- 1Department of Orthopedics, The Second Xiangya Hospital, Central South University, Changsha, China
- 2Hunan Key Laboratory of Tumor Models and Individualized Medicine, The Second Xiangya Hospital, Central South University, Changsha, China
- 3Department of Dermatology, The Second Xiangya Hospital, Central South University, Hunan Key Laboratory of Medical Epigenomics, Changsha, China
Tremendous advances in tissue engineering and regenerative medicine have revealed the potential of fabricating biomaterials to solve the dilemma of bone and articular defects by promoting osteochondral and cartilage regeneration. Three-dimensional (3D) bioprinting is an innovative fabrication technology to precisely distribute the cell-laden bioink for the construction of artificial tissues, demonstrating great prospect in bone and joint construction areas. With well controllable printability, biocompatibility, biodegradability, and mechanical properties, hydrogels have been emerging as an attractive 3D bioprinting material, which provides a favorable biomimetic microenvironment for cell adhesion, orientation, migration, proliferation, and differentiation. Stem cell-based therapy has been known as a promising approach in regenerative medicine; however, limitations arise from the uncontrollable proliferation, migration, and differentiation of the stem cells and fortunately could be improved after stem cells were encapsulated in the hydrogel. In this review, our focus was centered on the characterization and application of stem cell-laden hydrogel-based 3D bioprinting for bone and cartilage tissue engineering. We not only highlighted the effect of various kinds of hydrogels, stem cells, inorganic particles, and growth factors on chondrogenesis and osteogenesis but also outlined the relationship between biophysical properties like biocompatibility, biodegradability, osteoinductivity, and the regeneration of bone and cartilage. This study was invented to discuss the challenge we have been encountering, the recent progress we have achieved, and the future perspective we have proposed for in this field.
1 Introduction
Bone and cartilage defects, a worldwide health problem leaving heavy social and family burdens, originate from diverse causes like trauma, degenerative diseases, congenital defects, tumor, and infection (osteomyelitis) (Qasim et al., 2019). More than 900 million reconstructive surgery operations are performed annually in response to all these leading causes of bone defects, far more than originally thought (Grasman et al., 2015). Bone and cartilage are two essential components of the body’s skeletal system, with the self-repair property under internal and external stimulation. But the self-remodeling process could only meet the demand of adapting to the body burden and repairing old micro-damaged tissues. Moreover, the solutions to repair bone defects including orthopedic procedures (bone grafts or substitute surgery) and medication (Gage et al., 20132018) could not implement successfully for bone and cartilage reconstruction. Therefore, the need of bone and cartilage reconstruction has assigned an extremely great market value to bone grafts and related materials (Zhang et al., 2018), while the liberalization for these orthopedic procedures must be achieved. In this case, the engineering of soft and hard tissues for the repair of bone and cartilage tissues has emerged as a trend that occupied a key factor to boost the market value (Pereira et al., 2018).
Three-dimensional (3D) printing was first introduced by Hull and Warfel (1986). As he described, thin layers of a material could be cured with ultraviolet light and then be printed in layers to form a solid 3D structure. Nowadays, it has been considered as a scientific hot button in the tissue engineering and biomedical field (Jain et al., 2019). Three-dimensional bioprinting is an evolutionary form of tissue engineering, which allows many cells and biomaterials to be dispensed with micrometer precision (Murphy and Atala, 2014; Moroni et al., 2018). Various 3D bioprinting technologies have been reported for the fabrication of different kinds of biological structures such as blood vessels, liver, bone, and heart (Zhu et al., 2016). Especially, scientists have produced promising prototypes of the clinically and mechanically robust bone with a functional bone marrow (Zhang et al., 2019a). Therefore, 3D bioprinting technology for bone and cartilage tissue engineering has evolved into the most promising therapeutic strategy the reconstruction (Qasim et al., 2019; Ashammakhi et al., 2019; Daly et al., 2017; Huang et al., 2019) (Figure 1). Three-dimensional bioprinting refers to using hydrogels to create complex constructs in a rapid and customizable manner, which has the capability of controlling cell distribution, high-resolution cell deposition, scalability, and cost-effectiveness. More importantly, the elements for 3D bioprinting should be characterized with low viscosity, porous, nontoxic, biodegradable, biocompatible, and promoting cell differentiation and tissue regeneration (Polo-Corrales et al., 2014; Tao et al., 2020). Hydrogels play the most essential role in 3D bioprinting because they could not only be elaborately functionalized or modified to replicate the physicochemical properties of multiple tissues (Gaharwar et al., 2014) but also provide a 3D environment similar to that of the native extracellular matrix (ECM) and deliver biological molecules like growth factors, drugs, and cells (Hayashi et al., 2009; Askari et al., 2021). Hydrogels can be composed of natural polymers and synthetic polymers. Alginate, agarose, hyaluronic acid (HA), collagen, and fibroin are representative examples for the former group, and poly(ethylene glycol) (PEG), polymer oligo(poly(ethylene glycol) fumarate) (OPF), and polyvinyl alcohol (PVA) are some salient materials for the latter group (Park et al., 2004; Mauck et al., 2006; Yamaoka et al., 2006; Markstedt et al., 2015; Hong et al., 2020; Wu et al., 2020; Zhao et al., 2020; Lamparelli et al., 2021; Liu et al., 2021). An ideal hydrogel network for bone and cartilage engineering should support cell growth/proliferation, maintain phenotypes of chondrocytes/osteoblasts, and promote chondrogenic/osteogenic differentiation of stem cells for recapitulation of the osteochondral interface or cartilage tissues (Yang et al., 2017). Bioprinted cells are crucial for correct functioning of the fabricated construct. More importantly, the cell type and the number are key important factors in bioprinting. Stem cells are a promising cell type because of their ability to proliferate in an undifferentiated but a multipotent state (self-renewal) and their capability to generate multiple functional tissue-specific cell phenotypes (Rastogi and Kandasubramanian, 2019). Modified hydrogels could mimic the microenvironment to guide the differentiation and maturation of stem cells into functional tissue constructs, which have great potential to challenge the regeneration of bone and cartilage defects by promoting osteogenesis and chondrogenesis (Xu et al., 2019a). The most commonly studied stem cells include mesenchymal stem cells (MSCs), induced pluripotent stem cells, embryonic stem cells (ESCs), and peripheral blood mononuclear cells (PBMCs) (Xu et al., 2019a; Antich et al., 2020; Guo et al., 2020; Fryhofer et al., 2021). Levato R et al. obtained MSC-laden polylactic acid microcarriers via static culture or spinner flask expansion and encapsulating MSCs in gelatin methacrylamide–gellan gum bioinks. This bioprinting approach could not only enhance the stiffness of the hydrogel constructs but also promote cell adhesion, osteogenic differentiation, and bone matrix deposition by MSCs (Levato et al., 2014). Thus, stem cell-laden hydrogel-based 3D bioprinting is a remarkable system to provide promising therapeutic strategies for bone and cartilage reconstruction.
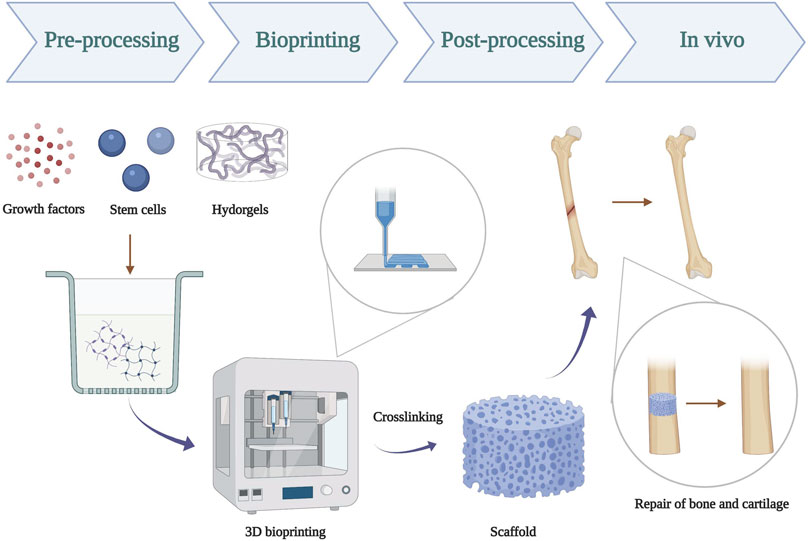
FIGURE 1. Schematic illustration of 3D bioprinting of hydrogels scaffold for repair of bone and cartilage defect. Pre-processing: prepare a mixture using hydrogel, stem cells, and growth factors; bioprinting: successful 3D bioprinting of biomaterials with physiological cell density in a designed way; post-processing: crosslinking of bioprinted constructs by UV-ray.
Unlike conventional 3D printing techniques that have been used to print temporary cell-free scaffolds for use in surgery, 3D bioprinting is a relatively new technology compatible with depositing living cells. Three-dimensional bioprinting is being developed not only for transplantation but also for use in drug discovery, analysis of chemical, biological and toxicological agents, and basic research. Nowadays, this platform has been applied to bone and cartilage tissue engineering and is expected to solve the problematic issues and help to meet the future demands of cartilage and bone tissue repair. This review focused on the characterization and application of stem cell-laden hydrogel-based 3D bioprinting for bone and cartilage tissue engineering. We first introduce the current prevailing 3D bioprinting materials, techniques, and main process. Then, emphasis is put on the process and application of different subtypes of hydrogel scaffolds with various stem cells or growth factors in repairing bone and cartilage defects. Then we specifically summarize the cellular and molecular mechanisms of osteogenesis/chondrogenesis in bone and cartilage repairing. Finally, we discussed the challenges we are encountering and proposed some advice and prospects on improving the stem cell-laden hydrogel system for the field of bone and cartilage tissue bioprinting.
2 Strategy of Fabrication of Stem Cell-Laden Hydrogel-Based 3D Bioprinting
2.1 Materials for 3D Bioprinting
The “raw materials” of bioprinting are formulations of printable biomaterials known as “bioinks” (Barrs et al., 2020). Hydrogels present immense superiority in tissue engineering and have emerged as the most common biomaterials used for 3D bioprinting. In 1954, Wichterle and Lim synthesized the first hydrogel (Ruiz et al., 2008). Nowadays, the most widely used definition for hydrogel is “hydrogel is a water-swollen and cross-linked polymeric network, produced by the simple reaction of one or more monomer/polymer/cross-linker units.” They possess a highly hydrated polymeric structure, which can be easily modified in response to various physical and biological stimuli such as temperature, light, pH, ions, and other biochemical signals (Xu et al., 2008; Gaharwar et al., 2014). As aforementioned, hydrogels can be further categorized as natural or synthetic, depending on their source. Naturally derived hydrogels originate from a biological source, with the advantage of inherent bioactivity. Generally, researchers show a preference for natural hydrogels in bone and cartilage tissue engineering because of their incomparable properties of biocompatibility, the biodegradability of hydrogel that is similar to the native bone or cartilage. Synthetic hydrogels are based on hydrated networks of polymers synthesized using chemical methods (Qin et al., 2018). Synthetic hydrogels are favored for their user-defined functionalities because they can be tailored with specific chemical and physical properties to meet the specific application requirement. Cross-linking is a key procedure in controlling these properties of the printed constructs, and various cross-linking methods have been applied for 3D bioprinting of hydrogels, such as covalent bonding, photopolymerization, thermo-gelation, cryo-gelation, and other noncovalent bonding (Huang et al., 2017). However, challenges in the use of synthetic hydrogels include poor biocompatibility, toxic degradation products, and loss of mechanical properties during degradation. Synthetic hydrogels are attractive for 3D bioprinting due to the ease of controlling their physicochemical properties during synthesis.
A wide range of hydrogels possess relevant properties and superiorities in different tissue engineering applications. Moreover, an ideal hydrogel should have proper biocompatibility, mechanical, rheological, biological, and chemical characteristics (Lee et al., 2015) because they act as a structural scaffold and provide a microenvironment for encapsulated cells (Khetan et al., 2013; Gjorevski et al., 2016). First, biocompatibility refers to the coexistence of the transplant and endogenous tissues, which is a basic design parameter for bioprinted tissues (Yilmaz et al., 2019). In addition, the hydrogel scaffold should be mechanically strong to create an environment that is compatible with cellular activities, such as cell viability, migration, and proliferation. Specially, it is important for bone and cartilage tissue engineering because these tissues mainly rely on the mechanical properties to provide solid physical support. These mechanical properties include strain, shear stress, compressive modulus, and mass swelling ratio. Moreover, scientists have made great effort to strengthen the mechanical properties of the hydrogel scaffold. For example, hydroxyapatite and graphene oxide were used to provide adequate mechanical properties for bone regeneration (Sivashankari and Prabaharan, 2020). Rheological character is the flow properties of materials under external forces, which is essential for fidelity and cell viability (Knowlton et al., 2018). Biological and chemical characteristics refer the tissue-specific modification of the printed scaffolds, which are crucial because they are related to cell growth, differentiation, and organization by direct contact. Moreover, osteoconduction for scaffold and osteoinduction for bioinks are important in bone and cartilages tissue engineering.
The choice of cells is crucial for the correct functioning of bioprinted construct. Current options for 3D bioprinting cells involve either the deposition of multiple primary cell types into patterns that represent the native tissue or printing stem cells that can proliferate and differentiate into required cell types. Stem cells are widely used because they may enhance the imitation ability of materials secondary to their unique features such as their paracrine activity and the immune-privileged status. Isolated cells can be stabilized by cross-linking during or immediately after the bioprinting process to form the final structure of the intended construct. More importantly, it is well established that the features of materials have a large influence on bioprinted cells, for example, cell attachment, size, shape, proliferation, and differentiation. Maintaining cell viability and bioactivity should be kept in sight to obtain a good biocompatibility for bioprinted constructs. In this regard, scientists have made great effort to decrease the force damages during the printing process and design an ideal scaffold to preserve cell viability. In addition, a variety of growth factors/peptides have been incorporated into bioinks to obtain superior properties for the bioprinted construct.
2.2 Strategies for 3D Bioprinting
Currently, 3D bioprinting techniques could be divided into four main categories: extrusion-based bioprinting, inkjet-based bioprinting, laser-assisted bioprinting, and stereolithography-based bioprinting (Figure 2). Extrusion-based bioprinting (EBB) is the most popular technique to build hydrogel scaffolds, this method uses pneumatic-, piston-, or screw-driven actuators to extrude bioinks through a nozzle onto a printing substrate. Almost all types of hydrogel pre-polymer solutions of varying viscosity as well as aggregates with high cell density can be printed with extrusion bioprinters. One of the advantages of this technique is the high structural integrity because of continuous deposition and a wide range of speed (Skardal and Atala, 2015). In addition, it has a high flexibility and enables the production of 3D bioprinted constructs with high cell density and viability (Ostrovidov et al., 2019). While beneficial, this method is limited by printing resolution, which is about 100 um (Derakhshanfar et al., 2018). Inkjet-based bioprinting consists of the thermal, piezoelectric, electrostatic, acoustic, hydrodynamic, and microvalves mechanisms, using either electrical heating to produce air-piezoelectric-pressure pulses to propel droplets from the nozzle. The drop-by-drop bioink deposition through the nozzle is synchronized with a motorized stage, allowing the fabrication of 3D constructs (Gudapati et al., 2016). The advantages of inkjet-based bioprinting include low cost, high printing speed, and high cell viability. However, it usually requires low viscosity (<10 mPa s) for bioinks (Matai et al., 2020). Laser-assisted printing is a platform that forces bioinks onto a collector substrate with pressures generated by lasers focused on an absorbing substrate (Murphy and Atala, 2014). It is a costly and fast printing technique, and it can achieve high cell viability. It allows high precision in bioink deposition; however, it has more requirements, such as rapid gelation kinetics and relatively low flow rates. Stereolithography-based bioprinting is based on light to selectively solidify bioinks in a layer-by-layer process that additively builds up objects (Moroni et al., 2018). This technology offers superior speed, resolution, scalability, and flexibility for printing a 3D structure with micrometer resolution (Ligon et al., 2017). Importantly, EBB is the most commonly used bioprinting technique in bone and cartilages tissue engineering. For example, biphasic calcium phosphate (BCP) and matrigel/alginate hydrogel composites were synthesized by EBB to induce osteogenesis of incorporated MSCs as an osteoinductive bone filler at the area of bone defects (Fedorovich et al., 2011). In addition, volumetric 3D bioprinting is a light-mediated technique, which enables excellent cell viability, structural fidelity, and tissue maturation potential (Rizzo et al., 2021). It has been reported that volumetric tomographic bioprinting of a bone model could promote osteocytic differentiation, which would be a powerful tool for biofabrication of 3D bone-like tissues (Gehlen et al., 2021).
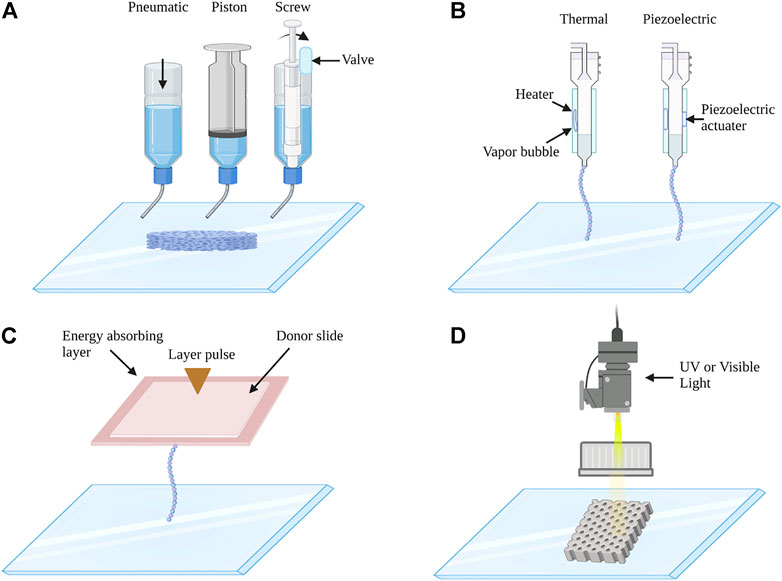
FIGURE 2. Schematic images of (A) extrusion-based, (B) inkjet-based, (C) laser-assisted, and (D) stereolithography 3D bioprinting system. (A) Extrusion-based 3D bioprinting: extrusion bioprinters use pneumatics, piston, or screw force to continuously extrude a liquid cell-hydrogel solution. (B) Inkjet-based 3D bioprinting: the printer heads are deformed by a thermal or piezoelectric actuator and squeezed to generate droplets of a controllable size. (C) Laser-assisted 3D bioprinting: laser bioprinters use a laser to vaporize a region in the donor layer (top) forming a bubble that propels a suspended bioink to fall onto the substrate. (D) Stereolithography 3D bioprinting: stereolithographic printers use a digital UV or visible light projector to selectively cross-link bioinks plane-by-plane.
2.3 3D Bioprinting Process
Bioprinters cannot print without instruction. As aforementioned, appropriate bioinks are important for creating bioprinted tissues successfully. In addition, a correct design and planning of printing paths, control the bioprinter and post-printing operations are necessary. The bioprinting process is complex and error-prone; therefore, many researchers have utilized computer-aided design/computer-aided manufacturing technologies to obtain anatomically correct tissues (Jung et al., 2016). Computer-aided design can accelerate the speed of the whole bioprinting process, and computer-aided manufacturing can guarantee the quality of what is printed (Mandrycky et al., 2016).
A fundamental step for the transition to clinical application is the good integration of various bioinks, which mimic the specific geometry of tissues of interest. The general bioprinting process is as follows (Qasim et al., 2019): designers draw the specific design taking into account the function that this structure should have in vivo, different operating temperatures, and appropriate printing times, in which to insert the cells by computer-aided design (Grasman et al., 2015); designers prepare and load appropriate bioinks (Gage et al., 20132018); and the bioprinter builds structures and follows the predesigned path by computer automation systems. Furthermore, post-printing modification is often used to increase or trim the scaffold performance. Such modifications not only improve differentiation and growth of stem cells in vitro but also enhance histocompatibility after transplantation. Therefore, post-printing is important to maintain the bioprinted structures viable. Moreover, some special approaches to fabricate tensegrity structures acting as an external stabilizations system without further process after printing, which can pave the way toward more algorithmic designs of 3D bioprinting (O’Bryan et al., 2017; Lee et al., 2020a; Chiesa et al., 2020).
Three-dimensional bioprinting could combine functions and properties of various hydrogels to generate high-resolution, multi-component living constructs, which developed exciting perspectives in the area of stem cell therapy for tissue engineering (Negro et al., 2018). Three-dimensional bioprinting represents a formidable technology in tissue engineering (Matai et al., 2020). However, a lot of problems and challenges remain to be solved. For instance, the limited materials and printing systems could not meet the stringent demand of hydrogel composite systems. We need to do further research to find an ideal material with high cross-linking efficiency. Moreover, substandard technology would bring out agglomeration of reinforcements inside the hydrogel matrix, resulting in poor performance of the hydrogel composite. Moreover, it becomes more complex to find out the most optimal bioink design in case of 3D bioprinting of bone, as it is a tough task to strike the correct balance between biocompatibility and mechanical strength. Thus, we need to take a greater effort to improve 3D printing techniques of hydrogel composites in the aspects of the material design and printing systems.
3 Hydrogel for 3D Bioprinting
As aforementioned, 3D bioprinting of hydrogel has been increasingly applied in tissue engineering and regenerative medicine over the past years (Yue et al., 2015; Naahidi et al., 2017). Moreover, it is a very attractive carrier for encapsulating cells because of the hydrophilic nature with the high water content, heightening the application of stem cell-laden hydrogel in the tissue engineering area (Li and Mooney, 2016). Accordingly, the ideal stem cell-laden hydrogel that is used for 3D bioprinting should possess the following capacity: 1) well printability—the ability to produce the 3D structure scaffold with high shape integrity and fidelity; 2) proper degradability—the scaffold printed and implemented in the bone or cartilage should be degraded in a speed similar to the native extracellular environment; 3) sufficient mechanical property—enough mechanical property can not only support the structure but also enhance cell viability and stimulate the differentiation of stem cells; 4) bioactivation—both in vivo and in vitro bioprinting materials should be nontoxic and no immunogenic effect to boost the cytocompatibility; and 5) differentiation of the encapsulated stem cells—the stem cells that are loaded in hydrogel should undergo osteogenic or chondrogenic differentiation with the stimuli of the mimetic environment, such as the growth factor (Schuurman et al., 2013; Li et al., 2017a; Wang et al., 2018).
However, another two intrinsic features restrict the usage of hydrogel, one is the weak mechanical property, which hampers the shape fidelity of hydrogel and the 3D printing process, the other is the performance of being prone to degradation as a bioink (Jang et al., 2018). To address these issues, the strategies have emerged to reinforce the mechanical property and bioactive feature of the biomaterial. This part overviews the recent progress of the 3D printing scaffold with stem cell-laden as the representative of the polysaccharide-based natural hydrogels (alginate, agarose, and HA) and protein-based natural hydrogels (gelatin, collagen, and silk) (Table 1).
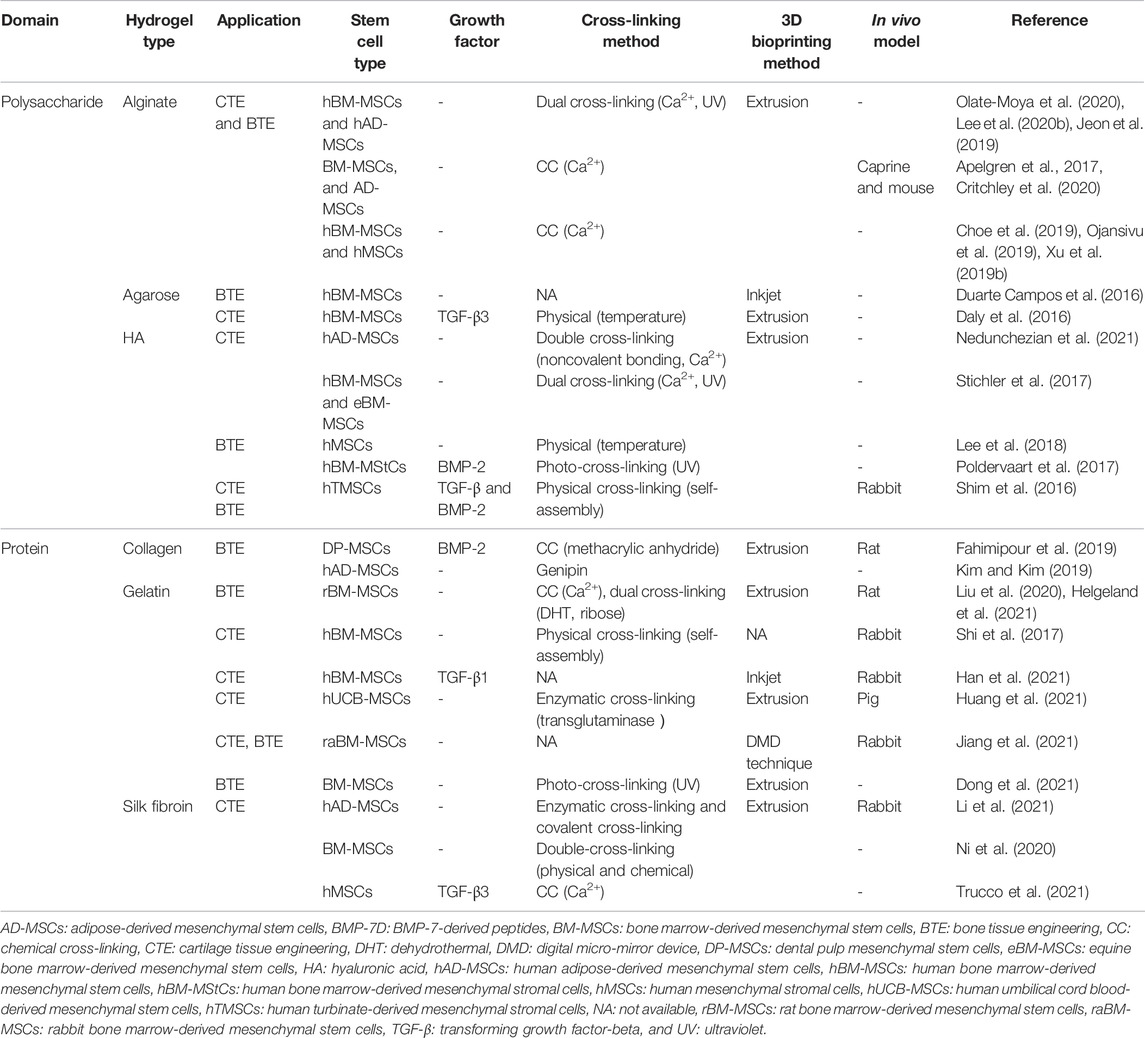
TABLE 1. Hydrogel, stem cell, growth factor, cross-linking method, and 3D bioprinting method used for CTE and BTE.
3.1 Alginate-Based Hydrogel and Their Derivatives
Alginate, a kind of polysaccharide, consists of two different uronic acids that occur naturally in the cell wall of algae and capsule of Azotobacter and Pseudomonas, and therefore can be obtained from brown seaweed and the bacterial ones (Trica et al., 2019; Ahmad Raus et al., 2020). Owing to its promising physiological properties such as biocompatibility, biodegradability, and the capability of forming gel, alginate has been widely applied in various biomedical areas, like wound dressing, drug delivery, and tissue engineering (Reakasame and Boccaccini, 2018; Rastogi and Kandasubramanian, 2019) Supplement with divalent cations such as Ca2+ turns the alginate into an ideal bioink for 3D printing (Song et al., 2011).
Stem cell-laden alginate-based 3D bioprinting structures have been used in different tissues including bones, cartilage, cardiac, and blood vessels (Choe et al., 2019; Chu et al., 2021; De Santis et al., 2021). However, two main disadvantages challenge the development: one is the loss of the shape fidelity and integrity after being printed due to the weak mechanical property and the other is the impairment to the adhesion and proliferation of the stem cell. To address the issues, distinct biomaterials were blended with alginate forming alginate composite to improve the property and meet the requirement of tissue engineering (Venkatesan et al., 2015; Apelgren et al., 2017; Hernández-González et al., 2020).
Compared to the pure alginate hydrogel, mixing type I collagen (COL I) and agarose into alginate could enhance the mechanical strength, while the COL I could also facilitate cell adhesion, proliferation, and expression of cartilage-specific genes (Yang et al., 2018). To improve printability and structural stability, Goeun Choe et al. added the graphene oxide into the alginate as a composite for bioink, revealing that the addition of graphene oxide could enhance printing and structure, viability and proliferation of MSCs, and osteogenic differentiation. As a result, the best balance of alginate and graphene oxide were identified as 3% and 0.5 mg ml−1, respectively (Choe et al., 2019) (Table 2). Similarly, adding graphene oxide into a tailor-made alginate-based hydrogel not only highlighted the shape fidelity and resolution of 3D scaffolds but also induced chondrogenic differentiation of human adipose-derived mesenchymal stem cells (hAD-MSCs) (Olate-Moya et al., 2020). Another attempt was to combine alginate and the decellularized extracellular matrix (dECM) derived from bone tissue of porcine, with an appropriate concentration of dECM; consequently, the printability and viability of hAD-MSCs encapsulated in the scaffold as well as the osteogenic differentiation was greatly improved (Lee et al., 2020b).
In addition, supplementation with other material, especially modified alginate might be another selection. The stem cell-laden dual cross-linkable alginate microgels consist of oxidized and methacrylated alginate (OMA) directly assembled into the 3D structure and cryopreserved for long-term storage, while the stem cells could maintain equivalent after recovery compared with the freshly processed stem cells, which has provided a new paradigm for 3D printing (Jeon et al., 2019).
Although the physiology of a weak mechanical property and inferior capability for cell attachment and proliferation has affected the tissue engineering application (Ahmad Raus et al., 2020), several strategies have been explored to improve the embarrassing situation, including 1) supplementing with other materials such as graphene oxide (Li et al., 2020a) and polycaprolactone (PCL) (Critchley et al., 2020); 2) modifying the alginate-based bioink (Ojansivu et al., 2019); and 3) optimizing the fabrication method of stem cell-laden 3D-printed hydrogel (Xu et al., 2019b).
3.2 Agarose-Based Hydrogel and Their Derivatives
Agarose, a sort of natural polysaccharide, is extracted from red algae and composed of alternating β-d-galactopyranosyl and 3,6-anhydro-α-l-galactopyranosyl units (Krömmelbein et al., 2021). Agarose, along with its blend-based hydrogel, has been extensively used in cartilage formation and bone regeneration because of its good biocompatibility and biodegradability (Tabata et al., 2003; Zarrintaj et al., 2018). Moreover, the porous structure, tunable mechanical strength as well as the stiffness of agarose-based hydrogel facilitate the tuning of 3D scaffolds for cell culture, allowing agarose to be a promising cell-laden 3D printing hydrogel (Ulrich et al., 2010; López-Marcial et al., 2018; Salati et al., 2020).
The agarose and alginate hydrogel composites demonstrated similar effects in mechanical and rheological properties compared to pluronic hydrogel, a kind of hydrogel with well-printed capability, but exhibited better cell viability and matrix production during a 28-day culture period (López-Marcial et al., 2018). To address the problem of the dissatisfactory bioink and the limited size of the printed construct, Daniela et al. encapsulated the hMSC and MG-63 cells into agarose hydrogel and submerged in high-density fluorocarbon when printing, manufacturing the stem cell-laden scaffold with variable shapes and sizes, and maintaining viable cells after 21 days culture (Duarte Campos et al., 2013). To balance the contradiction between 3D printability and optimal cytocompatibility, Marius et al. developed a novel blend hydrogel of agarose and Col I with the encapsulation of human umbilical artery smooth muscle cells (HUASMCs), finding the blend hydrogel with a concentration of 0.5% agarose, and 0.2% Col I exhibited better stiffness, printing accuracy, and cell spreading and attachment (Köpf et al., 2016).
By contrast with native agarose, the carboxylated agarose-derived hydrogel has been regarded as a more appropriate stem cell-laden scaffold because of the significant higher human MSC survival rate (95:62%) (Forget et al., 2017). Neha Arya et al. explored that a human articular chondrocyte-laden extrudable carboxylated agarose-derived hydrogel, and discovered that the stiffness of carboxylated agarose-derived hydrogel and integrin-binding peptide sequence (GGGGRGDSP) could affect chondrocyte differentiation, indicating carboxylated agarose served as high suitable bioink in the cartilage area (Arya et al., 2019). To fabricate materials like the osteochondral interface that could imitate anisotropic tissues, Merve Kuzucu et al. worked out an extrusion-based 3D bioprinting platform based on carboxylated agarose hydrogel, and cell centration gradient and the stiffness gradient scaffold could be printed with this bioink (Kuzucu et al., 2021), laying the foundation for the manufacture of tissue with gradients, such as cartilage.
Though agarose-based hydrogel is an ideal cell-laden 3D printing hydrogel, the immigration and differentiation of stem cells encapsulated in agarose-based hydrogel alone is difficult. To address this problem, Daniela et al. added Col I into the agarose hydrogel, and discovered that the addition of collagen I promoted the cell spreading and osteogenic differentiation, and the effect was more obvious with the increase of collagen I in a range (Duarte Campos et al., 2016). Beyond that, mechanical compression might be another selection to induce osteogenesis. Dynamic mechanical compression could induce osteogenic differentiation of human synovium-derived mesenchymal stem cells (SMSCs) encapsulated in agarose and maintain the cartilage phenotype, providing an approach for stem cell-laden hydrogel-based cartilage therapy was found by Ge et al. (2021). Specifically, it is easier for the MSCs seeded in 3D-printed agarose hydrogel to differentiate into hyaline-like cartilage after 28-day culturing, with the presence of the transforming growth factor-beta 3 (TGF-β3), similar to alginate, while the differentiation fate of MSCs in GelMA tended to be fibrocartilage (Daly et al., 2016), providing a reference of bioink selection when printing different cartilaginous tissues.
3.3 Hyaluronic Acid-Based Hydrogel and Their Derivatives
Hyaluronic acid (HA), a high molecular hydrophilic natural glycosaminoglycan, is abundant in the ECM of many tissues, as well as the most abundant component in cartilage (Fraser et al., 1997; Yang et al., 2017). HA exerts its biological functions via antioxidant properties, biocompatibility, the presence of cell receptors, and so on (Nedunchezian et al., 2021). HA can also facilitate cell attachment, migration, and regulate differentiation of MSCs, making HA-based hydrogel a very promising stem cell-laden material for bone tissue engineering (BTE) and cartilage tissue engineering (CTE) (Zhu et al., 2006).
However, the limited viscoelastic properties of natural HA during the bioprinting process precludes its application in the 3D-printed area, while chemical modification such as methacrylate or glycidyl methacrylate makes it suitable for cross-linking, which could meet the requirement of 3D printing. For example, the mechanical stiffness and shape stability of a modified methacrylated HA-based 3D-printed scaffold could be strengthened by photo-cross-linking, evaluated by the assessment of rheology and mechanical tests, and the cell viability of human bone marrow-derived mesenchymal stromal cells incorporated into the modified hydrogel maintained 64.4% after 21-day culturing, while the osteogenesis of human bone marrow-derived mesenchymal stromal cells could be further enhanced (Poldervaart et al., 2017). Given this, researchers applied two approaches to enlarge the usage of HA-based hydrogel and their derivatives, and were applying the cross-linking strategy to increase the mechanical property; the other was integrating biological functions into physical structures by mixture with other modifications or materials.
Swathi Nedunchezian et al. developed a kind of adipose-derived mesenchymal stem cell (AD-MSC)-laden HA hydrogel-based 3D bioprinting applied for chondrogenic engineering by the double cross-linked strategy (Nedunchezian et al., 2021). Specifically, AD-MSC-laden HA was partially cross-linked into HA–biotin–streptavidin (HBS) hydrogel through noncovalent bonding via biotin and streptavidin to enhance the viscoelastic property and shape fidelity, and then the partially cross-linked hydrogel was printed to a 3D scaffold after mixed with sodium alginate and immersed in CaCl2 solution subsequently to heighten the final stability through the second step ion transfer cross-linking. As a result, the AD-MSC-laden HA-based 3D scaffold exhibited improved printability, shape integrity, cell viability, and chondrogenic formation compared to the HA hydrogel. Similarly, allyl-functionalized poly(glycidol)s (P(AGE-co-G)) was added into thiol-functionalized HA as a cytocompatible cross-linker, and the chemical cross-linking could be induced by UV via thiol-ene coupling generated by thiol and allyl groups of the two materials. The hybrid hydrogel loaded with the 3D-printed equine or human bone marrow-derived mesenchymal stem cell (BM-MSC) scaffold demonstrated higher cell viability and promising chondrogenesis after 21 days of observation, tested by live/dead cell staining, safranin-O staining for glycosaminoglycans (GAG) and immunohistochemistry (IHC) for aggrecan, Col I, and type collagen II (Col II) (Stichler et al., 2017). However, Jin-Hyung Shim et al. exploited a novel 3D bioprinting multilayered mechanical stable scaffold via the host–guest chemistry-based strategy according to the two preprocessed hydrogel supramolecular HA and atelocollagen with human turbinate-derived mesenchymal stromal cells (hTMSCs) encapsulated, avoiding the affection to the cell viability of the chemical agent and physical stimulation during cross-linking or the post-printing cross-linking process, and the in vivo study of this hTMSC-laden hydrogel-based 3D-printed multilayered structures for knee articular cartilage injury of rabbits proved it to be an appropriate and promoted approach according to the gross morphology, Hematoxylin and eosin staining (H&E staining), safranin-O staining, and immunohistochemistry for Col II and type collagen X (Col X) (Shim et al., 2016).
Another disadvantage of HA-based hydrogel is the long-term gelation process. In terms of this issue, Jaeyeon Lee et al. produced a hybrid bioink with tyramine-conjugated for improved printability, mechanical integrity, and fast gelation while BMP-7-derived peptides (BMP-7D) and osteopontin immobilized for osteogenesis, with shorter gelation time (<200 s), higher hMSC viability encapsulated in the 3D-printed scaffold (>90%), and favorable osteogenic differentiation (Lee et al., 2018).
3.4 Collagen-Based Hydrogel and Their Derivatives
As the most abundant protein family of the ECM, collagen accounts for two-thirds of the dry mass of adult articular cartilage (Eyre, 2004). Specifically, Col II makes up the most proportion of the articular cartilage and is accompanied by the other minor collagens to provide the tensile strength and physical property of the matrix of the cartilage (Luo et al., 2017), while bone is made of an organic matrix that consists of about 90% of Col I, which contributes to the prominent mechanical properties (Depalle et al., 2021). Both the bone marrow-derived mesenchymal stem cell (BM-MSC)-embedded Col I hydrogel and the blend collagen (Col I/II = 3:1) hydrogel exhibited chondrogenic differentiation; however, the GAG production is higher in the blend collagen hydrogel group, suggesting the enhancement of Col II in the production of GAG in vitro, while histochemical staining demonstrated that the blend collagen hydrogel group manifested a favorable effect of cartilage repair of the defects in the rabbit’s femur after 13 weeks treatment (Kilmer et al., 2020). Compared to MSCs in 2D culture in a collagen hydrogel, the 3D culture exhibited a stronger capability of differentiation of MSCs into osteoblasts both in vitro and in vivo of rat (Naito et al., 2013).
Though stem cells encapsulated in collagen hydrogel are widely used in BTE and CTE, 3D printing of stem cells and collagen remains challenged, whose obstacles rely on stabilizing the soft and dynamic biomaterial, and achieving the shape fidelity of the complex scaffold required the 3D printing process (Lee et al., 2019). Researchers have developed different approaches including additive manufacturing techniques and complex structure design to improve it. To reinforce the support of the scaffold, tricalcium phosphate (TCP)-based bioceramic was added by the additive layer manufacturing technique, both the compressive strength and the compressive modulus increased, forming an ideal scaffold construct (Fahimipour et al., 2019). The water solubility and photochemical cross-linking ability of collagen derivatives were greatly improved after modified with glycidyl methacrylate, demonstrating the advantage of enhancing cell adhesion, proliferation, and promoting osteogenic differentiation of BM-MSCs, and providing another approach to fabricate stem cell-laden collagen 3D bioprinting (Zhang et al., 2019b). The mechanical stiffness and printed constructs could be improved by adding thermo-responsive agarose into Col I while the 3D-printed agarose and collagen blend hydrogels with a high collagen ratio favor the osteogenic differentiation of human bone marrow-derived mesenchymal stem cells (hBM-MSCs) in it, demonstrated by two-photon microscopy, alizarin red staining (ARS), and real-time polymerase chain reaction (RT-PCR) (Duarte Campos et al., 2016). In addition to the mechanical properties, inducing chondrogenesis and osteogenesis has been another challenge in this area. Various studies reported the impact of physical–chemical cues to hydrogel materials on differentiation (Yang et al., 2021), while the effect of 3D scaffold microstructure on stem cell differentiation remains to be seen. Yang et al. designed two BM-MSC-laden collagen hydrogels and named them fibrous network and porous network, respectively, according to the microstructure of collagen, and the fibrous network induced more chondrogenic differentiation of the encapsulated BM-MSCs, revealing that the microstructure of the hydrogel may be a pivotal feature for BM-MSC chondrogenic differentiation (Yang et al., 2019).
Furthermore, collagen can cover the shortage of another kind of materials according to its advantage. For example, as a promising material for bone regeneration, the application of bioactive hydroxyapatite (HAP) was limited by inefficient mineralization, mechanical instability, and incomplete osteointegration, but was greatly improved by the HAP and collagen hybrid fiber hydrogel, promoting the adhesion, proliferation, and osteogenic differentiation of rabbit BM-MSCs in vitro and osteoinductivity and mineralization (Li et al., 2020b).
Collagen hydrogel exhibits unique superiority based on its similarity to the ECM of the native bone and cartilage. Though some disadvantages still exist, different approaches have been developed to address the shortcoming: 1) design and process of the appropriate bioink can be mechanically modified, 2) creation and production of focus on the microstructure of the scaffold, and 3) optimized fabrication method and techniques (Kim and Kim, 2019; Marques et al., 2019).
3.5 Gelatin-Based Hydrogel and Their Derivatives
Gelatin is a hydrolysate of collagen, as well as a major component in most tissues including bone and cartilage (Yue et al., 2015). As a derivative of collagen, gelatin inherits its superior advantages of good biocompatibility, degradability, and bioactivity of collagen as a biomedical scaffold. In addition, the lower immunogenicity and more feasible compatibility by comparison with collagen amplify the application of gelatin-based hydrogel as a cell-laden scaffold (Lien et al., 2009; Yue et al., 2015; Gao et al., 2019). Above all, the sequence arginine–glycine–aspartic among the gelatin could interact with stem cells, hence facilitating cell proliferation, adhesion, and migration (Echave et al., 2017; Bello et al., 2020).
The instability of the physically cross-linked gelatin-based hydrogel precluded its application, while the chemical cross-linking gelatin-based hydrogel demonstrated greater potential despite the inevitable disadvantage of weakness and degradability (Sakai et al., 2009). To make up for the weak strength and rapid degradability of gelatin, silk fibroin was blended in, and the appropriate mechanical and degradable properties could be adjusted by the concentration and ratios of gelatin and silk fibroin to fit in the native cartilage environment (Rodriguez et al., 2017). By comparison, the gelatin–silk fibroin bioink with a ratio of 2:1 was proven to be favorable (Huang et al., 2014). Furthermore, to optimize the function of 3D-printed BM-MSC-laden gelatin–silk fibroin, conjugating the BM-MSC affinity peptide F7 onto the 3D-printed scaffold enhanced the BM-MSC homing in vitro and in vivo, as shown of the predominant chondrogenic differentiation of the encapsulated BM-MSCs of the scaffold (Shi et al., 2017). Here is a study that applied both of the strategies mentioned earlier. Transglutaminase cross-links gelatin to stabilize the structure to sustain the 3D scaffold, while HAP was blended to strengthen the hydrogel, then the human umbilical cord blood-derived mesenchymal stem cells (hUCB-MSC) encapsulated HAP doped, enzyme cross-linked gelatin hydrogel-based 3D-printed scaffold exhibited an effect of the scaffold enhancing proliferation and chondrogenic differentiation of hUCB-MSC in vitro and cartilage regeneration in vivo, revealing the potential application of 3D-printed gelatin hydrogels in tissue engineering (Huang et al., 2021). In addition to silk fibroin and HAP, poly(lactic-co-glycolic acid), alginate, and nanosilicate can also be addictive to improve the property of gelatin (Liu et al., 2020; Han et al., 2021).
Apart from adding other materials, modulating the modification method could be another strategy. Yu et al. applied melt electro-writing technology to print BMSC laden high porosity and high precision scaffolds to enhance cartilage repair (Han et al., 2021). While Helgeland et al. (2021) compared three different cross-linking methods including dehydrothermal (DHT), ribose glycation, and dual cross-linking (DHT and ribose treatments), and revealed that dual cross-linking exhibited the great advantage of promoting cell proliferation and rBMSC differentiation of 3D-printed gelatin scaffolds with promising application in cartilage tissue engineering. Mentioning modification of gelatin, GelMA, a chemical cross-linking method of grafting methacryloyl by photopolymerization, is bound to cover. The modulation can be disposed under mild conditions contributing to the fabrication of stem cell-laden GelMA hydrogel and the improved formability and downregulated immunogenicity broaden the application of GelMA (Jiang et al., 2021). A composite hydrogel composed of 15% (w/v) GelMA and 8% (w/v) laponite that demonstrated favorable printability and biocompatibility was developed, promoting BMSC proliferation and osteogenic differentiation, offering a candidate of bioink in bone tissue engineering (Dong et al., 2021).
The good biocompatibility and insufficient mechanical property of gelatin hydrogel is not absolute. A study about the effect on the physical performance of different concentration of gelatin in cell-laden 3D-printed scaffold showed that the scaffold with low-gelatin concentration (0.8% alginate) displayed good cell viability and cell morphology, same as described before, while high-concentration scaffold (2.3% alginate) preserved the scaffold shape fidelity and mechanical property but significantly impaired cell viability (Zhang et al., 2019c). In consideration of the improved physical property tunable technique, we usually focus on the low-gelatin concentration scaffold.
3.6 Silk Fibroin-Based Hydrogel and Their Derivatives
Silk fibroin is a fibrous protein mainly produced by silkworms and spiders, in the form of aqueous protein solution (Melke et al., 2016). Due to the remarkable mechanical property, impressive biocompatibility, and slow and tunable degradability, silk fibroin has been widely applied in tissue engineering as a biomedical material (Rockwood et al., 2011). Based on the diverse structure of the silk fibroin fabrication, the silk fibroin scaffold can be divided into films, mats, artificial fibers, sponges, and hydrogels (Yan et al., 2012; Jacobsen et al., 2017; Zhang et al., 2017; Yin and Xiong, 2018). Among them, with a 3D polymer network, hydrogel could be cross-linked physically and chemically processing for cell seeding and encapsulation, demonstrating great potential in the development of the cell-laden biomaterial area (Ahmed, 2015).
For instance, with induction of high temperature, low PH, vortexing, sonication, high ionic strength, freeze gelation, or electrogelation, silk fibroin can transform into a hydrogel form (Kim et al., 2004; Wang et al., 2008; Yucel et al., 2009; Bhardwaj et al., 2011). Though the silk fibroin hydrogel scaffold with an ideal mechanical property was processed according to the methods aforementioned, the cells encapsulated in them cannot fit in the environment of the structure produced by these techniques, as the biocompatibility was an imperative factor for processing of the cell-laden scaffold (Piluso et al., 2020). Under the circumstances, chemically cross-linking silk fibroin hydrogel maybe a better selection. For example, the degradable and biocompatible silk fibroin hydrogel have been acquired by riboflavin (Piluso et al., 2020) and HRP (Ribeiro et al., 2018). However, the physiologically fabricated silk fibroin hydrogel would lose the mechanical property and impaired the biomedical application (Jonker et al., 2012). In terms of this issue, some strategies have successfully increased the mechanical performance, such as adding functionally complementary bioink and optimizing the printing process (Kapoor and Kundu, 2016; Trucco et al., 2021).
Li et al. (2021)fabricated a silk fibroin-based 3D-printed macroporous hydrogel scaffold though HRP-medicated cross-linking of silk fibroin and gelatin under physiochemical condition, with marvelous structure fidelity, remarkable mechanical property, tunnel degradability, and a cell aggregate seeding method applied to improve the MSC inoculation efficiency in it, promoting osteogenic differentiation and articular cartilage repair in the rabbit model. Ni et al. (2020) developed a BM-MSC-laden silk fibroin hydrogel-based 3D printing with a double-network scaffold, and mechanical property including fracture strength, breaking elongation, and compressive reproducibility greatly increased with satisfied cell viability, proliferation, and chondrogenic differentiation, revealing the promise of silk fibroin for cartilage tissue engineering.
Another shortcoming of silk fibroin is the deficiency of the cell adhesion sequence resulting in poor interaction between stem cells and biomaterial (Hasturk et al., 2020). Based on this problem, combination with the collagen, poor mechanical properties and cell-mediated shrinkage could be a solution. Buitrago et al. (2018) designed a hybrid silk fibroin/collagen hydrogel, whose scaffold structure, mechanical property, and cellular behavior were greatly enhanced compared to the pure silk fibroin or collagen hydrogel.
4 Various Stem Cells Encapsulated in Hydrogel for 3D Bioprinting
Three-dimensional bioprinting has been a very promising approach in regeneration medicine due to the superiority of this technique with precisely control of construction of the complexed structures of tissues and organs (Murphy and Atala, 2014). However, selection of the appropriate bioink to print an ideal tissue such as bone or cartilage is still a problem that is confusing us. Hydrogel is emerging as a versatile biomaterial among the variety of biomaterials because of its good cytocompatibility, bioactivity, and degradability (Stephanopoulos et al., 2013), and various in vitro and in vivo studies have proven that the cell-laden hydrogels have opened the new possibility for reconstruction of osteochondral and cartilage tissues (Yang et al., 2017). Specifically, the cells embedded in the hydrogel can be human or animal chondrocytes, mesenchymal stem/stromal cells (MSCs), and so on, and the stem/stromal cells loaded in the hydrogel exhibited incomparable advantage due to the abundant cell sources, the multidirectional differentiated ability as well as the paracrine activities and the immune-privileged status that can imitate the heterogeneity of the tissues (Roseti et al., 2018). This part will center on the various stem cells encapsulated in hydrogel for 3D bioprinting.
4.1 BM-MSCs
Bone marrow-derived mesenchymal stem/stromal cells (BM-MSCs) are heterogeneous population cells with the potential of multilineage differentiation and are mainly isolated from bone marrow tissue. The BM-MSCs were first discovered and the most prevalent MSCs in clinical practice (Strioga et al., 2012). For example, Costantini et al. (2016)bioprinted a hybrid hydrogel composed of GelMA, chondroitin sulfate amino ethyl methacrylate, and hyaluronic acid methacrylate (HAMA) with high density hBM-MSCs (107/ml), while the cell viability was higher than 85% demonstrated by a live/dead assay 3 h after printing, and UV cross-linking and the chondrogenic and osteogenic differentiation was ideally validated by immunocytochemistry (ICC) and RT-qPCR of aggrecan, collagen I, II, and X (Table 3). Alternatively, De Moor et al. (2020) combined spheroids of BM-MSCs with GelMA as the boink, and the viability and cartilage phenotype of the BM-MSC spheroids were maintained after bioprinting and 42 days culture, which is demonstrated by the increase in GAG, COL II, and decrease in Col I (Table 3). To enhance the osteogenesis, Wenhai Zhang et al. fabricated a 3D-printed BM-MSC-laden HA (Me-HA)/PCL with dual small molecule (RVS, SrRn) loaded, and both of the molecules upregulated the expression of ALP, runt-related transcription factor 2 (RUNX2), osteocalcin (OCN), and collagen 1A1 (Col 1A1) of BM-MSCs, while the osteogenic differentiation of the combination of the two molecules was more significant. Consistently, more newly formed bone can be observed in the rat bone defect model after 8 weeks implantation of the dual small molecule-loaded 3D scaffold (Zhang et al., 2020a). Challenges still remained in the clinical translation of the implantation of the stem cell-laden 3D scaffold because of the avascular structure, especially in the large osteochondral defect. To address this problem, Daly et al. (2018) developed a 3D-printed BM-MSC-laden GelMA hydrogel that guided vascularization during endochondral bone repair, revealing that this BM-MSC-laden 3D scaffold could not only facilitate vascular network formation but also promote bone formation.
4.2 AD-MSCs
Adipose derived mesenchymal stem cells (AD-MSCs) are isolated from adipose tissue, consequently the abundant source and the large amount of the stem cells as well as the suitable biological characteristics greatly broaden the clinical application (Strioga et al., 2012; Wang and Liu, 2018). And it is known that AD-MSCs have been widely used in bioprinting organs and tissues as a bioink by virtue of the multilineage differentiation (Wang and Liu, 2018). Swathi et al. developed a bioink of modified HA-based hydrogel with AD-MSCs encapsulated, and printed a 3D scaffold with this bioink. MTT assay was used to assess the cell viability for 1-, 4-, and 7-day culturing, observing an increase of the AD-MSC viability laden on the scaffold compared to the non-3D-printed group. However, the expression of the chondrogenic marker gene (SOX-9, aggrecan, Col I, Col II) significantly increased at day 5 and sGAG enlarged from days 7–14 in the AD-MSCs of the scaffold, revealing the potential chondrogenic differentiation of AD-MSCs (Nedunchezian et al., 2021).
As we all know, hydrogel is usually physically or chemically cross-linked to achieve a stable structure to sustain the proliferation and differentiation of the stem cells. It must be admitted that the impair of the improper cross-linking is really lethal to the cell viability. Lee et al. proposed an alginate-based hydrogel encapsulated with AD-MSCs, and cross-linked via UV exposure with the dose (0–6.0 J/cm2); however, the higher dose (2.4 J/cm2) greatly suppressed the cell viability with a dose-dependent effect, while the dose of UV less than 2.4 J/cm2 exhibited high cell viability (90%) (Lee et al., 2020b). In vivo, the AD-MSC-laden gelatin–alginate-based 3D-printed scaffold was implanted into the dorsal area of the BALB/c nude mice subcutaneously for 8 weeks, and the results of RT-PCR, immunofluorescent staining and western blotting showed the significant increase in the osteogenic gene (OSX, RUNX 2, and OCN), demonstrating obvious bone formation (Wang et al., 2016). Though proliferation and differentiation of the AD-MSCs laden in 3D-printed hydrogel were demonstrated, they cannot last long, with a peak at day 7 and then decreased gradually during a 16-day observation (Narayanan et al., 2016).
4.3 Dental Pulp Stem Cells
Human dental pulp stem cells (hDPSCs) are generally isolated from the teeth that are extracted by the dentist, and demonstrate great promise in BTE because of the low-cost and easy accessibility (Fernandes et al., 2020). Buyuksungur et al. (2021) bioprinted a 3D scaffold via PCL and GelMA that carries hDPSCs, and then the stable composite 3D structure exhibited excellent cell viability during a 21-day dynamic test of live/dead cell assay and ideal osteogenic differentiation as well as mineralization validated by immunofluorescent staining of OPN, OCN, and alizarin red staining, respectively. To induce the osteogenic differentiation of hDPSCs in vivo, amorphous magnesium phosphates (AMPs) was doped in the ECM-based hydrogel, observing high cell viability, mineralization, and osteogenic markers in the absence of growth factors in vitro and significant increase in bone formation and bone density at bone defect of the rats skull at 4 and 8 weeks, tested by micro-CT, H&E staining, Masson’s trichrome staining (MTS), paving the way for clinical translation of the hDPSCs-laden hydrogel-based 3D scaffold (Dubey et al., 2020). Furthermore, the additive growth factor such as BMP-2 may be another strategy to induce the differentiation of hDPSCs-laden in the scaffold. Park et al. tethered a novel BMP peptide to GelMA-based hydrogel, and printed a scaffold with this bioink. Though nearly half of the conjugated BMP peptide missed after 3 weeks culture, the BMP peptide-tethering scaffold exhibited much more calcification than the non-BMP peptide scaffold group, suggesting a promising approach to induce differentiation of stem cells laden on the 3D structure (Park et al., 2020).
4.4 Umbilical Vein Endothelial Cells
Human umbilical vein endothelial cells (hUVECs) are a kind of endothelial cells isolated from human umbilical cord veins after the child’s birth (Lei et al., 2016). It is reported that HUVECs expressed many endothelial biomarkers related to the vascular homeostasis, thereby HUVECs were applied into angiogenesis as well as vascularization of tissue engineering, such as bone tissue (Kocherova et al., 2019). HUVECs are the most common endothelial cells explored as a biomaterial and have been successfully differentiated into 3D structure alone or cocultured with other cells (Bersini et al., 2016; Kocherova et al., 2019). Chen et al. (2018) fabricated a hybrid scaffold via HUVECs-laden alginate–gelatin hydrogel and polydopamine-modified calcium silicate (PDACS)/PCL, finding a high viability, increased proliferation as well as angiogenesis and osteogenesis of HUVECs encapsulated on the scaffold. In fact, it is the angiogenesis of hUVECs that could couple with the osteogenesis of other kind of stem cells rather than the direct osteogenic differentiation of hUVECs made it applied in bone tissue engineering. For example, to fabricate a complex bone construct with vasculature that mimic the large-scale bone tissue, Byambaa et al. (2017) cocultured hUVEC and hBM-MSCs with a ratio of 2:1 in the GelMA-based hydrogel before bioprinting, then formed a capillary-like network inside the printed construct rely on the synergistic interactions of the cocultured hUVEC and hBM-MSCs in the system, leading to a hopeful approach for the treatment of vascularized bone tissue regeneration.
5 Growth Factors/Peptides Promote Osteogenesis and Chondrogenesis of Stem Cells That Laden on the 3D-Printed Hydrogel
5.1 Mechanisms of Growth Factors on Osteogenesis and Chondrogenesis
In bone remodeling sites, MSCs renew and proliferate, and then are committed to adipogenesis, chondrogenesis, and/or osteogenesis. Osteogenesis occurs in the intramembranous and endochondral ossification pathway (Berendsen and Olsen, 2015), and chondrogenesis precedes endochondral ossification (Figure 3). MSCs aggregate and form mesenchymal condensations through the interaction with surrounding epithelial cells and ECM components like syndecan, tenascin, fibronectin, and versican (Choocheep et al., 2010). Neural cell adhesion molecule (N-CAM) and neural cadherin (N-cadherin) are required for mesenchymal condensations and chondrogenesis (Delise and Tuan, 2002). Growth factors also stimulate condensation and overt chondrogenic differentiation, including the tumor growth factor-beta (TGF-β) subfamily (Jin et al., 2010), fibroblast growth factor 2 (FGF-2) (Zhang et al., 2020b), and bone morphogenetic proteins (BMPs) (Jin et al., 2006). Chondrocytes proliferate and form a perichondrium surrounding condensations, secret type 2a1 collagen, and the aggrecan-rich matrix to enlarge the cartilage before hypertrophy and terminal differentiation, and express specific genetic program controlled by the transcription factors SRY box transcription factor 9 (SOX9) and runt-related transcription factor 2 (RUNX2). SOX9 is required in chondrocyte differentiation, and the deficiency of SOX9 exhibits severe chondrodysplasia (Akiyama et al., 2002). RUNX2 expressed in pre-hypertrophic chondrocytes promotes chondrocyte hypertrophy; however, highly-expressed RUNX2 in perichondrial cells exerts antagonistic function on chondrocyte proliferation and hypertrophy(182). Additionally, homeodomain (HOX) transcription factors (BARX2, NKX3-2, MSX1, MSX2, PAX1, and PAX9) (Hinoi et al., 2006), TGF subfamily (Pizette and Niswander, 2000), insulin-like growth factors (IGFs) (Oh and Chun, 2003), and FGF (Danišovič et al., 2012) are also crucial for chondrogenesis. Differentiated chondrocytes either proliferate in cartilage elements or exhibit hypertrophic maturation for subsequent endochondral ossification. Articular chondrocytes secrete aggrecan and lubricin for joint smoothness and low level of collagen 2 with the expression of SOX9, SOX5, and SOX6 but the switch off of RUNX2 expression (Lefebvre and Smits, 2005). Hypertrophic chondrocytes mineralize the surrounding matrix, secrete the vascular endothelial growth factor (VEGF) and other factors to attract vessels and chondroclasts, and induce the transforming of perichondrial cells to osteoblasts, which is regulated by a series of cell and stage-specific molecular pathways, called endochondral ossification. Osteogenic molecular pathways regulate chondrocyte pre-hypertrophy, including the Indian hedgehog-the parathyroid hormone-related peptide (Ihh–PTHrP) axis, BMPs, Wnt-β-catenin canonical pathway, and MAPK signaling pathway (Deschaseaux et al., 2009). Mechanically, Ihh, PTHrP, and BMPs synergistically promote osteoblastic differentiation through inducing RUNX2 and downstream of RUNX2-Osterix (Krishnan et al., 2001). Conversely, PTHrP suppresses hypertrophic maturation to control bone formation in a negative feedback manner. The Wnt pathway stimulates proliferation, promotes osteoblast differentiation, and inhibits adipogenesis or chondrogenesis potential. BMPs and growth factors like the epidermal growth factor (EGF) and FGF can trigger the MAPK pathway that facilitates osteoblast maturation by phosphorylating the osteoblast-specific transcription factors and enhancing the function of RUNX2 and Dlx5 (Ge et al., 2007; Ulsamer et al., 2008). Notably, SOX9 serves as a negative regulator of chondrocyte hypertrophy (Akiyama et al., 2004), and RUNX2 emerges as a key effector of hypertrophic maturation (Dong et al., 2006). Finally, chondrocytes undergo terminal differentiation after the hypertrophy stage under the facilitation of activating the transcription factor 3 (ATF3) (James et al., 2006), RUNX2, and c-Maf (MacLean et al., 2003), and increase the expression of matrix metalloproteinase 13 (MMP13), osteopontin (spp1), and alkaline phosphate (Adams and Shapiro, 2002).
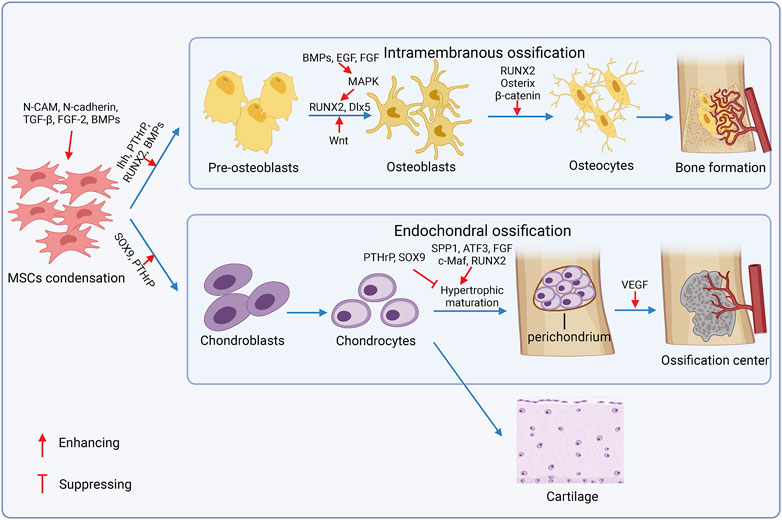
FIGURE 3. Mechanisms of osteogenesis and chondrogenesis. In bone remodeling sites, MSCs aggregate and form mesenchymal condensations. Then, bone is formed in two ways: intramembranous ossification and endochondral ossification. During the intramembranous ossification process, MSCs are differentiated into pre-osteoblasts, then they lost proliferation capacity and mature into osteoblasts, which secret alkaline phosphatase and osteocalcin that participate in the secretion, maturation, and mineralization of the extracellular matrix (ECM). In endochondral ossification, differentiated chondrocytes either proliferate in cartilage elements or exhibit hypertrophic maturation for subsequent endochondral ossification.
During the intramembranous ossification process, MSCs are differentiated into pre-osteoblasts, then they lost proliferation capacity and are matured into osteoblasts, in which secret alkaline phosphatase and osteocalcin participate in the secretion, maturation, and mineralization of ECM, under the mutual exclusive and fine-tuned control of transcription factors (Chen et al., 2016; Infante and Rodríguez, 2018). Eventually, osteoblasts either become osteocytes or inactive bone-lining cells (BLCs), or die by apoptosis. The transcription factors, such as RUNX2, osterix, SP7, and β-catenin, have played a key role in the osteogenesis (Nakashima et al., 2002; Komori, 2006). RUNX2 guides lineage commitment of MSCs to osteogenesis instead of adipogenesis and chondrogenesis. RUNX2, osterix, and β-catenin regulate osteoblast terminal differentiation (Komori, 2006). Moreover, the MAF bZIP transcription factor (MAF) was upregulated in osteogenic MSCs. MAF promotes osteogenesis synergized with RUNX2 and suppresses adipogenesis by restraining peroxisome proliferator activated receptor gamma (PPARγ) that directs adipogenic lineage commitment of MSCs (Nishikawa et al., 2010). The core-binding factor subunit beta (CBFβ) forms a complex with RUNX2 to activate the Wnt/β-catenin pathway and repress adipogenesis-related gene expression, as a result, maintaining osteogenesis and suppressing adipogenesis (Nishikawa et al., 2010). Moreover, forkhead box P1 (FOXP1) interacts with CCAAT enhancer binding protein beta (CEBPβ) required for adipogenesis, and represses the activation of the NOTCH pathway to promote biased osteogenesis and inhibit adipogenesis (Li et al., 2017b).
5.2 Effect of Growth Factors/Peptides on Osteogenesis and Chondrogenesis of Stem Cells Laden on the 3D-Printed Hydrogel
As aforementioned, some growth factors/peptides were mixed into the hydrogel to induce the osteogenesis and chondrogenesis of 3D scaffold-loaded stem cells. As the classical growth factors are widely used in bone tissue engineering, BMPs attract great focus and have been proven by the Food and Drug Administration (FDA). Poldervaart et al. (2013). designed a BMP-2 sustained release system loaded in the stem cell-laden hydrogel-based 3D construct, and the continuous release of BMP was detected in a high level during 3 weeks culture while the increased osteogenesis and bone formation were observed in the mice and rat models. In addition, assembling BMP-2 into the scaffold is another strategy to maintain the sustained release. Du et al. (2015) assembled a kind of collagen-binding domain-bone morphogenetic protein 2 (CBD BMP-2)-collagen microfibers, and this hydrogel mixed with the BM-MSCs were printed as the bioink. Similarly, stable release of BMP was observed under a confocal microscope, and osteogenic differentiation of BM-MSCs was more effective. BMP-7, a member of the BMP family that could regulate the early staged signals of osteogenesis and bone formation, was immobilized on the 3D-printed hydrogel, and greatly promoted osteogenic differentiation via the activation of SMAD (Lee et al., 2018). To address the issue of fast degradation of the BMPs, Park et al. (2020) developed a synthetic BMP-2 mimetic peptide and tethered it into hydrogel before bioprinting, which showed robust acceleration of the hDPSC osteogenic differentiation. For cartilage regeneration, TGF-β1 was tethered to a HA-based bioink to induce the chondrogenic differentiation of BM-MSCs, showing increased chondrogenic gene expression, ECM deposition, and activated early TGF-β1 signaling (Hauptstein et al., 2022). In a rabbit osteochondral cartilage defect model, an interleukin-4 (IL-4)-loaded hydrogel-based 3D-printed scaffold was implanted, with 8- and 16-week observation, and the IL-4-loaded scaffold significantly promoted the formation of neocartilage and neobone tissues according to the assessment of safranin-O, Col II immunohistochemical staining, and micro-CT (Gong et al., 2020).
6 Conclusion and Perspective
3D bioprinting refers to a promising and an innovative technique that can be used to fabricate the 3D scaffold with the bioink composed of cells and materials and demonstrates great potential in creating complex tissue constructs (Jeon et al., 2022). Hydrogels are deemed ideal and appealing materials with regard to its controllable biological and biophysical properties to construct a desirable 3D-bioprinted ECM for the attachment, proliferation, migration, and differentiation of the cells loaded in (Gao et al., 2019). Diverse kinds of cells have been implied in bone and cartilage tissue engineering while chondrocytes and stem cells are the most potential cell types among them. Although chondrocytes are the intrinsic cells of the cartilage and could repair the osteochondral damages, the limited supply, long expansion time, and the possibility of differentiation into fibroblast restrict the application of them. On the other hand, the abundant source, the easy isolation procedure, the large quantity of the stem cells, and the suitable biological characteristics including the adjustable differentiation into osteoblasts and chondrocytes greatly broaden the clinical prospect (Xu et al., 2020). Meanwhile, the advances of the stem cell therapy in the regenerative medicine stimulated the progress of the stem cell-laden hydrogel as the bioink of 3D bioprinting. As a result, the 3D bioprinting technique and hydrogels combine with stem cells that could better meet the demand of the clinical requirement and have significantly facilitated the regeneration of bone and cartilage tissue engineering over the past decade (Ni et al., 2020; Buyuksungur et al., 2021; Ge et al., 2021; Huang et al., 2021; Nedunchezian et al., 2021; Jeon et al., 2022).
Stem cell-laden hydrogel-based 3D bioprinting has provided new opportunities for bone and cartilage tissue repair. Backbone of this system consists of biomaterials, stem cells, bioprinting methods, and their interactive microenvironment. Compared with conventional 2D tissue engineering, 3D bioprinting optimizes the structural and living conditions of stem cells. Over the past decades, emerging research studies have focused on the advancement of this technique regarding to its core elements to make it a substitute or better alternative over autologous bone and cartilage tissue. However, current strategies are still of limitations.
Constructing biomaterial with suitable biochemical and functional properties (such as printability, biocompatibility, biodegradability, and maintenance of cell viability) remains challenging (Figure 4). Some features of natural biomaterials may hinder their ideal usage in 3D bioprinting. For example, the natural alginate shows superior biocompatibility and biodegradability but lacks sufficient mechanical strength (Jang et al., 2018). Hybrid materials may help to overcome such limitation by producing more versatile items. For example, alginate hydrogel could maintain long-term strength after cross-linking with Ca2+, while gelatin could stabilize the 3D structure at the primary stage; hence, alginate–gelatin-mixed hydrogel has been extensively researched in tissue engineering because of the ideal biocompatibility and the improved mechanical property (Axpe and Oyen, 2016; Chawla et al., 2020). Meanwhile, adding Col I to agarose significantly increases cell spreading and differentiation (Duarte Campos et al., 2016). However, this is not the ultimate solution. Though combination of two kinds of hydrogel could make up the shortcoming, they are still far away from the requirement of the product. For instance, low-density alginate/gelatin (0.8% alginate)-mixed hydrogel-based scaffold demonstrates great priority in facilitating mineralization, maintaining cell viability, and inducing osteogenic differentiation of BM-MSCs, but the lack of mechanical property, shape fidelity, and printability is inevitable. On the contrary, the high-density alginate/gelatin (1.8% alginate) based-3D printing exhibits improved mechanical features and printability, but largely impairs the cell viability (Zhang et al., 2020c).
A further challenge is to establish appropriate interaction between materials and the cellular component. 3D bioprinting provides space for cell encapsulation and deposition, but lacks the control of biological and biomechanical heterogeneity. Thus, the microenvironment may be inappropriate for cell growth and differentiation into desired cartilage or bone tissue. Solutions for this limitation are to create concentration gradients using 3D bioprinting, for example, the mixing nozzles that can print materials with tunable gradients (Ober et al., 2015). In some cases, the printing patterns are more complicated, such as vascularized bone compositing with the hard mineral structure and the soft organic matrix. Cui et al. have developed a dual-3D bioprinting platform consisting with a fused deposition modeling 3D bioprinter and a stereolithography 3D bioprinter. With the addition of regional immobilization of bioactive factors, the printed system support controlled and provided continuous stimulus for vascularized bone regeneration (Cui et al., 2016). Notably, such interaction may vary in different individuals, influenced by the local destructional area, inflammatory factors, oxygen, etc. Therefore, in addition to the improvement of biomaterials, we should also focus on the interaction between biomaterials and cellular components, especially the in vivo model.
Selection and production of appropriate stem cell is another challenge for stem cell-laden hydrogel-based 3D bioprinting. Bone and cartilage are mesodermal-derived tissue and thus the multipotent stem cell populations are important source for their biofabrication. Stem cell has multiple differentiation potential and immune advantages over adult cells, but it demands regenerative ability to the ideal phenotype. It has been noticed that MSCs tend to display a hypertrophic phenotype that leads to endochondral bone formation (Eslaminejad and Malakooty Poor, 2014). Thus, the control of regenerative tendency is demanding. Otherwise, different types of stem cell exhibit dissimilar propensity of differentiation in certain scaffold. For instance, BM-MSCs tend to differentiate into chondrogenic cells and osteogenic cells in gelatin while AD-MSCs prone to osteogenesis. Notably, stem cell expansion in vitro is complicated. Cell senescence is inevitable following multiple expansion rounds (De Coppi et al., 2007). As a cell candidate, iPSC has shown greater accessibility and expandability. However, the risk of tumorigenicity and mutations has limited its clinical application (Lee et al., 2013; Simonson et al., 2015). The attempt to establish an iPSC bank may help to overcome this issue. Meanwhile, novel protocols for primary cell isolation and expansion are highly requested to support cell sourcing.
In addition, evolution in printing technique advances the bioprinting. Three-dimensional bioprinting produces scaffold that provides spatial environment closer to human tissue for stem cells. However, the current 3D bioprinting cannot meet the diverse needs simultaneously because the scaffold is fixable upon designing and printing. In 2014, Skylar Tibbits demonstrated the 4D printing which counted time as a new dimension (Wan et al., 2020). By adopting stimuli-responsive materials, 4D printing scaffold is flexible and able to change their configuration in response to different stimuli. Its usage in cell-laden bioprinting substantially improves the adjustment and function of implant. We propose that 4D bioprinting is an upcoming trend for bone and cartilage tissue engineering. Despite the high cost of stimuli-responsive and programmable biomaterials, stem cell-laden hydrogel-based 4D bioprinting are opening new avenue for bone and cartilage repair.
Author Contributions
ZY: writing—original draft and editing. PY: writing—original draft. ZL: writing—review and editing, and visualization. WZ, LM, and CF: review and editing. CT: writing—review and editing, supervision, and funding acquisition. ZL: writing—review and editing, supervision, and funding acquisition. All authors contributed to the writing and revision of the manuscript, knew the content of it, and approved its submission.
Funding
This work was supported by the National Natural Science Foundation of China under [Grant Nos. 81902745 and 82172500].
Conflict of Interest
The authors declare that the research was conducted in the absence of any commercial or financial relationships that could be construed as a potential conflict of interest.
Publisher’s Note
All claims expressed in this article are solely those of the authors and do not necessarily represent those of their affiliated organizations, or those of the publisher, the editors, and the reviewers. Any product that may be evaluated in this article, or claim that may be made by its manufacturer, is not guaranteed or endorsed by the publisher.
References
Adams, C. S., and Shapiro, I. M. (2002). The Fate of the Terminally Differentiated Chondrocyte: Evidence for Microenvironmental Regulation of Chondrocyte Apoptosis. Crit. Rev. Oral Biol. Med. 13 (6), 465–473. doi:10.1177/154411130201300604
Ahmad Raus, R., Wan Nawawi, W. M. F., and Nasaruddin, R. R. (2020). Alginate and Alginate Composites for Biomedical Applications. Asian J. Pharm. Sci. 16 (3), 280–306. doi:10.1016/j.ajps.2020.10.001
Ahmed, E. M. (2015). Hydrogel: Preparation, Characterization, and Applications: A Review. J. Adv. Res. 6 (2), 105–121. doi:10.1016/j.jare.2013.07.006
Akiyama, H., Chaboissier, M.-C., Martin, J. F., Schedl, A., and de Crombrugghe, B. (2002). The Transcription Factor Sox9 Has Essential Roles in Successive Steps of the Chondrocyte Differentiation Pathway and Is Required for Expression of Sox5 and Sox6. Genes Dev. 16 (21), 2813–2828. doi:10.1101/gad.1017802
Akiyama, H., Lyons, J. P., Mori-Akiyama, Y., Yang, X., Zhang, R., Zhang, Z., et al. (2004). Interactions between Sox9 and β-catenin Control Chondrocyte Differentiation. Genes Dev. 18 (9), 1072–1087. doi:10.1101/gad.1171104
Antich, C., de Vicente, J., Jiménez, G., Chocarro, C., Carrillo, E., Montañez, E., et al. (2020). Bio-inspired Hydrogel Composed of Hyaluronic Acid and Alginate as a Potential Bioink for 3D Bioprinting of Articular Cartilage Engineering Constructs. Acta Biomater. 106, 114–123. doi:10.1016/j.actbio.2020.01.046
Apelgren, P., Amoroso, M., Lindahl, A., Brantsing, C., Rotter, N., Gatenholm, P., et al. (2017). Chondrocytes and Stem Cells in 3D-Bioprinted Structures Create Human Cartilage In Vivo. PLoS One 12 (12), e0189428. doi:10.1371/journal.pone.0189428
Arya, N., Forget, A., Sarem, M., and Shastri, V. P. (2019). RGDSP Functionalized Carboxylated Agarose as Extrudable Carriers for Chondrocyte Delivery. Mater. Sci. Eng. C 99, 103–111. doi:10.1016/j.msec.2019.01.080
Ashammakhi, N., Hasan, A., Kaarela, O., Byambaa, B., Sheikhi, A., Gaharwar, A. K., et al. (2019). Advancing Frontiers in Bone Bioprinting. Adv. Healthc. Mat. 8 (7), 1801048. doi:10.1002/adhm.201801048
Askari, M., Afzali Naniz, M., Kouhi, M., Saberi, A., Zolfagharian, A., and Bodaghi, M. (2021). Recent Progress in Extrusion 3D Bioprinting of Hydrogel Biomaterials for Tissue Regeneration: a Comprehensive Review with Focus on Advanced Fabrication Techniques. Biomater. Sci. 9 (3), 535–573. doi:10.1039/d0bm00973c
Axpe, E., and Oyen, M. (2016). Applications of Alginate-Based Bioinks in 3D Bioprinting. Ijms 17 (12), 1976. doi:10.3390/ijms17121976
Barrs, R. W., Jia, J., Silver, S. E., Yost, M., and Mei, Y. (2020). Biomaterials for Bioprinting Microvasculature. Chem. Rev. 120 (19), 10887–10949. doi:10.1021/acs.chemrev.0c00027
Bello, A. B., Kim, D., Kim, D., Park, H., and Lee, S.-H. (2020). Engineering and Functionalization of Gelatin Biomaterials: From Cell Culture to Medical Applications. Tissue Eng. Part B Rev. 26 (2), 164–180. doi:10.1089/ten.TEB.2019.0256
Berendsen, A. D., and Olsen, B. R. (2015). Bone Development. Bone 80, 14–18. doi:10.1016/j.bone.2015.04.035
Bersini, S., Gilardi, M., Arrigoni, C., Talò, G., Zamai, M., Zagra, L., et al. (2016). Human In Vitro 3D Co-culture Model to Engineer Vascularized Bone-Mimicking Tissues Combining Computational Tools and Statistical Experimental Approach. Biomaterials 76, 157–172. doi:10.1016/j.biomaterials.2015.10.057
Bhardwaj, N., Chakraborty, S., and Kundu, S. C. (2011). Freeze-gelled Silk Fibroin Protein Scaffolds for Potential Applications in Soft Tissue Engineering. Int. J. Biol. Macromol. 49 (3), 260–267. doi:10.1016/j.ijbiomac.2011.04.013
Buitrago, J. O., Patel, K. D., El-Fiqi, A., Lee, J.-H., Kundu, B., Lee, H.-H., et al. (2018). Silk Fibroin/collagen Protein Hybrid Cell-Encapsulating Hydrogels with Tunable Gelation and Improved Physical and Biological Properties. Acta Biomater. 69, 218–233. doi:10.1016/j.actbio.2017.12.026
Buyuksungur, S., Hasirci, V., and Hasirci, N. (2021). 3D Printed Hybrid Bone Constructs of PCL and Dental Pulp Stem Cells Loaded GelMA. J. Biomed. Mater Res. 109 (12), 2425–2437. doi:10.1002/jbm.a.37235
Byambaa, B., Annabi, N., Yue, K., Trujillo‐de Santiago, G., Alvarez, M. M., Jia, W., et al. (2017). Bioprinted Osteogenic and Vasculogenic Patterns for Engineering 3D Bone Tissue. Adv. Healthc. Mat. 6 (16), 1700015. doi:10.1002/adhm.201700015
Chawla, D., Kaur, T., Joshi, A., and Singh, N. (2020). 3D Bioprinted Alginate-Gelatin Based Scaffolds for Soft Tissue Engineering. Int. J. Biol. Macromol. 144, 560–567. doi:10.1016/j.ijbiomac.2019.12.127
Chen, Q., Shou, P., Zheng, C., Jiang, M., Cao, G., Yang, Q., et al. (2016). Fate Decision of Mesenchymal Stem Cells: Adipocytes or Osteoblasts? Cell Death Differ. 23 (7), 1128–1139. doi:10.1038/cdd.2015.168
Chen, Y.-W., Shen, Y.-F., Ho, C.-C., Yu, J., Wu, Y.-H. A., Wang, K., et al. (2018). Osteogenic and Angiogenic Potentials of the Cell-Laden Hydrogel/mussel-Inspired Calcium Silicate Complex Hierarchical Porous Scaffold Fabricated by 3D Bioprinting. Mater. Sci. Eng. C 91, 679–687. doi:10.1016/j.msec.2018.06.005
Chiesa, I., Ligorio, C., Bonatti, A. F., De Acutis, A., Smith, A. M., Saiani, A., et al. (2020). Modeling the Three-Dimensional Bioprinting Process of β-Sheet Self-Assembling Peptide Hydrogel Scaffolds. Front. Med. Technol. 2, 571626. doi:10.3389/fmedt.2020.571626
Choe, G., Oh, S., Seok, J. M., Park, S. A., and Lee, J. Y. (2019). Graphene Oxide/alginate Composites as Novel Bioinks for Three-Dimensional Mesenchymal Stem Cell Printing and Bone Regeneration Applications. Nanoscale 11 (48), 23275–23285. doi:10.1039/c9nr07643c
Choocheep, K., Hatano, S., Takagi, H., Watanabe, H., Kimata, K., Kongtawelert, P., et al. (2010). Versican Facilitates Chondrocyte Differentiation and Regulates Joint Morphogenesis. J. Biol. Chem. 285 (27), 21114–21125. doi:10.1074/jbc.M109.096479
Chu, Y., Huang, L., Hao, W., Zhao, T., Zhao, H., Yang, W., et al. (2021). Long-term Stability, High Strength, and 3D Printable Alginate Hydrogel for Cartilage Tissue Engineering Application. Biomed. Mat. 16 (6), 064102. doi:10.1088/1748-605X/ac2595
Costantini, M., Idaszek, J., Szöke, K., Jaroszewicz, J., Dentini, M., Barbetta, A., et al. (2016). 3D Bioprinting of BM-MSCs-Loaded ECM Biomimetic Hydrogels for In Vitro Neocartilage Formation. Biofabrication 8 (3), 035002. doi:10.1088/1758-5090/8/3/035002
Critchley, S., Sheehy, E. J., Cunniffe, G., Diaz-Payno, P., Carroll, S. F., Jeon, O., et al. (2020). 3D Printing of Fibre-Reinforced Cartilaginous Templates for the Regeneration of Osteochondral Defects. Acta Biomater. 113, 130–143. doi:10.1016/j.actbio.2020.05.040
Cui, H., Zhu, W., Nowicki, M., Zhou, X., Khademhosseini, A., and Zhang, L. G. (2016). Hierarchical Fabrication of Engineered Vascularized Bone Biphasic Constructs via Dual 3D Bioprinting: Integrating Regional Bioactive Factors into Architectural Design. Adv. Healthc. Mat. 5 (17), 2174–2181. doi:10.1002/adhm.201600505
Daly, A. C., Critchley, S. E., Rencsok, E. M., and Kelly, D. J. (2016). A Comparison of Different Bioinks for 3D Bioprinting of Fibrocartilage and Hyaline Cartilage. Biofabrication 8 (4), 045002. doi:10.1088/1758-5090/8/4/045002
Daly, A. C., Freeman, F. E., Gonzalez-Fernandez, T., Critchley, S. E., Nulty, J., and Kelly, D. J. (2017). 3D Bioprinting for Cartilage and Osteochondral Tissue Engineering. Adv. Healthc. Mat. 6 (22), 1700298. doi:10.1002/adhm.201700298
Daly, A. C., Pitacco, P., Nulty, J., Cunniffe, G. M., and Kelly, D. J. (2018). 3D Printed Microchannel Networks to Direct Vascularisation during Endochondral Bone Repair. Biomaterials 162, 34–46. doi:10.1016/j.biomaterials.2018.01.057
Danišovič, Ľ., Varga, I., and Polák, Š. (2012). Growth Factors and Chondrogenic Differentiation of Mesenchymal Stem Cells. Tissue Cell 44 (2), 69–73. doi:10.1016/j.tice.2011.11.005
De Coppi, P., Bartsch, G., Siddiqui, M. M., Xu, T., Santos, C. C., Perin, L., et al. (2007). Isolation of Amniotic Stem Cell Lines with Potential for Therapy. Nat. Biotechnol. 25 (1), 100–106. doi:10.1038/nbt1274
De Moor, L., Fernandez, S., Vercruysse, C., Tytgat, L., Asadian, M., De Geyter, N., et al. (2020). Hybrid Bioprinting of Chondrogenically Induced Human Mesenchymal Stem Cell Spheroids. Front. Bioeng. Biotechnol. 8, 484. doi:10.3389/fbioe.2020.00484
De Santis, M. M., Alsafadi, H. N., Tas, S., Bölükbas, D. A., Prithiviraj, S., Da Silva, I. A. N., et al. (2021). Extracellular‐Matrix‐Reinforced Bioinks for 3D Bioprinting Human Tissue. Adv. Mater. 33 (3), 2005476. doi:10.1002/adma.202005476
Delise, A. M., and Tuan, R. S. (2002). Analysis of N-Cadherin Function in Limb Mesenchymal Chondrogenesis In Vitro. Dev. Dyn. 225 (2), 195–204. doi:10.1002/dvdy.10151
Depalle, B., McGilvery, C. M., Nobakhti, S., Aldegaither, N., Shefelbine, S. J., and Porter, A. E. (2021). Osteopontin Regulates Type I Collagen Fibril Formation in Bone Tissue. Acta Biomater. 120, 194–202. doi:10.1016/j.actbio.2020.04.040
Derakhshanfar, S., Mbeleck, R., Xu, K., Zhang, X., Zhong, W., and Xing, M. (2018). 3D Bioprinting for Biomedical Devices and Tissue Engineering: A Review of Recent Trends and Advances. Bioact. Mater. 3 (2), 144–156. doi:10.1016/j.bioactmat.2017.11.008
Deschaseaux, F., Sensébé, L., and Heymann, D. (2009). Mechanisms of Bone Repair and Regeneration. Trends Mol. Med. 15 (9), 417–429. doi:10.1016/j.molmed.2009.07.002
Dong, L., Bu, Z., Xiong, Y., Zhang, H., Fang, J., Hu, H., et al. (2021). Facile Extrusion 3D Printing of Gelatine methacrylate/Laponite Nanocomposite Hydrogel with High Concentration Nanoclay for Bone Tissue Regeneration. Int. J. Biol. Macromol. 188, 72–81. doi:10.1016/j.ijbiomac.2021.07.199
Dong, Y.-F., Soung, D. Y., Schwarz, E. M., O'Keefe, R. J., and Drissi, H. (2006). Wnt Induction of Chondrocyte Hypertrophy through the Runx2 Transcription Factor. J. Cell. Physiol. 208 (1), 77–86. doi:10.1002/jcp.20656
Du, M., Chen, B., Meng, Q., Liu, S., Zheng, X., Zhang, C., et al. (2015). 3D Bioprinting of BMSC-Laden Methacrylamide Gelatin Scaffolds with CBD-BMP2-Collagen Microfibers. Biofabrication 7 (4), 044104. doi:10.1088/1758-5090/7/4/044104
Duarte Campos, D. F., Blaeser, A., Buellesbach, K., Sen, K. S., Xun, W., Tillmann, W., et al. (2016). Bioprinting Organotypic Hydrogels with Improved Mesenchymal Stem Cell Remodeling and Mineralization Properties for Bone Tissue Engineering. Adv. Healthc. Mat. 5 (11), 1336–1345. doi:10.1002/adhm.201501033
Duarte Campos, D. F., Blaeser, A., Weber, M., Jäkel, J., Neuss, S., Jahnen-Dechent, W., et al. (2013). Three-dimensional Printing of Stem Cell-Laden Hydrogels Submerged in a Hydrophobic High-Density Fluid. Biofabrication 5 (1), 015003. doi:10.1088/1758-5082/5/1/015003
Dubey, N., Ferreira, J. A., Malda, J., Bhaduri, S. B., and Bottino, M. C. (2020). Extracellular Matrix/Amorphous Magnesium Phosphate Bioink for 3D Bioprinting of Craniomaxillofacial Bone Tissue. ACS Appl. Mat. Interfaces 12 (21), 23752–23763. doi:10.1021/acsami.0c05311
Echave, M. C., Burgo, L. S., Pedraz, J. L., and Orive, G. (2017). Gelatin as Biomaterial for Tissue Engineering. Cpd 23 (24), 3567–3584. doi:10.2174/0929867324666170511123101
Eslaminejad, M. B., and Malakooty Poor, E. (2014). Mesenchymal Stem Cells as a Potent Cell Source for Articular Cartilage Regeneration. Wjsc 6 (3), 344–354. doi:10.4252/wjsc.v6.i3.344
Eyre, D. R. (2004). Collagens and Cartilage Matrix Homeostasis. Clin. Orthop. Relat. Res. 427 (427 Suppl. l), S118–S122. doi:10.1097/01.blo.0000144855.48640.b9
Fahimipour, F., Dashtimoghadam, E., Mahdi Hasani-Sadrabadi, M., Vargas, J., Vashaee, D., Lobner, D. C., et al. (2019). Enhancing Cell Seeding and Osteogenesis of MSCs on 3D Printed Scaffolds through Injectable BMP2 Immobilized ECM-Mimetic Gel. Dent. Mater. 35 (7), 990–1006. doi:10.1016/j.dental.2019.04.004
Fedorovich, N. E., Wijnberg, H. M., Dhert, W. J. A., and Alblas, J. (2011). Distinct Tissue Formation by Heterogeneous Printing of Osteo- and Endothelial Progenitor Cells. Tissue Eng. Part A 17 (15-16), 2113–2121. doi:10.1089/ten.TEA.2011.0019
Fernandes, T. L., Cortez de SantAnna, J. P., Frisene, I., Gazarini, J. P., Gomes Pinheiro, C. C., Gomoll, A. H., et al. (2020). Systematic Review of Human Dental Pulp Stem Cells for Cartilage Regeneration. Tissue Eng. Part B Rev. 26 (1), 1–12. doi:10.1089/ten.TEB.2019.0140
Forget, A., Blaeser, A., Miessmer, F., Köpf, M., Campos, D. F. D., Voelcker, N. H., et al. (2017). Mechanically Tunable Bioink for 3D Bioprinting of Human Cells. Adv. Healthc. Mat. 6 (20), 1700255. doi:10.1002/adhm.201700255
Fraser, J. R. E., Laurent, T. C., and Laurent, U. B. G. (1997). Hyaluronan: its Nature, Distribution, Functions and Turnover. J. Intern Med. 242 (1), 27–33. doi:10.1046/j.1365-2796.1997.00170.x
Fryhofer, G. W., Zlotnick, H. M., Stoeckl, B. D., Farrell, M. J., Steinberg, D. R., and Mauck, R. L. (2021). Fabrication and Maturation of Integrated Biphasic Anatomic Mesenchymal Stromal Cell‐laden Composite Scaffolds for Osteochondral Repair and Joint Resurfacing. J. Orthop. Res. 39, 2323–2332. doi:10.1002/jor.24969
Gage, J., Liporace, A., Egol, A., and McLaurin, M. (20132018). Management of Bone Defects in Orthopedic Trauma. Bull. Hosp. Jt. Dis. (2013) 76 (1), 4–8.
Gaharwar, A. K., Peppas, N. A., and Khademhosseini, A. (2014). Nanocomposite Hydrogels for Biomedical Applications. Biotechnol. Bioeng. 111 (3), 441–453. doi:10.1002/bit.25160
Gao, F., Xu, Z., Liang, Q., Li, H., Peng, L., Wu, M., et al. (2019). Osteochondral Regeneration with 3D‐Printed Biodegradable High‐Strength Supramolecular Polymer Reinforced‐Gelatin Hydrogel Scaffolds. Adv. Sci. 6 (15), 1900867. doi:10.1002/advs.201900867
Ge, C., Xiao, G., Jiang, D., and Franceschi, R. T. (2007). Critical Role of the Extracellular Signal-Regulated Kinase-MAPK Pathway in Osteoblast Differentiation and Skeletal Development. J. cell Biol. 176 (5), 709–718. doi:10.1083/jcb.200610046
Ge, Y., Li, Y., Wang, Z., Li, L., Teng, H., and Jiang, Q. (2021). Effects of Mechanical Compression on Chondrogenesis of Human Synovium-Derived Mesenchymal Stem Cells in Agarose Hydrogel. Front. Bioeng. Biotechnol. 9, 697281. doi:10.3389/fbioe.2021.697281
Gehlen, J., Qiu, W., Müller, R., and Qin, X.-H. (2021). Volumetric Tomographic 3D Bioprinting of Heterocellular Bone-like Tissues in Seconds. bioRxiv. doi:10.1101/2021.11.14.468504
Gjorevski, N., Sachs, N., Manfrin, A., Giger, S., Bragina, M. E., Ordóñez-Morán, P., et al. (2016). Designer Matrices for Intestinal Stem Cell and Organoid Culture. Nature 539 (7630), 560–564. doi:10.1038/nature20168
Gong, L., Li, J., Zhang, J., Pan, Z., Liu, Y., Zhou, F., et al. (2020). An Interleukin-4-Loaded Bi-layer 3D Printed Scaffold Promotes Osteochondral Regeneration. Acta Biomater. 117, 246–260. doi:10.1016/j.actbio.2020.09.039
Grasman, J. M., Zayas, M. J., Page, R. L., and Pins, G. D. (2015). Biomimetic Scaffolds for Regeneration of Volumetric Muscle Loss in Skeletal Muscle Injuries. Acta Biomater. 25, 2–15. doi:10.1016/j.actbio.2015.07.038
Gudapati, H., Dey, M., and Ozbolat, I. (2016). A Comprehensive Review on Droplet-Based Bioprinting: Past, Present and Future. Biomaterials 102, 20–42. doi:10.1016/j.biomaterials.2016.06.012
Guo, J. L., Li, A., Kim, Y. S., Xie, V. Y., Smith, B. T., Watson, E., et al. (2020). Click Functionalized, Tissue‐specific Hydrogels for Osteochondral Tissue Engineering. J. Biomed. Mater Res. 108 (3), 684–693. doi:10.1002/jbm.a.36848
Han, Y., Jia, B., Lian, M., Sun, B., Wu, Q., Sun, B., et al. (2021). High-precision, Gelatin-Based, Hybrid, Bilayer Scaffolds Using Melt Electro-Writing to Repair Cartilage Injury. Bioact. Mater. 6 (7), 2173–2186. doi:10.1016/j.bioactmat.2020.12.018
Hasturk, O., Jordan, K. E., Choi, J., and Kaplan, D. L. (2020). Enzymatically Crosslinked Silk and Silk-Gelatin Hydrogels with Tunable Gelation Kinetics, Mechanical Properties and Bioactivity for Cell Culture and Encapsulation. Biomaterials 232, 119720. doi:10.1016/j.biomaterials.2019.119720
Hauptstein, J., Forster, L., Nadernezhad, A., Groll, J., Teßmar, J., and Blunk, T. (2022). Tethered TGF-Β1 in a Hyaluronic Acid-Based Bioink for Bioprinting Cartilaginous Tissues. Ijms 23 (2), 924. doi:10.3390/ijms23020924
Hayashi, C., Hasegawa, U., Saita, Y., Hemmi, H., Hayata, T., Nakashima, K., et al. (2009). Osteoblastic Bone Formation Is Induced by Using Nanogel-Crosslinking Hydrogel as Novel Scaffold for Bone Growth Factor. J. Cell. Physiol. 220 (1), 1–7. doi:10.1002/jcp.21760
Helgeland, E., Rashad, A., Campodoni, E., Goksøyr, Ø., Pedersen, T. Ø., Sandri, M., et al. (2021). Dual-crosslinked 3D Printed Gelatin Scaffolds with Potential for Temporomandibular Joint Cartilage Regeneration. Biomed. Mat. 16, 035026. doi:10.1088/1748-605X/abe6d9
Hernández-González, A. C., Téllez-Jurado, L., and Rodríguez-Lorenzo, L. M. (2020). Alginate Hydrogels for Bone Tissue Engineering, from Injectables to Bioprinting: A Review. Carbohydr. Polym. 229, 115514. doi:10.1016/j.carbpol.2019.115514
Hinoi, E., Bialek, P., Chen, Y.-T., Rached, M.-T., Groner, Y., Behringer, R. R., et al. (2006). Runx2 Inhibits Chondrocyte Proliferation and Hypertrophy through its Expression in the Perichondrium. Genes Dev. 20 (21), 2937–2942. doi:10.1101/gad.1482906
Hong, H., Seo, Y. B., Kim, D. Y., Lee, J. S., Lee, Y. J., Lee, H., et al. (2020). Digital Light Processing 3D Printed Silk Fibroin Hydrogel for Cartilage Tissue Engineering. Biomaterials 232, 119679. doi:10.1016/j.biomaterials.2019.119679
Huang, H., Zhang, X., Hu, X., Shao, Z., Zhu, J., Dai, L., et al. (2014). A Functional Biphasic Biomaterial Homing Mesenchymal Stem Cells for In Vivo Cartilage Regeneration. Biomaterials 35 (36), 9608–9619. doi:10.1016/j.biomaterials.2014.08.020
Huang, J., Huang, Z., Liang, Y., Yuan, W., Bian, L., Duan, L., et al. (2021). 3D Printed Gelatin/hydroxyapatite Scaffolds for Stem Cell Chondrogenic Differentiation and Articular Cartilage Repair. Biomater. Sci. 9 (7), 2620–2630. doi:10.1039/d0bm02103b
Huang, Q., Zou, Y., Arno, M. C., Chen, S., Wang, T., Gao, J., et al. (2017). Hydrogel Scaffolds for Differentiation of Adipose-Derived Stem Cells. Chem. Soc. Rev. 46 (20), 6255–6275. doi:10.1039/c6cs00052e
Huang, Y., Seitz, D., König, F., Müller, P. E., Jansson, V., and Klar, R. M. (2019). Induction of Articular Chondrogenesis by Chitosan/Hyaluronic-Acid-Based Biomimetic Matrices Using Human Adipose-Derived Stem Cells. Ijms 20 (18), 4487. doi:10.3390/ijms20184487
Hull, M. T., and Warfel, K. A. (1986). Basal Lamina at the Epithelial-Connective Tissue Junction in the Rat Forestomach, Esophagus, Tongue and Palate: Scanning Electron Microscopic Study. Scan Electron Microsc. 1986 (Pt 4), 1395–1401.
Infante, A., and Rodríguez, C. I. (2018). Osteogenesis and Aging: Lessons from Mesenchymal Stem Cells. Stem Cell Res. Ther. 9 (1), 244. doi:10.1186/s13287-018-0995-x
Jacobsen, M. M., Li, D., Gyune Rim, N., Backman, D., Smith, M. L., and Wong, J. Y. (2017). Silk-fibronectin Protein Alloy Fibres Support Cell Adhesion and Viability as a High Strength, Matrix Fibre Analogue. Sci. Rep. 7, 45653. doi:10.1038/srep45653
Jain, A., Bansal, K. K., Tiwari, A., Rosling, A., and Rosenholm, J. M. (2019). Role of Polymers in 3D Printing Technology for Drug Delivery - an Overview. Cpd 24 (42), 4979–4990. doi:10.2174/1381612825666181226160040
James, C. G., Woods, A., Underhill, T. M., and Beier, F. (2006). The Transcription Factor ATF3 Is Upregulated during Chondrocyte Differentiation and Represses Cyclin D1 and A Gene Transcription. BMC Mol. Biol. 7, 30. doi:10.1186/1471-2199-7-30
Jang, T.-S., Jung, H.-D., Pan, H. M., Han, W. T., Chen, S., and Song, J. (2018). 3D Printing of Hydrogel Composite Systems: Recent Advances in Technology for Tissue Engineering. Int. J. Bioprint 4 (1), 126. doi:10.18063/IJB.v4i1.126
Jeon, O., Lee, Y. B., Hinton, T. J., Feinberg, A. W., and Alsberg, E. (2019). Cryopreserved Cell-Laden Alginate Microgel Bioink for 3D Bioprinting of Living Tissues. Mater. Today Chem. 12, 61–70. doi:10.1016/j.mtchem.2018.11.009
Jeon, O., Lee, Y. B., Lee, S. J., Guliyeva, N., Lee, J., and Alsberg, E. (2022). Stem Cell-Laden Hydrogel Bioink for Generation of High Resolution and Fidelity Engineered Tissues with Complex Geometries. Bioact. Mater. 15, 185–193. doi:10.1016/j.bioactmat.2021.11.025
Jiang, G., Li, S., Yu, K., He, B., Hong, J., Xu, T., et al. (2021). A 3D-Printed PRP-GelMA Hydrogel Promotes Osteochondral Regeneration through M2 Macrophage Polarization in a Rabbit Model. Acta Biomater. 128, 150–162. doi:10.1016/j.actbio.2021.04.010
Jin, E.-J., Park, K. S., Kim, D., Lee, Y.-S., Sonn, J. K., Jung, J. C., et al. (2010). TGF-β3 Inhibits Chondrogenesis by Suppressing Precartilage Condensation through Stimulation of N-Cadherin Shedding and Reduction of cRREB-1 Expression. Mol. Cells 29 (4), 425–432. doi:10.1007/s10059-010-0078-z
Jin, E. J., Lee, S. Y., Choi, Y. A., Jung, J. C., Bang, O. S., and Kang, S. S. (2006). BMP-2-enhanced Chondrogenesis Involves P38 MAPK-Mediated Down-Regulation of Wnt-7a Pathway. Mol. Cells 22 (3), 353–359.
Jonker, A. M., Löwik, D. W. P. M., and van Hest, J. C. M. (2012). Peptide- and Protein-Based Hydrogels. Chem. Mat. 24 (5), 759–773. doi:10.1021/cm202640w
Jung, J. W., Lee, J.-S., and Cho, D.-W. (2016). Computer-aided Multiple-Head 3D Printing System for Printing of Heterogeneous Organ/tissue Constructs. Sci. Rep. 6, 21685. doi:10.1038/srep21685
Kapoor, S., and Kundu, S. C. (2016). Silk Protein-Based Hydrogels: Promising Advanced Materials for Biomedical Applications. Acta Biomater. 31, 17–32. doi:10.1016/j.actbio.2015.11.034
Khetan, S., Guvendiren, M., Legant, W. R., Cohen, D. M., Chen, C. S., and Burdick, J. A. (2013). Degradation-mediated Cellular Traction Directs Stem Cell Fate in Covalently Crosslinked Three-Dimensional Hydrogels. Nat. Mater 12 (5), 458–465. doi:10.1038/nmat3586
Kilmer, C. E., Battistoni, C. M., Cox, A., Breur, G. J., Panitch, A., and Liu, J. C. (2020). Collagen Type I and II Blend Hydrogel with Autologous Mesenchymal Stem Cells as a Scaffold for Articular Cartilage Defect Repair. ACS Biomater. Sci. Eng. 6 (6), 3464–3476. doi:10.1021/acsbiomaterials.9b01939
Kim, U.-J., Park, J., Li, C., Jin, H.-J., Valluzzi, R., and Kaplan, D. L. (2004). Structure and Properties of Silk Hydrogels. Biomacromolecules 5 (3), 786–792. doi:10.1021/bm0345460
Kim, W., and Kim, G. (2019). Collagen/bioceramic-based Composite Bioink to Fabricate a Porous 3D hASCs-Laden Structure for Bone Tissue Regeneration. Biofabrication 12 (1), 015007. doi:10.1088/1758-5090/ab436d
Knowlton, S., Anand, S., Shah, T., and Tasoglu, S. (2018). Bioprinting for Neural Tissue Engineering. Trends Neurosci. 41 (1), 31–46. doi:10.1016/j.tins.2017.11.001
Kocherova, I., Bryja, A., Mozdziak, P., Angelova Volponi, A., Dyszkiewicz-Konwińska, M., Piotrowska-Kempisty, H., et al. (2019). Human Umbilical Vein Endothelial Cells (HUVECs) Co-culture with Osteogenic Cells: From Molecular Communication to Engineering Prevascularised Bone Grafts. Jcm 8 (10), 1602. doi:10.3390/jcm8101602
Komori, T. (2006). Regulation of Osteoblast Differentiation by Transcription Factors. J. Cell. Biochem. 99 (5), 1233–1239. doi:10.1002/jcb.20958
Köpf, M., Campos, D. F. D., Blaeser, A., Sen, K. S., and Fischer, H. (2016). A Tailored Three-Dimensionally Printable Agarose-Collagen Blend Allows Encapsulation, Spreading, and Attachment of Human Umbilical Artery Smooth Muscle Cells. Biofabrication 8 (2), 025011. doi:10.1088/1758-5090/8/2/025011
Krishnan, V., Ma, Y. L., Moseley, J. M., Geiser, A. G., Friant, S., and Frolik, C. A. (2001). Bone Anabolic Effects of Sonic/indian Hedgehog Are Mediated by Bmp-2/4-dependent Pathways in the Neonatal Rat Metatarsal Model. Endocrinology 142 (2), 940–947. doi:10.1210/endo.142.2.7922
Krömmelbein, C., Mütze, M., Konieczny, R., Schönherr, N., Griebel, J., Gerdes, W., et al. (2021). Impact of High-Energy Electron Irradiation on Mechanical, Structural and Chemical Properties of Agarose Hydrogels. Carbohydr. Polym. 263, 117970. doi:10.1016/j.carbpol.2021.117970
Kuzucu, M., Vera, G., Beaumont, M., Fischer, S., Wei, P., Shastri, V. P., et al. (2021). Extrusion-Based 3D Bioprinting of Gradients of Stiffness, Cell Density, and Immobilized Peptide Using Thermogelling Hydrogels. ACS Biomater. Sci. Eng. 7 (6), 2192–2197. doi:10.1021/acsbiomaterials.1c00183
Lamparelli, E. P., Lovecchio, J., Ciardulli, M. C., Giudice, V., Dale, T. P., Selleri, C., et al. (2021). Chondrogenic Commitment of Human Bone Marrow Mesenchymal Stem Cells in a Perfused Collagen Hydrogel Functionalized with hTGF-Β1-Releasing PLGA Microcarrier. Pharmaceutics 13 (3), 399. doi:10.3390/pharmaceutics13030399
Lee, A., Hudson, A. R., Shiwarski, D. J., Tashman, J. W., Hinton, T. J., Yerneni, S., et al. (2019). 3D Bioprinting of Collagen to Rebuild Components of the Human Heart. Science 365 (6452), 482–487. doi:10.1126/science.aav9051
Lee, A. S., Tang, C., Rao, M. S., Weissman, I. L., and Wu, J. C. (2013). Tumorigenicity as a Clinical Hurdle for Pluripotent Stem Cell Therapies. Nat. Med. 19 (8), 998–1004. doi:10.1038/nm.3267
Lee, H., Jang, Y., Choe, J. K., Lee, S., Song, H., Lee, J. P., et al. (2020). 3D-printed Programmable Tensegrity for Soft Robotics. Sci. Robot. 5 (45), eaay9024. doi:10.1126/scirobotics.aay9024
Lee, H. J., Kim, Y. B., Ahn, S. H., Lee, J.-S., Jang, C. H., Yoon, H., et al. (2015). A New Approach for Fabricating Collagen/ECM-Based Bioinks Using Preosteoblasts and Human Adipose Stem Cells. Adv. Healthc. Mat. 4 (9), 1359–1368. doi:10.1002/adhm.201500193
Lee, J., Hong, J., Kim, W., and Kim, G. H. (2020). Bone-derived dECM/alginate Bioink for Fabricating a 3D Cell-Laden Mesh Structure for Bone Tissue Engineering. Carbohydr. Polym. 250, 116914. doi:10.1016/j.carbpol.2020.116914
Lee, J., Lee, S.-H., Kim, B. S., Cho, Y.-S., and Park, Y. (2018). Development and Evaluation of Hyaluronic Acid-Based Hybrid Bio-Ink for Tissue Regeneration. Tissue Eng. Regen. Med. 15 (6), 761–769. doi:10.1007/s13770-018-0144-8
Lefebvre, V., and Smits, P. (2005). Transcriptional Control of Chondrocyte Fate and Differentiation. Birth Defect Res. C 75 (3), 200–212. doi:10.1002/bdrc.20048
Lei, J., Peng, S., Samuel, S. B., Zhang, S., Wu, Y., Wang, P., et al. (2016). A Simple and Biosafe Method for Isolation of Human Umbilical Vein Endothelial Cells. Anal. Biochem. 508, 15–18. doi:10.1016/j.ab.2016.06.018
Levato, R., Visser, J., Planell, J. A., Engel, E., Malda, J., and Mateos-Timoneda, M. A. (2014). Biofabrication of Tissue Constructs by 3D Bioprinting of Cell-Laden Microcarriers. Biofabrication 6 (3), 035020. doi:10.1088/1758-5082/6/3/035020
Li, H., Liu, P., Xu, S., Li, Y., Dekker, J. D., Li, B., et al. (2017). FOXP1 Controls Mesenchymal Stem Cell Commitment and Senescence during Skeletal Aging. J. Clin. investigation 127 (4), 1241–1253. doi:10.1172/jci89511
Li, H., Tan, Y. J., Leong, K. F., and Li, L. (2017). 3D Bioprinting of Highly Thixotropic Alginate/Methylcellulose Hydrogel with Strong Interface Bonding. ACS Appl. Mat. Interfaces 9 (23), 20086–20097. doi:10.1021/acsami.7b04216
Li, J., Liu, X., Crook, J. M., and Wallace, G. G. (2020). 3D Printing of Cytocompatible Graphene/Alginate Scaffolds for Mimetic Tissue Constructs. Front. Bioeng. Biotechnol. 8, 824. doi:10.3389/fbioe.2020.00824
Li, J., and Mooney, D. J. (2016). Designing Hydrogels for Controlled Drug Delivery. Nat. Rev. Mater 1 (12), 16071. doi:10.1038/natrevmats.2016.71
Li, Q., Xu, S., Feng, Q., Dai, Q., Yao, L., Zhang, Y., et al. (2021). 3D Printed Silk-Gelatin Hydrogel Scaffold with Different Porous Structure and Cell Seeding Strategy for Cartilage Regeneration. Bioact. Mater. 6 (10), 3396–3410. doi:10.1016/j.bioactmat.2021.03.013
Li, X., Chen, M., Wang, P., Yao, Y., Han, X., Liang, J., et al. (2020). A Highly Interweaved HA-SS-nHAp/collagen Hybrid Fibering Hydrogel Enhances Osteoinductivity and Mineralization. Nanoscale 12 (24), 12869–12882. doi:10.1039/d0nr01824d
Lien, S.-M., Ko, L.-Y., and Huang, T.-J. (2009). Effect of Pore Size on ECM Secretion and Cell Growth in Gelatin Scaffold for Articular Cartilage Tissue Engineering. Acta Biomater. 5 (2), 670–679. doi:10.1016/j.actbio.2008.09.020
Ligon, S. C., Liska, R., Stampfl, J., Gurr, M., and Mülhaupt, R. (2017). Polymers for 3D Printing and Customized Additive Manufacturing. Chem. Rev. 117 (15), 10212–10290. doi:10.1021/acs.chemrev.7b00074
Liu, B., Li, J., Lei, X., Cheng, P., Song, Y., Gao, Y., et al. (2020). 3D-bioprinted Functional and Biomimetic Hydrogel Scaffolds Incorporated with Nanosilicates to Promote Bone Healing in Rat Calvarial Defect Model. Mater. Sci. Eng. C 112, 110905. doi:10.1016/j.msec.2020.110905
Liu, X., Gaihre, B., George, M. N., Miller, A. L., Xu, H., Waletzki, B. E., et al. (2021). 3D Bioprinting of Oligo(poly[ethylene Glycol] Fumarate) for Bone and Nerve Tissue Engineering. J. Biomed. Mater Res. 109 (1), 6–17. doi:10.1002/jbm.a.37002
López-Marcial, G. R., Zeng, A. Y., Osuna, C., Dennis, J., García, J. M., and O’Connell, G. D. (2018). Agarose-Based Hydrogels as Suitable Bioprinting Materials for Tissue Engineering. ACS Biomater. Sci. Eng. 4 (10), 3610–3616. doi:10.1021/acsbiomaterials.8b00903
Luo, Y., Sinkeviciute, D., He, Y., Karsdal, M., Henrotin, Y., Mobasheri, A., et al. (2017). The Minor Collagens in Articular Cartilage. Protein Cell 8 (8), 560–572. doi:10.1007/s13238-017-0377-7
MacLean, H. E., Kim, J. I., Glimcher, M. J., Wang, J., Kronenberg, H. M., and Glimcher, L. H. (2003). Absence of Transcription Factor C-Maf Causes Abnormal Terminal Differentiation of Hypertrophic Chondrocytes during Endochondral Bone Development. Dev. Biol. 262 (1), 51–63. doi:10.1016/s0012-1606(03)00324-5
Mandrycky, C., Wang, Z., Kim, K., and Kim, D.-H. (2016). 3D Bioprinting for Engineering Complex Tissues. Biotechnol. Adv. 34 (4), 422–434. doi:10.1016/j.biotechadv.2015.12.011
Markstedt, K., Mantas, A., Tournier, I., Martínez Ávila, H., Hägg, D., and Gatenholm, P. (2015). 3D Bioprinting Human Chondrocytes with Nanocellulose-Alginate Bioink for Cartilage Tissue Engineering Applications. Biomacromolecules 16 (5), 1489–1496. doi:10.1021/acs.biomac.5b00188
Marques, C. F., Diogo, G. S., Pina, S., Oliveira, J. M., Silva, T. H., and Reis, R. L. (2019). Collagen-based Bioinks for Hard Tissue Engineering Applications: a Comprehensive Review. J. Mater Sci. Mater Med. 30 (3), 32. doi:10.1007/s10856-019-6234-x
Matai, I., Kaur, G., Seyedsalehi, A., McClinton, A., and Laurencin, C. T. (2020). Progress in 3D Bioprinting Technology for Tissue/organ Regenerative Engineering. Biomaterials 226, 119536. doi:10.1016/j.biomaterials.2019.119536
Mauck, R. L., Yuan, X., and Tuan, R. S. (2006). Chondrogenic Differentiation and Functional Maturation of Bovine Mesenchymal Stem Cells in Long-Term Agarose Culture. Osteoarthr. Cartil. 14 (2), 179–189. doi:10.1016/j.joca.2005.09.002
Melke, J., Midha, S., Ghosh, S., Ito, K., and Hofmann, S. (2016). Silk Fibroin as Biomaterial for Bone Tissue Engineering. Acta Biomater. 31, 1–16. doi:10.1016/j.actbio.2015.09.005
Moroni, L., Boland, T., Burdick, J. A., De Maria, C., Derby, B., Forgacs, G., et al. (2018). Biofabrication: A Guide to Technology and Terminology. Trends Biotechnol. 36 (4), 384–402. doi:10.1016/j.tibtech.2017.10.015
Murphy, S. V., and Atala, A. (2014). 3D Bioprinting of Tissues and Organs. Nat. Biotechnol. 32 (8), 773–785. doi:10.1038/nbt.2958
Naahidi, S., Jafari, M., Logan, M., Wang, Y., Yuan, Y., Bae, H., et al. (2017). Biocompatibility of Hydrogel-Based Scaffolds for Tissue Engineering Applications. Biotechnol. Adv. 35 (5), 530–544. doi:10.1016/j.biotechadv.2017.05.006
Naito, H., Yoshimura, M., Mizuno, T., Takasawa, S., Tojo, T., and Taniguchi, S. (2013). The Advantages of Three-Dimensional Culture in a Collagen Hydrogel for Stem Cell Differentiation. J. Biomed. Mat. Res. 101 (10), 2838–2845. doi:10.1002/jbm.a.34578
Nakashima, K., Zhou, X., Kunkel, G., Zhang, Z., Deng, J. M., Behringer, R. R., et al. (2002). The Novel Zinc Finger-Containing Transcription Factor Osterix Is Required for Osteoblast Differentiation and Bone Formation. Cell 108 (1), 17–29. doi:10.1016/s0092-8674(01)00622-5
Narayanan, L. K., Huebner, P., Fisher, M. B., Spang, J. T., Starly, B., and Shirwaiker, R. A. (2016). 3D-Bioprinting of Polylactic Acid (PLA) Nanofiber-Alginate Hydrogel Bioink Containing Human Adipose-Derived Stem Cells. ACS Biomater. Sci. Eng. 2 (10), 1732–1742. doi:10.1021/acsbiomaterials.6b00196
Nedunchezian, S., Banerjee, P., Lee, C.-Y., Lee, S.-S., Lin, C.-W., Wu, C.-W., et al. (2021). Generating Adipose Stem Cell-Laden Hyaluronic Acid-Based Scaffolds Using 3D Bioprinting via the Double Crosslinked Strategy for Chondrogenesis. Mater. Sci. Eng. C 124, 112072. doi:10.1016/j.msec.2021.112072
Negro, A., Cherbuin, T., and Lutolf, M. P. (2018). 3D Inkjet Printing of Complex, Cell-Laden Hydrogel Structures. Sci. Rep. 8 (1), 17099. doi:10.1038/s41598-018-35504-2
Ni, T., Liu, M., Zhang, Y., Cao, Y., and Pei, R. (2020). 3D Bioprinting of Bone Marrow Mesenchymal Stem Cell-Laden Silk Fibroin Double Network Scaffolds for Cartilage Tissue Repair. Bioconjugate Chem. 31 (8), 1938–1947. doi:10.1021/acs.bioconjchem.0c00298
Nishikawa, K., Nakashima, T., Takeda, S., Isogai, M., Hamada, M., Kimura, A., et al. (2010). Maf Promotes Osteoblast Differentiation in Mice by Mediating the Age-Related Switch in Mesenchymal Cell Differentiation. J. Clin. Invest. 120 (10), 3455–3465. doi:10.1172/jci42528
Ober, T. J., Foresti, D., and Lewis, J. A. (2015). Active Mixing of Complex Fluids at the Microscale. Proc. Natl. Acad. Sci. U.S.A. 112 (40), 12293–12298. doi:10.1073/pnas.1509224112
O’Bryan, C. S., Bhattacharjee, T., Hart, S., Kabb, C. P., Schulze, K. D., Chilakala, I., et al. (2017). Self-assembled Micro-organogels for 3D Printing Silicone Structures. Sci. Adv. 3 (5), e1602800. doi:10.1126/sciadv.1602800
Oh, C.-D., and Chun, J.-S. (2003). Signaling Mechanisms Leading to the Regulation of Differentiation and Apoptosis of Articular Chondrocytes by Insulin-like Growth Factor-1. J. Biol. Chem. 278 (38), 36563–36571. doi:10.1074/jbc.M304857200
Ojansivu, M., Rashad, A., Ahlinder, A., Massera, J., Mishra, A., Syverud, K., et al. (2019). Wood-based Nanocellulose and Bioactive Glass Modified Gelatin-Alginate Bioinks for 3D Bioprinting of Bone Cells. Biofabrication 11 (3), 035010. doi:10.1088/1758-5090/ab0692
Olate-Moya, F., Arens, L., Wilhelm, M., Mateos-Timoneda, M. A., Engel, E., and Palza, H. (2020). Chondroinductive Alginate-Based Hydrogels Having Graphene Oxide for 3D Printed Scaffold Fabrication. ACS Appl. Mat. Interfaces 12 (4), 4343–4357. doi:10.1021/acsami.9b22062
Ostrovidov, S., Salehi, S., Costantini, M., Suthiwanich, K., Ebrahimi, M., Sadeghian, R. B., et al. (2019). 3D Bioprinting in Skeletal Muscle Tissue Engineering. Small 15 (24), 1805530. doi:10.1002/smll.201805530
Park, J. H., Gillispie, G. J., Copus, J. S., Zhang, W., Atala, A., Yoo, J. J., et al. (2020). The Effect of BMP-Mimetic Peptide Tethering Bioinks on the Differentiation of Dental Pulp Stem Cells (DPSCs) in 3D Bioprinted Dental Constructs. Biofabrication 12 (3), 035029. doi:10.1088/1758-5090/ab9492
Park, Y., Lutolf, M. P., Hubbell, J. A., Hunziker, E. B., and Wong, M. (2004). Bovine Primary Chondrocyte Culture in Synthetic Matrix Metalloproteinase-Sensitive Poly(ethylene Glycol)-Based Hydrogels as a Scaffold for Cartilage Repair. Tissue Eng. 10 (3-4), 515–522. doi:10.1089/107632704323061870
Pereira, R. F., Barrias, C. C., Bártolo, P. J., and Granja, P. L. (2018). Cell-instructive Pectin Hydrogels Crosslinked via Thiol-Norbornene Photo-Click Chemistry for Skin Tissue Engineering. Acta Biomater. 66, 282–293. doi:10.1016/j.actbio.2017.11.016
Piluso, S., Flores Gomez, D., Dokter, I., Moreira Texeira, L., Li, Y., Leijten, J., et al. (2020). Rapid and Cytocompatible Cell-Laden Silk Hydrogel Formation via Riboflavin-Mediated Crosslinking. J. Mat. Chem. B 8 (41), 9566–9575. doi:10.1039/d0tb01731k
Pizette, S., and Niswander, L. (2000). BMPs Are Required at Two Steps of Limb Chondrogenesis: Formation of Prechondrogenic Condensations and Their Differentiation into Chondrocytes. Dev. Biol. 219 (2), 237–249. doi:10.1006/dbio.2000.9610
Poldervaart, M. T., Goversen, B., de Ruijter, M., Abbadessa, A., Melchels, F. P. W., Öner, F. C., et al. (2017). 3D Bioprinting of Methacrylated Hyaluronic Acid (MeHA) Hydrogel with Intrinsic Osteogenicity. PLoS One 12 (6), e0177628. doi:10.1371/journal.pone.0177628
Poldervaart, M. T., Wang, H., van der Stok, J., Weinans, H., Leeuwenburgh, S. C. G., Öner, F. C., et al. (2013). Sustained Release of BMP-2 in Bioprinted Alginate for Osteogenicity in Mice and Rats. PLoS One 8 (8), e72610. doi:10.1371/journal.pone.0072610
Polo-Corrales, L., Latorre-Esteves, M., and Ramirez-Vick, J. E. (2014). Scaffold Design for Bone Regeneration. J. Nanosci. Nanotech. 14 (1), 15–56. doi:10.1166/jnn.2014.9127
Qasim, M., Chae, D. S., and Lee, N. Y. (2019). Advancements and Frontiers in Nano-Based 3D and 4D Scaffolds for Bone and Cartilage Tissue Engineering. Ijn 14, 4333–4351. doi:10.2147/IJN.S209431
Qin, X.-H., Wang, X., Rottmar, M., Nelson, B. J., and Maniura-Weber, K. (2018). Near-Infrared Light-Sensitive Polyvinyl Alcohol Hydrogel Photoresist for Spatiotemporal Control of Cell-Instructive 3D Microenvironments. Adv. Mat. 30 (10), 1705564. doi:10.1002/adma.201705564
Rastogi, P., and Kandasubramanian, B. (2019). Review of Alginate-Based Hydrogel Bioprinting for Application in Tissue Engineering. Biofabrication 11 (4), 042001. doi:10.1088/1758-5090/ab331e
Reakasame, S., and Boccaccini, A. R. (2018). Oxidized Alginate-Based Hydrogels for Tissue Engineering Applications: A Review. Biomacromolecules 19 (1), 3–21. doi:10.1021/acs.biomac.7b01331
Ribeiro, V. P., da Silva Morais, A., Maia, F. R., Canadas, R. F., Costa, J. B., Oliveira, A. L., et al. (2018). Combinatory Approach for Developing Silk Fibroin Scaffolds for Cartilage Regeneration. Acta Biomater. 72, 167–181. doi:10.1016/j.actbio.2018.03.047
Rizzo, R., Ruetsche, D., Liu, H., and Zenobi‐Wong, M. (2021). Optimized Photoclick (Bio)Resins for Fast Volumetric Bioprinting. Adv. Mat. 33, 2102900. doi:10.1002/adma.202102900
Rockwood, D. N., Preda, R. C., Yücel, T., Wang, X., Lovett, M. L., and Kaplan, D. L. (2011). Materials Fabrication from Bombyx mori Silk Fibroin. Nat. Protoc. 6 (10), 1612–1631. doi:10.1038/nprot.2011.379
Rodriguez, M. J., Brown, J., Giordano, J., Lin, S. J., Omenetto, F. G., and Kaplan, D. L. (2017). Silk Based Bioinks for Soft Tissue Reconstruction Using 3-dimensional (3D) Printing with In Vitro and In Vivo Assessments. Biomaterials 117, 105–115. doi:10.1016/j.biomaterials.2016.11.046
Roseti, L., Cavallo, C., Desando, G., Parisi, V., Petretta, M., Bartolotti, I., et al. (2018). Three-Dimensional Bioprinting of Cartilage by the Use of Stem Cells: A Strategy to Improve Regeneration. Materials 11 (9), 1749. doi:10.3390/ma11091749
Ruiz, R., Kang, H., Detcheverry, F. A., Dobisz, E., Kercher, D. S., Albrecht, T. R., et al. (2008). Density Multiplication and Improved Lithography by Directed Block Copolymer Assembly. Science 321 (5891), 936–939. doi:10.1126/science.1157626
Sakai, S., Hirose, K., Taguchi, K., Ogushi, Y., and Kawakami, K. (2009). An Injectable, In Situ Enzymatically Gellable, Gelatin Derivative for Drug Delivery and Tissue Engineering. Biomaterials 30 (20), 3371–3377. doi:10.1016/j.biomaterials.2009.03.030
Salati, M. A., Khazai, J., Tahmuri, A. M., Samadi, A., Taghizadeh, A., Taghizadeh, M., et al. (2020). Agarose-Based Biomaterials: Opportunities and Challenges in Cartilage Tissue Engineering. Polymers 12 (5), 1150. doi:10.3390/polym12051150
Schuurman, W., Levett, P. A., Pot, M. W., van Weeren, P. R., Dhert, W. J. A., Hutmacher, D. W., et al. (2013). Gelatin-methacrylamide Hydrogels as Potential Biomaterials for Fabrication of Tissue-Engineered Cartilage Constructs. Macromol. Biosci. 13 (5), 551–561. doi:10.1002/mabi.201200471
Shi, W., Sun, M., Hu, X., Ren, B., Cheng, J., Li, C., et al. (2017). Structurally and Functionally Optimized Silk-Fibroin-Gelatin Scaffold Using 3D Printing to Repair Cartilage Injury In Vitro and In Vivo. Adv. Mat. 29 (29), 1701089. doi:10.1002/adma.201701089
Shim, J.-H., Jang, K.-M., Hahn, S. K., Park, J. Y., Jung, H., Oh, K., et al. (2016). Three-dimensional Bioprinting of Multilayered Constructs Containing Human Mesenchymal Stromal Cells for Osteochondral Tissue Regeneration in the Rabbit Knee Joint. Biofabrication 8 (1), 014102. doi:10.1088/1758-5090/8/1/014102
Simonson, O. E., Domogatskaya, A., Volchkov, P., and Rodin, S. (2015). The Safety of Human Pluripotent Stem Cells in Clinical Treatment. Ann. Med. 47 (5), 370–380. doi:10.3109/07853890.2015.1051579
Sivashankari, P. R., and Prabaharan, M. (2020). Three-dimensional Porous Scaffolds Based on Agarose/chitosan/graphene Oxide Composite for Tissue Engineering. Int. J. Biol. Macromol. 146, 222–231. doi:10.1016/j.ijbiomac.2019.12.219
Skardal, A., and Atala, A. (2015). Biomaterials for Integration with 3-D Bioprinting. Ann. Biomed. Eng. 43 (3), 730–746. doi:10.1007/s10439-014-1207-1
Song, S.-J., Choi, J., Park, Y.-D., Hong, S., Lee, J. J., Ahn, C. B., et al. (2011). Sodium Alginate Hydrogel-Based Bioprinting Using a Novel Multinozzle Bioprinting System. Artif. Organs 35 (11), 1132–1136. doi:10.1111/j.1525-1594.2011.01377.x
Stephanopoulos, N., Ortony, J. H., and Stupp, S. I. (2013). Self-Assembly for the Synthesis of Functional Biomaterials. Acta Mater. 61 (3), 912–930. doi:10.1016/j.actamat.2012.10.046
Stichler, S., Böck, T., Paxton, N., Bertlein, S., Levato, R., Schill, V., et al. (2017). Double Printing of Hyaluronic Acid/poly(glycidol) Hybrid Hydrogels with Poly( ε -caprolactone) for MSC Chondrogenesis. Biofabrication 9 (4), 044108. doi:10.1088/1758-5090/aa8cb7
Strioga, M., Viswanathan, S., Darinskas, A., Slaby, O., and Michalek, J. (2012). Same or Not the Same? Comparison of Adipose Tissue-Derived versus Bone Marrow-Derived Mesenchymal Stem and Stromal Cells. Stem Cells Dev. 21 (14), 2724–2752. doi:10.1089/scd.2011.0722
Tabata, M., Shimoda, T., Sugihara, K., Ogomi, D., Serizawa, T., and Akashi, M. (2003). Osteoconductive and Hemostatic Properties of Apatite Formed On/in Agarose Gel as a Bone-Grafting Material. J. Biomed. Mat. Res. 67B (2), 680–688. doi:10.1002/jbm.b.10063
Tao, F., Cheng, Y., Shi, X., Zheng, H., Du, Y., Xiang, W., et al. (2020). Applications of Chitin and Chitosan Nanofibers in Bone Regenerative Engineering. Carbohydr. Polym. 230, 115658. doi:10.1016/j.carbpol.2019.115658
Trica, B., Delattre, C., Gros, F., Ursu, A. V., Dobre, T., Djelveh, G., et al. (2019). Extraction and Characterization of Alginate from an Edible Brown Seaweed (Cystoseira Barbata) Harvested in the Romanian Black Sea. Mar. Drugs 17 (7), 405. doi:10.3390/md17070405
Trucco, D., Sharma, A., Manferdini, C., Gabusi, E., Petretta, M., Desando, G., et al. (2021). Modeling and Fabrication of Silk Fibroin-Gelatin-Based Constructs Using Extrusion-Based Three-Dimensional Bioprinting. ACS Biomater. Sci. Eng. 7 (7), 3306–3320. doi:10.1021/acsbiomaterials.1c00410
Ulrich, T. A., Jain, A., Tanner, K., MacKay, J. L., and Kumar, S. (2010). Probing Cellular Mechanobiology in Three-Dimensional Culture with Collagen-Agarose Matrices. Biomaterials 31 (7), 1875–1884. doi:10.1016/j.biomaterials.2009.10.047
Ulsamer, A., Ortuño, M. J., Ruiz, S., Susperregui, A. R. G., Osses, N., Rosa, J. L., et al. (2008). BMP-2 Induces Osterix Expression through Up-Regulation of Dlx5 and its Phosphorylation by P38. J. Biol. Chem. 283 (7), 3816–3826. doi:10.1074/jbc.M704724200
Venkatesan, J., Bhatnagar, I., Manivasagan, P., Kang, K.-H., and Kim, S.-K. (2015). Alginate Composites for Bone Tissue Engineering: a Review. Int. J. Biol. Macromol. 72, 269–281. doi:10.1016/j.ijbiomac.2014.07.008
Wan, Z., Zhang, P., Liu, Y., Lv, L., and Zhou, Y. (2020). Four-dimensional Bioprinting: Current Developments and Applications in Bone Tissue Engineering. Acta Biomater. 101, 26–42. doi:10.1016/j.actbio.2019.10.038
Wang, L. L., Highley, C. B., Yeh, Y. C., Galarraga, J. H., Uman, S., and Burdick, J. A. (2018). Three‐dimensional Extrusion Bioprinting of Single‐ and Double‐network Hydrogels Containing Dynamic Covalent Crosslinks. J. Biomed. Mat. Res. 106 (4), 865–875. doi:10.1002/jbm.a.36323
Wang, X.-F., Song, Y., Liu, Y.-S., Sun, Y.-c., Wang, Y.-g., Wang, Y., et al. (2016). Osteogenic Differentiation of Three-Dimensional Bioprinted Constructs Consisting of Human Adipose-Derived Stem Cells In Vitro and In Vivo. PLoS One 11 (6), e0157214. doi:10.1371/journal.pone.0157214
Wang, X., Kluge, J. A., Leisk, G. G., and Kaplan, D. L. (2008). Sonication-induced Gelation of Silk Fibroin for Cell Encapsulation. Biomaterials 29 (8), 1054–1064. doi:10.1016/j.biomaterials.2007.11.003
Wang, X., and Liu, C. (2018). 3D Bioprinting of Adipose-Derived Stem Cells for Organ Manufacturing. Adv. Exp. Med. Biol. 1078, 3–14. doi:10.1007/978-981-13-0950-2_1
Wu, Y., Yu, C., Xing, M., Wang, L., and Guan, G. (2020). Surface Modification of Polyvinyl Alcohol (PVA)/polyacrylamide (PAAm) Hydrogels with Polydopamine and REDV for Improved Applicability. J. Biomed. Mat. Res. 108 (1), 117–127. doi:10.1002/jbm.b.34371
Xu, B., Ye, J., Yuan, F.-Z., Zhang, J.-Y., Chen, Y.-R., Fan, B.-S., et al. (2020). Advances of Stem Cell-Laden Hydrogels with Biomimetic Microenvironment for Osteochondral Repair. Front. Bioeng. Biotechnol. 8, 247. doi:10.3389/fbioe.2020.00247
Xu, J., Feng, Q., Lin, S., Yuan, W., Li, R., Li, J., et al. (2019). Injectable Stem Cell-Laden Supramolecular Hydrogels Enhance In Situ Osteochondral Regeneration via the Sustained Co-delivery of Hydrophilic and Hydrophobic Chondrogenic Molecules. Biomaterials 210, 51–61. doi:10.1016/j.biomaterials.2019.04.031
Xu, K., Wang, J., Chen, Q., Yue, Y., Zhang, W., and Wang, P. (2008). Spontaneous Volume Transition of Polyampholyte Nanocomposite Hydrogels Based on Pure Electrostatic Interaction. J. Colloid Interface Sci. 321 (2), 272–278. doi:10.1016/j.jcis.2008.02.024
Xu, Y., Peng, J., Richards, G., Lu, S., and Eglin, D. (2019). Optimization of Electrospray Fabrication of Stem Cell-Embedded Alginate-Gelatin Microspheres and Their Assembly in 3D-Printed Poly(ε-Caprolactone) Scaffold for Cartilage Tissue Engineering. J. Orthop. Transl. 18, 128–141. doi:10.1016/j.jot.2019.05.003
Yamaoka, H., Asato, H., Ogasawara, T., Nishizawa, S., Takahashi, T., Nakatsuka, T., et al. (2006). Cartilage Tissue Engineering Using Human Auricular Chondrocytes Embedded in Different Hydrogel Materials. J. Biomed. Mat. Res. 78A (1), 1–11. doi:10.1002/jbm.a.30655
Yan, L.-P., Oliveira, J. M., Oliveira, A. L., Caridade, S. G., Mano, J. F., and Reis, R. L. (2012). Macro/microporous Silk Fibroin Scaffolds with Potential for Articular Cartilage and Meniscus Tissue Engineering Applications. Acta Biomater. 8 (1), 289–301. doi:10.1016/j.actbio.2011.09.037
Yang, J., Li, Y., Liu, Y., Li, D., Zhang, L., Wang, Q., et al. (2019). Influence of Hydrogel Network Microstructures on Mesenchymal Stem Cell Chondrogenesis In Vitro and In Vivo. Acta Biomater. 91, 159–172. doi:10.1016/j.actbio.2019.04.054
Yang, J., Tang, Z., Liu, Y., Luo, Z., Xiao, Y., and Zhang, X. (2021). Comparison of Chondro-Inductivity between Collagen and Hyaluronic Acid Hydrogel Based on Chemical/physical Microenvironment. Int. J. Biol. Macromol. 182, 1941–1952. doi:10.1016/j.ijbiomac.2021.05.188
Yang, J., Zhang, Y. S., Yue, K., and Khademhosseini, A. (2017). Cell-laden Hydrogels for Osteochondral and Cartilage Tissue Engineering. Acta Biomater. 57, 1–25. doi:10.1016/j.actbio.2017.01.036
Yang, X., Lu, Z., Wu, H., Li, W., Zheng, L., and Zhao, J. (2018). Collagen-alginate as Bioink for Three-Dimensional (3D) Cell Printing Based Cartilage Tissue Engineering. Mater. Sci. Eng. C 83, 195–201. doi:10.1016/j.msec.2017.09.002
Yilmaz, B., Tahmasebifar, A., and Baran, E. T. (2019). Bioprinting Technologies in Tissue Engineering. Adv. Biochem. Eng. Biotechnol. 171, 279–319. doi:10.1007/10_2019_108
Yin, Y., and Xiong, J. (2018). Finite Element Analysis of Electrospun Nanofibrous Mats under Biaxial Tension. Nanomaterials 8 (5), 348. doi:10.3390/nano8050348
Yucel, T., Cebe, P., and Kaplan, D. L. (2009). Vortex-induced Injectable Silk Fibroin Hydrogels. Biophysical J. 97 (7), 2044–2050. doi:10.1016/j.bpj.2009.07.028
Yue, K., Trujillo-de Santiago, G., Alvarez, M. M., Tamayol, A., Annabi, N., and Khademhosseini, A. (2015). Synthesis, Properties, and Biomedical Applications of Gelatin Methacryloyl (GelMA) Hydrogels. Biomaterials 73, 254–271. doi:10.1016/j.biomaterials.2015.08.045
Zarrintaj, P., Manouchehri, S., Ahmadi, Z., Saeb, M. R., Urbanska, A. M., Kaplan, D. L., et al. (2018). Agarose-based Biomaterials for Tissue Engineering. Carbohydr. Polym. 187, 66–84. doi:10.1016/j.carbpol.2018.01.060
Zhang, J., Liu, Z., Li, Y., You, Q., Yang, J., Jin, Y., et al. (2020). FGF-2-Induced Human Amniotic Mesenchymal Stem Cells Seeded on a Human Acellular Amniotic Membrane Scaffold Accelerated Tendon-To-Bone Healing in a Rabbit Extra-articular Model. Stem cells Int. 2020, 1–14. doi:10.1155/2020/4701476
Zhang, J., Wehrle, E., Adamek, P., Paul, G. R., Qin, X.-H., Rubert, M., et al. (2020). Optimization of Mechanical Stiffness and Cell Density of 3D Bioprinted Cell-Laden Scaffolds Improves Extracellular Matrix Mineralization and Cellular Organization for Bone Tissue Engineering. Acta Biomater. 114, 307–322. doi:10.1016/j.actbio.2020.07.016
Zhang, J., Wehrle, E., Vetsch, J. R., Paul, G. R., Rubert, M., and Müller, R. (2019). Alginate Dependent Changes of Physical Properties in 3D Bioprinted Cell-Laden Porous Scaffolds Affect Cell Viability and Cell Morphology. Biomed. Mat. 14 (6), 065009. doi:10.1088/1748-605X/ab3c74
Zhang, K., Wang, S., Zhou, C., Cheng, L., Gao, X., Xie, X., et al. (2018). Advanced Smart Biomaterials and Constructs for Hard Tissue Engineering and Regeneration. Bone Res. 6, 31. doi:10.1038/s41413-018-0032-9
Zhang, L., Yang, G., Johnson, B. N., and Jia, X. (2019). Three-dimensional (3D) Printed Scaffold and Material Selection for Bone Repair. Acta Biomater. 84, 16–33. doi:10.1016/j.actbio.2018.11.039
Zhang, T., Chen, H., Zhang, Y., Zan, Y., Ni, T., Liu, M., et al. (2019). Photo-crosslinkable, Bone Marrow-Derived Mesenchymal Stem Cells-Encapsulating Hydrogel Based on Collagen for Osteogenic Differentiation. Colloids Surfaces B Biointerfaces 174, 528–535. doi:10.1016/j.colsurfb.2018.11.050
Zhang, W., Chen, L., Chen, J., Wang, L., Gui, X., Ran, J., et al. (2017). Silk Fibroin Biomaterial Shows Safe and Effective Wound Healing in Animal Models and a Randomized Controlled Clinical Trial. Adv. Healthc. Mat. 6 (10), 1700121. doi:10.1002/adhm.201700121
Zhang, W., Shi, W., Wu, S., Kuss, M., Jiang, X., Untrauer, J. B., et al. (2020). 3D Printed Composite Scaffolds with Dual Small Molecule Delivery for Mandibular Bone Regeneration. Biofabrication 12 (3), 035020. doi:10.1088/1758-5090/ab906e
Zhao, X., Shan, P., Liu, H., Li, D., Cai, P., Li, Z., et al. (2020). Poly(ethylene Glycol)s with a Single Cinnamaldehyde Acetal Unit for Fabricating Acid-Degradable Hydrogel. Front. Chem. 8, 839. doi:10.3389/fchem.2020.00839
Zhu, H., Mitsuhashi, N., Klein, A., Barsky, L. W., Weinberg, K., Barr, M. L., et al. (2006). The Role of the Hyaluronan Receptor CD44 in Mesenchymal Stem Cell Migration in the Extracellular Matrix. Stem Cells 24 (4), 928–935. doi:10.1634/stemcells.2005-0186
Zhu, W., Ma, X., Gou, M., Mei, D., Zhang, K., and Chen, S. (2016). 3D Printing of Functional Biomaterials for Tissue Engineering. Curr. Opin. Biotechnol. 40, 103–112. doi:10.1016/j.copbio.2016.03.014
Glossary
3D Three-dimensional
ABS Alcian blue staining
AD-MSCs Adipose-derived mesenchymal stem cells
AGG Aggrecan
ALP Alkaline phosphatase
AMPs Amorphous magnesium phosphates
ARS Alizarin red staining
BaG Bioactive glass
BCP Biphasic calcium phosphate
BMP-2 Bone morphogenetic protein-2
BMP-7D Bone morphogenetic protein -7-derived peptides
BM-MSCs Bone marrow-derived mesenchymal stem cells
β-TCP Beta-tricalcium phosphate powder
BTE Bone tissue engineering
CB[6] Cucurbit[6] uril
CD Chondrogenic differentiation
CNF Cellulose nanofibrils
Col I Collagen type I
COL 1A1 Collagen type I alpha 1
Col II Collagen type II
Col X Collagen type X,
CTE Cartilage tissue engineering
DAH Diaminohexane
DHT Dehydrothermal
DMD Digital micro-mirror device
DMMB Dimethylmethylene blue
DP-MSCs Dental pulp mesenchymal stem cells
DPSCs Dental pulp stem cells
eBM-MSCs Equine bone marrow-derived mesenchymal stem cells
ECM Extracellular matrix
ESCs Embryonic stem cells
FGF Fibroblast growth factor
GAG Glycosaminoglycans
GelMA Methacrylated gelatin
GNPs Gold nanoparticles
HA Hyaluronic acid
HAMA Hyaluronic acid methacrylate
H&E staining Hematoxylin and eosin staining
HAP Hydroxyapatite
hAD-MSCs Human adipose-derived mesenchymal stem cells
hBM-MSCs Human bone marrow-derived mesenchymal stem cells
hBM-MStCs Human bone marrow-derived mesenchymal stromal cells
hMSCs Human mesenchymal stromal cells
HPMC-MA Hydroxy propyl methyl cellulose of methacrylation
hUCB-MSCs Human umbilical cord blood-derived mesenchymal stem cells
hTMSCs Human turbinate-derived mesenchymal stromal cells
ICC Immunocytochemistry
IHC Immunohistochemistry
Ma-dECM Methacrylated decellularized extracellular matrix
MAF MAF bZIP transcription factor
MeHA Methacrylated hyaluronic acid
MPEG-b-PCL Methoxy PEG-block-PCL
MSCs Mesenchymal stem cells
MTS Masson’s trichrome staining
NA Not available
OCN Osteocalcin
OD Osteogenic differentiation
OMA Oxidized and methacrylated alginate
OPF Oligo(poly(ethylene glycol) fumarate
OPN Osteopontin
OTE Osteochondral tissue engineering
P(AGE-co-G) Poly(allyl glycidyl ether-co-glycidyl)
PBMCs Peripheral blood mononuclear cells
PCL Polycaprolactone
PEG Poly(ethylene glycol)
PLA Polylactic acid
PLGA Poly(lactic-co-glycolic acid)
PSRS Picrosirius red staining
PVA Polyvinyl alcohol
rBM-MSCs Rat bone marrow-derived mesenchymal stem cells
raBM-MSCs Rabbit bone marrow-derived mesenchymal stem cells
RUNX 2 Runt-related transcription factor 2
sGAG Sulfated glycosaminoglycans
SOX9 SRY box transcription factor 9
TGF-β Transforming growth factor-beta
UVECs Umbilical vein endothelial cells
UV Ultraviolet
VEGF Vascular endothelial growth factor
Keywords: stem cell, hydrogel, 3D bioprinting, bone, cartilage
Citation: Yang Z, Yi P, Liu Z, Zhang W, Mei L, Feng C, Tu C and Li Z (2022) Stem Cell-Laden Hydrogel-Based 3D Bioprinting for Bone and Cartilage Tissue Engineering. Front. Bioeng. Biotechnol. 10:865770. doi: 10.3389/fbioe.2022.865770
Received: 30 January 2022; Accepted: 18 April 2022;
Published: 17 May 2022.
Edited by:
Weili Fu, Sichuan University, ChinaReviewed by:
Xiao-Hua Qin, ETH Zürich, SwitzerlandChaoming Xie, Southwest Jiaotong University, China
Copyright © 2022 Yang, Yi, Liu, Zhang, Mei, Feng, Tu and Li. This is an open-access article distributed under the terms of the Creative Commons Attribution License (CC BY). The use, distribution or reproduction in other forums is permitted, provided the original author(s) and the copyright owner(s) are credited and that the original publication in this journal is cited, in accordance with accepted academic practice. No use, distribution or reproduction is permitted which does not comply with these terms.
*Correspondence: Zhihong Li, bGl6aGlob25nQGNzdS5lZHUuY24=; Chao Tu, dHVjaGFvQGNzdS5lZHUuY24=
†These authors have contributed equally to this work