- The Eighth Affiliated Hospital, Sun Yat-sen University, Shenzhen, Guangdong, China
Immunotherapy is a revolutionary and promising approach to cancer treatment. However, traditional cancer immunotherapy often has the disadvantages of limited immune response rate, poor targeting, and low treatment index due to systemic administration. Hydrogels are drug carriers with many advantages. They can be loaded and transported with immunotherapeutic agents, chemical anticancer drugs, radiopharmaceuticals, photothermal agents, photosensitizers, and other therapeutic agents to achieve controlled release of drugs, extend the retention time of drugs, and thus successfully trigger anti-tumor effects and maintain long-term therapeutic effects after administration. This paper reviews recent advances in injectable hydrogel-based cancer immunotherapy, including immunotherapy alone, immunotherapy with combination chemotherapy, radiotherapy, phototherapy, and DNA hydrogel-based immunotherapy. Finally, we review the potential and limitations of injectable hydrogels in cancer immunotherapy.
Introduction
As early as the 19th century, someone put forward the idea of using our immune system to classify and damage cancer cells, known as tumor immunotherapy (Wiemann and Starnes, 1994). Immunotherapy is one type of treatment that uses regulators or drugs to initiate and regulate the immune response to destroy malignant cells. Cancer immunotherapy is a revolutionary effective cancer therapy method next to traditional operation, chemotherapeutic and radiotherapeutic (Topalian et al., 2015; Nam et al., 2019). It has changed people’s way of thinking from directly destroying cancer cells to recognizing and attacking cancer cells by triggering the host’s own anti-cancer immunity. There are more than 30 different types of immunotherapies approved by FAD, mainly immunosuppressants, tumor vaccines, cytokines, chimeric antigen receptor T cell (CAR-T) therapies, and immune checkpoint blockade (ICB) (Postow et al., 2015; Riley et al., 2019a; Sterner and Sterner, 2021). Different from conventional treatment approaches, such as surgery, radiotherapy, chemotherapeutic, etc., which are merely effective for resident solid cancers, immunotherapeutic can destroy resident and remote metastatic cancers, and inhibit cancer recurrence. However, traditional cancer immunotherapy methods often have disadvantages such as expensive cost (Zhao et al., 2020), low therapeutic index, poor targeting, high drug resistance, and serious immune-related adverse events (IRAEs), which are primarily ascribed to high doses or multiple injections of immunotherapy drugs (Blattman and Greenberg, 2004; Milling et al., 2017; Senapati et al., 2018; Riley et al., 2019b; Zhao et al., 2019; Haanen et al., 2020). The traditional immunotherapy strategy is often a systemic administration of immune drugs. Unfortunately, only a small number of drugs administered intravenously can reach the target of cancer cells in their body, while the remaining circulating drugs may damage normal cells. Therefore, to improve the efficacy of each dose and reduce side effects, appropriate nanomaterials are selected as drug carriers to overcome the biological barrier and achieve enhanced uptake of immunotherapeutic agents in cancer cells (Riley et al., 2019b; Zhao et al., 2019; Yan et al., 2020). Among them, hydrogel, due to its high biocompatibility, allows for a multi-purpose design to provide both sensing and therapy (Oliva et al., 2017).
Over recent years, hydrogels have been widely studied by scholars for its excellent physical, chemical and biological performances and potential application prospect. They are highly crosslinked, water-swelling networks of hydrophilic three-dimensional polymers that retain a lot of water in its 3D network (Buwalda et al., 2014). Hydrogels formed in situ has recently become one of the most favorable biomedical ingredients and can be sprayed directly or delivered into the body. In situ hydrogels have shown several advantages, including fewer intrusive handling, easy use, effortless cell embedding, and proficient encapsulation (Liao et al., 2022). The application of hydrogels in many aspects has shown great promise. So far, it has been applied as typical biocompatible materials (Huang et al., 2017; Tong and Yang, 2018), wound repair (Liu et al., 2007; Tavakoli and Klar, 2020), drug delivery (Dimatteo et al., 2018; Mathew et al., 2018), cell therapeutic (Sivaraj et al., 2021), tumor therapeutic (Yu et al., 2018a; Bu et al., 2019) and extra areas.
Hydrogel is an appealing “intelligent” drug delivery system, which can deliver a variety of bioactive agents to specific locations, and has the ability of controllable and supportable release (Singh and Peppas, 2014; Li and Mooney, 2016). By implanting hydrogels, immunotherapy drugs are delivered in the body so that they can circulate in the blood for longer periods, accumulate effectively in tumors, increase drug concentration, and actively target cancer cells to improve the efficacy of anti-tumor immunotherapy (Jin et al., 2018). Therefore, injectable hydrogels can effectively overcome the above obstacles of systemic administration. At present, injectable hydrogels have become a significant part of tumor immunotherapy (Yu et al., 2018a).
Injectable hydrogels for cancer immunotherapy
Various immunotherapy drugs that can cause anti-tumor immune responses have been developed recently, but the inefficiency of drug delivery and serious side effects of these anti-cancer drugs throughout the body have hindered their use in cancer immunotherapy (Kim et al., 2022). It can specifically deliver and continuously release drugs with therapeutic effects at the target site, which not only reduces the systemic toxicity caused by systemic circulation but also improves the administration efficiency, therefore, the efficacy of cancer treatment is significantly improved (Elstad and Fowers, 2009; Wu et al., 2016; Shin and Kwon, 2017; Tiwari et al., 2018; Attama et al., 2022). What’s more, it enables the regulated release of the medicine, minimizing the non-specific distribution of the drug in healthy tissue (Hamidi et al., 2008; Li and Mooney, 2016; Xue et al., 2017; Riley et al., 2019b; Xie et al., 2021; Li Y. et al., 2022). This controlled drug delivery action based on polymer hydrogels with high spatiotemporal precision has been used in cancer immunotherapeutics (Thambi et al., 2016; Duong et al., 2020). The recent research progress of cancer immunotherapy based on injectable hydrogels, including immunotherapy alone, immunotherapy combined with chemotherapy, radiotherapy, phototherapy, and immunotherapy based on DNA hydrogel are currently being explored (Figure 1).
Sole immunotherapy based on hydrogel delivery
Immunotherapy drugs have presented huge promise for cancer, however, their widespread application in clinical practice is challenging (Zhao et al., 2019; Xie et al., 2021). Lately, the local administration of immunotherapeutic drugs based on the action of hydrogel drug carriers has attracted wide attention, and we will summarize the function of immune therapy drugs combined with hydrogels (Table 1).
Hydrogels based on a single immunotherapeutic agent are used in immunotherapy
Immunotherapeutic agents mainly include antigens, cytokines, adjuvants, checkpoint inhibitors, and immune cells, which can be individually loaded and transported by the hydrogel (Figure 2). Ovalbumin (OVA) can provoke robust OVA-specific immunity and has a good immune effect (Lee S.-J. et al., 2019; Habibi et al., 2020). According to the study, researchers devised a DNA hydrogel with auxiliary activity (Umeki et al., 2015). By conjugation of ethylenediamine (ED) and cationized OVA, complexes with hexapod structure DNA (ED-OVA) were developed with a slower release of OVA compared to unmodified OVA and induced higher IFN-γ production. Cytosine-guanine (CpG) dinucleotides is a positive immune adjuvant, and the integration of ED-OVA and immune-stimulating DNA injection hydrogels containing it is conducive to effective binding of DCs, thus improving the efficiency of antigen presentation.
Cytokines are a powerful weapon in immunotherapy, however, their half-life in patients is quite short. To overcome this major obstacle, Shimizu et al. loaded IL-12 into the cholesterol-containing pullulan (CHP) hydrogel to ensure its slow release of cytokines (Shimizu et al., 2008). A mouse model of CSA1M fibrosarcoma was established, and the hydrogel was injected subcutaneously into mice, which was found to cause a persisted increase in the concentration of IL-12 in serum. In addition, interleukin-15 (IL-15) is also a vital cytokine indispensable and has vast potential as tumor immunotherapy (Robinson and Schluns, 2017). Santi et al. prepared hydrogel microspheres (MS), which were connected to IL-15, to maintain IL-15 in vivo for a long time, namely ultra-long-acting IL-15 (Hangasky et al., 2022). It was found that the half-life of cytokines released from the library was prolonged, showing moderate tumor growth inhibition and effective and lasting anti-tumor activity.
Hydrogels load and transport immune checkpoint inhibitors to induce immune checkpoint blockade, which is a reliable immune tactic for the therapy of tumors. Chen and Liu’s group developed an injectable hydrogel based on betamethasone phosphate (BetP) for topical delivery of aPD-L1 (Chen et al., 2020). The study found that this steroid-based carrier-free system could reprogram the tumor microenvironment (TME) into anti-tumor TME, and it will continuously release aPD-L1 to combinational enhance the immunity system. The results showed that the gel administration of aPD-L1 greatly extended the retention time in the tumor. Gu’s group has developed a library of heat-sensitive and reactive oxygen species (ROS)-sensitive peptide hydrogels for sustained delivery of aPD-L1 and dextro-1-methyltryptophan (D-1MT), and modulates ROS concentration in the TME, facilitating the release of immunotherapeutic drugs that enhance efficacy against melanoma in vivo (Yu et al., 2018b). In addition, Yang’s group developed injectable supramolecular hydrogels for the topical delivery of DPPA-1 peptides and doxorubicin (DOX). DPPA-1 peptides can block the PD-1/PD-L1 pathway to enhance the T cell-mediated immune response (Liu et al., 2021). Similarly, Shi et al.’s study demonstrated that aPD-L1 and Dox combined hydrogel therapy with B16F10 melanoma models significantly restrained cancer progression and extended animal survival (Shi et al., 2021).
Dendritic cells (DCs) can activate the key APCs of immature T cells, which are effective initiators of the immune response (Banchereau et al., 2000; Steinman and Banchereau, 2007; Gardner et al., 2020). Treatment based on autologous dendritic cell injection has become a well-studied strategy (Bol et al., 2016; Santos and Butterfield, 2018; Bol et al., 2019; Perez and De Palma, 2019). Irvine et al. prepared an injectable hydrogel by combining alginate microsphere with DCs. The purpose was to achieve long-term storage of dendritic cells at a determined location, capture cytokines secreted by dendritic cells, and start the natural T cell immune response (Hori et al., 2008). The DCs vaccine is a promising and powerful immunotherapy for cancer, but it has drawbacks that cannot be ignored, the most critical one is that the transplanted DC dies, or is lost to a non-cancerous site, and rarely returns to the lymph nodes (Palucka and Banchereau, 2013; Abraham and Mitchell, 2016; Cornel et al., 2018), thus causing the T cell response is not strong enough. In recent years, there are still many studies on hydrogel-based dendritic cell vaccines. For example, Wang’s study showed that the hydrogel containing DC, aPD-1, and tumor antigen caused a robust immune response, showing excellent antitumor immunotherapeutic effect (Yang et al., 2018). Recently, Zheng and his colleagues devised a Sendai virus (SeV) based hydrogel vaccine (SHV), which can effectively inhibit the occurrence of melanoma (Zheng et al., 2021). In addition, to overcome the limitations of rapid spread and short half-life of therapeutic drugs at the tumor site (Chang et al., 2011; Liau et al., 2018; Goradel et al., 2021), Yun et al. used gelatin hydrogels to co-deliver onco-lytic adenoviruses AD. (Oh et al., 2017) This study discovered that, compared with single cure, the effect of this method on tumor growth was significantly increased. For hydrogels containing immune cells, some key questions should be considered to facilitate their potential applications. For example, these hydrogels require good biodegradability to achieve DC release.
The biological activity of a single immunotherapeutic drug is limited, so this strategy is not ideal for tumor treatment. Therefore, scientists have considered delivering multiple immunotherapeutic agents at the same time.
Hydrogels based on multiple immunotherapeutic agents are used in immunotherapy
Recently, the PCL-PEG-PCL thermos-sensitive hydrogel encapsulated GM-CSF and OVA NPs to improve antigen absorption efficacy (Sun et al., 2018). It could trigger an effective immune response. In another study, Chen et al. co-encapsulated nano-hydroxyapatite (nHA) and GM-CSF into a biocompatible heat-sensitive hydrogel (Chen et al., 2022). Interestingly, the biological activity of nHA and GM-CSF can not only be very kept in hydrogel, but the addition of nHA can also weaken the erupt emancipate of GM-CSF, which is conducive to the continuously release of GM-CSF and realize enhanced and extended anti-melanoma immunity.
In another study, Wang et al. developed a PEG-b-poly (L-alanine) hydrogel, in which the tumor cell lysate, GM-CSF, and a dual immune checkpoint inhibitor (anti-CTLA-4/PD-1 antibodies) were easily loaded into (Song et al., 2019). It disclosed that the continuous release of cancer antigen and GM-CSF continuously recruited and stimulated DCs, and provoked robust T cell response. Immune checkpoint therapy boosted T cell response, and also upregulated the creation of IgG and cytokine secretion. Importantly, hydrogel combination therapy has a better immunotherapeutic effect on melanoma and 4T-1 tumor than using the vaccine alone or blocking immune checkpoints alone.
Overall, the co-delivery of hydrogel-based multiple immune therapeutics generally results in higher efficacy in treating tumors than a single drug (Blidner et al., 2020; Ramos-Casals et al., 2020). In addition, the design of such intelligently delivered composite hydrogels is often complex, which is a test for researchers.
Chemotherapy-combinational immunotherapy
Chemotherapy is a cancer treatment in which various chemicals, such as chemotherapy drugs, are used to directly kill cancer cells (Anand et al., 2022). Chemotherapeutics is considered one of the most widespread approaches for treating malignancies (Liu et al., 2020; Xie et al., 2020; Yin et al., 2020). In addition, it has been shown that several chemotherapy agents that cause apoptosis of tumor cells can induce immunogenic cell death (ICD), thereby converting non-immunogenic tumors into immunogenic phenotypes, resulting in anti-tumor immune responses (Johnson et al., 2017; Wang Q. et al., 2018; Chattopadhyay et al., 2020; Wang-Bishop et al., 2020). However, chemotherapeutics is also associated with several general side effects unfortunately, such as bone marrow suppression and neurotoxicity, which often limit the effectiveness of chemotherapy drugs. Surprisingly, hydrogels can effectively reduce side effects by releasing drugs locally (Fan et al., 2019). Strategies using injectable hydrogels for local co-delivery of chemotherapy drugs and immunotherapy drugs have been applied to trigger long-term effective anti-malignant immune responses.
Combination of chemotherapy with stimulant-mediated immunotherapy
Polymer-based hydrogels can be loaded with multiple chemotherapy drugs for cancer at the same time, and the efficacy can be improved through drug combinations. Melittin is a very potent anti-cancer agent, however, hemolysis is the main limitation of its application (Rady et al., 2017; Wang et al., 2022). To overcome this limitation, Yang et al. devised a peptide hydrogel encapsulated with DOX and Melittin-RADA32 (Jin et al., 2018). It can stimulate DCs, specifically consume M2 TAMs and recruit activated NK cells from tumors to further protect cells from residual tumors. It has significant anti-tumor effects on subcutaneous and metastatic tumors in vivo.
DOX is a potent anti-tumor cytotoxic chemotherapy drug and a representative ICD inducer that can lead to apoptosis of cancer cells (Hu et al., 2021). There has been a lot of research on the effective treatment of cancer using DOX as a chemotherapy drug loaded into hydrogels along with immunotherapeutic agents. Recently, Lv et al. devised an injectable hydrogel for the programmed delivery of DOX and cytosine-phosphate-guanine (CpG) nanoparticles guided by dual fluorescence imaging (Dong et al., 2020). CpG as an immune adjuvant can successfully stimulate an antitumor immune response by interacting with Toll-like receptor 9 (TLR9), and according to previous studies, the amalgamation of DOX and CpG is one of the most useful companions of chemotherapeutic immunotherapy (Kumagai et al., 2008; Wang Z. et al., 2017; Yu et al., 2017; Xia et al., 2018; Kheirolomoom et al., 2019). CpG self-crosslinked nanoparticles from hydrogels ensure long-term immunostimulant effects of dual continuous delivery systems. In addition to inducing apoptosis of tumor cells by doxorubicin, this hydrogel actively modulated the tumor microenvironment, which will construct a more favorable treatment response.
Imiquimod (R837) is also an immune adjuvant that activates TLRs and nuclear factor-kappa B (NF-κB) (Schon and Schon, 2007). Previously, Zhang et al. prepared a novel near-infrared (NIR) in situ resistant tumor vaccine by combining hyaluronic acid-modified polydopamine nanoparticles (HA-PDA NPs) with immunoadjuvants R837 and DOX into a thermosensitive hydrogel (Zhang et al., 2021). Due to the long retention time at the tumor site, after a single injection of near-infrared radiation, the NPs are endowed with multiple photothermal ablation characteristics. What’s more, this pattern enables it to stimulate DC maturation and trigger a robust anti-tumor immune response. Due to membrane permeability, nanoparticles can successfully enter the cancer tissues, generate tumor-associated antigens in situ, induce DC maturation and secrete related cytokines in vitro. Recently, Zhang et al. devised an injectable hydrogel loading DOX and R837 for the synergistic therapy of melanoma (Li J. et al., 2022). It efficiently inhibits melanoma growth and metastasis in vivo by DOX-based ICD, and R837-based immune responses are secreted by DC maturation, M1 type macrophage activation, TNF-α, and IFN-γ. This shows that this continuous release system can provide effective cancer treatment strategies, showing the potential of precise targeting tumor therapy.
Injectable hydrogels can be utilized to load and release chemotherapy agents and cytokines for chemoimmunotherapeutic. Chen et al. prepared an in situ forming hydrogel for the joint delivery of IL-15 and cisplatin (Wu et al., 2017). This peptide-based hydrogel can reduce systemic toxicity as a topical drug delivery vehicle. It can mediate the S-phase cell cycle block and increase CD8+ T cells. In another study in the same group, DOX-IL-2-IFN-γ co-loaded hydrogel was used for the chemical immunotherapy of melanoma. The antitumor effect was enhanced by expanding the proportion of apoptosis and G2/S phage cycle block (Lv et al., 2018).
In addition to the above stimulants, other immunomodulators also can be combined with hydrogels for amplified tumor immunotherapy. For example, In the article by Luan et al., the L-norvaline-based immunomodulatory gel is reported, which can effectively block the arginase 1 (ARG1) pathway (Ren et al., 2021). ARG1 can impair the synthesis of the T cell receptor (TCR) chain, contributing to reactive inactivated T cells (Bronte and Zanovello, 2005; Rodriguez et al., 2007; Geiger et al., 2016). The reactive cleavage of the hydrogel peptide bond ensures the controlled release of L-norvaline, thereby efficiently blocking the ARG1 pathway. DOX was further introduced to the hydrogel to trigger the ICD. It was found that this strategy showed a strong immunotherapeutic effect. Recently, Zhang et al. developed biomimetic nanobubbles loading DOX (DOX@LINV) for combined immunochemotherapy by blending synthetic liposomes with tumor-derived nanovesicles (TNVs) (Hu et al., 2021). Overall, DOX@LINV was found to enhance the penetration of effector immune cells and improve the immune suppressive TME.
Combination of chemotherapy with checkpoint blockade immunotherapy
Injectable hydrogels are widely employed in the combined delivery of chemotherapy agents and immune checkpoint inhibitors for combined chemoimmunotherapy. The immune checkpoint inhibitors provoke a combined antitumor immune response by intervening with suppressive T cell signaling (Jenkins et al., 2018).
In cancer immunotherapy, it significantly enhances treatment response for immune checkpoint inhibitors to aim at the CTLA-4 and PD-1/PD-L1 axes (Littman, 2015; Klevorn and Teague, 2016; Robert, 2020; Naimi et al., 2022). For instance, Gu and others obtained ROS-responsive hydrogels by crosslinking polyvinyl alcohol (PVA) for co-delivery of chemotherapy gemcitabine and aPD-L1 (Wang C. et al., 2018). The designed hydrogels can be used to load and release therapeutic drugs when implanted at the tumor site because ROS is very abundant. This study showed that this type of gel scaffold promoted immunogenic tumor phenotyping and enhanced the anti-tumor response by local release of aPD-L1. In another study, a dual bioresponsive gel depot for co-deliver aPD1 and Zebularine is developed (Ruan et al., 2019). The results showed that this synergetic therapeutic improved tumor cells’ immunogenicity.
Additionally, Yang et al. fabricated an injectable thermosensitive hydrogel loading DOX and PD-L1 agonist peptide (DPPA-1) (HDU hydrogels) (Liu et al., 2021). The HDU hydrogel steadily emitted encapsulated DOX and DPPA-1 to stimulate an antitumor immune response. DPPA-1 is based on the local blockade of PD-1/PD-L1 pathway, thereby enhancing the immune response mediated by T cells and reducing toxicity (Figure 3). In another study, Cui’s group established a prodrug hydrogel for locally delivering ICBs to further boost antitumor immunity (Wang et al., 2020). Their research results show that this hydrogel can be used as a continuous response emancipation library in TME, inducing powerful PD-1 to block the immune response.
Chemotherapy combined with injectable hydrogels containing immune checkpoint inhibitors is more effective than hydrogels loading a single immune checkpoint inhibitor (Table 2). Despite this, most synthetic hydrogels with complex structures should be carefully evaluated for long-term toxicity. In addition, the load ratio of agents should be elevated to achieve the maximum therapeutic benefit (Xie et al., 2021).
Radiotherapy-combinational immunotherapy
Radiation therapy(RT), a method of treating tumors by killing cancer cells with ionizing radiation of high-energy rays, is widely used in treatment because of its immediate and sustained response, accompanied by moderate inflammatory changes (Formenti and Demaria, 2013). X-rays, γ rays, and heavy ions are common types of radiation used in cancer radiation therapy (Baskar et al., 2012). Radiation has previously been presented to trigger an antitumor immune response, and radiotherapy has an immune-modulatory effect on the TME and adjacent normal tissues (Reits et al., 2006; Galluzzi et al., 2017; Guipaud et al., 2018; Cytlak et al., 2022). The influence of radiation therapy on the tumor immune system depends on the immune environment, dose, and the fractionation of radiation therapy (Romano et al., 2021). To overcome these problems, researchers have devised a protocol for loading radioactive material onto hydrogels (Table 3).
Liu et al. designed an in situ formed approach in which 131I radioisotope-labeled catalase (Cat) is encapsulated on alginate-based hydrogels to instigate radioimmunotherapy (Chao et al., 2018). Compared with brachytherapy, it shows a significantly superior therapeutic effect. Radioisotope-labeled enzymes are trapped within the tumor along with immunoadjuvants, consequently, radioisotope therapy (RIT), which is hypoxia-relieving, has an excellent local tumor-killing effect on the primary tumor.
Certain forms of chemotherapy drugs and ionizing radiation are known to induce ICD (Galluzzi et al., 2013). If immune adjuvants are present in the tumor, this anti-tumor immunity is amplified further (Steinhagen et al., 2011). However, since clinical chemotherapy/radiation therapy is often repeated in the form of low doses, it is unreasonable to administer immunoadjuvants to cancers at chemotherapy/radiation therapy dosage (Sun et al., 2021). Therefore, recently, Liu et al. devised an intelligent hydrogel that conjugates ALG to an ATP-specific aptamer, which is crossed with CpG oligonucleotides subsequently and forms an alginate-based hydrogel in situ during the intratumoral injection (Sun et al., 2021). By irradiating CT26 mouse colon cancer cells with an X-ray dose gradient, it was found that intracellular ATP concentrations increased significantly. This suggests that X-rays can trigger the release of ATP while inducing ICD in tumor cells. ATP then competitively binds to apt to trigger CpG release, thereby enhancing anti-tumor immunity after ICD induction therapy. It was found that the in situ smart hydrogel based on ALG significantly enhanced anticancer immune responses and boosted the overall therapeutic effect of repeated chemotherapy combined with immunotherapy. Thus, this smart hydrogel can release immune adjuvants synchronized with radiotherapy, and other experiments have also proved that this smart hydrogel achieved a significant synergistic reaction and inhibited tumor regeneration and metastasis.
Another study of radiotherapy combined with immunotherapy was recently published. Liu and others modulated immunosuppressive tumor microenvironment (ITM) and overcome radioactivity by coupling the Toll-like receptor agonist TLR7/8a with a radiation-sensitive hydrogel (Smac-TLR7/8 hydrogel) to adjust TAMs repolarization from M2 type into M1 type (Zhang et al., 2022). In the C57BL/6 mouse model, after treatment with reasonable doses of γ irradiation, it was found that TMs could be repolarized to the M1 type by stimulating the NF-κB pathway, which significantly improved the radiotherapeutic effect on mouse tumors. What’s more, macrophage repolarization induced immune responses, promoted the recruitment of tumor-infiltrating lymphocytes, and decreased regulatory T cells, effectively alleviating the ITM. This study showed that this novel strategy, both hydrogel-based radiation therapy combined with immunotherapy, reconstructed ITM through repolarizing TAMs, effectively improving RT efficacy, and overcoming radioactivity (Figure 4).
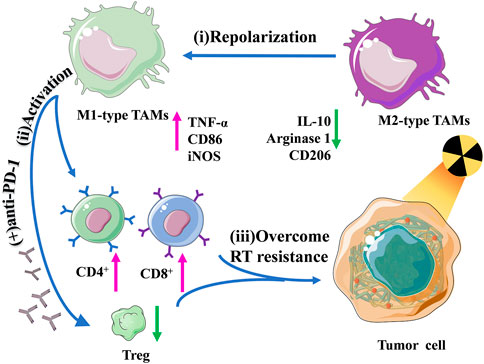
FIGURE 4. Schematic illustration of the regulation of macrophage repolarization to overcome radiation resistance by the Smac-TLR7/8 hydrogel.
Phototherapy-combinational immunotherapy
Photosensitizer-based photoactivation therapy has been determined as a harmless way of tumor ablation for many tumor indications. There are mainly two methods: photodynamic therapy (PDT) causing local chemical damage, and photothermal therapy (PTT) causing local thermal damage (Li X. et al., 2020). In addition, PTT and PDT perform a vital part in activating anti-tumor immune responses because they can initiate ICD, thereby increasing tumor immunogenicity (Ng et al., 2018; Li X. et al., 2020). The use of nanomaterials as carriers combined with phototherapy and immunotherapy can magnify the immune response and enhance the curative effect, therefore, several hydrogels have been exploited for phototherapeutic combination immunotherapeutic for the treatment of cancer.
Photothermal therapy (PTT) -combinational immunotherapy
PTT is a hopeful approach that employs ingredients with extraordinary photothermal conversion efficiency to transform illumination energy into heat (Huang et al., 2006; Yang et al., 2010; Huang P. et al., 2019). The PTT-induced hyperthermic effect defeats cancer cells by damaging cell membranes and generating DNA injury, thereby causing cell necrosis, cell apoptosis as well ICD (Hildebrandt et al., 2002; Li Y. et al., 2020; Gao et al., 2020). By filling it and immunotherapeutic agents into hydrogel carriers, PTT combination immunotherapy can be achieved.
PTT has been reported to promote the discharge of the protein antigens from tumor by inducing immunogenic cell death, which exposes large amounts of autoantigens after PTT treatment, thereby enhancing a robust immune response (Ng et al., 2018; Wang et al., 2019). Wang et al. presented a heat-sensitive nanogels loading MnO2 NPs to activate anti-tumor immune responses (Fan et al., 2021). In particular, the catechol group is introduced into the structure of the heat-sensitive nanogel, and its strong adhesion gives it the capability to capture the antigens produced by PTT. After intertumoral injection of hydrogel, it continuously releases antigens to realize improved and extended immune stimulation, inducing both strong cytotoxicity and ICD. It indicated that the use of PTT based on injection of adhesive hydrogel resulted in complete ablation of the solid tumor and efficient inhibition of distal and regenerated cancer cells.
The combination of photothermal effect and immune adjuvant will result in a more effective immunotherapeutic effect, there has been a lot of research on PTT-combination immunotherapy based on hydrogels loaded with immunoadjuvants (Guo et al., 2014). Nishikawa’s group devised a compound immune-stimulatory hydrogel consisting of hexapod DNA with CpG sequence and Au NPs (Yata et al., 2017). Laser irradiation l leads to hexapods release, which effectively stimulates the stimulation of macrophages and DCs, and stimulates the emancipation of pro-inflammatory cytokines.
In another study using CpG as an immune adjuvant, Lv and coworkers proposed a conjugated hydrogel with CpG self-crosslinked NPs and IR820 for combined PTT and immunotherapeutic (Dong et al., 2019). It can advance continuous release by blocking rapid degradation of CpG, with CpG-mediated immunostimulatory effects of TLR-9 activation. In the B16 melanoma mouse model, after injection of CpG NPs/IR820 hydrogel and irradiation treatment, DC maturation and CD8+ T cell activation were moderately enhanced, and the anti-tumor immune effect was enhanced. More specifically, auto-fluorescent CpG NPs/IR820 hydrogels do not need additional fluorescence and are used for image-guided synergistic PTT and immunotherapeutic using a dual fluorescence imaging technique. It provides a platform full of possibilities and hopes for precision cancer diagnosis and therapy. Furthermore, Wei et al. designed a local injectable platform to achieve combined PTT and immunotherapy, which was formed by the photothermal agent Indocyanine Green (ICG), the TLR-7/8 agonist (R848), and the TLR-9 agonist CPG ODN (Jia et al., 2020). NIR triggered the thermally responsive hydrogel PLEL to release the immune components CPG ODN and R848, which, together with tumor-associated antigens, induce effective and sustained anti-tumor immunity as carcinoma in situ vaccines for postoperative immunotherapy.
Apart from immune adjuvants, strategies for immune checkpoint blocking can also be introduced into injectable hydrogels for photothermal immunotherapeutics. As Sun et al. proposed an all-in-one and fully controlled combination strategy, NIR photothermal agents IR820 and aPD-L1 were loaded into a lipid gel library with excellent thermally reversible gel-sol phase transition properties to achieve native symbiotic PTT-assisted immunotherapeutic (Huang L. et al., 2019). This study shows that symbiotic mild photothermal-assisted immunotherapy is an operational and potential approach for curing “cold” tumors. Other than that Li et al. proposed a personalized cancer vaccine (PVAX) for postoperative immunotherapeutics, by loading tumor cells with a BRD4 inhibitor and ICG into the hydrogel-based matrix (Wang T. et al., 2018). PVAX can advance the growth of DCs, and trigger tumor penetration of cytotoxic T lymphocytes.
PTT can also be used in combination with chemotherapy for cancer immunotherapy. For instance, Liu’s group developed a Ag2S QD/DOX/Bestatin@PC10ARGD peptide gel for breast cancer treatment as a lasting-release material (Hou et al., 2020). The experiments were carried out and it presented that the laser-irradiated hydrogel could stimulate anti-tumor immunity, relieve tumor pressure, and inhibit primary tumor and lung metastasis. At the same time, the safer low-temperature several laser irradiation approaches showed more effective tumor-killing performance. In summary, the strategy of combining PTT with other therapies based on injectable hydrogels effectively improves the effect of cancer treatment and is a promising and potential option.
Photodynamic therapy (PDT)-combinational immunotherapy
Lately, PDT has fascinated widespread notice owing to its little toxicity, great spatiotemporal selectivity, minimally invasive, and considerable therapeutic effect (Zhang and Li, 2018; Shu et al., 2021). It can not only exactly destroy cancer cells, but also initiate ICD, providing anti-tumor immunity. Regrettably, the inherent nature and complex limitations of TMEs drastically reduce the efficacy of PDT (Ji et al., 2022). Emerging smart polymer hydrogels have been developed, loaded with immunotherapeutic agents through the combined action of PDT and immunotherapy, cleverly modulating the pharmacokinetics of drugs and TME, thus improving the anti-tumor efficacy.
Oxygen is essential for PDT to perform therapeutic roles, but the hypoxic TME present in most solid tumors will significantly restrain the efficiency of PDT (Yang et al., 2017; Min et al., 2021). To relieve hypoxic TME, Liu et al. used PEGDA as a polymer matrix to construct a light-triggered gelation procedure encompassing catalase modified by the photosensitizer (Meng et al., 2019). First, the photosensitizer Chlorin e6 (Ce6), which is extensively used in clinical, is coupled with catalase (CAT), and then the resulting coupling is mixed with the polymer matrix PEGDA and R837-loaded PLGA nanoparticles (RPNPs) as immune adjuvants. The retained CAT in the hydrogel reverses immunosuppressive TME by decomposing endogenous H2O2 of tumors to produce O2 leading to sustained remission of tumor hypoxia. Subsequently, tumor cell fragments after PDT-triggered ICDs can pretend TAAs and elicit a strong anti-tumor immune response alongside with RPNPs. In addition, this hybrid hydrogel can offer the chance to continually stimulate the immune response by triggering PDT through several times of lighting, which contribute to an extensively improved immune response. Studies have also found that further combination with a CTLA-4 checkpoint blockade could inhibit the evolution of distant metastatic cancers.
Sun’s team developed a continuous luminescent immune hydrogel by introducing a persistent luminescent material (PLM) and immunoadjuvant R837 into alginate-Ca2+ to convert solids into gels (Shu et al., 2021). PLM is a unique property that can stimulate continuous and repetitive PDT (Lécuyer et al., 2016; Wang J. et al., 2017; Wu et al., 2020; Wang et al., 2021). PLM, R837 and alginate-Ca2+ solutions were mixed to synthesize a rechargeable immune hydrogel (PRA) with a homogeneous structure. The designed PRA has great biocompatibility and excellent injectability, and can be effortlessly inserted into the tumor site, which the continuous luminescence efficiency of PLM can reach 100%. The experiments have exposed that DCs are activated, resulting in increased emission of TNF-α and IL-6, and a significant amplification of the anti-cancer immune response. It showed that the reliable anti-tumor immune effect is obtained by hydrogel-based PDT synergistic immunotherapy.
Recently, Li and others reported the use of NIR dual-response precursor hydrogels for synergistic immunotherapy for cancer (Ding et al., 2022). This prodrug hydrogel is formed by calcium-induced iron oxide (Fe3O4) nanoparticles modified with the photosensitizer protoporphyrin IX (PpIX) and aPD-L1 prodrug nanoparticles crosslinked through ROS-responsive linkers. This hydrogel enabled the on-demand discharge of aPD-L1 during photoactivation. This type of prodrug hydrogel could stimulate ICD by mediating photokinetic and chemokinetic therapy and expand the efficiency of aPD-L1-mediated immune checkpoint blockade. Together, this study provided a double-response hydrogel strategy for precise tumor immunotherapy.
Sometimes, two combinations of therapies still do not meet the therapeutic effect, so researchers try to use multiple treatments to enhance the efficiency and achieve the purpose of treatment. For example, Cao and Liu et al. devised and formulated a hydrogel based on DOX, polypeptide PC10A, and MoS2 nanosheet (Figure 5). (Jin et al., 2020) Firstly, positively charged DOX and negatively charged PC10A are filled on the exterior of MoS2 nanosheets by electrostatic adsorption, and then hybrid PC10A/DOX/MoS2 NPs are ‘dissolved’ in PC10A hydrogel to form an injectable hydrogel. The experiments showed that it has excellent photothermal efficiency. MoS2 nanosheets are materials with both PTT and PDT effects. It was found that the amount of DC, CD4+ T cells, and CD8+ T cells increased, and the combination of these treatments greatly increased the number of tumor cell apoptosis. In conclusion, this study showed that the combination therapy meaningfully enhanced the impact of tumor inhibition and inhibited the development of primary tumors and remote metastasis.
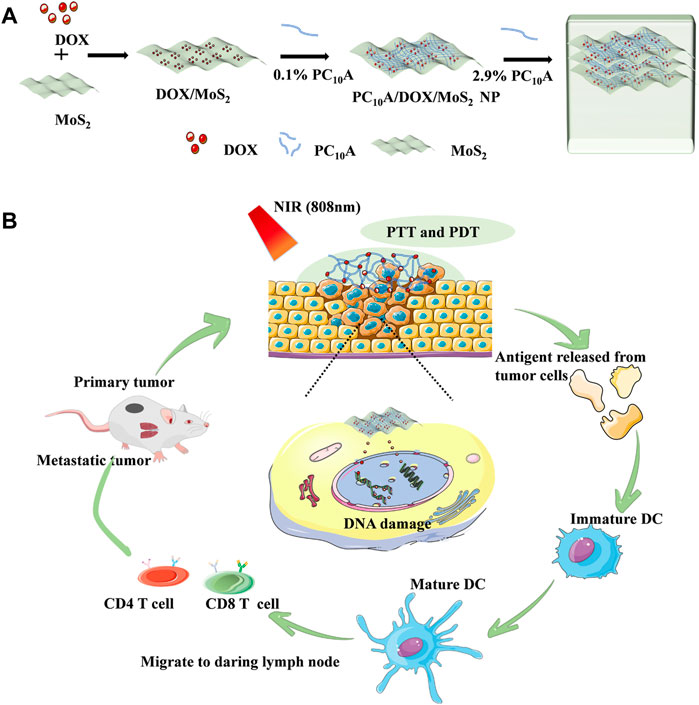
FIGURE 5. Schematic illustration of injectable hydrogels with both PTT and PDT effects based on DOX, peptide PC10A and MoS2 nanosheets. (A) Schematic illustration of the preparation for PC10A/DOX/MoS2 hydrogel; (B) Chemo-photothermal-photodynamic therapy.
In summary, phototherapy-activated ICDs can stimulate immune responses, hydrogel-mediated combination phototherapeutic and immunotherapeutic generally provide better efficiency than hydrogel-mediated immunotherapeutic (Table 4). Compared to hydrogel-mediated combination chemoimmunotherapy, this approach likewise showed excellent controllability in space and time.
DNA hydrogel and cancer immunotherapy
Gene therapy is a developing targeted therapy approach for the treatment of malignant tumors. It aims to prevent tumor proliferation and metastasis by specifically targeting genes closely related to tumor genesis, progression, and prognosis. However, currently, there are few studies about hydrogels for gene therapy combined with immunotherapy.
The Achilles heel of gene therapy is gene conveyance, which has been difficult to achieve the desired success as a result of the shortage of an operational delivery system, prolonged-expression, and host immune response (Verma and Somia, 1997). However, today, the research on excellent delivery systems is more extensive and deep, which can help overcome this problem and make the future of gene therapy full of possibilities. Current great advances in the development of DNA-based nanostructure technology, it has several advantages as a drug delivery procedure, such as its high water-solubility, stability, and biodegradability (Chhabra et al., 2010; Nishikawa et al., 2010; Nishikawa et al., 2011; Rangnekar and LaBean, 2014). DNA hydrogels have the advantages of outstanding biocompatibility, adjustable mechanical properties, controllable phase transitions, and simple preparation, and have broad prospects as suitable carriers (Mo et al., 2021). As an excellent drug delivery platform, DNA hydrogel can deliver different drugs, including functional macromolecules, small molecule drugs and inorganic materials, and plays an important role in cancer chemotherapy, immunotherapy and gene therapy (Figure 6). (Mo et al., 2021) Several studies of immunotherapy based on DNA hydrogel delivery will be presented next.
Nishikawa and others fabricated a novel greatly immunostimulatory DNA component by combing six very effective CpG motifs into X-DNA and formulated hydrogel by ligating with DNA ligase, and doxorubicin(DXR) was inserted into DNA subsequently (Nishikawa et al., 2011). This kind of DNA was found to be more operational than its component or CpG counterpart. The anti-tumor ability of the synthesized hydrogel was examined in colon 26/Luc cells, and CpG transmitted an immune stimulation signal to TLR9-positive immune cells to slow-release DXR.
Li et al. demonstrated a DNA supramolecular structure hydrogel vaccine (DSHV) consisting of the TLR9 agonist unmethylated CpG, and tetanus toxoid-derived amino acid peptide P30 co-loaded into the DNA hydrogel network (Shao et al., 2018). The amino acid peptide P30 is a helper T cell epitope that has been shown to conjugate to the MUC1 glycopeptide antigen and can cause a super strong immune response and induce a stronger immune response and is considered a very hopeful therapeutic antitumor vaccine (Cai et al., 2013). This study found that the DSHV system can usefully recruit and trigger APCs. The process in vitro can be imagined by fluorescence microscopy. In vivo experiments, by intraperitoneal or subcutaneous injection of BALB/c mice, it was found that the DSHV system can simulate the role of lymph nodes (LNs). At the same time, antigenic peptides exert a strong immune effect and synergistic anti-tumor immune effect in DNA supramolecular hydrogel.
It’It is well known that CRISPR-associated protein 9 is a potential genome editing tool, which is a complex of RNA-guided DNA endonuclease single-stranded guide RNA (sgRNA) and can enable Cas9 to cut DNA sequences precisely (Anders et al., 2014; O'Connell et al., 2014; Jiang and Doudna, 2017). In a particular study, researchers explored the usage of Cas9/sgRNA to regulate the emancipation of DNA aptamers from the hydrogel. Wu’s group used Cas9/sgRNA’s specific double-stranded DNA editing capability which can emancipate PD-1 aptamers programmatically (Lee J. et al., 2019). PD-1 DNA aptamers act as immune checkpoint inhibitors to generate hydrogels comprising it and sgRNA targeting sequence by rolling loop amplification (RCA). When mingled with Cas9/sgRNA, it may lose gel property and accurately release PD-1 aptamer sequences, and gel electrophoresis confirmed that this program release mode can successfully stimulate the occupation of splenocytes to secrete cytokines. Furthermore, molecular imaging showed higher anti-tumor effects and survival rates. It indicated that the combination therapy of the DNA-based hydrogel plus Cas9/sgRNA exerted anti-cancer effects by supporting the initiation of the immune system. This study showed that hydrogel has powerful anti-cancer immunotherapy potential, and also demonstrates a promising future in which gene editing plays a therapeutic role in synergistic with immunotherapy to treat cancer.
The results of these studies show that DNA can be operated as a transmission system of immunostimulatory signal and antitumor agents, and has a promising application value. It is supposed that the cooperation of gene therapy and immunotherapy based on hydrogel will achieve a big step forward and help human beings climb the mountain of “cancer”.
Conclusion and outlook
In this article, the research progress of injectable hydrogel in cancer immunotherapy was discussed. The biocompatibility, injectability, degradability, easy encapsulation, stimuli-responsive, and local sustained release of hydrogels make them promising cancer immunotherapy materials (Bu et al., 2019). Single or multiple cancer immunotherapies and combination therapies can trigger and enhance a strong immune response, which can effectually restrain the growth of solid tumors. However, there are still some unavoidable problems with this treatment strategy. First, individual heterogeneity of patients may be the cause of cancer treatment failure, so there is also a need to strengthen research on the immune system, innovate and optimize injectable hydrogels to achieve personalized ideal treatment. Secondly, synthetic polymer-based hydrogels have the potential to cause chronic inflammation and immune response, and it is also necessary to continuously monitor the toxicity and biocompatibility of synthetic hydrogels and their degradation products (Merkle, 2015; Piluso et al., 2017). Thirdly, how to accurately inject hydrogels into deep tumors is a huge challenge. Multiple imaging techniques for deep tumor administration should be developed to guide the injection process. At last, the dosage and release rate of immunotherapeutic agents administered in vivo still needs to be further explored, and in clinical practice, the dose and rate of administration should be strictly controlled. Fifthly, the vast majority of existing research is based on mouse models, to achieve clinical application and experiments on other large animal models.
In short, hydrogels are materials with excellent prospects for cancer immunotherapeutic, and although there are many challenges to be faced in the future, we believe that with the unremitting efforts of scientists, we will be able to develop an ideal hydrogel delivery system to maximize the immune effect to treat cancer, and this biggest “threat” to human life and health will 1 day be defeated.
Author contributions
YuL, YZ, and JL conceived this review. CL, YiL, LL, and LX searched the literature, wrote the review, and prepared the table. YiL and CL prepared the figures. YZ, YuL, and JL revised the article. All authors are involved in the revision and approved the submitted manuscript.
Funding
This work was supported by the Medical Scientific Research Foundation of Guangdong Province of China (NO. 20211100).
Conflict of interest
The authors declare that the research was conducted in the absence of any commercial or financial relationships that could be construed as a potential conflict of interest.
Publisher’s note
All claims expressed in this article are solely those of the authors and do not necessarily represent those of their affiliated organizations, or those of the publisher, the editors and the reviewers. Any product that may be evaluated in this article, or claim that may be made by its manufacturer, is not guaranteed or endorsed by the publisher.
References
Abraham, R. S., and Mitchell, D. A. (2016). Gene-modified dendritic cell vaccines for cancer. Cytotherapy 18 (11), 1446–1455. doi:10.1016/j.jcyt.2016.09.009
Anand, U., Dey, A., Chandel, A. K. S., Sanyal, R., Mishra, A., Pandey, D. K., et al. (2022). Cancer chemotherapy and beyond: Current status, drug candidates, associated risks and progress in targeted therapeutics. Genes & Dis. doi:10.1016/j.gendis.2022.02.007
Anders, C., Niewoehner, O., Duerst, A., and Jinek, M. (2014). Structural basis of PAM-dependent target DNA recognition by the Cas9 endonuclease. Nature 513 (7519), 569–573. doi:10.1038/nature13579
Attama, A. A., Nnamani, P. O., Onokala, O. B., Ugwu, A. A., and Onugwu, A. L. (2022). Nanogels as target drug delivery systems in cancer therapy: A review of the last decade. Front. Pharmacol. 13, 874510. doi:10.3389/fphar.2022.874510
Banchereau, J., Briere, F., Caux, C., Davoust, J., Lebecque, S., Liu, Y. J., et al. (2000). Immunobiology of dendritic cells. Annu. Rev. Immunol. 18, 767–811. doi:10.1146/annurev.immunol.18.1.767
Baskar, R., Lee, K. A., Yeo, R., and Yeoh, K.-W. (2012). Cancer and radiation therapy: Current advances and future directions. Int. J. Med. Sci. 9 (3), 193–199. doi:10.7150/ijms.3635
Blattman, J. N., and Greenberg, P. D. (2004). Cancer immunotherapy: A treatment for the masses. Science 305 (5681), 200–205. doi:10.1126/science.1100369
Blidner, A. G., Choi, J., Cooksley, T., Dougan, M., Glezerman, I., Ginex, P., et al. (2020). Cancer immunotherapy-related adverse events: Causes and challenges. Support. Care Cancer 28 (12), 6111–6117. doi:10.1007/s00520-020-05705-5
Bol, K. F., Schreibelt, G., Gerritsen, W. R., de Vries, I. J. M., and Figdor, C. G. (2016). Dendritic cell-based immunotherapy: State of the art and beyond. Clin. Cancer Res. 22 (8), 1897–1906. doi:10.1158/1078-0432.CCR-15-1399
Bol, K. F., Schreibelt, G., Rabold, K., Wculek, S. K., Schwarze, J. K., Dzionek, A., et al. (2019). The clinical application of cancer immunotherapy based on naturally circulating dendritic cells. J. For Immunother. Cancer 7 (1), 109. doi:10.1186/s40425-019-0580-6
Bronte, V., and Zanovello, P. (2005). Regulation of immune responses by L-arginine metabolism. Nat. Rev. Immunol. 5 (8), 641–654. doi:10.1038/nri1668
Bu, L.-L., Yan, J., Wang, Z., Ruan, H., Chen, Q., Gunadhi, V., et al. (2019). Advances in drug delivery for post-surgical cancer treatment. Biomaterials 219, 119182. doi:10.1016/j.biomaterials.2019.04.027
Buwalda, S. J., Boere, K. W., Dijkstra, P. J., Feijen, J., Vermonden, T., and Hennink, W. E. (2014). Hydrogels in a historical perspective: From simple networks to smart materials. J. Control Release 190, 254–273. doi:10.1016/j.jconrel.2014.03.052
Cai, H., Chen, M.-S., Sun, Z.-Y., Zhao, Y.-F., Kunz, H., and Li, Y.-M. (2013). Self-adjuvanting synthetic antitumor vaccines from MUC1 glycopeptides conjugated to T-cell epitopes from tetanus toxoid. Angewandte Chemie Int. Ed. Engl. 52 (23), 6106–6110. doi:10.1002/anie.201300390
Chang, C.-N., Huang, Y.-C., Yang, D.-M., Kikuta, K., Wei, K.-J., Kubota, T., et al. (2011). A phase I/II clinical trial investigating the adverse and therapeutic effects of a postoperative autologous dendritic cell tumor vaccine in patients with malignant glioma. J. Clin. Neurosci. 18 (8), 1048–1054. doi:10.1016/j.jocn.2010.11.034
Chao, Y., Xu, L., Liang, C., Feng, L., Xu, J., Dong, Z., et al. (2018). Combined local immunostimulatory radioisotope therapy and systemic immune checkpoint blockade imparts potent antitumour responses. Nat. Biomed. Eng. 2 (8), 611–621. doi:10.1038/s41551-018-0262-6
Chattopadhyay, S., Liu, Y.-H., Fang, Z.-S., Lin, C.-L., Lin, J.-C., Yao, B.-Y., et al. (2020). Synthetic immunogenic cell death mediated by intracellular delivery of STING agonist nanoshells enhances anticancer chemo-immunotherapy. Nano Lett. 20 (4), 2246–2256. doi:10.1021/acs.nanolett.9b04094
Chen, M., Tan, Y., Dong, Z., Lu, J., Han, X., Jin, Q., et al. (2020). Injectable anti-inflammatory nanofiber hydrogel to achieve systemic immunotherapy post local administration. Nano Lett. 20 (9), 6763–6773. doi:10.1021/acs.nanolett.0c02684
Chen, Z., Deng, J., Cao, J., Wu, H., Feng, G., Zhang, R., et al. (2022). Nano-hydroxyapatite-evoked immune response synchronized with controllable immune adjuvant release for strengthening melanoma-specific growth inhibition. Acta Biomater. 145, 159–171. doi:10.1016/j.actbio.2022.04.002
Chhabra, R., Sharma, J., Liu, Y., Rinker, S., and Yan, H. (2010). DNA self-assembly for nanomedicine. Adv. Drug Deliv. Rev. 62 (6), 617–625. doi:10.1016/j.addr.2010.03.005
Cornel, A. M., van Til, N. P., Boelens, J. J., and Nierkens, S. (2018). Strategies to genetically modulate dendritic cells to potentiate anti-tumor responses in hematologic malignancies. Front. Immunol. 9, 982. doi:10.3389/fimmu.2018.00982
Cytlak, U. M., Dyer, D. P., Honeychurch, J., Williams, K. J., Travis, M. A., and Illidge, T. M. (2022). Immunomodulation by radiotherapy in tumour control and normal tissue toxicity. Nat. Rev. Immunol. 22 (2), 124–138. doi:10.1038/s41577-021-00568-1
Dimatteo, R., Darling, N. J., and Segura, T. (2018). In situ forming injectable hydrogels for drug delivery and wound repair. Adv. Drug Deliv. Rev. 127, 167–184. doi:10.1016/j.addr.2018.03.007
Ding, M., Fan, Y., Lv, Y., Liu, J., Yu, N., Kong, D., et al. (2022). A prodrug hydrogel with tumor microenvironment and near-infrared light dual-responsive action for synergistic cancer immunotherapy. Acta Biomater. 149, 334–346. doi:10.1016/j.actbio.2022.06.041
Dong, X., Liang, J., Yang, A., Qian, Z., Kong, D., and Lv, F. (2019). Fluorescence imaging guided CpG nanoparticles-loaded IR820-hydrogel for synergistic photothermal immunotherapy. Biomaterials 209, 111–125. doi:10.1016/j.biomaterials.2019.04.024
Dong, X., Yang, A., Bai, Y., Kong, D., and Lv, F. (2020). Dual fluorescence imaging-guided programmed delivery of doxorubicin and CpG nanoparticles to modulate tumor microenvironment for effective chemo-immunotherapy. Biomaterials 230, 119659. doi:10.1016/j.biomaterials.2019.119659
Duong, H. T. T., Thambi, T., Yin, Y., Kim, S. H., Nguyen, T. L., Phan, V. H. G., et al. (2020). Degradation-regulated architecture of injectable smart hydrogels enhances humoral immune response and potentiates antitumor activity in human lung carcinoma. Biomaterials 230, 119599. doi:10.1016/j.biomaterials.2019.119599
Elstad, N. L., and Fowers, K. D. (2009). OncoGel (ReGel/paclitaxel)-clinical applications for a novel paclitaxel delivery system. Adv. Drug Deliv. Rev. 61 (10), 785–794. doi:10.1016/j.addr.2009.04.010
Fan, D. Y., Tian, Y., and Liu, Z. J. (2019). Injectable hydrogels for localized cancer therapy. Front. Chem. 7, 675. doi:10.3389/fchem.2019.00675
Fan, M., Jia, L., Pang, M., Yang, X., Yang, Y., Kamel Elyzayati, S., et al. (2021). Injectable adhesive hydrogel as photothermal-derived antigen reservoir for enhanced anti-tumor immunity. Adv. Funct. Mater. 31 (20), 2010587. doi:10.1002/adfm.202010587
Formenti, S. C., and Demaria, S. (2013). Combining radiotherapy and cancer immunotherapy: A paradigm shift. J. Natl. Cancer Inst. 105 (4), 256–265. doi:10.1093/jnci/djs629
Galluzzi, L., Buqué, A., Kepp, O., Zitvogel, L., and Kroemer, G. (2017). Immunogenic cell death in cancer and infectious disease. Nat. Rev. Immunol. 17 (2), 97–111. doi:10.1038/nri.2016.107
Galluzzi, L., Kepp, O., and Kroemer, G. (2013). Immunogenic cell death in radiation therapy. Oncoimmunology 2 (10), e26536. doi:10.4161/onci.26536
Gao, J., Wang, W.-Q., Pei, Q., Lord, M. S., and Yu, H.-J. (2020). Engineering nanomedicines through boosting immunogenic cell death for improved cancer immunotherapy. Acta Pharmacol. Sin. 41 (7), 986–994. doi:10.1038/s41401-020-0400-z
Gardner, A., de Mingo Pulido, Á., and Ruffell, B. (2020). Dendritic cells and their role in immunotherapy. Front. Immunol. 11, 924. doi:10.3389/fimmu.2020.00924
Geiger, R., Rieckmann, J. C., Wolf, T., Basso, C., Feng, Y., Fuhrer, T., et al. (2016). L-arginine modulates T cell metabolism and enhances survival and anti-tumor activity. Cell 167 (3), 829–842.e13. doi:10.1016/j.cell.2016.09.031
Goradel, N. H., Baker, A. T., Arashkia, A., Ebrahimi, N., Ghorghanlu, S., and Negahdari, B. (2021). Oncolytic virotherapy: Challenges and solutions. Curr. Problems Cancer 45 (1), 100639. doi:10.1016/j.currproblcancer.2020.100639
Guipaud, O., Jaillet, C., Clément-Colmou, K., François, A., Supiot, S., and Milliat, F. (2018). The importance of the vascular endothelial barrier in the immune-inflammatory response induced by radiotherapy. Br. J. Radiology 91 (1089), 20170762. doi:10.1259/bjr.20170762
Guo, L., Yan, D. D., Yang, D., Li, Y., Wang, X., Zalewski, O., et al. (2014). Combinatorial photothermal and immuno cancer therapy using chitosan-coated hollow copper sulfide nanoparticles. ACS Nano 8 (6), 5670–5681. doi:10.1021/nn5002112
Haanen, J., Ernstoff, M. S., Wang, Y., Menzies, A. M., Puzanov, I., Grivas, P., et al. (2020). Autoimmune diseases and immune-checkpoint inhibitors for cancer therapy: Review of the literature and personalized risk-based prevention strategy. Ann. Oncol. 31 (6), 724–744. doi:10.1016/j.annonc.2020.03.285
Habibi, N., Christau, S., Ochyl, L. J., Fan, Z., Hassani Najafabadi, A., Kuehnhammer, M., et al. (2020). Engineered ovalbumin nanoparticles for cancer immunotherapy. Adv. Ther. 3 (10), 2000100. doi:10.1002/adtp.202000100
Hamidi, M., Azadi, A., and Rafiei, P. (2008). Hydrogel nanoparticles in drug delivery. Adv. Drug Deliv. Rev. 60 (15), 1638–1649. doi:10.1016/j.addr.2008.08.002
Hangasky, J. A., Chen, W., Dubois, S. P., Daenthanasanmak, A., Müller, J. R., Reid, R., et al. (2022). A very long-acting IL-15: Implications for the immunotherapy of cancer. J. For Immunother. Cancer 10 (1), e004104. doi:10.1136/jitc-2021-004104
Hildebrandt, B., Wust, P., Ahlers, O., Dieing, A., Sreenivasa, G., Kerner, T., et al. (2002). The cellular and molecular basis of hyperthermia. Crit. Rev. Oncology/hematology 43 (1), 33–56. doi:10.1016/s1040-8428(01)00179-2
Hori, Y., Winans, A. M., Huang, C. C., Horrigan, E. M., and Irvine, D. J. (2008). Injectable dendritic cell-carrying alginate gels for immunization and immunotherapy. Biomaterials 29 (27), 3671–3682. doi:10.1016/j.biomaterials.2008.05.033
Hou, X.-L., Dai, X., Yang, J., Zhang, B., Zhao, D.-H., Li, C.-Q., et al. (2020). Injectable polypeptide-engineered hydrogel depot for amplifying the anti-tumor immune effect induced by chemo-photothermal therapy. J. Mater. Chem. B 8 (37), 8623–8633. doi:10.1039/d0tb01370f
Hu, M., Zhang, J., Kong, L., Yu, Y., Hu, Q., Yang, T., et al. (2021). Immunogenic hybrid nanovesicles of liposomes and tumor-derived nanovesicles for cancer immunochemotherapy. ACS Nano 15 (2), 3123–3138. doi:10.1021/acsnano.0c09681
Huang, L., Li, Y., Du, Y., Zhang, Y., Wang, X., Ding, Y., et al. (2019a). Mild photothermal therapy potentiates anti-PD-L1 treatment for immunologically cold tumors via an all-in-one and all-in-control strategy. Nat. Commun. 10 (1), 4871. doi:10.1038/s41467-019-12771-9
Huang, P., Wang, X., Liang, X., Yang, J., Zhang, C., Kong, D., et al. (2019b). Nano-micro-and macroscale drug delivery systems for cancer immunotherapy. Acta Biomater. 85, 1–26. doi:10.1016/j.actbio.2018.12.028
Huang, Q., Zou, Y., Arno, M. C., Chen, S., Wang, T., Gao, J., et al. (2017). Hydrogel scaffolds for differentiation of adipose-derived stem cells. Chem. Soc. Rev. 46 (20), 6255–6275. doi:10.1039/c6cs00052e
Huang, X., El-Sayed, I. H., Qian, W., and El-Sayed, M. A. (2006). Cancer cell imaging and photothermal therapy in the near-infrared region by using gold nanorods. J. Am. Chem. Soc. 128 (6), 2115–2120. doi:10.1021/ja057254a
Jenkins, R. W., Barbie, D. A., and Flaherty, K. T. (2018). Mechanisms of resistance to immune checkpoint inhibitors. Br. J. Cancer 118 (1), 9–16. doi:10.1038/bjc.2017.434
Ji, B., Wei, M., and Yang, B. (2022). Recent advances in nanomedicines for photodynamic therapy (PDT)-driven cancer immunotherapy. Theranostics 12 (1), 434–458. doi:10.7150/thno.67300
Jia, Y. P., Shi, K., Yang, F., Liao, J. F., Han, R. X., Yuan, L. P., et al. (2020). Multifunctional nanoparticle loaded injectable thermoresponsive hydrogel as NIR controlled release platform for local photothermal immunotherapy to prevent breast cancer postoperative recurrence and metastases. Adv. Funct. Mater. 30 (25), 2001059. doi:10.1002/adfm.202001059
Jiang, F., and Doudna, J. A. (2017). CRISPR-Cas9 structures and mechanisms. Annu. Rev. Biophysics 46, 505–529. doi:10.1146/annurev-biophys-062215-010822
Jin, H., Wan, C., Zou, Z., Zhao, G., Zhang, L., Geng, Y., et al. (2018). Tumor ablation and therapeutic immunity induction by an injectable peptide hydrogel. ACS Nano 12 (4), 3295–3310. doi:10.1021/acsnano.7b08148
Jin, R., Yang, J., Ding, P., Li, C., Zhang, B., Chen, W., et al. (2020). Antitumor immunity triggered by photothermal therapy and photodynamic therapy of a 2D MoS2 nanosheet-incorporated injectable polypeptide-engineered hydrogel combinated with chemotherapy for 4T1 breast tumor therapy. Nanotechnology 31 (20), 205102. doi:10.1088/1361-6528/ab72b9
Johnson, T. S., McGaha, T., and Munn, D. H. (2017). Chemo-Immunotherapy: Role of indoleamine 2,3-dioxygenase in defining immunogenic versus tolerogenic cell death in the tumor microenvironment. Adv. Exp. Med. Biol. 1036, 91–104. doi:10.1007/978-3-319-67577-0_7
Kheirolomoom, A., Silvestrini, M. T., Ingham, E. S., Mahakian, L. M., Tam, S. M., Tumbale, S. K., et al. (2019). Combining activatable nanodelivery with immunotherapy in a murine breast cancer model. J. Control. Release Official J. Control. Release Soc. 303, 42–54. doi:10.1016/j.jconrel.2019.04.008
Kim, J., Choi, Y., Kim, D. H., Yoon, H. Y., and Kim, K. (2022). Injectable hydrogel-based combination cancer immunotherapy for overcoming localized therapeutic efficacy. Pharmaceutics 14 (9), 1908. doi:10.3390/pharmaceutics14091908
Klevorn, L. E., and Teague, R. M. (2016). Adapting cancer immunotherapy models for the real world. Trends Immunol. 37 (6), 354–363. doi:10.1016/j.it.2016.03.010
Kumagai, Y., Takeuchi, O., and Akira, S. (2008). TLR9 as a key receptor for the recognition of DNA. Adv. Drug Deliv. Rev. 60 (7), 795–804. doi:10.1016/j.addr.2007.12.004
Lécuyer, T., Teston, E., Ramirez-Garcia, G., Maldiney, T., Viana, B., Seguin, J., et al. (2016). Chemically engineered persistent luminescence nanoprobes for bioimaging. Theranostics 6 (13), 2488–2523. doi:10.7150/thno.16589
Lee, J., Le, Q.-V., Yang, G., and Oh, Y.-K. (2019a). Cas9-edited immune checkpoint blockade PD-1 DNA polyaptamer hydrogel for cancer immunotherapy. Biomaterials 218, 119359. doi:10.1016/j.biomaterials.2019.119359
Lee, S.-J., Kim, J.-J., Kang, K.-Y., Paik, M.-J., Lee, G., and Yee, S.-T. (2019b). <p>Enhanced anti-tumor immunotherapy by silica-coated magnetic nanoparticles conjugated with ovalbumin</p>. Int. J. Nanomedicine 14, 8235–8249. doi:10.2147/IJN.S194352
Li, J., Luo, G., Zhang, C., Long, S., Guo, L., Yang, G., et al. (2022a). In situ injectable hydrogel-loaded drugs induce anti-tumor immune responses in melanoma immunochemotherapy. Mater. Today Bio 14, 100238. doi:10.1016/j.mtbio.2022.100238
Li, J., and Mooney, D. J. (2016). Designing hydrogels for controlled drug delivery. Nat. Rev. Mater 1 (12), 16071. doi:10.1038/natrevmats.2016.71
Li, X., Lovell, J. F., Yoon, J., and Chen, X. (2020a). Clinical development and potential of photothermal and photodynamic therapies for cancer. Nat. Rev. Clin. Oncol. 17 (11), 657–674. doi:10.1038/s41571-020-0410-2
Li, Y., Liu, X., Pan, W., Li, N., and Tang, B. (2020b). Photothermal therapy-induced immunogenic cell death based on natural melanin nanoparticles against breast cancer. Chem. Commun. Camb. Engl. 56 (9), 1389–1392. doi:10.1039/c9cc08447a
Li, Y., Yang, H. Y., and Lee, D. S. (2022b). Biodegradable and injectable hydrogels in biomedical applications. Biomacromolecules 23 (3), 609–618. doi:10.1021/acs.biomac.1c01552
Liao, Y., Xie, L., Ye, J., Chen, T., Huang, T., Shi, L., et al. (2022). Sprayable hydrogel for biomedical applications. Biomater. Sci. 10 (11), 2759–2771. doi:10.1039/d2bm00338d
Liau, L. M., Ashkan, K., Tran, D. D., Campian, J. L., Trusheim, J. E., Cobbs, C. S., et al. (2018). Correction to: First results on survival from a large Phase 3 clinical trial of an autologous dendritic cell vaccine in newly diagnosed glioblastoma. J. Transl. Med. 16 (1), 179. doi:10.1186/s12967-018-1552-1
Littman, D. R. (2015). Releasing the brakes on cancer immunotherapy. Cell 162 (6), 1186–1190. doi:10.1016/j.cell.2015.08.038
Liu, D., Hong, Y., Li, Y., Hu, C., Yip, T.-C., Yu, W.-K., et al. (2020). Targeted destruction of cancer stem cells using multifunctional magnetic nanoparticles that enable combined hyperthermia and chemotherapy. Theranostics 10 (3), 1181–1196. doi:10.7150/thno.38989
Liu, M., Cao, Z., Zhang, R., Chen, Y., and Yang, X. (2021). Injectable supramolecular hydrogel for locoregional immune checkpoint blockade and enhanced cancer chemo-immunotherapy. ACS Appl. Mater Interfaces 13 (29), 33874–33884. doi:10.1021/acsami.1c08285
Liu, Y., Shu, X. Z., and Prestwich, G. D. (2007). Reduced postoperative intra-abdominal adhesions using Carbylan-SX, a semisynthetic glycosaminoglycan hydrogel. Fertil. Steril. 87 (4), 940–948. doi:10.1016/j.fertnstert.2006.07.1532
Lv, Q., He, C., Quan, F., Yu, S., and Chen, X. (2018). DOX/IL-2/IFN-γ co-loaded thermo-sensitive polypeptide hydrogel for efficient melanoma treatment. Bioact. Mater. 3 (1), 118–128. doi:10.1016/j.bioactmat.2017.08.003
Mathew, A. P., Uthaman, S., Cho, K. H., Cho, C. S., and Park, I. K. (2018). Injectable hydrogels for delivering biotherapeutic molecules. Int. J. Biol. Macromol. 110, 17–29. doi:10.1016/j.ijbiomac.2017.11.113
Meng, Z., Zhou, X., Xu, J., Han, X., Dong, Z., Wang, H., et al. (2019). Light-triggered in situ gelation to enable robust photodynamic-immunotherapy by repeated stimulations. Adv. Mater. Deerf. Beach, Fla 31 (24), e1900927. doi:10.1002/adma.201900927
Merkle, H. P. (2015). Drug delivery's quest for polymers: Where are the frontiers? Eur. J. Pharm. Biopharm. 97, 293–303. doi:10.1016/j.ejpb.2015.04.038
Milling, L., Zhang, Y., and Irvine, D. J. (2017). Delivering safer immunotherapies for cancer. Adv. Drug Deliv. Rev. 114, 79–101. doi:10.1016/j.addr.2017.05.011
Min, Y., Roche, K. C., Tian, S., Eblan, M. J., McKinnon, K. P., Caster, J. M., et al. (2021). Author Correction: Antigen-capturing nanoparticles improve the abscopal effect and cancer immunotherapy. Nat. Nanotechnol. 16 (6), 743–744. doi:10.1038/s41565-021-00864-w
Mo, F., Jiang, K., Zhao, D., Wang, Y., Song, J., and Tan, W. (2021). DNA hydrogel-based gene editing and drug delivery systems. Adv. Drug Deliv. Rev. 168, 79–98. doi:10.1016/j.addr.2020.07.018
Naimi, A., Mohammed, R. N., Raji, A., Chupradit, S., Yumashev, A. V., Suksatan, W., et al. (2022). Tumor immunotherapies by immune checkpoint inhibitors (ICIs); the pros and cons. Cell Commun. Signal. CCS 20 (1), 44. doi:10.1186/s12964-022-00854-y
Nam, J., Son, S., Park, K. S., Zou, W., Shea, L. D., and Moon, J. J. (2019). Cancer nanomedicine for combination cancer immunotherapy. Nat. Rev. Mater. 4 (6), 398–414. doi:10.1038/s41578-019-0108-1
Ng, C. W., Li, J., and Pu, K. (2018). Recent progresses in phototherapy-synergized cancer immunotherapy. Adv. Funct. Mater. 28 (46), 1804688. doi:10.1002/adfm.201804688
Nishikawa, M., Mizuno, Y., Mohri, K., Matsuoka, N., Rattanakiat, S., Takahashi, Y., et al. (2011). Biodegradable CpG DNA hydrogels for sustained delivery of doxorubicin and immunostimulatory signals in tumor-bearing mice. Biomaterials 32 (2), 488–494. doi:10.1016/j.biomaterials.2010.09.013
Nishikawa, M., Rattanakiat, S., and Takakura, Y. (2010). DNA-based nano-sized systems for pharmaceutical and biomedical applications. Adv. Drug Deliv. Rev. 62 (6), 626–632. doi:10.1016/j.addr.2010.03.006
O'Connell, M. R., Oakes, B. L., Sternberg, S. H., East-Seletsky, A., Kaplan, M., and Doudna, J. A. (2014). Programmable RNA recognition and cleavage by CRISPR/Cas9. Nature 516 (7530), 263–266. doi:10.1038/nature13769
Oh, E., Oh, J.-E., Hong, J., Chung, Y., Lee, Y., Park, K. D., et al. (2017). Optimized biodegradable polymeric reservoir-mediated local and sustained co-delivery of dendritic cells and oncolytic adenovirus co-expressing IL-12 and GM-CSF for cancer immunotherapy. J. Control. Release 259, 115–127. doi:10.1016/j.jconrel.2017.03.028
Oliva, N., Conde, J., Wang, K., and Artzi, N. (2017). Designing hydrogels for on-demand therapy. Acc. Chem. Res. 50 (4), 669–679. doi:10.1021/acs.accounts.6b00536
Palucka, K., and Banchereau, J. (2013). Dendritic-cell-based therapeutic cancer vaccines. Immunity 39 (1), 38–48. doi:10.1016/j.immuni.2013.07.004
Perez, C. R., and De Palma, M. (2019). Engineering dendritic cell vaccines to improve cancer immunotherapy. Nat. Commun. 10 (1), 5408. doi:10.1038/s41467-019-13368-y
Piluso, S., Soultan, A. H., and Patterson, J. (2017). Molecularly engineered polymer-based systems in drug delivery and regenerative medicine. Curr. Pharm. Des. 23 (2), 281–294. doi:10.2174/1381612822666161021104239
Postow, M. A., Callahan, M. K., and Wolchok, J. D. (2015). Immune checkpoint blockade in cancer therapy. J. Clin. Oncol. 33 (17), 1974–1982. doi:10.1200/JCO.2014.59.4358
Rady, I., Siddiqui, I. A., Rady, M., and Mukhtar, H. (2017). Melittin, a major peptide component of bee venom, and its conjugates in cancer therapy. Cancer Lett. 402, 16–31. doi:10.1016/j.canlet.2017.05.010
Ramos-Casals, M., Brahmer, J. R., Callahan, M. K., Flores-Chávez, A., Keegan, N., Khamashta, M. A., et al. (2020). Immune-related adverse events of checkpoint inhibitors. Nat. Rev. Dis. Prim. 6 (1), 38. doi:10.1038/s41572-020-0160-6
Rangnekar, A., and LaBean, T. H. (2014). Building DNA nanostructures for molecular computation, templated assembly, and biological applications. Accounts Chem. Res. 47 (6), 1778–1788. doi:10.1021/ar500023b
Reits, E. A., Hodge, J. W., Herberts, C. A., Groothuis, T. A., Chakraborty, M., Wansley, E. K., et al. (2006). Radiation modulates the peptide repertoire, enhances MHC class I expression, and induces successful antitumor immunotherapy. J. Exp. Med. 203 (5), 1259–1271. doi:10.1084/jem.20052494
Ren, X., Wang, N., Zhou, Y., Song, A., Jin, G., Li, Z., et al. (2021). An injectable hydrogel using an immunomodulating gelator for amplified tumor immunotherapy by blocking the arginase pathway. Acta Biomater. 124, 179–190. doi:10.1016/j.actbio.2021.01.041
Riley, R. S., June, C. H., Langer, R., and Mitchell, M. J. (2019a). Delivery technologies for cancer immunotherapy. Nat. Rev. Drug Discov. 18 (3), 175–196. doi:10.1038/s41573-018-0006-z
Riley, R. S., June, C. H., Langer, R., and Mitchell, M. J. (2019b). Delivery technologies for cancer immunotherapy. Nat. Rev. Drug Discov. 18 (3), 175–196. doi:10.1038/s41573-018-0006-z
Robert, C. (2020). A decade of immune-checkpoint inhibitors in cancer therapy. Nat. Commun. 11 (1), 3801. doi:10.1038/s41467-020-17670-y
Robinson, T. O., and Schluns, K. S. (2017). The potential and promise of IL-15 in immuno-oncogenic therapies. Immunol. Lett. 190, 159–168. doi:10.1016/j.imlet.2017.08.010
Rodriguez, P. C., Quiceno, D. G., and Ochoa, A. C. (2007). L-arginine availability regulates T-lymphocyte cell-cycle progression. Blood 109 (4), 1568–1573. doi:10.1182/blood-2006-06-031856
Romano, E., Honeychurch, J., and Illidge, T. M. (2021). Radiotherapy-immunotherapy combination: How will we bridge the gap between pre-clinical promise and effective clinical delivery? Cancers 13 (3), 457. doi:10.3390/cancers13030457
Ruan, H., Hu, Q., Wen, D., Chen, Q., Chen, G., Lu, Y., et al. (2019). A dual-bioresponsive drug-delivery depot for combination of epigenetic modulation and immune checkpoint blockade. Adv. Mater. Deerf. Beach, Fla.) 31 (17), e1806957. doi:10.1002/adma.201806957
Santos, P. M., and Butterfield, L. H. (2018). Dendritic cell-based cancer vaccines. J. Immunol. 200 (2), 443–449. doi:10.4049/jimmunol.1701024
Schon, M. P., and Schon, M. (2007). Imiquimod: Mode of action. Br. J. Dermatol 157, 8–13. doi:10.1111/j.1365-2133.2007.08265.x
Senapati, S., Mahanta, A. K., Kumar, S., and Maiti, P. (2018). Controlled drug delivery vehicles for cancer treatment and their performance. Signal Transduct. Target Ther. 3, 7. doi:10.1038/s41392-017-0004-3
Shao, Y., Sun, Z.-Y., Wang, Y., Zhang, B.-D., Liu, D., and Li, Y.-M. (2018). Designable immune therapeutical vaccine system based on DNA supramolecular hydrogels. ACS Appl. Mater. Interfaces 10 (11), 9310–9314. doi:10.1021/acsami.8b00312
Shi, Y., Li, D., He, C., and Chen, X. (2021). Design of an injectable polypeptide hydrogel depot containing the immune checkpoint blocker anti-PD-L1 and doxorubicin to enhance antitumor combination therapy. Macromol. Biosci. 21 (6), e2100049. doi:10.1002/mabi.202100049
Shimizu, T., Kishida, T., Hasegawa, U., Ueda, Y., Imanishi, J., Yamagishi, H., et al. (2008). Nanogel DDS enables sustained release of IL-12 for tumor immunotherapy. Biochem. Biophysical Res. Commun. 367 (2), 330–335. doi:10.1016/j.bbrc.2007.12.112
Shin, D. H., and Kwon, G. S. (2017). Pre-clinical evaluation of a themosensitive gel containing epothilone B and mTOR/Hsp90 targeted agents in an ovarian tumor model. J. Control. Release Official J. Control. Release Soc. 268, 176–183. doi:10.1016/j.jconrel.2017.10.022
Shu, G., Zhu, W., Jiang, Y., Li, X., Pan, J., Zhang, X., et al. (2021). Persistent luminescence immune hydrogel for photodynamic-immunotherapy of tumors in vivo. Adv. Funct. Mater. 31 (36), 2104472. doi:10.1002/adfm.202104472
Singh, A., and Peppas, N. A. (2014). Hydrogels and scaffolds for immunomodulation. Adv. Mater. Deerf. Beach, Fla.) 26 (38), 6530–6541. doi:10.1002/adma.201402105
Sivaraj, D., Chen, K., Chattopadhyay, A., Henn, D., Wu, W., Noishiki, C., et al. (2021). Hydrogel scaffolds to deliver cell therapies for wound healing. Front. Bioeng. Biotechnol. 9, 660145. doi:10.3389/fbioe.2021.660145
Song, H., Yang, P., Huang, P., Zhang, C., Kong, D., and Wang, W. (2019). Injectable polypeptide hydrogel-based co-delivery of vaccine and immune checkpoint inhibitors improves tumor immunotherapy. Theranostics 9 (8), 2299–2314. doi:10.7150/thno.30577
Steinhagen, F., Kinjo, T., Bode, C., and Klinman, D. M. (2011). TLR-based immune adjuvants. Vaccine 29 (17), 3341–3355. doi:10.1016/j.vaccine.2010.08.002
Steinman, R. M., and Banchereau, J. (2007). Taking dendritic cells into medicine. Nature 449 (7161), 419–426. doi:10.1038/nature06175
Sterner, R. C., and Sterner, R. M. (2021). CAR-T cell therapy: Current limitations and potential strategies. Blood Cancer J. 11 (4), 69. doi:10.1038/s41408-021-00459-7
Sun, L., Shen, F., Tian, L., Tao, H., Xiong, Z., Xu, J., et al. (2021). ATP-responsive smart hydrogel releasing immune adjuvant synchronized with repeated chemotherapy or radiotherapy to boost antitumor immunity. Adv. Mater. Deerf. Beach, Fla.) 33 (18), e2007910. doi:10.1002/adma.202007910
Sun, Z., Liang, J., Dong, X., Wang, C., Kong, D., and Lv, F. (2018). Injectable hydrogels coencapsulating granulocyte-macrophage colony-stimulating factor and ovalbumin nanoparticles to enhance antigen uptake efficiency. ACS Appl. Mater Interfaces 10 (24), 20315–20325. doi:10.1021/acsami.8b04312
Tavakoli, S., and Klar, A. S. (2020). Advanced hydrogels as wound dressings. Biomolecules 10 (8), 1169. doi:10.3390/biom10081169
Thambi, T., Phan, V. H., and Lee, D. S. (2016). Stimuli-sensitive injectable hydrogels based on polysaccharides and their biomedical applications. Macromol. Rapid Commun. 37 (23), 1881–1896. doi:10.1002/marc.201600371
Tiwari, A. P., Hwang, T. I., Oh, J.-M., Maharjan, B., Chun, S., Kim, B. S., et al. (2018). pH/NIR-responsive polypyrrole-functionalized fibrous localized drug-delivery platform for synergistic cancer therapy. ACS Appl. Mater. Interfaces 10 (24), 20256–20270. doi:10.1021/acsami.7b17664
Tong, X., and Yang, F. (2018). Recent progress in developing injectable matrices for enhancing cell delivery and tissue regeneration. Adv. Healthc. Mater 7 (7), e1701065. doi:10.1002/adhm.201701065
Topalian, S. L., Drake, C. G., and Pardoll, D. M. (2015). Immune checkpoint blockade: A common denominator approach to cancer therapy. Cancer Cell 27 (4), 450–461. doi:10.1016/j.ccell.2015.03.001
Umeki, Y., Mohri, K., Kawasaki, Y., Watanabe, H., Takahashi, R., Takahashi, Y., et al. (2015). Induction of potent antitumor immunity by sustained release of cationic antigen from a DNA-based hydrogel with adjuvant activity. Adv. Funct. Mater. 25 (36), 5758–5767. doi:10.1002/adfm.201502139
Verma, I. M., and Somia, N. (1997). Gene therapy - promises, problems and prospects. Nature 389 (6648), 239–242. doi:10.1038/38410
Wang, A., Zheng, Y., Zhu, W., Yang, L., Yang, Y., and Peng, J. (2022). Melittin-based nano-delivery systems for cancer therapy. Biomolecules 12 (1), 118. doi:10.3390/biom12010118
Wang, C., Wang, J., Zhang, X., Yu, S., Wen, D., Hu, Q., et al. (2018a). In situ formed reactive oxygen species-responsive scaffold with gemcitabine and checkpoint inhibitor for combination therapy. Sci. Transl. Med. 10 (429), eaan3682. doi:10.1126/scitranslmed.aan3682
Wang, F., Xu, D., Su, H., Zhang, W., Sun, X., Monroe, M. K., et al. (2020). Supramolecular prodrug hydrogelator as an immune booster for checkpoint blocker-based immunotherapy. Sci. Adv. 6 (18), eaaz8985. doi:10.1126/sciadv.aaz8985
Wang, J., Li, Y., Mao, R., Wang, Y., Yan, X., and Liu, J. (2017a). Persistent luminescent nanoparticles as energy mediators for enhanced photodynamic therapy with fractionated irradiation. J. Mater. Chem. B 5 (29), 5793–5805. doi:10.1039/c7tb00950j
Wang, M., Song, J., Zhou, F., Hoover, A. R., Murray, C., Zhou, B., et al. (2019). NIR-triggered phototherapy and immunotherapy via an antigen-capturing nanoplatform for metastatic cancer treatment. Adv. Sci. (Weinheim, Baden-Wurttemberg, Ger. 6 (10), 1802157. doi:10.1002/advs.201802157
Wang, Q., Ju, X., Wang, J., Fan, Y., Ren, M., and Zhang, H. (2018b). Immunogenic cell death in anticancer chemotherapy and its impact on clinical studies. Cancer Lett. 438, 17–23. doi:10.1016/j.canlet.2018.08.028
Wang, Q., Liu, N., Hou, Z., Shi, J., Su, X., and Sun, X. (2021). Radioiodinated persistent luminescence nanoplatform for radiation-induced photodynamic therapy and radiotherapy. Adv. Healthc. Mater. 10 (5), e2000802. doi:10.1002/adhm.202000802
Wang, T., Wang, D., Yu, H., Feng, B., Zhou, F., Zhang, H., et al. (2018c). A cancer vaccine-mediated postoperative immunotherapy for recurrent and metastatic tumors. Nat. Commun. 9 (1), 1532. doi:10.1038/s41467-018-03915-4
Wang, Z., Zhang, Y., Liu, Z., Dong, K., Liu, C., Ran, X., et al. (2017b). A bifunctional nanomodulator for boosting CpG-mediated cancer immunotherapy. Nanoscale 9 (37), 14236–14247. doi:10.1039/c7nr04396a
Wang-Bishop, L., Wehbe, M., Shae, D., James, J., Hacker, B. C., Garland, K., et al. (2020). Potent STING activation stimulates immunogenic cell death to enhance antitumor immunity in neuroblastoma. J. For Immunother. Cancer 8 (1), e000282. doi:10.1136/jitc-2019-000282
Wiemann, B., and Starnes, C. O. (1994). Coley's toxins, tumor necrosis factor and cancer research: A historical perspective. Pharmacol. Ther. 64, 529–564. doi:10.1016/0163-7258(94)90023-x
Wu, S., Li, Y., Ding, W., Xu, L., Ma, Y., and Zhang, L. (2020). Recent advances of persistent luminescence nanoparticles in bioapplications. Nano-micro Lett. 12 (1), 70. doi:10.1007/s40820-020-0404-8
Wu, X., He, C., Wu, Y., and Chen, X. (2016). Synergistic therapeutic effects of Schiff's base cross-linked injectable hydrogels for local co-delivery of metformin and 5-fluorouracil in a mouse colon carcinoma model. Biomaterials 75, 148–162. doi:10.1016/j.biomaterials.2015.10.016
Wu, X., Wu, Y., Ye, H., Yu, S., He, C., and Chen, X. (2017). Interleukin-15 and cisplatin co-encapsulated thermosensitive polypeptide hydrogels for combined immuno-chemotherapy. J. Control. Release 255, 81–93. doi:10.1016/j.jconrel.2017.04.011
Xia, Q., Gong, C., Gu, F., Wang, Z., Hu, C., Zhang, L., et al. (2018). Functionalized multi-walled carbon nanotubes for targeting delivery of immunostimulatory CpG oligonucleotides against prostate cancer. J. Biomed. Nanotechnol. 14 (9), 1613–1626. doi:10.1166/jbn.2018.2605
Xie, L., Jin, W., Zuo, X., Ji, S., Nan, W., Chen, H., et al. (2020). Construction of small-sized superparamagnetic Janus nanoparticles and their application in cancer combined chemotherapy and magnetic hyperthermia. Biomaterials Sci. 8 (5), 1431–1441. doi:10.1039/c9bm01880h
Xie, Z., Shen, J., Sun, H., Li, J., and Wang, X. (2021). Polymer-based hydrogels with local drug release for cancer immunotherapy. Biomed. Pharmacother. 137, 111333. doi:10.1016/j.biopha.2021.111333
Xue, B., Wang, W., Qin, J. J., Nijampatnam, B., Murugesan, S., Kozlovskaya, V., et al. (2017). Highly efficient delivery of potent anticancer iminoquinone derivative by multilayer hydrogel cubes. Acta Biomater. 58, 386–398. doi:10.1016/j.actbio.2017.06.004
Yan, S., Luo, Z., Li, Z., Wang, Y., Tao, J., Gong, C., et al. (2020). Improving cancer immunotherapy outcomes using biomaterials. Angew. Chem. Int. Ed. Engl. 59 (40), 17332–17343. doi:10.1002/anie.202002780
Yang, G., Xu, L., Chao, Y., Xu, J., Sun, X., Wu, Y., et al. (2017). Hollow MnO2 as a tumor-microenvironment-responsive biodegradable nano-platform for combination therapy favoring antitumor immune responses. Nat. Commun. 8 (1), 902. doi:10.1038/s41467-017-01050-0
Yang, K., Zhang, S., Zhang, G., Sun, X., Lee, S.-T., and Liu, Z. (2010). Graphene in mice: Ultrahigh in vivo tumor uptake and efficient photothermal therapy. Nano Lett. 10 (9), 3318–3323. doi:10.1021/nl100996u
Yang, P., Song, H., Qin, Y., Huang, P., Zhang, C., Kong, D., et al. (2018). Engineering dendritic-cell-based vaccines and PD-1 blockade in self-assembled peptide nanofibrous hydrogel to amplify antitumor T-cell immunity. Nano Lett. 18 (7), 4377–4385. doi:10.1021/acs.nanolett.8b01406
Yata, T., Takahashi, Y., Tan, M., Nakatsuji, H., Ohtsuki, S., Murakami, T., et al. (2017). DNA nanotechnology-based composite-type gold nanoparticle-immunostimulatory DNA hydrogel for tumor photothermal immunotherapy. Biomaterials 146, 136–145. doi:10.1016/j.biomaterials.2017.09.014
Yin, S., Gao, Y., Zhang, Y., Xu, J., Zhu, J., Zhou, F., et al. (2020). Reduction/oxidation-responsive hierarchical nanoparticles with self-driven degradability for enhanced tumor penetration and precise chemotherapy. ACS Appl. Mater. Interfaces 12 (16), 18273–18291. doi:10.1021/acsami.0c00355
Yu, C., An, M., Li, M., and Liu, H. (2017). Immunostimulatory properties of lipid modified CpG oligonucleotides. Mol. Pharm. 14 (8), 2815–2823. doi:10.1021/acs.molpharmaceut.7b00335
Yu, S., He, C., and Chen, X. (2018a). Injectable hydrogels as unique platforms for local chemotherapeutics-based combination antitumor therapy. Macromol. Biosci. 18 (12), e1800240. doi:10.1002/mabi.201800240
Yu, S., Wang, C., Yu, J., Wang, J., Lu, Y., Zhang, Y., et al. (2018b). Injectable bioresponsive gel depot for enhanced immune checkpoint blockade. Adv. Mater 30 (28), e1801527. doi:10.1002/adma.201801527
Zhang, L., Zhang, J., Xu, L., Zhuang, Z., Liu, J., Liu, S., et al. (2021). NIR responsive tumor vaccine in situ for photothermal ablation and chemotherapy to trigger robust antitumor immune responses. J. Nanobiotechnology 19 (1), 142. doi:10.1186/s12951-021-00880-x
Zhang, Q., and Li, L. (2018). Photodynamic combinational therapy in cancer treatment. J. B.U.ON, Official J. Balkan Union Oncol. 23 (3), 561–567.
Zhang, Y., Feng, Z., Liu, J., Li, H., Su, Q., Zhang, J., et al. (2022). Polarization of tumor-associated macrophages by TLR7/8 conjugated radiosensitive peptide hydrogel for overcoming tumor radioresistance. Bioact. Mater. 16, 359–371. doi:10.1016/j.bioactmat.2021.12.033
Zhao, Z., Ukidve, A., Krishnan, V., Fehnel, A., Pan, D. C., Gao, Y., et al. (2020). Systemic tumour suppression via the preferential accumulation of erythrocyte-anchored chemokine-encapsulating nanoparticles in lung metastases. Nat. Biomed. Eng. 5, 441–454. doi:10.1038/s41551-020-00644-2
Zhao, Z., Zheng, L., Chen, W., Weng, W., Song, J., and Ji, J. (2019). Delivery strategies of cancer immunotherapy: Recent advances and future perspectives. J. Hematol. Oncol. 12 (1), 126. doi:10.1186/s13045-019-0817-3
Keywords: injectable hydrogel, cancer immunotherapy, delivery system, chemotherapy, radiotherapy, phototherapy, DNA hydrogel
Citation: Liu C, Liao Y, Liu L, Xie L, Liu J, Zhang Y and Li Y (2023) Application of injectable hydrogels in cancer immunotherapy. Front. Bioeng. Biotechnol. 11:1121887. doi: 10.3389/fbioe.2023.1121887
Received: 12 December 2022; Accepted: 20 January 2023;
Published: 03 February 2023.
Edited by:
Weiwei Wu, Xidian University, ChinaReviewed by:
Bingxia Zhao, Southern Medical University, ChinaChristopher Synatschke, Max Planck Institute for Polymer Research, Germany
Ning Tang, Xidian University, China
Copyright © 2023 Liu, Liao, Liu, Xie, Liu, Zhang and Li. This is an open-access article distributed under the terms of the Creative Commons Attribution License (CC BY). The use, distribution or reproduction in other forums is permitted, provided the original author(s) and the copyright owner(s) are credited and that the original publication in this journal is cited, in accordance with accepted academic practice. No use, distribution or reproduction is permitted which does not comply with these terms.
*Correspondence: Junbo Liu, MTcxNDQxNjU4QHFxLmNvbQ==; Yumao Zhang, eS56aGFuZ3ltQGdtYWlsLmNvbQ==; Yuzhen Li, bGl5emg1NkBtYWlsLnN5c3UuZWR1LmNu
†These authors have contributed equally to this work