- 1Division of Neurosurgery, Hamilton Health Sciences, McMaster University Faculty of Health Sciences, Hamilton, ON, Canada
- 2Department of Radiology, Hamilton Health Sciences, McMaster University Faculty of Health Sciences, Hamilton, ON, Canada
Introduction
Body scanners and operating microscopes have become standard of care for diagnostic imaging and operative planning in neurosurgical oncology. Recently, the preclinical development of novel nanoscale materials for use in enhancing imaging and visualization of brain tumors in vivo have led to translational platforms that offer clinicians the potential for improving surgical outcomes and tailoring personalized treatment regimens. In this piece written by three physician-scientists with over 30 years of combined expertise in neurosurgery, neuroradiology, neuro-oncology, and CNS nanotherapeutics, we provide our collective opinion regarding the emerging uses of nanotechnology in our respective subspecialties.
Nanotechnology in Neuroradiology
Common neuroimaging modalities such as computed tomography (CT) and magnetic resonance imaging (MRI) provide anatomical details of the brain and spine. Both CT and MRI scans provide ∼25–100 μm resolution of neural structures (Kaviarasi et al., 2019). CT uses X-rays while MRI uses radiowaves and magnetic fields for image acquisition and iodine- or gadolinium (Gd)-based contrast dye agents, respectively, for further enhancement and delineation of lesions such as higher grade tumors, vascular lesions, or traumatic brain injuries that cause leakiness of the blood-brain barrier (BBB) (Martina et al., 2005; Bauer et al., 2014). Tracer-based imaging modalities such as positron emission spectroscopy (PET) and single-photon emission computerized tomography (SPECT) have limits of resolution between 2 and 10 mm (Moses, 2011; Bailey and Willowson, 2013) and rely on costly, injectable radioactive tracers to detect diseased cells.
Nanotechnology for neuroimaging largely remains in early stage preclinical development. Functionalized nanoparticles containing iron oxide or gold or quantum dots have been tested in mouse models of stroke and brain tumors, demonstrating enhanced visualization of tumor foci, thrombi, or infarcted brain tissues (reviewed in Kaviarasi et al., 2019) (Kaviarasi et al., 2019). In particular, high resolution magnetic particle imaging (MPI) utilizing superparamagnetic iron oxide nanoparticles to acquire quantitative three-dimensional, in vivo real-time imaging shows promise in the fields of vascular, tumor, and cell labeling and tracking (reviewed in Wu et al., 2019) (Wu et al., 2019). Furthermore, the use of artificial intelligence and machine learning to deconvolute neural networks with brain mapping will further compliment the uses of nanotechnology in molecular neuroimaging (Lui et al., 2020; Yao et al., 2020).
More recently, investigators have begun to use nanotechnology to improve the functionality and safety of Gd-based contrast agents for MRI. While Gd is the most frequently used metal ion for MRI scanning due to its high magnetic moment causing significant relaxation rates of nearby water protons, allowing for enhanced anatomical resolution and the visualization of higher-grade primary and metastatic brain tumors, the known renal toxicity of free Gd ions have led to the development of Gd-chelates such as Gd-DPTA (Magnevist, Schering AG), which rapidly clears the contrast agent through the kidneys to decrease the risk of kidney injury (Wang, 2011; Weidman et al., 2015; Russo et al., 2017). However, chelation reduces the relaxivity of Gd by decreasing the number of sites for water proton exchange, thus decreasing contrast enhancement. Several nanotechnologies have been shown to increase the relaxivity of Gd, including: 1) Geometric confinement of Magnevist, gadofullerenes, or gadonanotubes in porous silicon nanoparticles (Ananta et al., 2010); 2) Encapsulating Gd-DPTA in hyaluronic acid hydrogel nanoparticles to increase its hydrodenticity (Russo et al., 2017); and 3) Rigidification of Gd by constraining it as a tricyclic tetraazatriacetic Gd chelate (Port et al., 2006), all three methods of which have been shown to significantly increase proton relaxivity compared to that of clinically available Gd-based contrast agents.
Another emerging use of nanotechnology is in the field of molecular imaging, including PET and SPECT, which with their high sensitivity of detection, is being co-apted into multimodal imaging systems (PET/CT, SPECT/CT, PET/MRI) to allow for early cancer detection and/or personalized treatment algorithms (Weissleder, 2006; James and Gambhir, 2012; Goel et al., 2017). 18F-fluorodeoxyglucose (18F-FDG) is the most common radionuclide that is used as a PET tracer that is taken up by rapidly metabolizing cells such as malignant tumor cells, however its specificity for tumor cells is low–this has led to the development of nanomaterials that can be deployed as targeted molecular imaging probes simultaneously functionalized to target tumor cells and deliver therapeutic payloads (Weissleder, 2006; Hong et al., 2009). 124I-labeled gold nanostars have recently been shown to serve as a subcellular tracker for PET scanning in a preclinical mouse model of glioma with the potential for translational applications (Liu et al., 2019), while 99mTc-labeled peptide targeting nanoprobes have shown promise in preclinical studies for glioma SPECT imaging (Zhao, 2016). The ability to combine these targeted molecular probes with multimodal imaging has the potential to improve the characterization of brain tumors at the time of diagnosis and throughout a patient’s course of treatment.
Nanotechnology in Neurosurgery
The use of nanotechnology in neurosurgical procedures has gained much more maturity than in neuroradiology, with the application of nanomaterials across each subspecialty of neurosurgery. Nano-roughened titanium interbody cages that stimulate osteoblastic activation and osteointegration (Gittens et al., 2012); bioactive nanofiber scaffolds as carriers for recombinant human bone morphogenic protein (Lee et al., 2015), and polyethyl ether ketone nanocomposite polymers that assist in implant technologies for patients with osteoporotic bone (Li, 2012), are commonly used in spinal fusion surgeries (reviewed in Viswanathan et al., 2019) (Viswanathan et al., 2019).
Intra-operative fluorescence-guided surgery using the fluorescent pro-agent 5-aminolevulenic acid (5-ALA) and the fluorescent dye indocyanine green (ICG), both of which have demonstrated good penetrance across the blood-brain barrier (BBB), have shown promise in improving the extent of maximal safe surgical resection of brain tumors (Valdés et al., 2015; Teng et al., 2021). Similarly, a phase one safety trial of BLZ-100, a 36 amino acid synthetic peptide chlorotoxin derived from scorpion venom conjugated to ICG (Tozuleristide), has established dose-limiting toxicities and enhanced intra-operative fluorescence of newly diagnosed or recurrent adult gliomas (Patil et al., 2019). The increased prevalence of fluorescence-guided intraoperative surgical resection of brain tumors have been aided by the development of several intraoperative imaging systems, such as: The commonly available Leica OH6 microscope (Wetzlar, Germany) which is equipped with the FL800TM module that has a high-sensitivity near-infrared (NIR) camera and 820–860 nm filter that detects NIR fluorescence and generates a black and white image that cannot be overlaid with visible light microscopy images (Cho, 2018; Cho et al., 2018); The Zeiss CONVIVO® confocal laser endomicroscopy digital biopsy probe that allows for in situ visualization of fluorescein-labelled tissues and allows for seamless interfacing with their KINEVO® 900 robotic visualization system combining fluorescent views of tissue microstructure with surgical views under the operating microscope (Zeiss, Oberkochen, Germany); And finally, the FDA-approved VisionSense IridiumTM handheld NIR exoscope (VisionSense, Philadelphia, PA) which is less commonly used in neurosurgery but more widely used in plastic surgery and reconstructive general surgery procedures, and allows for overlay of fluorescence and white light images in real time (AV, 2016; Cho et al., 2018). These intra-operative nanoscale imaging materials and fluorescence imaging systems have the potential to maximize the extent of safe surgical resection of highly malignant brain tumors with hopes of prolonging patient survival.
Nanomaterials that promote osteoblastic stem cell growth in intervertebral cages made out of titanium or polyetheretherketone (PEEK) have dramatically improved the fusion rates in spine surgery (Girasole et al., 2013). Nanofibrous poly (D,L-lactide-co-e-caprolactone) balloons have been used in verteplasty procedures to fill compressed fractured vertebra (Sun et al., 2013). Finally, experimental collagen scaffolds of collagen-binding brain-derived neurotrophic factor have been shown therapeutic promise in a canine model of spinal cord injury (Andrychowski et al., 2013).
Nanotechnology in Neuro-Oncology
Currently, the food and drug administration (FDA) has approved albumin-bound paclitaxel and pegylated liposomal doxorubicin for used as systemic anti-cancer therapies. These nanomedicines do not cross the BBB and therefore are not appropriate for use in the neuro-oncology space. A variety of nanoparticles have been developed in the preclinical testing phases that have shown promising results to be able to circumvent the BBB for delivery of small molecule inhibitors, chemotherapies, antibody-drug conjugates, and gene therapies in murine models of gliomas (Glaser et al., 2017; Grabowska et al., 2019). We recently reported enhanced safety and efficacy of nanoliposomes functionalized with transferrin, which aids in receptor-mediated endocytosis across the BBB and targeting to glioma cells via the expression of transferrin receptors on the surfaces of both endothelial and glioma cells, in the delivery of combination anti-cancer therapies to murine models of gliomas (Lam et al., 2018). However, all these nanoscale materials are still restricted to preclinical studies and have yet to translate into the clinical setting for the treatment of patients with brain tumors.
Recent advances in photon beam radiation therapy (RT) techniques for the treatment of brain tumors have allowed for the ability to deliver high doses of radiation precisely to different regions of the brain. Treatment planning algorithms such as intensity- or volume-modulated radiotherapy/arc therapy (Sheu et al., 2019), when applied to techniques such as stereotactic radiosurgery and fractionated RT (Scaringi et al., 2018), have allowed radiation oncologists to deliver highly conformed doses of radiation to tumors while sparing adjacent normal neurovasculature with promising preliminary outcomes in maintaining high local control rates (Ruggieri et al., 2018). However, RT treatments are associated with acute and late central nervous system toxicities, including headaches, vomiting, motor/sensory neuropathy, cognitive deficits, and seizures (Brown et al., 2016; Chen et al., 2017). One emerging modality to avoid these toxicities is proton beam therapy, which uses positively charged elementary particles to deposit a sharp peak of energy (the Bragg Peak) to the target tumor volume with minimal exit dose to decrease the rates of acute and late toxicities and increases the therapeutic ratio of RT (Sherman et al., 2016; Indelicato et al., 2019; Weber et al., 2020). However, it has also been postulated that the high transfer of energy at the distal range of the beam may be also cause toxicities (Peeler et al., 2016; Haas-Kogan et al., 2018). To further increase the therapeutic window and minimize dose-related toxicities, the development of nanoscintillators, down-conversion NPs that absorb x-rays and emit a wide-range of photons, have been used as photosensitizers in photodynamic therapy to produce high amounts of reactive oxygen species when delivered locally into tumor tissues, creating cytotoxicity and tumor cell killing (Castano et al., 2006; Celli et al., 2010). These include rare-earth lanthanum/iron/ceramide-based composite NPs that have shown promise in reducing radiation treatment-induced toxicities and improving therapeutic benefits in preclinical models of brain tumors (Bulin et al., 2020).
Current Limitations of Nanotechnology for Use in the Human Central Nervous System
Limitations to successful translation of most benchtop nanotechnologies into the clinic have been due to the potential systemic toxicities of NPs. FDA-approved pegylated liposomal doxorubicin (Doxil®) has increased systemic circulation times, reduced cardiotoxicity, and demonstrated similar efficacy compared to conventional doxorubicin for the treatment of metastatic breast cancer (O’Brien et al., 2004). Compared to other types of nanotechnologies composed of organic, inorganic, or metal components, liposomes and other lipid-based NPs appear to have the lowest toxicity profiles in vivo (Puri et al., 2009). However, patients taking Doxil® have reported increased incidences of hand-foot syndrome and developed cutaneous squamous cell carcinoma after repeated use (Anselmo and Mitragotri, 2019; Pease et al., 2019). A recent systematic review of 14 randomized clinical trials comparing the efficacy of liposomal encapsulated cytotoxic therapies to equivalent conventional formulations did not show improved efficacy in humans despite showing significantly increased survival in tumor-bearing mice (Petersen et al., 2016). This review exemplifies deficiencies in understanding the pharmacokinetics and pharmacodynamics of nanomedicines in humans and the limitations for clinical use. Little is known regarding the long-term effects of NP deposition in human organs, however animal studies have shown increased cellular oxidative stress in different organs (Dick et al., 2003; Donaldson, 2004). Other purported mechansims of toxicity include generation of DNA damage, protein structural and functional modifications, and disruption of membrane integrity (reviewed in Najahi-Missaoui et al., 2021) (Najahi-Missaoui et al., 2020). Furthermore, most preclinical studies have manufactured NPs under non-GLP (Good Laboratory Practices)/non-GMP (Good Manufacturing Practice) laboratory environments in relatively small batches, thus without knowledge of how large scale-up production of NPs can affect the quality and consistency of the final product, it becomes difficult to assess the safety and efficacy of these nanomedicines in humans.
Preclinical studies have also shown mechanisms of neurotoxicity associated with nanomaterials. Interactions of nanomaterials with glial cells and neurons have led to oxidative burst activity in microglia (Long et al., 2006; Ze et al., 2013; Shrivastava et al., 2014), inflammation due to the release of cytokines and tumor necrosis factor-α by microglia (Li et al., 2009; Ze et al., 2014), DNA damage (Golbamaki et al., 2015; Ren et al., 2016), and apoptosis (Márquez-Ramírez et al., 2012; Ganguly et al., 2018). Our experience with glioma-targeted liposomal NP delivery of cytotoxic therapies across the BBB in mouse models of glioma has demonstrated successful accumulation of transferrin-functionalized NPs on the surface of glioma tumors (Lam et al., 2018). Intravital multiphoton imaging through a cranial window into the brains of these glioma bearing mice (Figure 1A, glioma tumor in green) immediately after systemic injection of fluorescence-conjugated liposomal NPs demonstrates the presence of NPs flowing through an adjacent tumor vessel (Figure 1A, white arrows pointing to liposomal NPs in red). Twenty-four hours after systemic injection, imaging demonstrated accumulation of NPs on the surface of the tumor (Figure 1B, tumor signal in green, liposomal NPs in red). Red fluorescence signal is seen in cells surrounding the tumor with a bulls-eye appearance, likely suggestive of scavenger uptake of NPs by resident microglia, which would serve as a mechanism for the eventual degradation and clearance of NPs from the CNS milieu (Figure 1).
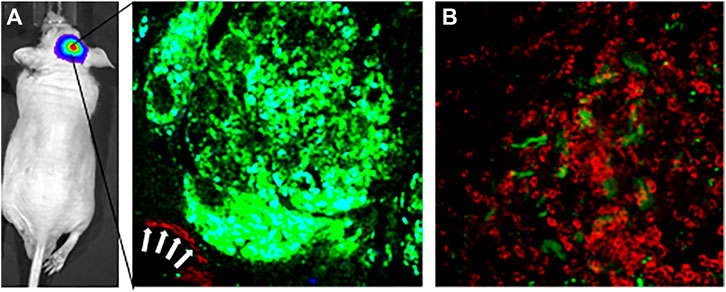
FIGURE 1. Intravital multiphoton imaging demonstrates delivery of fluorescent tumor-targeting liposomal nanoparticles across the blood-brain barrier to a glioma brain tumor in an intracranial orthotopic xenograft mouse model of glioma. (A) Left panel-intravital multiphoton image through a cranical window of a GFP-expressing glioma tumor. White arrows show Cy5.5-conjugated liposomal nanoparticles coursing throught a blood vessel adjacent to the tumor. Imaged immediately after tail vein injection of nanoparticles. (B) Intravital mutiphoton image taken 24 h following tail vein injection showing accumulation of Cy5.5-conjugated liposomal nanoparticles on the surface of the brain tumor. Accumulation Cy5.5 signal in cells with a bulls-eye center suggestive of uptake of nanoparticles by resident brain microglia.
New Horizons
Despite the potential for nanoscale applications to revolutionize the fields of neuroradiology, neuro-oncology, and neurosurgery, the largely impenetrable BBB remains to be a major hindrance in the effective delivery of payload into the CNS. To circumvent the BBB, researchers have exploited both intranasal and intrathecal delivery routes for direct delivery into the CNS. The nasal mucosa provides a direct route into the brain via the olfactory epithelium through the cribriform plate of the skull base (Tashima, 2020). Most intranasal medications have been marketed for local or systemic delivery to the nose or the upper respiratory tract for indications such as allergic rhinitis. More recently, micellar, liposomal, and chitosan NPs have been used to deliver insulin intranasally into the brain for the treatment of Alzheimer’s disease (reviewed in Tashima et al., 2020), paving the road for the possibility of using insulin as a backbone conjugate linker to carry other small molecules intranasally into the CNS space to treat other CNS disorders (Tashima, 2020).
Intrathecal delivery of therapeutics can also circumvent systemic barriers to CNS drug delivery to achieve high concentrations of drugs in the CSF while averting systemic exposure and have shown promise in both the clinical and preclinical space for the treatment of intraventricular tumors and leptomeningeal disease (Sandberg et al., 2012; Bottros and Christo, 2014; Chen et al., 2015; Sandberg et al., 2015). A recent study characterizing the biodistribution of 100 nm fluorescent-conjugated PEGylated NPs delivered into the cisterna magna of healthy mice found that these NPs were evenly distributed throughout the subarachnoid space along the brain and spinal cord with retention in the leptomeninges for up to 3 weeks–however, there was minimal penetration into the brain parenchyma (Householder et al., 2019). This points towards the selective use of intrathecal delivery for the treatment of diseases with an affinity for the meninges, such as leptomeningeal carcinomatosis, for which implantable intraventricular devices such as the NeuroinfuseTM multicatheter infusion device (Renishaw, United Kingdom) are currently in human clinical trials (Chen et al., 2015), or the need to further functionalize nanoparticles to allow for cell type-specific delivery within the brain.
Finally, the field of theranostics–combining therapeutic and diagnostic capabilities into a single NPs delivery system have gradually come into the forefront of personalized medicine, potentially offering time and cost savings while improving patient outcomes (Lammers et al., 2010; Wang et al., 2014). Multifunctionalized theranostic NPs that contain imaging agents such as iron oxide, chemotherapeutic agents such as doxorubicin, and tumor cell-targeting moieties such as folate, have the ability to allow for real-time imaging and tracking of therapeutic responses for the treatment of brain tumors (reviewed in d’Angelo et al., 2019) (d’Angelo et al., 2019). Superparamagnetic iron oxide and doxorubicin loaded into chitosan NPs have the ability to be internalized by C6 glioma cells, demonstrating a decline in T2 relaxation times in vitro during MRI scanning, paving the road for further preclinical testing of theranostic NPs for the treatment of brain tumors (Gholami et al., 2019). The ability to deliver multimodal NPs using novel routes of delivery into the CNS space could further the field of personalized medicine for the treatment of brain tumors.
Discussion
From a neurosurgeon’s perspective, we often implant foreign materials into the brain, including Ommaya reservoirs (Magill et al., 2020), external ventricular catheters (Dey et al., 2015), and deep brain stimulator electrodes (Engel et al., 2018), composed of inert soft plastics, as well biodegradable wafers impregnated with the cytotoxic agent carmustine (Gliadel®) into the surgical resection cavity for the treatment of gliomas (Chowdhary et al., 2015). Some reported side-effects of these implantable devices include migration within the brain leading to inadvertent hemorrhage and neurologic injury, brain swelling due to foreign body reactions, seizures, and scarring. Extrapolating our experience using macromolecular intracranial implants, there is a need to design early phase clinical trials to thoroughly determine the safety of all novel nanomaterials in the CNS leading into larger phase II/III randomized trials to assess the efficacy of these materials for their intended CNS applications.
Conclusion
Nanomaterials for use in CNS applications is a rapidly emerging space with much potential for improving the care of patients with neurological and neurosurgical issues. There remains a large translational gap between benchtop to clinical studies but the increasing involvement of clinician scientists with an interest in nanomaterials research will undoubtedly narrow that gap.
Author Contributions
FL wrote the manuscript. FS and EK edited the manuscript. FL, FS, and EK conceived the content and structure of the manuscript.
Conflict of Interest
The authors declare that the research was conducted in the absence of any commercial or financial relationships that could be construed as a potential conflict of interest.
Publisher’s Note
All claims expressed in this article are solely those of the authors and do not necessarily represent those of their affiliated organizations, or those of the publisher, the editors and the reviewers. Any product that may be evaluated in this article, or claim that may be made by its manufacturer, is not guaranteed or endorsed by the publisher.
References
Ananta, J. S., Godin, B., Sethi, R., Moriggi, L., Liu, X., Serda, R. E., et al. (2010). Geometrical Confinement of Gadolinium-Based Contrast Agents in Nanoporous Particles Enhances T1 Contrast. Nat. Nanotech 5 (11), 815–821. doi:10.1038/nnano.2010.203
Andrychowski, J., Frontczak-Baniewicz, M., Sulejczak, D., Kowalczyk, T., Chmielewski, T., Czernicki, Z., et al. (2013). Original Article Nanofiber Nets in Prevention of Cicatrisation in Spinal Procedures. Experimental Study. fn 2 (2), 147–157. doi:10.5114/fn.2013.35958
Anselmo, A. C., and Mitragotri, S. (2019). Nanoparticles in the Clinic: An Update. Bioeng. Transl Med. 4 (3), e10143. doi:10.1002/btm2.10143
Av, D. S., (2016). Review of Fluorescence Guided Surgery Systems: Identification of Key Performance Capabilities beyond Indocyanine green Imaging. J. Biomed. Opt. 21 (8), 80901.
Bailey, D. L., and Willowson, K. P. (2013). An Evidence-Based Review of Quantitative SPECT Imaging and Potential Clinical Applications. J. Nucl. Med. 54 (1), 83–89. doi:10.2967/jnumed.112.111476
Bauer, A. Q., Kraft, A. W., Wright, P. W., Snyder, A. Z., Lee, J.-M., and Culver, J. P. (2014). Optical Imaging of Disrupted Functional Connectivity Following Ischemic Stroke in Mice. Neuroimage 99, 388–401. doi:10.1016/j.neuroimage.2014.05.051
Bottros, M. M., and Christo, P. J. (2014). Current Perspectives on Intrathecal Drug Delivery. J. Pain Res. 7, 615–626. doi:10.2147/JPR.S37591
Brown, P. D., Jaeckle, K., Ballman, K. V., Farace, E., Cerhan, J. H., Anderson, S. K., et al. (2016). Effect of Radiosurgery Alone vs Radiosurgery with Whole Brain Radiation Therapy on Cognitive Function in Patients with 1 to 3 Brain Metastases. JAMA 316 (4), 401–409. doi:10.1001/jama.2016.9839
Bulin, A. L., Broekgaarden, M., Chaput, F., Baisamy, V., Garrevoet, J., Busser, B., et al. (2020). Radiation Dose‐Enhancement Is a Potent Radiotherapeutic Effect of Rare‐Earth Composite Nanoscintillators in Preclinical Models of Glioblastoma. Adv. Sci. 7 (20), 2001675. doi:10.1002/advs.202001675
Castano, A. P., Mroz, P., and Hamblin, M. R. (2006). Photodynamic Therapy and Anti-tumour Immunity. Nat. Rev. Cancer 6 (7), 535–545. doi:10.1038/nrc1894
Celli, J. P., Spring, B. Q., Rizvi, I., Evans, C. L., Samkoe, K. S., Verma, S., et al. (2010). Imaging and Photodynamic Therapy: Mechanisms, Monitoring, and Optimization. Chem. Rev. 110 (5), 2795–2838. doi:10.1021/cr900300p
Chen, L., Shen, C., Redmond, K. J., Page, B. R., Kummerlowe, M., Mcnutt, T., et al. (2017). Use of Stereotactic Radiosurgery in Elderly and Very Elderly Patients with Brain Metastases to Limit Toxicity Associated with Whole Brain Radiation Therapy. Int. J. Radiat. Oncology*Biology*Physics 98 (4), 939–947. doi:10.1016/j.ijrobp.2017.02.031
Chen, T. C., Napolitano, G. R., Adell, F., Schönthal, A. H., and Shachar, Y. (2015). Development of the Metronomic Biofeedback Pump for Leptomeningeal Carcinomatosis: Technical Note. Jns 123 (2), 362–372. doi:10.3171/2014.10.jns14343
Cho, S. S., Zeh, R., Pierce, J. T., Salinas, R., Singhal, S., and Lee, J. Y. K. (2018). Comparison of Near-Infrared Imaging Camera Systems for Intracranial Tumor Detection. Mol. Imaging Biol. 20 (2), 213–220. doi:10.1007/s11307-017-1107-5
Chowdhary, S. A., Ryken, T., and Newton, H. B. (2015). Survival Outcomes and Safety of Carmustine Wafers in the Treatment of High-Grade Gliomas: a Meta-Analysis. J. Neurooncol. 122 (2), 367–382. doi:10.1007/s11060-015-1724-2
d'Angelo, M., Castelli, V., Benedetti, E., Antonosante, A., Catanesi, M., Dominguez-Benot, R., et al. (2019). Theranostic Nanomedicine for Malignant Gliomas. Front. Bioeng. Biotechnol. 7, 325. doi:10.3389/fbioe.2019.00325
Dey, M., Stadnik, A., Riad, F., Zhang, L., McBee, N., Kase, C., et al. (2015). Bleeding and Infection with External Ventricular Drainage. Neurosurgery 76 (3), 291–301. ; discussion 301. doi:10.1227/neu.0000000000000624
Dick, C. A. J., Brown, D. M., Donaldson, K., and Stone, V. (2003). The Role of Free Radicals in the Toxic and Inflammatory Effects of Four Different Ultrafine Particle Types. Inhalation Toxicol. 15 (1), 39–52. doi:10.1080/08958370304454
Donaldson, K., (2004). Nanotoxicology. Occup. Environ. Med. 61 (9), 727–728. doi:10.1136/oem.2004.013243
Engel, K., Huckhagel, T., Gulberti, A., Pötter-Nerger, M., Vettorazzi, E., Hidding, U., et al. (2018). Towards Unambiguous Reporting of Complications Related to Deep Brain Stimulation Surgery: A Retrospective Single-center Analysis and Systematic Review of the Literature. PLoS One 13 (8), e0198529. doi:10.1371/journal.pone.0198529
Ganguly, P., Breen, A., and Pillai, S. C. (2018). Toxicity of Nanomaterials: Exposure, Pathways, Assessment, and Recent Advances. ACS Biomater. Sci. Eng. 4 (7), 2237–2275. doi:10.1021/acsbiomaterials.8b00068
Gholami, L., Tafaghodi, M., Abbasi, B., Daroudi, M., and Kazemi Oskuee, R. (2019). Preparation of Superparamagnetic Iron Oxide/doxorubicin Loaded Chitosan Nanoparticles as a Promising Glioblastoma Theranostic Tool. J. Cel Physiol 234 (2), 1547–1559. doi:10.1002/jcp.27019
Girasole, G., Muro, G., Mintz, A., and Chertoff, J. (2013). Transforaminal Lumbar Interbody Fusion Rates in Patients Using a Novel Titanium Implant and Demineralized Cancellous Allograft Bone Sponge. Int. J. Spine Surg. 7 (1), e95–e100. doi:10.1016/j.ijsp.2013.08.001
Gittens, R. A., Olivares-Navarrete, R., McLachlan, T., Cai, Y., Hyzy, S. L., Schneider, J. M., et al. (2012). Differential Responses of Osteoblast Lineage Cells to Nanotopographically-Modified, Microroughened Titanium-Aluminum-Vanadium alloy Surfaces. Biomaterials 33 (35), 8986–8994. doi:10.1016/j.biomaterials.2012.08.059
Glaser, T., Han, I., Wu, L., and Zeng, X. (2017). Targeted Nanotechnology in Glioblastoma Multiforme. Front. Pharmacol. 8, 166. doi:10.3389/fphar.2017.00166
Goel, S., England, C. G., Chen, F., and Cai, W. (2017). Positron Emission Tomography and Nanotechnology: A Dynamic Duo for Cancer Theranostics. Adv. Drug Deliv. Rev. 113, 157–176. doi:10.1016/j.addr.2016.08.001
Golbamaki, N., Rasulev, B., Cassano, A., Marchese Robinson, R. L., Benfenati, E., Leszczynski, J., et al. (2015). Genotoxicity of Metal Oxide Nanomaterials: Review of Recent Data and Discussion of Possible Mechanisms. Nanoscale 7 (6), 2154–2198. doi:10.1039/c4nr06670g
Grabowska, M., Grześkowiak, B. F., Szutkowski, K., Wawrzyniak, D., Głodowicz, P., Barciszewski, J., et al. (2019). Nano-mediated Delivery of Double-Stranded RNA for Gene Therapy of Glioblastoma Multiforme. PLoS One 14 (3), e0213852. doi:10.1371/journal.pone.0213852
Haas-Kogan, D., Indelicato, D., Paganetti, H., Esiashvili, N., Mahajan, A., Yock, T., et al. (2018). National Cancer Institute Workshop on Proton Therapy for Children: Considerations Regarding Brainstem Injury. Int. J. Radiat. Oncology*Biology*Physics 101 (1), 152–168. doi:10.1016/j.ijrobp.2018.01.013
Hong, H., Zhang, Y., Sun, J., and Cai, W. (2009). Molecular Imaging and Therapy of Cancer with Radiolabeled Nanoparticles. Nano Today 4 (5), 399–413. doi:10.1016/j.nantod.2009.07.001
Householder, K. T., Dharmaraj, S., Sandberg, D. I., Wechsler-Reya, R. J., and Sirianni, R. W. (2019). Fate of Nanoparticles in the central Nervous System after Intrathecal Injection in Healthy Mice. Sci. Rep. 9 (1), 12587. doi:10.1038/s41598-019-49028-w
Indelicato, D. J., Rotondo, R. L., Uezono, H., Sandler, E. S., Aldana, P. R., Ranalli, N. J., et al. (2019). Outcomes Following Proton Therapy for Pediatric Low-Grade Glioma. Int. J. Radiat. Oncology*Biology*Physics 104 (1), 149–156. doi:10.1016/j.ijrobp.2019.01.078
James, M. L., and Gambhir, S. S. (2012). A Molecular Imaging Primer: Modalities, Imaging Agents, and Applications. Physiol. Rev. 92 (2), 897–965. doi:10.1152/physrev.00049.2010
Kaviarasi, S., Yuba, E., Harada, A., and Krishnan, U. M. (2019). Emerging Paradigms in Nanotechnology for Imaging and Treatment of Cerebral Ischemia. J. Controlled Release 300, 22–45. doi:10.1016/j.jconrel.2019.02.031
Lam, F. C., Morton, S. W., Wyckoff, J., Vu Han, T.-L., Hwang, M. K., Maffa, A., et al. (2018). Enhanced Efficacy of Combined Temozolomide and Bromodomain Inhibitor Therapy for Gliomas Using Targeted Nanoparticles. Nat. Commun. 9 (1), 1991. doi:10.1038/s41467-018-04315-4
Lammers, T., Kiessling, F., Hennink, W. E., and Storm, G. (2010). Nanotheranostics and Image-Guided Drug Delivery: Current Concepts and Future Directions. Mol. Pharmaceutics 7 (6), 1899–1912. doi:10.1021/mp100228v
Lee, S. S., Hsu, E. L., Mendoza, M., Ghodasra, J., Nickoli, M. S., Ashtekar, A., et al. (2015). Gel Scaffolds of BMP-2-Binding Peptide Amphiphile Nanofibers for Spinal Arthrodesis. Adv. Healthc. Mater. 4 (1), 131–141. doi:10.1002/adhm.201400129
Li, K., (2012). Sintered Hydroxyapatite/polyetheretherketone Nanocomposites: Mechanical Behavior and Biocompatibility. Adv. Eng. Mater. 14, 155–165. doi:10.1002/adem.201080145
Li, X.-b., Zheng, H., Zhang, Z.-r., Li, M., Huang, Z.-y., Schluesener, H. J., et al. (2009). Glia Activation Induced by Peripheral Administration of Aluminum Oxide Nanoparticles in Rat Brains. Nanomedicine: Nanotechnology, Biol. Med. 5 (4), 473–479. doi:10.1016/j.nano.2009.01.013
Liu, Y., Carpenter, A. B., Pirozzi, C. J., Yuan, H., Waitkus, M. S., Zhou, Z., et al. (2019). Non-invasive Sensitive Brain Tumor Detection Using Dual-Modality Bioimaging Nanoprobe. Nanotechnology 30 (27), 275101. doi:10.1088/1361-6528/ab0e9c
Long, T. C., Saleh, N., Tilton, R. D., Lowry, G. V., and Veronesi, B. (2006). Titanium Dioxide (P25) Produces Reactive Oxygen Species in Immortalized Brain Microglia (BV2): Implications for Nanoparticle Neurotoxicity. Environ. Sci. Technol. 40 (14), 4346–4352. doi:10.1021/es060589n
Lui, Y. W., Chang, P. D., Zaharchuk, G., Barboriak, D. P., Flanders, A. E., Wintermark, M., et al. (2020). Artificial Intelligence in Neuroradiology: Current Status and Future Directions. AJNR Am. J. Neuroradiol 41 (8), E52–E59. doi:10.3174/ajnr.A6681
Magill, S. T., Choy, W., Nguyen, M. P., and McDermott, M. W. (2020). Ommaya Reservoir Insertion: A Technical Note. Cureus 12 (4), e7731. doi:10.7759/cureus.7731
Márquez-Ramírez, S. G., Delgado-Buenrostro, N. L., Chirino, Y. I., Iglesias, G. G., and López-Marure, R. (2012). Titanium Dioxide Nanoparticles Inhibit Proliferation and Induce Morphological Changes and Apoptosis in Glial Cells. Toxicology 302 (2-3), 146–156. doi:10.1016/j.tox.2012.09.005
Martina, A. D., Meyer-Wiethe, K., Allémann, E., and Seidel, G. (2005). Ultrasound Contrast Agents for Brain Perfusion Imaging and Ischemic Stroke Therapy. J. Neuroimaging 15 (3), 217–232. doi:10.1111/j.1552-6569.2005.tb00314.x
Moses, W. W. (2011). Fundamental Limits of Spatial Resolution in PET. Nucl. Instr. Methods Phys. Res. Section A: Acc. Spectrometers, Detectors Associated Equipment 648 (Suppl. 1), S236–S240. doi:10.1016/j.nima.2010.11.092
Najahi-Missaoui, W., Arnold, R. D., and Cummings, B. S. (2020). Safe Nanoparticles: Are We There yet. Int. J. Mol. Sci. 22 (1). doi:10.3390/ijms22010385
O'Brien, M. E., Wigler, N., Inbar, M., Rosso, R., Grischke, E., Santoro, A., et al. (2004). Reduced Cardiotoxicity and Comparable Efficacy in a Phase III Trial of Pegylated Liposomal Doxorubicin HCl (CAELYX/Doxil) versus Conventional Doxorubicin for First-Line Treatment of Metastatic Breast Cancer. Ann. Oncol. 15 (3), 440–449. doi:10.1093/annonc/mdh097
Patil, C. G., Walker, D. G., Miller, D. M., Butte, P., Morrison, B., Kittle, D. S., et al. (2019). Phase 1 Safety, Pharmacokinetics, and Fluorescence Imaging Study of Tozuleristide (BLZ-100) in Adults with Newly Diagnosed or Recurrent Gliomas. Neurosurg. 85 (4), E641–E649. doi:10.1093/neuros/nyz125
Pease, D. F., Peterson, B. A., Gilles, S., Hordinsky, M. K., Bohjanen, K. A., and Skubitz, K. M. (2019). Development of Cutaneous Squamous Cell Carcinoma after Prolonged Exposure to Pegylated Liposomal Doxorubicin and Hand-Foot Syndrome: a Newly Recognized Toxicity. Cancer Chemother. Pharmacol. 84 (1), 217–221. doi:10.1007/s00280-019-03849-8
Peeler, C. R., Mirkovic, D., Titt, U., Blanchard, P., Gunther, J. R., Mahajan, A., et al. (2016). Clinical Evidence of Variable Proton Biological Effectiveness in Pediatric Patients Treated for Ependymoma. Radiother. Oncol. 121 (3), 395–401. doi:10.1016/j.radonc.2016.11.001
Petersen, G. H., Alzghari, S. K., Chee, W., Sankari, S. S., and La-Beck, N. M. (2016). Meta-analysis of Clinical and Preclinical Studies Comparing the Anticancer Efficacy of Liposomal versus Conventional Non-liposomal Doxorubicin. J. Controlled Release 232, 255–264. doi:10.1016/j.jconrel.2016.04.028
Port, M., Raynal, I., Vander Elst, L., Muller, R. N., Dioury, F., Ferroud, C., et al. (2006). Impact of Rigidification on Relaxometric Properties of a Tricyclic Tetraazatriacetic Gadolinium Chelate. Contrast Media Mol. Imaging 1 (3), 121–127. doi:10.1002/cmmi.99
Puri, A., Loomis, K., Smith, B., Lee, J.-H., Yavlovich, A., Heldman, E., et al. (2009). Lipid-based Nanoparticles as Pharmaceutical Drug Carriers: from Concepts to Clinic. Crit. Rev. Ther. Drug Carrier Syst. 26 (6), 523–580. doi:10.1615/critrevtherdrugcarriersyst.v26.i6.10
Ren, C., Hu, X., Li, X., and Zhou, Q. (2016). Ultra-trace Graphene Oxide in a Water Environment Triggers Parkinson's Disease-like Symptoms and Metabolic Disturbance in Zebrafish Larvae. Biomaterials 93, 83–94. doi:10.1016/j.biomaterials.2016.03.036
Ruggieri, R., Naccarato, S., Mazzola, R., Ricchetti, F., Corradini, S., Fiorentino, A., et al. (2018). Linac-based VMAT Radiosurgery for Multiple Brain Lesions: Comparison between a Conventional Multi-Isocenter Approach and a New Dedicated Mono-Isocenter Technique. Radiat. Oncol. 13 (1), 38. doi:10.1186/s13014-018-0985-2
Russo, M., Ponsiglione, A. M., Forte, E., Netti, P. A., and Torino, E. (2017). Hydrodenticity to Enhance Relaxivity of Gadolinium-DTPA within Crosslinked Hyaluronic Acid Nanoparticles. Nanomedicine 12 (18), 2199–2210. doi:10.2217/nnm-2017-0098
Sandberg, D. I., Peet, M. M., Johnson, M. D., Cole, P., Koru-Sengul, T., and Luqman, A. W. (2012). Chemotherapy Administration Directly into the Fourth Ventricle in a Nonhuman Primate Model. Ped 9 (5), 530–541. doi:10.3171/2012.1.peds11410
Sandberg, D. I., Rytting, M., Zaky, W., Kerr, M., Ketonen, L., Kundu, U., et al. (2015). Methotrexate Administration Directly into the Fourth Ventricle in Children with Malignant Fourth Ventricular Brain Tumors: a Pilot Clinical Trial. J. Neurooncol. 125 (1), 133–141. doi:10.1007/s11060-015-1878-y
Scaringi, C., Agolli, L., and Minniti, G. (2018). Technical Advances in Radiation Therapy for Brain Tumors. Anticancer Res. 38 (11), 6041–6045. doi:10.21873/anticanres.12954
Sherman, J. C., Colvin, M. K., Mancuso, S. M., Batchelor, T. T., Oh, K. S., Loeffler, J. S., et al. (2016). Neurocognitive Effects of Proton Radiation Therapy in Adults with Low-Grade Glioma. J. Neurooncol. 126 (1), 157–164. doi:10.1007/s11060-015-1952-5
Sheu, T., Briere, T. M., Olanrewaju, A. M., and McAleer, M. F. (2019). Intensity Modulated Radiation Therapy versus Volumetric Arc Radiation Therapy in the Treatment of Glioblastoma-Does Clinical Benefit Follow Dosimetric Advantage. Adv. Radiat. Oncol. 4 (1), 50–56. doi:10.1016/j.adro.2018.09.010
Shrivastava, R., Raza, S., Yadav, A., Kushwaha, P., and Flora, S. J. S. (2014). Effects of Sub-acute Exposure to TiO2, ZnO and Al2O3nanoparticles on Oxidative Stress and Histological Changes in Mouse Liver and Brain. Drug Chem. Toxicol. 37 (3), 336–347. doi:10.3109/01480545.2013.866134
Sun, G., Wei, D., Liu, X., Chen, Y., Li, M., He, D., et al. (2013). Novel Biodegradable Electrospun Nanofibrous P(DLLA-CL) Balloons for the Treatment of Vertebral Compression Fractures. Nanomedicine: Nanotechnology, Biol. Med. 9 (6), 829–838. doi:10.1016/j.nano.2012.12.003
Tashima, T. (2020). Shortcut Approaches to Substance Delivery into the Brain Based on Intranasal Administration Using Nanodelivery Strategies for Insulin. Molecules 25 (21). doi:10.3390/molecules25215188
Teng, C. W., Huang, V., Arguelles, G. R., Zhou, C., Cho, S. S., Harmsen, S., et al. (2021). Applications of Indocyanine green in Brain Tumor Surgery: Review of Clinical Evidence and Emerging Technologies. Neurosurg. Focus 50 (1), E4. doi:10.3171/2020.10.focus20782
Valdés, P. A., Jacobs, V., Harris, B. T., Wilson, B. C., Leblond, F., Paulsen, K. D., et al. (2015). Quantitative Fluorescence Using 5-aminolevulinic Acid-Induced Protoporphyrin IX Biomarker as a Surgical Adjunct in Low-Grade Glioma Surgery. Jns 123 (3), 771–780. doi:10.3171/2014.12.jns14391
Viswanathan, V. K., Rajaram Manoharan, S. R., Subramanian, S., and Moon, A. (2019). Nanotechnology in Spine Surgery: A Current Update and Critical Review of the Literature. World Neurosurg. 123, 142–155. doi:10.1016/j.wneu.2018.11.035
Wang, D., Lin, B., and Ai, H. (2014). Theranostic Nanoparticles for Cancer and Cardiovascular Applications. Pharm. Res. 31 (6), 1390–1406. doi:10.1007/s11095-013-1277-z
Wang, Y. X. (2011). Superparamagnetic Iron Oxide Based MRI Contrast Agents: Current Status of Clinical Application. Quant Imaging Med. Surg. 1 (1), 35–40. doi:10.3978/j.issn.2223-4292.2011.08.03
Weber, D. C., Lim, P. S., Tran, S., Walser, M., Bolsi, A., Kliebsch, U., et al. (2020). Proton Therapy for Brain Tumours in the Area of Evidence-Based Medicine. Bjr 93 (1107), 20190237. doi:10.1259/bjr.20190237
Weidman, E. K., Dean, K. E., Rivera, W., Loftus, M. L., Stokes, T. W., and Min, R. J. (2015). MRI Safety: a Report of Current Practice and Advancements in Patient Preparation and Screening. Clin. Imaging 39 (6), 935–937. doi:10.1016/j.clinimag.2015.09.002
Weissleder, R. (2006). Molecular Imaging in Cancer. Science 312 (5777), 1168–1171. doi:10.1126/science.1125949
Wu, L. C., Zhang, Y., Steinberg, G., Qu, H., Huang, S., Cheng, M., et al. (2019). A Review of Magnetic Particle Imaging and Perspectives on Neuroimaging. AJNR Am. J. Neuroradiol 40 (2), 206–212. doi:10.3174/ajnr.a5896
Yao, A. D., Cheng, D. L., Pan, I., and Kitamura, F. (2020). Deep Learning in Neuroradiology: A Systematic Review of Current Algorithms and Approaches for the New Wave of Imaging Technology. Radiol. Artif. Intelligence 2 (2), e190026. doi:10.1148/ryai.2020190026
Ze, Y., Sheng, L., Zhao, X., Hong, J., Ze, X., Yu, X., et al. (2014). TiO2 Nanoparticles Induced Hippocampal Neuroinflammation in Mice. PLoS One 9 (3), e92230. doi:10.1371/journal.pone.0092230
Ze, Y., Zheng, L., Zhao, X., Gui, S., Sang, X., Su, J., et al. (2013). Molecular Mechanism of Titanium Dioxide Nanoparticles-Induced Oxidative Injury in the Brain of Mice. Chemosphere 92 (9), 1183–1189. doi:10.1016/j.chemosphere.2013.01.094
Keywords: nanotechnology, neurosurgery, neuro-oncology, neuroradiology, neuro-imaging
Citation: Lam FC, Salehi F and Kasper EM (2022) Integrating Nanotechnology in Neurosurgery, Neuroradiology, and Neuro-Oncology Practice—The Clinicians’ Perspective. Front. Bioeng. Biotechnol. 10:801822. doi: 10.3389/fbioe.2022.801822
Received: 25 October 2021; Accepted: 11 January 2022;
Published: 09 February 2022.
Edited by:
Qingxin Mu, University of Washington, United StatesReviewed by:
Enza Torino, University of Naples Federico II, ItalyErik Dreaden, School of Medicine Emory University, United States
Copyright © 2022 Lam, Salehi and Kasper. This is an open-access article distributed under the terms of the Creative Commons Attribution License (CC BY). The use, distribution or reproduction in other forums is permitted, provided the original author(s) and the copyright owner(s) are credited and that the original publication in this journal is cited, in accordance with accepted academic practice. No use, distribution or reproduction is permitted which does not comply with these terms.
*Correspondence: Fred C. Lam, ZmxhbTFAbm9ydGh3ZWxsLmVkdQ==