- 1School of Life Science and Food Engineering, Huaiyin Institute of Technology, Huaian, China
- 2State Key Laboratory of Microbial Metabolism, School of Life Sciences and Biotechnology, Shanghai Jiao Tong University, Shanghai, China
- 3National Center of Technology Innovation for Synthetic Biology, Tianjin, China
- 4Tecnologico de Monterrey, School of Engineering and Sciences, Monterrey, Mexico
Kluyveromyces marxianus is an emerging non-conventional food-grade yeast that is generally isolated from diverse habitats, like kefir grain, fermented dairy products, sugar industry sewage, plants, and sisal leaves. A unique set of beneficial traits, such as fastest growth, thermotolerance, and broad substrate spectrum (i.e., hemi-cellulose hydrolysates, xylose, l-arabinose, d-mannose, galactose, maltose, sugar syrup molasses, cellobiose, and dairy industry) makes this yeast a particularly attractive host for applications in a variety of food and biotechnology industries. In contrast to Saccharomyces cerevisiae, most of the K. marxianus strains are apparently Crabtree-negative or having aerobic-respiring characteristics, and unlikely to endure aerobic alcoholic fermentation. This is a desirable phenotype for the large-scale biosynthesis of products associated with biomass formation because the formation of ethanol as an undesirable byproduct can be evaded under aerobic conditions. Herein, we discuss the current insight into the potential applications of K. marxianus as a robust yeast cell factory to produce various industrially pertinent enzymes, bioethanol, cell proteins, probiotic, fructose, and fructo-oligosaccharides, and vaccines, with excellent natural features. Moreover, the biotechnological improvement and development of new biotechnological tools, particularly CRISPR–Cas9-assisted precise genome editing in K. marxianus are delineated. Lastly, the ongoing challenges, concluding remarks, and future prospects for expanding the scope of K. marxianus utilization in modern biotechnology, food, feed, and pharmaceutical industries are also thoroughly vetted. In conclusion, it is critical to apprehend knowledge gaps around genes, metabolic pathways, key enzymes, and regulation for gaining a complete insight into the mechanism for producing relevant metabolites by K. marxianus.
1 Introduction
Yeasts are a heterogeneous group of eukaryotic fungi that have diverse applications in biotechnological, food, environmental, and pharmaceutical fields since the ancient times (Bilal et al., 2020; Mamaev and Zvyagilskaya, 2021). Yeasts are endowed with a set of unique advantageous attributes, such as high production, growth on a variety of feedstocks, ability to compartmentalize reactions in organelles, potential to execute many posttranslational modifications, easy cultivation in small vessels or large-scale bioreactors, facile product separation, and resistance to infectious agents (i.e., bacteriophages) (Cheng et al., 2018; Wang et al., 2020; Staniszewski and Kordowska-Wiater, 2021). All these traits render yeasts, particularly attractive candidates for broad-spectrum applications in various industries (Wagner and Alper, 2016). Although many yeast species have been isolated and identified, the industrial or biotechnological applications are restricted to only a few species, primarily belonging to Pichia pastoris, Saccharomyces cerevisiae, Candida utilis, Yarrowia lipolytica, and Kluyveromyces marxianus and its asexual forms (e.g., K. bulgaricus, K. fragilis, C. kefyr, and C. pseudotropicalis) (Lane and Morrissey, 2010; Wagner and Alper, 2016; Chi et al., 2019). Among these, S. cerevisiae holds a prominent place and a largely exploited microbial platform in the biotechnological field owing to its well-annotated genome, availability, well-known physiological traits, ease of use, and genetic manipulability (Lyu et al., 2021). In addition to this traditional yeast, various other non-conventional yeasts with advantageous features for industrial bioprocesses including P. pastoris, K. lactis, H. polymorpha, and Y. lipolytica have also gained importance (Gellissen et al., 2005; Wagner and Alper, 2016). Among these non-conventional strains, the Kluyveromyces genus has recently emerged as a representative candidate for many applications in food, environment, and biotechnology Duan et al., 2019; Zhou et al., 2018; Hua et al., 2019).
In contrast to S. cerevisiae, most of the K. marxianus strains are Crabtree-negative or having aerobic-respiring characteristics, and unlikely to endure aerobic alcoholic fermentation. This could be an advantageous characteristic for the large-scale biosynthesis of compounds whose products are associated with biomass formation because the formation of ethanol as an undesirable byproduct can be evaded under aerobic conditions (Wagner and Alper, 2016). Moreover, K. marxianus can metabolize a broad spectrum of low-cost feedstocks, such as cheese whey or dairy industry wastes owing to its exceptional physiological features and aptitude to produce heterologous proteins. This thermotolerant yeast is capable of growing at elevated temperatures, with a fast growth efficiency of 0.99/h at 40°C (Banat et al., 1992). The fermentation process at high temperatures can markedly decrease the cooling cost and contamination risk. Simultaneous saccharification and fermentation of lignocellulosic and other polysaccharide-based feedstocks at higher temperature can augment the enzyme efficacy and reducing the enzymes-associated costs. All these desired traits, such as fastest growth, thermotolerance, and broad substrate spectrum (i.e., hemi-cellulose hydrolysates, xylose, l-arabinose, d-mannose, galactose, maltose, sugar syrup molasses, cellobiose, dairy industry waste, such as cheese whey, lactose, and galactose) constitutes K. marxianus, a versatile host for applications in the food, feed, and pharmaceutical industries (Löser et al., 2013; Morrissey et al., 2015; Mo et al., 2019a; Mo et al., 2019b; Lyu et al., 2021).
Increasing worldwide demands for biomolecules to be used in foods and other products necessitate exploiting the K. marxianus potential in biotechnological applications, including enzymes production, ethanol fermentation, single-cell protein, vaccine preparation, and probiotics (Figure 1). However, the lack of fundamental information about its genetics and physiology is the major drawback for its advancement (Morrissey et al., 2015). This scenario is quickly changing, where significant progress is being devoted to optimizing cultivation conditions and fermentation processes. Moreover, evolutionary or rational engineering approaches have also been applied to develop new strains with unique attributes.
Studies regarding the yeast, K. marxianus, are increasing in the recent years. Based on transcriptome analysis, Diniz et al. (2017) analyzed the metabolic flow through the central metabolic pathways and found impaired under the ethanol stress. They observed that the genes involved in ribosome biogenesis are downregulated and gene-encoding heat shock proteins are upregulated. These results can provide evidence to restructure K. marxianus to obtain higher ethanol tolerance of K. marxianus than S. cerevisiae, which makes K. marxinus a more robust host producing ethanol than S. cerevisiae. To widen the substrate range, Anandharaj et al. (2020) constructed K. marxianus to express the largest cellulosome complex on the cell surface of K. marxianus, where 63 enzymes were displayed on the surface, enabling this yeast to efficiently degrade cellulosic substrates and accumulate 3.09 and 8.61 g/L of ethanol from avicel and phosphoric acid-swollen cellulose, respectively. Recently, K. marxianus was rationally engineered to produce aromatic products, such as 2-phenylethanol (2-PE), by a series of metabolic engineering strategies, including modification and overexpression of the pathway to be resistant to feedback inhibition, for overproducing phenylalanine de novo from synthetic minimal medium (Rajkumar and Morrissey, 2020). Gene resources and transcriptome data of K. marxianus are particularly useful for fundamental and applied research for innovative applications, bearing beneficial properties of temperature resistance, wide-range bioconversion ability, and production of recombinant proteins (Lertwattanasakul et al., 2015). In this review, we provide the current insight into the potential applications of K. marxianus for producing an array of various industrially pertinent bioproducts. The CRISPR–Cas9-assisted precise genome editing in K. marxianus is also delineated for broadening the scope of K. marxianus in biotechnology.
2 Applications in Biotechnological Products
2.1 Enzyme Production
Kluyveromyces marxianus is regarded as an emerging host platform for synthesizing extracellular proteins owing to its capability to grow on an array of cheap feedstocks, including whey, molasses, and spent sulphite liquor (Fonseca et al., 2008). It is also capable of growing on various polysaccharides, such as inulin and pectin (Hensing et al., 1994). K. marxianus exhibits inherent ability to produce enzymes because all complex materials stated earlier can be extracellularly hydrolyzed to monomers (Chi et al., 2011). The K. marxianus growth on inulin and sucrose occurs via the catalytic action of extracellular enzymes, mainly inulinase (Rouwenhorst et al., 1988). The degradation of lactose by β-galactosidases excretion is promising because of simultaneous synthesis of biologically active ingredients, biomass, and enzyme biocatalysts of industrial importance (Fonseca et al., 2008; Chi et al., 2011). A recent summary of different enzyme productions by K. marxianus strains is depicted in Table 1. Figure 2 shows the schematic illustrations of uptake mechanism and metabolism of lactose in K. marxianus.
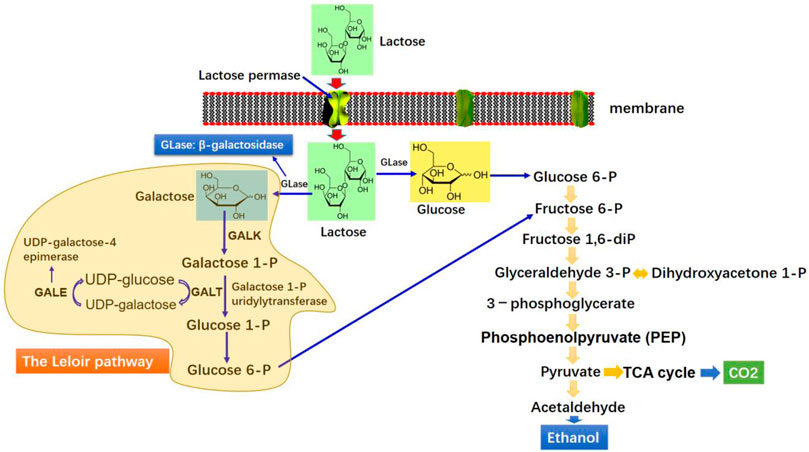
FIGURE 2. Schematic illustration of the catabolic pathway of lactose and ethanol production by K. marxianus.
2.1.1 Inulinases
Inulinase (EC 3.2.1.7) is extensively employed to hydrolyze inulin for producing fructose, fructo-oligosaccharides, and bioethanol, which are the imperative ingredients in pharmaceutical and food industries. Various microbial strains, such as yeast: K. marxianus (Selvakumar and Pandey, 1999), filamentous fungi: Aspergillus fumigatus, A. niger, Rhizopus sp., Penicillium sp. (Ohta et al., 2002; Moriyama and Ohta, 2007; Kango, 2008), and bacteria: Staphylococcus sp., Bacillus sp., Streptomyces sp., Pseudomonas, and Xanthomonas (Selvakumar and Pandey, 1999; Kalil et al., 2005; Gao et al., 2009) have been used to produce inulinases. Nonetheless, K. marxianus has been considered the most prodigious host to produce a commercial scale inulinase among all the inulinase-producing microbial strains (Kalil et al., 2005; Zhang et al., 2012).
Recently, Hoshida et al. (2018) investigated the biosynthesis of extracellular proteins by K. marxianus using culture media comprising various sugars, such as xylose, glucose, and galactose. Inactivation of INU1 in DMKU3–1042 resulted in the disappearance of extracellular protein and growth retardation in inulin and sucrose media, representing the extracellular protein as an inulinase (sucrase). Among the 16 K. marxianus strains analyzed, six strains produced a high level of inulinase in xylose than glucose-based fermentation medium. Nevertheless, a lower activity of INU1 promoter in the xylose medium than glucose medium suggests the controlling of elevated inulinase production in a posttranscriptional manner. Cultures with more agitation also led to produce high titer of inulinase, indicating that oxygen supplementation might influence the inulinase production. Overall, both xylose and oxygen supply improve the titer of extracellular inulinase. An inulinase gene (INU1 gene) was overexpressed in K. marxianus to attain hyperproduction of inulinase and ultrahigh-fructose syrup. Overexpression was accomplished by codon optimization following assortment of an appropriate promoter, and inulinase enzyme was synthesized by a high-cell density fermentation mode. Results revealed that codon optimization of the native INU1 gene generated an engineered strain KM-N70 (harboring the optimized INU1N gene) that was able to synthesize a high titer of the inulinase (251.4 U/ml). The inulinase activity reached 338.5 U/ml by a recombinant KM-KN16 strain carrying the optimized INU1N gene with a native TPS1 promoter from K. marxianus KM-0. KM-KN16 strain produced 374.3 U/ml of inulinase within 24 h in 10-L fermentation, while the inulinase titer reached 896.1 U/ml by the recombinant KM-KN16 strain during high-cell density fed-batch fermentation. Furthermore, over 90% of inulin was degraded to glucose and fructose monomers in tubers of Jerusalem artichoke extract by the catalytic action of inulinase preparation in 8 h. Together, the outcomes render recombinant KM-KN16 as the highest producer of inulinase than other bacterial, fungal, and yeast strains (Zhang et al., 2019).
By increasing the glucose concentration from 10 to 20 g/L, the activity of inulinase by K. marxianus KM-0 was greatly enhanced; however, further glucose increments to 80 m/L decreased the inulinase activity that might be ascribed to the repressive effect of glucose on the biosynthesis of enzyme. Transcriptional repressor Mig1 encoded by MIG1 is likely to play a major role for glucose repression. Nevertheless, recombinant K. marxianus KM-69 (inactivated MIG1 gene) yielded a higher inulinase titer (101.7 U/ml) than non-modified K. marxianus KM-0 strain (84.3 U/ml). Overexpressing native inulinase gene from KM-0 into KM-69 additionally improved the inulinase titer to 119.3 U/ml (Zhou et al., 2014). For further enhancing the production of inulinase by the yeast, derepression of glucose repression, and overexpression of the native inulinase gene are important. After disruption of the MIG1 gene in the K. marxianus KM-0, the resultant glucose-derepressed mutant (KM-69) yielded 94.6 U/ml of inulinase in the inulin-based fermentation medium within 36 h. Afterward, the overexpression of a native inulinase gene in the KM-69 mutant further increased the level of inulinase. During the 10-L fermentation, the engineered KM-526 strain produced 133.5 U/ml within a short period of 24 h. Inulinase secreted by recombinant KM-526 effectively catalyzed the transformation of inulin into monosaccharides compared to K. marxianus KM-0-derived counterpart. Therefore, disruption of the MIG1 gene together with native INU1 gene overexpression is meaningful for enhancing the yield of inulinase in K. marxianus KM-0 in short duration, indicating its usefulness in food industries (Zhou et al., 2014).
2.1.2 β-Galactosidases
β-Galactosidases (EC 3.2.1.23) are among the most widely utilized biocatalysts in the food industry. These are also referred to as lactase involved in hydrolyzing lactose to a mixture of glucose and galactose. These enzymes have enormous applications in the food and pharmaceutical industries and can be applied for whey saccharification and milk treatment to reduce lactose (Singh et al., 2016). Lactose reduction is primarily useful for the individuals with inherited disorders for lactose breakdown, such as the black people in Central Africa, Brazil, and United States (Bayless et al., 2017). A plethora of Kluyveromyces strains have been described as large-scale producers of β-galactosidases. A number of different approaches with diverse cultivation strategies have been attempted for the biosynthesis of β-galactosidases from cheese whey and molasses (Morrissey et al., 2015; Padilla et al., 2015). Indispensable food processing-related activities like hydrolytic and transgalactosylic activities are generally executed by the use of commercial β-galactosidases (Pivarnik et al., 1995). Enzyme-mediated hydrolysis is implicated for lactose reduction in milk in the food sector, whereas the transgalactosylation is carried out for synthesizing galactose and galacto-oligosaccharides-containing products (Oliveira et al., 2011). Padilla et al. (2015) reported the successful lactulose oligosaccharides production via transgalactosylated whey permeate isomerization using the β-galactosidases from K. marxianus and K. lactis.
The expression of β-galactosidases in K. marxianus is regulated by its natural inducers, lactose, and galactose (Liu et al., 2021). Nonetheless, β-galactosidases production depends on the concentration of substrate. The repressive mechanism was superimposed to the substrate inducing effects when the strain was exposed to an elevated level of d-glucose, or lactose, resulting in reduced β-galactosidases activity. This may be attributed to the accumulation of glycolysis metabolite intermediate when microbial cells assimilate d-glucose or lactose with a high rate (Martins et al., 2002). Zhou et al. (2013) observed the suppressed biosynthesis of β-galactosidase in the presence of glucose; however, the production level was restored by the elimination of the MIG1 gene. After disruption of the MIG1 gene, the resulting K. marxianus KM-15 strain accumulated a high titer of β-galactosidase compared to the native strain. Under the optimized processing conditions, galactosidase activity by the disruptant KM-15 reached 121 U/ml within 60 h. The fermentative potential of okara (soybean residue) feedstock was explored to produce β-glucosidase by K. marxianus (Su et al., 2021). It was found that okara notably induced the synthesis of β-glucosidase reaching 4.5 U/mg under the optimal fermentation conditions of okara 30 g/L, temperature 35°C, inoculum size 10%, and cultivation duration 98 h. The enzyme was a dimer of 66 kDa with two different subunits (44 and 22 kDa). It presented high-acid–alkali resistance, thermal stability, and inhibition tolerance to DMSO (10–50%) (Su et al., 2021).
2.1.3 Lipases
Lipases or triacylglycerol acyl hydrolases (EC 3.1.1.3) are a fascinating class of hydrolytic enzymes, which mediate the hydrolysis as well as synthesis of esters. Generally, they catalyze the breakdown of acyl glycerides that are prerequisite for lipids (triacylglycerol) bioconversion (Vakhlu, 2006). Lipases are endowed with a set of unique attributes including regiospecificity, stereospecificity, substrate specificity, and capability of performing reactions at the interface of water soluble and insoluble systems (Sharma et al., 2002). Similar to proteases or carbohydrases, microbial originated lipases are of prodigious industrial importance owing to high stability than animal or plant counterparts and bulk production at low cost (Vakhlu, 2006). Moreover, lipases from microbial sources offer broad spectrum biocatalytic activities, rapid microbial growth on cheap media, easy genetic amendments, and lack of seasonal fluctuations (Šiekštelė et al., 2015). Among the bacterial and fungal strains, fungal lipases are widely explored, thanks to their unique features, such as efficient extraction procedure, substrate specificity, thermal and pH tolerance, and activity preservation in organic media (Sarmah et al., 2018).
Although the production of lipase is predominant among yeasts, only a few species are able to secrete lipases with desired characteristics and inadequate amount for industrial exploitation. Y. lipolytica, Candida rugosa, Candida utilis, Candida antarctica, Saccharomyces, and K. marxianus have been found as the yeasts with high-lipase synthesizing capabilities (Sarmah et al., 2018). Deive et al., 2003) assessed the capability of K. marxianus for the production of lipases and evaluated the influence of surfactants and various lipidic compounds on the synthesis and secretion of enzyme. A complex liquid medium containing different inducers, like fatty acids and triacylglycerols supported the maximum production of lipases by K. marxianus. The utmost enzyme titer (80 U/ml in 3 days) was recorded in the medium with the inclusion of 5 g tributyrin/L and 2 g urea/L. However, the inclusion of surfactants does not have favorable effect on the production.
In addition to physicochemical parameters like temperature, pH, and dissolved oxygen, medium composition can also significantly affect the lipase production from K. marxianus. For example, Stergiou et al. (2012) evaluated the optimization of various processing factors that influence the production of extracellular lipase by K. marxianus IFO 0288. Results revealed that the production was 18-fold increased using optimal cultivation (35°C, pH 6.5, 150 rpm) and nutritional (0.5% olive oil) conditions after the 65-h fermentation of olive oil as a growth substrate. In another study, K. marxianus 83F presented higher lipase production activity in Serro Minas cheese, indicating it as a promising starter culture for cheese production owing to desired sensory features (Cardoso et al., 2015). Due to the presence of high fatty acids contents, avocado oil was tested as an inducing agent to synthesize lipolytic enzymes from K. marxianus L-2029. For determining the best avocado oil levels in the yeast medium, induction was performed for 24 h using different concentrations. K. marxianus L-2029 accumulated the highest productivity of extracellular lipase at 3.5% v/v avocado oil, pH 6, and 36°C (Martinez-Corona et al., 2019). In another study, the same authors carried out a bioinformatics analysis of genes from K. marxianus L-2029 for the identification and characterization of extracellular lipases. By in silico translation, amino acid sequence of 10 putative lipases was obtained ranging between 389 and 773 amino acids. Among these, two putative proteins exhibited a signal peptide, 33 and 25 amino acids long for KmLIP3p and KmYJR107Wp, and a molecular mass of 58.23 and 44.53 kDa, respectively. From the 3D models of K. marxianus putative L-2029 lipases, the conserved pentapeptide was determined to be GHSLG for KmYJR107Wp and GTSMG for KmLIP3p. The phylogenetic analysis of all K. marxianus lipases demonstrated evolutionary affinities with lipases from abH23.01, abH23.02, and abH15.03 and families (Martínez-Corona et al., 2020). In fact, microbial lipases are an interesting class of biocatalysts with prospective applications in medical and pharmaceutical, detergent, food, baking, dairy, flavor, organic synthesis, paper manufacturing, perfumery, cosmetics, leather, biosurfactant, agrochemical, oleochemical, bioremediation, and biosensors related industries (Sarmah et al., 2018).
2.1.4 Pectinases
Endo-polygalacturonases (EC 3.2.1.15), generally referred to as pectinases, catalyzes the hydrolysis of heteropolysaccharide pectins that comprise the major structural component of the plant cell walls. Due to cell wall degradation abilities, pectinases find increasing applications in the wine and juice manufacturing (Alimardani-Theuil et al., 2011). Among the pectinase extracted from plants and various microbial strains including filamentous fungi, yeasts, and bacteria, yeast pectinases have gained considerable importance in the recent past. Pectinolytic yeasts can synthesize diverse types of pectinolytic enzymes depending on the genetic background and environmental conditions. Four yeast species, S. cerevisiae, S. fragilis, Torulopsis kefir, and K. marxianus have been largely studied to produce pectinolytic enzymes. These enzymes include pectin lyases (PL), polygalacturonase (PG), pectate lyase (PaL), and pectinesterase (PE) based on the pH, temperature, substrate availabilities. For example, Kluyveromyces, Saccharomyces, and Candida synthesize PG (i.e., endopolygalacturonase), while PG and PE are the major enzymes secreted by Rhodotorula (Alimardani-Theuil et al., 2011). Oliveira et al. (2012) investigated the impact of various pectic feedstocks, temperature, pH, and glucose on the polygalacturonase biosynthesis by K. marxianus CCMB 322. The enzyme secreted in the culture filtrate of K. marxianus CCMB 322 was found to be partially constitutive, exhibiting optimal temperature and pH of 70°C and 7.36, respectively. It retained over 90% of its initial activity for 50 min at a high temperature of 50°C. It was found that supplementation of glucose in the medium can affect the regulation of polygalacturonase synthesis. In order to determine optimal pH, temperature, and incubation time for pectinase production by K. wickerhamii, Moyo et al. (2003) applied a central composite rotatable design. pH and temperature were found to be the most influential factors that affect the enzyme synthesis. The best enzyme yield was recorded at pH 5.0 and 32°C after fermentation duration of 91 h. The pectinolytic enzyme produced was referred to as polygalacturonase (PG), thermotolerant at different temperatures. Its activity was enhanced by Ca2+ ions; however, Zn2+, Mg2+, Na+, Mn2+, and Co2+ ions inhibited the activity (Moyo et al., 2003).
2.2 Bioethanol Production
Fermentative production of bioethanol at elevated temperatures has gained attention because the rapid fermentation rate at high temperature is likely to minimize the cooling cost, circumvent the contamination risk, and enables simultaneous saccharification and fermentation (Nonklang et al., 2008). The optimal K. marxianus growth at a high temperature is particularly alluring to facilitate cooling during the extensive level fermentations for which transferring heat is a restrictive factor. Current industrial production of ethanol mainly employs S. cerevisiae due to its superior production titer and tolerability to high ethanol concentrations (about 120 g/L). Nevertheless, the optimal temperature of this yeast is relatively low (25–30°C) (Qiu and Jiang, 2017). Therefore, much interest has recently been geared to explore yeast species that are capable of producing ethanol at higher temperatures, and K. marxianus appears a robust isolate for this purpose (Figure 2) (Madeira-Jr and Gombert, 2018). Table 2 gives a recent summary of ethanol production by various K. marxianus strains. K. marxianus species are thermotolerant and can grow at 47 (Nonklang et al., 2008; da Silva et al., 2018), 49 (Hughes et al., 1984), and even 52°C (Banat et al., 1992). It is able to produce ethanol at temperature above 40°C (Madeira-Jr and Gombert, 2018), and assimilate a wide range of cheap substrate, including molasses, whey permeates (Hang et al., 2003; Ozmihci and Kargi, 2007; Martínez et al., 2017), and corn silage juice to synthesize ethanol. Given all these features, K. marxianus is appreciated as a prospective alternative to S. cerevisiae for commercial scale ethanol production.
Production of ethanol from non-food substrate is favorably suggested for avoiding criticism with food supply. Nutrients enriched whey can be considered a non-food low-cost substrate for producing ethanol by Kluyveromyces spp. Tesfaw et al. (2021) achieved the optimized ethanol production from crude whey using a newly isolated K. marxianus ETP87 strain. The non-sterilized and sterilized whey were utilized as feedstocks to assess the K. marxianus ETP87 competition with lactic acid and other microflora. The use of sterilized whey resulted in a slightly higher amount of ethanol than non-sterilized counterpart. Likewise, a substantially higher ethanol titer was achieved from whey without pH adjustment, and yeast accumulated the maximum ethanol between 30 and 35°C. It was capable of producing high level of ethanol until 45°C, and ethanol titer was drastically reduced when temperature was increased at 50°C. Inclusion of ammonium sulfate and peptone stimulated the ethanol production, while yeast extract and urea have negative effect on the ethanol productivity. Based on a circular economy concept, Tinôco et al. (2021) produced cellulosic ethanol by K. marxianus CCT 7735. Following acid–base pretreatment and hydrolysis at 50°C for 3 days, the saccharified sweet sorghum was subjected to ethanol production at three different temperatures (37, 42, and 45°C). Precisely, 17.83 g/L ethanol was produced after 24 h at 42°C with a corresponding yield of 2769.8 L/hasorghum, which is double compared to corn straw.
In a recent study, Madeira-Jr and Gombert (2018) demonstrated that the production of bioethanol at elevated temperatures (∼48°C) by K. marxianus NCYC 3396 using sugarcane can reduce cooling costs, risk of contamination, use of antibiotics, water usages, consumption of H2SO4 in cell reprocessing, and the use of energy in distillation, which ultimately reduce the bioethanol production cost in Brazilian biorefinery. Using K. marxianus, they achieved a yield of 0.40 g ethanol/g glucose at 48°C that was similar to S. cerevisiae CEN.PK113-7D at 37°C. Though the ethanol titer (0.43 g/g glucose) was comparable by K. marxianus K213 and S. cerevisiae using glucose at 30°C, S. cerevisiae lost its ethanol production ability when temperature raised to 45°C, while K. marxianus K213 retained the same conversion efficacy (0.43 g/g glucose) at this temperature (Yan et al., 2015). Murari et al. (2019) also corroborated that temperature (32.5–35°C) was the most influential parameter to produce ethanol from cheese whey by K. marxianus URM7404 following pH (4.8–5.3) and lactose concentration (61–65 g/L).
Raw starch-degrading enzyme (RSDE) is being employed in the ethanolic fermentation bioprocess for hydrolyzing starchy materials, such as cassava chips and cassava starch (Lomthong et al., 2015; Lomthong et al., 2016). Using RSDE reduces processing costs and energy consumption because the hydrolysis of starch granules is carried out below the gelatinization temperature than traditional starch degradation that occurs at 80–100°C (Cinelli et al., 2015; Lomthong et al., 2018). In a recent study, Lomthong et al. (2016) produced RSDE by Laceyella sacchari LP175 and applied to hydrolyze cassava chips at 50°C. The resulting sugar syrup was used as a fermentative substrate for bioethanol production. The saccharification and hydrolysis of cassava chips was carried out at lower temperature without heating-fostered ethanol fermentation by K. marxianus DMKU-KS07 at 42°C (Trakarnpaiboon et al., 2017). Similarly, molasses is enriched with high sucrose contents and other nutrients, which are conducive for microbial growth to produce bioethanol. Arshad et al. (2017) utilized molasse substrate for very high gravity (VHG) bioethanol by S. cerevisiae, yielding the highest ethanol titer to be 122 g/L. In contrast to other feedstocks, molasses present the benefits of bioethanol production without any pretreatment and saccharification (Chotineeranat et al., 2010). Nevertheless, a high concentration of metal ions impedes the activity of microbial enzymes and might lower ethanol yield (Chotineeranat et al., 2010; Wattanagonniyom et al., 2017). Under non-sterile conditions, Lomthong et al. (2021) evaluated the improvement of VHG bioethanol titer using molasses and cassava chip co-substrate hydrolysates by K. marxianus DMKU-KS07 at 42°C. Results showed a high bioethanol concentration of 118 g/L with ethanol yield (YP/S) at 0.44 g EtOH/g total sugar and productivity of 2.19 g/L/h by simultaneous saccharification and fermentation at 42°C for 12 h.
2.3 Single-Cell Proteins
Single-cell proteins (SCPs) are referred to as dietary single-cell microorganisms, whose protein extracts or biomass are originated from mixed or pure bacterial cultures, yeasts, mushrooms, or microalgae. These microorganisms can be utilized as food ingredients, dietary supplements, or high protein foods for animal and human consumption (Ritala et al., 2017). Thus, extensive biosynthesis of microbial biomasses could be useful to replace animal- and plant-derived proteins for food or feed owing to high-protein contents, fast proliferation rate, and the aptitude to assimilate a wide spectrum of carbon containing waste materials (Karimi et al., 2018; Karim et al., 2020). Given a shorter microbial life cycle, SCPs can be attained rapidly than that of the proteins produced from agricultural sources. Amid the SCP producers, yeasts have appeared as emerging cell factories due to tiny particle size, large protein content, facile handling, and comparatively minimum production costs. K. marxianus is a promising candidate with considerable potential for SCP biosynthesis and thus extensively employed as a feed organism in many countries. Compared to S. cerevisiae, K. marxianus displays a high specific growth efficiency and highest biomass yield in aerobic continuous fermentation. Yadav et al. (2014a) utilized K. marxianus GQ 506972 to produce SCP with a simultaneous COD elimination of cheese whey. They achieved 6 g/L of biomass along with over 50% removal of COD at pH 3.5 and 40°C after 36 h of batch fermentation. Increase in the inoculum level led to increased biomass production of 15 g/L and 80% removal of COD. The same authors also appraised the efficiency of the mixed culture system for obtaining high-quality SCP and enhancing the COD removal in continuous and batch fermentations of whey under cultivation conditions of pH 3.5 and 40°C (Yadav et al., 2014b). The coculture (C. krusei and K. marxianus) gives rise to 19% greater biomass yield and 33% higher productivity with concurrent 8.8% elimination of COD than the single culture in the batch system. The SCP produced from coculture contained essential amino acids and a desired protein content, indicating a mixed culture prodigious approach for producing SCP and wastewater treatment (Yadav et al., 2014a; 2014b). Yadav et al. (2016) produced food-grade SCPs at pH 6.5 and 30°C using a coculture system of S. cerevisiae and K. marxianus. In comparison to single culture (84%), the mixed culture resulted in 92% of total whey proteins under the optimal conditions. Many microorganisms including K. marxianus, C. kefyr, and S. cerevisiae have been grown in the food wastes via SSF. Among these, K. marxianus contains the maximum concentration of fat and protein, and thus might be utilized to enrich livestock feed (Aggelopoulos et al., 2014).
2.4 Probiotic Role
Probiotics are the living microorganisms that confer health benefits on the host by administrating inadequate amounts (Hill et al., 2014). These include Lactobacillus, Clostridia, Bifidobacterium, Enterococcus, Faecalibacterium, and Propinonibacterium (Altieri, 2016). Although S. cerevisiae var. boulardii has also been considered a probiotic since many years (Moré and Swidsinski, 2015), growing interest is diverted in recent years to explore the probiotic potential of non-conventional yeasts. K. marxianus is regarded as a promising probiotic due to its modification capacity in the adhesion, cell immunity, and human gut microbiota. In addition, it also possesses anti-inflammatory, antioxidant, and hypocholesterolemic features (Xie et al., 2015; Cho et al., 2018). It might be able to survive in the digestive tract reaching the intestines to serve as prebiotics due to acid and bile tolerance present in the gastrointestinal environment. K. marxianus isolated from kefir presented cholesterol-lowering capability, reducing 30% of cholesterol even greater than K. lactis ATCC 34440 and S. cerevisiae ATCC 6037 (Cho et al., 2018). K. marxianus AS4, obtained from dairy products, that is, cheese and yogurts, has shown excellent resistance to acidic pH, high bile salts, and superior antimicrobial activity. It also revealed outstanding anticancer activity against gastrointestinal tumor cells due to the secretion of biologically active metabolites, downregulating Bcl-2 gene, upregulating a pro-apoptotic protein, and BAD and CASP9 gene expression system (Saber et al., 2017). Among various yeast strains with probiotic efficiency, K. marxianus JYC2614 showed the best tolerance to bile salt and acid, as well as good cell surface hydrophobicity and satisfactory auto-aggregation (Hsiung et al., 2021). Recently, Goktas et al. (2021) determined the probiotic properties of yeast species isolated from kefir samples and compared with a commercial probiotic yeast S. boulardii. In contrast to S. boulardii, Kefir isolates, K. marxianus and S. cerevisiae, showed hydrophobicity, high auto-aggregation, and antioxidant potentialities. They also demonstrated potent antifungal activities against some mold species. Motey et al. (2020) assessed the probiotic characteristics of K. marxianus and S. cerevisiae, which were isolated and identified from the West African milk products nunu, lait caillé, and a cereal-based product mawè. Isolates were capable of growing in bile salts with the highest specific growth rate (μmax) of 0.58–1.50 hour−1. Adhesion of yeast to Caco-2 cells was found to be strain-specific varying between 8.0 and 36.2%. At the gastrointestinal pH environment, S. cerevisiae and K. marxianus also maintained the pHi homeostasis and increased transepithelial electrical resistance across the Caco-2 monolayers, indicating their appreciable probiotic efficiency in West African fermented products. In another work, K. marxianus PTCC = 5189 was utilized for the production of probiotic yoghurt, which was then contaminated with Penicillium notatum PTCC = 5074 and Aspergillus parasiticus PTCC = 5018. The contaminants and changes in the count of probiotic yeasts during the storage at 4°C at 28 days were assessed and subjected to comparison with the control. Because of K. marxianus, the numbers of yoghurt adulterating molds were significantly reduced. K. marxianus population was satisfactory after 28 days of storage for providing a probiotic product with 7.35 log CFU/gr (p < 0.01), indicating its usefulness to produce probiotic yoghurt on an industrial scale (Oroojzadeh, 2020).
Wang et al. (2017) studied the impacts of K. marxianus addition on intestinal structure, immune responses, and growth attributes in broiler chickens. Experimental results revealed that increasing supplementation of K. marxianus resulted in reduced feed conversion efficiency but a linear improvement in relative thymus weight, increased serum IgG, and lysozyme levels. Better feed conversion rate and immune function along with intestinal structure in broilers by K. marxianus supplementation might be ascribed to the better structure of ileal microbes.
2.5 Fructo-Oligosaccharides Production
Fructose is a potential alternate sweetener to sucrose owing to its high sweeting capacity (1.5–2 times than sucrose) and could increase the absorption of iron in children. Fructo-oligosaccharides (FOS) are considered a prospective source of dietary fiber with bifidogenic effects. They are utilized as food ingredients owing to their health beneficial properties such as maintaining a good balance in the intestinal flora and promoting the multiplication of bifidobacterial community in the intestine. Recently, they have granted the “GRAS” status by the FDA (Flores-Gallegos et al., 2015). Both FOS and fructose can be produced through the enzymatic degradation of inulin; however, the synthesis was typically carried out at an elevated temperature (around 60°C) because of inadequate solubility of inulin at room temperature. Therefore, it is of great significance to characterize and isolate thermotolerant inulinases for catalyzing the hydrolysis of inulin at higher temperatures (Flores-Gallegos et al., 2015). Inulin is regarded as a good feedstock for producing inulo-oligosaccharides by endoinulinase-mediated catalytic reactions, but lack of solubility in cold water or slight solubility (only 5%) in water at 55°C remains a major challenge for its hydrolysis. This fact necessitates the exploration of inulinase that are capable of working at higher temperatures for industrial applicability. In this perspective, K. marxianus can be considered a robust candidate for FOS production. Struyf et al. (2017) developed a yeast-based approach by an inulinase-producing K. marxianus strain that resulted in over 90% fructan reduction in the final product, whereas only 56% fructan was reduced in the case of S. cerevisiae strain as a control. In order to develop a high-performing endo-inulinase for FOS biosynthesis from inulin, an inulin-binding module (IBM) was integrated into C- or N- terminal of the enzyme. The C-terminal fusion one (Eninu-IBM) with high activity, thermal stability, and inulin binding efficiency was used to catalyze inulin break down at high-temperature in a 10-L bioreactor. Results obtained high purity FOS (91.4%) with FOS titer, productivity, and yield of 717.3 g/L, 358.6 g/L/h and 0.912 gFOS/g inulin, respectively (Mao et al., 2019). Risso et al. (2012) investigated the FOS production by carrier-supported inulinase from K. marxianus NRRL Y-7571 in aqueous and aqueous-organic systems using sucrose as a substrate. In the case of an aqueous–organic system, the utmost yield of FOS (18.2 ± S0.9 wt%) was achieved at optimal condition of pH 5.0, 40°C, aqueous/organic ratio (25/75 wt%), enzyme activity (4 U/ml), and sucrose concentration of 60% (w/w), whereas the highest yield of FOS was achieved to be 14.6 ± 0.9 wt% for the aqueous system.
Various yeasts, including C. kefyr, Debaryomyces antarelli, and K. fragilis or K. marxianus have been used to produce inulinases (Beluche et al., 1980; Negoro and Kito, 1973; Holyavka et al., 2016). Among these, K. marxianus is preferred for fructose biosynthesis because of their growth on fructans, such as inulin; thus, inulinases can hydrolyze plant-originated fructans (Chi et al., 2011). A considerable amount of inulin is present in the tubers of various plants, such as dahlia, yacon, chicory, and Jerusalem artichoke. Biosynthesis of fructose by enzyme-assisted hydrolysis of plant extract-derived oligo- and polysaccharides by K. marxianus-immobilized inulinases could be a gainful strategy for large scale production of sugar (Holyavka et al., 2016). Singh et al. (2016) applied duolite A568-immobilized extracellular exoinulinase from K. marxianus YS-1 for the production of high-fructose syrup. The catalytic action of biocatalyst generated 39.2 and 40.2 g/L of fructose in 4 h using raw and pure inulins, respectively. In addition, inulinases secreted by K. marxianus using xylose medium is another appealing way for producing elevated titer of fructose syrup (Hoshida et al., 2018).
2.6 Vaccine Candidates
Porcine circovirus (PCV), belongs to the genus Circovirus, family Circoviridae, is among the smallest animal virus consisting of a single-stranded circular DNA (1.7–2 kb). Three genotypes of PCV include PCV1, PCV2, and PCV3. Among these, PCV1 was initially identified and characterized in infected PK15 cells (Tischer et al., 1974). This virus was considered non-pathogenic with widespread infection in all swine-producing areas (Allan et al., 2012). PCV2 is a pervasive kind of virus with considerable pathogenicity and closely related to the postweaning multisystemic wasting syndrome (PMWS), as well as other porcine circovirus diseases (PCVDs), such as respiratory disorder, enteric disease, reproductive failure, porcine dermatitis, and nephropathy syndrome (PDNS), resulting in massive economic losses in the swine industries (Chae 2005; Gillespie et al., 2009; Cruz et al., 2018). PCV3 is a new PCV genotype, which was initially recognized from piglets with cardiac pathology and multisystemic inflammation in the United States (Phan et al., 2016).
Vaccination is regarded as the best strategy for reducing the death rate and improving the cultivation of porcine circovirus disease-affected pig populations (Fachinger et al., 2008). PCV2 virus-like particles (VLPs) are a kind of subunit vaccine, which imitate PCV2 virus morphology and exhibit advantageous features over the inactivated vaccine and live-attenuated vaccines for their safety, non-replicating, and particulate structure (Wu et al., 2012). A number of host platforms, such as insect–baculovirus, E. coli, P. pastoris, S. cerevisiae, and silkworm pupae, have been explored and attempted to express CAP protein for obtaining PCV2 VLPs (Wu et al., 2012; Tu et al., 2013; Nainys et al., 2014; Masuda et al., 2018); however, the cost-effective production remain a major hindrance. Duan et al. (2019) reported PCV2 VLPs preparation by expressing PCV2d CAP protein in K. marxianus with a high yield of 1.91 g/L in a 5-L bioreactor. The yield obtained significantly surpassed the PCV2 VLPs achieved by E. coli, P. pastoris, and baculovirus–insect cell. The PCV2 VLPs was capable of inducing a high titer of anti-PCV2 IgG antibodies in mice serum together with decreasing the virus titers in spleens and livers of the tested mice. Spontaneously assembly of CAP protein into VLPs showed the prospects of cost-effective preparation of PCV2 vaccines. Recently, Yang et al. (2021) used K. marxianus to evaluate the expression and downstream processing of PPV VLPs. The VP2 protein from Kresse strain was subjected to expression following optimization by the codon bias of K. marxianus. After 48-h fermentation, the PPV VLPs yield reached 2.5 g/L in a 5-L fermenter. Two strategies, including cation exchange chromatography with Sephacryl® S-500 HR chromatography and anion exchange chromatography following cross-flow diafiltration were used for the purification of VLPs from the supernatants. Administration of mice with purified PPV VLPs (95% purity) resulted in the induction of high titers of IgG antibodies in sera, showing hemagglutination inhibitions on both guinea and swine pig erythrocytes. Cytokine’s detection and spleen lymphocyte proliferation suggest K. marxianus-induced PPV VLPs triggered the humoral and immune immunity responses in mice.
3 Biotechnological Improvement and Developing New Biotechnological Toolsets
Isolation of K. marxianus strains from different sources display substantial differences in physiological traits as well as productivity and yield of platform biomaterials and commodity chemicals. Notwithstanding such difference, biotechnology tools, and adaptation to stress, metabolic, or genetic engineering are promising to improve the inherent abilities of K. marxianus (Nurcholis et al., 2020). Sharma et al. (2017) carried out evolutionary adaptation on K. marxianus NIRE-K3 at 45°C using xylose as a solitary carbon source, yielding a new derivative with considerable enhancement in xylose assimilation, and yields and productivities of xylitol and ethanol. Likewise, an osmotolerant yeast strain with higher lactose assimilation capability from K. marxianus MTCC 1389, a strain with high ethanol biosynthesis and fast growth in lactose containing whey, was obtained by adapting to a high level of lactose (Saini et al., 2017). Kim et al. (2019) developed a thermally stable derivative with glucose and xylose co-fermentation capability by the alleviation of catabolite repression through evolutionary approach using 2-deoxyglucose. Increased activity of xylosidase was reported via evolutionary adaptation by cultivating K. marxianus in a xylose-containing medium (Behera et al., 2016). All these positive outcomes encourage additional in-depth evaluation of evolutionary adaptation approaches. Nevertheless, identification of key mutation points in these mutants remains to be challenging due to the lack of sufficient genomic data for the wild-type strains. Therefore, it is remarkably imperative to acquire comprehensive information on mutation points. A 2-deoxyglucose-tolerant K. marxianus DMKU-3-1042-derived mutant has a single-point missense mutation in RAG5 that causes reduced RAG1 expression and increased expression of INU1 (Nguyen et al., 2015; Nurcholis et al., 2019). By ethanol-driven laboratory evolution, Mo et al. (2019) acquired a mutant that possesses high ethanol yield at elevated temperature and ethanol conditions. They proposed a positive relationship between the ethanol production and tolerance by yeast and inferred that improved ethanol resistance is not due to a meaningful mutations or ploidy change but attributed to transcriptional reprogramming based on transcriptome and genome analyses.
Li et al. (2018) tailored K. marxianus DMKU3–1042 by introducing one amino acid substitution (K31E) of the TATA-binding protein Spt15. This strategy causes differential expression of many genes related to ethanol tolerance. Transcriptional factors Msn2 and Hsf1 of K. marxianus can improve both ethanol fermentation and cell growth of S. cerevisiae at higher temperatures (Li et al., 2017). Hua et al. (2019) developed a xylose and glucose co-utilizing strain by integrating gene knockout mutations of SNF1 or HXK1 with overexpressing xylose-specific transporter genes and xylose reductase. Cernak et al. (2018) created a heterothallic K. marxianus strain by genetic engineering to carry three important properties of thermo-resistance, lipid biosynthesis, and easy transformation of exogenous DNA. All these traits might result in the development of K. marxianus as a robust industrial platform to produce an array of bio-renewable chemicals. In another study, various promoter combinations (PACO1 and PMDH1) and secretion signal peptides (Scα-MFss and KmINUss) were used to construct strains bearing either plasmid-borne or genomically integrated constructs for secretion of a single-chain antibody (scFv) (Nambu-Nishida et al., 2018).
4 Mechanistic Insights Into the CRISPR–Cas9 System
The CRISPR–Cas system was first discovered to offer an immunological weapon for archaea and bacteria against invasive bacteriophages (viruses) and mobile genetic elements (Lander 2016; Mojica and Montoliu 2016). Based on effector module organizations, these systems were characterized into two different classes (six types). Among these, the type II CRISPR system from Streptococcus pyogenes is being well characterized and studied (Makarova et al., 2017). It is also one of the commonly applied toolboxes for genetic engineering of yeast strains. Cas9 protein is an RNA-assisted endonuclease that catalyze the cleavage of DNA double strands with two active parts— the RuvC and HNH domains. Due to the identification of Cas9 in bacterium, fusion of a nucleus localization sequence (NLS) to Cas9 is required to facilitate targeting the nucleus genomes of eukaryotes (Löbs et al., 2017a). Single-guide RNA (sgRNA) is another necessary constituent that guides Cas9 to targeting sites. The canonical sgRNA contains a trans-activating crRNA (tracrRNA) and a CRISPR-targeting RNA (crRNA). The first 20 base pairs complementary sequence at 5′ end of crRNA is important for Cas9 endonuclease functioning, and three-nucleotide protospacer adjacent motif (PAM). NGG must be found immediately at 3′ end of the desired locus in genome (Murugan et al., 2017). After sgRNA direction, Cas9 targets the genome-specific sequence with PAM, resulting in the cleavage of both DNA strands (Wang et al., 2015). Following DSBs introduction, the DNA repairing process is proceeded for preventing the cell death. Generally, non-homologous end joining (NHEJ) repair generates gene disruption by insertion or deletion (indel) mutation, whereas homologous recombination (HR) repair facilitates the substitution or insertion of target sequences with the presence of donor DNA.
5 Genome Editing in K. marxianus by the CRISPR–Cas9-Mediated System
Based on encouraging experiences of S. cerevisiae, the CRISPR–Cas9 system has now been widely applied in various non-conventional yeast strains. Although additional improvement and optimization are required, this system exhibit tremendous promise in genome manipulation and editing in yeasts. K. marxianus is well recognized as a perfect host for the biosynthesis of a plethora of chemicals and bioactive compounds due to its fastest growth rate and Crabtree-negative properties (Wagner and Alper, 2016). Since NHEJ is likely to play a major role in K. marxianus, comparatively longer homologous arms are required to edit HR (Hong et al., 2007). A stop codon was introduced in NHEJ core genes of cell type-specific regulator (Nej1) and DNA ligase 4 (Dnl4) by altering C to T at 16 to 19 bp upstream of PAM for repressing the effect of NHEJ. This results in 100% correct HR-mediated genome editing at the URA3 site applying a zeocin selection marker that harbor 1-kb homologous arms, leading to 4-fold improvement than that to native counterpart (Nambu-Nishida et al., 2017). Application of a wide-host range CRISPR–Cas9 system in K. marxianus haploid and diploid strains caused over 80% disruption of ADE2 with 24% HR-based repair (Juergens et al., 2018). This low efficiency of HR substantiates the major role of NHEJ in DSB repair. Löbs et al. (2017b) characterized the functional genes related to the biosynthesis of ethanol and ethyl acetate in K. marxianus by applying the CRISPR–Cas9 system. Three different kinds of hybrid pol III promoters, such as SCR1-tRNAGly, SNR52-tRNAGly, and RPR1-tRNAGly, were utilized to confirm the functional expression of various sgRNAs. Among the promoters, RPR1-tRNAGly presented the maximum editing efficiency (66%). Screening of alcohol-O-acetyltransferase (ATF) and alcohol dehydrogenase (ADH) genes corroborated that ADH7 plays a main contribution as an alternate pathway to biosynthesize ethyl acetate. This work provides an excellent example that the CRISPR–Cas9 system has the capability of rapidly constructing gene disruption sets to characterize metabolic pathways and genes associated with the biosynthesis of valued chemicals.
The wild-type K. marxianus yeast is used to produce aroma and fermented products; however, the lack of standardized toolsets for metabolic and pathway engineering hinders its potential as a microbial cell factory. In this regard, Rajkumar et al. (2019) brought together and characterized an assortment of native K. marxianus-specific parts and vectors to express multiple genes for its genetic engineering and synthetic biology. More than 30 inducible and constitutive promoters, centromeres, terminators, and a number of known autonomously replicating sequence (ARS) elements were chosen from transcriptomic and genomic data and presented as a part of this assortment (Figure 3). All parts were cloned following the MoClo/Yeast Tool Kit standard for fast construction. They also selected various chromosomal integration sites for quick screening and developed a single-plasmid CRISPR/Cas9-based platform for genome editing and gene targeting for the rational creation of auxotrophic strains to broaden the scope of selective markers for K. marxianus. Overall, this collection is considered as a valued toolset for the research community interested in K. marxianus to build next-generation cell factories.
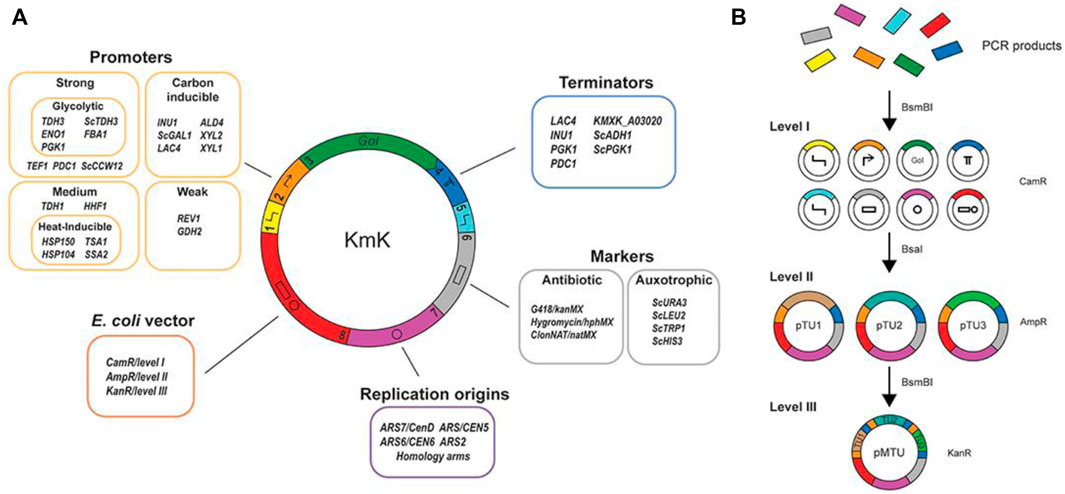
FIGURE 3. Collection of biological parts and synthetic biology tools for Kluyveromyces marxianus. Reproduced with permission from Rajkumar et al. (2019); an open-access article distributed under the terms of the Creative Commons Attribution License (CC BY).
6 Concluding Remarks and Future Perspective
Thanks to recent advances in biotechnological toolsets and strategies, K. marxianus has become an attractive non-conventional yeast compared to S. cerevisiae (a model yeast) for application in food, environment, and biotechnology. This yeast is endowed with a number of exceptional attributes, such as thermal tolerance, high ethyl acetate synthesis, ability to utilize various low-cost carbon sources (i.e., xylose, lactose, and inulin), fast growth rate, and short replication time. In addition, its survival ability in the presence of high temperature and elevated concentration of ethanol indicates its enhanced adaptation capability. The tolerance capacity of K. marxianus has been demonstrated in numerous literature reports against stress conditions, such as acidic pH, high temperature, high ethanol concentration, and severe oxygen-depleted condition, which might be advantageous features for future industrial exploitability. Furthermore, the production of high-value fragrance and flavor biomolecules, single-cell proteins, single-cell bio-oil, and protein makes K. marxianus an interesting tool for food industries by expanding the product portfolio. Nevertheless, most of the investigations on K. marxianus have been conducted using liquid fermentation (LF) that is likely to inhibit microbial cells because of a high concentration of the final product in the fermenting medium. In order to address this setback, various approaches like medium standardization, development of new strains, cell immobilization, and removal of product were generally employed utilizing synthetic media. Due to low water content, small reactor capacity, and no additional stirring, solid-state fermentation (SSF) using waste biomasses is preferred over liquid fermentation, but longer retention time and requirement of large amounts of inoculum are the associated challenges in liquid fermentation. Thus, additional studies are needed for engineering new techniques and suitable reactor design for industrial-scale application of SSF. Currently, the exploitation of K. marxianus for bioethanol production using lignocellulosic biomass has gained considerable importance due to abundant accessibility of lignocelluloses. Nonetheless, the lignocellulosic depolymerization and fermentation might be hard owing to its complex architecture. In future, studies exploring various new and ecofriendly pretreatment approaches could be useful for making biomass accessibility to the microbial strains. Moreover, immobilization and evolutionary adaptation techniques are likely to enhance the efficiency of high-value products and commodity chemicals via economically feasible fermentative processes.
Based on literature assessment, it can be contended that the use of K. marxianus in industrial setting would be an exciting era. In contrast, relatively scarce information is available on the diversity of K. marxianus species at the physiological, metabolic, and genetic levels that necessitate novel engineering tools to biosynthesize a plethora of valuable products based on transcriptomic, genomic, and metabolic modeling data. It is also possible to reveal the mechanisms underlying the fast growth (it is believed to be fastest growing yeast on the Earth), thermoresistant, and high ability to secret proteins. It is meaningful to apprehend lack of knowledge around genes, metabolic pathways, key enzymes, and their regulation for a complete insight into mechanism for producing relevant metabolites by K. marxianus. Exploitation of coordination between functional diversities and genetic variation at the level of species might be a potential research prospect for advancing this field. In conclusion, it can be envisioned that K. marxianus is useful in a large number of biotechnological applications, but an in-depth acquaintance with the genetic diversity remains to be elucidated. In this review, we outline the diverse applications of K. marxianus in different industrial sectors that would pave the way for exploring new opportunities in the food, feed, biotechnological, environmental, and pharmaceutical industries.
Author Contributions
All authors listed have made a substantial, direct, and intellectual contribution to the work and approved it for publication.
Funding
This work was supported by Tianjin Synthetic Biotechnology Innovation Capacity Improvement Action (grant No. TSBICIP-KJGG-012-02).
Conflict of Interest
The authors declare that the research was conducted in the absence of any commercial or financial relationships that could be construed as a potential conflict of interest.
Publisher’s Note
All claims expressed in this article are solely those of the authors and do not necessarily represent those of their affiliated organizations, or those of the publisher, the editors, and the reviewers. Any product that may be evaluated in this article, or claim that may be made by its manufacturer, is not guaranteed or endorsed by the publisher.
References
Aggelopoulos, T., Katsieris, K., Bekatorou, A., Pandey, A., Banat, I. M., and Koutinas, A. A. (2014). Solid State Fermentation of Food Waste Mixtures for Single Cell Protein, Aroma Volatiles and Fat Production. Food Chem. 145, 710–716. doi:10.1016/j.foodchem.2013.07.105
Alimardani-Theuil, P., Gainvors-Claisse, A., and Duchiron, F. (2011). Yeasts: An Attractive Source of Pectinases-From Gene Expression to Potential Applications: A Review. Process Biochem. 46 (8), 1525–1537. doi:10.1016/j.procbio.2011.05.010
Allan, G., Krakowka, S., Ellis, J., and Charreyre, C. (2012). Discovery and Evolving History of Two Genetically Related but Phenotypically Different Viruses, Porcine Circoviruses 1 and 2. Virus. Res. 164 (1-2), 4–9. doi:10.1016/j.virusres.2011.09.013
Alonso-Vargas, M., Téllez-Jurado, A., Gómez-Aldapa, C. A., Ramírez-Vargas, M. D. R., Conde-Báez, L., Castro-Rosas, J., et al. (2022). Optimization of 2-Phenylethanol Production from Sweet Whey Fermentation Using Kluyveromyces Marxianus. Fermentation 8 (2), 39. doi:10.3390/fermentation8020039
Altieri, C. (2016). Dairy Propionibacteria as Probiotics: Recent Evidences. World J. Microbiol. Biotechnol. 32 (10), 172–178. doi:10.1007/s11274-016-2118-0
Alves, É. D. P., Morioka, L. R. I., and Suguimoto, H. H. (2019). Comparison of Bioethanol and Beta‐galactosidase Production by Kluyveromyces and Saccharomyces Strains Grown in Cheese Whey. Int. J. Dairy Technology 72 (3), 409–415.
Anandharaj, M., Lin, Y.-J., Rani, R. P., Nadendla, E. K., Ho, M.-C., Huang, C.-C., ..., , and Cheng, J.-F. (2020). Constructing a Yeast to Express the Largest Cellulosome Complex on the Cell Surface. Proc. Natl. Acad. Sci. 117 (5), 2385–2394. doi:10.1073/pnas.1916529117
Arshad, M., Hussain, T., Iqbal, M., and Abbas, M. (2017). Enhanced Ethanol Production at Commercial Scale from Molasses Using High Gravity Technology by Mutant. S. Cerevisiaebrazilian Journal Microbiology 48, 403–409. doi:10.1016/j.bjm.2017.02.003
Banat, I. M., Nigam, P., and Marchant, R. (1992). Isolation of Thermotolerant, Fermentative Yeasts Growing at 52 C and Producing Ethanol at 45 °C and 50 °C. World J. Microbiol. Biotechnol. 8 (3), 259–263. doi:10.1007/bf01201874
Bayless, T. M., Brown, E., and Paige, D. M. (2017). Lactase Non-persistence and Lactose Intolerance. Curr. Gastroenterol. Rep. 19 (5), 23. doi:10.1007/s11894-017-0558-9
Behera, S., Sharma, N. K., Arora, R., and Kumar, S. (2016). Effect of Evolutionary Adaption on Xylosidase Activity in Thermotolerant Yeast Isolates Kluyveromyces Marxianus NIRE-K1 and NIRE-K3. Appl. Biochem. Biotechnol. 179 (7), 1143–1154. doi:10.1007/s12010-016-2055-2
Bilal, M., Xu, S., Iqbal, H. M., and Cheng, H. (2020). Yarrowia Lipolytica as an Emerging Biotechnological Chassis for Functional Sugars Biosynthesis. Crit. Rev. Food Sci. Nutr., 1–18. doi:10.1080/10408398.2020.1739000
Braga, A. R. C., Gomes, P. A., and Kalil, S. J. (2012). Formulation of Culture Medium with Agroindustrial Waste for β-galactosidase Production from Kluyveromyces Marxianus ATCC 16045. Food Bioproc. Technol. 5 (5), 1653–1663. doi:10.1007/s11947-011-0511-0
Cardoso, V. M., Borelli, B. M., Lara, C. A., Soares, M. A., Pataro, C., Bodevan, E. C., et al. (2015). The Influence of Seasons and Ripening Time on Yeast Communities of a Traditional Brazilian Cheese. Food Res. Int. 69, 331–340. doi:10.1016/j.foodres.2014.12.040
Cernak, P., Estrela, R., Poddar, S., Skerker, J. M., Cheng, Y. F., Carlson, A. K., et al. (2018). Engineering Kluyveromyces Marxianus as a Robust Synthetic Biology Platform Host. MBio 9 (5), e01410–18. doi:10.1128/mBio.01410-18
Chae, C. (2005). A Review of Porcine Circovirus 2-associated Syndromes and Diseases. Vet. J. 169 (3), 326–336. doi:10.1016/j.tvjl.2004.01.012
Cheng, H., Wang, S., Bilal, M., Ge, X., Zhang, C., Fickers, P., et al. (2018). Identification, Characterization of Two NADPH-dependent Erythrose Reductases in the Yeast Yarrowia Lipolytica and Improvement of Erythritol Productivity Using Metabolic Engineering. Microb. Cel. factories 17 (1), 1–12. doi:10.1186/s12934-018-0982-z
Chi, P., Wang, S., Ge, X., Bilal, M., Fickers, P., and Cheng, H. (2019). Efficient D-Threitol Production by an Engineered Strain of Yarrowia Lipolytica Overexpressing Xylitol Dehydrogenase Gene from Scheffersomyces Stipitis. Biochem. Eng. J. 149, 107259. doi:10.1016/j.bej.2019.107259
Chi, Z. M., Zhang, T., Cao, T. S., Liu, X. Y., Cui, W., and Zhao, C. H. (2011). Biotechnological Potential of Inulin for Bioprocesses. Bioresour. Technology 102 (6), 4295–4303. doi:10.1016/j.biortech.2010.12.086
Cho, Y. J., Kim, D. H., Jeong, D., Seo, K. H., Jeong, H. S., Lee, H. G., et al. (2018). Characterization of Yeasts Isolated from Kefir as a Probiotic and its Synergic Interaction with the Wine Byproduct Grape Seed Flour/extract. Lwt 90, 535–539. doi:10.1016/j.lwt.2018.01.010
Chotineeranat, S., Wansuksri, R., Piyachomkwan, K., Chatakanonda, P., Weerathaworn, P., and Sriroth, K. (2010). Effect of Calcium Ions on Ethanol Production from Molasses by Saccharomyces cerevisiae. Sugar Tech. 12 (2), 120–124. doi:10.1007/s12355-010-0024-6
Chupaza, M. H., Park, Y. R., Kim, S. H., Yang, J. W., Jeong, G. T., and Kim, S. K. (2021). Bioethanol Production from Azolla filiculoides by Saccharomyces cerevisiae, Pichia Stipitis, Candida Lusitaniae, and Kluyveromyces Marxianus. Appl. Biochem. Biotechnol. 193 (2), 502–514. doi:10.1007/s12010-020-03437-0
Cinelli, B. A., Castilho, L. R., Freire, D. M., and Castro, A. M. (2015). A Brief Review on the Emerging Technology of Ethanol Production by Cold Hydrolysis of Raw Starch. Fuel 150, 721–729. doi:10.1016/j.fuel.2015.02.063
Cruz, T. F., Magro, A. J., de Castro, A. M., Pedraza-Ordoñez, F. J., Tsunemi, M. H., Perahia, D., et al. (2018). In Vitro and In Silico Studies Reveal Capsid-Mutant Porcine Circovirus 2b with Novel Cytopathogenic and Structural Characteristics. Virus. Res. 251, 22–33. doi:10.1016/j.virusres.2018.04.019
da Silva, F. L., de Oliveira Campos, A., dos Santos, D. A., Magalhães, E. R. B., de Macedo, G. R., and dos Santos, E. S. (2018). Valorization of an Agroextractive Residue—Carnauba Straw—For the Production of Bioethanol by Simultaneous Saccharification and Fermentation (SSF). Renew. Energ. 127, 661–669. doi:10.1016/j.renene.2018.05.025
Deive, F. J., Costas, M., and Longo, M. A. (2003). Production of a Thermostable Extracellular Lipase by Kluyveromyces Marxianus. Biotechnol. Lett. 25 (17), 1403–1406. doi:10.1023/a:1025049825720
Diniz, R. H. S., Villada, J. C., Alvim, M. C. T., Vidigal, P. M. P., Vieira, N. M., Lamas-Maceiras, M., ..., , and da Silveira, W. B. (2017). Transcriptome Analysis of the Thermotolerant Yeast Kluyveromyces Marxianus CCT 7735 under Ethanol Stress. Appl. Microbiol. Biotechnol. 101 (18), 6969–6980. doi:10.1007/s00253-017-8432-0
Duan, J., Yang, D., Chen, L., Yu, Y., Zhou, J., and Lu, H. (2019). Efficient Production of Porcine Circovirus Virus-like Particles Using the Nonconventional Yeast Kluyveromyces Marxianus. Appl. Microbiol. Biotechnol. 103 (2), 833–842. doi:10.1007/s00253-018-9487-2
Fachinger, V., Bischoff, R., Jedidia, S. B., Saalmüller, A., and Elbers, K. (2008). The Effect of Vaccination against Porcine Circovirus Type 2 in Pigs Suffering from Porcine Respiratory Disease Complex. Vaccine 26 (11), 1488–1499. doi:10.1016/j.vaccine.2007.11.053
Fonseca, G. G., Heinzle, E., Wittmann, C., and Gombert, A. K. (2008). The Yeast Kluyveromyces Marxianus and its Biotechnological Potential. Appl. Microbiol. Biotechnol. 79 (3), 339–354. doi:10.1007/s00253-008-1458-6
Gao, W., Bao, Y., Liu, Y., Zhang, X., Wang, J., and An, L. (2009). Characterization of Thermo-Stable Endoinulinase from a New Strain Bacillus Smithii T7. Appl. Biochem. Biotechnol. 157 (3), 498–506. doi:10.1007/s12010-008-8313-1
Gellissen, G., Kunze, G., Gaillardin, C., Cregg, J. M., Berardi, E., Veenhuis, M., et al. (2005). New Yeast Expression Platforms Based on Methylotrophic Hansenula Polymorpha and Pichia pastoris and on Dimorphic Arxula Adeninivorans and Yarrowia Lipolytica–a Comparison. FEMS yeast Res. 5 (11), 1079–1096. doi:10.1016/j.femsyr.2005.06.004
Gillespie, J., Opriessnig, T., Meng, X. J., Pelzer, K., and Buechner‐Maxwell, V. (2009). Porcine Circovirus Type 2 and Porcine Circovirus‐associated Disease. J. Vet. Intern. Med. 23 (6), 1151–1163. doi:10.1111/j.1939-1676.2009.0389.x
Goktas, H., Dikmen, H., Demirbas, F., Sagdic, O., and Dertli, E. (2021). Characterisation of Probiotic Properties of Yeast Strains Isolated from Kefir Samples. Int. J. Dairy Technology 74 (4), 715–722. doi:10.1111/1471-0307.12802
Gomes, M. A., dos Santos Rocha, M. S. R., Barbosa, K. L., de Abreu, Í. B. S., de Oliveira Pimentel, W. R., Silva, C. E. D. F., et al. (2021). Agricultural Coconut Cultivation Wastes as Feedstock for Lignocellulosic Ethanol Production by Kluyveromyces Marxianus. Waste Biomass Valor. 12, 1–9. doi:10.1007/s12649-021-01345-w
Hang, Y. D., Woodams, E. E., and Hang, L. E. (2003). Utilization of Corn Silage Juice by Klyuveromyces Marxianus. Bioresour. Technol. 86 (3), 305–307. doi:10.1016/s0960-8524(02)00170-0
Hensing, M., Vrouwenvelder, H., Hellinga, C., Baartmans, R., and Van Dijken, H. (1994). Production of Extracellular Inulinase in High-Cell-Density Fed-Batch Cultures of Kluyveromyces Marxianus. Appl. Microbiol. Biotechnol. 42 (4), 516–521. doi:10.1007/bf00173914
Hill, C., Guarner, F., Reid, G., Gibson, G. R., Merenstein, D. J., Pot, B., ..., , and Sanders, M. E. (2014). Expert Consensus Document: The International Scientific Association for Probiotics and Prebiotics Consensus Statement on the Scope and Appropriate Use of the Term Probiotic. Nat. Rev. Gastroenterol. Hepatol. 11, 506. doi:10.1038/nrgastro.2014.66
Hong, J., Wang, Y., Kumagai, H., and Tamaki, H. (2007). Construction of Thermotolerant Yeast Expressing Thermostable Cellulase Genes. J. Biotechnol. 130 (2), 114–123. doi:10.1016/j.jbiotec.2007.03.008
Hoshida, H., Kidera, K., Takishita, R., Fujioka, N., Fukagawa, T., and Akada, R. (2018). Enhanced Production of Extracellular Inulinase by the Yeast Kluyveromyces Marxianus in Xylose Catabolic State. J. Biosci. Bioeng. 125 (6), 676–681. doi:10.1016/j.jbiosc.2017.12.024
Hsiung, R. T., Fang, W. T., LePage, B. A., Hsu, S. A., Hsu, C. H., and Chou, J. Y. (2021). In Vitro properties of Potential Probiotic Indigenous Yeasts Originating from Fermented Food and Beverages in Taiwan. Probiotics Antimicrob. Proteins 13, 113–124. doi:10.1007/s12602-020-09661-8
Hua, Y., Wang, J., Zhu, Y., Zhang, B., Kong, X., Li, W., et al. (2019). Release of Glucose Repression on Xylose Utilization in Kluyveromyces Marxianus to Enhance Glucose-Xylose Co-utilization and Xylitol Production from Corncob Hydrolysate. Microb. Cel. factories 18 (1), 1–18. doi:10.1186/s12934-019-1068-2
Hughes, D. B., Tudroszen, N. J., and Moye, C. J. (1984). The Effect of Temperature on the Kinetics of Ethanol Production by a Thermotolerant Strain of Kluveromyces Marxianus. Biotechnol. Lett. 6 (1), 1–6. doi:10.1007/bf00128221
Juergens, H., Varela, J. A., Gorter de Vries, A. R., Perli, T., Gast, V. J., Gyurchev, N. Y., ..., , and Daran, J. M. G. (2018). Genome Editing in Kluyveromyces and Ogataea Yeasts Using a Broad-Host-Range Cas9/gRNA Co-expression Plasmid. FEMS yeast Res. 18 (3), foy012. doi:10.1093/femsyr/foy012
Kalil, S. J., Maugeri-Filho, F., and Rodrigues, M. I. (2005). Ion Exchange Expanded Bed Chromatography for the Purification of an Extracelular Inulinase from Kluyveromyces Marxianus. Process Biochem. 40 (2), 581–586. doi:10.1016/j.procbio.2004.01.034
Kango, N. (2008). Production of Inulinase Using Tap Roots of Dandelion (Taraxacum officinale) by Aspergillus niger. J. Food Eng. 85 (3), 473–478. doi:10.1016/j.jfoodeng.2007.08.006
Karim, A., Gerliani, N., and Aïder, M. (2020). Kluyveromyces Marxianus: An Emerging Yeast Cell Factory for Applications in Food and Biotechnology. Int. J. Food Microbiol. 333, 108818. doi:10.1016/j.ijfoodmicro.2020.108818
Karimi, S., Mahboobi Soofiani, N., Mahboubi, A., and Taherzadeh, M. J. (2018). Use of Organic Wastes and Industrial By-Products to Produce Filamentous Fungi with Potential as Aqua-Feed Ingredients. Sustainability 10 (9), 3296. doi:10.3390/su10093296
Kaushik, A., Yadav, G., and Saini, J. K. (2022). Simultaneous Saccharification and Fermentation of Sequential Dilute Acid‐alkali Pretreated Cotton (Gossypium Hirsutum L.) Stalk for Cellulosic Ethanol Production. J. Chem. Technology Biotechnol. 97 (2), 534–542.
Kim, S. B., Kwon, D. H., Park, J. B., and Ha, S. J. (2019). Alleviation of Catabolite Repression in Kluyveromyces Marxianus: the Thermotolerant SBK1 Mutant Simultaneously Coferments Glucose and Xylose. Biotechnol. biofuels 12 (1), 1–9. doi:10.1186/s13068-019-1431-x
Lane, M. M., and Morrissey, J. P. (2010). Kluyveromyces Marxianus: a Yeast Emerging from its Sister's Shadow. Fungal Biol. Rev. 24 (1-2), 17–26. doi:10.1016/j.fbr.2010.01.001
Lertwattanasakul, N., Kosaka, T., Hosoyama, A., Suzuki, Y., Rodrussamee, N., Matsutani, M., ..., , and Yamada, M. (2015). Genetic Basis of the Highly Efficient Yeast Kluyveromyces Marxianus: Complete Genome Sequence and Transcriptome Analyses. Biotechnol. biofuels 8 (1), 1–14. doi:10.1186/s13068-015-0227-x
Li, P., Fu, X., Li, S., and Zhang, L. (2018). Engineering TATA-Binding Protein Spt15 to Improve Ethanol Tolerance and Production in Kluyveromyces Marxianus. Biotechnol. biofuels 11 (1), 1–13. doi:10.1186/s13068-018-1206-9
Li, P., Fu, X., Zhang, L., Zhang, Z., Li, J., and Li, S. (2017). The Transcription Factors Hsf1 and Msn2 of Thermotolerant Kluyveromyces Marxianus Promote Cell Growth and Ethanol Fermentation of Saccharomyces cerevisiae at High Temperatures. Biotechnol. biofuels 10 (1), 1–13. doi:10.1186/s13068-017-0984-9
Liu, B., Wu, P., Zhou, J., Yin, A., Yu, Y., and Lu, H. (2021). Characterization and Optimization of the LAC4 Upstream Region for Low Leakage Expression in Kluyveromyces Marxianus. Yeast. doi:10.1002/yea.3682
Löbs, A. K., Engel, R., Schwartz, C., Flores, A., and Wheeldon, I. (2017a). CRISPR–Cas9-enabled Genetic Disruptions for Understanding Ethanol and Ethyl Acetate Biosynthesis in Kluyveromyces Marxianus. Biotechnol. biofuels 10 (1), 1–14. doi:10.1186/s13068-017-0854-5
Löbs, A. K., Schwartz, C., and Wheeldon, I. (2017b). Genome and Metabolic Engineering in Non-conventional Yeasts: Current Advances and Applications. Synth. Syst. Biotechnol. 2 (3), 198–207. doi:10.1016/j.synbio.2017.08.002
Lomthong, T., Chotineeranat, S., Cioci, G., Laville, E., Duquesne, S., Choowongkomon, K., et al. (2018). Molecular Cloning and Sequencing of Raw Starch Degrading Gene from Laceyella Sacchari LP175 and its Functional Expression in Escherichia coli. Chiang Mai J. Sci. 45, 1634–1648.
Lomthong, T., Chotineeranat, S., and Kitpreechavanich, V. (2015). Production and Characterization of Raw Starch Degrading Enzyme from a Newly Isolated Thermophilic Filamentous Bacterium, Laceyella Sacchari LP175. Starch‐Stärke 67 (3-4), 255–266. doi:10.1002/star.201400150
Lomthong, T., Lertwattanasakul, N., and Kitpreechavanich, V. (2016). Production of Raw Starch Degrading Enzyme by the Thermophilic Filamentous Bacterium Laceyella Sacchari LP175 and its Application for Ethanol Production from Dried Cassava Chips. Starch‐Stärke 68 (11-12), 1264–1274. doi:10.1002/star.201600018
Lomthong, T., Netprasom, P., Kancharu, N., Jitmala, K., Areesirisuk, A., Trakarnpaiboon, S., et al. (2021). Very High Gravity (VHG) Bioethanol Production Using Modified Simultaneous Saccharification and Fermentation of Raw Cassava Chips with Molasses by Kluyveromyces Marxianus DMKU-KS07. Waste Biomass Valor. 12 (7), 3683–3693. doi:10.1007/s12649-020-01257-1
Löser, C., Urit, T., Stukert, A., and Bley, T. (2013). Formation of Ethyl Acetate from Whey by Kluyveromyces Marxianus on a Pilot Scale. J. Biotechnol. 163 (1), 17–23. doi:10.1016/j.jbiotec.2012.10.009
Lyu, Y., Wu, P., Zhou, J., Yu, Y., and Lu, H. (2021). Protoplast Transformation of Kluyveromyces Marxianus. Biotechnol. J. 16, 2100122. doi:10.1002/biot.202100122
Madeira-, J. V., and Gombert, A. K. (2018). Towards High-Temperature Fuel Ethanol Production Using Kluyveromyces Marxianus: On the Search for Plug-In Strains for the Brazilian Sugarcane-Based Biorefinery. Biomass and bioenergy 119, 217–228. doi:10.1016/j.biombioe.2018.09.010
Mahmoud, A. E., Fathy, S. A., Rashad, M. M., Ezz, M. K., and Mohammed, A. T. (2018). Purification and Characterization of a Novel Tannase Produced by Kluyveromyces Marxianus Using Olive Pomace as Solid Support, and its Promising Role in Gallic Acid Production. Int. J. Biol. macromolecules 107, 2342–2350. doi:10.1016/j.ijbiomac.2017.10.117
Makarova, K. S., Zhang, F., and Koonin, E. V. (2017). SnapShot: Class 2 CRISPR-Cas Systems. Cell 168 (1), 328. doi:10.1016/j.cell.2016.12.038
Mamaev, D., and Zvyagilskaya, R. (2021). Yarrowia Lipolytica: a Multitalented Yeast Species of Ecological Significance. FEMS Yeast Res. 21 (2), foab008. doi:10.1093/femsyr/foab008
Mao, W., Han, Y., Wang, X., Zhao, X., Chi, Z., Chi, Z., et al. (2019). A New Engineered Endo-Inulinase with Improved Activity and Thermostability: Application in the Production of Prebiotic Fructo-Oligosaccharides from Inulin. Food Chem. 294, 293–301. doi:10.1016/j.foodchem.2019.05.062
Martínez, O., Sánchez, A., Font, X., and Barrena, R. (2017). Valorization of Sugarcane Bagasse and Sugar Beet Molasses Using Kluyveromyces Marxianus for Producing Value-Added Aroma Compounds via Solid-State Fermentation. J. Clean. Prod. 158, 8–17. doi:10.1016/j.jclepro.2017.04.155
Martínez‐Corona, R., Vázquez Marrufo, G., Cortés Penagos, C., Madrigal‐Pérez, L. A., and González‐Hernández, J. C. (2020). Bioinformatic Characterization of the Extracellular Lipases from Kluyveromyces Marxianus. Yeast 37 (1), 149–162.
Martinez-Corona, R., Banderas-Martínez, F. J., Pérez-Castillo, J. N., Cortes-Penagos, C., and González-Hernández, J. C. (2019). Avocado Oil as an Inducer of the Extracellular Lipase Activity of Kluyveromyces Marxianus L-2029. Food Sci. Technology 40, 121–129. doi:10.1590/fst.06519
Martins, D. B. G., de Souza, C. G., Simoes, D. A., and de Morais, M. A. (2002). The β-galactosidase Activity in Kluyveromyces Marxianus CBS6556 Decreases by High Concentrations of Galactose. Curr. Microbiol. 44 (5), 379–382. doi:10.1007/s00284-001-0052-2
Masuda, A., Lee, J. M., Miyata, T., Sato, T., Hayashi, S., Hino, M., ..., , and Kusakabe, T. (2018). Purification and Characterization of Immunogenic Recombinant Virus-like Particles of Porcine Circovirus Type 2 Expressed in Silkworm Pupae. J. Gen. Virol. 99 (7), 917–926. doi:10.1099/jgv.0.001087
Mo, W., Ren, H., Yang, X., Luo, T., Lu, W., Zhou, J., and Lv, H. (2019a). Comparative Genomic Analysis Reveals Metabolic Mechanisms for Kluyveromyces Marxianus’ Fast Growth during Evolution. doi:10.21203/rs.2.18764/v1
Mo, W., Wang, M., Zhan, R., Yu, Y., He, Y., and Lu, H. (2019b). Kluyveromyces Marxianus Developing Ethanol Tolerance during Adaptive Evolution with Significant Improvements of Multiple Pathways. Biotechnol. biofuels 12 (1), 1–15. doi:10.1186/s13068-019-1393-z
Mojica, F. J., and Montoliu, L. (2016). On the Origin of CRISPR-Cas Technology: from Prokaryotes to Mammals. Trends Microbiology 24 (10), 811–820. doi:10.1016/j.tim.2016.06.005
Moré, M. I., and Swidsinski, A. (2015). Saccharomyces Boulardii CNCM I-745 Supports Regeneration of the Intestinal Microbiota after Diarrheic Dysbiosis–A Review. Clin. Exp. Gastroenterol. 8, 237. doi:10.2147/ceg.s85574
Moriyama, S., and Ohta, K. (2007). Functional Characterization and Evolutionary Implication of the Internal 157-Amino-Acid Sequence of an Exoinulinase from Penicillium Sp. Strain TN-88. J. Biosci. Bioeng. 103 (4), 293–297. doi:10.1263/jbb.103.293
Morrissey, J. P., Etschmann, M. M., Schrader, J., and de Billerbeck, G. M. (2015). Cell Factory Applications of the Yeast Kluyveromyces Marxianus for the Biotechnological Production of Natural Flavour and Fragrance Molecules. Yeast 32 (1), 3–16. doi:10.1002/yea.3054
Motey, G. A., Johansen, P. G., Owusu‐Kwarteng, J., Ofori, L. A., Obiri‐Danso, K., Siegumfeldt, H., ..., , and Jespersen, L. (2020). Probiotic Potential of Saccharomyces cerevisiae and Kluyveromyces Marxianus Isolated from West African Spontaneously Fermented Cereal and Milk Products. Yeast 37 (9-10), 403–412. doi:10.1002/yea.3513
Moyo, S., Gashe, B. A., Collison, E. K., and Mpuchane, S. (2003). Optimising Growth Conditions for the Pectinolytic Activity of Kluyveromyces Wickerhamii by Using Response Surface Methodology. Int. J. Food Microbiol. 85 (1-2), 87–100. doi:10.1016/s0168-1605(02)00503-2
Murari, C. S., Machado, W. R. C., Schuina, G. L., and Del Bianchi, V. L. (2019). Optimization of Bioethanol Production from Cheese Whey Using Kluyveromyces Marxianus URM 7404. Biocatal. Agric. Biotechnol. 20, 101182. doi:10.1016/j.bcab.2019.101182
Murugan, K., Babu, K., Sundaresan, R., Rajan, R., and Sashital, D. G. (2017). The Revolution Continues: Newly Discovered Systems Expand the CRISPR-Cas Toolkit. Mol. Cel. 68 (1), 15–25. doi:10.1016/j.molcel.2017.09.007
Nainys, J., Lasickiene, R., Petraityte-Burneikiene, R., Dabrisius, J., Lelesius, R., Sereika, V., ..., , and Gedvilaite, A. (2014). Generation in Yeast of Recombinant Virus-like Particles of Porcine Circovirus Type 2 Capsid Protein and Their Use for a Serologic Assay and Development of Monoclonal Antibodies. BMC Biotechnol. 14 (1), 1–11. doi:10.1186/s12896-014-0100-1
Nambu-Nishida, Y., Nishida, K., Hasunuma, T., and Kondo, A. (2017). Development of a Comprehensive Set of Tools for Genome Engineering in a Cold-And Thermo-Tolerant Kluyveromyces Marxianus Yeast Strain. Scientific Rep. 7 (1), 1–8. doi:10.1038/s41598-017-08356-5
Nambu-Nishida, Y., Nishida, K., Hasunuma, T., and Kondo, A. (2018). Genetic and Physiological Basis for Antibody Production by Kluyveromyces Marxianus. AMB Express 8 (1), 1–9. doi:10.1186/s13568-018-0588-1
Nguyen, M. T., Lertwattanasakul, N., Rodrussamee, N., Limtong, S., Kosaka, T., and Yamada, M. (2015). A Kluyveromyces Marxianus 2-Deoxyglucose-Resistant Mutant with Enhanced Activity of Xylose Utilization. Int. Microbiol. 18 (4), 235–244. doi:10.2436/20.1501.01.255
Nonklang, S., Abdel-Banat, B. M., Cha-Aim, K., Moonjai, N., Hoshida, H., Limtong, S., ..., , and Akada, R. (2008). High-temperature Ethanol Fermentation and Transformation with Linear DNA in the Thermotolerant Yeast Kluyveromyces Marxianus DMKU3-1042. Appl. Environ. Microbiol. 74 (24), 7514–7521. doi:10.1128/aem.01854-08
Nurcholis, M., Lertwattanasakul, N., Rodrussamee, N., Kosaka, T., Murata, M., and Yamada, M. (2020). Integration of Comprehensive Data and Biotechnological Tools for Industrial Applications of Kluyveromyces Marxianus. Appl. Microbiol. Biotechnol. 104 (2), 475–488. doi:10.1007/s00253-019-10224-3
Nurcholis, M., Nitiyon, S., Rodrussamee, N., Lertwattanasakul, N., Limtong, S., Kosaka, T., et al. (2019). Functional Analysis of Mig1 and Rag5 as Expressional Regulators in Thermotolerant Yeast Kluyveromyces Marxianus. Appl. Microbiol. Biotechnol. 103 (1), 395–410. doi:10.1007/s00253-018-9462-y
Ohta, K., Suetsugu, N., and Nakamura, T. (2002). Purification and Properties of an Extracellular Inulinase from Rhizopus Sp. Strain TN-96. J. Biosci. Bioeng. 94 (1), 78–80. doi:10.1016/s1389-1723(02)80120-7
Oliveira, C., Guimarães, P. M., and Domingues, L. (2011). Recombinant Microbial Systems for Improved β-galactosidase Production and Biotechnological Applications. Biotechnol. Adv. 29 (6), 600–609. doi:10.1016/j.biotechadv.2011.03.008
Oliveira, R. D. Q., Valasques Junior, G. L., Uetanabaro, A. P. T., Koblitz, M. G. B., Góes-Neto, A., Rosa, C. A., et al. (2012). Influence of Carbon Source, pH, and Temperature on the Polygalacturonase Activity of Kluyveromyces Marxianus CCMB 322. Food Sci. Technology 32, 499–505. doi:10.1590/s0101-20612012005000087
Oroojzadeh, P. (2020). Evaluating the Effect of Probiotic Yeast Kluyveromyces Marxianus on Survival of Penicillium. Notatum and Aspergillus. Parasiticus in Set Yoghurt. Tabriz, Iran: Tabriz University of Medical Sciences, Faculty of Nutrition and Food Sciences. Doctoral dissertation.
Ozmihci, S., and Kargi, F. (2007). Comparison of Yeast Strains for Batch Ethanol Fermentation of Cheese–Whey Powder (CWP) Solution. Lett. Appl. Microbiol. 44 (6), 602–606. doi:10.1111/j.1472-765x.2007.02132.x
Padilla, B., Frau, F., Ruiz-Matute, A. I., Montilla, A., Belloch, C., Manzanares, P., et al. (2015). Production of Lactulose Oligosaccharides by Isomerisation of Transgalactosylated Cheese Whey Permeate Obtained by β-galactosidases from Dairy Kluyveromyces. J. Dairy Res. 82 (3), 356–364. doi:10.1017/s0022029915000217
Palacios, A., Ilyina, A., Ramos-González, R., Aguilar, C. N., Martínez-Hernández, J. L., Segura-Ceniceros, E. P., ..., , and Ruiz, H. A. (2021). Ethanol Production from Banana Peels at High Pretreated Substrate Loading: Comparison of Two Operational Strategies. Biomass Convers. Biorefinery 11 (5), 1587–1596. doi:10.1007/s13399-019-00562-7
Papathoti, N. K., Laemchiab, K., Megavath, V. S., Keshav, P. K., Numparditsub, P., Le Thanh, T., et al. (2021). Augmented Ethanol Production from Alkali-Assisted Hydrothermal Pretreated Cassava Peel Waste. Energy Sourc. A: Recovery, Utilization, Environ. Effects, 1–11. doi:10.1080/15567036.2021.1928338
Phan, T. G., Giannitti, F., Rossow, S., Marthaler, D., Knutson, T. P., Li, L., et al. (2016). Detection of a Novel Circovirus PCV3 in Pigs with Cardiac and Multi-Systemic Inflammation. Virol. J. 13 (1), 1–8. doi:10.1186/s12985-016-0642-z
Piemolini-Barreto, L. T., Antônio, R. V., and Echeverrigaray, S. (2015). Comparison of a Pectinolytic Extract of Kluyveromyces Marxianus and a Commercial Enzyme Preparation in the Production of Ives (Vitis Labrusca) Grape Juice. World J. Microbiol. Biotechnol. 31 (5), 755–762. doi:10.1007/s11274-015-1828-z
Pivarnik, L. F., Senecal, A. G., and Rand, A. G. (1995). Hydrolytic and Transgalactosylic Activities of Commercial β-galactosidase (Lactase) in Food Processing. Adv. Food Nutr. Res. 38, 1–102. doi:10.1016/s1043-4526(08)60083-2
Qiu, Z., and Jiang, R. (2017). Improving Saccharomyces cerevisiae Ethanol Production and Tolerance via RNA Polymerase II Subunit Rpb7. Biotechnol. biofuels 10 (1), 1–13. doi:10.1186/s13068-017-0806-0
Rajkumar, A. S., and Morrissey, J. P. (2020). Rational Engineering of Kluyveromyces Marxianus to Create a Chassis for the Production of Aromatic Products. Microb. Cel. factories 19 (1), 1–19. doi:10.1186/s12934-020-01461-7
Rajkumar, A. S., Varela, J. A., Juergens, H., Daran, J. M. G., and Morrissey, J. P. (2019). Biological Parts for Kluyveromyces Marxianus Synthetic Biology. Front. Bioeng. Biotechnol. 7, 97. doi:10.3389/fbioe.2019.00097
Risso, F. V. A., Mazutti, M. A., Treichel, H., Costa, F., Maugeri, F., and Rodrigues, M. I. (2012). Assessment of Fructooligosaccharides Production from Sucrose in Aqueous and Aqueous-Organic Systems Using Immobilized Inulinase from Kluyveromyces Marxianus NRRL Y-7571. Food Sci. Technology 32 (2), 245–249. doi:10.1590/s0101-20612012005000030
Ritala, A., Häkkinen, S. T., Toivari, M., and Wiebe, M. G. (2017). Single Cell Protein—State-Of-The-Art, Industrial Landscape, and Patents 2001–2016. Front. Microbiol. 8, 2009. doi:10.3389/fmicb.2017.02009
Rouwenhorst, R. J., Visser, L. E., Van Der Baan, A. A., Scheffers, W. A., and Van Dijken, J. P. (1988). Production, Distribution, and Kinetic Properties of Inulinase in Continuous Cultures of Kluyveromyces Marxianus CBS 6556. Appl. Environ. Microbiol. 54 (5), 1131–1137. doi:10.1128/aem.54.5.1131-1137.1988
Saber, A., Alipour, B., Faghfoori, Z., and Khosroushahi, A. Y. (2017). Secretion Metabolites of Dairy Kluyveromyces Marxianus AS41 Isolated as Probiotic, Induces Apoptosis in Different Human Cancer Cell Lines and Exhibit Anti-pathogenic Effects. J. Funct. Foods 34, 408–421. doi:10.1016/j.jff.2017.05.007
Saini, P., Beniwal, A., Kokkiligadda, A., and Vij, S. (2017). Evolutionary Adaptation of Kluyveromyces Marxianus Strain for Efficient Conversion of Whey Lactose to Bioethanol. Process Biochem. 62, 69–79. doi:10.1016/j.procbio.2017.07.013
Sarmah, N., Revathi, D., Sheelu, G., Yamuna Rani, K., Sridhar, S., Mehtab, V., et al. (2018). Recent Advances on Sources and Industrial Applications of Lipases. Biotechnol. Prog. 34 (1), 5–28. doi:10.1002/btpr.2581
Selvakumar, P., and Pandey, A. (1999). Solid State Fermentation for the Synthesis of Inulinase from Staphylococcus Sp. And Kluyveromyces Marxianus. Process Biochem. 34 (8), 851–855. doi:10.1016/s0032-9592(99)00008-4
Sharma, N. K., Behera, S., Arora, R., and Kumar, S. (2017). Evolutionary Adaptation of Kluyveromyces Marxianus NIRE-K3 for Enhanced Xylose Utilization. Front. Energ. Res. 5, 32. doi:10.3389/fenrg.2017.00032
Sharma, R., Soni, S. K., Vohra, R. M., Gupta, L. K., and Gupta, J. K. (2002). Purification and Characterisation of a Thermostable Alkaline Lipase from a New Thermophilic Bacillus Sp. RSJ-1. Process Biochem. 37 (10), 1075–1084. doi:10.1016/s0032-9592(01)00316-8
Šiekštelė, R., Veteikytė, A., Tvaska, B., and Matijošytė, I. (2015). Yeast Kluyveromyces Lactis as Host for Expression of the Bacterial Lipase: Cloning and Adaptation of the New Lipase Gene from Serratia Sp. J. Ind. Microbiol. Biotechnol. 42 (10), 1309–1317.
Singh, R., Kumar, M., Mittal, A., and Mehta, P. K. (2016). Microbial Enzymes: Industrial Progress in 21st century. 3 Biotech. 6 (2), 1–15. doi:10.1007/s13205-016-0485-8
Staniszewski, A., and Kordowska-Wiater, M. (2021). Probiotic and Potentially Probiotic Yeasts—Characteristics and Food Application. Foods 10 (6), 1306. doi:10.3390/foods10061306
Stergiou, P. Y., Foukis, A., Sklivaniti, H., Zacharaki, P., Papagianni, M., and Papamichael, E. M. (2012). Experimental Investigation and Optimization of Process Variables Affecting the Production of Extracellular Lipase by Kluyveromyces Marxianus IFO 0288. Appl. Biochem. Biotechnol. 168 (3), 672–680. doi:10.1007/s12010-012-9808-3
Struyf, N., Laurent, J., Verspreet, J., Verstrepen, K. J., and Courtin, C. M. (2017). Saccharomyces cerevisiae and Kluyveromyces Marxianus Cocultures Allow Reduction of Fermentable Oligo-, Di-, and Monosaccharides and Polyols Levels in Whole Wheat Bread. J. Agric. Food Chem. 65 (39), 8704–8713. doi:10.1021/acs.jafc.7b02793
Su, M., Hu, Y., Cui, Y., Wang, Y., Yu, H., Liu, J., ..., , and Piao, C. (2021). Production of β-glucosidase from Okara Fermentation Using Kluyveromyces Marxianus. J. Food Sci. Technology 58 (1), 366–376. doi:10.1007/s13197-020-04550-y
Tesfaw, A., Oner, E. T., and Assefa, F. (2021). Evaluating Crude Whey for Bioethanol Production Using Non-Saccharomyces Yeast, Kluyveromyces Marxianus. SN Appl. Sci. 3 (1), 1–8. doi:10.1007/s42452-020-03996-1
Tinôco, D., Genier, H. L. A., and da Silveira, W. B. (2021). Technology Valuation of Cellulosic Ethanol Production by Kluyveromyces Marxianus CCT 7735 from Sweet Sorghum Bagasse at Elevated Temperatures. Renew. Energ. 173, 188–196. doi:10.1016/j.renene.2021.03.132
Tischer, I., Rasch, R., and Tochtermann, G. J. Z. B. (1974). Characterization of Papovavirus and Picornavirus-like Particles in Permanent Pig Kidney Cell Lines. Zenibl. Bukt. 226 (2), 153–167.
Trakarnpaiboon, S., Srisuk, N., Piyachomkwan, K., Sakai, K., and Kitpreechavanich, V. (2017). Enhanced Production of Raw Starch Degrading Enzyme Using Agro-Industrial Waste Mixtures by Thermotolerant Rhizopus Microsporus for Raw Cassava Chip Saccharification in Ethanol Production. Prep. Biochem. Biotechnol. 47 (8), 813–823. doi:10.1080/10826068.2017.1342264
Tu, Y., Wang, Y., Wang, G., Wu, J., Liu, Y., Wang, S., et al. (2013). High-level Expression and Immunogenicity of a Porcine Circovirus Type 2 Capsid Protein through Codon Optimization in Pichia pastoris. Appl. Microbiol. Biotechnol. 97 (7), 2867–2875. doi:10.1007/s00253-012-4540-z
Vakhlu, J. (2006). Yeast Lipases: Enzyme Purification, Biochemical Properties and Gene Cloning. Electron. J. Biotechnol. 9 (1), 0. doi:10.2225/vol9-issue1-fulltext-9
Wagner, J. M., and Alper, H. S. (2016). Synthetic Biology and Molecular Genetics in Non-conventional Yeasts: Current Tools and Future Advances. Fungal Genet. Biol. 89, 126–136. doi:10.1016/j.fgb.2015.12.001
Wang, J., Li, J., Zhao, H., Sheng, G., Wang, M., Yin, M., et al. (2015). Structural and Mechanistic Basis of PAM-dependent Spacer Acquisition in CRISPR-Cas Systems. Cell 163 (4), 840–853. doi:10.1016/j.cell.2015.10.008
Wang, N., Chi, P., Zou, Y., Xu, Y., Xu, S., Bilal, M., ..., , and Cheng, H. (2020). Metabolic Engineering of Yarrowia Lipolytica for Thermoresistance and Enhanced Erythritol Productivity. Biotechnol. biofuels 13 (1), 1–20. doi:10.1186/s13068-020-01815-8
Wang, W., Li, Z., Lv, Z., Zhang, B., Lv, H., and Guo, Y. (2017). Effects of Kluyveromyces Marxianus Supplementation on Immune Responses, Intestinal Structure and Microbiota in Broiler Chickens. PloS one 12 (7), e0180884. doi:10.1371/journal.pone.0180884
Wattanagonniyom, T., Lee, W. C., Tolieng, V., Tanasupawat, S., and Akaracharanya, A. (2017). Co-fermentation of Cassava Waste Pulp Hydrolysate with Molasses to Ethanol for Economic Optimization. Ann. Microbiol. 67 (2), 157–163. doi:10.1007/s13213-016-1245-z
Wu, P. C., Lin, W. L., Wu, C. M., Chi, J. N., Chien, M. S., and Huang, C. (2012). Characterization of Porcine Circovirus Type 2 (PCV2) Capsid Particle Assembly and its Application to Virus-like Particle Vaccine Development. Appl. Microbiol. Biotechnol. 95 (6), 1501–1507. doi:10.1007/s00253-012-4015-2
Xie, Y., Zhang, H., Liu, H., Xiong, L., Gao, X., Jia, H., ..., , and Han, T. (2015). Hypocholesterolemic Effects of Kluyveromyces Marxianus M3 Isolated from Tibetan Mushrooms on Diet-Induced Hypercholesterolemia in Rat. Braz. J. Microbiol. 46, 389–395. doi:10.1590/s1517-838246220131278
Yadav, J. S. S., Bezawada, J., Ajila, C. M., Yan, S., Tyagi, R. D., and Surampalli, R. Y. (2014a). Mixed Culture of Kluyveromyces Marxianus and Candida Krusei for Single-Cell Protein Production and Organic Load Removal from Whey. Bioresour. Technol. 164, 119–127. doi:10.1016/j.biortech.2014.04.069
Yadav, J. S. S., Bezawada, J., Elharche, S., Yan, S., Tyagi, R. D., and Surampalli, R. Y. (2014b). Simultaneous Single-Cell Protein Production and COD Removal with Characterization of Residual Protein and Intermediate Metabolites during Whey Fermentation by K. Marxianus. Bioproc. Biosyst. Eng. 37 (6), 1017–1029. doi:10.1007/s00449-013-1072-6
Yadav, J. S. S., Yan, S., Ajila, C. M., Bezawada, J., Tyagi, R. D., and Surampalli, R. Y. (2016). Food-grade Single-Cell Protein Production, Characterization and Ultrafiltration Recovery of Residual Fermented Whey Proteins from Whey. Food Bioproducts Process. 99, 156–165. doi:10.1016/j.fbp.2016.04.012
Yan, J., Wei, Z., Wang, Q., He, M., Li, S., and Irbis, C. (2015). Bioethanol Production from Sodium Hydroxide/hydrogen Peroxide-Pretreated Water Hyacinth via Simultaneous Saccharification and Fermentation with a Newly Isolated Thermotolerant Kluyveromyces Marxianu Strain. Bioresour. Technol. 193, 103–109. doi:10.1016/j.biortech.2015.06.069
Yang, D., Chen, L., Duan, J., Yu, Y., Zhou, J., and Lu, H. (2021). Investigation of Kluyveromyces Marxianus as a Novel Host for Large‐scale Production of Porcine Parvovirus Virus‐like Particles. Microb. Cel Factories 20 (1), 1–13. doi:10.1186/s12934-021-01514-5
Yardimci, G. O., and Cekmecelioglu, D. (2018). Assessment and Optimization of Xylanase Production Using Co-cultures of Bacillus Subtilis and Kluyveromyces Marxianus. 3 Biotech. 8 (7), 1–10. doi:10.1007/s13205-018-1315-y
Zhang, S., Yang, F., Wang, Q., Hua, Y., and Zhao, Z. K. (2012). High-level Secretory Expression and Characterization of the Recombinant Kluyveromyces Marxianus Inulinase. Process Biochem. 47 (1), 151–155. doi:10.1016/j.procbio.2011.10.002
Zhang, Y., Li, Y. F., Chi, Z., Liu, G. L., Jiang, H., Hu, Z., et al. (2019). Inulinase Hyperproduction by Kluyveromyces Marxianus through Codon Optimization, Selection of the Promoter, and High-Cell-Density Fermentation for Efficient Inulin Hydrolysis. Ann. Microbiol. 69 (6), 647–657. doi:10.1007/s13213-019-01457-8
Zhou, H. X., Xin, F. H., Chi, Z., Liu, G. L., and Chi, Z. M. (2014). Inulinase Production by the Yeast Kluyveromyces Marxianus with the Disrupted MIG1 Gene and the Over-expressed Inulinase Gene. Process Biochem. 49 (11), 1867–1874. doi:10.1016/j.procbio.2014.08.001
Zhou, H. X., Xu, J. L., Chi, Z., Liu, G. L., and Chi, Z. M. (2013). β-Galactosidase Over-production by a Mig1 Mutant of Kluyveromyces Marxianus KM for Efficient Hydrolysis of Lactose. Biochem. Eng. J. 76, 17–24. doi:10.1016/j.bej.2013.04.010
Keywords: Kluyveromyces marxianus, genome editing, food, enzymes, bioethanol
Citation: Bilal M, Ji L, Xu Y, Xu S, Lin Y, Iqbal HMN and Cheng H (2022) Bioprospecting Kluyveromyces marxianus as a Robust Host for Industrial Biotechnology. Front. Bioeng. Biotechnol. 10:851768. doi: 10.3389/fbioe.2022.851768
Received: 10 January 2022; Accepted: 23 March 2022;
Published: 20 April 2022.
Edited by:
Farshad Darvishi, Alzahra University, IranReviewed by:
Mamoru Yamada, Yamaguchi University, JapanMario Andrea Marchisio, Tianjin University, China
Copyright © 2022 Bilal, Ji, Xu, Xu, Lin, Iqbal and Cheng. This is an open-access article distributed under the terms of the Creative Commons Attribution License (CC BY). The use, distribution or reproduction in other forums is permitted, provided the original author(s) and the copyright owner(s) are credited and that the original publication in this journal is cited, in accordance with accepted academic practice. No use, distribution or reproduction is permitted which does not comply with these terms.
*Correspondence: Hairong Cheng, Y2hycXJxQHNqdHUuZWR1LmNu; Muhammad Bilal, YmlsYWx1YWZAaHlpdC5lZHUuY24=