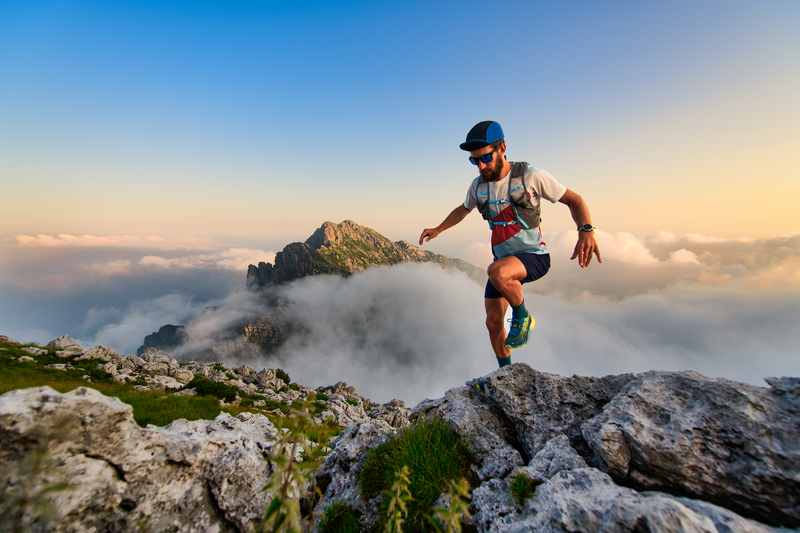
95% of researchers rate our articles as excellent or good
Learn more about the work of our research integrity team to safeguard the quality of each article we publish.
Find out more
REVIEW article
Front. Bioeng. Biotechnol. , 08 June 2022
Sec. Synthetic Biology
Volume 10 - 2022 | https://doi.org/10.3389/fbioe.2022.907861
This article is part of the Research Topic Insights in Synthetic Biology 2021: Novel Developments, Current Challenges, and Future Perspectives View all 16 articles
Engineering microorganisms to grow on alternative feedstocks is crucial not just because of the indisputable biotechnological applications but also to deepen our understanding of microbial metabolism. One-carbon (C1) substrate metabolism has been the focus of extensive research for the prominent role of C1 compounds in establishing a circular bioeconomy. Methanol in particular holds great promise as it can be produced directly from greenhouse gases methane and carbon dioxide using renewable resources. Synthetic methylotrophy, i.e. introducing a non-native methanol utilization pathway into a model host, has therefore been the focus of long-time efforts and is perhaps the pinnacle of metabolic engineering. It entails completely changing a microorganism’s lifestyle, from breaking up multi-carbon nutrients for growth to building C-C bonds from a single-carbon molecule to obtain all metabolites necessary to biomass formation as well as energy. The frontiers of synthetic methylotrophy have been pushed further than ever before and in this review, we outline the advances that paved the way for the more recent accomplishments. These include optimizing the host’s metabolism, “copy and pasting” naturally existing methylotrophic pathways, “mixing and matching” enzymes to build new pathways, and even creating novel enzymatic functions to obtain strains that are able to grow solely on methanol. Finally, new approaches are contemplated to further advance the field and succeed in obtaining a strain that efficiently grows on methanol and allows C1-based production of added-value compounds.
Synthetic methylotrophy refers to the design and engineering of methanol assimilation pathways into established non-methylotrophic production hosts making use of their vast biotechnological potential (Becker et al., 2015) and providing access to methanol as feedstock.
To date, attempts to introduce methylotrophy into biotechnologically relevant microbes have been described for Escherichia coli (Muller et al., 2015; Whitaker et al., 2017), Corynebacterium glutamicum (Lessmeier et al., 2015; Witthoff et al., 2015), Pseudomonas putida (Koopman et al., 2009), Saccharomyces cerevisiae (Dai et al., 2017) and Yarrowia lipolytica (Vartiainen et al., 2019). Efforts to engineer this non-native nutrient catabolism have relied on four different levels of engineering (Erb et al., 2017). In the first level, existing pathways are used to allow methanol assimilation in the non-methylotrophic host (Espinosa et al., 2020). In the next level, synthetic methylotrophs were obtained by “copying and pasting” naturally occurring methanol assimilation pathways (Muller, et al., 2015; Dai, et al., 2017). In the third level, synthetic methanol assimilation pathways were developed by “mixing and matching” enzymes from these methanol assimilation pathways (De Simone et al., 2020). In the last level, novel methanol assimilation pathways were created from known or new enzyme mechanisms (Siegel et al., 2015). However, none of these synthetic strains was able to grow on methanol alone.
If the implementation of methylotrophy in non-native methylotrophs can be seen as a quite straightforward approach, researchers quickly realised that the complexity of methylotrophy could not be reduced to a simple metabolic transplant. In contrast with heterotrophic metabolism where multi-carbon substrates are essentially broken down to obtain metabolites and biomass building blocks, in methylotrophy all carbon-carbon bonds essential to life must be built from a single carbon (C1) molecule. Furthermore, in all these implemented pathways carbon is assimilated in the form of formaldehyde, a central but highly toxic intermediate that can lead to cell death in case of imbalance between dissimilation and assimilation. In the engineering strategies based on naturally occurring methanol assimilation pathways, formaldehyde assimilation is achieved by a cyclic process and requires a C1-acceptor that enables the formation of a C-C bond (Wendisch et al., 2021). The efficiency of methanol assimilation is then determined by the capability of the cells to produce and regenerate the C1-acceptor, especially when C1 is assimilated as formaldehyde. In contrast, the novel pathways, created from novel reactions, are linear and thus independent from any C1-acceptor. However, as formaldehyde is the only intermediate, a high concentration of formaldehyde is required to support a sustainable rate of formaldehyde condensation that enables the formation of a C-C bond. The efficiency of methanol assimilation is then determined by the capability of the cells to tolerate a higher level of formaldehyde. To overcome these obstacles, different rational and evolutionary engineering strategies have been applied during the last decade. The ultimate growth phenotype was recently achieved by Chen et al., 2020 and Kim et al., 2020 who observed growth on methanol as the sole carbon source by a synthetic methylotrophic E. coli (Chen et al., 2020; Kim et al., 2020). This review proposes to present the various strategies implemented to reprogram the food diet of non-methylotrophic production hosts.
In this approach, existing pathways within the natural host are engineered (i.e. through gene deletion or overexpression) to improve their overall capacity towards a given function. A few studies have followed this approach to implement synthetic methylotrophy.
In S. cerevisiae, when grown with methanol (plus yeast extract), the final optical density increased by 39% relative to medium without methanol. In addition, 13C from labeled methanol was incorporated into intracellular metabolites (60% of the acetyl-coA pool was fully labeled). Based on these observations, Espinosa et al. decided to further boost the assimilation of methanol in S. cerevisiae by adaptive laboratory evolution (ALE). Genomic analysis of the resulting evolved strains helped in the identification of beneficial mutations allowing improvement of methanol utilization. These mutations were implemented in a wild type strain and the reconstructed strain exhibited a higher biomass yield on methanol (a 21% increase in OD600) than the parental strain. In addition, a 13C-methanol tracer analysis revealed that the reconstructed strain had a higher percentage of fully 13C-labelled intracellular metabolites (33% of fructose-1,6-bisphosphate and 60% of acetyl-CoA fully labelled) compared with the parental strain (Espinosa, et al., 2020).
It is interesting to notice that other non-methylotrophic microorganisms possess endogenous enzymes that may confer a native capacity for methylotrophy. Endogenous methanol oxidizing activities, which may stem from promiscuous alcohol dehydrogenases, have been observed in Y. lipolitica (Koopman, et al., 2009) and P. putida (Vartiainen, et al., 2019). Bacillus subtilis possesses a Ribulose Monophosphate (RuMP) pathway which may act as a detoxification system for formaldehyde (Yasueda et al., 1999). However, no studies report the use of ALE to optimize the native methylotrophic metabolism of those microorganisms.
In this more advanced approach, naturally occurring metabolic pathways for methanol assimilation are implemented in another host and optimized by shaping the metabolic network of the host to fit the acquired property. Aerobic natural methylotrophy is supported by four different metabolic pathways found among methylotrophic bacteria and yeast: the RuMP pathway, the Xylulose Monophosphate (XuMP) pathway, the Calvin-Benson-Bassham (CBB) cycle and the Serine cycle (Wendisch, et al., 2021). In thoses studies aiming at “copying and pasting” existing methylotrophic pathways into non-methylotrophic hosts, the RuMP pathway has been used far more frequently than the others.
In this pathway only three heterologous enzymes, i.e. a methanol dehydrogenase (Mdh), a 3-hexulose-6-phophate synthase (Hps) and a 6-phospho-3-hexuloisomerase (Phi) are needed to integrate the methylotrophic module (Yurimoto et al., 2009). The challenging parts often consist in connecting methanol assimilation to the host central metabolism and in ensuring proper C1-acceptor regeneration.
The oxidation of methanol to formaldehyde, the first step in methanol assimilation, is ensured by an Mdh. Depending on the electron acceptor, Mdh enzymes can be classified into three groups: the periplasmic pyrrolo-quinoline-quinone (PQQ)-dependent Mdh found in Gram-negative methylotrophs, the cytoplasmic NAD-dependent Mdh common in Gram-positive methylotrophs and the cytoplasmic NDMA (N,N-dimethyl-4-nitrosoaniline)-dependent Mdh found in Mycobacterium and that uses mycofactocin as an in vivo electron acceptor (Heux et al., 2018; Dubey et al., 2019). The PQQ- and NDMA-dependent Mdhs are often multimeric proteins (Keltjens et al., 2014; Dubey, et al., 2019) and require the synthesis of PQQ and mycofactocin through complex biosynthetic pathways absent in a lot of hosts. The PQQ-dependent Mdh requires oxygen and specific cellular locations for its proper function while the NAD-dependent Mdh is located in the cytoplasm and functions under both aerobic and anaerobic conditions. For all these reasons, the choice of a NAD-dependent Mdh appeared to be the simplest way to implement synthetic methylotrophy. The mdh gene from Bacillus methanolicus was the first to be used (Lessmeier, et al., 2015; Muller, et al., 2015; Witthoff, et al., 2015). However, its efficiency towards methanol conversion remains relatively low and the same states for several reported variants. Thereby it required a high amount of methanol to yield high amounts of intracellular labelling (25% 13C-enrichment in phosphoenolpyruvate (PEP) with 1M of 13C-methanol) (Muller, et al., 2015). Scientists therefore aimed to find new NAD-dependent Mdhs with better kinetic parameters by screening Mdhs coming from different donor organisms. Among them, an alcohol dehydrogenase from the non-methylotrophic Bacillus stearothermophilus was found to use methanol as a substrate with a 10 times lower Km value (Sheehan et al., 1988). Whitaker and colleagues used this Mdh combined with Hps and Phi from B. methanolicus in E. coli and showed that the combination resulted in a 43% increase in biomass yield and a 10 times higher 13C-enrichment in intracellular metabolites compared to the association using the Mdh from B. methanolicus in a media containing 16 times less methanol (i.e. 60 mM vs 1M) but yeast extract (Whitaker, et al., 2017). Another alcohol dehydrogenase from Cupriavidus necator was found to exhibit similar activity towards methanol at 30°C to the Mdh from B. methanolicus at 45°C (its optimal temperature for activity). To further improve the kinetic parameters of Mdh, engineering strategies were applied. By using directed molecular evolution, a variant of the Mdh from C. necator with a catalytic efficiency for methanol 6-fold higher than the wild-type was obtained (Wu et al., 2016). In another study, by using phage assisted evolution on the Mdh2 of B. methanolicus, a two times higher methanol incorporation was observed in the resulting strain compared to the strain expressing the native Mdh2 (Roth et al., 2019).
Cellular redox state directly affects the Mdh activity since high NADH/NAD+ ratios are unfavourable to methanol oxidation. Results obtained after ALE experiments aiming at improving methanol assimilation in E. coli showed mutations in the nadR gene encoding for a transcriptional regulator of genes involved in NAD+ transport and de novo synthesis (Meyer et al., 2018). The activity of this repressor was found to be reduced, highlighting the importance of the NADH/NAD+ ratio during growth on methanol. To balance the redox state of the cell, authors also showed that when the NAD-dependent malate dehydrogenase gene maldh was knocked-out, growth was improved on methanol and gluconate. This was also observed in E. coli during growth on methanol and yeast extract even if methanol assimilation was not improved (Rohlhill et al., 2020). In a similar way, the methanol oxidation rate was improved when Mdh was coupled with a “NADH sink” by using lactate dehydrogenase to recycle NADH into NAD+ (Price et al., 2016).
The implementation of a methylotrophic pathway in E. coli was done by testing a series of Hps and Phi candidates from different donor organisms in vitro and in vivo (Muller, et al., 2015; Whitaker, et al., 2017; Fan et al., 2018). In all these studies, Hps and Phi from B. methanolicus were the best performing enzymes both in vitro and in vivo. In C. glutamicum, methanol utilization has been achieved by expressing Mdh from B. methanolicus together with hxlA (3-hexulose-phosphate synthase) and hxlB (6-phospho-3-hexuloisomerase) from B. subtilis (Lessmeier, et al., 2015; Witthoff, et al., 2015). In the resulting strains, the incorporation of 13C-label from 13C-methanol into central metabolites was detected, demonstrating the in vivo operation of the synthetic methanol utilization pathway (Lessmeier, et al., 2015; Witthoff, et al., 2015). However, expression of the same genes in S. cerevisiae failed to allow methanol consumption and cell growth in a minimal medium containing methanol as the sole carbon source (Dai, et al., 2017). In the bacterium P. putida, introduction of the hps and phi genes from Bacillus brevis allowed the strain to utilize methanol and formaldehyde as auxiliary substrates (Koopman, et al., 2009). The Hps and Phi expressing strain showed a two times higher biomass yield compared to the control strain when grown on a medium containing formaldehyde plus glucose. Furthermore, the strain was also able to grow when replacing formaldehyde by methanol while the control strain did not reach steady state under these conditions. However, authors did not show any evidence that a functional RuMP was operating in vivo. Overall, these studies demonstrate that the efficiency of Hps and Phi is not only linked with their origin but also with the host in which they are expressed. Improvement of Hps and Phi catalytic efficiency has been obtained by fusing both enzymes together and this will be discussed in the section Spatial engineering.
In order to drive the metabolism towards methanol assimilation, a common strategy is to delete one or more genes encoding for the formaldehyde detoxification pathway to avoid a carbon loss as CO2 (Whitaker, et al., 2017; Bennett et al., 2018; Vartiainen, et al., 2019; De Simone, et al., 2020; Rohlhill, et al., 2020). The importance of this mutation has been confirmed in ALE experiments where all the evolved strains with improved methylotrophic capacity had a deletion in one or more genes of the formaldehyde detoxification operon (Chen et al., 2018; Meyer, et al., 2018; Chen, et al., 2020).
Another strategy to stimulate methanol assimilation was to favour the recycling of the C1-acceptor (i.e. Ribulose-5-phosphate, Ru5P). In synthetic methylotrophs, Ru5P regeneration is ensured by the non-oxidative part of the pentose phosphate pathway (PPP). In the natural methylotroph B. methanolicus, it was shown that pfk, rpe, tkt, glpX, and fba (i.e. part of the sedoheptulose-1,7-biphosphatase (SBPase) variant of the RuMP pathway) are key genes involved in Ru5P regeneration. Their deletion resulted in the loss of the capacity of the bacteria to grow on methanol (Brautaset et al., 2004). Therefore, enhancing the host’s capacity to regenerate Ru5P by overexpressing heterologous enzymes appears critical to achieve efficient synthetic methylotrophy. To boost Ru5P regeneration, Bennett et al. overexpressed PPP enzymes by integrating pfk, rpe, tkt, glpX and fba from B. methanolicus under a strong inducible promoter into the E. coli chromosome (Bennett, et al., 2018). Even if culture media had to be supplemented with yeast extract, the resulting strain achieved a 20% improvement in biomass production during growth on methanol compared to the parental strain and 59% of 13C-enrichment was reached in PEP. PEP is an interesting metabolite to follow methanol assimilation and Ru5P recycling. Indeed, the more carbons in PEP are labelled, the more methanol has been assimilated and so the more Ru5P has been recycled. Overexpressing the PPP enzymes does not suffice, as a fine balance must be found between the amounts of methanol carbon directed towards recycling vs biomass formation. Woolston et al. used iodoacetate to block glyceraldehyde-3-phosphate dehydrogenase (Ga3PDH). Authors hypothesised that by inhibiting this reaction, which connects the RuMP pathway to lower glycolysis, larger intermediate pools of the upper glycolytic and RuMP pathways could be maintained in starved cells. This strategy led to a higher methanol incorporation into central metabolites in E. coli. The 13C-enrichment in fructose-6-phosphate (F6P) reached 27.5% when iodoacetate was present. Moreover, the intracellular concentrations of F6P, Ru5P and sedoheptulose-7-phosphate (S7P) were higher (Woolston et al., 2018). Rohlhill et al. went one step further by modulating the expression of rpe and tkt using the native formaldehyde-inducible promoter Pfrm of E. coli. When combined with the disruption of the malate dehydrogenase, methanol carbon incorporation into intracellular metabolites was improved, but yeast extract was still needed during the experiment (Rohlhill, et al., 2020).
To boost both assimilation and recycling, Chen et al. first integrated two operons in E. coli’s genome. The first one included mdh, hps and phi genes while the second one was composed by the same mdh and phi genes plus the hps, tkt and tal genes from various organisms. Adding the tkt and tal genes in the operon helped enhancing Ru5P recycling (Chen, et al., 2020). Moreover, after the first ALE experiment, using Ensemble Modelling for Robustness Analysis (EMRA), authors identified that the high activity of phosphofructokinase and Ga3PDH was channelling the flux away from the RuMP cycle, which tended to unbalance the metabolic system. To reduce the activity of these two enzymes, pfkA was knockout and the gapA gene was replaced by another gapC from E. coli BL21 encoding for a less efficient Ga3PDH than the native one.
Natural methylotrophs have developed complex regulatory networks allowing them to express the genes required for the methanol metabolism via sensing methanol and/or formaldehyde (Jakobsen et al., 2006). In contrast, synthetic methylotrophs do not possess such genetic machinery to recognize methanol. Therefore, attempts to make synthetic methylotrophs sensing methanol have been carried out. A chimeric two-component system was created in E. coli, MxaYZ, by fusing the periplasmic methanol sensing domain MxaY of the Mdh from Paracoccus denitrificans with the cytoplasmic catalytic transmitter domain of EnvZ from E. coli (Ganesh et al., 2017). MxaYZ sensed extracellular methanol and could activate the expression of a fluorescent reporter. Formaldehyde on the other hand is naturally encountered by E. coli via the formaldehyde-inducible promoter (Pfrm) which activates the dissimilatory pathway to avoid any intracellular formaldehyde accumulation. Several examples of engineered Pfrm in synthetic methylotrophs have been described (Denby et al., 2016; Rohlhill et al., 2017). In E. coli, Pfrm is situated upstream to the frmRAB operon and is repressed by FrmR, a transcriptional repressor. When formaldehyde is present in the cells, it interacts with FrmR and triggers conformational changes, leading to the dissociation of FrmR from its recognized DNA motif within Pfrm and to the derepression of the transcription of the operon (Denby, et al., 2016). When placed upstream the mdh, hps and phi operon, both the native and engineered Pfrm enabled improved biomass production in the engineered strain (Rohlhill, et al., 2017). In another study, FrmR and Pfrm were used to build a formaldehyde biosensor in E. coli and to modulate the expression of mdh, hps and phi (Woolston, et al., 2018).
Despite these efforts, rational engineering strategies were not sufficient to ensure full synthetic C1-assimilation in microorganisms. Rational engineering had to be associated with evolutionary engineering to improve the microorganism’s performance. However, as no growth on pure methanol was initially observed in the synthetic methylotrophs, the strategy adopted by several groups was to first build an “auxotrophic” strain in which methanol is necessary to ensure growth when another carbon source (i.e. glucose, xylose, ribose, gluconate or pyruvate) is present and then to subject the strain to ALE (Chen, et al., 2018; Meyer, et al., 2018; Bennett et al., 2020b; Chen, et al., 2020; Keller et al., 2020).
To ensure methanol is co-utilized with the other carbon source, cells are engineered in order to gain a benefit in utilizing methanol. To do so, the common strategy was to design a strain dependent on methanol utilization for growth while the other carbon source is used only to ensure Ru5P production. This is achieved by blocking either the conversion of glucose to F6P or the Ru5P catabolic pathways depending on the carbon source used (Figure 1). That way, cells have no other choice than producing F6P from the co-assimilation of methanol and the other carbon source while ensuring a high pool of the key Ru5P. This strategy was applied by Meyer et al. to engineer an E. coli methanol dependent-strain (∆rpiAB∆edd∆maldh) co-utilizing gluconate (Meyer, et al., 2018). Similarly, Chen et al. engineered an E. coli strain (∆rpiAB) co-utilizing xylose and another one (∆rpe) co-utilizing ribose (Chen, et al., 2018). Later, to build their fully methylotrophic strain, Chen et al. adopted the same strategy than in 2018 by deleting rpiAB but switched from E. coli BL21 to E. coli K12 BW25113 to facilitate the genetic engineering efforts (Chen, et al., 2020). To enable the co-utilization of methanol and glucose, Bennet et al. first deleted the phosphoglucose isomerase (pgi) that converts glucose-6-phosphate (G6P) to F6P, so that G6P is only used by the oxidative part of the PPP to directly fill the pool of Ru5P. The frmA gene, encoding for formaldehyde dehydrogenase, was also deleted in order to push formaldehyde towards assimilation (Bennett, et al., 2018). Later, authors went ahead by knocking-out edd and rpiAB in their ∆frmA∆pgi E. coli strain to completely abolish glucose assimilation but amino acids supplementation in the cultivation medium was then required (Bennett, et al., 2020b). Keller et al. used a different strategy by applying first a flux balance analysis and identifying candidate deletions in silico that could lead to methanol-auxotrophy with a complete RuMP cycle and a high degree of methanol incorporation. Authors investigated two out of 1,200 candidate strains, one with a deletion of fructose-1,6-bisphosphatase (fbp) and another with triosephosphate isomerase (tpiA) deleted. Those strains were methanol dependent and showed a 99% fractional incorporation of methanol into RuMP cycle metabolites (Keller, et al., 2020).
FIGURE 1. Targeted genes to construct methanol-dependent strains in E. coli. Adapted from (Wang et al., 2020b). Engineered synthetic methanol-dependent strain co-assimilating gluconate (in light blue) by (Meyer, et al., 2018); co-assimilating glucose (in blue) by (Bennett, et al., 2020b); co-assimilating ribose by (Chen, et al., 2018) or xylose by (Chen, et al., 2018; Chen, et al., 2020) (in purple); co-assimilating pyruvate (in dark blue) by (Keller, et al., 2020). Enzymes are written in bold. edd, 6-phosphogluconate isomerase; fadh, formaldehyde dehydrogenase; hps, 3-hexulose-6-phosphate synthase; maldh, malate dehydrogenase; mdh, methanol dehydrogenase; pgi, glucose-6-phosphate isomerase; phi, 6-phospho-3-hexuloisomerase; rpe, ribulose-3-phosphate epimerase; rpi, ribose-5-phosphate isomerase. Ac-CoA, acetyl-CoA; E4P, erythrose-4-phosphate; F6P, fructose-6-phosphate; FBP, fructose-1,6-biphosphate; GAP, glyceraldehyde-3-phosphate; DHAP, dihydroxyacetone-phosphate, H6P, hexulose-6-phosphate; KDPG, 2-keto-3-deoxy-6-phosphogluconate; 6 PG, 6-phosphogluconate; R5P, ribose-5-phosphate; Ru5P, ribulose-5-phosphate; S7P, sedoheptulose-7-phosphate; SBP, sedoheptulose-1,7-biphosphate; Xu5P, xylulose-5-phosphate.
If most of the attempts were done in E. coli, methanol dependent strains were also obtained in C. glutamicum by generating ∆aldh∆fadH∆rpi and ∆aldh∆fadH∆rpe mutants, respectively using xylose or gluconate and ribose or gluconate as co-substrates (Hennig et al., 2020; Tuyishime et al., 2020). The aldH and fadH genes are respectively coding for an acetaldehyde dehydrogenase and a formaldehyde dehydrogenase, so their deletion prevented formaldehyde oxidation to CO2. More recently, synthetic methanol auxotrophy has also been engineered in Bacillus subtilis by deleting rpiAB and rpe (Gao et al., 2022).
By engineering methanol-dependent strains, methanol assimilation is coupled with cell growth thus opening the way to ALE to improve methanol assimilation. ALE is used on microorganisms cultivated under defined conditions for weeks to months in order to select improved phenotypes on relevant carbon sources. Microorganisms can either be cultured in shake flasks with sequential serial passages or cells can be grown in chemostats with a controlled environment in which one component of the medium is limiting. Shake flasks have the great advantage to enable to run many culture conditions in parallel but pH, oxygenation and cell density can vary during the experiment. By using a chemostat, these parameters as well as growth rate can be closely monitored and kept constant, and higher cell densities can be reached. However, the operation costs and the know-how of this device are higher than for shake flasks (Dragosits et al., 2013).
Mutants evolving towards a faster growth can be selected, and methanol utilization can be tested by measuring the incorporation of label in intracellular metabolites from 13C-methanol (Table 1). Meyer et al. decided to knock out maldh in a ∆rpiAB∆edd background after in silico analysis, and to apply ALE to this E. coli strain. A mutant co-utilizing methanol and gluconate at a growth rate of µ = 0.08 h−1 and with a methanol uptake rate of 13 mmol. gCDW−1. h−1 - which is close to the one reported for natural methylotrophs—was selected. In this condition, 21% of the PEP carbon atoms came from methanol (Meyer, et al., 2018). When Chen et al. used ALE on their ∆rpiAB strain, they succeeded to select a strain with a growth rate of µ = 0.17 h−1. This strain was able to produce butanol from the co-consumption of methanol and xylose, and 22% of butanol carbons came from methanol (Chen, et al., 2018). After the ALE experiment, Bennet et al. isolated an E. coli strain that was growing on glucose and methanol with a specific growth rate of µ = 0.15 h−1 and without the need for amino acid supplementation. The strain was able to produce acetone, for which 22% of carbon atoms derived from methanol (Bennett, et al., 2020b). An ALE experiment was successful for Chen et al. as they obtained an evolved methylotrophic E. coli strain that exhibited a growth rate of µ = 0.08 h−1 on pure methanol (Chen, et al., 2020). However, no proof of in vivo functionality of the introduced methanol pathway was shown.
TABLE 1. Overview of 13C-methanol incorporation level at the intracellular level or in final products in strains after ALE experiment.
Tuyshime et al. performed ALE experiments on their C. glutamicum mutant and selected a strain growing on xylose and methanol at a growth rate of 0.03 h−1 with a methanol uptake rate of 0.86 mmol. gCDW−1. h−1 (Tuyishime et al., 2018). Based on transcriptome analysis, further work was done on this strain to explore the metabolic regulation that operated during methylotrophic conditions (Fan et al., 2021). Results demonstrated that the evolved C. glutamicum used the SBPase variant to recycle Ru5P as fba and glpX were upregulated while tal was downregulated. Nitrate was also shown to serve as a complementary electron acceptor during aerobic methanol metabolism as the operon narKGHJI was upregulated. This operon encodes for a respiratory nitrate reductase which is usually active during anaerobic growth. Amino acids biosynthesis was also shown to limit growth on methanol as genes involved in the biosynthesis of several L-aspartate derived amino acids were downregulated. This strain was subjected to a second ALE experiment which enabled to select a new evolved strain exhibiting a growth rate of 0.052 h−1 on xylose and methanol as well as a higher tolerance towards methanol (Wang et al., 2020a). In another study, Henning et al. selected, in C. glutamicum, an evolved ∆aldh∆fadH∆rpe strain co-utilizing methanol and gluconate. This strain was able to produce cadaverine, for which 43% of carbon atoms came from methanol. After a new round of ALE experiments started from the previous strain, another one was selected on ribose and methanol. This strain grew without yeast extract and exhibited a specific growth rate of µ = 0.10 h−1 (Hennig, et al., 2020).
As in nature, during ALE genomic changes occur to generate an improved phenotype enabling cells to cope with their environment. It is of great interest to get insight on which genes are essential (or non-essential) for methanol assimilation by investigating the mutations and their consequences. Mutations found in evolved strains can be classified in three categories, mutations affecting: 1) the dissimilatory pathway and the recycling pathways; 2) the redox and energy balance; 3) the substrate uptake and other enzymes connected to metabolism (Wang et al., 2020b).
Mutations in the frm operon genes leading to the inactivation of the formaldehyde dissimilatory pathway were found in all the evolved E. coli strains (Chen, et al., 2018; Meyer, et al., 2018; Chen, et al., 2020). These mutations are consistent with the fact that efficient methanol-dependent growth required formaldehyde to be entirely redirected towards C1-assimilation. Mutations leading to pykF and zwf inactivation possibly enabled to increase the F6P pool size for Ru5P regeneration via the PPP (Chen et al., 2018). Similarly, the deoD mutation was thought to increase the Ru5P pool size for formaldehyde assimilation (Chen, et al., 2018).
Mutations were found in nadR (Meyer, et al., 2018), cyaA (Chen, et al., 2018) in E. coli and mtrA in C. glutamicum (Tuyishime, et al., 2018). The enzymes encoded by these genes are involved in the redox state or in the energy supply of the cell. The nadR and mtrA genes encode for enzymes involved in the balance of the redox state. Both mutations are thought to lead to the increase of NAD+ availability in the cell, thereby most probably promoting methanol oxidation. cyaA encodes for an enzyme producing cyclic AMP (cAMP) from ATP. The transcription of most of the TCA cycle enzymes is activated by cAMP. Most probably a cyaA mutation would lower the TCA cycle activity and consequently NAD(P)H production, at a beneficial cost for methanol oxidation. Moreover, inactivation of frmA and fdoG (encoding a formate dehydrogenase) for the synthetic methylotrophic strain, help limiting further the production of NADH (Chen, et al., 2020).
Mutations in gnd encoding for 6-phosphogluconate dehydrogenase were found in a strain assimilating methanol better than the parental one but still requiring xylose for growth (Chen, et al., 2020). GntR and AltR are transcriptional regulators and mutations in genes encoding these proteins were found respectively in the E. coli strain co-utilizing methanol and gluconate and C. glutamicum strain co-utilizing methanol and xylose (Meyer, et al., 2018; Tuyishime, et al., 2018). GntR represses two enzymes involved in gluconate uptake, therefore gntR mutations may have improved gluconate uptake. Similarly, AltR regulates among other genes the expression of xylB encoding for a xylulose kinase and adhA encoding for an alcohol dehydrogenase functioning as a methanol dehydrogenase in C. glutamicum. Therefore, an altR mutation may have modified xylB and adhA expression leading to improved co-utilization of methanol and xylose by increasing substrate uptake. In the strain exhibiting higher tolerance towards methanol, two mutations in cgl0653 and cgl0833, respectively encoding for an O-acetyl-L-homoserine sulfhydrylase and for a methanol-induced membrane-bound transporter were found (Wang, et al., 2020a).
Once produced, formaldehyde has to be quickly condensed with Ru5P to avoid its toxic effect. To optimize formaldehyde assimilation, another strategy has been proposed that consists in enhancing substrate channelling by co-localizing the enzymes in a delimited space. Orita et al. were the first to address this question by fusing hps and phi from Mycobacterium gastri (Orita et al., 2007). In vivo assays showed that when expressed in E. coli, the fusion protein of Hps and Phi leads to an increased growth rate on formaldehyde than when the proteins were separated. Later, Price et al. advanced the field by engineering a supramolecular enzyme complex with Mdh from B. methanolicus and Hps and Phi from Mycobacterium gastri (Price, et al., 2016). They took advantage of the decameric structure of Mdh to design a supramolecular complex able to self-assemble by using SH3-ligand in order to “plug” on it the fusion protein of Hps and Phi previously described by Orita et al. This engineered complex enabled a faster conversion of methanol into F6P compared to unassembled proteins in vitro. In the same way, Fan et al. used an alternative strategy to fuse Mdh from B. stearothermophilus with Hps and Phi from B. methanolicus by using flexible linkers (GGGGS)n. They also demonstrated an improvement in methanol conversion to F6P in vitro (Fan, et al., 2018).
Dai et al. introduced the XuMP pathway in the non-methylotrophic yeast S. cerevisae by integrating an alcohol oxidase, a catalase, a dihydroxyacetone synthase (Das) and a dihydroxyacetone kinase (DhaK) from Pichia pastoris in its genome (Dai, et al., 2017). By using the native peroxisome targeting peptide of P. pastoris, enzymes were addressed to the S. cerevisae peroxisome. When yeast extract was added to the medium, methanol consumption was further improved from 1.04 g/L to 2.35 g/L, suggesting that Xu5P recycling was enhanced by yeast extract addition. However, no proof of in vivo functionality of the introduced methanol pathway was shown.
In the yeast Y. lipolitica, the expression of a dihydroxyacetone synthase from Candida boidini restored the formaldehyde tolerance of a formaldehyde sensitive strain (i.e. deleted for the FaDH) but was not enough to convert methanol into biomass (Vartiainen, et al., 2019).
Different from the above strategies that mainly build on existing pathways, the “mix and match” approach allowed combining existing enzymes from different microbial sources into a synthetic pathway and implementing it in a host. Considering the methylotrophic metabolic diversity, we can estimate that there are more than 500 unique methanol assimilation pathways from methanol to biomass (Heux, et al., 2018). Therefore, such an approach is perfectly adapted to explore this large space of metabolic solutions while identifying the most powerful combinations. At the moment, only four hybrid pathways have been studied (Figure 2).
FIGURE 2. Hybrid pathways implemented for C1-assimilation. (A) Homoserine cycle pathway (B) Modified serine cycle pathway (C) Methanol condensation cycle pathway (D) Hybrid Mdh-Das pathway. Adapted from (Tuyishime, et al., 2020). Enzymes are written in bold. Acdc, acetaldehyde dehydrogenase; Agt, Alanine-glyoxylate aminotransferase; Dhak, DHA kinase; FaDH, formaldehyde dehydrogenase; FtfL, formate-H4F ligase; Fsa, Fructose-6-aldolase; Gdh, glutamate dehydrogenase; Gpt; Glutamate-pyruvate transaminase; Hal, 4-hydroxy-2-oxobutanoate aldolase; Hat, 4-hydroxy-2-oxobutanoate aminotransferase; Hsk, homoserine kinase; Lta, threonine aldolase; MaDH, malate dehydrogenase; Mcl, malyl-CoA lyase; Mdh, methanol dehydrogenase; Mthfs, methylene-H4F dehydrogenase; Mtk, malate thiokinase; Ppc, phosphoenolpyruvate carboxylase; Pps, phosphoenolpyruvate synthetase; Rpe, ribulose-3-phosphate epimerase; Rpi, ribose-5-phosphate isomerase; Sal, serine aldolase; SdaA, serine deaminase; Sdh, serine, dehydratase; Shmt, serine-H4F hydroxymethyltransferase; Tal, transaldolase; Tkt1 & Tkt2, transketolase type 1 & 2; Ts, threonine synthase; Ala, alanine; E4P, erythrose-4-phosphate; F6P, fructose-6-phosphate; GAP, glyceraldehyde-3-phosphate; Glu, glutamate; H6P, hexulose-6-phosphate; OAA, oxaloacetate; Oxo-Glu, 2-oxoglutarate; PEP, phosphoenolpyruvate; Pyr, pyruvate; R5P, ribose-5-phosphate; Ru5P, ribulose-5-phosphate; S7P, sedoheptulose-7-phosphate; SBP, sedoheptulose-1,7-biphosphate; Xu5P, xylulose-5-phosphate.
Yu and Liao implemented a modified serine cycle in E. coli (Figure 2B). Methanol is assimilated via the H4F-dependent pathway by being converted into formate. Rather than “copy-pasting” the serine cycle, the authors decided to select a set of enzymes to replace key reactions in the natural pathway by other known reactions from other microorganisms to fit the activity of this pathway with E. coli metabolism. The E. coli metabolic network was modified to become dependent on methanol for growth. Once the operation of the two modules was confirmed (i.e. formate conversion to CH2H4F and the modified serine cycle), the complete pathway was tested in vivo by using 13C-methanol and xylose. Label derived from methanol was found in intracellular metabolites (Yu et al., 2018). Later, He et al. designed a complete homoserine cycle based on E. coli native enzymes and the promiscuous activity of the formaldehyde aldolase (Figure 2A). Unlike the serine cycle, here the C1 intermediate for assimilation is formaldehyde. A codon-optimized Mdh from C. glutamicum was used to oxidise methanol to formaldehyde. Then, the E. coli native metabolic network was modified in order to design a strain in which methanol assimilation is required for serine biosynthesis. The functionality of the homoserine cycle was tested in vivo with labelling experiments by using E. coli auxotrophic strains in which the complete formaldehyde dissimilation pathway was blocked. However, optimization is still required to achieve E. coli growth using the homoserine cycle (He et al., 2020).
In 2014, Bogorad et al. designed a non-natural pathway enabling methanol assimilation named methanol condensation cycle (MCC) (Figure 2C) (Bogorad et al., 2014). In the MCC pathway, existing enzymes from various microorganisms were combined to convert methanol into acetyl-CoA. These enzymes do not work together in nature. The MCC pathway was first constructed in silico and then tested in a cell-free environment. This pathway ensures the conservation of phosphates in the catalytic cycle as well as the balance of the redox state. The MCC was found to be functional and acetyl-CoA production was achieved in vitro. Acetyl-CoA is a precursor for the production of biofuels (i.e. ethanol and butanol). However, there is no report of the in vivo functionality of the MCC pathway yet.
The hybrid Mdh-Das (HMD) pathway (Figure 2D) was built in E. coli using an iterative combination of dry and wet lab approaches to design, implement and optimize this metabolic trait (De Simone, et al., 2020). Through in silico modelling, a new route that “mixed and matched” two methylotrophic enzymes, a bacterial methanol dehydrogenase (Mdh) and a dihydroxyacetone synthase (Das) from yeast was designed. To identify the best combination of enzymes to introduce into E. coli, a library of 266 pathway variants containing different combinations of Mdh and Das homologues was built and then screened using high-throughput 13C-labeling experiments. The highest level of incorporation of methanol into central metabolism intermediates (i.e. 22% into PEP) was obtained using a variant composed of a Mdh from Acinetobacter gerneri and a codon-optimized version of Pichia angusta Das. Finally, the activity of the HMD pathway was further improved by engineering strategic metabolic targets identified using omics and modelling approaches. The final synthetic strain had 1.5 to 5.9 times higher methanol assimilation in intracellular metabolites and proteinogenic amino acids than the starting strain but was still unable to grow on methanol as the sole carbon and energy source (De Simone, et al., 2020).
A chimeric methanol assimilation pathway was engineered in Y. lipolytica by introducing both the RuMP and the XumP pathways (Wang et al., 2021). In this strain, methanol was oxidised by the Mdh from B. stearothermophilus. For the RuMP pathway, Hps and Phi from B. methanolicus were selected while Das and DhaK from P. pastoris were chosen. The formaldehyde dissimilation pathway was blocked by deleting FLD1 the gene, the homologue of frmA in E. coli. Recycling of the C1-acceptors, Ru5P and Xu5P, was enhanced by the overexpression of several key genes of the glycolysis and the PPP (i.e. TKL1, PFK, FBA, and RPE1) as well as glpX from B. methanolicus that encodes for a SBPase and a FBPase. After ALE experiments, two evolved strains with improved methanol assimilation were obtained. Interestingly, in one strain, only the expression of the genes of the RuMP pathway was upregulated while in the other one the expression of both RuMP and XuMP pathways was increased. This highlights the capacity of the microbial strain to invoke several strategies in response to externally introduced genetic perturbations.
Creating new reactions that do not exist in nature is the ultimate strategy to fully explore the metabolic solution space. With the advances in enzyme engineering and de novo-enzyme design, it becomes possible to create these reactions and integrate them into a synthetic pathway to perform a novel function. So far, only four studies have implemented synthetic methylotrophic pathways that involve novel catalytic transformations (Figure 3).
FIGURE 3. Synthetic linear pathways implemented for C1-assimilation. (A) Formolase pathway, (B) Synthetic acetyl-CoA pathway, (C) 2-hydroxyacyl-CoA lyase pathway, (D) Reductive glycine pathway. Adapted from (Tuyishime, et al., 2020). Enzymes are written in bold. Acps, acetylphosphate synthase; Acr, acyl-CoA reductase; Adh, aldehyde dehydrogenase; Dak, dihydroxyacetone kinase; FaDH, formaldehyde dehydrogenase; Fch, methenyl H4F-cyclohydrolase; Fls, formolase; FtfL, formate-H4F ligase; GalS, glycolaldehyde synthase; GlyA, serine hydroxymethyltransferase; Hacl, 2-hydroxyacyl-CoA lyase; Mdh, methanol dehydrogenase; MtdA, methylene-H4F dehydrogenase/methylene-H4MPT dehydrogenase; Pta, phosphate acetyltransferase; SdaA, serine deaminase. DHA, dihydroxyacetone; DHAP, dihydroxyacetone phosphate.
Wang et al. were the first in succeeding to implement a linear synthetic pathway. This pathway relies on two enzymes, a NAD-dependent Mdh and a formolase (Fls) (Figure 3A). Fls is a synthetic, computationally designed enzyme (Siegel, et al., 2015) that condensates three molecules of formaldehyde to produce one molecule of DHA. The cooperation of the Mdh from B. methanolicus MGA3 or PB1 were first tested with Fls in vitro. From this experiment, authors decided to introduce the Mdh from B. methanolicus PB1 combined with Fls in an E. coli strain deleted for frmA. To improve the performance of this strain, ALE was used. By using 13C-methanol, the resulting evolved strain was shown to have a higher level of labelling in the proteinogenic amino acids than the parental strain but failed to growth on pure methanol (Wang et al., 2017).
The synthetic acetyl-CoA (SACA) is a linear pathway that was designed and constructed by Lu et al. (Figure 3B). This pathway was first designed to produce acetyl-CoA from formaldehyde and relies on three enzymes. A glycolaldehyde synthase (GalS) condensates two molecules of formaldehyde to produce one molecule of glycolaldehyde. Then, glycolaldehyde is converted into acetyl-phosphate by the acetyl-phosphate synthase. Acetyl-phosphate is subsequently used to produce acetyl-CoA by the phosphate acetyltransferase. The authors first designed GalS and checked for glycolaldehyde production. Then, they optimized GalS by using directed evolution to improve the kinetic properties of the enzyme. The SACA pathway was functional in vitro and the authors then decided to implement the pathway in E. coli. The functionality of the pathway was tested in vivo in rich media. By combining the SACA pathway with the Mdh from B. stearothermophilus, a slight improvement in the final OD was observed. When tested on minimal media by using 13C-methanol, label was also found in some proteinogenic amino acids after 26 h but no growth was observed (Lu et al., 2019).
Based on a computational analysis of metabolic reactions from MetaCyc and Atlas databases, Yang et al. designed a new formaldehyde assimilation pathway named the glycolaldehyde assimilation (GAA) pathway (Yang et al., 2019). This pathway was tested in vitro using engineered versions of GalS and transaldolase B and reached a carbon yield of 88%. However, no proof of in vivo functionality of this pathway was shown. More recently a new GalS was engineered based on a newly discovered glyoxylate carboligase enzyme found in E. coli (Jo et al., 2022). One of the variants showed a 10 times higher affinity and a 2 times higher catalytic efficiency for formaldehyde compared to the previously described GalS (Lu, et al., 2019; Yang, et al., 2019). When this optimized GalS was associated in vitro to a lactaldehyde reductase (FucO), 66% of the formaldehyde was converted into ethylene glycol (Jo, et al., 2022).
The 2-hydroxyacyl-CoA lyase (Hacl) is a synthetic pathway designed by Chou et al. where Hacl condenses formaldehyde with formyl-CoA to produce glycolyl-CoA (Figure 3C). Glycolyl-CoA is subsequently converted to glycolaldehyde by an acyl-CoA reductase (Acr). Finally, a aldehyde dehydrogenase produces glycolate from glycolaldehyde. Hacl is a mammalian enzyme involved in α-oxidation. However, after a BLAST research limited to prokaryotes, the authors identified and tested one Hacl from Rhodospiralleles bacterium (RuHacl) exhibiting the condensation activity of formaldehyde with formyl-CoA. They tested the pathway in vivo in an E. coli strain deleted for ∆frmA, ∆fdhF∆fdnG∆fdoG and ∆glcD to avoid any competitive reactions using formaldehyde, formate or gluconate. The strain produced 0.5 g/L of glycolate corresponding to a yield of 67%. After enzyme engineering using directed evolution, a variant of RuHacl with improved enzyme kinetics was selected. By expressing this variant, E. coli produced 1.2 g/L of glycolate. However, the pathway has not been tested yet in vitro or in vivo on methanol with the addition of a Mdh (Chou et al., 2019). Later, the Hacl pathway was used to establish the formyl-CoA elongation (FORCE) pathway (Chou et al., 2021). The FORCE pathway was designed as an orthogonal platform for C1 utilization. Based on thermodynamics and stoichiometric analyses, different FORCE pathways were evaluated. Several were tested both in vitro and in vivo in E. coli. The conversion of formate, formaldehyde and methanol into glycolate, ethylene glycol and glycerate was demonstrated among other products.
It was shown that Hacls were poorly produced in E. coli, which could limit its efficiency (Chou, et al., 2019; Burgener et al., 2020). Together with Hacl, Oxalyl-CoA decarboxylase (Oxc) are members of a family enzyme catalyzing the condensation of formyl-CoA with formaldehyde to produce glycolyl-CoA. Oxc was thus repurposed to improve its glycolyl-CoA synthase activity under physiological conditions (Nattermann et al., 2021). Oxc from Methylorubrum extorquens (MeOxc) was subjected to several rounds of iterative site mutagenesis. A quadruple variant MeOxc4 was selected showing affinity towards formaldehyde similar to natural formaldehyde converting-enzymes and with an improvement for the carboligation activity of 200-fold compared to wild-type MeOxc. To test this enzyme in vivo, RuHacl was replaced with MeOxc4 in the E. coli strain previously designed for whole-cell bioconversion (Chou, et al., 2019; Nattermann, et al., 2021). Glycolate production was two-fold higher using MeOxc4 than RuHacl. With higher production rates in E. coli, this enzyme offers great possibilities for the engineering of new linear C1 pathways.
Kim et al. designed a fourth linear pathway: the reductive glycine pathway which, combined with Mdh, enabled methanol assimilation in E. coli (Figure 3D). In this pathway, formate is the key C1-intermediate that enables carbon assimilation (Kim, et al., 2020). The authors introduced the H4F-pathway from Methylobacter extorquens associated with a glycine cleavage system in an E. coli strain auxotrophic for serine, glycine and C1 moieties (∆serA∆kbl∆ltaE∆aceA). It has been previously described that even if growth could not be supported on formate, the latter compound could be still assimilated in E. coli via the H4F-dependent pathway (Tashiro et al., 2018). Kim et al. optimized the operation of this pathway first on formate. Then, the authors optimized the strain using ALE before implementing Mdh. The selected strain was able to grow on formate and CO2 at a growth rate of µ = 0.086 h−1. In this strain, methanol to be assimilated is first oxidized to formaldehyde by Mdh and then formaldehyde is oxidized to formate via the native GSH-dependent pathway of E. coli encoded by the operon frmRAB. Several Mdhs were tested and only the Mdh from B. stearothermophilus supported growth on methanol and CO2 at a growth rate of µ = 0.013 h−1. To confirm that the methanol pathway was functional, they confirmed that a frmA deletion blocked growth on methanol. By using 13C-methanol, authors showed that methanol carbon atoms were recovered in proteinogenic amino acids. The labelling pattern found was the same than when the strain grew on 13C-formate and 12CO2.
Among all the attempts to engineer synthetic methylotrophy in the platform host microorganisms cited above, it is very interesting to notice that synthetic methylotrophic growth was only achieved in E. coli and using both a natural cyclic pathway (Chen, et al., 2020) and a synthetic linear methylotrophic pathway (Kim, et al., 2020). In both examples, rational and evolutionary approaches have been combined. Even if different NAD-dependent Mdhs were used, both are exhibiting improved kinetic parameters for methanol oxidation (i.e. engineered version of Mdh from C. necator (Chen, et al., 2020) and Mdh from B. stearothermophilus (Kim, et al., 2020)). Moreover, in both cases, auxotrophic strains were first designed prior to applying ALE, which has been determinant to reach the final phenotype. Indeed, when comparing the flux distribution within the metabolic network of the native methylotroph B. methanolicus in both methylotrophic and non-methylotrophic conditions (Delepine et al., 2020), we quickly realized that the number of metabolic adaptations required to enable growth on methanol is too high to be solely achieved by a targeted engineering approach. ALE thus appears as the most reasonable strategy to achieve the complete reorganisation of the central metabolism required to fit a methylotrophic lifestyle. In all these studies (Chen, et al., 2018; Meyer, et al., 2018; Bennett, et al., 2020b; Chen, et al., 2020; Keller, et al., 2020), ALE helped in rerouting carbon fluxes towards methanol assimilation by limiting competing pathways and improving substrate uptakes in order to enhance efficient biomass production while adjusting the energy and redox state of the cell. Nevertheless, sometimes rational engineering was necessary in between two rounds of ALE to obtain a fully methylotrophic lifestyle (Chen, et al., 2020). The length of ALE varied according to the study but at least 6 months were necessary to select a strain growing solely on methanol. When looking at the genetic adaptations level, ALE revealed that some are very specific of the co-assimilated carbon source used while others are more general, e.g. the mutations in the genes involved in redox homeostasis or formaldehyde detoxification. It would be very interesting to reconstruct a strain with those mutations in order to identify the minimal set of mutations required for building a true synthetic methylotroph.
However, despite these encouraging accomplishments, synthetic methylotrophy remains a major challenge as the observed growth rates are largely sub-optimal and can barely be considered as active growth. Doubling times of 50 h on a mixture of methanol-CO2 and 9 h on methanol (corresponding to growth rates of 0.01 and 0.08 h−1, respectively) are far from the performance of natural methylotrophs that is 0.20 h−1 for Bacillus methanollicus at 37°C (Müller et al., 2014); 0.10–0.15 h−1 for Pichia Pastoris at 25°C (Tomàs-Gamisans et al., 2018); and 0.17 h−1 for M. extorquens at 28°C (Kiefer et al., 2009). Both strains are not yet fully adapted to these non-native carbon sources and further work is necessary. So far, efforts to engineer this non-native substrate catabolism have all taken the straightforward approach of metabolic pathway overexpression, ignoring coordination with the overall cellular processes that include central metabolism, stress-response and cell doubling. However, natural systems use genome-scale regulatory networks, called regulons, which coordinate nutrient catabolism with the larger cellular infrastructure. Therefore, nutrient metabolism cannot be considered as a hermetic process and engineering non-native carbon source utilisation should promote regulation processes together with metabolic pathway diversification and improvement for an efficient and dynamic cellular coordination. Indeed, recent studies demonstrated that targeting regulation of amino acid biosynthesis, an essential process to biomass formation and which is negatively impacted when E. coli grows on methanol, can improve methanol assimilation levels (Bennett et al., 2020a; Bennett et al., 2021).
In addition, synthetic biologists have left out the exploration of the spatial dimension for metabolic engineering in prokaryotes, as they do not have any particular subcellular organization. However, a study revealed that around 17% of bacteria contain a bacterial microcompartment (BMC) locus in their genome and that microcompartments are found in 23 different phyla (Axen et al., 2014) including E. coli. In addition, compartmentalization of the methanol assimilation pathway in peroxisomes has proven to be efficient in methylotrophic yeasts to protect themselves against toxicity of reactive intermediates (i.e. formaldehyde and H2O2) while improving the reaction efficiencies as enzymes are in close vicinity to their substrates and intermediates (van der Klei et al., 2006). Furthermore, compartmentalization allows to build an orthogonal network structure operating with minimal interactions to native metabolic and regulatory networks. Finally, in literature, tools to repurpose BMCs are described (Lau et al., 2018; Lee et al., 2019). For all these reasons, compartmentalizing the methanol assimilatory pathways seems to be an approach worthy of consideration to establish synthetic methylotrophy in prokaryotes.
Changing a lifestyle from heterotrophic to methylotrophic represents major engineering that have been partially achieved. In 4–10 years from now, it is likely that the next generation of synthetic methylotrophs will be further developed for the production of chemicals (e.g. bulk chemicals, fuels) from methanol, after which commercial production may be feasible. However, there is undoubtedly a long way ahead to achieve a synthetic strain able to use methanol for the production of both biomass and chemicals.
All authors listed have made a substantial, direct, and intellectual contribution to the work and approved it for publication.
CMV was supported by the PostAgreenskills Fund from INRAE.
The authors declare that the research was conducted in the absence of any commercial or financial relationships that could be construed as a potential conflict of interest.
All claims expressed in this article are solely those of the authors and do not necessarily represent those of their affiliated organizations, or those of the publisher, the editors and the reviewers. Any product that may be evaluated in this article, or claim that may be made by its manufacturer, is not guaranteed or endorsed by the publisher.
Axen, S. D., Erbilgin, O., and Kerfeld, C. A. (2014). A Taxonomy of Bacterial Microcompartment Loci Constructed by a Novel Scoring Method. PLoS Comput. Biol. 10, e1003898. doi:10.1371/journal.pcbi.1003898
Becker, J., and Wittmann, C. (2015). Advanced Biotechnology: Metabolically Engineered Cells for the Bio-Based Production of Chemicals and Fuels, Materials, and Health-Care Products. Angew. Chem. Int. Ed. 54, 3328–3350. doi:10.1002/anie.201409033
Bennett, R. K., Agee, A., Gerald Har, J. R., von Hagel, B., Siu, K.-H., Antoniewicz, M. R., et al. (2020a). Triggering the Stringent Response Enhances Synthetic Methanol Utilization in Escherichia coli. Metab. Eng. 61, 1–10. doi:10.1016/j.ymben.2020.04.007
Bennett, R. K., Dillon, M., Gerald Har, J. R., Agee, A., von Hagel, B., Rohlhill, J., et al. (2020b). Engineering Escherichia coli for Methanol-dependent Growth on Glucose for Metabolite Production. Metab. Eng. 60, 45–55. doi:10.1016/j.ymben.2020.03.003
Bennett, R. K., Gonzalez, J. E., Whitaker, W. B., Antoniewicz, M. R., and Papoutsakis, E. T. (2018). Expression of Heterologous Non-oxidative Pentose Phosphate Pathway from Bacillus Methanolicus and Phosphoglucose Isomerase Deletion Improves Methanol Assimilation and Metabolite Production by a Synthetic Escherichia coli Methylotroph. Metab. Eng. 45, 75–85. doi:10.1016/j.ymben.2017.11.016
Bogorad, I. W., Chen, C.-T., Theisen, M. K., Wu, T.-Y., Schlenz, A. R., Lam, A. T., et al. (2014). Building Carbon-Carbon Bonds Using a Biocatalytic Methanol Condensation Cycle. Proc. Natl. Acad. Sci. U.S.A. 111, 15928–15933. doi:10.1073/pnas.1413470111
Brautaset, T., Jakobsen, Ø. M., Flickinger, M. C., Valla, S., and Ellingsen, T. E. (2004). Plasmid-dependent Methylotrophy in Thermotolerant Bacillus Methanolicus. J. Bacteriol. 186, 1229–1238. doi:10.1128/jb.186.5.1229-1238.2004
Burgener, S., Cortina, N. S., and Erb, T. J. (2020). Oxalyl‐CoA Decarboxylase Enables Nucleophilic One‐Carbon Extension of Aldehydes to Chiral α‐Hydroxy Acids. Angew. Chem. Int. Ed. 59, 5526–5530. doi:10.1002/anie.201915155
Chen, C. T., Chen, F. Y., Bogorad, I. W., Wu, T. Y., Zhang, R., Lee, A. S., et al. (2018). Synthetic Methanol Auxotrophy of Escherichia coli for Methanol-dependent Growth and Production. Metab. Eng. 49, 257–266. doi:10.1016/j.ymben.2018.08.010
Chen, F. Y.-H., Jung, H.-W., Tsuei, C.-Y., and Liao, J. C. (2020). Converting Escherichia coli to a Synthetic Methylotroph Growing Solely on Methanol. Cell. 182, 933–946. doi:10.1016/j.cell.2020.07.010
Chou, A., Clomburg, J. M., Qian, S., and Gonzalez, R. (2019). 2-Hydroxyacyl-CoA Lyase Catalyzes Acyloin Condensation for One-Carbon Bioconversion. Nat. Chem. Biol. 15, 900–906. doi:10.1038/s41589-019-0328-0
Chou, A., Lee, S. H., Zhu, F., Clomburg, J. M., and Gonzalez, R. (2021). An Orthogonal Metabolic Framework for One-Carbon Utilization. Nat. Metab. 20213, 1385–1399. doi:10.1038/s42255-021-00453-0
Dai, Z., Gu, H., Zhang, S., Xin, F., Zhang, W., Dong, W., et al. (2017). Metabolic Construction Strategies for Direct Methanol Utilization in Saccharomyces cerevisiae, Bioresour. Technol., 245, 1407–1412. doi:10.1016/j.biortech.2017.05.100
De Simone, A., Vicente, C. M., Peiro, C., Gales, L., Bellvert, F., Enjalbert, B., et al. (2020). Mixing and Matching Methylotrophic Enzymes to Design a Novel Methanol Utilization Pathway in E. coli. Metab. Eng. 61, 315–325. doi:10.1016/j.ymben.2020.07.005
Delépine, B., López, M. G., Carnicer, M., Vicente, C. M., Wendisch, V. F., and Heux, S. (2020). Charting the Metabolic Landscape of the Facultative Methylotroph Bacillus Methanolicus. mSystems 5, 5. doi:10.1128/mSystems.00745-20
Denby, K. J., Iwig, J., Bisson, C., Westwood, J., Rolfe, M. D., Sedelnikova, S. E., et al. (2016). The Mechanism of a Formaldehyde-Sensing Transcriptional Regulator. Sci. Rep. 6, 38879. doi:10.1038/srep38879
Dragosits, M., and Mattanovich, D. (2013). Adaptive Laboratory Evolution - Principles and Applications for Biotechnology. Microb. Cell. Fact. 12, 64. doi:10.1186/1475-2859-12-64
Dubey, A. A., and Jain, V. (2019). Mycofactocin Is Essential for the Establishment of Methylotrophy in Mycobacterium Smegmatis. Biochem. Biophysical Res. Commun. 516, 1073–1077. doi:10.1016/j.bbrc.2019.07.008
Erb, T. J., Jones, P. R., and Bar-Even, A. (2017). Synthetic Metabolism: Metabolic Engineering Meets Enzyme Design. Curr. Opin. Chem. Biol. 37, 56–62. doi:10.1016/j.cbpa.2016.12.023
Espinosa, M. I., Gonzalez-Garcia, R. A., Valgepea, K., Plan, M. R., Scott, C., Pretorius, I. S., et al. (2020). Adaptive Laboratory Evolution of Native Methanol Assimilation in Saccharomyces cerevisiae. Nat. Commun. 11, 5564. doi:10.1038/s41467-020-19390-9
Fan, L., Wang, Y., Qian, J., Gao, N., Zhang, Z., Ni, X., et al. (2021). Transcriptome Analysis Reveals the Roles of Nitrogen Metabolism and Sedoheptulose Bisphosphatase Pathway in Methanol-dependent Growth of Corynebacterium Glutamicum. Microb. Biotechnol. 14, 1797–1808. doi:10.1111/1751-7915.13863
Fan, L., Wang, Y., Tuyishime, P., Gao, N., Li, Q., Zheng, P., et al. (2018). Engineering Artificial Fusion Proteins for Enhanced Methanol Bioconversion. ChemBioChem 19, 2465–2471. doi:10.1002/cbic.201800424
Ganesh, I., Vidhya, S., Eom, G. T., and Hong, S. H. (2017). Construction of Methanol-Sensing Escherichia coli by the Introduction of a Paracoccus Denitrificans MxaY-Based Chimeric Two-Component System. J. Microbiol. Biotechnol. 27, 1106–1111. doi:10.4014/jmb.1611.11070
Gao, B., Zhao, N., Deng, J., Gu, Y., Jia, S., Hou, Y., et al. (2022). Constructing a Methanol-dependent Bacillus Subtilis by Engineering the Methanol Metabolism. J. Biotechnol. 343, 128–137. doi:10.1016/j.jbiotec.2021.12.005
He, H., Höper, R., Dodenhöft, M., Marlière, P., and Bar-Even, A. (2020). An Optimized Methanol Assimilation Pathway Relying on Promiscuous Formaldehyde-Condensing Aldolases in E. coli. Metab. Eng. 60, 1–13. doi:10.1016/j.ymben.2020.03.002
Hennig, G., Haupka, C., Brito, L. F., Rückert, C., Cahoreau, E., Heux, S., et al. (2020). Methanol-Essential Growth of Corynebacterium Glutamicum: Adaptive Laboratory Evolution Overcomes Limitation Due to Methanethiol Assimilation Pathway. Ijms 21, 3617. doi:10.3390/ijms21103617
Heux, S., Brautaset, T., Vorholt, J. A., Wendisch, V. F., and Portais, J. C. (2018). “Synthetic Methylotrophy: Past, Present, and Future,” in Methane Biocatalysis: Paving the Way to Sustainability (Cham: Springer), 133–151. doi:10.1007/978-3-319-74866-5_9
Jakobsen, Ø. M., Benichou, A., Flickinger, M. C., Valla, S., Ellingsen, T. E., and Brautaset, T. (2006). Upregulated Transcription of Plasmid and Chromosomal Ribulose Monophosphate Pathway Genes Is Critical for Methanol Assimilation Rate and Methanol Tolerance in the Methylotrophic Bacterium Bacillus Methanolicus. J. Bacteriol. 188, 3063–3072. doi:10.1128/jb.188.8.3063-3072.2006
Jo, H.-J., Kim, J.-H., Kim, Y.-N., Seo, P.-W., Kim, C.-Y., Kim, J.-W., et al. (2022). Glyoxylate Carboligase-Based Whole-Cell Biotransformation of Formaldehyde into Ethylene Glycol via Glycolaldehyde. Green Chem. 24, 218–226. doi:10.1039/D1GC03549E
Keller, P., Noor, E., Meyer, F., Reiter, M. A., Anastassov, S., Kiefer, P., et al. (2020). Methanol-dependent Escherichia coli Strains with a Complete Ribulose Monophosphate Cycle. Nat. Commun. 11, 5403. doi:10.1038/s41467-020-19235-5
Keltjens, J. T., Pol, A., Reimann, J., and Op den Camp, H. J. M. (2014). PQQ-dependent Methanol Dehydrogenases: Rare-Earth Elements Make a Difference. Appl. Microbiol. Biotechnol. 98, 6163–6183. doi:10.1007/s00253-014-5766-8
Kiefer, P., Buchhaupt, M., Christen, P., Kaup, B., Schrader, J., and Vorholt, J. A. (2009). Metabolite Profiling Uncovers Plasmid-Induced Cobalt Limitation under Methylotrophic Growth Conditions. PLoS One 4, e7831. doi:10.1371/journal.pone.0007831
Kim, S., Lindner, S. N., Aslan, S., Yishai, O., Wenk, S., Schann, K., et al. (2020). Growth of E. coli on Formate and Methanol via the Reductive glycine Pathway. Nat. Chem. Biol. 16, 538–545. doi:10.1038/s41589-020-0473-5
Koopman, F. W., de Winde, J. H., and Ruijssenaars, H. J. (2009). C1 Compounds as Auxiliary Substrate for Engineered Pseudomonas Putida S12. Appl. Microbiol. Biotechnol. 83, 705–713. doi:10.1007/s00253-009-1922-y
Kyle Bennett, R., Agee, A., Har, J. R. G., Hagel, B., Antoniewicz, M. R., and Papoutsakis, E. T. (2021). Regulatory Interventions Improve the Biosynthesis of Limiting Amino Acids from Methanol Carbon to Improve Synthetic Methylotrophy in Escherichia coli. Biotechnol. Bioeng. 118, 43–57. doi:10.1002/bit.27549
Lau, Y. H., Giessen, T. W., Altenburg, W. J., and Silver, P. A. (2018). Prokaryotic Nanocompartments Form Synthetic Organelles in a Eukaryote. Nat. Commun. 9, 1311. doi:10.1038/s41467-018-03768-x
Lee, M. J., Palmer, D. J., and Warren, M. J. (2019). Biotechnological Advances in Bacterial Microcompartment Technology. Trends Biotechnol. 37, 325–336. doi:10.1016/j.tibtech.2018.08.006
Lessmeier, L., Pfeifenschneider, J., Carnicer, M., Heux, S., Portais, J.-C., and Wendisch, V. F. (2015). Production of Carbon-13-Labeled Cadaverine by Engineered Corynebacterium Glutamicum Using Carbon-13-Labeled Methanol as Co-substrate. Appl. Microbiol. Biotechnol. 99, 10163–10176. doi:10.1007/s00253-015-6906-5
Lu, X., Liu, Y., Yang, Y., Wang, S., Wang, Q., Wang, X., et al. (2019). Constructing a Synthetic Pathway for Acetyl-Coenzyme A from One-Carbon through Enzyme Design. Nat. Commun. 10, 1378. doi:10.1038/s41467-019-09095-z
Meyer, F., Keller, P., Hartl, J., Gröninger, O. G., Kiefer, P., and Vorholt, J. A. (2018). Methanol-essential Growth of Escherichia coli. Nat. Commun. 9, 1508. doi:10.1038/s41467-018-03937-y
Müller, J. E. N., Litsanov, B., Bortfeld-Miller, M., Trachsel, C., Grossmann, J., Brautaset, T., et al. (2014). Proteomic Analysis of the Thermophilic Methylotroph Bacillus Methanolicus MGA3. Proteomics 14, 725–737. doi:10.1002/pmic.201300515
Müller, J. E. N., Meyer, F., Litsanov, B., Kiefer, P., Potthoff, E., Heux, S., et al. (2015). Engineering Escherichia coli for Methanol Conversion. Metab. Eng. 28, 190–201. doi:10.1016/j.ymben.2014.12.008
Nattermann, M., Burgener, S., Pfister, P., Chou, A., Schulz, L., Lee, S. H., et al. (2021). Engineering a Highly Efficient Carboligase for Synthetic One-Carbon Metabolism. ACS Catal. 11, 5396–5404. doi:10.1021/acscatal.1c01237
Orita, I., Sakamoto, N., Kato, N., Yurimoto, H., and Sakai, Y. (2007). Bifunctional Enzyme Fusion of 3-Hexulose-6-Phosphate Synthase and 6-Phospho-3-Hexuloisomerase. Appl. Microbiol. Biotechnol. 76, 439–445. doi:10.1007/s00253-007-1023-8
Price, J. V., Chen, L., Whitaker, W. B., Papoutsakis, E., and Chen, W. (2016). Scaffoldless Engineered Enzyme Assembly for Enhanced Methanol Utilization. Proc. Natl. Acad. Sci. U.S.A. 113, 12691–12696. doi:10.1073/pnas.1601797113
Rohlhill, J., Gerald Har, J. R., Antoniewicz, M. R., and Papoutsakis, E. T. (2020). Improving Synthetic Methylotrophy via Dynamic Formaldehyde Regulation of Pentose Phosphate Pathway Genes and Redox Perturbation. Metab. Eng. 57, 247–255. doi:10.1016/j.ymben.2019.12.006
Rohlhill, J., Sandoval, N. R., and Papoutsakis, E. T. (2017). Sort-Seq Approach to Engineering a Formaldehyde-Inducible Promoter for Dynamically Regulated Escherichia coli Growth on Methanol. ACS Synth. Biol. 6, 1584–1595. doi:10.1021/acssynbio.7b00114
Roth, T. B., Woolston, B. M., Stephanopoulos, G., and Liu, D. R. (2019). Phage-Assisted Evolution of Bacillus Methanolicus Methanol Dehydrogenase 2. ACS Synth. Biol. 8, 796–806. doi:10.1021/acssynbio.8b00481
Sheehan, M. C., Bailey, C. J., Dowds, B. C. A., and McConnell, D. J. (1988). A New Alcohol Dehydrogenase, Reactive towards Methanol, from Bacillus Stearothermophilus. Biochem. J. 252, 661–666. doi:10.1042/bj2520661
Siegel, J. B., Smith, A. L., Poust, S., Wargacki, A. J., Bar-Even, A., Louw, C., et al. (2015). Computational Protein Design Enables a Novel One-Carbon Assimilation Pathway. Proc. Natl. Acad. Sci. U.S.A. 112, 3704–3709. doi:10.1073/pnas.1500545112
Tashiro, Y., Hirano, S., Matson, M. M., Atsumi, S., and Kondo, A. (2018). Electrical-biological Hybrid System for CO2 Reduction. Metab. Eng. 47, 211–218. doi:10.1016/j.ymben.2018.03.015
Tomàs-Gamisans, M., Ferrer, P., and Albiol, J. (2018). Fine-tuning the P. Pastoris iMT1026 Genome-Scale Metabolic Model for Improved Prediction of Growth on Methanol or Glycerol as Sole Carbon Sources. Microb. Biotechnol. 11, 224–237. doi:10.1111/1751-7915.12871
Tuyishime, P., and Sinumvayo, J. P. (2020). Novel Outlook in Engineering Synthetic Methylotrophs and Formatotrophs: a Course for Advancing C1-Based Chemicals Production. World J. Microbiol. Biotechnol. 36, 118. doi:10.1007/s11274-020-02899-y
Tuyishime, P., Wang, Y., Fan, L., Zhang, Q., Li, Q., Zheng, P., et al. (2018). Engineering Corynebacterium Glutamicum for Methanol-dependent Growth and Glutamate Production. Metab. Eng. 49, 220–231. doi:10.1016/j.ymben.2018.07.011
van der Klei, I. J., Yurimoto, H., Sakai, Y., and Veenhuis, M. (2006). The Significance of Peroxisomes in Methanol Metabolism in Methylotrophic Yeast. Biochimica Biophysica Acta (BBA) - Mol. Cell. Res. 1763, 1453–1462. doi:10.1016/j.bbamcr.2006.07.016
Vartiainen, E., Blomberg, P., Ilmén, M., Andberg, M., Toivari, M., and Penttilä, M. (2019). Evaluation of Synthetic Formaldehyde and Methanol Assimilation Pathways in Yarrowia Lipolytica. Fungal Biol. Biotechnol. 6, 27. doi:10.1186/s40694-019-0090-9
Wang, G., Olofsson-Dolk, M., Hansson, F. G., Donati, S., Li, X., Chang, H., et al. (2021). Engineering Yeast Yarrowia Lipolytica for Methanol Assimilation. ACS Synth. Biol. 10, 3537–3550. doi:10.1021/acssynbio.1c00464
Wang, X., Wang, Y., Liu, J., Li, Q., Zhang, Z., Zheng, P., et al. (2017). Biological Conversion of Methanol by Evolved Escherichia coli Carrying a Linear Methanol Assimilation Pathway. Bioresour. Bioprocess. 4, 41. doi:10.1186/s40643-017-0172-6
Wang, Y., Fan, L., Tuyishime, P., Liu, J., Zhang, K., Gao, N., et al. (2020a). Adaptive Laboratory Evolution Enhances Methanol Tolerance and Conversion in Engineered Corynebacterium Glutamicum. Commun. Biol. 3, 217. doi:10.1038/s42003-020-0954-9
Wang, Y., Fan, L., Tuyishime, P., Zheng, P., and Sun, J. (2020b). Synthetic Methylotrophy: A Practical Solution for Methanol-Based Biomanufacturing. Trends Biotechnol. 38, 650–666. doi:10.1016/j.tibtech.2019.12.013
Wendisch, V. F., Kosec, G., Heux, S., and Brautaset, T. (2021). Aerobic Utilization of Methanol for Microbial Growth and Production. Adv. Biochem. Eng. Biotechnol. Nov. 11. doi:10.1007/10_2021_177
Whitaker, W. B., Jones, J. A., Bennett, R. K., Gonzalez, J. E., Vernacchio, V. R., Collins, S. M., et al. (2017). Engineering the Biological Conversion of Methanol to Specialty Chemicals in Escherichia coli. Metab. Eng. 39, 49–59. doi:10.1016/j.ymben.2016.10.015
Witthoff, S., Schmitz, K., Niedenführ, S., Nöh, K., Noack, S., Bott, M., et al. (2015). Metabolic Engineering of Corynebacterium Glutamicum for Methanol Metabolism. Appl. Environ. Microbiol. 81, 2215–2225. doi:10.1128/AEM.03110-14
Woolston, B. M., King, J. R., Reiter, M., Van Hove, B., and Stephanopoulos, G. (2018). Improving Formaldehyde Consumption Drives Methanol Assimilation in Engineered E. coli. Nat. Commun. 9, 2387. doi:10.1038/s41467-018-04795-4
Wu, T.-Y., Chen, C.-T., Liu, J. T.-J., Bogorad, I. W., Damoiseaux, R., and Liao, J. C. (2016). Characterization and Evolution of an Activator-independent Methanol Dehydrogenase from Cupriavidus Necator N-1. Appl. Microbiol. Biotechnol. 100, 4969–4983. doi:10.1007/s00253-016-7320-3
Yang, X., Yuan, Q., Luo, H., Li, F., Mao, Y., Zhao, X., et al. (2019). Systematic Design and In Vitro Validation of Novel One-Carbon Assimilation Pathways. Metab. Eng. 56, 142–153. doi:10.1016/j.ymben.2019.09.001
Yasueda, H., Kawahara, Y., and Sugimoto, S.-i. (1999). Bacillus Subtilis yckG and yckF Encode Two Key Enzymes of the Ribulose Monophosphate Pathway Used by Methylotrophs, and yckH Is Required for Their Expression. J. Bacteriol. 181, 7154–7160. doi:10.1128/jb.181.23.7154-7160.1999
Yu, H., and Liao, J. C. (2018). A Modified Serine Cycle in Escherichia coli Coverts Methanol and CO2 to Two-Carbon Compounds. Nat. Commun. 9 (9), 3992. doi:10.1038/s41467-018-06496-4
Keywords: synthetic methylotrophs, methanol, metabolic engineering, modelling, biotechnology
Citation: Peiro C, Vicente CM, Jallet D and Heux S (2022) From a Hetero- to a Methylotrophic Lifestyle: Flash Back on the Engineering Strategies to Create Synthetic Methanol-User Strains. Front. Bioeng. Biotechnol. 10:907861. doi: 10.3389/fbioe.2022.907861
Received: 30 March 2022; Accepted: 16 May 2022;
Published: 08 June 2022.
Edited by:
Jean Marie François, Institut Biotechnologique de Toulouse (INSA), FranceReviewed by:
Hiroya Yurimoto, Kyoto University, JapanCopyright © 2022 Peiro, Vicente, Jallet and Heux. This is an open-access article distributed under the terms of the Creative Commons Attribution License (CC BY). The use, distribution or reproduction in other forums is permitted, provided the original author(s) and the copyright owner(s) are credited and that the original publication in this journal is cited, in accordance with accepted academic practice. No use, distribution or reproduction is permitted which does not comply with these terms.
*Correspondence: Stephanie Heux, c3RlcGhhbmllLmhldXhAaW5zYS10b3Vsb3VzZS5mcg==
Disclaimer: All claims expressed in this article are solely those of the authors and do not necessarily represent those of their affiliated organizations, or those of the publisher, the editors and the reviewers. Any product that may be evaluated in this article or claim that may be made by its manufacturer is not guaranteed or endorsed by the publisher.
Research integrity at Frontiers
Learn more about the work of our research integrity team to safeguard the quality of each article we publish.