- 1Department of Ultrasound Diagnosis, The Second Xiangya Hospital, Central South University, Changsha, China
- 2Research Center of Ultrasonography, The Second Xiangya Hospital, Central South University, Changsha, China
Many cancers have evolved various mechanisms to evade immunological surveillance, such as the inhibitory immune checkpoint of the CD47-SIRPα signaling pathway. By targeting this signaling pathway, researchers have developed diverse nanovehicles with different loaded drugs and modifications in anticancer treatment. In this review, we present a brief overview of CD47-SIRPα interaction and nanomedicine. Then, we delve into recent applications of the CD47-SIRPα interaction as a target for nanomedicine-based antitumor treatment and its combination with other targeting pathway drugs and/or therapeutic approaches.
Introduction
The CD47-SIRPα signaling axis plays an important role in antitumor immunology, tissue homeostasis and remodeling (Logtenberg et al., 2020). Upregulated expression of CD47 on tumor cells increases the interaction with SIRPα on the myeloid cell membrane, leading to a release of the “don’t eat me” signal to evade the phagocytosis of myeloid cells, which is one of the primary mechanisms of cancer and disease formulation (Oldenborg et al., 2000; Jaiswal et al., 2009; Willingham et al., 2012; Hayat et al., 2020; Logtenberg et al., 2020). Hence, increasing studies have focused on the CD47-SIRPα interaction to achieve better therapeutic efficacy for cancer and other diseases (Ho et al., 2015; Petrova et al., 2017; Yanagita et al., 2017). Nevertheless, similar to other conventional medical treatments, the disadvantages of systemic administration of CD47-SIRPα blockade, such as nontargeting distribution, side effects, and short half-life period, have limited its translation to clinical use. These disadvantages can be abated by nanotechnology, which also offers nanotemedicine a promising opportunity to develop. In this review, we will discuss the recent achievements of CD47-SIRPα interaction-based antitumor nanomedicine from the following three aspects: CD47-SIRPα interaction, an overview of nanomedicine, and the role of the CD47-SIRPα checkpoint in nanomedicine-based anticancer treatment.
Overview of the CD47-SIRPα Checkpoint
CD47 Structure
The CD47 protein, a member of the membrane protein IG superfamily, is ubiquitously expressed on varieties of types of cellular membranes, especially on senile erythrocytes and cancer cells (Hayat et al., 2020). Its molecular structure includes a single IgV-like extracellular domain at the N terminus, a highly hydrophobic stretch with five membrane-spanning sections and an alternative splicing cytoplasmic domain at its C terminus (Brown and Frazier, 2001; Mushegian, 2002). By interacting with integrin and TSP-1, CD47 is involved in a variety of physiological processes, such as migration, adhesion, proliferation, differentiation (Lindberg et al., 1996; Liu et al., 2001; Lymn et al., 2002). As an inhibitory receptor, CD47 can bind with SIRPα to not only inhibit phagocytosis by phagocytes but also inhibit the activation and maturation of dendritic cells (DCs) (Oldenborg et al., 2000; Latour et al., 2001; Lutz and Bogdanova, 2013). In addition, CD47-SIRPα also regulates neuron development and bone remodeling (Barclay, 2009; Maile et al., 2011).
SIRPα Structure
SIRPα, a member of the Ig superfamily (IgSF), consists of three Ig-like extracellular domains at its N terminus four tyrosine phosphorylation sites and two immunoreceptor tyrosine inhibitory motifs (ITIMs) in its cytoplasmic domain (Adams et al., 1998; Hayat et al., 2020). In contrast to the ubiquitous expression of CD47, SIRPα is limitedly expressed on macrophages, monocytes, granulocytes and neurons (Adams et al., 1998).
CD47-SIRPα Interaction
The N-terminal Ig-like extracellular domain of SIRPα binds with the N-terminal IgV-like extracellular domain of CD47, resulting in the phosphorylation of ITIM of SIRPα and recruitment and activation of protein tyrosine phosphatases, especially Src homology 2 including SHP-1 and SHP-2, dephosphorylation of the downstream molecule ITAM, the accumulation of myosin IIA damage in the phagocytic synapse, releasing the “don’t eat me” signal, leading to an inhibition of phagocytosis (Hayat et al., 2020; Logtenberg et al., 2020; Figure 1).
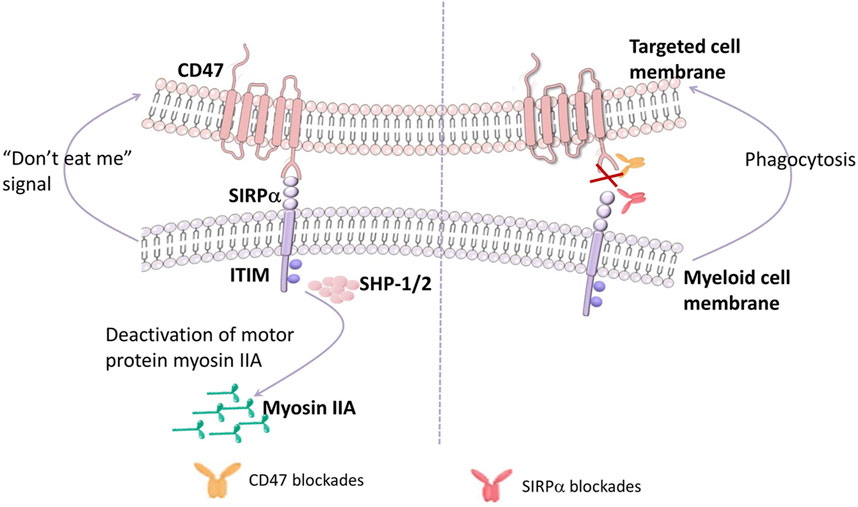
FIGURE 1. CD47 interacts with SIRPα. The N-terminal Ig-like extracellular domain of SIRPα on myeloid cells binding to the N-terminal IgV-like extracellular domain of CD47 on targeted cells induces the phosphorylation of ITIM of SIRPα, then recruits and activates the protein tyrosine phosphatases, especially Src homology 2 (including SHP-1 and SHP-2). Upon activated SHP-1/2 engagement, myosin IIA dephosphorylation Occurs, releasing the “don’t eat me” signal, leading to an inhibition of phagocytosis. On the contrary, inhibiting the CD47-SIRPα pathway activates the phagocytosis by myeloid cells.
Homeostasis of cells and tissues depends on a balance of regulation of pro-phagocytic signals [calreticulin (CRT)-low density lipoprotein-receptor related protein-1 (LRP-1), Fcγ, complement receptor] and anti-phagocytic signals (CD47-SIRPα) (Oldenborg et al., 2001; Chao et al., 2010a). Chao et al. demonstrated that CRT plays a leading role in pro-phagocytic signals and is essential for anti-CD47 antibody therapy in multiple human cancers. In their study, the in vitro phagocytosis assays were performed by incubating primary human normal cells and cancer cells with human macrophages with a therapeutic dosage of anti-CD47 antibody, and showed that primary cancer cells were obviously phagocytized, whereas no phagocytosis of normal cells was observed, suggesting that blocking the CD47-SIRPα is not the only rationale for pro-phagocytosis (Chao et al., 2010a). Note that the anti-CD47 antibody with intact Fcγ should be utilized with caution given the pro-phagocytosis role of Fcγ, which could increase systemic toxicity by enhancing antigen sink effects (Ingram et al., 2017).
Increasing studies have concluded that the antitumor effect mediated by blocking the CD47-SIRPα interaction mainly owes to the activation of innate immune responses [including phagocytosis by macrophages and the antibody dependent cellular cytotoxicity (ADCC) by neutrophil granulocytes] (Jaiswal et al., 2009). However, it is important to note that the results of these works were based on xenograft models, which may favor innate immune responses to kill tumor cells with some unique features (Majeti et al., 2009; Chao et al., 2010b; Willingham et al., 2012). Liu et al. used syngeneic immune-competent mouse models to exclude these effects (Liu et al., 2015a). In this study, the mouse anti-CD47 antibody showed an evident antitumor effect, especially by intratumoral delivery, and the therapeutic effect was diminished when CD8+ T cells were depleted. In addition, CD47-SIRPα blockade activates the maturation of DCs and boosts DC-mediated antigen cross-presentation and cytotoxic T lymphocyte induction. Hence, the CD47-SIRPα signaling axis is an inhibitory checkpoint that bridges innate and adaptive immunity for tumor evasion.
Strategies for Inhibiting the CD47-SIRPα Interaction
According to the different signaling pathway blocking sites, the strategies for inhibiting the CD47-SIRPα interaction can be divided into three types: molecules that inhibit the CD47 protein on the tumor cells, molecules that inhibit SIRPα protein on the myeloid cells, and inhibitors of the glutaminyl-peptide cyclotransferase-like (QPCTL) enzyme, which is necessary for the maturation of CD47 protein (Logtenberg et al., 2020).
The strategy targeting CD47 on tumor cells has been the most commonly studied. For instance, Hu5F9-G4, a humanized anti-CD47 antibody with a human immunoglobulin G4, has been proved a potent antitumor effect in preclinical experiments and clinical trials. In a malignant pediatric brain tumor-bearing mouse model, administration of Hu5F9 evidently inhibited tumor growth and showed significant survival benefit (Gholamin et al., 2017). However, CD47 is not only over-expressed on cancer cells, but also expressed on normal cells, such as ethrocytes. Therefore, administration of CD47 blocking agents would lead to anemia and “antigen sink” effect. In the development of CD47 targeting agents, multiple approaches have been employed to solve these problems, such as change in the mode of administration (Liu et al., 2015b; Advani et al., 2018; Sikic et al., 2019); dual targeting bispecific antibodies of CD47 (Dheilly et al., 2017; Shi et al., 2020; Wang et al., 2021); CD47 antibodies/SIRPα fusion proteins (Petrova et al., 2017; Meng et al., 2019; Puro et al., 2020; Andrejeva et al., 2021). From the efficacy of view, dual targeting bispecific antibodies are more promising. Wang et al. designed a CD47-PD-L1 bi-specific antibody, named IB322 (Wang et al., 2021). As a dual inhibitor of innate and adaptive immune checkpoint, IB322 efficiently triggered the tumor cell phagocytosis by macrophages and killing effect by T cells and induced complete tumor regression in vivo. Moreover, IB322 showed negligible RBCs depletion and was well tolerated in non-human primates.
Compared with the wide expression of CD47, SIRPα is restrictedly expressed on myeloid cells and neurons (Adams et al., 1998). Hence, biologicals that target SIRPα do not suffer from amenia and “antigen sink” issues (Yanagita et al., 2017; Voets et al., 2019). For example, Ho et al. developed an engineered, high-affinity, CD47 variant (termed Vecro-CD47), which could remarkably increase the affinity to wild-type (WT) SIRPα and disrupt the CD47-SIRPα interaction, thereby promoting macrophage phagocytosis of tumor cells (Ho et al., 2015). Voets et al. developed a humanized mAb ADU-1805, which inhibits the CD47-SIRPα signaling pathway by closely binding with SIRPα, showing similar antitumor efficacy as the anti-CD47 antibody with good safety in vitro and in vivo (Voets et al., 2019). However, researches and clinical trials focused on SIRPα blocking target are fewer than that on CD47 blocking target.
Inhibitors of QPCTL enzyme is another promising strategy that does not result in anemia easily and “antigen sink” issues (Ingram et al., 2017). Logtenberg et al. reported that both genetically and pharmacologically blocking QPCTL activity enhanced antibody-dependent cellular phagocytosis (ADCP) and ADCC of tumor cells (Logtenberg et al., 2019). Moreover, the intervention of QPCTL activity can alter the immunosuppressive tumor microenvironment (monocyte skewed, myCAF, TGF-β) to a proinflammatory (macrophage skewed, iCAF, IFN) milieu, and enhances the therapeutic effect of anti-PD-L1 therapy (Bresser et al., 2022).
To date, over 20 CD47/SIRPα blocking agents have been employed in clinical trials (summarized in Table 1), involving in both hematological malignancies and solid tumors. However, the current clinical trial data of QPCTL inhibitors in antitumor treatment are lacking.
Although promising, the CD47-SIRPα blocking agents still face some challenges that restrict their translation to clinical settings. For instance, the ubiquitous expression of CD47 indicates that large dose or frequent administration of anti-CD47 antibodies is required (eg: antigen sink effect) (Chen et al., 2022), suggesting the efficacy of anti-CD47 antibodies treatment is relatively low. With regard to targeting bispecific antibodies of CD47 and CD47 antibodies/SIRPα fusion protein technology, while promising, it requires complex design and isolation (Chen et al., 2013; Labrijn et al., 2019). Therefore, the cost of these therapies is usually unaffordable for patients, which limits their clinical applications (Chen et al., 2013; Labrijn et al., 2019). As for SIRPα targeting strategy, it constantly fail to induce ADCP and ADCC against cancer cells when administrated alone due to immune cells target (Chen et al., 2013; Ring et al., 2017; Labrijn et al., 2019; Chen et al., 2022). The demand for safer and more efficient drug delivery is therefore increasing.
Nanomedicine, defined as the application of nanotechnology, can meet this need. Nanotechnology enables therapeutic drugs to target sites with high spatial and temporal resolution, prolonged half-life and great convenience for combination therapy. Therefore, CD47-SIRPα targeting based nanomedicine holds great potential in antitumor field, which will be reviewed in more detail in the following sections.
Overview of Nanomedicine
The efficacy of drugs has been limited, due to nonspecific distribution, side effects and short circulation time, offering an evolutionary opportunity for nanomedicine to circumvent these drawbacks and improve therapeutic efficacy. Diverse applications of nanomedicine have been investigated in multiple areas, such as drug delivery, vaccine development, diagnosis, and imaging tools (Pelaz et al., 2017). In this section, we mainly focus on the application of nanomedicine in drug delivery.
Type of Nanoparticles
Nanoparticles (NPs) are important components of nanomedicine. The unique characteristics of NPs, such as large surface-volume ratio, small size, capacity to encapsulate various drugs, and tunable surface chemistry, provides themselves a large variety of advantages, including multivalent surface modification, efficient navigation in vivo, increased intracellular trafficking and sustained release of drug payloads (Xu et al., 2015). Currently, diverse types of NPs exist, including liposomes (Huynh et al., 2009; Wang et al., 2016; Olusanya et al., 2018; Yang et al., 2021), micelles (Torchilin, 2007; Tawfik et al., 2020), poly (lactic-co-glycolic acid) (PLGA) (Sadat Tabatabaei Mirakabad et al., 2014; Rezvantalab et al., 2018), graphene (Diez-Pascual, 2020), graphene oxide (Kinnear et al., 2017; Diez-Pascual, 2020), protein nanoparticles (Lohcharoenkal et al., 2014; Jain et al., 2018), extracellular vesicles (EVs) (S et al., 2013; Si et al., 2022; Logozzi et al., 2021), exosomes (De La Peña et al., 2009; Nie et al., 2020; Xia et al., 2020; Logozzi et al., 2021), magnetic NPs (MNPs) (Colombo et al., 2012; Wu et al., 2019; Farzin et al., 2020), mesoporous silica NPs (MSNPs) (Fu et al., 2013; Wang et al., 2015; Pelaz et al., 2017; Rastegari et al., 2021), and metal-organ frameworks (MOFs) (Zheng et al., 2016; Wu and Yang, 2017; Xing et al., 2020), Ferritin (Lee et al., 2017; Cho et al., 2018; Sun et al., 2021). Detailed information about the charaterizations, advantages and disadvantages of each type of NP is summarized in Table 2. As summarized in Table 2, while each nanocarrier possesses unique merits, they still face certain problems that restrict their optimal performance in the drug delivery system.
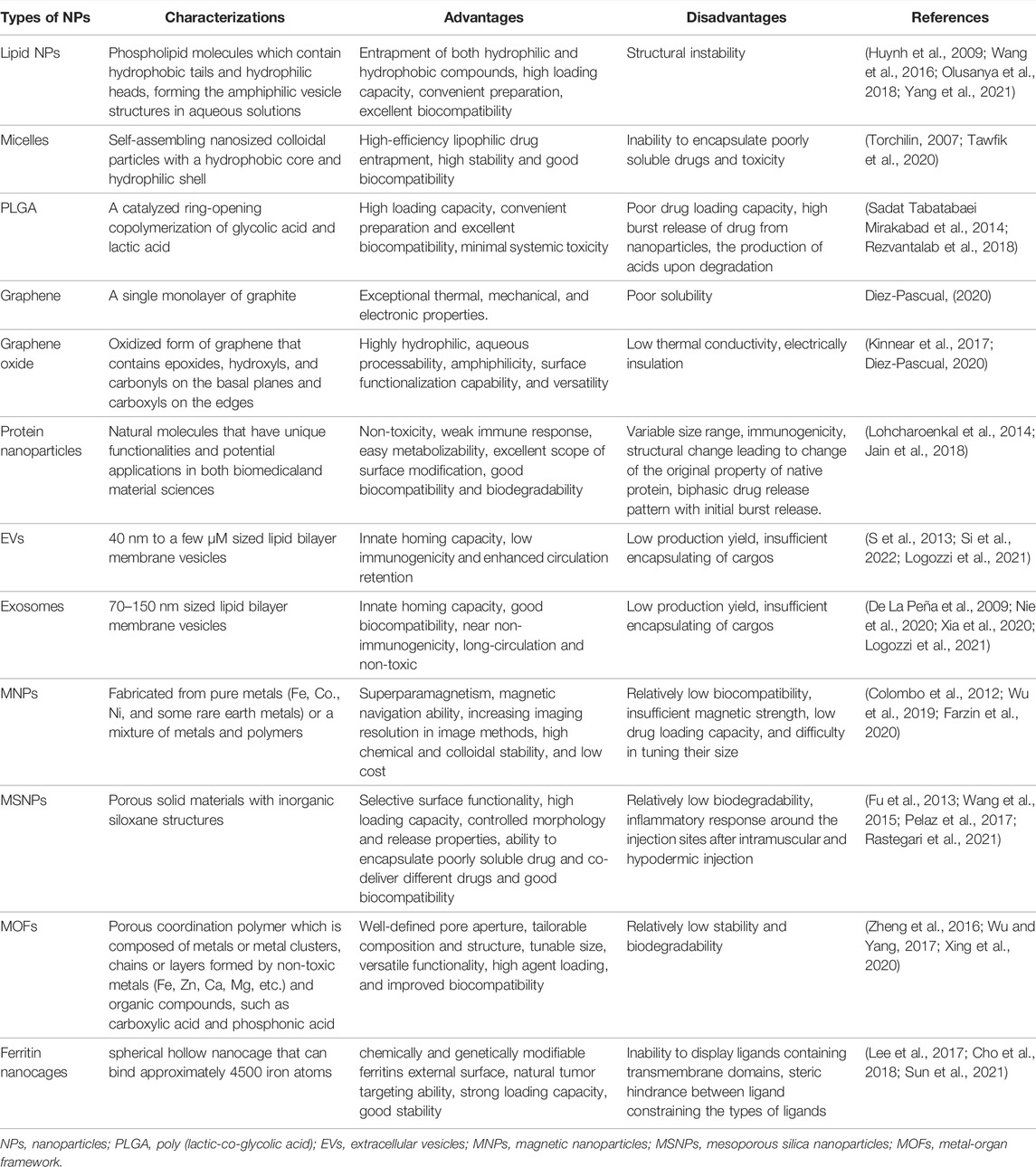
TABLE 2. Detailed information about the charaterizations, advantages and disadvantages of each type of nanomaterials.
Targeted Delivery Capacity of NPs
Targeted delivery capacity, including passive targeting and active targeting, is a key feature of nanomedicine and has been vastly studied.
Passive targeting is achieved by the enhanced permeability and retention (EPR) effect, prolonging the half-life of NPs in the body circulation (Maeda et al., 1999; Fang et al., 2003). Thus, prolonging the circulation time of NPs is a good strategy to increase their accumulation at the tumor site. The stealth modification of NPs is of great importance. Camouflaging the surface with polyethylene glycol (PEG) is the most common way to create a protective layer for encapsulated cargo by reducing the absorption of plasma proteins and extending the half-life of NPs (Gref et al., 2000). In addition to PEGylation, new biomaterials and drug delivery strategies have been developed to prolong the circulation time of NPs, including zwitterionic polymer-coating (Zhu et al., 2014), minimal self-peptides (e.g., CD47-derived self-peptides) (Rodriguez et al., 2013), and biomimetic membrane-coating (Hu et al., 2011). Nevertheless, passive targeting is far to reach the requirement of therapeutic efficacy. Due to the phagocytosis of mononuclear phagocytes, the majority (more than 90%) of NPs are inevitably entrapped by reticuloendithelial organs, such as liver and spleen (Albanese et al., 2012).
To overcome this severe drawback, measures that make nanomedicine actively target the disease site should be taken to increase the accumulation of drugs at the target site and subsequently enhance the therapeutic efficacy. Active targeting is a strategy to achieve the goal of orientation in space and simultaneously eliminates the off-target effect in normal tissues by intentionally guiding NPs to the disease site. A common approach to active targeting is to decorate the appropriate ligands to the surface of NPs. These ligands interact with the surface receptor of target cells inducing receptor-mediated endocytosis (Chen et al., 2017). Target agents can be broadly categorized as proteins (mainly antibodies and their fragments), nucleic acids (aptamers), or other receptor ligands (Peer et al., 2007).
Controlled Drug Release
To release the drug at a specific site and time, various efforts have been made to develop stimuli-responsive NPs, which further enhance the therapeutic efficacy (Li et al., 2020). These stimuli-responsive NPs can be stimulated by either endogenous stimuli-responsive strategies, such as pH variation, redox, enzyme, hypoxia, or exogenous stimuli-responsive strategies, such as light, ultrasound, magnetic field, temperature (Tawfik et al., 2020). However, these single stimuli-responsive strategies still face some challenges. For example, several temperatue- and light-responsive agents can damage normal cells and even tissues and organs (Wu et al., 2018). Due to insufficient H2O2 levels in tumor tissues, the nonspecificity and low therapeutic efficiency of H2O2-responsive nanoplatforms are also key challenges for clinical translation (Chang et al., 2017). Nanoassemblies activated by both exogenous stimuli and endogenous stimuli have gained tremendous attention by virtue of the enhanced encapsulated payload and the higher accuracy of spatiotemporal release. For instance, chen et al. developed a photothermal-PH-hypoxia responsive multifunctional nanoplatform (TENAB NP) for cancer photochemotherapy, for synergistic chemo-phototherapy with minimized skin photosensitization (Chen et al., 2019a). In this multistimuli responsive drug delivery system, tirapazamine, the hypoxia-specific prodrug, and ENAB, the pH-responsive photosensitizer, were encapsulated into the phase change materials (LASA), a mixture of linoleic acid and stearyl alcohol.
Role of the CD47-SIRPα Checkpoint in Nanomedicine-Based Disease Treatment
As previously described, systemic administration of CD47-SIRPα blocking agents has led to remarkable achievements, but the concomitant side effects (e.g., anemia) and limitations have restricted their translation to clinical use. To address these issues, nanotechnology has been introduced to reduce the side effects and enhance the stability and efficacy of the drug and the possibility of controlled release. In this section, we review the recent advances in the role of the CD47-SIRPα checkpoint in nanomedicine-based disease treatment.
Blocking the “Don’t Eat Me” Signal of the CD47-SIRPα Interaction
The upregulated CD47 on tumor cells increases its interaction with SIRPα on macrophages, resulting in an evasion of immunological surveillance and the muturation of DCs (Willingham et al., 2012; Jaiswal et al., 2009; Liu et al., 2015a; Liu et al., 2017). This inhibitory checkpoint paves the way for therapeutic strategies involving blocking this interaction to enhance innate and adaptive immunity for tumor killing (Figure 2). For instance, Koh et al. constructed an exosome through surface engineering with SIRPα variants termed SIRPα-exosomes, which can bind to both human and mouse CD47 as antagonists. The therapeutic efficacy of these engineered exosomes was verified in HT27 tumor-bearing mice (including immunodeficient and immunocompetent mice). Systemic administration of SIRPα-exosomes induced significant regression of tumor growth in immunocompetent mice, while tumor growth was slightly reduced in immunodeficient mice, suggesting that T-cell immunity might be essential to maximize the antitumor effect of CD47 blockade therapy (Koh et al., 2017). Ramesh et al. developed a multivalent lipid-based phagocytosis nanoenhancer with the conjugation of anti-CD47 and anti-SIRPα antibodies (LPN). LPN treatment showed remarkable tumor growth suppression and increased survival in B16F10 tumor-bearing mice with no systemic toxicity (Ramesh et al., 2020).
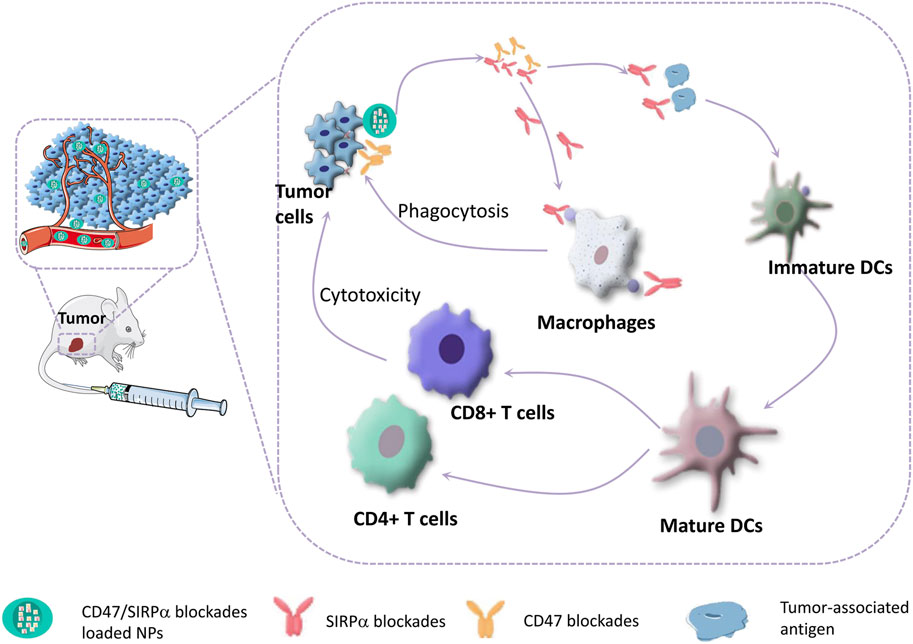
FIGURE 2. CD47-SIRPα blockades can bridge innate and adaptive antitumor immunity. Blocking of CD47-SIRPα signaling axis can activate macrophages to phagocytize tumor cells, and promote the maturation of DCs, which enhances DC-mediated tumor-associated antigen presentation, thereby triggering T-cell mediated destruction of tumor cells.
However, cancers are very complex diseases involving multiple pathways, and their progression is associated with various continuous mutations in cell lines. In addition, in order to survive, tumor cells mutate as chemotherapy progresses, thereby resulting in intrinsic and acquired resistance to chemotherapeutics (Iyer et al., 2013). Hence, the inhibition of the CD47-SIRPα signaling pathway is not sufficient to fight against tumor, and often requires a combination of blockades of different pathways, genes or chemotherapeutics (Xu et al., 2015). The advantages of nanomedicine, such as the capacity of encapsulating different drugs, targeted delivery and controlled release, offer a great opportunity for combination therapy for tumors. Some examples to show how the combination of blockade of the CD47-SIRPα interaction and other therapeutics or modalities works in nanomedicine are listed in Table 3 and explained in the next section.
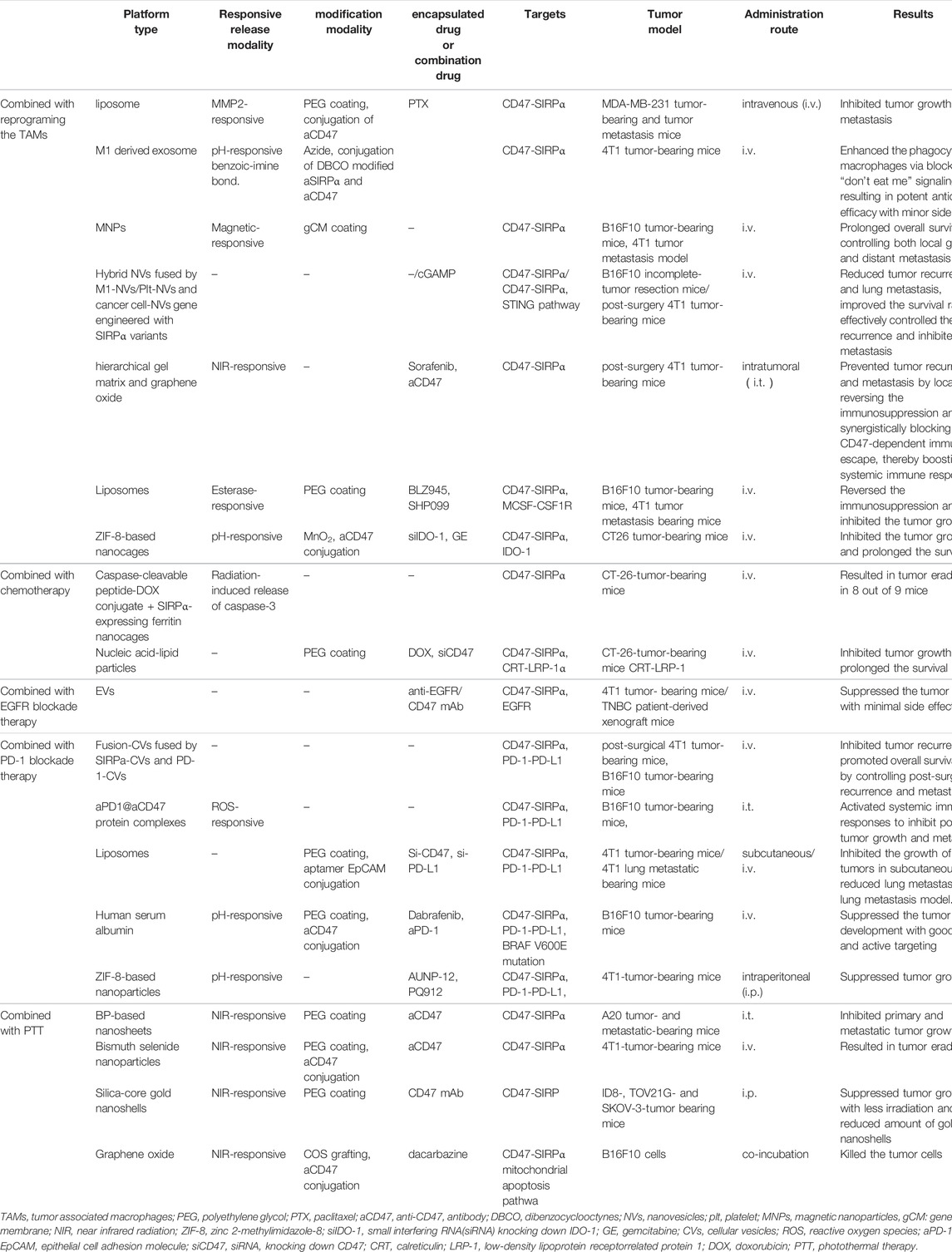
TABLE 3. Examples of nanoparticle-based combination therapy utilized blocking CD47-SIRPα signal axis.
Recent advances in tumor immunology suggest that the antitumor effect of blocking the CD47-SiRPα signaling pathway may be discounted by the immunosuppressive tumor microenvironment (TME) (Noy and Pollard, 2014; Chen et al., 2019b). In particular, colony-stimulating factors, secreted by tumor cells, are abundant in the TME, polarizing TAMs to the tumorigenic M2 phenotype (Noy and Pollard, 2014; Chen et al., 2019b). M2 TAMs can recruit regulatory T cells (Tregs) and secrete proinflammatory cytokines, all of which impair the activation of CD47 blockers against tumor T-cell immunity (Mantovani et al., 2017; Kulkarni et al., 2018; Feng et al., 2019). In this context, blocking the CD47-SIRPα signaling axis while polarizing tumorigenic M2- to anti-tumor M1-phenotype TAMs can improve the antitumor effect of CD47 immune checkpoint inhibitors (Ramesh et al., 2019; Rao et al., 2020a; Rao et al., 2020b; Chen et al., 2020; Nie et al., 2020; Chen et al., 2021; Huang et al., 2021). For example, Rao et al. have developed a genetically engineered cell-membrane-coated magnetic nanovehicle (gCM-MNs). The gCM shell genetically overexpressing SIRPα variants with prominent affinity and efficiently inhibits the CD47-SIRPα signaling axis, which can also protect the MN core from macrophage phagocytosis. The MN core promotes M2 TAM repolarization, synergistically triggering potent macrophage immune responses. Moreover, the MN core delivers the gCMs into tumor tissues under magnetic navigation, effectively promoting their tumor accumulation. In melanoma and triple-negative breast cancer models, gCM-MNs remarkably extended overall survival by inhibiting local tumor growth and distant metastasis (Rao et al., 2020a; Figure 3).
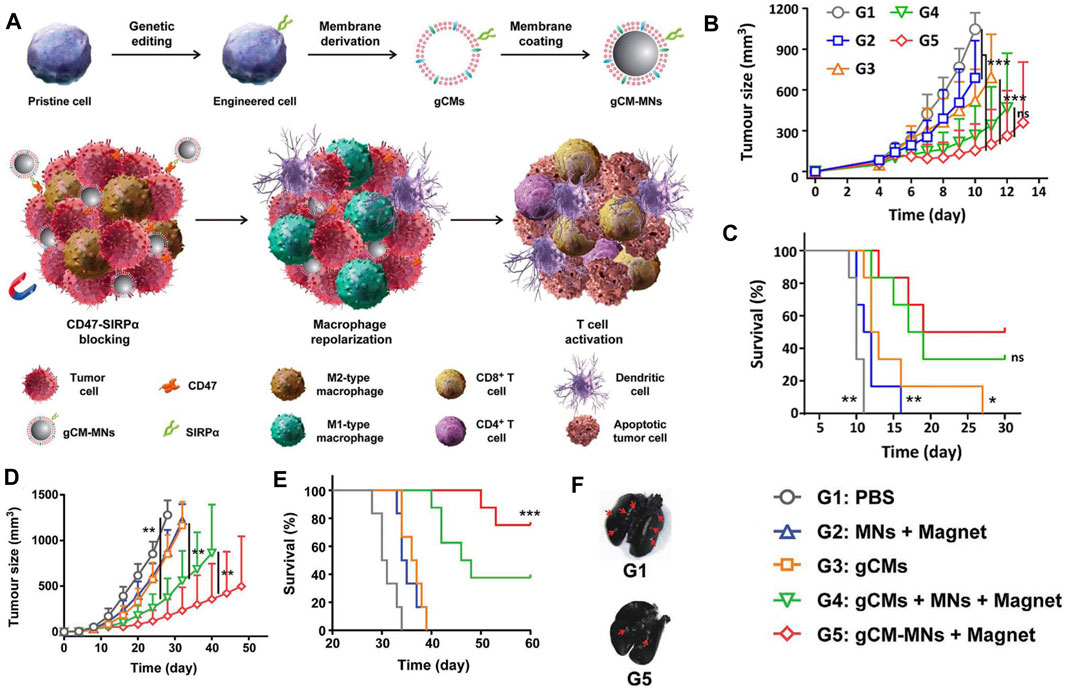
FIGURE 3. (A) Scheme of genetically edited cell-membrane-coated magnetic nanoparticles (gCM-MNs) elicits potent macrophage immune responses for cancer immunotherapy. (B,C) gCM-MNs inhibit B16F10 tumor growth. (B) Tumor growth kinetics after indicated treatments. (C) Survival curves for different treatment groups. (D–F) gCM-MNs suppress 4T1 tumor growth and lung metastasis. (D) Average tumor growth kinetics after indicated treatments. (E) Survival curves for different treatment groups. (F) Ink-stained lung photographs for different treatment groups. The red arrowheads indicate tumor foci in the lung. Adapted with permission [59]. Copyright 2020. Wiley.
Many studies have investigated the efficacy of chemotherapy as an adjuvant to immunotherapy, suggesting that the most significant potential mechanism of such adjunct is immunogenic cell death (ICD) (Zitvogel et al., 2008; RA and BW, 2005; Hou et al., 2013). ICD has a number of clearly defined physiological characteristics, including cell surface CRT expression, release of DAMPs such as adenosine triphosphate and heat shock proteins, and release of high mobility group box 1 (Liu et al., 2016). Among these factors, the surface expression of CRT is considered to be the single most important element of ICD (Abdel-Bar et al., 2021). As previously mentioned, the effect of CRT exposure, serving as an “eat me” signal, is considered to be counterbalanced and potentially dampened by CD47 expression (Chao et al., 2010a). Moreover, the upregulated expression of CD47 on the surface of tumor cells makes it an active targeting site for tumor cells, facilitating nonspecific distributed ICD inducing drugs to target tumor tissues and reduce systemic toxicity (Tang et al., 2021). Therefore, codelivery, which simultaneously removes an inhibitory signal and introduces an activating signal, can produce an enhanced antitumor effect (Abdel-Bar et al., 2021; Lee et al., 2021). For example, Abdel-Bar et al. reported a stable nucleic acid-lipid particle (SNALP) formulation with the simultaneous delivery of an ICD inducing drug (Dox) with small interfering RNA (siRNA) knocking down CD47 (siCD47) for synergistic enhancement of ICD. In a CT-26-tumor-bearing mouse model, SNALPs synergistically inhibited tumor growth and prolonged the survival (Abdel-Bar et al., 2021).
EGFR is overexpressed in various solid tumors, such as breast, renal, colon, head and neck cancer (Normanno et al., 2001; Gazdar, 2009; Yu et al., 2013) Hence, EGFR targeting strategy is a promising way for antitumor treatment (Mendelsohn and Baselga, 2003; Yu et al., 2013; Sabbah et al., 2020). CD47 is also overexpressed on the surface of multiple tumor cells. Therefore, dual targeting to EGFR and CD47 strategy can efficiently target and inhibit tumor growth. For example, Si et al. constructed anti-EGFR/CD47 mAb marked EV which showed a high anti-TNBC efficacy with negligible toxicity in both 4T1 tumor-bearing mouse models and TNBC patient-derived xenograft models (Si et al., 2022).
Programmed cell death-ligand 1 (PD-L1) blockade therapy has achieved exciting success in the clinic (Pardoll, 2012). PD-L1, which is highly expressed in many tumor cells, sends a “don’t find me” signal to the adaptive immune system, inhibiting T-cell activation by engaging the PD-1 receptor (Parsa et al., 2007). CD47 sends a “don’t eat me” signal to the innate immune system, inhibiting the phagocytosis of macrophages by engaging SIRPα (Hayat et al., 2020; Logtenberg et al., 2020; Zhang et al., 2020). Hence, dual-blockade of PD-L1 and CD47 can activate potent antitumor effects via both innate and adaptive immune responses (Chen et al., 2019c; Lian et al., 2019; Meng et al., 2021; Pham et al., 2021; Zhao et al., 2021). For example, Meng et al. designed genetically programmable fusion cellular vesicles (Fus-CVs), which were fused by SIRPα variants and PD-1 variants. This bispecific targeting design improves the targeting of tumor cells while reducing the adverse off-target effect on normal cells. In malignant melanoma and mammary carcinoma models, Fus-CVs synergistically suppressed postsurgery tumor recurrence and metastasis, thereby improving overall survival (Meng et al., 2021).
PTT is a promising cancer treatment modality. PTT-induced hyperthermia can be controlled through the local use of photosensitizers and minimally invasive near-infrared (NIR) radiation to reduce damage to untargeted tissues (Sica et al., 2006; Chu and Dupuy, 2014; Chen et al., 2015). Recently, increasing studies have demonstrated that hyperthermia can induce dying tumor cells to release massive amounts of cytokines, such as IL-1β and TNF-α, promoting the immune responses of macrophages, NK cells and T lymphocytes. Yet, it is difficult to completely eradicate large tumors with conventional PTT due to residual tumor mass at the treatment margins (Mantovani et al., 2006; Chu and Dupuy, 2014; Shim et al., 2017). Therefore, researchers combined CD47 blockers with PTT to synergistically enhance the antitumor effect (Wu et al., 2015; Guo et al., 2019; Xie et al., 2020; Zhan et al., 2021). For example, Guo et al. reported bismuth selenide nanoparticles conjugated with anti-CD47 antibody and coated with PEG (Ab-PEG-Bi2Se3). In the 4T1 tumor-bearing model, Ab-PEG-Bi2Se3 plus PTT synergistically eradicate the tumor (Guo et al., 2019).
Utilizing the “Don’t Eat Me” Signal of the CD47-SIRPα Interaction
Reducing the capture of NPs by reticuloendothelial organs (such as liver, spleen, and lung) and extending their circulation time in the blood to accumulate more NPs in tumor tissues have been challenges. Currently, there are many approaches to prolong the half-life of NPs in blood, such as PEG surface modification and bionic membrane coating techniques described above. However, these approaches still have some disadvantages and limitations. Therefore, more suitable alternatives are urgently needed. The CD47 protein, as a “self” marker, can evade phagocytosis by the CD47-SIRPα interaction (Logtenberg et al., 2020). With regard to the pivotal role of CD47 in the regulation of immune responses, the present paper outlines emerging methods for the production of bioinert biomaterials and NPs using CD47 (Gheibi Hayat et al., 2019; Figure 4) Examples of stealth functionalization by CD47 mimicry utilized for antitumor nanomedicine are listed in Table 4 (Kamerkar et al., 2017; Shim et al., 2017; Jiang et al., 2018; Song et al., 2019; Wang et al., 2019; Belhadj et al., 2020; Cheng et al., 2021; Tang et al., 2021; Xie et al., 2021; Zhang et al., 2021). For instance, Tang et al. designed a precise delivery nanomedicine to M2 macrophages by combining “eat me/don’t eat me” signals and verified its role in antitumor therapy in an A20 subcutaneous tumor mouse model. In this delivery system, CD47-derived self-peptide ligand and galactose ligand were introduced on liposomes to reduce the phagocytosis of M1 macrophages and enhance the uptake of M2 macrophages, respectively. Cleavable phospholipid-PEG covering on the surface of liposomes can be removed by the redox microenvironment upon transcytosis through the tumor endothelium and re-expose the self-peptide and galactose. Therefore, this nanocarrier can precisely target M2-type TAMs. In addition, DOX loaded into liposomes further enhances its antitumor effect (Tang et al., 2021; Figure 5)
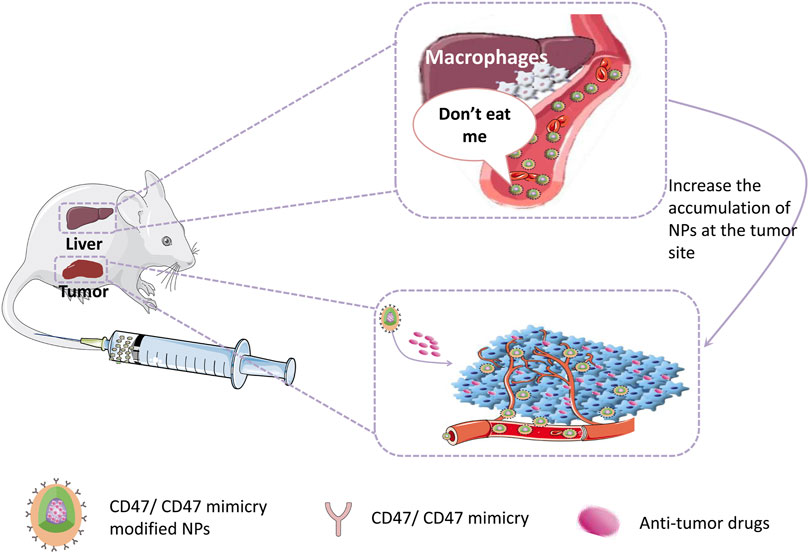
FIGURE 4. CD47/CD47mimicry modified NPs can evade the phagocytic by reticuloendothelial system which increases their half-life in circulation and the accumulation at the tumor site, hence enhancing the therapeutic effect.
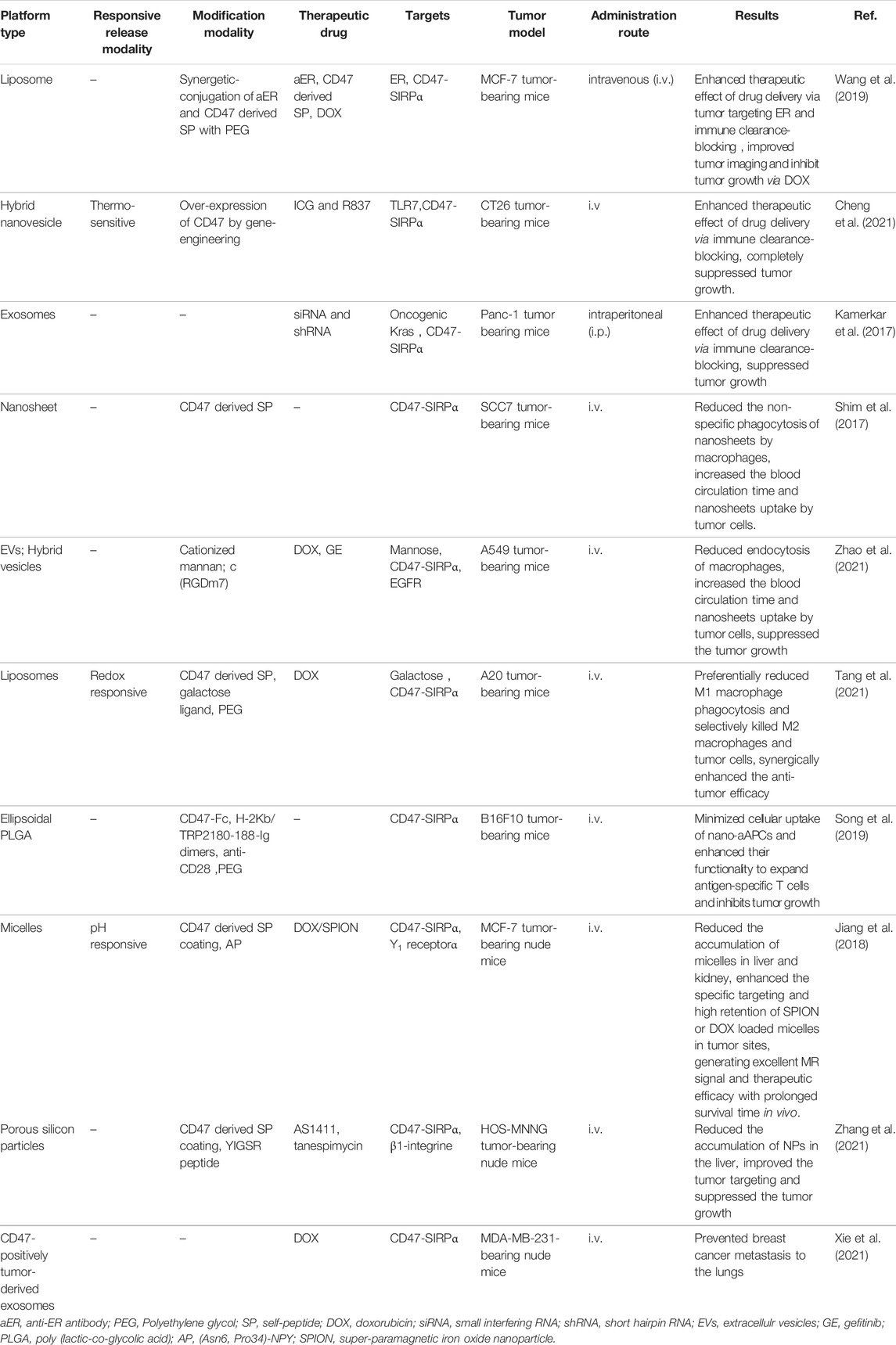
TABLE 4. Examples of nanoparticle-based combination therapy utilized activating CD47-SIRPα signal axis.
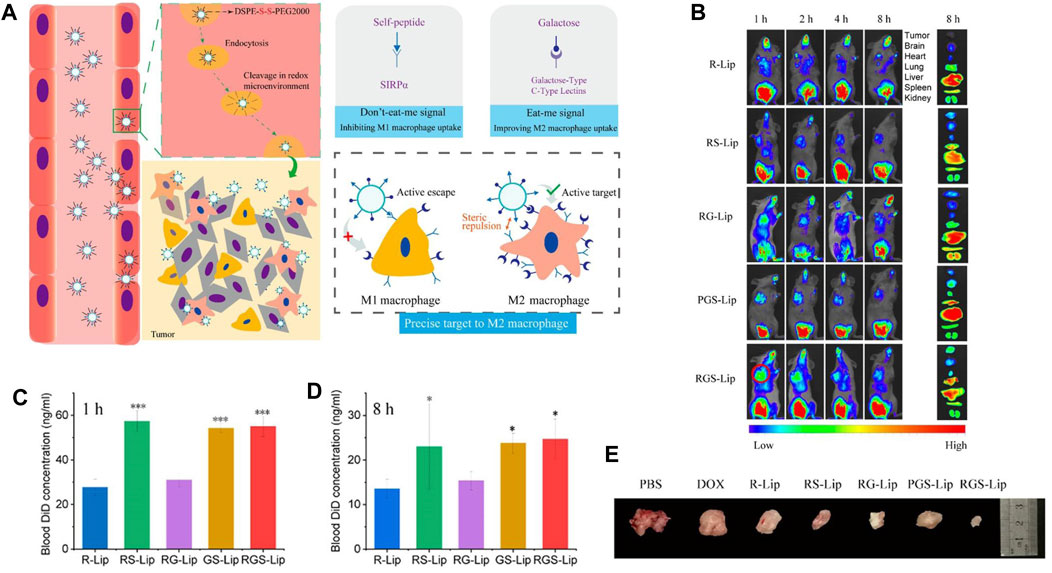
FIGURE 5. (A) Schematic diagram depicting precise delivery of nanomedicine to M2 macrophages. (B–D) RGS-Lip prolongs the circulation time and increases the accumulation of liposomes in the tumor. (B) In vivo and ex vivo fluorescence images of liposomes injected into mice. Blood DiD concentration at (C) 1 h and (D) 8 h. (E) Photographs of A20 subcutaneous tumors at the end of treatment. Adapted with permission [98]. Copyright 2020. American Chemical Society.
Conclusion and Limitations
CD47 is an inhibitory immune checkpoint that is highly expressed on tumor cells, binding with SIRPα on myeloid cells and thereby releasing a “don’t eat me” signal, inhibiting phagocytosis. On one hand, blocking the CD47-SIRPα signaling axis can activate macrophage phagocytosis of tumor cells and enhance the antigen presenting function of DC, subsequently bridging innate immune responses with the adaptive immune responses. Therefore, tumor immunotherapy focusing on the CD47-SIRPα axis has recently garnished significant attention. However, due to nonspecific targeting, systemic administration of CD47-SIRPα blockades can cause severe side effects, which provides the development of nanomedicine a great opportunity. On the other hand, researchers make use of this negative regulatory effect of the CD47-SIRPα axis to decorate the NPs with a stealthy function, markedly increasing both circulation time and drug uptake by tumor cells. Due to the high plasticity and selectivity of nanomaterials, they can be used as therapeutic agents (such as CD47-rich vesicles) and drug delivery vehicles of any site of the CD47-SiRPα signaling axis. It offers great convenience for the realization of targeted therapy, combination therapy and the improvement of antitumor effect.
Although anticancer nanomedicine focusing on this signaling axis has extensive prospects, there are still many challenges to be overcome to realize full practical applications. For example, understanding of the CD47-SiRPα signal axis is not thorough enough, such as how to properly regulate the intensity of this signal axis in the spatiotemporal category. In addition, the synthesis of ideal NPs is complex and difficult. As mentioned earlier, small changes in any part of the NP manufacturing process can lead to large changes in the performance of nanomedicines. This sensitivity requires the knowledge of nanomaterials and rigor in the fabrication process. The harm of nanomaterials cannot be neglected. Last but not least, regarding combination therapy, control of the drug loading ratio and the spatiotemporal order of drug release are problems to be solved.
Nevertheless, these challenges can also be opportunities. With the deepening of researchers’ knowledge of the CD47-SiRPα pathway and tumor nanomedical science, the perfect combination of increasingly mature nanotechnology and body pathology and physiology in the future will achieve better clinical transformation.
Data AvailabilityStatement
The original contributions presented in the study are included in the article/Supplementary Material, further inquiries can be directed to the corresponding author.
Ethics Statement
All the procedures in this case were conducted according to guidelines and according to clinical practice.
Author Contributions
CN contributed to the conception and design of the work. HL participated to manuscript writing. All authors contributed to the article and approved the submitted version.
Funding
This project was funded by the National Natural Science Foundation of China (81974267) and the Science and Technology Innovation Program of Hunan Province (2021RC3033).
Conflict of Interest
The authors declare that the research was conducted in the absence of any commercial or financial relationships that could be construed as a potential conflict of interest.
Publisher’s Note
All claims expressed in this article are solely those of the authors and do not necessarily represent those of their affiliated organizations, or those of the publisher, the editors and the reviewers. Any product that may be evaluated in this article, or claim that may be made by its manufacturer, is not guaranteed or endorsed by the publisher.
References
Abdel-Bar, H. M., Walters, A. A., Lim, Y., Rouatbi, N., Qin, Y., Gheidari, F., et al. (2021). An "eat Me" Combinatory Nano-Formulation for Systemic Immunotherapy of Solid Tumors. Theranostics 11 (18), 8738–8754. doi:10.7150/thno.56936
Adams, S., van der Laan, L. J., Vernon-Wilson, E., Renardel de Lavalette, C., Döpp, E. A., and Dijkstra, C. D., Signal-regulatory Protein Is Selectively Expressed by Myeloid and Neuronal Cells. J. Immun., 161(4), 1853–1859. (1998).
Advani, R., Flinn, I., Popplewell, L., Forero, A., Bartlett, N. L., Ghosh, N., et al. (2018). CD47 Blockade by Hu5F9-G4 and Rituximab in Non-hodgkin's Lymphoma. N. Engl. J. Med. 379 (18), 1711–1721. doi:10.1056/NEJMoa1807315
Albanese, A., Tang, P. S., and Chan, W. C. (2012). The Effect of Nanoparticle Size, Shape, and Surface Chemistry on Biological Systems. Annu. Rev. Biomed. Eng. 14, 1–16. doi:10.1146/annurev-bioeng-071811-150124
Andrejeva, G., Capoccia, B. J., Hiebsch, R. R., Donio, M. J., Darwech, I. M., Puro, R. J., et al. (2021). Novel SIRPalpha Antibodies that Induce Single-Agent Phagocytosis of Tumor Cells while Preserving T Cells. J. Immunol. 206 (4), 712–721. doi:10.4049/jimmunol.2001019
Barclay, A. N. (2009). Signal Regulatory Protein Alpha (SIRPα)/CD47 Interaction and Function. Curr. Opin. Immunol. 21 (1), 47–52. doi:10.1016/j.coi.2009.01.008
Belhadj, Z., He, B., Deng, H., Song, S., Zhang, H., Wang, X., et al. (2020). A Combined "eat Me/don't Eat Me" Strategy Based on Extracellular Vesicles for Anticancer Nanomedicine. J. Extracell Vesicles 9 (1), 1806444. doi:10.1080/20013078.2020.1806444
Bresser, K., Logtenberg, M. E. W., Toebes, M., Proost, N., Sprengers, J., Siteur, B., et al. (2022). QPCTL Regulates Macrophage and Monocyte Abundance and Inflammatory Signatures in the Tumor Microenvironment. Oncoimmunology 11 (1), 2049486. doi:10.1080/2162402X.2022.2049486
Brown, E., and Frazier, W. A. (2001). Integrin-associated Protein (CD47) and its Ligands. Trends. Cel Biol. 11 (3), 130–135. doi:10.1016/s0962-8924(00)01906-1
Chang, K., Liu, Z., Fang, X., Chen, H., Men, X., Yuan, Y., et al. (2017). Enhanced Phototherapy by Nanoparticle-Enzyme via Generation and Photolysis of Hydrogen Peroxide. Nano Lett. 17 (7), 4323–4329. doi:10.1021/acs.nanolett.7b01382
Chao, M. P., Alizadeh, A. A., Tang, C., Myklebust, J. H., Varghese, B., Gill, S., et al. (2010). Anti-CD47 Antibody Synergizes with Rituximab to Promote Phagocytosis and Eradicate Non-hodgkin Lymphoma. Cell 142 (5), 699–713. doi:10.1016/j.cell.2010.07.044
Chao, M. P., Jaiswal, S., Weissman-Tsukamoto, R., Alizadeh, A. A., Gentles, A. J., Volkmer, J., et al. (2010). Calreticulin Is the Dominant Pro-phagocytic Signal on Multiple Human Cancers and Is Counterbalanced by CD47. Sci. Transl Med. 2 (63), 63ra94. doi:10.1126/scitranslmed.3001375
Chen, C., Li, A., Sun, P., Xu, J., Du, W., Zhang, J., et al. (2020). Efficiently Restoring the Tumoricidal Immunity against Resistant Malignancies via an Immune Nanomodulator. J. Control. Release 324, 574–585. doi:10.1016/j.jconrel.2020.05.039
Chen, D., Tang, Y., Zhu, J., Zhang, J., Song, X., Wang, W., et al. (2019). Photothermal-pH-hypoxia Responsive Multifunctional Nanoplatform for Cancer Photo-Chemo Therapy with Negligible Skin Phototoxicity. Biomaterials 221, 119422. doi:10.1016/j.biomaterials.2019.119422
Chen, H., Di, Y., Chen, D., Madrid, K., Zhang, M., Tian, C., et al. (2015). Combined Chemo- and Photo-thermal Therapy Delivered by Multifunctional Theranostic Gold Nanorod-Loaded Microcapsules. Nanoscale 7 (19), 8884–8897. doi:10.1039/c5nr00473j
Chen, M., Daddy, J. C. K., Xiao, Y., Ping, Q., and Zong, L. (2017). Advanced Nanomedicine for Rheumatoid Arthritis Treatment: Focus on Active Targeting. Expert Opin. Drug Deliv. 14 (10), 1141–1144. doi:10.1080/17425247.2017.1372746
Chen, M., Miao, Y., Qian, K., Zhou, X., Guo, L., Qiu, Y., et al. (2021). Detachable Liposomes Combined Immunochemotherapy for Enhanced Triple-Negative Breast Cancer Treatment through Reprogramming of Tumor-Associated Macrophages. Nano Lett. 21 (14), 6031–6041. doi:10.1021/acs.nanolett.1c01210
Chen, Q., Chen, G., Chen, J., Shen, J., Zhang, X., Wang, J., et al. (2019). Bioresponsive Protein Complex of aPD1 and aCD47 Antibodies for Enhanced Immunotherapy. Nano Lett. 19 (8), 4879–4889. doi:10.1021/acs.nanolett.9b00584
Chen, Q., Wang, C., Zhang, X., Chen, G., Hu, Q., Li, H., et al. (2019). In Situ sprayed Bioresponsive Immunotherapeutic Gel for post-surgical Cancer Treatment. Nat. Nanotechnol 14 (1), 89–97. doi:10.1038/s41565-018-0319-4
Chen, X., Zaro, J. L., and Shen, W. C. (2013). Fusion Protein Linkers: Property, Design and Functionality. Adv. Drug Deliv. Rev. 65 (10), 1357–1369. doi:10.1016/j.addr.2012.09.039
Chen, Y. C., Shi, W., Shi, J. J., and Lu, J. J. (2022). Progress of CD47 Immune Checkpoint Blockade Agents in Anticancer Therapy: a Hematotoxic Perspective. J. Cancer Res. Clin. Oncol. 148 (1), 1–14. doi:10.1007/s00432-021-03815-z
Cheng, L., Zhang, X., Tang, J., Lv, Q., and Liu, J. (2021). Gene-engineered Exosomes-Thermosensitive Liposomes Hybrid Nanovesicles by the Blockade of CD47 Signal for Combined Photothermal Therapy and Cancer Immunotherapy. Biomaterials 275, 120964. doi:10.1016/j.biomaterials.2021.120964
Cho, E., Nam, G. H., Hong, Y., Kim, Y. K., Kim, D. H., Yang, Y., et al. (2018). Comparison of Exosomes and Ferritin Protein Nanocages for the Delivery of Membrane Protein Therapeutics. J. Control. Release 279, 326–335. doi:10.1016/j.jconrel.2018.04.037
Chu, K. F., and Dupuy, D. E. (2014). Thermal Ablation of Tumours: Biological Mechanisms and Advances in Therapy. Nat. Rev. Cancer 14 (3), 199–208. doi:10.1038/nrc3672
Colombo, M., Carregal-Romero, S., Casula, M. F., Gutierrez, L., Morales, M. P., Bohm, I. B., et al. (2012). Biological Applications of Magnetic Nanoparticles. Chem. Soc. Rev. 41 (11), 4306–4334. doi:10.1039/c2cs15337h
De La Peña, H., Madrigal, J. A., Rusakiewicz, S., Bencsik, M., Cave, G. W. V., Selman, A., et al. (2009). Artificial Exosomes as Tools for Basic and Clinical Immunology. J. Immunological Methods 344 (2), 121–132. doi:10.1016/j.jim.2009.03.011
Dheilly, E., Moine, V., Broyer, L., Salgado-Pires, S., Johnson, Z., Papaioannou, A., et al. (2017). Selective Blockade of the Ubiquitous Checkpoint Receptor CD47 Is Enabled by Dual-Targeting Bispecific Antibodies. Mol. Ther. 25 (2), 523–533. doi:10.1016/j.ymthe.2016.11.006
Diez-Pascual, A. M. (2020). Antibacterial Action of Nanoparticle Loaded Nanocomposites Based on Graphene and its Derivatives: A Mini-Review. Int. J. Mol. Sci. 21 (10). 3563. doi:10.3390/ijms21103563
Fang, J., Sawa, T., and Maeda, H. (2003). Factors and Mechanism of "EPR" Effect and the Enhanced Antitumor Effects of Macromolecular Drugs Including SMANCS. Adv. Exp. Med. Biol. 519, 29–49. doi:10.1007/0-306-47932-X_2
Farzin, A., Etesami, S. A., Quint, J., Memic, A., and Tamayol, A. (2020). Magnetic Nanoparticles in Cancer Therapy and Diagnosis. Adv. Healthc. Mater. 9 (9). 147. doi:10.1002/adhm.201901058
Feng, M., Jiang, W., Kim, B. Y. S., Zhang, C. C., Fu, Y. X., and Weissman, I. L. (2019). Phagocytosis Checkpoints as New Targets for Cancer Immunotherapy. Nat. Rev. Cancer 19 (10), 568–586. doi:10.1038/s41568-019-0183-z
Fu, C., Liu, T., Li, L., Liu, H., Chen, D., and Tang, F. (2013). The Absorption, Distribution, Excretion and Toxicity of Mesoporous Silica Nanoparticles in Mice Following Different Exposure Routes. Biomaterials 34 (10), 2565–2575. doi:10.1016/j.biomaterials.2012.12.043
Gazdar, A. F. (2009). Activating and Resistance Mutations of EGFR in Non-small-cell Lung Cancer: Role in Clinical Response to EGFR Tyrosine Kinase Inhibitors. Oncogene 28 (Suppl. 1), S24–S31. doi:10.1038/onc.2009.198
Gheibi Hayat, S. M., Bianconi, V., Pirro, M., and Sahebkar, A. (2019). Stealth Functionalization of Biomaterials and Nanoparticles by CD47 Mimicry. Int. J. Pharm. 569, 118628. doi:10.1016/j.ijpharm.2019.118628
Gholamin, S., Mitra, S. S., Feroze, A. H., Liu, J., Kahn, S. A., and Zhang, M. (2017). Disrupting the CD47-Sirpα Anti-phagocytic axis by a Humanized Anti-CD47 Antibody Is an Efficacious Treatment for Malignant Pediatric Brain Tumors. Sci. Transl 9 (381), eaaf2968. doi:10.1126/scitranslmed.aaf2968
Gref, R., Lück, M., Quellec, P., Marchand, M., Dellacherie, E., and Harnisch, S. (2000). ‘Stealth’ corona-core Nanoparticles Surface Modified by Polyethylene Glycol (PEG) Influences of the corona (PEG Chain Length and Surface Density) and of the Core Composition on Phagocytic Uptake and Plasma Protein Adsorptio. Colloids Surf. B Biointerfaces 18 (3-4), 301–313. doi:10.1016/s0927-7765(99)00156-3
Guo, Z., Liu, Y., Zhou, H., Zheng, K., Wang, D., Jia, M., et al. (2019). CD47-targeted Bismuth Selenide Nanoparticles Actualize Improved Photothermal Therapy by Increasing Macrophage Phagocytosis of Cancer Cells. Colloids Surf. B Biointerfaces 184, 110546. doi:10.1016/j.colsurfb.2019.110546
Hayat, S. M. G., Bianconi, V., Pirro, M., Jaafari, M. R., Hatamipour, M., and Sahebkar, A. (2020). CD47: Role in the Immune System and Application to Cancer Therapy. Cell Oncol. 43 (1), 19–30. doi:10.1007/s13402-019-00469-5
Ho, C. C. M., Guo, N., Sockolosky, J. T., Ring, A. M., Weiskopf, K., Özkan, E., et al. (2015). "Velcro" Engineering of High Affinity CD47 Ectodomain as Signal Regulatory Protein α (SIRPα) Antagonists that Enhance Antibody-dependent Cellular Phagocytosis. J. Biol. Chem. 290 (20), 12650–12663. doi:10.1074/jbc.M115.648220
Hou, W., Zhang, Q., Yan, Z., Chen, R., Zeh, H. J., Kang, R., et al. (2013). Strange Attractors: DAMPs and Autophagy Link Tumor Cell Death and Immunity. Cell Death Dis 4, e966. doi:10.1038/cddis.2013.493
Hu, C. M., Zhang, L., Aryal, S., Cheung, C., Fang, R. H., and Zhang, L. (2011). Erythrocyte Membrane-Camouflaged Polymeric Nanoparticles as a Biomimetic Delivery Platform. Proc. Natl. Acad. Sci. U S A. 108 (27), 10980–10985. doi:10.1073/pnas.1106634108
Huang, L., Zhang, Y., Li, Y., Meng, F., Li, H., Zhang, H., et al. (2021). Time-Programmed Delivery of Sorafenib and Anti-CD47 Antibody via a Double-Layer-Gel Matrix for Postsurgical Treatment of Breast Cancer. Nanomicro Lett. 13 (1), 141. doi:10.1007/s40820-021-00647-x
Huynh, N. T., Passirani, C., Saulnier, P., and Benoit, J. P. (2009). Lipid Nanocapsules: a New Platform for Nanomedicine. Int. J. Pharm. 379 (2), 201–209. doi:10.1016/j.ijpharm.2009.04.026
Ingram, J. R., Blomberg, O. S., Sockolosky, J. T., Ali, L., Schmidt, F. I., Pishesha, N., et al. (2017). Localized CD47 Blockade Enhances Immunotherapy for Murine Melanoma. Proc. Natl. Acad. Sci. U S A. 114 (38), 10184–10189. doi:10.1073/pnas.1710776114
Iyer, A. K., Singh, A., Ganta, S., and Amiji, M. M. (2013). Role of Integrated Cancer Nanomedicine in Overcoming Drug Resistance. Adv. Drug Deliv. Rev. 65 (13-14), 1784–1802. doi:10.1016/j.addr.2013.07.012
Jain, A., Singh, S. K., Arya, S. K., Kundu, S. C., and Kapoor, S. (2018). Protein Nanoparticles: Promising Platforms for Drug Delivery Applications. ACS Biomater. Sci. Eng. 4 (12), 3939–3961. doi:10.1021/acsbiomaterials.8b01098
Jaiswal, S., Jamieson, C. H. M., Pang, W. W., Park, C. Y., Chao, M. P., Majeti, R., et al. (2009). CD47 Is Upregulated on Circulating Hematopoietic Stem Cells and Leukemia Cells to Avoid Phagocytosis. Cell 138 (2), 271–285. doi:10.1016/j.cell.2009.05.046
Jiang, Z., Tian, Y., Shan, D., Wang, Y., Gerhard, E., Xia, J., et al. (2018). pH Protective Y1 Receptor Ligand Functionalized Antiphagocytosis BPLP-WPU Micelles for Enhanced Tumor Imaging and Therapy with Prolonged Survival Time. Biomaterials 170, 70–81. doi:10.1016/j.biomaterials.2018.04.002
Kamerkar, S., LeBleu, V. S., Sugimoto, H., Yang, S., Ruivo, C. F., Melo, S. A., et al. (2017). Exosomes Facilitate Therapeutic Targeting of Oncogenic KRAS in Pancreatic Cancer. Nature 546 (7659), 498–503. doi:10.1038/nature22341
Kinnear, C., Moore, T. L., Rodriguez-Lorenzo, L., Rothen-Rutishauser, B., and Petri-Fink, A. (2017). Form Follows Function: Nanoparticle Shape and its Implications for Nanomedicine. Chem. Rev. 117 (17), 11476–11521. doi:10.1021/acs.chemrev.7b00194
Koh, E., Lee, E. J., Nam, G. H., Hong, Y., Cho, E., Yang, Y., et al. (2017). Exosome-SIRPalpha, a CD47 Blockade Increases Cancer Cell Phagocytosis. Biomaterials 121, 121–129. doi:10.1016/j.biomaterials.2017.01.004
Kulkarni, A., Chandrasekar, V., Natarajan, S. K., Ramesh, A., Pandey, P., Nirgud, J., et al. (2018). A Designer Self-Assembled Supramolecule Amplifies Macrophage Immune Responses against Aggressive Cancer. Nat. Biomed. Eng. 2 (8), 589–599. doi:10.1038/s41551-018-0254-6
Labrijn, A. F., Janmaat, M. L., Reichert, J. M., and Parren, P. (2019). Bispecific Antibodies: a Mechanistic Review of the Pipeline. Nat. Rev. Drug Discov. 18 (8), 585–608. doi:10.1038/s41573-019-0028-1
Latour, S., Tanaka, H., Demeure, C., Mateo, V., Rubio, M., Brown, E. J., et al. (2001). Bidirectional Negative Regulation of Human T and Dendritic Cells by CD47 and its Cognate Receptor Signal-Regulator Protein-α: Down-Regulation of IL-12 Responsiveness and Inhibition of Dendritic Cell Activation. J. Immunol. 167 (5), 2547–2554. doi:10.4049/jimmunol.167.5.2547
Lee, N. K., Choi, J. U., Kim, H. R., Chung, S. W., Ko, Y. G., Cho, Y. S., et al. (2021). Caspase-cleavable Peptide-Doxorubicin Conjugate in Combination with CD47-Antagonizing Nanocage Therapeutics for Immune-Mediated Elimination of Colorectal Cancer. Biomaterials 277, 121105. doi:10.1016/j.biomaterials.2021.121105
Lee, N. K., Lee, E. J., Kim, S., Nam, G. H., Kih, M., Hong, Y., et al. (2017). Ferritin Nanocage with Intrinsically Disordered Proteins and Affibody: a Platform for Tumor Targeting with Extended Pharmacokinetics. J. Control Release 267, 172–180. doi:10.1016/j.jconrel.2017.08.014
Li, F., Qin, Y., Lee, J., Liao, H., Wang, N., Davis, T. P., et al. (2020). Stimuli-responsive Nano-Assemblies for Remotely Controlled Drug Delivery. J. Control. Release 322, 566–592. doi:10.1016/j.jconrel.2020.03.051
Lian, S., Xie, R., Ye, Y., Xie, X., Li, S., Lu, Y., et al. (2019). Simultaneous Blocking of CD47 and PD-L1 Increases Innate and Adaptive Cancer Immune Responses and Cytokine Release. EBioMedicine 42, 281–295. doi:10.1016/j.ebiom.2019.03.018
Lindberg, F. P., Bullard, D. C., Caver, T. E., Gresham, H. D., Beaudet, A. L., and Brown, E. J. (1996). Decreased Resistance to Bacterial Infection and Granulocyte Defects in IAP-Deficient Mice. Science 274 (5288), 795–798. doi:10.1126/science.274.5288.795
Liu, C. C., Leclair, P., Monajemi, M., Sly, L. M., Reid, G. S., and Lim, C. J. (2016). Alpha-Integrin Expression and Function Modulates Presentation of Cell Surface Calreticulin. Cel Death Dis 7, e2268. doi:10.1038/cddis.2016.176
Liu, J., Wang, L., Zhao, F., Tseng, S., Narayanan, C., Shura, L., et al. (2015). Pre-Clinical Development of a Humanized Anti-CD47 Antibody with Anti-cancer Therapeutic Potential. PLoS One 10 (9), e0137345. doi:10.1371/journal.pone.0137345
Liu, X., Kwon, H., Li, Z., and Fu, Y. X. (2017). Is CD47 an Innate Immune Checkpoint for Tumor Evasion? J. Hematol. Oncol. 10 (1), 12. doi:10.1186/s13045-016-0381-z
Liu, X., Pu, Y., Cron, K., Deng, L., Kline, J., Frazier, W. A., et al. (2015). CD47 Blockade Triggers T Cell-Mediated Destruction of Immunogenic Tumors. Nat. Med. 21 (10), 1209–1215. doi:10.1038/nm.3931
Liu, Y., Merlin, D., Burst, S. L., Pochet, M., Madara, J. L., and Parkos, C. A. (2001). The Role of CD47 in Neutrophil Transmigration. J. Biol. Chem. 276 (43), 40156–40166. doi:10.1074/jbc.M104138200
Logozzi, M., Di Raimo, R., Mizzoni, D., and Fais, S. (2021). What We Know on the Potential Use of Exosomes for Nanodelivery. Semin. Cancer Biol. 579X (21), 00229-7. doi:10.1016/j.semcancer.2021.09.005
Logtenberg, M. E. W., Jansen, J. H. M., Raaben, M., Toebes, M., Franke, K., Brandsma, A. M., et al. (2019). Glutaminyl Cyclase Is an Enzymatic Modifier of the CD47- SIRPalpha axis and a Target for Cancer Immunotherapy. Nat. Med. 25 (4), 612–619. doi:10.1038/s41591-019-0356-z
Logtenberg, M. E. W., Scheeren, F. A., and Schumacher, T. N. (2020). The CD47-Sirpα Immune Checkpoint. Immunity 52 (5), 742–752. doi:10.1016/j.immuni.2020.04.011
Lohcharoenkal, W., Wang, L., Chen, Y. C., and Rojanasakul, Y. (2014). Protein Nanoparticles as Drug Delivery Carriers for Cancer Therapy. Biomed. Res. Int. 2014, 180549. doi:10.1155/2014/180549
Lutz, H. U., and Bogdanova, A. (2013). Mechanisms Tagging Senescent Red Blood Cells for Clearance in Healthy Humans. Front. Physiol. 4, 387. doi:10.3389/fphys.2013.00387
Lymn, J. S., Patel, M. K., Clunn, G. F., Rao, S. J., Gallagher, K. L., and Hughes, A. D. (2002). Thrombospondin-1 Differentially Induces Chemotaxis and DNA Synthesis of Human Venous Smooth Muscle Cells at the Receptor-Binding Level. J. Cel Sci 115 (Pt 22), 4353–4360. doi:10.1242/jcs.00119
Maeda, H., Wu, J., Sawa, T., Matsumura, Y., and Hori, K. (1999). Tumor Vascular Permeability and the EPR Effect in Macromolecular Therapeutics: a Review. J. Control. Release 65 (1-2), 271–284. doi:10.1016/s0168-3659(99)00248-5
Maile, L. A., DeMambro, V. E., Wai, C., Aday, A. W., Capps, B. E., Beamer, W. G., et al. (2011). An Essential Role for the Association of CD47 to SHPS-1 in Skeletal Remodeling. J. Bone Miner Res. 26 (9), 2068–2081. doi:10.1002/jbmr.441
Majeti, R., Chao, M. P., Alizadeh, A. A., Pang, W. W., Jaiswal, S., Gibbs, K. D., et al. (2009). CD47 Is an Adverse Prognostic Factor and Therapeutic Antibody Target on Human Acute Myeloid Leukemia Stem Cells. Cell 138 (2), 286–299. doi:10.1016/j.cell.2009.05.045
Mantovani, A., Marchesi, F., Malesci, A., Laghi, L., and Allavena, P. (2017). Tumour-associated Macrophages as Treatment Targets in Oncology. Nat. Rev. Clin. Oncol. 14 (7), 399–416. doi:10.1038/nrclinonc.2016.217
Mantovani, A., Schioppa, T., Porta, C., Allavena, P., and Sica, A. (2006). Role of Tumor-Associated Macrophages in Tumor Progression and Invasion. Cancer Metastasis Rev. 25 (3), 315–322. doi:10.1007/s10555-006-9001-7
Mendelsohn, J., and Baselga, J. (2003). Status of Epidermal Growth Factor Receptor Antagonists in the Biology and Treatment of Cancer. J. Clin. Oncol. 21 (14), 2787–2799. doi:10.1200/JCO.2003.01.504
Meng, Q. F., Zhao, Y., Dong, C., Liu, L., Pan, Y., Lai, J., et al. (2021). Genetically Programmable Fusion Cellular Vesicles for Cancer Immunotherapy. Angew. Chem. Int. Ed. Engl. 60 (50), 26320–26326. doi:10.1002/anie.202108342
Meng, Z., Wang, Z., Guo, B., Cao, W., and Shen, H. (2019). TJC4, a Differentiated Anti-CD47 Antibody with Novel Epitope and RBC Sparing Properties. Blood 134 (Suppl. ment_1), 4063. doi:10.1182/blood-2019-122793
Mushegian, A. (2002). Refining Structural and Functional Predictions for Secretasome Components by Comparative Sequence Analysis. Proteins 47 (1), 69–74. doi:10.1002/prot.10073
Nie, W., Wu, G., Zhang, J., Huang, L. L., Ding, J., Jiang, A., et al. (2020). Responsive Exosome Nano-Bioconjugates for Synergistic Cancer Therapy. Angew. Chem. Int. Ed. Engl. 59 (5), 2018–2022. doi:10.1002/anie.201912524
Normanno, N., Bianco, C., De Luca, A., and Salomon, D. S. (2001). The Role of EGF-Related Peptides in Tumor Growth. Front. Biosci. 6, D685–D707. doi:10.2741/normano
Noy, R., and Pollard, J. W. (2014). Tumor-associated Macrophages: from Mechanisms to Therapy. Immunity 41 (1), 49–61. doi:10.1016/j.immuni.2014.06.010
Oldenborg, P.-A., Zheleznyak, A., Fang, Y.-F., Lagenaur, C. F., Gresham, H. D., and Lindberg, F. P. (2000). Role of CD47 as a Marker of Self on Red Blood Cells. Science 288 (5473), 2051–2054. doi:10.1126/science.288.5473.2051
Oldenborg, P. A., Gresham, H. D., and Lindberg, F. P. (2001). CD47-signal Regulatory Protein α (Sirpα) Regulates Fcγ and Complement Receptor–Mediated Phagocytosis. J. Exp. Med. 193 (7), 855–861. doi:10.1084/jem.193.7.855
Olusanya, T., Haj Ahmad, R., Ibegbu, D., Smith, J., and Elkordy, A. (2018). Liposomal Drug Delivery Systems and Anticancer Drugs. Molecules 23 (4). doi:10.3390/molecules23040907
Pardoll, D. M. (2012). The Blockade of Immune Checkpoints in Cancer Immunotherapy. Nat. Rev. Cancer 12 (4), 252–264. doi:10.1038/nrc3239
Parsa, A. T., Waldron, J. S., Panner, A., Crane, C. A., Parney, I. F., Barry, J. J., et al. (2007). Loss of Tumor Suppressor PTEN Function Increases B7-H1 Expression and Immunoresistance in Glioma. Nat. Med. 13 (1), 84–88. doi:10.1038/nm1517
Peer, D., Karp, J. M., Hong, S., Farokhzad, O. C., Margalit, R., and Langer, R. (2007). Nanocarriers as an Emerging Platform for Cancer Therapy. Nat. Nanotechnol 2 (12), 751–760. doi:10.1038/nnano.2007.387
Pelaz, B., Alexiou, C., Alvarez-Puebla, R. A., Alves, F., Andrews, A. M., Ashraf, S., et al. (2017). Diverse Applications of Nanomedicine. ACS Nano 11 (3), 2313–2381. doi:10.1021/acsnano.6b06040
Petrova, P. S., Viller, N. N., Wong, M., Pang, X., Lin, G. H. Y., Dodge, K., et al. (2017). TTI-621 (SIRPαFc): A CD47-Blocking Innate Immune Checkpoint Inhibitor with Broad Antitumor Activity and Minimal Erythrocyte Binding. Clin. Cancer Res. 23 (4), 1068–1079. doi:10.1158/1078-0432.CCR-16-1700
Pham, L. M., Poudel, K., Phung, C. D., Nguyen, T. T., Pandit, M., Nguyen, H. T., et al. (2021). Preparation and Evaluation of Dabrafenib-Loaded, CD47-Conjugated Human Serum Albumin-Based Nanoconstructs for Chemoimmunomodulation. Colloids Surf. B Biointerfaces 208, 112093. doi:10.1016/j.colsurfb.2021.112093
Puro, R. J., Bouchlaka, M. N., Hiebsch, R. R., Capoccia, B. J., Donio, M. J., Manning, P. T., et al. (2020). Development of AO-176, a Next-Generation Humanized Anti-CD47 Antibody with Novel Anticancer Properties and Negligible Red Blood Cell Binding. Mol. Cancer Ther. 19 (3), 835–846. doi:10.1158/1535-7163.MCT-19-1079
Ra, L., and Bw, R. (2005). Immunotherapy and Chemotherapy-Aa Practical Partnership. Nat. Rev. Cancer 5 (5), 397–405. doi:10.1038/nrc1613
Ramesh, A., Kumar, S., Nandi, D., and Kulkarni, A. (2019). CSF1R- and SHP2-Inhibitor-Loaded Nanoparticles Enhance Cytotoxic Activity and Phagocytosis in Tumor-Associated Macrophages. Adv. Mater. 31 (51), e1904364. doi:10.1002/adma.201904364
Ramesh, A., Kumar, S., Nguyen, A., Brouillard, A., and Kulkarni, A. (2020). Lipid-based Phagocytosis Nanoenhancer for Macrophage Immunotherapy. Nanoscale 12 (3), 1875–1885. doi:10.1039/c9nr08670f
Rao, L., Wu, L., Liu, Z., Tian, R., Yu, G., Zhou, Z., et al. (2020). Hybrid Cellular Membrane Nanovesicles Amplify Macrophage Immune Responses against Cancer Recurrence and Metastasis. Nat. Commun. 11 (1), 4909. doi:10.1038/s41467-020-18626-y
Rao, L., Zhao, S. K., Wen, C., Tian, R., Lin, L., Cai, B., et al. (2020). Activating Macrophage-Mediated Cancer Immunotherapy by Genetically Edited Nanoparticles. Adv. Mater. 32 (47), e2004853. doi:10.1002/adma.202004853
Rastegari, E., Hsiao, Y. J., Lai, W. Y., Lai, Y. H., Yang, T. C., Chen, S. J., et al. (2021). An Update on Mesoporous Silica Nanoparticle Applications in Nanomedicine. Pharmaceutics 13 (7). 1067. doi:10.3390/pharmaceutics13071067
Rezvantalab, S., Drude, N. I., Moraveji, M. K., Guvener, N., Koons, E. K., Shi, Y., et al. (2018). PLGA-based Nanoparticles in Cancer Treatment. Front. Pharmacol. 9, 1260. doi:10.3389/fphar.2018.01260
Ring, N. G., Herndler-Brandstetter, D., Weiskopf, K., Shan, L., Volkmer, J. P., George, B. M., et al. (2017). Anti-SIRPalpha Antibody Immunotherapy Enhances Neutrophil and Macrophage Antitumor Activity. Proc. Natl. Acad. Sci. U S A. 114 (49), E10578–E10585. doi:10.1073/pnas.1710877114
Rodriguez, P. L., Harada, T., Christian, D. A., Pantano, D. A., Tsai, R. K., and Discher, D. E. (2013). Minimal "Self" Peptides that Inhibit Phagocytic Clearance and Enhance Delivery of Nanoparticles. Science 339 (6122), 971–975. doi:10.1126/science.1229568
S, E. L. A., Mager, I., Breakefield, X. O., and Wood, M. J. (2013). Extracellular Vesicles: Biology and Emerging Therapeutic Opportunities. Nat. Rev. Drug Discov. 12 (5), 347–357. doi:10.1038/nrd3978
Sabbah, D. A., Hajjo, R., and Sweidan, K. (2020). Review on Epidermal Growth Factor Receptor (EGFR) Structure, Signaling Pathways, Interactions, and Recent Updates of EGFR Inhibitors. Curr. Top. Med. Chem. 20 (10), 815–834. doi:10.2174/1568026620666200303123102
Sadat Tabatabaei Mirakabad, F., Nejati-Koshki, K., Akbarzadeh, A., Yamchi, M. R., Milani, M., Zarghami, N., et al. (2014). PLGA-based Nanoparticles as Cancer Drug Delivery Systems. Asian Pac. J. Cancer Prev. 15 (2), 517–535. doi:10.7314/apjcp.2014.15.2.517
Shi, R., Chai, Y., Duan, X., Bi, X., Huang, Q., Wang, Q., et al. (2020). The Identification of a CD47-Blocking "hotspot" and Design of a CD47/PD-L1 Dual-specific Antibody with Limited Hemagglutination. Signal. Transduct Target. Ther. 5 (1), 16. doi:10.1038/s41392-020-0121-2
Shim, G., Miao, W., Ko, S., Park, G. T., Kim, J. Y., Kim, M. G., et al. (2017). Immune-camouflaged Graphene Oxide Nanosheets for Negative Regulation of Phagocytosis by Macrophages. J. Mater. Chem. B 5 (32), 6666–6675. doi:10.1039/c7tb00648a
Si, Y., Chen, K., Ngo, H. G., Guan, J. S., Totoro, A., Zhou, Z., et al. (2022). Targeted EV to Deliver Chemotherapy to Treat Triple-Negative Breast Cancers. Pharmaceutics 14 (1). 146. doi:10.3390/pharmaceutics14010146
Sica, A., Schioppa, T., Mantovani, A., and Allavena, P. (2006). Tumour-associated Macrophages Are a Distinct M2 Polarised Population Promoting Tumour Progression: Potential Targets of Anti-cancer Therapy. Eur. J. Cancer 42 (6), 717–727. doi:10.1016/j.ejca.2006.01.003
Sikic, B. I., Lakhani, N., Patnaik, A., Shah, S. A., Chandana, S. R., and Rasco, D. (2019). First-in-human, First-In-Class Phase I Trial of the Anti-cd47 Antibody Hu5f9-G4 in Patients with Advanced Cancers. J. Clin. Oncol. 37 (12), 946–953. doi:10.1200/JCO.18.02018
Song, S., Jin, X., Zhang, L., Zhao, C., Ding, Y., Ang, Q., et al. (2019). PEGylated and CD47-Conjugated Nanoellipsoidal Artificial Antigen-Presenting Cells Minimize Phagocytosis and Augment Anti-tumor T-Cell Responses. Int. J. Nanomedicine 14, 2465–2483. doi:10.2147/IJN.S195828
Sun, X., Hong, Y., Gong, Y., Zheng, S., and Xie, D. (2021). Bioengineered Ferritin Nanocarriers for Cancer Therapy. Int. J. Mol. Sci. 22 (13). 1. doi:10.3390/ijms22137023
Tang, Y., Tang, Z., Li, P., Tang, K., Ma, Z., Wang, Y., et al. (2021). Precise Delivery of Nanomedicines to M2 Macrophages by Combining "Eat Me/Don't Eat Me" Signals and its Anticancer Application. ACS Nano 15 (11), 18100–18112. doi:10.1021/acsnano.1c06707
Tawfik, S. M., Azizov, S., Elmasry, M. R., Sharipov, M., and Lee, Y. I. (2020). Recent Advances in Nanomicelles Delivery Systems. Nanomaterials (Basel) 11 (1). doi:10.3390/nano11010070
Torchilin, V. P. (2007). Micellar Nanocarriers: Pharmaceutical Perspectives. Pharm. Res. 24 (1), 1–16. doi:10.1007/s11095-006-9132-0
Voets, E., Parade, M., Lutje Hulsik, D., Spijkers, S., Janssen, W., Rens, J., et al. (2019). Functional Characterization of the Selective Pan-Allele Anti-SIRPalpha Antibody ADU-1805 that Blocks the SIRPalpha-CD47 Innate Immune Checkpoint. J. Immunother. Cancer 7 (1), 340. doi:10.1186/s40425-019-0772-0
Wang, M., Zuris, J. A., Meng, F., Rees, H., Sun, S., Deng, P., et al. (2016). Efficient Delivery of Genome-Editing Proteins Using Bioreducible Lipid Nanoparticles. Proc. Natl. Acad. Sci. U S A. 113 (11), 2868–2873. doi:10.1073/pnas.1520244113
Wang, Y., Ni, H., Zhou, S., He, K., Gao, Y., Wu, W., et al. (2021). Tumor-selective Blockade of CD47 Signaling with a CD47/PD-L1 Bispecific Antibody for Enhanced Anti-tumor Activity and Limited Toxicity. Cancer Immunol. Immunother. 70 (2), 365–376. doi:10.1007/s00262-020-02679-5
Wang, Y., Wang, Z., Qian, Y., Fan, L., Yue, C., Jia, F., et al. (2019). Synergetic Estrogen Receptor-Targeting Liposome Nanocarriers with Anti-phagocytic Properties for Enhanced Tumor Theranostics. J. Mater. Chem. B 7 (7), 1056–1063. doi:10.1039/c8tb03351j
Wang, Y., Zhao, Q., Han, N., Bai, L., Li, J., Liu, J., et al. (2015). Mesoporous Silica Nanoparticles in Drug Delivery and Biomedical Applications. Nanomedicine 11 (2), 313–327. doi:10.1016/j.nano.2014.09.014
Willingham, S. B., Volkmer, J.-P., Gentles, A. J., Sahoo, D., Dalerba, P., Mitra, S. S., et al. (2012). The CD47-Signal Regulatory Protein Alpha (SIRPa) Interaction Is a Therapeutic Target for Human Solid Tumors. Proc. Natl. Acad. Sci. U.S.A. 109 (17), 6662–6667. doi:10.1073/pnas.1121623109
Wu, C. C., Yang, Y. C., Hsu, Y. T., Wu, T. C., Hung, C. F., and Huang, J. T. (2015). Nanoparticle-induced Intraperitoneal Hyperthermia and Targeted Photoablation in Treating Ovarian Cancer. Oncotarget 6 (29), 26861–26875. doi:10.18632/oncotarget.4766
Wu, K., Su, D., Liu, J., Saha, R., and Wang, J. P. (2019). Magnetic Nanoparticles in Nanomedicine: a Review of Recent Advances. Nanotechnology 30 (50), 502003. doi:10.1088/1361-6528/ab4241
Wu, M. X., and Yang, Y. W. (2017). Metal-Organic Framework (MOF)-Based Drug/Cargo Delivery and Cancer Therapy. Adv. Mater. 29 (23). 1. doi:10.1002/adma.201606134
Wu, W., Luo, L., Wang, Y., Wu, Q., Dai, H. B., Li, J. S., et al. (2018). Endogenous pH-Responsive Nanoparticles with Programmable Size Changes for Targeted Tumor Therapy and Imaging Applications. Theranostics 8 (11), 3038–3058. doi:10.7150/thno.23459
Xia, Y., Rao, L., Yao, H., Wang, Z., Ning, P., and Chen, X. (2020). Engineering Macrophages for Cancer Immunotherapy and Drug Delivery. Adv. Mater. 32 (40). 1. doi:10.1002/adma.202002054
Xie, X., Lian, S., Zhou, Y., Li, B., Lu, Y., Yeung, I., et al. (2021). Tumor-derived Exosomes Can Specifically Prevent Cancer Metastatic Organotropism. J. Control. Release 331, 404–415. doi:10.1016/j.jconrel.2021.01.030
Xie, Z., Peng, M., Lu, R., Meng, X., Liang, W., Li, Z., et al. (2020). Black Phosphorus-Based Photothermal Therapy with aCD47-Mediated Immune Checkpoint Blockade for Enhanced Cancer Immunotherapy. Light Sci. Appl. 9, 161. doi:10.1038/s41377-020-00388-3
Xing, Q., Pan, Y., Hu, Y., and Wang, L. (2020). Review of the Biomolecular Modification of the Metal-Organ-Framework. Front. Chem. 8. 642. doi:10.3389/fchem.2020.00642
Xu, X., Ho, W., Zhang, X., Bertrand, N., and Farokhzad, O. (2015). Cancer Nanomedicine: from Targeted Delivery to Combination Therapy. Trends Mol. Med. 21 (4), 223–232. doi:10.1016/j.molmed.2015.01.001
Yanagita, T., Murata, Y., Tanaka, D., Motegi, S.-i., Arai, E., Daniwijaya, E. W., et al. (2017). Anti-Sirpα Antibodies as a Potential New Tool for Cancer Immunotherapy. JCI Insight 2 (1), e89140. doi:10.1172/jci.insight.89140
Yang, B., Song, B. P., Shankar, S., Guller, A., and Deng, W. (2021). Recent Advances in Liposome Formulations for Breast Cancer Therapeutics. Cell Mol Life Sci 78 (13), 5225–5243. doi:10.1007/s00018-021-03850-6
Yu, Y. L., Chou, R. H., Liang, J. H., Chang, W. J., Su, K. J., Tseng, Y. J., et al. (2013). Targeting the EGFR/PCNA Signaling Suppresses Tumor Growth of Triple-Negative Breast Cancer Cells with Cell-Penetrating PCNA Peptides. PLoS One 8 (4), e61362. doi:10.1371/journal.pone.0061362
Zhan, X., Teng, W., Sun, K., He, J., Yang, J., Tian, J., et al. (2021). CD47-mediated DTIC-Loaded Chitosan Oligosaccharide-Grafted nGO for Synergistic Chemo-Photothermal Therapy against Malignant Melanoma. Mater. Sci. Eng. C Mater. Biol. Appl. 123, 112014. doi:10.1016/j.msec.2021.112014
Zhang, F., Zhang, Y., Kong, L., Luo, H., Zhang, Y., Makila, E., et al. (2021). Multistage Signal-Interactive Nanoparticles Improve Tumor Targeting through Efficient Nanoparticle-Cell Communications. Cell Rep 35 (8), 109131. doi:10.1016/j.celrep.2021.109131
Zhang, W., Huang, Q., Xiao, W., Zhao, Y., Pi, J., Xu, H., et al. (2020). Advances in Anti-tumor Treatments Targeting the CD47/SIRPalpha Axis. Front. Immunol. 11, 18. doi:10.3389/fimmu.2020.00018
Zhao, W., Hu, X., Li, W., Li, R., Chen, J., Zhou, L., et al. (2021). M2-Like TAMs Function Reversal Contributes to Breast Cancer Eradication by Combination Dual Immune Checkpoint Blockade and Photothermal Therapy. Small 17 (13), e2007051. doi:10.1002/smll.202007051
Zheng, H., Zhang, Y., Liu, L., Wan, W., Guo, P., Nystrom, A. M., et al. (2016). One-pot Synthesis of Metal-Organic Frameworks with Encapsulated Target Molecules and Their Applications for Controlled Drug Delivery. J. Am. Chem. Soc. 138 (3), 962–968. doi:10.1021/jacs.5b11720
Zhu, Y., Sundaram, H. S., Liu, S., Zhang, L., Xu, X., Yu, Q., et al. (2014). A Robust Graft-To Strategy to Form Multifunctional and Stealth Zwitterionic Polymer-Coated Mesoporous Silica Nanoparticles. Biomacromolecules 15 (5), 1845–1851. doi:10.1021/bm500209a
Keywords: cd47, SIRPα, tumor, immunotherapy, nanomedicine
Citation: Liao H and Niu C (2022) Role of CD47-SIRPα Checkpoint in Nanomedicine-Based Anti-Cancer Treatment. Front. Bioeng. Biotechnol. 10:887463. doi: 10.3389/fbioe.2022.887463
Received: 01 March 2022; Accepted: 04 April 2022;
Published: 26 April 2022.
Edited by:
Cristina Fornaguera, Institut Químic de Sarrià, SpainReviewed by:
Jinjin Chen, Tufts University, United StatesYoosoo Yang, Korea Institute of Science and Technology (KIST), South Korea
Copyright © 2022 Liao and Niu. This is an open-access article distributed under the terms of the Creative Commons Attribution License (CC BY). The use, distribution or reproduction in other forums is permitted, provided the original author(s) and the copyright owner(s) are credited and that the original publication in this journal is cited, in accordance with accepted academic practice. No use, distribution or reproduction is permitted which does not comply with these terms.
*Correspondence: Chengcheng Niu, bml1Y2hlbmdjaGVuZ0Bjc3UuZWR1LmNu