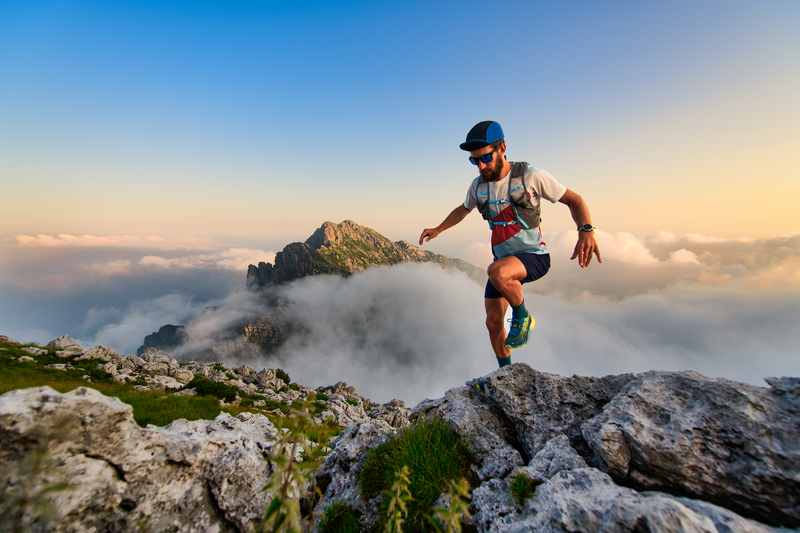
95% of researchers rate our articles as excellent or good
Learn more about the work of our research integrity team to safeguard the quality of each article we publish.
Find out more
REVIEW article
Front. Bioeng. Biotechnol. , 30 May 2022
Sec. Nanobiotechnology
Volume 10 - 2022 | https://doi.org/10.3389/fbioe.2022.880214
This article is part of the Research Topic Advanced Nanotechnology for Reactive Oxygen Species (ROS)-mediated Therapies View all 5 articles
Nanozymes are inorganic nanostructures whose enzyme mimic activities are increasingly explored in disease treatment, taking inspiration from natural enzymes. The catalytic ability of nanozymes to generate reactive oxygen species can be used for designing effective antimicrobials and antitumor therapeutics. In this context, composite nanozymes are advantageous, particularly because they integrate the properties of various nanomaterials to offer a single multifunctional platform combining photodynamic therapy (PDT), photothermal therapy (PTT), and chemodynamic therapy (CDT). Hence, recent years have witnessed great progress in engineering composite nanozymes for enhanced pro-oxidative activity that can be utilized in therapeutics. Therefore, the present review traverses over the newer strategies to design composite nanozymes as pro-oxidative therapeutics. It provides recent trends in the use of composite nanozymes as antibacterial, antibiofilm, and antitumor agents. This review also analyzes various challenges yet to be overcome by pro-oxidative composite nanozymes before being used in the field.
Nanozymes are a class of nanomaterials possessing intrinsic enzyme activity, which puts them at the center of attraction for diagnostics and therapeutic applications. Engineering of nanozymes for high catalytic activity, easy synthesis, cost-effectiveness, stability, and sometimes reusability makes them superior to natural enzymes (Haung et al., 2019; Meng et al., 2019). Ever since 2007, when ferromagnetic nanozymes were first reported to have peroxidase (POD) mimicking activity, nanozymes possessing oxidase (OXD), superoxide dismutase (SOD), catalase (CAT), esterase, or nuclease-like activities were synthesized and used in environmental or biomedical applications (Wang X. et al., 2016a; Yu et al., 2020; Yang et al, 2021; Gomma, 2021). The utilization of nanozymes in therapeutics relies on the use of their pro-oxidative and antioxidative activities. Nanozymes exhibiting POD- and OXD like properties possess the capability of converting H2O2 into reactive oxygen species (ROS) such as hydroxyl radical (•OH), singlet oxygen (1O2), and superoxide anion (•O2−) (Xiong et al., 2019; Chong et al., 2021). These radicals are highly oxidative molecules capable of interacting with proteins and lipids in the cell membrane of living cells, causing intense damage to the organelles and inducing apoptosis or necrosis-mediated cell death (Yang et al., 2020). In addition to the pro-oxidative catalytic activity, nanozymes also display antioxidative catalytic activities such as SOD and CAT, which mediate the scavenging of ROS generated in the cell and prevent cells from oxidative damage. Both these activities of nanozymes are harnessed in therapeutics such as wound disinfection, tumor therapy, nervous disorders, etc. (Yang et al., 2021). The treatment regime mediated by nanozyme-generated oxidative radicals is termed CDT (Xu et al., 2020). The use of nanozyme pro-oxidative potential has shown immense potential as nanoantibiotics for treating bacterial infections, inhibiting biofilms, wound disinfection, and healing and in tumor therapy (Wang H. et al., 2019). Despite this, their successful translation from the laboratory to the clinic is not yet achieved due to some limitations such as poor efficacy in catalyzing low concentration of H2O2, non-reusability of nanozymes, most nanozymes are unable to engage effectively with target cells, intrinsic shortcoming of ROS due to its short lifetime (less than 200 ns), and a small diffusion distance (approximately 20 nm). In addition, the nanozyme’s non-compatibility with specific tissue environments such as highly moist wounds and weekly acidic and hypoxic tumor microenvironment (TME) restricts their practical applicability.
To circumvent such challenges, nanozymes can be engineered by tailoring their size, shape, surface, etc. One effective strategy for engineering pro-oxidative nanozymes is to produce a hybrid or alloy of more than one component. Such composite nanozymes not only inherit the individual properties of each component and overcome the shortcomings but can also integrate other functionalities (photothermal effect, photodynamic effect, etc.) to design intelligent, multifunctional nanoplatforms for disease therapy. Various advantages offered by composite nanozymes are depicted in Figure 1. Recent reviews have discussed the synthesis strategies of different nanozymes and their overall applications in diverse disciplines (Liu et al., 2020; Huang et al., 2018) or toward detection, imaging, and biomedicine development (Liang and Yan, 2019). However, focused reviews encompassing composite nanozyme-based nanoplatforms with augmented ROS capability and their applicability in therapeutics are scarce. Recently, Li Y. et al. (2021) reviewed nanozyme-based composite materials for antibacterial and antibiofilm application. Nevertheless, considering the potential embedded in the composite nanozyme-mediated ROS-based disease therapeutics and the volume of research articles published in this area, it is highly relevant to review the recent trends in this specific domain. Hence, in this review, recent advancements in designing composite nanozymes to upregulate their ROS generating potential are discussed. The strategies for making them multifunctional nanoplatforms and conferring newer properties to enhance their applicability as ROS-mediated disease treatment regimens are reviewed here. We illustrate various composite nanozymes with pro-oxidative activity used in therapeutic applications and also discuss their advantages and future prospects. We believe that this review will help in future investigations on composite nanozymes with augmented pro-oxidative potential in disease therapy.
Nanotechnology has presented an opportunity to design disease therapeutics utilizing enzyme-based natural defense mechanisms in living cells such as those mediated by xanthine peroxidase, myeloperoxidase, and haloperoxidase (Chen et al., 2018). Such defense routes bestow protection by highly damaging oxide radicals against the pathogenic microorganism. Natural enzymes act as antibacterial agents by ROS production that irreversibly kills the bacteria. For example, in fresh milk, an enzyme called lactoperoxidase acts as an antibacterial agent by catalyzing H2O2 and oxidizing thiocyanate (SCN−) to produce hypothionite ions (OSCN−) (Davidson et al., 2015). Nanomaterials mimic natural enzymes in action and not only display pro- or antioxidative catalytic activity but also mimic hydrolase-like activity (Jiang et al.,2019). For instance, nanomaterials exhibiting DNAse (Chen et al., 2016) and phospholipase activity (Khulbe et al., 2020) cause hydrolytic cleavage of extracellular DNA (eDNA) and phospholipids, respectively, and display antibiofilm activity. Hence, a brief understanding of the mechanism of different enzyme mimetic actions of nanozymes becomes important toward designing novel nanomaterials.
Peroxidases are a group of enzymes which catalyze the oxidation of various substrates (TMB, OPD, and ABTS) in the presence of H2O2 to generate reactive-free radicals (Scheme 1A). Until now, various nanomaterials such as carbon-based, metals, metal oxides, and metal sulfides are known to display POD mimic activity (Yang et al., 2020). Fe3O4 nanoparticles were the first to demonstrate POD-like activity through a Ping–Pong kinetic mechanism (Jiang et al., 2021). POD-catalyzed oxidation of TMB/H2O2 is a two-step electron transfer process. The first step yields a TMB radical cation through single-electron oxidation. Two of these intermediate radical cations combine to form a blue-colored charge-transfer complex (= 370 and 652 nm). In the second electron transfer step, the cation radical is further oxidized to yield a TMB diimine derivative (TMBDI; λ = 450 nm). In this way, the absorption spectrum of the oxidation products of TMB usually shows three absorption bands (Scheme 1B). Following more or less similar reaction kinetics, POD mimic composite nanozymes catalyze the breakdown of H2O2 to produce toxic ROS (•OH, •O2) that have a promising role in therapeutics.
SCHEME 1. (A) Reaction catalyzed by peroxidase mimic nanozymes (Bhaskar et al., 2015). (B) Schematic for POD-catalyzed two-electron oxidation of TMB into TMBDI. Adapted and reprinted with permission from Liu et al. (2021).
Glutathione peroxidase also belongs to the peroxidase family and protects organisms from oxidative damage. It oxidizes glutathione (GSH) in the presence of H2O2 to glutathione disulfide (GSSG) and H2O (Scheme 2). These nanozymes also exhibit a Ping–Pong catalytic mechanism wherein the nanozyme first reacts with H2O2 to form an intermediate which then oxidizes GSH to GSSG. Glutathione (GSH) is a tripeptide present in bacteria, as an antioxidant defensive system, and protects bacteria from oxidative stress by scavenging reactive-free radicals. Similarly, nanozymes with both GSH peroxidase and POD-like activity could be used as effective therapeutics in killing bacterial and cancer cells. For instance, nickel disulfide (ND) composite nanozyme with glutathione peroxidase–like activity depleted GSH and weakened the bacterial defense system, whereas its POD activity generated high ROS that irreversibly damaged bacteria (Wang et al., 2020). Similarly, the ultrasmall SnFe2O4 nanozyme possessed both POD and glutathione peroxidase activity. The SnFe2O4 nanozyme depleted GSH levels in cancer cells, and high ROS production could efficiently kill the cancerous cells (Feng et al., 2021).
SCHEME 2. Reaction catalyzed by glutathione peroxidase mimic nanozymes (Barrington, 2013).
Haloperoxidases also belong to the peroxidase family and catalyze the oxidation of halide ions to hypohalous acid by H2O2. Nanozyme catalytic activity has also been shown to eliminate biofilms in the marine environment. The V2O5 nanowire, with the assistance of H2O2, converted halide ions (Cl−, Br−) to produce hypohalous acid, which caused oxidative stress to the bacteria and could protect the ships against microbial adhesion in the ocean. The local vanadium coordination geometry of the exposed lattice planes of V2O5 nanowires was similar to that of the active site of natural vanadium haloperoxidase. Vanadium atoms could function as catalytic reactive sites to produce intermediate peroxo species due to their strong affinity for H2O2, a critical step in the enzymatic reaction. As a result, the halide ions attack the more susceptible and less electron-rich oxygen atoms of the peroxo complex intermediate with the production of hypohalous acid (Scheme 3A). The strong oxidizing ability of hypohalous acid can irreversibly kill bacteria (Huang et al., 2019). Likewise, haloperoxidase mimicking CeO2-x nanorods (NRs) were reported to catalyze oxidative bromination of organic signaling molecules which regulate quorum sensing mechanisms in bacteria and lead to antibiofouling (Scheme 3B). This signifies the therapeutic potential of haloperoxidase mimic nanozymes for antibacterial applications (Herget et al., 2017; Hu et al., 2018).
SCHEME 3. (A) Reaction catalyzed by haloperoxidase mimic nanozymes, (B) Fabrication of ceria nanofibrous mat. The hybrid mat can catalyze Br− with H2O2 to HOBr to prevent bacterial adhesion on its surface. Adapted and reprinted with permission from Hu et al. (2018), Herget et al. (2017).
Oxidase is an enzyme having a key role in cellular metabolism and processes that catalyze oxidation of various substrates by utilizing molecular oxygen to form ROS (H2O2 or •O2) (Scheme 4A). The oxidase family is categorized according to the acting groups of donors, including sulfur groups, amino groups, CH-OH groups, Ph-OH groups, and ferrous ions (Chong et al., 2021). Among these, the OXD-mimetic nanozymes that act on amino groups are widely studied. The generation of intermediates (e.g., singlet oxygen, oxygen superoxide anion) and the electron transfer process have a significant effect on the OXD mimic property of nanozymes. Cheng et al. reported OXD-mimic activity of nanoceria where O2 molecules are first adsorbed onto defect-rich sites of nanoceria and converted into O2·− under acidic conditions (Eq. 1). Subsequently, TMB was oxidized and Ce4+ present on the surface was reduced to Ce3+ (Eq. 3). As the main intermediate, the in situ produced O2·− finally regenerated Ce4+ via the oxidation of Ce3+, accompanied by the generation of water (Eq. 4). Alternatively, the oxidation of TMB could be directly initiated by O2·− as well (Eq. 2) (Cheng et al., 2016). Recently, various nanostructures displaying OXD activity were reported to have potential application in therapeutics. Wang et al. designed Co4S3/Co(OH)2 HNTs to mimic OXD-like activity that can catalyze O2 to generate ROS such as •O2. It displays excellent antibacterial properties against gram-negative bacteria (E. coli and P. aeruginosa) and gram-positive bacteria (S. sciuri and Bacillus) and avoids H2O2 toxicity (Wang et al., 2020).
SCHEME 4. (A) Reaction catalyzed by oxidase mimic nanozymes (Li et al., 2020). (B) Equations 1–4 represent proposed mechanism of oxidase mimic nanoceria. Adapted and reprinted with permission from (Cheng et al. (2016).
Deoxyribonuclease (DNase), a type of nuclease, cleaves phosphodiester linkage in DNA molecules and degrades it into fragments (Scheme 5). DNases can be used for gene editing, DNA repair, or as antibiotics in the treatment of skin diseases and bacterial infections (Fang and Liu, 2020). A DNase-mimetic artificial enzyme (DMAE) synthesized by incorporating cerium (IV) ion complexes on the Au component of Fe3O4/SiO2 core/shell nanoparticles showed excellent DNase-like activity against bacterial biofilms. With its greater penetration inside the biofilm, DMAE caused 80% eDNA cleavage within the biofilm and prevented upto 90% bacterial adhesion (Chen et al., 2016). Recently, it was reported that the combination of POD and DNAse activity in a single nanozyme (MOF-2.5Au-Ce) could achieve total elimination of the biofilm and prevention of secondary biofilm formation (Liu et al., 2019).
SCHEME 5. Reaction catalyzed by DNase mimic nanozymes (Laukova et al., 2020).
Phospholipase is an enzyme that catalyzes the hydrolytic cleavage of phospholipids at various sites (Scheme 6). A major component of the bacterial cell membrane is phospholipid, which plays a key role in biofilm formation, and its cleavage could disrupt biofilms. Phospholipase-mimetic ceria-based nanozyme could hydrolyze the phospholipids in bacterial cell membranes and bacterial biofilms efficiently (Khulbe et al., 2020). The study also demonstrated the potential of nanozyme in preventing bacterial colonization on the surface of urinary catheters. However, scarce literature is available for applying phospholipase mimetic nanozymes for therapeutic applications.
SCHEME 6. Depiction of various cleavage sites of a phospholipid by different phospholipases, PLA1-cleaves sn-1 acyl chain; PLA2-cleaves the sn-2acyl chain; PLC-cleaves before phosphate, releasing diacylglycerol and phosphate-containing group; PLD- cleaves after phosphate, releasing phosphatidic acid and alcohol. Adapted and reprinted with permission from Khulbe et al. (2020).
Table 1 presents a comparison of the aforementioned enzyme mimic activities displayed by nanozymes that kill bacteria or cancerous cells through different mechanisms. Some of these such as DNAse and phospholipase mimic nanozymes are relatively less explored. The design of a nanozyme that could exhibit and utilize more than one type of these pro-oxidative activities to kill its target cell may have high potential as a therapeutic agent.
TABLE 1. Comparison of various pro-oxidative enzyme mimic activities exhibited by composite nanozymes.
Nanozymes, particularly new-generation hybrid nanostructures, have aroused increasing attention by virtue of their superior pro-oxidative potential compared to natural enzymes and have shown practical applications such as antibacterial, antibiofilm, and antitumor in therapeutics. Currently, a number of hybrid or composite nanozymes (Figure 2) are being designed by integrating their pro-oxidative potential with other functionalities and using them as multifunctional nanoplatforms in disease therapeutics as discussed here.
Metal-based nanoparticles are well known to mimic POD and OXD activity, with promising applications in therapeutics. Metal-based nanozymes are in vogue owing to their easy synthesis and facile surface modification and convenience to hybridize with other elements and tailor their shape-size, high stability, biocompatibility, and electromagnetic functionality (Li M. et al., 2020; Shi et al., 2020). For instance, Xi et al. have shown that the doping of Cu in hollow carbon spheres (Cu-HCS) accelerated their POD activity (Xi et al., 2019). The catalytic activity of the nanohybrid was dependent on the state of the metal (Cu0/Cu2+) rather than its content or size as CuO-HCS displayed eight times lower catalytic activity than Cu-HCS, despite a very high Cu concentration in the former. Moreover, CuO-HCS nanozymes showed antibacterial activity against only Gram-negative bacteria in the absence of H2O2 through the release of Cu2+ ions, whereas Cu-HCS displayed broad-spectrum antibacterial activity at low H2O2 concentrations through ROS generation. Metallic nanocomposites such as PEG-Cu2Se HNCs nanozyme was used as a multifunctional nanoplatform for antitumor application. It displayed enhanced antitumor activity due to synergistic effects of Fenton-like reactions with CDT and PTT without any long-term toxicity (Wang et al., 2019). Noble metals (Ag, Au, Pd, and Pt) exhibit strong catalytic activity, which have been used for designing nanohybrids and have application in cancer therapy, immunological assays, and antibacterial agents (Cai and Yang, 2020; Sharifi et al., 2020). Bifunctionalized mesoporous silica-supported gold nanoparticles (MSN-Au NPs) displayed dual-enzyme activity (POD and OXD) to generate ROS such as • OH, 1O2, and •O2−. As a result, the MSN-Au NPs exhibited effective antibacterial and antibiofilm properties (Tao and Ju, 2015). Mirhossein et al. fabricated core-shell Au@Co-Fe hybrid nanoparticles (Au@Co-Fe NPs) in which all nanoparticles such as Au, Fe, and Cu possess POD activity. Thus, these NPs in combination displayed enhanced enzyme activity and exhibited ROS-mediated excellent antibacterial activity against E. coli, P. aeruginosa, S. aureus, and B. cereus (Mirhosseini et al., 2020). Along with antibacterial activity, Au nanozyme also acts as an imaging agent and can be used for imaging infections associated with the biofilm. Therefore, a hybrid of AuNPs provides new scope for diagnosis and therapeutic applications for bacterial diseases. Ag nanoparticles provide good optical response and are easily miscible in any alloy composition. Hence using Ag with Pd nanoparticles with POD mimic activity could prove to be promising for tumor treatment. Li et al. demonstrated that AgPd@BSA/DOX nanocomposite possessed enhanced POD-like activity and better photothermal conversion efficiency (PCE) under NIR light irradiation as than individual Ag or Pd metal. Ag/Pd nanocomposite also functioned as a nanovehicle for carrying doxorubicin drugs, which were released under NIR light, induced hyperthermia and by a synergistic mechanism, and acted as an effective therapeutic agent. It was demonstrated that AgPd@BSA/DOX could catalyze conversion of H2O2, which is overexpressed in the TME, to generate a high amount of •OH, causing cell cycle arrest of cancerous cells, apoptosis, and senescence (Li L. et al., 2020). Another bimetallic nanozyme silk fibroin capped AuPt responded to tumor hypoxia and its reducing environment to treat tumors with high efficiency. AuPt@SF (APS) decomposed glucose to produce abundant H2O2 and oxidized intratumoral GSH to resist ROS depletion and simultaneously catalyzed O2 and H2O2 to generate ROS such as superoxide radicals (•O2−) and hydroxyl radicals (•OH), respectively (Yang W. et al., 2021a).
Recently, various metal oxides such as Fe3O4 (Fu et al., 2017), MnO2 (Liu et al., 2021), and CeO2 (Zhu et al., 2021) with significant pro-oxidative enzymatic activity have been utilized for therapeutic applications. Among different metal oxide–based nanozymes, Fe3O4 nanoparticles (NP) received great attention owing to their excellent POD-like activity. Vallabani et al. constructed an ATP-triggered citrate-coated Fe3O4 NP with POD mimetic activity. Generally, most nanozymes are active under an acidic condition toward H2O2 breakdown, and this limits the practical application of nanozymes at physiological pH, but in this nanoalloy, ATP formed a complex with Fe2+ in the presence of H2O2 and generated ROS such as •OH, which promoted chronic wound healing at pH near 6.5–8.5. This nanocomposite displayed high antibacterial/antibiofilm activity (Vallabani et al., 2020). Karim et al., found that photoactive CuO nanorods (NRs) with POD-mimetic activity could act as a potent antibacterial agent. The catalytic activity increased approximately 20-fold due to strong affinity with H2O2, producing large amounts of •OH at a low concentration of H2O2 molecules (Karim et al., 2018). Wei et al. fabricated a defect-rich rough surface Fe3O4@MoS2-Ag composite nanozyme with POD mimicking activity. Fe3O4@MoS2-Ag generated ROS and leaked Ag+ killed ∼69.4% E. coli cells. It also displayed photothermal response under NIR activation to achieve outstanding synergistic disinfection (∼100%). The magnetic properties of Fe3O4 made it feasible to recycle it (Wei F. et al., 2021).
Currently, manganese dioxide (MnO2), a transition metal oxide, in combination with other components, is widely explored for cancer therapy with special and unique physicochemical properties. The MnO2 nanostructure is highly sensitive to the TME and rapidly degrades in the reduced and acidic environment, which is why it can be used as a tumor-specific drug vehicle. In addition, MnO2 is shown to catalyze H2O2 overproduced in tumor cells to produce O2 in situ and overcome TME hypoxia (Wu M. et al., 2019b; Zhu et al., 2020). Zhu et al. constructed a composite core–shell-structured nanozyme (MS-ICG@MnO2@PEG) having indocyanine green (ICG) loaded as a photosensitizer in the MnO2 shell for photodynamic therapy (PDT) clubbed with ROS-mediated chemodynamic therapy (Zhu et al., 2020). MnO2 also catalyzed intratumoral glutathione (GSH) to convert Mn4+ oxidation state into Mn2+ oxidation state, which simultaneously decomposed H2O2 by POD-like activity to form highly reactive •OH. Mn2+ being water-soluble were excreted easily from the body without causing toxicity. Li et al. fabricated MnO2/IrO2-PVP nanocomposite and loaded it with a photosensitizer Chlorin e6 (Ce6) that specifically responded to the TME. MnO2 in tumors catalyzed the production of O2 by H2O2 to alleviate hypoxia condition and also reacted with H+ and performed MRI function, whereas IrO2 possessed photothermal activity that converted O2 formed in the tumor to toxic singlet oxygen upon light irradiation, thereby enhancing the PDT. Thus MnO2/IrO2-PVP nanocomposite by synergistic mechanism displayed outstanding antitumor therapy (Li J. et al., 2021).
In recent years, experiments indicate that metal-sulfide nanomaterials with enzyme-like activity exhibit excellent antibacterial properties. Xu et al. reported a strategy for converting garlic-derived natural organosulfur compounds into a nano-iron sulfide that exhibited excellent antibacterial activity. It was shown that nano-iron sulfide with POD- and CAT-like activities can catalyze the oxidation of H2O2 to generate highly toxic hydrogen polysulfide. Nano-iron sulfide exhibited 500-fold increased antibacterial efficacy and also eliminated biofilms on human caries and promoted wound healing (Xu et al., 2018). Yin et al. synthesized molybdenum disulfide nanoflowers functionalized with polyethylene glycol that possessed high POD and PTT activity under NIR absorption. PEG-MoS2 NFs generated high ROS and disrupted the membrane of drug-resistant and endospore-forming bacteria and promoted wound healing (Yin et al., 2016).
The metal-organic framework (MOF) is a type of porous crystalline material designed by incorporating metal-containing nodes with organic ligands linked through coordination bonds (Wang et al., 2020; Ding S. et al., 2020). The different types of metal nodes/organic ligands with tailorable hollow cavities and open channels in MOFs provide high surface area, ease of pore tuning, property adjustability, and structural diversity. Facile modification of metal and ligand makes MOFs a promising host for immobilizing various metals, metal oxides, quantum dots, biomacromolecules, etc. (Zhao W. et al., 2019; Chen et al., 2018). Similar to natural enzymes, MOF cavities provide a hydrophobic environment; ordered arrangement of active catalytic sites offers highly dense substrates and mimics the enzyme activity (Lee et al., 2009; Cai and Yang, 2020). MOF-based composite nanozymes with various functional ligands have been fabricated to generate either ROS or reactive nitrogen species (RNS) for highly efficient antibacterial therapy. Cheng et al. designed biomimetic L-Arg/GOx@CuBDC with glucose oxidase (GOx), POD, and nitric oxide synthetase mimetic activity as an excellent antibacterial agent (inactivation efficacy ≥97%) for both gram-positive and gram-negative bacteria. The mechanism of L-Arg/GOx@CuBDC action was based on a double-cascade reaction system which under aerobic conditions first converted endogenous glucose to gluconic acid and H2O2, which acted as an initiation reaction for generating ROS. In the case of the RNS cascade reaction, NO produced by oxidation of L-Arg in the presence of H2O2 quickly reacted with •O2− (ROS) to generate ONOO− which was highly toxic (Cheng et al., 2020). Ma et al. designed IL@MIL-101(Fe)@BSA-AuNCs nanocomposite NPs mimicking POD-like activity and also possessed dual-modality imaging properties. Under microwave irradiation, MIL-101 (Fe) catalyzed H2O2 to generate •OH and treat tumor cells with high efficiency. The BSA-Au NCs were used for detecting dynamic distribution processes and diagnosing tumor sites with high specificity (Ma et al., 2019).
Carbon-based nanomaterials that possess catalytic activities include graphene, carbon nanotubes, fullerene, and carbon dots (Sun H. et al., 2020), and their derivatives find wide applications in diverse fields due to their outstanding electronic, optical, thermal, mechanical properties, low cost, biosafety, and multienzyme mimicking activities (Wang X. et al., 2016b; Ding H. et al., 2020). Fan et al. fabricated a nitrogen-doped porous carbon nanosphere mimicking multienzyme activity to target and destruct tumor cells with very high efficacy. Here, ferritin was integrated to guide nanozyme for specifically targeting HFn receptor (TfR1)–positive tumors and killing tumor tissue through abundant ROS generation. More importantly, the porosity of the nanocomposite provided numerous active sites for the substrate and improved and enhanced the catalytic activity (Fan K. et al., 2018). Graphitic carbon nitride nanosheets (g-C3N4 NSs), a carbon-based nontoxic semiconductor, exhibited POD mimetic activity and showed good potential as an antibacterial. The nanocomposite g-C3N4@Au NPs with POD mimetic activity were prepared by integrating Au NPs which stabilized the free radical and exerted a positive synergistic coupling effect. They showed potent antibacterial activity against multidrug resistant S. aureus and antibiofilm activity in the presence of a low amount of H2O2. In addition, the g-C3N4@Au Band-Aid was designed to combat in vivo bacterial infection and promote wound healing in the presence of ultralow concentrations of H2O2 (10 µM) (Wang et al., 2017). An alloy of carbon nanozymes also displayed photothermal activity under NIR and not only acted as an antibacterial agent but also enhanced their catalytic activities. Xi et al. fabricated N-doped sponge-like carbon spheres (N-SCSs) with multienzyme mimetic activity and photothermal response for synergetic antibacterial therapy. Under NIR, the catalytic activity of N-SCSs increased due to the laser irradiation that exposed a more active surface. When bacteria were kept with N-SCSs for 20 min and then irradiated with NIR light again for 10 min, the antibacterial performance increased toward E. coli (>−3.0 Lg (CFU ml−1)) and S. aureus (>−2.0 Lg (CFUml−1) (Xi et al., 2019).
Hydrogels are known to maintain a moist environment and could act as a barrier for microbes around the wound interface. Newer techniques have been used to integrate ROS-generating composite nanozymes with hydrogels for sustained and efficient therapeutic applications. A PVA hydrogel incorporated with rGO/MoS2/Ag3PO4 composites was synthesized to have enhanced photothermal and photocatalytic function and was used for rapid and effective treatment of bacterial infection in chronic wound healing (Zhang et al., 2019). The mechanical property and swelling ratio of hydrogels were significantly improved with rGO. In another study, ZnO QDs@GO NCs were introduced into chitosan hydrogels and designed as a multifunctional platform. Zn2+ produced with the dissolution of ZnO QDs by lysosomal acid was absorbed by bacteria, leading to inhibition of respiratory enzymes and ROS generation, whereas GO acted as a photosensitizer under NIR irradiation. This multifunctional hydrogel showed excellent wound healing and antibacterial applications in moist conditions (Liang et al., 2019). Similarly, poly-2-dimethylaminoethyl methacrylate (PDMAA) hydrogels were decorated with multifunctionality by encapsulating ROS generating hollow carbon nanoparticles and aloe-emodin (AE antibiotic extracted from Aloe leaves) within them. NIR-triggered ROS generation caused immediate bacterial killing, and continuous release of AE from the gel showed long-term effects and accelerated recovery of an infected wound (Xi et al., 2018).
Excessive amounts of free copper for therapeutic applications induced toxicity, which could be reduced with the utilization of hydrogels. Qiu et al. constructed the hydrogel-based artificial enzyme comprising copper and amino acids with good biocompatibility and peroxidase mimetic activity, exhibiting broad-spectrum antibacterial activities against both drug-resistant Gram-positive bacteria and Gram-negative bacteria. Furthermore, this system was prepared to function as a wound dressing, which could combat wound pathogens effectively and promote wound healing by stimulating angiogenesis and collagen deposition (Qiu et al., 2020). Sang et al. reported that MoS2-hydrogel mimicking peroxidase nanozyme could efficiently capture bacteria and realize excellent antibacterial and wound healing efficiency compared to traditional nanozyme. In addition, the system removed dead bacteria from the wound and reduced the incidence of inflammation (Sang et al., 2019). A comparison of the advantages and shortcomings of the abovediscussed classes of composite nanozymes is compared in Table 2.
Composite nanozymes can be variously tailored to generate strong ROS that can be subsequently used in antitumor, antibacterial, or wound healing applications. A number of factors can be considered to design an effective composite nanozyme with strong pro-oxidant potential (Figure 3).
FIGURE 3. Engineering composite nanozymes with enhanced pro-oxidative (A). Defect-rich surface of composite nanozymes enhance the affinity of composite nanozymes to bacteria. Adapted and reprinted with permission from reference (Wang et al., 2020). (B). Multicatalytic action of composite nanozymes perform cascade reaction and exert superior activity. Adapted and reprinted with permission from the reference (Wei et al., 2020) (C). Conjugation of CXCR4 to Fe3O4@Pt@E5 specifically target cancerous cells and synergistically treat AML (Kong et al., 2021) (D). Multifunctionality such as photothermal/chemodynamic/pharmaco of WS2QDs synergistically kill and eradicate bacteria Adapted and reprinted with permission from reference (Xu et al., 2020).
The choice of materials used to synthesize hybrid or composite nanozymes with high ROS generating ability is highly crucial. H2O2 is considered an effective antibacterial disinfectant. However, a biologically safe level of H2O2 can only be used for generating •OH radicals. Hence, it is imperative to design highly efficient catalytic nanozymes that can catalyze the conversion of very low levels of H2O2. Composite nanozymes can solve this problem to a great extent. Some materials such as AuNPs exert a synergistic coupling effect by generating more ROS and stabilizing the generating radicals. For instance, Wang et al. integrated AuNPs with g-C3N4 because -NH2 and -NH groups on g-C3N4 nanosheets served as effective Lewis bases to chelate metal ions and showed high affinity for Au3+, leading to the growth of AuNPs on g-C3N4 nanosheets. The composite nanozyme possessed excellent peroxidase ability as compared to only AuNPs and g-C3N4 nanosheets, which was due to the stabilization of •OH radicals generated by the breakdown of H2O2 by AuNPs via partial electron exchange interaction (Wang et al., 2017). Ultrasmall AuNPs possess high catalytic activity but are prone to aggregation, which can be reduced by producing hybrids with 2D MOFs as the latter can impose fast kinetics and effectively lower mass-transfer resistance of catalytic reactions (Hu et al., 2020).
The adsorption energy of metallic NPs dispersed on any solid support and the electron transfer capability of the nanocomposite greatly influences their catalytic ability. Wang et al. prepared PdFe nanostructure decorated graphdiyne nanosheet (PdFe/GDY) as a peroxidase mimic because Pd when alloyed with Fe or GDY was previously shown to have the optimum adsorption energy required for accelerated H2O2 decomposition and stability of oxygen-containing radicals, whereas GDY could act as a promising support due to bonding between its π electron pair and empty d-orbitals of Pd atoms. PdFe/GDY showed surprisingly high peroxidase activity compared to HRP. This was owing to more available adsorption sites on PdFe/GDY for TMB and H2O2 and low adsorption energy (-0.62 eV) of •OH on the Pd, leading to an increase in H2O2 decomposition (Wang et al., 2021).
Recently, the effect of doping transition metals (Zn, Ni, and Co) on the catalytic performance of Fe3O4 nanozymes was evaluated (Vetr et al., 2018), and CoFe2O4 NPs showed the highest catalytic activity. Later, Wang et al. showed that Co-doped Fe3O4 nanozymes not only possessed high peroxidase activity but had a 100-fold higher affinity for H2O2 than Fe3O4 nanozymes that could catalyze ultralow concentrations (10 nM) of H2O2. Though Co possesses a similar size as Fe atoms, its higher redox potential Co3+/Co2+ (1.30 V) than that of Fe3+/Fe2+ (0.771 V) and ability to produce more catalytically active sites and substrate-binding sites on nanozyme could be responsible for the high peroxidase activity of Co@Fe3O4 nanozyme (Wang Y. et al., 2019).
Similarly, Jiang et al. have synthesized B-doped core-shell Fe@BC nanozymes, where the Fe core was covered by over ten layers of B-doped carbon shells. The DFT calculations proved that the high peroxidase activity of the nanozyme was attributed to B doping in BCO2 that provides more electrons to H2O2 to promote O–O bond breakdown and a low-energy barrier (-1.16ev) for H2O2 conversion (Jiang et al., 2021). N- doping also contributed to enhancing the peroxidase activity of Fe3C/N-doped graphitic carbon nanomaterials (Fe3C/NeC) (Li Y. et al., 2020). Nanocomposites made of wide band-gap materials (ZnO) or those possessing crystal defects such as a large number of valence band holes or conduction band electrons possess the capability of generating effective ROS in the cells (Liang et al., 2019).
The catalytic action of nanozymes is affected by a number of factors inherent to the nanozyme such as size, shape, and surface charge, some of which have been explained in recent reviews (Navyatha et al., 2021). The ROS generating capability of nanozymes is strongly affected by the oxidation or valence state of the atom, structural defects, surface roughness, etc. These factors become important in designing a composite nanozyme when its ROS generating capability is intended to be of therapeutic use. Because these properties of nanozymes could affect the bacteria capturing ability of the nanozyme, they generate surface-stabilized ROS, which helps in overcoming the short life span and slow diffusion rate of ROS. It further could make the nanozymes efficiently catalyze much lower and safer H2O2 concentrations. Defect engineering means modifying the band edges and band-gap energies which increases the catalytic performance of the nanozyme (Twilton et al., 2017), and the rugged nano-morphology provides a trap for bacterial adsorption. Inspired by this, Wang et al. synthesized a defect-rich adhesive MoS2/rGO vertical heterostructure as a multienzyme antibacterial mimic (Figure 3A) (Wang et al., 2020). The microwave-assisted hydrothermal method introduced many surface defects with double vacancies for S and Mo. At acidic pH, the nanozyme possessed POD-, CAT-, and OXD-like activities, and the strong ROS species could show excellent antibacterial effects in situ. Recent experimental approaches show that oxygen vacancy engineering can create trapping sites for electrons and can be used to improve the catalytic activity of nanomaterials (Huang et al., 2019). Such sites can increase electron-hole recombinations, resulting in the enhanced photothermal performance of nanomaterials (Wang L. et al., 2019a; Wang et al., 2020). Peng et al. reported the first-time use of oxygen vacancy engineering in simultaneously enhancing ROS production and photothermal performance of the MnO2@Au nanostructure. The bonding of Au atoms to (O) atoms of MnO2 creates an oxygen vacancy in the MnO2 lattice. The nanozyme produced highly toxic superoxide radical (•O2−) and photothermal activity along with GSH depletion capacity, allowing photothermal/oxidation synergistic theranostics TME-regulation (Peng et al., 2020).
Recently, composite nanozymes possessing multiple catalytic actions, including pro-oxidative and antioxidative functions, have been synthesized. However, their catalytic activities can be tuned by optimizing their pH of action. Some pro-oxidative nanozymes can possess both OXD- and POD-like activity and could act as superior nanozymes by generating both •OH and •O2− radicals. For instance, Fe and N co-doping in hollow carbon spheres endowed them with appreciable POD-, CAT-, and SOD-like activities (Fan et al., 2020) POD-like activity generates •OH radicals under weak acidic conditions and is effectively used for bacteria-infected wound healing, whereas CAT/SOD activity scavenged generated H2O2 and •O2 under near neutral conditions and used for noninfectious inflammatory bowel disease. Similarly, pH-switchable Co(OH)2/FeOOH/WO3 nanoflowers displayed POD activity at acidic pH (<7) and CAT activity at basic pH (>7) and were used for PDT-integrated tumor therapy (Alizadeh et al., 2021).
Nanozymes are also used as multienzyme nanoreactors which display cascade catalytic reactions and are used in tumor therapy (Liu et al., 2020). For instance, GOx activity was integrated with the ROS generating ability of nanozymes and was used for ROS-mediated tumor cell killing along with inducing starvation conditions in the cells. IrRu-GOx@PEG NPs were loaded with natural GOx, which converted tumor-sensitive glucose to H2O2 and killed tumor cells due to starvation by depleting the nutrient source. Second, IrRu NPs catalyzed endogenously produced H2O2 to highly toxic singlet oxygen 1O2 (that mediates oxidative damage), and O2 released oxygen helped in continuing the reactions of starvation therapy (Figure 3B) (Wei et al., 2020). Another such cascade nanoreactor, Pd@Pt-GOx/HA, was modified with an outer layer of hyaluronic acid, which could block various catalytic activities of the nanozyme, reduce its cytotoxicity to normal cells, and specifically target CD44-overexpressed tumors (Ming et al., 2020). The HA layer gets decomposed by hyaluronidase in tumor cells and exposes the nanozyme’s catalytic sites. Ultrasmall trimetallic (Pd, Cu, and Fe) alloy nanozyme (PCF-a NEs) displayed glutathione oxidase and POD cascade reactions in circumneutral pH, which was used for tumor CDT in combination with photothermal ablation and ultrasound (Jana et al., 2021).
Introducing surface coatings, chelating ions, or antibiotics on the surface can increase the stability and enzyme mimetic activity of nanostructures. The use of tannic acid for reducing Au (III) to form AuNPs and subsequent chelation with Cu2+ ions was used to fabricate a shell-coated Au@TACu nanozyme with increased peroxidase activity and excellent photothermal performance (Liu et al., 2020). Apart from the dual ROS and PTT effects, the acidic TME caused the dissolution of the TACu shell and the released Cu2+ depleted the overexpressed GSH, augmenting the oxidative stress–mediated killing of tumor cells. It is well known that CXCR4/CXCL12 interaction leads to acute myeloid leukemia (AML) after chemotherapy and hence the strategy of conjugating the CXCR4 antagonist on the Fe3O4@Pt composite nanozyme’s surface interferes with this axis (Figure 3C) (Kong et al., 2021). This approach, along with the synergistic action of ROS generated by nanozymes, caused AML cell apoptosis and prevented their migration from bone marrow to other tissues, which in turn prolonged AML mouse survival.
An important aspect of composite nanozyme designing also encompasses endowing them with some additional features in addition to ROS generating ability to enhance their usability and efficacy. For instance, Mu et al. reported reusable nanozymes by synthesizing a super-paramagnetic NiCo2O4-Au composite that can be easily separated from the media with a magnet. Their antibacterial activity remained intact for three cycles of separation (Mu et al., 2021). MnOx-PLGA@PDA nanoparticles (PP-MnOx) were synthesized as a multifunctional antitumor platform by integrating the oxidase mimic potential of MnOx and its ability to act as a sensitive T1-weighted magnetic resonance imaging agent with the cytotoxic effect of artesunate encapsulated within the PLGA core (Xi et al., 2021). The multifunctionality feature of composite nanozymes can also be augmented by combining their ROS generating ability with PTT and PDT. The synergistic actions of these modalities have shown accelerated antitumor and antibacterial effects. For effective CDT, nanozymes catalyzing the oxidation of intracellular glutathione were also designed.
Light has been shown to activate the enzyme-like properties of nanozymes (Liu et al., 2019). Liu et al. have studied the effect of surface plasmon resonance (SPR) on activating the POD-like activity of CeO2 for antibacterial action. Au@CeO2 nanozymes were synthesized with low oxidase and peroxidase-like activity, which was enhanced three times after 808-nm laser irradiation as compared to only CeO2 NPs or Au nanorods at weekly acidic pH (Liu et al., 2021). Xu et al. synthesized a multifunctional platform enabling photothermal/chemodynamic/pharmaco-synergistic antibacterial action by encapsulating tungsten sulfide quantum dot (WS2QD) nanozyme and vancomycin antibiotic in a thermal-sensitive liposome. POD mimic activity of WS2QD generated radicals; OXD mimic activity catalyzed GSH oxidation, causing its depletion; and thermal sensitivity improved catalytic activity and liposome rupture, facilitating targeted drug delivery (Figure 3D) (Xu et al., 2020).
Thus, the first strategy for designing efficient ROS-generating composite nanozymes should be to judiciously choose constituent elements that can enhance electron transfer to generate ROS, provide low adsorption energy to stabilize the generated radicals on the nanozymes surface, possess higher redox potentials and high affinity, could act as support for POD mimic elements, or could allow plasmon-induced electron transfer to other constituent elements. These properties, besides being intrinsic characteristics of an element, can also be improved to some extent by modifications in synthesis conditions (reactants and stabilizers used, method of synthesis, pH, temperature of synthesis, calcinations etc.) of the composite nanozymes. Modifications in synthesis conditions can directly influence their surface topography and morphology, for example, creating surface defects, roughness, oxygen vacancies, electron-hole pairs, making them porous, or having pseudopodia-like extensions can directly impact the intrinsic catalytic behavior of the composite nanozymes. Since catalysis is a surface phenomenon, such modifications can have nanozymes with improved Km and Vmax values, which can be used to catalyze low H2O2 concentrations or in situ produced H2O2. In addition, the overall bacterial/tumor cell killing ability of composite nanozymes can be accelerated by exploring multienzyme mimic synergistic activities within a nanozyme and prohibiting the antagonistic catalytic activity. For instance, heteroatom doping in carbon nanomaterials confers multimimic activity besides enhancing the enzymatic efficiency. Making cascade nanozymes and integration with PTT or PDT can also enhance their specific cell killing ability.
Composite nanozymes designed recently show both intrinsic peroxidase- and oxidase-like activity to generate ROS •OH, •O2−, and H2O2, which can vividly damage more lipids, amino acids, and polysaccharides in the bacterial membrane and also kill cancerous cells due to their oxidative and electrophilic nature (Li D. et al., 2020). Hence, the pro-oxidative potential of these nanozymes has been used for diverse therapeutic applications, as discussed ahead.
Composite nanozymes have shown broad-spectrum nanoantibiotic effects by killing gram-positive, gram-negative, and multidrug resistant bacteria at low and biologically safe H2O2 concentrations (Chen et al., 2020). Cai et al. designed POD and OXD mimics of Pd@Ir nanohybrid which killed bacteria by causing oxidative stress (Figure 4A) (Cai et al., 2019). Composite formation of CuS with graphene oxide was carried out to synthesize a needle-shaped nanozyme that could puncture bacterial membranes in addition to toxic •OH generation through its POD and OXD mimic activity (Wang et al., 2020). It could effectively eliminate MRSA and accelerate MRSA-infected wound healing. Nanostructures are also being explored as efficient photocatalysts for antibacterial photocatalytic therapy. For instance, an all-organic heterostructure g-C3N4 nanosheet loaded with self-assembled perylene-3,4,9,10-tetracarboxylic diimide (PDINH) was synthesized and shown to generate active species such as •O2−, 1O2, and •OH upon visible-IR light irradiation (Wang L. et al., 2019b). The active radicals diffused and efficiently killed both gram-positive and gram-negative bacteria. The photocatalyst accelerated the healing of bacteria-infected skin and promoted collagen regeneration. Mixed-metal oxides (Co-Al-Ce) prepared by compounding CeO2 with Co3O4 and CoAl2O4 showed maximum POD activity at 2.5% Ce and 200°C calcination temperature (Chen et al., 2020). It exhibited maximum antibacterial activity against Gram-negative bacteria at near-neutral pH. For efficient wound healing, it is important to regulate infection-related host responses besides eradicating bacteria from the wound site. Hemin@Phmg-TA-MSN was demonstrated as a dual-functional nanomedicine (Wei Z. et al., 2021). Graphdiyne-hemin (GDY-hemin) composite was synthesized as a stable nanozyme displaying bacterial cell killing at the wound site and accelerated wound healing mediated by ROS produced by its POD activity (Ali et al., 2021). The UiO-66-NH2/CS composite membrane exhibited POD activity and could generate active oxygen (•OH) before UV irradiation at a trace amount of H2O2. With UV irradiation, Zr4+ changes to Zr3+, and after removal of UV light, Zr3+ loses electrons to form Zr4+, which was used to produce H2O2 and •O2−. The membrane exhibits high antibacterial activity and has potential in wound dressings (Wang et al., 2021). Table 3 enlists composite nanozymes with promising potential as antibacterial or antibiofilm agents.
FIGURE 4. Schematic representation of composite nanozymes as effective antibacterial and antibiofilm agents. (A). Mechanism of enzyme mimic Pd@Ir nanostructures for potential antibacterial therapy. Adapted and reprinted with permission from the reference (Cai et al., 2019). HA: humic acid. (B). Dual-catalytic activity of Dex-IONP-GOx for the disruption and inhibition of the bacterial biofilm. Adapted and reprinted with permission from the reference (Huang et al., 2021).
TABLE 3. Various composite nanozymes with demonstrated use as antibacterial and wound healing agent through ROS.
One of the strategies to prevent biofilm formation on surfaces is to incorporate antibacterial and anti-adhesive coatings on these surfaces. In this context, the strategy of using nanomaterials for designing super hydrophobic (Ren et al., 2018), and more stable hydrophilic/superhydrophilic surfaces (Valencia et al., 2018) was explored. But these coatings were susceptible to damage from complex environmental changes. To address this issue, the recent approach is to design smart responsive materials as anti-adhesive materials and possessing the switchable function of being antibacterial (Ghasemlou et al., 2019). Very recently, Lin et al. designed a light and thermoresponsive adjustable anti-adhesion surface using light-responsive titanium oxide nanotubes as a base. Thermo-responsive P (vinylcaprolactam (VCL)–co-polyethylene glycol-methacrylate (PEGMA)–co-alkyl-dimethyl tertiary amine (QAS)–co-vinyltrimethoxysilane (VTMO)) copolymer was grafted on TiO2 surface along with antibacterial QAS constituent and anti-adhesive PEGMA polymer (Lin et al., 2021). Hence, due to VCL-triggered conformational change, bacterial antiadhesion was enhanced by thermoresponse.
Streptococcus mutans thrives well in sugar-rich and acidic microenvironments associated with dental caries. The antagonist action of commensals such as S. oralis is limited due to low concentrations of intrinsic H2O2 production and its confined effect. Hence, a bifunctional nanohybrid system possessing dual-catalytic activity was designed by conjugating GOx on dextran-coated iron oxide NPs (Figure 4B) (Huang et al., 2021). GOx catalyzed abundant glucose available in dental caries to H2O2 and dextran coated iron oxide NPs converted it to generate ROS that killed bacteria and degraded the EPS matrix. The nanohybrid system acted as a targeted antibiofilm agent in vivo compared to the gold standard drug chlorhexidine.
Nanozymes display strong ROS-mediated antitumor potential, and considerable endeavors are made to realize this potential. However, the complex TME limits their therapeutic efficacy and drives the need for a TME-specific and endogenously responsive approach for efficiently utilizing nanocatalytic therapy. The TME is characterized by a weakly acidic environment, high glutathione (GSH) concentrations, overproduction of H2O2 (50–100 × 10−6 m) hypoxia, and an immunosuppressive environment. High GSH concentrations could offshoot the pro-oxidative nanocatalytic effect of nanozymes (Dong et al., 2020). To realize this, Fan et al., developed yolk-shell gold@carbon nanozymes with a synergistic action of photothermal-enhanced catalytic activity (Figure 5) (Fan L. et al., 2018). An et al., synthesized MnO2@HMCu2–xS nanocomposite with photothermal-enhanced GSH depletion ability exhibiting tumor destruction (An et al., 2019). Dong et al. have integrated hyperthermia-enhanced dual-enzyme mimic activity (POD and CAT mimic) with GSH depletion capability in cerium-based nanozyme (PEG/Ce-Bi@DMSN) for improved tumor ablation (Dong et al., 2020). Fe-based NPs show Fenton reaction for •OH radical generation, but the reduction of Fe3+ by H2O2 is hampered due to weakly acidic TME. The pH dependency of nanozyme was attempted to be overcome by using NIR energy (Nie et al., 2019) and co-delivery of nanocatalysts and iron species (Zhao P. et al., 2019) to drive Fe3+ reduction; however, electron delivery between Fe2+ and Fe3+ was not efficient at mild acidic pH. The hurdle was overcome recently when Fe, Al, and N co-incorporated graphitic nanozyme (Fe/Al-GNE) were synthesized to boost electron transfer capacity (Li J. et al., 2020), Al functioned as an electron pump to transfer electrons to Fe3+ and the graphitic sheet accelerated the process of electron delivery. Thus, Fe/Al-GNE nanozymes could efficiently catalyze excess H2O2 in the TME, inducing apoptosis of tumor cells. Yang et al. constructed bimetallic NPs on a metal organic framework to be used as hosts instead of Fenton agents. Among them, Cu-Pd@MIL-101 exhibited excellent POD-like activity along with GSH depletion ability, which synergistically favored CDT for tumors. Hypoxic TME favors tumor growth, which could be minimized by constant oxygen supply (Yang P. et al., 2021c). To this end, Ru@MnO2 nanozymes were coated with erythrocyte membrane that increases its biocompatibility and circulation time in blood (Zhu et al., 2021). These nanozymes catalyzed endogenous H2O2 to generate O2 to relieve hypoxia and support PTT and CDT. With an intention to overcome immunosuppressive TME, TGF-β inhibitor (TI) was loaded into PEGylated iron manganese silicate nanoparticles (MSN-PEG-TI) (Xu et al., 2020). Both IMSN and TI promoted polarization of macrophages from M2 to M1 and induced H2O2 regeneration, which accelerated the POD-like activities of IMSN nanozyme. This immunomodulation-enhanced nanozyme activity showed a potent antitumor effect on multicellular tumor spheroids (MCTS) and in vivo CT26-tumor-bearing mouse models. A sufficient level of endogenous H2O2 was needed to produce •OH radicals for efficient tumor therapy, and it was achieved by developing nanoplatforms with cascade catalytic abilities as described in Section 4.3. However, the extremely short half-life (∼1 µs) of •OH restricts its future application. This problem was addressed by constructing Fe3O4 nanocrystals coated with Schwertmannite (Sch) matrix (Sch@Fe3O4), which possessed the ability to generate SO4•- radicals from SO42- when attacked by •OH radicals in a cascade reaction (Wu et al., 2021). Fe3O4 hollow core generates •OH in Fenton’s reaction, which is converted to SO4•- (half-life of ∼30 µs) by nanoscale cellular pseudopodia-shaped Sch grown on the Fe3O4 core. The combined effect of two radicals, along with PTT and GSH depletion mediated by L-buthionine sulfoximine (BSO) molecules loaded in the hollow Fe3O4 cores, showed efficient tumor cell death.
FIGURE 5. Schematic of representation of yolk-shell gold@carbon nanozymes with intrinsic enzyme mimic activity and photothermal response as an effective antitumor agent Adapted and reprinted with permission from (Fan, 2018).
The antitumor potential of nanozymes is also realized by integrating MnOx nanozymes with artesunate-loaded drug carrier Poly-(lactic-glycolic acid) PLGA to form PLGA@PDA nanoparticles (PP-MnOx NPs) (Xi et al., 2021). PDA was used to link nanozyme with PLGA. The nanozyme exhibited OXD-mimicking activity to catalyze the conversion of O2 and generate ROS. Mechanistic insight showed that the electrons released during conversion of Mn2+/Mn3+ on the surface of nanozyme to Mn4+ were trapped by O2 to form H2O2. Activation of mitochondrial apoptotic pathways due to synergistic action of ROS and sustained release of the drug artesunate leads to efficient tumor cell death. The ROS generating capability of Fe3(PO4)2 8H2O–CDs (carbon nanodots)–FA hybrid nanoflowers (hNFs) was found to effectively kill cancerous cell lines in the presence of exogenous H2O2 and in combination with ascorbic acid mediated endogenously produced H2O2 (Guo et al., 2019). In another approach, the ROS generating capability of Au–Ag@HA NPs was combined with a radiation paradigm for effectively killing tumor cells (Chong et al., 2020). Ionizing radiation boosted •OH radical production and Ag+ release at the tumor site, thereby synergistically killing 4T1 breast cancer cells. Urchin-like Fe–MIL-88B–NH2@PFC-1-GOx (MPG) nanoparticles were constructed as versatile nanoplatform to synergistically offer CDT, sonodynamic therapy, and starvation therapy to effectively kill tumor cells (Hu et al., 2021). Table 4 enlists such composite nanozymes with demonstrated anticancerous potential mediated through ROS.
As discussed above, composite nanozymes have presented themselves as multifunctional catalytic agents for disease therapy. Despite the numerous advantages offered by composite nanozymes, their translation from the laboratory to field is far from reality because of the limitations and challenges faced by them. The first and foremost limitation is their low selectivity toward the target cell, which could raise concern about their toxicity and off-target effects. Second, composite nanozymes at times display multiple enzyme mimic activities, such as pro-oxidative (peroxidase and oxidase) and antioxidative activity (superoxide dismutase and catalase) at the same time, which could interfere with desired activity in the living system or may cause a reverse effect. Third, the optimal catalytic activity of many composite nanozymes is restricted to acidic pH, which is not compatible with the physiological and biological conditions. The peroxidase activity of nanozymes is also dependent on the use of H2O2, which itself could be toxic beyond a threshold. Hence, pro-oxidative nanozymes that do not depend on the use of H2O2 would be more suitable and welcomed. Another important issue with composite nanozymes is their biosafety. Composite nanozymes intended to be used for biomedical applications are engineered to interact with cells/tissue. However, broad focus remains on the therapeutic performance of composite nanozymes while ignoring their biosafety assessment. For instance, inorganic nanoparticles mimicking enzyme-like activity frequently accumulate in the reticuloendothelial system (RES), resulting in low passive targeting specificity and long-term toxicity, limiting their use in clinical trials (Yang et al., 2019). Some nanozymes such as nickel disulfide showed good biodegradable properties but had relatively long blood circulation times, which limits their practical applications (Wang et al., 2020). The size, composition, surface charge, dose, and functional groups of composite nanozymes affect their kinetics, specificity, and toxicity. For instance, increasing the size of graphene oxide can improve the potential for bacteria killing but poses toxicity to normal cells and tissues (Mei et al., 2021). Metal-based composite nanozymes not only display good therapeutic effects but also impose a potential health issue due to ionic dissolution such as Zn2+ and Cu2+ that interact with biomolecules such as proteins and enzymes, inactivating them and causing metal poisoning to cells and tissue (Halbus et al., 2017).
Hence, it is equally critical to conduct a systematic assessment of nanozyme biosafety in terms of assessing their absorption, biodistribution, metabolism, clearance mechanism, pharmacokinetics, H2O2 concentration, and long-term toxicity in addition to their therapeutic effect in in vivo studies. Furthermore, long-term toxicity studies involving particle size, shape, and surface chemistry are required to ensure the nanozymes are suitable for in vivo biological applications. In addition, research into nano-bio interfaces, nanozyme immunotoxicity, genotoxicity, and neurotoxicity from molecules to organisms is still in progress and has to be thoroughly investigated. Because these nanomaterials are designed to interact with cells, it is critical that these interactions do not have a negative impact on the human body.
In this review, the recent developments in using the intrinsic ROS generating ability of composite nanozymes for various therapeutic applications are presented and understood. Composite nanozymes score over other nanozymes in offering unique inherent properties of constituent elements that synergistically enhance their applicability. For instance, Ni nanoparticles barely oxidize the enzyme substrate, whereas Pd NPs possess multienzyme mimic activity, and NiPd nanoparticles exhibit higher catalytic activity than any of these alone (Wang Q. et al., 2016). The pro-oxidative composite nanozymes have found increased applicability as antibacterial, antibiofilm, and antitumor agents. It is due to their ability to alleviate limitations of existing ROS generating nanozymes such as 1) inefficiency to produce significant levels of ROS to kill bacteria at biologically safe low H2O2 concentrations; 2) single-modal nanozymes cannot effectively eradicate resistant bacteria or abnormal cells, 3) inefficient capture of H2O2 or generated radicals on bacterial surface; and 4) limited catalytic activity in the TME.
The composite nanozymes provide flexibility in their design and synthesis by offering a diverse choice of elements to be used for support or as dispersed nanoparticles. Tailoring nanozymes to closely mimic natural enzymes can be used as a strategy to design efficient composite structures. For instance, Zhang et al. have used covalent organic frameworks (COFs) to tailor the pore microenvironment around active centers and enhance the catalytic ability of MOFs (Zhang et al., 2021). The pseudopodia-like structure of the COF enabled the nanozyme platform to capture bacteria efficiently through multivalent interactions between hairy bacteria and spiky COFs. The various strategies discussed for engineering composite nanozymes in this review can be used for accelerating their ROS generating ability, making them multifunctional (photothermal, optical, photodynamic, and chemodynamic activity), adhesive, reusable, and compatible to act at neutral or near-neutral pH and in hypoxic TME, efficient enough to eliminate multidrug resistant bacteria/biofilm and targeted tumor cell killing. Hydrogel-based composite nanozyme can especially accelerate wound healing and disinfection.
Despite the reporting of various composite nanozymes with enhanced ROS ability and superior therapeutic applications, their translation from the laboratory to market is yet to be achieved. Hence, composite nanozymes need to be engineered to overcome the challenges discussed in Section 6. As this field is still in its infancy and evolving, we expect that the following new paradigms in engineering composite nanozymes could contribute toward addressing these challenges in the near future. 1) The catalytic efficiency of the nanoenzyme could be improved by modifying its lattice (spatial) structure so as to increase active catalytic centers, defect-rich active edges or oxygen vacancies etc. 2) In order to restrict the intrinsic antagonistic catalytic activity of a composite nanozyme, its exact molecular mechanism for electron movements within the nano-composite may be studied toward identifying specific inhibitors that could suppress the antagonistic catalysis. For example, the carbonyl groups on the carbon nanotubes are active sites for their POD mimic activity, while hydroxyl and carboxyl groups act as competitive sites. A study indicated that oxygenated group–enriched carbon nanotubes (o-CNTs) could be modified to show POD activity by blocking the carboxyl group and hydroxyl group. The modified o-CNTs were proved to demonstrate improved antibacterial effects when used in disinfection (Karim et al., 2018). 3) To solve the problem of less specificity and off-target activity, stimuli-responsive nanozymes (such as pH-responsive, hypoxia-responsive, light-responsive, and ultrasonic (US) responsive) could be developed to control the nanozyme’s activation and inactivation. An US switchable nanoplatform (Pd@Pt-T790) system was proposed for the controllable generation of catalytic oxygen and sonosensitizer-mediated reactive oxygen species by ultrasound activation, thereby overcoming the hypoxia-associated barrier and augmenting sonodynamic therapy efficacy. Modification of T790 onto Pd@Pt could block the catalase mimic of Pd@Pt, and upon US irradiation, the nanozyme activity was effectively recovered to catalyze the decomposition of endogenous H2O2 into O2. Such “blocking and activating” enzyme activity was specifically important for decreasing the adverse effects and toxicity of nanozymes on normal cells and has the potential to realize active, controllable, and disease loci–specific composite nanozyme (Sun D. et al., 2020). To overcome the working pH restriction, one strategy has been to use some trigger to accelerate the catalytic action of composite nanozymes at biological pH. From this stand point citrate coated Fe3O4 NP by utilizing adenosine triphosphate disodium salt (ATP) was used as a synergistic agent to make this nanozyme work at neutral pH as discussed in Section 3.2 (Vallabani et al., 2020). Furthermore for selectivity and specificity, ligand attachment to the nanocomposite could be beneficial for guiding the nanozyme to target cells such as ferritin attachment to nitrogen-doped porous carbon nanospheres, which guides nanocomposite into lysosomes and boosts reactive oxygen species generation in a tumor-specific manner (Fan et al., 2018). In addition, improving the catalytic specificity of nanozymes through artificial molecular imprinting is also a way to realize selectivity. 4) To address the dilemma of biosafety, surface modification or coating is one of the strategies, so it is critical to carefully select an appropriate modifying or coating agent and equip the composite nanozymes for increased biosafety. Also, externally applied H2O2 toxicity could be avoided by the rational design of cascade nanozymes utilizing in situ produced substrates such as H2O2 and generating ROS. Yan et al. reported an H2O2-free cascade reaction system by integrating CaO2 and hemin-loading graphene (H-G) into alginate (CaO2/H-G@alginate). In this nanocomposite, CaO2 reacts with the water infiltrated into depots from the interstitial tissues to produce calcium hydroxide [Ca(OH)2] and H2O2. Second, H-G decomposed H2O2 into hROS. The alginate depots not only offer a confined environment for enhanced reaction efficiency but also improve biocompatibility (Yan et al., 2013). Future research may also use various computational tools and molecular simulation software to help elucidate the catalytic reaction mechanism of nanozymes and establish a structure–function relationship toward designing better nanozymes. Taken together, the pro-oxidative potential of composite nanozymes can be enhanced and harnessed to produce cost-effective, biocompatible, and safe therapeutic agents.
SM contributed toward the collection of literature, writing the manuscript, figures, table preparation, and editing. SN conceptualized the idea, designed the methodology, supervised the process of reviewing and editing the manuscript, and overall monitoring of the manuscript.
The authors declare that the research was conducted in the absence of any commercial or financial relationships that could be construed as a potential conflict of interest.
All claims expressed in this article are solely those of the authors and do not necessarily represent those of their affiliated organizations, or those of the publisher, the editors, and the reviewers. Any product that may be evaluated in this article, or claim that may be made by its manufacturer, is not guaranteed or endorsed by the publisher.
The authors are grateful to MNNIT Allahabad for supporting the research activities carried out by our team and the support they received in compiling this article.
The Supplementary Material for this article can be found online at: https://www.frontiersin.org/articles/10.3389/fbioe.2022.880214/full#supplementary-material
Ali, A., Liu, J., Zhou, H., Liu, T., Ovais, M., Liu, H., et al. (2021). Graphdiyne-hemin-mediated Catalytic System for Wound Disinfection and Accelerated Wound Healing. Mat. Chem. Front. 5 (5), 6041–6051. doi:10.1039/d1qm00490e
Alizadeh, N., Salimi, A., Sham, T.-K., Bazylewski, P., Fanchini, G., Fathi, F., et al. (2021). Hierarchical Co(OH)2/FeOOH/WO3 Ternary Nanoflowers as a Dual-Function Enzyme with pH-Switchable Peroxidase and Catalase Mimic Activities for Cancer Cell Detection and Enhanced Photodynamic Therapy. Chem. Eng. J. 417, 129134. doi:10.1016/j.cej.2021.129134
An, P., Gao, Z., Sun, K., Gu, D., Wu, H., You, C., et al. (2019). Photothermal-enhanced Inactivation of Glutathione Peroxidase for Ferroptosis Sensitized by an Autophagy Promotor. ACS Appl. Mat. Interfaces 11, 42988–42997. doi:10.1021/acsami.9b16124
An, Q., Sun, C., Li, D., Xu, K., Guo, J., and Wang, C. (2013). Peroxidase-Like Activity of Fe3O4@Carbon Nanoparticles Enhances Ascorbic Acid-Induced Oxidative Stress and Selective Damage to PC-3 Prostate Cancer Cells. ACS Appl. Mat. Interfaces 5, 13248–13257. doi:10.1021/am4042367
Barrington, R. (2013). Selenium: Depletion and Repletion. Available at: http://www.robert.barrington.net/selenium-depletion-and-repletion/ (Accessed April 10, 2021 ).
Cai, S., Jia, X., Han, Q., Yan, X., Yang, R., and Wang, C. (2017). Porous Pt/Ag Nanoparticles with Excellent Multifunctional Enzyme Mimic Activities and Antibacterial Effects. Nano Res. 10, 2056–2069. doi:10.1007/s12274-016-1395-0
Cai, S., and Yang, R. (2020). Nanozymology Nanostructure Science and Technology Springer. Singapore: Sharifi.
Cai, T., Fang, G., Tian, X., Yin, J. J., Chen, C., Yin, J.-J., et al. (2019). Optimization of Antibacterial Efficacy of Noble-Metal-Based Core-Shell Nanostructures and Effect of Natural Organic Matter. ACS Nano 13, 12694–12702. doi:10.1021/acsnano.9b04366
Chen, C., Wang, Y., and Zhang, D. (2020). Bifunctional Nanozyme Activities of Layered Double Hydroxide Derived Co-al-ce Mixed Metal Oxides for Antibacterial Application. J. Ocean. Limnol. 38, 1233–1245. doi:10.1007/s00343-020-0041-6
Chen, J., Shu, Y., Li, H., Xu, Q., and Hu, X. (2018). Nickel Metal-Organic Framework 2D Nanosheets with Enhanced Peroxidase Nanozyme Activity for Colorimetric Detection of H2O2. Talanta 189, 254–261. doi:10.1016/j.nantod.2019.05.00710.1016/j.talanta.2018.06.075
Chen, S., Quan, Y., Yu, Y.-L., and Wang, J.-H. (2017). Graphene Quantum Dot/silver Nanoparticle Hybrids with Oxidase Activities for Antibacterial Application. ACS Biomater. Sci. Eng. 3, 313–321. doi:10.1021/acsbiomaterials.6b00644
Chen, Z., Ji, H., Liu, C., Bing, W., Wang, Z., and Qu, X. (2016). A Multinuclear Metal Complex Based DNase-Mimetic Artificial Enzyme: Matrix Cleavage for Combating Bacterial Biofilms. Angew. Chem. 128, 10890–10894. doi:10.1002/ange.201605296
Chen, Z., Wang, Z., Ren, J., and Qu, X. (2018b). Enzyme Mimicry for Combating Bacteria and Biofilms. Acc. Chem. Res. 51, 789–799. doi:10.1021/acs.accounts.8b00011
Cheng, H., Lin, S., Muhammad, F., Lin, Y.-W., and Wei, H. (2016). Rationally Modulate the Oxidase-like Activity of Nanoceria for Self-Regulated Bioassays. ACS Sens. 1, 1336–1343. doi:10.1021/acssensors.6b00500
Cheng, X., Zhang, S., Liu, H., Chen, H., Zhou, J., Chen, Z., et al. (2020). Biomimetic Metal-Organic Framework Composite-Mediated Cascade Catalysis for Synergistic Bacteria Killing. ACS Appl. Mat. Interfaces 12, 36996–37005. doi:10.1021/acsami.0c12159
Chong, Y., Huang, J., Xu, X., Yu, C., Ning, X., Fan, S., et al. (2020). Hyaluronic Acid-Modified Au-Ag Alloy Nanoparticles for Radiation/Nanozyme/Ag+ Multimodal Synergistically Enhanced Cancer Therapy. Bioconjugate Chem. 31, 1756–1765. doi:10.1021/acs.bioconjchem.0c00224
Chong, Y., Liu, Q., and Ge, C. (2021). Advances in Oxidase-Mimicking Nanozymes: Classification, Activity Regulation and Biomedical Applications. Nano Today 37, 101076. doi:10.1016/j.nantod.2021.101076
Davidson, P. M., Cekmer, H. B., Monu, E. A., and Monu, E. A. (2015). The Use of Natural Antimicrobials in Food. Knoxville, TN, USA: University of Tennessee, 1–27. doi:10.1016/b978-1-78242-034-7.00001-3
Ding, H., Hu, B., Zhang, B., Zhang, H., Yan, X., Nie, G., et al. (2020). Carbon-based Nanozymes for Biomedical Applications. Nano Res. 14, 570–583. doi:10.1007/s12274-020-3053-9
Ding, S., He, L., Bian, X.-w., and Tian, G. (2020). Metal-organic Frameworks-Based Nanozymes for Combined Cancer Therapy. Nano Today 35, 100920. doi:10.1016/j.nantod.2020.100920
Dong, S., Dong, Y., Jia, T., Liu, S., Liu, J., Yang, D., et al. (2020). GSH‐Depleted Nanozymes with Hyperthermia‐Enhanced Dual Enzyme‐Mimic Activities for Tumor Nanocatalytic Therapy. Adv. Mat. 32, 2002439. doi:10.1002/adma.202002439
Fan, K., Xi, J., Fan, L., Wang, P., Zhu, C., Tang, Y., et al. (2018). In Vivo guiding Nitrogen-Doped Carbon Nanozyme for Tumor Catalytic Therapy. Nat. Commun. 9, 1–11. doi:10.1038/s41467-018-03903-8
Fan, L., Sun, P., Huang, Y., Xu, Z., Lu, X., Xi, J., et al. (2020). One-Pot Synthesis of Fe/N-Doped Hollow Carbon Nanospheres with Multienzyme Mimic Activities against Inflammation. ACS Appl. Bio Mat. 3, 1147–1157. doi:10.1021/acsabm.9b01079
Fan, L., Xu, X., Zhu, C., Han, J., Gao, L., Xi, J., et al. (2018). Tumor Catalytic-Photothermal Therapy with Yolk-Shell Gold@Carbon Nanozymes. ACS Appl. Mat. Interfaces 10, 4502–4511. doi:10.1021/acsami.7b17916
Fang, R., and Liu, J. (2020). Cleaving DNA by Nanozymes. J. Mat. Chem. B 8, 7135–7142. doi:10.1039/D0TB01274B
Feng, L., Liu, B., Wang, d., Qian, C., Zhou, W., Liu, J., et al. (2021). An Ultrasmall SnFe2O4 Nanozyme with Endogenous Oxygen Generation and Glutathione Depletion for Synergistic Cancer Therapy. Adv. Funct. Mater. 31, 2006216. doi:10.1002/adfm.202006216
Feng, W., Han, Z., Wang, R., Gao, X., Hu, P., Yue, W., et al. (2019). Nanocatalysts‐augmented and Photothermal‐enhanced Tumor‐specific Sequential Nanocatalytic Therapy in Both NIR‐I and NIR‐II Biowindows. Adv. Mat. 31, 1805919. doi:10.1002/adma.201805919
Fu, S., Wang, S., Zhang, X., Qu, A., Liu, Z., Yu, X., et al. (2017). Structural Effect of Fe3O4 Nanoparticles on Peroxidase-like Activity for Cancer Therapy. Colloids Surf. B Biointerfaces 154, 239–245. doi:10.1016/j.colsurfb.2017.03.038
Fu, S., Yang, R., Zhang, L., Lu, W., Du, G., Cao, Y., et al. (2020). Biomimetic CoO@ AuPt Nanozyme Responsive to Multiple Tumor Microenvironmental Clues for Augmenting Chemodynamic Therapy. Biomaterials 257, 120279. doi:10.1016/j.biomaterials.2020.120279
Gao, S., Lin, H., Zhang, H., Yao, H., Chen, Y., and Shi, J. (2019). Nanocatalytic Tumor Therapy by Biomimetic Dual Inorganic Nanozyme‐catalyzed Cascade Reaction. Adv. Sci. 6, 1801733. doi:10.1002/advs.201801733
Garg, B., Bisht, T., and Ling, Y.-C. (2015). Graphene-Based Nanomaterials as Efficient Peroxidase Mimetic Catalysts for Biosensing Applications: An Overview. Molecules 20, 14155–14190. doi:10.3390/molecules200814155
Ghasemlou, M., Daver, F., Lvanova, E. P., Rhim, W., and Adhikari, B. (2019). Switchable Dual-Function and Bioresponsive Materials to Control Bacterial Infections. ACS Appl. Mat. Interfaces 11, 22897–22914. doi:10.1021/acsami.9b05901
Gomaa, E. Z. (2021). Nanozymes: A Promising Horizon for Medical and Environmental Applications. J. Clus. Sci. 1–23. doi:10.1007/s10876-021-02079-4
Guo, J., Wang, Y., and Zhao, M. (2019). Target-directed Functionalized Ferrous Phosphate-Carbon Dots Fluorescent Nanostructures as Peroxidase Mimetics for Cancer Cell Detection and ROS-Mediated Therapy. Sens. Actuators. B Chem. 297, 126739. doi:10.1016/j.snb.2019.126739
Halbus, A. F., Horozov, T. S., and Panouv, V. N. (2017). Colloid Particle Formulations for Antimicrobial Applications. Adv. colloid interface Sci. 249, 134–148. doi:10.1016/j.cis.2017.05.012
Haung, Y., Ren, J., and Qu, X. (2019). Nanozymes: Classification, Catalytic Mechanisms, Activity Regulation, and Applications. Che. Rev. 119, 4357–4412. doi:10.1021/acs.chemrev.8b00672
He, L., Ni, Q., Mu, J., Fan, W., Liu, L., Wang, Z., et al. (2020). Solvent-assisted Self-Assembly of a Metal–Organic Framework Based Biocatalyst for Cascade Reaction Driven Photodynamic Therapy. J. Am. Chem Soc. 142, 6822–6832. doi:10.1021/jacs.0c02497
Herget, K., Hubach, P., Pusch, S., Deglmann, P., Götz, H., Gorelik, T. E., et al. (2017). Haloperoxidase Mimicry by CeO2− X Nanorods Combats Biofouling. Adv. Mater 29, 1603823. doi:10.1002/adma.201603823
Hu, C., Wang, J., Liu, S., Cai, L., Zhou, Y., Liu, X., et al. (2020). Hyaluronic Acid-Modified Au–Ag Alloy Nanoparticles for Radiation/Nanozyme/Ag+ Multimodal Synergistically Enhanced Cancer Therapy. Bioconj. Chem. 31, 1756–1765. doi:10.1021/acsami.0c19584
Hu, M., Korschelt, L., Viel, M., Wiesmann, N., Kappl, M., Brieger, J., et al. (2018). Nanozymes in Nanofibrous Mats with Haloperoxidase-like Activity to Combat Biofouling. ACS Appl. Mat. Interfaces. 10, 44722–44730. doi:10.1021/acsami.8b16307
Hu, W., Younis, M. R., Zhou, Y., Wang, C., and Xia, X. H. (2020). Situ Fabrication of Ultrasmall Gold nanoparticles/2D MOFs Hybrid as Nanozyme for Antibacterial Therapy. Small 16, 2000553. doi:10.1002/smll.202000553
Huang, Q., Zhang, S., Zhang, H., Han, Y., Liu, H., Ren, F., et al. (2019). Boosting the Radiosensitizing and Photothermal Performance of Cu2–X Se Nanocrystals for Synergetic Radiophotothermal Therapy of Orthotopic Breast Cancer. ACS Nano 113, 1342–1353. doi:10.1021/acsnano.8b06795
Huang, Y., Liu, Y., Shah, S., Kim, D., Soro, A. S., Ito, T., et al. (2021). Precision Targeting of Bacterial Pathogen via Bi-functional Nanozyme Activated by Biofilm Microenvironment. Biomaterials 268, 120581. doi:10.1016/j.biomaterials.2020.120581
Jana, D., Wang, D., Bindra, A. K., Guo, Y., Liu, J., and Zhao, Y. (2021). Ultrasmall Alloy Nanozyme for Ultrasound-And Near-Infrared Light-Promoted Tumor Ablation. ACS Nano 15, 7774–7782. doi:10.1021/acsnano.1c01830
Jiang, D., Ni, D., Rosenkrans, Z. T., Huang, P., Yan, X., and Cai, W. (2019). Nanozyme: New Horizons for Responsive Biomedical Applications. Chem. Soc. Rev. 48, 3683–3704. doi:10.1039/C8CS00718G
Jiang, X., Liu, K., Li, Q., Liu, M., Yang, M., and Chen, X. (2021). B-doped Core–Shell Fe@ BC Nanozymes: Active Site Identification and Bacterial Inhibition. Chem. Comm. 202 (57), 1623–1626. doi:10.1039/D0CC06692C
Jin, S., Weng, L., Li, Z., Yang, Z., Zhu, L., Shi, J., et al. (2020). Nanoscale Dual-Enzyme Cascade Metal–Organic Frameworks through Biomimetic Mineralization as ROS Generators for Synergistic Cancer Therapy. J. Mat. Chem. B 8, 4620–4626. doi:10.1039/D0TB00357C
Karim, N., Singh, M., Weeerathunge, P., Bian, P., Zheng, R., Dekiwadia, C., et al. (2018). Visible-light-triggered Reactive-Oxygen-Species-Mediated Antibacterial Activity of Peroxidase-Mimic CuO Nanorods. ACS Appl. Nano, Mat. 1, 1694–1704. doi:10.1021/acsanm.8b00153
Khulbe, K., Karmakar, K., Ghosh, S., Chandra, K., Chakravortty, D., and Mugesh, G. (2020). Nanoceria-Based Phospholipase-Mimetic Cell Membrane Disruptive Antibiofilm Agents. ACS Appl. Bio Mater 3, 4316–4328. doi:10.1021/acsabm.0c00363
Kong, F., Bai, H., Ma, M., Wang, C., Xu, H., Gu, N., et al. (2021). Fe3O4@ Pt Nanozymes Combining with CXCR4 Antagonists to Synergistically Treat Acute Myeloid Leukemia. Nano Today 37, 101106. doi:10.1016/j.nantod.2021.101106
Laukova, L., Konecna, B., Janovicova, L., Vlkova, B., and Celec, P. (2020). Deoxyribonucleases and Their Applications in Biomedicine. Biomolecules. 10, 1036. doi:10.3390/biom10071036
Lee, J. Y., Farha, O. K., Roberts, J., Sheidt, K. A., Nguyen, S. B. T., and Hupp, J. T. (2009). Metal–organic Framework Materials as Catalysts. Chem. Soc. Rev. 38, 1450. doi:10.1039/B807080F
Li, D., Guo, Q., Ding, L., Zhang, W., Cheng, L., Wang, Y., et al. (2020). Bimetallic CuCo2S4 Nanozymes with Enhanced Peroxidase Activity at Neutral Ph for Combating Burn Infections. Chem. Bio. Chem. 21, 2620–2627. doi:10.1002/cbic.202000066
Li, J., Lei, Y., Qing, Z., Zou, Z., Zhang, Y., Sun, H., et al. (2020). Al Centre-Powered Graphitic Nanozyme with High Catalytic Efficiency for pH-independent Chemodynamic Therapy of Cancer. Chem. Comm. 56, 6285–6288. doi:10.1039/D0CC01331E
Li, J., Zhou, C., Zhang, J., Xu, F., Zheng, Y., Wang, S., et al. (2021). Photo-induced Tumor Therapy Using MnO2/IrO2-PVP Nano-Enzyme with TME-Responsive Behaviors. Colloids Surf. B Biointerfaces 205, 111852. doi:10.1016/j.colsurfb.2021.111852
Li, L., Liu, H., Bian, J., Zhang, X., Fu, Y., Li, Z., et al. (2020). Ag/Pd Bimetal Nanozyme with Enhanced Catalytic and Photothermal Effects for ROS/hyperthermia/chemotherapy Triple-Modality Antitumor Therapy. Chem. Eng. J. 397, 125438. doi:10.1016/j.cej.2020.125438
Li, M., Zhang, H., Hou, Y., Wang, X., Xue, C., Li, W., et al. (2020). State-of-the-art Iron-Based Nanozymes for Biocatalytic Tumor Therapy. Nanoscale Horiz. 5, 202–217. doi:10.1039/C9NH00577C
Li, S., Shang, L., Xu, B., Wang, S., Gu, K., Wu, Q., et al. (2019). A Nanozyme with Photo‐enhanced Dual Enzyme‐like Activities for Deep Pancreatic Cancer Therapy. Angew. Chem. 131, 12754–12761. doi:10.1002/ange.201904751
Li, Y., Ma, W., Sun, J., Lin, M., Niu, Y., Yang, X., et al. (2020). Electrochemical Generation of Fe3C/N-Doped Graphitic Carbon Nanozyme for Efficient Wound Healing In Vivo. Carbon 159, 149–160. doi:10.1016/j.carbon.2019.11.093
Li, Y., Zhu, W., and Li, J. (2021). Research Progress in Nanozyme-Based Composite Materials for Fighting against Bacteria and Biofilms. Colloids Surf. B 198, 111465. doi:10.1016/j.colsurfb.2020.111465
Liang, M., and Yan, X. (2019). Nanozymes: from New Concepts, Mechanisms, and Standards to Applications. Acc. Chem. Res. 52, 2190–2200. doi:10.1021/acs.accounts.9b00140
Liang, Y., Wang, M., Zhang, Z., Ren, G., Liu, Y., Wu, S., et al. (2019). Facile Synthesis of ZnO QDs@ GO-CS Hydrogel for Synergetic Antibacterial Applications and Enhanced Wound Healing. Chem. Eng. J. 378, 122043. doi:10.1016/j.cej.2019.122043
Lin, J., Hu, J., Wang, W., Liu, K., Zhou, C., Liu, Z., et al. (2021). Thermo and Light-Responsive Strategies of Smart Titanium-Containing Composite Material Surface for Enhancing Bacterially Anti-adhesive Property. Chem. Eng. J. 407, 125783. doi:10.1016/j.cej.2020.125783
Liu, C., Zhang, M., Geng, H., Zhang, P., Zhang, Z., Zhou, Y., et al. (2021). NIR Enhanced Peroxidase-like Activity of Au@ CeO2 Hybrid Nanozyme by Plasmon-Induced Hot Electrons and Photothermal Effect for Bacteria Killing. Appl. Catal. B Environ. 295, 120317. doi:10.1016/j.apcatb.2021.120317
Liu, L., Wang, C., Li, Y., Qui, L., Zhou, S., Cui, P., et al. (2021). Manganese Dioxide Nanozyme for Reactive Oxygen Therapy of Bacterial Infection and Wound Healing. Biomater. Sci. 2021 (9), 5965–5976. doi:10.1039/D1BM00683E
Liu, Q., Zhang, A., Wang, R., Zhang, Q., and Cui, D. (2021). A Review on Metal- and Metal Oxide-Based Nanozymes: Properties, Mechanisms, and Applications. Nano-Micro Lett. 13, 1–53. doi:10.1007/s40820-021-00674-8
Liu, S., Xu, J., Xing, Y., Yan, T., Yu, S., Sun, H., et al. (2021). Nanozymes as Efficient Tools for Catalytic Therapeutics. View, 3(2):20200147. doi:10.1002/VIW.20200147
Liu, X., Yan, Z., Zhang, Y., Liu, Z., Sun, Y., Ren, J., et al. (2019). Two-dimensional Metal–Organic Framework/enzyme Hybrid Nanocatalyst as a Benign and Self-Activated Cascade Reagent for In Vivo Wound Healing. ACS Nano 13, 5222–5230. doi:10.1021/acsnano.8b09501
Liu, Y., Shi, Q., Zhang, Y., Jing, J., and Pei, J. (2020). One-step Facile Synthesis of Au@ Copper–Tannic Acid Coordination Core–Shell Nanostructures as Photothermally-Enhanced ROS Generators for Synergistic Tumour Therapy. New J. Chem. 44, 19262–19269. doi:10.1039/D0NJ04460A
Liu, Y., Zhou, M., Cao, W., Wang, X., Wang, Q., and Li, S. (2019). Light-responsive Metal–Organic Framework as an Oxidase Mimic for Cellular Glutathione Detection. Anal. Chem. 91, 8170–8175. doi:10.1021/acs.analchem.9b00512
Liu, Z., Wang, F., Ren, J., and Qu, X. (2019). A Series of MOF/Ce-based Nanozymes with Dual Enzyme-like Activity Disrupting Biofilms and Hindering Recolonization of Bacteria. Biomaterials 208, 21–31. doi:10.1016/j.biomaterials.2019.04.007
Ma, X., Ren, X., Guo, X., Fu, C., Wu, Q., Tan, L., et al. (2019). Multifunctional Iron-Based Metal− Organic Framework as Biodegradable Nanozyme for Microwave Enhancing Dynamic Therapy. Biomaterials,214 119223. doi:10.1016/j.biomaterials.2019.119223
Maji, S. K., Mandal, A. K., Nguyen, K. T., Borah, P., and Zhao, Y. (2015). Cancer Cell Detection and Therapeutics Using Peroxidase-Active Nanohybrid of Gold Nanoparticle-Loaded Mesoporous Silica-Coated Grapheme. ACS Appl. Mat. Interfaces 7, 9807–9816. doi:10.1021/acsami.5b01758
Mei, L., Zhu, S., Liu, Y., Yin, W., Yin, W., Gu, Z., et al. (2021). An Overview of the Use of Nanozymes in Antibacterial Applications. Chem. Eng. J. 418, 129431. doi:10.1016/j.cej.2021.129431
Meng, X., Fan, K., and Yan, X. (2019). Nanozymes: an Emerging Field Bridging Nanotechnology and Enzymology. Sci. China Life Sci. 62, 1543–1546. doi:10.1007/s11427-019-1557-8
Ming, J., Zhu, T., Yang, W., Shi, Y., Haung, D., Li, J., et al. (2020). Pd@ Pt-GOx/HA as a Novel Enzymatic Cascade Nanoreactor for High-Efficiency Starving-Enhanced Chemodynamic Cancer Therapy. ACS Appl. Mat. Interfaces 12, 51249–51262. doi:10.1021/acsami.0c15211
Mirhosseini, M., Far, A. S., Hakimian, F., Haghiralsadat, B. F., Fatemi, S. K., and Dashtestani, F. (2020). Core-shell Au@ Co-fe Hybrid Nanoparticles as Peroxidase Mimetic Nanozyme for Antibacterial Application. Process Biochem. 95, 131. doi:10.1016/j.procbio.2020.05.003
Mu, Q., Sun, Y., Guo, A., Xu, X., Qin, B., and Cai, A. (2021). A Bifunctionalized NiCo2O4-Au Composite: Intrinsic Peroxidase and Oxidase Catalytic Activities for Killing Bacteria and Disinfecting Wound. J. Hazard. Mater. 402, 123939. doi:10.1016/j.jhazmat.2020.123939
Navyatha, B., Singh, S., and Nara, S. (2021). AuPeroxidase Nanozymes: Promises and Applications in Biosensing. Biosens. Bioelectron. Biosens. Bioelectron. 175, 112882. doi:10.1016/j.bios.2020.112882
Nie, X., Xia, L., Wang, H. L., Chen, G., Wu, B., Zeng, T. Y., et al. (2019). Photothermal Therapy Nanomaterials Boosting Transformation of Fe (III) into Fe (II) in Tumor Cells for Highly Improving Chemodynamic Therapy. ACS Appl. Mat. Iinterfaces 11, 31735–31742. doi:10.1021/acsami.9b11291
Peng, C., Liu, J., and Guo, L. (2020). Oxygen Vacancy-Enhanced Photothermal Performance and Reactive Oxygen Species Generation for Synergistic Tumour Therapy. Chem. Comm. 56, 11259–11262. doi:10.1039/D0CC0253610.1039/d0cc02536d
Qiu, H., Pu, F., Liu, Z., Liu, X., Dong, K., Liu, C., et al. (2020). Hydrogel-based Artificial Enzyme for Combating Bacteria and Accelerating Wound Healing. Nano Res. 13, 496–502. doi:10.1007/s12274-020-2636-9
Ren, T., Yang, M., Wang, K., Zhang, Y., and He, J. (2018). CuO Nanoparticles-Containing Highly Transparent and Superhydrophobic Coatings with Extremely Low Bacterial Adhesion and Excellent Bactericidal Property. ACS Appl.Mater. Interfaces 10, 25717–25725. doi:10.1021/acsami.8b09945
Sang, Y., Li, W., Liu, H., and Zhang, L. (2019). Construction of Nanozyme‐hydrogel for Enhanced Capture and Elimination of Bacteria. Adv. Funct. Mat. 29, 1900518. doi:10.1002/adfm.201900518
Shan, J., Li, X., Yang, K., Xiu, W., Wen, W., Zhang, Y., et al. (2019). Efficient Bacteria Killing by Cu2WS4 Nanocrystals with Enzyme-like Properties and Bacteria-Binding Ability. ACS Nano 13, 13797–13808. doi:10.1021/acsnano.9b03868
Shan, J., Yang, K., Xiu, W., Qui, Q., Dai, S., Yuwen, L., et al. (2020). Cu2MoS4 Nanozyme with NIR‐II Light Enhanced Catalytic Activity for Efficient Eradication of Multidrug‐Resistant Bacteria. Small 6, 2001099. doi:10.1002/smll.202001099
Sharifi, M., Hosseinali, S. H., Yousefvand, P., Salihi, A., Shekha, M. S., and Aziz, F. M. (2020). Gold Nanozyme: Biosensing and Therapeutic Activities. Mater Sci. Eng. C Mater Biol. Appl. 108, 110422. doi:10.1016/j.msec.2019.110422
Shi, C., Li, Y., and Gu, N. (2020). Iron‐Based Nanozymes in Disease Diagnosis and Treatment Chem. Bio. Chem. 21, 2722. doi:10.1002/cbic.202000094
Sun, D., Pang, X., Cheng, Y., Ming, J., Xiang, S., Zhang, C., et al. (2020). Ultrasound-switchable Nanozyme Augments Sonodynamic Therapy against Multidrug-Resistant Bacterial Infection. ACS Nano 14, 2063–2076. doi:10.1021/acsnano.9b08610.1021/acsnano.9b08667
Sun, H., Ren, R., and Qu, X. (2020). Nanostructure Science and Technology. Singapore: Springer, 171. doi:10.1007/978-981-15-1490-6_7
Tao, Y., and Ju, E. (2015). Bifunctionalized Mesoporous Silica‐supported Gold Nanoparticles: Intrinsic Oxidase and Peroxidase Catalytic Activities for Antibacterial Applications. Adv. Mat. 27, 1097. doi:10.1002/adma.201405105
Tripathi, K. M., Ahn, H. T., Chung, M., Le, X. A., Saini, D., Bhati, A., et al. (2020). N, S, and P-Co-Doped Carbon Quantum Dots: Intrinsic Peroxidase Activity in a Wide pH Range and its Antibacterial Applications. ACS Biomater. Sci.Eng. 6, 5527–5537. doi:10.1021/acsbiomaterials.0c00831
Twilton, J., Le, C., Zhang, P., Shaw, H. S., Evans, S. W., and Macmilan, D. W. C. (2017). The Merger of Transition Metal and Photocatalysis. Nat. Rev. Chem. 11, 1–19. doi:10.1038/s41570-017-0052
Valencia, L., Kumar, S., Jalvo, B., Mautner, A., Alvarez, G. S., and Mathew, A. P. (2018). Fully Bio-Based Zwitterionic Membranes with Superior Antifouling and Antibacterial Properties Prepared via Surface-Initiated Free-Radical Polymerization of Poly (Cysteine Methacrylate). J. Mat. Chem. A 6, 16361–16370. doi:10.1039/C8TA06095A
Vallabani, N. V. S., Vinu, A., Singh, S., and Karakoti, A. (2020). Tuning the ATP-Triggered Pro-oxidant Activity of Iron Oxide-Based Nanozyme towards an Efficient Antibacterial Strategy. J. colloid. Interface Sci. 567, 154. doi:10.1016/j.jcis.2020.01.099
Vetr, F., Moradi‐Shoeili, Z., and Özkar, S. (2018). Oxidation of O‐phenylenediamine to 2, 3‐diaminophenazine in the Presence of Cubic Ferrites MFe2O4 (M= Mn, Co, Ni, Zn) and the Application in Colorimetric Detection of H2O2. Appl. Organo. Chem. 32, e4465. doi:10.1002/aoc.4465
Wan, X., Song, L., Pan, W., Zhong, H., Li, N., and Tang, B. (2020). Tumor-targeted Cascade Nanoreactor Based on Metal–Organic Frameworks for Synergistic Ferroptosis–Starvation Anticancer Therapy. ACS Nano 14, 11017–11028. doi:10.1021/acsnano.9b07789
Wang, D., Jana, D., and Zhao, Y. (2020i). Metal–organic Framework Derived Nanozymes in Biomedicine. Acc. Chem. Res. 53, 1389–1400. doi:10.1021/acs.accounts.0c00268
Wang, H., Cheng, L., Ma, S., Ding, L., Zhang, W., Xu, Z., et al. (2020h). Self-assembled Multiple-Enzyme Composites for Enhanced Synergistic Cancer Starving–Catalytic Therapy. ACS Appl. Mat. Interfaces. 12, 20191–20201. doi:10.1002/adma.201805919
Wang, H., Wan, K., and Shi, X. (2019). Recent Advances in Nanozyme Research. Adv. Mat. 31, 1805368. doi:10.1002/adma.201805368
Wang, J., Wang, Y., Zhang, D., and Chen, C. (2020a). Intrinsic Oxidase-like Nanoenzyme Co4S3/Co(OH)2 Hybrid Nanotubes with Broad-Spectrum Antibacterial Activity. ACS Appl. Mat. Interfaces. 12, 29614. doi:10.1021/acsami.0c05141
Wang, J., Wang, Y., Zhang, D., Xu, C., and Xing, R. (2020b). Dual Response Mimetic Enzyme of Novel Co4S3/Co3O4 Composite Nanotube for Antibacterial Application. J. Haz. Mat. 392, 122278. doi:10.1016/j.jhazmat.2020.122278
Wang, L., Gao, F., Wang, A., Chen, X., Li, H., Zhang, X., et al. (2020c). Defect‐Rich Adhesive Molybdenum Disulfide/rGO Vertical Heterostructures with Enhanced Nanozyme Activity for Smart Bacterial Killing Application. Adv. Mat. 32, 2005423. doi:10.1002/adma.202005423
Wang, L., Guan, S., Weng, Y., Xu, S. M., Lu, H., Meng, X., et al. (2019a). Highly Efficient Vacancy-Driven Photothermal Therapy Mediated by Ultrathin MnO2 Nanosheets. ACS Appl. Mat. Interfaces 1111, 6267–6275. doi:10.1021/acsami.8b20639
Wang, L., Li, B., Yang, H., Lin, Z., Chen, Z., Li, Z., et al. (2020d). Efficient Elimination of Multidrug-Resistant Bacteria Using Copper Sulfide Nanozymes Anchored to Graphene Oxide Nanosheets. Nano Res. 13, 2156. doi:10.1007/s12274-020-2824-7
Wang, L., Zhang, X., Yu, X., Gao, F., Shen, Z., Zhang, X., et al. (2019b). An All‐organic Semiconductor C3N4/PDINH Heterostructure with Advanced Antibacterial Photocatalytic Therapy Activity. Adv. Mat. 31, 1901965. doi:10.1002/adma.201901965
Wang, Q., Zhang, L., Shang, C., and Dong, S. (2016). Triple-enzyme Mimetic Activity of Nickel–Palladium Hollow Nanoparticles and Their Application in Colorimetric Biosensing of Glucose. Chem. Comm. 52, 5410–5413. doi:10.1039/C6CC00194G
Wang, T., Zhang, H., Liu., H., Yuan, Q., Ren, F., Han, Y., et al. (2020j). Boosting H2O2‐guided Chemodynamic Therapy of Cancer by Enhancing Reaction Kinetics through Versatile Biomimetic Fenton Nanocatalysts and the Second Near‐infrared Light Irradiation. Adv. Func. Mater 2020 (30), 1906128. doi:10.1002/adfm.201906128
Wang, T., Bai, Q., Zhu, Z., Xiao, H., Jiang, F., Du, F., et al. (2021a). Graphdiyne-supported Palladium-Iron Nanosheets: A Dual-Functional Peroxidase Mimetic Nanozyme for Glutathione Detection and Antibacterial Application. Chem. Eng. J. 413, 127537. doi:10.1016/j.cej.2020.127537
Wang, X., Fan, L., Sun, Y., Wang, X., Zhong, X., Shi, Q., et al. (2020e). Biodegradable Nickel Disulfide Nanozymes with GSH-Depleting Function for High-Efficiency Photothermal-Catalytic Antibacterial Therapy. Iscience 23, 101281. doi:10.1016/j.isci.2020.101281
Wang, X., Hu, Y., and Wei, H. (2016a). Nanozymes in Bionanotechnology: from Sensing to Therapeutics and beyond. Inorg. Chem. Front. 3, 41–60. doi:10.1039/C5QI00240K
Wang, X., Guo, W., Hu, Y., Wu, J., and Wei, H. (2016b). Nanozymes: Next Wave of Artificial Enzymes New York. NY: USA Springer.
Wang, X., Xiong, T., Cui, M., Guan, X., Yuan, J., Wang, Z., et al. (2020f). Targeted Self-Activating Au-Fe3O4 Composite Nanocatalyst for Enhanced Precise Hepatocellular Carcinoma Therapy via Dual Nanozyme-Catalyzed Cascade Reactions. Appl. Mater. Today 21, 100827. doi:10.1016/j.apmt.2020.100827
Wang, X., Zhong, X., Lei, H., Geng, Y., Zhao, Q., Gong, F., et al. (2019c). Hollow Cu2Se Nanozymes for Tumor Photothermal-Catalytic Therapy. Chem. Mat. Chem. Mat. 31, 6174–6186. doi:10.1021/acs.chemmater.9b01958
Wang, X., Zhou, X., Yang, K., Li, Q., Wan, R., Hu, G., et al. (2021b). Peroxidase-and UV-Triggered Oxidase Mimetic Activities of the UiO-66-NH 2/chitosan Composite Membrane for Antibacterial Properties. Biomater. Sci. 9, 2647–2657. doi:10.1039/d0bm01960g
Wang, Y., Chen, C., Zhang, D., and Wang, J. (2020g). Bifunctionalized Novel Co-V MMO Nanowires: Intrinsic Oxidase and Peroxidase like Catalytic Activities for Antibacterial Application. Appl. Catal. B Envir. 261, 118256. doi:10.1016/j.apcatb.2019.118256
Wang, Y., Li, H., and Guo, L. (2019). A Cobalt-Doped Iron Oxide Nanozyme as a Highly Active Peroxidase for Renal Tumor Catalytic Therapy. RSC Adv. 9, 18815–18822. doi:10.1039/C8RA05487H
Wang, Z., Dong, K., Liu, Z., Zhang, Y., Chen, Z., Sun, H., et al. (2017). Activation of Biologically Relevant Levels of Reactive Oxygen Species by Au/g-C3n4 Hybrid Nanozyme for Bacteria Killing and Wound Disinfection. Biomaterials 113, 145–157. doi:10.1016/j.biomaterials.2016.10.041
Wang, Z., Zhang, Y., Ju, E., Liu, Z., Cao, F., Chen, Z., et al. (2018). Biomimetic Nanoflowers by Self-Assembly of Nanozymes to Induce Intracellular Oxidative Damage against Hypoxic Tumors. Nat. Comm. 9, 1–14. doi:10.1038/s41467-018-05798-x
Wei, C., Liu, Y., Zhu, X., Chen, X., Zhou, Y., Yuan, G., et al. (2020). Iridium/ruthenium Nanozyme Reactors with Cascade Catalytic Ability for Synergistic Oxidation Therapy and Starvation Therapy in the Treatment of Breast Cancer. Biomaterials 238, 119848. doi:10.1016/j.biomaterials.2020.119848
Wei, F., Cui, X., Wang, Z., Dong, C., Li, J., Han, X., et al. (2021). Recoverable Peroxidase-like Fe3O4@ MoS2-Ag Nanozyme with Enhanced Antibacterial Ability Chem. Eng. J. 408, 127240. doi:10.1016/j.cej.2020.127240
Wei, Z., Zhang, Y., Wang, L., Wang, Z., Chen, S., Bao, J., et al. (2021). Photoenhanced Dual-Functional Nanomedicine for Promoting Wound Healing: Shifting Focus from Bacteria Eradication to Host Microenvironment Modulation. ACS Appl. Mat. Interfaces 13, 32316–32331. doi:10.1021/acsami.1c08875
Wen, M., Ouyang, J., Wei, C., Li, H., Chen, W., and Liu, Y. N. (2019). Artificial Enzyme Catalyzed Cascade Reactions: Antitumor Immunotherapy Reinforced by NIR‐II Light. Ang. Chem. Inter. Ed. 58, 17425–17432. doi:10.1002/anie.201909729
Wu, H., Liu, L., Song, L., Ma, M., Gu, N., and Zhang, Y. (2019a). Enhanced Tumor Synergistic Therapy by Injectable Magnetic Hydrogel Mediated Generation of Hyperthermia and Highly Toxic Reactive Oxygen Species. ACS Nano 13, 4013–14023. doi:10.1021/acsnano.9b06134
Wu, M., Hou, P., Dong, L., Cai, L., Chen, Z., Zhao, M., et al. (2019b). Manganese Dioxide Nanosheets: from Preparation to Biomedical Applications. Int. J. Nanomedicine 14, 4781. doi:10.2147/ijn.s207666
Wu, S., Wang, P., Qin, J., Pei, Y., and Wang, Y. (2021). GSH‐Depleted Nanozymes with Dual‐Radicals Enzyme Activities for Tumor Synergic Therapy. Adv. Funct. Mater.,31(31) 2102160. doi:10.1002/adfm.202102160
Xi, J., Haung, Y., Chen, J., Zhang, J., Gao, L., Fan, L., et al. (2021). Artesunate-loaded Poly (Lactic-co-glycolic Acid)/polydopamine-Manganese Oxides Nanoparticles as an Oxidase Mimic for Tumor Chemo-Catalytic Therapy. Int.J. Bio. Macromol. 181, 72–81. doi:10.1016/j.ijbiomac.2021.03.124
Xi, J., Wei, G., An, L., Xu, Z., and Fan, L. (2019a). Copper/carbon Hybrid Nanozyme: Tuning Catalytic Activity by the Copper State for Antibacterial Therapy Nano. lett 9, 7645–7654. doi:10.1021/acs.nanolett.9b02242
Xi, J., Wei, G., Wu, Q., Xu, Z., Liu, Y., Han, J., et al. (2019b). Light-enhanced Sponge-like Carbon Nanozyme Used for Synergetic Antibacterial Therapy. Biomater. Sci. 7, 4131. doi:10.1039/C9BM00705A
Xi, J., Wu, Q., Xu, Z., Wang, Y., Zhu, B., Fan, L., et al. (2018). Aloe-Emodin/carbon Nanoparticle Hybrid Gels with Light-Induced and Long-Term Antibacterial Activity. ACS Biomater. Sci. Eng. 4, 4391–4400. doi:10.1021/acsbiomaterials.8b00972
Xiong, X., Haung, Y., Lin, C., and Liu, X. Y. (2019). Recent Advances in Nanoparticulate Biomimetic Catalysts for Combating Bacteria and Biofilms. Nanoscale 11, 22206–22215. doi:10.1039/C9NR05054J
Xu, B., Cui, Y., Wang, W., Li, S., Lyu, C., Wang, W., et al. (2020a). Immunomodulation‐Enhanced Nanozyme‐Based Tumor Catalytic Therapy. Adv. Mat. 32, 2003563. doi:10.1002/adma.202003563
Xu, M., Hu, Y., Xiao, Y., Zhang, Y., Sun, K., Wu, T., et al. (2020b). Near-infrared-controlled Nanoplatform Exploiting Photothermal Promotion of Peroxidase-like and OXD-like Activities for Potent Antibacterial and Anti-biofilm Therapies. ACS Appl. Mat. Interfaces 12(45)50260. doi:10.1021/acsami.0c14451
Xu, Z., Qiu, Z., Liu, Q., Huang, Y., Li, D., Shen, X., et al. (2018). Converting Organosulfur Compounds to Inorganic Polysulfides against Resistant Bacterial Infections. Nat. Commun. 2018 (9), 1–13. doi:10.1038/s41467-018-06164-7
Xu, Z., Sun, P., Zhang, J., Lu, X., Fan, L., Xi, J., et al. (2020c). High-efficiency Platinum–Carbon Nanozyme for Photodynamic and Catalytic Synergistic Tumor Therapy. Chem. Eng. J. 399, 125797. doi:10.1016/j.cej.2020.125797
Yan, Z., Bing, W., Ding, C., Dong, K., Ren, J., and Qu, X. (2018). AH2O2-free Depot for Treating Bacterial Infection: Localized Cascade Reactions to Eradicate Biofilms In Vivo. NanWoscale, 10 17656–17662. doi:10.1039/C8NR03963A
Yang, D., Chen, Z., Gao, Z., Tammina, S. K., and Yang, Y. (2020). Nanozymes Used for Antimicrobials and Their Applications. Coll. Sur. B Biointerfaces. 195, 111252. doi:10.1016/j.colsurfb.2020.111252
Yang, G., Phua, S. Z. F., Bindra, A. K., and Zhao, Y. (2019). Degradability and Clearance of Inorganic Nanoparticles for Biomedical Applications. Adv. Mater. 31, 1805730. doi:10.1002/adma.201805730
Yang, P., Tao, J., Chen, F., Chen, Y., He, J., Shen, K., et al. (2021c). Multienzyme‐Mimic Ultrafine Alloyed Nanoparticles in Metal Organic Frameworks for Enhanced Chemodynamic Therapy. Small 17, 2005865. doi:10.1002/smll.202005865
Yang, R., Fu, S., Li, R., Zhang, L., Xu, Z., Cao, Y., et al. (2021b). Facile Engineering of Silk Fibroin Capped AuPt Bimetallic Nanozyme Responsive to Tumor Microenvironmental Factors for Enhanced Nanocatalytic Therapy. Theranostics 11, 107. doi:10.7150/thno.50486
Yang, W., Yang, X., Zhu, L., Chu, H., and Li, X. (2021a). Nanozymes: Activity Origin, Catalytic Mechanism, and Biological Application. Coord. Chem. Rev. 448, 214170. doi:10.1016/j.ccr.2021.214170
Yim, G., Kim, C. Y., Kang, S., Min, D. H., and Kang, K. (2020). Intrinsic Peroxidase-Mimicking Ir Nanoplates for Nanozymatic Anticancer and Antibacterial Treatment. ACS Appl. Mat. Interfaces 12, 41062–41070. doi:10.1021/acsami.0c10981
Yin, W., Yu, J., Lv, F., Yan, L., Zheng, L. R., Gu, Z., et al. (2016). Functionalized Nano-MoS 2 with Peroxidase Catalytic and Near-Infrared Photothermal Activities for Safe and Synergetic Wound Antibacterial Applications ACS Nano 1, 11000–11011. doi:10.1021/acsnano.6b05810
Yu, Z., Lou, R., Pan, W., Li, N., and Tang, B. (2020). Nanoenzymes in Disease Diagnosis and Therapy. Chem. Comm. 56, 15513. doi:10.1039/D0CC05427E
Zhang, C., Wang, J., Chi, R., and Shi, J. (2019). Reduced Graphene Oxide Loaded with MoS2 and Ag3PO4 Nanoparticles/PVA Interpenetrating Hydrogels for Improved Mechanical and Antibacterial Properties. Mat. Des. 183, 108166. doi:10.1016/j.matdes.2019.108166
Zhang, L., Liu, Z., Deng, Q., Sang, Y., Dong, K., Ren, J., et al. (2021). Nature‐Inspired Construction of MOF@ COF Nanozyme with Active Sites in Tailored Microenvironment and Pseudopodia‐Like Surface for Enhanced Bacterial Inhibition. Ang. Chem. Inter. Ed. 60, 3469–3474. doi:10.1002/anie.202012487
Zhao, P., Tang, Z., Chen, X., He, Z., He, X., Zhang, M., et al. (2019). Ferrous-cysteine–phosphotungstate Nanoagent with Neutral pH Fenton Reaction Activity for Enhanced Cancer Chemodynamic Therapy. Mat. Horiz. 6, 369–374. doi:10.1039/C8MH01176A
Zhao, W., Li, G., and Tang, Z. (2019). Metal-organic Frameworks as Emerging Platform for Supporting Isolated Single-Site Catalysts. Nano Today 2019 (27), 178–197. doi:10.1016/j.nantod.2019.05.007
Zhao, Y., Ding, B., Xiao, X., Jiang, F., Wang, M., Hou, Z., et al. (2020). Virus-like Fe3O4@ Bi2S3 Nanozymes with Resistance-free Apoptotic Hyperthermia-Augmented Nanozymitic Activity for Enhanced Synergetic Cancer Therapy. ACS Appl. Mat. Interfaces 12, 11320–11328. doi:10.1021/acsami.9b20661
Zhu, D., Zhu, X. H., Ren, S. Z., Lu, Y. D., and Zhu, H. L. (2020a). Manganese Dioxide (MnO2) Based Nanomaterials for Cancer Therapies and Theranostics. J. Drug Target. 2020, 1–37. doi:10.1080/1061186X.2020.1815209
Zhu, W., Wang, L., Li, Q., Jiao, L., Yu, X., Gao, X., et al. (2021a). Will the Bacteria Survive in the CeO2 Nanozyme-H2o2 System? Molecules 26, 3747. doi:10.3390/molecules26123747
Zhu, X., Chen, X., Huo, D., Cen, J., Jia, Z., Liu, Y., et al. (2021b). A Hybrid Nanozymes In Situ Oxygen Supply Synergistic Photothermal/chemotherapy of Cancer Management. Biomaterials Sci. 9, 5330–5343. doi:10.1039/D1BM00667C
Keywords: nanozyme, pro-oxidative, therapeutic, antibacterial, antitumor
Citation: Maddheshiya S and Nara S (2022) Recent Trends in Composite Nanozymes and Their Pro-Oxidative Role in Therapeutics. Front. Bioeng. Biotechnol. 10:880214. doi: 10.3389/fbioe.2022.880214
Received: 21 February 2022; Accepted: 20 April 2022;
Published: 30 May 2022.
Edited by:
Wooram Park, Sungkyunkwan University, South KoreaReviewed by:
Jianlong Wang, Northwest A&F University, ChinaCopyright © 2022 Maddheshiya and Nara. This is an open-access article distributed under the terms of the Creative Commons Attribution License (CC BY). The use, distribution or reproduction in other forums is permitted, provided the original author(s) and the copyright owner(s) are credited and that the original publication in this journal is cited, in accordance with accepted academic practice. No use, distribution or reproduction is permitted which does not comply with these terms.
*Correspondence: Seema Nara, c2VlbWFuYXJhQG1ubml0LmFjLmlu, c2VlbWFuYXJhQGdtYWlsLmNvbQ==
Disclaimer: All claims expressed in this article are solely those of the authors and do not necessarily represent those of their affiliated organizations, or those of the publisher, the editors and the reviewers. Any product that may be evaluated in this article or claim that may be made by its manufacturer is not guaranteed or endorsed by the publisher.
Research integrity at Frontiers
Learn more about the work of our research integrity team to safeguard the quality of each article we publish.