- 1Department of Neurosurgery, Qilu Hospital, Cheeloo College of Medicine and Institute of Brain and Brain-Inspired Science, Shandong University, Jinan, China
- 2Jinan Microecological Biomedicine Shandong Laboratory and Shandong Key Laboratory of Brain Function Remodeling, Jinan, China
Neural tissue is an important soft tissue; for instance, craniofacial nerves govern several aspects of human behavior, including the expression of speech, emotion transmission, sensation, and motor function. Therefore, nerve repair to promote functional recovery after craniofacial soft tissue injuries is indispensable. However, the repair and regeneration of craniofacial nerves are challenging due to their intricate anatomical and physiological characteristics. Currently, nerve transplantation is an irreplaceable treatment for segmental nerve defects. With the development of emerging technologies, transplantation donors have become more diverse. The present article reviews the traditional and emerging alternative materials aimed at advancing cutting-edge research on craniofacial nerve repair and facilitating the transition from the laboratory to the clinic. It also provides a reference for donor selection for nerve repair after clinical craniofacial soft tissue injuries. We found that autografts are still widely accepted as the first options for segmental nerve defects. However, allogeneic composite functional units have a strong advantage for nerve transplantation for nerve defects accompanied by several tissue damages or loss. As an alternative to autografts, decellularized tissue has attracted increasing attention because of its low immunogenicity. Nerve conduits have been developed from traditional autologous tissue to composite conduits based on various synthetic materials, with developments in tissue engineering technology. Nerve conduits have great potential to replace traditional donors because their structures are more consistent with the physiological microenvironment and show self-regulation performance with improvements in 3D technology. New materials, such as hydrogels and nanomaterials, have attracted increasing attention in the biomedical field. Their biocompatibility and stimuli-responsiveness have been gradually explored by researchers in the regeneration and regulation of neural networks.
Introduction
Although the surface area of the head and neck accounts for approximately 12 percent of the entire body, they are widely populated with craniofacial nerves, which perform various functions. As part of peripheral nerves, craniofacial nerves are responsible for extremely complex sensory and motor functions, including olfaction, vision, hearing, taste, touch, chewing, eye movement, and balance. It is important to pay attention to nerve repair during craniofacial injuries. The causes of craniofacial nerve injury are complex and diverse. Trauma, infection, inflammation, tumors, and iatrogenic factors can cause reversible or irreversible nerve injuries (Jandali and Revenaugh, 2019; Pan et al., 2020). Therefore, the complicated anatomical structure and physiological functions of the craniofacial nerves make their regeneration a challenge.
In 1943, Herbert Seddon proposed a classification method based on the severity and prognosis of peripheral nerve injuries, including neurapraxia, axonotmesis, and neurotmesis (Kaya and Sarikcioglu, 2015). Based on Seddon’s classification, Sunderland proposed five categories according to the extent of axonal injury (Sunderland, 1951) (Table 1). The degree and type of nerve injury should be considered in surgical repair. The location, length, shape, and cross-sectional area of the donor and the damaged nerves are important considerations in donor selection. Patient preferences should be assessed, as nerve harvesting may result in loss of function at the donor site (Altafulla et al., 2019). However, autologous nerve transplantation is still the preferred treatment for segmental nerve defects that are difficult for primary repair. However, imperfect results have suppressed the advantages of autologous transplantation as the first-line option, which include the formation of neuroma, loss of sensation at the donor site, infection, and pain caused by the nerve stump. This has encouraged researchers to explore additional treatment options for nerve transplantation (Szynkaruk et al., 2013; Carvalho et al., 2020). Allotransplantation allows a wide range of donor sources for nerve transplantation. Among these, the complex functional unit containing nerve tissue facilitates nerve function recovery in multiple tissue injuries. Meanwhile, there is the risk of long-term immunosuppression after transplantation and infection, especially under circumstances where the structures of cranial and facial tissues are complex and the bacterial flora is diverse (Husain et al., 2003; Singh et al., 2005; Cuellar-Rodriguez et al., 2009). In recent years, the advantages of nerve conduits combined with tissue engineering have been realized in the field of nerve transplantation (Zor et al., 2010; Szynkaruk et al., 2013; Roche et al., 2015a; Long et al., 2021). Researchers are incorporating absorbable and non-absorbable materials, such as silica gel, polyhydroxy acid, poly (lactate-glycolic acid) graphene foam, and polycaprolactone, in these new conduits (Han et al., 2019; Bahremandi Tolou et al., 2021). Improving the physiological performance of emerging grafts by combining cells, such as Schwann cells (SC), or growth factors has become an important research trend (Huard et al., 1998; Evans, 2001; Murrell et al., 2005; Matsumine et al., 2016; Watanabe et al., 2017). These new explorations have stimulated the interest of researchers in using novel materials, such as hydrogels and nanomaterials, for nerve regeneration (Oprych et al., 2016; Celikkin et al., 2017; Fan et al., 2017). Their superior biocompatibility allows simulation of the extracellular matrix (ECM) and microenvironment in vivo to promote adhesion and survival of neural cells. Sensitive responses to external stimuli make it possible to use external factors (light, electricity, magnetic field, temperature, etc.) to achieve the directional control of implant materials (Riggio et al., 2014; Marcus et al., 2016; Abatangelo et al., 2020).
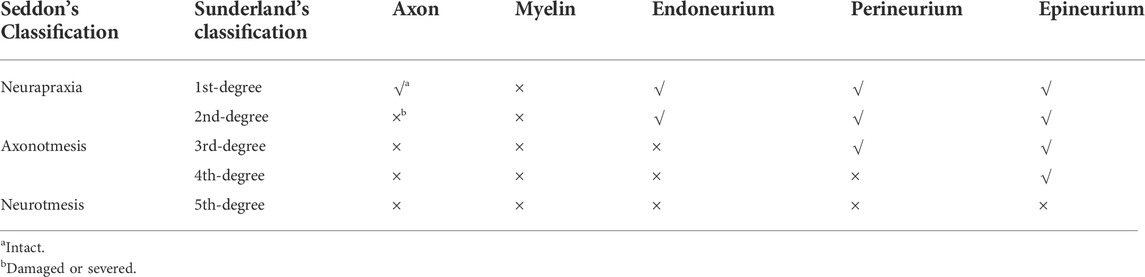
TABLE 1. Seddon’s and Sunderland’s classification of peripheral nerve injuries. Peripheral nerve injuries are divided into three categories in Seddon’s classification, including neurapraxia, axonotmesis and neurotmesis. Based on Seddon’s classification, Sunderland classified five different levels in detail according to the extent of a xonal injury. Neurapraxia in Seddon’s classification is equivalent to the first degree injury in Sunderland’s classification. Neurotmesis is equivalent to the fifth degree nerve injury and axonotmesis is equivalent to the second-to-fourth-degree nerve injury.
To drive cutting-edge research on craniofacial nerve repair and its progression from the laboratory to the clinic, we reviewed the selection of donors for repair and regeneration of craniofacial nerve injury, especially segmental defects. The main focus is on nerves that are easily damaged and have obvious effects on human function, such as the facial nerve, trigeminal nerve and its branches (mandibular nerve, inferior alveolar nerve), branches of the vagus nerve (recurrent laryngeal nerve), optic nerve, and oculomotor nerve. The present article has summarized the following areas: autografts, allografts, xenografts, and nerve conduits. Allografts have been subdivided into allogeneic composite functional units and decellularized tissues, as required. Finally, the two most widely used novel materials (hydrogels and nanomaterials) in the biomedical field are described in detail to promote the application of novel materials for craniofacial nerve repair (Figure 1).
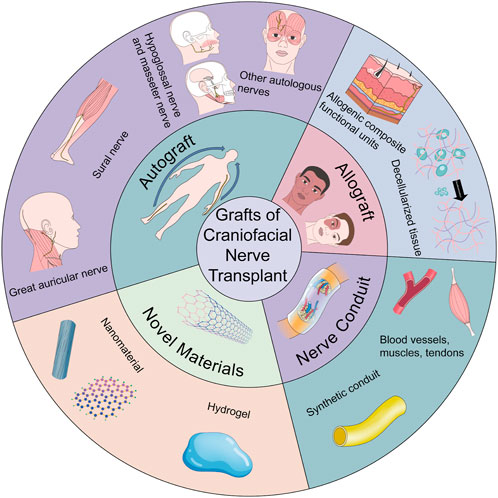
FIGURE 1. The brief description on donors for nerve transplantation in craniofacial soft tissue injuries.
Autografts
Given the underlying principles, end-to-end repair is not feasible for peripheral nerve regeneration in some cases, such as excessive nerve tension, delayed repair, or obvious segmental nerve defects. Autogenous nerve transplantation is the first-line treatment for this condition. In addition to better biocompatibility, autografts can provide cells (e.g., SCs) and cellular components that can promote nerve growth. They have not been associated with rejection and have a lower infection rate than allografts (Moore et al., 2015). However, autografts have some unavoidable disadvantages, including trauma and sensory defects as a result of harvesting the donor nerve and pain caused by the nerve stump. Therefore, the selection of autografts should be based on the principles of easy acquisition, less trauma, and a match with the size at the recipient site (Trehan et al., 2016). Common clinical donors for craniofacial nerve autotransplantation include the great auricular nerve (GAN), sural nerve, hypoglossal and masseter nerves, antebrachial cutaneous nerve, and motor nerve to the vastus lateralis.
Great auricular nerve
The GAN is a branch of the cervical plexus that arises from the second and third cervical nerves and passes through the posterior margin of the sternocleidomastoid muscle (SCM). It proceeds forward and upward and divides into two branches that are dominant in the areas of the mandible, auricula, and earlobe (Humphrey and Kriet, 2008; Altafulla et al., 2019). GAN has more than one marker. Traditionally, the McKinney spot, which is 6.5 cm below the external auditory canal and 0.5 cm behind the external jugular vein, has been the anatomical marker for distinguishing the GAN during surgical localization (McKinney and Katrana, 1980). The greater auricular point of the GAN at the posterior edge of the SCM has also been described as an important landmark (Raikos et al., 2017). Another marker, Erb’s point, is situated 2–3 cm above the clavicle and at the same level as the carotid nodule (Tubbs et al., 2007a). Researchers have delineated another marker for locating the mastoid branch of the GAN, which is 9 cm outside the external occipital eminence and 1 cm above the mastoid cusp (Tubbs et al., 2007b). Unlike traditional landmarks, this point uses bony structures that are easy to localize rather than deep blood vessels or muscles.
Transplantation of the craniofacial nerve and its branches with the GAN as a donor can be used for several types of nerve repair, including facial nerve repair, jaw reconstruction and restoration of perioral sensation, recurrent laryngeal nerve reconstruction after thyroidectomy to preserve vocal function, promoting corneal nerve regeneration, and restoration of sensation as a surgical method for neurotrophic keratosis (LaBanc et al., 1987; Yoshimura et al., 2013; Sun et al., 2015; Benkhatar et al., 2018). For facial nerve reconstruction, the GAN is used to reconstruct nerve damage caused by resection of craniofacial masses, such as facial nerve schwannomas, endolymphatic sac tumor, facial nerve hemangioma, skull base tumor, and nerve defects or function loss caused by trauma and inflammatory diseases (Stephanian et al., 1992; Katoh et al., 1998; Chi et al., 2006; De Ceulaer et al., 2012; Hou et al., 2012; Marchioni et al., 2014; Sai et al., 2019). Selecting the GAN as the donor for facial nerve repair has advantages, such as proximity to the surgical region, which helps avoid a second site of surgery, and easy localization because it is a clear marker. The GAN is also utilized as a nerve graft to reconstruct the inferior alveolar nerve for mandibular defects resulting from trauma or mandibular resection caused by a tumor. Yoshimura et al. performed segmental mandibular resection of a large ossifying fibroma and immediate reconstruction with the iliac bone and GAN. Postoperative neurosensory examination revealed sensitivity recovery of the dental pulp and mental region (Yoshimura et al., 2013). GAN transplantation for repairing craniofacial nerve defects has shown relatively stable effects in long-term clinical applications; the most commonly used nerve donor is the GAN without blood vessels.
However, vascularized nerve grafts may promote nerve regeneration better than non-vascularized nerve grafts when they are longer and nerve recovery conditions are poor (e.g., in areas with poor vascularization). Koshima et al. used a vascularized GAN graft for the primary repair of facial nerve defects. The nerve regeneration results were satisfactory, and there were fewer complications at the donor site. The main reason for the better effects of this transplantation is that blood supply can be restored immediately after surgery using a vascularized nerve graft (Koshima et al., 2004). Therefore, vascularized grafts have more significant early effects on nerve repairs. Corneal nerve fibers arise predominantly from the ophthalmic branch of the trigeminal nerve. Neurotrophic keratitis primarily results from herpes virus invasion, corneal surgery, diabetes, and orbital or intracranial surgery-related trigeminal nerve damage. Corneal neuralization is increasingly being used to restore corneal innervation after trigeminal nerve injury (Benkhatar et al., 2018). End-to-end anastomosis of the GAN and contralateral supratrochlear nerve (Benkhatar et al., 2018) or implantation of sensory fibers from the ipsilateral GAN in the precorneal stroma (Jowett and Pineda Ii, 2019) is effective in restoring corneal perception in adults.
Sural nerve
The sural nerve is a sensory nerve consisting of branches of the tibial nerve and common peroneal nerve. It passes through the deep fascia and descends laterally to the Achilles tendon before advancing to the lateral malleolus and the distal side of the calcaneus (Im et al., 2022). In craniofacial nerve transplantation, sural nerves are often used to restore sensory disturbances and malocclusions in the mandibular region as a result of inferior alveolar nerve (IAN) defects caused by tumor resection or trauma (LaBanc et al., 1987; DeLeonibus et al., 2017) and facial paralysis caused by facial nerve injury (Biglioli et al., 2018; Jeong et al., 2018; Baccarani et al., 2019; Rashid et al., 2019; Yoshioka, 2020; Chang et al., 2021; Sakuma et al., 2021). Traditionally, end-to-end suturing of the sural nerve with the nerve stump via interposition transplantation has been a common method for repairing nerve defects (Rashid et al., 2019; Matos Cruz and De Jesus, 2022). Rashid et al. used sural nerves to repair facial nerve defects caused by parotid gland tumor resection. The results demonstrate that sural nerve interposition transplantation is easy to perform and has a good effect on segmental facial nerve defects (Rashid et al., 2019). However, nerve sharing or cross nerve transplantation shows greater advantages when the proximal end of the recipient nerve is not available or the defect site is too long. LaBanc et al. reported a case of the successful restoration of the sensation of the lower lip by transplanting the autogenous sural nerve to the region between the ipsilateral GAN and distal IAN using the principle of nerve sharing (LaBanc et al., 1987). In 2009, researchers presented a new method of repairing the paralyzed eyelid, which involves transferring the facial nerve on the undamaged side to supply the upper and lower eyelids with two sural nerve grafts. Biglioli modified this technique by repairing the upper eyelid using cross grafting of the facial and sural nerves, with the lower eyelid suspended upward by the fascia lata. Eyelid closure and the blink reflex were effectively observed in 14 patients with eyelid paralysis who underwent this surgery (Biglioli et al., 2018). Therefore, nerve sharing and cross nerve transplantation have shown significant advantages in patients with long nerve defects. The muscle-nerve-muscle neurotisation technique also embodies the principle of nerve sharing. For example, the sural nerve was attached to the bilateral frontalis muscle to facilitate innervation from the healthy to the affected frontalis muscle. Postoperative nerve conduction and muscle response were normal, and spontaneous and simultaneous contraction of the bilateral frontalis muscles was successfully observed 4 months after surgery (Chang et al., 2021). Similar to the GAN, the sural nerve plays a significant role during corneal neuralization in neurotrophic keratopathy and can successfully promote the restoration of corneal sensation (Weis et al., 2018; Elalfy et al., 2021; Charlson et al., 2022). However, previous studies have found that there is no obvious distinction between the repair ability of the sural nerve and GAN for corneal neuralization; however, it can provide more options for adult patients with neurotrophic keratopathy. In this situation, the patient’s choice and physical condition may be decisive factors.
Hypoglossal nerve and masseter nerve
The hypoglossal and masseter nerves have similar functions in nerve transplantation but are not as widely used as sural nerves and the GAN, which are mainly used as donors for facial nerve anastomosis in facial resuscitation (Bayrak et al., 2017; Yetiser, 2018; Yoshioka, 2018). Anastomosis of the hypoglossal and facial nerves is one of the earliest methods used for facial resuscitation. Complete disconnection of the hypoglossal nerve and anastomosis with the main trunk of the facial nerve are the main steps during the surgery. These can provide sufficient facial tension and motor stimulation but may result in lateral tongue atrophy and difficulties with speech, chewing, and swallowing (Jandali and Revenaugh, 2019). However, anastomosis of the masseter and facial nerves can reduce the postoperative complications of dysarthria and dysphagia (Bayrak et al., 2017). The masseter nerve has several advantages, such as a stronger motor impulse, fewer complications of the donor defect, and faster recovery of innervation (Sakthivel et al., 2020). Therefore, the masseter nerve presents greater advantages than the hypoglossal nerve as a donor for facial nerve anastomosis. However, a single transfer of the hypoglossal or masseteric nerve can cause significant postoperative mass movement because both are single motor sources reinnervating the entire muscular system (Yoshioka, 2018). The joint transfers between the hypoglossal and masseteric nerves may solve this problem and promote nerve repair and motor coordination. Kuta et al. used the masseter nerve to selectively innervate the midface and restore the vitality of the remaining region of the face in combination with hypoglossal nerve transplantation. Apparent improvements in facial movement and resting facial tone were observed without mass movement after surgery (Kuta and Taylor, 2022). Yoshioka N et al. performed joint nerve transfers and cross grafting of the facial nerves. The masseter and hypoglossal nerves were transferred to the zygomatic and cervicofacial branches of the facial nerves, respectively. Patients obtained symmetrical resting lips and could raise their mouth corners voluntarily without impaired chewing function, tongue atrophy, or significant mass movement (Yoshioka, 2018). Therefore, double nerve transfer involving the hypoglossal and masseter nerves has more advantages in restoring facial tension, symmetry, and coordinated movement.
Other autologous nerves
In addition to the commonly used donor nerves mentioned above, the antebrachial cutaneous nerve and motor nerve to the vastus lateralis also have the potential to be used in the repair of the craniofacial nerve, especially for facial nerve repair (Revenaugh et al., 2012; Jandali and Revenaugh, 2019). Rodriguez-Lorenzo et al. observed complete restoration of nerve function after using the platysma motor nerve to repair the segmental defect of the marginal mandibular nerve caused by resection of soft tissue sarcoma of the jaw (Rodriguez-Lorenzo et al., 2016). This approach has been proven to be more effective in recovering laryngeal local function than performing laryngeal reinnervation utilizing the ansa cervicalis, but it has not shown significant advantages compared with the traditional vocal fold medialization laryngoplasty. Comparative studies are underway (Paniello et al., 2011; Wang et al., 2011). Li et al. reconstructed the parotid gland defects by innovatively constructing the vascularized latissimus dorsi nerve flap. The facial symmetry and smiling improved postoperatively, and it was confirmed that vascularized nerve donors can induce better nerve recovery than single nerve donors (Li S. S. et al., 2021). The supratrochlear or supraorbital nerve has also been reported for corneal neutralization. However, using the GAN can minimize further loss of facial sensation in persons who have suffered sensory deficits relative to the transfer of the supratrochlear or supraorbital nerves. However, this carries the risk of a sub-optimal blink reflex recovery (Jowett and Pineda Ii, 2019). In general, more clinical cases and research are needed to confirm the advantages and disadvantages of these rare or modified grafts as alternatives to traditional transplant donors.
Allografts
Allogeneic composite functional units
Craniofacial nerve composite allograft transplantations are used to repair severe facial injuries such as severe trauma, deep burns, and multiple tissue defects after tumor resection. The ideal reconstructive surgery allows the repair of damaged tissue and restoration of motor and sensory functions to promote optimal functional recovery of the recipient site. However, autologous transplantation does not guarantee ideal functional and aesthetic recovery of complex craniofacial defects (Devauchelle et al., 2006). Therefore, optimizing nerve regeneration by utilizing allogeneic composite functional units is beneficial for overall functional recovery (Zor et al., 2010; Roche et al., 2015a). In allogeneic composite functional units, nerve components are part of the functional unit rather than independent grafts. The functional unit includes the skin, muscle, bone, fat, and lymph nodes, in addition to nerve tissue (Broyles et al., 2014). The midface, lower face, auricle, and periorbital and perinasal regions are common functional units (Figure 2). The main nerves involved are the trigeminal and facial nerves and their branches, such as the maxillary, mandibular, buccal, and zygomatic branches of the facial nerve (De Letter et al., 2017; Lassus et al., 2018; Kauke et al., 2021). Devauchelle et al. were the first to perform the human face allograft. In addition to soft tissue reconstruction, the bilateral infraorbital and mental-sensitive nerves were anastomosed. The mandibular branch of the facial nerve was used to innervate the mimic muscle. Postoperative sensory function and verbal ability improved rapidly, and the muscle contractile function gradually recovered. These results not only verify the feasibility of allogeneic complex tissue transplantation for repairing severe facial injury but also provide important technical guidance for the extensive development of human craniofacial allografts (Devauchelle et al., 2006). At present, the consistency of the donor and injury sites is the main consideration in the selection of transplant units for composite transplantation. For example, a patient suffered a severe facial defect, resulting in a large soft tissue defect on one side of the face with severe damage to the upper jaw, both eyes, bottom of the mouth, and left side of the jaw, and all the teeth. After the clinical evaluation, the lower parts of the face were used as functional units for transplantation. The main nerves of the unit contain the facial nerves and branches. In addition, the functional unit includes the maxilla, mandible, blood vessels, and skin of the middle and lower two-thirds of the face (Roche et al., 2015b). Eyelid defects for various reasons often cause chronic eyelid discomfort, corneal ulcers, and visual impairment. Complex ocular and periorbital defects may occur after traumatic avulsion, burns, or cancer resection. It is difficult to achieve better functional and aesthetic restorations using traditional reconstruction methods (Vasilic et al., 2010; Siemionow et al., 2018). In this case, a periorbital functional unit allograft may be a better option. The researchers used fresh cadavers to develop a new model of composite eyeball-periorbital transplants. The optic nerve, oculomotor nerve, and trochlear nerve were included to promote the recovery of visual acuity and eye movement. The edge of the composite flap included the lower and lateral margins of the orbit, nasal dorsum, and the upper edge of the eyebrow. This study confirmed the feasibility of composite eyeball-periorbital transplantation and provided a new option for the reconstruction of complex periorbital malformations, as well as a new vascularized composite allograft model for whole-eye transplantation (Siemionow et al., 2018). In brief, as the donor of a craniofacial nerve allograft is mostly a composite functional unit, the main requirement for selection is consistency with the injury site. Currently, there are more preclinical studies on the functional units of craniofacial nerve transplantation, particularly facial transplantation. However, these studies have mainly focused on the feasibility of functional units centered on single organs, such as periorbital and auricular functional units, which are different from half-facial or full facial allografts that have been clinically tested (Figure 2). The corresponding nerves mainly involve the buccal and zygomatic branches of the facial nerve, the eye branch of the trigeminal nerve, and the GAN (Ulusal et al., 2005; Mathes et al., 2014; Gao et al., 2017). These studies confirmed the feasibility of using complex functional subunits for transplantation and provided more allograft options for complex craniofacial injuries.
In addition to difficulties in donor acquisition, craniofacial nerve allografts are associated with adverse consequences of long-term immunosuppression, especially infectious complications. Infection in craniofacial allografts follows a process similar to that of solid organ transplantation, but there are differences. The incidence of infectious complications was lower than that of solid organ transplantation (Roche et al., 2015a). Meanwhile, the graft, as a functional unit, has a unique source of bacterial flora. Craniofacial functional transplant units often include organs such as the oral cavity, nasal cavity, sinus, and upper respiratory mucosa, which may be sources of several pathogenic microorganisms. For instance, the oral mucosa can easily be colonized by streptococcus, candida, and several anaerobic bacteria. There is an increased risk of opportunistic infection if the graft contains sinuses or nasal mucosa that may contain fungal spores (Husain et al., 2003; Singh et al., 2005; Cuellar-Rodriguez et al., 2009). A mass of donor-derived bare skin may carry groups of pathogenic bacteria such as staphylococcus, gram-negative bacteria, and anaerobic bacteria (Gordon et al., 2011). This increases the requirements for the prevention of infections after transplantation. Therefore, it is necessary to develop a comprehensive program for infection prevention according to the type of functional unit used before transplantation. In conclusion, repairing complex craniofacial defects with an allogeneic composite functional unit has benefits and risks in craniofacial nerve transplantation. It can provide an ideal function and aesthetic effect that autologous transplantation cannot; however, it is also associated with facial immune rejection and the risk of infection caused by immunosuppression and special bacterial flora.
Decellularized tissues
Decellularized tissues are obtained using physical, chemical, or enzymatic hydrolysis to destroy the bonds between cells and the extracellular matrix (ECM), remove the cellular components in the natural tissue, and retain the ECM, including key structural and functional proteins. This is associated with low immune rejection and provides a favorable microenvironment for cell growth. Therefore, decellularized tissue is considered a new graft for promoting nerve repair and regeneration. In essence, it can still be considered a type of allograft if the decellularized tissue is from the same species. Current studies confirm that decellularized tissue can remove components that inhibit the growth of nerve axons and retain growth-promoting proteins, which play a crucial role in promoting nerve repair (Buckenmeyer et al., 2020; Sun et al., 2020).
Although mature decellularization techniques for the sciatic nerve, spinal cord, and other tissues have been used to repair various nerve injuries, few attempts have been made to use decellularized tissues for craniofacial nerve repairs. Current decellularized products for clinical use are made from the purified ECM of human peripheral nerves after decellularization. It retains bundle structure and contains molecules crucial to the growth of axons, such as laminin (Hall, 1986). These decellularized tissues have been applied successfully to peripheral nerve regeneration with satisfactory effects as a mature substitute for nerve transplantation (Szynkaruk et al., 2013). There have been increased attempts to use decellularized tissues, with the main focus on the repair of trigeminal nerve injury in the field of craniofacial nerve transplantation (Zuniga, 2015; Salomon et al., 2016; Yampolsky et al., 2017). Yampolsky et al. supported the hypothesis that decellularized graft can be used to effectively repair short-distance (<2 cm) trigeminal nerve defects based on the outcomes of trigeminal nerve reconstruction (Yampolsky et al., 2017). Salomon et al. used decellularized nerve grafts as ECM scaffolds to repair the long-span (>30 mm) defects of the inferior alveolar nerve (IAN) and also observed good recovery after surgery (Salomon et al., 2016). In 2019, treated human decellularized allograft nerve was utilized to recover corneal perception in patients suffering from neurotrophic keratopathy by transplanting it to the supraorbital nerve, supratrochlear nerve, or IAN and transferring it to the affected eye. The study found that patients regained corneal perception and the integrity of corneal epithelial cells (Leyngold et al., 2019). However, some researchers have different perspectives, arguing that there is not sufficient evidence to support better outcomes with the use of decellularized tissue to repair long nerve defects than those of autografts or allografts (Moore et al., 2011; Giusti et al., 2012; Saheb-Al-Zamani et al., 2013; Poppler et al., 2016). The decellularized tissue loses SCs in during immunogenicity removal, which are critical cells for axon regeneration. SCs from the nerve remnant at the recipient site must fill the graft for nerve regeneration after implantation (Poppler et al., 2016). Therefore, the researchers do not recommend the use of current decellularized products to repair crucial nerves, long-diameter nerves, or short-diameter sensory nerves with gaps longer than 3 cm (Moore et al., 2009; Rbia and Shin, 2017). However, the potential advantages of decellularized tissues cannot be ignored in the field of craniofacial nerve transplantation and neural transplantation in its entirety. They eliminate the limitations of limited donor sources and damage to the donor site in autologous nerve transplantation. They also provide a good growth environment for nerve cells and reduce the risk of immune rejection and infection caused by other allotransplants. Recently, researchers have attempted to enhance the effectiveness of decellularized nerve tissues by combining them with cells or growth factors. For example, Schwann cells (SCs), adipose stem cells (ADSCs), and bone marrow mesenchymal stem cells (BMMSCs) can be combined in decellularized tissues. Compared with a single graft, decellularized tissue combined with cells or growth factors showed better outcomes in promoting nerve regeneration (Nadim et al., 1990; De Ugarte et al., 2003; Chen et al., 2007).
Xenografts
There are no clinical applications of animal-derived xenografts for nerve repair. In skin transplantations, no skin substitutes can replace all the functions of intact human skin (Khan et al., 2020). However, xenotransplantation should still be considered given the shortage of donors and to reduce the injury to the donor site in autologous or allogeneic transplantation. For large soft tissue defects, autologous or allogeneic transplantation cannot meet the demand, and the need for xenotransplantation is more urgent. Therefore, research progress in xenotransplantation can promote xenotransplantation development in soft tissue injury nerve repair. At present, pigs are the main animal source of xenotransplantation tissue, with heart and kidney transplantation being the main operations (Hawthorne et al., 2021; Meier et al., 2021; Niu et al., 2021; Ryczek et al., 2021). The main disadvantage to xenotransplantation is antibody-mediated rejection (AMR). Based on previous research, the prevention and treatment of AMR include the following; preformed xenoantibodies are removed before transplantation, effectively avoiding hyperacute rejection (HAR) (Reichart and Längin, 2020). HAR is caused by the binding of preformed antibodies to porcine alpha 1,3- galactosyl- transferase (GAL). Similarly, complete knockout of the GAL gene in pig organs also prevents HAR. GAL gene-knockout pigs have been widely used in preclinical studies of xenotransplantation (Dai et al., 2002; Lai et al., 2002). With the development of gene editing technology, researchers have developed transgenic animals that express human complement regulators, such as CD40, to exert the immunomodulatory role of complement and thereby suppress immune rejection (Schmoeckel et al., 1996). Although systematic immunosuppressive therapy has achieved reliable efficacy, its serious side effects should not be ignored. Recent studies have suggested that immunological tolerance, based on regulatory T cells (Tregs), can significantly reduce the use of immunosuppressive agents (Romano et al., 2019). In conclusion, AMR and infection are still the primary problems that need to be faced and solved in future xenotransplantation for nerve repair of soft tissue injuries.
Nerve conduits
Autologous and allogeneic nerve transplantations have achieved great success as common methods of nerve repair. However, surgical complications include nerve defects at the donor site, limited donor sources, and easy induction of infection. Therefore, fabricating various artificial nerve conduits based on tissue engineering as a substitute for autografts is currently an area of focus in nerve regeneration research (Long et al., 2021). The common nerve conduits in recent studies consist of autogenous conduits and artificial nerve conduits. Autogenous conduits include the blood vessels, muscles, tendons, and other autologous tissues. Previous studies have shown that veins and arteries can provide support for the damaged nerves. As tissues promote nerve repair, their effects are similar to those of the autografts (Chiu and Strauch, 1990; Tang et al., 1993). There are also differences between arteries and veins as nerve conduits. Veins are useful because of their abundance and lower incidence of donor-site complications. However, separate venous conduits are at risk of collapse and impeding nerve regeneration (Wolford and Stevao, 2003). Therefore, researchers have modified the veins by filling them with nerve or muscle tissue, which prevents the wall from collapsing and provides ECM and growth factors (Edgar et al., 1984; Lundborg et al., 1994). However, Tang JB et al. suggested that venous conduits were unsuitable for primary repair of nerves with nerve gaps greater than 5.0 cm or with complex nerve injuries based on their findings (Tang et al., 1995). Skeletal muscle contains multiple types of collagens (e.g., type IV collagen and laminin), which could guide the regeneration of the axon (Edgar et al., 1984; Lein et al., 1992; Lundborg et al., 1994; Meek et al., 2004). Therefore, skeletal muscle could be a good candidate for a nerve conduit, and it could assist in nerve regeneration as well as autologous nerve transplant (Pereira et al., 1991a; Pereira et al., 1991b; Meek et al., 2004). Tendons can also be used as autologous nerve conduits because they can provide laminin, fibronectin, and components that can promote the growth of axons (Guizzardi et al., 1987; Banes et al., 1988). Similar to muscle tissue, tendons can, theoretically provide a good spatial structure for nerve growth. However, previous studies have revealed that the ability of tendons to promote nerve regeneration has not met expectations (Brandt et al., 1999). Therefore, tendons are not preferred candidate material for autologous conduits in the field of nerve transplantation when compared to blood vessels and muscle.
In addition to autologous tissue, synthetic materials are the focus of research on nerve conduits. Multiple synthetic materials, such as polyhydroxy acid, collagen, polylactic acid glycolic acid, polycaprolactone, and silk, have been explored as substitutes for interposition transplants (Bini et al., 2004; Rinker and Liau, 2011; Taras et al., 2011; Huang et al., 2012; Reid et al., 2013; Sarker et al., 2018). However, although synthetic nerve conduits can provide some mechanical support, they lack the active ingredients that could promote axon growth. Recent studies have considered a combination of nerve conduits with special cells and growth factors that can promote axon growth or myelin formation. Olfactory ensheathing cells (OEC) are glial cells that secrete many neurotrophic factors to promote axonal growth. Based on this characteristic, Lee et al. implanted OECs into a nerve conduit and transplanted them into a rat sciatic nerve. The outcome revealed that there was no obvious difference in nerve conduction velocity, muscle wet weight, and nerve density between OEC-containing nerve conduits and autologous nerve grafts (Lee J. Y. et al., 2021). Other components commonly combined with nerve conduits include SCs, olfactory stem cells, ADSC, BMMSCs, IGF-1, and transforming growth factor-β (TGF-β) (Huard et al., 1998; Evans, 2001; Murrell et al., 2005; Wang et al., 2016a; Matsumine et al., 2016; Watanabe et al., 2017). Among them, induced pluripotent stem cells (iPSC) are a promising source of cells for nerve repair. They can be induced from adult somatic cells and, therefore, avoid ethical concerns (Takahashi and Yamanaka, 2006; Takahashi et al., 2007; Huang et al., 2020). Under suitable conditions, iPSCs can differentiate into SCs and promote axonal regeneration and myelination (Pan et al., 2022). They can also differentiate into neural crest-like cells that produce and secrete neurotrophic factors such as nerve growth factor (NGF) or VEGF, which could improve microcirculation and act directly on axons and endogenous SCs (Mittal and Schrenk-Siemens, 2020). However, iPSCs have an increased risk of uncontrolled cell division and tumorigenesis. Exosomes have functions similar to those of maternal cells but without immunogenicity. Therefore, in nerve repair, researchers use iPSC-derived exosomes to replace iPSCs, which not only retain the ability to promote nerve repair but also avoid the risk of carcinogenesis and other biosafety issues (Yu B. et al., 2014; Sun et al., 2016; Pan et al., 2022).
Although synthetic conduits have shown unique advantages in peripheral nerve repair, they have not been widely applied in clinical practice. The conduits are still in the animal model stage, and in the field of craniofacial nerve transplantation research, the focus is on nerve injury repair in rats. For example, Long et al. cross-linked collagen tubes with heparin to construct a neural guidance pipeline for transporting nerve growth factor (NGF) and implanted it into facial nerve defects in rats. The short half-life of NGF was improved by the combination of NGF and collagen and was enhanced by heparin. Neural-guided conduits significantly promoted axonal growth and proliferation of SCs 3 months after surgery. The neurological recovery results were consistent with those of the autografts (Long et al., 2021). The characteristics of ADSCs are similar to the mesenchymal lineage and could differentiate into Schwann - like cells. ADSCs are easy to isolate and amplify. Based on these properties, a silicone tube containing ADSCs was inserted into the collagen gel and used to repair the gap in the rat nerve. Postoperative examination showed that the nerve repair ability of the synthetic material was nearly identical to that of the autologous nerve transplants (Watanabe et al., 2017). Therefore, in the field of craniofacial nerve repair, the effect of a nerve conduit is positive in animal models, especially in facial nerve injury repair. However, it is still necessary to research its application in other nerves, such as the trigeminal and glossopharyngeal nerves and other nerves that have important sensory or motor functions and are vulnerable to damage. However, more cases are needed to verify its clinical feasibility. Attention should be paid to nerve conduits, which have great potential as substitutes for autografts. Moreover, the rapid improvement in 3D printing has promoted the technological progress of nerve conduits. The 3D printing technology allows for the rapid fabrication of complex peripheral nerve conduits and enables multiple types of materials to be involved in the synthesis of the conduits, such as hydrogels, thermoplastics, thermosetting polyesters, and bioinks. This could provide more physiologically suitable grafts for nerve repair and a wider delivery system for precursor cells and biologically active substances (Ashraf et al., 2019; Yu et al., 2020; Bahremandi Tolou et al., 2021). Researchers have even introduced the concept of 4D printing into neural repair. They have proposed inducing dynamic reactions of the 3D-printed scaffold using temperature, pH, light, electricity, and other stimuli. This can realize intelligent control of the internal environment of the recipient site and regulate the differentiation and migration of nerve cells. Combined with these stimulation factors, the performance of synthetic conduits can be improved to regulate nerve repair better under physiological conditions (Zhu et al., 2017; Fantino et al., 2018; Uz et al., 2019) (Figure 3). In conclusion, it is worth exploring the important role of the conduit as an alternative to the autograft in craniofacial nerve transplantation and even in the field of peripheral nerve repair as a whole. Further research on the laboratory to clinical transformation of this technique is worthwhile.
Novel materials
The nerve conduit is aimed at replacing autografts. However, the repair of long nerve defects remains limited. Bioengineering and nanomedicine focus on the construction of bioactive materials and the surface modification of neural conduits or 3D biological scaffolds to promote the attachment and survival of cells and molecules. This could effectively promote the long-distance regeneration of axons (Sun et al., 2010; Pabari et al., 2014; Faroni et al., 2015; Mobasseri et al., 2015). Hydrogels and nanomaterials are two novel materials with the most potential for nerve repair. They are possible replacement materials for craniofacial nerve transplantation and the combined repair of craniofacial soft tissues because of their superior biocompatibility and stimuli-responsiveness.
Hydrogels
Hydrogels can be classified in several ways. Here, they are divided into natural and synthetic hydrogels, according to their source. Hydrogels are perfect carriers of active substances and are biodegradable. They can, therefore, provide structural and nutritional support for the growth of neural tissues in the early stage of repair but also provide suitable space for the growth of newly formed tissue through gradual degradation. Moreover, it has satisfactory biocompatibility compared with other materials. It can provide a growth environment similar to the extracellular matrix for nerve repair and guide the orderly regeneration of axons in specific directions, helping to rebuild damaged neural networks (Araújo et al., 2017; Celikkin et al., 2017; Fan et al., 2017) (Table 2). Natural hydrogels include hyaluronic acid (HA), fibrin, chitosan, and silk fibroin. HA can hydrate the ECM and regulate the dynamic balance of tissues as a polysaccharide component of the extracellular matrix (ECM). It also helps the matrix maintain the gel state and promotes the structural stability of the ECM by interacting with other proteins or polysaccharides (Abatangelo et al., 2020). Cross-linked HA hydrogels have been shown to promote significant axonal growth and to achieve sustained electrical activity and strong synaptic connections in vivo (Horn et al., 2007; Broguiere et al., 2016). Meanwhile, HA hydrogel scaffolds have also shown advantages in neural regeneration, including improving the survival rate of NSCs and SCs and promoting the differentiation of neural progenitor cells into neurons and glial cells. However, this property can be affected by various chemical modifications (Pan et al., 2009; Seidlits et al., 2010; Liang et al., 2013; Wang et al., 2013). Porous cross-linked HA hydrogels provide the possibility of accelerating nerve regeneration by promoting cell infiltration, assisting in angiogenesis, and inhibiting scar formation during repair (Hou et al., 2005). In line with HA hydrogels, other types of natural hydrogels, such as fibrin, collagen, chitosan, and keratin hydrogels, are also highly effective in promoting the differentiation of NSCs and axonal regeneration (Sierpinski et al., 2008; Mooney et al., 2010; Pace et al., 2014; Yao et al., 2016; Salehi et al., 2018; Yoo et al., 2020). They do, however, exhibit different properties due to their diverse composition. Degradation products such as chitosan hydrogels can protect nerve cells from toxic and oxidative damage and stimulate the proliferation of SCs (Zhou et al., 2008; Huang et al., 2015; Wang et al., 2016b). Sericin has significant neurotrophic effects as a kind of natural protein. Glycine and serine are degradation products of sericin and are important neurotransmitters that play indispensable roles in information transmission (Hernandes and Troncone, 2009).
In addition to natural materials, synthetic hydrogels have been widely used for nerve regeneration. Synthetic materials have strong plasticity and superior physical properties compared to natural materials. Polyethylene glycol hydrogel is an adhesive gelatinous material that acts as a fibrin sealant to enhance nerve repair. It can effectively reduce scar formation and contribute to axonal regeneration and myelination (Isaacs et al., 2009; Estrada et al., 2014). Hejcl et al. found that the positively charged 2-hydroxyethyl methacrylate (HEMA) hydrogel was structurally conducive to massive implantation of connective tissue. They observed that the porous structure of the 2-MEMA hydrogel was filled with blood vessels, nerve filaments, and Schwann cells, making it a good scaffold for axon growth (Hejcl et al., 2008). Although synthetic hydrogels have advantages in their physicochemical properties, they are inferior to natural materials in biocompatibility. Therefore, it is necessary to improve biocompatibility through the molecular modification of synthetic hydrogels or by fusing them with other materials. For example, researchers modified poly (2-hydroxyethyl methacrylate) using laminin-derived peptides to improve cell adhesion and promote the differentiation of NSCs (Kubinová et al., 2010). In conclusion, hydrogels have obtained positive results in the field of neural repair because of their good biocompatibility and plasticity. Adjusting the physicochemical properties of hydrogels to improve their nerve repair ability or using them as cellular or molecular carriers to promote nerve regeneration is still an important research direction. Research on the comprehensive ability of hydrogels to repair nerves, blood vessels, muscles, and skin is in great demand for craniofacial nerve transplantation and craniofacial multi-tissue combined repair.
Nanomaterials
Nanomaterials have inherent advantages for tissue repair. First, nanomaterials can be used as carriers of a wide range of drugs, bioactive substances, or genes because of their high surface-area-to-volume ratio (Kim and Hyeon, 2014). Secondly, the stimuli responsiveness is the intrinsic characteristic of nanometer materials. These materials can respond to changes in stimuli, including pH, temperature, light, and magnetic fields. In addition, the strength, surface morphology, and degradation behavior of nanomaterials can be regulated by different molecular or synthetic conditions to meet the requirements of tissue repair. In the field of nerve repair, nanomaterials do not only serve as fillers for nerve conduits to provide structural and nutritional support (Li et al., 2014; Meyer et al., 2016) but their inherent properties, such as conductivity, can also be taken advantage of to improve the physical and chemical properties of nerve conduits (Oprych et al., 2016). Meantime, nanoparticles can deliver molecules to cells in a directional manner (Chen et al., 2017). This section reviews the common nanomaterials and their properties in nerve repair to promote their application in craniofacial nerve transplantation and craniofacial repair. Nanoscale hydrogels were not repeated.
Carbon nanotubes (CNTs) are chemically stable and inert. They have electrical properties consistent with those of neural tissue. They also facilitate cell adhesion, differentiation, and growth. These characteristics support their application in neural repair (Hopley et al., 2014; Alshehri et al., 2016). CNTs have shown the general characteristics of repair materials, such as the ability to promote nerve cell differentiation and axon growth (Yu W. et al., 2014; Roberts et al., 2014; Ahn et al., 2015). They also show advantages in promoting nerve regeneration. For example, using carbon nanotubes as coatings for polydimethylsiloxane (PDMS) scaffolds enhances cell adhesion and viability while doubling the survival and proliferation rates of primary neurons and SCs (Kang et al., 2015). CNTs are superior conductive materials. Their conductive properties and unique nanoscale textures may affect the growth of cells and neurites. Researchers have found that long neurites tend to line up in the direction of the nanotubes on horizontal CNTs, which has important implications for using physical and electrical factors to direct axonal growth (Roberts et al., 2014). Tu Q et al. observed the effect of surface charge on neurites and its branches. The results showed that positively charged graphene oxide (GO) was more conducive to the growth and branching of neurites than neutral ions, zwitterions, or negatively charged GO (Tu et al., 2014). The application of metal nanoparticles, such as iron, gold, and silver, has attracted more attention in nerve repair. Superparamagnetism and photoreactivity are the two characteristics that distinguish metal nanoparticles from other materials. Superparamagnetic nanoparticles (SMNPs) can guide cells to specific locations by using an external magnetic field. This superparamagnetism can contribute to the directional growth of neurites during nerve repair (Riggio et al., 2014; Marcus et al., 2016). The distribution of nerve cells and the growth direction of primary neurons can be successfully regulated by controlling the external magnetic field gradient using magnetic iron nanoparticles as a medium. Therefore, as a remote control platform, magnetic nanoparticles (MNPs) are expected to become a new therapeutic agent for nerve injury (Marcus et al., 2016). Gold nanoparticles are widely used in biomedical research for their unique optical properties, as their absorption spectrum can vary in the range between visible and near-infrared. Irradiation of gold nanorods in NG108-15 neural cells with a 780 nm laser stimulated cell growth, especially neurite growth (Paviolo et al., 2013). Similarly, stimulation of gold nanorod-treated neural cells with 780 nm near-infrared light-induced intracellular calcium transients. This stimulatory effect may be related to the mechanisms of cell differentiation and axon growth under optical induction (Paviolo et al., 2014). Recently, the combination of nanomaterials and hydrogels has shown its advantages. Hybrid materials possess the biological affinity of hydrogels and the superior photoelectric, mechanical, thermal, and other physical properties of nanomaterials (He et al., 2020; Huang et al., 2021; Zheng et al., 2022). For example, combining CNTs with self-assembled peptides had better electrical conductivity and injectable properties, providing a practical method for promoting nerve repair (He et al., 2020). The fusion of pegylated CNTs with silk fibroin provided a flexible scaffold for photoacoustic nerve stimulation (Zheng et al., 2022). Table 3 summarizes the recent studies on nanomaterial binding hydrogels in peripheral nerve regeneration.
Recently, the advantages of nanoencapsulation have become increasingly apparent. Its application in neural repair is a topic of great interest. Nanoencapsulation uses an ultrathin external structure (<100 nm) to encapsulate viable cells or factors (Ariga and Fakhrullin, 2021). External materials come from a wide range of sources, such as polymers, hydrogels, and minerals (Lee H. et al., 2021). Its main advantage is its better physicochemical protection, which allows cells and factors to survive and function under harsh conditions such as ultra-low temperatures and toxic microenvironments (Park et al., 2014; Youn et al., 2017; Zhu et al., 2019; Wang et al., 2021). However, recent studies have extended the benefits of nanoencapsulation. Based on a metal-organic framework, Lee et al. combined Fe3+ and benzene-1,3, 5-tricarboxylic acid to wrap living cells. Thus, the catalytic efficiency of the enzymatic reactions was improved. Nanoshells have exogenous biochemical functions, such as converting toxic chemicals into nutrients (Lee et al., 2022). Polyphenols have strong anti-inflammatory properties, but their low bioavailability limits their application. The nano-embedding of polyphenols with different carriers can improve their bioavailability and reduce their degradability (Jayusman et al., 2022). In addition, as a natural barrier in the human body, the blood-brain barrier prevents harmful substances from entering the brain and also hinders the entry of most drugs. Lipid-based nanoparticles are good carriers for neuroprotective drugs and factors that cross the blood-brain barrier (Fernandes et al., 2021). In conclusion, we believe that nanoencapsulation can show its unique advantages in future neural repair research. Furthermore, the use of nanoencapsulation to create a system composed of physically separated but interacting cell hybrids is also a future research direction.
Although a variety of nanomaterials have shown potential in promoting nerve repair, their toxicity to cells and tissues cannot be ignored. These side effects arise mainly from two aspects: Reactive oxygen species (ROS) may be generated during the interactions between nanoparticles and cells. This leads to oxidative stress, which in turn threatens the growth of cells and tissues (Soto et al., 2007; Balasubramanyam et al., 2009; Radziun et al., 2011). However, the toxicity does not originate from the nanoparticles themselves but from the coating applied in the process of surface modification and functionalization (Rivet et al., 2012). In addition, there are specific side effects associated with different material types. CNTs have been shown to have effects on the reproductive system, affecting embryonic development and delaying pregnancy. These side effects have been proven to be concentration-dependent (Philbrook et al., 2011; Xu et al., 2015). In addition to causing oxidative stress, silver nanoparticles are prone to deposition in organs such as the kidney, liver, spleen, and lungs. In animal testing, it has been found to penetrate the blood-brain barrier and enter nerve cells, causing neuronal degeneration (Tang et al., 2009). Therefore, the neurotoxicity of nanomaterials should be fully considered in the selection, surface modification, and functionalization of these conduits.
Summary
Although there are many surgical methods and grafts for nerve repair in craniofacial soft tissue injuries, the ultimate purpose is to achieve functional and aesthetic normalization. Autografts are still the preferred choice for segmental nerve defects in clinical practice because their regeneration microenvironment is the most suitable for physiological conditions (Moore et al., 2015). Vascularization of the graft can promote an immediate postoperative blood supply and achieve better functional recovery compared to nerve grafts alone (Koshima et al., 2004; Li S. S. et al., 2021). At the same time, when it comes to motor nerve transfers, dual nerve transfers and combined transplantation of sensory and motor nerves are effective methods to reduce postoperative mass movement (Yoshioka, 2018; Kuta and Taylor, 2022). But the trauma and nerve defect complications at the donor site are inevitable. However, with the development of tissue engineering technology, artificial tissues or scaffolds may replace autografts. For complex craniofacial defects, using allogeneic composite functional units for transplantation is inevitable to achieve functional recovery (Zor et al., 2010; Roche et al., 2015a). The nerve tissue in this unit plays a major role in functional recovery, while the effect of the structural restoration of bone and other soft tissues is also indispensable. However, the limited source of donors and individual differences are inherent problems. Immunosuppression and infection have puzzled researchers for a long time. In particular, the complexity of cranial and facial tissues increases the risk of infection by specific bacterial flora from multiple sources (Husain et al., 2003; Singh et al., 2005; Cuellar-Rodriguez et al., 2009). Therefore, designing more reasonable allogeneic composite functional units by using animal models and controlling the infection during perioperative and early postoperative periods are urgent areas of study that need to be explored. Decellularized tissue is rarely rejected because of the removal of immunogenicity and has been widely used in peripheral nerves. However, decellularized tissue has not shown better results for functional recovery than autografts in clinical studies of the craniofacial nerves. Therefore, it should be applied with caution in large-caliber nerves and long defect gaps (Moore et al., 2009; Rbia and Shin, 2017). But its advantages of low immunogenicity and the absence of donor site complications should not be overlooked. Therefore, combining decellularized tissue with special additives (e.g., NSCs and growth factors) to produce new grafts that are more suitable for the physiological environment of nerve regeneration is an important part of current research. Traditional autologous nerve conduits (e.g., arteries, veins, muscles, and tendons) have shown good results in promoting nerve repair in clinical applications, but they do not show stronger repair ability than autologous nerve grafts and have defects leading to complications at the donor site (Chiu and Strauch, 1990; Tang et al., 1993; Tang et al., 1995; Brandt et al., 1999; Meek et al., 2004). Synthetic conduit materials have a wide range of sources and strong plasticity. Synthetic conduits have more physiologically appropriate structures and have become better delivery systems for a wide range of cells, growth factors, and drugs because of advances in tissue engineering, particularly 3D printing (Ashraf et al., 2019; Yu et al., 2020; Bahremandi Tolou et al., 2021). However, this technique has not been widely applied in nerve transplantation. Therefore, research to clinical transformation of synthetic conduits combined with 3D printing technology is undoubtedly the focus of current research. With the introduction of 4D printing, basic research should focus on achieving dynamic regulation of 3D scaffolds using stimulation factors such as temperature, pH, light, and electricity. This is conducive to the construction of new materials that can intelligently adjust the nerve repair microenvironment and promote nerve function normalization. The replacement of biological grafts with synthetic materials is new in the field of nerve transplantation. As representative novel materials, hydrogels and nanomaterials show excellent biocompatibility and stimuli-responsiveness (Kim and Hyeon, 2014; Araújo et al., 2017; Celikkin et al., 2017; Fan et al., 2017). Their ability to promote neural cell proliferation, inhibit scar formation, and accelerate the growth of neurites has been demonstrated. However, the toxicity and side effects (on the reproductive system and oxidative stress) of the material and modifying substances should be avoided. Future research should focus on improving and modifying nerve conduits using novel materials to promote the directional growth of axons and inhibit scar formation. This may solve the problem of repairing long nerve defects.
Author contributions
Data acquisition, analysis, and writing, SS, DL, HZ; review and editing, XL, SN, BH, CL, NY; project administration and funding acquisition, XL, SN, BH.
Fundings
This work was supported by grants from the National Natural Science Foundation of China (82172740, 82111530202, 81874082), Shandong Provincial Natural Science Foundation (ZR2021LSW008, ZR2020QH234), Clinical Research Center of Shandong University (No. 2020SDUCRCB002), Department of Science & Technology of Shandong Province (ZR2019ZD33 and 2020CXGC010903), Special Foundation for Taishan Scholars (ts20110814, tspd20210322), Research Project of Jinan Microecological Biomedicine Shandong Laboratory (JNL-2022003A and JNL-2022042C), Innovation Project of Jinan Science and Technology Bureau (2021GXRC065), and Rongxiang Regenerative Medical Fund (2019SDRX-19).
Acknowledgments
We would like to thank Editage (www.editage.cn) for English language editing.
Conflict of interest
The authors declare that the research was conducted in the absence of any commercial or financial relationships that could be construed as a potential conflict of interest.
Publisher’s note
All claims expressed in this article are solely those of the authors and do not necessarily represent those of their affiliated organizations, or those of the publisher, the editors and the reviewers. Any product that may be evaluated in this article, or claim that may be made by its manufacturer, is not guaranteed or endorsed by the publisher.
Abbreviations
ADSC, adipose stem cell; BDNF, brain-derived neurotrophic factor; BMMSC, bone marrow mesenchymal stem cell; CNT, carbon nanotube; ECM, extracellular matrix; EPO, erythropoietin; GAN, great auricular nerve; GO, graphene oxide; HA, hyaluronic acid; IAN, inferior alveolar nerve; IGF-1, insulin-like growth factor-1; MSC, mesenchymal stem cell; NGF, nerve growth factor; NSC, neural stem cell; OEC, olfactory ensheathing cell; ROS, reactive oxygen species; SC, Schwann cell; SCM, sternocleidomastoid muscle; TGF-β, transforming growth factor-β; VEGF, vascular endothelial growth factor.
References
Abatangelo, G., Vindigni, V., Avruscio, G., Pandis, L., and Brun, P. (2020). Hyaluronic acid: Redefining its role. Cells 9 (7), 1743. doi:10.3390/cells9071743
Ahn, H.-S., Hwang, J.-Y., Kim, M. S., Lee, J.-Y., Kim, J.-W., and Kim, H.-S. (2015). Carbon-nanotube-interfaced glass fiber scaffold for regeneration of transected sciatic nerve. Acta Biomater. 13, 324–334. doi:10.1016/j.actbio.2014.11.026
Alshehri, R., Ilyas, A. M., Hasan, A., Arnaout, A., Ahmed, F., and Memic, A. (2016). Carbon nanotubes in biomedical applications: Factors, mechanisms, and remedies of toxicity. J. Med. Chem. 59 (18), 8149–8167. doi:10.1021/acs.jmedchem.5b01770
Altafulla, J., Iwanaga, J., Lachkar, S., Prickett, J., Dupont, G., Yilmaz, E., et al. (2019). The great auricular nerve: Anatomical study with application to nerve grafting procedures. World Neurosurg. 125, e403–e407. doi:10.1016/j.wneu.2019.01.087
Araújo, M. R., Kyrylenko, S., Spejo, A. B., Castro, M. V., Ferreira Junior, R. S., Barraviera, B., et al. (2017). Transgenic human embryonic stem cells overexpressing FGF2 stimulate neuroprotection following spinal cord ventral root avulsion. Exp. Neurol. 294, 45–57. doi:10.1016/j.expneurol.2017.04.009
Ariga, K., and Fakhrullin, R. (2021). Nanoarchitectonics on living cells. RSC Adv. 11 (31), 18898–18914. doi:10.1039/d1ra03424c
Ashraf, R., Sofi, H. S., Beigh, M. A., and Sheikh, F. A. (2019). Recent trends in peripheral nervous regeneration using 3D biomaterials. Tissue Cell 59, 70–81. doi:10.1016/j.tice.2019.06.003
Askarzadeh, N., Nazarpak, M. H., Mansoori, K., Farokhi, M., Gholami, M., Mohammadi, J., et al. (2020). Bilayer cylindrical conduit consisting of electrospun polycaprolactone nanofibers and DSC cross-linked sodium alginate hydrogel to bridge peripheral nerve gaps. Macromol. Biosci. 20 (9), e2000149. doi:10.1002/mabi.202000149
Baccarani, A., Starnoni, M., Zaccaria, G., Anesi, A., Benanti, E., Spaggiari, A., et al. (2019). Obturator nerve split for gracilis free-flap double reinnervation in facial paralysis. Plast. Reconstr. Surg. Glob. Open 7 (6), e2106. doi:10.1097/gox.0000000000002106
Bahremandi Tolou, N., Salimijazi, H., Kharaziha, M., Faggio, G., Chierchia, R., and Lisi, N. (2021). A three-dimensional nerve guide conduit based on graphene foam/polycaprolactone. Mater Sci. Eng. C Mater Biol. Appl. 126, 112110. doi:10.1016/j.msec.2021.112110
Balasubramanyam, A., Sailaja, N., Mahboob, M., Rahman, M. F., Hussain, S. M., and Grover, P. (2009). In vivo genotoxicity assessment of aluminium oxide nanomaterials in rat peripheral blood cells using the comet assay and micronucleus test. Mutagenesis 24 (3), 245–251. doi:10.1093/mutage/gep003
Banes, A. J., Link, G. W., Bevin, A. G., Peterson, H. D., Gillespie, Y., Bynum, D., et al. (1988). Tendon synovial cells secrete fibronectin in vivo and in vitro. J. Orthop. Res. 6 (1), 73–82. doi:10.1002/jor.1100060110
Bayrak, S. B., Kriet, J. D., and Humphrey, C. D. (2017). Masseteric to buccal branch nerve transfer. Curr. Opin. Otolaryngol. Head. Neck Surg. 25 (4), 280–285. doi:10.1097/moo.0000000000000380
Benkhatar, H., Levy, O., Goemaere, I., Borderie, V., Laroche, L., and Bouheraoua, N. (2018). Corneal neurotization with a great auricular nerve graft: Effective reinnervation demonstrated by in vivo confocal microscopy. Cornea 37 (5), 647–650. doi:10.1097/ico.0000000000001549
Biglioli, F., Zago, M., Allevi, F., Ciprandi, D., Dell'Aversana Orabona, G., Pucciarelli, V., et al. (2018). Reanimation of the paralyzed lids by cross-face nerve graft and platysma transfer. J. Craniomaxillofac Surg. 46 (3), 521–526. doi:10.1016/j.jcms.2017.12.022
Bini, T. B., Gao, S., Xu, X., Wang, S., Ramakrishna, S., and Leong, K. W. (2004). Peripheral nerve regeneration by microbraided poly(L-lactide-co-glycolide) biodegradable polymer fibers. J. Biomed. Mater Res. A 68 (2), 286–295. doi:10.1002/jbm.a.20050
Brandt, J., Dahlin, L. B., and Lundborg, G. (1999). Autologous tendons used as grafts for bridging peripheral nerve defects. J. Hand Surg. Br. 24 (3), 284–290. doi:10.1054/jhsb.1999.0074
Broguiere, N., Isenmann, L., and Zenobi-Wong, M. (2016). Novel enzymatically cross-linked hyaluronan hydrogels support the formation of 3D neuronal networks. Biomaterials 99, 47–55. doi:10.1016/j.biomaterials.2016.04.036
Broyles, J. M., Alrakan, M., Ensor, C. R., Khalifian, S., Kotton, C. N., Avery, R. K., et al. (2014). Characterization, prophylaxis, and treatment of infectious complications in craniomaxillofacial and upper extremity allotransplantation: A multicenter perspective. Plast. Reconstr. Surg. 133 (4), 543e–551e. doi:10.1097/prs.0000000000000015
Buckenmeyer, M. J., Meder, T. J., Prest, T. A., and Brown, B. N. (2020). Decellularization techniques and their applications for the repair and regeneration of the nervous system. Methods 171, 41–61. doi:10.1016/j.ymeth.2019.07.023
Carvalho, C. R., Reis, R. L., and Oliveira, J. M. (2020). Fundamentals and current strategies for peripheral nerve repair and regeneration. Adv. Exp. Med. Biol. 1249, 173–201. doi:10.1007/978-981-15-3258-0_12
Celikkin, N., Rinoldi, C., Costantini, M., Trombetta, M., Rainer, A., and Święszkowski, W. (2017). Naturally derived proteins and glycosaminoglycan scaffolds for tissue engineering applications. Mater Sci. Eng. C Mater Biol. Appl. 78, 1277–1299. doi:10.1016/j.msec.2017.04.016
Chang, Y. C., Vanz, R. L., Aurenção, J. C., Burgues, T., and Schanaider, A. (2021). Unilateral frontalis muscle paralysis reanimated by contralateral frontalis muscle through sural nerve graft connecting the two muscles (muscle-nerve-muscle neurotization technique)-A case report. Microsurgery 41 (2), 181–185. doi:10.1002/micr.30665
Charlson, E. S., Pepper, J. P., and Kossler, A. L. (2022). Corneal neurotization via dual nerve autografting. Ophthalmic Plast. Reconstr. Surg. 38 (1), e17–e19. doi:10.1097/iop.0000000000002064
Chen, C. J., Ou, Y. C., Liao, S. L., Chen, W. Y., Chen, S. Y., Wu, C. W., et al. (2007). Transplantation of bone marrow stromal cells for peripheral nerve repair. Exp. Neurol. 204 (1), 443–453. doi:10.1016/j.expneurol.2006.12.004
Chen, Y., Xu, M., Guo, Y., Tu, K., Wu, W., Wang, J., et al. (2017). Targeted chimera delivery to ovarian cancer cells by heterogeneous gold magnetic nanoparticle. Nanotechnology 28 (2), 025101. doi:10.1088/0957-4484/28/2/025101
Chi, F. L., Wang, Z. M., Chen, Z. Y., and Wu, Y. Z. (2006). Diagnosis and management of facial nerve neuromas. Zhonghua Er Bi Yan Hou Tou Jing Wai Ke Za Zhi 41 (4), 262–265.
Chiu, D. T., and Strauch, B. (1990). A prospective clinical evaluation of autogenous vein grafts used as a nerve conduit for distal sensory nerve defects of 3 cm or less. Plast. Reconstr. Surg. 86 (5), 928–934. doi:10.1097/00006534-199011000-00015
Cuellar-Rodriguez, J., Avery, R. K., Lard, M., Budev, M., Gordon, S. M., Shrestha, N. K., et al. (2009). Histoplasmosis in solid organ transplant recipients: 10 years of experience at a large transplant center in an endemic area. Clin. Infect. Dis. 49 (5), 710–716. doi:10.1086/604712
Dai, Y., Vaught, T. D., Boone, J., Chen, S. H., Phelps, C. J., Ball, S., et al. (2002). Targeted disruption of the alpha1,3-galactosyltransferase gene in cloned pigs. Nat. Biotechnol. 20 (3), 251–255. doi:10.1038/nbt0302-251
De Ceulaer, J., Decat, M., and Reychler, H. (2012). Intraparotid facial nerve schwannoma: Case report and literature review. B-ent 8 (3), 225–228.
De Letter, M., Vanhoutte, S., Aerts, A., Santens, P., Vermeersch, H., Roche, N., et al. (2017). Facial nerve regeneration after facial allotransplantation: A longitudinal clinical and electromyographic follow-up of lip movements during speech. J. Plast. Reconstr. Aesthet. Surg. 70 (6), 729–733. doi:10.1016/j.bjps.2017.02.025
De Ugarte, D. A., Alfonso, Z., Zuk, P. A., Elbarbary, A., Zhu, M., Ashjian, P., et al. (2003). Differential expression of stem cell mobilization-associated molecules on multi-lineage cells from adipose tissue and bone marrow. Immunol. Lett. 89 (2-3), 267–270. doi:10.1016/s0165-2478(03)00108-1
DeLeonibus, A., Bassiri Gharb, B., Papay, F., Zins, J. E., and Rampazzo, A. (2017). Surgical management of mandibular intraosseous schwannomas. J. Craniofac Surg. 28 (4), e307–e311. doi:10.1097/scs.0000000000003557
Deng, P., Chen, F., Zhang, H., Chen, Y., and Zhou, J. (2022). Multifunctional double-layer composite hydrogel conduit based on chitosan for peripheral nerve repairing. Adv. Healthc. Mater, e2200115. doi:10.1002/adhm.202200115
Devauchelle, B., Badet, L., Lengelé, B., Morelon, E., Testelin, S., Michallet, M., et al. (2006). First human face allograft: Early report. Lancet 368 (9531), 203–209. doi:10.1016/s0140-6736(06)68935-6
Edgar, D., Timpl, R., and Thoenen, H. (1984). The heparin-binding domain of laminin is responsible for its effects on neurite outgrowth and neuronal survival. Embo J. 3 (7), 1463–1468. doi:10.1002/j.1460-2075.1984.tb01997.x
Elalfy, M., Maqsood, S., Hau, S., Kannan, R. Y., Nduka, C., Hamada, S., et al. (2021). Functional and structural changes following corneal neurotisation in the management of neurotrophic keratopathy: UK single centre series. Clin. Ophthalmol. 15, 2149–2160. doi:10.2147/opth.S298941
Entekhabi, E., Haghbin Nazarpak, M., Shafieian, M., Mohammadi, H., Firouzi, M., and Hassannejad, Z. (2021). Fabrication and in vitro evaluation of 3D composite scaffold based on collagen/hyaluronic acid sponge and electrospun polycaprolactone nanofibers for peripheral nerve regeneration. J. Biomed. Mater Res. A 109 (3), 300–312. doi:10.1002/jbm.a.37023
Estrada, V., Brazda, N., Schmitz, C., Heller, S., Blazyca, H., Martini, R., et al. (2014). Long-lasting significant functional improvement in chronic severe spinal cord injury following scar resection and polyethylene glycol implantation. Neurobiol. Dis. 67, 165–179. doi:10.1016/j.nbd.2014.03.018
Evans, G. R. (2001). Peripheral nerve injury: A review and approach to tissue engineered constructs. Anat. Rec. 263 (4), 396–404. doi:10.1002/ar.1120
Fan, C., Li, X., Xiao, Z., Zhao, Y., Liang, H., Wang, B., et al. (2017). A modified collagen scaffold facilitates endogenous neurogenesis for acute spinal cord injury repair. Acta Biomater. 51, 304–316. doi:10.1016/j.actbio.2017.01.009
Fantino, E., Roppolo, I., Zhang, D., Xiao, J., Chiappone, A., Castellino, M., et al. (2018). 3D printing/interfacial polymerization coupling for the fabrication of conductive hydrogel. Macromol. Mater. Eng. 303 (4), 1700356. doi:10.1002/mame.201700356
Faroni, A., Mobasseri, S. A., Kingham, P. J., and Reid, A. J. (2015). Peripheral nerve regeneration: Experimental strategies and future perspectives. Adv. Drug Deliv. Rev. 82-83, 160–167. doi:10.1016/j.addr.2014.11.010
Fernandes, F., Dias-Teixeira, M., Delerue-Matos, C., and Grosso, C. (2021). Critical review of lipid-based nanoparticles as carriers of neuroprotective drugs and extracts. Nanomater. (Basel) 11 (3). doi:10.3390/nano11030563
Gao, B., Li, B., Li, X., Bae, J., Xiao, K., Li, Q., et al. (2017). A murine model of orthotopic periorbital subunit transplantation. Burns 43 (2), 429–435. doi:10.1016/j.burns.2016.08.024
Giusti, G., Willems, W. F., Kremer, T., Friedrich, P. F., Bishop, A. T., and Shin, A. Y. (2012). Return of motor function after segmental nerve loss in a rat model: Comparison of autogenous nerve graft, collagen conduit, and processed allograft (AxoGen). J. Bone Jt. Surg. Am. 94 (5), 410–417. doi:10.2106/jbjs.K.00253
Gordon, C. R., Avery, R. K., Abouhassan, W., and Siemionow, M. (2011). Cytomegalovirus and other infectious issues related to face transplantation: Specific considerations, lessons learned, and future recommendations. Plast. Reconstr. Surg. 127 (4), 1515–1523. doi:10.1097/PRS.0b013e318208d03c
Guizzardi, S., Foidart, J. M., Leonardi, L., Strocchi, R., and Ruggeri, A. (1987). Evidence for basement membranes in rat tail tendon sheaths. Basic Appl. Histochem 31 (2), 177–181.
Hall, S. M. (1986). Regeneration in cellular and acellular autografts in the peripheral nervous system. Neuropathol. Appl. Neurobiol. 12 (1), 27–46. doi:10.1111/j.1365-2990.1986.tb00679.x
Han, G. H., Peng, J., Liu, P., Ding, X., Wei, S., Lu, S., et al. (2019). Therapeutic strategies for peripheral nerve injury: Decellularized nerve conduits and Schwann cell transplantation. Neural Regen. Res. 14 (8), 1343–1351. doi:10.4103/1673-5374.253511
Hawthorne, W. J., Thomas, A., and Burlak, C. (2021). Xenotransplantation literature update. November/DecemberXenotransplantation 28 (2), e12674. doi:10.1111/xen.12674
He, J., Zhang, N., Zhu, Y., Jin, R., and Wu, F. (2021). MSC spheroids-loaded collagen hydrogels simultaneously promote neuronal differentiation and suppress inflammatory reaction through PI3K-Akt signaling pathway. Biomaterials 265, 120448. doi:10.1016/j.biomaterials.2020.120448
He, L., Xiao, Q., Zhao, Y., Li, J., Reddy, S., Shi, X., et al. (2020). Engineering an injectable electroactive nanohybrid hydrogel for boosting peripheral nerve growth and myelination in combination with electrical stimulation. ACS Appl. Mater Interfaces 12 (47), 53150–53163. doi:10.1021/acsami.0c16885
Hejcl, A., Urdzikova, L., Sedy, J., Lesny, P., Pradny, M., Michalek, J., et al. (2008). Acute and delayed implantation of positively charged 2-hydroxyethyl methacrylate scaffolds in spinal cord injury in the rat: Laboratory investigation. J. Neurosurg. Spine SPI 8 (1), 67–73. doi:10.3171/spi-08/01/067
Hernandes, M. S., and Troncone, L. R. (2009). Glycine as a neurotransmitter in the forebrain: A short review. J. Neural Transm. (Vienna) 116 (12), 1551–1560. doi:10.1007/s00702-009-0326-6
Hopley, E. L., Salmasi, S., Kalaskar, D. M., and Seifalian, A. M. (2014). Carbon nanotubes leading the way forward in new generation 3D tissue engineering. Biotechnol. Adv. 32 (5), 1000–1014. doi:10.1016/j.biotechadv.2014.05.003
Horn, E. M., Beaumont, M., Shu, X. Z., Harvey, A., Prestwich, G. D., Horn, K. M., et al. (2007). Influence of cross-linked hyaluronic acid hydrogels on neurite outgrowth and recovery from spinal cord injury. J. Neurosurg. Spine 6 (2), 133–140. doi:10.3171/spi.2007.6.2.133
Hou, S., Xu, Q., Tian, W., Cui, F., Cai, Q., Ma, J., et al. (2005). The repair of brain lesion by implantation of hyaluronic acid hydrogels modified with laminin. J. Neurosci. Methods 148 (1), 60–70. doi:10.1016/j.jneumeth.2005.04.016
Hou, Z. H., Huang, D. L., Han, D. Y., Dai, P., Young, W. Y., and Yang, S. M. (2012). Surgical treatment of endolymphatic sac tumor. Acta Otolaryngol. 132 (3), 329–336. doi:10.3109/00016489.2011.640349
Huang, H. C., Hong, L., Chang, P., Zhang, J., Lu, S. Y., Zheng, B. W., et al. (2015). Chitooligosaccharides attenuate Cu2+-induced cellular oxidative damage and cell apoptosis involving Nrf2 activation. Neurotox. Res. 27 (4), 411–420. doi:10.1007/s12640-014-9512-x
Huang, L., Yang, X., Deng, L., Ying, D., Lu, A., Zhang, L., et al. (2021). Biocompatible chitin hydrogel incorporated with PEDOT nanoparticles for peripheral nerve repair. ACS Appl. Mater Interfaces 13 (14), 16106–16117. doi:10.1021/acsami.1c01904
Huang, W., Begum, R., Barber, T., Ibba, V., Tee, N. C., Hussain, M., et al. (2012). Regenerative potential of silk conduits in repair of peripheral nerve injury in adult rats. Biomaterials 33 (1), 59–71. doi:10.1016/j.biomaterials.2011.09.030
Huang, Z., Powell, R., Phillips, J. B., and Haastert-Talini, K. (2020). Perspective on Schwann cells derived from induced pluripotent stem cells in peripheral nerve tissue engineering. Cells 9 (11). doi:10.3390/cells9112497
Huard, J. M., Youngentob, S. L., Goldstein, B. J., Luskin, M. B., and Schwob, J. E. (1998). Adult olfactory epithelium contains multipotent progenitors that give rise to neurons and non-neural cells. J. Comp. Neurol. 400 (4), 469–486. doi:10.1002/(sici)1096-9861(19981102)400:4<469::aid-cne3>3.0.co;2-8
Humphrey, C. D., and Kriet, J. D. (2008). Nerve repair and cable grafting for facial paralysis. Facial Plast. Surg. 24 (2), 170–176. doi:10.1055/s-2008-1075832
Husain, S., Alexander, B. D., Munoz, P., Avery, R. K., Houston, S., Pruett, T., et al. (2003). Opportunistic mycelial fungal infections in organ transplant recipients: Emerging importance of non-Aspergillus mycelial fungi. Clin. Infect. Dis. 37 (2), 221–229. doi:10.1086/375822
Im, J. H., Shin, S. H., Lee, M. K., Lee, S. R., Lee, J. J., and Chung, Y. G. (2022). Evaluation of anatomical and histological characteristics of human peripheral nerves: As an effort to develop an efficient allogeneic nerve graft. Cell Tissue Bank. doi:10.1007/s10561-022-09998-0
Isaacs, J., Klumb, I., and McDaniel, C. (2009). Preliminary investigation of a polyethylene glycol hydrogel "nerve glue. J. Brachial Plex. Peripher Nerve Inj. 4, 16. doi:10.1186/1749-7221-4-16
Jandali, D., and Revenaugh, P. C. (2019). Facial reanimation: An update on nerve transfers in facial paralysis. Curr. Opin. Otolaryngol. Head. Neck Surg. 27 (4), 231–236. doi:10.1097/moo.0000000000000543
Jayusman, P. A., Nasruddin, N. S., Mahamad Apandi, N. I., Ibrahim, N., and Budin, S. B. (2022). Therapeutic potential of polyphenol and nanoparticles mediated delivery in periodontal inflammation: A review of current trends and future perspectives. Front. Pharmacol. 13, 847702. doi:10.3389/fphar.2022.847702
Jeong, J., Almansoori, A. A., Park, H. S., Byun, S. H., Min, S. K., Choung, H. W., et al. (2018). Per-oral cross-facial sural nerve graft for facial reanimation. Maxillofac. Plast. Reconstr. Surg. 40 (1), 22. doi:10.1186/s40902-018-0163-3
Jowett, N., and Pineda Ii, R. (2019). Corneal neurotisation by great auricular nerve transfer and scleral-corneal tunnel incisions for neurotrophic keratopathy. Br. J. Ophthalmol. 103 (9), 1235–1238. doi:10.1136/bjophthalmol-2018-312563
Kang, D.-W., Sun, F., Choi, Y. J., Zou, F., Cho, W.-H., Choi, B.-K., et al. (2015). Enhancement of primary neuronal cell proliferation using printing-transferred carbon nanotube sheets. J. Biomed. Mater. Res. Part A 103 (5), 1746–1754. doi:10.1002/jbm.a.35294
Katoh, M., Tada, M., Sawamura, Y., and Abe, H. (1998). Total removal of huge chondrosarcoma of the skull base with facial nerve resection and reconstruction. J. Clin. Neurosci. 5 (3), 342–345. doi:10.1016/s0967-5868(98)90075-3
Kauke, M., Panayi, A. C., Safi, A. F., Haug, V., Perry, B., Kollar, B., et al. (2021). Full facial retransplantation in a female patient-Technical, immunologic, and clinical considerations. Am. J. Transpl. 21 (10), 3472–3480. doi:10.1111/ajt.16696
Kaya, Y., and Sarikcioglu, L. (2015). Sir Herbert Seddon (1903-1977) and his classification scheme for peripheral nerve injury. Childs Nerv. Syst. 31 (2), 177–180. doi:10.1007/s00381-014-2560-y
Khan, A. A., Khan, I. M., Nguyen, P. P., Lo, E., Chahadeh, H., Cerniglia, M., et al. (2020). Skin graft techniques. Clin. Podiatr. Med. Surg. 37 (4), 821–835. doi:10.1016/j.cpm.2020.07.007
Kim, T., and Hyeon, T. (2014). Applications of inorganic nanoparticles as therapeutic agents. Nanotechnology 25 (1), 012001. doi:10.1088/0957-4484/25/1/012001
Koshima, I., Nanba, Y., Tsutsui, T., Takahashi, Y., and Itoh, S. (2004). New one-stage nerve pedicle grafting technique using the great auricular nerve for reconstruction of facial nerve defects. J. Reconstr. Microsurg 20 (5), 357–361. doi:10.1055/s-2004-829998
Kubinová, S., Horák, D., Kozubenko, N., Vanecek, V., Proks, V., Price, J., et al. (2010). The use of superporous Ac-CGGASIKVAVS-OH-modified PHEMA scaffolds to promote cell adhesion and the differentiation of human fetal neural precursors. Biomaterials 31 (23), 5966–5975. doi:10.1016/j.biomaterials.2010.04.040
Kuta, V., and Taylor, S. M. (2022). Supercharging the smile: A novel dual nerve transfer for facial reanimation. Plast. Reconstr. Surg. Glob. Open 10 (2), e4124. doi:10.1097/gox.0000000000004124
LaBanc, J. P., Epker, B. N., Jones, D. L., and Milam, S. (1987). Nerve sharing by an interpositional sural nerve graft between the great auricular and inferior alveolar nerve to restore lower lip sensation. J. Oral Maxillofac. Surg. 45 (7), 621–627. doi:10.1016/0278-2391(87)90275-8
Lai, L., Kolber-Simonds, D., Park, K. W., Cheong, H. T., Greenstein, J. L., Im, G. S., et al. (2002). Production of alpha-1,3-galactosyltransferase knockout pigs by nuclear transfer cloning. Science 295 (5557), 1089–1092. doi:10.1126/science.1068228
Lassus, P., Lindford, A., Vuola, J., Bäck, L., Suominen, S., Mesimäki, K., et al. (2018). The helsinki face transplantation: Surgical aspects and 1-year outcome. J. Plast. Reconstr. Aesthet. Surg. 71 (2), 132–139. doi:10.1016/j.bjps.2017.10.007
Lee, H., Kim, N., Rheem, H. B., Kim, B. J., Park, J. H., and Choi, I. S. (2021a). A decade of advances in single-cell nanocoating for mammalian cells. Adv. Healthc. Mater 10 (13), e2100347. doi:10.1002/adhm.202100347
Lee, H., Park, J., Kim, N., Youn, W., Yun, G., Han, S. Y., et al. (2022). Cell-in-Catalytic-Shell nanoarchitectonics: Catalytic empowerment of individual living cells by single-cell nanoencapsulation. Adv. Mater 34 (30), e2201247. doi:10.1002/adma.202201247
Lee, J. Y., Kim, Y. H., Kim, B. Y., Jang, D. H., Choi, S. W., Joen, S. H., et al. (2021b). Peripheral nerve regeneration using a nerve conduit with olfactory ensheathing cells in a rat model. Tissue Eng. Regen. Med. 18 (3), 453–465. doi:10.1007/s13770-020-00326-9
Lein, P. J., Banker, G. A., and Higgins, D. (1992). Laminin selectively enhances axonal growth and accelerates the development of polarity by hippocampal neurons in culture. Brain Res. Dev. Brain Res. 69 (2), 191–197. doi:10.1016/0165-3806(92)90159-t
Leyngold, I. M., Yen, M. T., Tian, J., Leyngold, M. M., Vora, G. K., and Weller, C. (2019). Minimally invasive corneal neurotization with acellular nerve allograft: Surgical technique and clinical outcomes. Ophthalmic Plast. Reconstr. Surg. 35 (2), 133–140. doi:10.1097/iop.0000000000001181
Li, A., Hokugo, A., Yalom, A., Berns, E. J., Stephanopoulos, N., McClendon, M. T., et al. (2014). A bioengineered peripheral nerve construct using aligned peptide amphiphile nanofibers. Biomaterials 35 (31), 8780–8790. doi:10.1016/j.biomaterials.2014.06.049
Li, H., Li, M., Liu, P., Wang, K., Fang, H., Yin, J., et al. (2021a). A multifunctional substance P-conjugated chitosan hydrochloride hydrogel accelerates full-thickness wound healing by enhancing synchronized vascularization, extracellular matrix deposition, and nerve regeneration. Biomater. Sci. 9 (11), 4199–4210. doi:10.1039/d1bm00357g
Li, S. S., Mangialardi, M. L., Nguyen, Q. T., Orosco, R. K., Honart, J. F., Qassemyar, Q., et al. (2021b). The chimeric scapulodorsal vascularized latissimus dorsi nerve flap for immediate reconstruction of total parotidectomy defects with facial nerve sacrifice: Building a new program and preliminary results from 25 cases. Ann. Plast. Surg. 86 (3), S379–s383. doi:10.1097/sap.0000000000002746
Liang, Y., Walczak, P., and Bulte, J. W. (2013). The survival of engrafted neural stem cells within hyaluronic acid hydrogels. Biomaterials 34 (22), 5521–5529. doi:10.1016/j.biomaterials.2013.03.095
Liu, S., Liu, Y., Zhou, L., Li, C., Zhang, M., Zhang, F., et al. (2021). XT-type DNA hydrogels loaded with VEGF and NGF promote peripheral nerve regeneration via a biphasic release profile. Biomater. Sci. 9 (24), 8221–8234. doi:10.1039/d1bm01377g
Long, Q., Wu, B., Yang, Y., Wang, S., Shen, Y., Bao, Q., et al. (2021). Nerve guidance conduit promoted peripheral nerve regeneration in rats. Artif. Organs 45 (6), 616–624. doi:10.1111/aor.13881
Lundborg, G., Dahlin, L., Danielsen, N., and Zhao, Q. (1994). Trophism, tropism, and specificity in nerve regeneration. J. Reconstr. Microsurg 10 (5), 345–354. doi:10.1055/s-2007-1006604
Marchioni, D., Soloperto, D., Genovese, E., Rubini, A., and Presutti, L. (2014). Facial nerve hemangioma of the geniculate ganglion: An endoscopic surgical approach. Auris Nasus Larynx 41 (6), 576–581. doi:10.1016/j.anl.2014.06.004
Marcus, M., Karni, M., Baranes, K., Levy, I., Alon, N., Margel, S., et al. (2016). Iron oxide nanoparticles for neuronal cell applications: Uptake study and magnetic manipulations. J. Nanobiotechnology 14 (1), 37. doi:10.1186/s12951-016-0190-0
Mathes, D. W., Edwards, J. A., Anzai, Y., and Neligan, P. C. (2014). A functional periorbital subunit allograft: Vascular, anatomic, and technical considerations for future subunit facial transplantation. J. Plast. Reconstr. Aesthet. Surg. 67 (10), 1371–1377. doi:10.1016/j.bjps.2014.05.046
Matos Cruz, A. J., and De Jesus, O. (2022). “Facial nerve repair,” in StatPearls (Treasure Island, FL: StatPearls Publishing LLC.). StatPearls PublishingCopyright © 2022.
Matsumine, H., Sasaki, R., Tabata, Y., Matsui, M., Yamato, M., Okano, T., et al. (2016). Facial nerve regeneration using basic fibroblast growth factor-impregnated gelatin microspheres in a rat model. J. Tissue Eng. Regen. Med. 10 (10), E559–e567. doi:10.1002/term.1884
McKinney, P., and Katrana, D. J. (1980). Prevention of injury to the great auricular nerve during rhytidectomy. Plast. Reconstr. Surg. 66 (5), 675–679. doi:10.1097/00006534-198011000-00001
Meek, M. F., Varejão, A. S., and Geuna, S. (2004). Use of skeletal muscle tissue in peripheral nerve repair: Review of the literature. Tissue Eng. 10 (7-8), 1027–1036. doi:10.1089/ten.2004.10.1027
Meier, R. P. H., Longchamp, A., Mohiuddin, M., Manuel, O., Vrakas, G., Maluf, D. G., et al. (2021). Recent progress and remaining hurdles toward clinical xenotransplantation. Xenotransplantation 28 (3), e12681. doi:10.1111/xen.12681
Meyer, C., Stenberg, L., Gonzalez-Perez, F., Wrobel, S., Ronchi, G., Udina, E., et al. (2016). Chitosan-film enhanced chitosan nerve guides for long-distance regeneration of peripheral nerves. Biomaterials 76, 33–51. doi:10.1016/j.biomaterials.2015.10.040
Mittal, K., and Schrenk-Siemens, K. (2020). Lessons from iPSC research: Insights on peripheral nerve disease. Neurosci. Lett. 738, 135358. doi:10.1016/j.neulet.2020.135358
Mobasseri, A., Faroni, A., Minogue, B. M., Downes, S., Terenghi, G., and Reid, A. J. (2015). Polymer scaffolds with preferential parallel grooves enhance nerve regeneration. Tissue Eng. Part A 21 (5-6), 1152–1162. doi:10.1089/ten.TEA.2014.0266
Mooney, R., Tawil, B., and Mahoney, M. (2010). Specific fibrinogen and thrombin concentrations promote neuronal rather than glial growth when primary neural cells are seeded within plasma-derived fibrin gels. Tissue Eng. Part A 16 (5), 1607–1619. doi:10.1089/ten.TEA.2009.0372
Moore, A. M., Kasukurthi, R., Magill, C. K., Farhadi, H. F., Borschel, G. H., and Mackinnon, S. E. (2009). Limitations of conduits in peripheral nerve repairs. Hand (N Y) 4 (2), 180–186. doi:10.1007/s11552-008-9158-3
Moore, A. M., MacEwan, M., Santosa, K. B., Chenard, K. E., Ray, W. Z., Hunter, D. A., et al. (2011). Acellular nerve allografts in peripheral nerve regeneration: A comparative study. Muscle Nerve 44 (2), 221–234. doi:10.1002/mus.22033
Moore, A. M., Wagner, I. J., and Fox, I. K. (2015). Principles of nerve repair in complex wounds of the upper extremity. Semin. Plast. Surg. 29 (1), 40–47. doi:10.1055/s-0035-1544169
Murrell, W., Féron, F., Wetzig, A., Cameron, N., Splatt, K., Bellette, B., et al. (2005). Multipotent stem cells from adult olfactory mucosa. Dev. Dyn. 233 (2), 496–515. doi:10.1002/dvdy.20360
Nadim, W., Anderson, P. N., and Turmaine, M. (1990). The role of Schwann cells and basal lamina tubes in the regeneration of axons through long lengths of freeze-killed nerve grafts. Neuropathol. Appl. Neurobiol. 16 (5), 411–421. doi:10.1111/j.1365-2990.1990.tb01277.x
Niu, D., Ma, X., Yuan, T., Niu, Y., Xu, Y., Sun, Z., et al. (2021). Porcine genome engineering for xenotransplantation. Adv. Drug Deliv. Rev. 168, 229–245. doi:10.1016/j.addr.2020.04.001
Oprych, K. M., Whitby, R. L., Mikhalovsky, S. V., Tomlins, P., and Adu, J. (2016). Repairing peripheral nerves: Is there a role for carbon nanotubes? Adv. Healthc. Mater 5 (11), 1253–1271. doi:10.1002/adhm.201500864
Pabari, A., Lloyd-Hughes, H., Seifalian, A. M., and Mosahebi, A. (2014). Nerve conduits for peripheral nerve surgery. Plast. Reconstr. Surg. 133 (6), 1420–1430. doi:10.1097/prs.0000000000000226
Pace, L. A., Plate, J. F., Mannava, S., Barnwell, J. C., Koman, L. A., Li, Z., et al. (2014). A human hair keratin hydrogel scaffold enhances median nerve regeneration in nonhuman primates: An electrophysiological and histological study. Tissue Eng. Part A 20 (3-4), 507–517. doi:10.1089/ten.TEA.2013.0084
Pan, D., Mackinnon, S. E., and Wood, M. D. (2020). Advances in the repair of segmental nerve injuries and trends in reconstruction. Muscle Nerve 61 (6), 726–739. doi:10.1002/mus.26797
Pan, J., Zhao, M., Yi, X., Tao, J., Li, S., Jiang, Z., et al. (2022). Acellular nerve grafts supplemented with induced pluripotent stem cell-derived exosomes promote peripheral nerve reconstruction and motor function recovery. Bioact. Mater 15, 272–287. doi:10.1016/j.bioactmat.2021.12.004
Pan, L., Ren, Y., Cui, F., and Xu, Q. (2009). Viability and differentiation of neural precursors on hyaluronic acid hydrogel scaffold. J. Neurosci. Res. 87 (14), 3207–3220. doi:10.1002/jnr.22142
Paniello, R. C., Edgar, J. D., Kallogjeri, D., and Piccirillo, J. F. (2011). Medialization versus reinnervation for unilateral vocal fold paralysis: A multicenter randomized clinical trial. Laryngoscope 121 (10), 2172–2179. doi:10.1002/lary.21754
Park, J. H., Kim, K., Lee, J., Choi, J. Y., Hong, D., Yang, S. H., et al. (2014). A cytoprotective and degradable metal-polyphenol nanoshell for single-cell encapsulation. Angew. Chem. Int. Ed. Engl. 53 (46), 12420–12425. doi:10.1002/anie.201405905
Paviolo, C., Haycock, J. W., Cadusch, P. J., McArthur, S. L., and Stoddart, P. R. (2014). Laser exposure of gold nanorods can induce intracellular calcium transients. J. Biophot. 7 (10), 761–765. doi:10.1002/jbio.201300043
Paviolo, C., Haycock, J. W., Yong, J., Yu, A., Stoddart, P. R., and McArthur, S. L. (2013). Laser exposure of gold nanorods can increase neuronal cell outgrowth. Biotechnol. Bioeng. 110 (8), 2277–2291. doi:10.1002/bit.24889
Pereira, J. H., Bowden, R. E., Gattuso, J. M., and Norris, R. W. (1991a). Comparison of results of repair of digital nerves by denatured muscle grafts and end-to-end sutures. J. Hand Surg. Br. 16 (5), 519–523. doi:10.1016/0266-7681(91)90107-y
Pereira, J. H., Palande, D. D., Subramanian, A., Narayanakumar, T. S., Curtis, J., and Turk, J. L. (1991b). Denatured autologous muscle graft in leprosy. Lancet 338 (8777), 1239–1240. doi:10.1016/0140-6736(91)92105-b
Philbrook, N. A., Walker, V. K., Afrooz, A. R. M. N., Saleh, N. B., and Winn, L. M. (2011). Investigating the effects of functionalized carbon nanotubes on reproduction and development in Drosophila melanogaster and CD-1 mice. Reprod. Toxicol. 32 (4), 442–448. doi:10.1016/j.reprotox.2011.09.002
Poppler, L. H., Ee, X., Schellhardt, L., Hoben, G. M., Pan, D., Hunter, D. A., et al. (2016). Axonal growth arrests after an increased accumulation of Schwann cells expressing senescence markers and stromal cells in acellular nerve allografts. Tissue Eng. Part A 22 (13-14), 949–961. doi:10.1089/ten.TEA.2016.0003
Prest, T. A., Yeager, E., LoPresti, S. T., Zygelyte, E., Martin, M. J., Dong, L., et al. (2018). Nerve-specific, xenogeneic extracellular matrix hydrogel promotes recovery following peripheral nerve injury. J. Biomed. Mater Res. A 106 (2), 450–459. doi:10.1002/jbm.a.36235
Radziun, E., Dudkiewicz Wilczyńska, J., Książek, I., Nowak, K., Anuszewska, E. L., Kunicki, A., et al. (2011). Assessment of the cytotoxicity of aluminium oxide nanoparticles on selected mammalian cells. Toxicol. Vitro 25 (8), 1694–1700. doi:10.1016/j.tiv.2011.07.010
Raikos, A., English, T., Yousif, O. K., Sandhu, M., and Stirling, A. (2017). Topographic anatomy of the great auricular point: Landmarks for its localization and classification. Surg. Radiol. Anat. 39 (5), 535–540. doi:10.1007/s00276-016-1758-y
Ramburrun, P., Kumar, P., Ndobe, E., and Choonara, Y. E. (2021). Gellan-xanthan hydrogel conduits with intraluminal electrospun nanofibers as physical, chemical and therapeutic cues for peripheral nerve repair. Int. J. Mol. Sci. 22 (21). doi:10.3390/ijms222111555
Rao, F., Wang, Y., Zhang, D., Lu, C., Cao, Z., Sui, J., et al. (2020). Aligned chitosan nanofiber hydrogel grafted with peptides mimicking bioactive brain-derived neurotrophic factor and vascular endothelial growth factor repair long-distance sciatic nerve defects in rats. Theranostics 10 (4), 1590–1603. doi:10.7150/thno.36272
Rashid, H. U., Rehman, I. U., Rashid, M., Yousaf, S., Khan, N., and Aqsa, A. (2019). Results of immediate facial nerve reconstruction in patients undergoing parotid tumour resection. J. Ayub Med. Coll. Abbottabad 31 (3), 340–345.
Rbia, N., and Shin, A. Y. (2017). The role of nerve graft substitutes in motor and mixed motor/sensory peripheral nerve injuries. J. Hand Surg. Am. 42 (5), 367–377. doi:10.1016/j.jhsa.2017.02.017
Reichart, B., and Längin, M. (2020). On the way (my way) to clinical xenogeneic heart transplantation. Presented at the 15th biannual IXA meeting, Munich, October 11, 2019. Xenotransplantation 27 (6), e12637. doi:10.1111/xen.12637
Reid, A. J., de Luca, A. C., Faroni, A., Downes, S., Sun, M., Terenghi, G., et al. (2013). Long term peripheral nerve regeneration using a novel PCL nerve conduit. Neurosci. Lett. 544, 125–130. doi:10.1016/j.neulet.2013.04.001
Revenaugh, P. C., Knott, D., McBride, J. M., and Fritz, M. A. (2012). Motor nerve to the vastus lateralis. Arch. Facial Plast. Surg. 14 (5), 365–368. doi:10.1001/archfacial.2012.195
Riggio, C., Calatayud, M. P., Giannaccini, M., Sanz, B., Torres, T. E., Fernández-Pacheco, R., et al. (2014). The orientation of the neuronal growth process can be directed via magnetic nanoparticles under an applied magnetic field. Nanomedicine Nanotechnol. Biol. Med. 10 (7), 1549–1558. doi:10.1016/j.nano.2013.12.008
Rinker, B., and Liau, J. Y. (2011). A prospective randomized study comparing woven polyglycolic acid and autogenous vein conduits for reconstruction of digital nerve gaps. J. Hand Surg. Am. 36 (5), 775–781. doi:10.1016/j.jhsa.2011.01.030
Rivet, C. J., Yuan, Y., Borca-Tasciuc, D. A., and Gilbert, R. J. (2012). Altering iron oxide nanoparticle surface properties induce cortical neuron cytotoxicity. Chem. Res. Toxicol. 25 (1), 153–161. doi:10.1021/tx200369s
Roberts, M. J., Leach, M. K., Bedewy, M., Meshot, E. R., Copic, D., Corey, J. M., et al. (2014). Growth of primary motor neurons on horizontally aligned carbon nanotube thin films and striped patterns. J. Neural Eng. 11 (3), 036013. doi:10.1088/1741-2560/11/3/036013
Roche, N. A., Blondeel, P. N., Vermeersch, H. F., Peeters, P. C., Lemmens, G. M., De Cubber, J., et al. (2015a). Long-term multifunctional outcome and risks of face vascularized composite allotransplantation. J. Craniofac Surg. 26 (7), 2038–2046. doi:10.1097/scs.0000000000002110
Roche, N. A., Vermeersch, H. F., Stillaert, F. B., Peters, K. T., De Cubber, J., Van Lierde, K., et al. (2015b). Complex facial reconstruction by vascularized composite allotransplantation: The first Belgian case. J. Plast. Reconstr. Aesthet. Surg. 68 (3), 362–371. doi:10.1016/j.bjps.2014.11.005
Rodriguez-Lorenzo, A., Jensson, D., Weninger, W. J., Schmid, M., Meng, S., and Tzou, C. H. (2016). Platysma motor nerve transfer for restoring marginal mandibular nerve function. Plast. Reconstr. Surg. Glob. Open 4 (12), e1164. doi:10.1097/gox.0000000000001164
Romano, M., Fanelli, G., Albany, C. J., Giganti, G., and Lombardi, G. (2019). Past, present, and future of regulatory T cell therapy in transplantation and autoimmunity. Front. Immunol. 10, 43. doi:10.3389/fimmu.2019.00043
Ryczek, N., Hryhorowicz, M., Zeyland, J., Lipiński, D., and Słomski, R. (2021). CRISPR/Cas technology in pig-to-human xenotransplantation research. Int. J. Mol. Sci. 22 (6). doi:10.3390/ijms22063196
Saheb-Al-Zamani, M., Yan, Y., Farber, S. J., Hunter, D. A., Newton, P., Wood, M. D., et al. (2013). Limited regeneration in long acellular nerve allografts is associated with increased Schwann cell senescence. Exp. Neurol. 247, 165–177. doi:10.1016/j.expneurol.2013.04.011
Sai, N., Han, W. J., Wang, M. M., Qin, X., Zhang, T., Shen, W. D., et al. (2019). Clinical diagnosis and surgical management of 110 cases of facial nerve schwannomas. Zhonghua Er Bi Yan Hou Tou Jing Wai Ke Za Zhi 54 (2), 101–109. doi:10.3760/cma.j.issn.1673-0860.2019.02.002
Sakthivel, P., Singh, C. A., Thakar, A., Thirumeni, G., Raveendran, S., and Sharma, S. C. (2020). Masseteric-facial nerve anastomosis: Surgical techniques and outcomes-A pilot Indian study. Indian J. Otolaryngol. Head. Neck Surg. 72 (1), 92–97. doi:10.1007/s12070-019-01758-z
Sakuma, H., Tanaka, I., Yazawa, M., and Oh, A. (2021). Dual-innervated multivector muscle transfer using two superficial subslips of the serratus anterior muscle for long-standing facial paralysis. Arch. Plast. Surg. 48 (3), 282–286. doi:10.5999/aps.2020.01599
Salehi, M., Naseri-Nosar, M., Ebrahimi-Barough, S., Nourani, M., Vaez, A., Farzamfar, S., et al. (2018). Regeneration of sciatic nerve crush injury by a hydroxyapatite nanoparticle-containing collagen type I hydrogel. J. Physiol. Sci. 68 (5), 579–587. doi:10.1007/s12576-017-0564-6
Salomon, D., Miloro, M., and Kolokythas, A. (2016). Outcomes of immediate allograft reconstruction of long-span defects of the inferior alveolar nerve. J. Oral Maxillofac. Surg. 74 (12), 2507–2514. doi:10.1016/j.joms.2016.05.029
Sarker, M. D., Naghieh, S., McInnes, A. D., Schreyer, D. J., and Chen, X. (2018). Regeneration of peripheral nerves by nerve guidance conduits: Influence of design, biopolymers, cells, growth factors, and physical stimuli. Prog. Neurobiol. 171, 125–150. doi:10.1016/j.pneurobio.2018.07.002
Schmoeckel, M., Nollert, G., Shahmohammadi, M., Young, V. K., Chavez, G., Kasper-König, W., et al. (1996). Prevention of hyperacute rejection by human decay accelerating factor in xenogeneic perfused working hearts. Transplantation 62 (6), 729–734. doi:10.1097/00007890-199609270-00005
Seidlits, S. K., Khaing, Z. Z., Petersen, R. R., Nickels, J. D., Vanscoy, J. E., Shear, J. B., et al. (2010). The effects of hyaluronic acid hydrogels with tunable mechanical properties on neural progenitor cell differentiation. Biomaterials 31 (14), 3930–3940. doi:10.1016/j.biomaterials.2010.01.125
Siemionow, M., Bozkurt, M., Zor, F., Kulahci, Y., Uygur, S., Ozturk, C., et al. (2018). A new composite eyeball-periorbital transplantation model in humans: An anatomical study in preparation for eyeball transplantation. Plast. Reconstr. Surg. 141 (4), 1011–1018. doi:10.1097/prs.0000000000004250
Sierpinski, P., Garrett, J., Ma, J., Apel, P., Klorig, D., Smith, T., et al. (2008). The use of keratin biomaterials derived from human hair for the promotion of rapid regeneration of peripheral nerves. Biomaterials 29 (1), 118–128. doi:10.1016/j.biomaterials.2007.08.023
Singh, N., Lortholary, O., Alexander, B. D., Gupta, K. L., John, G. T., Pursell, K., et al. (2005). Allograft loss in renal transplant recipients with cryptococcus neoformans associated immune reconstitution syndrome. Transplantation 80 (8), 1131–1133. doi:10.1097/01.tp.0000180530.17683.02
Soto, K., Garza, K. M., and Murr, L. E. (2007). Cytotoxic effects of aggregated nanomaterials. Acta Biomater. 3 (3), 351–358. doi:10.1016/j.actbio.2006.11.004
Stephanian, E., Sekhar, L. N., Janecka, I. P., and Hirsch, B. (1992). Facial nerve repair by interposition nerve graft: Results in 22 patients. Neurosurgery 31 (1), 73–76. discussion 77. doi:10.1227/00006123-199207000-00010
Sun, J. H., Li, G., Wu, T. T., Lin, Z. J., Zou, J. L., Huang, L. J., et al. (2020). Decellularization optimizes the inhibitory microenvironment of the optic nerve to support neurite growth. Biomaterials 258, 120289. doi:10.1016/j.biomaterials.2020.120289
Sun, L., Xu, R., Sun, X., Duan, Y., Han, Y., Zhao, Y., et al. (2016). Safety evaluation of exosomes derived from human umbilical cord mesenchymal stromal cell. Cytotherapy 18 (3), 413–422. doi:10.1016/j.jcyt.2015.11.018
Sun, M., McGowan, M., Kingham, P. J., Terenghi, G., and Downes, S. (2010). Novel thin-walled nerve conduit with microgrooved surface patterns for enhanced peripheral nerve repair. J. Mater Sci. Mater Med. 21 (10), 2765–2774. doi:10.1007/s10856-010-4120-7
Sun, Y., Liu, L., Han, Y., Xu, L., Zhang, D., and Wang, H. (2015). The role of great auricular-facial nerve neurorrhaphy in facial nerve damage. Int. J. Clin. Exp. Med. 8 (8), 12970–12976.
Sunderland, S. (1951). A classification of peripheral nerve injuries producing loss of function. Brain 74 (4), 491–516. doi:10.1093/brain/74.4.491
Szynkaruk, M., Kemp, S. W., Wood, M. D., Gordon, T., and Borschel, G. H. (2013). Experimental and clinical evidence for use of decellularized nerve allografts in peripheral nerve gap reconstruction. Tissue Eng. Part B Rev. 19 (1), 83–96. doi:10.1089/ten.TEB.2012.0275
Takahashi, K., Tanabe, K., Ohnuki, M., Narita, M., Ichisaka, T., Tomoda, K., et al. (2007). Induction of pluripotent stem cells from adult human fibroblasts by defined factors. Cell 131 (5), 861–872. doi:10.1016/j.cell.2007.11.019
Takahashi, K., and Yamanaka, S. (2006). Induction of pluripotent stem cells from mouse embryonic and adult fibroblast cultures by defined factors. Cell 126 (4), 663–676. doi:10.1016/j.cell.2006.07.024
Tang, J. B., Gu, Y. Q., and Song, Y. S. (1993). Repair of digital nerve defect with autogenous vein graft during flexor tendon surgery in zone 2. J. Hand Surg. Br. 18 (4), 449–453. doi:10.1016/0266-7681(93)90144-5
Tang, J. B., Shi, D., and Zhou, H. (1995). Vein conduits for repair of nerves with a prolonged gap or in unfavourable conditions: An analysis of three failed cases. Microsurgery 16 (3), 133–137. doi:10.1002/micr.1920160303
Tang, J., Xiong, L., Wang, S., Wang, J., Liu, L., Li, J., et al. (2009). Distribution, translocation and accumulation of silver nanoparticles in rats. J. Nanosci. Nanotechnol. 9 (8), 4924–4932. doi:10.1166/jnn.2009.1269
Taras, J. S., Jacoby, S. M., and Lincoski, C. J. (2011). Reconstruction of digital nerves with collagen conduits. J. Hand Surg. Am. 36 (9), 1441–1446. doi:10.1016/j.jhsa.2011.06.009
Trehan, S. K., Model, Z., and Lee, S. K. (2016). Nerve repair and nerve grafting. Hand Clin. 32 (2), 119–125. doi:10.1016/j.hcl.2015.12.002
Tu, Q., Pang, L., Chen, Y., Zhang, Y., Zhang, R., Lu, B., et al. (2014). Effects of surface charges of graphene oxide on neuronal outgrowth and branching. Analyst 139 (1), 105–115. doi:10.1039/c3an01796f
Tubbs, R. S., Loukas, M., Salter, E. G., and Oakes, W. J. (2007a). Wilhelm Erb and Erb's point. Clin. Anat. 20 (5), 486–488. doi:10.1002/ca.20385
Tubbs, R. S., Salter, E. G., Wellons, J. C., Blount, J. P., and Oakes, W. J. (2007b). Landmarks for the identification of the cutaneous nerves of the occiput and nuchal regions. Clin. Anat. 20 (3), 235–238. doi:10.1002/ca.20297
Ulusal, A. E., Ulusal, B. G., Hung, L. M., and Wei, F. C. (2005). Establishing a composite auricle allotransplantation model in rats: Introduction to transplantation of facial subunits. Plast. Reconstr. Surg. 116 (3), 811–817. doi:10.1097/01.prs.0000176249.27930.38
Uz, M., Donta, M., Mededovic, M., Sakaguchi, D. S., and Mallapragada, S. K. (2019). Development of gelatin and graphene-based nerve regeneration conduits using three-dimensional (3D) printing strategies for electrical transdifferentiation of mesenchymal stem cells. Industrial Eng. Chem. Res. 58 (18), 7421–7427. doi:10.1021/acs.iecr.8b05537
Vasilic, D., Barker, J. H., Blagg, R., Whitaker, I., Kon, M., and Gossman, M. D. (2010). Facial transplantation: An anatomic and surgical analysis of the periorbital functional unit. Plast. Reconstr. Surg. 125 (1), 125–134. doi:10.1097/PRS.0b013e3181c2a5cc
Wang, L., Li, Y., Yang, X. Y., Zhang, B. B., Ninane, N., Busscher, H. J., et al. (2021). Single-cell yolk-shell nanoencapsulation for long-term viability with size-dependent permeability and molecular recognition. Natl. Sci. Rev. 8 (4), nwaa097. doi:10.1093/nsr/nwaa097
Wang, L., Zhang, D., Ren, Y., Guo, S., Li, J., Ma, S., et al. (2022). Injectable hyaluronic acid hydrogel loaded with BMSC and NGF for traumatic brain injury treatment. Mater Today Bio 13, 100201. doi:10.1016/j.mtbio.2021.100201
Wang, M.-D., Zhai, P., Schreyer, D. J., Zheng, R.-S., Sun, X.-D., Cui, F.-Z., et al. (2013). Novel crosslinked alginate/hyaluronic acid hydrogels for nerve tissue engineering. Front. Mater. Sci. 7 (3), 269–284. doi:10.1007/s11706-013-0211-y
Wang, W., Chen, D., Chen, S., Li, D., Li, M., Xia, S., et al. (2011). Laryngeal reinnervation using ansa cervicalis for thyroid surgery-related unilateral vocal fold paralysis: A long-term outcome analysis of 237 cases. PLoS One 6 (4), e19128. doi:10.1371/journal.pone.0019128
Wang, Y., Zhao, X., Huojia, M., Xu, H., and Zhuang, Y. (2016a). Transforming growth factor-β3 promotes facial nerve injury repair in rabbits. Exp. Ther. Med. 11 (3), 703–708. doi:10.3892/etm.2016.2972
Wang, Y., Zhao, Y., Sun, C., Hu, W., Zhao, J., Li, G., et al. (2016b). Chitosan degradation products promote nerve regeneration by stimulating Schwann cell proliferation via miR-27a/FOXO1 Axis. Mol. Neurobiol. 53 (1), 28–39. doi:10.1007/s12035-014-8968-2
Watanabe, Y., Sasaki, R., Matsumine, H., Yamato, M., and Okano, T. (2017). Undifferentiated and differentiated adipose-derived stem cells improve nerve regeneration in a rat model of facial nerve defect. J. Tissue Eng. Regen. Med. 11 (2), 362–374. doi:10.1002/term.1919
Weis, E., Rubinov, A., Al-Ghoul, A. R., and Yau, F. M. (2018). Sural nerve graft for neurotrophic keratitis: Early results. Can. J. Ophthalmol. 53 (1), 24–29. doi:10.1016/j.jcjo.2017.10.044
Wolford, L. M., and Stevao, E. L. L. (2003). Considerations in nerve repair. Bayl. Univ. Med. Cent. Proc. 16 (2), 152–156. doi:10.1080/08998280.2003.11927897
Xu, S., Zhang, Z., and Chu, M. (2015). Long-term toxicity of reduced graphene oxide nanosheets: Effects on female mouse reproductive ability and offspring development. Biomaterials 54, 188–200. doi:10.1016/j.biomaterials.2015.03.015
Yampolsky, A., Ziccardi, V., and Chuang, S. K. (2017). Efficacy of acellular nerve allografts in trigeminal nerve reconstruction. J. Oral Maxillofac. Surg. 75 (10), 2230–2234. doi:10.1016/j.joms.2017.02.015
Yao, S., Liu, X., Yu, S., Wang, X., Zhang, S., Wu, Q., et al. (2016). Co-effects of matrix low elasticity and aligned topography on stem cell neurogenic differentiation and rapid neurite outgrowth. Nanoscale 8 (19), 10252–10265. doi:10.1039/c6nr01169a
Yetiser, S. (2018). Immediate hypoglossal-facial anastomosis in patients with facial interruption. J. Craniofac Surg. 29 (3), 648–650. doi:10.1097/scs.0000000000004150
Yoo, J., Park, J. H., Kwon, Y. W., Chung, J. J., Choi, I. C., Nam, J. J., et al. (2020). Augmented peripheral nerve regeneration through elastic nerve guidance conduits prepared using a porous PLCL membrane with a 3D printed collagen hydrogel. Biomater. Sci. 8 (22), 6261–6271. doi:10.1039/d0bm00847h
Yoshimura, H., Ohba, S., Nakamura, M., and Sano, K. (2013). Mandibular reconstruction using iliac bone and great auricular nerve grafts and oral rehabilitation using osseointegrated implants in a patient with a large ossifying fibroma: A 10-year follow-up study. J. Oral Maxillofac. Surg. 71 (12), 2176–2188. doi:10.1016/j.joms.2013.04.034
Yoshioka, N. (2018). Differential reanimation of the midface and lower face using the masseteric and hypoglossal nerves for facial paralysis. Oper. Neurosurg. Hagerst. 15 (2), 174–178. doi:10.1093/ons/opx217
Yoshioka, N. (2020). Hypoglossal-facial side-to-end neurorrhaphy with concomitant masseteric-zygomatic nerve branch coaptation and muscle transfer for facial reanimation: Technique and case report. Oper. Neurosurg. Hagerst. 19 (3), E230–e235. doi:10.1093/ons/opaa128
Youn, W., Ko, E. H., Kim, M. H., Park, M., Hong, D., Seisenbaeva, G. A., et al. (2017). Cytoprotective encapsulation of individual jurkat T cells within durable TiO(2) shells for T-cell therapy. Angew. Chem. Int. Ed. Engl. 56 (36), 10702–10706. doi:10.1002/anie.201703886
Yu, B., Zhang, X., and Li, X. (2014a). Exosomes derived from mesenchymal stem cells. Int. J. Mol. Sci. 15 (3), 4142–4157. doi:10.3390/ijms15034142
Yu, W., Jiang, X., Cai, M., Zhao, W., Ye, D., Zhou, Y., et al. (2014b). A novel electrospun nerve conduit enhanced by carbon nanotubes for peripheral nerve regeneration. Nanotechnology 25 (16), 165102. doi:10.1088/0957-4484/25/16/165102
Yu, X., Zhang, T., and Li, Y. (2020). 3D printing and bioprinting nerve conduits for neural tissue engineering. Polym. (Basel) 12 (8). doi:10.3390/polym12081637
Zheng, C., Yang, Z., Chen, S., Zhang, F., Rao, Z., Zhao, C., et al. (2021). Nanofibrous nerve guidance conduits decorated with decellularized matrix hydrogel facilitate peripheral nerve injury repair. Theranostics 11 (6), 2917–2931. doi:10.7150/thno.50825
Zheng, N., Fitzpatrick, V., Cheng, R., Shi, L., Kaplan, D. L., and Yang, C. (2022). Photoacoustic carbon nanotubes embedded silk scaffolds for neural stimulation and regeneration. ACS Nano 16 (2), 2292–2305. doi:10.1021/acsnano.1c08491
Zhou, S., Yang, Y., Gu, X., and Ding, F. (2008). Chitooligosaccharides protect cultured hippocampal neurons against glutamate-induced neurotoxicity. Neurosci. Lett. 444 (3), 270–274. doi:10.1016/j.neulet.2008.08.040
Zhu, W., George, J. K., Sorger, V. J., and Grace Zhang, L. (2017). 3D printing scaffold coupled with low level light therapy for neural tissue regeneration. Biofabrication 9 (2), 025002. doi:10.1088/1758-5090/aa6999
Zhu, W., Guo, J., Amini, S., Ju, Y., Agola, J. O., Zimpel, A., et al. (2019). SupraCells: Living mammalian cells protected within functional modular nanoparticle-based exoskeletons. Adv. Mater 31 (25), e1900545. doi:10.1002/adma.201900545
Zor, F., Bozkurt, M., Nair, D., and Siemionow, M. (2010). A new composite midface allotransplantation model with sensory and motor reinnervation. Transpl. Int. 23 (6), 649–656. doi:10.1111/j.1432-2277.2009.01032.x
Keywords: soft tissue injuries, nerve transplantation, Biomaterials, tissue engineering, Regenerative medicine, nerve conduit, stem cell, 3D printing
Citation: Sun S, Lu D, Zhong H, Li C, Yang N, Huang B, Ni S and Li X (2022) Donors for nerve transplantation in craniofacial soft tissue injuries. Front. Bioeng. Biotechnol. 10:978980. doi: 10.3389/fbioe.2022.978980
Received: 27 June 2022; Accepted: 16 August 2022;
Published: 07 September 2022.
Edited by:
Jiayuan Zhu, The First Affiliated Hospital of Sun Yat-sen University, ChinaReviewed by:
Jingang Xiao, Southwest Medical University, ChinaJulia Teixeira Oliveira, Federal University of Rio de Janeiro, Brazil
Copyright © 2022 Sun, Lu, Zhong, Li, Yang, Huang, Ni and Li. This is an open-access article distributed under the terms of the Creative Commons Attribution License (CC BY). The use, distribution or reproduction in other forums is permitted, provided the original author(s) and the copyright owner(s) are credited and that the original publication in this journal is cited, in accordance with accepted academic practice. No use, distribution or reproduction is permitted which does not comply with these terms.
*Correspondence: Shilei Ni, eXRmaXNobkAxMjYuY29t; Xingang Li, bGl4Z0BzZHUuZWR1LmNu
†These authors have contributed equally to this work