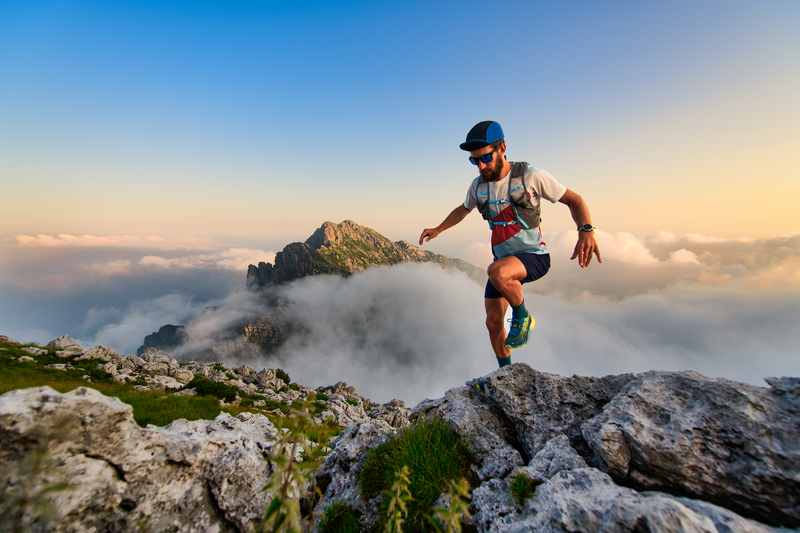
95% of researchers rate our articles as excellent or good
Learn more about the work of our research integrity team to safeguard the quality of each article we publish.
Find out more
REVIEW article
Front. Bioeng. Biotechnol. , 05 May 2022
Sec. Biomaterials
Volume 10 - 2022 | https://doi.org/10.3389/fbioe.2022.849831
This article is part of the Research Topic Cell and therapeutic delivery using Injectable hydrogels for tissue engineering applications View all 8 articles
While the soft mechanics and tunable cell interactions facilitated by hydrogels have attracted significant interest in the development of functional hydrogel-based tissue engineering scaffolds, translating the many positive results observed in the lab into the clinic remains a slow process. In this review, we address the key design criteria in terms of the materials, crosslinkers, and fabrication techniques useful for fabricating translationally-relevant tissue engineering hydrogels, with particular attention to three emerging fabrication techniques that enable simultaneous scaffold fabrication and cell loading: 3D printing, in situ tissue engineering, and cell electrospinning. In particular, we emphasize strategies for manufacturing tissue engineering hydrogels in which both macroporous scaffold fabrication and cell loading can be conducted in a single manufacturing step – electrospinning, 3D printing, and in situ tissue engineering. We suggest that combining such integrated fabrication approaches with the lessons learned from previously successful translational experiences with other hydrogels represents a promising strategy to accelerate the implementation of hydrogels for tissue engineering in the clinic.
Every year, a high number of deaths or disabilities results from the loss or damage of tissues and organs from injuries or diseases (Chapekar, 2000; Vos et al., 2020). According to the recent international report from the Global Observatory on Donation and Transplantation (GODT), >153,000 organs were transplanted worldwide in 2019, a 4.8% of increase over 2018; however, this number still represented only 10% of global needs. (Organ Donation and Transplantation Activities, 2021) While the transplantation of the damaged tissues or organs either with compatible donors or artificial devices can in part address this challenge (Lee and Mooney, 2001), the number of suitable donors is extremely limited, resulting in long-term wait lists for the patients. Side effects caused by immune/inflammatory responses to transplanted tissues pose additional challenges (Wang et al., 2007; Beyar, 2011; Black et al., 2018). Tissue engineering approaches, first defined in 1988 as the “application of the principles and methods of engineering and life sciences toward a fundamental understanding of structure-function relationship in normal and pathological mammalian tissues and the development of biological substitutes for the repair or regeneration of tissue or organ function”. (Skalak and Fox, 1988; Langer and Vacanti, 1993), aim to address these challenges by fabricating biomaterials into structural scaffolds to mimic the extracellular matrix of cells and provide support for cell proliferation and tissue regeneration; subsequent implantation of these scaffolds, either cell-free (to promote cell ingrowth from the native tissue) or cell-laden (to functionally regenerate native tissue with transplanted cells) leads to tissue regeneration, with scaffolds in most cases designed to degrade at a rate suitable to support new functional tissue formation for as long as required to enable native cell proliferation/organization but ultimately clear once the natively-produced ECM can support the tissue. More recently, tissue engineering has been more specifically defined as the use of cells, scaffolds, and growth factors to replace or regenerate damaged tissues, differentiating it from the broader field of regenerative medicine in which other strategies including gene therapy, cell-based therapies, and/or immunomodulation are leveraged in combination with tissue engineering strategies to regenerate tissues and/or organs (Frey et al., 2016; Han et al., 2020).
Hydrogels, networks of water-soluble polymers physically or chemically crosslinked to form a gel, have been widely used as vehicles to deliver cells to a designated location in the body (Hunt et al., 2014), scaffolds to encapsulate cells to improve cell adhesion or cell proliferation (Ayala et al., 2011), or fillers to fill defects and promote healing while preventing infection (Mooney and Vandenburgh, 2008). The use of hydrogels in this context is motivated by their soft biomechanics mimicking those of native soft tissues such as skin, muscle, fat, or nerve (Ma et al., 2003; Bian et al., 2009; Tibbitt and Anseth, 2009; Guillame-Gentil et al., 2010), tunable pore sizes, compatibility with the cellular environment, suppression of inflammatory responses, and ease of functionalization (Lee and Mooney, 2001; Drury and Mooney, 2003). However, challenges still exist that limit the use of hydrogels as functional tissue engineering scaffolds: 1) the mismatch between the pore (mesh) size of the gel network of conventional hydrogels (on the tens of nanometer scale) and the dimensions of cells (on the micron scale) poses challenges with promoting cell proliferation unless specific strategies to introduce the desired micro/microporosity are implemented; 2) the inherently lower modulus of most hydrogels relative to other types of biomaterials can cause challenges with stabilizing the macro or micro-porous structures or complex geometries typically sought to mimic the morphology of native extracellular matrix; and 3) the inherent cell repellency of most hydrogels (in particular actively cell-repellent/anti-fibrotic hydrogels such as poly (ethylene glycol) or zwitterionic hydrogels widely applied in tissue engineering contexts (Bai et al., 2014; Bernhard et al., 2017)) limits the degree of cell adhesion that is typically achieved. As such, to design effective hydrogel-based tissue engineering scaffolds, their chemical properties (e.g., degradation, crosslinking), physical properties (e.g., biomechanics, porosity, diffusion) and biological properties (e.g., cell type, growth factor and bioactive cues) must all be rationally designed.
Although multiple biomaterials and techniques such as combining natural and synthetic polymers to improve cell compatibility (Place et al., 2009), incorporating biomolecules or functional groups to improve cell adhesion (Zhu, 2010), or using advanced techniques to incorporate stable micro/nanostructures in scaffolds (De France et al., 2018) have been applied to design hydrogel-based scaffolds for specific cell types, the translation of hydrogels for clinical use remains a challenge (Bhatia, 2012; Caló and Khutoryanskiy, 2015); with only a few hydrogels (e.g., Apligraf®, AlloDerm®, and Juvéderm®) have been approved for use in the clinic (Gaharwar et al., 2020; Mandal et al., 2020). In this review, we will focus on the design and fabrication of hydrogels for tissue engineering with particular attention to their clinical translation, including recent advances in chemistry and fabrication approaches to better mimic the extracellular matrix of soft tissues. In particular, we emphasize strategies for manufacturing tissue engineering hydrogels in which both macroporous scaffold fabrication and cell loading can be conducted in a single manufacturing step – electrospinning, 3D printing, and in situ tissue engineering (Figure 1). We suggest that combining such integrated fabrication approaches with the lessons learned from previously successful translational experiences with other hydrogels represents a promising strategy to accelerate the implementation of hydrogels for tissue engineering in the clinic.
FIGURE 1. Design of functional hydrogels for tissue engineering. Selected constituent images reproduced with permission from references (Jin et al., 2011; Fuoco et al., 2014; Fang et al., 2016; Grigoryan et al., 2019; Mirdamadi et al., 2020; Li et al., 2021).
A key consideration in the design of functional hydrogels for tissue engineering is their ability to closely mimic the native extracellular matrix (ECM) of the targeted tissues (Tibbitt and Anseth, 2009; Tsang et al., 2010). The ECM plays an integral role in maintaining tissue homeostasis by regulating cell function, tissue architecture, and storing growth factors that regulate cell adhesion and interactions (del Bakhshayesh et al., 2019), thus serving as the key signaling strategy for cell differentiation, proliferation and migration (Unal and West, 2020). As such, many examples of the use of hydrogels in tissue engineering have applied native or modified ECM materials (e.g., collagen, gelatin, fibrin) that contain specific peptide sequences (e.g., RGD, YIGSR, and others) that can interact with cell surface receptors (e.g., integrins) to promote cell adhesion and tissue growth without additional functionalization (Lei et al., 2011). However, native ECM materials also contain other types of binding domains (e.g., immunoglobulin-like adhesion molecules) that can elicit other types of biological responses that can result in poor tissue growth or undesirable side-effects (e.g., inflammation or fibrosis), particularly if the components are in some way denatured during processing (Chen and Hunt, 2007; Unal and West, 2020). In contrast, synthetic hydrogels (e.g., poly (ethylene glycol)) minimize non-specific protein adsorption and thus immune/inflammatory responses but typically have poor cell adhesion to the hydrogel scaffold (Li et al., 2012). While grafting small adhesive peptides, commonly Arg-Gly-Asp (RGD) peptides, can in part overcome this challenge, such modifications represent an additional synthetic and purification steps that can increase the cost of material and complicate the regulatory approval process (Zhu, 2010).
The degradation and clearance rate of the polymers selected is also critical for promoting tissue growth in most tissue engineering approaches (Unal and West, 2020). As cells form functional tissues, hydrogel scaffolds are typically designed to degrade and clear from the body with minimal impact to surrounding tissues and organs. Native and modified ECM proteins like collagen, elastin, fibrin and hyaluronic acid (HA) are susceptible to enzyme-mediated degradation and are metabolized into biocompatible small molecules (Smeets et al., 2014). In contrast, most synthetic hydrogels are based on polymers with carbon-carbon backbones that cannot be metabolized, thus requiring the incorporation of hydrolytically labile segments or enzyme-sensitive linkages (e.g., peptide binding domains for native enzymes) to enable controlled degradation (Zhu, 2010), typically into oligomeric by-products with molecular weights appropriate for renal clearance (<60 × 103 g/mol) (Pasut and Veronese, 2007).
Finally, the compositional and morphological diversity (and thus range of accessible physicochemical properties) of a given hydrogel in relation to its target application should be considered in choosing the correct hydrogel material. The exceptional control that modern polymer chemistry techniques can impart on both the chemistry and the molecular structure of a synthetic polymer can enable precise tailoring of the physiochemical and mechanical properties of synthetic hydrogels in a way that is challenging to reproduce with naturally-derived polymers that typically have broad molecular weight distributions and complex variable compositions (Place et al., 2009). Synthetic polymers also offer higher reproducibility and significantly less potential for batch contamination given that they avoid the need for the complex purification protocols required to isolate natural polymers from their animal or plant source (Hutmacher, 2010; Unal and West, 2020); in particular, naturally derived and modified ECM components pose significant challenges in terms of the removal of contaminants such as proteins (e.g., non-tissue relevant structural proteins, immunity-triggering xenograft proteins), polyphenolics, endotoxins, RNA, and DNA which can trigger undesirable biological responses and thus significantly complicate practical clinical use (Gilpin and Yang, 2017; Montalbano et al., 2018).
Balancing these competing considerations makes the selection of the type of polymer(s) (natural or synthetic) and the specific polymer(s) within one of those two groups to design a hydrogel-based tissue engineering scaffold complex (Figure 2). In the following sections, we will outline the major types of natural and synthetic polymers used to form hydrogels, the major strategies available to crosslink those polymers to form hydrogels, and the relative advantages and disadvantages of each in the context of practical tissue engineering applications.
FIGURE 2. Venn diagram describing the key properties of natural and synthetic polymers most commonly used for fabricating hydrogel-based tissue scaffolds.
Collagen is a principal component of the ECM typically isolated from mammalian bone, cartilage, skin, tendons, and ligaments (Antoine et al., 2015). Collagen hydrogels, commonly based on type I collagen that constitutes the large majority of the total collagen found in the body, are typically formed through hydrogen bond-driven self-assembly into fibrils under physiological temperature irrespective of pH (Ferreira et al., 2012), although other approaches such as crosslinking with small molecules (e.g., glutaraldehyde, genipin, or EDC/NHS (1-ethyl-3-(3-dimethylaminopropyl) carbodiimide/N-hydroxysuccinimide)) or enzyme-induced crosslinking (e.g., microbial transglutaminase (MTG)) have also been reported (Garcia et al., 2007; Adamiak and Sionkowska, 2020). Like many naturally derived hydrogels, the amino acid sequence of collagen is easily recognized by host cells and can be degraded in vivo by naturally occurring collagenase (Seibel et al., 2006). Naturally occurring binding domains on collagen can promote cell adhesion and cell-cell interactions, allowing encapsulated cells to develop into functional tissues (Glowacki and Mizuno, 2008). Furthermore, the larger pore sizes typically observed following the self-assembly of collagen provide physical space for cell proliferation, although also making the gels susceptible to relatively rapid degradation in vivo that can limit accessible culturing times before native cell ECM production must take replace the structural properties of the delivered scaffold (Song et al., 2006; Ferreira et al., 2012). The production of collagen hydrogels is however associated with high manufacturing costs stemming from the time-consuming purification and isolation procedures required for collagen isolation (Nagai et al., 2004). Despite its primary role of structural support in the ECM, collagen-based hydrogels also typically have poor mechanical strength in comparison to many synthetic hydrogels (Antoine et al., 2015; Montalbano et al., 2018). Mixing collagen with other biomaterials (most typically other natural polymers such as alginate, gelatin, or fibrin) can in part improve such properties. For example, Montalbano et al. reported that collagen concentrations of 2.5 w/v% in collagen-alginate-fibrin thermoresponsive hydrogels promoted increased fibril homogeneity, faster gelation times, greater stability, and sustained cell morphology relative to collagen-only hydrogels, although care must be taken to maintain high nutrient transfer and metabolite diffusion in denser collagen networks (Montalbano et al., 2018).
Gelatin is an inexpensive and readily available material derived from denaturing the triple-helix structure of collagen into single strands (Elzoghby, 2013). Gelatin hydrogels undergo facile physical crosslinking under low temperatures and can hold significant amounts of water, favorable for nutrient transport (van den Bulcke et al., 2000). Since gelatin is a derivative of collagen, it also contains RGD binding motifs for cell adhesion and degrades into non-toxic resorbable products, with the denatured state lowering the antigenicity of gelatin compared to collagen (Elzoghby et al., 2012). However, the low stability of gelatin hydrogels at physiological temperatures in the absence of chemical crosslinks (or another type of temperature-insensitive physical crosslink) typically imparts poor mechanical strength (van den Bulcke et al., 2000). Grafting gelatin with methacrylic anhydride to create gelatin methacryloyl (commonly referred to as GelMA) that is UV photocrosslinkable offers an alternative to introduce covalent crosslinks into the gelatin scaffolds to improve their stability and has been widely applied for tissue scaffold development, although such functionalization requires additional synthetic grafting and subsequent polymerization (typically photopolymerization) steps. As with collagen, combinations of gelatin with other natural polymers are also often pursued to address the mechanical limitations of native gelatin hydrogels. For example, Shen et al. fabricated gelatin-chitosan hydrogels to recreate ideal scaffold degradation rates and pore sizes for cartilage formation, with the added gelatin enabling the reduced pore size, increased mechanical strength and increased elasticity required to grow cartilage (Shen et al., 2015).
Fibrin, formed by thrombin-mediated crosslinking of fibrinogen, plays a critical role in the regulation of tissue homeostasis and wound healing (Ehrbar et al., 2007; Ahmed et al., 2008). Similar to collagen and gelatin, fibrin contains multiple integrin and cell binding domains (including the RGD sequence most commonly implicated in integrin-mediated cell adhesion) (Janmey et al., 2009) and degrades into non-toxic by-products via enzymatic (plasmin-mediated) degradation (Janmey et al., 2009); unlike other natural hydrogels, fibrin networks rapidly self-crosslink via polycondensation reactions catalyzed by thrombin to form relatively stiffer gel matrices (Janmey, 1982) with the gelation time and the mechanics of the resulting fibrin hydrogels controllable by modifying thrombin concentrations (Janmey et al., 2009). However, consistent with its role in reversible clot formation, fibrin gels typically degrade rapidly in 15 days (Bensaı̈d et al., 2003; Noori et al., 2017). As such, combinations of fibrin with other biomaterials (e.g., medical biodegradable aliphatic polyurethane or alginate) have been reported to prolong the residence time of the hydrogel scaffold to better match the targeted rate of tissue regeneration (Lee et al., 2005; Deepthi and Jayakumar, 2018).
Hyaluronic acid (HA) is a linear polysaccharide that is found throughout the body but particularly in connective tissues (Price et al., 2007). HA plays a critical role in tissue hydration, nutrient diffusion, proteoglycan organization and cell differentiation (Tan et al., 2009). Similar to other natural polymers derived from the ECM, HA is degraded by host enzymes - specifically, hyaluronidase which is found in serum (Smeds and Grinstaff, 2001). HA is also upregulated in tissues with high growth rates and at wound sites due to the key role of HA in promoting cell spreading and proliferation (Burdick and Prestwich, 2011). Compared to other natural materials such as collagen and gelatin, HA is easily modified to diversify its mechanism of gelation, with a range of functional groups including thiol, hydrazide, aldehyde, and tyramine groups all reported to prepare crosslinked hydrogels (Burdick and Prestwich, 2011). For example, Park et al. prepared an injectable HA hydrogel using tetrazine-modified HA (HA-Tet) and transcyclooctene-modified HA (HA-TCO) that can be crosslinked in situ under physiological conditions via a Diels–Alder click reaction (Park et al., 2019). The in situ crosslinked HA hydrogel can enable chondrogenic differentiation of encapsulated human periodontal ligament stem cells (hPLSCs) as induced by cytomodulin-2 (CM) that was also covalently linked to HA (Park et al., 2019). However, the extremely high-water binding capacity of HA can limit the concentration of the polymer that can be used to fabricate hydrogels based on the high viscosity of the precursor polymers, thus also limiting the resulting mechanics of the resulting hydrogels.
Chitosan is a positively charged polysaccharide formed through the deacylation of chitin, the main structural component of crustacean exoskeletons (Islam et al., 2020). Although chitosan is not found natively in human ECM, the somewhat unique cationic charge of chitosan among carbohydrates can promote cell adhesion via electrostatic interactions, avoiding the common need for incorporating specific peptide motif cell binding sites into ECM-mimicking hydrogels. Chitosan hydrogels can be formed via physical crosslinking (typically hydrogen bonding, although such hydrogels are weak and can be highly pH-sensitive (Nicodemus and Bryant, 2008)), ionic complexation (e.g., with polyaspartic acid sodium salt or tripolyphosphate), or covalent crosslinking (e.g., with glutaraldehyde or formaldehyde), although the covalent crosslinkers typically used can pose significant toxicity challenges if residual crosslinker is not fully removed from the gel after crosslinking is complete (Hennink and van Nostrum, 2012; Cho et al., 2016). Chitosan is non-toxic (with proper purification), can have low immunogenicity (Nicodemus and Bryant, 2008), can adhere strongly to mucosal surfaces, and has some inherent anti-bacterial properties that may be beneficial to prevent post-implantation infections (Samprasit et al., 2015; Karava et al., 2020). However, native chitosan is insoluble at neutral pH (thus requiring the use of acidic solutions to fabricate hydrogels that can pose cell toxicity challenges). Chitosan is also not inherently biodegradable, although oxidative degradation of the glycosidic bonds can occur over time to break chitosan back down to oligomeric sugars (Einbu et al., 2007). Modified chitosan derivatives such as carboxymethyl chitosan can overcome the solubility problem but can partially dilute some of the benefits of chitosan in terms of cell adhesion or anti-bacterial properties. While chitosan has been used in a range of tissue engineering applications, these hydrogels have notably been found to promote bone formation by increasing alkaline phosphatase activity and calcium deposition in osteogenic mediums (Wang and Stegemann, 2010).
Alginate is a linear anionic polysaccharide isolated from brown algae that can rapidly form hydrogels upon exposure to divalent cations (Augst et al., 2006; Bidarra et al., 2014). Calcium is the most common crosslinking ion and facilitates relatively strong alginate gelation through the formation of an “egg crate” structure in which four alginate residues interact with a single calcium, although other alkali earth metal ions (in particular barium) can also be used as crosslinkers to modify the stability of the ionic crosslink in different environments (Bidarra et al., 2014; Matyash et al., 2014). Although ionic crosslinking mechanism is simple and highly cytocompatible, solutes present in the microenvironment can strongly influence alginate crosslinks and can result in poorly controlled degradation through ion exchange with the high concentration of monovalent ions in the physiological environment (Neves et al., 2020). Alginate also cannot inherently promote cell adhesion, with co-formulation with other ECM components that can be physically entrapped within the rapidly forming alginate-calcium hydrogel (e.g., collagen) and/or cell adhesion peptides grafted to alginate both found to improve adhesion (Moxon et al., 2019). Furthermore, while degradation of the hydrogel is facile via ion exchange, clearance of the alginate polymer itself can be slow if the molecular weight exceeds the renal cut-off, with only oxidation available to degrade the polymer itself in vivo (Lueckgen et al., 2019). Chemical modifications on the hydroxyl groups or carboxyl groups of alginate have been developed to improve the physiochemical and mechanical properties of alginate hydrogels (Neves et al., 2020), with methacrylation being the most popular method to enable the fabrication of dual ionic (calcium-induced gelation)/covalent (photogelation of methacrylate groups) crosslinked hydrogels that can achieve significantly higher moduli than either crosslinking approach can achieve alone (Samorezov et al., 2015).
Methylcellulose (MC) is a non-toxic, degradable, and thermoresponsive polymer derived from cellulose (Adamiak and Sionkowska, 2020). Given that the sol-gel transition temperature of MC is close to the body temperature, MC has been used to prepare thermoresponsive hydrogels that have been widely applied in tissue engineering, including in situ gelling systems for cell and biomolecule delivery, bioprinting inks, and surface modifications for cell adhesion (Adamiak and Sionkowska, 2020). The lower critical solution temperature (LCST) of MC can be adjusted by either changing the properties of MC (e.g., concentrations, degree of substitution, modifications) or the external environment (e.g., anions, solvent, electromagnetic fields) (Adamiak and Sionkowska, 2020). However, purely physically crosslinked MC hydrogels cannot provide a long-term mechanical support for cell encapsulation, requiring the co-formulation with other materials or chemical modifications to improve the mechanical properties and/or longevity of the MC-based scaffolds. For example, Shin et al. prepared a tyramine-modified MC hydrogel with improved mechanical properties by combining MC-driven thermally-induced crosslinking with photocrosslinking and demonstrated the utility of the material for 3D bioprinting (Shin et al., 2020), while the Shoichet group developed an injectable hydrogel blend composed of hyaluronan and methylcellulose (HA-MC) in which HA was added to improve the mechanics of the gel and promote shear thinning to aid in injectability at higher polymer concentrations without compromising the thermogelation capacity of the MC component (Tam et al., 2012; Ho et al., 2019).
Poly (ethylene glycol) (PEG) is a synthetic hydrophilic polymer used for a variety of biomedical applications due to its low cytotoxicity, non-immunogenicity and non-specific protein adhesion properties (LEE et al., 1995; Zhu, 2010). PEG-based hydrogels are typically formed by the free radical polymerization of bifunctional PEG diacrylate (PEGDA) or PEG dimethacrylate (PEGDMA) macromonomers, with the average pore size of the gel directly controllable based on the average chain length of the PEG chains between the crosslinker groups (Reid et al., 2015). Multi-arm PEG acrylates or end-functionalized PEG precursors (e.g., those crosslinkable by click chemistry with complementary functional groups) have also been formed that offer the potential to form ideal hydrogels with highly defined pore structures (Bakaic et al., 2015). As such, the degree of hydrogel structure control achievable with PEG is very high. However, the high cell repellency of hydrogels represents a drawback of this material for practical tissue engineering applications, typically requiring the incorporation of other entities such as grafted cell adhesion peptides, hydrophobic block copolymers (e.g., PEG-block-poly (ε-caprolactone), PEG-PCL) or thermoresponsive block copolymers (PEG-block-poly (propylene oxide), PEG-PPO); the latter two approaches also enhance the mechanics of the hydrogel via a dual covalent bonding/hydrophobic interaction crosslinking mechanism) (Zhu, 2010; Bakaic et al., 2015). The choice of crosslinking agent(s) can directly influence hydrogel degradation, swelling, porosity, and mechanical strength as desired for a specific tissue engineering application. For example, Liu et al. mixed non-degradable PEG-dithiol and degradable PEG-metalloproteinase crosslinkers in various ratios with 4-armed PEG-MAL to create enzymatically degradable PEG hydrogels with tunable degradation rates, pore size and mechanical strengths relevant to promote adipocyte and osteoblast differentiation (Liu et al., 2020). However, unless degradation is directly incorporated into the network (as in the Liu et al. example above), PEG is not inherently degradable aside from slow oxidation that may occur at the ether linkages in the main PEG chain. In addition, increasing concern about the development of PEG antibodies (estimated to exist in up to 89% of the patients (Sundy et al., 2011; Zhang et al., 2016) due to environmental exposure to PEG in personal care products and laxatives, among others) may result in an unanticipated immune response to a PEG-based material (Garay et al., 2012; Zhang et al., 2016). PEO-based block copolymers such as poly (ethylene oxide)-b-poly (propylene oxide)-b-poly (ethylene oxide) (PEO-PPO-PEO), commercially known as poloxamers, Pluronics®, Synperonics® or Lutrol®, are particularly notable given their potential to undergo thermal gelation at body temperature without the need for any external chemicals or post-treatment (Russo and Villa, 2019; Zarrintaj et al., 2020). The in situ gelation of poloxamers has been leveraged to create injectable hydrogels for cell delivery and tissue regeneration (Russo and Villa, 2019). However, the micelle-based gelation mechanism typically results in weaker hydrogels that can disassemble relatively quickly in vivo. Hybrid materials that combine poloxamers with other gelling polymers offer promise to address this challenge, often using the poloxamer to rapidly immobilize the hydrogel at the site of injection to allow further fixation to occur. For example, Suntornnond et al. mixed Pluronic F127 (poloxamer 407) with GelMA to prepare a printable hydrogel ink for fabricating vasculature-like structures (Suntornnond et al., 2017). The micelle formed by Pluronic improved the printability of the composite hydrogel ink at a wide range of temperatures while GelMA can be further crosslinked by UV light to improve the mechanical stability of printed structures (Suntornnond et al., 2017). The surfactant-like structure of poloxamers can however introduce challenges with preserving high cell viability within the scaffold, causing changes in the lipidic profile, or inducing renal toxicity (Dumortier et al., 2006) that may limit the translational potential of poloxamers.
Poly (vinyl alcohol) (PVA) has attracted attention for its high-water retention and superior mechanical strength to PEG, enabling its use in applications requiring stiffer scaffolds such as the replacement of articular cartilage (Schmedlen et al., 2002). PVA hydrogels are typically formed via a simple repeated freeze-thawing process, resulting in the formation of strong hydrogen bonded crosslinked networks with tunable pore sizes based on the rate and frequency of freezing used. PVA hydrogels can also be prepared using freeze-drying techniques by taking advantage of the same strong hydrogen bonding networks (Vrana et al., 2009); using either technique, elastic, non-toxic and stable hydrogels can be formed at room temperature. However, neither process is readily adaptable to injectable use like many other polymers described, which may limit the use of PVA to surgical implantation rather than injectable scaffold formation. PVA is a non-degradable polymer under physiological environment and does not inherently promote cell adhesion, which limits the applications in tissue regeneration. Moreover, the hydrogen bonding-based crosslinking strategy of PVA increases the difficulties of chemical modifications with other polymers that can address these drawbacks. The formation of composite PVA hydrogels by physically encapsulating natural ECM components such as collagen or gelatin (Wu et al., 2018) or 3 (Lim et al., 2013) have both been pursued to avoid this problem; in the latter case, a tyramine-functionalized poly (vinyl alcohol) (PVA-Tyr) polymer was synthesized that can be crosslinked via photopolymerization and degraded via hydrolysis of the ester bond linking the Tyr groups with PVA, with gelatin also incorporated via interactions of the tyrosine fractions of gelatin and tyramine groups of PVA-Tyr to enable improve cell adhesion (Lim et al., 2013).
Poly (N-isopropylacrylamide) (PNIPAAm) is an amphiphilic temperature-responsive smart polymer typically fabricated via free radical polymerization. PNIPAM has a lower critical solution temperature (LCST) of ∼32°C and therefore can form a hydrogel at physiological temperatures by thermally-driven self-assembly (Haq et al., 2017); however, the highly dehydrated state of the self-assembled polymers at 37°C has motivated covalent crosslinking of PNIPAM and/or the co-incorporation of more water-binding components into the hydrogel (e.g., by copolymerization of more hydrophilic comonomers or physical encapsulation of hygroscopic polymers (Jin et al., 2008; Ding et al., 2020)) to maintain higher water contents under physiological conditions. The more hydrophobic character of PNIPAM at body temperature can promote cell adhesion via hydrophobic interactions despite the lack of specific cell binding domains (Ashraf et al., 2016), although the collapse of the gel can result in relatively low pore sizes that may hinder cell growth and signaling (Atoufi et al., 2019). The main drawback of PNIPAM is the high toxicity of the NIPAM monomer (Joseph et al., 2021), which requires extensive purification of the hydrogel prior to practical use in vivo and has posed translational challenges with other PNIPAM-based technologies (Capella et al., 2019). PNIPAM is also not inherently degradable in vivo, although crosslinking PNIPAM oligomers that are renally clearable via hydrolytically labile bonds can address this degradation/clearance challenge (Patenaude and Hoare, 2012).
Poly (oligoethylene glycol methacrylate) (POEGMA) has a methacrylate-based backbone (making it polymerizable via free radical polymerization) and PEG-based side chains. By tuning the length of the PEG side chains, the polymer properties can be switched from being temperature-responsive (n = 2–3 ethylene oxide side chains) to being highly protein-repellent (n > 5–6 ethylene oxide repeat units) (Patenaude et al., 2014; Smeets et al., 2014); coupling this benefit with the capacity to include any type or number of functional group(s) in the polymer via simple free radical copolymerization and the noted lower (or absent) immune response to the material (Bakaic et al., 2015; Chen et al., 2021), POEGMA avoids many of the challenges of PEG while maintaining its beneficial non-toxic, non-immunogenic, and protein repellent properties. We have demonstrated that incorporating aldehyde and hydrazide moieties into POEGMA-based polymers enables the formation of injectable in situ-gelling and hydrolytically-labile hydrazone crosslinked hydrogels with highly tunable pore size, gelation time and mechanical strength without inducing any significant cytotoxic effects in vitro or in vivo; keeping the precursor polymer molecular weight < 40 kDa maintains the potential for clearance upon hydrolysis of the hydrazone crosslink even in the context of the non-degradable C-C backbone (Smeets et al., 2014; Bakaic et al., 2015). POEGMA offers an attractive alternative to PNIPAM and PEG because of its non-toxic degradation products and facile functionalizability, respectively (Smeets et al., 2014; Bakaic et al., 2015). In situ gelling thermosensitive POEGMA hydrogels that can undergo phase transitions at a range of physiologically relevant temperatures can be obtained by mixing di(ethylene glycol) methyl ether methacrylate (M(EO)2MA) with longer chain (n = 7–8 ethylene oxide repeat units) OEGMA monomers, with the transition temperature varying linearly with the mole percentage of each monomer (Lutz et al., 2006) while preserving a relatively sharp temperature response (unlike with PNIPAM, in which transitions become much broader when hydrophilic comonomers are incorporated) (Smeets et al., 2014; Xu et al., 2021). In addition, although POEGMA does not contain inherent binding cell binding domains, the thermo-reversible natural of POEGMA smart gels enables cell adhesion at temperatures above the volume phase transition temperature of the hydrogel (Xu et al., 2021). For example, Smeets et al. demonstrated good cell adhesion, mild inflammatory responses, and good stability (over several weeks) using hydrazone-crosslinked POEGMA hydrogels in vivo (Smeets et al., 2014).
Poly (2-hydroxyethyl methacrylate) (PHEMA) hydrogels were first introduced in the 1960s for use in contact lenses and have since been widely explored in tissue engineering given that they have many of the same advantages as PVA and PEG previously described (Xinming et al., 2008; Zare et al., 2021). However, unlike PEG, it can be freely copolymerized with other comonomers (making functionalization easier) and, unlike PVA, it is easily crosslinkable via a range of different strategies (making its degradability more controllable). Creating scaffolds that combine PHEMA with other natural (e.g., HA (Huang et al., 2013) or dextran (Meyvis et al., 2000)) and synthetic polymers can optimize the mechanical strength, non-immunogenicity, and physical properties of PHEMA gels to match those of living tissues (Bach et al., 2012). Similar to other synthetic hydrogels, PHEMA hydrogels are not inherently degradable in physiological conditions, requiring the incorporation of hydrolytic or enzyme-degradable segments, although such copolymerization is relatively facile with free radical copolymerization or derivatization of the alcohol side groups. (Meyvis et al., 2000). For example, Dragusin et al. prepared gelatin-pHEMA hydrogel scaffolds by photocrosslinking HEMA with methacrylamide-modified gelatin, with the incorporation of gelation improving the swelling properties, enhancing cell adhesion, and enabling enzymatic control over gel degradation (Dragusin et al., 2012). PHEMA hydrogels have also been noted to undergo calcification after long-term implantation, a potential benefit for bone tissue engineering but a potentially negative consideration for regenerating other soft tissues (Vijayasekaran et al., 2000).
Once the backbone polymer is chosen, the method of crosslinking the polymer chains together must be judiciously selected. Physical crosslinking and chemical crosslinking strategies are available, each with their own advantages and drawbacks in the context of tissue engineering. Compared to physical crosslinking, chemical crosslinking strategies typically result in linkages with more controllable degradation profiles and better mechanical properties; however, chemical crosslinking typically requires some kind of chemical derivatization of the backbone polymer and/or additional synthetic steps that may not be fully compatible with cells, unlike most physical crosslinking processes (Hennink and van Nostrum, 2012; Hu et al., 2019). In situ gelling in which the hydrogel gels upon injection in vivo via either physical crosslinking (i.e., ionic crosslinking, thermoresponsive phase transitions) or chemical crosslinking (i.e., the application of visible light/UV irradiation or click chemistry) offers particular promise for preparing injectable hydrogels for cell delivery in that such hydrogels avoid the need for surgical implantation, facilitating their practical clinical use (van Tomme et al., 2008). Herein, we will only briefly summarize the major categories of crosslinking approaches used for the fabrication of tissue engineering scaffolds; for more details on the options, we refer the interested reader to more in-depth reviews on hydrogel crosslinking strategies (Hennink and van Nostrum, 2012; Hu et al., 2019; Mueller et al., 2022). Note that, while the most common method(s) used to crosslink each polymer previously discussed were described in Sections 2.1 and 2.2, in principle any chemical crosslinking approach could be pursued with any of polymer by exploiting either native functional groups (e.g., all naturally-sourced polymers) or by introducing functional groups via, e.g., copolymerization (e.g., any synthetic polymer described); in principle, subsequent conversion of those functional groups to another functional group suitable for a specific crosslinking reaction could be conducted to crosslink any given polymer with any specific type of linkage. However, a few physical interactions (e.g., the egg crate Ca2+/alginate interaction or the freeze-thaw gelation of PVA) tend to be more specific to particular polymer types.
Networks of physically crosslinked hydrogels are generally formed through ionic/electrostatic interactions, hydrogen bonding, metal-ligand interactions, polymer chain entanglements, hydrophobic interactions, and/or host-guest interactions between polymers or between polymers and small molecule crosslinkers (Hennink and van Nostrum, 2012; Hu et al., 2019). Due to the absence of chemical crosslinkers and solvents, physical crosslinking can enable the preparation of cell compatible hydrogels under a mild environment (e.g., at room temperature); moreover, physical crosslinking typically does not require the functionalization of the gel precursor polymers and is often highly reversible, both beneficial to practical clinical translation (Hu et al., 2019). Ionic crosslinking interactions by which polysaccharides such as alginate (Bidarra et al., 2014), chitosan (Moura et al., 2011), and cellulose derivatives (Chang and Zhang, 2011; Du et al., 2019), can be crosslinked by cations (e.g., Ca2+, Mg2+, Fe3+) have been particularly widely leveraged to prepare hydrogels for tissue engineering given the high cell compatibility of the divalent cation crosslinkers, although as previously noted ion exchange with the ions in the physiological environment can make it challenging to control the degradation of the hydrogels (Anamizu and Tabata, 2019). Hydrogen bonding interactions, particularly following freeze-thaw processes that help enhance chain alignment and thus hydrogen bonding, have also been widely used, particularly using poly (vinyl alcohol) (PVA) as the main polymer and optionally including other polymers and/or nanoparticles (e.g., cellulose nanocrystals (CNCs)) with hydrogen bonding groups (Lin et al., 2020). However, given the highly aqueous environment of a hydrogel, only strongly hydrogen bonding precursors (such as PVA) will yield hydrogels with reasonable stability following implantation. Hydrophobic interactions, particularly those driven by thermoresponsive polymers that transition from being soluble at lower temperature to self-associative at higher temperature (e.g., methylcellulose (MC) and Pluronic-based materials) can enable gelation while also providing more hydrophobic adhesion sites for cells, although the degradation of such hydrogels can also be challenging to control (Park et al., 2017). Supramolecular host-guest interactions, often involving β-cyclodextrin (βCD) interactions with guest compounds such as poly (ethylene oxide) and adamantane, have also been widely used given their highly effective shear thinning/self-healing properties and facile tuning of matrix stiffness, although the materials available to form such hydrogels are limited to those that can form sufficiently strong inclusion complexes (Hörning et al., 2017; Liu et al., 2018). As such, in general, physical crosslinking can be highly beneficial for tissue engineering applications but can limit the types of materials that can be used and/or introduce challenges with tuning the degradation rate of the gel to that of the cell proliferation process.
Chemical crosslinking for the fabrication of tissue engineering matrices can typically be categorized into one of two main strategies: photocrosslinking and click chemistry. In photocrosslinking, light (e.g., most commonly UV light but in some cases visible light) is used to initiate the polymerization of unsaturated vinyl, acrylate, methacrylate or allylic groups conjugated to the gel precursor polymers, typically aided by water-soluble photoinitiators (e.g., Irgacure 2959) to promote free radical generation under lower intensities of light than would be required to form a gel via only hydrogen abstraction from the precursor polymers (Stephens-Altus et al., 2011). While photocrosslinking has been used successfully for a range of different cell types, the potential risks caused by UV radiation (e.g., DNA damage, aging, etc.) can still be concerns, particularly for less robust cell lines. Visible-light-initiated crosslinking such as ruthenium-catalyzed photocrosslinking under blue light with a wavelength of 458 nm can avoid these issues but can complicate the chemistry involved in the gelation process (Bjork et al., 2011; Fernandes-Cunha et al., 2017). Of note, in situ photopolymerization in which the scaffold is irradiated as it is administered in vivo has attracted increasing interest and has been used successfully for the repair of corneal wounds, in situ cartilage regeneration, and other applications (Smeds and Grinstaff, 2001; Burdick and Anseth, 2002; Leach et al., 2004).
Click chemistry, a range of reactions that can occur rapidly and spontaneously under physiological conditions without producing toxic by-products (i.e., no by-products or water) has also attracted significant increasing attention given that no additional crosslinkers/initiators/catalysts are required to prepare the hydrogels, no post-treatment is necessary, and (if delivered using a double barrel syringe and/or a static mixer) hydrogels can be administered directly into the body by simple injection to avoid the need for surgical implantation (Crescenzi et al., 2007). A range of chemistries including Diels–Alder reactions, Michael additions, oxime formation, Schiff base formation, disulfide formation, boronate ester formation and (with the addition of a low-intensity UV stimulus) thiol-ene reactions have been reported used to prepare multiple hydrogels under physiological conditions (Mueller et al., 2022).
A range of other chemical crosslinking methods based on di/multi-functional small molecule crosslinkers has been reported to prepare hydrogels (Kabiri et al., 2003; Hennink and van Nostrum, 2012; Hu et al., 2019). However, the inherent tissue toxicity many small molecule crosslinkers (in particular aldehydes such as glutaraldehyde and similar molecules) offers a potential risk for clinic use that is significantly mitigated by synthesizing polymeric analogues which tend to exhibit significantly lower toxicity. One possible exception to this rule is genipin, a natural aglycone extracted from plants that can crosslink amines (analogous to di/polyaldehydes like glutaraldehyde) while inducing significantly lower cytotoxicity (Yu et al., 2021); however, the strong purple color of this crosslinker may be non-ideal in some tissue engineering applications.
Enzymatic approaches can also be used to crosslink hydrogels, particularly beneficial in terms of leveraging naturally-occurring crosslinking strategies (e.g., in clotting) to create hydrogels with low inherent immunogenicity (Moreira Teixeira et al., 2012). Enzymatic reactions also typically occur under physiological conditions to enable the formation of in situ gelling hydrogels under physiological conditions in the presence of cells (Sperinde and Griffith, 1997; Jin et al., 2010; Moreira Teixeira et al., 2012). For example, Wang et al. reported an injectable gelatin-hydroxyphenylpropionic acid (Gtn-HPA) hydrogel that crosslinked by hydrogen peroxide (H2O2) and horseradish peroxidase (HRP) in which the stiffness of hydrogel was directly tunable by adjusting the concentrations of H2O2 and Gtn-HPA to optimize the proliferation of chondrocytes (Wang et al., 2014). However, the fast degradation and typically poor mechanical properties of enzymatically crosslinked hydrogels can limit their practical applications for tissue regeneration (Moreira Teixeira et al., 2012).
As another alternative, instead of using physical, chemical, or enzymatic processes to crosslink individual polymers together that were not previously networked together, ECM-mimetic hydrogel scaffolds can also be created by decellularizing native tissues. Decellularization refers to the removal of cells and other potential components that may introduce an immune/inflammatory response (e.g., cellular DNA, lipopolysaccharides) from native tissues using methods that do not disrupt the strong physical and/or chemical interactions in the native ECM (Saldin et al., 2017; Sackett et al., 2018), methods that may include chemical stimuli (i.e., surfactants or acids/bases to degrade cell membranes), enzymatic stimuli (e.g., trypsin, dispase, nucleases and phospholipase A2), physical stimuli (e.g., high pressure, supercritical carbon dioxide, or freeze-thaw cycles), or combinations thereof (Gilpin and Yang, 2017). The benefit of decellularization is that the internal structure and chemistry (including crosslinking) of the native ECM (ideally from the same tissue targeted for regeneration) is directly reproduced to grow the new tissue without the need to perform any additional crosslinking or structuring step. In addition, and critically given the poor availability of human donor tissues, non-human sources may still be useful for acquiring the implant scaffolds given the washing procedures used to remove potential immunogenic components (Fernández-Pérez and Ahearne, 2019). However, the challenges inherent in tissue sourcing and the extensive purification processes required to make decellularized scaffolds as well as the potential denaturation of some ECM components upon processing do offer some drawbacks to this strategy that can be avoided with the use of other scaffold building blocks.
To prepare functional tissue constructs using the materials and crosslinking approaches described, the micro- or nano- structure of the hydrogel scaffold must also be carefully controlled given the critical role that the ECM structure plays in regulating cell adhesion, migration, and proliferation as well as the degradation rate of the scaffold (Seliktar, 2012; Schulte et al., 2013; de France et al., 2018). For example, collagen, elastin and fibronectin all form nanofibrous structures in native ECM that play a key role in regulating cell behavior (Stevens and George, 2005), particularly in terms of promoting cell adhesion and spreading. The internal porous structure of a hydrogel is also critical to tune the transport of nutrients, gases, and wastes within the tissue and providing sufficient physical space for cells to communicate and ultimately form a tissue (Atala, 2014; de France et al., 2021). To obtain such structured hydrogels, traditional methods such as emulsion templating (Zhang and Cooper, 2005; Partap et al., 2006), gas foaming (Harris et al., 1998), salt leaching (Lee et al., 2005), and cryogelation (Vrana et al., 2009) have been used to prepare scaffolds with different pore size distributions (Figure 3). However, the required use of at least one of solvents, additives, or external energy in each of these strategies significantly impedes the potential for the direct encapsulation of cells and in vivo cell delivery; control over the pore size and shape is also very challenging with these methods, creating a situation in which cells in different parts of the scaffold receive different cues from their microenvironment. Photopatterning (Liu and Bhatia, 2002; Chan et al., 2010) and micro-molding (Hammer et al., 2014; Liu et al., 2014). can address these challenges but are low-throughput techniques that can significantly limit the size and/or the number of scaffolds that can be practically fabricated. In the following section, we will emphasize on three emerging fabrication techniques for preparing hydrogel-based tissue scaffolds with well-defined pore structures that are both scalable in the context of the materials and crosslinking strategies previously discussed as well as cell-friendly to enable simultaneous structure formation and cell loading (as is essential for effective translation): 3D bioprinting, cell electrospinning and in situ tissue engineering.
FIGURE 3. Schematic of techniques to fabricate hydrogel-based macroporous scaffolds for tissue engineering. (Inset) Spider plot of the relative advantages of different macroporous scaffold formation techniques (scale 1–5: 1 = least advantageous, 5 = most advantageous) Note that the cryogelation plot overlaps with the salt leaching plot such that it is not clearly visible in the graph.
Three-dimensional (3D) bioprinting is an additive manufacturing process that creates highly-complex tissue constructs using the layer-by-layer deposition of biomaterials onto a computer-controlled build platform (Pedde et al., 2017). 3D bioprinting allows for the fabrication of complex hydrogel geometries that can be loaded with active components such as drugs, growth factors, or viable cells directly during the fabrication process (Figure 4). The automated nature of 3D bioprinting enables the fabrication of both reproducible and scalable structures, both of which are key challenges with conventional structuring strategies. Many variations of 3D bioprinting including extrusion bioprinting (Ramesh et al., 2021), microfluidic bioprinting (Zhang et al., 2017), inkjet bioprinting (Li et al., 2020), digital light processing (DLP) bioprinting (Wang et al., 2021), laser-assisted bioprinting (Guillotin et al., 2010), stereolithography (SLA) bioprinting (Grigoryan et al., 2021), and embedded bioprinting (Shiwarski et al., 2021) have been developed, each of which has its own optimal set of bioink materials, advantages and disadvantages, and most promising clinical applications. For hydrogels, inkjet bioprinting is typically avoided given the high viscosity of many hydrogel-based bioinks; however, hydrogels with well-defined shapes and internal morphologies have been widely printed using each of the other techniques. Note that, in the following sections, the term “biomaterial ink” is used to refer to a biomaterial printed alone and then subsequently seeded with cells while the term “bioink” is used to refer to cases in which a biomaterial is (or could be) co-printed with one or more cell(s).
FIGURE 4. Schematic of ex vivo and in vivo bioprinting techniques (* represents the viscosity range of bioinks useful for each bioprinting technique).
In extrusion bioprinting, a hydrogel or hydrogel precursor bioink is extruded out of a narrow print-tip such as a needle or conical nozzle that is moving through space to build a 3D structure layer by layer. The flow of the hydrogel can be driven by pneumatics (Duarte Campos et al., 2019; Ouyang et al., 2020) or mechanical flow driven by a syringe pump or screw mechanism (Liu et al., 2017). The tunable rheological properties (Camci-Unal et al., 2013; Antich et al., 2020) and controllable gelation rates (Wang et al., 2018; Xu et al., 2018) of hydrogels make them well-suited for extrusion-based bioprinting, particularly for shear-thinning hydrogels that can maintain their shape upon extrusion; however, low-viscosity hydrogels with controllable gelation rates can also be used for extrusion bioprinting if gelation of the hydrogel can be induced during or immediately after extrusion such that the print will maintain its geometry (Ramesh et al., 2021).
The most prevalent bioink used in extrusion bioprinting is the alginate/calcium chloride system, performing the crosslinking either via a coaxial nozzle in which alginate and calcium chloride are delivered in separate streams (Zhang et al., 2013; Mirani et al., 2017) or by printing the alginate into a calcium chloride bath (Tabriz et al., 2015). However, most of the useful hydrogel materials outlined earlier do not inherently have rapid gelation mechanisms like calcium/alginate, driving the development of more advanced approaches such as using hybrid hydrogels consisting of homogeneous mixtures of multiple hydrogels or using coaxial extruders to create fibers with a distinct core and sheath from separate materials (Tamayol et al., 2015); in many cases, alginate-calcium is still used as the primary gelling component to entrap/encapsulate other functional components (Onoe et al., 2013). For example, Antich et al. bioprinted chondrocyte-laden hyaluronic acid (HA) in vitro by mixing alginate with HA to allow for rapid ionic crosslinking immediately after printing while maintaining a HA-rich hydrogel phase (Antich et al., 2020) while Liu et al. (2018) developed a coaxial bioprinting strategy with alginate in the sheath and GelMA, cells, and calcium chloride in the core channels (Liu et al., 2018); in the latter case, the rapidly crosslinked and comparatively strong alginate sheath allowed for the use of very low (1% w/v) GelMA concentrations in the core which are very desirable for cell proliferation but too mechanically fragile and slow to crosslink to print directly (Liu et al., 2018). A major challenge with extrusion bioprinting however is the shear stress in the nozzle which can be detrimental to cell viability and function. Higher viscosity hydrogels, that are often used for extrusion bioprinting due to their better printability, typically require higher pressures to extrude, leading to even higher shear stresses. In addition, the resolution of the extruded features in hydrogel-based bioinks is typically low (i.e., on the several tens to hundreds of micron length scale), much higher than the nanoscale features that predominantly regulate cell responses in the native ECM. As such, the development of new extrusion printing approaches/geometries to reduce the minimum printed feature size may be highly impactful. One potential strategy to address this drawback without requiring the development of entirely new printing strategies may be the use of jammed microgel-based bioinks, in which the size and softness of the hydrogel microparticles printed creates a specific self assembly/packing pattern that results in a specific pore size between the jammed microparticles on a length relevant to the dimensions of native ECM features. For example, Xin et al. demonstrated the thiol-ene photopolymerization of packed electrosprayed PEG-microgels (∼200 µ
Microfluidic chips, typically fabricated with polydimethylsiloxane (PDMS) (Colosi et al., 2016; Feng et al., 2019; Dickman et al., 2020) or micro-milled surfaces (Costantini et al., 2017), enable hydrogels to be mixed, crosslinked, or otherwise manipulated upstream from the print tip. The channel pattern on microfluidic chips is highly customizable, making microfluidic bioprinting a good strategy for addressing many difficult biofabrication problems. In addition, microfluidic bioprinting allows for seamless switching between different materials during fabrication using either programmable syringe pumps connected to the hydrogel inputs (Feng et al., 2019), or valves actuated by pneumatics (Colosi et al., 2016; Addario et al., 2020). Microfluidic bioprinting solutions that incorporate more complicated microfluidic geometries or channel junctions are commercially available from Fluicell and Aspect Biosystems. Fluicell’s microfluidic bioprinter and handheld biopen can fabricate 2D or 3D cell-laden structures with control over single-cell deposition (Jeffries et al., 2020), particularly useful for conducting pre-clinical testing of valuable drugs on cells in 3D environments. Aspect Biosystems’ microfluidic bioprinter allows for rapid switching between multiple cell-laden hydrogels and contains a microfluidic junction enabling mixing of crosslinkers with precursor polymers (e.g., alginate and calcium) shortly before extrusion (Figure 5A) (Dickman et al., 2020). By modifying the crosslinking and precursor polymer solutions, this microfluidic chip can also bioprint chemically crosslinkable thrombin (Abelseth et al., 2019; Lee et al., 2019) or photo-crosslinkable bioinks (Mirani et al., 2021). In general, microfluidics bioprinting benefits from the wide array of operations that can be performed on a microfluidic chip prior to bioprinting, allowing for highly controlled and dynamic mixing of multiple components over time. However, the fabrication, testing, and optimization of microfluidic chips is a lengthy process that requires expensive and specialized equipment and the microfluidic print heads can be considerably more expensive than other print heads, particularly in cases in which the print head itself is designed as a consumable unit.
FIGURE 5. Examples of emerging 3D printing approaches: (A) 3D bioprinting system with a microfluidic printhead that can load multiple biomaterials in different channels (Reproduced with permission from Dickman et al., 2020). (B) Schematic and images of FRESH printed alginate gels embedded in gelatin slurry bath. Scale bar = 1 cm (Reproduced with permission from Hinton et al., 2015). (C) Extruded FRESH printing of HA hydrogel into self-healing support hydrogel bath. Scale bar = 200 μm (Reproduced with permission from Highley et al., 2015).
In digital light processing (DLP) bioprinting, a photosensitive hydrogel is crosslinked by patterns of light in a layer-by-layer approach. Typically, the build platform is lowered into a hydrogel precursor solution placed on top of a transparent substrate and a light source. A thin layer of the hydrogel is exposed to a light pattern, the build platform is raised a small distance, and the process is repeated until the print is complete. The light pattern for each layer can be produced by a photomask (Shen et al., 2020), projector (Grigoryan et al., 2019; Mahdavi et al., 2020), or digital micromirror device (DMD) (Rujing and Larsen, 2017; Wang et al., 2018). A major benefit of this technique is that it harnesses the high spatial resolution of projectors or DMDs to produce constructs with spatial resolutions of <100 mm in the horizontal plane (Wang et al., 2018; Grigoryan et al., 2019; Magalhaes et al., 2020), a notable improvement compared to most extrusion bioprinting. Additionally, DLP bioprinting does not have issues with layer adhesion, is well suited to produce constructs with perfusable channels (Grigoryan et al., 2019), and can produce large constructs quickly as entire layers crosslink simultaneously. Stereolithography (SLA) bioprinting uses a similar concept except the light source is a laser which photo-crosslinks a single point at each moment in time instead of a single layer, using mirrors angled to reflect the laser across the corresponding areas of the build platform. While such an approach can create even more complex geometries with superior resolutions, it is correspondingly much slower given that only a single laser cross-section can be crosslinked at any given time, posing challenges with scale-up manufacturing of particularly larger tissue scaffolds. Aside from speed, the main constraint of DLP and SLA bioprinting is the limitation of hydrogel types that can be used. Although any polymer functionalized with a photopolymerizable functional group could in principle be bioprinted using DLP/SLA bioprinters, commonly used hydrogels for DLP or SLA bioprinting include gelatin methacryloyl (GelMA), poly (ethylene glycol) diacrylate (PEGDA) and hyaluronic acid methacrylate (HAMA) (Wang M. et al., 2021; Grigoryan et al., 2021). PEGDA currently provides the best spatial resolution of all biocompatible photo-crosslinkable hydrogels, however, it does not promote cell attachment and alone is not suitable as a matrix for tissue engineering applications. GelMA offers slightly lower resolution, but its high capacity for cell adhesion has resulted in successful DLP and SLA printing of corneal stroma (Mahdavi et al., 2020), cartilage (Lam et al., 2019), and vasculature (Tomov et al., 2021).
Soft hydrogels provide a favorable environment for cells to grow, proliferate and differentiate (He et al., 2016; Ramiah et al., 2020); however, the weak mechanics of such hydrogels results in challenges maintaining print fidelity/avoiding structure collapse when printed on a platform directly. Embedded bioprinting addresses this challenge via the use of gel-based support baths to support extrusion printing of soft hydrogels into higher-resolution 3D constructs (McCormack et al., 2020). Highly shear-thinning but viscous materials such as Carbopol (Bhattacharjee et al., 2015), gellan (Compaan et al., 2019), agarose (Mirdamadi et al., 2019), and gelatin microparticles (Hinton et al., 2015) have been most commonly reported as the support bath material. The freeform reversible embedding of suspended hydrogels (FRESH) printing process that uses gelatin microparticles as the support bath, pioneered in 2015 by the Feinberg, Angelini and Burdick research groups (Figures 5B,C), has been particularly successful in enabling the printing of inks with a broad range of rheological properties (Bhattacharjee et al., 2015; Highley et al., 2015; Hinton et al., 2015; Shiwarski et al., 2021). In this process, the bioink is printed directly into the gelatin support bath, crosslinked, and subsequently placed in physiological temperature to liquify the gelatin support bath to release the printed construct. The most common crosslinking strategies include ionic crosslinking [e.g., alginate/calcium (Compaan et al., 2019; Lindsay et al., 2019)], photocrosslinking [e.g., methacrylated or acrylated pre-polymer solutions (Ouyang et al., 2017)], and post-modification by changing the solution conditions [e.g., a change in pH for collagen gelation (Isaacson et al., 2018; Noor et al., 2019)]. Embedded bioprinting overcomes challenges with printing soft hydrogels and incorporating viable cells using a low-shear printing process but remains amenable to a relatively low diversity of bioinks, the potential for highly inhomogeneous crosslinking (particularly when the crosslinker is placed in the support bath), and scale-up to allow for larger printed constructs, particularly given the significant costs of some of the support bath materials.
In electrospinning, a high voltage difference is applied between a needle extruding a precursor polymer solution and a conductive collector. When the electrostatic repulsion forces of the charged polymer solution overcome the surface tension of the polymer solution, a fiber is ejected (Reneker and Yarin, 2008) that can be collected on a grounded conductive collector, which may include rotating drums, flat collectors, or parallel collectors (Li et al., 2003; Katta et al., 2004; Xu et al., 2016). The versatility and relatively low experimental complexity of the electrospinning setup makes it an accessible and feasible method of forming polymer nanofibers that have been widely used in a variety of tissue engineering applications (Greiner and Wendorff, 2007; Sill and von Recum, 2008). The recent development of portable handheld electrospinning devices (in contrast to conventional devices that require a large power supply) has further expanded the potential of this technique (Brako et al., 2018), enabling the direct in situ application of polymeric nanofibers (to-date including PCL, polystyrene (PS), poly (lactic acid) (PLA) and poly (vinylidene fluoride) (PVDF)) in the clinic for wound healing and other applications (Xu et al., 2015).
Electrospinning requires careful tuning of several parameters including polymer concentration, solvent, relative humidity, high voltage, collector, working distance, solution viscosity, and flow rate (Xue et al., 2019). When considering the electrospinning of hydrogels, crosslinking and gelation kinetics must also be considered, particularly relative to their effects on the polymer solution viscosity throughout the electrospinning process (Xu et al., 2016); in particular, it is imperative to maintain a flowable solution at the needle outlet but produce a stable crosslinked fiber (or a sufficiently viscous fiber that buys time for covalent or physical crosslinking to occur) at the collector. Some methods of hydrogel electrospinning instead elect to crosslink as a post-processing step of the nanofiber scaffold to avoid changes in viscosity to the electrospinning solution during the fabrication process itself; however, this choice adds an additional step to the process and may result in deformation of the original electrospun structure on the collector prior to the completion of the crosslinking process (Deng et al., 2018).
Electrospun hydrogels based on both natural polymers such as collagen, gelatin, dextran, alginate, HA, or chitosan as well as synthetic polymers such as POEGMA (Xu et al., 2016) have been reported, with other synthetic polymers such as poly (ethylene oxide) (PEO), poly (vinyl pyrrolidone) (PVP), or poly (vinyl acetate) (PVA) also in some cases included to promote chain entanglement in the precursor polymer solution and thus nanofiber formation instead of particle sprays. Stiffer degradable polymers such as poly (ε-caprolactone) (PCL) may also be added to increase the mechanical strength of the porous hydrogel scaffold, a key challenge with electrospinning (Koosha and Mirzadeh, 2015; Xu et al., 2016; Majidi et al., 2018; Wakuda et al., 2018; Askarzadeh et al., 2020). Table 1 summarizes different materials and methods used for electrospinning hydrogels. Common crosslinking methods include chemical crosslinking through saturation of the scaffold with the chemical crosslinking agent, photo-crosslinking with UV-light irradiation, and physical crosslinking such as the ionic crosslinking of calcium-alginate hydrogels (Gombotz, 1998; Hennink and van Nostrum, 2012), methods that will be further described in the following sections.
Methacrylated natural polymers such as alginate, gelatin and dextran have been electrospun to form photocrosslinked hydrogel scaffolds. Photocrosslinking can be used as the only crosslinking strategy or (depending on its speed) a secondary crosslinking step toward producing a multi-crosslinked hydrogel network (Chen et al., 2018). For example, alginate, GelMA, PEO, and the photoinitiator Irgacure 2959 were electrospun, placed in a CaCl2 bath (enabling rapid primary alginate-calcium ionic crosslinking) and subsequently exposed to 10 min of UV irradiation (enabling secondary GelMA photo-crosslinking). High cell viability (>90%) could be maintained coupled with an 8-fold increase of cell number in human iPSC-derived ventricular cardiomyocytes in 3D culture over 2 weeks of observation (Majidi et al., 2018).
For soft tissues, photocrosslinking can be used directly to form the hydrogels. For example, GelMA dissolved in the solvent hexafluoroisopropanol (HFIP) was reported to fabricate aligned electrospun nanofibers that were subsequently submerged in anhydrous alcohol containing Irgacure 2959 and crosslinked under UV light for 60 min to form hydrogel nanofibers (Chen et al., 2019). In vivo work showed that implantation of the GelMA scaffolds in rats enabled decreased glial scar tissue formation, increased vascularization, and increased neuronal development compared to electrospun gelatin fibers crosslinked with glutaraldehyde. The degree of crosslinking can also be tuned by adjusting the UV-light exposure time post-fabrication. Baker et al. (2015) similarly showed that DexMA could be electrospun to form a hydrogel scaffold with a range of scaffold moduli based on the UV exposure time. While the fiber scaffold stiffness did not affect cell viability, remodeling of the scaffold occurred to a much higher degree in the soft scaffolds (Baker et al., 2015). However, it should be noted that there is considerable debate over the degree to which UV irradiation may impact encapsulated cells (both in the short term and the long term). While the wavelength and the total dose (intensity + time) of the irradiation certainly does influence the degree to which UV irradiation may impact encapsulated cells, access to alternative crosslinking strategies (particularly for cells that have less robust viability in vitro) is recommended.
Electrospun hydrogels can also be formed by chemically crosslinking the polymer components via covalent bond forming chemistries. Common chemical crosslinking agents include N-(3-dimethylaminopropyl)-N′-ethylcarbodiimide hydrochloride (EDC, for crosslinking proteins), genipin (for crosslinking aminated polymers), glutaraldehyde (GTA, for crosslinking hydrazide and aminated polymers), and glyoxal (for crosslinking hydroxylated polymers) (Luo et al., 2018; Koosha et al., 2019). Such crosslinking agents are typically added either in liquid or vapor form following electrospinning to crosslink the hydrogel fibers (Sisson et al., 2009), with the typically rapid hydration rate of the electrospun hydrogel when exposed to a moist or saturated environment potentially deforming the fibers (via swelling) on the same time scale as crosslinking. In addition, the cytotoxicity of many of these chemical crosslinking agents is a significant concern, with many studies having been published seeking to define the optimum type and concentration of crosslinker for maintaining high cell viability (Hennink and van Nostrum, 2012; Luo et al., 2018; Campiglio et al., 2020). For example, Deng et al. (2018) examined the use of low concentrations of EDC co-electrospun recombinant human collagen (RHC), chitosan, and PEO in an acetic acid and ethanol solution to aid in solvent evaporation and dry fiber formation. The slow gelation that occurs at the low EDC concentration used results in the fabrication of hydrogel nanofibers with diameters of 168 ± 58 nm that facilitated improved seeded NIH 3T3 and human umbilical vein endothelial cell (HUVEC) cell viability when compared to the tissue culture dish control (Deng et al., 2018); however, the fabrication of thicker scaffolds (requiring longer electrospinning times) may be challenging when the crosslinker is added directly to the electrospinning solution. As another example, genipin, EDC-NHS, and glutaraldehyde were compared to assess which crosslinker best maintained the triple helical structure of electrospun collagen fibers (Luo et al., 2018), with the best cell proliferation observed using EDC-NHS as the crosslinker (although all three scaffolds exhibited improved cell proliferation relative to the control) (Luo et al., 2018). Similarly, Torres-Giner et al. electrospun collagen dissolved in HFP, post-crosslinked the fibers with EDC-NHS, and then seeded with the scaffold with MG-63 cells, enabling faster cell proliferation and far more cell growth over 21 days compared to a genipin-crosslinked scaffold (Torres-Giner et al., 2009). Genipin, GTA vapour, and glyceraldehyde have also been specifically compared by Sisson et al. as crosslinking agents for electrospun gelatin fibers, with glyceraldehyde-crosslinked nanofibers found to maintain the highest cell viability and growth (Sisson et al., 2009). However, any small molecule crosslinker that reacts non-bioorthogonally with proteins in or secreted from cells does offer some risk in terms of promoting undesired cell or (following implantation if the crosslinker is not thoroughly removed) tissue toxicity and should be used only judiciously.
Chemical crosslinking with GTA has also been explored in comparison to the physical crosslinking method of dehydrothermal treatment (DHT) for fabricating an electrospun collagen scaffold (Chen et al., 2021). The triple helix in the collagen fibers was better maintained when using chemical crosslinking methods, with the use of GTA avoiding the need for heat application as is required with the DHT method (Chen et al., 2021). Ammonia treatment of the collagen electrospun scaffold after fabrication to neutralize any remaining acetic acid further improved maintenance of the triple helix collagen structure in the fibers (Chen et al., 2021). Using a volatile crosslinker can also facilitate both penetration throughout the scaffold and post-purification of the scaffold to ensure the removal of unreacted crosslinker; for example, a PVA/collagen blend electrospun in HFIP and crosslinked under phosphoric acid vapor followed by GTA vapor promoting suitable seeded cell viabilities and transparency for corneal tissue engineering applications (Wu et al., 2018).
Covalent chemical crosslinking can also enable more efficient entrapment of additives into the electrospun hydrogels without significantly compromising the crosslinking and mechanical strength of the scaffold. For example, Hussein et al. (2020) electrospun HA, PVA, cellulose nanocrystals (CNCs), and l-arginine to form hydrogel fibers with the PVA component chemically crosslinked through the addition of anhydrous citric acid prior to electrospinning. CNC addition serves to increase the mechanical strength of the fibers while l-arginine promotes ECM collagen synthesis, angiogenesis, and epithelialization, promoting improved cell viabilities when cells were seeded on the scaffold. The scaffold also had anti-microbial properties related to l-arginine release and faster wound closure times compared to controls. However, the inclusion of multiple components in the electrospinning solution that may cross-interact in multiple ways can pose challenges with controlling the precursor solution viscosity and thus the uniformity of the electrospun hydrogel.
Enzymes can also be used as chemical crosslinking agents to minimize cytotoxicity. For example, a gelatin-hydroxyphenylpropanoic acid (Gel–HPA) scaffold electrospun in hexafluoroisopropanol (HFIP) was crosslinked via enzymatic oxidation of the HPA moieties with the addition of horseradish peroxidase and H2O2 (Nie et al., 2020). Enzymatic crosslinking scaffold preparations reported full scaffold degradation within 4 weeks of in vivo implantation with good cell penetration (Nie et al., 2020); however, the specific substrates required plus the cost of most enzymatic crosslinkers may limit the broad utility of this approach in the context of a larger manufacturing platform.
While the use of organic solvents can be a useful tool in the formation of electrospun nanofibers due to the ease of solvent evaporation between the nozzle and the collector, emerging studies have focused on electrospinning polymer solutions in water to remove the need for solvent removal before cell seeding and do not risk exposure of the seeded cells to cytotoxic crosslinking chemicals or photoinitiators. Altered experimental setups and polymer chemistries have both been explored as methods to reduce the need for electrospinning with organic solvents. First, the use of sacrificial sheaths can be used. Wakuda et al. (2018) reported a core-shell electrospinning approach in which the outer layer contained PVP while the inner layer consisted of collagen pre-crosslinked in a basic solution. Post-fabrication, the PVP layer was dissolved to leave the insoluble collagen, avoiding challenges associated with maintaining the anisotropic triple helix structure in organic solvents (Torres-Giner et al., 2009; Luo et al., 2018). HUVECs cultured on the surface of the formed collagen fibers followed the orientation of the fibers, unlike HUVECs seeded on collagen fibers electrospun in HFIP directly (Wakuda et al., 2018). Second, latent click or click-like chemistries can be used to lock the printed nanofiber in place after electrospinning. For example, Ji et al. (2006) electrospun thiolated HA with PEO as an electrospinning aid in DMEM, enabling chemical crosslinking via both disulfide formation and thiol-Michael addition following the post-fabrication addition of poly (ethylene glycol)-diacrylate (PEGDA). 3T3 fibroblasts seeded on the scaffolds were found to infiltrate the scaffold up to 32 mm below the scaffold surface and showed better morphology compared to 2D cell culture controls (Ji et al., 2006). However, this approach still required a post-treatment of a pre-electrospun nanofiber network. Third, reactive electrospinning techniques have been developed in which in situ-gelling polymer pairs are co-delivered through a double-barrel syringe directly into the electrospinning process. For example, Xu et al. (2016) electrospun aldehyde and hydrazide-functionalized POEGMA polymers from a double-barrel syringe tuned to have gelation times that ensured free flow of the polymers at the needle tip but rapid gelation upon electrospinning, leveraging the acceleration of gelation rate as water evaporates and the polymers concentrate in the emitted jet; as such, the nanofibers are sufficiently gelled upon hitting the collector that their structure can be maintained without the need for any post-processing/post-crosslinking step (Xu et al., 2016). Of note, conducting the process in a biosafety cabinet can also directly produce sterile scaffolds without any additional sterilization requirement. Both protein-repellent and thermo-responsive electrospun POEGMA scaffolds were prepared by varying the polymer component ratios, with the latter found to expand and contract reversibly to facilitate cell adhesion (at physiological temperature) but rapid cell delamination within 2 min upon swelling of the scaffold at 4°C that could serve as a replacement for typical trypsin-based cell delamination methods (Xu et al., 2021).
All examples described to this point required separate fabrication and cell loading steps, with the use of solvents, the drying of the scaffold, and/or the challenges with conducting electrospinning in a sterile environment all limiting cell survival in addition to the shear and electric field stress placed on cells during the electrospinning process. Relatively few examples exist of combining these steps together, which is highly beneficial to avoid the need to seed cells onto the scaffold post-fabrication and introduce more flexibility into the electrospinning process (akin to 3D printing) in terms of structuring different cell types within a single fabricated scaffold. The reactive electrospinning technique (Figure 6A) offers particular advantages in this regard given that no additional post-processing is required, enabling returning cells to an incubator in sterile conditions much faster than with other techniques, and the entire process is designed to run in water. The cell-friendly and high water-binding nature of the POEGMA electrospun fibers enabled high survivals of >80% of both NIH 3T3 fibroblasts and C2C12 myoblasts, survivals maintained even following cryoprotectant-free storage of the visibly “dry” scaffolds in liquid nitrogen over a 3 week period; in addition, 3-4x increases in cell number were observed over the 18-day observation period, showing how the otherwise cell-repellent POEGMA scaffold could support cell adhesion and proliferation due to the nanofiber structure formed in situ around the cells during electrospinning (Xu et al., 2018, 2020).
FIGURE 6. Strategies for cell electrospinning: (A) reactive electrospinning of 3T3 mouse fibroblasts and C2C12 mouse myoblasts in POEGMA hydrogel nanofibers; (B) cell-laden electrospinning of C2C12 myoblasts in fibrin scaffolds; (C) combining cell printing and cell electrospinning to encapsulate C2C12 myoblasts in alginate scaffolds. Reproduced with permission (Xu et al., 2018; Yeo and Kim, 2018; Guo et al., 2019).
A few other examples cell electrospinning have been reported using post-crosslinked nanofiber scaffolds, although successful cell electrospinning remains somewhat uncommon. In one such example, C2C12 cells were suspended in a mixture of fibrinogen and the electrospinning aid PEO and electrospun into a collection bath containing thrombin to crosslink the fibers into fibrin (Figure 6B) (Guo et al., 2019). Cell viability, and ultimate differentiation of the myoblasts into mature myotubes, was promoted through the electrospinning process by decreasing the voltage applied to 4.5 kV and by using more stable C2C12 cell aggregates rather than monodispersed cells (Guo et al., 2019). As another example (Figure 6C), C2C12 myoblasts were electrospun in water with alginate and PEO (electrospinning aid) and then crosslinked in a calcium ion/DMEM bath for 2 min to produce hydrogel nanofibers with aligned morphologies (Yeo and Kim, 2018). The C2C12 cells maintained cell viabilities above 90% for 7 days and grew along the directions of the aligned fibers, with a follow-up study showing similar efficacy with HUVEC cells (Yeo and Kim, 2020). Subsequent seeding of C2C12 cells on top of the alginate/PEO/HUVEC fibers resulted in faster myoblast maturation compared to the same scaffold without the HUVECs (Yeo and Kim, 2020). However, the design of electrospinning strategies compatible with cells is still in its infancy despite offering enormous potential to create ECM mimics that can reproduce the nanofibrous internal morphologies of native ECM.
While we have focused on the use of electrospinning as a method to enable simultaneous nanofiber production and cell encapsulation, microfluidics can also be used to generate fibers at somewhat larger length scales that, while potentially less directly biomimetic of nanofiber dimensions in native ECM, can still play useful roles in directing cell adhesion and proliferation responses. Hydrogel fibers can be prepared either by spinning hydrogel precursors from a microfluidic head composed of spinneret or capillaries or by using a PDMS microdevice with microchannels (Jun et al., 2014). Compared to electrospinning, the high degree of customization possible in a microfluidic chip can enable the fabrication of multiple types of internal structures inaccessible with other fabrication techniques (Jun et al., 2014; Sun et al., 2018). Cells can be encapsulated by mixing them together with the hydrogel precursors (as with electrospinning) or by merging cell-containing droplets with the fiber precursor droplets directly on-chip, minimizing the time over which the cells and the uncrosslinked precursor materials directly interact and thus potentially reducing any cytotoxicity related to such precursor materials (Guimarães et al., 2021; Wang et al., 2021). As one example of such an approach, Wang et al. fabricated one-step aqueous-droplet-filled hydrogel fibers as islet organoid carriers using a coaxial channel microfluidic chip in which droplets containing human induced pluripotent stem cells (hiPSC) loaded into the inner channel were encapsulated in an alginate hydrogel shell formed from middle and outer channels by ionic crosslinking of sodium alginate (NaA) with calcium chloride (CaCl2) (Wang et al., 2021). However, the diameter of fibers fabricated via microfluidic strategies typically ranges from a few micrometers to millimeters, far larger than the size of fibers (hundreds of nanometers to a few micrometers) found in native extracellular matrix (Jun et al., 2014). As such, while microfluidic-produced microfibers offer the potential for more complex morphologies and likely less aggressive chemical/physical fabrication conditions perhaps better suited for processing more sensitive cell lines into fibrous structures, electrospun scaffolds offer significantly better resolution to mimic the nanofibrous structure in native extracellular matrix.
Instead of conventional tissue engineering in which a cellularized scaffold is implanted at the defect site, in situ tissue engineering involves the injection of hydrogels (typically but not necessarily including cells) to the defect site to direct regeneration using a minimally-invasive technique (Gaharwar et al., 2020). In this context, the body’s own capacity for regeneration supported by the injected hydrogel that provides the required biophysical and biochemical cues to guide or stimulate functional restoration at the site of damaged tissues and/or deliver viable cells that can be used to create healthy tissue at a diseased tissue site (Pratt et al., 2004; Malek-Khatabi et al., 2020). The use of in situ-gelling hydrogels that can crosslink spontaneously upon injection (via physical interactions and/or click/click-like covalent bonding) is most common given that in situ gelation facilitates local injection of the hydrogel without the need for transplantation (Dias et al., 2020) (Figure 4). Also, in situ-gelling hydrogels can adapt the shape of the hydrogel directly for the defect site in the surgical room, particularly advantageous to treat irregularly shaped injury sites that may be difficult to fill accurately using an ex vivo-fabricated scaffold (Dias et al., 2020). The key design criteria for injectable hydrogels useful for in situ tissue engineering were described in a recent review by Young et al.: 1) to maintain the viability and function of encapsulated cells; 2) to reproduce the target tissue morphology, including its mechanical profile and adhesion to surrounding tissues; and 3) to tune the degradation rate to ensure that complete tissue replacement can occur on a clinically-relevant timescale (Young et al., 2019). Table 2 summarizes multiple materials and methods used for in situ tissue engineering.
While the direct injection of the in situ-gelling precursor polymers can be performed into any site, there is growing interest in leveraging in situ gelation chemistry with 3D bioprinting to perform in situ (or in vivo) printing (Singh et al., 2020), typically using either a robotic arm or a handheld device that could be used directly by healthcare professionals on the patient and thus eliminates the need for moving the printed tissue between the printer and the operating room (Duchi et al., 2017; di Bella et al., 2018; Hakimi et al., 2018) (Figure 7A–C). Such an approach leverages the reproduction of fine structures enabled by 3D bioprinting while eliminating the need for subsequent transplantation, which is challenging in the context of conventional 3D bioprinting due to 1) the potential for the disruption of both micro and macro-architectures (i.e., from swelling), 2) the challenges around maintaining structural fidelity and integrity upon handling and transport, 3) the risk of contamination and thus the need to maintain a highly sterile environment throughout the entire fabrication/transplant process, and 4) the requirement for good replicability and low error rates. In situ bioprinting has already been demonstrated to enable functional tissue regeneration in multiple tissue types including bone, skin, and cartilage. In the following sections, we will highlight key examples of strategies to address these challenges specific to in situ 3D bioprinting; however, any of these materials could also be directly injected to enable in situ tissue engineering using a more direct injection-based approach.
FIGURE 7. (A) Design of the handheld Biopen to print gelatin methacrylamide and hyaluronic acid methacrylate (HA-GelMa) hydrogel scaffolds with core-shell structure. Reproduced with permission (di Bella et al., 2018). (B) In situ bioprinting to fabricate HA hydrogels for chondral defect repair. Reproduced with permission (Li et al., 2017). (C) In situ formation of fibrin-HA/collagen sheet for skin tissue regeneration. Reproduced with permission (Hakimi et al., 2018). (D) In situ bioprinting of mesenchymal stromal cells and nano-hydroxyapatite collagen for in vivo bone tissue regeneration. Reproduced with permission (Keriquel et al., 2017).
Bone defects are conventionally treated using non-biological implants that either fill the missing bone part or support the remaining bone to provide enough mechanical strength for a limited time (Liu et al., 2017). However, such implants do not provide a long-lasting solution and will need to be surgically replaced over time, leading to potential complications. Keriquel et al. (2017) demonstrated the use of an in situ laser-assisted bioprinting process as a viable technique for printing mesenchymal stromal cells loaded in a collagen-nano hydroxyapatite hydrogel to promote functional bone regeneration in a murine calvaria defect model. Laser-assisted bioprinting (LAB) uses a near-infrared pulsed laser beam coupled to a scanning mirror and focusing system to enable high cell printing resolution and precision (Keriquel et al., 2017). While the proliferation of the printed cells was not significantly different between the ring and disk geometries tested, the disk geometry showed a significant increase in bone formation after 1 and 2 months (Keriquel et al., 2017) (Figure 7D). Alternately, Cohen et al. (2010) demonstrated the feasibility of in situ bioprinting using an extrusion-based bioprinter to print alginate-based hydrogels to treat chondral and osteochondral effected in a calf femur. Alginate was mixed with demineralized bone ECM and gelatin and printed directly into the osteochondral defect, enabling the functional repair of defects directly at the injury site with precise geometry constraints (mean surface errors of <0.1 mm) (Cohen et al., 2010). The inherent mechanical mismatch between bone ECM and a printable hydrogel should however be considered in this context, providing further impetus toward accelerating existing efforts to fabricate hydrogel-based bioinks with a higher modulus value without compromising printability.
Skin is the largest and most accessible organ and has thus become an ideal platform to validate in situ tissue engineering approaches to create skin substitutes, though only a few studies have been reported. Hakimi et al. developed a portable handheld extrusion-based skin printer that allowed for the co-printing of both ionically crosslinked biomaterials (i.e., alginate) and enzymatically crosslinked protein (i.e., fibrin) with dermal and epidermal cells to form cell-laden sheets of consistent thickness, width, and composition (Hakimi et al., 2018) (Figure 7C). The in situ deposition was validated in a murine wound model and a porcine full-thickness wound model, with the bioprinted structure observed to cover the full wound with a homogeneous layer that did not impede on normal re-epithelization or wound contraction (Keriquel et al., 2017). Alternately, Albanna et al. (2019) reported a mobile inkjet printer that facilitates the precise delivery of either autologous or allogeneic dermal fibroblasts and epidermal keratinocytes directly into an injured area. Using a thrombin-crosslinked fibrinogen hydrogel and an integrated imaging technology to pre-determine the topography of the wound, the direct bioprinting approach rapidly closed the wound, reduced wound contraction, and accelerated re-epithelialization in a murine full-thickness excisional wound model; the wound area at 1-wk post-surgery was 66% of the original wound area for the printed group compared to 95% of the control group, while the printed skin cells completely closed the wound by 3 weeks post-surgery compared to 5 weeks for the controls (Albanna et al., 2019). Developing strategies that can in situ print striated features with thinner dimensions (on the length scale of native skin) may further improve the efficacy of this therapeutic option.
Damaged cartilage cannot be easily treated due to its lack of vasculature and inability to self-repair by our body, with osteoarthritis being the most common chronic joint disease that leads to inflammation and degradation of cartilage (Liu et al., 2017). While existing treatments are available in the form of implantation and grafts, such treatments are highly invasive, expensive, and require revisions over time. Furthermore, the implantation of prefabricated tissue scaffolds often results in a significant geometric mismatch to the native tissue while lacking sufficient structural support and nutrient diffusion. O’Connell created a handheld pneumatic in situ extrusion device dubbed the “BioPen” that addresses these challenges by enabling printing of two inks in a collinear geometry (di Bella et al., 2018). High viability (>97%) of human adipose stem cells could be maintained after 1 week using methacrylated gelatin and hyaluronic acid hydrogel bioinks (O’Connell et al., 2016). The design of the BioPen has since been improved by the same group to allow for a multi-inlet extruder nozzle and a motorized extrusion system, permitting coaxial in situ bioprinting in which the core bioink encapsulated the stem cells and the shell bioink (mixed with the photoinitiator) (Figure 7A) is cured in situ via ultraviolet (UV) photocrosslinking in the chondral defect of a sheep knee joint (O’Connell et al., 2016; Duchi et al., 2017). The in vivo 3D-printed construct showed early formation of hyaline-like cartilage and better macroscopic/microscopic characteristics than the controls, with the coaxial mixing approach better protecting the cells from the printing process and any potential damaging effects of the free radicals generated during photocrosslinking (Duchi et al., 2017).
To be appropriate for clinical translation, a hydrogel must be biocompatible and have suitable mechanics and an appropriate degradation profile while addressing key biological challenges (e.g., effective regeneration while avoiding unwanted immune responses) and logistical challenges (e.g., sterilizability, transportation, and storage) associated with the targeted application. The majority of clinically approved injectable or implantable hydrogels are targeted for skin or joint regeneration or drug delivery applications (Mandal et al., 2020). Table 3 summarizes the various classes hydrogels that have been approved for clinical use. As shown, injectable or implantable hydrogels for cell therapies have not yet been approved for clinical use by the United States Food and Drug Administration (FDA) (Sivaraj et al., 2021); however, one cell-laden hydrogel scaffold has been approved for wound healing applications. Apligraf® is a bovine collagen-I hydrogel scaffold loaded with human neonatal fibroblasts that is covered on one side by a stratified layer of neonatal keratinocytes (Gentzkow et al., 1996). This tissue-engineered graft is approved for the treatment of chronic wounds such as diabetic foot ulcers (DFU) and venous leg ulcers (VLU) (Streit and Braathen, 2000; Edmonds, 2009). The hydrogel graft is typically applied to the wound site for a period of weeks and can be reapplied as needed at the discretion of the physician (Zaulyanov and Kirsner, 2007). A study investigating the persistence of Apligraf® in acute wounds concluded that the DNA from Apligraf’s cells was very minimally detected after 4 weeks and that the hydrogel did not exhibit features of engraftment (Griffiths et al., 2004); however, Apligraf® still shows clinical efficacy, making the cellular secretions of pro-healing cytokines the most likely mechanism of action (Streit and Braathen, 2000; Zaulyanov and Kirsner, 2007). A clinical study with over 100 participants that investigated anti-bovine collagen-I and anti-bovine serum antibodies as well as T-cell proliferation found no humoral or cellular immune response to Apligraf® in comparison with the control group (Eaglstein et al., 1999), suggesting its safety. Decellularized ECM scaffolds combined with cells have also been clinically approved for soft tissue repair (e.g., AlloDerm®, GRAFTJACKET Now®, and OrthADAPT® Bioimplant) but are generally very expensive given the extensive purification steps required to ensure sufficiently consistent batch-to-batch manufacturing of non-inflammatory decellularized matrix. A cell-loaded alginate-based matrix is the only currently non-ECM derived approved tissue regeneration hydrogel (AlgiMatrix®) but to-date is only used for 3D tissue models for in vitro drug screening. As such, despite the massive developmental efforts invested to-date in the design and fabrication of tissue engineering-based hydrogels, the translation of such hydrogels remains slow.
Clinically approved hydrogel therapies that do not include cells fall into four broad categories: natural hydrogels for cosmetic applications (Busso and Voigts, 2008; Lorenc, 2012; Sparavigna et al., 2014), natural or synthetic hydrogels for functional volume-filling purposes (Sack et al., 2018; Freitas et al., 2020), natural or synthetic hydrogels for osteoarthritic pain management (Miltner et al., 2002; Tnibar et al., 2015), or synthetic hydrogels for sustained drug delivery (Shore et al., 2011). The characteristics of such clinically approved hydrogels can serve as relevant case studies for understanding the regulatory and translational considerations that may be limiting for the translation of tissue engineering hydrogels. We will discuss two such examples: Juvéderm® and Bulkamid®.
Juvéderm® is a 1,4-butanediol diglycidyl ether (BDDE)-crosslinked hyaluronic acid (HA) gel clinically approved in 2006 in the United States for aesthetic treatment of dermal wrinkles and folds (Allemann and Baumann, 2008; Barr et al., 2015). Pre-clinical studies of chemically crosslinked HA showed that its degradation profile (Sall and Férard, 2007; Boulle et al., 2013) and the cytocompatibility of its degradation by-products (RD, 1996; Boulle et al., 2013) are suitable for clinical applications; following, numerous clinical trials have concluded that HA dermal fillers have significant advantages in longevity and biocompatibility over other treatment options (Duranti et al., 1998; Sparavigna et al., 2014; Barr et al., 2015). Slight variations of Juvéderm with different degrees of crosslinking and therefore different stiffnesses and longevity have also been approved and widely adopted for clinical use (Allemann and Baumann, 2008). Clinical translation of Juvéderm was bolstered by the fact that hyaluronic acid had already been used clinically for decades as a vitreous substitute (Pruett et al., 1979) as well as the approach to manufacture HA via bacterial fermentation processes, improving the batch-to-batch consistency of the HA product.
Bulkamid® is an injectable synthetic polyacrylamide hydrogel approved by the FDA for use in the United States in 2020 to treat stress urinary incontinence (SUI) by functioning as a bulking agent to add a total volume of up to 2 ml to the walls of the urethra (U.S. Food and Drug Administration, 2021; Kasi et al., 2016). In vitro and in vivo studies investigated the cytotoxicity (McCollister et al., 1965) and degradation (Caulfield et al., 2002) of polyacrylamide, informing the choice of the 2.5% (w/v) polyacrylamide concentration that was confirmed via multiple long-term clinical studies to be suitable in the targeted application (Lose et al., 2010; Pai and Al-Singary, 2015; Kasi et al., 2016). Although polyacrylamide was not previously approved for clinical use by the FDA, it had been in clinically used for breast augmentation in China since 1997 and years earlier in Ukraine (Margolis et al., 2015), albeit with reports of multiple adverse complications such as lumps, breast pain and induration, and in certain cases a foreign body response which destroyed the structure of adjacent muscle and gland (Qiao et al., 2005). The much smaller volume of gel required for Bulkamid injection (total of 2 ml in 3 to 4 locations) relative to breast injections (∼150 ml) and the structure of the urethra significantly suppresses this risk, even in the context of a non-degradable hydrogel like polyacrylamide.
In both cases, the clinical translation of Juvéderm and Bulkamid benefited from the pre-existing clinical use of the same hydrogel for a different application, motivating translational researchers to focus efforts on developing variations of hydrogels that are already approved for clinical use; this tendency is strongly reinforced by the high costs of funding a therapy from pre-clinical studies through to FDA approval. These barriers hinder innovative hydrogel formulations from entering the process of clinical translation, particularly in the area of tissue engineering in which both a material and a therapeutic (i.e., a regenerative cell) are key parts of the functional product. These barriers, and the regulatory challenges in dealing with regenerative tissue scaffolds, have been recognized by the FDA, which in 2017 acknowledged that as we enter a “new era of 3D printing of medical products regulatory issues related to the bioprinting of biological, cellular and tissue-based products” will have to adapt as the applications and practical implementations of tissue engineering continue to drift from the definitions of traditional therapies (U.S. Food and Drug Administration, 2017). This emerging regulatory framework must be navigated by researchers in this area with an emphasis on ensuring the manufacturability, sterilizability, and storage stability of any hydrogel formulation developed in addition to ensuring efficacy of the product for the intended application.
While the majority of discussion on translation has focused on the materials design, the cell sourcing and production of large number of functional cells for practical translation is perhaps an even more challenging problem given the limited potential of many cell types to be expanded in a lab and the potential for non-host transplants to induce potential immune response for patients. The ability of tissue engineering scaffolds to fully recapitulate the full complexity of the microenvironment some more sensitive cells need to function is also to-date weakly addressed in the area, at least using scalable methodologies for scaffold manufacturing. While not the main focus of this review, it is critical that methods to use the patient’s own cells (e.g., induced pluripotent stem cells from patients that can be reprogrammed to the desired phenotype) and/or to expand immune-compatible cell lines are developed and scaled to ensure consistency in the cell responses and thus reliable tissue regeneration responses.
Hydrogels as tissue engineering scaffolds offer enormous potential for clinical translation given their clear advantages in maintaining a hydrated environment for cells, mimicking the mechanics of soft tissues, and facilitating the presentation of appropriate interfaces and cell cues to promote tissue regeneration. However, the slow translation of these materials to the clinic goes beyond simple regulatory barriers. For natural polymer-based hydrogels, the effective scale up and management of batch-to-batch differences in natural polymers remains a huge challenge, as is addressing the often weaker mechanical properties of natural polymer-based hydrogels for promoting proper signaling to regrow stiffer tissues. For synthetic polymer-based hydrogels, challenges around degradability can be limiting (although the approval of Bulkamid suggests that this challenge is not inherently problematic for regulatory approval but rather related to the need to balance scaffold clearance with the rate of tissue regeneration). Combining natural and synthetic polymers into a single scaffold may offer benefits to leverage the benefits (and dilute the drawbacks) of each individual polymer type, although the inclusion of multiple materials is also likely to further complicate the regulatory process.
The three techniques described in depth that enable scaffold fabrication and cell loading in a single step (3D bioprinting, cell electrospinning, and in situ tissue engineering) in our view offer the most practical ways forward to commercialize cell-loaded tissue engineering hydrogels given that they all streamline multiple fabrication steps, make maintaining sterility much easier, and could in principle be applied directly in the operating room. However, each method still offers challenges, particularly in terms of broadening the types of materials that can be used for scaffold fabrication. For bioprinting, a relatively small number of bioink options is currently available due to the requirements of printing and the need to maintain precise crosslinking kinetics to fix the printed shape in place, although the emergence of embedded printing approaches (e.g., FRESH) can help to offset some of these challenges. The still relatively low feature resolution printable with high-throughput printing strategies like extrusion also offers a limitation of current bioprinting strategies for reproducing the nanofibrous structure of native ECM. Cell electrospinning can provide similar feature sizes to native ECM (i.e., in a range of hundreds of nanometers to a few micrometers) essential to provide cues for cell adhesion and proliferation but involves the exposure of cells to a strong electric field and (in typical processes) induces at least some degree of cell dehydration as the electrospun scaffolds are collected, both of which apply mechanical and/or osmotic stresses on cells that may compromise the viability of particularly more sensitive primary cell lines. Electrospinning directly on tissues (e.g., using hand-held devices) or into cell media instead of on dry collectors may address some of these challenges, while minimization of the applied voltage can ensure as high as possible cell membrane viability during the electrospinning process. In situ tissue engineering approaches offer a mechanism to directly administer cells and hydrogels into the body via injection but are more difficult to fabricate into controlled internal microstructures and morphologies optimized for cell growth. Methods to perform in situ gas foaming using biologically-safe foaming agents, incorporate sacrificial pore-forming materials that can be dissolved or otherwise in situ degraded over time to introduce tunable porosity, and/or provide alignment cues (e.g., via the incorporation of anisotropic nanoparticles that can be remotely aligned via non-invasive stimuli (de France et al., 2017)) all offer potential to improve the outcomes of such therapies, although further research is certainly required to make such approaches truly translatable. While each of these three processes offers in our opinion outstanding promise for translation, further developments in these processes (or the development of new processes) that place minimal stress on cells and can be used with the broadest possible set of materials would further expand the potential for practical translation of hydrogel-based tissue scaffolds to the clinic.
FX contributed to figures and paragraphs including introduction, biomaterials, conclusion and outlook. CD contributed to electrospinning section. ML contributed to the section of biomaterials for hydrogel preparation. EM contributed to 3D bioprinting and in situ tissue engineering. ES contributed to 3D bioprinting and Clinical Translation of Hydrogels. MA and TH outlined and revised the manuscript. All authors contributed to manuscript revision, read, and approved the submitted version.
The authors declare that the research was conducted in the absence of any commercial or financial relationships that could be construed as a potential conflict of interest.
All claims expressed in this article are solely those of the authors and do not necessarily represent those of their affiliated organizations, or those of the publisher, the editors and the reviewers. Any product that may be evaluated in this article, or claim that may be made by its manufacturer, is not guaranteed or endorsed by the publisher.
The authors gratefully acknowledge funding from the Natural Sciences and Engineering Research Council of Canada (Discovery grant RGPIN-2017-06455 to TH and RGPIN-2016-04024 to MA and ES).
Abelseth, E., Abelseth, L., de la Vega, L., Beyer, S. T., Wadsworth, S. J., and Willerth, S. M. (2019). 3D Printing of Neural Tissues Derived from Human Induced Pluripotent Stem Cells Using a Fibrin-Based Bioink. ACS Biomater. Sci. Eng. 5, 234–243. doi:10.1021/acsbiomaterials.8b01235
Adamiak, K., and Sionkowska, A. (2020). Current Methods of Collagen Cross-Linking: Review. Int. J. Biol. Macromolecules 161, 550–560. doi:10.1016/j.ijbiomac.2020.06.075
Addario, G., Djudjaj, S., Farè, S., Boor, P., Moroni, L., and Mota, C. (2020). Microfluidic Bioprinting towards a Renal In Vitro Model. Bioprinting 20, e00108. doi:10.1016/j.bprint.2020.e00108
Agarwal, C., Kumar, B., and Mehta, D. (2015). An Acellular Dermal Matrix Allograft (Alloderm) for Increasing Keratinized Attached Gingiva: A Case Series. J. Indian Soc. Periodontol. 19, 216. doi:10.4103/0972-124X.149938
Ahmed, T. A. E., Dare, E. v., and Hincke, M. (2008). Fibrin: A Versatile Scaffold for Tissue Engineering Applications. Tissue Eng. B: Rev. 14, 199–215. doi:10.1089/ten.teb.2007.0435
Albanna, M., Binder, K. W., Murphy, S. v., Kim, J., Qasem, S. A., Zhao, W., et al. (2019). In Situ Bioprinting of Autologous Skin Cells Accelerates Wound Healing of Extensive Excisional Full-Thickness Wounds. Sci. Rep. 9, 1856. doi:10.1038/s41598-018-38366-w
Anamizu, M., and Tabata, Y. (2019). Design of Injectable Hydrogels of Gelatin and Alginate with Ferric Ions for Cell Transplantation. Acta Biomater. 100, 184–190. doi:10.1016/j.actbio.2019.10.001
Antich, C., de Vicente, J., Jiménez, G., Chocarro, C., Carrillo, E., Montañez, E., et al. (2020). Bio-inspired Hydrogel Composed of Hyaluronic Acid and Alginate as a Potential Bioink for 3D Bioprinting of Articular Cartilage Engineering Constructs. Acta Biomater. 106, 114–123. doi:10.1016/J.ACTBIO.2020.01.046
Antoine, E. E., Vlachos, P. P., and Rylander, M. N. (2015). Tunable Collagen I Hydrogels for Engineered Physiological Tissue Micro-environments. PLOS ONE 10, e0122500. doi:10.1371/journal.pone.0122500
Ashraf, S., Park, H.-K., Park, H., and Lee, S.-H. (2016). Snapshot of Phase Transition in Thermoresponsive Hydrogel PNIPAM: Role in Drug Delivery and Tissue Engineering. Macromol. Res. 24, 297–304. doi:10.1007/s13233-016-4052-2
Askarzadeh, N., Nazarpak, M. H., Mansoori, K., Farokhi, M., Gholami, M., Mohammadi, J., et al. (2020). Bilayer Cylindrical Conduit Consisting of Electrospun Polycaprolactone Nanofibers and DSC Cross‐Linked Sodium Alginate Hydrogel to Bridge Peripheral Nerve Gaps. Macromol. Biosci. 20, 2000149–2000215. doi:10.1002/mabi.202000149
Atoufi, Z., Kamrava, S. K., Davachi, S. M., Hassanabadi, M., Saeedi Garakani, S., Alizadeh, R., et al. (2019). Injectable PNIPAM/Hyaluronic Acid Hydrogels Containing Multipurpose Modified Particles for Cartilage Tissue Engineering: Synthesis, Characterization, Drug Release and Cell Culture Study. Int. J. Biol. Macromolecules 139, 1168–1181. doi:10.1016/j.ijbiomac.2019.08.101
Augst, A. D., Kong, H. J., and Mooney, D. J. (2006). Alginate Hydrogels as Biomaterials. Macromolecular Biosci. 6, 623–633. doi:10.1002/mabi.200600069
Ayala, R., Zhang, C., Yang, D., Hwang, Y., Aung, A., Shroff, S. S., et al. (2011). Engineering the Cell-Material Interface for Controlling Stem Cell Adhesion, Migration, and Differentiation. Biomaterials 32, 3700–3711. doi:10.1016/j.biomaterials.2011.02.004
Bach, L. G., Islam, Md. R., Jeong, Y. T., Gal, Y. S., and Lim, K. T. (2012). Synthesis and Characterization of Chemically Anchored Adenosine with PHEMA Grafted Gold Nanoparticles. Appl. Surf. Sci. 258, 2816–2822. doi:10.1016/j.apsusc.2011.10.140
Bai, T., Sun, F., Zhang, L., Sinclair, A., Liu, S., Ella-Menye, J.-R., et al. (2014). Restraint of the Differentiation of Mesenchymal Stem Cells by a Nonfouling Zwitterionic Hydrogel. Angew. Chem. 126, 12943–12948. doi:10.1002/ange.201405930
Bakaic, E., Smeets, N. M. B., Badv, M., Dodd, M., Barrigar, O., Siebers, E., et al. (2018). Injectable and Degradable Poly(Oligoethylene Glycol Methacrylate) Hydrogels with Tunable Charge Densities as Adhesive Peptide-free Cell Scaffolds. ACS Biomater. Sci. Eng. 4, 3713–3725. doi:10.1021/acsbiomaterials.7b00397
Bakaic, E., Smeets, N. M. B., Dorrington, H., and Hoare, T. (2015). Off-the-shelf” Thermoresponsive Hydrogel Design: Tuning Hydrogel Properties by Mixing Precursor Polymers with Different Lower-Critical Solution Temperatures. RSC Adv. 5, 33364–33376. doi:10.1039/C5RA00920K
Baker, B. M., Trappmann, B., Wang, W. Y., Sakar, M. S., Kim, I. L., Shenoy, V. B., et al. (2015). Cell-mediated Fibre Recruitment Drives Extracellular Matrix Mechanosensing in Engineered Fibrillar Microenvironments. Nat. Mater. 14, 1262–1268. doi:10.1038/nmat4444
Barr, S. K., Benchetrit, A., Sapijaszko, M., and Andriessen, A. (2015). Clinical Evaluation of a Cross-Linked Hyaluronic Acid Dermal Filler Applied for Facial Augmentation. J. Drugs Dermatol. 1, 19–23.
Bauman, L. (2004). CosmoDerm/CosmoPlast (Human Bioengineered Collagen) for the Aging Face. Facial Plast. Surg. 20, 125–128. doi:10.1055/s-2004-861752
Baumann, L., and Baumann, L. (2008). Hyaluronic Acid Gel (Juvéderm™) Preparations in the Treatment of Facial Wrinkles and Folds. Cia Vol. 3, 629–634. doi:10.2147/CIA.S3118
Bensaı̈d, W., Triffitt, J. T., Blanchat, C., Oudina, K., Sedel, L., and Petite, H. (2003). A Biodegradable Fibrin Scaffold for Mesenchymal Stem Cell Transplantation. Biomaterials 24, 2497–2502. doi:10.1016/S0142-9612(02)00618-X
Bernhard, C., Roeters, S. J., Franz, J., Weidner, T., Bonn, M., and Gonella, G. (2017). Repelling and Ordering: the Influence of Poly(ethylene Glycol) on Protein Adsorption. Phys. Chem. Chem. Phys. 19, 28182–28188. doi:10.1039/C7CP05445A
Bernice, W., Richard, A. C., Joseph, P. C., and Vitaliy, V. K. (2012). Enhanced Viability of Corneal Epithelial Cells for Efficient Transport/storage Using a Structurally Modified Calcium Alginate Hydrogel. Regenerative Med. 7, 295. doi:10.2217/rme.12.7
Beyar, R. (2011). Challenges in Organ Transplantation. Rambam Maimonides Med. J. 2. doi:10.5041/RMMJ.10049
Bhatia, S. K. (2012). Engineering Biomaterials for Regenerative Medicine: Novel Technologies for Clinical Applications. Eng. Biomater. Regenerative MedicineNovel Tech. Clin. Appl., 1–352. doi:10.1007/978-1-4614-1080-5
Bhattacharjee, T., Zehnder, S. M., Rowe, K. G., Jain, S., Nixon, R. M., Sawyer, W. G., et al. (2015). Writing in the Granular Gel Medium. Sci. Adv. 1. doi:10.1126/sciadv.1500655
Bian, W., Liau, B., Badie, N., and Bursac, N. (2009). Mesoscopic Hydrogel Molding to Control the 3D Geometry of Bioartificial Muscle Tissues. Nat. Protoc. 4, 1522–1534. doi:10.1038/nprot.2009.155
Bidarra, S. J., Barrias, C. C., and Granja, P. L. (2014). Injectable Alginate Hydrogels for Cell Delivery in Tissue Engineering. Acta Biomater. 10, 1646–1662. doi:10.1016/j.actbio.2013.12.006
Bjork, J. W., Johnson, S. L., and Tranquillo, R. T. (2011). Ruthenium-catalyzed Photo Cross-Linking of Fibrin-Based Engineered Tissue. Biomaterials 32, 2479–2488. doi:10.1016/j.biomaterials.2010.12.010
Black, C. K., Termanini, K. M., Aguirre, O., Hawksworth, J. S., and Sosin, M. (2018). Solid Organ Transplantation in the 21st century. Ann. Translational Med. 6, 409. doi:10.21037/atm.2018.09.68
Boulle, K. D. E., Glogau, R., Kono, T., Nathan, M., Tezel, A., Roca-martinez, J., et al. (2013). A Review of the Metabolism of 1,4-Butanediol Diglycidyl Ether-Crosslinked Hyaluronic Acid Dermal Fillers. American Society for Dermatologic Surgery 39, 1758–1766. doi:10.1111/dsu.12301
Brako, F., Luo, C., Craig, D. Q. M., and Edirisinghe, M. (2018). An Inexpensive, Portable Device for Point-of-Need Generation of Silver-Nanoparticle Doped Cellulose Acetate Nanofibers for Advanced Wound Dressing. Macromolecular Mater. Eng. 303, 1700586. doi:10.1002/mame.201700586
Burdick, J. A., and Anseth, K. S. (2002). Photoencapsulation of Osteoblasts in Injectable RGD-Modified PEG Hydrogels for Bone Tissue Engineering. Biomaterials 23, 4315–4323. doi:10.1016/S0142-9612(02)00176-X
Burdick, J. A., and Prestwich, G. D. (2011). Hyaluronic Acid Hydrogels for Biomedical Applications. Adv. Mater. 23, H41–H56. doi:10.1002/adma.201003963
Busso, M., and Voigts, R. (2008). An Investigation of Changes in Physical Properties of Injectable Calcium Hydroxylapatite in a Carrier Gel when Mixed with Lidocaine and with Lidocaine/Epinephrine. Am. Soc. Dermatol. Surg. 34, S16–S24. doi:10.1111/J.1524-4725.2008.34238.X
Caldwell, A. S., Campbell, G. T., Shekiro, K. M. T., and Anseth, K. S. (2017). Clickable Microgel Scaffolds as Platforms for 3D Cell Encapsulation. Adv. Healthc. Mater. 6, 1700254. doi:10.1002/adhm.201700254
Caló, E., and Khutoryanskiy, V. v ( (2015). Biomedical Applications of Hydrogels: A Review of Patents and Commercial Products. Eur. Polym. J. 65, 252–267. doi:10.1016/j.eurpolymj.2014.11.024
Camci-Unal, G., Cuttica, D., Annabi, N., Demarchi, D., and Khademhosseini, A. (2013). Synthesis and Characterization of Hybrid Hyaluronic Acid-Gelatin Hydrogels. Biomacromolecules 14, 1085–1092. doi:10.1021/bm3019856
Campiglio, C. E., Ponzini, S., De Stefano, P., Ortoleva, G., Vignati, L., and Draghi, L. (2020). Cross-Linking Optimization for Electrospun Gelatin: Challenge of Preserving Fiber Topography. Polymers 12, 2472. doi:10.3390/polym12112472
Capella, V., Rivero, R. E., Liaudat, A. C., Ibarra, L. E., Roma, D. A., Alustiza, F., et al. (2019). Cytotoxicity and Bioadhesive Properties of Poly-N-Isopropylacrylamide Hydrogel. Heliyon 5, e01474. doi:10.1016/j.heliyon.2019.e01474
Chan, V., Zorlutuna, P., Jeong, J. H., Kong, H., and Bashir, R. (2010). Three-dimensional Photopatterning of Hydrogels Using Stereolithography for Long-Term Cell Encapsulation. Lab. A Chip 10, 2062–2070. doi:10.1039/c004285d
Chang, C., and Zhang, L. (2011). Cellulose-based Hydrogels: Present Status and Application Prospects. Carbohydr. Polym. 84, 40–53. doi:10.1016/j.carbpol.2010.12.023
Chapekar, M. S. (2000). Tissue Engineering: Challenges and Opportunities. J. Biomed. Mater. Res. 53, 617–620. doi:10.1002/1097-4636(2000)53:6<617::AID-JBM1>3.0.CO;2-C
Chen, B.-M., Cheng, T.-L., and Roffler, S. R. (2021a). Polyethylene Glycol Immunogenicity: Theoretical, Clinical, and Practical Aspects of Anti-polyethylene Glycol Antibodies. ACS Nano 15, 14022–14048. doi:10.1021/acsnano.1c05922
Chen, C., Tang, J., Gu, Y., Liu, L., Liu, X., Deng, L., et al. (2019). Bioinspired Hydrogel Electrospun Fibers for Spinal Cord Regeneration. Adv. Funct. Mater. 29. doi:10.1002/adfm.201806899
Chen, J., Xu, J., Wang, A., and Zheng, M. (2009). Scaffolds for Tendon and Ligament Repair: Review of the Efficacy of Commercial Products. Expert Rev. Med. Devices 6, 61–73. doi:10.1586/17434440.6.1.61
Chen, R., and Hunt, J. A. (2007). Biomimetic Materials Processing for Tissue-Engineering Processes. J. Mater. Chem. 17, 3974. doi:10.1039/b706765h
Chen, T., Bakhshi, H., Liu, L., Ji, J., and Agarwal, S. (2018). Combining 3D Printing with Electrospinning for Rapid Response and Enhanced Designability of Hydrogel Actuators. Adv. Funct. Mater. 28, 1800514. doi:10.1002/adfm.201800514
Chen, X., Meng, J., Xu, H., Shinoda, M., Kishimoto, M., Sakurai, S., et al. (2021b). Fabrication and Properties of Electrospun Collagen Tubular Scaffold Crosslinked by Physical and Chemical Treatments. Polymers 13, 755. doi:10.3390/polym13050755
Cheng, Y., Nada, A. A., Valmikinathan, C. M., Lee, P., Liang, D., Yu, X., et al. (2014). In Situ gelling Polysaccharide-Based Hydrogel for Cell and Drug Delivery in Tissue Engineering. J. Appl. Polym. Sci. 131, n/a. doi:10.1002/app.39934
Cho, I. S., Cho, M. O., Li, Z., Nurunnabi, M., Park, S. Y., Kang, S.-W., et al. (2016). Synthesis and Characterization of a New Photo-Crosslinkable Glycol Chitosan Thermogel for Biomedical Applications. Carbohydr. Polym. 144, 59–67. doi:10.1016/j.carbpol.2016.02.029
Cohen, D. L., Lipton, J. I., Bonassar, L. J., and Lipson, H. (2010). Additive Manufacturing for In Situ Repair of Osteochondral Defects. Biofabrication 2, 035004. doi:10.1088/1758-5082/2/3/035004
Colosi, C., Shin, S. R., Manoharan, V., Massa, S., Costantini, M., Barbetta, A., et al. (2016). Microfluidic Bioprinting of Heterogeneous 3D Tissue Constructs Using Low-Viscosity Bioink. Adv. Mater. 28, 677–684. doi:10.1002/adma.201503310
Compaan, A. M., Song, K., and Huang, Y. (2019). Gellan Fluid Gel as a Versatile Support Bath Material for Fluid Extrusion Bioprinting. ACS Appl. Mater. Inter. 11, 5714–5726. doi:10.1021/acsami.8b13792
Coons, D. A., and Alan Barber, F. (2006). Tendon Graft Substitutes???Rotator Cuff Patches. Sports Med. Arthrosc. Rev. 14, 185–190. doi:10.1097/00132585-200609000-00011
Costantini, M., Testa, S., Mozetic, P., Barbetta, A., Fuoco, C., Fornetti, E., et al. (2017). Microfluidic-enhanced 3D Bioprinting of Aligned Myoblast-Laden Hydrogels Leads to Functionally Organized Myofibers In Vitro and In Vivo. Biomaterials 131, 98–110. doi:10.1016/j.biomaterials.2017.03.026
Crescenzi, V., Cornelio, L., di Meo, C., Nardecchia, S., and Lamanna, R. (2007). Novel Hydrogels via Click Chemistry: Synthesis and Potential Biomedical Applications. Biomacromolecules 8, 1844–1850. doi:10.1021/bm0700800
de France, K. J., Xu, F., and Hoare, T. (2018). Structured Macroporous Hydrogels: Progress, Challenges, and Opportunities. Adv. Healthc. Mater. 7, 1700927. doi:10.1002/adhm.201700927
de France, K. J., Xu, F., Toufanian, S., Chan, K. J. W., Said, S., Stimpson, T. C., et al. (2021). Multi-scale Structuring of Cell-Instructive Cellulose Nanocrystal Composite Hydrogel Sheets via Sequential Electrospinning and thermal Wrinkling. Acta Biomater. 128, 250–261. doi:10.1016/j.actbio.2021.04.044
de France, K. J., Yager, K. G., Chan, K. J. W., Corbett, B., Cranston, E. D., and Hoare, T. (2017). Injectable Anisotropic Nanocomposite Hydrogels Direct In Situ Growth and Alignment of Myotubes. Nano Lett. 17, 6487–6495. doi:10.1021/acs.nanolett.7b03600
Deepthi, S., and Jayakumar, R. (2018). Alginate Nanobeads Interspersed Fibrin Network as In Situ Forming Hydrogel for Soft Tissue Engineering. Bioactive Mater. 3, 194–200. doi:10.1016/j.bioactmat.2017.09.005
del Bakhshayesh, A. R., Asadi, N., Alihemmati, A., Tayefi Nasrabadi, H., Montaseri, A., Davaran, S., et al. (2019). An Overview of Advanced Biocompatible and Biomimetic Materials for Creation of Replacement Structures in the Musculoskeletal Systems: Focusing on Cartilage Tissue Engineering. J. Biol. Eng. 13, 85. doi:10.1186/s13036-019-0209-9
Deng, A., Yang, Y., Du, S., and Yang, S. (2018). Electrospinning of In Situ Crosslinked Recombinant Human Collagen Peptide/chitosan Nanofibers for Wound Healing. Biomater. Sci. 6, 2197–2208. doi:10.1039/C8BM00492G
di Bella, C., Duchi, S., O’Connell, C. D., Blanchard, R., Augustine, C., Yue, Z., et al. (2018). In Situ handheld Three-Dimensional Bioprinting for Cartilage Regeneration. J. Tissue Eng. Regenerative Med. 12, 611–621. doi:10.1002/term.2476
Dias, J. R., Ribeiro, N., Baptista-Silva, S., Costa-Pinto, A. R., Alves, N., and Oliveira, A. L. (2020). In Situ Enabling Approaches for Tissue Regeneration: Current Challenges and New Developments. Front. Bioeng. Biotechnol. 8. doi:10.3389/fbioe.2020.00085
Dickman, C. T. D., Russo, V., Thain, K., Pan, S., Beyer, S. T., Walus, K., et al. (2020). Functional Characterization of 3D Contractile Smooth Muscle Tissues Generated Using a Unique Microfluidic 3D Bioprinting Technology. FASEB J. 34, 1652–1664. doi:10.1096/fj.201901063RR
Ding, H., Li, B., Liu, Z., Liu, G., Pu, S., Feng, Y., et al. (2020). Decoupled pH- and Thermo- Responsive Injectable Chitosan/PNIPAM Hydrogel via Thiol‐Ene Click Chemistry for Potential Applications in Tissue Engineering. Adv. Healthc. Mater. 9, 2000454. doi:10.1002/adhm.202000454
Dragusin, D.-M., van Vlierberghe, S., Dubruel, P., Dierick, M., van Hoorebeke, L., Declercq, H. A., et al. (2012). Novel Gelatin–PHEMA Porous Scaffolds for Tissue Engineering Applications. Soft Matter 8, 9589. doi:10.1039/c2sm25536g
Drury, J. L., and Mooney, D. J. (2003). Hydrogels for Tissue Engineering: Scaffold Design Variables and Applications. Biomaterials 24, 4337–4351. doi:10.1016/S0142-9612(03)00340-5
Du, H., Liu, W., Zhang, M., Si, C., Zhang, X., and Li, B. (2019). Cellulose Nanocrystals and Cellulose Nanofibrils Based Hydrogels for Biomedical Applications. Carbohydr. Polym. 209, 130–144. doi:10.1016/j.carbpol.2019.01.020
Duarte Campos, D. F., Rohde, M., Ross, M., Anvari, P., Blaeser, A., Vogt, M., et al. (2019). Corneal Bioprinting Utilizing Collagen-Based Bioinks and Primary Human Keratocytes. J. Biomed. Mater. Res. - A 107, 1945–1953. doi:10.1002/jbm.a.36702
Duchi, S., Onofrillo, C., O’Connell, C. D., Blanchard, R., Augustine, C., Quigley, A. F., et al. (2017). Handheld Co-axial Bioprinting: Application to In Situ Surgical Cartilage Repair. Scientific Rep. 7, 5837. doi:10.1038/s41598-017-05699-x
Dumortier, G., Grossiord, J. L., Agnely, F., and Chaumeil, J. C. (2006). A Review of Poloxamer 407 Pharmaceutical and Pharmacological Characteristics. Pharm. Res. 23, 2709–2728. doi:10.1007/s11095-006-9104-4
Duranti, F., Salti, G., Bovani, B., Calandra, M., and Rosati, M. L. (1998). Injectable Hyaluronic Acid Gel for Soft Tissue Augmentation: A Clinical and Histological Study. Am. Soc. Dermatol. Surg. 24, 1317–1325. doi:10.1111/j.1524-4725.1998.tb00007.x
Eaglstein, W. H., Alvarez, O. M., Auletta, M., Leffel, D., Rogers, G. S., Zitelli, J. A., et al. (1999). Acute Excisional Wounds Treated with a Tissue-Engineered Skin (Apligraf). Dermatol. Surg. 25, 195–201. doi:10.1046/J.1524-4725.1999.08186.X
Edmonds, M. (2009). Apligraf in the Treatment of Neuropathic Diabetic Foot Ulcers. doi:10.1177/1534734609331597
Ehrbar, M., Rizzi, S. C., Hlushchuk, R., Djonov, V., Zisch, A. H., Hubbell, J. A., et al. (2007). Enzymatic Formation of Modular Cell-Instructive Fibrin Analogs for Tissue Engineering. Biomaterials 28, 3856–3866. doi:10.1016/j.biomaterials.2007.03.027
Einbu, A., Grasdalen, H., and Vårum, K. M. (2007). Kinetics of Hydrolysis of Chitin/chitosan Oligomers in Concentrated Hydrochloric Acid. Carbohydr. Res. 342, 1055–1062. doi:10.1016/j.carres.2007.02.022
Elzoghby, A. O. (2013). Gelatin-based Nanoparticles as Drug and Gene Delivery Systems: Reviewing Three Decades of Research. J. Controlled Release 172, 1075–1091. doi:10.1016/j.jconrel.2013.09.019
Elzoghby, A. O., Samy, W. M., and Elgindy, N. A. (2012). Protein-based Nanocarriers as Promising Drug and Gene Delivery Systems. J. Controlled Release 161, 38–49. doi:10.1016/j.jconrel.2012.04.036
Fang, X., Xie, J., Zhong, L., Li, J., Rong, D., Li, X., et al. (2016). Biomimetic Gelatin Methacrylamide Hydrogel Scaffolds for Bone Tissue Engineering. J. Mater. Chem. B 4, 1070–1080. doi:10.1039/C5TB02251G
Feng, F., He, J., Li, J., Mao, M., and Li, D. (2019). Multicomponent Bioprinting of Heterogeneous Hydrogel Constructs Based on Microfluidic Printheads. Int. J. Bioprinting 5, 39. doi:10.18063/ijb.v5i2.202
Fernandes-Cunha, G. M., Lee, H. J., Kumar, A., Kreymerman, A., Heilshorn, S., and Myung, D. (2017). Immobilization of Growth Factors to Collagen Surfaces Using Pulsed Visible Light. Biomacromolecules 18, 3185–3196. doi:10.1021/acs.biomac.7b00838
Fernández-Pérez, J., and Ahearne, M. (2019). The Impact of Decellularization Methods on Extracellular Matrix Derived Hydrogels. Scientific Rep. 9, 14933. doi:10.1038/s41598-019-49575-2
Ferreira, A. M., Gentile, P., Chiono, V., and Ciardelli, G. (2012). Collagen for Bone Tissue Regeneration. Acta Biomater. 8, 3191–3200. doi:10.1016/j.actbio.2012.06.014
Freitas, A.-M. I., Mentula, M., Rahkola-Soisalo, P., Tulokas, S., and Mikkola, T. S. (2020). Tension-Free Vaginal Tape Surgery versus Polyacrylamide Hydrogel Injection for Primary Stress Urinary Incontinence: A Randomized Clinical Trial. J. Urol. 203, 372–378. doi:10.1097/JU.0000000000000517
Frey, B. M., Zeisberger, S. M., and Hoerstrup, S. P. (2016). Tissue Engineering and Regenerative Medicine - New Initiatives for Individual Treatment Offers. Transfus. Med. Hemotherapy 43, 318–319. doi:10.1159/000450716
Fuoco, C., Sangalli, E., Vono, R., Testa, S., Sacchetti, B., Latronico, M. V. G., et al. (2014). 3D Hydrogel Environment Rejuvenates Aged Pericytes for Skeletal Muscle Tissue Engineering. Front. Physiol. 5. doi:10.3389/fphys.2014.00203
Gaharwar, A. K., Singh, I., and Khademhosseini, A. (2020). Engineered Biomaterials for In Situ Tissue Regeneration. Nat. Rev. Mater. 5, 686–705. doi:10.1038/s41578-020-0209-x
Garay, R. P., El-Gewely, R., Armstrong, J. K., Garratty, G., and Richette, P. (2012). Antibodies against Polyethylene Glycol in Healthy Subjects and in Patients Treated with PEG-Conjugated Agents. Expert Opin. Drug Deliv. 9, 1319–1323. doi:10.1517/17425247.2012.720969
Garcia, Y., Collighan, R., Griffin, M., and Pandit, A. (2007). Assessment of Cell Viability in a Three-Dimensional Enzymatically Cross-Linked Collagen Scaffold. J. Mater. Sci. Mater. Med. 18, 1991–2001. doi:10.1007/s10856-007-3091-9
Gentzkow, G. D., Iwasaki, S. D., Hershon, K. S., Mengel, M., Prendergast, J. J., Ricotta, J. J., et al. (1996). Use of Dermagraft, a Cultured Human Dermis, to Treat Diabetic Foot Ulcers. Diabetes Care 19, 350–354. doi:10.2337/diacare.19.4.350
Gilpin, A., and Yang, Y. (20172017). Decellularization Strategies for Regenerative Medicine: From Processing Techniques to Applications. Biomed. Res. Int., 1–13. doi:10.1155/2017/9831534
Glineur, D., Hendrikx, M., Krievins, D., Stradins, P., Voss, B., Waldow, T., et al. (2018). A Randomized, Controlled Trial of Veriset™ Hemostatic Patch in Halting Cardiovascular Bleeding. Med. Devices: Evid. Res. 11, 65–75. doi:10.2147/MDER.S145651
Glowacki, J., and Mizuno, S. (2008). Collagen Scaffolds for Tissue Engineering. Biopolymers 89, 338–344. doi:10.1002/bip.20871
Godugu, C., Patel, A. R., Desai, U., Andey, T., Sams, A., and Singh, M. (2013). AlgiMatrixTM Based 3D Cell Culture System as an In-Vitro Tumor Model for Anticancer Studies. PLoS ONE 8, e53708. doi:10.1371/journal.pone.0053708
Gombotz, W. (1998). Protein Release from Alginate Matrices. Adv. Drug Deliv. Rev. 31, 267–285. doi:10.1016/S0169-409X(97)00124-5
Greiner, A., and Wendorff, J. H. (2007). Electrospinning: A Fascinating Method for the Preparation of Ultrathin Fibers. Angew. Chem. - Int. Edition 46, 5670–5703. doi:10.1002/anie.200604646
Griffiths, M., Ojeh, N., Livingstone, R., Price, R., and Navsaria, H. (2004). Survival of Apligraf in Acute Human Wounds, 1180–1195. Available at: https://home.liebertpub.com/ten. doi:10.1089/TEN.2004.10.1180
Grigoryan, B., Paulsen, S. J., Corbett, D. C., Sazer, D. W., Fortin, C. L., Zaita, A. J., et al. (2019). Multivascular Networks and Functional Intravascular Topologies within Biocompatible Hydrogels. Science 364, 458–464. doi:10.1126/science.aav9750
Grigoryan, B., Sazer, D. W., Avila, A., Albritton, J. L., Padhye, A., Ta, A. H., et al. (2021). Development, Characterization, and Applications of Multi-Material Stereolithography Bioprinting. Scientific Rep. 11, 3171. doi:10.1038/s41598-021-82102-w
Guillame-Gentil, O., Semenov, O., Roca, A. S., Groth, T., Zahn, R., Vörös, J., et al. (2010). Engineering the Extracellular Environment: Strategies for Building 2D and 3D Cellular Structures. Adv. Mater. 22, 5443–5462. doi:10.1002/adma.201001747
Guillotin, B., Souquet, A., Catros, S., Duocastella, M., Pippenger, B., Bellance, S., et al. (2010). Laser Assisted Bioprinting of Engineered Tissue with High Cell Density and Microscale Organization. Biomaterials 31, 7250–7256. doi:10.1016/j.biomaterials.2010.05.055
Guimarães, C. F., Gasperini, L., Marques, A. P., and Reis, R. L. (2021). 3D Flow-Focusing Microfluidic Biofabrication: One-Chip-Fits-All Hydrogel Fiber Architectures. Appl. Mater. Today 23, 101013. doi:10.1016/j.apmt.2021.101013
Guo, Y., Gilbert-Honick, J., Somers, S. M., Mao, H.-Q., and Grayson, W. L. (2019). Modified Cell-Electrospinning for 3D Myogenesis of C2C12s in Aligned Fibrin Microfiber Bundles. Biochem. Biophysical Res. Commun. 516, 558–564. doi:10.1016/j.bbrc.2019.06.082
Hakimi, N., Cheng, R., Leng, L., Sotoudehfar, M., Ba, P. Q., Bakhtyar, N., et al. (2018). Handheld Skin Printer: In Situ Formation of Planar Biomaterials and Tissues. Lab. A Chip 18, 1440–1451. doi:10.1039/C7LC01236E
Hammer, J., Han, L.-H., Tong, X., and Yang, F. (2014). A Facile Method to Fabricate Hydrogels with Microchannel-like Porosity for Tissue Engineering. Tissue Eng. C: Methods 20, 169–176. doi:10.1089/ten.tec.2013.0176
Han, F., Wang, J., Ding, L., Hu, Y., Li, W., Yuan, Z., et al. (2020). Tissue Engineering and Regenerative Medicine: Achievements, Future, and Sustainability in Asia. Front. Bioeng. Biotechnol. 8. doi:10.3389/fbioe.2020.00083
Haq, M. A., Su, Y., and Wang, D. (2017). Mechanical Properties of PNIPAM Based Hydrogels: A Review. Mater. Sci. Eng. C 70, 842–855. doi:10.1016/j.msec.2016.09.081
Harris, L. D., Baldwin, D. F., and Mooney, D. J. (1998). Open Pore Biodegradable Matrices Formed with Gas Foaming. J. Biomed. Mater. Res. 42 (32), 396–402. doi:10.1002/(sici)1097-4636(19981205)42:3<396::aid-jbm7>3.0.co;2-e
He, Y., Yang, F., Zhao, H., Gao, Q., Xia, B., and Fu, J. (2016). Research on the Printability of Hydrogels in 3D Bioprinting. Scientific Rep. 6, 29977. doi:10.1038/srep29977
Hedrick, S. G., Fagundes, M., Robison, B., Blakey, M., Renegar, J., Artz, M., et al. (2017). A Comparison between Hydrogel Spacer and Endorectal Balloon: An Analysis of Intrafraction Prostate Motion during Proton Therapy. J. Appl. Clin. Med. Phys. 18, 106–112. doi:10.1002/acm2.12051
Helary, C., Zarka, M., and Giraud-Guille, M. M. (2012). Fibroblasts within Concentrated Collagen Hydrogels Favour Chronic Skin Wound Healing. J. Tissue Eng. Regenerative Med. 6, 225–237. doi:10.1002/term.420
Hennink, W. E., and van Nostrum, C. F. (2012). Novel Crosslinking Methods to Design Hydrogels. Adv. Drug Deliv. Rev. 64, 223–236. doi:10.1016/j.addr.2012.09.009
Hennink, W. E., and van Nostrum, C. F. (2012). Novel Crosslinking Methods to Design Hydrogels. Adv. Drug Deliv. Rev. 64, 223–236. doi:10.1016/j.addr.2012.09.009
Highley, C. B., Rodell, C. B., and Burdick, J. A. (2015). Direct 3D Printing of Shear-Thinning Hydrogels into Self-Healing Hydrogels. Adv. Mater. 27, 5075–5079. doi:10.1002/adma.201501234
Hinton, T. J., Jallerat, Q., Palchesko, R. N., Park, J. H., Grodzicki, M. S., Shue, H.-J., et al. (2015). Three-dimensional Printing of Complex Biological Structures by Freeform Reversible Embedding of Suspended Hydrogels. Sci. Adv. 1. doi:10.1126/sciadv.1500758
Ho, M. T., Teal, C. J., and Shoichet, M. S. (2019). A Hyaluronan/methylcellulose-Based Hydrogel for Local Cell and Biomolecule Delivery to the central Nervous System. Brain Res. Bull. 148, 46–54. doi:10.1016/j.brainresbull.2019.03.005
Hörning, M., Nakahata, M., Linke, P., Yamamoto, A., Veschgini, M., Kaufmann, S., et al. (2017). Dynamic Mechano-Regulation of Myoblast Cells on Supramolecular Hydrogels Cross-Linked by Reversible Host-Guest Interactions. Scientific Rep. 7, 7660. doi:10.1038/s41598-017-07934-x
Hou, S., Lake, R., Park, S., Edwards, S., Jones, C., and Jeong, K. J. (2018). Injectable Macroporous Hydrogel Formed by Enzymatic Cross-Linking of Gelatin Microgels. ACS Appl. Bio Mater. 1, 1430–1439. doi:10.1021/acsabm.8b00380
Hu, W., Wang, Z., Xiao, Y., Zhang, S., and Wang, J. (2019). Advances in Crosslinking Strategies of Biomedical Hydrogels. Biomater. Sci. 7, 843–855. doi:10.1039/C8BM01246F
Huang, J., Ten, E., Liu, G., Finzen, M., Yu, W., Lee, J. S., et al. (2013). Biocomposites of pHEMA with HA/β-TCP (60/40) for Bone Tissue Engineering: Swelling, Hydrolytic Degradation, and In Vitro Behavior. Polymer 54, 1197–1207. doi:10.1016/j.polymer.2012.12.045
Hunt, J. A., Chen, R., van Veen, T., and Bryan, N. (2014). Hydrogels for Tissue Engineering and Regenerative Medicine. J. Mater. Chem. B 2, 5319–5338. doi:10.1039/C4TB00775A
Hussein, Y., El-Fakharany, E. M., Kamoun, E. A., Loutfy, S. A., Amin, R., Taha, T. H., et al. (2020). Electrospun PVA/hyaluronic acid/L-Arginine Nanofibers for Wound Healing Applications: Nanofibers Optimization and In Vitro Bioevaluation. Int. J. Biol. Macromolecules 164, 667–676. doi:10.1016/j.ijbiomac.2020.07.126
Hutmacher, D. W. (2010). Biomaterials Offer Cancer Research the Third Dimension. Nat. Mater. 9, 90–93. doi:10.1038/nmat2619
INTERNATIONAL REPORT ON (2021). Organ Donation and Transplantation Activities. Available at: www.transplant-observatory.org.
Isaacson, A., Swioklo, S., and Connon, C. J. (2018). 3D Bioprinting of a Corneal Stroma Equivalent. Exp. Eye Res. 173, 188–193. doi:10.1016/j.exer.2018.05.010
Ishikawa, M., Yoshioka, K., Urano, K., Tanaka, Y., Hatanaka, T., and Nii, A. (2014). Biocompatibility of Cross-Linked Hyaluronate (Gel-200) for the Treatment of Knee Osteoarthritis. Osteoarthritis and Cartilage 22, 1902–1909. doi:10.1016/j.joca.2014.08.002
Islam, Md. M., Shahruzzaman, Md., Biswas, S., Nurus Sakib, Md., and Rashid, T. U. (2020). Chitosan Based Bioactive Materials in Tissue Engineering Applications-A Review. Bioactive Mater. 5, 164–183. doi:10.1016/j.bioactmat.2020.01.012
Janmey, P. A. (1982). Kinetics of Formation of Fibrin Oligomers. I. Theory. Biopolymers 21, 2253–2264. doi:10.1002/bip.360211112
Janmey, P. A., Winer, J. P., and Weisel, J. W. (2009). Fibrin Gels and Their Clinical and Bioengineering Applications. J. R. Soc. Interf. 6, 1–10. doi:10.1098/rsif.2008.0327
Jeffries, G. D. M., Xu, S., Lobovkina, T., Kirejev, V., Tusseau, F., Gyllensten, C., et al. (2020). 3D Micro-organisation Printing of Mammalian Cells to Generate Biological Tissues. Scientific Rep. 10, 19529. doi:10.1038/s41598-020-74191-w
Ji, Y., Ghosh, K., Shu, X., Li, B., Sokolov, J., Prestwich, G., et al. (2006). Electrospun Three-Dimensional Hyaluronic Acid Nanofibrous Scaffolds. Biomaterials 27, 3782–3792. doi:10.1016/j.biomaterials.2006.02.037
Jin, G., Prabhakaran, M. P., and Ramakrishna, S. (2011). Stem Cell Differentiation to Epidermal Lineages on Electrospun Nanofibrous Substrates for Skin Tissue Engineering. Acta Biomater. 7, 3113–3122. doi:10.1016/j.actbio.2011.04.017
Jin, R., Moreira Teixeira, L. S., Dijkstra, P. J., van Blitterswijk, C. A., Karperien, M., and Feijen, J. (2010). Enzymatically-crosslinked Injectable Hydrogels Based on Biomimetic Dextran–Hyaluronic Acid Conjugates for Cartilage Tissue Engineering. Biomaterials 31, 3103–3113. doi:10.1016/j.biomaterials.2010.01.013
Jin, S., Liu, M., Chen, S., and Gao, C. (2008). Synthesis, Characterization and the Rapid Response Property of the Temperature Responsive PVP-G-PNIPAM Hydrogel. Eur. Polym. J. 44, 2162–2170. doi:10.1016/j.eurpolymj.2008.04.017
Joseph, B., Krishnan, S., Kavil, S. V., Pai, A. R., James, J., Kalarikkal, N., et al. (2021). Green Chemistry Approach for Fabrication of Polymer Composites. Sust. Chem. 2, 254–270. doi:10.3390/suschem2020015
Jun, Y., Kang, E., Chae, S., and Lee, S.-H. (2014). Microfluidic Spinning of Micro- and Nano-Scale Fibers for Tissue Engineering. Lab. Chip 14, 2145–2160. doi:10.1039/C3LC51414E
Kabiri, K., Omidian, H., Hashemi, S. A., and Zohuriaan-Mehr, M. J. (2003). Synthesis of Fast-Swelling Superabsorbent Hydrogels: Effect of Crosslinker Type and Concentration on Porosity and Absorption Rate. Eur. Polym. J. 39, 1341–1348. doi:10.1016/S0014-3057(02)00391-9
Karava, A., Lazaridou, M., Nanaki, S., Michailidou, G., Christodoulou, E., Kostoglou, M., et al. (2020). Chitosan Derivatives with Mucoadhesive and Antimicrobial Properties for Simultaneous Nanoencapsulation and Extended Ocular Release Formulations of Dexamethasone and Chloramphenicol Drugs. Pharmaceutics 12, 594. doi:10.3390/pharmaceutics12060594
Kasi, A. D., Pergialiotis, V., Perrea, D. N., Khunda, A., and Doumouchtsis, S. K. (2016). Polyacrylamide Hydrogel (Bulkamid®) for Stress Urinary Incontinence in Women: a Systematic Review of the Literature. Methods We searched MEDLINE 27, 367–375. doi:10.1007/s00192-015-2781-y
Katta, P., Alessandro, M., Ramsier, R. D., and Chase, G. G. (2004). Continuous Electrospinning of Aligned Polymer Nanofibers onto a Wire Drum Collector. Nano Lett. 4, 2215–2218. doi:10.1021/nl0486158
Keriquel, V., Oliveira, H., Rémy, M., Ziane, S., Delmond, S., Rousseau, B., et al. (2017). In Situ printing of Mesenchymal Stromal Cells, by Laser-Assisted Bioprinting, for In Vivo Bone Regeneration Applications. Scientific Rep. 7, 1778. doi:10.1038/s41598-017-01914-x
Koosha, M., and Mirzadeh, H. (2015). Electrospinning, Mechanical Properties, and Cell Behavior Study of Chitosan/PVA Nanofibers. J. Biomed. Mater. Res. - Part A 103, 3081–3093. doi:10.1002/jbm.a.35443
Koosha, M., Raoufi, M., and Moravvej, H. (2019). One-pot Reactive Electrospinning of Chitosan/PVA Hydrogel Nanofibers Reinforced by Halloysite Nanotubes with Enhanced Fibroblast Cell Attachment for Skin Tissue Regeneration. Colloids Surf. B: Biointerfaces 179, 270–279. doi:10.1016/j.colsurfb.2019.03.054
Lam, T., Dehne, T., Krüger, J. P., Hondke, S., Endres, M., Thomas, A., et al. (2019). Photopolymerizable Gelatin and Hyaluronic Acid for Stereolithographic 3D Bioprinting of Tissue‐engineered Cartilage. J. Biomed. Mater. Res. B: Appl. Biomater. 107, 2649–2657. doi:10.1002/jbm.b.34354
Langer, R., and Vacanti, J. P. (1993). Tissue Engineering. Science 260, 920–926. doi:10.1126/science.8493529
Leach, J. B., Bivens, K. A., Collins, C. N., and Schmidt, C. E. (2004). Development of Photocrosslinkable Hyaluronic Acid-Polyethylene Glycol-Peptide Composite Hydrogels for Soft Tissue Engineering. J. Biomed. Mater. Res. 70A, 74–82. doi:10.1002/jbm.a.30063
Lee, C., Abelseth, E., de la Vega, L., and Willerth, S. M. (2019). Bioprinting a Novel Glioblastoma Tumor Model Using a Fibrin-Based Bioink for Drug Screening. Mater. Today Chem. 12, 78–84. doi:10.1016/j.mtchem.2018.12.005
Lee, C. R., Grad, S., Gorna, K., Gogolewski, S., Goessl, A., and Alini, M. (2005a). Fibrin–Polyurethane Composites for Articular Cartilage Tissue Engineering: A Preliminary Analysis. Tissue Eng. 11, 1562–1573. doi:10.1089/ten.2005.11.1562
Lee, J., Lee, H., and Andrade, J. (1995). Blood Compatibility of Polyethylene Oxide Surfaces. Prog. Polym. Sci. 20, 1043–1079. doi:10.1016/0079-6700(95)00011-4
Lee, K. Y., and Mooney, D. J. (2001). Hydrogels for Tissue Engineering. Chem. Rev. 101, 1869–1880. doi:10.1021/cr000108x
Lee, S.-H., Park, C.-W., Lee, S.-G., and Kim, W.-K. (2013). Postoperative Cervical Cord Compression Induced by Hydrogel Dural Sealant (DuraSeal®). Korean J. Spine 10, 44. doi:10.14245/kjs.2013.10.1.44
Lee, S. B., Kim, Y. H., Chong, M. S., Hong, S. H., and Lee, Y. M. (2005b). Study of Gelatin-Containing Artificial Skin V: Fabrication of Gelatin Scaffolds Using a Salt-Leaching Method. Biomaterials 26, 1961–1968. doi:10.1016/j.biomaterials.2004.06.032
Lei, Y., Gojgini, S., Lam, J., and Segura, T. (2011). The Spreading, Migration and Proliferation of Mouse Mesenchymal Stem Cells Cultured inside Hyaluronic Acid Hydrogels. Biomaterials 32, 39–47. doi:10.1016/j.biomaterials.2010.08.103
Li, C., Kuss, M., Kong, Y., Nie, F., Liu, X., Liu, B., et al. (2021). 3D Printed Hydrogels with Aligned Microchannels to Guide Neural Stem Cell Migration. ACS Biomater. Sci. Eng. 7, 690–700. doi:10.1021/acsbiomaterials.0c01619
Li, D., Wang, Y., and Xia, Y. (2003). Electrospinning of Polymeric and Ceramic Nanofibers as Uniaxially Aligned Arrays. Nano Lett. 3, 1167–1171. doi:10.1021/nl0344256
Li, L., Yu, F., Shi, J., Shen, S., Teng, H., Yang, J., et al. (2017). In Situ repair of Bone and Cartilage Defects Using 3D Scanning and 3D Printing. Scientific Rep. 7, 9416. doi:10.1038/s41598-017-10060-3
Li, X., Liu, B., Pei, B., Chen, J., Zhou, D., Peng, J., et al. (2020). Inkjet Bioprinting of Biomaterials. Chem. Rev. 120, 10793–10833. doi:10.1021/acs.chemrev.0c00008
Li, Y., Rodrigues, J., and Tomas, H. (2012). Injectable and Biodegradable Hydrogels: Gelation{,} Biodegradation and Biomedical Applications. Chem. Soc. Rev. 41, 2193–2221. doi:10.1039/C1CS15203C
Lim, K. S., Alves, M. H., Poole-Warren, L. A., and Martens, P. J. (2013). Covalent Incorporation of Non-chemically Modified Gelatin into Degradable PVA-Tyramine Hydrogels. Biomaterials 34, 7097–7105. doi:10.1016/j.biomaterials.2013.06.005
Lin, F., Wang, Z., Chen, J., Lu, B., Tang, L., Chen, X., et al. (2020). A Bioinspired Hydrogen Bond Crosslink Strategy toward Toughening Ultrastrong and Multifunctional Nanocomposite Hydrogels. J. Mater. Chem. B 8, 4002–4015. doi:10.1039/D0TB00424C
Lindsay, C. D., Roth, J. G., LeSavage, B. L., and Heilshorn, S. C. (2019). Bioprinting of Stem Cell Expansion Lattices. Acta Biomater. 95, 225–235. doi:10.1016/j.actbio.2019.05.014
Linz, L. A., Burke, L. H., and Miller, L. A. (2013). Two Cross-Linked Porcine Dermal Implants in a Single Patient Undergoing Hernia Repair. Case Rep. 2013. doi:10.1136/bcr-2012-007562
Liu, L., Feng, X., Pei, Y., Wang, J., Ding, J., and Chen, L. (2018a). α-Cyclodextrin Concentration-Controlled Thermo-Sensitive Supramolecular Hydrogels. Mater. Sci. Eng. C 82, 25–28. doi:10.1016/j.msec.2017.08.045
Liu, M., Zeng, X., Ma, C., Yi, H., Ali, Z., Mou, X., et al. (2017a). Injectable Hydrogels for Cartilage and Bone Tissue Engineering. Bone Res. 5. doi:10.1038/boneres.2017.14
Liu, S., Cao, H., Guo, R., Li, H., Lu, C., Yang, G., et al. (2020). Effects of the Proportion of Two Different Cross-Linkers on the Material and Biological Properties of Enzymatically Degradable PEG Hydrogels. Polym. Degrad. Stab. 172, 109067. doi:10.1016/j.polymdegradstab.2019.109067
Liu, V. A., and Bhatia, S. N. (2002). Three-Dimensional Photopatterning of Hydrogels Containing Living Cells. Biomed. Microdevices 4, 257–266. doi:10.1023/A:1020932105236
Liu, W., Heinrich, M. A., Zhou, Y., Akpek, A., Hu, N., Liu, X., et al. (2017b). Extrusion Bioprinting of Shear-Thinning Gelatin Methacryloyl Bioinks. Adv. Healthc. Mater. 6, 1–11. doi:10.1002/adhm.201601451
Liu, W., Li, Y., Zeng, Y., Zhang, X., Wang, J., Xie, L., et al. (2014). Microcryogels as Injectable 3-D Cellular Microniches for Site-Directed and Augmented Cell Delivery. Acta Biomater. 10, 1864–1875. doi:10.1016/j.actbio.2013.12.008
Liu, W., Zhong, Z., Hu, N., Zhou, Y., Maggio, L., Miri, A. K., et al. (2018b). Coaxial Extrusion Bioprinting of 3D Microfibrous Constructs with Cell-Favorable Gelatin Methacryloyl Microenvironments. Biofabrication 10. doi:10.1088/1758-5090/aa9d44
Lorenc, Z. P. (2012). Techniques for the Optimization of Facial and Nonfacial Volumization with Injectable Poly-L-Lactic Acid. Aesth Plast. Surg. 36, 1222–1229. doi:10.1007/s00266-012-9920-3
Lose, G., Sørensen, H. C., Axelsen, S. M., Falconer, C., Lobodasch, K., and Safwat, T. (20102010). An Open Multicenter Study of Polyacrylamide Hydrogel (Bulkamid®) for Female Stress and Mixed Urinary Incontinence. Int. Urogynecol. J. 21 (12 21), 1471–1477. doi:10.1007/S00192-010-1214-1
Lueckgen, A., Garske, D. S., Ellinghaus, A., Mooney, D. J., Duda, G. N., and Cipitria, A. (2019). Enzymatically-degradable Alginate Hydrogels Promote Cell Spreading and In Vivo Tissue Infiltration. Biomaterials 217, 119294. doi:10.1016/j.biomaterials.2019.119294
Luo, X., Guo, Z., He, P., Chen, T., Li, L., Ding, S., et al. (2018). Study on Structure, Mechanical Property and Cell Cytocompatibility of Electrospun Collagen Nanofibers Crosslinked by Common Agents. Int. J. Biol. Macromolecules 113, 476–486. doi:10.1016/j.ijbiomac.2018.01.179
Lutz, J.-F., Akdemir, Ö., and Hoth, A. (2006). Point by Point Comparison of Two Thermosensitive Polymers Exhibiting a Similar LCST: Is the Age of Poly(NIPAM) over? J. Am. Chem. Soc. 128, 13046–13047. doi:10.1021/ja065324n
Ma, L., Gao, C., Mao, Z., Zhou, J., Shen, J., Hu, X., et al. (2003). Collagen/chitosan Porous Scaffolds with Improved Biostability for Skin Tissue Engineering. Biomaterials 24, 4833–4841. doi:10.1016/S0142-9612(03)00374-0
Magalhaes, L. S., Santos, F. E. P., Elias, C. de. M. V., Afewerki, S., Sousa, G. F., Furtado, A. S. A., et al. (2020). Printing 3D Hydrogel Structures Employing Low-Cost Stereolithography Technology. J. Funct. Biomater. 11. doi:10.3390/jfb11010012
Mahdavi, S. S., Abdekhodaie, M. J., Kumar, H., Mashayekhan, S., Baradaran-Rafii, A., and Kim, K. (2020). Stereolithography 3D Bioprinting Method for Fabrication of Human Corneal Stroma Equivalent. Ann. Biomed. Eng. 48, 1955–1970. doi:10.1007/s10439-020-02537-6
Majidi, S. S., Slemming-Adamsen, P., Hanif, M., Zhang, Z., Wang, Z., and Chen, M. (2018). Wet Electrospun Alginate/gelatin Hydrogel Nanofibers for 3D Cell Culture. Int. J. Biol. Macromolecules 118, 1648–1654. doi:10.1016/j.ijbiomac.2018.07.005
Malek-Khatabi, A., Javar, H. A., Dashtimoghadam, E., Ansari, S., Hasani-Sadrabadi, M. M., and Moshaverinia, A. (2020). In Situ bone Tissue Engineering Using Gene Delivery Nanocomplexes. Acta Biomater. 108, 326–336. doi:10.1016/j.actbio.2020.03.008
Mandal, A., Clegg, J. R., Anselmo, A. C., and Mitragotri, S. (2020). Hydrogels in the Clinic. Bioeng. Translational Med. 5. doi:10.1002/btm2.10158
Caulfield, Marcus. J., Qiao, Greg. G., and Solomon*, D. H. (2002). Some Aspects of the Properties and Degradation of Polyacrylamides. Chem. Rev. 102, 3067–3083. doi:10.1021/CR010439P
Margolis, N. E., Bassiri-Tehrani, B., Chhor, C., Singer, C., Hernandez, O., and Moy, L. (2015). Polyacrylamide Gel Breast Augmentation: Report of Two Cases and Review of the Literature. Clin. Imaging 39, 339–343. doi:10.1016/j.clinimag.2014.12.008
Martens, T. P., Godier, A. F. G., Parks, J. J., Wan, L. Q., Koeckert, M. S., Eng, G. M., et al. (2009). Percutaneous Cell Delivery into the Heart Using Hydrogels Polymerizing In Situ. Cel Transplant. 18, 297–304. doi:10.3727/096368909788534915
Matyash, M., Despang, F., Ikonomidou, C., and Gelinsky, M. (2014). Swelling and Mechanical Properties of Alginate Hydrogels with Respect to Promotion of Neural Growth. Tissue Eng. Part C: Methods 20, 401–411. doi:10.1089/ten.tec.2013.0252
McCollister, D. D., Hake, C. L., Sadek, S. E., and Rowe, V. K. (1965). Toxicologic Investigations of Polyacrylamides. Toxicol. Appl. Pharmacol. 7, 639–651. doi:10.1016/0041-008X(65)90119-5
McCormack, A., Highley, C. B., Leslie, N. R., and Melchels, F. P. W. (2020). 3D Printing in Suspension Baths: Keeping the Promises of Bioprinting Afloat. Trends Biotechnol. 38, 584–593. doi:10.1016/j.tibtech.2019.12.020
Meyvis, T. K. L., de Smedt, S. C., Demeester, J., and Hennink, W. E. (2000). Influence of the Degradation Mechanism of Hydrogels on Their Elastic and Swelling Properties during Degradation. Macromolecules 33, 4717–4725. doi:10.1021/ma992131u
Miltner, O., Schneider, U., Siebert, C. H., Niedhart, C., and Niethard, F. U. (2002). Efficacy of Intraarticular Hyaluronic Acid in Patients with Osteoarthritis—A Prospective Clinical Trial. Osteoarthritis and Cartilage 10, 680–686. doi:10.1053/JOCA.2002.0815
Mirani, B., Pagan, E., Currie, B., Siddiqui, M. A., Hosseinzadeh, R., Mostafalu, P., et al. (2017). An Advanced Multifunctional Hydrogel-Based Dressing for Wound Monitoring and Drug Delivery. Adv. Healthc. Mater. 6, 1–15. doi:10.1002/adhm.201700718
Mirani, B., Stefanek, E., Godau, B., Hossein Dabiri, S. M., and Akbari, M. (2021). Microfluidic 3D Printing of a Photo-Cross-Linkable Bioink Using Insights from Computational Modeling. ACS Biomater. Sci. Eng. 7, 3269–3280. doi:10.1021/acsbiomaterials.1c00084
Mirdamadi, E., Muselimyan, N., Koti, P., Asfour, H., and Sarvazyan, N. (2019). Agarose Slurry as a Support Medium for Bioprinting and Culturing Freestanding Cell-Laden Hydrogel Constructs. 3D Printing and Additive Manufacturing 6, 158–164. doi:10.1089/3dp.2018.0175
Mirdamadi, E., Tashman, J. W., Shiwarski, D. J., Palchesko, R. N., and Feinberg, A. W. (2020). FRESH 3D Bioprinting a Full-Size Model of the Human Heart. ACS Biomater. Sci. Eng. 6, 6453–6459. doi:10.1021/acsbiomaterials.0c01133
Moeinzadeh, S., Park, Y., Lin, S., and Yang, Y. P. (2021). In-situ Stable Injectable Collagen-Based Hydrogels for Cell and Growth Factor Delivery. Materialia 15, 100954. doi:10.1016/j.mtla.2020.100954
Montalbano, G., Toumpaniari, S., Popov, A., Duan, P., Chen, J., Dalgarno, K., et al. (2018). Synthesis of Bioinspired Collagen/alginate/fibrin Based Hydrogels for Soft Tissue Engineering. Mater. Sci. Eng. C 91, 236–246. doi:10.1016/j.msec.2018.04.101
Mooney, D. J., and Vandenburgh, H. (2008). Cell Delivery Mechanisms for Tissue Repair. Cell Stem Cell 2, 205–213. doi:10.1016/j.stem.2008.02.005
Moreira Teixeira, L. S., Feijen, J., van Blitterswijk, C. A., Dijkstra, P. J., and Karperien, M. (2012). Enzyme-catalyzed Crosslinkable Hydrogels: Emerging Strategies for Tissue Engineering. Biomaterials 33, 1281–1290. doi:10.1016/j.biomaterials.2011.10.067
Moura, M. J., Faneca, H., Lima, M. P., Gil, M. H., and Figueiredo, M. M. (2011). Situ Forming Chitosan Hydrogels Prepared via Ionic/Covalent Co-cross-linking. Biomacromolecules 12, 3275–3284. doi:10.1021/bm200731x
Moxon, S. R., Corbett, N. J., Fisher, K., Potjewyd, G., Domingos, M., and Hooper, N. M. (2019). Blended Alginate/collagen Hydrogels Promote Neurogenesis and Neuronal Maturation. Mater. Sci. Eng. C 104, 109904. doi:10.1016/j.msec.2019.109904
Mueller, E., Poulin, I., Bodnaryk, W. J., and Hoare, T. (2022). Click Chemistry Hydrogels for Extrusion Bioprinting: Progress, Challenges, and Opportunities. Biomacromolecules 23 (3), 619–640. doi:10.1021/acs.biomac.1c01105
Murphy, S. V. (2014). 3D Bioprinting of Tissues and Organs. Nat. Biotechnol. 32, 773–785. doi:10.1038/nbt.2958
Nagai, T., Izumi, M., and Ishii, M. (2004). Fish Scale Collagen. Preparation and Partial Characterization. Int. J. Food Sci. Tech. 39, 239–244. doi:10.1111/j.1365-2621.2004.00777.x
Neves, M. I., Moroni, L., and Barrias, C. C. (2020). Modulating Alginate Hydrogels for Improved Biological Performance as Cellular 3D Microenvironments. Front. Bioeng. Biotechnol. 8. doi:10.3389/fbioe.2020.00665
Nicodemus, G. D., and Bryant, S. J. (2008). Cell Encapsulation in Biodegradable Hydrogels for Tissue Engineering Applications. Tissue Eng. Part B: Rev. 14, 149–165. doi:10.1089/ten.teb.2007.0332
Nie, K., Han, S., Yang, J., Sun, Q., Wang, X., Li, X., et al. (2020). Enzyme-Crosslinked Electrospun Fibrous Gelatin Hydrogel for Potential Soft Tissue Engineering. Polymers 12, 1977. doi:10.3390/polym12091977
Noor, N., Shapira, A., Edri, R., Gal, I., Wertheim, L., and Dvir, T. (2019). 3D Printing of Personalized Thick and Perfusable Cardiac Patches and Hearts. Adv. Sci. 6, 1900344. doi:10.1002/advs.201900344
Noori, A., Ashrafi, S. J., Vaez-Ghaemi, R., Hatamian-Zaremi, A., and Webster, T. J. (2017). A Review of Fibrin and Fibrin Composites for Bone Tissue Engineering. Int. J. Nanomedicine 12, 4937–4961. doi:10.2147/IJN.S124671
O’Connell, C. D., di Bella, C., Thompson, F., Augustine, C., Beirne, S., Cornock, R., et al. (2016). Development of the Biopen: a Handheld Device for Surgical Printing of Adipose Stem Cells at a Chondral Wound Site. Biofabrication 8, 015019. doi:10.1088/1758-5090/8/1/015019
Onoe, H., Okitsu, T., Itou, A., Kato-Negishi, M., Gojo, R., Kiriya, D., et al. (2013). Metre-long Cell-Laden Microfibres Exhibit Tissue Morphologies and Functions. Nat. Mater. 12, 584–590. doi:10.1038/nmat3606
Ouyang, L., Armstrong, J. P. K., Lin, Y., Wojciechowski, J. P., Lee-reeves, C., Hachim, D., et al. (2020). Expanding and Optimizing 3D Bioprinting Capabilities Using Complementary Network Bioinks. Sci. Adv. 6, 1–14. doi:10.1126/sciadv.abc5529
Ouyang, L., Highley, C. B., Sun, W., and Burdick, J. A. (2017). A Generalizable Strategy for the 3D Bioprinting of Hydrogels from Nonviscous Photo‐crosslinkable Inks. Adv. Mater. 29, 1604983. doi:10.1002/adma.201604983
Pai, A., and Al-Singary, W. (2015). Durability, Safety and Efficacy of Polyacrylamide Hydrogel (Bulkamid®) in the Management of Stress and Mixed Urinary Incontinence: Three Year Follow up Outcomes. Cent. Eur. J. Urol. 68, 428. doi:10.5173/CEJU.2015.647
Park, H., Kim, M. H., Yoon, Y. il., and Park, W. H. (2017). One-pot Synthesis of Injectable Methylcellulose Hydrogel Containing Calcium Phosphate Nanoparticles. Carbohydr. Polym. 157, 775–783. doi:10.1016/j.carbpol.2016.10.055
Park, S. H., Seo, J. Y., Park, J. Y., Ji, Y. B., Kim, K., Choi, H. S., et al. (2019). An Injectable, Click-Crosslinked, Cytomodulin-Modified Hyaluronic Acid Hydrogel for Cartilage Tissue Engineering. NPG Asia Mater. 11, 30. doi:10.1038/s41427-019-0130-1
Partap, S., Rehman, I., Jones, J. R., and Darr, J. a. (2006). Supercritical Carbon Dioxide in Water” Emulsion-Templated Synthesis of Porous Calcium Alginate Hydrogels. Adv. Mater. 18, 501–504. doi:10.1002/adma.200501423
Pasut, G., and Veronese, F. M. (2007). Polymer–drug Conjugation, Recent Achievements and General Strategies. Prog. Polym. Sci. 32, 933–961. doi:10.1016/j.progpolymsci.2007.05.008
Patel, B., Wonski, B. T., Saliganan, D. M., Rteil, A., Kabbani, L. S., and Lam, M. T. (2021). Decellularized Dermis Extracellular Matrix Alloderm Mechanically Strengthens Biological Engineered tunica Adventitia-Based Blood Vessels. Scientific Rep. 11, 11384. doi:10.1038/s41598-021-91005-9
Patenaude, M., and Hoare, T. (2012). Injectable, Degradable Thermoresponsive Poly(N-Isopropylacrylamide) Hydrogels. ACS Macro Lett. 1, 409–413. doi:10.1021/mz200121k
Patenaude, M., Smeets, N. M. B., and Hoare, T. (2014). Designing Injectable, Covalently Cross-Linked Hydrogels for Biomedical Applications. Macromolecular Rapid Commun. 35, 598–617. doi:10.1002/marc.201300818
Pedde, R. D., Mirani, B., Navaei, A., Styan, T., Wong, S., Mehrali, M., et al. (2017). Emerging Biofabrication Strategies for Engineering Complex Tissue Constructs. Adv. Mater. 29, 1606061. doi:10.1002/adma.201606061
Place, E. S., George, J. H., Williams, C. K., and Stevens, M. M. (2009). Synthetic Polymer Scaffolds for Tissue Engineering. Chem. Soc. Rev. 38, 1139–1151. doi:10.1039/b811392k
Pratt, A. B., Weber, F. E., Schmoekel, H. G., Müller, R., and Hubbell, J. A. (2004). Synthetic Extracellular Matrices for In Situ Tissue Engineering. Biotechnol. Bioeng. 86, 27–36. doi:10.1002/bit.10897
Price, R. D., Berry, M. G., and Navsaria, H. A. (2007). Hyaluronic Acid: the Scientific and Clinical Evidence. J. Plast. Reconstr. Aesthet. Surg. 60, 1110–1119. doi:10.1016/j.bjps.2007.03.005
Pruett, R. C., Schepens, C. L., and Swann, D. A. (1979). Hyaluronic Acid Vitreous Substitute: A Six-Year Clinical Evaluation. Arch. Ophthalmol. 97, 2325–2330. doi:10.1001/ARCHOPHT.1979.01020020541006
Qiao, Q., Wang, X., Sun, J., Zhao, R., Liu, Z., Wang, Y., et al. (2005). Management for Postoperative Complications of Breast Augmentation by Injected Polyacrylamide Hydrogel. Aesthet. Plast. Surg. 29, 156–161. doi:10.1007/s00266-004-0099-0
Ramesh, S., Harrysson, O. L. A., Rao, P. K., Tamayol, A., Cormier, D. R., Zhang, Y., et al. (2021). Extrusion Bioprinting: Recent Progress, Challenges, and Future Opportunities. Bioprinting 21, e00116. doi:10.1016/j.bprint.2020.e00116
Ramiah, P., du Toit, L. C., Choonara, Y. E., Kondiah, P. P. D., and Pillay, V. (2020). Hydrogel-Based Bioinks for 3D Bioprinting in Tissue Regeneration. Front. Mater. 7. doi:10.3389/fmats.2020.00076
Rana, D., Zreiqat, H., Benkirane-Jessel, N., Ramakrishna, S., and Ramalingam, M. (2017). Development of Decellularized Scaffolds for Stem Cell-Driven Tissue Engineering. J. Tissue Eng. Regenerative Med. 11, 942–965. doi:10.1002/term.2061
Rd, I. (1996). NTP Summary Report on the Metabolism, Disposition, and Toxicity of 1,4-butanediol (CAS No. 110-63-4). Toxicity Rep. Ser. 1–28 (A1-8), B1–B5.
Reid, B., Gibson, M., Singh, A., Taube, J., Furlong, C., Murcia, M., et al. (2015). PEG Hydrogel Degradation and the Role of the Surrounding Tissue Environment. J. Tissue Eng. Regenerative Med. 9, 315–318. doi:10.1002/term.1688
Reneker, D. H., and Yarin, A. L. (2008). Electrospinning Jets and Polymer Nanofibers. Polymer 49, 2387–2425. doi:10.1016/j.polymer.2008.02.002
Rhodes, N. P., Hunt, J. A., Longinotti, C., and Pavesio, A. (2011). In Vivo Characterization of Hyalonect, a Novel Biodegradable Surgical Mesh. J. Surg. Res. 168, e31–e38. doi:10.1016/j.jss.2010.09.015
Riccio, M., Battiston, B., Pajardi, G., Corradi, M., Passaretti, U., Atzei, A., et al. (2010). Efficiency of Hyaloglide® in the Prevention of the Recurrence of Adhesions after Tenolysis of Flexor Tendons in Zone II: a Randomized, Controlled, Multicentre Clinical Trial. J. Hand Surg. (European Volume) 35, 130–138. doi:10.1177/1753193409342044
Rujing, Z., and Larsen, N. B. (2017). Stereolithographic Hydrogel Printing of 3D Culture Chips with Biofunctionalized Complex 3D Perfusion Networks, 4273–4282. doi:10.1039/c7lc00926g
Russo, E., and Villa, C. (2019). Poloxamer Hydrogels for Biomedical Applications. Pharmaceutics 11, 671. doi:10.3390/pharmaceutics11120671
Sack, K. L., Aliotta, E., Choy, J. S., Ennis, D. B., Davies, N. H., Franz, T., et al. (2018). Effect of Intra-myocardial Algisyl-LVR TM Injectates on Fibre Structure in Porcine Heart Failure. J. Mech. Behav. Biomed. Mater. 87, 172–179. doi:10.1016/j.jmbbm.2018.07.005
Sackett, S. D., Tremmel, D. M., Ma, F., Feeney, A. K., Maguire, R. M., Brown, M. E., et al. (2018). Extracellular Matrix Scaffold and Hydrogel Derived from Decellularized and Delipidized Human Pancreas. Scientific Rep. 8, 10452. doi:10.1038/s41598-018-28857-1
Saldin, L. T., Cramer, M. C., Velankar, S. S., White, L. J., and Badylak, S. F. (2017). Extracellular Matrix hydrogels from decellularized tissues: Structure and function. Acta Biomaterialia 49, 1–15. doi:10.1016/j.actbio.2016.11.068
Sall, I., and Férard, G. (2007). Comparison of the sensitivity of 11 crosslinked hyaluronic acid gels to bovine testis hyaluronidase. Polymer Degradation and Stability 92, 915–919. doi:10.1016/j.polymdegradstab.2006.11.020
Samorezov, J. E., Morlock, C. M., and Alsberg, E. (2015). Dual Ionic and Photo-Crosslinked Alginate Hydrogels for Micropatterned Spatial Control of Material Properties and Cell Behavior. Bioconjugate Chemistry 26, 1339–1347. doi:10.1021/acs.bioconjchem.5b00117
Samprasit, W., Kaomongkolgit, R., Sukma, M., Rojanarata, T., Ngawhirunpat, T., and Opanasopit, P. (2015). Mucoadhesive electrospun chitosan-based nanofibre mats for dental caries prevention. Carbohydrate Polymers 117, 933–940. doi:10.1016/j.carbpol.2014.10.026
Schmedlen, R. H., Masters, K. S., and West, J. L. (2002). Photocrosslinkable polyvinyl alcohol hydrogels that can be modified with cell adhesion peptides for use in tissue engineering. Biomaterials 23, 4325–4332. doi:10.1016/S0142-9612(02)00177-1
Schulte, V. A., Alves, D. F., Dalton, P. P., Moeller, M., Lensen, M. C., and Mela, P. (2013). Microengineered PEG hydrogels: 3D scaffolds for guided cell growth. Macromolecular Bioscience 13, 562–572. doi:10.1002/mabi.201200376
Seibel, M. J., Robins, S. P., and Bilezikian, J. P. (2006). Dynamics of Bone and Cartilage Metabolism. Second Edition. Elsevier. doi:10.1016/B978-0-12-088562-6.X5000-6
Seliktar, D. (2012). Designing Cell-Compatible Hydrogels for Biomedical Applications. Science 336, 1124. LP – 1128. Available at: http://science.sciencemag.org/content/336/6085/1124.abstract. doi:10.1126/science.1214804
Shen, Y., Tang, H., Huang, X., Hang, R., Zhang, X., Wang, Y., et al. (2020). DLP printing photocurable chitosan to build bio-constructs for tissue engineering. Carbohydrate Polymers 235, 115970. doi:10.1016/j.carbpol.2020.115970
Shen, Z.-S., Cui, X., Hou, R.-X., Li, Q., Deng, H.-X., and Fu, J. (2015). Tough biodegradable chitosan–gelatin hydrogels via in situ precipitation for potential cartilage tissue engineering. RSC Advances 5, 55640–55647. doi:10.1039/C5RA06835E
Shin, J. Y., Yeo, Y. H., Jeong, J. E., Park, S. A., and Park, W. H. (2020). Dual-crosslinked methylcellulose hydrogels for 3D bioprinting applications. Carbohydrate Polymers 238, 116192. doi:10.1016/j.carbpol.2020.116192
Shiwarski, D. J., Hudson, A. R., Tashman, J. W., and Feinberg, A. W. (2021). Emergence of FRESH 3D printing as a platform for advanced tissue biofabrication. APL Bioengineering 5, 010904. doi:10.1063/5.0032777
Shore, N., Cookson, M. S., and Gittelman, M. C. (2011). Long-term efficacy and tolerability of once-yearly histrelin acetate subcutaneous implant in patients with advanced prostate cancer. BJU International 109, 226–232. doi:10.1111/j.1464-410x.2011.10370.x
Sill, T. J., and von Recum, H. A. (20082006). Electrospinning: Applications in drug delivery and tissue engineering. Biomaterials 29, 1989. doi:10.1016/j.biomaterials.2008.01.011
Singh, S., Choudhury, D., Yu, F., Mironov, V., and Naing, M. W. (2020). In situ bioprinting – Bioprinting from benchside to bedside? Acta Biomaterialia 101, 14–25. doi:10.1016/j.actbio.2019.08.045
Sisson, K., Zhang, C., Farach-Carson, M. C., Chase, D. B., and Rabolt, J. F. (2009). Evaluation of cross-linking methods for electrospun gelatin on cell growth and viability. Biomacromolecules 10, 1675–1680. doi:10.1021/bm900036s
Sivaraj, D., Chen, K., Chattopadhyay, A., Henn, D., Wu, W., Noishiki, C., et al. (2021). Hydrogel Scaffolds to Deliver Cell Therapies for Wound Healing. Frontiers in Bioengineering and Biotechnology 9. doi:10.3389/FBIOE.2021.660145
Skalak, R., and Fox, C. F. (1988). Tissue engineering : proceedings of a workshop held at Granlibakken. California; Lake Tahoe, 26–29.
Smeds, K. A., and Grinstaff, M. W. (2001).Photocrosslinkable polysaccharides forin situ hydrogel formation. Journal of Biomedical Materials Research 54. 115–121. doi:10.1002/1097-4636(200101)54:1<115::aid-jbm14>3.0.co;2-q
Smeets, N. M. B., Bakaic, E., Patenaude, M., and Hoare, T. (2014). Injectable and tunable poly(ethylene glycol) analogue hydrogels based on poly(oligoethylene glycol methacrylate). Chemical communications 50, 3306–9. doi:10.1039/c3cc48514e
Smeets, N. M. B., Bakaic, E., Patenaude, M., and Hoare, T. (2014). Injectable poly(oligoethylene glycol methacrylate)-based hydrogels with tunable phase transition behaviours: Physicochemical and biological responses. Acta Biomaterialia 10, 4143–4155. doi:10.1016/j.actbio.2014.05.035
Smeets, N. M. B., Bakaic, E., Yavitt, F. M., Yang, F.-C., Rheinstädter, M. C., and Hoare, T. (2014). Probing the Internal Morphology of Injectable Poly(oligoethylene glycol methacrylate) Hydrogels by Light and Small-Angle Neutron Scattering. Macromolecules 47, 6017–6027. doi:10.1021/ma5011827
Snyder, S. J., Arnoczky, S. P., Bond, J. L., and Dopirak, R. (2009). Histologic Evaluation of a Biopsy Specimen Obtained 3 Months After Rotator Cuff Augmentation With GraftJacket Matrix. Arthroscopy: The Journal of Arthroscopic & Related Surgery 25, 329–333. doi:10.1016/j.arthro.2008.05.023
Song, E., Yeon Kim, S., Chun, T., Byun, H.-J., and Lee, Y. M. (2006). Collagen scaffolds derived from a marine source and their biocompatibility. Biomaterials 27, 2951–2961. doi:10.1016/j.biomaterials.2006.01.015
Sparavigna, A., Fino, P., Tenconi, B., Giordan, N., Amorosi, V., and Scuderi, N. (2014). A new dermal filler made of cross-linked and auto-cross-linked hyaluronic acid in the correction of facial aging defects. Journal of Cosmetic Dermatology 13, 307–314. doi:10.1111/JOCD.12116
Sperinde, J. J., and Griffith, L. G. (1997). Synthesis and Characterization of Enzymatically-Cross-Linked Poly(ethylene glycol) Hydrogels. Macromolecules 30, 5255–5264. doi:10.1021/ma970345a
Stephens-Altus, J. S., Sundelacruz, P., Rowland, M. L., and West, J. L. (2011). Development of bioactive photocrosslinkable fibrous hydrogels. Journal of Biomedical Materials Research Part A 98A, 167–176. doi:10.1002/jbm.a.33095
Stevens, M. M., and George, J. H. (2005). Exploring and Engineering the Cell Surface Interface. Science 310, 1135–1138. doi:10.1126/science.1106587
Streit, M., and Braathen, L. R. (2000). Apligraf - A living human skin equivalent for the treatment of chronic wounds. International Journal of Artificial Organs 23, 831–833. doi:10.1177/039139880002301208
Sun, T., Li, X., Shi, Q., Wang, H., Huang, Q., and Fukuda, T. (2018). Microfluidic Spun Alginate Hydrogel Microfibers and Their Application in Tissue Engineering. Gels 4, 38. doi:10.3390/gels4020038
Sundy, J. S., Baraf, H. S. B., Yood, R. A., Edwards, N. L., Gutierrez-Urena, S. R., Treadwell, E. L., et al. (2011). Efficacy and Tolerability of Pegloticase for the Treatment of Chronic Gout in Patients Refractory to Conventional Treatment: Two Randomized Controlled Trials. JAMA 306, 711–720. doi:10.1001/jama.2011.1169
Suntornnond, R., Tan, E. Y. S., An, J., and Chua, C. K. (2017). A highly printable and biocompatible hydrogel composite for direct printing of soft and perfusable vasculature-like structures. Scientific Reports 7, 16902. doi:10.1038/s41598-017-17198-0
Tabriz, A. G., Hermida, M. A., Leslie, N. R., and Shu, W. (2015). Three-dimensional bioprinting of complex cell laden alginate hydrogel structures. Biofabrication 7, 045012. doi:10.1088/1758-5090/7/4/045012
Tam, R. Y., Cooke, M. J., and Shoichet, M. S. (2012). A covalently modified hydrogel blend of hyaluronan–methyl cellulose with peptides and growth factors influences neural stem/progenitor cell fate. Journal of Materials Chemistry 22, 19402–19411. doi:10.1039/C2JM33680D
Tamayol, A., Najafabadi, A. H., Aliakbarian, B., Arab-Tehrany, E., Akbari, M., Annabi, N., et al. (2015). Hydrogel Templates for Rapid Manufacturing of Bioactive Fibers and 3D Constructs. Advanced Healthcare Materials 4, 2146–2153. doi:10.1002/adhm.201500492
Tan, H., Ramirez, C. M., Miljkovic, N., Li, H., Rubin, J. P., and Marra, K. G. (2009). Thermosensitive injectable hyaluronic acid hydrogel for adipose tissue engineering. Biomaterials 30, 6844–6853. doi:10.1016/j.biomaterials.2009.08.058
Tang, Y., Tong, X., Conrad, B., and Yang, F. (2020). Injectable and in situ crosslinkable gelatin microribbon hydrogels for stem cell delivery and bone regeneration in vivo. Theranostics 10, 6035–6047. doi:10.7150/thno.41096
Tekin, A. C. (2013). Hyalonect in the treatment of pseudarthrosis. Acta Orthopaedica et Traumatologica Turcica 47, 379–386. doi:10.3944/AOTT.2013.2875
Tibbitt, M. W., and Anseth, K. S. (2009). Hydrogels as extracellular matrix mimics for 3D cell culture. Biotechnology and Bioengineering 103, 655–663. doi:10.1002/bit.22361
Tnibar, A., Schougaard, H., Camitz, L., Rasmussen, J., Koene, M., Jahn, W., et al. (2015). An international multi-centre prospective study on the efficacy of an intraarticular polyacrylamide hydrogel in horses with osteoarthritis: a 24 months follow-up. Acta Veterinaria Scandinavica 57. doi:10.1186/S13028-015-0110-6
Tomov, M. L., Perez, L., Ning, L., Chen, H., Jing, B., Mingee, A., et al. (2021). A 3D Bioprinted In Vitro Model of Pulmonary Artery Atresia to Evaluate Endothelial Cell Response to Microenvironment. Advanced Healthcare Materials 10, 2100968. doi:10.1002/adhm.202100968
Torres-Giner, S., Gimeno-Alcañiz, J. v., Ocio, M. J., and Lagaron, J. M. (2009). Comparative Performance of Electrospun Collagen Nanofibers Cross-linked by Means of Different Methods. ACS Applied Materials & Interfaces 1, 218–223. doi:10.1021/am800063x
Tsang, K. Y., Cheung, M. C. H., Chan, D., and Cheah, K. S. E. (2010). The developmental roles of the extracellular matrix: Beyond structure to regulation. Cel and Tissue Research 339, 93–110. doi:10.1007/s00441-009-0893-8
Unal, A. Z., and West, J. L. (2020). Synthetic ECM: Bioactive Synthetic Hydrogels for 3D Tissue Engineering. Bioconjugate Chemistry 31, 2253–2271. doi:10.1021/acs.bioconjchem.0c00270
U.S. Food and Drug Administration (2021). Premarket Approval (PMA) - Bulkamid Urethral Bulking System. PMA Number: P170023. Available at: https://www.accessdata.fda.gov/scripts/cdrh/cfdocs/cfpma/pma.cfm?id=P170023.
U.S. Food and Drug Administration (2017). Statement by FDA Commissioner Scott Gottlieb, M.D., on FDA ushering in new era of 3D printing of medical products; provides guidance to manufacturers of medical devices. Fda Statement. Available at: fda.gov/news-events/press-announcements/statement-fda-commissioner-scott-gottlieb-md-fda-ushering-new-era-3d-printing-medical-products.
van den Bulcke, A. I., Bogdanov, B., de Rooze, N., Schacht, E. H., Cornelissen, M., and Berghmans, H. (2000). Structural and Rheological Properties of Methacrylamide Modified Gelatin Hydrogels. Biomacromolecules 1, 31–38. doi:10.1021/bm990017d
van Tomme, S. R., Storm, G., and Hennink, W. E. (2008). In situ gelling hydrogels for pharmaceutical and biomedical applications. International Journal of Pharmaceutics 355, 1–18. doi:10.1016/j.ijpharm.2008.01.057
Vijayasekaran, S., Chirila, V., Robertson, T. A., Lou, X., Fitton, J. H., Hicks, C. R., et al. (2000). Calcification of poly(2-hydroxyethyl methacrylate) hydrogel sponges implanted in the rabbit cornea: A 3-month study. Journal of Biomaterials Science, Polymer Edition 11, 599–615. doi:10.1163/156856200743896
Vrana, N. E., O’Grady, A., Kay, E., Cahill, P. A., and McGuinness, G. B. (2009). Cell encapsulation within PVA-based hydrogels via freeze-thawing: a one-step scaffold formation and cell storage technique. Journal of tissue engineering and regenerative medicine 3, 567–572. doi:10.1002/term10.1002/term.193
Wakuda, Y., Nishimoto, S., Suye, S., and Fujita, S. (2018). Native collagen hydrogel nanofibres with anisotropic structure using core-shell electrospinning. Scientific Reports 8, 6248. doi:10.1038/s41598-018-24700-9
Wang, H., Liu, H., Zhang, X., Wang, Y., Zhao, M., Chen, W., et al. (2021a). One-Step Generation of Aqueous-Droplet-Filled Hydrogel Fibers as Organoid Carriers Using an All-in-Water Microfluidic System. ACS Applied Materials & Interfaces 13, 3199–3208. doi:10.1021/acsami.0c20434
Wang, L.-S., Du, C., Toh, W. S., Wan, A. C. A., Gao, S. J., and Kurisawa, M. (2014). Modulation of chondrocyte functions and stiffness-dependent cartilage repair using an injectable enzymatically crosslinked hydrogel with tunable mechanical properties. Biomaterials 35, 2207–2217. doi:10.1016/j.biomaterials.2013.11.070
Wang, L., and Stegemann, J. P. (2010). Thermogelling chitosan and collagen composite hydrogels initiated with β-glycerophosphate for bone tissue engineering. Biomaterials 31, 3976–3985. doi:10.1016/j.biomaterials.2010.01.131
Wang, M., Li, W., Mille, L. S., Ching, T., Luo, Z., Tang, G., et al. (2021b). Digital Light Processing Based Bioprinting with Composable Gradients. Advanced Materials. doi:10.1002/adma.202107038
Wang, S., Zinderman, C., Wise, R., and Braun, M. (2007). Infections and human tissue transplants: review of FDA MedWatch reports 2001–2004. Cell and Tissue Banking 8, 211–219. doi:10.1007/s10561-007-9034-3
Wang, X., Dai, X., Zhang, X., Ma, C., Li, X., Xu, T., et al. (2018a). 3D bioprinted glioma cell-laden scaffolds enriching glioma stem cells via epithelial – mesenchymal transition. Journal of Biomedical Materials Research Part A 107A, 383–391. doi:10.1002/jbm.a.36549
Wang, Z., Kumar, H., Tian, Z., Jin, X., Holzman, J. F., Menard, F., et al. (2018b). Visible Light Photoinitiation of Cell-Adhesive Gelatin Methacryloyl Hydrogels for Stereolithography 3D Bioprinting. Applied Materials & Interfaces 10, 26859–26869. doi:10.1021/acsami.8b06607
Weil, L. (2008). Augmented Brostrom Repair Using Biologic Collagen Implant: Report on 9 Consecutive Patients. The Foot & Ankle Journal 1. doi:10.3827/faoj.2008.0107.0004
Wu, Z., Kong, B., Liu, R., Sun, W., and Mi, S. (2018). Engineering of Corneal Tissue through an Aligned PVA/Collagen Composite Nanofibrous Electrospun Scaffold. Nanomaterials 8, 124. doi:10.3390/nano8020124
Xin, S., Wyman, O. M., and Alge, D. L. (2018). Assembly of PEG Microgels into Porous Cell-Instructive 3D Scaffolds via Thiol-Ene Click Chemistry. Advanced Healthcare Materials 7, 1800160. doi:10.1002/adhm.201800160
Xinming, L., Yingde, C., Lloyd, A. W., Mikhalovsky, S. v., Sandeman, S. R., Howel, C. A., et al. (2008). Polymeric hydrogels for novel contact lens-based ophthalmic drug delivery systems: A review. Contact Lens and Anterior Eye 31, 57–64. doi:10.1016/j.clae.2007.09.002
Xu, F., Dodd, M., Sheardown, H., and Hoare, T. (2018a). Single-Step Reactive Electrospinning of Cell-Loaded Nanofibrous Scaffolds as Ready-to-Use Tissue Patches. Biomacromolecules 19, 4182–4192. doi:10.1021/acs.biomac.8b00770
Xu, F., Gough, I., Dorogin, J., Sheardown, H., and Hoare, T. (2020). Nanostructured degradable macroporous hydrogel scaffolds with controllable internal morphologies via reactive electrospinning. Acta Biomaterialia. doi:10.1016/j.actbio.2019.12.038
Xu, F., Lam, A., Pan, Z., Randhawa, G., Lamb, M., Sheardown, H., et al. (2021). Fast Thermoresponsive Poly(oligoethylene glycol methacrylate) (POEGMA)-Based Nanostructured Hydrogels for Reversible Tuning of Cell Interactions. ACS Biomaterials Science & Engineering 7, 4258–4268. doi:10.1021/acsbiomaterials.0c01552
Xu, F., Sheardown, H., and Hoare, T. (2016). Reactive electrospinning of degradable poly(oligoethylene glycol methacrylate)-based nanofibrous hydrogel networks. Chemical Communications 52, 1451–1454. doi:10.1039/c5cc08053c
Xu, S.-C., Qin, C.-C., Yu, M., Dong, R.-H., Yan, X., Zhao, H., et al. (2015). A battery-operated portable handheld electrospinning apparatus. Nanoscale 7, 12351–12355. doi:10.1039/C5NR02922H
Xu, Y., Hu, Y., Liu, C., Yao, H., Liu, B., and Mi, S. (2018b). A novel strategy for creating tissue-engineered biomimetic blood vessels using 3D bioprinting technology. Materials 11. doi:10.3390/ma11091581
Xue, J., Wu, T., Dai, Y., and Xia, Y. (2019). Electrospinning and Electrospun Nanofibers: Methods, Materials, and Applications. Chemical Reviews 119, 5298–5415. doi:10.1021/acs.chemrev.8b00593
Yeo, M., and Kim, G. H. (2018). Anisotropically Aligned Cell-Laden Nanofibrous Bundle Fabricated via Cell Electrospinning to Regenerate Skeletal Muscle Tissue. Small 14, 1803491. doi:10.1002/smll.201803491
Yeo, M., and Kim, G. (2020). Micro/nano-hierarchical scaffold fabricated using a cell electrospinning/3D printing process for co-culturing myoblasts and HUVECs to induce myoblast alignment and differentiation. Acta Biomaterialia 107, 102–114. doi:10.1016/j.actbio.2020.02.042
Young, S. A., Riahinezhad, H., and Amsden, B. G. (2019). In situ forming, mechanically resilient hydrogels for cell delivery. Journal of Materials Chemistry B 7, 5742–5761. doi:10.1039/C9TB01398A
Yu, Y., Xu, S., Li, S., and Pan, H. (2021). Genipin-cross-linked hydrogels based on biomaterials for drug delivery: a review. Biomaterials Science 9, 1583–1597. doi:10.1039/D0BM01403F
Zare, M., Bigham, A., Zare, M., Luo, H., Rezvani Ghomi, E., and Ramakrishna, S. (2021). pHEMA: An Overview for Biomedical Applications. International Journal of Molecular Sciences 22, 6376. doi:10.3390/ijms22126376
Zarrintaj, P., Ramsey, J. D., Samadi, A., Atoufi, Z., Yazdi, M. K., Ganjali, M. R., et al. (2020). Poloxamer: A versatile tri-block copolymer for biomedical applications. Acta Biomaterialia 110, 37–67. doi:10.1016/j.actbio.2020.04.028
Zaulyanov, L., and Kirsner, R. S. (2007). A review of a bi-layered living cell treatment (Apligraf ®) in the treatment of venous leg ulcers and diabetic foot ulcers. Clinical Interventions in Aging 2, 93. doi:10.2147/CIIA.2007.2.1.93
Zhang, H., and Cooper, A. I. (2005). Synthesis and applications of emulsion-templated porous materials. Soft Matter 1, 107–113. doi:10.1039/B502551F
Zhang, P., Sun, F., Liu, S., and Jiang, S. (2016). Anti-PEG antibodies in the clinic: Current issues and beyond PEGylation. Journal of Controlled Release 244, 184–193. doi:10.1016/j.jconrel.2016.06.040
Zhang, Y. S., Pi, Q., and van Genderen, A. M. (2017). Microfluidic Bioprinting for Engineering Vascularized Tissues and Organoids. Journal of Visualized Experiments. doi:10.3791/55957
Zhang, Y., Yu, Y., Chen, H., and Ozbolat, I. T. (2013). Characterization of printable cellular micro-fluidic channels for tissue engineering. Biofabrication 5, 025004. doi:10.1088/1758-5082/5/2/025004
Zhu, D., Li, Z., Huang, K., Caranasos, T. G., Rossi, J. S., and Cheng, K. (2021). Minimally invasive delivery of therapeutic agents by hydrogel injection into the pericardial cavity for cardiac repair. Nature Communications 12, 1412. doi:10.1038/s41467-021-21682-7
Zhu, J. (2010a). Bioactive modification of poly(ethylene glycol) hydrogels for tissue engineering. Biomaterials 31, 4639–4656. doi:10.1016/j.biomaterials.2010.02.044
Keywords: Hydrogels, Tissue Engineering, Bioprinting, Electrospinning, Biomaterials
Citation: Xu F, Dawson C, Lamb M, Mueller E, Stefanek E, Akbari M and Hoare T (2022) Hydrogels for Tissue Engineering: Addressing Key Design Needs Toward Clinical Translation. Front. Bioeng. Biotechnol. 10:849831. doi: 10.3389/fbioe.2022.849831
Received: 06 January 2022; Accepted: 12 April 2022;
Published: 05 May 2022.
Edited by:
David K. Mills, Louisiana Tech University, United StatesReviewed by:
Silvia Fare’, Politecnico di Milano, ItalyCopyright © 2022 Xu, Dawson, Lamb, Mueller, Stefanek, Akbari and Hoare. This is an open-access article distributed under the terms of the Creative Commons Attribution License (CC BY). The use, distribution or reproduction in other forums is permitted, provided the original author(s) and the copyright owner(s) are credited and that the original publication in this journal is cited, in accordance with accepted academic practice. No use, distribution or reproduction is permitted which does not comply with these terms.
*Correspondence: Mohsen Akbari, bWFrYmFyaUB1dmljLmNh; Todd Hoare, aG9hcmV0ckBtY21hc3Rlci5jYQ==
Disclaimer: All claims expressed in this article are solely those of the authors and do not necessarily represent those of their affiliated organizations, or those of the publisher, the editors and the reviewers. Any product that may be evaluated in this article or claim that may be made by its manufacturer is not guaranteed or endorsed by the publisher.
Research integrity at Frontiers
Learn more about the work of our research integrity team to safeguard the quality of each article we publish.