- Key Laboratory of Combinatorial Biosynthesis and Drug Discovery (Ministry of Education), School of Pharmaceutical Sciences, Wuhan University, Wuhan, China
Drug-carrying nanoparticles have obtained great attention for disease treatments due to the fact that they can improve drug solubility, provide drug protection and prolong release duration, thus enhancing drug bioavailability and increasing therapeutic efficacy. Although nanoparticles containing drugs can be administered via different routes such as oral, intravenous and ocular, transdermal delivery of nanoparticles mediated by microneedles has attracted considerable interest due to the capability of circumventing enzymatic degradation caused by gastrointestinal track, and increasing patient compliance by reducing pain associated with hypodermic injection. In this review, we first introduce four types of nanoparticles that were used for drug delivery, and then summarize strategies that have been employed to facilitate delivery of drug-loaded nanoparticles via microneedles. Finally, we give a conclusion and provide our perspectives on the potential clinical translation of microneedle-facilitated nanoparticles delivery.
Introduction
Due to the unique advantages, such as protection from enzyme degradation, prolonged half-life of drugs, desired targetability, ability to achieve sustained release, nanoparticles have been extensively used for delivering a wide variety of drugs that are applied for multiple disease treatments, such as diabetes, wound healing and cancers (Baetke et al., 2015). Although drug-loaded nanoparticles can be administered via different routes for therapies such as oral, intravenous and ocular administration, transdermal delivery of nanoparticles mediated by microneedles (MNs) has drawn considerable attention due to the capability of circumventing enzymatic degradation caused by gastrointestinal track, and increasing patient compliance by reducing pain associated with hypodermic injection (Table 1). MNs are array of micro-scale needles that can penetrate the outmost skin layer, termed stratum corneum, and enter the skin to achieve transdermal drug delivery in a minimally invasive manner (Prausnitz, 2017). Using MNs, many kinds of drugs have been successfully and efficiently delivered into the skin, such as levonorgestrel (Li et al., 2019), insulin (Zhu et al., 2020), calcitonin (Tas et al., 2012) and influenza vaccine (Stinson et al., 2021). As portable and minimally invasive devices, MN patches that contain hundreds of MNs connecting with supporting layers can effectively overcome the barrier of stratum corneum to facilitate transdermal delivery of nanoparticles that are far greater than pure drugs, either by producing reversible microchannels for enhancing skin permeation of topically applied nanoparticles (e.g., solid MNs), or by getting dissolved under the skin to achieve direct delivery of nanoparticles in the skin (e.g., coated MNs and dissolvable MNs) (Figure 1). Although the topic about transdermal delivery of nanoparticles via MNs has been recently reported by some review papers (Chen et al., 2020; Alimardani et al., 2021; Ruan and Zhang, 2021; Salwa et al., 2021), this work put a different emphasis on this subject that include the design and summarization of drug-carrying nanoparticles integrated with MNs. In this review, we first introduce the four types of nanoparticles capable of carrying drugs, including nanocrystals, lipid nanoparticles, polymeric nanoparticles and inorganic nanoparticles, and then describe MN-based strategies that have been adopted to aid transdermal delivery of these nanoparticles for drug delivery. Finally, we provide our perspectives on the potential translation of MNs-mediated delivery of nanoparticles in the skin.
Nanoparticles for Drug Delivery Mediated by Microneedles
There have been a great number of drug-carrying nanoparticles that are developed for transdermal drug delivery mediated by MNs, either for localized delivery or for systemic release to treat a variety of diseases, such as skin cancer, contraception, diabetes or cardiovascular diseases (Chen et al., 2020; Salwa et al., 2021; Vora et al., 2021) (Table 2). These nanoparticles can be classified into four types based on their fabricating materials, including nanocrystals that are made of pure drugs, lipid-based nanoparticles that are fabricated with lipids, polymeric nanoparticles that are comprised of natural or synthetic polymers, and inorganic nanoparticles that are composed of inorganic materials (e.g., silicas, metals).
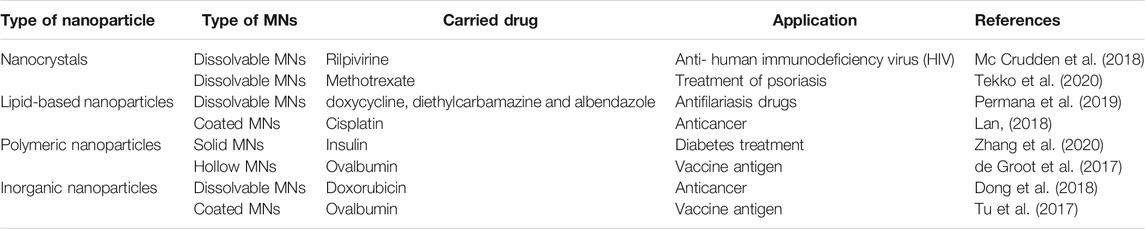
TABLE 2. The representative applications of transdermal delivery of drug-loaded nanoparticles via MNs.
Nanocrystals
Generally, low bioavailability and small absorption of poorly soluble drugs represent major problems in the pharmaceutical drug development (Savjani et al., 2012). Numerous efforts have been made to increase the solubility and biodistribution of poorly soluble drugs (Nagarwal et al., 2011), among which nanocrystal technology plays an important role in addressing the problems associated with low solubility of drugs. Nanocrystals are carrier-free drug particles within nanometer size range, and have increased dissolution rate due to increased surface area, thus possessing enhanced bioavailability (Srivalli and Mishra, 2015). Moreover, owing to the structure of non-polymer covering, nanocrystals have high drug loading capability (as high as 100%), which makes them extremely attractive for treatments of the diseases that usually require high drug doses. A further characteristic of nanocrystals is that they can achieve sustained release of the drug for an extended period at the administration site due to the slow dissolution in the aqueous environment (Permana et al., 2020).
Lipid-Based Nanoparticles
Lipid-based nanoparticles are most typically spherical vesicles with single or multi-lipid bilayers that encapsulate aqueous droplets, and the lipid-based nanoparticles mainly include: liposomes, nanoemulsions, solid lipid nanoparticles (SLNs), nanostructured lipid carriers (NLCs) (Vitorino et al., 2014). Due to the lipophicity of the nanoparticles, they are beneficial to increase solubility of poorly water-soluble drugs, thereby enhancing bioavailability. Besides, lipid layers of nanoparticles can fuse with stratum corneum lipids to further improve the drug transport through the skin (Kuntsche et al., 2008). As one kind of the most prevalent lipid-based nanocarriers, liposomes are usually made of phospholipids that can form unilamellar and multilamellar vesicular structures, which makes liposomes suitable to carry and deliver hydrophilic, hydrophobic or lipophilic drugs (Mitchell et al., 2021).
Polymeric Nanoparticles
Polymeric nanoparticles used for drug delivery offer many special advantages over other kinds of nanoparticles, including increased target ability after surface modification, improved biocompatibility, reduced cytotoxicity and prolonged drug release duration (Begines and Ortiz, 2020). A variety of natural or synthetic polymers have been used in polymeric nanoparticle formulations, such as poly (D, l-lactide-co-glycolide) (PLGA), poly (lactic acid) (PLA), polyethylene glycol (PEG), polyacrylates, chitosan, alginate, gelatin and albumin (De Jong and Borm, 2008). Among the above polymers, PLGA is the most frequently used hydrophobic polymer because of its excellent biocompatibility and slow biodegradation rate, which makes it appealing for the development of controlled release formulations (Naves et al., 2017), and PEG is the most commonly used hydrophilic polymer with non-immunogenic, biocompatible and flexible nature, which makes it suitable for the delivery of hydrophilic drugs or bioactive molecules that usually require mild condition during encapsulation process (Jeyhani et al., 2019).
Inorganic Nanoparticles
Inorganic nanoparticles are non-toxic, hydrophilic, biocompatible and highly stable, which makes them ideal for drug delivery. Inorganic nanoparticles for drug delivery mainly include mesoporous silica nanoparticles (MSNs), superparamagnetic iron oxides (SPIONs), quantum dots and metallic nanoparticles. MSNs have been widely used as controlled release carriers for drugs due to their large specific surface area, regular pore structure, adjustable pore size and good biocompatibility. Meanwhile, their unique mesoporous structure can prevent drugs from enzyme degradation or early release. In addition, the porous surface is covered with a large number of silica hydroxyl groups, which enables mesoporous silica nanoparticles to be functionalized by post-modification with a variety of polymers or specific drugs, forming intelligent drug control systems (Choi et al., 2021). SPIONs are kind of magnetic nanoparticles that can be guided by the direction of external magnetic field. Besides, they can also be used as contrast agents for magnetic resonance imaging (MRI) for diagnosis of diseases (Zhu et al., 2017). Quantum dots are semiconductor nanomaterials with diameters between 2 and 100 nm, usually prepared from III–V or group II–VI elements. Quantum dot excitation light has wide band range, narrow emission spectrum width, high fluorescence intensity, good stability, long life and certain antibacterial activity (Ren et al., 2020), which makes it have a good application prospect in wound healing (Salleh and Fauzi, 2021), drug transport (Fakhri et al., 2017), fluorescent biosensors (Hu et al., 2021) and disease diagnosis (Mansuriya and Altintas, 2021). In recent years, metallic nanoparticles have attracted growing interest in drug delivery, and the modification and functionalization of metallic nanoparticles with specific functional groups allow them to bind to antibodies, drugs and other ligands, making metallic nanoparticles promising in biomedical applications (Patra et al., 2018).
Strategies of MN-Mediated Transdermal Delivery of Nanoparticles
Although many research have confirmed the benefits of nanoparticles as drug reservoirs for transdermal drug delivery, a lot of evidences demonstrate that nanoparticles still stay in the upper layer of stratum corneum and are restricted to deep penetration in the skin after topical application (Lademann et al., 2007; Sahle et al., 2017). In order to address the issue, minimally invasive MN-based strategies have been developed to facilitate transdermal delivery of therapeutics-loaded nanoparticles, including topical application of nanoparticles after MN penetration, transdermal delivery via coated MNs, transdermal delivery via dissolvable MNs, and transdermal delivery by the combination of iontophoresis and MNs.
Topical Application Through the MN-Punctured Pores
The most typical strategy of MN-mediated transdermal delivery of nanoparticles is the topical application of a formulation containing nanoparticles after MNs pretreatment, which created microscopic puncture holes in the skin allowing nanoparticles to diffuse through the skin (Figure 2) (Eneko et al., 2016). Zhang et al. used confocal laser scanning microscopy to visualize the distribution of fluorescent PLGA nanoparticles in the skin through the microchannels produced by solid MNs application, and they observed a great number of nanoparticles could travel and deposit in Epidermis located below the stratum corneum, demonstrating the enhanced transdermal delivery of nanoparticles facilitated by solid MNs pretreatment (Zhang et al., 2010). This strategy has the advantage of enhancing skin permeation of nanoparticles, while it still suffers from limited drug amount that can be transported through the skin (Alimardani et al., 2021).

FIGURE 2. A schematic representation of solid MNs pretreatment for increasing the permeability of nanoparticles by creating micro-holes across the skin (Eneko et al., 2016).
Transdermal Delivery of Nanoparticles by Hollow MNs
Hollow MNs are also beneficial for transdermal delivery of nanoparticles, which allows for continuous delivery of liquid nanoparticle formulations, like nanoparticle suspensions, into the skin through the inserted hollow needles. Such kind of MNs is possibly capable of precisely delivering larger amounts of nanoparticles with spatial and temporal resolution compared to solid MNs (Roxhed et al., 2008). For example, Mir et al. developed a liquid injection system (AdminPen®) by combining bacterial enzyme-responsive nanoparticles with hollow MNs. In vivo skin insertion and dermatokinetic studies suggested that the system delivered about 8.5 times higher concentrations of the drug, carvacrol (CAR), in the form of NPs as compared with topically applied hydrogel containing pure CAR, indicating a great potential of increasing transdermal delivery of nanoparticles by hollow MNs (Mir et al., 2020). In spite of the capability of enabling transdermal delivery of precise and increased nanoparticles facilitated by hollow MNs, such strategy is compromised by the use of complicated setups.
Transdermal Delivery of Nanoparticles via Coated MNs
Coated MNs that contain a nanoparticle formulation at the surface can completely dissolve the coating and subsequently deliver the nanoparticles at the administration site upon skin insertion. Coated MNs are generally made of solid MNs and surface coatings that can be prepared by various methods such as dip coating (Ma and Gill, 2014), spray coating (Ning et al., 2020) and other sophisticated methods (Tort and Mutlu Agardan, 2020). For example, DeMuth et al. designed PLGA MNs coated with cationic poly (β-amino ester) (PBAE) and negatively charged interbilayer-cross-linked multilamellar lipid vesicles (ICMVs) for delivery of protein antigen and the antigen adjuvant (DeMuth et al., 2012). This coating of PBAE and ICMV rapidly transferred from the MNs to the skin after MNs insertion, leading to an efficient delivery of antigens to antigen-presenting cells and inducing a robust immune response (Figure 3) (DeMuth et al., 2012). Although this method is very straightforward, but it still suffers from limited amount of nanoparticles that can be delivered into the skin via coated MNs (Kim et al., 2012).
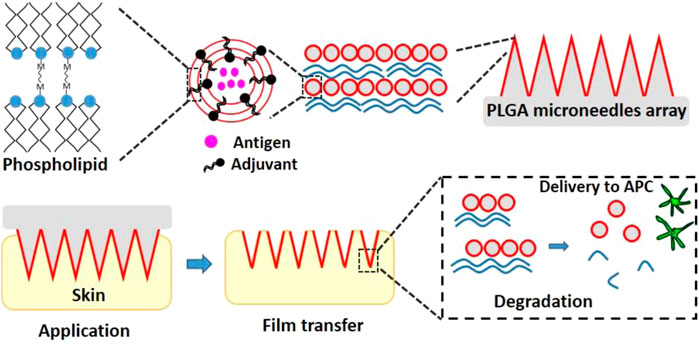
FIGURE 3. The schematic graph of PLGA MNs coated with PBAE and ICMVs for co-delivery of the antigen and adjuvant (DeMuth et al., 2012).
Transdermal Delivery of Nanoparticles via Dissolvable MNs
The grim situation of poorly soluble drugs in the topical application has encouraged the combination of drug nanocrystals or drug-loaded nanoparticles with dissolvable MNs (Figure 4) (Lee et al., 2014). Unlike coated MNs, dissolvable MNs can get dissolution of the whole MNs and then release the encapsulated nanocrystals or nanoparticles at the administration site under skin, which makes dissolvable MNs be able to deliver more nanoparticles compared with coated MNs. For example, methotrexate sodium salt (MTX Na), a drug for psoriasis treatment, is poorly water soluble and hard to be used topically. Such drug could be made into nanocrystals and incorporated into dissolvable MNs. After skin insertion, the MTX nanocrystal-carrying MNs exhibited desired drug delivery efficiency, showing approximately 322-fold higher accumulation in the skin 24 h after administration than free MTX. In vivo studies in rats revealed that 72 h after administration, there was still about 12.5% of the MTX nanocrystals deposited in the skin, suggesting a localized and sustained drug delivery facilitated by the strategy of dissolvable MNs and nanotechnology (Tekko et al., 2020).
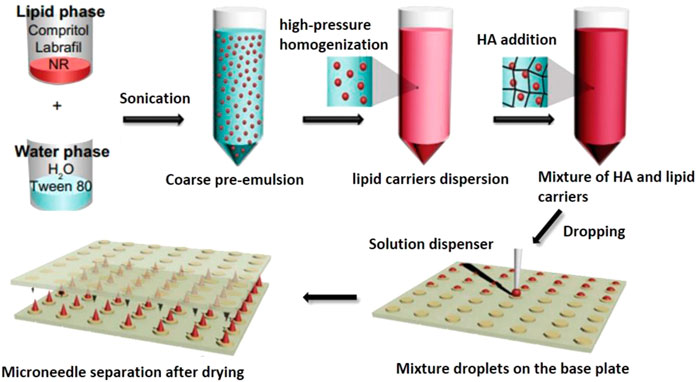
FIGURE 4. The schematic graph of the synthesis of HA MNs containing lipophilic NR-loaded lipid nanoparticles. Abbreviation: HA (hyaluronic acid), NR (Nile red), NLCs (nanostructured lipid carrier) (Lee et al., 2014).
Combination of Iontophoresis With MNs for Transdermal Delivery of Nanoparticles
Electric-field related methods have been used as auxiliary means for better drug transport (Niamlang and Sirivat, 2009). Transdermal iontophoresis is a physically noninvasive method that involves applying a low electrical potential gradient across the skin to facilitate the passage of charged or polar substances through the skin (Katikaneni et al., 2009). However, the use of iontophoresis alone still has limited improvement and do not significantly promote drug penetration from stratum corneum to deeper layers (e.g., Epidermis and dermis), especially for those biological macromolecules such as proteins or DNA. The strategy of combining iontophoresis with MNs can significantly improve the transdermal delivery efficiency as well as broaden the diversity of drugs ranging from small chemicals to large molecules or drug-loaded nanoparticles (Katikaneni et al., 2009; Lanke et al., 2009; Vemulapalli et al., 2012; Gaware et al., 2019). For example, Chen et al. investigated the transdermal delivery of insulin-loaded nanovesicles driven by iontophoresis through microchannels created by solid MNs (Chen et al., 2009). Facilitated by MNs puncture, in vivo permeation study exhibited 86.1–166.7 times higher drug permeability than that without MNs pretreatment. Further, under the influence of a forward current, the positive charged nanovesicles accelerated the movement towards deeper site of skin through the microchannels created by MNs and showed 3.4–7.1 times higher than nanovesicles with MNs pretreatment alone, suggesting the greatly improved delivery efficiency of nanoparticles when using MNs and iontophoresis together. Although this method possesses significantly enhanced efficiency for transdermal delivery of drug-carrying nanoparticles, it is only restricted to polar substance and has limited effect for neutral nanoparticles compared with the approach of MNs pretreatment alone.
Conclusion and Perspectives
Due to the unique advantages, drug-carrying nanoparticles have been demonstrated to be valuable drug delivery systems in a variety of biomedical applications, and some drug-loaded nanoparticles have even been applied for clinical use (Mitchell et al., 2021). Although nanoparticles can be administered via different routes, like oral taken and intravenous injection, MN-mediated transdermal delivery of nanoparticles has attracted considerable interest since this administration method can significantly improve drug bioavailability while avoiding pain associated with hypodermic injection. In this review, we introduced four types of currently used nanoparticles for drug delivery and summarized the strategies that had been explored to facilitate the transdermal delivery of drug-loaded nanoparticles.
The development of microfabrication technology and nanotechnology will enhance drug stability during preparation of nanoparticles and fabrication of MNs, increase drug amount for each MN patch, and promote transdermal drug delivery efficiency after skin insertion. Also, special designs (e.g., core-shell structure) can be incorporated in the nanoparticle-encapsulated MNs, and facilitate the delivery systems to achieve sustained release of drugs for an extended period under the skin (e.g., 3 or 6 months), which will make the systems appealing for the treatment of chronic diseases by reducing dosing frequency and increasing patient compliance, such as type 2 diabetes, cancer, obesity, psoriasis or spinal cord injury. It is optimistically envisioned that expanded academic research in MNs and nanoparticles will accelerate clinical translation of MN-mediated delivery of nanoparticles for transdermal drug delivery.
Author Contributions
XJ and HZ did the literature research and wrote the manuscript. XJ developed or collected the figures. WL reviewed, edited, and supervised. All authors contributed to the article and approved the submitted version.
Conflict of Interest
The authors declare that the research was conducted in the absence of any commercial or financial relationships that could be construed as a potential conflict of interest.
Publisher’s Note
All claims expressed in this article are solely those of the authors and do not necessarily represent those of their affiliated organizations, or those of the publisher, the editors and the reviewers. Any product that may be evaluated in this article, or claim that may be made by its manufacturer, is not guaranteed or endorsed by the publisher.
Acknowledgments
The authors are very thankful for financial supports by the National Natural Science Foundation of China (NSFC, No. 52103182), the Fundamental Research Funds for the Central Universities (No. 2042021kf0073) and start-up package of Wuhan University.
References
Alimardani, V., Abolmaali, S. S., Yousefi, G., Rahiminezhad, Z., Abedi, M., Tamaddon, A., et al. (2021). Microneedle Arrays Combined with Nanomedicine Approaches for Transdermal Delivery of Therapeutics. Jcm 10 (2), 181. doi:10.3390/jcm10020181
Baetke, S. C., Lammers, T., and Kiessling, F. (2015). Applications of Nanoparticles for Diagnosis and Therapy of Cancer. Bjr 88 (1054), 20150207. doi:10.1259/bjr.20150207
Begines, B., Ortiz, T., Pérez-Aranda, M., Martínez, G., Merinero, M., Argüelles-Arias, F., et al. (2020). Polymeric Nanoparticles for Drug Delivery: Recent Developments and Future Prospects. Nanomaterials 10 (7), 1403. doi:10.3390/nano10071403
Chen, H., Zhu, H., Zheng, J., Mou, D., Wan, J., Zhang, J., et al. (2009). Iontophoresis-driven Penetration of Nanovesicles through Microneedle-Induced Skin Microchannels for Enhancing Transdermal Delivery of Insulin. J. Controlled Release 139 (1), 63–72. doi:10.1016/j.jconrel.2009.05.031
Chen, M., Quan, G., Sun, Y., Yang, D., Pan, X., and Wu, C. (2020). Nanoparticles-encapsulated Polymeric Microneedles for Transdermal Drug Delivery. J. Controlled Release 325, 163–175. doi:10.1016/j.jconrel.2020.06.039
Choi, G., Fitriasari, E. I., and Park, C. (2021). Electro-Mechanochemical Gating of a Metal-Phenolic Nanocage for Controlled Guest-Release Self-Powered Patches and Injectable Gels. ACS Nano 15 (9), 14580–14586. doi:10.1021/acsnano.1c04276
Choi, Y., Lee, S. G., Jeong, J. H., Lee, K. M., Jeong, K. H., Yang, H., et al. (2014). Nanostructured Lipid Carrier-Loaded Hyaluronic Acid Microneedles for Controlled Dermal Delivery of a Lipophilic Molecule. Ijn 9, 289–299. doi:10.2147/ijn.s54529
C. Nagarwal, R., Kumar, R., Dhanawat, M., Das, N., and K. Pandit, J. (2011). Nanocrystal Technology in the Delivery of Poorly Soluble Drugs: an Overview. Cdd 8 (4), 398–406. doi:10.2174/156720111795767988
de Groot, A. M., Du, G., Mönkäre, J., Platteel, A. C. M., Broere, F., Bouwstra, J. A., et al. (2017). Hollow Microneedle-Mediated Intradermal Delivery of Model Vaccine Antigen-Loaded PLGA Nanoparticles Elicits Protective T Cell-Mediated Immunity to an Intracellular Bacterium. J. Controlled Release 266, 27–35. doi:10.1016/j.jconrel.2017.09.017
De Jong, W. H., and Borm, P. J. (2008). Drug Delivery and Nanoparticles: Applications and Hazards. Ijn 3 (2), 133–149. doi:10.2147/ijn.s596
DeMuth, P. C., Moon, J. J., Suh, H., Hammond, P. T., and Irvine, D. J. (2012). Releasable Layer-By-Layer Assembly of Stabilized Lipid Nanocapsules on Microneedles for Enhanced Transcutaneous Vaccine Delivery. ACS Nano 6 (9), 8041–8051. doi:10.1021/nn302639r
Dong, L., Li, Y., Li, Z., Xu, N., Liu, P., Du, H., et al. (2018). Au Nanocage-Strengthened Dissolving Microneedles for Chemo-Photothermal Combined Therapy of Superficial Skin Tumors. ACS Appl. Mater. Inter. 10 (11), 9247–9256. doi:10.1021/acsami.7b18293
Fakhri, A., Tahami, S., and Nejad, P. A. (2017). Preparation and Characterization of Fe 3 O 4 -Ag 2 O Quantum Dots Decorated Cellulose Nanofibers as a Carrier of Anticancer Drugs for Skin Cancer. J. Photochem. Photobiol. B: Biol. 175, 83–88. doi:10.1016/j.jphotobiol.2017.08.032
Gaware, S. A., Rokade, K. A., Bala, P., and Kale, S. N. (2019). Microneedles of Chitosan‐porous Carbon Nanocomposites: Stimuli (pH and Electric Field)‐initiated Drug Delivery and Toxicological Studies. J. Biomed. Mater. Res. 107 (8), 1582–1596. doi:10.1002/jbm.a.36672
Hu, O., Li, Z., Tong, Y., Wang, Q., and Chen, Z. (2021). DNA Functionalized Double Quantum Dots-Based Fluorescence Biosensor for One-step Simultaneous Detection of Multiple microRNAs. Talanta 235, 122763. doi:10.1016/j.talanta.2021.122763
Jeyhani, M., Gnyawali, V., Abbasi, N., Hwang, D. K., and Tsai, S. S. H. (2019). Microneedle-assisted Microfluidic Flow Focusing for Versatile and High Throughput Water-In-Water Droplet Generation. J. Colloid Interf. Sci. 553, 382–389. doi:10.1016/j.jcis.2019.05.100
Katikaneni, S., Badkar, A., Nema, S., and Banga, A. K. (2009). Molecular Charge Mediated Transport of a 13kD Protein across Microporated Skin. Int. J. Pharmaceutics 378 (1-2), 93–100. doi:10.1016/j.ijpharm.2009.05.050
Kim, Y.-C., Park, J.-H., and Prausnitz, M. R. (2012). Microneedles for Drug and Vaccine Delivery. Adv. Drug Deliv. Rev. 64 (14), 1547–1568. doi:10.1016/j.addr.2012.04.005
Kuntsche, J., Bunjes, H., Fahr, A., Pappinen, S., Rönkkö, S., Suhonen, M., et al. (2008). Interaction of Lipid Nanoparticles with Human Epidermis and an Organotypic Cell Culture Model. Int. J. Pharmaceutics 354 (1-2), 180–195. doi:10.1016/j.ijpharm.2007.08.028
Lademann, J., Richter, H., Teichmann, A., Otberg, N., Blume-Peytavi, U., Luengo, J., et al. (2007). Nanoparticles - An Efficient Carrier for Drug Delivery into the Hair Follicles. Eur. J. Pharmaceutics Biopharmaceutics 66 (2), 159–164. doi:10.1016/j.ejpb.2006.10.019
Lan, X., She, J., Lin, D.-a., Xu, Y., Li, X., Yang, W.-f., et al. (2018). Microneedle-Mediated Delivery of Lipid-Coated Cisplatin Nanoparticles for Efficient and Safe Cancer Therapy. ACS Appl. Mater. Inter. 10 (39), 33060–33069. doi:10.1021/acsami.8b12926
Lanke, S., Kolli, C., Strom, J., and Banga, A. (2009). Enhanced Transdermal Delivery of Low Molecular Weight Heparin by Barrier Perturbation. Int. J. Pharmaceutics 365 (1-2), 26–33. doi:10.1016/j.ijpharm.2008.08.028
Larrañeta, E., Lutton, R. E. M., Woolfson, A. D., and Donnelly, R. F. (2016). Microneedle Arrays as Transdermal and Intradermal Drug Delivery Systems: Materials Science, Manufacture and Commercial Development. Mater. Sci. Eng. R: Rep. 104 (6), 1–32. doi:10.1016/j.mser.2016.03.001
Li, W., Terry, R. N., Tang, J., Feng, M. R., Schwendeman, S. P., and Prausnitz, M. R. (2019). Rapidly Separable Microneedle Patch for the Sustained Release of a Contraceptive. Nat. Biomed. Eng. 3 (3), 220–229. doi:10.1038/s41551-018-0337-4
Ma, Y., and Gill, H. S. (2014). Coating Solid Dispersions on Microneedles via a Molten Dip‐Coating Method: Development and In Vitro Evaluation for Transdermal Delivery of a Water‐Insoluble Drug. J. Pharm. Sci. 103 (11), 3621–3630. doi:10.1002/jps.24159
Mansuriya, B. D., and Altintas, Z. (2021). Enzyme-Free Electrochemical Nano-Immunosensor Based on Graphene Quantum Dots and Gold Nanoparticles for Cardiac Biomarker Determination. Nanomaterials 11 (3), 578. doi:10.3390/nano11030578
Mc Crudden, M. T. C., Larrañeta, E., Clark, A., Jarrahian, C., Rein-Weston, A., Lachau-Durand, S., et al. (2018). Design, Formulation and Evaluation of Novel Dissolving Microarray Patches Containing a Long-Acting Rilpivirine Nanosuspension. J. Controlled Release 292, 119–129. doi:10.1016/j.jconrel.2018.11.002
Mir, M., Permana, A. D., Tekko, I. A., McCarthy, H. O., Ahmed, N., Rehman, A. U., et al. (2020). Microneedle Liquid Injection System Assisted Delivery of Infection Responsive Nanoparticles: A Promising Approach for Enhanced Site-specific Delivery of Carvacrol against Polymicrobial Biofilms-Infected Wounds. Int. J. Pharmaceutics 587, 119643. doi:10.1016/j.ijpharm.2020.119643
Mitchell, M. J., Billingsley, M. M., Haley, R. M., Wechsler, M. E., Peppas, N. A., and Langer, R. (2021). Engineering Precision Nanoparticles for Drug Delivery. Nat. Rev. Drug Discov. 20 (2), 101–124. doi:10.1038/s41573-020-0090-8
Naves, L., Dhand, C., Almeida, L., Rajamani, L., Ramakrishna, S., and Soares, G. (2017). Poly(lactic-co-glycolic) Acid Drug Delivery Systems through Transdermal Pathway: An Overview. Prog. Biomater. 6 (1-2), 1–11. doi:10.1007/s40204-017-0063-0
Niamlang, S., and Sirivat, A. (2009). Electric Field Assisted Transdermal Drug Delivery from Salicylic Acid-Loaded Polyacrylamide Hydrogels. Drug Deliv. 16 (7), 378–388. doi:10.1080/10717540903090601
Ning, X., Wiraja, C., Lio, D. C. S., and Xu, C. (2020). A Double‐Layered Microneedle Platform Fabricated through Frozen Spray‐Coating. Adv. Healthc. Mater. 9 (10), 2000147. doi:10.1002/adhm.202000147
Patra, J. K., Das, G., Fraceto, L. F., Campos, E. V. R., Rodriguez-Torres, M. D. P., Acosta-Torres, L. S., et al. (2018). Nano Based Drug Delivery Systems: Recent Developments and Future Prospects. J. Nanobiotechnol 16 (1), 71. doi:10.1186/s12951-018-0392-8
Permana, A. D., Paredes, A. J., Volpe-Zanutto, F., Anjani, Q. K., Utomo, E., and Donnelly, R. F. (2020). Dissolving Microneedle-Mediated Dermal Delivery of Itraconazole Nanocrystals for Improved Treatment of Cutaneous Candidiasis. Eur. J. Pharmaceutics Biopharmaceutics 154, 50–61. doi:10.1016/j.ejpb.2020.06.025
Permana, A. D., Tekko, I. A., McCrudden, M. T. C., Anjani, Q. K., Ramadon, D., McCarthy, H. O., et al. (2019). Solid Lipid Nanoparticle-Based Dissolving Microneedles: A Promising Intradermal Lymph Targeting Drug Delivery System with Potential for Enhanced Treatment of Lymphatic Filariasis. J. Controlled Release 316, 34–52. doi:10.1016/j.jconrel.2019.10.004
Prausnitz, M. R. (2017). Engineering Microneedle Patches for Vaccination and Drug Delivery to Skin. Annu. Rev. Chem. Biomol. Eng. 8, 177–200. doi:10.1146/annurev-chembioeng-060816-101514
Ren, Y., Yu, X., Li, Z., Liu, D., and Xue, X. (2020). Fabrication of pH-Responsive TA-Keratin Bio-Composited Hydrogels Encapsulated with Photoluminescent GO Quantum Dots for Improved Bacterial Inhibition and Healing Efficacy in Wound Care Management: In Vivo Wound Evaluations. J. Photochem. Photobiol. B: Biol. 202, 111676. doi:10.1016/j.jphotobiol.2019.111676
Roxhed, N., Griss, P., and Stemme, G. (2008). Membrane-sealed Hollow Microneedles and Related Administration Schemes for Transdermal Drug Delivery. Biomed. Microdevices 10 (2), 271–279. doi:10.1007/s10544-007-9133-8
Ruan, S., Zhang, Y., and Feng, N. (2021). Microneedle-mediated Transdermal Nanodelivery Systems: A Review. Biomater. Sci. 9 (24), 8065–8089. doi:10.1039/d1bm01249e
Sahle, F. F., Giulbudagian, M., Bergueiro, J., Lademann, J., and Calderón, M. (2017). Dendritic Polyglycerol and N-Isopropylacrylamide Based Thermoresponsive Nanogels as Smart Carriers for Controlled Delivery of Drugs through the Hair Follicle. Nanoscale 9 (1), 172–182. doi:10.1039/c6nr06435c
Salleh, A., and Fauzi, M. B. (2021). The In Vivo, In Vitro and in Ovo Evaluation of Quantum Dots in Wound Healing: A ReviewVitro and in Ovo Evaluation of Quantum Dots in Wound Healing: A Review. Polymers 13 (2), 191. doi:10.3390/polym13020191
Salwa, , , Chevala, N. T., Jitta, S. R., Marques, S. M., Vaz, V. M., and Kumar, L. (2021). Polymeric Microneedles for Transdermal Delivery of Nanoparticles: Frontiers of Formulation, Sterility and Stability Aspects. J. Drug Deliv. Sci. Technol. 65 (3), 102711. doi:10.1016/j.jddst.2021.102711
Savjani, K. T., Gajjar, A. K., and Savjani, J. K. (20122012). Drug Solubility: Importance and Enhancement Techniques. ISRN Pharmaceutics 2012, 1–10. doi:10.5402/2012/195727
Srivalli, K., and Mishra, B. (2015). Drug Nanocrystals: Four Basic Prerequisites for Formulation Development and Scale-Up. Cdt 16 (2), 136–147. doi:10.2174/1389450115666141120114036
Stinson, J. A., Boopathy, A. V., Cieslewicz, B. M., Zhang, Y., Hartman, N. W., Miller, D. P., et al. (2021). Enhancing Influenza Vaccine Immunogenicity and Efficacy through Infection Mimicry Using Silk Microneedles. Vaccine 39 (38), 5410–5421. doi:10.1016/j.vaccine.2021.07.064
Tas, C., Mansoor, S., Kalluri, H., Zarnitsyn, V. G., Choi, S.-O., Banga, A. K., et al. (2012). Delivery of salmon Calcitonin Using a Microneedle Patch. Int. J. Pharmaceutics 423 (2), 257–263. doi:10.1016/j.ijpharm.2011.11.046
Tekko, I. A., Permana, A. D., Vora, L., Hatahet, T., McCarthy, H. O., and Donnelly, R. F. (2020). Localised and Sustained Intradermal Delivery of Methotrexate Using Nanocrystal-Loaded Microneedle Arrays: Potential for Enhanced Treatment of Psoriasis. Eur. J. Pharm. Sci. 152, 105469. doi:10.1016/j.ejps.2020.105469
Tort, S., Mutlu Agardan, N. B., Han, D., and Steckl, A. J. (2020). In Vitro and In Vivo Evaluation of Microneedles Coated with Electrosprayed Micro/nanoparticles for Medical Skin Treatments. J. Microencapsulation 37 (7), 517–527. doi:10.1080/02652048.2020.1809725
Tu, J., Du, G., Reza Nejadnik, M., Mönkäre, J., van der Maaden, K., Bomans, P. H. H., et al. (2017). Mesoporous Silica Nanoparticle-Coated Microneedle Arrays for Intradermal Antigen Delivery. Pharm. Res. 34 (8), 1693–1706. doi:10.1007/s11095-017-2177-4
Vemulapalli, V., Bai, Y., Kalluri, H., Herwadkar, A., Kim, H., Davis, S. P., et al. (2012). In Vivo iontophoretic Delivery of salmon Calcitonin across Microporated Skin. J. Pharm. Sci. 101 (8), 2861–2869. doi:10.1002/jps.23222
Vitorino, C., Almeida, A., Sousa, J., Lamarche, I., Gobin, P., Marchand, S., et al. (2014). Passive and Active Strategies for Transdermal Delivery Using Co-encapsulating Nanostructured Lipid Carriers: In Vitro vs. In Vivo Studies. Eur. J. Pharmaceutics Biopharmaceutics 86 (2), 133–144. doi:10.1016/j.ejpb.2013.12.004
Vora, L. K., Moffatt, K., Tekko, I. A., Paredes, A. J., Volpe-Zanutto, F., Mishra, D., et al. (2021). Microneedle Array Systems for Long-Acting Drug Delivery. Eur. J. Pharmaceutics Biopharmaceutics 159, 44–76. doi:10.1016/j.ejpb.2020.12.006
Zhang, P., Zhang, Y., and Liu, C.-G. (2020). Polymeric Nanoparticles Based on Carboxymethyl Chitosan in Combination with Painless Microneedle Therapy Systems for Enhancing Transdermal Insulin Delivery. RSC Adv. 10, 24319–24329. doi:10.1039/D0RA04460A
Zhang, W., Gao, J., Zhu, Q., Zhang, M., Ding, X., Wang, X., et al. (2010). Penetration and Distribution of PLGA Nanoparticles in the Human Skin Treated with Microneedles. Int. J. Pharmaceutics 402 (1-2), 205–212. doi:10.1016/j.ijpharm.2010.09.037
Zhu, L., Zhou, Z., Mao, H., and Yang, L. (2017). Magnetic Nanoparticles for Precision Oncology: Theranostic Magnetic Iron Oxide Nanoparticles for Image-Guided and Targeted Cancer Therapy. Nanomedicine 12 (1), 73–87. doi:10.2217/nnm-2016-0316
Keywords: microneedle, transdermal delivery, nanoparticles, controlled release, drug delivery
Citation: Jiang X, Zhao H and Li W (2022) Microneedle-Mediated Transdermal Delivery of Drug-Carrying Nanoparticles. Front. Bioeng. Biotechnol. 10:840395. doi: 10.3389/fbioe.2022.840395
Received: 21 December 2021; Accepted: 20 January 2022;
Published: 11 February 2022.
Edited by:
Gang Ruan, Nanjing University, ChinaReviewed by:
Yixiao Cui, Boehringer Ingelheim, United StatesJun Wang, Nanjing Medical University, China
Qingguo Xu, Virginia Commonwealth University, United States
Copyright © 2022 Jiang, Zhao and Li. This is an open-access article distributed under the terms of the Creative Commons Attribution License (CC BY). The use, distribution or reproduction in other forums is permitted, provided the original author(s) and the copyright owner(s) are credited and that the original publication in this journal is cited, in accordance with accepted academic practice. No use, distribution or reproduction is permitted which does not comply with these terms.
*Correspondence: Wei Li, d2VpbGkubW5Ad2h1LmVkdS5jbg==