- Department of Orthopedics, Orthopedic Research Institute, West China Hospital, Sichuan University, Chengdu, China
Background: The treatment of cartilage damage is a hot topic at present, and cell therapy is an emerging alternative therapy. Stem cells derived from peripheral blood have become the focus of current research due to the ease of obtaining materials and a wide range of sources.
Methods: We used a text search strategy using the [“mesenchymal stem cells” (MeSH term) OR “MSC” OR “BMMSC” OR “PBMSC” OR” PBMNC” OR “peripheral blood stem cells”] AND (cartilage injury [MeSH term] OR “cartilage” OR “chondral lesion”). After searching the literature, through the inclusion and exclusion criteria, the last included articles were systematically reviewed.
Result: We found that peripheral blood-derived stem cells have chondrogenic differentiation ability and can induce chondrogenic differentiation and repair in vivo and have statistical significance in clinical and imaging prognosis. It is an improvement of academic differences. Compared with the bone marrow, peripheral blood is easier to obtain, widely sourced, and simple to obtain. In the future, peripheral blood will be a more potential cell source for cell therapy in the treatment of cartilage damage.
Conclusion: Stem cells derived from peripheral blood can repair cartilage and are an important resource for the treatment of cartilage damage in the future. The specific mechanism and way of repairing cartilage need further study.
1 Introduction
Cartilage is a special, low-friction articular surface tissue that is essential for weight absorption and smooth gliding of the articulating surfaces in diarthrodial joints, whose primary function is to absorb, cushion, and protect the underlying bone from the forces that arise when the joint is being used. Chondral lesions can lead to direct contact with bone, ultimately leading to osteoarthritis (Rackwitz et al., 2014). Due to the lack of native blood vessels and lymphatic return, the spontaneous healing capacity of cartilage is low and is generally replaced by fibrocartilage (Frisch et al., 2017a). The newly generated fibrocartilage can withstand far less mechanical stress than the original cartilage tissue (Hunziker, 2002). Numerous studies have reported that the newly formed fibrocartilage tends to deteriorate over time (Orth et al., 2014). Therefore, the treatment of chondral lesions is currently an important research topic in traumatology.
Conservative treatment of cartilage damage usually includes corticosteroids, nonsteroidal anti-inflammatory drugs, hyaluronan, and polysulfated glycosaminoglycan (Ferris et al., 2011). However, the abovementioned drugs can only control the symptoms and cannot prevent the occurrence of osteoarthritis (Frisbie et al., 2009). Marrow stimulation techniques, including microfracture and microdrilling, have been widely reported as promoting chondral healing, with microfracture being the most commonly performed (Madry et al., 2011). It penetrates the underlying subchondral bone marrow through drilling, allowing bone marrow mesenchymal stem cells (MSC) and other progenitor cells to enter the cartilage defect for repair and present good clinical outcomes (Bieback et al., 2008). However, after bone marrow stimulation, the joint normally covered by hyaline cartilage is repaired by fibrocartilage, which is biochemically and mechanically inferior to hyaline cartilage (Saris et al., 2009; Seol et al., 2012; Jiang and Tuan, 2015). Continued stress can lead to tissue degeneration and deteriorating results in the long term (Vinatier et al., 2009). Therefore, improving the quality of prosthetic tissue has become a new issue.
The application of autologous mesenchymal stem cells in the joint cavity shows the effect of enhancing cartilage repair in a lasting way (Saw et al., 2013; Skowroński and Rutka, 2013; Reissis et al., 2016). Thus, lately researchers have focused on cell therapy as a therapeutic alternative (Brittberg et al., 1994). There are many sources of mesenchymal stem cells, including bone marrow, adipose tissue, skin, or peripheral blood, or from an umbilical cord donor (Kassis et al., 2006; Larochelle et al., 2006; Huang et al., 2009). While bone marrow (BM) MSCs show a decline in the number and differentiation potential of MSCs with aging or transformation in long-term in vitro culture, the peripheral blood mononuclear cell fraction has been shown to enhance cartilage repair in an ovine osteochondral defect model (Emadedin et al., 2012; Hopper et al., 2015a). The use of peripheral blood may provide workable and less invasive translational procedures as this resource also contains MSC with the same potency for chondrogenic differentiation as that of bone marrow MSC (Zvaifler et al., 2000; Huang et al., 2009; Raghunath et al., 2010; Al Faqeh et al., 2012). The purpose of this systematic review is to evaluate the potential of peripheral blood-derived stem cells in the treatment of cartilage injury by collecting relevant literature on the treatment of cartilage injury with peripheral blood-derived stem cells in the past two decades, including in vitro and in vivo experimental articles.
2 Materials and Methods
2.1 Data Sources and Search Strategy
We conducted a systematic review based on the PRISMA (Preferred Reporting Items for Systematic Review and Meta-Analysis) guidelines (Moher et al., 2009). We used a text search strategy using the [“mesenchymal stem cells” (MeSH term) OR “MSC” OR “BMMSC” OR “PBMSC” OR” PBMNC” OR “peripheral blood stem cells”] AND [cartilage injury (MeSH term) OR “cartilage” OR “chondral lesion”]. Specifically, we searched the PubMed, Embase, and OVID databases from inception to 20 April 2022. We also assessed the bibliographies of identified studies to seek additional articles. We did not add language restrictions.
Along with the database search, we examined the references of included studies and previously published systematic reviews to identify additional studies. We also checked the International Clinical Trials Registry Platform Search Portal and ClinicalTrials.gov (https://clinicaltrials.gov/) to identify the currently ongoing or recently completed trials.
2.2 Inclusion and Exclusion Criteria
2.2.1 Inclusion
1. Any basic English-language scientific studies of the PB-derived primitive cells that exhibited chondrogenic or multipotent mesenchymal differentiation abilities.
2. in vivo animals using PB as a source of chondrogenic progenitor cells for cartilage regeneration were also included.
3. Human studies using PB as a source of chondrogenic progenitor cells for cartilage regeneration were also included.
4. Any study that has at least one outcome that can be documented.
2.3 Exclusion
Any studies of primitive cells that were not chondrogenic or not derived from the PB and in vivo studies that only used non-PB sources were excluded.
2.4 Quality Assessment
The risk of bias graph in Review Manager 5.3 was used to evaluate the methodologic quality of included RCT studies in this systematic review. This seven-element checklist qualitatively assesses various aspects of trial quality (random sequence generation, allocation concealment, blinding of participant and personnel, blinding of outcome assessment, incomplete outcome data, selective reporting, and other bias) using an ordinal scoring system comprising high risk, low risk, or unclear risk response options for each statement in Review Manager 5.3. A higher score obtained with the Review Manager 5.3 is indicative of higher methodological study quality. We did not assess publication bias with a funnel chart because we had less than 10studies for each comparison in this review.
QUADAS (Quality Assessment of Diagnostic Accuracy Studies) was used to evaluate the methodologic quality of other studies. The detailed items of the scale are as follows:
1. Was a consecutive or random sample of patients enrolled? Yes/No/Unclear
2. Was a case-control design avoided? Yes/No/Unclear
3. Did the study avoid inappropriate exclusions? Yes/No/Unclear
4. Could the selection of patients have introduced bias? RISK: LOW/HIGH/UNCLEAR
5. Is there a concern that the included patients do not match the review question? CONCERN: LOW/HIGH/UNCLEAR
6. Were the index test results interpreted without the knowledge of the results of the reference standard? Yes/No/Unclear
7. If a threshold was used, was it prespecified? Yes/No/Unclear
8. Could the conduct or interpretation of the index test have introduced bias? RISK: LOW /HIGH/UNCLEAR
9. Is there a concern that the index test, its conduct, or interpretation differ from the review question? CONCERN: LOW /HIGH/UNCLEAR
10. Is the reference standard likely to correctly classify the target condition? Yes/No/Unclear
11. Were the reference standard results interpreted without the knowledge of the results of the index test? Yes/No/Unclear
12. Could the reference standard, its conduct, or its interpretation have introduced bias? RISK: LOW /HIGH/UNCLEAR
13. Is there a concern that the target condition, as defined by the reference standard, does match the review questions? CONCERN: LOW /HIGH/UNCLEAR
14. Was there an appropriate interval between index test(s) and reference standard? Yes/No/Unclear
15. Did all patients receive reference standard? Yes/No/Unclear
16. Did patients receive the same reference standard? Yes/No/Unclear
17. Were all patients included in the analysis? Yes/No/Unclear
18. Could the patient flow have introduced bias? RISK: LOW /HIGH/UNCLEAR
2.5 Data Extraction
A single reviewer screened all the citations and abstracts generated by the literature search and applied the selection criteria. Identified randomized trials were assessed for inclusion by two reviewers. Any disagreement between them on the eligibility of certain studies was resolved through discussion with a third reviewer. The titles of journals and names of authors were not masked during the study selection process.
Each investigator independently extracted the following data:
1. Study characteristics, including species, number, character of included species, type of study, evaluation method, injury site, and degree of damage.
2. Experimental details including cell source, cultivation and extraction methods, cell character markers, number of cells, cell implantation method, and surgical approach.
3. Experimental results and adverse events.
3 Result
3.1 Basic Characteristic
According to our retrieval strategy abovementioned, we retrieved a total of 3,076 articles. After a brief reading of the abstracts and titles, duplicate articles and irrelevant articles were excluded, and a total of 296 articles were reviewed in detail (Figure 1). After excluding articles that do not contain related stem cells, we ultimately included 24 articles between 2008 and 2022 for the systematic review (Jancewicz et al., 1995; Pufe et al., 2008; Saw et al., 2011; Casado et al., 2012; Chong et al., 2012; Kim et al., 2012; Skowroński et al., 2012; Saw et al., 2013; Skowroński and Rutka, 2013; Turajane et al., 2013; Broeckx et al., 2014a; Fu et al., 2014a; Broeckx et al., 2014b; Fu et al., 2014b; Turajane et al., 2014; Hopper et al., 2015b; Frisch et al., 2017b; Broeckx et al., 2019a; Broeckx et al., 2019b; Monckeberg et al., 2019; Ying et al., 2020; Henson et al., 2021). The data from 24 studies were analyzed, including seven fully in vitro studies and 17 in vivo studies (Table 1). The experimental subject includes humans, sheep, rabbits, and horses. A total of nine articles included in vitro experiments, all (100%) of which confirmed the tendency of peripheral blood-derived stem cells to differentiate into chondrocytes. In terms of cell sources, 10 articles used G-CSF-stimulated PBMSCs, four articles used chondro-induced PBMSCs, and eight articles directly used the peripheral blood stem cells after apheresis or gradient centrifugation. In vivo experiments include three comparative studies, one prospective study, three RCTs, five case reports, one preliminary study, and one pilot study. Cartilage defects in nine of the studies were graded with ICRS and were all greater than grade 3. All characteristics of the included literature are listed in Tables 1, 2, 3. Figures 2, 3 demonstrates the basic experimental procedure. Table 1 and Figure 4 show the methodological quality evaluation results. The detailed results of the quality evaluation are shown in Figure 4 and Table 4.
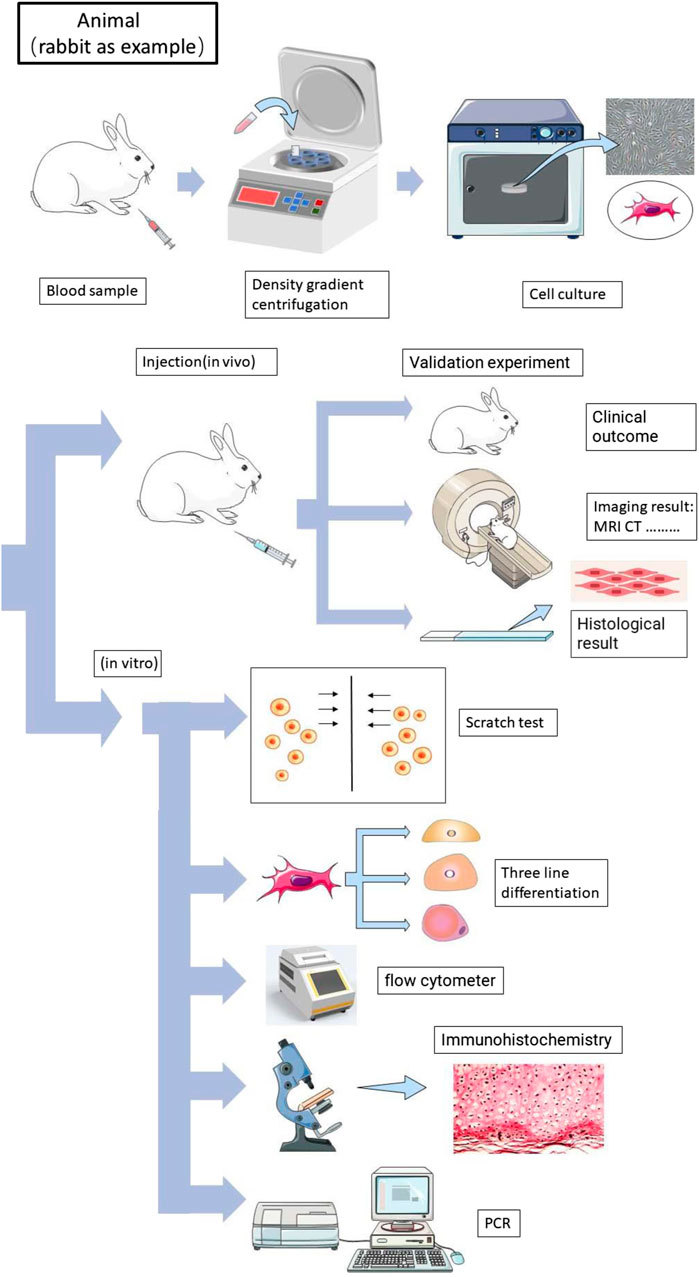
FIGURE 3. The general process of animal and in vitro peripheral blood-derived stem cell experiments (rabbit as an example).
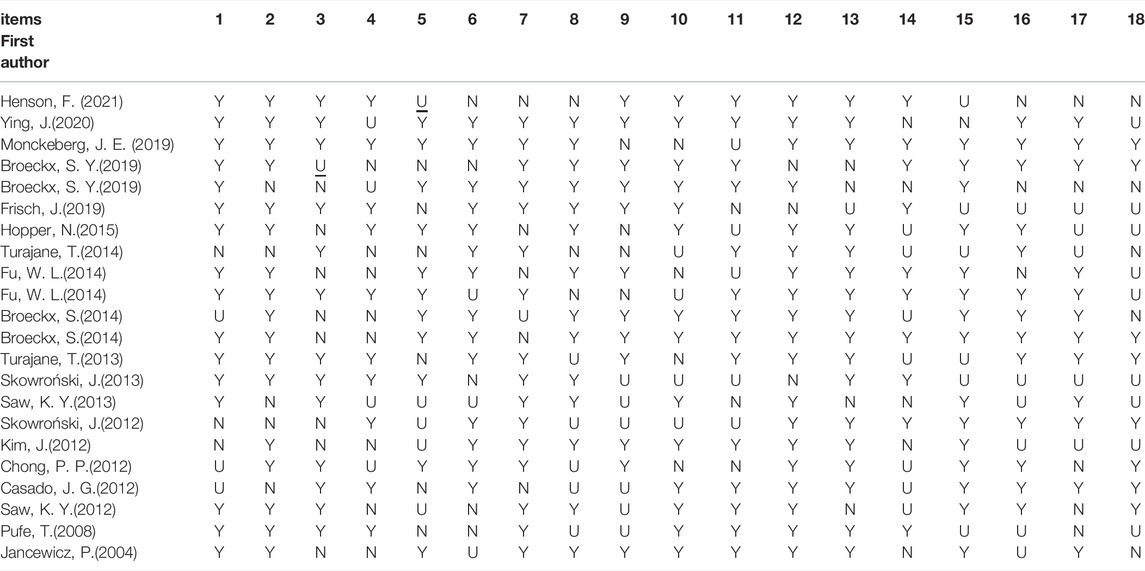
TABLE 4. QUADAS quality assessment of other study(Y =Yes, N=No, and U=Unclear) based on the items that are described in the method section.
3.2 PBMSC in Humans
We included nine studies with human subjects, including one prospective study, three comparative studies, 4 case reports, and one RCTs (Jancewicz et al., 1995; Saw et al., 2011; Skowroński et al., 2012; Saw et al., 2013; Skowroński and Rutka, 2013; Fu et al., 2014b; Turajane et al., 2014; Monckeberg et al., 2019; Ying et al., 2020). A total of 225 people were included. Most injuries are concentrated in the patella and femoral condyle, and a few in the hip joint. Cartilage damage in all patients included in the study was degenerative. Except for the study conducted by Ying et al. (2020), which did not report the degree of cartilage damage, the rest of the studies reported that cartilage damage and the ICRS grade was greater than grade 3. The evaluation methods include International Knee Documentation Committee score (IKDC), visual analog scale (VAS), and International Cartilage Repair Society morphologic score system (ICRS) for subjective scoring; X-ray and Magnetic Resonance Imaging (MRI) for imaging examination; and tissue biopsy and Immunohistochemistry for laboratory examination. Seven studies used the G-CSF-stimulated peripheral blood stem cells, and two studies used apheresis peripheral blood stem cells. The preparation process uses red blood cell lysis and gradient centrifugation, which has been proven to be effective in isolating PBMSCs (Kim et al., 2012). All studies used the intra-articular injection for cell implantation. Five articles report on postoperative treatment, including drug therapy: acetaminophen, NSAIDs, and Dexmedetomidine, and different types of rehabilitation programs. Seven studies reported clinical outcomes, except Ying, J (Ying et al., 2020), who reported no significant difference in HHSs between the two groups at 36 months followup. However, clinical results of the remaining six studies reported a significant improvement in clinical scores (KOOS, VAS, The Western Ontario, and McMaster Universities Osteoarthritis Index (WOMAC)) after the peripheral blood-derived stem cells were injected into the defect site. Similarly, in the imaging results and laboratory test results, except for Ying, J, all the other reported studies showed a statistically significant improvement after peripheral blood stem cell transplantation. In terms of adverse events, except for a case of deep vein thrombosis reported by Saw, K. Y, which is a high-risk event, all the other adverse events are low-risk events, including fever and joint adhesion (Saw et al., 2013). The detailed information is shown in Table 2.
3.3 PBMSC in Animals
Six animal studies were included in our systematic review, including two RCTs, one comparative study, one controlled laboratory study, one preliminary study, and one pilot study (Broeckx et al., 2014a; Fu et al., 2014a; Broeckx et al., 2014b; Broeckx et al., 2019a; Monckeberg et al., 2019; Henson et al., 2021), and subjects included sheep, horses, and rabbits. The lesions are mainly concentrated in the lower extremity joints or the metacarpophalangeal joints. The cartilage defects of the experimental subjects of Fu, W. L (Fu et al., 2014a), Henson, F. (Henson et al., 2021), and Broeckx, S. Y (Broeckx et al., 2019b) were all using experimental modeling, and the cartilage defects of the experimental subjects of other researchers were all caused by degenerative diseases. Grade of cartilage damage was not reported. Two studies used the G-CSF-stimulated peripheral blood stem cells, and four studies used chondrogenic induced PBMSCs. Gradient centrifugation was used for cell isolation in all experiments, and plastic adhesion was also used in some experiments. All studies did not impose strict requirements on the postoperative rehabilitation of experimental animals and did not limit their range and intensity of activities. Only Broeckx, S. Y. in the 2019 experiment gave experimental animals postoperative drug treatment for sedation and analgesia. In experiments where flow cytometry was performed, Henson, F. et al. (2021) detected: CD34, CD45, CD73, CD90,and CD 105, and Broeckx, S. Y. et al. (2019) detected: CD45, MHC II, CD29, CD44, and CD90, Fu, W. L. et al. (2014) detected: CD44, CD45, and MHC II. All studies used intra-articular injection for cell implantation. In the postoperative results, the clinical results showed a consistent trend of improvement, whether it was objective indicators or subjective scores. In the imaging results, except for the study done by Broeckx, S. Y in 2019, the radiographic changes were not significantly different. The rest showed improvements after the use of peripheral blood-derived stem cells. In the absence of laboratory validation, only three articles showed increased levels of cartilage-related matrix or components around damaged cartilage tissue, such as type II collagen and cartilage oligomeric matrix protein. The detailed information is shown in Table 1.
3.4 PBMSC In Vitro
In this systematic review, we included a total of seven articles from in vitro studies (Pufe et al., 2008; Casado et al., 2012; Chong et al., 2012; Kim et al., 2012; Turajane et al., 2014; Hopper et al., 2015b; Frisch et al., 2017b). Among them, the donors of three experiments were humans, the donors of one experiment were pigs, and the peripheral blood donors were not specified in the remaining experiments. The validation methods for in vitro experiments include scratch experiments, immunohistochemistry, flow cytometry, RT-PCR, and more. The authors described the peripheral blood-derived stem cells used in the article, including the peripheral blood mononuclear cells, PBMSC, autologous G-CSF activated PB, and peripheral blood stem cells (PBSCs). CD105+ was found in all experiments with flow cytometry results, but CD34+ was found in all experiments by Turajane, T. et al., may indicate that the cells used in the experiments are the nonmesenchymal presence of stem cells. Other experiments uncovered the secretion of many chemokines, which may also be largely involved in the induction of cartilage repair. In terms of results, all studies have proved that the peripheral blood-derived stem cells can differentiate into cartilage and have the potential to repair cartilage damage. Hopper, N, and Turajane, T. all found the upregulation of SOX-9 in their experiments, indicating that the peripheral blood-derived stem cells have a regulatory effect on cartilage differentiation. The formation of the extrachondral matrix was found in all in vitro studies, which is important for cartilage repair. The detailed information is shown in Table 3.
4 Discussion
According to the research on stem cells derived from peripheral blood in vitro, they have the same or similar chondrogenic differentiation ability as that of the bone marrow mesenchymal stem cells in the process of culture and passage in vitro, as Chong, P. P. showed in his research. (Chong et al., 2012; Gong et al., 2021). Combined with the human and animal research reports on its improved in vivo results, this systematic review shows that peripheral blood-derived stem cells have chondrogenic differentiation ability and can induce chondrogenic differentiation and repair in vivo, and have statistical significance in the clinical and imaging prognosis. There is improvement of academic differences. Compared with bone marrow, the peripheral blood is easier to obtain, widely sourced, and simple to obtain. In the future, peripheral blood will be a more potential cell source for cell therapy in the treatment of cartilage damage.
However, some studies have contrary results. In the study of Ying, J. et al. (Ying et al., 2020), peripheral blood-derived stem cells did not show improvement in the clinical and imaging results in the treatment of femoral head necrosis, and combined treatment in histology. The bone destruction in the group was more severe than that in the control group. But a previous study showed that combination therapy with an intra-arterial infusion of PBSCs showed improved the outcomes in patients with early and mid-stage necrosis of the femoral head (Schmitt-Sody et al., 2008; Mao et al., 2015). Considering the advantages of PBSC in easily harvesting and stimulating neovascularization and osteogenesis in the damaged skeletal tissue, PBSC transplantation is a selective approach for the treatment of ONFH (Zhang et al., 2016). In this study, it was used to treat patients with femoral head necrosis with cartilage cap separation, which has exceeded the early and middle stages and is an advanced stage disease (Xiong et al., 2016). At this stage, the active expression of osteoclasts and the widespread occurrence of inflammatory responses lead to irreversible necrosis of the femoral head, which may require more complex mechanisms to explain (Feng et al., 2010). Femoral head necrosis is a complex pathophysiological process involving cartilage, subchondral bone, bone, and surrounding tissues. The repair mechanism of cartilage damaged by the peripheral blood stem cells alone may not be able to offset the overall damage caused by the inflammatory response. Moreover, in this study conducted by Ying, J. et al., although the injection of PBSCs into the internal circumflex artery did not improve the survival rate of femoral head necrosis, it had a good effect on relieving pain and improving the joint function. This result can also reflect that peripheral blood-derived stem cells have a repairing effect on intra-articular cartilage damage, although it cannot be reflected in the histology of this study (Hopson and Siverhus, 1988). This makes us think that in the treatment of some diseases with more complex mechanisms than simple cartilage damage, the use of stem cells derived from peripheral blood alone may not have a good prognosis, and more combined treatment or surgical treatment is needed. But not being able to cure the disease is not the same as denying its effect on the repair of cartilage damage Figure 5.
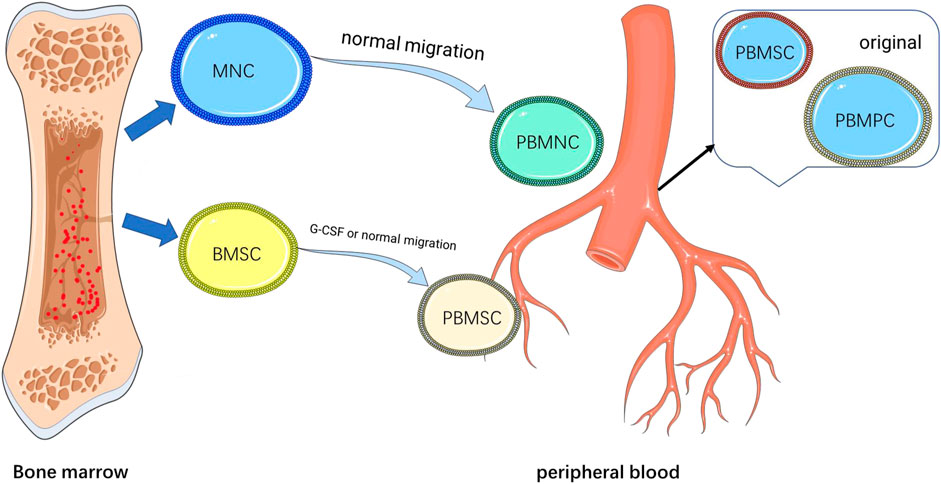
FIGURE 5. Basic biology of blood-derived stem cells. MNC, mononuclear cells; BMSC, Bone marrow mesenchymal stem cells; PBMSC, peripheral blood mesenchymal stem cells; PBMNC, peripheral blood mononuclear cells; and PBMPC, peripheral blood mesenchymal progenitor cells.
The cell types and potential repair mechanisms are detailed in Figure 5, 6. At present, the cell source used in most research is G-CSF activated PB or chondrogenic-induced PBMSCs. It has been demonstrated in the previous literature that G-CSF and CXCR4 antagonists can mobilize mesenchymal stem cells into peripheral blood (Pelus, 2008; Kolonin and Simmons, 2009). It can improve the success rate of subsequent mesenchymal stem cell culture, and the density of mesenchymal stem cells is also an important feature to evaluate cartilage repair. Moreover, in the other literature, a simple injection of G-CSF can make bone marrow and peripheral blood mesenchymal stem cells home to the joint cavity and help cartilage regeneration (Sasaki et al., 2017; Turajane et al., 2017). The literature included in this systematic review also showed that G-CSF activated PB has the potential for chondrogenic differentiation and repair and is a good alternative resource. While chondrogenic-induced PBMSCs secrete more extrachondral matrix including aggrecan, type II collagen, and cartilage oligomeric matrix protein when cultured in vitro, which reflects better proliferation ability (Broeckx et al., 2014a) and has been shown in one study to better adhere to cartilage in explant cultures (Spaas et al., 2015). TGF-β, one of the cartilage-stimulating growth factors used in the current study for predifferentiation of chondrocyte differentiation, can reduce the expression of MHC (Berglund et al., 2017). This can reduce the occurrence of inflammatory reactions and reduce the chance of immune rejection (Schnabel et al., 2014). The two preparation methods have their advantages, but there is no research to compare the advantages and disadvantages of the two methods to give guiding opinions. Future research can combine the advantages of the two methods, and it is believed that a more effective new preparation method can be obtained.
Stem cells have many advantages and can effectively treat cartilage damage; for example, they have strong self-renewal capacity, pluripotency, and plasticity. However, the properties of MSCs may be altered by various elements of the local microenvironment that influence differentiation, may cause reduced chondrogenic activity or differentiation into other tissues, so they may suffer from disadvantages such as eventual hypertrophy or tumorigenesis (Chen and Tuan, 2008; Vinatier et al., 2009; Koh et al., 2014; Pandey et al., 2022). However, in the studies we included, adverse events were mild and there was no worsening change in the imaging findings. This may indicate that stem cells derived from peripheral blood have stable differentiation (Chong et al., 2012). This also proves our point that peripheral blood-derived stem cells are an important source of cells to repair cartilage damage.
This article also has certain limitations. In the selection of literature, due to the continuous updating of preparation methods and repair mechanisms, we only included relevant literature after 2008, excluding some studies in older periods, which may make the research results subject to influence. In the statistics of cell phenotype, no further analysis was performed for the events whose cells highly expressed CD34+ and some studies did not express the mesenchymal stem cell marker CD105+. This means that, in some of the included studies, it is not only mesenchymal stem cells that perform cartilage repair, but may also be mononuclear cells or other stem cells in peripheral blood. Therefore, here, we refer to them as the peripheral blood-derived stem cells and use this fully as a resource for cartilage repair.
5 Conclusion
Stem cells derived from peripheral blood have the ability to repair cartilage and are an important resource for the treatment of cartilage damage in the future. The specific mechanism and way of repairing cartilage need further study.
Data Availability Statement
The original contributions presented in the study are included in the article/Supplementary Material. Further inquiries can be directed to the corresponding author.
Author Contributions
All authors contributed to the study conception and design. Material preparation, data collection, and analysis were performed by YZ. The first draft of the manuscript was written by YZ and all authors commented on previous versions of the manuscript. All authors read and approved the final manuscript.
Conflict of Interest
The authors declare that the research was conducted in the absence of any commercial or financial relationships that could be construed as a potential conflict of interest.
Publisher’s Note
All claims expressed in this article are solely those of the authors and do not necessarily represent those of their affiliated organizations, or those of the publisher, the editors, and the reviewers. Any product that may be evaluated in this article, or claim that may be made by its manufacturer, is not guaranteed or endorsed by the publisher.
References
Al Faqeh, H., Nor Hamdan, B. M. Y., Chen, H. C., Aminuddin, B. S., and Ruszymah, B. H. I. (2012). The Potential of Intra-articular Injection of Chondrogenic-Induced Bone Marrow Stem Cells to Retard the Progression of Osteoarthritis in a Sheep Model. Exp. Gerontol. 47 (6), 458–464. doi:10.1016/j.exger.2012.03.018
Berglund, A. K., Fisher, M. B., Cameron, K. A., Poole, E. J., and Schnabel, L. V. (2017). Transforming Growth Factor-Β2 Downregulates Major Histocompatibility Complex (MHC) I and MHC II Surface Expression on Equine Bone Marrow-Derived Mesenchymal Stem Cells without Altering Other Phenotypic Cell Surface Markers. Front. Vet. Sci. 4, 84. doi:10.3389/fvets.2017.00084
Bieback, K., Kern, S., Kocaömer, A., Ferlik, K., and Bugert, P. (2008). Comparing Mesenchymal Stromal Cells from Different Human Tissues: Bone Marrow, Adipose Tissue and Umbilical Cord Blood. Biomed. Mater Eng. 18 (1 Suppl. l), S71–S76.
Brittberg, M., Lindahl, A., Nilsson, A., Ohlsson, C., Isaksson, O., and Peterson, L. (1994). Treatment of Deep Cartilage Defects in the Knee with Autologous Chondrocyte Transplantation. N. Engl. J. Med. 331 (14), 889–895. doi:10.1056/nejm199410063311401
Broeckx, S., Suls, M., Beerts, C., Vandenberghe, A., Seys, B., Wuertz-Kozak, K., et al. (2014). Allogenic Mesenchymal Stem Cells as a Treatment for Equine Degenerative Joint Disease: a Pilot Study. Cscr 9 (6), 497–503. doi:10.2174/1574888x09666140826110601
Broeckx, S. Y., Martens, A. M., Bertone, A. L., Van Brantegem, L., Duchateau, L., Van Hecke, L., et al. (2019). The Use of Equine Chondrogenic‐induced Mesenchymal Stem Cells as a Treatment for Osteoarthritis: A Randomised, Double‐blinded, Placebo‐controlled Proof‐of‐concept Study. Equine Vet. J. 51 (6), 787–794. doi:10.1111/evj.13089
Broeckx, S. Y., Seys, B., Suls, M., Vandenberghe, A., Mariën, T., Adriaensen, E., et al. (2019). Equine Allogeneic Chondrogenic Induced Mesenchymal Stem Cells Are an Effective Treatment for Degenerative Joint Disease in Horses. Stem cells Dev. 28 (6), 410–422. doi:10.1089/scd.2018.0061
Broeckx, S., Zimmerman, M., Crocetti, S., Suls, M., Mariën, T., Ferguson, S. J., et al. (2014). Regenerative Therapies for Equine Degenerative Joint Disease: a Preliminary Study. PloS one 9 (1), e85917. doi:10.1371/journal.pone.0085917
Casado, J. G., Gomez-Mauricio, G., Alvarez, V., Mijares, J., Tarazona, R., Bernad, A., et al. (2012). Comparative Phenotypic and Molecular Characterization of Porcine Mesenchymal Stem Cells from Different Sources for Translational Studies in a Large Animal Model. Vet. Immunol. Immunopathol. 147 (1-2), 104–112. doi:10.1016/j.vetimm.2012.03.015
Chen, F. H., and Tuan, R. S. (2008). Mesenchymal Stem Cells in Arthritic Diseases. Arthritis Res. Ther. 10 (5), 223. doi:10.1186/ar2514
Chong, P.-P., Selvaratnam, L., Abbas, A. A., and Kamarul, T. (2012). Human Peripheral Blood Derived Mesenchymal Stem Cells Demonstrate Similar Characteristics and Chondrogenic Differentiation Potential to Bone Marrow Derived Mesenchymal Stem Cells. J. Orthop. Res. 30 (4), 634–642. doi:10.1002/jor.21556
Emadedin, M., Aghdami, N., Taghiyar, L., Fazeli, R., Moghadasali, R., Jahangir, S., et al. (2012). Intra-articular Injection of Autologous Mesenchymal Stem Cells in Six Patients with Knee Osteoarthritis. Arch. Iran. Med. 15 (7), 422–428. doi:10.12157/AIM.0010
Feng, Y., Yang, S.-H., Xiao, B.-J., Xu, W.-H., Ye, S.-N., Xia, T., et al. (2010). Decreased in the Number and Function of Circulation Endothelial Progenitor Cells in Patients with Avascular Necrosis of the Femoral Head. Bone 46 (1), 32–40. doi:10.1016/j.bone.2009.09.001
Ferris, D. J., Frisbie, D. D., McIlwraith, C. W., and Kawcak, C. E. (2011). Current Joint Therapy Usage in Equine Practice: a Survey of Veterinarians 2009. Equine veterinary J. 43 (5), 530–535. doi:10.1111/j.2042-3306.2010.00324.x
Frisbie, D. D., Kisiday, J. D., Kawcak, C. E., Werpy, N. M., and McIlwraith, C. W. (2009). Evaluation of Adipose-Derived Stromal Vascular Fraction or Bone Marrow-Derived Mesenchymal Stem Cells for Treatment of Osteoarthritis. J. Orthop. Res. 27 (12), 1675–1680. doi:10.1002/jor.20933
Frisch, J., Orth, P., Rey-Rico, A., Venkatesan, J. K., Schmitt, G., Madry, H., et al. (2017). Peripheral Blood Aspirates Overexpressing IGF-IviarAAV Gene Transfer Undergo Enhanced Chondrogenic Differentiation Processes. J. Cell. Mol. Med. 21 (11), 2748–2758. doi:10.1111/jcmm.13190
Frisch, J., Orth, P., Venkatesan, J. K., Rey-Rico, A., Schmitt, G., Kohn, D., et al. (2017). Genetic Modification of Human Peripheral Blood Aspirates Using Recombinant Adeno-Associated Viral Vectors for Articular Cartilage Repair with a Focus on Chondrogenic Transforming Growth Factor-β Gene Delivery. Stem cells Transl. Med. 6 (1), 249–260. doi:10.5966/sctm.2016-0149
Fu, W.-L., Ao, Y.-F., Ke, X.-Y., Zheng, Z.-Z., Gong, X., Jiang, D., et al. (2014). Repair of Large Full-Thickness Cartilage Defect by Activating Endogenous Peripheral Blood Stem Cells and Autologous Periosteum Flap Transplantation Combined with Patellofemoral Realignment. Knee 21 (2), 609–612. doi:10.1016/j.knee.2013.10.010
Fu, W.-L., Zhou, C.-Y., and Yu, J.-K. (2014). A New Source of Mesenchymal Stem Cells for Articular Cartilage Repair. Am. J. Sports Med. 42 (3), 592–601. doi:10.1177/0363546513512778
Gong, J., Fairley, J., Cicuttini, F. M., Hussain, S. M., Vashishtha, R., Chou, L., et al. (2021). Effect of Stem Cell Injections on Osteoarthritis-Related Structural Outcomes: A Systematic Review. J. Rheumatol. 48 (4), 585–597. doi:10.3899/jrheum.200021
Henson, F., Lydon, H., Birch, M., Brooks, R., and McCaskie, A. (2021). Using Apheresis‐derived Cells to Augment Microdrilling in the Treatment of Chondral Defects in an Ovine Model. J. Orthop. Res. 39 (7), 1411–1422. doi:10.1002/jor.24889
Hopper, N., Henson, F., Brooks, R., Ali, E., Rushton, N., and Wardale, J. (2015). Peripheral Blood Derived Mononuclear Cells Enhance Osteoarthritic Human Chondrocyte Migration. Arthritis Res. Ther. 17 (1), 199. doi:10.1186/s13075-015-0709-z
Hopper, N., Wardale, J., Brooks, R., Power, J., Rushton, N., and Henson, F. (2015). Peripheral Blood Mononuclear Cells Enhance Cartilage Repair in In Vivo Osteochondral Defect Model. PloS one 10 (8), e0133937. doi:10.1371/journal.pone.0133937
Hopson, C. N., and Siverhus, S. W. (1988). Ischemic Necrosis of the Femoral Head. Treatment by Core Decompression. J. Bone & Jt. Surg. 70 (7), 1048–1051. doi:10.2106/00004623-198870070-00013
Huang, Y.-C., Yang, Z.-M., Chen, X.-H., Tan, M.-Y., Wang, J., Li, X.-Q., et al. (2009). Isolation of Mesenchymal Stem Cells from Human Placental Decidua Basalis and Resistance to Hypoxia and Serum Deprivation. Stem Cell. Rev Rep 5 (3), 247–255. doi:10.1007/s12015-009-9069-x
Hunziker, E. B. (2002). Articular Cartilage Repair: Basic Science and Clinical Progress. A Review of the Current Status and Prospects. Osteoarthr. Cartil. 10 (6), 432–463. doi:10.1053/joca.2002.0801
Jancewicz, P., Dzienis, W., Pietruczuk, M., Skowroński, J., and Bielecki, M. (1995). Osteochondral Defects of the Talus Treated by Mesenchymal Stem Cell Implantation-Eearly Results. Rocz. Akad. Med. Bialymst 49 (Suppl. 1), 25–27.
Jiang, Y., and Tuan, R. S. (2015). Origin and Function of Cartilage Stem/progenitor Cells in Osteoarthritis. Nat. Rev. Rheumatol. 11 (4), 206–212. doi:10.1038/nrrheum.2014.200
Kassis, I., Zangi, L., Rivkin, R., Levdansky, L., Samuel, S., Marx, G., et al. (2006). Isolation of Mesenchymal Stem Cells from G-CSF-Mobilized Human Peripheral Blood Using Fibrin Microbeads. Bone Marrow Transpl. 37 (10), 967–976. doi:10.1038/sj.bmt.1705358
Kim, J., Shin, J. M., Jeon, Y. J., Chung, H. M., and Chae, J.-I. (2012). Proteomic Validation of Multifunctional Molecules in Mesenchymal Stem Cells Derived from Human Bone Marrow, Umbilical Cord Blood and Peripheral Blood. PloS one 7 (5), e32350. doi:10.1371/journal.pone.0032350
Koh, Y. G., Choi, Y. J., Kwon, O. R., and Kim, Y. S. (2014). Second-Look Arthroscopic Evaluation of Cartilage Lesions after Mesenchymal Stem Cell Implantation in Osteoarthritic Knees. Am. J. Sports Med. 42 (7), 1628–1637. doi:10.1177/0363546514529641
Kolonin, M. G., and Simmons, P. J. (2009). Combinatorial Stem Cell Mobilization. Nat. Biotechnol. 27 (3), 252–253. doi:10.1038/nbt0309-252
Larochelle, A., Krouse, A., Metzger, M., Orlic, D., Donahue, R. E., Fricker, S., et al. (2006). AMD3100 Mobilizes Hematopoietic Stem Cells with Long-Term Repopulating Capacity in Nonhuman Primates. Blood 107 (9), 3772–3778. doi:10.1182/blood-2005-09-3592
Madry, H., Grün, U. W., and Knutsen, G. (2011). Cartilage Repair and Joint Preservation. Dtsch. Arzteblatt Int. 108 (40), 669–677. doi:10.3238/arztebl.2011.0669
Mao, Q., Wang, W., Xu, T., Zhang, S., Xiao, L., Chen, D., et al. (2015). Combination Treatment of Biomechanical Support and Targeted Intra-arterial Infusion of Peripheral Blood Stem Cells Mobilized by Granulocyte-Colony Stimulating Factor for the Osteonecrosis of the Femoral Head: a Randomized Controlled Clinical Trial. J. bone mineral Res. official J. Am. Soc. Bone Mineral Res. 30 (4), 647–656.
Moher, D., Liberati, A., Tetzlaff, J., and Altman, D. G. (2009). Preferred Reporting Items for Systematic Reviews and Meta-Analyses: the PRISMA Statement. PLoS Med. 6 (7), e1000097. doi:10.1371/journal.pmed.1000097
Monckeberg, J. E., Rafols, C., Apablaza, F., Gerhard, P., and Rosales, J. (2019). Intra-articular Administration of Peripheral Blood Stem Cells with Platelet-Rich Plasma Regenerated Articular Cartilage and Improved Clinical Outcomes for Knee Chondral Lesions. Knee 26 (4), 824–831. doi:10.1016/j.knee.2019.05.008
Orth, P., Rey-Rico, A., Venkatesan, J. K., Madry, H., and Cucchiarini, M. (2014). Current Perspectives in Stem Cell Research for Knee Cartilage Repair. Stem Cells Cloning 7, 1–17. doi:10.2147/SCCAA.S42880
Pandey, V., Madi, S., and Gupta, P. (2022). The Promising Role of Autologous and Allogeneic Mesenchymal Stromal Cells in Managing Knee Osteoarthritis. What Is beyond Mesenchymal Stromal Cells? J. Clin. Orthop. trauma 26, 101804. doi:10.1016/j.jcot.2022.101804
Pelus, L. M. (2008). Peripheral Blood Stem Cell Mobilization: New Regimens, New Cells, where Do We Stand. Curr. Opin. Hematol. 15 (4), 285–292. doi:10.1097/moh.0b013e328302f43a
Pufe, T., Petersen, W., Fändrich, F., Varoga, D., Wruck, C. J., Mentlein, R., et al. (2008). Programmable Cells of Monocytic Origin (PCMO): a Source of Peripheral Blood Stem Cells that Generate Collagen Type II-Producing Chondrocytes. J. Orthop. Res. 26 (3), 304–313. doi:10.1002/jor.20516
Rackwitz, L., Djouad, F., Janjanin, S., Nöth, U., and Tuan, R. S. (2014). Functional Cartilage Repair Capacity of De-differentiated, Chondrocyte- and Mesenchymal Stem Cell-Laden Hydrogels In Vitro. Osteoarthr. Cartil. 22 (8), 1148–1157. doi:10.1016/j.joca.2014.05.019
Raghunath, J., Sutherland, J., Salih, V., Mordan, N., Butler, P. E., and Seifalian, A. M. (2010). Chondrogenic Potential of Blood-Acquired Mesenchymal Progenitor Cells. J. Plastic, Reconstr. Aesthetic Surg. 63 (5), 841–847. doi:10.1016/j.bjps.2009.01.063
Reissis, D., Tang, Q. O., Cooper, N. C., Carasco, C. F., Gamie, Z., Mantalaris, A., et al. (2016). Current Clinical Evidence for the Use of Mesenchymal Stem Cells in Articular Cartilage Repair. Expert Opin. Biol. Ther. 16 (4), 535–557. doi:10.1517/14712598.2016.1145651
Saris, D. B., Vanlauwe, J., Victor, J., Almqvist, K. F., Verdonk, R., Bellemans, J., et al. (2009). Treatment of Symptomatic Cartilage Defects of the Knee: Characterized Chondrocyte Implantation Results in Better Clinical Outcome at 36 Months in a Randomized Trial Compared to Microfracture. Am. J. Sports Med. 37 Suppl 1 (Suppl. 1), 10s–19s. doi:10.1177/0363546509350694
Sasaki, T., Akagi, R., Akatsu, Y., Fukawa, T., Hoshi, H., Yamamoto, Y., et al. (2017). The Effect of Systemic Administration of G-CSF on a Full-Thickness Cartilage Defect in a Rabbit Model MSC Proliferation as Presumed Mechanism. Bone & Jt. Res. 6 (3), 123–131. doi:10.1302/2046-3758.63.bjr-2016-0083
Saw, K.-Y., Anz, A., Merican, S., Tay, Y.-G., Ragavanaidu, K., Jee, C. S. Y., et al. (2011). Articular Cartilage Regeneration with Autologous Peripheral Blood Progenitor Cells and Hyaluronic Acid after Arthroscopic Subchondral Drilling: a Report of 5 Cases with Histology. Arthrosc. J. Arthrosc. Relat. Surg. 27 (4), 493–506. doi:10.1016/j.arthro.2010.11.054
Saw, K.-Y., Anz, A., Siew-Yoke Jee, C., Merican, S., Ching-Soong Ng, R., Roohi, S. A., et al. (2013). Articular Cartilage Regeneration with Autologous Peripheral Blood Stem Cells versus Hyaluronic Acid: a Randomized Controlled Trial. Arthrosc. J. Arthrosc. Relat. Surg. 29 (4), 684–694. doi:10.1016/j.arthro.2012.12.008
Schmitt-Sody, M., Kirchhoff, C., Mayer, W., Goebel, M., and Jansson, V. (2008). Avascular Necrosis of the Femoral Head: Inter- and Intraobserver Variations of Ficat and ARCO Classifications. Int. Orthop. 32 (3), 283–287.
Schnabel, L. V., Pezzanite, L. M., Antczak, D. F., Felippe, M. J. B., and Fortier, L. A. (2014). Equine Bone Marrow-Derived Mesenchymal Stromal Cells Are Heterogeneous in MHC Class II Expression and Capable of Inciting an Immune Response In Vitro. Stem Cell. Res. Ther. 5 (1), 13. doi:10.1186/scrt402
Seol, D., McCabe, D. J., Choe, H., Zheng, H., Yu, Y., Jang, K., et al. (2012). Chondrogenic Progenitor Cells Respond to Cartilage Injury. Arthritis & Rheumatism 64 (11), 3626–3637. doi:10.1002/art.34613
Skowroński, J., and Rutka, M. (2013). Osteochondral Lesions of the Knee Reconstructed with Mesenchymal Stem Cells - Results. Ortop. Traumatol. Rehabil. 15 (3), 195–204. doi:10.5604/15093492.1058409
Skowroński, J., Skowroński, R., and Rutka, M. (2012). Cartilage Lesions of the Knee Treated with Blood Mesenchymal Stem Cells - Results. Ortop. Traumatol. Rehabil. 14 (6), 569–577. doi:10.5604/15093492.1012404
Spaas, J. H., Broeckx, S. Y., Chiers, K., Ferguson, S. J., Casarosa, M., Van Bruaene, N., et al. (2015). Chondrogenic Priming at Reduced Cell Density Enhances Cartilage Adhesion of Equine Allogeneic MSCs - a Loading Sensitive Phenomenon in an Organ Culture Study with 180 Explants. Cell. Physiol. Biochem. 37 (2), 651–665. doi:10.1159/000430384
Turajane, T., Chaveewanakorn, U., Fongsarun, W., Aojanepong, J., and Papadopoulos, K. I. (2017). Avoidance of Total Knee Arthroplasty in Early Osteoarthritis of the Knee with Intra-articular Implantation of Autologous Activated Peripheral Blood Stem Cells versus Hyaluronic Acid: A Randomized Controlled Trial with Differential Effects of Growth Factor Addition. Stem Cells Int. 2017, 8925132. doi:10.1155/2017/8925132
Turajane, T., Chaweewannakorn, U., Larbpaiboonpong, V., Aojanepong, J., Thitiset, T., Honsawek, S., et al. (2013). Combination of Intra-articular Autologous Activated Peripheral Blood Stem Cells with Growth Factor Addition/ Preservation and Hyaluronic Acid in Conjunction with Arthroscopic Microdrilling Mesenchymal Cell Stimulation Improves Quality of Life and Regenerates Articular Cartilage in Early Osteoarthritic Knee Disease. J. Med. Assoc. Thai 96 (5), 580–588.
Turajane, T., Thitiset, T., Honsawek, S., Chaveewanakorn, U., Aojanepong, J., and Papadopoulos, K. I. (2014). Assessment of Chondrogenic Differentiation Potential of Autologous Activated Peripheral Blood Stem Cells on Human Early Osteoarthritic Cancellous Tibial Bone Scaffold. Musculoskelet. Surg. 98 (1), 35–43. doi:10.1007/s12306-013-0303-y
Vinatier, C., Bouffi, C., Merceron, C., Gordeladze, J., Brondello, J.-M., Jorgensen, C., et al. (2009). Cartilage Tissue Engineering: towards a Biomaterial-Assisted Mesenchymal Stem Cell Therapy. Cscr 4 (4), 318–329. doi:10.2174/157488809789649205
Xiong, M. Y., Liu, L. Q., Liu, S. Q., Liu, Z. H., and Gao, H. F. (2016). Effects of Osteoprotegerin, RANK and RANKL on Bone Destruction and Collapse in Avascular Necrosis Femoral Head. Am. J. Transl. Res. 8 (7), 3133–3140.
Ying, J., Wang, P., Ding, Q., Shen, J., O'Keefe, R. J., Chen, D., et al. (2020). Peripheral Blood Stem Cell Therapy Does Not Improve Outcomes of Femoral Head Osteonecrosis with Cap‐Shaped Separated Cartilage Defect. J. Orthop. Res. 38 (2), 269–276. doi:10.1002/jor.24471
Zhang, Y., Yin, J., Ding, H., Zhang, C., and Gao, Y.-S. (2016). Vitamin K2 Ameliorates Damage of Blood Vessels by Glucocorticoid: a Potential Mechanism for its Protective Effects in Glucocorticoid-Induced Osteonecrosis of the Femoral Head in a Rat Model. Int. J. Biol. Sci. 12 (7), 776–785. doi:10.7150/ijbs.15248
Keywords: peripheral blood-derived stem cells, cartilage injuries, PBMSC, BMSC, PBMNCs
Citation: Zhu Y and Fu W (2022) Peripheral Blood-Derived Stem Cells for the Treatment of Cartilage Injuries: A Systematic Review. Front. Bioeng. Biotechnol. 10:956614. doi: 10.3389/fbioe.2022.956614
Received: 30 May 2022; Accepted: 22 June 2022;
Published: 22 July 2022.
Edited by:
Jun Lin, First Affiliated Hospital of Soochow University, ChinaReviewed by:
Hang Lin, University of Pittsburgh, United StatesDong Jiang, Peking University Third Hospital, China
Copyright © 2022 Zhu and Fu. This is an open-access article distributed under the terms of the Creative Commons Attribution License (CC BY). The use, distribution or reproduction in other forums is permitted, provided the original author(s) and the copyright owner(s) are credited and that the original publication in this journal is cited, in accordance with accepted academic practice. No use, distribution or reproduction is permitted which does not comply with these terms.
*Correspondence: Weili Fu, Zm94d2luMjAwOEAxNjMuY29t