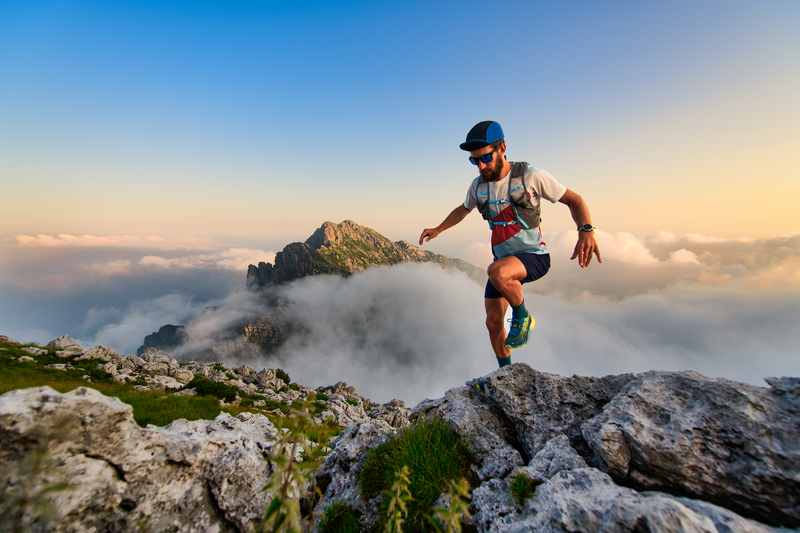
95% of researchers rate our articles as excellent or good
Learn more about the work of our research integrity team to safeguard the quality of each article we publish.
Find out more
ORIGINAL RESEARCH article
Front. Bioeng. Biotechnol. , 23 June 2022
Sec. Biomaterials
Volume 10 - 2022 | https://doi.org/10.3389/fbioe.2022.874931
This article is part of the Research Topic 3D-printed Biomaterials in Osteochondral Repair View all 15 articles
Polylactic glycolic acid copolymer (PLGA) has been widely used in tissue engineering due to its good biocompatibility and degradation properties. However, the mismatched mechanical and unsatisfactory biological properties of PLGA limit further application in bone tissue engineering. Calcium sulfate (CaSO4) is one of the most promising bone repair materials due to its non-immunogenicity, well biocompatibility, and excellent bone conductivity. In this study, aiming at the shortcomings of activity-lack and low mechanical of PLGA in bone tissue engineering, customized-designed 3D porous PLGA/CaSO4 scaffolds were prepared by 3D printing. We first studied the physical properties of PLGA/CaSO4 scaffolds and the results showed that CaSO4 improved the mechanical properties of PLGA scaffolds. In vitro experiments showed that PLGA/CaSO4 scaffold exhibited good biocompatibility. Moreover, the addition of CaSO4 could significantly improve the migration and osteogenic differentiation of MC3T3-E1 cells in the PLGA/CaSO4 scaffolds, and the PLGA/CaSO4 scaffolds made with 20 wt.% CaSO4 exhibited the best osteogenesis properties. Therefore, calcium sulfate was added to PLGA could lead to customized 3D printed scaffolds for enhanced mechanical properties and biological properties. The customized 3D-printed PLGA/CaSO4 scaffold shows great potential for precisely repairing irregular load-bearing bone defects.
Although bone is a tissue with superior self-healing potential, massive irregular bone defects created by trauma, tumor resection, or infection remain a challenge in the clinic (Kuss et al., 2017; Ye et al., 2018; Nulty et al., 2021; Yang et al., 2021; Ye et al., 2021). Autologous bone is considered an ideal material for the treatment of large bone defects due to its retention of osteoblasts and bioactive molecules, including growth factors with osteogenic induction properties (Cheng et al., 2018; Pförringer et al., 2018). However, there are some problems with autologous bone, such as limited donor bone and the risk of bleeding and infection during collection (Ishikawa et al., 2017; Lee et al., 2020). Allografts can also be used to treat bone defects to compensate for the limitations of autografts, but carry the risk of immune rejection (Lai et al., 2019). Thus, we needed to construct new materials as substitutes for autografts and allografts.
The success of load-bearing materials is largely dependent on physical and chemical properties that are known to drive cellular response and it is of great importance to construct a scaffold with an ability to promote cells proliferation, adhesion, migration, and differentiation for bone regeneration (Kim et al., 2017; Li H. et al., 2020). With the rapid development of bone tissue engineering, the interconnected porous scaffolds prepared by 3D printing technology to simulate the extracellular matrix of living bone are showing obvious advantages (Cui et al., 2018). More importantly, the 3D printing technology could fabricate custom-fit scaffolds based on a computed tomography scan of the defect site to repair irregular bone defects with complex geometry (Lai et al., 2019; Wu et al., 2021; Zhu et al., 2022). Besides, the customized design of 3D scaffolds can not only achieve the perfect match between the material and the bone defect but also regulate the structure of the material and the arrangement of cells in the microstructure, which is more conducive to promoting the growth and differentiation of cells and supporting the bone tissue regeneration process (Han et al., 2022).
In recent years, a variety of materials including polymers (Oryan and Sahvieh, 2017; Cui et al., 2019; Ranganathan et al., 2019; Lavanya et al., 2020; Hu et al., 2021; Yu et al., 2021), nanomaterials (Xia et al., 2018; Lu et al., 2019; Singh et al., 2019) metal materials (Lai et al., 2019; Tan et al., 2021), ceramic (Gao et al., 2017; Ma H. et al., 2018; Adithya et al., 2020), and other biological materials (Zimmermann and Ritchie, 2015; Daly et al., 2017; Bose and Sarkar, 2020; Cao et al., 2022; Pang et al., 2022) have been widely used in 3D printing technology to fabricate scaffolds for bone defect repair.
Among a variety of materials for bone tissue engineering, polylactic glycolic acid copolymer (PLGA) has been approved by US Food and Drug Administration (FDA) for human use due to its good biocompatibility and biodegradability (Jia et al., 2016). However, although PLGA is widely used in various tissue engineering applications, it still has the problems of mismatched mechanical and unsatisfactory biological properties owing to the low stiffness between PLGA-based implants and natural bones and the hydrophobic surface of PLGA-based scaffolds (Zou et al., 2020; Jin et al., 2021; Oizumi et al., 2021; Wei et al., 2021; Zhao et al., 2021). Many researchers address these problems by introducing inorganic material in the modification of PLGA-based scaffolds. To overcome the disadvantages of poor mechanical properties and osteogenic properties of PLGA, Zhu TT designed PLGA/nHA scaffolds to repair large bone defects and achieved good results (Zhu et al., 2022). Lai Y X constructed bone repair scaffolds by adding TCP to improve the mechanical properties of PLGA (Lai et al., 2019). In addition, PCL and bioglass are also used to improve the performance of PLGA (Cheng et al., 2018; Qian et al., 2019).
Calcium sulfate (CaSO4) is a commercial bone graft replacement material with a long history of application in a variety of medical applications, such as bone defect filling and tissue regeneration guidance. Calcium sulfate as a bone graft material has the advantages of the minimal inflammatory response, complete degradation, osteoconductive, and Ca2+ released during dissolution may promote osteogenic differentiation (Arun Kumar et al., 2016). As a synthetic bone graft material, CaSO4 could induce a biological reaction similar to that generated during bone remodeling, creating a calcium-rich environment in the implanted area (Zhou et al., 2014; Aquino-Martínez et al., 2017). Moreover, as an inorganic material, CaSO4 could enhance the mechanical strength and hydrophilicity of the polymeric scaffolds (Ye et al., 2018).
Herein we aim to develop 3D-printed customized scaffolds with proper mechanical and bioactivity properties for repairing irregular bone defects. In this study, we incorporated CaSO4 powder into PLGA and then fabricated 3D porous PLGA/CaSO4 scaffolds using fused deposition modeling (FDM) system (Scheme 1). The prepared PLGA, PLGA/10%CaSO4, PLGA/20%CaSO4, and PLGA/30%CaSO4 scaffolds all had a Customized 3D porous structure. We found that the addition of CaSO4 improved the mechanical properties of PLGA scaffolds, and with the increase of the CaSO4 ratio, the scaffolds could stand more pressure. Moreover, in vitro experiments showed that all scaffolds had good biocompatibility, and the PLGA/CaSO4 scaffolds improved the migration of MC3T3-E1 cells compared with PLGA scaffolds. Furthermore, PLGA/CaSO4 scaffolds significantly improved the osteogenic differentiation of MC3T3-E1 cells, and PLGA/20%CaSO4 scaffolds exhibited the best osteogenic properties. The customized-designed 3D porous PLGA/CaSO4 with satisfactory mechanical and proper biological are expected to solve the problems of PLGA scaffolds and be further used for irregular bone defects.
SCHEME 1. Schematic illustration of the fabrication process for PLGA/CaSO4 scaffolds. The rabbit radius was first scanned by micro-CT and a bone tissue model was constructed by 3D reconstruction. Then the scaffold was designed using CAD software, and the CAD data was transferred to a 3D printer to fabricate PLGA/CaSO4 scaffolds. The PLGA/CaSO4 scaffolds could promote cell proliferation, migration, and osteogenesis.
Polylactic glycolic acid copolymer (PLGA, MW = 15,000 g/mol) was purchased from Dai Gang Biology Co., Ltd. (Jinan, China). Anhydrous calcium sulfate (CaSO4, MW = 136.14 g/mol) was obtained from Macklin Biochemical Co., Ltd. (Shanghai, China).
We scanned the rabbit radius bone by microcomputed tomography (micro-CT). Then the CT scan data of bone was imported into computer-aided design (CAD) software to establish a customized bone defect model, and generate the model file. The CAD data was utilized to design the scaffolds with the desired shape and 3D porous structure and then transferred to a 3D printer.
The PLGA/CaSO4 scaffolds in different proportions were fabricated by a biological 3D printer (Livprint® N series, Medprin, Guangzhou, China) layer by layer (Supplementary Figure S1). Firstly, PLGA and CaSO4 (CaSO4 accounts for 0, 10, 20, and 30 wt% of the quality of PLGA) powder were added into the beaker and then stirred evenly at 200°C (Du et al., 2018; Nulty et al., 2021). The mixture was then injected into the 3D printer and the scaffold was printed by following the constructed bone model. The nozzle temperature was 180°C, and the temperature of supporting substrates during FDM printing was 120°C.
The surface morphology and pore size of the calcium sulfate powder and the scaffolds were observed using an Scanning Electron Microscopy (SEM) (Sigma 300, ZEISS, Germany). After being frozen in a refrigerator, freeze-dried in a lyophilizer, and coated with gold, the scaffolds were analyzed by SEM.
The hydrophilicity of each scaffold was measured using a contact angle measurement system (ASUMI GIKEN Limited, Tokyo, Japan). A droplet of deionized water was deposited on the scaffold. Then, the image of the static liquid deposition was obtained within a few seconds and the contact angles were measured. Three samples were assessed for each group to ensure reproducibility and the average value.
The Fourier transform infrared spectroscopy [(FTIR), Nicolet iS10, Thermo Fisher Scientific, United States] was used to evaluate the changes in the chemical structures of the scaffolds.
X-ray diffraction (XRD) patterns were obtained using an Ultima IV X-ray diffractometer (Rigaku, Japan) in the range of 10°–80°.
The mechanical properties of the scaffold were evaluated by a universal machine (RGT-3, Shenzhen Reger Instrument Co., Ltd., China) with a constant speed of 1 mm/min. Three repeated measurements were made for each scaffold (Ye et al., 2018; Ma et al., 2020).
The swelling ratio of different scaffolds was weighed and placed in centrifuge tubes with 5 ml of simulated body fluid (SBF), and then placed on a shaker (37°C, 150 rpm/min). After 24 h, the scaffolds were taken out, removed the surface water by filter paper, and weighed. The swelling ratio was determined by using Eq. 1:
where Ww and Wd are the wet and dry weights, respectively.
The initial weight of each scaffold was recorded and placed in a centrifuge tube containing 5 ml of SBF. The tubes were placed in a shaker stirring at a speed of 150 rpm at 37°C. Scaffolds were removed from the tubes every 3 days and weighted, and then replaced with the SBF solution (Li et al., 2021). The percentage of degradation was calculated using Eq. 2:
Where Wi is the initial weight of the samples and Wt is the weight at each time interval.
Human umbilical vein endothelial cells (HUVECs) were purchased from Cyagen Biotechnology (United States), and cultured with Dulbecco’s Modified Eagle’s Medium (DMEM high glucose, Gibco, United States) containing 10% fetal bovine serum (FBS, Biological Industries, United States) and 1% penicillin-streptomycin (Gibco, United States) at 37°C in a humidified and 5% CO2 incubator.
Cell Counting Kit-8 (CCK-8) assay was used to evaluate the cell viability for proliferation in the scaffold. HUVEC cells were seeded in a 24-well plate at a density of 1 × 104 cells per well and incubated with different scaffolds for 1, 2, and 3 days, respectively (Kim DS et al., 2021). The scaffolds were removed from the plate and the media solution was replaced with 300 μl CCK-8 solution (Biosharp, China) in each well and incubated for 2 h. 100 μL of the supernatant was removed to a 96-well plate and the OD value was measured with a microplate (Multiskan GO, Thermo Fisher Scientific, United States).
Live/dead staining was used to evaluate the cytocompatibility of the scaffolds. The HUVECs were co-cultured with different scaffolds for 1, 2, and 3 days. After being washed with PBS, Calcein-AM/palladium staining solution (Bestbio, China) was added to each well for 30 min at room temperature. A fluorescence microscope (DMI4000, Leica, Germany) was employed to record the fluorescent images of HUVEC cells.
Healthy human blood containing EDTA was collected and diluted with PBS in a ratio of 4:5. Then the different scaffolds were immersed in 1.8 ml of PBS in each group and incubated at 37°C for 30 min, and 2 ml of ddH2O and PBS were set as positive and negative controls, respectively. Next, 0.2 ml of the diluted whole blood was added to each scaffold sample, and the scaffolds were incubated at 37°C for 1 h. Then, the samples were centrifuged at room temperature (3,000 rpm, 5 min). The supernatant was removed from the samples and the absorbance at 545 nm was measured with a microplate reader (Ye et al., 2018). The hemolysis rate (HR) was calculated using Eq. 3:
where ODs, ODp, and ODn are the OD values of the scaffold, positive control, and negative control groups, respectively.
Mouse embryo osteoblast precursor cells (MC3T3-E1) were purchased from Cyagen Biotechnology (United States), and cultured with Minimum essential medium alpha (MEM-α, Gibco, United States) containing 1% penicillin-streptomycin and 10% fetal bovine serum at 37°C in a humidified and 5% CO2 incubator.
For the cell migration assay, MC3T3-E1 cells were seeded in 12-well plates at a density of 5 × 104 cells per well. After the cells were cultured to confluence, a straight scratch was made with a 200 μl pipette tip, and then the scaffolds were directly co-culture with MC3T3-E1 cells for 12 h and stained with Calcein-AM/PI kit for 30 min at room temperature. After removing the free dyes, the distance of the scratch was visualized with a fluorescence microscope and the wound healing rate was calculated using Eq. 4:
where A0h and A24h are the initial distance and the gap after 24 h of coculture, respectively.
The migration of MC3T3-E1 cells was also tested using a transwell assay. Briefly, 80 μl of Matrigel (Corning, United States) was added to the upper chambers of a 24-well transwell plate (Corning; pore size = 8 µm) and gelatinized for 2 h at 37°C. The scaffolds were completely immersed in MEM-α culture medium, which contained 10% FBS, and 1% penicillin-streptomycin at a concentration of 10 mg/ml. The samples were maintained in a shaker at 37°C with a speed of 120 rpm to obtain the extract solutions. 600 μl extracted liquid from each scaffold containing 20% FBS was added to the lower chamber. Then, MC3T3-E1 were seeded in the upper chambers at a density of 1 × 105 cells per well. After incubation at 37°C for 24 h, the Matrigel was erased with a swab, and MC3T3-E1 migrated to the opposite side of the membrane were fixed with 4% paraformaldehyde for 30 min and stained with 0.5% crystal violet (Macklin, China) for 1 h. Three random fields from each plate were recorded using an optical microscope. The stained MC3T3-E1 were lysed in 95% ethanol for 1 h to measure the OD value at 590 nm using a microplate reader.
Cytoskeleton staining was used to evaluate cell morphology on the scaffold surface. Briefly, MC3T3-E1 cells were incubated with different scaffolds at a density of 1 × 104 per well in a 24-well plate. After incubation for 3 days, cells were fixed with 4% paraformaldehyde for 2 h and then permeabilized with 0.1% Triton X-100 (Sigma-Aldrich, United States) for 5 min at room temperature. After washed with PBS, the cells were stained with Actin Cytoskeleton/Focal Adhesion Staining Kit (FAK 100, Sigma-Aldrich, United States) for 1 h and DAPI (Solarbio, China) for 5 min at room temperature. The reaction was stopped by removing the DAPI solution and washing it with PBS, the cells were visualized with confocal laser scanning microscopy (CLSM) (TCS SP-2, Leica, Germany).
The Alkaline Phosphatase (ALP) activity assay was performed to analyze the effect of scaffolds on the early osteogenic differentiation of cells. For ALP staining, MC3T3-E1 cells were seeded in a 6-well plate at a density of 1 × 104 cells per well and incubated with different scaffolds for 7 and 14 days, respectively. The ALP activity assay was then performed with the BCIP/NBT alkaline phosphatase color development kit (Beyotime, China) according to the manufacturer’s instructions. After removing the ALP stain working solution and washing with PBS. Stained MC3T3-E1 cells were visualized with an inverted research microscope (DMI4000, Leica, Germany).
The ALP activity was also employed to evaluate the effect of scaffolds on the osteogenic differentiation of cells. MC3T3-E1cells were seeded in a 6-well plate at a density of 1 × 104 cells per well and incubated with different scaffolds for 7 and 14 days, respectively. After the incubation, cells were washed with PBS and lysed using 0.2% Triton X-100 for 12 h at 4°C. ALP activity was determined using an ALP detection kit (P0321, Beyotime Biotechnology, China). The total protein content in the samples was determined by the BCA protein assay kit (Thermo Fisher Scientific, United States) with the same protocol above. The relative ALP activity was finally normalized to the corresponding total protein content.
For the Alizarin red assay, MC3T3-E1 cells were fixed with 4% paraformaldehyde for 30 min at room temperature after culturing with scaffolds for 7 and 14 days. Then the cells were stained with 400 μl Alizarin red S solution (ARS, Sigma, United States) for 3 h. The plates were then observed with a microscope.
MC3T3-E1 cells were seeded in a 6-well plate at a density of 1 × 104 cells per well and incubated with different scaffolds for 7 and 14 days, respectively. The osteogenesis-related genes include osteoprotein (OPN), osteocalcin (OCN), runt-related transcription factor 2 (RUNX2), type I collagen (COL-1), and bone morphogenetic protein-2 (BMP-2) were analyzed by real-time quantitative polymerase chain reaction (RT-qPCR). Total RNA was obtained from the MC3T3-E1 cells with a total RNA extraction kit (Accurate Biology, China) and reversed transcribed into complementary DNA with the PrimeScript TM reagent kit (Takara, Japan). The gene expression levels were quantified using an ABI Prism 7000 machine (Thermo Fisher Scientific, United States) with TB Green Premix Ex Taq II (Takara, Japan). Primers were presented in Supplementary Table S1.
Analysis was performed using SPSS 19.0 software (IBM, United States). A two-tailed Student’s t-test was used in the comparison between the two groups. One-way analysis of variance (ANOVA) followed by Tukey’s multiple comparisons was carried out in the comparison among more than two groups (*p < 0.05, **p < 0.01, and ***p < 0.005). All the data are expressed with mean ± standard deviation.
The shape, size, and morphology of the CaSO4 particles are closely related to the formation of 3D printed scaffolds. The morphology of CaSO4 particles was evaluated using SEM. As shown in Figure 1, the particle size of CaSO4 was about 2.0–20.0 µm, and the particles were evenly dispersed without agglomeration. CaSO4 particles are a new type of fiber with high mechanical strength compared with polymers due to the near-perfect crystal structures (Fm et al., 2021), so they could be used as a potential reinforcement material for PLGA.
We successfully constructed customized-designed PLGA, PLGA/10%CaSO4, PLGA/20%CaSO4, and PLGA/30%CaSO4 scaffolds by a 3D printer in the FDM system, respectively (Figure 2A). SEM was employed to observe the microstructure and surface morphology of the scaffolds. As shown in Figure 2B, all the scaffolds had a three-dimensional network structure and there was no statistical difference between the pore size of different scaffolds (Figure 2E). The scaffolds had a regular structure with interconnected pores of about 400 μm. The interconnected macropores facilitate the diffusion of oxygen and nutrients, providing sufficient space for the proliferation, adhesion, migration, and differentiation of cells (Zhao et al., 2018; Yan et al., 2019; Gu et al., 2021; Kim H. D. et al., 2021). High-resolution SEM showed that the surface of the pure PLGA scaffold was smooth. However, with the incorporation of calcium sulfate, the roughness of the surface was improved (Sivashanmugam et al., 2017), which facilitated cell adhesion and migration (Kim D.-S. et al., 2021).
FIGURE 2. Characterization of the scaffolds. (A) Representative pictures of 3D printed PLGA, PLGA/10%CaSO4, PLGA/20%CaSO4, and PLGA/30%CaSO4 scaffolds. Scale bar = 5 mm. (B) SEM images of the side of the different scaffolds. Scale bar = 200 and 100 μm, respectively. (C) Water contact angle images of different scaffolds. (D) Contact angle (degree) of PLGA, PLGA/10%CaSO4, PLGA/20%CaSO4, and PLGA/30%CaSO4 scaffolds, respectively. (E) The pore size of each scaffold. Data are presented as mean ± SD (n = 3); *p < 0.05, **p < 0.01, ***p < 0.001.
The water contact angle test was performed to evaluate the surface hydrophilicity of the scaffolds. Figure 2C showed the contact angle images of each scaffold. Due to the intrinsic hydrophobicity of PLGA (Zhu et al., 2022), the water contact angle of the PLGA scaffold was (94.88 ± 3.20°), while the contact angle of PLGA/10%CaSO4, PLGA/20%CaSO4, and PLGA/30%CaSO4 were (86.57 ± 3.30°), (85.33 ± 5.47°), and (84.75 ± 4.91°), respectively (Figure 2D). The incorporation of CaSO4 decreased the contact angle of the scaffolds. Thus, CaSO4 improved the hydrophilicity of the composite scaffold. Considering that the hydrophilicity of the materials played an important role in protein absorption and cell proliferation (Liu et al., 2018), the improvement in the hydrophilicity of the PLGA/CaSO4 scaffold may determine the subsequent cellular behavior.
Excellent mechanical properties are essential for scaffolds. Hence, we performed compress tests on different groups of scaffolds. As shown in Figure 3A, PLGA scaffolds had minimum compress stress. Figure 3B showed the compressive strength of the scaffolds. The compressive strength of the PLGA scaffold was 6.95 MPa. After adding different CaSO4 content, the compression stress of the scaffolds had improved, which were 14.27, 16.54, and 20.21 MPa, respectively. The scaffolds made with 30% CaSO4 exhibited maximum compressive stress. When CaSO4 is combined with H2O, a hydration reaction can occur to form needle-like calcium sulfate dihydrate whiskers. These whiskers bridge and stack with each other to solidify into deposits of a certain shape and strength, which has better mechanical properties (Chiang et al., 2021). Therefore, CaSO4 could enhance the mechanical properties when combined with polymer materials, enable the material to withstand greater deformation, and give it better mechanical properties (Zhao et al., 2008). The result indicated that the addition of CaSO4 could greatly enhance the mechanical properties of the PLGA scaffold to bear loads.
FIGURE 3. Mechanical performance of the 3D printed scaffolds. (A) The stress-strain curves. (B) The compress stress. Data are presented as mean ± SD (n = 3); *p < 0.05, **p < 0.01, ***p < 0.001.
The changes in the chemical structure of the composite before and after the addition of CaSO4 could be observed in the FTIR spectra. As shown in Supplementary Figure S2. PLGA spectrum showed an intense peak of characteristic carbonyl (C=O) at 1,850 cm−1. In the same spectrum could also be observed a characteristic peak of the C-C(=O)-O at 1,150 cm−1 (Fernandes et al., 2017; Chen et al., 2021). After adding CaSO4, the PLGA/CaSO4 peaks became less intense and narrow, showing a decrease in the PLGA ratio. The XRD patterns (Supplementary Figure S3) of the PLGA/CaSO4 scaffolds showed characteristic crystalline peaks at 15, 25, 30, 31, and 48° corresponding to (200), (020), (002), (102), and (302) planes of CaSO4 (Sindhura et al., 2014; Zhu et al., 2022). These characteristic peaks of the CaSO4 in the scaffolds intensified with the increasing proportion of the CaSO4, thereby indicating the successful doping of the CaSO4 into the PLGA/CaSO4 scaffold. The swelling ratio represents the ability of the material to absorb water. As shown in Supplementary Figure S4, the swelling ratio of the different scaffolds was similar and had no statistical difference (p > 0.05), indicating that the addition of CaSO4 could not change the swelling of the scaffolds.
The biodegradability of materials is highly beneficial for clinical applications since it could be able to prevent damage caused by secondary surgical removal (Li et al., 2021). An ideal biomimetic scaffold composed of biodegradable materials should provide proper mechanical support while degrading to non-toxic products being excreted from the body ultimately. The weight loss of scaffolds in SBF was evaluated to study the in vitro degradation (Figure 4). We found that all scaffolds degrade slowly in the first 6 days, which meant that the scaffold could provide effective support and protection for bone in the initial stage of bone defect repair (Qian et al., 2014; Tan et al., 2021; Xue et al., 2021). Subsequently, the degradation rate of the scaffolds accelerated in all groups. PLGA degraded fastest and could be completely degraded within 24 days, which was inconsistent with the rate of bone repair. This indicated that PLGA could not be used as a scaffold alone to repair bone defects (Qian et al., 2014; Jia et al., 2016; Lai et al., 2019; Dos Santos et al., 2020; Kim H. D. et al., 2021). The degradation rate of PLGA/CaSO4 scaffolds was decreased than that of PLGA. PLGA was completely degraded in 4 weeks and the degradation products were polylactic acid and glycolic acid. CaSO4 was difficult to dissolve in water and it can combine with H2O to form calcium sulfate whiskers (Chiang et al., 2021). The whiskers were interconnected and stacked together to make their structure even tighter, which made CaSO4 have a relatively slow degradation rate and can be completely degraded within 6 weeks. What’s more, CaSO4 mixed with PLGA can form a dense solid, which can slow down the degradation rate (Amirthalingam et al., 2021). The addition of CaSO4 slowed down the degradation rate of PLGA (Arun Kumar et al., 2016). This indicated that CaSO4 improved the degradation performance of PLGA scaffolds. The bone-bonding ability and in vivo bone bioactivity of bone repair materials could be evaluated by examining the ability of apatite to form on its surface in SBF (Kokubo and Takadama, 2006). Chan et al. (2004) observed that calcium sulfate could form apatite on the surface both in SBF and in vivo. The findings indicated that calcium sulfate precipitation as carbonate-containing hydroxyapatite and its surface apatite formation in SBF could enhance the acellular and bone bioactivity. Hence, the addition of CaSO4 would promote the formation of apatite on the scaffolds and enhance the acellular bioactivity.
Biocompatibility is an important indicator for the clinical use of biomaterials in orthopedics. Therefore, biocompatibility assessment is an important part of biomedical materials and a primary requirement for the development of biomaterials. First, HUVECs proliferation in different scaffolds was evaluated by CCK-8 assay. As shown in Figure 5B, the scaffolds had good cell viability after incubating with HUVEC cells for 1, 2, and 3 days. However, PLGA/30%CaSO4 scaffolds had the lowest cell viability rate. This might be because Ca2+ was the second messenger in cells, and excessive Ca2+ affects cell signaling, thereby inhibiting cell proliferation and migration (Teparat-Burana et al., 2015). We further evaluated the biocompatibility by live/dead staining assay (Figure 5A). HUVEC cells indicated by green fluorescence were still alive after incubating with different scaffolds for 1, 2, and 3 days. The number of cells in control, PLGA, PLGA/10%CaSO4, PLGA/20%CaSO4, and PLGA/30%CaSO4 groups increased with the culturing time, suggesting that the scaffolds had non-toxicity to HUVEC cells. Hemolysis rate is another indicator to evaluate the biocompatibility of materials (Li et al., 2021). Hemolysis experiment results showed that the supernatant in the positive control group turned red because the relatively low osmotic pressure caused a large number of erythrocytes to rupture (Figure 5C) (Li et al., 2021). While the supernatant in the negative control group and the scaffold groups was still clear, demonstrating that almost no red blood cells were broken. In addition, the hemolysis ratio of the different scaffolds was less than 5%, which met the requirements for the hemolysis rate of medical materials (Tang et al., 2020). These observations suggested that PLGA, PLGA/10%CaSO4, PLGA/20%CaSO4, and PLGA/30%CaSO4 scaffolds had good biocompatibility.
FIGURE 5. In vitro biocompatibility. (A) Representative fluorescence images for HUVEC cells cultured with different scaffolds. Live cells were stained by calcein-AM (green color). Scale bar = 200 μm. (B) CCK-8 assay for HUVECs cultured with the PLGA, PLGA/10%CaSO4, PLGA/20%CaSO4, and PLGA/30%CaSO4 scaffolds, respectively. (C) In vitro hemolysis of different scaffolds and Hemolytic rate (%). Data are presented as mean ± SD (n = 3); *p < 0.05, **p < 0.01, ***p < 0.001.
The repair of bone defects depends on the proliferation and migration of cells, so the ideal bone repair material should be able to promote the migration of osteoblasts. Wound healing assay and transwell assay were used to simulate the effect of scaffolds on osteoblast migration. As shown in Figure 6A, compared with control, PLGA, PLGA/10%CaSO4, and PLGA/30%CaSO4 groups, PLGA/20%CaSO4 could significantly promote MC3T3-E1 cells migration. Figure 6C showed that the PLGA/20%CaSO4 group had the highest cell migration rate (61.16%). The transwell assay (Figure 6B) also showed that PLGA/20%CaSO4 group had the highest number of cell migrations per field (Figure 6D). What’s more, compared with other groups, PLGA/20%CaSO4 group had a higher OD value (Figure 6E). Calcium sulfate as a bone graft material had osteoconductive properties, completely degradable. CaSO4 promotes cell migration in a concentration-dependent manner. It recruits cells to migrate to sites with high concentrations of CaSO4. But CaSO4 to promote cell migration requires a suitable concentration range. When calcium levels exceed this range, cell migration is inhibited (Arun Kumar et al., 2016; Aquino-Martínez et al., 2017). What’s more, excessive calcium ions will affect cell proliferation, and cell migration will also be affected when the number of cell proliferation is reduced (Teparat-Burana et al., 2015), which was consistent with the results of in vitro biocompatibility. The PLGA/20%CaSO4 scaffold had the highest cell viability, while the cell viability in PLGA/30%CaSO4 decreased compared with 20%. Therefore, scaffolds in the 20% group had a strong ability to promote cell migration, which plays a critical role in bone reconstruction.
FIGURE 6. In vitro cell migration. (A) In vitro wound healing assay of MC3T3-E1 cell. Scale bar = 100 μm. (B) Transwell assay of MC3T3-E1 cells. Scale bar = 100 μm. (C) Cell migration rate (%) of cells. (D) Cell migration number of MC3T3-E1 cells per field. (E) Absorbance at 590 nm. Data are presented as mean ± SD (n = 3); *p < 0.05, **p < 0.01, ***p < 0.001.
We also carried out the cytoskeleton staining to evaluate the cell extension and adhesion on the scaffolds (Figure 7), which showed spindle MC3T3-E1 cells presented well-stretch morphology and favorable proliferation status on PLGA/10%CaSO4, PLGA/20%CaSO4, and PLGA/30%CaSO4 scaffolds than on PLGA scaffold. Moreover, the number of adhered cells on the surface of the PLGA/10%CaSO4, PLGA/20%CaSO4, and PLGA/30%CaSO4 scaffolds were more than that of PLGA scaffolds, and the number and distribution density of actin microfilaments in the cytoskeleton were also more than those on the surface of PLGA scaffolds. Spreading and differentiation of cells were particularly affected by microscopic roughness and hydrophilicity. Hydrophilic biomaterial surfaces could promote cell growth and improve biocompatibility. Besides, CaSO4 could promote cell proliferation, extension, and adhesion (Phang et al., 2004). Thus, the PLGA/CaSO4 scaffold constructed by adding CaSO4 with PLGA had good properties to promote cell expansion and adhesion due to the improvement of surface hydrophilicity.
Osteogenic differentiation property is the key to evaluating the success of a biomaterial (Huan and Chang, 2007; Ma L. et al., 2018; Wang et al., 2018; Freeman et al., 2019). We detected the osteogenic differentiation of the different scaffolds based on ALP staining, ALP activity assay, alizarin red staining, and osteogenesis-related genes expression. As an important early osteogenic enzyme during osteogenesis, the activity of ALP was represented as a typical marker of osteogenic differentiation (Yin et al., 2019; Li M. et al., 2020; Zhai et al., 2021). ALP staining result was shown in Figure 8A. It was found that the PLGA/20%CaSO4 exhibited deeper dyeing than other groups on day 14. This indicated that more ALP was produced in the PLGA/20%CaSO4 group. The ALP activity of the MC3T3-E1 cells in the PLGA/20%CaSO4 and PLGA/30%CaSO4 groups was significantly higher than in other groups on day 7 (Figure 8C). And the ALP activity in the PLGA/20%CaSO4 group was significantly higher than in other groups on day 14. Therefore, compared with other groups, PLGA/20%CaSO4 group can effectively promote the production of ALP, which was consistent with the above results of ALP staining. Figure 8B showed the results of Alizarin staining on days 7 and 14. Compared with the control group and PLGA group, PLGA/10%CaSO4, PLGA/20%CaSO4, and PLGA/30%CaSO4 group scaffolds could promote the production of calcium nodules. Cells cultured with PLGA/20%CaSO4 scaffold had the largest red staining area and the most calcium nodules compared with the other groups.
FIGURE 8. In vitro osteogenesis capability of the scaffolds. (A) ALP staining. Scale bar = 200 μm. (B) Staining area of Alizarin red. Scale bar = 200 μm. (C) The ALP activity of the MC3T3-E1 cells co-cultured with the scaffolds on days 7 and 14. ALP level was significantly high in the PLGA/20%CaSO4 scaffolds compared to the other scaffolds. (D–H) Relative mRNA expression of the osteogenic genes (OPN, OCN, RUNX-2, Collagen I, and BMP-2). Data are presented as mean ± SD (n = 3); *p < 0.05, **p < 0.01, ***p < 0.001.
Moreover, we examined the expression levels of several critical osteogenic genes including OPN, OCN, RUNX-2, Collagen I, and BMP-2. As shown in Figures 8D–H, the expression levels of BMP-2, Col-1, RUXN-2, and OCN genes in PLGA/20%CaSO4 and PLGA/30%CaSO4 groups were higher than in other groups on day 7, and the expression level of OPN in the PLGA/20%CaSO4 group was the highest at day 7. And the expression levels of all these genes in the PLGA/20%CaSO4 group were higher than in other groups on day 14. These results suggested that CaSO4 could promote osteogenic differentiation in vitro, which could make up for the lack of osteogenic induction activity of PLGA (Du et al., 2018; Kim D.-S. et al., 2021). And PLGA/20%CaSO4 scaffold had the best osteogenic performance among all the scaffolds we constructed.
Calcium sulfate is the most commonly used bone repair material in clinical, which can cause changes in local calcium ions concentration after implantation, thereby regulating the process of bone tissue regeneration. As an intracellular second messenger and an important signaling molecule, Ca2+ controls many key processes in cells, including proliferation, migration, and differentiation (Teparat-Burana et al., 2015). Ca2+ is released during CaSO4 degradation Ca2+ can bind to calmodulin (CaM) to Ca2+-CaM form complexes and promote osteoblast differentiation and matrix mineralization through corresponding signaling pathways (Cao et al., 2022). The favorable osteogenic property of CaSO4 could improve the insufficient osteoinductive activity of polymer materials (Du et al., 2018; Kim H. D. et al., 2021). As mentioned above, we think that the incorporation of CaSO4 in the PLGA scaffolds provides Ca2+ ions that might enhance the osteogenic differentiation of cells. In addition, the prepared PLGA/CaSO4 scaffolds improved the hydrophilicity of the scaffold surface, which was more conducive to cell proliferation and adhesion on the scaffold, and further promoted osteogenic differentiation.
We fabricated PLGA/CaSO4 scaffolds of different proportions by 3D printing and then evaluated their properties. Physical performance tests showed that adding CaSO4 into the PLGA scaffold improved the mechanical properties of the scaffold and made the surface of the scaffold rougher. In vitro cytotoxicity experiments showed that PLGA, PLGA/10%CaSO4, PLGA/20%CaSO4, and PLGA/30%CaSO4 groups had good biocompatibility. In addition, the PLGA/20%CaSO4 scaffold also promoted the migration of MC3T3-E1 cells. In vitro osteogenic experiments showed that PLGA/10%CaSO4, PLGA/20%CaSO4, and PLGA/30%CaSO4 scaffolds had osteogenic properties. Among them, PLGA/20%CaSO4 scaffolds significantly promoted the new bone formation in vitro. The whisker formed by the combination of CaSO4 and H2O can make its structure even tighter. The mechanical and degradation properties of PLGA were improved when combined with CaSO4 thanks to its tight structure. The rough surface of CaSO4 was conducive to cell migration and extension, so PLGA/CaSO4 scaffolds could promote cell migration compared with PLGA scaffolds. And CaSO4 could also make up for the lack of osteogenic induction activity of PLGA due to its good osteogenesis properties. Therefore, CaSO4 could significantly improve the performance of the PLGA scaffold. Among them, PLGA/20%CaSO4 showed the best overall performance. In summary, PLGA/20%CaSO4 scaffolds were promising for bone tissue engineering applications.
The original contributions presented in the study are included in the article/Supplementary Material, further inquiries can be directed to the corresponding authors.
TL: Conceptualization, Methodology, Data curation, Visualization, and Writing-original draft preparation. ZhL: Conceptualization, Software, Investigation, and Methodology. LZ: Conceptualization, Methodology, Data curation. ZC: Supervision, Project administration, and Data curation. ZeL: Conceptualization, Investigation, Methodology, and Data curation. BL: Supervision, Project administration, and Data curation. ZF: Conceptualization, Investigation, Methodology, and Data curation. PJ: Supervision, Project administration, and Data curation. JZ: Supervision, Project administration, and Data curation. ZW: Supervision, Project administration, and Data curation. HW: Project administration and Investigation. XX: Supervision, Funding acquisition, Writing—review and editing. XY: Supervision, Funding acquisition, Writing—review and editing. YZ: Supervision, Funding acquisition, Writing—review and editing.
This work was supported by the Research Program of PLA (No. 20WQ030 and 2021NZC026); Natural Science Foundation of Guangdong Province of China (No. 2021A1515011545); Science and Technology Innovation Strategy Special Fund of Guangdong Province (No. 2021B1111610007); the National Natural Science Foundation of China (No. 81972080); the Research Foundation of Medical Science and Technology of Guangdong Province (No. 2020112693915296); the Science and Technology Planning Project of Guangdong Province (No. 2017B030314139).
The authors declare that the research was conducted in the absence of any commercial or financial relationships that could be construed as a potential conflict of interest.
All claims expressed in this article are solely those of the authors and do not necessarily represent those of their affiliated organizations, or those of the publisher, the editors and the reviewers. Any product that may be evaluated in this article, or claim that may be made by its manufacturer, is not guaranteed or endorsed by the publisher.
The Supplementary Material for this article can be found online at: https://www.frontiersin.org/articles/10.3389/fbioe.2022.874931/full#supplementary-material
Adithya, S. P., Sidharthan, D. S., Abhinandan, R., Balagangadharan, K., and Selvamurugan, N. (2020). Nanosheets-incorporated Bio-Composites Containing Natural and Synthetic Polymers/ceramics for Bone Tissue Engineering. Int. J. Biol. Macromol. 164, 1960–1972. doi:10.1016/j.ijbiomac.2020.08.053
Amirthalingam, S., Lee, S. S., Rajendran, A. K., Kim, I., Hwang, N. S., and Rangasamy, J. (2021). Addition of Lactoferrin and Substance P in a chitin/PLGA-CaSO4 Hydrogel for Regeneration of Calvarial Bone Defects. Mater. Sci. Eng. C 126, 112172. doi:10.1016/j.msec.2021.112172
Aquino-Martínez, R., Angelo, A. P., and Pujol, F. V. (2017). Calcium-containing Scaffolds Induce Bone Regeneration by Regulating Mesenchymal Stem Cell Differentiation and Migration. Stem Cell Res. Ther. 8 (1), 265. doi:10.1186/s13287-017-0713-0
Arun Kumar, R., Sivashanmugam, A., Deepthi, S., Bumgardner, J. D., Nair, S. V., and Jayakumar, R. (2016). Nano-fibrin Stabilized CaSO 4 Crystals Incorporated Injectable Chitin Composite Hydrogel for Enhanced Angiogenesis & Osteogenesis. Carbohydr. Polym. 140, 144–153. doi:10.1016/j.carbpol.2015.11.074
Bose, S., and Sarkar, N. (2020). Natural Medicinal Compounds in Bone Tissue Engineering. Trends Biotechnol. 38 (4), 404–417. doi:10.1016/j.tibtech.2019.11.005
Cao, R., Xu, Y., Xu, Y., Brand, D. D., Zhou, G., Xiao, K., et al. (2022). Development of Tri‐Layered Biomimetic Atelocollagen Scaffolds with Interfaces for Osteochondral Tissue Engineering. Adv. Healthc. Mater. 8, e2101643. doi:10.1002/adhm.202101643
Chan, H., Mijares, D., and Ricci, J. L. (2004). “In Vitro dissolution of Calcium Sulfate: Evidence of Bioactivity,” in Transactions-7th World Biomaterials Congress, Sydney, Australia, 17-21 May, 2004.
Chen, I.-C., Su, C.-Y., Lai, C.-C., Tsou, Y.-S., Zheng, Y., and Fang, H.-W. (2021). Preparation and Characterization of Moldable Demineralized Bone Matrix/Calcium Sulfate Composite Bone Graft Materials. Jfb 12 (4), 56. doi:10.3390/jfb12040056
Cheng, T., Qu, H., Zhang, G., and Zhang, X. (2018). Osteogenic and Antibacterial Properties of Vancomycin-Laden Mesoporous Bioglass/PLGA Composite Scaffolds for Bone Regeneration in Infected Bone Defects. Artif. Cells, Nanomedicine, Biotechnol. 46 (8), 1–13. doi:10.1080/21691401.2017.1396997
Chiang, C.-C., Hsieh, M.-K., Wang, C.-Y., Tuan, W.-H., and Lai, P.-L. (2021). Cytotoxicity and Cell Response of Preosteoblast in Calcium Sulfate-Augmented PMMA Bone Cement. Biomed. Mat. 16 (5), 055014. doi:10.1088/1748-605X/ac1ab5
Cui, Y., Zhu, T., Li, A., Liu, B., Cui, Z., Qiao, Y., et al. (2018). Porous Particle-Reinforced Bioactive Gelatin Scaffold for Large Segmental Bone Defect Repairing. ACS Appl. Mat. Interfaces 10 (8), 6956–6964. doi:10.1021/acsami.7b19010
Cui, Z.-K., Kim, S., Baljon, J. J., Wu, B. M., Aghaloo, T., and Lee, M. (2019). Microporous Methacrylated Glycol Chitosan-Montmorillonite Nanocomposite Hydrogel for Bone Tissue Engineering. Nat. Commun. 10 (1), 3523. doi:10.1038/s41467-019-11511-3
Daly, A. C., Freeman, F. E., Gonzalez-Fernandez, T., Critchley, S. E., Nulty, J., and Kelly, D. J. (2017). 3D Bioprinting for Cartilage and Osteochondral Tissue Engineering. Adv. Healthc. Mat. 6 (22), 1700298. doi:10.1002/adhm.201700298
Dos Santos, V. I., Merlini, C., Aragones, Á., Cesca, K., and Fredel, M. C. (2020). In Vitro evaluation of Bilayer Membranes of PLGA/hydroxyapatite/β-tricalcium Phosphate for Guided Bone Regeneration. Mater. Sci. Eng. C 112, 110849. doi:10.1016/j.msec.2020.110849
Du, X., Yu, B., Pei, P., Ding, H., Yu, B., and Zhu, Y. (2018). 3D Printing of pearl/CaSO4composite Scaffolds for Bone Regeneration. J. Mat. Chem. B 6 (3), 499–509. doi:10.1039/c7tb02667f
Fernandes, K. R., Magri, A. M. P., Kido, H. W., Parisi, J. R., Assis, L., Fernandes, K. P. S., et al. (2017). Biosilicate/PLGA Osteogenic Effects Modulated by Laser Therapy: In Vitro and In Vivo Studies. J. Photochem. Photobiol. B. 173, 258–265. doi:10.1016/j.jphotobiol.2017.06.002
Fm, A., Chang, C., and Yw, C. (2021). Mechanical Behavior of Calcium Sulfate Whisker-Reinforced Paraffin/gypsum Composites. Constr. Build. Mater. 305, 124795. doi:10.1016/j.conbuildmat.2021.124795
Freeman, S., Ramos, R., AlexisChando, P., Zhou, L., Reeser, K., Jin, S., et al. (2019). A Bioink Blend for Rotary 3D Bioprinting Tissue Engineered Small-Diameter Vascular Constructs. Acta Biomater. 95, 152–164. doi:10.1016/j.actbio.2019.06.052
Gao, C., Feng, P., Peng, S., and Shuai, C. (2017). Carbon Nanotube, Graphene and Boron Nitride Nanotube Reinforced Bioactive Ceramics for Bone Repair. Acta Biomater. 61, 1–20. doi:10.1016/j.actbio.2017.05.020
Gu, J., Zhang, Q., Geng, M., Wang, W., Yang, J., Khan, A. u. R., et al. (2021). Construction of Nanofibrous Scaffolds with Interconnected Perfusable Microchannel Networks for Engineering of Vascularized Bone Tissue. Bioact. Mater. 6 (10), 3254–3268. doi:10.1016/j.bioactmat.2021.02.033
Han, P., Gomez, G. A., Duda, G. N., Ivanovski, S., and Poh, P. S. P. (2022). Scaffold Geometry Modulation of Mechanotransduction and its Influence on Epigenetics. Acta Biomater. 15 (22), S174200021–S174270616. doi:10.1016/j.actbio.2022.01.020
Hu, J., Wang, Z., Miszuk, J. M., Zhu, M., Lansakara, T. I., Tivanski, A. V., et al. (2021). Vanillin-bioglass Cross-Linked 3D Porous Chitosan Scaffolds with Strong Osteopromotive and Antibacterial Abilities for Bone Tissue Engineering. Carbohydr. Polym. 271, 118440. doi:10.1016/j.carbpol.2021.118440
Huan, Z., and Chang, J. (2007). Self-setting Properties and In Vitro Bioactivity of Calcium Sulfate Hemihydrate-Tricalcium Silicate Composite Bone Cements. Acta Biomater. 3 (6), 952–960. doi:10.1016/j.actbio.2007.05.003
Ishikawa, K., Kawachi, G., Tsuru, K., and Yoshimoto, A. (2017). Fabrication of Calcite Blocks from Gypsum Blocks by Compositional Transformation Based on Dissolution-Precipitation Reactions in Sodium Carbonate Solution. Mater. Sci. Eng. C 72, 389–393. doi:10.1016/j.msec.2016.11.093
Jia, P., Chen, H., Kang, H., Qi, J., Zhao, P., Jiang, M., et al. (2016). Deferoxamine Released from Poly(lactic-Co-Glycolic Acid) Promotes Healing of Osteoporotic Bone Defect via Enhanced Angiogenesis and Osteogenesis. J. Biomed. Mat. Res. 104 (10), 2515–2527. doi:10.1002/jbm.a.35793
Jin, S., Xia, X., Huang, J., Yuan, C., Zuo, Y., Li, Y., et al. (2021). Recent Advances in PLGA-Based Biomaterials for Bone Tissue Regeneration. Acta Biomater. 127, 56–79. doi:10.1016/j.actbio.2021.03.067
Kim, D.-S., Lee, J.-K., Kim, J. H., Lee, J., Kim, D. S., An, S., et al. (2021). Advanced PLGA Hybrid Scaffold with a Bioactive PDRN/BMP2 Nanocomplex for Angiogenesis and Bone Regeneration Using Human Fetal MSCs. Sci. Adv. 7 (50), eabj1083. doi:10.1126/sciadv.abj1083
Kim, H. D., Amirthalingam, S., Kim, S. L., Lee, S. S., Rangasamy, J., and Hwang, N. S. (2017). Biomimetic Materials and Fabrication Approaches for Bone Tissue Engineering. Adv. Healthc. Mat. 6 (23), 1700612. doi:10.1002/adhm.201700612
Kim, H. D., Hong, X., An, Y. H., Park, M. J., Kim, D. G., Greene, A. K., et al. (2021). A Biphasic Osteovascular Biomimetic Scaffold for Rapid and Self‐Sustained Endochondral Ossification. Adv. Healthc. Mater. 10 (13), 2100070. doi:10.1002/adhm.202100070
Kokubo, T., and Takadama, H. (2006). How Useful Is SBF in Predicting In Vivo Bone Bioactivity? Biomaterials 27 (15), 2907–2915. doi:10.1016/j.biomaterials.2006.01.017
Kuss, M. A., Harms, R., Wu, S., Wang, Y., Untrauer, J. B., Carlson, M. A., et al. (2017). Short-term Hypoxic Preconditioning Promotes Prevascularization in 3D Bioprinted Bone Constructs with Stromal Vascular Fraction Derived Cells. RSC Adv. 7 (47), 29312–29320. doi:10.1039/c7ra04372d
Lai, Y., Li, Y., Cao, H., Long, J., Wang, X., Li, L., et al. (2019). Osteogenic Magnesium Incorporated into PLGA/TCP Porous Scaffold by 3D Printing for Repairing Challenging Bone Defect. Biomaterials 197, 207–219. doi:10.1016/j.biomaterials.2019.01.013
Lavanya, K., Chandran, S. V., Balagangadharan, K., and Selvamurugan, N. (2020). Temperature- and pH-Responsive Chitosan-Based Injectable Hydrogels for Bone Tissue Engineering. Mater. Sci. Eng. C 111, 110862. doi:10.1016/j.msec.2020.110862
Lee, C. S., Hwang, H. S., Kim, S., Fan, J., Aghaloo, T., and Lee, M. (2020). Inspired by Nature: Facile Design of Nanoclay-Organic Hydrogel Bone Sealant with Multifunctional Properties for Robust Bone Regeneration. Adv. Funct. Mat. 30 (43), 2003717. doi:10.1002/adfm.202003717
Li, H., Wang, H., Pan, J., Li, J., Zhang, K., Duan, W., et al. (2020). Nanoscaled Bionic Periosteum Orchestrating the Osteogenic Microenvironment for Sequential Bone Regeneration. ACS Appl. Mat. Interfaces 12 (33), 36823–36836. doi:10.1021/acsami.0c06906
Li, M., Zhang, Z., Liang, Y., He, J., and Guo, B. (2020). Multifunctional Tissue-Adhesive Cryogel Wound Dressing for Rapid Nonpressing Surface Hemorrhage and Wound Repair. ACS Appl. Mat. Interfaces 12 (32), 35856–35872. doi:10.1021/acsami.0c08285
Li Z., Z., Li, B., Li, X., Lin, Z., Chen, L., Chen, H., et al. (2021). Ultrafast In-Situ Forming Halloysite Nanotube-Doped Chitosan/oxidized Dextran Hydrogels for Hemostasis and Wound Repair. Carbohydr. Polym. 267, 118155. doi:10.1016/j.carbpol.2021.118155
Liu, P., Sun, L., Liu, P., Yu, W., Zhang, Q., Zhang, W., et al. (2018). Surface Modification of Porous PLGA Scaffolds with Plasma for Preventing Dimensional Shrinkage and Promoting Scaffold-Cell/tissue Interactions. J. Mat. Chem. B 6 (46), 7605–7613. doi:10.1039/c8tb02374c
Lu, H.-T., Lu, T.-W., Chen, C.-H., and Mi, F.-L. (2019). Development of Genipin-Crosslinked and Fucoidan-Adsorbed Nano-Hydroxyapatite/hydroxypropyl Chitosan Composite Scaffolds for Bone Tissue Engineering. Int. J. Biol. Macromol. 128, 973–984. doi:10.1016/j.ijbiomac.2019.02.010
Ma, H., Feng, C., Chang, J., and Wu, C. (2018). 3D-printed Bioceramic Scaffolds: From Bone Tissue Engineering to Tumor Therapy. Acta Biomater. 79, 37–59. doi:10.1016/j.actbio.2018.08.026
Ma, L., Cheng, S., Ji, X., Zhou, Y., Zhang, Y., Li, Q., et al. (2020). Immobilizing Magnesium Ions on 3D Printed Porous Tantalum Scaffolds with Polydopamine for Improved Vascularization and Osteogenesis. Mater. Sci. Eng. C 117, 111303. doi:10.1016/j.msec.2020.111303
Ma, L., Wang, X., Zhao, N., Zhu, Y., Qiu, Z., Li, Q., et al. (2018). Integrating 3D Printing and Biomimetic Mineralization for Personalized Enhanced Osteogenesis, Angiogenesis, and Osteointegration. ACS Appl. Mat. Interfaces 10 (49), 42146–42154. doi:10.1021/acsami.8b17495
Nulty, J., Freeman, F. E., Browe, D. C., Burdis, R., Ahern, D. P., Pitacco, P., et al. (2021). 3D Bioprinting of Prevascularised Implants for the Repair of Critically-Sized Bone Defects. Acta Biomater. 126, 154–169. doi:10.1016/j.actbio.2021.03.003
Oizumi, I., Hamai, R., Shiwaku, Y., Mori, Y., Anada, T., Baba, K., et al. (2021). Impact of Simultaneous Hydrolysis of OCP and PLGA on Bone Induction of a PLGA-OCP Composite Scaffold in a Rat Femoral Defect. Acta Biomater. 124, 358–373. doi:10.1016/j.actbio.2021.01.048
Oryan, A., and Sahvieh, S. (2017). Effectiveness of Chitosan Scaffold in Skin, Bone and Cartilage Healing. Int. J. Biol. Macromol. 104 (Pt A), 1003–1011. doi:10.1016/j.ijbiomac.2017.06.124
Pang, L., Zhao, R., Chen, J., Ding, J., Chen, X., Chai, W., et al. (2022). Osteogenic and Anti-tumor Cu and Mn-Doped Borosilicate Nanoparticles for Syncretic Bone Repair and Chemodynamic Therapy in Bone Tumor Treatment. Bioact. Mater. 12, 1–15. doi:10.1016/j.bioactmat.2021.10.030
Pförringer, D., Harrasser, N., Mühlhofer, H., Kiokekli, M., Stemberger, A., van Griensven, M., et al. (2018). Osteoinduction and -conduction through Absorbable Bone Substitute Materials Based on Calcium Sulfate: In Vivo Biological Behavior in a Rabbit Model. J. Mater Sci. Mater Med. 29 (2), 17. doi:10.1007/s10856-017-6017-1
Phang, M. Y., Ng, M. H., Tan, K. K., Aminuddin, B. S., Ruszymah, B. H., and Fauziah, O. (2004). Evaluation of Suitable Biodegradable Scaffolds for Engineered Bone Tissue. Med. J. Malays. 59 (Suppl. B), 198–199.
Qian, J., Xu, W., Yong, X., Jin, X., and Zhang, W. (2014). Fabrication and In Vitro Biocompatibility of Biomorphic PLGA/nHA Composite Scaffolds for Bone Tissue Engineering. Mater. Sci. Eng. C 36, 95–101. doi:10.1016/j.msec.2013.11.047
Qian, Y., Zhou, X., Zhang, F., Diekwisch, T. G. H., Luan, X., and Yang, J. (2019). Triple PLGA/PCL Scaffold Modification Including Silver Impregnation, Collagen Coating, and Electrospinning Significantly Improve Biocompatibility, Antimicrobial, and Osteogenic Properties for Orofacial Tissue Regeneration. ACS Appl. Mat. Interfaces 11 (41), 37381–37396. doi:10.1021/acsami.9b07053
Ranganathan, S., Balagangadharan, K., and Selvamurugan, N. (2019). Chitosan and Gelatin-Based Electrospun Fibers for Bone Tissue Engineering. Int. J. Biol. Macromol. 133, 354–364. doi:10.1016/j.ijbiomac.2019.04.115
Sindhura Reddy, N., Sowmya, S., Bumgardner, J. D., Chennazhi, K. P., Biswas, R., and Jayakumar, R. (2014). Tetracycline Nanoparticles Loaded Calcium Sulfate Composite Beads for Periodontal Management. Biochimica Biophysica Acta (BBA) - General Subj. 1840 (6), 2080–2090. doi:10.1016/j.bbagen.2014.02.007
Singh, B. N., Veeresh, V., Mallick, S. P., Jain, Y., Sinha, S., Rastogi, A., et al. (2019). Design and Evaluation of Chitosan/chondroitin Sulfate/nano-Bioglass Based Composite Scaffold for Bone Tissue Engineering. Int. J. Biol. Macromol. 133, 817–830. doi:10.1016/j.ijbiomac.2019.04.107
Sivashanmugam, A., Charoenlarp, P., Deepthi, S., Rajendran, A., Nair, S. V., Iseki, S., et al. (2017). Injectable Shear-Thinning CaSO4/FGF-18-Incorporated Chitin-PLGA Hydrogel Enhances Bone Regeneration in Mice Cranial Bone Defect Model. ACS Appl. Mat. Interfaces 9 (49), 42639–42652. doi:10.1021/acsami.7b15845
Tan, S., Wang, Y., Du, Y., Xiao, Y., and Zhang, S. (2021). Injectable Bone Cement with Magnesium-Containing Microspheres Enhances Osteogenesis via Anti-inflammatory Immunoregulation. Bioact. Mater. 6 (10), 3411–3423. doi:10.1016/j.bioactmat.2021.03.006
Tang, Q., Lim, T., Wei, X.-J., Wang, Q.-Y., Xu, J.-C., Shen, L.-Y., et al. (2020). A Free-Standing Multilayer Film as a Novel Delivery Carrier of Platelet Lysates for Potential Wound-Dressing Applications. Biomaterials 255, 120138. doi:10.1016/j.biomaterials.2020.120138
Teparat-Burana, T., Onsiri, N., and Jantarat, J. (2015). Cytotoxicity and Migration of Fibroblasts on Two Types of Calcium Sulfate Dihydrate. J. Invest. Clin. Dent. 8 (1), e12177. doi:10.1111/jicd.12177
Wang, L., Zhu, L.-x., Wang, Z., Lou, A.-j., Yang, Y.-x., Guo, Y., et al. (2018). Development of a Centrally Vascularized Tissue Engineering Bone Graft with the Unique Core-Shell Composite Structure for Large Femoral Bone Defect Treatment. Biomaterials 175, 44–60. doi:10.1016/j.biomaterials.2018.05.017
Wei, J., Yan, Y., Gao, J., Li, Y., Wang, R., Wang, J., et al. (2021). 3D-printed Hydroxyapatite Microspheres Reinforced PLGA Scaffolds for Bone Regeneration. Mater. Sci. Eng. C 23, 112618. doi:10.1016/j.msec.2021.112618
Wu, N., Liu, J., Ma, W., Dong, X., Wang, F., Yang, D., et al. (2021). Degradable Calcium Deficient Hydroxyapatite/poly(lactic-Glycolic Acid Copolymer) Bilayer Scaffold through Integral Molding 3D Printing for Bone Defect Repair. Biofabrication 13 (2), 025005. doi:10.1088/1758-5090/abcb48
Xia, Y., Sun, J., Zhao, L., Zhang, F., Liang, X.-J., Guo, Y., et al. (2018). Magnetic Field and Nano-Scaffolds with Stem Cells to Enhance Bone Regeneration. Biomaterials 183, 151–170. doi:10.1016/j.biomaterials.2018.08.040
Xue, Y., Zhu, Z., Zhang, X., Chen, J., Yang, X., Gao, X., et al. (2021). Accelerated Bone Regeneration by MOF Modified Multifunctional Membranes through Enhancement of Osteogenic and Angiogenic Performance. Adv. Healthc. Mat. 10 (6), 2001369. doi:10.1002/adhm.202001369
Yan, Y., Chen, H., Zhang, H., Guo, C., Yang, K., Chen, K., et al. (2019). Vascularized 3D Printed Scaffolds for Promoting Bone Regeneration. Biomaterials 190-191, 97–110. doi:10.1016/j.biomaterials.2018.10.033
Yang, G., Liu, H., Cui, Y., Li, J., Zhou, X., Wang, N., et al. (2021). Bioinspired Membrane Provides Periosteum-Mimetic Microenvironment for Accelerating Vascularized Bone Regeneration. Biomaterials 268, 120561. doi:10.1016/j.biomaterials.2020.120561
Ye Li, Li., Xu, J., Mi, J., He, X., Pan, Q., Zheng, L., et al. (2021). Biodegradable Magnesium Combined with Distraction Osteogenesis Synergistically Stimulates Bone Tissue Regeneration via CGRP-FAK-VEGF Signaling axis. Biomaterials 275, 120984. doi:10.1016/j.biomaterials.2021.120984
Ye, X., Li, L., Lin, Z., Yang, W., Duan, M., Chen, L., et al. (2018). Integrating 3D-Printed PHBV/Calcium Sulfate Hemihydrate Scaffold and Chitosan Hydrogel for Enhanced Osteogenic Property. Carbohydr. Polym. 202, 106–114. doi:10.1016/j.carbpol.2018.08.117
Yin, S., Zhang, W., Zhang, Z., and Jiang, X. (2019). Recent Advances in Scaffold Design and Material for Vascularized Tissue‐Engineered Bone Regeneration. Adv. Healthc. Mat. 8 (10), 1801433. doi:10.1002/adhm.201801433
Yu, Y., Yu, X., Tian, D., Yu, A., and Wan, Y. (2021). Thermo-responsive Chitosan/silk Fibroin/amino-Functionalized Mesoporous Silica Hydrogels with Strong and Elastic Characteristics for Bone Tissue Engineering. Int. J. Biol. Macromol. 182, 1746–1758. doi:10.1016/j.ijbiomac.2021.05.166
Zhai, Y., Schilling, K., Wang, T., ElKhatib, M., Vinogradov, S., Brown, E. B., et al. (2021). Spatiotemporal Blood Vessel Specification at the Osteogenesis and Angiogenesis Interface of Biomimetic Nanofiber-Enabled Bone Tissue Engineering. Biomaterials 276, 121041. doi:10.1016/j.biomaterials.2021.121041
Zhao, D., Zhu, T., Li, J., Cui, L., Zhang, Z., Zhuang, X., et al. (2021). Poly(lactic-co-glycolic Acid)-Based Composite Bone-Substitute Materials. Bioact. Mater. 6 (2), 346–360. doi:10.1016/j.bioactmat.2020.08.016
Zhao, F., Lei, B., Li, X., Mo, Y., Wang, R., Chen, D., et al. (2018). Promoting In Vivo Early Angiogenesis with Sub-micrometer Strontium-Contained Bioactive Microspheres through Modulating Macrophage Phenotypes. Biomaterials 178, 36–47. doi:10.1016/j.biomaterials.2018.06.004
Zhao, W., Chang, J., and Zhai, W. (2008). Self-setting Properties and In Vitro Bioactivity of Ca3SiO5/CaSO4.1/2H2O Composite Cement. J. Biomed. Mat. Res. 85A (2), 336–344. doi:10.1002/jbm.a.31523
Zhou, Z., Buchanan, F., Mitchell, C., and Dunne, N. (2014). Printability of Calcium Phosphate: Calcium Sulfate Powders for the Application of Tissue Engineered Bone Scaffolds Using the 3D Printing Technique. Mater. Sci. Eng. C 38, 1–10. doi:10.1016/j.msec.2014.01.027
Zhu, T., Jiang, M., Zhang, M., Cui, L., Yang, X., Wang, X., et al. (2022). Biofunctionalized Composite Scaffold to Potentiate Osteoconduction, Angiogenesis, and Favorable Metabolic Microenvironment for Osteonecrosis Therapy. Bioact. Mater. 9, 446–460. doi:10.1016/j.bioactmat.2021.08.005
Zimmermann, E. A., and Ritchie, R. O. (2015). Bone as a Structural Material. Adv. Healthc. Mat. 4 (9), 1287–1304. doi:10.1002/adhm.201500070
Keywords: bone defect, 3D printing scaffold, polylactic glycolic acid copolymer, calcium sulfate, mechanical properties, biological properties
Citation: Liu T, Li Z, Zhao L, Chen Z, Lin Z, Li B, Feng Z, Jin P, Zhang J, Wu Z, Wu H, Xu X, Ye X and Zhang Y (2022) Customized Design 3D Printed PLGA/Calcium Sulfate Scaffold Enhances Mechanical and Biological Properties for Bone Regeneration. Front. Bioeng. Biotechnol. 10:874931. doi: 10.3389/fbioe.2022.874931
Received: 13 February 2022; Accepted: 11 May 2022;
Published: 23 June 2022.
Edited by:
Changchun Zhou, Sichuan University, ChinaCopyright © 2022 Liu, Li, Zhao, Chen, Lin, Li, Feng, Jin, Zhang, Wu, Wu, Xu, Ye and Zhang. This is an open-access article distributed under the terms of the Creative Commons Attribution License (CC BY). The use, distribution or reproduction in other forums is permitted, provided the original author(s) and the copyright owner(s) are credited and that the original publication in this journal is cited, in accordance with accepted academic practice. No use, distribution or reproduction is permitted which does not comply with these terms.
*Correspondence: Xuemeng Xu, eHV4dWVtZW5nQDE2My5jb20=; Xiangling Ye, eXhsMjAxNjAyMjhAMTYzLmNvbQ==; Ying Zhang, eWluZ196aGFuZzEyMUAxNjMuY29t
†These authors have contributed equally to this work
Disclaimer: All claims expressed in this article are solely those of the authors and do not necessarily represent those of their affiliated organizations, or those of the publisher, the editors and the reviewers. Any product that may be evaluated in this article or claim that may be made by its manufacturer is not guaranteed or endorsed by the publisher.
Research integrity at Frontiers
Learn more about the work of our research integrity team to safeguard the quality of each article we publish.