- 1School of Medicine, University of Nottingham, Nottingham, United Kingdom
- 2Pain Centre Versus Arthritis, University of Nottingham, Nottingham, United Kingdom
- 3School of Life Sciences, University of Nottingham, Nottingham, United Kingdom
- 4Department of Molecular Medicine, University of Pavia, Pavia, Italy
The increasing prevalence of joint disease, and in particular osteoarthritis (OA), calls for novel treatment strategies to prevent disease progression in addition to existing approaches focusing mainly on the relief of pain symptoms. The inherent properties of mesenchymal stem cells (MSCs) make them an attractive candidate for novel tissue repair strategies, as these progenitors have the potential to differentiate into chondrocytes needed to replace degraded cartilage and can exert a modulating effect on the inflammatory environment of the diseased joint. However, the inflammatory environment of the joint may affect the ability of these cells to functionally integrate into the host tissue and exert beneficial effects, as hinted by a lack of success seen in clinical trials. Identification of factors and cell signalling pathways that influence MSC function is therefore critical for ensuring their success in the clinic, and here the effects of inflammatory mediators on bone marrow-derived MSCs were evaluated. Human MSCs were cultured in the presence of inflammatory mediators typically associated with OA pathology (IL-1β, IL-8, IL-10). While exposure to these factors did not produce marked effects on MSC proliferation, changes were observed when the mediators were added under differentiating conditions. Results collected over 21 days showed that exposure to IL-1β significantly affected the differentiation response of these cells exposed to chondrogenic and osteogenic conditions, with gene expression analysis indicating changes in MAPK, Wnt and TLR signalling pathways, alongside an increased expression of pro-inflammatory cytokines and cartilage degrading enzymes. These results highlight the value of MSCs as a preclinical model to study OA and provide a basis to define the impact of factors driving OA pathology on the therapeutic potential of MSCs for novel OA treatments.
Introduction
Although osteoarthritis is the most prevalent form of joint disease, the molecular mechanisms underpinning OA pathogenesis are still elusive (Chen et al., 2017; He et al., 2020). Consequently, strategies to prevent disease progression and preserve cartilage are lacking, with treatment mainly relying on the pain relief provided by pharmacological or surgical interventions (Charlesworth et al., 2019; Grässel and Muschter, 2020; National Institute for Health and Care Excellence, 2020). Given that endogenous cartilage regeneration is limited (Heng et al., 2004; Kock et al., 2012), novel approaches aiming to restore joint tissue through the use of mesenchymal stem cells (MSCs) are emerging. These progenitors can differentiate into osteoblasts and chondrocytes, and therefore have the potential to promote the regeneration of damaged cartilage (Hwang et al., 2021; Zhu et al., 2021; Xiang et al., 2022). Pre-clinical studies have revealed that while endogenous populations of synovial MSCs can contribute to cartilage repair following chondrogenesis (Harrell et al., 2019), the exogenous delivery of MSCs may result in a reduction in cartilage degradation and pain (Chapman et al., 2017; Harrell et al., 2019; Wang et al., 2022). OA also has a strong inflammatory component (Sokolove and Lepus, 2013), with synovial infiltration of immune cells such as macrophages, T cells and B cells (De Lange-Brokaar et al., 2012; Zhao et al., 2020). MSCs have been shown to act on cells of both the innate and adaptive immune systems and promote an anti-inflammatory environment, through cell-cell contact as well as paracrine mechanisms (Gao et al., 2016; Wang et al., 2018; Song et al., 2020; Zhao et al., 2020). Therefore, MSCs may present an important therapeutic potential as they could promote a regenerative microenvironment primed for cartilage repair; however, retention of these cells in an osteoarthritic joint in vivo remains uncertain (Mancuso et al., 2019).
As such, MSCs have been the focus of many clinical trials assessing their efficacy and safety in humans. While these trials appear to support the safety of MSC-based therapies and indicate some modulation of OA through a reduction in pain, it is not yet possible to ascertain the efficacy of MSC treatment in OA due to a current lack of reproducibility and long-term follow-up, as well as high-risk bias (Iijima et al., 2018). Recent meta-analyses have exposed a high variability between trial methods, including MSC source, preparation, cell density, and implantation techniques (Iijima et al., 2018; Wang et al., 2020; Zhu et al., 2021). Current studies have also been unable to demonstrate a positive impact of MSC treatment on cartilage repair (Gupta et al., 2016; Matas et al., 2019; Wang et al., 2020), which may be partly linked to the OA environment in which these cells are introduced.
Activation of the immune response in the OA joint results in the production of destructive inflammatory mediators and proteases (Sokolove and Lepus, 2013). This is, in part, regulated by the expression of toll-like receptors (Barreto et al., 2020), which are also important modulators of MSC differentiation and immunoregulation (DelaRosa et al., 2012; Sangiorgi and Panepucci, 2016; Shirjang et al., 2017). Upregulated inflammatory mediators include the pro-inflammatory cytokine IL-1β, which is markedly increased in the chondrocytes and synovium of OA patients (Kapoor et al., 2011; Wojdasiewicz et al., 2014). IL-1β levels have been shown to have a positive correlation with OA changes (Daheshia and Yao, 2008; Denoble et al., 2011). Similarly, the pro-inflammatory chemokine IL-8 (or CXCL8) is high in the synovium of OA patients (Kaneko et al., 2000; Pierzchala et al., 2011; Molnar et al., 2021) and its level is linked to clinical severity (García-Manrique et al., 2021). IL-10 is also present in the osteoarthritic joint, but is considered an anti-inflammatory cytokine (Iannone et al., 2001) known to inhibit inflammatory arthritis progression in mice (Choi et al., 2008). This cytokine was also shown to stimulate the production of proteoglycans in articular cartilage (Van Roon et al., 1996), suggesting that it could exert an overall mitigating effect in OA by improving both cartilage and immunological parameters. Since the OA joint presents an altered level of inflammatory mediators, the present study aimed to investigate the effects of leading interleukins IL-1β, IL-8 and IL-10 on MSCs in vitro, focusing on their impact on cell differentiation and the possible underlying mechanisms of action.
Methods
Reagents were purchased from Thermo Fisher Scientific (Hemel Hempstead, United Kingdom) unless otherwise stated.
Cell Culture
Immortalised human bone marrow MSCs (Macri-Pellizzeri et al., 2018) and human primary bone marrow-derived MSCs (BMSCs, Lonza, United Kingdom) were cultured in standard medium (SM) consisting of low glucose (1 g/L) Dulbecco’s Modified Eagle Medium (DMEM) supplemented with 10% (v/v) fetal bovine serum, 1% (v/v) non-essential amino acids, 1% (v/v) L-glutamine and 1% (v/v) penicillin-streptomycin. Cells were passaged using 0.25% (v/v) trypsin/0.02% (v/v) EDTA. Recombinant human IL-1β (Abnova), recombinant human IL-8 (R&D systems) or recombinant human IL-10 (Source Bioscience) were reconstituted according to manufacturer’s guidelines and added to the medium at dose ranges based on previous studies (De Jager et al., 2003; Meijer et al., 2003; Rutgers et al., 2010) as indicated in the different experiments. Cells were kept at 37.5°C, 5% CO2, and the medium was changed every 2–3 days.
Cell Proliferation and Viability
Cells were seeded into well plates and subsequently counted and passaged every 48 h. Cell proliferation was evaluated using the Countess II FL automated system. Cell metabolic activity was analysed using the PrestoBlue Cell Viability reagent, according to the manufacturer’s instructions.
Osteogenic Differentiation
Cells were seeded at a density of 4,000 cells/cm2 onto tissue culture plastic. After 24 h, SM was removed and osteogenic medium (OM), with or without recombinant IL-1β, IL-8 or IL-10, was added to induce osteoblast differentiation. Osteogenic medium consisted of SM supplemented with 100 nM dexamethasone (Sigma-Aldrich), 0.05 mM l-ascorbic acid-2-phosphate (Sigma-Aldrich) and 10 mM β-glycerophosphate (Sigma-Aldrich). The medium was changed every 2–3 days for 21 days.
Alkaline Phosphatase Assay and Alizarin Red S Staining
Early osteogenic differentiation was assessed on days 10 and 14 by alkaline phosphatase activity and measured using p-nitrophenyl phosphate tablets (Sigma-Aldrich), according to manufacturer’s instructions. Mineral deposition was assessed on days 10, 14 and 21 as previously described (Macri-Pellizzeri et al., 2018), using 1% Alizarin Red solution and imaged using an Eclipse TS100 inverted microscope (Nikon). Alizarin Red signal was quantified using an Infinite 200 microplate reader at 405 nm.
Chondrogenic Differentiation
Cells were pelleted in 1.5 ml Eppendorf tubes at 1 × 106 cells/tube. After 24 h, SM was removed and chondrogenic medium (CM), with or without recombinant IL-1β, IL-8 or IL-10, was added to induce chondrocyte differentiation. Chondrogenic medium consisted of high glucose (4.5 g/L) DMEM supplemented with 1% (v/v) non-essential amino acids, 1% (v/v) L-glutamine, 1% (v/v) penicillin-streptomycin, 110 mg/L (w/v) sodium pyruvate, 0.1 μM dexamethasone, 50 μM l-ascorbic acid-2-phosphate, 40 μg/ml (w/v) L-proline, 1% (v/v) ITS supplement and 10 ng/ml TGF-β1 (Prosser et al., 2019). The medium was changed three times a week for 21 days.
DMMB Assay and PicoGreen Assay
Chondrogenic differentiation was assessed through the measurement of sulfated glycosaminoglycans (sGAGs) extracted using a papain extraction reagent made of 0.2 M sodium phosphate buffer with 8 mg/ml (w/v) sodium acetate (Sigma-Aldrich), 4 mg/ml (w/v) EDTA (Acros Organics), 0.8 mg/ml (w/v) cysteine hydrochloride (Sigma-Aldrich) and 100 μg/ml papain (Sigma-Aldrich), at pH 6.4. Pellets were incubated in 1 ml papain extraction reagent at 65°C for 18 h, then centrifuged at 10,000 × g for 10 min. The supernatant was decanted for use with the Blyscan Sulfated Glycosaminoglycan Assay kit (Biocolor Life Science Assays, United Kingdom) according to the manufacturer’s instructions. sGAG concentration was normalised to the nucleic acid concentration, measured with the QuantiT PicoGreen® dsDNA kit according to the manufacturer’s instructions.
TaqMan Low Density Arrays
MSCs were washed twice with PBS and suspended in Tri-Reagent for subsequent RNA extraction using the RNeasy Micro Kit (Qiagen) according to the manufacturer’s instructions. RNA concentration and purity were assessed using a Nanodrop ND-1000 spectrophotometer before performing cDNA synthesis using a High Capacity RNA-to-cDNA Kit, according to manufacturer’s instructions. Custom TaqMan Low Density Array (TLDA) 384-well microfluidic cards with a 96 gene × 4 sample layout from Thermo Fisher Scientific were used for the analysis of MSC gene expression. Where possible, probes spanning an exon boundary and outside of the 5′ untranslated region were chosen. The cards were loaded with 200 ng of cDNA diluted in 50 μl RNase free water and 50 μl of TaqMan Universal Master Mix II with uracil-N-glycosylase in each reservoir. cDNA was dispersed across the wells by centrifugation at 200 × g for two 1 min spins, after which the plate was sealed and run in a 7900HT Fast Real-Time PCR System using SDS RQ Manager Software (Applied Biosystems). Gene expression was calculated as fold change from untreated cells (Pfaffl, 2001), normalised to the geometric mean of the two most stable reference genes (Vandesompele et al., 2002).
Statistical Analysis
All data are presented as mean ± SEM. Statistical analysis was performed using GraphPad Prism Software (https://www.graphpad.com), using a two-tailed Student’s t-test with significance as *p < 0.05; **p < 0.01; ***p < 0.001; ****p < 0.0001.
Results
MSC Response to Interleukin Treatment in vitro
To evaluate the effect of IL-1β, IL-8 and IL-10 on MSCs, cells were cultured with increasing doses of each interleukin, and their proliferative capacity and metabolic activity were assessed over 10 days. Both sets of measurements indicated that the proliferative capacity (Figure 1A) and metabolic activity (Figure 1B) of cells exposed to increasing doses of any of the mediators remained overall unchanged compared to cells cultured in SM.
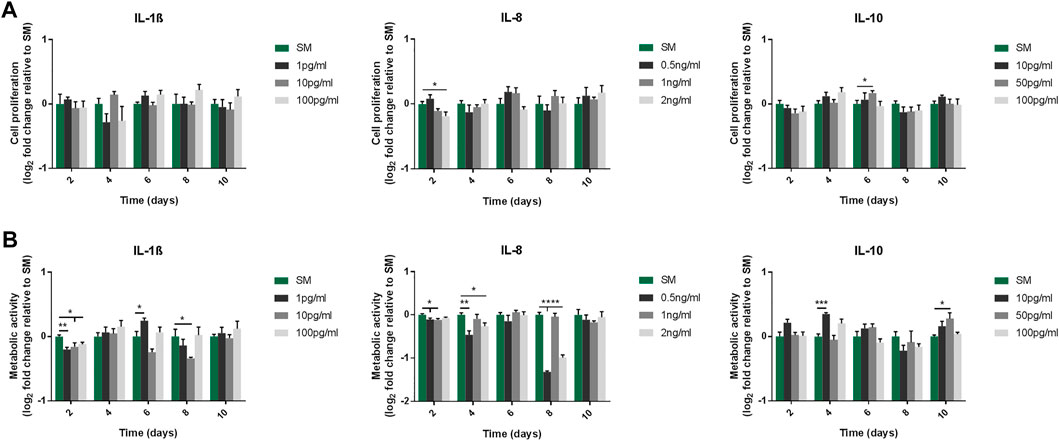
FIGURE 1. Proliferative capacity (A) and metabolic activity (B) of MSC cultures following exposure to IL-1β, IL-8 and IL-10 for 10 days. (A) Proliferative capacity measured by cell counts. Doses used were 1/10/100 pg/ml for IL-1β, 0.5/1/2 ng/ml for IL-8, and 10/50/100 pg/ml for IL-10. Data shown as log2 fold change compared to SM control condition. Error bars represent SEM. n = 4, *p < 0.05, **p < 0.01, ***p < 0.001, ****p < 0.0001.
Effects of Interleukin Supplementation on Mesenchymal Stem Cell Chondrogenic Differentiation
The effect of the interleukins on MSC chondrogenic differentiation was analysed by treating pellet cultures maintained under pro-chondrogenic conditions, and measuring the level of sulfated glycosaminoglycans (sGAGs) after 21 days of differentiation. The concentration of sGAGs was normalised to DNA content to account for possible variation in cell numbers in the different samples analysed. Whilst the addition of IL-8 (2 ng/ml) and IL-10 (100 pg/ml) did not significantly alter the chondrogenic response compared to CM control, exposure to IL-1β (100 pg/ml) significantly decreased the chondrogenic response, as indicated by the decrease in sGAGs measured at day 21 (0.79 ± 0.07 fold change compared to CM) (Figure 2).
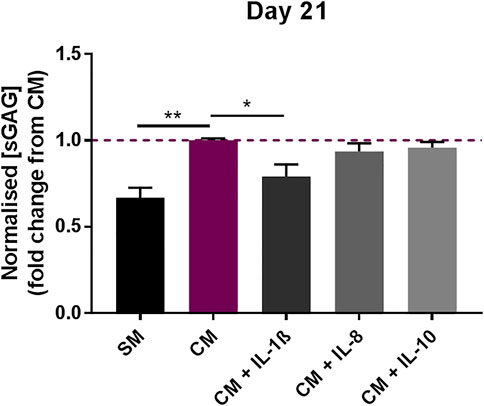
FIGURE 2. Effects of IL-1β (100 pg/ml), IL-8 (2 ng/ml) and IL-10 (100 pg/ml) supplementation on MSC chondrogenic response. sGAG concentration in MSC pellet cultures in the presence or absence of IL-1β, IL-8 and IL-10 measured after 21 days, normalised to DNA content. Data shown as fold change compared to CM control condition. Error bars represent SEM. n = 4, *p < 0.05, **p < 0.01.
Effects of Interleukin Supplementation on Mesenchymal Stem Cell Osteogenic Differentiation
To analyse the impact of the interleukins on the MSC osteogenic response, the levels of alkaline phosphatase activity (ALP), used as early marker, and mineral deposition, used as late marker, were measured in osteogenic cultures supplemented with each molecule. The addition of IL-1β (100 pg/ml) significantly increased ALP activity in MSCs compared to OM alone at both time-points analysed (1.13 ± 0.02 fold change compared to OM at day 7 and 1.14 ± 0.02 fold change compared to OM at day 10) (Figure 3A). This was mirrored by an increase in mineralisation measured at day 14 (1.55 ± 0.14 fold change compared to OM) and then lost by day 21 (Figures 3B,C). By contrast, supplementation with IL-8 (2 ng/ml) did not significantly alter the levels of ALP activity or mineral deposition in MSCs compared to OM controls. IL-10 (100 pg/ml) supplementation did show a modest increase in ALP activity by day 10 only (1.07 ± 0.01 fold change compared to OM), while mineral deposition showed a transient decrease at day 14 (0.52 ± 0.12 fold change compared to OM), which recovered by day 21.
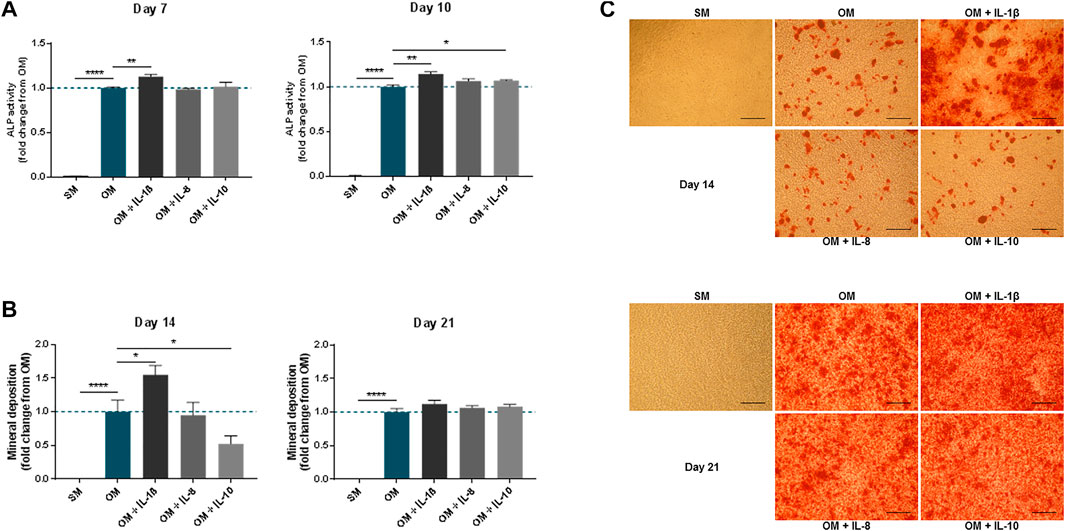
FIGURE 3. Effects of IL-1β, IL-8 or IL-10 supplementation on MSC osteogenic medium. ALP activity (A) and mineral deposition (B) measured in MSC cultures in the presence or absence of IL-1β (100 pg/ml), IL-8 (2 ng/ml) or IL-10 (100 pg/ml) measured over up to 3 weeks. (C) Representative images of Alizarin Red staining at days 14 and 21. Scale bar = 150 μm. Data shown as fold change compared to the OM control condition. Error bars represent SEM. n ≥ 4, *p < 0.05, **p < 0.01, ***p < 0.001, ****p < 0.0001.
Effects of IL-1β Supplementation on Primary Cultures
To further verify the effect of the interleukins on MSCs, inflammatory mediators (IL-1β, IL-8, IL-10) were applied to primary human BMSCs treated in osteogenic conditions for 11 days. As observed in the immortalised cell line, IL-1β supplementation (100 pg/ml) in primary cells led to a significant increase in mineral deposition (2.91 ± 0.15 fold change compared to OM), while IL-8 (2 ng/ml) and IL-10 (100 pg/ml) did not produce any notable changes (Figure 4).
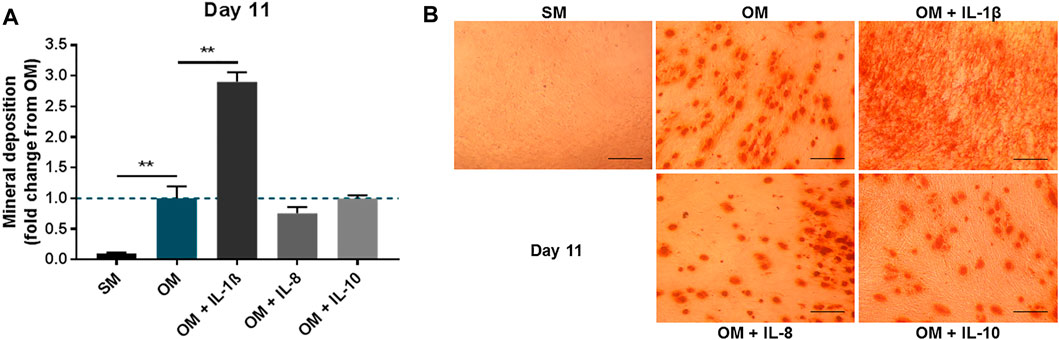
FIGURE 4. Effects of IL-1β, IL-8 or IL-10 supplementation on primary MSC cultures treated in osteogenic medium. (A) Mineral deposition in cultures treated in the presence or absence of IL-1β (100 pg/ml), IL-8 (2 ng/ml) or IL-10 (100 pg/ml) measured after 11 days. (B) Representative images of Alizarin Red staining at day 11. Scale bar = 150 μm. Data shown as fold change compared to the OM control condition. Error bars represent SEM. n = 3, **p < 0.01.
Dose-Response of IL-1β on Osteogenic Differentiation
The effect of IL-1β on MSC osteogenic response was further refined using doses ranging from 1 pg/ml to 1,000 pg/ml (Figure 5). An increase in ALP activity in response to IL-1β was observed at day 7 and day 10 for all doses, although beyond 10 pg/ml the response was largely similar (Day 7: 1 pg/ml 1.14 ± 0.04, 10 pg/ml 1.29 ± 0.02, 100 pg/ml 1.28 ± 0.06, 1,000 pg/ml 1.35 ± 0.03 fold change compared to OM; Day 10: 1 pg/ml 1.16 ± 0.03, 10 pg/ml 1.23 ± 0.02, 100 pg/ml 1.24 ± 0.02, 1,000 pg/ml 1.19 ± 0.04 fold change compared to OM). Analysis of mineral deposition at day 14 showed a positive response (Figure 5C), with a significant increase in OM conditions supplemented with IL-1β at 100 pg/ml (2.12 ± 0.06 fold change compared to OM) (Figure 5B). Interestingly, while mineral deposition did not show significant differences with any of the IL-1β doses at day 10 (Figure 5B), brightfield microscopy observation revealed that mineral deposition had begun in discrete areas of cultures exposed to IL-1β (Figure 5C).
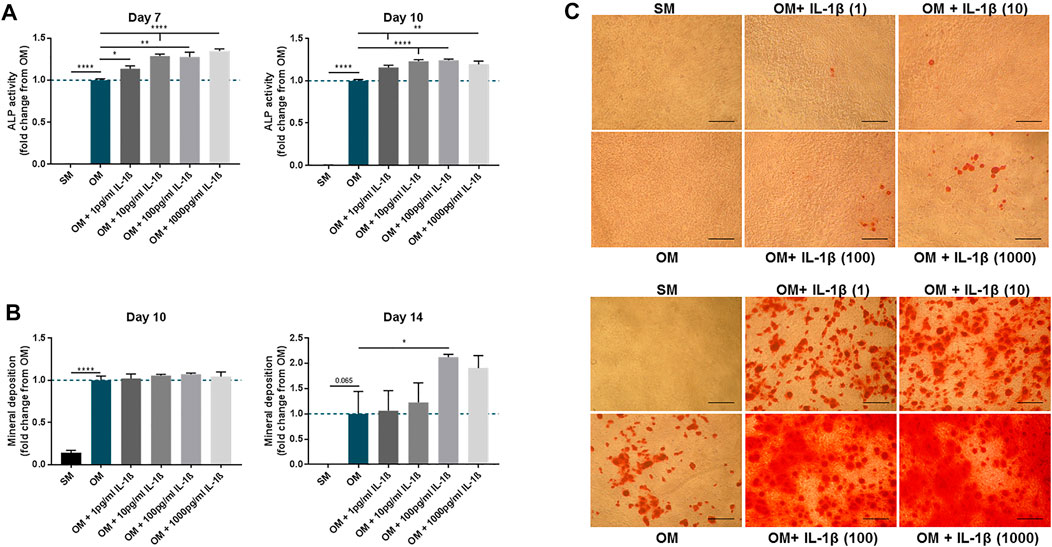
FIGURE 5. Dose-response of IL-1β (1–1,000 pg/ml) on MSC cultures undergoing osteogenic treatment. (A) ALP activity measured at day 7 and 10. (B) Mineral deposition measured at day 10 and 14. (C) Representative images of Alizarin Red staining at day 10 (upper panel) and 14 (lower panel). Scale bar = 150 μm. Data shown as fold change compared to the OM control condition. Error bars represent SEM. n = 4, *p < 0.05, **p < 0.01, ***p < 0.001, ****p < 0.0001.
Effects of IL-1β Supplementation on MSC Gene Expression
To further characterise the response of MSCs to IL-1β, gene expression was assessed after 10 days of treatment under SM conditions in vitro, focusing on genes linked to inflammation and differentiation (Figure 6). Expression of pro-inflammatory mediators was increased with IL-1β treatment (IL1B 90.53 ± 29.07, CXCL8 518.4 ± 158.3, CCL2 21.97 ± 7.85, IL6 21.47 ± 1.62 fold change compared to SM), alongside expression of immunomodulatory genes (PTGS2 19.74 ± 2.59, IDO1 36.24 ± 7.28, HGF 7.8 ± 3.44 fold change compared to SM). Whilst IL-1β supplementation upregulated the expression of ALPL, RUNX2, BGLAP and SPP1, which are associated with osteogenic differentiation (ALPL 2.02 ± 0.47, RUNX2 1.95 ± 0.2, BGLAP 3.75 ± 0.94, SPP1 19.69 ± 7.37 fold change compared to SM), expression of the chondrogenic marker ACAN was decreased (0.1 ± 0.01 fold change compared to SM) and that of the cartilage degrading enzyme MMP13 was increased (6.45 ± 1.62 fold change compared to SM). Furthermore, expression of toll-like receptor genes, including TLR1, TLR3 and TLR4, which are important for the secretion of inflammatory mediators (DelaRosa et al., 2012; Sangiorgi and Panepucci, 2016; Shirjang et al., 2017; Barreto et al., 2020), were all increased (TLR1 40.72 ± 31.05, TLR3 12.08 ± 4.38, TLR4 3.38 ± 0.96 fold change compared to SM).
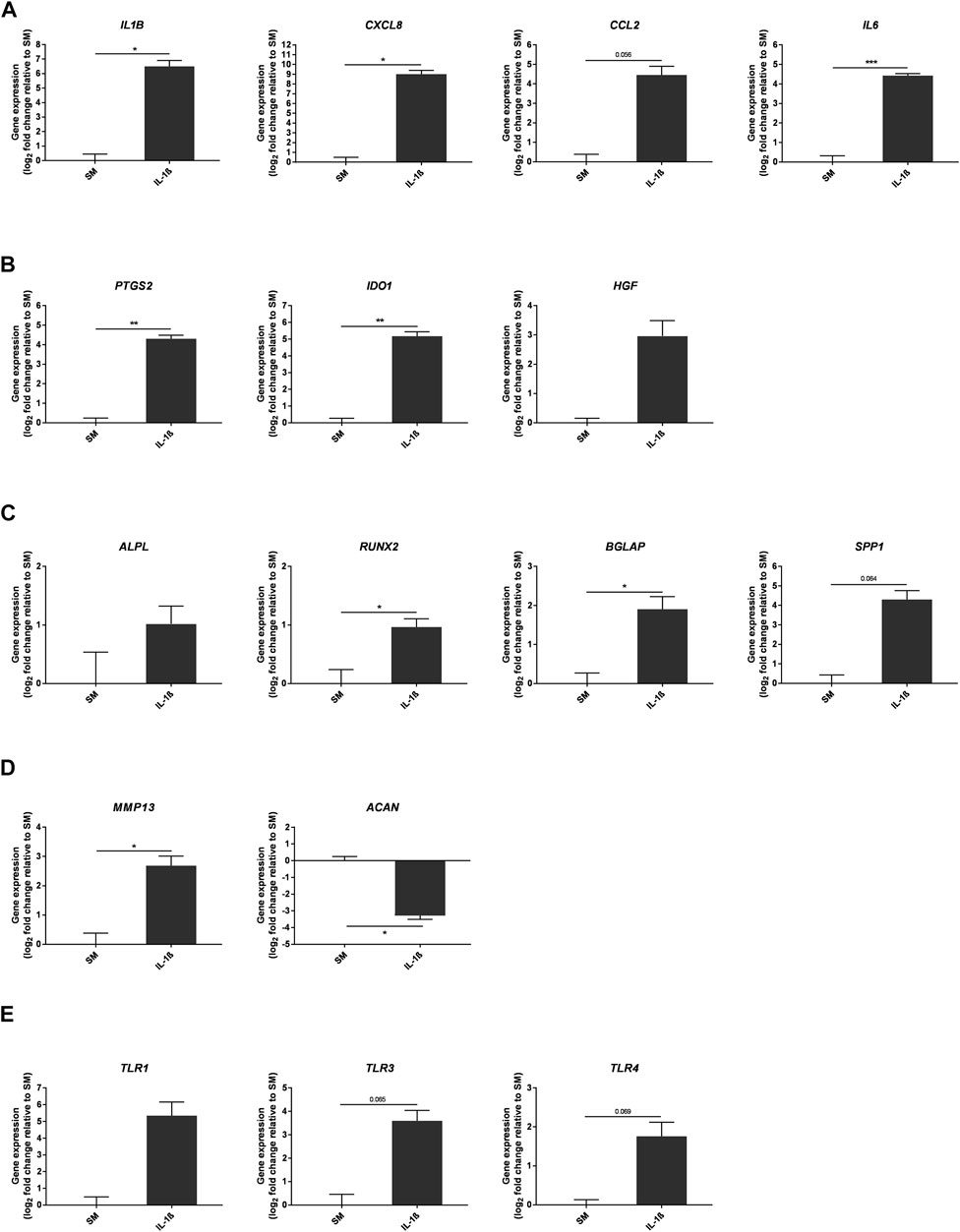
FIGURE 6. Gene expression changes in response to IL-1β (100 pg/ml) added to MSC cultures in SM over 10 days. Gene expression of pro-inflammatory (A) and immunomodulatory (B) mediators, osteogenic (C) and chondrogenic (D) factors, and toll-like receptors (E). Data shown as log2 fold change compared to SM control condition. Error bars represent SEM. n = 3, *p < 0.05, **p < 0.01, ***p < 0.001.
Effects of IL-1β Supplementation on Mesenchymal Stem Cell Gene Expression During Chondrogenic Differentiation
The effect of IL-1β supplementation (100 pg/ml) on gene expression was also measured during chondrogenic differentiation of MSCs in CM medium (Figure 7). As expected, the expression of ACAN was increased at day 10 of the chondrogenic differentiation protocol (22.6 ± 15.22 fold change compared to SM); however the presence of IL-1β negatively impacted ACAN expression (9.12 ± 3.4 fold change compared to SM). In addition, expression of catabolic genes including cartilage degrading enzymes such as MMP1, MMP3, and the growth factor FGF2 were increased upon supplementation with IL-1β (MMP1 2.09 ± 0.95, MMP3 5.98 ± 3.96, FGF2 1.22 ± 0.25 fold change compared to SM). In contrast, genes encoding anabolic growth factors such as TGFB3 and IGF1 showed a marked decrease in expression upon IL-1β supplementation (TGFB3 0.25 ± 0.05, IGF1 0.1 ± 0.02 fold change compared to SM). Of note, the expression of genes encoding for chondrocyte hypertrophy markers such as RUNX2, ALPL and MMP13 showed an upward trend following IL-1β supplementation (RUNX2 1.92 ± 0.45, ALPL 144.4 ± 115.9, MMP13 9.18 ± 1.84 fold change compared to SM), alongside increased expression of MAPK1, MAPK9 and TLR2, which are associated with increased expression of matrix metalloproteinases (MMPs) and pro-inflammatory mediators (MAPK1 1.2 ± 0.06, MAPK9 2.17 ± 0.3, TLR2 28.18 ± 6.44 fold change compared to SM) (Wang et al., 2011; DelaRosa et al., 2012; Mariani et al., 2014; Sangiorgi and Panepucci, 2016; Barreto et al., 2020).
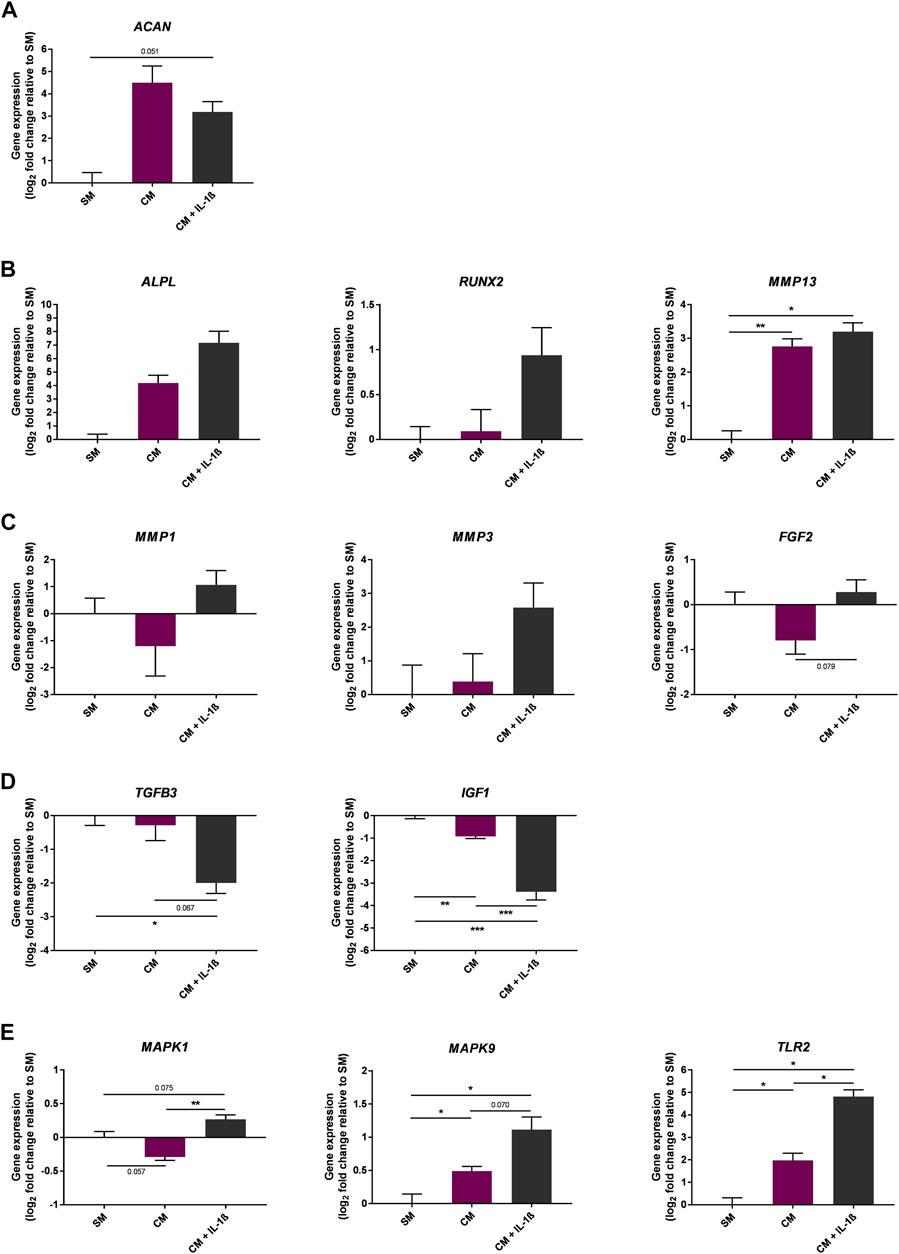
FIGURE 7. Gene expression changes in response to IL-1β (100 pg/ml) added to MSC cultures in CM over 10 days. Gene expression of chondrogenic (A) and chondrogenic hypertrophy (B) markers, catabolic (C) and anabolic (D) markers, and MAPK enzymes and toll-like receptors (E). Data shown as log2 fold change compared to SM control condition. Error bars represent SEM. n = 3, *p < 0.05, **p < 0.01, ***p < 0.001.
Effects of IL-1β Supplementation on Mesenchymal Stem Cell Gene Expression During Osteogenic Differentiation
To further determine the effect of IL-1β on MSC osteogenesis in vitro, gene expression was assessed in cells at day 10 of osteogenic differentiation (Figure 8). The early osteogenic differentiation marker ALPL increased in response to osteogenic conditions (29.96 ± 13.85 fold change compared to SM), including with supplementation of IL-1β (100 pg/ml) (30.56 ± 9.72 fold change compared to SM). In contrast, expression of BGLAP, which is important for the late mineral deposition stage, was increased following supplementation of IL-1β (2.31 ± 2.14 fold change compared to SM), as was the expression of genes encoding inflammatory mediators (CXCL8 236.7 ± 229.7, IL6 2.37 ± 0.62, IDO1 11.75 ± 7.45 fold change compared to SM). Expression of genes involved in the regulation of bone remodelling and the production of inflammatory mediators (MAPK3, MAPK14, TLR2, TLR3, WNT5A) (Jaiswal et al., 2000; Sonomoto et al., 2012; Yamashita et al., 2012; Artigas et al., 2014; Qi et al., 2014; Muthukuru and Darveau, 2015; Kobayashi et al., 2016; Sangiorgi and Panepucci, 2016; Shirjang et al., 2017; Amarasekara et al., 2021) were also altered in the presence of IL-1β (MAPK3 1.04 ± 0.1, MAPK14 1.2 ± 0.02, TLR2 66.38 ± 61.57, TLR3 4 ± 1.9, WNT5A 3.76 ± 1.09 fold change compared to SM).
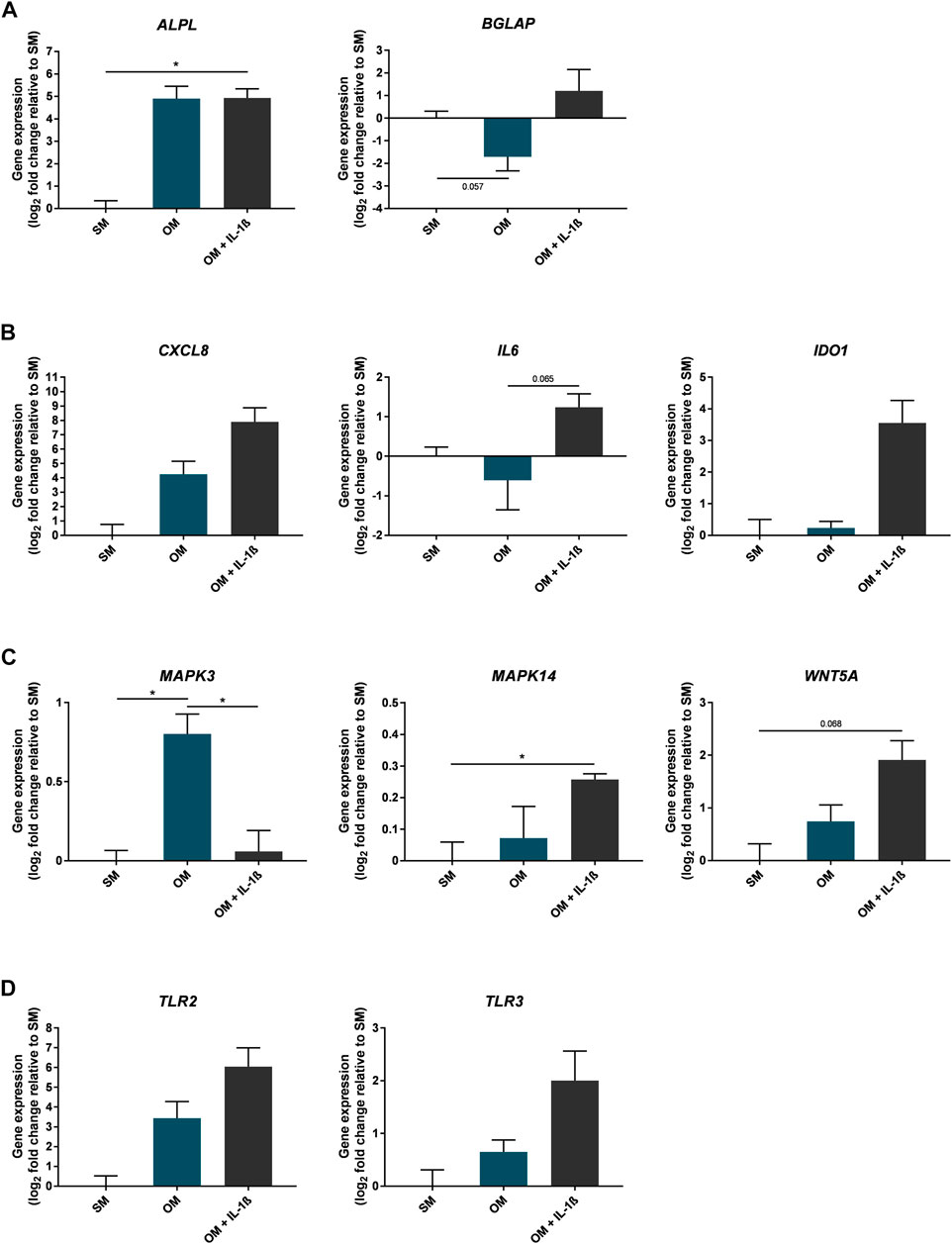
FIGURE 8. Gene expression changes in response to IL-1β (100 pg/ml) added to MSC cultures in osteogenic medium over 10 days. Gene expression of osteogenic (A), inflammatory mediators (B), MAPK enzymes and Wnt signalling factors (C), and toll-like receptors (D). Data shown as log2 fold change compared to SM control condition. Error bars represent SEM. n = 3, *p < 0.05.
Discussion
The intrinsic regenerative properties of MSCs may allow these cells to repair cartilage, as well as dampen inflammation in the joint due to their recognised local immunosuppressant effects (Gao et al., 2016; Wang et al., 2018; Harrell et al., 2019; Song et al., 2020; Zhao et al., 2020; Hwang et al., 2021; Zhu et al., 2021; Xiang et al., 2022). However, MSC transplantation attempts in OA clinical trials have thus far produced limited evidence of cartilage regeneration, despite reported improvements in pain and physical function (Iijima et al., 2018; Wang et al., 2020; Zhu et al., 2021). This might be due to the hostile local microenvironment encountered in the OA joint, where the presence of inflammatory mediators such as interleukins can contribute to disease progression (Kapoor et al., 2011; Wojdasiewicz et al., 2014; Molnar et al., 2021). Although the effect of inflammatory mediators on transplanted cells remains unclear, their role in altering the phenotype and differentiation potential of endogenous MSC populations in the OA joint has been suggested (Murphy et al., 2002; Coleman et al., 2010; Jayasuriya et al., 2018), thereby limiting the therapeutic potential of exogenous MSCs to mitigate disease progression. On that basis, analysing how specific mediators present in the affected joint impact on MSC function is required to inform novel cell-based therapies for OA. The present study investigated how exposure to IL-1β (Westacott et al., 1990), IL-8 (Kaneko et al., 2000) and IL-10 (Helmark et al., 2010), which are upregulated in the OA joint, affected MSC phenotype and potential by measuring parameters of cell proliferation, differentiation and gene expression. Results demonstrated that while standard concentrations of IL-8 and IL-10 did not affect the proliferation or differentiation capacity of MSCs, the addition of IL-1β significantly affected the differentiation of MSCs in vitro.
IL-1β Effect in Chondrogenic Conditions
Exposure to IL-1β was observed to impede the chondrogenic response of MSCs in vitro, with a notable reduction in sGAG production. This was consistent with decreased ACAN expression compared to untreated controls, supporting the association between reduced GAG synthesis and lower proteoglycan gene expression (Pfander et al., 2004). In parallel to this negative effect on chondrogenesis, IL-1β is also thought to exert some negative effects in the joint through degradation of extracellular matrix (ECM) components, a key feature of OA cartilage breakdown (Martel-Pelletier, 2004; Goldring and Marcu, 2009). This is supported by the increased expression of the growth factor FGF2, reported to have catabolic effects in human articular chondrocytes (Li et al., 2012), as well as cartilage degrading enzymes including MMP1, MMP3 and MMP13, upon IL-1β treatment. In the OA joint, secretion of these proteases indicates the progression of chondrocytes towards a state of hypertrophy, which subsequently stimulates endochondral ossification processes linked to the development of painful osteophytes (Van der Kraan and Van den Berg, 2012). Although in vitro systems of chondrogenic differentiation are intrinsically limited by the spontaneous progression of MSCs towards the hypertrophic stage (Zhang et al., 2010; Chen et al., 2015), data presented here show that exposure to IL-1β-rich conditions upregulated chondrocyte hypertrophy markers such as RUNX2, ALPL and MMP13 in MSCs. Gene expression analysis also highlighted changes in MAPK1, MAPK9 and TLR2 expression following IL-1β exposure, thereby indicating alterations to signalling pathways known to augment expression of MMPs and pro-inflammatory mediators (Wang et al., 2011; Mariani et al., 2014; Sangiorgi and Panepucci, 2016; Barreto et al., 2020). In parallel, IL-1β treatment reduced the expression of TGFB3 and IGF1, known to promote the synthesis of ECM proteins (Bohme et al., 1992; Tang et al., 2009) and contribute to cartilage maintenance in the healthy joint. The consequences of such changes should now be further explored, considering the ensuing risk of transplanted MSCs contributing to cartilage degradation when exposed to an OA environment.
IL-1β Effect in Osteogenic Conditions
In addition to cartilage degradation, abnormal bone remodelling is also a key feature of OA pathogenesis (Donell, 2019; Hu et al., 2020), and is linked to increased pain in this condition (Nwosu et al., 2016; Hu et al., 2021). Here, IL-1β was observed to significantly enhance the osteogenic response of MSC cultures, with a notable induction of ALP activity and mineral deposition. These findings were corroborated by the upregulation of BGLAP expression, a gene involved in the later mineralisation stage of osteogenesis (Birmingham et al., 2012). IL-1β addition to osteogenic medium also increased WNT5A expression. This is in line with previous observations of a pro-osteogenic effect of IL-1β on human MSCs, reported to be mediated primarily through the Wnt-5a/receptor tyrosine kinase-like orphan receptor 2 pathway (Sonomoto et al., 2012). Both canonical and non-canonical Wnt signalling play an important role in bone formation (Kim et al., 2015; Kobayashi et al., 2016; Maeda et al., 2019). However, in the OA joint, osteoblasts display reduced canonical Wnt/β-catenin signalling and increased non-canonical Wnt signalling via Wnt5a (Martineau et al., 2017), a ligand also linked to enhanced osteoclastogenesis and the production of inflammatory mediators (Yamashita et al., 2012; Roberts et al., 2020). Similarly, TLR2, TLR3 and MAPK14 showed increased expression upon IL-1β exposure, and are associated with increased osteogenesis (Artigas et al., 2014; Qi et al., 2014; Muthukuru and Darveau, 2015), whilst simultaneously promoting the production of pro-inflammatory mediators linked to osteoclastogenesis (Patil et al., 2004; Sangiorgi and Panepucci, 2016; Amarasekara et al., 2021). These include IL-8 (CXCL8) and IL-6, which both showed increased gene expression upon IL-1β exposure in this study. The observed decrease in MAPK3 expression upon IL-1β exposure compared to OM could be linked to the accelerated time-course of osteogenic response observed in these cells, as expression of this signalling molecule is important during early differentiation stages before rapidly returning to baseline (Jaiswal et al., 2000; Li et al., 2016). Taken together, this could implicate osteogenic MSCs exposed to IL-1β as a contributing factor in the increased rate of bone resorption and remodelling observed in OA.
IL-1β Effect in Undifferentiated Conditions and Relevance for Immune Modulation
This preferential drive towards osteogenesis was also observed when IL-1β was applied to MSCs in their undifferentiated state. IL-1β exposure under standard culture conditions led to increased expression of genes involved in bone remodelling, including ALPL, RUNX2, BGLAP and SPP1, whilst genes involved in cartilage anabolism (ACAN) and catabolism (MMP13) were decreased or increased, respectively. It can thus be hypothesised that IL-1β primes MSCs towards the osteogenic lineage, which could have a detrimental outcome for MSCs transplanted into the OA joint for cartilage regeneration. Furthermore, since IL-1β treatment of undifferentiated MSCs also increased expression of genes encoding pro-inflammatory mediators such as IL-1β, IL-6, IL-8 and MCP-1, it can be hypothesised that MSCs exposed to an IL-1β-rich environment could secrete increased levels of mediators that contribute to inflammatory joint disease (Kapoor et al., 2011; Wojdasiewicz et al., 2014; Molnar et al., 2021). The upregulated expression of PTGS2 (which encodes COX-2) in IL-1β-treated MSCs supports this view since both IL-6 and COX-2 are linked to pain in OA (Laine et al., 2008; Lin et al., 2017). Upregulation of IDO1 and HGF, also observed here in MSCs exposed to IL-1β, has previously been correlated to OA disease. In particular, increased HGF has been observed in OA osteoblasts (Abed et al., 2015), and IDO1 is overexpressed in the synovial fluid of OA patients (Alahdal et al., 2020), supporting the hypothesis that the response to IL-1β observed in MSC cultures aligns with pathological features seen in OA.
Based on the present observations, it will be useful to extend the scope of the analysis and, in particular, to assess differentiation in MSCs exposed to combinations of different mediators which are found in the OA joint. The present report analysed the effect of single factors over a defined time-scale in culture, which could be extended in subsequent studies to monitor the longer term effect on MSC response, adding further differentiation markers detectable through protein expression assays to refine the phenotypic characterisation.
Clinical Relevance
This study provides further evidence of the value of MSCs as a preclinical model, to evaluate specific pathophysiological factors and assess their impact on cell populations of therapeutic interest. The results presented now call for further investigations with increased experimental complexity, through the combined application of multiple inflammatory mediators present in the OA joint, to assess possible synergistic or antagonistic effects.
In conclusion, these results underline that IL-1β, a pro-inflammatory mediator associated with OA pathology, directly impacts the differentiation potential of MSCs in vitro, through a reduction in their chondrogenic capacity combined with a notable induction of osteogenesis. This suggests that the exposure of transplanted MSCs to this interleukin in the OA joint could promote subchondral bone remodelling rather than cartilage regeneration. Gene expression analysis highlighted changes in TLR, MAPK and Wnt signalling pathways and revealed increased expression of genes encoding pro-inflammatory mediators and cartilage-degrading enzymes, further highlighting the likely detrimental impact of the local inflammatory OA environment on transplanted MSCs. Given the widespread presence of IL-1β in the OA joint, it could indeed be hypothesised that the OA microenvironment may limit the therapeutic potential of MSCs, and favour their participation in the progression of OA pathology. These observations call for further investigation into the effects of the OA microenvironment on candidate therapeutic cell populations, in order to evaluate the expected benefit of these cell-based approaches.
Data Availability Statement
The original contributions presented in the study are included in the article, further relevant inquiries can be directed to the corresponding author.
Author Contributions
SM and TT performed the experiments and analyses. VC and VS designed and supervised the project. SM and VS wrote the manuscript, with input from all. All authors contributed to the manuscript.
Funding
This work was supported by the Biotechnology and Biological Sciences Research Council [grant number BB/J014508/1], and by the Pain Centre Versus Arthritis at the University of Nottingham. VS is supported by a grant from the Italian Ministry of University and Research (MUR) to the Department of Molecular Medicine of the University of Pavia under the initiative “Dipartimenti di Eccellenza (2019–2022)”.
Conflict of Interest
The authors declare that the research was conducted in the absence of any commercial or financial relationships that could be construed as a potential conflict of interest.
Publisher’s Note
All claims expressed in this article are solely those of the authors and do not necessarily represent those of their affiliated organizations, or those of the publisher, the editors and the reviewers. Any product that may be evaluated in this article, or claim that may be made by its manufacturer, is not guaranteed or endorsed by the publisher.
Acknowledgments
We thank N. De Melo for valuable input for the TLDA data analysis.
References
Abed, E., Bouvard, B., Martineau, X., Jouzeau, J.-Y., Reboul, P., and Lajeunesse, D. (2015). Elevated Hepatocyte Growth Factor Levels in Osteoarthritis Osteoblasts Contribute to Their Altered Response to Bone Morphogenetic Protein-2 and Reduced Mineralization Capacity. Bone 75, 111–119. doi:10.1016/j.bone.2015.02.001
Alahdal, M., Duan, L., Ouyang, H., and Wang, D. (2020). The Role of Indoleamine 2,3 Dioxygenase 1 in the Osteoarthritis. Am. J. Transl. Res. 12, 2322–2343.
Amarasekara, D. S., Kim, S., and Rho, J. (2021). Regulation of Osteoblast Differentiation by Cytokine Networks. Ijms 22, 2851. doi:10.3390/ijms22062851
Artigas, N., Ureña, C., Rodríguez-Carballo, E., Rosa, J. L., and Ventura, F. (2014). Mitogen-activated Protein Kinase (MAPK)-regulated Interactions between Osterix and Runx2 Are Critical for the Transcriptional Osteogenic Program. J. Biol. Chem. 289, 27105–27117. doi:10.1074/jbc.M114.576793
Barreto, G., Manninen, M., and K. Eklund, K. (2020). Osteoarthritis and Toll-like Receptors: when Innate Immunity Meets Chondrocyte Apoptosis. Biology 9, 65. doi:10.3390/biology9040065
Birmingham, E., Niebur, G. L., Niebur, G., McHugh, P., Shaw, G., Barry, F., et al. (2012). Osteogenic Differentiation of Mesenchymal Stem Cells Is Regulated by Osteocyte and Osteoblast Cells in a Simplified Bone Niche. eCM 23, 13–27. doi:10.22203/eCM.v023a02
Böhme, K., Conscience-Egli, M., Tschan, T., Winterhalter, K. H., and Bruckner, P. (1992). Induction of Proliferation or Hypertrophy of Chondrocytes in Serum-free Culture: the Role of Insulin-like Growth Factor-I, Insulin, or Thyroxine. J. Cell Biol. 116, 1035–1042. doi:10.1083/jcb.116.4.1035
Chapman, V., Markides, H., Sagar, D. R., Xu, L., Burston, J. J., Mapp, P., et al. (2017). Therapeutic Benefit for Late, but Not Early, Passage Mesenchymal Stem Cells on Pain Behaviour in an Animal Model of Osteoarthritis. Stem Cells Int. 2017, 1–11. doi:10.1155/2017/2905104
Charlesworth, J., Fitzpatrick, J., Perera, N. K. P., and Orchard, J. (2019). Osteoarthritis- a Systematic Review of Long-Term Safety Implications for Osteoarthritis of the Knee. BMC Musculoskelet. Disord. 20, 151. doi:10.1186/s12891-019-2525-0
Chen, D., Shen, J., Zhao, W., Wang, T., Han, L., Hamilton, J. L., et al. (2017). Osteoarthritis: toward a Comprehensive Understanding of Pathological Mechanism. Bone Res. 5, 16044. doi:10.1038/boneres.2016.44
Chen, S., Fu, P., Cong, R., Wu, H., and Pei, M. (2015). Strategies to Minimize Hypertrophy in Cartilage Engineering and Regeneration. Genes & Dis. 2, 76–95. doi:10.1016/j.gendis.2014.12.003
Choi, J.-J., Yoo, S.-A., Park, S.-J., Kang, Y.-J., Kim, W.-U., Oh, I.-H., et al. (2008). Mesenchymal Stem Cells Overexpressing Interleukin-10 Attenuate Collagen-Induced Arthritis in Mice. Clin. Exp. Immunol. 153, 269–276. doi:10.1111/j.1365-2249.2008.03683.x
Coleman, C. M., Curtin, C., Barry, F. P., O'Flatharta, C., and Murphy, J. M. (2010). Mesenchymal Stem Cells and Osteoarthritis: Remedy or Accomplice? Hum. Gene Ther. 21, 1239–1250. doi:10.1089/hum.2010.138
Daheshia, M., and Yao, J. Q. (2008). The Interleukin 1β Pathway in the Pathogenesis of Osteoarthritis. J. Rheumatol. 35, 2306–2312. doi:10.3899/jrheum.080346
De Jager, W., te Velthuis, H., Prakken, B. J., Kuis, W., and Rijkers, G. T. (2003). Simultaneous Detection of 15 Human Cytokines in a Single Sample of Stimulated Peripheral Blood Mononuclear Cells. Clin. Vaccine Immunol. 10, 133–139. doi:10.1128/cdli.10.1.133-139.2003
De Lange-Brokaar, B. J. E., Ioan-Facsinay, A., Van Osch, G. J. V. M., Zuurmond, A.-M., Schoones, J., Toes, R. E. M., et al. (2012). Synovial Inflammation, Immune Cells and Their Cytokines in Osteoarthritis: a Review. Osteoarthr. Cartil. 20, 1484–1499. doi:10.1016/j.joca.2012.08.027
DelaRosa, O., Dalemans, W., and Lombardo, E. (2012). Toll-like Receptors as Modulators of Mesenchymal Stem Cells. Front. Immun. 3, 182. doi:10.3389/fimmu.2012.00182
Denoble, A. E., Huffman, K. M., Stabler, T. V., Kelly, S. J., Hershfield, M. S., McDaniel, G. E., et al. (2011). Uric Acid Is a Danger Signal of Increasing Risk for Osteoarthritis through Inflammasome Activation. Proc. Natl. Acad. Sci. U.S.A. 108, 2088–2093. doi:10.1073/pnas.1012743108
Donell, S. (2019). Subchondral Bone Remodelling in Osteoarthritis. EFORT Open Rev. 4, 221–229. doi:10.1302/2058-5241.4.180102
Gao, F., Chiu, S. M., Motan, D. A. L., Zhang, Z., Chen, L., Ji, H.-L., et al. (2016). Mesenchymal Stem Cells and Immunomodulation: Current Status and Future Prospects. Cell Death Dis. 7–e2062. doi:10.1038/cddis.2015.327
García-Manrique, M., Calvet, J., Orellana, C., Berenguer-Llergo, A., Garcia-Cirera, S., Llop, M., et al. (2021). Synovial Fluid but Not Plasma Interleukin-8 Is Associated with Clinical Severity and Inflammatory Markers in Knee Osteoarthritis Women with Joint Effusion. Sci. Rep. 11, 5258. doi:10.1038/s41598-021-84582-2
Goldring, M. B., and Marcu, K. B. (2009). Cartilage Homeostasis in Health and Rheumatic Diseases. Arthritis Res. Ther. 11, 224. doi:10.1186/ar2592
Grässel, S., and Muschter, D. (2020). Recent Advances in the Treatment of Osteoarthritis. F1000Res 9, 325. doi:10.12688/f1000research.22115.1
Gupta, P. K., Chullikana, A., Rengasamy, M., Shetty, N., Pandey, V., Agarwal, V., et al. (2016). Efficacy and Safety of Adult Human Bone Marrow-Derived, Cultured, Pooled, Allogeneic Mesenchymal Stromal Cells (Stempeucel): Preclinical and Clinical Trial in Osteoarthritis of the Knee Joint. Arthritis Res. Ther. 18, 301. doi:10.1186/s13075-016-1195-7
Harrell, C. R., Markovic, B. S., Fellabaum, C., Arsenijevic, A., and Volarevic, V. (2019). Mesenchymal Stem Cell-Based Therapy of Osteoarthritis: Current Knowledge and Future Perspectives. Biomed. Pharmacother. 109, 2318–2326. doi:10.1016/j.biopha.2018.11.099
He, Y., Li, Z., Alexander, P. G., Ocasio-Nieves, B. D., Yocum, L., Lin, H., et al. (2020). Pathogenesis of Osteoarthritis: Risk Factors, Regulatory Pathways in Chondrocytes, and Experimental Models. Biology 9, 194. doi:10.3390/biology9080194
Helmark, I. C., Mikkelsen, U. R., Børglum, J., Rothe, A., Petersen, M. C., Andersen, O., et al. (2010). Exercise Increases Interleukin-10 Levels Both Intraarticularly and Peri-Synovially in Patients with Knee Osteoarthritis: a Randomized Controlled Trial. Arthritis Res. Ther. 12, R126. doi:10.1186/ar3064
Heng, B. C., Cao, T., and Lee, E. H. (2004). Directing Stem Cell Differentiation into the Chondrogenic Lineage In Vitro. Stem Cells 22, 1152–1167. doi:10.1634/stemcells.2004-0062
Hu, W., Chen, Y., Dou, C., and Dong, S. (2020). Microenvironment in Subchondral Bone: Predominant Regulator for the Treatment of Osteoarthritis. Ann. Rheum. Dis. 80, 413–422. doi:10.1136/annrheumdis-2020-218089
Hu, Y., Chen, X., Wang, S., Jing, Y., and Su, J. (2021). Subchondral Bone Microenvironment in Osteoarthritis and Pain. Bone Res. 9, 20. doi:10.1038/s41413-021-00147-z
Hwang, J. J., Rim, Y. A., Nam, Y., and Ju, J. H. (2021). Recent Developments in Clinical Applications of Mesenchymal Stem Cells in the Treatment of Rheumatoid Arthritis and Osteoarthritis. Front. Immunol. 12, 631291. doi:10.3389/fimmu.2021.631291
Iannone, F., De Bari, C., Dell'Accio, F., Covelli, M., Cantatore, F. P., Patella, V., et al. (2001). Interleukin-10 and Interleukin-10 Receptor in Human Osteoarthritic and Healthy Chondrocytes. Clin. Exp. Rheumatol. 19, 139–145.
Iijima, H., Isho, T., Kuroki, H., Takahashi, M., and Aoyama, T. (2018). Effectiveness of Mesenchymal Stem Cells for Treating Patients with Knee Osteoarthritis: a Meta-Analysis toward the Establishment of Effective Regenerative Rehabilitation. NPJ Regen. Med. 3, 15. doi:10.1038/s41536-018-0041-8
Jaiswal, R. K., Jaiswal, N., Bruder, S. P., Mbalaviele, G., Marshak, D. R., and Pittenger, M. F. (2000). Adult Human Mesenchymal Stem Cell Differentiation to the Osteogenic or Adipogenic Lineage Is Regulated by Mitogen-Activated Protein Kinase. J. Biol. Chem. 275, 9645–9652. doi:10.1074/jbc.275.13.9645
Jayasuriya, C. T., Hu, N., Li, J., Lemme, N., Terek, R., Ehrlich, M. G., et al. (2018). Molecular Characterization of Mesenchymal Stem Cells in Human Osteoarthritis Cartilage Reveals Contribution to the OA Phenotype. Sci. Rep. 8, 7044. doi:10.1038/s41598-018-25395-8
Kaneko, S., Satoh, T., Chiba, J., Ju, C., Inoue, K., and Kagawa, J. (2000). Interleukin-6 and Interleukin-8 Levels in Serum and Synovial Fluid of Patients with Osteoarthritis. Cytokines, Cell. Mol. Ther. 6, 71–79. doi:10.1080/13684730050515796
Kapoor, M., Martel-Pelletier, J., Lajeunesse, D., Pelletier, J.-P., and Fahmi, H. (2011). Role of Proinflammatory Cytokines in the Pathophysiology of Osteoarthritis. Nat. Rev. Rheumatol. 7, 33–42. doi:10.1038/nrrheum.2010.196
Kim, J.-A., Choi, H.-K., Kim, T.-M., Leem, S.-H., and Oh, I.-H. (2015). Regulation of Mesenchymal Stromal Cells through Fine Tuning of Canonical Wnt Signaling. Stem Cell Res. 14, 356–368. doi:10.1016/j.scr.2015.02.007
Kobayashi, Y., Uehara, S., Udagawa, N., and Takahashi, N. (2016). Regulation of Bone Metabolism by Wnt Signals. J. Biochem. 159, 387–392. doi:10.1093/jb/mvv124
Kock, L., Van Donkelaar, C. C., and Ito, K. (2012). Tissue Engineering of Functional Articular Cartilage: the Current Status. Cell Tissue Res. 347, 613–627. doi:10.1007/s00441-011-1243-1
Laine, L., White, W. B., Rostom, A., and Hochberg, M. (2008). COX-2 Selective Inhibitors in the Treatment of Osteoarthritis. Seminars Arthritis Rheumatism 38, 165–187. doi:10.1016/j.semarthrit.2007.10.004
Li, C.-S., Zheng, Z., Su, X.-X., Wang, F., Ling, M., Zou, M., et al. (2016). Activation of the Extracellular Signal-Regulated Kinase Signaling Is Critical for Human Umbilical Cord Mesenchymal Stem Cell Osteogenic Differentiation. BioMed Res. Int. 2016, 1–10. doi:10.1155/2016/3764372
Li, X., Ellman, M. B., Kroin, J. S., Chen, D., Yan, D., Mikecz, K., et al. (2012). Species-specific Biological Effects of FGF-2 in Articular Cartilage: Implication for Distinct Roles within the FGF Receptor Family. J. Cell. Biochem. 113, 2532–2542. doi:10.1002/jcb.24129
Lin, Y., Liu, L., Jiang, H., Zhou, J., and Tang, Y. (2017). Inhibition of Interleukin-6 Function Attenuates the Central Sensitization and Pain Behavior Induced by Osteoarthritis. Eur. J. Pharmacol. 811, 260–267. doi:10.1016/j.ejphar.2017.06.032
Macri-Pellizzeri, L., De Melo, N., Ahmed, I., Grant, D., Scammell, B., and Sottile, V. (2018). Live Quantitative Monitoring of Mineral Deposition in Stem Cells Using Tetracycline Hydrochloride. Tissue Eng. Part C. Methods 24, 171–178. doi:10.1089/ten.tec.2017.0400
Maeda, K., Kobayashi, Y., Koide, M., Uehara, S., Okamoto, M., Ishihara, A., et al. (2019). The Regulation of Bone Metabolism and Disorders by Wnt Signaling. Ijms 20, 5525. doi:10.3390/ijms20225525
Mancuso, P., Raman, S., Glynn, A., Barry, F., and Murphy, J. M. (2019). Mesenchymal Stem Cell Therapy for Osteoarthritis: the Critical Role of the Cell Secretome. Front. Bioeng. Biotechnol. 7, 9. doi:10.3389/fbioe.2019.00009
Mariani, E., Pulsatelli, L., and Facchini, A. (2014). Signaling Pathways in Cartilage Repair. Ijms 15, 8667–8698. doi:10.3390/ijms15058667
Martel-Pelletier, J. (2004). Pathophysiology of Osteoarthritis. Osteoarthr. Cartil. 12, 31–33. doi:10.1016/j.joca.2003.10.002
Martineau, X., Abed, É., Martel-Pelletier, J., Pelletier, J.-P., and Lajeunesse, D. (2017). Alteration of Wnt5a Expression and of the Non-canonical Wnt/PCP and Wnt/PKC-Ca2+ Pathways in Human Osteoarthritis Osteoblasts. PLoS One 12, e0180711. doi:10.1371/journal.pone.0180711
Matas, J., Orrego, M., Amenabar, D., Infante, C., Tapia-Limonchi, R., Cadiz, M. I., et al. (2019). Umbilical Cord-Derived Mesenchymal Stromal Cells (MSCs) for Knee Osteoarthritis: Repeated MSC Dosing Is Superior to a Single MSC Dose and to Hyaluronic Acid in a Controlled Randomized Phase I/II Trial. Stem Cells Transl. Med. 8, 215–224. doi:10.1002/sctm.18-0053
Meijer, H., Reinecke, J., Becker, C., Tholen, G., and Wehling, P. (2003). The Production of Anti-inflammatory Cytokines in Whole Blood by Physico-Chemical Induction. Inflamm. Res. 52, 404–407. doi:10.1007/s00011-003-1197-1
Molnar, V., Matišić, V., Kodvanj, I., Bjelica, R., Jeleč, Ž., Hudetz, D., et al. (2021). Cytokines and Chemokines Involved in Osteoarthritis Pathogenesis. Ijms 22, 9208. doi:10.3390/ijms22179208
Murphy, J. M., Dixon, K., Beck, S., Fabian, D., Feldman, A., and Barry, F. (2002). Reduced Chondrogenic and Adipogenic Activity of Mesenchymal Stem Cells from Patients with Advanced Osteoarthritis. Arthritis & Rheumatism 46, 704–713. doi:10.1002/art.10118
Muthukuru, M., and Darveau, R. P. (2014). TLR Signaling that Induces Weak Inflammatory Response and SHIP1 Enhances Osteogenic Functions. Bone Res. 2, 14031. doi:10.1038/boneres.2014.31
National Institute for Health and Care Excellence (2020). Osteoarthritis: Care and Management (Clinical Guidance [CG177]). Available at: https://www.nice.org.uk/guidance/cg177.
Nwosu, L. N., Chapman, V., Walsh, D. A., and Kraus, V. B. (2016). Subchondral Bone Biomarkers as Predictors of OA Pain Progression; Linking Structural Pathology and Pain. Osteoarthr. Cartil. 24, S51. doi:10.1016/j.joca.2016.01.116
Patil, C., Zhu, X., Rossa, C., Kim, Y. J., and Kirkwood, K. L. (2004). p38 MAPK Regulates IL‐1β Induced IL‐6 Expression through mRNA Stability in Osteoblasts. Immunol. Investig. 33, 213–233. doi:10.1081/imm-120034231
Pfaffl, M. W. (2001). A New Mathematical Model for Relative Quantification in Real-Time RT-PCR. Nucleic Acids Res. 29, 45e–45. doi:10.1093/nar/29.9.e45
Pfander, D., Heinz, N., Rothe, P., Carl, H. D., and Swoboda, B. (2004). Tenascin and Aggrecan Expression by Articular Chondrocytes Is Influenced by Interleukin 1 : a Possible Explanation for the Changes in Matrix Synthesis during Osteoarthritis. Ann. Rheumatic Dis. 63, 240–244. doi:10.1136/ard.2002.003749
Pierzchala, A. W., Kusz, D. J., and Hajduk, G. (2011). CXCL8 and CCL5 Expression in Synovial Fluid and Blood Serum in Patients with Osteoarthritis of the Knee. Arch. Immunol. Ther. Exp. 59, 151–155. doi:10.1007/s00005-011-0115-4
Prosser, A., Scotchford, C., Roberts, G., Grant, D., and Sottile, V. (2019). Integrated Multi-Assay Culture Model for Stem Cell Chondrogenic Differentiation. Ijms 20, 951. doi:10.3390/ijms20040951
Qi, C., Xiaofeng, X., and Xiaoguang, W. (2014). Effects of Toll-like Receptors 3 and 4 in the Osteogenesis of Stem Cells. Stem Cells Int. 2014, 1–7. doi:10.1155/2014/917168
Roberts, J. L., Liu, G., Paglia, D. N., Kinter, C. W., Fernandes, L. M., Lorenzo, J., et al. (2020). Deletion of Wnt5a in Osteoclasts Results in Bone Loss through Decreased Bone Formation. Ann. N.Y. Acad. Sci. 1463, 45–59. doi:10.1111/nyas.14293
Rutgers, M., Saris, D. B., Dhert, W. J., and Creemers, L. B. (2010). Cytokine Profile of Autologous Conditioned Serum for Treatment of Osteoarthritis, In Vitro Effects on Cartilage Metabolism and Intra-articular Levels after Injection. Arthritis Res. Ther. 12, R114. doi:10.1186/ar3050
Sangiorgi, B., and Panepucci, R. A. (2016). Modulation of Immunoregulatory Properties of Mesenchymal Stromal Cells by Toll-like Receptors: Potential Applications on GVHD. Stem Cells Int. 2016, 1–10. doi:10.1155/2016/9434250
Shirjang, S., Mansoori, B., Solali, S., Hagh, M. F., and Shamsasenjan, K. (2017). Toll-like Receptors as a Key Regulator of Mesenchymal Stem Cell Function: an Up-To-Date Review. Cell. Immunol. 315, 1–10. doi:10.1016/j.cellimm.2016.12.005
Sokolove, J., and Lepus, C. M. (2013). Role of Inflammation in the Pathogenesis of Osteoarthritis: Latest Findings and Interpretations. Ther. Adv. Musculoskelet. 5, 77–94. doi:10.1177/1759720X12467868
Song, N., Scholtemeijer, M., and Shah, K. (2020). Mesenchymal Stem Cell Immunomodulation: Mechanisms and Therapeutic Potential. Trends Pharmacol. Sci. 41, 653–664. doi:10.1016/j.tips.2020.06.009
Sonomoto, K., Yamaoka, K., Oshita, K., Fukuyo, S., Zhang, X., Nakano, K., et al. (2012). Interleukin-1β Induces Differentiation of Human Mesenchymal Stem Cells into Osteoblasts via the Wnt-5a/receptor Tyrosine Kinase-like Orphan Receptor 2 Pathway. Arthritis & Rheumatism 64, 3355–3363. doi:10.1002/art.34555
Tang, Q. O., Shakib, K., Heliotis, M., Tsiridis, E., Mantalaris, A., Ripamonti, U., et al. (2009). TGF-β3: A Potential Biological Therapy for Enhancing Chondrogenesis. Expert Opin. Biol. Ther. 9, 689–701. doi:10.1517/14712590902936823
Van der Kraan, P. M., and Van den Berg, W. B. (2012). Chondrocyte Hypertrophy and Osteoarthritis: Role in Initiation and Progression of Cartilage Degeneration? Osteoarthr. Cartil. 20, 223–232. doi:10.1016/j.joca.2011.12.003
Van Roon, J. A. G., Van Roy, J. L. A. M., Gmelig-Meyling, F. H. J., Lafeber, F. P. J. G., and Bijlsma, J. W. J. (1996). Prevention and Reversal of Cartilage Degradation in Rheumatoid Arthritis by Interleukin-10 and Interleukin-4. Arthritis & Rheumatism 39, 829–835. doi:10.1002/art.1780390516
Vandesompele, J., De Preter, K., Pattyn, F., Poppe, B., Van Roy, N., De Paepe, A., et al. (2002). Accurate Normalization of Real-Time Quantitative RT-PCR Data by Geometric Averaging of Multiple Internal Control Genes. Genome Biol. 3, 0034.1. doi:10.1186/gb-2002-3-7-research0034
Wang, G., Xing, D., Liu, W., Zhu, Y., Liu, H., Yan, L., et al. (2022). Preclinical Studies and Clinical Trials on Mesenchymal Stem Cell Therapy for Knee Osteoarthritis: A Systematic Review on Models and Cell Doses. Int J Rheum Dis 25, 532–562. doi:10.1111/1756-185X.14306
Wang, J., Zhou, L., Zhang, Y., Huang, L., and Shi, Q. (2020). Mesenchymal Stem Cells - a Promising Strategy for Treating Knee Osteoarthritis. Bone & Jt. Res. 9, 719–728. doi:10.1302/2046-3758.910.BJR-2020-0031.R3
Wang, M., Yuan, Q., and Xie, L. (2018). Mesenchymal Stem Cell-Based Immunomodulation: Properties and Clinical Application. Stem Cells Int. 2018, 1–12. doi:10.1155/2018/3057624
Wang, X., Li, F., Fan, C., Wang, C., and Ruan, H. (2011). Effects and Relationship of ERK1 and ERK2 in Interleukin-1β-Induced Alterations in MMP3, MMP13, Type II Collagen and Aggrecan Expression in Human Chondrocytes. Int. J. Mol. Med. 27, 583–589. doi:10.3892/ijmm.2011.611
Westacott, C. I., Whicher, J. T., Barnes, I. C., Thompson, D., Swan, A. J., and Dieppe, P. A. (1990). Synovial Fluid Concentration of Five Different Cytokines in Rheumatic Diseases. Ann. Rheumatic Dis. 49, 676–681. doi:10.1136/ard.49.9.676
Wojdasiewicz, P., Poniatowski, Ł. A., and Szukiewicz, D. (2014). The Role of Inflammatory and Anti-inflammatory Cytokines in the Pathogenesis of Osteoarthritis. Mediat. Inflamm. 2014, 1–19. doi:10.1155/2014/561459
Xiang, X.-N., Zhu, S.-Y., He, H.-C., Yu, X., Xu, Y., and He, C.-Q. (2022). Mesenchymal Stromal Cell-Based Therapy for Cartilage Regeneration in Knee Osteoarthritis. Stem Cell Res. Ther. 13, 14. doi:10.1186/s13287-021-02689-9
Yamashita, T., Takahashi, N., and Udagawa, N. (2012). New Roles of Osteoblasts Involved in Osteoclast Differentiation. Wjo 3, 175–181. doi:10.5312/wjo.v3.i11.175
Zhang, L., Su, P., Xu, C., Yang, J., Yu, W., and Huang, D. (2010). Chondrogenic Differentiation of Human Mesenchymal Stem Cells: a Comparison between Micromass and Pellet Culture Systems. Biotechnol. Lett. 32, 1339–1346. doi:10.1007/s10529-010-0293-x
Zhao, X., Zhao, Y., Sun, X., Xing, Y., Wang, X., and Yang, Q. (2020). Immunomodulation of MSCs and MSC-Derived Extracellular Vesicles in Osteoarthritis. Front. Bioeng. Biotechnol. 8, 575057. doi:10.3389/fbioe.2020.575057
Keywords: differentiation, mesenchymal progenitors, interleukins, inflammation, osteoarthritis, in vitro model
Citation: Marsh S, Constantin-Teodosiu T, Chapman V and Sottile V (2022) In vitro Exposure to Inflammatory Mediators Affects the Differentiation of Mesenchymal Progenitors. Front. Bioeng. Biotechnol. 10:908507. doi: 10.3389/fbioe.2022.908507
Received: 30 March 2022; Accepted: 23 May 2022;
Published: 22 June 2022.
Edited by:
Ahmed Lotfy, Beni-Suef University, EgyptCopyright © 2022 Marsh, Constantin-Teodosiu, Chapman and Sottile. This is an open-access article distributed under the terms of the Creative Commons Attribution License (CC BY). The use, distribution or reproduction in other forums is permitted, provided the original author(s) and the copyright owner(s) are credited and that the original publication in this journal is cited, in accordance with accepted academic practice. No use, distribution or reproduction is permitted which does not comply with these terms.
*Correspondence: V. Sottile, dmlyZ2luaWUuc290dGlsZUB1bmlwdi5pdA==