinyl radicals have been exploited for bulk polymer crosslinking, molecular labeling, and target identification and dazirine has been extensively exploited for surface modification of biomaterials (Ye et al., 2018).
The diazirine is applied to the conjugation and reinforces physical strength. In the study related to the conjugation, the diazirine conjugated GFOGER peptide showed enhanced cell (HT1080) adhesion and spreading when mixed with film (Malcor and Mallein-Gerin, 2022). And the diazirine conjugated elastic-like protein is formed a carbene intermediate and can be inserted rapidly into the located close conjugated protein releasing N2 (Raphel et al., 2012). The conjugated protein is made into a film that has 50 μm thickness, is sturdy enough to withstand a weight of 4.5 g, and can maintain the cell metabolism system (6 days) (Raphel et al., 2012). The rapid (60 s) gelation of diazirine led to an oral adhesive application that involved the chemical curing of bromo-diazirine to form carbene of bacterial cellulose (Singh et al., 2021). The rapid gelation (within 60 s) promoted low flowability with up to 35 kPa adhesiveness in wet conditions, which is suitable for mucoadhesive drug delivery (Singh et al., 2021).
Benzophenone, which consists of the carbonyl group and two benzene groups, forms two radicals when activated by ultraviolet (350–365 nm) light (Anderson and Castle, 2003; Neumann et al., 2013; Fu and Chang, 2019; Sharma et al., 2021; Xue et al., 2021). Benzophenone shapes triplet-state ketone and forms C-C bonding (Liu et al., 2020; Olson et al., 2020). Then, the formed radicals react with the neighboring C-H bond, extract protons of the carbon chain, and create methyl-radical at the surface (Neumann et al., 2013). The formed radical reacts with hydrogen bonds such as carbon, nitrogen, or oxygen (Figure 4B) (Yagci et al., 2010; Lago et al., 2015; Fu and Chang, 2019). The triplet-state, which is formed during the reactive process, is related to electron donation and diradical (Porter and Suppan, 1965). Thus, various solutions are involved in different contents of atoms and it can regulate bonding by using different solutions (Christensen et al., 2012). It unnecessary co-initiator because they performed photosensitizer and hydrogen donor (Temel et al., 2011). Additionally, its non-polar property allows benzophenone to be stable underwater (Ge et al., 2018). The tolerance for oxygen and water promotes its applicability in printing and coating materials (e.g., sunglasses coating and plastic colorings) (Keskin et al., 2018; Wang et al., 2018).
Benzophenone is a reinforcement or UV-supported agent (Yuk et al., 2016). The photo-crosslinking has been employed with chitosan/polyethylene oxide (PEO) fibers to transform PEO into a water-insoluble material (Kianfar et al., 2019). As a result, this enhances the solubility resistance (water or organic solvents) and thermal stability (Wang et al., 2018). The benzophenone introduction also helps the carboxyl group of the cellulose nanofibrils (CNF) to increase the tensile strength of the CNF gel, even in wet conditions (Orelma et al., 2016). Experimentally, the cured CNF gel (138 MPa cm3 g-1) showed high tensile strength compared with non-cured CNF gel (109 MPa cm3 g-1) (Orelma et al., 2016). Furthermore, the hydrogel-elastomer hybrid (polyacrylamide (PAAm)-alginate, PAAm-hyaluronan, PAAm-chitosan, polyethylene glycol diacrylate (PEGDA)-alginate and PEGDA-hyaluronan) can have minute structure and interface toughness increase due to benzophenone (200 to 900 Jm-2) (Yuk et al., 2016). Collagen-GAG biomaterial platform can regulate the mechanical properties independently by curing with benzophenone and identify metabolizing activity, proliferation, and gene expression of the adipose-derived mesenchymal stem cells (ASCs) based on mechanical properties (Banks et al., 2014). Also, collagen treated modified benzophenone (benzophenone dimer) can make sub-micrometer or micrometer scaffold through multiphoton excited photochemistry (Basu et al., 2005).
Owing to benzophenone and diazirine using a UV light for crosslinking, the cells are significantly less negatively affected corresponding UV range (350–380 nm) (Anderson and Castle, 2003). So, they are not caused the harmful effect to cells and not damage biological (Chou et al., 2011; Hill and Robertson, 2018).
The carboxy group deprotonation of cinnamic acid generates UV-sensitive cinnamate groups (Shi et al., 2009; Bobula et al., 2015; Zhang et al., 2020; Gadek et al., 2021). Those cinnamates are photo-dimerized through (2 + 2) photocycloaddition (Figure 5A). The photocrosslinking of cinnamate has a unique characteristic of reversible reactions. Photo-dimerization is dominant longer than 260 nm, whereas photocleavage happens less than 260 nm (Nakayama and Matsuda, 1992). It means that the formation and division of the photo-crosslinking can be adjusted by the wavelength range. Using this, latex-based sizing nanoparticles react reversibly to wavelengths, suggesting the emergence of new carriers in environmental and biomedical fields (Shi et al., 2008). It also manufactured a hydrogel film treated with cinnamoyl on the surface to prove its ability to heal itself using photoreaction (Froimowicz et al., 2011). In addition, the self-healing ability of bio-based lignin and glycerol-derived monomers with cinnamate groups was optimized to help design a new photocrosslinking-based self-healing product that responds environmentally friendly and reversibly (Sinha Roy et al., 2021).
FIGURE 5. Brief mechanism of (A) cyclobutane and (B) (2 + 2) photocycloaddition (Gupta et al., 2004; Sarkar et al., 2020).
In addition, cinnamate forms (2 + 2) cyclobutane and is attracting great attention because it can perform photo-crosslinking without a photoinitiator (Bobula et al., 2015; Schelkle et al., 2016). The irritation induces two olefins of the alkenyl group in the cinnamate and forms a cyclobutene capable of photo-crosslink (Gupta et al., 2004). During the cycloaddition process, enone is bonded with olefin. At this time, the negative end of the alkene group dipole with olefin and the β carbon of enone are combined (Figure 5B) (Corey et al., 1964). The β-alkenyl-substituted enone has a steric hindrance that determines the region and stereoselectivity of the polymer (Sarkar et al., 2020).
Since the olefin structure is present in the side chain, photo-crosslinking of the cinnamate is limited to the side chain polymer (Schelkle et al., 2016). However, (2 + 2) photo-reduction addition reactions are considered an efficient synthesis method because the molecular binding is fast and predictable (Hoffmann, 2008; Sanyal, 2010; Cardenas-Daw et al., 2012), especially because they occur easily in cinnamoyl (Rennert et al., 1967; Schelkle et al., 2016).
Using a series of advantages, cinnamic acid is often used in various ways for natural polymer synthesis. It is also welcomed in the photo-activated polymer (Balaji et al., 2003) and patterned polymer (Kim et al., 2009) areas because it exhibits stable reactivity without a photoinitiator and photosensitizer (Fertier et al., 2013).
Hyaluronan and trans-cinnamic acid were employed as photocurable derivatives to generate a water-insoluble microfiber (Bobula et al., 2015). The anhydride of cinnamic acid reacted with hyaluronan to be acylated (Bobula et al., 2015). As a similar manner, gellan gum films are negatively charged, enabling long-term electrostatic repulsion of bacteria (Lee et al., 2012). In addition to these, photocrosslinking was carried out with chitosan (Wu et al., 2007) and starch (Zhang et al., 2020), and physical or mechanical properties were improved to alleviate low durability problems. Octanoyl chitosan cinnamate synthesized using a regioselective variant of chitosan have been shown to form a stable monolayer by dispersing at the interface between air and water (Wu et al., 2007). The backbone of OCC maintained chirality in the film and facilitated optical characterization (Wu et al., 2007). Cinnamic acid-modified starch (CA-St) was used for the nanoprecipitation and photo-crosslinking of the colloidal particles to fabricate colloidal particles (CPs) (Zhang et al., 2020). The cycloaddition of cinnamic acid-modified starch CPs alleviates low durability problems in intravenous administration or drug loading/release (Zhang et al., 2020). Moreover, the hydrophobic moieties allow the starch to deliver relatively hydrophobic drug molecules (Zhang et al., 2020). Cinnamated-collagen made using EDC/NHS conjugating decreased storage modulus as the cinnamate content increased (Dong et al., 2005). Scaffold was produced using hydrogels using gelatin one-pot synthesis, which can be used appropriately for biomaterial applications because various trigger reactions exist and are not cytotoxic to fibroblasts (Gattás-Asfura et al., 2005).
Also, it was confirmed that the physical properties were improved by completing crosslinking within 60 min in both hydrated gel and dry film, and the potential applicability of collagen-based materials in drug delivery and tissue engineering was improved (Dong et al., 2005).
Norbornene is a cyclic alkene mainly used as a monomer and an intermediate for organic synthesis and is a particularly reactive ene compared to alkene due to the deformation of the inherent ring (Blasco et al., 2017). Norbornene moiety reacts with thiolated agents and forms crosslinkage, under UV light. Thiols react with various ene functional groups such as alkene, vinyl ether, and acrylate. Among them, however, norbornene moiety is commercially valuable because it is most reactive and progresses quickly (Hoyle and Bowman, 2010; Blasco et al., 2017; Nguyen et al., 2021). Norbornene is incorporated into natural polymers through amide reactions (Devaraj et al., 2008). Natural polymers incorporated with norbornene exhibit low solubility under acidic aqueous solvent conditions (Mũnoz et al., 2014; Alves et al., 2022). This is because the overall hydrophobicity was increased by the norbornene moiety, and the positive elective charge was reduced due to the conversion of the amine into the amide (Michel et al., 2019). In general, norbornene is incorporated into a natural polymer in the presence of carboxy anhydride (Devaraj et al., 2008). It has the advantage of improving the solubility of the natural polymer in the aqueous medium than before and is very stable in vivo (Devaraj et al., 2008; Michel et al., 2019). The mechanism of this thiol-norbornene photo-crosslinking reaction is initiated by a type I photoinitiator (typically LAP or Irgacure 2959), which is photo-decomposed into radicals by UV (UV-A, 320–400 nm) light (Figure 6A) (Hoorick et al., 2020). The decomposed photo-initiator radicals extract hydrogen atoms from the thiol of the thiol-containing molecule (R1-SH) and produce thiyl radicals (Lin et al., 2015). The produced thiyl radical crosses the norbornene carbon-carbon double bond of the norbornene-functionalized macromer (R2-norbornene), subsequently producing norbornene radical (Lin et al., 2015). Norbornene radical extracts hydrogen atoms from thiol complete thioether bonds, and regenerates thiyl radical (Figure 6B) (Cramer et al., 2003; Lin et al., 2015). This photo-crosslinking reaction proceeds at a stoichiometric rate until thiol or norbornene is depleted and is crosslinked step by step (Lin et al., 2015). Norbornene’s high reactivity to thiol radical and low reactivity to norbornene radical alleviates the non-specific photo-crosslinking (Gramlich et al., 2013).
FIGURE 6. (A) LAP decomposition by UV and (B) thiol–norbornene photo-click reaction step with a thiol–containing molecule (R1–SH) (Lin et al., 2015).
In rare cases, thiol-norbornene photo-crosslinking by visible light can occur, similar to the mechanism of UV-based photo-crosslinking systems, except that type II photoinitiator (non-cleavage-type) (e.g., eosin-Y, rose bengal) is used (Lin et al., 2015). Thiol-norbornene has been utilized as a photo-crosslinking moiety of natural biopolymer materials, including chitosan, gelatin, collagen, and pectin, especially for tissue engineering applications (Mũnoz et al., 2014; Pereira et al., 2018a; Michel et al., 2019; Guo et al., 2021).
As for chitosan, the norbornene moiety is acquired by amide bond formation, which is initiated by the reaction between chitosan and carbic anhydride [norbornene-derived chitosan (CS-nbn-COOH)] (Michel et al., 2019, 2020). In one research, thiolated diethylene glycol (HS-DEG-SH) was used as a crosslinker and Irgacure 2959 was utilized as a photoinitiator [UV-A (320–400 nm)] (Michel et al., 2019). The UV-A exposure time was between 20 s and 30 min (Michel et al., 2019). Similar procedures are applied to other natural polymers. For instance, in another study, the primary amine of gelatin reacted with carbic anhydride at 50°C for 2 h to form norbornene through amide bonding (Mũnoz et al., 2014). LAP was used as a photoinitiator and dithiothreitol (DTT) was added as a crosslinker (Guo et al., 2021). Pectin required VA-086 as a photoinitiator and dimethyl sulfoxide (DMSO) as a crosslinker in a different study (Pereira et al., 2018a). The manufactured hydrogel is a scaffold in tissue engineering and plays a role in skin wound healing, 3D printing, and tissue environment formation (Sun et al., 2011). Methacrylated collagen and GelMA hydrogel formation with random chain growth photopolymerization are unstable and yield high concentrations of initial radicals (Brinkman et al., 2003; Gaudet and Shreiber, 2012; Guo et al., 2021). Therefore, it is not ideal for cell-containing hydrogel formation and is rarely used in bioprinting (Lin et al., 2011; Mũnoz et al., 2014; Guo et al., 2021). Hydrogels formed from thiol-norbornene are suitable for cell-containing hydrogel formation in this respect because they can be photo-crosslinked at low radical concentrations with very fast reactions and induce cell proliferation (Guo et al., 2021). In particular, NorCol (norbornene-functionalized collage)/gelatin bio-ink showed good printability by controlling light and temperature at once, and cells showed great potential by showing excellent viability within bio-printed hydrogels (Guo et al., 2021). In addition, it is important to establish a pertinent in vitro culture system for liver cells to understand the mechanism of liver disease progression or recovery (Lau et al., 2012; Greene and Lin, 2015). At this time, norbornene-functionalized gelatin (GelNB) hydrogels can be used as a three-dimensional scaffold capable of designing parts of the cell-extracellular matrix (ECM) interactions important for cell survival and function (Lau et al., 2011; McCall and Anseth, 2012; Greene and Lin, 2015).
Aryl azide is a moiety containing azide groups (-N3) as a functional group or substituent derived from aromatic hydrocarbons. Aryl azide is mainly made by replacing diazonium salts or aryl halide with sodium azide (Liu and Tor, 2003). When exposed to light sources, aryl azide releases nitrogen molecules to produce electron-deficient nitrene that can be inserted into the C-H bond (Ono et al., 2000; Liu and Tor, 2003). It facilitates covalent bond formation between natural biopolymers (Zhu and Ma, 2004). The hydrogel is generated by UV irradiation, and the increase in its exposure time results in better mechanical properties and lower swelling ratio (Cho et al., 2016).
The mechanism of the aryl azide photo-crosslinking reaction starts with UV irradiation. The azide group (-N3) releases nitrogen molecules (N2) and is converted into highly reactive nitrene groups (Ono et al., 2000). Nitrene groups with two unshared electron pairs interact very quickly with the amino groups of the natural biopolymer to be bonded to form azo groups (-N=N-) and become crosslinked (Figure 7A) (Ono et al., 2000). Through this photo-crosslinking process, gelation is completed. If the proper reaction site is not nearby, nitrene is rearranged into the more stable ketenimine and has a disadvantage in that crosslinking efficiency is lost (Tanaka et al., 2008). The maximum absorption wavelength of azide is 250 nm, but the absorption amount is increased through substitution with aryl azide; thus, a wavelength of over 400 nm can be used (Ono et al., 2000; Tanaka et al., 2008). This wide-range reactivity allows the aryl azide compound to react with various biomolecules (Tanaka et al., 2008).
FIGURE 7. (A) Aryl azide moiety is converted into highly reactive nitrene by releasing N₂ under UV light (Tanaka et al., 2008) and (B) azide-chitosan-lactose (Az-CH-LA) obtained by photo-crosslinking (Az-CH) and dehydration condensation reaction (CH-LA) (Ono et al., 2001; Tanaka et al., 2008).
In one study, this crosslinking method was employed with an azide (p-azidebenzoic acid) conjugated chitosan (Ono et al., 2000). The rapid gelation [60 s, under UV (254 nm) light and Irgacure 2959] and dense network retarded the encapsulated drug release (Figure 7B) (Ono et al., 2000; Sydow et al., 2019). In tissue engineering, these photochemical biopolymers are used as scaffolds for potential drug and cell delivery to tissues and biological activity is enhanced by photo-crosslinking (Sydow et al., 2019).
The properties of photo-crosslinking can be used to detect protein-protein interaction (PPI) in living cells using the aryl azide moiety as a probe (Baruah et al., 2008; Pham et al., 2013; Mishra et al., 2020). These aryl azide ligases are incorporated into proteins and when irradiated with UV (300–360 nm), produce reactive species that interact with other proteins nearby, forming covalent bonds (Baruah et al., 2008; Mishra et al., 2020). It has advantages over other PPI detection methods in that it can detect endogenous protein interactions (Baruah et al., 2008; Mishra et al., 2020).
In addition, medical tissue adhesives use reactive groups to produce shared crosslinking, which includes aryl azide that exhibit spontaneous and highly reactive crosslinking as reactive groups (Ono et al., 2000; Nam and Mooney, 2021). Therefore, aryl azide photo-crosslinking hydrogel using UV is mainly used as a tissue adhesive with cell compatibility (Rickett et al., 2011; Nam and Mooney, 2021).
Triethanolamine (TEA) is a sacrificial electron donor involved in the photopolymerization reaction of eosin (Popielarz and Vogt, 2008; Wong et al., 2015). The red-colored eosin is a photocatalyst (photoinitiator) and generates radicals at a reactive moiety of natural polymers such as cellulose, gelatin, and chitosan (Lim et al., 2008; Haria and König, 2014). Photocrosslinking using radicals has the high stability of a shared crosslinked network and gelates the surroundings quickly (Shih and Lin, 2013).
The TEA transfers electrons to excited eosin, producing anionic and triethanolamine cation radicals once the eosin (eosin Y) is excited in a triplet state under visible light (400 nm < θ < 700 nm) (Noshadi et al., 2017). Subsequently, it leads to rapid proton loss from the triethanolamine radical cation (TEA·+) to neutral R-amino radical (TEA·) (Noshadi et al., 2017). The protons are then transferred to eosin anionic radicals to produce neutral eosin radicals (Figure 8) (Valdes-Aguilera et al., 1992; Noshadi et al., 2017). TEA radicals initiate polymerization with monomers with vinyl groups, such as poly (ethylene glycol) diacrylate (PEG-diacrylate) and vinylpyrrolidone (VP), and are also used to accelerate gelation of covalent monomers (e.g., N-vinylpyrrolidone or NVP) (Elbert and Hubbell, 2001; Popielarz and Vogt, 2008). A 3D -printed isomalt structure made of a carbohydrate glass material was coated through poly (ethylene glycol) diacrylate (PEGDA) and surface-initiated photopolymerization (Chen et al., 2020). Coating with Eosin/TEA photopolymerization had the advantage of isomalt miscibility and stability at high temperatures and showed the potential to create physiologically related tissues by facilitating the construction of biomimetic vascular structures in various hydrogels (Chen et al., 2020).
FIGURE 8. The mechanism of (A) eosin-based photopolymerization and its (B) hydrogel formation (Noshadi et al., 2017).
The Eosin Y-TEA reaction is co-initiator and co-monomers dependent, and the use of high TEA concentrations can cause undesirable cytotoxic effects on some sensitive cell types, so caution should be taken (Hao et al., 2014). Nevertheless, stability and safety are evaluated to prevail over the use of ultraviolet initiators because visible light initiators can reduce the risk to proteins and DNA (Avens et al., 2008). Gelatin-methacrylate (GelMA) bioink, whose viscosity was adjusted using silk fibroin particles, improved cell suspension and proved to be a biocompatible material through non-cytotoxic and high level of metabolic activity (Na et al., 2018). Since hydrogels cured under visible light showed stability for a long time (18 months) from exfoliation compared to ultraviolet (UV)-photocured hydrogels, visible light-induced photocrosslinking is promising to be applied to the 2D patterning of hydrogels (Kizilel et al., 2004). In PEGDA scaffolds consisting of 1-vinyl-2-pyrrolidone, we define non-toxic conditions for photoencapsulation of human mesenchymal stem cells, enhancing the viability of human mesenchymal stem cells and generating hydrogel scaffolds with tightly bridged networks (Bahney et al., 2011). Through this, it can be confirmed that it is desirable to apply it to biological applications such as cell encapsulation (Avens et al., 2008; Occhetta et al., 2015).
Due to these advantages, Eosin–coinitiator photocrosslinking has been used for various purposes such as encapsulation, drug testing, and biosensing in addition to bioink formulations for 3D bioprinters. In situ photocroslinkable hyaluronan, which encapsulated Chondrocytes, promoted the maintenance of cartilage phenotype and cartilage matrix synthesis, accumulated a significant amount of cartilage matrix, and was evaluated as a scaffold for repairing cartilage by accelerating healing in vivo osteochondral defects (Nettles et al., 2004). Photopolymerizable Dock-and-Lock hydrogel, which can be used as a scaffold to support fast self-assembled cells and drugs, can control moduli according to the duration of light exposure and can be used for various purposes depending on the unique physical properties of the gel (Lu et al., 2013) Visible ray-induced gelation of methacrylate materials (gelatin methacrylate (Gel-MA) and methacrylate alginate (Alg-MA)) showed high potential for surgical tissue sealing for in vivo systems (Charron et al., 2016; Kumar et al., 2022).
This review covered photo-reactive moieties and their mechanisms. Their application examples in tissue engineering were also dealt with. The whole part was classified into free-radical chain polymerization (tyrosine, tyramine, methacrylol, cinnamoyl, and eosin-based photopolymerization), thiol-ene photo-crosslinking (norbornene), and photo-mediated redox crosslinking (benzophenone, aryl azide, and diazirine) (Table 1) (Lim et al., 2020a; Lim et al., 2020b).
Since photo-crosslinking has unique and unparalleled merits in specific controllability and invasiveness, many moieties have been chemically incorporated into natural polymers to facilitate its photo-reactivity, as well as generate covalent networks. Especially, this photo-initiated crosslinking was welcomed by natural polymers due to their poor mechanical properties which deterred their wide applications (Sundaramurthi et al., 2014; Choi and Cha, 2019). Employing a variety of photocrosslinking moieties regulate not only mechanical, but also biological, and physicochemical properties with a wide range (Zhao et al., 2013).
The tissue matrixes that have variety mechanical properties through regulating the photocrosslinking affect cellular behavior like cell growth, differentiation, and proliferation (Camp et al., 2020). Thus, it can develop the scaffold that have variety mechanical properties and can biodegradable completely using union such as protein-protein binding, hydrogel-based polysaccharide, and gene binding. Moreover, photocrosslinking can applicate not only scaffolds that have various mechanical properties range such as bone, cartilage, tissue, and organ but also biocompatible drug delivery, biosensor, and bio-probes. Likewise, the photocrosslinking technique is promising technology for tissue engineering fields.
All authors listed have made a substantial, direct, and intellectual contribution to the work and approved it for publication.
The authors acknowledge the financial support from the basic science research program (NRF-2020R1C1C1006737) funded by the Ministry of Science and ICT, Korea; development of technology for biomaterialization of marine fisheries byproducts (KIMST-20220128) of Korea Institute of Marine Science & Technology Promotion (KIMST) funded by the Ministry of Oceans and Fisheries, Korea; and Inha University Research Grant.
The authors declare that the research was conducted in the absence of any commercial or financial relationships that could be construed as a potential conflict of interest.
All claims expressed in this article are solely those of the authors and do not necessarily represent those of their affiliated organizations, or those of the publisher, the editors and the reviewers. Any product that may be evaluated in this article, or claim that may be made by its manufacturer, is not guaranteed or endorsed by the publisher.
Álvarez-Hernández, M. H., Artés-Hernández, F., Ávalos-Belmontes, F., Castillo-Campohermoso, M. A., Contreras-Esquivel, J. C., Ventura-Sobrevilla, J. M., et al. (2018). Current scenario of adsorbent materials used in ethylene scavenging systems to extend fruit and vegetable postharvest life. Food Bioprocess Technol. 11, 511–525. doi:10.1007/s11947-018-2076-7
Alves, P. M., Pereira, R. F., Costa, B., Tassi, N., Teixeira, C., Leiro, V., et al. (2022). Thiol-norbornene photoclick chemistry for grafting antimicrobial peptides onto chitosan to create antibacterial biomaterials. ACS Appl. Polym. Mat. 4, 5012–5026. doi:10.1021/acsapm.2c00563
Anderson, W. A. C., and Castle, L. (2003). Benzophenone in cartonboard packaging materials and the factors that influence its migration into food. Food Addit. Contam. 20, 607–618. doi:10.1080/0265203031000109486
Applegate, M. B., Partlow, B. P., Coburn, J., Marelli, B., Pirie, C., Pineda, R., et al. (2016). Photocrosslinking of silk fibroin using riboflavin for ocular prostheses. Adv. Mat. 28, 2417–2420. doi:10.1002/adma.201504527
Avens, H. J., Randle, T. J., and Bowman, C. N. (2008). Polymerization behavior and polymer properties of eosin-mediated surface modification reactions. Polym. Guildf. 49, 4762–4768. doi:10.1016/j.polymer.2008.08.054
Bahney, C. S., Lujan, T. J., Hsu, C. W., Bottlang, M., West, J. L., and Johnstone, B. (2011). Visible light photoinitiation of mesenchymal stem cell-laden bioresponsive hydrogels. Eur. Cell. Mat. 22, 43–55. doi:10.22203/ecm.v022a04
Balaji, R., Grande, D., and Nanjundan, S. (2003). Studies on photocrosslinkable polymers having bromo-substituted pendant cinnamoyl group. React. Funct. Polym. 56, 45–57. doi:10.1016/s1381-5148(03)00031-2
Banks, J. M., Mozdzen, L. C., Harley, B. A. C., and Bailey, R. C. (2014). The combined effects of matrix stiffness and growth factor immobilization on the bioactivity and differentiation capabilities of adipose-derived stem cells. Biomaterials 35, 8951–8959. doi:10.1016/j.biomaterials.2014.07.012
Baruah, H., Puthenveetil, S., Choi, Y. A., Shah, S., and Ting, A. Y. (2008). An engineered aryl azide ligase for site-specific mapping of protein–protein interactions through photo-cross-linking. Angew. Chem. Int. Ed. Engl. 47, 7126–7129. doi:10.1002/ange.200802088
Basara, G., Yue, X., and Zorlutuna, P. (2019). Dual crosslinked gelatin methacryloyl hydrogels for photolithography and 3D printing. Gels 5, 34–48. doi:10.3390/gels5030034
Basu, S., Cunningham, L. P., Pins, G. D., Bush, K. A., Taboada, R., Howell, A. R., et al. (2005). Multiphoton excited fabrication of collagen matrixes cross-linked by a modified benzophenone dimer: Bioactivity and enzymatic degradation. Biomacromolecules 6, 1465–1474. doi:10.1021/bm049258y
Blasco, E., Wegener, M., and Barner-Kowollik, C. (2017). Photochemically driven polymeric network formation: Synthesis and applications, 29. Wiley Online Libr, 15. doi:10.1002/adma.201604005
Bobula, T., Bě͗ák, J., Buffa, R., Moravcová, M., Klein, P., Židek, O., et al. (2015). Solid-state photocrosslinking of hyaluronan microfibres. Carbohydr. Polym. 125, 153–160. doi:10.1016/j.carbpol.2015.02.027
Brinkman, W. T., Nagapudi, K., Thomas, B. S., and Chaikof, E. L. (2003). Photo-cross-linking of type I collagen gels in the presence of smooth muscle cells: Mechanical properties, cell viability, and function. Biomacromolecules 4, 890–895. doi:10.1021/bm0257412
Bupphathong, S., Quiroz, C., Huang, W., Chung, P. F., Tao, H. Y., and Lin, C. H. (2022). Gelatin methacrylate hydrogel for tissue engineering applications-a review on material modifications. Pharm. (Basel). 15, 171–196. doi:10.3390/ph15020171
Camp, C. P., Peterson, I. L., Knoff, D. S., Melcher, L. G., Maxwell, C. J., Cohen, A. T., et al. (2020). Non-cytotoxic dityrosine photocrosslinked polymeric materials with targeted elastic moduli. Front. Chem. 8, 173. doi:10.3389/fchem.2020.00173
Cardenas-Daw, C., Kroeger, A., Schaertl, W., Froimowicz, P., and Landfester, K. (2012). Reversible photocycloadditions, a powerful tool for tailoring (nano)materials. Macromol. Chem. Phys. 213, 144–156. doi:10.1002/macp.201100399
Chandrasekharan, A., Seong, K. Y., Yim, S. G., Kim, S., Seo, S., Yoon, J., et al. (2019). In situ photocrosslinkable hyaluronic acid-based surgical glue with tunable mechanical properties and high adhesive strength. J. Polym. Sci. A Polym. Chem. J. Polym. Sci. Pol. Chem. Chem. 57, 522–530. doi:10.1002/pola.29290
Charron, P. N., Fenn, S. L., Poniz, A., and Oldinski, R. A. (2016). Mechanical properties and failure analysis of visible light crosslinked alginate-based tissue sealants. J. Mech. Behav. Biomed. Mat. 59, 314–321. doi:10.1016/j.jmbbm.2016.02.003
Chen, L., Kenkel, S. M., Hsieh, P. H., Gryka, M. C., and Bhargava, R. (2020). Freeform three-dimensionally printed microchannels via surface-initiated photopolymerization combined with sacrificial molding. ACS Appl. Mat. 12, 50105–50112. doi:10.1021/acsami.0c12158
Cho, I. S., Cho, M. O., Li, Z., Nurunnabi, M., Park, S. Y., Kang, S. W., et al. (2016). Synthesis and characterization of a new photo-crosslinkable glycol chitosan thermogel for biomedical applications. Carbohydr. Polym. 144, 59–67. doi:10.1016/j.carbpol.2016.02.029
Choi, G., and Cha, H. J. (2019). Recent advances in the development of nature-derived photocrosslinkable biomaterials for 3D printing in tissue engineering. Biomater. Res. 23, 160–166. doi:10.1186/s40824-019-0168-8
Chou, A. I., and Nicoll, S. B. (2009). Characterization of photocrosslinked alginate hydrogels for nucleus pulposus cell encapsulation. J. Biomed. Mat. Res. 91, 187–194. doi:10.1002/jbm.a.32191
Chou, C., Uprety, R., Davis, L., Chin, J. W., and Deiters, A. (2011). Genetically encoding an aliphatic diazirine for protein photocrosslinking. Chem. Sci. 2, 480–483. doi:10.1039/c0sc00373e
Christensen, S. K., Chiappelli, M. C., and Hayward, R. C. (2012). Gelation of copolymers with pendent benzophenone photo-cross-linkers. Macromolecules 45, 5237–5246. doi:10.1021/ma300784d
Corey, E. J., Bass, J. D., LeMahieu, R., and Mitra, R. B. (1964). A study of the photochemical reactions of 2-cyclohexenones with substituted olefins. J. Am. Chem. Soc. 86, 5570–5583. doi:10.1021/ja01078a034
Correia, M., Neves-Petersen, M. T., Jeppesen, P. B., Gregersen, S., and Petersen, S. B. (2012). UV-Light exposure of insulin: Pharmaceutical implications upon covalent insulin dityrosine dimerization and disulphide bond photolysis. PLoS One 7, e50733. doi:10.1371/journal.pone.0050733
Cramer, N. B., Reddy, S. K., O’Brien, A. K., and Bowman, C. N. (2003). Thiol - ene photopolymerization mechanism and rate limiting step changes for various vinyl functional group chemistries. Macromolecules 36, 7964–7969. doi:10.1021/ma034667s
Cruise, G. M., Hegre, O. D., Scharp, D. S., and Hubbell, J. A. (1998). A sensitivity study of the key parameters in the interfacial photopolymerization of poly(ethylene glycol) diacrylate upon porcine islets. Biotechnol. Bioeng. 57, 655–665. doi:10.1002/(sici)1097-0290(19980320)57:6<655:aid-bit3>3.0.co;2-k
Daood, U., Iqbal, K., Nitisusanta, L. I., and Fawzy, A. S. (2013). Effect of chitosan/riboflavin modification on resin/dentin interface: Spectroscopic and microscopic investigations. J. Biomed. Mat. Res. - Part A. 101 A, 1846–1856. doi:10.1002/jbm.a.34482
Deseke, E., Nakatani, Y., and Ourisson, G. (1998). Intrinsic reactivities of amino acids towards photoalkylation with benzophenone - a study preliminary to photolabelling of the transmembrane protein glycophorin A. Eur. J. Org. Chem. 1998, 243–251. doi:10.1002/(sici)1099-0690(199802)1998:2<243:aid-ejoc243>3.0.co;2-i
Devaraj, N. K., Weissleder, R., and Hilderbrand, S. A. (2008). Tetrazine-based cycloadditions: Application to pretargeted live cell imaging. Bioconjug. Chem. 19, 2297–2299. doi:10.1021/bc8004446
Dey, K., Roy Chowdhury, S., Dykstra, E., Lu, H. P., Shinar, R., Shinar, J., et al. (2021). Effect of bis-diazirine-mediated photo-crosslinking on polyvinylcarbazole and solution-processed polymer LEDs. ACS Appl. Electron. Mat. 3, 3365–3371. doi:10.1021/acsaelm.1c00354
Dong, C. M., Wu, X., Caves, J., Rele, S. S., Thomas, B. S., and Chaikof, E. L. (2005). Photomediated crosslinking of C6-cinnamate derivatized type I collagen. Biomaterials 26, 4041–4049. doi:10.1016/j.biomaterials.2004.10.017
Elbert, D. L., and Hubbell, J. A. (2001). Conjugate addition reactions combined with free-radical cross-linking for the design of materials for tissue engineering. Biomacromolecules 2, 430–441. doi:10.1021/bm0056299
Elvin, C. M., Brownlee, A. G., Huson, M. G., Tebb, T. A., Kim, M., Lyons, R. E., et al. (2009). The development of photochemically crosslinked native fibrinogen as a rapidly formed and mechanically strong surgical tissue sealant. Biomaterials 30, 2059–2065. doi:10.1016/j.biomaterials.2008.12.059
Fancy, D. A., and Kodadek, T. (1999). Chemistry for the analysis of protein-protein interactions: Rapid and efficient cross-linking triggered by long wavelength light. Proc. Natl. Acad. Sci. U. S. A. 96, 6020–6024. doi:10.1073/pnas.96.11.6020
Fernández, M., and Orozco, J. (2021). Advances in functionalized photosensitive polymeric nanocarriers. Polym 13, 2464–2506. doi:10.3390/polym13152464
Fertier, L., Koleilat, H., Stemmelen, M., Giani, O., Joly-Duhamel, C., Lapinte, V., et al. (2013). The use of renewable feedstock in UV-curable materials – a new age for polymers and green chemistry. Prog. Polym. Sci. 38, 932–962. doi:10.1016/j.progpolymsci.2012.12.002
Froimowicz, P., Klinger, D., and Landfester, K. (2011). Photoreactive nanoparticles as nanometric building blocks for the generation of self-healing hydrogel thin films. Chem. – A Eur. J. 17, 12465–12475. doi:10.1002/chem.201100685
Fu, X., and Chang, Z. (2019). Biogenesis, quality control, and structural dynamics of proteins as explored in living cells via site-directed photocrosslinking. Protein Sci. 28, 1194–1209. doi:10.1002/pro.3627
Gadek, M., Strachota, B., and Matějka, L. (2021). Photosynthesis of polymer networks with controlled properties by dimerization of cinnamoyl groups. Polym. Int. 70, 1225–1233. doi:10.1002/pi.6185
Gattás-Asfura, K. M., Weisman, E., Andreopoulos, F. M., Micic, M., Muller, B., Sirpal, S., et al. (2005). Nitrocinnamate-functionalized gelatin: Synthesis and “smart” hydrogel formation via photo-cross-linking. Biomacromolecules 6, 1503–1509. doi:10.1021/bm049238w
Gaudet, I. D., and Shreiber, D. I. (2012). Characterization of methacrylated type-I collagen as a dynamic, photoactive hydrogel. Biointerphases 7, 25. doi:10.1007/s13758-012-0025-y
Ge, S. S., Chen, B., Wu, Y. Y., Long, Q. S., Zhao, Y. L., Wang, P. Y., et al. (2018). Current advances of carbene-mediated photoaffinity labeling in medicinal chemistry. RSC Adv. 8, 29428–29454. doi:10.1039/c8ra03538e
Gillespie, J. M. (1972). Proteins rich in glycine and tyrosine from keratins. Comp. Biochem. Physiol. B 41, 723–734. doi:10.1016/0305-0491(72)90085-5
Gong, D., Lin, Q., Shao, Z., Chen, X., and Yang, Y. (2020). Preparing 3D-printable silk fibroin hydrogels with robustness by a two-step crosslinking method. RSC Adv. 10, 27225–27234. doi:10.1039/d0ra04789a
Gramlich, W. M., Kim, I. L., and Burdick, J. A. (2013). Synthesis and orthogonal photopatterning of hyaluronic acid hydrogels with thiol-norbornene chemistry. Biomaterials 34, 9803–9811. doi:10.1016/j.biomaterials.2013.08.089
Greene, T., and Lin, C. C. (2015). Modular cross-linking of gelatin-based thiol-norbornene hydrogels for in vitro 3D culture of hepatocellular carcinoma cells. ACS Biomater. Sci. Eng. 1, 1314–1323. doi:10.1021/acsbiomaterials.5b00436
Guo, K., Wang, H., Li, S., Zhang, H., Li, S., Zhu, H., et al. (2021). Collagen-based thiol-norbornene photoclick bio-ink with excellent bioactivity and printability. ACS Appl. Mat. Interfaces. 13, 7037–7050. doi:10.1021/acsami.0c16714
Gupta, P., Trenor, S. R., Long, T. E., and Wilkes, G. L. (2004). In situ photo-cross-linking of cinnamate functionalized poly(methyl methacrylate-co-2-hydroxyethyl acrylate) fibers during electrospinning. Macromolecules 37, 9211–9218. doi:10.1021/ma048844g
Han, C., Zhang, H., Wu, Y., He, X., and Chen, X. (2020). Dual-crosslinked hyaluronan hydrogels with rapid gelation and high injectability for stem cell protection. Sci. Rep. 10, 14997–7. doi:10.1038/s41598-020-71462-4
Hao, Y., Shih, H., Muňoz, Z., Kemp, A., and Lin, C. C. (2014). Visible light cured thiol-vinyl hydrogels with tunable degradation for 3D cell culture. Acta Biomater. 10, 104–114. doi:10.1016/j.actbio.2013.08.044
Haria, D. P., and König, B. (2014). Synthetic applications of eosin Y in photoredox catalysis. Chem. Commun. 50, 6688–6699. doi:10.1039/c4cc00751d
Hasany, M., Talebian, S., Sadat, S., Ranjbar, N., Mehrali, M., Wallace, G. G., et al. (2021). Synthesis, properties, and biomedical applications of alginate methacrylate (ALMA)-based hydrogels: Current advances and challenges. Appl. Mat. Today. 24, 101150–101170. doi:10.1016/j.apmt.2021.101150
He, Y., Li, Y., Sun, Y., Zhao, S., Feng, M., Xu, G., et al. (2021). A double-network polysaccharide-based composite hydrogel for skin wound healing. Carbohydr. Polym. 261, 117870–117881. doi:10.1016/j.carbpol.2021.117870
Hill, J. R., and Robertson, A. A. B. (2018). Fishing for drug targets: A focus on diazirine photoaffinity probe synthesis. J. Med. Chem. 61, 6945–6963. doi:10.1021/acs.jmedchem.7b01561
Hoffmann, N. (2008). Photochemical reactions as key steps in organic synthesis. Chem. Rev. 108, 1052–1103. doi:10.1021/cr0680336
Hoorick, J. V., Dobos, A., Markovic, M., Gheysens, T., Damme, L. V., Gruber, P., et al. (2020). Thiol-norbornene gelatin hydrogels: Influence of thiolated crosslinker on network properties and high definition 3D printing. Biofabrication 13, 015017.
Houée-Lévin, C., Bobrowski, K., Horakova, L., Karademir, B., Schöneich, C., Davies, M. J., et al. (2015). Exploring oxidative modifications of tyrosine: An update on mechanisms of formation, advances in analysis and biological consequences. Free Radic. Res. 49, 347–373. doi:10.3109/10715762.2015.1007968
Hoyle, C. E., and Bowman, C. N. (2010). Thiol–ene click chemistry, 49. Wiley Online Libr., 1540–1573.
Huang, R., Choe, E., and Min, D. B. (2004). Kinetics for singlet oxygen formation by riboflavin photosensitization and the reaction between riboflavin and singlet oxygen. J. Food Sci. 69, C726–C732. doi:10.1111/j.1365-2621.2004.tb09924.x
Huang, Y. C., Liu, Z. H., Kuo, C. Y., and Chen, J. P. (2022a). Photo-crosslinked hyaluronic acid/carboxymethyl cellulose composite hydrogel as a dural substitute to prevent post-surgical adhesion. Int. J. Mol. Sci. 23, 6177–6195. doi:10.3390/ijms23116177
Huang, Y., Sun, G., Lyu, L., Li, Y., Li, D., Fan, Q., et al. (2022b). Dityrosine-inspired photocrosslinking technique for 3D printing of silk fibroin-based composite hydrogel scaffolds. Soft Matter 18, 3705–3712. doi:10.1039/d1sm01817e
Jakubovska, J., Tauraitė, D., and Meškys, R. (2018). A versatile method for the UVA-induced cross-linking of acetophenone- or benzophenone-functionalized DNA. Sci. Rep. 8, 16484–16510. doi:10.1038/s41598-018-34892-9
Jeon, O., Bouhadir, K. H., Mansour, J. M., and Alsberg, E. (2009). Photocrosslinked alginate hydrogels with tunable biodegradation rates and mechanical properties. Biomaterials 30, 2724–2734. doi:10.1016/j.biomaterials.2009.01.034
Keskin, D., Mokabbar, T., Pei, Y., and Rijn, V. P. (2018). The relationship between bulk silicone and benzophenone-initiated hydrogel coating properties. Polym. (Basel) 10, 534–548. doi:10.3390/polym10050534
Kianfar, P., Vitale, A., Vacche, D. S., and Bongiovanni, R. (2019). Photo-crosslinking of chitosan/poly(ethylene oxide) electrospun nanofibers. Carbohydr. Polym. 217, 144–151. doi:10.1016/j.carbpol.2019.04.062
Kim, E. H., Han, G. D., Noh, S. H., Kim, J. W., Lee, J. G., Ito, Y., et al. (2017). Photo-reactive natural polymer derivatives for medical application. J. Ind. Eng. Chem. 54, 1–13. doi:10.1016/j.jiec.2017.05.029
Kim, E., Seok, J. M., Bae, S. B., Park, S. A., and Park, W. H. (2021a). Silk fibroin enhances cytocompatibilty and dimensional stability of alginate hydrogels for light-based three-dimensional bioprinting. Biomacromolecules 22, 1921–1931. doi:10.1021/acs.biomac.1c00034
Kim, H., Kang, B., Cui, X., Lee, S. H., Lee, K., Cho, D. W., et al. (2021b). Light-activated decellularized extracellular matrix-based bioinks for volumetric tissue analogs at the centimeter scale. Adv. Funct. Mat. 31, 2011252. doi:10.1002/adfm.202011252
Kim, S. H., Hong, H., Ajiteru, O., Sultan, M. T., Lee, Y. J., Lee, J. S., et al. (2021c). 3D bioprinted silk fibroin hydrogels for tissue engineering. Nat. Protoc. 16, 5484–5532. doi:10.1038/s41596-021-00622-1
Kim, T. G., Jeong, E. H., Lim, S. C., Kim, S. H., Kim, G. H., Kim, S. H., et al. (2009). PMMA-based patternable gate insulators for organic thin-film transistors. Synth. Met. 159, 749–753. doi:10.1016/j.synthmet.2008.11.027
Kizilel, S., Pérez-Luna, V. H., and Teymour, F. (2004). Photopolymerization of poly (ethylene glycol) diacrylate on eosin-functionalized surfaces. Langmuir 20, 8652–8658. doi:10.1021/la0496744
Ko, H. F., Sfeir, C., and Kumta, P. N. (2010). Novel synthesis strategies for natural polymer and composite biomaterials as potential scaffolds for tissue engineering. Philos. Trans. Math. Phys. Eng. Sci. 368, 1981–1997. doi:10.1098/rsta.2010.0009
Kono, H., Uno, T., Tsujisaki, H., Matsushima, T., and Tajima, K. (2020). Nanofibrillated bacterial cellulose modified with (3-aminopropyl)trimethoxysilane under aqueous conditions: Applications to poly(methyl methacrylate) fiber-reinforced nanocomposites. ACS Omega 5, 29561–29569. doi:10.1021/acsomega.0c04533
Kuboe, S., Yoda, M., Ogata, A., Kitade, Y., Tomari, Y., and Ueno, Y. (2010). Diazirine-containing RNA photocrosslinking probes for the study of siRNA-protein interactions. Chem. Commun. (Camb). 46, 7367. doi:10.1039/c0cc02450c
Kumar, H., Ambhorkar, P., Foulds, I., Golovin, K., and Kim, K. (2022). A kinetic model for predicting imperfections in bioink photopolymerization during visible-light stereolithography printing. Addit. Manuf. 55, 102808. doi:10.1016/j.addma.2022.102808
Kurioz, Y., Reznikov, Y., Tereshchenko, O., Gerus, I., Buluy, O., Ha, K. R., et al. (2008). Highly sensitive photoaligning materials on a base of cellulose-cinnamates. Mol. Cryst. Liq. Cryst. 480, 81–90. doi:10.1080/15421400701825458
Lago, M. A., Rodríguez-Bernaldo de Quirós, A., Sendón, R., Bustos, J., Nieto, M. T., and Paseiro, P. (2015). Photoinitiators: A food safety review. Food Addit. Contam. - Chem. Anal. Control Expo. Risk Assess. 32, 779–798. doi:10.1080/19440049.2015.1014866
Lau, T. T., Lee, L. Q. P., Leong, W., and Wang, D. A. (2012). Formation of model hepatocellular aggregates in a hydrogel scaffold using degradable genipin crosslinked gelatin microspheres as cell carriers. Biomed. Mat. 7, 065003. doi:10.1088/1748-6041/7/6/065003
Lau, T. T., Wang, C., Png, S. W., Su, K., and Wang, D. A. (2011). Genipin-crosslinked microcarriers mediating hepatocellular aggregates formation and functionalities. J. Biomed. Mat. Res. A 96, 204–211. doi:10.1002/jbm.a.32975
Lee, J., Ju, M., Cho, O. H., Kim, Y., and Nam, K. T. (2019). Tyrosine-rich peptides as a platform for assembly and material synthesis. Adv. Sci. (Weinh). 6, 1801255. doi:10.1002/advs.201801255
Lee, M. W., Tsai, H. F., Wen, S. M., and Huang, C. H. (2012). Photocrosslinkable gellan gum film as an anti-adhesion barrier. Carbohydr. Polym. 90, 1132–1138. doi:10.1016/j.carbpol.2012.06.064
Lepage, M. L., Simhadri, C., Liu, C., Takaffoli, M., Bi, L., Crawford, B., et al. (2019). A broadly applicable cross-linker for aliphatic polymers containing C–H bonds. Science 366, 875–878. doi:10.1126/science.aay6230
Li, P., Müller, M., Chang, M. W., Frettlöh, M., and Schönherr, H. (2017). Encapsulation of autoinducer sensing reporter bacteria in reinforced alginate-based microbeads. ACS Appl. Mat. Interfaces. 9, 22321–22331. doi:10.1021/acsami.7b07166
Li, Q., Wang, D., and Elisseeff, J. H. (2003). Heterogeneous-phase reaction of glycidyl methacrylate and chondroitin sulfate: Mechanism of ring-opening-transesterification competition. Macromolecules 36, 2556–2562. doi:10.1021/ma021190w
Lim, D. W., Nettles, D. L., Setton, L. A., and Chilkoti, A. (2008). In situ cross-linking of elastin-like polypeptide block copolymers for tissue repair. Biomacromolecules 9, 222–230. doi:10.1021/bm7007982
Lim, J., Choi, G., Joo, K. I., Cha, H. J., and Kim, J. (2021). Embolization of vascular malformations via in situ photocrosslinking of mechanically reinforced alginate microfibers using an optical-fiber-integrated microfluidic device. Adv. Mat. 33, 2006759. doi:10.1002/adma.202006759
Lim, K. S., Abinzano, F., Bernal, P. N., Sanchez, A. A., Atienza-Roca, P., Otto, I. A., et al. (2020a). One-step photoactivation of a dual-functionalized bioink as cell carrier and cartilage-binding glue for chondral regeneration. Adv. Healthc. Mat. 9, 1901792. doi:10.1002/adhm.201901792
Lim, K. S., Galarraga, J. H., Cui, X., Lindberg, G. C. J., Burdick, J. A., and Woodfield, T. B. F. (2020b). Fundamentals and applications of photo-cross-linking in bioprinting. Chem. Rev. 120, 10662–10694. doi:10.1021/acs.chemrev.9b00812
Lin, C. C., Ki, C. S., and Shih, H. (2015). Thiol–norbornene photoclick hydrogels for tissue engineering applications. J. Appl. Polym. Sci. 132, 41563. doi:10.1002/app.41563
Lin, C. C., Raza, A., and Shih, H. (2011). PEG hydrogels formed by thiol-ene photo-click chemistry and their effect on the formation and recovery of insulin-secreting cell spheroids. Biomaterials 32, 9685–9695. doi:10.1016/j.biomaterials.2011.08.083
Liu, C., Hua, J., Ng, P. F., and Fei, B. (2021). Photochemistry of bioinspired dityrosine crosslinking. J. Mat. Sci. Technol. 63, 182–191. doi:10.1016/j.jmst.2020.02.086
Liu, J., Qu, M., Wang, C., Xue, Y., Huang, H., Chen, Q., et al. (2022a). A dual-cross-linked hydrogel patch for promoting diabetic wound healing. Small 18, 2106172. doi:10.1002/smll.202106172
Liu, J., Su, C., Chen, Y., Tian, S., Lu, C., Huang, W., et al. (2022b). Current understanding of the applications of photocrosslinked hydrogels in biomedical engineering. Gels 8, 216–242. doi:10.3390/gels8040216
Liu, Q., and Tor, Y. (2003). Simple conversion of aromatic amines into azides. Org. Lett. 5, 2571–2572. doi:10.1021/ol034919+
Liu, S., Brunel, D., Sun, K., Zhang, Y., Chen, H., Xiao, P., et al. (2020). Novel photoinitiators based on benzophenone-triphenylamine hybrid structure for LED photopolymerization. Macromol. Rapid Commun. 41, 2000460. doi:10.1002/marc.202000460
Lu, H. D., Soranno, D. E., Rodell, C. B., Kim, I. L., and Burdick, J. A. (2013). Secondary photocrosslinking of injectable shear-thinning dock-and-lock hydrogels. Adv. Healthc. Mat. 2, 1028–1036. doi:10.1002/adhm.201200343
Maiz-Fernández, S., Pérez-Álvarez, L., Silván, U., Vilas-Vilela, J. L., and Lanceros-Mendez, S. (2022). Photocrosslinkable and self-healable hydrogels of chitosan and hyaluronic acid. Int. J. Biol. Macromol. 216, 291–302. doi:10.1016/j.ijbiomac.2022.07.004
Malcor, J. D., and Mallein-Gerin, F. (2022). Biomaterial functionalization with triple-helical peptides for tissue engineering. Acta Biomater. 148, 1–21. doi:10.1016/j.actbio.2022.06.003
Marizza, P., Abrami, M., Keller, S. S., Posocco, P., Laurini, E., Goswami, K., et al. (2016). Synthesis and characterization of UV photocrosslinkable hydrogels with poly(N-vinyl-2-pyrrolidone): Determination of the network mesh size distribution. Int. J. Polym. Mat. Polym. 65, 516–525. doi:10.1080/00914037.2015.1129964
McCall, J. D., and Anseth, K. S. (2012). Thiol-ene photopolymerizations provide a facile method to encapsulate proteins and maintain their bioactivity. Biomacromolecules 13, 2410–2417. doi:10.1021/bm300671s
Messager, L., Portecop, N., Hachet, E., Lapeyre, V., Pignot-Paintrand, I., Catargi, B., et al. (2013). Photochemical crosslinking of hyaluronic acid confined in nanoemulsions: Towards nanogels with a controlled structure. J. Mat. Chem. B 1, 3369. doi:10.1039/c3tb20300j
Michel, S. E. S., Dutertre, F., Denbow, M. L., Galan, M. C., and Briscoe, W. H. (2019). Facile synthesis of chitosan-based hydrogels and microgels through thiol-ene photoclick cross-linking. ACS Appl. Bio Mat. 2, 3257–3268. doi:10.1021/acsabm.9b00218
Michel, S. E. S., Rogers, S. E., Briscoe, W. H., and Galan, M. C. (2020). Tunable thiol-ene photo-cross-linked chitosan-based hydrogels for biomedical applications. ACS Appl. Bio Mat. 3, 8075–8083. doi:10.1021/acsabm.0c01171
Mihaila, S. M., Gaharwar, A. K., Reis, R. L., Marques, A. P., Gomes, M. E., and Khademhosseini, A. (2012). Photocrosslinkable kappa-carrageenan hydrogels for tissue engineering applications. Adv. Healthc. Mater 2, 895–907. doi:10.1002/adhm.201200317
Min, K. I., Kim, D. H., Lee, H. J., Lin, L., and Kim, D. P. (2018). Direct synthesis of a covalently self-assembled peptide nanogel from a tyrosine-rich peptide monomer and its biomineralized hybrids. Angew. Chem. Int. Ed. Engl. 57, 5630–5634. doi:10.1002/anie.201713261
Min, K. I., Yun, G., Jang, Y., Kim, K. R., Ko, Y. H., Jang, H. S., et al. (2016). Covalent self-assembly and one-step photocrosslinking of tyrosine-rich oligopeptides to form diverse nanostructures. Angew. Chem. Int. Ed. 55, 6925–6928. doi:10.1002/anie.201601675
Mishra, P. K., Yoo, C. M., Hong, E., and Rhee, H. W. (2020). Photo-crosslinking: An emerging chemical tool for investigating molecular networks in live cells. Chembiochem 21, 924–932. doi:10.1002/cbic.201900600
Mu, X., Sahoo, J. K., Cebe, P., and Kaplan, D. L. (2020). Photo-crosslinked silk fibroin for 3D printing. Polymers 12, 2936–2953. doi:10.3390/polym12122936
Mukherjee, S., Fang, M., Kok, W. M., Kapp, E. A., Thombare, V. J., Huguet, R., et al. (2019). Establishing signature fragments for identification and sequencing of dityrosine cross-linked peptides using ultraviolet photodissociation mass spectrometry. Anal. Chem. 91, 12129–12133. doi:10.1021/acs.analchem.9b02986
Mũnoz, Z., Shih, H., and Lin, C. C. (2014). Gelatin hydrogels formed by orthogonal thiol–norbornene photochemistry for cell encapsulation. Biomater. Sci. 2, 1063–1072. doi:10.1039/c4bm00070f
Murale, D. P., Hong, S. C., Haque, M. M., and Lee, J. S. (2017). Photo-affinity labeling (PAL) in chemical proteomics: A handy tool to investigate protein-protein interactions (PPIs). Proteome Sci. 15, 14. doi:10.1186/s12953-017-0123-3
Musolino, S. F., Pei, Z., Bi, L., DiLabio, G. A., and Wulff, J. E. (2021). Structure-function relationships in aryl diazirines reveal optimal design features to maximize C-H insertion. Chem. Sci. 12, 12138–12148. doi:10.1039/d1sc03631a
Na, K., Shin, S., Lee, H., Shin, D., Baek, J., Kwak, H., et al. (2018). Effect of solution viscosity on retardation of cell sedimentation in DLP 3D printing of gelatin methacrylate/silk fibroin bioink. J. Ind. Eng. Chem. 61, 340–347. doi:10.1016/j.jiec.2017.12.032
Nakayama, Y., and Matsuda, T. (1992). Preparation and characteristics of photocrosslinkable hydrophilic polymer having cinnamate moiety. J. Polym. Sci. Part A Polym. Chem. 30, 2451–2457. doi:10.1002/pola.1992.080301119
Nam, S., and Mooney, D. (2021). Polymeric tissue adhesives. Chem. Rev. 121, 11336–11384. doi:10.1021/acs.chemrev.0c00798
Navarro, J., Swayambunathan, J., Santoro, M., and Fisher, J. (2018). Assessment of the effects of energy density in crosslinking of keratin-based photo-sensitive resin. Int. Semin. Biomed. Eng. 2018, 18144107. doi:10.1109/sib.2018.8467744
Nettles, D. L., Vail, T. P., Morgan, M. T., Grinstaff, M. W., and Setton, L. A. (2004). Photocrosslinkable hyaluronan as a scaffold for articular cartilage repair. Ann. Biomed. Eng. 32, 391–397. doi:10.1023/b:abme.0000017552.65260.94
Neumann, M. G., Schmitt, C. C., Goi, B. E., and Pinto, L. F. A. (2013). Photodegradation of polystyrene films containing UV-visible sensitizers. J. Polym. Sci. 2, 39–47.
Nguyen, K. D. Q., Crespo-Ribadeneyra, M., Picot, O., Colak, B., and Gautrot, J. E. (2021). Ultrafast photo-crosslinking of thiol-norbornene opaque silicone elastomer nanocomposites in air. ACS Appl. Polym. Mat. 3, 5373–5385. doi:10.1021/acsapm.1c00440
Noè, C., Tonda-Turo, C., Chiappone, A., Sangermano, M., and Hakkarainen, M. (2020). Light processable starch hydrogels. Polym. (Basel) 12, 1359–1372. doi:10.3390/polym12061359
Noshadi, I., Hong, S., Sullivan, K. E., Shirzaei Sani, E., Portillo-Lara, R., Tamayol, A., et al. (2017). In vitro and in vivo analysis of visible light crosslinkable gelatin methacryloyl (GelMA) hydrogels. Biomater. Sci. 5, 2093–2105. doi:10.1039/c7bm00110j
Occhetta, P., Visone, R., Russo, L., Cipolla, L., Moretti, M., and Rasponi, M. (2015). VA-086 methacrylate gelatine photopolymerizable hydrogels: A parametric study for highly biocompatible 3D cell embedding. J. Biomed. Mat. Res. 103, 2109–2117. doi:10.1002/jbm.a.35346
Olson, R. A., Korpusik, A. B., and Sumerlin, B. S. (2020). Enlightening advances in polymer bioconjugate chemistry: Light-based techniques for grafting to and from biomacromolecules. Chem. Sci. 11, 5142–5156. doi:10.1039/d0sc01544j
Ono, K., Ishihara, M., Ozeki, Y., Deguchi, H., Sato, M., Saito, Y., et al. (2001). Experimental evaluation of photocrosslinkable chitosan as a biologic adhesive with surgical applications. Surgery 130, 844–850. doi:10.1067/msy.2001.117197
Ono, K., Saito, Y., Yura, H., Ishikawa, K., Kurita, A., Akaike, T., et al. (2000). Photocrosslinkable chitosan as a biological adhesive. J. Biomed. Mat. Res. 49, 289–295. doi:10.1002/(sici)1097-4636(200002)49:2<289:aid-jbm18>3.0.co;2-m
Orelma, H., Vuoriluoto, M., Johansson, L. S., Campbell, J. M., Filpponen, I., Biesalski, M., et al. (2016). Preparation of photoreactive nanocellulosic materials: Via benzophenone grafting. RSC Adv. 6, 85100–85106. doi:10.1039/c6ra15015b
Park, T. Y., Yang, Y. J., Ha, D. H., Cho, D. W., and Cha, H. J. (2019). Marine-derived natural polymer-based bioprinting ink for biocompatible, durable, and controllable 3D constructs. Biofabrication 11 (035001), 035001–035013. doi:10.1088/1758-5090/ab0c6f
Partlow, B. P., Applegate, M. B., Omenetto, F. G., and Kaplan, D. L. (2016). Dityrosine cross-linking in designing biomaterials. ACS Biomater. Sci. Eng. 2, 2108–2121. doi:10.1021/acsbiomaterials.6b00454
Pei, M., Mao, J., Xu, W., Zhou, Y., and Xiao, P. (2019). Photocrosslinkable chitosan hydrogels and their biomedical applications. J. Polym. Sci. Part A Polym. Chem. 57, 1862–1871. doi:10.1002/pola.29305
Pereira, R. F., Barrias, C. C., Bártolo, P. J., and Granja, P. L. (2018a). Cell-instructive pectin hydrogels crosslinked via thiol-norbornene photo-click chemistry for skin tissue engineering. Acta Biomater. 66, 282–293. doi:10.1016/j.actbio.2017.11.016
Pereira, R. F., Sousa, A., Barrias, C. C., Bártolo, P. J., and Granja, P. L. (2018b). A single-component hydrogel bioink for bioprinting of bioengineered 3D constructs for dermal tissue engineering. Mat. Horiz. 5, 1100–1111. doi:10.1039/c8mh00525g
Perin, F., Mota, C., Mancini, I., Motta, A., and Maniglio, D. (2022). Photo-enzymatic dityrosine crosslinking for bioprinting. Polymer 252, 124941–124945. doi:10.1016/j.polymer.2022.124941
Pham, N. D., Parker, R. B., and Kohler, J. J. (2013). Photocrosslinking approaches to interactome mapping. Curr. Opin. Chem. Biol. 17, 90–101. doi:10.1016/j.cbpa.2012.10.034
Popielarz, R., and Vogt, O. (2008). Effect of coinitiator type on initiation efficiency of two-component photoinitiator systems based on eosin. J. Polym. Sci. Part A Polym. Chem. 46, 3519–3532. doi:10.1002/pola.22688
Porter, G., and Suppan, P. (1965). Primary photochemical processes in aromatic molecules. Part 12.—excited states of benzophenone derivatives. J. Chem. Soc. Faraday Trans. 61, 1664–1673. doi:10.1039/tf9656101664
Raphel, J., Parisi-Amon, A., and Heilshorn, S. C. (2012). Photoreactive elastin-like proteins for use as versatile bioactive materials and surface coatings. J. Mat. Chem. 22, 19429–19437. doi:10.1039/c2jm31768k
Raven, D. J., Earland, C., and Little, M. (1971). Occurrence of dityrosine in Tussah silk fibroin and keratin. Biochim. Biophys. Acta - Proteins Proteom. 251, 96–99. doi:10.1016/0005-2795(71)90065-1
Reis, A. V., Fajardo, A. R., Schuquel, I. T. A., Guilherme, M. R., Vidotti, G. J., Rubira, A. F., et al. (2009). Reaction of glycidyl methacrylate at the hydroxyl and carboxylic groups of poly(vinyl alcohol) and poly(acrylic acid): Is this reaction mechanism still unclear? J. Org. Chem. 74, 3750–3757. doi:10.1021/jo900033c
Rennert, J., Ruggiero, E., and Rapp, J. (1967). The non-radiative dissipation of excitation energy in solid cinnamic acid by dimer formation, 6. Wiley Online Libr., 29–34.
Rickett, T. A., Amoozgar, Z., Tuchek, C. A., Park, J., Yeo, Y., and Shi, R. (2011). Rapidly photo-cross-linkable chitosan hydrogel for peripheral neurosurgeries. Biomacromolecules 12, 57–65. doi:10.1021/bm101004r
Roberts, J. J., Naudiyal, P., Lim, K. S., Poole-Warren, L. A., and Martens, P. J. (2016). A comparative study of enzyme initiators for crosslinking phenol-functionalized hydrogels for cell encapsulation. Biomater. Res. 20, 30. doi:10.1186/s40824-016-0077-z
Samani, S., Bonakdar, S., Farzin, A., Hadjati, J., and Azami, M. (2020). A facile way to synthesize a photocrosslinkable methacrylated chitosan hydrogel for biomedical applications. Int. J. Polym. Mat. Polym. Biomater. 70, 730–741. doi:10.1080/00914037.2020.1760274
Sando, L., Kim, M., Colgrave, M. L., Ramshaw, J. A. M., Werkmeister, J. A., and Elvin, C. M. (2010). Photochemical crosslinking of soluble wool keratins produces a mechanically stable biomaterial that supports cell adhesion and proliferation. J. Biomed. Mat. Res. A 95A, 901–911. doi:10.1002/jbm.a.32913
Sanyal, A. (2010). Diels–alder cycloaddition-cycloreversion: A powerful combo in materials design. Macromol. Chem. Phys. 211, 1417–1425. doi:10.1002/macp.201000108
Sarkar, D., Bera, N., and Ghosh, S. (2020). [2+2] Photochemical cycloaddition in organic synthesis. Eur. J. Org. Chem. 2020, 1310–1326. doi:10.1002/ejoc.201901143
Schelkle, K. M., Bender, M., Beck, S., Jeltsch, K. F., Stolz, S., Zimmermann, J., et al. (2016). Photo-cross-linkable polymeric optoelectronics based on the [2 + 2] cycloaddition reaction of cinnamic acid. Macromolecules 49, 1518–1522. doi:10.1021/acs.macromol.5b02407
Schulz, A., Gepp, M. M., Stracke, F., von Briesen, H., Neubauer, J. C., and Zimmermann, H. (2019). Tyramine-conjugated alginate hydrogels as a platform for bioactive scaffolds. J. Biomed. Mat. Res. A 107, 114–121. doi:10.1002/jbm.a.36538
Sharma, S., Sudhakara, P., Singh, J., Ilyas, R. A., Asyraf, M. R. M., and Razman, M. R. (2021). Critical review of biodegradable and bioactive polymer composites for bone tissue engineering and drug delivery applications. Polym. (Basel) 13, 2623–2687. doi:10.3390/polym13162623
Shi, D., Liu, X., Xiang, F., Chen, M., Yang, C., and Akashi, M. (2009). Studies on preparation and fluorescent properties of a novel photo-sensitive nanoparticle composed of europium ion and cinnamic acid derivative. Macromol. Chem. Phys. 210, 2063–2069. doi:10.1002/macp.200900209
Shi, D., Matsusaki, M., Kaneko, T., and Akashi, M. (2008). Photo-cross-linking and cleavage induced reversible size change of bio-based nanoparticles. Macromolecules 41, 8167–8172. doi:10.1021/ma800648e
Shih, H., and Lin, C. C. (2013). Visible-light-mediated thiol-ene hydrogelation using eosin-y as the only photoinitiator. Macromol. Rapid Commun. 34, 269–273. doi:10.1002/marc.201200605
Singh, J., Steele, T. W. J., and Lim, S. (2021). Fibrillated bacterial cellulose liquid carbene bioadhesives for mimicking and bonding oral cavity surfaces. J. Mat. Chem. B 10, 2570–2583. doi:10.1039/d1tb02044g
Sinha Roy, P., Mention, M. M., Turner, M. A. P., Brunissen, F., Stavros, V. G., Garnier, G., et al. (2021). Bio-based photo-reversible self-healing polymer designed from lignin. Green Chem. 23, 10050–10061. doi:10.1039/d1gc02957f
Smeds, K. A., Pfister-Serres, A., Miki, D., Dastgheib, K., Inoue, M., Hatchell, D. L., et al. (2001). Photocrosslinkable polysaccharides for in situ hydrogel formation. J. Biomed. Mat. Res. 54, 115–121. doi:10.1002/1097-4636(200101)54:1<115:aid-jbm14>3.0.co;2-q
Sogawa, H., Katashima, T., and Numata, K. (2020). A covalently crosslinked silk fibroin hydrogel using enzymatic oxidation and chemoenzymatically synthesized copolypeptide crosslinkers consisting of a GPG tripeptide motif and tyrosine: Control of gelation and resilience. Polym. Chem. 11, 3152–3161. doi:10.1039/d0py00187b
Soucy, J. R., Sani, E. S., Lara, R. P., Diaz, D., Dias, F., Weiss, A. S., et al. (2018). Photocrosslinkable gelatin/tropoelastin hydrogel adhesives for peripheral nerve repair. Tissue. Eng. Part. A. 24, 17–18. doi:10.1089/ten.tea.2017.0502
Sun, G., Zhang, X., Shen, Y. I., Sebastian, R., Dickinson, L. E., Fox-Talbot, K., et al. (2011). Dextran hydrogel scaffolds enhance angiogenic responses and promote complete skin regeneration during burn wound healing. Proc. Natl. Acad. Sci. U. S. A. 108, 20976–20981. doi:10.1073/pnas.1115973108
Sundaramurthi, D., Krishnan, U. M., and Sethuraman, S. (2014). Electrospun nanofibers as scaffolds for skin tissue engineering. Polym. Rev. 54, 348–376. doi:10.1080/15583724.2014.881374
Sydow, S., Aniol, A., Hadler, C., and Menzel, H. (2019). Chitosan–azide nanoparticle coating as a degradation barrier in multilayered polyelectrolyte drug delivery systems. Biomolecules 9, 573. doi:10.3390/biom9100573
Tanaka, Y., Bond, M. R., and Kohler, J. J. (2008). Photocrosslinkers illuminate interactions in living cells. Mol. Biosyst. 4, 473. doi:10.1039/b803218a
Temel, G., Enginol, B., Aydin, M., Balta, D. K., and Arsu, N. (2011). Photopolymerization and photophysical properties of amine linked benzophenone photoinitiator for free radical polymerization. J. Photochem. Photobiol. A Chem. 219, 26–31. doi:10.1016/j.jphotochem.2011.01.012
Truong, M. Y., Dutta, N. K., Choudhury, N. R., Kim, M., Elvin, C. M., Nairn, K. M., et al. (2011). The effect of hydration on molecular chain mobility and the viscoelastic behavior of resilin-mimetic protein-based hydrogels. Biomaterials 32, 8462–8473. doi:10.1016/j.biomaterials.2011.07.064
Valdes-Aguilera, O., Pathak, C. P., Shi, J., Watson, D., and Neckers, D. C. (1992). Photopolymerization studies using visible light photoinitiators. Macromolecules 25, 541–547. doi:10.1021/ma00028a008
Wang, J., Yang, J., Atif, M., Bongiovanni, R., Li, G., Xue, Z., et al. (2018). One-component photoinitiator based on benzophenone and sesamol. Polym. Adv. Technol. 29, 2264–2272. doi:10.1002/pat.4337
Wang, L., Li, J., Zhang, D., Ma, S., Zhang, J., Gao, F., et al. (2020). Dual-enzymatically crosslinked and injectable hyaluronic acid hydrogels for potential application in tissue engineering. Rsc. Adv. 10, 2870–2876. doi:10.1039/c9ra09531d
West, A. V., Muncipinto, G., Wu, H. Y., Huang, A. C., Labenski, M. T., Jones, L. H., et al. (2021). Labeling preferences of diazirines with protein biomolecules. J. Am. Chem. Soc. 143, 6691–6700. doi:10.1021/jacs.1c02509
Wong, J., Kaastrup, K., Aguirre-Soto, A., and Sikes, H. D. (2015). A quantitative analysis of peroxy-mediated cyclic regeneration of eosin under oxygen-rich photopolymerization conditions. Polymer 69, 169–177. doi:10.1016/j.polymer.2015.05.043
Wu, H., and Kohler, J. (2019). Photocrosslinking probes for capture of carbohydrate interactions. Curr. Opin. Chem. Biol. 53, 173–182. doi:10.1016/j.cbpa.2019.09.002
Wu, Y., Hisada, K., Maeda, S., Sasaki, T., and Sakurai, K. (2007). Fabrication and structural characterization of the Langmuir–Blodgett films from a new chitosan derivative containing cinnamate chromophores. Carbohydr. Polym. 68, 766–772. doi:10.1016/j.carbpol.2006.08.016
Xue, T., Li, Y., Zhao, X., Nie, J., and Zhu, X. (2021). A facile synthesized benzophenone schiff-base ligand as efficient type II visible light photoinitiator. Prog. Org. Coat. 157, 106329. doi:10.1016/j.porgcoat.2021.106329
Yagci, Y., Jockusch, S., and Turro, N. J. (2010). Photoinitiated polymerization: Advances, challenges, and opportunities. Macromolecules 43, 6245–6260. doi:10.1021/ma1007545
Yano, S., Iwase, T., Shibata, M., Miyamoto, Y., Shimasaki, T., and Teramoto, N. (2020). Synthesis of photocrosslinkable copolymers of cinnamoyl group-modified methacrylate and 2-hydroxyethyl methacrylate, and fibroblast cell growth on their thin films. J. Photopolym. Sci. Technol. 32, 823–833. doi:10.2494/photopolymer.32.823
Ye, K., Sinawang, P. D., Tok, A. I. Y., and Marks, R. S. (2018). Photoinducible silane diazirine as an effective crosslinker in the construction of a chemiluminescent immunosensor targeting a model E. coli analyte. Sens. Actuators. B Chem. 256, 234–242. doi:10.1016/j.snb.2017.10.058
Yu, W., and Baskin, J. M. (2022). Photoaffinity labeling approaches to elucidate lipid–protein interactions. Curr. Opin. Chem. Biol. 69, 102173. doi:10.1016/j.cbpa.2022.102173
Yue, K., Li, X., Schrobback, K., Sheikhi, A., Annabi, N., Leijten, J., et al. (2017). Structural analysis of photocrosslinkable methacryloyl-modified protein derivatives. Biomaterials 139, 163–171. doi:10.1016/j.biomaterials.2017.04.050
Yuk, H., Zhang, T., Parada, G. A., Liu, X., and Zhao, X. (2016). Skin-inspired hydrogel-elastomer hybrids with robust interfaces and functional microstructures. Nat. Commun. 7, 12028. doi:10.1038/ncomms12028
Zhang, R., Chu, F., Hu, Y., Hu, H., Hu, Y., Liu, H., et al. (2020). Preparation of photo-crosslinking starch colloidal particles. Starch/Staerke. 72, 1900175–1900176. doi:10.1002/star.201900175
Zhao, W., Jin, X., Cong, Y., Liu, Y., and Fu, J. (2013). Degradable natural polymer hydrogels for articular cartilage tissue engineering. J. Chem. Technol. Biotechnol. 88, 327–339. doi:10.1002/jctb.3970
Zhou, L., Shi, H., Li, Z., and He, C. (2020). Recent advances in complex coacervation design from macromolecular assemblies and emerging applications. Macromol. Rapid. Commun. 41, 2000149. doi:10.1002/marc.202000149
Keywords: photo-reactive moiety, photo-crosslinking, photoinitiator, catalyst, polymerization
Citation: Moon SH, Hwang HJ, Jeon HR, Park SJ, Bae IS and Yang YJ (2023) Photocrosslinkable natural polymers in tissue engineering. Front. Bioeng. Biotechnol. 11:1127757. doi: 10.3389/fbioe.2023.1127757
Received: 20 December 2022; Accepted: 15 February 2023;
Published: 02 March 2023.
Edited by:
Kunyu Zhang, South China University of Technology, ChinaReviewed by:
Qianyu Lin, Institute of Materials Research and Engineering (A∗STAR), SingaporeCopyright © 2023 Moon, Hwang, Jeon, Park, Bae and Yang. This is an open-access article distributed under the terms of the Creative Commons Attribution License (CC BY). The use, distribution or reproduction in other forums is permitted, provided the original author(s) and the copyright owner(s) are credited and that the original publication in this journal is cited, in accordance with accepted academic practice. No use, distribution or reproduction is permitted which does not comply with these terms.
*Correspondence: Yun Jung Yang, eWoueWFuZ0BpbmhhLmFjLmty
†These authors have contributed equally to this work
‡ORCID: Seo Hyung Moon, orcid.org/0000-0003-1365-5017; Hye Jin Hwang, orcid.org/0000-0002-9045-8845; Hye Ryeong Jeon, orcid.org/0000-0002-9380-1661; Sol Ji Park, orcid.org/0000-0002-0620-0754; Yun Jung Yang, orcid.org/0000-0003-0684-5218
Disclaimer: All claims expressed in this article are solely those of the authors and do not necessarily represent those of their affiliated organizations, or those of the publisher, the editors and the reviewers. Any product that may be evaluated in this article or claim that may be made by its manufacturer is not guaranteed or endorsed by the publisher.
Research integrity at Frontiers
Learn more about the work of our research integrity team to safeguard the quality of each article we publish.