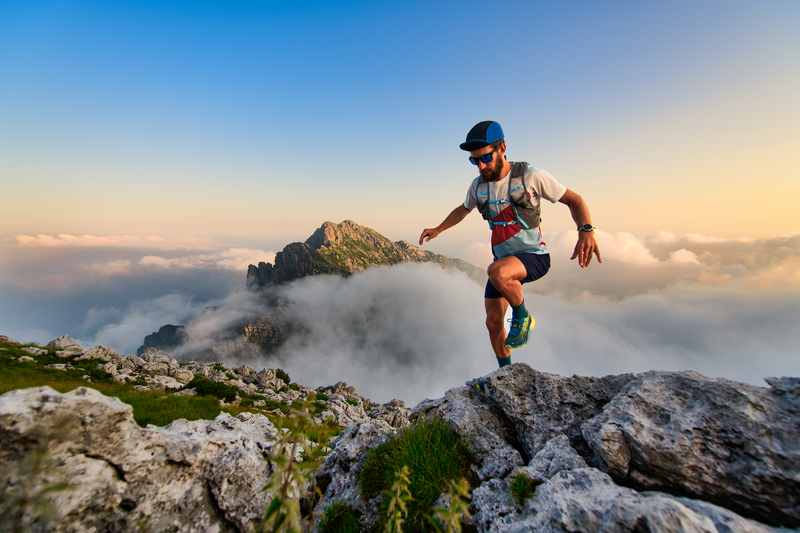
95% of researchers rate our articles as excellent or good
Learn more about the work of our research integrity team to safeguard the quality of each article we publish.
Find out more
MINI REVIEW article
Front. Bioeng. Biotechnol. , 19 July 2022
Sec. Biomaterials
Volume 10 - 2022 | https://doi.org/10.3389/fbioe.2022.954699
This article is part of the Research Topic Biomedical Applications of Natural Polymers View all 15 articles
Soft tissues such as skin, muscle, and tendon are easily damaged due to injury from physical activity and pathological lesions. For soft tissue repair and regeneration, biomaterials are often used to build scaffolds with appropriate structures and tailored functionalities that can support cell growth and new tissue formation. Among all types of scaffolds, natural polymer-based scaffolds attract much attention due to their excellent biocompatibility and tunable mechanical properties. In this comprehensive mini-review, we summarize recent progress on natural polymer-based scaffolds for soft tissue repair, focusing on clinical translations and materials design. Furthermore, the limitations and challenges, such as unsatisfied mechanical properties and unfavorable biological responses, are discussed to advance the development of novel scaffolds for soft tissue repair and regeneration toward clinical translation.
Soft tissue injury is generally caused by traumatic or pathological lesions where muscle or connective tissues get damaged (Henriksen et al., 2017). Biomaterials are used to replace the impaired soft tissue or function as scaffolds to facilitate tissue regeneration to repair the damaged soft tissue (Keane and Badylak, 2014; Gaharwar et al., 2020). Though the direct replacement of the damaged soft tissue using inert implants or autologous grafts is still commonly applied in current clinical practices, some adverse effects exist, such as chronic pain and implant-related complications (Entekhabi et al., 2021; Liang et al., 2021; Rodrigues and Raz, 2022). In comparison, scaffolds in two-dimensional or three-dimensional forms can be used as templates for tissue regeneration. The cells can bind to the scaffolds and then proliferate and differentiate (Avolio et al., 2017; Turnbull et al., 2020; Abdollahiyan et al., 2021; Bianchi et al., 2021; Masson-Meyers and Tayebi, 2021). In addition, growth factors can be incorporated into the scaffolds to advance tissue regrowth and repair (Hormozi et al., 2017; Kakudo et al., 2020; González-Pérez et al., 2021). To meet the clinical needs, the scaffolds for soft tissue repair should have tissue-matching mechanical properties, excellent biocompatibility, and appropriate biodegradability. Both synthetic polymers and natural polymers have been used to fabricate scaffolds. The synthetic polymers include polylactic acid (PLA), polyglycolic acid (PGA), poly (lactic-co-glycolic acid) (PLGA), and poly-e-caprolactone (PCL), while the natural polymers include proteins and polysaccharides (Janoušková, 2018; Rao et al., 2018). Compared with synthetic polymers, natural polymers such as collagen, fibrin, silk protein, chitosan, and hyaluronic acid generally present better biocompatibility but limited processability (Naghieh et al., 2017; Caillol, 2020; Taghipour et al., 2020). With the rapid development of processing technology in recent years, more natural polymer-based scaffolds have been successfully fabricated and applied in biomedical applications. This mini-review summarizes the status of the natural polymer-based scaffold in clinical translation and the advanced processing techniques used for making scaffolds for soft tissue repair.
Over the past decades, many natural polymer-based scaffolds for soft tissue repair have been developed for biomedical applications and some of them are commercially available. Table 1 summarizes the natural polymer-based scaffolds either commercially available or in clinical trials. These developed scaffolds are primarily composed of fibrinogen, collagen, silk, and alginate. Through advanced processing, these materials can be fabricated into functional scaffolds for various applications, including wound repair, hernia repair, cartilage repair, and blood vessel grafting. In some cases, the repairing efficacy can be improved by incorporating bioactive materials such as growth factors and antibacterial agents in the scaffolds. In recent years, silk-based scaffolds have attracted much attention due to their excellent mechanical properties and biocompatibility (Zhao and Li, 2011; Zhou et al., 2018; Mao et al., 2021; Zhao et al., 2021).
An ideal scaffold for soft tissue repair should meet the requirements for specific applications, including good biocompatibility, suitable mechanical properties, satisfied porosity, and controlled degradability. (Arbade et al., 2020) (Janoušková, 2018) The satisfied pore size for soft engineering is ∼5–200 μM. (Janoušková, 2018) Regarding the mechanical properties, constructing scaffolds with matching mechanical properties to native soft tissues is very critical. Human tissues span a broad spectrum of mechanical properties, where stiffness of soft tissues typically ranges from 1 kPa (e.g., brain) to ∼1 MPa (e.g., nerve and cartilage). (Guimarães et al., 2020) Over the past decades, numerous approaches have been developed for fabricating natural polymer-based scaffolds, such as electrospinning, freeze-drying, and 3D printing. In this section, we provide a general overview of these approaches and discuss their use in processing natural polymers into functional scaffolds.
Electrospinning offers a convenient approach to fabricate fiber-based scaffolds for soft tissue repair. Nanofibers can be fabricated through electrospinning from polymer solutions under a high electrical field and further organized into porous nanofiber-based mats. When designing an ideal electrospun scaffold for soft tissue repair, some critical factors need to be considered. These factors include biocompatibility, mechanical properties, porosity, and the ability to regulate cellular behavior (Zhong et al., 2022). Many studies have been reported on fabrication of natural polymer-based scaffolds using electrospinning. Lee et al. fabricated electrospun nanofibrous gelatin sheets and investigated the influence of electron beam (e-beam) irradiation doses on the molecular weight, morphology, pore structure, and cell proliferation profiles of the sheets (Lee et al., 2017). In addition, electrospinning using a core-shell nozzle was employed to make collagen/polyvinylpyrrolidone (PVP) core-shell nanofibers where the collagen was encapsulated within a shell of PVP. The PVP shell was then washed away in a basic ethanol solution to yield anisotropic collagen nanofibers which mimics the structures of the native extracellular matrix (Wakuda et al., 2018). It is worth mentioning here that the structure of the nanofiber-based mats mimics the structure of the natural extracellular matrix, providing a biomimetic microenvironment for cells to proliferate and differentiate (Nie et al., 2020). Moreover, some strategies have been developed to enhance the physical properties and biofunctions of the scaffolds. These scaffolds have been widely used in several soft tissue engineering, such as skin, vascular tissue, cavernous nerve (CN) and cardiac tissues (Ehrmann, 2021). For instance, Uibo et al. demonstrated that the scaffold composed of salmon fibrinogen and chitosan could promote wound healing without any complications (Laidmäe et al., 2018). Jadbabaei et al. developed a novel approach to enhance the electrospinnability of sodium alginate and made alginate-PVA polymeric scaffolds for skin tissue engineering applications (Jadbabaei et al., 2021). Zhang et al. successfully fabricated silk-based scaffolds for cavernous nerve (CN) regeneration using coaxial electrospinning. The scaffolds with a core of RSF-VEGF and a shell of RSF-BDNF promoted the regeneration of cavernous nerves and were able to converse into nerve guidance conduit to facilitate nerve regeneration (Figure 1A) (Zhang et al., 2016). Recombinant spider silk protein (pNSR32) and gelatin (Gt) were also used to enhance the cytocompatibility of electrospun PCL scaffolds. The pNSR32/PCL/Gt composite scaffolds show potential for small-caliber vascular tissue engineering (Xiang et al., 2018). Wu et al. designed a scaffold for cardiac tissue regeneration to guide the orientation of the cells by mimicking the anisotropic cardiac structure (Wu Y. et al., 2017). The scaffold within a hydrogel shell was composed of aligned electrospun conductive nanofibers (NEYs-NET) which contained the polycaprolactone, silk fibroin, and carbon nanotubes. Cardiomyocytes (CMs) were aligned along the nanofibers on each layer of the 3D nanofibrous scaffold in the stable hydrogel environment (Figure 1B). Overall, the electrospinning technique allows researchers to fabricate ECM-mimic nanofibrous scaffolds with tunable fiber diameters, surface areas, porosity depending on different technique factors, such as solution viscosity and work voltage. Furthermore, electrospinning provides the feasibility for introducing and incorporating bioactive molecules for soft tissue repair and regeneration (Arbade et al., 2019).
FIGURE 1. The fabrication of natural polymer-based scaffolds via various methods: (A,B) Electrospinning. (C) Freeze-drying and (D) 3D printing. The pictures got permissions from (Zhang et al., 2016), (Wu Y. et al., 2017), (Feng et al., 2021), (Luo et al., 2022), respectively.
Freeze-drying is an easy and eco-friendly method that can be readily used to fabricate 3D scaffolds with microporous structures. For fabrication of natural polymer-based scaffolds, natural polymers were first dissolved in water to obtain aqueous solutions, followed by freeze-drying. During freeze-drying, the water in the frozen sample undergoes sublimation under a high vacuum, leading to the scaffolds with porous structures. The pore size in the scaffolds depends on the type of natural polymers and the concentration of the solution. The porous structures generated via freeze-drying benefit the cells to attach, differentiate, proliferate, and mass transport. Indurkar et al. showed that the physical parameters of the scaffolds, such as surface roughness, porosity, interconnectivity, and contact angle influence the transport of nutrition and waste products (Indurkar et al., 2020). Furthermore, Afjoul et al. prepared alginate-gelatin scaffolds through freezing dry and revealed that the ratio of alginate to gelatin affects swelling, biodegradation, cell culture, and mechanical properties of the scaffolds. The optimized scaffolds showed good biocompatibility and satisfied outcomes of wound healing in rats (Afjoul et al., 2020). In another study, Chen et al. prepared a hybrid cobalt-doped alginate/waterborne polyurethane 3D porous scaffold with nano-topology of a “coral reef-like” rough surface via two-step freeze-drying (Chen et al., 2021). The “coral reef-like” rugged surface topology and bioactive cobalt dopant synergistically promote the neurite outgrowth and up-regulate the synaptophysin expression of neuron-like cells PC12 on the scaffold. In addition, two types of cellulose-derived materials, oxidized cellulose and carboxymethyl cellulose (CMC), were mixed with collagen to fabricate scaffolds through freeze drying. The prepared scaffolds showed good mechanical properties, hemostasis, and antibacterial properties (Kacvinská et al., 2022). Protein-based scaffolds have also been developed. For example, dual-crosslinked silk fibroin scaffolds with EGDE have been developed, where the researchers showed that an appropriate dosage of crosslinking agent was critical to achieve good mechanical properties, in vivo degradability, and mild immune responses in soft tissue engineering (Mao et al., 2021). The scaffold notably relieved the inflammatory response of microglial cells BV2 with the transformation from pro-inflammatory (M1) to anti-inflammatory (M2) phenotype. Regarding better control of the structure, morphology, and density of scaffolds, Jiang et al. developed chitosan scaffolds with tunable microchannels by combining a 3D printing-assisted microfiber templates-leaching approach and a freeze-drying approach (Jiang et al., 2021). Moreover, Feng et al. fabricated a novel chitosan scaffold with lamellar structures by mimicking the layered structure of the attached gingiva using a bidirectional freeze-drying method (Feng et al., 2021). The bio-inspired lamellar chitosan scaffold (LCS) with ordered porous structure showed excellent mechanical properties, good cell-compatibility and could promote the vessel formation and gingival tissue regeneration in vivo. In addition, the LCS is found to be capable of inducing macrophage differentiation to M2 macrophages, which is thought to play an important role in tissue regeneration (Figure 1C). Also, the microstructure of the scaffolds could be controlled by optimizing the mold and freezing parameters for a certain application. Brougham et al. developed organ-specific collagen-based scaffolds geometries for tissue engineering applications, where the geometries of the scaffolds could be tailored by adjusting the mold patterns and freezing parameters (Brougham et al., 2017). In a brief summary, freeze-drying is a good method for natural polymer-based scaffold fabrication since it is easily applied to obtain porous structures without a high temperature or a washing step though the fabrication time is relatively long (Boffito et al., 2014).
Three-dimensional (3D) printing is a technique that can be used to fabricate biomedical scaffolds in a controlled way (Pérez-Köhler et al., 2021). Compared with traditional thermal-based 3D printing, 3D bioprinting combines 3D printing with living cells or other non-living biological materials (e.g., growth factors, drugs) to construct scaffolds for tissue engineering and tissue regeneration. 3D bioprinting allows researchers to design 3D tissue-mimicking scaffolds which provide tailored cellular environments to facilitate the growth and proliferation of cells (Kim et al., 2016; Perez-Puyana et al., 2020). A broad range of natural polymer-based scaffolds have been fabricated using 3D bioprinting and some studies have been reported. Regarding the materials used for making the bioinks, a variety of materials have been used including collagen, gelatin, alginate, silk fibroin, and extra cellular matrix (ECM). For example, Jang et al. fabricated the artificial skin based on decellularized ECM derived from porcine skin via 3D bioprinting method (Jang et al., 2021). The 3D printed artificial skin exhibited rapid re-epithelialization and facilitate tissue regeneration on a mouse chimney wound model, showing great potential of clinical translation. In addition, alginate-based scaffolds with the features of high cell viability and low concentration alginate for potential nerve tissue engineering application were developed (Naghieh et al., 2019). Moreover, Tijore et al. developed a 3D bioprinting microchannel gelatin hydrogel that promoted human mesenchymal stem cells (hMSCs) myocardial commitment and supports native cardiomyocytes (CMs) contractile functionality (Tijore et al., 2018). Luo et al. used gelatin and alginate to fabricate scaffolds with microporous structures and interconnected microchannels using 3D bioprinting (Figure 1D) (Luo et al., 2022). The fabricated scaffold could support vascularization and growth of new tissues, promoting wound healing. Furthermore, Wang et al. fabricated a hybrid hydrogel system using a combination of decellularized extracellular matrix (dECM-G) and photo-crosslinkable gelatin methacrylate (GelMA) for nerve regeneration (Wang et al., 2022). The system showed good printability and structural fidelity for facilitating neurite growth and cell migration. Fabrication of scaffolds with designed microstructures to guide cell growth also attracts a lot of attention recently. Wu et al. precisely controlled architectures of micro-structured and stretchable chitosan hydrogels for guided cell growth (Wu Q. et al., 2017). The hybrid bioink prepared with gelatin, sodium alginate, and carbon nanotubes were used to fabricate cylindrical scaffolds through a combination of the vertical directional extrusion of printing nozzle and axial rotation of stepper motor module for blood vessel regeneration (Li et al., 2020).
Natural polymer-based scaffolds have been rapidly developed and applied in soft tissue repair in the past few decades. Some products are now commercially available and in clinical use. However, some limitations are associated with the current products, such as unsatisfied mechanical properties, uncontrolled degradability, and unfavorable immune response. Some critical points need to be considered when developing high-performance scaffolds that better meet clinical needs. Firstly, advanced processing approaches are required to achieve high-quality processing of natural polymers. For collagen-based materials, how to maintain their bioactivity during processing is a challenge. Secondly, rational materials design and advanced fabrication technologies are needed since the structures and properties of the scaffolds should be tailored for different applications. For instance, for treating pelvic organ prolapse, porous scaffolds with robust mechanical properties and controlled biodegradability are required. In some applications, an aligned scaffold is preferred to allow the cells to grow directionally. Moreover, enhancing the biocompatibility and mimicking the biological functions of the extracellular matrix should be considered. Integrins and cadherins can be grafted to the scaffolds since they are serving as adhesion molecules for migration and localization of cells. Furthermore, patient-oriented scaffold design with the assistance of the 3D printing fabrication technique is of great potential to offer precise repair. Thirdly, scaffolds with bioresponsiveness or biofunctions are promising since such scaffolds allow better tissue repair control. For example, scaffolds with the incorporation of antibiotics can effectively prevent infections during the tissue regeneration process. In addition, growth factors can be incorporated into scaffolds to facilitate tissue repair. Lastly, a comprehensive understanding of the materials-cell interactions is needed to support the development of novel functional scaffolds. The fundamental research would lay a solid foundation for novel material designs, the development of advanced fabrication techniques, and clinical translations.
MC, RJ, ND, XZ, and XL wrote the manuscript; XL and CG conceived the idea of the study, supervised, and wrote the manuscript.
This work was supported by The National Health Commission of the People’s Republic of China Research Fund (WKJ-ZJ-2215) and National Natural Science Foundation of China (No. 52103129).
The authors declare that the research was conducted in the absence of any commercial or financial relationships that could be construed as a potential conflict of interest.
All claims expressed in this article are solely those of the authors and do not necessarily represent those of their affiliated organizations, or those of the publisher, the editors and the reviewers. Any product that may be evaluated in this article, or claim that may be made by its manufacturer, is not guaranteed or endorsed by the publisher.
The Supplementary Material for this article can be found online at: https://www.frontiersin.org/articles/10.3389/fbioe.2022.954699/full#supplementary-material
Abdollahiyan, P., Oroojalian, F., and Mokhtarzadeh, A. (2021). The triad of nanotechnology, cell signalling, and scaffold implantation for the successful repair of damaged organs: An overview on soft-tissue engineering. J. Control. Release 332, 460–492. doi:10.1016/j.jconrel.2021.02.036
Afjoul, H., Shamloo, A., and Kamali, A. (2020). Freeze-gelled alginate/gelatin scaffolds for wound healing applications: An in vitro, in vivo study. Mater. Sci. Eng. C 113, 110957. doi:10.1016/j.msec.2020.110957
Al-Maawi, S., Herrera-Vizcaíno, C., Orlowska, A., Willershausen, I., Sader, R., Miron, R. J., et al. (2019). Biologization of collagen-based biomaterials using liquid-platelet-rich fibrin: New insights into clinically applicable tissue engineering. Mater. (Basel) 12 (23), 3993. doi:10.3390/ma12233993
Arbade, G. K., Kumar, V., Tripathi, V., Menon, A., Bose, S., Patro, T. U., et al. (2019). Emblica officinalis-loaded poly(ε-caprolactone) electrospun nanofiber scaffold as potential antibacterial and anticancer deployable patch. New J. Chem. 43 (19), 7427–7440. doi:10.1039/C9NJ01137D
Arbade, G. K., Srivastava, J., Tripathi, V., Lenka, N., and Patro, T. U. (2020). Enhancement of hydrophilicity, biocompatibility and biodegradability of poly(ε-caprolactone) electrospun nanofiber scaffolds using poly(ethylene glycol) and poly(L-lactide-co-ε-caprolactone-co-glycolide) as additives for soft tissue engineering. J. Biomaterials Sci. Polym. Ed. 31 (13), 1648–1670. doi:10.1080/09205063.2020.1769799
Avolio, E., Alvino, V. V., Ghorbel, M. T., and Campagnolo, P. (2017). Perivascular cells and tissue engineering: Current applications and untapped potential. Pharmacol. Ther. 171, 83–92. doi:10.1016/j.pharmthera.2016.11.002
Bajuri, M. Y., Sabri, S., Mazli, N., Sarifulnizam, F. A., and Mohd Apandi, H. (2021). Osteochondral injury of the talus treated with cell-free hyaluronic acid-based scaffold (Hyalofast®) - a reliable solution. Cureus 13 (9), e17928. doi:10.7759/cureus.17928
Bianchi, E., Ruggeri, M., Rossi, S., Vigani, B., Miele, D., Bonferoni, M. C., et al. (2021). Innovative strategies in tendon tissue engineering. Pharmaceutics 13 (1), 89. doi:10.3390/pharmaceutics13010089
Boffito, M., Sartori, S., and Ciardelli, G. (2014). Polymeric scaffolds for cardiac tissue engineering: Requirements and fabrication technologies. Polym. Int. 63 (1), 2–11. doi:10.1002/pi.4608
Brougham, C. M., Levingstone, T. J., Shen, N., Cooney, G. M., Jockenhoevel, S., Flanagan, T. C., et al. (2017). Freeze-drying as a novel biofabrication method for achieving a controlled microarchitecture within large, complex natural biomaterial scaffolds. Adv. Healthc. Mat. 6 (21), 1700598. doi:10.1002/adhm.201700598
Caillol, S. (2020). Special issue "natural polymers and biopolymers II. Molecules 26 (1), 112. doi:10.3390/molecules26010112
Chen, Y., Long, X., Lin, W., Du, B., Yin, H., Lan, W., et al. (2021). Bioactive 3D porous cobalt-doped alginate/waterborne polyurethane scaffolds with a coral reef-like rough surface for nerve tissue engineering application. J. Mat. Chem. B 9 (2), 322–335. doi:10.1039/d0tb02347g
Clavé, A., Potel, J. F., Servien, E., Neyret, P., Dubrana, F., Stindel, E., et al. (2016). Third-generation autologous chondrocyte implantation versus mosaicplasty for knee cartilage injury: 2-year randomized trial. J. Orthop. Res. 34 (4), 658–665. doi:10.1002/jor.23152
Ehrmann, A. (2021). Non-toxic crosslinking of electrospun gelatin nanofibers for tissue engineering and biomedicine-A review. Polym. (Basel) 13 (12), 1973. doi:10.3390/polym13121973
Entekhabi, E., Haghbin Nazarpak, M., Shafieian, M., Mohammadi, H., Firouzi, M., Hassannejad, Z., et al. (2021). Fabrication and in vitro evaluation of 3D composite scaffold based on collagen/hyaluronic acid sponge and electrospun polycaprolactone nanofibers for peripheral nerve regeneration. J. Biomed. Mat. Res. A 109 (3), 300–312. doi:10.1002/jbm.a.37023
Eviana Putri, N. R., Wang, X., Chen, Y., Li, X., Kawazoe, N., Chen, G., et al. (2020). Preparation of PLGA-collagen hybrid scaffolds with controlled pore structures for cartilage tissue engineering. Prog. Nat. Sci. Mater. Int. 30 (5), 642–650. doi:10.1016/j.pnsc.2020.07.003
Feng, Y., Gao, H. L., Wu, D., Weng, Y. T., Wang, Z. Y., Yu, S. H., et al. (2021). Biomimetic lamellar chitosan scaffold for soft gingival tissue regeneration. Adv. Funct. Mat. 31 (43), 2105348. doi:10.1002/adfm.202105348
Gaharwar, A. K., Singh, I., and Khademhosseini, A. (2020). Engineered biomaterials for in situ tissue regeneration. Nat. Rev. Mat. 5 (9), 686–705. doi:10.1038/s41578-020-0209-x
Gang, Y., Yansha, Q., Yan, L., Lu, W., and Hongbing, H. (2021). Preparation and characterization of polylactic acid-caprolactone/fibrinogen nanofiber based hernia mesh. J. Text. Res. 42 (01), 40–45. doi:10.13475/j.fzxb.20200301106
Gao, F., Xu, Z., Liang, Q., Li, H., Peng, L., Wu, M., et al. (2019). Osteochondral regeneration with 3D-printed biodegradable high-strength supramolecular polymer reinforced-gelatin hydrogel scaffolds. Adv. Sci. (Weinh). 6 (15), 1900867. doi:10.1002/advs.201900867
González-Pérez, F., Ibáñez-Fonseca, A., Alonso, M., and Rodríguez-Cabello, J. C. (2021). Combining tunable proteolytic sequences and a VEGF-mimetic peptide for the spatiotemporal control of angiogenesis within Elastin-Like Recombinamer scaffolds. Acta Biomater. 130, 149–160. doi:10.1016/j.actbio.2021.06.005
Ha, J. H., Jeong, Y., Koo, Y. T., Jeon, S., Chung, J., Kim, S., et al. (2020). Effect of collagen matrix on postoperative palatal fistula in cleft palate repair. Sci. Rep. 10 (1), 15236. doi:10.1038/s41598-020-72046-y
Haaparanta, A. M., Järvinen, E., Cengiz, I. F., Ellä, V., Kokkonen, H. T., Kiviranta, I., et al. (2014). Preparation and characterization of collagen/PLA, chitosan/PLA, and collagen/chitosan/PLA hybrid scaffolds for cartilage tissue engineering. J. Mat. Sci. Mat. Med. 25 (4), 1129–1136. doi:10.1007/s10856-013-5129-5
Henriksen, N. A., Jensen, K. K., and Jorgensen, L. N. (2017). “The biology of hernia formation,” in Textbook of hernia. Editors W. W. Hope, W. S. Cobb, and G. L. Adrales (Cham: Springer International Publishing), 1–5. doi:10.1007/978-3-319-43045-4_1
Hormozi, M., Assaei, R., and Boroujeni, M. B. (2017). The effect of aloe vera on the expression of wound healing factors (TGFβ1 and bFGF) in mouse embryonic fibroblast cell: In vitro study. Biomed. Pharmacother. 88, 610–616. doi:10.1016/j.biopha.2017.01.095
Indurkar, A., Bangde, P., Gore, M., Agrawal, A. K., Jain, R., Dandekar, P., et al. (2020). Fabrication of guar gum-gelatin scaffold for soft tissue engineering. Carbohydr. Polym. Technol. Appl. 1, 100006. doi:10.1016/j.carpta.2020.100006
Jadbabaei, S., Kolahdoozan, M., Naeimi, F., and Ebadi-Dehaghani, H. (2021). Preparation and characterization of sodium alginate–PVA polymeric scaffolds by electrospinning method for skin tissue engineering applications. RSC Adv. 11 (49), 30674–30688. doi:10.1039/D1RA04176B
Jang, K. S., Park, S. J., Choi, J. J., Kim, H. N., Shim, K. M., Kim, M. J., et al. (2021). Therapeutic efficacy of artificial skin produced by 3D bioprinting. Mater. (Basel) 14 (18), 5177. doi:10.3390/ma14185177
Janoušková, O. (2018). Synthetic polymer scaffolds for soft tissue engineering. Physiol. Res. 67, S335–S348. doi:10.33549/physiolres.933983
Jiang, Z., Zhang, K., Du, L., Cheng, Z., Zhang, T., Ding, J., et al. (2021). Construction of chitosan scaffolds with controllable microchannel for tissue engineering and regenerative medicine. Mater. Sci. Eng. C 126, 112178. doi:10.1016/j.msec.2021.112178
Kacvinská, K., Trávničková, M., Vojtová, L., Poláček, P., Dorazilová, J., Kohoutek, M., et al. (2022). Porous cellulose-collagen scaffolds for soft tissue regeneration: Influence of cellulose derivatives on mechanical properties and compatibility with adipose-derived stem cells. Res. Square). doi:10.21203/rs.3.rs-1187939/v1
Kakudo, N., Morimoto, N., Ogawa, T., and Kusumoto, K. (2020). Effects of fibroblast growth factor-2 combined with a collagen/gelatin sponge for adipogenesis in the mouse subcutis. Ann. Plast. Surg. 84 (2), 216–221. doi:10.1097/sap.0000000000002046
Kanatlı, U., Eren, A., Eren, T. K., and Vural, A. (2017). Treatment of osteochondral lesions of the talus with cell-free polymer-based scaffold in single-step arthroscopic surgery. Arthrosc. Tech. 6 (5), e1727–e1734. doi:10.1016/j.eats.2017.06.043
Kayaalp, M. E., Cirdi, Y. U., Kopf, S., and Becker, R. (2021). Prone-positioned knee arthroscopy for isolated retropatellar cartilage defects with gel-type autologous chondrocyte implantation. Oper. Orthop. Traumatol. 33 (5), 436–444. doi:10.1007/s00064-021-00710-1
Keane, T. J., and Badylak, S. F. (2014). “"Biomaterials for tissue engineering applications,” in Seminars in pediatric surgery (Elsevier), 112–118. doi:10.1053/j.sempedsurg.2014.06.010
Kim, J. E., Kim, S. H., and Jung, Y. (2016). Current status of three-dimensional printing inks for soft tissue regeneration. Tissue Eng. Regen. Med. 13 (6), 636–646. doi:10.1007/s13770-016-0125-8
Kim, J. S., Choi, J., Ki, C. S., and Lee, K. H. (2021). 3D silk fiber construct embedded dual-layer PEG hydrogel for articular cartilage repair - in vitro assessment. Front. Bioeng. Biotechnol. 9, 653509. doi:10.3389/fbioe.2021.653509
Kim, W. J., Yun, H.-S., and Kim, G. H. (2017). An innovative cell-laden α-TCP/collagen scaffold fabricated using a two-step printing process for potential application in regenerating hard tissues. Sci. Rep. 7 (1), 3181. doi:10.1038/s41598-017-03455-9
Laidmäe, I., Ērglis, K., Cēbers, A., Janmey, P. A., and Uibo, R. (2018). Salmon fibrinogen and chitosan scaffold for tissue engineering: In vitro and in vivo evaluation. J. Mat. Sci. Mat. Med. 29 (12), 182. doi:10.1007/s10856-018-6192-8
Lee, J. B., Ko, Y. G., Cho, D., Park, W. H., and Kwon, O. H. (2017). Modification and optimization of electrospun gelatin sheets by electron beam irradiation for soft tissue engineering. Biomater. Res. 21, 14. doi:10.1186/s40824-017-0100-z
Lee, J. H., and Park, J. H. (2020). Can genoss DES™ stand out in the crowd of stents? Korean Circ. J. 50 (4), 328. doi:10.4070/kcj.2020.0040
Li, L., Qin, S., Peng, J., Chen, A., Nie, Y., Liu, T., et al. (2020). Engineering gelatin-based alginate/carbon nanotubes blend bioink for direct 3D printing of vessel constructs. Int. J. Biol. Macromol. 145, 262–271. doi:10.1016/j.ijbiomac.2019.12.174
Liang, S., Chen, J., Zhang, Y., Ma, Y. D., Ma, C. C., Ye, Y., et al. (2021). Long-term mesh-related complications after total pelvic reconstruction surgery with tension-free transvaginal mesh. Zhonghua Yi Xue Za Zhi 101 (24), 1908–1914. doi:10.3760/cma.j.cn112137-20210306-00575
Liao, J., Qu, Y., Chu, B., Zhang, X., and Qian, Z. (2015). Biodegradable CSMA/PECA/graphene porous hybrid scaffold for cartilage tissue engineering. Sci. Rep. 5, 9879. doi:10.1038/srep09879
Luo, Y., Zhang, T., and Lin, X. (2022). 3D printed hydrogel scaffolds with macro pores and interconnected microchannel networks for tissue engineering vascularization. Chem. Eng. J. 430, 132926. doi:10.1016/j.cej.2021.132926
Mao, Z., Bi, X., Ye, F., Du, P., Shu, X., Sun, L., et al. (2021). The relationship between crosslinking structure and silk fibroin scaffold performance for soft tissue engineering. Int. J. Biol. Macromol. 182, 1268–1277. doi:10.1016/j.ijbiomac.2021.05.058
Masson-Meyers, D. S., and Tayebi, L. (2021). Vascularization strategies in tissue engineering approaches for soft tissue repair. J. Tissue Eng. Regen. Med. 15 (9), 747–762. doi:10.1002/term.3225
Naghieh, S., Foroozmehr, E., Badrossamay, M., and Kharaziha, M. (2017). Combinational processing of 3D printing and electrospinning of hierarchical poly(lactic acid)/gelatin-forsterite scaffolds as a biocomposite: Mechanical and biological assessment. Mater. Des. 133, 128–135. doi:10.1016/j.matdes.2017.07.051
Naghieh, S., Sarker, M. D., Abelseth, E., and Chen, X. (2019). Indirect 3D bioprinting and characterization of alginate scaffolds for potential nerve tissue engineering applications. J. Mech. Behav. Biomed. Mat. 93, 183–193. doi:10.1016/j.jmbbm.2019.02.014
Nie, K., Han, S., Yang, J., Sun, Q., Wang, X., Li, X., et al. (2020). Enzyme-crosslinked electrospun fibrous gelatin hydrogel for potential soft tissue engineering. Polym. (Basel) 12 (9), 1977. doi:10.3390/polym12091977
Niemeyer, P., Pestka, J. M., Kreuz, P. C., Erggelet, C., Schmal, H., Suedkamp, N. P., et al. (2008). Characteristic complications after autologous chondrocyte implantation for cartilage defects of the knee joint. Am. J. Sports Med. 36 (11), 2091–2099. doi:10.1177/0363546508322131
Parmaksiz, M., Elçin, A. E., and Elçin, Y. M. (2019). Decellularized bovine small intestinal submucosa-PCL/hydroxyapatite-based multilayer composite scaffold for hard tissue repair. Mater. Sci. Eng. C 94, 788–797. doi:10.1016/j.msec.2018.10.011
Pérez-Köhler, B., Benito-Martínez, S., Gómez-Gil, V., Rodríguez, M., Pascual, G., Bellón, J. M., et al. (2021). New insights into the application of 3D-printing technology in hernia repair. Mater. (Basel) 14 (22), 7092. doi:10.3390/ma14227092
Perez-Puyana, V., Jiménez-Rosado, M., Romero, A., and Guerrero, A. (2020). Polymer-based scaffolds for soft-tissue engineering. Polym. (Basel) 12 (7), 1566. doi:10.3390/polym12071566
Rao, S. H., Harini, B., Shadamarshan, R. P. K., Balagangadharan, K., and Selvamurugan, N. (2018). Natural and synthetic polymers/bioceramics/bioactive compounds-mediated cell signalling in bone tissue engineering. Int. J. Biol. Macromol. 110, 88–96. doi:10.1016/j.ijbiomac.2017.09.029
Rodrigues, P., and Raz, S. (2022). The burden of reoperations and timeline of problems in 1, 530 cases of mesh-related complications. Urol. Int. 106 (3), 235–242. doi:10.1159/000514389
Rokn, A., Zare, H., and Haddadi, P. (2020). Use of mucograft collagen Matrix(®) versus free gingival graft to augment keratinized tissue around teeth: A randomized controlled clinical trial. Front. Dent. 17 (5), 1–8. doi:10.18502/fid.v17i1.3965
Taghipour, Y. D., Hokmabad, V. R., Del Bakhshayesh, A. R., Asadi, N., Salehi, R., Nasrabadi, H. T., et al. (2020). The application of hydrogels based on natural polymers for tissue engineering. Curr. Med. Chem. 27 (16), 2658–2680. doi:10.2174/0929867326666190711103956
Tanaka, T., Abe, Y., Cheng, C. J., Tanaka, R., Naito, A., Asakura, T., et al. (2020). Development of small-diameter elastin-silk fibroin vascular grafts. Front. Bioeng. Biotechnol. 8, 622220. doi:10.3389/fbioe.2020.622220
Tijore, A., Irvine, S. A., Sarig, U., Mhaisalkar, P., Baisane, V., Venkatraman, S., et al. (2018). Contact guidance for cardiac tissue engineering using 3D bioprinted gelatin patterned hydrogel. Biofabrication 10 (2), 025003. doi:10.1088/1758-5090/aaa15d
Turnbull, G., Clarke, J., Picard, F., Zhang, W., Riches, P., Li, B., et al. (2020). 3D biofabrication for soft tissue and cartilage engineering. Med. Eng. Phys. 82, 13–39. doi:10.1016/j.medengphy.2020.06.003
Wakuda, Y., Nishimoto, S., Suye, S. I., and Fujita, S. (2018). Native collagen hydrogel nanofibres with anisotropic structure using core-shell electrospinning. Sci. Rep. 8 (1), 6248. doi:10.1038/s41598-018-24700-9
Wang, T., Han, Y., Wu, Z., Qiu, S., Rao, Z., Zhao, C., et al. (2022). Tissue-specific hydrogels for three-dimensional printing and potential application in peripheral nerve regeneration. Tissue Eng. Part A 28 (3-4), 161–174. doi:10.1089/ten.TEA.2021.0093
Wu, Q., Maire, M., Lerouge, S., Therriault, D., and Heuzey, M.-C. (2017a). 3D printing of microstructured and stretchable chitosan hydrogel for guided cell growth. Adv. Biosyst. 1 (6), 1700058. doi:10.1002/adbi.201700058
Wu, Y., Wang, L., Guo, B., and Ma, P. X. (2017b). Interwoven aligned conductive nanofiber yarn/hydrogel composite scaffolds for engineered 3D cardiac anisotropy. ACS Nano 11 (6), 5646–5659. doi:10.1021/acsnano.7b01062
Xiang, P., Wang, S. S., He, M., Han, Y. H., Zhou, Z. H., Chen, D. L., et al. (2018). The in vitro and in vivo biocompatibility evaluation of electrospun recombinant spider silk protein/PCL/gelatin for small caliber vascular tissue engineering scaffolds. Colloids Surfaces B Biointerfaces 163, 19–28. doi:10.1016/j.colsurfb.2017.12.020
Yang, Z., Wu, Y., Li, C., Zhang, T., Zou, Y., Hui, J. H. P., et al. (2012). Improved mesenchymal stem cells attachment and in vitro cartilage tissue formation on chitosan-modified poly(L-lactide-co-epsilon-caprolactone) scaffold. Tissue Eng. Part A 18 (3-4), 242–251. doi:10.1089/ten.TEA.2011.0315
Zhang, Y., Huang, J., Huang, L., Liu, Q., Shao, H., Hu, X., et al. (2016). Silk fibroin-based scaffolds with controlled delivery order of VEGF and BDNF for cavernous nerve regeneration. ACS Biomater. Sci. Eng. 2 (11), 2018–2025. doi:10.1021/acsbiomaterials.6b00436
Zhao, H., and Li, M. (2011). “Preparation of braided silk as a tubular tissue engineering scaffold,” in 7th China international silk conference on inheritance and innovation - modern silk road (STAFA-ZURICH: Trans Tech Publications Ltd), 95–99. doi:10.4028/www.scientific.net/AMR.175-176.95
Zhao, Y., Zhu, Z. S., Guan, J., and Wu, S. J. (2021). Processing, mechanical properties and bio-applications of silk fibroin-based high-strength hydrogels. Acta Biomater. 125, 57–71. doi:10.1016/j.actbio.2021.02.018
Zhong, H., Huang, J., Wu, J., and Du, J. (2022). Electrospinning nanofibers to 1D, 2D, and 3D scaffolds and their biomedical applications. Nano Res. 15 (2), 787–804. doi:10.1007/s12274-021-3593-7
Keywords: soft tissue repair, natural polymers, scaffolds, clinical translation, materials processing
Citation: Chen M, Jiang R, Deng N, Zhao X, Li X and Guo C (2022) Natural polymer-based scaffolds for soft tissue repair. Front. Bioeng. Biotechnol. 10:954699. doi: 10.3389/fbioe.2022.954699
Received: 27 May 2022; Accepted: 29 June 2022;
Published: 19 July 2022.
Edited by:
Yongsheng Yu, Chinese Academy of Sciences, ChinaReviewed by:
Gajanan Arbade, National Centre for Cell Science, IndiaCopyright © 2022 Chen, Jiang, Deng, Zhao, Li and Guo. This is an open-access article distributed under the terms of the Creative Commons Attribution License (CC BY). The use, distribution or reproduction in other forums is permitted, provided the original author(s) and the copyright owner(s) are credited and that the original publication in this journal is cited, in accordance with accepted academic practice. No use, distribution or reproduction is permitted which does not comply with these terms.
*Correspondence: Xiangjuan Li, Mzg2NTAxNjc4QHFxLmNvbSYjeDAyMDBhOw==; Chengchen Guo, Z3VvY2hlbmdjaGVuQHdlc3RsYWtlLmVkdS5jbg==
†These authors have contributed equally to this work
Disclaimer: All claims expressed in this article are solely those of the authors and do not necessarily represent those of their affiliated organizations, or those of the publisher, the editors and the reviewers. Any product that may be evaluated in this article or claim that may be made by its manufacturer is not guaranteed or endorsed by the publisher.
Research integrity at Frontiers
Learn more about the work of our research integrity team to safeguard the quality of each article we publish.