- 1Periodicals Agency, Zhejiang Sci-Tech University, Hangzhou, China
- 2School of Economics and Management, Zhejiang Sci-Tech University, Hangzhou, China
- 3Library, Zhejiang University of Technology, Hangzhou, China
Background: Artificial muscles are an active research area now.
Methods: A bibliometric analysis was performed to evaluate the development of artificial muscles based on research papers and patents. A detailed overview of artificial muscles’ scientific and technological innovation was presented from aspects of productive countries/regions, institutions, journals, researchers, highly cited papers, and emerging topics.
Results: 1,743 papers and 1,925 patents were identified after retrieval in Science Citation Index-Expanded (SCI-E) and Derwent Innovations Index (DII). The results show that China, the United States, and Japan are leading in the scientific and technological innovation of artificial muscles. The University of Wollongong has the most publications and Spinks is the most productive author in artificial muscle research. Smart Materials and Structures is the journal most productive in this field. Materials science, mechanical and automation, and robotics are the three fields related to artificial muscles most. Types of artificial muscles like pneumatic artificial muscles (PAMs) and dielectric elastomer actuator (DEA) are maturing. Shape memory alloy (SMA), carbon nanotubes (CNTs), graphene, and other novel materials have shown promising applications in this field.
Conclusion: Along with the development of new materials and processes, researchers are paying more attention to the performance improvement and cost reduction of artificial muscles.
1 Introduction
Artificial muscle is a generic term for a class of materials and devices that can reversibly contract, expand, or rotate within one component due to an external stimulus (Mirvakili and Hunter, 2018). Due to external stimuli (voltage (Frank et al., 2022), current (Hao et al., 2019), temperature (Tada and Yoshida, 2021), pressure (Nuchkrua and Leephakpreeda, 2022), light (Li et al., 2020a; Yu et al., 2022), humidity (Zhou et al., 2018), etc.), artificial muscles can be deformed by changes in their own structure to produce the three basic actions of reversible expansion (Wang et al., 2021a), rotation (Wang H Q et al., 2020), and retraction (Oh et al., 2022). Due to the advantages of versatility, usability and high power-to-weight ratio compared to conventional motor drives (Wang et al., 2021b), artificial muscles have shown great potential for applications (Phan et al., 2021; Zakeri and Zakeri, 2022).
The study of artificial muscles emerged from intensive explorations on natural muscle and the maturation of bionic technology. The natural muscle is a soft biological actuator with excellent drive capability, compliance and self-repair ability. Drawing on the principles of natural muscle, researchers have developed film artificial muscles (Chang et al., 2021) and fiber artificial muscles (Zhang et al., 2022a) according to their macroscopic presentation. Graphene fibers (Wang R et al., 2019), carbon nanotube fibers (Jang et al., 2020), nylon fibers (Saharan et al., 2020), spandex fibers (Zhang et al., 2022b) and other artificial fibers, as well as silk (Lin et al., 2020), cotton thread (Li Y et al., 2020), and other natural fibers that constitute artificial muscles are closer to biological muscle in many properties, with good prospects for development, and have become the major direction of scientific and technological innovation of artificial muscles. After decades of development, artificial muscles have come to play a pivotal role in robotics (Dragone et al., 2022; Khalid et al., 2022), artificial limbs (Chen et al., 2020), exoskeletons (Kimura S et al., 2021) and other fields. A large number of applications in various fields have been developed, such as soft robotic swimmer (Lin et al., 2019), microhydraulic actuation for surgical instruments (Moers et al., 2012), micro flying robots (Xu et al., 2020), switch (Leng et al., 2021a), bowel peristaltic pump (Nakamura, 2022), and artificial insect (Yang et al., 2020). With the further integration with material science (Bastola and Hossain, 2021), mechanical engineering and automatic, chemical engineering, robotics and other disciplines, artificial muscles will be more widely adopted in medical (Aiyuan et al., 2021; Yarali et al., 2022), industry, military (Khan et al., 2019) and daily life (Paskett et al., 2021), and will make greater contributions to human beings.
Common review methods include systematic literature review, bibliometric methods, and meta-analysis methods. A systematic literature review can provide an in-depth and systematic review of a topic but require high academic credentials and usually only cover a small amount of literature (Snyder, 2019). Meta-analysis can extract data from a large amount of literature for a combined study of quantitative analysis and qualitative analysis on a topic (Junni et al., 2013). Bibliometric can analyze the overview of research on a topic by literature based on literature components like title, abstract, keywords, etc. The development of computer-aided analysis software makes it easy to be scaled from the micro to macro-level (Porter et al., 2019a). These three methods are not orthogonal and which one to use depends on the goals of the review and the magnitude and nature of the literature being reviewed (Donthu et al., 2021). As a knowledge-generating system that can analyze research activities and figure out future research directions in different fields based on thousands of documents (Xiang et al., 2022), bibliometric method is suitable for this study.
Bibliometrics was defined as “the application of mathematics and statistical methods to books and other media of communication” (OECD, 2022). The development of bibliometric methods can be traced back to (Lotka, 2022). Pareto published the first paper on bibliometrics (Lotka, 1926). In the following decades, Zipf, (1932), Bradford (Brookes and Bradford, 1985), Price (de Solla Price, 1974), et al. developed new bibliometric methods. Nowadays, bibliometric methodology has become an important method for review studies. It’s used in quantitative research in many fields such as materials science (Porter et al., 2019b), mechanical engineering (Li et al., 2020b; Wang et al., 2022), computer science (Darko et al., 2020; Bai et al., 2021), industry economy (Muhuri et al., 2019; Zhang et al., 2021), economics (Rousseau and Rousseau, 2021), environmental sciences (Jin et al., 2023), education (Villa et al., 2019), psychology (Tam and Milfont, 2020), public policy (Ruzhong et al., 2022; Solomon et al., 2019), and public health (Fang et al., 2021; Fang et al., 2022; He et al., 2022).
To all our knowledge, this paper is the first one to use bibliometric methods in the scientific and technological innovation of artificial muscles. Bao et al. (2018) published a bibliometric paper on soft robotics in 2018. A data-driven review is presented by Jumet et al. (2022) that addresses the recent surge of research of soft robot. But their research target and approach are different from our paper.
2 Methods
Our study consists of three steps. Firstly, subject, scope and objectives of the study were defined. Secondly, research data were collected. Finally, a bibliometric analysis was conducted and the results were reported.
As the first bibliometric analysis of artificial muscles, the aims of this paper are to provide a comprehensive retrospective of the progress of artificial muscles, to demonstrate the current state of artificial muscle development and to explore the future direction of artificial muscles, so the research data should include academic papers published in international mainstream journals and patent literature registered by major institutions.
The search term “artificial muscle” or “pneumatic muscle” or “bionic arm” or “bionic muscle” or “rehabilitation manipulator” was designed to retrieve research data. Two databases, Science Citation Index-Expanded (SCI-E) and Derwent Innovations Index (DII) in Web of Science (WoS) were selected as data sources. SCI-E is one of the most recognized indexing databases in the world with more than 9,500 world-renowned journals and 52 million records. DII consists of the Derwent World Patents Index (DWPI) and the Patents Citation Index (PCI), covering 96% of the world’s patent data since 1963.1,895 papers (articles and reviews) were collected from SCI-E and a search in DII got 1,970 patents with the defined research term. The retrieval date is 20 May 2022 and the retrieval field is restricted to “topic” (the title, abstract, and keywords of papers in SCI-E and title and abstract of patents in DII). Then, we carried out extended searches with search terms such as “IPMC”, “soft actuator”, and “soft robot” and cleaned the data. After removing duplicate records and some weakly relevant records by manual scanning, a total of 1,743 papers and 1,913 patents were retained and used for the follow-up analysis.
The bibliometric method was used in the data analysis of this paper. The analyzed items include bibliometric indicators such as total publications (TP) and total citations (TC), and scientific mapping such as collaborative network maps and co-word analysis maps.
We used Derwent Data Analyzer (DDA, version 10.0 build 27330) as the data cleaning and analytical tool. Like BibExcel, BibCOMB, Loet Tools, VOSviewer, Citespace, and HistCite, DDA is a data-mining tool that converts patent data, research paper and business intelligence into visualized, actionable insight. The data of papers and patents retrieved were cleaned, organized, and presented in tables and DDA charts (Fang et al., 2022). Tables were applied to show the output, collaboration and influence of journals, and authors, as well as highly cited papers and emerging topics; a chart that is a composite of line and stacked bar charts was used to illustrate the publication trend and contributions of countries/regions; pie maps were used to demonstrate the numerical relationships and co-occurrence between institutions and terms; a bubble chart was adopted to show the development trends of topics; a cluster map was used to display the field distribution of artificial muscle research and applications.
3 Results
The 1,743 papers were published in 492 journals and were spread over 37 years since 1961. A total of 5,207 authors from 70 countries/regions and 1,175 institutions contributed to the research of artificial muscles. As an interdisciplinary study, the 1,743 artificial muscle research papers, including 1,654 articles and 89 reviews, came from 97 WoS categories including multidisciplinary materials science, robotics, and instruments and instrumentation, etc. An average citation of 33 per paper, 57,240 total times cited, and 66,592 cited reference build the knowledge network of artificial muscles.
There are 925 patentees and 2,965 inventors who have contributed artificial muscle patents since 1975. The 1,913 patents retrieved were from 33 countries/regions, distributed in 271 International Patent Classifications (IPC) and 216 DWPI classifications.
3.1 Production and countries/regions’ contributions
The variation of the papers and patent output reflects the process of scientific and technological innovation on a theme. National scientific and technological innovation output is a major indicator of a country’s contribution to world science and technology. Figure 1 shows the yearly production of artificial muscles and contributions of the top 10 productive countries/regions since 2000. The paper output and patent output of the 10 countries/regions with the most papers output are presented in the form of stacked bar graphs, and the global output of papers and patents for artificial muscles are presented in the form of line graphs.
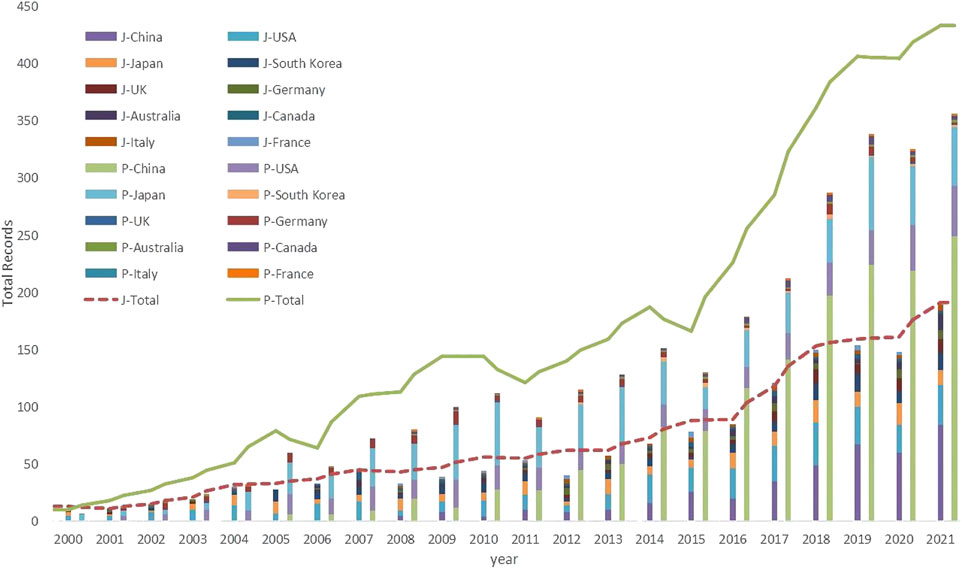
FIGURE 1. The global production of artificial muscle scientific and technological innovation and top 10 productive countries/regions contributions by year. Note(s): J-refers to the output of research papers, and P- refers to the output of technology patents.
The earliest research literature on artificial muscles available in the SCI-E database is a paper published in The Lancet in 1961, describing a pneumatic artificial muscle invented by McKibben (Agerholm and Lord, 1961). A United States patent on fabric tendons artificial skeletal muscles (US 3882551) is the earliest artificial muscle patent in DII, disclosed in 1975.
In the decades that followed, the development of both academic research and technological applications of artificial muscles was in the gestation phase, without significant growth in related achievements. This situation did not improve until the advent of the 21st century. The number of patents and papers both exceeded 10 in 2000, and then exhibited a steady upward trend, with the growth of patents being particularly pronounced. The number of outcomes of academic research and technological applications of artificial muscles reached new heights in 2016, both exceeding or approaching 100. Since then, the growth of papers and patents on artificial muscles has become more significant, with over doubling in the 6 years from 2016 to 2021.
It is noteworthy that the top three countries/regions in terms of artificial muscle paper output, China, the United States and Japan, accounted for nearly 3/5 of the total papers, while the top 10 countries/regions in terms of output contributed nearly 4/5 of the artificial muscle papers (Table 1). The distribution of papers citation times was similar to output. The distribution of the number of patents is slightly different from that of papers. A number of artificial muscle patents are global patents or European patents. The three top-ranked countries/regions, China, Japan and the United States, have a higher percentage of patent applications than papers, reaching more than 4/5 of the total. At the same time, the specific rankings of patents and papers differ somewhat, such as the United Kingdom contributed more papers but disadvantaged in terms of patents compared to Germany.
It can also be noted from Figure 1 and Table 1 that the United States and Japan are the two veteran countries/regions in the field of artificial muscles and have been ahead of other countries/regions in both patent applications and paper publications for years. Among the emerging countries, South Korea entered this field earlier and started to catch up with the United States and Japan in 2005. China is today the leading country in artificial muscle research and application, with the number of patents and papers surpassing the United States in 2010 and 2015 (except for 2016), respectively. Other artificial muscles research forces that have emerged successively in recent years include India, Iran, and others.
3.2 Contribution and collaboration of institutions
To show the main forces in the field of artificial muscles’ scientific and technological innovation and to reveal the collaboration between these institutions, we give information of the 20 institutions with the highest output of papers and patents of artificial muscle (Figure 2A). These 20 institutions contribute nearly one-third of the papers and papers citations on artificial muscles. The larger the proportion of an institution in the circle, the more its paper or patent output; the thicker the line between two institutions, the higher the frequency of collaboration between them. The main forces of academic research on artificial muscles are universities from different countries. Three universities, the University of Wollongong from Australia, Zhejiang University from China, and Sungkyunkwan University from South Korea, are the top three institutions in terms of paper output. Other institutions in the top ten are Harbin Institute of Technology, the University of Auckland, Chinese Academy of Sciences, Huazhong University of Science and Technology, Tokyo Institution of Technology, the University of Texas Dallas, and MIT. Among the major institutions filing artificial muscle patents, enterprises have a large share. Enterprises among the top 10 institutions are Tokai Rubber Industries Ltd., Matsushita Denki Sangyo KK, Eamex Corp, and LINTEC America Inc. Jiaxing Institute (University Jiaxing), Three Chinese universities, Harbin Institute of Technology, China Jiliang University, and Zhejiang University of Technology, rank the top 3 in the number of patent applications.
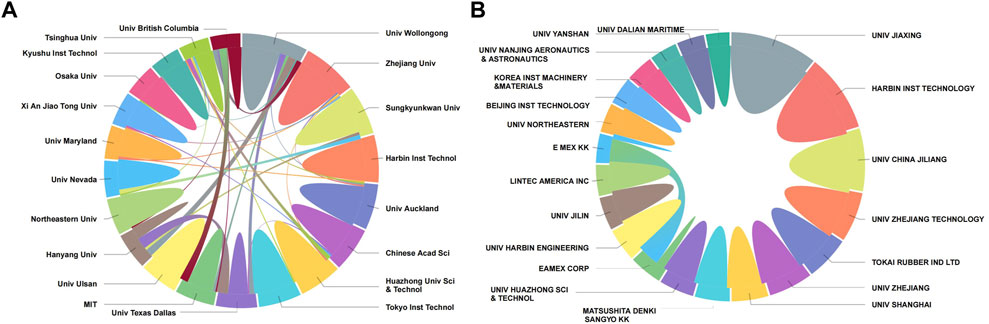
FIGURE 2. Pie map on productions and collaborations of the top 20 most productive institutions in artificial muscles (A) the top 20 productive institutions from a paper perspective; (B) the top 20 productive institutions from a patent perspective.
It can also be seen from Figure 2 that the frequency of academic research collaborations among the major institutions of artificial muscles is higher than that of technical applications. There are stable academic research collaboration ties among Hanyang University and the University of Texas Dallas, the University of British Columbia and MIT, the University of Wollongong and Hanyang University, and Sungkyunkwan University and the University of Nevada. Collaborative patenting among major institutions is rare, with collaboration between E MEX KK (TOYT-C) and EAMEX Corporation being one of the few cases.
With the help of temporal analysis, it can also be observed that the University of Wollongong, MIT, and Tokyo Institution of Technology were the mainstay of early artificial muscles research. Among these three institutions, the University of Wollongong has maintained the top position in the number of papers so far, MIT and Tokyo Institution of Technology have produced few papers in recent years but Tokyo Institution of Technology has had a revival in recent years. Nankai University is the representative of emerging research institutions in artificial muscles, with a rapid growth in the number of papers since 2020. Other emerging research institutions are Hohai University, Zhejiang University, etc.
Companies such as Tokai Rubber Industries Ltd., Matsushita Denki Sangyo KK, and EAMEX Corporation were among the first to layout patents on artificial muscles, but in recent years universities have begun to occupy an increasingly large share of the patent map. The current top-ranking institute in terms of the number of patents, Jiaxing University, started to lay out artificial muscle patents since 2017, and its patent applications are mainly about the design of vices, clamps, gripping heads, and manipulators’ products. It is notable that AISIN Corporation is not in the first 20 in the ranking of patent application count, but the action of manipulators’ patent layout is considerable in recent years.
3.3 Contribution of authors
The contribution of a researcher is usually measured by his or her publications and citation frequency. The top 20 authors in terms of number of published papers and their representative papers are presented in Table 2. Spinks from the University of Wollongong in Australia, Ahn from Ulsan University in South Korea and Kim from Nevada University in the United States ranked in the top three. In terms of frequency of citations, Baughman from the University of Texas Dallas, and Okuzaki from the University of Yamanashi occupy the top two positions, and Spinks ranks third. Spinks also has the highest h-index in the field of artificial muscles, followed by Xie from Univ Auckland, New Zealand and Kim. The high-producing scholars of artificial muscles papers are mainly from South Korea, Japan, and the United States, and there are also some high influence scholars from Australia, Belgium, New Zealand, etc.
Other influential scholars in recent years include Baughman from the University of Texas Dallas (Mu et al., 2019; Chu et al., 2021), Mirvakili (Mirvakili and Hunter, 2017) from MIT, Shepherd (Li G R et al., 2021) from Cornell University, etc. The first study on twisted artificial muscle was jointly reported by Spinks and Baughman, then he reported a series of important studies of twisted artificial muscles (Wang R et al., 2020; Zhou D et al., 2021). Mirvakili has published papers in journals such as Advanced Materials, Science Robotics, and ACS Applied Materials & Interfaces. Shepherd’s research on topics such as 3D printing antagonistic systems of artificial muscle has been published in Science Advances, Macromolecular Rapid Communications, Bioinspiration & Biomimetics, etc.
It is noticeable that some teams focusing on artificial muscle research have emerged, such as the term of Choi, Koo, Do Nam, and Hyungpil, the term of Lefeber and Van Ham, and the term of Kaneto and Takashima.
3.4 An analysis of leading journals
Academic journals are the platform for scientific research achievements presentation, and high-quality research works are usually published in journals with high academic reputation. The top 20 most productive journals in artificial muscle research were listed in Table 3. Among these 20 journals, the category that appears mostly is robotics.
In terms of the number of published artificial muscle papers, Smart Materials and Structures, Sensors and Actuators A-Physical, IEEE-ASME Transactions on Mechatronics, Advanced Robotics, and Actuators are in the top 5. For the citation of papers, Advanced Materials, Smart Materials and Structures, Sensors and Actuators A-Physical, IEEE-ASME Transactions on Mechatronics, and Synthetic Metals ranked in the top 5. Different from the number ranking, Advanced Materials received 2,734 citations with only 21 papers, surpassing Smart Materials and Structures’ 2,108 citations with 100 papers. Advanced Functional Materials is also on the rise. Regarding average citation per paper, Advanced Materials, Advanced Functional Materials, Synthetic Metals, IEEE-ASME Transactions on Mechatronics, and ACS Applied Materials & Interfaces performed the best. For h-index, Smart Materials and Structures, Advanced Materials, Sensors and Actuators A-Physical, IEEE-ASME Transactions on Mechatronics, Sensors and Actuators B-Chemical are in the top 5.
If only considering the number of artificial muscle papers published in the last 3 years, Smart Materials and Structures is still in the first place. The top ranking journals are Smart Materials and Structures, Actuators, IEEE Robotics and Automation Letters, IEEE Access, Sensors and Actuators A Physical, Soft Robotics, Bioinspiration Biomimetics, Journal of Robotics and Mechatronics, and Polymers. Physical, Soft Robotics, Bioinspiration Biomimetics, Journal of Robotics and Mechatronics and Polymers, Actuators, IEEE Robotics and Automation Letters, IEEE Access, and Polymers are representatives of the emerging journals. In recent years, more papers on artificial muscles have also been published in Science Robotics, Advanced Materials Technologies, Science Advances, etc. Synthetic Metals, Electroactive Polymerica, Journal of Electroanalytical Chemistry, and Macromolecules have decreased the publication of artificial muscle papers in recent years.
The top 20 journals in terms of number of publications include five and four journals from Elsevier and IEEE, respectively. Five major publishers, Elsevier, IEEE, IOP, Springer, and Wiley, published more than half of the artificial muscle research papers. Elsevier ranked first with 282 papers. MDPI is the representative of emerging publishers.
3.5 An analysis of prominent fields
As a Frontier scientific problem, artificial muscle research covers a wide range of fields. Figure 3 shows the WoS category clustering map of the artificial muscle papers and the DWPI category clustering map of the patents. The size of a dot represents the number of papers, and the thickness of the line between the dots indicates the intensity of the interaction between the two fields. Multidisciplinary materials science, instruments instrumentation and robotics are the major fields of artificial muscle research, making up more than half of the total papers. In the patent clustering circle pack diagram, the DWPI patent categories are shown in concentric circles. The large circles indicate high-level categories, and the smaller circles in the circles indicate related categories of lower levels. The size of each circle indicates the number of documents in that category.
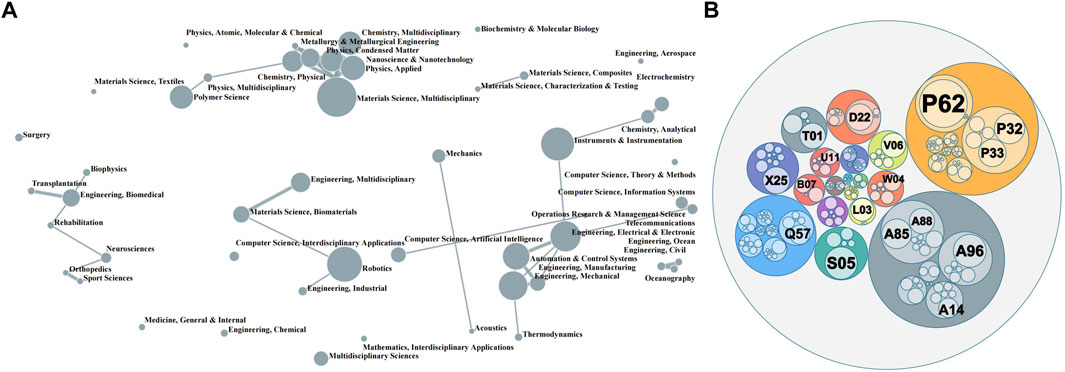
FIGURE 3. Cluster map of artificial muscle scientific and technological innovation fields (A) cluster of papers’ WoS categories; (B) cluster of patents’ DWPI categories.
Research on artificial muscles is concentrated in three clusters, the materials science cluster consisting of multidisciplinary materials science, applied physics, multidisciplinary chemistry, and others, the mechanical and automation cluster consisting of instruments and instrumentation, electrical and electronic engineering, mechanical engineering, automation and control systems, etc, and the robotics cluster consisting of robotics, biomaterials materials science, interdisciplinary applications computer science, and industrial engineering. There are also some more independent fields including textile, surgery, aerospace engineering, ocean engineering, civil oceanography engineering and so on.
The largest cluster of artificial muscle patents is the general engineering cluster, followed by the polymers chemical cluster and the instrumentation cluster. Among the general engineering clusters, hand tools (P62), prosthesis (P32), and medical aids (P33) appear most frequently. The instrumentation cluster is mainly focused on electrical medical equipment (S05). In addition, industrial electric equipment (X25), bandages (D22), and digital computers (T01) also have a sizeable patent portfolio.
3.6 Development of various kinds of artificial muscle
Various kinds of artificial muscle have been investigated. On the basis of different stimuli, artificial muscles can be classified as electrical stimulation, photo stimulation, chemical stimulation, humidity stimulation, magnetic stimulation, pressure stimulation, etc. These artificial muscles can be classified into many types depending on the power mechanism and material. For example, electro-active polymer (EAP) can be classified to ionic EAP, electronic EAP, and electro-thermal EAP by stimulation mechanism, and nanoparticle-Based actuators are represented by actuators using new materials such as carbon nanotubes, graphene and fullerenes. Figure 4 illustrates the evolution of research and applications of various artificial muscles. Since the number of papers before 1994 and patents before 2001 are minor, the papers and patents in the figure were dated back to 1994 and 2001, respectively. The artificial muscles selected in this paper are PAMs, DEAs, SMAs, IPMCs, carbon nanotube (CNT) electroactive polymers, textile fiber based actuators, twisted fiber actuators, graphene based actuators, natural skeletal muscles, piezoelectric actuators and other artificial muscles.
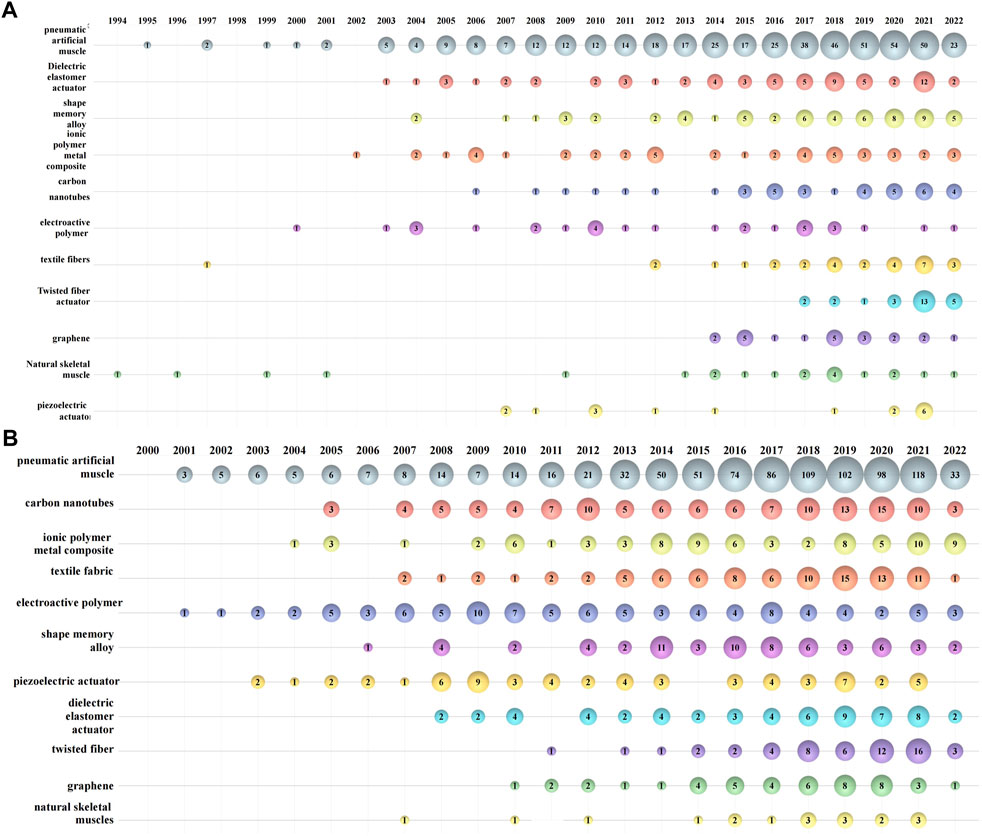
FIGURE 4. Bubble chart of various artificial muscle development (A) development in papers (B) development in patents.
PAMs is the major artificial muscle research and application topic for its high compliance, safety, and force (Abu Mohareb et al., 2021; Liang et al., 2022a), with the same long history as natural skeletal muscle. Since Pelrine et al. investigated the large strains, fracture toughness, and fast response of acrylic and silicone elastomers compared to a natural muscle in 2000 (Pelrine et al., 2000), DEA related papers have been studied extensively, but the technology development is rare. SMA artificial muscles are a kind of artificial muscles based on the shape memory effect of alloys originating from a phase transition due to temperature variation (Park et al., 2020), and researchers are working to overcome the limitation of low working frequencies impose on its application (Choi et al., 2022). CNTs have been used widely in the field of artificial muscles in recent years due to the excellent microwave thermal effect and mechanical properties (Aziz et al., 2021), especially in the area of patent applications. Twisted fiber actuator and graphene are the popular emerging materials in recent years (Ghosh and Karak, 2021; Kimura D et al., 2021), and the related research and development is hot. Unlike natural skeletal muscle, about which the scientific and technological innovation scale is getting smaller, textile fibers have been growing in patents in the field of artificial muscles. Piezoelectric artificial muscles have relatively few papers and many patents but their high power density and simple structure (Kanik et al., 2014; Mokhtari et al., 2021) make them a possible hot spot for future artificial muscle research and development.
3.7 A discussion of most discussed terms
The top 20 terms of the paper and patent outputs in the field of artificial muscles are shown in Figure 5A, correspondingly. In them, the terms of papers come from the cleaned author keywords of papers and the terms of patents come from the results of natural language processing (NLP) of patent abstracts. The more a topic occupies in the circle, the more its paper or patent output; the thicker the line between two topics, the higher the frequency of their common occurrence.
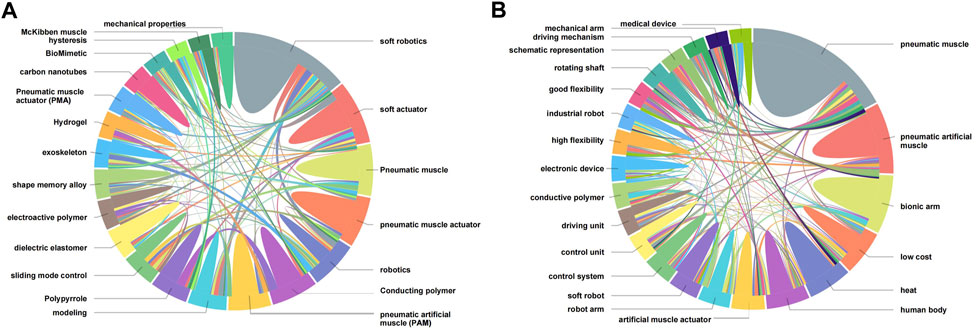
FIGURE 5. Pie map of the top 20 terms of artificial muscle (A) author keywords of papers; (B) abstract NLP of patents.
As seen in Figure 5A, the academic research on artificial muscles focuses on the research and development of novel materials. Hot research terms include soft robotics, soft actuator, pneumatic muscle, pneumatic muscle actuator, robotics, conducting polymer, PAMs, modeling muscles, sliding mode control, DEA, electroactive polymer, modeling muscles, sliding mode control, dielectric elastomer, electroactive polymer, etc. Due to the strong correlation between artificial muscle research and soft robotic research, soft robotic research has connected most of the artificial muscle research terms. PAMs, as the most commonly used artificial muscle type, has also led to the development of similar term research.
As far as the scientific and technological innovation of artificial muscles goes, researchers have focused more on the performance and functional structure of artificial muscle products in the patent application process. PAMs, bionic arm, low cost, heat human, body artificial muscle actuator, robot arm, soft robot control system, control unit, driving unit, conductive polymer electronic device, high flexibility, industrial robot, and good flexibility are the most involved terms in artificial muscles patents. The pursuit of higher performance, more application scenarios, and lower production costs is the main reason for the discrepancy between the artificial muscle technological innovation terms and the academic research terms.
3.8 An analysis of scientific and technological innovation frontiers
To reveal the scientific and technological innovation frontiers of artificial muscles, we analyzed the ESI highly cited papers, highly cited patents, hot paper, and emerging terms. The ESI highly cited papers are the top 1% cited papers in the research field published in the past 10 years. Usually, a paper selected as an ESI highly cited paper indicates that its research direction is the current focus of the academic community, and the ESI highly cited papers can indicate the focus of a research theme. The ESI highly cited papers on artificial muscle research since 2012 is shown in Table 4.
Reviews can provide a systematic overview of the progress of a research direction, and articles can report the latest original findings in a timely manner. The 27 highly cited papers, especially the 15 articles, revealed the frontiers of artificial muscle research. In Takashima et al. 2012 designed a photoresponsive supramolecular actuator by integrating host-guest interactions and photoswitching ability in a hydrogel. Zang et al. reported an approach to reversibly control the crumpling and unfolding of large-area graphene sheets used as artificial muscles actuators in 2013. Park et al. designed a wearable robotic device powered by PAMs in ankle-foot rehabilitation in 2014. In Yang et al. (2015) studied the optical, magnetic, and electrical properties of ReSe2, which enabled an application of artificial muscles actuators. Li et al. (2017) reported an artificial muscle in 2017, which can be driven by fluids at negative pressures. Diels-Alder polymers were used to develop artificial muscle applications of self-healing pneumatic actuators by Terryn et al. Acome et al. described a class of hydraulically amplified self-healing electrostatic actuators in 2018. Gu et al. reported a soft wall-climbing robot based on muscle-like actuators. A soft robot that senses environment and adaptively performs soft crawling with artificial muscles is reported by Wang et al. (2018). Lee et al. reported an organic optoelectronic sensorimotor synapse for light-interactive actuation of an artificial muscle actuator. In Kanik et al. (2019) applied a high-throughput iterative fiber-drawing technique to create strain-programmable artificial muscles. Qin et al. described a general in situ polymerization strategy for the fabrication of anisotropic hydrogels. Umrao et al. reported an MXene artificial muscle with ultrafast rise time. In Sun et al. (2020) reported a new adaptive control method of PAMs systems.
Besides the ESI Highly Cited Papers, there are also papers published over 10 years ago that are still frequently cited by researchers in recent years. A paper published in 1996 tested the McKibben artificial muscle pneumatic actuator first developed in the 1950s, which became a classic work of McKibben artificial muscle research, and is still of great reference value to researchers today (Chou and Hannaford, 1996). The principles involved in a 2004 paper by Madden et al. (2004) that reviewed the physical principles of artificial muscle technology are still widely used today. The DEA research progress reported by Brochu et al., in 2010 is still of great reference value to researchers today (Brochu and Pei, 2010) and a chemomechanical system based on a synthetic polymer gel was reported by OSADA et al. (1992) opening a new window of artificial muscle R&D. The same goes for Mirfakhrai’s research in 2007 on the mechanisms and performance related to polymer artificial muscle technology (Mirfakhrai et al., 2007), Pelrine’s paper on elastomeric dielectric materials with compliant electrodes (Pelrine et al., 2000), and Thomsen’s work on liquid crystal elastomers with mechanical properties of a muscle (Thomsen et al., 2001). It is notable that the research themes of high-level journal papers are more likely to be of sustained interest to researchers. For instance, a 2005 research paper published in Nature on motors capable of inducing mechanical motion (van Delden et al., 2005) and a paper on torsional CNTs artificial muscles in Science (Foroughi et al., 2011) are still frequently cited today.
Unlike ESI highly cited papers, ESI hot papers reflect the latest Frontier issues in the field. ESI hot papers are papers published in the past 2 years that have received a number of citations in the most recent 3-month period that places them in the top .1% of papers in the same field. Hot papers generally conduct research geared toward interdisciplinary Frontier issues. Artificial muscles-related topics currently have a paper published in Nature in 2021 that was selected as an ESI hot paper, exploring the application of artificial muscles in extreme environments. The paper designs a soft robot with artificial muscles, power and control electronics distributed on a polymer matrix that can swim freely in a deep ocean high pressure environment like a deep ocean fish (Li S et al., 2021).
When we talk about the technological innovation of artificial muscles, highly cited patent is an important reference indicator (Table 5). The industry’s most interesting artificial muscle technologies include: process for producing artificial muscles electric conductive polymers (WO2004014987, WO200158973), SNA fibre actuator (US5092901), carbon nanotube actuator (WO2005102924, WO2007033438), artificial muscle hydrogel polymer (US5100933, WO2008079440), and magnetorheological fluid in artificial muscle tissue (US6168634), artificial muscle-skeletal mechanism (US4776852), bilayered structure artificial muscles (JP2006297005). Highly cited patents for artificial muscles are mainly from two scenarios: implementation of products and improvement of technologies. The current high-priority artificial muscle products are mainly from the medicine and healthcare fields, such as wearing hand function rehabilitation training robot (CN101181176, CN101433491), flexible bladder device (US6223648), artificial sphincter (US2003212306, US2005004425), apparatus for augmenting near vision accommodation (US2003028248), cardiac repair artificial muscle sheath (US4176411), implantable cardiac defibrillation devices (US5578069), and artificial anatomic lumen structure model (WO2006083963).
To help researchers identify emerging research areas worthy of attention in the field, we calculated emerging topics in artificial muscle scientific and technological innovation with DDA (Table 6). The DDA Emerging Trends Indicator tracks the evolution of subject terms based on four key factors: novelty, persistence, degree of community, and growth, demonstrating a temporal sequence of subject evolution with some predictive power (Porter et al., 2020). The Emerging Trends Indicator is calculated using words or phrases mined from the titles and abstracts of patents from the past 10 years to detect emerging subject terms based on a benchmark of the previous 3 years (Li M et al., 2021).
There are nine emerging topics in the field of artificial muscle patents. Soft robots and robotic devices are the main applications of artificial muscles and are receiving attention from researchers from China, India, South Korea, Japan, the United States, and other countries. As a key connector for key parts such as knee joints, waist joints, and lower limb joints, artificial muscles have lots of applications in pneumatic system-based simulated robot systems. A continuous and stable power supply is a prerequisite for artificial muscles to work, and power supply devices are a necessary panel for patent layout. In order to enable robots to effectively work in more extreme environments, researchers in China, South Korea, Russia, and other countries have carried out research and development of artificial muscles to cope with fluid pressure. Compared with biological muscle, the reaction speed of artificial muscles has always been slow, which restricts the application of artificial muscles, and researchers in China, the United States, and other countries have also been working to obtain a more rapid reaction speed of artificial muscles.
Another way to identify Frontier issues is to observe the variation of topics across time. We counted the 20 most frequently occurring terms in papers and patents from 2020 to 2021 and compared them to their frequency of occurrence during 2018–2019 (Table 7). The growth topics of artificial muscle research in the last 2 years are SMA, twisted fiber actuator, CNTs, piezoelectric actuator, etc. Liquid crystal elastomer is one of the directions that deserve special attention (Lee et al., 2022; Liu G et al., 2022). The evolution of artificial muscle applications is relatively smooth and bionic arm patent applications show a declining trend. Twisted fiber (Leng et al., 2021b) and soft robot (Mirvakili et al., 2020) are the topics with more patent growth since 2020.
4 Discussion
The scientific and technological innovation of artificial muscles has a long history, but the real rise did not start until 20 years ago. Since 2000, there has been a great deal of interest and invest of science and technical issues in artificial muscles. It is still far from a large scale application of artificial muscles. Researchers are enthusiastically devoting themselves to the research of artificial muscles and are achieving an increasing number of results.
4.1 Rapid development and shifting focus
There are high expectations for artificial muscles. The research shows that many institutions, especially enterprises, have started the patent layout of artificial muscles early, and research results will be applied for patent protection as soon as they are considered to have the potential to be transformed into practical applications. From the characteristics of the achievements, papers regarding artificial muscles strive to achieve innovation in materials and methods, and constantly open up new horizons in artificial muscle research, while patents pay attention to cost, effectiveness and function, so that artificial muscles can be applied to people’s lives and industrial production as soon as possible.
The rapid expansion of the scale of scientific and technological innovation achievements is a perfect proof of the rapid development of artificial muscle technology. From 2000 to 2021, the number of research papers and patents on artificial muscle grew from 13 to 10 to 191 and 433, respectively. In the past 20 years, more than 5,000 researchers from over 1,000 institutions have been involved in the research on artificial muscles, with the research results being distributed in 492 academic journals. Artificial muscle research has covered the areas of materials science, robotics, instruments and instrumentation, electrical and electronic engineering, involving nearly one hundred categories. In terms of technological innovation, materials science, mechanics and automation, and robotics are the triumvirate driving the artificial muscle technological innovation, with the participation of dozens of fields such as textile science, surgery, and aeronautics.
We can also find that the center of artificial muscle science and technological innovation is migrating to emerging countries, especially to universities in emerging countries. The United States, Japan, Germany and other developed countries are the pioneers of artificial muscle research and development, but the global scientific and technological innovation center is migrating to emerging countries/regions. Research institutions and scholars from China, South Korea, India, and Iran have grown to be a significant force in artificial muscle R&D. However, countries such as the United States and Japan still have enormous influence and maintain the lead in terms of citations of papers and the attractiveness of research institutions. In terms of technological innovation, the current applications of artificial muscles are mainly in the field of health and medical devices, but the involvement of companies is modest. As for basic research, research papers are mainly from university researchers. For application development, artificial muscle patent applicants are also increasingly being from universities rather than companies.
4.2 Soft actuators and robots have been the focus
Robots have entered the manufacturing and daily life of people from science fiction. Autonomous robots include drive, energy, sensory and control systems that mimic various systems containing high complexity in animals and other living organisms (Aubin et al., 2022). With the refinement and miniaturization of autonomous robot design and scale, soft actuators are gradually replacing traditional actuators to provide higher integration and energy density for robots. The advent of artificial muscles has allowed soft actuators to better mimic biological muscles, adapt to their surroundings, and collaborate with the various components of the robots (Zou et al., 2021). Artificial muscles made of elastomeric dielectric materials can deform in response to light, heat, electricity, and magnetic stimuli to actuate soft robots. The use of soft materials allows soft robots to be lighter than conventional robots and to work safely with humans, as well as to adapt to different shapes autonomously.
Nowadays, artificial muscles are the core components of intelligent and interactive soft robotics systems. Researchers even proposed the concept of “artificial super muscles” to give artificial muscles better properties for more complex human-computer interaction and integration drives (Higueras-Ruiz et al., 2022). In the last 2 years, the performance metrics of artificial muscles have been upgraded and have continued to gain new applications in the field of soft robotics. We can see the refinement of artificial muscle powered insect-sized robots (Wang Z et al., 2021; Kim D et al., 2022), the improvement of artificial muscle driven water-walking robots (Zhou X et al., 2021; Kim S et al., 2022), soft tension robots for exploring unknown spaces (Kobayashi et al., 2022; Zhou et al., 2022a), the enhancement of artificial muscle driven soft crawling robots (Liu et al., 2021; Wu et al., 2022), the realization of rehabilitation assistance training robot based on pneumatic artificial muscles (Wang and Xu, 2021; Chu et al., 2022; Tsai and Chiang, 2022), control optimization and modeling of artificial muscle-actuated endo-exoskeleton robots (Chen et al., 2022; Lin et al., 2021; Liu H et al., 2022; Yang et al., 2022), and the application of SNA in soft wearable robots (Jeong et al., 2022).
Soft robots also occupy an influential position in the technological invention of artificial muscles. As seen from the highly cited patents, the citation frequency of medical robot patents such as artificial skeletal mechanism for robotic actuator, wearing hand function rehabilitation training robot is rising rapidly over time. Emerging artificial muscle patents are mainly on robot device and soft robot. The latest patents on versatility, performance, and reliability improved artificial muscles, artificial muscle lock mechanism, cut-off valves, and liquid pressure control device of the robot device are highlighted by companies, universities and research institutions.
4.3 Emergence of smart materials
Artificial muscles are sometimes considered to be and equivalent to smart muscles, but not all artificial muscle types actually belong to the category of smart materials. A variety of artificial muscles have been manufactured from metals, polymers, ceramics, and diverse composite materials, many of which do not have intelligent components. The familiar PAMs are non-smart muscles that do not contain any smart materials. Similarly, hydraulic artificial muscles are not smart, but as one of the most mature artificial muscle technologies, they are widely used, and organizations such as Soft Robotics Inc. and Soft Gripping have patented and manufactured a variety of soft robots. Research on various artificial muscles made of smart materials, such as CNTs and SMAs, has been extremely active in recent years.
PAMs remain one of the most widely used artificial muscles. In recent years, researchers have made new progress in the direction of three-dimensional printing miniature piezoelectric actuators (Cui et al., 2019), energy harvesting and sensing soft piezoelectric materials, capacitive wearable haptic sensing devices (Ozioko et al., 2020), humanoid arm robots actuated by PAMs (Liang et al., 2022b), multi-directional bending PAMs (Xiao et al., 2021a; Xiao et al., 2021b), amplified piezoelectric actuators based on piezoelectric hydraulic pumps (Park and Cha, 2019), origami micro-manipulators for minimally invasive surgery (Suzuki and Wood, 2020), and non-tethered flapping wing micro-vehicles (Shi et al., 2020). The number of patents for artificial intelligence and soft robots based on PAMs is also growing rapidly.
PAMs, hydraulic artificial muscles, and other non-smart artificial muscles have enabled soft actuators with better mechanical properties to be fully integrated with various electronic devices to make soft actuators and robots more intelligent, a new trend in research in recent years. Smart artificial muscles should have the ability to sense various external stimuli such as temperature, pressure, light, magnetism, color, sound, etc. and respond quickly and autonomously. Smart artificial muscles include DEAs, IPMCs, etc. Each smart material has its application area.
In recent years, researchers have made a lot of achievements in the scientific and technological innovation of intelligent artificial muscles. Among them, novel artificial muscles such as twisted and coiled artificial muscles (TCAMs) and alkene-carbon artificial muscles are particularly noteworthy.
TCAMs have attracted attention in the field of smart actuators and robotics due to their low time lag, high operating density, and low manufacturing difficulty. They are made of twisted and coiled polymer fibers that form a spring-like structure and can contract or expand tremendously when heated. In the past few years, researchers have reported a variety of TCAMs, including TCAMs Driven Soft Crawling Robot (Wu et al., 2022), Modeling framework for macroscopic dynamics of twisted and coiled polymer actuator driven by Joule heating focusing on energy and convective heat transfer (Masuya et al., 2017), force enhanced multi-twisted and coiled actuator (Zhou et al., 2022b), CN-polypyrrole coated twisted and coiled yarn artificial muscles (Aziz et al., 2020), spandex fibers and SMA Skeleton TCAMs (Zhang and Yang et al., 2022), etc. Twisted and coiled soft actuators used for special clothing, twisted and coiled carbon nanotube yarn annealing and reinforcement process, and TCAMs array devices have been applied for patents on their technologies, providing a foundation for commercial production.
Alkene-carbon materials such as CNT and graphene have outstanding advantages in terms of tensile strength, thermal conductivity, electrical conductivity and specific surface area (Plisko and Bildyukevich, 2014), and have promising prospects for applications. In recent years, alkene carbon material artificial muscles programmable response deformation (Kim et al., 2020), multi-directional actuation (Oh et al., 2021; Ling et al., 2020), integrated actuation and motion sensing (Zhao et al., 2020; Wang H Q et al., 2020), laser-induced graphene soft actuators (Ling et al., 2020), bilayer membrane structure temperature sensing actuator (Xiao Y W et al., 2021), and other directions have shown new progress, which are expected to meet the demand for more scenarios of artificial muscle applications.
Artificial muscles have not been able to achieve a full performance advantage over conventional actuators. Artificial muscles with large output stresses, such as piezoelectric actuators, are usually limited in output strain. The opposite is the case of dielectric elastomers. Some of the novel artificial muscles have achieved a better balance between stress and strain, yet face a trade-off between actuation rate and efficiency (Greco et al., 2022). Various smart materials have different drive modes, physical properties and manufacturing processes, all with pros and cons. Therefore, before the emergence of a more comprehensive performance smart actuator, it is necessary to determine the type of artificial muscle according to the application scenario so as to obtain the best environmental sensitivity and intelligent interaction capability.
4.4 The future of artificial muscles
The application of artificial muscles in a wide range is already visible, including some interesting frontiers such as programmable biomimetic actuators (Dong et al., 2019), shape-color-switchable thermochromic actuators (Wang X et al., 2019), space robots (Ashby et al., 2022), and micro vehicles (Chen and Zhang, 2019). All signs indicate that artificial muscles are competitive in replacing conventional actuators in areas that require strong human-machine interaction and strong adaptability to the surrounding environment now. Researchers are also looking ahead to more thrilling applications of artificial muscles, especially for more intelligent robots that adapt to complex environments. The combination of artificial muscle technology with artificial intelligence technology, wireless communication technology and novel energy storage technology is expected to allow intelligent robots to take the place of humans in scientific research activities in every extreme environment, down to the Marianas Trench, up to Mount Everest, and far to the Moon and Mars. Meanwhile, micro-robots with artificial muscle technology are highly anticipated. It is hoped that such micro-robots can autonomously complete various in vivo tests and treatments to relieve patients’ pain and save more lives.
Recent research results also show that although the scientific and technological innovation of artificial muscles is gaining momentum, and some of the properties of artificial muscles have surpassed those of biological muscles, no artificial muscle has completely replaced biological muscles, and there are still many issues that need to be solved. The first issue is the cost as the transformation of graphene, CNTs, SMAs, SMPs, and other artificial muscle materials into artificial muscles is still very costly, and there is still a big gap from mass production. The next issue is reliability. Temperature difference drive, solvent adsorption drive or electrochemical drive can not solve the problem of slow response speed and long recovery time of artificial muscles. In addition, the sustainability also needs to be improved, the energy conversion efficiency of existing artificial muscles is still far below that of traditional electric motors or heat engines. Researchers have proposed to improve artificial muscles by appropriately cooling the fibers, increasing Joule heat to assist adsorption, preparing non-metallic IPMC, and increasing the ion diffusion rate of electrolytes, but they have not yet been widely employed. To better reproduce natural muscle functionalities, researchers treat artificial muscle as a dynamic non-linear system and introduced various methods such as adaptive control law, adaptive fuzzy-sliding mode control method, learning-based adaptive robust control framework, etc, but there is still a lot of distance from lib to life. People are also continually updating technology and improving materials in the hope that artificial muscles can actuate soft robots better with programmable motions (gripping, twisting, and three-dimensional bending) (Nuchkrua and Leephakpreeda, 2022) in the near future. The future research and development of artificial muscles still needs to strengthen the cross-fertilization of subjects such as material science, computer science, machinery and automation, improve the synergy of enterprises, universities, and research institutions, continuously upgrade materials, improve performance, and reduce costs.
According to the needs of production and life of human beings, the target of artificial muscle material improvement and technology enhancement should be to improve the response speed, enhance cycle stability and significantly reduce manufacturing costs to achieve billions of drive cycle mechanical applications and meet the needs of billions of people worldwide for soft robots, medical devices, and kinds of artificial intelligence applications.
5 Conclusion
Studies of artificial muscles represent a rapidly growing field. This paper uses bibliometric methods to provide a brief review of the development of artificial muscles based on papers and patent data, retrospect and analyze the progress and status of artificial muscles based on SMAs, CNTs, graphene, and other materials, and prospects the future development of artificial muscles. This paper will help researchers to grasp the overall picture of artificial muscle research, follow the frontiers of scientific and technological innovation, determine research directions and select journals for submission, and help enterprises in patent layout.
There are still some limitations in this study. It is a reasonable and common research method to use papers to refer to scientific research and patents to refer to technological innovation (Jin et al., 2023), but patents need to be transformed into products to complete R&D even patents covered more than 90% of the latest technological information in the world (Milanez et al., 2014), so there are different views. The bibliometric method can help researchers to get a comprehensive picture of a scientific issue, but biases can occur for some reasons. For this study, the pre-defined retrieval formula and the restricted retrieval field cannot cover the artificial muscles papers and patents completely and accurately, although we carefully designed the retrieval process according to the specifications and introduced human intervention to remedy this.
Author contributions
Conceptualization, YJ; methodology, YJ; software, YW and HF; data curation, XY; writing—original draft preparation, YJ and FS; writing—review and editing, HF and XY; visualization, FS; supervision, YW; project administration, YW. All authors have read and agreed to the published version of the manuscript.
Conflict of interest
The authors declare that the research was conducted in the absence of any commercial or financial relationships that could be construed as a potential conflict of interest.
Publisher’s note
All claims expressed in this article are solely those of the authors and do not necessarily represent those of their affiliated organizations, or those of the publisher, the editors and the reviewers. Any product that may be evaluated in this article, or claim that may be made by its manufacturer, is not guaranteed or endorsed by the publisher.
References
Abu Mohareb, S., Alsharkawi, A., and Zgoul, M. (2021). Hysteresis modeling of a PAM system using ANFIS. Actuators 10, 280. doi:10.3390/act10110280
Acome, E., Mitchell, S. K., Morrissey, T. G., Emmett, M. B., Benjamin, C., King, M., et al. (2018). Hydraulically amplified self-healing electrostatic actuators with muscle-like performance. Science 359 (6371), 61–65. doi:10.1126/science.aao6139
Agerholm, M., and Lord, A. (1961). The artificial muscle of McKibben. Lancet 1 (717), 660–661. doi:10.1016/s0140-6736(61)91676-2
Aiyuan, L., Xinyu, S., and Wanfu, Y. (2021). Application and research progress of silk fibroin scaffold in musculoskeletal tissue engineering. J. Silk 58 (11), 18–22. doi:10.3969/j.issn.1001-7003.2021.11.004
Anderson, I. A., Gisby, T. A., McKay, T. G., O'Brien, B. M., and Calius, E. P. (2012). Multi-functional dielectric elastomer artificial muscles for soft and smart machines. J. Appl. Phys. 112, 041101. doi:10.1063/1.4740023
Ashby, J., Rosset, S., Henke, E. F. M., and Anderson, I. A. (2022). One soft step: Bio-Inspired artificial muscle mechanisms for space applications. Front. Robotics Ai 8, 792831. doi:10.3389/frobt.2021.792831
Aubin, C. A., Gorissen, B., Milana, E., Buskohl, P. R., Lazarus, N., Slipher, G. A., et al. (2022). Towards enduring autonomous robots via embodied energy. Nature 602 (7897), 393–402. doi:10.1038/s41586-021-04138-2
Aziz, S., Martinez, J. G., Foroughi, J., Spinks, G. M., and Jager, E. (2020). Artificial muscles from hybrid carbon nanotube-polypyrrole-coated twisted and coiled yarns. Macromol. Mat. Eng. 305 (11), 2000421. doi:10.1002/mame.202000421
Aziz, S., Villacorta, B., Naficy, S., Salahuddin, B., Gao, S., Baigh, T. A., et al. (2021). A microwave powered polymeric artificial muscle. Appl. Mater. Today 23, 101021. doi:10.1016/j.apmt.2021.101021
Bai, W. Y., Fang, H., Wang, Y. W., Zeng, Q., Hu, G. Y., Bao, G. J., et al. (2021). Academic insights and perspectives in 3D printing: A bibliometric review. Appl. Sciences-Basel 11 (18), 8298. doi:10.3390/app11188298
Bao, G., Fang, H., Chen, L., Wan, Y., Xu, F., Yang, Q., et al. (2018). Soft robotics: Academic insights and perspectives through bibliometric analysis. Soft Robot. 5 (3), 229–241. doi:10.1089/soro.2017.0135
Bastola, A. K., and Hossain, M. (2021). The shape - morphing performance of magnetoactive soft materials. Mater. Des. 211, 110172. doi:10.1016/j.matdes.2021.110172
Brochu, P., and Pei, Q. (2010). Advances in dielectric elastomers for actuators and artificial muscles. Macromol. Rapid Comm. 31 (1), 10–36. doi:10.1002/marc.200900425
Brookes, B. C., and Bradford, S. C. (1985). Sources of information on specific subjects by S.C. Bradford. J. Inf. Sci. 10 (4), 173–175. doi:10.1177/016555158501000406
Bruns, C. J., and Stoddart, J. F. (2014). Rotaxane-based molecular muscles. Accounts Chem. Res. 47 (7), 2186–2199. doi:10.1021/ar500138u
Chang, J., Zhou, X., Zhao, Q., Cao, W., Zhang, M., and Yuan, J. (2021). Reduced graphene oxide-poly (ionic liquid) composite films of high mechanical performance. Front. Mater. 8, 635987. doi:10.3389/fmats.2021.635987
Chen, C., and Zhang, T. (2019). A review of design and fabrication of the bionic flapping wing micro air vehicles. Micromachines-Basel. 10, 144. doi:10.3390/mi10020144
Chen, C., Lien, W., Chen, C., and Wu, Y. (2020). Implementation of an upper-limb exoskeleton robot driven by pneumatic muscle actuators for rehabilitation. Actuators 9, 106. doi:10.3390/act9040106
Chen, J., Leung, F. K., Stuart, M. C. A., Kajitani, T., Fukushima, T., van der Giessen, E., et al. (2018). Artificial muscle-like function from hierarchical supramolecular assembly of photoresponsive molecular motors. Nat. Chem. 10 (2), 132–138. doi:10.1038/NCHEM.2887
Chen, P., Yuan, T. W., Yu, Y., and Liu, Y. W. (2022). Design and modeling of a novel soft parallel robot driven by endoskeleton pneumatic artificial muscles. Front. Mech. Eng. 17 (2), 22. doi:10.1007/s11465-022-0678-2
Chen, S., Costil, R., Leung, F. K., and Feringa, B. L. (2021). Self-Assembly of photoresponsive molecular amphiphiles in aqueous media. Angew. Chem. Int. Ed. 60 (21), 11604–11627. doi:10.1002/anie.202007693
Choi, K., Park, S. J., Won, M., and Park, C. H. (2022). Soft fabric muscle based on thin diameter SMA springs. Smart Mat. Struct. 31, 055020. doi:10.1088/1361-665X/ac6550
Chou, C. P., and Hannaford, B. (1996). Measurement and modeling of McKibben pneumatic artificial muscles. IEEE Trans. Robotics Automation 12 (1), 90–102. doi:10.1109/70.481753
Chu, H. T., Hu, X. H., Wang, Z., Mu, J. K., Li, N., Zhou, X. S., et al. (2021). Unipolar stroke, electroosmotic pump carbon nanotube yarn muscles. Science 371 (6528), 494–498. doi:10.1126/science.abc4538
Chu, W. L., Lin, C. J., and Chen, Y. Y. (2022). Redundant robot with pneumatic artificial muscles for rehabilitation works using iterative learning control. Appl. Sciences-Basel 12 (17), 8419. doi:10.3390/app12178419
Cianchetti, M., Laschi, C., Menciassi, A., and Dario, P. (2018). Biomedical applications of soft robotics. Nat. Rev. Mater. 3 (6), 143–153. doi:10.1038/s41578-018-0022-y
Cui, H. C., Hensleigh, R., Yao, D. S., Maurya, D., Kumar, P., Kang, M. G., et al. (2019). Three-dimensional printing of piezoelectric materials with designed anisotropy and directional response. Nat. Mat. 18 (3), 234–241. doi:10.1038/s41563-018-0268-1
Darko, A., Chan, A., Adabre, M. A., Edwards, D. J., Hosseini, M. R., and Ameyaw, E. E. (2020). Artificial intelligence in the AEC industry: Scientometric analysis and visualization of research activities. Autom. Constr. 112, 103081. doi:10.1016/j.autcon.2020.103081
de Solla Price, D. (1974). Gears from the Greeks: The antikythera mechanism: A calendar computer from ca. 80 B. C. Trans. Am. Philosophical Soc. 64 (7), 1–70. doi:10.2307/1006146
Dong, Y., Wang, J., Guo, X. K., Yang, S. S., Ozen, M. O., Chen, P., et al. (2019). Multi-stimuli-responsive programmable biomimetic actuator. Nat. Commun. 10, 4087. doi:10.1038/s41467-019-12044-5
Donthu, N., Kumar, S., Mukherjee, D., Pandey, N., and Lim, W. M. (2021). How to conduct a bibliometric analysis: An overview and guidelines. J. Bus. Res. 133, 285–296. doi:10.1016/j.jbusres.2021.04.070
Dragone, D., Randazzini, L., Capace, A., Nesci, F., Cosentino, C., Amato, F., et al. (2022). Design, computational modelling and experimental characterization of bistable hybrid soft actuators for a controllable-compliance joint of an exoskeleton rehabilitation robot. Actuators 11 (322), 32. doi:10.3390/act11020032
Fang, H., He, L. G., He, H. D., Wang, X. L., Wang, Y. Y., Ge, H. W., et al. (2022). The 100 most-cited articles in castration-resistant prostate cancer: A bibliometric analysis. J. Mens Health 18 (1). doi:10.31083/jomh.2021.053
Fang, H., Jing, Y., Chen, J., Wu, Y. Q., and Wan, Y. H. (2021). Recent trends in sedentary time: A systematic literature review. Healthcare 9 (8), 969. doi:10.3390/healthcare9080969
Foroughi, J., Spinks, G. M., Wallace, G. G., Oh, J., Kozlov, M. E., Fang, S., et al. (2011). Torsional carbon nanotube artificial muscles. Science 334 (6055), 494–497. doi:10.1126/science.1211220
Frank, Z., Al-Rubaiai, M., Tan, X., and Kim, K. J. (2022). A study of electroactive polyvinyl chloride (PVC) gel actuators through the use of the electric modulus formalism and cyclic linear voltage sweeps. Smart Mat. Struct. 31, 035020. doi:10.1088/1361-665X/ac4e51
Ghosh, T., and Karak, N. (2021). Interpenetrating polymer network/functionalized-reduced graphene oxide nanocomposite: As an advanced functional material. J. Appl. Polym. Sci. 138, 50499. doi:10.1002/app.50499
Greco, C., Kotak, P., Pagnotta, L., and Lamuta, C. (2022). The evolution of mechanical actuation: From conventional actuators to artificial muscles. Int. Mat. Rev. 67, 575–619. doi:10.1080/09506608.2021.1971428
Gu, G., Zou, J., Zhao, R., Zhao, X., and Zhu, X. (2018). Soft wall-climbing robots. Sci. Robotics 3, eaat2874. doi:10.1126/scirobotics.aat2874
Hao, M., Wang, Y., Zhu, Z., He, Q., Zhu, D., and Luo, M. (2019). A compact review of IPMC as soft actuator and sensor: Current trends, challenges, and potential solutions from our recent work. Front. Robotics Ai 6, 129. doi:10.3389/frobt.2019.00129
Harada, A., Takashima, Y., and Nakahata, M. (2014). Supramolecular polymeric materials via cyclodextrin–guest interactions. Accounts Chem. Res. 47 (7), 2128–2140. doi:10.1021/ar500109h
He, L. G., Wang, X. L., Li, C. J., Wan, Y. H., and Fang, H. (2022). Bibliometric analysis of the 100 top-cited articles on immunotherapy of urological cancer. Hum. Vacc. Immunother. 18 (1), 2035552. doi:10.1080/21645515.2022.2035552
Higueras-Ruiz, D. R., Nishikawa, K., Feigenbaum, H., and Shafer, M. (2022). What is an artificial muscle? A comparison of soft actuators to biological muscles. Bioinspir. Biomim. 17, 011001. doi:10.1088/1748-3190/ac3adf
Hines, L., Petersen, K., Lum, G. Z., and Sitti, M. (2017). Soft actuators for small-scale robotics. Adv. Mat. 29, 1603483. doi:10.1002/adma.201603483
Ho, P. H. A., and Ahn, K. K. (2011). Hybrid control of a pneumatic artificial muscle (PAM) robot arm using an inverse NARX fuzzy model. Eng. Appl. Artif. Intel. 24 (4), 697–716. doi:10.1016/j.engappai.2010.11.007
Hussain, S., Xie, S. Q., and Jamwal, P. K. (2013). Adaptive impedance control of a robotic orthosis for gait rehabilitation. IEEE Trans. Cybern. 43 (3), 1025–1034. doi:10.1109/TSMCB.2012.2222374
Ismail, Y. A., Martinez, J. G., Al Harrasi, A. S., Kim, S. J., and Otero, T. F. (2011). Sensing characteristics of a conducting polymer/hydrogel hybrid microfiber artificial muscle. Sens. Actuat. B-Chem. 160 (1), 1180–1190. doi:10.1016/j.snb.2011.09.044
Ismail, Y. A., Shin, S. R., Shin, K. M., Yoon, S. G., Shon, K., Kim, S. I., et al. (2008). Electrochemical actuation in chitosan/polyaniline microfibers for artificial muscles fabricated using an in situ polymerization. Sens. Actuat. B-Chem. 129 (2), 834–840. doi:10.1016/j.snb.2007.09.083
Jang, Y., Kim, S. M., Spinks, G. M., and Kim, S. J. (2020). Carbon nanotube yarn for fiber-shaped electrical sensors, actuators, and energy storage for smart systems. Adv. Mat. 32, 19026. doi:10.1002/adma.201902670
Jeong, J., Hyeon, K., Jang, S., Chung, C., Hussain, S., Ahn, S., et al. (2022). Soft wearable robot with shape memory alloy (SMA)-Based artificial muscle for assisting with elbow flexion and forearm supination/pronation. IEEE Robotics Automation Lett. 7 (3), 6028–6035. doi:10.1109/LRA.2022.3161700
Jin, L. L., Sun, X. Z., Ren, H. Q., and Huang, H. (2023). Hotspots and trends of biological water treatment based on bibliometric review and patents analysis. J. Environ. Sci.-China. 125, 774–785. doi:10.1016/j.jes.2022.03.037
Jo, C., Pugal, D., Oh, I., Kim, K. J., and Asaka, K. (2013). Recent advances in ionic polymer–metal composite actuators and their modeling and applications. Prog. Polym. Sci. 38 (7), 1037–1066. doi:10.1016/j.progpolymsci.2013.04.003
Jumet, B., Bell, M. D., Sanchez, V., and Preston, D. J. (2022). A Data-Driven review of soft robotics. Adv. Intell. Syst. 4 (4), 2100163. doi:10.1002/aisy.202100163
Jung, K., Lee, J., Cho, M., Koo, J. C., Nam, J., Lee, Y., et al. (2007). Development of enhanced synthetic elastomer for energy-efficient polymer actuators. Smart Mat. Struct. 16 (2), S288–S294. doi:10.1088/0964-1726/16/2/S13
Junni, P., Sarala, R., Taras, V., and Tarba, S. (2013). A meta-analysis of the effect of organizational ambidexterity on performance. Acad. Manag. Proc. 2013, 17601. doi:10.5465/AMBPP.2013.17601abstract
Kaneto, K., Kaneko, M., Min, Y., and Macdiarmid, A. G. (1995). Artificial muscle: Electromechanical actuators using polyaniline films. Synth. Met. 71 (1-3), 2211–2212. doi:10.1016/0379-6779(94)03226-V
Kanik, M., Aktas, O., Sen, H. S., Durgun, E., and Bayindir, M. (2014). Spontaneous high piezoelectricity in poly(vinylidene fluoride) nanoribbons produced by iterative thermal size reduction technique. ACS Nano 8 (9), 9311–9323. doi:10.1021/nn503269b
Kanik, M., Orguc, S., Varnavides, G., Kim, J., Benavides, T., Gonzalez, D., et al. (2019). Strain-programmable fiber-based artificial muscle. Science 365 (6449), 145–150. doi:10.1126/science.aaw2502
Khalid, M. Y., Arif, Z. U., Ahmed, W., Umer, R., Zolfagharian, A., and Bodaghi, M. (2022). 4D printing: Technological developments in robotics applications. Sens. Actuat. A-Phys 343, 113670. doi:10.1016/j.sna.2022.113670
Khan, A., Alamry, K. A., and Jain, R. K. (2019). Polypyrrole nanoparticles-based soft actuator for artificial muscle applications. RSC Adv. 9 (68), 39721–39734. doi:10.1039/c9ra06900c
Kim, D., Gwon, M., Kim, B., Ortega-Jimenez, V. M., Han, S., Kang, D., et al. (2022). Design of a biologically inspired Water-Walking robot powered by artificial muscle. Micromachines-Basel 13, 6274. doi:10.3390/mi13040627
Kim, H., Ahn, S. K., Mackie, D. M., Kwon, J., Kim, S. H., Choi, C., et al. (2020). Shape morphing smart 3D actuator materials for micro soft robot. Mat. Today. 41, 243–269. doi:10.1016/j.mattod.2020.06.005
Kim, J., Jeon, J., Kim, H., Lim, H., and Oh, I. (2014). Durable and water-floatable ionic polymer actuator with hydrophobic and asymmetrically laser-scribed reduced graphene oxide paper electrodes. ACS Nano 8 (3), 2986–2997. doi:10.1021/nn500283q
Kim, S., Hsiao, Y. H., Chen, Y., Mao, J., and Chen, Y. (2022). FireFly: An Insect-Scale aerial robot powered by electroluminescent soft artificial muscles. IEEE Robotics Automation Lett. 7 (3), 6950–6957. doi:10.1109/LRA.2022.3179486
Kimura, D., Irisawa, T., Takagi, K., Tahara, K., Sakurai, D., Watanabe, H., et al. (2021). Mechanism for anisotropic thermal expansion of polyamide fibers. Sens. Actuat. B-Chem. 344, 130262. doi:10.1016/j.snb.2021.130262
Kimura, S., Suzuki, R., Machida, K., Kashima, M., Okui, M., Nishihama, R., et al. (2021). Development of an Exoskeleton-Type assist suit utilizing variable stiffness control devices based on human joint characteristics. Actuators 10, 17. doi:10.3390/act10010017
Kobayashi, R., Nabae, H., Endo, G., and Suzumori, K. (2022). Soft tensegrity robot driven by thin artificial muscles for the exploration of unknown spatial configurations. IEEE Robotics Automation Lett. 7 (2), 5349–5356. doi:10.1109/LRA.2022.3153700
Lee, J., Bae, J., Hwang, J. H., Choi, M., Kim, Y. S., Park, S., et al. (2022). Robust and reprocessable artificial muscles based on liquid crystal elastomers with dynamic thiourea bonds. Adv. Funct. Mat. 32, 2110360. doi:10.1002/adfm.202110360
Lee, Y., Oh, J. Y., Xu, W., Kim, O., Kim, T. R., Kang, J., et al. (2018). Stretchable organic optoelectronic sensorimotor synapse. Sci. Adv. 4, eaat7387. doi:10.1126/sciadv.aat7387
Leng, X., Hu, X., Zhao, W., An, B., Zhou, X., and Liu, Z. (2021b). Recent advances in twisted-fiber artificial muscles. Adv. Intell. Syst. 3, 2000185. doi:10.1002/aisy.202000185
Leng, X., Zhou, X., Liu, J. Y., Xiao, Y. C., Sun, J. K., Li, Y. W., et al. (2021a). Tuning the reversibility of hair artificial muscles by disulfide cross-linking for sensors, switches, and soft robotics. Mater. Horizons 8 (5), 1538–1546. doi:10.1039/d1mh00234a
Li, G. R., Chen, X. P., Zhou, F. H., Liang, Y. M., Xiao, Y. H., Cao, X., et al. (2021). Self-powered soft robot in the mariana trench. Nature 591 (7848), 66–71. doi:10.1038/s41586-020-03153-z
Li, L., Lu, J. F., Fang, H., Yin, Z. C., Wang, T., Wang, R. H., et al. (2020b). Lattice Boltzmann method for Fluid-Thermal systems: Status, hotspots, trends and outlook. IEEE Access 8, 27649–27675. doi:10.1109/ACCESS.2020.2971546
Li, L., Zhou, X., and Liu, Z. F. (2020a). Recent advances in photoactuators and their applications in intelligent bionic movements. Adv. Opt. Mat. 8 (18), 2000886. doi:10.1002/adom.202000886
Li, M., Porter, A. L., Suominen, A., Burmaoglu, S., and Carley, S. (2021). An exploratory perspective to measure the emergence degree for a specific technology based on the philosophy of swarm intelligence. Technol. Forecast. Soc. Change 166 (1), 120621. doi:10.1016/j.techfore.2021.120621
Li, S., Bai, H., Liu, Z., Zhang, X., Huang, C., Wiesner, L. W., et al. (2021). Digital light processing of liquid crystal elastomers for self-sensing artificial muscles. Sci. Adv. 7, eabg3677. doi:10.1126/sciadv.abg3677
Li, S., Vogt, D. M., Rus, D., and Wood, R. J. (2017). Fluid-driven origami-inspired artificial muscles. P. Natl. Acad. Sci. U. S. A. 114 (50), 13132–13137. doi:10.1073/pnas.1713450114
Li, Y., Leng, X., Sun, J., Zhou, X., Wu, W., Chen, H., et al. (2020). Moisture-sensitive torsional cotton artificial muscle and textile. Chin. Phys. B 29, 048103. doi:10.1088/1674-1056/ab7745
Liang, D. K., Sun, N., Wu, Y., Chen, Y., Fang, Y., and Liu, L. (2022b). Energy-based motion control for pneumatic artificial muscle actuated robots with experiments. IEEE Trans. Ind. Electron. 69 (7), 7295–7306. doi:10.1109/TIE.2021.3095788
Liang, D. K., Sun, N., Wu, Y. M., Liu, G. D., and Fang, Y. C. (2022a). Fuzzy-Sliding mode control for humanoid arm robots actuated by pneumatic artificial muscles with unidirectional inputs, saturations, and dead zones. IEEE Trans. Ind. Inf. 18 (5), 3011–3021. doi:10.1109/TII.2021.3111655
Lin, C., Sie, T., Chu, W., Yau, H., and Ding, C. (2021). Tracking control of pneumatic artificial Muscle-Activated robot arm based on Sliding-Mode control. Actuators 10, 66. doi:10.3390/act10030066
Lin, S., Wang, Z., Chen, X., Ren, J., and Ling, S. (2020). Ultrastrong and highly sensitive fiber microactuators constructed by Force-Reeled silks. Adv. Sci. 7, 1902743. doi:10.1002/advs.201902743
Lin, Z., Hess, A., Yu, Z., Cai, S., and Gao, T. (2019). A fluid–structure interaction study of soft robotic swimmer using a fictitious domain/active-strain method. J. Comput. Phys. 376, 1138–1155. doi:10.1016/j.jcp.2018.10.015
Ling, Y., Pang, W. B., Li, X. P., Goswami, S., Xu, Z., Stroman, D., et al. (2020). Laser-Induced graphene for electrothermally controlled, mechanically guided, 3D assembly and Human-Soft actuators interaction. Adv. Mat. 32 (17), 1908475. doi:10.1002/adma.201908475
Liu, G., Sun, N., Liang, D., Chen, Y., Yang, T., and Fang, Y. (2022). Neural network-based adaptive command filtering control for pneumatic artificial muscle robots with input uncertainties. Control Eng. Pract. 118, 104960. doi:10.1016/j.conengprac.2021.104960
Liu, H., Tian, H., Li, X., Chen, X., Zhang, K., Shi, H., et al. (2022). Shape-programmable, deformation-locking, and self-sensing artificial muscle based on liquid crystal elastomer and low–melting point alloy. Sci. Adv. 8, eabn5722. doi:10.1126/sciadv.abn5722
Liu, Z., Zhang, R., Xiao, Y., Li, J., Chang, W., Qian, D., et al. (2021). Somatosensitive film soft crawling robots driven by artificial muscle for load carrying and multi-terrain locomotion. Mater. Horizons 8 (6), 1783–1794. doi:10.1039/d1mh00457c
Lotka, A. J. (1926). The frequency distribution of scientific productivity. J. Frankl. Inst. 202 (2), 271. doi:10.1016/S0016-0032(26)91166-6
Madden, J., Vandesteeg, N. A., Anquetil, P. A., Madden, P., Takshi, A., Pytel, R. Z., et al. (2004). Artificial muscle technology: Physical principles and naval prospects. IEEE J. Ocean. Eng. 29 (3), 706–728. doi:10.1109/JOE.2004.833135
Madsen, F. B., Daugaard, A. E., Hvilsted, S., and Skov, A. L. (2016). The current state of silicone-based dielectric elastomer transducers. Macromol. Rapid Comm. 37 (5), 378–413. doi:10.1002/marc.201500576
Masuya, K., Ono, S., Takagi, K., and Tahara, K. (2017). Modeling framework for macroscopic dynamics of twisted and coiled polymer actuator driven by Joule heating focusing on energy and convective heat transfer. Sens. Actuat. A-Phys. 267, 443–454. doi:10.1016/j.sna.2017.10.016
McCracken, J. M., Donovan, B. R., and White, T. J. (2020). Materials as machines. Adv. Mat. 32, 1906564. doi:10.1002/adma.201906564
Milanez, D. H., de Faria, L., Do Amaral, R. M., Leiva, D. R., and Gregolin, J. (2014). Patents in nanotechnology: An analysis using macro-indicators and forecasting curves. Scientometrics 101 (2), 1097–1112. doi:10.1007/s11192-014-1244-4
Mirfakhrai, T., Madden, J. D. W., and Baughman, R. H. (2007). Polymer artificial muscles. Mat. Today 10 (4), 30–38. doi:10.1016/S1369-7021(07)70048-2
Mirvakili, S. M., and Hunter, I. W. (2018). Artificial muscles: Mechanisms, applications, and challenges. Adv. Mat. 30, 1704407. doi:10.1002/adma.201704407
Mirvakili, S. M., and Hunter, I. W. (2017). Fast torsional artificial muscles from NiTi twisted yarns. ACS Appl. Mat. Inter. 9 (19), 16321–16326. doi:10.1021/acsami.7b02335
Mirvakili, S. M., Sim, D., Hunter, I. W., and Langer, R. (2020). Actuation of untethered pneumatic artificial muscles and soft robots using magnetically induced liquid-to-gas phase transitions. Sci. Robotics 5, eaaz4239. doi:10.1126/scirobotics.aaz4239
Moers, A. J. M., De Volder, M. F. L., and Reynaerts, D. (2012). Integrated high pressure microhydraulic actuation and control for surgical instruments. Biomed. Microdevices 14 (4), 699–708. doi:10.1007/s10544-012-9650-y
Mokhtari, F., Azimi, B., Salehi, M., Hashemikia, S., and Danti, S. (2021). Recent advances of polymer-based piezoelectric composites for biomedical applications. J. Mech. Behav. Biomed. 122, 104669. doi:10.1016/j.jmbbm.2021.104669
Mu, J. K., de Andrade, M. J., Fang, S. L., Wang, X. M., Gao, E. L., Li, N., et al. (2019). Sheath-run artificial muscles. Science 365 (6449), 150–155. doi:10.1126/science.aaw2403
Muhuri, P. K., Shukla, A. K., and Abraham, A. (2019). Industry 4.0: A bibliometric analysis and detailed overview. Eng. Appl. Artif. Intel. 78, 218–235. doi:10.1016/j.engappai.2018.11.007
Nakamura, T. (2022). Peristaltic mixing pump based on bowel peristalsis using pneumatic artificial rubber muscles and prospects for practical applications. J. Robotics Mechatronics 34 (2SI), 276–278. doi:10.20965/jrm.2022.p0276
Nakamura, T., Tanaka, D., and Maeda, H. (2011). Joint stiffness and position control of an artificial muscle manipulator for instantaneous loads using a mechanical equilibrium model. Adv. Robot. 25 (3-4), 387–406. doi:10.1163/016918610X552169
Nuchkrua, T., and Leephakpreeda, T. (2022). Novel compliant control of a pneumatic artificial muscle driven by hydrogen pressure under a varying environment. IEEE Trans. Ind. Electron 69 (7), 7120–7129. doi:10.1109/TIE.2021.3102486
OECD (2022). Bibliometrics: OECD glossary of statistical terms. Available at: https://stats.oecd.org/glossary/.
Oh, J. H., Anas, M., Barnes, E., Moores, L. C., and Green, M. J. (2021). Site-specific selective bending of actuators using radio frequency heating. Adv. Eng. Mat. 23 (5), 2000873. doi:10.1002/adem.202000873
Oh, S., Tabassian, R., Thangasamy, P., Mahato, M., Van Hiep, N., Nam, S., et al. (2022). Cooling-accelerated nanowire-nitinol hybrid muscle for versatile prosthetic hand and biomimetic retractable claw. Adv. Funct. Mat. 32, 2111145. doi:10.1002/adfm.202111145
Osada, Y., Okuzaki, H., and Hori, H. (1992). A polymer gel with electrically driven motility. Nature 355 (6357), 242–244. doi:10.1038/355242a0
Ozioko, O., Navaraj, W., Hersh, M., and Dahiya, R. (2020). Tacsac: A wearable haptic device with capacitive touch-sensing capability for tactile display. Sensors-Basel 20 (17), 4780. doi:10.3390/s20174780
Park, S. J., Kim, U., and Park, C. H. (2020). A novel fabric muscle based on shape memory alloy springs. Soft Robot. 7 (3), 321–331. doi:10.1089/soro.2018.0107
Park, T., and Cha, Y. (2019). Soft mobile robot inspired by animal-like running motion. Sci. Rep.-UK 9, 14700. doi:10.1038/s41598-019-51308-4
Park, Y., Chen, B., Perez-Arancibia, N. O., Young, D., Stirling, L., Wood, R. J., et al. (2014). Design and control of a bio-inspired soft wearable robotic device for ankle-foot rehabilitation. Bioinspir. Biomim. 9, 016007. doi:10.1088/1748-3182/9/1/016007
Paskett, M. D., Brinton, M. R., Hansen, T. C., George, J. A., Davis, T. S., Duncan, C. C., et al. (2021). Activities of daily living with bionic arm improved by combination training and latching filter in prosthesis control comparison. J. Neuroeng. Rehabil. 18 (451), 45. doi:10.1186/s12984-021-00839-x
Pelrine, R., Kornbluh, R., Joseph, J., Heydt, R., Pei, Q. B., and Chiba, S. (2000). High-field deformation of elastomeric dielectrics for actuators. Mater. Sci. Eng. C-Biomimetic Supramol. Syst. 11 (2), 89–100. doi:10.1016/S0928-4931(00)00128-4
Phan, P. T., Hoang, T. T., Thai, M. T., Low, H., Davies, J., Lovell, N. H., et al. (2021). Smart surgical sutures using soft artificial muscles. Sci. Rep.-UK 11, 22420. doi:10.1038/s41598-021-01910-2
Plisko, T. V., and Bildyukevich, A. V. (2014). Debundling of multiwalled carbon nanotubes in N, N-dimethylacetamide by polymers. Colloid Polym. Sci. 292 (10), 2571–2580. doi:10.1007/s00396-014-3305-x
Porter, A. L., Chiavetta, D., and Newman, N. C. (2020). Measuring tech emergence: A contest. Technol. Forecast. Soc. Change 159, 120176. doi:10.1016/j.techfore.2020.120176
Porter, A. L., Garner, J., Newman, N. C., Carley, S. F., Youtie, J., Kwon, S., et al. (2019a). National nanotechnology research prominence. Technol. Analysis Strategic Manag. 31 (1), 25–39. doi:10.1080/09537325.2018.1480013
Porter, A. L., Schoeneck, D. J., Youtie, J., Solomon, G., Kwon, S., and Carley, S. F. (2019b). Learning about learning: Patterns of sharing of research knowledge among Education, Border, and Cognitive Science fields. Scientometrics 118 (3), 1093–1117. doi:10.1007/s11192-019-03012-3
Qin, H., Zhang, T., Li, N., Cong, H., and Yu, S. (2019). Anisotropic and self-healing hydrogels with multi-responsive actuating capability. Nat. Commun. 10, 2202. doi:10.1038/s41467-019-10243-8
Robinson, R. M., Kothera, C. S., Sanner, R. M., and Wereley, N. M. (2016). Nonlinear control of robotic manipulators driven by pneumatic artificial muscles. IEEE-ASME Trans. Mech. 21 (1), 55–68. doi:10.1109/TMECH.2015.2483520
Romasanta, L. J., Lopez-Manchado, M. A., and Verdejo, R. (2015). Increasing the performance of dielectric elastomer actuators: A review from the materials perspective. Prog. Polym. Sci. 51, 188–211. doi:10.1016/j.progpolymsci.2015.08.002
Rousseau, S., and Rousseau, R. (2021). Bibliometric techniques and their use in business and economics research. J. Econ. Surv. 35 (5), 1428–1451. doi:10.1111/joes.12415
Ruzhong, C., Yang, Z., Die, W., Xinru, L., and Hua, G. (2022). Visual analysis of cultural and creative industry policy research based on bibliometrics. J. Silk 59 (3), 76–84. doi:10.3969/j.issn.1001-7003.2022.03.011
Saharan, L., Wu, L., and Tadesse, Y. (2020). Modeling and simulation of robotic finger powered by nylon artificial muscles. J. Mech. Robot. 12, 0145011. doi:10.1115/1.4044740
Shi, M. Y., Holmes, A. S., and Yeatman, E. M. (2020). Piezoelectric wind velocity sensor based on the variation of galloping frequency with drag force. Appl. Phys. Lett. 116 (26), 264101. doi:10.1063/5.0012244
Snyder, H. (2019). Literature review as a research methodology: An overview and guidelines. J. Bus. Res. 104, 333–339. doi:10.1016/j.jbusres.2019.07.039
Solomon, G., Youtie, J., Carley, S., and Porter, A. L. (2019). What people learn about how people learn: An analysis of citation behavior and the multidisciplinary flow of knowledge. Res. Policy 48 (9), 103835. doi:10.1016/j.respol.2019.103835
Sun, N., Liang, D., Wu, Y., Chen, Y., Qin, Y., and Fang, Y. (2020). Adaptive control for pneumatic artificial muscle systems with parametric uncertainties and unidirectional input constraints. IEEE Trans. Ind. Inf. 16 (2), 969–979. doi:10.1109/TII.2019.2923715
Suzuki, H., and Wood, R. J. (2020). Origami-inspired miniature manipulator for teleoperated microsurgery. Nat. Mach. Intell. 2 (8), 437–446. doi:10.1038/s42256-020-0203-4
Tada, K., and Yoshida, T. (2021). Effect of temperature on electrical Resistance-Length characteristic of electroactive supercoiled polymer artificial muscle. IEICET. Electron. E104C (6), 192–193. doi:10.1587/transele.2020OMS0002
Takashima, Y., Hatanaka, S., Otsubo, M., Nakahata, M., Kakuta, T., Hashidzume, A., et al. (2012). Expansion–contraction of photoresponsive artificial muscle regulated by host–guest interactions. Nat. Commun. 3, 1270. doi:10.1038/ncomms2280
Tam, K. P., and Milfont, T. L. (2020). Towards cross-cultural environmental psychology: A state-of-the-art review and recommendations. J. Environ. Psychol. 71, 101474. doi:10.1016/j.jenvp.2020.101474
Terryn, S., Brancart, J., Lefeber, D., Van Assche, G., and Vanderborght, B. (2017). Self-healing soft pneumatic robots. Sci. Robotics 2, eaan4268. doi:10.1126/scirobotics.aan4268
Thomsen, D. L., Keller, P., Naciri, J., Pink, R., Jeon, H., Shenoy, D., et al. (2001). Liquid crystal elastomers with mechanical properties of a muscle. Macromolecules 34 (17), 5868–5875. doi:10.1021/ma001639q
Tsai, T., and Chiang, M. (2022). A lower limb rehabilitation assistance training robot system driven by an innovative pneumatic artificial muscle system. Soft Robot. 9, 1031–1039. doi:10.1089/soro.2020.0216
Tu, D. C. T., and Ahn, K. K. (2006). Nonlinear PID control to improve the control performance of 2 axes pneumatic artificial muscle manipulator using neural network. Mechatronics 16 (9), 577–587. doi:10.1016/j.mechatronics.2006.03.011
Umrao, S., Tabassian, R., Kim, J., Nguyen, V. H., Zhou, Q., Nam, S., et al. (2019). MXene artificial muscles based on ionically cross-linked Ti3C2T x electrode for kinetic soft robotics. Sci. Robotics 4, eaaw7797. doi:10.1126/scirobotics.aaw7797
van Delden, R. A., ter Wiel, M., Pollard, M. M., Vicario, J., Koumura, N., and Feringa, B. L. (2005). Unidirectional molecular motor on a gold surface. Nature 437 (7063), 1337–1340. doi:10.1038/nature04127
Vanderborght, B., Albu-Schaeffer, A., Bicchi, A., Burdet, E., Caldwell, D. G., Carloni, R., et al. (2013). Variable impedance actuators: A review. Robot. Auton. Syst. 61 (12), 1601–1614. doi:10.1016/j.robot.2013.06.009
Villa, S. M., Mazzola, V. M., Santaniello, T., Locatelli, E., Maturi, M., Migliorini, L., et al. (2019). Soft Piezoionic/Piezoelectric nanocomposites based on Ionogel/BaTiO3 nanoparticles for low frequency and directional discriminative pressure sensing. ACS Macro Lett. 8 (4), 414–420. doi:10.1021/acsmacrolett.8b01011
Wang, C., Sim, K., Chen, J., Kim, H., Rao, Z., Li, Y., et al. (2018). Soft ultrathin electronics innervated adaptive fully soft robots. Adv. Mat. 30, 1706695. doi:10.1002/adma.201706695
Wang, H. Q., Gao, D. C., and Lee, P. S. (2021b). Recent progress in artificial muscles for interactive soft robotics. Adv. Mat. 33 (19), 2003088. doi:10.1002/adma.202003088
Wang, H. Q., York, P., Chen, Y. F., Russo, S., Ranzani, T., Walsh, C., et al. (2021a). Biologically inspired electrostatic artificial muscles for insect-sized robots. Int. J. Robot. Res. 40 (6-7), 895–922. doi:10.1177/02783649211002545
Wang, Q., Yang, Y. T., Fang, H., and Wan, Y. H. (2022). Input saturation: Academic insights and future trends. Int. J. Syst. Sci. 53 (6), 1138–1152. doi:10.1080/00207721.2021.1989726
Wang, R., Fang, S. L., Xiao, Y. C., Gao, E. L., Jiang, N., Li, Y. W., et al. (2019). Torsional refrigeration by twisted, coiled, and supercoiled fibers. Science 366 (6462), 216–221. doi:10.1126/science.aax6182
Wang, R., Shen, Y., Qian, D., Sun, J., Zhou, X., Wang, W., et al. (2020). Tensile and torsional elastomer fiber artificial muscle by entropic elasticity with thermo-piezoresistive sensing of strain and rotation by a single electric signal. Mater. Horizons 7 (12), 3305–3315. doi:10.1039/d0mh01003k
Wang, X., Jiao, N., Tung, S., and Liu, L. (2019). Photoresponsive graphene composite bilayer actuator for soft robots. ACS Appl. Mat. Inter. 11 (33), 30290–30299. doi:10.1021/acsami.9b09491
Wang, X. Q., Chan, K. H., Cheng, Y., Ding, T. P., Li, T. T., Achavananthadith, S., et al. (2020). Somatosensory, Light-Driven, Thin-Film robots capable of integrated perception and motility. Adv. Mat. 32 (21), 2000351. doi:10.1002/adma.202000351
Wang, Y., and Xu, Q. (2021). Design and testing of a soft parallel robot based on pneumatic artificial muscles for wrist rehabilitation. Sci. Rep.-UK 11, 12731. doi:10.1038/s41598-020-80411-0
Wang, Z., Hou, X., Duan, N., Ren, Y., and Yan, F. (2021). Shape- and color-switchable polyurethane thermochromic actuators based on metal-containing ionic liquids. ACS Appl. Mat. Inter. 13 (24), 28878–28888. doi:10.1021/acsami.1c06422
Wu, C. B., Zhang, Z., and Zheng, W. (2022). A twisted and coiled polymer artificial muscles driven soft crawling robot based on enhanced antagonistic configuration. Machines 10 (2), 142. doi:10.3390/machines10020142
Xiang, C., Yang, H., Sun, Z., Xue, B., Hao, L., Rahoman, M. D. A., et al. (2017). The design, hysteresis modeling and control of a novel SMA-fishing-line actuator. Smart Mat. Struct. 26, 037004. doi:10.1088/1361-665X/aa5b03
Xiang, F., Xiaopeng, W., and Xiaoxiao, Q. (2022). Bibliometric analysis of literatures on textile and clothing footprint based on CiteSpace. Adv. Text. Technol. 30 (1), 9–17. doi:10.19398/j.att.20201100
Xiao, W., Hu, D., Chen, W., Yang, G., and Han, X. (2021b). Design, characterization and optimization of multi-directional bending pneumatic artificial muscles. J. Bionic Eng. 18 (6), 1358–1368. doi:10.1007/s42235-021-00077-w
Xiao, W., Hu, D., Chen, W., Yang, G., and Han, X. (2021a). Modeling and analysis of bending pneumatic artificial muscle with multi-degree of freedom. Smart Mat. Struct. 30, 095018. doi:10.1088/1361-665X/ac1939
Xiao, Y. W., Lin, J., Xiao, J., Weng, M. C., Zhang, W., Zhou, P. D., et al. (2021). A multi-functional light-driven actuator with an integrated temperature-sensing function based on a carbon nanotube composite. Nanoscale 13 (12), 6259–6265. doi:10.1039/d0nr09210j
Xu, H., Han, C., Liu, S., Hao, X., Rao, Y., Gong, Z., et al. (2020). Sodium alginate-chitosan hydrogel-based soft ionic artificial muscle with different moisture content. Ionics 26 (12), 6371–6378. doi:10.1007/s11581-020-03773-5
Yang, S., Wang, C., Sahin, H., Chen, H., Li, Y., Li, S., et al. (2015). Tuning the optical, magnetic, and electrical properties of ReSe2 by nanoscale strain engineering. Nano Lett. 15 (3), 1660–1666. doi:10.1021/nl504276u
Yang, S. Y., Cho, K. H., Kim, Y., Song, M., Jung, H. S., Yoo, J. W., et al. (2017). High performance twisted and coiled soft actuator with spandex fiber for artificial muscles. Smart Mat. Struct. 26, 105025. doi:10.1088/1361-665X/aa84e4
Yang, T., Chen, Y., Sun, N., Liu, L., Qin, Y., and Fang, Y. (2022). Learning-based error-constrained motion control for pneumatic artificial muscle-actuated exoskeleton robots with hardware experiments. IEEE Trans. Autom. Sci. Eng. 19, 3700–3711. doi:10.1109/TASE.2021.3131034
Yang, X., Chang, L., and Perez-Arancibia, N. O. (2020). An 88-milligram insect-scale autonomous crawling robot driven by a catalytic artificial muscle. Sci. Robotics 5, eaba0015. doi:10.1126/scirobotics.aba0015
Yarali, E., Baniasadi, M., Zolfagharian, A., Chavoshi, M., Arefi, F., Hossain, M., et al. (2022). Magneto-/electro-responsive polymers toward manufacturing, characterization, and biomedical/soft robotic applications. Appl. Mater. Today 26, 101306. doi:10.1016/j.apmt.2021.101306
Yu, Y., Li, L., Liu, E., Han, X., Wang, J., Xie, Y., et al. (2022). Light-driven core-shell fiber actuator based on carbon nanotubes/liquid crystal elastomer for artificial muscle and phototropic locomotion. Carbon 187, 97–107. doi:10.1016/j.carbon.2021.10.071
Zakeri, R., and Zakeri, R. (2022). Bio inspired general artificial muscle using hybrid of mixed electrolysis and fluids chemical reaction (HEFR). Sci. Rep.-UK 12, 3627. doi:10.1038/s41598-022-07799-9
Zang, J., Ryu, S., Pugno, N., Wang, Q., Tu, Q., Buehler, M. J., et al. (2013). Multifunctionality and control of the crumpling and unfolding of large-area graphene. Nat. Mat. 12 (4), 321–325. doi:10.1038/NMAT3542
Zare, M., Prabhakaran, M. P., Parvin, N., and Ramakrishna, S. (2019). Thermally-induced two-way shape memory polymers: Mechanisms, structures, and applications. Chem. Eng. J. 374, 706–720. doi:10.1016/j.cej.2019.05.167
Zhang, G., Huang, J., Leng, X., Bai, J., and Liu, Z. (2022a). Recent progress in fiber artificial muscles. Acta Polym. Sin. 53 (2), 119–132. doi:10.11777/j.issn1000-3304.2021.21240
Zhang, G., Yang, G. L., Zheng, T. J., Shen, W. J., Wang, Y., Zhang, G. L., et al. (2022b). Design and modeling of a compound twisted and coiled actuator based on spandex fibers and an SMA skeleton. IEEE Robotics Automation Lett. 7 (2), 1439–1446. doi:10.1109/LRA.2021.3137567
Zhang, Y., Porter, A. L., Cunningham, S., Chiavetta, D., and Newman, N. (2021). Parallel or intersecting lines? Intelligent bibliometrics for investigating the involvement of data science in policy analysis. IEEE Trans. Eng. Manage. 68 (5), 1259–1271. doi:10.1109/TEM.2020.2974761
Zhao, H. T., Hu, R., Li, P., Gao, A. Z., Sun, X. T., Zhang, X. H., et al. (2020). Soft bimorph actuator with real-time multiplex motion perception. Nano Energy 76, 104926. doi:10.1016/j.nanoen.2020.104926
Zhou, D., Liu, Y. X., Deng, J., Sun, J., and Fu, Y. (2022a). Force enhanced Multi-Twisted and coiled actuator and its application in temperature Self-Adaptive tensegrity mechanisms. IEEE-ASME Trans. Mech. 27, 3964–3976. doi:10.1109/TMECH.2022.3150761
Zhou, D., Liu, Y. X., Tang, X. T., Sun, J., and Deng, J. (2022b). A lightweight and multimotion crawling tensegrity robot driven by twisted artificial muscles. IEEE Trans. Ind. Electron. 69 (11), 11447–11457. doi:10.1109/TIE.2021.3125646
Zhou, D., Zuo, W., Tang, X., Deng, J., and Liu, Y. (2021). A multi-motion bionic soft hexapod robot driven by self-sensing controlled twisted artificial muscles. Bioinspir. Biomim. 16, 045003. doi:10.1088/1748-3190/ac0121
Zhou, P., Chen, L., Yao, L., Weng, M., and Zhang, W. (2018). Humidity- and light-driven actuators based on carbon nanotube-coated paper and polymer composite. Nanoscale 10 (18), 8422–8427. doi:10.1039/c7nr09580e
Zhou, X., Fang, S. L., Leng, X. Q., Liu, Z. F., and Baughman, R. H. (2021). The power of fiber twist. Accounts Chem. Res. 54 (11), 2624–2636. doi:10.1021/acs.accounts.1c00112
Zipf, G. K. (1932). Selected studies of the principle of relative frequency in language. Cambridge, Mass: Harvard University Press.
Keywords: artificial muscle, bibliometric analysis, visualized analysis, patent, scientific and technological innovation
Citation: Jing Y, Su F, Yu X, Fang H and Wan Y (2023) Advances in artificial muscles: A brief literature and patent review. Front. Bioeng. Biotechnol. 11:1083857. doi: 10.3389/fbioe.2023.1083857
Received: 29 October 2022; Accepted: 03 January 2023;
Published: 19 January 2023.
Edited by:
Wei Chen, Hong Kong Polytechnic University, Hong Kong SAR, ChinaReviewed by:
Ying Hu, Hefei University of Technology, ChinaZunfeng Liu, Nankai University, China
Longfei Chang, Hefei University of Technology, China
Copyright © 2023 Jing, Su, Yu, Fang and Wan. This is an open-access article distributed under the terms of the Creative Commons Attribution License (CC BY). The use, distribution or reproduction in other forums is permitted, provided the original author(s) and the copyright owner(s) are credited and that the original publication in this journal is cited, in accordance with accepted academic practice. No use, distribution or reproduction is permitted which does not comply with these terms.
*Correspondence: Yuan Jing, amluZ3l1YW5AenN0dS5lZHUuY24=