- 1Herbal and Traditional Medicines Research Center, Kerman University of Medical Sciences, Kerman, Iran
- 2Brain Cancer Research Core (BCRC), Universal Scientific Education and Research Network (USERN), Kerman, Iran
- 3Department of Pharmaceutics, Faculty of Pharmacy and Pharmaceutical Sciences, Tehran Medical Sciences, Islamic Azad University, Tehran, Iran
- 4Department of Biology, Faculty of Science, Alzahra University, Tehran, Iran
- 5Department of Plant Biology, Faculty of Biological Science, Tarbiat Modares University, Tehran, Iran
- 6Medical Mycology and Bacteriology Research Center, Kerman University of Medical Sciences, Kerman, Iran
- 7Student Research Committee, Kerman University of Medical Sciences, Kerman, Iran
- 8Pharmaceutical Sciences and Cosmetic Products Research Center, Kerman University of Medical Sciences, Kerman, Iran
- 9Department of Pharmaceutical Biotechnology, Faculty of Pharmacy, Kerman University of Medical Sciences, Kerman, Iran
Nanotechnology is one of the most promising technologies available today, holding tremendous potential for biomedical and healthcare applications. In this field, there is an increasing interest in the use of polymeric micro/nanofibers for the construction of biomedical structures. Due to its potential applications in various fields like pharmaceutics and biomedicine, the electrospinning process has gained considerable attention for producing nano-sized fibers. Electrospun nanofiber membranes have been used in drug delivery, controlled drug release, regenerative medicine, tissue engineering, biosensing, stent coating, implants, cosmetics, facial masks, and theranostics. Various natural and synthetic polymers have been successfully electrospun into ultrafine fibers. Although biopolymers demonstrate exciting properties such as good biocompatibility, non-toxicity, and biodegradability, they possess poor mechanical properties. Hybrid nanofibers from bio and synthetic nanofibers combine the characteristics of biopolymers with those of synthetic polymers, such as high mechanical strength and stability. In addition, a variety of functional agents, such as nanoparticles and biomolecules, can be incorporated into nanofibers to create multifunctional hybrid nanofibers. Due to the remarkable properties of hybrid nanofibers, the latest research on the unique properties of hybrid nanofibers is highlighted in this study. Moreover, various established hybrid nanofiber fabrication techniques, especially the electrospinning-based methods, as well as emerging strategies for the characterization of hybrid nanofibers, are summarized. Finally, the development and application of electrospun hybrid nanofibers in biomedical applications are discussed.
1 Introduction
Currently, nanomaterials do not have an internationally accepted definition. Based on nanomaterials characteristics, they can be designed as nanoparticles (NPs), nanowires, nanotubes, nanofibers, and nanorods (Barhoum et al., 2019a). Recently, nanofibers have drawn significant interest among many researchers in different fields. The unique properties of nanofibers, including high surface area-to-volume ratio, tunable porosity, and superior mechanical and physicochemical properties, make them an ideal candidate for applications that require large surface areas (Yousefzadeh and Ghasemkhah, 2019). Since the production of nanofibers has gained increasing attention in recent years, several conventional scaffold fabrication techniques have been developed. Some of the most commonly used methods include the sonochemical method (Lee et al., 2019b), template-based synthesis (Zhao et al., 2021b), self-assembly (Jiang et al., 2021), electrospinning (Teo and Ramakrishna, 2006; Zahmatkeshan et al., 2019), and polymerization (Bhowmick et al., 2014).
In recent years, electrospinning has gained a great deal of attention to create nanofibers. The major reasons for the popularity of the electrospinning technique are its cost-efficiency, ability to manufacture continuous nanofibers, high flexibility, and simplicity in setting up and controlling nanofiber diameters, compositions, and orientations based on the desired application (Gugulothu et al., 2019). Many materials, such as natural and synthetic polymers, metals, and metal oxides, carbon-based and composite nanomaterials, can be utilized for electrospun nanofiber production (Kenry and Lim, 2017; Barhoum et al., 2019a). Nanofibers have been classified according to their composition (e.g., polymers, metals, metal oxides, ceramics, carbon, and hybrids), size (e.g., diameter, length, porosity), and morphology (e.g., nonporous, mesoporous, hollow, core-shell, biocomponent, multi-component) (Barhoum et al., 2019b).
A wide variety of biodegradable and biocompatible polymers (natural and synthetic polymers) can be combined to create hybrid mats (Khamrai et al., 2019; Ndlovu et al., 2021a). Blending polymers can improve the low mechanical properties of natural polymers and the low biocompatibility of synthetic polymers (Khamrai et al., 2019; Sionkowska, 2021). In addition, different functional agents, such as drugs, biomolecules, and NPs, can be incorporated into the polymeric matrix to produce unique hybrid nanofibers. By combining these materials with polymers in a nanofiber matrix, multifunctional nanocomposites with improved mechanical, chemical, and electrical properties as well as superior biocompatibility and biodegradability, can be created.
In this literature review, the recent research performed on the fabrication and characterization of hybrid electrospun nanofibers, not limited to different blended polymers but considering a broader set of polymers with functional agents, such as drugs, biomolecules, and NPs, is discussed. Also, a summary of the biomedical applications of electrospun hybrid nanofibers in drug delivery, tissue engineering, wound healing, and biosensors is provided. Finally, recent challenges in mechanical strength, degradation, and industrial mass fabrication of electrospun nanofibers, along with prospects of hybrid nanofibers for tissue engineering and biomedical applications, are discussed.
2 Hybrid nanofibers fabrication techniques
Hybrid nanofibers have received significant attention owing to their unique properties. These materials show unique magnetic, optoelectrical, and biological properties essential for a wide range of applications in optics, energy generation and storage, environment, medicine, and biotechnology (He et al., 2014; Zeng et al., 2014; Ghajarieh et al., 2021). Various techniques can be used to fabricate hybrid nanofiber-based structures. However, this study has focused on electrospinning methods. These methods enable the production of two-dimensional (2D) as well as three-dimensional (3D) nanofibrous structures, which is of considerable significance. Notably, some exceptional characteristics of hybrid nanofibers have resulted from incorporating functional agents into nanofibrous structures. This section discusses the main electrospinning-based methods to create hybrid nanofibers and immobilization techniques for functional agents, particularly NPs.
2.1 Electrospinning methods
Currently, many efforts have been made in the direction of up-scaling the production and improving the nanofiber properties. Among various fabrication techniques, electrospinning is a promising method that offers the opportunity to produce nanofibers using different materials in various fibrous assemblies. In recent years, electrospinning received much attention in both academics and industry due to its simplicity, applicability over a wide range of materials, and low cost (Alghoraibi and Alomari, 2018).
Electrospinning is a dry spinning technique used to fabricate continuous nanofibers (Barhoum et al., 2019b). Fibers are drawn from a melt or liquid polymer solution with electrostatic force, and nanofiber networks are generated in one step (Tucker et al., 2012). It is considered a suitable flexible technique for producing electrostatic fibers on a large scale (Barhoum et al., 2019a; Gugulothu et al., 2019). In this method, the size of fibers can be simply controlled from nanometer to micrometer (Gugulothu et al., 2019).
This technology can generate nanofibers with a high surface-to-volume ratio and a structure similar to the extracellular matrix (ECM), receiving much attention for research in biomedicine (Liu et al., 2021b). Several electrospinning methods have been patented in the past 20 years (Barhoum et al., 2019a). Electrospinning can produce many forms of nanofibers, including smooth nanofibers, branched nanofibers, core-shell nanofibers, ribbon-like nanofibers, porous nanofibers, and nanofibers with fractal surface structures (Yousefzadeh and Ghasemkhah, 2019).
Extensive use of electrospinning in different industries and high-tech fields has led to infrastructure for the mass production of related equipment, making it commercially available alongside nanofibrous material (Blakney et al., 2013). This has made nanofibers available for future clinical use after Food and Drug Administration (FDA) approval (Blakney et al., 2013). There are various electrospinning-based techniques to fabricate 2D and 3D hybrid nanofiber structures for different applications. The conventional electrospinning methods used for 2D nanostructures, including blend electrospinning, coaxial electrospinning, emulsion electrospinning, and side-by-side electrospinning, along with main 3D electrospinning techniques, are described below.
2.1.1 conventional electrospinning methods
2.1.1.1 Blend electrospinning
Blend electrospinning is considered the simplest method, based on mixing different polymers or polymers with functional agents to prepare a single fluid for electrospinning (Figure 1A). In blend electrospinning, drug encapsulation can be achieved in one step by dissolving or dispersing a drug in the polymeric mixture prior to electrospinning. This leads to a prolonged-release profile of the drug under specific conditions. It is noteworthy that the physicochemical properties of polymers strongly affect the functionality and release rate of the encapsulated drug by direct interaction between polymers and drugs. The solubility of a drug is an important issue that should be considered in this method. Insufficient solubility can result in the migration of drug molecules towards the fibers’ surface, leading to the drug’s burst release. This challenge can be overcome by maintaining the balance between hydrophilic and hydrophobic properties between drugs and polymers (Kumar, 2017; Keirouz et al., 2020).
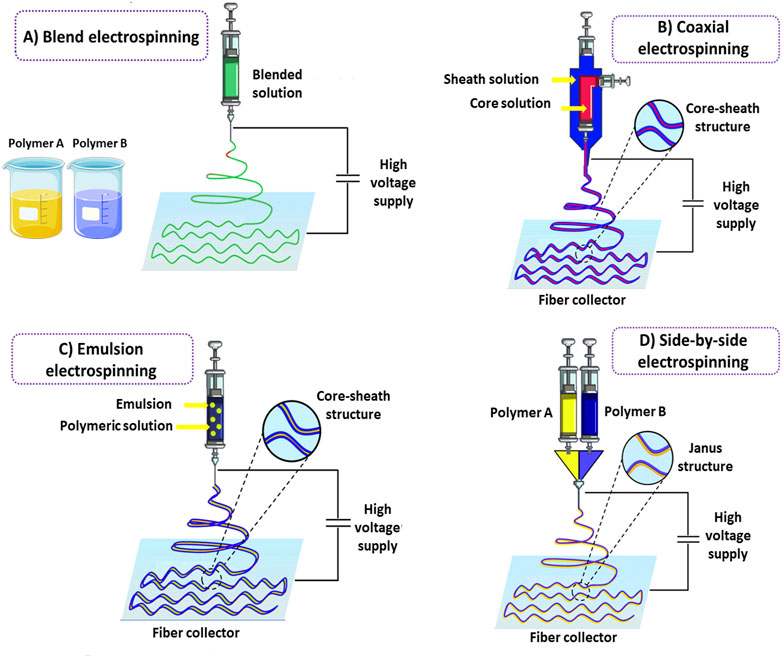
FIGURE 1. Schematic illustration of conventional electrospinning methods for hybrid nanofibers fabrication. Blend electrospinning (A). Co-axial electrospinning (B). Emulsion electrospinning (C). Side-by-side electrospinning (D).
2.1.1.2 Coaxial electrospinning
In order to enhance the efficiency of nanofibers, many modifications have been performed on electrospinning techniques through the years. Developing core-sheath nanofibers utilizing coaxial or triaxial electrospinning is one of these modifications. In this strategy, a polymeric nanofiber in the core is covered by another polymer as a shell (Naeimirad et al., 2018) (Figure 1B). Nanofibers fabricated by the triaxial electrospinning method contain three layers: core, middle, and shell (Yu et al., 2015). Layer by layer, the structure of nanofibers in this method can modify drug profile release and prevent any damage to the drugs incorporated into the core part of the nanofibers. The shell phase can act as a physical barrier, providing prolonged release kinetics and avoiding drug degradation following direct exposure to the external environment (Wang and Windbergs, 2019).
Interestingly, two different release patterns from one delivery system can be reached by incorporating different drugs into the core and shell phases. Coaxial or triaxial electrospinning is the most common technique for preparing hybrid nanofibers. This technique is based on a concurrent flow of a core and sheath solution from separate capillaries to form a nanofiber (Qin, 2017). In this technique, multiple drugs can be incorporated into different nanofiber layers, overcoming incompatibilities (Lu et al., 2016). Furthermore, core-sheath nanofibers made by coaxial electrospinning have higher drug loading efficiency than nanofibers prepared by blend electrospinning, and the possibility of initial burst release is also lower in this kind of nanofibers. The ability of this technique to prepare nanofibers from unspinnable solutions is considered another advantage compared to blend electrospinning (Pant et al., 2019).
2.1.1.3 Emulsion electrospinning
Emulsion electrospinning is one of the most straightforward techniques for preparing hybrid nanofibers. Many scientists have developed nanofibers using emulsion electrospinning to incorporate different therapeutic agents into fibers in the form of core-sheath structures. Emulsion electrospinning has some superiorities compared to traditional techniques, such as allowing the incorporation of lipophilic compounds in low-cost hydrophilic polymers with no need for organic solvents, which are highly limited in food systems. Emulsion electrospinning has a similar setup to blend electrospinning in which immiscible solutions are concurrently spun to produce core-sheath structures. In this method, initially, the emulsification of bioactive agents is performed by adding surfactant into the solution to form a water-in-oil emulsion and thereafter, the as-prepared emulsion is mixed with a polymer solution (Nikmaram et al., 2017). Evaporation of the continuous phase during electrospinning increases the viscosity. The emerging viscosity gradient leads to the migration of the aqueous phase droplets containing bioactive agents towards the jet’s center. The droplets are combined under the electric field resulting from the mutual dielectrophoresis that forms column-like structures and ultimately generates a fiber with a core-sheath structure (McClellan and Landis, 2016; Nikmaram et al., 2017) (Figure 1C). The applied voltage levels, flow rate, and spinning distance significantly affect emulsion-based hybrid nanofibers (Zhang and Wang, 2012). It should be noted that in some cases, the interface tension between the organic and aqueous phases of the emulsion can destroy the bioactive agents incorporated into the electrospun nanofibers (Wang et al., 2012a). Hence, emulsion electrospinning may not be appropriate for loading sensitive bioactive agents into nanofibers.
2.1.1.4 Side-by-side electrospinning
The side-by-side electrospinning technique is a two-compartment system commonly used to prepare Janus nanofibers (Figure 1D). In Janus nanofibers, the composition of the two sides of the structure is different. In contrast to the core-sheath structure, both components directly interact with the surrounding environment, which can be beneficial for developing novel structures. Unlike coaxial electrospinning, two chambers containing polymers are separated in the side-by-side electrospinning strategy. In this method, versatile nanofibers with different widths and interface areas can be developed by designing the structure of the spinneret and regulating the electrospinning parameters (Wang et al., 2020a; Li et al., 2021a). The interaction between fluid dynamics, electrodynamics, and rheology is one of the most challenging issues in the side-by-side synchronization of two fluid flows from the spinneret to the collector under an electrical field (Wang et al., 2019).
2.1.2 3D nanofiber-based scaffolds’ fabrication techniques
Electrospinning not only plays an important role in the construction of 2D nanomaterials, but also in the production of 3D scaffolds. Conventional electrospinning techniques use a charged nozzle containing polymer solution(s) at a certain distance from a static collector and form 2D mats. The 2D electrospinning processes produce tightly packed nanofiber scaffolds with only surface pores due to the sheet-like assembly. These scaffolds have a limited thickness and are unable to infiltrate cells or diffuse nutrients. Thus, researchers try to develop 3D electrospun nanofiber scaffolds to better mimic the ECM’s architecture and morphology. The advantages of electrospinning techniques for fabricating 3D nanofibers include control over morphology and tuning of fiber size and scaffold porosity. Moreover, 3D structures offer a greater surface area than 2D mats, which makes them very promising for various applications (e.g., catalysis, water filtration, energy harvesting, tissue engineering, and drug development) (Radacsi and Nuansing, 2020a). However, the main challenge is scaling up.
Considerable effort has been devoted to fabricating 3D scaffolds, employing auxiliary equipment or modified electrospinning apparatuses. The most commonly used techniques for 3D electrospun scaffolds are multilayer electrospinning, wet electrospinning, template-assisted electrospinning, electrospinning with post-processing (gas-foaming, freeze-drying, and electrospraying), self-assembly electrospinning, and electrospinning combined with 3D printing.
2.1.2.1 Multilayer electrospinning
Multilayer electrospinning is one of several promising strategies to fabricate 3D hybrid structures to better mimic the morphological and physicochemical properties of tissues and organs. Multilayer scaffolds can be fabricated by electrospinning nanofibers onto other electrospun fiber mats in a layer-by-layer pattern. Indeed, multilayer electrospinning is the stacking of electrospun layers by sequential electrospinning processes or co-electrospinning (Radacsi and Nuansing, 2020a). Adjusting the fiber diameter, porosity, composition, structure, and mechanical properties of the layers within the scaffold is made possible with multilayer electrospinning. This technology can improve the function of scaffolds for biomedical purposes by enhancing cell adhesion, proliferation, and migration (Sun et al., 2014; Radacsi and Nuansing, 2020a). Therefore, it is highly desirable for tissue engineering, particularly tissues with multilayer structures such as blood vessels and skin (Law et al., 2017). Besides tissue engineering, this approach can be an appropriate choice for developing controlled-release drug delivery systems. For instance, Teno et al. (2022) developed a multilayer system for buccal drug delivery of ciprofloxacin by means of blend electrospinning. In this study, a reservoir layer (ciprofloxacin, poly-ε-caprolactone (PCL) and poly (lactic acid) (PLA)), a mucoadhesive layer (polyethylene oxide (PEO)/PCL/Eudragit RS100) and a backing layer (PCL) were assembled independently to obtain a sustained drug delivery system (Figure 2A). The multilayer strategy exhibited outstanding antimicrobial effects over time and a strong adhesion patch time—for an average of 7 h in volunteers. These results showed the high potential of multilayer electrospun patches as platforms to treat oral infections.
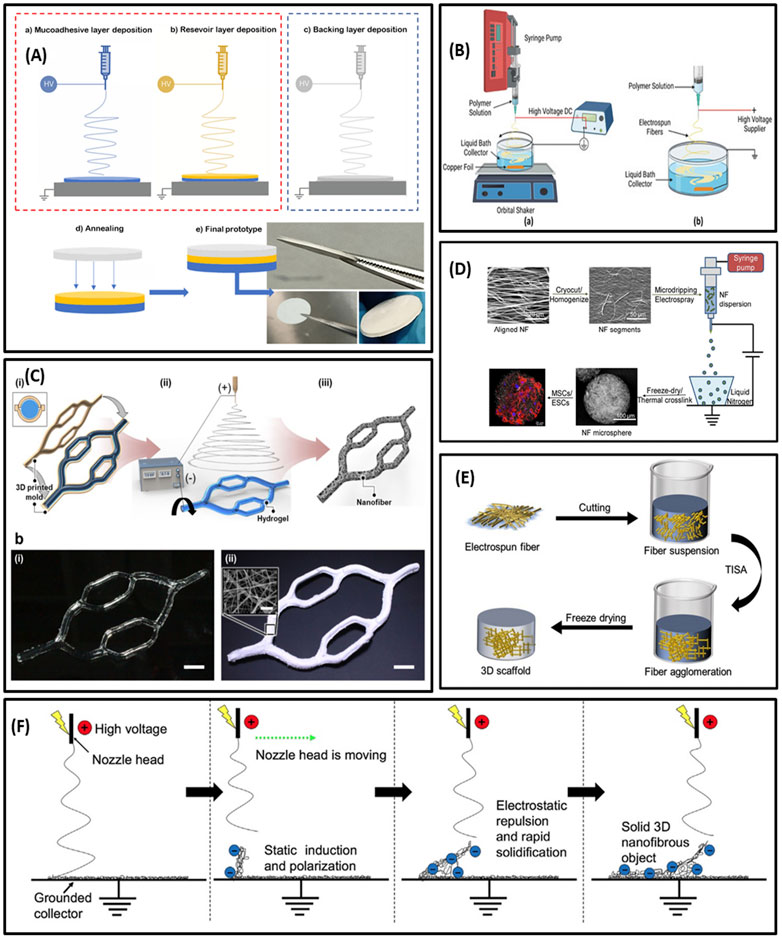
FIGURE 2. (A) Schematic illustration of multilayering electrospinning (A); electrospinning of adhesive layer (a), electrospinning of reservoir layer over the adhesive layer (b); backing layer electrospun separately from the previous layers (c); attachment of the backing layer to the bilayer patch by low-temperature annealing (d); resultant multilayer membrane (e). (B) Illustration of wet electrospinning. (C) Schematic of template-assisted electrospinning; diagram of template (hydrogel)-assisted electrospinning with the two sequential processes of 3D printing (a–i) and electrospinning (a-ii); photographs of the 3D hydrogel collector (b–i) and the 3D PCL nanofiber macrostructure (bii). (D) Schematic summary of nanofiber microspheres fabrication. (E) Illustration of freeze-drying post-processing. (F) Diagram of self-assembly electrospinning. (A) Reprinted with permission from (Teno et al., 2022). Copyright 2022 Multidisciplinary Digital Publishing Institute. (B) Reprinted with permission from (Xu et al., 2022). Copyright 2022 Multidisciplinary Digital Publishing Institute. (C) Reprinted with permission from (Eom et al., 2020). Copyright 2020 American Chemical Society. (D) Reprinted with permission from (Boda et al., 2018). Copyright 2018 American Chemical Society. (E) Reprinted with permission from (Yang et al., 2021). Copyright 2021 Multidisciplinary Digital Publishing Institute. (F) Reprinted with permission from (Vong et al., 2018). Copyright 2018 Royal Society of Chemistry.
2.1.2.2 Wet electrospinning
Wet or liquid electrospinning is derived from the conventional electrospinning process. In a wet electrospinning process, a liquid/coagulation bath in a metal container is replaced with a solid collector in the electrospinning setup (Figure 2B) (Keirouz et al., 2020). The collector type is essential in electrospinning and significantly impacts the scaffold’s 3D geometry and surface topography. In order to coagulate the electrospun polymers, the liquid bath contains a nonsolvent for the polymers. As the electrospun polymers reach the non-solvent bath, they may precipitate or coagulate. Wet electrospinning can be performed with non-solvent liquids such as water, ethanol, mixed water/ethanol, methanol, tertiary-butyl alcohol, hexane, and subcritical CO2 fluid (Taskin et al., 2020). Hydrophilic polymers such as gelatin, alginate, and chitosan need post-processing to withstand aqueous conditions (Sa and Kornev, 2011). Therefore, post-modifications are required, including surface coating, cross-linking, molding, and freeze-drying with functional moieties in the liquid bath (Jing et al., 2019). Many different 3D hybrid nanofibers like PCL/PEO, cellulose acetate/gelatin have been collected in the coagulation bath by using the wet electrospinning technique (Chen et al., 2019b).
2.1.2.3 Template-assisted electrospinning
Template-assisted electrospinning, based on modifying the shape of the collector, is another technique used for fabricating 3D scaffolds. In this method, utilizing an insulated poly (methyl methacrylate) (PMMA) mask on the collecting copper plate to focus the collection of fibers makes it possible to synthesize scaffolds with 3 mm thickness in a short time (Gao et al., 2017). In order to develop customized 3D structures, the architecture of the fiber collector plates may also be modified. Typically, template-assisted collectors are designed by a computer-aided design (CAD) program (Fukunishi et al., 2017) or conventional textiles (Şenel Ayaz et al., 2014). Scaffolds with various shapes such as honeycomb-like (Nedjari et al., 2014), helical spring (Hejazi et al., 2017), metal pin (Kim et al., 2018), and micropatterned structures (Rogers et al., 2014) can be manufactured with this method for different purposes. Additionally, biomimetic collectors, such as auricle-shaped (Walser et al., 2016) and vascular-like (Hammer et al., 2014) collectors, can be designed for tissue engineering applications.
Interestingly, in a recent study, a 3D hydrogel structure as a grounded collector was used instead of the conventional electroconductive collector (Eom et al., 2020). The multi-bifurcated 3D gelatin cylindrical structure was precisely fabricated by 3D printing (Figure 2C). During the electrospinning process, mobile ions in the hydrogel led to concentrating the electric field towards the grounded hydrogel collector and acting like an electroconductive collector. This concentrated electric field permitted the nanofibers to be deposited on the surface of the hydrogel collector, thus electrospun nanofibers precisely replicated the 3D hydrogel collector’s shape and formed a 3D tailored nanofiber macrostructure embedding the hydrogel. Additionally, diverse beneficial features of hydrogels, such as thermally reversible sol−gel transition and exceptional biocompatibility, offer new potential in developing 3D nanofiber macrostructures. For instance, the thermally reversible sol−gel transition of gelatin permitted the elective removal of the 3D hydrogel collector after electrospinning. Furthermore, the remarkable biocompatibility and high-water content of hydrogels eased the loading of biomolecules and cells inside 3D nanofiber macrostructures (Eom et al., 2020).
In some cases, the template may be added to or shaped during the electrospinning process. For example, it has been shown that microcrystals created during low-temperature electrospinning under high humidity can act as removable pore templates between the fibers, leading to the fabrication of highly porous 3D scaffolds. In a similar study, NaCl crystals enhanced nanofibrous scaffolds’ pore size and increased cell proliferation. This technology can also be combined with microfluidic devices to improve cell culture conditions under constant flow (Chen et al., 2016a).
2.1.2.4 Electrospinning with post-processing
3D hybrid scaffolds can also be made from 2D structures using conventional methods followed by post-processing approaches, such as electrospraying, short fiber freeze-drying, gas-foaming, and various other methods. Therefore, post-processing approaches mostly rely on electrospun nanofibers and scarcely need significant changes in the conventional electrospinning setup.
2.1.2.4.1 Gas-foaming
The gas-foaming technique is a simple and versatile post-processing electrospinning approach that enables the conversion of 2D mats to 3D scaffolds. This method is based on in situ gas foaming within the pores of the 2D nanofibrous mats as the driving force. In this approach, gas bubbles produced in situ via a chemical reaction (e.g., the decomposition of sodium borohydride) or the addition of an inert gas (e.g., carbon dioxide) lead to the creation of highly porous 3D scaffolds (Jiang et al., 2015; Jiang et al., 2018).
Sodium borohydride (NaBH4) is the most used foaming agent in the gas-foaming process. However, it needs to be dissolved in water to produce the necessary hydrogen through a chemical reaction with water. Therefore, NaBH4 is not applicable to the processing of water-soluble materials. Also, owing to the strong reactivity of NaBH4, materials with weak mechanical stability may be destroyed during foaming. In such cases, using carbon dioxide is an appropriate choice for gas-foaming post-treatment. In this process, polymeric material along with carbon dioxide is placed in a chamber at an increasing pressure until the dissolution of the gas in the polymer. When the pressure is relieved, large pores are formed thermodynamically (Pina et al., 2015). This method leads to a highly-interconnected porous structure and significantly enhances the porosity which is beneficial for cell infiltration (Lin et al., 2020; Chen et al., 2021). The porosity and mechanical strength of scaffolds can be modulated by adjusting the chemical reaction’s rate, resulting in gas generation or controlling gas pressure. Also, changing the temperature, pressure, and rates of parameter reduction can control pore sizes (Garg et al., 2012).
2.1.2.4.2 Electrospraying
In addition to aerogels and scaffolds, microspheres can be a promising option for loading various growth factors, cells, and biologics for tissue engineering applications (Hossain et al., 2015). In contrast to implantable scaffolds, injectable microspheres can be utilized to heal irregular defects via minimally invasive procedures without the need for invasive surgery (Zhang et al., 2016). Conventional methods for microsphere fabrication (e.g., self-assembly and thermally-induced phase separation) are not applicable to a wide variety of materials (Liu et al., 2011). Hence, alternative approaches for fabricating microspheres are needed.
Nanofibrous microspheres can be prepared by combining electrospraying and electrospinning technology. This strategy is based on the assembly of short electrospun nanofibers into microspheres by electrospraying. For instance, Boda et al. (2018) developed nanofiber microspheres by combining electrospinning with electrospraying. They cut nanofibers into short nanofibers and homogenized them at a low temperature to form a homogeneous dispersion in water. The resulting dispersion was electrosprayed to attain nanofiber microspheres (Figure 2D).
2.1.2.4.3 Short nanofiber assembly (freeze-drying)
Using short electrospun nanofibers as building blocks for constructing 3D scaffolds is a promising approach to adjusting the physicochemical properties and mechanical flexibility of the scaffolds. In this method, electrospun fibers are initially cut into short fibers and homogeneously dispersed in a solution using an ultrasonic homogenizer. Afterward, the solution containing short nanofibers is freeze-dried to prepare highly porous 3D sponges or aerogels (Chen et al., 2016b) (Figure 2E). Different cross-linking strategies can be applied to regulate the mechanical properties and biological functions of the scaffold. Cross-linking among short fibers can be accomplished by chemical cross-linking or temperature-mediated cross-linking. In chemical cross-linking, the functional groups on the surface of nanofibers are exploited to form cross-linking agents-mediated covalent bond formation, whereas in temperature-mediated cross-linking, increasing the temperature can lead to thermal annealing mediated physical cross-linking among short nanofibers (Chen et al., 2022).
2.1.2.5 Self-assembly electrospinning
Since desired structures can be created in a single step, 3D nanofibrous scaffolds self-assembly via electrospinning is of interest. In this process, 3D structures result from the fast solidification of the nanofibers, leading to a self-standing object (Figure 2F). This incidence also depends on electrostatic induction and polarization of the deposited nanofibers. Therefore, charging the top part of the deposited nanofiber mats to negative via an electric field is necessary for providing a favored deposition area to attract the positively charged jet ejected from the electrospinning nozzle. Considering the negative charge of the fibers in the top part of mats, they will repel each other, leading to the creation of 3D spongy scaffolds (Sun et al., 2012; Mi et al., 2017).
The structure and pore size of scaffolds can be controlled by modulating the polarizability of electrospun fibers by including some conductive additives such as phosphoric acid (H3PO4) in the polymeric solution. In other words, adding such compounds to the polymeric mixtures can induce repulsive forces between nanofibers during the electrospinning process, resulting in 3D porous structures (Chin and Chang, 1989; Farrokhi-Rad, 2016). It is noteworthy that manipulating the electric field can also affect the structures of resulting 3D mats. For instance, in a recent study performed by Yan et al. (2011), the self-assembly of PCL electrospun nanofibers into 3D honeycomb structures was explored. In order to interpret this incidence, they hypothesized that it may be related to the gradients in the applied electric field.
2.1.2.6 Combining 3D printing with electrospinning
3D bioprinting or additive manufacturing (AM) technology can bridge the gap between artificial tissue scaffolds and natural tissues (Zhang et al., 2017b). 3D printing improves scaffolds’ pore size, interconnections, and mechanical strength by layering the materials together (Yang et al., 2022). This technique has been used in various technical and biomedical applications, including medical and aviation devices (composite, metal, or plastic), personalized clothes, and grafts (Su et al., 2021). The 3D printing technique comprises three basic components: hardware (the 3D printer), software, and materials.
Additionally, CAD-based 3D printing technology has recently gained considerable attention, allowing the fabrication of cellular, acellular, and hybrid scaffolds (Abel et al., 2020). 3D bioprinting has made major advances in recent years. However, it is still associated with many limitations, hindering its clinical application. The most crucial challenge is the low mechanical strength of 3D printed scaffolds, which restrict their biomedical application, especially in hard tissue engineering. Furthermore, the variety of materials, and the resolution of scaffolds that can be printed, are limited (Potyondy et al., 2021; Yang et al., 2022).
To overcome this challenge, the combination of 3D printing with electrospinning can produce multifunctional hybrid nanofibers with highly porous interconnected structures and improved mechanical properties (Yang et al., 2022). Different methods for combining these two techniques include electrospinning onto 3D printed scaffolds, 3D printing onto electrospun fibers, alternate use of 3D printing and electrospinning, 3D printing with short fiber inkgenerated from electrospun nanofibers, decorating/infusing 3D printed scaffolds with electrospun nanofiber segments, fabrication of electrospun scaffolds on 3D printed collectors/templates, combination al use of different components prepared by electrospinning and 3D printing, electrohydrodynamic (EHD) printing, and a platform combining 3D printing and electrospinning techniques (Yang et al., 2022).
2.2 Functionalization of nanofibers
The properties of hybrid nanofibers made by blending only polymers are limited, and much can be performed to improve their biological, mechanical, electrical, or optical features. The mentioned properties can be improved by immobilizing functional agents in nanofibers and forming multifunctional hybrid nanofibers. These functional agents include inorganic/organic NPs, biomolecules (e.g., growth factors, hormones, and nucleic acid), drug molecules, etc. The immobilization of various functional moieties in nanofiber matrix can considerably improve mechanical, physicochemical, and biological properties (Foraida et al., 2017; Nirwan et al., 2022).
2.2.1 Functionalization using nanoparticles
Immobilization of NPs in hybrid nanofibers provides exceptional properties, combining NPs’ advantages with polymer properties. These nanofibers may also have more functions, including photothermal properties, magnetic responses, biosensing, antibacterial properties, and drug delivery capabilities. Furthermore, NPs can improve nanofibers’ physicochemical and mechanical properties and stability (Kalia and Haldorai, 2015).
An increasing number of published articles on nanofiber-nanoparticle hybrids show the high potential of such multifunctional nanofibers for different applications (Kalia and Haldorai, 2015). Diverse types of NPs have successfully been used for this aim, including metal (Li et al., 2017), metal oxide (Pinto et al., 2016), carbon nanotubes, and polymeric NPs (Tuğcu-Demiröz et al., 2021).
The functionalization of nanofibers with NPs can be achieved using different methods, including direct solubilization of the NPs in an electrospinning solution (Wang et al., 2018a), reduction of the precursor for NPs in situ, growth of NPs on nanofibers (Zhang et al., 2018), or electrospraying NPs on nanofibers’ surfaces (Nekounam et al., 2020).
Co-blending NPs with a polymer solution is the most common method to fabricate nanofiber-nanoparticle hybrids. In this method, NPs will be uniformly distributed on the surface or inside the nanofibers. Notably, during this process, the interfacial interaction between NPs and polymer solution can strongly influence the spinnability, composite fiber morphology, and mechanical properties. The aggregation of NPs in the working solution can lead to needle blocking during the electrospinning process, and NPs will not be distributed in the nanofibers, reducing the mechanical properties and functionality of composite fiber membranes. To minimize this effect, NPs can be combined with a polymer solution through stirring and sonication, separately dissolving the NPs and polymer in different solvents or adding a certain amount of surfactant into the working solution (Rasekh and Raisi, 2021; Jiang et al., 2022; Ren et al., 2022).
In addition to the co-blending strategy, NPs can be loaded into nanofibers by combining other processes with electrospinning technology. For instance, combining electrospinning with plasma technology can lead to the formation of NPs on the surface of nanofibers containing the precursor for NPs (Annur et al., 2015). The electrospray or magnetron sputtering technology also allows the uniform coverage of NPs on the surface of nanofibers (Enculescu et al., 2021; Park et al., 2021).
Multifluid electrospinning, such as coaxial or side-by-side electrospinning, can also be used for preparing nanofiber-nanoparticle hybrid scaffolds. In these methods, polymer solution and NPs are loaded into separate syringes to reduce the possibility of NPs aggregation inside the polymer solution (Zhang et al., 2022a).
3 Effective parameters in electrospinning
Different parameters can influence the electrospinning process. Therefore, adjusting these parameters is essential to achieve desired nanofibers. The main effective parameters in the electrospinning process include: 1) physicochemical properties of the system (nature of the polymer, solution viscosity, conductivity, and surface tension) (Madruga and Kipper, 2022), 2) process parameters (voltage, receiving distance, and flow rate) (Zhang et al., 2022b), and 3) environmental factors (temperature and humidity) (Wang et al., 2021b). The mentioned parameters’ effects are presented in Table 1.
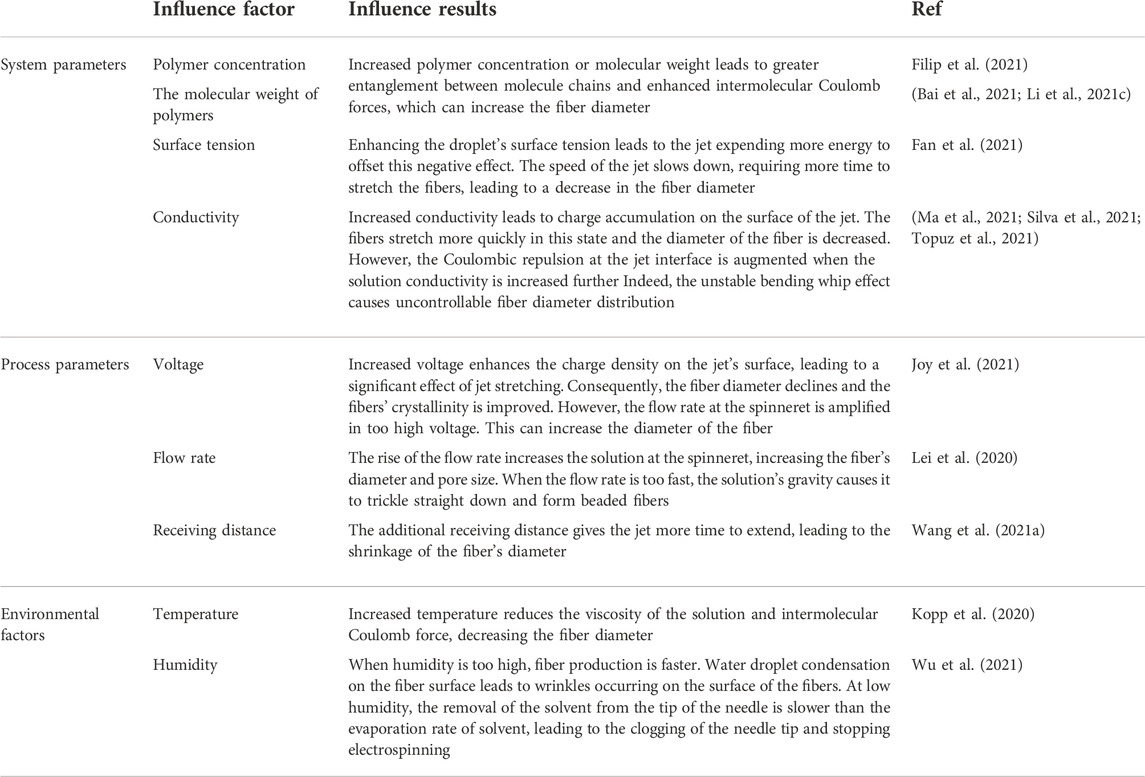
TABLE 1. Effect of different parameters on the electrospinning process (Zhang et al., 2022a).
4 Polymers in electrospun nanofibers
Various polymers from different sources can be combined and spun to fabricate hybrid nanofibers. Selection of the polymers is a critical step for producing nanofibers with specific characteristics suited to particular applications. The ideal polymer for biomedical applications must be biodegradable, safe, biocompatible, moderately hydrophilic, and have proper mechanical strength. These polymers can be obtained from natural or synthetic sources, each having different advantages and disadvantages. The polymer type must be selected based on the end-use of the nanofibers (Makadia and Siegel, 2011). Table 2 lists the polymers most commonly used in nanofiber production.
Natural polymers are a popular choice in electrospinning for biomedical applications. They can come from animal or plant resources or other living organisms, making them mostly biodegradable, biocompatible, and non-toxic with low antigenicity (Juncos Bombin et al., 2020). Additionally, they may have biological effects such as antimicrobial activities, anti-inflammatory, and hemostatic effects. Compared with synthetic polymers, natural polymers are more costly and harder to process during electrospinning (Juncos Bombin et al., 2020). It is noteworthy that synthetic polymers have better thermal stability, electrospinning, and mechanical properties. Synthetic polymers are favored over bio-based polymers for specific applications, as they can be applied to develop nanofibers with optimum mechanical and degradation characteristics. They can be combined with natural polymers to adjust their mechanical performance and degradation rate (El-Aassar et al., 2021).
5 Characterization of a hybrid nanofiber
The high quality of nanofibers can be assured during the production process by characterizing nanofibers according to test metrics that correlate with the material’s functional properties. Single fiber characterization provides fundamental data for understanding the relationship between nanofiber’s structure and properties. Also, depending on their ultimate utilization, various methods have been developed to characterize nanofiber scaffolds (Lopez Marquez et al., 2022). For example, electrical conductivity and electrochemical reactivity are necessary when nanofibers are intended to be used as sensors (Liu et al., 2019). If the nanofibers are synthesized for air filtration, fibers’ permeability, porosity, and particle penetration are significant characteristics (Lu et al., 2021). In the following section, we summarize various characterization techniques in order to gain a better understanding of nanofibers’ function.
5.1 Morphological characterization
5.1.1 Porosity
Porosity refers to the empty spaces between nanofiber components. These pores are located on the surface or inside of nanofibers, making them very lightweight and providing a large surface area (Roodbar Shojaei et al., 2019; Yousefzadeh and Ghasemkhah, 2019). Porous nanofibers can be used in the fields of filtration, gas separation, energy, sensor, and tissue engineering. According to the American Society for Testing and Materials (ASTM), the porosity of nanofibers can be measured by different methods such as mercury porosimetry, liquid extrusion porosimetry, capillary flow porometry (CFP), and nuclear magnetic resonance (NMR) (Roodbar Shojaei et al., 2019). Porosity is an important parameter when selecting the scaffold for tissue engineering. In the study conducted by Ghasemi-Mobarakeh et al. (2008), the porosity of hybrid nanofibers (PCL/gelatin) was calculated by the CFP method. According to the results, the pore diameter decreased with increasing gelatin content. In another study, the porosity of 3D scaffolds manufactured by co-electrospinning of poly (hydroxybutyrate-co-hydroxy valerate (PHBV)/PCL), as the first layer, and diatom shells (DS) incorporated pullulan (PUL), as the second layer, was measured by mercury porosimetry. The calculated value was 42.17% (Dalgic et al., 2019).
5.1.2 Diameter and size distribution of electrospun nanofibers
Geometric characterizations are able to determine the diameter, size distribution, orientation, and morphology of the fabricated fibers. Morphological features of nanofibers can be measured by scanning electron microscopy (SEM), energy dispersive X-ray spectroscopy (EDX), atomic force microscopy (AFM), and transmission electron microscopy (TEM).
SEM is the most commonly used method due to its availability and ease of use. By measuring apparent density from the SEM images and comparing it to the bulk density of the electrospun polymers, SEM can reveal information about the fiber diameter/alignment, pore diameter, and porosity of scaffolds (Lopez Marquez et al., 2022). In conjunction with SEM, an EDX is also used to analyze chemical composition near the sample’s surface (Tomlins, 2015). However, there is a probability of accuracy reduction for fibers with <200 nm in diameter because the electron beam can destroy these fibers. Moreover, coating non-conductive samples with conductive metals may result in questionable accuracy in thin fibers. Based on the mentioned reasons, atomic force microscopy (AFM) or transmission electron microscopy (TEM) are better options for determining the morphology of nanofibers, especially tiny fibers (Ramakrishna, 2005). AFM provides information about the surface of the nanofiber’s topographical, morphological, mechanical, and physicochemical properties (Lopez Marquez et al., 2022). It has been reported that scaffold stiffness can modulate cell function and remodeling (Zhu et al., 2019; Yi et al., 2022). AFM can be used to measure and interpret scaffold stiffness; thus, it can be helpful in tissue engineering and regenerative medicine. TEM imaging is a leading method for studying hybrid nanofibers, especially core-sheath nanostructures. This method is based on different amounts of electron transmission by each polymer. It can be used on individual fibers, especially for fibers containing nanomaterials (Lopez Marquez et al., 2022).
5.2 Mechanical characterization of the nanofibers
5.2.1 Tensile
The tensile test is one of the most frequently used methods for determining nanofiber’s mechanical properties. Tensile tests define factors such as young’s modulus, tensile strength, and strain at the break of polymeric fibers. Previous studies have reported that hybrid scaffolds exhibit better mechanical strength (Karbasi et al., 2016; Hou et al., 2019).
5.3 Structural evaluation of nanofibers
5.3.1 X-ray diffraction (XRD)
XRD spectroscopy is a non-destructive and well-established method to detect changes in crystallinity after electrospinning. The crystallinity of many different hybrid electrospun scaffolds has been clarified using the XRD method (Yuan and Zhang, 2012; Manjumeena et al., 2015; Yang et al., 2015; Lv et al., 2021). The crystallization level can explain the relationship between fiber’s diameter and drug encapsulation efficiency in core-sheath electrospun nanofibers (He et al., 2015). Since the drug/polymer system has a limited time to recrystallize during electrospinning, non-crystalline solid dispersions are more likely to form (Preem et al., 2017). Amorphous solid dispersions of drugs are commonly confirmed using the XRD method after blend electrospinning (Lopez et al., 2014; Tamm et al., 2016; Preem et al., 2017).
5.4 Chemical characterization of nanofibers
5.4.1 Fourier transform infra-red (FTIR)
Information about nanofiber composition and chemical characterizations can be gleaned from FTIR analysis (Huang et al., 2000). Furthermore, FTIR can be used to detect inter and intramolecular bonding, polymer-polymer, and polymers-drug interactions (Preem et al., 2017; Zhao et al., 2021a). Additionally, the effect of surface functionalization (Karuppannan et al., 2022) and cross-linking (Baştürk and Kahraman, 2012; Jia et al., 2022) of hybrid nanofibers can be evaluated by this method.
5.4.2 Raman spectroscopy
Raman spectroscopy is typically complemented by infrared (IR) spectroscopy (Horzum et al., 2019). Raman spectroscopy has been widely used to investigate the considerable variations and other low-frequency modes in the distribution of polymers within polymeric nanofiber matrices and to assess the effects of carbonaceous or nanostructured materials on the morphology and physical properties of the electrospun nanofibers (Chipara et al., 2013; Kotzianová et al., 2016; Roodbar Shojaei et al., 2019).
5.4.3 Water contact angle measurement
Wettability or hydrophilicity of the surface is important for understanding the outer surface chemistry of electrospun nanofibers. Of note, the hydrophilicity of nanofiber surface is vital in biological studies due to better cell attachment and proliferation (Unnithan et al., 2014; Kalia and Haldorai, 2015).
5.5 Thermal evaluation techniques
Thermal properties of hybrid electrospun nanofibers can be evaluated by differential scanning calorimeter (DSC) and thermogravimetric analysis (TGA) methods (Aykut et al., 2019).
5.5.1 Differential scanning calorimeter (DSC)
DSC consists of a cooling or heating test to maintain the sample and reference at a constant temperature. The DSC can be used to determine the solid-state properties of active pharmaceutical agents and the encapsulation efficiency of crystalline compounds and identify the configuration of the macromolecules in nanofibers and their interactions (e.g., plasticizing and hydrogen bonding) (Balogh et al., 2014; Lopez et al., 2014; Deng et al., 2018; Islam et al., 2019).
5.5.2 Thermogravimetric analysis (TGA)
TGA is employed to determine the encapsulation efficiency of hybrid nanofibers, the stability, and the solvents evaporation of formulations by measuring material weight (loss or gain) as a function of altering the heating temperature (Waghmare et al., 2018; Islam et al., 2019; Ye et al., 2021).
6 Biomedical applications of hybrid nanofibers
Biocompatibility and mechanical properties are important parameters in biomedical applications. The previously mentioned positive characteristics of electrospun nanofibers, high porosity, versatility, and high surface-to-volume ratio, make them a good choice for different biomedical applications such as drug delivery, tissue engineering, wound dressing, and biosensors (Liu et al., 2021b) (Figure 3).
6.1 Drug delivery
To date, various drug delivery systems have been developed to enhance the clinical efficiency of drugs and decrease their toxic effects compared to conventional dosage forms. Some characteristics of an ideal drug delivery system include high loading capacity and encapsulation efficiency, ease of operation, low cost, and controlled drug release. All of the mentioned criteria can be achieved using electrospun nanofibers (Torres-Martinez et al., 2018).
Electrospun nanofibers have received a great deal of attention among different drug delivery systems due to their favorable characteristics. A wide variety of materials and drugs, ranging from small molecule drugs, such as antibiotics, to macromolecules, like proteins and DNA, can be loaded into electrospun nanofibers for improving their bioavailability or attaining controlled release (Torres-Martinez et al., 2018). Different factors can affect the drug release from nanofibers which should be considered in designing drug-loaded nanofibers. For instance, the degradation and dissolution rate of the polymeric matrix can significantly influence drug release, which is associated with water solubility of components and structural properties of nanofibers such as fiber diameter, specific surface area, size and total volume of pores, and crystallinity of polymeric matrix. The nature of the drug and its compatibility with the matrix should also be taken into consideration as one of the practical factors in the drug release profile (Hrib et al., 2015).
In recent years, a large part of studies in the area of controlled-release drug delivery has focused on developing stimuli-responsive systems. The drug release in these systems is based on response to changes in environmental parameters, including pH, temperature, light, electrical, and magnetic fields (Contreras-Cáceres et al., 2019). Electrospun nanofibers are appropriate candidates for developing stimuli-responsive systems because they can be easily manipulated to tailor the drug release rate. In the following section, an overview of drug-loaded hybrid nanofibers is presented. Table 3 provides a summary of electrospun hybrid nanofibers used for drug delivery.
6.1.1 Anti-inflammatory drug
Anti-inflammatory agents refer to compounds that can suppress inflammation and swelling symptoms and may have analgesic and antipyretic effects. Loading anti-inflammatory agents into nanofibers has been of great interest for researchers due to the low water-solubility of such molecules and the desired effect of almost immediate relief. In a recent study, nanofiber-based scaffolds were prepared with a polymeric mixture (PLA and PCL) and dexamethasone, the steroid anti-inflammatory drug, to decrease immune response in tissue engineering. They showed a controlled release of dexamethasone over time, and stem cells could also successfully attach and proliferate on the nanofibers in vitro (Vacanti et al., 2012). In another study, electrospun nanofibers were developed as Guided Tissue Regeneration (GTR) membrane by PCL and a blend of polyvinyl alcohol (PVA)/collagen/Ibuprofen (Ibu) (Limoee et al., 2019). The membrane indicated satisfying mechanical properties, and Ibu release was sustained and controlled. In a recent study, to achieve pH and thermo-responsive release of ketoprofen, poly (N-isopropyl acrylamide) (PNIPAAm), as a thermo-sensitive polymer, and Eudragit® L100-55 (EL100-55), as a pH-sensitive polymer, were used to prepare electrospun nanofibers (Li et al., 2018). They showed that the release of ketoprofen was dependent on pH and temperature.
6.1.2 Antibiotics
Microbial infection is considered one of the most challenging issues in medicine that currently threatens world health. In addition, antimicrobial resistance is accelerating due to the overuse of antibiotics. Therefore, designing an efficient drug delivery system for antibiotics with selective action in the infection site to prevent overdosage and antimicrobial resistance is necessary. Eren Boncu et al. (2020) prepared electrospun nanofibers composed of poly (lactic-co-glycolic acid) (PLGA) and PCL loaded with linezolid for prophylaxis of skeletal prosthesis-related infections. They showed that nanofibers could effectively improve healing in damaged and infected areas in the rat model by providing an optimal dosage of the drug in the intended site. They could reduce the need for antibiotic administration by around 37-fold compared to usual methods by providing controlled drug release. This approach is a cost-effective treatment that can avoid the progression of antibiotic resistance (Eren Boncu et al., 2020). In a recent study, nanofibers were fabricated by electrospinning a blend of PVA and chitosan incorporated with moxifloxacin (Liu et al., 2021a). According to the obtained results, the as-prepared nanofibers could significantly inhibit the growth of Staphylococcus aureus and Pseudomonas aeruginosa. They possessed better antibacterial activity than the control group (Liu et al., 2021a).
6.1.3 Antitumor drugs
Despite all the advances in oncology, cancer is among the deadliest diseases in the world. Cancer treatment by chemotherapy is generally based on the administration of cytotoxic agents to suppress the growth of cancerous cells. It is clear that severe adverse effects accompany the administration of cytotoxic drugs. Thus, developing a localized drug delivery system can be a promising approach to promote the efficiency of conventional chemotherapeutic agents by restricting the action of drugs mostly to tumor tissues and reducing systemic adverse effects. Tumors have specific features that can be considered for developing delivery systems with high specificity towards cancer cells. For instance, due to the increased metabolic rate in tumor cells and high lactic acid production resulting from the glycolytic pathway, the tumor microenvironment is acidic compared to normal tissues. Hence, the pH value difference can be taken into consideration for the fabrication of responsive materials to release loaded cargos only in an acidic environment such as tumors. Zhang et al. (2020) designed a pH-responsive scaffold based on electrospun nanofibers for 5-fluorouracil (5-FU). To achieve pH-responsive release of the drug, 5-FU was covalently attached to keratin, and the resulting polymer was blended with PLA for electrospinning and preparing nanofibrous scaffolds for localized delivery of 5-FU. Fabricated scaffolds showed potent antitumor effects following a rapid release of drugs in the first hours. However, it could not provide continuous release of the drug for a prolonged time. Notably, the prolonged release of the cargo in the tumor site is necessary to eradicate the tumor (Zhang et al., 2020).
Carbon nanotubes (CNTs) have the potential to be utilized for improving mechanical, structural, and drug delivery properties in electrospun nanofibers. In a recent study performed by Qi et al. (2016), doxorubicin (DOX) was chemically attached to the surface of multi-walled carbon nanotubes (MWCNTs). After optimization, the drug was encapsulated up to 83.7%, and as-prepared MWCNTS@DOX particles were blended with a PLGA polymer solution to synthesize a hybrid nanofiber by electrospinning. The incorporation of MWCNTs into PLGA nanofibers not only did not change the morphology of the PLGA nanofibers, but also enhanced their mechanical properties. In addition, this hybrid system could decrease burst DOX release and provide sustained release of DOX over 42 days. One of the strategies to kill cancer cells is increasing temperature locally in the tumor tissue, known as hyperthermia. This can be achieved using magnetic NPs, which are categorized into magnetic alloy NPs (MANPs) and magnetic metal oxide NPs (MMONPs). These NPs are able to accumulate in the tumor tissue and produce heat under a magnetic field (Peiravi et al., 2022).
In a recent study, an implantable hybrid magnetic nanofibers device was designed to be used for magnetic hyperthermia and pH-dependent anticancer drug release in the tumor (Sasikala et al., 2016). For this aim, Fe3O4 NPs were mixed with a PLGA solution to prepare the electrospinning solution. After electrospinning, a shell of polydopamine was grown through a simple immersion on the surface of as-prepared magnetic nanofibers. The polydopamine-based shell with numerous catechol moieties on the surface of nanofibers was able to attach to bortezomib (BTZ), an anticancer agent. According to the results, they found this smart system highly beneficial owing to its higher therapeutic efficacy and low toxicity towards normal cells and also the potential of magnetic NPs for repeated hyperthermia application and controlled drug release in the tumor tissue (Sasikala et al., 2016).
In a similar study, Radmansouri et al. (2018) developed DOX-loaded chitosan/cobalt ferrite/titanium oxide nanofibers to achieve pH-dependent DOX release along with hyperthermia to treat melanoma. Hybrid nanofibers were prepared by co-blending chitosan with cobalt ferrite NPs, titanium oxide NPs, and DOX. According to their drug-release study, the fast release of DOX from nanofibers was seen at low pH by alternating the magnetic field. Also, cytotoxicity results exhibited considerable cell death in the combination of chemotherapy and hyperthermia.
6.1.4 Cardiovascular drugs
Cardiovascular diseases, such as stroke, heart failure, and hypertensive heart disease, are the leading cause of death globally.
Different therapeutic approaches have been developed for the treatment of cardiovascular diseases. For example, arterial stents are the most used approach for coronary heart disease. Arterial stents are used to keep the artery open and prevent its obstruction to maintain continuous blood flow (McGinty, 2014). In order to enhance the efficiency of this approach, Kersani et al. (2020a) fabricated hybrid electrospun nanofibers loaded with simvastatin to cover self-expandable NiTiNOL stents. Nanofibers were produced by electrospinning a polymeric mixture containing chitosan and β-cyclodextrin (CD), forming a polyelectrolyte complex for loading simvastatin. These nanofibers showed high drug loading capacity along with excellent mechanical properties. However, more preclinical tests are necessary to investigate the biocompatibility of these implants (Kersani et al., 2020a).
Nicorandil is a vasodilator that can be administered for angina pectoris, a chest pain resulting from episodes of transient myocardial ischemia. In a recent study, this drug was loaded into electrospun nanofibers for sublingual administration to minimize mucosal ulceration (i.e., the main adverse effect of sublingual administration of nicorandil) and enhance the bioavailability of the drug (Singh et al., 2016). Polymeric nanofibers were prepared with hyaluronic acid, PVA, and vitamin B12. In this study, hyaluronic acid was used for its viscoelastic properties, which ensure the sustained release of a loaded drug with an extended retention time at the site of administration (Joshi et al., 2016) and vitamin B12 was employed due to its promising positive effects on mucosal ulceration (Kalia et al., 2016). According to histopathological results, there was no evidence of mucosal ulceration at the application site, and the preclinical safety of the as-prepared nanofibers was proved. Results showed that these biocompatible nanofibers had a high potential for sublingual administration of nicorandil and improved its low bioavailability (Singh et al., 2016).
6.1.5 Ophthalmic drugs
Eyes are repeatedly washed by tears to eliminate irritants and help the immune system (Farandos et al., 2015). The usual approach for treating ocular diseases is using eye drops which are the saline solutions of drugs used directly over the eye. However, rapid turnover of the tear film, small available surface for drug absorption, and several physiological barriers lead to poor bioavailability of eye drops. Therefore, designing solid delivery systems for ocular diseases receives a great deal of attention due to their potential for improving the bioavailability of drugs resulting from reduced clearance of the solid drug delivery systems compared to liquid ones (Mishima et al., 1966; Everitt and Avorn, 1990). Grimaudo and Concheiro (2020) formulated nanofibers made of hyaluronan and polyvinylpyrrolidone (PVP) for the ocular delivery of ferulic acid (an antioxidant) and ε-polylysine (an antimicrobial peptide). According to cytocompatibility assays, the nanofibrous scaffolds showed no hemorrhage or coagulation, and there was no difference between the scaffolds and saline solution as the control. Hence, it was regarded as non-irritant. In addition, they exhibited potent antibacterial effects against p. aeruginosa and S. aureus. Considering the fast erosion of scaffolds, drug release was fast, taking place within 20 min. Thus, this designed nanofibrous scaffold was applicable for short-term medication (Grimaudo and Concheiro, 2020).
6.2 Tissue engineering
Tissue engineering is an emerging field in biomedicine that applies the principles of biomedical sciences and engineering to develop biological alternatives to restore, preserve or ameliorate tissue functions. In order to create an efficient tissue, the designed structures should simulate ECM, enable oxygen and nutrient circulation as well as eliminate metabolic waste during the process of tissue regeneration. A lot of effort has recently been put into providing 3D scaffolds for tissue engineering. Among these, electrospinning is one of the most promising methods. In recent years, many nanofiber-based scaffolds have been designed for tissue engineering (Rahmati et al., 2021). This section presents the application of nanofiber-based scaffolds for different tissue regeneration.
6.2.1 Bone tissue engineering
Bone tissue engineering refers to designing scaffolds for delivering therapeutic agents and cells to the damaged tissue to provoke bone regeneration (Qu et al., 2019). In the design of scaffolds for bone tissue engineering, there are some considerations, including 1) the size of porosity, 2) appropriate mechanical properties and adjustable biodegradation kinetics, 3) interconnected open porosity for growth factors, 4) biocompatibility of materials used for scaffolds, and 5) sterile and suitable environment for cell growth (Qu et al., 2019).
In other words, designed scaffolds for bone tissue engineering should not only provide structure and mechanical robustness for the tissue but also mimic the ECM and stimulate bone repair. Electrospun nanofibers can provide many of the above criteria for bone tissue engineering. Different natural or synthetic polymers can be applied for designing bone tissues, such as chitosan, alginate, PCL, and polyglycolic acid (PGA).
For instance, Rachmiel et al. (2021) developed a composite nanofibrous scaffold by core-shell electrospinning of PCL and hyaluronic acid containing a short self-assembling peptide. Hyaluronic acid is one of the major ECM components responsible for modulating many biological processes. It has been stated that incorporating hyaluronic acid can enhance cell migration and proliferation in the prepared scaffolds. However, hyaluronic acid has low mechanical strength and a significant amount of free charge carriers (Shin et al., 2016). Therefore, electrospinning of pure hyaluronic acid would be challenging, and it needs to be combined with other polymers to fabricate electrospun nanofiber scaffolds. In order to modify the mechanical properties of hyaluronic acid, a low-molecular-weight hydrogelator, Fmoc-phenylalanine-arginine-glycine-aspartic acid (FmocFRGD), was employed in addition to utilizing PCL. Short synthetic peptides containing the RGD sequence can efficiently mimic ECM, promoting bone growth and accelerating cellular differentiation into osteoblasts. These favorable properties of RGD sequence can be potentiated by incorporating them into natural polymers such as hyaluronic acid (Alipour et al., 2020). In this study, the prepared scaffolds showed similar morphology to ECM and could facilitate osteogenesis (Rachmiel et al., 2021).
Wang et al. (2018b) developed a novel bioactive scaffold for regulating bone remodeling and promoting bone regeneration using mesoporous silica nanoparticles (MSNs) embedded into electrospun PCL/gelatin nanofibers. Based on the synergism effects of silicate and alendronate (ALN) − promoting bone formation with silicate and inhibiting the bone-resorbing process by ALN −, ALN was loaded into MSNs. The scaffold was successfully fabricated by co-electrospinning of acetic acid-mediated PCL/gelatin homogeneous polymeric mixture containing well-dispersed MSNs loaded with ALN. The release profile of the designed scaffolds showed that the ALN@MSN-loaded nanofibers achieved the dual release of ALN and silicate (Figure 4). In addition, in vivo study exhibited that the healing time in test groups was three times faster than in the control group. It seems that as-prepared electrospun nanofibers have a high potential for clinical use (Wang et al., 2018b). Table 4 summarizes recent studies on hybrid electrospun nanofibers applied in bone tissue engineering.
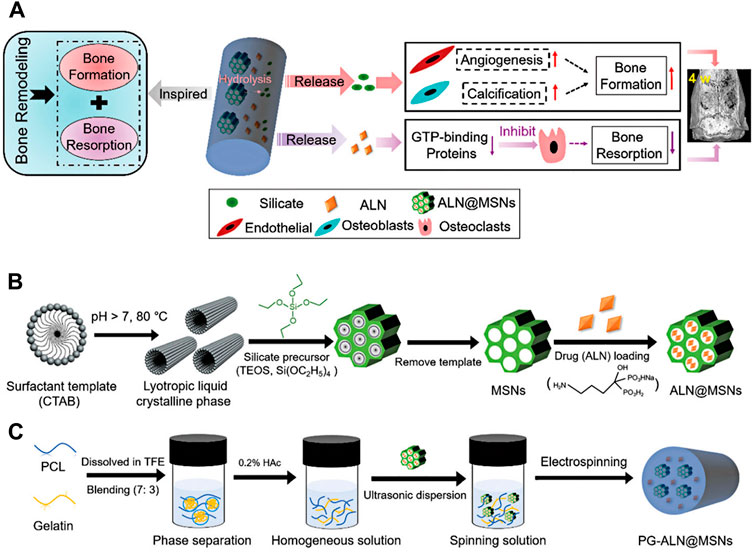
FIGURE 4. Schematic illustration of PG-ALN@MSN fabrication process and effects of prepared nanofibers on bone repair. The mechanism of action of PG-ALN@MSN for improving the bone healing process (A). Preparation of ALN@MSNs (B). Electrospinning of PG-ALN@MSN nanofibers (C). Adapted with permission from (Wang et al., 2018b). Copyright 2018 Royal Society of Chemistry.
6.2.2 Cartilage tissue engineering
Articular cartilage defects are considered one of the challenging issues in medicine. It can progress to osteoarthritis associated with high healthcare costs and negatively influence patients’ lives. Conventional therapeutic strategies are ineffective and mainly focus on relieving the pain or delaying tissue degradation. To this end, tissue engineering opens the door to treating articular cartilage defects. To date, various scaffolds with variable degrees of efficiency have been developed for such disorders. Among them, nanofibrous scaffolds have a high potential in cartilage engineering.
Chondroitin sulfate (CHS) is a major component of cartilage that plays a critical role in regulating many chondrocytes signaling pathways (Tang et al., 2019). Moreover, CHS can act as an antioxidant and immune regulating agent (Zou et al., 2020). Irani et al. (2020) fabricated electrospun hybrid nanofibers composed of PVA/gelatin/CHS with different concentrations of CHS (10%, 15%, 20%). They exhibited that a medium concentration of CHS (15%) had better biocompatibility than other test groups. In addition, they showed that after 21 days, cultured mesenchymal stem cells (MSCs) on the prepared scaffold could produce type II collagen. However, the mechanical properties of the scaffolds were not investigated in this study (Irani et al., 2020).
3D scaffolds are more desirable for tissue engineering than 2D scaffolds (He et al., 2020). Hence, Li et al. (2019) developed 3D spongy scaffolds by adding a decellularized cartilage matrix (DCECM) into gelatin/PCL electrospun nanofibers by utilizing the homogenization and freeze-drying method for cartilage tissue engineering. The resulting fabricated scaffold had good mechanical properties, stability, and biocompatibility. In addition, the DCEM components in the scaffold could enhance the proliferation rate of chondrocytes along with the secretion of collagen and glycosaminoglycan, leading to the early maturation of cartilage lacunae (Li et al., 2019). Table 5 provides an overview of major hybrid nanofibrous scaffolds used for cartilage tissue engineering applications.
6.2.3 Vascular tissue engineering
Nanofibers can also be applied for vascular tissue engineering and renovating blood vessels for clinical applications. The high porosity of nanofibers facilitates nutrient and gaseous transports leading to angiogenesis and vascular regeneration (Fraisl et al., 2009). In designing vascular scaffolds, some critical parameters should be taken into consideration by researchers, including the tensile stiffness, elasticity, and compressibility of a blood vessel (Thottappillil and Nair, 2015). Due to the high versatility of electrospun nanofibers, they can be used for developing tubular scaffolds for vascular tissue engineering. For instance, Yazdanpanah et al. (2015) fabricated nanofibrous scaffolds with a blend of PLA and gelatin. Considering the tensile test results, nanofibrous PLA/gelatin scaffolds improved mechanical strength and estimated burst pressure compared to layered PLA/gelatin and gelatin scaffolds (Yazdanpanah et al., 2015). In another study, Yang et al. (2020b) used electrospinning to fabricate nanofibrous scaffolds based on PCL and fibrin. They showed that a 20:80 PCL/fibrin graft in rats had good hemocompatibility and cytocompatibility along with satisfying mechanical properties and stability for 9 months.
Interestingly, the PCL/fibrin scaffold exhibited a better response to vasoconstrictors and vasodilators as the native artery compared to the pure PCL graft. In addition, similar to the native artery, a PCL/fibrin scaffold could increase cell infiltration, tissue regeneration, and deposition of ECM proteins, including collagen, elastin, and GAGs. They suggested PCL/fibrin scaffold as a promising tissue engineering material for vascular grafts (Yang et al., 2020b). Table 6 summarizes the major hybrid nanofibrous scaffolds utilized in vascular tissue engineering applications.
6.2.4 Cardiac tissue engineering
Myocardial infarction is one of the most prevalent disorders, leading to cardiomyocyte death due to an interruption in nutrient and oxygen transport in the myocardium. Following the infraction, the myocardium usually loses its regeneration ability. Therefore, implanting tissue-engineered myocardium into the damaged tissue is the easiest approach to compensate for tissue damage resulting from the infarction. The main challenge in myocardial tissue regeneration is providing an environment similar to native cardiac tissue (Weinberger et al., 2017; Lodrini and Goumans, 2021). Electrospun nanofiber-based scaffolds have been explored for cardiac tissue engineering. They are able to simulate myocardium ECM structure to increase cell adhesion, viability, and tissue regeneration. High conductivity and elasticity to mimic cardiac function are necessary for developing cardiac tissue scaffolds (Rahmati et al., 2021). Conductive nanofibrous scaffolds are highly desirable for cardiomyocytes-based bioactuators. However, few studies have worked on such scaffolds.
Developing conductive nanofibrous scaffolds by electrospinning would be beneficial for cardiomyocytes-based bioactuators, but such scaffolds have rarely been reported. The work performed by Rahmati et al. (2021) presented a conductive nanofibrous sheet with tunable conductivity based on polylactide and polyaniline (PANI) via electrospinning. These conductive nanofibrous sheets had the ability to enhance cardiomyocytes’ maturation and spontaneous beating. In addition, they could form cardiomyocyte-based 3D bioactuators with tubular and folding shapes, which indicated their great potential in cardiac tissue engineering and bioactuators’ applications (Rahmati et al., 2021).
Wang et al. (2017) designed conductive nanofibers based on PANI, an electroconductive polymer, and PLA composition as a cardiac tissue scaffold. The prepared PANI/PLA hybrid nanofibers displayed suitable biocompatibility and increased cellular interaction and spontaneous beating of primary cardiomyocytes. Furthermore, a satisfying amount of cardiomyocytes-based 3D bioactuators with tubular and folding shapes were formed.
Dippold et al. (2016) formulated elastic nanofibers composed of poly (glycerol sebacate) (PGS) and zein for cardiac engineering. Results exhibited that adding zein to PGS could augment the mechanical properties of PGS, and the fibers had good biocompatibility as well as stability for cardiac tissue engineering. In a more recent study, Kumar et al. (2020) prepared an aligned PCL/gelatin coaxial nanofiber patch via the electrospinning method. The cells on cardiac patches had synchronous contraction with a fast response to therapeutic agents. Therefore, they suggested the designed patches for in vitro drug screening in cardiotoxicity studies (Kumar et al., 2020). Table 7 summarizes the major hybrid nanofibrous scaffolds utilized in vascular tissue engineering applications.
6.2.5 Nerve tissue engineering
Peripheral nerve injury is one of the most common disorders which lead to devastating consequences with loss of motor and sensory function and poor quality of life. Currently, conventional treatments focus on nerve autografting to restore damaged nerves. Despite all the advances in neurology, this process has limitations, such as low efficiency and mismatching between the damaged and donor nerves. In addition, although peripheral nerves can regenerate to some extent, the results are not satisfying for serious injuries. Therefore, there is a need to find a novel approach instead of nerve autografting (Behtaj et al., 2022).
Nerve guidance conduits (NGCs) can be a promising strategy as an alternative to nerve autografts for improving functional outcomes. NGCs are tubular biostructures used to bridge nerve injury sites. Thus, they act as a guide and protective microenvironment for the regeneration and restoration of function in the target area (Behtaj et al., 2022). Designed NGS should mimic ECM to promote NGS interaction with nerves to induce neural repairment. Electrospun nanofibrous scaffolds have a high potential to replicate the native fibrous ECM and create an appropriate environment guiding nerve regeneration. In order to enhance the efficiency of nerve regeneration, the topological structure, bioelectricity, surface characteristics, permeability, degradation rate, and mechanical properties of scaffolds should be optimized (Behtaj et al., 2022).
For instance, Tian et al. (2016) fabricated a surface-modified, electrically conductive, aligned nanofibrous scaffold composed of PLA and polypyrrole (Ppy) for nerve regeneration. The effects of prepared electrospun nanofibers on neuronal differentiation using PC12 cells were assessed. In order to increase cell attachment to the nanofibers, they provided a hydrophilic surface with a Poly-ornithine coating. They showed that conductively aligned nanofibers based on PLA and Ppy guided PC12 cells’growth along the fiber direction and were advantageous for neurite outgrowth. Indeed, aligned fibers could promote cell proliferation, differentiation, and neurite outgrowth more than random fibers. Moreover, external electrical stimulation (40 mV) improved neurite outgrowth (Tian et al., 2016). Table 8 presents a summary of electrospun nanofibers used for nerve regeneration.
6.3 Wound healing
Wound healing is a complex process with different stages, including hemostasis, inflammation, proliferation, and remodeling. One of the critical steps in wound management is wound dressing to shield the wound from external risk factors and accelerate the healing process (Chen et al., 2020a; El Ayadi et al., 2020). Characteristics of an ideal wound dressing include: 1) absorbs excess exudate, 2) prevents infection, 3) keeps the moisture of the wound site, 4) ease of gas exchange, 5) is biocompatible and degradable, 6) does not stick to the wound, and easy to remove, 7) induces angiogenesis and tissue remodeling (Das et al., 2019; Fahimirad and Ajalloueian, 2019; Kanikireddy et al., 2020). Currently, different wound dressings exist in the market (e.g., hydrogels, foams, films, and nanofibers). Nanofiber membranes have received much attention among wound dressings due to their unique feature, high porosity, small pores, and large surface area. This structural feature of nanofibers can prevent pathogens’ growth in a wound’s environment and ensure gas and liquid molecules’ exchange. Electrospun nanofibers show high potential as wound dressings (Liu et al., 2021b). Table 9 offers an overview of electrospun hybrid nanofibers applied for wound dressing.
6.3.1 Types of wounds
Wounds refer to skin deformities or tissue discontinuities caused by physical or thermal injury or underlying ailments. Generally, wounds are divided into acute and chronic wounds. Acute wounds are usually caused by surface burns, chemical, and mechanical injuries, etc. Acute wounds can go through the normal process of wound healing. On the other hand, chronic wounds cannot go through this process and may be open for more than 1 month (Tottoli et al., 2020; Smet et al., 2021). Chronic wounds are commonly caused by particular diseases like diabetes. It is noteworthy that chronic wounds are susceptible to infection and inflammation, disturbing the process of wound healing. Chronic wounds are an important medical issue, putting a heavy burden on health care systems (Homaeigohar and Boccaccini, 2020; Smet et al., 2021).
6.3.2 Electrospun nanofiber for wound healing
6.3.2.1 Cell delivery
Full-thickness skin wounds that may result from different injuries can lead to many functional problems and strongly affect the quality of life. In these kinds of wounds, the skin cannot regenerate spontaneously due to the severity of damage to skin tissue. The standard treatment in these cases is thin split-thickness skin autografts. In this method, scar formation and donor site availability are the major hurdles. Where skin autografting is not possible, allografts or porcine xenografts are used, which are highly likely to reject the graft. Dermal cell or stem cell transplantation via electrospun dressings is a promising strategy for treating severe wounds (Gizaw et al., 2019; Behere and Ingavle, 2022). Different synthetic and natural polymers can be used to fabricate nanofiber-based scaffolds for skin tissue regeneration. In view of low cell attachment, proliferation, or infiltration in using only synthetic polymers, copolymerizations and blending with other hydrophilic polymers, especially combining with the skin tissue components − such as collagen, hyaluronic acid, and fibronectin—is highly recommended (Behere and Ingavle, 2022). For instance, in a recent study, Mirzaei-Parsa et al. (2019) developed nanofiber-based scaffolds for adipose-derived stem cells (ADSCs) as a wound dressing by electrospinning of PCL/fibrinogen. They reported that ADSCs seeded on nanofibers showed the best results for promoting re-epithelialization, angiogenesis, and collagen remodeling compared to the control and other tested groups (Mirzaei-Parsa et al., 2019).
In order to prevent infection in the damaged area, particularly for severe and chronic wounds, maintaining a skin-balanced microbiome is helpful. Probiotic strains have shown considerable healing properties for burn wounds. The potential of using probiotics for accelerating wound healing led to the development of probiotic (Enterococcus mundtii QAUEM2808)-functionalized PVA/PVP/glycerol electrospun scaffolds for wound dressing (Khan et al., 2019). They exhibited that obtained scaffolds could enhance probiotic survival with no disturbance in the function of probiotics. The scaffolds were degraded over time in a simulated wound fluid which is crucial for activating probiotic strains to inhibit Gram-positive and Gram-negative pathogens. Additionally, the scaffolds could accelerate re-epithelialization, collagen deposition, and hair follicle formation. Furthermore, they prevented bacterial infection and provided interference benefits in a second-degree contact burn on the dorsum of male BALB/c mice (Khan et al., 2019).
6.3.2.2 Growth factors
Due to the important role of growth factors in skin regeneration, using growth factors in wound dressings can accelerate the healing process. Lai et al. (2014) designed collagen and hyaluronic acid inter-stacking nanofibrous scaffolds containing four angiogenic growth factors, including vascular endothelial growth factor (VEGF), platelet-derived growth factor (PDGF), basic fibroblast growth factor (bFGF), and epidermal growth factor (EGF). It has been revealed that the delivery of EGF and bFGF in the early stage can improve epithelialization and vasculature sprouting. In contrast, the release of PDGF and VEGF in the late stage can stimulate blood vessel maturation (Lai et al., 2014).
Thus, for earlier release of EGF and bFGF, they were freely added into the polymeric matrix, whereas PDGF and VEGF were loaded into gelatin NPs to provide extended release of these growth factors in the damaged site. The mechanical properties of prepared scaffolds were the same as native skin and could provide sustained release of growth factors over 1 month. The scaffolds indicated elevated collagen deposition and angiogenesis on streptozotocin (STZ) induced diabetic rats and accelerated wound closure rate. Altogether, the composite nanofibrous electrospun scaffolds with a stage-wise release pattern of multiple angiogenic factors can be a promising candidate for chronic wound healing (Lai et al., 2014).
In order to provide prolonged release of growth factors in the damaged area, transgene expression of growth factors in dermal cells has been developed (Laiva et al., 2018). Nanofibers have the potential to be employed for the delivery of DNA. There are different strategies to incorporate plasmid DNA into fibrous scaffolds. Among them, core-sheath electrospun structures and DNA condensation techniques are the most efficient strategies that result in the desired DNA release profile (Afsharian and Rahimnejad, 2021). For instance, plasmid bFGF (pbFGF) polyplex with poly (ethylene imine) were incorporated into poly (dl-lactide)-poly-(ethylene glycol) (PELA)/PEG nanofibers with core-sheath structures by emulsion electrospinning for skin wound healing in diabetic rats (Yang et al., 2012). The sustained release of pbFGF could considerably improve wound recovery rate, vascularization, collagen deposition, and maturation, leading to complete re-epithelialization and formation of skin appendages.
6.3.2.3 Drugs
In order to accelerate the process of wound healing and regeneration, nanofibers can be loaded with different drugs such as antibiotics, pain relievers, etc. For instance, Varshosaz et al. (2020) prepared a wound dressing membrane based on hybrid nanofibers using modified polybutylene adipate-co-terephthalate and gelatin, loaded with doxycycline, by using the double electrospinning technique. In these nanofibrous structures, polybutylene adipate-co-terephthalate was used to enhance the mechanical properties, and gelatin was applied to provide a water-soluble part inside the scaffold to control the release and achieve better ECM mimicry. Moreover, to improve cell adhesion, prepared electrospun nanofibers were modified by RGD, a peptide with a critical role in cell adhesion to the ECM. Also, the presence of doxycycline in nanofibers could prevent bacterial infection in the wound environment, particularly strains of S. aureus and p. aeruginosa. The nanofibers showed considerable positive effects on the wound healing process within 3 days after initiation of the treatment. However, the used polymers in the composition of nanofibers did not show noticeable improvement in the scaffold’s mechanical properties (Varshosaz et al., 2020).
In another study, pH-responsive coaxial nanofibers were developed to co-deliver two drugs—lidocaine hydrochloride used for pain relief and curcumin as an anti-inflammatory agent (Guo et al., 2020). In this study, the composition of chitosan/PEO was used as a shell part for loading lidocaine, and PCL containing curcumin was placed at the core. Additionally, sodium bicarbonate was used within the core layer to provide a pH-responsive release of curcumin. The pH-responsive release of drugs in this system was achieved by employing chitosan and sodium bicarbonate. Under an acidic environment, chitosan was protonated, and lidocaine was released. Also, sodium bicarbonate generated CO2 by reacting with hydrogen ions, resulting in many holes in nanofiber structures and curcumin release. This novel design led to an immediate release of lidocaine to decrease pain, and when the inflammatory phase started—leading to a more acidic wound environment—the release of curcumin was intensified. It is noteworthy that curcumin could exert antibacterial effects against E. coli and S. aureus (Guo et al., 2020).
Burns are another major skin damage that may be life-threatening or influence the quality of life. Usually, skin damages caused by burning are more susceptible to bacterial infection due to loss of skin integrity. In a recent study, Hadisi et al. (2020) developed novel core-shell nanofibers with hyaluronic acid and silk protein. Hyaluronic acid was selected among various natural polymers because of its high potential in accelerating wound healing by modulating inflammatory responses, angiogenesis, and cell migration. However, due to its insufficient mechanical properties and fast degradation, it had to be combined with another polymer with high mechanical resistance. Therefore, silk protein was chosen to solve the mentioned drawbacks of hyaluronic acid. In addition, zinc oxide was added to the blend of hyaluronic and silk protein owing to its antibacterial effects. Prepared hybrid nanofibers showed noticeable healing effects and antibacterial properties against E. coli and S. aureus in a scratch assay in vitro and in vivo (Hadisi et al., 2020).
6.3.2.4 Herbal compounds
Bromelain, a mixture of proteolytic enzymes derived from pineapple tissues, is a natural debriding agent which can remove fibrin clots in the burned area. Furthermore, bromelain can improve and accelerate the healing process in the damaged area. In a recent study done by Bayat et al. (2019), PEO/chitosan nanofibers were loaded with bromelain by the electrospinning method. These nanofibers showed considerable healing effects and decreased burned lesions in the animal model. Complete regeneration of skin was achieved when applying these fibrous nanostructures (Bayat et al., 2019).
Zhang et al. (2019a) prepared a nanofibrous electrospun based on silk fibroin (SF) and gelatin polymers loaded with Astragaloside IV (AS-IV) for promoting wound healing and relieving scars in vivo in an acute trauma model. AS-IV is a natural active component with antioxidant, immunostimulant, anti-inflammatory, and angiogenic effects. Results showed that the SF/gelatin/AS-IV nanofibers could potentiate wound recovery and angiogenesis. Additionally, they prevented scar complications. Quercetin is a flavonoid derived from rutin with remarkable antioxidant activity found in fruits and vegetables. Quercetin and rutin have a high potential to be used for wound healing, considering their favorable characteristics, including antioxidant, anti-inflammatory, and antibacterial activities and pain management.
Zhou et al. (2021) prepared a membrane of electrospun fibers composed of PCL, chitosan oligosaccharides, and quercetin/rutin to heal burned skin. Developed nanofibers exhibited high antibacterial and antioxidant effects in the wound environment. Generally, this study showed PCL/chitosan/Quercetin/Rutin nanofibers’ potential for developing burn dressings.
Lawsone (Law) is a quinone derived from the leaves of the henna plant. In a recent study, lawsone was incorporated into PCL/gelatin nanofiber by the coaxial electrospinning technique and tested for wound healing activity. This compound was successfully entrapped into the core polymer. As a result of wound healing activity, PCL/gelatin/Law mats of 0.5% and 1% increased the expression of transforming growth factor-beta (TGF-β1) and collagen type 1(COL1) genes as well as rat wound re-epithelialization in vivo (Adeli-Sardou et al., 2019).
Lavender oil is an essential oil with various pharmacological effects, including antibacterial, antifungal, carminative (smooth muscle relaxant), sedative, and wound healing properties for burns and insect bites (Cavanagh and Wilkinson, 2002). Hajiali et al. (2016) prepared electrospun nanofibers with a blend of sodium alginate (SA) and PEO embedded with lavender oil. The SA-PEO/lavender oil fibers could effectively inhibit bacterial colonies and also inflammation, resulting in a considerable decrease in interleukin 6 (IL-6) (66%) and IL-8 (49%) concentrations. The SA-PEO/lavender oil nanofibers in the animal model could also moderate cytokine production and inflammation and improve wound recovery without leaving marks or spots on the skin (Hajiali et al., 2016).
6.4 Biosensors
Biosensors are analytical devices that play an important role in both research and the market—owing to their potential to detect various substrates for clinical diagnostics (Li et al., 2021b), food analysis (Ali et al., 2020), and environmental monitoring (Hara and Singh, 2021). Biosensors have some advantages compared to conventional sensors, such as portability, selectivity, and sensitivity. In addition, they can offer a fast, facile, and cost-effective assay of analytes (Dincer et al., 2019). Biosensors consist of three main parts: 1) a biorecognition element for sensing the analyte, 2) a transducer for converting the biological response into a quantitative signal, and 3) a signal capture for measuring signals derived from biological interactions (Figure 5). Indeed, biosensors are based on biological interactions between recognizing biomolecules and target analytes and converting them to measurable signals. The functionality and efficacy of biosensors are associated with the materials used to immobilize bio-receptors (Cavalcante et al., 2021). In this process, the biomolecules should fix on the transducer’s surface, and their functionality should be maintained during the assay (Scouten et al., 1995). In recent years, nanomaterials have received increasing attention for engineering electrode surfaces into appropriate immobilization matrices (Guan et al., 2008). For example, high surface area and ease of surface functionalization in nanofibers make them an attractive candidate for the development of biosensors. The large surface area of nanofibers can increase biosensors’ sensitivity by enhancing the possibilities of interaction with analytes (Mercante et al., 2017).
Moreover, the high porosity of nanofibers leads to low mass transport resistance, thereby elevating analyte diffusion through the sensing layer (Zhang et al., 2017a). According to aimed application, the mechanical properties, hydrophilicity, and stability of nanofibers should be modified by adjusting electrospinning parameters and materials used. In order to enhance the interaction site for the target analyte and biosensors’ sensitivity, the composition of nanofibers should be modulated. In this regard, various synthetic and natural polymers have been used for designing nanofiber-based biosensors. Along with synthetic and natural polymers, conductive polymers including poly (3,4-ethylene dioxythiophene) (PEDOT) (Yang et al., 2014), poly (pyrrole) (PPy) (Hazarika and Kumar, 2017), and PANI (Scagion et al., 2016) can also be combined with other macromolecules to provide the obtained nanofibers with electrical, electrochemical and electromechanical properties. Nanostructures, such as inorganic NPs (Zhu et al., 2013), graphene quantum dots (Zhang et al., 2015), CNTs (Li et al., 2014), and reduced graphene oxide (rGO) (Pavinatto et al., 2018), can be added to polymeric matrices to improve nanofiber-based biosensors’ transducer performance or increase their capability for sensing analytes by modulating mechanical, optical, electrical, and thermal characteristics of nanofibers (Mercante et al., 2017). However, optimizing the material composition in these cases is challenging due to the high tendency of NPs for aggregation, which can disturb the sensing function of biosensors (Mercante et al., 2017).
7 Conclusion and future perspectives
In recent years, electrospinning technology has attracted increasing attention for fabricating micro or nanofibers. Electrospun nanofibers have some outstanding characteristics, including high surface-to-volume ratios, high porosity, low cost, easy fabrication and surface functionalization, and adjustable fiber morphology, making them attractive for biomedical use. A wide range of polymers with different sources can be applied for hybrid nanofiber fabrication. Generally, polymers are divided into natural and synthetic polymers. Biopolymers are non-toxic with higher biocompatibility and biodegradability compared to synthetic polymers.
Additionally, they may possess unique biological activities such as antibacterial, antifungal, and anticancer effects. Despite all their benefits, biopolymers have poor mechanical properties compared to synthetic polymers. Hence, biopolymers and synthetic polymers can be combined and make hybrid nanofibers to benefit from the advantages of both polymers. There are several techniques to prepare electrospun hybrid nanofibers with different structures. Furthermore, functional agents such as drugs, biomolecules, and NPs can be incorporated into a polymeric matrix to fabricate multifunctional hybrid nanofibers.
The main and basic setup to fabricate hybrid nanofibers include: 1) blend electrospinning, 2) coaxial or triaxial electrospinning, 3) emulsion electrospinning, and 4) side-by-side electrospinning. The future will bring more advanced and complex multi-fluid electrospinning technologies for producing nanofibers with unique collective properties.
Electrospun nanofibers are ideal candidates for various biomedical and healthcare applications, especially the antimicrobial treatment of bacteria-related biofilms, orthopedic implant-related infections, tissue scaffolds, drug delivery, wound dressings, and biosensors. However, some challenges should be considered to improve the functionality of nanofibers. For instance: 1) the pore size of nanofibrous scaffolds is too small, making cell infiltration difficult. 2) The mechanical strength of electrospun nanofiber-based scaffolds is usually not enough for hard tissue regeneration. 3) Controlling in vivo degradation of nanofibers is a complicated process 4) Industrial fabrication of electrospun hybrid nanofibers remains challenging. It is noteworthy that although nanofibers have shown promising results in preclinical studies, many clinical studies are still needed to ensure their clinical applications.
In the future, it is necessary to improve the function of electrospun nanofibers and develop new functional nanofibers to provide more technical support for biomedical use. As a result of the trend and prospects, nanofiber-miniaturized systems are increasingly used for next-generation biomedicine platforms in clinical and point-of-care studies. In addition, the combination of 3D printing and computational simulation with electrospinning is the potential trend. Ultimately, we believe all these will be achieved in the future with the development of science and technology.
Author contributions
The main idea for this work was conceived by HF and MA-S. Fabrication methods were written by BA, MA-S, and JT. Characterization was written by NG and MA-S. BA wrote the biomedical applications of hybrid nanofibers. Figures were produced by BA. SB contributed in writing the fabrication and biomedical application sections. She also checked the English proficiency of the manuscript.
Conflict of interest
The authors declare that the research was conducted in the absence of any commercial or financial relationships that could be construed as a potential conflict of interest.
Publisher’s note
All claims expressed in this article are solely those of the authors and do not necessarily represent those of their affiliated organizations, or those of the publisher, the editors and the reviewers. Any product that may be evaluated in this article, or claim that may be made by its manufacturer, is not guaranteed or endorsed by the publisher.
References
Abdal-Hay, A., Bartnikowski, M., Hamlet, S., and Ivanovski, S. (2018). Electrospun biphasic tubular scaffold with enhanced mechanical properties for vascular tissue engineering. Mater. Sci. Eng. C 82, 10–18. doi:10.1016/j.msec.2017.08.041
Abel, S. B., Ballarin, F. M., and Abraham, G. A. J. N. (2020). Combination of electrospinning with other techniques for the fabrication of 3D polymeric and composite nanofibrous scaffolds with improved cellular interactions. Nanotechnology 31, 172002. doi:10.1088/1361-6528/ab6ab4
Adeli, H., Khorasani, M. T., and Parvazinia, M. (2019). Wound dressing based on electrospun PVA/chitosan/starch nanofibrous mats: Fabrication, antibacterial and cytocompatibility evaluation and in vitro healing assay. Int. J. Biol. Macromol. 122, 238–254. doi:10.1016/j.ijbiomac.2018.10.115
Adeli-Sardou, M., Yaghoobi, M. M., Torkzadeh-Mahani, M., and Dodel, M. (2019). Controlled release of lawsone from polycaprolactone/gelatin electrospun nano fibers for skin tissue regeneration. Int. J. Biol. Macromol. 124, 478–491. doi:10.1016/j.ijbiomac.2018.11.237
Afsharian, Y. P., and Rahimnejad, M. (2021). Bioactive electrospun scaffolds for wound healing applications: A comprehensive review. Polym. Test. 93, 106952. doi:10.1016/j.polymertesting.2020.106952
Agarwal, Y., Rajinikanth, P. S., Ranjan, S., Tiwari, U., Balasubramnaiam, J., Pandey, P., et al. (2021). Curcumin loaded polycaprolactone-/polyvinyl alcohol-silk fibroin based electrospun nanofibrous mat for rapid healing of diabetic wound: An in-vitro and in-vivo studies. Int. J. Biol. Macromol. 176, 376–386. doi:10.1016/j.ijbiomac.2021.02.025
Aggarwal, U., Goyal, A. K., and Rath, G. (2017). Development and characterization of the cisplatin loaded nanofibers for the treatment of cervical cancer. Mater. Sci. Eng. C 75, 125–132. doi:10.1016/j.msec.2017.02.013
Ahmadian, S., Ghorbani, M., and Mahmoodzadeh, F. (2020). Silver sulfadiazine-loaded electrospun ethyl cellulose/polylactic acid/collagen nanofibrous mats with antibacterial properties for wound healing. Int. J. Biol. Macromol. 162, 1555–1565. doi:10.1016/j.ijbiomac.2020.08.059
Ahn, H., Ju, Y. M., Takahashi, H., Williams, D. F., Yoo, J. J., Lee, S. J., et al. (2015). Engineered small diameter vascular grafts by combining cell sheet engineering and electrospinning technology. Acta Biomater. 16, 14–22. doi:10.1016/j.actbio.2015.01.030
Alghoraibi, I., and Alomari, S. (2018). “Different methods for nanofiber design and fabrication,” in Handbook of nanofibers. Editors A. BARHOUM, M. BECHELANY, and A. MAKHLOUF (Cham: Springer International Publishing).
Ali, A. A., Altemimi, A. B., Alhelfi, N., and Ibrahim, S. A. (2020). Application of biosensors for detection of pathogenic food bacteria: A review. Biosensors 10, 58. doi:10.3390/bios10060058
Alipour, M., Baneshi, M., Hosseinkhani, S., Mahmoudi, R., Jabari Arabzadeh, A., Akrami, M., et al. (2020). Recent progress in biomedical applications of RGD-based ligand: From precise cancer theranostics to biomaterial engineering: A systematic review. J. Biomed. Mat. Res. A 108, 839–850. doi:10.1002/jbm.a.36862
Alvarez-Perez, M. A., Guarino, V., Cirillo, V., and Ambrosio, L. (2010). Influence of gelatin cues in PCL electrospun membranes on nerve outgrowth. Biomacromolecules 11, 2238–2246. doi:10.1021/bm100221h
Amaraweera, S. M., Gunathilake, C., Gunawardene, O. H. P., Fernando, N. M. L., Wanninayaka, D. B., Dassanayake, R. S., et al. (2021). Development of starch-based materials using current modification techniques and their applications: A review. Molecules 26, 6880. doi:10.3390/molecules26226880
Amiri, N., Ajami, S., Shahroodi, A., Jannatabadi, N., Amiri Darban, S., Fazly Bazzaz, B. S., et al. (2020). Teicoplanin-loaded chitosan-PEO nanofibers for local antibiotic delivery and wound healing. Int. J. Biol. Macromol. 162, 645–656. doi:10.1016/j.ijbiomac.2020.06.195
Annur, D., Wang, Z.-K., Liao, J.-D., and Kuo, C. (2015). Plasma-synthesized silver nanoparticles on electrospun chitosan nanofiber surfaces for antibacterial applications. Biomacromolecules 16, 3248–3255. doi:10.1021/acs.biomac.5b00920
Awasthi, G. P., Kaliannagounder, V. K., Maharjan, B., Lee, J. Y., Park, C. H., and Kim, C. S. (2020). Albumin-induced exfoliation of molybdenum disulfide nanosheets incorporated polycaprolactone/zein composite nanofibers for bone tissue regeneration. Mater. Sci. Eng. C 116, 111162. doi:10.1016/j.msec.2020.111162
BostancI, A., Tanik, N. A., and Aykut, Y. (2019). Cellulose monoacetate/Nafion (CMA/N) hybrid nanofibers as interface for electrochemical DNA biosensors. TEKSTİL ve KONFEKSİYON 29, 228–236. doi:10.32710/tekstilvekonfeksiyon.501435
Bai, J., Wang, S., Li, Y., Wang, Z., and Tang, J. (2021). Effect of chemical structure and molecular weight on the properties of lignin-based ultrafine carbon fibers. Int. J. Biol. Macromol. 187, 594–602. doi:10.1016/j.ijbiomac.2021.07.149
Balogh, A., Drávavölgyi, G., Faragó, K., Farkas, A., Vigh, T., Sóti, P. L., et al. (2014). Plasticized drug-loaded melt electrospun polymer mats: Characterization, thermal degradation, and release kinetics. J. Pharm. Sci. 103, 1278–1287. doi:10.1002/jps.23904
Barhoum, A., Pal, K., Rahier, H., Uludag, H., Kim, I. S., and Bechelany, M. (2019a). Nanofibers as new-generation materials: From spinning and nano-spinning fabrication techniques to emerging applications. Appl. Mater. Today 17, 1–35. doi:10.1016/j.apmt.2019.06.015
Barhoum, A., Rasouli, R., Yousefzadeh, M., Rahier, H., and Bechelany, M. (2019b). “Nanofiber technologies: History and development,” in Handbook of nanofibers. Editors A. BARHOUM, and A. MAKHLOUF (Cham: Springer).
Baştürk, E., and Kahraman, M. V. (2012). Thermal and morphological properties of PVA/4-vinylbenzene boronic acid hybrid nanofibrous. Polym. Compos. 33, 829–837. doi:10.1002/pc.22219
Bayat, S., Amiri, N., Pishavar, E., Kalalinia, F., Movaffagh, J., and Hashemi, M. (2019). Bromelain-loaded chitosan nanofibers prepared by electrospinning method for burn wound healing in animal models. Life Sci. 229, 57–66. doi:10.1016/j.lfs.2019.05.028
Bayer, I. S. (2020). Hyaluronic acid and controlled release: A review, Molecules 25, 2649. doi:10.3390/molecules25112649
Begum, R., Perriman, A. W., Su, B., Scarpa, F., and Kafienah, W. (2018). Electrospun cellulose-silk composite nanofibres direct mesenchymal stem cell chondrogenesis in the absence of biological stimulation. bioRxiv, 434316. doi:10.1101/434316
Behere, I., and Ingavle, G. (2022). In vitro and in vivo advancement of multifunctional electrospun nanofiber scaffolds in wound healing applications: Innovative nanofiber designs, stem cell approaches, and future perspectives. J. Biomed. Mat. Res. A 110, 443–461. doi:10.1002/jbm.a.37290
Behtaj, S., Ekberg, J. A. K., and St John, J. A. (2022). Advances in electrospun nerve guidance conduits for engineering neural regeneration. Pharmaceutics, 14, 219. doi:10.3390/pharmaceutics14020219
Bhaarathy, V., Venugopal, J., Gandhimathi, C., Ponpandian, N., Mangalaraj, D., and Ramakrishna, S. (2014). Biologically improved nanofibrous scaffolds for cardiac tissue engineering. Mater. Sci. Eng. C 44, 268–277. doi:10.1016/j.msec.2014.08.018
Bhowmick, B., Mollick, M. M. R., Mondal, D., Maity, D., Bain, M. K., Bera, N. K., et al. (2014). Poloxamer and gelatin gel guided polyaniline nanofibers: Synthesis and characterization. Polym. Int. 63, 1505–1512. doi:10.1002/pi.4657
Blakney, A. K., Ball, C., Krogstad, E. A., and Woodrow, K. A. (2013). Electrospun fibers for vaginal anti-HIV drug delivery. Antivir. Res. 100, S9–S16. doi:10.1016/j.antiviral.2013.09.022
Boda, S. K., Chen, S., Chu, K., Kim, H. J., and Xie, J. (2018). Electrospraying electrospun nanofiber segments into injectable microspheres for potential cell delivery. ACS Appl. Mat. Interfaces 10, 25069–25079. doi:10.1021/acsami.8b06386
Castro, K. C., Campos, M. G. N., and Mei, L. H. I. (2021). Hyaluronic acid electrospinning: Challenges, applications in wound dressings and new perspectives. Int. J. Biol. Macromol. 173, 251–266. doi:10.1016/j.ijbiomac.2021.01.100
Cavalcante, F. T. T., Defalcão, A., Souza, D. A. S., Rocha, T. G., de Sousa, I. G., Cavalcante, A. L. G., et al. (2021). Designing of nanomaterials-based enzymatic biosensors: Synthesis, properties, and applications. Electrochem 2, 149–184. doi:10.3390/electrochem2010012
Cavanagh, H. M., and Wilkinson, J. M. (2002). Biological activities of lavender essential oil. Phytother. Res. 16, 301–308. doi:10.1002/ptr.1103
Chen, C., Mehl, B. T., Sell, S. A., and Martin, R. S. (2016a). Use of electrospinning and dynamic air focusing to create three-dimensional cell culture scaffolds in microfluidic devices. Analyst 141, 5311–5320. doi:10.1039/c6an01282e
Chen, K., Wang, F., Liu, S., Wu, X., Xu, L., and Zhang, D. (2020a). In situ reduction of silver nanoparticles by sodium alginate to obtain silver-loaded composite wound dressing with enhanced mechanical and antimicrobial property. Int. J. Biol. Macromol. 148, 501–509. doi:10.1016/j.ijbiomac.2020.01.156
Chen, W., Ma, J., Zhu, L., Morsi, Y., Ei-Hamshary, H., al-Deyab, S. S., et al. (2016b). Superelastic, superabsorbent and 3D nanofiber-assembled scaffold for tissue engineering. Colloids Surfaces B Biointerfaces 142, 165–172. doi:10.1016/j.colsurfb.2016.02.050
Chen, W., Wang, C., Gao, Y., Wu, Y., Wu, G., Shi, X., et al. (2020b). Incorporating chitin derived glucosamine sulfate into nanofibers via coaxial electrospinning for cartilage regeneration. Carbohydr. Polym. 229, 115544. doi:10.1016/j.carbpol.2019.115544
Chen, W., Xu, Y., Li, Y., Jia, L., Mo, X., Jiang, G., et al. (2020c). 3D printing electrospinning fiber-reinforced decellularized extracellular matrix for cartilage regeneration. Chem. Eng. J. 382, 122986. doi:10.1016/j.cej.2019.122986
Chen, W., Xu, Y., Liu, Y., Wang, Z., Li, Y., Jiang, G., et al. (2019a). Three-dimensional printed electrospun fiber-based scaffold for cartilage regeneration. Mater. Des. 179, 107886. doi:10.1016/j.matdes.2019.107886
Chen, Y., Dong, X., Shafiq, M., Myles, G., Radacsi, N., and Mo, X. (2022). Recent advancements on three-dimensional electrospun nanofiber scaffolds for tissue engineering. Adv. Fiber Mat. 4, 959–986. doi:10.1007/s42765-022-00170-7
Chen, Y., Jia, Z., Shafiq, M., Xie, X., Xiao, X., Castro, R., et al. (2021). Gas foaming of electrospun poly(L-lactide-co-caprolactone)/silk fibroin nanofiber scaffolds to promote cellular infiltration and tissue regeneration. Colloids Surfaces B Biointerfaces 201, 111637. doi:10.1016/j.colsurfb.2021.111637
Chen, Y., Taskin, M. B., Zhang, Z., Su, Y., Han, X., and Chen, M. (2019b). Bioadhesive anisotropic nanogrooved microfibers directing three-dimensional neurite extension. Biomater. Sci. 7, 2165–2173. doi:10.1039/c8bm01603h
Chin, D.-T., and Chang, H. H. (1989). On the conductivity of phosphoric acid electrolyte. J. Appl. Electrochem. 19, 95–99. doi:10.1007/bf01039396
Ching, K. Y., Andriotis, O., Sengers, B., and Stolz, M. (2021). Genipin crosslinked chitosan/PEO nanofibrous scaffolds exhibiting an improved microenvironment for the regeneration of articular cartilage. J. Biomater. Appl. 36, 503–516. doi:10.1177/08853282211002015
Chipara, D. M., Macossay, J., Ybarra, A. V. R., Chipara, A. C., Eubanks, T. M., and Chipara, M. (2013). Raman spectroscopy of polystyrene nanofibers—multiwalled carbon nanotubes composites. Appl. Surf. Sci. 275, 23–27. doi:10.1016/j.apsusc.2013.01.116
Contreras-Cáceres, R., Cabeza, L., Perazzoli, G., Díaz, A., López-Romero, J. M., Melguizo, C., et al. (2019). Electrospun nanofibers: Recent applications in drug delivery and cancer therapy. Nanomaterials 9, 656. doi:10.3390/nano9040656
Dalgic, A. D., Atila, D., Karatas, A., Tezcaner, A., Keskin, D. J. M. S., and C, E. (2019). Diatom shell incorporated PHBV/PCL-pullulan co-electrospun scaffold for bone tissue engineering. Mater. Sci. Eng. C 100, 735–746. doi:10.1016/j.msec.2019.03.046
Das, A., Uppaluri, R., and Das, C. (2019). Feasibility of poly-vinyl alcohol/starch/glycerol/citric acid composite films for wound dressing applications. Int. J. Biol. Macromol. 131, 998–1007. doi:10.1016/j.ijbiomac.2019.03.160
Deng, L., Zhang, X., Li, Y., Que, F., Kang, X., Liu, Y., et al. (2018). Characterization of gelatin/zein nanofibers by hybrid electrospinning. Food Hydrocoll. 75, 72–80. doi:10.1016/j.foodhyd.2017.09.011
Dincer, C., Bruch, R., Costa-Rama, E., Fernández-Abedul, M. T., Merkoçi, A., Manz, A., et al. (2019). Disposable sensors in diagnostics, food, and environmental monitoring. Adv. Mat. 31, 1806739. doi:10.1002/adma.201806739
Dippold, D., Tallawi, M., Tansaz, S., Roether, J. A., and Boccaccini, A. R. (2016). Novel electrospun poly(glycerol sebacate)–zein fiber mats as candidate materials for cardiac tissue engineering. Eur. Polym. J. 75, 504–513. doi:10.1016/j.eurpolymj.2015.12.030
Duan, N., Geng, X., Ye, L., Zhang, A., Feng, Z., Guo, L., et al. (2016). A vascular tissue engineering scaffold with core-shell structured nano-fibers formed by coaxial electrospinning and its biocompatibility evaluation. Biomed. Mat. 11, 035007. doi:10.1088/1748-6041/11/3/035007
el Ayadi, A., Jay, J. W., and Prasai, A. (2020). Current approaches targeting the wound healing phases to attenuate fibrosis and scarring. Int. J. Mol. Sci. 21, 1105. doi:10.3390/ijms21031105
el Fawal, G., Hong, H., Mo, X., and Wang, H. (2021). Fabrication of scaffold based on gelatin and polycaprolactone (PCL) for wound dressing application. J. Drug Deliv. Sci. Technol. 63, 102501. doi:10.1016/j.jddst.2021.102501
el-Aassar, M. R., Ibrahim, O. M., and al-Oanzi, Z. H. (2021). Biotechnological applications of polymeric nanofiber platforms loaded with diverse bioactive materials, 13. Basel: Polymers.
Enculescu, M., Costas, A., Evanghelidis, A., and Enculescu, I. (2021). Fabrication of ZnO and TiO2 nanotubes via flexible electro-spun nanofibers for photocatalytic applications. Nanomaterials 11, 1305. doi:10.3390/nano11051305
Eom, S., Park, S. M., Hong, H., Kwon, J., Oh, S.-R., Kim, J., et al. (2020). Hydrogel-assisted electrospinning for fabrication of a 3D complex tailored nanofiber macrostructure. ACS Appl. Mat. Interfaces 12, 51212–51224. doi:10.1021/acsami.0c14438
Eren Boncu, T., Uskudar Guclu, A., Catma, M. F., Savaser, A., Gokce, A., and Ozdemir, N. (2020). In vitro and in vivo evaluation of linezolid loaded electrospun PLGA and PLGA/PCL fiber mats for prophylaxis and treatment of MRSA induced prosthetic infections. Int. J. Pharm. X. 573, 118758. doi:10.1016/j.ijpharm.2019.118758
Esmaeili, A., and Haseli, M. (2017). Electrospinning of thermoplastic carboxymethyl cellulose/poly(ethylene oxide) nanofibers for use in drug-release systems. Mater. Sci. Eng. C 77, 1117–1127. doi:10.1016/j.msec.2017.03.252
Everitt, D. E., and Avorn, J. (1990). Systemic effects of medications used to treat glaucoma. Ann. Intern. Med. 112, 120–125. doi:10.7326/0003-4819-112-2-120
Fahimirad, S., Abtahi, H., Satei, P., Ghaznavi-Rad, E., Moslehi, M., and Ganji, A. (2021). Wound healing performance of PCL/chitosan based electrospun nanofiber electrosprayed with curcumin loaded chitosan nanoparticles. Carbohydr. Polym. 259, 117640. doi:10.1016/j.carbpol.2021.117640
Fahimirad, S., and Ajalloueian, F. (2019). Naturally-derived electrospun wound dressings for target delivery of bio-active agents. Int. J. Pharm. X. 566, 307–328. doi:10.1016/j.ijpharm.2019.05.053
Fan, Y., Tian, X., Zheng, L., Jin, X., Zhang, Q., Xu, S., et al. (2021). Yeast encapsulation in nanofiber via electrospinning: Shape transformation, cell activity and immobilized efficiency. Mater. Sci. Eng. C 120, 111747. doi:10.1016/j.msec.2020.111747
Farandos, N. M., Yetisen, A. K., Monteiro, M. J., Lowe, C. R., and Yun, S. H. (2015). Contact lens sensors in ocular diagnostics. Adv. Healthc. Mat. 4, 792–810. doi:10.1002/adhm.201400504
Farrokhi-Rad, M. (2016). Electrophoretic deposition of hydroxyapatite nanoparticles in different alcohols: Effect of Tris (tris(hydroxymethyl)aminomethane) as a dispersant. Ceram. Int. 42, 3361–3371. doi:10.1016/j.ceramint.2015.10.130
Filip, P., Zelenkova, J., and Peer, P. (2021). Electrospinning of a copolymer PVDF-co-HFP solved in DMF/acetone: Explicit relations among viscosity, polymer concentration, DMF/acetone ratio and mean nanofiber diameter. Polymers 13, 3418. doi:10.3390/polym13193418
Foraida, Z. I., Kamaldinov, T., Nelson, D. A., Larsen, M., and Castracane, J. (2017). Elastin-PLGA hybrid electrospun nanofiber scaffolds for salivary epithelial cell self-organization and polarization. Acta Biomater. 62, 116–127. doi:10.1016/j.actbio.2017.08.009
Fraisl, P., Mazzone, M., Schmidt, T., and Carmeliet, P. (2009). Regulation of angiogenesis by oxygen and metabolism. Dev. Cell. 16, 167–179. doi:10.1016/j.devcel.2009.01.003
Frohbergh, M. E., Katsman, A., Botta, G. P., Lazarovici, P., Schauer, C. L., Wegst, U. G. K., et al. (2012). Electrospun hydroxyapatite-containing chitosan nanofibers crosslinked with genipin for bone tissue engineering. Biomaterials 33, 9167–9178. doi:10.1016/j.biomaterials.2012.09.009
Fukunishi, T., Best, C. A., Sugiura, T., Opfermann, J., Ong, C. S., Shinoka, T., et al. (2017). Preclinical study of patient-specific cell-free nanofiber tissue-engineered vascular grafts using 3-dimensional printing in a sheep model. J. Thorac. Cardiovasc. Surg. 153, 924–932. doi:10.1016/j.jtcvs.2016.10.066
Gao, J., Luo, J., and Xiong, J. (2017). A facile method for tailoring the three-dimensional porous nanofibrous scaffolds by the dual electrode electrospinning. Mater. Lett. 209, 384–387. doi:10.1016/j.matlet.2017.08.032
Garg, T., Singh, O., Arora, S., and Murthy, R. (2012). Scaffold: A novel carrier for cell and drug delivery. Crit. Rev. Ther. Drug Carr. Syst. 29, 1–63. doi:10.1615/critrevtherdrugcarriersyst.v29.i1.10
Ghajarieh, A., Habibi, S., and Talebian, A. (2021). Biomedical applications of nanofibers. Russ. J. Appl. Chem. 97, 847–872. doi:10.1134/s1070427221070016
Ghasemi-Mobarakeh, L., Prabhakaran, M. P., Morshed, M., Nasr-Esfahani, M.-H., and Ramakrishna, S. (2008). Electrospun poly(ɛ-caprolactone)/gelatin nanofibrous scaffolds for nerve tissue engineering. Biomaterials 29, 4532–4539. doi:10.1016/j.biomaterials.2008.08.007
Ghorbani, M., Nezhad-Mokhtari, P., and Ramazani, S. (2020). Aloe vera-loaded nanofibrous scaffold based on Zein/Polycaprolactone/Collagen for wound healing. Int. J. Biol. Macromol. 153, 921–930. doi:10.1016/j.ijbiomac.2020.03.036
Gizaw, M., Faglie, A., Pieper, M., Poudel, S., and Chou, S. F. (2019). The role of electrospun fiber scaffolds in stem cell therapy for skin tissue regeneration. Med. One 4, e190002. doi:10.20900/mo.20190002
Grimaudo, M. A., Concheiro, A., and Alvarez-Lorenzo, C. (2020). Crosslinked hyaluronan electrospun nanofibers for ferulic acid ocular delivery. Pharmaceutics, 12, 274. doi:10.3390/pharmaceutics12030274
Guan, G., Liu, B., Wang, Z., and Zhang, Z. (2008). Imprinting of molecular recognition sites on nanostructures and its applications in chemosensors. Sensors 8, 8291–8320. doi:10.3390/s8128291
Gugulothu, D., Barhoum, A., Nerella, R., Ajmer, R., and Bechelany, M. (2019). “Fabrication of nanofibers: Electrospinning and non-electrospinning techniques,” in Handbook of nanofibers. Editors A. BARHOUM, M. BECHELANY, and A. MAKHLOUF (Cham: Springer).
Guo, H., Tan, S., Gao, J., and Wang, L. (2020). Sequential release of drugs form a dual-delivery system based on pH-responsive nanofibrous mats towards wound care. J. Mat. Chem. B 8, 1759–1770. doi:10.1039/c9tb02522g
Hadisi, Z., Farokhi, M., Bakhsheshi-Rad, H. R., Jahanshahi, M., Hasanpour, S., Pagan, E., et al. (2020). Hyaluronic acid (HA)-Based silk fibroin/zinc oxide core–shell electrospun dressing for burn wound management. Macromol. Biosci. 20, 1900328. doi:10.1002/mabi.201900328
Haghjooy Javanmard, S., Anari, J., Zargar Kharazi, A., and Vatankhah, E. (2016). In vitro hemocompatibility and cytocompatibility of a three-layered vascular scaffold fabricated by sequential electrospinning of PCL, collagen, and PLLA nanofibers. J. Biomater. Appl. 31, 438–449. doi:10.1177/0885328216652068
Hajiali, H., Summa, M., Russo, D., Armirotti, A., Brunetti, V., Bertorelli, R., et al. (2016). Alginate-lavender nanofibers with antibacterial and anti-inflammatory activity to effectively promote burn healing. J. Mat. Chem. B 4, 1686–1695. doi:10.1039/c5tb02174j
Hajikhani, M., Emam-Djomeh, Z., and Askari, G. (2021). Fabrication and characterization of mucoadhesive bioplastic patch via coaxial polylactic acid (PLA) based electrospun nanofibers with antimicrobial and wound healing application. Int. J. Biol. Macromol. 172, 143–153. doi:10.1016/j.ijbiomac.2021.01.051
Hammer, J., Han, L.-H., Tong, X., and Yang, F. (2014). A facile method to fabricate hydrogels with microchannel-like porosity for tissue engineering. Tissue Eng. Part C. Methods 20, 169–176. doi:10.1089/ten.tec.2013.0176
Hara, T. O., and Singh, B. (2021). Electrochemical biosensors for detection of pesticides and heavy metal toxicants in water: Recent trends and progress. ACS Es. Throughput Water 1, 462–478. doi:10.1021/acsestwater.0c00125
Hasan, A., Deeb, G., Atwi, K., Soliman, S., and Hasan, A. (2015). Land contestation in Karachi and the impact on housing and urban development. Environ. Urban. 16-18, 217–230. doi:10.1177/0956247814567263
Hazarika, J., and Kumar, A. (2017). Scalable and low cost synthesis of highly conducting polypyrrole nanofibers using oil–water interfacial polymerization under constant stirring. J. Phys. Chem. B 121, 6926–6933. doi:10.1021/acs.jpcb.7b03179
He, M., Xue, J., Geng, H., Gu, H., Chen, D., Shi, R., et al. (2015). Fibrous guided tissue regeneration membrane loaded with anti-inflammatory agent prepared by coaxial electrospinning for the purpose of controlled release. Appl. Surf. Sci. 335, 121–129. doi:10.1016/j.apsusc.2015.02.037
He, X., Cheng, L., Wang, Y., Zhao, J., Zhang, W., and Lu, C. (2014). Aerogels from quaternary ammonium-functionalized cellulose nanofibers for rapid removal of Cr (VI) from water. Carbohydr. Polym. 111, 683–687. doi:10.1016/j.carbpol.2014.05.020
He, X., Fu, W., Feng, B., Wang, H., Liu, Z., Yin, M., et al. (2013). Electrospun collagen-poly(L-lactic acid-co-ε-caprolactone) membranes for cartilage tissue engineering. Regen. Med. 8, 425–436. doi:10.2217/rme.13.29
He, Y., Chen, G., Tu, H., Liu, X., Yan, J., Wang, X., et al. (2020). 3D-HA scaffold functionalized by extracellular matrix of stem cells promotes bone repair. Int. J. Nanomedicine 15, 5825–5838. doi:10.2147/IJN.S259678
Hedayatyanfard, K., Bagheri Khoulenjani, S., Abdollahifar, M. A., Amani, D., Habibi, B., Zare, F., et al. (2020). Chitosan/PVA/Doxycycline film and nanofiber accelerate diabetic wound healing in a rat model. Iran. J. Pharm. Res. 19, 225–239. doi:10.22037/ijpr.2020.112620.13859
Hejazi, F., Mirzadeh, H., Contessi, N., Tanzi, M. C., and Fare, S. (2017). Novel class of collector in electrospinning device for the fabrication of 3D nanofibrous structure for large defect load-bearing tissue engineering application. J. Biomed. Mat. Res. A 105, 1535–1548. doi:10.1002/jbm.a.35822
Hernández-Rangel, A., and Martin-Martinez, E. S. (2021). Collagen based electrospun materials for skin wounds treatment. J. Biomed. Mat. Res. A 109, 1751–1764. doi:10.1002/jbm.a.37154
Homaeigohar, S., and Boccaccini, A. R. (2020). Antibacterial biohybrid nanofibers for wound dressings. Acta Biomater. 107, 25–49. doi:10.1016/j.actbio.2020.02.022
Honarpardaz, A., Irani, S., Pezeshki-Modaress, M., Zandi, M., and Sadeghi, A. (2019). Enhanced chondrogenic differentiation of bone marrow mesenchymal stem cells on gelatin/glycosaminoglycan electrospun nanofibers with different amount of glycosaminoglycan. J. Biomed. Mat. Res. A 107, 38–48. doi:10.1002/jbm.a.36501
Horzum, N., Demir, M. M., Muñoz-Espí, R., and Crespy, D. (2019). Green electrospinning. Berlin: De Gruyter.
Hossain, K. M. Z., Patel, U., and Ahmed, I. (2015). Development of microspheres for biomedical applications: A review. Prog. Biomater. 4, 1–19. doi:10.1007/s40204-014-0033-8
Hou, J., Yun, J., and Byun, H. (2019). Fabrication and characterization of modified graphene oxide/PAN hybrid nanofiber. Membranes, 9, 122. doi:10.3390/membranes9090122
Hrib, J., Sirc, J., Hobzova, R., Hampejsova, Z., Bosakova, Z., Munzarova, M., et al. (2015). Nanofibers for drug delivery - incorporation and release of model molecules, influence of molecular weight and polymer structure. Beilstein J. Nanotechnol. 6, 1939–1945. doi:10.3762/bjnano.6.198
Hu, J., Li, H. Y., Williams, G. R., Yang, H. H., Tao, L., and Zhu, L. M. (2016). Electrospun poly(N-isopropylacrylamide)/Ethyl cellulose nanofibers as thermoresponsive drug delivery systems. J. Pharm. Sci. 105, 1104–1112. doi:10.1016/s0022-3549(15)00191-4
Huang, C., Yang, G., Zhou, S., Luo, E., Pan, J., Bao, C., et al. (2020). Controlled delivery of growth factor by hierarchical nanostructured core–shell nanofibers for the efficient repair of critical-sized rat calvarial defect. ACS Biomater. Sci. Eng. 6, 5758–5770. doi:10.1021/acsbiomaterials.0c00837
Huang, L., Mcmillan, R. A., Apkarian, R. P., Pourdeyhimi, B., Conticello, V. P., and Chaikof, E. L. (2000). Generation of synthetic elastin-mimetic small diameter fibers and fiber networks. Macromolecules 33, 2989–2997. doi:10.1021/ma991858f
Huang, T. Y., Wang, G. S., Tseng, C. C., and Su, W. T. (2019). Epidermal cells differentiated from stem cells from human exfoliated deciduous teeth and seeded onto polyvinyl alcohol/silk fibroin nanofiber dressings accelerate wound repair. Mater. Sci. Eng. C 104, 109986. doi:10.1016/j.msec.2019.109986
Huang, W., Ling, S., Li, C., Omenetto, F. G., and Kaplan, D. L. (2018). Silkworm silk-based materials and devices generated using bio-nanotechnology. Chem. Soc. Rev. 47, 6486–6504. doi:10.1039/c8cs00187a
Iacob, A. T., Drăgan, M., Ionescu, O. M., Profire, L., Ficai, A., Andronescu, E., et al. (2020). An overview of biopolymeric electrospun nanofibers based on polysaccharides for wound healing management. Pharmaceutics 12, 983. doi:10.3390/pharmaceutics12100983
Irani, S., Honarpardaz, A., Choubini, N., Pezeshki-Modaress, M., and Zandi, M. (2020). Chondro-inductive nanofibrous scaffold based gelatin/polyvinyl alcohol/chondroitin sulfate for cartilage tissue engineering. Polym. Adv. Technol. 31, 1395–1402. doi:10.1002/pat.4869
Islam, M. S., Ang, B. C., Andriyana, A., and Afifi, A. M. (2019). A review on fabrication of nanofibers via electrospinning and their applications. SN Appl. Sci. 1, 1248. doi:10.1007/s42452-019-1288-4
Ji, W., Yang, F., Ma, J., Bouma, M. J., Boerman, O. C., Chen, Z., et al. (2013). Incorporation of stromal cell-derived factor-1α in PCL/gelatin electrospun membranes for guided bone regeneration. Biomaterials 34, 735–745. doi:10.1016/j.biomaterials.2012.10.016
Jia, X., Li, X., Zhao, J., Kong, B., Wang, H., Liu, Q., et al. (2022). Fabrication and characterization of crosslinked pea protein isolated/pullulan/allicin electrospun nanofiber films as potential active packaging material. Food Packag. Shelf Life 33, 100873. doi:10.1016/j.fpsl.2022.100873
Jiang, J., Carlson, M. A., Teusink, M. J., Wang, H., Macewan, M. R., and Xie, J. (2015). Expanding two-dimensional electrospun nanofiber membranes in the third dimension by a modified gas-foaming technique. ACS Biomater. Sci. Eng. 1, 991–1001. doi:10.1021/acsbiomaterials.5b00238
Jiang, J., Chen, S., Wang, H., Carlson, M. A., Gombart, A. F., and Xie, J. (2018). CO2-expanded nanofiber scaffolds maintain activity of encapsulated bioactive materials and promote cellular infiltration and positive host response. Acta Biomater. 68, 237–248. doi:10.1016/j.actbio.2017.12.018
Jiang, Q., Liu, X., Liang, G., and Sun, X. (2021). Self-assembly of peptide nanofibers for imaging applications. Nanoscale 13, 15142–15150. doi:10.1039/d1nr04992e
Jiang, X., Zhang, S., Zou, B., Li, G., Yang, S., Zhao, Y., et al. (2022). Electrospun CoSe@NC nanofiber membrane as an effective polysulfides adsorption-catalysis interlayer for Li-S batteries. Chem. Eng. J. 430, 131911. doi:10.1016/j.cej.2021.131911
Jing, X., Li, H., Mi, H.-Y., Liu, Y.-J., Tan, Y.-M. J. I., and Research, E. C. (2019). Fabrication of three-dimensional fluffy nanofibrous scaffolds for tissue engineering via electrospinning and CO2 escaping foaming. Ind. Eng. Chem. Res. 58, 9412–9421. doi:10.1021/acs.iecr.9b00935
John, J. V., Choksi, M., Chen, S., Boda, S. K., Su, Y., Mccarthy, A., et al. (2019). Tethering peptides onto biomimetic and injectable nanofiber microspheres to direct cellular response. Nanomedicine Nanotechnol. Biol. Med. 22, 102081. doi:10.1016/j.nano.2019.102081
Joshi, D., Garg, T., Goyal, A. K., and Rath, G. (2016). Advanced drug delivery approaches against periodontitis. Drug Deliv. (Lond). 23, 363–377. doi:10.3109/10717544.2014.935531
Joy, N., Anuraj, R., Viravalli, A., Dixit, H. N., and Samavedi, S. (2021). Coupling between voltage and tip-to-collector distance in polymer electrospinning: Insights from analysis of regimes, transitions and cone/jet features. Chem. Eng. Sci. 230, 116200. doi:10.1016/j.ces.2020.116200
Juncos Bombin, A. D., Dunne, N. J., and Mccarthy, H. O. (2020). Electrospinning of natural polymers for the production of nanofibres for wound healing applications. Mater. Sci. Eng. C 114, 110994. doi:10.1016/j.msec.2020.110994
Kai, D., Prabhakaran, M. P., Jin, G., and Ramakrishna, S. (2011). Guided orientation of cardiomyocytes on electrospun aligned nanofibers for cardiac tissue engineering. J. Biomed. Mat. Res. 98, 379–386. doi:10.1002/jbm.b.31862
Kalalinia, F., Taherzadeh, Z., Jirofti, N., Amiri, N., Foroghinia, N., Beheshti, M., et al. (2021). Evaluation of wound healing efficiency of vancomycin-loaded electrospun chitosan/poly ethylene oxide nanofibers in full thickness wound model of rat. Int. J. Biol. Macromol. 177, 100–110. doi:10.1016/j.ijbiomac.2021.01.209
Kalantari, K., Afifi, A. M., Jahangirian, H., and Webster, T. J. (2019). Biomedical applications of chitosan electrospun nanofibers as a green polymer - Review. Carbohydr. Polym. 207, 588–600. doi:10.1016/j.carbpol.2018.12.011
Kalia, V., Garg, T., Rath, G., and Goyal, A. K. (2016). Development and evaluation of a sublingual film of the antiemetic granisetron hydrochloride. Artif. Cells Nanomed. Biotechnol. 44, 842–846. doi:10.3109/21691401.2014.984303
Kanikireddy, V., Varaprasad, K., Jayaramudu, T., Karthikeyan, C., and Sadiku, R. (2020). Carboxymethyl cellulose-based materials for infection control and wound healing: A review. Int. J. Biol. Macromol. 164, 963–975. doi:10.1016/j.ijbiomac.2020.07.160
Karbasi, S., Fekrat, F., Semnani, D., Razavi, S., and Zargar, E. N. (2016). Evaluation of structural and mechanical properties of electrospun nano-micro hybrid of poly hydroxybutyrate-chitosan/silk scaffold for cartilage tissue engineering. Adv. Biomed. Res. 5, 180. doi:10.4103/2277-9175.194802
Karuppannan, S. K., Dowlath, M. J. H., Ramalingam, R., Musthafa, S. A., Ganesh, M. R., Chithra, V., et al. (2022). Quercetin functionalized hybrid electrospun nanofibers for wound dressing application. Mater. Sci. Eng. B 285, 115933. doi:10.1016/j.mseb.2022.115933
Kataria, K., Gupta, A., Rath, G., Mathur, R. B., and Dhakate, S. R. (2014). In vivo wound healing performance of drug loaded electrospun composite nanofibers transdermal patch. Int. J. Pharm. 469, 102–110. doi:10.1016/j.ijpharm.2014.04.047
Keirouz, A., Chung, M., Kwon, J., Fortunato, G., and Radacsi, N. (2020). 2D and 3D electrospinning technologies for the fabrication of nanofibrous scaffolds for skin tissue engineering: A review. WIREs Nanomed. Nanobiotechnol. 12, e1626. doi:10.1002/wnan.1626
Kersani, D., Mougin, J., Lopez, M., Degoutin, S., Tabary, N., Cazaux, F., et al. (2020a). Stent coating by electrospinning with chitosan/poly-cyclodextrin based nanofibers loaded with simvastatin for restenosis prevention. Eur. J. Pharm. Biopharm. 150, 156–167. doi:10.1016/j.ejpb.2019.12.017
Kersani, D., Mougin, J., Lopez, M., Degoutin, S., Tabary, N., Cazaux, F., et al. (2020b). Stent coating by electrospinning with chitosan/poly-cyclodextrin based nanofibers loaded with simvastatin for restenosis prevention. Eur. J. Pharm. Biopharm. 150, 156–167. doi:10.1016/j.ejpb.2019.12.017
Khamrai, M., Banerjee, S. L., Paul, S., Samanta, S., and Kundu, P. P. (2019). Curcumin entrapped gelatin/ionically modified bacterial cellulose based self-healable hydrogel film: An eco-friendly sustainable synthesis method of wound healing patch. Int. J. Biol. Macromol. 122, 940–953. doi:10.1016/j.ijbiomac.2018.10.196
Khan, M. A., Hussain, Z., Ali, S., Qamar, Z., Imran, M., and Hafeez, F. Y. (2019). Fabrication of electrospun probiotic functionalized nanocomposite scaffolds for infection control and dermal burn healing in a mice model. ACS Biomater. Sci. Eng. 5, 6109–6116. doi:10.1021/acsbiomaterials.9b01002
Khosravimelal, S., Chizari, M., Farhadihosseinabadi, B., Moosazadeh Moghaddam, M., and Gholipourmalekabadi, M. (2021). Fabrication and characterization of an antibacterial chitosan/silk fibroin electrospun nanofiber loaded with a cationic peptide for wound-dressing application. J. Mat. Sci. Mat. Med. 32, 114. doi:10.1007/s10856-021-06542-6
Kijeńska, E., Prabhakaran, M. P., Swieszkowski, W., Kurzydlowski, K. J., and Ramakrishna, S. (2012). Electrospun bio-composite P(LLA-CL)/collagen I/collagen III scaffolds for nerve tissue engineering. J. Biomed. Mat. Res. B Appl. Biomater. 100B, 1093–1102. doi:10.1002/jbm.b.32676
Kijeńska, E., Prabhakaran, M. P., Swieszkowski, W., Kurzydlowski, K. J., and Ramakrishna, S. (2014). Interaction of Schwann cells with laminin encapsulated PLCL core–shell nanofibers for nerve tissue engineering. Eur. Polym. J. 50, 30–38. doi:10.1016/j.eurpolymj.2013.10.021
Kim, J. I., Kim, J. Y., and Park, C. H. (2018). Fabrication of transparent hemispherical 3D nanofibrous scaffolds with radially aligned patterns via a novel electrospinning method. Sci. Rep. 8, 3424–3513. doi:10.1038/s41598-018-21618-0
Kopp, A., Smeets, R., Gosau, M., Kröger, N., Fuest, S., Köpf, M., et al. (2020). Effect of process parameters on additive-free electrospinning of regenerated silk fibroin nonwovens. Bioact. Mater. 5, 241–252. doi:10.1016/j.bioactmat.2020.01.010
Kotzianová, A., Řebíček, J., Pokorný, M., Hrbáč, J., and Velebný, V. (2016). Raman spectroscopy analysis of biodegradable electrospun nanofibers prepared from polymer blends. Monatsh. Chem. 147, 919–923. doi:10.1007/s00706-015-1639-9
Kuang, G., Zhang, Z., Liu, S., Zhou, D., Lu, X., Jing, X., et al. (2018). Biphasic drug release from electrospun polyblend nanofibers for optimized local cancer treatment. Biomater. Sci. 6, 324–331. doi:10.1039/c7bm01018d
Kuang, H., Wang, Y., Shi, Y., Yao, W., He, X., Liu, X., et al. (2020). Construction and performance evaluation of Hep/silk-PLCL composite nanofiber small-caliber artificial blood vessel graft. Biomaterials 259, 120288. doi:10.1016/j.biomaterials.2020.120288
Kumar, M. R. (2017). Handbook of polyester drug delivery systems. New York: Jenny Stanford Publishing.
Kumar, N., Sridharan, D., Palaniappan, A., Dougherty, J. A., Czirok, A., Isai, D. G., et al. (2020). Scalable biomimetic coaxial aligned nanofiber cardiac patch: A potential model for "clinical trials in a dish. Front. Bioeng. Biotechnol. 8, 567842. doi:10.3389/fbioe.2020.567842
Lai, H.-J., Kuan, C.-H., Wu, H.-C., Tsai, J.-C., Chen, T.-M., Hsieh, D.-J., et al. (2014). Tailored design of electrospun composite nanofibers with staged release of multiple angiogenic growth factors for chronic wound healing. Acta Biomater. 10, 4156–4166. doi:10.1016/j.actbio.2014.05.001
Laiva, A. L., O'Brien, F. J., and Keogh, M. B. (2018). Innovations in gene and growth factor delivery systems for diabetic wound healing. J. Tissue Eng. Regen. Med. 12, e296–e312. doi:10.1002/term.2443
Law, J. X., Liau, L. L., Saim, A., Yang, Y., and Idrus, R. (2017). Electrospun collagen nanofibers and their applications in skin tissue engineering. Tissue Eng. Regen. Med. 14, 699–718. doi:10.1007/s13770-017-0075-9
Lee, H.-J., Abueva, C. D. G., Padalhin, A. R., and Lee, B.-T. (2019a). Soya protein isolate-polyethylene oxide electrospun nanofiber membrane with bone marrow-derived mesenchymal stem cell for enhanced bone regeneration. J. Biomater. Appl. 34, 1142–1149. doi:10.1177/0885328219891614
Lee, S. H., Park, C., Park, J. W., Kim, S. J., Im, S. S., and Ahn, H. (2019b). Synthesis of conducting polymer-intercalated vanadate nanofiber composites using a sonochemical method for high performance pseudocapacitor applications. J. Power Sources 414, 460–469. doi:10.1016/j.jpowsour.2019.01.031
Lee, S. J., Heo, D. N., Park, J. S., Kwon, S. K., Lee, J. H., Lee, J. H., et al. (2015). Characterization and preparation of bio-tubular scaffolds for fabricating artificial vascular grafts by combining electrospinning and a 3D printing system. Phys. Chem. Chem. Phys. 17, 2996–2999. doi:10.1039/c4cp04801f
Lei, L., Chen, S., Nachtigal, C. J., Moy, T. F., Yong, X., and Singer, J. P. (2020). Homogeneous gelation leads to nanowire forests in the transition between electrospray and electrospinning. Mat. Horiz. 7, 2643–2650. doi:10.1039/d0mh00872a
Li, D., Wang, M., Song, W.-L., Yu, D.-G., and Bligh, S. W. A. (2021a). Electrospun Janus beads-on-A-string structures for different types of controlled release profiles of double drugs. Biomolecules 11, 635. doi:10.3390/biom11050635
Li, H., Sang, Q., Wu, J., Williams, G. R., Wang, H., Niu, S., et al. (2018). Dual-responsive drug delivery systems prepared by blend electrospinning. Int. J. Pharm. 543, 1–7. doi:10.1016/j.ijpharm.2018.03.009
Li, J., Mei, H., Zheng, W., Pan, P., Sun, X. J., Li, F., et al. (2014). A novel hydrogen peroxide biosensor based on hemoglobin-collagen-CNTs composite nanofibers. Colloids Surfaces B Biointerfaces 118, 77–82. doi:10.1016/j.colsurfb.2014.03.035
Li, K., Nejadnik, H., and Daldrup-Link, H. E. (2017). Next-generation superparamagnetic iron oxide nanoparticles for cancer theranostics. Drug Discov. Today 22, 1421–1429. doi:10.1016/j.drudis.2017.04.008
Li, P., Lee, G.-H., Kim, S. Y., Kwon, S. Y., Kim, H.-R., and Park, S. (2021b). From diagnosis to treatment: Recent advances in patient-friendly biosensors and implantable devices. ACS Nano 15, 1960–2004. doi:10.1021/acsnano.0c06688
Li, S., Kong, L., and Ziegler, G. R. (2021c). Electrospinning of octenylsuccinylated starch-pullulan nanofibers from aqueous dispersions. Carbohydr. Polym. 258, 116933. doi:10.1016/j.carbpol.2020.116933
Li, Y., Liu, Y., Xun, X., Zhang, W., Xu, Y., and Gu, D. (2019). Three-dimensional porous scaffolds with biomimetic microarchitecture and bioactivity for cartilage tissue engineering. ACS Appl. Mat. Interfaces 11, 36359–36370. doi:10.1021/acsami.9b12206
KENRY, , and LIM, C. T. (2017). Nanofiber technology: Current status and emerging developments. Prog. Polym. Sci. 70, 1–17. doi:10.1016/j.progpolymsci.2017.03.002
Limoee, M., Moradipour, P., Godarzi, M., Arkan, E., and Behbood, L. (2019). Fabrication and in-vitro investigation of polycaprolactone - (polyvinyl alcohol/collagen) hybrid nanofiber as anti-inflammatory guided tissue regeneration membrane. Curr. Pharm. Biotechnol. 20, 1122–1133. doi:10.2174/1389201020666190722161004
Lin, W., Chen, M., Qu, T., Li, J., and Man, Y. (2020). Three-dimensional electrospun nanofibrous scaffolds for bone tissue engineering. J. Biomed. Mat. Res. 108, 1311–1321. doi:10.1002/jbm.b.34479
Liu, C., Huang, Y., Pang, M., Yang, Y., Li, S., Liu, L., et al. (2015). Tissue-engineered regeneration of completely transected spinal cord using induced neural stem cells and gelatin-electrospun poly (lactide-Co-Glycolide)/Polyethylene glycol scaffolds. PLOS ONE 10, e0117709. doi:10.1371/journal.pone.0117709
Liu, G., Gu, Z., Hong, Y., Cheng, L., and Li, C. (2017). Electrospun starch nanofibers: Recent advances, challenges, and strategies for potential pharmaceutical applications. J. Control. Release 252, 95–107. doi:10.1016/j.jconrel.2017.03.016
Liu, Q., Ouyang, W.-C., Zhou, X.-H., Jin, T., and Wu, Z.-W. (2021a). Antibacterial activity and drug loading of moxifloxacin-loaded poly(vinyl alcohol)/chitosan electrospun nanofibers. Front. Mat. 8, 643428. doi:10.3389/fmats.2021.643428
Liu, T., Guo, Y., Zhang, Z., Miao, Z., Zhang, X., and Su, Z. (2019). Fabrication of hollow CuO/PANI hybrid nanofibers for non-enzymatic electrochemical detection of H2O2 and glucose. Sensors Actuators B Chem. 286, 370–376. doi:10.1016/j.snb.2019.02.006
Liu, X., Jin, X., and Ma, P. X. (2011). Nanofibrous hollow microspheres self-assembled from star-shaped polymers as injectable cell carriers for knee repair. Nat. Mat. 10, 398–406. doi:10.1038/nmat2999
Liu, X., Xu, H., Zhang, M., and Yu, D. G. (2021b). Electrospun medicated nanofibers for wound healing: Review. Membr. (Basel) 11, 770. doi:10.3390/membranes11100770
Liu, Y., Jiang, C., Li, S., and Hu, Q. (2016). Composite vascular scaffold combining electrospun fibers and physically-crosslinked hydrogel with copper wire-induced grooves structure. J. Mech. Behav. Biomed. Mater. 61, 12–25. doi:10.1016/j.jmbbm.2016.01.002
Lodrini, A. M., and Goumans, M. J. (2021). Cardiomyocytes cellular phenotypes after myocardial infarction. Front. Cardiovasc. Med. 8, 750510. doi:10.3389/fcvm.2021.750510
Lopez, F. L., Shearman, G. C., Gaisford, S., and Williams, G. R. (2014). Amorphous formulations of indomethacin and griseofulvin prepared by electrospinning. Mol. Pharm. 11, 4327–4338. doi:10.1021/mp500391y
Lopez Marquez, A., Gareis, I. E., Dias, F. J., Gerhard, C., and Lezcano, M. F. J. P. (2022). Methods to characterize electrospun scaffold morphology: A critical review. Polym. (Basel). 14, 467. doi:10.3390/polym14030467
López-Calderón, H. D., Avilés-Arnaut, H., Galán-Wong, L. J., Almaguer-Cantú, V., Laguna-Camacho, J. R., Calderón-Ramón, C., et al. (2020). Electrospun polyvinylpyrrolidone-gelatin and cellulose acetate Bi-layer scaffold loaded with gentamicin as possible wound dressing. Polymers 12, 2311. doi:10.3390/polym12102311
Lu, T., Cui, J., Qu, Q., Wang, Y., Zhang, J., Xiong, R., et al. (2021). Multistructured electrospun nanofibers for air filtration: A review. ACS Appl. Mat. Interfaces 13, 23293–23313. doi:10.1021/acsami.1c06520
Lu, Y., Huang, J., Yu, G., Cardenas, R., Wei, S., Wujcik, E. K., et al. (2016). Coaxial electrospun fibers: Applications in drug delivery and tissue engineering. WIREs Nanomed. Nanobiotechnol. 8, 654–677. doi:10.1002/wnan.1391
Lv, H., Guo, S., Zhang, G., He, W., Wu, Y., and Yu, D. G. (2021). Electrospun Structural Hybrids of Acyclovir-Polyacrylonitrile at Acyclovir for Modifying Drug Release, 13. Basel: Polymers.
Ma, J., Xu, C., Yu, H., Feng, Z., Yu, W., Gu, L., et al. (2021). Electro-encapsulation of probiotics in gum Arabic-pullulan blend nanofibres using electrospinning technology. Food Hydrocoll. 111, 106381. doi:10.1016/j.foodhyd.2020.106381
Ma, Y., Wang, X., Zong, S., Zhang, Z., Xie, Z., Huang, Y., et al. (2015). Local, combination chemotherapy in prevention of cervical cancer recurrence after surgery by using nanofibers co-loaded with cisplatin and curcumin. RSC Adv. 5, 106325–106332. doi:10.1039/c5ra17230f
Madruga, L. Y. C., and Kipper, M. J. (2022). Expanding the repertoire of electrospinning: New and emerging biopolymers, techniques, and applications. Adv. Healthc. Mat. 11, 2101979. doi:10.1002/adhm.202101979
Mahanta, B., Mary, S. A., Bhaduri, A., and Giridev, V. (2014). Electrospun PVA/keratin nanofibrous scaffold and its application in neural repair. Trends Biomaterials Artif. Organs 28, 188–196.
Makadia, H. K., and Siegel, S. J. (2011). Poly lactic-co-glycolic acid (PLGA) as biodegradable controlled drug delivery carrier. Polymers 3, 1377–1397. doi:10.3390/polym3031377
Manjumeena, R., Elakkiya, T., Duraibabu, D., Feroze Ahamed, A., Kalaichelvan, P. T., and Venkatesan, R. (2015). 'Green' biocompatible organic-inorganic hybrid electrospun nanofibers for potential biomedical applications. J. Biomater. Appl. 29, 1039–1055. doi:10.1177/0885328214550011
Marins, N. H., Lee, B. E. J., E Silva, R. M., Raghavan, A., Villarreal Carreño, N. L., and Grandfield, K. (2019). Niobium pentoxide and hydroxyapatite particle loaded electrospun polycaprolactone/gelatin membranes for bone tissue engineering. Colloids Surfaces B Biointerfaces 182, 110386. doi:10.1016/j.colsurfb.2019.110386
Mcclellan, P., and Landis, W. J. (2016). Recent applications of coaxial and emulsion electrospinning methods in the field of tissue engineering. Biores. Open Access 5, 212–227. doi:10.1089/biores.2016.0022
Mcginty, S. (2014). A decade of modelling drug release from arterial stents. Math. Biosci. 257, 80–90. doi:10.1016/j.mbs.2014.06.016
Mercante, L. A., Scagion, V. P., Migliorini, F. L., Mattoso, L. H. C., and Correa, D. S. (2017). Electrospinning-based (bio)sensors for food and agricultural applications: A review. TrAC Trends Anal. Chem. 91, 91–103. doi:10.1016/j.trac.2017.04.004
Mi, H.-Y., Jing, X., Huang, H.-X., and Turng, L.-S. (2017). Instantaneous self-assembly of three-dimensional silica fibers in electrospinning: Insights into fiber deposition behavior. Mater. Lett. 204, 45–48. doi:10.1016/j.matlet.2017.05.128
Mirzaeei, S., Taghe, S., Asare-Addo, K., and Nokhodchi, A. (2021). Polyvinyl alcohol/chitosan single-layered and polyvinyl alcohol/chitosan/eudragit RL100 multi-layered electrospun nanofibers as an ocular matrix for the controlled release of ofloxacin: An in vitro and in vivo evaluation. AAPS PharmSciTech 22, 170. doi:10.1208/s12249-021-02051-5
Mirzaei-Parsa, M. J., Ghanbari, H., Alipoor, B., Tavakoli, A., Najafabadi, M. R. H., and Faridi-Majidi, R. (2019). Nanofiber-acellular dermal matrix as a bilayer scaffold containing mesenchymal stem cell for healing of full-thickness skin wounds. Cell. Tissue Res. 375, 709–721. doi:10.1007/s00441-018-2927-6
Mishima, S., Gasset, A., Klyce, S. D., and Baum, J. L. (1966). Determination of tear volume and tear flow. Investig. Ophthalmol. 5, 264
Movahedi, M., Asefnejad, A., Rafienia, M., and Khorasani, M. T. (2020). Potential of novel electrospun core-shell structured polyurethane/starch (hyaluronic acid) nanofibers for skin tissue engineering: In vitro and in vivo evaluation. Int. J. Biol. Macromol. 146, 627–637. doi:10.1016/j.ijbiomac.2019.11.233
Murugan, S., and Parcha, S. R. (2021). Fabrication techniques involved in developing the composite scaffolds PCL/HA nanoparticles for bone tissue engineering applications. J. Mat. Sci. Mat. Med. 32, 93. doi:10.1007/s10856-021-06564-0
Naeimirad, M., Zadhoush, A., Kotek, R., Esmaeely Neisiany, R., Nouri Khorasani, S., and Ramakrishna, S. (2018). Recent advances in core/shell bicomponent fibers and nanofibers: A review. J. Appl. Polym. Sci. 135, 46265. doi:10.1002/app.46265
Najafi, S., Gholipour-Kanani, A., Eslahi, N., and Bahrami, S. H. (2021). Study on release of cardamom extract as an antibacterial agent from electrospun scaffold based on sodium alginate. J. Text. Inst. 112, 1482–1490. doi:10.1080/00405000.2020.1825164
Najafiasl, M., Osfouri, S., Azin, R., and Zaeri, S. (2020). Alginate-based electrospun core/shell nanofibers containing dexpanthenol: A good candidate for wound dressing. J. Drug Deliv. Sci. Technol. 57, 101708. doi:10.1016/j.jddst.2020.101708
Nandakumar, A., Yang, L., Habibovic, P., and van Blitterswijk, C. (2010). Calcium phosphate coated electrospun fiber matrices as scaffolds for bone tissue engineering. Langmuir 26, 7380–7387. doi:10.1021/la904406b
Ndlovu, S., Ngece, K., Sibusiso, A., and Aderibigbe, B. (2021a). Gelatin-based hybrid scaffolds: Promising wound dressings. Polymers 13, 2959. doi:10.3390/polym13172959
Ndlovu, S. P., Ngece, K., Alven, S., and Aderibigbe, B. A. (2021b). Gelatin-Based Hybrid Scaffolds: Promising Wound Dressings. 13. Basel: Polymers.
Nedjari, S., Eap, S., Hébraud, A., Wittmer, C. R., Benkirane-Jessel, N., and Schlatter, G. (2014). Electrospun honeycomb as nests for controlled osteoblast spatial organization. Macromol. Biosci. 14, 1580–1589. doi:10.1002/mabi.201400226
Nekounam, H., Allahyari, Z., Gholizadeh, S., Mirzaei, E., Shokrgozar, M. A., and Faridi-Majidi, R. (2020). Simple and robust fabrication and characterization of conductive carbonized nanofibers loaded with gold nanoparticles for bone tissue engineering applications. Mater. Sci. Eng. C 117, 111226. doi:10.1016/j.msec.2020.111226
Nikmaram, N., Roohinejad, S., Hashemi, S., Koubaa, M., Barba, F. J., Abbaspourrad, A., et al. (2017). Emulsion-based systems for fabrication of electrospun nanofibers: Food, pharmaceutical and biomedical applications. RSC Adv. 7, 28951–28964. doi:10.1039/c7ra00179g
Nirwan, V. P., Kowalczyk, T., Bar, J., Buzgo, M., Filová, E., and Fahmi, A. (2022). Advances in electrospun hybrid nanofibers for biomedical applications. Nanomaterials 12, 1829. doi:10.3390/nano12111829
Pant, B., Park, M., and Park, S.-J. (2019). Drug delivery applications of core-sheath nanofibers prepared by coaxial electrospinning: A review. Pharmaceutics 11, 305. doi:10.3390/pharmaceutics11070305
Park, K., Kang, S., Park, J.-W., and Hwang, J. (2021). Fabrication of silver nanowire coated fibrous air filter medium via a two-step process of electrospinning and electrospray for anti-bioaerosol treatment. J. Hazard. Mater. 411, 125043. doi:10.1016/j.jhazmat.2021.125043
Pavinatto, A., Mercante, L. A., Facure, M. H. M., Pena, R. B., Sanfelice, R. C., Mattoso, L. H. C., et al. (2018). Ultrasensitive biosensor based on polyvinylpyrrolidone/chitosan/reduced graphene oxide electrospun nanofibers for 17α – ethinylestradiol electrochemical detection. Appl. Surf. Sci. 458, 431–437. doi:10.1016/j.apsusc.2018.07.035
Peiravi, M., Eslami, H., Ansari, M., and Zare-Zardini, H. (2022). Magnetic hyperthermia: Potentials and limitations. J. Indian Chem. Soc. 99, 100269. doi:10.1016/j.jics.2021.100269
Peng, Y., Ma, Y., Bao, Y., Liu, Z., Chen, L., Dai, F., et al. (2021). Electrospun PLGA/SF/artemisinin composite nanofibrous membranes for wound dressing. Int. J. Biol. Macromol. 183, 68–78. doi:10.1016/j.ijbiomac.2021.04.021
Phromviyo, N., Lert-Itthiporn, A., Swatsitang, E., and Chompoosor, A. (2015). Biodegradable poly(vinyl alcohol)/polyoxalate electrospun nanofibers for hydrogen peroxide-triggered drug release. J. Biomaterials Sci. Polym. Ed. 26, 975–987. doi:10.1080/09205063.2015.1069781
Pina, S., Oliveira, J. M., and Reis, R. L. (2015). Natural-based nanocomposites for bone tissue engineering and regenerative medicine: A review. Adv. Mat. 27, 1143–1169. doi:10.1002/adma.201403354
Pinto, T. V., Costa, P., Sousa, C. M., Sousa, C. A. D., Pereira, C., Silva, C. J. S. M., et al. (2016). Screen-printed photochromic textiles through new inks based on SiO2@naphthopyran nanoparticles. ACS Appl. Mat. Interfaces 8, 28935–28945. doi:10.1021/acsami.6b06686
Potyondy, T., Uquillas, J. A., Tebon, P. J., Byambaa, B., Hasan, A., Tavafoghi, M., et al. (2021). Recent advances in 3D bioprinting of musculoskeletal tissues, 13, 022001. doi:10.1088/1758-5090/abc8de
Prabhakaran, M. P., Ghasemi-Mobarakeh, L., Jin, G., and Ramakrishna, S. (2011). Electrospun conducting polymer nanofibers and electrical stimulation of nerve stem cells. J. Biosci. Bioeng. 112, 501–507. doi:10.1016/j.jbiosc.2011.07.010
Prabhakaran, M. P., Nair, A. S., Kai, D., and Ramakrishna, S. (2012). Electrospun composite scaffolds containing poly(octanediol-co-citrate) for cardiac tissue engineering. Biopolymers 97, 529–538. doi:10.1002/bip.22035
Prabhakaran, M. P., Vatankhah, E., and Ramakrishna, S. (2013). Electrospun aligned PHBV/collagen nanofibers as substrates for nerve tissue engineering. Biotechnol. Bioeng. 110, 2775–2784. doi:10.1002/bit.24937
Prabhakaran, M. P., Venugopal, J. R., and Ramakrishna, S. (2009). Mesenchymal stem cell differentiation to neuronal cells on electrospun nanofibrous substrates for nerve tissue engineering. Biomaterials 30, 4996–5003. doi:10.1016/j.biomaterials.2009.05.057
Preem, L., Mahmoudzadeh, M., Putrinš, M., Meos, A., Laidmäe, I., Romann, T., et al. (2017). Interactions between chloramphenicol, carrier polymers, and bacteria-implications for designing electrospun drug delivery systems countering wound infection. Mol. Pharm. 14, 4417–4430. doi:10.1021/acs.molpharmaceut.7b00524
Qi, R.-L., Tian, X.-J., Guo, R., Luo, Y., Shen, M.-W., Yu, J.-Y., et al. (2016). Controlled release of doxorubicin from electrospun MWCNTs/PLGA hybrid nanofibers. Chin. J. Polym. Sci. 34, 1047–1059. doi:10.1007/s10118-016-1827-z
Qin, X. (2017). “Coaxial electrospinning of nanofibers,” in Electrospun nanofibers. Editor M. AFSHARI (Cambridge, UK: Woodhead Publishing).
Qu, H., Fu, H., Han, Z., and Sun, Y. (2019). Biomaterials for bone tissue engineering scaffolds: A review. RSC Adv. 9, 26252–26262. doi:10.1039/c9ra05214c
Rachmiel, D., Anconina, I., Rudnick-Glick, S., Halperin-Sternfeld, M., Adler-Abramovich, L., and Sitt, A. (2021). Hyaluronic acid and a short peptide improve the performance of a PCL electrospun fibrous scaffold designed for bone tissue engineering applications. Int. J. Mol. Sci. 22, 2425. doi:10.3390/ijms22052425
Radacsi, N., and Nuansing, W. (2020). “Fabrication of 3D and 4D polymer micro- and nanostructures based on electrospinning,” in 3D and 4D printing of polymer nanocomposite materials. Editors K. K. Sadasivuni, K. Deshmukh, and M. A. Almaadeed (Amesterdam: Elsevier), 191–229.
Radmansouri, M., Bahmani, E., Sarikhani, E., Rahmani, K., Sharifianjazi, F., and Irani, M. (2018). Doxorubicin hydrochloride - loaded electrospun chitosan/cobalt ferrite/titanium oxide nanofibers for hyperthermic tumor cell treatment and controlled drug release. Int. J. Biol. Macromol. 116, 378–384. doi:10.1016/j.ijbiomac.2018.04.161
Rahmati, M., Mills, D. K., Urbanska, A. M., Saeb, M. R., Venugopal, J. R., Ramakrishna, S., et al. (2021). Electrospinning for tissue engineering applications. Prog. Mater. Sci. 117, 100721. doi:10.1016/j.pmatsci.2020.100721
Ramakrishna, S. (2005). An introduction to electrospinning and nanofibers. Singapore: World Scientific.
Rasekh, A., and Raisi, A. (2021). Electrospun nanofibrous polyether-block-amide membrane containing silica nanoparticles for water desalination by vacuum membrane distillation. Sep. Purif. Technol. 275, 119149. doi:10.1016/j.seppur.2021.119149
Rashtchian, M., Hivechi, A., Bahrami, S. H., Milan, P. B., and Simorgh, S. (2020). Fabricating alginate/poly(caprolactone) nanofibers with enhanced bio-mechanical properties via cellulose nanocrystal incorporation. Carbohydr. Polym. 233, 115873. doi:10.1016/j.carbpol.2020.115873
Rastogi, P., and Kandasubramanian, B. (2019). Review of alginate-based hydrogel bioprinting for application in tissue engineering. Biofabrication 11, 042001. doi:10.1088/1758-5090/ab331e
Ren, S., Zhou, Y., Zheng, K., Xu, X., Yang, J., Wang, X., et al. (2022). Cerium oxide nanoparticles loaded nanofibrous membranes promote bone regeneration for periodontal tissue engineering. Bioact. Mater. 7, 242–253. doi:10.1016/j.bioactmat.2021.05.037
Rethinam, S., Wilson Aruni, A., Vijayan, S., Munusamy, C., and Gobi, N. (2019). Enhanced bone regeneration using an electrospun nanofibrous membrane – a novel approach. J. Drug Deliv. Sci. Technol. 53, 101163. doi:10.1016/j.jddst.2019.101163
Ribeiro, A. S., Costa, S. M., Ferreira, D. P., Calhelha, R. C., Barros, L., Stojković, D., et al. (2021). Chitosan/nanocellulose electrospun fibers with enhanced antibacterial and antifungal activity for wound dressing applications. React. Funct. Polym. 159, 104808. doi:10.1016/j.reactfunctpolym.2020.104808
Riva, L., Fiorati, A., and Punta, C. (2021). Synthesis and application of cellulose-polyethyleneimine composites and nanocomposites: A concise review. Materials (Basel), 14, 473. doi:10.3390/ma14030473
Rodrigues, A., and Emeje, M. (2012). Recent applications of starch derivatives in nanodrug delivery. Carbohydr. Polym. 87, 987–994. doi:10.1016/j.carbpol.2011.09.044
Rogers, C. M., Morris, G. E., Gould, T. W., Bail, R., Toumpaniari, S., Harrington, H., et al. (2014). A novel technique for the production of electrospun scaffolds with tailored three-dimensional micro-patterns employing additive manufacturing. Biofabrication 6, 035003. doi:10.1088/1758-5082/6/3/035003
Roodbar Shojaei, T., Hajalilou, A., Tabatabaei, M., Mobli, H., and Aghbashlo, M. (2019). “Characterization and evaluation of nanofiber materials,” in Handbook of nanofibers. Editors A. BARHOUM, M. BECHELANY, and A. S. H. MAKHLOUF (Cham: Springer International Publishing).
Sa, V., and Kornev, K. G. (2011). A method for wet spinning of alginate fibers with a high concentration of single-walled carbon nanotubes. Carbon N. Y. 49, 1859–1868. doi:10.1016/j.carbon.2011.01.008
Saini, P., Arora, M., and Kumar, M. (2016). Poly(lactic acid) blends in biomedical applications. Adv. Drug Deliv. Rev. 107, 47–59. doi:10.1016/j.addr.2016.06.014
Samadian, H., Zamiri, S., Ehterami, A., Farzamfar, S., Vaez, A., Khastar, H., et al. (2020). Electrospun cellulose acetate/gelatin nanofibrous wound dressing containing berberine for diabetic foot ulcer healing: In vitro and in vivo studies. Sci. Rep. 10, 8312. doi:10.1038/s41598-020-65268-7
Sambudi, N. S., Sathyamurthy, M., Lee, G. M., and Park, S. B. (2015). Electrospun chitosan/poly(vinyl alcohol) reinforced with CaCO3 nanoparticles with enhanced mechanical properties and biocompatibility for cartilage tissue engineering. Compos. Sci. Technol. 106, 76–84. doi:10.1016/j.compscitech.2014.11.003
Sasikala, A. R. K., Unnithan, A. R., Yun, Y.-H., Park, C. H., and Kim, C. S. (2016). An implantable smart magnetic nanofiber device for endoscopic hyperthermia treatment and tumor-triggered controlled drug release. Acta Biomater. 31, 122–133. doi:10.1016/j.actbio.2015.12.015
Scagion, V. P., Mercante, L. A., Sakamoto, K. Y., Oliveira, J. E., Fonseca, F. J., Mattoso, L. H. C., et al. (2016). An electronic tongue based on conducting electrospun nanofibers for detecting tetracycline in milk samples. RSC Adv. 6, 103740–103746. doi:10.1039/c6ra21326j
Scouten, W. H., Luong, J. H. T., and Stephen Brown, R. (1995). Enzyme or protein immobilization techniques for applications in biosensor design. Trends Biotechnol. 13, 178–185. doi:10.1016/s0167-7799(00)88935-0
Sedghi, R., Shaabani, A., and Sayyari, N. (2020). Electrospun triazole-based chitosan nanofibers as a novel scaffolds for bone tissue repair and regeneration. Carbohydr. Polym. 230, 115707. doi:10.1016/j.carbpol.2019.115707
Semitela, Â., Girão, A. F., Fernandes, C., Ramalho, G., Bdikin, I., Completo, A., et al. (2020). Electrospinning of bioactive polycaprolactone-gelatin nanofibres with increased pore size for cartilage tissue engineering applications. J. Biomater. Appl. 35, 471–484. doi:10.1177/0885328220940194
Şenel Ayaz, H. G., Perets, A., Ayaz, H., Gilroy, K. D., Govindaraj, M., Brookstein, D., et al. (2014). Textile-templated electrospun anisotropic scaffolds for regenerative cardiac tissue engineering. Biomaterials 35, 8540–8552. doi:10.1016/j.biomaterials.2014.06.029
Shanmugavel, S., Reddy, V. J., Ramakrishna, S., Lakshmi, B. S., and Dev, V. R. G. (2013). Precipitation of hydroxyapatite on electrospun polycaprolactone/aloe vera/silk fibroin nanofibrous scaffolds for bone tissue engineering. J. Biomater. Appl. 29, 46–58. doi:10.1177/0885328213513934
Shimomura, K., Rothrauff, B. B., Hart, D. A., Hamamoto, S., Kobayashi, M., Yoshikawa, H., et al. (2019). Enhanced repair of meniscal hoop structure injuries using an aligned electrospun nanofibrous scaffold combined with a mesenchymal stem cell-derived tissue engineered construct. Biomaterials 192, 346–354. doi:10.1016/j.biomaterials.2018.11.009
Shin, E. J., and Choi, S. M. (2018). Advances in waterborne polyurethane-based biomaterials for biomedical applications. Adv. Exp. Med. Biol. 1077, 251–283. doi:10.1007/978-981-13-0947-2_14
Shin, Y. C., Shin, D. M., Lee, E. J., Lee, J. H., Kim, J. E., Song, S. H., et al. (2016). Hyaluronic acid/PLGA core/shell fiber matrices loaded with EGCG beneficial to diabetic wound healing. Adv. Healthc. Mat. 5, 3035–3045. doi:10.1002/adhm.201600658
Silva, J. C., Udangawa, R. N., Chen, J., Mancinelli, C. D., Garrudo, F. F. F., Mikael, P. E., et al. (2020). Kartogenin-loaded coaxial PGS/PCL aligned nanofibers for cartilage tissue engineering. Mater. Sci. Eng. C 107, 110291. doi:10.1016/j.msec.2019.110291
Silva, P. M., Prieto, C., Lagarón, J. M., Pastrana, L. M., Coimbra, M. A., Vicente, A. A., et al. (2021). Food-grade hydroxypropyl methylcellulose-based formulations for electrohydrodynamic processing: Part I – role of solution parameters on fibre and particle production. Food Hydrocoll. 118, 106761. doi:10.1016/j.foodhyd.2021.106761
Singh, B., Garg, T., Goyal, A. K., and Rath, G. (2016). Development, optimization, and characterization of polymeric electrospun nanofiber: A new attempt in sublingual delivery of nicorandil for the management of angina pectoris. Artif. Cells Nanomed. Biotechnol. 44, 1498–1507. doi:10.3109/21691401.2015.1052472
Sionkowska, A. (2021). Collagen blended with natural polymers: Recent advances and trends. Prog. Polym. Sci. 122, 101452. doi:10.1016/j.progpolymsci.2021.101452
Smet, S., Probst, S., Holloway, S., Fourie, A., Beele, H., and Beeckman, D. (2021). The measurement properties of assessment tools for chronic wounds: A systematic review. Int. J. Nurs. Stud. 121, 103998. doi:10.1016/j.ijnurstu.2021.103998
Song, W., Seta, J., Chen, L., Bergum, C., Zhou, Z., Kanneganti, P., et al. (2017). Doxycycline-loaded coaxial nanofiber coating of titanium implants enhances osseointegration and inhibits Staphylococcus aureus infection. Biomed. Mat. 12, 045008. doi:10.1088/1748-605x/aa6a26
Su, Y., Toftdal, M. S., le Friec, A., Dong, M., Han, X., and Chen, M. J. S. S. (2021). 3D electrospun synthetic extracellular matrix for tissue regeneration. Small Sciences 1, 2100003. doi:10.1002/smsc.202100003
Sun, B., Long, Y.-Z., Yu, F., Li, M.-M., Zhang, H.-D., Li, W.-J., et al. (2012). Self-assembly of a three-dimensional fibrous polymer sponge by electrospinning. Nanoscale 4, 2134–2137. doi:10.1039/c2nr11782g
Sun, B., Long, Y. Z., Zhang, H. D., Li, M. M., Duvail, J. L., Jiang, X. Y., et al. (2014). Advances in three-dimensional nanofibrous macrostructures via electrospinning. Prog. Polym. Sci. 39, 862–890. doi:10.1016/j.progpolymsci.2013.06.002
Taghe, S., Mehrandish, S., and Mirzaeei, S. (2022). Preparation of azithromycin nanofibers as controlled release ophthalmic drug carriers using electrospinning technique: In vitro and in vivo characterization. Adv. Pharm. Bull. 12, 346–355. doi:10.34172/apb.2022.033
Tallawi, M., Dippold, D., Rai, R., D'Atri, D., Roether, J. A., Schubert, D. W., et al. (2016). Novel PGS/PCL electrospun fiber mats with patterned topographical features for cardiac patch applications. Mater. Sci. Eng. C 69, 569–576. doi:10.1016/j.msec.2016.06.083
Tamm, I., Heinämäki, J., Laidmäe, I., Rammo, L., Paaver, U., Ingebrigtsen, S. G., et al. (2016). Development of suberin fatty acids and chloramphenicol-loaded antimicrobial electrospun nanofibrous mats intended for wound therapy. J. Pharm. Sci. 105, 1239–1247. doi:10.1016/j.xphs.2015.12.025
Tan, Z., Wang, H., Gao, X., Liu, T., and Tan, Y. (2016). Composite vascular grafts with high cell infiltration by co-electrospinning. Mater. Sci. Eng. C 67, 369–377. doi:10.1016/j.msec.2016.05.067
Tang, C., Holt, B. D., Wright, Z. M., Arnold, A. M., Moy, A. C., and Sydlik, S. A. (2019). Injectable amine functionalized graphene and chondroitin sulfate hydrogel with potential for cartilage regeneration. J. Mat. Chem. B 7, 2442–2453. doi:10.1039/c8tb02967a
Taskin, M. B., Klausen, L. H., Dong, M., and Chen, M. (2020). Emerging wet electrohydrodynamic approaches for versatile bioactive 3D interfaces. Nano Res. 13, 315–327. doi:10.1007/s12274-020-2635-x
Teno, J., Pardo-Figuerez, M., Figueroa-Lopez, K. J., Prieto, C., and Lagaron, J. M. (2022). Development of multilayer ciprofloxacin hydrochloride electrospun patches for buccal drug delivery. J. Funct. Biomater. 13, 170. doi:10.3390/jfb13040170
Teo, W. E., and Ramakrishna, S. J. N. (2006). A review on electrospinning design and nanofibre assemblies. Nanotechnol. , 17, R89. doi:10.1088/0957-4484/17/14/R01
Thottappillil, N., and Nair, P. D. (2015). Scaffolds in vascular regeneration: Current status. Vasc. Health Risk Manag. 11, 79–91. doi:10.2147/vhrm.s50536
Tian, L., Prabhakaran, M. P., Hu, J., Chen, M., Besenbacher, F., and Ramakrishna, S. (2016). Synergistic effect of topography, surface chemistry and conductivity of the electrospun nanofibrous scaffold on cellular response of PC12 cells. Colloids Surfaces B Biointerfaces 145, 420–429. doi:10.1016/j.colsurfb.2016.05.032
Topuz, F., Abdulhamid, M. A., Holtzl, T., and Szekely, G. (2021). Nanofiber engineering of microporous polyimides through electrospinning: Influence of electrospinning parameters and salt addition. Mater. Des. 198, 109280. doi:10.1016/j.matdes.2020.109280
Torres-Martinez, E. J., Cornejo Bravo, J. M., Serrano Medina, A., Pérez González, G. L., and Villarreal Gómez, L. J. (2018). A summary of electrospun nanofibers as drug delivery system: Drugs loaded and biopolymers used as matrices. Curr. Drug Deliv. 15, 1360–1374. doi:10.2174/1567201815666180723114326
Tottoli, E. M., Dorati, R., Genta, I., Chiesa, E., Pisani, S., and Conti, B. (2020). Skin wound healing process and new emerging technologies for skin wound care and regeneration. Pharmaceutics 12, 735. doi:10.3390/pharmaceutics12080735
Tran, T., Hernandez, M., Patel, D., and Wu, J. (2015). Temperature and pH responsive microfibers for controllable and variable ibuprofen delivery. Adv. Mater. Sci. Eng. 2015, 1–6. doi:10.1155/2015/180187
Tucker, N., Stanger, J. J., Staiger, M. P., Razzaq, H., and Hofman, K. (2012). The history of the science and technology of electrospinning from 1600 to 1995. J. Eng. Fibers Fabr. 7, 155892501200702. doi:10.1177/155892501200702s10
Tuğcu-Demiröz, F., Saar, S., Kara, A. A., YıLDıZ, A., Tunçel, E., and Acartürk, F. (2021). Development and characterization of chitosan nanoparticles loaded nanofiber hybrid system for vaginal controlled release of benzydamine. Eur. J. Pharm. Sci. 161, 105801. doi:10.1016/j.ejps.2021.105801
Unalan, I., Endlein, S. J., Slavik, B., Buettner, A., Goldmann, W. H., Detsch, R., et al. (2019). Evaluation of electrospun poly(ε-caprolactone)/gelatin nanofiber mats containing clove essential oil for antibacterial wound dressing. Pharmaceutics 11, 570. doi:10.3390/pharmaceutics11110570
Unnithan, A. R., Gnanasekaran, G., Sathishkumar, Y., Lee, Y. S., and Kim, C. S. J. C. P. (2014). Electrospun antibacterial polyurethane–cellulose acetate–zein composite mats for wound dressing. Carbohydr. Polym. 102, 884–892. doi:10.1016/j.carbpol.2013.10.070
Vacanti, N. M., Cheng, H., Hill, P. S., Guerreiro, J. D., Dang, T. T., Ma, M., et al. (2012). Localized delivery of dexamethasone from electrospun fibers reduces the foreign body response. Biomacromolecules 13, 3031–3038. doi:10.1021/bm300520u
Varshosaz, J., Arabloo, K., Sarrami, N., Ghassami, E., Yazdani Kachouei, E., Kouhi, M., et al. (2020). RGD peptide grafted polybutylene adipate-co-terephthalate/gelatin electrospun nanofibers loaded with a matrix metalloproteinase inhibitor drug for alleviating of wounds: An in vitro/in vivo study. Drug Dev. Ind. Pharm. 46, 484–497. doi:10.1080/03639045.2020.1730397
Vashisth, P., Raghuwanshi, N., Srivastava, A. K., Singh, H., Nagar, H., and Pruthi, V. (2017). Ofloxacin loaded gellan/PVA nanofibers - synthesis, characterization and evaluation of their gastroretentive/mucoadhesive drug delivery potential. Mater. Sci. Eng. C 71, 611–619. doi:10.1016/j.msec.2016.10.051
Vicini, S., Mauri, M., Vita, S., and Castellano, M. (2018). Alginate and alginate/hyaluronic acid membranes generated by electrospinning in wet conditions: Relationship between solution viscosity and spinnability. J. Appl. Polym. Sci. 135, 46390. doi:10.1002/app.46390
Vong, M., Speirs, E., Klomkliang, C., Akinwumi, I., Nuansing, W., and Radacsi, N. (2018). Controlled three-dimensional polystyrene micro- and nano-structures fabricated by three-dimensional electrospinning. RSC Adv. 8, 15501–15512. doi:10.1039/c7ra13278f
Waghmare, V. S., Wadke, P. R., Dyawanapelly, S., Deshpande, A., Jain, R., and Dandekar, P. (2018). Starch based nanofibrous scaffolds for wound healing applications. Bioact. Mat. 3, 255–266. doi:10.1016/j.bioactmat.2017.11.006
Walser, J., Stok, K. S., Caversaccio, M. D., and Ferguson, S. J. (2016). Direct electrospinning of 3D auricle-shaped scaffolds for tissue engineering applications. Biofabrication 8, 025007. doi:10.1088/1758-5090/8/2/025007
Wang, C., Tong, S. N., Tse, Y. H., and Wang, M. (2012a). Conventional electrospinning vs. emulsion electrospinning: A comparative study on the development of nanofibrous drug/biomolecule delivery vehicles. Adv. Mater. Res. 410, 118121. doi:10.4028/www.scientific.net/AMR.410.118
Wang, C., Wang, J., Zeng, L., Qiao, Z., Liu, X., Liu, H., et al. (2019). Fabrication of electrospun polymer nanofibers with diverse morphologies. Molecules 24, 834. doi:10.3390/molecules24050834
Wang, C., Zuo, Q., Wang, L., Long, B., Salleh, K. M., Anuar, N. I. S., et al. (2021a). Diameter optimization of polyvinyl alcohol/sodium alginate fiber membranes using response surface methodology. Mater. Chem. Phys. 271, 124969. doi:10.1016/j.matchemphys.2021.124969
Wang, H., Feng, Y., Fang, Z., Yuan, W., and Khan, M. (2012b). Co-electrospun blends of PU and PEG as potential biocompatible scaffolds for small-diameter vascular tissue engineering. Mater. Sci. Eng. C 32, 2306–2315. doi:10.1016/j.msec.2012.07.001
Wang, J., and Windbergs, M. (2019). Controlled dual drug release by coaxial electrospun fibers – impact of the core fluid on drug encapsulation and release. Int. J. Pharm. 556, 363–371. doi:10.1016/j.ijpharm.2018.12.026
Wang, L., Wu, Y., Hu, T., Guo, B., and Ma, P. X. (2017). Electrospun conductive nanofibrous scaffolds for engineering cardiac tissue and 3D bioactuators. Acta Biomater. 59, 68–81. doi:10.1016/j.actbio.2017.06.036
Wang, M., Li, D., Li, J., Li, S., Chen, Z., Yu, D.-G., et al. (2020a). Electrospun Janus zein–PVP nanofibers provide a two-stage controlled release of poorly water-soluble drugs. Mater. Des. 196, 109075. doi:10.1016/j.matdes.2020.109075
Wang, S., Yan, F., Ren, P., Li, Y., Wu, Q., Fang, X., et al. (2020b). Incorporation of metal-organic frameworks into electrospun chitosan/poly (vinyl alcohol) nanofibrous membrane with enhanced antibacterial activity for wound dressing application. Int. J. Biol. Macromol. 158, 9–17. doi:10.1016/j.ijbiomac.2020.04.116
Wang, X.-X., Yu, G.-F., Zhang, J., Yu, M., Ramakrishna, S., and Long, Y.-Z. (2021b). Conductive polymer ultrafine fibers via electrospinning: Preparation, physical properties and applications. Prog. Mater. Sci. 115, 100704. doi:10.1016/j.pmatsci.2020.100704
Wang, Y., Cui, W., Chou, J., Wen, S., Sun, Y., and Zhang, H. (2018a). Electrospun nanosilicates-based organic/inorganic nanofibers for potential bone tissue engineering. Colloids Surfaces B Biointerfaces 172, 90–97. doi:10.1016/j.colsurfb.2018.08.032
Wang, Y., Cui, W., Zhao, X., Wen, S., Sun, Y., Han, J., et al. (2018b). Bone remodeling-inspired dual delivery electrospun nanofibers for promoting bone regeneration. Nanoscale 11, 60–71. doi:10.1039/c8nr07329e
Wang, Z., Lin, M., Xie, Q., Sun, H., Huang, Y., Zhang, D., et al. (2016). Electrospun silk fibroin/poly(lactide-co-ε-caprolactone) nanofibrous scaffolds for bone regeneration. Int. J. Nanomedicine 11, 1483–1500. doi:10.2147/ijn.s97445
Wei, J., Hu, J., Li, M., Chen, Y., and Chen, Y. (2014). Multiple drug-loaded electrospun PLGA/gelatin composite nanofibers encapsulated with mesoporous ZnO nanospheres for potential postsurgical cancer treatment. RSC Adv. 4, 28011–28019. doi:10.1039/c4ra03722g
Weinberger, F., Mannhardt, I., and Eschenhagen, T. (2017). Engineering cardiac muscle tissue: A maturating field of research. Circ. Res. 120, 1487–1500. doi:10.1161/circresaha.117.310738
Wilk, S., and Benko, A. (2021). Advances in fabricating the electrospun biopolymer-based biomaterials. J. Funct. Biomater. 12, 26. doi:10.3390/jfb12020026
Wong, C. S., Liu, X., Xu, Z., Lin, T., and Wang, X. (2013). Elastin and collagen enhances electrospun aligned polyurethane as scaffolds for vascular graft. J. Mat. Sci. Mat. Med. 24, 1865–1874. doi:10.1007/s10856-013-4937-y
Wu, L., Zhang, Y., Yu, H., Jia, Y., Wang, X., and Ding, B. (2021). Self-assembly of polyethylene oxide and its composite nanofibrous membranes with cellular network structure. Compos. Commun. 27, 100759. doi:10.1016/j.coco.2021.100759
Xu, T., Yao, Q., Miszuk, J. M., Sanyour, H. J., Hong, Z., Sun, H., et al. (2018). Tailoring weight ratio of PCL/PLA in electrospun three-dimensional nanofibrous scaffolds and the effect on osteogenic differentiation of stem cells. Colloids Surfaces B Biointerfaces 171, 31–39. doi:10.1016/j.colsurfb.2018.07.004
Xu, X., Chen, X., Wang, Z., and Jing, X. (2009). Ultrafine PEG–PLA fibers loaded with both paclitaxel and doxorubicin hydrochloride and their in vitro cytotoxicity. Eur. J. Pharm. Biopharm. 72, 18–25. doi:10.1016/j.ejpb.2008.10.015
Xu, Y., Guo, P., and Akono, A.-T. (2022). Novel wet electrospinning inside a reactive pre-ceramic gel to yield advanced nanofiber-reinforced geopolymer composites. Polymers 14, 3943. doi:10.3390/polym14193943
Xue, J., Feng, B., Zheng, R., Lu, Y., Zhou, G., Liu, W., et al. (2013). Engineering ear-shaped cartilage using electrospun fibrous membranes of gelatin/polycaprolactone. Biomaterials 34, 2624–2631. doi:10.1016/j.biomaterials.2012.12.011
Yan, G., Yu, J., Qiu, Y., Yi, X., Lu, J., Zhou, X., et al. (2011). Self-assembly of electrospun polymer nanofibers: A general phenomenon generating honeycomb-patterned nanofibrous structures. Langmuir 27, 4285–4289. doi:10.1021/la1047936
Yang, C., Shao, Q., Han, Y., Liu, Q., He, L., Sun, Q., et al. (2021). Fibers by electrospinning and their emerging applications in bone tissue engineering. Appl. Sci. 11, 9082. doi:10.3390/app11199082
Yang, D. L., Faraz, F., Wang, J. X., and Radacsi, N. (2022). Combination of 3D printing and electrospinning techniques for biofabrication. Adv. Mater. Technol. 7, 2101309. doi:10.1002/admt.202101309
Yang, G., Kampstra, K. L., and Abidian, M. R. (2014). High performance conducting polymer nanofiber biosensors for detection of biomolecules. Adv. Mat. 26, 4954–4960. doi:10.1002/adma.201400753
Yang, J., Wang, K., Yu, D.-G., Yang, Y., Bligh, S. W. A., and Williams, G. R. (2020a). Electrospun Janus nanofibers loaded with a drug and inorganic nanoparticles as an effective antibacterial wound dressing. Mater. Sci. Eng. C 111, 110805. doi:10.1016/j.msec.2020.110805
Yang, L., Li, X., Wang, D., Mu, S., Lv, W., Hao, Y., et al. (2020b). Improved mechanical properties by modifying fibrin scaffold with PCL and its biocompatibility evaluation. J. Biomaterials Sci. Polym. Ed. 31, 658–678. doi:10.1080/09205063.2019.1710370
Yang, S., Li, X., Liu, P., Zhang, M., Wang, C., and Zhang, B. (2020c). Multifunctional chitosan/polycaprolactone nanofiber scaffolds with varied dual-drug release for wound-healing applications. ACS Biomater. Sci. Eng. 6, 4666–4676. doi:10.1021/acsbiomaterials.0c00674
Yang, T., Yang, H., Zhen, S. J., and Huang, C. Z. (2015). Hydrogen-bond-mediated in situ fabrication of AgNPs/agar/PAN electrospun nanofibers as reproducible SERS substrates. ACS Appl. Mat. Interfaces 7, 1586–1594. doi:10.1021/am507010q
Yang, W., Yang, F., Wang, Y., Both, S. K., and Jansen, J. A. (2013). In vivo bone generation via the endochondral pathway on three-dimensional electrospun fibers. Acta Biomater. 9, 4505–4512. doi:10.1016/j.actbio.2012.10.003
Yang, Y., Xia, T., Chen, F., Wei, W., Liu, C., He, S., et al. (2012). Electrospun fibers with plasmid bFGF polyplex loadings promote skin wound healing in diabetic rats. Mol. Pharm. 9, 48–58. doi:10.1021/mp200246b
Yao, Q., Cosme, J. G. L., Xu, T., Miszuk, J. M., Picciani, P. H. S., Fong, H., et al. (2017). Three dimensional electrospun PCL/PLA blend nanofibrous scaffolds with significantly improved stem cells osteogenic differentiation and cranial bone formation. Biomaterials 115, 115–127. doi:10.1016/j.biomaterials.2016.11.018
Yazdanpanah, A., Tahmasbi, M., Amoabediny, G., Nourmohammadi, J., Moztarzadeh, F., and Mozafari, M. (2015). Fabrication and characterization of electrospun poly-L-lactide/gelatin graded tubular scaffolds: Toward a new design for performance enhancement in vascular tissue engineering. Prog. Nat. Sci. Mater. Int. 25, 405–413. doi:10.1016/j.pnsc.2015.09.009
Ye, K., Liu, D., Kuang, H., Cai, J., Chen, W., Sun, B., et al. (2019). Three-dimensional electrospun nanofibrous scaffolds displaying bone morphogenetic protein-2-derived peptides for the promotion of osteogenic differentiation of stem cells and bone regeneration. J. Colloid Interface Sci. 534, 625–636. doi:10.1016/j.jcis.2018.09.071
Ye, L., Lv, Y., Zhao, Y., Zhou, Z., Shen, Y., and Jiang, L. (2021). Encapsulation of fragrances in micron-size silk fibroin carriers via coaxial electrohydrodynamic techniques. Mater. Chem. Phys. 260, 124167. doi:10.1016/j.matchemphys.2020.124167
Yi, B., Xu, Q., and Liu, W. (2022). An overview of substrate stiffness guided cellular response and its applications in tissue regeneration. Bioact. Mater. 15, 82–102. doi:10.1016/j.bioactmat.2021.12.005
Yin, J., and Xu, L. (2020). Batch preparation of electrospun polycaprolactone/chitosan/aloe vera blended nanofiber membranes for novel wound dressing. Int. J. Biol. Macromol. 160, 352–363. doi:10.1016/j.ijbiomac.2020.05.211
Yousefzadeh, M., and Ghasemkhah, F. (2019). Design of porous, core-shell, and hollow nanofibers.” in Handbook of nanofibers. Cham: Springer.
Yu, D.-G., Li, X.-Y., Wang, X., Yang, J.-H., Bligh, S. W. A., and Williams, G. R. (2015). Nanofibers fabricated using triaxial electrospinning as zero order drug delivery systems. ACS Appl. Mat. Interfaces 7, 18891–18897. doi:10.1021/acsami.5b06007
Yu, F., Li, M., Yuan, Z., Rao, F., Fang, X., Jiang, B., et al. (2018). Mechanism research on a bioactive resveratrol&ndash;PLA&ndash;gelatin porous nano-scaffold in promoting the repair of cartilage defect. Int. J. Nanomedicine 13, 7845–7858. doi:10.2147/ijn.s181855
Yu, H., Yang, P., Jia, Y., Zhang, Y., Ye, Q., and Zeng, S. (2016). Regulation of biphasic drug release behavior by graphene oxide in polyvinyl pyrrolidone/poly(ε-caprolactone) core/sheath nanofiber mats. Colloids Surfaces B Biointerfaces 146, 63–69. doi:10.1016/j.colsurfb.2016.05.052
Yuan, W., and Zhang, K.-Q. (2012). Structural evolution of electrospun composite fibers from the blend of polyvinyl alcohol and polymer nanoparticles. Langmuir 28, 15418–15424. doi:10.1021/la303312q
Zahmatkeshan, M., Adel, M., Bahrami, S., Esmaeili, F., Rezayat, S. M., Saeedi, Y., et al. (2019). Polymer-based nanofibers: Preparation, fabrication, and applications.” in Handbook of nanofibers. Springer.
Zamanlui, S., Mahmoudifard, M., Soleimani, M., Bakhshandeh, B., Vasei, M., and Faghihi, S. (2018). Enhanced chondrogenic differentiation of human bone marrow mesenchymal stem cells on PCL/PLGA electrospun with different alignments and compositions. Int. J. Polym. Mater. Polym. Biomaterials 67, 50–60. doi:10.1080/00914037.2017.1297941
Zeng, L., Pan, F., Li, W., Jiang, Y., Zhong, X., and Yu, Y. (2014). Free-standing porous carbon nanofibers–sulfur composite for flexible Li–S battery cathode. Nanoscale 6, 9579–9587. doi:10.1039/c4nr02498b
Zhang, D., Li, L., Shan, Y., Xiong, J., Hu, Z., Zhang, Y., et al. (2019a). In vivo study of silk fibroin/gelatin electrospun nanofiber dressing loaded with astragaloside IV on the effect of promoting wound healing and relieving scar. J. Drug Deliv. Sci. Technol. 52, 272–281. doi:10.1016/j.jddst.2019.04.021
Zhang, J., Li, J., Xu, C., XI, Z., Ren, Y., Song, Q., et al. (2020). Novel pH-sensitive drug-loaded electrospun nanofibers based on regenerated keratin for local tumor chemotherapy. Text. Res. J. 90, 2336–2349. doi:10.1177/0040517520919920
Zhang, J., Wang, X.-X., Zhang, B., Ramakrishna, S., Yu, M., Ma, J.-W., et al. (2018). In situ assembly of well-dispersed Ag nanoparticles throughout electrospun alginate nanofibers for monitoring human breath—smart fabrics. ACS Appl. Mat. Interfaces 10, 19863–19870. doi:10.1021/acsami.8b01718
Zhang, K., Bai, X., Yuan, Z., Cao, X., Jiao, X., Li, Y., et al. (2019b). Layered nanofiber sponge with an improved capacity for promoting blood coagulation and wound healing. Biomaterials 204, 70–79. doi:10.1016/j.biomaterials.2019.03.008
Zhang, M., Zhao, X., Zhang, G., Wei, G., and Su, Z. (2017a). Electrospinning design of functional nanostructures for biosensor applications. J. Mat. Chem. B 5, 1699–1711. doi:10.1039/c6tb03121h
Zhang, P., Zhao, X., Ji, Y., Ouyang, Z., Wen, X., Li, J., et al. (2015). Electrospinning graphene quantum dots into a nanofibrous membrane for dual-purpose fluorescent and electrochemical biosensors. J. Mat. Chem. B 3, 2487–2496. doi:10.1039/c4tb02092h
Zhang, X., and Wang, M. (2012). Effects of emulsion electrospinning parameters on the morphology and structure of core-shell structured PLLA fibers. Adv. Mater. Res. 410, 386389. doi:10.4028/www.scientific.net/AMR.410.386
Zhang, X., Xie, L., Wang, X., Shao, Z., and Kong, B. (2022b). Electrospinning super-assembly of ultrathin fibers from single- to multi-Taylor cone sites. Appl. Mater. Today 26, 101272. doi:10.1016/j.apmt.2021.101272
Zhang, Y. S., Yue, K., Aleman, J., Mollazadeh-Moghaddam, K., Bakht, S. M., Yang, J., et al. (2017b). 3D bioprinting for tissue and organ fabrication. Ann. Biomed. Eng. 45, 148–163. doi:10.1007/s10439-016-1612-8
Zhang, Z., Eyster, T. W., and Ma, P. X. (2016). Nanostructured injectable cell microcarriers for tissue regeneration. Nanomedicine 11, 1611–1628. doi:10.2217/nnm-2016-0083
Zhang., M., Song., W., Tang., Y., Xu, ., X., Huang, Y., and Yu, D. (2022a). Polymer-based nanofiber–nanoparticle hybrids and their medical applications. Polymers 14, 351. doi:10.3390/polym14020351
Zhao, K., Lu, Z.-H., Zhao, P., Kang, S.-X., Yang, Y.-Y., and Yu, D.-G. (2021a). Modified tri–axial electrospun functional core–shell nanofibrous membranes for natural photodegradation of antibiotics. Chem. Eng. J. 425, 131455. doi:10.1016/j.cej.2021.131455
Zhao, W., Zhang, Y., Wang, X., Lu, H., Liu, G., Wei, J., et al. (2021b). Carbon-nanotube-templated carbon nanofibers with improved mechanical performance. J. Appl. Phys. 129, 044303. doi:10.1063/5.0031159
Zhao, Y.-H., Niu, C.-M., Shi, J.-Q., Wang, Y.-Y., Yang, Y.-M., and Wang, H.-B. (2018). Novel conductive polypyrrole/silk fibroin scaffold for neural tissue repair. Neural Regen. Res. 13, 1455. doi:10.4103/1673-5374.235303
Zheng, R., Wang, X., Xue, J., Yao, L., Wu, G., Yi, B., et al. (2021). Regeneration of subcutaneous cartilage in a swine model using autologous auricular chondrocytes and electrospun nanofiber membranes under conditions of varying gelatin/PCL ratios. Front. Bioeng. Biotechnol. 9, 752677. doi:10.3389/fbioe.2021.752677
Zhou, G., Liu, S., Ma, Y., Xu, W., Meng, W., Lin, X., et al. (2017). Innovative biodegradable poly(L-lactide)/collagen/hydroxyapatite composite fibrous scaffolds promote osteoblastic proliferation and differentiation. Int. J. Nanomedicine 12, 7577–7588. doi:10.2147/ijn.s146679
Zhou, L., Cai, L., Ruan, H., Zhang, L., Wang, J., Jiang, H., et al. (2021). Electrospun chitosan oligosaccharide/polycaprolactone nanofibers loaded with wound-healing compounds of Rutin and Quercetin as antibacterial dressings. Int. J. Biol. Macromol. 183, 1145–1154. doi:10.1016/j.ijbiomac.2021.05.031
Zhu, H., du, M., Zhang, M., Wang, P., Bao, S., Fu, Y., et al. (2013). Facile and green fabrication of small, mono-disperse and size-controlled noble metal nanoparticles embedded in water-stable polyvinyl alcohol nanofibers: High sensitive, flexible and reliable materials for biosensors. Sensors Actuators B Chem. 185, 608–619. doi:10.1016/j.snb.2013.05.062
Zhu, X., Ni, S., Xia, T., Yao, Q., Li, H., Wang, B., et al. (2015). Anti-neoplastic cytotoxicity of SN-38-Loaded PCL/gelatin electrospun composite nanofiber scaffolds against human glioblastoma cells in vitro. J. Pharm. Sci. 104, 4345–4354. doi:10.1002/jps.24684
Zhu, Y., Li, X., Janairo, R. R. R., Kwong, G., Tsou, A. D., Chu, J. S., et al. (2019). Matrix stiffness modulates the differentiation of neural crest stem cells in vivo. J. Cell. Physiol. 234, 7569–7578. doi:10.1002/jcp.27518
Keywords: electrospinning, hybrid nanofibers, biomedical applications, wound healing, characterization
Citation: Abadi B, Goshtasbi N, Bolourian S, Tahsili J, Adeli-Sardou M and Forootanfar H (2022) Electrospun hybrid nanofibers: Fabrication, characterization, and biomedical applications. Front. Bioeng. Biotechnol. 10:986975. doi: 10.3389/fbioe.2022.986975
Received: 05 July 2022; Accepted: 16 November 2022;
Published: 01 December 2022.
Edited by:
Mehdi Razavi, University of Central Florida, United StatesReviewed by:
Xiaoran Li, Donghua University, ChinaYilei Zhang, University of Canterbury, New Zealand
Copyright © 2022 Abadi, Goshtasbi, Bolourian, Tahsili, Adeli-Sardou and Forootanfar. This is an open-access article distributed under the terms of the Creative Commons Attribution License (CC BY). The use, distribution or reproduction in other forums is permitted, provided the original author(s) and the copyright owner(s) are credited and that the original publication in this journal is cited, in accordance with accepted academic practice. No use, distribution or reproduction is permitted which does not comply with these terms.
*Correspondence: Mahboubeh Adeli-Sardou, bV9hZGVsaTYyMjAwMkB5YWhvby5jb20=; Hamid Forootanfar, aF9mb3Jvb3RhbmZhckBrbXUuYWMuaXI=