- 1College of Life Science and Technology, Beijing University of Chemical Technology, Beijing, China
- 2College of Bioscience and Resources Environment, Beijing University of Agriculture, Beijing, China
The recent decline of the international biodiesel industry has led to decreased production and therefore increased the price of glycerol, which is a major by-product of biodiesel but a substrate for production of 3-hydroxypropionic acid (3-HP), that is, glycerol as a feedstock has no advantage over glucose in price. Hence, we engineered glucose to the glycerol pathway and improved 3-HP production by CRISPR interference (CRISPRi). To begin with, we cloned the genes encoding glycerol 3-phosphate dehydrogenase (gpd1) and glycerol 3-phosphatase (gpp2) from Saccharomyces cerevisiae, which jointly catalyze glucose into glycerol. The genes gpd1 and gpp2 were co-expressed in K. pneumoniae with the dCas9 gene integrated in genome, and this recombinant strain produced 2 g/L glycerol in the shake flask. To minimize the glucose consumption by competing pathways including the EMP pathway, glycerol oxidation pathway, and by-products pathways, we developed an CRISPRi system in aforementioned recombinant K. pneumoniae strain to inhibit the expression of the glyceraldehyde-3-phosphate dehydrogenase gene (gapA) and 2,3-butanediol production gene (budA), resulting in a bi-functional strain harboring both glucose-to-glycerol pathway and CRISPRi system. Reverse transcription and quantitative PCR (RT-qPCR) results showed that this engineered CRISPRi system transcriptionally inhibited gapA and budA by 82% and 24%, respectively. In shake flask cultivation, this bi-functional strain produced 2.8 g/L glycerol using glucose as the carbon source, which was 46.6% increase compared to the strain without the engineered CRISPRi system. Moreover, this bi-functional strain produced 0.78 g/L 3-HP using glucose as the sole carbon source. In fed-batch cultivation, this bi-functional strain produced 1.77 g/L 3-HP. This study provides insights for co-utilization of distinct carbon sources.
Introduction
As an isomer of lactic acid, 3-hydroxypropionic acid (3-HP) is chemically active (de Fouchecour et al., 2018; Matsakas et al., 2018). As such, 3-HP is a versatile platform compound from which a variety of economically important chemicals can be derived. Currently, industrial production of 3-HP relies mainly on chemical synthesis (Della Pina et al., 2011). However, chemical synthesis not only relies on non-renewable resources but also leads to excessive by-products and severe environmental pollution (Della Pina et al., 2011). In recent years, microbial fermentation has emerged as an alternative to chemical synthesis. For instance, 3-HP can be produced by diverse wild-type or engineered strains, including Escherichia coli (Mohan Raj et al., 2009), S. cerevisiae (Semkiv et al., 2017), Corynebacterium glutamicum (Chen et al., 2017), Lactobacillus reuteri (Luo et al., 2011), and K. pneumoniae (Li et al., 2016). In aforementioned host microbes, 3-HP biosynthesis can be roughly divided into three types: glycerol-based (Kumar and Park, 2018), glucose-based (Straathof et al., 2005), and acetate-based 3-HP pathways (Zhao and Tian, 2021). The glycerol-based 3-HP synthesis involves coenzyme A-dependent pathway and coenzyme A-independent pathway (Jers et al., 2019). The CoA-independent 3-HP production from glycerol requires only two-step catalysis by glycerol dehydrogenase (GDH) (EC 4.2.1.30) and acetaldehyde dehydrogenase (ALDH) (EC 1.2.1.3). Therefore, this 3-HP pathway is of great attractiveness. However, the ALDHs in wild-type strains show low activity, and only trace amount of 3-HP can be generated (Raj et al., 2010; Ko et al., 2012). Fortunately, when the tac promoter was used to express PuuC, an ALDH native to K. pneumoniae, 3-HP was overproduced from glycerol (Li et al., 2016; Zhao et al., 2019). To produce 3-HP from glucose through the CoA-independent pathway, a glucose-to-glycerol pathway needs to be engineered. To do so, it is necessary to clone the genes encoding glycerol 3-phosphate dehydrogenase (GPD1, EC 1.1.1.8) and glycerol 3-phosphatase (GPP2, EC 3.1.3.21) from S. cerevisiae (Zheng et al., 2008). In addition, a host strain tolerant to glycerol is required. Of candidate host strains, K. pneumoniae is promising in this regard (Zhao et al., 2019), as it can efficiently metabolize glycerol. Although glycerol is the most used carbon source for production of 3-HP (Nguyen-Vo et al., 2018), its price is high due to the recent decline of the biodiesel industry.
In S. cerevisiae, glucose is catalyzed into glycerol by GPD1 and GPP2 (Chen et al., 2014; Kildegaard et al., 2016). In wild-type microbes, glucose conversion to glycerol is rather limited and high-level production of 3-HP is thus challenging. The reason behind is that glucose uptake is mainly accomplished by the phosphoenolpyruvate-dependent glucose uptake system (PTS), and glycolysis pathway (EMP) consumes a large amount of glucose (Chen et al., 2017). Moreover, in the presence of glucose, the Crabtree effect inhibits the conversion of glycerol to 3-HP (Joseph et al., 1981). When metabolic pathways were optimized and glucose was used as the carbon source, the maximum 3-HP titer of engineered E. coli reached 37.6 g/L (Heo et al., 2019). Despite a lot of studies, the low 3-HP production constrains its commercialization (Ashok et al., 2011). Hence, global regulation is required to improve the conversion rate of glucose to 3-HP.
CRISPR interference (CRISPRi, Figure 1B) is a powerful tool for modulation of multiple genes (Qi et al., 2013). In fact, fermentation titer is a quantitative trait controlled by multiple genes. The guide RNAs in the CRISPR system can direct dCas9 to user-defined genetic loci, leading to suppression of single or multiple genes and alternation of metabolites formation (Jakounas et al., 2015; Liu et al., 2019; Xu and Qi, 2019). For instance, lactic acid production can be attenuated by an engineered CRISPRi system targeting D-lactate dehydrogenase gene in K. pneumoniae (Wang et al., 2018). Conversely, aconitic acid production can be improved by an engineered CRISPRi system that simultaneously targeting pyruvate kinase (PK) in the glycolytic pathway (EMP pathway) and isocitrate dehydrogenase (IDH) in the tricarboxylic acid cycle (TCA cycle) pathway in E. coli (Li et al., 2020). These studies highlight the potential of the CRISPRi system in allocating metabolic flux.
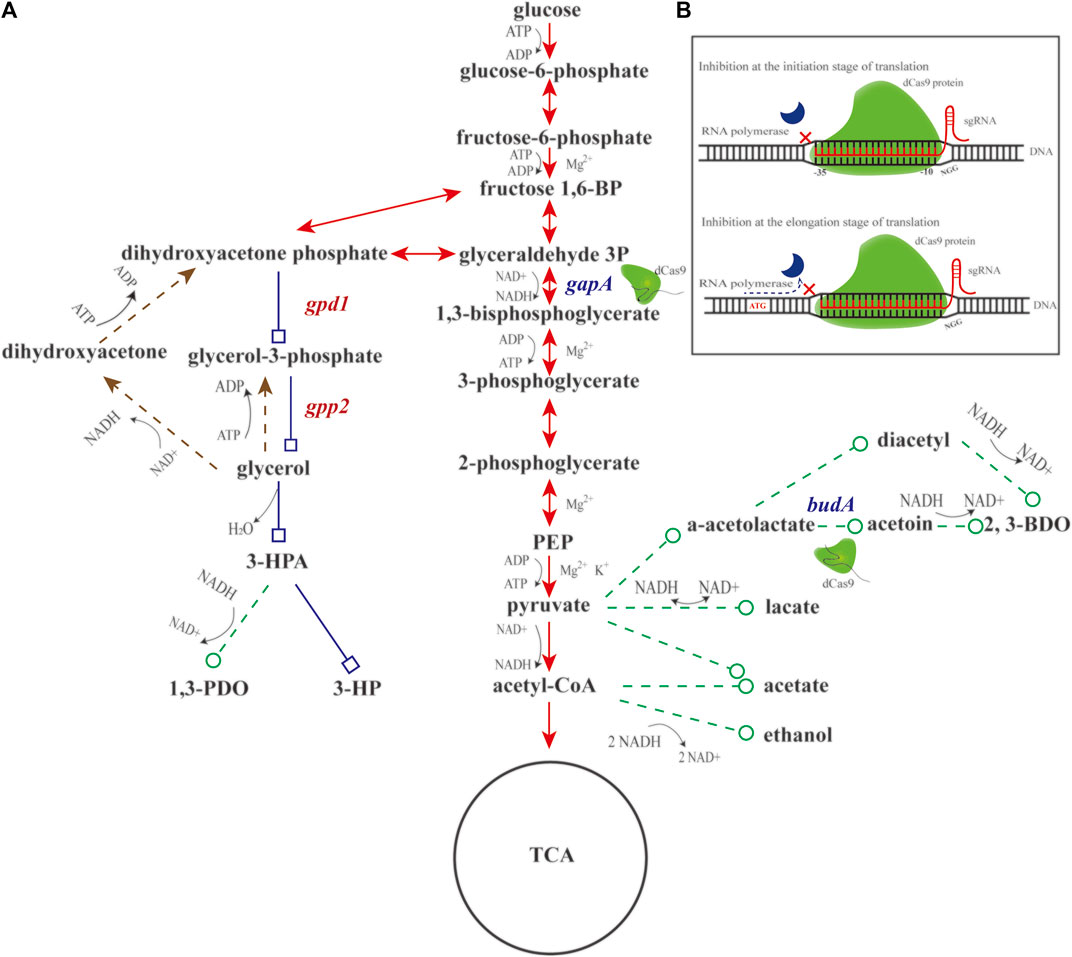
FIGURE 1. Schematic diagram of engineering glucose-to-glycerol pathway and CRISPRi system in K. pneumoniae. (A) Diagram of engineering glucose-to-glycerol pathway. (B) Diagram of the CRISPRi system. Triangular arrow of solid line denotes the EMP pathway. Triangular arrow of dotted line denotes the glycerol oxidation pathway. Square arrow of solid line denotes the engineered glucose to 3-hydroxypropionic acid (3-HP) pathway, and circular arrow of dotted line denotes by-product pathways. gapA and budA are two native genes inhibited by CRISPRi. gpd1 and gpp2 are two heterologous genes introduced for glycerol production. Fructose 1,6-BP, fructose 1,6-diphosphate; glyceraldehyde 3P, glyceraldehyde-3-phosphate; PEP, phosphoenolpyruvate; 3-HPA, 3-hydroxypropionaldehyde; 3-HP, 3-hydroxypropionic acid; 1,3-PDO, 1,3-propanediol; 2,3-BDO, 2,3-butanediol; gpd1, glycerol 3-phosphate dehydrogenase; gpp2, glycerol 3-phosphatase; gapA, glyceraldehyde-3-phosphate dehydrogenase; and budA, α-acetolactate decarboxylase.
Given aforementioned information, we conjecture that engineering a glucose-to-glycerol pathway in K. pneumoniae enables 3-HP production using glucose as carbon source. In addition, the CRISPRi system might be engineered to reallocate the metabolic flux toward 3-HP and other metabolites (Figure 1A). In doing so, based on the previous work, two genes, gpd1 (GenBank accession No. Z74071.1) and gpp2 (GenBank accession No. DQ332890.1) were used to construct the shortest pathway for conversion of glucose to 3-HP. In addition, the CRISPRi system was developed to regulate the pathways affecting 3-HP production. To boost glucose conversion to glycerol, we engineered a CRISPRi system targeting glyceraldehyde-3-phosphate dehydrogenase gene (gapA) and α-acetolactate decarboxylase gene (budA). Reverse transcription and quantitative PCR (RT-qPCR) was performed to examine the expression of gpd1, gpp2, gapA, and budA. Shake flask cultivation of the recombinant strains were to determine the production of glycerol, 3-HP, acetic acid, lactic acid, 1,3-propanediol (1,3-PDO), and 2,3-butanediol (2,3-BDO). Fed-batch cultivation of the strain was to examine the production of 3-HP.
Materials and Methods
Strains, Vectors, and Medium
The recombinant K. pneumoniae strain with dCas9 integrated in genome (KP-dCas9) and plasmid ptac-15A was preserved in laboratory (Zhao et al., 2021). The plv plasmid was provided by Professor George Guoqiang Chen from Tsinghua University (Lv et al., 2015). E. coli Top 10 was purchased from Biomed, China. The recombinant K. pneumoniae strain with dCas9 integrated in genome was used as the host strain for the development of the CRISPRi system and co-overexpression of gpd1 and gpp2 genes for the conversion of glucose-to-glycerol (Supplementary Table S1). E. coli Top 10 was employed for vector construction. The primers used in this study are listed in Supplementary Table S2. S. cerevisiae was used as the donor strain of genes gpd1 and gpp2. The original T7 promoter in vector pET-28A was replaced by the tac promoter, resulting in a vector designated ptac-15A, which was used to overexpress gpd1 and gpp2. In vector construction experiments, E. coli Top 10 was grown in Luria-Bertani (LB) medium containing the following components per liter: NaCl 10 g, tryptone 10 g, yeast extract 5 g, and chloramphenicol 34 mg. In CRISPRi and fermentation experiments, K. pneumoniae strain was grown in fermentation medium containing the following components per liter: glucose, 20 g; (NH4)2SO4, 4 g; K2HPO4·3H2O, 3.4 g; KH2PO4, 1.3 g; MgSO4·7H2O, 0.5 g; yeast extract, 3 g; CaCO3, 0.1 g; 1.25 ml of trace element solution; and chloramphenicol 34 mg. The trace element solution contained the following components per liter: CuCl2·2H2O, 1.88 g; FeSO4, 32 g; CoCl2·6H2O, 1.88 g; ZnCl2·6H2O, 2.72 g; Na2MoO4, 0.02 g; MnCl2·4H2O, 0.68 g; H3BO3, 0.24 g; and 40 ml concentrated HCl. Restriction enzymes, Taq DNA polymerase, and T4 DNA ligase were purchased from New England Biolabs (Beijing, China). Primer synthesis and DNA sequencing were performed by Biomed Co., Ltd. RT-qPCR was completed by RuiBiotech Co., Ltd.
Construction of Recombinants
To overexpress GPD1 and GPP2 in KP-dCas9, their coding genes gpd1 (GenBank accession No. Z74071.1) and gpp2 (GenBank accession No. DQ332890.1) genes were cloned by PCR from S. cerevisiae genome. Next, the gpd1 gene was cloned into ptac-15A at Noc I/Nde I sites, leading to a vector designated ptac-G1; the gpp2 gene was cloned into ptac-15A at Nde I/Nhe I sites, leading to a vector designated ptac-G2. Ligating gpd1 with the tac promoter and the RBS sequence from ptac-G1 to vector ptac-G2 at Not I/Xho I sites led to a vector designated ptac-G12 (Supplementary Figure S2). Transformation of vectors ptac-15A and ptac-G12 into competent KP-dCas9 cells led to recombinant strains KP-15A and KP-G12, respectively (Supplementary Table S1). KP-dCas9 was transformed with the constructed plasmid by electro-transformation (0.2 cm, 2.5 kV, and time duration >0.5 ms). E. coli was transformed with the constructed plasmid by heat shock. Positive clones were confirmed by colony PCR and DNA sequencing.
To develop the CRISPRi system in KP-dCas9, the CRISPRi vectors targeting gapA or budA were constructed by replacement of the sgRNA sequence in vector plv-sgRNA (Supplementary Table S1, plv-gapA-1, plv-gapA-2, plv-gapA-3, plv-budA-1, plv-budA-2, and plv-budA-3). Briefly, two complementary single-stranded target sequences were chemically synthesized and annealed to form a 23 bp double-stranded DNA owning cohesive ends matching the BspQ I-digested vector (Table 1). Subsequent ligation resulted in desired CRISPRi vectors. For each gapA or budA, three candidate guide RNAs targeting the different regions were synthesized to ensure efficient inhibition, and the sgRNA sequences, tet promoter and an RBS sequence from plv-vector were cloned into ptac-G12 at Eag I/Bmt I sites (Supplementary Table S1, ptac-GS, ptac-GG1, ptac-GG2, ptac-GG3, ptac-GB1, ptac-GB2, and ptac-GB3). Transforming vectors ptac-GS, ptac-GG1, ptac-GG2, ptac-GG3, ptac-GB1, ptac-GB2, and ptac-GB3 into competent KP-dCas9 led to recombinant strains KP-GS, KP-GG1, KP-GG2, KP-GG3, KP-GB1, KP-GB2, and KP-GB3, respectively (Supplementary Table S1). Last, RT-qPCR was conducted to determine the most inhibited gene. The best-performing gapA and budA sgRNAs were cloned using isocaudamers NgoM IV and Xma I and then ligated in tandem. The sgRNA expression cassettes were digested by Eag I and NgoM IV, while the plasmid was digested by Eag I and Xma I, leading to vector named ptac-GGB. Transforming the vector ptac-GGB into competent KP-dCas9 led to the recombinant strain KP-GGB (Supplementary Table S1; Supplementary Figure S3).
RT-qPCR Analysis of CRISPR Interference
To examine the transcription levels of gpd1, gpp2, gapA, and budA, RT-qPCR was performed. Briefly, strains including KP-dCas9, KP-G12, KP-GS, KP-GG1, KP-GG2, KP-GG3, KP-GB1, KP-GB2, KP-GB3, and KP-GGB were cultivated in medium for 24 h and harvested by centrifugation at 4°C, 12,000 rpm. Then, the harvested bacteria were lysed with TRIzol reagent. The RNA samples were used as template to synthesize cDNAs through reverse transcription. RT-qPCR was carried out by Applied Biosystems™ 7900HT Fast Real-Time PCR System (Thermo Fisher) with SYBR Green addition. The RT-qPCR data were analyzed using the ΔΔCT method with 16S rRNA as an internal control. All experiments were performed in triplicate.
Shake Flask and Bioreactor Cultivation
In shake flask cultivation, strains KP-dCas9, KP-15A, KP-GS, KP-GG1, KP-GB2, and KP-GGB were pre-cultured in 4 ml LB medium overnight. Next, these strains were independently grown in 250-ml shake flasks each containing 100 ml 3-HP–producing medium and 8.5 mg chloramphenicol at 37°C and 150 rpm. After 3 h cultivation, IPTG at a final concentration of 0.5 mM was added to induce the expression of gpd1 and gpp2. Dehydrated tetracycline (aTc) at a final concentration of 2 μM was added to induce sgRNA expression. Fermentation broth was sampled every 3 h to examine cell growth, glucose consumption, and metabolites formation.
In fed-batch cultivation, the recombinant strain was grown in a 5 L bioreactor (Baoxing, China) containing 3 L fermentation medium to produce 3-HP. The strain KP-GB2 was pre-cultured in 100 ml of LB medium overnight at 37°C and subsequently inoculated into a bioreactor, containing antibiotics and IPTG. Air was supplied at 1.5 vvm, and agitation speed was set at 400 rpm. The temperature was set at 37°C, and the pH was maintained at 7.0 by adding 5 M NaOH. A total of 700 ml of 500 g/L glucose solution was added at a rate of 4.5 g/h to maintain cell growth after the glucose in medium was exhausted. The samples were taken out every 6 h to examine cell growth, glucose consumption, and metabolites formation.
Analytical Methods
Cell concentrations were measured by using microplate reader at 600 nm with 200 μL fermentation broth and 1,800 μL ddH2O added in a cuvette. To measure metabolites, fermentation broth was centrifuged at 12,000 rpm for 10 min to remove bacteria. Major metabolites including 3-HP, lactic acid, and acetic acid in supernatant were analyzed by using the high-performance liquid chromatography (HPLC) system (Shimadzu, Kyoto, Japan) equipped with a C18 column and an SPD-20A UV detector at 210 nm. The column was maintained at 25°C. The mobile phase was 0.05% phosphoric acid at a flow rate of 0.8 ml/min. 1,3-PDO and 2,3-BDO were analyzed by GC (Persee). Analytically pure 1,3-PDO and 2,3-BDO were used as standard for quantification. Residual glucose concentration was measured by an SBA-40E biosensor analyzer (Biology Institute of Shandong Academy of Science, China). A new immobilized enzyme membrane was installed on the electrode, 500 ml standard buffer was prepared with ddH2O, and the three-in-one standard solution of SBA was prepared (three materials mentioned earlier are provided by the instrument manufacturer). Glucose concentration in the supernatant was diluted to less than 1 g/L. After 25 μL standard solution was injected into the sampling port stabilization instrument with a microinjector, the glucose content in the sample was measured successively, and each sample was measured three times. Glycerol concentration was measured by the glycerin test kit (APPLYGEN Beijing). All samples were filtered through a 0.22 μm membrane filter.
Result
Determination of Plasmids
The genes gpd1 and gpp2 were cloned from S. cerevisiae and independently subcloned into vector ptac-15A, resulting in vectors ptac-G1 and ptac-G2, respectively (Figures 2A–C). Next, PCR was conducted to obtain ptac-G1 as template, gpd1 fragment, tac promoter, and operon (Figure 2D). These fragments were then inserted into ptac-G2 to construct ptac-G12 (Figure 2E). The two genes gpd1 and gpp2 were expressed using respective tac promoter, and their expression was induced by IPTG.
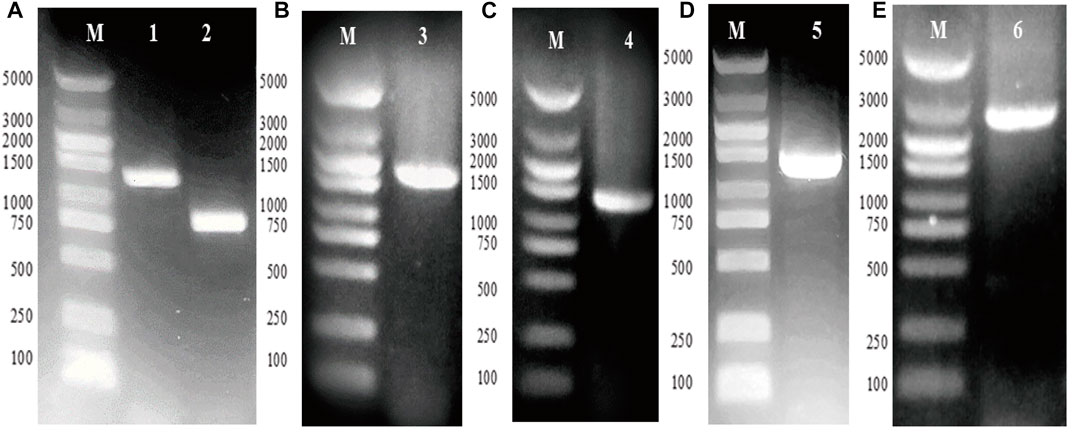
FIGURE 2. Construction of plasmid ptac-G12 that co-expressing gpd1 and gpp2 under tac promoter. G1 denotes gpd1 gene encoding glycerol 3-phosphate dehydrogenase; G2 denotes gpp2 gene encoding glycerol 3-phosphatase; and G12 denotes co-expression of gpd1 and gpp2. (A) PCR amplification of the gpd1 and gpp2 genes from S. cerevisiae. (B) Colony PCR of plasmid ptac-gpd1. (C) Colony PCR of plasmid ptac-gpp2. (D) PCR amplification of ptac-gpd1 expression cassette. (E) Colony PCR of gpd1 and gpp2. M, Marker; lane 1, gpd1 gene; lane 2, gpp2 gene; lane 3, plasmid ptac-gpd1 expression cassette; lane 4, plasmid ptac-gpp2 expression cassette; lane 5, plasmid ptac-gpd1 expression cassette; and lane 6, colony PCR of the co-expressed gpd1 and gpp2 in tandem.
Glycerol Production by Engineered K. pneumoniae
The recombinant K. pneumoniae strain was cultured in a shake flask to verify glycerol production. To construct glucose-to-glycerol pathway, gpd1 and gpp2 were co-expressed in strain KP-dCas9, which harbored the engineered CRISPRi system. In doing so, we constructed a recombinant strain named KP-G12, and the strains KP-15A and KP-dCas9 were used as controls. RT-qPCR results showed that the transcription levels of gpd1 and gpp2 were significantly increased in KP-G12 compared to KP-dCas9 (Figures 3A,B). In 24 h cultivation, glucose consumption was slowed down due to the expression of heterologous genes (Figure 3C), and the strain KP-G12 showed a significant decrease in OD600 (Figure 3D). The strain containing vector ptac-G12 produced 2 g/L glycerol, with 0.1 g/g yield on glycerol (g of glucose)−1. In contrast, no glycerol was produced by strains KP-dCas9 and KP-15A (Figure 3E,F), indicating that gpd1 and gpp2 catalyzed glucose into glycerol in K. pneumoniae.
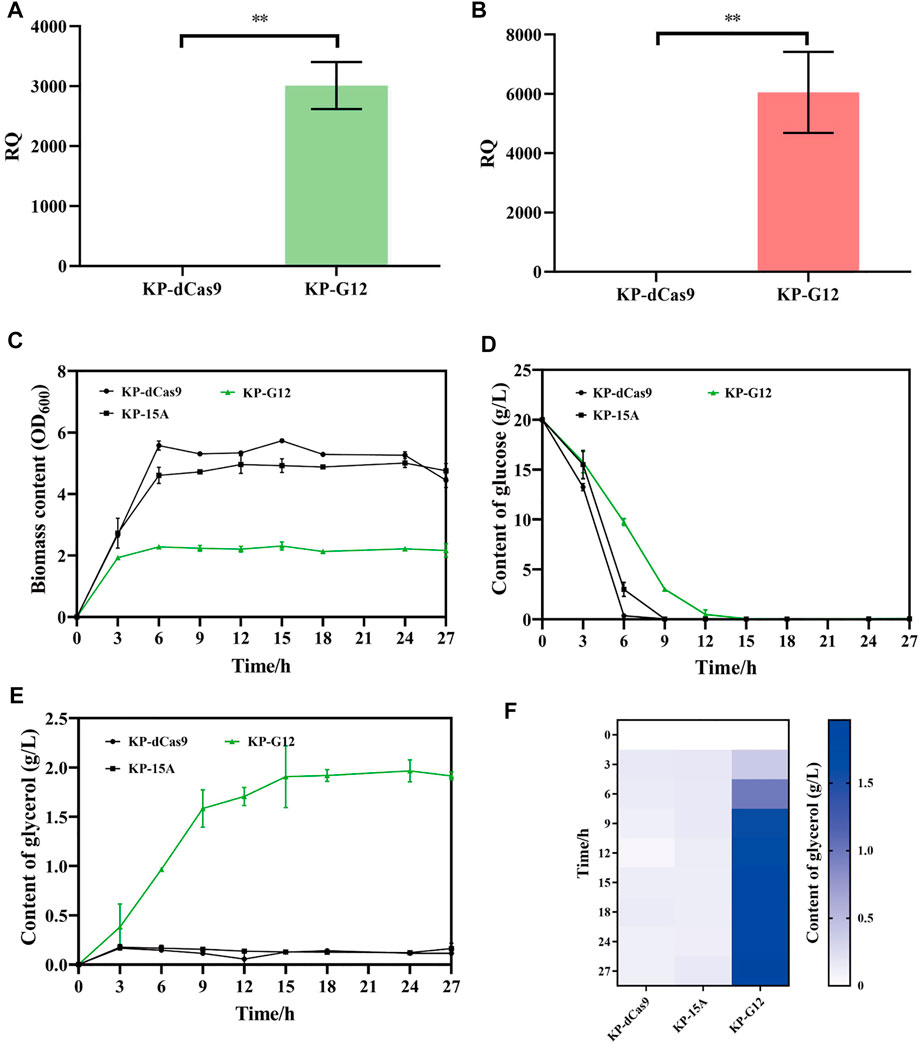
FIGURE 3. Performance of strains in shake flasks. (A) Relative quantity of gpd1 gene transcription. (B) Relative quantity of gpp2 gene transcription. KP-dCas9 indicates the recombinant K. pneumoniae with dCas9 integrated in genome, and its 16S rRNA serves as the internal reference gene. (C) Glucose level after 24 h fermentation. (D) OD600 after 24 h fermentation. (E) Line chart represents the glycerol level. (F) Heatmap of the glycerol level. KP-dCas9 indicates the recombinant K. pneumoniae with dCas9 integrated in genome. KP-15A indicates the recombinant K. pneumoniae harboring plasmid ptac-15A and serves as a control. KP-G12 indicates the recombinant K. pneumoniae harboring plasmid ptac-G12 co-expressing gpd1 and gpp2 genes. RQ, relative quantity of transcription. *p < 0.05 and **p < 0.01.
CRISPR Interference System Inhibiting EMP Downstream Pathway and 2,3-BDO Production
To optimize the CRISPRi system, we prepared several guide RNAs named G1, G2, G3, B1, B2, and B3 that target the different regions of gapA and budA (Supplementary Figures S1A,B,G). The aforementioned six sgRNAs and one non-target sgRNA were independently ligated to vector ptac-G12 (Supplementary Figures S1C,D; Figure 4A). Transforming these vectors into KP-dCas9 led to recombinant strains KP-GG1, KP-GG2, KP-GG3, KP-GB1, KP-GB2, KP-GB3, and KP-GS, respectively. The strain KP-GS was used as the control. RT-qPCR results showed that compared to KP-GS, the strains KP-GG1 and KP-GB2 showed significant decrease in the expression of target genes (Figures 4A,B). The gapA gene in strain KP-GG1was transcriptionally inhibited by 60%, and the budA gene in strain KP-GB2 was transcriptionally inhibited by 54%. When budA-2 was connected in series with gapA-1, gpd1, and gpp2 (Supplementary Figures S1E,F), the resulting plasmid was named GGB, and the corresponding recombinant strain was named KP-GGB. In strain KP-GGB, the inhibitory rates of the CRISPRi system on genes gapA and budA reached 82 and 24%, respectively.
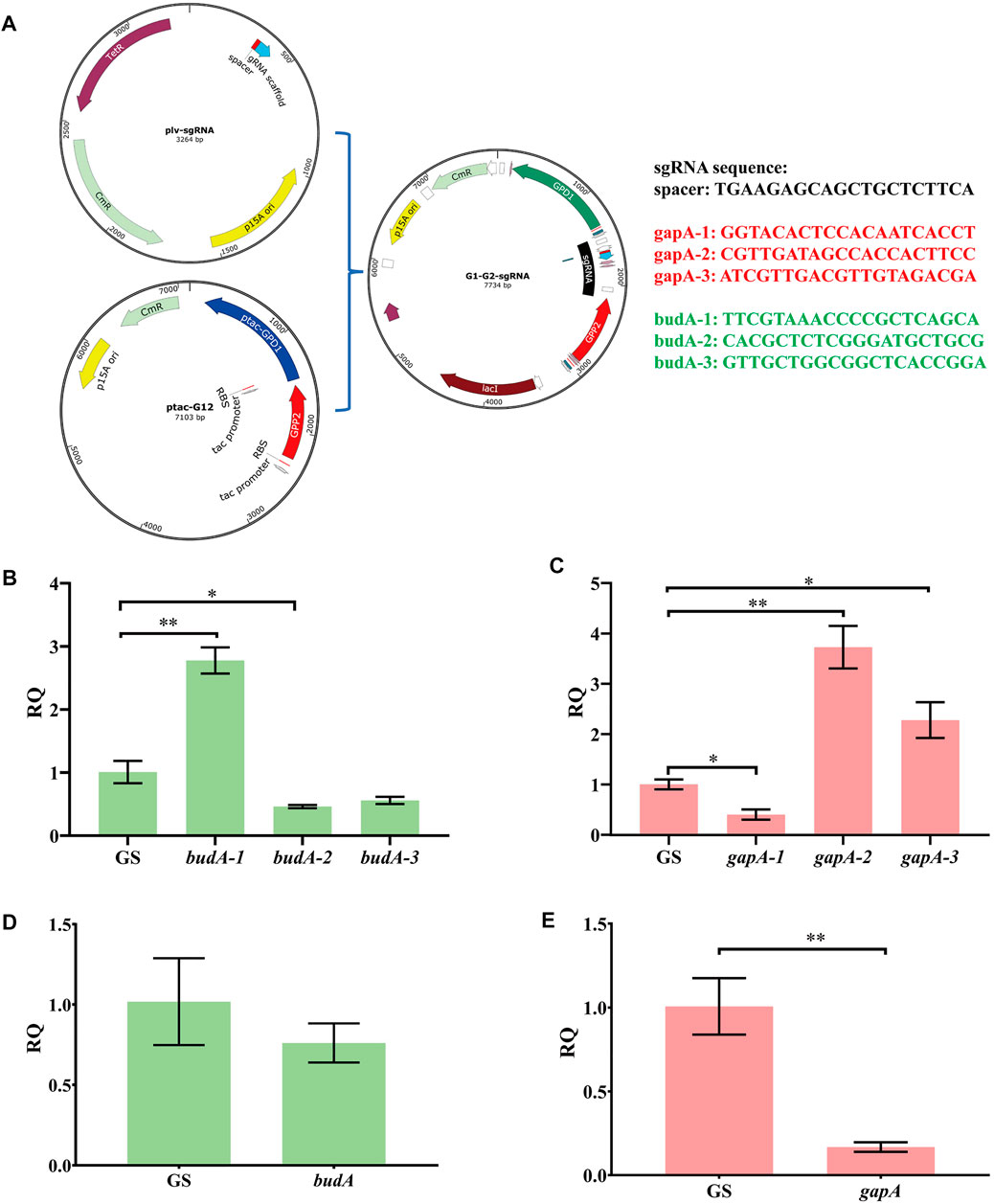
FIGURE 4. Relative transcription quantity of K. pneumoniae with dCas9 integrated in genome and an engineered CRISPRi system. (A) Schematic diagram of linking sgRNA to plasmid ptac-G12 co-expressing gpd1 and gpp2. (B) RT-qPCR analysis of CRISPRi inhibition on different regions of the budA gene. budA-1, budA-2, and budA-3 represent different regions of budA gene. GS, recombinant K. pneumoniae harboring the non-targeting CRISPRi system. (C) RT-qPCR analysis of CRISPRi inhibition on different regions of gapA gene. gapA-1, gapA-2, and gapA-3 represent different regions of the budA gene. (D) RT-qPCR analysis of transcription inhibition on the budA gene by the CRISPRi system carrying the sgRNAs for both budA and gapA. (E) RT-qPCR analysis of transcription inhibition on the gapA gene by the CRISPRi system carrying the sgRNAs for both budA and gapA. gapA-1, gapA-2, and gapA-3 denote different regions of the gapA gene. RQ, relative quantity of transcription. *p < 0.05 and **p < 0.01.
Shake Flask Cultivation of the CRISPR Interference Strains
To maximize 3-HP and minimize 2,3-BDO, the glycerol synthesis genes gpd1 and gpp2 were co-expressed, and two genes gapA and budA were inhibited by the CRISPRi system. RT-qPCR results showed that the strains KP-GG1, KP-GB2, and KP-GGB demonstrated the strongest CRISPR inhibition under micro-oxygen conditions as compared to the strains KP-GS, KP-dCas9, and KP-15A. In 24 h cultivation, these engineered strains showed retarded cell growth and reduced glucose consumption. All engineered K. pneumoniae strains produced glycerol, but the difference was not significant. For 2,3-BDO production, the strain KP-15A presented the highest titer (8.39 ± 0.33 g/L), while the strain KP-GB2 presented the lowest titer (1.185 ± 0.045 g/L). In addition, the strain KP-GB2 produced the highest 3-HP (0.734 ± 0.032 g/L). Interestingly, no significant differences were observed in the production of acetic acid, lactic acid, and 1,3-PDO in all strains.
Fed-Batch Cultivation of CRISPR Interference Strains
Now that the strain co-expressing gpd1 and gpp2 was able to synthesize glycerol from glucose and the strain KP-GB2 showed the highest 3-HP level in the shake flask, the strain was then cultivated in a 5 L bioreactor. Results showed that the strain did not consume glycerol until 12 h fermentation, at this time the glucose was exhausted. When glucose was supplemented, the glycerol level was further improved, indicating that the strain did not consume glycerol until glucose was exhausted. In addition, only a small amount of 2,3-BDO was produced in the initial stage of fermentation. However, in the middle and late stages of fermentation, 2,3-BDO production was increased presumably due to the rising pH value of medium and the failure of aTc induction in an alkaline environment, which hampered the inhibition of the CRISPRi system on by-product pathways. Due to the engineered glycerol synthesis pathway, the strain KP-GB2 produced 1.77 ± 0.04 g/L 3-HP in 72 h, with productivity of 0.025 g/L/h. This low productivity may be ascribed to the engineered CRISPRi system and genes gpd1 and gpp2, which imposed a heavy burden on cell growth. Indeed, the maximum OD600 was only 12.135 ± 0.005.
Discussion
In this study, the glycerol synthesis pathway was engineered in K. pneumoniae, and the resulting strain was subjected to CRISPRi-dependent gene regulation (Figure 2). By co-expressing gpd1 and gpp2 and simultaneously inhibiting gapA and budA, the levels of both glycerol and 3-HP were improved (Figure 4, Figure 5). In addition, the by-products and glucose flux in the EMP pathway were reduced. The aforementioned results confirmed the engineered glucose to the 3-HP pathway in K. pneumoniae. In K. pneumoniae, glycerol-based 3-HP synthesis requires only two reactions, and the theoretical conversion rate is therefore high. After introducing gpd1 and gpp2 into K. pneumoniae, glucose-based production of 3-HP requires only four enzyme genes including gpd, gpp2, gdh, and aldH (e.g., puuC). As for the low 3-HP production, it was ascribed to the presence of glucose. In particular, the Crabtree effect hindered glycerol utilization. Future studies may focus on how to attenuate the Crabtree effect. To address this issue, an orthogonal expression system could be engineered to express glycerol utilization genes and minimize the Crabtree effect so that glycerol can be efficiently converted to 3-HP while cell growth is not compromised. Clearly, profound understanding of the dha regulon and Crabtree effect is a prerequisite for achieving this goal.
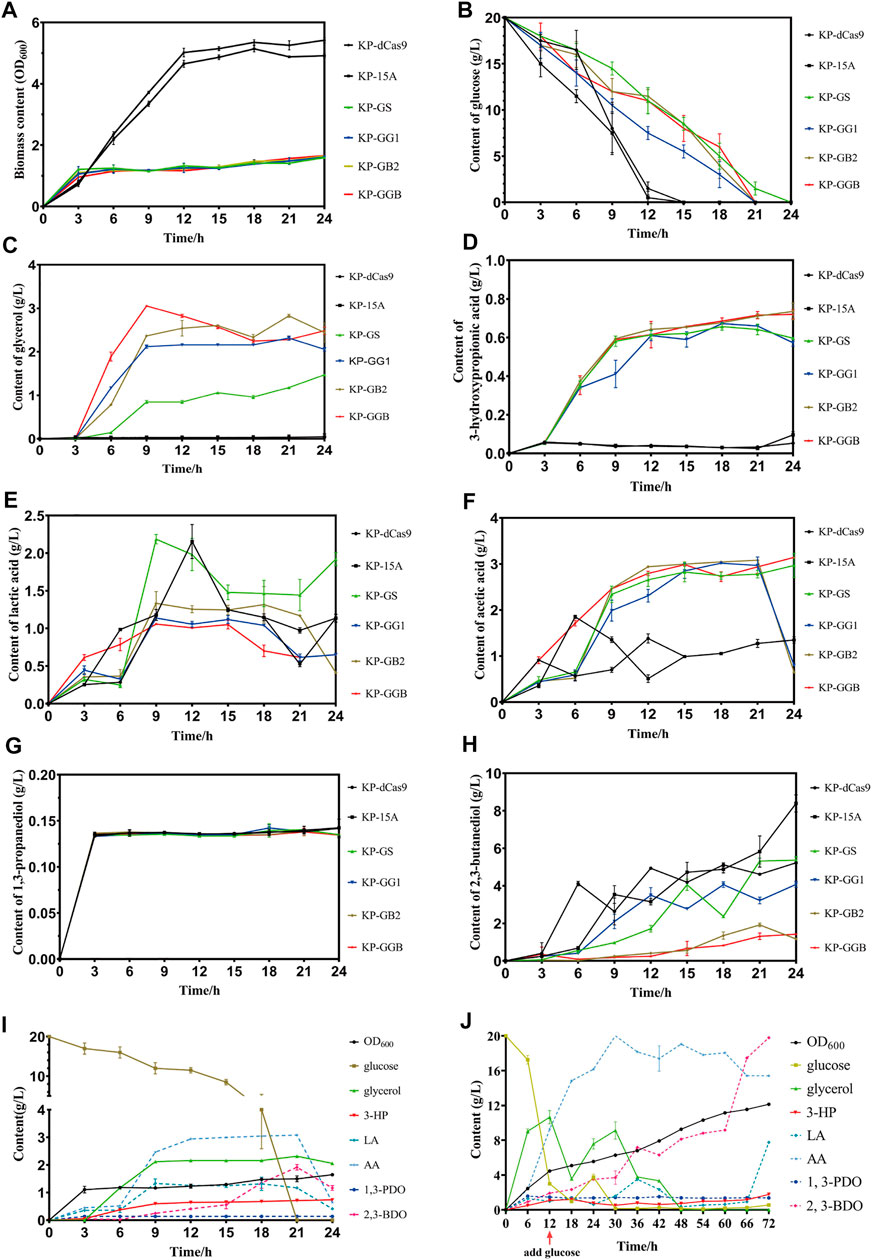
FIGURE 5. Fermentation of strain harboring CRISPRi system to repress by-product pathways. (A) Biomass (OD600) of the CRISPRi strain in the shake flask. (B) Glucose consumption of CRISPRi strain in the shake flask. (C) Glycerol production by CRISPRi strain in the shake flask. (D) 3-Hydroxypropionic acid production by CRISPRi strain in the shake flask. (E) Lactic acid production by CRISPRi strain in the shake flask. (F) Acetic acid production by CRISPRi strain in the shake flask. (G) 1,3-Propanediol production of CRISPRi strain in the shake flask. (H) 2,3-Butanediol production by CRISPRi strain in the shake flask. (I) Metabolites of CRISPRi strain KP-GB2 in the shake flask. (J) Metabolites of CRISPRi strain KP-GB2 in fed-batch culture. KP-dCas9, the recombinant K. pneumoniae strain with dCas9 integrated in genome; KP-15A, the recombinant K. pneumoniae strain harboring empty vector ptac-15A; KP-GS, the recombinant K. pneumoniae with dCas9 integrated in genome, and harboring vector ptac-GS co-expressing non-target sgRNA, gpd1 and gpp2; KP-GG1, recombinant K. pneumoniae with dCas9 integrated in genome of and ptac-GG1 plasmid co-expressing gpd1, gpp2, and gapA-targeting sgRNA; KP-GB2, the recombinant K. pneumoniae with dCas9 integrated in genome and vector ptac-GB2 co-expressing gpd1, gpp2, and budA-targeting sgRNA; KP-GGB, the recombinant K. pneumoniae with dCas9 integrated in genome and plasmid ptac-GGB co-expressing gpd1, gpp2, and sgRNAs targeting gapA and budA. 3-HP, 3-hydroxypropionic acid; LA, lactic acid; AA, acetic acid; 1,3-PDO, 1,3-propanediol; and 2,3-BDO, 2,3-butanediol.
The Crabtree effect and Pasteur effect (Winkler et al., 2003) are two important regulatory mechanisms affecting glucose metabolism. The Crabtree effect is a common problem encountered by mixed carbon source fermentation. Removal of the Crabtree effect enables bacteria to co-utilize glucose and other carbon sources. The Pasteur effect entangles the choice of aeration during fermentation. Aerobic respiration increases bacterial growth but reduces the activity of phosphofructokinase through feedback inhibition, thereby inhibiting the EMP pathway. In fed-batch cultivation, it is necessary to maintain different oxygen levels in bioreactor for different purposes. If the Crabtree effect can be attenuated and aeration can be adjusted in fed-batch cultivation, the strain will use glucose as a carbon source for growth, which will in turn improve the production of glycerol and 3-HP. However, it is extremely challenging to completely decouple glucose pathways from glycerol pathways, and co-utilization of different carbon sources may be feasible to maintain active cell growth.
CRISPRi is usually not lethal to host cell, as it does not digest DNA. In contrast, gene knockout tools such as CRISPR editing, RecA homologous recombination, and Red homologous recombination are in most cases lethal to host cells. In present study, CRISPRi was developed to inhibit gapA, an enzyme catalyzes 3-glyceraldehyde triphosphate into 1,3-diphosphoglyceric acid. CRISPRi was also used to inhibit budA, an enzyme required for 2,3-BDO production in K. pneumoniae (Yang et al., 2014). RT-qPCR results showed that the engineered CRISPRi systems could significantly inhibit the expression of budA and gapA. In shake flask cultivation, the CRISPRi strain demonstrated slight enhancement in the glycerol level but significant decrease in 2,3-BDO production. However, the inhibitory effect on budA was significantly reduced when two groups of sgRNAs were linked in tandem. This might be ascribed to the low expression of dCas9, which was insufficient for binding two target genes simultaneously. Plasmid-based overexpression of dCas9 and multi-copy integration of dCas9 into genome may be feasible solutions. Overall, this study demonstrated the feasibility of glucose-to-glycerol as well as CRISPRi-dependent gene regulation in K. pneumoniae. We anticipate that better understanding of the metabolic networks is crucial for high-level production of 3-HP, 1,3-PDO, 2,3-BDO, and beyond.
Data Availability Statement
The original contributions presented in the study are included in the article/Supplementary Material; further inquiries can be directed to the corresponding author.
Author Contributions
PT conceived the protocol. HL and PZ performed experiments. HL and PT wrote the manuscript. All authors read and approved the manuscript.
Funding
This study was funded by grants from the National Key Research and Development Program of China (2018YFA0901800) and Hebei Program for the Development of Science and Technology Guided by Central Universities (206Z2902G).
Conflict of Interest
The authors declare that the research was conducted in the absence of any commercial or financial relationships that could be construed as a potential conflict of interest.
Publisher’s Note
All claims expressed in this article are solely those of the authors and do not necessarily represent those of their affiliated organizations, or those of the publisher, the editors, and the reviewers. Any product that may be evaluated in this article, or claim that may be made by its manufacturer, is not guaranteed or endorsed by the publisher.
Acknowledgments
We thank Professor Guoqiang Chen in Tsinghua University for providing CRISPRi plasmid plv.
Supplementary Material
The Supplementary Material for this article can be found online at: https://www.frontiersin.org/articles/10.3389/fbioe.2022.908431/full#supplementary-material
References
Ashok, S., Raj, S. M., Rathnasingh, C., and Park, S. (2011). Development of Recombinant Klebsiella pneumoniae dhaT Strain for the Co-production of 3-hydroxypropionic Acid and 1,3-Propanediol from Glycerol. Appl. Microbiol. Biotechnol. 90, 1253–1265. doi:10.1007/s00253-011-3148-z
Chen, Y., Bao, J., Kim, I.-K., Siewers, V., and Nielsen, J. (2014). Coupled Incremental Precursor and Co-factor Supply Improves 3-Hydroxypropionic Acid Production in Saccharomyces cerevisiae. Metab. Eng. 22, 104–109. doi:10.1016/j.ymben.2014.01.005
Chen, Z., Huang, J., Wu, Y., Wu, W., Zhang, Y., and Liu, D. (2017). Metabolic Engineering of Corynebacterium Glutamicum for the Production of 3-hydroxypropionic Acid from Glucose and Xylose. Metab. Eng. 39, 151–158. doi:10.1016/j.ymben.2016.11.009
de Fouchecour, F., Sanchez-Castaneda, A. K., Saulou-Berion, C., and Spinnler, H. E. (2018). Process Engineering for Microbial Production of 3-hydroxypropionic Acid. Biotechnol. Adv. 36, 1207–1222. doi:10.1016/j.biotechadv.2018.03.020
Della Pina, C., Falletta, E., and Rossi, M. (2011). A Green Approach to Chemical Building Blocks. The Case of 3-hydroxypropanoic Acid. Green Chem. 13, 1624–1632. doi:10.1039/c1gc15052a
Heo, W., Kim, J. H., Kim, S., Kim, K. H., Kim, H. J., and Seo, J.-H. (2019). Enhanced Production of 3-hydroxypropionic Acid from Glucose and Xylose by Alleviation of Metabolic Congestion Due to Glycerol Flux in Engineered Escherichia coli. Bioresour. Technol. 285, 121320. doi:10.1016/j.biortech.2019.121320
Jakočiūnas, T., Bonde, I., Herrgård, M., Harrison, S. J., Kristensen, M., Pedersen, L. E., et al. (2015). Multiplex Metabolic Pathway Engineering Using CRISPR/Cas9 in Saccharomyces cerevisiae. Metab. Eng. 28, 213–222. doi:10.1016/j.ymben.2015.01.008
Jers, C., Kalantari, A., Garg, A., and Mijakovic, I. (2019). Production of 3-hydroxypropanoic Acid from Glycerol by Metabolically Engineered Bacteria. Front. Bioeng. Biotechnol. 7, 124. doi:10.3389/fbioe.2019.00124
Joseph, E., Danchin, A., and Ullmann, A. (1981). Regulation of Galactose Operon Expression: Glucose Effects and Role of Cyclic Adenosine 3',5'-monophosphate. J. Bacteriol. 146, 149–154. doi:10.1128/jb.146.1.149-154.1981
Kildegaard, K. R., Jensen, N. B., Schneider, K., Czarnotta, E., Özdemir, E., Klein, T., et al. (2016). Engineering and Systems-Level Analysis of Saccharomyces cerevisiae for Production of 3-Hydroxypropionic Acid via Malonyl-CoA Reductase-Dependent Pathway. Microb. Cell Fact. 15, 53. doi:10.1186/s12934-016-0451-5
Ko, Y., Ashok, S., Zhou, S., Kumar, V., and Park, S. (2012). Aldehyde Dehydrogenase Activity Is Important to the Production of 3-hydroxypropionic Acid from Glycerol by Recombinant Klebsiella pneumoniae. Process Biochem. 47, 1135–1143. doi:10.1016/j.procbio.2012.04.007
Kumar, V., and Park, S. (2018). Potential and Limitations of Klebsiella pneumoniae as a Microbial Cell Factory Utilizing Glycerol as the Carbon Source. Biotechnol. Adv. 36, 150–167. doi:10.1016/j.biotechadv.2017.10.004
Li, Y., Wang, X., Ge, X., and Tian, P. (2016). High Production of 3-hydroxypropionic Acid in Klebsiella pneumoniae by Systematic Optimization of Glycerol Metabolism. Sci. Rep. 6, 26932. doi:10.1038/srep26932
Li, Q., Zhao, P., Yin, H., Liu, Z., Zhao, H., and Tian, P. (2020). CRISPR Interference-Guided Modulation of Glucose Pathways to Boost Aconitic Acid Production in Escherichia coli. Microb. Cell Fact. 19, 174. doi:10.1186/s12934-020-01435-9
Liu, K., Yuan, X., Liang, L., Fang, J., Chen, Y., He, W., et al. (2019). Using CRISPR/Cas9 for Multiplex Genome Engineering to Optimize the Ethanol Metabolic Pathway in Saccharomyces cerevisiae. Biochem. Eng. J. 145, 120–126. doi:10.1016/j.bej.2019.02.017
Luo, L. H., Seo, J.-W., Baek, J.-O., Oh, B.-R., Heo, S.-Y., Hong, W.-K., et al. (2011). Identification and Characterization of the Propanediol Utilization Protein PduP of Lactobacillus Reuteri for 3-hydroxypropionic Acid Production from Glycerol. Appl. Microbiol. Biotechnol. 89, 697–703. doi:10.1007/s00253-010-2887-6
Lv, L., Ren, Y.-L., Chen, J.-C., Wu, Q., and Chen, G.-Q. (2015). Application of CRISPRi for Prokaryotic Metabolic Engineering Involving Multiple Genes, a Case Study: Controllable P(3HB-co-4HB) Biosynthesis. Metab. Eng. 29, 160–168. doi:10.1016/j.ymben.2015.03.013
Matsakas, L., Hrůzová, K., Rova, U., and Christakopoulos, P. (2018). Biological Production of 3-hydroxypropionic Acid: An Update on the Current Status. Fermentation 4, 13. doi:10.3390/fermentation4010013
Mohan Raj, S., Rathnasingh, C., Jung, W.-C., and Park, S. (2009). Effect of Process Parameters on 3-hydroxypropionic Acid Production from Glycerol Using a Recombinant Escherichia coli. Appl. Microbiol. Biotechnol. 84, 649–657. doi:10.1007/s00253-009-1986-8
Nguyen-Vo, T. P., Ainala, S. K., Kim, J.-R., and Park, S. (2018). Analysis and Characterization of Coenzyme B-12 Biosynthetic Gene Clusters and Improvement of B-12 Biosynthesis in Pseudomonas Denitrificans ATCC 13867. Fems Microbiol. Lett. 365 (21), 1–7. doi:10.1093/femsle/fny211
Qi, L. S., Larson, M. H., Gilbert, L. A., Doudna, J. A., Weissman, J. S., Arkin, A. P., et al. (2013). Repurposing CRISPR as an RNA-Guided Platform for Sequence-specific Control of Gene Expression. Cell 152, 1173–1183. doi:10.1016/j.cell.2013.02.022
Raj, S. M., Rathnasingh, C., Jung, W.-C., Selvakumar, E., and Park, S. (2010). A Novel NAD(+)-dependent Aldehyde Dehydrogenase Encoded by the puuC Gene of Klebsiella pneumoniae DSM 2026 that Utilizes 3-Hydroxypropionaldehyde as a Substrate. Biotechnol. Bioproc E 15, 131–138. doi:10.1007/s12257-010-0030-2
Semkiv, M. V., Dmytruk, K. V., Abbas, C. A., and Sibirny, A. A. (2017). Metabolic Engineering for High Glycerol Production by the Anaerobic Cultures of Saccharomyces cerevisiae. Appl. Microbiol. Biotechnol. 101, 4403–4416. doi:10.1007/s00253-017-8202-z
Straathof, A. J. J., Sie, S., Franco, T. T., and Van Der Wielen, L. A. M. (2005). Feasibility of Acrylic Acid Production by Fermentation. Appl. Microbiol. Biotechnol. 67, 727–734. doi:10.1007/s00253-005-1942-1
Wang, J., Zhao, P., Li, Y., Xu, L., and Tian, P. (2018). Engineering CRISPR Interference System in Klebsiella pneumoniae for Attenuating Lactic Acid Synthesis. Microb. Cell Fact. 17, 56. doi:10.1186/s12934-018-0903-1
Winkler, B. S., Sauer, M. W., and Starnes, C. A. (2003). Modulation of the Pasteur Effect in Retinal Cells: Implications for Understanding Compensatory Metabolic Mechanisms. Exp. Eye Res. 76, 715–723. doi:10.1016/s0014-4835(03)00052-6
Xu, X., and Qi, L. S. (2019). A CRISPR-dCas Toolbox for Genetic Engineering and Synthetic Biology. J. Mol. Biol. 431, 34–47. doi:10.1016/j.jmb.2018.06.037
Yang, T. H., Rathnasingh, C., Lee, H. J., and Seung, D. (2014). Identification of Acetoin Reductases Involved in 2,3-butanediol Pathway in Klebsiella Oxytoca. J. Biotechnol. 172, 59–66. doi:10.1016/j.jbiotec.2013.12.007
Zhao, P., and Tian, P. (2021). Biosynthesis Pathways and Strategies for Improving 3-hydroxypropionic Acid Production in Bacteria. World J. Microbiol. Biotechnol. 37, 117. doi:10.1007/s11274-021-03091-6
Zhao, P., Ma, C., Xu, L., and Tian, P. (2019). Exploiting Tandem Repetitive Promoters for High-Level Production of 3-hydroxypropionic Acid. Appl. Microbiol. Biotechnol. 103, 4017–4031. doi:10.1007/s00253-019-09772-5
Zhao, P., Li, Q., Tian, P., and Tan, T. (2021). Switching Metabolic Flux by Engineering Tryptophan Operon-Assisted CRISPR Interference System in Klebsiella pneumoniae. Metab. Eng. 65, 30–41. doi:10.1016/j.ymben.2021.03.001
Keywords: Klebsiella pneumoniae, glucose, glycerol, 3-hydroxypropionic acid, CRISPR interference
Citation: Liu H, Zhao P and Tian P (2022) Engineering Glucose-to-Glycerol Pathway in Klebsiella pneumoniae and Boosting 3-Hydroxypropionic Acid Production Through CRISPR Interference. Front. Bioeng. Biotechnol. 10:908431. doi: 10.3389/fbioe.2022.908431
Received: 30 March 2022; Accepted: 23 May 2022;
Published: 30 June 2022.
Edited by:
Guang Zhao, Shandong University, ChinaReviewed by:
Fei Tao, Shanghai Jiao Tong University, ChinaMingfeng Cao, Xiamen University, China
Zhen Kang, Jiangnan University, China
Copyright © 2022 Liu, Zhao and Tian. This is an open-access article distributed under the terms of the Creative Commons Attribution License (CC BY). The use, distribution or reproduction in other forums is permitted, provided the original author(s) and the copyright owner(s) are credited and that the original publication in this journal is cited, in accordance with accepted academic practice. No use, distribution or reproduction is permitted which does not comply with these terms.
*Correspondence: Pingfang Tian, dGlhbnBmQG1haWwuYnVjdC5lZHUuY24=