- Shulan International Medical College, Zhejiang Shuren University, Hangzhou, China
Severe Acute Respiratory Syndrome Coronavirus 2 (SARS-CoV-2) is still in an epidemic situation, which poses a serious threat to the safety of people and property. Rapid diagnosis and isolation of infected individuals are one of the important methods to control virus transmission. Existing lateral flow immunoassay techniques have the advantages of rapid, sensitive, and easy operation, and some new options have emerged with the continuous development of nanotechnology. Such as lateral flow immunoassay test strips based on colorimetric-fluorescent dual-mode and gold nanoparticles, Surface Enhanced Raman Scattering, etc., these technologies have played an important role in the rapid diagnosis of COVID-19. In this paper, we summarize the current research progress of lateral flow immunoassay in the field of Severe Acute Respiratory Syndrome Coronavirus 2 infection diagnosis, analyze the performance of Severe Acute Respiratory Syndrome Coronavirus 2 lateral flow immunoassay products, review the advantages and limitations of different detection methods and markers, and then explore the competitive CRISPR-based nucleic acid chromatography detection method. This method combines the advantages of gene editing and lateral flow immunoassay and can achieve rapid and highly sensitive lateral flow immunoassay detection of target nucleic acids, which is expected to be the most representative method for community and clinical point-of-care testing. We hope that researchers will be inspired by this review and strive to solve the problems in the design of highly sensitive targets, the selection of detection methods, and the enhancement of CRISPR technology, to truly achieve rapid, sensitive, convenient, and specific detection of novel coronaviruses, thus promoting the development of novel coronavirus diagnosis and contributing our modest contribution to the world’s fight against epidemics.
1 Introduction
2019 Coronavirus disease (COVID-19) is an infectious disease caused by Severe Acute Respiratory Syndrome Coronavirus 2 (SARS-CoV-2) (2022a). The once-in-a-century pandemic epidemic caused by COVID-19 (2020c) has taken a huge toll on lives and livelihoods, disrupting health systems, economies, and societies (2020b). According to WHO, as of 2 September 2022, there have been more than 600 million confirmed cases of COVID-19 worldwide, including more than 6.47 million deaths (2022e).
SARS-CoV-2 is a betacoronavirus, an enveloped virus containing a large nucleoprotein-encapsidated positive sense RNA genome (Ke et al., 2020). SARS-CoV-2 contains four structural proteins: spike (S), membrane (M), nucleocapsid (N), and envelope (E) proteins. The N protein is mainly involved in RNA packaging and virus particle release and is a highly immunogenic and abundantly expressed protein during the infection process and is therefore commonly used in serotype analysis (Zeng et al., 2020; Liu et al., 2006); the S protein exhibits virus specificity mediates virus-host cell adhesion and invades the organism by recognizing and binding angiotensin-converting enzyme 2 (ACE2) (Wang et al., 2020; V'Kovski et al., 2021; Kadam et al., 2021). The process is illustrated in the following figure (Figure 1). The organization of the SARS-CoV-2 genomeis 5′-leader-UTR- replicase-S (Spike)-E (Envelope)-M (Membrane)-N (Nucleocapsid)-3′UTR-poly (A) tail with accessory genes interspersed within the structural genes at the 3′end of the genome (Malik, 2020). After infection with SARS-CoV-2, an immune response occurs in the human body to produce immunoglobulins corresponding to the antigen. Studies have shown that SARS-CoV-2 is highly infectious (Harrison et al., 2020), and the production of variants (Harvey et al., 2021; Tao et al., 2021) and the increase in asymptomatic infections (Johansson et al., 2021; Tanriover et al., 2021) have growth its transmission rate. Infection with SARS-CoV-2 affects multiple organs, as ACE2 is present in large numbers in human lung and small intestine epithelial cells, making the respiratory (Mandal et al., 2021; Stavem et al., 2021) and digestive (Aiyegbusi et al., 2021) systems susceptible to viral attack and disease, and it has also been documented that ACE2 is also expressed in the bile ducts, thus predisposing to liver injury (Hamming et al., 2004; Yang et al., 2020). Besides, ACE 2/angiotensin-(1–7)/MAS can counteract the negative effects of the renin-angiotensin system, however, ACE 2 downregulation after infection can lead to multi-organ damage, such as cardiovascular (Dweck et al., 2020; Puntmann et al., 2020) and neurological (Moghimi et al., 2021) diseases, among others (Ni et al., 2020). Currently, the gold standard for the detection of SARS-CoV-2 is real-time reverse transcription-polymerase chain reaction (RT-PCR), which has high specificity and relatively high sensitivity and accuracy of detection, and can accurately diagnose the disease during the latent phase and determine the virulence and mutation site of the virus, and has an irreplaceable position in the diagnosis of COVID-19 (Corman et al., 2020; Hoehl et al., 2020; Rothe et al., 2020; Kampf et al., 2021; Rahbari et al., 2021). However, it is time-consuming and requires high equipment and personnel, which makes the virus spread much faster than the diagnosis and isolation, and cannot meet the requirements of rapid detection in epidemic prevention and control (Safiabadi Tali et al., 2021b; Gupta et al., 2021). Therefore, there is an urgent need to develop a rapid detection method for COVID-19 to effectively curb the spread of the epidemic.
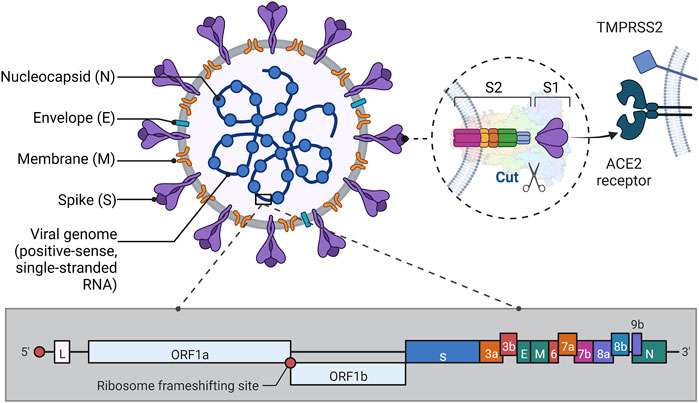
FIGURE 1. Structure of SARS-CoV-2. Illustrative scheme: This figure shows the approximate protein structure of SARS-CoV-2 and the gene fragment of RNA, in addition to depicting the way S1 binds to the ACE2 receptor to invade the organism. As can be seen in the figure, except for the N protein which is wrapped around the nucleic acid, the E, M, and S proteins are anchored to the envelope, each in its way. Among them, the S protein consists of two subunits, S1 and S2. S1 protein has a receptor binding domain (RBD) on it that binds to ACE2 on the host cell. S2 protein has a more complex structure and its role is to fuse the virus to the host cell membrane. In the pre-fusion conformation, the S1 and S2 subunits remain non-covalently bound. When the novel coronavirus wants to enter the host cell, the S protein shifts from the closed to the open state, thus mediating entry into the host cell (Lan et al., 2020; Shang et al., 2020; Walls et al., 2020). In addition, when RBD is structured and ACE2, it is activated by proteases such as TMPRSS2 on the host cell membrane, which causes the S1/S2 enzymatic cleavage site to be cut, thus facilitating the fusion of the virus with the host cell membrane (Zhou et al., 2020). The genetic structure of the virus mentioned in the paper is also briefly shown in Fig. The RNA genome of SARS-CoV-2 consists of 14 open reading frames (ORFs) (Khailany et al., 2020). Where ORF1a and ORF1b overlap at the (-1) ribosomal frameshift, this part contains about two-thirds of the genome and is mainly used for processing and synthesis of non-borrowed proteins. The remaining one-third of the genome in which the different ORFs also overlap each other is mainly used to encode four structural proteins (Arya et al., 2021). (This figure created with BioRender.com).
Lateral flow immunoassay (LFIA) is an assay developed by applying the principles of chromatographic chromatography and specific binding of antigen and antibody (Nguyen et al., 2020). LFIA strips consist of a sample pad, a releasing pad, nitrocellulose (NC) membrane, an absorbent pad, and a polyvinyl chloride backing. The principle is that after the sample is added to the sample pad, the test material binds to the nano marker on the releasing pad by capillary action, thus forming a coupling and binding to the corresponding ligand in the detection line on the NC membrane to show changes in color or light, which is used for qualitative or quantitative detection of SARS-CoV-2, and finally, the remaining liquid reaches the aspiration pad (Islam and Iqbal, 2020; Mahmoudinobar et al., 2021). Some studies have shown that LFIA still has advantages over the gold standard, such as rapid and accurate detection of patients in the period of shedding virus, and can be used for primary screening with the advantage of its rapid detection, thus reducing the waste of resources, under the full consideration of diagnostic accuracy, which also depends on various factors such as time to result, acceptable complexity of the test, and acceptance by the tested person (Dinnes et al., 2020; Yamayoshi et al., 2020). However, the performance of LFIA is definitely inferior to that of the gold standard, and researchers need to make efforts to shorten the detection window and improve the specificity to promote the development of LFIA testing (Tuaillon et al., 2020). Since Faulk (Faulk and Taylor, 1971) pioneered LFIA technology, it has been widely used in many fields such as food testing (Xu et al., 2021) and microbial detection (Zeng et al., 2021). With the continuous development of nanotechnology, the LFIA technology has also been extended with different detection modes, which can be classified into colloidal gold nanoparticle-based immunoassay (Qin et al., 2021), automated fluorescence immunoassay (Kang et al., 2021b), magnetic particle immunoassay (Bai et al., 2020), etc. according to the different markers.
After the outbreak of COVID-19, LFIA technique has taken the advantage in the rapid detection of COVID-19 because of its rapid, cheap, and convenient operation (Porte et al., 2020), which can effectively help control the epidemic and cut off the infection chain; it can also solve the problem that many countries cannot perform large-scale PCR detection due to the backward technology (2020a). At present, LFIA has been widely used in the detection of antigens (Pollock et al., 2021), antibodies (Fulford et al., 2021), and nucleic acid (Zou et al., 2021) of SARS-CoV-2 according to different detection targets, and some LFIA test strips have been marketed and used. In addition, new nanotechnologies are being applied to LFIA to improve the specificity and sensitivity of COVID-19 detection. In this paper, we will review the applications of LFIA combined with nanotechnology for the rapid detection of SARS-CoV-2 antigen, antibody, and nucleic acid based on LFIA and summarize the different detection modes; we will also outline and compare the LFIA products for the rapid detection of SARS-CoV-2 in China and abroad; Additionally, we will summarize the development of LFIA for the rapid detection of SARS-CoV-2. Finally, the development of LFIA for rapid SARS-CoV-2 detection is summarized.
2 LFIA technique for SARS-CoV-2 antibody detection
Antibodies are immunoglobulins produced by B cells or plasma cells by the immune system under the stimulation of antigens that bind specifically to antigens and are mainly classified into five categories: immunoglobulin M (IgM), immunoglobulin G (IgG), immunoglobulin A (IgA), immunoglobulin D (IgD) and immunoglobulin E (IgE) (Post et al., 2020). Therefore, after the invasion of a pathogen microorganism, the antibodies produced can be used as biomarkers for LFIA technology to determine whether it is infected. For example, its use in the diagnosis of pathogenic microbial infections such as Coccidioides (Azeem et al., 2022), Chlamydia trachomatis (Gwyn et al., 2019), and West Nile virus (Rebollo et al., 2018) has had many applications, so LFIA techniques based on SARS-CoV-2 antibodies for the diagnosis of COVID-19 have been widely followed.
2.1 Colloidal gold immunoassay technique for SARS-CoV-2 antibody detection
Colloidal gold immunoassay is the traditional LFIA technique, which uses gold nanoparticles as markers and is most widely used in SARS-CoV-2 antibody detection. Colorimetric analysis is a method that uses the color of the measured solution itself, or the color presented by the addition of reagents, to determine the concentration of the measured substance in the solution by observing and comparing the color depth of the solution by eye (or visual colorimeter), or by measuring it with a photoelectric colorimeter. The colorimetric method includes visual colorimetry and photoelectric colorimetry (Fernandes et al., 2020). Visual colorimetry is the most direct and simple method to determine the negative or positive diagnostic result in LFIA. For example, the following two individuals performed the test using the visual colorimetric method. Wang et al. (Wang et al., 2021a) designed a colloidal gold immunoassay kit for the detection of two antibodies, IgM and IgG, to the SARS-CoV-2 N protein. The colloidal gold of their test strips was modified with SARS-CoV-2 N antigen obtained from the expression system of pCMVp-NEO-BAN vector + HEK293 cells, and mouse anti-human IgM and IgG were immobilized on the NC membrane. When the results were positive, colloidal gold SARS-CoV-2 recombinant antigen-antibody complexes bound to IgM or IgG detection lines and showed a purplish red color. The study detected a total of 128 SARS-CoV-2 positive and negative cases, and the samples were taken from the serum of the subjects, and the final sensitivity and specificity were found to be 88.66% and 90.63%, respectively. In addition, 1061 non-SARS-CoV-2 infected persons aged 21–71 years were analyzed, and the false positive rates of IgM and IgG obtained were .75% and 0%, respectively. Cavalera et al. (2021) designed a double detection line of colloidal gold immunoassay test strips for N protein IgA, IgM, and IgG codetection. The tested antibody extracted from serum on the releasing pad binds to the nanogold particles labeled with N protein and biotin, then sequentially passes through the detection line with SPA and N protein fixed and binds to it, then binds to biotin and avidin on the quality control line, and finally completes the detection by the naked eye and portable scanner within 20 min. The test strips were analyzed from 147 clinical samples (62 RT-PCR positive samples and 85 RT-PCR negative samples), resulting in diagnostic sensitivity and specificity of 94.6% and 100%, respectively. Thus, it seems that the performance of the visual colorimetric method for the detection of SARS-CoV-2 antibodies is good, and from the preparation method, the preparation method of LFIA kits based on colloidal gold are more or less the same, and the main difference in the performance of the above two groups of products is the substances labeled on colloidal gold. The former N antigen is obtained by pCMVp-NEO-BAN vector expression, and the latter by pSER vector expression, both are commonly used expression vectors, which can stabilize and highly express the antigen of the target gene, while the difference between the two needs to be further explored by scholars (Rosati et al., 2004). The latter also uses biotin-labeled nanogold, which can induce aggregation of colloidal particles and thus improve the specificity of the assay, so this can also be considered as one of the reasons for the more advantageous performance of the LFIA kit designed by Cavalera et al. and worthy of study by researchers.
Chemiluminescence immunoassay (CLIA) is a kind of labeled antibody technology, which labels antibodies or antigens with substances such as chemiluminescent agents, catalytic luminescent enzymes, or products indirectly involved in the luminescence reaction, etc. When the labeled antibody or labeled antigen binds to the corresponding antigen or antibody, the luminescent substrate is affected by the luminescent agent, catalytic enzymes, or products involved, and a redox reaction occurs, in which visible light is released or the reaction excites fluorescent substances to emit light, and finally detected by spectrophotometer (Oishee et al., 2021). Roda et al. (2021) reported an optical/chemiluminescence-based colloidal gold immunoassay test strip for the SARS-CoV-2 IgA’s semi-quantitative detection. The test strip was immobilized with N protein antigen on the detection line and staphylococcal protein A (SPA) on the quality control line. The optical method uses gold nanoparticles modified with mouse anti-human IgA to label the antibody and a smartphone camera to image the color band formed at the detection line for signal transduction and quantification. The chemical method uses mouse anti-human IgA modified horseradish peroxidase to label the antibody and a portable device with a CCD camera to capture and analyze the chemiluminescent signal. The method can be performed on saliva or serum samples and results can be obtained within 15 min. Ten SARS-CoV-2 negative samples and 25 positive serum samples were compared with RT-PCR, and the false positive and false negative rates were 0% and 40%, respectively, besides the sensitivity of the chemiluminescence assay was 10 times higher than that of the optical method.
Surface-enhanced Raman spectroscopy scattering (SERS) originates from the presence of a strong local plasma electromagnetic field on a rough surface at the nanoscale, and when molecules are adsorbed on the surface of a metal structure their scattering cross section is dramatically amplified by the local electromagnetic field of the metal surface, resulting in a 1010 to 1011fold increase in the Raman intensity of the molecules (Camden et al., 2008). Compared with gold nanoparticle-based colorimetric methods, the SERS immunochromatographic technique takes advantage of the high sensitivity and accuracy of SERS, allowing for a sensitivity increase of typically 3 to 4 orders of magnitude (Wang et al., 2017; Deng et al., 2021). SERS method is often combined with Au or Ag (Sultangaziyev et al., 2022). For example, Chen et al. (2021c) developed a surface-enhanced Raman scattering based on the LFIA strip to simultaneous detect IgM and IgG. The test strips require serum as the sample, and use 4-nitrobenzenethiol modified Au-conjugated COVID-19 recombinant antigen as gap-enhanced Raman nanotags, and mouse anti-human IgM, mouse anti-human IgG, and sheep anti-chicken IgY as two detection lines and one quality control line, respectively. Compared with the conventional nanotags, the surface-enhanced Raman scattering signal of gap-enhanced Raman nanotags was improved by 30-fold, and the sensitivity of the measured IgM and IgG was excellent, 1 nm/ml and .1 nm/ml, respectively. Liu et al. (Liu H. et al., 2021a) proposed a LFIA test strip based on the SiO2@Ag SERS label for the quantitative detection of IgM/IgG antibodies to the S protein of SARS-CoV-2. The nano-marker was fabricated using the DTNB modification method, and the bilayer DTNB-modified SiO2@Ag nanoparticles were made by redox method after coating nanogold on silicon monoxide, and then attached to S protein to capture the antibody. The marker exhibited excellent SERS signal, high stability, and good monodispersity. The SERS signal intensity of the SiO2@Ag-spike protein-anti-novel coronavirus IgM/IgG immunocomplex formed by the capture on the detection line can be recorded by a portable Raman instrument. The method can be performed on serum samples and yield results within 25 min. The researchers obtained 100% accuracy and specificity by testing 49 SARS-CoV-2 negative samples against 19 positive serum samples and comparing them with RT-PCR, and the detection limit was 1 pg/ml, which is 800 times more sensitive than the conventional colloidal gold-based LFIA method. From the experimental results of the researchers, the LFIA strips have more excellent sensitivity and specificity mainly attributed to the study of bilayer DTNB-modified SiO2@Ag nanoparticles, and the SERS signal is greatly increased after the bilayer DTNB modification and the surface attachment of Ag coating, so this method can also be studied in the design of markers for the SERS method in the future. Further analysis, scholars can also be inspired by the screening of different nanoparticle platforms for the SERS method. These different nanoparticle fabrication methods can be used to evolve the structure by changing the particle shape or designing different materials or platforms to achieve the ultimate goal of signal enhancement, and thus the method of Liu et al. is superior to the experimental results (Serafinelli et al., 2022). In addition, there are various ways to design probes by the SERS method, such as double DTNB modified magnetic SERS probes with the structure of Fe3O4/DTNB@Ag/DTNB, or SERS probes prepared by immobilizing the Raman reporter molecule of malachite green isothiocyanate or coupling 4- amino thiophenol to the surface of the Raman reporter molecule to form SERS probes, etc (Huang et al., 2020). All of these probes showed good sensitivity and can provide researchers with a reference in LFIA-based detection of new coronaviruses.
With the increasing number of people receiving the COVID-19 vaccine, there is an emerging trend for antibody testing for SARS-CoV-2 to assess vaccine efficacy. Liu et al. (2021b) also developed a colloidal gold immunoassay strip for combined lgM-lgG detection, which was used to test the effect of antibodies in vaccine recipients and thus evaluates the effectiveness of inactivated neocoronavirus vaccine. In this method, goat anti-human IgG and IgM were immobilized on the NC membrane, and goat anti-mouse IgG, S protein and N protein were modified on the surface of colloidal gold. The blood of the subject was taken, and if it produced IgG and IgM of S protein, and N protein due to vaccination, then when detected, the antibody combined with S protein, and N protein on the surface of colloidal gold, and then passed through the IgG and IgM detection line successively, and combined with the corresponding antibodies to form protein complexes, and finally the quality control line combined with the colloidal gold particles modified with goat anti-mouse IgG, so that both the detection line and the quality control line appeared red. The method was used to test clinical samples from three inactivated vaccine recipients and one non-inactivated vaccine recipient. The small sample size makes this study limited, but it provides a reference for future evaluation of vaccine efficacy.
2.2 SARS-CoV-2 antibody detection method based on other nanomaterials
With the continuous development of nanotechnology, many novel materials have been used for LFIA and have shown good sensitivity and specificity. In addition to traditional fluorescent nanoparticles, materials such as quantum dots and dye-loaded polymers are becoming increasingly popular (Banga Ndzouboukou et al., 2021).
Immunofluorescence techniques are based on the principle of antigen-antibody reaction, in which a known antigen (or antibody) labeled with a fluorescent moiety is first used as a probe to capture the corresponding antibody (or antigen) and thus localize the antibody (or antigen) to be measured using a fluorescent detector (Im et al., 2019). Traditional fluorescence-based LFIA techniques commonly use fluorescent latex particles as markers, as in the study conducted by Kang et al. (2021a) The investigators designed a kit for the co-testing of IgM with IgG. This technique uses the RBD of S1 to make SARS-CoV-2 recombinant antigen, and then the fluorescent latex particles modified with this antigen are used as markers. Mouse anti-human IgM and IgG as detection lines, and goat anti-chicken IgY as quality control lines, respectively, and provides rapid results within 10 min after addition of whole blood, serum or plasma samples. In addition, the technique achieved semiquantitative detection with the help of a single channel or multichannel immunofluorescence analyzer, and the sensitivity and specificity measured in 733 COVID-19 confirmed sample compared with RT-PCR were high, 89.22% and 96.86%, respectively. The method was designed in the traditional way of immunofluorescence technique, which also obtained better sensitivity and specificity. However, there are two drawbacks, firstly, it did not use the advantages of the fluorescence technique to achieve the quantification of SARS-CoV-2. Secondly, although many samples were used for validation, they lacked the support of COVID-19-negative samples. Therefore, in combination with the above, the relevant similar products still need to enhance the fluorescent markers and do a comprehensive clinical validation, to achieve a quantitative assay with good performance and simplicity.
Quantum dots (QDs) are widely used to replace conventional fluorescent nanoparticles due to their wide excitation range, good photostability, and high fluorescence quantum yield. When QDs are irradiated by light pulses, they produce a variety of colors to mark the substance to be measured (Chang et al., 2021). In addition, when multiple QDs are irradiated and excited by a laser of a certain wavelength, multiple colors can be observed and multiple measurements can be performed simultaneously (Matea et al., 2017). And it can achieve more accurate quantitative detection compared to the fluorescence detection method. Li et al. (2022) developed a fluorescent LFIA based on QDs nanoparticles to detect the N protein-specific antibodies, representing the majority of conventional QDs for the detection of SARS-CoV-2. This study used ZnCdSe/ZnS QDs-conjugated N proteins as probes, SPA as a capture molecule, and specific antibodies for N protein as target points. If the subject is antibody positive, the complex of specific antibodies bound to the QDs-conjugated N proteins will be captured by the SPA and fluorescence will be shown, and the remaining QDs probe will bind to the antibody at the quality control line, which can be quantified by the fluorescence immunoassay analyzer. The method requires only 1 μl of serum as a sample and can be performed within 20 min. The analysis of five clinical samples showed high sensitivity and good specificity with a detection limit of 48.84 ng/ml. However, the performance advantages over assays such as SERS cannot be demonstrated. However, it was found that some new techniques of QDs for SARS-CoV-2 detection could be borrowed for LFIA, thus providing excellent new options for POCT continuously. For example, a QDs method for the quantitative detection of SARS-CoV-2 IgG reported in the study by Moabelo et al. has an excellent detection limit of 4 pg/ml, which is a cause for concern (Moabelo et al., 2021). The method is a highly sensitive QDs-linked immunoassay based on QDs nanoparticles and magnetic iron oxide (MnFe3O4) nanomicrospheres. With MnFesO4-coupled mouse anti-human IgG as the capture probe and QDs-coupled rabbit anti-human IgG as the detection probe, these three form a sandwich structure thus emitting fluorescence when antibodies are present in the serum (Guo et al., 2020). This method coincides with the principle of LFIA. If the capture probe and detection probe are fixed on the binding pad and detection line respectively, and the substances at the buffer and quality control line are investigated more deeply, it is expected to make more commercial and intuitive LFIA test strips, which also provide another possibility for SARS-CoV-2 related detection.
In addition to this, several methods have been used for the detection of SARS-CoV-2 antibodies. For example, Faezeh et al. (Ghorbanizamani et al., 2021) developed a homemade marker based on the principle of dye-loaded polymersome for anti-body test strips for SARS-CoV-2. In this study, a methoxy polyethylene glycol-b-polycaprolactone diblock copolymers were used to prepare the polymer and loaded with a Komas blue dye, thereby labeling the antibody in the sample. If the result is positive, the detection line, which has been immobilized with the purified antigen, binds to the antibody of the sample due to the principle of competitive LFIA and finally reaches the rabbit anti-human IgG-coated quality control line to bind to the remaining polymer, presenting two blue bands. The assay is diagnostic by visual colorimetry and can be completed within 9 min. By testing 60 clinical samples and comparing them with RT-PCR, it yielded a good sensitivity of 92.2% as well as a false positive rate of 0% and a false negative rate of 15.5%.
Antibody assays have attracted many researchers and are widely used because of their cheap production, low developmental skills, and ease of operation, which has led to a wide variety of methods (Chen et al., 2021b). The detection of different types of immunoglobulins allows us to obtain more information about the duration of infection of the infected person (Mallano et al., 2022). However, antibody testing has several limitations. First, because antibodies are present in the blood, the available data require whole blood, serum, or plasma as the sample for antibody detection, which makes sampling more difficult and makes LFIA much less easy to perform (Harritshøj et al., 2021). Secondly, after antigen invasion, there is a difference in the time of production and duration of different antibodies with a certain lag, so it is not conducive to the initial screening of infected patients (Hakki et al., 2022). In addition, the false-positive rate of antibody testing, especially the false-negative rate, is high compared to that of nucleic acid testing, which is not conducive to accurate diagnosis and precise control of virus transmission. However, researchers have tried to improve the sensitivity and specificity of the test by various methods, and it has been shown that the combined IgM-IgG test is effective in improving sensitivity and specificity due to the increased variety of antibodies detected and the expanded range of antibody production processes (Paces et al., 2020; Shen et al., 2020; Kang et al., 2021b). In addition, as the number of people receiving the SARS-CoV-2 vaccine increases, the need to assess the effectiveness of the vaccine and the body’s resistance to SARS-CoV-2 is high, and antibody testing may be a good method. The choice of different methods for antibody detection has been carried out by scholars with the common aim of finding more sensitive, specific or convenient detection methods.
3 LFIA technique for SARS-CoV-2 antigen detection
Antigens are substances that induce the body to produce antibodies, such as most proteins, bacteria, and viruses can be used as antigens. Although, the current LFIA technique of antigen is less studied in the diagnosis of COVID-19, the LFIA technique used for antigen detection, such as Cryptococcus (Zeng et al., 2021), Ebola virus (Wonderly et al., 2019), hepatitis C virus (Reddy et al., 2020), etc., has a certain clinical application basis, so the feasibility of LFIA antigen detection of SARS-CoV-2 exists. Investigations have shown that various proteins such as N, S, and RBD in SARS-CoV-2 as antigenic targets have been applied by some researchers in LFIA techniques.
Colloidal gold has certainly been used in the detection of antigens in LFIA. Peng et al. (2021) modified the traditional method of colloidal gold and developed an LFIA test strip for the rapid detection of NP antigens using colloidal gold nanoparticles after copper deposition. The investigators placed a colloidal gold probe conjugated with mouse anti-N protein monoclonal antibody 1 on a binding pad, and then immobilized mouse monoclonal antibody 2 and goat anti-mouse IgG on the detection and quality control lines, respectively, to form an LFIA test strip. The special feature is that after the reaction of the substance in the test strip is completed, it is immersed in a solution containing Cu2+ and sodium ascorbate, and after a series of reactions copper can be deposited on the strip with colloidal gold binding, thus enhancing the signal. The method can be completed in about 20 min for qualitative detection, while the copper deposition process takes only 3 min, but can amplify the signal to 3 times the traditional one, and its detection limit is 0.01 μg/ml. Thus, it can be seen that LFIA after copper deposition has the advantages of low cost, high efficiency, and high sensitivity, and if it can be further validated and improved in clinical samples, it may become a new choice for SARS-CoV-2 antigen detection. Of course, similar methods are more common, such as silver staining, biotin-affinin system, and enzyme catalysis (Mertens et al., 2020; Ibrahim et al., 2021). All these methods, when combined with the colloidal gold method, can be used to improve the sensitivity of LFIA test strips and can be used as a reference by researchers when developing them.
Immunofluorescence techniques have also been applied in this regard. For example, the commonly used fluorescent microsphere labeling method. Zhang et al. (2020) developed a LFIA test strip based on fluorescent microspheres for the detection of the N protein of SARS-CoV-2. In this study, a fluorescent microsphere monoclonal antibody complex for N protein was prepared and mouse monoclonal antibody and goat anti-rabbit IgG were immobilized on NC membrane as detection and quality control lines, respectively. A nasal or oropharyngeal swab was taken from the subject, and if the result was positive, the N protein in the sample first bound to the fluorescent microsphere monoclonal antibody complex immobilized on the releasing pad, and then to the monoclonal antibody on the detection line to form a double antibody sandwich complex. The remaining fluorescent microsphere-modified complex continues to move forward to the quality control line to bind to the goat anti-rabbit secondary antibody, thus generating a fluorescent signal in both lines, which is finally quantified by a fluorometric LFIA analyzer. In this study, by testing 990 different clinical samples, the specificity of the method was 100.00% and 97.29% for healthy controls and patients with other respiratory diseases, respectively, and the sensitivity was 67.15% and 7.02% for progressive and cured cases, respectively, and the detection limit of the N protein was 100 ng/ml. Some researchers have improved the fluorescent microspheres, thus greatly improving the sensitivity. Mao et al. (2022) developed a SARS-CoV-2 N protein LFIA test strip based on p-toluenesulfonyl-modified fluorescent microspheres. The p-toluenesulfonyl-modified nanomaterials can provide sulfonyl esters, which can covalently link antibodies or other ligands containing primary amino or sulfhydryl groups, allowing the nanomaterials to be more tightly linked to the antibody on the detection line, thus improving the capture rate of the target analyte and achieving a detection limit of 0.01 ng/ml for its test strips. LFIA qualitative test strips can increase the sensitivity by such a large amount without major differences in the use of the test strips, and also have the advantages of being more affordable, which can be used as one of the methods for the development of new crown antigen detection products. However, a certain dose of p-toluenesulfonyl is toxic and can be absorbed through the skin, and developers need to consider such issues, such as the need to explore the issue of dose and toxicity when mass-producing and marketing the product, to protect it well, and to use it in a ventilated area (Metcalf and Kearns, 1941).
Some researchers have also designed a colorimetric and fluorescent dual-mode detection method. For example, Han et al. (2022) developed a dual-mode LFIA test strip based on colloidal gold and QDs for neo-coronavirus S protein detection. Their markers were silica coated with a mixture of a monolayer of 20 nm colloidal gold and QDs. The method has a detection limit of 1 and 0.033 ng/ml for S protein detection by the colorimetric and fluorescence functions of the companion biosensor, respectively. This similar dual-mode method has the advantage of being selectively dual-functional. The visual colorimetric method can be performed first when the working environment and equipment are not ideal or for rapid screening of suspected SARS-CoV-2 infection, etc.; when sensitive and quantitative detection of viral infection is required at the early stage of detection, the accompanying biosensor can be used for fluorescence determination.
Compared with the above methods, SERS still presents a greater advantage. Liu et al. (2022) designed an LFIA test strip based on SERS tags modified with Fe3O4/DTNB@Au/DTNB nanoparticles for the quantitative detection of SARS-CoV-2 antigen. Its probe utilizes magnetic Fe3O4 to adsorb pathogens directly onto the surface. The process is to gradually attach Au nanoparticles to the magnetic Fe3O4 and then modify it twice with DTNB, in which it is immobilized with PVP and NH2OH-HCl. The team developed the design of LFIA test strips for triple testing, taking into account the impact of multiple viruses on disease determination, in which the detection limit of new coronavirus is 8 pg/ml. The obvious sensitivity advantage of the SERS method can be seen from its detection limit, but what attracts us more here is the design of the pathogen probe for triple testing. The team used a magnetic probe to simplify the probe structure and avoid the interference of impurities in the sample. A double DTNB modification was also performed, which, from experimental results and related investigations, correlates with a greatly enhanced detection signal.
In addition to these popular studies above, other methods for LFIA detection of SARS-CoV-2 have also been investigated. For example, An antigen detection LFIA test strip was designed by Faezeh et al. (Ghorbanizamani et al., 2021). This antigen detection method labels the antigen with a polymer prepared from glycol-b-polycaprolactone diblock copolymers loaded with Thomas Brilliant Blue, and the quality control line is immobilized with rabbit anti-human IgG. But differs in that it utilizes the double antibody sandwich principle to capture the antigen by immobilizing a purified antibody at the detection line. In addition, the test requires a nasal swab as the sample, and it takes only about 5 min to complete the diagnosis by the naked eye, and more detailed antigen information can be obtained after instrumental analysis such as electrophoresis and mass spectrometry. By testing 60 clinical samples, the measured sensitivity was 93%, and the false-positive and false-negative rates were 0% and 14%, respectively. Lee et al. (2021) developed a LFIA test strip for the detection of S1 protein. First, unlike other products used for antibody detection, this test strip consists of angiotensin converting enzyme 2 to capture S1 protein, which then binds to S1 antibody to form protein complexes. The study sample was taken from a nasal swab and the detection limit was 1. 86 × 105 copies/mL.
Although there are various probes for antigen detection, most of them use the double antibody sandwich principle. It has many advantages: first, it usually requires only qualitative judgment with the naked eye, and the samples are mainly nasal or oropharyngeal swabs, so the requirements for equipment and sampling are lower and the operation is easier (Safiabadi Tali et al., 2021a). Second, the antigen detection of SARS-COV-2 itself, without a window period, can be used for early screening of infection. However, studies have shown that its sensitivity and specificity are lower than those of nucleic acid and antibody tests, and it increases the probability of false positives and false negatives (Kowada, 2021). In addition, the risk of infection in antigen detection is a problem that cannot be ignored, but it is ideal for point-of-care testing (POCT) because it can be performed with only nasopharyngeal swabs and LFIA kits, and if it is accompanied by relevant monitoring software, it can greatly save labor and material resources, control the spread of the epidemic, and obtain large data related to the epidemic, and it can facilitate self-testing, thus reducing psychological anxiety and worry.
4 LIFA technique for SARS-CoV-2 nucleic acid detection
Nucleic acids are carriers of biological genetic information and are divided into ribonucleic acid (RNA) and deoxyribonucleic acid (DNA), which is one of the important indicators for determining SARS-CoV-2 infection. Common LFIA nucleic acid techniques detect nucleic acids through signals generated by combining nucleic acids with capture and detection probes. This method has been used in many clinical applications, such as for the detection of pathogenic microorganisms like Pseudomonas aeruginosa (Choi et al., 2016) and hepatitis B virus (Yu et al., 2020), so it can also be tried for the nucleic acid detection of SARS-CoV-2. In addition, with the prevalence of RT-PCR as the gold standard for the diagnosis of COVID-19, many scholars have been thinking of methods that can replace it for more rapid and accurate detection, which has incubated the idea of combining various rapid nucleic acid amplification techniques with LFIA. In addition, recently, the CRISPR-based SARS-CoV-2 nucleic acid detection has received much attention, which combines gene editing technology with traditional LFIA and provides a new idea for nucleic acid detection.
4.1 SARS-CoV-2 nucleic acid detection method based on non-CRISPR technology
Zhang et al. (2021) investigated a laminar test paper incorporating reverse transcript loop-mediated isothermal amplification (RT-LAMP) for the visual detection of the N and ORF1ab genes of SARS-CoV-2. The method divides the detection process into two steps. In step 1, RT-LAMP is applied to efficiently and rapidly amplify RNA without specialized equipment. After treating the sample to be tested with lysate, RT-LAMP-based amplification of fluorescein FITC-labeled dUTP, outer primers biotin-labeled inner primers are performed to generate labeled amplification products. In step 2, LFIA test strips are applied for detection. The paper is inserted into the reaction tube and if the result is positive, the amplification product and the streptavidin-coated particle complex can be captured by the anti-FITC antibody at the detection line and show a red band. The whole detection process can be completed within 40 min, and the amplified test strips only need 3 min to complete the chromatography and obtain the results, and the sample required for the test is a nasal or pharyngeal swab. The detection limits of the N and ORF1ab genes of SARS-CoV-2 pseudovirus were less than 2 copies/μL, and the results of 12 clinical RNA samples (8 RT-PCR-positive and 4 RT-PCR-negative samples) were compared with RT-PCR and showed 100% concordance. Zhu et al. (2020) devised a combined multiplex reverse transcription loop-mediated isothermal amplification (mRT-LAMP) and lateral flow biosensor (LFB) assay named mRT-LAMP-LFB for the genes of N and ORF1ab to diagnose COVID-19. The approximate reaction procedure is to design two primer sets, with primers for the ORF1ab gene fluorescein-biotin labeling and digoxigenin-biotin labeling for the primers of the N gene; then many double-stranded amplicons with these markers attached can be generated under mRT-LAMP at a constant temperature of 63°C; finally, the resulting solution is then transferred to LFB, the essence of which is LFIA test strips, and the biotin on the amplification products can be bound to dynein streptavidin-coated nanoparticles (SA-DNPs), which can amplify the signal. The anti-fluorescein antibody and anti-digoxin antibody immobilized on detection line 1 and detection line 2, respectively, can capture the amplification product, so that it can be judged under the naked eye, and it is positive when both detection lines and quality control lines show red color. The method is qualitative in less than 1 h and is highly sensitive and specific with a predicted cost of $5.5. It has a detection limit of 12 copies/reaction (24 copies/μL) and does not cross-react with non-SARS-CoV-2 templates. Its accuracy was 100% by testing 33 positive patients and 96 negative patients. What this method shows us, in addition to its excellent performance, is the reliability of the assay after the detection of clinical samples, the accuracy of the results brought by the genetic double detection of N and ORF1ab, the low requirements for operation and instrumentation, and the careful estimation of the cost of the product. And comparing the two LAMP-related methods mentioned above, the advantages do not seem to be comparable. However, some aspects can be considered for improvement, for example: although both perform dual gene assays, the Zhang et al. design does not seem to fully highlight the advantages of the dual gene assay, and one can try to apply different markers to label the two genes as in the method designed by Zhu et al. and also design separate bands for both genes on the test strips, thus making the presented results clearer.
Yu et al. (2020) developed a novel LFIA technique that allows RT-PCR to obtain the desired nucleic acid products for simultaneous detection of RdRp, ORF3a, and N genes. After reverse transcription and amplification, the resulting nucleic acid is added dropwise to the sample pads of LFIA strips and passed successively through the detection line containing capture probes for RdRp, ORF3a, and N genes, and the signal is finally read by a microfluorescence detector. The method was applied to 162 clinical samples and compared with RT-PCR, showing 99.4% concordance and a detection limit of 10 copies/reaction (2 copies/μL).
Chen et al. (2021a) designed a LFIA test strip based on a Duplex Reverse Transcription-Multienzyme Isothermal Rapid Amplification (RT-MIRA) for the visualization of the N and ORF1ab genes of SARS-CoV-2. The method eliminates the need for RNA extraction and requires only 2 steps for the detection of nasal or pharyngeal swab samples. Firstly, RT-MIRA is applied to amplify the dual target of SARS-CoV-2 in a single reaction. Secondly, the amplification products are captured on LFIA strips by colloidal gold probes made of ORF1ab and N genes combined with a digoxigenin ligand and a FAM at the 5′ end, respectively, and as the amplification products flow along the strips, the ORF1ab and N genes are successively detected. The ORF1ab gene and the N gene were detected on the two detection lines immobilized with anti-digoxigenin and anti-FAM antibodies and showed a red band. The method takes only about 25 min to complete the assay, including 3 min of strip chromatography time. The 95% detection limits for the N gene and ORF1ab gene were 0.049 copies/μl and 0.050 copies/μl, respectively. 243 clinical samples were tested using the RT-MIRA chromatography system, and the results showed 100% agreement with the Real Time Quantitative PCR results.
Zou et al. (2021) combined an isothermal non-enzymatic signal amplification system and LFIA technology to develop a catalytic hairpin assembly-LFIA assay system witch for the detection of the N and ORF1ab genes of SARS-CoV-2.The investigators labeled the 5′ ends of complementary single-stranded hairpin DNA with digoxin and biotin, respectively, to make probes, and sprayed streptavidin and fluorophore Alexa Fluor 647 double-labeled polyethylene nanoparticles on the detection line, thus serving as part of the catalytic hairpin assembly-LFIA detection system. The method uses catalytic hairpin assembly to amplify SARS-CoV-2 RNA extracted from nasal or pharyngeal swabs, and after LFIA test strips, the fluorescence signal is detected by a fluorescence detection device, which ultimately allows quantitative detection within 90 min. The method was applied to 15 clinical samples and compared with RT-PCR, showing 100% concordance and a detection limit of 2 copies/μl.
Recently, antisense oligonucleotides (ASOs) can be used to target the N gene of SARS-CoV-2 (Borghei et al., 2022). Moitra et al. (2020) reported a gold nanoparticle-based turbidimetric assay, in which sulfhydryl-modified ASOs were covered on gold nanoparticles, and when the N gene of SARS-CoV-2 was encountered, the gold nanoparticles selectively aggregated due to their plasmon resonance properties (Jain et al., 2006). Then RNaseH is added to separate the RNA strand from the RNA- Dna heterodimer, which enables the aggregation between the gold nanoparticles again, thus forming the aggregation phenomenon visible to the naked eye, which is judged to be positive for COVID-19. The reaction does not require any complex instrumentation and can yield rapid qualitative results within 10 min, and the experimental limit of detection for RNA with SARSCoV-2 viral load is .18 ng/uL. The advantages of this method in terms of time, portability, and cost effectiveness are undoubtedly outstanding in the genetic testing of COVID-19 and deserve our consideration. In addition this method may provide a reference in the production of similar LFIA test strips, if the modified nanogold particles are attached to the detection line, whether it can also visually show the results visible to the naked eye, and, if the probe can be designed on the binding pad, whether it will increase its specificity, etc. Of course, this is just an idea and further research is needed, but it is certain that if a design similar to LFIA test strips is used, it can promote commercialization and not be discarded in the form of solution after the test is completed, which can improve biosafety assurance. When it comes to ASOs, it is important to mention the electrochemical biosensor chip pioneered by Alafeef et al. (2020) that can perform the assay in an ultra-fast and convenient manner. This is an electrochemical biosensor chip consisting of properly designed ASOs combined with graphene and gold nanoparticles for the detection of the N-gene of SARS-CoV-2, which can be read by hand only in 5 min to produce results with high specificity, sensitivity, and accuracy. The method uses gold nanoparticles covered with optimized concentrations of four ASOs probes to selectively target the viral N gene with a detection limit of 6.9 copies/μl. Moreover, the team found that the sensitivity of ASOs directly affixed to the surface of gold nanoparticles is much less than when covered on the surface, providing a reference value for researchers when designing ASOs-related nano markers. In addition, since the WHO considers the RdRp gene to be relatively more analytical than the N gene in detecting neocrown pneumonia infection (Corman et al., 2020). Kumar et al. (2022) conducted a study on the visual detection of the RdRp gene based on gold nanoparticles. The principle is that hybridization of the oligonucleotide probe of the RdRp gene with the RNA target of SARS-CoV-2 of patients leads to salt-induced aggregation and a pink-to-blue color change of gold nanoparticles for visualization purposes. The method can be completed qualitatively in less than 30 min, and by testing 136 clinically positive samples and comparing them with RT-PCR, it yielded a sensitivity and specificity of 85.29% and 94.12%, respectively, with a limit of detection of .5 ng. This series of oligonucleotides on the probe allows for a significant reduction in detection time, and results can be obtained by simple methods such as colorimetry, with a very low instrument. The personnel requirements are very low, which provides a good reference value for nanoprobes for our related gene assays.
4.2 SARS-CoV-2 nucleic acid detection method based on CRISPR technology
CRISPR-Cas is a species-adaptive immune system (Hille et al., 2018) with natural and extraordinary gene editing (Alkhnbashi et al., 2020) and cleavage capabilities for disease (Jolany Vangah et al., 2020) and microbial detection (Medina-Aparicio et al., 2018). Currently, this system has been found valuable by researchers for SARS-CoV-2 nucleic acid detection, which mainly contains studies related to CRISPR-Cas9, Cas12a, and Cas13a (Figure 2). All three systems consist of large single multi-domain proteins (Koonin et al., 2017), and the CRISPR-Cas9 system is widely used to cleave target DNA (Hryhorowicz et al., 2017) in vitro by crRNA guidance. In recent years, the discovery of the ability of Cas12a and Cas13a to non-specifically degrade DNA (Zetsche et al., 2015) and RNA (Gootenberg et al., 2017), respectively, upon activation has revolutionized the nucleic acid field (Koonin et al., 2017).
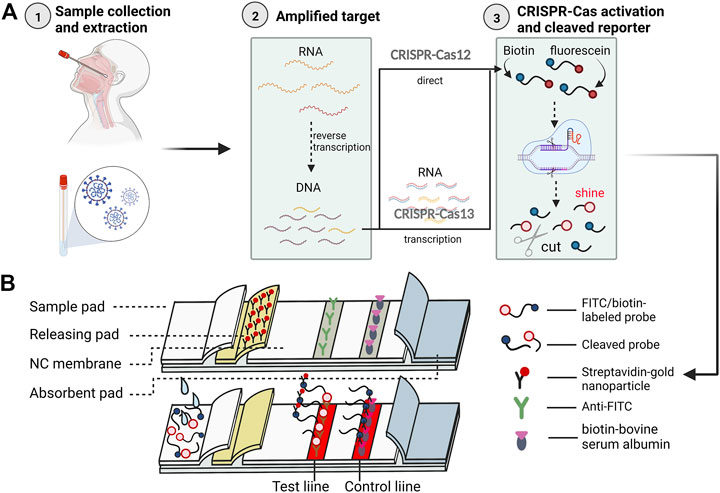
FIGURE 2. The CRISPR-Cas system for the whole process of COVID-19 immunochromatography detection. Illustrative scheme:The figure briefly summarizes the technical flow of most current CRISPR combined nucleic acid amplification and LFIA using the method designed by Zhu et al. (2021) (A) In total, there are three major steps before using immunochromatographic test strips. First, a nasal or pharyngeal swab is used to collect a sample from the subject and extract RNA. second, the nucleic acid is amplified, and the reverse transcription amplification method is generally chosen, in which the extracted RNA is specifically amplified after binding to a probe modified with a fluorescent moiety (fluorescein) and a quenching moiety (biotin), during which the target RNA is converted into a target for more DNA. Then the CRISPR-Cas system is applied to cut the target gene motif, but because Cas12 and Cas13 cut RNA and DNA respectively, the nucleic acid also needs to be transcribed to RNA when Cas13 is selected. Third, the CRISPR-Cas system cuts and a probe with the target RNA or DNA bound to the Cas protein in advance is used to identify the target nucleic acid The CRISPR-Cas system is activated when the target nucleic acid, which has been modified by fluorescent and quenching motifs, binds to the probe and cleaves the nucleic acid sequence non-selectively. The final target nucleic acid sequence is cleaved and the fluorophore fluoresces, allowing the detection of the signal (Ramachandran and Santiago, 2021). (B) The test strip located at the top is a schematic diagram of the structure of the product, and the one at the bottom is presented after receiving a positive sample. The above processed products were transferred to LFIA strips for examination, and the cleaved and uncleaved nucleic acid fragments were first labeled with colloidal gold and then flowed to detection and quality control lines immobilized with anti-biotin and anti-luciferin antibodies, respectively, so that the aggregation of uncleaved nucleic acid fragments could show a red band, while the cleaved nucleic acid fragments could be detected by a fluorescence detector. (This figure created with BioRender.com).
Qin et al. (Zhang et al., 2022) chose a CRISPR/cas13-based fluorescent nanoparticle SARS-CoV-2 detection method and performed compliance experiments. This study combined a cas13-based nucleic acid detection strip with quantum dot microspheres for the detection of the S gene of SARS-CoV-2. First, sufficient target nucleic acid was obtained by reverse-transcription recombinase-aided amplification, and then the CRISPR/Cas13 reaction was applied to specifically identify and cleave the amplification product, followed by flowing the cleaved fragment to the detection line, mixing it with sheep anti-fitc IgG-labeled quantum dot microsphere antibody and generating a fluorescent signal, which could finally be detected in a intelligent miniature fluorescence detector. The method requires samples of pharyngeal swabs, anal swabs, or sputum, and the reaction time in the test strips is 15 min, and the overall detection time does not exceed 1 h. After testing 87 clinical cases and comparing with RT-PCR, p < .05 was obtained, so the method is not statistically different from PCR, and the sensitivity and specificity are 100%.
Sun et al. (2021) combined reverse transcription and Reverse Transcription and Recombinase Polymerase Isothermal Amplification (RT-RPA) with CRISPR-Cas12a technology to develop a LFIA strip to detect the N and RdRp genes of SARS-CoV-2. This technique firstly uses RT-RPA to thermostatically amplify the extracted nucleic acids to a certain amount and then uses CRISPR-Cas12a to cleave the FAM-biotin reporter molecule and continue to amplify it, then uses the designed OR-DETECTR to generate a fluorescent signal and finally performs fluorescence detection. The method selected pharyngeal swabs as samples, and OR-DETECTR took about 50 min. After comparing 50 negative samples with PCR, the results were completely consistent, and the detection limit was 2.5 copies/μL. Yu et al. (Wang et al., 2021b) reported the CALIBURN nucleic acid detection platform, which also utilizes RT-RPA with CRISPR-Cas12a technology for the detection of the E and N genes of SARS-CoV-2, with the entire platform reflecting a process of approximately 1 h. After testing 63 clinical samples using this method and comparing it to RT-PCR, a false-negative rate of 7.9% was obtained; in addition, 57 negative results of 57 negative samples indicated a specificity of 100%.
Zhu et al. (2021) designed a method for MCCD detection of the SARS-CoV-2 N gene and ORF1ab gene based on the RT-MCDA and CRISPR-Cas12a system. After adding primers modified with PAM sites, the method amplifies nucleic acids by RT-MCDA, followed by excitation of the CRISPR-Cas12a/CrRNA system to cleave biotin and fluorescein isothiocyanate (FITC)-modified nucleic acids, and then use the lateral flow biosensor detection. Streptavidin-immobilized gold nanoparticles are immobilized on the releasing pad of the LFIA strips, and anti-FITC and biotin-bovine serum albumin are immobilized on the detection and quality control lines of the NC membrane, respectively, to serve as capture reagents (Figure 2B). The method was able to produce results within 1 h. After testing 114 samples (37 RT-PCR-positive and 41 RT-PCR-negative samples), 100% sensitivity and specificity were obtained when compared with RT-PCR results.
Xiong et al. (2021) combined the CRISPR/Cas9 system with RT-RPA technology to develop a simultaneous rapid triple-linelateral flow assay for the E and ORF1ab double genes of SARS-CoV-2. In this study, the viral E and ORF1ab genes were first amplified using the multiplex RT-RPA technique, and the two forward amplification products were modified using biotin and digoxin, respectively; then two sgRNAs (sgRNA1 and sgRNA2) containing variable recognition sequences and constant scaffold sequences were designed for targeting the completed E and ORF1ab genes, respectively. The 2 Cas9/sgRNAs hybridized to the biotin-labeled E gene amplicon and the digoxigenin-labeled ORF1ab gene amplicon, respectively, and the hybridization complex flowed through the LFIA test paper with the gold nanoparticles-DNA probe on the releasing pad first hybridized to the gold nanoparticles-DNA probe via nucleic acid hybridization. The gold nanoparticles-DNA probe first binds to the scaffold sequence of Cas9/sgRNA by nucleic acid hybridization on the releasing pad and then is captured successively on two detection lines immobilized with streptavidin and anti-digoxin antibodies, from which a red band appears due to the aggregation of gold nanoparticles. The detection limits of the test strips for both E and ORF1ab genes of SARS-CoV-2 were 4 copies/μl. By testing 64 clinical samples (35 RT-PCR-positive and 29 RT-PCR-negative samples) and comparing them with the RT-PCR results, the negative and positive prediction rates were 100% and 97. 14%, respectively.
LFIA for nucleic acid detection combines the advantages of nucleic acid amplification and LFIA, finding a balance between accuracy and simplicity. It is simpler, shorter, and less costly than RT-PCR, requires a simple sample of the nasal or pharyngeal swabs, and has a high degree of consistency between results. Moreover, like the antigen assay, this method also targets SARS-CoV-2 itself without a window period, making it suitable for early screening of infection. However, compared to antibody and antigen assays, this method still has many shortcomings, such as a long detection time, requires relevant equipment, and cannot be self-tested at home; the development is difficult; it is easy to contaminate and difficult to remove, and produce false-positive results.
At present, CRISPR technology in the detection of LFIA of SARS-CoV-2 mainly combines various simple nucleic acid amplification techniques, and then uses the CRISP-Cas12/Cas13 system to shear the amplification products, and finally uses LFIA to visualize the results, which can be seen that the combination of simple nucleic acid amplification method is widely recognized by scholars, and can take into account the portability and high performance. High performance. In particular, the combination of isothermal amplification technology and CRISPR technology allows amplification to be performed in a thermostatic thermos, greatly reducing the requirement for equipment. In addition, there are also researchers developing amplification-free nucleic acid detection based on the CRISPR-Cas system, which can shorten the detection time and reduce the difficulty of detection (Fozouni et al., 2021). Due to the gene editing function of CRISPR, its related products can have the following advantages: firstly, it can form very small gene fragments, which can make it more sensitive and specific; secondly, if combined with markers and other applications, it can achieve site-specific detection of mutations, while most of the functions of RT-PCR can be achieved and it is more convenient and faster than it (Gootenberg et al., 2017). With the continuous spread of SARS-CoV-2, virus virulence will decrease, mutation types will increase, and infectiousness will increase, so rapid diagnosis must be the best way to control the spread of the epidemic in the future, and only by detecting viral load and mutation types can we effectively grasp the viral transmission and related treatment plan of the diseased (Araf et al., 2022). CRISPR technology has the most comprehensive advantages and the ability to detect viral mutations, so it is necessary to strengthen the research related to CRISPR technology. However, it also has certain R&D difficulties, such as overcoming the problems of isothermal amplification and CRISPR/Cas reactions interfering with each other and being prone to aerosol generation during transfer, which need to be continuously solved by researchers (Sun et al., 2021).
5 Comparison of the detection performance of the LFIA strips
This review discusses the specificity (SP), sensitivity (SN), positive predictive rate (PPV), negative predictive rate (NPV), detection limit, and convenience of the test by discussing the performance of some of the widely used LFIA test strips with U.S. Food and Drug Administration emergency use authority, and comparing the advantages and disadvantages for the selection of COVID-19 in different scenarios. It also reflects the overall situation of the performance of the LFIA test strips in the market. Due to the differences in the performance of the test strips by various research teams, the results of this paper are divided into two parts: antibody, antigen, and nucleic acid (Table 1 and Table 2).
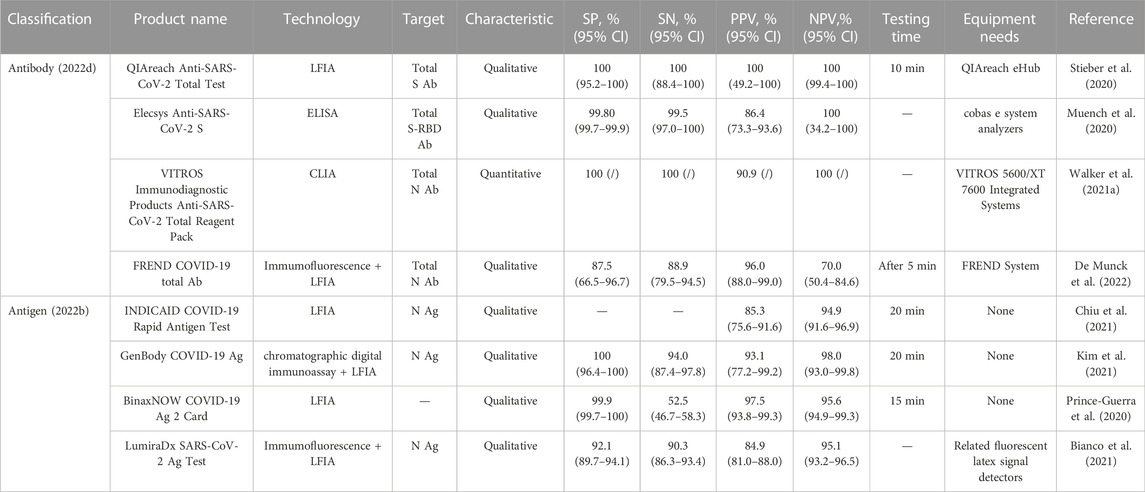
TABLE 1. Comparison of the performance of some of the LFIC test strips authorized for antibody and antigen detection by the U.S. Food and Drug Administration emergency use.
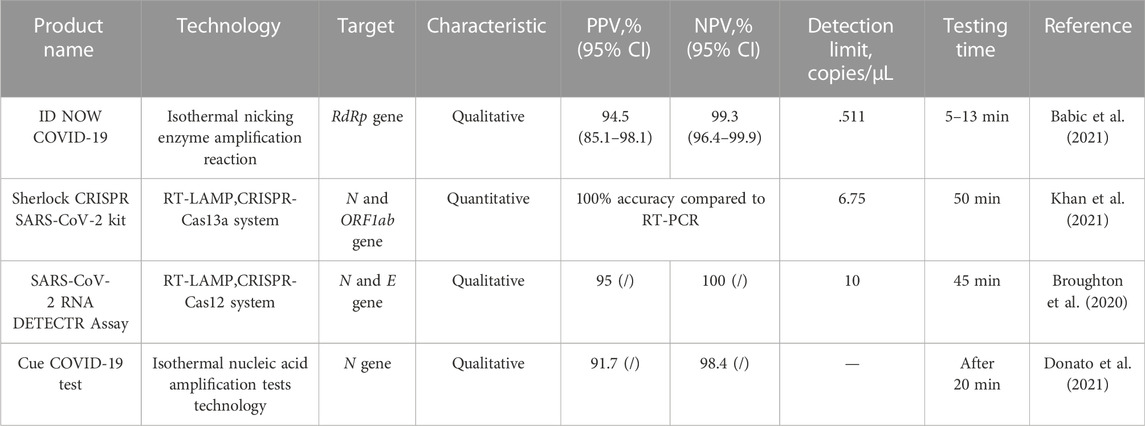
TABLE 2. Comparison of the performance of some of the LFIC test strips authorized for nucleic acid (2022c) by the U.S. Food and Drug Administration emergency use.
The data in both tables below are compared to the gold standard RT-PCR results. Although there is a small difference in cycle threshold values of the samples measured by each experiment, the comparison of the data has high value. Table 1 shows the results that analyzed a certain number of adults or children. The convenience of the assay is reflected by the time required to add the sample and the time required to read the results; the equipment requirements refer to the need for ancillary testing equipment in addition to the LFIA strips. Table 2 shows the results that analyzed a certain number of symptomatic or asymptomatic patients with COVID-19 or other respiratory infectious diseases. The time reflects the convenience of the assay, specifically the time between the addition of the sample and the reading of the results, but the nucleic acid test products all require supporting equipment and are technically demanding. In addition, it presents information about the product in terms of the R&D company, the test target, and the amplification or gene editing technology, which gives some insight into the product being compared and may also help people to keep track of the market.
WHO indicates that it recommends COVID-19 assays with a sensitivity of ≥ 80% and specificity of ≥ 97% (Peeling et al., 2021). Therefore, our team used this as a measure and selected data from the relevant studies with the largest known sample sizes for analysis for reference. First, although the above products utilize different markers, many are not commercially available to describe their specific composition and rationale and therefore are of little reference significance in the discussion. Secondly, we have performed the following analyses by examining the differences in detection principles. Most of the antibody and antigen assays are LFIA-related applications, which can be seen to be very popular in the market, and the principles of such assays are qualitative products. It can also be found that it is often used in combination with other methods such as immunofluorescence, and the methods used in combination have associated supporting detection equipment, although more complex than the visual colorimetric method and more demanding equipment, its performance, especially the sensitivity has been significantly improved. This is probably because the combined method draws on the common advantages of both, optimizes the markers, and is supported by the equipment, and its detected signal is certainly larger than that of visual colorimetric methods. The remaining methods are ELISA and chemiluminescence, of which ELISA does not show significant advantages, while chemiluminescence is the best of the products used and is attributed to its markers, reaction system, and supporting equipment, which gives it the ability to be quantified, thus effectively improving the relevant performance. Moreover, the amount of samples collected is not too small and the variety is comprehensive, so it can be used as a more reliable method for POCT (Walker et al., 2021b). Among the nucleic acid detection methods, a variety of principles are used, and their overall performance is basically better than that of antigen and antibody detection methods and following WHO recommendations, however, the isothermal nicking enzyme amplification reaction method is qualitative, but its detection limit and detection time have great advantages. In addition, the choice of detection target is also closely related to the performance of the product. Among them, antibodies and antigens for N protein and N gene are the most selected, which is also associated with its high stability, where the advantage of multiplex detection is shown in nucleic acid detection, and the performance of multi-gene detection is better than that of a single gene, in addition, it is also mentioned above that RdRp gene is more capable of analyzing new coronavirus infection, so this is most likely the reason for its low detection limit, so the choice of a target can be considered depending on the situation The choice of RdRp gene. Overall, qualitative products currently dominate the market, and their purpose is generally for rapid home detection of COVID-19, without more comprehensive quantitative or mutational analysis, so products at this stage are more inclined to improve convenience while ensuring performance.
6 Discussion
Above all, LFIA test strips for SARS-CoV-2 antibody, antigen and nucleic acid detection are designed in a variety of ways. Researchers usually spend their efforts on the selection of many assays and markers, depending on their detection principles, mainly to achieve the ultimate goal of improving the performance and social value of the assay.
First, antibody-related assays often choose colloidal gold in the choice of markers, in addition to fluorescent microspheres, QDs, and dyes; the assays include immunofluorescence, QDs, chemiluminescence, and SERS, in addition to the commonly used colorimetric methods. By analyzing the assay performance, antibody assays are often qualitative, although QDs, chemiluminescence, and SERS methods are commonly used for quantification. The sensitivity of antibody detection is around 90%–95%, the specificity is around 95%–100%, and the detection limit for quantification varies from 1pg/ml-1 ng/ml, with the SERS method performing the best. Speaking of its application, because antibodies need to be produced gradually after the body has been stimulated by the pathogen for some time, with a window period, and because many people have now been vaccinated and their bodies produce antibodies, the detection of antibodies is not a mainstream method for the diagnosis of COVID-19. However, in the future, we can mainly carry out matters such as the evaluation of the duration of infection and the potency of antibodies in patients. From this point of view, the double test and quantitative test of IgM and IgG are very necessary, and we can continue the research from the aspect of SERS or QDs.
Second, the detection of COVID-19 using antigens is a direct test with no window period. There are various methods, still colorimetric, fluorescence, QDs, and SERS methods. Performance, sensitivity, specificity, etc. are lower than those of antibodies. And the qualitative study of antigen detection started long ago, and the method has become mature, and recently gradually changed to quantitative research, but such studies, although the results are good, lack clinical samples to verify, so the follow-up should continue. Because of the current epidemic situation, China and other places are still trying to control the epidemic, and some places are gradually liberalizing their policies, and there is no telling when the post-epidemic era will return, which makes home self-testing and POCT very important directions for development. Among them, home self-testing generally requires less comprehensive results and there is no instrument to perform quantitative testing, so it is recommended to use relevant qualitative methods; POCT for clinical use often requires more reliable and comprehensive results, so quantitative testing is preferable.
Third, the nucleic acid test also has no window period and can be used for the diagnosis of neo-coronaviruses. Compared with the antigen test for SARS-CoV-2, the most significant change is that the performance of each has been improved, and the requirements for instruments, environment, and personnel have been upgraded, so it is intermediate between the gold standard and the antigen test, which is only applicable but very suitable for community or clinical POCT testing. The main methods of nucleic acid detection are an amplification of target genes, often by RT-LAMP, RT-PCR, RT-RAA, RT-PAR, RT-MIRA, and CHA, followed by cleavage or no cleavage by CRISPR technology, and then the reaction products are moved to LFIA for visualization and qualitative or quantitative detection. Their detection limits are around 2–20 copies/μl, and some can be lower than 0.05 copies/μl. In addition, there are a few non-amplified assays that are commonly used for ASOs or cut directly by CRISPR technology. The markers used in these assays are similar and relatively plain, often colloidal gold or fluorescein, digoxigenin-biotin.
Afterwards, based on the discussion of the different assays, we can again find the markers commonly used in these methods and the advantages of each. Four of the most commonly seen methods in the text will be listed here. The first is the colorimetric method, in which commonly used markers must be colloidal gold and products derived from colloidal gold, such as biotin-labeled colloidal gold and copper deposited after the signal can be enhanced, although which substance is better, but also need to be compared in specific cases. And other methods can be combined with colloidal gold to achieve the visualization of the results of the very convenient operation. In addition, colloidal gold is also commonly used in the SERS method. The second is the immunofluorescence method, the commonly used markers for fluorescent microspheres, some researchers have reported p-toluenesulfonyl modified fluorescent microspheres, so the sensitivity is greatly increased. The third is the QDs method, whose commonly used markers are QDs, although less often modified with QDs, although its combination with magnetic MnFe3O4 can make the detection limit greatly reduced. The fourth is the SERS method, which requires binding to metal ions and therefore uses modified Au or Ag as markers in addition, such as 4-NBT-modified Au, double-layer DTNB-modified SiO2@Ag, and double-layer DTNB-modified Fe3O4@Au, etc. The detection limits of the two markers using the double-layer DTNB modification are similar and extremely low. Then we compared the lowest detection limits of the four methods, which were: colloidal gold after copper deposition—0.01 μg/ml; p-toluenesulfonyl-modified fluorescent microspheres—0.01 ng/ml; QDs-conjugated magnetic MnFe3O4 --4pg/ml; double-layer DTNB-modified SiO2@Ag—1 pg/ml. Thus the related colorimetric and fluorescent immunoassays are more suitable for the antigen detection of SARS-CoV-2 in R&D homes, and the QDs and SERS method is more suitable for quantitative antibody detection of SARS-CoV-2, and high standard of antigen and nucleic acid clinical POCT. In addition, the probes for nucleic acid assays are more rudimentary and their performance is often more related to the design of the primers.
Finally, according to the comparison of the situation of the marketed products for SARS-CoV-2 detection with the LFIA products under study, it can be found that the detection methods, most of the marketed products choose conventional colorimetric, fluorescent, RT-LAMP, and other methods. Secondly, some products with relevant clinical sample validation are compared, in which the new products under study that have been clinically validated use the traditional assay methods. The performance of the marketed products was not as good as that of the new products under study, except for antibody detection products, while antigens and nucleic acids were comparable to the marketed products in the comparison. In addition, the performance of antibody assays in marketed products tends to be lower than that of antigen and nucleic acid assays, especially for negative predictive values, but new products under investigation show the opposite. In response to the above analysis, the most likely reason for the low negative predictive value of the antibody test-related products is that the test was performed after vaccination. The difference in the performance of antibody and antigen assays is most likely because the antibody products under study are better designed in terms of markers, etc., and that the research is still basically theoretical while there are still problems with the actual window period, and the clinical validation of the products is less comprehensive than that of the marketed products. Finally, QDs, SERS, and other products with excellent performance have not been clinically validated, and it is hoped that researchers can screen good technologies from them to utilize them in practice so that the detection technology of new coronaviruses can be more prosperous. In addition, there are listed products and research products that have several common features worth mentioning, the detection of the RdRp gene and the use of related enzymes in nucleic acid amplification technology can greatly improve the detection performance.
7 Conclusion and outlook
The LFIA technique is simple, inexpensive, and suitable for the rapid detection of SARS-CoV-2. Currently, several flowmetric immunochromatographic assays have been reported for different targets of SARS-CoV-2 antibodies, antigens, and nucleic acids, and we illustrate the current development and future directions of LFIA technology by listing some of the typical methods. The advantages and disadvantages of the methods and markers used for the three types of tests: antigen, antibody, and nucleic acid, are discussed to provide a framework and reference for researchers in the development of LFIA test strips for SARS-CoV-2. Finally, we summarize the advantages and disadvantages between the three different targets and their application scope, as shown in Table 3.
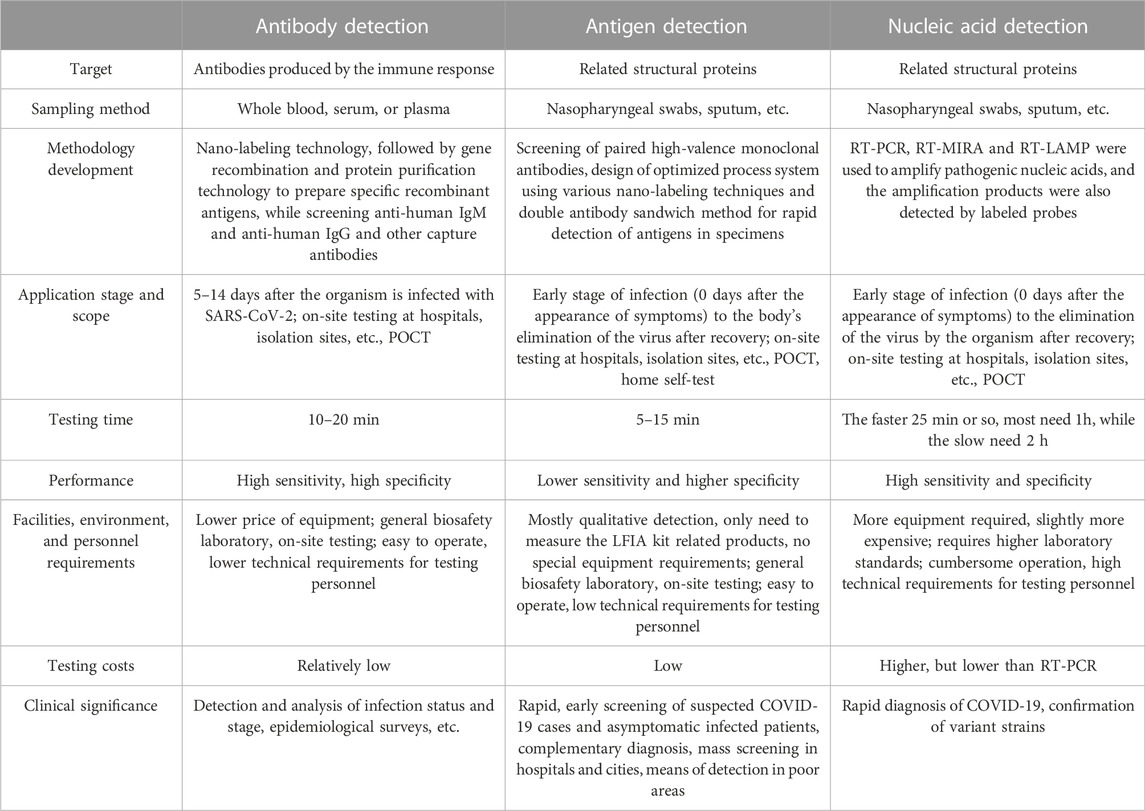
TABLE 3. Differences, advantages, and disadvantages of LFIA for antibody, antigen and nucleic acid detection.
Currently, LFIA techniques for antibodies, antigens, and nucleic acids have their characteristics and advantages, but it is difficult to integrate the four aspects of accuracy, convenience, rapidity, and affordable price. Therefore, in the future COVID-19 assay, we can consider switching different detection modes according to different application scenarios, and this method is very suitable for the actual situation and future development direction, and we hope that it can be carried out systematically. Specifically, the detection of antibodies is suitable for determining the duration of infection and the assessment of antibody potency in patients, and highly sensitive probes or SERS, QDs, and other methods can be selected for quantitative studies; antigen detection should play its greatest advantage of portability and carry out qualitative home detection with high sensitivity and specificity in colloidal gold and other aspects; the advantages of nucleic acid detection are mainly good performance, fast detection time compared with gold standards and low instrument requirements, suitable for the community and hospital-based POCT testing with high requirements. Finally, the related application of CRISPR technology in nucleic acid detection has a good prospect for development because of its good performance and its ability to cut genes and analyze SARS-CoV-2 gene and variant strains with technical support, which is the closest to the gold standard and the most comprehensive detection method at present.
Although, RT-PCR remains the current gold standard for the diagnosis of COVID-19, LFIA test strips also have promising applications. If a high-performance and less operationally and equipment-demanding CRISPR method LFIA system can be developed, it is expected to be the most representative method for clinical POCT, which can provide rapid and reliable results for diagnosis, analysis, and dosing of COVID-19. According to a survey of the literature on the use of CRISPR for SARS-CoV-2 nucleic acid LFIA, both Cas12 and Cas13 systems can be used for this assay, and the combination of the two systems is expected to improve performance by obtaining more comprehensive information on the SARS-CoV-2 gene at the time of detection, although the non-specific shearing of the gene by both systems may disrupt. However, since both systems are non-specific for gene shearing, they may destroy other targets to be tested, and interference between analytes and cross-reactivity pose challenges for multiplex assays. In addition, the use of isothermal amplification has greatly reduced the dependence of nucleic acid detection on instruments, and CRISPR technology continues to try to integrate with isothermal amplification technology. We hope that researchers can make good use of isothermal amplification technology and overcome the problems in the direction of multiplex detection technology of Cas12 and Cas13 systems, to develop the POCT test strips of SARS-CoV-2 based on CRISPR nucleic acid chromatography technology, and truly achieve rapid, sensitive, convenient, and specific detection of SARS-CoV-2, to promote the development of COVID-19 diagnosis and contribute our modest contribution to the world’s fight against epidemics.
Author contributions
JH and SZ critically analyzed the literature, drew graphs and wrote the article. XL is responsible for resourcing, writing-review and editing, oversight, project management, and funding acquisition. The rest of the authors were involved in creating tables, reviewing literature, and touching up the article. All authors contributed to the article and approved the submitted version.
Funding
This study was financially supported by the Zhejiang provincial basic public welfare research program (LGF21H200007) and 2022 National Student Innovation and Entrepreneurship Training Program Project (Item Number: 202211842047). These funders did not participate in the designing, performing or reporting in the current study.
Conflict of interest
The authors declare that the research was conducted in the absence of any commercial or financial relationships that could be construed as a potential conflict of interest.
Publisher’s note
All claims expressed in this article are solely those of the authors and do not necessarily represent those of their affiliated organizations, or those of the publisher, the editors and the reviewers. Any product that may be evaluated in this article, or claim that may be made by its manufacturer, is not guaranteed or endorsed by the publisher.
References
Aiyegbusi, O. L., Hughes, S. E., Turner, G., Rivera, S. C., Mcmullan, C., Chandan, J. S., et al. (2021). Symptoms, complications and management of long COVID: A review. J. R. Soc. Med. 114, 428–442. doi:10.1177/01410768211032850
Alafeef, M., Dighe, K., Moitra, P., and Pan, D. (2020). Rapid, ultrasensitive, and quantitative detection of SARS-CoV-2 using antisense oligonucleotides directed electrochemical biosensor chip. ACS Nano. 14, 17028–17045. doi:10.1021/acsnano.0c06392
Alkhnbashi, O. S., Meier, T., Mitrofanov, A., Backofen, R., and Voß, B. (2020). CRISPR-Cas bioinformatics. Methods. 172, 3–11. doi:10.1016/j.ymeth.2019.07.013
Araf, Y., Akter, F., Tang, Y. D., Fatemi, R., Parvez, M. S. A., Zheng, C., et al. (2022). Omicron variant of SARS-CoV-2: Genomics, transmissibility, and responses to current COVID-19 vaccines. J. Med. Virol. 94, 1825–1832. doi:10.1002/jmv.27588
Arya, R., Kumari, S., Pandey, B., Mistry, H., Bihani, S. C., Das, A., et al. (2021). Structural insights into SARS-CoV-2 proteins. J. Mol. Biol. 433, 166725. doi:10.1016/j.jmb.2020.11.024
Azeem, A., Quimby, D., Krajicek, B., and Horne, J. (2022). (Ig)Easy diagnosis of disseminated coccidioidomycosis. BMJ. Case. Rep. 15 15, e248894. doi:10.1136/bcr-2022-248894
Babic, N., Garner, K. S., Hirschhorn, J. W., Zebian, R., and Nolte, F. S. (2021). Evaluation Of Abbott ID NOW COVID-19 POC Test Performance Characteristics And Integration In The Regional Health Network Workflows To Improve Health Care Delivery. Clin. Biochem. S0009-9120, 00320–00329. doi:10.1016/j.clinbiochem.2021.12.003
Bai, Z., Wei, H., Yang, X., Zhu, Y., Peng, Y., Yang, J., et al. (2020). Rapid enrichment and ultrasensitive detection of influenza A virus in human specimen using magnetic quantum dot nanobeads based test strips. Sens. Actuators. B. Chem. 325, 128780. doi:10.1016/j.snb.2020.128780
Bianco, G., Boattini, M., Barbui, A. M., Scozzari, G., Riccardini, F., Coggiola, M., et al. (2021). Evaluation of an antigen-based test for hospital point-of-care diagnosis of SARS-CoV-2 infection. J. Clin. Virol. 139, 104838. doi:10.1016/j.jcv.2021.104838
Borghei, Y. S., Samadikhah, H. R., and Hosseinkhani, S. (2022). Exploitation of N-gene of SARS-CoV-2 to develop a new rapid assay by ASOs@AuNPs. Anal. Chem. 94, 13616–13622. doi:10.1021/acs.analchem.2c03544
Broughton, J. P., Deng, X., Yu, G., Fasching, C. L., Servellita, V., Singh, J., et al. (2020). CRISPR-Cas12-based detection of SARS-CoV-2. Nat. Biotechnol. 38, 870–874. doi:10.1038/s41587-020-0513-4
Camden, J. P., Dieringer, J. A., Wang, Y., Masiello, D. J., Marks, L. D., Schatz, G. C., et al. (2008). Probing the structure of single-molecule surface-enhanced Raman scattering hot spots. J. Am. Chem. Soc. 130, 12616–12617. doi:10.1021/ja8051427
Cavalera, S., Colitti, B., Rosati, S., Ferrara, G., Bertolotti, L., Nogarol, C., et al. (2021). A multi-target lateral flow immunoassay enabling the specific and sensitive detection of total antibodies to SARS COV-2. Talanta. 223, 121737. doi:10.1016/j.talanta.2020.121737
Chang, H., Kim, J., Lee, S. H., Rho, W. Y., Lee, J. H., Jeong, D. H., et al. (2021). Luminescent nanomaterials (II). Adv. Exp. Med. Biol. 1309, 97–132. doi:10.1007/978-981-33-6158-4_5
Chen, H., Sun, C., Wang, Y., Gao, X., You, J., Yu, W., et al. (2021a). Rapid detection of SARS-CoV-2 using Duplex reverse transcription-multienzyme isothermal rapid amplification in a point-of-care testing. Front. Cell. Infect. Microbiol. 11, 678703. doi:10.3389/fcimb.2021.678703
Chen, J., Niu, Z., Li, H., Tang, D., and Zhang, P. (2021b). Analysis of nucleic acid and antibody detection results for SARS-CoV-2 infection. Arch. Iran. Med. 24, 427–433. doi:10.34172/aim.2021.61
Chen, S., Meng, L., Wang, L., Huang, X., Ali, S., Chen, X., et al. (2021c). SERS-based lateral flow immunoassay for sensitive and simultaneous detection of anti-SARS-CoV-2 IgM and IgG antibodies by using gap-enhanced Raman nanotags. Sens. Actuators. B. Chem. 348, 130706. doi:10.1016/j.snb.2021.130706
Chiu, R. Y. T., Kojima, N., Mosley, G. L., Cheng, K. K., Pereira, D. Y., Brobeck, M., et al. (2021). Evaluation of the INDICAID COVID-19 rapid antigen test in symptomatic populations and asymptomatic community testing. Microbiol. Spectr. 9, e0034221. doi:10.1128/spectrum.00342-21
Choi, J. R., Liu, Z., Hu, J., Tang, R., Gong, Y., Feng, S., et al. (2016). Polydimethylsiloxane-paper hybrid lateral flow assay for highly sensitive point-of-care nucleic acid testing. Anal. Chem. 88, 6254–6264. doi:10.1021/acs.analchem.6b00195
Corman, V. M., Landt, O., Kaiser, M., Molenkamp, R., Meijer, A., Chu, D. K., et al. (2020). Detection of 2019 novel coronavirus (2019-nCoV) by real-time RT-PCR. Euro Surveill. 25 25, 2000045. doi:10.2807/1560-7917.ES.2020.25.3.2000045
Deng, Y., Jiang, H., Li, X., and Lv, X. (2021). Recent advances in sensitivity enhancement for lateral flow assay. Mikrochim. Acta. 188, 379. doi:10.1007/s00604-021-05037-z
Dinnes, J., Deeks, J. J., Adriano, A., Berhane, S., Davenport, C., Dittrich, S., et al. (2020). Rapid, point-of-care antigen and molecular-based tests for diagnosis of SARS-CoV-2 infection. Cochrane Database Syst. Rev. 8, CD013705. doi:10.1002/14651858.CD013705
Donato, L. J., Trivedi, V. A., Stransky, A. M., Misra, A., Pritt, B. S., Binnicker, M. J., et al. (2021). Evaluation of the Cue Health point-of-care COVID-19 (SARS-CoV-2 nucleic acid amplification) test at a community drive through collection center. Diagn. Microbiol. Infect. Dis. 100, 115307. doi:10.1016/j.diagmicrobio.2020.115307
Dweck, M. R., Bularga, A., Hahn, R. T., Bing, R., Lee, K. K., Chapman, A. R., et al. (2020). Global evaluation of echocardiography in patients with COVID-19. Eur. Heart. J. Cardiovasc. Imaging. 21, 949–958. doi:10.1093/ehjci/jeaa178
Faulk, W. P., and Taylor, G. M. (1971). Communication to the editors. Immunochemistry. 8, 1081–1083. doi:10.1016/0019-2791(71)90496-4
Fernandes, G. M., Silva, W. R., Barreto, D. N., Lamarca, R. S., Lima Gomes, P. C. F., Flávio DaPetruci, S. J., et al. (2020). Novel approaches for colorimetric measurements in analytical chemistry - a review. Anal. Chim. Acta. 1135, 187–203. doi:10.1016/j.aca.2020.07.030
Fozouni, P., Son, S., Díaz De León Derby, M., Knott, G. J., Gray, C. N., D'ambrosio, M. V., et al. (2021). Amplification-free detection of SARS-CoV-2 with CRISPR-Cas13a and mobile phone microscopy. Cell. 184, 323–333. doi:10.1016/j.cell.2020.12.001
Fulford, T. S., Van, H., Gherardin, N. A., Zheng, S., Ciula, M., Drummer, H. E., et al. (2021). A point-of-care lateral flow assay for neutralising antibodies against SARS-CoV-2. EBioMedicine. 74, 103729. doi:10.1016/j.ebiom.2021.103729
Ghorbanizamani, F., Tok, K., Moulahoum, H., Harmanci, D., Hanoglu, S. B., Durmus, C., et al. (2021). Dye-loaded polymersome-based lateral flow assay: Rational design of a COVID-19 testing platform by repurposing SARS-CoV-2 antibody cocktail and antigens obtained from positive human samples. Acs. Sens. 6, 2988–2997. doi:10.1021/acssensors.1c00854
Gootenberg, J. S., Abudayyeh, O. O., Lee, J. W., Essletzbichler, P., Dy, A. J., Joung, J., et al. (2017). Nucleic acid detection with CRISPR-Cas13a/C2c2. Science. 356, 438–442. doi:10.1126/science.aam9321
Guo, J., Wang, Y., Niu, S., Li, H., Tian, Y., Yu, S., et al. (2020). Highly sensitive fluorescence-linked immunosorbent assay for the determination of human IgG in serum using quantum dot nanobeads and magnetic Fe(3)O(4) nanospheres. ACS Omega. 5, 23229–23236. doi:10.1021/acsomega.0c02987
Gupta, N., Augustine, S., Narayan, T., O'riordan, A., Das, A., Kumar, D., et al. (2021). Point-of-Care PCR assays for COVID-19 detection. Biosens. (Basel). 11, 141. doi:10.3390/bios11050141
Gwyn, S., Mkocha, H., Randall, J. M., Kasubi, M., and Martin, D. L. (2019). Optimization of a rapid test for antibodies to the Chlamydia trachomatis antigen Pgp3. Diagn. Microbiol. Infect. Dis. 93, 293–298. doi:10.1016/j.diagmicrobio.2018.11.001
Hakki, S., Zhou, J., Jonnerby, J., Singanayagam, A., Barnett, J. L., Madon, K. J., et al. (2022). Onset and window of SARS-CoV-2 infectiousness and temporal correlation with symptom onset: A prospective, longitudinal, community cohort study. Lancet Respir. Med. 10, 1061–1073. doi:10.1016/s2213-2600(22)00226-0
Hamming, I., Timens, W., Bulthuis, M. L., Lely, A. T., Navis, G., and Van Goor, H. (2004). Tissue distribution of ACE2 protein, the functional receptor for SARS coronavirus. A first step in understanding SARS pathogenesis. J. Pathol. 203, 631–637. doi:10.1002/path.1570
Han, H., Wang, C., Yang, X., Zheng, S., Cheng, X., Liu, Z., et al. (2022). Rapid field determination of SARS-CoV-2 by a colorimetric and fluorescent dual-functional lateral flow immunoassay biosensor. Sens. Actuators B Chem. 351, 130897. doi:10.1016/j.snb.2021.130897
Harrison, A. G., Lin, T., and Wang, P. (2020). Mechanisms of SARS-CoV-2 transmission and pathogenesis. Trends. Immunol. 41, 1100–1115. doi:10.1016/j.it.2020.10.004
Harritshøj, L. H., Gybel-Brask, M., Afzal, S., Kamstrup, P. R., Jørgensen, C. S., Thomsen, M. K., et al. (2021). Comparison of 16 serological SARS-CoV-2 immunoassays in 16 clinical laboratories. J. Clin. Microbiol. 59. doi:10.1128/jcm.02596-20
Harvey, W. T., Carabelli, A. M., Jackson, B., Gupta, R. K., Thomson, E. C., Harrison, E. M., et al. (2021). SARS-CoV-2 variants, spike mutations and immune escape. Nat. Rev. Microbiol. 19, 409–424. doi:10.1038/s41579-021-00573-0
Hille, F., Richter, H., Wong, S. P., Bratovič, M., Ressel, S., and Charpentier, E. (2018). The biology of CRISPR-cas: Backward and forward. Cell. 172, 1239–1259. doi:10.1016/j.cell.2017.11.032
Hoehl, S., Rabenau, H., Berger, A., Kortenbusch, M., Cinatl, J., Bojkova, D., et al. (2020). Evidence of SARS-CoV-2 infection in returning travelers from wuhan, China. N. Engl. J. Med. 382, 1278–1280. doi:10.1056/nejmc2001899
Hryhorowicz, M., Lipiński, D., Zeyland, J., and Słomski, R. (2017). CRISPR/Cas9 immune system as a tool for genome engineering. Arch. Immunol. Ther. Exp. Warsz. 65, 233–240. doi:10.1007/s00005-016-0427-5
Huang, L., Tian, S., Zhao, W., Liu, K., Ma, X., and Guo, J. (2020). Multiplexed detection of biomarkers in lateral-flow immunoassays. Analyst. 145, 2828–2840. doi:10.1039/c9an02485a
Ibrahim, N., Jamaluddin, N. D., Tan, L. L., and Yusof, Mohd, (2021). A review on the development of gold and silver nanoparticles-based biosensor as a detection strategy of emerging and pathogenic RNA virus. Sensors (Basel). 21. doi:10.3390/s21155114
Im, K., Mareninov, S., Diaz, M. F. P., and Yong, W. H. (2019). An introduction to performing immunofluorescence staining. Methods Mol. Biol. 1897, 299–311. doi:10.1007/978-1-4939-8935-5_26
Islam, K. U., and Iqbal, J. (2020). An update on molecular diagnostics for COVID-19. Front. Cell. Infect. Microbiol. 10, 560616. doi:10.3389/fcimb.2020.560616
Jain, P. K., Lee, K. S., El-Sayed, I. H., and El-Sayed, M. A. (2006). Calculated absorption and scattering properties of gold nanoparticles of different size, shape, and composition: Applications in biological imaging and biomedicine. J. Phys. Chem. B 110, 7238–7248. doi:10.1021/jp057170o
Johansson, M. A., Quandelacy, T. M., Kada, S., Prasad, P. V., Steele, M., Brooks, J. T., et al. (2021). SARS-CoV-2 transmission from people without COVID-19 symptoms. JAMA. Netw. Open. 4, e2035057. doi:10.1001/jamanetworkopen.2020.35057
Jolany Vangah, S., Katalani, C., Booneh, H. A., Hajizade, A., Sijercic, A., and Ahmadian, G. (2020). CRISPR-based diagnosis of infectious and noninfectious diseases. Biol. Proced. Online. 22, 22. doi:10.1186/s12575-020-00135-3
Kadam, S. B., Sukhramani, G. S., Bishnoi, P., Pable, A. A., and Barvkar, V. T. (2021). SARS-CoV-2, the pandemic coronavirus: Molecular and structural insights. J. Basic Microbiol. 61, 180–202. doi:10.1002/jobm.202000537
Kampf, G., Lemmen, S., and Suchomel, M. (2021). Ct values and infectivity of SARS-CoV-2 on surfaces. Lancet Infect. Dis. 21, e141. doi:10.1016/s1473-3099(20)30883-5
Kang, K., Huang, L., Ouyang, C., Du, J., Yang, B., Chi, Y., et al. (2021a). Development, performance evaluation, and clinical application of a Rapid SARS-CoV-2 IgM and IgG Test Kit based on automated fluorescence immunoassay. J. Med. Virol. 93, 2838–2847. doi:10.1002/jmv.26696
Kang, K., Huang, L., Ouyang, C., Du, J., Yang, B., Chi, Y., et al. (2021b). Development, performance evaluation, and clinical application of a Rapid SARS-CoV-2 IgM and IgG Test Kit based on automated fluorescence immunoassay. J. Med. Virol. 93, 2838–2847. doi:10.1002/jmv.26696
Ke, Z., Oton, J., Qu, K., Cortese, M., Zila, V., Mckeane, L., et al. (2020). Structures and distributions of SARS-CoV-2 spike proteins on intact virions. Nature. 588, 498–502. doi:10.1038/s41586-020-2665-2
Khailany, R. A., Safdar, M., and Ozaslan, M. (2020). Genomic characterization of a novel SARS-CoV-2. Gene Rep. 19, 100682. doi:10.1016/j.genrep.2020.100682
Khan, W. A., Barney, R. E., and Tsongalis, G. J. (2021). CRISPR-cas13 enzymology rapidly detects SARS-CoV-2 fragments in a clinical setting. J. Clin. Virol. 145, 105019. doi:10.1016/j.jcv.2021.105019
Kim, D., Lee, J., Bal, J., Seo, S. K., Chong, C. K., Lee, J. H., et al. (2021). Development and clinical evaluation of an immunochromatography-based rapid antigen test (GenBody™ COVAG025) for COVID-19 diagnosis. Viruses. 13, 796. doi:10.3390/v13050796
Koonin, E. V., Makarova, K. S., and Zhang, F. (2017). Diversity, classification and evolution of CRISPR-Cas systems. Curr. Opin. Microbiol. 37, 67–78. doi:10.1016/j.mib.2017.05.008
Kowada, A. (2021). Greater public health impact of COVID-19 antigen detection tests. Bmc. Med. 19, 82. doi:10.1186/s12916-021-01956-z
Kumar, V., Mishra, S., Sharma, R., Agarwal, J., Ghoshal, U., Khanna, T., et al. (2022). Development of RNA-based assay for rapid detection of SARS-CoV-2 in clinical samples. Intervirology. 65, 181–187. doi:10.1159/000522337
Lan, J., Ge, J., Yu, J., Shan, S., Zhou, H., Fan, S., et al. (2020). Structure of the SARS-CoV-2 spike receptor-binding domain bound to the ACE2 receptor. Nature. 581, 215–220. doi:10.1038/s41586-020-2180-5
Lee, J. H., Choi, M., Jung, Y., Lee, S. K., Lee, C. S., Kim, J., et al. (2021). A novel rapid detection for SARS-CoV-2 spike 1 antigens using human angiotensin converting enzyme 2 (ACE2). Biosens. Bioelectron. 171, 112715. doi:10.1016/j.bios.2020.112715
Li, Z., Wang, A., Zhou, J., Chen, Y., Liu, H., Liu, Y., et al. (2022). A universal fluorescent immunochromatography assay based on quantum dot nanoparticles for the rapid detection of specific antibodies against SARS-CoV-2 nucleocapsid protein. Int. J. Mol. Sci. 23. doi:10.3390/ijms23116225
Liu, H., Dai, E., Xiao, R., Zhou, Z., Zhang, M., Bai, Z., et al. (2021a). Development of a SERS-based lateral flow immunoassay for rapid and ultra-sensitive detection of anti-SARS-CoV-2 IgM/IgG in clinical samples. Sens. Actuators B Chem. 329, 129196. doi:10.1016/j.snb.2020.129196
Liu, J., Yan, W., Liu, Z., Han, Y., Xia, Y., and Yu, J. (2021b). A colloidal gold-based immunochromatographic strip for rapid detection of SARS-CoV-2 antibodies after vaccination. Med. Nov. Technol. Devices. 11, 100084. doi:10.1016/j.medntd.2021.100084
Liu, S. J., Leng, C. H., Lien, S. P., Chi, H. Y., Huang, C. Y., Lin, C. L., et al. (2006). Immunological characterizations of the nucleocapsid protein based SARS vaccine candidates. Vaccine. 24, 3100–3108. doi:10.1016/j.vaccine.2006.01.058
Liu, Z., Wang, C., Zheng, S., Yang, X., Han, H., Dai, Y., et al. (2022). Simultaneously ultrasensitive and quantitative detection of influenza A virus, SARS-CoV-2, and respiratory syncytial virus via multichannel magnetic SERS-based lateral flow immunoassay. Nanomedicine. 47, 102624. doi:10.1016/j.nano.2022.102624
Mahmoudinobar, F., Britton, D., and Montclare, J. K. (2021). Protein-based lateral flow assays for COVID-19 detection. Protein. Eng. Des. Sel. 34 34, gzab010. doi:10.1093/protein/gzab010
Mallano, A., Ascione, A., and Flego, M. (2022). Antibody response against SARS-CoV-2 infection: Implications for diagnosis, treatment and vaccine development. Int. Rev. Immunol. 41, 393–413. doi:10.1080/08830185.2021.1929205
Mandal, S., Barnett, J., Brill, S. E., Brown, J. S., Denneny, E. K., Hare, S. S., et al. (2021). 'Long-COVID': A cross-sectional study of persisting symptoms, biomarker and imaging abnormalities following hospitalisation for COVID-19. Thorax. 76, 396–398. doi:10.1136/thoraxjnl-2020-215818
Mao, M., Wu, F., Shi, X., Huang, Y., and Ma, L. (2022). Ultrasensitive detection of COVID-19 virus N protein based on p-toluenesulfonyl modified fluorescent microspheres immunoassay. Biosens. (Basel). 12 12, 437. doi:10.3390/bios12070437
Matea, C. T., Mocan, T., Tabaran, F., Pop, T., Mosteanu, O., Puia, C., et al. (2017). Quantum dots in imaging, drug delivery and sensor applications. Int. J. Nanomedicine. 12, 5421–5431. doi:10.2147/ijn.s138624
Medina-Aparicio, L., Dávila, S., Rebollar-Flores, J. E., Calva, E., and Hernández-Lucas, I. (2018). The CRISPR-Cas system in Enterobacteriaceae. Pathog. Dis. 76. doi:10.1093/femspd/fty002
Mertens, P., De Vos, N., Martiny, D., Jassoy, C., Mirazimi, A., Cuypers, L., et al. (2020). Development and potential usefulness of the COVID-19 Ag respi-strip diagnostic assay in a pandemic context. Front. Med. (Lausanne). 7, 225. doi:10.3389/fmed.2020.00225
Metcalf, R. L., and Kearns, C. W. (1941). %J journal of economic EntomologyThe toxicity and repellent action of some derivatives of picramic acid and of toluenesulfonyl chloride to the greenhouse leaf tier. J. Econ. Entomology. 34, 306–309. doi:10.1093/jee/34.2.306
Moabelo, K. L., Martin, D. R., Fadaka, A. O., Sibuyi, N. R. S., Meyer, M., and Madiehe, A. M. (2021). Nanotechnology-based strategies for effective and rapid detection of SARS-CoV-2. Mater. (Basel). 14, 7851. doi:10.3390/ma14247851
Moghimi, N., Di Napoli, M., Biller, J., Siegler, J. E., Shekhar, R., Mccullough, L. D., et al. (2021). The neurological manifestations of post-acute sequelae of SARS-CoV-2 infection. Curr. Neurol. Neurosci. Rep. 21, 44. doi:10.1007/s11910-021-01130-1
Moitra, P., Alafeef, M., Dighe, K., Frieman, M. B., and Pan, D. (2020). Selective naked-eye detection of SARS-CoV-2 mediated by N gene targeted antisense oligonucleotide capped plasmonic nanoparticles. ACS Nano. 14, 7617–7627. doi:10.1021/acsnano.0c03822
Muench, P., Jochum, S., Wenderoth, V., Ofenloch-Haehnle, B., Hombach, M., Strobl, M., et al. (2020). Development and validation of the elecsys anti-SARS-CoV-2 immunoassay as a highly specific tool for determining past exposure to SARS-CoV-2. J. Clin. Microbiol. 58 (10), e01694–20. doi:10.1128/jcm.01694-20
Munck, D., Peeters, B., Huyghe, E., Goossens, H., Ieven, M., et al. (2022). Performance of the FREND™ COVID-19 IgG/IgM Duo point-of-care test for SARS-CoV-2 antibody detection. Acta. Clin. belg. 77, 647–652. doi:10.1080/17843286.2021.1940776
Ndzouboukou, B., Zhang, Y. D., and Fan, X. L. (2021). Recent developments in SARS-CoV-2 neutralizing antibody detection methods. Curr. Med. Sci. 41, 1052–1064. doi:10.1007/s11596-021-2470-7
Nguyen, V. T., Song, S., Park, S., and Joo, C. (2020). Recent advances in high-sensitivity detection methods for paper-based lateral-flow assay. Biosens. Bioelectron. 152, 112015. doi:10.1016/j.bios.2020.112015
Ni, W., Yang, X., Yang, D., Bao, J., Li, R., Xiao, Y., et al. (2020). Role of angiotensin-converting enzyme 2 (ACE2) in COVID-19. Crit. Care. 24, 422. doi:10.1186/s13054-020-03120-0
Oishee, M. J., Ali, T., Jahan, N., Khandker, S. S., Haq, M. A., Khondoker, M. U., et al. (2021). COVID-19 pandemic: Review of contemporary and forthcoming detection tools. Infect. Drug Resist. 14, 1049–1082. doi:10.2147/idr.s289629
Paces, J., Strizova, Z., Smrz, D., and Cerny, J. (2020). COVID-19 and the immune system. Physiol. Res. 69, 379–388. doi:10.33549/physiolres.934492
Peeling, R. W., Olliaro, P. L., Boeras, D. I., and Fongwen, N. (2021). Scaling up COVID-19 rapid antigen tests: Promises and challenges. Lancet Infect. Dis. 21, e290–e295. doi:10.1016/s1473-3099(21)00048-7
Peng, T., Jiao, X., Liang, Z., Zhao, H., Zhao, Y., Xie, J., et al. (2021). Lateral flow immunoassay coupled with copper enhancement for rapid and sensitive SARS-CoV-2 nucleocapsid protein detection. Biosens. (Basel). 12, 13. doi:10.3390/bios12010013
Pollock, N. R., Jacobs, J. R., Tran, K., Cranston, A. E., Smith, S., O'kane, C. Y., et al. (2021). Performance and implementation evaluation of the abbott BinaxNOW rapid antigen test in a high-throughput drive-through community testing site in Massachusetts. J. Clin. Microbiol. 59 (5), e00083–21. doi:10.1128/jcm.00083-21
Porte, L., Legarraga, P., Vollrath, V., Aguilera, X., Munita, J. M., Araos, R., et al. (2020). Evaluation of a novel antigen-based rapid detection test for the diagnosis of SARS-CoV-2 in respiratory samples. Int. J. Infect. Dis. 99, 328–333. doi:10.1016/j.ijid.2020.05.098
Post, N., Eddy, D., Huntley, C., Van Schalkwyk, M. C. I., Shrotri, M., Leeman, D., et al. (2020). Antibody response to SARS-CoV-2 infection in humans: A systematic review. PLoS. One. 15, e0244126. doi:10.1371/journal.pone.0244126
Prince-Guerra, J. L., Almendares, O., Nolen, L. D., Gunn, J. K. L., Dale, A. P., Buono, S. A., et al. . Evaluation of abbott BinaxNOW rapid antigen test for SARS-CoV-2 infection at two community-based testing sites — pima county, Arizona, november 3–17, 2020. MMWR. Morb. Mortal. Wkly. Rep. 70, 100–105. doi:10.15585/mmwr.mm7003e3
Puntmann, V. O., Carerj, M. L., Wieters, I., Fahim, M., Arendt, C., Hoffmann, J., et al. (2020). Outcomes of cardiovascular magnetic resonance imaging in patients recently recovered from coronavirus disease 2019 (COVID-19). JAMA. Cardiol. 5, 1265–1273. doi:10.1001/jamacardio.2020.3557
Qin, J., Lu, Q., Wang, C., Luo, J., and Yang, M. (2021). Colloidal gold-based lateral flow immunoassay with inline cleanup for rapid on-site screening of carbendazim in functional foods. Anal. Bioanal. Chem. 413, 3725–3735. doi:10.1007/s00216-021-03321-8
Rahbari, R., Moradi, N., and Abdi, M. (2021). rRT-PCR for SARS-CoV-2: Analytical considerations. Clin. Chim. Acta. 516, 1–7. doi:10.1016/j.cca.2021.01.011
Ramachandran, A., and Santiago, J. G. (2021). CRISPR enzyme kinetics for molecular diagnostics. Anal. Chem. 93, 7456–7464. doi:10.1021/acs.analchem.1c00525
Rebollo, B., Pérez, T., Camuñas, A., Pérez-Ramírez, E., Llorente, F., Sánchez-Seco, M. P., et al. (2018). A monoclonal antibody to DIII E protein allowing the differentiation of West Nile virus from other flaviviruses by a lateral flow assay. J. Virol. Methods. 260, 41–44. doi:10.1016/j.jviromet.2018.06.016
Reddy, A., Bosch, I., Salcedo, N., Herrera, B. B., De Puig, H., Narváez, C. F., et al. (2020). Development and validation of a rapid lateral flow E1/E2-antigen test and ELISA in patients infected with emerging asian strain of chikungunya virus in the americas. Viruses. 12, 971. doi:10.3390/v12090971
Roda, A., Cavalera, S., Di Nardo, F., Calabria, D., Rosati, S., Simoni, P., et al. (2021). Dual lateral flow optical/chemiluminescence immunosensors for the rapid detection of salivary and serum IgA in patients with COVID-19 disease. Biosens. Bioelectron. 172, 112765. doi:10.1016/j.bios.2020.112765
Rosati, S., Profiti, M., Lorenzetti, R., Bandecchi, P., Mannelli, A., Ortoffi, M., et al. (2004). Development of recombinant capsid antigen/transmembrane epitope fusion proteins for serological diagnosis of animal lentivirus infections. J. Virol. Methods. 121, 73–78. doi:10.1016/j.jviromet.2004.06.001
Rothe, C., Schunk, M., Sothmann, P., Bretzel, G., Froeschl, G., Wallrauch, C., et al. (2020). Transmission of 2019-nCoV infection from an asymptomatic contact in Germany. N. Engl. J. Med. 382, 970–971. doi:10.1056/nejmc2001468
Serafinelli, C., Fantoni, A., Alegria, E., and Vieira, M. (2022). Plasmonic metal nanoparticles hybridized with 2D nanomaterials for SERS detection: A review. Biosens. (Basel) 12. doi:10.3390/bios12040225
Shang, J., Wan, Y., Luo, C., Ye, G., Geng, Q., Auerbach, A., et al. (2020). Cell entry mechanisms of SARS-CoV-2. Proc. Natl. Acad. Sci. U. S. A. 117, 11727–11734. doi:10.1073/pnas.2003138117
Shen, B., Zheng, Y., Zhang, X., Zhang, W., Wang, D., Jin, J., et al. (2020). Clinical evaluation of a rapid colloidal gold immunochromatography assay for SARS-Cov-2 IgM/IgG. Am. J. Transl. Res. 12, 1348–1354.
Stavem, K., Ghanima, W., Olsen, M. K., Gilboe, H. M., and Einvik, G. (2021). Persistent symptoms 1.5-6 months after COVID-19 in non-hospitalised subjects: A population-based cohort study. Thorax. 76, 405–407. doi:10.1136/thoraxjnl-2020-216377
Stieber, F., Howard, J., Rao, S. N., Kawamura, L. M., Manissero, D., Love, J., et al. (2020). First performance report of QIAreach™ Anti-SARS-CoV-2 Total Test, an innovative nanoparticle fluorescence digital detection platform. J. Clin. Virol. 133, 104681. doi:10.1016/j.jcv.2020.104681
Sultangaziyev, A., Ilyas, A., Dyussupova, A., and Bukasov, R. (2022). Trends in application of SERS substrates beyond Ag and Au, and their role in bioanalysis. Biosens. (Basel). 12, 967. doi:10.3390/bios12110967
Sun, Y., Yu, L., Liu, C., Ye, S., Chen, W., Li, D., et al. (2021). One-tube SARS-CoV-2 detection platform based on RT-RPA and CRISPR/Cas12a. J. Transl. Med. 19, 74. doi:10.1186/s12967-021-02741-5
Tali, S., Leblanc, J. J., Sadiq, Z., Oyewunmi, O. D., Camargo, C., et al. (2021a). Tools and techniques for Severe Acute respiratory Syndrome coronavirus 2 (SARS-CoV-2)/COVID-19 detection. Clin. Microbiol. Rev. 34 (3), e00228–20. doi:10.1128/CMR.00228-20
Tali, S., Leblanc, J. J., Sadiq, Z., Oyewunmi, O. D., Camargo, C., et al. (2021b). Tools and techniques for Severe Acute respiratory Syndrome coronavirus 2 (SARS-CoV-2)/COVID-19 detection. Clin. Microbiol. Rev. 34 (3), e00228–20. doi:10.1128/CMR.00228-20
Tanriover, M. D., Fathi, A., Raspe, M., Novais, A. G., and Uyaroğlu, O. A. (2021). Characteristics and management of asymptomatic SARS-CoV-2 infections. J. Basic Clin. Physiol. Pharmacol. 33, 1–7. doi:10.1515/jbcpp-2021-0159
Tao, K., Tzou, P. L., Nouhin, J., Gupta, R. K., De Oliveira, T., Kosakovsky Pond, S. L., et al. (2021). The biological and clinical significance of emerging SARS-CoV-2 variants. Nat. Rev. Genet. 22, 757–773. doi:10.1038/s41576-021-00408-x
Tuaillon, E., Bolloré, K., Pisoni, A., Debiesse, S., Renault, C., Marie, S., et al. (2020). Detection of SARS-CoV-2 antibodies using commercial assays and seroconversion patterns in hospitalized patients. J. Infect. 81, e39–e45. doi:10.1016/j.jinf.2020.05.077
U.S. Food & Drug Administration (2022b). In vitro diagnostics EUAs - antigen diagnostic tests for SARS-CoV-2. Available at: https://www.fda.gov/medical-devices/coronavirus-disease-2019-covid-19-emergency-use-authorizations-medical-devices/in-vitro-diagnostics-euas-antigen-diagnostic-tests-sars-cov-2 (Accessed September 15, 2022).
U.S. Food & Drug Administration (2022c). In vitro diagnostics EUAs - molecular diagnostic tests for SARS-CoV-2. Available at: https://www.fda.gov/medical-devices/coronavirus-disease-2019-covid-19-emergency-use-authorizations-medical-devices/in-vitro-diagnostics-euas-molecular-diagnostic-tests-sars-cov-2 (Accessed September 15, 2022).
U.S. Food & Drug Administration (2022d). In vitro diagnostics EUAs - serology and other adaptive immune response tests for SARS-CoV-2. Available at: https://www.fda.gov/medical-devices/coronavirus-disease-2019-covid-19-emergency-use-authorizations-medical-devices/in-vitro-diagnostics-euas-serology-and-other-adaptive-immune-response-tests-sars-cov-2 (Accessed September 15, 2022).
V'kovski, P., Kratzel, A., Steiner, S., Stalder, H., and Thiel, V. (2021). Coronavirus biology and replication: Implications for SARS-CoV-2. Nat. Rev. Microbiol. 19, 155–170. doi:10.1038/s41579-020-00468-6
Walker, G. J., Naing, Z., Ospina Stella, A., Yeang, M., Caguicla, J., Ramachandran, V., et al. (2021a). SARS coronavirus-2 microneutralisation and commercial serological assays correlated closely for some but not all enzyme immunoassays. Viruses. 13, 247. doi:10.3390/v13020247
Walker, G. J., Naing, Z., Ospina Stella, A., Yeang, M., Caguicla, J., Ramachandran, V., et al. (2021b). SARS coronavirus-2 microneutralisation and commercial serological assays correlated closely for some but not all enzyme immunoassays. Viruses. 13, 247. doi:10.3390/v13020247
Walls, A. C., Park, Y. J., Tortorici, M. A., Wall, A., Mcguire, A. T., and Veesler, D. (2020). Structure, function, and antigenicity of the SARS-CoV-2 spike glycoprotein. Cell. 181, 281–292. doi:10.1016/j.cell.2020.02.058
Wang, H., Li, X., Li, T., Wang, L., Wang, L., Lin, J., et al. (2021a). Development of a SARS-CoV-2 rapid antibody detection kit and study on dynamic changes in antibodies in infected patients. Clin. Respir. J. 15, 499–505. doi:10.1111/crj.13331
Wang, M. Y., Zhao, R., Gao, L. J., Gao, X. F., Wang, D. P., and Cao, J. M. (2020). SARS-CoV-2: Structure, biology, and structure-based therapeutics development. Front. Cell. Infect. Microbiol. 10, 6. doi:10.3389/fcimb.2020.00006
Wang, X., Choi, N., Cheng, Z., Ko, J., Chen, L., and Choo, J. (2017). Simultaneous detection of dual nucleic acids using a SERS-based lateral flow assay biosensor. Anal. Chem. 89, 1163–1169. doi:10.1021/acs.analchem.6b03536
Wang, Y., Liu, D., Lin, H., Chen, D., Sun, J., Xie, Y., et al. (2021b). Development of a broadly applicable cas12a-linked beam unlocking reaction for sensitive and specific detection of respiratory pathogens including SARS-CoV-2. Acs. Chem. Biol. 16, 491–500. doi:10.1021/acschembio.0c00840
Wonderly, B., Jones, S., Gatton, M. L., Barber, J., Killip, M., Hudson, C., et al. (2019). Comparative performance of four rapid Ebola antigen-detection lateral flow immunoassays during the 2014-2016 Ebola epidemic in West Africa. PLoS. One. 14 14, e0212113. doi:10.1371/journal.pone.0212113
World Health Organization (2022a). Coronavirus disease (COVID-19). Available at: https://www.who.int/zh/health-topics/coronavirus#tab=tab_1 (Accessed September 10, 2022).
World Health Organization (2020a). Laboratory testing strategy recommendations for COVID-19: Interim guidance, 21 march 2020. Available at: https://apps.who.int/iris/handle/10665/331509 (Accessed September 10, 2022).
World Health Organization (2022e). WHO coronavirus (COVID-19) dashboard. Available at: https://covid19.who.int/(Accessed September 2, 2022).
World Health Organization (2020c). WHO director-general opening remarks at the member state briefing on the COVID-19 pandemic evaluation - 9 july 2020. Available at: https://www.who.int/zh/director-general/speeches/detail/who-director-general-opening-remarks-at-the-member-state-briefing-on-the-covid-19-pandemic-evaluation–9-july-2020 (Accessed September 10, 2022).
World Health Organization (2020b). WHO director-general's introductory remarks for the launch of the gpmb 2020 annual report: A world in disorder. Available at: https://www.who.int/zh/director-general/speeches/detail/who-director-general-s-introductory-remarks-for-the-launch-of-the-gpmb-2020-annual-report-a-world-in-disorder (Accessed September 10, 2022).
Xiong, E., Jiang, L., Tian, T., Hu, M., Yue, H., Huang, M., et al. (2021). Simultaneous dual-gene diagnosis of SARS-CoV-2 based on CRISPR/Cas9-Mediated lateral flow assay. Angew. Chem. Int. Ed. Engl. 60, 5307–5315. doi:10.1002/anie.202014506
Xu, Y., Ma, B., Chen, E., Yu, X., Ye, Z., Sun, C., et al. (2021). Dual fluorescent immunochromatographic assay for simultaneous quantitative detection of citrinin and zearalenone in corn samples. Food. Chem. 336, 127713. doi:10.1016/j.foodchem.2020.127713
Yamayoshi, S., Sakai-Tagawa, Y., Koga, M., Akasaka, O., Nakachi, I., Koh, H., et al. (2020). Comparison of rapid antigen tests for COVID-19. Viruses. 12, 1420. doi:10.3390/v12121420
Yang, R. X., Zheng, R. D., and Fan, J. G. (2020). Etiology and management of liver injury in patients with COVID-19. World J. Gastroenterol. 26, 4753–4762. doi:10.3748/wjg.v26.i32.4753
Yu, S., Nimse, S. B., Kim, J., Song, K. S., and Kim, T. (2020). Development of a lateral flow strip membrane assay for rapid and sensitive detection of the SARS-CoV-2. Anal. Chem. 92, 14139–14144. doi:10.1021/acs.analchem.0c03202
Zeng, H. Q., Zhang, X. B., Cai, X. Y., Yang, D. Y., Lin, L., Chen, M. J., et al. (2021). Diagnostic value of bronchoalveolar lavage fluid cryptococcal antigen-lateral flow immunochromatographic assay for pulmonary cryptococcosis in non-HIV patients. Diagn. Microbiol. Infect. Dis. 99, 115276. doi:10.1016/j.diagmicrobio.2020.115276
Zeng, W., Liu, G., Ma, H., Zhao, D., Yang, Y., Liu, M., et al. (2020). Biochemical characterization of SARS-CoV-2 nucleocapsid protein. Biochem. Biophys. Res. Commun. 527, 618–623. doi:10.1016/j.bbrc.2020.04.136
Zetsche, B., Gootenberg, J. S., Abudayyeh, O. O., Slaymaker, I. M., Makarova, K. S., Essletzbichler, P., et al. (2015). Cpf1 is a single RNA-guided endonuclease of a class 2 CRISPR-Cas system. Cell. 163, 759–771. doi:10.1016/j.cell.2015.09.038
Zhang, C., Zheng, T., Wang, H., Chen, W., Huang, X., Liang, J., et al. (2021). Rapid one-pot detection of SARS-CoV-2 based on a lateral flow assay in clinical samples. Anal. Chem. 93, 3325–3330. doi:10.1021/acs.analchem.0c05059
Zhang, C., Zhou, L., Du, K., Zhang, Y., Wang, J., Chen, L., et al. (2020). Foundation and clinical evaluation of a new method for detecting SARS-CoV-2 antigen by fluorescent microsphere immunochromatography. Front. Cell. Infect. Microbiol. 10, 553837. doi:10.3389/fcimb.2020.553837
Zhang, Q., Li, J., Li, Y., Tan, G., Sun, M., Shan, Y., et al. (2022). SARS-CoV-2 detection using quantum dot fluorescence immunochromatography combined with isothermal amplification and CRISPR/Cas13a. Biosens. Bioelectron. 202, 113978. doi:10.1016/j.bios.2022.113978
Zhou, L., Niu, Z., Jiang, X., Zhang, Z., Zheng, Y., Wang, Z., et al. (2020). SARS-CoV-2 targets by the pscRNA profiling of ACE2, TMPRSS2 and furin proteases. iScience. 23, 101744. doi:10.1016/j.isci.2020.101744
Zhu, X., Wang, X., Han, L., Chen, T., Wang, L., Li, H., et al. (2020). Multiplex reverse transcription loop-mediated isothermal amplification combined with nanoparticle-based lateral flow biosensor for the diagnosis of COVID-19. Biosens. Bioelectron. 166, 112437. doi:10.1016/j.bios.2020.112437
Zhu, X., Wang, X., Li, S., Luo, W., Zhang, X., Wang, C., et al. (2021). Rapid, ultrasensitive, and highly specific diagnosis of COVID-19 by CRISPR-based detection. Acs. Sens. 6, 881–888. doi:10.1021/acssensors.0c01984
Zou, M., Su, F., Zhang, R., Jiang, X., Xiao, H., Yan, X., et al. (2021). Rapid point-of-care testing for SARS-CoV-2 virus nucleic acid detection by an isothermal and nonenzymatic Signal amplification system coupled with a lateral flow immunoassay strip. Sens. Actuators. B. Chem. 342, 129899. doi:10.1016/j.snb.2021.129899
Keywords: SARS-CoV-2, lateral flow immunoassay, nanotechnology, CRISPR, COVID-19, antibody, antigen, nucleic acid
Citation: He J, Zhu S, Zhou J, Jiang W, Yin L, Su L, Zhang X, Chen Q and Li X (2023) Rapid detection of SARS-CoV-2: The gradual boom of lateral flow immunoassay. Front. Bioeng. Biotechnol. 10:1090281. doi: 10.3389/fbioe.2022.1090281
Received: 05 November 2022; Accepted: 13 December 2022;
Published: 10 January 2023.
Edited by:
Monica Neagu, Victor Babes National Institute of Pathology (INCDVB), RomaniaReviewed by:
Agustin Valenzuela-Fernandez, University of La Laguna, SpainHongxing Liu, First Affiliated Hospital of Guangzhou Medical University, China
Copyright © 2023 He, Zhu, Zhou, Jiang, Yin, Su, Zhang, Chen and Li. This is an open-access article distributed under the terms of the Creative Commons Attribution License (CC BY). The use, distribution or reproduction in other forums is permitted, provided the original author(s) and the copyright owner(s) are credited and that the original publication in this journal is cited, in accordance with accepted academic practice. No use, distribution or reproduction is permitted which does not comply with these terms.
*Correspondence: Xiaoping Li, bGkteHBAempzcnUuZWR1LmNu