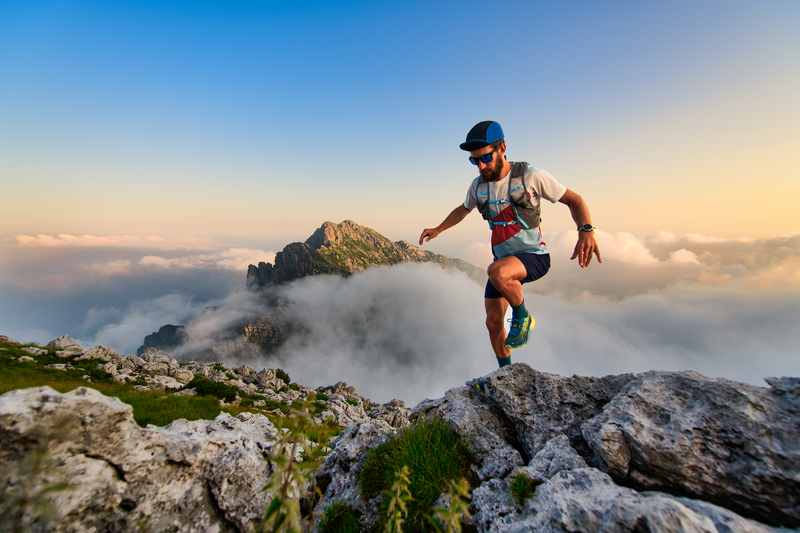
94% of researchers rate our articles as excellent or good
Learn more about the work of our research integrity team to safeguard the quality of each article we publish.
Find out more
REVIEW article
Front. Bioeng. Biotechnol. , 12 May 2022
Sec. Nanobiotechnology
Volume 10 - 2022 | https://doi.org/10.3389/fbioe.2022.837172
Bone regeneration in large segmental defects depends on the action of osteoblasts and the ingrowth of new blood vessels. Therefore, it is important to promote the release of osteogenic/angiogenic growth factors. Since the discovery of heparin, its anticoagulant, anti-inflammatory, and anticancer functions have been extensively studied for over a century. Although the application of heparin is widely used in the orthopedic field, its auxiliary effect on bone regeneration is yet to be unveiled. Specifically, approximately one-third of the transforming growth factor (TGF) superfamily is bound to heparin and heparan sulfate, among which TGF-β1, TGF-β2, and bone morphogenetic protein (BMP) are the most common growth factors used. In addition, heparin can also improve the delivery and retention of BMP-2 in vivo promoting the healing of large bone defects at hyper physiological doses. In blood vessel formation, heparin still plays an integral part of fracture healing by cooperating with the platelet-derived growth factor (PDGF). Importantly, since heparin binds to growth factors and release components in nanomaterials, it can significantly facilitate the controlled release and retention of growth factors [such as fibroblast growth factor (FGF), BMP, and PDGF] in vivo. Consequently, the knowledge of scaffolds or delivery systems composed of heparin and different biomaterials (including organic, inorganic, metal, and natural polymers) is vital for material-guided bone regeneration research. This study systematically reviews the structural properties and auxiliary functions of heparin, with an emphasis on bone regeneration and its application in biomaterials under physiological conditions.
Research into anticoagulant drugs has provided substantial evidence that heparin and its derivatives play an important role in the treatment of venous thromboembolism (VTE) (Piran and Schulman, 2016). Heparin was discovered in 1916, and from 1937 it was in use for the prevention of pulmonary embolism and later for the treatment of acute VTE. The binding of heparin to antithrombin Ⅲ enhances antithrombin III activity. This results in the formation of molecular complexes including thrombin and serine proteinases which inhibit the activity of coagulation factors Ⅱ and Ⅹ. Heparin exhibits rapid, long-lasting anticoagulation activity and low bioavailability, especially at low dosages (Zee et al., 2017). Low molecular weight heparin (LMWH) is derived from the depolymerization of heparin, and it was introduced as an anticoagulation drug approximately 10 years ago. Compared with unfractionated heparin (UFH), the advantages of using LMWH include a stronger anticoagulation effect, increased bioavailability, reduced risk of bleeding, and an enhanced safety profile. Moreover, the use of LMWH is known to reduce the risk of thrombocytopenia (HIT) compared with UFH, thereby allowing it to be used in pregnant women to treat VTE (Cosmi, 2016; Thaler et al., 2015). Furthermore, administering LMWH removes the need of coagulation tests as opposed to when UFH is used to treat VTE.
Long-term use of heparin has been linked to potential osteoporosis, which leads to poor bone healing (Schulman and Hellgren-Wångdahl, 2002; Alban, 2012). Miur et al. demonstrated that both UFH and LMWH reduced bone formation by decreasing osteoblasts and the osteoid surface, but only UFH increased bone resorption by increasing the osteoclast surface (Muir et al., 1996; Muir et al., 1997). The long-term use of UFH has been found to impede bone mineral density (BMD) resulting in increased risk of fracture, whereas LMWH treatment did not show any obvious effects on fracture risk (Schulman and Hellgren-Wångdahl, 2002; Pettilä et al., 1999; Monreal et al., 1994). Recent findings suggest that heparin can serve as an effective tool to modify bone substitute materials since the incorporation of heparin with biomaterials can improve bone tissue bioengineering. Current biomaterial delivery vehicles for carrying therapeutic growth factors (GFs) have limitations which lead to low affinity for GFs. Therefore, improved biomaterials capable of providing localized and persistent release of bioactive proteins are essential for bone regeneration (Hettiaratchi et al., 2014). Heparin is an attractive vehicle for GF delivery due to its ability to reversibly bind positively charged proteins required to maintain bioactivity and sustained release of GFs. However, the main challenge is how to ensure the grafted tissue substitutes with a sufficient blood supply. Interestingly, the application of heparin–chitosan coating can significantly enhance blood perfusion and re-endothelialization in the early stages of transplantation (Sun et al., 2011). Therefore, heparin is considered an important bioactive component in bone substitute material design. In the current review, we have summarized the structural properties and function of heparin and the role of heparin on bone regeneration, specifically its application in biomaterial development.
The molecular weight (MW) of heparin ranges between 3,000 and 30,000 Daltons. It is proposed that L-iduronic acid (IdoA), mainly IdoA2SO3, is the major aldehydic acid which heparin is composed of, while D-glucuronic acid (GlcA) and N-acetyl glucosamine (GlcNAc) are components of the heparin chain (Carlsson and Kjellén, 2012; Nahain et al., 2018). These studies, together with structural analyses of purified heparin, established the idea that heparin displayed structural microheterogeneity. Furthermore, studies have also described structural differences of heparin between species such as porcine and bovine (Bett et al., 2017). Also, the content and size of IdoA2SO3 and IdoA in heparin are different, which results in different lengths of the heparin chains (Crijns et al., 2021). Antithrombin (AT), a serine protease inhibitor, functions by binding to serine residues within serine proteases leading to their inactivation. AT-mediated inhibition was also found. Indeed, the ability of AT to inhibit serine protease could be significantly enhanced by heparin (Onishi et al., 2016). The anticoagulation activity of heparin is primarily based on its capacity to bind with AT, thereby increasing the AT-derived inhibitory effects on coagulants by several orders of magnitude. The inhibitory effects of heparin and AT vary and depends on the affinity of heparin with AT which can be variable. The antithrombin-binding region of heparin was identified as a pentasaccharide sequence, with the structure GlcNAc6S-GlcA-GlcNS3S6S-IdoA2S-GlcNS6S, with the 3-O-sulfate and 6-O-sulfate structures involved specifically in AT binding (Alekseeva et al., 2019). In summary, heparin exists as a linear, unbranched, and deeply sulfated GAG, which primarily consists of the helical structure (Mulloy et al., 1993).
Heparin is mainly biosynthesized at the endoplasmic reticulum and Golgi apparatus of mast cells in the liver, intestine, and lungs. The anticoagulation activity of heparin is derived from its inhibitory effect on multiple proteins in the coagulation cascade. Heparin binds to AT and facilitates the inhibition of coagulation proteins including Factor Ⅱa and Xa. Upon heparin binding, AT undergoes a conformational change exposing a reactive loop that is subjected to factor Xa and thrombin to catalyze their inactivation. Heparin binds with AT and thrombin to form a ternary complex, which requires heparin to have at least 18 polysaccharide residues (Lane et al., 1984; Jonas and Quartermain, 2001).
Heparan sulfate (HS) is a linear polysaccharide composed of repeated disaccharide units, increased sulfation, and covalently linked to a core protein to form heparin sulfate proteoglycan. HS can bind to a variety of bioactive molecules, including GFs [such as heparin-binding epidermal growth factor (HB-EGF), fibroblast growth factor (FGF), and transforming growth factor-β (TGF-β)], chemokines, and morphogenetic proteins, which are linked to the core protein and function (Li and Kusche-Gullberg 2016). HS bound with GFs protects from protease degradation and promotes their ability to bind to their cognate receptors, thereby enhancing and prolonging the activity of GFs. HS has been used in fracture models to promote bone healing, suggesting it may be a useful tool in the treatment of non-union or delayed union fractures (Nozawa et al., 2018).
LMWH, which have an average MW ranging between 2 and 5 kDa have more desirable characteristics compared to UFH, including a longer plasma half-life, better bioavailability at low doses, and more predictable dose–response effects (Schwartz, 1990). The inhibitory effect of LMWH is mainly exerted via FXa and has little effect on thrombin in contrast to heparin. For this reason, LMWH can result in effective anticoagulation and reduced thrombocytopenia compared with heparin, which, due to its powerful thrombin-inhibitory effect, is the main cause of thrombocytopenia. This suggests that the use of LMWH has great value in clinical application (Gray et al., 2008). LMWH is expressed at lower levels in macrophages, endothelial cells, platelets, osteoblasts, platelet factor 4, and plasma proteins, which can reduce many problems generated by heparin, such as short plasma half-life, HIT, and osteoporosis (Mikhailov et al., 1999).
Ultralow molecular weight heparins (ULMWHs) consist of small heparin chains, many of which are homogeneous compounds, with a MW ranging between 1.5 and 3.5 kDa (Chandarajoti et al., 2016). ULMWHs are demonstrated to have increased bioavailability, longer plasma half-lives, reduced risk of bleeding, and osteoporosis (Hao et al., 2011) and were shown in one clinical study to have a greater safety margin reducing the risk of bleeding (Rico et al., 2011). ULMWHs are suggested to be more suitable than UFH for long-term clinical treatment and can even be administered subcutaneously to prevent or treat VTE (Alquwaizani et al., 2013).
Heparins are heterogenous in sequence and consist predominantly of a disaccharide repeating structure. The IdoA residue is responsible for specific orientation of the O- and S- groups, which allows heparin to bind to a variety of important proteins, such as cytokines, growth factors, and morphogenetic proteins (Mulloy and Linhardt, 2001). The interaction of heparin with heparin-binding proteins depends on the ionic and hydrogen bonds between its sulfur group and the amino group of the protein. Protein bound with heparin can participate in biological processes to promote tissue growth and development (e.g., binding growth factors or morphogenetic proteins to stimulate bone regeneration) (Välimäki et al., 2021). The binding of heparin to proteins depends on the distance between basic amino acids as opposed to the configuration of the heparin-binding site. However, basic amino acids, arginine and lysine, were also found to be essential for hydrogen bonding. In addition, van der Waals forces and electrostatic interactions are required for the binding of heparin to proteins (Margalit et al., 1993).
Surface functionalization is very important for heparin-modified biomaterials. The ways in which heparin-functionalized surfaces can be formed includes 1) covalent binding of partial deamination of UFH molecules to the substrate of the amine-containing functional group by reductive amine (Biran and Pond, 2017); 2) the coating surface was treated with cationic polyamines, and then heparin was connected to the polyamines through ion interaction (Biran and Pond, 2017); 3) heparin binds to the material through covalent interaction and ion interaction (Diez-Escudero et al., 2018); 4) heparin and light reagent applied to the material under ultraviolet irradiation produced covalent-fixed heparin surface (Charron et al., 2022). The modification and pretreatment of heparin resulted in better performance, such as the reaction of heparin and 2-aminoethyl methacrylate to prepare heparin methacrylate containing two carboxyl groups; heparin cultivated with N-(3-dimethylaminopropyl)-N'-ethylcarbodiimide/N-hydroxysulfosuccinimide (EDC/ Sulfo-NHS) solution on ice (about 2–4°C) for 15 min can activate the heparin carboxylic group (Prokoph et al., 2012); low molecular weight heparin sodium solution was prepared by ionic water; heparin-AL (aldehyde-modified heparin) was obtained by reaction of NaIO4 with amino-glycerol–modified heparin (Roberts et al., 2016). The heparin aqueous solution was mixed and incubated with the material (e.g., chitosan) aqueous solution to the desired concentration, then the unbound heparin was eluted with PBS or balanced salt solution to obtain the preliminary heparin-modified materials. Heparin-bound growth factors (e.g., PDGF-BB, BMP-2, and TGF-β1) were immobilized to prevent their diffusion from the matrix, while heparin was immobilized on the surface of materials (Wang et al., 2015). Moreover, aqueous solution of materials (such as chitosan) were combined with aqueous heparin using magnetic stirring and ultrasonic treatment; free heparin and chitosan were removed by washing with distilled water; then the nanomaterials were obtained by supercentrifugation (14000rMP, 15min) (Tan et al., 2011).
The interaction between GFs [such as TGF-β, FGF-1, and bone morphogenetic protein 2 (BMP-2)] and heparin is mostly dependent on alkaline residues within GFs, ionic interaction of acid sulfate, and carboxylate groups of heparin (Hettiaratchi et al., 2020). As such, heparin and GFs can be modified and assembled into biomaterials to be delivered to the injured site for bone regeneration. The use of heparin (UFH or LMWH) in tissue engineering includes heparin-functionalized surfaces (via electrostatic interaction, physical encapsulation, or chemical fixation), nanohydrogels, polymer nanoparticles, heparin nanogel layers, and polyelectrolyte composite nanoparticles (Farokhzad and Langer, 2009). In recent years, heparin-modified nanomaterials have attracted more attention due to their improved stability, enhanced cell uptake capacity, and ability to facilitate targeted therapy. Heparin covers the surface of nanoparticles and sometimes becomes the structural unit of the materials (Gallagher et al., 2020). Heparin nanoparticles (synthesized by the emulsion method) can provide higher protein affinity and improved controlled release of GFs. Modification of heparin can greatly reduce the damage of secondary and tertiary protein structures (Liang and Kiick, 2014). In addition, composite nanoparticles can be prepared by mixing oppositely charged polymer solutions with polypositive/anionic solutions. These composite heparin-containing nanoparticles can be combined with high porosity nanostructures, such as chitosan, for binding, stabilization, and release of GFs (Laner-Plamberger et al., 2021).
Currently, heparins used in bone tissue engineering mainly include UFH, LMWH, and HS. They exhibit similar biological properties, such as the storage and release of GFs although their chemical structures are different (Banik et al., 2021). Among them, LMWH is a popular choice in bone tissue engineering because of its excellent efficacy, reduced side effects, and optimized molecular size. For example, in a collagen sponge with LMWH-binding fibrin carrier system, LMWH polyelectrolyte carrier was designed for a more controlled release of slower growth factor (Facchetti et al., 2021). Figure 1 depicting several forces at which heparin interacts with growth factors.
FIGURE 1. Heparin binds to growth factors in several ways. At least 300 membrane-associated proteins are known to bind to heparin (here, growth factors represent osteogenic proteins). Their interactions can be categorized into four classes: (A) electrostatic interactions: electrostatic interactions dominate most protein–heparin interactions. The negatively charged sulfate or hydroxyl groups in heparin are attracted to the positively charged lysine or arginine residues in the protein, thereby holding the molecules together. (B) Hydrogen bonds: polar residues, especially asparagine and glutamine, usually form hydrogen bonds with sulfate groups. For example, in FGF-1 and FGF-2, all three polar residues are involved in hydrogen bonding with sulfate groups, and the hydrogen bonding contributes most of the binding free energy. (C) Van der Waals forces: van der Waals force between heparin and protein molecules and include inducement force, orientation force, and dispersion force. (D) Hydrophobicity: the energy effect caused by the electrostatic forces and hydrogen bonds of polar groups in heparin and proteins cause polar groups to come together.
As an effective anticoagulant, heparin has been used clinically for over 80 years, and it is still the primary medicine for prevention and treatment of VTE (Aláez-Versón et al., 2017; Torri and Naggi, 2016). With deep vein thrombosis and pulmonary embolism, the choice of heparin dosage depends on disease progression, heparin administered, and pre-existing medical problems. The subcutaneous dose is approximately 170–200 IU/kg for LMWH and 230–300 IU/kg for UFH (Konstantinides, 2014). These can be administered as a single dose or divided into two smaller doses delivered twice a day. After initial treatment, patients will be transitioned to vitamin K agonists (VKAs) or new oral anticoagulant treatments. The treatment is usually continued for 3 months or longer to guarantee a reduced risk of deep vein thrombosis (DVT) (Wells et al., 2014). LMWH is the treatment of choice for pregnant patients as it requires only twice-daily injections. VKAs are prohibited during pregnancy because of their teratogenic effects resulting in fetal abnormalities in the first trimester and risk of intracranial bleeding in fetuses in the third trimester with the capacity to cross the blood–brain barrier (Konstantinides 2014; Wells et al., 2014). Similarly, twice-daily administration of LMWH stabilizes plasma levels. Compared with patients who experience unprovoked VTE, patients with cancer are four times more likely to develop VTE. Therefore, the initial treatment of LMWH or VKAs should be extended to 3–6 months. Patients with non-progressed cancer can continue treatment indefinitely (Du et al., 2019). Heparin also reduced the clotting response and may reduce the risk of stroke by treating or preventing atrial fibrillation-related thromboembolic complications (Ezekowitz et al., 2018).
The non-anticoagulant properties of heparin are mainly reflected in its anticancer, anti-inflammation, and antivirus activities. Heparin and its derivatives inhibit the progression of cancer by generating antimetastatic effects as well as alleviating the hypercoagulable condition in cancer patients (Klerk et al., 2005). Heparin binds to P-selectin glycoprotein ligand-1 (PSGL-1) and blocks platelets to bind to cancer cells via mucin ligands on the cell surface of cancer cells, thus blocking their subsequent interaction with endothelium and reducing metastasis and tumor colonization (Mousa and Mohamed, 2004; Wahrenbrock et al., 2003; Borsig et al., 2001). Another common route through which cancer metastases occurs is via the lymphatic system. Recently, researchers have found a heparin derivative—a conjugation of LMWH and four bis-deoxycholates, which can reduce lymphangiogenesis by inhibiting vascular endothelial growth factor C (VEGF-C)–mediated phosphorylation of vascular endothelial growth factor receptor 3 (VEGFR-3) (Choi J. U et al., 2017). LMWH has been shown to improve 3- and 6-month survival in cancer patients (Icli et al., 2007; Prandoni et al., 1992; Gould et al., 1999), and heparin-like glycosaminoglycans (HLGAGs) have been reported to have similar effects (Kakkar et al., 2004). Several publications have demonstrated non-anticoagulation effects of heparin, such as binding to inflammatory factors, neutralization of the complement factor C5a, prevention of neutrophil chemotaxis and migration of leukocytes, and isolation of acute inflammatory proteins. Heparin can interact with many proteins involved in the pro-inflammatory and pro-coagulant cascade to guard against sepsis-associated inflammation and coagulation abnormalities (Li and Ma, 2017; Gockel et al., 2022; Lippi et al., 2022). In addition, in animal models of chronic myocarditis, heparin was found to be associated with low rates of myocardial inflammation and collagen deposition (Frizelle et al., 1992). The polyanion properties of heparin allow its binding to a variety of proteins, thus becoming an efficient inhibitor of viral attachment and plays a unique role in certain infectious diseases (including herpes simplex virus, Zika virus, and SARS-associated Vero cells) (Pouyan et al., 2021; Ghezzi et al., 2017). Other effects of heparin are summarized in Table 1.
Bone is a dynamic tissue which constantly undergoes a life-long remodeling process and comprises osteoblast-driven bone formation and osteoclast-driven bone resorption. Long-term use of heparin can lead to many adverse effects such as hemorrhage, HIT, general hypersensitivity reactions, elevated transaminase levels, and osteoporosis (Hemker, 2016; Arepally and Cines, 2020; Signorelli et al., 2019; Ludwig et al., 2006). Here, we focus on the effects of heparin on bone metabolism. Current studies demonstrate controversial results, wherein heparin was found to inhibit bone formation and contributed to the development of osteoporosis (Signorelli et al., 2019), while in another study, it induced osteogenesis by binding to BMP-2 and PDGF (Smith et al., 2018; Rindone et al., 2019). This part of the review will center on the effects of heparin on bone remodeling.
Long-term use of heparin has been shown to result in increased risk of osteoporosis and fracture due to decreasing BMD. Clinical studies demonstrated long-term use of UFH (> for 6 months) resulted in a significant decrease in BMD. A BMD analysis of 123 pregnant women with antiphospholipid syndrome treated with either UFH (n = 46) or LMWH (n = 77) revealed a 4% reduction in lumbar BMD during pregnancy. In addition, in a small case-control study, measurements of proximal femoral density decreased by ≥ 10% in 5 out of 14 pregnant women (35.7%). No one in the control group exhibited a decrease in BMD (p = 0.04), with the difference still statistically significant 6 months after delivery (p = 0.03) (Barbour et al., 1994). Similarly, Douketis et al. and Dahlman et al. found that long-term heparin therapy reduced BMD by 7 and 5%, respectively (Douketis et al., 1996; Dahlman et al., 1994).
The effect of LMWH on BMD is controversial. Some researchers found that using dalteparin did not correlate with reduced BMD during pregnancy (Rodger et al., 2007), and there was no discernible difference between LMWH-related bone loss and loss attributed to the physiological process of pregnancy (Carlin et al., 2004). Casele et al., also reported that the rate of bone loss in pregnant women receiving thromboprophylaxis was between 2 and 5%, and the results were similar for those administered with LMWH or UFH (H. Casele et al., 2006). However, a more significant loss in BMD was observed in patients receiving enoxaparin for a year or longer (Nelson-Piercy et al., 1997; Casele and Laifer, 2000; Greer and Nelson-Piercy, 2005). In addition, LMWH administration for at least 3 months also associated with bone loss and fractures (Carlin et al., 2004; Pettilä et al., 2002).
The mechanism underlying heparin-facilitated osteoporosis is only partially understood. Studies demonstrate that UFH and LMWH can both reduce osteoblast or osteoid formation (alkaline phosphatase is decreased), but only UFH increased osteoclast formation resulting in bone resorption (Muir et al., 1996). This can be largely attributed to the differences in the MW of heparin. Heparin fragments with an average MW < 3000 Daltons minimally affect osteoblast differentiation or bone mineralization. In addition, heparin can bind to osteoprotegerin (OPG) preventing its interaction with receptor activator of nuclear factor-κB ligand (RANKL), which impedes the OPG-RANKL axis while facilitating RANKL–RANK interactions to enhance osteoclastogenesis. Moreover, UFH with a high MW is more likely to form an obstacle in the space and has a higher affinity with OPG than LMWH (Li et al., 2016).
Thus far, approximately a third of the TGF-β superfamily members including TGF-β1, TGF-β2, BMP-2, growth and differentiation factors (GDFs), and glial cell-line–derived neurotropic factor (GDNF) along with its two close homologues have been found to bind to heparin and HS (Rider and Mulloy, 2017). Most TGF-β family members show enhanced capacity to promote bone formation. Thus, heparin or HS indirectly facilitates osteogenesis by binding with the members of the TGF-β family.
TGF-β1, a universal growth factor, is involved in proliferation, migration, and differentiation. In bone, TGF-β1 signaling is a crucial key factor in cartilage and bone formation, remodeling, and maintenance. TGF-β1 promotes proliferation and chemotactic attraction of osteoblasts, enhances initial stages of osteogenic differentiation, facilitates production of extracellular matrix (ECM) proteins, stimulates type II collagen expression, stimulates chondrocyte precursors proteoglycan synthesis, reduces osteoblast apoptosis, and inhibits hematopoietic precursors (Janssens et al., 2005; Xu et al., 2020). TGF-β1 induces transcription factor Runx2 (a major driver of bone formation) in combination with BMPs during early stages of osteogenic differentiation (Liu et al., 2019). On the other hand, TGF-β1 also participates in regulating the recruitment of osteoclast precursors into the bone environment, which regulates differentiation and maturation of osteoclasts, bone resorption, and osteoclast apoptosis (Lee et al., 2019). Indeed, TGF-β1 enhances the osteogenic effect by promoting osteoblasts and inhibiting osteoclasts, but this effect can be influenced by many factors. Specifically, in vivo, the skeletal environment and the presence of other cytokines can alter the effects of TGF-β1 signaling (Bendinelli et al., 2019; Xie et al., 2020).
Heparin and highly sulfated HS can bind tightly to TGF-β1 enhancing the activity of TGF-β1. Unlike conventional cytokine–receptor interactions, this enhancement is due to the antagonistic action of α2-macroglobulin binding and inactivation of TGF-β1 (Gigi et al., 2012). In a study investigating fibrin extradomain-A (ED-A)–containing fibronectin (ED-A FN) and TGF-β–binding protein 1 (LTBP-1), Klingberg et al. proposed that the ED-A domain within ED-A FN promoted direct binding of heparin with LTBP-1. Furthermore, potential TGF-β1 binding was enhanced by heparin-mediated protein interactions within the FNIII12-13-14 (HepII) region in fibronectin. TGF-β1 activation was significantly reduced when the function of the ED-A domain was blocked with antibodies and competitive peptides (Klingberg et al., 2018). Together, this suggests that the heparin-enhanced TGF-β1 activity can be induced by specific domains in fibronectin. The structure and properties of heparin are crucial for TGF-β1 activity for many physiological processes. A competitive analysis of optical biosensors (Biacore) suggests that N- and 6-O-sulfate groups may play central roles in binding TGF-β1, whereas the 2-O-sulfate groups play a minor or a negligible role (Lee J et al., 2015). Thus, the effect of heparin on the TGF-β1 activity depends on the sulfated form of the polysaccharide. Furthermore, during osteogenic differentiation of human mesenchymal stem cells (hMSCs), cells treated with 1 ng/ml TGF-β1 and 10 μg/ml heparin expressed higher levels of TGF-β1 downstream effectors, phospho-SMAD2, and phospho-SMAD3 compared with TGF-β1 alone However, higher doses of heparin (40 μg/ml) or TGF-β1 (5 ng/ml) did not enhance phosphorylation of SMAD2 and SMAD3. This suggests that the role of heparin is to prolong, instead of enhance, TGF-β1–induced SMAD signaling. This supports the opinion that heparin protects TGF-β1 from inactivation by binding to α2-macroglobulin (McCaffrey et al., 1989; Lee J et al., 2015). In addition, heparin significantly stimulated TGF-β1 production at concentrations ranging from 50 to 400 ug/mL during valvular interstitial cell (VIC) differentiation in the presence of heparin-modified substrates resulting in changes in cell morphology as well as promoting tissue growth by increasing the absorption of serum proteins, especially TGF-β1 (Cushing et al., 2005).
Since the discovery of BMP-2 as an effective osteoinductive cytokine in 1988, the BMP superfamily is well studied in vertebrate bone biology (Salazar et al., 2016). In bone tissue engineering, BMP-2 plays a critical role due to its strong osteoinduction capability (Hashimoto et al., 2020) and has been observed to interact with heparin. Glycoaminoglycans (GAGs) enhance the BMP activity and BMP-2 and has been shown to be more selective for heparin than similar GAG polysaccharides. The size of heparin and degree of sulfation is crucial for the binding and activity of BMP-2. BMP-2 can bind with heparin hexasaccharides (dp6) and octasaccharides (dp8), but decasaccharides (dp10) have been found to be the shortest chain length to activate effective heparin-dependent cellular reactions (Smith et al., 2018). In addition, the effect of 6-O-sulfation on BMP-2 binding and activity was very important, whereas the effect of 2-O-sulfation was not clear. The activity of BMP-2 in the presence of higher levels of HS was at least four times higher than when less HS was present (Tellier et al., 2015). In addition, induction of BMP-2 activity is mainly due to the stimulation of 6-O-sulfated chitosan (6SCS), and 2-N-sulfate was the lower activated subgroup. A low dose of 2-N, 6-O-sulfated chitosan (26SCS) significantly enhanced the alkaline phosphatase (ALP; an osteogenic protein) activity and BMP-2–induced mineralization as well has increased mRNA expression of ALP and osteocalcin (OCN). Increased chain-length and enhanced sulfation of 26SCS resulted in an increased ALP activity. Moreover, in the presence of 26SCS, Noggin (a BMP-2 antagonist) did not inhibit the BMP-2 activity. 26SCS showed a stronger synchronous effect on the biological activity of BMP-2 at low dosage. Compared with BMP-2 alone, the combination with 26SCS contributed to a larger amount of ectopic bone formation. Together, this suggests that 26SCS is a stronger stimulator of BMP-2 activity resulting in osteoblast differentiation (Zhou et al., 2009).
Hyper physiological doses of BMP-2 have been used clinically for bone regeneration in non-union fractures, large bone defects, and spondylodesis. Its application has been limited as it degrades quickly and causes side effects such as abnormal bone formation (heterotopic ossification). In combination with heparin, the dosage used can be reduced but increases the osteoinductivity of BMP-2 (Terauchi et al., 2019). The use of heparin microparticles (HMP) has been demonstrated to improve BMP-2 retention in vivo and improve protein transfer to bone defects due to its strong affinity with BMP-2. Consequently, heterotopic ossification was decreased and there was improved spatial localization of bone formation in large bone defects (Hettiaratchi et al., 2020). In vitro experiments demonstrated that heparin-dependent osteoblast differentiation was stimulated by homodimers (BMP-2 or BMP-4) and heterodimers (BMP-2/6 or BMP-2/7). This was suggested to be due to heparin continually supplying ligands to signal receptors expressed on cell membranes, thereby enhancing activity of BMP homodimers and heterodimers (Takada et al., 2003). In an in vivo study, Zhao et al. treated C2C12 myoblast cells with heparin to investigate the molecular mechanisms underlying the stimulation of the BMP activity. Heparin treatment augmented gene expression of BMP-2 and phosphorylation of SMAD1/5/8 at 24 h. Furthermore, degradation of BMP-2 was inhibited, half-life of BMP-2 was increased 20-fold, and Noggin failed to inhibit BMP-2 from binding to heparin. The combination of BMP-2 and heparin enhanced mineralized bone tissue compared with using BMP-2 alone. Therefore, heparin prevents degradation of BMPs and augments their osteogenesis effect in vitro and in vivo (Zhao et al., 2006). The negative regulator of BMPs, Noggin, is able to bind heparin sulfate proteoglycan (HSPGs) on the surface of cells resulting in localization at the plasma membrane where it retains its antagonistic functions and can bind to BMP-4. As such, the interaction between Noggin and HSPGs regulates the formation of the BMP activity gradient in vivo (Paine-Saunders et al., 2002).
MSCs are pluripotent stromal stem cells that play a significant role in bone healing. Heparin has been found to enhance WNT and FGF signal transduction in human embryonic stem cells (hESCs) to upregulate cell proliferation (Furue et al., 2008; Sasaki et al., 2008). Heparin increases WNT-induced signalling in osteoblast differentiation (Ling et al., 2010) and affects the differentiation of bone precursors as well as having a crucial role in stereotyping and osteogenic/adipogenic differentiation of primary human bone marrow stromal cells (hBMSCs). Simann et al. demonstrated that heparin treatment significantly increase mRNA expression and activity of ALP as well as enhancing mineralization and augmented levels of BMP-4 mRNA. In addition, heparin treatment partially inhibited adipogenesis differentiation and transformation of MSCs by decreasing the expression of adipogenesis markers and reducing the formation of lipid droplets. The authors showed heparin-mediated osteogenesis signal transduction not only on BMP pathways but also through reducing mRNA levels of the WNT pathway inhibitor, dickkopf1 (DKK1), and sclerostin (SOST), hence indirectly promoting bone formation (Simann et al., 2015). Therefore, heparin not only promotes osteogenic differentiation in vitro but also inhibits adipogenic differentiation and transformation. Different doses of heparin have distinctive effects on proliferation and pluripotency of hMSCs. Treatment of hMSCs with low doses of heparin (<200 ng/ml) exhibited pleiotropic effects on proliferation and signalling growth and differentiation pathways (including TGFβ/BMP, FGFs, and WNT). However, at high doses of heparin (≥100 μg/ ml), cell growth was inhibited, cell size increased (including nuclear area), and hMSCs became more senescent (Ling et al., 2016).
Various types of heparin analogues have been shown to participate in bone regeneration. A heparin-like synthetic polymer derived from dextran called RGTA was able to enhance the bioactivity of the heparin-binding growth factor (HBGF) in vitro and interact with HBGF released at the wound site and stimulate bone healing. RGTA increased the activity of ALP and parathyroid hormone reactive adenylate cyclase in MC3T3 pre-osteoblasts. RGTA was also able to enhance the ALP activity stimulated by BMP-2 and increases the response to parathyroid hormone stimulated by BMP-2 (Blanquaert et al., 1999). Furthermore, RGTA alone or in combination with HBGF stimulated the expression of osteoblast phenotypic characteristics. When HS was cocultured in the osteogenic medium, HS core protein gene expression, in particular glypican-3, was increased (Haupt et al., 2009). Heparin-induced bone formation is therefore dependent on specific HS chains, especially those containing glypican-3. Cell surface HSPGs are involved in BMP-induced osteogenesis by regulating the BMP activity and gradient formation. Studies have shown that HSPGs directly control the BMP-2–mediated transdifferentiation of C2C12 myoblasts into osteoblasts and regulate the osteogenic activity of BMP-2 by sequestering BMP-2 and mediate its internalization (Jiao et al., 2007). It was suggested by Fisher et al., that exogenous HS significantly increased the ability of BMP-2 to activate chondrogenesis and chondrogenic gene expression and decreased concentration of BMP-2 required to activate chondrogenesis. In addition, HS stimulated BMP-2–mediated SMAD1/5/8 phosphorylation, suggesting that HS increases the interaction of BMP-2 with its receptors. Heparinase treatment to degrade endogenous HSPG enhanced the chondrogenic capacity of BMP-2. Together, these results suggest that exogenous HS or heparinase can augment the chondrogenic capacity of BMP-2 by interfering with the interaction between BMP-2 and endogenous HSPG (Fisher et al., 2006).
Different sources of HS have pleotropic effects. HS and heparin are derived from the bone marrow stromal cell line; HS-5 increase BMP-2–stimulated osteogenesis in C2C12 myoblasts by increasing ALP activation and OCN mRNA expression, respectively. Furthermore, HS significantly enhanced BMP-2–stimulated bone formation in vitro and in vivo by elongating the half-life of BMP-2, decreasing the antagonism of Noggin, and regulating the distribution of BMP-2 on the cell surface. This suggests that bone marrow–derived HS is highly effective in bone formation and is better suited for bone regeneration by improving the delivery and bioavailability of BMP-2 (Bramono et al., 2012).
Bone morphogenetic protein 4 (BMP-4) is known to be involved in the process of bone formation both in vitro and in vivo (Sun et al., 2021; Zhang et al., 2020). Interaction of heparin to the heparin-binding domain (HBD) has been demonstrated to enhance the osteogenic function of BMP-4, and the synthetic peptide sequence homologous to the residues 15–24 of HBD within BMP-4 can bind to heparin to exert osteogenic properties. hMSCs treated with the HBD peptide showed increased ALP expression and calcium phosphate crystal formation similar to the osteogenic effect of BMP-4. Similarly, the HBD peptide could increase the expression of osteoblast-specific genes, including ALP, osteopontin (OPN), and OCN, and induce osteoblast differentiation via the phosphorylation of ERK1/2 in a concentration-dependent manner. Treatment with heparinase blocked HBD peptide-induced osteogenic differentiation and inhibited phosphorylation of ERK1/2. This revealed that the HBD peptide may stimulate osteoblastic differentiation by binding to cell surface heparin and activating ERK1/2 signaling. In summary, the osteogenic efficacy of the HBD sequence of BMP-4 is similar to BMP-4 (Choi et al., 2010).
Chondroitin sulfate (CS) is a family of sulfated GAGs of which chondroitin sulfate E (CS-E) plays and important role in regulating the differentiation and mineralization of osteoblasts by binding to BMP-4. Compared with heparin, CS-E enhanced ALP activity and mineralization as well as cell growth and collagen deposition. The administration of exogenous (soluble) BMP-4 further enhanced the mineralization ability of CS-E (Miyazaki et al., 2008). Thus, signaling and activation of BMP-4 are regulated by both exogenous and endogenous GAG, which was depended on sulfate residues of GAG (Khan et al., 2008). The extracellular level of BMP is regulated by endocytosis, which reduces the amount of BMP-4 and reduces the osteogenic role of BMP-4. HSPGs on the cell surface are the main receptor for the internalization of BMP-4. Treatment with heparinase (to reduce HSPG synthesis) or supplementation with heparin (to inhibit BMP-4 binding to HSPG) can reduce BMP-4 internalization and increase BMP-4 concentration. Heparin thus promotes bone formation by increasing extracellular BMP-4 concentration in vivo (Kim C. L et al., 2018).
4.2.2.1.4 BMP-7Bone morphogenetic protein 7 (BMP-7), also termed osteogenic protein (OP-1), is a member of the TGF-β superfamily. In the clinic, BMP-7 is used as a GF to accelerate bone healing, and clinical trials suggest that BMP-7 treatment may be an alternative to autologous bone graft, the gold standard for treating non-union fractures (Kim Y et al., 2018; Sun et al., 2020). BMP-7 is also a HBGF that exerts unique osteogenic effects in the presence of heparin. HS and heparin chains bind specifically to BMP-7. Researchers used heparinase to neutralize HS on the cell surface to downregulate BMP-7–mediated phosphorylation of SMAD in osteoblasts. Chlorate inhibition of HS sulfation also resulted in disruption of SMAD phosphorylation. Thus, the combination of BMP-7 and HS on the cell surface is required for BMP-7 signaling (Irie et al., 2003). Similarly, heparin and HS also enhance BMP bioactivity. It was found that the activity of BMP-2/4/6/7 was enhanced by heparin, of which activity of BMP-2/6 and BMP-2/7 heterodimers increased more significantly. Complex formation produced by sulfated polysaccharides with BMP was mediated via negatively charged polysaccharide chains or basic amino acid chains of BMP in the culture medium and continued to provide ligands for its signal receptors (Takada et al., 2003).
Heparin and BMP-7 also stimulate the development of embryonic cartilage. Macias et al., used heparin as a carrier to implant BMP-2 and BMP-7 in chicken embryos and found that BMP-7 was strongly expressed in the perichondrium of developing cartilage, while BMP-2 mainly acted on the joint space. In addition, they may have a direct role in limb morphology, such as regulating the number and distribution of undifferentiated prechondrogenic mesenchyme and controlling the initial position of long bone cartilage (Macias et al., 1997). BMP-7 is expressed in sclerotome, hypertrophied chondrocytes, osteoblasts, and periosteum in human embryos. In addition to bone formation, it also maintains a high affinity for basement membrane components and plays other important regulatory roles in the embryo (Vukicevic et al., 1994).
Different binding sites of heparin and BMP-7 can regulate the retention time of BMP-7, and an increased retention time has shown advantages in clinical application. The N-terminus of BMP-7 was replaced by the heparin-binding area of BMP-2, which increased the binding ability of the new protein to heparin. Moreover, in vitro, 100 ng/ml of BMP-7 increased the binding ability of heparin by approximately 20% compared with the untreated group (Nematollahi et al., 2013). Soluble BMP-7 protein was transferred into the periplasmic space of Escherichia coli, resulting in a monomer of approximately 16 kDa, which at a concentration of 500 ng/ml, binds to 50% more heparin than wild type. Therefore, BMP-7 with abundant binding sites may be more effective in osteogenesis (Nematollahi et al., 2012). The in vivo signal transduction process of BMP is illustrated in Figure 2:
FIGURE 2. Bone morphogenic protein (BMP) signaling in vivo: TGF-β superfamily members include activin, inhibin, Mullerian inhibitor substance, and bone morphogenetic proteins (BMPs). BMP is the largest subfamily, with more than 30 BMP ligands in humans. BMPs transduce signals through a polymeric cell surface complex of which there are two classes, type I and type Ⅱ receptors. Both type I and type II receptors are single-channel transmembrane proteins with an intracellular serine/threonine kinase domain. Type II receptors phosphorylate type I receptors after ligand assembly, and the activated type I receptor recruit specific SMAD1/5/8 (blue pathway) and SMAD2/3 (yellow pathway), which combine with SMAD4 to form trimers which translocate to the nucleus. SMADs bind to DNA and recruit chromatin remodeling factors and tissue-specific transcription factors to regulate gene expression.
Blood vessels are essential for the development, growth, and remodeling of bone tissue. Anatomically, blood vessels provide bone tissue with nutrients, GFs, and other substances that enhance bone development and reconstruction. Blood vessels also transport the metabolites of bone tissue and ensure the stability of the bone microenvironment (Peng Y et al., 2020). Platelet-derived growth factor-BB (PDGF-BB) has been suggested to be beneficial for bone formation since it is demonstrated to stimulate angiogenesis during bone regeneration (Xie H et al., 2014) and local treatment of PDGF-BB increased angiogenesis and stimulated bone healing (Gao et al., 2021).
PDGF-BB contains a C-terminal heparin-binding sequence which binds heparin and has been shown to be important for the recruitment of pericytes during vascular development, since deletion of the heparin-binding sequence inhibited PDGF-BB retention and pericyte recruitment in vivo. The degree of sulfation is an inhibiting factor for the activity of PDGF-BB. Decreased N- sulfation reduced PDGF-BB binding in vitro and resulted in pericyte detachment or delayed pericyte migration as well as diminish PDGF-BB signaling directed cell migration. Therefore, the recruitment of pericytes requires an appropriate N-sulfate domain to retain PDGF-BB and stimulate PDGF-BB signaling (Abramsson et al., 2007). PGDF functions by activating a specific tyrosine kinase of the PDGF-α/β receptor. Low concentrations of heparin enhanced PDGF-BB–stimulated PDGF-α receptor phosphorylation in a dose-dependent manner. In CHO667 cells, heparin disaccharide treatment induced maximum (6-fold) phosphorylation of the PDGF-α receptor. Heparin enhanced PDGF-BB–induced mitogen-activated protein kinase (MAPK) and Akt activation and increased the chemotaxis of CHO667 cells to PDGF-BB. Therefore, heparin regulates PDGF-α phosphorylation and downstream signal transduction induced by PDGF-BB (Rolny et al., 2002).
Scaffolds for bone regeneration need to induce the inward growth of blood vessels. Porous scaffolds with a covalently bound heparin coating were demonstrated to be effective for in vivo delivery of PDGF-BB and VEGF. Both VEGF and heparin increased vascular ingrowth at 10 days; however, after 2 months, PDGF-BB–mediated delivery (but not VEGF) resulted in a significant increase in vascularization compared with heparin alone. Therefore, the use of a porous scaffold covalently combined to heparin resulted in a differential release of VEGF and PDGF-BB resulting in rapid and sustained vascular regeneration in the scaffold (Davies et al., 2012). In another study, heparin-bound fibrin was found to continuously release GFs that had a high affinity to heparin (Yang et al., 2011). Furthermore, heparin could potentially be used as a carrier for PDGF-BB continuous release in bone defects. PDGF-BB release was found to be prolonged by heparin-conjugated poly(lactic-co-glycolic acid) nanospheres, resulting in accelerated angiogenesis at the wound site (La and Yang, 2015).
In another study, recombinant human platelet-derived growth factor (rhPDGF-BB) was fixed on the surface of heparinized titanium (Hep-Ti). When the rhPDGF-BB complex was combined with heparin, ALP activation and OCN mRNA levels increased. Moreover, it showed anti-inflammatory properties demonstrated by the downregulation of TNF-α and IL-6 at the transcript level (Huh et al., 2011). In addition, studies have shown that physiological concentrations of PDGF-BB can directly enhance the osteogenic effect of adipose stem cells (ASCs). Heparin-conjugated decellularized bone matrix promoted binding to PDGF-BB and after 3 months enhanced bone formation when grafts were implanted into critical-size skull defects in rats. Therefore, heparin-bound decellularized bone matrix can promote osteogenic signal transduction from PDGF-BB to ASC and stimulate ASC-mediated bone regeneration (Rindone et al., 2019). The in vivo signal pathway transduction of PDGF is illustrated in Figure 3.
FIGURE 3. Platelet-derived growth factor (PDGF) signaling pathway in vivo. The PDGFR family includes PDGFRα and PDGFRβ. Activation of the receptor complex is associated with ligand binding leading to dimerization of PDGFRα and PDGFRβ forming homodimers or heterodimers. Ligands PDGF-C and D are secreted as inactive homodimers and require cleavage by tPA (PDGF-C), uPA (PDGF-D), or matriptase to be activated. PDGF-AA binds and activates only PDGFR-αα homodimers, while PDGF-BB can bind and activate PDGFR homodimers or heterodimers. PDGF-CC binds and activates PDGFR-αα and PDGFR-αβ. PDGF-DD activates PDGFR-ββ and, in some cases, PDGFR-αβ (Demoulin and Essaghir, 2014). Upon activation, intracellular tyrosine kinase domains of PDGFR autophosphorylate activating intracellular signaling pathways such as the RAS-MAPK and P13K pathways.
Fibroblast growth factor 2 (FGF-2), a classic member of FGF-1 subfamily, is expressed from a variety of cells and regulates proliferation, differentiation, migration, and cell survival. FGF-2 is also a major player in bone development, bone formation, and fracture repair (Yamaguchi and Rossant, 1995). Not only does it act as a strong mesoderm inducer during embryogenesis, but its receptor is also strongly expressed in developing bones (Kimelman and Kirschner, 1987). It is also expressed continuously in osteoblasts and stored in the ECM (Hurley et al., 1994).
FGF-2 binding and activation of its cognate receptor tyrosine kinases (FGFR-1/2/3/4) results in pleotropic effects: binding of FGF-2 to FGFR via the HS glycosaminoglycan binding site stimulates proliferation of BMSCs via the ERK1/2 pathway (Choi et al., 2008); FGF-2 signaling promotes Runx2 activity through FGF-2–mediated activation of the MAPK pathway (Long 2011). FGF-2 induces osteoblast and chondrocyte differentiation through the ERK1/2 pathway (Lai et al., 2001). The bioactivity of FGF-2 can be controlled through its binding to HS to promote the formation of ligand–receptor complexes. FGF-2–interacting HS variant (HS2), isolated from embryos, increased the proliferation and capacity of hMSC (Dombrowski et al., 2009). In addition, HS8 obtained from HS affinity isolation from porcine mucous membranes has higher FGF-2 binding affinity, upregulating FGF signaling, and hMSC proliferation via FGFR-1 (Wijesinghe et al., 2017). Therefore, affinity-purified HS variants have better FGF-2 binding ability and potentially improved bone regeneration performance.
Bone tissue engineering is an excellent method to treat bone defects, and its efficiency has been improved with the development of orthopedic nanomaterials. Electrospun nanofibrous scaffolds and hydrogel scaffolds simulate natural ECM of bone tissue, enhance vitality and function of cells, promote formation of osteoblasts, and stimulate process of vascularization (Qasim et al., 2019). Nano/nanocomposites for bone regeneration form a class of easily absorbable orthopedic fillers by combining nanoscale bioactivity with biopolymeric and degradable matrix structures. Its design was based on a variety of materials, including metal (including nano zirconia, silver nanoparticles, and nano titanium dioxide), ceramics (nano hydroxyapatite and nano silicon dioxide), natural polymers (including chitosan, collagen, cellulose, silk fibroin, and alginate), high-molecular polymers [polycaprolactone (PCL), poly(lactic-co-glycolic) acid (PLGA), polyethylene glycol (PEG), poly(lactic acid) (PLA)] (Bharadwaz and Jayasuriya, 2020), and carbon-based nanomaterials such as graphene and its derivatives, carbon nanotubes and carbon dots (Peng Z et al., 2020). The modification of nanomaterials (such as large surface area, enhanced mechanical strength and stability, improved cell and drug adhesion, and delivery) is more favorable to the development of unique therapeutic strategies for specific bone defects (Peng Z et al., 2020). In addition, nanomaterial particles are essential in cell labeling and drug and gene delivery. Indeed, it can highlight special potential for mesenchymal stem cells, local release, and timing control in bone tissue (directly affecting osteoblasts and osteoclasts), and as a carrier of genetic material to overcome the limitations of traditional methods (Tautzenberger et al., 2012). Magnetic composite scaffolds formed by magnetic nanoparticles (MNP)–activated multiple signaling pathways (including MAPK, integrin, BMP, and NF-κB), resulting in a 2- to 3-fold increase in osteogenic differentiation, angiogenesis, and bone regeneration (Xia et al., 2018).
Due to the inherent nature of heparin to reversibly bind positively charged proteins, it is able to mediate continuous delivery of GFs (in particular VEGF, PDGF-BB, FGF, and BMP-2/4/6/7) while maintaining protein bioactivity (Biran and Pond, 2017; Hettiaratchi et al., 2017). In combination with biomaterials, heparin promotes angiogenesis and bone formation in bone tissue engineering, a well-researched field that aims to improve delivery of GFs for bone regeneration (Hettiaratchi et al., 2017; Rindone et al., 2019).
The use of heparin as a delivery mode may contribute to solving the limitations of nanomaterials used in bone engineering such as low growth factor retention or reduced growth factor activity. Nanomaterials with coated or electrodeposited heparin showed stable GF release without damaging the nanostructure and internal folding skeleton. Furthermore, these scaffolds were better than conventional scaffolds in bridging tissue gaps and reproducing the characteristics of the extracellular environment (Lee et al., 2017). In addition, magnetostrictive nanoparticles and biomimetic heparin coatings can change their shape according to the properties of the external magnetic field to better adapt to changes in the environment of bone regeneration (Guillot-Ferriols, et al., 2020). Nanosilver, antibiotics, or specific genes can cooperate with heparin composite nanomaterials and have excellent antibacterial and anti-inflammatory effects, or can enhance osteogenic signaling activation (Li et al., 2021; Coelho et al., 2015; Xie C. M et al., 2014). Different bonding modes between heparin and nanomaterials (e.g., nanocrystalline hydroxyapatite) produced different heparin chain conformations, which will affect the stability of heparin on nanomaterials and subsequent release of GFs (Konar et al., 2019). Therefore, the assembly of heparin and nanomaterials can control the release of growth factors by changing the way they interact with each other.
It is critical to develop effective carrier systems for therapeutic compounds for bone tissue engineering applications (Zhang et al., 2018). The ideal carrier should be biocompatible, efficient in drug-release, and preserve the compound bioavailability. The combination of heparin with the carrier should provide a stable, persistent, and targeted-controlled release of the drug (Liang and Kiick, 2014). Currently, various nanomaterials are being used for bone repair, including metallic, inorganic, organic, and natural polymers (Sakiyama-Elbert 2014) with heparin incorporated through surface coating, covalent binding, and biomimetic delivery (Hettiaratchi et al., 2014; Johnson et al., 2011; Kim et al., 2015a).
The binding of heparin to titanium (Ti) surfaces has been extensively studied. The heparin/BMP-2/Ti complex enhanced osteogenic activation of MG-63 cells, and the heparin/BMP-2/Ti complex increased ALP activity and calcium deposition compared with pristine Ti surfaces (Lee S. Y et al., 2013). Similarly, Kim et al. demonstrated that Ti/Hep/BMP-2 increased OCN and OPN levels in differentiated osteoblasts. Moreover, BMD and bone to implant contact ratio were significantly greater with Ti/Hep/BMP-2 substrates compared to Ti in vivo (Kim S. E et al., 2014). The aminated titanium exhibited enhanced heparin binding and augmented release kinetics of BMP2 over a 28-day period compared with Ti only resulting in superior osteoinductivity (Kim E. C et al., 2014). In addition, downregulation of TNF-α and IL-6 mRNA was also detected in cells grown on Ti/Hep/BMP-2 compared with those on heparin-grafted Ti. This suggests that Ti/Hep/BMP-2 should also have beneficial anti-inflammatory effects on osteogenesis (Kim et al., 2011). In another study, the addition of gentamicin sulfate to Ti/Hep/BMP-2 significantly inhibited bacterial infection and enhanced ALP activity and calcium deposition of osteoblasts. Therefore, dual drug-eluting (antibiotic and bone-inducing protein) Ti substrates are beneficial for improving the prognosis of orthopedic implants (Lee D. W et al., 2012).
Silver- and GF-doped hydroxyapatite-coated Ti implants showed enhanced osteoinduction capacity and antibacterial properties. BMP-2, chitosan, and heparin were adsorbed on the coat by electrostatic attraction to ensure continuous release of BMP-2 and silver ions, and it demonstrated excellent antibacterial capacity against Staphylococcus epidermis and Escherichia coli, as well as efficient osteoinduction (Xie C. M et al., 2014). Therefore, GFs binding to antimicrobial agents on the surface of metal implants is a simple and effective method to promote bone formation.
Bioinorganic non-metallic materials include bioglass, bioceramic, bioactive cement, and bioceramic cement. They are characterized by excellent chemical stability, histocompatibility, high compressive strength (which plays a role in bone healing through surface modification), covalent action, and biomimetic delivery of heparin (Fahimipour et al., 2019).
Mesoporous bioactive glass (MBG) is an excellent bioceramic for bone transplantation. To recapitulate an ECM-like surface, scaffolds using decellularized matrix from porcine small intestinal submucosal (SIS) provides a plane that can enhance self-renewal, proliferation, and differentiation of stem cells. By heparinizing MBG/SIS scaffolds and conjugating P28, a BMP-2–related peptide (MGB/SIS-Hep-P28), the release of P28 was extended (40 days), resulting in increased cell proliferation, viability, ALP activation, and enhanced expression of osteogenesis-related genes (including Runx2, OCN, OPN, and ALP) in vitro. Furthermore, MGB/SIS-Hep-P28 scaffolds significantly increased bone formation of rat calvarial critical-size skull defects compared with controls in vivo (Zhou et al., 2020).
Similarly, loading heparin/collagen multilayer films on bidirectional calcium phosphate (BCP) allows for the programmed release of BMP-2. The heparin/collagen multilayer on BCP reduced the initial release of BMP-2 by >50% and loaded more BMP-2 during the differentiation phase of osteogenic cells. This suggests that heparin loading on the surface of orthopedic biomaterials is more favorable for the stability and durability of bone formation (Han et al., 2021).
One promising strategy for promoting angiogenesis in bone tissue is local and continuous release of angiogenic factors. Varying the amounts of heparin within the heparin-modified biocement (Bio D)/collagen type I (Col-I) complexes resulted in diverse effects on the release of VEGF. Increasing heparin inhibited the initial burst of release in a concentration-dependent manner and promoted VEGF activity and improved BioD/coll biological properties. In addition, the heparin-modified BioD/coll composite showed a finer microstructure, with smaller heparin particles and higher specific surface area, making it more favorable as a bone graft substitute for bone healing (Lode et al., 2008).
The performance of heparin can be affected by how it is coupled to a substrate. Goonasekera et al., assessed the impact of how heparin attached to the surface of hydroxyapatite particles affected the rate of BMP-2 release. When heparin was covalently attached to the hydroxyapatite surface via a (3-aminopropyl)triethoxysilane (APTES) layer, the release rate of BMP-2 was 31% after 7 days. This is compared with release rates of 16 and 5% when heparin was adsorbed to APTES-modified particles or pure hydroxyapatite, respectively. Consequently, the release curve and total amount of BMP-2 can be controlled by changing the attachment mode between heparin and a biomaterial (Goonasekera et al., 2015).
Another method of enhancing the regeneration of bone via heparin-mediated mechanisms is to conjugate heparin with 1-amino-1,1-diphosphonate methane (aminoBP) to enhance its affinity to hydroxyapatite. Increasing the number of conjugated aminoBPs resulted in a > 2-fold increase in the affinity of heparin for hydroxyapatite in vitro. Moreover, aminoBP-heparin conjugants were investigated, and they could enhance bone mineral affinity of bFGF and BMP-2. The authors found that conjugated heparin increased bone mineral affinity of these GFs in an aminoBP-dependent manner indicating that combining aminoBP with heparin improves the affinity of osteogenic GFs for hydroxyapatite (Gittens et al., 2004).
Biomimetic delivery systems are important for bone regeneration. Heparin was covalently connected to cross-linked type I collagen–coated tricalcium phosphate/hydroxyapatite and loaded with BMP-2 to form a local continuous delivery system of BMP-2 to improve bone regeneration for the treatment of large bone defects (Hannink et al., 2013). Delivery of BMP-7 from a bioactive glass/heparin/gelatin nanocomposite scaffold in rat calvarial critical-size skull defects induced fully mature new bone at the site of injury at 12 weeks suggesting synergistic effects of cells, scaffolds, and growth factors in bone regeneration (Kargozar et al., 2017). Jo et al., hypothesized sequential delivery of BMP-2 and BMP-7 would enhance bone regeneration more effectively than BMP-2 alone. The sequential delivery of BMP-2/7 with heparinized collagen membranes showed significant induced new bone formation in rat calvarial defects compared with single delivery of BMP-2 or BMP-7 (Jo et al., 2015). Another composite scaffold is CaCO3 microspheres which possess osteoinductivity, rough surfaces, and specific binding sites for BMP-2. When encapsulated in heparin fibrin hydrogel, osteogenic differentiation of BMSCs was augmented (Gong et al., 2019). In summary, different delivery systems can affect bone regeneration by regulating GF delivery (Wang et al., 2018).
Alginate sulfate, a synthetic heparin structure mimic, has similar bioactivity to heparin and is used in three-dimensional (3D)-printed scaffolds. This 3D micropatterning method can be applied to different heparin-binding GFs (including FGF-2, VEGF, TGF-β, and BMP) while retaining the natural degradation and cellular compatibility of hydrogels. Stem cells loaded in micropattern hydrogels exhibited spatially localized growth and differentiation responses corresponding to various GFs patterns, suggesting the adaptability of using 3D micropatterning to control stem cell behavior in bone tissue engineering (Jeon et al., 2018).
Biosynthetic organic materials are widely used in the field of orthopedics with heparin-coated organic materials demonstrating benefits such as sustained protein release, favorable biocompatibility, high growth factor loading rate, and precise control (Hamideh et al., 2020; Nakamura et al., 2020).
Improvements in the development of coacervate particles have included the electrostatic complexation between poly(ethylene arginyl aspartate diglyceride) (PEAD) polycations and anionic heparin (termed “coacersomes”) to avoid aggregation and regulate the release of BMP-2. The coacersomes demonstrated biocompatibility with human dermal fibroblasts, a high loading efficiency (> 96%) for encapsulated BMP-2, sustained release of up to 28 days, and increased osteogenic differentiation of hMSCs (Jo et al., 2019).
Although PCL fiber scaffolds are inert, the surface incorporation of heparin and BMP-2 (PCL/Hep/BMP-2 scaffold) can promote rapid and stable integration of adjacent bone tissue. In these scaffolds, the concentration of BMP-2 increased systematically with the incorporation of heparin, and its efficacy was preserved through covalent binding, facilitating MSC proliferation and increasing ALP activity, deposition of bone sialoprotein, OPN, and calcium minerals deposition (Gadalla and Goldstein, 2020).
Insufficient vascularization is an important limitation in engineering porous scaffolds in tissue engineering. Heparin cross-linked with N-hydroxysuccinimide and N-(3-di-methylaminopropyl)-N'-ethylcarbodiimide resulted in demineralized bone matrices (DBM) being able to bind more VEGF, and this achieved localized and sustained delivery compared with non-cross-linked scaffolds. VEGF was biologically active when bound to heparinized DBMs demonstrated by increased proliferation of endothelial cells and improved angiogenesis thus augmenting vascularization and bone regeneration (Chen et al., 2010).
Currently, the biomimetic delivery systems of heparin/organic polymer materials have been studied extensively using hydrogels (Sievers et al., 2019), scaffolds (Jeon et al., 2007), fibers (Lü et al., 2018), collagen (Vantucci et al., 2021), and nanospheres (Reguera-Nuñez et al., 2014). Multiple organic materials loaded with GFs at the bone defect have achieved long-term sustained release in the target area and induced bone formation. In Li et al., PEAD and heparin were complexed to form a novel platform to release and deliver BMP-2, which promoted differentiation of myogenic stem cells into an osteogenic lineage (Li et al., 2013). Thin film materials assembled by using the layer-by-layer (LBL) method are highly adaptable and versatile in terms of the type of substrates and polyelectrolytes (such as GFs) that can be used for bone tissue engineering. Polyelectrolytes provide the initial charge necessary for multilayer construction with counter polyelectrolytes such as heparin being able to enhance the activity of GFs by protecting their ligands. This produced the highest loading efficiency for the tested GFs (Damanik et al., 2019) suggesting the LBL assembly method can improve the delivery system of biological molecules for bone tissue engineering applications.
The addition of a heparin-based hydrogel to porous cylinder poly (L-lactide-co-ε-caprolactone) (PLCL) scaffolds effectively accelerated the maturation and differentiation of fibrochondrocytes, and was used for local release of fibrochondrocytes and BMP-2 to the fibrochondral region (Lee et al., 2011). Photocrosslinkable biomaterials such as alginate saline gel (Hep-ALG) have been harnessed to develop a controlled, prolonged release of BMP-2 with the addition of heparin to the hydrogel (Jeon et al., 2011). Another hydrogel system containing hyaluronic acid which included heparin also supported osteoblast growth (> 8 weeks) and sustained BMP-2 release (> 35 days) in rats (Rath et al., 2011). Compared with hydrogels without heparin, it inhibited the initial burst of BMP-2 and maintained BMP-2 activity for up to 28 days (Bhakta et al., 2012). Other scaffolds use sulfonated hydrogels to mimic heparin [such as poly(vinylsulfonic acid) (PVSA) or poly-4-styrenesulfonic acid (PSS)] which was found to effectively isolate and stabilize BMP-2 to enhance bone induction activity (Kim S et al., 2018).
When hMSCs were cultured on top of heparin-conjugated PLGA (Hep-PLGA) scaffolds loaded with BMP-7/TGF-β3 nanocomplexes, they showed cartilage formation macroscopically and histologically (Crecente-Campo et al., 2017). BMP-2 released by Hep-PLGA stimulated ALP activity for approximately 14 days, increased bone formation area 9-fold, and induced calcium content 4-fold compared with unmodified heparin PLGA scaffolds (Jeon et al., 2007). PCL/PLGA scaffolds were conjugated with heparin and dopamine to form a scaffold and coated with BMP-2. In vitro studies with osteoblast-like MG-63 cells cultured on the scaffolds demonstrated significantly enhanced ALP activity, calcium deposition and bone formation on BMP-2/Hep-DOPA/PCL/PLGA scaffolds compare to those without dopamine. It is suggested that dopamine may enhance the osteogenic effects in conjunction with heparin (Kim T. H et al., 2014). Hep/PCL/gelatin scaffolds also provide a controlled release of PDGF-BB and prolong the bioactivity of the molecule, therefore facilitating angiogenesis (Lee J et al., 2012). Another delivery system involves using polyelectrolyte multilayer films to release FGF-2 locally, precisely, and continuously. The presence of counter polyanion, HS in the multilayer structure enhanced FGF-2 osteogenic activation (Macdonald et al., 2010).
The GF delivery carrier formed by the heparinization modification of polylactic acid (PLA) is termed fiber particles (Hep-FP). Hep-FPs showed stable BMP-2 binding and sustained release, augmented ALP activity, hMSC mineralization, and higher BMD formation in the defect area (Shin et al., 2015). Combination of heparin-mediated bFGF into PCL/gelatin fiber mesh also had the ability to direct bone regeneration (Lee J. H et al., 2015). VEGF and heparin were fixed in PLGA nanofibers, which induced vascular formation of MSCs and were more favorable for bone regeneration (Lü et al., 2018).
Nano-microspheres, nanosponges, and nanocomplexes can effectively bind several heparin-binding GFs (including BMP-2, PDGF-BB, VEGF, FGF-2). Most of them are prepared with heparin and PLGA. BMP-2 or BMP-7 incorporated into microspheres remain biologically active and effectively initiate functional cellular responses (Hettiaratchi et al., 2014; Reguera-Nuñez et al., 2014), which significantly induced ALP activity, calcium deposition, OCN and OPN expression (Kim et al., 2015b; Liu et al., 2016). The GF delivered by PDGF-BB nanoparticles accelerated the generation of blood vessels, meeting the key requirements of bone regeneration (d'Angelo et al., 2010; La and Yang, 2015). Meanwhile, the release of FGF-2 was prolonged by heparin nanosponges and stimulated the growth and differentiation of hBMSCs (Choi W. I et al., 2017; Shin et al., 2014).
Natural polymer materials include chitosan, collagen, silk protein and cellulose. The chitosan/agarose/gelatin (CAG) scaffold was modified with heparin and loaded with stromal cell derived factor 1 (SDF-1) and BMP-2. SDF-1 and BMP-2 released retained bioactivity and induced the sustained recruitment and differentiation of MSCs (Wang et al., 2018). Heparin-modified collagen scaffolds can promote the transmission of BMP-2 to bone defects and reduce heterotopic ossification in critically sized femoral defects in a rat model (Hettiaratchi et al., 2020). BMP-2 released by heparin-conjugated fibrin (HCF) induced higher BMP-2 retention, ALP activity and BMD compared with collagen sponges (Yang et al., 2012).
The heparin analogue, dextran sulfate (DS) is covalently linked to chitosan to form nanoparticles. Heparin-binding proteins (SDF-1α VEGF, FGF-2, BMP-2.) were added, and SDF-1α and VEGF demonstrated full activity and sustained heat stability. The other GFs exhibited good osteogenic effects due to their heparin-binding sites (Zaman et al., 2016). On the other hand, neither heparin nor BMP-2 alone promoted bone growth within collagen scaffolds. BMP-2 collagen scaffolds complexed with heparin stimulated new bone regeneration with similar mechanical properties to intact bone (Johnson et al., 2011).
Heparinized chitosan has a protective effect on BMP bioactivity and can resist the physiological stressors related to fracture healing. It enhanced osteogenesis by inhibiting Noggin activity and attracted BMSCs (Kim et al., 2020). Moreover, it exhibited synergetic effects between BMP-2 and VEGF, which effectively augmented bone formation stimulating osteogenesis and angiogenesis (Zhang S et al., 2019). Heparinized chitosan can stably adsorb FGF-2 to the surface and release it into the bone defect (Zomer Volpato et al., 2012). In addition, stabilizing FGF-2 in the interior of chitosan with a heparin-based nanoparticle complex can preserve its activity and stimulate BMSCs (Place et al., 2016). The gelatin/chitosan frozen gel surrounding the heparin/gelatin frozen gel exhibited different drug release kinetics. Early release of VEGF and continuous delivery of BMP-4 can induce successful osteogenic differentiation in vitro. The dual release leads to the enhanced effect of bone regeneration (Lee et al., 2020). In addition, whitlockite enhanced VEGF secretion of human adipose stem cells inoculated with heparin/gelatin. The sustained release of VEGF was observed, which promoted angiogenesis to enhance bone formation in vivo and significantly increased bone regeneration (Kim et al., 2019).
The BMP-2 delivery using heparin-conjugated collagen sponges (HCS) had a low initial burst and then a sustained BMP-2 release. Over time, HCS-BMP scaffolds guided more efficient bone regeneration within the defect, as well as ossification outside the defect (Kim et al., 2016). When combined with a matrix derived SDF-1α, the concentration of BMP-2 could be reduced since SDF-1α could enhance the osteoinduction ability of BMP-2 (Zwingenberger et al., 2016). Compared with the conventional collagen scaffold used in clinic, the mixed scaffold composed of heparin, BMP-2, nanofiber, and fibronectin was more effective in bridging the gap (Lee S. S et al., 2013). Heparin-conjugated collagen also acted as a carrier to deliver PDGF-BB, which stimulated angiogenesis. VEGF-preloaded heparin collagen scaffolds also promoted the formation and stabilization of prevascular structures (Quade et al., 2017). Therefore, heparinized collagen coupled with GF can significantly improve angiogenesis and bone regeneration.
HCF systems provided long-term release of BMP thus enhancing bone regeneration, stimulating ALP activity, increasing OCN level and the ratio of calcium to phosphorus in the regenerated bone, as well as producing more mature and highly mineralized bone than bare fiber gel (Chung et al., 2007). In addition, the controlled delivery of recombinant BMP-2 stimulated by HCF inhibited the formation of adipose tissue in the defect area and enhanced mineralization, thus greatly alleviating the side effects of adipose bone marrow formation caused by high concentration of recombinant BMP-2 (Lee J. S et al., 2015).
Combining multiple composite materials has been found to produce better results than using individual materials. Combining inorganic and natural polymer materials provides enhanced mechanical properties as well as thermal stability. Heparin coupled to a strontium-substituted hydroxyapatite/silk scaffold was found to increase proliferation and adhesion of BMSCs, upregulate expression of osteogenic genes including OCN and OPN, and increase BMD, thereby enhancing new bone regeneration (Yan et al., 2018).
Hydroxyapatite was coated with collagen to mimic the composition of bone and enhanced cell attachment, proliferation, nutrient transport, and infiltration of new bone tissue. Combining heparin and a mineralized collagen matrix is important to maintain the activity and sustained release of loaded BMP-2 and VEGF. It can stimulate angiogenesis and bone regeneration through GF binding and favorable release properties (Knaack et al., 2014).
Hybrid materials comprising inorganic and metallic materials promoted increased bone volume, bone volume/tissue volume, and better bone remodeling characteristics than the individual materials (Yang et al., 2015). Incorporation of metal particles such as silver into hydroxyapatite coating can increase the antibacterial properties in addition to osteogenesis. Furthermore, chitosan can not only stabilize chelation but reduce the toxicity of silver ions (Xie C. M et al., 2014).
The incorporation of gold nanoparticles into poly-l-lysine heparin membranes showed enhanced mechanical properties (Qi et al., 2017). The macroporous scaffold composed of chitosan, hydroxyapatite, heparin and polyvinyl alcohol resulted in a more uniform matrix structure, and has the mechanical properties to promote bone regeneration (Sultankulov et al., 2019). In summary, the combinations of the appropriate materials have synergistic GF induction properties and provide a better microenvironment for bone healing. Therefore, multifunctional membranes as bone induction coatings for biomaterials have far-reaching significance. The effects of heparin on GFs are summarized in Figure 4.
FIGURE 4. Cartoon representation of the different ways heparin interacts with growth factors: The heparin oligosaccharide unit (highlighted by the dashed oval) is located relative to the heparin-binding site within the proteins structure of the growth factors. (A) Heparin-induced dimerization of heparin-binding protein. Numerous members of the FGF family interact with heparin to form dimers. Protein–heparin interactions drive the dimerization in the absence of protein interactions at the dimer interface. (B) Heparin acts as a scaffold for protein–protein interactions for efficient binding and regulation between proteins. The eutectic structures of FGF-1, FGFR2, and heparin-derived decosaccharides are shown here. (C) Heparin acts as an allosteric regulator. The unique pentasaccharide within heparin binds to antithrombin and induces allosteric changes mitigating the actions of serine protein C inhibitors which inhibit antithrombin activity. (D) Interaction between heparin and growth factors (such as BMP and VEGF) is dependent on salt concentration. Binding affinity increases with increasing oligosaccharide length. (E) Ability of heparin to bind proteins to specific locations in tissues. The length and flexibility of the heparin chain allows bound growth factors (e.g., FGF-2) to move unidirectionally along the chain and ensure localization of the bound growth factors.
In this review, we have summarized the recent advances of how heparin is utilized in bone tissue engineering, including the regulation of osteogenic GFs and bone regeneration materials. We highlighted the use of heparin as a multifunctional material modifier to promote angiogenesis and osteogenesis. Heparin provides a range of features (including the regulation of GFs BMP, FGF-2, PDGF-BB, and TGF-β and their applications in biomaterials) that are important for effective GF release, angiogenesis, and bone formation. In addition to heparin, there are numerous other substances or molecules that can bind GFs for bone regeneration. For example, bisphosphonate-loaded nanomaterials achieved controlled and sustained delivery of GFs (Fanord et al., 2011). In addition, molecules such as peptides (e.g., AspSerSer6) or plasmid DNA can effectively deliver transcription factors and GFs to induce osteoblasts and thereby enhancing bone formation (Zhang et al., 2012). Moreover, interleukin-1 receptor antagonist-binding nanomaterials reduced inflammation and reversed bone turnover (Fernandes et al., 2008). However, the use of heparin is a more promising area than the aforementioned methods because of its improved safety, degradability, surface modification capacity, and easier space application.
The specific binding site within heparin that mediates interaction with a variety of osteogenic GFs improves neovascularization and bone tissue regeneration. Compared with other anticoagulant drugs, heparin-modified bone regenerative materials have higher osteogenic activity, which is favorable for the delivery and retention of therapeutic GFs. This is closely related to the fact that heparin is a negatively charged natural polysaccharide with a specific binding site that binds GFs, which prevents their degradation and also has anti-inflammatory and angiogenic effects. Heparin-modified biomaterials (particles, fibers, films, and 3D scaffolds) can be used as GF vehicles to transport drugs/GFs expressing angiogenic and osteogenic capabilities, augmenting kinetic release and decreasing side effects due to the inherent biological activity of heparin. By effectively stimulating bone formation through the release of therapeutic drugs/GFs, the delivery of heparin modified materials may serve as a more widespread platform for bone and cartilage regeneration. We also discussed the control of heparin on the initial burst of GFs and the importance on sustaining the subsequent release. More focus should center on the relative merits of these GFs and their application forms (as particles or scaffolds), dosing for different cell lines or animal models as well as optimizing the specific combination of GFs and materials to achieve synergistic effects while managing release kinetics. Future research should concentrate on the development of heparin-modified materials with inflammatory components that simultaneously promote the coupling of angiogenesis and osteogenesis.
Although heparin-modified material carriers have many advantages, limitations need to be addressed. For example, the drug conjugated to heparin does not maintain the same biological characteristics in vivo after delivery, the effective charge may be unstable in vivo, and chemical conjugation may also affect the non-covalent interaction of the carrier in some heparin-modified materials. Therefore, multilayer slow-release systems can be designed to make the release of drugs or GFs more stable. Furthermore, the addition of a constant magnetic field to the outside of the material may promote directional force to ensure steadier drug release. The bioactivity of GFs plays a critical role in bone regeneration, and the application of heparin in biomaterials is the key to maintaining GF activity. Heparin can effectively protect the biological activity of GFs at the site of bone injury and ensure a controlled release during the treatment for maximum effects. Therefore, heparin modification is a promising approach in bone tissue engineering biomaterial development. In addition, directions for future research should include but not limited to investigating the optimal components for stimulating bone formation: 1) the particular effects of heparin-specific therapeutic GF dissolution and its relevant concentrations on angiogenesis; 2) develop standard in vitro models to characterize multifunctional biomaterial systems that reflect the participation of the immune system in vivo; 3) heparin-modified composite scaffold with multiple GFs and multiple materials; and 4) develop appropriate animal models to study heparin-induced new bone tissue and blood vessels. It is likely that in the future, advances in the understanding of osteogenic regulation of heparin and the advances in composite material research will further enhance the appeal of using heparin clinically for the treatment of bone tissue defects.
JW and LX wrote original draft; JW made the figures; XW and LX revised and edited the manuscript. All authors have read and agreed to the published version of the manuscript.
This work was funded by the National Natural Science Foundation of China (Grant no. 86060620, 31960209 and 31760266), “Thousand” Level Project of Training High-Level Innovative Talents in Guizhou Province (Grant no. fzc20200612), Guizhou Science and Technology Fund Project (Grant no. (2020)1Y093), and the Zunyi Science and Technology Fund Project (Grant no. Zun Shi Ke He HZ Zi 2021–40). and Doctoral Science Research Startup Funding of Zunyi Medical University (Grant no. F-934 and no. 2017–01).
The authors declare that the research was conducted in the absence of any commercial or financial relationships that could be construed as a potential conflict of interest.
All claims expressed in this article are solely those of the authors and do not necessarily represent those of their affiliated organizations, or those of the publisher, the editors, and the reviewers. Any product that may be evaluated in this article, or claim that may be made by its manufacturer, is not guaranteed or endorsed by the publisher.
Abramsson, A., Kurup, S., Busse, M., Yamada, S., Lindblom, P., Schallmeiner, E., et al. (2007). Defective N-Sulfation of Heparan Sulfate Proteoglycans Limits PDGF-BB Binding and Pericyte Recruitment in Vascular Development. Genes Dev. 21 (3), 316–331. doi:10.1101/gad.398207
Aláez-Versón, C. R., Lantero, E., and Fernàndez-Busquets, X. (2017). Heparin: New Life for an Old Drug. Nanomedicine 12 (14), 1727–1744. doi:10.2217/nnm-2017-0127
Alban, S. (2012). Adverse Effects of Heparin. Handb. Exp. Pharmacol. 207, 211–263. doi:10.1007/978-3-642-23056-1_10
Alekseeva, A., Urso, E., Mazzini, G., and Naggi, A. (2019). Heparanase as an Additional Tool for Detecting Structural Peculiarities of Heparin Oligosaccharides. Molecules 24 (23), 4403. doi:10.3390/molecules24234403
Alquwaizani, M., Buckley, L., Adams, C., and Fanikos, J. (2013). Anticoagulants: A Review of the Pharmacology, Dosing, and Complications. Curr. Emerg. Hosp. Med. Rep. 1 (2), 83–97. doi:10.1007/s40138-013-0014-6
Arepally, G. M., and Cines, D. B. (2020). Pathogenesis of Heparin-Induced Thrombocytopenia. Transl. Res. 225, 131–140. doi:10.1016/j.trsl.2020.04.014
Arepally, G. M. (2017). Heparin-induced Thrombocytopenia. Blood 129 (21), 2864–2872. doi:10.1182/blood-2016-11-709873
Banik, N., Yang, S.-B., Kang, T.-B., Lim, J.-H., and Park, J. (2021). Heparin and its Derivatives: Challenges and Advances in Therapeutic Biomolecules. Ijms 22 (19), 10524. doi:10.3390/ijms221910524
Barbour, L. A., Kick, S. D., Steiner, J. F., LoVerde, M. E., Heddleston, L. N., Lear, J. L., et al. (1994). A Prospective Study of Heparin-Induced Osteoporosis in Pregnancy Using Bone Densitometry. Am. J. Obstetrics Gynecol. 170 (3), 862–869. doi:10.1016/s0002-9378(94)70299-3
Bendinelli, P., Maroni, P., Dall’Olio, V., Matteucci, E., and Desiderio, M. A. (2019). Bone Metastasis Phenotype and Growth Undergo Regulation by Micro-environment Stimuli: Efficacy of Early Therapy with HGF or TGFβ1-type I Receptor Blockade. Ijms 20 (10), 2520. doi:10.3390/ijms20102520
Bett, C., Grgac, K., Long, D., Karfunkle, M., Keire, D. A., Asher, D. M., et al. (2017). A Heparin Purification Process Removes Spiked Transmissible Spongiform Encephalopathy Agent. Aaps J. 19 (3), 765–771. doi:10.1208/s12248-017-0047-y
Bhakta, G., Rai, B., Lim, Z. X. H., Hui, J. H., Stein, G. S., van Wijnen, A. J., et al. (2012). Hyaluronic Acid-Based Hydrogels Functionalized with Heparin that Support Controlled Release of Bioactive BMP-2. Biomaterials 33 (26), 6113–6122. doi:10.1016/j.biomaterials.2012.05.030
Bharadwaz, A., and Jayasuriya, A. C. (2020). Recent Trends in the Application of Widely Used Natural and Synthetic Polymer Nanocomposites in Bone Tissue Regeneration. Mater. Sci. Eng. C 110, 110698. doi:10.1016/j.msec.2020.110698
Biran, R., and Pond, D. (2017). Heparin Coatings for Improving Blood Compatibility of Medical Devices. Adv. Drug Deliv. Rev. 112, 12–23. doi:10.1016/j.addr.2016.12.002
Blanquaert, F., Barritault, D., and Caruelle, J.-P. (199919990). Effects of Heparan-like Polymers Associated with Growth Factors on Osteoblast Proliferation and Phenotype Expression. J. Biomed. Mat. Res. 44 (1), 63–72. doi:10.1002/(sici)1097-4636(199901)44:1<63::aid-jbm7>3.0.co;2-s
Bleich, H. L., Boro, E. S., and Rosenberg, R. D. (1975). Actions and Interactions of Antithrombin and Heparin. N. Engl. J. Med. 292 (3), 146–151. doi:10.1056/nejm197501162920307
Borsig, L., Wong, R., Feramisco, J., Nadeau, D. R., Varki, N. M., and Varki, A. (2001). Heparin and Cancer Revisited: Mechanistic Connections Involving Platelets, P-Selectin, Carcinoma Mucins, and Tumor Metastasis. Proc. Natl. Acad. Sci. U.S.A. 98 (6), 3352–3357. doi:10.1073/pnas.061615598
Bramono, D. S., Murali, S., Rai, B., Ling, L., Poh, W. T., Lim, Z. X., et al. (2012). Bone Marrow-Derived Heparan Sulfate Potentiates the Osteogenic Activity of Bone Morphogenetic Protein-2 (BMP-2). Bone 50 (4), 954–964. doi:10.1016/j.bone.2011.12.013
Carlin, A. J., Farquharson, R. G., Quenby, S. M., Topping, J., and Fraser, W. D. (2004). Prospective Observational Study of Bone Mineral Density during Pregnancy: Low Molecular Weight Heparin versus Control. Hum. Reprod. 19 (5), 1211–1214. doi:10.1093/humrep/deh115
Carlsson, P., and Kjellén, L. (2012). Heparin Biosynthesis. Handb. Exp. Pharmacol. 207, 23–41. doi:10.1007/978-3-642-23056-1_2
Casele, H., Haney, E. I., James, A., Rosene-Montella, K., and Carson, M. (2006). Bone Density Changes in Women Who Receive Thromboprophylaxis in Pregnancy. Am. J. Obstetrics Gynecol. 195 (4), 1109–1113. doi:10.1016/j.ajog.2006.06.080
Casele, H. L., and Laifer, S. A. (2000). Prospective Evaluation of Bone Density in Pregnant Women Receiving the Low Molecular Weight Heparin Enoxaparin Sodium. J. Matern. Fetal Med. 9 (2), 122–125. doi:10.1002/(sici)1520-6661(200003/04)9:2<122::Aid-mfm7>3.0.Co;2-q
Cassinelli, G., and Naggi, A. (2016). Old and New Applications of Non-anticoagulant Heparin. Int. J. Cardiol. 212 (Suppl. 1), S14–S21. doi:10.1016/s0167-5273(16)12004-2
Chandarajoti, K., Liu, J., and Pawlinski, R. (2016). The Design and Synthesis of New Synthetic Low-Molecular-Weight Heparins. J. Thromb. Haemost. 14 (6), 1135–1145. doi:10.1111/jth.13312
Charron, P. N., Garcia, L. M., Tahir, I., and Floreani, R. A. (2022). Bio-inspired Green Light Crosslinked Alginate-Heparin Hydrogels Support HUVEC Tube Formation. J. Mech. Behav. Biomed. Mater. 125, 104932. doi:10.1016/j.jmbbm.2021.104932
Chen, L., He, Z., Chen, B., Yang, M., Zhao, Y., Sun, W., et al. (2010). Loading of VEGF to the Heparin Cross-Linked Demineralized Bone Matrix Improves Vascularization of the Scaffold. J. Mater Sci. Mater Med. 21 (1), 309–317. doi:10.1007/s10856-009-3827-9
Choi, J. U., Chung, S. W., Al-Hilal, T. A., Alam, F., Park, J., Mahmud, F., et al. (2017). A Heparin Conjugate, LHbisD4, Inhibits Lymphangiogenesis and Attenuates Lymph Node Metastasis by Blocking VEGF-C Signaling Pathway. Biomaterials 139, 56–66. doi:10.1016/j.biomaterials.2017.05.026
Choi, S.-C., Kim, S.-J., Choi, J.-H., Park, C.-Y., Shim, W.-J., and Lim, D.-S. (2008). Fibroblast Growth Factor-2 and -4 Promote the Proliferation of Bone Marrow Mesenchymal Stem Cells by the Activation of the PI3K-Akt and ERK1/2 Signaling Pathways. Stem Cells Dev. 17 (4), 725–736. doi:10.1089/scd.2007.0230
Choi, W. I., Sahu, A., Vilos, C., Kamaly, N., Jo, S.-M., Lee, J. H., et al. (2017). Bioinspired Heparin Nanosponge Prepared by Photo-Crosslinking for Controlled Release of Growth Factors. Sci. Rep. 7 (1), 14351. doi:10.1038/s41598-017-14040-5
Choi, Y. J., Lee, J. Y., Park, J. H., Park, J. B., Suh, J. S., Choi, Y. S., et al. (2010). The Identification of a Heparin Binding Domain Peptide from Bone Morphogenetic Protein-4 and its Role on Osteogenesis. Biomaterials 31 (28), 7226–7238. doi:10.1016/j.biomaterials.2010.05.022
Chung, Y.-I., Ahn, K.-M., Jeon, S.-H., Lee, S.-Y., Lee, J.-H., and Tae, G. (2007). Enhanced Bone Regeneration with BMP-2 Loaded Functional Nanoparticle-Hydrogel Complex. J. Control. Release 121 (1-2), 91–99. doi:10.1016/j.jconrel.2007.05.029
Coelho, C. C., Sousa, S. R., and Monteiro, F. J. (2015). Heparinized Nanohydroxyapatite/collagen Granules for Controlled Release of Vancomycin. J. Biomed. Mat. Res. 103 (10), 3128–3138. doi:10.1002/jbm.a.35454
Cosmi, B. (2016). Management of Idiopathic Venous Thromboembolism. Expert Rev. Cardiovasc. Ther. 14 (12), 1371–1384. doi:10.1080/14779072.2016.1248406
Crecente-Campo, J., Borrajo, E., Vidal, A., and Garcia-Fuentes, M. (2017). New Scaffolds Encapsulating TGF-Β3/bmp-7 Combinations Driving Strong Chondrogenic Differentiation. Eur. J. Pharm. Biopharm. 114, 69–78. doi:10.1016/j.ejpb.2016.12.021
Crijns, H., Adyns, L., Ganseman, E., Cambier, S., Vandekerckhove, E., Pörtner, N., et al. (2021). Affinity and Specificity for Binding to Glycosaminoglycans Can Be Tuned by Adapting Peptide Length and Sequence. Ijms 23 (1), 447. doi:10.3390/ijms23010447
Cushing, M. C., Liao, J.-T., and Anseth, K. S. (2005). Activation of Valvular Interstitial Cells Is Mediated by Transforming Growth Factor-β1 Interactions with Matrix Molecules. Matrix Biol. 24 (6), 428–437. doi:10.1016/j.matbio.2005.06.007
Dahlman, T. C., Sjöberg, H. E., and Ringertz, H. (1994). Bone Mineral Density during Long-Term Prophylaxis with Heparin in Pregnancy. Am. J. Obstet. Gynecol. 170 (5 Pt 1), 1315–1320. doi:10.1016/s0002-9378(94)70149-0
Damanik, F. F. R., Brunelli, M., Pastorino, L., Ruggiero, C., van Blitterswijk, C., Rotmans, J., et al. (2019). Sustained Delivery of Growth Factors with High Loading Efficiency in a Layer by Layer Assembly. Biomater. Sci. 8 (1), 174–188. doi:10.1039/c9bm00979e
d’Angelo, I., Garcia-Fuentes, M., Parajó, Y., Welle, A., Vántus, T., Horváth, A., et al. (2010). Nanoparticles Based on PLGA:poloxamer Blends for the Delivery of Proangiogenic Growth Factors. Mol. Pharm. 7 (5), 1724–1733. doi:10.1021/mp1001262
Davies, N. H., Schmidt, C., Bezuidenhout, D., and Zilla, P. (2012). Sustaining Neovascularization of a Scaffold through Staged Release of Vascular Endothelial Growth Factor-A and Platelet-Derived Growth Factor-BB. Tissue Eng. Part A 18 (1-2), 26–34. doi:10.1089/ten.tea.2011.0192
Demoulin, J.-B., and Essaghir, A. (2014). PDGF Receptor Signaling Networks in Normal and Cancer Cells. Cytokine & Growth Factor Rev. 25 (3), 273–283. doi:10.1016/j.cytogfr.2014.03.003
Diez-Escudero, A., Espanol, M., Bonany, M., Lu, X., Persson, C., and Ginebra, M.-P. (2018). Heparinization of Beta Tricalcium Phosphate: Osteo-Immunomodulatory Effects. Adv. Healthc. Mat. 7 (5), 1700867. doi:10.1002/adhm.201700867
Dombrowski, C., Song, S. J., Chuan, P., Lim, X., Susanto, E., Sawyer, A. A., et al. (2009). Heparan Sulfate Mediates the Proliferation and Differentiation of Rat Mesenchymal Stem Cells. Stem Cells Dev. 18 (4), 661–670. doi:10.1089/scd.2008.0157
Dong, Z. R., Sun, D., Yang, Y. F., Zhou, W., Wu, R., Wang, X. W., et al. (2020). TMPRSS4 Drives Angiogenesis in Hepatocellular Carcinoma by Promoting HB‐EGF Expression and Proteolytic Cleavage. Hepatology 72 (3), 923–939. doi:10.1002/hep.31076
Douketis, J. D., Ginsberg, J. S., Burrows, R. F., Duku, E. K., Webber, C. E., and Brill-Edwards, P. (1996). The Effects of Long-Term Heparin Therapy during Pregnancy on Bone Density. A Prospective Matched Cohort Study. Thromb. Haemost. 75 (2), 254–257. doi:10.1055/s-0038-1650255
Du, S., Yu, Y., Xu, C., Xiong, H., Yang, S., and Yao, J. (2019). LMWH and its Derivatives Represent New Rational for Cancer Therapy: Construction Strategies and Combination Therapy. Drug Discov. Today 24 (10), 2096–2104. doi:10.1016/j.drudis.2019.06.011
Ezekowitz, M. D., Pollack, C. V., Halperin, J. L., England, R. D., VanPelt Nguyen, S., Spahr, J., et al. (2018). Apixaban Compared to Heparin/vitamin K Antagonist in Patients with Atrial Fibrillation Scheduled for Cardioversion: the EMANATE Trial. Eur. Heart J. 39 (32), 2959–2971. doi:10.1093/eurheartj/ehy148
Facchetti, D., Hempel, U., Martocq, L., Smith, A. M., Koptyug, A., Surmenev, R. A., et al. (2021). Heparin Enriched-WPI Coating on Ti6Al4V Increases Hydrophilicity and Improves Proliferation and Differentiation of Human Bone Marrow Stromal Cells. Ijms 23 (1), 139. doi:10.3390/ijms23010139
Fahimipour, F., Dashtimoghadam, E., Mahdi Hasani-Sadrabadi, M., Vargas, J., Vashaee, D., Lobner, D. C., et al. (2019). Enhancing Cell Seeding and Osteogenesis of MSCs on 3D Printed Scaffolds through Injectable BMP2 Immobilized ECM-Mimetic Gel. Dent. Mater. 35 (7), 990–1006. doi:10.1016/j.dental.2019.04.004
Fanord, F., Fairbairn, K., Kim, H., Garces, A., Bhethanabotla, V., and Gupta, V. K. (2011). Bisphosphonate-modified Gold Nanoparticles: a Useful Vehicle to Study the Treatment of Osteonecrosis of the Femoral Head. Nanotechnology 22 (3), 035102. doi:10.1088/0957-4484/22/3/035102
Farokhzad, O. C., and Langer, R. (2009). Impact of Nanotechnology on Drug Delivery. ACS Nano 3 (1), 16–20. doi:10.1021/nn900002m
Fernandes, J. C., Wang, H., Jreyssaty, C., Benderdour, M., Lavigne, P., Qiu, X., et al. (2008). Bone-protective Effects of Nonviral Gene Therapy with Folate-Chitosan DNA Nanoparticle Containing Interleukin-1 Receptor Antagonist Gene in Rats with Adjuvant-Induced Arthritis. Mol. Ther. 16 (7), 1243–1251. doi:10.1038/mt.2008.99
Fisher, M. C., Li, Y., Seghatoleslami, M. R., Dealy, C. N., and Kosher, R. A. (2006). Heparan Sulfate Proteoglycans Including Syndecan-3 Modulate BMP Activity during Limb Cartilage Differentiation. Matrix Biol. 25 (1), 27–39. doi:10.1016/j.matbio.2005.07.008
Font Tellado, S., Chiera, S., Bonani, W., Poh, P. S. P., Migliaresi, C., Motta, A., et al. (2018). Heparin Functionalization Increases Retention of TGF-β2 and GDF5 on Biphasic Silk Fibroin Scaffolds for Tendon/ligament-To-Bone Tissue Engineering. Acta Biomater. 72, 150–166. doi:10.1016/j.actbio.2018.03.017
Frizelle, S., Schwarz, J., Huber, S. A., and Leslie, K. (1992). Evaluation of the Effects of Low Molecular Weight Heparin on Inflammation and Collagen Deposition in Chronic Coxsackievirus B3-Induced Myocarditis in A/J Mice. Am. J. Pathol. 141 (1), 203–209.
Furue, M. K., Na, J., Jackson, J. P., Okamoto, T., Jones, M., Baker, D., et al. (2008). Heparin Promotes the Growth of Human Embryonic Stem Cells in a Defined Serum-free Medium. Proc. Natl. Acad. Sci. U.S.A. 105 (36), 13409–13414. doi:10.1073/pnas.0806136105
Gadalla, D., and Goldstein, A. S. (2020). Improving the Osteogenicity of PCL Fiber Substrates by Surface-Immobilization of Bone Morphogenic Protein-2. Ann. Biomed. Eng. 48 (3), 1006–1015. doi:10.1007/s10439-019-02286-1
Gallagher, Z. J., Fleetwood, S., Kirley, T. L., Shaw, M. A., Mullins, E. S., Ayres, N., et al. (2020). Heparin Mimic Material Derived from Cellulose Nanocrystals. Biomacromolecules 21 (3), 1103–1111. doi:10.1021/acs.biomac.9b01460
Gao, S.-y., Lin, R.-b., Huang, S.-h., Liang, Y.-j., Li, X., Zhang, S.-e., et al. (2021). PDGF-BB Exhibited Therapeutic Effects on Rat Model of Bisphosphonate-Related Osteonecrosis of the Jaw by Enhancing Angiogenesis and Osteogenesis. Bone 144, 115117. doi:10.1016/j.bone.2019.115117
Ghezzi, S., Cooper, L., Rubio, A., Pagani, I., Capobianchi, M. R., Ippolito, G., et al. (2017). Heparin Prevents Zika Virus Induced-Cytopathic Effects in Human Neural Progenitor Cells. Antivir. Res. 140, 13–17. doi:10.1016/j.antiviral.2016.12.023
Gigi, R., Salai, M., Dolkart, O., Chechik, O., Katzburg, S., Stern, N., et al. (2012). The Effects of Direct Factor Xa Inhibitor (Rivaroxaban) on the Human Osteoblastic Cell Line SaOS2. Connect. Tissue Res. 53 (6), 446–450. doi:10.3109/03008207.2012.711867
Gittens, S. A., Bagnall, K., Matyas, J. R., Löbenberg, R., and Uludaǧ, H. (2004). Imparting Bone Mineral Affinity to Osteogenic Proteins through Heparin-Bisphosphonate Conjugates. J. Control. Release 98 (2), 255–268. doi:10.1016/j.jconrel.2004.05.001
Gockel, L. M., Nekipelov, K., Ferro, V., Bendas, G., and Schlesinger, M. (2022). Tumour Cell-Activated Platelets Modulate the Immunological Activity of CD4+, CD8+, and NK Cells, Which Is Efficiently Antagonized by Heparin. Cancer Immunol. Immunother. 2022. doi:10.1007/s00262-022-03186-5
Gong, Y., Zhang, Y., Cao, Z., Ye, F., Lin, Z., and Li, Y. (2019). Development of CaCO3 Microsphere-Based Composite Hydrogel for Dual Delivery of Growth Factor and Ca to Enhance Bone Regeneration. Biomater. Sci. 7 (9), 3614–3626. doi:10.1039/c9bm00463g
Goonasekera, C. S., Jack, K. S., Bhakta, G., Rai, B., Luong-Van, E., Nurcombe, V., et al. (2015). Mode of Heparin Attachment to Nanocrystalline Hydroxyapatite Affects its Interaction with Bone Morphogenetic Protein-2. Biointerphases 10 (4), 04a308. doi:10.1116/1.4933109
Gould, M. K., Dembitzer, A. D., Doyle, R. L., Hastie, T. J., and Garber, A. M. (1999). Low-Molecular-Weight Heparins Compared with Unfractionated Heparin for Treatment of Acute Deep Venous Thrombosis. Ann. Intern Med. 130 (10), 800–809. doi:10.7326/0003-4819-130-10-199905180-00003
Greer, I. A., and Nelson-Piercy, C. (2005). Low-molecular-weight Heparins for Thromboprophylaxis and Treatment of Venous Thromboembolism in Pregnancy: a Systematic Review of Safety and Efficacy. Blood 106 (2), 401–407. doi:10.1182/blood-2005-02-0626
Guillot-Ferriols, M., Rodríguez-Hernández, J. C., Correia, D. M., Carabineiro, S. A. C., Lanceros-Méndez, S., Gómez Ribelles, J. L., et al. (2020). Poly(vinylidene) Fluoride Membranes Coated by Heparin/collagen Layer-By-Layer, Smart Biomimetic Approaches for Mesenchymal Stem Cell Culture. Mater. Sci. Eng. C 117, 111281. doi:10.1016/j.msec.2020.111281
Hamideh, R. A., Akbari, B., Fathi, P., Misra, S. K., Sutrisno, A., Lam, F., et al. (2020). Biodegradable MRI Visible Drug Eluting Stent Reinforced by Metal Organic Frameworks. Adv. Healthc. Mat. 9 (14), 2000136. doi:10.1002/adhm.202000136
Han, S., Paeng, K.-W., Park, S., Jung, U.-W., Cha, J.-K., and Hong, J. (2021). Programmed BMP-2 Release from Biphasic Calcium Phosphates for Optimal Bone Regeneration. Biomaterials 272, 120785. doi:10.1016/j.biomaterials.2021.120785
Hannink, G., Geutjes, P. J., Daamen, W. F., and Buma, P. (2013). Evaluation of Collagen/heparin Coated TCP/HA Granules for Long-Term Delivery of BMP-2. J. Mater Sci. Mater Med. 24 (2), 325–332. doi:10.1007/s10856-012-4802-4
Hao, L., Zhang, Q., Yu, T., Yu, L., and Cheng, Y. (2011). Modulation of Ultra-low-molecular-weight Heparin on [Ca2+]i in Nervous Cells. Brain Res. Bull. 86 (5-6), 355–359. doi:10.1016/j.brainresbull.2011.08.018
Hashimoto, K., Kaito, T., Furuya, M., Seno, S., Okuzaki, D., Kikuta, J., et al. (2020). In Vivo dynamic Analysis of BMP-2-Induced Ectopic Bone Formation. Sci. Rep. 10 (1), 4751. doi:10.1038/s41598-020-61825-2
Haupt, L. M., Murali, S., Mun, F. K., Teplyuk, N., Mei, L. F., Stein, G. S., et al. (2009). The Heparan Sulfate Proteoglycan (HSPG) Glypican-3 Mediates Commitment of MC3T3-E1 Cells toward Osteogenesis. J. Cell. Physiol. 220 (3), 780–791. doi:10.1002/jcp.21825
Hemker, H. C. (2016). A Century of Heparin: Past, Present and Future. J. Thromb. Haemost. 14 (12), 2329–2338. doi:10.1111/jth.13555
Hettiaratchi, M. H., Krishnan, L., Rouse, T., Chou, C., McDevitt, T. C., and Guldberg, R. E. (2020). Heparin-mediated Delivery of Bone Morphogenetic Protein-2 Improves Spatial Localization of Bone Regeneration. Sci. Adv. 6 (1), eaay1240. doi:10.1126/sciadv.aay1240
Hettiaratchi, M. H., Miller, T., Temenoff, J. S., Guldberg, R. E., and McDevitt, T. C. (2014). Heparin Microparticle Effects on Presentation and Bioactivity of Bone Morphogenetic Protein-2. Biomaterials 35 (25), 7228–7238. doi:10.1016/j.biomaterials.2014.05.011
Hettiaratchi, M. H., Rouse, T., Chou, C., Krishnan, L., Stevens, H. Y., Li, M.-T. A., et al. (2017). Enhanced In Vivo Retention of Low Dose BMP-2 via Heparin Microparticle Delivery Does Not Accelerate Bone Healing in a Critically Sized Femoral Defect. Acta Biomater. 59, 21–32. doi:10.1016/j.actbio.2017.06.028
Huh, J.-B., Lee, J.-Y., Lee, K.-L., Kim, S.-E., Yun, M.-J., Shim, J.-S., et al. (2011). Effects of the Immobilization of Heparin and rhPDGF-BB to Titanium Surfaces for the Enhancement of Osteoblastic Functions and Anti-inflammation. J. Adv. Prosthodont 3 (3), 152–160. doi:10.4047/jap.2011.3.3.152
Hurley, M. M., Abreu, C., Gronowicz, G., Kawaguchi, H., and Lorenzo, J. (1994). Expression and Regulation of Basic Fibroblast Growth Factor mRNA Levels in Mouse Osteoblastic MC3T3-E1 Cells. J. Biol. Chem. 269 (12), 9392–9396. doi:10.1016/s0021-9258(17)37121-1
Icli, F., Akbulut, H., Utkan, G., Yalcin, B., Dincol, D., Isikdogan, A., et al. (2007). Low Molecular Weight Heparin (LMWH) Increases the Efficacy of Cisplatinum Plus Gemcitabine Combination in Advanced Pancreatic Cancer. J. Surg. Oncol. 95 (6), 507–512. doi:10.1002/jso.20728
Irie, A., Habuchi, H., Kimata, K., and Sanai, Y. (2003). Heparan Sulfate Is Required for Bone Morphogenetic Protein-7 Signaling. Biochem. Biophysical Res. Commun. 308 (4), 858–865. doi:10.1016/s0006-291x(03)01500-6
Ishihara, J., Ishihara, A., Starke, R. D., Peghaire, C. R., Smith, K. E., McKinnon, T. A. J., et al. (2019). The heparin binding domain of von Willebrand factor binds to growth factors and promotes angiogenesis in wound healing. Blood 133 (24), 2559–2569. doi:10.1182/blood.2019000510
Janssens, K., ten Dijke, P., Janssens, S., and Van Hul, W. (2005). Transforming Growth Factor-β1 to the Bone. Endocr. Rev. 26 (6), 743–774. doi:10.1210/er.2004-0001
Jeon, O., Lee, K., and Alsberg, E. (2018). Spatial Micropatterning of Growth Factors in 3D Hydrogels for Location-specific Regulation of Cellular Behaviors. Small 14 (25), 1800579. doi:10.1002/smll.201800579
Jeon, O., Powell, C., Solorio, L. D., Krebs, M. D., and Alsberg, E. (2011). Affinity-based Growth Factor Delivery Using Biodegradable, Photocrosslinked Heparin-Alginate Hydrogels. J. Control. Release 154 (3), 258–266. doi:10.1016/j.jconrel.2011.06.027
Jeon, O., Song, S. J., Kang, S.-W., Putnam, A. J., and Kim, B.-S. (2007). Enhancement of Ectopic Bone Formation by Bone Morphogenetic Protein-2 Released from a Heparin-Conjugated poly(L-Lactic-Co-Glycolic Acid) Scaffold. Biomaterials 28 (17), 2763–2771. doi:10.1016/j.biomaterials.2007.02.023
Jiao, X., Billings, P. C., O'Connell, M. P., Kaplan, F. S., Shore, E. M., and Glaser, D. L. (2007). Heparan Sulfate Proteoglycans (HSPGs) Modulate BMP2 Osteogenic Bioactivity in C2C12 Cells. J. Biol. Chem. 282 (2), 1080–1086. doi:10.1074/jbc.M513414200
Jo, H., Gajendiran, M., and Kim, K. (2019). Development of Polymer Coacersome Structure with Enhanced Colloidal Stability for Therapeutic Protein Delivery. Macromol. Biosci. 19 (12), 1900207. doi:10.1002/mabi.201900207
Jo, J.-Y., Jeong, S.-I., Shin, Y.-M., Kang, S.-S., Kim, S.-E., Jeong, C.-M., et al. (2015). Sequential Delivery of BMP-2 and BMP-7 for Bone Regeneration Using a Heparinized Collagen Membrane. Int. J. Oral Maxillofac. Surg. 44 (7), 921–928. doi:10.1016/j.ijom.2015.02.015
Johnson, M. R., Boerckel, J. D., Dupont, K. M., and Guldberg, R. E. (2011). Functional Restoration of Critically Sized Segmental Defects with Bone Morphogenetic Protein-2 and Heparin Treatment. Clin. Orthop. Relat. Res. 469 (11), 3111–3117. doi:10.1007/s11999-011-2012-x
Jonas, S., and Quartermain, D. (2001). Low Molecular Weight Heparin and the Treatment of Ischemic Stroke. Animal Results, the Reasons for Failure in Human Stroke Trials, Mechanisms of Action, and the Possibilities for Future Use in Stroke. Ann. N. Y. Acad. Sci. 939, 268–270.
Kakkar, A. K., Levine, M. N., Kadziola, Z., Lemoine, N. R., Low, V., Patel, H. K., et al. (2004). Low Molecular Weight Heparin, Therapy with Dalteparin, and Survival in Advanced Cancer: the Fragmin Advanced Malignancy Outcome Study (FAMOUS). Jco 22 (10), 1944–1948. doi:10.1200/jco.2004.10.002
Kamhi, E., Joo, E. J., Dordick, J. S., and Linhardt, R. J. (2013). Glycosaminoglycans in Infectious Disease. Biol. Rev. 88 (4), 928–943. doi:10.1111/brv.12034
Kargozar, S., Hashemian, S. J., Soleimani, M., Milan, P. B., Askari, M., Khalaj, V., et al. (2017). Acceleration of Bone Regeneration in Bioactive Glass/gelatin Composite Scaffolds Seeded with Bone Marrow-Derived Mesenchymal Stem Cells Over-expressing Bone Morphogenetic Protein-7. Mater. Sci. Eng. C 75, 688–698. doi:10.1016/j.msec.2017.02.097
Khan, S. A., Nelson, M. S., Pan, C., Gaffney, P. M., and Gupta, P. (2008). Endogenous Heparan Sulfate and Heparin Modulate Bone Morphogenetic Protein-4 Signaling and Activity. Am. J. Physiology-Cell Physiology 294 (6), C1387–C1397. doi:10.1152/ajpcell.00346.2007
Kim C. L, C. L., Jung, M. Y., Kim, Y. S., Jang, J. W., and Lee, G. M. (2018). Improving the Production of Recombinant Human Bone Morphogenetic Protein‐4 in Chinese Hamster Ovary Cell Cultures by Inhibition of Undesirable Endocytosis. Biotechnol. Bioeng. 115 (10), 2565–2575. doi:10.1002/bit.26798
Kim, E.-C., Kim, T.-H., Jung, J.-H., Hong, S. O., and Lee, D.-W. (2014). Enhanced Osteogenic Differentiation of MC3T3-E1 on rhBMP-2-Immobilized Titanium via Click Reaction. Carbohydr. Polym. 103, 170–178. doi:10.1016/j.carbpol.2013.12.023
Kim, I., Lee, S. S., Kim, S. H. L., Bae, S., Lee, H., and Hwang, N. S. (2019). Osteogenic Effects of VEGF‐Overexpressed Human Adipose‐Derived Stem Cells with Whitlockite Reinforced Cryogel for Bone Regeneration. Macromol. Biosci. 19 (5), 1800460. doi:10.1002/mabi.201800460
Kim, R. Y., Lee, B., Park, S.-N., Ko, J.-H., Kim, I. S., and Hwang, S. J. (2016). Is Heparin Effective for the Controlled Delivery of High-Dose Bone Morphogenetic Protein-2? Tissue Eng. Part A 22 (9-10), 801–817. doi:10.1089/ten.TEA.2015.0537
Kim, S., Cui, Z.-K., Kim, P. J., Jung, L. Y., and Lee, M. (2018). Design of Hydrogels to Stabilize and Enhance Bone Morphogenetic Protein Activity by Heparin Mimetics. Acta Biomater. 72, 45–54. doi:10.1016/j.actbio.2018.03.034
Kim, S. E., Kim, C.-S., Yun, Y.-P., Yang, D. H., Park, K., Kim, S. E., et al. (2014). Improving Osteoblast Functions and Bone Formation upon BMP-2 Immobilization on Titanium Modified with Heparin. Carbohydr. Polym. 114, 123–132. doi:10.1016/j.carbpol.2014.08.005
Kim, S. E., Song, S.-H., Yun, Y. P., Choi, B.-J., Kwon, I. K., Bae, M. S., et al. (2011). The Effect of Immobilization of Heparin and Bone Morphogenic Protein-2 (BMP-2) to Titanium Surfaces on Inflammation and Osteoblast Function. Biomaterials 32 (2), 366–373. doi:10.1016/j.biomaterials.2010.09.008
Kim, S. E., Yun, Y.-P., Lee, J. Y., Shim, J.-S., Park, K., and Huh, J.-B. (2015). Co-delivery of Platelet-Derived Growth Factor (PDGF-BB) and Bone Morphogenic Protein (BMP-2) Coated onto Heparinized Titanium for Improving Osteoblast Function and Osteointegration. J. Tissue Eng. Regen. Med. 9 (12), E219–E228. doi:10.1002/term.1668
Kim, S. E., Yun, Y.-P., Shim, K.-S., Park, K., Choi, S.-W., Shin, D. H., et al. (2015). Fabrication of a BMP-2-Immobilized Porous Microsphere Modified by Heparin for Bone Tissue Engineering. Colloids Surfaces B Biointerfaces 134, 453–460. doi:10.1016/j.colsurfb.2015.05.003
Kim, S., Fan, J., Lee, C.-S., Chen, C., Bubukina, K., and Lee, M. (2020). Heparinized Chitosan Stabilizes the Bioactivity of BMP-2 and Potentiates the Osteogenic Efficacy of Demineralized Bone Matrix. J. Biol. Eng. 14, 6. doi:10.1186/s13036-020-0231-y
Kim, T.-H., Yun, Y.-P., Park, Y.-E., Lee, S.-H., Yong, W., Kundu, J., et al. (2014). In Vitro and In Vivo Evaluation of Bone Formation Using Solid Freeform Fabrication-Based Bone Morphogenic Protein-2 Releasing PCL/PLGA Scaffolds. Biomed. Mat. 9 (2), 025008. doi:10.1088/1748-6041/9/2/025008
Kim, Y., Kang, B.-J., Kim, W., Yun, H.-s., and Kweon, O.-k. (2018). Evaluation of Mesenchymal Stem Cell Sheets Overexpressing BMP-7 in Canine Critical-Sized Bone Defects. Ijms 19 (7), 2073. doi:10.3390/ijms19072073
Kimelman, D., and Kirschner, M. (1987). Synergistic Induction of Mesoderm by FGF and TGF-β and the Identification of an mRNA Coding for FGF in the Early xenopus Embryo. Cell 51 (5), 869–877. doi:10.1016/0092-8674(87)90110-3
Kishibe, J., Yamada, S., Okada, Y., Sato, J., Ito, A., Miyazaki, K., et al. (2000). Structural Requirements of Heparan Sulfate for the Binding to the Tumor-Derived Adhesion Factor/angiomodulin that Induces Cord-like Structures to ECV-304 Human Carcinoma Cells. J. Biol. Chem. 275 (20), 15321–15329. doi:10.1074/jbc.275.20.15321
Klerk, C. P. W., Smorenburg, S. M., Otten, H.-M., Lensing, A. W. A., Prins, M. H., Piovella, F., et al. (2005). The Effect of Low Molecular Weight Heparin on Survival in Patients with Advanced Malignancy. Jco 23 (10), 2130–2135. doi:10.1200/jco.2005.03.134
Klingberg, F., Chau, G., Walraven, M., Boo, S., Koehler, A., Chow, M. L., et al. (2018). The ED-A Domain Enhances the Capacity of Fibronectin to Store Latent TGF-β Binding Protein-1 in the Fibroblast Matrix. J. Cell Sci. 131 (5). doi:10.1242/jcs.201293
Knaack, S., Lode, A., Hoyer, B., Rösen-Wolff, A., Gabrielyan, A., Roeder, I., et al. (2014). Heparin Modification of a Biomimetic Bone Matrix for Controlled Release of VEGF. J. Biomed. Mat. Res. 102 (10), 3500–3511. doi:10.1002/jbm.a.35020
Konar, M., Sahoo, J. K., and Sahoo, H. (2019). Impact of Bone Extracellular Matrix Mineral Based Nanoparticles on Structure and Stability of Purified Bone Morphogenetic Protein 2 (BMP-2). J. Photochem. Photobiol. B Biol. 198, 111563. doi:10.1016/j.jphotobiol.2019.111563
Konstantinides, S. V., Konstantinides, S., Torbicki, A., Agnelli, G., Danchin, N., Fitzmaurice, D., et al. (2014). 2014 ESC Guidelines on the Diagnosis and Management of Acute Pulmonary Embolism. Kardiol. Pol. 72 (45), 997–1053. doi:10.1093/eurheartj/ehu283
La, W.-G., and Yang, H. S. (2015). Heparin-conjugated Poly(lactic-Co-Glycolic Acid) Nanospheres Enhance Large-Wound Healing by Delivering Growth Factors in Platelet-Rich Plasma. Artif. Organs 39 (4), 388–394. doi:10.1111/aor.12389
Lai, C.-F., Chaudhary, L., Fausto, A., Halstead, L. R., Ory, D. S., Avioli, L. V., et al. (2001). Erk Is Essential for Growth, Differentiation, Integrin Expression, and Cell Function in Human Osteoblastic Cells. J. Biol. Chem. 276 (17), 14443–14450. doi:10.1074/jbc.M010021200
Lane, D. A., Denton, J., Flynn, A. M., Thunberg, L., and Lindahl, U. (1984). Anticoagulant Activities of Heparin Oligosaccharides and Their Neutralization by Platelet Factor 4. Biochem. J. 218 (3), 725–732. doi:10.1042/bj2180725
Laner-Plamberger, S., Oeller, M., Rohde, E., Schallmoser, K., and Strunk, D. (2021). Heparin and Derivatives for Advanced Cell Therapies. Ijms 22 (21), 12041. doi:10.3390/ijms222112041
Lee, B., Oh, Y., Jo, S., Kim, T.-H., and Ji, J. D. (2019). A Dual Role of TGF-β in Human Osteoclast Differentiation Mediated by Smad1 versus Smad3 Signaling. Immunol. Lett. 206, 33–40. doi:10.1016/j.imlet.2018.12.003
Lee, D.-W., Yun, Y.-P., Park, K., and Kim, S. E. (2012). Gentamicin and Bone Morphogenic Protein-2 (BMP-2)-Delivering Heparinized-Titanium Implant with Enhanced Antibacterial Activity and Osteointegration. Bone 50 (4), 974–982. doi:10.1016/j.bone.2012.01.007
Lee J, J., Yoo, J. J., Atala, A., and Lee, S. J. (2012). The Effect of Controlled Release of PDGF-BB from Heparin-Conjugated Electrospun PCL/gelatin Scaffolds on Cellular Bioactivity and Infiltration. Biomaterials 33 (28), 6709–6720. doi:10.1016/j.biomaterials.2012.06.017
Lee, J.-h., Lee, Y. J., Cho, H.-j., Kim, D. W., and Shin, H. (2015). The Incorporation of bFGF Mediated by Heparin into PCL/gelatin Composite Fiber Meshes for Guided Bone Regeneration. Drug Deliv. Transl. Res. 5 (2), 146–159. doi:10.1007/s13346-013-0154-y
Lee, J.-S., Lee, S.-K., Kim, B.-S., Im, G.-I., Cho, K.-S., and Kim, C.-S. (2015). Controlled Release of BMP-2 Using a Heparin-Conjugated Carrier System Reducesin Vivoadipose Tissue Formation. J. Biomed. Mat. Res. 103 (2), 545–554. doi:10.1002/jbm.a.35207
Lee, J., Choi, W. I., Tae, G., Kim, Y. H., Kang, S. S., Kim, S. E., et al. (2011). Enhanced Regeneration of the Ligament-Bone Interface Using a Poly(l-Lactide-Co-ε-Caprolactone) Scaffold with Local Delivery of cells/BMP-2 Using a Heparin-Based Hydrogel. Acta Biomater. 7 (1), 244–257. doi:10.1016/j.actbio.2010.08.017
Lee, J., Wee, S., Gunaratne, J., Chua, R. J. E., Smith, R. A. A., Ling, L., et al. (2015). Structural Determinants of Heparin-Transforming Growth Factor-β1 Interactions and Their Effects on Signaling. Glycobiology 25 (12), 1491–1504. doi:10.1093/glycob/cwv064
Lee, S.-Y., Yun, Y.-P., Song, H.-R., Chun, H. J., Yang, D. H., Park, K., et al. (2013). The Effect of Titanium with heparin/BMP-2 Complex for Improving Osteoblast Activity. Carbohydr. Polym. 98 (1), 546–554. doi:10.1016/j.carbpol.2013.05.095
Lee S. S, S. S., Huang, B. J., Kaltz, S. R., Sur, S., Newcomb, C. J., Stock, S. R., et al. (2013). Bone Regeneration with Low Dose BMP-2 Amplified by Biomimetic Supramolecular Nanofibers within Collagen Scaffolds. Biomaterials 34 (2), 452–459. doi:10.1016/j.biomaterials.2012.10.005
Lee, S. S., Fyrner, T., Chen, F., Álvarez, Z., Sleep, E., Chun, D. S., et al. (2017). Sulfated Glycopeptide Nanostructures for Multipotent Protein Activation. Nat. Nanotech 12 (8), 821–829. doi:10.1038/nnano.2017.109
Lee, S. S., Kim, J. H., Jeong, J., Kim, S. H. L., Koh, R. H., Kim, I., et al. (2020). Sequential Growth Factor Releasing Double Cryogel System for Enhanced Bone Regeneration. Biomaterials 257, 120223. doi:10.1016/j.biomaterials.2020.120223
Li, B., Lu, D., Chen, Y., Zhao, M., and Zuo, L. (2016). Unfractionated Heparin Promotes Osteoclast Formation In Vitro by Inhibiting Osteoprotegerin Activity. Ijms 17 (4), 613. doi:10.3390/ijms17040613
Li, D., Yang, Z., Luo, Y., Zhao, X., Tian, M., and Kang, P. (2021). Delivery of MiR335‐5p‐Pendant Tetrahedron DNA Nanostructures Using an Injectable Heparin Lithium Hydrogel for Challenging Bone Defects in Steroid‐Associated Osteonecrosis. Adv. Healthc. Mater. 11, 2101412. doi:10.1002/adhm.202101412
Li, H., Johnson, N. R., Usas, A., Lu, A., Poddar, M., Wang, Y., et al. (2013). Sustained Release of Bone Morphogenetic Protein 2 via Coacervate Improves the Osteogenic Potential of Muscle-Derived Stem Cells. Stem Cells Transl. Med. 2 (9), 667–677. doi:10.5966/sctm.2013-0027
Li, J.-P., and Kusche-Gullberg, M. (2016). Heparan Sulfate: Biosynthesis, Structure, and Function. Int. Rev. Cell Mol. Biol. 325, 215–273. doi:10.1016/bs.ircmb.2016.02.009
Li, X., and Ma, X. (2017). The Role of Heparin in Sepsis: Much More Than Just an Anticoagulant. Br. J. Haematol. 179 (3), 389–398. doi:10.1111/bjh.14885
Liang, Y., and Kiick, K. L. (2014). Heparin-functionalized Polymeric Biomaterials in Tissue Engineering and Drug Delivery Applications. Acta Biomater. 10 (4), 1588–1600. doi:10.1016/j.actbio.2013.07.031
Ling, L., Camilleri, E. T., Helledie, T., Samsonraj, R. M., Titmarsh, D. M., Chua, R. J., et al. (2016). Effect of Heparin on the Biological Properties and Molecular Signature of Human Mesenchymal Stem Cells. Gene 576 (1 Pt 2), 292–303. doi:10.1016/j.gene.2015.10.039
Ling, L., Dombrowski, C., Foong, K. M., Haupt, L. M., Stein, G. S., Nurcombe, V., et al. (2010). Synergism between Wnt3a and Heparin Enhances Osteogenesis via a Phosphoinositide 3-kinase/Akt/RUNX2 Pathway. J. Biol. Chem. 285 (34), 26233–26244. doi:10.1074/jbc.M110.122069
Linhardt, R. J., St Ange, K., Dordick, J. S., and Linhardt, R. J. (2016). Heparin and Anticoagulation. Front. Biosci. 21, 1372–1392. doi:10.2741/4462
Lippi, G., Henry, B. M., and Favaloro, E. J. (2022). The Benefits of Heparin Use in COVID-19: Pleiotropic Antiviral Activity beyond Anticoagulant and Anti-inflammatory Properties. Semin. Thromb. Hemost. 2022. doi:10.1055/s-0042-1742740
Liu, X., Xu, C., Tian, Y., Sun, Y., Zhang, J., Bai, J., et al. (2019). RUNX2 Contributes to TGF-β1-Induced Expression of Wdr72 in Ameloblasts during Enamel Mineralization. Biomed. Pharmacother. 118, 109235. doi:10.1016/j.biopha.2019.109235
Liu, Y., Deng, F., Zhang, L., Deng, L., Sun, H., Xu, J., et al. (2016). Sustained Dual Release of Placental Growth Factor-2 and Bone Morphogenic Protein-2 from Heparin-Based Nanocomplexes for Direct Osteogenesis. Ijn 11, 1147–1158. doi:10.2147/ijn.S100156
Lode, A., Reinstorf, A., Bernhardt, A., Wolf‐Brandstetter, C., König, U., and Gelinsky, M. (2008). Heparin Modification of Calcium Phosphate Bone Cements for VEGF Functionalization. J. Biomed. Mat. Res. 86A (3), 749–759. doi:10.1002/jbm.a.31581
Long, F. (2011). Building Strong Bones: Molecular Regulation of the Osteoblast Lineage. Nat. Rev. Mol. Cell Biol. 13 (1), 27–38. doi:10.1038/nrm3254
Lü, L., Deegan, A., Musa, F., Xu, T., and Yang, Y. (2018). The Effects of Biomimetically Conjugated VEGF on Osteogenesis and Angiogenesis of MSCs (Human and Rat) and HUVECs Co-culture Models. Colloids Surfaces B Biointerfaces 167, 550–559. doi:10.1016/j.colsurfb.2018.04.060
Ludwig, R. J., Schindewolf, M., Utikal, J., Lindhoff-Last, E., and Boehncke, W. H. (2006). Management of Cutaneous Type IV Hypersensitivity Reactions Induced by Heparin. Thromb. Haemost. 96 (5), 611–617.
Macdonald, M. L., Rodriguez, N. M., Shah, N. J., and Hammond, P. T. (2010). Characterization of Tunable FGF-2 Releasing Polyelectrolyte Multilayers. Biomacromolecules 11 (8), 2053–2059. doi:10.1021/bm100413w
Macias, D., Ganan, Y., Sampath, T. K., Piedra, M. E., Ros, M. A., and Hurle, J. M. (1997). Role of BMP-2 and OP-1 (BMP-7) in Programmed Cell Death and Skeletogenesis during Chick Limb Development. Development 124 (6), 1109–1117. doi:10.1242/dev.124.6.1109
Margalit, H., Fischer, N., and Ben-Sasson, S. A. (1993). Comparative Analysis of Structurally Defined Heparin Binding Sequences Reveals a Distinct Spatial Distribution of Basic Residues. J. Biol. Chem. 268 (26), 19228–19231. doi:10.1016/s0021-9258(19)36503-2
McCaffrey, T. A., Falcone, D. J., Brayton, C. F., Agarwal, L. A., Welt, F. G., and Weksler, B. B. (1989). Transforming Growth Factor-Beta Activity Is Potentiated by Heparin via Dissociation of the Transforming Growth Factor-Beta/alpha 2-macroglobulin Inactive Complex. J. Cell Biol. 109 (1), 441–448. doi:10.1083/jcb.109.1.441
Mikhailov, D., Young, H. C., Linhardt, R. J., and Mayo, K. H. (1999). Heparin Dodecasaccharide Binding to Platelet Factor-4 and Growth-Related Protein-α. J. Biol. Chem. 274 (36), 25317–25329. doi:10.1074/jbc.274.36.25317
Miyazaki, T., Miyauchi, S., Tawada, A., Anada, T., Matsuzaka, S., and Suzuki, O. (2008). Oversulfated Chondroitin Sulfate-E Binds to BMP-4 and Enhances Osteoblast Differentiation. J. Cell. Physiol. 217 (3), 769–777. doi:10.1002/jcp.21557
Monreal, M., Lafoz, E., Olive, A., del Rio, L., and Vedia, C. (1994). Comparison of Subcutaneous Unfractionated Heparin with a Low Molecular Weight Heparin (Fragmin) in Patients with Venous Thromboembolism and Contraindications to Coumarin. Thromb. Haemost. 71 (1), 7–11. doi:10.1055/s-0038-1642376
Mousa, S., and Mohamed, S. (2004). Inhibition of Endothelial Cell Tube Formation by the Low Molecular Weight Heparin, Tinzaparin, Is Mediated by Tissue Factor Pathway Inhibitor. Thromb. Haemost. 92 (3), 627–633. doi:10.1160/th04-02-0069
Muir, J., Andrew, M., Hirsh, J., Weitz, J., Young, E., Deschamps, P., et al. (1996). Histomorphometric Analysis of the Effects of Standard Heparin on Trabecular Bone In Vivo. Blood 88 (4), 1314–1320. doi:10.1182/blood.v88.4.1314.bloodjournal8841314
Muir, J. M., Hirsh, J., Weitz, J. I., Andrew, M., Young, E., and Shaughnessy, S. G. (1997). A Histomorphometric Comparison of the Effects of Heparin and Low-Molecular-Weight Heparin on Cancellous Bone in Rats. Blood 89 (9), 3236–3242. doi:10.1182/blood.v89.9.3236
Mulloy, B., Barrowcliffe, T., and Gray, E. (2008). Heparin and Low-Molecular-Weight Heparin. Thromb. Haemost. 99 (5), 807–818. doi:10.1160/th08-01-0032
Mulloy, B., Forster, M. J., Jones, C., and Davies, D. B. (1993). N.m.r. And Molecular-Modelling Studies of the Solution Conformation of Heparin. Biochem. J. 293 (Pt 3Pt 3), 849–858. doi:10.1042/bj2930849
Mulloy, B., and Linhardt, R. J. (2001). Order Out of Complexity - Protein Structures that Interact with Heparin. Curr. Opin. Struct. Biol. 11 (5), 623–628. doi:10.1016/s0959-440x(00)00257-8
Nahain, A. A., Ignjatovic, V., Monagle, P., Tsanaktsidis, J., and Ferro, V. (2018). Heparin Mimetics with Anticoagulant Activity. Med. Res. Rev. 38 (5), 1582–1613. doi:10.1002/med.21489
Nakamura, S., Ando, N., Ishihara, M., and Sato, M. (2020). Development of Novel Heparin/Protamine Nanoparticles Useful for Delivery of Exogenous Proteins In Vitro and In Vivo. Nanomaterials 10 (8), 1584. doi:10.3390/nano10081584
Nelson, R., Cecconi, O., Roberts, W., Aruffo, A., Linhardt, R., and Bevilacqua, M. (1993). Heparin Oligosaccharides Bind L- and P-Selectin and Inhibit Acute Inflammation. Blood 82 (11), 3253–3258. doi:10.1182/blood.v82.11.3253.3253
Nelson-Piercy, C., Letsky, E. A., and de Swiet, M. (1997). Low-molecular-weight Heparin for Obstetric Thromboprophylaxis: Experience of Sixty-Nine Pregnancies in Sixty-One Women at High Risk. Am. J. Obstetrics Gynecol. 176 (5), 1062–1068. doi:10.1016/s0002-9378(97)70403-4
Nematollahi, L., Khalaj, V., Babazadeh, S. M., Rahimpour, A., Jahandar, H., Davami, F., et al. (2012). Periplasmic Expression of a Novel Human Bone Morphogenetic Protein-7 Mutant in Escherichia coli. Avicenna J. Med. Biotechnol. 4 (4), 178–185.
Nematollahi, L., Mahboudi, F., Rahimpour, A., Jahandar, H., and Khalai, V. (2013). “[A novel human bone morphogenetic protein-7 variant with an enriched heparin-binding site].”. Mol Biol (Mosk) 47 (3), 453–460. doi:10.7868/s0026898413030105
Nozawa, S., Inubushi, T., Irie, F., Takigami, I., Matsumoto, K., Shimizu, K., et al. (2018). Osteoblastic Heparan Sulfate Regulates Osteoprotegerin Function and Bone Mass. JCI Insight 3 (3). doi:10.1172/jci.insight.89624
Paine-Saunders, S., Viviano, B. L., Economides, A. N., and Saunders, S. (2002). Heparan Sulfate Proteoglycans Retain Noggin at the Cell Surface. J. Biol. Chem. 277 (3), 2089–2096. doi:10.1074/jbc.M109151200
Peng, Y., Wu, S., Li, Y., and Crane, J. L. (2020). Type H Blood Vessels in Bone Modeling and Remodeling. Theranostics 10 (1), 426–436. doi:10.7150/thno.34126
Peng, Z., Zhao, T., Zhou, Y., Li, S., Li, J., and Leblanc, R. M. (2020). Bone Tissue Engineering via Carbon‐Based Nanomaterials. Adv. Healthc. Mat. 9 (5), 1901495. doi:10.1002/adhm.201901495
Peter, K., Schwarz, M., Conradt, C., Nordt, T., Moser, M., Kübler, W., et al. (1999). Heparin Inhibits Ligand Binding to the Leukocyte Integrin Mac-1 (CD11b/CD18). Circulation 100 (14), 1533–1539. doi:10.1161/01.cir.100.14.1533
Pettilä, V., Kaaja, R., Leinonen, P., Ekblad, U., Kataja, M., and Ikkala, E. (1999). Thromboprophylaxis with Low Molecular Weight Heparin (Dalteparin) in Pregnancy. Thromb. Res. 96 (4), 275–282. doi:10.1016/s0049-3848(99)00110-3
Pettilä, V., Leinonen, P., Markkola, A., Hiilesmaa, V., and Kaaja, R. (2002). Postpartum Bone Mineral Density in Women Treated for Thromboprophylaxis with Unfractionated Heparin or LMW Heparin. Thromb. Haemost. 87 (2), 182–186.
Piran, S., and Schulman, S. (2016). Management of Venous Thromboembolism: an Update. Thromb. J. 14 (Suppl. 1), 23. doi:10.1186/s12959-016-0107-z
Place, L. W., Sekyi, M., Taussig, J., and Kipper, M. J. (2016). Two-Phase Electrospinning to Incorporate Polyelectrolyte Complexes and Growth Factors into Electrospun Chitosan Nanofibers. Macromol. Biosci. 16 (3), 371–380. doi:10.1002/mabi.201500288
Pouyan, P., Nie, C., Bhatia, S., Wedepohl, S., Achazi, K., Osterrieder, N., et al. (2021). Inhibition of Herpes Simplex Virus Type 1 Attachment and Infection by Sulfated Polyglycerols with Different Architectures. Biomacromolecules 22 (4), 1545–1554. doi:10.1021/acs.biomac.0c01789
Prandoni, P., Carta, M., Cogo, A., Ruol, A., Vigo, M., Casara, D., et al. (1992). Comparison of Subcutaneous Low-Molecular-Weight Heparin with Intravenous Standard Heparin in Proximal Deep-Vein Thrombosis. Lancet 339 (8791), 441–445. doi:10.1016/0140-6736(92)91054-c
Prokoph, S., Chavakis, E., Levental, K. R., Zieris, A., Freudenberg, U., Dimmeler, S., et al. (2012). Sustained Delivery of SDF-1α from Heparin-Based Hydrogels to Attract Circulating Pro-angiogenic Cells. Biomaterials 33 (19), 4792–4800. doi:10.1016/j.biomaterials.2012.03.039
Qasim, M., Chae, D. S., and Lee, N. Y. (2019). Advancements and Frontiers in Nano-Based 3D and 4D Scaffolds for Bone and Cartilage Tissue Engineering. Ijn 14, 4333–4351. doi:10.2147/ijn.S209431
Qi, W., Yan, J., Sun, H., and Wang, H. (2017). Multifunctional Nanocomposite Films for Synergistic Delivery of bFGF and BMP-2. ACS Omega 2 (3), 899–909. doi:10.1021/acsomega.6b00420
Quade, M., Knaack, S., Knaack, S., Weber, D., König, U., Paul, B., et al. (2017). Heparin Modification of a Biomimetic Bone Matrix Modulates Osteogenic and Angiogenic Cell Response In Vitro. eCM 33, 105–120. doi:10.22203/eCM.v033a08
Rabenstein, D. L. (2002). Heparin and Heparan Sulfate: Structure and Function. Nat. Prod. Rep. 19 (3), 312–331. doi:10.1039/b100916h
Rath, S. N., Pryymachuk, G., Bleiziffer, O. A., Lam, C. X. F., Arkudas, A., Ho, S. T. B., et al. (2011). Hyaluronan-based Heparin-Incorporated Hydrogels for Generation of Axially Vascularized Bioartificial Bone Tissues: In Vitro and In Vivo Evaluation in a PLDLLA-TCP-PCL-Composite System. J. Mater Sci. Mater Med. 22 (5), 1279–1291. doi:10.1007/s10856-011-4300-0
Reguera-Nuñez, E., Roca, C., Hardy, E., de la Fuente, M., Csaba, N., and Garcia-Fuentes, M. (2014). Implantable Controlled Release Devices for BMP-7 Delivery and Suppression of Glioblastoma Initiating Cells. Biomaterials 35 (9), 2859–2867. doi:10.1016/j.biomaterials.2013.12.001
Rico, S., Antonijoan, R. M., Gich, I., Borrell, M., Fontcuberta, J., Monreal, M., et al. (2011). Safety Assessment and Pharmacodynamics of a Novel Ultra Low Molecular Weight Heparin (RO-14) in Healthy Volunteers - A First-Time-In-Human Single Ascending Dose Study. Thrombosis Res. 127 (4), 292–298. doi:10.1016/j.thromres.2010.12.009
Rider, C., and Mulloy, B. (2017). Heparin, Heparan Sulphate and the TGF-β Cytokine Superfamily. Molecules 22 (5), 713. doi:10.3390/molecules22050713
Rindone, A. N., Kachniarz, B., Achebe, C. C., Riddle, R. C., O'Sullivan, A. N., Dorafshar, A. H., et al. (2019). Heparin‐Conjugated Decellularized Bone Particles Promote Enhanced Osteogenic Signaling of PDGF‐BB to Adipose‐Derived Stem Cells in Tissue Engineered Bone Grafts. Adv. Healthc. Mat. 8 (10), 1801565. doi:10.1002/adhm.201801565
Roberts, J. J., Farrugia, B. L., Green, R. A., Rnjak-Kovacina, J., and Martens, P. J. (2016). In Situ formation of Poly(vinyl Alcohol)-Heparin Hydrogels for Mild Encapsulation and Prolonged Release of Basic Fibroblast Growth Factor and Vascular Endothelial Growth Factor. J. Tissue Eng. 7, 204173141667713. doi:10.1177/2041731416677132
Rodger, M. A., Kahn, S. R., Cranney, A., Hodsman, A., Kovacs, M. J., Clement, A. M., et al. (2007). Long-term Dalteparin in Pregnancy Not Associated with a Decrease in Bone Mineral Density: Substudy of a Randomized Controlled Trial. J. Thromb. Haemost. 5 (8), 1600–1606. doi:10.1111/j.1538-7836.2007.02634.x
Rolny, C., Spillmann, D., Lindahl, U., and Claesson-Welsh, L. (2002). Heparin Amplifies Platelet-Derived Growth Factor (PDGF)- BB-Induced PDGF α-Receptor but Not PDGF β-Receptor Tyrosine Phosphorylation in Heparan Sulfate-Deficient Cells. J. Biol. Chem. 277 (22), 19315–19321. doi:10.1074/jbc.M111805200
Sakiyama-Elbert, S. E. (2014). Incorporation of Heparin into Biomaterials. Acta Biomater. 10 (4), 1581–1587. doi:10.1016/j.actbio.2013.08.045
Salazar, V. S., Gamer, L. W., and Rosen, V. (2016). BMP Signalling in Skeletal Development, Disease and Repair. Nat. Rev. Endocrinol. 12 (4), 203–221. doi:10.1038/nrendo.2016.12
Sasaki, N., Okishio, K., Ui-Tei, K., Saigo, K., Kinoshita-Toyoda, A., Toyoda, H., et al. (2008). Heparan Sulfate Regulates Self-Renewal and Pluripotency of Embryonic Stem Cells. J. Biol. Chem. 283 (6), 3594–3606. doi:10.1074/jbc.M705621200
Schulman, S., and Hellgren-Wångdahl, M. (2002). Pregnancy, Heparin and Osteoporosis. Thromb. Haemost. 87 (2), 180–181.
Schwartz, B. S. (1990). Heparin: what Is it? How Does it Work? Clin. Cardiol. 13 (4 Suppl. 6), Vi12–5. doi:10.1002/clc.1990.13.s6.12
Shin, D. A., Pennant, W. A., Yoon, D. H., Ha, Y., and Kim, K. N. (2014). Co-transplantation of Bone Marrow-Derived Mesenchymal Stem Cells and Nanospheres Containing FGF-2 Improve Cell Survival and Neurological Function in the Injured Rat Spinal Cord. Acta Neurochir. 156 (2), 297–303. doi:10.1007/s00701-013-1963-y
Shin, Y. M., La, W.-G., Lee, M. S., Yang, H. S., and Lim, Y.-M. (2015). Extracellular Matrix-Inspired BMP-2-Delivering Biodegradable Fibrous Particles for Bone Tissue Engineering. J. Mat. Chem. B 3 (42), 8375–8382. doi:10.1039/c5tb01310k
Shute, J. K., Puxeddu, E., and Calzetta, L. (2018). Therapeutic Use of Heparin and Derivatives beyond Anticoagulation in Patients with Bronchial Asthma or COPD. Curr. Opin. Pharmacol. 40, 39–45. doi:10.1016/j.coph.2018.01.006
Sievers, J., Zschoche, S., Dockhorn, R., Friedrichs, J., Werner, C., and Freudenberg, U. (2019). Temperature-Induced Mechanomodulation of Interpenetrating Networks of Star Poly(ethylene Glycol)-Heparin and Poly(N-Isopropylacrylamide). ACS Appl. Mat. Interfaces 11 (45), 41862–41874. doi:10.1021/acsami.9b11719
Signorelli, S. S., Scuto, S., Marino, E., Giusti, M., Xourafa, A., and Gaudio, A. (2019). Anticoagulants and Osteoporosis. Ijms 20 (21), 5275. doi:10.3390/ijms20215275
Simann, M., Schneider, V., Le Blanc, S., Dotterweich, J., Zehe, V., Krug, M., et al. (2015). Heparin Affects Human Bone Marrow Stromal Cell Fate: Promoting Osteogenic and Reducing Adipogenic Differentiation and Conversion. Bone 78, 102–113. doi:10.1016/j.bone.2015.04.039
Smith, R. A. A., Murali, S., Rai, B., Lu, X., Lim, Z. X. H., Lee, J. J. L., et al. (2018). Minimum Structural Requirements for BMP-2-Binding of Heparin Oligosaccharides. Biomaterials 184, 41–55. doi:10.1016/j.biomaterials.2018.08.056
Spivak-Kroizman, T., Lemmon, M. A., Dikic, I., Ladbury, J. E., Pinchasi, D., Huang, J., et al. (1994). Heparin-induced Oligomerization of FGF Molecules Is Responsible for FGF Receptor Dimerization, Activation, and Cell Proliferation. Cell 79 (6), 1015–1024. doi:10.1016/0092-8674(94)90032-9
Sultankulov, B., Berillo, D., Kauanova, S., Mikhalovsky, S., Mikhalovska, L., and Saparov, A. (2019). Composite Cryogel with Polyelectrolyte Complexes for Growth Factor Delivery. Pharmaceutics 11 (12), 650. doi:10.3390/pharmaceutics11120650
Sun, J., Lyu, J., Xing, F., Chen, R., Duan, X., and Xiang, Z. (2020). A Biphasic, Demineralized, and Decellularized Allograft Bone‐hydrogel Scaffold with a Cell‐based BMP ‐7 Delivery System for Osteochondral Defect Regeneration. J. Biomed. Mater Res. 108 (9), 1909–1921. doi:10.1002/jbm.a.36954
Sun, X.-j., Peng, W., Yang, Z.-l., Ren, M.-l., Zhang, S.-c., Zhang, W.-g., et al. (2011). Heparin-chitosan-coated Acellular Bone Matrix Enhances Perfusion of Blood and Vascularization in Bone Tissue Engineering Scaffolds. Tissue Eng. Part A 17 (19-20), 2369–2378. doi:10.1089/ten.TEA.2011.0027
Sun, X., Ma, Z., Zhao, X., Jin, W., Zhang, C., Ma, J., et al. (2021). Three-dimensional Bioprinting of Multicell-Laden Scaffolds Containing Bone Morphogenic Protein-4 for Promoting M2 Macrophage Polarization and Accelerating Bone Defect Repair in Diabetes Mellitus. Bioact. Mater. 6 (3), 757–769. doi:10.1016/j.bioactmat.2020.08.030
Takada, T., Katagiri, T., Ifuku, M., Morimura, N., Kobayashi, M., Hasegawa, K., et al. (2003). Sulfated Polysaccharides Enhance the Biological Activities of Bone Morphogenetic Proteins. J. Biol. Chem. 278 (44), 43229–43235. doi:10.1074/jbc.M300937200
Tan, Q., Tang, H., Hu, J., Hu, Y., Zhou, X., Tao, R., et al. (2011). Controlled Release of Chitosan/heparin Nanoparticle-Delivered VEGF Enhances Regeneration of Decellularized Tissue-Engineered Scaffolds. Ijn 6, 929–942. doi:10.2147/ijn.S18753
Tang, H., Wang, B., Tan, L., Deng, D., Lu, T., Zhou, C., et al. (2015). Novel Stable Cytokine Delivery System in Physiological pH Solution: Chitosan Oligosaccharide/heparin Nanoparticles. Ijn 10, 3417–3427. doi:10.2147/ijn.S82091
Tautzenberger, A., Kovtun, A., and Ignatius, A. (2012). Nanoparticles and Their Potential for Application in Bone. Ijn 7, 4545–4557. doi:10.2147/ijn.S34127
Tellier, L. E., Miller, T., McDevitt, T. C., and Temenoff, J. S. (2015). Hydrolysis and Sulfation Pattern Effects on Release of Bioactive Bone Morphogenetic Protein-2 from Heparin-Based Microparticles. J. Mat. Chem. B 3 (40), 8001–8009. doi:10.1039/c5tb00933b
Terauchi, M., Tamura, A., Tonegawa, A., Yamaguchi, S., Yoda, T., and Yui, N. (2019). Polyelectrolyte Complexes between Polycarboxylates and BMP-2 for Enhancing Osteogenic Differentiation: Effect of Chemical Structure of Polycarboxylates. Polymers 11 (8), 1327. doi:10.3390/polym11081327
Thaler, J., Pabinger, I., and Ay, C. (2015). Anticoagulant Treatment of Deep Vein Thrombosis and Pulmonary Embolism: The Present State of the Art. Front. Cardiovasc. Med. 2, 30. doi:10.3389/fcvm.2015.00030
Torri, G., and Naggi, A. (2016). Heparin Centenary - an Ever-Young Life-Saving Drug. Int. J. Cardiol. 212 (Suppl. 1), S1–S4. doi:10.1016/s0167-5273(16)12001-7
Välimäki, S., Liu, Q., Schoonen, L., Vervoort, D. F. M., Nonappa, V. Linko., Linko, V., et al. (2021). Engineered Protein Cages for Selective Heparin Encapsulation. J. Mat. Chem. B 9 (5), 1272–1276. doi:10.1039/d0tb02541k
Vantucci, C. E., Krishan, L., Cheng, A., Prather, A., Roy, K., and Guldberg, R. E. (2021). BMP-2 Delivery Strategy Modulates Local Bone Regeneration and Systemic Immune Responses to Complex Extremity Trauma. Biomater. Sci. 9 (5), 1668–1682. doi:10.1039/d0bm01728k
Vukicevic, S., Latin, V., Chen, P., Batorsky, R., Reddi, A. H., and Sampath, T. K. (1994). Localization of Osteogenic Protein-1 (Bone Morphogenetic Protein-7) during Human Embryonic Development: High Affinity Binding to Basement Membranes. Biochem. Biophysical Res. Commun. 198 (2), 693–700. doi:10.1006/bbrc.1994.1100
Wahrenbrock, M., Borsig, L., Le, D., Varki, N., and Varki, A. (2003). Selectin-mucin Interactions as a Probable Molecular Explanation for the Association of Trousseau Syndrome with Mucinous Adenocarcinomas. J. Clin. Invest. 112 (6), 853–862. doi:10.1172/jci1888210.1172/jci200318882
Wang, B., Guo, Y., Chen, X., Zeng, C., Hu, Q., Yin, W., et al. (2018). Nanoparticle-modified Chitosan-Agarose-Gelatin Scaffold for Sustained Release of SDF-1 and BMP-2. Ijn 13, 7395–7408. doi:10.2147/ijn.S180859
Wells, P. S., Forgie, M. A., and Rodger, M. A. (2014). Treatment of Venous Thromboembolism. Jama 311 (7), 717–728. doi:10.1001/jama.2014.65
Wijesinghe, S. J., Ling, L., Murali, S., Qing, Y. H., Hinkley, S. F. R., Carnachan, S. M., et al. (2017). Affinity Selection of FGF2-Binding Heparan Sulfates for Ex Vivo Expansion of Human Mesenchymal Stem Cells. J. Cell. Physiol. 232 (3), 566–575. doi:10.1002/jcp.25454
Xia, Y., Sun, J., Zhao, L., Zhang, F., Liang, X.-J., Guo, Y., et al. (2018). Magnetic Field and Nano-Scaffolds with Stem Cells to Enhance Bone Regeneration. Biomaterials 183, 151–170. doi:10.1016/j.biomaterials.2018.08.040
Xie, C.-M., Lu, X., Wang, K.-F., Meng, F.-Z., Jiang, O., Zhang, H.-P., et al. (2014). Silver Nanoparticles and Growth Factors Incorporated Hydroxyapatite Coatings on Metallic Implant Surfaces for Enhancement of Osteoinductivity and Antibacterial Properties. ACS Appl. Mat. Interfaces 6 (11), 8580–8589. doi:10.1021/am501428e
Xie, H., Cui, Z., Wang, L., Xia, Z., Hu, Y., Xian, L., et al. (2014). PDGF-BB Secreted by Preosteoclasts Induces Angiogenesis during Coupling with Osteogenesis. Nat. Med. 20 (11), 1270–1278. doi:10.1038/nm.3668
Xie, J., Li, B., Yao, B., Zhang, P., Wang, L., Lu, H., et al. (2020). Transforming Growth Factor-β1-Regulated Fas/FasL Pathway Activation Suppresses Nucleus Pulposus Cell Apoptosis in an Inflammatory Environment. Biosci. Rep. 40 (2). doi:10.1042/bsr20191726
Xu, J., Liu, J., Gan, Y., Dai, K., Zhao, J., Huang, M., et al. (2020). High‐Dose TGF‐β1 Impairs Mesenchymal Stem Cell-Mediated Bone Regeneration via Bmp2 Inhibition. J. Bone Min. Res. 35 (1), 167–180. doi:10.1002/jbmr.3871
Yamaguchi, T. P., and Rossant, J. (1995). Fibroblast Growth Factors in Mammalian Development. Curr. Opin. Genet. Dev. 5 (4), 485–491. doi:10.1016/0959-437x(95)90053-j
Yan, S., Feng, L., Zhu, Q., Yang, W., Lan, Y., Li, D., et al. (2018). Controlled Release of BMP-2 from a Heparin-Conjugated Strontium-Substituted Nanohydroxyapatite/Silk Fibroin Scaffold for Bone Regeneration. ACS Biomater. Sci. Eng. 4 (9), 3291–3303. doi:10.1021/acsbiomaterials.8b00459
Yang, D. H., Lee, D.-W., Kwon, Y.-D., Kim, H. J., Chun, H. J., Jang, J. W., et al. (2015). Surface Modification of Titanium with Hydroxyapatite-Heparin-BMP-2 Enhances the Efficacy of Bone Formation and Osseointegrationin Vitroandin Vivo. J. Tissue Eng. Regen. Med. 9 (9), 1067–1077. doi:10.1002/term.1973
Yang, H. S., La, W.-G., Cho, Y.-M., Shin, W., Yeo, G.-D., and Kim, B.-S. (2012). Comparison between Heparin-Conjugated Fibrin and Collagen Sponge as Bone Morphogenetic Protein-2 Carriers for Bone Regeneration. Exp. Mol. Med. 44 (5), 350–355. doi:10.3858/emm.2012.44.5.039
Yang, H. S., Shin, J., Bhang, S. H., Shin, J.-Y., Park, J., Im, G.-I., et al. (2011). Enhanced Skin Wound Healing by a Sustained Release of Growth Factors Contained in Platelet-Rich Plasma. Exp. Mol. Med. 43 (11), 622–629. doi:10.3858/emm.2011.43.11.070
Yu, S., Geng, Q., Ma, J., Sun, F., Yu, Y., Pan, Q., et al. (2013). Heparin-binding EGF-like Growth Factor and miR-1192 Exert Opposite Effect on Runx2-Induced Osteogenic Differentiation. Cell Death Dis. 4 (10), e868. doi:10.1038/cddis.2013.363
Zaman, P., Wang, J., Blau, A., Wang, W., Li, T., Kohane, D., et al. (2016). Incorporation of Heparin-Binding Proteins into Preformed Dextran Sulfate-Chitosan Nanoparticles. Ijn 11, 6149–6159. doi:10.2147/ijn.S119174
Zee, A. A., van Lieshout, K., van der Heide, M., Janssen, L., and Janzing, H. M. (2017). Low Molecular Weight Heparin for Prevention of Venous Thromboembolism in Patients with Lower-Limb Immobilization. Cochrane Database Syst. Rev. 2017 (8), Cd006681. doi:10.1002/14651858.CD006681.pub4
Zhang, G., Guo, B., Wu, H., Tang, T., Zhang, B.-T., Zheng, L., et al. (2012). A Delivery System Targeting Bone Formation Surfaces to Facilitate RNAi-Based Anabolic Therapy. Nat. Med. 18 (2), 307–314. doi:10.1038/nm.2617
Zhang, M., Shi, J., Xie, M., Wen, J., Niibe, K., Zhang, X., et al. (2020). Recapitulation of Cartilage/bone Formation Using iPSCs via Biomimetic 3D Rotary Culture Approach for Developmental Engineering. Biomaterials 260, 120334. doi:10.1016/j.biomaterials.2020.120334
Zhang, S., Chen, J., Yu, Y., Dai, K., Wang, J., and Liu, C. (2019). Accelerated Bone Regenerative Efficiency by Regulating Sequential Release of BMP-2 and VEGF and Synergism with Sulfated Chitosan. ACS Biomater. Sci. Eng. 5 (4), 1944–1955. doi:10.1021/acsbiomaterials.8b01490
Zhang, W., Falcon, B., Murzin, A. G., Fan, J., Crowther, R. A., Goedert, M., et al. (2019). Heparin-induced Tau Filaments Are Polymorphic and Differ from Those in Alzheimer's and Pick's Diseases. Elife 8. doi:10.7554/eLife.43584
Zhang, Y., Sun, T., and Jiang, C. (2018). Biomacromolecules as Carriers in Drug Delivery and Tissue Engineering. Acta Pharm. Sin. B 8 (1), 34–50. doi:10.1016/j.apsb.2017.11.005
Zhao, B., Katagiri, T., Toyoda, H., Takada, T., Yanai, T., Fukuda, T., et al. (2006). Heparin Potentiates the In Vivo Ectopic Bone Formation Induced by Bone Morphogenetic Protein-2. J. Biol. Chem. 281 (32), 23246–23253. doi:10.1074/jbc.M511039200
Zhou, H., Qian, J., Wang, J., Yao, W., Liu, C., Chen, J., et al. (2009). Enhanced Bioactivity of Bone Morphogenetic Protein-2 with Low Dose of 2-N, 6-O-Sulfated Chitosan In Vitro and In Vivo. Biomaterials 30 (9), 1715–1724. doi:10.1016/j.biomaterials.2008.12.016
Zhou, J., Xiong, Z., Liu, M., Yang, L., Yao, S., Chen, K., et al. (2020). Creation of Bony Microenvironment with Extracellular Matrix Doped-Bioactive Ceramics to Enhance Osteoblast Behavior and Delivery of Aspartic Acid-Modified BMP-2 Peptides. Ijn 15, 8465–8478. doi:10.2147/ijn.S272571
Zomer Volpato, F., Almodóvar, J., Erickson, K., Popat, K. C., Migliaresi, C., and Kipper, M. J. (2012). Preservation of FGF-2 Bioactivity Using Heparin-Based Nanoparticles, and Their Delivery from Electrospun Chitosan Fibers. Acta Biomater. 8 (4), 1551–1559. doi:10.1016/j.actbio.2011.12.023
Zwingenberger, S., Langanke, R., Vater, C., Lee, G., Niederlohmann, E., Sensenschmidt, M., et al. (2016). The Effect of SDF-1α on Low Dose BMP-2 Mediated Bone Regeneration by Release from Heparinized Mineralized Collagen Type I Matrix Scaffolds in a Murine Critical Size Bone Defect Model. J. Biomed. Mat. Res. 104 (9), 2126–2134. doi:10.1002/jbm.a.35744
Keywords: heparin, nanomaterial, osteogenic, bone regeneration, bone morphogenic protein-2, bone morphogenic protein-4, heparan sulfate
Citation: Wang J, Xiao L, Wang W, Zhang D, Ma Y, Zhang Y and Wang X (2022) The Auxiliary Role of Heparin in Bone Regeneration and its Application in Bone Substitute Materials. Front. Bioeng. Biotechnol. 10:837172. doi: 10.3389/fbioe.2022.837172
Received: 16 December 2021; Accepted: 13 April 2022;
Published: 12 May 2022.
Edited by:
Valeria Chiono, Polytechnic University of Turin, ItalyReviewed by:
Fengxuan Han, Soochow University, ChinaCopyright © 2022 Wang, Xiao, Wang, Zhang, Ma, Zhang and Wang. This is an open-access article distributed under the terms of the Creative Commons Attribution License (CC BY). The use, distribution or reproduction in other forums is permitted, provided the original author(s) and the copyright owner(s) are credited and that the original publication in this journal is cited, in accordance with accepted academic practice. No use, distribution or reproduction is permitted which does not comply with these terms.
*Correspondence: Yi Zhang, emhhbmd5aXptckAxNjMuY29t; Xin Wang, bGNod3hAYWxpeXVuLmNvbQ==
†These authors have contributed equally to this work and share first authorship
Disclaimer: All claims expressed in this article are solely those of the authors and do not necessarily represent those of their affiliated organizations, or those of the publisher, the editors and the reviewers. Any product that may be evaluated in this article or claim that may be made by its manufacturer is not guaranteed or endorsed by the publisher.
Research integrity at Frontiers
Learn more about the work of our research integrity team to safeguard the quality of each article we publish.