- 1School of Biological Sciences, Universiti Sains Malaysia, Penang, Malaysia
- 2PETRONAS Research Sdn. Bhd., Selangor, Malaysia
The search for biodegradable plastics has become the focus in combating the global plastic pollution crisis. Polyhydroxyalkanoates (PHAs) are renewable substitutes to petroleum-based plastics with the ability to completely mineralize in soil, compost, and marine environments. The preferred choice of PHA synthesis is from bacteria or archaea. However, microbial production of PHAs faces a major drawback due to high production costs attributed to the high price of organic substrates as compared to synthetic plastics. As such, microalgal biomass presents a low-cost solution as feedstock for PHA synthesis. Photoautotrophic microalgae are ubiquitous in our ecosystem and thrive from utilizing easily accessible light, carbon dioxide and inorganic nutrients. Biomass production from microalgae offers advantages that include high yields, effective carbon dioxide capture, efficient treatment of effluents and the usage of infertile land. Nevertheless, the success of large-scale PHA synthesis using microalgal biomass faces constraints that encompass the entire flow of the microalgal biomass production, i.e., from molecular aspects of the microalgae to cultivation conditions to harvesting and drying microalgal biomass along with the conversion of the biomass into PHA. This review discusses approaches such as optimization of growth conditions, improvement of the microalgal biomass manufacturing technologies as well as the genetic engineering of both microalgae and PHA-producing bacteria with the purpose of refining PHA production from microalgal biomass.
1 Introduction
The universal plastic pollution emergency is edging towards an alarming irreversible “tipping point”. In 2019, approximately 370 million tons of plastic were produced worldwide, marking the highest expansion rate since the introduction of plastics for everyday use in the 1900s (Group, 2020). Owing to their high stability, synthetic petroleum-based plastics resist degradation, and inadvertently remain in the ecosystem for hundreds to thousands of years to come (Barnes et al., 2009). A projected annual flux of 4.8–12.7 million metric tons of plastic waste are discharged to ocean bodies (Jambeck et al., 2015), causing adverse injury to wildlife (Marn et al., 2020), damage to ecosystems (Lamb et al., 2018) and also harming human health (Thompson et al., 2009). In light of the recent COVID-19 pandemic, global plastic pollution has seen a surge in numbers with increased use of single-use plastics including personal protective equipment (Silva et al., 2021). In efforts to curb this predicament, biosynthetic and biodegradable plastics were introduced to the mass public.
Among the biodegradable polymers available, polyhydroxyalkanoates (PHAs) are suitable substitutes for some conventional plastics that offer unique benefits. Not only do these polymers possess similar mechanical properties to petroleum-based plastics such as polypropylene (PP), but they are also the only polymer that is 100% biodegradable (Khanna and Srivastava, 2005). PHAs are produced through microbial fermentation wherein carbon sources are metabolized into PHA and aggregate intracellularly in granules (Yu et al., 2006; Mathuriya et al., 2017). Over 300 species of microbes including Gram-negative and Gram-positive bacteria along with archaea and algae were reported to have PHA synthesizing capabilities (Grigore et al., 2019). The most commonly employed bacteria for PHA production are Cupriavidus necator (Sohn et al., 2021), Bacillus (Mohapatra et al., 2017) recombinant Escherichia coli (Leong et al., 2014), and Pseudomonas (Mozejko-Ciesielska et al., 2019).
However, the large-scale application of PHA is plagued by high market prices compared to conventional plastics. The current commercial production of PHA costs up to €2.2 to 5.0 per kg, while conventional PP costs only €1.0 per kg (Liu et al., 2021). Such a huge difference is attributed to the production cost wherein PHA synthesis employs pure cultures and expensive carbon substrates while conventional plastics is economical due to the larger capacity of manufacturing a broad range of applications (Ong et al., 2018). Substrate prices account for 30–50% of the overall PHA cost (Choi and Lee, 1997). Thus, the search for suitable candidates for cost-effective feedstock that have high efficiency and high yield is necessary to ensure the success of PHA production on an industrial scale.
With high potential as feedstock biofactories, recent research has focused on the use of microalgal biomass as carbon source for PHA production because of the high carbohydrate yield and the lack of lignin which facilitates low-cost retrieval of fermentable sugars (Ghosh et al., 2019). Microalgae encompass a wide range of unicellular photosynthetic microorganisms and are ubiquitously found in all aquatic environments including freshwater and saltwater bodies with adapted tolerance to a wide range of abiotic and biotic stress (Rani et al., 2021). In terms of metabolism, microalgae are not only photoautotrophic but can adapt to heterotrophy or even mixotrophy depending on the environment (Mitra et al., 2012). While microalgae’s photosynthetic mechanism is analogous to that of terrestrial plants, the presence of pyrenoids for carbon dioxide fixation (Machingura and Moroney, 2018) in addition to aqueous habitats that enable easy access to growth requirements allow microalgae to yield biomass with efficiencies of at least two magnitudes higher than customary agricultural generation (Packer, 2009; Weyer et al., 2010). PHA production directly from microalgae has also been researched but the yield remains low. As such, the proposed “two module system” whereby microalgal biomass is used as feedstock is a promising solution to the financial plight of expensive substrates for PHA production (Afreen et al., 2021).
Despite this, to ensure the success of large-scale PHA synthesis using microalgal biomass, a few critical issues have to be taken into consideration such as 1) identifying microalgae strains along with determining optimum growth conditions to ensure maximum growth rates for greater biomass production, 2) employing microalgae cultivation systems together with microalgae harvesting techniques that are both economical and necessitate less management, and 3) selecting the ideal bacteria that work in tandem with the biomass produced by microalgae. Considering this, the following sections will discuss the recent advancement in refining PHA synthesis from microalgal biomass as an industry-scale production that holds economic competitiveness against conventional plastics as well as non-microalgae production systems.
2 Microalgae and Bacterial PHA Synthesis
Many studies have been reported on the development of efficient processes for the production of PHA. Microalgae are attractive because of their ability to fix CO2 directly to produce biomass. The cultivation of microalgae is especially attractive in tropical countries because of suitable climatic conditions.
2.1 Role of Microalgae in the Bacterial Biosynthesis of PHA
The commercial production of PHAs is commonly carried out in large scale by heterotrophic bacteria. While certain natural microalgae species are also able to generate PHAs under stress conditions, the yield is relatively low, ranging from 5 wt% (Synechocystis sp. PCC6803) (Sudesh et al., 2002) to a maximum PHA production of 69 wt% (Nostoc muscorum Agardh) (Serif et al., 2018) of dry microalgal biomass. Even with genetic engineering, microalgal production of PHA reached a peak of 85 wt% (Aulosira fertilissima CCC444) which is lower than bacterial PHA production that can reach over 95 wt% (Cupriavidus necator) of cell dry weight (CDW). However, bacterial PHA production is hindered by high price of carbon substrate. This can be solved by utilizing the more economical microalgal biomass for bacterial PHA production.
PHAs are categorized into three clusters depending on their lengths. Short chain length (scl)-PHAs contain three to five carbon atoms with the most commonly synthesized scl-PHA being the homopolymer of poly(3-hydroxybutyrate) [P(3HB)]. In contrast, medium chain length (mcl)-PHAs contain six to 15 carbon atoms. On the other hand, PHA copolymers consist of a combination of different monomer types. For instance, poly(3-hydroxyhexanoate-co-3-hydroxyoctanoate) [P(3HHx-co-3HO)] is a copolymer of mcl monomers while poly(3-hydroxybutyrate-co-3-hydroxyhexanoate) [P(3HB-co-3HHx)] is a combination of scl and mcl monomers. In particular, scl-mcl-PHAs are highly sought for their similar biophysical properties to common plastics such as negligible water solubility with high resistance towards moisture and hydrolytic degradation (Surendran et al., 2020). The most common types of bacterial PHAs produced using microalgal feedstock are the scl copolymer poly(3-hydroxybutyrate-co-3-hyroxyvalerate) [P(3HB-co-3HV)] and poly-(R)-3-hydroxybutyrate (P3HB) (Table 1). With 60% crystallinity, P3HB, is thought to be a suitable substitute to PP. It has a melting temperature of 175°C and transition temperature of 0–9°C (Madadi et al., 2021). On the other hand, P(3HB-co-3HV) is more flexible with a melting temperature between 148 and 168°C and transition temperature of −5.5 to −2.2°C, making it more commercially profitable (Samantaray and Mallick, 2012).
The PHA synthases of heterotrophic bacteria fall under four classes; PHA synthases of class I, III, and IV polymerize scl monomers, whereas class II polymerizes mcl monomers. Due to this, heterotrophic bacteria can utilize a wide range of substrates such as monosaccharides, starch, glycerol, and fatty acids for PHA production, many of which can be derived from microalgal biomass. PHAs of different composition are produced depending on the carbon substrate supplemented as well as the PHA-producing microorganism (Surendran et al., 2020).
In general, microalgal biomass is rich in various proteins (10–47 wt% of CDW), starch components (10–20 wt% of CDW), and amylopectin (80–90 wt% of CDW), cellulose, and lipids (20–50 wt% of CDW) (Wang et al., 2016; Sun et al., 2018a; Madadi et al., 2021). Table 2 depicts the macromolecule composition of different microalgal strains. Microalgal carbohydrates are the most commonly used carbon sources from microalgal biomass for PHA-synthesizing bacteria. For instance, defatted Chlorella biomass was pretreated to yield fermentable sugars which were fed to PHA-synthesizing bacteria to produce P(3HB-co-3HV) (Naduthodi et al., 2019). Furthermore, various microalgal strains contain monosaccharides including glucose, mannose, rhamnose, galactose, arabinose, and xylose. Sucrose has also been extracted from Desmodesums and Scenedesmus (Smachetti et al., 2020). Many bacteria such as Azeobacter vinelandii and Alcaligenes latus can synthesize PHA from sucrose (Winnacker, 2019). A. latus can also utilize starch to synthesize P3HB (Chen, 2009). Galactose and glucose can be utilized by bacteria such as Hydrogenophaga pseudoflava DSM 1034 and Pseudomonas hydrogenovora (Koller et al., 2008; Povolo et al., 2013). Recently, crude glycerol from the algal biodiesel industry has been found to be a suitable feedstock for PHA production. PHB was produced by Halomonas daqingensis and Halomonas ventosae when fed with algal biodiesel waste residue that is rich in glycerol (Dubey and Mishra, 2021).
2.2 Choosing the Right Bacterium
To make full use of the microalgal biomass, it is crucial to determine suitable PHA-producing bacteria that can utilize the most of the microalgal nutrients. The general population of PHA-producing microorganisms are able to utilize simple sugars and some are able to consume triglycerides while hydrocarbon utilization for PHA synthesis is rare (Jiang et al., 2016). C. necator is one of the commonly used bacterium for industrial PHA synthesis and is deemed as the model organism for PHA metabolism (Reinecke and Steinbuechel, 2009). It stores PHA up to 96 wt% of its CDW when given excess carbon source while being starved of nitrogen or phosphate. The use of genetic manipulation has further increased the commercial potential of C. necator in PHA synthesis. The glucose-utilizing mutant, C. necator NCIMB 11599, was able to accumulate PHA up to 49 wt% of CDW using the brown algae Laminaria japonica biomass as carbon source (Muhammad et al., 2020). Another strain, C. necator PTCC 1615, successfully utilized brown seaweed Sargassum sp. as feedstock for PHB production (Azizi et al., 2017). C. necator KCTC 2649 was able to produce 75.4 wt% of CDW of PHA by using 10% (w/v) of defatted Chlorella biomass (Khomlaem et al., 2021). Likewise, C. necator TISTR 1335 was fed a combination of Chlorella sp. biomass co-digested with sugarcane leaves to produce 60.9 wt% of PHA which contributes to the zero-waste generation concept (Sitthikitpanya et al., 2021).
Halophilic bacteria have gained attention as a candidate for PHA synthesis owing to their unique growth conditions that reduces the chances of contamination. Members of the halophilic Halomonadaceae family are able to amass large quantities of PHAs from different carbon sources (Abd El-Malek et al., 2021). Recently, production of biodiesel from microalgae is gaining worldwide attention as it has been shown to be the only renewable biodiesel source able to meet the global demand for transport fuels. A major by-product of this production is crude glycerol. H. daqingensis was found to be able to synthesize PHA by utilizing glycerol-rich algal biodiesel waste residue as the sole carbon source (Dubey and Mishra, 2021). Moreover, Halomonas pacifica ASL10 and Halomonas salifodiane successfully produced PHAs from the macroalgae Pterocladia capillaceais and Corallina mediterraneais as well as Arthrospira (Abd El-Malek et al., 2021).
The Paracoccus species is another potential workhorse for PHA production. In addition to the ability to switch between autotrophic and heterotrophic growth, this Gram-negative species is methylotrophic with denitrifying capabilities and as such is commonly used to treat wastewaters (Kim et al., 2015). Paracoccus denitrificans and Paracoccus pantotrophus are well studied for their ability to accumulate PHA by utilizing numerous carbon sources, such as glycerol, methanol, n-penthanol, and CO2 (Kim et al., 2015; Chang et al., 2020). Using L. japonica biomass, Paracoccus sp. LL1 was able to synthesize PHA as well as carotenoids (Muhammad et al., 2020). Fascinatingly, this same species was able to utilize defatted Chlorella biomass to produce 37.4 wt% of CDW of PHA and 6.08 mg·L−1 of carotenoids (Khomlaem et al., 2021). These findings highlight the compatibility of using microalgal biomass as feedstock for PHA production in Paracoccus.
The wild type bacterium E. coli is unable to synthesize PHA. However, transmutating the bacterium with PhaC gene allows for the production of PHA. This principle is applied for E. coli XL1-Blue harbouring phaCAB from C. necator (Spiekermann et al., 1999). By employing the aqueous fraction from an algal wet lipid extraction technique as the medium, PHB production of this particular strain saw an increase of 51% (Sathish et al., 2014). Furthermore, this same recombinant strain was also able to utilize wastewater microalgae to produce the PHB with a maximum accumulation of 31 wt% of the CDW (Rahman et al., 2015).
PHA synthesis is not only limited to Gram-negative bacteria. The Gram-positive Bacillus are also known for their PHA production, although not all genus can accumulate PHA in their cells under limiting growth environments. Bacillus pumilus (E10) isolated from the wastewaters of University of Santa Cruz do Sul was able to utilize the hydrolysate of A. platensis biomass in conjunction with glucose and glycerol to produce PHB. The soil bacterium, Bacillus megaterium ALA2, could make use of defatted Chlorella biomass and L. japonica biomass with PHA production of 29.7 wt% and 32 wt% of CDW, respectively (Muhammad et al., 2020; Khomlaem et al., 2021).
3 Microalgae as Feedstock Biofactories
Like most plants, microalgae can grow photoautotrophically but at higher rates which makes them attractive as a source of biomass. In addition, the cellulose in microalgae is more accessible compared to plants. Therefore, much interest and efforts have been directed to the production and use of microalgae biomass as feedstock in various processes.
3.1 Wild Type Microalgae
Different microalgae give rise to various types of biomasses. Choosing the right microalgae is crucial in determining the maximum biomass productivity and relative composition of the biomass constituents which then define the end product of the downstream PHA synthesis. Presently, the common wild types that provide high biomass productivity include Arthrospira, Chlorella, and Chlamydomonas reinhardtii. Figure 1 shows the morphologies of A. platensis, C. reinhardtii, and Synechocystis sp. strain PCC6803.
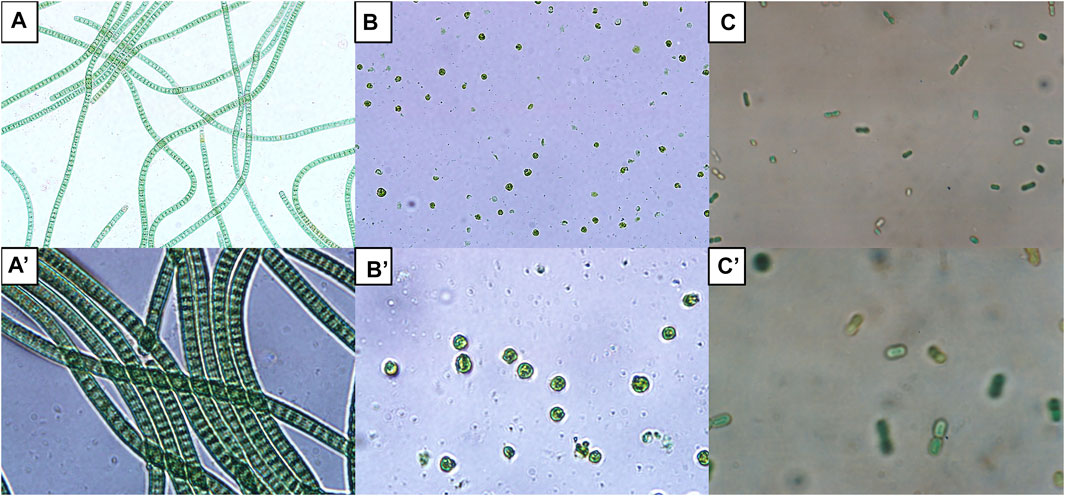
FIGURE 1. Morphology of some microalgae commonly used for biomass generation viewed under light microscope. Spirulina (Arthrospira platensis) UMACC 161 (A) total magnification of ×400 and (A′) total magnification of ×1000; Chlamydomonas reinhardtii (B) total magnification of ×400 and (B′) total magnification of ×1000; Synechocystis sp. strain PCC6803 (C) total magnification of ×400 and (C′) total magnification of ×1000.
3.1.1 Arthrospira
Formerly known as Spirulina, Arthrospira are filamentous cyanobacteria that thrive in salt lakes but can also be found in freshwaters (Ciferri and Tiboni, 1985). They are generally cultivated as nutritional supplements for their rich protein and vitamin content (Marles et al., 2011). It has been shown that Arthrospira can be produced up to 15,000 tons of dry weight annually (Lu et al., 2011). Members of Arthrospira can withstand extreme alkaline and saline conditions which makes their cultivation culture free from common contaminants and are thus typically used as microalgae cultures for open ponds cultivation (Feng et al., 2018). Despite its significantly lower lipid content (4–6%), Arthrospira biomass tend to accumulate large amounts of carbohydrate under nutrient stress condition (∼up to 70%). In light of this, Arthrospira offers a suitable candidate as feedstock for bacterial PHA synthesis (Heasman et al., 2000).
3.1.2 Chlorella
Chlorella are among the pioneer algae used for commercial applications in open ponds (Guccione et al., 2014). They are spherical single-celled green microalgae. Similar to Arthrospira, Chlorella biomass has been used as dietary supplements. These microalgae offer a fast biomass growth rate with daily productivity of 25 g·m−2 and annual production of more than 2,000 tons (Zhou et al., 2011; Deshmukh et al., 2021). The composition of Chlorella is rich in saturated and unsaturated C18 fatty acids which is comparable to vegetable oils and are often used as oil substitutes (Liang et al., 2009).
3.1.3 Nannochloropsis
Nannochloropsis are a genus of microalgae found in both freshwater and brackish water environments. Members of Nannochloropsis have long been used in biopharmaceutical applications for their bioactive compounds such as eicosapentaenoic acid that provide positive health benefits (Rodolfi et al., 2009; Hulatt et al., 2017). Recently, much interest has focused on Nannochloropsis as aquaculture feed and biodiesel production for their rapid growth rate, high lipid content, and resistance towards various irradiation conditions (Ma et al., 2014). The annual growth rate of Nannochloropsis is approximately 0.16 g·L−1∙d−1, peaking at 0.37 g·L−1∙d−1 (Quinn et al., 2012).
3.1.4 Phaeodactylum tricornutum
P. tricornutum is a marine diatom and the sole species of the genus Phaeodactylum. They are unique diatoms as they can survive without silicon and thus lack silicified frustules. It is rich in fucoxanthin, eicosapentaenoic acid, and chrysolaminarin that have extensive beneficial health effects (Zhang et al., 2018). P. tricornutum has a biomass composition of 7.85% carbohydrates, 38.40% proteins, and 9.08% lipids as well as a maximum biomass density of 0.6 g·L−1 and 1.0 g·L−1 when grown in open ponds and photobioreactors, respectively (Branco-Vieira et al., 2020). With a fully sequenced whole genome, P. tricornutum is a model photosynthetic representative for non-green algae. For instance, the synthesis of carbohydrate metabolism pathway was discovered using P. tricornutum which aids in the understanding of improving carbohydrate accumulation (Kroth et al., 2008).
3.2 Bioengineering Enhances Biomass Production
While optimization of microalgae growth factors is known to deliver peak biomass productivities of wild-type microalgae, bioengineering pushes these limits to overcome disadvantages that come with specific microalgae strains. Genetic engineering or genetic modification directly alters an organism’s genes via biotechnology tools. In terms of microalgae, genetic engineering aims to increase biomass productivities by modifying genes associated with photosynthesis, resistance towards extreme conditions and metabolism.
Currently, about 30 microalgae species have fully sequenced genomes. Among them are Chlorella vulgaris (Guarnieri et al., 2018), C. reinhardtii (Merchant et al., 2007), Dunaliella salina (Polle et al., 2017), Synechocystis sp. strain PCC6803 (Kaneko and Tabata, 1997), and Scenedesmus obliquus (Starkenburg et al., 2017). The pioneer of microalgae DNA modification was C. reinhardtii by Rochaix and van Dillewijn over 30 years ago (Rochaix and Van Dillewijn, 1982). Since then, more tools were developed to enhance the yield of C. reinhardtii but few of these approaches are viable for other microalgae.
Bioengineering methods widely employed for gene sequence modification in microalgae include Clustered Regularly Interspaced Short Palindromic Repeats—CRISPR associated with the protein 9 (CRISPR–Cas9) (Shin et al., 2016), zinc-finger nuclease (ZFN) (Sizova et al., 2013), Transcription Activator-Like Effector Nucleases (TALENs) (Daboussi et al., 2014) whereas RNA interference techniques such as microRNAs (RNAi) and short interfering RNAs (siRNA) are used to activate or repress expression of certain genes (Kim et al., 2015).
Compared to TALENs and ZFN, the CRISPR approach had higher applicability, allowing modulation of multiple gene expressions. However, the use of CRISPR editing for microalgae is obstructed by the toxicity of the Cas9 nuclease which results in a 10% mutation rate (Jiang et al., 2014). To overcome this, Cas9 protein-gRNA ribonucleoproteins (RNPs) provided an alternative to the lethal Cas9 (Jiang et al., 2014). Cas9 RNPs were successfully delivered into C. reinhardtii with an improved mutation rate of almost 100-fold compared to the general Cas9 approach (Shin et al., 2016). Following this success, RNPs were adopted for gene editing of other microalgae including Nannochloropsis oceanica IMET1 (Naduthodi et al., 2019), P. tricornutum (Serif et al., 2018), and Tetraselmis sp. (Chang et al., 2020).
Regardless of the recent developments in genetic engineering techniques for microalgae, bioengineering microalgae is still at its infancy stage with only a few fully sequenced genome species in addition to their complicated anatomy and physiology that hinder most genetic engineering tools. With the advancement and evolving CRISPR technology and the combination of different genetic tools, it is expected that genetic engineering will reach a breakthrough for more efficient developments of commercially-sustainable genetically engineered microalgae.
4 Optimization of Microalgal Growth Conditions
Microalgae development is affected by both biotic and abiotic parameters. Biotic parameters comprise of stresses from pathogens including viruses, detrimental bacteria, fungi as well as other microalgae. Contrariwise, abiotic factors cover parameters, such as light quantity and quality, pH, salinity, temperature, carbon dioxide, dissolved oxygen and availability of nutrients. While regulating biotic parameters is essential for healthy growth, different algae species require specific abiotic factors. Therefore, optimization of these growth requirements is required to improve biomass output. Paliwal et al. has documented how abiotic stress is used for maximizing lipid and fatty acid production (Paliwal et al., 2017).
4.1 Light
Photosynthetic organisms utilize light as the main source of energy. The most crucial aspect in microalgae growth is arguably light in the form of light limitation, saturation, and inhibition (Gatamaneni et al., 2018). The photosynthesis process necessitates both dark and light phases. In the presence of light, the light energy is absorbed by microalgae and assimilated into adenosine triphosphate (ATP) which is utilized for biomass synthesis throughout the dark cycle. As such, the dispensation of light to the cultivation system requires optimization in terms of the system’s geometric design and orientation (Fernández et al., 2001; Tredici et al., 2015). In light limiting environments, microalgae growth is proportional to the increase of light intensity. On the other hand, light saturation conditions diminish photosynthesis as the absorption of photons surpasses electron turnover (Chang et al., 2017). Further light over-exposure leads to permanent impairment to the photosynthetic system in a phenomenon termed photo-inhibition (Tredici and Zittelli, 1998). Most microalgae have a saturated photosynthesis rate at 100–500 μE∙m−2∙s−1 and any excess light exposure will cause the microalgae to be photo-inhibited (Vejrazka et al., 2012).
Artificial or natural (solar) light sources can be utilized in cultivation systems. While solar energy is the most economically and readily available source, artificial light is preferred in high value-added cultures for the accurate regulation of photoperiod and control of the light spectrum (Schulze et al., 2014). Among the many artificial lights available such as halogen lamps and fluorescent lights, light-emitting diodes (LEDs) allows for the best modulation of light with different wavelengths (Schulze et al., 2016). The photosynthetically active radiation (PAR) that most microalgae thrive under is at the wavelength range of 380–750 nm (white light), wavelength range of 420–470 nm (blue light) and wavelength range of approximately 660 nm (red light) (Fu et al., 2013; Schulze et al., 2014). Red to far-red lights result in increased microalgae growth rate with smaller cells and reduced nutrient consumption. Blue light modifies gene expression and specific metabolic pathways resulting in an increased nutrient intake with larger cells but slower growths (Schulze et al., 2016).
Microalgae will light acclimate throughout the production phase in batch cultures; being high light (HL) acclimated consecutively after introduction with new cultures while being low light (LL) acclimated by the end of the batch cycle at high cell density (Grobbelaar et al., 1996). As a result, mega-scale microalgal cultivation systems can retain biomass at low light by maintaining biomass at high concentrations. Alternatively, small-scale microalgal cultivation systems can also employ the microalgal photo-acclimated state to attain exponentially high produce. Using a multi-compartment photobioreactor, the first layer of microalgae facing the light source were HL acclimated and consecutive layers of microalgae became gradually more LL acclimated. This continuous flow photobioreactor design takes advantage of microalgae that are acclimated simultaneously at different light conditions with productivity rates of almost 40% more than the conventional single-layered perpendicular plate reactor (Grobbelaar and Kurano, 2003).
Recent years have also seen an increase in studies on other novel strategies to boost light utilization by microalgae and thereby increasing productivities. Table 3 shows a number of these approaches. In raceway ponds, addition of light filters increased the productivity of Chlorella (32.6% increase in biomass productivity) and D. salina (68% cell weight increment) (Cheng et al., 2015b; Nwoba et al., 2021). When grown in photobioreactors with light/dark regulation, Chlorella experienced a 21.6% increase in biomass productivity. The use of light-splitting/light-harvesting additives also aids in increase in biomass productivities. Silicon dioxide nanoparticles increased biomass productivity of Scenedesmus by 22.3% while calcium carbonate crystals raised biomass activity of Neochloris oleoabundans by 31.5% (Hong et al., 2019; Ren et al., 2020).
Rise in light intensity yielded an increase in carbohydrate generation with light intensity in the range of 30–400 μmol·m−2·s−1 boosts the accumulation of carbohydrates. For instance, S. obliquus CNW-N proved that there was a positive association between biomass/carbohydrate productivities and light intensity prior to photoinhibition; high light intensity of 420 μmol·m−2·s−1 led to peak carbohydrate productivity of 0.322 g·L−1∙d−1 which was higher than that of Tetraselmis subcordiformis (0.256 g·L−1∙d−1) (Zheng et al., 2011; Ho et al., 2012).
4.2 Temperature
Temperature regulates biochemical processes of microalgae, particularly the gross photosynthetic rate through cellular division, which in turn affects biomass production. Culturing microalgae at lower temperatures than optimum will affect photosynthesis as carbon assimilation activity is reduced while overheating degrades photosynthetic proteins which lowers photosynthetic rates and thereby shrinks microalgae cells (Anjos et al., 2013). Optimal temperatures for most microalgae species range between 20 and 35°C (Xu et al., 2010; Cho et al., 2011) but certain thermophilic species such as Anacystis nidulans can tolerate up to 40°C (Caïa et al., 2018).
Microalgae typically absorb radiated heat from the light source. Additionally, microalgae growth is highly exothermic with over 95% of light absorbed converted into heat (Cheng et al., 2015a). Small-scale microalgae cultivation systems may not require temperature control as heat is released to the environment through convection when the surrounding setting is cold enough. However, mega-scale outdoor cultivation of microalgae is constantly exposed to solar radiation which directly heats cultures (Chiang et al., 2011). In essence, closed systems are inclined to overheat while open systems experience high water evaporation rates under intense irradiance (Gonzalez-Camejo et al., 2020). Since higher temperatures are more lethal to microalgae than lower temperatures, culture cooling is employed particularly with closed photobioreactors. Nevertheless, lower temperatures (<10°C) result in reduced biomass productivities (Thompson, 1996). Furthermore, temperature highly affects enzymes involved in starch production such as starch synthase and sucrose synthase. When temperature was increased from 5 to 20°C, carbohydrate content in C. vulgaris SO-26 plummeted from 70 to 50% (Madadi et al., 2021). As such, depending on the surrounding climate, heating during winter and cooling during summers are advantageous to microalgae culturing.
Much effort was made to identify the best method to prevent overheating of cultures in large-scale settings. The typical approach is using water sprays to sprinkle the surface of the photobioreactors with heated or cooled liquid but this is only suitable for sites of low air humidity (Cheng et al., 2019b). Heat exchangers are also widely employed to dissipate excess heat to large water bodies. Both open and closed cultivation systems in temperate regions can be housed in greenhouses (Gerardi, 2015; Cheng et al., 2019a).
Recent technologies have allowed for in-depth studies on the effects of temperature on microalgae culture conditions; be it in a controlled laboratory setting, outdoor systems, photobioreactor simulations, and theoretical models. For instance, cultivation of Arthrospira platensis in winter saw an increase of phycocyanin productivity when the microalgal was grown in a thermally-insulated photobioreactor complimented by photovoltaic plate incorporation (Gensemer et al., 1993). Another study reported increased photosynthetic conversion efficiency of over 7% from cultures with Scenedesmus and Chlorella species when grown in a photobioreactor fixed with a double-wall hose structure combined with temperature control in a closed system (Anderson and Morel, 1978). Furthermore, thermal modeling aids in simulating and optimizing various reactor designs. One such model delved into the effect of different flat-plate reactor designs which identified accurate predictions of monthly energy consumption needed to regulate the temperature of microalgae cultures (Rotatore and Colman, 1991).
4.3 Carbon Dioxide
Microalgal biomass production is unique as microalgae are capable of fixing carbon dioxide (CO2). As mentioned, the CO2 fixing efficiency of microalgae is higher than terrestrial plants. Consequently, carbon dioxide is one of the limiting reactants in photosynthesis. Concentrations of CO2 needed for peak photosynthetic efficiency is 1–5 vol% (Solimeno et al., 2015). Given this, the available CO2 in the air of only 0.04 vol% is insufficient for high productivity (Xu et al., 2010). CO2 can be supplied as atmospheric air, commercially purified CO2, raw flue gas or via supplementation of salts, for instance, bicarbonates (Cho et al., 2011).
The demand for CO2 for microalgae growth is at its highest during the day when photosynthetic activity is active, while there is zero demand at night. CO2 delivery approaches must manage these demands that fluctuate seasonally and diurnally. Open raceway ponds and closed systems often use spargers or diffusers to deliver CO2 (Chiang et al., 2011; Anjos et al., 2013). The sparger injects CO2 in gas bubbles at the bottom of the pond while a paddlewheel circulates CO2 throughout the microalgae culture (Cheng et al., 2015a). By studying CO2 transfer rates in an open algal pond, it was found that decelerating the paddle wheel rotation speed to 13 rpm decreases CO2 and losses up to 61% (Caïa et al., 2018). Additionally, to achieve the maximum CO2 utilization efficiency, CO2 must be extracted from gas bubbles before the bubbles escape to the pond surface. Sumps were introduced at the point of gas injection to lengthen the retention time of bubbles (Thompson, 1996). Another strategy was to minimize the bubble size. Microbubbles of diameters not larger than 100 μm have a higher surface-to-volume ratio and rise slowly to the surface of culture, allowing more CO2 to disperse throughout the medium (Gonzalez-Camejo et al., 2020). Novel photobioreactors designs have considered these factors when integrating technologies to increase CO2 utilization efficiency. Some examples include the jet-aerated tangential swirling-flow plate photobioreactor (Cheng et al., 2019a) that condenses bubble diameter and CO2 microbubbles dissolver (CMD) (Cheng et al., 2019b) that facilitates dissolved CO2 in photobioreactors.
4.4 Hydrogen Potential (pH)
Hydrogen potential (pH) is known to affect not just the microalgae but also influences the mineral and carbon dioxide solubility of the media. Most microalgae tolerate a pH range of 6–10 (Yang et al., 2011). At extremely acidic conditions, the absorption rate of nutrients and trace metals is altered which might cause metal toxicity (Anderson and Morel, 1978; Gensemer et al., 1993). On the other hand, extremely alkaline conditions cause enzyme degradation as well as lowering microalgae affinity towards free CO2 (Rotatore and Colman, 1991). During microalgal photosynthesis at optimal pH, available bicarbonate in the medium is transformed into CO2 which releases hydroxyl ions. These excess hydroxyl ions increase the pH of the medium (Gerardi, 2015). Carbon at alkaline conditions is present in the form of carbonates and is not favored by microalgae (Solimeno et al., 2015). Supplementation of CO2 acidifies the microalgal medium by altering carbonate balance (Galloway and Krauss, 1961). Consequently, controlled injection of CO2 is required to maintain optimal pH levels of the medium. The employment of sensors to monitor pH levels has also benefitted microalgae cultivation as seen in a model that observed C. reinhardtii cells (Ifrim et al., 2014). Such pH monitors can also aid in determining photosynthetic productivity (Gonzalez-Camejo et al., 2020).
4.5 Nutrients
Understanding the key nutrients for microalgae growth would aid in maximizing biomass productivity as well as the production of favored synthesis. Microalgae demonstrate significant fluctuations in biochemical compositions when cultured under different limiting nutrients. Nutrient requirements of microalgae are calculated based on the formula of CO0.48H1.83N0.11P0.01 (Ifrim et al., 2014). The three non-mineral nutrients essential for photosynthesis are carbon, oxygen, and hydrogen. Carbon is required in bulk as it is a major component of all organic substances including proteins, carbohydrates, lipids and even nucleic acids (Ifrim et al., 2014). Autotrophic microalgae entail inorganic carbon sources in the forms of CO2, carbonate, and bicarbonate while heterotrophic microalgae can use acetate or glucose (Ifrim et al., 2014).
Nitrogen is the second most element in the microalgal biomass which makes up for 7–20% of the dry cell weight (Ifrim et al., 2014). It is the building block of all structural and functional proteins. Nitrogen-depleted microalgae are inclined to carbohydrate synthesis (Ifrim et al., 2014). By limiting NaNO3, NaH2PO4, metals, and vitamins on Tetraselmis sp., starch content peaked at 42% of CDW (Dammak et al., 2017). Similarly, starch content of C. vulgaris and Chlorella zofingiensis increased over 40% and 66%, respectively when starved of nitrogen (Dragone et al., 2011). Nitrogen limitation was also shown to be the most superior inducer of carbohydrate accumulation compared to limiting sulphur or phosphorus (Brányiková et al., 2011; Yuan et al., 2018). Parachlorella kessleri that were starved off of nitrogen, phosphorus or sulphur experienced a spike in carbohydrate content for the first few days before dropping. This was then followed by lipid accumulation. The initial reaction might be due to carbohydrate synthesis as energy reserves in response to the initial stress before the same energy is utilized for lipid synthesis if starvation was prolonged (Shaikh et al., 2019).
Phosphorus is a core macronutrient involved in ATP biosynthesis, nucleic acid formation, growth and cellular maintenance. Starvation of inorganic phosphate in Chlorella sp. FC2IITG exhibited high carbohydrate content up to 47.35 wt% of CDW (Muthuraj et al., 2014). Deprivation of phosphate on Leptolyngbya limnetica and Oscillatoria obscura resulted in increased carbohydrate levels of 44.5 wt% and 40.4 wt% of CDW, respectively which were 45% more than carbohydrate levels under nitrogen limitation (Kushwaha et al., 2018).
Additionally, trace elements such as sulphur, copper, and manganese play significant roles in microalgae growth. Sulphur starvation stops cellular metabolism while amassing compounds such as carbohydrates. C. vulgaris was reported to amass 60% of carbohydrates when sulphur was limited (Brányiková et al., 2011). Starving of manganese and potassium also instigated an increase in carbohydrate content in C. reinhardtii (Markou et al., 2012). Calcium and magnesium limitation also increased carbohydrate concentration of Chlorella sorokiniana by 50% without compromising biomass productivity (Hanifzadeh et al., 2018). Conversely, exposure to high copper concentration induced carbohydrate production in diatoms Cylindrotheca fusiformis and Gymnodimium sp. (Pistocchi et al., 2000). Likewise, high carbohydrate accumulation was reported when iron was in excess in tandem with nitrogen limitation and high light illumination (Yeesang and Cheirsilp, 2011).
4.6 Chemical Modulators
In addition to moderating microalgal growth parameters, an alternative technique in refining biomass production is the provision of chemicals. Chemical modulators do not compromise microalgal growth that is observed in nutrient deficiency nor does it necessitate specific data on molecular targets that is required in the genetic engineering method. Instead, chemical modulators are naturally occurring molecules in microalgae that rely on phenotypic screening to target the cellular functions, growth, and metabolism of the microalgae. For instance, a large scale phenotypic screening of 42 chemicals on their functions in microalgal lipid metabolism identified 12 modulators that enhanced over 100% of intracellular lipid levels in addition to successfully up-scaling the use of two chemicals, propyl gallate and butylated hydroxyanisole (Franz et al., 2013). Similarly, 10 chemical modulators were screened on Scenedesmus dimorphus UTEX1237 and 6 were found to enhance carbohydrate productivity: butylated hydroxyanisole, forskolin, acetylcholine, brefeldin, propyl gallate, and jasmonic acid (Paliwal and Jutur, 2021). In the same study, butylated hydroxyanisole, forskolin, acetylcholine, brefeldin and propyl gallate are economically feasible. Additionally, naphthoxyacetic acid or jasmonic acid enhanced lipid accumulation of Schizochytrium sp. S31 by 11.16% and 12.71%, respectively (Wang et al., 2018). As chemical modulators either act directly on a target enzyme or functions as signal molecules, a novel approach termed “chemical modulator based adaptive laboratory evolution” was developed for Crypthecodinium cohnii (Diao et al., 2019). By using the chemical sesamol, this study found that quenching of reactive oxygen species enhanced central carbohydrate and energy metabolism. Conversely, the application of γ-Aminobutyric acid (GABA) along with nitrogen starvation was found to improve starch content of Tetraselmis subcordiformis by 23.4% and starch yield by 28.6% presumably by reducing reactive oxygen species (Ran et al., 2020). Considering their low-cost, chemical modulators are appropriate treatments for sustainable microalgal biorefinery.
5 Microalgal Biomass Manufacturing Technology
5.1 Mass Cultivation of Microalgal Biomass
There are generally two classes of microalgae cultivation systems available: open raceway pond (RWP) and closed photobioreactor (PBR). Closed systems allow for precise control of growth parameters as well as minimal risk of biological and non-biological contamination. Alternatively, open systems make use of simple designs, natural solar illumination, and lower operating costs. Table 4 depicts a summarized comparison between the available microalgae cultivation systems.
5.1.1 Open Raceway Pond (RWP)
The RWP is the most widely employed open system design. In addition to being more cost-effective than closed PBR, the system has a simplistic operation, requires low energy and is easily scalable (Costa and De Morais, 2014). RWPs are generally assembled with a paddle wheel to circulate microalgae in a series of continuous loops wherein nutrients and fresh microalgal broth are added to the front of the wheel while harvesting occurs behind the wheel (Mata et al., 2010). This design is considered to be the most energy-efficient pond cultivation design as it only requires a single paddle wheel for agitation of a 5-hectare pond (Rogers et al., 2014). The wheel gently mixes the pond culture with high mixing efficiency which minimizes injury to the flocculated microalgae (Morris et al., 1974). However, RWPs suffer from excessive water loss to the environment which affects the CO2 utilization efficiency (Tan et al., 2018). There is also relatively low light penetrance throughout the microalgae cultures which creates a “dark zone” at the bottom of the pond (Hadiyanto et al., 2013). Furthermore, the nature of RWPs that is subject to environmental conditions results in inconsistent microalgae growth rates and a high risk in contamination (Chisti, 2007).
Current RWP designs are excavated at a shallow depth of not more than 20 cm for efficient light capture in addition to reducing hydraulic power consumption of the paddle wheel (De La Obra et al., 2017). However, this depth expedites a larger surface area to incorporate large culture volumes, which increases evaporation. While evaporation aids in preventing overheating of the cultivation medium, it is essential for the cultivation water to be refilled regularly. Transparent light-scattering columns (LSC) can mitigate both of these issues. Vertically immersed LSCs not only enhanced brightness throughout the depth of the pond but also decreased up to 13.6% evaporation loss by decreasing the surface area between the air-liquid layer (Sirikulrat et al., 2021).
Open ponds are exposed to the environment and are thus vulnerable to contamination. To combat this, microalgae strains that require specific environments are preferred such as A. platensis that thrive in extreme alkaline states or D. salina that can tolerate high salinity (Cheng et al., 2019b). Organic pollutants and unwanted microbes can also be removed via solar photo-Fenton by employing iron and hydrogen peroxide to irradiate water (Polo-López and Pérez, 2021).
Dead zones or stagnant zones occur due to imperfect mixing of the culture, leading to sedimentation and anaerobic conditions (Hadiyanto et al., 2013). Such an environment promotes the growth of unwanted anaerobic microbes which results in a drastic drop in biomass production (Becker, 1994). The liquid velocity needed to minimize dead zones must be at least 0.1 m s−1 (Weissman et al., 1988). The application of flow deflectors and wing baffles at each bend of the pond promotes higher consistency of the velocity flow throughout the pond (Hadiyanto et al., 2013).
5.1.2 Photobioreactors (PBR)
PBRs are enclosed vessels supplied with artificial light (Kilham et al., 1997). A PBR is made up of four main phases; the solid phase of microalgal cells, the liquid phase with culture medium, the gaseous phase consisting of CO2 and O2, and a light-radiation field (Wu et al., 2013). The closed systems were originally introduced to solve complications from the open system ponds (Chisti, 2007). PBRs are compact and space-efficient which does not require large land masses (Wu et al., 2013). The system also allows for a highly controlled growth environment (Wu et al., 2013). This makes the microalgal cultures less prone to contamination as well as allows for optimized growth conditions which results in higher biomass production. Designs of efficient PBRs require optimization of the mixing state for improved effective CO2 mass transfer, strengthening the flashlight effect whereby cultures experience the transition between light and dark regions, maintenance of a good nutrient distribution, and prevention of culture sedimentation (Ugwu et al., 2008). Regardless, utilization of PBRs is restricted by its limited scalability and immense capital costs and high capital (Ciferri and Tiboni, 1985).
Tubular PBRs are the most commonly used closed system design. They are constructed as transparent long tubes known as solar collectors which are organized in vertical, inclined, helix, or horizontal positions for efficient light capture (Tarantino, 2003). Microalgal culture is circulated in a constant loop from the reservoir to the solar collector via a mechanical pump or an airlift structure (Chisti, 2007). The recycling of microalgal culture allows the exchange of CO2 and O2 while sustaining the mixing process (Marles et al., 2011). To avoid photooxidation, the culture is also continuously directed to a degassing column to eliminate the collected O2 while cooling water is propelled into the column as a temperature regulator (Chisti, 2007). In efforts to further optimize the consumption of dissolved O2, a novel electrochemical tubular PBR that employs anion-exchange membrane alkaline fuel cells coupled with Pt40Ru20 as the cathode catalyst was able to reduce dissolved O2 content from 20.0 to 10.72 mg·L−1 in 45 min (Feng et al., 2018). Another design addresses the drawback of low CO2 dissolution which leads to deficient carbon sources and thereby inhibiting microalgal growth. Here, the use of ZIF8-SE medium comprising of zeolitic imidazolate framework-8 (ZIF-8) nanoparticles increased CO2 mass transfer resulting in a 25.5% increase in biomass yield (Xu et al., 2021). Similarly, an innovative spiral-ascending CO2 dissolver was established to enhance the CO2 mass transfer and extend gas−liquid contact time. This tubular PBR design markedly improved biomass accumulation by 40.8% (Xu et al., 2020).
Flat-plate PBRs are transparent rectangular-shaped compartments with a depth of 1–5 cm that are positioned horizontally or vertically (Lu et al., 2011). The plate is thin with a thickness of 16 mm to allow optimum radiance penetration (Deshmukh et al., 2021). Mixing of microalgal culture is achieved by an airlift system (Zhou et al., 2011). Flat-plate PBRs boost a high total surface area for efficient light illumination as well as low O2 accumulation. However, poor aeration and short penetration depths result in lower yields compared to conventional tubular PBRs. To resolve this, a novel flat-plate PBR utilized double paddlewheels to promote mass transfer and increased horizontal fluid velocity between light/dark zones. This addition of paddlewheels augmented the microalgal growth rate by 121.1% (Cheng et al., 2018). Besides this, a novel jet-aerated tangential swirling-flow plate PBR design was shown to reduce average bubble diameter by 80.2% and enhance mass transfer coefficient by 4.6 times (Cheng et al., 2019a).
The column PBR is designed as clear cylindrical tubing fitted with a gas sparger that mixes and agitates the microalgal culture by propelling in air bubbles (Mohan et al., 2019). Typical column PBR designs include only the sparger and no other internal structures. This system allows for strong gas-liquid mass transfer, highly efficient CO2 usage and release of O2, inexpensive capital cost, and low shear forces (Singh and Sharma, 2012; Moreno-Garcia et al., 2017). Unfortunately, the cylindrical structure has limited efficiency of light utilization and therefore requires high energy to achieve adequate lighting (Huang et al., 2017). Due to this, the tubing cylinder diameter is limited to 0.2 m to while the maximum height is 4 m for structural support (Wang et al., 2012; Moreno-Garcia et al., 2017). By attaching an internal light column, a novel column PBR design enhanced light intensity in the column and increased biomass production by 82.4% (Li et al., 2018). Another design employed a serial lantern-shaped draft tube that improves flashing light in column PBR (Ye et al., 2018). This yielded a 74% increment in biomass production.
5.2 Microalgal Biomass Harvesting Techniques
Harvesting is the process of separating microalgae from their growth media. This generally involves the elimination of water from the microalgal medium which thereby concentrates the biomass. Due to the minute sizes of microalgae (diameters of 3–30 m), their cell density is similar to water which poses a challenge in the recovery process (Moreno-Garrido, 2008). Harvesting cost accounts for at least 20% of total microalgal biomass cost and can even reach up to 90% of total costs for open RWPs (Rodolfi et al., 2009; Hulatt et al., 2017). As such, the selection of a suitable harvesting method must consider the overall energy consumption and properties of the chosen microalgal, cell size and density, final product specifications and reusability of the culture medium (Quinn et al., 2012; Ma et al., 2014).
5.2.1 Centrifugation
Centrifugation separates microalgal cells from the media depending on the particle size and density, microalgal species and type of centrifuge used (Heasman et al., 2000; Soomro et al., 2016). This technique offers many advantages including high cell separating efficiency (over 90%), chemical-free biomass and applies to all microalgae.
Disc stack centrifuges are the most utilized industrial centrifuge for commercially valuable algal products (Hulatt et al., 2017). With high centrifugation forces of 4,000 to 14,000 times gravitational force, this method has a low separation time and is ideal for extracting particles of sizes between 3 and 30 µm and low concentrations of 0.02–0.05% of microalgae cultures with a maximum of 15% solids (Milledge and Heaven, 2013). Moreover, a disc stack centrifuge effectively separated solid/liquid, liquid/liquid and liquid/liquid/solid by applying high centrifugal forces in a single continuous course (Sharples and Doman Road, 1991). As a result, these centrifuges require higher energy consumption than other centrifuges. Calculations from using a Westfalia HSB400 disc-bowl centrifuge observed that energy used for centrifugation is four times the energy produced by the subsequent algal biodiesel product (Milledge and Heaven, 2013). To improve energy efficiency, the culture is pre-concentrated to 0.5% of the dry weight through a series of separation techniques. Another recommendation includes utilizing the entire biomass for energy production instead of only the lipid fraction (Amaro et al., 2017). Furthermore, 90% of energy consumption by the disc stack centrifuge can be reduced by optimizing three main factors: particle size, the rotational speed, and the outer radius of the centrifuge bucket (Abu-Shamleh and Najjar, 2020).
The decanter centrifuge consists of a horizontal conical bowl with a screw conveyor that rotates at high speeds to separate particles based on weight (Show et al., 2013). It was designed to handle high solid concentrations of up to 22% but necessitates massive energy utilization (Mohn, 1980). The multi-chamber centrifuge is made up of tubular bowls positioned coaxially to accumulate particles depending on the sizes in each chamber. This centrifuge design can separate up to 20% of solid concentration. However, the multi-chamber centrifuge requires manual cleaning of solids that is tedious, making it impractical for large-scale harvesting (Weatherley, 2013). The hydrocyclones is a cylindrical section attached to a conical base where microalgae culture is introduced from the top and cells move to the bottom in a cyclonic manner. Finer particles are discharged through the overflow pipe while larger particles are removed through the underflow (Show and Lee, 2014). Hydrocyclones can only handle low solid concentrations with low harvesting efficiency for particles of less than 400 μm in diameter (Gregg et al., 2009).
Overall, centrifugation comes with drawbacks that are time-consuming with high energy utilization as well as high capital and maintenance costs (Dassey and Theegala, 2013). Additionally, high gravitational force during centrifugation is physically damaging to cells which subsequently lowers yield for microalgae with delicate cell walls (Heasman et al., 2000). While centrifugation is effective for high-value products, the costs outweigh the yield for low-value products (Monte et al., 2018). Furthermore, centrifugation is unfeasible for saline environment usage as the high maintenance requirements would add to the already high cost (Najjar and Abu-Shamleh, 2020). Consequently, laboratory centrifugation was deemed more appropriate for concentrations of biomass over 30 mg·L−1 (Horowitz, 1986).
5.2.2 Filtration
During filtration, microalgae are passed through a semipermeable membrane via gravity, pressure or vacuum force that strains microalgae cells from liquid media; extracting algal biomass (Amaro et al., 2017). This chemical-free technique can sieve through large amounts of cells with little physical damage to cells (Wicaksana et al., 2012). Conversely, filters are prone to rapid fouling and clogging which lowers throughput and increases maintenance costs (Milledge and Heaven, 2013).
Filtration under high pressure or vacuum is ideal to separate microalgae strains that are large such as A. platensis but is ineffective in recovering species that are smaller than 10 μm, for instance, Dunaliella and Chlorella (Hulatt et al., 2017). The pore size of the membrane determines the type of filter: micro-filters have pores larger than 10 μm, micro-filters range between 0.1 and 10 μm, ultrafilters have sizes of 0.02–0.2 μm, and reverse osmosis filter pores are smaller than 0.001 μm while tangential flow filters, vacuum filters, and pressure filters are few examples of relatively new filtration methods (Milledge and Heaven, 2013).
Most common microalgae are between the sizes of 5–6 μm (Edzwald, 1993), making micro-filters the most suitable membrane. Tangential flow and pressure filtrations are energy-saving techniques, providing output with higher energy than the initial energy consumed during the dewatering process (Danquah et al., 2009). Tangential flow filtration has a removal efficiency of up to 89% (Ma et al., 2014). On the other hand, ultrafiltration is suitable for long-term harvesting with better flux over time and fouling resistance compared to conventional micro-filtration (De Baerdemaeker et al., 2013). However, ultrafiltration has low energy efficiency and is expensive with high operating and maintenance costs (Ma et al., 2014). Belt filtration is successful in the water treatment industry especially for the separation of Arthrospira with low overall costs (Mohn, 1980). A novel electrochemical membrane filtration method was also successful in removing microorganisms and biomass separation. In addition, this technique was also shown to degrade pollutants and enhance membrane defouling better than hydraulic or backwash approaches (Merchant et al., 2007).
Membrane fouling is the most significant issue faced in the filtration process. Organic matter from algae cells tends to deposit onto the membrane resulting in a thick cake layer (Marbelia et al., 2016). Hydrophilic membranes are more resistant to fouling than hydrophobic membranes (Sun et al., 2014). Backwashing with subsequent forward flushing for 20 min was most effective in removing fouling in ultrafilters while sodium hydroxide (0.02 N) and sodium hypochlorite (100 mg·L−1) was applied to maximize flux recovery (Liang et al., 2008). In situ pre-oxidation method creates a porous and loose cake layer that increases flux but comes with extreme cell breakage and more release of organic matter (Qu et al., 2015). This can be mitigated by employing immobilizing catalysts to confine oxidation within the membrane interface. For instance, zero-valent iron nanoparticles were adhered to the membrane to activate peroxymonosulfate that oxidizes organic matter (Huang et al., 2020). The use of oxidation techniques also allows permeates to be recycled, reducing microalgae cultivation costs as well as water footprint. D. salina permeate recovered from ultrafiltration was successfully recycled after treatment with ultraviolet radiation and hydrogen peroxide (Guarnieri et al., 2018).
5.2.3 Flocculation
The negative charge on microalgae surfaces prevents cells from self-aggregating which complicates the harvesting process. Flocculation uses organic and inorganic flocculants that neutralize this negative charge and promotes the accumulation of the microalgal cells. Flocculants carrying positive charge are supplemented to algae culture to absorb the negative charge on cell surfaces. This removes the electrostatic repulsion between cell particles and cells start to coagulate. Three critical aspects affect flocculation efficiency: surface charge neutralization, adsorption, and adsorption bridging (Polle et al., 2017). This approach is an appropriate harvesting technique for large-scale microalgae harvesting of a wide range of microalgae species (Hulatt et al., 2017). Flocculation is employed as the initial procedure in concentrating dilute suspensions of 0.5 g·L−1 of dry matter up to 100 times to a concentrate of 50 g·L−1. Subsequent mechanical harvesting techniques such as centrifugation will then result in an algal paste with 25% of dry biomass (Wileman et al., 2012). This combination makes the total energy utilization acceptable as the particles have agglomerated into large sizes, thus less processing water volume is required (Schlesinger et al., 2012).
Chemical flocculation has a low cost with easily obtainable chemical flocculants such as alum and ferric chloride (Bracharz et al., 2018). Metal salts yield separation of up to 95% of microalgal biomass but tend to remain in the biomass residue (Chatsungnoen and Chisti, 2016). These multivalent salts are influenced by their electronegativity and solubility; the more electronegative the ion, the faster the coagulation (Barros et al., 2015). Such chemicals are also highly toxic to the environment and thus require an additional removal treatment step which increases production cost (Uduman et al., 2010). Moreover, inorganic and synthetic flocculants have serious implications on human health including Alzheimer’s disease and other neurodegenerative disorders (Kumar et al., 2019b). As such, an extra pretreatment step is required to remove chemical residues from the harvested biomass. On the other hand, positively charged biopolymers such as chitosan are safer alternatives but only function at low pH, limiting this flocculation method to only acidic dwelling microalgae (Chang and Lee, 2012). A novel time-saving, economical, and scalable chemical flocculation method using potash alum at pH lower than 8.5 or with the addition of hydrochloric acid for cultures of pH over 8.5 was found to have a harvesting efficiency of up to 98.7%. This rapid chemical flocculation with multiple recycling lowered harvesting costs as low as $0.06 per kg of dry algal biomass compared to the typical harvesting cost of $3.3 (Mehta and Chakraborty, 2021).
In auto-flocculation, microalgae flocculate due to environmental stress such as nitrogen fluctuation, changes in pH, or photosynthetic CO2 depletion (Schenk et al., 2008). This process utilizes natural gravity settling that is inexpensive and less damaging to cells compared to centrifugation. Increasing pH in the presence of calcium and magnesium ions induces this phenomenon to yield high biomass recovery with over 90% efficiency (García-Pérez et al., 2014). This process is aided by the addition of 1M sodium hydroxide (Singh and Patidar, 2018). However, this natural occurrence only occurs in certain microalgae and is known to be slow and unreliable. Additionally, the use of sodium hydroxide is undesirable due to the tedious process control and alteration of cell composition (Schenk et al., 2008).
Bioflocculation is another safer and eco-friendly alternative to chemical flocculation. Bioflocculants are produced by microalgae, for instance, the external polysaccharides (EPS) of bacteria, certain microalgae, and fungi are used to flocculate algae in suspension (Hulatt et al., 2017). Bacteria with flocculant EPS are typically added to the microalgae culture supplemented with a suitable organic carbon source to prevent altering biomass productivity (Udayan et al., 2022). As this is a chemical-free process, there is no need for pre-treatment for the recovered biomass. For instance, the bacteria Solibacillus silvestris harvested N. oceanica at 88% efficiency while the fungi Aspergillus oryzae flocculated C. vulgaris with a removal efficiency of over 97% (Wan et al., 2013; Zhou et al., 2013). A novel polymeric bioflocculant from Streptomyces has also been identified. Using 0.5% of this bioflocculant (ABF), the flocculation rate was 99.18% within 10 min on Nannochloropsis (Sivasankar et al., 2020).
5.2.4 Flotation
Flotation takes advantage of air or gas bubbles that adhere to microalgae cells to carry the suspended cells to the surface of the liquid media for harvesting (Laamanen et al., 2016). This technique has a simple operating procedure, a relatively high harvesting efficiency for both marine and freshwater microalgae as well as high processing throughput while being economical (Ndikubwimana et al., 2016). Furthermore, flotation can be a rapid process for certain microalgae species with low density and self-floating properties (Edzwald, 1993). Flotation often necessitates flocculants and is therefore used in tandem with flocculation (Rubio et al., 2002).
Dissolved air flotation (DAF) produces air bubbles by saturating the culture with compressed air before releasing the culture at high pressure (Edzwald, 1993). DAF utilizes minute bubbles of sizes between 10 and 100 µm (Edzwald, 1993). Chemical flocculation often precedes DAF to produce pure effluents. DAF boosts up to 95% removal efficiency when using surface-modified bubbles with cationic polymer (Henderson et al., 2009). A novel dissolved air flotation process that utilizes positively charged bubbles (PosiDAF) supplemented with the algal organic matter has a cell separation of over 90% (Rao et al., 2018). Regardless of DAF’s efficiency, it is still obstructed by high energy requirements due to the required high pressures and usage of chemicals (Hanotu et al., 2012).
Dispersed air flotation (DiAF) employs a sparger to continuously produce air bubbles of sizes 700 to 1,500 µm (Alhattab and Brooks, 2017). This technique has lower energy demand at the cost of expensive equipment and high-pressure drop for producing bubbles (Shin et al., 2016). Natural and synthetic collectors, for instance sodium dodecylsulfate, cetyl trimethylammonium bromide (CTAB), saponin, and chitosan have been used to support DiAF with high algal removal efficiency (Kurniawati et al., 2014). A surfactant-aided DiAF for Chlorella saccharophila and the CTAB had a recovery efficiency of 95% (Alhattab and Brooks, 2020). Marine microalgae have also been successfully harvested with a 23-fold rise in algal concentration and more than 99% recovery efficiency using an advanced flotation machine with dodecyl pyridinium chloride (Garg et al., 2014).
In electro-flotation, electrolysis generates microbubbles from electrodes to capture microalgae cells (Baierle et al., 2015). This method is chemical-free and can be applied to most microalgae species. It also simultaneously disrupts cells, enables recycling of culture media, has low process time, and continuous operation (Baierle et al., 2015; Krishnamoorthy et al., 2021). However, it is heavy on energy consumption with the need to frequently replace electrodes due to fouling (Singh and Patidar, 2018). A recent electrochemical dewatering approach was proposed using boron-doped diamond and aluminium electrodes for harvesting Scenedesmus quadricauda (Ryu et al., 2018). This technique that induces bioaggregation by combining floc-forming microorganisms and microalgae is an effective substitute for chemical flocculation, as it reduces toxic metal coagulant pollutants produced from electrochemical harvesting. Another low-cost novel electro-flotation design employs an Arduino-based magnetic stirrer, wherein a short distance between electrodes, medium mixing rates of 200 rpm with 50 W could decant as much as 100% of algal biomass from 500 ml of media (Sanchez-Galvis et al., 2020).
5.3 Drying of Microalgal Biomass
Microalgal biomass obtained directly from dewatering is usually dried before subsequent downstream processes as the dry solid content is low (Starkenburg et al., 2017). Drying inhibits microbial spoilage, increases the shelf life of the biomass, and lessens the costs of packing, handling, transportation and storage of the microalgal biomass (Bennamoun et al., 2013). An ideal drying method should dehydrate cells while reducing as much deterioration as possible to the delicate microalgal cells. Moreover, the time taken and costs incurred for the drying process must also be taken into consideration (Hosseinizand et al., 2018).
Traditional solar drying is the most common and cost-saving method as it utilizes direct energy from the sun (Marles et al., 2011). Drying from solar energy does not alter the composition of the microalgal biomass, as such is ideal for downstream processing of PHAs. However, it requires long drying periods with large drying surface areas, in addition to difficult quality maintenance due to biomass degradation and a high risk of bacterial contamination in open conditions as well as overheating (Shin et al., 2016). Open solar drying was also found to decrease as much as 40% in polyunsaturated fatty acids from Derbesia tenuissima biomass (Sizova et al., 2013). Closed solar drying systems that employ solar water heating systems have been developed that increase environmental temperatures to 60°C. Such drying methods can dry biomass to 10% water content within 5 h (Rochaix and Van Dillewijn, 1982). On the other hand, freeze-drying preserves cell constituents. The freeze-drying process was able to retain over 90% of protein composition (Shin et al., 2016). Regardless, this method aggregates more cells in crystal form resulting in a smaller cell surface area of contact with the extracting solvent, which would have significant effects on the cell wall integrity (Sizova et al., 2013). Spray drying is also utilized for many microalgae species (Hosseinizand et al., 2018). Here, atomized water droplets are sprayed into a vertical tower while hot gas is passed down and the dried biomass is collected from the tower base (Soeder, 1980). While drying is achieved within seconds, high pressure from the atomization procedure could damage cells and cause degradation (Show et al., 2019). Due to this atomization process, spray drying requires high energy demand and high capital (Chen et al., 2015).
Regardless of the many drying techniques available, the mechanism of how remaining water in the microalgal biomass affects nutrient extraction such as carbohydrates is not fully understood. There is much debate on whether this step is necessary. Water creates a barrier that prevents the effective nutrient mass transfer from the cells to the extraction solvent and is thus thought to be an indispensable process (Amaro et al., 2017). On the other hand, another hypothesis claims that the presence of water in the biomass improves nutrient extraction efficiency and can be removed (Medina et al., 1998). Indeed, nutrient extraction such as carbohydrates and lipids from wet microalgal biomass devoid of drying has been fruitful (Vieira et al., 2021). Since the drying step requires at least 89% of energy demand and incurs up to 75% of total processing cost, eliminating this step could prove economical and time-saving for large-scale production of microalgal biomass (Taher et al., 2014).
6 Pretreatment Methods for Microalgal Biomass
The microalgal carbohydrates are mainly in the form of cellulose and soluble polysaccharides in the cell wall, and starch in the plastids, with low hemicellulose content and absence of lignin. These carbohydrates need to be converted into fermentable carbon sources prior to microbial fermentation (Chen et al., 2013; Khan et al., 2018; Saratale et al., 2018). There are different pretreatment methods that have been studied to break down the algae carbohydrates into simple sugars, which can be majorly classified into physical, chemical, and biological pretreatments as listed in Table 5. The physical method utilizes direct steps for cell breakage which poses little issues to the environment but is plagued with high production cost due to high energy consumption that off-balances energy recovered from the biomass. Conversely, the chemical and biological methods require low energy utilization. The chemical method improves cell disintegration at a faster pace with lower energy demand but also comes with a high risk of chemical contamination towards the resulting biomass as well as the environment. While enzymatic pretreatments are more environmental-friendly with simple procedures without the need for complicated machinery, they incur high costs and long waiting periods. Therefore, the type of pretreatment chosen is important to efficiently convert these carbohydrates to sugars as the cell wall compositions vary according to microalgal species (Sankaran et al., 2020; Constantino et al., 2021; Debnath et al., 2021; Sirohi et al., 2021).
Physical pretreatment involves either mechanical, radiation, or electrical techniques (Sankaran et al., 2020; Debnath et al., 2021; Sirohi et al., 2021). It was found that total reducing sugars concentration was significantly affected by temperature whereas the concentration only slightly increased upon increasing the sonication time as shown from the sonification of Chlamydomonas mexicana biomass. Hence, the optimum sonication conditions for C. mexicana were set at 50°C and 15 min, while taking into account the energy and time consumption, which released 7.4 wt% of total reducing sugars of dry cell weight (Eldalatony et al., 2016).
Chemical pretreatment is usually performed via alkaline, acid, and ionic liquids, coupled with other reaction conditions such as high temperature and pressure to hydrolyze the microalgal cell wall (Sankaran et al., 2020; Constantino et al., 2021; Debnath et al., 2021; Sirohi et al., 2021). Through hydrothermal acid pretreatment, 50 g·L−1 of lyophilized Chlorella sorokiniana and C. reinhardtii biomass were added separately into 4 vol% of sulfuric acid solution before being autoclaved at 121°C for 30 min, which resulted in an increase of reducing sugar yield to 7% and 1%, respectively (Constantino et al., 2021). The application of ozone pretreatment on a mixed microalgal biomass under increasing dosages of 0.25, 0.5, 1.0, 1.5, and 2.0 g of applied ozone/g of dry weight biomass, without supplementary enzymatic hydrolysis led to microalgal cell breakage. However, the glucose conversion yields were still insignificant at less than 0.5 wt% of total carbohydrate (Keris-Sen and Gurol, 2017).
The most commonly used biological pretreatment employ the use of enzymes and hydrolytic microorganism, which can be microbes or fungi (Sankaran et al., 2020; Debnath et al., 2021; Sirohi et al., 2021). Occasionally, the microalgal biomass is pretreated by means of either physical or chemical methods prior to using this strategy (Sankaran et al., 2020). Enzymatic hydrolysis of C. mexicana performed using cellulase from Trichoderma reesei after sonication improved the yield to 28.05 wt% of total reducing sugars of dry cell weight. The previous sonication step partially hydrolysed the microalgal biomass, making it more susceptible to enzymatic hydrolysis (Eldalatony et al., 2016). Apart from physical pretreatment, chemical pretreatment can also partially disintegrate both the C. sorokiniana and C. reinhardtii biomass, resulting in higher yields of reducing sugar, from 7% to 1%, to 47% and 25%, respectively, when further two-step enzymatic saccharification was conducted on the chemically pretreated biomass using amyloglucosidase (600 U·g−1 biomass) (Constantino et al., 2021). Therefore, a combination of pretreatment methods can improve the release of reducing sugars.
7 In a Nutshell: Future Prospects and Conclusion
Current microalgae productions only focus on an individual area such as emphasizing energy yield or boosting the value of the resultant bioproducts. To further ensure that microalgal biomass is able to compete with other carbon feedstocks, model biorefinery outlines focusing on reducing energy requirement, economical cost, and maximizing biomass constituents have been proposed (Figure 2). A biorefinery is termed as “an establishment that assimilates biomass conversion operations and equipment to yield fuels, power, and chemicals from biomass” (Jiang et al., 2016). The microalgae biorefinery system layout should take into consideration local conditions, regional climate, economics, infrastructure, and available resources. For instance, as many countries implement their own COVID-associated economic border restrictions, many sectors including the logistics industry have faced negative impacts.
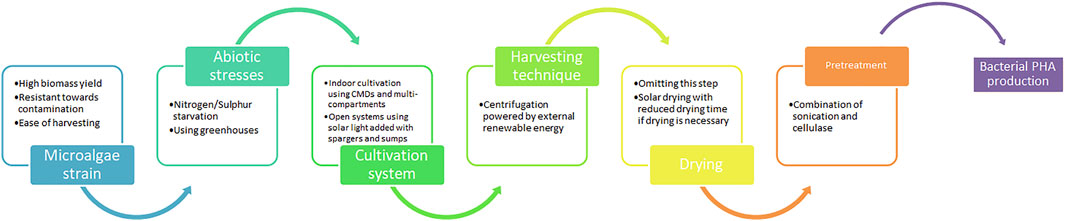
FIGURE 2. A model biorefinery process chain focusing on reducing energy requirement, economical cost. and maximizing biomass constituents.
The initial and most crucial step is to identify the strain of microalgae, either through species screening or genetic manipulations, that not only provides consistently high yields but also other necessary traits beneficial to the microalgal biomass manufacturing technology. For example, most natural microalgae cannot endure long-term open pond cultivation due to the high risk to contamination by fast-growing microbes (Assunção and Malcata, 2020). Introduction of genetically engineered microalgae with the ability to utilize limited nutrients and resistance towards field contamination could be an option.
As seen in Chapter 4, abiotic stresses can be utilized to modulate microalgal metabolite profiles and nutrients from the subsequent biomass attained depending on the desired end products. Nitrogen or phosphorus limitation has been the most efficient stress to amass high levels of carbohydrates. Similarly, for indoor cultivation of microalgae, usage of CMDs to increase CO2 utilization efficiency as well as a multi-compartment with different light acclimatation will increase productivity rates. Open systems are more economical with the advantage of using solar light added with spargers and sumps to disperse CO2. Both indoor and outdoor systems would benefit from using greenhouses for microalgae cultivation throughout the year to stabilize temperature.
On the other hand, there is no single harvesting technique that is compatible with all types of microalgae. While flocculation and coagulants are inexpensive and effective, the contaminants reduce the quality and quantity of the recovered biomass. Conversely, physical harvesting necessitates high operating cost and time which is uneconomical. Further research is required for more economical and eco-friendly optimum extractions. A suggested method is to reconstruct physical harvesting to be powered on external renewable energy such as solar panels or wind grids to offset the high energy requirement and decrease costs. Similarly, while it is possible to forgo the drying step, it is eminent for solar drying to be coupled with technology that reduces the drying time without sacrificing biomass quality in the event that drying is exigent.
As proven, the microalgal biomass manufacturing industry has made big strides to overcome obstacles that are bottlenecks for other feedstock production. Microalgae cultivation is possible with low-cost, energy-efficient RWP and PBR designs while economical harvesting techniques with high yields are available for large scale production. The flexible nature of microalgae to dwell in both freshwater and saltwater environments is advantageous for intensive algae production systems. On top of that, fertile land is unnecessary for microalgae growth and they can thrive even in industrial effluents; therefore, not only is there no competition for food production resources (Duffy et al., 2009), microalgae have also been applied as wastewater treatment (Liu et al., 2020). In regards to this, emerging studies have suggested cultivating microalgae in wastewater without nutrient supplementation which eliminates the need for media preparation (Karemore and Sen, 2015; Daneshvar et al., 2018). Additionally, industrial flue gas has been successfully utilized as carbon source for microalgal biomass production (Gentili, 2014).
Bacterial PHA production from microalgal biomass is a concept that is amassing more attention globally (Mishra et al., 2014). Research on the types of polymers that is obtained from microalgal biomass is needed in efforts to reduce single-use plastics. With the current post-COVID economy shifting towards sustainable energy, microalgae offer photosynthetic biomass for bacterial PHA synthesis as a substitute renewable feedstock which can be cultivated with limited natural resources with the extra benefits of phycoremediation besides CO2 sequestration.
Author Contributions
Conceptualization: FHPT and KS. Writing—original draft, review and editing, data curation: FHPT and NN. Funding acquisition: KS. Manuscript revision: KS. All authors contributed to the article and approved the submitted version.
Conflict of Interest
Author NN was employed by company PETRONAS Research Sdn. Bhd.
The remaining authors declare that the research was conducted in the absence of any commercial or financial relationships that could be construed as a potential conflict of interest.
Publisher’s Note
All claims expressed in this article are solely those of the authors and do not necessarily represent those of their affiliated organizations, or those of the publisher, the editors and the reviewers. Any product that may be evaluated in this article, or claim that may be made by its manufacturer, is not guaranteed or endorsed by the publisher.
Acknowledgments
We would like to thank all our collaborators and colleagues for the discussion and the work conducted in this lab. This work was supported by PETRONAS Sdn. Bhd. Spirulina (Arthrospira) platensis UMACC 161 was obtained from Prof. Phang Siew Moi and Dr. Ng Fong Lee of Universiti Malaya. The authors also thank Prof. Nazalan Najimudin and Mr. Ahmad Faisal Bin Mohamad of Universiti Sains Malaysia for their help in obtaining Chlamydomonas reinhardtii cultures.
References
Abd El-Malek, F., Rofeal, M., Farag, A., Omar, S., and Khairy, H. (2021). Polyhydroxyalkanoate Nanoparticles Produced by Marine Bacteria Cultivated on Cost Effective Mediterranean Algal Hydrolysate Media. J. Biotechnol. 328, 95–105. doi:10.1016/j.jbiotec.2021.01.008
Abomohra, A. E.-F., Eladel, H., El-Esawi, M., Wang, S., Wang, Q., He, Z., et al. (2018). Effect of Lipid-Free Microalgal Biomass and Waste Glycerol on Growth and Lipid Production of Scenedesmus Obliquus: Innovative Waste Recycling for Extraordinary Lipid Production. Bioresour. Technology 249, 992–999. doi:10.1016/j.biortech.2017.10.102
Abomohra, A. E.-F., Shang, H., El-Sheekh, M., Eladel, H., Ebaid, R., Wang, S., et al. (2019). Night Illumination Using Monochromatic Light-Emitting Diodes for Enhanced Microalgal Growth and Biodiesel Production. Bioresour. Technology 288, 121514. doi:10.1016/j.biortech.2019.121514
Abu-Shamleh, A., and Najjar, Y. S. H. (2020). Optimization of Mechanical Harvesting of Microalgae by Centrifugation for Biofuels Production. Biomass and Bioenergy 143, 105877. doi:10.1016/j.biombioe.2020.105877
Adamczyk, M., Lasek, J., and Skawińska, A. (2016). CO2 Biofixation and Growth Kinetics of Chlorella vulgaris and Nannochloropsis gaditana. Appl. Biochem. Biotechnol. 179, 1248–1261. doi:10.1007/S12010-016-2062-3
Afreen, R., Tyagi, S., Singh, G. P., and Singh, M. (2021). Challenges and Perspectives of Polyhydroxyalkanoate Production From Microalgae/Cyanobacteria and Bacteria as Microbial Factories: An Assessment of Hybrid Biological System. Front. Bioeng. Biotechnol. 9, 109. doi:10.3389/fbioe.2021.624885
Alhattab, M., and Brooks, M. S.-L. (2017). Dispersed Air Flotation and Foam Fractionation for the Recovery of Microalgae in the Production of Biodiesel. Separation Sci. Technology 52, 2002–2016. doi:10.1080/01496395.2017.1308957
Alhattab, M., and Brooks, M. S.-L. (2020). Optimization of Chlorella saccharophila Harvesting by Surfactant-Aided Dispersed Air Flotation for Biodiesel Production Processes. Biomass and Bioenergy 134, 105472. doi:10.1016/j.biombioe.2020.105472
Alkotaini, B., Koo, H., and Kim, B.S. (2016). Production of Polyhydroxyalkanoates by Batch and Fed-Batch Cultivations of Bacillus megaterium from Acid-Treated Red Algae. Korean J. Chem. Eng. 33, 1669–1673. doi:10.1007/s11814-015-0293-6
Amaro, H. M., Sousa-Pinto, I., Malcata, F. X., and Guedes, A. C. (2017). “Microalgal Fatty Acids-From Harvesting Until Extraction,” in Microalgae-Based Biofuels and Bioproducts (Amsterdam: Elsevier), 369–400. doi:10.1016/b978-0-08-101023-5.00016-9
Anderson, D. M., and Morel, F. M. M. (1978). Copper Sensitivity of Gonyaulax tamarensis 1. Limnol. Oceanogr. 23, 283–295. doi:10.4319/lo.1978.23.2.0283
Anjos, M., Fernandes, B. D., Vicente, A. A., Teixeira, J. A., and Dragone, G. (2013). Optimization of CO2 Bio-Mitigation by Chlorella vulgaris. Bioresour. Technology 139, 149–154. doi:10.1016/j.biortech.2013.04.032
Assunção, J., and Malcata, F. X. (2020). Enclosed “Non-conventional” Photobioreactors for Microalga Production: A Review. Algal Res. 52, 102107. doi:10.1016/j.algal.2020.102107
Azizi, N., Najafpour, G., and Younesi, H. (2017). Acid Pretreatment and Enzymatic Saccharification of Brown Seaweed for Polyhydroxybutyrate (PHB) Production Using Cupriavidus necator. Int. J. Biol. Macromolecules 101, 1029–1040. doi:10.1016/j.ijbiomac.2017.03.184
Baer, S., Heining, M., Schwerna, P., Buchholz, R., and Hübner, H. (2016). Optimization of Spectral Light Quality for Growth and Product Formation in Different Microalgae Using a Continuous Photobioreactor. Algal Res. 14, 109–115. doi:10.1016/j.algal.2016.01.011
Baierle, F., John, D. K., Souza, M. P., Bjerk, T. R., Moraes, M. S. A., Hoeltz, M., et al. (2015). Biomass from Microalgae Separation by Electroflotation with Iron and Aluminum Spiral Electrodes. Chem. Eng. J. 267, 274–281. doi:10.1016/j.cej.2015.01.031
Banerjee, S., Ray, A., and Das, D. (2021). Optimization of Chlamydomonas reinhardtii Cultivation with Simultaneous CO2 Sequestration and Biofuels Production in a Biorefinery Framework. Sci. Total Environ. 762, 143080. doi:10.1016/j.scitotenv.2020.143080
Barat, W. O., Kawaroe, M., and Bengen, D. G. (2017). Correlation Between Paddle Wheel Operation Time with Microalgae (Nannochloropsis sp.) Growth Rate and Lipid Contents in Open Raceway Ponds. Omni-Akuatika 13, 110–116. doi:10.20884/1.oa.2017.13.1.76
Barnes, D. K. A., Galgani, F., Thompson, R. C., and Barlaz, M. (2009). Accumulation and Fragmentation of Plastic Debris in Global Environments. Phil. Trans. R. Soc. B 364, 1985–1998. doi:10.1098/rstb.2008.0205
Barros, A. I., Gonçalves, A. L., Simões, M., and Pires, J. C. M. (2015). Harvesting Techniques Applied to Microalgae: A Review. Renew. Sustainable Energ. Rev. 41, 1489–1500. doi:10.1016/j.rser.2014.09.037
Becker, E. W. (1994). Microalgae: Biotechnology and Microbiology. Cambridge: Cambridge University Press.
Bennamoun, L., Arlabosse, P., and Léonard, A. (2013). Review on Fundamental Aspect of Application of Drying Process to Wastewater Sludge. Renew. Sustainable Energ. Rev. 28, 29–43. doi:10.1016/j.rser.2013.07.043
Bera, A., Dubey, S., Bhayani, K., Mondal, D., Mishra, S., and Ghosh, P.K. (2015). Microbial Synthesis of Polyhydroxyalkanoate Using Seaweed-Derived Crude Levulinic Acid as Co-Nutrient. Int. J. Biol. Macromol. 72, 487–494. doi:10.1016/j.ijbiomac.2014.08.037
Bracharz, F., Helmdach, D., Aschenbrenner, I., Funck, N., Wibberg, D., Winkler, A., et al. (2018). Harvest of the Oleaginous Microalgae Scenedesmus obtusiusculus by Flocculation from Culture Based on Natural Water Sources. Front. Bioeng. Biotechnol. 6, 200. doi:10.3389/fbioe.2018.00200/full
Branco-Vieira, M., San Martin, S., Agurto, C., Freitas, M. A., Martins, A. A., Mata, T. M., et al. (2020). Biotechnological Potential of Phaeodactylum tricornutum for Biorefinery Processes. Fuel 268, 117357. doi:10.1016/j.fuel.2020.117357
Brányiková, I., Maršálková, B., Doucha, J., Brányik, T., Bišová, K., Zachleder, V., et al. (2011). Microalgae—Novel Highly Efficient Starch Producers. Biotechnol. Bioeng. 108, 766–776. doi:10.1002/bit.23016
Caia, M., Bernard, O., and Béchet, Q. (2018). Optimizing CO2 Transfer in Algal Open Ponds. Algal Res. 35, 530–538. doi:10.1016/j.algal.2018.09.009
Canelli, G., Neutsch, L., Carpine, R., Tevere, S., Giuffrida, F., Rohfritsch, Z., et al. (2020). Chlorella vulgaris in a Heterotrophic Bioprocess: Study of the Lipid Bioaccessibility and Oxidative Stability. Algal Res. 45, 101754. doi:10.1016/j.algal.2019.101754
Chang, J.-S., Show, P.-L., Ling, T.-C., Chen, C.-Y., Ho, S.-H., Tan, C.-H., et al. (2017). “Photobioreactors,” in Current Developments in Biotechnology and Bioengineering (Amsterdam: Elsevier), 313–352. doi:10.1016/b978-0-444-63663-8.00011-2
Chang, K. S., Kim, J., Park, H., Hong, S.-J., Lee, C.-G., and Jin, E. (2020). Enhanced Lipid Productivity in AGP Knockout Marine Microalga Tetraselmis sp. Using a DNA-Free CRISPR-Cas9 RNP Method. Bioresour. Technology 303, 122932. doi:10.1016/j.biortech.2020.122932
Chang, Y.-R., and Lee, D.-J. (2012). Coagulation-Membrane Filtration of Chlorella vulgaris at Different Growth Phases. Drying Technology 30, 1317–1322. doi:10.1080/07373937.2012.662710
Chatsungnoen, T., and Chisti, Y. (2016). Harvesting Microalgae by Flocculation-Sedimentation. Algal Res. 13, 271–283. doi:10.1016/j.algal.2015.12.009
Chen, C.-L., Chang, J.-S., and Lee, D.-J. (2015). Dewatering and Drying Methods for Microalgae. Drying Technol. 33, 443–454. doi:10.1080/07373937.2014.997881
Chen, C.-Y., Zhao, X.-Q., Yen, H.-W., Ho, S.-H., Cheng, C.-L., Lee, D.-J., et al. (2013). Microalgae-Based Carbohydrates for Biofuel Production. Biochem. Eng. J. 78, 1–10. doi:10.1016/j.bej.2013.03.006
Chen, G.-Q. (2009). A Microbial Polyhydroxyalkanoates (PHA) Based Bio-and Materials Industry. Chem. Soc. Rev. 38, 2434–2446. doi:10.1039/b812677c
Chen, J.-H., Chen, C.-Y., Hasunuma, T., Kondo, A., Chang, C.-H., Ng, I.-S., et al. (2019). Enhancing Lutein Production With Mixotrophic Cultivation of Chlorella sorokiniana MB-1-M12 Using Different Bioprocess Operation Strategies. Bioresour. Technol. 278, 17–25. doi:10.1016/j.biortech.2019.01.041
Cheng, J., Lai, X., Ye, Q., Guo, W., Xu, J., Ren, W., et al. (2019a). A Novel Jet-Aerated Tangential Swirling-Flow Plate Photobioreactor Generates Microbubbles that Enhance Mass Transfer and Improve Microalgal Growth. Bioresour. Technology 288, 121531. doi:10.1016/j.biortech.2019.121531
Cheng, J., Xu, J., Lu, H., Ye, Q., Liu, J., and Zhou, J. (2018). Generating Cycle Flow Between Dark and Light Zones with Double Paddlewheels to Improve Microalgal Growth in a Flat Plate Photo-Bioreactor. Bioresour. Technology 261, 151–157. doi:10.1016/j.biortech.2018.04.022
Cheng, J., Xu, J., Ye, Q., Lai, X., Zhang, X., and Zhou, J. (2019b). Strengthening Mass Transfer of Carbon Dioxide Microbubbles Dissolver in a Horizontal Tubular Photo-Bioreactor for Improving Microalgae Growth. Bioresour. Technology 277, 11–17. doi:10.1016/j.biortech.2019.01.019
Cheng, J., Yang, Z., Huang, Y., Huang, L., Hu, L., Xu, D., et al. (2015a). Improving Growth Rate of Microalgae in a 1191m2 Raceway Pond to Fix CO2 from Flue Gas in a Coal-Fired Power Plant. Bioresour. Technology 190, 235–241. doi:10.1016/j.biortech.2015.04.085
Cheng, J., Yang, Z., Ye, Q., Zhou, J., and Cen, K. (2015b). Enhanced Flashing Light Effect with Up-Down Chute Baffles to Improve Microalgal Growth in a Raceway Pond. Bioresour. Technology 190, 29–35. doi:10.1016/j.biortech.2015.04.050
Chi, N. T. L., Mathimani, T., Manigandan, S., Shanmugam, S., Ha, N. T., Nhung, T. C., et al. (2022). Small Scale Photobioreactor, Outdoor Open Pond Cultivation of Chlorella sp. and Harvesting at Log and Stationary Growth Phase Towards Lipids and Methyl Ester Production. Fuel 319, 123813. doi:10.1016/j.fuel.2022.123813
Chiang, C.-L., Lee, C.-M., and Chen, P.-C. (2011). Utilization of the Cyanobacteria Anabaena sp. CH1 in Biological Carbon Dioxide Mitigation Processes. Bioresour. Technology 102, 5400–5405. doi:10.1016/j.biortech.2010.10.089
Chisti, Y. (2007). Biodiesel from Microalgae. Biotechnol. Adv. 25, 294–306. doi:10.1016/j.biotechadv.2007.02.001
Cho, S., Luong, T. T., Lee, D., Oh, Y.-K., and Lee, T. (2011). Reuse of Effluent Water from a Municipal Wastewater Treatment Plant in Microalgae Cultivation for Biofuel Production. Bioresour. Technology 102, 8639–8645. doi:10.1016/j.biortech.2011.03.037
Choi, J.-I., and Lee, S. Y. (1997). Process Analysis and Economic Evaluation for Poly(3-Hydroxybutyrate) Production by Fermentation. Bioproc. Eng. 17, 335–342. doi:10.1007/s004490050394
Ciferri, O., and Tiboni, O. (1985). The Biochemistry and Industrial Potential of Spirulina. Annu. Rev. Microbiol. 39, 503–526. doi:10.1146/annurev.mi.39.100185.002443
Constantino, A., Rodrigues, B., Leon, R., Barros, R., and Raposo, S. (2021). Alternative Chemo-Enzymatic Hydrolysis Strategy Applied to Different Microalgae Species for Bioethanol Production. Algal Res. 56, 102329. doi:10.1016/j.algal.2021.102329
Costa, J. A. V., and De Morais, M. G. (2014). “An Open Pond System for Microalgal Cultivation,” in Biofuels from Algae (Amsterdam: Elsevier), 1–22. doi:10.1016/b978-0-444-59558-4.00001-2
Daboussi, F., Leduc, S., Marechal, A., Dubois, G., Guyot, V., Perez-Michaut, C., et al. (2014). Genome Engineering Empowers the Diatom Phaeodactylum tricornutum for Biotechnology. Nat. Commun. 5 (1), 1–7. doi:10.1038/ncomms4831
Dammak, M., Hadrich, B., Miladi, R., Barkallah, M., Hentati, F., Hachicha, R., et al. (2017). Effects of Nutritional Conditions on Growth and Biochemical Composition of Tetraselmis sp. Lipids Health Dis. 16, 1–13. doi:10.1186/s12944-016-0378-1
Daneshvar, E., Antikainen, L., Koutra, E., Kornaros, M., and Bhatnagar, A. (2018). Investigation on the Feasibility of Chlorella vulgaris Cultivation in a Mixture of Pulp and Aquaculture Effluents: Treatment of Wastewater and Lipid Extraction. Bioresour. Technology 255, 104–110. doi:10.1016/j.biortech.2018.01.101
Danquah, M. K., Ang, L., Uduman, N., Moheimani, N., and Forde, G. M. (2009). Dewatering of Microalgal Culture for Biodiesel Production: Exploring Polymer Flocculation and Tangential Flow Filtration. J. Chem. Technol. Biotechnol. 84, 1078–1083. doi:10.1002/jctb.2137
Dassey, A. J., and Theegala, C. S. (2013). Harvesting Economics and Strategies Using Centrifugation for Cost Effective Separation of Microalgae Cells for Biodiesel Applications. Bioresour. Technology 128, 241–245. doi:10.1016/j.biortech.2012.10.061
De Baerdemaeker, T., Lemmens, B., Dotremont, C., Fret, J., Roef, L., Goiris, K., et al. (2013). Benchmark Study on Algae Harvesting with Backwashable Submerged Flat Panel Membranes. Bioresour. Technol. 129, 582–591. doi:10.1016/j.biortech.2012.10.153
De La Obra, I., Ponce-Robles, L., Miralles-Cuevas, S., Oller, I., Malato, S., and Sánchez Pérez, J. A. (2017). Microcontaminant Removal in Secondary Effluents by Solar Photo-Fenton at Circumneutral pH in Raceway Pond Reactors. Catal. Today 287, 10–14. doi:10.1016/j.cattod.2016.12.028
Debnath, C., Bandyopadhyay, T. K., Bhunia, B., Mishra, U., Narayanasamy, S., and Muthuraj, M. (2021). Microalgae: Sustainable Resource of Carbohydrates in Third-Generation Biofuel Production. Renew. Sustainable Energ. Rev. 150, 111464. doi:10.1016/j.rser.2021.111464
Desai, R. K., Fernandez, M. S., Wijffels, R. H., and Eppink, M. H. M. (2019). Mild Fractionation of Hydrophilic and Hydrophobic Components from Neochloris oleoabundans Using Ionic Liquids. Front. Bioeng. Biotechnol. 7, 284. doi:10.3389/fbioe.2019.00284
Deshmukh, A. R., Aloui, H., Khomlaem, C., Negi, A., Yun, J.-H., Kim, H.-S., et al. (2021). Biodegradable Films Based on Chitosan and Defatted Chlorella Biomass: Functional and Physical Characterization. Food Chem. 337, 127777. doi:10.1016/j.foodchem.2020.127777
Diao, J., Song, X., Cui, J., Liu, L., Shi, M., Wang, F., et al. (2019). Rewiring Metabolic Network by Chemical Modulator Based Laboratory Evolution Doubles Lipid Production in Crypthecodinium cohnii. Metab. Eng. 51, 88–98. doi:10.1016/j.ymben.2018.10.004
Do, C. V. T., Dinh, C. T., Dang, M. T., Tran, T. D., and Le, T. G. (2022). A Novel Flat-Panel Photobioreactor for Simultaneous Production of Lutein and Carbon Sequestration by Chlorella sorokiniana TH01. Bioresour. Technol. 345, 126552. doi:10.1016/j.biortech.2021.126552
Dragone, G., Fernandes, B. D., Abreu, A. P., Vicente, A. A., and Teixeira, J. A. (2011). Nutrient Limitation as a Strategy for Increasing Starch Accumulation in Microalgae. Appl. Energy 88, 3331–3335. doi:10.1016/j.apenergy.2011.03.012
Dubey, S., and Mishra, S. (2021). Efficient Production of Polyhydroxyalkanoate Through Halophilic Bacteria Utilizing Algal Biodiesel Waste Residue. Front. Bioeng. Biotechnol. 9, 624859. doi:10.3389/fbioe.2021.624859
Duffy, J. E., Canuel, E. A., Adey, W., and Swaddle, J. P. (2009). Biofuels: Algae. Science 326, 1345. doi:10.1126/science.326.5958.1345-a
Edzwald, J. K. (1993). Algae, Bubbles, Coagulants, and Dissolved Air Flotation. Water Sci. Technol. 27, 67–81. doi:10.2166/wst.1993.0207
Eldalatony, M. M., Kabra, A. N., Hwang, J.-H., Govindwar, S. P., Kim, K.-H., Kim, H., et al. (2016). Pretreatment of Microalgal Biomass for Enhanced Recovery/Extraction of Reducing Sugars and Proteins. Bioproc. Biosyst. Eng. 39, 95–103. doi:10.1007/s00449-015-1493-5
Feng, H., Zhang, B., He, Z., Wang, S., Salih, O., and Wang, Q. (2018). Study on Co-Liquefaction of Spirulina and Spartina alterniflora in Ethanol-Water Co-Solvent for Bio-Oil. Energy 155, 1093–1101. doi:10.1016/j.energy.2018.02.146
Fernández, F. A., Sevilla, J. F., Pérez, J. S., Grima, E. M., and Chisti, Y. (2001). Airlift-Driven External-Loop Tubular Photobioreactors for Outdoor Production of Microalgae: Assessment of Design and Performance. Chem. Eng. Sci. 56, 2721–2732. doi:10.1016/S0009-2509(00)00521-2
Ferreira, A. S., Mendonça, I., Póvoa, I., Carvalho, H., Correia, A., Vilanova, M., et al. (2021). Impact of Growth Medium Salinity on Galactoxylan Exopolysaccharides of Porphyridium purpureum. Algal Res. 59, 102439. doi:10.1016/j.algal.2021.102439
Franz, A. K., Danielewicz, M. A., Wong, D. M., Anderson, L. A., and Boothe, J. R. (2013). Phenotypic Screening with Oleaginous Microalgae Reveals Modulators of Lipid Productivity. ACS Chem. Biol. 8, 1053–1062. doi:10.1021/cb300573r
Fu, W., Guðmundsson, Ó., Paglia, G., Herjólfsson, G., Andrésson, Ó. S., Palsson, B. Ø., et al. (2013). Enhancement of Carotenoid Biosynthesis in the Green Microalga Dunaliella salina with Light-Emitting Diodes and Adaptive Laboratory Evolution. Appl. Microbiol. Biotechnol. 97, 2395–2403. doi:10.1007/s00253-012-4502-5
Galloway, R. A., and Krauss, R. W. (1961). The Effect of CO2 on pH in Culture Media for Algae. Plant Cel. Physiol. 2, 331–337. doi:10.1093/oxfordjournals.pcp.a077689
Gao, K., Liu, Q., Gao, Z., Xue, C., Qian, P., Dong, J., et al. (2021). A Dilution Strategy Used to Enhance Nutrient Removal and Biomass Production of Chlorella sorokiniana in Frigon Wastewater. Algal Res. 58, 102438. doi:10.1016/j.algal.2021.102438
García-Pérez, J. S., Beuckels, A., Vandamme, D., Depraetere, O., Foubert, I., Parra, R., et al. (2014). Influence of Magnesium Concentration, Biomass Concentration and pH on Flocculation of Chlorella vulgaris. Algal Res. 3, 24–29. doi:10.1016/j.algal.2013.11.016
Garg, S., Wang, L., and Schenk, P. M. (2014). Effective Harvesting of Low Surface-Hydrophobicity Microalgae by Froth Flotation. Bioresour. Technology 159, 437–441. doi:10.1016/j.biortech.2014.03.030
Gatamaneni, B. L., Orsat, V., and Lefsrud, M. (2018). Factors Affecting Growth of Various Microalgal Species. Environ. Eng. Sci. 35, 1037–1048. doi:10.1089/ees.2017.0521
Gensemer, R. W., Smith, R. E. H., and Duthie, H. C. (1993). Comparative Effects of pH and Aluminum on Silica-Limited Growth and Nutrient Uptake in Asterionella ralfsii Var. Americana (Bacillariophyceae)1. J. Phycol. 29, 36–44. doi:10.1111/j.1529-8817.1993.tb00277.x
Gentili, F. G. (2014). Microalgal Biomass and Lipid Production in Mixed Municipal, Dairy, Pulp and Paper Wastewater Together with Added Flue Gases. Bioresour. Technology 169, 27–32. doi:10.1016/j.biortech.2014.06.061
Ghosh, S., Gnaim, R., Greiserman, S., Fadeev, L., Gozin, M., and Golberg, A. (2019). Macroalgal Biomass Subcritical Hydrolysates for the Production of Polyhydroxyalkanoate (PHA) by Haloferax mediterranei. Bioresour. Technology 271, 166–173. doi:10.1016/j.biortech.2018.09.108
González-Camejo, J., Robles, A., Seco, A., Ferrer, J., and Ruano, M. V. (2020). On-Line Monitoring of Photosynthetic Activity Based on pH Data to Assess Microalgae Cultivation. J. Environ. Manage. 276, 111343. doi:10.1016/j.jenvman.2020.111343
Gouveia, L., Graça, S., Sousa, C., Ambrosano, L., Ribeiro, B., Botrel, E. P., et al. (2016). Microalgae Biomass Production Using Wastewater: Treatment and Costs: Scale-Up Considerations. Algal Res. 16, 167–176. doi:10.1016/j.algal.2016.03.010
Gregg, M., Rigby, R., and Hallegraeff, G. (2009). Review of Two Decades of Progress in the Development of Management Options for Reducing or Eradicating Phytoplankton, Zooplankton and Bacteria in Ship's Ballast Water. Ai 4, 521–565. doi:10.3391/ai.2009.4.3.14
Grigore, M. E., Grigorescu, R. M., Iancu, L., Ion, R.-M., Zaharia, C., and Andrei, E. R. (2019). Methods of Synthesis, Properties and Biomedical Applications of Polyhydroxyalkanoates: a Review. J. Biomater. Sci. Polym. Edition 30, 695–712. doi:10.1080/09205063.2019.1605866
Grobbelaar, J. U., and Kurano, N. (2003). Use of Photoacclimation in the Design of a Novel Photobioreactor to Achieve High Yields in Algal Mass Cultivation. J. Appl. Phycology 15, 121–126. doi:10.1023/A:1023802820093
Grobbelaar, J. U., Nedbal, L., and Tichý, V. (1996). Influence of High Frequency Light/Dark Fluctuations on Photosynthetic Characteristics of Microalgae Photoacclimated to Different Light Intensities and Implications for Mass Algal Cultivation. J. Appl. Phycol. 8, 335–343. doi:10.1007/BF02178576
Guarnieri, M. T., Levering, J., Henard, C. A., Boore, J. L., Betenbaugh, M. J., Zengler, K., et al. (2018). Genome Sequence of the Oleaginous Green Alga, Chlorella vulgaris UTEX 395. Front. Bioeng. Biotechnol. 6, 37. doi:10.3389/fbioe.2018.00037
Guccione, A., Biondi, N., Sampietro, G., Rodolfi, L., Bassi, N., and Tredici, M. R. (2014). Chlorella for Protein and Biofuels: From Strain Selection to Outdoor Cultivation in a Green Wall Panel Photobioreactor. Biotechnol. Biofuels 7, 1–12. doi:10.1186/1754-6834-7-84
Hadiyanto, H., Elmore, S., Van Gerven, T., and Stankiewicz, A. (2013). Hydrodynamic Evaluations in High Rate Algae Pond (HRAP) Design. Chem. Eng. J. 217, 231–239. doi:10.1016/j.cej.2012.12.015
Hanifzadeh, M., Garcia, E. C., and Viamajala, S. (2018). Production of Lipid and Carbohydrate From Microalgae Without Compromising Biomass Productivities: Role of Ca and Mg. Renewable Energy 127, 989–997. doi:10.1016/j.renene.2018.05.012
Hanotu, J., Bandulasena, H. C. H., and Zimmerman, W. B. (2012). Microflotation Performance for Algal Separation. Biotechnol. Bioeng. 109, 1663–1673. doi:10.1002/bit.24449
Heasman, M., Diemar, J., O'connor, W., Sushames, T., and Foulkes, L. (2000). Development of Extended Shelf-Life Microalgae Concentrate Diets Harvested by Centrifugation for Bivalve Molluscs - a Summary. Aquac. Res. 31, 637–659. doi:10.1046/j.1365-2109.2000.00492.x
Henderson, R. K., Parsons, S. A., and Jefferson, B. (2009). The Potential for Using Bubble Modification Chemicals in Dissolved Air Flotation for Algae Removal. Separation Sci. Technology 44, 1923–1940. doi:10.1080/01496390902955628
Ho, S.-H., Chen, C.-Y., and Chang, J.-S. (2012). Effect of Light Intensity and Nitrogen Starvation on CO2 Fixation and Lipid/Carbohydrate Production of an Indigenous Microalga Scenedesmus obliquus CNW-N Bioresour. Technol. 113, 244–252. doi:10.1016/j.biortech.2011.11.133
Ho, S.-H., Chan, M.-C., Liu, C.-C., Chen, C.-Y., Lee, W.-L., Lee, D.-J., et al. (2014). Enhancing Lutein Productivity of an Indigenous Microalga Scenedesmus obliquus FSP-3 Using Light-Related Strategies. Bioresour. Technology 152, 275–282. doi:10.1016/j.biortech.2013.11.031
Hong, M. E., Yu, B. S., Patel, A. K., Choi, H. I., Song, S., Sung, Y. J., et al. (2019). Enhanced Biomass and Lipid Production of Neochloris oleoabundans Under High Light Conditions by Anisotropic Nature of Light-Splitting CaCO3 Crystal. Bioresour. Technology 287, 121483. doi:10.1016/j.biortech.2019.121483
Horowitz, A. J. (1986). Comparison of Methods for the Concentration of Suspended Sediment in River Water for Subsequent Chemical Analysis. Environ. Sci. Technol. 20, 155–160. doi:10.1021/es00144a007
Hosseinizand, H., Sokhansanj, S., and Lim, C. J. (2018). Studying the Drying Mechanism of Microalgae Chlorella vulgaris and the Optimum Drying Temperature to Preserve Quality Characteristics. Drying Technology 36, 1049–1060. doi:10.1080/07373937.2017.1369986
Huang, Q., Jiang, F., Wang, L., and Yang, C. (2017). Design of Photobioreactors for Mass Cultivation of Photosynthetic Organisms. Engineering 3, 318–329. doi:10.1016/J.ENG.2017.03.020
Huang, R., Liu, Z., Yan, B., Li, Y., Li, H., Liu, D., et al. (2020). Interfacial Catalytic Oxidation for Membrane Fouling Mitigation during Algae-Laden Water Filtration: Higher Efficiency without Algae Integrity Loss. Separation Purif. Technology 251, 117366. doi:10.1016/j.seppur.2020.117366
Hulatt, C. J., Wijffels, R. H., Bolla, S., and Kiron, V. (2017). Production of Fatty Acids and Protein by Nannochloropsis in Flat-Plate Photobioreactors. PLoS One 12, e0170440. doi:10.1371/journal.pone.0170440
Ifrim, G. A., Titica, M., Cogne, G., Boillereaux, L., Legrand, J., and Caraman, S. (2014). Dynamic pH Model for Autotrophic Growth of Microalgae in Photobioreactor: A Tool for Monitoring and Control Purposes. Aiche J. 60, 585–599. doi:10.1002/aic.14290
Jambeck, J. R., Geyer, R., Wilcox, C., Siegler, T. R., Perryman, M., Andrady, A., et al. (2015). Plastic Waste Inputs from Land into the Ocean. Science 347, 768–771. doi:10.1126/science.1260352
Jiang, G., Hill, D., Kowalczuk, M., Johnston, B., Adamus, G., Irorere, V., et al. (2016). Carbon Sources for Polyhydroxyalkanoates and an Integrated Biorefinery. Ijms 17, 1157. doi:10.3390/ijms17071157
Jiang, W., Brueggeman, A. J., Horken, K. M., Plucinak, T. M., and Weeks, D. P. (2014). Successful Transient Expression of Cas9 and Single Guide RNA Genes in Chlamydomonas reinhardtii. Eukaryotic cell 13 (11), 1465–1469. doi:10.1128/EC.00213-14
Kaneko, T., and Tabata, S. (1997). Complete Genome Structure of the Unicellular Cyanobacterium Synechocystis sp. PCC6803. Plant Cel. Physiol. 38, 1171–1176. doi:10.1093/oxfordjournals.pcp.a029103
Karemore, A., and Sen, R. (2015). Green Integrated Process for Mitigation of Municipal and Industrial Liquid and Solid Waste Mixes for Enhanced Microalgal Biomass and Lipid Synthesis for Biodiesel. RSC Adv. 5, 70929–70938. doi:10.1039/C5RA11670H
Keris-Sen, U. D., and Gurol, M. D. (2017). Using Ozone for Microalgal Cell Disruption to Improve Enzymatic Saccharification of Cellular Carbohydrates. Biomass and Bioenergy 105, 59–65. doi:10.1016/j.biombioe.2017.06.023
Khalili, A., Najafpour, G. D., Amini, G., and Samkhaniyani, F. (2015). Influence of Nutrients and LED Light Intensities on Biomass Production of Microalgae Chlorella vulgaris. Biotechnol. Bioproc. E 20, 284–290. doi:10.1007/s12257-013-0845-8
Khan, M. I., Shin, J. H., and Kim, J. D. (2018). The Promising Future of Microalgae: Current Status, Challenges, and Optimization of a Sustainable and Renewable Industry for Biofuels, Feed, and Other Products. Microb. Cel. Fact. 17, 1–21. doi:10.1186/s12934-018-0879-x
Khanna, S., and Srivastava, A. K. (2005). Recent Advances in Microbial Polyhydroxyalkanoates. Process Biochem. 40, 607–619. doi:10.1016/j.procbio.2004.01.053
Khomlaem, C., Aloui, H., and Kim, B. S. (2021). Biosynthesis of Polyhydroxyalkanoates from Defatted Chlorella Biomass as an Inexpensive Substrate. Appl. Sci. 11, 1094. doi:10.3390/app11031094
Kilham, S., Kreeger, D., Goulden, C., and Lynn, S. (1997). Effects of Nutrient Limitation on Biochemical Constituents of Ankistrodesmus falcatus. Freshw. Biol. 38, 591–596. doi:10.1046/j.1365-2427.1997.00231.x
Kim, E.-J., Ma, X., and Cerutti, H. (2015). Gene Silencing in Microalgae: Mechanisms and Biological Roles. Bioresour. Technology 184, 23–32. doi:10.1016/j.biortech.2014.10.119
Kim, S., Moon, M., Kwak, M., Lee, B., and Chang, Y. K. (2018). Statistical Optimization of Light Intensity and CO2 Concentration for Lipid Production Derived from Attached Cultivation of Green Microalga Ettlia sp. Sci. Rep. 8, 1–13. doi:10.1038/s41598-018-33793-1
Kim, Z.-H., Park, Y.-S., Ryu, Y.-J., and Lee, C.-G. (2017). Enhancing Biomass and Fatty Acid Productivity of Tetraselmis sp. In Bubble Column Photobioreactors by Modifying Light Quality Using Light Filters. Biotechnol. Bioproc. E 22, 397–404. doi:10.1007/s12257-017-0200-6
Koller, M., Bona, R., Chiellini, E., Fernandes, E. G., Horvat, P., Kutschera, C., Hesse, P., and Braunegg, G. (2008). Polyhydroxyalkanoate Production From Whey by Pseudomonas hydrogenovora. Bioresour. Technol. 99, 4854–4863. doi:10.1016/j.biortech.2007.09.049
Krishnamoorthy, N., Unpaprom, Y., Ramaraj, R., Maniam, G. P., Govindan, N., Arunachalam, T., et al. (2021). Recent Advances and Future Prospects of Electrochemical Processes for Microalgae Harvesting. J. Environ. Chem. Eng. 9, 105875. doi:10.1016/j.jece.2021.105875
Kroth, P. G., Chiovitti, A., Gruber, A., Martin-Jezequel, V., Mock, T., Parker, M. S., et al. (2008). A Model for Carbohydrate Metabolism in the Diatom Phaeodactylum tricornutum Deduced From Comparative Whole Genome Analysis. PLoS One 3, e1426. doi:10.1371/journal.pone.0001426
Kumar, A. K., Sharma, S., Dixit, G., Shah, E., and Patel, A. (2020). Techno-Economic Analysis of Microalgae Production With Simultaneous Dairy Effluent Treatment Using a Pilot-Scale High Volume V-Shape Pond System. Renew.e Energy 145, 1620–1632. doi:10.1016/j.renene.2019.07.087
Kumar, A. K., Sharma, S., Patel, A., Dixit, G., and Shah, E. (2019a). Comprehensive Evaluation of Microalgal Based Dairy Effluent Treatment Process for Clean Water Generation and Other Value Added Products. Int. J. Phytoremediation 21, 519–530. doi:10.1080/15226514.2018.1537248
Kumar, N., Banerjee, C., Kumar, N., and Jagadevan, S. (2019b). A Novel Non-Starch Based Cationic Polymer as Flocculant for Harvesting Microalgae. Bioresour. Technology 271, 383–390. doi:10.1016/j.biortech.2018.09.073
Kurniawati, H. A., Ismadji, S., and Liu, J. C. (2014). Microalgae Harvesting by Flotation Using Natural Saponin and Chitosan. Bioresour. Technology 166, 429–434. doi:10.1016/j.biortech.2014.05.079
Kushwaha, D., Upadhyay, S., and Mishra, P. K. (2018). Growth of Cyanobacteria: Optimization for Increased Carbohydrate Content. Appl. Biochem. Biotechnol. 184, 1247–1262. doi:10.1007/s12010-017-2620-3
Laamanen, C. A., Ross, G. M., and Scott, J. A. (2016). Flotation Harvesting of Microalgae. Renew. Sustainable Energ. Rev. 58, 75–86. doi:10.1016/j.rser.2015.12.293
Lamb, J. B., Willis, B. L., Fiorenza, E. A., Couch, C. S., Howard, R., Rader, D. N., et al. (2018). Plastic Waste Associated with Disease on Coral Reefs. Science 359, 460–462. doi:10.1126/science.aar3320
Leong, Y. K., Show, P. L., Ooi, C. W., Ling, T. C., and Lan, J. C.-W. (2014). Current Trends in Polyhydroxyalkanoates (PHAs) Biosynthesis: Insights from the Recombinant Escherichia coli. J. Biotechnol. 180, 52–65. doi:10.1016/j.jbiotec.2014.03.020
Li, J.-Y., Wang, S.-Y., Ni, Z.-Y., Xu, X.-H., Cheng, L.-H., and Yue, A. (2018). Improving Microalgal Growth in Column Photobioreactor with Internal Light Column. Bioresour. Technology Rep. 4, 181–185. doi:10.1016/j.biteb.2018.10.006
Li, T., Chen, Z., Wu, J., Wu, H., Yang, B., Dai, L., et al. (2020a). The Potential Productivity of the Microalga, Nannochloropsis oceanica SCS-1981, in a Solar Powered Outdoor Open Pond as an Aquaculture Feed. Algal Res. 46, 101793. doi:10.1016/j.algal.2020.101793
Li, X., Sun, H., Mao, X., Lao, Y., and Chen, F. (2020b). Enhanced Photosynthesis of Carotenoids in Microalgae Driven by Light-Harvesting Gold Nanoparticles. ACS Sustainable Chem. Eng. 8, 7600–7608. doi:10.1021/acssuschemeng.0c00315
Liang, H., Gong, W., Chen, J., and Li, G. (2008). Cleaning of Fouled Ultrafiltration (UF) Membrane by Algae During Reservoir Water Treatment. Desalination 220, 267–272. doi:10.1016/j.desal.2007.01.033
Liang, Y., Sarkany, N., and Cui, Y. (2009). Biomass and Lipid Productivities of Chlorella vulgaris under Autotrophic, Heterotrophic and Mixotrophic Growth Conditions. Biotechnol. Lett. 31, 1043–1049. doi:10.1007/s10529-009-9975-7
Liao, Q., Li, L., Chen, R., and Zhu, X. (2014). A Novel Photobioreactor Generating the Light/Dark Cycle to Improve Microalgae Cultivation. Bioresour. Technology 161, 186–191. doi:10.1016/j.biortech.2014.02.119
Lin, Q., and Lin, J. (2011). Effects of Nitrogen Source and Concentration on Biomass and Oil Production of a Scenedesmus rubescens Like Microalga. Bioresour. Technol. 102, 1615–1621. doi:10.1016/j.biortech.2010.09.008
Liu, H., Kumar, V., Jia, L., Sarsaiya, S., Kumar, D., Juneja, A., et al. (2021). Biopolymer Poly-Hydroxyalkanoates (PHA) Production from Apple Industrial Waste Residues: A Review. Chemosphere 284, 131427. doi:10.1016/j.chemosphere.2021.131427
Liu, J., Pemberton, B., Lewis, J., Scales, P. J., and Martin, G. J. O. (2020). Wastewater Treatment Using Filamentous Algae - A Review. Bioresour. Technology 298, 122556. doi:10.1016/j.biortech.2019.122556
López, M. G.-M., Sánchez, E. D. R., López, J. C., Fernández, F. A., Sevilla, J. F., Rivas, J., Guerrero, M., and Grima, E. M. (2006). Comparative Analysis of the Outdoor Culture of Haematococcus pluvialis in Tubular And Bubble Column Photobioreactors. J. Biotechnol. 123, 329–342. doi:10.1016/j.jbiotec.2005.11.010
Lu, Y.-M., Xiang, W.-Z., and Wen, Y.-H. (2011). Spirulina (Arthrospira) Industry in Inner Mongolia of China: Current Status and Prospects. J. Appl. Phycol. 23, 265–269. doi:10.1007/s10811-010-9552-4
Ma, Y., Wang, Z., Yu, C., Yin, Y., and Zhou, G. (2014). Evaluation of the Potential of 9 Nannochloropsis Strains for Biodiesel Production. Bioresour. Technology 167, 503–509. doi:10.1016/j.biortech.2014.06.047
Machingura, M. C., and Moroney, J. V. (2018). Closing the Circle. Elife 7, e42507. doi:10.7554/eLife.42507
Madadi, R., Maljaee, H., Serafim, L. S., and Ventura, S. P. (2021). Microalgae as Contributors to Produce Biopolymers. Mar. Drugs 19, 466. doi:10.3390/md19080466
Marbelia, L., Mulier, M., Vandamme, D., Muylaert, K., Szymczyk, A., and Vankelecom, I. F. J. (2016). Polyacrylonitrile Membranes for Microalgae Filtration: Influence of Porosity, Surface Charge and Microalgae Species on Membrane Fouling. Algal Res. 19, 128–137. doi:10.1016/j.algal.2016.08.004
Markou, G., Angelidaki, I., and Georgakakis, D. (2012). Microalgal Carbohydrates: An Overview of the Factors Influencing Carbohydrates Production, and of Main Bioconversion Technologies for Production of Biofuels. Appl. Microbiol. Biotechnol. 96, 631-645. doi:10.1007/s00253-012-4398-0
Marles, R. J., Barrett, M. L., Barnes, J., Chavez, M. L., Gardiner, P., Ko, R., et al. (2011). United States Pharmacopeia Safety Evaluation of Spirulina. Crit. Rev. Food Sci. Nutr. 51, 593–604. doi:10.1080/10408391003721719
Marn, N., Jusup, M., Kooijman, S. A. L. M., and Klanjscek, T. (2020). Quantifying Impacts of Plastic Debris on Marine Wildlife Identifies Ecological Breakpoints. Ecol. Lett. 23, 1479–1487. doi:10.1111/ele.13574
Mata, T. M., Martins, A. A., and Caetano, N. S. (2010). Microalgae for Biodiesel Production and Other Applications: A Review. Renew. Sustainable Energ. Rev. 14, 217–232. doi:10.1016/j.rser.2009.07.020
Mathuriya, A. S., Yakhmi, J. V., Martínez, L., Kharissova, O., and Kharisov, B. (2017). “Polyhydroxyalkanoates: Biodegradable Plastics and Their Applications,” in Handbook of Ecomaterials (Berlin: Springer), 1–29. doi:10.1007/978-3-319-48281-1_84-1
Medina, A. R., Grima, E. M., Giménez, A. G., and González, M. J. I. (1998). Downstream Processing of Algal Polyunsaturated Fatty Acids. Biotechnol. Adv. 16, 517–580. doi:10.1016/S0734-9750(97)00083-9
Mehta, A. K., and Chakraborty, S. (2021). A Rapid, Low-Cost Flocculation Technology for Enhanced Microalgae Harvesting. Bioresour. Technology Rep. 16, 100856. doi:10.1016/j.biteb.2021.100856
Merchant, S. S., Prochnik, S. E., Vallon, O., Harris, E. H., Karpowicz, S. J., Witman, G. B., et al. (2007). The Chlamydomonas Genome Reveals the Evolution of Key Animal and Plant Functions. Science 318, 245–250. doi:10.1126/science.1143609
Milledge, J. J., and Heaven, S. (2013). A Review of the Harvesting of Micro-Algae for Biofuel Production. Rev. Environ. Sci. Biotechnol. 12, 165–178. doi:10.1007%252Fs11157-012-9301-z10.1007/s11157-012-9301-z
Mishra, S. C. P., Ghosh, P. K., Gandhi, M. R., Bhattacharya, S., Maiti, S., Upadhyay, S. C., et al. (2014). Engine Worthy Fatty Acid Methyl Ester (Biodiesel) From Naturally Occuring Marine Microalgal Mats and Marine Microalgae Cultured in Open Salt Pans Together with Value Addition of Co-Products. Alexandria, VA: Google Patents.
Mitra, D., Van Leeuwen, J., and Lamsal, B. (2012). Heterotrophic/Mixotrophic Cultivation of Oleaginous Chlorella vulgaris on Industrial Co-Products. Algal Res. 1, 40–48. doi:10.1016/j.algal.2012.03.002
Mohan, S. V., Rohit, M. V., Subhash, G. V., Chandra, R., Devi, M. P., Butti, S. K., et al. (2019). “Algal Oils as Biodiesel,” in Biofuels from Algae (Amsterdam: Elsevier), 287–323. doi:10.1016/b978-0-444-64192-2.00012-3
Mohapatra, S., Maity, S., Dash, H. R., Das, S., Pattnaik, S., Rath, C. C., et al. (2017). Bacillus and Biopolymer: Prospects and Challenges. Biochem. Biophys. Rep. 12, 206–213. doi:10.1016/j.bbrep.2017.10.001
Mohn, F. (1980). “Experiences and Strategies in the Recovery of Biomass from Mass Cultures of Microalgae,” in Algae Biomass: Production and Use (Amsterdam: Elsevier), 548–571.
Monte, J., Sá, M., Galinha, C. F., Costa, L., Hoekstra, H., Brazinha, C., et al. (2018). Harvesting of Dunaliella salina by Membrane Filtration at Pilot Scale. Separation Purif. Technology 190, 252–260. doi:10.1016/j.seppur.2017.08.019
Moreno-Garcia, L., Adjallé, K., Barnabé, S., and Raghavan, G. S. V. (2017). Microalgae Biomass Production for a Biorefinery System: Recent Advances and the Way Towards Sustainability. Renew. Sustainable Energ. Rev. 76, 493–506. doi:10.1016/j.rser.2017.03.024
Moreno-Garrido, I. (2008). Microalgae Immobilization: Current Techniques and Uses. Bioresour. Technology 99, 3949–3964. doi:10.1016/j.biortech.2007.05.040
Morris, I., Glover, H. E., and Yentsch, C. S. (1974). Products of Photosynthesis by Marine Phytoplankton: The Effect of Environmental Factors on the Relative Rates of Protein Synthesis. Mar. Biol. 27, 1–9. doi:10.1007/BF00394754
Mozejko-Ciesielska, J., Szacherska, K., and Marciniak, P. (2019). Pseudomonas Species as Producers of Eco-Friendly Polyhydroxyalkanoates. J. Polym. Environ. 27, 1151–1166. doi:10.1007/s10924-019-01422-1
Muhammad, M., Aloui, H., Khomlaem, C., Hou, C. T., and Kim, B. S. (2020). Production of Polyhydroxyalkanoates and Carotenoids through Cultivation of Different Bacterial Strains Using Brown Algae Hydrolysate as a Carbon Source. Biocatal. Agric. Biotechnol. 30, 101852. doi:10.1016/j.bcab.2020.101852
Muthuraj, M., Kumar, V., Palabhanvi, B., and Das, D. (2014). Evaluation of indigenous Microalgal Isolate Chlorella sp. FC2 IITG as a Cell Factory for Biodiesel Production and Scale up in Outdoor Conditions. J. Ind. Microbiol. Biotechnol. 41, 499–511. doi:10.1007/s10295-013-1397-9
Naduthodi, M. I. S., Mohanraju, P., Südfeld, C., D’Adamo, S., Barbosa, M. J., and Van Der Oost, J. (2019). CRISPR-Cas Ribonucleoprotein Mediated Homology-Directed Repair for Efficient Targeted Genome Editing in Microalgae Nannochloropsis oceanica IMET1. Biotechnol. Biofuels 12, 1–11. doi:10.1186/s13068-019-1401-3
Nair, A., and Chakraborty, S. (2020). Synergistic Effects Between Autotrophy and Heterotrophy in Optimization of Mixotrophic Cultivation of Chlorella sorokiniana in Bubble-Column Photobioreactors. Algal Res. 46, 101799. doi:10.1016/j.algal.2020.101799
Najjar, Y. S. H., and Abu-Shamleh, A. (2020). Harvesting of Microalgae by Centrifugation for Biodiesel Production: A Review. Algal Res. 51, 102046. doi:10.1016/j.algal.2020.102046
Ndikubwimana, T., Chang, J., Xiao, Z., Shao, W., Zeng, X., Ng, I.-S., et al. (2016). Flotation: A Promising Microalgae Harvesting and Dewatering Technology for Biofuels Production. Biotechnol. J. 11, 315–326. doi:10.1002/biot.201500175
Nwoba, E. G., Rohani, T., Raeisossadati, M., Vadiveloo, A., Bahri, P. A., and Moheimani, N. R. (2021). Monochromatic Light Filters to Enhance Biomass and Carotenoid Productivities of Dunaliella salina in Raceway Ponds. Bioresour. Technology 340, 125689. doi:10.1016/j.biortech.2021.125689
Olguín, E. J., Galicia, S., Mercado, G., and Pérez, T. (2003). Annual Productivity of Spirulina (Arthrospira) and Nutrient Removal in a Pig Wastewater Recycling Process Under Tropical Conditions. J. Appl. Phycol. 15, 249–257. doi:10.1023/A:1023856702544
Olivieri, G., Gargano, I., Andreozzi, R., Marotta, R., Marzocchella, A., Pinto, G., and Pollio, A. (2013). Effects of Photobioreactors Design and Operating Conditions on Stichococcus bacillaris Biomass and Biodiesel Production. Biochem. Eng. J. 74, 8–14. doi:10.1016/j.bej.2013.02.006
Ong, S. Y., Zainab-L, I., Pyary, S., and Sudesh, K. (2018). A Novel Biological Recovery Approach for PHA Employing Selective Digestion of Bacterial Biomass in Animals. Appl. Microbiol. Biotechnol. 102, 2117–2127. doi:10.1007/s00253-018-8788-9
Packer, M. (2009). Algal Capture of Carbon Dioxide; Biomass Generation as a Tool for Greenhouse Gas Mitigation with Reference to New Zealand Energy Strategy and Policy. Energy Policy 37, 3428–3437. doi:10.1016/j.enpol.2008.12.025
Paliwal, C., and Jutur, P. P. (2021). Dynamic Allocation of Carbon Flux Triggered by Task-Specific Chemicals Is an Effective Non-Gene Disruptive Strategy for Sustainable and Cost-Effective Algal Biorefineries. Chem. Eng. J. 418, 129413. doi:10.1016/j.cej.2021.129413
Paliwal, C., Mitra, M., Bhayani, K., Bharadwaj, S.V., Ghosh, T., Dubey, S., and Mishra, S. (2017). Abiotic Stresses as Tools for Metabolites in Microalgae. Bioresour. Technol. 244, 1216–1226. doi:10.1016/j.biortech.2017.05.058
Pistocchi, R., Mormile, M., Guerrini, F., Isani, G., and Boni, L. (2000). Increased Production of Extra-and Intracellular Metal-Ligands in Phytoplankton Exposed to Copper and Cadmium. J. Appl. Phycol. 12, 469–477. doi:10.1023/a:1008162812651
Pleissner, D., Lindner, A. V., and Händel, N. (2021). Heterotrophic Cultivation of Galdieria sulphuraria Under Non-Sterile Conditions in Digestate and Hydrolyzed Straw. Bioresour. Technology 337, 125477. doi:10.1016/j.biortech.2021.125477
Polle, J. E. W., Barry, K., Cushman, J., Schmutz, J., Tran, D., Hathwaik, L. T., et al. (2017). Draft Nuclear Genome Sequence of the Halophilic and Beta-Carotene-Accumulating green Alga Dunaliella salina Strain CCAP19/18. Genome Announc 5, e01105–01117. doi:10.1128/genomeA.01105-17
Polo-López, M. I., and Sánchez Pérez, J. A. (2021). Perspectives of the Solar Photo-Fenton Process against the Spreading of Pathogens, Antibiotic-Resistant Bacteria and Genes in the Environment. Curr. Opin. Green Sustainable Chem. 27, 100416. doi:10.1016/j.cogsc.2020.100416
Povolo, S., Romanelli, M. G., Basaglia, M., Ilieva, V. I., Corti, A., Morelli, A., Chiellini, E., and Casella, S. (2013). Polyhydroxyalkanoate Biosynthesis by Hydrogenophaga pseudoflava DSM1034 From Structurally Unrelated Carbon Sources. New Biotechnol. 30, 629–634. doi:10.1016/j.nbt.2012.11.019
Qu, F., Du, X., Liu, B., He, J., Ren, N., Li, G., et al. (2015). Control of Ultrafiltration Membrane Fouling Caused by Microcystis Cells with Permanganate Preoxidation: Significance of In Situ Formed Manganese Dioxide. Chem. Eng. J. 279, 56–65. doi:10.1016/j.cej.2015.05.009
Quinn, J. C., Yates, T., Douglas, N., Weyer, K., Butler, J., Bradley, T. H., et al. (2012). Nannochloropsis Production Metrics in a Scalable Outdoor Photobioreactor for Commercial Applications. Bioresour. Technology 117, 164–171. doi:10.1016/j.biortech.2012.04.073
Rahman, A., Putman, R. J., Inan, K., Sal, F. A., Sathish, A., Smith, T., et al. (2015). Polyhydroxybutyrate Production Using a Wastewater Microalgae Based media. Algal Res. 8, 95–98. doi:10.1016/j.algal.2015.01.009
Ran, W., Xiang, Q., Pan, Y., Xie, T., Zhang, Y., and Yao, C. (2020). Enhancing Photosynthetic Starch Production by γ-Aminobutyric Acid Addition in a Marine Green Microalga Tetraselmis Subcordiformis Under Nitrogen Stress. Ind. Eng. Chem. Res. 59, 17103–17112. doi:10.1021/acs.iecr.0c00398
Rani, S., Gunjyal, N., Ojha, C. S. P., and Singh, R. P. (2021). Review of Challenges for Algae-Based Wastewater Treatment: Strain Selection, Wastewater Characteristics, Abiotic, and Biotic Factors. J. Hazard. Tox. Radioact. Waste 25, 03120004. doi:10.1061/(asce)hz.2153-5515.0000578
Rao, N. R. H., Yap, R., Whittaker, M., Stuetz, R. M., Jefferson, B., Peirson, W. L., et al. (2018). The Role of Algal Organic Matter in the Separation of Algae and Cyanobacteria Using the Novel “Posi”-Dissolved Air Flotation Process. Water Res. 130, 20–30. doi:10.1016/j.watres.2017.11.049
Reinecke, F., and Steinbüchel, A. (2009). Ralstonia eutropha Strain H16 as Model Organism for PHA Metabolism and for Biotechnological Production of Technically Interesting Biopolymers. J. Mol. Microbiol. Biotechnol. 16, 91–108. doi:10.1159/000142897
Rempel, A., Machado, T., Treichel, H., Colla, E., Margarites, A. C., and Colla, L. M. (2018). Saccharification of Spirulina Platensis Biomass Using Free and Immobilized Amylolytic Enzymes. Bioresour. Technology 263, 163–171. doi:10.1016/j.biortech.2018.04.114
Ren, H.-Y., Dai, Y.-Q., Kong, F., Xing, D., Zhao, L., Ren, N.-Q., et al. (2020). Enhanced Microalgal Growth and Lipid Accumulation by Addition of Different Nanoparticles Under Xenon Lamp Illumination. Bioresour. Technology 297, 122409. doi:10.1016/j.biortech.2019.122409
Rochaix, J.-D., and Van Dillewijn, J. (1982). Transformation of the Green Alga Chlamydomonas reinhardii with Yeast DNA. Nature 296, 70–72. doi:10.1038/296070a0
Rodolfi, L., Chini Zittelli, G., Bassi, N., Padovani, G., Biondi, N., Bonini, G., et al. (2009). Microalgae for Oil: Strain Selection, Induction of Lipid Synthesis and Outdoor Mass Cultivation in a Low-Cost Photobioreactor. Biotechnol. Bioeng. 102, 100–112. doi:10.1002/bit.22033
Rogers, J. N., Rosenberg, J. N., Guzman, B. J., Oh, V. H., Mimbela, L. E., Ghassemi, A., et al. (2014). A Critical Analysis of Paddlewheel-Driven Raceway Ponds for Algal Biofuel Production at Commercial Scales. Algal Res. 4, 76–88. doi:10.1016/j.algal.2013.11.007
Rotatore, C., and Colman, B. (1991). The Acquisition and Accumulation of Inorganic Carbon by the Unicellular Green Alga Chlorella ellipsoidea. Plant Cel. Environ. 14, 377–382. doi:10.1111/j.1365-3040.1991.tb00946.x
Ruangsomboon, S., Prachom, N., and Sornchai, P. (2017). Enhanced Growth and Hydrocarbon Production of Botryococcus braunii KMITL 2 by Optimum Carbon Dioxide Concentration and Concentration-Dependent Effects on its Biochemical Composition and Biodiesel Properties. Bioresour. Technology 244, 1358–1366. doi:10.1016/j.biortech.2017.06.042
Rubio, J., Souza, M. L., and Smith, R. W. (2002). Overview of Flotation as a Wastewater Treatment Technique. Minerals Eng. 15, 139–155. doi:10.1016/S0892-6875(01)00216-3
Ryu, B.-G., Kim, J., Han, J.-I., Kim, K., Kim, D., Seo, B.-K., et al. (2018). Evaluation of an Electro-Flotation-Oxidation Process for Harvesting Bio-Flocculated Algal Biomass and Simultaneous Treatment of Residual Pollutants in Coke Wastewater Following an Algal-Bacterial Process. Algal Res. 31, 497–505. doi:10.1016/j.algal.2017.06.012
Samantaray, S., and Mallick, N. (2012). Production and Characterization of Poly-β-Hydroxybutyrate (PHB) Polymer From Aulosira fertilissima. J. Appl. Phycol. 24, 803–814. doi:10.1007/s10811-011-9699-7
Sanchez-Galvis, E. M., Cardenas-Gutierrez, I. Y., Contreras-Ropero, J. E., García-Martínez, J. B., Barajas-Solano, A. F., and Zuorro, A. (2020). An Innovative Low-Cost Equipment for Electro-Concentration of Microalgal Biomass. Appl. Sci. 10, 4841. doi:10.3390/app10144841
Sandmann, M., Smetana, S., Heinz, V., and Rohn, S. (2021). Comparative Life Cycle Assessment of a Mesh Ultra-Thin Layer Photobioreactor and a Tubular Glass Photobioreactor for the Production of Bioactive Algae Extracts. Bioresour. Technol. 340, 125657.
Sankaran, R., Parra Cruz, R. A., Pakalapati, H., Show, P. L., Ling, T. C., Chen, W.-H., et al. (2020). Recent Advances in the Pretreatment of Microalgal and Lignocellulosic Biomass: A Comprehensive Review. Bioresour. Technology 298, 122476. doi:10.1016/j.biortech.2019.122476
Saratale, R. G., Kumar, G., Banu, R., Xia, A., Periyasamy, S., and Saratale, G. D. (2018). A Critical Review on Anaerobic Digestion of Microalgae and Macroalgae and Co-Digestion of Biomass for Enhanced Methane Generation. Bioresour. Technology 262, 319–332. doi:10.1016/j.biortech.2018.03.030
Sathish, A., Glaittli, K., Sims, R. C., and Miller, C. D. (2014). Algae Biomass Based Media for Poly(3-Hydroxybutyrate) (PHB) Production by Escherichia coli. J. Polym. Environ. 22, 272–277. doi:10.1007/s10924-014-0647-x
Sawant, S. S., Salunke, B. K., and Kim, B. S. (2018). Consolidated Bioprocessing for Production of Polyhydroxyalkanotes From Red Algae Gelidium amansii. Int. J. Biol. Macromol. 109, 1012–1018. doi:10.1016/j.ijbiomac.2017.11.084
Schenk, P. M., Thomas-Hall, S. R., Stephens, E., Marx, U. C., Mussgnug, J. H., Posten, C., et al. (2008). Second Generation Biofuels: High-Efficiency Microalgae for Biodiesel Production. Bioenerg. Res. 1, 20–43. doi:10.1007/s12155-008-9008-8
Schlesinger, A., Eisenstadt, D., Bar-Gil, A., Carmely, H., Einbinder, S., and Gressel, J. (2012). Inexpensive Non-Toxic Flocculation of Microalgae Contradicts Theories; Overcoming a Major Hurdle to Bulk Algal Production. Biotechnol. Adv. 30, 1023–1030. doi:10.1016/j.biotechadv.2012.01.011
Schulze, P. S. C., Barreira, L. A., Pereira, H. G. C., Perales, J. A., and Varela, J. C. S. (2014). Light Emitting Diodes (LEDs) Applied to Microalgal Production. Trends Biotechnol. 32, 422–430. doi:10.1016/j.tibtech.2014.06.001
Schulze, P. S. C., Pereira, H. G. C., Santos, T. F. C., Schueler, L., Guerra, R., Barreira, L. A., et al. (2016). Effect of Light Quality Supplied by Light Emitting Diodes (LEDs) on Growth and Biochemical Profiles of Nannochloropsis oculata and Tetraselmis chuii. Algal Res. 16, 387–398. doi:10.1016/j.algal.2016.03.034
Seo, Y. H., Lee, Y., Jeon, D. Y., and Han, J.-I. (2015). Enhancing the Light Utilization Efficiency of Microalgae Using Organic Dyes. Bioresour. Technology 181, 355–359. doi:10.1016/j.biortech.2015.01.031
Serif, M., Dubois, G., Finoux, A.-L., Teste, M.-A., Jallet, D., and Daboussi, F. (2018). One-Step Generation of Multiple Gene Knock-Outs in the Diatom Phaeodactylum tricornutum by DNA-Free Genome Editing. Nat. Commun. 9, 1–10. doi:10.1038/s41467-018-06378-9
Shabani, M. (2016). CO2 Bio-Sequestration by Chlorella vulgaris and Spirulina platensis in Response to Different Levels of Salinity and CO2. Proc. Int. Acad. Ecol. Environ. Sci. 6, 53.
Shaikh, K. M., Nesamma, A. A., Abdin, M. Z., and Jutur, P. P. (2019). Molecular Profiling of an Oleaginous Trebouxiophycean Alga Parachlorella kessleri Subjected to Nutrient Deprivation for Enhanced Biofuel Production. Biotechnol. Biofuels 12, 1–15. doi:10.1186/s13068-019-1521-9
Sharples, A.-L., and Doman Road, C. (1991). Decanter and Disc-Stack Centrifuge Systems. Filtr. Sep. 28, 387–388. doi:10.1016/0015-1882(91)80258-7
Shin, S.-E., Lim, J.-M., Koh, H. G., Kim, E. K., Kang, N. K., Jeon, S., et al. (2016). CRISPR/Cas9-Induced Knockout and Knock-In Mutations in Chlamydomonas reinhardtii. Sci. Rep. 6, 1–15. doi:10.1038/srep27810
Show, K.-Y., and Lee, D.-J. (2014). “Algal Biomass Harvesting,” in Biofuels from Algae (Amsterdam: Elsevier), 85–110. doi:10.1016/b978-0-444-59558-4.00005-x
Show, K.-Y., Lee, D.-J., and Chang, J.-S. (2013). Algal Biomass Dehydration. Bioresour. Technology 135, 720–729. doi:10.1016/j.biortech.2012.08.021
Show, K.-Y., Yan, Y.-G., and Lee, D.-J. (2019). “Algal Biomass Harvesting and Drying,” in Biofuels from Algae (Amsterdam: Elsevier), 135–166. doi:10.1016/b978-0-444-64192-2.00007-x
Silambarasan, S., Logeswari, P., Sivaramakrishnan, R., Kamaraj, B., Lan Chi, N. T., and Cornejo, P. (2021). Cultivation of Nostoc sp. LS04 in Municipal Wastewater for Biodiesel Production and Their Deoiled Biomass Cellular Extracts as Biostimulants for Lactuca sativa Growth Improvement. Chemosphere 280, 130644. doi:10.1016/j.chemosphere.2021.130644
Silva, A. L. P., Prata, J. C., Walker, T. R., Duarte, A. C., Ouyang, W., Barcelò, D., et al. (2021). Increased Plastic Pollution Due to COVID-19 Pandemic: Challenges and Recommendations. Chem. Eng. J. 405, 126683. doi:10.1016/j.cej.2020.126683
Singh, G., and Patidar, S. K. (2018). Microalgae Harvesting Techniques: A Review. J. Environ. Manage. 217, 499–508. doi:10.1016/j.jenvman.2018.04.010
Singh, R. N., and Sharma, S. (2012). Development of Suitable Photobioreactor for Algae Production - A Review. Renew. Sustainable Energ. Rev. 16, 2347–2353. doi:10.1016/j.rser.2012.01.026
Sirikulrat, K., Pekkoh, J., and Pumas, C. (2021). Illumination System for Growth and Net Energy Ratio Enhancement of Arthrospira (Spirulina) platensis Outdoor Cultivation in Deep Raceway Pond. Bioresour. Technology Rep. 14, 100661. doi:10.1016/j.biteb.2021.100661
Sirohi, R., Ummalyma, S. B., Sagar, N. A., Sharma, P., Awasthi, M. K., Badgujar, P. C., et al. (2021). Strategies and Advances in the Pretreatment of Microalgal Biomass. J. Biotechnol. 341, 63–75. doi:10.1016/j.jbiotec.2021.09.010
Sitthikitpanya, N., Sittijunda, S., Khamtib, S., and Reungsang, A. (2021). Co-Generation of Biohydrogen and Biochemicals from Co-Digestion of Chlorella sp. Biomass Hydrolysate with Sugarcane Leaf Hydrolysate in an Integrated Circular Biorefinery Concept. Biotechnol. Biofuels 14, 1–16. doi:10.1186/s13068-021-02041-6
Sivasankar, P., Poongodi, S., Lobo, A. O., and Pugazhendhi, A. (2020). Characterization of a Novel Polymeric Bioflocculant from Marine Actinobacterium Streptomyces sp. and its Application in Recovery of Microalgae. Int. Biodeterioration Biodegradation 148, 104883. doi:10.1016/j.ibiod.2020.104883
Sizova, I., Greiner, A., Awasthi, M., Kateriya, S., and Hegemann, P. (2013). Nuclear Gene Targeting in Chlamydomonas using Engineered Zinc‐Finger Nucleases. Plant J. 73, 873-882. doi:10.1111/tpj.12066
Smachetti, M. E. S., Coronel, C. D., Salerno, G. L., and Curatti, L. (2020). Sucrose-to-Ethanol Microalgae-Based Platform Using Seawater. Algal Res. 45, 101733. doi:10.1016/j.algal.2019.101733
Soeder, C. J. (1980). Massive Cultivation of Microalgae: Results and Prospects. Hydrobiologia 72, 197–209. doi:10.1007/bf00016247
Sohn, Y. J., Son, J., Jo, S. Y., Park, S. Y., Yoo, J. I., Baritugo, K.-A., et al. (2021). Chemoautotroph Cupriavidus necator as a Potential Game-Changer for Global Warming and Plastic Waste Problem: A Review. Bioresour. Technology 340, 125693. doi:10.1016/j.biortech.2021.125693
Solimeno, A., Samsó, R., Uggetti, E., Sialve, B., Steyer, J.-P., Gabarró, A., et al. (2015). New Mechanistic Model to Simulate Microalgae Growth. Algal Res. 12, 350–358. doi:10.1016/j.algal.2015.09.008
Song, Y., Cheng, J., Lai, X., Guo, W., and Yang, W. (2021). Developing a Three-Dimensional Tangential Swirl Plate Photobioreactor to Enhance Mass Transfer and Flashlight Effect for Microalgal CO2 Fixation. Chem. Eng. Sci. 244, 116837. doi:10.1016/j.ces.2021.116837
Soomro, R. R., Ndikubwimana, T., Zeng, X., Lu, Y., Lin, L., and Danquah, M. K. (2016). Development of a Two-Stage Microalgae Dewatering Process - A Life Cycle Assessment Approach. Front. Plant Sci. 7, 113. doi:10.3389/fpls.2016.00113
Spiekermann, P., Rehm, B. H. A., Kalscheuer, R., Baumeister, D., and Steinbüchel, A. (1999). A Sensitive, Viable-Colony Staining Method Using Nile Red for Direct Screening of Bacteria that Accumulate Polyhydroxyalkanoic Acids and Other Lipid Storage Compounds. Arch. Microbiol. 171, 73–80. doi:10.1007/s002030050681
Starkenburg, S. R., Polle, J. E. W., Hovde, B., Daligault, H. E., Davenport, K. W., Huang, A., et al. (2017). Draft Nuclear Genome, Complete Chloroplast Genome, and Complete Mitochondrial Genome for the Biofuel/Bioproduct Feedstock Species Scenedesmus obliquus Strain DOE0152z. Genome Announc. 5, e00617–00617. doi:10.1128/genomeA.00617-17
Sudesh, K., Taguchi, K., and Doi, Y. (2002). Effect of Increased PHA Synthase Activity on Polyhydroxyalkanoates Biosynthesis in Synechocystis sp. PCC6803. Int. J. Biol. Macromolecules 30, 97–104. doi:10.1016/S0141-8130(02)00010-7
Sun, H., Ren, Y., Lao, Y., Li, X., and Chen, F. (2020). A Novel Fed-Batch Strategy Enhances Lipid and Astaxanthin Productivity without Compromising Biomass of Chromochloris zofingiensis. Bioresour. Technology 308, 123306. doi:10.1016/j.biortech.2020.123306
Sun, X.-M., Ren, L.-J., Zhao, Q.-Y., Ji, X.-J., and Huang, H. (2018a). Microalgae for the Production of Lipid and Carotenoids: A Review with Focus on Stress Regulation and Adaptation. Biotechnol. Biofuels 11, 1–16. doi:10.1186/s13068-018-1275-9
Sun, X., Wang, C., Tong, Y., Wang, W., and Wei, J. (2014). Microalgae Filtration by UF Membranes: Influence of Three Membrane Materials. Desalination Water Treat. 52, 5229–5236. doi:10.1080/19443994.2013.813103
Sun, Y., Huang, Y., Liao, Q., Fu, Q., and Zhu, X. (2016). Enhancement of Microalgae Production by Embedding Hollow Light Guides to a Flat-Plate Photobioreactor. Bioresour. Technology 207, 31–38. doi:10.1016/j.biortech.2016.01.136
Sun, Y., Liao, Q., Huang, Y., Xia, A., Fu, Q., Zhu, X., et al. (2018b). Application of Growth-Phase Based Light-Feeding Strategies to Simultaneously Enhance Chlorella vulgaris Growth and Lipid Accumulation. Bioresour. Technology 256, 421–430. doi:10.1016/j.biortech.2018.02.045
Surendran, A., Lakshmanan, M., Chee, J. Y., Sulaiman, A. M., Thuoc, D. V., and Sudesh, K. (2020). Can Polyhydroxyalkanoates be Produced Efficiently From Waste Plant and Animal Oils? Front. Bioeng. Biotechnol. 8, 169. doi:10.3389/fbioe.2020.00169
Taher, H., Al-Zuhair, S., Al-Marzouqi, A. H., Haik, Y., and Farid, M. (2014). Effective Extraction of Microalgae Lipids from Wet Biomass for Biodiesel Production. Biomass and Bioenergy 66, 159–167. doi:10.1016/j.biombioe.2014.02.034
Tan, X.-B., Zhao, X.-C., and Yang, L.-B. (2019). Strategies for Enhanced Biomass and Lipid Production by Chlorella pyrenoidosa Culture in Starch Processing Wastewater. J. Clean. Prod. 236, 117671. doi:10.1016/j.jclepro.2019.117671
Tan, X. B., Lam, M. K., Uemura, Y., Lim, J. W., Wong, C. Y., and Lee, K. T. (2018). Cultivation of Microalgae for Biodiesel Production: A Review on Upstream and Downstream Processing. Chin. J. Chem. Eng. 26, 17–30. doi:10.1016/j.cjche.2017.08.010
Tarantino, L. (2003). Agency Response Letter GRAS Notice No. GRN000127. Washington, DC: FDA Home page.
Thompson, G. A. (1996). Lipids and Membrane Function in Green Algae. Biochim. Biophys. Acta Mol. Cell. 1302, 17–45. doi:10.1016/0005-2760(96)00045-8
Thompson, R. C., Moore, C. J., Vom Saal, F. S., and Swan, S. H. (2009). Plastics, the Environment and Human Health: Current Consensus and Future Trends. Phil. Trans. R. Soc. B 364, 2153–2166. doi:10.1098/rstb.2009.0053
Tiwari, O. N., Mondal, A., Bhunia, B., Bandyopadhyay, T. k., Jaladi, P., Oinam, G., et al. (2019). Purification, Characterization and Biotechnological Potential of New Exopolysaccharide Polymers Produced by Cyanobacterium Anabaena sp. CCC 745. Polymer 178, 121695. doi:10.1016/j.polymer.2019.121695
Tredici, M. R., Bassi, N., Prussi, M., Biondi, N., Rodolfi, L., Chini Zittelli, G., et al. (2015). Energy Balance of Algal Biomass Production in a 1-ha “Green Wall Panel” Plant: How to Produce Algal Biomass in a Closed Reactor Achieving a High Net Energy Ratio. Appl. Energ. 154, 1103–1111. doi:10.1016/j.apenergy.2015.01.086
Tredici, M. R., and Zittelli, G. C. (1998). Efficiency of Sunlight Utilization: Tubular versus Flat Photobioreactors. Biotechnol. Bioeng. 57, 187–197. doi:10.1002/(sici)1097-0290(19980120)57:2<187::aid-bit7>3.0.co;2-j
Udayan, A., Sirohi, R., Sreekumar, N., Sang, B.-I., and Sim, S. J. (2022). Mass Cultivation and Harvesting of Microalgal Biomass: Current Trends and Future Perspectives. Bioresour. Technology 344, 126406. doi:10.1016/j.biortech.2021.126406
Uduman, N., Qi, Y., Danquah, M. K., Forde, G. M., and Hoadley, A. (2010). Dewatering of Microalgal Cultures: A Major Bottleneck to Algae-Based Fuels. J. Renew. Sustainable Energ. 2, 012701. doi:10.1063/1.3294480
Ugwu, C. U., Aoyagi, H., and Uchiyama, H. (2008). Photobioreactors for Mass Cultivation of Algae. Bioresour. Technology 99, 4021–4028. doi:10.1016/j.biortech.2007.01.046
Vejrazka, C., Janssen, M., Streefland, M., and Wijffels, R. H. (2012). Photosynthetic Efficiency of Chlamydomonas reinhardtii in Attenuated, Flashing Light. Biotechnol. Bioeng. 109, 2567–2574. doi:10.1002/bit.24525
Vieira, B. B., Soares, J., Amorim, M. L., Bittencourt, P. V. Q., de Cássia Superbi, R., De Oliveira, E. B., et al. (2021). Optimized Extraction of Neutral Carbohydrates, Crude Lipids and Photosynthetic Pigments from the Wet Biomass of the Microalga Scenedesmus Obliquus BR003. Separation Purif. Technology 269, 118711. doi:10.1016/j.seppur.2021.118711
Wan, C., Zhao, X.-Q., Guo, S.-L., Asraful Alam, M., and Bai, F.-W. (2013). Bioflocculant Production from Solibacillus silvestris W01 and its Application in Cost-Effective Harvest of Marine Microalga Nannochloropsis oceanica by Flocculation. Bioresour. Technology 135, 207–212. doi:10.1016/j.biortech.2012.10.004
Wang, B., Lan, C. Q., and Horsman, M. (2012). Closed Photobioreactors for Production of Microalgal Biomasses. Biotechnol. Adv. 30, 904–912. doi:10.1016/j.biotechadv.2012.01.019
Wang, K., Mandal, A., Ayton, E., Hunt, R., Zeller, M. A., and Sharma, S. (2016). “Modification of Protein Rich Algal-Biomass to Form Bioplastics and Odor Removal,” in Protein Byproducts (Amsterdam: Elsevier), 107–117. doi:10.1016/b978-0-12-802391-4.00006-9
Wang, K., Sun, T., Cui, J., Liu, L., Bi, Y., Pei, G., et al. (2018). Screening of Chemical Modulators for Lipid Accumulation in Schizochytrium sp. S31. Bioresour. Technology 260, 124–129. doi:10.1016/j.biortech.2018.03.104
Weissman, J. C., Goebel, R. P., and Benemann, J. R. (1988). Photobioreactor Design: Mixing, Carbon Utilization, and Oxygen Accumulation. Biotechnol. Bioeng. 31, 336–344. doi:10.1002/bit.260310409
Wen, X., Du, K., Wang, Z., Peng, X., Luo, L., Tao, H., et al. (2016). Effective Cultivation of Microalgae for Biofuel Production: A Pilot-Scale Evaluation of a Novel Oleaginous Microalga Graesiella sp. WBG-1. Biotechnology for biofuels 9, 1–12. doi:10.1186/s13068-016-0541-y
Weyer, K. M., Bush, D. R., Darzins, A., and Willson, B. D. (2010). Theoretical Maximum Algal Oil Production. Bioenerg. Res. 3, 204–213. doi:10.1007/s12155-009-9046-x
Wicaksana, F., Fane, A. G., Pongpairoj, P., and Field, R. (2012). Microfiltration of Algae (Chlorella sorokiniana): Critical Flux, Fouling and Transmission. J. Membr. Sci. 387-388, 83–92. doi:10.1016/j.memsci.2011.10.013
Wileman, A., Ozkan, A., and Berberoglu, H. (2012). Rheological Properties of Algae Slurries for Minimizing Harvesting Energy Requirements in Biofuel Production. Bioresour. Technology 104, 432–439. doi:10.1016/j.biortech.2011.11.027
Winnacker, M. (2019). Polyhydroxyalkanoates: Recent Advances in Their Synthesis and Applications. Eur. J. Lipid Sci. Technol. 121, 1900101. doi:10.1002/ejlt.201900101
Wu, Y.-H., Yu, Y., and Hu, H.-Y. (2013). Potential Biomass Yield Per Phosphorus and Lipid Accumulation Property of Seven Microalgal Species. Bioresour. Technology 130, 599–602. doi:10.1016/j.biortech.2012.12.116
Xu, J., Cheng, J., Wang, Y., Yang, W., Park, J.-Y., Kim, H., et al. (2021). Strengthening CO2 Dissolution with Zeolitic Imidazolate Framework-8 Nanoparticles to Improve Microalgal Growth in a Horizontal Tubular Photobioreactor. Chem. Eng. J. 405, 126062. doi:10.1016/j.cej.2020.126062
Xu, J., Cheng, J., Xin, K., Xu, J., and Yang, W. (2020). Developing a Spiral-Ascending CO2 Dissolver to Enhance CO2 Mass Transfer in a Horizontal Tubular Photobioreactor for Improved Microalgal Growth. ACS Sustainable Chem. Eng. 8, 18926–18935. doi:10.1021/acssuschemeng.0c06124
Xu, Y., Isom, L., and Hanna, M. A. (2010). Adding Value to Carbon Dioxide from Ethanol Fermentations. Bioresour. Technology 101, 3311–3319. doi:10.1016/j.biortech.2010.01.006
Yang, J., Xu, M., Zhang, X., Hu, Q., Sommerfeld, M., and Chen, Y. (2011). Life-Cycle Analysis on Biodiesel Production from Microalgae: Water Footprint and Nutrients Balance. Bioresour. Technology 102, 159–165. doi:10.1016/j.biortech.2010.07.017
Ye, Q., Cheng, J., Guo, W., Xu, J., Li, K., and Zhou, J. (2018). Serial Lantern-Shaped Draft Tube Enhanced Flashing Light Effect for Improving CO2 Fixation with Microalgae in a Gas-Lift Circumflux Column Photobioreactor. Bioresour. Technology 255, 156–162. doi:10.1016/j.biortech.2018.01.127
Yeesang, C., and Cheirsilp, B. (2011). Effect of Nitrogen, Salt, and Iron Content in the Growth Medium and Light Intensity on Lipid Production by Microalgae Isolated From Freshwater Sources in Thailand. Bioresour. Technol. 102, 3034–3040. doi:10.1016/j.biortech.2010.10.013
Yu, L., Dean, K., and Li, L. (2006). Polymer Blends and Composites from Renewable Resources. Prog. Polym. Sci. 31, 576–602. doi:10.1016/j.progpolymsci.2006.03.002
Yuan, Y., Liu, H., Li, X., Qi, W., Cheng, D., Tang, T., et al. (2018). Enhancing Carbohydrate Productivity of Chlorella sp. AE10 in Semi-Continuous Cultivation and Unraveling the Mechanism by Flow Cytometry. Appl. Biochem. Biotechnol. 185, 419–433. doi:10.1007/s12010-017-2667-1
Yustinadiar, N., Manurung, R., and Suantika, G. (2020). Enhanced Biomass Productivity of Microalgae Nannochloropsis sp. in an Airlift Photobioreactor Using Low-Frequency Flashing Light With Blue LED. Bioresources and Bioprocessing 7, 1–15. doi:10.1186/s40643-020-00331-9
Zhang, K., Kurano, N., and Miyachi, S. (2002). Optimized Aeration by Carbon Dioxide Gas for Microalgal Production and Mass Transfer Characterization in a Vertical Flat-Plate Photobioreactor. Bioprocess Biosyst. Eng. 25, 97–101. doi:10.1007/s00449-002-0284-y
Zhang, W., Wang, F., Gao, B., Huang, L., and Zhang, C. (2018). An Integrated Biorefinery Process: Stepwise Extraction of Fucoxanthin, Eicosapentaenoic Acid and Chrysolaminarin From the Same Phaeodactylum tricornutum Biomass. Algal Res. 32, 193–200. doi:10.1016/j.algal.2018.04.002
Zheng, Y., Chen, Z., Lu, H., and Zhang, W. (2011). Optimization of Carbon Dioxide Fixation and Starch Accumulation by Tetraselmis subcordiformis in a Rectangular Airlift Photobioreactor. Afr. J. Biotechnol. 10, 1888–1901. doi:10.5897/AJB10.1620
Zhou, N., Zhang, Y., Wu, X., Gong, X., and Wang, Q. (2011). Hydrolysis of Chlorella Biomass for Fermentable Sugars in the Presence of HCl and MgCl2. Bioresour. Technology 102, 10158–10161. doi:10.1016/j.biortech.2011.08.051
Keywords: microalgae, biomass, microalgal biomass production, polyhydroxyalkanoates (PHA), microbial PHA synthesis, photoautotrophy
Citation: Tan FHP, Nadir N and Sudesh K (2022) Microalgal Biomass as Feedstock for Bacterial Production of PHA: Advances and Future Prospects. Front. Bioeng. Biotechnol. 10:879476. doi: 10.3389/fbioe.2022.879476
Received: 19 February 2022; Accepted: 06 April 2022;
Published: 12 May 2022.
Edited by:
Tuck Seng Wong, The University of Sheffield, United KingdomReviewed by:
Chetan Paliwal, International Centre for Genetic Engineering and Biotechnology, IndiaMesut Bekirogullari, Siirt University, Turkey
Copyright © 2022 Tan, Nadir and Sudesh. This is an open-access article distributed under the terms of the Creative Commons Attribution License (CC BY). The use, distribution or reproduction in other forums is permitted, provided the original author(s) and the copyright owner(s) are credited and that the original publication in this journal is cited, in accordance with accepted academic practice. No use, distribution or reproduction is permitted which does not comply with these terms.
*Correspondence: Kumar Sudesh, a3N1ZGVzaEB1c20ubXk=