- 1Department of Mechanical Engineering, University of Colorado Boulder, Boulder, CO, United States
- 2McGowan Institute for Regenerative Medicine, University of Pittsburgh, Pittsburgh, PA, United States
- 3Department of Mechanical Engineering, North Dakota State University, Fargo, ND, United States
Vascular grafts are widely used for vascular surgeries, to bypass a diseased artery or function as a vascular access for hemodialysis. Bioengineered or tissue-engineered vascular grafts have long been envisioned to take the place of bioinert synthetic grafts and even vein grafts under certain clinical circumstances. However, host responses to a graft device induce adverse remodeling, to varied degrees depending on the graft property and host’s developmental and health conditions. This in turn leads to invention or failure. Herein, we have mapped out the relationship between the design constraints and outcomes for vascular grafts, by analyzing impairment factors involved in the adverse graft remodeling. Strategies to tackle these impairment factors and counteract adverse healing are then summarized by outlining the research landscape of graft innovations in three dimensions—cell technology, scaffold technology and graft translation. Such a comprehensive view of cell and scaffold technological innovations in the translational context may benefit the future advancements in vascular grafts. From this perspective, we conclude the review with recommendations for future design endeavors.
1 Introduction
Vascular grafts are widely used for vascular surgeries. Their uses occur in a variety of forms, including vascular access for hemodialysis, as well as surgical bypass for diseased coronary artery, cerebral artery, or peripheral artery in the abdomen (e.g., aorto-iliac bypass) or in the leg (e.g., femoro-popliteal bypass), routing blood flow around an area of blockage or narrowing. A vascular graft can be a native artery or vein, or fabricated from synthetic or natural biomaterials. The current gold standard for a graft is autologous blood vessels such as a saphenous vein, which has better clinical outcomes than biomaterials-based grafts (Caliskan et al., 2020), even those made with tissue engineering approaches (Zilla et al., 2020). A vein fistula for dialysis access, for example, shows better primary patency than a polytetrafluoroethylene (PTFE) graft (Stegmayr et al., 2021). Similarly, for femoro-popliteal bypass, the 5-year patency rate of a vein graft is higher than a PTFE graft (van der Slegt et al., 2014). However, a quality vein suitable for grafting may be unavailable for many patients, particularly elders (Conte, 2012; AbuRahma, 2018). Also, to become a dialysis access, a vein must undergo a prolonged process to gain sufficient strength until its maturation into artery-like property. The maturation of a vein fistula requires several weeks up to months, during which compromised dialysis access may deteriorate patients’ health (Robbin et al., 2016). To make it worse, the incidence of fistula non-maturation varies between 20 and 60% (Kosa et al., 2015; Siddiqui et al., 2017).
Thus, bioengineered or tissue-engineered vascular grafts (TEVGs) have long been envisioned to take the place of synthetic grafts and even vein grafts under certain clinical circumstances. Selective TEVGs have gradually progressed into clinical trials, for both adult patients (Lawson et al., 2016; Kirkton et al., 2019) and pediatric patients with congenital heart diseases (Hibino et al., 2010; Drews et al., 2017; Sugiura et al., 2018; Drews et al., 2020), showing some promises despite yet limited successes. The clinical potentials of TEVGs, acellular or cellular, still require tremendous efforts to unveil (Zilla et al., 2020; Fang et al., 2021a). One possible route is to cater the TEVG development to a specific grafting need or a patient cohort, in which factors that impair healing or regeneration around the vascular graft can be well defined and targeted for graft design and engineering.
To this end, this review starts with analyzing healing- or regeneration-impairment factors, which are tackled by recent graft innovations. To withstand or override the influences of impairment factors, pro-healing and/or pro-regenerative environments could be precisely defined and built into a graft through structural optimizations, signal molecule incorporations, and integration of bioactive materials, cells or cell products. As illustrated in Figure 1, major healing-impairing factors are identified according to their roles in causing the dysfunction of a graft and/or neighboring blood vessels. A number of studies have been designed to reveal cause-effect relationships and unravel underlying molecular mechanisms. To counteract these impairing factors, a variety of strategies that promote design optimization, graft healing or regeneration have emerged. We focused a literature review on those published in recent years and placed them in the landscape of graft innovations in translation, scaffold and cell technologies. Essential to the future graft development is a continual effort to balance the multi-dimensional graft innovations with the fundamental mechanistic understandings underlying adverse graft remodeling.
2 Factors impairing healing or regeneration around vascular grafts
In clinic, graft outcomes in the treatment of vascular diseases are often determined by the size and location of the devices as well as the patients’ developmental and health conditions, besides the graft type (i.e., native vessel vs. synthetic graft). Herein, we examined these graft design constraints and associated clinical outcomes, by analyzing relevant impairment factors such as flow-graft interactions, cellular and molecular environments around grafts, and graft bioinertness.
2.1 Graft location, size and compliance: Related to flow-graft interactions
Abnormal blood flow in the graft has long been known as a key impairment factor that alters graft healing and overall patency (Schwarz et al., 2021). Computational flow dynamics tools, with the inputs of graft location, diameter, length and/or compliance together with graft design, have been applied to predict not only flow conditions but also consequent remodeling of a graft (Lu et al., 2014; Ramachandra et al., 2017; Donadoni et al., 2020). Variations in these physical characteristics of a graft delicately influence local fluid stresses in a blood vessel or a graft. Abnormal hemodynamic stresses may further result in impaired graft endothelialization, impaired graft healing and endothelial disruption in neighboring vessels (Chistiakov et al., 2017). Irregularities in the wall shear stress are known to induce endothelial dysfunctions, leading to negative clinical outcomes such as intimal hyperplasia, wherein smooth muscle cells (SMCs) grow to restrict the lumen of a vessel or a graft (Chistiakov et al., 2017; Ng et al., 2017; Totorean et al., 2019). Interestingly, in the case of dialysis access graft, hyperplasia develops predominantly in the vein or anastomotic regions, particularly toe and heel of distal anastomosis, where flow disturbances and altered hemodynamic factors are most prevalent (Sottiurai et al., 1989; Bassiouny et al., 1992). Quantitatively, low shear stress values (<.5 Pa) or oscillatory values (±.1–.2 Pa), as opposed to physiological values (1–2 Pa), were shown to induce endothelial dysfunction and subsequent hyperplasia development (Dhawan et al., 2010). The graft location determines flow environments around a graft (Chiu and Chien, 2011); abnormal flow patterns might occur in arteries with branches or curvatures or grafts such as looped vascular access or brachial arterial grafts as compared to aortic grafts.
The graft diameter is key to reach a hemodynamic balance. Bypass grafts with unfavorable diameter ratios to the original vessel can result in competitive flow, ultimately leading to graft failure. Competitive flows occur when a partially stenotic blood vessel is bypassed with a graft, but still retains some of its throughput, thereby competing with the newly grafted path and causing irregular flow through it (Gaudino et al., 2016). A high competitive flow decreases the amount of blood that reaches the graft, creating low shear stresses that increase the levels of hyperplasia at the graft’s anastomosis (Jin and Liu, 2019). Clinical findings suggest that more stenotic vessels with lower competitive flow, compared to less stenotic vessels, were better tolerated by coronary bypasses, and arterial grafts were more susceptible to competitive flow-induced impairment than venous grafts (Nordgaard et al., 2010; Gaudino et al., 2016; Sabik Joseph, 2016). The presence of chronic competitive flow is inversely related to internal mammary graft patency (Binns et al., 1989; Sabik et al., 2003). Intriguingly, saphenous vein graft patency did not decrease with increasing competitive flow (Ding et al., 2012; Swillens et al., 2012; Sugiura et al., 2018). Such difference could be attributed to cell and tissue differences between venous and arterial grafts (Harskamp et al., 2016; Sabik Joseph, 2016). Thus, the graft diameter in comparison with the original vessel is an important consideration for reducing flow-induced impairment, in particular for some graft types.
The roles of graft compliance and length in determining the graft patency are also well known (Skovrind et al., 2019). Compliance mismatch was recognized as a major contributor to graft failure (Tiwari et al., 2003). Though the influence of graft length on patency rates was insignificant for a similar grafting application (Tinica et al., 2018; Bosiers et al., 2020), applications that require long grafts, such as femoral artery lesion and hemodialysis access, often yielded low patency rates (Connors et al., 2011; Boutrous et al., 2019). This may be attributed to a wide range of biomechanical forces across a long graft with a large surface area. These forces consist of a complex loading combination from varying amounts of radial deformation, bending, extension/contraction, and torsion/flexion, which significantly impact the cell-graft interactions.
2.2 Patient’s conditions: Related to cellular and molecular environments around grafts
The graft failure in the cases of coronary bypass, dialysis access, or stent graft have been variably associated to age, diabetes mellitus, hypertension or other health conditions (Gaudino et al., 2017; AbuRahma, 2018; Misskey et al., 2018). Sex may serve as a possible compounding factor (Blum et al., 2021). Diabetes mellitus in particular causes a high risk of platelet aggregation in the blood and increases the release of von Willebrand factor, which promotes platelet aggregation and results in significant endothelial damage and arterial stiffening (Creager et al., 2003). This vascular intima damage coupled with the pathophysiological mechanism of diabetes leads to the increased formation of thrombosis (Reddy et al., 2013), and thus sooner obstruction of a graft such as arteriovenous fistula (Afsar and Elsurer, 2012). The primary failure of fistulas is associated with an enormous overall cost. Interestingly, arteriovenous fistula showed even higher primary failure rates than synthetic grafts in patients with diabetes mellites (Qin et al., 2016). To make it worse, failure of a fistula reduces the number of possible sites for an alternate access, exposing the patients to risks from salvaging attempts (Schinstock et al., 2011). Therefore, using hemodialysis access for diabetic patients as an example, it is clear that patients’ health conditions complicate cellular and molecular environments around grafts, worsening the ultimate fate of grafts, in particular biological grafts. For above-knee femoropopliteal bypass, diseases including diabetes and ischemia significantly reduced graft patency in both vein and PTFE grafts, but showing similar reduction rates for both types of grafts (Klinkert et al., 2004). Furthermore, during atherosclerosis such as coronary artery diseases, systemic biological factors (e.g., diabetes mellitus, dyslipidemia, sex, age, hypertension, and smoking) and local biological mechanisms (e.g., increased oxidative stress, vascular inflammation, and endothelial dysfunction) all contribute to the initial plaque formation and subsequent progression (Gaudino et al., 2017). These classic cardiovascular risk factors, particularly diabetes mellitus, seem to play a role in determining graft failure. For instance, the impact of diabetes mellitus on arterial and venous bypass grafts has been investigated by several groups, with most but not all, showing a detrimental effect on graft patency (Gaudino et al., 2017).
Besides comorbid diseases, the patency of a biological graft, compared to an inert synthetic graft, might be more influenced by cellular and molecular environments associated with older age. Older patient’s arteries tend to be more easily calcified making them more prone to abnormal shear stress, inflammation and other endothelial dysfunction (Tesauro et al., 2017). Due to significant comorbidities in elderly patients, a vein fistula is more likely to fail due to the deteriorating condition of blood vessels (Miller et al., 1999; Ponce, 2001; Lazarides et al., 2007). The use of synthetic PTFE grafts instead of vein fistula in elderly patients and those with compromised vessels might improve primary patency rates (Staramos et al., 2000; Rooijens et al., 2005). Clinical data point to advanced age as a significant factor in adversely affecting outcome of dialysis graft (Sugimoto et al., 2003; Moist et al., 2012). Meanwhile, almost half of all patients initiating dialysis are 65 years of age or older in the United States and the percentage of elderly patients is even higher in Canada and Europe (Ahmed and Catic, 2018), which makes the age as an important consideration for the graft design. It is increasingly recognized that an advanced age is associated with adverse graft remodeling and differentially altered remodeling of vein fistula and synthetic graft, likely through aged-related changes in vascular cells and molecular environments initiated by increases in pro-inflammatory cytokines, pro-calcification signaling, advanced glycation end products, and/or extracellular matrix stiffness. Similarly, when a bioengineered graft or TEVG include a cellular and/or molecular component, its design should consider adverse environments related to clinical conditions such as aging. In the case of vein fistula for dialysis access, its risk of failure is greatest among older patients and/or obese patients (Lok et al., 2006; Peterson et al., 2008; Shingarev et al., 2011).
2.3 Bioinertness of grafts
Graft bioinertness, or lack of biointegration, is another major impairment factor for long-term graft patency. It is mainly related to commercial grafts such as those made of PTFE or Dacron, but could be variedly applicable to different TEVGs, particularly prior to the completion of vascular tissue regeneration. Previous review articles have elaborated the main issues with the graft inertness, which include thrombosis, inflammation and intimal hyperplasia of a graft. Infection can also be a serious issue (Kirkton et al., 2018), in particular for dialysis graft requiring continuous needle access (Halbert et al., 2020; Kostokis and Loukopoulos, 2020; Stegmayr et al., 2021). A generally accepted root cause of these issues is the poor interaction of cells and the non-specific adsorption of proteins onto the graft, which lead to platelet aggregation but fail to form organized endothelium and/or matured smooth muscle. To circumvent these issues, bioengineering strategies including chemical coatings, materials modifications with peptides, proteins or other biomolecules, and seedings of endothelial cells or stem cells on inert or degradable grafts, have been utilized, with the goal of better integrating grafts with the native vasculature (Hielscher et al., 2018; Wang et al., 2020; Ham et al., 2021; Liu et al., 2021; Yu et al., 2021). Results with these strategies demonstrated improved endothelialization and/or inhibited thrombosis, inflammation, intimal hyperplasia, or infection. More recent approaches involve degradable, bioactive materials, as described below.
3 Counteracting adverse remodeling with regenerative signals
To tackle above-mentioned factors involved in adverse graft remodeling, we summarize a three-thronged approach being taken in this field. A focused literature review of recent publications is taken to map out the research landscape of graft innovations in three dimensions, namely innovations in cell technology, scaffold technology and translation (Figure 2). Each referred study is a data point plotted on three axes in the attempt to show the relationship of the study in the context of the three innovations. Thus, a comprehensive perspective is taken for a combined view of both cell and scaffold technological innovations in the translational context.
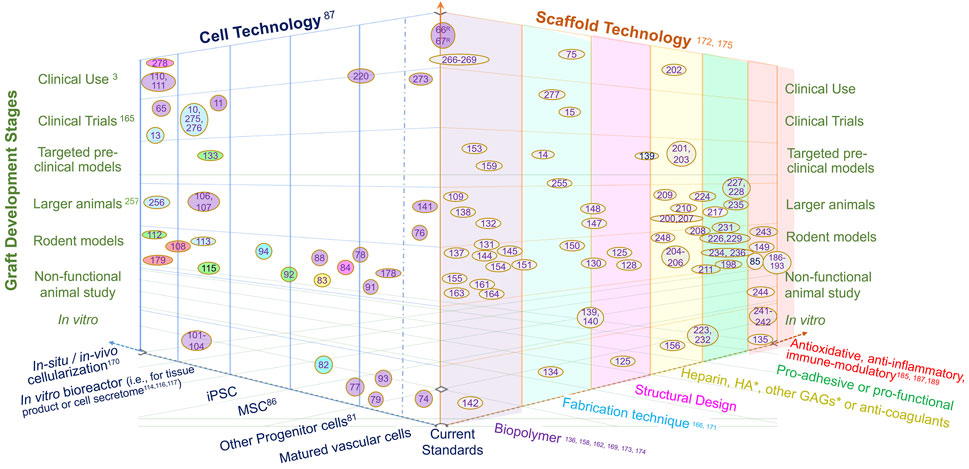
FIGURE 2. The landscape of vascular graft research in three dimensions, namely cell technology, scaffold technology and development stage. The citations of references used here are the numbered references listed in numerical order as they appear in the paper, as shown in Supplementary Material. The reference literatures are mapped in this landscape by locating research studies in the 3-dimensional space with reviews with various scopes labeled on the axes. The colors of oval boxes in the space of cell technology are correlated to the axis scaffold, showing the scaffold technology utilizied in these studies. Herein, “Current standards” refer to autologous graft (vein or artery) or bioinert graft (mostly PTFE-based); “Targeted preclinical models” refer to animal studies targeted at a clinical application such as dialysis access, Fontan graft, bypass for coronary, femoral or other arteries; “Larger animal” refer to animal models larger than rodents, such as rabbit, sheep, pig, dog and balloon; “Non-functional animal study” refer to non-vascular implants (often subcutaneous) or those used in ex vivo circulation; “In situ or in vivo cellularization” refer to the cellularization and/or tissue production by implanting acellular polymer; “Matured vascular cells” refer to vascular endothelial, smooth muscle and fibroblast cells; “Other progenitor cells” including endothelial progenitor cell and smooth muscle progenitor cell; “Biopolymer” includes biodegradable polymer , biologically-derived polymer, decellularized ECM or vessel, bioinert polymer, etc. *HA, hyaluronic acid; GAGs, glycosaminnoglycans.
3.1 Cell technology
The contribution of cell technology to counteract adverse remodeling is to enhance graft acceptance and integration with native vessels, including attenuating chronic inflammation, reducing thrombogenicity, and promoting fast healing and vascular regeneration. The cell technology includes the technology to isolate, culture and use different types of cells for vascular graft applications, the technology to recruit cells into grafts, and the technology to produce cell products crucial for regeneration. Herein, a brief overview is taken on each cell type used for vascular grafting, highlighting recent innovations in tissue bank, immune-tolerant cells, and bioreactors. Recent cell technology has been expanded to include the use of cell secretomes, while on the other end adding a range of in situ or in vivo cellularization technique into the realm of cell technology.
Autologous matured vascular cells, such as SMCs, endothelial cells and fibroblasts (McAllister et al., 2009; Syedain et al., 2011; Amensag and McFetridge, 2012), were employed for the TEVG creation in early preclinical and clinical studies. They are not immunogenic, but their disadvantages include invasive harvest of vascular cells, limited availability, varied quality, and low potential of replication and regeneration.
Progenitor cells, compared to matured cells, are isolated using less invasive procedures, from blood, bone marrow, umbilical cord, adipose or other tissues, and they demonstrate greater proliferative capacities despite their scarcity in tissues. For example, bone marrow-derived smooth muscle progenitor cells, compared to adult vascular SMCs, produced stronger and tougher TEVGs in vitro, and consequently progenitor seeded-grafts produced more organized elastin when implanted in vivo as jugular replacements in lambs (Swartz et al., 2005; Liu et al., 2007). Similarly, endothelial progenitor cells have been used to seed TEVG (Melchiorri et al., 2016; Muniswami et al., 2020; Tamma et al., 2020), but their high heterogeneity and rare presence could complicate the translation process (Munisso and Yamaoka, 2020). Mesenchymal stem cell (MSC), a type of progenitor cell with the potential of generating various vascular cell types (Gong and Niklason, 2008), lacks major histocompatibility complexes or other immune-stimulatory molecules while secreting anti-thrombotic and anti-inflammatory molecules, such as IL-10 (Fukunishi et al., 2018; Mirhaidari et al., 2020), and TGF-β receptor 1 (Lee et al., 2016). These characteristics make MSC an excellent allogeneic cell candidate for the TEVG production (Wang et al., 2016; Afra and Matin, 2020). In particular, adipose-derived MSCs are advantageous for their easy extraction in high quantities and high regenerative potency in a way unaffected by age (Harris et al., 2011; Zhang et al., 2011; Krawiec et al., 2016; Krawiec et al., 2017; Haskett et al., 2018). Finally, other new types of progenitor cells such as pericardial effusion-derived progenitor cells (Wang et al., 2021) can be potential candidates for cellularizing vascular grafts.
Induced pluripotent stem cells (iPSCs) have the potential of generating patient-specific SMCs, endothelial cells, and fibroblasts for TEVGs (Generali et al., 2019; Luo et al., 2020; Shi et al., 2021). The availability of iPSC-derived cell sources may eliminate the problems with variation in the cell quality and address the timing issue for “off-the-shelf” availability, both of which are concerns relevant to progenitor cells. Nevertheless, a bottleneck of using human iPSC derivatives is their tumorigenicity and immunogenicity (de Almeida et al., 2013). Thus, human leukocyte antigen-matched iPSC tissue banks would be a valuable source for personalized grafts (Jin et al., 2016; Petrus-Reurer et al., 2021). Additionally, genetically engineered master human iPSC lines, which give rise to immune-tolerant (immune-evasive or immune-suppressive) iPSC derivatives (Riolobos et al., 2013), might yield universal “off-the-shelf” cell sources for hypo-immunogenic TEVGs.
Cell seeding in vitro often requires an incubation time ranging from days to months in a dish or in a sophisticated bioreactor. Bioreactor designs vary from a rotary bioreactor for stem cell differentiation (Xing et al., 2017; Li et al., 2019a), a closed apparatus for standardizing cell technology (Kurobe et al., 2015), a biaxial stretching system for tissue matrix maturation (Huang et al., 2015; Huang et al., 2016), a semi-automated cell seeding device (Cunnane et al., 2020a), to a perfusion bioreactor for applying flow stresses (Melchiorri et al., 2016) and individualized graft conditioning (Håkansson et al., 2021). Another recent innovation in the in vitro cell seeding technology is to exploit 3D bioprinting techniques for printing patterned cell-laden hydrogels, utilizing a mixture of cells and hydrogel as the bioink to create vascular grafts with precisely-defined structures.
Recently, in situ or in vivo cellularization, utilizing human (or animal) body as a bioreactor, added a novel option into the cell technology for vascular grafts. This approach starts with placing a tube composed of Teflon (Wang et al., 2019a; Qiu et al., 2021), silicone (Fujita et al., 2020) or collagen (Nakayama et al., 2020), as a subcutaneous or peritoneal cavity implant or in an ex vivo location, allowing fibrous tissues to grow and form an tubular structure, which is then explanted and subsequently implanted as an autologous graft (Chen et al., 2019; Nakayama et al., 2020) or as an allograft after decellularization (Wang et al., 2019a; Fujita et al., 2020; Qiu et al., 2021). Preclinical or clinical studies showed some encouraging outcomes.
Last but not least is that the future cell technology for vascular grafts will surely see an increased use of stem cell secretomes (Chen et al., 2018; Cunnane et al., 2018; Cunnane et al., 2020b), as their therapeutic and regenerative values are being unveiled (Ahangar et al., 2020; Baruah and Wary, 2020; Nikfarjam et al., 2020; Tang et al., 2021) and recently exploited in other cardiovascular implants (Li et al., 2019b; Baruah and Wary, 2020; Hu et al., 2021).
3.2 Scaffold technology
The scaffold technology encompasses the techniques to improve the graft structure and mechanics and those to improve the biological integration. The former includes design innovations involving novel computational tools, biopolymers, or scaffold fabrication techniques ranging from decellularization to electrospinning and 3D printing. The scaffold technology for improved biological integration often involves the incorporation of a range of bioactive functional biomolecules on the surface or within a biopolymer, in order to modulate immune responses and/or to promote regeneration.
3.2.1 Structural optimization and scaffold fabrication
As described in Section 2.1, the patency of vascular grafts is largely determined by the structural and mechanical interactions between blood flow and vascular graft, which continuously alter neotissue growth and graft remodeling. The neotissue growth and graft remodeling in turn change the flow-graft interactions. Therefore, computational models simulate such interactions as well as advanced statistical analyses of a parametric cause-effect relationship have been exploited to accelerate the rational optimization of vascular grafts (Keshavarzian et al., 2019; Tamimi et al., 2019; Khosravi et al., 2020). Recently, data-informed models have been developed to improve graft designs, even toward patient-specific design (Best et al., 2018), for desired graft performances in terms of lumen diameter, extracellular matrix (ECM) production, and levels of inflammation (Best et al., 2019; Drews et al., 2020). Model parameters comprise not only graft compliance, pore size, fiber diameter and degradation rate (Szafron et al., 2019; Tamimi et al., 2019; Furdella et al., 2021), but possibly also polymer chemistry, cytokine and other biological inputs (Szafron et al., 2018; Khosravi et al., 2020). Importantly, model outputs were compared to experimental outcomes and further predicting outcomes, which thus unravel mechano-biological mechanisms of neovessel formation and graft degradation in vivo. For example, models have been used to guide the design of mechanocompatible grafts (Furdella et al., 2021), to examine the role of inflammation activity using immuno-compromised animals (Szafron et al., 2018), or to parametrically explore graft narrowing (Drews et al., 2020; Khosravi et al., 2020). Besides computational models, statistical methods were used to reveal the roles of specialized design variables such as braiding angle, braiding density and coating structure in the graft development (Zbinden et al., 2020). Collectively, these studies have confirmed the well-accepted tissue engineering principle—the successful neovessel formation requires neotissue development to balance the scaffold degradation. However, such balance varies greatly with the animal model (Fukunishi et al., 2020). Lack of a balanced coordination over the time can result in adverse remodeling such as excessive degradation, impaired ECM synthesis, elevated inflammation and neotissue overgrowth in the lumen, leading to aneurysm (Best et al., 2019) or stenosis (Drews et al., 2020), and ultimately graft failure (Stowell et al., 2020). Figure 3 illustrates graft wall remodeling in various scenarios, where physiological routes ended up with partial or complete regeneration.
In parallel with the development of scaffold optimization tools, equally important is a rich availability of scaffold fabrication techniques and biopolymer choices, both of which promote parametric control for optimizations. Some recent developments in the graft fabrication include: 1) Shortening the production time for cell sheet self-assembly method (von Bornstädt et al., 2018); 2) loading drugs, anti-thrombogenic or pro-regenerative molecules for electrospun grafts or 3D printed grafts (Zhang et al., 2019; Domínguez-Robles et al., 2021); 3) refining decellularization protocols for reduced immunological responses (Schneider et al., 2018; Valencia-Rivero et al., 2019; Kimicata et al., 2020; Lopera Higuita et al., 2021); 4) improving the precision of pore generation in scaffold (Zhen et al., 2021); 5) enhancing recellularization for allogenic or xenogenic decellularized grafts (Dahan et al., 2017; Lin et al., 2019; Fayon et al., 2021); 6) expediting degradation with scaffold composition (Fukunishi et al., 2021) or textile technique (Fukunishi et al., 2019) to enhance matrix remodeling; 7) mimicking the structure and/or composition of vascular ECM using electrochemical fabrication (Nguyen et al., 2018) or an automated technology combining dip-spinning with solution blow spinning (Akentjew et al., 2019); 8) creating patient-specific grafts (Fukunishi et al., 2017); and 9) hybrid approaches, for example, combining electrospinning with decellularized matrices (Gong et al., 2016; Ran et al., 2019; Wu et al., 2019; Yang et al., 2019).
Biopolymers used for the graft construction kept diversifying the graft repertoire. For instance, the recent addition of shape memory polymer as a self-enclosable external support to a graft reduced stenosis (Yi et al., 2021). Currently, graft biopolymers consist of biologically-derived biomaterials such as silk fibroin (Zamani et al., 2017; Gupta et al., 2020; Tanaka et al., 2020), collagen (Li et al., 2017; Copes et al., 2019) and fibrin (Syedain et al., 2017), and synthetic polymers mostly degradable polymers such as polycaprolactone (Mrówczyński et al., 2014), polyglycolic acid (Sugiura et al., 2017), polyurethane (Zhen et al., 2021; Fathi-Karkan et al., 2022), polycarbonate urethane (Eilenberg et al., 2020), polylactic acid, and poly (l-lactide-co-ε-caprolactone) (Zhu et al., 2018). Hybrid graft materials composed of both biological polymers and synthetic polymers further leveraged the benefits offered by both materials for the mechanics and biological recognition of a graft. For further information, a number of reviews have provided historical views and in-depth analyses of graft fabrication techniques and graft polymers (Hiob et al., 2017; Pagel and Beck-Sickinger, 2017; Song et al., 2018; Chandra and Atala, 2019; Gentile et al., 2020; Niklason and Lawson, 2020; Syedain et al., 2020; Yuan et al., 2020; Gupta and Mandal, 2021; Heng et al., 2021; Zhang et al., 2021).
By and large, a long-term, dynamic, mechanistic view is taken into the design parameters and optimizations for vascular grafts, while continuous advancements in fabrication techniques and materials allow diverse choices and better controls over design parameters. A central finding in the graft optimization is that dynamically evolving mechanical properties can dictate the levels of inflammation and stress shielding of cells, and in turn affect mechanobiologically-mediated ECM production, remodeling and degradation (Szafron et al., 2019; Tamimi et al., 2019; Khosravi et al., 2020; Furdella et al., 2021). Therefore, adjusting the material chemistry and fabrication technique for desired dynamic profiles of graft mechanics in a specific clinical setting would be essential to future improvements.
3.2.2 Starting right at the onset—molecular designs for anti-thrombotic and anti-inflammatory control
Events immediately occurring upon vascular grafting in the body involve endothelial injury platelet activation, and acute inflammation. Properly regulating these early events is crucial to the fate of a graft, either promoting regeneration or preventing failure from thrombosis or intimal hyperplasia (Roh et al., 2010; Tseng et al., 2014; Wissing et al., 2017). Talacua et al. (2015) showed that modulating the initial inflammatory phase in the first days remarkably improved the 3-month implantation outcome. In fact, the immune response is always pivotal to tissue regeneration: on one hand, it is indispensable for proper healing; on the other hand, extensive, prolonged inflammation prevent regeneration (Julier et al., 2017). Ideally, after acute inflammation, a vascular graft would start healing, gradually reach long-term structural stability, restore the normal vascular flow, and regenerate vascular functions. However, when a graft undergoes a healing process with a prolonged presence of inflammatory cells and non-vascular replacement tissues, fibrous formation and fibrous encapsulation may dominate, compromising graft mechanics, vascular flow and flow-dependent vascular cell functions and tissue regeneration (Boccafoschi et al., 2014).
Critical in the initial healing process is the macrophage activity, which determines ultimate graft remodeling or regeneration (Rodriguez-Soto et al., 2021). Macrophage functions are often performed through macrophage polarization (M1/M2) as well as their secreted factors including cytokines acting directly or indirectly on the vascular cells to regulate arteriogenesis (Boccafoschi et al., 2014). Cytokines such as IL-4 and IL-10 have been implicated in the transition of macrophages from a pro-inflammatory phenotype to an anti-inflammatory, pro-healing phenotype in vivo (Browne and Pandit, 2015). The biomaterials form, crosslinking level, degradability, hydrophilicity, topography, and materials choice of an implant can all affect the immune system, triggering varied inflammation (Boccafoschi et al., 2014; Browne and Pandit, 2015). Macrophage infiltration and polarization provides a strategy for predicting, detecting, and inhibiting stenosis in TEVGs (Szafron et al., 2018).
Because of the pivotal roles of macrophages at the onset of grafting (Zhang and King, 2022), recent strategies towards regulating macrophage functions include the use of immunomodulatory agents, drugs, cytokines, and cells such as progenitor cells. Immunomodulatory agents such as losartan (an angiotensin-II type1 receptor antagonist), zoledronate (a bisphosphonate), and aspirin-triggered resolvin D1, for example, significantly reduce macrophage infiltration or expedite inflammation resolution, thus attenuating graft stenosis and/or promoting regeneration (Ruiz-Rosado et al., 2018; Shi et al., 2019a; Chang et al., 2021). Grafts loaded with immunomodulatory cytokines, such as IL-4 (Tan et al., 2019) or human Wharton’s jelly matrix (rich in immunomodulatory cytokines) (Gupta et al., 2021), enhanced M2 macrophage percentage, which suppressed intimal hyperplasia (Tan et al., 2019) or promoted functional regeneration (Gupta et al., 2021). Similarly, immunomodulatory cells, either by in vitro cell seeding or by circulating cell recruitment via ligands immobilized to a graft, successfully regulated macrophage polarization and inflammation process. Fukunishi et al., for instance, seeded mononuclear cells onto electrospun TEVG scaffolds, which reduced platelet activation and attenuated graft stenosis through a higher M2 macrophage percentage and lower macrophage infiltration (Fukunishi et al., 2018). Lorentz et al. loaded microparticles with monocyte recruitment factor (C-C motif chemokine ligand 2) to induce tissue remodeling of vascular grafts (Lorentz et al., 2021). Shafiq et al. (2018) used Substance P and stromal cell–derived factor-1α peptide, both of which encouraged the recruitment of bone marrow cells to initiate healing and anti-inflammatory response. Similarly, Kim et al. (2019) also used Substance P for cell recruitment and accelerated regeneration.
Thrombosis is another early adverse event for a vascular graft and is caused by the lack of a functional endothelium which prevents the aggregation of blood components. Due to the essential roles of endothelial cells in the regulation of thrombogenesis, the in vitro endothelialization of a vascular graft was used prior to implantation. But for acellular grafts, if antithrombotic therapy is not used (Fang et al., 2021b), anti-fouling polymers or anticoagulants such as heparin must be attached to the graft surface for thrombogenic reduction through the prevention of platelet adhesion and/or activation (Radke et al., 2018). Long, flexible hydrophilic polymer chains can offer protein-repellent backgrounds for anti-coagulation (Thalla et al., 2012; Strang et al., 2014); multi-armed hydrophilic polymers further allow other functional molecules to be clickable to the graft surface (Iglesias-Echevarria et al., 2021). Binding of heparin to vascular grafts continues to be the most effective and widely-used method to prevent thrombosis, meanwhile heparin has anti-inflammatory effect and inhibitory effect on intima hyperplasia (Poterucha et al., 2017). Heparin binding can be via chemical conjugations or physical methods, but chemical bonding may compromise mechanical compatibility (Jiang et al., 2016). Regardless of graft materials or fabrication methods, heparinized grafts, including ePTFE grafts (Samson et al., 2016; Freeman et al., 2018), electrospun grafts (Qiu et al., 2017; Xu et al., 2018; Shi et al., 2019b; Matsuzaki et al., 2021; Zhu et al., 2021), and decelluarized grafts (Kong et al., 2019), all outperformed those without heparin in terms of graft patency, showing critical roles of heparin in the thromboresistance and in situ endothelialization. Besides heparin, anticoagulation methods include the incorporation of anti-thrombotic drugs (Lee et al., 2022), hyaluronic acid (Dimitrievska et al., 2020) or more sophisticated ECM secreted by thrombospondin-2 knockout cells on decellularized aorta grafts (Kristofik et al., 2017).
In summary, regulating the platelet activity and immune response to a graft is pivotal to initiate graft healing and determine regeneration—galvanize or depress it. The final stage of immune responses involves the coordination of macrophages, endothelial cells and SMCs. The migration and proliferation of the latter two result in the regeneration of new functional arterial tissues. Disruption to the collaborative efforts of these cells as well as any negative exogenous factors such as infection, stresses or environmental factors, can lead to thrombosis and hyperplasia, and eventually graft failure. Therefore, factors influencing graft inflammation at the onset may largely determine its long-term patency. Additional factors affecting the long-term graft patency include physiochemical properties of biomaterials, such as hydrophobicity, stiffness and bioinertness. The current graft material, PTFE, for example, in some cases, induces severe graft calcification, resulting in suboptimal mid-term and long-term outcomes.
3.2.3 Biological functionalization of a graft for vascular regeneration
Due to indispensable, protective roles of endothelial cells throughout the vasculature, it is widely accepted that the endothelialization of a vascular graft is of the utmost importance to graft designers. Reinstitution of a continuous endothelium monolayer on the lumen surface of a graft is the best way to avoid the activation of coagulation cascade and progression of intimal hyperplasia (Heath, 2017). Four endothelialization mechanisms have been identified (Figure 4). The pros and cons of each are summarized in Table 1. Transanastomotic growth refers to the migration of matured endothelial cells from the native vessel onto the luminal surface of the graft across the anastomoses, where a vascular graft is connected to a native vessel. It served as a major endothelialization mechanism in animal models, but did not extend beyond 1 cm in humans (Zilla et al., 2007; Zilla et al., 2020). Therefore, to endothelialize the middle section of a longer graft, other mechanisms must be considered. Transmural growth occurs through cellular and vascular infiltration from an adjacent blood vessel (Pennel et al., 2013). Blood-borne growth is through cells in the circulation with the capacity of differentiation into endothelial phenotypes, such as endothelial progenitor cells. Such endothelialization modality requires a strong binding between the circulating cell and the graft surface, which may be offered by progenitor-specific antibodies or ligand (Lu et al., 2013; Hao et al., 2020) or adhesive peptides (Choi et al., 2016; Hao et al., 2017; Liu et al., 2021) immobilized on the lumen surface. Since all three in situ mechanisms are still insufficient to create a continuous endothelium throughout a long vascular graft in human patients, ex vivo or in vitro seeding of matured vascular cells (Dahan et al., 2017), progenitor/stem cells (Olausson et al., 2012; Ardila et al., 2019), or genetically modified autologous cells expressing fibulin-5 and VEGF, onto the graft lumen have been employed (Sánchez et al., 2018).
For in situ endothelialization and in situ smooth muscle regeneration, strategies to functionalize graft lumen or an inner layer, have been under continuous development to improve the regeneration speed and quality. To encourage the adhesion, migration, differentiation and proliferation of matured or progenitor-derived vascular cells, bioactive molecules utilized for graft functionalization include: (a) growth factors, (b) genes, (c) peptides, and (d) antioxidants. Figure 5 illustrates functionalization strategies for graft endothelialization.
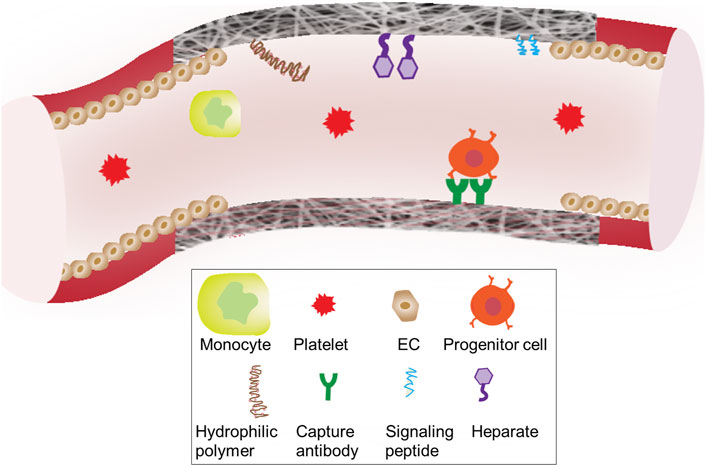
FIGURE 5. Surface engineering strategies of grafts Biomolecules conjugated to the surface graft materials are designed to interact with the blood components including blood cells, progenitor cells and platelets.
One of the most adopted growth factors is VEGF (Koobatian et al., 2016; Wang et al., 2019b). Despite an early study showing undesired inflammation and intimal hyperplasia on a decellularized vascular graft with a coating of FGF and VEGF (Heidenhain et al., 2011), a number of recent studies using these or other angiogenic or stem cell-homing molecules demonstrated their positive influences on graft performances. For example, the incorporation of triple factors, VEGF, bFGF, and SDF-1α into a two-layered graft was found to improve endothelialization, SMC layer formation, and thus graft patency compared with VEGF alone (Antonova et al., 2021). Allogeneic decellularized vascular scaffold grafted with VEGF, bFGF, and heparin exhibited similar mechanical properties to the natural vessels, and positive expression of factor VIII and α2-actin (Kong et al., 2019). Smith et al. (2019) and Smith et al. (2020) developed a vascular graft based on small intestinal submucosa with immobilized heparin and VEGF on the graft lumen to capture VEGF receptor-expressing circulating angiogenic cells from the blood, which promoted the formation of artery-mimic structure with endothelial and medial layers. Besides VEGF, other signaling mediators, including brain-derived neurotrophic factors (Zeng et al., 2012), dickkopf-3 glycoprotein (Issa Bhaloo et al., 2018), and SDF-1α (Shafiq et al., 2018; Wang et al., 2019c), were used to improve graft patency through progenitor cell homing and/or paracrine mechanisms for graft endothelialization and smooth muscle formation. Furthermore, these cell-recruiting molecules were used in combination with anticoagulant and immunomodulatory molecules. For example, the PTFE graft covalently coupled with heparin, SDF-1α and CD47 reduced thrombogenicity, facilitated the recruitment of progenitor cells, and alleviated the immune responses (Gao et al., 2017). Indeed, fast, high-quality in situ vascular regeneration could benefit from an exquisite graft design orchestrating the functions of multiple signaling molecules.
The gene incorporation into a graft can also effectively enhance vascular regeneration. Genes such as miRNAs and vectors encoding VEGF receptor-2 ligands (Hytönen et al., 2019) were used instead of functional proteins or peptides. In particular, miRNAs, such as miRNA-126 which accelerates the synthesis of VEGF and FGF and miRNA-145 which modulates the contractile SMC phenotype, were loaded into grafts: miRNA-126 alone was found to accelerate graft endothelialization (Zhou et al., 2016), while dual miRNA loading further improved contractile SMC regeneration and promoted vascular ECM formation (Wen et al., 2020). However, the lack of cell-specific transfection and release kinetics as well as potential toxicity and inflammation are several challenges for the gene approach.
The antioxidant approach could address both regenerative need for a functional endothelium and therapeutic need for inhibiting neointimal growth or calcification, which is related to oxidative stress-caused cellular damage (Gaudino et al., 2017; Washington and Bashur, 2017). A natural antioxidant, oxide (NO) is consistently secreted by endothelial cells, providing essential signaling to prevent thrombosis, SMC proliferation or intimal hyperplasia (Napoli et al., 2006; Strijdom et al., 2009; Devine et al., 2020). Therefore, NO-producing mechanisms, including NO-releasing coating and NO-generating coating such as NO-catalytic bioactive coating that generates NO from endogenous S-nitrosothiols, are integrated into vascular grafts (Wang et al., 2015; Tang et al., 2018; Dou et al., 2020; Li et al., 2020; Enayati et al., 2021) and stents (Yang et al., 2015; Yang et al., 2020), to promote endothelialization and reduce restenosis. Another type of antioxidant used was poly (1,8-octamethylenecitrate-co-cysteine), which was found to inhibit the calcification of decellularized grafts (Jiang et al., 2017).
4 Translation of vascular grafts for clinics: Success measures
Essential to the future development is continual efforts to balance the mechanistic understandings underlying adverse graft remodeling in clinic with the innovations in grafting technologies. Translational innovations are as important as innovations in cell technology and scaffold technology. Assessment tests and preclinical models for vascular grafts are to be properly matched to the targeted clinical application (Zilla et al., 2020), in terms of the anatomic structure such as graft diameter, length and looping, graft functions such as regular needle access for dialysis grafts, and regeneration potential related to clinical use, patient’s age and disease condition. Ideally, grafts with regenerated tissues could also simulate vasoreactivity and pharmacological responses of a native vessel, for the long-term success (Strobel et al., 2018).
4.1 Device function tests
Related to tubular vascular implants, FDA guidance documents have specified testing criteria for a number of physical and mechanical properties of grafts, including porosity, burst strength, compliance, elastic modulus, water permeability, compliance, and kink diameter (ANSI, 2010). Vascular grafts for a specific application such as arteriovenous graft access may require additional guidelines for function testing (Vascular Access Society, 2021). For example, when the synthetic grafts are placed a loop configuration, e.g., for vascular access, preventing graft kinking is crucial (Wu et al., 2020). Nevertheless, all the test guidelines refer to traditional, permanent graft materials. When biodegradable materials, synthetic or natural, are used to construct grafts, it may be necessary to test the temporal changes of physical and mechanical properties, in order to avoid devastating events such as aneurysm. Besides physical and mechanical properties, in vitro device tests also include biological function assessment. As TEVG improvements focus on the graft development by attending to the main causes of graft failure—thrombus formation and intimal hyperplasia, in vitro thrombogenicity tests as well as proliferation and migration tests of vascular cells have been widely employed.
4.2 Preclinical assessment with animals
The goal of TEVG regeneration studies is to translate new findings to human clinical therapy, which relies on animal models to generate enormous data useful to the translation. Ideally, the selection of animal model considers the animal’s analog close to human patients in terms of similarities in anatomical, structural, pathological and/or functional aspects. Immunodeficient animal models further allow long-term accommodation of humanized vascular grafts (Itoh et al., 2019). To evaluate the biochemical, structural and functional similarities between a TEVG implant and a native artery in animals, measures taken on the implants include ultrasonic scan, flow Doppler and X-ray/angiography, as well as on explant analyses, such as mechanics (i.e., strength, compliance), histological analyses such as geometry (i.e., lumen size, thickness), layer structure, cells (e.g., inflammatory cells, circulating cells, vascular endothelial cells and SMC, cells related to fibrotic or calcified remodeling), ECM (e.g., elastin, collagen types I/III/IV), and biomolecules through gene and/or protein assays.
A closer analog to clinical conditions allows one to better predict graft healing and regeneration in clinic. A huge discrepancy exists between endothelialization outcomes in animal models and those occurring in clinic (Zilla et al., 2007; Sánchez et al., 2018; Cai et al., 2021). Despite increased graft assessments in different animal models, most studies still use young, healthy small animals. It could be attributed to drastic differences in the vascular anatomy and physiology between human and rodents, which are used for evaluations in the majority of animal studies. The re-endothelization of a vein graft was reported to complete after several weeks of post-surgery in rodents, but re-endothelization duration in human took much longer. More important than the late presence of an endothelium on TEVG in human is a mismatch in the endothelialization mechanism—mainly trans-anastomotic mechanism in short grafts for rodents versus mainly transmural or blood-borne endothelialization in much longer grafts for humans (Zilla et al., 2007). In the clinical context of PTFE grafts, trans-anastomotic endothelialization covers only the immediate peri-anastomotic graft region in patients. Based on the analysis in a senescent non-human primate model, Zilla et al. (2007) and Zilla et al. (2020), concluded that a major issue with the development of prosthetic vascular grafts lied in wrong animal models, which led to wrong questions and no healing. Large animal models such as sheep, pigs, and baboons, though less available and more costly, mimic human physiology, in terms of vascular anatomy, thrombogenicity, inflammatory responses and regenerative potentials (Fukunishi et al., 2016; Rothuizen et al., 2016; Ju et al., 2017; Ma et al., 2017; Syedain et al., 2017; Liu et al., 2018; Schleimer et al., 2018; Fang et al., 2021b).
Overall, the design of future preclinical studies would better address the clinical relevance, for example, dialysis access (Gage and Lawson, 2017; Ong et al., 2017) and meso-Rex bypass (Maxfield et al., 2017), by providing further insight into the mechanisms through experimental designs that are aligned with the purpose and scope of specific clinical applications. In selecting animal models that prematurely terminate adverse remodeling events, the factors that impair vascular regeneration around grafts in clinic may remain largely overlooked. Besides the type of animal models, the significant “impairment factors” as listed in Section 2 include age and disease conditions, e.g., diabetes and ischemia. Additionally, temporal and spatial characterizations of the host responses in animals would be crucial to improve our mechanistic understandings of TEVG remodeling.
4.3 Clinical trials and potential commercialization values
Clinical trials are a true success measure and a determinant to the commercial value of any graft innovation. Prior trials have been performed on three types of grafts: Biological grafts (i.e., xenografts or allografts), bioreactor-manufactured grafts, and readily implantable grafts. Though trial results are encouraging and enlightening for future TEVG strategies, the overall success is still limited.
Biological grafts, referring to acellular blood vessels from human or animal (e.g., bovine) origin, have undergone clinical trials for decades. Bovine carotid artery grafts, such as those commercialized as Artegraft™, were used as arteriovenous fistula in patients undergoing chronic hemodialysis. These grafts outperformed PTFE grafts, though incrementally (Hutchin et al., 1975; Kennealey et al., 2011; Arhuidese et al., 2017). Lindsey et al. (2018) did a 15-year follow-up study on Artegaft used in lower extremity bypass surgery of 120 patients, yielding positive results for patency and salvage limb rates. Recently, the concern regarding the immunologic sensitization of artery xenografts in patients was also addressed (Dyer-Kindy et al., 2020). Similar to an artery graft, a vein xenograft such as bovine vein-derived ProCol™ demonstrated their candidacy as an alternative to synthetic grafts through clinical trials in both hemodialysis patients and patients with critical limb ischemia for 14–20 months (Hatzibaloglou et al., 2004; Schmidli et al., 2004). Vein allografts derived from human cadaveric veins were also a possible alternative. Cryopreserved allografts were used in 90 patients, showing similar patency, more resistant to infection but significantly more susceptible to aneurysms, when compared to PTFE grafts (Madden et al., 2004). More recently, 15 vein allografts seeded with autologous endothelial cells were used in coronary artery bypass surgery with 12 patients, showing patency up to 32 months (Herrmann et al., 2019). Autologous stem cells were also used to recellularize vein allografts and successfully tried on one pediatric patient for vascular vein shunts without the need for immunosuppression (Olausson et al., 2012). Overall, decades of clinical trials have shown that an artery- or vein-based xenografts or allografts can be considered as an alternative to synthetic PTFE or Dacron grafts. However, significant improvements over these graft products, in particular decellularization and recellularization as well as deproteinization and surface engineering to address complications- and/or rejections-derived from immune responses (Merola et al., 2017), are needed before they may gain a bit market share.
Bioreactor-manufactured grafts produce TEVG by culturing human autologous cells and/or allogeneic cells with or without a scaffold in a bioreactor with controlled physical, mechanical and/or biological environments. Clinical trials have illuminated promises and challenges with current methods. Lawson et al. (2016) seeded allogeneic human VSMCs onto polyglycolic acid scaffold, subjected the cell-loaded scaffold to pulsatile cyclic distension for 8 weeks before decellularization for final acellular implant. The patency of these grafts (Humacyte™) on 60 hemodialysis patients was 63% at 6 months and 28% at 18 months, with no evidence of immune response or aneurysm formation. From this trial, 16 tissue samples were acquired from 16 to 200 weeks for the characterization of vascular remodeling, showing recellularization of grafts by non-inflammatory host progenitor and vascular cells (Kirkton et al., 2019). A recent follow-up report provided 5-year (phase II) results on 11 patients who completed the study (Jakimowicz et al., 2022). Results showed that one patient maintained primary patency, and 10 maintained secondary patency. Like PTFE grafts, such acellular grafts showed better clinical outcomes when used as above-knee, femoral-to-popliteal arterial bypass conduits in 20 patients (Gutowski et al., 2020). As an example for scaffold-free cell-engineered graft, Cytograft™ employed patients’ fibroblasts to create an autologous TEVG through a prolonged fabrication procedure, either finishing with autologous endothelial seeding or implanting as it is. Its clinical trial with hemodialysis grafts on ten patients showed 1-month patency of 78% and 6-month patency of 60% (McAllister et al., 2009). Later, L’Heureux’s group modified the graft production into an “off-the-shelf” design—allogeneic Lifeline™ graft. Prior to implantation in patients for hemodialysis access, frozen cell-sheet based scaffolds were thawed, rehydrated and seeded with autologous endothelial cells the luminal side. All three implanted grafts worked well till 11 months (Wystrychowski et al., 2014).
More readily implantable grafts are desirable in clinic, as they require minimal or no need of cell culture time, relying on in vivo degradation, remodeling, regeneration and maturation. One recent approach is embedding a biotube in subcutaneous spaces of a patient for months, using patient’s body to fabricate a graft. This has been used successfully as a pulmonary artery substitute in pediatric patients (Kato et al., 2016; Fujita et al., 2020; Nakatsuji et al., 2021; Higashita et al., 2022). Another approach was employing biodegradable scaffolds in combination with autologous bone marrow mononuclear cells. Their clinical trials investigated these TEVGs in congenital heart surgery (Sugiura et al., 2018). Researchers observed postoperative growth of a TEVG as well as frequent graft narrowing in pediatric patients, but there was no graft-related mortality during the 11-year follow-up period.
5 Challenge and future
In conclusion, we reviewed the studies in the context of three innovations, i.e., cell technology, scaffold technology, and translational stages. Regenerative medicine has demonstrated great promises in the TEVG context. Future endeavors require the technological innovations to be coupled with translational innovations, in order to tackle the adverse remodeling of vascular grafts (Figure 6). This is critical to our fundamental understandings of the regenerative mechanisms and eventual transfer of the understandings into improved products for clinical practices. The complex nature of vascular remodeling and regeneration mechanisms in the spatial and temporal dimensions demands more sophisticated designs of in vitro studies and animal models. A combined approach employing both experimental and computational tools could significantly expedite the determination of optimal design parameters in a disease- or host-specific condition. From a perspective of clinical translation and graft commercialization, the considerations about costs and graft processing logistics become more and more important and are also highlighted in other recent reviews and studies. To address scientific questions to the point of clinical relevance, it is critical to highlight and increase the use of appropriate cell models, such as 3D cell models in diseased conditions, appropriate animal models, such as senescent animals, diseased animals, animals with slow vascular regenerative rates. Additionally, to more quantitatively and systematically understand the mechanisms, it would be helpful to standardize protocols such as sampling times across studies to obtain critical mechanobiological modeling inputs such as material degradation, inflammation, growth/remodeling/regeneration parameters.
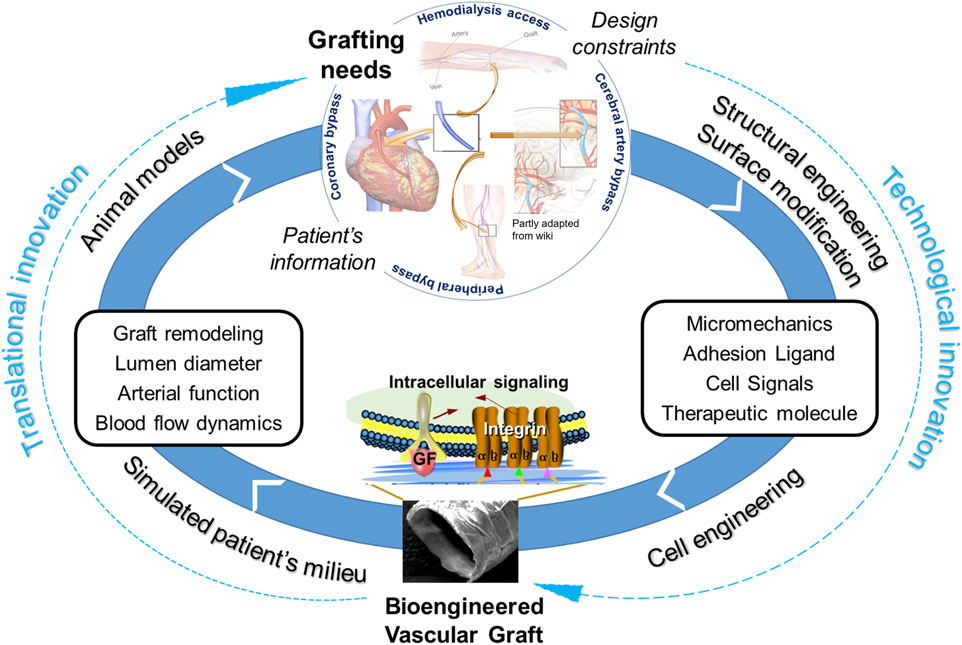
FIGURE 6. Coupling of technological innovations with translational innovations to tackle adverse remodeling of vascular grafts for clinical applications.
Also, it is expected that the adaptation and integration of bioengineered or tissue-engineered vascular grafts to human patients are heterogeneous because of cells’ varied ability to adapt to new microenvironments. A comparable case of graft heterogeneity in clinic is the use of vein grafts. Some veins are poor remodelers due to lack of adaptation, which had a 13-fold increased risk of failure at 2 years compared with “robust remodelers” (Owens et al., 2015). One factor in this lack of adaptation is biological incompatibility between cells and host endothelium. Cellular heterogeneity arises due to reasons not fully understood yet. For example, iPSC-derived arterial endothelial cells exhibit functional differences from iPSC-derived venous endothelial cells, including nitric oxide production and elongation under shear stress (Rosa et al., 2019). At present, the limitation of cellular heterogeneity is also a direct consequence of difficulty in identifying and precisely isolating progenitor cells which has limited their use in TEVGs. Therefore, improving methods of cell isolation and differentiation as well as reducing heterogeneity between cells in grafts and host vessels could be a solution to better integrate TEVGs into native vasculature.
We acknowledge several limitations of this review study, which include the lack of in-depth analyses and justifications of graft design, fabrication, biomaterials, and molecular mechanisms underlying graft failure and integration.
Author contributions
WT, SK, and PB drafted and wrote the manuscript, and PS edited it.
Funding
We acknowledge the funding received from NHLBI (R01HL119371), AHA (19TPA34850168) and the University of Colorado at Boulder.
Conflict of interest
The authors declare that the research was conducted in the absence of any commercial or financial relationships that could be construed as a potential conflict of interest.
Publisher’s note
All claims expressed in this article are solely those of the authors and do not necessarily represent those of their affiliated organizations, or those of the publisher, the editors and the reviewers. Any product that may be evaluated in this article, or claim that may be made by its manufacturer, is not guaranteed or endorsed by the publisher.
Supplementary Material
The Supplementary Material for this article can be found online at: https://www.frontiersin.org/articles/10.3389/fbioe.2022.1097334/full#supplementary-material
Abbreviations
ECM, extracellular matrix; iPSCs, induced pluripotent stem cells; MSC, mesenchymal stem cell; NO, nitric oxide; PTFE, polytetrafluoroethylene; SMC, smooth muscle cell; TEVG, tissue-engineered vascular grafts.
References
AbuRahma, A. F. (2018). When are endovascular and open bypass treatments preferred for femoropopliteal occlusive disease? Ann. Vasc. Dis. 11 (1), 25–40. doi:10.3400/avd.ra.18-00001
Afra, S., and Matin, M. M. (2020). Potential of mesenchymal stem cells for bioengineered blood vessels in comparison with other eligible cell sources. Cell Tissue Res. 380 (1), 1–13. doi:10.1007/s00441-019-03161-0
Afsar, B., and Elsurer, R. (2012). The primary arteriovenous fistula failure—a comparison between diabetic and non-diabetic patients: Glycemic control matters. Int. Urol. Nephrol. 44 (2), 575–581. doi:10.1007/s11255-011-9978-x
Ahangar, P., Mills, S. J., and Cowin, A. J. (2020). Mesenchymal stem cell secretome as an emerging cell-free alternative for improving wound repair. Int. J. Mol. Sci. 21 (19), 7038. doi:10.3390/ijms21197038
Ahmed, F. A., and Catic, A. G. (2018). Decision-making in geriatric patients with end-stage renal disease: Thinking beyond nephrology. J. Clin. Med. 8 (1), 5. doi:10.3390/jcm8010005
Akentjew, T. L., Terraza, C., Suazo, C., Maksimcuka, J., Wilkens, C. A., Vargas, F., et al. (2019). Rapid fabrication of reinforced and cell-laden vascular grafts structurally inspired by human coronary arteries. Nat. Commun. 10 (1), 3098. doi:10.1038/s41467-019-11090-3
Amensag, S., and McFetridge, P. S. (2012). Rolling the human amnion to engineer laminated vascular tissues. Tissue Eng. Part C Methods 18 (11), 903–912. doi:10.1089/ten.tec.2012.0119
ANSI 2010. ANSI/AAMI/ISO 7198:1998/2001 (R2010) - cardiovascular implants - tubular vascular prostheses. Accessed December 15, 2021. Available at: https://webstore.ansi.org/standards/aami/ansiaamiiso719819982001r2010.
Antonova, L., Kutikhin, A., Sevostianova, V., Velikanova, E., Matveeva, V., Glushkova, T., et al. (2021). bFGF and SDF-1α improve in vivo performance of VEGF-incorporating small-diameter vascular grafts. Pharm. Basel Switz. 14 (4), 302. doi:10.3390/ph14040302
Ardila, D. C., Liou, J. J., Maestas, D., Slepian, M., Badowski, M., Wagner, W., et al. (2019). Surface modification of electrospun scaffolds for endothelialization of tissue-engineered vascular grafts using human cord blood-derived endothelial cells. J. Clin. Med. 8 (2), E185. doi:10.3390/jcm8020185
Arhuidese, I., Reifsnyder, T., Islam, T., Karim, O., Nejim, B., Obeid, T., et al. (2017). Bovine carotid artery biologic graft outperforms expanded polytetrafluoroethylene for hemodialysis access. J. Vasc. Surg. 65 (3), 775–782. doi:10.1016/j.jvs.2016.10.080
Baruah, J., and Wary, K. K. (2020). Exosomes in the regulation of vascular endothelial cell regeneration. Front. Cell Dev. Biol. 7, 353. doi:10.3389/fcell.2019.00353
Bassiouny, H. S., White, S., Glagov, S., Choi, E., Giddens, D. P., and Zarins, C. K. (1992). Anastomotic intimal hyperplasia: Mechanical injury or flow induced. J. Vasc. Surg. 15 (4), 708–717. doi:10.1016/0741-5214(92)90019-5
Best, C., Strouse, R., Hor, K., Pepper, V., Tipton, A., Kelly, J., et al. (2018). Toward a patient-specific tissue engineered vascular graft. J. Tissue Eng. 9, 204173141876470. doi:10.1177/2041731418764709
Best, C. A., Szafron, J. M., Rocco, K. A., Zbinden, J., Dean, E. W., Maxfield, M. W., et al. (2019). Differential outcomes of venous and arterial tissue engineered vascular grafts highlight the importance of coupling long-term implantation studies with computational modeling. Acta Biomater. 94, 183–194. doi:10.1016/j.actbio.2019.05.063
Binns, R. L., Ku, D. N., Stewart, M. T., Ansley, J. P., and Coyle, K. A. (1989). Optimal graft diameter: Effect of wall shear stress on vascular healing. J. Vasc. Surg. 10 (3), 326–337. doi:10.1016/0741-5214(89)90449-7
Blum, K. M., Roby, L. C., Zbinden, J. C., Chang, Y. C., Mirhaidari, G. J. M., Reinhardt, J. W., et al. (2021). Sex and Tamoxifen confound murine experimental studies in cardiovascular tissue engineering. Sci. Rep. 11(1), 8037. doi:10.1038/s41598-021-87006-3
Boccafoschi, F., Mosca, C., and Cannas, M. (2014). Cardiovascular biomaterials: When the inflammatory response helps to efficiently restore tissue functionality? J. Tissue Eng. Regen. Med. 8 (4), 253–267. doi:10.1002/term.1526
Bosiers, M. J., Panuccio, G., Bisdas, T., Stachmann, A., Donas, K. P., Torsello, G., et al. (2020). Longer bridging stent-grafts in iliac branch endografting does not worsen outcome and expands its applicability, even in concomitant diseased hypogastric arteries. J. Cardiovasc Surg. (Torino) 61 (2), 191–195. doi:10.23736/S0021-9509.18.10504-0
Boutrous, M. L., Alvarez, A. C., Okoye, O. T., Laws, J. C., Jacobs, D. L., and Smeds, M. R. (2019). Stent-graft length is associated with decreased patency in treatment of central venous stenosis in hemodialysis patients. Ann. Vasc. Surg. 59, 225–230. doi:10.1016/j.avsg.2019.01.024
Browne, S., and Pandit, A. (2015). Biomaterial-mediated modification of the local inflammatory environment. Front. Bioeng. Biotechnol. 3, 67. doi:10.3389/fbioe.2015.00067
Cai, Q., Liao, W., Xue, F., Wang, X., Zhou, W., Li, Y., et al. (2021). Selection of different endothelialization modes and different seed cells for tissue-engineered vascular graft. Bioact. Mater 6 (8), 2557–2568. doi:10.1016/j.bioactmat.2020.12.021
Caliskan, E., de Souza, D. R., Böning, A., Liakopoulos, O. J., Choi, Y. H., Pepper, J., et al. (2020). Saphenous vein grafts in contemporary coronary artery bypass graft surgery. Nat. Rev. Cardiol. 17 (3), 155–169. doi:10.1038/s41569-019-0249-3
Chandra, P., and Atala, A. (2019). Engineering blood vessels and vascularized tissues: Technology trends and potential clinical applications. Clin. Sci. Lond Engl. 133 (9), 1115–1135. doi:10.1042/CS20180155
Chang, Y. C., Li, J., Mirhaidari, G., Zbinden, J., Barker, J., Blum, K., et al. (2021). Zoledronate alters natural progression of tissue-engineered vascular grafts. FASEB J. Off. Publ. Fed. Am. Soc. Exp. Biol. 35 (10), e21849. doi:10.1096/fj.202001606RR
Chen, C. L., Guo, H. R., Wang, Y. J., Chang, H. T., Pan, C. Y., Tuan-Mu, H. Y., et al. (2019). Combination of inductive effect of lipopolysaccharide and in situ mechanical conditioning for forming an autologous vascular graft in vivo. Sci. Rep. 9 (1), 10616. doi:10.1038/s41598-019-47054-2
Chen, W., Yang, M., Bai, J., Li, X., Kong, X., Gao, Y., et al. (2018). Exosome-modified tissue engineered blood vessel for endothelial progenitor cell capture and targeted siRNA delivery. Macromol. Biosci. 18 (2), 1700242. doi:10.1002/mabi.201700242
Chistiakov, D. A., Orekhov, A. N., and Bobryshev, Y. V. (2017). Effects of shear stress on endothelial cells: Go with the flow. Acta Physiol. 219 (2), 382–408. doi:10.1111/apha.12725
Chiu, J. J., and Chien, S. (2011). Effects of disturbed flow on vascular endothelium: Pathophysiological basis and clinical perspectives. Physiol. Rev. 91 (1), 327–387. doi:10.1152/physrev.00047.2009
Choi, W. S., Joung, Y. K., Lee, Y., Bae, J. W., Park, H. K., Park, Y. H., et al. (2016). Enhanced patency and endothelialization of small-caliber vascular grafts fabricated by coimmobilization of heparin and cell-adhesive peptides. ACS Appl. Mater Interfaces 8 (7), 4336–4346. doi:10.1021/acsami.5b12052
Connors, G., Todoran, T. M., Engelson, B. A., Sobieszczyk, P. S., Eisenhauer, A. C., and Kinlay, S. (2011). Percutaneous revascularization of long femoral artery lesions for claudication: Patency over 2.5 years and impact of systematic surveillance. Catheter Cardiovasc Interv. Off. J. Soc. Card. Angiogr. Interv. 77 (7), 1055–1062. doi:10.1002/ccd.22802
Conte, M. S. (2012). Diabetic revascularization: Endovascular versus open bypass—do we have the answer? Semin. Vasc. Surg. 25 (2), 108–114. doi:10.1053/j.semvascsurg.2012.04.004
Copes, F., Pien, N., Van Vlierberghe, S., Boccafoschi, F., and Mantovani, D. (2019). Collagen-based tissue engineering strategies for vascular medicine. Front. Bioeng. Biotechnol. 7, 166. doi:10.3389/fbioe.2019.00166
Creager, M. A., Lüscher, T. F., Beckman, J. A., and Cosentino, T. F. (2003). Diabetes and vascular disease:pathophysiology, clinical consequences, and medical therapy: Part I. Circulation 108(12), 1527–1532. doi:10.1161/01.CIR.0000091257.27563.32
Cunnane, E. M., Lorentz, K. L., Ramaswamy, A. K., Gupta, P., Mandal, B. B., O’Brien, F. J., et al. (2020). Extracellular vesicles enhance the remodeling of cell-free silk vascular scaffolds in rat aortae. ACS Appl. Mater Interfaces 12 (24), 26955–26965. doi:10.1021/acsami.0c06609
Cunnane, E. M., Lorentz, K. L., Soletti, L., Ramaswamy, A. K., Chung, T. K., Haskett, D. G., et al. (2020). Development of a semi-automated, bulk seeding device for large animal model implantation of tissue engineered vascular grafts. Front. Bioeng. Biotechnol. 8, 597847. doi:10.3389/fbioe.2020.597847
Cunnane, E. M., Weinbaum, J. S., O’Brien, F. J., and Vorp, D. A. (2018). Future perspectives on the role of stem cells and extracellular vesicles in vascular tissue regeneration. Front. Cardiovasc Med. 5, 86. doi:10.3389/fcvm.2018.00086
Dahan, N., Sarig, U., Bronshtein, T., Baruch, L., Karram, T., Hoffman, A., et al. (2017). Dynamic autologous reendothelialization of small-caliber arterial extracellular matrix: A preclinical large animal study. Tissue Eng. Part A 23 (1-2), 69–79. doi:10.1089/ten.TEA.2016.0126
de Almeida, P. E., Ransohoff, J. D., Nahid, A., and Wu, J. C. (2013). Immunogenicity of pluripotent stem cells and their derivatives. Circ. Res. 112 (3), 549–561. doi:10.1161/CIRCRESAHA.111.249243
Devine, R., Goudie, M. J., Singha, P., Schmiedt, C., Douglass, M., Brisbois, E. J., et al. (2020). Mimicking the endothelium: Dual action heparinized nitric oxide releasing surface. ACS Appl. Mater Interfaces 12 (18), 20158–20171. doi:10.1021/acsami.9b22277
Dhawan, S. S., Nanjundappa, R. P. A., Branch, J. R., Taylor, W. R., Quyyumi, A. A., Jo, H., et al. (2010). Shear stress and plaque development. Expert Rev. Cardiovasc Ther. 8 (4), 545–556. doi:10.1586/erc.10.28
Dimitrievska, S., Wang, J., Lin, T., Weyers, A., Bai, H., Qin, L., et al. (2020). Glycocalyx-like hydrogel coatings for small diameter vascular grafts. Adv. Funct. Mater 30 (23), 1908963. doi:10.1002/adfm.201908963
Ding, J., Liu, Y., Wang, F., and Bai, F. (2012). Impact of competitive flow on hemodynamics in coronary surgery: Numerical study of ITA-LAD model. Comput. Math. Methods Med. 2012, 1–7. doi:10.1155/2012/356187
Domínguez-Robles, J., Shen, T., Cornelius, V. A., Corduas, F., Mancuso, E., Donnelly, R. F., et al. (2021). Development of drug loaded cardiovascular prosthesis for thrombosis prevention using 3D printing. Mater Sci. Eng. C Mater Biol. Appl. 129, 112375. doi:10.1016/j.msec.2021.112375
Donadoni, F., Pichardo-Almarza, C., Homer-Vanniasinkam, S., Dardik, A., and Díaz-Zuccarini, V. (2020). Multiscale, patient-specific computational fluid dynamics models predict formation of neointimal hyperplasia in saphenous vein grafts. J. Vasc. Surg. Cases Innov. Tech. 6 (2), 292–306. doi:10.1016/j.jvscit.2019.09.009
Dou, J., Wang, Y., Jin, X., Li, P., Wang, L., Yuan, J., et al. (2020). PCL/sulfonated keratin mats for vascular tissue engineering scaffold with potential of catalytic nitric oxide generation. Mater Sci. Eng. C Mater Biol. Appl. 107, 110246. doi:10.1016/j.msec.2019.110246
Drews, J. D., Miyachi, H., and Shinoka, T. (2017). Tissue-engineered vascular grafts for congenital cardiac disease: Clinical experience and current status. Trends Cardiovasc Med. 27 (8), 521–531. doi:10.1016/j.tcm.2017.06.013
Drews, J. D., Pepper, V. K., Best, C. A., Szafron, J. M., Cheatham, J. P., Yates, A. R., et al. (2020). Spontaneous reversal of stenosis in tissue-engineered vascular grafts. Sci. Transl. Med. 12 (537), eaax6919. doi:10.1126/scitranslmed.aax6919
Dyer-Kindy, L. M., Heelan Gladden, A. A., Gralla, J., McCormick, N. D., Jenks, C., Cooper, J., et al. (2020). Relationship between bovine carotid artery grafts for hemodialysis access and human leukocyte antigen sensitization. Hemodial. Int. Int. Symp. Home Hemodial. 24 (1), 36–42. doi:10.1111/hdi.12784
Eilenberg, M., Enayati, M., Ehebruster, D., Grasl, C., Walter, I., Messner, B., et al. (2020). Long term evaluation of nanofibrous, bioabsorbable polycarbonate urethane grafts for small diameter vessel replacement in rodents. Eur. J. Vasc. Endovasc. Surg. 59 (4), 643–652. doi:10.1016/j.ejvs.2019.11.004
Enayati, M., Schneider, K. H., Almeria, C., Grasl, C., Kaun, C., Messner, B., et al. (2021). S-nitroso human serum albumin as a nitric oxide donor in drug-eluting vascular grafts: Biofunctionality and preclinical evaluation. Acta Biomater. 134, 276–288. doi:10.1016/j.actbio.2021.07.048
Fang, S., Ahlmann, A. H., Langhorn, L., Hussein, K., Sorensen, J. A., Guan, X., et al. (2021). Small diameter polycaprolactone vascular grafts are patent in sheep carotid bypass but require antithrombotic therapy. Regen. Med. 25, 117–130. doi:10.2217/rme-2020-0171
Fang, S., Ellman, D. G., and Andersen, D. C. (2021). Review: Tissue engineering of small-diameter vascular grafts and their in vivo evaluation in large animals and humans. Cells 10 (3), 713. doi:10.3390/cells10030713
Fathi-Karkan, S., Banimohamad-Shotorbani, B., Saghati, S., Rahbarghazi, R., and Davaran, S. (2022). A critical review of fibrous polyurethane-based vascular tissue engineering scaffolds. J. Biol. Eng. 16 (1), 6. doi:10.1186/s13036-022-00286-9
Fayon, A., Menu, P., and El Omar, R. (2021). Cellularized small-caliber tissue-engineered vascular grafts: Looking for the ultimate gold standard. Npj Regen. Med. 6 (1), 46–11. doi:10.1038/s41536-021-00155-x
Freeman, J., Chen, A., Weinberg, R. J., Okada, T., Chen, C., and Lin, P. H. (2018). Sustained thromboresistant bioactivity with reduced intimal hyperplasia of heparin-bonded polytetrafluoroethylene propaten graft in a chronic canine femoral artery bypass model. Ann. Vasc. Surg. 49, 295–303. doi:10.1016/j.avsg.2017.09.017
Fujita, S., Yamagishi, M., Kanda, K., Maeda, Y., Inoue, T., Yamanami, M., et al. (2020). Histology and mechanics of in vivo tissue-engineered vascular graft for children. Ann. Thorac. Surg. 110 (3), 1050–1054. doi:10.1016/j.athoracsur.2020.03.069
Fukunishi, T., Best, C. A., Ong, C. S., Groehl, T., Reinhardt, J., Yi, T., et al. (2018). Role of bone marrow mononuclear cell seeding for nanofiber vascular grafts. Tissue Eng. Part A 24 (1-2), 135–144. doi:10.1089/ten.TEA.2017.0044
Fukunishi, T., Best, C. A., Sugiura, T., Opfermann, J., Ong, C. S., Shinoka, T., et al. (2017). Preclinical study of patient-specific cell-free nanofiber tissue-engineered vascular grafts using 3-dimensional printing in a sheep model. J. Thorac. Cardiovasc Surg. 153 (4), 924–932. doi:10.1016/j.jtcvs.2016.10.066
Fukunishi, T., Best, C. A., Sugiura, T., Shoji, T., Yi, T., Udelsman, B., et al. (2016). Tissue-engineered small diameter arterial vascular grafts from cell-free nanofiber PCL/chitosan scaffolds in a sheep model. PLOS ONE 11 (7), e0158555. doi:10.1371/journal.pone.0158555
Fukunishi, T., Ong, C. S., He, Y. J., Inoue, T., Zhang, H., Steppan, J., et al. (2021). Fast-Degrading tissue-engineered vascular grafts lead to increased extracellular matrix cross-linking enzyme expression. Tissue Eng. Part A 27 (21-22), 1368–1375. doi:10.1089/ten.TEA.2020.0266
Fukunishi, T., Ong, C. S., Lui, C., Pitaktong, I., Smoot, C., Harris, J., et al. (2019). formation of neoarteries with optimal remodeling using rapidly degrading textile vascular grafts. Tissue Eng. Part A 25 (7-8), 632–641. doi:10.1089/ten.TEA.2018.0167
Fukunishi, T., Ong, C. S., Yesantharao, P., Best, C. A., Yi, T., Zhang, H., et al. (2020). Different degradation rates of nanofiber vascular grafts in small and large animal models. J. Tissue Eng. Regen. Med. 14 (2), 203–214. doi:10.1002/term.2977
Furdella, K. J., Higuchi, S., Behrangzade, A., Kim, K., Wagner, W. R., and Vande Geest, J. P. (2021). In-vivo assessment of a tissue engineered vascular graft computationally optimized for target vessel compliance. Acta Biomater. 123, 298–311. doi:10.1016/j.actbio.2020.12.058
Gage, S. M., and Lawson, J. H. (2017). Bioengineered hemodialysis access grafts. J. Vasc. Access 18, 56–63. doi:10.5301/jva.5000692
Gao, A., Hang, R., Li, W., Zhang, W., Li, P., Wang, G., et al. (2017). Linker-free covalent immobilization of heparin, SDF-1α, and CD47 on PTFE surface for antithrombogenicity, endothelialization and anti-inflammation. Biomaterials 140, 201–211. doi:10.1016/j.biomaterials.2017.06.023
Gaudino, M., Antoniades, C., Benedetto, U., Deb, S., Di Franco, A., Di Giammarco, G., et al. (2017). Mechanisms, consequences, and prevention of coronary graft failure. Circulation 136 (18), 1749–1764. doi:10.1161/CIRCULATIONAHA.117.027597
Gaudino, M., Niccoli, G., and Scalone, G. (2016). “Competitive flow and coronary artery bypass grafts,” in Coronary graft failure: State of the art. Editors I. C. Ţintoiu, M. J. Underwood, S. P. Cook, H. Kitabata, and A. Abbas (Germany: Springer International Publishing), 277–284. doi:10.1007/978-3-319-26515-5_25
Generali, M., Casanova, E. A., Kehl, D., Wanner, D., Hoerstrup, S. P., Cinelli, P., et al. (2019). Autologous endothelialized small-caliber vascular grafts engineered from blood-derived induced pluripotent stem cells. Acta Biomater. 97, 333–343. doi:10.1016/j.actbio.2019.07.032
Gentile, P., Sterodimas, A., Pizzicannella, J., Dionisi, L., De Fazio, D., Calabrese, C., et al. (2020). Systematic review: Allogenic use of stromal vascular fraction (SVF) and decellularized extracellular matrices (ECM) as advanced therapy medicinal products (ATMP) in tissue regeneration. Int. J. Mol. Sci. 21 (14), E4982. doi:10.3390/ijms21144982
Gong, W., Lei, D., Li, S., Huang, P., Qi, Q., Sun, Y., et al. (2016). Hybrid small-diameter vascular grafts: Anti-expansion effect of electrospun poly ε-caprolactone on heparin-coated decellularized matrices. Biomaterials 76, 359–370. doi:10.1016/j.biomaterials.2015.10.066
Gong, Z., and Niklason, L. E. (2008). Small-diameter human vessel wall engineered from bone marrow-derived mesenchymal stem cells (hMSCs). FASEB J. 22 (6), 1635–1648. doi:10.1096/fj.07-087924
Gupta, P., Chaudhuri, G. R., Janani, G., Agarwala, M., Ghosh, D., Nandi, S. K., et al. (2021). Functionalized silk vascular grafts with decellularized human wharton’s jelly improves remodeling via immunomodulation in rabbit jugular vein. Adv. Healthc. Mater 10 (19), e2100750. doi:10.1002/adhm.202100750
Gupta, P., Lorentz, K. L., Haskett, D. G., Cunnane, E. M., Ramaswamy, A. K., Weinbaum, J. S., et al. (2020). Bioresorbable silk grafts for small diameter vascular tissue engineering applications: In vitro and in vivo functional analysis. Acta Biomater. 105, 146–158. doi:10.1016/j.actbio.2020.01.020
Gupta, P., and Mandal, B. B. (2021). Tissue-engineered vascular grafts: Emerging trends and technologies. Adv. Funct. Mater 31 (33), 2100027. doi:10.1002/adfm.202100027
Gutowski, P., Gage, S. M., Guziewicz, M., Ilzecki, M., Kazimierczak, A., Kirkton, R. D., et al. (2020). Arterial reconstruction with human bioengineered acellular blood vessels in patients with peripheral arterial disease. J. Vasc. Surg. 72 (4), 1247–1258. doi:10.1016/j.jvs.2019.11.056
Håkansson, J., Simsa, R., Bogestål, Y., Jenndahl, L., Gustafsson-Hedberg, T., Petronis, S., et al. (2021). Individualized tissue-engineered veins as vascular grafts: A proof of concept study in pig. J. Tissue Eng. Regen. Med. 15 (10), 818–830. doi:10.1002/term.3233
Halbert, R. J., Nicholson, G., Nordyke, R. J., Pilgrim, A., and Niklason, L. (2020). Patency of ePTFE arteriovenous graft placements in hemodialysis patients: Systematic literature review and meta-analysis. Kidney360. 1 (12), 1437–1446. doi:10.34067/KID.0003502020
Ham, H. O., Haller, C. A., Su, G., Dai, E., Patel, M. S., Liu, D. R., et al. (2021). A rechargeable anti-thrombotic coating for blood-contacting devices. Biomaterials 276, 121011. doi:10.1016/j.biomaterials.2021.121011
Hao, D., Fan, Y., Xiao, W., Liu, R., Pivetti, C., Walimbe, T., et al. (2020). Rapid endothelialization of small diameter vascular grafts by a bioactive integrin-binding ligand specifically targeting endothelial progenitor cells and endothelial cells. Acta Biomater. 108, 178–193. doi:10.1016/j.actbio.2020.03.005
Hao, D., Xiao, W., Liu, R., Kumar, P., Li, Y., Zhou, P., et al. (2017). Discovery and characterization of a potent and specific peptide ligand targeting endothelial progenitor cells and endothelial cells for tissue regeneration. ACS Chem. Biol. 12 (4), 1075–1086. doi:10.1021/acschembio.7b00118
Harris, L. J., Abdollahi, H., Zhang, P., McIlhenny, S., Tulenko, T. N., and DiMuzio, P. J. (2011). Differentiation of adult stem cells into smooth muscle for vascular tissue engineering. J. Surg. Res. 168 (2), 306–314. doi:10.1016/j.jss.2009.08.001
Harskamp, R. E., Alexander, J. H., Ferguson, T. B., Hager, R., Mack, M. J., Englum, B., et al. (2016). Frequency and predictors of internal mammary artery graft failure and subsequent clinical outcomes: Insights from the project of ex-vivo vein graft engineering via transfection (PREVENT) IV trial. Circulation 133 (2), 131–138. doi:10.1161/CIRCULATIONAHA.115.015549
Haskett, D. G., Saleh, K. S., Lorentz, K. L., Josowitz, A. D., Luketich, S. K., Weinbaum, J. S., et al. (2018). An exploratory study on the preparation and evaluation of a “same-day” adipose stem cell-based tissue-engineered vascular graft. J. Thorac. Cardiovasc Surg. 156 (5), 1814–1822. doi:10.1016/j.jtcvs.2018.05.120
Hatzibaloglou, A., Velissaris, I., Kaitzis, D., Grekas, D., Avdelidou, A., and Kiskinis, D. (2004). ProCol® vascular bioprosthesis for vascular access: Midterm results. J. Vasc. Access 5 (1), 16–18. doi:10.1177/112972980400500104
Heath, D. E. (2017). Promoting endothelialization of polymeric cardiovascular biomaterials. Macromol. Chem. Phys. 218 (8), 1600574. doi:10.1002/macp.201600574
Heidenhain, C., Veeravoorn, A., Vachkov, B., Weichert, W., Schmidmaier, G., Wildemann, B., et al. (2011). Fibroblast and vascular endothelial growth factor coating of decellularized vascular grafts stimulates undesired giant cells and graft encapsulation in a rat model. Artif. Organs 35 (1), E1–E10. doi:10.1111/j.1525-1594.2010.01072.x
Heng, J. W., Yazid, M. D., Abdul Rahman, M. R., and Sulaiman, N. (2021). Coatings in decellularized vascular scaffolds for the establishment of a functional endothelium: A scoping review of vascular graft refinement. Front. Cardiovasc Med. 8, 677588. doi:10.3389/fcvm.2021.677588
Herrmann, F. E. M., Lamm, P., Wellmann, P., Milz, S., Hagl, C., and Juchem, G. (2019). Autologous endothelialized vein allografts in coronary artery bypass surgery – long term results. Biomaterials 212, 87–97. doi:10.1016/j.biomaterials.2019.05.019
Hibino, N., McGillicuddy, E., Matsumura, G., Ichihara, Y., Naito, Y., Breuer, C., et al. (2010). Late-term results of tissue-engineered vascular grafts in humans. J. Thorac. Cardiovasc Surg. 139 (2), 431–436. doi:10.1016/j.jtcvs.2009.09.057
Hielscher, D., Kaebisch, C., Braun, B. J. V., Gray, K., and Tobiasch, E. (2018). Stem cell sources and graft material for vascular tissue engineering. Stem Cell Rev. Rep. 14 (5), 642–667. doi:10.1007/s12015-018-9825-x
Higashita, R., Miyazaki, M., Oi, M., and Ishikawa, N. (2022). First-in-human results of an in-body tissue architecture-induced tissue-engineered vascular graft “Biotube” for application in distal bypass for chronic limb-threatening ischemia. J. Vasc. Surg. Cases Innov. Tech. 8, 488–493. doi:10.1016/j.jvscit.2022.07.007
Hiob, M. A., She, S., Muiznieks, L. D., and Weiss, A. S. (2017). Biomaterials and modifications in the development of small-diameter vascular grafts. ACS Biomater. Sci. Eng. 3 (5), 712–723. doi:10.1021/acsbiomaterials.6b00220
Hu, S., Li, Z., Shen, D., Zhu, D., Huang, K., Su, T., et al. (2021). Exosome-eluting stents for vascular healing after ischaemic injury. Nat. Biomed. Eng. 5 (10), 1174–1188. doi:10.1038/s41551-021-00705-0
Huang, A. H., Balestrini, J. L., Udelsman, B. V., Zhou, K. C., Zhao, L., Ferruzzi, J., et al. (2016). Biaxial stretch improves elastic fiber maturation, collagen arrangement, and mechanical properties in engineered arteries. Tissue Eng. Part C Methods 22 (6), 524–533. doi:10.1089/ten.TEC.2015.0309
Huang, A. H., Lee, Y. U., Calle, E. A., Boyle, M., Starcher, B. C., Humphrey, J. D., et al. (2015). Design and use of a novel bioreactor for regeneration of biaxially stretched tissue-engineered vessels. Tissue Eng. Part C Methods 21 (8), 841–851. doi:10.1089/ten.TEC.2014.0287
Hutchin, P., Jacobs, J. R., Devin, J. B., Shaughnessy, S., and Roland, A. S. (1975). Bovine graft arteriovenous fistulas for maintenance hemodialysis. Surg. Gynecol. Obstet. 141 (2), 255–258.
Hytönen, J. P., Leppänen, O., Taavitsainen, J., Korpisalo, P., Laidinen, S., Alitalo, K., et al. (2019). Improved endothelialization of small-diameter ePTFE vascular grafts through growth factor therapy. Vasc. Biol. 1 (1), 1–9. doi:10.1530/VB-18-0001
Iglesias-Echevarria, M., Johnson, R., Rafuse, M., Ding, Y., and Tan, W. (2021). Vascular grafts with tailored stiffness and a ligand environment via multiarmed polymer sheath for expeditious regeneration. ACS Appl. Bio Mater 4 (1), 545–558. doi:10.1021/acsabm.0c01114
Issa Bhaloo, S., Wu, Y., Le Bras, A., Yu, B., Gu, W., Xie, Y., et al. (2018). Binding of dickkopf-3 to CXCR7 enhances vascular progenitor cell migration and degradable graft regeneration. Circ. Res. 123 (4), 451–466. doi:10.1161/CIRCRESAHA.118.312945
Itoh, M., Mukae, Y., Kitsuka, T., Arai, K., Nakamura, A., Uchihashi, K., et al. (2019). Development of an immunodeficient pig model allowing long-term accommodation of artificial human vascular tubes. Nat. Commun. 10 (1), 2244. doi:10.1038/s41467-019-10107-1
Jakimowicz, T., Przywara, S., Turek, J., Pilgrim, A., Macech, M., Zapotoczny, N., et al. (2022). Five year outcomes in patients with end stage renal disease who received a bioengineered human acellular vessel for dialysis access. EJVES Vasc. Forum 54, 58–63. doi:10.1016/j.ejvsvf.2022.01.003
Jiang, B., Suen, R., Wang, J. J., Zhang, Z. J., Wertheim, J. A., and Ameer, G. A. (2016). Mechanocompatible polymer-extracellular matrix composites for vascular tissue engineering. Adv. Healthc. Mater 5 (13), 1594–1605. doi:10.1002/adhm.201501003
Jiang, B., Suen, R., Wang, J. J., Zhang, Z. J., Wertheim, J. A., and Ameer, G. A. (2017). Vascular scaffolds with enhanced antioxidant activity inhibit graft calcification. Biomaterials 144, 166–175. doi:10.1016/j.biomaterials.2017.08.014
Jin, C., and Liu, Y. (2019). Influence of competitive flow caused by different stenosis on coronary artery bypass hemodynamics and PIV study. Mol. Cell Biomech. 16 (S1)–52. doi:10.32604/mcb.2019.05728
Jin, X., Lin, T., and Xu, Y. (2016). Stem cell therapy and immunological rejection in animal models. Curr. Mol. Pharmacol. 9 (4), 284–288. doi:10.2174/1874467208666150928153511
Ju, Y. M., Ahn, H., Arenas-Herrera, J., Kim, C., Abolbashari, M., Atala, A., et al. (2017). Electrospun vascular scaffold for cellularized small diameter blood vessels: A preclinical large animal study. Acta Biomater. 59, 58–67. doi:10.1016/j.actbio.2017.06.027
Julier, Z., Park, A. J., Briquez, P. S., and Martino, M. M. (2017). Promoting tissue regeneration by modulating the immune system. Acta Biomater. 53, 13–28. doi:10.1016/j.actbio.2017.01.056
Kato, N., Yamagishi, M., Kanda, K., Miyazaki, T., Maeda, Y., Yamanami, M., et al. (2016). First successful clinical application of the in vivo tissue-engineered autologous vascular graft. Ann. Thorac. Surg. 102 (4), 1387–1390. doi:10.1016/j.athoracsur.2016.06.095
Kennealey, P. T., Elias, N., Hertl, M., Ko, D. S., Saidi, R. F., Markmann, J. F., et al. (2011). A prospective, randomized comparison of bovine carotid artery and expanded polytetrafluoroethylene for permanent hemodialysis vascular access. J. Vasc. Surg. 53 (6), 1640–1648. doi:10.1016/j.jvs.2011.02.008
Keshavarzian, M., Meyer, C. A., and Hayenga, H. N. (2019). In SilicoTissue engineering: A coupled agent-based finite element approach. Tissue Eng. Part C Methods 25 (11), 641–654. doi:10.1089/ten.TEC.2019.0103
Khosravi, R., Ramachandra, A. B., Szafron, J. M., Schiavazzi, D. E., Breuer, C. K., and Humphrey, J. D. (2020). A computational bio-chemo-mechanical model of in vivo tissue-engineered vascular graft development. Integr. Biol. Quant. Biosci. Nano Macro 12 (3), 47–63. doi:10.1093/intbio/zyaa004
Kim, D., Chung, J. J., Jung, Y., and Kim, S. H. (2019). The effect of Substance P/Heparin conjugated PLCL polymer coating of bioinert ePTFE vascular grafts on the recruitment of both ECs and SMCs for accelerated regeneration. Sci. Rep. 9 (1), 17083. doi:10.1038/s41598-019-53514-6
Kimicata, M., Swamykumar, P., and Fisher, J. P. (2020). Extracellular matrix for small-diameter vascular grafts. Tissue Eng. Part A 26 (23-24), 1388–1401. doi:10.1089/ten.tea.2020.0201
Kirkton, R. D., Prichard, H. L., Santiago-Maysonet, M., Niklason, L. E., Lawson, J. H., and Dahl, S. L. M. (2018). Susceptibility of ePTFE vascular grafts and bioengineered human acellular vessels to infection. J. Surg. Res. 221, 143–151. doi:10.1016/j.jss.2017.08.035
Kirkton, R. D., Santiago-Maysonet, M., Lawson, J. H., Tente, W. E., Dahl, S. L. M., Niklason, L. E., et al. (2019). Bioengineered human acellular vessels recellularize and evolve into living blood vessels after human implantation. Sci. Transl. Med. 11 (485), eaau6934. doi:10.1126/scitranslmed.aau6934
Klinkert, P., Post, P. N., Breslau, P. J., and van Bockel, J. H. (2004). Saphenous vein versus PTFE for above-knee femoropopliteal bypass. A review of the literature. Eur. J. Vasc. Endovasc. Surg. 27 (4), 357–362. doi:10.1016/j.ejvs.2003.12.027
Kong, X., Kong, C., Wen, S., and Shi, J. (2019). The use of heparin, bFGF, and VEGF 145 grafted acellular vascular scaffold in small diameter vascular graft. J. Biomed. Mater Res. B Appl. Biomater. 107 (3), 672–679. doi:10.1002/jbm.b.34160
Koobatian, M. T., Row, S., Smith, R. J., Koenigsknecht, C., Andreadis, S. T., and Swartz, D. D. (2016). Successful endothelialization and remodeling of a cell-free small-diameter arterial graft in a large animal model. Biomaterials 76, 344–358. doi:10.1016/j.biomaterials.2015.10.020
Kosa, S. D., Al-Jaishi, A. A., Moist, L., and Lok, C. E. (2015). Preoperative vascular access evaluation for haemodialysis patients. Cochrane Database Syst. Rev. 9, CD007013. doi:10.1002/14651858.CD007013.pub2
Kostokis, I. D., and Loukopoulos, I. (2020). Comparison between bovine carotid artery graft and polytetrafluoroethylene graft for haemodialysis vascular access: A systemic review and meta-analysis. J. Vasc. Surg. 72 (5), 1814. doi:10.1016/j.jvs.2020.08.012
Krawiec, J. T., Liao, H. T., Kwan, L. L., D'Amore, A., Weinbaum, J. S., Rubin, J. P., et al. (2017). Evaluation of the stromal vascular fraction of adipose tissue as the basis for a stem cell-based tissue-engineered vascular graft. J. Vasc. Surg. 66 (3), 883–890. doi:10.1016/j.jvs.2016.09.034
Krawiec, J. T., Weinbaum, J. S., Liao, H. T., Ramaswamy, A. K., Pezzone, D. J., Josowitz, A. D., et al. (2016). In VivoFunctional evaluation of tissue-engineered vascular grafts fabricated using human adipose-derived stem cells from high cardiovascular risk populations. Tissue Eng. Part A 22 (9-10), 765–775. doi:10.1089/ten.TEA.2015.0379
Kristofik, N. J., Qin, L., Calabro, N. E., Dimitrievska, S., Li, G., Tellides, G., et al. (2017). Improving in vivo outcomes of decellularized vascular grafts via incorporation of a novel extracellular matrix. Biomaterials 141, 63–73. doi:10.1016/j.biomaterials.2017.06.025
Kurobe, H., Maxfield, M. W., Naito, Y., Cleary, M., Stacy, M. R., Solomon, D., et al. (2015). Comparison of a closed system to a standard open technique for preparing tissue-engineered vascular grafts. Tissue Eng. Part C Methods 21 (1), 88–93. doi:10.1089/ten.tec.2014.0160
Lawson, J. H., Glickman, M. H., Ilzecki, M., Jakimowicz, T., Jaroszynski, A., Peden, E. K., et al. (2016). Bioengineered human acellular vessels for dialysis access in patients with end-stage renal disease: Two phase 2 single-arm trials. Lancet Lond Engl. 387 (10032), 2026–2034. doi:10.1016/S0140-6736(16)00557-2
Lazarides, M. K., Georgiadis, G. S., Antoniou, G. A., and Staramos, D. N. (2007). A meta-analysis of dialysis access outcome in elderly patients. J. Vasc. Surg. 45 (2), 420–426. doi:10.1016/j.jvs.2006.10.035
Lee, K. S., Kayumov, M., Emechebe, G. A., Kim, D. W., Cho, H. J., Jeong, Y. J., et al. (2022). A comparative study of an anti-thrombotic small-diameter vascular graft with commercially available e-PTFE graft in a porcine carotid model. Tissue Eng. Regen. Med. 19 (3), 537–551. doi:10.1007/s13770-021-00422-4
Lee, Y. U., de Dios Ruiz-Rosado, J., Mahler, N., Best, C. A., Tara, S., Yi, T., et al. (2016). TGF-β receptor 1 inhibition prevents stenosis of tissue-engineered vascular grafts by reducing host mononuclear phagocyte activation. FASEB J. Off. Publ. Fed. Am. Soc. Exp. Biol. 30 (7), 2627–2636. doi:10.1096/fj.201500179R
Li, N., Rickel, A. P., Sanyour, H. J., and Hong, Z. (2019). Vessel graft fabricated by the on-site differentiation of human mesenchymal stem cells towards vascular cells on vascular extracellular matrix scaffold under mechanical stimulation in a rotary bioreactor. J. Mater Chem. B 7 (16), 2703–2713. doi:10.1039/c8tb03348j
Li, P., Wang, Y., Jin, X., Dou, J., Han, X., Wan, X., et al. (2020). Catalytic generation of nitric oxide from poly(ε-caprolactone)/phosphobetainized keratin mats for a vascular tissue engineering scaffold. Langmuir ACS J. Surf. Colloids 36 (16), 4396–4404. doi:10.1021/acs.langmuir.0c00579
Li, X., Ma, T., Sun, J., Shen, M., Xue, X., Chen, Y., et al. (2019). Harnessing the secretome of adipose-derived stem cells in the treatment of ischemic heart diseases. Stem Cell Res. Ther. 10, 196. doi:10.1186/s13287-019-1289-7
Li, X., Xu, J., Nicolescu, C. T., Marinelli, J. T., and Tien, J. (2017). Generation, endothelialization, and microsurgical suture anastomosis of strong 1-mm-Diameter collagen tubes. Tissue Eng. Part A 23 (7-8), 335–344. doi:10.1089/ten.tea.2016.0339
Lin, C. H., Hsia, K., Tsai, C. H., Ma, H., Lu, J. H., and Tsay, R. Y. (2019). Decellularized porcine coronary artery with adipose stem cells for vascular tissue engineering. Biomed. Mater Bristol Engl. 14 (4), 045014. doi:10.1088/1748-605X/ab2329
Lindsey, P., Echeverria, A., Cheung, M., Kfoury, E., Bechara, C. F., and Lin, P. H. (2018). Lower extremity bypass using bovine carotid artery graft (artegraft): An analysis of 124 cases with long-term results. World J. Surg. 42 (1), 295–301. doi:10.1007/s00268-017-4161-x
Liu, J. Y., Swartz, D. D., Peng, H. F., Gugino, S. F., Russell, J. A., and Andreadis, S. T. (2007). Functional tissue-engineered blood vessels from bone marrow progenitor cells. Cardiovasc Res. 75 (3), 618–628. doi:10.1016/j.cardiores.2007.04.018
Liu, R. H., Ong, C. S., Fukunishi, T., Ong, K., and Hibino, N. (2018). Review of vascular graft studies in large animal models. Tissue Eng. Part B Rev. 24 (2), 133–143. doi:10.1089/ten.teb.2017.0350
Liu, Y., Mahara, A., Kambe, Y., Hsu, Y. I., and Yamaoka, T. (2021). Endothelial cell adhesion and blood response to hemocompatible peptide 1 (HCP-1), REDV, and RGD peptide sequences with free N-terminal amino groups immobilized on a biomedical expanded polytetrafluorethylene surface. Biomater. Sci. 9 (3), 1034–1043. doi:10.1039/d0bm01396j
Lok, C. E., Allon, M., Moist, L., Oliver, M. J., Shah, H., and Zimmerman, D. (2006). Risk equation determining unsuccessful cannulation events and failure to maturation in arteriovenous fistulas (REDUCE FTM I). J. Am. Soc. Nephrol. 17 (11), 3204–3212. doi:10.1681/ASN.2006030190
Lopera Higuita, M., Lopera Giraldo, J. F., Sarrafian, T. L., and Griffiths, L. G. (2021). Tissue engineered bovine saphenous vein extracellular matrix scaffolds produced via antigen removal achieve high in vivo patency rates. Acta Biomater. 134, 144–159. doi:10.1016/j.actbio.2021.06.034
Lorentz, K. L., Gupta, P., Shehabeldin, M. S., Cunnane, E. M., Ramaswamy, A. K., Verdelis, K., et al. (2021). CCL2 loaded microparticles promote acute patency in silk-based vascular grafts implanted in rat aortae. Acta Biomater. 135, 126–138. doi:10.1016/j.actbio.2021.08.049
Lu, D. Y., Chen, E. Y., Wong, D. J., Yamamoto, K., Protack, C. D., Williams, W. T., et al. (2014). Vein graft adaptation and fistula maturation in the arterial environment. J. Surg. Res. 188 (1), 162–173. doi:10.1016/j.jss.2014.01.042
Lu, S., Zhang, P., Sun, X., Gong, F., Yang, S., Shen, L., et al. (2013). Synthetic ePTFE grafts coated with an anti-CD133 antibody-functionalized heparin/collagen multilayer with rapid in vivo endothelialization properties. ACS Appl. Mater Interfaces 5 (15), 7360–7369. doi:10.1021/am401706w
Luo, J., Qin, L., Zhao, L., Gui, L., Ellis, M. W., Huang, Y., et al. (2020). Tissue-engineered vascular grafts with advanced mechanical strength from human iPSCs. Cell Stem Cell 26 (2), 251–261. doi:10.1016/j.stem.2019.12.012
Ma, X., He, Z., Li, L., Liu, G., Li, Q., Yang, D., et al. (2017). Development and in vivo validation of tissue-engineered, small-diameter vascular grafts from decellularized aortae of fetal pigs and canine vascular endothelial cells. J. Cardiothorac. Surg. 12 (1), 101. doi:10.1186/s13019-017-0661-x
Madden, R. L., Lipkowitz, G. S., Browne, B. J., and Kurbanov, A. (2004). Experience with cryopreserved cadaveric femoral vein allografts used for hemodialysis access. Ann. Vasc. Surg. 18 (4), 453–458. doi:10.1007/s10016-004-0055-0
Matsuzaki, Y., Miyamoto, S., Miyachi, H., Iwaki, R., Shoji, T., Blum, K., et al. (2021). Improvement of a novel small-diameter tissue-engineered arterial graft with heparin conjugation. Ann. Thorac. Surg. 111 (4), 1234–1241. doi:10.1016/j.athoracsur.2020.06.112
Maxfield, M. W., Stacy, M. R., Kurobe, H., Tara, S., Yi, T., Cleary, M. A., et al. (2017). Novel application and serial evaluation of tissue-engineered portal vein grafts in a murine model. Regen. Med. 12 (8), 929–938. doi:10.2217/rme-2017-0021
McAllister, T. N., Maruszewski, M., Garrido, S. A., Wystrychowski, W., Dusserre, N., Marini, A., et al. (2009). Effectiveness of haemodialysis access with an autologous tissue-engineered vascular graft: A multicentre cohort study. Lancet Lond Engl. 373 (9673), 1440–1446. doi:10.1016/S0140-6736(09)60248-8
Melchiorri, A. J., Bracaglia, L. G., Kimerer, L. K., Hibino, N., and Fisher, J. P. (2016). In vitro endothelialization of biodegradable vascular grafts via endothelial progenitor cell seeding and maturation in a tubular perfusion system bioreactor. Tissue Eng. Part C Methods 22 (7), 663–670. doi:10.1089/ten.TEC.2015.0562
Merola, J., Jane-Wit, D. D., and Pober, J. S. (2017). Recent advances in allograft vasculopathy. Curr. Opin. Organ Transpl. 22 (1), 1–7. doi:10.1097/MOT.0000000000000370
Miller, P. E., Tolwani, A., Luscy, C. P., Deierhoi, M. H., Bailey, R., Redden, D. T., et al. (1999). Predictors of adequacy of arteriovenous fistulas in hemodialysis patients. Kidney Int. 56 (1), 275–280. doi:10.1046/j.1523-1755.1999.00515.x
Mirhaidari, G. J. M., Barker, J. C., Zbinden, J. C., Santantonio, B. M., Chang, Y., Best, C. A., et al. (2020). Tissue engineered vascular graft recipient interleukin 10 status is critical for preventing thrombosis. Adv. Healthc. Mater 9 (24), e2001094. doi:10.1002/adhm.202001094
Misskey, J., Faulds, J., Sidhu, R., Baxter, K., Gagnon, J., and Hsiang, Y. (2018). An age-based comparison of fistula location, patency, and maturation for elderly renal failure patients. J. Vasc. Surg. 67 (5), 1491–1500. doi:10.1016/j.jvs.2017.08.080
Moist, L. M., Lok, C. E., Vachharajani, T. J., Xi, W., AlJaishi, A., Polkinghorne, K. R., et al. (2012). Optimal hemodialysis vascular access in the elderly patient. Semin. Dial. 25 (6), 640–648. doi:10.1111/sdi.12037
Mrówczyński, W., Mugnai, D., de Valence, S., Tille, J. C., Khabiri, E., Cikirikcioglu, M., et al. (2014). Porcine carotid artery replacement with biodegradable electrospun poly-e-caprolactone vascular prosthesis. J. Vasc. Surg. 59 (1), 210–219. doi:10.1016/j.jvs.2013.03.004
Munisso, M. C., and Yamaoka, T. (2020). Circulating endothelial progenitor cells in small-diameter artificial blood vessel. J. Artif. Organs 23 (1), 6–13. doi:10.1007/s10047-019-01114-6
Muniswami, D. M., Reddy, L. V. K., Amirtham, S. M., Babu, S., Raj, A. N., Sen, D., et al. (2020). Endothelial progenitor/stem cells in engineered vessels for vascular transplantation. J. Mater Sci. Mater Med. 31 (12), 119. doi:10.1007/s10856-020-06458-7
Nakatsuji, H., Yamagishi, M., Maeda, Y., Itatani, K., Fujita, S., Hongu, H., et al. (2021). Midterm results of pulmonary artery plasty with in vivo tissue-engineered vascular grafts. Interact. Cardiovasc Thorac. Surg. 32 (6), 956–959. doi:10.1093/icvts/ivab019
Nakayama, Y., Kaneko, Y., Okumura, N., and Terazawa, T. (2020). Initial 3-year results of first human use of an in-body tissue-engineered autologous “Biotube” vascular graft for hemodialysis. J. Vasc. Access 21 (1), 110–115. doi:10.1177/1129729819852550
Napoli, C., de Nigris, F., Williams-Ignarro, S., Pignalosa, O., Sica, V., and Ignarro, L. J. (2006). Nitric oxide and atherosclerosis: An update. Nitric Oxide Biol. Chem. 15 (4), 265–279. doi:10.1016/j.niox.2006.03.011
Ng, J., Bourantas Christos, V., Ryo, T., Ang, H. Y., Tenekecioglu, E., Serruys, P. W., et al. (2017). Local hemodynamic forces after stenting. Arterioscler. Thromb. Vasc. Biol. 37 (12), 2231–2242. doi:10.1161/ATVBAHA.117.309728
Nguyen, T. U., Shojaee, M., Bashur, C. A., and Kishore, V. (2018). Electrochemical fabrication of a biomimetic elastin-containing bi-layered scaffold for vascular tissue engineering. Biofabrication 11 (1), 015007. doi:10.1088/1758-5090/aaeab0
Nikfarjam, S., Rezaie, J., Zolbanin, N. M., and Jafari, R. (2020). Mesenchymal stem cell derived-exosomes: A modern approach in translational medicine. J. Transl. Med. 18 (1), 449. doi:10.1186/s12967-020-02622-3
Niklason, L. E., and Lawson, J. H. (2020). Bioengineered human blood vessels. Science 370 (6513), eaaw8682. doi:10.1126/science.aaw8682
Nordgaard, H., Swillens, A., Nordhaug, D., Kirkeby-Garstad, I., Van Loo, D., Vitale, N., et al. (2010). Impact of competitive flow on wall shear stress in coronary surgery: Computational fluid dynamics of a LIMA–lad model. Cardiovasc Res. 88 (3), 512–519. doi:10.1093/cvr/cvq210
Olausson, M., Patil, P. B., Kuna, V. K., Chougule, P., Hernandez, N., Methe, K., et al. (2012). Transplantation of an allogeneic vein bioengineered with autologous stem cells: A proof-of-concept study. Lancet Lond Engl. 380 (9838), 230–237. doi:10.1016/S0140-6736(12)60633-3
Ong, C. S., Fukunishi, T., Liu, R. H., Nelson, K., Zhang, H., Wieczorek, E., et al. (2017). Bilateral arteriovenous shunts as a method for evaluating tissue-engineered vascular grafts in large animal models. Tissue Eng. Part C Methods 23 (11), 728–735. doi:10.1089/ten.TEC.2017.0217
Owens, C. D., Gasper, W. J., Rahman, A. S., and Conte, M. S. (2015). Vein graft failure. J. Vasc. Surg. 61 (1), 203–216. doi:10.1016/j.jvs.2013.08.019
Pagel, M., and Beck-Sickinger, A. G. (2017). Multifunctional biomaterial coatings: Synthetic challenges and biological activity. Biol. Chem. 398 (1), 3–22. doi:10.1515/hsz-2016-0204
Pennel, T., Zilla, P., and Bezuidenhout, D. (2013). Differentiating transmural from transanastomotic prosthetic graft endothelialization through an isolation loop-graft model. J. Vasc. Surg. 58 (4), 1053–1061. doi:10.1016/j.jvs.2012.11.093
Peterson, W. J., Barker, J., and Allon, M. (2008). Disparities in fistula maturation persist despite preoperative vascular mapping. Clin. J. Am. Soc. Nephrol. 3 (2), 437–441. doi:10.2215/CJN.03480807
Petrus-Reurer, S., Romano, M., Howlett, S., Jones, J. L., Lombardi, G., and Saeb-Parsy, K. (2021). Immunological considerations and challenges for regenerative cellular therapies. Commun. Biol. 4 (1), 798. doi:10.1038/s42003-021-02237-4
Ponce, P. (2001). Vascular access for dialysis in the elderly. Int. Urol. Nephrol. 33 (3), 571–573. doi:10.1023/A:1019550307514
Poterucha, T. J., Libby, P., and Goldhaber, S. Z. (2017). More than an anticoagulant: Do heparins have direct anti-inflammatory effects? Thromb. Haemost. 117 (3), 437–444. doi:10.1160/TH16-08-0620
Qin, H., Jia, P., and Liu, H. (2016). Nursing strategies for patients with chronic renal failure undergoing maintenance hemodialysis treatment by arteriovenous fistula. Iran. J. Public Health 45 (10), 1270–1275.
Qiu, X., Lee, B. L. P., Ning, X., Murthy, N., Dong, N., and Li, S. (2017). End-point immobilization of heparin on plasma-treated surface of electrospun polycarbonate-urethane vascular graft. Acta Biomater. 51, 138–147. doi:10.1016/j.actbio.2017.01.012
Qiu, X., Lee, B. L. P., Wong, S. Y., Ding, X., Xu, K., Zhao, W., et al. (2021). Cellular remodeling of fibrotic conduit as vascular graft. Biomaterials 268, 120565. doi:10.1016/j.biomaterials.2020.120565
Radke, D., Jia, W., Sharma, D., Fena, K., Wang, G., Goldman, J., et al. (2018). Tissue engineering at the blood-contacting surface: A review of challenges and strategies in vascular graft development. Adv. Healthc. Mater 7 (15), e1701461. doi:10.1002/adhm.201701461
Ramachandra, A. B., Humphrey, J. D., and Marsden, A. L. (2017). Gradual loading ameliorates maladaptation in computational simulations of vein graft growth and remodelling. J. R. Soc. Interface 14 (130), 20160995. doi:10.1098/rsif.2016.0995
Ran, X., Ye, Z., Fu, M., Wang, Q., Wu, H., Lin, S., et al. (2019). Design, preparation, and performance of a novel bilayer tissue-engineered small-diameter vascular graft. Macromol. Biosci. 19 (3), e1800189. doi:10.1002/mabi.201800189
Reddy, M. A., Tak Park, J., and Natarajan, R. (2013). Epigenetic modifications in the pathogenesis of diabetic nephropathy. Semin. Nephrol. 33(4), 341–353. doi:10.1016/j.semnephrol.2013.05.006
Riolobos, L., Hirata, R. K., Turtle, C. J., Wang, P. R., Gornalusse, G. G., Zavajlevski, M., et al. (2013). HLA engineering of human pluripotent stem cells. Mol. Ther. 21 (6), 1232–1241. doi:10.1038/mt.2013.59
Robbin, M. L., Greene, T., Cheung, A. K., Allon, M., Berceli, S. A., Kaufman, J. S., et al. (2016). Arteriovenous fistula development in the first 6 Weeks after creation. Radiology 279 (2), 620–629. doi:10.1148/radiol.2015150385
Rodriguez-Soto, M. A., Suarez Vargas, N., Riveros, A., Camargo, C. M., Cruz, J. C., Sandoval, N., et al. (2021). Failure analysis of TEVG’s I: Overcoming the initial stages of blood material interaction and stabilization of the immune response. Cells 10 (11), 3140. doi:10.3390/cells10113140
Roh, J. D., Sawh-Martinez, R., Brennan, M. P., Jay, S. M., Devine, L., Rao, D. A., et al. (2010). Tissue-engineered vascular grafts transform into mature blood vessels via an inflammation-mediated process of vascular remodeling. Proc. Natl. Acad. Sci. U. S. A. 107 (10), 4669–4674. doi:10.1073/pnas.0911465107
Rooijens, P. P. G. M., Burgmans, J. P. J., Yo, T. I., Hop, W., de Smet, A., van den Dorpel, M., et al. (2005). Autogenous radial-cephalic or prosthetic brachial-antecubital forearm loop avf in patients with compromised vessels? A randomized, multicenter study of the patency of primary hemodialysis access. J. Vasc. Surg. 42 (3), 481–487. doi:10.1016/j.jvs.2005.05.025
Rosa, S., Praça, C., Pitrez, P. R., Gouveia, P. J., Aranguren, X. L., Ricotti, L., et al. (2019). Functional characterization of iPSC-derived arterial- and venous-like endothelial cells. Sci. Rep. 9 (1), 3826. doi:10.1038/s41598-019-40417-9
Rothuizen, T. C., Damanik, F. F. R., Lavrijsen, T., Visser, M. J., Hamming, J. F., Lalai, R. A., et al. (2016). Development and evaluation of in vivo tissue engineered blood vessels in a porcine model. Biomaterials 75, 82–90. doi:10.1016/j.biomaterials.2015.10.023
Ruiz-Rosado, J. de D., Lee, Y. U., Mahler, N., Yi, T., Robledo-Avila, F., Martinez-Saucedo, D., et al. (2018). Angiotensin II receptor I blockade prevents stenosis of tissue engineered vascular grafts. J. Fed. Am. Soc. Exp. Biol. 15, 6822–6832. Published online June. doi:10.1096/fj.201800458
Sabik, J. F., Lytle, B. W., Blackstone, E. H., Khan, M., Houghtaling, P. L., and Cosgrove, D. M. (2003). Does competitive flow reduce internal thoracic artery graft patency? Ann. Thorac. Surg. 76 (5), 1490–1497. doi:10.1016/S0003-4975(03)01022-1
Sabik Joseph, F. (2016). Should coronary artery bypass grafting Be performed in patients with moderate stenosis of the left anterior descending coronary artery? Circulation 133 (2), 111–113. doi:10.1161/CIRCULATIONAHA.115.020084
Samson, R. H., Morales, R., Showalter, D. P., Lepore, M. R., and Nair, D. G. (2016). Heparin-bonded expanded polytetrafluoroethylene femoropopliteal bypass grafts outperform expanded polytetrafluoroethylene grafts without heparin in a long-term comparison. J. Vasc. Surg. 64 (3), 638–647. doi:10.1016/j.jvs.2016.03.414
Sánchez, P. F., Brey, E. M., and Briceño, J. C. (2018). Endothelialization mechanisms in vascular grafts. J. Tissue Eng. Regen. Med. 12 (11), 2164–2178. doi:10.1002/term.2747
Schinstock, C. A., Albright, R. C., Williams, A. W., Dillon, J. J., Bergstralh, E. J., Jenson, B. M., et al. (2011). Outcomes of arteriovenous fistula creation after the fistula first initiative. Clin. J. Am. Soc. Nephrol. 6 (8), 1996–2002. doi:10.2215/CJN.11251210
Schleimer, K., Jalaie, H., Afify, M., Woitok, A., Barbati, M. E., Hoeft, K., et al. (2018). Sheep models for evaluation of novel patch and prosthesis material in vascular surgery: Tips and tricks to avoid possible pitfalls. Acta Vet. Scand. 60, 42. doi:10.1186/s13028-018-0397-1
Schmidli, J., Savolainen, H., Heller, G., Widmer, M., Then-Schlagau, U., Baumgartner, I., et al. (2004). Bovine mesenteric vein graft (ProCol) in critical limb ischaemia with tissue loss and infection. Eur. J. Vasc. Endovasc. Surg. Off. J. Eur. Soc. Vasc. Surg. 27 (3), 251–253. doi:10.1016/j.ejvs.2003.12.001
Schneider, K. H., Enayati, M., Grasl, C., Walter, I., Budinsky, L., Zebic, G., et al. (2018). Acellular vascular matrix grafts from human placenta chorion: Impact of ECM preservation on graft characteristics, protein composition and in vivo performance. Biomaterials 177, 14–26. doi:10.1016/j.biomaterials.2018.05.045
Schwarz, E. L., Kelly, J. M., Blum, K. M., Hor, K. N., Yates, A. R., Zbinden, J. C., et al. (2021). Hemodynamic performance of tissue-engineered vascular grafts in Fontan patients. NPJ Regen. Med. 6 (1), 38. doi:10.1038/s41536-021-00148-w
Shafiq, M., Zhang, Q., Zhi, D., Wang, K., Kong, D., Kim, D. H., et al. (2018). In situ blood vessel regeneration using SP (substance P) and SDF (stromal cell–derived factor)-1α peptide eluting vascular grafts. Arterioscler. Thromb. Vasc. Biol. 38 (7), e117–e134. doi:10.1161/ATVBAHA.118.310934
Shi, J., Chen, S., Wang, L., Zhang, X., Gao, J., Jiang, L., et al. (2019). Rapid endothelialization and controlled smooth muscle regeneration by electrospun heparin-loaded polycaprolactone/gelatin hybrid vascular grafts. J. Biomed. Mater Res. B Appl. Biomater. 107 (6), 2040–2049. doi:10.1002/jbm.b.34295
Shi, J., Zhang, X., Jiang, L., Zhang, L., Dong, Y., Midgley, A. C., et al. (2019). Regulation of the inflammatory response by vascular grafts modified with Aspirin-Triggered Resolvin D1 promotes blood vessel regeneration. Acta Biomater. 97, 360–373. doi:10.1016/j.actbio.2019.07.037
Shi, X., He, L., Zhang, S. M., and Luo, J. (2021). Human iPS cell-derived tissue engineered vascular graft: Recent advances and future directions. Stem Cell Rev. Rep. 17 (3), 862–877. doi:10.1007/s12015-020-10091-w
Shingarev, R., Maya, I. D., Barker-Finkel, J., and Allon, M. (2011). Arteriovenous graft placement in predialysis patients: A potential catheter-sparing strategy. Am. J. Kidney Dis. 58 (2), 243–247. doi:10.1053/j.ajkd.2011.01.026
Siddiqui, M. A., Ashraff, S., and Carline, T. (2017). Maturation of arteriovenous fistula: Analysis of key factors. Kidney Res. Clin. Pract. 36 (4), 318–328. doi:10.23876/j.krcp.2017.36.4.318
Skovrind, I., Harvald, E. B., Juul Belling, H., Jørgensen, C. D., Lindholt, J. S., and Andersen, D. C. (2019). Concise review: Patency of small-diameter tissue-engineered vascular grafts: A meta-analysis of preclinical trials. Stem Cells Transl. Med. 8 (7), 671–680. doi:10.1002/sctm.18-0287
Smith, R. J., Yi, T., Nasiri, B., Breuer, C. K., and Andreadis, S. T. (2019). Implantation of VEGF-functionalized cell-free vascular grafts: Regenerative and immunological response. FASEB J. 33 (4), 5089–5100. doi:10.1096/fj.201801856R
Smith, R. J., Nasiri, B., Kann, J., Yergeau, D., Bard, J. E., Swartz, D. D., et al. (2020). Endothelialization of arterial vascular grafts by circulating monocytes. Nat. Commun. 11 (1), 1622. doi:10.1038/s41467-020-15361-2
Song, H. H. G., Rumma, R. T., Ozaki, C. K., Edelman, E. R., and Chen, C. S. (2018). Vascular tissue engineering: Progress, challenges, and clinical promise. Cell Stem Cell 22 (3), 340–354. doi:10.1016/j.stem.2018.02.009
Sottiurai, V. S., Yao, J. S. T., Batson, R. C., Sue, S. L., Jones, R., and Nakamura, Y. A. (1989). Distal anastomotic intimal hyperplasia: Histopathologic character and biogenesis. Ann. Vasc. Surg. 3 (1), 26–33. doi:10.1016/S0890-5096(06)62381-9
Staramos, D. N., Lazarides, M. K., Tzilalis, V. D., Ekonomou, C. S., Simopoulos, C. E., and Dayantas, J. N. (2000). Patency of autologous and prosthetic arteriovenous fistulas in elderly patients. Eur. J. Surg. 166 (10), 777–781. doi:10.1080/110241500447407
Stegmayr, B., Willems, C., Groth, T., Martins, A., Neves, N. M., Mottaghy, K., et al. (2021). Arteriovenous access in hemodialysis: A multidisciplinary perspective for future solutions. Int. J. Artif. Organs 44 (1), 3–16. doi:10.1177/0391398820922231
Stowell, C. E. T., Li, X., Matsunaga, M. H., Cockreham, C. B., Kelly, K. M., Cheetham, J., et al. (2020). Resorbable vascular grafts show rapid cellularization and degradation in the ovine carotid. J. Tissue Eng. Regen. Med. 14 (11), 1673–1684. doi:10.1002/term.3128
Strang, A. C., Knetsch, M. L. W., Idu, M. M., Bisoendial, R. J., Kramer, G., Speijer, D., et al. (2014). Superior in vivo compatibility of hydrophilic polymer coated prosthetic vascular grafts. J. Vasc. Access 15 (2), 95–101. doi:10.5301/jva.5000166
Strijdom, H., Chamane, N., and Lochner, A. (2009). Nitric oxide in the cardiovascular system: A simple molecule with complex actions. Cardiovasc J. Afr. 20 (5), 303–310.
Strobel, H. A., Hookway, T. A., Piola, M., Fiore, G. B., Soncini, M., Alsberg, E., et al. (2018). Assembly of tissue-engineered blood vessels with spatially controlled heterogeneities. Tissue Eng. Part A 24 (19-20), 1492–1503. doi:10.1089/ten.tea.2017.0492
Sugimoto, K., Higashino, T., Kuwata, Y., Imanaka, K., Hirota, S., and Sugimura, K. (2003). Percutaneous transluminal angioplasty of malfunctioning brescia-cimino arteriovenous fistula: Analysis of factors adversely affecting long-term patency. Eur. Radiol. 13 (7), 1615–1619. doi:10.1007/s00330-002-1764-9
Sugiura, T., Matsumura, G., Miyamoto, S., Miyachi, H., Breuer, C. K., and Shinoka, T. (2018). Tissue-engineered vascular grafts in children with congenital heart disease: Intermediate term follow-up. Semin. Thorac. Cardiovasc Surg. 30 (2), 175–179. doi:10.1053/j.semtcvs.2018.02.002
Sugiura, T., Tara, S., Nakayama, H., Yi, T., Lee, Y. U., Shoji, T., et al. (2017). Fast-degrading bioresorbable arterial vascular graft with high cellular infiltration inhibits calcification of the graft. J. Vasc. Surg. 66 (1), 243–250. doi:10.1016/j.jvs.2016.05.096
Swartz, D. D., Russell, J. A., and Andreadis, S. T. (2005). Engineering of fibrin-based functional and implantable small-diameter blood vessels. Am. J. Physiol. Heart Circ. Physiol. 288 (3), H1451–H1460. doi:10.1152/ajpheart.00479.2004
Swillens, A., De Witte, M., Nordgaard, H., Lovstakken, L., Van Loo, D., Trachet, B., et al. (2012). Effect of the degree of LAD stenosis on “competitive flow” and flow field characteristics in LIMA-to-LAD bypass surgery. Med. Biol. Eng. Comput. 50 (8), 839–849. doi:10.1007/s11517-012-0927-3
Syedain, Z. H., Graham, M. L., Dunn, T. B., O’Brien, T., Johnson, S. L., Schumacher, R. J., et al. (2017). A completely biological “off-the-shelf” arteriovenous graft that recellularizes in baboons. Sci. Transl. Med. 9 (414), eaan4209. doi:10.1126/scitranslmed.aan4209
Syedain, Z. H., Maciver, R., and Tranquillo, R. T. (2020). Vascular grafts and valves that animate, made from decellularized biologically-engineered tissue tubes. J. Cardiovasc Surg. (Torino) 61 (5), 577–585. doi:10.23736/S0021-9509.20.11615-X
Syedain, Z. H., Meier, L. A., Bjork, J. W., Lee, A., and Tranquillo, R. T. (2011). Implantable arterial grafts from human fibroblasts and fibrin using a multi-graft pulsed flow-stretch bioreactor with noninvasive strength monitoring. Biomaterials 32 (3), 714–722. doi:10.1016/j.biomaterials.2010.09.019
Szafron, J. M., Khosravi, R., Reinhardt, J., Best, C. A., Bersi, M. R., Yi, T., et al. (2018). Immuno-driven and mechano-mediated neotissue formation in tissue engineered vascular grafts. Ann. Biomed. Eng. 46 (11), 1938–1950. doi:10.1007/s10439-018-2086-7
Szafron, J. M., Ramachandra, A. B., Breuer, C. K., Marsden, A. L., and Humphrey, J. D. (2019). Optimization of tissue-engineered vascular graft design using computational modeling. Tissue Eng. Part C Methods 25 (10), 561–570. doi:10.1089/ten.tec.2019.0086
Talacua, H., Smits, A. I. P. M., Muylaert, D. E. P., van Rijswijk, J. W., Vink, A., Verhaar, M. C., et al. (2015). In situ tissue engineering of functional small-diameter blood vessels by host circulating cells only. Tissue Eng. Part A 21 (19-20), 2583–2594. doi:10.1089/ten.TEA.2015.0066
Tamimi, E. A., Ardila, D. C., Ensley, B. D., Kellar, R. S., and Vande Geest, J. P. (2019). Computationally optimizing the compliance of multilayered biomimetic tissue engineered vascular grafts. J. Biomech. Eng. 141 (6), 0610031–06100314. doi:10.1115/1.4042902
Tamma, R., Ruggieri, S., Annese, T., and Ribatti, D. (2020). “Vascular wall as source of stem cells able to differentiate into endothelial cells,” in Cell Biology and translational medicine, volume 7: Stem Cells and therapy: Emerging approaches. Advances in experimental medicine and biology. Editor K. Turksen (Germany: Springer International Publishing), 29–36. doi:10.1007/5584_2019_421
Tan, R. P., Chan, A. H. P., Wei, S., Santos, M., Lee, B. S., Filipe, E. C., et al. (2019). Bioactive materials facilitating targeted local modulation of inflammation. JACC Basic Transl. Sci. 4 (1), 56–71. doi:10.1016/j.jacbts.2018.10.004
Tanaka, T., Abe, Y., Cheng, C. J., Tanaka, R., Naito, A., and Asakura, T. (2020). Development of small-diameter elastin-silk fibroin vascular grafts. Front. Bioeng. Biotechnol. 8, 622220. doi:10.3389/fbioe.2020.622220
Tang, D., Chen, S., Hou, D., Gao, J., Jiang, L., Shi, J., et al. (2018). Regulation of macrophage polarization and promotion of endothelialization by NO generating and PEG-YIGSR modified vascular graft. Mater Sci. Eng. C Mater Biol. Appl. 84, 1–11. doi:10.1016/j.msec.2017.11.005
Tang, Y., Zhou, Y., and Li, H. J. (2021). Advances in mesenchymal stem cell exosomes: A review. Stem Cell Res. Ther. 12 (1), 71. doi:10.1186/s13287-021-02138-7
Tesauro, M., Mauriello, A., Rovella, V., Annicchiarico-Petruzzelli, M., Cardillo, C., Melino, G., et al. (2017). Arterial ageing: From endothelial dysfunction to vascular calcification. J. Intern Med. 281 (5), 471–482. doi:10.1111/joim.12605
Thalla, P. K., Contreras-García, A., Fadlallah, H., Barrette, J., De Crescenzo, G., Merhi, Y., et al. (2012). A versatile star PEG grafting method for the generation of nonfouling and nonthrombogenic surfaces. Biomed. Res. Int. 2013, 1–12. doi:10.1155/2013/962376
Tinica, G., Chistol, R. O., Enache, M., Constantin, M. M. L., Ciocoiu, M., and Furnica, C. (2018). Long-term graft patency after coronary artery bypass grafting: Effects of morphological and pathophysiological factors. Anatol. J. Cardiol. 20 (5), 275–282. doi:10.14744/AnatolJCardiol.2018.51447
Tiwari, A., Cheng, K. S., Salacinski, H., Hamilton, G., and Seifalian, A. M. (2003). Improving the patency of vascular bypass grafts: The role of suture materials and surgical techniques on reducing anastomotic compliance mismatch. Eur. J. Vasc. Endovasc. Surg. 25 (4), 287–295. doi:10.1053/ejvs.2002.1810
Totorean, A. F., and Hudrea, I. C. (2019). “Local hemodynamics in coronary bypass in the presence of competitive flow and different diameter ratios between graft and host artery,” in World Congress on medical Physics and biomedical engineering 2018. IFMBE proceedings. Editors L. Lhotska, L. Sukupova, I. Lacković, and G. S. Ibbott (Germany: Springer), 767–771. doi:10.1007/978-981-10-9035-6_141
Tseng, C. N., Karlöf, E., Chang, Y. T., Lengquist, M., Rotzius, P., Berggren, P. O., et al. (2014). Contribution of endothelial injury and inflammation in early phase to vein graft failure: The causal factors impact on the development of intimal hyperplasia in murine models. PLOS ONE 9 (6), e98904. doi:10.1371/journal.pone.0098904
Valencia-Rivero, K. T., Cruz, J. C., Wagner-Gutierrez, N., D’Amore, A., Miranda, M. C., Lopez, R., et al. (2019). Evaluation of microscopic Structure−Function relationships of PEGylated small intestinal submucosa vascular grafts for arteriovenous connection. ACS Appl. Bio Mater 2 (9), 3706–3721. doi:10.1021/acsabm.9b00158
van der Slegt, J., Steunenberg, S. L., Donker, J. M. W., Veen, E. J., Ho, G. H., de Groot, H. G., et al. (2014). The current position of precuffed expanded polytetrafluoroethylene bypass grafts in peripheral vascular surgery. J. Vasc. Surg. 60 (1), 120–128. doi:10.1016/j.jvs.2014.01.062
Vascular Access Society 2021. Guidelines | vascular access society. Accessed December 13, 2021. Available at: https://www.vascularaccesssociety.com/education/guidelines.
von Bornstädt, D., Wang, H., Paulsen, M. J., Goldstone, A. B., Eskandari, A., Thakore, A., et al. (2018). Rapid self-assembly of bioengineered cardiovascular bypass grafts from scaffold-stabilized, tubular bilevel cell sheets. Circulation 138 (19), 2130–2144. doi:10.1161/CIRCULATIONAHA.118.035231
Wang, D., Wang, X., Zhang, Z., Wang, L., Li, X., Xu, Y., et al. (2019). Programmed release of multimodal, cross-linked vascular endothelial growth factor and heparin layers on electrospun polycaprolactone vascular grafts. ACS Appl. Mater Interfaces 11 (35), 32533–32542. doi:10.1021/acsami.9b10621
Wang, D., Xu, Y., Wang, L., Wang, X., Ren, C., Zhang, B., et al. (2020). Expanded poly(tetrafluoroethylene) blood vessel grafts with embedded reactive oxygen species (ROS)-Responsive antithrombogenic drug for elimination of thrombosis. ACS Appl. Mater Interfaces 12 (26), 29844–29853. doi:10.1021/acsami.0c07868
Wang, J. N., Kan, C. D., Lin, S. H., Chang, K. C., Tsao, S., and Wong, T. W. (2021). Potential of autologous progenitor cells and decellularized porcine artery matrix in construction of tissue-engineered vascular grafts. Organogenesis 17, 72–84. doi:10.1080/15476278.2021.1963603
Wang, L., Hu, J., Sorek, C. E., Chen, E. Y., Ma, P. X., and Yang, B. (2016). Fabrication of tissue-engineered vascular grafts with stem cells and stem cell-derived vascular cells. Expert Opin. Biol. Ther. 16 (3), 317–330. doi:10.1517/14712598.2016.1118460
Wang, T., Dong, N., Yan, H., Wong, S. Y., Zhao, W., Xu, K., et al. (2019). Regeneration of a neoartery through a completely autologous acellular conduit in a minipig model: A pilot study. J. Transl. Med. 17 (1), 24. doi:10.1186/s12967-018-1763-5
Wang, W., Liu, D., Li, D., Du, H., Zhang, J., You, Z., et al. (2019). Nanofibrous vascular scaffold prepared from miscible polymer blend with heparin/stromal cell-derived factor-1 alpha for enhancing anticoagulation and endothelialization. Colloids Surf. B Biointerfaces 181, 963–972. doi:10.1016/j.colsurfb.2019.06.065
Wang, Y., Chen, S., Pan, Y., Gao, J., Tang, D., Kong, D., et al. (2015). Rapid in situ endothelialization of a small diameter vascular graft with catalytic nitric oxide generation and promoted endothelial cell adhesion. J. Mater Chem. B 3 (47), 9212–9222. doi:10.1039/C5TB02080H
Washington, K. S., and Bashur, C. A. (2017). Delivery of antioxidant and anti-inflammatory agents for tissue engineered vascular grafts. Front. Pharmacol. 8, 659. doi:10.3389/fphar.2017.00659
Wen, M., Zhi, D., Wang, L., Cui, C., Huang, Z., Zhao, Y., et al. (2020). Local delivery of dual MicroRNAs in trilayered electrospun grafts for vascular regeneration. ACS Appl. Mater Interfaces 12 (6), 6863–6875. doi:10.1021/acsami.9b19452
Wissing, T. B., Bonito, V., Bouten, C. V. C., and Smits, A. I. P. M. (2017). Biomaterial-driven in situ cardiovascular tissue engineering—A multi-disciplinary perspective. NPJ Regen. Med. 2, 18. doi:10.1038/s41536-017-0023-2
Wu, D. J., van Dongen, K., Szymczyk, W., Besseling, P. J., Cardinaels, R. M., Marchioli, G., et al. (2020). Optimization of anti-kinking designs for vascular grafts based on supramolecular materials. Front. Mater 7, 220. doi:10.3389/fmats.2020.00220
Wu, P., Nakamura, N., Morita, H., Nam, K., Fujisato, T., Kimura, T., et al. (2019). A hybrid small-diameter tube fabricated from decellularized aortic intima-media and electrospun fiber for artificial small-diameter blood vessel. J. Biomed. Mater Res. A 107 (5), 1064–1070. doi:10.1002/jbm.a.36631
Wystrychowski, W., McAllister, T. N., Zagalski, K., Dusserre, N., Cierpka, L., and L’Heureux, N. (2014). First human use of an allogeneic tissue-engineered vascular graft for hemodialysis access. J. Vasc. Surg. 60 (5), 1353–1357. doi:10.1016/j.jvs.2013.08.018
Xing, Q., Qian, Z., Tahtinen, M., Yap, A. H., Yates, K., and Zhao, F. (2017). Aligned nanofibrous cell-derived extracellular matrix for anisotropic vascular graft construction. Adv. Healthc. Mater 6 (10), 1601333. doi:10.1002/adhm.20160133310.1002/adhm.201601333
Xu, Z., Gu, Y., Li, J., Feng, Z., Guo, L., Tong, Z., et al. (2018). Vascular remodeling process of heparin-conjugated poly(ε-caprolactone) scaffold in a rat abdominal aorta replacement model. J. Vasc. Res. 55 (6), 338–349. doi:10.1159/000494509
Yang, Y., Gao, P., Wang, J., Tu, Q., Bai, L., Xiong, K., et al. (2020). Endothelium-mimicking multifunctional coating modified cardiovascular stents via a stepwise metal-catechol-(amine) surface engineering strategy. Research 2020, 1–20. doi:10.34133/2020/9203906
Yang, Y., Lei, D., Zou, H., Huang, S., Yang, Q., Li, S., et al. (2019). Hybrid electrospun rapamycin-loaded small-diameter decellularized vascular grafts effectively inhibit intimal hyperplasia. Acta Biomater. 97, 321–332. doi:10.1016/j.actbio.2019.06.037
Yang, Z., Yang, Y., Xiong, K., Li, X., Qi, P., Tu, Q., et al. (2015). Nitric oxide producing coating mimicking endothelium function for multifunctional vascular stents. Biomaterials 63, 80–92. doi:10.1016/j.biomaterials.2015.06.016
Yi, S. W., Shin, Y. M., Lee, J. B., Park, J. Y., Kim, D., Baek, W., et al. (2021). Dilation-responsive microshape programing prevents vascular graft stenosis. Small 17 (18), 2007297. doi:10.1002/smll.202007297
Yu, L., Newton, E. R., Gillis, D. C., Sun, K., Cooley, B. C., Keith, A. N., et al. (2021). Coating small-diameter ePTFE vascular grafts with tunable poly(diol-co-citrate-co-ascorbate) elastomers to reduce neointimal hyperplasia. Biomater. Sci. 9 (15), 5160–5174. doi:10.1039/d1bm00101a
Yuan, H., Chen, C., Liu, Y., Lu, T., and Wu, Z. (2020). Strategies in cell-free tissue-engineered vascular grafts. J. Biomed. Mater Res. A 108 (3), 426–445. doi:10.1002/jbm.a.36825
Zamani, M., Khafaji, M., Naji, M., Vossoughi, M., Alemzadeh, I., and Haghighipour, N. (2017). A biomimetic heparinized composite silk-based vascular scaffold with sustained antithrombogenicity. Sci. Rep. 7 (1), 4455. doi:10.1038/s41598-017-04510-1
Zbinden, J. C., Blum, K. M., Berman, A. G., Ramachandra, A. B., Szafron, J. M., Kerr, K. E., et al. (2020). Effects of braiding parameters on tissue engineered vascular graft development. Adv. Healthc. Mater 9 (24), 2001093. doi:10.1002/adhm.202001093
Zeng, W., Wen, C., Wu, Y., Li, L., Zhou, Z., Mi, J., et al. (2012). The use of BDNF to enhance the patency rate of small-diameter tissue-engineered blood vessels through stem cell homing mechanisms. Biomaterials 33 (2), 473–484. doi:10.1016/j.biomaterials.2011.09.066
Zhang, F., and King, M. W. (2022). Immunomodulation strategies for the successful regeneration of a tissue-engineered vascular graft. Adv. Healthc. Mater 11 (12), 2200045. doi:10.1002/adhm.202200045
Zhang, P., Moudgill, N., Hager, E., Tarola, N., DiMatteo, C., McIlhenny, S., et al. (2011). Endothelial differentiation of adipose-derived stem cells from elderly patients with cardiovascular disease. Stem Cells Dev. 20 (6), 977–988. doi:10.1089/scd.2010.0152
Zhang, Q., Bosch-Rué, È., Pérez, R. A., and Truskey, G. A. (2021). Biofabrication of tissue engineering vascular systems. Apl. Bioeng. 5 (2), 021507. doi:10.1063/5.0039628
Zhang, X., Shi, J., Chen, S., Dong, Y., Zhang, L., Midgley, A. C., et al. (2019). Polycaprolactone/gelatin degradable vascular grafts simulating endothelium functions modified by nitric oxide generation. Regen. Med. 14 (12), 1089–1105. doi:10.2217/rme-2019-0015
Zhen, L., Creason, S. A., Simonovsky, F. I., Snyder, J. M., Lindhartsen, S. L., Mecwan, M. M., et al. (2021). Precision-porous polyurethane elastomers engineered for application in pro-healing vascular grafts: Synthesis, fabrication and detailed biocompatibility assessment. Biomaterials 279, 121174. doi:10.1016/j.biomaterials.2021.121174
Zhou, F., Jia, X., Yang, Y., Yang, Q., Gao, C., Hu, S., et al. (2016). Nanofiber-mediated microRNA-126 delivery to vascular endothelial cells for blood vessel regeneration. Acta Biomater. 43, 303–313. doi:10.1016/j.actbio.2016.07.048
Zhu, M., Wu, Y., Li, W., Dong, X., Chang, H., Wang, K., et al. (2018). Biodegradable and elastomeric vascular grafts enable vascular remodeling. Biomaterials 183, 306–318. doi:10.1016/j.biomaterials.2018.08.063
Zhu, T., Gu, H., Zhang, H., Wang, H., Xia, H., Mo, X., et al. (2021). Covalent grafting of PEG and heparin improves biological performance of electrospun vascular grafts for carotid artery replacement. Acta Biomater. 119, 211–224. doi:10.1016/j.actbio.2020.11.013
Zilla, P., Bezuidenhout, D., and Human, P. (2007). Prosthetic vascular grafts: Wrong models, wrong questions and no healing. Biomaterials 28 (34), 5009–5027. doi:10.1016/j.biomaterials.2007.07.017
Keywords: vascular grafts, adverse remodeling, impairment factor, scaffold technology, cell technology
Citation: Tan W, Boodagh P, Selvakumar PP and Keyser S (2023) Strategies to counteract adverse remodeling of vascular graft: A 3D view of current graft innovations. Front. Bioeng. Biotechnol. 10:1097334. doi: 10.3389/fbioe.2022.1097334
Received: 13 November 2022; Accepted: 23 December 2022;
Published: 10 January 2023.
Edited by:
Zhen Ma, Syracuse University, United StatesReviewed by:
Xi Lou, University of Alabama at Birmingham, United StatesKatsuhiro Hosoyama, Tohoku University, Japan
Copyright © 2023 Tan, Boodagh, Selvakumar and Keyser. This is an open-access article distributed under the terms of the Creative Commons Attribution License (CC BY). The use, distribution or reproduction in other forums is permitted, provided the original author(s) and the copyright owner(s) are credited and that the original publication in this journal is cited, in accordance with accepted academic practice. No use, distribution or reproduction is permitted which does not comply with these terms.
*Correspondence: Wei Tan, wtan@colorado.edu