- 1BVBGR-LR11ES31, Higher Institute of Biotechnology of Sidi Thabet (ISBST), University of Manouba, Ariana, Tunisia
- 2Department of Engineering, German University of Technology in Oman, Muscat, Oman
- 3APVA-LR16ES20, National School of Engineers of Sfax (ENIS), University of Sfax, Sfax, Tunisia
Microbial polyhydroxyalkanoates (PHA) are biodegradable and biocompatible bio-based polyesters, which are used in various applications including packaging, medical and coating materials. In this study, an extremophilic hydrocarbonoclastic bacterium, previously isolated from saline sediment in the Tunisian desert, has been investigated for PHA production. The accumulation of intracellular PHA granules in Halomonas desertis G11 was detected by Nile blue A staining of the colonies. To achieve maximum PHA yield by the strain G11, the culture conditions were optimized through response surface methodology (RSM) employing a Box-Behnken Design (BBD) with three independent variables, namely, substrate concentration (1–5%), inoculum size (1–5%) and incubation time (5–15 days). Under optimized conditions, G11 strain produced 1.5 g/L (68% of DCW) of PHA using glycerol as a substrate. Application of NMR (1H and 13C) and FTIR spectroscopies showed that H. desertis accumulated PHA is a poly-3-hydroxybutyrate-co-3-hydroxyvalerate (PHBV). The genome analysis revealed the presence of typical structural genes involved in PHBV metabolism including phaA, phaB, phaC, phaP, phaZ, and phaR, coding for acetyl-CoA acetyltransferase, acetoacetyl-CoA reductase, class I polyhydroxyalkanoates synthases, phasin, polyhydroxyalkanoates depolymerase and polyhydroxyalkanoates synthesis repressor, respectively. Glycerol can be metabolized to 1) acetyl-CoA through the glycolysis pathway and subsequently converted to the 3HB monomer, and 2) to propionyl-CoA via the threonine biosynthetic pathway and subsequently converted to the 3HV monomer. In silico analysis of PhaC1 from H. desertis G11 indicated that this enzyme belongs to Class I PHA synthase family with a “lipase box”-like sequence (SYCVG). All these characteristics make the extremophilic bacterium H. desertis G11 a promising cell factory for the conversion of bio-renewable glycerol to high-value PHBV.
Introduction
Fossil fuel-based plastics play a crucial role in modern lifestyle (Chae and An, 2018). Approximately 400 million tons of plastic were produced globally in 2018. More than 10 million metric tons of plastic ended up into the oceans during 2018 alone. An estimated 13 billion tons of plastic waste will reach the environment by 2050 in the absence of any improvements in current plastic waste management practices (Shams et al., 2021). Synthetic plastics have significant negative impacts on ecosystems, biota, environment, economy, and human health (Sharma and Chatterjee, 2017). As a result, there is a growing demand for bioplastics such as polyhydroxyalkanoates (PHA), polylactic acid, biopolyamide, biopolyethylene and biopolypropylene that provide new solutions in terms of life cycle and raw materials (Akinwumi et al., 2019). The conversion of biowastes to PHAs is considered as a possible eco-friendly alternative to petro-plastics and are currently gaining a lot of interest in the field of industrial applications and waste management (Kourmentza et al., 2017; Patel et al., 2021). Global bioplastic production has increased from 2.1 metric tons in 2020 to about 2.4 metric tons in 2022 (Dubey and Mishra, 2021) and the market opportunity for PHA bioplastic could reach US$100 million by 2024 (Surendran et al., 2020).
PHA are linear polyesters that consist of hydroxy fatty acids and are synthesized by a wide range of different Gram-positive and Gram-negative bacteria, as well as in some haloarchaeal species (Bugnicourt et al., 2014; Han et al., 2015; Kalia et al., 2021). Among the PHA producing microorganisms, the most-studied were Pseudomonas oleovorans, P. aeruginosa and P. putida, Bacillus megaterium, B. cereus, Ralstonia eutropha, and Haloferax mediterranei (Poli et al., 2011; Kalia et al., 2021). PHAs are usually produced when the microorganismes are cultured in the presence of excess carbon sources and limited nitrogen, phosphorus, sulfur, or oxygen (Surendran et al., 2020). They are stored as reserve foods as either inclusion bodies or within calcium (Ca) or polyphosphate complexes. In a few cases, PHA can accumulate to 90% of the total cell dry weight (CDW), which helps the microorganism survive under environmental stress conditions (Kalia et al., 2021). PHA can be classified in three main categories: short-chain-length PHA (scl-PHA) consists of 3-5 carbons atoms [3-hydroxybutyrate (3HB), and 3-hydroxyvalerate (3HV)]; medium-chain-length PHA (mcl-PHA) that consists of 6–14 carbons atoms [3-hydroxyhexanoate (3HHx), 3-hydroxyheptanoate (3HHp), and 3-hydroxytetradecanoate (3HTD)…] and also mixtures of both scl-PHA and mcl-PHA (Leong et al., 2014; Tan et al., 2014). More than 150 different monomers have been identified in PHA chains under various fermentation conditions, resulting in PHA with different characteristics (Lee et al., 2019). Due to their properties similar to those of petrochemical thermoplastics and combined properties of biodegradability and biocompatibility, PHA have gained considerable importance as promising candidates for biologically-derived plastics as well as biomaterials (Han et al., 2010, 2015, 2017; Kourmentza et al., 2017; Mitra et al., 2020).
Poly (3-hydroxybutyrate-co-3-hydroxyvalerate), PHBV, is a biopolyester with good mechanical properties and biodegradability, with broad applications in a wide variety of sectors (food and medical packaging, drug release and transport systems, hygiene products, etc.) due to its excellent properties such as absorption capacity, low cytotoxicity, piezoelectricity, and thermoplasticity compared to brittle PHB (Tan et al., 2014; Rivera-Briso and Serrano, 2018; Chen et al., 2019). As reported by Ibrahim et al. (2020), PHBV is a very promising copolymer that has great potential to replace conventional non-degradable polymers and has a great sustainability potential in circular economy development strategy. For most industrial uses of PHBV, the 3HV fraction should be at least in the range of 10–20% and usually chemical precursors such as propionate and valerate were added to obtain such 3HV fraction (Hermann-Krauss et al., 2013). Increasing 3HV content enhances the biodegradability and reduces the crystallinity and melting point of the bio-copolymer (Policastro et al., 2021). However, even though it owns environmental advantages and more suitable properties compared to other bioplastics, the main problem facing commercial production is the high production costs and low productivity rate. Therefore, the current challenge for researchers is the implementation of efficient and low cost PHBV production processes (Albuquerque and Malafaia, 2018; Patel et al., 2021 a and b; Policastro et al., 2021). Therefore, many approaches have been developed to minimize the production cost such as 1) the use of agricultural wastes and industrial by-products (e.g., fruit peels, bagasse, waste plant oils, crude glycerol, cheese whey, etc.) as inexpensive carbon sources with widespread availability and renewability (Kulkarni et al., 2015; Patel et al., 2015; de Paula et al., 2017; Ray et al., 2018:; Surendran et al., 2020), 2) the application of experimental designs and response surface methodology as statistical tools for the optimization of microbial biomass and PHA production using different cultivation modes (batch, fed-batch, and continuous fermentations) (El-malek et al., 2020; Singh et al., 2021), 3) the use of extremophilic bacteria as efficient PHA-producing strains (Cai et al., 2011; Yin et al., 2015; Kourmentza et al., 2017; Chen et al., 2018; Patel S. K. S. et al., 2021; Dubey and Mishra 2021). As an example, PHA production can be more environmentally friendly low-cost feed like using greenhouse gases (biogas) by thermophilic methanotrophs such as under non-sterile conditions (Patel S. K. S. et al., 2021).
Microbial halophiles are considered to be a promising cell factory for high-value biomolecules synthesis (extremolytes, extremozymes, biosurfactants, bioplastics, etc.) due to their unique characteristics of thriving under extremes of temperature, salinity, pH, and solvent conditions (Mitra et al., 2020). Extremely halotolerant species (up to 20% salinity) of the genus Halomonas, mostly associated with saline or hypersaline environments such as ocean waters and hypersaline lakes have shown PHA production performance under open and non-sterile conditions allowing the development of continuous bioprocesses without contamination (Kourmentza et al., 2017; Neifar et al., 2019; Athmika et al., 2021). The Chinese company Bluepha produces both PHB and PHBV from a halophilic Halomonas strain (Mitra et al., 2020). The genomes of PHA-producing extremophilic strains were studied via bioinformatics and genetic engineering tools for the expression of the PHA synthesis genes in non-producing strains exposing less restrictive growth and production conditions (Cai et al., 2011; Thomas et al., 2020). Policastro et al. (2021) reviewed the most relevant studies on PHBV production by mesophilic and extremophilic strains and the strategies used for costs reduction. Halophilic and halotolerant Halomonas strains such as Halomonas sp. TD-0, Halomonas sp. SF2003, Halomonas i4786, H. pacifica ASL10 and H. salifodiane ASL11 have been reported as promising candidates able to produce short chain length PHA up to 80 wt% of cell dry weight (CDW), using conventional carbon sources as well as carbonaceous by-products from food wastes (Elain et al., 2016; El-Malek et al., 2020; Thomas et al., 2020; Dubey and Mishra, 2021).
Thus, the present study investigates the ability of the halophilic bacterium Halomonas desertis G11, isolated from the largest salt-lake in Tunisian Sahara (Neifar et al., 2019), to directly produce PHBV from a low-cost and non-toxic substrate and without addition of PHV precursors. The study focused on the use of glycerol as a carbon substrate taking into account that it is one of the promising substrates for large-scale production of PHA due to the growing production of biodiesel as an alternative renewable energy source (Kumar et al., 2015; Ray et al., 2018; Kondaveeti et al., 2019). The culture conditions were optimized using experimental design and response surface methodologies. This work will also contribute to a better understanding of PHA metabolism and PHA synthase functionality of G11 strain by genomic and structural approaches.
Materials and Methods
Sources of Bacterial Strain and Genome Sequences
The extremophilic hydrocarbonoclastic bacterium Halomonas desertis G11 used in this study was isolated from hypersaline sediment of Chott El-Djerid of southern Tunisia (Neifar et al., 2019; Riahi et al., 2020). It has an optimum growth at 5–10% NaCl and pH 8-10. The complete genomic sequences of G11 have been deposited in GenBank under the accession number LYXG00000000. The genomic and proteomic sequence data of the strain G11 was downloaded from the Universal Protein Resource UniProt database (https://www.uniprot.org/uniprot/?query=halomonas+g11&sort=score) and the Integrated Microbial Genomes and Microbiomes (IMG/M) platform (https://img.jgi.doe.gov/cgi-bin/m/main.cgi?section=TaxonDetail&page=taxonDetail&taxon_oid=2751185866).
Detection of PHA Accumulation in Halomonas desertis G11 After Nile Blue a Staining
Detection of intracellular accumulation of PHA was performed according to the Nile blue A staining method (Ostle and Holt, 1982). Halomonas desertis G11 was grown in modified high medium (HM) containing 10 g/L glycerol, 2 g/L yeast extract, 50 g/L NaCl; 0.25 g/L MgSO4·7H2O, 0.09 g/L CaCl2·2H2O, 0.5 g/L KCl, 0.25 g/L KH2PO4, 2 g/L granulated agar, 0.06 g/L NaBr, and Nile blue A (dissolved in dimethylsulfoxide) with a final concentration of 0.5 mg dye per L of medium (Hertadi et al., 2017). After 4 days of bacterial incubation at 30°C, the agar plates were exposed to ultraviolet light to visualize the fluorescence (Bhuwal et al., 2013; Mascarenhas and Aruna, 2017).
Experimental Conditions for PHA Production and Recovery
The PHA production by H. desertis G11 was carried out in modified liquid HM medium (Hertadi et al., 2017) using glycerol as a carbon substrate (concentrations 1–5%). 250 ml shake flask cultures (100 ml working volumes) were incubated at 30°C with an orbital agitation of 120 rpm. Bacterial growth was followed by monitoring optical densities (ODs) at 600 nm wavelength. Cells were washed with distilled water and then dried until constant cell weight was achieved. After incubation, G11 cells were recovered from cultures through centrifugation at 6,000 rpm for 15 min and the biomass was used for PHA extraction. Approximately 5 ml chloroform was added to 20 mg biomass and then incubated for 24 h at 60°C. The residual bacterial biomass was separated by filtration; as described by Kucera et al. (2018). PHA was precipitated by the addition of 2× volume of methanol. The white precipitate formed was filtered, dried in an oven at 60°C under vacuum for 24 h, weighed and the yield of PHA was expressed as % PHA formed on dry cell weight basis (Kulkarni et al., 2010; Hertadi et al., 2017). The purified PHA was used for subsequent analysis.
Optimization of PHA Production by Response Surface Methodology
Three-factor three-level Box-Behnken design (BBD) and response surface methodology (RSM) were used to determine the optimal culture conditions for PHA production by H. desertis G11. The BBD space and experimental domain were shown in Figure 1. The BBD is a type of nearly rotatable second-order design, which means that the model has a reasonably stable distribution of the prediction variance throughout the experimental domain. As a result, it is more efficient and cost-effective than the full 3k factorial design. It requires three levels for each factor instead of five as in the central composite design (CCD), which results in fewer experimental trials, and is more convenient and less expensive than CCD. Another advantage of the BBD is that it does not contain combinations in which all factors are simultaneously set at their extreme levels. These designs are therefore useful for avoiding experiments performed under such conditions for which unsatisfactory results could be observed (Montgomery, 2005).
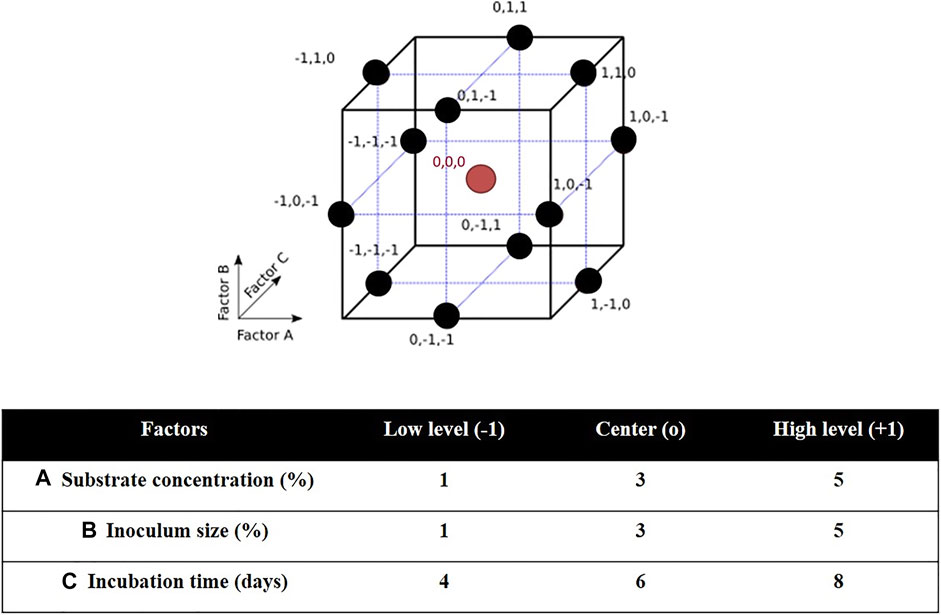
FIGURE 1. Characteristics of Box–Behnken design (BBD): BBD space for three factors and BBD experimental domain.
The relationship between the response R and the three quantitative factors A, B, and C was approximated by the following second-order polynomial equation:
Where R is the predictive measured response as PHA production yield (g/L); A, B, and C represent coded values of substrate concentration (%); inoculum size (%); and incubation time (days).; e0 is the intercept, e1, e2, e3, e11, e22 and e33 are the regression coefficients.
The accuracy and fitness of the above model were analyzed by ANOVA and evaluated by the coefficient of determination (R2) and F value. The optimum levels of studied factors for maximum PHA production were obtained by solving the regression equation, analyzing the contour plots and 3D surface plots. For validation, additional experiments were performed under these optimal conditions.
Characterization of Produced PHA by Spectroscopy Analyses
Produced PHA was analyzed by Fourier Transform Infrared (FT-IR) and Nuclear Magnetic Resonance (NMR) spectroscopies. The purified polymer (2 mg) of PHA was subjected to FTIR analysis to characterize the presence of specific functional groups. The spectrum was recorded using a FTIR spectrometer (Nicolet IR200 FT-IR) with a spectral range 4,000 and 400 cm−1. For 1H and 13C NMR analysis, PHA samples were dissolved in deuterated chloroform (CDCl3) and analyzed on a 400 MHz NMR spectrophotometer (Divyashree and Shamala, 2009; El-malek et al., 2020). The composition of PHB and PHV in the copolymer was calculated from the area ratio of absorption peaks in methyl groups corresponding to the HB and HV groups (1.2 and 0.9 ppm, respectively) as reported by Kulkarni et al. (2010).
Identification of PHA Related Genes by Genomic Analysis
Genome annotation was performed using the Rapid Annotations using Subsystems Technology (RAST, http://rast.nmpdr.org/) server (Aziz et al., 2008) to identify genes involved in the metabolic pathways of PHA. The gene predictions were manually verified by BLAST searches against the protein databases NCBI (http://www.ncbi.nlm.nih.gov/) and the UniProt database (http://www.uniprot.org/).
Phylogenetic Analysis and Classification of H. desertis PHA Synthase
Multiple alignments and phylogeny analysis were performed with a group of characterized PHA synthases from several PHA-producing strains selected from the UniProt database. A Neighbour Joining starting tree was generated from this alignment through Mega-X. The identification of PhaC1 box lipase consensus sequence of H. desertis G11 was conducted using BioEdit software using the ClustalW multiple alignment tool (Thomas et al., 2020).
Analysis of the Primary Amino Acid Sequences and Structure Prediction of PHA Synthase PhaC1
The primary structure of the PhaC1 was analyzed using the ExPASy-ProtParam tool (Gasteiger et al., 2005). Its secondary structure was predicted using the self-optimized prediction method (SOPMA), Phyre2 (http://www.sbg.bio.ic.ac.uk/phyre2) (Kelley et al., 2015) and PredictProtein (http://www.predictprotein.org/). The 3D models of the enzymes were constructed using I-TASSER homology modeling servers (Yang and Zhang, 2015). I-TASSER generates five three-dimensional structures for each model. The coordinates of the refined model were evaluated by MolProbity (Davis et al., 2007). Models were evaluated by Coach (Yang et al., 2013), a protein-ligand binding site prediction software and visualized using the PyMOL molecular graphics system.
Statistical Analysis
The regression analysis, estimation of the coefficient, generation and data treatments, plotting 2D and 3-D plots of BBD were performed using the experimental design software NemrodW (Mathieu et al., 2000).
Results
Preliminary Assessment of PHA Accumulation
The simple and highly sensitive Nile blue A staining method was used to detect PHA accumulation in growing H. desertis colonies. As revealed in Supplementary Figure S1, the strain G11 showed bright orange fluorescence indicating PHA accumulation.
Experimental Design and Statistical Modeling of PHA Production
H. desertis G11 was able to utilize glycerol as a carbon source for growth and PHA synthesis (Table 1). BBD and RSM were applied to determine the optimal conditions for PHA production. The variables and their levels were selected based on preliminary tests and bibliographic results. The BBD along with corresponding experimental and predicted responses of PHA and biomass productions, was shown in Table 1. The following quadratic equation was obtained by regression analysis to predict PHA production by the strain G11.
Where, R is the PHA production yields (g/L), and A, B, and C are the factors coded values of substrate concentration (%), inoculum size (%) and incubation period (days), respectively.
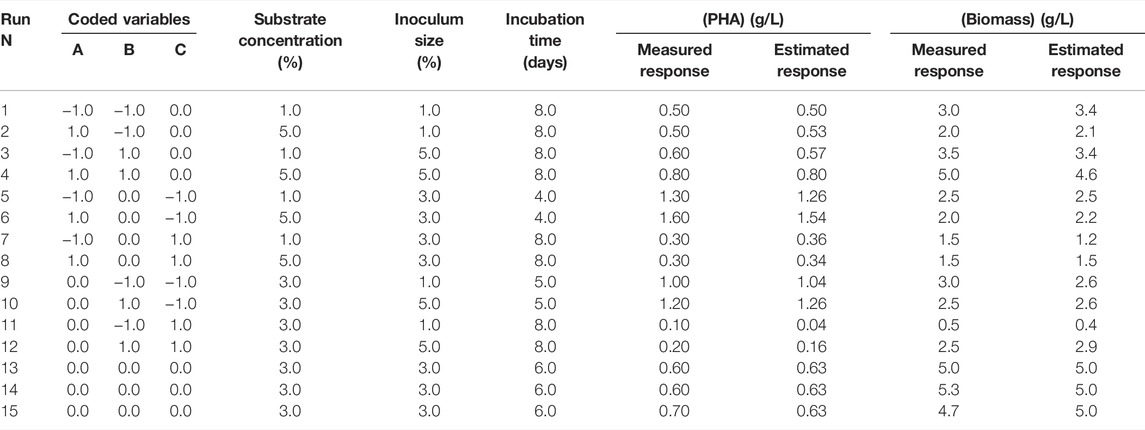
TABLE 1. Experimental conditions of BDD in natural variables and the corresponding experimental and theoretical PHA production responses.
Analysis of variance (ANOVA) results of this quadratic model was presented in Supplementary Table S1. The regression model for PHA production was highly significant with p-values < 0.001%. The quality of fit of the generated equation was further confirmed by the values of the coefficient of multiple determinations (R2) and the adjusted coefficient of multiple determinations (Adj. R2) of 0.989 and 0.968, respectively. As reported by Nygaard et al. (2019), the experimental design model has a very high fit correlation between observed and predicted response values if R2 > 0.9 and a high fit correlation if 0.7 < R2 < 0.9. The statistically significant model terms for H. desertis PHA production, are the linear regression coefficients (e2 and e3) and the quadratic regression coefficients (e11, e22, and e33) (Supplementary Table S2). The contour plots and response surface curves for the predicted PHA production yields were shown in Figure 2. They provided useful information about interactions between factors on the PHA accumulation and allowed an easy prediction of the optimal levels of each factor for maximum PHA production.
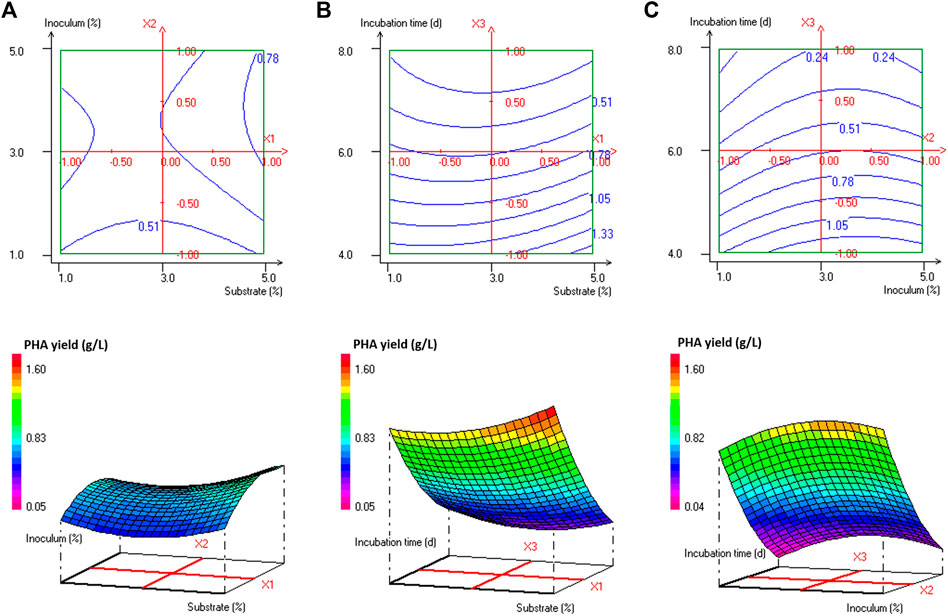
FIGURE 2. Response surface and contour plots illustrating the effect of (A) substrate concentration and inoculum size at 6 days’ incubation time, (B) substrate concentration and incubation time at 3% inoculum size, and (C) inoculum size and incubation time at 3% substrate concentration on PHA production (g/L) by H. desertis G11 using glycerol as substrate.
The production of PHA by the strain G11 is enhanced by increasing the inoculum size (Figures 2A,C) and decreasing the incubation time (Figures 2B,C). As a result, based on the response surface and contour plots (Figure 2) as well as the optimal scheme (Figure 3), the optimum operating culture conditions, carried out using NemrodW software, were found to be: PHA production of 1.54 ± 0.07 g/L obtained with 5% of glycerol concentration, 4 days for incubation time and 3% of inoculum size. These optimal conditions for PHA production correspond to a biomass production of 2.25 ± 0.37 g/L. Supplementary experiments were carried out under the selected optimal conditions. They led to PHA and biomass productions equal to 1.51 ± 0.06 and 2.23 ± 0.32 g/L respectively, which were in close agreement with the predicted values.
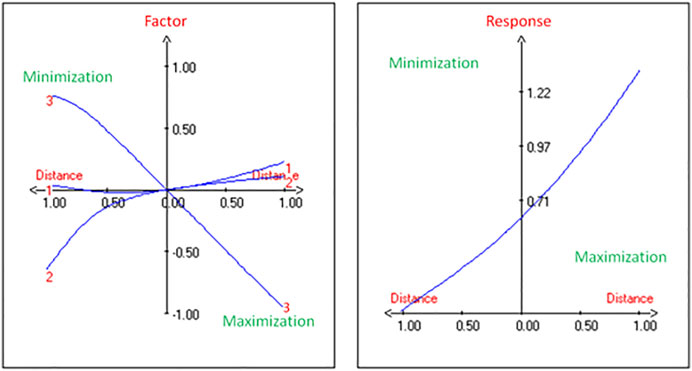
FIGURE 3. Determination of maximum PHA yield from H. desertis G11 through optimum schemes generated by NemrodW software.
Characterization of Recovered PHA
The presence of the functional groups in the extracted PHA was analyzed by FTIR and NMR spectroscopies (Figure 4) and compared with previous studies (Supplementary Table S3).
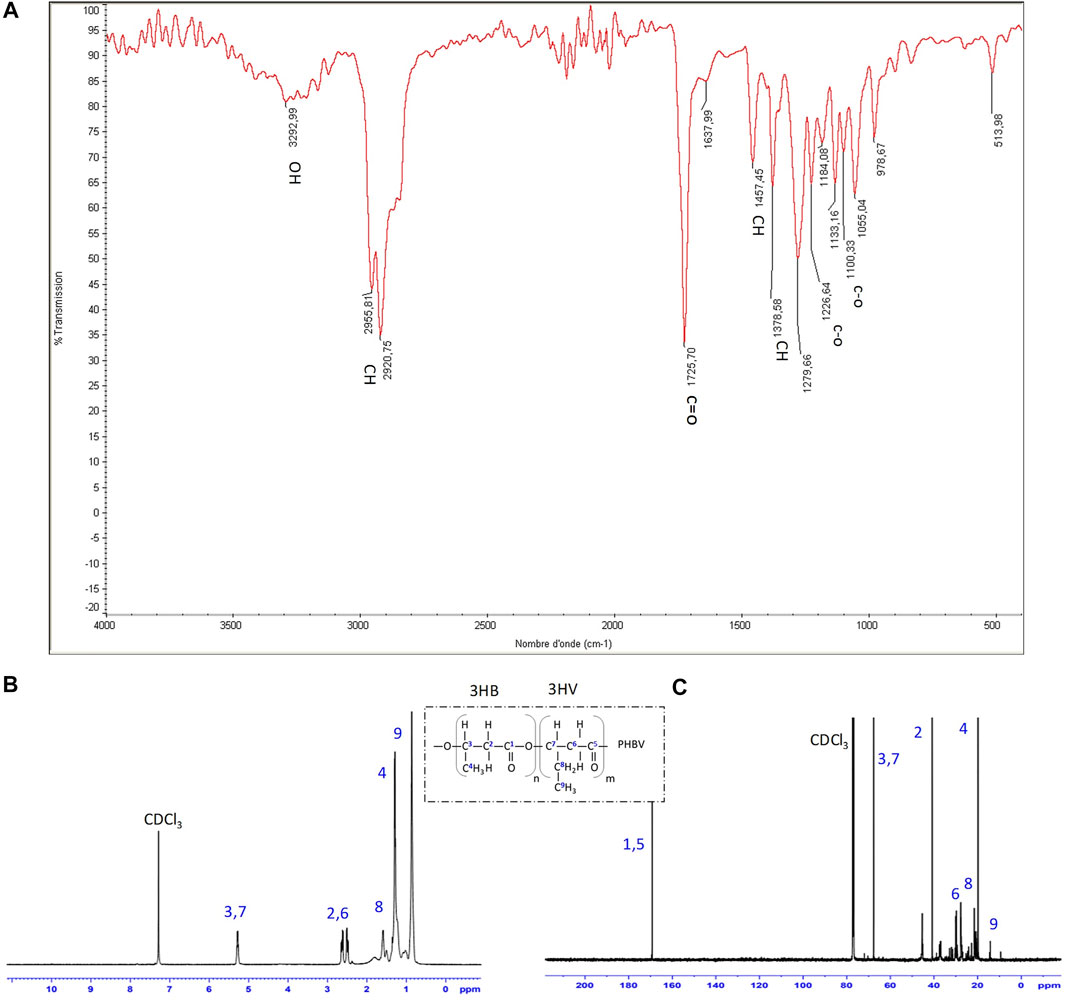
FIGURE 4. (A) FTIR (B) 1H-NMR and (C) 13C-NMR spectra of purified PHBV isolated from strain G11 grown on glycerol (5%, v/w) as a carbon source.
FTIR and NMR spectra of PHA produced by H. desertis G11 corresponded to a copolymer, PHBV. The FTIR spectrum of PHBV showed an absorption peak at approximately 1,279.66 cm−1 corresponding to the saturated ester linkage of C-O-C groups. The absorption peak at 1,378.58 cm−1 and 1,457.45 cm−1 corresponded to the respective stretching and bending mode of the vibration of the methyl (–CH3) group. The peaks at 2.920.75/2955.81, 1725.70, and 3,292.99 cm−1 were the respective characteristic peaks of methane (–CH), ester carbonyl (C=O), and hydroxyl (–OH) groups (Figure 4, Supplementary Table S3).
The proton and carbon nuclear magnetic resonance analysis (Figure 4, Supplementary Table S3) confirmed that this indigenously synthesized polymer was composed of 3-hydroxybutyrate (3-HB) and 3-hydroxyvalerate (3-HV) monomers. The chemical shifts of the major signals assigned to the different types of proton atoms [CH3 (HB) at 1.253 ppm, CH3 (HV) at 0.878 ppm, CH2 (HV) at 1.606 ppm, CH2 (HV-HB main) at 2.307 ppm, and CH (HV and HB bulk structure) at 5.340 ppm] and carbon atoms [C=O (HB) at 169.12 ppm, C=O (HV) at 169.29 ppm, CH2 (HB) at 40.75 ppm, CH2 (HV) at 38.75 ppm, CH (HB) at 67.4 ppm, CH3 (HB) at 19.74 ppm and CH3 (HV) at 9.31 ppm] in the PHBV structure agree with those obtained by Kemavongse et al. (2008) and showed not only uniformity in results but also a substantial degree of purity in terms of peaks for the extracted PHBV. The molar percentages of HB and HV units in H. desertis PHA copolymer were 47.96% and 52.04%, respectively.
Identification and Evolutionary Analysis of PHA Relevant Genes From H. desertis G11
The reported whole-genome sequence of H. desertis G11 was used for gene annotations using the RAST server’s rapid annotation platform. The relevant genes for PHA production were identified through homologous alignments against the public annotation databases using BLAST program. A complete pathway for converting glycerol to PHBV was identified in the G11 genome (Figure 5). After a cascade of metabolizing enzymes involving the conversion of glycerol to acetyl CoA, the formation of PHBV from its precursors, acetyl-CoA and propionyl-CoA was involved three enzymes (PhaA, PhaB and PhaC1). In H. desertis G11 cells, glycerol can be consumed and metabolized to propionyl-CoA via the threonine biosynthetic pathway and subsequently converted to the 3HV monomer (Figure 5). Ketothiolase (PhaA) catalyzed the condensation of two acetyl-CoA or condensation of acetyl-CoA and propionyl-CoA. The resulting intermediates were reduced to 3-hydroxybutyryl-CoA and 3-ketovaleryl-CoA by NADPH dependent acetoacetyl-CoA reductase (PhaB). The hydroxyl acyl-CoA monomers were then incorporated into the growing biopolymer chain by PHA synthase (PhaC1).
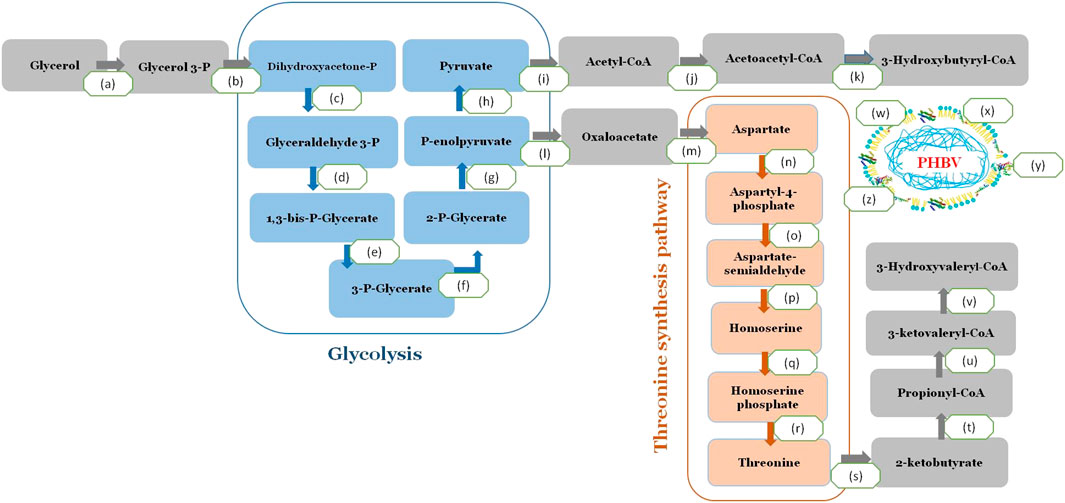
FIGURE 5. A complete pathway for converting glycerol to PHBV in H. desertis genome. (a) Glycerol kinase, EC 2.7.1.30, glpK, A0A1A0F938, (b) Glycerol-3-phosphate dehydrogenase, EC 1.1.5.3 (A0A1A0F0A7) (c) Triosephosphate isomerase, EC 5.3.1.1 (A0A1A0FLG5) (d) Glyceraldehyde-3-phosphate dehydrogenase, EC 1.2.1.12, (A0A1A0FJ82, A0A1A0FBT9, A0A1A0FMC5, A0A1A0F5L9) (e) Phosphoglycerate kinase, EC 2.7.2.3 (A0A1A0F555) (f) 2,3-bisphosphoglycerate-independent phosphoglycerate mutase, EC 5.4.2.12 (A0A1A0FLZ7) (g) Enolase, EC 4.2.1.11 (A0A1A0EWM0), (h) Pyruvate kinase, EC 2.7.1.40 (A0A1A0EQ50, A0A1A0FJ83) (i) Pyruvate dehydrogenase, EC 1.2.4.1 (A0A1A0FHI0) (j) 3-ketoacyl-CoA thiolase (phaA), EC 2.3.1.16 (A0A1A0FJC6) Acetyl-CoA acetyltransferase (EC 2.3.1.9) (A0A1A0FLF6, WP_066316105.1) (k) acetoacetyl-CoA reductase EC 1.1.1.36 (phaB) (A0A1A0FCW9, WP_066319309.1), (l) Class I poly(R)-hydroxyalkanoic acid synthase, EC 2.3.1-(phaC) (A0A1A0ERQ1), (m) Polyhydroxyalkanoate depolymerase (EC 3.1.1.75/76) (A0A1A0FL46) (PhaZ), (n) Phasin family proteins (A0A1A0ERX1, A0A1A0FMH7, A0A1A0EQ92A0EQ92) and (o) Polyhydroxyalkanoate synthesis repressor (PhaR) (A0A1A0EWK0), (p) Homoserine dehydrogenase EC 1.1.1.3, (q) homoserine kinase EC 2.7.1.39, (r) threonine synthase EC 4.2.3.1, (s) threonine deaminase EC 4.3.1.19.
Besides genes that are directly involved in PHA biosynthesis, other genes of PHA metabolism were annotated in the genome of G11 strain such as the PHA depolymerase (phaZ) that degrades PHA granules for subsequent reuse, the non catalytic PHA granule associated proteins, phasins that regulate the number and size of the PHA granules and the transcriptional repressor protein, PhaR, that regulates expression of phasin genes and releases repression upon binding PHA.
In Silico Study of PHA Synthase PhaC1 of H. desertis G11
PHA synthase is the crucial enzyme for PHA biosynthesis which polymerizes monomeric hydroxyalkanoate substrates into PHA. They are classified into four classes based on their substrate specificity and subunit composition (Zou et al., 2017). In this study, a phylogenetic tree was constructed based on the amino acid sequences of PhaC1 from H. desertis G11 with other bacterial PHA synthases described in the literature and included in the Uniprot database. Analysis of PhaC1 of the strain G11 showed that this enzyme was consistent with class I PHA synthases from other Halomonas species (Figure 6A).
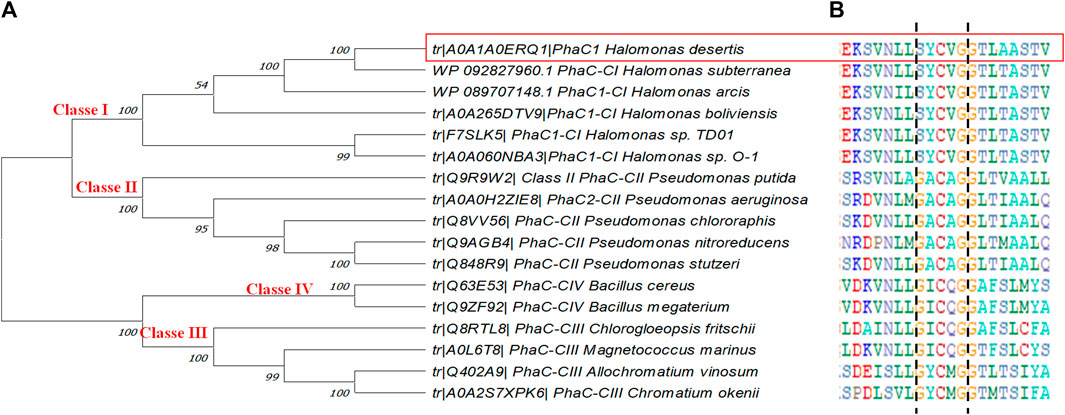
FIGURE 6. (A) Phylogenetic tree of PhaC1 from H. desertis G11 with reported PHA synthases. The tree was constructed using Mega-X software. (B) Multiple alignments of partial amino acid sequences of PHA synthases exposing lipase box-like patterns from different bacterial species. All the sequences are available on the Universal Protein Resource (UniProt) database. Highlighted sequences correspond to PhaC1 of H. desertis G11.
Predicted amino acid composition (615 amino acid residues) of PhaC1 from H. desertis G11 showed 135 charged amino acids, 81 acidic (Asp and Glu), and 54 basic amino acids (His, Arg, and Lys), respectively. The molecular weight of the protein was estimated at 69.67 kDa with a pI of 4.93. SOPMA analysis indicates that the predicted PHA synthase I protein possesses 45.04% alpha-helices, 12.85% extended strand, 6.50% Beta turn and 35.61% Random coil. Scratch Protein Predictor analysis suggests that the protein is globular and predicted one disulfide bridge formed (2 Cys residues at position 324 and 335) contribute to the structural stability of the protein.
The alignment of amino acid sequences of selected PHA synthases have been allowed the identification of lipase box-like patterns composed of Serine-Tyrosine-Cysteine-valine-Glycine (S-Y-C-V-G) (Figure 6B). Halomonas strains have a similar sequence (S-X-C-X-G) at the active site where X is an arbitrary amino acid.
Through a structural analysis, PhaC1 of H. desertis G11 is folded into a globular structure which belongs to the α/β hydrolase super-family and comprises the N-terminal domain which is important for stabilizing dimeric PhaC and the C-terminal catalytic (CAT) domain-containing the CAP subdomain (residues 364–483) and α/β core subdomains which possesses a catalytic pocket comprising of a catalytic triad (Cys-Asp-His) at its core (Figure 7A). The enzyme composed of 13 β-strands (β1 (Gly230-Asn236), β2 (Leu238-Tyr244), β3 (Pro255-Val250), β4 (Val288-Trp290), β5 (Val329-Tyr334), β6 (Val357-Met363), β7 (Arg400-Ala403), β8 (441-Ala446), β9(Ser484-Lys490), β10(Ala511-Gly517), β11 (Gly534-Thr537), β12 (Thr553-Glu550), β13(Val593-Ala597)) and 28 α-helices (α1 (Glu16-Leu39), α2 (Ser50-Met67), α3 (Leu72-Gln75), α4 (Thr76-Ala96), α5 (Lys114-Thr119), α6 (His123-Asp143), α7 (Ser146-Met167), α8 (Asn176-Glu185), α9 (Asn190-Ala206), α10 (Tyr 266-Leu271), α11 (Gln273-Ser276), α12 (Met277-Gln284), α13 (Pro298-His301), α14 (Trp305-Cys324), α15 (Cys335-Arg352), α16 (Asp374-Asn380), α17 (Leu414-Glu428), α18 (Phe432-Thr439), α19 (Gly447-Glu459), α20 (Lys461-Glu461), α21 (Leu477-Ile480), α22 (Lys496-Leu505), α23 (Gly519-Val525), α24 (Pro528-Lys530), α25 (His545-Thr551), α26 (Trp560-Asn570), α27 (Arg600-Lys603), α28 (Pro607-Glu612)). The core subdomain comprises 13 strands (β1-β13) and 8 helices (α1-α8). The CAP subdomain is connected from β7 and back to the core domain through β8. The catalytic triad (Cys335, His520, and Asp492) is covered by the CAP subdomain which blocks the substrate access through the lid loop structure (a helix-loop-helix motif) (Figure 7B). Once the acyl-CoA enters the catalytic pocket of the enzyme, the catalytic His activates the nucleophilic Cys, which subsequently attacks the thiol group of acyl-CoA. The catalytic Asp is proposed to activate the 3-OH group of acyl-CoA to attack the second incoming substrate for the elongation process (Yuan et al., 2001; Tian et al., 2005; Wahab et al., 2006).
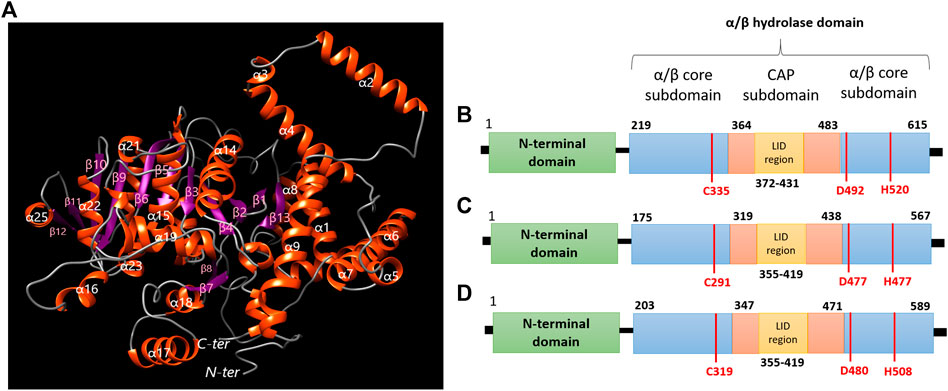
FIGURE 7. (A) Predicted 3D structure modeling information of polyhydroxyalkanoate synthases of H. desertis G11 generated by Phyre2 server. (B) Domain organization of class I PhaCHd from H. desertis G11 compared to (C) PhaCCs from Chromobacterium sp. USM2 and (D) PhaCCn from Cupriavidus necator.
Discussion
Halophilic microorganisms especially Halomonas spp., have been attracted a lot of interest, recently due to their potential to produce exoenzymes, exopolysaccharides, osmolytes, biosurfactants, and bioplastics (Neifar et al., 2019). In this work, we report the capacity of the hydrocarbonoclastic, plant growth-promoting halophilic bacterium H. desertis G11 (Naili et al., 2018; Neifar et al., 2019; Riahi et al., 2020) to produce PHA. The ability of this strain to grow at NaCl concentrations up to 20% with an optimum at 5%–10% allowing an open and continuous fermentation process without contamination and to produce many compatible organic solutes such as osmoregulated periplasmic glucans, choline-glycine betaine, and ectoine hydroxyectoine make it a well-equipped bacterium to cope with the osmotic stress (Neifar et al., 2019).
In this study, the potential of halophile H. desertis G11 to produce PHA was firstly revealed using Nile bleu A staining plate assay (Ostle and Holt, 1982) under saline condition (5% NaCl). The bright pink fluorescence displayed by the bacterial colonies under UV light indicates intracellular accumulation of PHA by G11. Although the mechanism of selective PHA staining by Nile blue is not yet fully understood, it has been suggested that the dye diffuses through the bacterial membrane and labels cytoplasmic PHA granules by binding to lipid molecules in the surrounding layer. Thus, this method has been adapted for many years, for its affinity to bind to PHA granules and has therefore been accepted as a presumptive test for PHA storage in bacteria. This method has been applied to other strains such as Bacillus subtilis MANA18 (Al-Hamdani and Jaber, 2017) and Vibrio sp. BTTC26 (Raghul. 2012). The Halomonas PHA granules have a diameter range of 0.2–0.5 µM as reported by Muhammadi et al. (2015) and Obruca et al. (2020). The use of lipophilic dye staining and TEM and SEM microscopy techniques have been applied to detect PHA granules in other Halomonas species including Halomonas sp. SF2003, H. boliviensis LC1, Halomonas sp. TD01, H. pacifica ASL10 and H. salifodiane ASL11 and H. hydrothermalis (Quillaguaman et al., 2006; Fu et al., 2014; Thomas et al., 2019; El-malek et al., 2020; Obruca et al., 2020).
PHA production by H. desertis G11 was assayed in submerged cultures using glycerol as a substrate. Statistical analysis and model fitting of the optimization of PHA production was performed using BBD and RSM. The optimum PHA production (1.5 g/L; 68% of DCW) was obtained at 4 days while longer incubation time showed decreasing trend. Incubation period for PHA production by different organisms reported so far was longer than 24 h (Kulkarni et al., 2010). H. boliviensis required 33 h incubation period for highest PHA accumulation (Quillaguaman et al., 2006). Other species of Halomonas such as H. profoundus, H. elongate and Halomonas marina required 3 days for PHA production (Mothes et al., 2008; Simon-Colin et al., 2008; Biswas et al., 2009). In contrast, a longer incubation time of 120 h was required by the halophilic strain H. elongata to produce similar PHA content (50–60 wt%) (Mothes et al., 2008; Biswas et al., 2009).
Halophiles are of particular interest since they eliminate the need to maintain aseptic conditions and enable continuous production in saline (even sea) water and they serve as feasible genetic sources for industrially important compounds like biopolymers, osmolytes and extremozymes (Chen et al., 2018; Rodriguez-Perez et al., 2018). Several halophilic microorganisms demonstrated their ability to produce PHA. Most of them belong to the family Halomonadaceae (Haba et al., 2011). The genus Halomonas is known to accumulate scl-PHA (Table 2). Most Halomonas sp. has a NaCl requirement of 3%–15% for optimal growth and this concentration is sufficient enough to prevent microbial contamination. Halomonas species including H. marina, H. boliviensis, H. hydrothermalis, Halomonas sp. TD01, H. elongata DSM 2581, and H. campaniensis, isolated from different saline habitats in the word, have been reported as high polymer accumulators from different sugars, sodium acetate and butyric acid, and agricultural residues (Mothes et al., 2007; Van-Thuoc et al., 2008; Biswas et al., 2009; Quillaguamán et al., 2010; Tao et al., 2017; Cristea et al., 2018).
The use of glycerol as a substrate allows higher PHBV content with reduced cost, it does not consume phosphoenolpyruvate of the PTS transporter system compared with other sugars, more oxaloacetate and energy can be saved for PHBV production (Jincy et al., 2013; Tan et al., 2014). As crude glycerol contains high salt concentration, ranging from 3 to 7% w/v, the possibility of using halophilic bacteria could potentially provide an attractive production system for PHA (Van-Thuoc et al., 2015). Glycerol has previously been reported as an ideal carbon source for PHA production in some vibrios (Chien et al., 2007; Wei et al., 2011; Raghul. 2012), Ralstonia eutropha (Taidi et al., 1994). In addition, results with Bacillus megaterium demonstrated the valorization of glycerol for PHB production (Naranjo et al., 2013). They were able to produce 4.8 g/L of PHB using 2% purified glycerol under controlled conditions. Jincy et al. (2013) performed statistical optimization for PHB production (0.60 g/L) using 2% crude glycerol by Bacillus firmus NII 0830. Studies also revealed the ability of several Halomonas strains to accumulate PHA from glycerol among them, H. campisalis ATCC 700597T synthesizes PHB with a yield of 24.4%, H. meridiana NBRC 15608T which gives about 25% by weight of PHB. Similarly, Halomonas sp. KM-1 accumulates about 34% of PHB and 63.6% at 3% and 10% glycerol respectively (Kawata and Aiba, 2010). More recently, Dubey and Mishra (2021) reported a PHA content of 0.20 ± 0.02 g/100 ml in H. daqingensis and 0.21 ± 0.01 g/100 ml in H. ventosae when cultivated in 3% algal biodiesel waste residue, 5% NaCl supplementation at 35°C within 48 h of incubation. These result points to the future use of a cheap industrial by-product such as glycerol from biodiesel waste in the industrial production of PHA by the halophilic Halomonas species.
The chemical structures of the purified polymer were analyzed by FT-IR and 1H and 13C NMR. The assigned signals in the corresponding spectra agree with earlier studies (Kemavongse et al., 2008; Shamala et al., 2009; Kulkarni et al., 2011; Getachew and Woldesenbet, 2016; El-malek et al., 2020), confirming the chemical structure of PHBV. Based on the peak areas of the CH3 of HB unit and the CH3 of HV unit, the mole fraction of 3HV was determined as 52.04 mol %. It has been reported that Halomonas species can accumulate PHB, PHBV and poly (3-hydroxybutyrate-co-4-hydroxybutyrate) (P3HB4HB) (Ren et al., 2018; Chen et al., 2019; Ye et al., 2020). The copolymer PHBV is of high commercial interest due to its favorable properties compared to PHB (Tian et al., 2001; Chen. 2009; Ferre-Guell and Winterburn, 2018). Most PHBV producers require co-substrates such as propionic acid or valeric acid. H. profundus produces PHBV with different molar fractions of 3HV in the presence of valeric acid and propionic acid (Simon-Colin et al., 2008). Similarly, a prerequisite to the formation of 3HV-CoA in E. coli is the intracellular presence of propionyl-CoA as a precursor (Srirangan et al., 2016). The supplementation of precursors due to the lack of intracellular propionyl-CoA as a precursor in the biotransformation systems is toxic which not only inhibit cell growth but also increase production cost but also makes PHBV production difficult to control and thus increases production cost (Steinbüchel and Lutke-Eversloh, 2003). CRISPR/Cas9 method was used to engineer the TCA cycle in H. bluephagenesis on its chromosome for production of PHBV from glucose as a sole carbon source (Yu et al., 2020). The recombinant Halomonas TD08 is able to produce PHBV consisting of 4–6 mol% 3HV, from various carbohydrates as the sole carbon source (Tan et al., 2014). The copolymer formation without addition of precursors or genetic engineering tools has been reported in a few microorganisms such as Bacillus cereus SPV, Rhodobacter rubber, Nocardia corallina, Cupriavidus necator SH-69, Agrobacterium sp. strain SH1 and GW-014, Rhodospirillum Rubum, and H. campisalis MCM B-1027 (Valappil et al., 2008; Kulkarni et al., 2010; Policastro et al., 2021). To our knowledge, the moderately haloalkalitolerant strain, H. campisalis isolated from alkaline and saline Lonar Lake (Buldhana India) was the only Halomonas strain reported to accumulate PHBV (10.4 mol% HV) using a simple carbon source without addition of any precursors (Kulkarni et al., 2010).
The metabolic pathways relevant to glycerol assimilation and PHBV biosynthesis was predicted by genome analysis of H. desertis G11. In the predicted pathways, glycerol can be metabolized to two essential precursors acetyl-CoA through glycolysis pathway and converted to the 3HB monomer and to propionyl-CoA via threonine biosynthetic pathway and converted to 3HV monomer. These precursors lead to PHBV formation by three crucial enzymes. The genes encoding these three key enzymes of PHBV biosynthesis (PhaA, PhaB, and PhaC) are frequently organized in a single operon, as in C. necator or Pseudomonas sp. (Babel et al., 2001; Luengo et al., 2003). This organization is absent in other bacteria such as Halomonas sp. SF 2003. Idem, the genes encoding the three key enzymes of PHA biosynthesis pathway are not clustered in an operon in G11 strain genome but are scattered all over the genome. It has been reported that the organization of the biosynthetic genes depends on the bacterial species and also on the class of PHA synthases.
Based on phylogenetic analyses performed in this study, PhaC1 present in the genome of H. desertis G11 belongs to class I. Similar results were found with Halomonas sp. TD01 (Cai et al., 2011), Halomonas sp. SF2003 (Thomas et al., 2020) and H. sp. O-1 (Ilham et al., 2014). Multiple alignments of PHA synthases of several Halomonas species allowed identifying different PhaC box consensus sequences (Thomas et al., 2020). Analysis of CI-PhaC amino acids sequences of different halophiles PHA-producing Halomonas strains showed similar lipase box-like sequence (Ser-X-Cys-X-Gly) (figX). From the database analysis the most common described pattern is Glycine-X-Cysteine-X-Glycine (G-X-C-X-G) which can be modified to (GS)-X-C-X-(GA)-(GA) (Nambu et al., 2019). The PhaCHd has a lipase box-like sequence, S-X-C-X-G located between the residue 371 and the residue 375.
Structural analysis by different web servers for protein modeling (Phyre2, iterative TASSER, Swiss-Model), generated only two reliable three-dimensional protein structure models for Pha C1 of H. desertis G11 (Figure 7). The abilities of both strains Chromobacterium sp. USM2 and C. necator to produce PHBV from different carbon sources, have been previously reported (Obruca et al., 2010; Aramvash et al., 2016; Chek et al., 2017). Obruca et al. (2010) investigated the production of PHBV by Cupriavidus necator H16 in fed-batch mode from waste rapeseed oil using propanol as a precursor of 3-hydroxyvalerate. Aramvash et al. (2016) studied the effects of using a combination of substrates (fructose, propanol, citric acid, acetic acid, propionic acid and beef extract) on the PHBV production. The results of batch PHBV optimization by RSM, indicated that fructose in combination with propanol and beef extract, showed better PHBV yield, as compared to that of propionic acid. Chek et al. (2017) published a high-resolution crystal structure of the catalytic domain of homodimer Class I PHA synthase from Chromobacterium sp. USM2. This enzyme produces poly (3-hydroxybutyrate-co-3-hydroxyhexanoate) copolymer and poly (3-hydroxybutyrate-co-3-hydroxyvalerate-co-3-hydroxyhexanoate) terpolymer, which are good materials for industry due to their softness and flexibility (Jia et al., 2000; Amara and Rehm 2003; Tian et al., 2005).
Conclusion
The production of PHA by the halophilic strain H. desertis G11 on glycerol-based medium under saline condition was successfully optimized through statistical approach (BBD and RSM) indicating that G11 is an efficient PHA producer (1.5 g/L; 68% of DCW) with a high potential for industry applications. The NMR and FTIR spectra showed the presence of required functional groups in biosynthesized PHBV based on chemical shifts and bands observed. Genomic analysis revealed the presence of the key genes involved in the biosynthesis and biodegradation of PHA including phaA, phaB, phaC, phaZ, phaP and phaR. Through multiple sequences alignments with its homologs, H. desertis PhaC1 can be classified as a class I PHA synthase. Threonine pathway seems to have a key role in PHV synthesis.
The promising potential to use crude glycerol as low-cost substrate, in addition to its capacity to grow in front of stressful conditions and to produce PHA copolymers, make H. desertis G11 a versatile strain with a high potential for biotechnological use. The future research will be focused on 1) scaling up of the optimized process in bioreactor (fed batch and continuous processes) using biodiesel waste as carbon substrate; 2) studying the thermomechanical and visco-elastic properties of produced PHA; 3) valorizing low-cost substrates for the production of PHA under non-sterile high-salting conditions, 4) studying the role of PHA synthase in the synthesis of PHBV by H. desertis G11 using X-ray crystallography and exploiting the phaC gene for genetic and enzyme engineering, and 5) developing suitable genetic tools for manipulating the H. desertis G11 as a platform for synthesizing polymers.
Data Availability Statement
The datasets presented in this study can be found in online repositories. The names of the repository/repositories and accession number(s) can be found in the article/Supplementary Material.
Author Contributions
MN, YS, ASM, and AC conceived the original idea and supervised the project. DE-H isolated and characterized the PHA-producing strain. KH, MJ, HC, YS, and MN conducted FTIR, RMN and microscopic analyses and examined the data. KH, AS, HC, YS, and MN designed, conducted, and statistically analyzed the experimental design. MN, KH, AO, AM, ASM, and AC performed genomic and functional analyses. All authors contributed to the preparation and final approval of the manuscript.
Conflict of Interest
The authors declare that the research was conducted in the absence of any commercial or financial relationships that could be construed as a potential conflict of interest.
Publisher’s Note
All claims expressed in this article are solely those of the authors and do not necessarily represent those of their affiliated organizations, or those of the publisher, the editors and the reviewers. Any product that may be evaluated in this article, or claim that may be made by its manufacturer, is not guaranteed or endorsed by the publisher.
Acknowledgments
The authors acknowledge financial support from the European Union in the ambit of the project MADFORWATER (H2020, GA 688320) and the Tunisian Ministry of Higher Education and Scientific Research in the ambit of the laboratory project LR11ES31.
Supplementary Material
The Supplementary Material for this article can be found online at: https://www.frontiersin.org/articles/10.3389/fbioe.2022.878843/full#supplementary-material
References
Akinwumi, I. I., Domo-Spiff, A. H., and Salami, A. (2019). Marine Plastic Pollution and Affordable Housing challenge: Shredded Waste Plastic Stabilized Soil for Producing Compressed Earth Bricks. Case Stud. Construction Mater. 11, e00241. doi:10.1016/j.cscm.2019.e00241
Al-Hamdani, M. A., and Jaber, N. N. (2017). Production of Polyhydroxy Alkanoates by New Strain Bacillus Subtilis MANA18. Basrah J. Vet. Res. 16, 263–277.
Albuquerque, P. B. S., and Malafaia, C. B. (2018). Perspectives on the Production, Structural Characteristics and Potential Applications of Bioplastics Derived from Polyhydroxyalkanoates. Int. J. Biol. Macromolecules 107, 615–625. doi:10.1016/j.ijbiomac.2017.09.026
Amara, A. A., and Rehm, B. H. A. (2003). Replacement of the Catalytic Nucleophile Cysteine-296 by Serine in Class II Polyhydroxyalkanoate Synthase from Pseudomonas Aeruginosa-Mediated Synthesis of a New Polyester: Identification of Catalytic Residues. Biochem. J. 374, 413–421. doi:10.1042/bj20030431
Aramvash, A., Hajizadeh-Turchi, S., Moazzeni-Zavareh, F., Gholami-Banadkuki, N., Malek-Sabet, N., and Akbari-Shahabi, Z. (2016). Effective Enhancement of Hydroxyvalerate Content of PHBV in Cupriavidus Necator and its Characterization. Int. J. Biol. Macromolecules 87, 397–404. doi:10.1016/j.ijbiomac.2016.03.002
Athmika, S. D., Ghate, S. D., Arun, A. B., Rao, S. S., and Kumar, S. T. A. (2021). Genome Analysis of a Halophilic Bacterium Halomonasmalpeensis YU-PRIM-29t Reveals its Exopolysaccharide and Pigment Producing Capabilities. Sci. Rep 11, 1749. doi:10.1038/s41598-021-81395-1
Aziz, R. K., Bartels, D., Best, A. A., DeJongh, M., Disz, T., Edwards, R. A., et al. (2008). The RAST Server: Rapid Annotations Using Subsystems Technology. BMC Genomics 9, 75. doi:10.1186/1471-2164-9-75
Babel, W., Ackermann, J. U., and Breuer, U. (2001). Physiology, Regulation, and Limits of the Synthesis of poly(3HB). Adv. Biochem. Eng. Biotechnol. 71, 125–157. doi:10.1007/3-540-40021-4_4
Bhuwal, A. K., Singh, G., Aggarwal, N. K., Goyal, V., and Yadav, A. (2013). Isolation and Screening of Polyhydroxyalkanoates Producing Bacteria from Pulp, Paper, and Cardboard Industry Wastes. Int. J. Biomater. 2013, 752821. doi:10.1155/2013/752821
Biswas, A., Patra, A., and Paul, A. (2009). Production of Poly-3-Hydroxyalkanoic Acids by a Moderately Halophilic Bacterium, Halomonas marina HMA 103 Isolated from Solar Saltern of Orissa, India. Acta Microbiol. Immunol. Hung. 56, 125–143. doi:10.1556/amicr.56.2009.2.9
Bloembergen, S., Holden, D. A., Hamer, G. K., Bluhm, T. L., and Marchessault, R. H. (1986). Studies of Composition and Crystallinity of Bacterial Poly(β-Hydroxybutyrate-Co-β-Hydroxyvalerate). Macromolecules 11, 2865–2871. doi:10.1021/ma00165a034
Bugnicourt, E., Cinelli, P., Lazzeri, A., and Alvarez, V. (2014). Polyhydroxyalkanoate (PHA): Review of Synthesis, Characteristics, Processing and Potential Applications in Packaging. Express Polym. Lett. 8, 791–808. doi:10.3144/expresspolymlett.2014.82
Cai, L., Tan, D., Aibaidula, G., Dong, X., Chen, J. C., Tian, W. D., et al. (2011). Comparative Genomics Study of Polyhydroxyalkanoates (PHA) and Ectoine Relevant Genes from Halomonas Sp. TD01 Revealed Extensive Horizontal Gene Transfer Events and Co-evolutionary Relationships. Microb. Cel Fact 10, 88. doi:10.1186/1475-2859-10-88
Chae, Y., and An, Y. J. (2018). Current Research Trends on Plastic Pollution and Ecological Impacts on the Soil Ecosystem: A Review. Environ. Pollut. 240, 387–395. doi:10.1016/j.envpol.2018.05.008
Chek, M. F., Kim, S.-Y., Mori, T., Arsad, H., Samian, M. R., Sudesh, K., et al. (2017). Structure of Polyhydroxyalkanoate (PHA) Synthase PhaC from Chromobacterium Sp. USM2, Producing Biodegradable Plastics. Sci. Rep. 7, 5312. doi:10.1038/s41598-017-05509-4
Chen, G.-Q., and Jiang, X.-R. (2017). Engineering Bacteria for Enhanced Polyhydroxyalkanoates (PHA) Biosynthesis. Synth. Syst. Biotechnol. 2, 192–197. doi:10.1016/j.synbio.2017.09.001
Chen, G. Q. (20092010). “A Microbial Polyhydroxyalkanoates (PHA) Based Bio- and Materials Industry,” in Plastics from Bacteria: Natural Functions and Applications (Berlin/Heidelberg, Germany; Münster, Germany: Springer), 121–132. doi:10.1007/978-3-642-03287-5_6
Chen, G. Q., and Albertsson, A. C. (2019). Polyhydroxyalkanoates and Other Biopolymers. Biomacromolecules 20, 3211–3212. doi:10.1021/acs.biomac.9b01044
Chen, X., Li, L., Wei, T., Venerus, D. C., and Torkelson, J. M. (2018). Reprocessable Polyhydroxyurethane Network Composites: Effect of Filler Surface Functionality on Cross-Link Density Recovery and Stress Relaxation. ACS Appl. Mater. Inter. 11, 2398–2407. doi:10.1021/acsami.8b19100
Chen, Y., Chen, X. Y., Du, H. T., Zhang, X., Ma, Y. M., Chen, J. C., et al. (2019). Chromosome Engineering of the TCA Cycle in Halomonas Bluephagenesis for Production of Copolymers of 3-hydroxybutyrate and 3-hydroxyvalerate (PHBV). Metab. Eng. 54, 69–82. doi:10.1016/j.ymben.2019.03.006
Chien, C. C., Chen, C. C., Choi, M. H., Kung, S. S., and Wei, Y. H. (2007). Production of Poly-β-Hydroxybutyrate (PHB) by Vibrio Spp. Isolated from marine Environment. J. Biotechnol. 132, 259–263. doi:10.1016/j.jbiotec.2007.03.002
Cristea, A., Baricz, A., Leopold, N., Floare, C. G., Borodi, G., Kacso, I., et al. (2018). Polyhydroxybutyrate Production by an Extremely Halotolerant Halomonas Elongate Strain Isolated from the Hypersaline Meromictic Fără Fund Lake (Transylvanian Basin, Romania). J. Appl. Microbiol. 125, 1343–1357. doi:10.1111/jam.14029
Davis, I. W., Leaver-Fay, A., Chen, V. B., Block, J. N., Kapral, G. J., Wang, X., et al. (2007). MolProbity: All-Atom Contacts and Structure Validation for Proteins and Nucleic Acids. Nucleic Acids Res. 35 (Suppl. 2), W375–W383. doi:10.1093/nar/gkm216
de Paula, F. C., Kakazu, S., de PaulaGomez, C. B. C. J. G. C., and Contiero, J. (2017). Polyhydroxyalkanoate Production from Crude Glycerol by Newly Isolated Pandoraea Sp. J. King Saud Univ. – Sci. 29 (2), 166–173. doi:10.1016/j.jksus.2016.07.002
Divyashree, M. S., and Shamala, T. R. (2009). Effect of Gamma Irradiation on Cell Lysis and Polyhydroxyalkanoate Produced by Bacillus Flexus. Radiat. Phys. Chem. 78, 147–152. doi:10.1016/j.radphyschem.2008.08.010
Dubey, S., and Mishra, S. (2021). Efficient Production of Polyhydroxyalkanoate through Halophilic Bacteria Utilizing Algal Biodiesel Waste Residue. Front. Bioeng. Biotechnol. 9, 624859. doi:10.3389/fbioe.2021.624859
El-malek, F. A., Farag, A., Omar, S., and Khairy, H. (2020). Polyhydroxyalkanoates (PHA) from Halomonas pacifica ASL10 and Halomonas Salifodiane ASL11 Isolated from Mariout Salt Lakes. Int. J. Biol. Macromol. 161, 1318–1328. doi:10.1016/j.ijbiomac.2020.07.258
Elain, A., Le Grand, A., Corre, Y-M., Le Fellic, M., Hachet, N., Tilly, V. L., et al. (2016). Valorisation of Local Agro-Industrial Processing Waters as Growth media for Polyhydroxyalkanoates (PHA) Production. Ind. Crop Prod. 80, 1–5. doi:10.1016/j.indcrop.2015.10.052
Ferre-Güell, A., and Winterburn, J. (2018). Production of the Copolymer Poly(3-Hydroxybutyrate-Co-3-Hydroxyvalerate) with Varied Composition Using Different Nitrogen Sources with Haloferax Mediterranei. Extremophiles 21, 1037–1047. doi:10.1021/acs.biomac.7b01788
Fu, X.-Z., Tan, D., Aibaidula, G., Wu, Q., Chen, J.-C., and Chen, G.-Q. (2014). Development of Halomonas TD01 as a Host for Open Production of Chemicals. Metab. Eng. 23, 78–91. doi:10.1016/j.ymben.2014.02.006
Gasteiger, E., Hoogland, C., Gattiker, A., Duvaud, S., Wilkins, M. R., Appel, R. D., et al. (2005). “Protein Identification and Analysis Tools on the ExPASy Server,” in The Proteomics Protocols Handbook. Editor J. M. Walker (Totowa, NJ: Humana), 571–607. doi:10.1385/1-59259-890-0:571
Getachew, A., and Woldesenbet, F. (2016). Production of Biodegradable Plastic by Polyhydroxybutyrate (PHB) Accumulating Bacteria Using Low Cost Agricultural Waste Material. BMC Res. Notes 9, 509. doi:10.1186/s13104-016-2321-y
Haba, R. R., Sánchez-Porro, C., and Ventosa, A. (2011). Taxonomy, Phylogeny, and Biotechnological Interest of the Family Halomonadaceae. Halophiles and hypersaline environments 27, 64. doi:10.1007/978-3-642-20198-1_310.1007/978-3-662-45796-2_3
Han, J., Hou, J., Liu, H., Cai, S., Feng, B., Zhou, J., et al. (2010). Wide Distribution Among Halophilic Archaea of a Novel Polyhydroxyalkanoate Synthase Subtype with Homology to Bacterial Type III Synthases. Appl. Environ. Microbiol. 76, 7811–7819. doi:10.1128/AEM.01117-10
Han, J., Wu, L. P., Hou, J., Zhao, D., and Xiang, H. (2015). Biosynthesis, Characterization, and Hemostasis Potential of Tailor-Made Poly (3-Hydroxybutyrate-Co-3-Hydroxyvalerate) Produced by Haloferax Mediterranei. Biomacromolecules 16, 578–588. doi:10.1021/bm5016267
Han, J., Wu, L. P., Liu, X. B., Hou, J., Zhao, L. L., Chen, J. Y., et al. (2017). Biodegradation and Biocompatibility of Haloarchaea-Produced Poly(3-Hydroxybutyrate-Co-3-Hydroxyvalerate) Copolymers. Biomaterials 139, 172–186. doi:10.1016/j.biomaterials.2017.06.006
Haywood, G. W., Anderson, A. J., Williams, D. R., Daws, E. A., and Ewing, D. F. (1991). Accumulation of a Poly(hydroxyalkanoate) Copolymer Containing Primarily 3-hydroxyvalerate from Simple Carbohydrate Substrates by Rhodococcus Sp. NCIMB 40126. Int. J. Biol. Macromol. 13, 83–88. doi:10.1016/0141-8130(91)90053-w
Hermann-Krauss, C., Koller, M., Muhr, A., Fasl, H., Stelzer, F., and Braunegg, G. (2013). Archaeal Production of Polyhydroxyalkanoate (PHA) Co- and Terpolyesters from Biodiesel Industry-Derived By-Products. Archaea. doi:10.1155/2013/129268
Hertadi, R., Kurnia, K., Falahudin, W., and Puspasari, M. (2017). Poly-hydroxybutyrate (PHB) Production by Halomonas Elongata BK AG 18 Indigenous from Salty Mud Crater at Central Java Indonesia. Malaysian J. Microbiol. 13, 1. doi:10.21161/mjm.88416
Ibrahim, M. I., Alsafadi, D., Alamry, K. A., and Hussein, M. A. (2020). Properties and Applications of Poly(3-Hydroxybutyrate-Co-3- -hydroxyvalerate) Biocomposites. J. Polym. Environ. 29, 1010–1030. doi:10.1007/s10924-020-01946-x
Ilham, M., Nakanomori, S., Kihara, T., Hokamura, A., Matsusaki, H., Tsuge, T., et al. (2014). Characterization of Polyhydroxyalkanoate Synthases from Halomonas Sp. O-1 and Halomonas Elongata DSM2581: Site-Directed Mutagenesis and Recombinant Expression. Polym. Degrad. Stab. 109, 416–423. doi:10.1016/j.polymdegradstab.2014.04.024
Jia, Y., Kappock, T. J., Frick, T., Sinskey, A. J., and Stubbe, J. (2000). Lipases Provide a New Mechanistic Model for Polyhydroxybutyrate (PHB) Synthases: Characterization of the Functional Residues in Chromatium Vinosum PHB Synthase. Biochemistry 39, 3927–3936. doi:10.1021/bi9928086
Jincy, M., Sindhu, R., Pandey, A., and Binod, P. (2013). Bioprocess Development for Utilizing Biodiesel Industry Generated Crude Glycerol for Production of Poly-3-Hydroxybutyrate. J. Sci. Ind. Res. 72, 596–602.
Kalia, V. C., Singh Patel, S. K., Shanmugam, R., and Lee, J.-K. (2021). Polyhydroxyalkanoates: Trends and Advances toward Biotechnological Applications. Bioresour. Technol. 326, 124737. doi:10.1016/j.biortech.2021.1247310.1016/j.biortech.2021.124737
Kawata, Y., and Aiba, S. I. (2010). Poly (3-hydroxybutyrate) Production by Isolated Halomonas Sp. KM-1 Using Waste Glycerol. Biosci. Biotechnol. Biochem. 74, 175–177. doi:10.1271/bbb.90459
Kelley, L. A., Mezulis, S., Yates, C. M., Wass, M. N., and Sternberg, M. J. (2015). The Phyre2 Web portal for Protein Modeling, Prediction and Analysis. Nat. Protoc. 10, 845–858. doi:10.1038/nprot.2015.053
Kemavongse, K., Prasertsan, P., Upaichit, A., and Methacanon, P. (2008). Poly-b-hydroxyalkanoate Production by Halotolerant Rhodobacter Sphaeroides U7. World J. Microbiol. Biotechnol. 24, 2073–2085. doi:10.1007/s11274-008-9712-8
Kondaveeti, S., Kim, I-W., Otari, S., Patel, S. K. S., Pagolu, R., Losetty, V., et al. (2019). Co-generation of Hydrogen and Electricity from Biodiesel Process Effluents. Int. J. Hydrog. Ener. 44 (50), 27285–27296. doi:10.1016/j.ijhydene.2019.08.258
Kourmentza, C., Plácido, J., Venetsaneas, N., Burniol-Figols, A., Varrone, C., Gavala, H. N., et al. (2017). Recent Advances and Challenges towards Sustainable Polyhydroxyalkanoate (PHA) Production. Bioengineering 4, E55. doi:10.3390/bioengineering4020055
Kucera, D., Pernicová, I., Kovalcik, A., Koller, M., Mullerova, L., Sedlacek, P., et al. (2018). Characterization of the Promising Poly(3-Hydroxybutyrate) Producing Halophilic Bacterium Halomonas Halophila. Bioresour. Technol. 256, 552–556. doi:10.1016/j.biortech.2018.02.062
Kulkarni, S. O., Kanekar, P. P., Jog, J. P., Sarnaik, S. S., and Nilegaonkar, S. S. (2015). Production of Copolymer, Poly (Hydroxybutyrate-co-hydroxyvalerate) by Halomonas Campisalis MCM B-1027 Using Agro-Wastes. Int. J. Biol. Macromol. doi:10.1016/j.ijbiomac.2014.09.028
Kulkarni, S. O., Kanekar, P. P., Jog, J. P., Patil, P. A., Nilegaonkar, S. S., Sarnaik, S. S., et al. (2011). Characterisation of Copolymer, Poly (Hydroxybutyrate-Co-Hydroxyvalerate) (PHB-Co-PHV) Produced by Halomonas Campisalis (MCM B-1027), its Biodegradability and Potential Application. Bioresour. Technol. 102, 6625–6628. doi:10.1016/j.biortech.2011.03.054
Kulkarni, S. O., Kanekar, P. P., Nilegaonkar, S. S., Sarnaik, S. S., and Jog, J. P. (2010). Production and Characterization of a Biodegradable Poly (Hydroxybutyrate-co-hydroxyvalerate) (PHB-Co-PHV) Copolymer by Moderately Haloalkalitolerant Halomonas Campisalis MCM B-1027 Isolated from Lonar Lake, India. Bioresour. Technol. 101 (24), 9765–9771. doi:10.1016/j.biortech.2010.07.089
Kumar, P., Ray, S., Patel, S. K., Lee, J. K., and Kalia, V. C. (2015). Bioconversion of Crude Glycerol to Polyhydroxyalkanoate by Bacillus Thuringiensis under Non-limiting Nitrogen Conditions. Int. J. Biol. Macromol. 78, 9–16. doi:10.1016/j.ijbiomac.2015.03.046
Lee, Y., Cho, I. J., Choi, S. Y., and Lee, S. Y. (2019). Systems Metabolic Engineering Strategies for Non-natural Microbial Polyester Production. Biotechnol. J. 14, e1800426. doi:10.1002/biot.201800426
Leong, Y. K., Show, P. L., Ooi, C. W., Ling, T. C., and Lan, J. C. W. (2014). Current Trends in Polyhydroxyalkanoates (PHAs) Biosynthesis: Insights from the Recombinant Escherichia coli. J. Biotechnol. 180, 52–65. doi:10.1016/j.jbiotec.2014.03.020
Levett, I., Birkett, G., Davies, N., Bell, A., Langford, A., and Laycock, B. (2016). Techno-economic Assessment of Poly-3-Hydroxybutyrate (PHB) Production from Methane - the Case for Thermophilic Bioprocessing. J. Environ. Chem. Eng. 4, 3724–3733. doi:10.1016/j.jece.2016.07.033
Luengo, J. M., Garcí, A. B., Sandoval, A., Naharro, G., and Olivera, E. A. R. (2003). Bioplastics from Microorganisms. Curr. Opin. Microbiol. 6, 251–260. doi:10.1016/S1369-5274(03)00040-7
Mascarenhas, J., and Aruna, K. (2017). Screening of Polyhydroxyalkonates (PHA) Accumulating Bacteria from Diverse Habitats. J. Glob. Biosci. 6, 4835–4848.
Mitra, R., Xu, T., Xiang, H., and Han, J. (2020). Current Developments on Polyhydroxyalkanoates Synthesis by Using Halophiles as a Promising Cell Factory. Microb. Cel Fact. 19, 86. doi:10.1186/s12934-020-01342-z
Mothes, G., Schnorpfeil, C., and Ackermann, J. (2007). Production of PHB from Crude Glycerol. Eng. Life Sci. 7, 475–479. doi:10.1002/elsc.200620210
Mothes, G., Schubert, T., Harms, H., and Maskow, T. (2008). Biotechnological Coproduction of Compatible Solutes and Polyhydroxyalkanoates Using the Genus Halomonas. Eng. Life Sci. 8, 658–662. doi:10.1002/elsc.200800097
Muhammadi, S., Afzal, M., and Hameed, S. (2015). Bacterial Polyhydroxyalkanoates-Eco-Friendly Next Generation Plastic: Production, Biocompatibility, Biodegradation, Physical Properties and Applications. Green. Chem. Lett. Rev. 8, 56–77. doi:10.1080/17518253.2015.1109715
Naili, F., Neifar, M., Elhidri, D., Cherif, H., Bejaoui, B., Aroua, M., et al. (2018). Optimization of the Effect of PGPR–Based Biofertlizer on Wheat Growth and Yield. Biom. Biostat. Int. J. 7, 226–232. doi:10.15406/bbij.2018.07.00213
Nambu, Y., Ishii-Hyakutake, M., Harada, K., Mizuno, S., and Tsuge, T. (2019). Expanded Amino Acid Sequence of the PhaC Box in the Active center of Polyhydroxyalkanoate Synthases. FEBS Lett. 594, 710–716. doi:10.1002/1873-3468.13651
Naranjo, J. M., Posada, J. A., Higuita, J. C., and Cardona, C. A. (2013). Valorization of Glycerol through the Production of Biopolymers: the PHB Case Using Bacillus Megaterium. Bioresour. Technol. 133, 38–44. doi:10.1016/j.biortech.2013.01.129
Neifar, M., Chouchane, H., Najjari, A., El Hidri, D., Mahjoubi, M., Ghedira, K., et al. (2019). Genome Analysis Provides Insights into Crude Oil Degradation and Biosurfactant Production by Extremely Halotolerant Halomonas Desertis G11 Isolated from Chott El-Djerid Salt-lake in Tunisian Desert. Genomics 111, 1802–1814. doi:10.1016/j.ygeno.2018.12.003
Nygaard, D., Yashchuk, O., and Hermida, E. B. (2019). Evaluation of Culture Medium on Poly(3-Hydroxybutyrate) Production by Cupriavidus Necator ATCC 17697: Application of the Response Surface Methodology. Heliyon 5, e01374. doi:10.1016/j.heliyon.2019.e01374
Obruca, S., Marova, I., Snajdar, O., Mravcova, L., and Svoboda, Z. (2010). Production of Poly(3-Hydroxybutyrate-Co-3-Hydroxyvalerate) by Cupriavidus Necator from Waste Rapeseed Oil Using Propanol as a Precursor of 3-hydroxyvalerate. Biotechnol. Lett. 32 (12), 1925–1932. doi:10.1007/s10529-010-0376-8
Obruca, S., Sedlacek, P., Slaninova, E., Fritz, I., Daffert, C., Meixner, K., et al. (2020). Novel Unexpected Functions of PHA Granules. Appl. Microbiol. Biotechnol. 104, 4795–4810. doi:10.1007/s00253-020-10568-1
Ostle, A. G., and Holt, J. G. (1982). Nile Blue a as a Fluorescent Stain for Poly-Beta-Hydroxybutyrate. Appl. Environ. Microbiol. 44, 238–241. doi:10.1128/aem.44.1.238-241.1982
Patel, S. K., Kumar, P., Singh, M., Lee, J. K., and Kalia, V. C. (2015). Integrative Approach to Produce Hydrogen and Polyhydroxybutyrate from Biowaste Using Defined Bacterial Cultures. Bioresour. Technol. 176, 136–141. doi:10.1016/j.biortech.2014.11.029
Patel, S. K. S., Das, D., Kim, S. C., Cho, B.-K., Kalia, V. C., and Lee, J.-K. (2021a). Integrating Strategies for Sustainable Conversion of Waste Biomass into Dark-Fermentative Hydrogen and Value-Added Products. Ren. Sust. Ene. Rev. 150, 111491. doi:10.1016/j.rser.2021.1114910.1016/j.rser.2021.111491
Patel, S. K. S., Shanmugam, R., Lee, J. K., Kalia, V. C., and Kim, I. W. (2021b). Biomolecules Production from Greenhouse Gases by Methanotrophs. Ind. J. Microbiol. 61 (4), 449–457. doi:10.1007/s12088-021-00986-8
Poli, A., Di Donato, P., Abbamondi, G. R., and Nicolaus, B. (2011). Synthesis, Production, and Biotechnological Applications of Exopolysaccharides and Polyhydroxyalkanoates by Archaea. Archaea 2011, 693253. (Vancouver, B.C.). doi:10.1155/2011/693253
Policastro, G., Panico, A., and Fabbricino, M. (2021). Improving Biological Production of Poly(3-Hydroxybutyrate-Co-3-Hydroxyvalerate) (PHBV) Co-polymer: a Critical Review. Rev. Environ. Sci. Biotechnol. 20, 479–513. doi:10.1007/s11157-021-09575-z
Quillaguaman, J., Delgado, O., Mattiasson, B., and Hatti-Kaul, R. (2006). Poly(beta-hydroxybutyrate) Production by a Moderate Halophile, Halomonas Boliviensis LC1. Enzym. Microb. Technol. 38, 148–154. doi:10.1016/j.enzmictec.2005.05.013
Quillaguamán, J., Guzmán, H., Van-Thuoc, D., and Hatti-Kaul, R. (2010). Synthesis and Production of Polyhydroxyalkanoates by Halophiles: Current Potential and Future Prospects. Appl. Microbiol. Biotechnol. 85, 1687–1696. doi:10.1007/s00253-009-2397-6
Raghul, S. S. (2012). Characterization of Polyhydroxyalkanoates Accumulating Vibrios from marine Benthic Environments and Production Studies of Polyhydroxyalkanoates by Vibrio Sp. BTKB33. Doctoral dissertation, Cochin University of Science and Technology. Available at: http://dyuthi.cusat.ac.in/purl/3100.
Ray, S., Sharma, R., and Kalia, V. C. (2018). Co-utilization of Crude Glycerol and Biowastes for Producing Polyhydroxyalkanoates. Ind. J. Microbiol. 58 (1), 33–38. doi:10.1007/s12088-017-0702-0
Ren, Y., Ling, C., Hajnal, I., Wu, Q., and Chen, G. Q. (2018). Construction of Halomonas Bluephagenesis Capable of High Cell Density Growth for Efcient PHA Production. Appl. Microbiol. Biotechnol. 102, 4499–4510. doi:10.1007/s00253-018-8931-7
Riahi, L., Cherif, H., Miladi, S., Neifar, M., Bejaoui, B., Chouchane, H., et al. (2020). Use of Plant Growth Promoting Bacteria as an Efficient Biotechnological Tool to Enhance the Biomass and Secondary Metabolites Production of the Industrial Crop Pelargonium Graveolens L'Hér. Under Semi-controlled Conditions. Ind. Crops Prod. 154, 112721. doi:10.1016/j.indcrop.2020.112721
Rivera-Briso, A. L., and Serrano-Aroca, Á. (2018). Poly(3-hydroxybutyrate-co-3-hydroxyvalerate): Enhancement Strategies for Advanced Applications. Polymers 10, 732. doi:10.3390/polym10070732
Rodriguez-Perez, S., Serrano, A., Pantión, A. A., and Alonso-Fariñas, B. (2018). Challenges of Scaling-Up PHA Production from Waste Streams. A Review. J. Environ. Manag. 205, 215–230. doi:10.1016/j.jenvman.2017.09.083
Shamala, T. R., Divyashree, M. S., Davis, R., Kumari, K. L., Vijayendra, S. V. N., and Raj, B. (2009). Production and Characterization of Bacterial Polyhydroxyalkanoate Copolymers and Evaluation of Their Blends by Fourier Transform Infrared Spectroscopy and Scanning Electron Microscopy. Indian J. Microbiol. 49, 251–258. doi:10.1007/s12088-009-0031-z
Shams, M., Alam, I., and Mahbub, M. S. (2021). Plastic Pollution during COVID-19: Plastic Waste Directives and its Long-Term Impact on the Environment. Environ. Adv. 5, 100119. doi:10.1016/j.envadv.2021.100119
Sharma, S., and Chatterjee, S. (2017). Microplastic Pollution, a Threat to marine Ecosystem and Human Health: a Short Review. Environ. Sci. Pollut. Res. 24, 21530–21547. doi:10.1007/s11356-017-9910-8
Simon-Colin, C., Raguénès, G., Cozien, J., and Guezennec, J. (2008). Halomonas Profundus Sp. nov., a New PHA-Producing Bacterium Isolated from a Deep-Sea Hydrothermal Vent Shrimp. J. Appl. Microbiol. 104, 1425–1432. doi:10.1111/j.1365-2672.2007.03667.x
Singh, S., Sithole, B., Lekha, P., Permaul, K., and Govinden, R. (2021). Optimization of Cultivation Medium and Cyclic Fed-Batch Fermentation Strategy for Enhanced Polyhydroxyalkanoate Production by Bacillus thuringiensis Using a Glucose-Rich Hydrolyzate. Bioresour. Bioproc. 8, 11. doi:10.1186/s40643-021-00361-x
Srirangan, K., Liu, X., Tran, T. T., Charles, T. C., Moo-Young, M., and Chou, C. P. (2016). Engineering of Escherichia coli for Direct and Modulated Biosynthesis of Poly(3- Hydroxybutyrate-Co-3-Hydroxyvalerate) Copolymer Using Unrelated Carbon Sources. Sci. Rep. 6, 36470. doi:10.1038/srep36470
Stanley, A., Kumar, P. H. N., Mutturi, S., and Vijayendra, S. V. N. (2018). Fed-batch Strategies for Production of PHA Using a Native Isolate of Halomonas Venusta KT832796 Strain. Appl. Biochem. Biotechnol. 184, 935–952. doi:10.1007/s12010-017-2601-6
Steinbüchel, A., and Lütke-Eversloh, T. (2003). Metabolic Engineering and Pathway Construction for Biotechnological Production of Relevant Polyhydroxyalkanoates in Microorganisms. Biochem. Eng. J. 16, 81–96. doi:10.1016/s1369-703x(03)00036-6
Surendran, A., Lakshmanan, M., Chee, J. Y., Sulaiman, A. M., Thuoc, D. V., and Sudesh, K. (2020). Can Polyhydroxyalkanoates Be Produced Efficiently from Waste Plant and Animal Oils? Front. Bioeng. Biotechnol. 8, 169. doi:10.3389/fbioe.2020.00169
Taidi, B., Anderson, A. J., Dawes, E. A., and Byrom, D. (1994). Effect of Carbon Source and Concentration on the Molecular-Mass of Poly(3-Hydroxybutyrate) Produced by Methylobacterium Extorquens and Alcaligenes Eutrophus. Appl. Microbiol. Biotechnol. 40, 786–790. doi:10.1007/bf00173975
Tan, D., Wu, Q., Chen, J. C., and Chen, G. Q. (2014). Engineering Halomonas TD01 for the Low-Cost Production of Polyhydroxyalkanoates. Metab. Eng. 26, 34–47. doi:10.1016/j.ymben.2014.09.001
Tan, D., Xue, Y.-S., Aibaidula, G., and Chen, G.-Q. (2011). Unsterile and Continuous Production of Polyhydroxybutyrate by Halomonas TD01. Bioresour. Technol. 102, 8130–8136. doi:10.1016/j.biortech.2011.05.068
Tao, W., Lv, L., and Chen, G. Q. (2017). Engineering Halomonas Species TD01 for Enhanced Polyhydroxyalkanoates Synthesis via CRISPRi. Microb. Cel Fact. 16, 48. doi:10.1186/s12934-017-0655-3
Thomas, T., Elain, A., Bazire, A., and Bruzaud, S. (2019). Complete Genome Sequence of the Halophilic PHA-Producing Bacterium Halomonas Sp. SF2003: Insights into its Biotechnological Potential. World J. Microbiol. Biotechnol. 35, 50. doi:10.1007/s11274-019-2627-8
Thomas, T., Sudesh, K., Bazire, A., Elain, A., Tan, H. T., and Lim, H. (2020). PHA Production and PHA Synthases of the Halophilic Bacterium Halomonas Sp. SF2003. Bioengineering 7, 29. doi:10.3390/bioengineering7010029
Tian, G., Wu, Q., Sun, S., Noda, I., and Chen, G. Q. (2001). Study of Thermal Melting Behavior of Microbial Polyhydroxyalkanoate Using Two-Dimensional Fourier Transform Infrared Correlation Spectroscopy. Appl. Spectrosc. 55, 888–893. doi:10.1366/0003702011952686
Tian, J., Sinskey, A. J., and Stubbe, J. (2005). Class III Polyhydroxybutyrate Synthase: Involvement in Chain Termination and Reinitiation. Biochemistry 44, 8369–8377. doi:10.1021/bi050331u
Valappil, S. P., Rai, R., Bucke, C., and Roy, I. (2008). Polyhydroxyalkanoate Biosynthesis in Bacillus Cereus SPV under Varied Limiting Conditions and an Insight into the Biosynthetic Genes Involved. J. Appl. Microbiol. 104, 1624–1635. doi:10.1111/j.1365-2672.2007.03678.x
Van-Thuoc, D., Huu-Phong, T., Minh-Khuong, D., and Hatti-Kaul, R. (2015). Poly(3-hydroxybutyrate-co-3-hydroxyvalerate) Production by a Moderate Halophile Yangia Sp. ND199 Using Glycerol as a Carbon Source. Appl. Biochem. Biotechnol. 175, 3120–3132. doi:10.1007/s12010-015-1479-4
Van-Thuoc, D., Quillaguamán, J., Mamo, G., and Matiason, B. (2008). Utilization of Agricultural Residues for Poly(3-Hydroxybutyrate) Production by Halomonas Boliviensis LC1. J. Appl. Microbiol. 104, 420–428. doi:10.1111/j.1365-2672.2007.03553.x
Wahab, H. A., Khairudin, A., Samian, M. R., and Najimudin, N. (2006). Sequence Analysis and Structure Prediction of Type II Pseudomonas Sp. USM 4–55 PHA Synthase and an Insight into its Catalytic Mechanism. BMC. Struct. Biol. 6, 6–23. doi:10.1186/1472-6807-6-23
Wei, Y. H., Chen, W. C., Wu, H. S., and Janarthanan, O. M. (2011). Biodegradable and Biocompatible Biomaterial, Polyhydroxybutyrate, Produced by an Indigenous Vibrio Sp. BM-1 Isolated from marine Environment. Mar. Drugs 9, 615–624. doi:10.3390/md9040615
Yang, J., Roy, A., and Zhang, Y. (2013). Protein–ligand Binding Site Recognition Using Complementary Binding-specific Substructure Comparison and Sequence Profile Alignment. Bioinformatics 29, 2588–2595. doi:10.1093/bioinformatics/btt447
Yang, J., and Zhang, Y. (2015). I-TASSER Server: New Development for Protein Structure and Function Predictions. Nucleic Acids Res. 43, W174–W181. doi:10.1093/nar/gkv342
Ye, J., Hu, D., Yin, J., Huang, W., Xiang, R., Zhang, L., et al. (2020). Stimulus Response-Based Fne-Tuning of Polyhydroxyalkanoate Pathway in Halomonas. Metab. Eng. 57, 85–95. doi:10.1016/j.ymben.2019.10.007
Yin, J., Chen, J. C., Wu, Q., and Chen, G. Q. (2015). Halophiles, Coming Stars for Industrial Biotechnology. Biotechnol. Adv. 33, 1433–1442. doi:10.1016/j.biotechadv.2014.10.008
Yu, L. P., Yan, X., Zhang, X., Chen, X. B., Wu, Q., and Jiang, X. R. (2020). Biosynthesis of Functional Polyhydroxyalkanoates by Engineered Halomonas Bluephagenesis. Metab. Eng. 59, 119–130. doi:10.1016/j.ymben.2020.02.005
Yuan, W., Jia, Y., Tian, J., Snell, K. D., Muh, U., and Sinskey, A. J. (2001). Class I and III Polyhydroxyalkanoate Synthases from Ralstonia Eutropha and Allochromatium Vinosum: Characterization and Substrate Specificity Studies. Arch. Biochem. Biophys. 394, 87–98. doi:10.1006/abbi.2001.2522
Keywords: Halomonas desertis G11, halophilic bacterium, polyhydroxyalkanoates, genome annotation, PHA synthase
Citation: Hammami K, Souissi Y, Souii A, Ouertani A, El-Hidri D, Jabberi M, Chouchane H, Mosbah A, Masmoudi AS, Cherif A and Neifar M (2022) Extremophilic Bacterium Halomonas desertis G11 as a Cell Factory for Poly-3-Hydroxybutyrate-co-3-Hydroxyvalerate Copolymer’s Production. Front. Bioeng. Biotechnol. 10:878843. doi: 10.3389/fbioe.2022.878843
Received: 18 February 2022; Accepted: 06 April 2022;
Published: 23 May 2022.
Edited by:
Suchada Chanprateep Napathorn, Chulalongkorn University, ThailandReviewed by:
Sanjay Kumar Singh Patel, Konkuk University, South KoreaAlfred Fernández-Castané, Aston University, United Kingdom
Copyright © 2022 Hammami, Souissi, Souii, Ouertani, El-Hidri, Jabberi, Chouchane, Mosbah, Masmoudi, Cherif and Neifar. This is an open-access article distributed under the terms of the Creative Commons Attribution License (CC BY). The use, distribution or reproduction in other forums is permitted, provided the original author(s) and the copyright owner(s) are credited and that the original publication in this journal is cited, in accordance with accepted academic practice. No use, distribution or reproduction is permitted which does not comply with these terms.
*Correspondence: Mohamed Neifar, bW9oYW1lZC5uYWlmYXJAZ21haWwuY29t