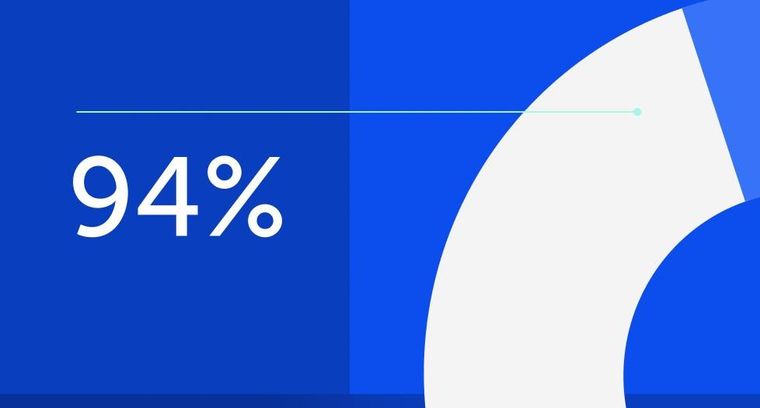
94% of researchers rate our articles as excellent or good
Learn more about the work of our research integrity team to safeguard the quality of each article we publish.
Find out more
REVIEW article
Front. Bioeng. Biotechnol., 31 August 2022
Sec. Tissue Engineering and Regenerative Medicine
Volume 10 - 2022 | https://doi.org/10.3389/fbioe.2022.911281
Sandwiched between articular cartilage and subchondral bone, the calcified cartilage layer (CCL) takes on both biomechanical and biochemical functions in joint development and ordinary activities. The formation of CCL is not only unique in articular cartilage but can also be found in the chondro-osseous junction adjacent to the growth plate during adolescence. The formation of CCL is an active process under both cellular regulation and intercellular communication. Abnormal alterations of CCL can be indications of degenerative diseases including osteoarthritis. Owing to the limited self-repair capability of articular cartilage and core status of CCL in microenvironment maintenance, tissue engineering reconstruction of CCL in damaged cartilage can be of great significance. This review focuses on possible tissue engineering reconstruction methods targeting CCL for further OA treatment.
In the structure of joints, the calcified cartilage layer (CCL), which is located between articular cartilage and subchondral bone, is bounded by the upper tidemark and the lower cement line. Multiple studies have suggested that CCL is significant either in the embryonic development of joints or normal joint activities. CCL alteration would disrupt its conventional physiological functions such as mechanical loading transmission, diffusion of materials, and cartilage calcification. When in metabolic musculoskeletal disorders, such as osteoarthritis (OA), CCL is altered accompanied by abnormal disruption of articular cartilage and subchondral bone due to biomechanical and biochemical changes. Thus, it is not sufficient to repair either articular cartilage or subchondral bone in OA treatment, and CCL regeneration is also critical. Tissue engineering reconstruction is suggested as a promising OA intervention. In this review, we summarize the structure and physiological function of CCL, as well as the pathological alteration in OA. Finally, we explore possible tissue engineering reconstruction methods targeting CCL for further OA treatment.
Articular cartilage consists of four highly organized zones: superficial, middle (transitional), deep (radial), and calcified layers (Buckwalter and Mankin, 1998; Sophia Fox et al., 2009). The chondrocyte phenotype and extracellular matrix (ECM) structure differ in each layer (Burr, 2004). The chondro-osseous junctional region, CCL, is defined as the mineralized cartilage which lies between the hyaline cartilage bounded by the upper tidemark and subchondral bone bounded by the lower cement line (Clark, 1990). Compared with the cement line, the tidemark appears to have more significant biomechanical functions (Madry et al., 2010), so we would state the role of the tidemark in the following sections.
As a fundamental structure in bone physiology (Wang et al., 2009a), CCL consists of dispersed, hypertrophic chondrocytes within lacunae in the calcified matrix which is composed of type I collagen, sodium hyaluronate, and nanohydroxyapatite in varying proportions (Zhou et al., 2022). Zhang, Ying et al. reported that the percentage of the dry weight of type II collagen as an organic compound of CCL was 20.16% ± 0.96%, lower than that of the hyaline cartilage layer (61.39% ± 0.38%); the percentage of the dry weight of hydroxyapatite (HA) as an inorganic compound was 65.09% ± 2.31%, less than that of subchondral bone (85.78% ± 3.42%) (Zhang et al., 2012). Inside CCL, perpendicular chondrocyte-derived collagen type II fibers become structurally cemented to collagen type I osteoid deposited by osteoblasts (Hoemann et al., 2012). The Fourier transform infrared spectroscopic imaging (FTIR-I) analysis reveals that the collagen content undergoes a stepwise increase from cartilage to bone, while proteoglycan only culminates within CCL (Khanarian et al., 2014).
Mature articular cartilage is integrated with subchondral bone through an approximately 20–250-μm-thick CCL (Hoemann et al., 2012). In one study of normal human femoral condyles, Wang et al. (2009a) reported that the mean thickness of CCL was 104.162 ± 0.87 μm, and the cell density of CCL was 51.25 ± 21.26 cells/mm2, which was much lower than that of the hyaline cartilage (152.54 ± 35.77 cells/mm2). They also discovered two junctional interfaces of CCL, with the “ravine-engomphosis”-shaped upper interface and “comb-anchor”-shaped lower interface, indicating the structural integration of the cartilage.
During embryonic development, CCL formation begins with ossification, which is the process in which mesenchymal tissues are gradually replaced by osseous tissues (Burr, 2004). Ossification is an indispensable way to form mature bones, and it includes two types: intramembranous and endochondral. The endochondral ossification begins with mesenchymal tissue transforming into a cartilage intermediate, which is later replaced by the bone under calcification—the deposition of calcium in the osseous tissue (Nishimura et al., 2012).
The endochondral bone growth occurs at the metaphyseal side of the growth plate, the proliferative area where cartilage continues to proliferate and be replaced by the bone in the diaphysis. There are also two chondro-osseous junctions between the epiphysis and diaphysis growth plate cartilage. The epiphyseal growth plate cartilage–bone interface has the tidemark, while the metaphyseal growth plate cartilage–subchondral bone interface has not (Kazemi and Williams, 2021). This indicates that the formation of CCL and tidemark in articular cartilage can be a common physiological process as the growth plate in endochondral ossification.
According to the study by Müller-Gerbl et al. (1987), the height of CCL occupies a relatively constant proportion of articular cartilage, and it is achieved by the balance between the progression of the tidemark into noncalcified cartilage and changing into a bone by vascular invasion and bony remodeling (Oegema et al., 1997). This phenomenon can be associated with bone growth by the function of the growth plate (Figure 1). During its formation, the cells that form CCL have properties similar to the cells of the growth plate. At maturity, the rate of osteoblast activity exceeds that of epiphyseal cartilage enlargement, and epiphyseal closure occurs. Thus, growth plate cartilage disappears and is replaced by a distinct epiphyseal line. The two aforementioned events take place sequentially along the timeline. In adults, calcified cartilage remains quiescent but can be reactivated both with the aging process and pathological process including OA (Oegema et al., 1997).
FIGURE 1. Origin and development of CCL and the growth plate. (1) Secondary ossification centers have formed. The initial structure of articular cartilage and growth plate comes from the same cartilaginous tissue. (2) Osteoblasts invade the lower boundary of the growth plate, replacing the cartilage with a bone; at the same rate, the growth plate enlarges through interstitial growth at the upper boundary by chondrocyte division and enlargement. The epiphysis is pushed away from the diaphysis, and the bone length increases. In contrast, the height of the calcified cartilage layer is maintained by the balance between tidemark advancement and bone transformation. (3) Following epiphyseal closure in adolescence, the calcified cartilage layer remains quiescent but can be reactivated in the ageing process and OA. (4) Microscopic three-dimensional structure of CCL.
The tidemark, the junction of mature calcified and uncalcified articular cartilage, was first reported by H. T. Fawns and J. W. Landells in 1953 (Fawns and Landells, 1953). It is defined as a clear hematoxyphil line up to 10 μm thickness from a photomicrographic view (Gannon and Sokoloff, 1999). Nevertheless, it is not only simply an undulating line across a joint but also a complicated three-dimensional structure that follows uncalcified prolongations, penetrates the calcified cartilage, and impinges on subjacent bone or marrow spaces (Lyons et al., 2006). Since articular cartilage is an avascular, alymphatic, and aneural connective tissue, this prolongation could probably provide a route for both nourishment and interaction within the chondro-osseous junction.
The mature mineralization front is delineated by a thin approximately 5 μm undulating tidemark structure that forms at the base of the articular cartilage. Under static compressive loading, articular cartilage distortion could come to an abrupt end at the tidemark. It is said that a tidemark can act as a tethering mechanism for collagen fibrils in unmineralized cartilage (Huber et al., 2000), and alternations of orientation and packing density of the collagen framework in the tidemark region could be observed under load-bearing conditions (Broom and Poole, 1982). Orientation alternations of collagen fibers could have stress buffer effects, and it can be concluded that the tidemark has the function of stress transmission and corporation from compliant articular cartilage to rigid calcified cartilage.
Chen et al. (2011) reported that the tidemark appeared as a dense wavy line at the interface between calcified and hypertrophic layers of the condylar cartilage. They also found that thickness and distribution of the tidemark are loading-related. They showed a highly wavy tidemark surface in the load-bearing areas and a relatively flat and smooth surface in the non-load-bearing areas (Clark, 1990). Furthermore, the thickness of the tidemark was significantly higher in load-bearing areas than in non-load-bearing areas. Tidemark duplication represents the progression or reactivation of CCL. Although mostly identified in OA, this can be a common phenomenon in the normal articulation of all species (Oegema et al., 1997).
From the traditional view, the mechanical transition from calcified cartilage to the subchondral bone undergoes a continuum of increasing stiffness (Hargrave-Thomas et al., 2015). Once the stiffness is discontinuous, shear stress will occur. This discontinuity exists between the cartilage and subchondral bone. As a transition layer, CCL can minimize the shear stress (Radin and Rose, 1986). Sandwiched between cartilage and subchondral bone, CCL shows transitional mechanical properties by stress facilitation (Broom and Poole, 1982). It is reported that calcified cartilage is 10–100 times more rigid than the cartilage but 10 times softer than the bone (Mente and Lewis, 1994), while alternation of this stiffness gradient is closely related to early degenerative changes in articular cartilage (Hargrave-Thomas et al., 2015).
Specifically, the transmission of force may be achieved through the network of branching collagen fibrils in CCL (Redler et al., 1975). The vertical collagen fibers transmit and dissipate stress, while the tidemark and cement line expand the contact area; thus, CCL fixes uncalcified cartilage on subchondral bone tightly and diffuses the load effectively, which contributes to the load-bearing capabilities of the joint (Green et al., 1970; Oegema et al., 1997; Zizak et al., 2003).
Additionally, it is reported that the mineral content in the calcified cartilage area increases exponentially, which is even higher than that in the bone (Khanarian et al., 2014). Compared with calcified cartilage, the bone requires less mineral content to achieve a similar elastic modulus (Gupta et al., 2005). This may explain that the elastic modulus of calcified cartilage is equivalent to that of subchondral bone in other studies (Burr and Gallant, 2012). With such high stiffness, it can be explained that CCL is always the first fraction zone when sudden trauma occurs (Keinan-Adamsky et al., 2005). Moreover, under repetitive traumatic injuries, including overload-related osteochondrosis, the collapse of CCL is also observed (Zhang et al., 2012). Above all, we can conclude that tidemark and CCL play a significant part in force transmission and structural stabilization.
In addition to taking on the biomechanical coupling role between articular cartilage and subchondral bone, calcified cartilage also assists biochemical communication (Boushell et al., 2017). With a lower diffusion coefficient than the uncalcified region, CCL acts as a barrier that limits diffusion and restrains vascular invasion from the subchondral bone (Boushell et al., 2017). Also, the low permeability of CCL could help stabilize the hypoxic environment of articular cartilage. However, the least diffusion capacity of the pathway upward does not equal none. The study by K.P. Arkill demonstrated that calcified cartilage permits small solutes to transport, and subchondral circulation may contribute much to the nourishment of the deep cartilage region (Arkill and Winlove, 2008). In this way, articular cartilage may get nutrition supplements both from superficial synovial fluid and the subchondral area. Based on fluorescence loss induced by photobleaching (FLIP), it is revealed through electron microscopy that the calcified cartilage matrix contained uncalcified regions (22% volume proportion) that are either large patches or myriad small regions among mineral deposition, which may function as transport pathways (Pan et al., 2009). In addition, since osteochondral vascularity is an indicator of pathological changes, calcified cartilage can restrain vascular invasion from subchondral bone to normally avascular hyaline cartilage as a direct physical barrier (Walsh et al., 2007; Staines et al., 2013).
Recent studies have shown that there is a network of nanochannels in both the calcified cartilage and bone, which may contribute to the transport of ions and small molecules. These nanochannels have a significant impact on the crosstalk between the cartilage and bone (Pouran et al., 2021; Tang et al., 2022). Pouran et al. (2021) found a three-dimensional continuous transition of mineralization architecture from the noncalcified cartilage to the calcified cartilage. They identified that the nanopore structure varies gradually with a radius of 10.71 ± 6.45 nm in calcified cartilage to 39.1 ± 26.17 nm in the subchondral bone plate, which indicated that connectivity of nanopores in calcified cartilage is highly compromised compared to the subchondral bone plate. Tang et al. (2022) also revealed densely packed nanochannels smaller than bone canaliculi (≈10–50 nm diameter) within the calcified cartilage and bone extracellular matrices but absent in the cement line. These novel discoveries emphasize the transportive role of CCL and the existence of possible direct signal pathways between articular cartilage and subchondral bone.
Crystal deposition, governed by a combination of physicochemical, cellular, and matrix factors, plays an essential role in the calcification of the cartilage (Bullough and Jagannath, 1983). The accumulation of calcium phosphate precipitates both inside and outside chondrocytes leads to cartilage calcification (Boskey, 2002). Deriving from observations of a particular cellular activity in the calcification front of articular cartilage, this process is correlated with extracellular matrix vesicles (Bullough and Jagannath, 1983). Calcification of the growth plate has similar underlying mechanisms as that of articular cartilage. Anderson (1969) also indicated matrix vesicles of different sizes (estimated 300 A to estimated 1 micro) and shapes derived from cells that are identified in the epiphyseal cartilage matrix, which may contribute to the initiation of cartilage calcification. More specifically, matrix vesicles enriched with microRNAs may possess significant regulatory functions on growth plate chondrocytes (Asmussen et al., 2021). Moreover, matrix vesicles presented with phospholipids also appear to be involved in the initial production of calcium HA crystals through the formation of phospholipid:Ca:Pi complexes (Boyan et al., 1989). Some other factors also participate in this process. Poole et al. (1984) emphasized that chondrocalcin may play a vital role in cartilage calcification by its unique affinity for HA. The inhibitory R2 fraction separated from protein–polysaccharide complexes are found to be degraded in the transient calcification zone, which may undergo the regulation of endochondral calcification (Pita et al., 1970). Regional cellular interactions of chondrocytes also regulate cell mineralization and matrix organization, and it is suggested that suppression of cartilage calcification under chondrocyte interaction is mediated by PTHrP (Jiang et al., 2008).
Hypertrophic chondrocytes derived from bone marrow mesenchymal stem cells (BMSCs) synthesized type X collagen and calcified the extracellular matrix around them (Yang et al., 2018). In the intermediate calcified process during endochondral ossification, along with the calcification of the matrix composed of type II collagen, type X correlates with type II collagen and may prevent the initial calcification of these fibrils (Poole et al., 1989; Kirsch and Wuthier, 1994).
OA is a chronic joint degenerative disease with unbalanced bone homeostasis. In the progression of OA, uncalcified cartilage, CCL, and subchondral bone together form one functional unit as a whole. Subchondral bone is commonly divided into the subchondral bone plate and subchondral cancellous bone and undertakes primary pathological changes during early OA stages. Secondary articular cartilage alternations, including fibrillations, are always an indication of the severity and late OA stages.
During early OA stages, constant remodeling of subchondral bone accomplished by a flourish of vascularity and active metabolism occurs (Aho et al., 2017). Meanwhile, microcracks in CCL take on both adaptive and repairing mechanisms to maintain cartilage integrity by recruiting osteocytes in the subchondral bone plate and initiating focal remodeling (Goldring, 2012; Zarka et al., 2019; Kaspiris et al., 2022). The increased remodeling rate undermines CCL and subchondral bone (Table 1). During the late stage of OA, subchondral cancellous bone continues to be osteopenic, while CCL and the subchondral bone plate thicken (Burr and Gallant, 2012). As OA progresses, tidemark duplication occurs, followed by fibrillation and deterioration of articular cartilage (Figure 2).
FIGURE 2. CCL in normal and OA joints with the progression of OA, tidemark advances, and calcified cartilage layer gets thicker, while noncalcified articular cartilage attenuates. Under repetitive stress, microcracks in the calcified cartilage layer take place, which promotes vascular invasion and associated repairing mechanisms. Moreover, sclerosis of both the subchondral plate and calcified cartilage aggravates too.
Recently, extensive research on mechanisms of OA-associated cartilage calcification has been published. DLX5-ALPL-IBSP-ENPP1 signal axis (Jiang et al., 2022), peripheral inhibition of SNS activity (Rösch et al., 2022), and overexpression of TUFT1 gene (Sliz et al., 2017) promote OA-specific cartilage calcification. β2-adrenoceptor deficiency results in increased CCL thickness in the murine model study (Rösch et al., 2021). Pharmaceutically, the long-term application of dexamethasone increases the calcium content of CCL in both murine model and OA patients, which can also predispose OA and accelerate OA progression (Chen et al., 2021). In the contrast, a list of molecules that perform chondroprotective roles through inhibition of cartilage calcification is also found, including ciliary protein intraflagellar transport protein 88 (IFT88) (Coveney et al., 2022), betaine (Yajun et al., 2021), gasotransmitter hydrogen sulfide (H2S) (Nasi et al., 2021), and Tougu Xiaotong capsule (Li et al., 2013). Phosphocitrate (PC) and its analogs can restrain endochondral ossification by suppressing the hypertrophic phenotype (Hartlev et al., 2018). Both OA meniscal cells and chondrocytes have calcifying potentials (Kiraly et al., 2017), and recruitment of osteoclasts contributes to CCL microcracks and subjacent bone loss (Bertuglia et al., 2016).
As for the composition of CCL during OA progression, both collagen and proteoglycan contents undergo a decline, while the calcium/phosphate ratio is unchanged (Fan et al., 2022). Also, stiffened collagen fibrils are found in the cartilage–bone interface (Wen et al., 2012). When it comes to material properties, it is common sense that stiffness is always positively related to the mineral content. According to the study of Finnilä et al. (2022), CCL associated with pathological tidemark duplication has more mineral content than surrounding CCL portions, and CCL itself has more mineral crystals than adjoining bones. However, CCL still has a lower modulus, i.e., less stiffness, no matter in early or late OA stages, were former for increased bone remodeling and the latter for impairment of osteoblast regulation of calcification (Burr and Gallant, 2012) (Table 2).
The thickness of CCL combined with the roughness of the tidemark and cement line can also be used to predict the reversibility of OA. In mild OA, the thickness first increases and later decreases, which indicates a reversible sign of OA pathological change. However, in moderate OA, the thickness continually increases, which indicates irreversibility (Deng et al., 2016). In addition to gross morphological and microstructural changes of CCL, specific biomarkers of CCL including calcium-binding protein, cadherin-binding protein, and carbohydrate metabolism-related proteins can also reflect the severity of OA (Fan et al., 2022).
Bone–cartilage crosstalk exists in both normal joints and OA joints. CCL was traditionally considered an impermeable structure until 1994. Milz and Putz, (1994) first put forward the existence of channels between the uncalcified cartilage and the subchondral region. In normal joints, the transport of measurable solutes across CCL was detected by Pan et al. (2012). The vascular plexus is distributed along the undulations of the cartilage–bone interface reaches and perforates CCL, and finally provides nourishment to the hyaline cartilage (Pan et al., 2012). As mentioned previously, the 3D structure of the uncalcified cartilage also penetrates CCL and makes direct contractions with subchondral bones.
In OA, the bone–cartilage crosstalk capacity is elevated through channels mentioned previously, combined with microcracks (Burr and Schaffler, 1997; Burr and Radin, 2003) and vascular flourishment (Imhof et al., 2000; Pan et al., 2012). Small solutes and signaling molecules with calcifying potentials or joint harmfulness, as well as osteoblasts, all can transverse upward through those possible pathways in CCL. Thus, CCL may contribute to OA pathogenesis through this role of bone–cartilage crosstalk. Knee loading is found to perform the role of suppressing the differentiation of osteoclasts from bone marrow-derived cells and subsequent bone resorption of osteoclasts from the subjacent bone through the crosstalk (Li et al., 2016).
The rigidity of calcified cartilage, cortical end plate, and subchondral bone determines that microcracks and fissures after repetitive loading commonly occur in those sites. Since microinjuries may elicit a repairing mechanism, with the invasion of fibrovascular tissue, osteoclasts, and osteoblasts, the chondro-osseous junction undergoes remodeling, and blood vessels intrude. Subsequently, tidemarks duplicate (Imhof et al., 2000). The vascular canals in calcified cartilage promote fluid pressurization and affect hydraulic permeability of the subchondral bone plate. Increased interstitial fluid could affect the microenvironment in the chondro-osseous junction which leads to OA pathogenesis. According to Hwang et al. (2008), increased fluid exudation has deleterious consequences for cartilage and correlates with stages of OA. Thus, the increased hydraulic permeability secondary to angiogenesis enhances interstitial fluid flow, which also strengthens the bone–cartilage crosstalk.
Studies showed impaired CCL along with cartilage and subchondral bone defects in multiple bone disorders, including trauma, aging, and OA (Huey et al., 2012). The osteochondral structures, such as cartilage, have limited self-repair ability, which may lead to disease progression and eventually cause disabilities without effective treatments (Chen et al., 2009). The pathological changes of OA are featured with damaged cartilage and subchondral bone forming disturbed fissure and chondro-osseous junctions. Considering the physiological role of preventing cartilage vascularization and mineralization, the regeneration of the calcified layer may be critical for osteochondral defect restoration and OA treatment (Yang et al., 2018). In the following parts, we introduce the strategies of CCL regeneration (shown in Table 3) and its potential role in OA treatment.
Conventional strategies treating osteochondral injury, including subchondral drilling, microfracture, autologous chondrocyte implantation (ACI) and matrix-associated ACI (MACI), and autologous and allogeneic osteochondral transplantation, remain unsatisfying. Subchondral drilling and microfracture are beneficial for cartilage defect restoration through increasing osteochondral vascular supply. The limitation of these processes is that the fibrocartilage, which is functionally inferior to the cartilage, would be gradually formed instead of the cartilage in the injured sites (Beiser and Kanat, 1990; Sledge, 2001; Gobbi et al., 2020). ACI and MACI are reported to be applied clinically in articular cartilage regeneration with satisfactory outcomes (Gobbi et al., 2009; Kon et al., 2009; Saris et al., 2014). However, drawbacks such as chondrocyte source shortage, periosteal hypertrophy and ablation, insufficient regenerative cartilage, and graft delamination are repeatedly reported in ACI or MACI (Harris et al., 2011), as well as the low effectiveness for aged patients (Yang et al., 2017). Autologous and allogeneic osteochondral transplantation plays a vital role in massive osteochondral reconstruction and shows a good prognosis (Czitrom et al., 1986). However, the defective graft sources restrict its application, such as donor site morbidity of autografts, immune rejection, and disease spread of allografts (Yang et al., 2017).
Recent studies suggested that osteochondral tissue engineering and cartilage tissue engineering (OTE and CTE) have a promising performance in addressing the aforementioned limitations (Yang et al., 2017) with two main approaches regarding osteochondral interface and full-thickness cartilage regeneration, including cell-based and scaffold-based strategies (Liu et al., 2021; Petrovova et al., 2021) (Table 4). The principle of tissue engineering repair is the integration of grafts with the surrounding cartilage and subchondral bone. Digestion reagents, chemotactic agents, and biomaterial-based approaches are applied for cartilage–cartilage integration. In addition, CCL regeneration is critical for stable and functional cartilage–bone integration (Boushell et al., 2017). Lack of CCL was reported to contribute to decreased interfacial shear strength between the bone and cartilage (Kandel et al., 2006). Studies focused on CCL regeneration showed us encouraging results; however, due to the complex composition and structure of CCL, its regeneration remains challenging (Yang et al., 2018).
The cell-based strategy had been applied in previous studies. Kandel et al. (1999) successfully formed calcified cartilage with interface-relevant deep zone chondrocytes (DZCs), type II collagen, and mineralization media. Allan et al. (2007) completed a similar attempt, suggesting that DZCs are a promising cell source for CCL formation.
The scaffold-based approach has proved superior to the cell-based strategy because it requires less chondrocytes, and the functional properties of each tissue type can be readily achieved (Boushell et al., 2017). The scaffold ordinarily consists of hydrogels of natural (polysaccharides and proteins) or synthetic polymers [polyethylene glycol (PEG), polyethylene glycol fumarate (OPF), and polylactic-co-glycolic acid (PLGA), etc.] or their hybrids due to the satisfactory biocompatibility, biodegradability, and bioactivity (Holland et al., 2005; Wang et al., 2010; Nguyen et al., 2012; Luo et al., 2018). The biological properties of the scaffold could be optimized through chemical modifications. The incorporation of inorganic particles such as phosphate and silicate could improve the mechanical stiffness, osteoconductivity, and osteoinductivity of hydrogels (Yang et al., 2017). You et al. (2018) found that HA in alginate hydrogel could stimulate the secretion of the calcified matrix in chondrocytes. Similarly, Khanarian et al. (2012) introduced a scaffold of HA and alginate hydrogel which was reported to promote the DZC-mediated formation of a calcified cartilage-like matrix. Jiang et al. (2010) produced a continuous structure containing calcified cartilage with a scaffold composed of agarose hydrogel and PLGA/45S5 bioactive glass composite microspheres. In addition, layered OPF hydrogels and fibrin/PLGA hydrogels also showed the ability for CCL reconstruction (Wang et al., 2010; Steinmetz et al., 2015).
A multi-phased graft can guide the simultaneous regeneration of bone, cartilage, and a calcified cartilage intermediate. The early generation monolayer or bilayer scaffolds lacking the “boundary layer structure” (which acts as natural CCL) had the problem of excessive growth of the cartilage down into subchondral bone in the repair of osteochondral defects (Galperin et al., 2013), while many studies introduced tri-layered scaffolds with natural CCL and suggested excellent outcomes (Huang et al., 2021). Heymer et al. (2009) introduced a tri-layered scaffold consisting of a hydrophobic interface that separates cartilage and bone portions that are comprised of collagen I fibers with incorporated hyaluronan or polylactic acid, respectively. Kon et al. (2009) developed an acellular tri-layered osteochondral scaffold that controls the spatial distribution of calcium phosphate and collagen to recapitulate the cartilage-to-bone transition.
The implantation of BMSCs in the scaffold was reported to be indispensable for the regeneration of the biomimetic calcified layer (Cheng et al., 2011). Growth factors, such as TGF-β1 or BMP-2, could induce cartilage differentiation of BMSCs in type I collagen hydrogel (Nöth et al., 2007), while controlled delivery of these factors temporally and spatially might influence the BMSCs/scaffold structure in biomimetic calcified layer reconstruction (Wang et al., 2009b), but the huge cost, instability, and immunogenicity of these factors limited their application in clinical treatments (Mitchell et al., 2021). However, it has been suggested that growth factors were not necessary for this process because the type I collagen hydrogel scaffold might provide a suitable microenvironment for chondrogenesis (Zheng et al., 2010). Cai et al. (2020) restored a complete calcified interface and reconstructed cartilage homeostasis with BMSCs and tissue-induced injectable type I collagen hydrogel scaffold. In particular, the anatomical structure and physiological function of the cartilage repaired by this method were similar to the surrounding natural tissues. In another study, Cheng et al. (2011) constructed a tri-layered scaffold containing the chondrogenic layer, osteogenic layer, and middle undifferentiated BMSC–collagen layer. Then, they successfully formed a continuous and complete CCL with this scaffold. Yang et al. (2018) reported that both osteogenic differentiation and chondrogenic differentiation of BMSCs could be effectively promoted by Ica-HA/Col hydrogel in vitro, as well as the synthesis of type X collagen, which is an important marker for the formation of the calcified layer. Moreover, the in vivo experiment on a rabbit model showed that this hydrogel might contribute to the reconstruction of osteochondral sites. Recently, novel strategies have been applied focusing on the scaffold. Three-dimensional bioprinting has significant advantages in dealing with complex shapes and structures, with great potential in the reconstruction of CCL (Sheehy et al., 2013). Kosik-Kozioł et al. (2019) developed a 3D bioprinting hydrogel model using alginate, gelatin methacrylamide, and β-tricalcium phosphate (β-TCP) particles as a bioink. They also found that this model could promote the formation of the calcified zone by modulating the differentiation of BMSCs. Similarly, with PLGA and β-TCP, Li et al. (2018) constructed calcified layers of their multilayer scaffold through low-temperature 3D bioprinting technology. However, some challenges remain to be addressed, such as the low resolution of the printed matter, which limits its printing precision, and long processing time, which may reduce cell viability (Yang et al., 2017).
Novel scaffold designs utilizing a compositional gradient eliminate the need to integrate distinct layers. Harley et al. (2010) reported a gradient scaffold using liquid-phase co-synthesis with an unmineralized collagen II-chondroitin-6-sulfate cartilage region and a mineralized collagen I-chondroitin-6-sulfate bone region. The solutions are interdiffused at the interface, which could lead to a gradual transition in the composition between cartilage and bone sites. The design of multiphase osteochondral scaffold III: fabrication of layered scaffolds with continuous interfaces (Salerno et al., 2012).
This review summarized the physiological roles of CCL and the ongoing studies focusing on CCL regeneration. CCL is suggested to play a significant role in force transmission, maintaining the stability of the osteochondral structure, and assisting the biochemical communication between the bone and cartilage. With the progress of OA, the normal structure and function of calcified cartilage are disrupted, and cartilage vascularization and mineralization occur, which is inconducive to osteochondral defect restoration. Therefore, the morphological, biochemical, and proteomic changes of CCL can reflect the progression of OA. CCL may contribute to OA pathogenesis through the role of bone–cartilage crosstalk which can be facilitated by both cartilage damage and vascular invasion in OA. However, the specific role of CCL in the occurrence and development of OA and its mechanism are still not very clear and need further research.
The regeneration of CCL is particularly important for osteochondral defect restoration, but it is still challenging. Compared with traditional regenerative strategies, tissue engineering shows great advantages in biocompatibility, strategy flexibility, repair integrity, and stability. The scaffold-based strategies have made successful attempts in the reconstruction of calcified cartilage both in vivo and in vitro. Chemical modification, the incorporation of inorganic particles, and the application of 3D bioprinting technology have flexibly improved the property of tissue engineering scaffolds, showing great research prospects, but the current research mainly focused on in vitro and in vivo experiments in small animals and not yet available for human. Maybe the organ culture models in large animal or human tissues could offer a promising integrated modality and are expected to address this limitation (Boushell et al., 2017). In addition, further elucidation of the role of intercellular interactions (like matrix vesicles mentioned previously) in maintaining the homeostasis of the osteochondral microenvironment and the occurrence and development of OA will be conducive to the development of new treatments.
WW and RY collected the data, decided on the content, and wrote the manuscript. WX and YuZ prepared the figures and tables. SA, YL, and YaZ conceptualized this review and revised the draft. All authors approved the final version of the manuscript and agreed to be accountable for all aspects of the work.
This work was supported by the National Key R&D Program of China (2019YFA0111900), the National Natural Science Foundation of China (Nos. 81874030, 82072506, 82102600), the National Clinical Research Center for Geriatric Disorders (2021LNJJ05), the Provincial Clinical Medical Technology Innovation Project of Hunan (2020SK53709), the Administration of Traditional Chinese Medicine of Hunan Province (2021075), the Innovation-Driven Project of Central South University (2020CX045), the Hunan Yong Talents of Science and Technology (2021RC3025), the Wu Jieping Medical Foundation (320.6750.2020-03-14), the Independent Exploration, Innovation Project for Postgraduate Students of Central South University (2021zzts1024) and the Hunan Provincial Innovation Foundation for Postgraduate (CX20210360).
The authors declare that the research was conducted in the absence of any commercial or financial relationships that could be construed as a potential conflict of interest.
All claims expressed in this article are solely those of the authors and do not necessarily represent those of their affiliated organizations, or those of the publisher, the editors, and the reviewers. Any product that may be evaluated in this article, or claim that may be made by its manufacturer, is not guaranteed or endorsed by the publisher.
Aho, O. M., Finnilä, M., Thevenot, J., Saarakkala, S., and Lehenkari, P. (2017). Subchondral bone histology and grading in osteoarthritis. PLoS One 12 (3), e0173726. doi:10.1371/journal.pone.0173726
Allan, K. S., Pilliar, R. M., Wang, J., Grynpas, M. D., and Kandel, R. A. (2007). Formation of biphasic constructs containing cartilage with a calcified zone interface. Tissue Eng. 13 (1), 167–177. doi:10.1089/ten.2006.0081
Anderson, H. C. (1969). Vesicles associated with calcification in the matrix of epiphyseal cartilage. J. Cell Biol. 41 (1), 59–72. doi:10.1083/jcb.41.1.59
Arkill, K. P., and Winlove, C. P. (2008). Solute transport in the deep and calcified zones of articular cartilage. Osteoarthr. Cartil. 16 (6), 708–714. doi:10.1016/j.joca.2007.10.001
Asmussen, N. C., Cohen, D. J., Lin, Z., McClure, M. J., Boyan, B. D., and Schwartz, Z. (2021). Specific MicroRNAs found in extracellular matrix vesicles regulate proliferation and differentiation in growth plate chondrocytes. Calcif. Tissue Int. 109 (4), 455–468. doi:10.1007/s00223-021-00855-y
Beiser, I. H., and Kanat, I. O. (1990). Subchondral bone drilling: A treatment for cartilage defects. J. Foot Surg. 29 (6), 595–601.
Bertuglia, A., Lacourt, M., Girard, C., Beauchamp, G., Richard, H., and Laverty, S. (2016). Osteoclasts are recruited to the subchondral bone in naturally occurring post-traumatic equine carpal osteoarthritis and may contribute to cartilage degradation. Osteoarthr. Cartil. 24 (3), 555–566. doi:10.1016/j.joca.2015.10.008
Boskey, A. L. (2002). Pathogenesis of cartilage calcification: Mechanisms of crystal deposition in cartilage. Curr. Rheumatol. Rep. 4 (3), 245–251. doi:10.1007/s11926-002-0072-3
Boushell, M. K., Hung, C. T., Hunziker, E. B., Strauss, E. J., and Lu, H. H. (2017). Current strategies for integrative cartilage repair. Connect. Tissue Res. 58 (5), 393–406. doi:10.1080/03008207.2016.1231180
Boyan, B. D., Schwartz, Z., Swain, L. D., and Khare, A. (1989). Role of lipids in calcification of cartilage. Anat. Rec. Hob. 224 (2), 211–219. doi:10.1002/ar.1092240210
Broom, N. D., and Poole, C. A. (1982). A functional-morphological study of the tidemark region of articular cartilage maintained in a non-viable physiological condition. J. Anat. 135 (1), 65–82.
Buckwalter, J. A., and Mankin, H. J. (1998). Articular cartilage: Tissue design and chondrocyte-matrix interactions. Instr. Course Lect. 47, 477–486.
Bullough, P. G., and Jagannath, A. (1983). The morphology of the calcification front in articular cartilage. Its significance in joint function. J. Bone Jt. Surg. Br. volume 65 (1), 72–78. doi:10.1302/0301-620x.65b1.6337169
Burr, D. B. (2004). Anatomy and physiology of the mineralized tissues: Role in the pathogenesis of osteoarthrosis. Osteoarthr. Cartil. 12, S20–S30. doi:10.1016/j.joca.2003.09.016
Burr, D. B., and Gallant, M. A. (2012). Bone remodelling in osteoarthritis. Nat. Rev. Rheumatol. 8 (11), 665–673. doi:10.1038/nrrheum.2012.130
Burr, D. B., and Radin, E. L. (2003). Microfractures and microcracks in subchondral bone: Are they relevant to osteoarthrosis? Rheumatic Dis. Clin. N. Am. 29 (4), 675–685. doi:10.1016/s0889-857x(03)00061-9
Burr, D. B., and Schaffler, M. B. (1997). The involvement of subchondral mineralized tissues in osteoarthrosis: Quantitative microscopic evidence. Microsc. Res. Tech. 37 (4), 343–357. doi:10.1002/(sici)1097-0029(19970515)37:4<343::aid-jemt9>3.0.co;2-l
Cai, H., Wang, P., Xu, Y., Yao, Y., Liu, J., Li, T., et al. (2020). BMSCs-assisted injectable Col I hydrogel-regenerated cartilage defect by reconstructing superficial and calcified cartilage. Regen. Biomater. 7 (1), 35–45. doi:10.1093/rb/rbz028
Chen, H., Sun, J., Hoemann, C. D., Lascau-Coman, V., Ouyang, W., McKee, M. D., et al. (2009). Drilling and microfracture lead to different bone structure and necrosis during bone-marrow stimulation for cartilage repair. J. Orthop. Res. 27 (11), 1432–1438. doi:10.1002/jor.20905
Chen, L., Ni, Z., Huang, J., Zhang, R., Zhang, J., Zhang, B., et al. (2021). Long term usage of dexamethasone accelerating the initiation of osteoarthritis via enhancing the extracellular matrix calcification and apoptosis of chondrocytes. Int. J. Biol. Sci. 17 (15), 4140–4153. doi:10.7150/ijbs.64152
Chen, R., Chen, S., Chen, X. M., and Long, X. (2011). Study of the tidemark in human mandibular condylar cartilage. Arch. Oral Biol. 56 (11), 1390–1397. doi:10.1016/j.archoralbio.2011.04.007
Cheng, H. W., Luk, K. D., Cheung, K. M., and Chan, B. P. (2011). In vitro generation of an osteochondral interface from mesenchymal stem cell-collagen microspheres. Biomaterials 32 (6), 1526–1535. doi:10.1016/j.biomaterials.2010.10.021
Clark, J. M. (1990). The structure of vascular channels in the subchondral plate. J. Anat. 171, 105–115.
Coveney, C. R., Zhu, L., Miotla-Zarebska, J., Stott, B., Parisi, I., Batchelor, V., et al. (2022). Role of ciliary protein intraflagellar transport protein 88 in the regulation of cartilage thickness and osteoarthritis development in mice. Arthritis Rheumatol. 74 (1), 49–59. doi:10.1002/art.41894
Czitrom, A. A., Langer, F., McKee, N., and Gross, A. E. (1986). Bone and cartilage allotransplantation. A review of 14 years of research and clinical studies. Clin. Orthop. Relat. Res. 208, 141–145. doi:10.1097/00003086-198607000-00028
Deng, B., Wang, F., Yin, L., Chen, C., Guo, L., Chen, H., et al. (2016). Quantitative study on morphology of calcified cartilage zone in OARSI 0∼4 cartilage from osteoarthritic knees. Curr. Res. Transl. Med. 64 (3), 149–154. doi:10.1016/j.retram.2016.01.009
Fan, X., Wu, X., Trevisan Franca De Lima, L., Stehbens, S., Punyadeera, C., Webb, R., et al. (2022). The deterioration of calcified cartilage integrity reflects the severity of osteoarthritis-A structural, molecular, and biochemical analysis. FASEB J. 36 (2), e22142. doi:10.1096/fj.202101449r
Fawns, H. T., and Landells, J. W. (1953). Histochemical studies of rheumatic conditions. I. Observations on the fine structures of the matrix of normal bone and cartilage. Ann. Rheum. Dis. 12 (2), 105–113. doi:10.1136/ard.12.2.105
Finnilä, M. A. J., Das Gupta, S., Turunen, M. J., Hellberg, I., Turkiewicz, A., Lutz-Bueno, V., et al. (2022). Mineral crystal thickness in calcified cartilage and subchondral bone in healthy and osteoarthritic human knees. J. Bone Min. Res, 1–11. doi:10.1002/jbmr.4642
Galperin, A., Oldinski, R. A., Florczyk, S. J., Bryers, J. D., Zhang, M., and Ratner, B. D. (2013). Integrated bi-layered scaffold for osteochondral tissue engineering. Adv. Healthc. Mat. 2 (6), 872–883. doi:10.1002/adhm.201200345
Gannon, F. H., and Sokoloff, L. (1999). Histomorphometry of the aging human patella: Histologic criteria and controls. Osteoarthr. Cartil. 7 (2), 173–181. doi:10.1053/joca.1998.0206
Gobbi, A., Kon, E., Berruto, M., Filardo, G., Delcogliano, M., Boldrini, L., et al. (2009). Patellofemoral full-thickness chondral defects treated with second-generation autologous chondrocyte implantation: Results at 5 years' follow-up. Am. J. Sports Med. 37 (6), 1083–1092. doi:10.1177/0363546509331419
Gobbi, A., Lane, J. G., and Dallo, I. (2020). Editorial commentary: Cartilage restoration-what is currently available? Arthrosc. J. Arthrosc. Relat. Surg. 36 (6), 1625–1628. doi:10.1016/j.arthro.2020.04.001
Goldring, S. R. (2012). Alterations in periarticular bone and cross talk between subchondral bone and articular cartilage in osteoarthritis. Ther. Adv. Musculoskelet. Dis. 4 (4), 249–258. doi:10.1177/1759720x12437353
Green, W. T., Martin, G. N., Eanes, E. D., and Sokoloff, L. (1970). Microradiographic study of the calcified layer of articular cartilage. Arch. Pathol. 90 (2), 151–158.
Gupta, H. S., Schratter, S., Tesch, W., Roschger, P., Berzlanovich, A., Schoeberl, T., et al. (2005). Two different correlations between nanoindentation modulus and mineral content in the bone-cartilage interface. J. Struct. Biol. X. 149 (2), 138–148. doi:10.1016/j.jsb.2004.10.010
Hargrave-Thomas, E., van Sloun, F., Dickinson, M., Broom, N., and Thambyah, A. (2015). Multi-scalar mechanical testing of the calcified cartilage and subchondral bone comparing healthy vs early degenerative states. Osteoarthr. Cartil. 23 (10), 1755–1762. doi:10.1016/j.joca.2015.05.012
Harley, B. A., Lynn, A. K., Wissner-Gross, Z., Bonfield, W., Yannas, I. V., and Gibson, L. J. (2010). Design of a multiphase osteochondral scaffold III: Fabrication of layered scaffolds with continuous interfaces. J. Biomed. Mat. Res. A 92 (3), 1078–1093. doi:10.1002/jbm.a.32387
Harris, J. D., Siston, R. A., Brophy, R. H., Lattermann, C., Carey, J. L., and Flanigan, D. C. (2011). Failures, re-operations, and complications after autologous chondrocyte implantation--a systematic review. Osteoarthr. Cartil. 19 (7), 779–791. doi:10.1016/j.joca.2011.02.010
Hartlev, L. B., Klose-Jensen, R., Thomsen, J. S., Nyengaard, J. R., Boel, L. W. T., Laursen, M. B., et al. (2018). Thickness of the bone-cartilage unit in relation to osteoarthritis severity in the human hip joint. RMD Open 4 (2), e000747. doi:10.1136/rmdopen-2018-000747
Heymer, A., Bradica, G., Eulert, J., and Nöth, U. (2009). Multiphasic collagen fibre-PLA composites seeded with human mesenchymal stem cells for osteochondral defect repair: An in vitro study. J. Tissue Eng. Regen. Med. 3 (5), 389–397. doi:10.1002/term.175
Hoemann, C. D., Lafantaisie-Favreau, C. H., Lascau-Coman, V., Chen, G., and Guzmán-Morales, J. (2012). The cartilage-bone interface. J. Knee Surg. 25 (2), 085–098. doi:10.1055/s-0032-1319782
Holland, T. A., Bodde, E. W., Baggett, L. S., Tabata, Y., Mikos, A. G., and Jansen, J. A. (2005). Osteochondral repair in the rabbit model utilizing bilayered, degradable oligo(poly(ethylene glycol) fumarate) hydrogel scaffolds. J. Biomed. Mat. Res. A 75 (1), 156–167. doi:10.1002/jbm.a.30379
Huang, Y., Fan, H., Gong, X., Yang, L., and Wang, F. (2021). Scaffold with natural calcified cartilage zone for osteochondral defect repair in minipigs. Am. J. Sports Med. 49 (7), 1883–1891. doi:10.1177/03635465211007139
Huber, M., Trattnig, S., and Lintner, F. (2000). Anatomy, biochemistry, and physiology of articular cartilage. Invest. Radiol. 35 (10), 573–580. doi:10.1097/00004424-200010000-00003
Huey, D. J., Hu, J. C., and Athanasiou, K. A. (2012). Unlike bone, cartilage regeneration remains elusive. Science 338 (6109), 917–921. doi:10.1126/science.1222454
Hwang, J., Bae, W. C., Shieu, W., Lewis, C. W., Bugbee, W. D., and Sah, R. L. (2008). Increased hydraulic conductance of human articular cartilage and subchondral bone plate with progression of osteoarthritis. Arthritis Rheum. 58 (12), 3831–3842. doi:10.1002/art.24069
Imhof, H., Breitenseher, M., Kainberger, F., Rand, T., and Trattnig, S. (1999). Importance of subchondral bone to articular cartilage in health and disease. Top. Magn. Reson. Imaging 10 (3), 180–192. doi:10.1097/00002142-199906000-00002
Imhof, H., Sulzbacher, I., Grampp, S., Czerny, C., Youssefzadeh, S., and Kainberger, F. (2000). Subchondral bone and cartilage disease: A rediscovered functional unit. Invest. Radiol. 35 (10), 581–588. doi:10.1097/00004424-200010000-00004
Jiang, J., Leong, N. L., Mung, J. C., Hidaka, C., and Lu, H. H. (2008). Interaction between zonal populations of articular chondrocytes suppresses chondrocyte mineralization and this process is mediated by PTHrP. Osteoarthr. Cartil. 16 (1), 70–82. doi:10.1016/j.joca.2007.05.014
Jiang, J., Tang, A., Ateshian, G. A., Guo, X. E., Hung, C. T., and Lu, H. H. (2010). Bioactive stratified polymer ceramic-hydrogel scaffold for integrative osteochondral repair. Ann. Biomed. Eng. 38 (6), 2183–2196. doi:10.1007/s10439-010-0038-y
Jiang, S., Zhang, C., Lu, Y., and Yuan, F. (2022). The molecular mechanism research of cartilage calcification induced by osteoarthritis. Bioengineered 13 (5), 13082–13088. doi:10.1080/21655979.2022.2078025
Kandel, R. A., Grynpas, M., Pilliar, R., Lee, J., Wang, J., Waldman, S., et al. (2006). Repair of osteochondral defects with biphasic cartilage-calcium polyphosphate constructs in a sheep model. Biomaterials 27 (22), 4120–4131. doi:10.1016/j.biomaterials.2006.03.005
Kandel, R., Hurtig, M., and Grynpas, M. (1999). Characterization of the mineral in calcified articular cartilagenous tissue formed in vitro. Tissue Eng. 5 (1), 25–34. doi:10.1089/ten.1999.5.25
Kaspiris, A., Chronopoulos, E., Vasiliadis, E., Khaldi, L., Melissaridou, D., Iliopoulos, I. D., et al. (2022). Sex, but not age and bone mass index positively impact on the development of osteochondral micro-defects and the accompanying cellular alterations during osteoarthritis progression. Chronic Dis. Transl. Med. 8 (1), 41–50. doi:10.1002/cdt3.16
Kazemi, M., and Williams, J. L. (2021). Properties of cartilage-subchondral bone junctions: A narrative review with specific focus on the growth plate. Cartilage 13 (2), 16s–33s. doi:10.1177/1947603520924776
Keinan-Adamsky, K., Shinar, H., and Navon, G. (2005). The effect of detachment of the articular cartilage from its calcified zone on the cartilage microstructure, assessed by 2H-spectroscopic double quantum filtered MRI. J. Orthop. Res. 23 (1), 109–117. doi:10.1016/j.orthres.2004.06.005
Khanarian, N. T., Boushell, M. K., Spalazzi, J. P., Pleshko, N., Boskey, A. L., and Lu, H. H. (2014). FTIR-I compositional mapping of the cartilage-to-bone interface as a function of tissue region and age. J. Bone Min. Res. 29 (12), 2643–2652. doi:10.1002/jbmr.2284
Khanarian, N. T., Jiang, J., Wan, L. Q., Mow, V. C., and Lu, H. H. (2012). A hydrogel-mineral composite scaffold for osteochondral interface tissue engineering. Tissue Eng. Part A 18 (5-6), 533–545. doi:10.1089/ten.tea.2011.0279
Kiraly, A. J., Roberts, A., Cox, M., Mauerhan, D., Hanley, E., and Sun, Y. (2017). Comparison of meniscal cell-mediated and chondrocyte-mediated calcification. Open Orthop. J. 11, 225–233. doi:10.2174/1874325001711010225
Kirsch, T., and Wuthier, R. E. (1994). Stimulation of calcification of growth plate cartilage matrix vesicles by binding to type II and X collagens. J. Biol. Chem. 269 (15), 11462–11469. doi:10.1016/s0021-9258(19)78146-0
Kon, E., Gobbi, A., Filardo, G., Delcogliano, M., Zaffagnini, S., and Marcacci, M. (2009). Arthroscopic second-generation autologous chondrocyte implantation compared with microfracture for chondral lesions of the knee: Prospective nonrandomized study at 5 years. Am. J. Sports Med. 37 (1), 33–41. doi:10.1177/0363546508323256
Kosik-Kozioł, A., Costantini, M., Mróz, A., Idaszek, J., Heljak, M., Jaroszewicz, J., et al. (2019). 3D bioprinted hydrogel model incorporating β-tricalcium phosphate for calcified cartilage tissue engineering. Biofabrication 11 (3), 035016. doi:10.1088/1758-5090/ab15cb
Li, X., Lang, W., Ye, H., Yu, F., Li, H., Chen, J., et al. (2013). Tougu Xiaotong capsule inhibits the tidemark replication and cartilage degradation of papain-induced osteoarthritis by the regulation of chondrocyte autophagy. Int. J. Mol. Med. 31 (6), 1349–1356. doi:10.3892/ijmm.2013.1341
Li, X., Yang, J., Liu, D., Li, J., Niu, K., Feng, S., et al. (2016). Knee loading inhibits osteoclast lineage in a mouse model of osteoarthritis. Sci. Rep. 6, 24668. doi:10.1038/srep24668
Li, Z., Jia, S., Xiong, Z., Long, Q., Yan, S., Hao, F., et al. (2018). 3D-printed scaffolds with calcified layer for osteochondral tissue engineering. J. Biosci. Bioeng. 126 (3), 389–396. doi:10.1016/j.jbiosc.2018.03.014
Liu, M., Ke, X., Yao, Y., Wu, F., Ye, S., Zhang, L., et al. (2021). Artificial osteochondral interface of bioactive fibrous membranes mediating calcified cartilage reconstruction. J. Mat. Chem. B 9 (37), 7782–7792. doi:10.1039/d1tb01238j
Luo, Q., Shan, Y., Zuo, X., and Liu, J. (2018). Anisotropic tough poly(vinyl alcohol)/graphene oxide nanocomposite hydrogels for potential biomedical applications. RSC Adv. 8 (24), 13284–13291. doi:10.1039/c8ra00340h
Lyons, T. J., McClure, S. F., Stoddart, R. W., and McClure, J. (2006). The normal human chondro-osseous junctional region: Evidence for contact of uncalcified cartilage with subchondral bone and marrow spaces. BMC Musculoskelet. Disord. 7, 52. doi:10.1186/1471-2474-7-52
Madry, H., van Dijk, C. N., and Mueller-Gerbl, M. (2010). The basic science of the subchondral bone. Knee Surg. Sports Traumatol. Arthrosc. 18 (4), 419–433. doi:10.1007/s00167-010-1054-z
Mente, P. L., and Lewis, J. L. (1994). Elastic modulus of calcified cartilage is an order of magnitude less than that of subchondral bone. J. Orthop. Res. 12 (5), 637–647. doi:10.1002/jor.1100120506
Milz, S., and Putz, R. (1994). Lückenbildungen der subchondralen Mineralisierungszone des Tibiaplateaus. Osteologie.
Mitchell, M. J., Billingsley, M. M., Haley, R. M., Wechsler, M. E., Peppas, N. A., and Langer, R. (2021). Engineering precision nanoparticles for drug delivery. Nat. Rev. Drug Discov. 20 (2), 101–124. doi:10.1038/s41573-020-0090-8
Müller-Gerbl, M., Schulte, E., and Putz, R. (1987). The thickness of the calcified layer of articular cartilage: A function of the load supported? J. Anat. 154, 103–111.
Nasi, S., Ehirchiou, D., Bertrand, J., Castelblanco, M., Mitchell, J., Ishii, I., et al. (2021). The gasotransmitter hydrogen sulfide (H(2)S) prevents pathologic calcification (PC) in cartilage. Antioxidants (Basel) 10 (9), 1433. doi:10.3390/antiox10091433
Nguyen, Q. T., Hwang, Y., Chen, A. C., Varghese, S., and Sah, R. L. (2012). Cartilage-like mechanical properties of poly (ethylene glycol)-diacrylate hydrogels. Biomaterials 33 (28), 6682–6690. doi:10.1016/j.biomaterials.2012.06.005
Nishimura, R., Hata, K., Ono, K., Amano, K., Takigawa, Y., Wakabayashi, M., et al. (2012). Regulation of endochondral ossification by transcription factors. Front. Biosci. 17 (7), 2657–2666. doi:10.2741/4076
Nöth, U., Rackwitz, L., Heymer, A., Weber, M., Baumann, B., Steinert, A., et al. (2007). Chondrogenic differentiation of human mesenchymal stem cells in collagen type I hydrogels. J. Biomed. Mat. Res. A 83 (3), 626–635. doi:10.1002/jbm.a.31254
Oegema, T. R., Carpenter, R. J., Hofmeister, F., and Thompson, R. C. (1997). The interaction of the zone of calcified cartilage and subchondral bone in osteoarthritis. Microsc. Res. Tech. 37 (4), 324–332. doi:10.1002/(sici)1097-0029(19970515)37:4<324::aid-jemt7>3.0.co;2-k
Pan, J., Wang, B., Li, W., Zhou, X., Scherr, T., Yang, Y., et al. (2012). Elevated cross-talk between subchondral bone and cartilage in osteoarthritic joints. Bone 51 (2), 212–217. doi:10.1016/j.bone.2011.11.030
Pan, J., Zhou, X., Li, W., Novotny, J. E., Doty, S. B., and Wang, L. (2009). In situ measurement of transport between subchondral bone and articular cartilage. J. Orthop. Res. 27 (10), 1347–1352. doi:10.1002/jor.20883
Petrovova, E., Tomco, M., Holovska, K., Danko, J., Kresakova, L., Vdoviakova, K., et al. (2021). PHB/CHIT scaffold as a promising biopolymer in the treatment of osteochondral defects-an experimental animal study. Polym. (Basel) 13 (8), 1232. doi:10.3390/polym13081232
Pita, J. C., Cuervo, L. A., Madruga, J. E., Muller, F. J., and Howell, D. S. (1970). Evidence for a role of proteinpolysaccharides in regulation of mineral phase separation in calcifying cartilage. J. Clin. Invest. 49 (12), 2188–2197. doi:10.1172/jci106437
Poole, A. R., Matsui, Y., Hinek, A., and Lee, E. R. (1989). Cartilage macromolecules and the calcification of cartilage matrix. Anat. Rec. Hob. 224 (2), 167–179. doi:10.1002/ar.1092240207
Poole, C. A., Flint, M. H., and Beaumont, B. W. (1984). Morphological and functional interrelationships of articular cartilage matrices. J. Anat. 138, 113–138.
Pouran, B., Raoof, A., de Winter, D. A. M., Arbabi, V., Bleys, R., Beekman, F. J., et al. (2021). Topographic features of nano-pores within the osteochondral interface and their effects on transport properties -a 3D imaging and modeling study. J. Biomech. 123, 110504. doi:10.1016/j.jbiomech.2021.110504
Radin, E. L., and Rose, R. M. (1986). Role of subchondral bone in the initiation and progression of cartilage damage. Clin. Orthop. Relat. Res. 213, 34–40. doi:10.1097/00003086-198612000-00005
Redler, I., Mow, V. C., Zimny, M. L., and Mansell, J. (1975). The ultrastructure and biomechanical significance of the tidemark of articular cartilage. Clin. Orthop. Relat. Res. 112, 357–362. doi:10.1097/00003086-197510000-00038
Rösch, G., El Bagdadi, K., Muschter, D., Taheri, S., Dorn, C., Meurer, A., et al. (2022). Sympathectomy aggravates subchondral bone changes during osteoarthritis progression in mice without affecting cartilage degeneration or synovial inflammation. Osteoarthr. Cartil. 30 (3), 461–474. doi:10.1016/j.joca.2021.11.016
Rösch, G., Muschter, D., Taheri, S., El Bagdadi, K., Dorn, C., Meurer, A., et al. (2021). β2-Adrenoceptor deficiency results in increased calcified cartilage thickness and subchondral bone remodeling in murine experimental osteoarthritis. Front. Immunol. 12, 801505. doi:10.3389/fimmu.2021.801505
Salerno, A., Iannace, S., and Netti, P. A. (2012). Graded biomimetic osteochondral scaffold prepared via CO2 foaming and micronized NaCl leaching. Mater. Lett. 82, 137–140. doi:10.1016/j.matlet.2012.05.072
Saris, D., Price, A., Widuchowski, W., Bertrand-Marchand, M., Caron, J., Drogset, J. O., et al. (2014). Matrix-applied characterized autologous cultured chondrocytes versus microfracture: Two-year follow-up of a prospective randomized trial. Am. J. Sports Med. 42 (6), 1384–1394. doi:10.1177/0363546514528093
Sheehy, E. J., Vinardell, T., Buckley, C. T., and Kelly, D. J. (2013). Engineering osteochondral constructs through spatial regulation of endochondral ossification. Acta biomater. 9 (3), 5484–5492. doi:10.1016/j.actbio.2012.11.008
Sledge, S. L. (2001). Microfracture techniques in the treatment of osteochondral injuries. Clin. Sports Med. 20 (2), 365–378. doi:10.1016/s0278-5919(05)70311-2
Sliz, E., Taipale, M., Welling, M., Skarp, S., Alaraudanjoki, V., Ignatius, J., et al. (2017). TUFT1, a novel candidate gene for metatarsophalangeal osteoarthritis, plays a role in chondrogenesis on a calcium-related pathway. PLoS One 12 (4), e0175474. doi:10.1371/journal.pone.0175474
Sophia Fox, A. J., Bedi, A., and Rodeo, S. A. (2009). The basic science of articular cartilage: Structure, composition, and function. Sports Health. 1 (6), 461–468. doi:10.1177/1941738109350438
Staines, K. A., Pollard, A. S., McGonnell, I. M., Farquharson, C., and Pitsillides, A. A. (2013). Cartilage to bone transitions in health and disease. J. Endocrinol. 219 (1), R1–r12. doi:10.1530/joe-13-0276
Steinmetz, N. J., Aisenbrey, E. A., Westbrook, K. K., Qi, H. J., and Bryant, S. J. (2015). Mechanical loading regulates human MSC differentiation in a multi-layer hydrogel for osteochondral tissue engineering. Acta biomater. 21, 142–153. doi:10.1016/j.actbio.2015.04.015
Tang, T., Landis, W., Raguin, E., Werner, P., Bertinetti, L., Dean, M., et al. (2022). A 3D network of nanochannels for possible ion and molecule transit in mineralizing bone and cartilage. Weinheim: Wiley-VCH GmbH.
Walsh, D. A., Bonnet, C. S., Turner, E. L., Wilson, D., Situ, M., and McWilliams, D. F. (2007). Angiogenesis in the synovium and at the osteochondral junction in osteoarthritis. Osteoarthr. Cartil. 15 (7), 743–751. doi:10.1016/j.joca.2007.01.020
Wang, F., Ying, Z., Duan, X., Tan, H., Yang, B., Guo, L., et al. (2009). Histomorphometric analysis of adult articular calcified cartilage zone. J. Struct. Biol. X. 168 (3), 359–365. doi:10.1016/j.jsb.2009.08.010
Wang, W., Li, B., Yang, J., Xin, L., Li, Y., Yin, H., et al. (2010). The restoration of full-thickness cartilage defects with BMSCs and TGF-beta 1 loaded PLGA/fibrin gel constructs. Biomaterials 31 (34), 8964–8973. doi:10.1016/j.biomaterials.2010.08.018
Wang, X., Wenk, E., Zhang, X., Meinel, L., Vunjak-Novakovic, G., and Kaplan, D. L. (2009). Growth factor gradients via microsphere delivery in biopolymer scaffolds for osteochondral tissue engineering. J. Control. Release 134 (2), 81–90. doi:10.1016/j.jconrel.2008.10.021
Wen, C. Y., Wu, C. B., Tang, B., Wang, T., Yan, C. H., Lu, W. W., et al. (2012). Collagen fibril stiffening in osteoarthritic cartilage of human beings revealed by atomic force microscopy. Osteoarthr. Cartil. 20 (8), 916–922. doi:10.1016/j.joca.2012.04.018
Yajun, W., Jin, C., Zhengrong, G., Chao, F., Yan, H., Weizong, W., et al. (2021). Betaine attenuates osteoarthritis by inhibiting osteoclastogenesis and angiogenesis in subchondral bone. Front. Pharmacol. 12, 723988. doi:10.3389/fphar.2021.723988
Yang, J., Liu, Y., He, L., Wang, Q., Wang, L., Yuan, T., et al. (2018). Icariin conjugated hyaluronic acid/collagen hydrogel for osteochondral interface restoration. Acta Biomater. 74, 156–167. doi:10.1016/j.actbio.2018.05.005
Yang, J., Zhang, Y. S., Yue, K., and Khademhosseini, A. (2017). Cell-laden hydrogels for osteochondral and cartilage tissue engineering. Acta biomater. 57, 1–25. doi:10.1016/j.actbio.2017.01.036
You, F., Chen, X., Cooper, D. M. L., Chang, T., and Eames, B. F. (2018). Homogeneous hydroxyapatite/alginate composite hydrogel promotes calcified cartilage matrix deposition with potential for three-dimensional bioprinting. Biofabrication 11 (1), 015015. doi:10.1088/1758-5090/aaf44a
Zarka, M., Hay, E., Ostertag, A., Marty, C., Chappard, C., Oudet, F., et al. (2019). Microcracks in subchondral bone plate is linked to less cartilage damage. Bone 123, 1–7. doi:10.1016/j.bone.2019.03.011
Zhang, Y., Wang, F., Tan, H., Chen, G., Guo, L., and Yang, L. (2012). Analysis of the mineral composition of the human calcified cartilage zone. Int. J. Med. Sci. 9 (5), 353–360. doi:10.7150/ijms.4276
Zheng, L., Fan, H. S., Sun, J., Chen, X. N., Wang, G., Zhang, L., et al. (2010). Chondrogenic differentiation of mesenchymal stem cells induced by collagen-based hydrogel: An in vivo study. J. Biomed. Mat. Res. A 93 (2), 783–792. doi:10.1002/jbm.a.32588
Zhou, H., Yuan, L., Xu, Z., Yi, X., Wu, X., Mu, C., et al. (2022). Mimicking the composition and structure of the osteochondral tissue to fabricate a heterogeneous three-layer scaffold for the repair of osteochondral defects. ACS Appl. Bio Mat. 5 (2), 734–746. doi:10.1021/acsabm.1c01152
Keywords: calcified cartilage layer, tidemark, osteoarthritis, scaffolds, engineering reconstruction
Citation: Wang W, Ye R, Xie W, Zhang Y, An S, Li Y and Zhou Y (2022) Roles of the calcified cartilage layer and its tissue engineering reconstruction in osteoarthritis treatment. Front. Bioeng. Biotechnol. 10:911281. doi: 10.3389/fbioe.2022.911281
Received: 02 April 2022; Accepted: 28 July 2022;
Published: 31 August 2022.
Edited by:
Ralph Müller, ETH Zürich, SwitzerlandReviewed by:
Miguel Castilho, University Medical Center Utrecht, NetherlandsCopyright © 2022 Wang, Ye, Xie, Zhang, An, Li and Zhou. This is an open-access article distributed under the terms of the Creative Commons Attribution License (CC BY). The use, distribution or reproduction in other forums is permitted, provided the original author(s) and the copyright owner(s) are credited and that the original publication in this journal is cited, in accordance with accepted academic practice. No use, distribution or reproduction is permitted which does not comply with these terms.
*Correspondence: Senbo An, YW5zZW5ib0BzZGZtdS5lZHUuY24=; Yusheng Li, bGl5dXNoZW5nQGNzdS5lZHUuY24=; Yang Zhou, emhvdXlhbmdjY2hAMTYzLmNvbQ==
†These authors have contributed equally to this work
Disclaimer: All claims expressed in this article are solely those of the authors and do not necessarily represent those of their affiliated organizations, or those of the publisher, the editors and the reviewers. Any product that may be evaluated in this article or claim that may be made by its manufacturer is not guaranteed or endorsed by the publisher.
Research integrity at Frontiers
Learn more about the work of our research integrity team to safeguard the quality of each article we publish.