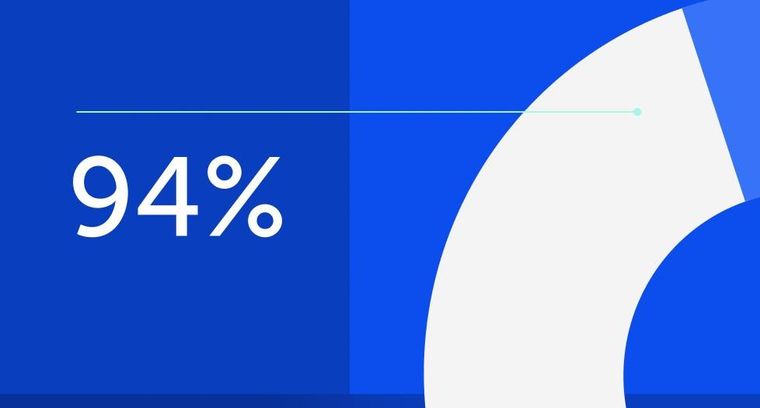
94% of researchers rate our articles as excellent or good
Learn more about the work of our research integrity team to safeguard the quality of each article we publish.
Find out more
ORIGINAL RESEARCH article
Front. Bioeng. Biotechnol., 22 July 2022
Sec. Bioprocess Engineering
Volume 10 - 2022 | https://doi.org/10.3389/fbioe.2022.960476
This article is part of the Research TopicHighly Efficient Bioconversion of Biomass Wastes: From Theoretical to IndustrialView all 8 articles
Pig manure is a reservoir of antibiotics and antibiotic resistance genes (ARGs). The effect of biochar on the variations in physicochemical properties, bacterial communities, antibiotics, ARGs, and mobile genetic elements (MGEs) of compost product during co-composting of pig manure and corn straw have been investigated in this study. Compared with the control treatment (CK), biochar addition accelerated the increase in pile temperature and prolonged the high temperature period (>55°C) for 2 days. Under biochar influence, organic matter degradation, NH4+-N conversion and NO3−-N production was accelerated, and dissolved total organic carbon (DOC) and dissolved total nitrogen (DTN) utilization by microorganisms were enhanced. Biochar addition altered the microbial community and promoted the vital activity of Actinobacteria in the later composting stage. The antibiotics removal efficiency (except danofloxacin and enrofloxacin) was accelerated in the early composting stage (1–14 days) by biochar addition, the pile temperature had a positive effect on antibiotics removal, and the total antibiotics removal efficiency in CK and CK+Biochar treatments was 69.58% and 78.67% at the end of the composting process, respectively. The absolute abundance of most of the ARGs in the CK+Biochar treatment was lower than that in the CK treatment during composting, and the ARGs removal mainly occurred in the early (1–14 days) and later (28–50 days) stages. Biochar addition reduced the absolute abundance of MGEs (intI1, intI2) in the compost product, and most of the ARGs had a significant positive correlation with MGEs. Network analysis and redundancy analysis showed that ARGs and MGEs occurred in various host bacteria (Firmicutes, Actinobacteria, Bacteroidetes, Proteobacteria, and Halanaerobiaeota), and that DTN and NH4+-N are the main factors regulating the changes in bacterial communities, antibiotics, ARGs, and MGEs during composting. Moreover, MGEs contributed the most to the variation in ARGs. In summary, biochar addition during composting accelerated antibiotics removal and inhibited accumulation and transmission of ARGs. The results of this study could provide theoretical and technical support for biochar application for antibiotics and ARGs removal during livestock and poultry manure composting.
In the past decade, livestock and poultry manure production in China exceeded 20 billion tons, and the proportion of pig manure production surpassed 30% (Wang et al., 2021a). Antibiotics are widely used in livestock and poultry farming to promote animal growth and disease prevention (Looft et al., 2012), and in China, 97,000 tons of antibiotics are used every year. The amount of some antibiotics (fluoroquinolones, macrolides, etc.) used in pig farming is more than 50% of the total use of certain antibiotics in the farming industry (Zhang et al., 2015). However, antibiotics are incompletely absorbed in animal tissues, with 30%–90% of antibiotics excreted with animal feces and urine (Heuer et al., 2011; Cheng et al., 2019). The residual concentration of tetracycline (TC) in pig manure was noted to be the highest (Zhang et al., 2013). The discharged antibiotics may impose selective pressure on microorganisms in the environment, thereby inducing the production of antibiotic-resistant microorganisms as well as antibiotic resistance genes (ARGs) (Wei et al., 2020). Previous study had detected all known types of ARGs in pig manures from some pig farms (Zhu et al., 2013). These manures are often used as organic fertilizers in farmland, resulting in antibiotics and ARGs entering the soil and penetrating into the deeper layers of the soil over time, and even into groundwater (Xie et al., 2018; Gu et al., 2019; Menz et al., 2019). The diversity and abundance of ARGs in soil have been found to significantly increase (Chen et al., 2016), especially in farmland (Mu et al., 2015), after long-term application of untreated livestock manure. Besides, ARGs spread in the environment through horizontal gene transfer (HGT) regulated by MGEs (Su et al., 2015; Zhang et al., 2017), which further threaten human health.
Microorganisms, antibiotics, ARGs, and mobile genetic elements (MGEs) are closely related, and composting has been reported to remove antibiotics and ARGs from pig manure (Li et al., 2020; Cheng et al., 2021; Wang et al., 2021c). After composting, the removal rates of tetracyclines, sulfonamides, and macrolides antibiotics have been found to reach more than 90% (Kim et al., 2012), with some antibiotics (chlortetracycline (CTC), sulfadiazine) being completely removed (Selvam et al., 2012b). Antibiotics exert selective pressure on ARGs, and some ARGs persist even after complete degradation of antibiotics (Jechalke et al., 2014a). During pig manure composting, the relative abundances of tetracycline and sulfonamide resistance genes have been found to significantly decrease (Selvam et al., 2012a). However, composting cannot remove all ARGs, and different ARGs exhibit distinct trends during the composting process. For example, at the end of pig manure composting, the absolute abundance of tetC was significantly higher than the initial level (Zhang et al., 2017), whereas the abundance of tetX remained unchanged (Wang et al., 2015). ARGs can be transmitted between different microorganisms through MGEs (Cui et al., 2020), leading to the emergence of multi-drug-resistant microorganisms. Composting can effectively remove MGEs (Gou et al., 2018; Zhu et al., 2019), thereby inhibiting HGT of ARGs and reducing multi-drug resistance of antibiotic-resistant bacteria. A large number of pathogenic microorganisms isolated from pig manure have been reported to contain ARGs (Fang et al., 2015). Continuous high temperature during composting can lead to the inactivation of pathogenic microorganisms (Qian et al., 2016b), thereby promoting ARGs removal. Some studies have reported that continuous high temperature could promote the removal of ARGs during composting (Wang et al., 2016; Xie et al., 2016; Youngquist et al., 2016), and facilitate antibiotics removal through degradation and adsorption (Arikan et al., 2007). However, some studies have indicated that the effect of high temperature on ARGs removal are not ideal (Huang et al., 2019; Wu et al., 2020). It can be seen that whether high temperature can remove ARGs is controversial. Besides, it should be noted that most of these previous studies had been conducted to explore the effects of aerobic composting on antibiotics in pig manure, and that the antibiotics had been artificially added to pig manure (Qian et al., 2016a; Song et al., 2020; Gou et al., 2021). The knowledge about the effect of composting on antibiotics and ARGs in realistic pig manure environment is still limited.
During pig manure composting, some conditioners are usually added to regulate the moisture content (MC) and C/N ratio of the pile. Corn straw is one of the effective materials that can regulate the MC and C/N ratio of the pile. Biochar is a carbon-rich material, produced by the pyrolysis of carbon-based biomass under nitrogen-rich conditions. Biochar has been applied as an additive in composting (Qiu et al., 2021). Addition of biochar makes it easier for the compost pile temperature to rise above 70°C (Czekała et al., 2016); as a result, organic matter degradation is accelerated at the thermophilic stage of composting and nitrification is enhanced at the maturity stage of composting (Sánchez-García et al., 2015). Besides, biochar can reduce nitrogen loss and gas emission during composting (Awasthi et al., 2017), accelerate the composting process, alter the number and diversity of microorganisms during composting (Huang et al., 2020), and improve the living environment of the microorganisms and enhance their activity (Sanchez-Monedero et al., 2018). Different types of biochar prepared using diverse materials can have varying degrees of effects on bacterial communities during composting (Cui et al., 2016), which may affect the changes in ARGs. Antibiotics with animal feces into the environment caused antibiotics pollution (Sarmah et al., 2006). Furthermore, it will aggravate environmental pressure, induce the formation of microbial resistance, increase the probability of pathogen resistance, accelerate the generation and dissemination of ARGs, and pose a serious threat to human health and aquaculture (Duan et al., 2017). ARGs in the environment may invade animals or human body along the food chain or contact transmission, thereby increasing the host’s resistance to antibiotics, resulting in antibiotics cannot achieve normal therapeutic effect, but also lead to difficult to treat human and animal diseases, threatening human life and health. However, the effects of biochar on antibiotics and ARGs removal during pig manure and corn straw co-composting have been rarely reported.
Accordingly, in the present study, a mixed composting system of biochar, pig manure, and corn straw was established outdoor, with a compost volume of 2.10 m × 1.30 m × 0.75 m, and the changes in the physicochemical properties, bacterial community, antibiotics, ARGs, and MGEs in the composting system and their relationships were systematically investigated. The objectives of this study were to 1) explore the effects of biochar on the physicochemical properties of compost product during composting; 2) investigate whether biochar addition has a significant effect on the removal of antibiotics and ARGs during the composting process; 3) identify potential hosts of ARGs and MGEs; and 4) determine the dominant factors affecting antibiotics and ARGs removal during composting.
The raw materials used for composting included pig manure, corn straw (1–2 cm), and corn-straw biochar. Pig manure and corn straw were collected from Nongshengyuan Family Farm, Jinzhong City, Shanxi Province, China. Corn-straw biochar was prepared by the pyrolysis of corn straw at 500°C for 2 h under nitrogen-rich conditions and provided by Henan Lize Environmental Protection Technology Company. The physicochemical properties of the raw materials are shown in Table 1.
Composting experiments were performed in open air, and CK and CK+Biochar two treatments were established. For CK treatment, pig manure (fresh weight) and corn straw (fresh weight) were mixed at a ratio of 4:1, the pile weight was 375 kg, and the MC and C/N ratio were adjusted to 70% and 25, respectively. The CK+Biochar treatment was prepared in the same procedure and 10% biochar (ratio of fresh weight of biochar to total fresh weight of pig manure and corn straw) was introduced to the pile. Because the volume of the compost pile is close to the pilot scale, each treatment has only one pile in this study. Supplementary Figure S1 shows the actual pile images of the CK and CK+Biochar treatments on day 1. It must be noted that except on day 1, the MC of the compost was not adjusted throughout the entire composting process. The pile was turned over on days 1, 3, 6, 10, 14, 21, 28, 35, 42, and 50, and samples were immediately collected at multiple points from the center of the pile and separated into two parts. One part of the samples was stored at 4°C for physicochemical properties analysis, such as MC, pH, electrical conductivity (EC), total organic matter (OM), total organic carbon (TOC), dissolved total organic carbon (DOC), total nitrogen (TN), dissolved total nitrogen (DTN), NH4+-N, NO3−-N, and seed germination index (GI) analyses, while the other part of the samples collected on days 1, 6, 14, 21, 28, 42, and 50 was stored at −20°C for bacterial community, antibiotics, ARGs, and MGEs analyses. The temperature (T) of the materials at a distance of 30 cm from the surface of the pile (represents the pile temperature) was measured at the front, middle, and back of the pile by using a thermometer at 9:00 a.m. every day.
The fresh samples were dried in an oven at 105°C for 24 h to determine the MC. The pH and EC were measured using a pH meter (PHS-3C, Shanghai, China) and conductivity meter (DDS-11A, Shanghai, China), respectively. The DOC and DTN contents were ascertained using total organic carbon analyzer (multi N/C 3100, Germany), NH4+-N and NO3−-N were measured using a flow analyzer (AMS Alliance, France), and TOC and TN contents were detected using the K2CrO4 oxidation method and Kjeldahl method. The OM content was calculated from the mass loss of dry matter after 4 h of combustion in a muffle furnace at 550°C. The GI was evaluated as described using cabbage seeds. The electrically conducting property of the biochar was investigated according to the method described by Fu et al. (2021), and the pore structure of the biochar was determined using an automated specific surface area and pore analyzer (TriStar II 3020 model, United States).
The concentrations of 14 antibiotics, including four tetracyclines, two sulfonamides, five fluoroquinolones, two macrolides, and one lincosamide [lincomycin (LCM)] were measured by liquid chromatography–mass spectrometry (LC-MS 8030, Japan). The compost samples were pretreated using the method described by Wang et al. 2021b. The chromatographic conditions were as follows: Shim-pack GIST C18 column (100 mm × 2.1 mm, 2 µm); flow rate, 0.3 ml/min; injection volume, 5 μl; column temperature, 40°C; operation time, 17 min; mobile phase A, 0.2% formic acid-water; and mobile phase B, acetonitrile. The gradient washing procedure is shown in Supplementary Table S1. The mass spectrometry conditions were as follows: ionization mode, ESI positive ion mode; scanning mode, multiple reaction monitoring (MRM); collision gas, argon; atomization gas, 3 L/min nitrogen; dry gas, 15 L/min nitrogen; heating module temperature, 400°C; and DL temperature, 250°C. The antibiotics were quantified with calibration standards, and the average recoveries of tetracyclines, fluoroquinolones, macrolides, sulfadiazine (SD), sulfamethazine (SMR) and LCM in the piles were 90.83 ± 6.12%, 94.28 ± 10.45%, 70.50 ± 21.43%, 73.07%, 92.06% and 103.21%, respectively.
The DNA was extracted using rapid DNA extraction kit (TIANNAMP DNA Kit, TIANGEN, China) according to the manufacturer’s instructions. A total of 20 ARGs (tetA, tetB, tetC, tetG, tetM, tetO, tetQ, tetW, tetX, tetZ, sul1, sul2, gyrA, qnrS, ermB, ermC, ermF, ermT, mefA, mphA) and two MGEs (intI1, intI2) were quantified by PCR using StepOnePlus™ real-time PCR system (Thermo, United States). The primer sequences used for the amplification of ARGs are presented in Supplementary Table S2. The thermal cycling steps for quantitative PCR (qPCR) amplification were employed as described previously (Yin et al., 2017), and the absolute abundance of ARGs and MGEs was defined as the copy number of ARG or MGE per unit of the composting sample (copies/g).
For the analysis of the bacterial communities in the composting system, the V3-V4 region of the 16S rRNA gene was amplified by PCR using the primers 341F/806R. The PCR amplification products were detected by 2% agarose gel electrophoresis, and the target fragment was recovered using the gel recovery kit (Qiagen). The purified PCR product was quantified by Qbit fluorescence quantification system using TruSeq®DNA PCR-free sample preparation kit. The amplified products were sequenced with Illumina MiSeq-PE300 sequencing platform. All the raw sequence data have been deposited in the NCBI SRA database (Accession number: PRJNA846099).
The data obtained in this study were analyzed using Microsoft Excel 2016 and SPSS version 25.0 software. The abundance heat map of the top 35 microorganisms at the genus level was plotted using TB tools. Network depictions were constructed using Cytoscape 3.9.0. Redundancy analysis (RDA) was performed using CANOCO 5.0, and structural equation model (SEM) was established using Amos Graphics.
As shown in Figure 1A, the OM content in the CK and CK+Biochar treatments presented a downward trend during composting process. Compared with the CK treatment, the OM degradation rate in the CK+Biochar treatment was faster during 1–10 days, which was owing to the unique surface structure of biochar that provided a suitable environment for microbial activities and promoted decomposition and metabolism of OM (Sánchez-García et al., 2015). Most of the microorganisms that degrade OM are aerobic, and the biochar addition improved the aeration conditions during the composting process and facilitated contact between microorganisms and oxygen. The biochar used in the present study can conduct electricity (Supplementary Figure S2A), which could accelerate the electron transfer in the oxidation process of OM, thereby promoting OM degradation.
During composting, the temperatures of both the CK and CK+Biochar treatments showed similar trends with obvious warming, high-temperature, and cooling period (Figure 1B). However, the high temperature period (>55°C for 22 days) in the CK+Biochar treatment was longer than that noted in the CK treatment (>55°C for 20 days), which is consistent with the results of a previous study (Awasthi et al., 2020). The temperature increased above 60°C on days 3 and 8 in the CK+Biochar and CK treatments, respectively, which could be attributed to the additional nutrients and suitable living environment provided by the biochar for microbial activities, facilitating OM degradation and release of heat (Wang et al., 2013). However, the temperature of the CK and CK+Biochar treatments decreased below 40°C on days 32 and 28, respectively. Nevertheless, both the CK and CK+Biochar treatments in the present study fulfilled the safety regulations in “Sanitation of manure without harm,” which require the pile temperature to be >50°C for at least 10 days.
During composting, the MC of the CK and CK+Biochar treatments showed a downward trend (Figure 1C), exhibiting 68.78% and 72.36% decrease at the end of composting, respectively. The presence of biochar can accelerate water loss from the pile, which might be owing to the increase in the porosity of the pile (Liu et al., 2017) and optimization of the pore structure, promoting air circulation in the pile and accelerating evaporation of water (Iqbal et al., 2010). Although the increase in temperature was more rapid in the CK+Biochar treatment, the decrease in MC was slower, when compared with those noted in the CK treatment, during the early stage of composting (days 1–6), implying that temperature increase was not the main reason for moisture loss from the pile.
The pH change during composting was demonstrated in Figure 1D. The pH of both CK and CK+Biochar treatments first exhibited an increasing trend, followed by a decreasing trend, and finally stabilized. The pH values increased up to 8.73 and 8.54 in the CK and CK+Biochar treatments, respectively, on day 10 of the composting process. The volatile emission of NH3 was predominant during the early stage of composting (Mao et al., 2018; Ravindran et al., 2019), leading to an increase in the pH value. When compared with the initial pH value, the pH at the end of the composting process was not significantly different in both CK and CK+Biochar treatments, suggesting that the effect of biochar was not significant on pH variation before and after composting.
At the end of the composting process, the EC of both CK and CK+Biochar treatments was lower than 4 mS/cm (Figure 1E), indicating that the final compost product in both the treatments was not phytotoxic (Mao et al., 2018). In the CK treatment, the rapid decline in MC during the first 3 days may be the main reason for the slight increase in EC, which was 3.14 mS/cm higher than the initial value. In contrast, the EC of the CK+Biochar treatment was 2.81 mS/cm lower than the initial value. These results indicated that addition of biochar decreased EC and enhanced the quality of the final compost products.
The DOC content in the CK and CK+Biochar treatments showed the opposite trend in the early stage of composting (Figure 2A). The rapid decrease in the DOC content in the CK+Biochar treatment could mainly be attributed to the quick rise in temperature resulting in an increase in microbial activity (Khan et al., 2014). After 10 days of composting, the DOC content in the CK treatment started to decrease and the microbial utilization of water-soluble organic matter accelerated, probably owing to the increased activity of thermophilic microorganisms caused by high temperatures at this stage. At the beginning of the composting, the DTN content in the CK+Biochar treatment decreased significantly faster than that in the CK treatment (Figure 2B), which was owing to the rapid increase in the pile temperature of the CK+Biochar treatment. After 10 days of composting, the DTN content in the CK treatment decreased faster, which was owing to the increased activity of thermophilic microorganisms at this stage. At the end of the composting process, the DOC content decreased by 49.54% and 59.22%, and the DTN content declined by 62.94% and 68.13% in the CK and CK+Biochar treatments, respectively. These results indicated that biochar facilitated the utilization of DOC and DTN by microorganisms.
In the present study, the compost substrate contained a large number of organic nitrogen compounds, which generated NH4+-N under the action of microorganisms, leading to the increase in the NH4+-N content in the early stage of composting. As shown in Figure 2C, the NH4+-N content in the CK and CK+Biochar treatments exhibited a decreasing trend, which is not consistent with the previously reported results (Agyarko-Mintah et al., 2017). This may be owing to the high frequency of turning of the pile during the composting period in this study, which promoted oxygen exposure to the microorganisms. As NH4+-N is more likely to be converted to NO3−-N by microorganisms in the presence of high level of oxygen in the environment (Canfield et al., 2010), a large amount of NO3−-N was produced in both CK and CK+Biochar treatments in the early stage of composting (Figure 2D). However, addition of biochar increased the porosity of the pile and promoted air flow, resulting in the rapid conversion of NH4+-N in the CK+Biochar treatment, compared with that in the CK treatment. The NO3−-N content in both CK and CK+Biochar treatments reached the maximum level on day 10, presenting 62.34% and 163.70% increase, respectively, which indicated that biochar could facilitate the vital activity of nitrifying bacteria. This effect of biochar might be caused by the specific pore structure of the biochar and the adsorption sites on its surface, which are beneficial to the adsorption of NH3 and NH4+, and provide a suitable environment for the metabolic activities of nitrifying bacteria. After 10 days of composting, the NO3−-N content began to decrease after reaching its peak, possibly as a result of increase in the proportion of denitrifying bacteria, which promote the conversion of NO3−-N to N2 and N2O (Cáceres et al., 2018). Moreover, the temperature of the pile gradually increased to 70°C at this stage, which inhibited the activity of nitrifying bacteria. However, in the maturity stage of composting, the NO3−-N content in both CK and CK+Biochar treatments increased owing to the recovery of nitrifying bacterial activity as a result of decrease in temperature (Zainudin et al., 2020).
At the end of composting, the GI value was used to evaluate the final compost products. The GI values of the final compost products of the CK and CK+Biochar treatments were 106.82% and 171.88%, respectively. GI value above 80% implies that the final compost products are fully mature without phytotoxicity (Huang et al., 2006). The results of the present study showed that biochar addition increased the GI value and improved the quality of the final compost products.
As shown in Figure 3A, bacterial communities evolved during the composting process. Firmicutes, Actinobacteria, Bacteroidetes, and Proteobacteria were the predominant bacterial phyla, accounting for 57.59%–97.16% of the total bacterial community, with Firmicutes being the major bacterial phylum in the early stage of composting. From days 1 to 21, the temperature of the CK and CK+Biochar treatments mostly remained above 50°C and Firmicutes were dominant in both the treatments, indicating that these bacteria could survive at high temperatures. Firmicutes play a key role in cellulose degradation (Lebuhn et al., 2014), and can decompose corn straw in the early stage of composting and accelerate maturation of the compost. At the genus level, Romboutsia, Clostridium_sensu_stricto_1, Terrisporobacter, and Turicibacter were predominant Firmicutes in both the treatments (Figure 3B). After 21 days of composting, the abundance of Firmicutes gradually declined, probably owing to the decrease in pile temperature, which was not suitable for the survival of most of the Firmicutes.
FIGURE 3. (A) Relative abundance of microorganisms at phylum level. (B) Abundance heat map of the top 35 microorganisms at genus level in different composting stages.
It can be observed from Figure 3A that Actinobacteria accounted for a relatively low proportion in the total bacterial community from days 1 to 21, because the high temperature in the early stage of composting could have inhibited the metabolic activities of Actinobacteria, which are thermosensitive microorganisms (Zhu et al., 2017b). However, during the maturity stage of composting, the temperature of the pile gradually decreased, and the activity of Actinobacteria increased, resulting in a slow increase in their proportion in the total bacterial community. At the genus level, Brevibacterium, Microbacterium, Brachybacterium, Georgenia, Longispora, Saccharomonospora, and Corynebacterium were the predominant Actinobacteria (Figure 3B), with Brevibacterium, Microbacterium, and Brachybacterium accounting for the highest proportion and presenting variation trend similar to that of Actinobacteria during composting. Furthermore, the abundance of Actinobacteria in the CK+Biochar treatment was higher than that in the CK treatment, with Brevibacterium, Microbacterium, and Brachybacterium being the dominant genera. These results demonstrated that the presence of biochar in the later composting stage stimulated the activity of Actinobacteria. When compared with the CK treatment, the low water content and faster water dissipation rate in the CK+Biochar treatment led to higher activity of Actinobacteria, because Actinobacteria are capable of generating spores to survive under low water condition (Zhou et al., 2019).
Throughout the composting process, the abundance of Bacteroidetes showed the opposite trend in the CK and CK+Biochar treatments (Figure 3A). On day 1, the abundance of Bacteroidetes in the CK+Biochar treatment (14.61%) was higher than that in the CK treatment (2.42%). However, in the first 6 days of composting, the abundance of Bacteroidetes gradually decreased in the two treatments, owing to the high pile turnover frequency. Bacteroidetes are anaerobic microorganisms, and oxygen supplementation can reduce their metabolic activities (López-González et al., 2021). The decrease in the abundance of Bacteroidetes in the CK+Biochar treatment was higher than that in the CK treatment, because biochar improved the pore structure of the pile and promoted the reaction of microorganisms to air contact. Bacteroides, Moheibacter, and Proteiniphilum belong to the phylum Bacteroidetes, and are the most sensitive to oxygen. In particular, Moheibacter was active during the highest temperature period (6–14 days), probably because of its thermophilic characteristic. At the end of the composting, the abundance of Bacteroidetes in the CK and CK+Biochar treatments was 47.56% and 0.86%, respectively, and this difference mainly occurred in the maturity stage. During the maturity stage of composting, the abundance of Bacteroidetes in the CK treatment increased, whereas that in the CK+Biochar treatment sharply decreased, especially in the last 8 days of composting, which was predominantly owing to the changes in the abundances of Anseongella and Membranicola (Figure 3B).
Although the abundance of Proteobacteria gradually increased in both CK and CK+Biochar treatments in the early stage of composting, the reproduction rate and abundance of Proteobacteria was higher in the CK+Biochar treatment. It has been reported that Proteobacteria have a key role in nitrogen transformation processes such as oxidation of ammonia in compost (Maeda et al., 2011). Thus, the NH4+-N content in the CK+Biochar treatment rapidly decreased in the early stage of composting, whereas the NO3−-N content was higher (Figure 2). In the later stage of composting, the higher abundance of Proteobacteria led to higher NO3−-N content in the CK treatment.
Figure 4 presents the changes of tetracyclines (TC), oxytetracycline (OTC), CTC, doxycycline (DOX), sulfonamides (SD, SMR), fluoroquinolones [ofloxacin (OFX), ciprofloxacin (CIP), difloxacin (DIF), danofloxacin (DAN), enrofloxacin (ENR)], macrolides [tilmicosin (TILM), tylosin (TYL)], and lincosamides (lincomycin (LCM)) antibiotics during composting in the CK and CK+Biochar treatments. At the end of composting, the total antibiotics removal rates in the CK and CK + Biochar treatments were 69.58% and 78.67%, respectively. The antibiotics removal mainly occurred in the early stage of composting (1–14 days), and the total antibiotics removal rates in the CK and CK+Biochar treatments were 64.24% and 71.84%, respectively. At the same time, temperature had a significant negative correlation with TC, OTC, CTC, DC, SD, SMR, OFX, CIP, ENR, TYL, TILM, and LCM concentration in the first 14 days of composting (Table 2), indicating that the increase in temperature was beneficial to antibiotics removal. This finding is consistent with that reported in previous studies, which revealed that high temperature could promote the degradation of antibiotics (Arikan et al., 2007; Dorival-García et al., 2013; Liu et al., 2015). The addition of biochar accelerated the increase in pile temperature, and consequently, the antibiotics removal rate in the CK+Biochar treatment was higher in the early stage of composting.
TABLE 2. Pearson’s correlation coefficient between temperature and antibiotics at 1–14 days of composting.
During the first 6 days of composting, 50.93%, 74.77%, 63.85% 66.13%, and 95.82% of TC, OTC, CTC, DOX, and TILM were removed in the CK+Biochar treatment, respectively, which were higher than those noted in the CK treatment (30.53%, 58.70%, 50.79%, 21.00%, and 68.28%, respectively). Biodegradation is an important pathway for the removal of tetracyclines antibiotics during pig manure composting (Wang et al., 2015). It must be noted that tetracyclines antibiotics are easily adsorbed by the medium in the environment (Tolls, 2001; Thiele-Bruhn and Beck, 2005), and addition of biochar, which has a rich pore structure and large number of binding sites, can facilitate the adsorption of tetracyclines antibiotics on the biochar surface and pores, and enhance the degradation of tetracyclines antibiotics by microorganisms adhering to the biochar. During 1–14 days of composting, the TYL removal rates in the CK and CK+Biochar treatments were 77.08% and 94.44%, respectively. TYL and TILM are amphoteric compounds with positive charges, which can form ion bonds with negatively charged components, such as the biochar surface, during composting (Tan et al., 2020); as a result, their removal was accelerated with biochar addition. At the end of composting, the removal rates of SD, SMR, OFX, CIP, DIF, and LCM in the CK treatment were 55.88%, 77.10%, 48.65%, 52.86%, 38.21%, and 40.23%, respectively, which were lower than those noted in the CK+Biochar treatment (68.32%, 84.75%, 87.24%, 64.73%, 62.55%, and 66.40%, respectively). In the presence of organic matter, high temperatures can lead to the production of more adsorption sites, decreasing the extractable concentration of sulfonamides antibiotics (Ezzariai et al., 2018). Biochar addition can inhibit the production of CO2 and reduce the loss of organic matter (Liu et al., 2017), thereby promoting the removal of sulfonamides in pig manure. The molecular structure of quinolone antibiotics comprises a large number of carboxyl, amino, and aromatic rings, which are easily adsorbed by humus (Kümmerer, 2009). At the end of composting, the total humic acid contents of CK and CK + Biochar treatments increased by 24.16% (from 62.02 g/kg to 77.01 g/kg) and 47.96% (from 64.78 g/kg to 95.85 g/kg), respectively. Biochar promotes the formation of humus during composting. Biodegradation is considered to be the main mechanism of LCM degradation (Alexandrino et al., 2017; Ren et al., 2018) and biochar can adsorb LCM for more than 200 days, exhibiting strong adsorption performance (Liu et al., 2019), which facilitates biodegradation of LCM. Bacillus, Saccharomonospora, and Georgenia presented significant negative correlations with TC, DOX, SD, SMR, DIF, TYL, and LCM concentrations (Supplementary Figure S4), indicating that these microorganisms can degrade antibiotics. Addition of biochar improved the activity of these microorganisms, thereby promoting the degradation of antibiotics during composting. In CK and CK+Biochar treatments, the concentration of DAN increased from 28.47 to 32.00 μg/kg and 23.49 to 32.32 μg/kg, and the concentration of ENR increased from 82.50 to 105.49 μg/kg and 68.06 to 146.32 μg/kg. Actinobacteria is considered to be the main producer of antibiotics (D'Costa et al., 2006), and Microbacterium and Brachybacterium, which belong to the phylum Actinobacteria, were significantly positively correlated with DAN and ENR concentrations, and their growth and reproduction during composting led to an increase in DAN and ENR concentrations. The results of this study revealed the high efficiency of antibiotic removal from co-composting of pig manure and corn straw with biochar. The removal rates of macrolide antibiotics were higher than that of other antibiotics at the early composting stage due to the differences in the types and chemical structures of antibiotics. Moreover, microorganisms are also important factors affecting the change of antibiotic concentration during composting.
Figure 5 shows the changes in the absolute abundance of ARGs (tetracyclines, sulfonamides, fluoroquinolones, macrolides, and lincosamides resistance genes) during composting. On day 1, biochar addition increased the absolute abundance of ARGs, similar to that reported in a previous study (Zhou et al., 2021). The reason for the increase in the absolute abundance of ARGs following biochar addition may be the higher abundance of Bacteroidetes and Proteobacteria, the major host bacteria of ARGs (Stegmann et al., 2015; Luo et al., 2017), in the CK+Biochar treatment, when compared with those in the CK treatment. However, with the progress in the composting process, the absolute abundance of the total ARGs increased by 81.24 times in the CK treatment, which was higher than that noted in the CK+Biochar treatment (0.48 times), indicating that biochar addition was beneficial to inhibit the increase in the absolute abundance of ARGs.
After 50 days of composting, the absolute abundance of tetracycline resistance genes changed in varying degrees of changes. In the CK treatment, only tetO abundance decreased by 49.63% after the composting process because anaerobic bacteria are the major hosts of this ARG (Wang et al., 2015), whereas the absolute abundances of the other nine tetracycline resistance genes increased at varying degrees, with tetA and tetM presenting the highest and lowest increase in abundance, respectively (3.63×103 and 1.52 times, respectively). In contrast, in the CK+Biochar treatment, the absolute abundances of tetM, tetO, tetW, and tetX decreased by 92.83%, 46.30%, 24.15%, and 57.86%, respectively, whereas those of tetA, tetC, tetG, and tetZ increased by 7.8, 0.4, 11.6, and 500 times, respectively, and tetB and tetQ were undetectable after the composting process. These findings indicated that biochar addition led to higher removal of tetracycline resistance genes from the compost products.
The absolute abundances of sulfonamide resistance genes sul1 and sul2 increased by 3.80×103 and 6.56 × 103 times, respectively, in the CK treatment, and 33.95 and 4.06 times, respectively, in the CK+Biochar treatment, revealing that biochar addition to the composting system significantly decreased sul1 and sul2 abundances, predominantly after 42 days of composting. Similarly, Guo et al. (2021) also found that ARGs were mainly removed in the mature stage of pig manure composting.
The fluoroquinolones resistance genes, gyrA and qnrS, were not detected in the final product of the CK treatment, whereas their absolute abundances decreased by 54.02% and 22.05%, respectively, in the CK+Biochar treatment, indicating that biochar addition might have promoted the growth and reproduction of bacteria possessing gyrA and qnrS. The absolute abundance of macrolide and lincosamide resistance genes in the CK treatment increased at varying degrees at the end of the composting process, with ermF and ermC presenting the highest and lowest increase of 2.54×103 and 5.12 times, respectively. In the CK+Biochar treatment, the absolute abundances of ermB and mefA decreased at the end of composting, with their removal rates reaching 90.77% and 53.51%, respectively, whereas those of ermC, ermF, ermT, and mphA increased with the progress of the composting process.
In summary, biochar addition to the composting system promoted the removal of ARGs by prolonging and maintaining the duration of high-temperature period, which resulted in the killing of some heat-sensitive bacteria possessing ARGs. As shown in Figure 5, the absolute abundance of ARGs in the CK and CK+Biochar treatments gradually increased after the start of composting, which could be owing to elevated temperatures facilitating increase in the activities of Firmicutes that carry and spread ARGs (Huerta et al., 2013). Addition of biochar promoted ARGs removal in the later stages of composting (42–50 days), due to the decrease in the relative abundance of Bacteroidetes and Proteobacteria. It has been reported that additional carbon sources can lead to a transient increase in the abundance of ARGs, followed by a decrease (Duan et al., 2018), and can degrade antibiotics, eliminate pathogens, and reduce the diversity and relative abundance of ARGs.
As HGT is considered to be an important mechanism for the spread and proliferation of ARGs in various environmental media (Thomas and Nielsen, 2005), the present study explored the changes in the absolute abundances of intI1 and intI2 during pig manure composting (Table 3). At the end of the composting process, the absolute abundance of intI1 in the CK and CK+Biochar treatments increased by 3.48 × 107 and 6.69 × 104 times, respectively. It has been reported that biochar addition could inhibit the increase in intI1 abundance during composting (Li et al., 2017). The absolute abundance of intI2 showed the opposite trend, presenting an increase of 1.51 × 105 times in the CK treatment and a decrease of 34.75% in the CK + Biochar treatment. Biochar contains a large number of micropores (Supplementary Figure S2B), which can enhance the spatial distance between microorganisms and reduce the possibility of mutual contact between microorganisms. As HGT of ARGs mainly occurs through interaction between microorganisms (Martínez et al., 2015), biochar could reduce the occurrence of HGT, resulting in the removal of ARGs. In the present study, a significant positive correlation was observed between intI1 and intI2 and most of the ARGs during the composting process, indicating that intI1 and intI2 played an important role in the spread of ARGs.
Network analysis was used in this study to determine the potential host bacteria of ARGs and MGEs (Figure 6). A total of 24 genus-level bacteria were significantly associated with 20 ARGs and 2 MGEs (p < 0.05). It has been reported that a significant positive correlation between ARGs and bacterial communities could provide new insights into the potential host bacteria of ARGs (Zhu et al., 2017a). A total of 24 bacterial genera, belonging to Firmicutes, Actinobacteria, Bacteroidetes, Proteobacteria, and Halanaerobiaeota, were found to be potential host bacteria of ARGs and MGEs. Lactobacillus was observed to be a potential host of gyrA and tetM, and the removal of gyrA and tetM during the composting process could be attributed to the decrease in the abundance of Lactobacillus, which is consistent with the findings of previous studies suggesting that Lactobacillus is a potential host of ARGs (Bonham et al., 2017). As the host bacteria of tetZ are Brachybacterium, Saccharomonospora, Microbacterium, and Brevibacterium, the increase in the abundance of tetZ was mainly owing to the proliferation of these four bacteria. These results showed that ARGs existed in various possible host bacteria, which is consistent with the previously reported findings that indicated that the succession of bacterial communities led to variations in ARGs abundance (Sun et al., 2018). Furthermore, Parapedobacter, Anseongella, Georgenia, Pusillimonas, Galbibacter, Membranicola, Alcanivorax, Longispora, Saccharomonospora, and Sphingobacterium were noted to carry more ARGs and MGEs (at least five or more ARGs and MEGs), with Parapedobacter possessing 12 kinds of ARGs and MGEs. Besides, the hosts of some ARGs were not only one type of bacteria, but a variety of bacteria; for instance, tetG was found to have as many as nine potential host bacteria. The correlation between ARGs and different potential hosts varied, which was mainly related to the spread of ARGs among bacteria through HGT (Forsberg et al., 2014). The use of multiple antibiotics can lead to the emergence of multi-resistant microorganisms.
FIGURE 6. Network analysis based on co-occurrence of ARGs, MGEs, and their potential host bacteria. The red node represents ARGs, yellow node denotes MGEs, green node indicates bacteria at the genus level, and red line implies significant (p < 0.05) positive correlation (r > 0). The thicker the line is, the stronger is the positive correlation.
Network analysis showed that intI1 and intI2 have the same potential hosts, namely, Parapedobacter, Alcanivorax, Pusillimonas, Anseongella, and Membranicola (Figure 6). These bacteria were significantly associated with a variety of ARGs (p < 0.05) and were the hub of ARGs for HGT, consistent with that reported in a previous study suggesting that MGEs are associated with multidrug resistance genes (Jechalke et al., 2014b). The variations in the abundances of intI1 and intI2 during the composting process were strongly affected by bacterial communities, which also supported the view that the changes in the bacterial communities could affect the proliferation and propagation of ARGs.
RDA was used to determine the effects of environmental factors on antibiotics, ARGs, MGEs, and bacterial communities during the composting process (Figure 7). The first two axes (RDA1 and RDA2) explained 57.31% of the changes in the bacterial communities, antibiotics, ARGs, and MGEs during composting. Among the environmental factors selected in this study, DTN and NH4+-N presented the highest contribution of 22.60% and 21.80%, respectively, and showed significant correlation with Firmicutes and Proteobacteria (p < 0.05). This result is consistent with the findings of a previous study that demonstrated that nitrogen content is closely related to microbial growth (Chen et al., 2014). Furthermore, a significant positive correlation was observed between DTN and NH4+-N contents and tetracyclines antibiotics, sulfonamides antibiotics, OFX, CIP, DIF, macrolides antibiotics, and LCM, indicating that biochar addition facilitated antibiotics removal from swine manure by promoting DTN utilization and NH4+-N transformation by microorganisms. In contrast, a significant negative correlation was noted between DTN and sul1 as well as NH4+-N and sul1, sul2, tetA, and tetG (Supplementary Figure S3), which could explain the observed increase in the absolute abundance of sul1, sul2, tetA, and tetG during the composting process. Furthermore, OM, MC, DOC, TOC, and C/N exhibited significant correlations with some antibiotics, ARGs, and microorganisms, indicating that the changes in the environmental factors were critical to the composting process (Supplementary Figure S3).
FIGURE 7. RDA of the relationship between environmental factors (red arrows) and ARGs, MGEs (blue arrows), antibiotics (purple arrows), and phylum-level bacteria (green arrows) during composting.
To further explore how antibiotics and ARGs were affected by other driving factors in the composting process, the direct and indirect relationships between different variables were examined by establishing a SEM (Figure 8). The results showed that the composting properties (λ = 0.663, p < 0.001) and community composition (λ = 0.250, p < 0.05) had significant effects on the variations in the antibiotics levels during composting. The composting properties were the dominant factors that determined the degradation status of antibiotics. Moreover, the variations in the ARGs abundances during composting were mainly influenced by antibiotics, bacterial community composition, and MGEs, with MGEs (λ = 0.692, p < 0.001) being the highest contributor to the changes in ARGs, followed by antibiotics (λ = −0.228, p < 0.05) and bacterial community composition (λ = −0.194, p < 0.05). This finding is consistent with those reported in previous studies that demonstrated that the contribution of MGEs to changes in ARGs was higher than that of bacterial community composition during composting (Ma et al., 2017; Wu et al., 2017). The effect of composting properties on ARGs was mainly indirect via the influence of antibiotics (λ = 0.663, p < 0.001), bacterial community composition (λ = 0.414, p < 0.01), and HGT dominated by MGEs (λ = −0.757, p < 0.01) on the changes in ARGs during composting. Moreover, addition of biochar, directly and indirectly, affected the changes in antibiotics and ARGs during composting by altering the composting properties.
FIGURE 8. (A) SEM reflecting direct and indirect relationships between compost physicochemical properties, antibiotics, bacterial communities, MGEs, and ARGs. GFI = 0.953. (B) Total effect of standardization estimated based on SEM. Red arrow indicates positive correlation and blue arrow denotes negative correlation. *p < 0.05, **p < 0.01, ***p < 0.001.
During pig manure and corn straw co-composting, biochar addition can: 1) accelerate the pile temperature, prolong the high-temperature period (>55°C), and reduce the phytotoxicity of the compost product; 2) promote the transformation of NH4+-N and formation of NO3−-N in the early stage of composting, and improve the DOC and DTN utilization rates; 3) accelerate the degradation of OM and water dissipation, and improved the GI value of the final composting product; and 4) quicken the degradation of antibiotics at the early stage of composting, change the bacterial community structure during composting, and promote the removal of ARGs and MGEs. Temperature was closely related to the degradation of antibiotics in the early stage of composting, and the composting properties affected the removal of antibiotics during composting. The bacterial communities and MGEs were the main factors driving the variations in ARGs, and biochar inhibited HGT of ARGs by reducing the abundance of MGEs. Thus, biochar addition can reduce the environmental risk of antibiotics and ARGs in the compost product.
The sequences data reported in this study have been deposited in NCBI SRA with the accession number PRJNA846099 (https://www.ncbi.nlm.nih.gov/sra/PRJNA846099).
ZT and FL designed the experiments; ZT performed the experiments; ZT and FL analyzed the experimental results; FL, YT, WB, JQ, HL, JZ, and SX reviewed the manuscript; ZT wrote the manuscript; JD provided composting materials and test sites for this study. All the authors agreed to be accountable for the content of the work.
This work was supported by the Key R&D Program Projects in Shanxi Province, China (201903D221015); the Shanxi Province “1331 Project” funded project (20211331-15); the Shanxi Province Applied Basic Research Project (201901D211353), the Natural Science Foundation for Young Scientists of Shanxi Province, China (201901D211355).
The authors thank Shanghai Origin Biotechnology Co., Ltd. for providing technical support, and JD for offering experimental sites and compost raw materials for this study.
The authors declare that the research was conducted in the absence of any commercial or financial relationships that could be construed as a potential conflict of interest.
All claims expressed in this article are solely those of the authors and do not necessarily represent those of their affiliated organizations, or those of the publisher, the editors and the reviewers. Any product that may be evaluated in this article, or claim that may be made by its manufacturer, is not guaranteed or endorsed by the publisher.
The Supplementary Material for this article can be found online at: https://www.frontiersin.org/articles/10.3389/fbioe.2022.960476/full#supplementary-material
Agyarko-Mintah, E., Cowie, A., Singh, B. P., Joseph, S., Van Zwieten, L., Cowie, A., et al. (2017). Biochar increases nitrogen retention and lowers greenhouse gas emissions when added to composting poultry litter. Waste Manag. 61, 138–149. doi:10.1016/j.wasman.2016.11.027
Alexandrino, D. A. M., Mucha, A. P., Almeida, C. M. R., Gao, W., Jia, Z., Carvalho, M. F., et al. (2017). Biodegradation of the veterinary antibiotics enrofloxacin and ceftiofur and associated microbial community dynamics. Sci. Total Environ. 581-582, 359–368. doi:10.1016/j.scitotenv.2016.12.141
Arikan, O. A., Sikora, L. J., Mulbry, W., Khan, S. U., and Foster, G. D. (2007). Composting rapidly reduces levels of extractable oxytetracycline in manure from therapeutically treated beef calves. Bioresour. Technol. 98 (1), 169–176. doi:10.1016/j.biortech.2005.10.041
Awasthi, M. K., Wang, Q., Chen, H., Wang, M., Ren, X., Zhao, J., et al. (2017). Evaluation of biochar amended biosolids co-composting to improve the nutrient transformation and its correlation as a function for the production of nutrient-rich compost. Bioresour. Technol. 237, 156–166. doi:10.1016/j.biortech.2017.01.044
Awasthi, M. K., Duan, Y., Awasthi, S. K., Liu, T., Chen, H., Pandey, A., et al. (2020). Emerging applications of biochar: Improving pig manure composting and attenuation of heavy metal mobility in mature compost. J. Hazard. Mat. 389, 122116. doi:10.1016/j.jhazmat.2020.122116
Bonham, K. S., Wolfe, B. E., and Dutton, R. J. (2017). Extensive horizontal gene transfer in cheese-associated bacteria. Elife 6, e22144. doi:10.7554/eLife.22144
Cáceres, R., Malińska, K., and Marfà, O. (2018). Nitrification within composting: a review. Waste Manag. 72, 119–137. doi:10.1016/j.wasman.2017.10.049
Canfield, D. E., Glazer, A. N., and Falkowski, P. G. (2010). The evolution and future of earth's nitrogen cycle. Science 330 (6001), 192–196. doi:10.1126/science.1186120
Chen, Y., Zhou, W., Li, Y., Zhang, J., Zeng, G., Huang, A., et al. (2014). Nitrite reductase genes as functional markers to investigate diversity of denitrifying bacteria during agricultural waste composting. Appl. Microbiol. Biotechnol. 98 (9), 4233–4243. doi:10.1007/s00253-014-5514-0
Chen, Q., An, X., Li, H., Su, J., Ma, Y., Zhu, Y. G., et al. (2016). Long-term field application of sewage sludge increases the abundance of antibiotic resistance genes in soil. Environ. Int. 92-93, 1–10. doi:10.1016/j.envint.2016.03.026
Cheng, D., Feng, Y., Liu, Y., Xue, J., and Li, Z. (2019). Dynamics of oxytetracycline, sulfamerazine, and ciprofloxacin and related antibiotic resistance genes during swine manure composting. J. Environ. Manage. 230, 102–109. doi:10.1016/j.jenvman.2018.09.074
Cheng, D., Liu, Y., Shehata, E., Feng, Y., Lin, H., Xue, J., et al. (2021). In-feed antibiotic use changed the behaviors of oxytetracycline, sulfamerazine, and ciprofloxacin and related antibiotic resistance genes during swine manure composting. J. Hazard. Mat. 402, 123710. doi:10.1016/j.jhazmat.2020.123710
Cui, E., Wu, Y., Zuo, Y., and Chen, H. (2016). Effect of different biochars on antibiotic resistance genes and bacterial community during chicken manure composting. Bioresour. Technol. 203, 11–17. doi:10.1016/j.biortech.2015.12.030
Cui, P., Bai, Y., Li, X., Peng, Z., Chen, D., Wu, Z., et al. (2020). Enhanced removal of antibiotic resistance genes and mobile genetic elements during sewage sludge composting covered with a semi-permeable membrane. J. Hazard. Mat. 396, 122738. doi:10.1016/j.jhazmat.2020.122738
Czekała, W., Malińska, K., Cáceres, R., Janczak, D., Dach, J., Lewicki, A., et al. (2016). Co-composting of poultry manure mixtures amended with biochar-The effect of biochar on temperature and C-CO2 emission. Bioresour. Technol. 200, 921–927. doi:10.1016/j.biortech.2015.11.019
D'Costa, V. M., McGrann, K. M., Hughes, D. W., and Wright, G. D. (2006). Sampling the antibiotic resistome. Science 311 (5759), 374–377. doi:10.1126/science.1120800
Dorival-García, N., Zafra-Gómez, A., Navalón, A., González, J., and Vílchez, J. L. (2013). Removal of quinolone antibiotics from wastewaters by sorption and biological degradation in laboratory-scale membrane bioreactors. Sci. Total Environ. 442, 317–328. doi:10.1016/j.scitotenv.2012.10.026
Duan, M., Li, H., Gu, J., Tuo, X., Sun, W., Qian, X., et al. (2017). Effects of biochar on reducing the abundance of oxytetracycline, antibiotic resistance genes, and human pathogenic bacteria in soil and lettuce. Environ. Pollut. 224, 787–795. doi:10.1016/j.envpol.2017.01.021
Duan, M., Gu, J., Wang, X., Li, Y., Zhang, S., Yin, Y., et al. (2018). Effects of genetically modified cotton stalks on antibiotic resistance genes, Inti1, and Inti2 during pig manure composting. Ecotoxicol. Environ. Saf. 147, 637–642. doi:10.1016/j.ecoenv.2017.09.023
Ezzariai, A., Hafidi, M., Khadra, A., Aemig, Q., El Fels, L., Barret, M., et al. (2018). Human and veterinary antibiotics during composting of sludge or manure: global perspectives on persistence, degradation, and resistance genes. J. Hazard. Mat. 359, 465–481. doi:10.1016/j.jhazmat.2018.07.092
Fang, H., Wang, H., Cai, L., and Yu, Y. (2015). Prevalence of antibiotic resistance genes and bacterial pathogens in long-term manured greenhouse soils as revealed by metagenomic survey. Environ. Sci. Technol. 49 (2), 1095–1104. doi:10.1021/es504157v
Forsberg, K. J., Patel, S., Gibson, M. K., Lauber, C. L., Knight, R., Fierer, N., et al. (2014). Bacterial phylogeny structures soil resistomes across habitats. Nature 509 (7502), 612–616. doi:10.1038/nature13377
Fu, T., Shangguan, H., Wu, J., Tang, J., Yuan, H., Zhou, S., et al. (2021). Insight into the synergistic effects of conductive biochar for accelerating maturation during electric field-assisted aerobic composting. Bioresour. Technol. 337, 125359. doi:10.1016/j.biortech.2021.125359
Gou, M., Hu, H. W., Zhang, Y. J., Wang, J. T., Hayden, H., Tang, Y. Q., et al. (2018). Aerobic composting reduces antibiotic resistance genes in cattle manure and the resistome dissemination in agricultural soils. Sci. Total Environ. 612, 1300–1310. doi:10.1016/j.scitotenv.2017.09.028
Gou, C., Wang, Y., Zhang, X., Zhong, R., and Gao, Y. (2021). Effects of chlorotetracycline on antibiotic resistance genes and the bacterial community during cattle manure composting. Bioresour. Technol. 323, 124517. doi:10.1016/j.biortech.2020.124517
Gu, D., Feng, Q., Guo, C., Hou, S., Lv, J., Zhang, Y., et al. (2019). Occurrence and risk assessment of antibiotics in manure, soil, wastewater, groundwater from livestock and poultry farms in Xuzhou, China. Bull. Environ. Contam. Toxicol. 103 (4), 590–596. doi:10.1007/s00128-019-02692-0
Guo, W., Huang, C., Xi, B., Tang, Z., Tan, W., Li, W., et al. (2021). The maturity period is the main stage of antibiotic resistance genes reduction in aerobic composting process of swine manure in sub-scale farms. Bioresour. Technol. 319, 124139. doi:10.1016/j.biortech.2020.124139
Heuer, H., Schmitt, H., and Smalla, K. (2011). Antibiotic resistance gene spread due to manure application on agricultural fields. Curr. Opin. Microbiol. 14 (3), 236–243. doi:10.1016/j.mib.2011.04.009
Huang, G. F., Wu, Q. T., Wong, J. W. C., and Nagar, B. B. (2006). Transformation of organic matter during Co-composting of pig manure with sawdust. Bioresour. Technol. 97 (15), 1834–1842. doi:10.1016/j.biortech.2005.08.024
Huang, X., Zheng, J., Tian, S., Liu, C., Liu, L., Wei, L., et al. (2019). Higher temperatures do not always achieve better antibiotic resistance gene removal in anaerobic digestion of swine manure. Appl. Environ. Microbiol. 85 (7), e02878–18. doi:10.1128/aem.02878-18
Huang, K., Chen, J., Guan, M., Xia, H., and Lin, L. (2020). Effects of biochars on the fate of antibiotics and their resistance genes during vermicomposting of dewatered sludge. J. Hazard. Mat. 397, 122767. doi:10.1016/j.jhazmat.2020.122767
Huerta, B., Marti, E., Gros, M., López, P., Pompêo, M., Armengol, J., et al. (2013). Exploring the links between antibiotic occurrence, antibiotic resistance, and bacterial communities in water supply reservoirs. Sci. Total Environ. 456-457, 161–170. doi:10.1016/j.scitotenv.2013.03.071
Iqbal, M. K., Shafiq, T., and Ahmed, K. (2010). Characterization of bulking agents and its effects on physical properties of compost. Bioresour. Technol. 101 (6), 1913–1919. doi:10.1016/j.biortech.2009.10.030
Jechalke, S., Heuer, H., Siemens, J., Amelung, W., and Smalla, K. (2014a). Fate and effects of veterinary antibiotics in soil. Trends Microbiol. 22 (9), 536–545. doi:10.1016/j.tim.2014.05.005
Jechalke, S., Schreiter, S., Wolters, B., Dealtry, S., Heuer, H., Smalla, K., et al. (2014b). Widespread dissemination of class 1 integron components in soils and related ecosystems as revealed by cultivation-independent analysis. Front. Microbiol. 4, 420. doi:10.3389/fmicb.2013.00420
Khan, N., Clark, I., Sánchez-Monedero, M. A., Shea, S., Meier, S., Bolan, N., et al. (2014). Maturity indices in Co-composting of chicken manure and sawdust with biochar. Bioresour. Technol. 168, 245–251. doi:10.1016/j.biortech.2014.02.123
Kim, K. R., Owens, G., Ok, Y. S., Park, W. K., Lee, D. B., Kwon, S. I., et al. (2012). Decline in extractable Antibiotics in manure-based composts during composting. Waste Manag. 32 (1), 110–116. doi:10.1016/j.wasman.2011.07.026
Kümmerer, K. (2009). Antibiotics in the aquatic environment–a review-Part I. Chemosphere 75 (4), 417–434. doi:10.1016/j.chemosphere.2008.11.086
Lebuhn, M., Hanreich, A., Klocke, M., Schlüter, A., Bauer, C., Pérez, C. M., et al. (2014). Towards molecular biomarkers for biogas production from lignocellulose-rich substrates. Anaerobe 29, 10–21. doi:10.1016/j.anaerobe.2014.04.006
Li, H., Duan, M., Gu, J., Zhang, Y., Qian, X., Ma, J., et al. (2017). Effects of bamboo charcoal on antibiotic resistance genes during chicken manure composting. Ecotoxicol. Environ. Saf. 140, 1–6. doi:10.1016/j.ecoenv.2017.01.007
Li, K., Cao, R., Mo, S., Yao, R., Ren, Z., Wu, J., et al. (2020). Swine manure composting with compound microbial inoculants: removal of antibiotic resistance genes and their associations with microbial community. Front. Microbiol. 11, 592592. doi:10.3389/fmicb.2020.592592
Liu, B., Li, Y., Zhang, X., Feng, C., Gao, M., Shen, Q., et al. (2015). Effects of composting process on the dissipation of extractable sulfonamides in swine manure. Bioresour. Technol. 175, 284–290. doi:10.1016/j.biortech.2014.10.098
Liu, N., Zhou, J., Han, L., Ma, S., Sun, X., Huang, G., et al. (2017). Role and multi-scale characterization of bamboo biochar during poultry manure aerobic composting. Bioresour. Technol. 241, 190–199. doi:10.1016/j.biortech.2017.03.144
Liu, C. H., Chuang, Y.-H., Li, H., Boyd, S. A., Teppen, B. J., Gonzalez, J. M., et al. (2019). Long-term sorption of lincomycin to biochars: the intertwined roles of pore diffusion and dissolved organic carbon. Water Res. 161, 108–118. doi:10.1016/j.watres.2019.06.006
Looft, T., Johnson, T. A., Allen, H. K., Bayles, D. O., Alt, D. P., Stedtfeld, R. D., et al. (2012). In-feed antibiotic effects on the swine intestinal microbiome. Proc. Natl. Acad. Sci. U. S. A. 109 (5), 1691–1696. doi:10.1073/pnas.1120238109
López-González, J. A., Estrella-González, M. J., Lerma-Moliz, R., Jurado, M. M., Suárez-Estrella, F., López, M. J., et al. (2021). Industrial composting of sewage sludge: Study of the bacteriome, sanitation, and antibiotic-resistant strains. Front. Microbiol. 12, 784071. doi:10.3389/fmicb.2021.784071
Luo, G., Li, B., Li, L.-G., Zhang, T., and Angelidaki, I. (2017). Antibiotic resistance genes and correlations with microbial community and metal resistance genes in full-scale biogas reactors as revealed by metagenomic analysis. Environ. Sci. Technol. 51 (7), 4069–4080. doi:10.1021/acs.est.6b05100
Ma, L., Li, A. D., Yin, X. L., and Zhang, T. (2017). The prevalence of integrons as the carrier of antibiotic resistance genes in natural and man-made environments. Environ. Sci. Technol. 51 (10), 5721–5728. doi:10.1021/acs.est.6b05887
Maeda, K., Hanajima, D., Toyoda, S., Yoshida, N., Morioka, R., Osada, T., et al. (2011). Microbiology of nitrogen cycle in animal manure compost. Microb. Biotechnol. 4 (6), 700–709. doi:10.1111/j.1751-7915.2010.00236.x
Mao, H., Lv, Z., Sun, H., Li, R., Zhai, B., Wang, Z., et al. (2018). Improvement of biochar and bacterial powder addition on gaseous emission and bacterial community in pig manure compost. Bioresour. Technol. 258, 195–202. doi:10.1016/j.biortech.2018.02.082
Martínez, J. L., Coque, T. M., and Baquero, F. (2015). What is a resistance gene? ranking risk in resistomes. Nat. Rev. Microbiol. 13 (2), 116–123. doi:10.1038/nrmicro3399
Menz, J., Olsson, O., and Kümmerer, K. (2019). Antibiotic residues in livestock manure: does the Eu risk assessment sufficiently protect against microbial toxicity and selection of resistant bacteria in the environment? J. Hazard. Mat. 379, 120807. doi:10.1016/j.jhazmat.2019.120807
Mu, Q., Li, J., Sun, Y., Mao, D., Wang, Q., Luo, Y., et al. (2015). Occurrence of sulfonamide-tetracycline-plasmid-mediated quinolone- and macrolide-resistance genes in livestock feedlots in northern China. Environ. Sci. Pollut. Res. 22 (9), 6932–6940. doi:10.1007/s11356-014-3905-5
Qian, X., Sun, W., Gu, J., Wang, X. J., Sun, J. J., Yin, Y. N., et al. (2016a). Variable effects of oxytetracycline on antibiotic resistance gene abundance and the bacterial community during aerobic composting of cow manure. J. Hazard. Mat. 315, 61–69. doi:10.1016/j.jhazmat.2016.05.002
Qian, X., Sun, W., Gu, J., Wang, X. J., Zhang, Y. J., Duan, M. L., et al. (2016b). Reducing antibiotic resistance genes, integrons, and pathogens in dairy manure by continuous thermophilic composting. Bioresour. Technol. 220, 425–432. doi:10.1016/j.biortech.2016.08.101
Qiu, X., Zhou, G., Chen, L., and Wang, H. (2021). Additive quality influences the reservoir of antibiotic resistance genes during chicken manure composting. Ecotoxicol. Environ. Saf. 220, 112413. doi:10.1016/j.ecoenv.2021.112413
Ravindran, B., Nguyen, D. D., Chaudhary, D. K., Chang, S. W., Kim, J., Lee, S. R., et al. (2019). Influence of biochar on physico-chemical and microbial community during swine manure composting process. J. Environ. Manage. 232, 592–599. doi:10.1016/j.jenvman.2018.11.119
Ren, S., Guo, X., Lu, A., Guo, X., Wang, Y., Sun, G., et al. (2018). Effects of Co-composting of lincomycin mycelia dregs with furfural slag on lincomycin degradation, maturity and microbial communities. Bioresour. Technol. 265, 155–162. doi:10.1016/j.biortech.2018.05.087
Sánchez-García, M., Alburquerque, J. A., Sánchez-Monedero, M. A., Roig, A., and Cayuela, M. L. (2015). Biochar accelerates organic matter degradation and enhances N mineralisation during composting of poultry manure without a relevant impact on gas emissions. Bioresour. Technol. 192, 272–279. doi:10.1016/j.biortech.2015.05.003
Sanchez-Monedero, M. A., Cayuela, M. L., Roig, A., Jindo, K., Mondini, C., Bolan, N., et al. (2018). Role of biochar as an additive in organic waste composting. Bioresour. Technol. 247, 1155–1164. doi:10.1016/j.biortech.2017.09.193
Sarmah, A. K., Meyer, M. T., and Boxall, A. B. A. (2006). A global perspective on the use, sales, exposure pathways, occurrence, fate and effects of veterinary antibiotics (vas) in the environment. Chemosphere 65 (5), 725–759. doi:10.1016/j.chemosphere.2006.03.026
Selvam, A., Xu, D., Zhao, Z., and Wong, J. W. (2012a). Fate of tetracycline, sulfonamide and fluoroquinolone resistance genes and the changes in bacterial diversity during composting of swine manure. Bioresour. Technol. 126, 383–390. doi:10.1016/j.biortech.2012.03.045
Selvam, A., Zhao, Z., and Wong, J. W. (2012b). Composting of swine manure spiked with sulfadiazine, chlortetracycline and ciprofloxacin. Bioresour. Technol. 126, 412–417. doi:10.1016/j.biortech.2011.12.073
Song, T., Zhu, C., Xue, S., Li, B., Ye, J., Geng, B., et al. (2020). Comparative effects of different antibiotics on antibiotic resistance during swine manure composting. Bioresour. Technol. 315, 123820. doi:10.1016/j.biortech.2020.123820
Stegmann, E., Frasch, H. J., Kilian, R., and Pozzi, R. (2015). Self-resistance mechanisms of actinomycetes producing lipid II-targeting antibiotics. Int. J. Med. Microbiol. 305 (2), 190–195. doi:10.1016/j.ijmm.2014.12.015
Su, J. Q., Wei, B., Ou-Yang, W. Y., Huang, F. Y., Zhao, Y., Xu, H. J., et al. (2015). Antibiotic resistome and its association with bacterial communities during sewage sludge composting. Environ. Sci. Technol. 49 (12), 7356–7363. doi:10.1021/acs.est.5b01012
Sun, W., Gu, J., Wang, X., Qian, X., and Tuo, X. (2018). Impacts of biochar on the environmental risk of antibiotic resistance genes and mobile genetic elements during anaerobic digestion of cattle farm wastewater. Bioresour. Technol. 256, 342–349. doi:10.1016/j.biortech.2018.02.052
Tan, Z., Yuan, S., Hong, M., Zhang, L., and Huang, Q. (2020). Mechanism of negative surface charge formation on biochar and its effect on the fixation of soil Cd. J. Hazard. Mat. 384, 121370. doi:10.1016/j.jhazmat.2019.121370
Thiele-Bruhn, S., and Beck, I. C. (2005). Effects of sulfonamide and tetracycline antibiotics on soil microbial activity and microbial biomass. Chemosphere 59 (4), 457–465. doi:10.1016/j.chemosphere.2005.01.023
Thomas, C. M., and Nielsen, K. M. (2005). Mechanisms of, and barriers to, horizontal gene transfer between bacteria. Nat. Rev. Microbiol. 3 (9), 711–721. doi:10.1038/nrmicro1234
Tolls, J. (2001). Sorption of veterinary pharmaceuticals in soils: A review. Environ. Sci. Technol. 35 (17), 3397–3406. doi:10.1021/es0003021
Wang, B., Huang, Y., Liu, W., Chen, S., Zhu, J., Belzile, N., et al. (2021a). Returning excrement from livestock, poultry, and humans to farmland as nutrient resources for crop growth: assessment of rural China. Process Saf. Environ. Prot. 146, 412–423. doi:10.1016/j.psep.2020.09.001
Wang, C., Lu, H., Dong, D., Deng, H., Strong, P. J., Wang, H., et al. (2013). Insight into the effects of biochar on manure composting: evidence supporting the relationship between N2O emission and denitrifying community. Environ. Sci. Technol. 47 (13), 7341–7349. doi:10.1021/es305293h
Wang, J., Ben, W., Zhang, Y., Yang, M., and Qiang, Z. (2015). Effects of thermophilic composting on oxytetracycline, sulfamethazine, and their corresponding resistance genes in swine manure. Environ. Sci. Process. Impacts 17 (9), 1654–1660. doi:10.1039/c5em00132c
Wang, R., Zhang, J., Sui, Q., Wan, H., Tong, J., Chen, M., et al. (2016). Effect of red mud addition on tetracycline and copper resistance genes and microbial community during the full scale swine manure composting. Bioresour. Technol. 216, 1049–1057. doi:10.1016/j.biortech.2016.06.012
Wang, G., Li, G., Chang, J., Kong, Y., Jiang, T., Wang, J., et al. (2021b). Enrichment of antibiotic resistance genes after sheep manure aerobic heap composting. Bioresour. Technol. 323, 124620. doi:10.1016/j.biortech.2020.124620
Wang, J., Gu, J., Wang, X., Song, Z., Dai, X., Guo, H., et al. (2021c). Enhanced removal of antibiotic resistance genes and mobile genetic elements during swine manure composting inoculated with mature compost. J. Hazard. Mat. 411, 125135. doi:10.1016/j.jhazmat.2021.125135
Wei, H., Ma, J., Su, Y., and Xie, B. (2020). Effect of nutritional energy regulation on the fate of antibiotic resistance genes during composting of sewage sludge. Bioresour. Technol. 297, 122513. doi:10.1016/j.biortech.2019.122513
Wu, D., Huang, X. H., Sun, J. Z., Graham, D. W., and Xie, B. (2017). Antibiotic resistance genes and associated microbial community conditions in aging landfill systems. Environ. Sci. Technol. 51 (21), 12859–12867. doi:10.1021/acs.est.7b03797
Wu, N., Xie, S., Zeng, M., Xu, X., Li, Y., Liu, X., et al. (2020). Impacts of pile temperature on antibiotic resistance, metal resistance and microbial community during swine manure composting. Sci. Total Environ. 744, 140920. doi:10.1016/j.scitotenv.2020.140920
Xie, W., Yang, X., Li, Q., Wu, L., Shen, Q., Zhao, F., et al. (2016). Changes in antibiotic concentrations and antibiotic resistome during commercial composting of animal manures. Environ. Pollut. 219, 182–190. doi:10.1016/j.envpol.2016.10.044
Xie, W. Y., Shen, Q., and Zhao, F. J. (2018). Antibiotics and antibiotic resistance from animal manures to soil: a review. Eur. J. Soil Sci. 69 (1), 181–195. doi:10.1111/ejss.12494
Yin, Y., Gu, J., Wang, X., Song, W., Zhang, K., Sun, W., et al. (2017). Effects of copper addition on copper resistance, antibiotic resistance genes, and Intl1 during swine manure composting. Front. Microbiol. 8, 344. doi:10.3389/fmicb.2017.00344
Youngquist, C. P., Mitchell, S. M., and Cogger, C. G. (2016). Fate of antibiotics and antibiotic resistance during digestion and composting: a review. J. Environ. Qual. 45 (2), 537–545. doi:10.2134/jeq2015.05.0256
Zainudin, M. H., Mustapha, N. A., Maeda, T., Ramli, N., Sakai, K., Hassan, M., et al. (2020). Biochar enhanced the nitrifying and denitrifying bacterial communities during the composting of poultry manure and rice straw. Waste Manag. 106, 240–249. doi:10.1016/j.wasman.2020.03.029
Zhang, Y., Zhang, C., Parker, D. B., Snow, D. D., Zhou, Z., Li, X., et al. (2013). Occurrence of antimicrobials and antimicrobial resistance genes in beef cattle storage ponds and swine treatment lagoons. Sci. Total Environ. 463-464, 631–638. doi:10.1016/j.scitotenv.2013.06.016
Zhang, Q. Q., Ying, G. G., Pan, C. G., Liu, Y. S., and Zhao, J. L. (2015). Comprehensive evaluation of antibiotics emission and fate in the river basins of China: source analysis, multimedia modeling, and linkage to bacterial resistance. Environ. Sci. Technol. 49 (11), 6772–6782. doi:10.1021/acs.est.5b00729
Zhang, L., Gu, J., Wang, X., Sun, W., Yin, Y., Sun, Y., et al. (2017). Behavior of antibiotic resistance genes during Co-composting of swine manure with Chinese medicinal herbal residues. Bioresour. Technol. 244, 252–260. doi:10.1016/j.biortech.2017.07.035
Zhou, G., Xu, X., Qiu, X., and Zhang, J. (2019). Biochar influences the succession of microbial communities and the metabolic functions during rice straw composting with pig manure. Bioresour. Technol. 272, 10–18. doi:10.1016/j.biortech.2018.09.135
Zhou, G., Qiu, X., Wu, X., and Lu, S. (2021). Horizontal gene transfer is a key determinant of antibiotic resistance genes profiles during chicken manure composting with the addition of biochar and zeolite. J. Hazard. Mat. 408, 124883. doi:10.1016/j.jhazmat.2020.124883
Zhu, Y. G., Johnson, T. A., Su, J. Q., Qiao, M., Guo, G. X., Stedtfeld, R. D., et al. (2013). Diverse and abundant antibiotic resistance genes in Chinese swine farms. Proc. Natl. Acad. Sci. U. S. A. 110 (9), 3435–3440. doi:10.1073/pnas.1222743110
Zhu, B., Chen, Q., Chen, S., and Zhu, Y. G. (2017a). Does organically produced lettuce harbor higher abundance of antibiotic resistance genes than conventionally produced? Environ. Int. 98, 152–159. doi:10.1016/j.envint.2016.11.001
Zhu, Y., Wang, Y., Jiang, X., Zhou, S., Wu, M., Pan, M., et al. (2017b). Microbial community compositional analysis for membrane bioreactor treating antibiotics containing wastewater. Chem. Eng. J. 325, 300–309. doi:10.1016/j.cej.2017.05.073
Keywords: biochar, antibiotics, antibiotic resistance genes, mobile genetic elements, compost
Citation: Tong Z, Liu F, Tian Y, Zhang J, Liu H, Duan J, Bi W, Qin J and Xu S (2022) Effect of biochar on antibiotics and antibiotic resistance genes variations during co-composting of pig manure and corn straw. Front. Bioeng. Biotechnol. 10:960476. doi: 10.3389/fbioe.2022.960476
Received: 03 June 2022; Accepted: 30 June 2022;
Published: 22 July 2022.
Edited by:
Jun Zhou, Nanjing Tech University, ChinaReviewed by:
Binghua Yan, Hunan Agricultural University, ChinaCopyright © 2022 Tong, Liu, Tian, Zhang, Liu, Duan, Bi, Qin and Xu. This is an open-access article distributed under the terms of the Creative Commons Attribution License (CC BY). The use, distribution or reproduction in other forums is permitted, provided the original author(s) and the copyright owner(s) are credited and that the original publication in this journal is cited, in accordance with accepted academic practice. No use, distribution or reproduction is permitted which does not comply with these terms.
*Correspondence: Fenwu Liu, bGl1ZmVud3VAc3hhdS5lZHUuY24=
Disclaimer: All claims expressed in this article are solely those of the authors and do not necessarily represent those of their affiliated organizations, or those of the publisher, the editors and the reviewers. Any product that may be evaluated in this article or claim that may be made by its manufacturer is not guaranteed or endorsed by the publisher.
Research integrity at Frontiers
Learn more about the work of our research integrity team to safeguard the quality of each article we publish.