- 1NHC Key Laboratory of Reproductive Health and Medical Genetics (China Medical University) and Liaoning Key Laboratory of Reproductive Health, Liaoning Research Institute of Family Planning (The Affiliated Reproductive Hospital of China Medical University), Shenyang, China
- 2Department of Obstetrics and Gynecology, Shengjing Hospital of China Medical University, Shenyang, China
Pelvic floor dysfunction (PFD) is a highly prevalent urogynecology disorder affecting many women worldwide, with symptoms including pelvic organ prolapse (POP), stress urinary incontinence (SUI), fecal incontinence, and overactive bladder syndrome (OAB). At present, the clinical treatments of PFD are still conservative and symptom-based, including non-surgical treatment and surgery. Surgical repair is an effective and durable treatment for PFD, and synthetic and biological materials can be used to enforce or reinforce the diseased tissue. However, synthetic materials such as polypropylene patches caused a series of complications such as mesh erosion, exposure, pain, and inflammation. The poor mechanical properties and high degradation speed of the biomaterial meshes resulted in poor anatomical reduction effect and limitation to clinical application. Therefore, the current treatment options are suboptimal. Recently, tissue-engineered repair material (TERM) has been applied to repair PFD and could markedly improve the prognosis of POP and SUI repair surgery in animal models. We review the directions and progression of TERM in POP and SUI repair. Adipose-derived stem cells (ADSCs) and endometrial mesenchymal stem cells (eMSCs) appear to be suitable cell types for scaffold seeding and clinical implantation. The multidisciplinary therapy approach to tissue engineering is a promising direction for tissue repair. More and longer follow-up studies are needed before determining cell types and materials for PFD repair.
Introduction
Pelvic floor dysfunction (PFD) is a highly prevalent urogynecology disorder, affecting about 30%∼50% of middle-aged and elderly women. The main manifestations of PFD are pelvic organ prolapse (POP), stress urinary incontinence (SUI), fecal incontinence, and overactive bladder syndrome (OAB). POP and SUI mostly occur in parous women and are caused by childbirth-associated pelvic floor injury. The prevalence of PFD varies in different geographical regions. It is estimated that one in three and one in nine women are affected by SUI and POP, respectively, and POP and SUI may coexist in up to 80% of women with prolapse (Farmer et al., 2020). Studies have predicted that the number of women with at least one PFD will increase by 55.8% from 2010 to 2050 (Wu et al., 2009).
At present, the clinical treatments of PFD are still conservative and symptom-based, including non-surgical treatment and surgery. Non-surgical treatment mainly consists of a manual approach, stimulation, or relaxation technique (Quaghebeur et al., 2021). Surgery was considered to be the most effective and durable treatment, which includes autologous tissue repair and synthetic mesh implantation. In general, autologous tissue repair showed good integration within host tissues, a minimal to moderate inflammatory response, and a moderate degree of collagen production and underwent a degree of remodeling over the long term, but a high recurrence rate (Gigliobianco et al., 2015; Lo et al., 2015). Polypropylene patches are the most used synthetic meshes in implantation surgery, which offer several advantages including lack of transmission of infectious diseases, ease of availability, sustainable tensile strength, and good mechanical properties. However, its nondegradable nature and poor histocompatibility caused a series of problems such as mesh erosion, exposure, pain, infection, pronounced inflammation, massive cell infiltration, and collagen production, and these led to the withdrawal of transvaginal polypropylene mesh from the market and its banning in some countries (Elmer et al., 2009; Gigliobianco et al., 2015). Biomaterial meshes have been used recently for their good histocompatibility, but the poor mechanical properties and high degradation speed resulted in poor anatomical reduction effect and limitation to clinical application. Therefore, the current treatment options are suboptimal, and alternative methods are needed to promote the repair of PFD.
The defects of the pelvic floor support, which are due to the interaction between the muscles and connective tissues within the pelvis, are the main pathophysiology of PFD. They are mainly manifested as altered elastin/collagen metabolism and connective tissue abnormalities (Jin et al., 2016a), such as (1) the interruption in elastin homeostasis caused by abnormal degradation/synthesis of elastin and (2) the changes in the total content and the ratio of subtypes of collagen and the deficient crosslinking of collagens. Therefore, the goal of PFD therapy is to support weakened tissue of the pelvic floor and promote tissue remodeling, thus achieving the effect of improving pelvic floor functions.
Tissue-engineered repair material (TERM) is a multidisciplinary therapy approach that seeks to use a combination of cells, materials, and sometimes additional genes, drugs, or growth factors, to regenerate diseased tissues. The purpose of the cell component, gene, and growth factor is to accelerate repair and promote regeneration of damaged or lost tissue, while the material provides physical support and niches to deliver cells, drugs, and growth factors to the tissue, which, in turn, provides a platform to control the local pharmacokinetics of growth factors and the proliferation and differentiation of transplanted stem cells (Mangir et al., 2019; Zhao et al., 2021). Using TERM for PFD treatment has obvious advantages: first, the scaffolds can support weakened tissue and will not cause so many complications for its degradable nature and good histocompatibility; second, the seeding cells will drive tissue remodeling, ultimately providing a permanent repair; third, the bioactive factors, such as growth factors, drugs, and estrogen can speed up repair and improve the microenvironment. So, TERM is a promising treatment for PFD. However, the choice of cells and scaffolds is crucial to the success of TERM. Therefore, different scaffolds and cells have been studied to create a TERM for the treatment of PFD. So, we reviewed the research results of TERM applied in PFD treatment and drew the structure diagram of TERM (Figure 1).
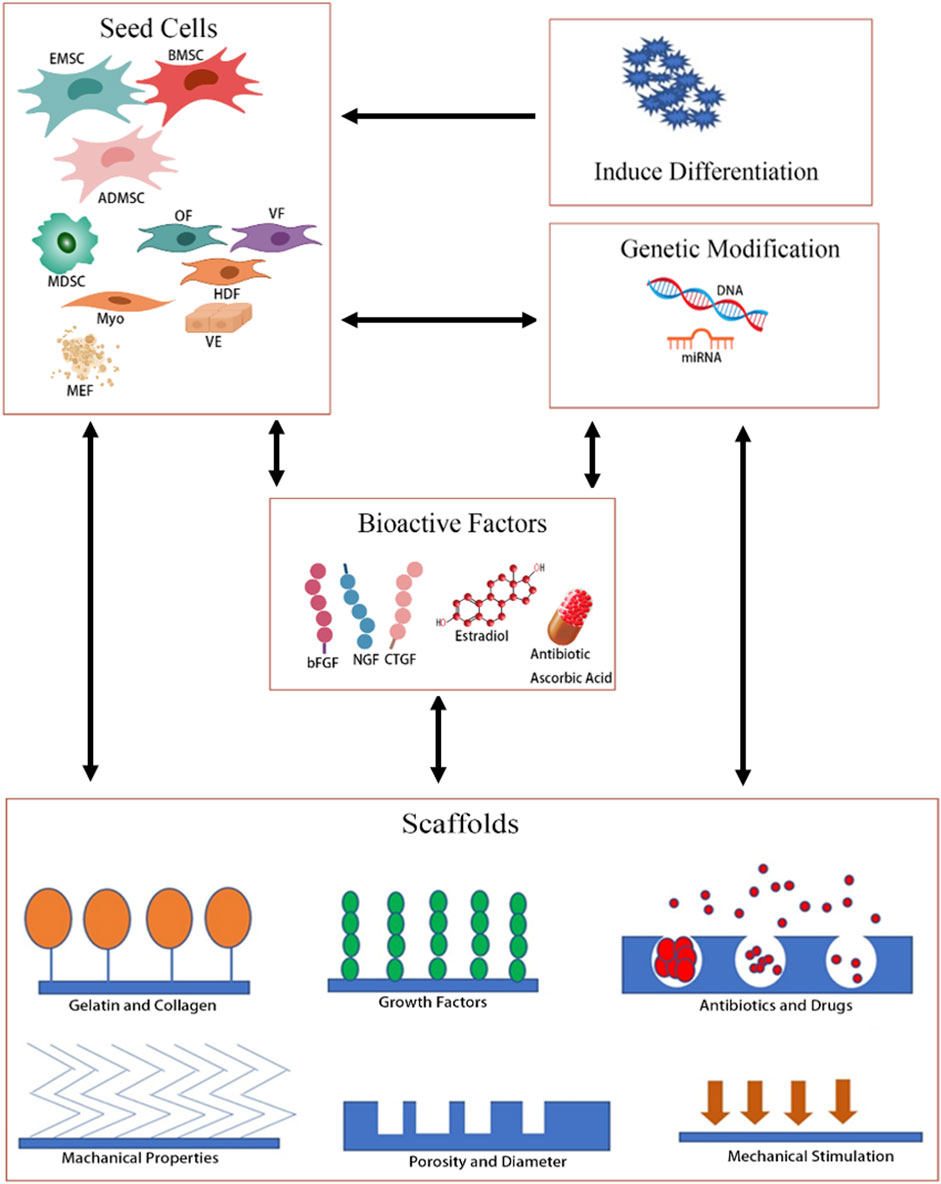
FIGURE 1. Overview of TERMs of different strategies for PFD. OF, oral fibroblast; VF, vaginal fibroblast; HDF, human dermal fibroblast; VE, vaginal epithelial; Myo, myoblasts; MFF, muscle fiber fragment.
Seed cells
Seed cells are the premise of tissue engineering; they could be obtained from a wide range of sources, including autologous, allogeneic, and heterologous tissues. Stem cells and differentiated cells have been reported as the seed cells for pelvic floor repair. Mesenchymal stem cells (MSCs) are the most widely used stem cells, including adipose-derived stem cells (ADSCs), bone marrow-derived mesenchymal stem cells (BMSCs), endometrial mesenchymal stem cells (eMSCs), and muscle-derived stem cells (MDSCs). The differentiated cells include fibroblasts, myoblasts, and smooth muscle cells. Among them, ADSCs and eMSCs are the most promising cells for experimental research and clinical treatment of PFD (Table 1).
ADSCs are considered beneficial for PFD treatment by in vitro and in vivo studies because ADSCs can be obtained in large quantities during single liposuction and without general anesthesia and are easy to culture and expand in vitro (Chen H. et al., 2021a; Wang et al., 2021). ADSCs not only exhibit self-renewal and multilineage differentiation, but they also have confirmed proliferative efficiency and low donor morbidity (Sterodimas et al., 2010). In addition, there is a relatively low occurrence of surgical interference in collecting them as compared with other derived stem cells. Some studies suggested that ADSCs have equal or superior therapeutic potential compared to BMSCs. ADSCs were used to treat SUI in many clinical trials by urethral injection showing better results and minimal side effects and complications (Barakat et al., 2020). Roman et al. (2014) showed that hADSCs appear to be suitable cell types to combine with biodegradable scaffolds for the treatment of SUI and POP.
eMSCs are a rare population of perivascular MSCs in the endometrial layer of the uterus and are another ideal source of autologous therapeutic cells for the treatment of POP using novel tissue engineering approaches for the following reasons: (1) eMSCs exhibit excellent regenerative capacity in the endometrial lining following menstruation and high proliferative capacity in vitro. (2) They can be easily obtained from menstrual blood or endometrial biopsies, even from the post-menopausal uterus; the procurement method is minimally invasive, without the need for an anesthetic, resulting in minimum pain and morbidity. (3) eMSCs can be purified using a unique marker, SUSD2 (Masuda et al., 2012). (4) A small molecule TGF-β receptor inhibitor (A83-01) can maintain clonogenic SUSD2+eMSCs and prevents spontaneous fibroblast differentiation during culture expansion (Gurung et al., 2015). (5) eMSCs could reduce the foreign body reaction to the degradable mesh by modulating inflammatory cytokine secretion and changing the ratio of M1/M2 of macrophages (Darzi et al., 2018).
BMSCs are among the best characterized of all stem cells studied so far, with great differentiation ability and the ability to secrete factors beneficial to tissue repair. BMSCs are used in the repair of various diseases and injuries with good efficacy in many studies. Among them, the treatment of PFD by BMSCs was also studied in vitro and in vivo and the results showing good repair effects, whether through local cell injection or tissue engineering combined with biological scaffolds (Jin et al., 2016a; Jin et al., 2016b; Zhao et al., 2017; Zhao et al., 2018; Zhao et al., 2021). However, BMSCs carry disadvantages such as painful isolation procedures, the need for general anesthesia, relatively scarce numbers, and low cell yields, and they, especially, cannot be easily expanded in middle-aged people. So these shortcomings limited the clinical use of BMSCs.
MDSCs are isolated from muscle biopsies and differentiated in vitro and in vivo into skeletal myotubes, osteoblasts, chondrocytes, neural cells, and smooth muscle cells. There are only small studies investigating PFD treatment using MDSCs with or without scaffolds. The clinical study showed that the quality of life in patients with SUI improved significantly 2−4 years after the MDSC injection procedure (Stangel-Wojcikiewicz et al., 2014; Stangel-Wojcikiewicz et al., 2016). In vitro studies demonstrated that urethral striated muscle-derived stem/progenitor cells (uMDSCs) could form myotubes when treated with micro-energy acoustic pulses or low-intensity extracorporeal shock waves (Wang B. et al., 2018a; Cui et al., 2019). Animal experiments showed that MDSC injection improved the function of urination in rats with intrinsic sphincter deficiency and increased the expression of myosin and α-smooth muscle actin (α-SMA) (Cui et al., 2018). However, the acquisition of MDSCs requires surgical anesthesia and invasive operation, resulting in pain and low cell yield, which limits its clinical application.
Additionally, some other differentiated cells were also used for repairing damaged tissue due to PFD, such as vaginal fibroblasts, epithelial cells, smooth muscle cells, and human oral fibroblasts. However, the use of these differentiated cells in tissue engineering repair is significantly less than that of MSCs, for MSCs have a particular advantage over differentiated cells.
Scaffolds
The ideal scaffolds for tissue engineering should meet the following conditions: (1) the scaffolds should be biocompatible, integrate well into the patient’s tissues, and reflect the properties of the tissues into which it is implanted. (2) The property of scaffolds should be stable, with proper porosity and pore size, conducive to cell adhesion, proliferation, no toxicity, and no immunity. (3) The scaffolds should be strong enough to provide structural support and remain relatively elastic to cope with the forces experienced with routine events such as coughing or sneezing and become reversibly stronger at higher strain, similar to the native healthy fascia. (4) Materials proposed for grafts should degrade in vivo, provoke an acute inflammatory response, undergo tissue remodeling, allow cell permeability, and exhibit mechanical robustness at the point of implantation (Gigliobianco et al., 2015). PFD is a chronic disorder with no optimal standards for the repair or treatment; an ideal mesh would be desirable for degradable biomaterials to last 6–12 months to ensure sufficient tissue re-organization with desired stiffness. The scaffolds in TERM for PFD were mainly synthetic materials in a large number of in vitro and in vivo studies, and they are all FDA-approved materials, such as poly-L-lactic acid (PLA), polyglycolide (PGA), poly lactic-co-glycolic acid (PLGA), and polycaprolactone (PCL). Different materials have their own advantages and disadvantages, and they had been reported in the study of PFD treatment by combining with different cells (Table 1).
PLA scaffold mimics the architecture of native fascia tissues, produces better extracellular matrix components for cell attachment and proliferation in vitro, and integrates well in native tissue with good cell infiltration, neovascularization, and macrophage type 2 (M2) response (Roman et al., 2019). However, PLA is too brittle and degrades very slowly in vivo, and the acidity and high crystallinity of its degradation byproducts often triggered inflammatory reactions. Relatively few studies were reported on the effect of PLA or PLA-added cells on PFD (Mangir et al., 2016; Roman et al., 2016; Mangir et al., 2019).
PCL is an FDA-approved polyester with excellent thermal stability, good biocompatibility, and low immunogenicity, it is easy to process and susceptible to surface modifications, and it has been widely used as biomaterials for tissue engineering purposes, including pelvic meshes (Siddiqui et al., 2018). However, the hydrophobicity of PCL impedes cell adhesion and limits the degradation rate. So many PCL composites were studied for PFD treatment, such as PCL/PLA, PCL/PGA, PCL/PEG (Ren et al., 2022), PLGA/PCL (Vashaghian et al., 2017; Vashaghian et al., 2019; Chen YP. et al., 2021b), and UPy-PCL(Hympanova et al., 2018), and the results showed that PCL/PEG and PLGA/PCL were better than the others, the degradation rate of PCL/PLA and PCL/PGA were too high, and UPy-PCL had a high failure rate.
PLGA scaffold is approved by the FDA (U.S. Food and Drug Administration, United States)/EMA (European Medicines Agency) and extensively explored. PLGA holds a prominent position in a variety of tissue repairs due to its biocompatibility and controllable biodegradability. PLGA scaffold has been successfully applied in pelvic floor repair (Oe et al., 2022) and bladder replacement alone (Salem et al., 2020) (Rocha et al., 2022) or PLGA-based composites, such as PLGA-NPs (Jin et al., 2016a; Jin et al., 2016b), PLGA/PCL (Qian et al., 2016; Vashaghian et al., 2016), PLGA-MPEG (Jangö et al., 2017), and PLACL/gelatin (Mukherjee et al., 2019). Among them, PLGA/PCL has been studied the most in the treatment of pelvic floor repair.
In addition, other scaffolds are also used for PFD repair, such as PGA, PLTG, PU, and PLCL/Fg (Hou et al., 2014; Wu et al., 2016; Wang et al., 2017; Wang X. et al., 2018b; Masudi and Abdelrahman, 2021). However, the relevant studies are limited. PGA is beneficial to using as sling material for SUI treatment because of its low stiffness.
Bioactive factors
The appropriate microenvironment for tissue regeneration is another key factor of an ideal tissue engineering scaffold. So some bioactive factors were added to scaffolds to induce stem cell proliferation and differentiation, promote cell–material interaction, enhance mechanical and biological properties, and antisepsis, etc. PFD-related factors include basic fibroblast growth factor (bFGF), nerve growth factor (NGF), connective tissue growth factor (CTGF), estrogen, 17-β-estradiol, ascorbic acid, ascorbate-2-phosphate, and antibiotics. Jin et al. demonstrated that bFGF significantly promoted the production of collagen and elastin from elastin-expressing BMSCs in vitro and in vivo, and co-injection of PLGA-loaded bFGF NP and elastin-expressing BMSCs into the PFD rats significantly improved the outcome of urodynamic tests (Jin et al., 2016a). In situ gel-forming bulking agent, containing NGF and bFGF, provided regeneration of damaged nerves and smooth muscles and enhanced biological function around the urethra (Oh et al., 2015). The solid PCL-CTGF mesh delivering rMSC demonstrated improved biomechanical properties and no complications after implantation in a rat model of a weakened vaginal wall after 8−24 weeks (Hansen et al., 2020). Studies have shown that estrogen released from PLA mesh promotes more ECM production involving collagen I, collagen III, and elastin and doubled new blood vessel formation in the chorioallantoic membrane (Mangir et al., 2019). Shafaat et al. (2018) reported that 17-β-estradiol-releasing PU scaffolds showed increased ultimate tensile strength and produced more ECM and blood vessels. However, the controversial therapeutic effect of estrogen was also reported (Wu et al., 2020). Ascorbic acid and ascorbate-2-phosphate in the PLA scaffold could increase its hydrophilicity and strength and promote the production of collagen from human dermal fibroblasts (Mangir et al., 2016). Ren et al. (2022) coated the PCL/PEG composite meshes with azithromycin and elicited the antibacterial properties of the meshes; the results showed that it is effective in suppressing the growth of S. aureus bacteria and will be supporting cell attachment and proliferation.
The cross-talk between scaffolds and cells
Cell therapy using MSCs and surgery with synthetic polymer scaffolds alone could somewhat improve the PFD symptoms, and cell-based tissue engineering has even more dramatic therapeutic effects. So, how and why therapeutic cells together with scaffolds enhance therapeutic outcomes is the key factor. From a clinical perspective, the cross-talk between scaffolds and cells may be imperative for enhancing the therapeutic effect of PFD (Mukherjee et al., 2019).
The cell–biomaterial interactions between eMSCs and nanofiber meshes of PLACL/gelatin in vitro and in vivo were evaluated by Mukherjee et al. (2019), and the results showed: (1) mesh properties influence eMSC size, proliferation rate, adhesion, migration, structure, and expression of F-actin and vinculin protein in vitro. PLACL/gelatin nanofiber meshes provide suitable scaffolding where cells can interact with the mesh, as well as with each other and the host cells. (2) PLACL/gelatin significantly enhanced eMSC retention and infiltration properties in vivo. Many clinical trials of cell therapies suggested that mere injection of therapeutic cells is not sufficient to cure diseases (Galipeau and Sensebe 2018). One reason is that there are no enough cells in the damaged site, so retention of MSCs in the transplantation site is a key factor for cellular infiltration and tissue integration. (3) eMSCs control ECM formation and degradation of nanofiber meshes in vivo, allowing time for tissue strengthening before the scaffold fully disappears. (4) eMSCs influence the macrophage-based foreign body response to nanofiber meshes in vivo. Wang X. et al. (2018b) reported that the addition of ADSCs onto both PLA and PLTG scaffolds led to a significant increase in stiffness and maximum stress of the scaffolds. A similar phenomenon was also observed in other experiments using human oral fibroblasts (OFs) and the hADSC effects on PLA scaffold (Roman et al., 2014). Significant temporal changes in tensile properties and clear differences in collagen organization with time between eMSC-seeded and -unseeded scaffolds were observed (Edwards et al., 2015). PLA combined with PU greatly improved the interaction of cells with this material (Hillary et al., 2016).
So, the cross-talk between scaffolds and cells is of great significance in tissue engineering. The scaffolds could provide support for cell growth, promote cell proliferation, and prevent cell migration. At the same time, the cells can affect the property of the biomaterials, such as stiffness, elasticity, and degradation rate, especially for PFD, it would be desirable for degradable biomaterials to last 6–12 months until new tissue of desired stiffness has been regenerated, so regulating the degradation of biomaterials is the key to TERM for PFD (Hillary et al., 2016).
Tissue-engineered repair material for stress urinary incontinence
SUI is the involuntary urine leakage associated with increased intra-abdominal pressure on the bladder during activities, such as coughing, laughing, sneezing, exercising, impact movements, or squatting (Wallace et al., 2019). The urine leakage of SUI happens in the absence of detrusor contraction. The pathophysiology of SUI is not well known and multifactorial, including pregnancies with subsequent vaginal deliveries, nerve damage, intrinsic sphincteric deficiency (ISD), body mass index (BMI), advancing age, hormonal changes, and smoking. The current treatment options mainly include conservative, pharmacological, and surgical treatments, and the efficacy is generally unsatisfactory. TERM has provided a variety of opportunities to restore the damaged sphincter function in patients with SUI (Table 2).
The suburethral sling implantation is the mainstay of surgical treatment for SUI. However, these approaches only supply passive support for the urethral lumen without restoring the damaged sphincter function. Also, tissue rejection, urethral erosion, and infection are the major problems and headaches of the currently used slings. Ying Wang tried to explore the possibility of generating a sling complex in vitro by seeding ADSCs onto PGA under constant strain; the results showed that the ADSC-PGA construct appeared as a sling-like structure with a cord-like shape during the first 4 weeks of in vitro culture, and the ADSC-PGA sling exhibited to be relatively thicker, smoother, much thinner, and mature at 12 weeks and had gradually increased stress/strain curves, max load, and Young’s modulus values over time. The collagen fibers and myoblasts’ expression increased in a time-dependent manner (Wang et al., 2017). A tissue-engineered sling was constructed by seeding GFP-transfected ADSCs on PGA fibers, and with the induction of 5-azacytidine for 4 weeks, the sling was then implanted in the SUI rat model established by the vaginal balloon dilatation method and bilateral ovariectomy. LPP increased significantly and reached nearly close to baseline normal levels, 2 months after implantation. ADSCs promote more collagen matrix formation and are integrated better with the urethral sphincter (Wang et al., 2016). To find materials with more appropriate mechanical properties for a sling, which can also support cell integration, scaffolds of different materials were compared; the results showed that PU Z1 and PU Z3 coped well with dynamic strain and maintained their elasticity after 7 days of sustained cyclical distention (Hillary et al., 2016). PLA is superior at supporting cellular interactions and new matrix production, but the mechanical properties changed after only 7 days of dynamic strain. PU/PLA was weaker and stiffer than PU or PPL but significantly improved the interaction of the scaffolds with cells and promoted the total collagen expression of hADSCs cultured on the scaffolds, suggesting that a combination of materials could be more suitable for successful implantation and longer survival for the management of SUI. Zhang et al. (2016) fabricated a novel electrospun nanoyarn with higher porosity, larger pore size, and aligned fibers/yarns than the nanofiber scaffold. A tissue-engineered sling was formed by seeding myoblasts on nanoyarn, and the myoblasts proliferated greatly on the nanoyarn scaffold and infiltrated deeply into it after 7 days; nanoyarn myoblasts produced more type 1 and 3 collagen and elastin and improved muscular tissue development, suggesting myoblast–nanoyarn sling could be a promising tissue-engineered sling for SUI.
Bulking agents are another common treatment for SUI, which allows minimally invasive treatment and low medical expenses. However, the migration and/or degradation of the injected bulking agents shortened the lasting of long-term effectiveness in the therapeutic period. An alternative approach based on cells/bioactive molecules and biodegradable biomaterials can induce the regeneration of target tissues and improve the sphincter function. Oh et al. (2015) developed an injectable bioactive bulking agent for the SUI rat model by loading dual growth factor (NGF and bFGF) in situ hydrogel/PCL bead mixture, and the results showed that the PCL beads were located stably at the applied site without migration. The sequential release of the growth factors from the bulking agent promoted the regeneration of damaged nerves and smooth muscles and thus enhanced biological function around the urethra. Jin et al. (2016a) formulated the bFGF-loaded PLGA nanoparticle (bFGF-loaded PLGA NPs) system to achieve the sustained release of bFGF and employed this system in elastin–over-expressing BMSC culture in vitro and in the rat vaginal distention translational model in vivo. The results showed that bFGF-loaded PLGA NPs could markedly stimulate the differentiation of elastin–over-expressing BMSCs to fibroblasts and then produce more collagen and elastin in vitro and in vivo. Also, the combination using of a bFGF-loaded PLGA NP system and elastin–over-expressing BMSCs in the rat model could alleviate the PFD symptoms, reverse the decreased void volume and bladder void pressure, and rescue the decreased peak bladder pressure. In another study, Jin et al. (2016b) demonstrated that inhibition of miR-29a-3p in BMSCs along with bFGF-PLGA-NP injection can markedly increase the expression and secretion of elastin, which consequently largely improved the urodynamic parameters and urodynamics and promoted the therapeutic effect of BMSCs on PFD rats.
Tissue-engineered repair material for pelvic organ prolapse
POP is defined as the descent of one or more of the pelvic organs (vaginal wall, bladder, uterus, and vaginal apex) from their natural positions in the pelvis because of the weakening of the pelvic floor and the organ support structure (Iglesia and Smithling 2017). POP mainly results from pregnancy/vaginal birth, which is due to the strain, overstretching, and tearing of the pelvic floor ligaments, muscles, and connective tissues (Wallace et al., 2019). Surgery treatment with synthetic meshes is the main method for POP therapy, but the surgical outcomes do not meet patients’ needs. Many studies demonstrated that TERM consisting of cells/drugs/growth factors/biodegradable scaffolds could improve the therapeutic effect (Table 2).
Jango et al. (2015) employed MPEG-PLGA (methoxypolyethyleneglycol polylactic-co-glycolic acid) scaffold seeded with autologous muscle fiber fragments (MFFs) to treat a rat abdominal wall defect model; the results demonstrated that MPEG-PLGA scaffold is a safe carrier for MFFs, and MFFs affect the regenerative repair process, but the MPEG-PLGA+MFF group showed a trend toward higher stiffness in the physiologically more important low stiffness zone, which is not ideal from a clinical point of view. An estradiol-releasing electrospun PLA mesh containing different concentrations of estradiol was designed, and the results showed that ADMSCs cultured on estradiol-releasing PLA meshes produced more ECM and formed more new blood vessels and outgrew the pro-angiogenic cells (Mangir et al., 2019). Meanwhile, the estradiol-releasing PLA mesh with ADMSCs was more elastic and stronger than control PLA meshes. PU scaffolds with 17-β-estradiol significantly increased the ultimate tensile strength of scaffolds, and hADMSCs on estradiol-releasing PU scaffolds showed more ECM production, higher angiogenic potential, and good cellular infiltration and tissue integration (Shafaat et al., 2018). Ascorbic acid (AA) and ascorbate-2-phosphate (A2P) containing PLA scaffolds were significantly more hydrophilic and stronger than PLA scaffolds and had approximately two times higher UTS, strain, and YM values than pure PLA. Human dermal fibroblasts seeded on AA or A2P containing PLA scaffolds produced more collagen (Mangir et al., 2016). Four different PLA scaffolds with various degrees of fiber alignment were constructed to mimic the three-dimensional architecture of human fascia, and the results showed that the bulk density, Young’s modulus, and UTS were significantly increased from PLA-random to PLA-aligned scaffolds, and ADSCs grew well on scaffolds with aligned fibers, produced the largest amount of total collagen, and maintained the strength and stiffness without changes after 2 weeks of culture in vitro (Roman et al., 2016). The scPLCL is a potential scaffold material for vaginal tissue engineering, vaginal epithelial (VE), and stromal cells (SCs) that attached and maintained viability on scPLCL (Sartoneva et al., 2018). The comparison of PLGA/PCL films with different fiber diameters suggested that the fiber diameter affects cell behavior, mechanical properties, and cellular infiltration ECM deposition (Vashaghian et al., 2016).
Conclusion
The clinical experience and studies in vitro or in vivo suggest that tissue engineering technology can provide successful outcomes when used in the surgical management of pelvic floor disorders. These data provide valuable evidence for clinical application in the urogynecological setting. However, different cells, scaffolds, or cell-combined scaffolds have their own advantages and disadvantages, which can produce different therapeutic effects on PFD. The question is which is most desirable? Therefore, more studies with longer follow-up are needed to select the most effective and safe cells and materials for PFD repair.
Author contributions
ML contributed to the literature search and drafting of the manuscript; YL contributed to drawing pictures and making tables; and JC designed and reviewed the manuscript. All the authors reviewed the manuscript and approved it for publication.
Funding
This research was supported by the following funding schemes: Scientific Research Funds Project of Higher Education Institutions of Liaoning Province, under No. LJKZ0791 and the Young and Middle-Aged Science and Technology Innovation Talents Support Program of Shenyang, under No. RC210454.
Conflict of interest
The authors declare that the research was conducted in the absence of any commercial or financial relationships that could be construed as a potential conflict of interest.
Publisher’s note
All claims expressed in this article are solely those of the authors and do not necessarily represent those of their affiliated organizations, or those of the publisher, the editors, and the reviewers. Any product that may be evaluated in this article, or claim that may be made by its manufacturer, is not guaranteed or endorsed by the publisher.
References
Barakat, B., Franke, K., Schakaki, S., Hijazi, S., Hasselhof, V., and Vogeli, T. A. (2020). Stem cell applications in regenerative medicine for stress urinary incontinence: A review of effectiveness based on clinical trials. Arab. J. Urol.18, 194–205. doi:10.1080/2090598x.2020.1750864
Chen, H., Li, Z., Lin, M., Lv, X., Wang, J., Wei, Q., et al. (2021a). MicroRNA-124-3p affects myogenic differentiation of adipose-derived stem cells by targeting Caveolin-1 during pelvic floor dysfunction in Sprague Dawley rats. Ann. Transl. Med.9, 161. doi:10.21037/atm-20-8212
Chen, Y. P., Lo, T. S., Lin, Y. T., Chien, Y. H., Lu, C. J., and Liu, S. J. (2021b). Fabrication of drug-eluting polycaprolactone/poly(lactic-co-glycolic acid) prolapse mats using solution-extrusion 3D printing and coaxial electrospinning techniques. Polym. (Basel)13, 2295. doi:10.3390/polym13142295
Cui, K., Kang, N., Banie, L., Zhou, T., Liu, T., Wang, B., et al. (2019). Microenergy acoustic pulses induced myogenesis of urethral striated muscle stem/progenitor cells. Transl. Androl. Urol.8, 489–500. doi:10.21037/tau.2019.08.18
Cui, L., Meng, Q., Wen, J., Gao, Z., Yan, Z., Tian, Y., et al. (2018). A functional comparison of treatment of intrinsic sphincter deficiency with muscle-derived and adipose tissue-derived stem cells. IUBMB Life70, 976–984. doi:10.1002/iub.1896
Darzi, S., Deane, J. A., Nold, C. A., Edwards, S. E., Gough, D. J., Mukherjee, S., et al. (2018). Endometrial mesenchymal stem/stromal cells modulate the macrophage response to implanted polyamide/gelatin composite mesh in immunocompromised and immunocompetent mice. Sci. Rep.8, 6554. doi:10.1038/s41598-018-24919-6
Edwards, S. L., Ulrich, D., White, J. F., Su, K., Rosamilia, A., Ramshaw, J. A., et al. (2015). Temporal changes in the biomechanical properties of endometrial mesenchymal stem cell seeded scaffolds in a rat model. Acta Biomater.13, 286–294. doi:10.1016/j.actbio.2014.10.043
Elmer, C., Blomgren, B., Falconer, C., Zhang, A., and Altman, D. (2009). Histological inflammatory response to transvaginal polypropylene mesh for pelvic reconstructive surgery. J. Urol.181, 1189–1195. doi:10.1016/j.juro.2008.11.030
Farmer, Z. L., Dominguez-Robles, J., Mancinelli, C., Larraneta, E., and Lamprou, D. A. (2020). Urogynecological surgical mesh implants: New trends in materials, manufacturing and therapeutic approaches. Int. J. Pharm. X.585, 119512. doi:10.1016/j.ijpharm.2020.119512
Galipeau, J., and Sensebe, L. (2018). Mesenchymal stromal cells: Clinical challenges and therapeutic opportunities. Cell Stem Cell22, 824–833. doi:10.1016/j.stem.2018.05.004
Gigliobianco, G., Regueros, S. R., Osman, N. I., Bissoli, J., Bullock, A. J., Chapple, C. R., et al. (2015). Biomaterials for pelvic floor reconstructive surgery: How can we do better?Biomed. Res. Int.2015, 1–20. doi:10.1155/2015/968087
Gurung, S., Werkmeister, J. A., and Gargett, C. E. (2015). Inhibition of transforming growth factor-beta receptor signaling promotes culture expansion of undifferentiated human endometrial mesenchymal stem/stromal cells. Sci. Rep.5, 15042. doi:10.1038/srep15042
Hansen, S. G., Taskin, M. B., Chen, M., Wogensen, L., Vinge Nygaard, J., and Axelsen, S. M. (2020). Electrospun nanofiber mesh with fibroblast growth factor and stem cells for pelvic floor repair. J. Biomed. Mat. Res.108, 48–55. doi:10.1002/jbm.b.34364
Hillary, C. J., Roman, S., Bullock, A. J., Green, N. H., Chapple, C. R., and MacNeil, S. (2016). Developing repair materials for stress urinary incontinence to withstand dynamic distension. PLoS One11, e0149971. doi:10.1371/journal.pone.0149971
Hou, M., Wu, Q., Dai, M., Xu, P., Gu, C., Jia, X., et al. (2014). Fabrication of electrospun thermoplastic polyurethane blended poly (l-lactide-co-e-caprolactone) microyarn scaffolds for engineering of female pelvic-floor tissue. Biomed. Mat.10, 015005. doi:10.1088/1748-6041/10/1/015005
Hympanova, L., Mori da Cunha, M., Rynkevic, R., Wach, R. A., Olejnik, A. K., Dankers, P. Y. W., et al. (2018). Experimental reconstruction of an abdominal wall defect with electrospun polycaprolactone-ureidopyrimidinone mesh conserves compliance yet may have insufficient strength. J. Mech. Behav. Biomed. Mat.88, 431–441. doi:10.1016/j.jmbbm.2018.08.026
Jango, H., Gras, S., Christensen, L., and Lose, G. (2015). Muscle fragments on a scaffold in rats: A potential regenerative strategy in urogynecology. Int. Urogynecol. J.26, 1843–1851. doi:10.1007/s00192-015-2782-x
Jangö, H., Gräs, S., Christensen, L., and Lose, G. (2017). Tissue-engineering with muscle fiber fragments improves the strength of a weak abdominal wall in rats. Int. Urogynecol J.28, 223–229. doi:10.1007/s00192-016-3091-8
Jin, M., Chen, Y., Zhou, Y., Mei, Y., Liu, W., Pan, C., et al. (2016a). Transplantation of bone marrow-derived mesenchymal stem cells expressing elastin alleviates pelvic floor dysfunction. Stem Cell Res. Ther.7, 51. doi:10.1186/s13287-016-0308-1
Jin, M., Wu, Y., Wang, J., Ye, W., Wang, L., Yin, P., et al. (2016b). MicroRNA-29 facilitates transplantation of bone marrow-derived mesenchymal stem cells to alleviate pelvic floor dysfunction by repressing elastin. Stem Cell Res. Ther.7, 167. doi:10.1186/s13287-016-0428-7
Lo, T. S., Pue, L. B., Hung, T. H., Wu, P. Y., and Tan, Y. L. (2015). Long-term outcome of native tissue reconstructive vaginal surgery for advanced pelvic organ prolapse at 86 months: Hysterectomy versus hysteropexy. J. Obstet. Gynaecol. Res.41, 1099–1107. doi:10.1111/jog.12678
Mangir, N., Bullock, A. J., Roman, S., Osman, N., Chapple, C., and MacNeil, S. (2016). Production of ascorbic acid releasing biomaterials for pelvic floor repair. Acta Biomater.29, 188–197. doi:10.1016/j.actbio.2015.10.019
Mangir, N., Hillary, C. J., Chapple, C. R., and MacNeil, S. (2019). Oestradiol-releasing biodegradable mesh stimulates collagen production and angiogenesis: An approach to improving biomaterial integration in pelvic floor repair. Eur. Urol. Focus5, 280–289. doi:10.1016/j.euf.2017.05.004
Masuda, H., Anwar, S. S., Buhring, H. J., Rao, J. R., and Gargett, C. E. (2012). A novel marker of human endometrial mesenchymal stem-like cells. Cell Transpl.21, 2201–2214. doi:10.3727/096368911x637362
Masudi, S. T., and Abdelrahman, A. (2021). Evaluating tissue-engineered repair material for pelvic floor dysfunction: A comparison of in vivo response to meshes implanted in rats. Int. Urogynecol J.doi:10.1007/s00192-022-05143-3
Mukherjee, S., Darzi, S., Rosamilia, A., Kadam, V., Truong, Y., Werkmeister, J. A., et al. (2019). Blended nanostructured degradable mesh with endometrial mesenchymal stem cells promotes tissue integration and anti-inflammatory response in vivo for pelvic floor application. Biomacromolecules20, 454–468. doi:10.1021/acs.biomac.8b01661
Oe, Y., Ogino, S., Kobayashi, M., Kojima, H., Terukina, T., Kanazawa, T., et al. (2022). Testosterone sustained release microspheres for the treatment of fecal incontinence. J. Pharm. Sci.111, 2322–2329. doi:10.1016/j.xphs.2022.03.008
Oh, S. H., Bae, J. W., Kang, J. G., Kim, I. G., Son, J. Y., Lee, J. Y., et al. (2015). Dual growth factor-loaded in situ gel-forming bulking agent: Passive and bioactive effects for the treatment of urinary incontinence. J. Mat. Sci. Mat. Med.26, 33. doi:10.1007/s10856-014-5365-3
Qian, Y., Chen, H., Xu, Y., Yang, J., Zhou, X., Zhang, F., et al. (2016). The preosteoblast response of electrospinning PLGA/PCL nanofibers: Effects of biomimetic architecture and collagen I. Int. J. Nanomed.11, 4157–4171. doi:10.2147/ijn.s110577
Quaghebeur, J., Petros, P., Wyndaele, J. J., and De Wachter, S. (2021). Pelvic-floor function, dysfunction, and treatment. Eur. J. Obstet. Gynecol. Reprod. Biol. X.265, 143–149. doi:10.1016/j.ejogrb.2021.08.026
Ren, J., Murray, R., Wong, C. S., Qin, J., Chen, M., Totsika, M., et al. (2022). Development of 3D Printed Biodegradable Mesh with Antimicrobial Properties for Pelvic Organ Prolapse. Polym. (Basel)14, 763. doi:10.3390/polym14040763
Rocha, C. V., Goncalves, V., da Silva, M. C., Banobre-Lopez, M., and Gallo, J. (2022). PLGA-based composites for various biomedical applications. Int. J. Mol. Sci.23, 2034. doi:10.3390/ijms23042034
Roman, S., Mangera, A., Osman, N. I., Bullock, A. J., Chapple, C. R., and MacNeil, S. (2014). Developing a tissue engineered repair material for treatment of stress urinary incontinence and pelvic organ prolapse-which cell source?Neurourol. Urodyn.33, 531–537. doi:10.1002/nau.22443
Roman, S., Mangir, N., Bissoli, J., Chapple, C. R., and MacNeil, S. (2016). Biodegradable scaffolds designed to mimic fascia-like properties for the treatment of pelvic organ prolapse and stress urinary incontinence. J. Biomater. Appl.30, 1578–1588. doi:10.1177/0885328216633373
Roman, S., Mangir, N., and MacNeil, S. (2019). Designing new synthetic materials for use in the pelvic floor: What is the problem with the existing polypropylene materials?Curr. Opin. Urol.29, 407–413. doi:10.1097/mou.0000000000000623
Salem, S. A., Rashidbenam, Z., Jasman, M. H., Ho, C. C. K., Sagap, I., Singh, R., et al. (2020). Incorporation of smooth muscle cells derived from human adipose stem cells on poly(lactic-co-glycolic acid) scaffold for the reconstruction of subtotally resected urinary bladder in athymic rats. Tissue Eng. Regen. Med.17, 553–563. doi:10.1007/s13770-020-00271-7
Sartoneva, R., Kuismanen, K., Juntunen, M., Karjalainen, S., Hannula, M., Kyllonen, L., et al. (2018). Porous poly- l -lactide-co-ɛ-caprolactone scaffold: A novel biomaterial for vaginal tissue engineering. R. Soc. open Sci.5, 180811. doi:10.1098/rsos.180811
Shafaat, S., Mangir, N., Regureos, S. R., Chapple, C. R., and MacNeil, S. (2018). Demonstration of improved tissue integration and angiogenesis with an elastic, estradiol releasing polyurethane material designed for use in pelvic floor repair. Neurourol. Urodyn.37, 716–725. doi:10.1002/nau.23510
Siddiqui, N., Asawa, S., Birru, B., Baadhe, R., and Rao, S. (2018). PCL-based composite scaffold matrices for tissue engineering applications. Mol. Biotechnol.60, 506–532. doi:10.1007/s12033-018-0084-5
Stangel-Wojcikiewicz, K., Jarocha, D., Piwowar, M., Jach, R., Uhl, T., Basta, A., et al. (2014). Autologous muscle-derived cells for the treatment of female stress urinary incontinence: A 2-year follow-up of a polish investigation. Neurourol. Urodyn.33, 324–330. doi:10.1002/nau.22404
Stangel-Wojcikiewicz, K., Piwowar, M., Jach, R., Majka, M., and Basta, A. (2016). Quality of life assessment in female patients 2 and 4 years after muscle-derived cell transplants for stress urinary incontinence treatment. Ginekol. Pol.87, 183–189. doi:10.17772/gp/61330
Sterodimas, A., de Faria, J., Nicaretta, B., and Pitanguy, I. (2010). Tissue engineering with adipose-derived stem cells (ADSCs): Current and future applications. J. Plast. Reconstr. Aesthet. Surg.63, 1886–1892. doi:10.1016/j.bjps.2009.10.028
Vashaghian, M., Diedrich, C. M., Zandieh-Doulabi, B., Werner, A., Smit, T. H., and Roovers, J. P. (2019). Gentle cyclic straining of human fibroblasts on electrospun scaffolds enhances their regenerative potential. Acta Biomater.84, 159–168. doi:10.1016/j.actbio.2018.11.034
Vashaghian, M., Ruiz-Zapata, A. M., Kerkhof, M. H., Zandieh-Doulabi, B., Werner, A., Roovers, J. P., et al. (2017). Toward a new generation of pelvic floor implants with electrospun nanofibrous matrices: A feasibility study. Neurourol. Urodyn.36, 565–573. doi:10.1002/nau.22969
Vashaghian, M., Zandieh-Doulabi, B., Roovers, J. P., and Smit, T. H. (2016). Electrospun matrices for pelvic floor repair: Effect of fiber diameter on mechanical properties and cell behavior. Tissue Eng. Part A22, 1305–1316. doi:10.1089/ten.tea.2016.0194
Wallace, S. L., Miller, L. D., and Mishra, K. (2019). Pelvic floor physical therapy in the treatment of pelvic floor dysfunction in women. Curr. Opin. Obstet. Gynecol.31, 485–493. doi:10.1097/gco.0000000000000584
Wang, B., Zhou, J., Banie, L., Reed-Maldonado, A. B., Ning, H., Lu, Z., et al. (2018a). Low-intensity extracorporeal shock wave therapy promotes myogenesis through PERK/ATF4 pathway. Neurourol. Urodyn.37, 699–707. doi:10.1002/nau.23380
Wang, X., Chen, Y., Fan, Z., and Hua, K. (2018b). Comparing different tissue-engineered repair materials for the treatment of pelvic organ prolapse and urinary incontinence: Which material is better?Int. Urogynecol. J.29, 131–138. doi:10.1007/s00192-017-3406-4
Wang, X., Chen, Y., Fan, Z., and Hua, K. (2021). Evaluating tissue-engineered repair material for pelvic floor dysfunction: a comparison of in vivo response to meshes implanted in ratsInt. Urogynecol. J.33, 2143–2150. doi:10.1007/s00192-021-05008-1
Wang, Y., Shi, G. W., Wang, J. H., Cao, N. L., and Fu, Q. (2016). Adipose-derived stem cells seeded on polyglycolic acid for the treatment of stress urinary incontinence. World J. Urol.34, 1447–1455. doi:10.1007/s00345-015-1757-3
Wang, Y., Wang, W., Wang, X., Wang, Y., Wang, J., Fu, Q., et al. (2017). Tissue-engineered sling with adipose-derived stem cells under static mechanical strain. Exp. Ther. Med.14, 1337–1342. doi:10.3892/etm.2017.4705
Wu, J. M., Hundley, A. F., Fulton, R. G., and Myers, E. R. (2009). Forecasting the prevalence of pelvic floor disorders in U.S. Women: 2010 to 2050. Obstetrics Gynecol.114, 1278–1283. doi:10.1097/aog.0b013e3181c2ce96
Wu, X., Jia, Y., Sun, X., and Wang, J. (2020). Tissue engineering in female pelvic floor reconstruction. Eng. Life Sci.20, 275–286. doi:10.1002/elsc.202000003
Wu, X., Wang, Y., Zhu, C., Tong, X., Yang, M., Yang, L., et al. (2016). Preclinical animal study and human clinical trial data of co-electrospun poly(L-lactide-co-caprolactone) and fibrinogen mesh for anterior pelvic floor reconstruction. Int. J. Nanomed.11, 389–397. doi:10.2147/ijn.s88803
Zhang, K., Guo, X., Li, Y., Fu, Q., Mo, X., Nelson, K., et al. (2016). Electrospun nanoyarn seeded with myoblasts induced from placental stem cells for the application of stress urinary incontinence sling: An in vitro study. Colloids Surfaces B Biointerfaces144, 21–32. doi:10.1016/j.colsurfb.2016.03.083
Zhao, B., Hu, M., Wu, H., Ren, C., Chen, J., Zhang, X., et al. (2018). Peroxisome proliferator-activated receptor-gamma and its related pathway in bone marrow mesenchymal stem cell differentiation co-cultured with mechanically stretched ligament fibroblasts. Int. J. Mol. Med.42, 219–227. doi:10.3892/ijmm.2018.3578
Zhao, B., Hu, M., Wu, H., Ren, C., Wang, J., and Cui, S. (2017). Tenascin-C expression and its associated pathway in BMSCs following co-culture with mechanically stretched ligament fibroblasts. Mol. Med. Rep.15, 2465–2472. doi:10.3892/mmr.2017.6329
Keywords: pelvic floor dysfunction, tissue-engineered repair material, mesenchymal stem cells, scaffolds, pelvic organ prolapses, stress urine incontinence
Citation: Lin M, Lu Y and Chen J (2022) Tissue-engineered repair material for pelvic floor dysfunction. Front. Bioeng. Biotechnol. 10:968482. doi: 10.3389/fbioe.2022.968482
Received: 14 June 2022; Accepted: 09 August 2022;
Published: 06 September 2022.
Edited by:
Jianshe Hu, Northeastern University, ChinaReviewed by:
Chengjin Ye, Texas Biomedical Research Institute, United StatesWanhua Xie, Shenyang Medical College, China
Copyright © 2022 Lin, Lu and Chen. This is an open-access article distributed under the terms of the Creative Commons Attribution License (CC BY). The use, distribution or reproduction in other forums is permitted, provided the original author(s) and the copyright owner(s) are credited and that the original publication in this journal is cited, in accordance with accepted academic practice. No use, distribution or reproduction is permitted which does not comply with these terms.
*Correspondence: Yongping Lu, dmFsZW5zdTQyM0AxNjMuY29t; Jing Chen, Y2hlbmpAc2otaG9zcGl0YWwub3Jn