- 1Department of Oncology, Shengjing Hospital of China Medical University, Shenyang, China
- 2Department of Thoracic Surgery, Shengjing Hospital of China Medical University, Shenyang, China
Spatially- and/or temporally-controlled drug release has always been the pursuit of drug delivery systems (DDSs) to achieve the ideal therapeutic effect. The abnormal pathophysiological characteristics of the tumor microenvironment, including acidosis, overexpression of special enzymes, hypoxia, and high levels of ROS, GSH, and ATP, offer the possibility for the design of stimulus-responsive DDSs for controlled drug release to realize more efficient drug delivery and anti-tumor activity. With the help of these stimulus signals, responsive DDSs can realize controlled drug release more precisely within the local tumor site and decrease the injected dose and systemic toxicity. This review first describes the major pathophysiological characteristics of the tumor microenvironment, and highlights the recent cutting-edge advances in DDSs responding to the tumor pathophysiological environment for cancer therapy. Finally, the challenges and future directions of bio-responsive DDSs are discussed.
1 Introduction
With the aggravation of population aging and the increase in life stress, the incidence and death rate of malignant tumors is increasing (Berben et al., 2021). According to the results from Global Cancer Statistics 2018 (Bray et al., 2018), there are approximately 18.1 million new cancer cases and approximately 9.6 million deaths due to cancer worldwide. In clinical practice, conventional cancer therapeutic strategies mainly include surgery, radiotherapy, chemotherapy, and immunotherapy (Lissoni et al., 2009). However, some patients lose the opportunity for surgery when they are diagnosed at a later stage (Wang et al., 2013). Additionally, considering the application limitation of radiotherapy and immunotherapy including tumor types, response rates, and high cost, traditional chemotherapy still plays an irreplaceable role in cancer therapy and will not be obsolete any time soon (Baxevanos and Mountzios, 2018; Sadeghi Rad et al., 2021; Fang, 2022). However, due to their low tumor accumulation, multidrug resistance, and lack of tumor selectivity, cytotoxic chemotherapy drugs and molecular targeted drugs commonly used in clinical practice often have serious side effects for normal tissues, which is one of the main formidable challenges faced by clinical chemotherapy (Shi et al., 2017; Hejmady et al., 2020). Currently, the application of drug delivery systems (DDSs), such as polymers, liposomes, dendrimers, biomolecular nanoparticles, and inorganic nanoparticles, has become a hot research topic in the field of chemotherapy because of their advantages in pharmacokinetics, targeting accumulation, high therapeutic efficiency, and lower organ toxicity (Ensign et al., 2014; Navya et al., 2019). Although DDS have many advantages compared to free drugs, the therapeutic effect remains unsatisfactory due to the heterogeneity of the EPR effect (Fang et al., 2020; Islam et al., 2021). For example, Doxil, the first FDA-approved nanomedicine, showed decreased cardiotoxicity in clinical use, but the overall clinical prognosis of patients with cancer treated with Doxil was not significantly improved (Barenholz, 2012). To address this dilemma, researchers have focused their attention on the design of various responsive DDSs according to different stimulus signals in the tumor microenvironment to realize rapid intratumoral or intracellular drug release and enhance tumor cell killing efficacy (Fang et al., 2011; Dou et al., 2020).
The tumor microenvironment mainly consists of extracellular matrix, fibroblasts, tumor blood vessels, lymphatic ducts, and immune cells, which reciprocally interact with tumor cells to promote their growth and metastasis (Arneth, 2019; Anderson and Simon, 2020). Due to persistent genetic mutations and particularly rapid proliferation, the tumor microenvironment is characterized by unique pathophysiological indicators compared to normal tissue, such as acidosis, overexpression of special enzymes, hypoxia, and high levels of reactive oxygen species (ROS), GSH, and ATP, all of which can be used as endogenous triggers for the design of responsive DDSs (Vaupel et al., 2019; Thomas et al., 2020; Park et al., 2021). In the current review, these hallmarks of the tumor microenvironment are first summarized in detail, before classifying responsive DDSs into several categories according to these pathological features (Scheme 1). Finally, the potential challenges and future development directions of responsive DDSs are discussed. We believe that this overview will guide the rapid development of this research field.
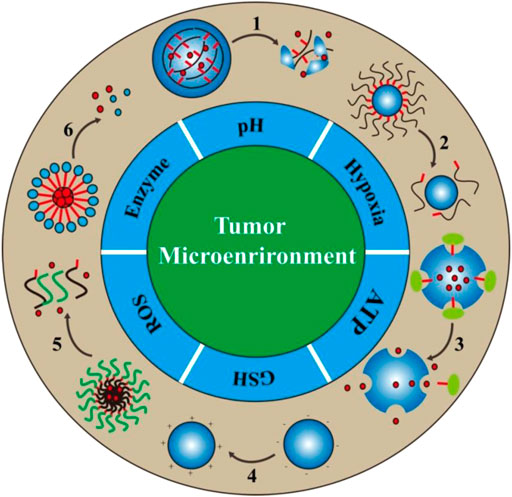
SCHEME 1. Schematic illustration of the design/action mechanism of responsive DDSs based on the abnormal pathophysiological characteristics in the tumor microenvironment. There are six major tumor specific features in the tumor microenvironment that are usually utilized for the design of bio-responsive drug delivery systems. The numbers in the scheme represent different drug release processes, 1: Degradation, 2: Detachment, 3: Uncap, 4: Charge reversal, 5: Dissociation, 6: Separation.
2 Main Pathophysiological Features of the Tumor Microenvironment
Acidosis is the most typical pathophysiological feature of the tumor microenvironment (Ji et al., 2019). The formation of this acidic state is usually attributed to the specific metabolic manner of tumor cells, known as the “Warburg effect,” in which tumor cells produce abundant lactate via the anaerobic glycolytic pathway even in the presence of sufficient oxygen (Hanahan, 2022). Increasing evidence has indicated the acidosis is associated with angiogenesis, tumor metastasis, drug resistance, and immune escape (Damgaci et al., 2018). The hypoxia present in some regions of tumor tissue is another important pathophysiological feature. The speed of angiogenesis cannot fully match the rapid proliferation of tumor cells, which leads to the formation of hypoxic regions in solid tumors (Fukumura and Jain, 2007; Petrova et al., 2018). Besides, as a feedback loop, cancer cells also secrete many proangiogenic factors to promote tumor angiogenesis, which results in disordered growth of the vasculature and further aggravates tumor hypoxia (Schito, 2019). Under hypoxic conditions, tumor cells undergo adaptive gene mutation, such as the acquisition of multidrug resistance, metastasis, suppressed immune response, and angiogenesis, all of which further accelerate tumor progression (Chan and Giaccia, 2007; Rankin and Giaccia, 2016). Growing in such a hostile environment (acidosis, hypoxia), tumor and stromal cells may also overexpress some specific enzymes, including MMP-2, MMP-9, FAP-α, legumain, uPA, and some elastases (Wang et al., 1999; Zhang et al., 2019a; Lian and Ji, 2020). Additionally, specific metabolic pathways and regulatory routes induce the excessive production of substances such as ROS, GSH, and ATP (Li et al., 2020a; Deng and Walther, 2020; Tao and Yin, 2020; Cao et al., 2021). Taken together, these unique features of the tumor microenvironment can be reasonably used for the design of stimulus-responsive DDSs. Table 1 summarizes the biological stimuli in the tumor microenvironment and their levels compared to those in normal tissue.
3 Internal Stimulus-Responsive DDS
The specific pathophysiological features or differentially expressed substances in the tumor microenvironment provide a possibility for the design of stimulus-responsive DDSs to realize controlled drug release in local tumor tissue (Mura et al., 2013). By doing this, the pharmacokinetic properties, tumor accumulation efficacy, and toxic effects of existing drugs can be reasonably optimized. According to the difference in internal stimulus signals, the following sections summarize the internal stimulus-responsive DDS from six aspects: pH-responsive DDS, ROS-responsive DDS, enzyme-responsive DDS, ATP-responsive DDS, GSH-responsive DDS, and hypoxia-responsive DDS.
3.1 pH-Responsive DDS
The lower pH in the tumor microenvironment is probably the most widely used stimulus for the design of responsive DDSs. The design of pH-sensitivity can be realized by the degradation of acid-cleavable bonds or the protonation of ionizable groups (Shin et al., 2021). Thus, we will review pH-responsive DDSs from the following two aspects: pH-responsive DDSs with acid-cleavable bonds, and protonation-based pH-responsive DDSs. The most commonly used acid-sensitive moieties with different responding pH ranges are summarized in Table 2.

TABLE 2. Acid-labile moieties and examples. Adapted with permission from (Shin et al., 2021).
3.1.1 pH-Responsive DDS With Acid-Cleavable Bonds
Various acid-sensitive bonds have been extensively investigated and used for the design of pH-responsive DDSs for site-specific delivery of different anti-tumor therapeutics. Compared to free drugs, nanomedicine possesses properties such as prolonged circulation time, tumor targeting accumulation, and reduced side effects. Thus, nanoparticles conjugated with chemotherapeutics are a powerful method to optimize the pharmacokinetic properties of free drugs. How to realize controlled drug release in a specific environment, such as the tumor microenvironment and lysosomes, is a popular research area. Hydrazone linkage is one of the most common acid-cleavable bonds and has been widely used in various biological and clinical applications, such as drug conjugation and drug delivery (Sonawane et al., 2017). Bae et al. designed a supramolecular assembly in which doxorubicin was chemically conjugated onto the hydrophobic group via hydrazine. They demonstrated that this self-assembling polymeric micelle could release doxorubicin in a pH-dependent manner in vitro and function in a highly controlled manner within cells (Bae et al., 2003). Geest’s group developed a pH-sensitive hydrazone-linked Doxorubicin Nanogel (Nanogeldox) using polymeric-activated ester as a scaffold (Van Driessche et al., 2018). In vitro results revealed that Nanogeldox showed a pH-dependent drug release profile, with enhanced tumor accumulation and tumor growth reduction in a zebrafish embryo tumor model, without obvious systemic toxicity. Zhang’s group constructed a pH-sensitive polymeric complex micelle, which was simultaneously chemically conjugated with DTX via a hydrazine bond and encapsulated with DTX (P123-d–hyd–DTX). The results indicated that P123-d–hyd–DTX showed highly pH-responsive release properties and enhanced anti-tumor activity in an in vitro B16F10 cell assay, as well as in an in vivo xenograft B16F10 melanoma model, with no obvious adverse effects. Furthermore, P123-d–hyd–DTX exhibited greater tumor cell killing efficacy than commercial drugs (Su et al., 2016). Taken together, these studies revealed the superiority of hydrazone-based nanoparticle-drug conjugations in antitumor applications due to their controlled drug release, enhanced tumor accumulation, antitumor efficacy, and decreased side effects. In addition to the conjugation with chemotherapeutics, hydrazine can also be used for constructing nanoparticles to realize controlled drug release. Cai’s group reported a drug-loading hollow silica nanoparticle (HMSN–PA–HA) whose surface was coated by a hyaluronic acid layer via hydrazine as bridge to block rapid drug release. HMSN–PA–HA could effectively accumulate at tumor sites by HA and CD44 interactions and release loaded drugs when hydrazine linkers between HA agents and HMSNs were broken, triggered by the low pH in the tumor microenvironment (Dai et al., 2016). He’ group constructed a pH sensitive liposomal drug delivery system (Cl-Lip) using PEG5K-Hydrazone-PE for pH responsiveness and DSPE-PEG2K-R8 for cellular endocytosis. Cl-Lip showed a rapid drug release in vitro in an acid environment and pH-dependent cellular uptake and cytotoxicity (Zhang et al., 2015). Moreover, similar to hydrazone, imine and oxime linkages with C=N bonds can also be used to construct pH-responsive DDSs. Yang’ group synthesized an amphiphilic polymer (PEG-b-C18) conjugated by a benzoic-imine linker to form an acid-sensitive micelle for drug delivery. Their results verified that the micelle is stable at physiological pH and hydrolyzes under acidic conditions (Ding et al., 2009). Tzakos’ group constructed a peptide-drug conjugate (PDC) using oxime bond as an acid-labile programmable linker. They used gemcitabine as the anticancer unit and gonadotropin releasing hormone (GnRH) as the cancer-targeting unit. The acid-dependent gemcitabine release was monitored in vitro in cell culture and in human plasma using LC-MS/MS (Vrettos et al., 2021) Acetals/ketals are also frequently used as acid-sensitive bonds to synthesize prodrugs or pH-sensitive nanoparticles due to their specificity for the acidic environment. Li et al. prepared acetal-based pH-sensitive polymeric microspheres loaded with the anticancer drug doxorubicin hydrochloride. In vitro results demonstrated the microspheres showed pH-dependent drug release. Moreover, the results of in vivo anticancer experiments indicated that this microsphere showed better antitumor efficiency and prolonged life-span than free doxorubicin, with no obvious cardiotoxicity (Li et al., 2018). Zhong’s group developed 2-[3-[5-amino-1-carboxypentyl]-ureido] pentanedioic acid (Acupa)-decorated pH-responsive chimeric polymersomes (Acupa-CPs) to targeted delivery protein drugs to PSMA-positive LNCaP cells. Meanwhile, an acid-responsive acetal bonds in the vesicular membrane enabled the formed nanocarrier to rapidly release the loaded proteins at mildly acidic pH (Li et al., 2015) Both Zhong’s and Sun’s groups developed acetal-linked paclitaxel prodrug nanoparticles that possessed pH-dependent drug-release properties and enhanced in vitro tumor cell killing efficacy (Gu et al., 2013; Zhai et al., 2017). Nishiyama’s group prepared the polymer gemcitabine conjugate through cyclic acetal linkage (P-GEM). P-GEM released GEM in response to acidic environments such as the endosome/lysosome and realized a higher tumor growth suppression effect compared to free GEM, without adverse side effects (Takemoto et al., 2020). Similar to acetals, ketal has also been widely used to construct pH-sensitive nanostructures. Guo’s group developed various ketal-based acid-sensitive prodrugs or nanomedicines, including an amphiphilic etoposide-ketal-glycoside prodrug, which could be fabricated into excipient-free nanoparticles and be activated by the dual action of glycosidase and acid in the lysosome of tumor cells. The authors demonstrated its efficacy in both in vitro cell experiments and an in vivo A549 xenograft tumor model (Yu et al., 2020). They also chemically conjugated the anticancer agent paclitaxel with different lengths of poly (ethylene glycol) (PEG) via an acyclic-ketal linkage to form an amphipathic structure that self-assembled into acid-activated nanoparticles (PKPs). The in vitro and in vivo results demonstrated that PKP nanoparticles showed acid-dependent drug release, improved pharmacokinetics, and superior antitumor efficacy (Mu et al., 2020). They also conjugated the anticancer nucleoside drug gemcitabine to the polyketal backbone via pH-sensitive ketal linkage and prepared pH-sensitive prodrug nanoparticles (Zhong et al., 2020). Orthoester has been widely applied for constructing pH-sensitive nanomedicines for cancer therapy due to its good biocompatibility. Tang’s group developed a pH-triggered hyaluronic acid nanogel system (HA-NGs) using orthoester as a linkage. HA-NGs exhibited pH-dependent degradation and drug release in vitro, enhanced tumor killing efficacy, and excellent tumor homing capability due to hyaluronic acid and CD44 interaction (Yang et al., 2017a). They also developed acid-degradable carboxymethyl chitosan nanogels, bromelain nanoparticles, and chitosan nanogels via an orthoester linkage, all of which were shown to have pH-triggered drug-release properties and improved tumor cell killing (Zha et al., 2017; Li et al., 2019a; Wang et al., 2020a; Wang et al., 2021). ß-thiopropionate, containing ester bonds, is also used for the preparation of acid-labile polymers. Chen’s group synthesized a biodegradable PAMAM-OH derivative (PAMSPF) containing an acid-labile b-thiopropionate bond for gene delivery. Approximately 95.8% of the loaded DNA in PAMSPF was rapidly released after incubation under acidic conditions. PAMSPF exhibited high transfection efficiency in KB and HepG2 cell lines (Chen et al., 2016). Acidosis is a universal feature of the tumor microenvironment and is suitable for the design of nanomedicines with controlled drug release features against almost all kinds of cancer.
3.1.2 Protonation-Based pH-Responsive DDS
In addition to pH-sensitive chemical bonds, materials with de/protonation properties under different pH conditions can also be used for the design of pH-responsive nanocarriers, e.g., poly (β-amino ester) (PBAE), poly (2-(dimethylamino) ethyl methacrylate) (PDMAEMA), poly (histidine) (poly (His)), and poly (2-ethyl-2-oxazoline) (PEOz). Wang’s group developed a pH-responsive lipid polymer hybrid nanoparticle (FA/PBAE/DTX-NPs) consisting of two portions, a docetaxel-loaded polymer inner core assembled from poly (β-amino ester), and a lipid outer shell of DSPE-PEG2000, FA-DSPE-PEG2000, and lecithin. The in vitro results indicated that FA/PBAE/DTX-NPs can efficiently target to 4T1 tumor cells and release drugs in a pH-dependent manner on demand. The in vivo results also showed that FA/PBAE/DTX-NPs possessed tumor targeting ability, significant antitumor effects, and minimal systemic toxicity in tumor-bearing mice (Zhang et al., 2021). Poly (β-amino ester) can also be used as a hydrophobic chain to construct amphipathic polymer, which can self-assemble into micelles and disassemble under acidic conditions. Li et al. synthesized a PEG-Poly (-Amino Ester) copolymer to develop a pH-responsive drug-loaded micelle (Thz/PPM), which was shown to be an effective pH-responsive DDS (Li et al., 2020b). Lin’s group synthesized a triblock copolymer MPEG-b-PBAE-b-PLA to construct a pH-responsive drug-loaded micelle (Yang et al., 2018). PDMAEMA was also used as pH-responsive block to realize controlled drug release. Meier’s group prepared a PDMS-b-PDMAEMA block copolymer via atom transfer radical polymerization, which could self-assemble into micelles in solution and encapsulate chemotherapeutic doxorubicin, with a pH-dependent drug release feature (Car et al., 2014). Tang’s group covered PDMAEMA polymer on the surface of drug-loaded hollow mesoporous silica nanoparticles to control drug release. Histidine has been reported as a pH-responsive element due to its pKa of ∼6.5 (Yu et al., 2013). Roy’s group developed a histidine modified star-shaped PLGA (sPLGA-His) to co-deliver the chemotherapeutics docetaxel and disulfiram. sPLGA-His was demonstrated to have pH-dependent drug release, lysosome escape, deep tumor penetration, and enhanced tumor cell killing (Swetha et al., 2021). Gu’s group synthesized an amphiphilic triblock copolymer methoxy-poly (ethylene glycol)-poly (l-histidine)-poly (l-lactide) (mPEG-PH-PLLA) to form pH-sensitive nanoparticles (Liu et al., 2012). The changes in the diameter and surface charge revealed that such copolymer nanoparticles have a pH-responsive feature. As a potential replacement for PEG, PEOz (poly (2-ethyl-2-oxazoline)) has its own advantages, including good stability and biocompatibility, higher reactive group substitution rate and pH-responsiveness. Liu et al. developed a platelet membrane–lipid hybrid DDS (platesome) by co-extrusion of pH-responsive Dox-loaded liposomes and platelet membrane nanovesicles, in which pH-responsiveness was realized by insertion of pH-sensitive lipid DSPE-PEOz. The introduction of platelet membrane endowed the nanoparticles with tumor targeting capability (Figure 1). Their results demonstrated that the platesome could release drugs in a pH-dependent manner and efficiently target and kill tumor cells (Liu et al., 2019). Wang et al. prepared a PEOz-b-PLA micelle and investigated its possible mechanisms of pH-sensitivity and cellular internalization in detail. They attributed the pH-responsiveness to the ionization of the tertiary amide groups along the PEOz chain at pH lower than its pKa (Wang et al., 2017). Li’s group used the polymer material poly (2-ethyl-2-oxazoline)-poly (l-lactide) (PEOz-PLA) to prepare self-assembled micelles, which showed pH-dependent drug release in vitro (Su et al., 2019). Xu et al. synthesized a novel material poly (2-ethyl-2-oxazoline)-cholesterol hemisuccinate (PEtOz-CHEMS) which will change from hydrophilic to hydrophobic under acidic conditions and can be used for the construction of pH-sensitive liposomes. They prepared the dox-loaded pH-responsive liposomes (PEtOz-L) and verified its pH-induced increase in particle size and drug release (Xu et al., 2015). For the protonation-based pH-responsive DDS, the responsiveness not only realizes spatially and/or temporally controlled drug release but also has the possibility of improving lysosome escape for enhanced antitumor activity. In addition, polyelectrolyte-based nanocarriers also play an important role in development of pH-responsive DDS. Suo’ group developed the Doxorubicin/cisplatin co-loaded hyaluronic acid/chitosan-based nanoparticles that exhibited pH-dependent drug release in vitro and improved synergistic anti-cancer activity (Wang et al., 2019a). Liu’s group prepared chitosan-based micelles (DOX/CS-PEG) for the intracellular delivery of anti-cancer drug doxorubicin to realize pH-responsive drug release in a lysosome environment. DOX/CS-PEG achieved a cumulative release ratio of 72.76% at pH 5.0 and only 2.32% at pH 7.4 in vitro and showed significant tumor cell killing efficacy (Zhao et al., 2016).
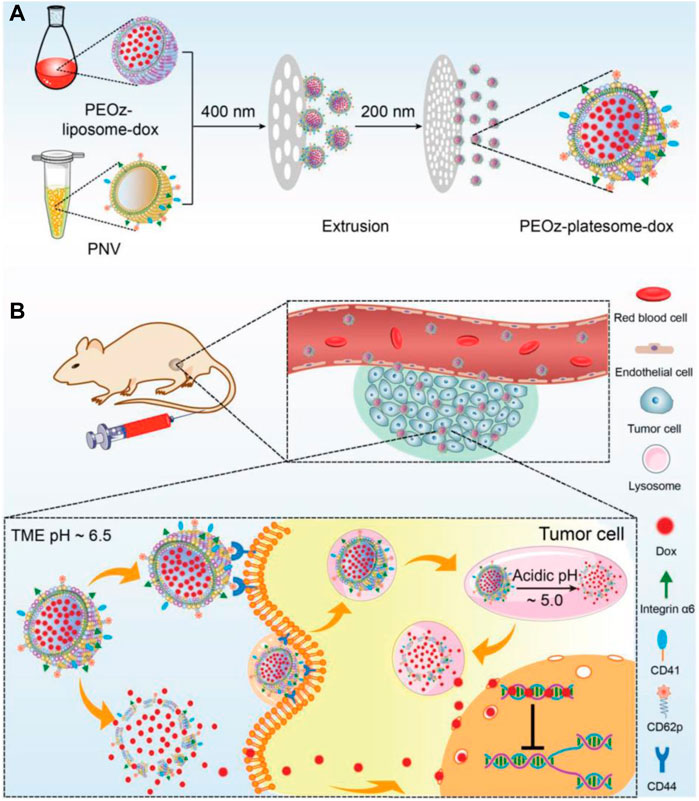
FIGURE 1. Schematic illustration of the preparation of PEOz-platesome-dox (A) PEOz-platesome-dox was generated by coextrusion of PEOz-liposome-dox and PNV (platelet membrane nanovesicles). (B) After intravenous injection, PEOz-platesome-dox is expected to target the tumor through molecular interactions between platelet membrane and tumor cell substituents, such as platelet CD62p and its cognate receptor, tumoral CD44. The incorporation of pH-sensitive lipids into the platesome would allow its cargo (dox) to be rapidly released at the tumor site in response to the acidic pH of the tumor microenvironment and/or lysosomal compartments. The released dox kills tumor cells by inhibiting the cellular DNA replication. Adapted with permission from (Liu et al., 2019).
Acidity is the most basic feature for most tumors. In the tumor microenvironment, there are obvious pH gradients (pH = ∼6.8 in the TME and pH = 4–5 in lysosomes) in favor of the design of DDSs for delivering different drugs with different mechanisms of action. However, the acidity of tumors varies widely in different tumors or different stages of the same tumor. Thus, the designed pH-responsive DDS is difficult to have universal applicability. The evaluation of pH levels in different tumors or different stage of the same tumor to establish a standard database is a promising approach to overcome above-mentioned problems.
3.2 ROS-Responsive DDS
Asides from the acidic conditions, the tumor microenvironment also overproduces ROS, which can also be used as a stimulus to design a responsive DDS. Massive efforts have been made in the development of ROS-responsive drug-release systems, most of which are based on thioether-based materials, diselenide linkages, thioketal-based materials, boronic ester-based, and polyoxalate-based materials (Ye et al., 2019; Cao et al., 2021). The typical examples of ROS-responsive DDS are summarized in Table 3.
Thioether-based materials achieve ROS-responsiveness through hydrophilic-hydrophobic transformation in structure. Since Hubbell’s group first copolymerized oxidation-convertible poly (propylene sulfide) (PPS) with polyethylene glycol (PEG) to form nanovesicles for drug delivery, various ROS-responsive DDSs have been developed (Napoli et al., 2004). Hasegawa’s group synthesized three micelles containing different thioether groups (3-methylthiopropylamide (TPAM), thiomorpholine amide (TMAM), and 4-(methylthio) benzylamide (TPhAM)) within the core to form TP, TM, and TPh micelles, respectively. They compared the stability of the three micelles in human liver cancer (HepG2) cells and human umbilical vein endothelial cells (HUVECs) and demonstrated that the TP micelles showed the highest oxidation sensitivity, while the TPh micelles presented the lowest reactivity toward H2O2. They also investigated the cytotoxicity of these three micelles after dox loading and reached a result that was consistent with the oxidation sensitivity (van der Vlies et al., 2022). He’s group also constructed three ROS-responsive amphiphilic copolymers of mPEG-poly (ester-thioether), mPEG-poly (thioketalester), and mPEG-poly (thioketal-ester-thioether), and three fabricated polymeric nanoparticles using thioketal and thioether, two moieties with ROS sensitivity. They demonstrated the ROS-responsive behavior of the three nanoparticles by NMR, DLS, SEM and in vitro drug release profiles, and demonstrated that all of them showed enhanced cellular uptake and anticancer efficacy (Xu et al., 2019). Wang’s group synthesized an amphiphilic copolymer consisting of polyethylene glycol and polyphosphoester with thioether groups in the side chain (mPEG-b-PMSPEP), self-assembling into nanoparticles with sensitivity for H2O2. They co-encapsulated the photosensitizer chlorin e6 (Ce6) and anticancer drug paclitaxel (PTX) into PMSPEP nanoparticles and realized photo-accelerated release of PTX in vitro. The cytotoxicity test also demonstrated the enhanced tumor cell killing efficacy of PMSPEP nanoparticles compared to photodynamic therapy (Wang et al., 2019b). Aside from polymeric nanoparticles, lipid-based nanoparticles are one of the most applied nanocarriers. Du et al. developed a series of phosphatidylcholine-containing thioether moieties (S-PCs) with different chain lengths. Using these phosphatidylcholines, they prepared S-PC-based stealth liposomes and investigated their ROS responsiveness in vitro and in vivo, showing enhanced tumor cell killing efficacy in comparison with nonresponsive liposome (Du et al., 2019).
Another important ROS-sensitive motif used for engineering-responsive nanomedicine is the diselenide linkage, which also shows specific biological functions. Xu’s group developed various diselenide-based nanostructures for cancer therapy, one of which was a diselenide/porphyrin-containing hyperbranched polymer that self-assembled into a nanostructure (PSe-Por) in solution. When PSe-Por was exposed to visible light, its structure depolymerized; surprisingly, the oxidized products presented cytotoxicity to cancer cells without the help of any cytotoxic molecule (Sun et al., 2017). In another work, they demonstrated that diselenide in diselenide–pemetrexed assemblies could be oxidized to seleninic acid, which showed the capability to suppress the expression of human leukocyte antigen E (HLA-E) in cancer cells for immune therapy (Figure 2). Besides, the produced seleninic acid also suppressed the expression of vascular endothelial growth factor (VEGF) and matrix metalloproteinase-2 (MMP-2) to achieve anti-angiogenesis therapy (Li et al., 2020c). Lang’s group also showed that diselenide-based polymers without drugs (PSeSeTMC) had high therapeutic efficacy in a controlled manner by regulating the contents of diselenide with no obvious side effects (Wei et al., 2021). Shao and co-workers incorporated diselenide linkage into mesoporous silica nanoparticles for X-ray and ROS responsiveness. They used this nanosystem to deliver chemotherapeutics and protein drugs and realized controlled drug release for cancer therapy (Shao et al., 2018; Shao et al., 2020). Park’s group developed diselenide-crosslinked and dox-loaded micelles (dox-DCMs) using polyethylene glycol (PEG) and polypeptide derivatives as copolymer building components to realize controlled drug release in the tumor site. The in vivo anti-tumor results indicated that dox-DCMs could significantly accumulate in tumor tissue and suppress tumor growth (Deepagan et al., 2016).
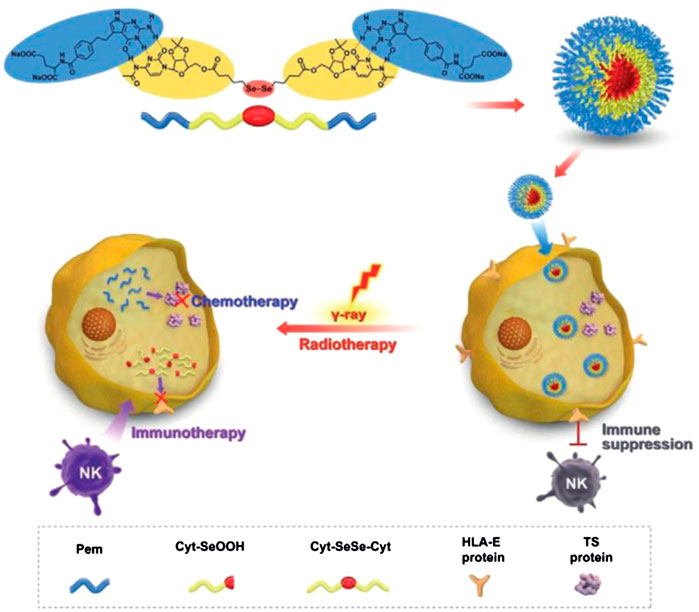
FIGURE 2. Schematic illustration of diselenide-pemetrexed assemblies for combined cancer immunotherapy with radiotherapy and chemotherapy. Under γ-radiation, diselenides are transformed into seleninic acid, pemetrexed is released from the assemblies, and combination therapy including immunotherapy, radiotherapy and chemotherapy is consequently achieved. Adapted with permission from (Li et al., 2020c).
Thioketal linkage is one of the most important ROS-cleavable motifs used for constructing ROS-responsive nanomedicines or prodrugs. Wang’s group developed a ROS-activatable drug-polymer conjugate in which the chemotherapeutic doxorubicin (dox) was chemically conjugated to the polyphosphoesters (PPEs) via a thioketal bond (PPE-TK-dox). Then, the photosensitizer Ce6 was encapsulated into the self-assembly of PPE-TK-dox in solution to obtain Ce6@PPE-TK-dox. When Ce6@PPE-TK-dox in tumor tissue was illuminated under 660-nm red light, the produced ROS cleaved the thioketal bond and triggered the rapid release of dox to kill tumor cells (Pei et al., 2019). Using a similar strategy, Pan et al. prepared a PEG doxorubicin (PEG-dox) prodrug using a thioketal moiety as the linker, which also self-assembled into nanoparticles in solution. The in vitro results indicated that the synthesized nanoparticles showed ROS-responsive properties (Pan et al., 2020). Farokhzad’s group developed a ROS-responsive polyprodrug by crosslinking the anticancer drug mitoxantrone (MTO) via a thioketal moiety as the linker, self-assembling with a lipid structure to form polyprodrug NPs (polyMTO). Simultaneously, a tumor targeting peptide iRGD was modified onto the surface of polyMTO. The results revealed that polyMTO NPs showed a ROS-dependent release of intact therapeutic drug molecules and enhanced anti-cancer efficacy (Xu et al., 2017). Thioketal linkage is also widely used for constructing prodrugs due to their high sensitivity and stability (Chu et al., 2020; Hao et al., 2020). Polyoxalate-based nanoparticles were originally designed for the imaging of H2O2 due to their high selectivity and sensitivity. Only a few of them have been applied for tumor therapy. Höcherl and co-workers developed a novel self-immolative H2O2-sensitive polyprodrug material (PDEB NPs) through a one-pot synthesis method. PDEB NPs showed H2O2-dependent structure degradation and released chemotherapeutic drugs for enhanced tumor cell killing efficacy (Höcherl et al., 2017). In addition to the above-mentioned examples, other ROS-sensitive motifs, such as boronic ester, have also been used as bio-responsive materials for drug delivery in cancer therapy. Indeed, Ping’s group developed a ROS-responsive boronic vehicle with a lipid layer on the surface for siRNA delivery. This system not only protected siRNA in circulation from degradation, but also achieved the efficient systemic transportation of siRNA to the tumor site for effective knockdown of the targeted gene in vivo (Li et al., 2017a).
Elevated ROS level is not only an important feature in the tumor microenvironment but also a toxic substance that can induce the apoptosis of tumor cells. Therefore, the strategy inducing the production of ROS to kill tumor cells, e.g., photodynamic therapy, is suitable for the combination with ROS-responsive DDSs. The main limitation of this kind bio-responsive nanoparticle is the complicated manufacturing process which hinders its large-scale production and clinical translation. It is very important to develop response motifs with simple structure and easy preparation. In addition, the heterogeneity of ROS concentration is also an important challenge.
3.3 Enzyme-Responsive DDS
Enzymes play important roles in almost every step of cancer progression, including angiogenesis, cell proliferation, and metastasis (Muz et al., 2015; Conlon and Murray, 2019). In the specific tumor microenvironment, various enzymes are highly expressed in tumor tissue but have relatively low expression in normal tissues, which offers the possibility for the design of enzyme-responsive DDSs (Shahriari et al., 2019; Li et al., 2020d). The typical enzyme-responsive DDSs and examples are summarized in Table 4.
Matrix metalloproteinases (MMPs), mainly including MMP-2 and MMP-9, which are overexpressed in the tumor microenvironment, have long been closely implicated in tumor invasion and metastasis and can be applied as stimuli for the design of bio-responsive materials (Yao et al., 2018). Li et al. inserted an amphiphilic peptide with an MMP-2-cleavable site into the structure of the lipid bilayer to package the surface of dox-loaded nanoparticles. When the lipid-polymer hybrid nanoparticles enter the tumor tissue by EPR effects, the lipid layer is degraded by the overexpressed MMP-2 and the platelet antibody and dox-loaded inner core are released to mediate effects at different regions of the tumor microenvironment (Li et al., 2017b). Besides, peptides with an MMP-2-cleavable sequence are frequently used to link functional elements on the surface of nanoparticles to realize controlled release or exposure. Liu et al. prepared an MMP-2-sensitive PS-modified nanoparticle in which PS could be exposed and mediate the phagocytosis of tumor-associated macrophages (TAMs) when PEG coating was specifically cleaved by the overexpressed MMP-2 in the tumor site (Liu et al., 2020). Moreover, Cai’s group kinked phenylboronic acid conjugated human serum albumin (PBA-HSA) onto the surface of mesoporous silica nanoparticles (MSNs) via a MMP-2 peptide to realize controlled drug release (Liu et al., 2015). Furthermore, Ai’s group developed a hyaluronic acid-modified gold nanorod (HA-AuNR) surface-linked with an M2-type TAMs (M2-TAMs) depletion peptide via an MMP-2-sensitive peptide, termed HA-AuNR/M-M2pep. This combined PTT and M2-TAMs depletion strategy effectively inhibited tumor growth (Tian et al., 2021). Inspired by the natural substrates for enzymes found in living organisms, researchers have also developed many nanomedicines with intrinsic enzyme responsiveness using these substrates. Indeed, Huang’s group established an MMP-2-responsive nanoparticle using gelatin as the basic framework (Li et al., 2021). The nanoparticle (GD/Ce6@GNP) consists of a gelatin core encapsulating photosensitizer chlorin e6 (Ce6) and a polymeric shell chemically conjugated to mitochondria-targeted anticancer drugs (doxorubicin-glycyrrhetinic acid conjugates) on the surface. When arriving at tumor tissue through the EPR effect, GD/Ce6@GNP was significantly degraded, and the Ce6 and doxorubicin-glycyrrhetinic acid conjugates were released. Moreover, the results showed that O2 consumption induced by mitochondria targeting significantly enhanced the effect of PDT (Figure 3). An MMP-2-cleavable peptide can also be used as the linkage between the hydrophilic group and hydrophobic group of amphiphilic lipids or polymers for constructing nanoparticles. Wang et al. synthesized a block copolymer containing PEG, MMP-2-degradable peptide, cationic cell penetrating peptide polyarginine r9, and poly (ε-caprolactone) (PCL) to prepare a core-shell for siRNA delivery. The decoration of PEG endowed the nanostructure with prolonged circulating time in blood. When the nanoparticle accumulated at the tumor site, the PEG layer was cleaved and the exposed cell penetrating peptide R9 enhanced cellular uptake of siRNA (Wang et al., 2014). MMP-9 is another matrix metalloproteinase overexpressed in tumors that is used for the design of responsive nanomedicines. Surface modification of nanoparticles by hydrophilic moieties using responsive linkers is often used to optimize pharmacokinetic properties, prevent drug leakage in the circulation, and realize surface charge changes to enhance cellular uptake in the tumor area. Zhang’s group constructed an OMPE (glutamate-rich segment) modified cationic liposome (O-NP) using MMP-9-cleavable peptide as linker. The surface change of O-NP changed from positive to negative upon binding MMP-9 in tumor tissue, which results in the enhanced endocytosis of tumor cells (Han et al., 2021). Meiners’s group packaged mesoporous silica nanoparticles (MSNs) using avidin molecules via MMP-9 sensitive linkers and realized spatiotemporally controlled release of loaded cargoes. They demonstrated the effectiveness of their proof-of-concept design in vitro and in vivo experiments (van Rijt et al., 2015). Gao’s group modified the gold nanorods via an MMP-9-responsive zwitterionic stealth peptide consisting of a cell penetrating Tat sequence, an MMP-9 cleavable sequence, and a zwitterionic antifouling sequence. This modification can improve the systemic circulating time and significantly enhance cellular uptake post-cleavage by MMP-9 in the tumor microenvironment (Wu et al., 2019). Mallik’s group synthesized a collagen mimetic lipid-peptide hybrid nanovesicle in which the MMP-9-cleavable peptide was incorporated to realize controlled drug release (Kulkarni et al., 2014). Additionally, other MMPs, including MMP 7, MMP12, MMP13, and MMP14, can also be used for the design of controlled drug delivery systems (Zhang et al., 2019b).
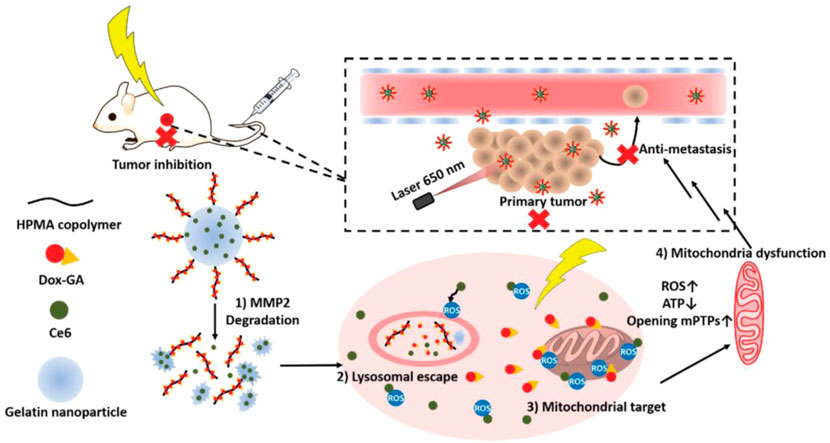
FIGURE 3. Schematic illustration of the stimuli-responsive nanoparticles combining photodynamic therapy and mitochondrial disruption to suppress tumor metastasis. When the nanoparticles arrived at tumor site, they collapsed in response to highly expressed matrix metallopeptidase 2 (MMP2) and released the Ce6 and polymer–Dox–GA conjugates. Then, Dox–GA was detached from the polymer in tumor lysosomes and escaped by Ce6-mediated photodynamic therapy under irradiation. Finally, Dox–GA subsequently targeted mitochondria to reduce the O2 level to maximize the efficacy of PDT. Adapted with permission from (Li et al., 2021)
Cathepsins are highly associated with cancer development and metastasis and can be used as the stimuli of responsive nanoparticles. Torre et al. synthesized the silica mesoporous nanoparticles, the surface of which was capped by cathepsin B-responsive peptide to realize controlled release of loaded cargoes. They verified their design by encapsulating safranin O or doxorubicin into the nanoparticles (de la Torre et al., 2014). Choi’s group chemically linked the hydrophilic group PEG with the hydrophobic group via a cathepsin B-responsive peptide to self-assemble into liposomes along with DOTAP (1,2-dioleoyl-3-trimethylammonium-propane (chloride salt)), DPPC (dipalmitoylphosphatidylcholine), and cholesterol. The prepared liposomes showed cathepsin B-dependent drug release and inhibited cancer cell proliferation in a zebrafish model (Lee et al., 2020). Besides, dendrimers have been extensively investigated in the biomedical field due to their high biocompatibility, easy modifiability, and drug loading capability. Lee’s group conjugated the chemotherapeutic dox with dendrimer-methoxy poly (ethylene glycol) (MPEG) via a cathepsin B-cleavable peptide to form responsive nanoparticles (Lee et al., 2015). Luo’s group also conjugated gemcitabine with a polyHPMA copolymer via a cathepsin B- cleavable peptide to form stimuli-responsive dendritic systems (Dai et al., 2018). Aside from cathepsin B, other cathepsins can also be used for constructing responsive DDSs, including cathepsin E and cathepsin L (Abd-Elgaliel et al., 2013). Hayman’s group developed a prodrug by coupling an acetylated lysine group to puromycin which can be activated by the endogenous protease cathepsin L. In vivo anti-tumor experiments indicated that the designed prodrug exhibited efficient tumor growth inhibition in cancer models with high cathepsin L activity (Ueki et al., 2013). Prostate-specific antigen (PSA), a type of serine protease that only exists around the prostate cells, is significantly overexpressed in the tumor microenvironment and can be used for the design of enzyme-responsive prodrugs or nanomedicines. Jones’s group first covalently linked doxorubicin with a peptide that is hydrolyzable by prostate-specific antigen to form an anti-cancer prodrug. This prodrug showed much cytotoxic activity in prostate-specific antigen overexpressing cell lines and tumor models (DeFeo-Jones et al., 2000). Niidome et al. modified gold nanorods using PEG chains by a peptide linker, a substrate for urokinase-type plasminogen activator (uPA). After arriving at tumor tissue with high uPA expression, the nanorods effectively released the PEG chain (Niidome et al., 2010). To overcome the pharmacokinetic deficiencies of a small peptide with tyrosine kinase with immunoglobulin and epidermal growth factor homology-2 (Tie2) inhibiting effects, Zhang et al. designed a dual-responsive amphiphilic peptide that self-assembled into nanoparticles in solution. The formed nanoformulation (P-T4) releases the small peptide at the tumor local site in response to legumain, which is commonly overexpressed in tumor tissue and inhibited tumor growth and metastasis (Zhang et al., 2019a). Additionally, trypsin, phospholipases and peroxidase can be used as triggers of responsive nanomedicines (Lu et al., 2016; Dou et al., 2020).
Tumor-associated enzymes are tumor-specific and highly associated with the type and stage of cancer. Future clinical applications require precise design and treatment. In addition, the enzyme activity assay within tumor tissue is also an important research field. The greatest challenge enzyme-responsive DDS faced is the complicated process for the introduction of enzyme-cleavable peptides. Besides, differences in enzyme expression between animal models and clinical tumor patients also need to be considered.
3.4 ATP-Responsive DDS
The concentration of ATP in tumor cells is extremely high due to the demand for rapid tumor growth, and, as such, can be used as an internal trigger for responsive nanomedicines. The two major mechanisms of ATP responsiveness are (I) competitive combination with the designed single stranded DNA (ssDNA) aptamers, and (II) structural degradation due to ATP consumption (Sun and Gu, 2016; Deng and Walther, 2020; Sameiyan et al., 2021). Gu’s group developed an ATP-responsive nanogel (Dox/NG) consisting of a DNA motif containing an ATP aptamer and its complementary strand with doxorubicin insertion, protamine, and a hyaluronic acid (HA)-crosslinked shell (Mo et al., 2014). In the presence of ATP, the ATP aptamer in Dox/NG competitively combined with ATP to form a stable tertiary structure and the loaded doxorubicin was released. When arriving at tumor tissue via the EPR effect and HA-mediated targeting, Dox/NG is degraded by HAase and releases the Dox-intercalated duplex. Subsequently, the Dox-intercalated duplex dissociates at a significantly higher level of ATP and releases dox to kill tumor cells (Figure 4). Gu’s group also induced graphene oxide (GO) aggregation through a combination of an ATP aptamer and two complementary single-stranded chains. This aggregation could be disrupted by the high concentration of ATP in tumor cells to release the loaded cargoes (Mo et al., 2015). Liu’s group developed an alginate-based hydrogel conjugated to the APT aptamer, which was hybridized with the immunoadjuvant CpG oligonucleotide. After intratumoral injection followed by chemotherapy or radiotherapy, alginate-based hydrogel can gradually release the immunoadjuvant CpG in a ATP-dependent manner to enhance the anti-tumor effect of immunogenic cell death (ICD) (Sun et al., 2021). Ramezani’s group developed a mesoporous silica nanosystem (dox@MSNs-Apts) modified with mucine-1 and ATP aptamers on the surface to form a Y-shaped DNA structure. As a gatekeeper, the ATP aptamer prevented the rapid release of dox from the pores of the MSNs. When encountering a high concentration of ATP after tumor cell endocytosis, ATP aptamers were competitively bonded with ATP, leading to the release of dox. Their results indicated that dox@MSNs-Apts showed greater cytotoxicity than the nanoparticles decorated with scrambled ATP aptamers (dox@MSNs-Apts scrambled) in C26 and MCF-7 cell lines (Bagheri et al., 2021). Willner’s group modified the metal-organic framework caged configurations (NMOFs) using the ATP aptamer for controlled drug release and the AS1411 aptamer for tumor targeting. Both aptamers and their complementary strands on the surface of NMOFs functioned as gatekeeper. Aptamer-decorated NMOFs release the loaded dye or drug in the presence of ATP (Chen et al., 2017a). Many studies have also indicated that ATP has high bonding affinity with Zn+, which can be used for detecting ATP. According to this phenomenon, nanoparticles with Zn+ in the structure might become unstable and degraded under ATP exposure. Chen’s group engineered self-assembled quantum dots (QDs)-phenolic nanoclusters (NCs) consisting of QDs, tannic acid (TA) and chemotherapeutics by metal-phenolic coordination. As they speculated, ATP could competitively bind to TA and dissolve the QD cluster (Song et al., 2018). Yang et al. also developed an ATP-responsive zeolitic imidazole gramework-90 nanoparticle according to a similar mechanism. Imidazole-2-carboxaldehyde, Zn2+, and protein self-assembled into nanoparticles in the solution. When ZIF-90/protein nanoparticles were exposed to ATP, their structure was degraded to release the loaded proteins (RNase and Cas9), thereby demonstrating the effectiveness of ZIF-90 nanoparticles. Additional biological processes that ATP is involved in also provide inspiration to develop ATP-responsive DDSs (Yang et al., 2019a). Yuan et al. used the cage-like protein GroEL to load chemotherapeutic dox, which could be activated by ATP molecules to release encapsulated dox. When incubated with ATP molecules, GroEL exhibited a rapid dox release profile in vitro, while in vivo anti-tumor experiments also indicated that this ATP-responsive delivery system could efficiently inhibit tumor growth (Yuan et al., 2018). The examples of ATP-responsive DDS of nanoparticles are summarized in Table 5.
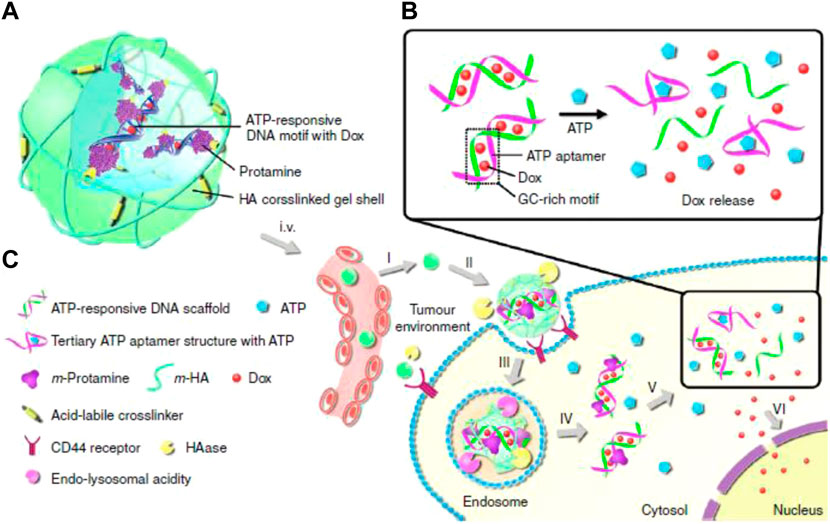
FIGURE 4. Schematic illustration of the ATP-triggered dox release system (A) The main components of Dox/NG: ATP-responsive DNA motif with Dox, protamine and a HA-crosslinked gel shell. (B) Mechanism of ATP-triggered release of Dox based on the structural change of duplex-to-aptamer. (C) ATP-responsive delivery of Dox by Dox/NG to nuclei for the targeted cancer therapy. I, accumulation of Dox/NG at the tumor site through passive and active targeting; II, specific binding to the overexpressing receptors on the tumor cells and degradation of HA shell by HAase rich in the tumor extracellular matrix; III, receptor-mediated endocytosis; IV, endosomal/lysosomal escape; V, ATP-triggered Dox release in the cytosol; VI, accumulation of Dox in the nucleus. Adapted with permission from (Mo et al., 2014).
ATP is the major energy source in each living cell in our body, especially in some tissue with high metabolism. Although the level of ATP in tumor tissue is relatively higher than that in other normal tissues, nonspecific drug release is still the major obstacle for ATP-responsive DDSs. Exploring the response strategy with ATP concentration selectivity is an important research direction.
3.5 GSH-Responsive DDS
Compared to normal tissues, the concentration of GSH in tumor tissues is much higher (∼four times), while the concentration of extracellular GSH (∼2–10 μM) is approximately 1,000 times higher than that in intracellular (∼2–10 mM) compartments, which can be used as an effective internal stimulus for responsive drug release (Guo et al., 2018; Raza et al., 2018; Mollazadeh et al., 2021). Mahato’s group synthesized redox-sensitive mPEG-co-P(Asp)-g-DC-g-S-S-GEM polymer, in which the chemotherapeutics gemcitabine (GEM) were conjugated by a disulfide bond. They also synthesized another similar polymer mPEGco- P(Asp)-g-TEPA-g-DC to form a complex along with miR-519c, which could reverse hypoxia-induced drug resistance in pancreatic cancer. Upon incubation with l-glutathione, approximately 90% of GEM was rapidly released. With surface modification by the epidermal growth factor receptor targeting peptide GE11, the prepared nanoparticles could efficiently accumulate in tumor tissue and significantly inhibit tumor growth (Xin et al., 2020). Qiu et al. conjugated the anti-cancer drug camptothecin (CPT) on the surface of AgNPs via a GSH-responsive disulfide bond to realize controlled drug release under glutathione (GSH) conditions (Qiu et al., 2018). Liu’s group linked the functional siRNA onto the surface of mesoporous silica nanoparticles (MSNs) by disulfide linkage, termed MSNs-SS-siRNA@Dox. The surface linkage of siRNA also served as a gatekeeper to prevent the burst release of loaded small molecular drugs. The introduction of the disulfide bond into the block structure endows nanoparticles with GSP-responsive properties (Zhao et al., 2017). Wu’s group developed redox-sensitive and CD44-targeted liposomes, consisting of a novel detachable polyethylene glycol (PEG 2000) conjugated to cholesterol through a bio-reducible disulfide linker (Chol-SS-mPEG), with SPC, cholesterol, cationic lipid DOTAP, DOPE, and hyaluronic acid (HA) non-covalently coated on the surface (Chi et al., 2017). The chemotherapeutic dox was also loaded (Chol-SSmPEG/HA-L), and an on vitro release investigation indicated the prepared lipid nanoparticles showed a burst drug release of 60% in the presence of 10 mM glutathione (GSH) compared to non-redox sensitive liposomes. Chol-SSmPEG/HA-L exhibited the most effective tumor suppression ratio in vivo (Figure 5). Zhang’s group also developed a liposome-based nanosystem (PTX/siRNA/SS-L) incorporating a redox-sensitive cationic oligopeptide lipid, which not only had a proton sponge effect to improve lysosomes escape, but also induced the disruption of nanostructure in the presence of GSH. PTX/siRNA/SS-L showed a synergistic inhibitory effect on tumor growth and pulmonary metastasis in a 4T1 tumor animal model (Chen et al., 2017b). Jiang’s group reported hyaluronic acid (HA) nanogels, which were crosslinked by a disulfide linker, with chemotherapeutic dox encapsulated into the nanogels. The results revealed that HA nanogels exhibited a high in vitro and in vivo RHAMM-mediated cellular uptake and drug delivery, leading to effective inhibition of tumor growth and metastasis (Yang et al., 2017b). Materials containing intrinsic disulfide bonds can be used for constructing a GSH-responsive drug delivery system. Lei et al. used 4,4′-dithiobisbenzoic acid (4,4′-DTBA) containing disulfide bond as an organic ligand to prepare a metal-organic framework nanostructure (MOF-Zr (DTBA) loaded with curcumin (CCM). MOF-Zr (DTBA) displayed faster release behavior in the presence of GSH in vitro and enhanced tumor growth inhibition compared to free CCM. Similar to other internal responsive strategies, GSH-triggered systems can also be achieved by fabricating a gatekeeper outside the nanoparticles (Lei et al., 2018). Shi’s group developed a GSH-responsive dox-loaded nanographene oxide (NGO-SS-mPEG). The decorated PEG on the surface was conjugated by a disulfide bond and served as a gatekeeper to prevent the release of the Dox. NGO-SS-mPEG showed faster drug release upon intracellular GSH stimulation than in the absence of GSH (Wen et al., 2012). Wang’s group also coated EPG on the surface of mesoporous silica nanoparticles (MSNs) via a disulfide bond to realize controlled dye release. These PEG modifications can also improve the biocompatibility of nanomaterials (Wang et al., 2015). Except for PEG molecules, which serve as the gatekeeper, low-molecular-weight heparin (LMWH) can be used to decorate nanoparticles via disulfide bonds (Tian et al., 2016). In addition, GSH-responsive polymersomes are another important drug delivery system. Hubbell’s group synthesized block copolymers of the hydrophile poly (ethylene glycol) (PEG) and the hydrophobe poly (propylene sulfide) (PPS) with disulfide linkage to fabricate nanoparticles for intracellular drug delivery (Cerritelli et al., 2007). Koul’ group synthesized an amphiphilic triblock copolymer poly (polyethylene glycol methacrylate)-poly (caprolactone)-s-s-poly (caprolactone)-poly (polyethylene glycol methacrylate) (pPEGMA-PCL-ss-PCL-pPEGMA) with a disulfide linkage that self-assembled into polymersomes with a size of ∼150 nm. The GSH-responsive polymersomes showed ∼59% drug release in pH 5.5 in the presence of 10 mM GSH and ∼85% tumor regression as compared to free doxorubicin (∼42%) (Kumar et al., 2015). In addition to disulfide bonds, ditelluride bonds are also used to construct GSH-responsive nanocarriers. Sun’ group synthesized a folic acid (FA) modified PEGylated polycaprolactone copolymer via a ditelluride linkage and prepared the Dox-loaded micelles (F-TeNPDOX). F-TeNPDOX was able to release drugs in a GSH-dependent manner through the degradation of ditelluride bonds and promoted drug accumulation and enhanced growth inhibition in 4T1 tumor in vivo (Pang et al., 2020). Xiang’ group reported a ditelluride-bridged mesoporous organosilica nanoparticle (DTeMSN) decorated with polyethylene glycol-curcumin (PEG-CCM) on the surface to realize GSH-responsive drug release. In this work, ditelluride bond was utilized as a linkage to prepare MSNs for the first time. DTeMSNs@Dox@PEG-CCM gradually degraded under redox conditions and allowed sustained Dox release. They also compared the redox responsive behavior of Te-Te-bridged MSNs and Se-Se-bridged MSNs (Xia et al., 2022). Zhang’ group fabricated a novel ultrasensitive redox-responsive system using ditelluride bond as a responsive element for the controlled release of doxorubicin (DOX). In this work, the ditelluride group was introduced for the first time into water-soluble copolymers. The synthesized copolymer nanoparticles released Dox in a GSH-dependent manner and exhibited specific tumor targeting and antitumor activity (Wang et al., 2016). The examples of GSH-responsive DDS of nanoparticles are summarized in Table 6.
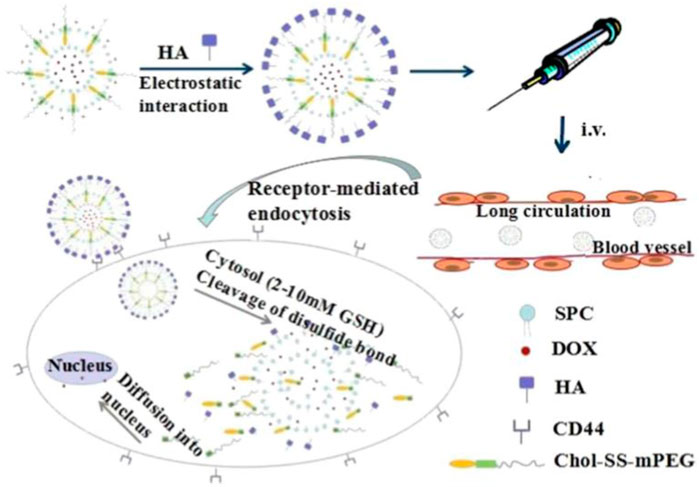
FIGURE 5. A Chol-SS-mPEG and HA dual-functionalized liposome (Chol-SS-mPEG/HA-L) designed for long circulation followed by receptor-mediated endocytosis and GSH-triggered cytoplasmic dox release. Adapted with permission from (Chi et al., 2017).
In general, high level of GSH is detected in the intracellular region, determining GSH-responsive DDS is just used for the delivery of drugs that work within tumor cells. Biological barriers including tumor blood vessels and cell membranes significantly hamper the efficacy of cellular internalization. Moreover, the concentration of GSH is just four times higher in tumor cells than that in normal cells, which makes the design of GSH-sensitive DDS difficult.
3.6 Hypoxia-Responsive DDS
The contradiction between the unsatisfied demand for nutrients and oxygen in the tumor region and the excessive tumor growth and metabolic strains leads to the formation of structurally defective and irregular tumor vasculature, which induces the hypoxic tumor microenvironment. Hypoxia is an important hallmark of tumors that is highly associated with angiogenesis, invasiveness, metastasis, and drug resistance, and can also be taken advantage of to design responsive DDSs (Thambi et al., 2016; Li et al., 2020e). Generally, three types of moieties can be used to realize hypoxia responsiveness, including azo linkers, nitrobenzyl alcohol, and nitroimidazoles (Zhou et al., 2020). Yang et al. prepared a hypoxia-responsive human serum albumin (HSA)-based nanosystem (HCHOA) by conjugating chlorin e6 (Ce6)-conjugated HSA (HC) and oxaliplatin prodrug-conjugated HSA (HO) via an azobenzene linker (Figure 6). In the circulation, HCHOA was stable and maintained a size of 100–150 nm, which could accumulate in tumor tissue through EPR effects. When arriving at tumor tissue and being exposed to the hypoxic tumor microenvironment, HCHOA quickly dissociates into HC and HO therapeutic nanoparticles with a diameter smaller than 10 nm, which showed enhanced tumor penetration capability. Their results demonstrated that HCHOA could efficiently target tumor tissue and presented a synergistic effect of photodynamic therapy and chemotherapy combination (Yang et al., 2019b). Torchilin’s group developed a hypoxia-responsive nanocarrier (PAPD) consisting of polyethyleneglycol 2000, polyethyleneimine (PEI), and 1,2-dioleyl-sn-glycero-3-phosphoethanolamine (DOPE), in which the PEG and PEI groups were linked by azobenzene. In the hypoxic tumor microenvironment, DOPE could detach the PEG group and expose the positively charged PEI to enhance cellular endocytosis and further transfection efficiency (Perche et al., 2014). Zhang’s group conjugated hydrophobic combretastatin A-4 (CA4) with hydrophilic irinotecan (IR) via an azobenzene (AZO) bond with hypoxia sensitivity to self-assemble into nanoparticles (IR–AZO–CA4/CP NPs) in solution. IR–AZO–CA4/CP NPs showed hypoxia-responsive properties and enhanced tumor penetration (Zhang et al., 2016). In addition to the azobenzene linker, the nitrobenzyl group is also widely used to synthesize hypoxia-responsive nanocarriers. Indeed, Xiao’s group prepared a hypoxia-responsive amphiphilic polymer by conjugating a hydrophobic small molecule, 4-nitrobenzyl (3-azidopropyl) carbamate onto the side chains of an mPEG-PPLG copolymer. The amphiphilic polymer self-assembled into nanoparticles loaded with dox (PPGN@dox). PPGN@dox presented a hypoxia-sensitive drug release behavior in vitro and increased antitumor activity in vivo via optimizing the pharmacokinetic properties. Nanoparticles with a positively charged surface exhibit enhanced tumor penetration capability; thus, nanoparticles with a negative charge in the circulation and positive charge in the tissue exhibit great potential to enhance anti-tumor activity (Zhang et al., 2020). Shi’s group developed a hypoxia-responsive nanocarrier, composed of a poly (caprolactone) core and a mixed shell of PEG and 4-nitrobenzyl chloroformate (NBCF)-modified polylysine (PLL). This nanocarrier can gradually increase the positive surface charge by responding to the hypoxic tumor microenvironment and penetrating deep into tumor tissues (Zhen et al., 2019). Nitroimidazole is another important hypoxia-responsive motif. Park’s group conjugated the carboxymethyl dextran with a hydrophobically modified 2-nitroimidazole derivative to establish hypoxia-responsive nanoparticles (HR-NPs), which simultaneously encapsulated dox. HR-NPs showed a hypoxia-dependent drug release profile in vitro and selectively accumulated in hypoxic tumor tissues to realize significant anti-tumor activity in vivo (Thambi et al., 2014). Zhu’s group incorporated nitroimidazole derivatives into the liposome structure to form hypoxia-responsive liposomal drug delivery system, termed dox-HR-LPs after encapsulation with dox. They demonstrated that dox-HR-LPs had high sensitivity to hypoxia and released dox in an oxygen-dependent manner. In vivo anti-tumor experiments also indicated that dox-HR-LPs exhibited enhanced therapeutic efficacy in both cell line-derived and patient-derived xenograft models (Li et al., 2019b). The typical examples of Hypoxia -responsive DDS are summarized in Table 7.
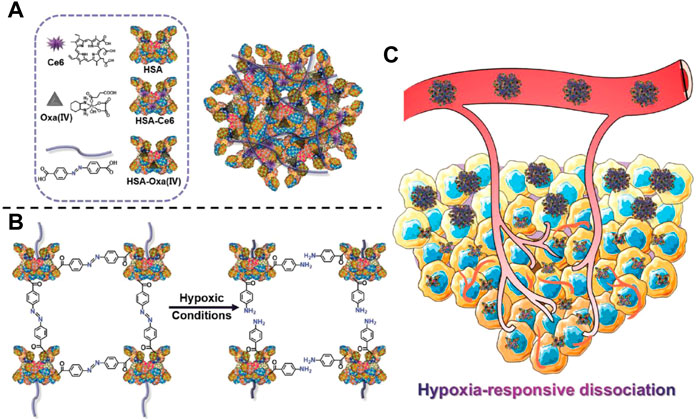
FIGURE 6. Schematic illustration of HCHOA and functional mechanism. (A) Schematic illustration of HCHOA and its components. (B) Scheme indicating the hypoxia-responsive dissociation of HCHOA. (C) Scheme showing that, in the microenvironment of tumor hypoxia, hypoxia-responsive HCHOA could disintegrate into individual HSA-based (Human Serum Albumin) complexes with improved tumor penetrating ability. Adapted with permission from (Yang et al., 2019b).
Hypoxia is a universal feature in different tumor types. However, hypoxia level in different area within the same tumor tissue is quite different. The hypoxia level in the central region of the tumor is usually much more severe than that in the peripheral region. Therefore, how to efficiently deliver hypoxia-responsive DDSs into the central region is an important problem. In addition, the relationship between the response rate and hypoxia level is also worth investigating.
4 Conclusion and Perspectives
Over the past decades, great advances have been achieved in the development of bio-responsive DDS and their applications in cancer therapy in preclinical studies. A rationally designed bio-responsive DDSs can overcome the difficulties encountered by many traditional chemotherapeutic drugs and provide a new way to treat tumors, which have important clinical application prospects, e.g., by improving the efficacy of traditional chemotherapy, controlling drug release, enhancing tumor specificity, improving pharmacokinetics/pharmacodynamics, reducing toxic side effects, and decreasing drug resistance. Despite the great efforts made in scientific publications, few bio-responsive DDSs have been successfully applied in clinical trials. Here, we summarize the recent progress of bio-responsive DDSs based on their specific characteristics in the tumor microenvironment (acidosis, overexpression of special enzymes, hypoxia and high levels of ROS, GSH and ATP), with the aim of providing a strong guideline for the design of bio-responsive DDS and their applications. Among these specific characteristics, most of them are universal in different tumor types, e.g., acidosis, hypoxia, ROS, GSH and ATP. Only the overexpressed enzymes are different and need to be selected according to tumor type.
In addition to the frequently applied DDS above-mentioned, multi-stimuli-responsive DDSs have been developed to overcome the dilemmas that single stimulus-responsive DDS face in terms of specificity and programmed release of different drugs, e.g., pH and enzyme combination, pH and ATP combination, pH and ROS combination, and pH and GSH combination (Jiang et al., 2019; Wang et al., 2020b; Du et al., 2020; Meng et al., 2020). To overcome the multiple biological barriers that hinder the drug delivery, Wang’ group described pH/cathepsin B hierarchical-responsive nanoconjugates (HRNs) which presented different states in different physiological environments. HRNs with an original size of 40 nm in the circulation were beneficial to tumor accumulation and dissociated into polymer conjugates with a size of 5 nm in an acidic tumor environment to facilitate deep tumor penetration. After being endocytosed into the lysosomes, the conjugates are cleaved by cathepsin B to release chemotherapeutics DTX into cytoplasm and inhibit cell proliferation. Moreover, this system can induce in vivo antitumor immune responses and is suitable for combination with immune therapy, e.g., a-PD-1 (programmed cell death 1) therapy. The results showed that HRNs in combination with a-PD-1 exhibited synergistic antitumor efficacy (Du et al., 2020). By endowing one nanocarrier with multi-stimuli-responsive properties, programmed drug delivery can be achieved. Liu’s group developed a pH/ROS/MMP-2 triple-responsive drug delivery system using the designed poly (ethylene glycol)-peptide-poly (ω-pentadecalactone-co-N-methyldiethyleneamine-co-3,3′-thiodipropionate) (PEG-M-PPMT) copolymer for enhanced tumor accumulation and deep penetration. In the presence of MMP2 in tumor the microenvironment, PEG-M-PPMT nanoparticles can partially shed PEG corona to form smaller particles and penetrate deep into the tumor tissue. Under the acidic endosomal pH conditions and high intracellular ROS levels, PEG-M-PPMT nanoparticles substantially swelled and released loaded drugs. All these properties of PEG-M-PPMT lead to an extraordinary combined chemo/photodynamic therapy (Shu et al., 2021). Zeolitic imidazole frameworks (ZIFs) have natural acid and ATP response features. Jiang et al. decorated ZIF90 nanoparticles using the Y1 receptor ligand [Asn6, Pro34]-NPY (AP) on the surface for tumor targeting. Combining targeted delivery and dual responsive release of DOX significantly improves the therapeutic efficacy of ZIF90 nanoparticles in MDA-MB-231 tumor bearing mouse (Jiang et al., 2019). Sun’ group integrated a pH-sensitive polymer octadecylamine-poly (aspartate-1-(3-aminopropyl) imidazole) (OA-P(Asp-API)), a ROS-generation agent and ß-Lapachone (Lap) into one nanosystem modified with RGD (iRGD)-modified ROS-responsive paclitaxel (PTX)-prodrug. The OA-P(Asp-API) in formed RLPA-NPs easily protonates in the endosome’s acidic environment and escape from endosome. The released lap produced ROS which triggered the release of PTX from PTX-prodrug to kill tumor cells (Li et al., 2020f). Chen’s group synthesized a GSH and pH dual sensitive polypeptide-DEX conjugate (L–SS–DEX) to self-assemble into nanoparticles. L–SS–DEX showed enhanced acid-triggered and GSH- triggered drug release in vitro and superior antitumor activity with an approximately 86% tumor suppression rate in comparison with 49% in the free DEX group (Ma et al., 2020). However, these combination strategies also increase the complexity of DDS and bring difficulties for their clinical application. Besides, external stimuli (magnetic fields, illumination and temperature) can also be used in combination with biological stimuli (Fang et al., 2021). Additionally, the low specificity of single stimuli, sluggish response speed, and poor biocompatibility of applied nanomaterials require further optimization. With the emergence of new technologies, such as nanomanufacturing technology and click chemistry, intelligent responsive DDSs with higher drug-forming potentials are expected to be developed. Although responsive DDSs based on the tumor microenvironment have been extensively explored, their clinical application still faces many challenges. First, there is a large heterogeneity among cancer patients, and the tumor microenvironment varies significantly in different types of tumors or different tumor growth stages (Pe’er et al., 2021). Therefore, preclinical models that better reflect tumor heterogeneity for evaluating bio-responsive DDSs should be established. Moreover, the complex interactions between the tumor extracellular matrix and tumor cells have not been fully clarified. Second, the in vivo degradability and biocompatibility of the materials have not been comprehensively verified in vivo experiments. The novel and multi-functional detecting systems need to be developed to monitor the pharmacokinetic process, toxicology-related characteristics, in vivo bio-distribution, and metabolism of drug delivery systems, which will greatly promote the development of intelligent drug delivery systems. Third, the biosafety including organ toxicity and immunogenicity of selected materials should be evaluated in depth. Fourth, how to precisely control the various physicochemical characteristics of complex drug delivery systems, e.g., size, shape, surface charge, stability, and functionalization, remains an important aspect for clinical translation. The structural and/or mechanistic complexity of multi-stimuli-responsive DDSs often restricts their clinical translation. A possible solution for this dilemma is the synthesis of motifs with two or more responsive properties to reduce their complexity. In addition, nanoparticles often have to travel the complex biological barriers to arrive at tumor cells, limiting the efficacy of nanomedicines (Niu et al., 2018; Feng et al., 2019). It is universally acknowledged that nanoparticles with a size of less than 50 nm have a short circulating half-life and can easily penetrate deep into tumor tissue but not tend to be trapped. In contrast, nanoparticles with sizes of 50–200 nm exhibit long circulating half-life and effective tumor accumulation and retention. One of the promising strategies to solve this issue is the development of multistage nanoparticles by the rational design of bio-responsive DDSs. Wang’s group reported a pH-responsive clustered nanoparticle to realize size changes from ∼100 nm in the circulation to ∼5 nm in the tumor tissue. By doing this, they achieved long blood circulation for effective tumor accumulation and deep tumor penetration. The functional process of this well-designed multistage nanosystem was demonstrated by penetration experiments using in vitro 3D tumor multicellular spheroids and an in vivo BxPC-3 xenograft tumor model (Li et al., 2016). Ren’s group synthesized an ultrafine single-chain tadpole polymer consisting of an intrachain crosslinked globule and a pH-sensitive linear polymer chain to fabricate polymer nanoparticles in solution, simultaneously encapsulating chemotherapeutic paclitaxel (PTX/MTAs). PTX/MTAs showed a size-changeable property in different pH condition and achieve deep tissue penetration in tumors (Song et al., 2019). He’s group developed a smart size-switchable nanoplatform (DGL/DOX@PP) by conjugating small dendrigraft poly-l-lysine (DGL) to poly (ethylene glycol)–poly (caprolactone) micelles via a matrix metalloproteinase 2 (MMP-2)-responsive peptide. When DGL/DOX@PP with a size of 100 nm accumulated in tumor tissue by the EPR effect and encountered overexpressed MMP2 in tumor microenvironment, small dox-loaded nanoparticles (∼30 nm) were rapidly released and penetrated into the deep area of tumor tissue (Cun et al., 2018). Many other stimulus-sensitive size shrinkable drug delivery systems have also been developed to overcome the tumor stromal barrier (Sun et al., 2015). Recent studies have indicated that immune cells in the tumor microenvironment also influence drug delivery. Weissleder’s group reported that tumor-associated macrophages (TAM) in the tumor microenvironment serve as a local drug depot that stores drug-loaded nanoparticles and are gradually released into to tumor cells (Miller et al., 2015). TAM depletion decreased the tumor accumulation of nanomedicine, indicating that we can optimize drug delivery efficacy by regulating immune cells in the tumor microenvironment.
Author Contributions
XC: Conceptualization, Methodology, Data curation, Writing—Original draft preparation. JC: Visualization, Investigation. RX: Writing—Reviewing and Editing, Funding acquisition.
Funding
This study was funded by the Natural Science Foundation of Liaoning Province (2021-MS-202).
Conflict of Interest
The authors declare that the research was conducted in the absence of any commercial or financial relationships that could be construed as a potential conflict of interest.
Publisher’s Note
All claims expressed in this article are solely those of the authors and do not necessarily represent those of their affiliated organizations, or those of the publisher, the editors and the reviewers. Any product that may be evaluated in this article, or claim that may be made by its manufacturer, is not guaranteed or endorsed by the publisher.
References
Abd-Elgaliel, W. R., Cruz-Monserrate, Z., Wang, H., Logsdon, C. D., and Tung, C.-H. (2013). Pancreatic Cancer-Associated Cathepsin E as a Drug Activator. J. Control. Release 167 (3), 221–227. doi:10.1016/j.jconrel.2013.02.007
Anderson, N. M., and Simon, M. C. (2020). The Tumor Microenvironment. Curr. Biol. 30 (16), R921–R925. doi:10.1016/j.cub.2020.06.081
Bae, Y., Fukushima, S., Harada, A., and Kataoka, K. (2003). Design of Environment-Sensitive Supramolecular Assemblies for Intracellular Drug Delivery: Polymeric Micelles that Are Responsive to Intracellular pH Change. Angew. Chem. Int. Ed. 42 (38), 4640–4643. doi:10.1002/anie.200250653
Bagheri, E., Alibolandi, M., Abnous, K., Taghdisi, S. M., and Ramezani, M. (2021). Targeted Delivery and Controlled Release of Doxorubicin to Cancer Cells by Smart ATP-Responsive Y-Shaped DNA Structure-Capped Mesoporous Silica Nanoparticles. J. Mat. Chem. B 9 (5), 1351–1363. doi:10.1039/d0tb01960g
Barenholz, Y. (2012). Doxil - the First FDA-Approved Nano-Drug: Lessons Learned. J. Control. Release 160 (2), 117–134. doi:10.1016/j.jconrel.2012.03.020
Baxevanos, P., and Mountzios, G. (2018). Novel Chemotherapy Regimens for Advanced Lung Cancer: Have We Reached a Plateau? Ann. Transl. Med. 6 (8), 139. doi:10.21037/atm.2018.04.04
Berben, L., Floris, G., Wildiers, H., and Hatse, S. (2021). Cancer and Aging: Two Tightly Interconnected Biological Processes. Cancers (Basel) 13 (6), 1400. doi:10.3390/cancers13061400
Bray, F., Ferlay, J., Soerjomataram, I., Siegel, R. L., Torre, L. A., and Jemal, A. (2018). Global Cancer Statistics 2018: GLOBOCAN Estimates of Incidence and Mortality Worldwide for 36 Cancers in 185 Countries. CA A Cancer J. Clin. 68 (6), 394–424. doi:10.3322/caac.21492
Cao, Z., Li, D., Wang, J., and Yang, X. (2021). Reactive Oxygen Species-Sensitive Polymeric Nanocarriers for Synergistic Cancer Therapy. Acta Biomater. 130, 17–31. doi:10.1016/j.actbio.2021.05.023
Car, A., Baumann, P., Duskey, J. T., Chami, M., Bruns, N., and Meier, W. (2014). pH-responsive PDMS-B-PDMAEMA Micelles for Intracellular Anticancer Drug Delivery. Biomacromolecules 15 (9), 3235–3245. doi:10.1021/bm500919z
Cerritelli, S., Velluto, D., Hubbell, J. A., and Peg-, S. S-P. P. S. (2007). PEG-SS-PPS: Reduction-Sensitive Disulfide Block Copolymer Vesicles for Intracellular Drug Delivery. Biomacromolecules 8 (6), 1966–1972. doi:10.1021/bm070085x
Chan, D. A., and Giaccia, A. J. (2007). Hypoxia, Gene Expression, and Metastasis. Cancer Metastasis Rev. 26 (2), 333–339. doi:10.1007/s10555-007-9063-1
Chen, K., Chen, Q., Wang, K., Zhu, J., Li, W., Li, W., et al. (2016). Synthesis and Characterization of a PAMAM-OH Derivative Containing an Acid-Labile β-thiopropionate Bond for Gene Delivery. Int. J. Pharm. 509 (1-2), 314–327. doi:10.1016/j.ijpharm.2016.05.060
Chen, W.-H., Yu, X., Liao, W.-C., Sohn, Y. S., Cecconello, A., Kozell, A., et al. (2017). ATP-responsive Aptamer-Based Metal-Organic Framework Nanoparticles (NMOFs) for the Controlled Release of Loads and Drugs. Adv. Funct. Mat. 27 (37), 1702102. doi:10.1002/adfm.201702102
Chen, X., Zhang, Y., Tang, C., Tian, C., Sun, Q., Su, Z., et al. (2017). Co-delivery of Paclitaxel and Anti-survivin siRNA via Redox-Sensitive Oligopeptide Liposomes for the Synergistic Treatment of Breast Cancer and Metastasis. Int. J. Pharm. 529 (1-2), 102–115. doi:10.1016/j.ijpharm.2017.06.071
Chi, Y., Yin, X., Sun, K., Feng, S., Liu, J., Chen, D., et al. (2017). Redox-sensitive and Hyaluronic Acid Functionalized Liposomes for Cytoplasmic Drug Delivery to Osteosarcoma in Animal Models. J. Control. Release 261, 113–125. doi:10.1016/j.jconrel.2017.06.027
Chu, B., Qu, Y., He, X., Hao, Y., Yang, C., Yang, Y., et al. (2020). ROS‐Responsive Camptothecin Prodrug Nanoparticles for On‐Demand Drug Release and Combination of Chemotherapy and Photodynamic Therapy. Adv. Funct. Mat. 30 (52), 2005918. doi:10.1002/adfm.202005918
Conlon, G. A., and Murray, G. I. (2019). Recent Advances in Understanding the Roles of Matrix Metalloproteinases in Tumour Invasion and Metastasis. J. Pathol. 247 (5), 629–640. doi:10.1002/path.5225
Cun, X., Li, M., Wang, S., Wang, Y., Wang, J., Lu, Z., et al. (2018). A Size Switchable Nanoplatform for Targeting the Tumor Microenvironment and Deep Tumor Penetration. Nanoscale 10 (21), 9935–9948. doi:10.1039/c8nr00640g
Dai, L., Zhang, Q., Shen, X., Sun, Q., Mu, C., Gu, H., et al. (2016). A pH-Responsive Nanocontainer Based on Hydrazone-Bearing Hollow Silica Nanoparticles for Targeted Tumor Therapy. J. Mat. Chem. B 4 (26), 4594–4604. doi:10.1039/c6tb01050d
Dai, Y., Ma, X., Zhang, Y., Chen, K., Tang, J. Z., Gong, Q., et al. (2018). A Biocompatible and Cathepsin B Sensitive Nanoscale System of Dendritic polyHPMA-Gemcitabine Prodrug Enhances Antitumor Activity Markedly. Biomater. Sci. 6 (11), 2976–2986. doi:10.1039/c8bm00946e
Damgaci, S., Ibrahim-Hashim, A., Enriquez-Navas, P. M., Pilon-Thomas, S., Guvenis, A., and Gillies, R. J. (2018). Hypoxia and Acidosis: Immune Suppressors and Therapeutic Targets. Immunology 154 (3), 354–362. doi:10.1111/imm.12917
de la Torre, C., Mondragón, L., Coll, C., Sancenón, F., Marcos, M. D., Martínez-Máñez, R., et al. (2014). Cathepsin-B Induced Controlled Release from Peptide-Capped Mesoporous Silica Nanoparticles. Chem. Eur. J. 20 (47), 15309–15314. doi:10.1002/chem.201404382
Deepagan, V. G., Kwon, S., You, D. G., Nguyen, V. Q., Um, W., Ko, H., et al. (2016). In Situ diselenide-crosslinked Polymeric Micelles for ROS-Mediated Anticancer Drug Delivery. Biomaterials 103, 56–66. doi:10.1016/j.biomaterials.2016.06.044
DeFeo-Jones, D., Garsky, V. M., Wong, B. K., Feng, D.-M., Bolyar, T., Haskell, K., et al. (2000). A Peptide-Doxorubicin 'prodrug' Activated by Prostate-specific Antigen Selectively Kills Prostate Tumor Cells Positive for Prostate-specific Antigen In Vivo. Nat. Med. 6 (11), 1248–1252. doi:10.1038/81351
Deng, J., and Walther, A. (2020). ATP-responsive and ATP-Fueled Self-Assembling Systems and Materials. Adv. Mater 32 (42), e2002629. doi:10.1002/adma.202002629
Ding, C., Gu, J., Qu, X., and Yang, Z. (2009). Preparation of Multifunctional Drug Carrier for Tumor-specific Uptake and Enhanced Intracellular Delivery through the Conjugation of Weak Acid Labile Linker. Bioconjugate Chem. 20 (6), 1163–1170. doi:10.1021/bc800563g
Dou, Y., Li, C., Li, L., Guo, J., and Zhang, J. (2020). Bioresponsive Drug Delivery Systems for the Treatment of Inflammatory Diseases. J. Control. Release 327, 641–666. doi:10.1016/j.jconrel.2020.09.008
Du, H., Zhao, S., Wang, Y., Wang, Z., Chen, B., Yan, Y., et al. (2020). pH/Cathepsin B Hierarchical‐Responsive Nanoconjugates for Enhanced Tumor Penetration and Chemo‐Immunotherapy. Adv. Funct. Mat. 30 (39), 2003757. doi:10.1002/adfm.202003757
Du, Y., He, W., Xia, Q., Zhou, W., Yao, C., and Li, X. (2019). Thioether Phosphatidylcholine Liposomes: A Novel ROS-Responsive Platform for Drug Delivery. ACS Appl. Mat. Interfaces 11 (41), 37411–37420. doi:10.1021/acsami.9b08901
Ensign, L. M., Cone, R., and Hanes, J. (2014). Nanoparticle-based Drug Delivery to the Vagina: a Review. J. Control. Release 190, 500–514. doi:10.1016/j.jconrel.2014.04.033
Fang, J. (2022). EPR Effect-Based Tumor Targeted Nanomedicine: A Promising Approach for Controlling Cancer. J. Pers. Med. 12 (1), 95. doi:10.3390/jpm12010095
Fang, J., Islam, W., and Maeda, H. (2020). Exploiting the Dynamics of the EPR Effect and Strategies to Improve the Therapeutic Effects of Nanomedicines by Using EPR Effect Enhancers. Adv. Drug Deliv. Rev. 157, 142–160. doi:10.1016/j.addr.2020.06.005
Fang, J., Nakamura, H., and Maeda, H. (2011). The EPR Effect: Unique Features of Tumor Blood Vessels for Drug Delivery, Factors Involved, and Limitations and Augmentation of the Effect. Adv. Drug Deliv. Rev. 63 (3), 136–151. doi:10.1016/j.addr.2010.04.009
Fang, Z., Shen, Y., and Gao, D. (2021). Stimulus-responsive Nanocarriers for Targeted Drug Delivery. New J. Chem. 45 (10), 4534–4544. doi:10.1039/d0nj05169a
Feng, X., Dixon, H., Glen‐Ravenhill, H., Karaosmanoglu, S., Li, Q., Yan, L., et al. (2019). Smart Nanotechnologies to Target Tumor with Deep Penetration Depth for Efficient Cancer Treatment and Imaging. Adv. Ther. 2 (10), 1900093. doi:10.1002/adtp.201900093
Fukumura, D., and Jain, R. K. (2007). Tumor Microenvironment Abnormalities: Causes, Consequences, and Strategies to Normalize. J. Cell. Biochem. 101 (4), 937–949. doi:10.1002/jcb.21187
Gu, Y., Zhong, Y., Meng, F., Cheng, R., Deng, C., and Zhong, Z. (2013). Acetal-linked Paclitaxel Prodrug Micellar Nanoparticles as a Versatile and Potent Platform for Cancer Therapy. Biomacromolecules 14 (8), 2772–2780. doi:10.1021/bm400615n
Guo, X., Cheng, Y., Zhao, X., Luo, Y., Chen, J., and Yuan, W.-E. (2018). Advances in Redox-Responsive Drug Delivery Systems of Tumor Microenvironment. J. Nanobiotechnol 16 (1), 74. doi:10.1186/s12951-018-0398-2
Han, Q. J., Lan, X. T., Wen, Y., Zhang, C. Z., Cleary, M., Sayyed, Y., et al. (2021). Matrix Metalloproteinase-9-Responsive Surface Charge-Reversible Nanocarrier to Enhance Endocytosis as Efficient Targeted Delivery System for Cancer Diagnosis and Therapy. Adv. Healthc. Mater 10 (9), e2002143. doi:10.1002/adhm.202002143
Hanahan, D. (2022). Hallmarks of Cancer: New Dimensions. Cancer Discov. 12 (1), 31–46. doi:10.1158/2159-8290.cd-21-1059
Hao, Y., Chen, Y., He, X., Yu, Y., Han, R., Li, Y., et al. (2020). Polymeric Nanoparticles with ROS‐Responsive Prodrug and Platinum Nanozyme for Enhanced Chemophotodynamic Therapy of Colon Cancer. Adv. Sci. 7 (20), 2001853. doi:10.1002/advs.202001853
Hejmady, S., Pradhan, R., Alexander, A., Agrawal, M., Singhvi, G., Gorain, B., et al. (2020). Recent Advances in Targeted Nanomedicine as Promising Antitumor Therapeutics. Drug Discov. Today 25 (12), 2227–2244. doi:10.1016/j.drudis.2020.09.031
Höcherl, A., Jäger, E., Jäger, A., Hrubý, M., Konefał, R., Janoušková, O., et al. (2017). One-pot Synthesis of Reactive Oxygen Species (ROS)-self-immolative Polyoxalate Prodrug Nanoparticles for Hormone Dependent Cancer Therapy with Minimized Side Effects. Polym. Chem. 8 (13), 1999–2004.
Huo, M., Yuan, J., Tao, L., and Wei, Y. (2014). Redox-responsive Polymers for Drug Delivery: from Molecular Design to Applications. Polym. Chem. 5 (5), 1519–1528. doi:10.1039/c3py01192e
Islam, R., Maeda, H., and Fang, J. (2021). Factors Affecting the Dynamics and Heterogeneity of the EPR Effect: Pathophysiological and Pathoanatomic Features, Drug Formulations and Physicochemical Factors. Expert Opin. Drug Deliv. 19, 1–14. doi:10.1080/17425247.2021.1874916
Ji, K., Mayernik, L., Moin, K., and Sloane, B. F. (2019). Acidosis and Proteolysis in the Tumor Microenvironment. Cancer Metastasis Rev. 38 (1-2), 103–112. doi:10.1007/s10555-019-09796-3
Jiang, Z., Wang, Y., Sun, L., Yuan, B., Tian, Y., Xiang, L., et al. (2019). Dual ATP and pH Responsive ZIF-90 Nanosystem with Favorable Biocompatibility and Facile Post-modification Improves Therapeutic Outcomes of Triple Negative Breast Cancer In Vivo. Biomaterials 197, 41–50. doi:10.1016/j.biomaterials.2019.01.001
Kulkarni, P. S., Haldar, M. K., Nahire, R. R., Katti, P., Ambre, A. H., Muhonen, W. W., et al. (2014). Mmp-9 Responsive PEG Cleavable Nanovesicles for Efficient Delivery of Chemotherapeutics to Pancreatic Cancer. Mol. Pharm. 11 (7), 2390–2399. doi:10.1021/mp500108p
Kumar, A., Lale, S. V., Mahajan, S., Choudhary, V., and Koul, V. (2015). ROP and ATRP Fabricated Dual Targeted Redox Sensitive Polymersomes Based on pPEGMA-PCL-Ss-PCL-pPEGMA Triblock Copolymers for Breast Cancer Therapeutics. ACS Appl. Mat. Interfaces 7 (17), 9211–9227. doi:10.1021/acsami.5b01731
Kumari, R., Sunil, D., and Ningthoujam, R. S. (2020). Hypoxia-responsive Nanoparticle Based Drug Delivery Systems in Cancer Therapy: An Up-To-Date Review. J. Control. Release 319, 135–156. doi:10.1016/j.jconrel.2019.12.041
Lee, S., Song, S. J., Lee, J., Ha, T. H., and Choi, J. S. (2020). Cathepsin B-Responsive Liposomes for Controlled Anticancer Drug Delivery in Hep G2 Cells. Pharmaceutics 12 (9), 876. doi:10.3390/pharmaceutics12090876
Lee, S. J., Jeong, Y. I., Park, H. K., Kang, D. H., Oh, J. S., Lee, S. G., et al. (2015). Enzyme-responsive Doxorubicin Release from Dendrimer Nanoparticles for Anticancer Drug Delivery. Int. J. Nanomedicine 10, 5489–5503. doi:10.2147/IJN.S87145
Lei, B., Wang, M., Jiang, Z., Qi, W., Su, R., and He, Z. (2018). Constructing Redox-Responsive Metal-Organic Framework Nanocarriers for Anticancer Drug Delivery. ACS Appl. Mat. Interfaces 10 (19), 16698–16706. doi:10.1021/acsami.7b19693
Li, D., Zhang, R., Liu, G., Kang, Y., and Wu, J. (2020). Redox-Responsive Self-Assembled Nanoparticles for Cancer Therapy. Adv. Healthc. Mater 9 (20), e2000605. doi:10.1002/adhm.202000605
Li, H.-J., Du, J.-Z., Du, X.-J., Xu, C.-F., Sun, C.-Y., Wang, H.-X., et al. (2016). Stimuli-responsive Clustered Nanoparticles for Improved Tumor Penetration and Therapeutic Efficacy. Proc. Natl. Acad. Sci. U.S.A. 113 (15), 4164–4169. doi:10.1073/pnas.1522080113
Li, J., Zhang, X., Zhao, M., Wu, L., Luo, K., Pu, Y., et al. (2018). Tumor-pH-Sensitive PLLA-Based Microsphere with Acid Cleavable Acetal Bonds on the Backbone for Efficient Localized Chemotherapy. Biomacromolecules 19 (7), 3140–3148. doi:10.1021/acs.biomac.8b00734
Li, M., Zhao, G., Su, W.-K., and Shuai, Q. (2020). Enzyme-Responsive Nanoparticles for Anti-tumor Drug Delivery. Front. Chem. 8, 647. doi:10.3389/fchem.2020.00647
Li, S., Hu, L., Li, D., Wang, X., Zhang, P., Wang, J., et al. (2019). Carboxymethyl Chitosan-Based Nanogels via Acid-Labile Ortho Ester Linkages Mediated Enhanced Drug Delivery. Int. J. Biol. Macromol. 129, 477–487. doi:10.1016/j.ijbiomac.2019.02.072
Li, S., Zhang, Y., Wang, J., Zhao, Y., Ji, T., Zhao, X., et al. (2017). Nanoparticle-mediated Local Depletion of Tumour-Associated Platelets Disrupts Vascular Barriers and Augments Drug Accumulation in Tumours. Nat. Biomed. Eng. 1 (8), 667–679. doi:10.1038/s41551-017-0115-8
Li, T., Pan, S., Gao, S., Xiang, W., Sun, C., Cao, W., et al. (2020). Diselenide-Pemetrexed Assemblies for Combined Cancer Immuno‐, Radio‐, and Chemotherapies. Angew. Chem. Int. Ed. 59 (7), 2700–2704. doi:10.1002/anie.201914453
Li, W., Sun, J., Zhang, X., Jia, L., Qiao, M., Zhao, X., et al. (2020). Synthesis and Characterization of pH-Responsive PEG-Poly(β-Amino Ester) Block Copolymer Micelles as Drug Carriers to Eliminate Cancer Stem Cells. Pharmaceutics 12 (2), 111. doi:10.3390/pharmaceutics12020111
Li, X., Yang, W., Zou, Y., Meng, F., Deng, C., and Zhong, Z. (2015). Efficacious Delivery of Protein Drugs to Prostate Cancer Cells by PSMA-Targeted pH-Responsive Chimaeric Polymersomes. J. Control. Release 220 (Pt B), 704–714. doi:10.1016/j.jconrel.2015.08.058
Li, X., Zhou, Z., Zhou, R., Yang, J., Zhou, M., Li, Q., et al. (2021). Stimuli‐Responsive Nanoparticles Combining Photodynamic Therapy and Mitochondria Disruption Suppressed Tumor Metastasis. Adv. Mater. Inter 8 (10), 2002200. doi:10.1002/admi.202002200
Li, Y., Bai, H., Wang, H., Shen, Y., Tang, G., and Ping, Y. (2017). Reactive Oxygen Species (ROS)-responsive Nanomedicine for RNAi-Based Cancer Therapy. Nanoscale 10 (1), 203–214. doi:10.1039/c7nr06689a
Li, Y., Chen, M., Yao, B., Lu, X., Song, B., Vasilatos, S. N., et al. (2020). Dual pH/ROS-Responsive Nanoplatform with Deep Tumor Penetration and Self-Amplified Drug Release for Enhancing Tumor Chemotherapeutic Efficacy. Small 16 (32), e2002188. doi:10.1002/smll.202002188
Li, Y., Jeon, J., and Park, J. H. (2020). Hypoxia-responsive Nanoparticles for Tumor-Targeted Drug Delivery. Cancer Lett. 490, 31–43. doi:10.1016/j.canlet.2020.05.032
Li, Y., Lu, A., Long, M., Cui, L., Chen, Z., and Zhu, L. (2019). Nitroimidazole Derivative Incorporated Liposomes for Hypoxia-Triggered Drug Delivery and Enhanced Therapeutic Efficacy in Patient-Derived Tumor Xenografts. Acta Biomater. 83, 334–348. doi:10.1016/j.actbio.2018.10.029
Lian, Z., and Ji, T. (2020). Functional Peptide-Based Drug Delivery Systems. J. Mat. Chem. B 8 (31), 6517–6529. doi:10.1039/d0tb00713g
Lissoni, P., Brivio, F., Fumagalli, L., Messina, G., Meregalli, S., Porro, G., et al. (2009). Effects of the Conventional Antitumor Therapies Surgery, Chemotherapy, Radiotherapy and Immunotherapy on Regulatory T Lymphocytes in Cancer Patients. Anticancer Res. 29 (5), 1847–1852.
Liu, G., Zhao, X., Zhang, Y., Xu, J., Xu, J., Li, Y., et al. (2019). Engineering Biomimetic Platesomes for pH-Responsive Drug Delivery and Enhanced Antitumor Activity. Adv. Mater 31 (32), e1900795. doi:10.1002/adma.201900795
Liu, J., Zhang, B., Luo, Z., Ding, X., Li, J., Dai, L., et al. (2015). Enzyme Responsive Mesoporous Silica Nanoparticles for Targeted Tumor Therapy In Vitro and In Vivo. Nanoscale 7 (8), 3614–3626. doi:10.1039/c5nr00072f
Liu, R., He, B., Li, D., Lai, Y., Tang, J. Z., and Gu, Z. (2012). Synthesis and Characterization of Poly(ethylene Glycol)-B-Poly(l-Histidine)-B-Poly(l-Lactide) with pH-Sensitivity. Polymer 53 (7), 1473–1482. doi:10.1016/j.polymer.2012.02.013
Liu, Y., Wang, J., Zhang, J., Marbach, S., Xu, W., and Zhu, L. (2020). Targeting Tumor-Associated Macrophages by MMP2-Sensitive Apoptotic Body-Mimicking Nanoparticles. ACS Appl. Mat. Interfaces 12 (47), 52402–52414. doi:10.1021/acsami.0c15983
Lu, Y., Aimetti, A. A., Langer, R., and Gu, Z. (2016). Bioresponsive Materials. Nat. Rev. Mater. 1 (1), 16075. doi:10.1038/natrevmats.2016.75
Ma, S., Song, W., Xu, Y., Si, X., Zhang, D., Lv, S., et al. (2020). Neutralizing Tumor-Promoting Inflammation with Polypeptide-Dexamethasone Conjugate for Microenvironment Modulation and Colorectal Cancer Therapy. Biomaterials 232, 119676. doi:10.1016/j.biomaterials.2019.119676
Meng, J., Jin, Z., Zhao, P., Zhao, B., Fan, M., and He, Q. (2020). A Multistage Assembly/disassembly Strategy for Tumor-Targeted CO Delivery. Sci. Adv. 6 (20), eaba1362. doi:10.1126/sciadv.aba1362
Miller, M. A., Zheng, Y.-R., Gadde, S., Pfirschke, C., Zope, H., Engblom, C., et al. (2015). Tumour-associated Macrophages Act as a Slow-Release Reservoir of Nano-Therapeutic Pt(IV) Pro-drug. Nat. Commun. 6, 8692. doi:10.1038/ncomms9692
Mo, R., Jiang, T., DiSanto, R., Tai, W., and Gu, Z. (2014). ATP-triggered Anticancer Drug Delivery. Nat. Commun. 5, 3364. doi:10.1038/ncomms4364
Mo, R., Jiang, T., Sun, W., and Gu, Z. (2015). ATP-responsive DNA-Graphene Hybrid Nanoaggregates for Anticancer Drug Delivery. Biomaterials 50, 67–74. doi:10.1016/j.biomaterials.2015.01.053
Mollazadeh, S., Mackiewicz, M., and Yazdimamaghani, M. (2021). Recent Advances in the Redox-Responsive Drug Delivery Nanoplatforms: A Chemical Structure and Physical Property Perspective. Mater. Sci. Eng. C 118, 111536. doi:10.1016/j.msec.2020.111536
Mu, J., Zhong, H., Zou, H., Liu, T., Yu, N., Zhang, X., et al. (2020). Acid-sensitive PEGylated Paclitaxel Prodrug Nanoparticles for Cancer Therapy: Effect of PEG Length on Antitumor Efficacy. J. Control. Release 326, 265–275. doi:10.1016/j.jconrel.2020.07.022
Mura, S., Nicolas, J., and Couvreur, P. (2013). Stimuli-responsive Nanocarriers for Drug Delivery. Nat. Mater 12 (11), 991–1003. doi:10.1038/nmat3776
Muz, B., de la Puente, P., Azab, F., and Azab, A. K. (2015). The Role of Hypoxia in Cancer Progression, Angiogenesis, Metastasis, and Resistance to Therapy. Hp 3, 83–92. doi:10.2147/hp.s93413
Napoli, A., Valentini, M., Tirelli, N., Müller, M., and Hubbell, J. A. (2004). Oxidation-responsive Polymeric Vesicles. Nat. Mater 3 (3), 183–189. doi:10.1038/nmat1081
Navya, P. N., Kaphle, A., Srinivas, S. P., Bhargava, S. K., Rotello, V. M., and Daima, H. K. (2019). Current Trends and Challenges in Cancer Management and Therapy Using Designer Nanomaterials. Nano Converg. 6 (1), 23. doi:10.1186/s40580-019-0193-2
Niidome, T., Ohga, A., Akiyama, Y., Watanabe, K., Niidome, Y., Mori, T., et al. (2010). Controlled Release of PEG Chain from Gold Nanorods: Targeted Delivery to Tumor. Bioorg. Med. Chem. 18 (12), 4453–4458. doi:10.1016/j.bmc.2010.04.070
Niu, Y., Zhu, J., Li, Y., Shi, H., Gong, Y., Li, R., et al. (2018). Size Shrinkable Drug Delivery Nanosystems and Priming the Tumor Microenvironment for Deep Intratumoral Penetration of Nanoparticles. J. Control. Release 277, 35–47. doi:10.1016/j.jconrel.2018.03.012
Pan, Q., Deng, X., Gao, W., Chang, J., Pu, Y., and He, B. (2020). ROS Triggered Cleavage of Thioketal Moiety to Dissociate Prodrug Nanoparticles for Chemotherapy. Colloids Surfaces B Biointerfaces 194, 111223. doi:10.1016/j.colsurfb.2020.111223
Pang, Z., Zhou, J., and Sun, C. (2020). Ditelluride-Bridged PEG-PCL Copolymer as Folic Acid-Targeted and Redox-Responsive Nanoparticles for Enhanced Cancer Therapy. Front. Chem. 8, 156. doi:10.3389/fchem.2020.00156
Park, H., Saravanakumar, G., Kim, J., Lim, J., and Kim, W. J. (2021). Tumor Microenvironment Sensitive Nanocarriers for Bioimaging and Therapeutics. Adv. Healthc. Mater 10 (5), e2000834. doi:10.1002/adhm.202000834
Pe'er, D., Ogawa, S., Elhanani, O., Keren, L., Oliver, T. G., and Wedge, D. (2021). Tumor Heterogeneity. Cancer Cell 39 (8), 1015–1017.
Pei, P., Sun, C., Tao, W., Li, J., Yang, X., and Wang, J. (2019). ROS-sensitive Thioketal-Linked Polyphosphoester-Doxorubicin Conjugate for Precise Phototriggered Locoregional Chemotherapy. Biomaterials 188, 74–82. doi:10.1016/j.biomaterials.2018.10.010
Perche, F., Biswas, S., Wang, T., Zhu, L., and Torchilin, V. P. (2014). Hypoxia-targeted siRNA Delivery. Angew. Chem. Int. Ed. 53 (13), 3362–3366. doi:10.1002/anie.201308368
Petrova, V., Annicchiarico-Petruzzelli, M., Melino, G., and Amelio, I. (2018). The Hypoxic Tumour Microenvironment. Oncogenesis 7 (1), 10. doi:10.1038/s41389-017-0011-9
Qiu, L., Zhao, L., Xing, C., and Zhan, Y. (2018). Redox-responsive Polymer prodrug/AgNPs Hybrid Nanoparticles for Drug Delivery. Chin. Chem. Lett. 29 (2), 301–304. doi:10.1016/j.cclet.2017.09.048
Rankin, E. B., and Giaccia, A. J. (2016). Hypoxic Control of Metastasis. Science 352 (6282), 175–180. doi:10.1126/science.aaf4405
Raza, A., Hayat, U., Rasheed, T., Bilal, M., and Iqbal, H. M. N. (2018). Redox-responsive Nano-Carriers as Tumor-Targeted Drug Delivery Systems. Eur. J. Med. Chem. 157, 705–715. doi:10.1016/j.ejmech.2018.08.034
Sadeghi Rad, H., Monkman, J., Warkiani, M. E., Ladwa, R., O'Byrne, K., Rezaei, N., et al. (2021). Understanding the Tumor Microenvironment for Effective Immunotherapy. Med. Res. Rev. 41 (3), 1474–1498. doi:10.1002/med.21765
Sameiyan, E., Bagheri, E., Dehghani, S., Ramezani, M., Alibolandi, M., Abnous, K., et al. (2021). Aptamer-based ATP-Responsive Delivery Systems for Cancer Diagnosis and Treatment. Acta Biomater. 123, 110–122. doi:10.1016/j.actbio.2020.12.057
Schito, L. (2019). Hypoxia-Dependent Angiogenesis and Lymphangiogenesis in Cancer. Adv. Exp. Med. Biol. 1136, 71–85. doi:10.1007/978-3-030-12734-3_5
Shahriari, M., Zahiri, M., Abnous, K., Taghdisi, S. M., Ramezani, M., and Alibolandi, M. (2019). Enzyme Responsive Drug Delivery Systems in Cancer Treatment. J. Control. Release 308, 172–189. doi:10.1016/j.jconrel.2019.07.004
Shao, D., Zhang, F., Chen, F., Zheng, X., Hu, H., Yang, C., et al. (2020). Biomimetic Diselenide-Bridged Mesoporous Organosilica Nanoparticles as an X-Ray-Responsive Biodegradable Carrier for Chemo-Immunotherapy. Adv. Mater 32 (50), e2004385. doi:10.1002/adma.202004385
Shao, D., Li, M., Wang, Z., Zheng, X., Lao, Y. H., Chang, Z., et al. (2018). Bioinspired Diselenide-Bridged Mesoporous Silica Nanoparticles for Dual-Responsive Protein Delivery. Adv. Mater 1, e1801198. doi:10.1002/adma.201801198
Shi, J., Kantoff, P. W., Wooster, R., and Farokhzad, O. C. (2017). Cancer Nanomedicine: Progress, Challenges and Opportunities. Nat. Rev. Cancer 17 (1), 20–37. doi:10.1038/nrc.2016.108
Shin, Y., Husni, P., Kang, K., Lee, D., Lee, S., Lee, E., et al. (2021). Recent Advances in pH- Or/and Photo-Responsive Nanovehicles. Pharmaceutics 13 (5), 725. doi:10.3390/pharmaceutics13050725
Shu, M., Tang, J., Chen, L., Zeng, Q., Li, C., Xiao, S., et al. (2021). Tumor Microenvironment Triple-Responsive Nanoparticles Enable Enhanced Tumor Penetration and Synergetic Chemo-Photodynamic Therapy. Biomaterials 268, 120574. doi:10.1016/j.biomaterials.2020.120574
Sonawane, S. J., Kalhapure, R. S., and Govender, T. (2017). Hydrazone Linkages in pH Responsive Drug Delivery Systems. Eur. J. Pharm. Sci. 99, 45–65. doi:10.1016/j.ejps.2016.12.011
Song, C., Lin, T., Zhang, Q., Thayumanavan, S., and Ren, L. (2019). pH-Sensitive Morphological Transitions in Polymeric Tadpole Assemblies for Programmed Tumor Therapy. J. Control. Release 293, 1–9. doi:10.1016/j.jconrel.2018.10.033
Song, X.-R., Li, S.-H., Guo, H., You, W., Tu, D., Li, J., et al. (2018). Enhancing Antitumor Efficacy by Simultaneous ATP-Responsive Chemodrug Release and Cancer Cell Sensitization Based on a Smart Nanoagent. Adv. Sci. 5 (12), 1801201. doi:10.1002/advs.201801201
Su, F., Yun, P., Li, C., Li, R., Xi, L., Wang, Y., et al. (2019). Novel Self-Assembled Micelles of Amphiphilic Poly(2-Ethyl-2-Oxazoline) -poly(L-Lactide) Diblock Copolymers for Sustained Drug Delivery. Colloids Surfaces A Physicochem. Eng. Aspects 566, 120–127. doi:10.1016/j.colsurfa.2019.01.015
Su, Z., Liang, Y., Yao, Y., Wang, T., and Zhang, N. (2016). Polymeric Complex Micelles Based on the Double-Hydrazone Linkage and Dual Drug-Loading Strategy for pH-Sensitive Docetaxel Delivery. J. Mat. Chem. B 4 (6), 1122–1133. doi:10.1039/c5tb02188j
Sun, C.-Y., Shen, S., Xu, C.-F., Li, H.-J., Liu, Y., Cao, Z.-T., et al. (2015). Tumor Acidity-Sensitive Polymeric Vector for Active Targeted siRNA Delivery. J. Am. Chem. Soc. 137 (48), 15217–15224. doi:10.1021/jacs.5b09602
Sun, C., Ji, S., Li, F., and Xu, H. (2017). Diselenide-Containing Hyperbranched Polymer with Light-Induced Cytotoxicity. ACS Appl. Mat. Interfaces 9 (15), 12924–12929. doi:10.1021/acsami.7b02367
Sun, L., Shen, F., Tian, L., Tao, H., Xiong, Z., Xu, J., et al. (2021). ATP-responsive Smart Hydrogel Releasing Immune Adjuvant Synchronized with Repeated Chemotherapy or Radiotherapy to Boost Antitumor Immunity. Adv. Mater 33 (18), e2007910. doi:10.1002/adma.202007910
Sun, W., and Gu, Z. (2016). ATP-responsive Drug Delivery Systems. Expert Opin. Drug Deliv. 13 (3), 311–314. doi:10.1517/17425247.2016.1140147
Swetha, K. L., Maravajjala, K. S., Sharma, S., Chowdhury, R., and Roy, A. (2021). Development of a Tumor Extracellular pH-Responsive Nanocarrier by Terminal Histidine Conjugation in a Star Shaped Poly(lactic-Co-Glycolic Acid). Eur. Polym. J. 147, 110337. doi:10.1016/j.eurpolymj.2021.110337
Takemoto, H., Inaba, T., Nomoto, T., Matsui, M., Liu, X., Toyoda, M., et al. (2020). Polymeric Modification of Gemcitabine via Cyclic Acetal Linkage for Enhanced Anticancer Potency with Negligible Side Effects. Biomaterials 235, 119804. doi:10.1016/j.biomaterials.2020.119804
Tao, B., and Yin, Z. (2020). Redox-Responsive Coordination Polymers of Dopamine-Modified Hyaluronic Acid with Copper and 6-Mercaptopurine for Targeted Drug Delivery and Improvement of Anticancer Activity against Cancer Cells. Polym. (Basel) 12 (5), 12. doi:10.3390/polym12051132
Thambi, T., Deepagan, V. G., Yoon, H. Y., Han, H. S., Kim, S.-H., Son, S., et al. (2014). Hypoxia-responsive Polymeric Nanoparticles for Tumor-Targeted Drug Delivery. Biomaterials 35 (5), 1735–1743. doi:10.1016/j.biomaterials.2013.11.022
Thambi, T., Park, J. H., and Lee, D. S. (2016). Hypoxia-responsive Nanocarriers for Cancer Imaging and Therapy: Recent Approaches and Future Perspectives. Chem. Commun. 52 (55), 8492–8500. doi:10.1039/c6cc02972h
Thomas, R. G., Surendran, S. P., and Jeong, Y. Y. (2020). Tumor Microenvironment-Stimuli Responsive Nanoparticles for Anticancer Therapy. Front. Mol. Biosci. 7, 610533. doi:10.3389/fmolb.2020.610533
Tian, D., Qin, F., Zhao, H., Zhang, C., Wang, H., Liu, N., et al. (2021). Bio-Responsive Nanoparticle for Tumor Targeting and Enhanced Photo-Immunotherapy. Colloids Surfaces B Biointerfaces 202, 111681. doi:10.1016/j.colsurfb.2021.111681
Tian, J., Han, M., Wang, Y., Qian, K., Ke, X., and Ci, T. (2016). Reduction-responsive Modification-Induced Higher Efficiency for Attenuation of Tumor Metastasis of Low Molecular Weight Heparin Functionalized Liposomes. RSC Adv. 6 (54), 49250–49262. doi:10.1039/c5ra27227k
Ueki, N., Lee, S., Sampson, N. S., and Hayman, M. J. (2013). Selective Cancer Targeting with Prodrugs Activated by Histone Deacetylases and a Tumour-Associated Protease. Nat. Commun. 4, 2735. doi:10.1038/ncomms3735
van der Vlies, A. J., Xu, J., Ghasemi, M., Bator, C., Bell, A., Rosoff-Verbit, B., et al. (2022). Thioether-Based Polymeric Micelles with Fine-Tuned Oxidation Sensitivities for Chemotherapeutic Drug Delivery. Biomacromolecules 23 (1), 77–88. doi:10.1021/acs.biomac.1c01010
Van Driessche, A., Kocere, A., Everaert, H., Nuhn, L., Van Herck, S., Griffiths, G., et al. (2018). pH-Sensitive Hydrazone-Linked Doxorubicin Nanogels via Polymeric-Activated Ester Scaffolds: Synthesis, Assembly, and In Vitro and In Vivo Evaluation in Tumor-Bearing Zebrafish. Chem. Mat. 30 (23), 8587–8596. doi:10.1021/acs.chemmater.8b03702
van Rijt, S. H., Bölükbas, D. A., Argyo, C., Datz, S., Lindner, M., Eickelberg, O., et al. (2015). Protease-mediated Release of Chemotherapeutics from Mesoporous Silica Nanoparticles to Ex Vivo Human and Mouse Lung Tumors. ACS Nano 9 (3), 2377–2389. doi:10.1021/nn5070343
Vaupel, P., Schmidberger, H., and Mayer, A. (2019). The Warburg Effect: Essential Part of Metabolic Reprogramming and Central Contributor to Cancer Progression. Int. J. Radiat. Biol. 95 (7), 912–919. doi:10.1080/09553002.2019.1589653
Vrettos, E. I., Karampelas, T., Sayyad, N., Kougioumtzi, A., Syed, N., Crook, T., et al. (2021). Development of Programmable Gemcitabine-GnRH Pro-drugs Bearing Linker Controllable "click" Oxime Bond Tethers and Preclinical Evaluation against Prostate Cancer. Eur. J. Med. Chem. 211, 113018. doi:10.1016/j.ejmech.2020.113018
Wang, D., Zhou, Y., Li, X., Qu, X., Deng, Y., Wang, Z., et al. (2017). Mechanisms of pH-Sensitivity and Cellular Internalization of PEOz-B-PLA Micelles with Varied Hydrophilic/Hydrophobic Ratios and Intracellular Trafficking Routes and Fate of the Copolymer. ACS Appl. Mat. Interfaces 9 (8), 6916–6930. doi:10.1021/acsami.6b16376
Wang, H.-X., Yang, X.-Z., Sun, C.-Y., Mao, C.-Q., Zhu, Y.-H., and Wang, J. (2014). Matrix Metalloproteinase 2-responsive Micelle for siRNA Delivery. Biomaterials 35 (26), 7622–7634. doi:10.1016/j.biomaterials.2014.05.050
Wang, J., Li, D., Tao, W., Lu, Y., Yang, X., and Wang, J. (2019). Synthesis of an Oxidation-Sensitive Polyphosphoester Bearing Thioether Group for Triggered Drug Release. Biomacromolecules 20 (4), 1740–1747. doi:10.1021/acs.biomac.9b00101
Wang, W., Abbruzzese, J. L., Evans, D. B., and Chiao, P. J. (1999). Overexpression of Urokinase-type Plasminogen Activator in Pancreatic Adenocarcinoma Is Regulated by Constitutively Activated RelA. Oncogene 18 (32), 4554–4563. doi:10.1038/sj.onc.1202833
Wang, X., Li, M., Hou, Y., Li, Y., Yao, X., Xue, C., et al. (2020). Tumor‐Microenvironment‐Activated In Situ Self‐Assembly of Sequentially Responsive Biopolymer for Targeted Photodynamic Therapy. Adv. Funct. Mat. 30 (40), 2000229. doi:10.1002/adfm.202000229
Wang, X., Xu, J., Xu, X., Fang, Q., and Tang, R. (2020). pH-sensitive Bromelain Nanoparticles by Ortho Ester Crosslinkage for Enhanced Doxorubicin Penetration in Solid Tumor. Mater. Sci. Eng. C 113, 111004. doi:10.1016/j.msec.2020.111004
Wang, X., Zheng, Y., Xue, Y., Wu, Y., Liu, Y., Cheng, X., et al. (2021). pH-sensitive and Tumor-Targeting Nanogels Based on Ortho Ester-Modified PEG for Improving the In Vivo Anti-tumor Efficiency of Doxorubicin. Colloids Surfaces B Biointerfaces 207, 112024. doi:10.1016/j.colsurfb.2021.112024
Wang, Y., Zhu, L., Wang, Y., Li, L., Lu, Y., Shen, L., et al. (2016). Ultrasensitive GSH-Responsive Ditelluride-Containing Poly(ether-Urethane) Nanoparticles for Controlled Drug Release. ACS Appl. Mat. Interfaces 8 (51), 35106–35113. doi:10.1021/acsami.6b14639
Wang, Y., Han, N., Zhao, Q., Bai, L., Li, J., Jiang, T., et al. (2015). Redox-responsive Mesoporous Silica as Carriers for Controlled Drug Delivery: a Comparative Study Based on Silica and PEG Gatekeepers. Eur. J. Pharm. Sci. 72, 12–20. doi:10.1016/j.ejps.2015.02.008
Wang, Y., Qian, J., Yang, M., Xu, W., Wang, J., Hou, G., et al. (2019). Doxorubicin/cisplatin Co-loaded Hyaluronic Acid/chitosan-Based Nanoparticles for In Vitro Synergistic Combination Chemotherapy of Breast Cancer. Carbohydr. Polym. 225, 115206. doi:10.1016/j.carbpol.2019.115206
Wang, Z., Zhang, G., Wu, J., and Jia, M. (2013). Adjuvant Therapy for Hepatocellular Carcinoma: Current Situation and Prospect. Drug Discov. Ther. 7 (4), 137–143. doi:10.5582/ddt.2013.v7.4.137
Wei, C., Liang, B., Li, Y., Yan, B., Zhou, Y., Liu, Y., et al. (2021). A Drug-free Therapeutic System for Cancer Therapy by Diselenide-Based Polymers Themselves. Adv. Healthc. Mater 10 (2), e2001471. doi:10.1002/adhm.202001471
Wen, H., Dong, C., Dong, H., Shen, A., Xia, W., Cai, X., et al. (2012). Engineered Redox-Responsive PEG Detachment Mechanism in PEGylated Nano-Graphene Oxide for Intracellular Drug Delivery. Small 8 (5), 760–769. doi:10.1002/smll.201101613
Wu, L., Lin, B., Yang, H., Chen, J., Mao, Z., Wang, W., et al. (2019). Enzyme-responsive Multifunctional Peptide Coating of Gold Nanorods Improves Tumor Targeting and Photothermal Therapy Efficacy. Acta Biomater. 86, 363–372. doi:10.1016/j.actbio.2019.01.026
Xia, X., Shi, J., Deng, Q., Xu, N., Huang, F., and Xiang, X. (2022). Biodegradable and Self-Fluorescent Ditelluride-Bridged Mesoporous Organosilica/polyethylene Glycol-Curcumin Nanocomposite for Dualresponsive Drug Delivery and Enhanced Therapy Efficiency. Mater. Today Chem. 23, 100660. doi:10.1016/j.mtchem.2021.100660
Xin, X., Kumar, V., Lin, F., Kumar, V., Bhattarai, R., Bhatt, V. R., et al. (2020). Redox-responsive Nanoplatform for Codelivery of miR-519c and Gemcitabine for Pancreatic Cancer Therapy. Sci. Adv. 6 (46), eabd6764. doi:10.1126/sciadv.abd6764
Xu, H., Hu, M., Yu, X., Li, Y., Fu, Y., Zhou, X., et al. (2015). Design and Evaluation of pH-Sensitive Liposomes Constructed by Poly(2-Ethyl-2-Oxazoline)-Cholesterol Hemisuccinate for Doxorubicin Delivery. Eur. J. Pharm. Biopharm. 91, 66–74. doi:10.1016/j.ejpb.2015.01.030
Xu, L., Zhao, M., Gao, W., Yang, Y., Zhang, J., Pu, Y., et al. (2019). Polymeric Nanoparticles Responsive to Intracellular ROS for Anticancer Drug Delivery. Colloids Surfaces B Biointerfaces 181, 252–260. doi:10.1016/j.colsurfb.2019.05.064
Xu, X., Saw, P. E., Tao, W., Li, Y., Ji, X., Bhasin, S., et al. (2017). ROS-responsive Polyprodrug Nanoparticles for Triggered Drug Delivery and Effective Cancer Therapy. Adv. Mater 29 (33), 1. doi:10.1002/adma.201700141
Yang, C., Li, C., Zhang, P., Wu, W., and Jiang, X. (2017). Redox Responsive Hyaluronic Acid Nanogels for Treating RHAMM (CD168) Over-expressive Cancer, Both Primary and Metastatic Tumors. Theranostics 7 (6), 1719–1734. doi:10.7150/thno.18340
Yang, C., Xue, Z., Liu, Y., Xiao, J., Chen, J., Zhang, L., et al. (2018). Delivery of Anticancer Drug Using pH-Sensitive Micelles from Triblock Copolymer MPEG-B-PBAE-B-PLA. Mater. Sci. Eng. C 84, 254–262. doi:10.1016/j.msec.2017.12.003
Yang, G., Phua, S. Z. F., Lim, W. Q., Zhang, R., Feng, L., Liu, G., et al. (2019). A Hypoxia-Responsive Albumin-Based Nanosystem for Deep Tumor Penetration and Excellent Therapeutic Efficacy. Adv. Mater 31 (25), e1901513. doi:10.1002/adma.201901513
Yang, G., Fu, S., Yao, W., Wang, X., Zha, Q., and Tang, R. (2017). Hyaluronic Acid Nanogels Prepared via Ortho Ester Linkages Show pH-Triggered Behavior, Enhanced Penetration and Antitumor Efficacy in 3-D Tumor Spheroids. J. Colloid Interface Sci. 504, 25–38. doi:10.1016/j.jcis.2017.05.033
Yang, X., Tang, Q., Jiang, Y., Zhang, M., Wang, M., and Mao, L. (2019). Nanoscale ATP-Responsive Zeolitic Imidazole Framework-90 as a General Platform for Cytosolic Protein Delivery and Genome Editing. J. Am. Chem. Soc. 141 (9), 3782–3786. doi:10.1021/jacs.8b11996
Yao, Q., Kou, L., Tu, Y., and Zhu, L. (2018). MMP-responsive 'Smart' Drug Delivery and Tumor Targeting. Trends Pharmacol. Sci. 39 (8), 766–781. doi:10.1016/j.tips.2018.06.003
Ye, H., Zhou, Y., Liu, X., Chen, Y., Duan, S., Zhu, R., et al. (2019). Recent Advances on Reactive Oxygen Species-Responsive Delivery and Diagnosis System. Biomacromolecules 20 (7), 2441–2463. doi:10.1021/acs.biomac.9b00628
Yu, F., Tang, X., and Pei, M. (2013). Facile Synthesis of PDMAEMA-Coated Hollow Mesoporous Silica Nanoparticles and Their pH-Responsive Controlled Release. Microporous Mesoporous Mater. 173, 64–69. doi:10.1016/j.micromeso.2013.02.012
Yu, N., Liu, T., Zhang, X., Gong, N., Ji, T., Chen, J., et al. (2020). Dually Enzyme- and Acid-Triggered Self-Immolative Ketal Glycoside Nanoparticles for Effective Cancer Prodrug Monotherapy. Nano Lett. 20 (7), 5465–5472. doi:10.1021/acs.nanolett.0c01973
Yuan, Y., Du, C., Sun, C., Zhu, J., Wu, S., Zhang, Y., et al. (2018). Chaperonin-GroEL as a Smart Hydrophobic Drug Delivery and Tumor Targeting Molecular Machine for Tumor Therapy. Nano Lett. 18 (2), 921–928. doi:10.1021/acs.nanolett.7b04307
Zha, Q., Wang, X., Cheng, X., Fu, S., Yang, G., Yao, W., et al. (2017). Acid-degradable Carboxymethyl Chitosan Nanogels via an Ortho Ester Linkage Mediated Improved Penetration and Growth Inhibition of 3-D Tumor Spheroids In Vitro. Mater. Sci. Eng. C 78, 246–257. doi:10.1016/j.msec.2017.04.098
Zhai, Y., Zhou, X., Jia, L., Ma, C., Song, R., Deng, Y., et al. (2017). Acetal-Linked Paclitaxel Polymeric Prodrug Based on Functionalized mPEG-PCL Diblock Polymer for pH-Triggered Drug Delivery. Polym. (Basel) 9 (12), 698. doi:10.3390/polym9120698
Zhang, H., Dong, S., Zhang, S., Li, Y., Li, J., Dai, Y., et al. (2021). pH-responsive Lipid Polymer Hybrid Nanoparticles (LPHNs) Based on Poly (β-Amino Ester) as a Promising Candidate to Resist Breast Cancers. J. Drug Deliv. Sci. Technol. 61, 102102. doi:10.1016/j.jddst.2020.102102
Zhang, L., Qi, Y., Min, H., Ni, C., Wang, F., Wang, B., et al. (2019). Cooperatively Responsive Peptide Nanotherapeutic that Regulates Angiopoietin Receptor Tie2 Activity in Tumor Microenvironment to Prevent Breast Tumor Relapse after Chemotherapy. ACS Nano 13 (5), 5091–5102. doi:10.1021/acsnano.8b08142
Zhang, L., Wang, Y., Yang, Y., Liu, Y., Ruan, S., Zhang, Q., et al. (2015). High Tumor Penetration of Paclitaxel Loaded pH Sensitive Cleavable Liposomes by Depletion of Tumor Collagen I in Breast Cancer. ACS Appl. Mat. Interfaces 7 (18), 9691–9701. doi:10.1021/acsami.5b01473
Zhang, P., Yang, H., Shen, W., Liu, W., Chen, L., and Xiao, C. (2020). Hypoxia-Responsive Polypeptide Nanoparticles Loaded with Doxorubicin for Breast Cancer Therapy. ACS Biomater. Sci. Eng. 6 (4), 2167–2174. doi:10.1021/acsbiomaterials.0c00125
Zhang, R., Li, Y., Zhang, M., Tang, Q., and Zhang, X. (2016). Hypoxia-responsive Drug-Drug Conjugated Nanoparticles for Breast Cancer Synergistic Therapy. RSC Adv. 6 (36), 30268–30276. doi:10.1039/c6ra01560c
Zhang, Z.-T., Huang-Fu, M.-Y., Xu, W.-H., and Han, M. (2019). Stimulus-responsive Nanoscale Delivery Systems Triggered by the Enzymes in the Tumor Microenvironment. Eur. J. Pharm. Biopharm. 137, 122–130. doi:10.1016/j.ejpb.2019.02.009
Zhao, S., Xu, M., Cao, C., Yu, Q., Zhou, Y., and Liu, J. (2017). A Redox-Responsive Strategy Using Mesoporous Silica Nanoparticles for Co-delivery of siRNA and Doxorubicin. J. Mat. Chem. B 5 (33), 6908–6919. doi:10.1039/c7tb00613f
Zhao, X., Yao, Y., Tian, K., Zhou, T., Jia, X., Li, J., et al. (2016). Leakage-free DOX/PEGylated Chitosan Micelles Fabricated via Facile One-step Assembly for Tumor Intracellular pH-Triggered Release. Eur. J. Pharm. Biopharm. 108, 91–99. doi:10.1016/j.ejpb.2016.08.018
Zhen, J., Tian, S., Liu, Q., Zheng, C., Zhang, Z., Ding, Y., et al. (2019). Nanocarriers Responsive to a Hypoxia Gradient Facilitate Enhanced Tumor Penetration and Improved Anti-tumor Efficacy. Biomater. Sci. 7 (7), 2986–2995. doi:10.1039/c9bm00461k
Zhong, H., Mu, J., Du, Y., Xu, Z., Xu, Y., Yu, N., et al. (2020). Acid-Triggered Release of Native Gemcitabine Conjugated in Polyketal Nanoparticles for Enhanced Anticancer Therapy. Biomacromolecules 21 (2), 803–814. doi:10.1021/acs.biomac.9b01493
Keywords: tumor microenvironment, bio-responsiveness, cancer therapy, drug delivery system, pathophysiological characteristics
Citation: Cong X, Chen J and Xu R (2022) Recent Progress in Bio-Responsive Drug Delivery Systems for Tumor Therapy. Front. Bioeng. Biotechnol. 10:916952. doi: 10.3389/fbioe.2022.916952
Received: 10 April 2022; Accepted: 09 June 2022;
Published: 29 June 2022.
Edited by:
Piera Di Martino, University of Camerino, ItalyReviewed by:
Monica Cristina Garcia, National University of Cordoba, ArgentinaBing Feng, Swiss Federal Institute of Technology Lausanne, Switzerland
Copyright © 2022 Cong, Chen and Xu. This is an open-access article distributed under the terms of the Creative Commons Attribution License (CC BY). The use, distribution or reproduction in other forums is permitted, provided the original author(s) and the copyright owner(s) are credited and that the original publication in this journal is cited, in accordance with accepted academic practice. No use, distribution or reproduction is permitted which does not comply with these terms.
*Correspondence: Ran Xu, eHVyMkBzai1ob3NwaXRhbC5vcmc=