- 1Department of Biomechanics, University of Nebraska at Omaha, Omaha, NE, United States
- 2School of Health and Kinesiology, University of Nebraska at Omaha, Omaha, NE, United States
- 3Department of Surgery, University of Nebraska Medical Center, Omaha, NE, United States
- 4Department of Biostatistics, University of Nebraska Medical Center, Omaha, NE, United States
Most of the terrestrial legged locomotion gaits, like human walking, necessitate energy dissipation upon ground collision. In humans, the heel mostly performs net-negative work during collisions, and it is currently unclear how it dissipates that energy. Based on the laws of thermodynamics, one possibility is that the net-negative collision work may be dissipated as heat. If supported, such a finding would inform the thermoregulation capacity of human feet, which may have implications for understanding foot complications and tissue damage. Here, we examined the correlation between energy dissipation and thermal responses by experimentally increasing the heel’s collisional forces. Twenty healthy young adults walked overground on force plates and for 10 min on a treadmill (both at 1.25 ms−1) while wearing a vest with three different levels of added mass (+0%, +15%, & +30% of their body mass). We estimated the heel’s work using a unified deformable segment analysis during overground walking. We measured the heel’s temperature immediately before and after each treadmill trial. We hypothesized that the heel’s temperature and net-negative work would increase when walking with added mass, and the temperature change is correlated with the increased net-negative work. We found that walking with +30% added mass significantly increased the heel’s temperature change by 0.72 ± 1.91
Introduction
The majority of the terrestrial legged locomotion gaits, like human walking, necessitate the dissipation of impact forces when the feet collide with the ground (Schmitt and Larson, 1995; Ruina et al., 2005; Lee et al., 2013; Miao et al., 2017; Usherwood, 2020; Zeininger et al., 2020). For instance, a large portion of energy dissipation (i.e., net-negative work) occurs immediately after the collision of the human heel with the ground. (Gefen et al., 2001; Chi and Schmitt, 2005; Takahashi et al., 2017; Baines et al., 2018; Honert and Zelik, 2019; Papachatzis et al., 2020). Such net-negative collision work is a vital feature of walking as the legs transition from one step to the next (Donelan et al., 2002a; Donelan et al., 2002b; Kuo, 2002, 2007; Kuo et al., 2005; Kuo and Donelan, 2010). Additionally, energy dissipation during the heel collision may highlight the heel’s protective mechanism to absorb impact and minimize trauma or injuries (Pain and Challis, 2001; Wearing et al., 2014). However, despite the importance of collisional energy losses during heel-strike, there remains a paucity of evidence on how the human heel dissipates (i.e., loses) mechanical energy. Consequently, a study of human bipedal walking is incomplete without understanding how the heel dissipates and utilizes net-negative work during heel-strike.
Conservation of energy suggests energy is a quantity that cannot be lost or created out of nothing. Therefore, the dissipated kinetic energy should be converted to heat and/or sound when the heel collides with the ground. Indeed, previous experiments have established that the collision between the heel and the ground produces an acoustic impact (Li et al., 1991; Ekimov and Sabatier, 2006; Visell et al., 2009; Larsson, 2014; Hung Au et al., 2021). Furthermore, principles of acoustics suggest that the amplitude of the produced sound should be proportional to the amplitude of the impact pressure. However, Hung Au et al., 2021 reported a statistically non-significant association between impact sound amplitude and vertical impact rate during running, with heel-strike running having the lowest peak sound amplitude (Hung Au et al., 2021). Thus, although energy dissipation as sound is plausible, it seems reasonable to predict that the heel’s collision energy during walking may be dissipated as heat.
Indeed, the principles of thermodynamics suggest that the heel’s net-negative collision work could be converted into heat and cause a temperature increase. In-vivo and in-vitro studies have shown that the heel exhibits viscoelastic behavior under vertical compression with energy presumably dissipated as heat (i.e., hysteresis) between the compressive loading-unloading cycles (Bennett and Ker, 1990; Aerts et al., 1995; Pain and Challis, 2001; Ledoux and Blevins, 2007; Pai and Ledoux, 2011; Behforootan et al., 2017). Energy dissipation as heat accords with previous data, which showed that the foot’s sites, including the heel, increase their skin temperature even by ∼5.0
gives a relationship between the generation or uptake of heat (
Therefore, the temperature change of the heel
Since a unit change in Kelvin (K) equals a unit change in Celsius (°C), the SI units are (°C).
Inserting the Eq. 2 into Eq. 3 yields:
where
For a given specific heat capacity
If indeed the heel’s energy is dissipated as heat, such knowledge would be a valuable tool for scientists studying the feet and legs of various species. For example, it could provide a foundation to study how mechanical energy of the legs and feet is converted or utilized by the body during terrestrial locomotion. In humans, such knowledge could allow us to understand factors that influence heel temperature regulation, leading to new insights into the prevention of foot skin complications, such as blisters, calluses, and ulcers which can be particularly unfavorable in patients with diabetes and peripheral artery disease (Armstrong et al., 2007; Maddah and Beigzadeh, 2020; van Netten et al., 2020; Chatzistergos et al., 2021).
To the best of our knowledge, no in-vivo studies have directly examined if the human heel dissipates net-negative mechanical work as heat. Therefore, this study examined the relationship between the heel’s mechanical energy and thermal responses during walking. We experimentally increased the human heel’s collision forces (via walking with added mass) (Papachatzis et al., 2020) and investigated its effect on the heel’s thermodynamic responses. We hypothesized that when participants walk with higher added body mass levels, the magnitudes of heel’s temperature change and net-negative work (i.e., energy dissipated) will increase. Additionally, we hypothesized that an increase in temperature is correlated with higher magnitudes of energy dissipated as net-negative work by the heel.
Materials and Methods
Participants
Twenty healthy young adults volunteered to participate in this research study (5 females, 15 males; age = 24.4 ± 2.8 years; height = 1.74 ± 0.07 m, mass = 83.6 ± 21.2 kg; means ± standard deviation). The sample size was based on a power analysis using published data comparing foot temperature differences between multiple walking cadences (Reddy et al., 2017). For an effect size of 0.81, a sample size of 19 participants will provide 80% power to detect comparable differences, with significance set to α = 0.016. Participants were free of cardiac and neurological pathologies (such as arrhythmia, heart attack, and stroke) and any musculoskeletal or pathological problems (such as osteoarthritis, bone fractures, etc.). The Institutional Review Board at the University of Nebraska Medical Center approved the experimental protocol, and all participants signed an informed consent form before participating in the experimental protocol.
Experimental Protocol
The participants walked barefoot over the ground and on a treadmill (10 min per trial) while carrying three different levels of added mass: +0; no added body mass, +15%, and +30% relative to their body mass. We chose the level of added mass comparable to previous studies (Griffin et al., 2003; Silder et al., 2013; Huang and Kuo, 2014; Mooney et al., 2014; Rice et al., 2017) and considered safety risks. We used the overground walking trials to collect foot mechanics data, whereas the treadmill trials measured the foot temperature data (Figure 1). The walking speed was controlled at 1.25 m s−1 for overground and treadmill walking. We monitored the speed using timing gates (Dashr Elite Kit, Lincoln, NE, United States) and verbal feedback for overground walking. The overground versus treadmill trials were randomized, and the order of the added mass trials within the overground/treadmill trials was also randomized.
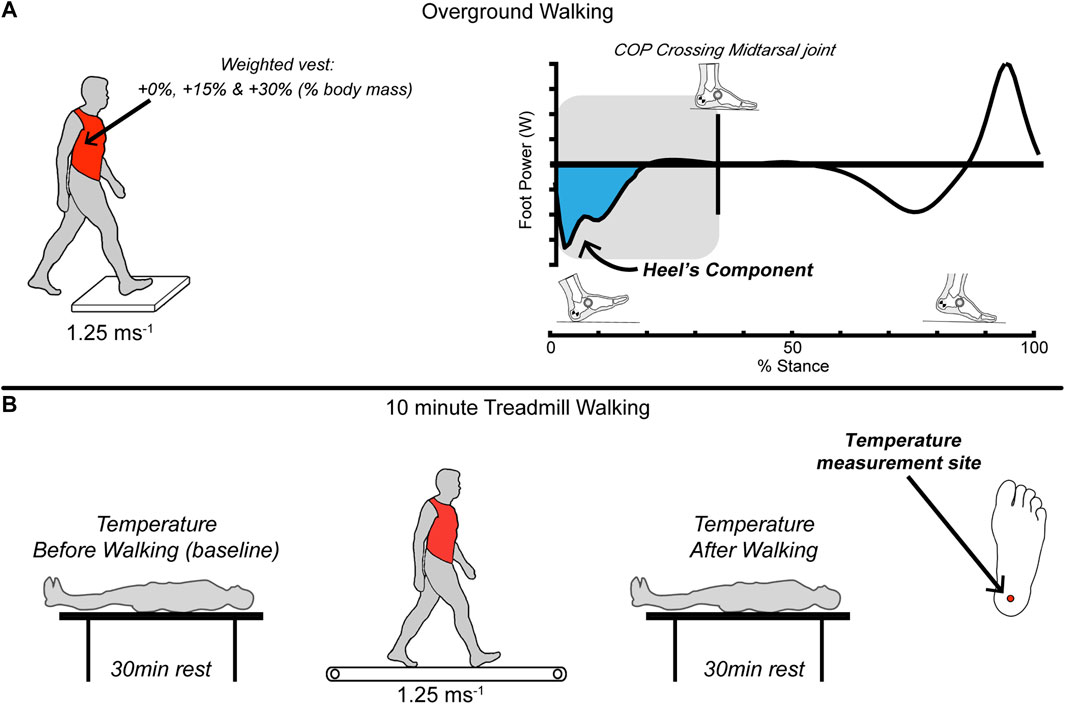
FIGURE 1. A total of 20 healthy young adults (N = 20) completed two barefoot randomized walking protocols: (A) overground on force plates and (B) 10 min on a treadmill (both at 1.25 m s−1). Participants carried (via weight vest) three different randomized levels of symmetrical loads: +0%; no added body mass, +15%, and +30% of their body mass. We used the overground walking trials to collect foot mechanics data (kinematic & kinetic), whereas the treadmill trials measured the foot temperature data. We quantified the mechanical power and work done by the foot using a unified-deformable analysis. We computed the work when the center-of-pressure was underneath the heel segment during the early stance phase to isolate the heel contribution. An estimate of the total work over the 10 min of treadmill walking was calculated by multiplying the average work per step measured in overground trials by the number of steps taken in 10 min of treadmill walking. Temperature measurements were taken immediately before and after each treadmill trial at the bottom of the right foot, including the heel pad. We computed the change in temperature of the heel before and after each walking trial.
Participants carried the added mass wearing a weighted vest (M.I.R. Pro Weighted Vest, San Jose, CA, United States). We used a weighted vest because, compared to other methods, the symmetrical distribution of the carrying loads reduces the muscular activity and postural sway and has a smaller effect on the anterior-posterior center-of-mass location (Datta and Ramanathan, 1971; Bobet and Norman, 1984; Simpson et al., 2012; Sahli et al., 2013; Liew et al., 2016).
Analysis: Foot and Heel Mechanics (Overground Walking)
The overground walking trials were performed over a ∼10 m long walkway consisting of five force plates aligned serially (AMTI Inc., Watertown, MA, United States), and ground reaction force data were collected at 1,080 Hz. We placed retro-reflective markers on bony landmarks of the feet, ankles, and knees while we used clusters to track the movement of the shank, thigh, and pelvis. We selected the landmarks based on Bruening et al. (2012), and we defined three segments within the foot (hindfoot, midfoot, and hallux). We used an eight-in an eight-infrared camera motion analysis system (Raptor-4S, Motion Analysis Corp., Mountain View, CA, United States) to capture the position of the retro-reflective markers on the lower extremity relative to the global reference system of the laboratory at 180 Hz. To minimize the error in the center of pressure (C.O.P.) estimates, we used the CalTester tool to assess the accuracy of the force platform when used in conjunction with the motion capture system (Holden et al., 2003), where we found an average error of 2.04 ± 1.35, 1.34 ± 1.23 and 1.36 ± 0.91 mm (means ± standard deviation of the five force plates) for the three axes.
We processed only the clean stance phases when the entire foot came in contact within the force plate’s borders without overlapping adjacent force plates or the floor. We analyzed approximately five stance phase data series (e.g., 3–7 per participant) for each walking trial for each participant. We averaged the stance data of each participant for each variable (e.g., negative, positive, and net work). We filtered the raw data by applying a second-order dual-pass low-pass Butterworth filter of 6 Hz for kinematic data and 25 Hz for kinetic data. A 20 N threshold for the vertical ground reaction force defined the start and the end time for each stance phase of walking. We collected, processed, and analyzed the data using Cortex motion analysis software (Motion Analysis Corp.), Visual3D (C-motion, Germantown, MD, United States), and MATLAB R2021a (MathWorks, Natick, MA, United States).
We quantified the mechanical power and work contribution (i.e., energy dissipation, absorption, return or generation) of the heel’s surrounding structures (e.g., fat pad) using computational methods described in our previous work (Papachatzis et al., 2020). Briefly, we quantified the mechanical power and work of all the structures distal to the hindfoot’s center of mass (i.e., structures of the entire foot) using a unified deformable segment analysis (Takahashi et al., 2017). Then, we determined the timing in which the C.O.P. crossed anteriorly to the midtarsal joint in the laboratory coordinate system. Based on a prior study (Bruening and Takahashi, 2018), we expected that the work performed when the C.O.P. was posterior to the midtarsal joint (i.e., C.O.P. was underneath the hindfoot segment) would include mostly contributions from structures underneath the hindfoot (e.g., heel pad).
For each stance phase, we integrated the mechanical power with respect to time to compute mechanical work (positive, negative, and net). To estimate work done by the foot during 10 min of walking, we multiplied the work per step computed from the overground trials by the number of steps estimated from the treadmill data (via the average step frequency observed). The stance phase was detected from the treadmill trials using a kinematics-based event detection algorithm using the heel and toe markers (Zeni et al., 2008).
Analysis: Foot and Heel Temperature (Treadmill Walking)
Heel pad temperature measurements were obtained from the right foot immediately before and after each treadmill trial (10 min) using a type T cooper thermocouple skin-surface probe (SST-2, Physitemp, Instruments, Clifton, NJ, United States) with an accuracy of ±0.1 (°C) connected to a 4-channel Extech SDL200 data logger thermometer with a sampling rate of 3,600 samples per second (Extech SDL200, Extech Instruments, Waltham, MA, United States). To ensure stable baseline temperature measurements before each added mass trial, we allowed an acclimatization period to adjust the feet of each participant to room temperature. Briefly, participants lay quietly on the treatment table for 30 min before each treadmill trial. At the start of this 30 min, 70% isopropyl alcohol was sprayed over the right foot, shank, and thigh, and 70% isopropyl alcohol wipes were used to disinfect the foot. We defined “baseline temperature” as the temperature of the recording site after the acclimatization period. All measurements were obtained while the subject was lying down on a standard treatment table.
While the focus of this study was quantifying heel pad temperature, we obtained additional temperature measurements from different sites of the plantar and dorsal surface of the foot and the anterior-posterior aspect of the shank and thigh. Specifically, we measured the temperature of the inferior aspect of the hallux, first and fifth metatarsal heads, the superior aspect of the first and fifth metatarsal heads; the temperature over the tibialis anterior muscle belly, posteriorly over the gastrocnemius medialis muscle belly, anteriorly over the vastus lateralis muscle belly and posteriorly over the semimembranosus muscle belly. Also, we measured the absolute tympanic temperature without any adjustments using a tympanic thermometer, placing it in the participant’s right ear. The thermometer had an accuracy of ±0.1 (°C), range (33.0–42.0°C), and recorded the temperature after ∼1 s (Covidien Genius 2, Cardinal Health, Dublin, Ireland). In addition, we measured the temperature of the treadmill belt surface by placing two contact thermometer probes on the treadmill’s surface in areas where the participants frequently stepped.
The probes of the contact thermometer were placed over the specific regions of interest until a stable measurement was obtained (∼20–30 s per site). Two investigators used the four probes simultaneously to minimize the recording time. The order of temperature measurement was as follows: all four plantar foot sites first, the two dorsal foot sites and two anterior sites (shank and thigh) second, the two posterior sites (shank and thigh) and core temperature third, and the two treadmill sites last. We also recorded the laboratory’s temperature and humidity at the end of the above temperature measurements. For all the treadmill walking trials, the participants had two reflective markers placed on each foot, one over the Achilles tendon insertion into the calcaneus and one on top of the hallucis over the nailbed.
We computed the change in temperature
Supplementary Experiment: Heel Temperature Measurements Validation
We performed an additional experiment to examine the validity of the heel temperature measurements acquired from the treadmill trials (see Supplementary Material). For this experiment, 12 participants walked barefoot for 10 min in a randomized order on a treadmill and on an overground track while carrying (via weight vest) two different levels of added mass: +0% (no added body mass) and +30% relative to their body mass. In addition, we controlled the walking speed for the treadmill and overground track trials at 1.25 m s−1. The order of the added mass levels was also randomized. We obtained all measurements precisely the same procedures described in the Analysis: Foot and Heel Temperature (Treadmill Walking) section.
Statistical Analysis: Hypotheses Testing
To determine the effect of the added mass on the heel temperature change after 10 min of barefoot walking on 10 min of negative and net-negative extrapolated barefoot work (dependent variables), we used repeated-measures ANOVAs (three levels of added mass). In addition, we used the Simulate technique to adjust p-values for multiple comparisons when we detected significant effects.
We used linear mixed models to examine the correlation between the heel’s mechanical work (negative or net-negative work; two different models) and temperature change while accounting for the repeated measures among participants due to different levels of added mass.
Statistical Analysis: Exploratory Analysis
We performed an exploratory analysis using linear mixed models to examine the correlation between the heel’s net-negative mechanical work extrapolated to 10 min of barefoot walking (predictor variables) and the heel’s temperature change (response variable). We used a manual variable backward selection procedure accounting for additional predictor variables: gender, body weight, height, foot length, impulse (vertical, mediolateral, and anterior-posterior) extrapolated to 10 min of walking, treadmill surface temperature after 10 min of walking, and heel temperature before the walking trials.
For all analyses, we used SAS version 9.4. The threshold for a significant effect was set to p-value ≤ 0.05, and residuals were examined to ensure model assumptions and fit.
Results
Effect of Added Body Mass on Heel’s Mechanical Work (Extrapolated to 10 min of Barefoot Walking)
Added body mass had a statistically significant effect on the heel’s negative and net-negative mechanical work extrapolated 10 min of barefoot walking (p = 0.004 and p = 0.007 respectively; Figure 2). Specifically, the magnitude of negative work significantly increased by 364.43 ± 404.06 J between +0% and +30% added mass (p = 0.003), but not between +0% and +15% added mass (p = 0.400) or between +15% and +30% added mass (p = 0.077). Additionally, the magnitude of net-negative work significantly increased by 326.94 ± 379.92 J between +0% and +30% added mass (p = 0.005), but not between +0% and +15% added mass (p = 0.505) or between +15% and +30% added mass (p = 0.084).
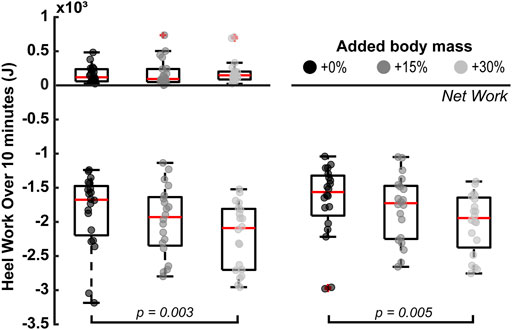
FIGURE 2. Walking with an additional 30% of body mass significantly increased the magnitudes of the extrapolated to 10 min, negative (p = 0.003) and net-negative (p = 0.005) work during the heel strike phase. The horizontal square brackets indicate the adjusted significant pair-wise comparisons (N = 20). We added jitter to the data on the x-axis only for visualization purposes, using the scatter function in MATLAB R2021a.
Effect of Added Body Mass on Heel’s Temperature Change After 10 min of Barefoot Walking
Added body mass had a statistically significant effect on the heel’s temperature change
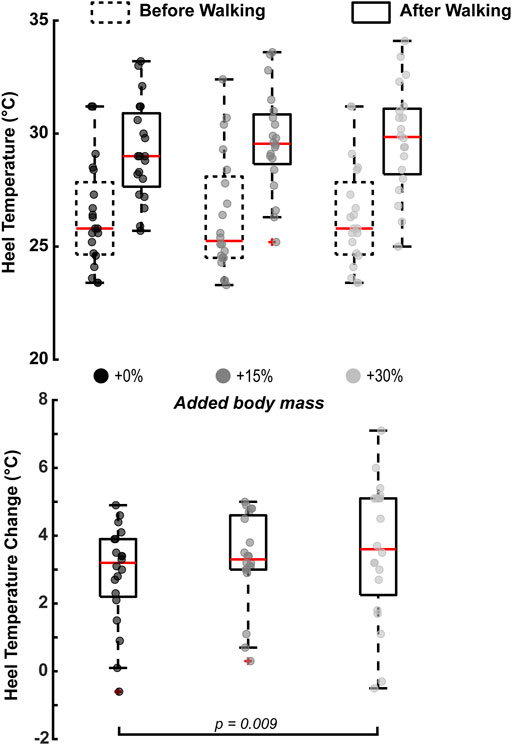
FIGURE 3. Walking with an additional 30% of body mass significantly increased the heel’s temperature change after 10 min of barefoot walking (p = 0.009). Heel’s temperature increased with the rest of the condition but was not statistically significant. The horizontal square brackets s indicate the adjusted significant pair-wise comparisons (N = 20). We added jitter to the data on the x-axis only for visualization purposes, using the scatter function in MATLAB R2021a.
Walking with added mass had no statistical effect (p = 0.322) on the tympanic (body) temperature change (+0%: 0.02 ± 0.22°C, +15%: 0.005 ± 0.30°C, +30%: 0.55 ± 2.33°C; means ± standard deviation).
We also examined the influence of the treadmill on the heel temperature measurements by comparing the temperature measurements obtained from the treadmill trials with temperature measurements obtained from overground trials. The difference in the heel’s temperature change between the two added mass conditions (i.e.,
Correlation Between Heel’s Temperature Change and Net-Negative Mechanical Work
There was no statistically significant correlation of negative or net-negative mechanical work extrapolated to 10 min of barefoot walking with heel’s temperature change after 10 min of barefoot walking (p = 0.383 and p = 0.277; respectively).
Exploratory Analysis: Additional Variables That Could Explain Heel Temperature Change
We adjusted for the gender, body weight, height, foot length, impulse, treadmill surface temperature after 10 min of walking, and heel temperature before the walking trial. The model of the analysis showed that only the temperature of the heel before walking (i.e., baseline) (p < 0.001) had a statistically significant effect on the heel’s temperature increase after 10 min of barefoot walking (Supplementary Table S1). Furthermore, the heel temperature before walking had a negative slope, indicating that the temperature change was less for a higher temperature before waking.
Discussion
The collision between the leg and the ground is a vital feature for most terrestrial legged locomotion gaits, particularly human walking (Ruina et al., 2005; Hutchinson et al., 2011; Miao et al., 2017; Panagiotopoulou et al., 2019; Clemente et al., 2020; Zeininger et al., 2020) where the heel is often associated with performing net-negative work upon ground contact (Gefen et al., 2001; Takahashi et al., 2017; Baines et al., 2018; Honert and Zelik, 2019; Papachatzis et al., 2020). Here, we examined the relationship between the heel’s mechanical work and its thermodynamic responses by experimentally increasing the collisional forces during walking. Our results showed that walking for 10 min with +30% added mass increased the magnitude of net-negative work that the heel dissipates by 326.94 ± 379.92 J (p = 0.005; Figure 2) and increased the heel’s temperature change by 0.72 ± 1.91
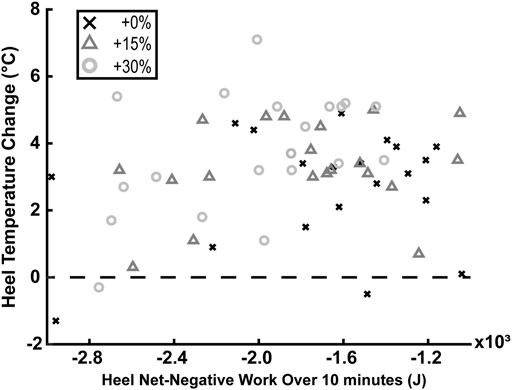
FIGURE 4. There was no statistically significant correlation between the heel’s net-negative mechanical work extrapolated to 10 min of barefoot walking and the heel’s temperature change after 10 min of barefoot walking (p = 0.277; N = 20). Values above the dashed line indicate that the temperature increased, while values below indicate the temperature decreased.
Work from several groups has established that skin temperature fluctuations trigger thermoregulatory responses to maintain the temperature within a normal physiological range (Taylor et al., 1984; Rowell, 1990; Pérgola et al., 1994; Charkoudian, 2003; Crandall and González-Alonso, 2010). In the absence of such responses, the skin temperature would have elevated excessively. For instance, Reddy et al. (2017) found an inverse association between the foot’s baseline temperature and foot temperature change after walking (Reddy et al., 2017). This finding corroborates the model of our exploratory analysis, which suggests that higher baseline heel temperature (i.e., before walking) was associated with a smaller increase in temperature (p < 0.001; Supplementary Table S1). In our study, a few participants had drastically elevated heel skin baseline temperature than others (range 8.4
While we did not observe a correlation between net-negative work and the temperature of the heel, we found that the heel’s temperature change increased when walking with added mass by 0.72 ± 1.91
Although we found an increase in the heel’s temperature during walking, this increase could be due to external heat stimuli, such as the treadmill surface. For instance, walking on a treadmill could have caused an irregular change in the heel’s temperature due to heating of the treadmill surface due to friction between the treadmill belt, the rollers, and the platform inside the treadmill’s deck. While our exploratory analysis suggested that the treadmill’s surface temperature had no significant effect on the heel’s temperature increase (p = 0.181; Supplementary Table S1), we performed an additional experiment to examine the validity of the heel’s temperature measurements acquired from the treadmill trials (see Supplementary Material). For this experiment, a subset of the participants walked barefoot for 10 min in a randomized order on a treadmill and on an overground track while carrying (via weight vest) two different levels of added mass: +0% (no added body mass) and +30% relative to their body mass. In addition, we controlled the walking speed for the treadmill and overground track trials at 1.25 m s−1. The supplementary experiment suggested that while the treadmill significantly affects the heel temperature measurements, the treadmill did not compromise our ability to detect temperature changes due to walking with added mass (Supplementary Figure S1). However, future experiments should consider the possible influence of the walking surface on the foot’s and heel’s temperature measurements. We also note that all of our participants walked barefoot. We chose barefoot walking because the mechanical characteristics of the shoe could influence our heel’s mechanical power and work calculations, which would have affected our ability to examine the mechanical-thermal relationships. Future studies should also consider the influence of footwear (e.g., shoes, orthoses, socks) on foot temperature responses during walking to increase the ecological validity.
In summary, our study revealed that the heel’s mechanical energy losses in healthy participants were not strongly correlated with the heel’s temperature changes. While this finding refuted our main hypothesis, it possibly highlights the agile control of the heel’s temperature by the vascular and sympathetic systems. Humans routinely perform tasks that require varying mechanical work demands during daily living, such as walking on slopes or stairs or continuously changing speeds. If all of the net-negative work done by the heel were dissipated as heat without additional thermoregulatory control mechanisms, performing basic daily activities may directly influence temperature increase, ultimately leading to skin complications, such as blisters and calluses. While our study involved healthy young participants, this study could be applicable for future studies that will examine impaired thermoregulation in individuals with neurovascular diseases (e.g., diabetes and peripheral artery disease) who are prone to foot complications (e.g., ulcers) (Armstrong et al., 2007; Maddah and Beigzadeh, 2020; van Netten et al., 2020; Chatzistergos et al., 2021). Our study provides a foundation to examine the link between mechanical energy, temperature responses, and the function of healthy and abnormal vascular and sympathetic systems in humans and/or animals. For example, future experiments may test alternative hypotheses related to energy dissipation mechanisms such as sound, shear forces, and skin blood flow, leading to novel insights for understanding how locomotion mechanics affect the thermoregulation of the foot. Thermoregulation is an essential homeostatic mechanism for mammals because most physiological processes include chemical reactions sensitive to temperature changes (Refinetti, 1999; Katschinski, 2004; Wooden and Walsberg, 2004). In the absence of such thermoregulation mechanisms, foot temperature could increase excessively during locomotor tasks, harming many terrestrial animals that have to cope with various terrains and environmental temperatures. Therefore, understanding the factors that influence healthy and dysfunctional thermoregulation will help us design new approaches for treating patients and identify new control mechanisms of terrestrial legged locomotion.
Conclusion
Our results indicated no strong correlation between the heel’s net-negative work and temperature in healthy participants. This finding is likely related to agile temperature control of our feet to prevent excessive temperature elevations that may predispose the tissue to damage. Such temperature control is likely due to the vascular and sympathetic systems, which may be compromised in various vascular diseases such as diabetes. Future studies are needed to uncover further the mechanisms of temperature responses, which may lead to novel insights for understanding the causes of foot complications such as diabetic ulcers and identify new control mechanisms for terrestrial legged locomotion.
Data Availability Statement
The datasets presented in this study can be found in online repositories. The names of the repository/repositories and accession number(s) can be found below: https://doi.org/10.6084/m9.figshare.19450421.v2.
Ethics Statement
The studies involving human participants were reviewed and approved by the Institutional Review Board at the University of Nebraska Medical Center. The patients/participants provided their written informed consent to participate in this study.
Author Contributions
NP and KT conceived and designed the research; NP performed experiments; NP analyzed data; KS assisted with the statistical analysis; NP and KT interpreted results of experiments; NP prepared figures; NP drafted manuscript; NP, DS, IP, KS, and KT edited and revised the manuscript; NP, DS, IP, KS, and KT approved the final version of the manuscript.
Funding
This project was supported by the University of Nebraska Collaboration Initiative, the Center for Research in Human Movement Variability of University of Nebraska at Omaha, and the National Institutes of Health (P20GM109090).
Conflict of Interest
The authors declare that the research was conducted in the absence of any commercial or financial relationships that could be construed as a potential conflict of interest.
Publisher’s Note
All claims expressed in this article are solely those of the authors and do not necessarily represent those of their affiliated organizations, or those of the publisher, the editors and the reviewers. Any product that may be evaluated in this article, or claim that may be made by its manufacturer, is not guaranteed or endorsed by the publisher.
Acknowledgments
The authors thank Jeffrey M. Patterson, Jose G. Anguiano-Hernandez, Ana L. Pineda-Gutierrez, Gregory J. Faber, and Andrew M. Kern for their experimental assistance.
Supplementary Material
The Supplementary Material for this article can be found online at: https://www.frontiersin.org/articles/10.3389/fbioe.2022.908725/full#supplementary-material
References
Aerts, P., Ker, R. F., De Clercq, D., Ilsley, D. W., and Alexander, R. M. (1995). The Mechanical Properties of the Human Heel Pad: A Paradox Resolved. J. Biomechanics 28, 1299–1308. doi:10.1016/0021-9290(95)00009-7
Armstrong, D. G., Holtz-Neiderer, K., Wendel, C., Mohler, M. J., Kimbriel, H. R., and Lavery, L. A. (2007). Skin Temperature Monitoring Reduces the Risk for Diabetic Foot Ulceration in High-Risk Patients. Am. J. Med. 120, 1042–1046. doi:10.1016/j.amjmed.2007.06.028
Baines, P. M., Schwab, A. L., and van Soest, A. J. (2018). Experimental Estimation of Energy Absorption during Heel Strike in Human Barefoot Walking. PLOS ONE 13, e0197428. doi:10.1371/journal.pone.0197428
Behforootan, S., Chatzistergos, P. E., Chockalingam, N., and Naemi, R. (2017). A Simulation of the Viscoelastic Behaviour of Heel Pad during Weight-Bearing Activities of Daily Living. Ann. Biomed. Eng. 45, 2750–2761. doi:10.1007/s10439-017-1918-1
Bennett, M. B., and Ker, R. F. (1990). The Mechanical Properties of the Human Subcalcaneal Fat Pad in Compression. J. Anat. 171, 131–138.
Bobet, J., and Norman, R. W. (1984). Effects of Load Placement on Back Muscle Activity in Load Carriage. Eur. J. Appl. Physiol. 53, 71–75. doi:10.1007/BF00964693
Bruening, D. A., Cooney, K. M., and Buczek, F. L. (2012). Analysis of a Kinetic Multi-Segment Foot Model Part II: Kinetics and Clinical Implications. Gait Posture 35, 535–540. doi:10.1016/j.gaitpost.2011.11.012
Bruening, D. A., and Takahashi, K. Z. (2018). Partitioning Ground Reaction Forces for Multi-Segment Foot Joint Kinetics. Gait posture 62, 111–116. doi:10.1016/j.gaitpost.2018.03.001
Burton, A. C. (1934). A New Technic for the Measurement of Average Skin Temperature over Surfaces of the Body and the Changes of Skin Temperature during Exercise. J. Nutr. 7, 481–496. doi:10.1093/jn/7.5.481
Chang, S. K., Arens, E., and Gonzalez, R. R. (1987). Determination of the Effect of Walking on the Forced Convective Heat Transfer Coefficient Using an Articulated Mannikin. Army Research Inst Of Environmental Medicine Natick Ma.
Charkoudian, N. (2003). Skin Blood Flow in Adult Human Thermoregulation: How it Works, when it Does Not, and Why. Mayo Clin. Proc. 78, 603–612. doi:10.4065/78.5.603
Chatzistergos, P., Naemi, R., and Chockalingam, N. (2021). “The Role of Tissue Biomechanics in Improving the Clinical Management of Diabetic Foot Ulcers,” in The Science, Etiology and Mechanobiology of Diabetes and its Complications (Elsevier), 123–141. doi:10.1016/b978-0-12-821070-3.00004-0
Chi, K.-J., and Schmitt, D. (2005). Mechanical Energy and Effective Foot Mass during Impact Loading of Walking and Running. J. Biomechanics 38, 1387–1395. doi:10.1016/j.jbiomech.2004.06.020
Clemente, C. J., Dick, T. J. M., Glen, C. L., and Panagiotopoulou, O. (2020). Biomechanical Insights into the Role of Foot Pads during Locomotion in Camelid Species. Sci. Rep. 10, 3856–3912. doi:10.1038/s41598-020-60795-9
Crandall, C. G., and González-Alonso, J. (2010). Cardiovascular Function in the Heat-Stressed Human. Acta Physiol. (Oxf) 199, 407–423. doi:10.1111/j.1748-1716.2010.02119.x
Datta, S. R., and Ramanathan, N. L. (1971). Ergonomic Comparison of Seven Modes of Carrying Loads on the Horizontal Plane. Ergonomics 14, 269–278. doi:10.1080/00140137108931244
Donelan, J. M., Kram, R., and Kuo, A. D. (2002a). Simultaneous Positive and Negative External Mechanical Work in Human Walking. J. Biomechanics 35, 117–124. doi:10.1016/S0021-9290(01)00169-5
Donelan, J. M., Kram, R., and Kuo, A. D. (2002b). Step-to-step Transition Costs. J. Exp. Biol. 205, 3717. doi:10.1242/jeb.205.23.3717
Ekimov, A., and Sabatier, J. M. (2006). Vibration and Sound Signatures of Human Footsteps in Buildings. J. Acoust. Soc. Am. 120, 762–768. doi:10.1121/1.2217371
Feldmann, A., Wili, P., Wili, P., Maquer, G., and Zysset, P. (2018). The Thermal Conductivity of Cortical and Cancellous Bone. eCM 35, 25–33. doi:10.22203/ecm.v035a03
Gefen, A., Megido-Ravid, M., and Itzchak, Y. (2001). In Vivo biomechanical Behavior of the Human Heel Pad during the Stance Phase of Gait. J. Biomechanics 34, 1661–1665. doi:10.1016/S0021-9290(01)00143-9
Giering, K., Lamprecht, I., and Minet, O. (1996, Specific Heat Capacities of Human and Animal Tissues.” in Specific Heat Capacities of Human and Animal Tissues. Editors. G. P. Delacretaz, R. W. Steiner, L. O. Svaasand, H. Albrecht, and T. H. Meier Spain: Barcelona, 188–197. doi:10.1117/12.229547
Gonzalez, A. E., Pineda Gutierrez, A., Kern, A. M., and Takahashi, K. Z. (2021). Association between Foot Thermal Responses and Shear Forces during Turning Gait in Young Adults. PeerJ 9, e10515. doi:10.7717/peerj.10515
Griffin, T. M., Roberts, T. J., and Kram, R. (2003). Metabolic Cost of Generating Muscular Force in Human Walking: Insights from Load-Carrying and Speed Experiments. J. Appl. physiology 95, 172–183. doi:10.1152/japplphysiol.00944.2002
Hall, M., Shurr, D. G., Zimmerman, M. B., and Saltzman, C. L. (2004). Plantar Foot Surface Temperatures with Use of Insoles. Iowa Orthop. J. 24, 72–75.
Holden, J. P., Selbie, W. S., and Stanhope, S. J. (2003). A Proposed Test to Support the Clinical Movement Analysis Laboratory Accreditation Process. Gait Posture 17, 205–213. doi:10.1016/S0966-6362(02)00088-7
Honert, E. C., and Zelik, K. E. (2019). Foot and Shoe Responsible for Majority of Soft Tissue Work in Early Stance of Walking. Hum. Mov. Sci. 64, 191–202. doi:10.1016/j.humov.2019.01.008
Huang, T. W., and Kuo, A. D. (2014). Mechanics and Energetics of Load Carriage during Human Walking. J. Exp. Biol. 217, 605–613. doi:10.1242/jeb.091587
Hung Au, I. P., Ng, L., Davey, P., So, M., Chan, B., Li, P., et al. (2021). Impact Sound across Rearfoot, Midfoot, and Forefoot Strike during Overground Running. J. Athl. Train. 56, 1362–1366. doi:10.4085/1062-6050-0708.20
Hutchinson, J. R., Delmer, C., Miller, C. E., Hildebrandt, T., Pitsillides, A. A., and Boyde, A. (2011). From Flat Foot to Fat Foot: Structure, Ontogeny, Function, and Evolution of Elephant "Sixth Toes". Science 334, 1699–1703. doi:10.1126/science.1211437
Jeong, H., Johnson, A. W., Feland, J. B., Petersen, S. R., Staten, J. M., and Bruening, D. A. (2021). Added Body Mass Alters Plantar Shear Stresses, Postural Control, and Gait Kinetics: Implications for Obesity. PLoS One 16, e0246605. doi:10.1371/journal.pone.0246605
Katschinski, D. M. (2004). On Heat and Cells and Proteins. Physiology 19, 11–15. doi:10.1152/nips.01403.2002
Kuo, A. D., and Donelan, J. M. (2010). Dynamic Principles of Gait and Their Clinical Implications. Phys. Ther. 90, 157–174. doi:10.2522/ptj.20090125
Kuo, A. D., Donelan, J. M., and Ruina, A. (2005). Energetic Consequences of Walking like an Inverted Pendulum: Step-to-step Transitions. Exerc. Sport Sci. Rev. 33, 88–97. doi:10.1097/00003677-200504000-00006
Kuo, A. D. (2002). Energetics of Actively Powered Locomotion Using the Simplest Walking Model. J. Biomechanical Eng. 124, 113–120. doi:10.1115/1.1427703
Kuo, A. D. (2007). The Six Determinants of Gait and the Inverted Pendulum Analogy: A Dynamic Walking Perspective. Hum. Mov. Sci. 26, 617–656. doi:10.1016/j.humov.2007.04.003
Larsson, M. (2014). Self-generated Sounds of Locomotion and Ventilation and the Evolution of Human Rhythmic Abilities. Anim. Cogn. 17, 1–14. doi:10.1007/s10071-013-0678-z
Ledoux, W. R., and Blevins, J. J. (2007). The Compressive Material Properties of the Plantar Soft Tissue. J. Biomechanics 40, 2975–2981. doi:10.1016/j.jbiomech.2007.02.009
Lee, D. V., Comanescu, T. N., Butcher, M. T., and Bertram, J. E. A. (2013). A Comparative Collision-Based Analysis of Human Gait. Proc. R. Soc. B 280, 20131779. doi:10.1098/rspb.2013.1779
Li, X., Logan, R. J., and Pastore, R. E. (1991). Perception of Acoustic Source Characteristics: Walking Sounds. J. Acoust. Soc. Am. 90, 3036–3049. doi:10.1121/1.401778
Liau, B.-Y., Wu, F.-L., Li, Y., Lung, C.-W., Mohamed, A. A., and Jan, Y.-K. (2021). Effect of Walking Speeds on Complexity of Plantar Pressure Patterns. Complexity 2021, 1336. doi:10.1155/2021/6571336
Liew, B., Morris, S., and Netto, K. (2016). The Effect of Backpack Carriage on the Biomechanics of Walking: a Systematic Review and Preliminary Meta-Analysis. J. Appl. biomechanics 32, 614–629. doi:10.1123/jab.2015-0339
Maddah, E., and Beigzadeh, B. (2020). Use of a Smartphone Thermometer to Monitor Thermal Conductivity Changes in Diabetic Foot Ulcers: a Pilot Study. J. Wound Care 29, 61–66. doi:10.12968/jowc.2020.29.1.61
Melnikov, V. R., Krzhizhanovskaya, V. V., Lees, M. H., and Sloot, P. M. A. (2020). The Impact of Pace of Life on Pedestrian Heat Stress: A Computational Modelling Approach. Environ. Res. 186, 109397. doi:10.1016/j.envres.2020.109397
Miao, H., Fu, J., Qian, Z., Ren, L., and Ren, L. (2017). How Does the Canine Paw Pad Attenuate Ground Impacts? A Multi-Layer Cushion System. Biol. Open 6, 1889–1896. doi:10.1242/bio.024828
Mooney, L. M., Rouse, E. J., and Herr, H. M. (2014). Autonomous Exoskeleton Reduces Metabolic Cost of Human Walking during Load Carriage. J. Neuroeng Rehabil. 11, 80–11. doi:10.1186/1743-0003-11-80
Pai, S., and Ledoux, W. R. (2011). The Quasi-Linear Viscoelastic Properties of Diabetic and Non-diabetic Plantar Soft Tissue. Ann. Biomed. Eng. 39, 1517–1527. doi:10.1007/s10439-011-0263-z
Pain, M. T. G., and Challis, J. H. (2001). The Role of the Heel Pad and Shank Soft Tissue during Impacts: a Further Resolution of a Paradox. J. Biomechanics 34, 327–333. doi:10.1016/S0021-9290(00)00199-8
Panagiotopoulou, O., Pataky, T. C., and Hutchinson, J. R. (2019). Foot Pressure Distribution in White Rhinoceroses (Ceratotherium simum) During Walking. PeerJ 7, e6881. doi:10.7717/peerj.6881
Papachatzis, N., Malcolm, P., Nelson, C. A., and Takahashi, K. Z. (2020). Walking with Added Mass Magnifies Salient Features of Human Foot Energetics. J. Exp. Biol. 223, jeb207472. doi:10.1242/jeb.207472
Pergola, P. E., Kellogg, D. L., Johnson, J. M., and Kosiba, W. A. (1994). Reflex Control of Active Cutaneous Vasodilation by Skin Temperature in Humans. Am. J. Physiology-Heart Circulatory Physiology 266, H1979–H1984. doi:10.1152/ajpheart.1994.266.5.H1979
Plagenhoef, S., Evans, F. G., and Abdelnour, T. (1983). Anatomical Data for Analyzing Human Motion. Res. Q. Exerc. sport 54, 169–178. doi:10.1080/02701367.1983.10605290
Reddy, P. N., Cooper, G., Weightman, A., Hodson-Tole, E., and Reeves, N. D. (2017). Walking Cadence Affects Rate of Plantar Foot Temperature Change but Not Final Temperature in Younger and Older Adults. Gait Posture 52, 272–279. doi:10.1016/j.gaitpost.2016.12.008
Refinetti, R. (1999). Amplitude of the Daily Rhythm of Body Temperature in Eleven Mammalian Species. J. Therm. Biol. 24, 477–481. doi:10.1016/s0306-4565(99)00077-7
Rice, H., Fallowfield, J., Allsopp, A., and Dixon, S. (2017). Influence of a 12.8-km Military Load Carriage Activity on Lower Limb Gait Mechanics and Muscle Activity. Ergonomics 60, 649–656. doi:10.1080/00140139.2016.1206624
Rowell, L. B. (1990). Hyperthermia: a Hyperadrenergic State. Hypertension 15, 505–507. doi:10.1161/01.HYP.15.5.505
Ruina, A., Bertram, J. E. A., and Srinivasan, M. (2005). A Collisional Model of the Energetic Cost of Support Work Qualitatively Explains Leg Sequencing in Walking and Galloping, Pseudo-elastic Leg Behavior in Running and the Walk-To-Run Transition. J. Theor. Biol. 237, 170–192. doi:10.1016/j.jtbi.2005.04.004
Sahli, S., Rebai, H., Ghroubi, S., Yahia, A., Guermazi, M., and Elleuch, M. H. (2013). The Effects of Backpack Load and Carrying Method on the Balance of Adolescent Idiopathic Scoliosis Subjects. Spine J. 13, 1835–1842. doi:10.1016/j.spinee.2013.06.023
Schmitt, D., and Larson, S. G. (1995). Heel Contact as a Function of Substrate Type and Speed in Primates. Am. J. Phys. Anthropol. 96, 39–50. doi:10.1002/ajpa.1330960105
Silder, A., Delp, S. L., and Besier, T. (2013). Men and Women Adopt Similar Walking Mechanics and Muscle Activation Patterns during Load Carriage. J. biomechanics 46, 2522–2528. doi:10.1016/j.jbiomech.2013.06.020
Simpson, K. M., Munro, B. J., and Steele, J. R. (2012). Does Load Position Affect Gait and Subjective Responses of Females during Load Carriage? Appl. Ergon. 43, 479–485. doi:10.1016/j.apergo.2011.07.005
Stucke, S., McFarland, D., Goss, L., Fonov, S., McMillan, G. R., Tucker, A., et al. (2012). Spatial Relationships between Shearing Stresses and Pressure on the Plantar Skin Surface during Gait. J. Biomechanics 45, 619–622. doi:10.1016/j.jbiomech.2011.11.004
Takahashi, K. Z., Worster, K., and Bruening, D. A. (2017). Energy Neutral: the Human Foot and Ankle Subsections Combine to Produce Near Zero Net Mechanical Work during Walking. Sci. Rep. 7, 15404–15409. doi:10.1038/s41598-017-15218-7
Taylor, W. F., Johnson, J. M., O'Leary, D., and Park, M. K. (1984). Effect of High Local Temperature on Reflex Cutaneous Vasodilation. J. Appl. Physiology 57, 191–196. doi:10.1152/jappl.1984.57.1.191
Usherwood, J. R. (2020). An Extension to the Collisional Model of the Energetic Cost of Support Qualitatively Explains Trotting and the Trot-Canter Transition. J. Exp. Zool. 333, 9–19. doi:10.1002/jez.2268
van Netten, J. J., Raspovic, A., Lavery, L. A., Monteiro-Soares, M., Rasmussen, A., Sacco, I. C. N., et al. (2020). Prevention of Foot Ulcers in the At-Risk Patient with Diabetes: a Systematic Review. Diabetes Metab. Res. Rev. 36, e3270. doi:10.1002/dmrr.3270
Visell, Y., Fontana, F., Giordano, B. L., Nordahl, R., Serafin, S., and Bresin, R. (2009). Sound Design and Perception in Walking Interactions. Int. J. Human-Computer Stud. 67, 947–959. doi:10.1016/j.ijhcs.2009.07.007
Wearing, S. C., Hooper, S. L., Dubois, P., Smeathers, J. E., and Dietze, A. (2014). Force-deformation Properties of the Human Heel Pad during Barefoot Walking. Med. Sci. sports Exerc. 46, 1588–1594. doi:10.1249/mss.0000000000000281
Wooden, K. M., and Walsberg, G. E. (2004). Body Temperature and Locomotor Capacity in a Heterothermic Rodent. J. Exp. Biol. 207, 41–46. doi:10.1242/jeb.00717
Yavuz, M., Brem, R. W., Davis, B. L., Patel, J., Osbourne, A., Matassini, M. R., et al. (2014). Temperature as a Predictive Tool for Plantar Triaxial Loading. J. biomechanics 47, 3767–3770. doi:10.1016/j.jbiomech.2014.09.028
Zeininger, A., Schmitt, D., and Wunderlich, R. E. (2020). Mechanics of Heel-Strike Plantigrady in African Apes. J. Hum. Evol. 145, 102840. doi:10.1016/j.jhevol.2020.102840
Keywords: foot, heel-strike, temperature, negative-work, locomotion, collision, energetics, biothermomechanics
Citation: Papachatzis N, Slivka DR, Pipinos II, Schmid KK and Takahashi KZ (2022) Does the Heel’s Dissipative Energetic Behavior Affect Its Thermodynamic Responses During Walking?. Front. Bioeng. Biotechnol. 10:908725. doi: 10.3389/fbioe.2022.908725
Received: 30 March 2022; Accepted: 06 June 2022;
Published: 27 June 2022.
Edited by:
Kristiaan D’Août, University of Liverpool, United KingdomCopyright © 2022 Papachatzis, Slivka, Pipinos, Schmid and Takahashi. This is an open-access article distributed under the terms of the Creative Commons Attribution License (CC BY). The use, distribution or reproduction in other forums is permitted, provided the original author(s) and the copyright owner(s) are credited and that the original publication in this journal is cited, in accordance with accepted academic practice. No use, distribution or reproduction is permitted which does not comply with these terms.
*Correspondence: Kota Z. Takahashi, a3Rha2FoYXNoaUB1bm9tYWhhLmVkdQ==