- 1Department of Periodontology, Shenzhen Stomatological Hospital (Pingshan), Southern Medical University, Shenzhen, Guangdong, China
- 2Department of Periodontology, Stomatological Hospital, Southern Medical University, Guangzhou, Guangdong, China
- 3State Key Laboratory of Military Stomatology and National Clinical Research Center for Oral Diseases and Shaanxi Engineering Research Center for Dental Materials and Advanced Manufacture and Department of Periodontology, School of Stomatology, Fourth Military Medical University, Xi’an, Shaanxi, China
- 4Department of Research and Development, Shaanxi Zhonghong Institute of Regenerative Medicine, Xi’an, Shaanxi, China
- 5Department of Orthodontics, Stomatological Hospital, Hebei Medical University, Shijiazhuang, Hebei, China
- 6Department of Stomatology, General Hospital of Tibetan Military Command, Lhasa, Tibet, China
- 7Department of Stomatology, Air Force Medical Center, Fourth Military Medical University, Beijing, China
In recent years, the incidence of critical-size bone defects has significantly increased. Critical-size bone defects seriously affect patients’ motor functions and quality of life and increase the need for additional clinical treatments. Bone tissue engineering (BTE) has made great progress in repairing critical-size bone defects. As one of the main components of bone tissue engineering, stem cell-based therapy is considered a potential effective strategy to regenerate bone tissues. However, there are some disadvantages including phenotypic changes, immune rejection, potential tumorigenicity, low homing efficiency and cell survival rate that restrict its wider clinical applications. Evidence has shown that the positive biological effects of stem cells on tissue repair are largely mediated through paracrine action by nanostructured extracellular vesicles (EVs), which may overcome the limitations of traditional stem cell-based treatments. In addition to stem cell-derived extracellular vesicles, the potential therapeutic roles of nonstem cell-derived extracellular vesicles in critical-size bone defect repair have also attracted attention from scholars in recent years. Currently, the development of extracellular vesicles-mediated cell-free regenerative medicine is still in the preliminary stage, and the specific mechanisms remain elusive. Herein, the authors first review the research progress and possible mechanisms of extracellular vesicles combined with bone tissue engineering scaffolds to promote bone regeneration via bioactive molecules. Engineering modified extracellular vesicles is an emerging component of bone tissue engineering and its main progression and clinical applications will be discussed. Finally, future perspectives and challenges of developing extracellular vesicle-based regenerative medicine will be given. This review may provide a theoretical basis for the future development of extracellular vesicle-based biomedicine and provide clinical references for promoting the repair of critical-size bone defects.
1 Introduction
Critical-size bone defects refer to the smallest intraosseous wound that cannot heal spontaneously (Falacho et al., 2021). The aetiologies include trauma, tumour and infection (Shang et al., 2020). In today’s rapidly ageing global population, the incidence of this disease has significantly increased. Critical-size bone defects considerably affect patients’ motor function and quality of life and increase the need for additional clinical treatments (Nauth et al., 2018). Currently, the clinical strategies for repairing critical-size bone defects mainly include autologous or allogeneic bone transplantation and synthetic biomaterial transplantation (Yu et al., 2022). Autologous bone transplantation is generally considered the gold standard for repairing critical-size bone defects; however, there are many limitations, including injury to the donor site, limited source, and unpredicted spontaneous resorption and disease transmission risk (Stahl and Yang, 2021). The clinical application of pure allogeneic bone transplantation also has some disadvantages, manifested by immune rejection (Behrend et al., 2016) or unwanted disease transmission (Baldwin et al., 2019).
Bone tissue engineering (BTE), which uses biomaterials with sophisticated biophysical or biochemical features to circumvent the constraints described above, has made great progress in repairing critical-size bone defects. BTE includes three basic components: biological scaffolds, seed cells and bone inductive factors (Roseti et al., 2017). Biological scaffolds are commonly referred to as bioactive materials, which can be combined with mesenchymal stem cells (MSCs) and their secreted cytokines to repair critical-size bone defects (Wu et al., 2021). MSCs are a heterogeneous mesenchymal stem cell subpopulation with the potential for multidirectional differentiation into mesoderm, ectoderm and endoderm lineages (Bianco and Gehron Robey, 2000). Therefore, stem cell therapy is considered to be a potential effective strategy to regenerate bone tissues (Silva et al., 2021). However, stem cell therapy also has some disadvantages that restrict its wider clinical applications, including phenotypic changes, immune rejection, potential tumorigenicity, low homing efficiency and cell survival rate (Sissung and Figg, 2020). These concerns, together with problems caused by storage and transport, further limit the application of MSCs in repairing critical-size bone defects (Nagelkerke et al., 2021). In addition, even after successful transplantation, factors such as donor age, passage times of in vitro expansion, culture conditions, cell transplantation procedures and recipient pathological microenvironments also have adverse effects on the survival and biological characteristics of cells (Rezaie et al., 2018). Many studies have attempted to overcome these shortcomings by genetically modifying MSCs and optimizing their culture conditions (García-Sánchez et al., 2019), but key limitations on the biological safety of MSCs still exist.
In recent years, cell-free regenerative medicine for critical-size bone defects has received extensive attention (Kang et al., 2022). Evidence has shown that the positive biological effects of MSCs on tissue repair are largely mediated through paracrine action by extracellular vesicles (EVs) rather than direct differentiation into parenchymal cells to repair or replace damaged tissues (Zhang J. et al., 2016). EVs are double phospholipid bilayer vesicles with nanostructures that bud from the membrane of their parent cells and play vital roles in cell-to-cell communications (Vizoso et al., 2017). Based on different diameters, compositions and sources, EVs mainly include exosomes, microvesicles and apoptotic bodies (Figure 1) (Zhou et al., 2022). Previously, the effects of EVs were mistaken for removing the generated waste of parent cells. Currently, EVs are reputed to be typical representatives of nanotherapeutics carrying abundant nucleic acids, proteins, lipid substances and bioactive small molecules derived from parent cells (Puddu et al., 2010). They transfer internal cargoes to target cells through ligand‒receptor interactions, endocytosis or direct membrane fusion to exert regulatory effects (Li Q. C. et al., 2022). Studies have shown that EVs obtained from MSCs have more ideal biocompatibility, effective cell interaction characteristics, exogenous cargo delivery and the ability to target specific tissues (van der Koog et al., 2022), and most importantly, they overcome the limitations of traditional MSC treatment (Malekpour et al., 2022). In recent years, in studies of bone regeneration and homeostasis, EVs have also become remarkable hotspots (Zara et al., 2011). EVs loaded with bioactive materials can significantly promote osteogenesis, angiogenesis and inflammation regulation, thus effectively repairing critical-size bone defects (Liang et al., 2022). Because EV subtypes do not have well-recognized specific markers, the International Society for Extracellular Vesicles recommends using “small EVs” for EV subtypes (Théry et al., 2018). However, most studies used the term “exosomes” in their experiments. In this review, to respect the cited literature, we reserve to use the term “exosomes” when the author gave a specific definition in the article. We use the generic term “EVs” when they were not clearly noted.
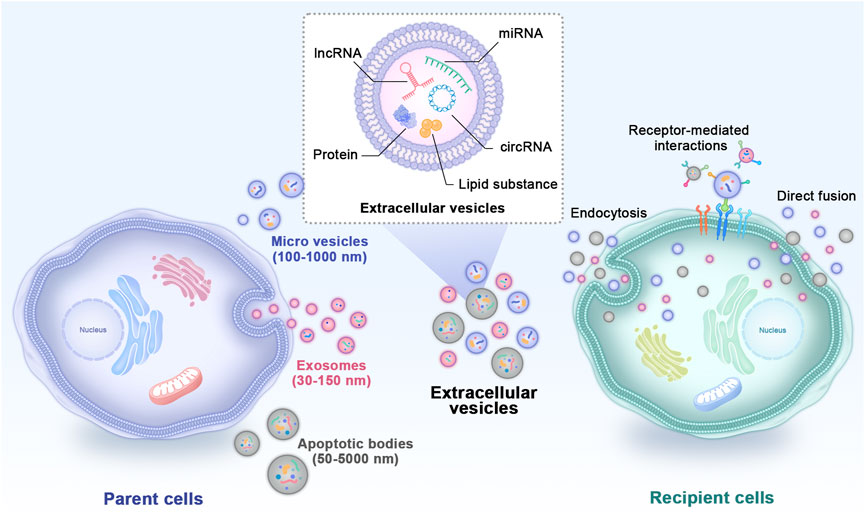
FIGURE 1. Types and internal cargoes of EVs and the way they act on target cells. EVs mainly include exosomes, microvesicles and apoptotic bodies. EVs carry abundant amounts of proteins, miRNAs, mRNAs, circRNAs and other biological molecules derived from parent cells. They transfer internal cargoes to target cells through ligand‒receptor interactions, endocytosis or direct membrane fusion. EVs: extracellular vesicles.
Although considerable research on EVs has focused on promoting the repair of critical-size bone defects, their development is still in the preliminary stage, and the specific mechanisms remain elusive. What is more, for critical-size bone defects, application of EVs alone could not fill defect spaces and guide bioactive molecules to repair and reconstruct the bone defect. Thus, some bioactive scaffolds are needed in EVs-based BTE application. Herein, the authors first review the research progress and possible mechanisms of EVs combined with BTE scaffolds to promote bone regeneration via bioactive molecules. Engineering modified EVs is an emerging component of BTE. Their main progress and opportunities will then be discussed. Finally, future perspectives and challenges of the developing EV-based regenerative medicine are given. Thus, this review may provide a theoretical basis for the future development of the EV-based biomedicine and provide clinical references for promoting the repair of critical-size bone defects.
2 Potential therapeutic applications of extracellular vesicles in bone repair
Tissue regenerative medicine aims to enhance the positive effects of stem cells delivered in vivo. To date, multiple studies have demonstrated that very few transplanted cells can be tracked in vivo after 4–8 weeks, suggesting that their regenerative ability is more likely to be associated with the indirect mechanisms of seed cells, including cytokine paracrine, immunoregulation, and signal transduction (Caplan, 2019). Based on that, researchers used cell aggregates with abundant extracellular matrix to replace dispersed stem cells, and the obtained results showed promising new bone formation in critical-size bone defects in osteoporosis rats (Shang et al., 2014). Although cell aggregates showed better results in preclinical experiments, limitations of cell-based therapy still rendered it taking a step from the research stage to clinical applications. Stem cell-based regenerative medicine has some disadvantages. These limitations, together with problems caused by storage and transport, further limit the application of MSCs in repairing critical-size bone defects (Sissung and Figg, 2020). EVs could overcome these shortages of stem cells while maintain similar functions in regenerating critical-size bone defects. Besides, effects of stem cells in BTE largely dependent on their paracrine action. Direct use of EVs may lead to more stable and predicable results. These are all reasons for applying EVs in cell-free regenerative medicine. In a femur fracture animal model without EV secretion, delayed bone healing was observed, while the healing situation was improved by injecting additional EVs (Furuta et al., 2016). Moreover, the application of MSC-derived EVs also shortened the recovery time, suggesting that EVs promote bone tissue regeneration. This finding highlighted the perspective of EV-mediated bone regenerative medicine (Gunawardena et al., 2019). Although the study of MSC-derived EVs in regenerative medicine is just at the outset, preclinical tests have shown more positive results and fewer adverse effects compared to applications of MSCs.
2.1 Parent cells of extracellular vesicles
EVs were originally observed in reticulocytes during their maturation and were treated as a way to remove waste transferrin receptors (Harding et al., 1983). Afterwards, the phenomenon of EV secretion was found not only in most human fluids but also in a variety of cell types (Johnstone et al., 1987). EVs take chemical or genetic materials to pass messages for cell‒cell communication. Thus, their function is more than that of superfluous membrane vesicles. The possible therapeutic roles of takeover stem cells are being further explored. Mounting evidence indicates that EVs can facilitate cell differentiation and viability and are actively involved in bone homeostasis through cargoes containing microRNAs (miRNAs), proteins and so on.
2.1.1 Mesenchymal stem cells-derived extracellular vesicles
During bone remodelling, the interaction between osteoblasts and osteoclasts is necessary to replace mechanically weak fibrous bone with mechanically strong lamellar bone. Takeuchi et al. (2019) confirmed that MSC-derived EVs manifested the same effects in critical-size bone defect repair with a complete MSC secretome, and both groups had almost half of the defect site covered with new bone tissues after 4 weeks of treatment by promoting angiogenesis and osteogenesis. MSC-derived exosomes, which are the most active vesicles from MSCs (Phan et al., 2018), have been identified as important messengers (Lai et al., 2010) and play a more vital immunoregulatory role than the parent cells (Del Fattore et al., 2015). It has been demonstrated that MSC-derived EVs can accelerate every step of bone defect repair, contributing to bone rehabilitation through immunoregulation. In the regenerative treatment of critical-size bone defects, MSC-derived EVs can stimulate the proliferation and angiogenesis of osteoblasts by delivering endogenous cargoes and inhibit osteoclast maturation (Qin et al., 2016a). Recent studies supported the view that MSC-derived EVs had comparable therapeutic properties compared with their parent cells (Kou et al., 2018). The expression of the protein spectrum showed that 1,927 of a total of 6,342 proteins were expressed in both MSCs and MSC-derived EVs (Anderson et al., 2016). Hence, EVs secreted by different MSCs may inherit matrilineal characteristics in bone regeneration and repair via similar mechanisms to parent cells (Tan et al., 2021). Therefore, selecting an applicable source of parent cells is extremely important for ideal applications of EVs in hard tissue regeneration for critical-size bone defects (Behera and Tyagi, 2018). The commonly applied parent MSCs of EVs mainly include bone marrow mesenchymal stem cells (BMMSCs) (Qin et al., 2016b), adipose-derived stem cells (ADSCs) (Li et al., 2018), umbilical cord mesenchymal stem cells (UCMSCs) (Zhang Y. et al., 2021), MSCs derived from induced pluripotent stem cells (iPS-MSCs) (Fu et al., 2012) and stem cells from human exfoliated deciduous teeth (SHEDs) (Wang M. et al., 2020).
BMMSCs are a major type of MSCs. Their bioactive molecules are now extensively investigated for better applications. Proteomic analyses indicated that as many as 1,533 proteins were involved in various biological actions (Baberg et al., 2019). Preclinical studies have also shown that BMMSC-derived EVs exerted favourable effects in promoting osteogenesis and angiogenesis of osteoblast precursor cells at the injury sites in a critical-size bone defect model, thus forming ideal bone mineralization (Zhang et al., 2022). Martins et al. (2016) discovered that hBMMSC-derived exosomes could promote osteogenic differentiation, as verified by early alkaline phosphatase (ALP) activation and bone morphogenetic protein 2 (BMP2) upregulation.
ADSCs are regarded as one of the most applicable cell sources for EVs because they can be more easily obtained and are widely distributed in the human body (Parker and Katz, 2006). In addition, these cells rapidly proliferate in vitro and retain stem cell phenotypes together with less susceptibility to ageing (Mirsaidi et al., 2012). These advantages make them favourable parent cells for increasing EV isolation (McIntosh et al., 2006). Although the osteogenic capacity of ADSCs is still controversial compared with BMMSCs, hADSC-derived exosomes have been proven to exhibit the capabilities of enhancing the proliferation, migration and osteogenic differentiation of hBMMSCs ex vivo and accelerating bone regeneration at critical-size bone defect sites in vivo (Liu A. et al., 2021).
UCMSCs Umbilical cords are cheap and exhaustless stem cell sources (Wang et al., 2004). hUCMSCs are primitive MSC subpopulations obtained from human postnatal waste tissues (Hendijani et al., 2014). Their collection process requires no invasive operation and has no ethical or moral issues like human embryonic stem cells (Zhao et al., 2010). Compared with other MSCs, hUCMSCs exhibit higher pluripotency (Wang et al., 2015), less immunorejection in vivo and no tumorigenic risk peculiarities (Can and Karahuseyinoglu, 2007). Instead of directly inducing osteogenic and chondrogenic differentiation, hUCMSCs improved bone regeneration by indirectly inducing angiogenesis. To date, hUCMSCs have shown significant clinical potential and have also attracted extensive attention from scholars in the bone regeneration field (Wang L. et al., 2020). In an animal model of critical-size bone defects of rat femurs, exosomes derived from hUCMSCs promoted angiogenesis at the injury site through hypoxia inducible factor-1α (HIF-1α) to achieve bone repair (Zhang et al., 2019).
iPS-MSCs Similar to hUCMSCs, there are neither immunorejection nor ethical problems in the clinical applications of iPSCs. However, some scholars have pointed out that using iPSCs may bring about tumorigenic risks (Jiang et al., 2016). Compared with iPSCs, iPS-MSCs can maintain their self-renewal capacity even after forty passages and have no tumorigenic risk (Lian et al., 2010). In treating bone defects, applications of iPS-MSCs have shown promising prospects in promoting bone repair and regeneration due to their strong proliferation and immunoregulation abilities (Fu et al., 2012). Because EVs from iPS-MSCs have nearly the same biological characteristics as the parent cell, iPS-MSCs have attracted increasing attention as a cell source to promote bone regeneration by using EVs (Zhang J. et al., 2016). In vivo studies reported that hiPS-MSC-derived exosomes enhanced osteogenesis in ovariectomized rats with critical-size calvarial defects (Qi et al., 2016). Moreover, iPS-MSC-derived exosomes may play a certain role in bone defect repair by promoting angiogenesis, as previous research has already demonstrated that they have significant therapeutic effects in treating ischaemic diseases (Hu et al., 2015).
SHEDs Deciduous teeth are the only organ that are replaceable and exfoliate naturally from the human body. Additionally, there are no ethical issues when harvesting stem cells from them, which makes these cells attractive for clinical therapy. SHEDs are not mature MSCs and have multidirectional differentiation potentials (Martinez Saez et al., 2016). Compared with BMMSCs, SHEDs showed increased proliferation ability because they secreted more growth factors, including fibroblast growth factor 2 and transforming growth factor-β2 (TGF-β2) (Nakamura et al., 2009). Studies have also demonstrated that SHED-derived exosomes combined with tricalcium phosphate (TCP) could improve alveolar bone repair by inducing angiogenesis and osteogenesis (Wu et al., 2019).
2.1.2 Non-mesenchymal stem cells-derived extracellular vesicles
MSCs differentiating into osteoblasts participate not only in endochondral ossification but also in the formation of intramembranous bones. These two procedures are essential for bone formation. In addition to the participation of MSCs, new bone formation and regeneration also require the functions of osteoblasts, osteoclasts, and chondrocytes. In particular, endothelial cells play a part in osteoblast maturation and angiogenesis (Qin et al., 2016a). A favourable microenvironment is essential for ideal osteogenesis and angiogenesis. It is well-known that immunoregulatory-related cells are of critical importance in maintaining an appropriate microenvironment for new bone and vascular formation. Thus, the potential therapeutic roles of non-MSC-derived EVs in critical-size bone defect repair have also attracted attention from scholars in recent years.
Osteoblasts are differentiated from BMMSCs. They synthesize and mineralize the bone matrix by releasing collagen and glycoprotein. Osteoblast-derived exosomes were confirmed to regulate Wnt and calcium signalling pathways and miRNAs to induce osteogenic differentiation of BMMSCs (Cui et al., 2016). Osteo-related miRNAs (miR-1192, miR-680 and miR-302a) were expressed in preosteoblast MC3T3-E1-derived exosomes, and the miRNAs in the target cells were also altered to promote their differentiation into osteoblasts (Cui et al., 2016). The effects of these exosomes have even been demonstrated to surpass the original extracellular matrix of parent cells in inducing the directional differentiation of stromal cells (Narayanan et al., 2018). In one study, the comprehensive analysis of EVs showed that the proteome profile changed continuously at different stages of osteoblast mineralization. At the later stage of mineralization, proteins with specific functions in promoting angiogenesis and bone development are enriched in EVs (Davies et al., 2019). A previous study confirmed that osteoblast-derived EVs could regulate osteoclast differentiation through the receptor activator of nuclear factor-κB ligand (RANKL)-RANK signalling pathway. Live cell imaging in the transgenic zebrafish fracture healing model also found internalization of osteoblast-derived EVs within osteoclasts (Kobayashi-Sun et al., 2020).
Osteocytes are terminally differentiated cells, occupying the largest proportion of cells that constitute bone tissues. Although soluble factors and signalling molecules secreted from osteocytes are considered to play key roles in the maintenance of bone homeostasis, current studies on EVs derived from osteocytes are very limited. It was confirmed that osteocyte-derived exosomes contained miRNAs that contributed to bone remodelling (Sato et al., 2017). Osteocytes are sensitive to mechanical stress and respond to external stimuli by regulating the internal cargoes of their exosomes. EVs derived from osteocytes can promote osteogenic differentiation when exposed to mechanical stress. RNA sequencing results suggested that the possible mechanisms were to upregulate miR-181b-5p, thereby inhibiting phosphatase and tensin homologue and thus activating the protein kinase B signalling pathway (Lv et al., 2020). In another similar study, osteocytes subjected to fluid shear stress were able to recruit more stromal progenitor cells through specific EVs, thus promoting local bone remodelling (Eichholz et al., 2020). Of note, the processes of repairing critical-size bone defects by osteocyte-derived EVs are negatively regulated by muscle. Myostatin secreted by muscle can upregulate the expression of miR-218 in exosomes derived from osteocytes, thereby blocking runt-related transcription factor 2 (Runx2) and Wnt signalling pathways and ultimately inhibiting the osteogenic differentiation of BMMSCs (Qin et al., 2017).
Endothelial cells Angiogenesis plays a key role in bone metabolism, in which endothelial cells comprise a single layer of the inner wall of blood vessels. The endothelial cells carry circulating blood macromolecules for the surrounding cells to guarantee their metabolism. Exosomes derived from endothelial cells highly directionally target bone tissues, inhibiting the development and activity of osteoclasts (Li X. et al., 2016). In a study of distraction osteogenesis, endothelial progenitor cell-derived exosomes promoted angiogenesis by upregulating miR-126, thereby accelerating the formation of new bones at the defect site (Jia et al., 2019). Another study also found that endothelial progenitor cell-derived EVs could directly act on BMMSCs to promote their osteogenic differentiation in vitro (Qin and Zhang, 2017).
Immune cells in the microenvironment of critical-size bone defects specifically activate osteoblasts or inhibit osteoclasts through paracrine action. EVs derived from immune cells trigger an immune response by presenting certain antigens. Dendritic cell (DC)-derived EVs were demonstrated to have a good osteogenic inductive effect (Cao et al., 2021), and the possible mechanisms were to regulate the Hippo signalling pathway and induce bone regeneration through exosomal miR-335 via LATS1 signalling (Cao et al., 2021). DC-derived exosomes also contain immunoregulatory cargoes TGF-β1 and interleukin (IL)-10, which enhance the recruitment of regulatory T-cell in inflammatory responses and ultimately inhibit bone loss caused by osteoclasts (Elashiry et al., 2020). Active DC-derived EVs may bring about the activation of humoral immune responses and CD4+/CD8+ T-cell, while immature DC-derived EVs were more likely to decrease systematic inflammation (Lindenbergh and Stoorvogel, 2018). In addition to DCs, exosomes from non-polarized M0, polarized M1 (proinflammatory phenotype) and M2 (anti-inflammatory phenotype) macrophages could specifically target BMMSCs to promote their directional osteogenic differentiation (Xia et al., 2020). Macrophage-derived exosomes can also regulate the gene expression of salt-inducible kinase 2/3 through miR-5106, thereby inducing osteoblast differentiation (Xiong et al., 2020). Effective regulation of the cargoes of EVs derived from immune cells will help scholars better understand the crosstalk between different cells in the microenvironment of critical-size bone defects in the future.
2.2 Applications of extracellular vesicles-loaded bioactive materials in bone tissue engineering
EV-mediated bone regeneration strategies have attracted increasing attention in the treatment of critical-size bone defects in recent years (Fernandez-Yague et al., 2015). Accumulating studies have shown that EVs alone or loaded in different scaffolds, such as hydrogels, can significantly promote local bone regeneration. Scaffold materials are required to fill defect spaces and guide bioactive molecules, such as EVs, to exert certain effects on bone defect repair and reconstruction, especially critical-size bone defects. EV-loaded scaffold materials have already achieved gratifying results in repairing animal models of critical-size bone defects. It effectively stimulates bone regeneration in critical-size skull defects (Qin et al., 2016b), promotes cartilage repair and subchondral bone regeneration in osteochondral defects (Zhang S. et al., 2016), and enhances angiogenesis in repairing femoral defects (Liu et al., 2017). In mouse animal models, EV-loaded scaffolds also showed a more ideal ability to promote bone regeneration and angiogenesis compared with the application of scaffold materials alone, suggesting that scaffolds rich in EVs are an attractive treatment alternative for repairing critical-size bone defects (Xie et al., 2017). Applications of different types of bioactive materials loaded with EVs in BTE are summarized in Table 1.
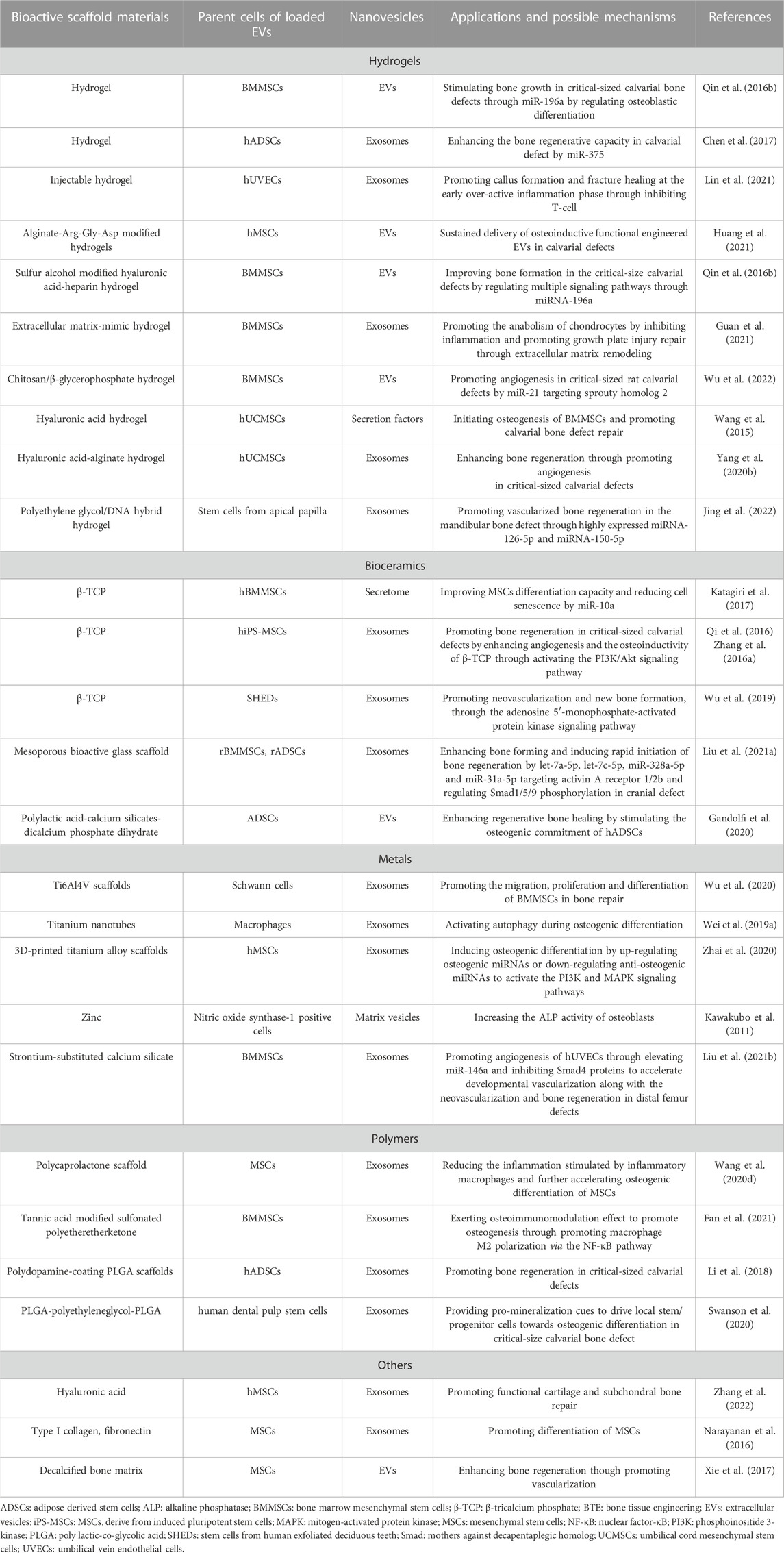
TABLE 1. Applications and possible mechanisms of different EVs-loaded bioactive scaffold materials in BTE.
2.2.1 Applications of extracellular vesicles-loaded hydrogels
As mentioned above, EVs are an ideal seed cell substitute in BTE. In preclinical or clinical applications, it can avert the safety and ethical problems caused by cell-based therapy, and it is also an effective bone inductive substance. However, there are also many limitations in the application of EVs alone in critical-size bone defect repair. The typical one is that due to the clearance of the reticuloendothelial system, EVs are quickly lost in vivo, and therefore, it is difficult to reach an effective therapeutic concentration locally. Thus, clinical applications require appropriate scaffold materials to ensure sustained release of EVs and maintain a local effective concentration of EVs (Riau et al., 2019).
Hydrogels with suitable biocompatibility and controlled release kinetics have been regarded as a preferred carrier material for delivering EVs to bone defect sites (Yan et al., 2020). Subcutaneous implantation of type I collagen hydrogel loaded with hBMMSC-derived exosomes showed better regenerative potential through the upregulation of Runx2 and osterix (Narayanan et al., 2016). EVs loaded in the hydrogel could also improve the bioactivity of this material, leading to the acceleration of human umbilical vein endothelial cell (UVEC) growth and differentiation and finally promoting bone regeneration at critical-size bone defect sites. Therapeutic applications of MSC-derived EVs loaded in hydrogels for treating critical-size calvarial defects have shown significant increases in bone volume, bone mineral density, and newly formed bone area (Qi et al., 2016). It was demonstrated that after being loaded into the hydrogels, EVs could be slowly released in a controlled manner. In addition, hydrogel biomaterials can be fully absorbed at defect sites while promoting osteogenic induction and enhancing bone remodelling (Yang S. et al., 2020). Recently, scholars have focused on constructing novel synthetic hydrogel composites to enhance their function as EV carriers to promote bone regeneration. Adding hydroxyapatite to hyaluronic acid and alginate, Yang S. et al. (2020) produced an injectable hydrogel, which could effectively promote osteoblast differentiation at the critical-size bone defect site after loading MSC exosomes. Another hotspot is how to optimize the controlled release of EVs loaded into hydrogels. Huang et al. (2021) generated engineered modified EVs and loaded them into photocrosslinked sodium alginate hydrogels. It was found that the release process of EVs could be sustained for as long as 7 days, and the biological functions of the loaded EVs did not change during the whole research period. Significant bone regeneration could be observed when applying this system to repair critical-size skull defects.
2.2.2 Applications of extracellular vesicles-loaded bioceramics
In the traditional MSC-based BTE used to treat bone defects, cells need to be directly injected into the target sites. After injection, the multidirectional differentiation potential of MSCs helps to regenerate bone tissues. However, because the injected MSCs lack mechanical support and cannot withstand local pressure, this method is generally not applicable, especially for critical-size bone defect repair (Korhonen and Jurvelin, 2010). To overcome this limitation, bioceramics have become a preferred scaffold material for transplanting cells or cytokines. Porous bioceramics have ideal mechanical strength, biocompatibility and biodegradability (Huang X. et al., 2020). Currently, there are absorbable bioceramics, such as β-TCP, and non-absorbable bioceramics, such as zirconia and alumina, applied in the clinic. Some bioceramics even have biological activities, such as hydroxyapatite glass ceramics. EVs derived from MSCs have similar biological characteristics to MSCs. Therefore, many scholars have loaded them into bioceramic scaffolds to improve the bioactivities of this material. It was found that loading EVs from BMMSCs into hydroxyapatite/TCP bioceramics could better induce new bone formation (Wang et al., 2015). Osteoconductive β-TCP could release hiPSC-MSC-derived exosomes in a controlled manner in vitro, thus stimulating the proliferation, migration and osteogenic differentiation of homing hBMMSCs in the injury sites to promote new bone formation. Application of this delivery system loaded with EVs in critical-size calvaria defects showed increased new bone formation through upregulating the expression of osteocalcin (OCN) and osteopontin along with increased levels of the vascular marker CD31 both in healthy (Zhang J. et al., 2016) and osteoporotic rats (Qi et al., 2016). Currently, scholars are also trying to better improve the survival microenvironment of EVs by adding minerals that can enhance the bioactivity of bioceramic materials so that EVs released from them can maintain favourable biological functions to play a bridge role in intercellular communications and promote osteogenic differentiation of stromal cells (Gandolfi et al., 2020). In a recent study, scholars used strontium to replace calcium silicate in the original scaffold materials and found that EVs loaded into new bioceramics could better exert osteogenic differentiation and angiogenesis potentials in zebrafish and rat femoral bone defect models, resulting in ideal regeneration of bone tissues and blood vessels at the defect sites. The possible mechanisms may be that composition changes upregulate the expression of miR-146a in EVs, which in turn inhibits the protein expression of mothers against decapentaplegic homologue (Smad) 4 and NF2 (Liu L. et al., 2021). In addition to modifying the compositions of bioceramics, decreasing the porous diameters would also create a better survival microenvironment for EVs. Micrometer-scale porous (.5–2 μm) graded mesoporous bioactive glass with osteogenesis, angiogenesis, and antimicrobial activities may provide BMMSC-derived exosomes with a larger surface area to meet the bone regeneration demand (Liu A. et al., 2021). In addition to optimizing the composition and construction of bioceramic materials, similar to hydrogels, scholars are also further exploring and improving the release kinetics of EVs loaded into them in vivo.
2.2.3 Applications of extracellular vesicles-loaded polymers
Synthetic biodegradable polymers are considered effective delivery carriers of EVs because of their unique advantages, such as adjustable release kinetics. Exosomes from ADSCs were loaded into polylactic-co-glycolic acid (PLGA) scaffolds with a polydopamine coating. In vitro, the sustained release of exosomes could be observed, while in vivo, it would promote bone regeneration of critical-size bone defects by enhancing homing of BMMSCs to the defect sites (Li et al., 2018). Scholars have also explored copolymer systems, such as PLGA-polyethylene glycol triblock, as a loading platform for the controlled release of EVs and found that its ability to induce osteogenesis is superior to that of exogenous direct delivery of EVs (Swanson et al., 2020). Polycaprolactone is another biomaterial with specificity for inducing bone regeneration. It has a degradable property. Loaded with EVs derived from BMMSCs, polycaprolactone could effectively repair bone defects in vivo (Wang X. et al., 2020). Polymer composites have the same elastic modulus as bone tissues, but their biological activity is weak compared with certain types of hydrogels or bioceramics. Therefore, the ability to repair critical-size bone defects in vivo could be further improved after loading with EVs.
2.2.4 Applications of extracellular vesicles-loaded metals
As the most traditional bone repair material, metal has good mechanical properties (Zhao et al., 2021). Titanium and its alloys are the most widely used metals in BTE. They have ideal biocompatibility, including non-toxicity, optimal porosity suitable for cell migration and proliferation, high mechanical strength and corrosion resistance. In addition, they are the only metals that have been proven to have osseointegration characteristics (Briguglio et al., 2019). Similar to polymers, many studies that used metals as scaffold materials for EVs have focused on improving their biological functions. Some scholars generated titanium scaffolds by selective laser sintering and 3D printing and then decorated them with EVs derived from hMSCs with preosteogenic differentiation for 4–20 days (Liang et al., 2020). Meanwhile, the adsorption of EVs on the scaffold material was enhanced by coating the scaffold surface with positively charged polylysine to mediate charge interaction. RNA sequencing results suggested that these treatments could simultaneously upregulate specific osteogenic miRNAs and downregulate anti-osteogenic miRNAs of loaded EVs to activate mitogen-activated protein kinase (MAPK) and phosphoinositide 3-kinase (PI3K)/Akt signals and finally obtained good bone induction effects in a rat radial bone defect model (Zhai et al., 2020). In addition, researchers have also tried to produce titanium alloys with porous properties and matching the elastic modulus of bone by 3D printing. After loading Schwann cell-derived exosomes, it was found that the modified titanium alloy had a better ability to repair critical-size bone defects (Wu et al., 2020). In another attempt to improve the function of implanted titanium, researchers loaded BMP2 prestimulated macrophage-derived exosomes into titanium nanotubes and found that surrounding BMMSCs had significantly enhanced osteogenic differentiation ability compared with applying scaffold materials alone (Wei F. et al., 2019). Polyetheretherketone, which has solid mechanical strength and favourable biocompatibility, such as transmissivity and anti-chemical corrosion, is now regarded as an alternative metal scaffold in BTE. After etching with concentrated sulfuric acid, the surface of polyethe retherketone showed a 3D porous structure, which was ideal for the loading and distribution of exosomes. Thus, this system was better at promoting osseointegration by regulating inflammatory responses. Detailed mechanisms include blocking nuclear factor-κB (NF-κB) signalling and enhancing macrophage M2 polarization, and these two processes are reversible (Wei R. et al., 2019). Moreover, tannic acid coated with polyphenol systems has been developed to form reversible hydrogen connections between EVs and scaffold materials, allowing the long-term release of EVs to be realized. The sustained release of exosomes would formulate an immune microenvironment ideal for bone regeneration (Liu and Xiong, 2021c).
In summary, EVs-based cell free regenerative medicine is playing a more and more important role in critical-size bone defect repair. MSC-derived EVs have similar effects to their parental cells while non-MSC-derived EVs have their own advantages in regulating microenvironment. In repairing critical-size bone defects, both MSC and non-MSC-derived EVs need the support of bioactive scaffold materials. These scaffold materials would not only maintain the shape of critical-size bone defect, but also help EVs to better exert their effects through different mechanisms. In future, according to different characteristics of diverse bioactive scaffold materials, more studies are needed to provide references for choosing more favorable EVs under different pathological or regenerative regulatory microenvironments.
3 Mechanisms of extracellular vesicles on critical-size bone defect repair
In treating bone defects, EVs can regulate osteogenesis, angiogenesis, and immune responses, inhibit osteoclast activity, and finally promote repair processes by transporting their internal cargoes to target cells via downstream signalling pathways (Figure 2) (Gao et al., 2018). A large number of bioactive substances are contained in EVs, such as nucleic acids, proteins and lipids. Among these bioactive molecules, miRNAs are the most common cargoes (Toh et al., 2017). MiRNAs exert critical effects in cell-to-cell crosstalk and regulate bone tissue repair and regeneration by posttranscriptional modification. In addition to promoting osteogenesis and angiogenesis, EVs take part in regulating immune responses through their cargoes to suppress the activities of osteoclasts during the repair of critical-size bone defects (Li R. et al., 2022). Nevertheless, because EVs contain many miRNAs, these miRNAs may interact with each other to form a complex network. In addition to miRNAs, EVs also contain many proteins, lipids and other types of nucleic acids, such as long non-coding RNAs, circular RNAs and mRNAs. Thus, elucidating the intrinsic molecular mechanisms of EVs in regenerating bone tissues is necessary.
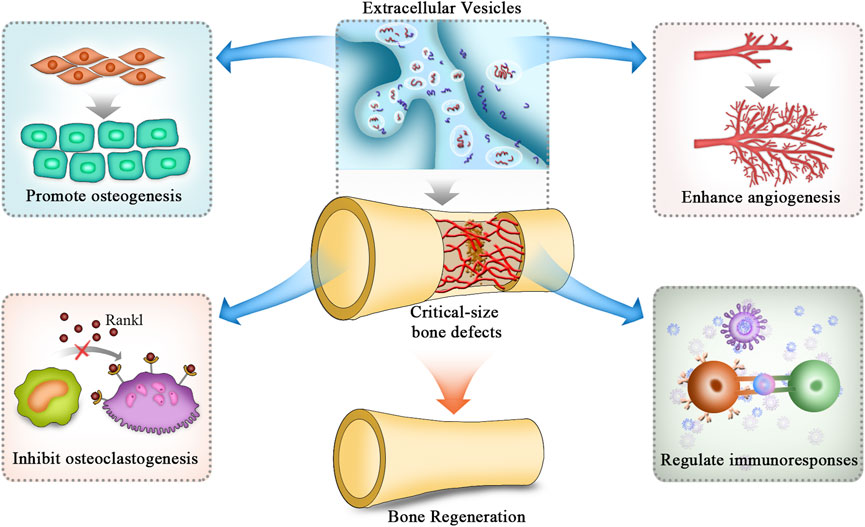
FIGURE 2. Mechanisms of EVs applied in BTE to repair critical-size bone defects. The mechanisms of EVs in repairing critical-size bone defects are mainly attributed to the promotion of osteogenic differentiation of stromal cells while inhibiting the differentiation of osteoclasts. In addition, they also promote angiogenesis and modulate inflammatory responses to provide a suitable environment for bone regeneration. EVs: extracellular vesicles.
3.1 Extracellular vesicles promote critical-size bone defect repair by enhancing osteogenesis
The crux to repair critical-size bone defects is to enhance the activities of osteoblasts while inhibiting those of osteoclasts in the local microenvironment. Researchers have already confirmed that EVs can enhance the homing of endogenous stromal cells to defect sites, thereby shortening the time of bone formation and mineralization (Chew et al., 2019). In addition, EVs can bind matrix proteins, such as type I collagen and fibronectin, to induce the development and maturation of osteoblasts both ex vivo and in vivo (Narayanan et al., 2016). Some scholars used EVs derived from hBMMSCs with preosteogenic induction for RNA sequencing. The obtained results indicated that these EVs contained miR-196a, miR-27a and miR-206, which are important for osteogenic differentiation. Among those miRNAs, miR-196a has the potential to induce the expression of ALP, OCN, osteopontin and Runx2, leading to the formation of more bone tissues in critical-size calvarial defects (Qin et al., 2016b). Moreover, the expression of osteogenic-related miRNAs, such as miR-146a-5p, miR-503-5p, miR-483-3p and miR-129-5p, were upregulated, and the expression of anti-osteogenic-related miRNAs, such as miR-32-5p, miR-133a-3p and miR-204-5p, were downregulated. These miRNAs could activate the PI3K/Akt and MAPK signalling pathways to exert the biological effects of promoting bone mineralization in the local microenvironment (Zhai et al., 2020).
EVs promoting osteogenesis also involve several other signalling pathways, including BMP/Smad, Wnt/β-Catenin, tensin homologue/PI3K/Akt and HIPPO. These signalling pathways have been confirmed by many studies to play active roles in promoting the repair of critical-size bone defects (Cao et al., 2021). In addition to miRNAs, EVs can transport long non-coding RNAs to stromal cells at bone defect sites and indirectly promote bone mineralization by downregulating genes that inhibit osteogenesis (Yang et al., 2019). In addition to MSC-derived EVs, Qi et al. (2016) also demonstrated that human iPS-MSC-derived exosomes significantly promoted osteogenesis and angiogenesis through their internal miRNAs. The application of iPSC-MSC-derived exosomes together with β-TCP scaffolds promoted bone regeneration in critical-sized calvarial defects in an ovariectomized rat model. The results of Li et al. (2018) showed that in the critical-size bone defect microenvironment, in addition to activating the extracellular regulated protein kinase 1/2 pathways, hADSC-derived exosomes could also promote the expression of vascular endothelial growth factor (VEGF) in MSCs through various miRNAs, thus indirectly creating a good microenvironment for bone tissue regeneration by promoting angiogenesis.
Other researchers analysed the proteomics of osteoblast-derived EVs. The obtained results showed that up to 786 proteins in EVs were involved in osteogenic-related signalling pathways, such as mammalian target of rapamycin and eukaryotic initiation factor-2 (Ge et al., 2015). Similar studies have focused on EVs derived from mouse MC3T3 cells. Proteomic analysis found that among the 1,536 proteins, 172 were closely related to bone development and functions (Ge et al., 2017). Therefore, EVs may improve the differentiation ability of stromal cells or osteoblast precursor cells by transporting cargoes such as miRNAs and proteins in the bone defect microenvironment and enhancing the repair of critical-size bone defects.
3.2 Extracellular vesicles promote critical-size bone defect repair by suppressing osteoclastogenesis
Under normal circumstances, the regeneration processes of critical-size bone defects involve the imbalance of bone resorption and bone formation; that is, the activity of osteoblasts needs to be more dynamic than that of osteoclasts (Wu et al., 2010). Therefore, understanding the mechanisms by which EVs regulate osteoclast differentiation and formation will also provide new concepts for treating critical-size bone defects. EVs in the BTE can regulate the bone marrow microenvironment and inhibit osteoclast activities through their internal proteins and miRNAs. Prostate cancer cell-derived exosomes could downregulate miR-214 in osteoclasts and block NF-κB signalling, therefore inhibiting osteoclast differentiation (Duan et al., 2019). In addition, these exosomes could also inhibit osteoclast proliferation and differentiation by reducing the expression of osteoclast markers such as tartrate-resistant acid phosphatase, cathepsin K and matrix metalloproteinase-9, ultimately regulating bone formation (Karlsson et al., 2016). ADSC-derived exosomes not only reduce the mRNA and protein expression of RANKL but also reduce the ratio of RANKL/osteoprotegerin, thereby inhibiting RANKL-involved osteoclast maturation (Ren et al., 2019). EVs derived from endothelial cells could effectively inhibit bone resorption at bone defect sites by reducing osteoclast activities (Song et al., 2019). In addition, osteoclast-derived EVs could act as mediators between osteoclasts and osteoblasts, regulating the osteogenic function of osteoblasts. Osteoclast exosomes are rich in miRNAs, which inhibit osteoblast activity. MiR-214-3p in osteoclast-derived exosomes could target osteoblasts, inhibiting osteoblast activities ex vivo and deteriorating bone formation in vivo, while in vivo injection of antagomir-214-3p could effectively reverse these negative effects (Li D. et al., 2016). Therefore, miR-214 or miR-214-3p in EVs not only serve as biological tags of bone mass reduction but also as therapeutic targets to promote bone regeneration. Researchers also found that RANKL could induce the expression of miR-23a-5p in osteoclast-derived exosomes. Because miR-23a-5p suppresses the expression of Runx2 and promotes Yes-associated protein-1-mediated anti-osteogenic signalling, RANKL may be a target protein that regulates EV-mediated osteoclastogenesis differentiation (Yang J. X. et al., 2020). In conclusion, EVs may regulate osteoclast activities, thus mediating the development of bone regeneration; and some specific miRNAs can be used as therapeutic targets for bone defect repair. However, compared with the functional study of EVs promoting osteogenesis, there are currently fewer studies on the effects of EVs on osteoclast differentiation. Therefore, it is necessary to conduct more in-depth and comprehensive research in the future.
3.3 Extracellular vesicles promote critical-size bone defect repair by enhancing angiogenesis
Bone contains a high density of vasculature. Numerous bone cells participate in maintaining normal functions of bone, while the biological activities of these bone cells rely on the ambient vasculature. There is increasing evidence that maintaining adequate local blood supply or maintaining continuously increased angiogenesis plays an important role in critical-size bone defect regeneration (Wang et al., 2017). Therefore, one of the basic strategies for promoting the repair of critical-size bone defects is to promote angiogenesis (Jia et al., 2016). Various studies have demonstrated that EVs enhance angiogenesis near tendon-bone junctions in vivo (Vizoso et al., 2017), which is shown by promoting the expression of angiogenic factors and tube formation and stimulating the proliferation and migration of endothelial cells (Zhang B. et al., 2021). It was also confirmed that endothelial progenitor cell-derived exosomes enhanced the proliferation, migration and angiogenesis of endothelial cells by delivering miR-126. Transduced miR-126 improved the vascular areas and thicknesses around the shin bones of rats and finally accelerated the bone repair process in the defect sites (Jia et al., 2019). Scholars also found that miR-21 in MSC-derived exosomes downregulates the expression of sprouty homologue 2 and promotes angiogenesis (Wu et al., 2022). It was reported that BMMSC-derived exosomes may independently activate the VEGF or Hippo signalling pathway by regulating cell-to-cell contact and activating cytoskeleton dynamics (Wang Z. et al., 2021). Another study performed by Takeuchi et al. (2019) showed that MSC-derived EVs enhanced tissue and vascular development in parietal bone defects in Wistar rats by upregulating the mRNA expression of VEGF, angiopoietin 1 and 2 together with osteogenesis-related collagen I, ALP, OCN and osteopontin. Zhang et al. (2015) found that hiPSC-derived exosomes could directly promote angiogenesis and collagen synthesis. Similar studies have also demonstrated that EVs derived from MSCs could upregulate the expression of functional angiogenesis molecules and enhance the migration of hUVECs, exhibiting a larger area of angiogenic tube formation (Zhang et al., 2020). A rat model of femoral fracture demonstrated that transplanting MSC-derived EVs to the defect sites could significantly enhance angiogenesis and osteogenesis, leading to better bone tissue regeneration. Other researchers found that exosomes from apical papilla stem cells promoted angiogenesis through miR-126-5p, which was indirectly manifested by increased expression of VEGF and angiopoietin-1 (Jing et al., 2022). Scholars have also demonstrated that hypoxia pretreatment can regulate the expression of bioactive substances in exosomes, thus promoting angiogenesis in different microenvironments. After hypoxia preconditioning, the expression of miR-126 in MSC-derived exosomes was upregulated, which further promoted angiogenesis, proliferation and migration of stromal cells, thus enhancing the process of fracture healing (Liu et al., 2020). In addition to hypoxia, the proangiogenic ability of exosome components is also enhanced by certain biomaterials. A biomaterial containing lithium induced miR-130a expression in exosomes, activated tensin homologue/Akt signalling pathways, and finally promoted angiogenesis in the bone defect microenvironment (Liu et al., 2019). In conclusion, by selecting appropriate EVs and regulating the expression of bioactive substances within them, EVs may be promising candidates for treating critical-size bone defects as proangiogenic vesicles.
3.4 Extracellular vesicles promote critical-size bone defect repair by regulating immune responses
The healing processes of bone defects are often accompanied by local inflammatory responses and imbalanced immune reactions. These changes will always result in unsuccessful scaffold implantation in bone defect treatments (Guder et al., 2020). Large numbers of inflammatory and immune cells are present in the microenvironment of bone defect sites. Mild inflammation-related responses are critical for bone defect regeneration (Lin et al., 2021). The functions of EVs in immune response regulation are diverse. EVs not only participate in the processes of anti-inflammatory responses, such as in collagen-induced arthritis mice (Cosenza et al., 2018) and antigen-induced synovitis pigs (Casado et al., 2017) but also modulate beneficial regenerative immune phenotypes, which are important in tissue repair as well as calcification (Lindenbergh and Stoorvogel, 2018). In addition, EVs can regulate the functions of T-cell, B cells, macrophages and other immune cells directly by membrane fusion or indirectly by transporting bioactive cargoes (Qian et al., 2021). It has already been demonstrated that MSC-derived exosomes are effective in suppressing immune responses by attenuating the proliferation of T-cell and B cells. Moreover, MSC-derived exosomes can also suppress the secretion of the proinflammatory cytokines tumour necrosis factor-α and IL-1β, together with increasing the excretion of the anti-inflammatory cytokine TGF-β in vitro (Chen et al., 2016), thus maintaining a favourable immune balance that was crucial for bone healing. Macrophages are part of innate immunity. They participate in removing pathogens and modulating inflammatory responses in the human body (Bozec and Soulat, 2017). Macrophage M2 polarization can enhance angiogenesis and bone tissue regeneration (Wasnik et al., 2018). EVs loaded into scaffolds could promote macrophage M2 polarization and suppress the inflammatory responses in the local microenvironment, as manifested by decreased mRNA and protein expression of the proinflammatory factors IL-6 and tumour necrosis factor-α (Guan et al., 2021). The inhibited inflammatory responses would indirectly boost vascularization, therefore facilitating osteoblast function and bone tissue mineralization and finally leading to favourable new bone tissue formation (Kim et al., 2021). This EV-mediated transplantation system has been recognized as a novel alternative for treating bone defects. Further mechanistic studies showed that the NF-κB pathway may play an important role in this process (Fan et al., 2021). To further confirm whether the bioactive molecules of MSC-derived exosomes play a key role in regulating immune responses, Li R. et al. (2022) analysed the role of miR-451a, which is highly expressed in ADSC-derived exosomes, in repairing rat skull defects. By transfecting miR-451a analogues and inhibitors into macrophages, it was found that miR-451a could directly regulate the mRNA expression of macrophage migration inhibitory factor by specifically binding to its 3′UTR, thereby promoting the polarization of the macrophage phenotype from M1 to M2. Moreover, the results of other studies also found that UVEC-derived exosomes, in which programmed cell death ligand 1 was overexpressed, could bind to programmed cell death-1 on the surface of T-cell, thereby inhibiting its activation. As a result, the osteogenic differentiation of MSCs was significantly enhanced after inhibiting the local hyperactivated inflammatory responses (Lin et al., 2021). Therefore, EVs are critical modulators of immune responses for regenerating new bone tissues.
In summary, the roles of EVs in repairing critical-size bone defects are attributed to 1) promoting osteogenic differentiation of stromal cells while inhibiting the differentiation of osteoclasts; 2) enhancing angiogenesis to provide a suitable environment for bone regeneration; and 3) modulating inflammatory responses to maintain a favourable immune microenvironment (Figure 2). Thus, EVs would be an ideal cell-free therapeutic component to treat critical-size bone defects. As we have reviewed, mechanistic studies of EV application in BTE are mainly performed from the aspect of internal cargoes of miRNAs and proteins. More studies focused on these are summarized in Table 2.
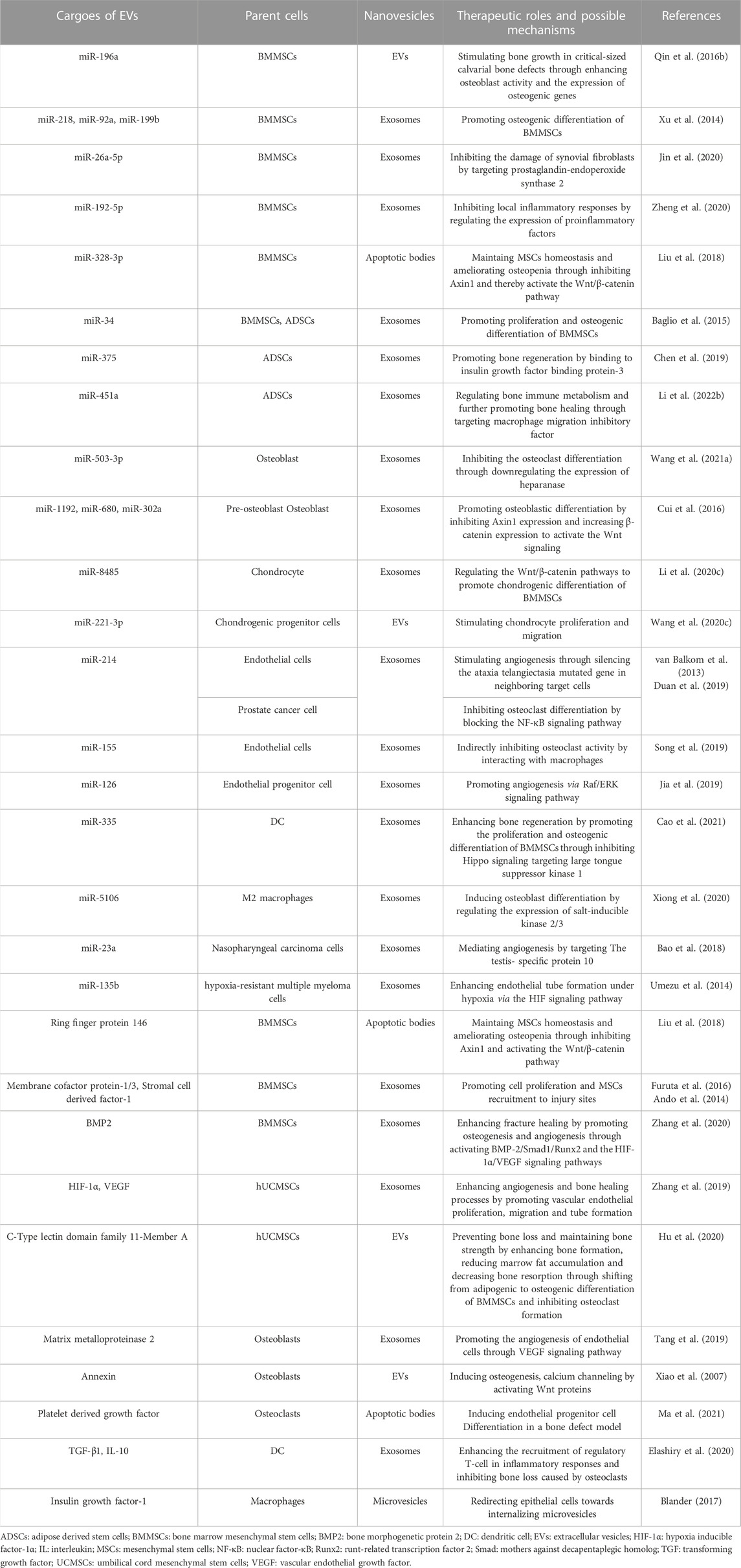
TABLE 2. Therapeutic roles and possible mechanisms of certain miRNAs and proteins of EVs in critical-size bone defects repair.
4 Application of engineering modified extracellular vesicles in bone tissue engineering
Although natural EVs exert diverse functions in regenerative medicine through multiple biological mechanisms, their limitations in repairing critical-size bone defects have gradually been recognized. Specifically, the targeting effect of natural EVs is poor. Most of the mature EVs delivered to bone defect sites will enter the circulatory system and cannot all accurately act on the target cells. In addition, not all EVs isolated from parent cells have therapeutic effects; thus, the number of effective natural EVs is far from sufficient. As a result, the bioactive molecules that are needed in different pathological circumstances cannot reach a therapeutic concentration. Although EVs are processed and assembled in parent cells and inherit similar biological characteristics to them, the bioactive molecules in natural EVs vary greatly, which will bring unstable efficacy in repairing critical-size bone defects. Therefore, engineering-modified EVs have been investigated to accelerate the bone regeneration process by applying multiple techniques that fall into two main categories in most circumstances: endogenous engineering strategies and exogenous engineering strategies. Advantages and disadvantages of some commonly used techniques are summarized in Table 3. Applications of engineered modified EVs can enhance therapeutic efficiency and more accurate targeting effects over natural EVs (Arenaccio et al., 2019).
4.1 Endogenous engineering modified extracellular vesicles
In the previous sections, the authors summarized the biological characteristics of EVs derived from different parent cells and their applications in BTE to repair critical-size bone defects. Studies have shown that except for selecting appropriate types of parent cells, modification of the parent cells before EV isolation through genetic strategies, preconditioning treatment and physical manipulations could also modify the biological characteristics of EVs (Jafari et al., 2020). These approaches are categorized as endogenous engineering modifications.
4.1.1 Genetic engineering strategies
Genetically modifying the natural procedures of protein synthesis of parent cells can endow the modified EVs with membrane modification and more desirable functions, such as precise targeting and individualized therapeutic performance, by regulating some targeted biomolecules. Compared with natural EVs, specific ligands can be added to the EV membrane to improve its targeting effects through genetic engineering (Hu et al., 2021). After transfection with the RUNX2 gene, the osteogenic differentiation of hBMMSCs was potentiated. Meanwhile, the differentiation induction capabilities of EVs derived from this gene-transfected cell were also enhanced in vitro (Martins et al., 2016). In a similar study, the expression of HIF-1α in parental MSCs was upregulated. The EVs derived from these cells exhibited better regenerative characteristics. The in vitro results indicated that EVs from HIF-1α-upregulated MSCs could better enhance matrix mineralization, ALP activity and osteogenesis-related gene expression in BMMSCs and promote the migration, proliferation and tube formation of HUVECs. Meanwhile, in vivo results indicated better bone tissue regeneration and neovascularization; therefore, the trabecula bone healing time was significantly shortened in rabbit necrotic femoral heads (Li et al., 2017). Another study also upregulated HIF-1α expression in BMMSCs. Their derived exosomes were loaded into a β-TCP scaffold to repair critical-size bone defects in rats. The obtained results also confirmed that compared with applying normal BMMSC-derived exosomes, exosomes derived from HIF-1α-upregulated BMMSCs considerably accelerated the regeneration of new bone tissues with favourable stromal cell neovascularization differentiation (Ying et al., 2020). Other researchers have tried to activate the endogenous BMP signalling pathway by downregulating the natural BMP antagonist noggin in the parent cells of EVs (Fan et al., 2016). They observed EVs obtained from noggin knockdown MSCs and found that a large number of endogenous aggregations of osteogenic-related molecules appeared in EVs. When these modified EVs were loaded into injectable ethylene glycol methacrylate chitosan hydrogels and transplanted into mouse critical-size calvarial defects, more desired bone regeneration could be observed (Fan et al., 2020).
4.1.2 Preconditioning treatment of parent cells
Preconditioning treatment of parent cells through conditioned culturing medium could improve the biological activities of EVs derived from them. It is an effective strategy to boost EV-mediated BTE in regenerating bone tissues. Preconditioning treatment can directly modify the DNA or RNA of the parent cells to obtain needed EVs rich in target cargoes. Narayanan et al. (2018) found that exosomes derived from MSCs that were precultured in osteogenic induction medium showed better capabilities of inducing osteogenic differentiation of other MSCs in vitro and of promoting neovascularization in 3D cultures in vivo. Liu A. et al. (2021) compared EVs derived from different types of stem cell sources and culturing media. The obtained results suggested that exosomes from rBMMSCs preconditioned with osteogenic induction medium exhibited the most desirable osteogenesis characteristics, and the ALP activity increased by more than 2 times compared with the blank control. In-depth bioinformatics analysis further confirmed that preconditioning rBMMSCs with osteogenic induction medium could upregulate the expression of multiple osteogenic-related miRNAs in their derived EVs such as let-7a-5p, let-7c-5p, miR-328a-5p and miR-31a-59. Mesoporous bioactive glass scaffold materials loaded with these modified exosomes could effectively repair rat skull defects in vivo. Another study found that exosomes derived from hBMMSCs preconditioned with dimethyloxaloylglycine, which stabilizes HIF-1α at a low concentration, could improve bone healing by boosting angiogenesis in critical-sized calvarial defects in rats (Liang et al., 2019). Moreover, Huang C. C. et al. (2020) demonstrated that in a rat skull critical-size defect model, compared with natural hBMMSC-derived EVs, BMP2 preconditioned hBMMSC-derived EVs had significantly enhanced bone regeneration ability. Other scholars used BMP2 to precondition mouse macrophages (RAW 264.7) and observed the osteogenic potential of their exosomes in bone regeneration applications (Wei F. et al., 2019). The obtained results showed that the preconditioned macrophage-derived EVs enhanced the osteogenic differentiation of BMMSCs. These results demonstrated that preconditioned parent cell-derived EVs could be better applied in bone regeneration.
Another widely applied preconditioning treatment of parent cells is a hypoxic environment. MSCs, which were preconditioned in a hypoxic environment, promoted angiogenesis capability. In addition, EVs derived from these cells also had better angiogenic characteristics and led to enhanced osteogenic capability. The in vitro experiments showed that these modified EVs could promote the proliferation and migration of HUVECs and tube formation. Due to the favourable angiogenic microenvironment, osteogenic differentiation of BMMSCs at the bone defect site was also enhanced, which was manifested by increased gene expression of serum OCN and ALP (Li et al., 2017). The in vivo experiments were performed using the steroid-induced avascular necrosis model in the femoral head. The obtained results indicated that the femoral head transplanted into EVs derived from hypoxia-preconditioned cells had higher vascular density and denser trabecular bone tissue formation (Li et al., 2017). The results of Sakaguchi et al. (2017) were similar. Researchers also found that exosomes derived from BMMSCs in a hypoxic environment could enhance the formation of tubular structures by HUVECs. Compared with natural exosomes, in vivo applications of modified EVs showed more favourable new bone formation.
4.1.3 Physical manipulation approaches
Physical manipulation could change the amount of EVs released from parent cells together with the type and amount of therapeutic cargoes in EVs. Generally, physical manipulation is performed by directly exerting physical forces on the parent cells by electroporation and extrusion (Richter et al., 2021), thereby optimizing EVs derived from them. Cui et al. (2022) reported bioinspired nanovesicles prepared from human iPSC-derived endothelial cells under hypoxia culture through an extrusion approach. Abundant membrane C-X-C motif chemokine receptor 4 conferred these nanovesicles bone-targeting ability and the endothelial homology facilitated the bone marrow endothelial cells tropism. Due to their unique endogenous miRNA cargoes, these nanovesicles re-educated bone marrow endothelial cells to secret cytokines favoring osteogenesis and anti-inflammation, leading to a better bone homeostasis. Researchers also used hydroxyapatite ceramics containing magnetic nanoparticles as a scaffold material to simultaneously load osteoblasts and osteoclasts. They found that magnetic nanoparticles could change the internal cargoes of osteoclast-derived exosomes, resulting in a decreased amount of osteoclast-derived exosomes absorbed by osteoblasts, thus weakening the negative regulatory effect of osteoclasts on osteoblasts. Osteoblasts showed enhanced proliferation and differentiation capabilities (Zhu et al., 2020). Further studies found that changes in the cargoes of exosomes derived from osteoclasts were mainly reflected in the magnetic nanoparticle-mediated upregulation of Rho kinase and the downregulation of ubiquitination and reactive oxygen species.
4.2 Exogenous engineering modified extracellular vesicles
To summarize briefly, exogenous engineering of EVs involves functional modification after their isolation, directly loading specific external cargoes into nanoscale EVs to deliver bioactive molecules with therapeutic effects to the local microenvironment, thereby promoting critical-size bone defect repair (Zha et al., 2021). Currently, engineered EVs can be prepared by incubation, electroporation, sonication, mechanical extrusion, recurrent freezing and thawing, and direct EV surface modification by applying covalent or non-covalent interactions (Liao et al., 2019). Using these techniques, the modified EVs would load a desired amount of therapeutic biomolecules, including small molecular drugs, nucleic acids, and proteins either inside or on the surface. In addition, these modified EVs specifically bind antibodies through covalent bonds on lipid membranes and ligands.
Liang et al. (2020) added a plasmid encoding BMP2 to EVs derived from rat MSCs to potentiate the bone inducibility of demineralized bone matrix. The specific method was to coat the membrane of EVs with polyethyleneimine, a cationic polymer usually applied to coat negatively charged DNA, and then add the plasmid BMP2 through a layer-by-layer self-assembly method. This easy technique constructed 100–1,000 nm diameter EV-polyethyleneimine/plasmid BMP2 complexes. Compared with the control group, EV complexes showed upregulated BMP2 gene transfection efficiency and lower toxicity to target cells in vitro. In addition, demineralized bone matrix loaded with these novel complexes exhibited increased bone regeneration induction capability after being implanted subcutaneously in rat and rabbit femoral head bone defect models.
VEGF plays a critical role in angiogenesis and BTE for bone tissue regeneration (Grosso et al., 2017). However, as a protein, VEGF has many problems in its direct delivery, including rapid degradation, uncontrolled release and unstable biological activities (Hu and Olsen, 2016). Zha et al. (2020) designed a matrix that could locally release VEGF plasmids for bone defect repair. They used EVs derived from rBMMSCs to transport the plasmid VEGF, which was equivalent to providing a protective layer for VEGF, thus avoiding the problems mentioned above, such as rapid degradation. Researchers first used the electroporation technique to temporarily create holes on the surface of EVs by an electric pulse to increase membrane permeability. After that, VEGF plasmids were loaded into EVs. The modified EVs successfully promoted vascularization and osteogenesis in rat skull defects. In addition, it could also effectively achieve vascularized bone regeneration in segmental bone defects (Zha et al., 2021).
Cui et al. (2021) developed an exosome delivery system based on exosomes secreted by iPSC-MSC. The engineered exosomes collaborated with the loaded siRNA of the SHN3 gene to enhance their therapeutic effects. Modification of a bone-targeting peptide endowed the engineered exosomes an ability to deliver siRNA to osteoblasts specifically. Silencing of the osteoblastic SHN3 gene enhanced osteogenic differentiation, and inhibited osteoclast formation. Furthermore, SHN3 gene silencing could facilitated vascularization, especially formation of type H vessels. This study demonstrated that exogenous engineered exosomes could serve as a promising therapy vesicle to kill three birds with one stone. Luo et al. (2019) also provided a promising method to enhance the ability of exosomes to target specific tissues. In their study, the surface of exosomes from bone marrow stromal cell was conjugated with a BMSC-specific aptamer, which delivers exosomes into BMSCs within bone marrow. Intravenous injection of the exosome-aptamer complex enhances bone mass in osteoporosis mice and accelerates bone regeneration in a femur fracture mouse model.
5 Future perspectives and challenges
With meticulous and thorough research exploring the biogenesis and biofunctions of EVs, natural and engineered modified EVs that possess various promising advantages, such as biological functions similar to those of parent cells, low immunorejection, desirable biocompatibility, and the capability to deliver individualized drugs, have shown exciting application prospects in diagnosing and treating diverse pathological conditions (Nagelkerke et al., 2021). The first phase I clinical trial on EVs derived from DCs was conducted for patients with malignant melanoma, and the obtained results indicated that their clinical application was safe (Escudier et al., 2005). Although this clinical safety evaluation trial started as early as 2005, further clinical studies focusing on the evaluation of the safety and effectiveness of EVs applied in tissue repair and regeneration are still in progress mainly in phases I and II. As an important part of BTE development, the progress of EVs in the future will largely determine the progress of BTE-led critical-size bone defect repair and regeneration. Certain achievements have been made in preclinical trials of EV-mediated nanotherapies, and the obtained results showed ideal effects in bone fracture healing (Zhang et al., 2019). However, scholars believe that there are still some unsolved theoretical challenges and important practical problems that should be addressed during future and wider clinical utilization (Figure 3). Nevertheless, challenges and difficulties could not hinder EVs from being a promising alternative for MSC-based therapy in bone regenerative medicine.
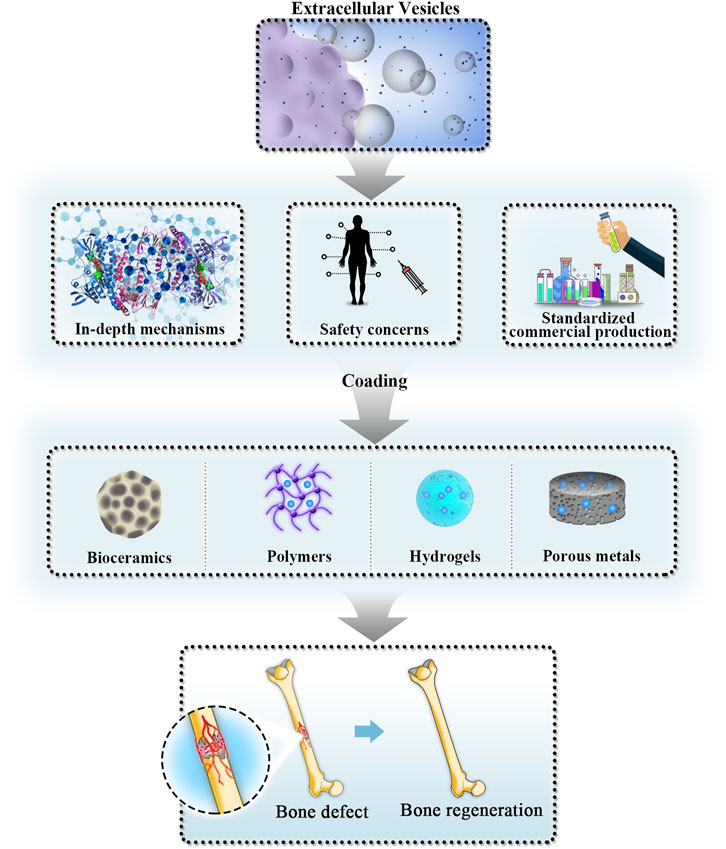
FIGURE 3. Future perspectives and challenges of EV clinical application in BTE. EVs have shown exciting application prospects in repairing critical-size bone defects. In the near future, scholars will focus on studying in-depth mechanisms, solving safety concerns and promoting standardized commercial production of EVs applied in BTE. With further development of bioactive scaffold materials, cell-free regenerative medicine in BTE will soon be widely used. BTE: bone tissue engineering; EVs: extracellular vesicles.
5.1 More in-depth mechanisms of extracellular vesicle-mediated bone defect regeneration
Although extensive studies have focused on various therapeutic mechanisms of natural or engineered modified EVs for different diseases, such as cancer and inflammatory diseases (Karlsson et al., 2016; Cosenza et al., 2018), the potential roles of specific functional units from EVs in bone defect regeneration have not been completely explained. In this review, the authors summarized some of the most widely raised clinical mechanisms of EVs as regenerative agents, such as immune regulation, as well as regulation of cell osteogenesis or angiogenesis. However, the detailed therapeutic mechanisms or related downstream targeted signalling cascades largely remain unclear and need to be further explored. These studies are of basic critical importance for scholars to comprehend the therapeutic effects of EVs in regenerative medicine and the biomedical applications of EVs.
Future studies at this stage will mainly focus on exploring the mechanisms of inducing bone regeneration and manufacturing more ideal scaffold materials. Theoretically, EVs regulate bone defect repair and regeneration through the interaction of their multiple internal cargoes (Arenaccio et al., 2019). However, most of the currently available studies focused on the roles of miRNAs in EVs, which indicated that the mechanisms of EVs in bone regeneration are far from being fully explored. It is also necessary to further elucidate the different types of effective active factors of EVs that promote osteogenic differentiation, angiogenesis, inflammation regulation and osteoclast inhibition. In addition, as previously mentioned, the in vivo application of EVs cannot be separated from the scaffold materials. In current studies, based on the combined applications of different scaffold materials, scholars have developed a variety of innovative methods to improve the delivery efficiency of EVs (Liao et al., 2019). However, problems such as ineffective delivery and lack of long-term retention of EVs still exist (Riau et al., 2019). In the future, with the development of innovative scaffold materials and standardization of the porosity value or size of biocompatible 3D porous scaffolds for bone defects, efficient transportation, sustained release and long-term storage of EVs will be achieved.
Furthermore, deeper investigations of in vivo bioeffects of EVs, such as distribution or bioelimination in blood circulation or in diverse pathological microenvironments, will also provide a deeper understanding of the pharmacokinetic and pharmacodynamic performances of EVs. This broader understanding will be beneficial to the proposal of EV-mediated targeted nanotherapeutic strategies.
5.2 Potential safety concerns of extracellular vesicles in clinical applications
As reviewed earlier, EVs have more favourable biocompatibility and enhanced biosafety over conventional stem cell therapy; however, the whole components of EVs, including some other unknown bioactive molecules, are still not completely clear (Phan et al., 2018). Although the Extracellular RNA Communication Consortium (Das et al., 2019) and the International Society for Extracellular Vesicles (Théry et al., 2018) have published guidelines to recommend researchers in standardizing the isolation and characterization procedures of EVs, the applications of EVs in the clinic would still lead to some unpredictable or undesirable biological effects. On the other hand, the heterogeneous constituents of EVs may lead to side effects that accumulate in non-target organs (Phan et al., 2018). Thus, more efforts to clarify the compositions of EVs are needed to avoid possible side effects during clinical applications in the human body.
Currently, the optimal therapeutic dose of EVs is still uncertain; thus, there would also be potential side effects after application. More preclinical studies are needed, especially on applying EVs in bone defect repair in large animals, to provide more safety references and a basis for the clinical promotion of EVs in later stages.
5.3 Standardized and commercialized production of extracellular vesicles
Effective isolation of EVs is the basis for their clinical application. From this point of view, standardized purification, preparation, production and preservation are essential. Increasing evidence indicates that EVs from different types of parent cells carry distinct bioactive molecules that play different biological functions in bone remodelling and regeneration (Tian et al., 2018). Even EVs derived from the same parent cell still have diverse sizes, component cargoes, proteins, and other biophysics or biochemical properties (Yan et al., 2020). Thus, only by solving the standardizing issues can we realize a safer clinical application of EVs.
Currently, differential ultracentrifugation and sequential ultrafiltration are the most commonly employed approaches to isolate EVs (Richter et al., 2021). However, these techniques have certain disadvantages, including being time-consuming, having low EV purity and output, and having unfavourable reproducibility (Théry et al., 2018). Commercialized isolation kits for EVs are now available but have not overcome the low efficiency limitation and are expensive. Therefore, the development of a standardized and time-saving isolation technique to harvest high-purity EVs at low costs would be a cornerstone to eventually achieve commercialized clinical application of EVs.
Accordingly, technology to accurately regulate the expression of bioactive molecules in EVs is crucial to achieve more desirable tissue repair. Therefore, we will further explore the engineering strategies of EVs through gene modification, specific preconditioning treatment, external gene/protein loading or combination to prepare EVs with enhanced bone induction ability. In addition to engineering modification of EVs, improving the loading efficiency of internal cargoes without interfering with the natural lipid and protein compositions of EVs is also an important issue that cannot be ignored.
6 Conclusion
Critical-size bone defects have become a serious threat to populations at both the individual and societal levels. The progress of stem cell therapy has led to promising results for remodelling and regeneration of bone tissues. However, stem cell therapy has some disadvantages that restrict its wider clinical applications. These disadvantages, together with problems caused by storage and transport, further limit the application of MSCs in repairing critical-size bone defects. An increasing amount of convincing evidence has demonstrated that most beneficial functions of stem cells are attributed to EVs, which gives rise to a new paradigm in which EVs secreted from both stem cells and other cells promote bone regeneration by modulating crosstalk between EVs and resident bone cells or the surrounding microenvironment. Although the utilization of EVs as bioactive nanotherapeutics in regenerative medicine has just started from the preliminary stage, the results of preclinical tests showed that applications of EVs would overcome limitations of stem cell-based therapies and possible side effects associated with the administration of stem cells. In addition, some unique advantages of EVs, including low immune rejection, feasibility to modify membrane constituents, ability to pass the blood‒brain barrier, convenient isolation, and lack of tumorigenicity, make the application of EVs a promising choice in biomedical treatment for critical-size bone defects.
Accumulated findings suggest that EV-mediated therapy may be an effective alternative to regulate the bone metabolism microenvironment. Here, the authors reviewed the molecular mechanisms of the effects of EVs on osteogenesis, osteoclast differentiation, angiogenesis, and immune reactions. It is well-recognized that EVs derived from different sources have different internal cargoes, such as inflammatory cytokines, signalling molecules, mRNAs, miRNAs and lncRNAs. The diverse capabilities of EVs come from different internal cargoes. Currently, scholars mainly focus on miRNAs, which play a critical role in EV-mediated critical-size bone defect regeneration. However, researchers had no information about critical proteins and other RNAs involved in the interactions between different EVs and EV-target cells, especially in distinct pathological circumstances. These problems must be of great interest to future preclinical and clinical studies aiming to explore the underlying therapeutic mechanisms of EVs. In addition to mechanistic studies, further functional studies of EV-mediated therapy are also needed, as EVs extracted ex vivo do not exactly mirror those extracted in vivo when parent cells are exposed to specific diseases or injury-related conditions.
Efficient delivery, bioactivity maintenance, and controlled/sustained release will become key factors for the safe and effective application of EVs in critical-size bone defect repair. Before resolving these issues, the isolation and characterization techniques need to be standardized first, but the field has yet to achieve consensus. Although scholars in EV-mediated BTE face numerous challenges, with continued advances in understanding EV biology and therapeutic mechanisms, together with the rapid development of related disciplines, such as bioengineering, chemistry, material science, and nanotechnology, it is believed that EVs will stimulate further explorations and inspirations for tissue engineering and efficient nanotherapeutics in the near future, eventually bringing the spring of cell-free regenerative medicine for critical-size bone defect repair.
Author contributions
All authors contributed to the review concept, design, manuscript preparation and writing. FL and TS conducted extensive literature searches, designed the figures and wrote the main part of the manuscript. YA and LM prepared the tables and edited the manuscript. YL contributed to the figure and table preparation. ZZ and FS both performed final revision of the manuscript.
Funding
This work was supported by the National Natural Science Foundation of China (No. 82201069), the Innovative Talent Promotion Plan of Shaanxi Province - Research Fund for Young Star of Science and Technology (No. 2021KJXX-24), the Postdoctoral Science Foundation of China (No. 2022M713578) and Guangdong Basic and Applied Basic Research Foundation (No. 2022A1515110175).
Conflict of interest
The authors declare that the research was conducted in the absence of any commercial or financial relationships that could be construed as a potential conflict of interest.
Publisher’s note
All claims expressed in this article are solely those of the authors and do not necessarily represent those of their affiliated organizations, or those of the publisher, the editors and the reviewers. Any product that may be evaluated in this article, or claim that may be made by its manufacturer, is not guaranteed or endorsed by the publisher.
Abbreviations
ADSCs, adipose-derived stem cells; ALP, alkaline phosphatase; BMMSCs, bone marrow mesenchymal stem cells; BMP2, bone morphogenetic protein 2; BTE, bone tissue engineering; DC, dendritic cell; EVs, extracellular vesicles; HIF-1α, hypoxia inducible factor-1α; IL, interleukin; iPS-MSCs, MSCs derived from induced pluripotent stem cells; MAPK, mitogen-activated protein kinase; MSCs, mesenchymal stem cells; NF-κB, nuclear factor-κB; OCN, osteocalcin; PI3K, phosphoinositide 3-kinase; PLGA, polylactic-co-glycolic acid; RANKL, receptor activator of nuclear factor-κB ligand; Runx2, runt-related transcription factor 2; SHEDs, stem cells from human exfoliated deciduous teeth; Smad4, mothers against decapentaplegic homologue 4; TCP, tricalcium phosphate; TGF, transforming growth factor; UCMSCs, umbilical cord mesenchymal stem cells; UVECs, umbilical vein endothelial cells; VEGF, vascular endothelial growth factor.
References
Anderson, J. D., Johansson, H. J., Graham, C. S., Vesterlund, M., Pham, M. T., Bramlett, C. S., et al. (2016). Comprehensive proteomic analysis of mesenchymal stem cell exosomes reveals modulation of angiogenesis via nuclear factor-KappaB signaling. Stem. Cells 34 (3), 601–613. doi:10.1002/stem.2298
Ando, Y., Matsubara, K., Ishikawa, J., Fujio, M., Shohara, R., Hibi, H., et al. (2014). Stem cell-conditioned medium accelerates distraction osteogenesis through multiple regenerative mechanisms. Bone 61, 82–90. doi:10.1016/j.bone.2013.12.029
Arenaccio, C., Chiozzini, C., Ferrantelli, F., Leone, P., Olivetta, E., and Federico, M. (2019). Exosomes in therapy: Engineering, pharmacokinetics and future applications. Curr. Drug. Targets 20 (1), 87–95. doi:10.2174/1389450119666180521100409
Baberg, F., Geyh, S., Waldera-Lupa, D., Stefanski, A., Zilkens, C., Haas, R., et al. (2019). Secretome analysis of human bone marrow derived mesenchymal stromal cells. Biochim. Biophys. Acta. Proteins. Proteom. 1867 (4), 434–441. doi:10.1016/j.bbapap.2019.01.013
Baglio, S. R., Rooijers, K., Koppers-Lalic, D., Verweij, F. J., Pérez Lanzón, M., Zini, N., et al. (2015). Human bone marrow- and adipose-mesenchymal stem cells secrete exosomes enriched in distinctive miRNA and tRNA species. Stem. Cell. Res. Ther. 6 (1), 127. doi:10.1186/s13287-015-0116-z
Baldwin, P., Li, D. J., Auston, D. A., Mir, H. S., Yoon, R. S., and Koval, K. J. (2019). Autograft, allograft, and bone graft substitutes: Clinical evidence and indications for use in the setting of orthopaedic trauma surgery. J. Orthop. Trauma. 33 (4), 203–213. doi:10.1097/BOT.0000000000001420
Bao, L., You, B., Shi, S., Shan, Y., Zhang, Q., Yue, H., et al. (2018). Metastasis-associated MiR-23a from nasopharyngeal carcinoma-derived exosomes mediates angiogenesis by repressing A novel target gene TSGA10. Oncogene 37 (21), 2873–2889. doi:10.1038/s41388-018-0183-6
Behera, J., and Tyagi, N. (2018). Exosomes: Mediators of bone diseases, protection, and therapeutics potential. Oncoscience 5 (5-6), 181–195. doi:10.18632/oncoscience.421
Behrend, C., Carmouche, J., Millhouse, P. W., Ritter, L., Moskal, J., Rubery, P., et al. (2016). Allogeneic and autogenous bone grafts are affected by historical donor environmental exposure. Clin. Orthop. Relat. Res. 474 (6), 1405–1409. doi:10.1007/s11999-015-4572-7
Bianco, P., and Gehron Robey, P. (2000). Marrow stromal stem cells. J. Clin. Investig. 105 (12), 1663–1668. doi:10.1172/JCI10413
Blander, J. M. (2017). The many ways tissue phagocytes respond to dying cells. Immunol. Rev. 277 (1), 158–173. doi:10.1111/imr.12537
Bozec, A., and Soulat, D. (2017). Latest perspectives on macrophages in bone homeostasis. Pflugers. Arch. 469 (3-4), 517–525. doi:10.1007/s00424-017-1952-8
Briguglio, F., Falcomatà, D., Marconcini, S., Fiorillo, L., Briguglio, R., and Farronato, D. (2019). The use of titanium mesh in guided bone regeneration: A systematic review. Int. J. Dent. 2019, 1–8. doi:10.1155/2019/9065423
Can, A., and Karahuseyinoglu, S. (2007). Concise review: Human umbilical cord stroma with regard to the source of fetus-derived stem cells. Stem Cells 25 (11), 2886–2895. doi:10.1634/stemcells.2007-0417
Cao, Z., Wu, Y., Yu, L., Zou, L., Yang, L., Lin, S., et al. (2021). Exosomal MiR-335 derived from mature dendritic cells enhanced mesenchymal stem cell-mediated bone regeneration of bone defects in athymic rats. Mol. Med. 27 (1), 20. doi:10.1186/s10020-021-00268-5
Caplan, A. I. (2019). There is No "stem cell mess. Tissue. Eng. Part b. Rev. 25 (4), 291–293. doi:10.1089/ten.TEB.2019.0049
Casado, J. G., Blázquez, R., Vela, F. J., Álvarez, V., Tarazona, R., and Sánchez-Margallo, F. M. (2017). Mesenchymal stem cell-derived exosomes: Immunomodulatory evaluation in an antigen-induced synovitis porcine model. Front. Vet. Sci. 4, 39. doi:10.3389/fvets.2017.00039
Chen, S., Tang, Y., Liu, Y., Zhang, P., Lv, L., Zhang, X., et al. (2019). Exosomes derived from miR-375-overexpressing human adipose mesenchymal stem cells promote bone regeneration. Cell. Prolif. 52 (5), e12669. doi:10.1111/cpr.12669
Chen, S., Zheng, Y., Zhang, S., Jia, L., and Zhou, Y. (2017). Promotion effects of miR-375 on the osteogenic differentiation of human adipose-derived mesenchymal stem cells. Stem. Cell. Rep. 8 (3), 773–786. doi:10.1016/j.stemcr.2017.01.028
Chen, W., Huang, Y., Han, J., Yu, L., Li, Y., Lu, Z., et al. (2016). Immunomodulatory effects of mesenchymal stromal cells-derived exosome. Immunol. Res. 64 (4), 831–840. doi:10.1007/s12026-016-8798-6
Chew, J., Chuah, S. J., Teo, K., Zhang, S., Lai, R. C., Fu, J. H., et al. (2019). Mesenchymal stem cell exosomes enhance periodontal ligament cell functions and promote periodontal regeneration. Acta. Biomater. 89, 252–264. doi:10.1016/j.actbio.2019.03.021
Cosenza, S., Toupet, K., Maumus, M., Luz-Crawford, P., Blanc-Brude, O., Jorgensen, C., et al. (2018). Mesenchymal stem cells-derived exosomes are more immunosuppressive than microparticles in inflammatory arthritis. Theranostics 8 (5), 1399–1410. doi:10.7150/thno.21072
Cui, Y., Guo, Y., Kong, L., Shi, J., Liu, P., Li, R., et al. (2021). A bone-targeted engineered exosome platform delivering siRNA to treat osteoporosis. Bioact. Mat. 10, 207–221. doi:10.1016/j.bioactmat.2021.09.015
Cui, Y., Li, Z., Guo, Y., Qi, X., Yang, Y., Jia, X., et al. (2022). Bioinspired nanovesicles convert the skeletal endothelium-associated secretory phenotype to treat osteoporosis. Acs. Nano. 16 (7), 11076–11091. doi:10.1021/acsnano.2c03781
Cui, Y., Luan, J., Li, H., Zhou, X., and Han, J. (2016). Exosomes derived from mineralizing osteoblasts promote ST2 cell osteogenic differentiation by alteration of MicroRNA expression. Febs. Lett. 590 (1), 185–192. doi:10.1002/1873-3468.12024
Das, S., Galas, D. J., Ansel, K. M., Bitzer, M., Breakefield, X. O., Charest, A., et al. (2019). Extracellular RNA communication ConsortiumThe extracellular RNA communication Consortium: Establishing foundational knowledge and technologies for extracellular RNA research. Cell. 177 (2), 231–242. doi:10.1016/j.cell.2019.03.023
Davies, O. G., Cox, S. C., Azoidis, I., McGuinness, A., Cooke, M., Heaney, L. M., et al. (2019). Osteoblast-derived vesicle protein content is temporally regulated during osteogenesis: Implications for regenerative therapies. Front. Bioeng. Biotechnol. 7, 92. doi:10.3389/fbioe.2019.00092
Del Fattore, A., Luciano, R., Pascucci, L., Goffredo, B. M., Giorda, E., Scapaticci, M., et al. (2015). Immunoregulatory effects of mesenchymal stem cell-derived extracellular vesicles on T lymphocytes. Cell. Transpl. 24 (12), 2615–2627. doi:10.3727/096368915X687543
Duan, Y., Tan, Z., Yang, M., Li, J., Liu, C., Wang, C., et al. (2019). PC-3-Derived exosomes inhibit osteoclast differentiation by downregulating miR-214 and blocking NF-κB signaling pathway. Biomed. Res. Int. 2019, 1–8. doi:10.1155/2019/8650846
Eichholz, K. F., Woods, I., Riffault, M., Johnson, G. P., Corrigan, M., Lowry, M. C., et al. (2020). Human bone marrow stem/stromal cell osteogenesis is regulated via mechanically activated osteocyte-derived extracellular vesicles. Stem. Cells. Transl. Med. 9 (11), 1431–1447. doi:10.1002/sctm.19-0405
Elashiry, M., Elashiry, M. M., Elsayed, R., Rajendran, M., Auersvald, C., Zeitoun, R., et al. (2020). Dendritic cell derived exosomes loaded with immunoregulatory cargo reprogram local immune responses and inhibit degenerative bone disease in vivo. J. Extracell. Vesicles. 9 (1), 1795362. doi:10.1080/20013078.2020.1795362
Escudier, B., Dorval, T., Chaput, N., André, F., Caby, M. P., Novault, S., et al. (2005). Vaccination of metastatic melanoma patients with autologous dendritic cell (DC) derived-exosomes: Results of the first phase I clinical trial. J. Transl. Med. 3 (1), 10. doi:10.1186/1479-5876-3-10
Falacho, R. I., Palma, P. J., Marques, J. A., Figueiredo, M. H., Caramelo, F., Dias, I., et al. (2021). Collagenated porcine heterologous bone grafts: Histomorphometric evaluation of bone formation using different physical forms in a rabbit cancellous bone model. Molecules 26 (5), 1339. doi:10.3390/molecules26051339
Fan, J., Im, C. S., Guo, M., Cui, Z. K., Fartash, A., Kim, S., et al. (2016). Enhanced osteogenesis of adipose-derived stem cells by regulating bone morphogenetic protein signaling antagonists and agonists. Stem. Cells. Transl. Med. 5 (4), 539–551. doi:10.5966/sctm.2015-0249
Fan, J., Lee, C. S., Kim, S., Chen, C., Aghaloo, T., and Lee, M. (2020). Generation of small RNA-modulated exosome mimetics for bone regeneration. Acs. Nano. 14 (9), 11973–11984. doi:10.1021/acsnano.0c05122
Fan, L., Guan, P., Xiao, C., Wen, H., Wang, Q., Liu, C., et al. (2021). Exosome-functionalized polyetheretherketone-based implant with immunomodulatory property for enhancing osseointegration. Bioact. Mat. 6 (9), 2754–2766. doi:10.1016/j.bioactmat.2021.02.005
Fernandez-Yague, M. A., Abbah, S. A., McNamara, L., Zeugolis, D. I., Pandit, A., and Biggs, M. J. (2015). Biomimetic approaches in bone tissue engineering: Integrating biological and physicomechanical strategies. Adv. Drug. Deliv. Rev. 84, 1–29. doi:10.1016/j.addr.2014.09.005
Fu, Q. L., Chow, Y. Y., Sun, S. J., Zeng, Q. X., Li, H. B., Shi, J. B., et al. (2012). Mesenchymal stem cells derived from human induced pluripotent stem cells modulate T-cell phenotypes in allergic rhinitis. Allergy 67 (10), 1215–1222. doi:10.1111/j.1398-9995.2012.02875.x
Fuhrmann, G., Serio, A., Mazo, M., Nair, R., and Stevens, M. M. (2015). Active loading into extracellular vesicles significantly improves the cellular uptake and photodynamic effect of porphyrins. J. Control. Release. 205, 35–44. doi:10.1016/j.jconrel.2014.11.029
Furuta, T., Miyaki, S., Ishitobi, H., Ogura, T., Kato, Y., Kamei, N., et al. (2016). Mesenchymal stem cell-derived exosomes promote fracture healing in a mouse model. Stem. Cells. Transl. Med. 5 (12), 1620–1630. doi:10.5966/sctm.2015-0285
Gandolfi, M. G., Gardin, C., Zamparini, F., Ferroni, L., Esposti, M. D., Parchi, G., et al. (2020). Mineral-doped poly(L-lactide) acid scaffolds enriched with exosomes improve osteogenic commitment of human adipose-derived mesenchymal stem cells. Nanomater. (Basel) 10 (3), 432. doi:10.3390/nano10030432
Gao, M., Gao, W., Papadimitriou, J. M., Zhang, C., Gao, J., and Zheng, M. (2018). Exosomes-the enigmatic regulators of bone homeostasis. Bone. Res. 6, 36. doi:10.1038/s41413-018-0039-2
García-Sánchez, D., Fernández, D., Rodríguez-Rey, J. C., and Pérez-Campo, F. M. (2019). Enhancing survival, engraftment, and osteogenic potential of mesenchymal stem cells. World. J. Stem. Cells 11 (10), 748–763. doi:10.4252/wjsc.v11.i10.748
Ge, M., Ke, R., Cai, T., Yang, J., and Mu, X. (2015). Identification and proteomic analysis of osteoblast-derived exosomes. Biochem. Biophys. Res. Commun. 467 (1), 27–32. doi:10.1016/j.bbrc.2015.09.135
Ge, M., Wu, Y., Ke, R., Cai, T., Yang, J., and Mu, X. (2017). Value of osteoblast-derived exosomes in bone diseases. J. Craniofac. Surg. 28 (4), 866–870. doi:10.1097/SCS.0000000000003463
Grosso, A., Burger, M. G., Lunger, A., Schaefer, D. J., Banfi, A., and Di Maggio, N. (2017). It takes two to tango: Coupling of angiogenesis and osteogenesis for bone regeneration. Front. Bioeng. Biotechnol. 5, 68. doi:10.3389/fbioe.2017.00068
Guan, P., Liu, C., Xie, D., Mao, S., Ji, Y., Lin, Y., et al. (2021). Exosome-loaded extracellular matrix-mimic hydrogel with anti-inflammatory property facilitates/promotes growth plate injury repair. Bioact. Mat. 10, 145–158. doi:10.1016/j.bioactmat.2021.09.010
Guder, C., Gravius, S., Burger, C., Wirtz, D. C., and Schildberg, F. A. (2020). Osteoimmunology: A current update of the interplay between bone and the immune system. Front. Immunol. 11, 58. doi:10.3389/fimmu.2020.00058
Gunawardena, T., Rahman, M. T., Abdullah, B., and Abu Kasim, N. H. (2019). Conditioned media derived from mesenchymal stem cell cultures: The next generation for regenerative medicine. J. Tissue. Eng. Regen. Med. 13 (4), 569–586. doi:10.1002/term.2806
Harding, C., Heuser, J., and Stahl, P. (1983). Receptor-mediated endocytosis of transferrin and recycling of the transferrin receptor in rat reticulocytes. J. Cell. Biol. 97 (2), 329–339. doi:10.1083/jcb.97.2.329
Hendijani, F., Sadeghi-Aliabadi, H., and Haghjooy Javanmard, S. (2014). Comparison of human mesenchymal stem cells isolated by explant culture method from entire umbilical cord and wharton's jelly matrix. Cell. Tissue. Bank. 15 (4), 555–565. doi:10.1007/s10561-014-9425-1
Hu, G. W., Li, Q., Niu, X., Hu, B., Liu, J., Zhou, S. M., et al. (2015). Exosomes secreted by human-induced pluripotent stem cell-derived mesenchymal stem cells attenuate limb ischemia by promoting angiogenesis in mice. Stem. Cell. Res. Ther. 6 (1), 10. doi:10.1186/scrt546
Hu, K., and Olsen, B. R. (2016). The roles of vascular endothelial growth factor in bone repair and regeneration. Bone 91, 30–38. doi:10.1016/j.bone.2016.06.013
Hu, Y., Li, X., Zhang, Q., Gu, Z., Luo, Y., Guo, J., et al. (2021). Exosome-guided bone targeted delivery of antagomir-188 as an anabolic therapy for bone loss. Bioact. Mater 6 (9), 2905–2913. doi:10.1016/j.bioactmat.2021.02.014
Hu, Y., Zhang, Y., Ni, C. Y., Chen, C. Y., Rao, S. S., Yin, H., et al. (2020). Human umbilical cord mesenchymal stromal cells-derived extracellular vesicles exert potent bone protective effects by clec11a-mediated regulation of bone metabolism. Theranostics 10 (5), 2293–2308. doi:10.7150/thno.39238
Huang, C. C., Kang, M., Lu, Y., Shirazi, S., Diaz, J. I., Cooper, L. F., et al. (2020a). Functionally engineered extracellular vesicles improve bone regeneration. Acta. Biomater. 109, 182–194. doi:10.1016/j.actbio.2020.04.017
Huang, C. C., Kang, M., Shirazi, S., Lu, Y., Cooper, L. F., Gajendrareddy, P., et al. (2021). 3D encapsulation and tethering of functionally engineered extracellular vesicles to hydrogels. Acta. Biomater. 126, 199–210. doi:10.1016/j.actbio.2021.03.030
Huang, X., Chen, Z., Zhao, G., Shi, J., Huang, G., Chen, F., et al. (2020b). Combined culture experiment of mouse bone marrow mesenchymal stem cells and bioceramic scaffolds. Exp. Ther. Med. 20 (5), 1. doi:10.3892/etm.2020.9147
Jafari, D., Shajari, S., Jafari, R., Mardi, N., Gomari, H., Ganji, F., et al. (2020). Designer exosomes: A new platform for Biotechnology therapeutics. BioDrugs 34 (5), 567–586. doi:10.1007/s40259-020-00434-x
Jia, P., Chen, H., Kang, H., Qi, J., Zhao, P., Jiang, M., et al. (2016). Deferoxamine released from poly(lactic-co-glycolic acid) promotes healing of osteoporotic bone defect via enhanced angiogenesis and osteogenesis. J. Biomed. Mat. Res. A 104 (10), 2515–2527. doi:10.1002/jbm.a.35793
Jia, Y., Zhu, Y., Qiu, S., Xu, J., and Chai, Y. (2019). Exosomes secreted by endothelial progenitor cells accelerate bone regeneration during distraction osteogenesis by stimulating angiogenesis. Stem. Cell. Res. Ther. 10 (1), 12. doi:10.1186/s13287-018-1115-7
Jiang, Z. Z., Liu, Y. M., Niu, X., Yin, J. Y., Hu, B., Guo, S. C., et al. (2016). Exosomes secreted by human urine-derived stem cells could prevent kidney complications from type I diabetes in rats. Stem. Cell. Res. Ther. 7, 24. doi:10.1186/s13287-016-0287-2
Jin, Z., Ren, J., and Qi, S. (2020). Human bone mesenchymal stem cells-derived exosomes overexpressing MicroRNA-26a-5p alleviate osteoarthritis via down-regulation of PTGS2. Int. Immunopharmacol. 78, 105946. doi:10.1016/j.intimp.2019.105946
Jing, X., Wang, S., Tang, H., Li, D., Zhou, F., Xin, L., et al. (2022). Dynamically bioresponsive DNA hydrogel incorporated with dual-functional stem cells from apical papilla-derived exosomes promotes diabetic bone regeneration. Acs. Appl. Mat. Interfaces. 14 (14), 16082–16099. doi:10.1021/acsami.2c02278
Johnsen, K. B., Gudbergsson, J. M., Skov, M. N., Christiansen, G., Gurevich, L., Moos, T., et al. (2016). Evaluation of electroporation-induced adverse effects on adipose-derived stem cell exosomes. Cytotechnology 68 (5), 2125–2138. doi:10.1007/s10616-016-9952-7
Johnstone, R. M., Adam, M., Hammond, J. R., Orr, L., and Turbide, C. (1987). Vesicle Formation during reticulocyte maturation. Association of plasma membrane activities with released vesicles (exosomes). J. Biol. Chem. 262 (19), 9412–9420. doi:10.1016/s0021-9258(18)48095-7
Kang, Y., Xu, C., Meng, L., Dong, X., Qi, M., and Jiang, D. (2022). Exosome-functionalized magnesium-organic framework-based scaffolds with osteogenic, angiogenic and anti-inflammatory properties for accelerated bone regeneration. Bioact. Mat. 18, 26–41. doi:10.1016/j.bioactmat.2022.02.012
Karlsson, T., Lundholm, M., Widmark, A., and Persson, E. (2016). Tumor cell-derived exosomes from the prostate cancer cell line TRAMP-C1 impair osteoclast formation and differentiation. PLoS One 11 (11), e0166284. doi:10.1371/journal.pone.0166284
Katagiri, W., Watanabe, J., Toyama, N., Osugi, M., Sakaguchi, K., and Hibi, H. (2017). Clinical study of bone regeneration by conditioned medium from mesenchymal stem cells after maxillary sinus floor elevation. Implant Dent. 26 (4), 607–612. doi:10.1097/ID.0000000000000618
Kawakubo, A., Matsunaga, T., Ishizaki, H., Yamada, S., and Hayashi, Y. (2011). Zinc as an essential trace element in the acceleration of matrix vesicles-mediated mineral deposition. Microsc. Res. Tech. 74 (12), 1161–1165. doi:10.1002/jemt.21009
Kim, D. S., Lee, J. K., Kim, J. H., Lee, J., Kim, D. S., An, S., et al. (2021). Advanced PLGA hybrid scaffold with a bioactive PDRN/BMP2 nanocomplex for angiogenesis and bone regeneration using human fetal MSCs. Sci. Adv. 7 (50), eabj1083. doi:10.1126/sciadv.abj1083
Kim, M. S., Haney, M. J., Zhao, Y., Mahajan, V., Deygen, I., Klyachko, N. L., et al. (2016). Development of exosome-encapsulated paclitaxel to overcome MDR in cancer cells. Nanomedicine 12 (3), 655–664. doi:10.1016/j.nano.2015.10.012
Kobayashi-Sun, J., Yamamori, S., Kondo, M., Kuroda, J., Ikegame, M., Suzuki, N., et al. (2020). Uptake of osteoblast-derived extracellular vesicles promotes the differentiation of osteoclasts in the zebrafish scale. Commun. Biol. 3 (1), 190. doi:10.1038/s42003-020-0925-1
Korhonen, R. K., and Jurvelin, J. S. (2010). Compressive and tensile properties of articular cartilage in axial loading are modulated differently by osmotic environment. Med. Eng. Phys. 32 (2), 155–160. doi:10.1016/j.medengphy.2009.11.004
Kou, X., Xu, X., Chen, C., Sanmillan, M. L., Cai, T., Zhou, Y., et al. (2018). The fas/fap-1/cav-1 complex regulates IL-1RA secretion in mesenchymal stem cells to accelerate wound healing. Sci. Transl. Med. 10 (432), eaai8524. doi:10.1126/scitranslmed.aai8524
Lai, R. C., Arslan, F., Lee, M. M., Sze, N. S., Choo, A., Chen, T. S., et al. (2010). Exosome secreted by MSC reduces myocardial ischemia/reperfusion injury. Stem. Cell. Res. 4 (3), 214–222. doi:10.1016/j.scr.2009.12.003
Li, D., Liu, J., Guo, B., Liang, C., Dang, L., Lu, C., et al. (2016a). Osteoclast-derived exosomal miR-214-3p inhibits osteoblastic bone formation. Nat. Commun. 7, 10872. doi:10.1038/ncomms10872
Li, H., Liu, D., Li, C., Zhou, S., Tian, D., Xiao, D., et al. (2017). Exosomes secreted from mutant-HIF-1α-modified bone-marrow-derived mesenchymal stem cells attenuate early steroid-induced avascular necrosis of femoral head in rabbit. Cell. Biol. Int. 41 (12), 1379–1390. doi:10.1002/cbin.10869
Li, Q. C., Li, C., Zhang, W., Pi, W., and Han, N. (2022a). Potential effects of exosomes and their MicroRNA carrier on osteoporosis. Curr. Pharm. Des. 28 (11), 899–909. doi:10.2174/1381612828666220128104206
Li, R., Li, D., Wang, H., Chen, K., Wang, S., Xu, J., et al. (2022b). Exosomes from adipose-derived stem cells regulate M1/M2 macrophage phenotypic polarization to promote bone healing via MiR-451a/MIF. Stem. Cell. Res. Ther. 13 (1), 149. doi:10.1186/s13287-022-02823-1
Li, W., Liu, Y., Zhang, P., Tang, Y., Zhou, M., Jiang, W., et al. (2018). Tissue-engineered bone immobilized with human adipose stem cells-derived exosomes promotes bone regeneration. Acs. Appl. Mat. Interfaces 10 (6), 5240–5254. doi:10.1021/acsami.7b17620
Li, X., Chen, C., Wei, L., Li, Q., Niu, X., Xu, Y., et al. (2016b). Exosomes derived from endothelial progenitor cells attenuate vascular repair and accelerate reendothelialization by enhancing endothelial function. Cytotherapy 18 (2), 253–262. doi:10.1016/j.jcyt.2015.11.009
Li, Z., Wang, Y., Xiang, S., Zheng, Z., Bian, Y., Feng, B., et al. (2020c). Chondrocytes-derived exosomal MiR-8485 regulated the wnt/β-catenin pathways to promote chondrogenic differentiation of BMSCs. Biochem. Biophys. Res. Commun. 523 (2), 506–513. doi:10.1016/j.bbrc.2019.12.065
Lian, Q., Zhang, Y., Zhang, J., Zhang, H. K., Wu, X., Zhang, Y., et al. (2010). Functional mesenchymal stem cells derived from human induced pluripotent stem cells attenuate limb ischemia in mice. Circulation 121 (9), 1113–1123. doi:10.1161/CIRCULATIONAHA.109.898312
Liang, B., Liang, J. M., Ding, J. N., Xu, J., Xu, J. G., and Chai, Y. M. (2019). Dimethyloxaloylglycine-stimulated human bone marrow mesenchymal stem cell-derived exosomes enhance bone regeneration through angiogenesis by targeting the AKT/mTOR pathway. Stem. Cell. Res. Ther. 10 (1), 335. doi:10.1186/s13287-019-1410-y
Liang, W., Han, B., Hai, Y., Sun, D., and Yin, P. (2022). Mechanism of action of mesenchymal stem cell-derived exosomes in the intervertebral disc degeneration treatment and bone repair and regeneration. Front. Cell. Dev. Biol. 9, 833840. doi:10.3389/fcell.2021.833840
Liang, Z., Luo, Y., and Lv, Y. (2020). Mesenchymal stem cell-derived microvesicles mediate BMP2 gene delivery and enhance bone regeneration. J. Mat. Chem. B 8 (30), 6378–6389. doi:10.1039/d0tb00422g
Liao, W., Du, Y., Zhang, C., Pan, F., Yao, Y., Zhang, T., et al. (2019). Exosomes: The next generation of endogenous nanomaterials for advanced drug delivery and therapy. Acta. Biomater. 86, 1–14. doi:10.1016/j.actbio.2018.12.045
Lin, Z., Xiong, Y., Meng, W., Hu, Y., Chen, L., Chen, L., et al. (2021). Exosomal PD-L1 induces osteogenic differentiation and promotes fracture healing by acting as an immunosuppressant. Bioact. Mat. 13, 300–311. doi:10.1016/j.bioactmat.2021.10.042
Lindenbergh, M., and Stoorvogel, W. (2018). Antigen presentation by extracellular vesicles from professional antigen-presenting cells. Annu. Rev. Immunol. 36, 435–459. doi:10.1146/annurev-immunol-041015-055700
Liu, A., Lin, D., Zhao, H., Chen, L., Cai, B., Lin, K., et al. (2021a). Optimized BMSC-derived osteoinductive exosomes immobilized in hierarchical scaffold via lyophilization for bone repair through bmpr2/acvr2b competitive receptor-activated Smad pathway. Biomaterials 272, 120718. doi:10.1016/j.biomaterials.2021.120718
Liu, D., Kou, X., Chen, C., Liu, S., Liu, Y., Yu, W., et al. (2018). Circulating apoptotic bodies maintain mesenchymal stem cell homeostasis and ameliorate osteopenia via transferring multiple cellular factors. Cell. Res. 28 (9), 918–933. doi:10.1038/s41422-018-0070-2
Liu, L., Liu, Y., Feng, C., Chang, J., Fu, R., Wu, T., et al. (2019). Lithium-containing biomaterials stimulate bone marrow stromal cell-derived exosomal miR-130a secretion to promote angiogenesis. Biomaterials 192, 523–536. doi:10.1016/j.biomaterials.2018.11.007
Liu, L., Yu, F., Li, L., Zhou, L., Zhou, T., Xu, Y., et al. (2021b). Bone marrow stromal cells stimulated by strontium-substituted calcium silicate ceramics: Release of exosomal miR-146a regulates osteogenesis and angiogenesis. Acta. Biomater. 119, 444–457. doi:10.1016/j.actbio.2020.10.038
Liu, W., Li, L., Rong, Y., Qian, D., Chen, J., Zhou, Z., et al. (2020). Hypoxic mesenchymal stem cell-derived exosomes promote bone fracture healing by the transfer of MiR-126. Acta. Biomater. 103, 196–212. doi:10.1016/j.actbio.2019.12.020
Liu, X., Li, Q., Niu, X., Hu, B., Chen, S., Song, W., et al. (2017). Exosomes secreted from human-induced pluripotent stem cell-derived mesenchymal stem cells prevent osteonecrosis of the femoral head by promoting angiogenesis. Int. J. Biol. Sci. 13 (2), 232–244. doi:10.7150/ijbs.16951
Liu, Y., and Xiong, D. (2021c). A tannic acid-reinforced PEEK-hydrogel composite material with good biotribological and self-healing properties for artificial joints. J. Mat. Chem. B 9 (38), 8021–8030. doi:10.1039/d1tb01357b
Luo, Z. W., Li, F. X., Liu, Y. W., Rao, S. S., Yin, H., Huang, J., et al. (2019). Aptamer-functionalized exosomes from bone marrow stromal cells target bone to promote bone regeneration. Nanoscale 11 (43), 20884–20892. doi:10.1039/c9nr02791b
Lv, P. Y., Gao, P. F., Tian, G. J., Yang, Y. Y., Mo, F. F., Wang, Z. H., et al. (2020). Osteocyte-derived exosomes induced by mechanical strain promote human periodontal ligament stem cell proliferation and osteogenic differentiation via the MiR-181b-5p/PTEN/AKT signaling pathway. Stem. Cell. Res. Ther. 11 (1), 295. doi:10.1186/s13287-020-01815-3
Ma, Q., Liang, M., Wu, Y., Luo, F., Ma, Z., Dong, S., et al. (2021). Osteoclast-derived apoptotic bodies couple bone resorption and formation in bone remodeling. Bone. Res. 9 (1), 5. doi:10.1038/s41413-020-00121-1
Malekpour, K., Hazrati, A., Zahar, M., Markov, A., Zekiy, A. O., Navashenaq, J. G., et al. (2022). The potential use of mesenchymal stem cells and their derived exosomes for orthopedic diseases treatment. Stem. Cell. Rev. Rep. 18 (3), 933–951. doi:10.1007/s12015-021-10185-z
Martinez Saez, D., Sasaki, R. T., Neves, A. D., and da Silva, M. C. (2016). Stem cells from human exfoliated deciduous teeth: A growing literature. Cells. Tissues. Organs 202 (5-6), 269–280. doi:10.1159/000447055
Martins, M., Ribeiro, D., Martins, A., Reis, R. L., and Neves, N. M. (2016). Extracellular vesicles derived from osteogenically induced human bone marrow mesenchymal stem cells can modulate lineage commitment. Stem. Cell. Rep. 6 (3), 284–291. doi:10.1016/j.stemcr.2016.01.001
McIntosh, K., Zvonic, S., Garrett, S., Mitchell, J. B., Floyd, Z. E., Hammill, L., et al. (2006). The immunogenicity of human adipose-derived cells: Temporal changes in vitro. Stem. Cells 24 (5), 1246–1253. doi:10.1634/stemcells.2005-0235
Mirsaidi, A., Kleinhans, K. N., Rimann, M., Tiaden, A. N., Stauber, M., Rudolph, K. L., et al. (2012). Telomere length, telomerase activity and osteogenic differentiation are maintained in adipose-derived stromal cells from senile osteoporotic SAMP6 mice. J. Tissue. Eng. Regen. Med. 6 (5), 378–390. doi:10.1002/term.440
Nagelkerke, A., Ojansivu, M., van der Koog, L., Whittaker, T. E., Cunnane, E. M., Silva, A. M., et al. (2021). Extracellular vesicles for tissue repair and regeneration: Evidence, challenges and opportunities. Adv. Drug. Deliv. Rev. 175, 113775. doi:10.1016/j.addr.2021.04.013
Nakamura, S., Yamada, Y., Katagiri, W., Sugito, T., Ito, K., and Ueda, M. (2009). Stem cell proliferation pathways comparison between human exfoliated deciduous teeth and dental pulp stem cells by gene expression profile from promising dental pulp. J. Endod. 35 (11), 1536–1542. doi:10.1016/j.joen.2009.07.024
Narayanan, K., Kumar, S., Padmanabhan, P., Gulyas, B., Wan, A., and Rajendran, V. M. (2018). Lineage-specific exosomes could override extracellular matrix mediated human mesenchymal stem cell differentiation. Biomaterials 182, 312–322. doi:10.1016/j.biomaterials.2018.08.027
Narayanan, R., Huang, C. C., and Ravindran, S. (2016). Hijacking the cellular mail: Exosome mediated differentiation of mesenchymal stem cells. Stem. Cells. Int. 2016, 1–11. doi:10.1155/2016/3808674
Nauth, A., Schemitsch, E., Norris, B., Nollin, Z., and Watson, J. T. (2018). Critical-size bone defects: Is there a consensus for diagnosis and treatment? J. Orthop. Trauma. 32 (1), S7–S11. S7–S11. doi:10.1097/BOT.0000000000001115
Parker, A. M., and Katz, A. J. (2006). Adipose-derived stem cells for the regeneration of damaged tissues. Expert. Opin. Biol. Ther. 6 (6), 567–578. doi:10.1517/14712598.6.6.567
Pascucci, L., Coccè, V., Bonomi, A., Ami, D., Ceccarelli, P., Ciusani, E., et al. (2014). Paclitaxel is incorporated by mesenchymal stromal cells and released in exosomes that inhibit in vitro tumor growth: A new approach for drug delivery. J. Control. Release 192, 262–270. doi:10.1016/j.jconrel.2014.07.042
Phan, J., Kumar, P., Hao, D., Gao, K., Farmer, D., and Wang, A. (2018). Engineering mesenchymal stem cells to improve their exosome efficacy and yield for cell-free therapy. J. Extracell. Vesicles. 7 (1), 1522236. doi:10.1080/20013078.2018.1522236
Puddu, P., Puddu, G. M., Cravero, E., Muscari, S., and Muscari, A. (2010). The involvement of circulating microparticles in inflammation, coagulation and cardiovascular diseases. Can. J. Cardiol. 26 (4), 140–145. doi:10.1016/s0828-282x(10)70371-8
Qi, X., Zhang, J., Yuan, H., Xu, Z., Li, Q., Niu, X., et al. (2016). Exosomes secreted by human-induced pluripotent stem cell-derived mesenchymal stem cells repair critical-sized bone defects through enhanced angiogenesis and osteogenesis in osteoporotic rats. Int. J. Biol. Sci. 12 (7), 836–849. doi:10.7150/ijbs.14809
Qian, X., An, N., Ren, Y., Yang, C., Zhang, X., and Li, L. (2021). Immunosuppressive effects of mesenchymal stem cells-derived exosomes. Stem. Cell. Rev. Rep. 17 (2), 411–427. doi:10.1007/s12015-020-10040-7
Qin, Y., Peng, Y., Zhao, W., Pan, J., Ksiezak-Reding, H., Cardozo, C., et al. (2017). Myostatin inhibits osteoblastic differentiation by suppressing osteocyte-derived exosomal MicroRNA-218: A novel mechanism in muscle-bone communication. J. Biol. Chem. 292 (26), 11021–11033. doi:10.1074/jbc.M116.770941
Qin, Y., Sun, R., Wu, C., Wang, L., and Zhang, C. (2016a). Exosome: A novel approach to stimulate bone regeneration through regulation of osteogenesis and angiogenesis. Int. J. Mol. Sci. 17 (5), 712. doi:10.3390/ijms17050712
Qin, Y., Wang, L., Gao, Z., Chen, G., and Zhang, C. (2016b). Bone marrow stromal/stem cell-derived extracellular vesicles regulate osteoblast activity and differentiation in vitro and promote bone regeneration in vivo. Sci. Rep. 6, 21961. doi:10.1038/srep21961
Qin, Y., and Zhang, C. (2017). Endothelial progenitor cell-derived extracellular vesicle-meditated cell-to-cell communication regulates the proliferation and osteoblastic differentiation of bone mesenchymal stromal cells. Mol. Med. Rep. 16 (5), 7018–7024. doi:10.3892/mmr.2017.7403
Ren, L., Song, Z. J., Cai, Q. W., Chen, R. X., Zou, Y., Fu, Q., et al. (2019). Adipose mesenchymal stem cell-derived exosomes ameliorate hypoxia/serum deprivation-induced osteocyte apoptosis and osteocyte-mediated osteoclastogenesis in vitro. Biochem. Biophys. Res. Commun. 508 (1), 138–144. doi:10.1016/j.bbrc.2018.11.109
Rezaie, J., Mehranjani, M. S., Rahbarghazi, R., and Shariatzadeh, M. A. (2018). Angiogenic and restorative abilities of human mesenchymal stem cells were reduced following treatment with serum from diabetes mellitus type 2 patients. J. Cell. Biochem. 119 (1), 524–535. doi:10.1002/jcb.26211
Riau, A. K., Ong, H. S., Yam, G., and Mehta, J. S. (2019). Sustained delivery system for stem cell-derived exosomes. Front. Pharmacol. 10, 1368. doi:10.3389/fphar.2019.01368
Richter, M., Vader, P., and Fuhrmann, G. (2021). Approaches to surface engineering of extracellular vesicles. Adv. Drug. Deliv. Rev. 173, 416–426. doi:10.1016/j.addr.2021.03.020
Roseti, L., Parisi, V., Petretta, M., Cavallo, C., Desando, G., Bartolotti, I., et al. (2017). Scaffolds for bone tissue engineering: State of the art and new perspectives. Mat. Sci. Eng. C. Mat. Biol. Appl. 78, 1246–1262. doi:10.1016/j.msec.2017.05.017
Sakaguchi, K., Katagiri, W., Osugi, M., Kawai, T., Sugimura-Wakayama, Y., and Hibi, H. (2017). Periodontal tissue regeneration using the cytokine cocktail mimicking secretomes in the conditioned media from human mesenchymal stem cells. Biochem. Biophys. Res. Commun. 484 (1), 100–106. doi:10.1016/j.bbrc.2017.01.065
Sato, M., Suzuki, T., Kawano, M., and Tamura, M. (2017). Circulating osteocyte-derived exosomes contain miRNAs which are enriched in exosomes from MLO-Y4 cells. Biomed. Rep. 6 (2), 223–231. doi:10.3892/br.2016.824
Sato, Y. T., Umezaki, K., Sawada, S., Mukai, S. A., Sasaki, Y., Harada, N., et al. (2016). Engineering hybrid exosomes by membrane fusion with liposomes. Sci. Rep. 6, 21933. doi:10.1038/srep21933
Shang, F., Ming, L., Zhou, Z., Yu, Y., Sun, J., Ding, Y., et al. (2014). The effect of licochalcone A on cell-aggregates ecm secretion and osteogenic differentiation during bone formation in metaphyseal defects in ovariectomized rats. Biomaterials 35 (9), 2789–2797. doi:10.1016/j.biomaterials.2013.12.061
Shang, F., Yu, Y., Liu, S., Ming, L., Zhang, Y., Zhou, Z., et al. (2020). Advancing application of mesenchymal stem cell-based bone tissue regeneration. Bioact. Mater. 6 (3), 666–683. doi:10.1016/j.bioactmat.2020.08.014
Silva, D., Paz, A., Portinho, C. P., Lima, E., Kliemann, L. M., and Collares, M. (2021). Reconstruction of parietal bone defects with adiposederived mesenchymal stem cells. Experimental study. Acta. Cir. Bras. 35 (12), e351201. doi:10.1590/ACB351201
Sissung, T. M., and Figg, W. D. (2020). Stem cell clinics: Risk of proliferation. Lancet. Oncol. 21 (2), 205–206. doi:10.1016/S1470-2045(19)30787-9
Song, H., Li, X., Zhao, Z., Qian, J., Wang, Y., Cui, J., et al. (2019). Reversal of osteoporotic activity by endothelial cell-secreted bone targeting and biocompatible exosomes. Nano. Lett. 19 (5), 3040–3048. doi:10.1021/acs.nanolett.9b00287
Stahl, A., and Yang, Y. P. (2021). Regenerative approaches for the treatment of large bone defects. Tissue. Eng. Part. b. Rev. 27 (6), 539–547. doi:10.1089/ten.TEB.2020.0281
Stickney, Z., Losacco, J., McDevitt, S., Zhang, Z., and Lu, B. (2016). Development of exosome surface display technology in living human cells. Biochem. Biophys. Res. Commun. 472 (1), 53–59. doi:10.1016/j.bbrc.2016.02.058
Swanson, W. B., Zhang, Z., Xiu, K., Gong, T., Eberle, M., Wang, Z., et al. (2020). Scaffolds with controlled release of pro-mineralization exosomes to promote craniofacial bone healing without cell transplantation. Acta. Biomater. 118, 215–232. doi:10.1016/j.actbio.2020.09.052
Takeuchi, R., Katagiri, W., Endo, S., and Kobayashi, T. (2019). Exosomes from conditioned media of bone marrow-derived mesenchymal stem cells promote bone regeneration by enhancing angiogenesis. PloS. One 14 (11), e0225472. doi:10.1371/journal.pone.0225472
Tan, S., Tjio, C., Wong, J., Wong, K. L., Chew, J., Hui, J., et al. (2021). Mesenchymal stem cell exosomes for cartilage regeneration: A systematic review of preclinical in vivo studies. Tissue. Eng. Part b. Rev. 27 (1), 1–13. doi:10.1089/ten.TEB.2019.0326
Tang, H., He, Y., Li, L., Mao, W., Chen, X., Ni, H., et al. (2019). Exosomal MMP2 derived from mature osteoblasts promotes angiogenesis of endothelial cells via VEGF/Erk1/2 signaling pathway. Exp. Cell. Res. 383 (2), 111541. doi:10.1016/j.yexcr.2019.111541
Théry, C., Witwer, K. W., Aikawa, E., Alcaraz, M. J., Anderson, J. D., Andriantsitohaina, R., et al. (2018). Minimal information for studies of extracellular vesicles 2018 (MISEV2018): A position statement of the international society for extracellular vesicles and update of the MISEV2014 guidelines. J. Extracell. Vesicles. 7 (1), 1535750. doi:10.1080/20013078.2018.1535750
Tian, T., Zhang, H. X., He, C. P., Fan, S., Zhu, Y. L., Qi, C., et al. (2018). Surface functionalized exosomes as targeted drug delivery vehicles for cerebral ischemia therapy. Biomaterials 150, 137–149. doi:10.1016/j.biomaterials.2017.10.012
Toh, W. S., Lai, R. C., Hui, J., and Lim, S. K. (2017). MSC exosome as A cell-free MSC therapy for cartilage regeneration: Implications for osteoarthritis treatment. Semin. Cell. Dev. Biol. 67, 56–64. doi:10.1016/j.semcdb.2016.11.008
Umezu, T., Tadokoro, H., Azuma, K., Yoshizawa, S., Ohyashiki, K., and Ohyashiki, J. H. (2014). Exosomal MiR-135b shed from hypoxic multiple myeloma cells enhances angiogenesis by targeting factor-inhibiting HIF-1. Blood 124 (25), 3748–3757. doi:10.1182/blood-2014-05-576116
van Balkom, B. W., de Jong, O. G., Smits, M., Brummelman, J., den Ouden, K., de Bree, P. M., et al. (2013). Endothelial cells require MiR-214 to secrete exosomes that suppress senescence and induce angiogenesis in human and mouse endothelial cells. Blood 121 (19), 3997–4006. doi:10.1182/blood-2013-02-478925
van der Koog, L., Gandek, T. B., and Nagelkerke, A. (2022). Liposomes and extracellular vesicles as drug delivery systems: A comparison of composition, pharmacokinetics, and functionalization. Adv. Healthc. Mat. 11 (5), e2100639. doi:10.1002/adhm.202100639
Vizoso, F. J., Eiro, N., Cid, S., Schneider, J., and Perez-Fernandez, R. (2017). Mesenchymal stem cell secretome: Toward cell-free therapeutic strategies in regenerative medicine. Int. J. Mol. Sci. 18 (9), 1852. doi:10.3390/ijms18091852
Wan, Y., Wang, L., Zhu, C., Zheng, Q., Wang, G., Tong, J., et al. (2018). Aptamer-conjugated extracellular nanovesicles for targeted drug delivery. Cancer. Res. 78 (3), 798–808. doi:10.1158/0008-5472.CAN-17-2880
Wang, H. S., Hung, S. C., Peng, S. T., Huang, C. C., Wei, H. M., Guo, Y. J., et al. (2004). Mesenchymal stem cells in the wharton's jelly of the human umbilical cord. Stem. Cells 22 (7), 1330–1337. doi:10.1634/stemcells.2004-0013
Wang, K. X., Xu, L. L., Rui, Y. F., Huang, S., Lin, S. E., Xiong, J. H., et al. (2015). The effects of secretion factors from umbilical cord derived mesenchymal stem cells on osteogenic differentiation of mesenchymal stem cells. PLoS. One. 10 (3), e0120593. doi:10.1371/journal.pone.0120593
Wang, L., Jia, P., Shan, Y., Hao, Y., Wang, X., Jiang, Y., et al. (2017). Synergistic protection of bone vasculature and bone mass by desferrioxamine in osteoporotic mice. Mol. Med. Rep. 16 (5), 6642–6649. doi:10.3892/mmr.2017.7451
Wang, L., Wang, J., Zhou, X., Sun, J., Zhu, B., Duan, C., et al. (2020a). A new self-healing hydrogel containing hucMSC-derived exosomes promotes bone regeneration. Front. Bioeng. Biotechnol. 8, 564731. doi:10.3389/fbioe.2020.564731
Wang, M., Li, J., Ye, Y., He, S., and Song, J. (2020b). SHED-Derived conditioned exosomes enhance the osteogenic differentiation of PDLSCs via Wnt and BMP signaling in vitro. Differentiation 111, 1–11. doi:10.1016/j.diff.2019.10.003
Wang, Q., Shen, X., Chen, Y., Chen, J., and Li, Y. (2021a). Osteoblasts-derived exosomes regulate osteoclast differentiation through MiR-503-3p/hpse Axis. Acta. Histochem. 123 (7), 151790. doi:10.1016/j.acthis.2021.151790
Wang, R., Jiang, W., Zhang, L., Xie, S., Zhang, S., Yuan, S., et al. (2020c). Intra-articular delivery of extracellular vesicles secreted by chondrogenic progenitor cells from MRL/MpJ superhealer mice enhances articular cartilage repair in A mouse injury model. Stem. Cell. Res. Ther. 11 (1), 93. doi:10.1186/s13287-020-01594-x
Wang, X., Ao, J., Lu, H., Zhao, Q., Ma, Y., Zhang, J., et al. (2020d). Osteoimmune modulation and guided osteogenesis promoted by barrier membranes incorporated with S-nitrosoglutathione (GSNO) and mesenchymal stem cell-derived exosomes</p>Nitrosoglutathione (GSNO) and mesenchymal stem cell-derived exosomes. Int. J. Nanomedicine. 15, 3483–3496. doi:10.2147/IJN.S248741
Wang, Z., Yuan, Y., Ji, X., Xiao, X., Li, Z., Yi, X., et al. (2021b). The hippo-TAZ Axis mediates vascular endothelial growth factor C in glioblastoma-derived exosomes to promote angiogenesis. Cancer. Lett. 513, 1–13. doi:10.1016/j.canlet.2021.05.002
Wasnik, S., Rundle, C. H., Baylink, D. J., Yazdi, M. S., Carreon, E. E., Xu, Y., et al. (2018). 1, 25-dihydroxyvitamin D suppresses M1 macrophages and promotes M2 differentiation at bone injury sites. JCI. Insight. 3 (17), e98773. doi:10.1172/jci.insight.98773
Wei, F., Li, M., Crawford, R., Zhou, Y., and Xiao, Y. (2019a). Exosome-integrated titanium oxide nanotubes for targeted bone regeneration. Acta. Biomater. 86, 480–492. doi:10.1016/j.actbio.2019.01.006
Wei, R., Wu, J., and Li, Y. (2019b). Macrophage polarization following three-dimensional porous PEEK. Mat. Sci. Eng. C. Mat. Biol. Appl. 104, 109948. doi:10.1016/j.msec.2019.109948
Wu, D., Chang, X., Tian, J., Kang, L., Wu, Y., Liu, J., et al. (2021). Bone mesenchymal stem cells stimulation by magnetic nanoparticles and A static magnetic field: Release of exosomal miR-1260a improves osteogenesis and angiogenesis. J. Nanobiotechnology. 19 (1), 209. doi:10.1186/s12951-021-00958-6
Wu, D., Qin, H., Wang, Z., Yu, M., Liu, Z., Peng, H., et al. (2022). Bone mesenchymal stem cell-derived sEV-encapsulated thermosensitive hydrogels accelerate osteogenesis and angiogenesis by release of exosomal miR-21. Front. Bioeng. Biotechnol. 9, 829136. doi:10.3389/fbioe.2021.829136
Wu, J., Chen, L., Wang, R., Song, Z., Shen, Z., Zhao, Y., et al. (2019). Exosomes secreted by stem cells from human exfoliated deciduous teeth promote alveolar bone defect repair through the regulation of angiogenesis and osteogenesis. Acs. Biomater. Sci. Eng. 5 (7), 3561–3571. doi:10.1021/acsbiomaterials.9b00607
Wu, X., Pang, L., Lei, W., Lu, W., Li, J., Li, Z., et al. (2010). Inhibition of sca-1-positive skeletal stem cell recruitment by alendronate blunts the anabolic effects of parathyroid hormone on bone remodeling. Cell. stem. Cell. 7 (5), 571–580. doi:10.1016/j.stem.2010.09.012
Wu, Z., Pu, P., Su, Z., Zhang, X., Nie, L., and Chang, Y. (2020). Schwann cell-derived exosomes promote bone regeneration and repair by enhancing the biological activity of porous Ti6Al4V scaffolds. Biochem. Biophys. Res. Commun. 531 (4), 559–565. doi:10.1016/j.bbrc.2020.07.094
Xia, Y., He, X. T., Xu, X. Y., Tian, B. M., An, Y., and Chen, F. M. (2020). Exosomes derived from M0, M1 and M2 macrophages exert distinct influences on the proliferation and differentiation of mesenchymal stem cells. PeerJ 8, e8970. doi:10.7717/peerj.8970
Xiao, Z., Camalier, C. E., Nagashima, K., Chan, K. C., Lucas, D. A., de la Cruz, M. J., et al. (2007). Analysis of the extracellular matrix vesicle proteome in mineralizing osteoblasts. J. Cell. Physiol. 210 (2), 325–335. doi:10.1002/jcp.20826
Xie, H., Wang, Z., Zhang, L., Lei, Q., Zhao, A., Wang, H., et al. (2017). Extracellular vesicle-functionalized decalcified bone matrix scaffolds with enhanced pro-angiogenic and pro-bone regeneration activities. Sci. Rep. 7, 45622. doi:10.1038/srep45622
Xiong, Y., Chen, L., Yan, C., Zhou, W., Yu, T., Sun, Y., et al. (2020). M2 macrophagy-derived exosomal miRNA-5106 induces bone mesenchymal stem cells towards osteoblastic fate by targeting salt-inducible kinase 2 and 3. J. Nanobiotechnology. 18 (1), 66. doi:10.1186/s12951-020-00622-5
Xu, J. F., Yang, G. H., Pan, X. H., Zhang, S. J., Zhao, C., Qiu, B. S., et al. (2014). Altered MicroRNA expression profile in exosomes during osteogenic differentiation of human bone marrow-derived mesenchymal stem cells. PLoS. One. 9 (12), e114627. doi:10.1371/journal.pone.0114627
Yan, H. C., Yu, T. T., Li, J., Qiao, Y. Q., Wang, L. C., Zhang, T., et al. (2020). The delivery of extracellular vesicles loaded in biomaterial scaffolds for bone regeneration. Front. Bioeng. Biotechnol. 8, 1015. doi:10.3389/fbioe.2020.01015
Yang, J. X., Xie, P., Li, Y. S., Wen, T., and Yang, X. C. (2020a). Osteoclast-derived miR-23a-5p-containing exosomes inhibit osteogenic differentiation by regulating Runx2. Cell. Signal. 70, 109504. doi:10.1016/j.cellsig.2019.109504
Yang, S., Zhu, B., Yin, P., Zhao, L., Wang, Y., Fu, Z., et al. (2020b). Integration of human umbilical cord mesenchymal stem cells-derived exosomes with hydroxyapatite-embedded hyaluronic acid-alginate hydrogel for bone regeneration. Acs. Biomater. Sci. Eng. 6 (3), 1590–1602. doi:10.1021/acsbiomaterials.9b01363
Yang, X., Yang, J., Lei, P., and Wen, T. (2019). LncRNA MALAT1 shuttled by bone marrow-derived mesenchymal stem cells-secreted exosomes alleviates osteoporosis through mediating MicroRNA-34c/SATB2 Axis. Aging 11 (20), 8777–8791. doi:10.18632/aging.102264
Ying, C., Wang, R., Wang, Z., Tao, J., Yin, W., Zhang, J., et al. (2020). BMSC-exosomes carry mutant HIF-1α for improving angiogenesis and osteogenesis in critical-sized calvarial defects. Front. Bioeng. Biotechnol. 8, 565561. doi:10.3389/fbioe.2020.565561
Yu, W., Li, S., Guan, X., Zhang, N., Xie, X., Zhang, K., et al. (2022). Higher yield and enhanced therapeutic effects of exosomes derived from MSCs in hydrogel-assisted 3D culture system for bone regeneration. Biomater. Adv. 133, 112646. doi:10.1016/j.msec.2022.112646
Zara, J. N., Siu, R. K., Zhang, X., Shen, J., Ngo, R., Lee, M., et al. (2011). High doses of bone morphogenetic protein 2 induce structurally abnormal bone and inflammation in vivo. Tissue. Eng. Part. a. 17 (9-10), 1389–1399. doi:10.1089/ten.TEA.2010.0555
Zha, Y., Li, Y., Lin, T., Chen, J., Zhang, S., and Wang, J. (2021). Progenitor cell-derived exosomes endowed with VEGF plasmids enhance osteogenic induction and vascular remodeling in large segmental bone defects. Theranostics 11 (1), 397–409. doi:10.7150/thno.50741
Zha, Y., Lin, T., Li, Y., Zhang, X., Wang, Z., Li, Z., et al. (2020). Exosome-mimetics as an engineered gene-activated matrix induces in-situ vascularized osteogenesis. Biomaterials 247, 119985. doi:10.1016/j.biomaterials.2020.119985
Zhai, M., Zhu, Y., Yang, M., and Mao, C. (2020). Human mesenchymal stem cell derived exosomes enhance cell-free bone regeneration by altering their miRNAs profiles. Adv. Sci. (Weinh). 7 (19), 2001334. doi:10.1002/advs.202001334
Zhang, B., Huang, J., Liu, J., Lin, F., Ding, Z., and Xu, J. (2021a). Injectable composite hydrogel promotes osteogenesis and angiogenesis in spinal fusion by optimizing the bone marrow mesenchymal stem cell microenvironment and exosomes secretion. Mat. Sci. Eng. C. Mat. Biol. Appl. 123, 111782. doi:10.1016/j.msec.2020.111782
Zhang, J., Guan, J., Niu, X., Hu, G., Guo, S., Li, Q., et al. (2015). Exosomes released from human induced pluripotent stem cells-derived MSCs facilitate cutaneous wound healing by promoting collagen synthesis and angiogenesis. J. Transl. Med. 13, 49. doi:10.1186/s12967-015-0417-0
Zhang, J., Liu, X., Li, H., Chen, C., Hu, B., Niu, X., et al. (2016a). Exosomes/tricalcium phosphate combination scaffolds can enhance bone regeneration by activating the PI3K/akt signaling pathway. Stem. Cell. Res. Ther. 7 (1), 136. doi:10.1186/s13287-016-0391-3
Zhang, L., Jiao, G., Ren, S., Zhang, X., Li, C., Wu, W., et al. (2020). Exosomes from bone marrow mesenchymal stem cells enhance fracture healing through the promotion of osteogenesis and angiogenesis in a rat model of nonunion. Stem. Cell. Res. Ther. 11 (1), 38. doi:10.1186/s13287-020-1562-9
Zhang, S., Chu, W. C., Lai, R. C., Lim, S. K., Hui, J. H., and Toh, W. S. (2016b). Exosomes derived from human embryonic mesenchymal stem cells promote osteochondral regeneration. Osteoarthr. Cartil. 24 (12), 2135–2140. doi:10.1016/j.joca.2016.06.022
Zhang, S., Wong, K. L., Ren, X., Teo, K., Afizah, H., Choo, A., et al. (2022). Mesenchymal stem cell exosomes promote functional osteochondral repair in a clinically relevant porcine model. Am. J. Sports. Med. 50 (3), 788–800. doi:10.1177/03635465211068129
Zhang, Y., Hao, Z., Wang, P., Xia, Y., Wu, J., Xia, D., et al. (2019). Exosomes from human umbilical cord mesenchymal stem cells enhance fracture healing through HIF-1α-mediated promotion of angiogenesis in a rat model of stabilized fracture. Cell. Prolif. 52 (2), e12570. doi:10.1111/cpr.12570
Zhang, Y., Xie, Y., Hao, Z., Zhou, P., Wang, P., Fang, S., et al. (2021b). Umbilical mesenchymal stem cell-derived exosome-encapsulated hydrogels accelerate bone repair by enhancing angiogenesis. Acs. Appl. Mat. Interfaces 13 (16), 18472–18487. doi:10.1021/acsami.0c22671
Zhao, L., Burguera, E. F., Xu, H. H., Amin, N., Ryou, H., and Arola, D. D. (2010). Fatigue and human umbilical cord stem cell seeding characteristics of calcium phosphate-chitosan-biodegradable fiber scaffolds. Biomaterials 31 (5), 840–847. doi:10.1016/j.biomaterials.2009.09.106
Zhao, R., Yang, R., Cooper, P. R., Khurshid, Z., Shavandi, A., and Ratnayake, J. (2021). Bone grafts and substitutes in dentistry: A review of current trends and developments. Molecules 26 (10), 3007. doi:10.3390/molecules26103007
Zhao, Z., McGill, J., Gamero-Kubota, P., and He, M. (2019). Microfluidic on-demand engineering of exosomes towards cancer immunotherapy. Lab. Chip 19 (10), 1877–1886. doi:10.1039/c8lc01279b
Zheng, J., Zhu, L., Chen, Y., Jia, N., and Zhu, W. (2020). Iok InBone marrow-derived mesenchymal stem cells-secreted exosomal MicroRNA-192-5p delays inflammatory response in rheumatoid arthritis. Int. Immunopharmacol 78, 105985. doi:10.1016/j.intimp.2019.105985
Zhou, X., Cao, H., Guo, J., Yuan, Y., and Ni, G. (2022). Effects of BMSC-derived EVs on bone metabolism. Pharmaceutics 14 (5), 1012. doi:10.3390/pharmaceutics14051012
Keywords: extracellular vesicles, critical-size bone defects, cell-free therapy, bioactive scaffold, bone tissue regeneration, engineering modification
Citation: Liu F, Sun T, An Y, Ming L, Li Y, Zhou Z and Shang F (2023) The potential therapeutic role of extracellular vesicles in critical-size bone defects: Spring of cell-free regenerative medicine is coming. Front. Bioeng. Biotechnol. 11:1050916. doi: 10.3389/fbioe.2023.1050916
Received: 18 October 2022; Accepted: 02 January 2023;
Published: 17 January 2023.
Edited by:
Xing Wang, Shanxi Medical University, ChinaCopyright © 2023 Liu, Sun, An, Ming, Li, Zhou and Shang. This is an open-access article distributed under the terms of the Creative Commons Attribution License (CC BY). The use, distribution or reproduction in other forums is permitted, provided the original author(s) and the copyright owner(s) are credited and that the original publication in this journal is cited, in accordance with accepted academic practice. No use, distribution or reproduction is permitted which does not comply with these terms.
*Correspondence: Fengqing Shang, c2hhbmdmZW5ncWluZzE5ODhAMTYzLmNvbQ==; Zhifei Zhou, Z2VvZmZyZXkyMDAyQHNpbmEuY29t
†These authors have contributed equally to this work and share first authorship