- College of Life Science, Xinyang Normal University, Xinyang, China
With growing concerns about environmental issues and sustainable economy, bioproduction of chemicals utilizing microbial cell factories provides an eco-friendly alternative to current petro-based processes. Creating high-performance strains (with high titer, yield, and productivity) through metabolic engineering strategies is critical for cost-competitive production. Commonly, it is inevitable to fine-tuning or rewire the endogenous or heterologous pathways in such processes. As an important pathway involved in the synthesis of many kinds of chemicals, the potential of the glyoxylate cycle in metabolic engineering has been studied extensively these years. Here, we review the metabolic regulation of the glyoxylate cycle and summarize recent achievements in microbial production of chemicals through tuning of the glyoxylate cycle, with a focus on studies implemented in model microorganisms. Also, future prospects for bioproduction of glyoxylate cycle-related chemicals are discussed.
Introduction
The glyoxylate cycle, also known as glyoxylate shunt (GS), was identified by Kornberg and Krebs in 1957, explaining how organisms could grow on acetate as the sole carbon source (Kornberg and Krebs, 1957). For substrates degraded exclusively to acetyl moieties (e.g., acetate, fatty acids, and ketogenic amino acids), this pathway provides a simple and efficient strategy for anaplerosis and gluconeogenesis, and, thus, cell growth. The glyoxylate cycle is generally regarded as an ancillary pathway of the TCA cycle, which is widely acknowledged as the central metabolic hub of the cell. This pathway comprises two dedicated enzymes: isocitrate lyase (ICL) and malate synthase (MS). ICL catalyzes the aldol cleavage of isocitrate to succinate and glyoxylate, while MS catalyzes the synthesis of malate from glyoxylate and acetyl-CoA. The overall effect of this pathway is the formation of one malate from two molecules of acetyl-CoA (Figure 1). It bypasses the oxidative decarboxylation steps of the TCA cycle and conserves carbon skeletons for biomass generation.
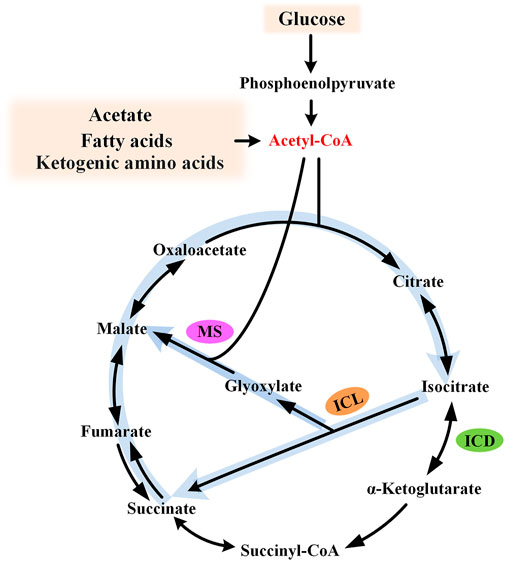
FIGURE 1. Diagram of the glyoxylate cycle. The glyoxylate cycle is shown by thick light blue arrows and the key intermediate acetyl-CoA is shown in red. ICL, isocitrate lyase; MS, malate synthase; and ICD, isocitrate dehydrogenase.
In addition to carbon assimilation, the glyoxylate cycle is also implicated in pathogenesis, antibiotic resistance, and oxidative stress tolerance. In view of its significance in metabolism and pathogenicity, the glyoxylate cycle has been extensively studied concerning the enzymology and metabolic regulation, particularly for Escherichia coli and the pathogenic bacterium Mycobacterium tuberculosis (Dolan and Welch, 2018). Undoubtedly, the gained knowledge lays a foundation for the bioproduction of related chemicals in metabolic engineering.
The glyoxylate cycle is involved in the synthesis of various chemicals. In recent years, studies on biosynthesis of organic acids, amino acids, and fatty acid-related products through GS engineering have been reported. Some of the outstanding results are presented in Table 1. To balance product output, reducing power regeneration, and cell growth, the glyoxylate cycle needs to be reinforced, weakened, fine-tuned, or dynamically controlled in different production cases. When heterologously expressed in some strains lacking this pathway, the GS amplified carbon source spectrum and enabled more metabolic flexibility, thus facilitating bioproduction (Kabisch et al., 2013; Schada von Borzyskowski et al., 2018; Shimizu et al., 2019).
In this review, we will briefly introduce the flux control of glyoxylate cycle in several different bacteria and recent achievements in biosynthesis of related chemicals. In addition, perspective for further study with the GS pathway will be presented.
Regulation of the glyoxylate cycle
The canonical regulation model in E. coli
A carbon flux is partitioned between the oxiditive TCA branch and GS at the isocitrate node, thereby, maintaining a balance of energy, reducing power, and gluconeogenic precursor production. In addition, glyoxylate is highly reactive or even toxic (due to the aldehyde group). Thus, tight regulation of the glyoxylate cycle is necessary.
In E. coli, ICL is a tetramer encoded by aceA. Its affinity for isocitrate is much lower than that of the NADP-dependent isocitrate dehydrogenase (ICD, encoded by icd). As a result, the control of ICD activity plays an important role in determining the flux of GS (LaPorte et al., 1985). The activity of ICD is primarily controlled by a kinase/phosphatase called AceK (encoded by aceK) (Figure 2A). When bacteria are grown on acetate, about 75% of ICD is inactivated by phosphorylation, thus, more isocitrate will be directed through the glyoxylate cycle. The differential kinase/phosphatase activity of AceK is allosterically regulated by several metabolites (e.g., isocitrate, PEP, and ATP), although the precise mechanism is still not fully clear (Ogawa et al., 2007). It is worth noting that PEP also uncompetitively inhibits ICL.
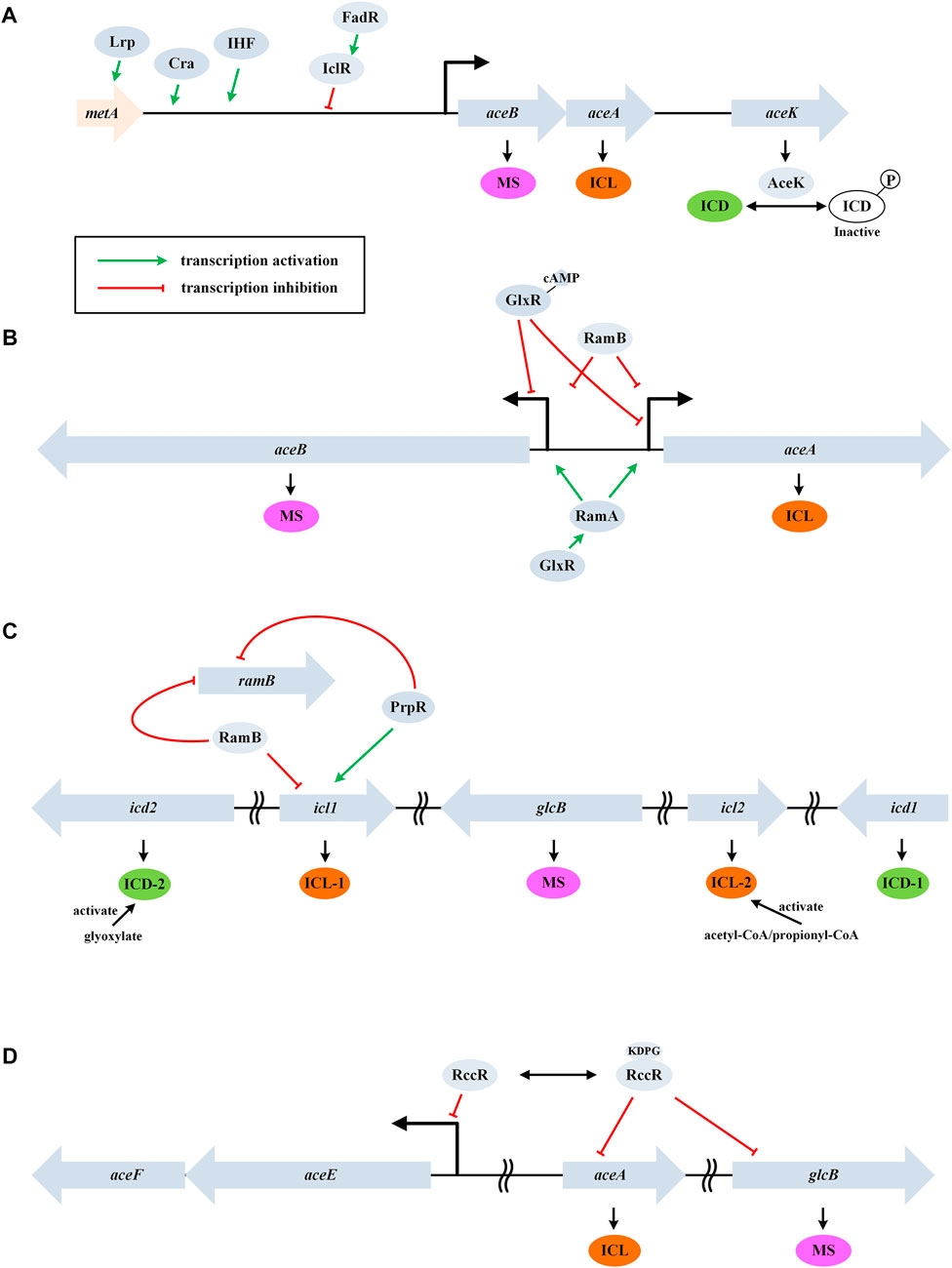
FIGURE 2. Control of the glyoxylate cycle in different bacteria. (A) GS regulation in the gram-negative E. coli. Genes aceB, aceA, and aceK form a tricistronic operon and AceK plays a critical role in controlling ICD activity through phosphorylation modification. (B) GS regulation in the gram-positive C. glutamicum. Genes aceA and aceB are transcribed divergently and a homologue of AceK is absent. (C) GS regulation in M. tuberculosis. This bacterium contains two isoforms of ICD and ICL. (D) GS regulation in P. fluorescens. RccR modulates pyruvate metabolism and GS via reciprocal regulation depending on the availability of KDPG. Genes aceE and aceF encode the pyruvate dehydrogenase components.
AceK-dependent regulation of the flux through the glyoxylate cycle is a feature associated with only the gram-negative bacteria (this enzyme is absent in nearly all the gram-positive bacteria) (Yates et al., 2011). In addition, ICL is negatively regulated by acetylation in E. coli (Castano-Cerezo et al., 2014). On the other hand, MS (encoded by aceB) shows high affinities for the substrates glyoxylate and acetyl-CoA, and thus inhibition or activation of MS plays only a minor role in controlling the glyoxylate cycle (Anstrom et al., 2003).
In E. coli, the open reading frames (ORFs) of aceB, aceA, and aceK form a tricistronic operon (aceBAK), which is positively controlled by a pleiotropic transcriptional regulator Cra and the integration host factor (IHF) and negatively controlled by IclR (isocitrate lyase regulator) (Cozzone, 1998) (Figure 2A). The expression of the aceBAK operon can also be enhanced by Lrp, a transcriptional activator of the upstream metA gene (Kroner et al., 2019). Glyoxyalte and PEP stabilize the inactive dimeric state of IclR, while pyruvate stabilizes the active tetrameric form (Lorca et al., 2007). Expression of IclR is under self-inhibition and is activated by FadR (which represses β-oxidation and activates biosynthesis of fatty acids) (Gui et al., 1996). The coordination of fatty acid metabolism and the glyoxylate cycle may indicate acetyl-CoA as a signaling molecule.
Control of GS in Corynebacterium glutamicum
Unlike the dimeric ICD enzyme from E. coli, the homologue (named IDH) is monomeric in C. glutamicum. The ORFs of aceA and aceB are transcribed divergently in C. glutamicum and a homologue of AceK is absent (Figure 2B). This organism does not encode an IclR homologue, while new types of transcription repressor RamB (regulator of acetate metabolism) and transcription activator RamA were characterized (Gerstmeir et al., 2004; Cramer et al., 2006). In the absence of acetate, RamB represses the expression of aceA, aceB, and pta-ack (encoding phosphotransacetylase and acetate kinase). The induction of glyoxylate cycle genes by acetate occurs independently of the presence or absence of glucose and other carbon source, which is different from E. coli.
Kim et al. reported a transcription repressor of aceB in C. glutamicum, which was designated as GlxR (Kim et al., 2004). The repression occurred in the presence of cAMP (e.g., when glucose medium was used). In addition, GlxR positively regulates ramA expression independent of the carbon source used (Toyoda et al., 2013) (Figure 2B). The glyoxylate cycle is also regulated through other mechanisms except for the transcriptional level. Maeda et al. found that RNase E/G (encoded by rneG) could cleave the aceA mRNA at the 3’ untranslated region, and the level of aceA mRNA was approximately 3-fold higher in the rneG mutant than in the wild type (Maeda and Wachi, 2012).
Control of GS in Mycobacterium tuberculosis and Pseudomonas fluorescens
Bioinformatic studies showed that only microorganisms capable of aerobic metabolism possess the glyoxylate cycle, and the genetic context of related genes were diversified among bacterial genera, indicating more complex regulation (Ahn et al., 2016). For example, a totally different flux rheostat model was characterized in the Mycobacterium spp. (Figure 2C) and a reciprocal regulation model was characterized in P. fluorescens (Figure 2D). Although application of these strains in metabolic engineering was limited due to the pathogenicity, the mechanisms of GS regulation may be instructive.
M. tuberculosis contains two isoforms of ICD (dimeric Mtb ICD-1 and monomeric Mtb ICD-2), two isoforms of ICL (E. coli-like ICL-1 and a less-studied ICL-2), and lacks an AceK homologue (Munoz-Elias and McKinney, 2005). The KMs of ICL and ICD were comparable, in contrast with the situation in E. coli (Murima et al., 2016). The activity of ICD-2 (the primary isoform under physiological conditions) is stimulated by glyoxylate thereby decreasing the flux through GS. In this way, the flux balance between the TCA cycle and GS was achieved via a rheostat model. On glucose, RamB specifically represses the transcription of icl1 and ramB itself (Micklinghoff et al., 2009). PrpR, a transcription factor involved in the methylcitrate pathway, can repress ramB expression and activate the expression of icl1 (Masiewicz et al., 2012). A recent study showed that ICL-2 was markedly activated by acetyl-CoA and propionyl-CoA at high lipid concentrations (Bhusal et al., 2019).
At the posttranslational level, ICL-1 was found to be acetylated on three lysine residues (K322, K331, and K392). Acetylation at position 392 increased ICL activity, whereas acetylation of K322 reduced its activity (Bi et al., 2017). In ICD, residues K30 and K129 are acetylated by Rv2170, and this leads to a 30% reduction in the enzyme activity (Lee et al., 2017).
In P. fluorescens, RccR, a homologue of the Entner–Doudoroff pathway regulator HexR, plays the key role in the GS control via reciprocal regulation. In the presence of glucose, RccR binds 2-keto-3-deoxy-6-phosphogluconate (KDPG) and represses the expression of aceA and glcB. In the absence of KDPG, it represses the expression of aceEF genes. In this way, strain can modulate pyruvate metabolism and GS/gluconeogenesis in response to carbon source availability (Campilongo et al., 2017).
In several studies, unpredictable up/down-regulation of GS was resulted from the fermentation process control or genetic manipulation of the unrelated pathway due to interactional metabolism (Martinez et al., 2010; Wei et al., 2016; Borja et al., 2019). These results provided us new understanding of this pathway, yet were not readily applicable in the targeted metabolic engineering practice. These approaches will not be discussed in the present review.
Production of organic acids
Succinate production
Succinate has been considered as a potential chemical platform with a wide range of applications in food, pharmacy, chemical, and agriculture. Many microorganisms naturally accumulate succinate using the reductive TCA branch:
However, the required NADH is typically generated by glucose heterofermentation, which reduces the theoretical yield to 1 mol/mol glucose (Figure 3). Ailen et al. developed a dual-route succinate production strategy through combining the reductive TCA part and the glyoxylate cycle (Sanchez et al., 2005). The lactate, ethanol, and acetate pathway were blocked to conserve NADH and acetyl-CoA. A heterologous pyruvate carboxylase (pyc) was overexpressed then to fix CO2 and increase the flux into the reductive TCA branch. Most importantly, conserved NADH and acetyl-CoA were channeled into the glyoxylate cycle by deletion of the repressor IclR. The engineered E. coli strain produced succinate at a yield of 1.6 mol/mol glucose under anaerobic conditions, which was close to the theoretical maximum (1.7 mol/mol glucose).
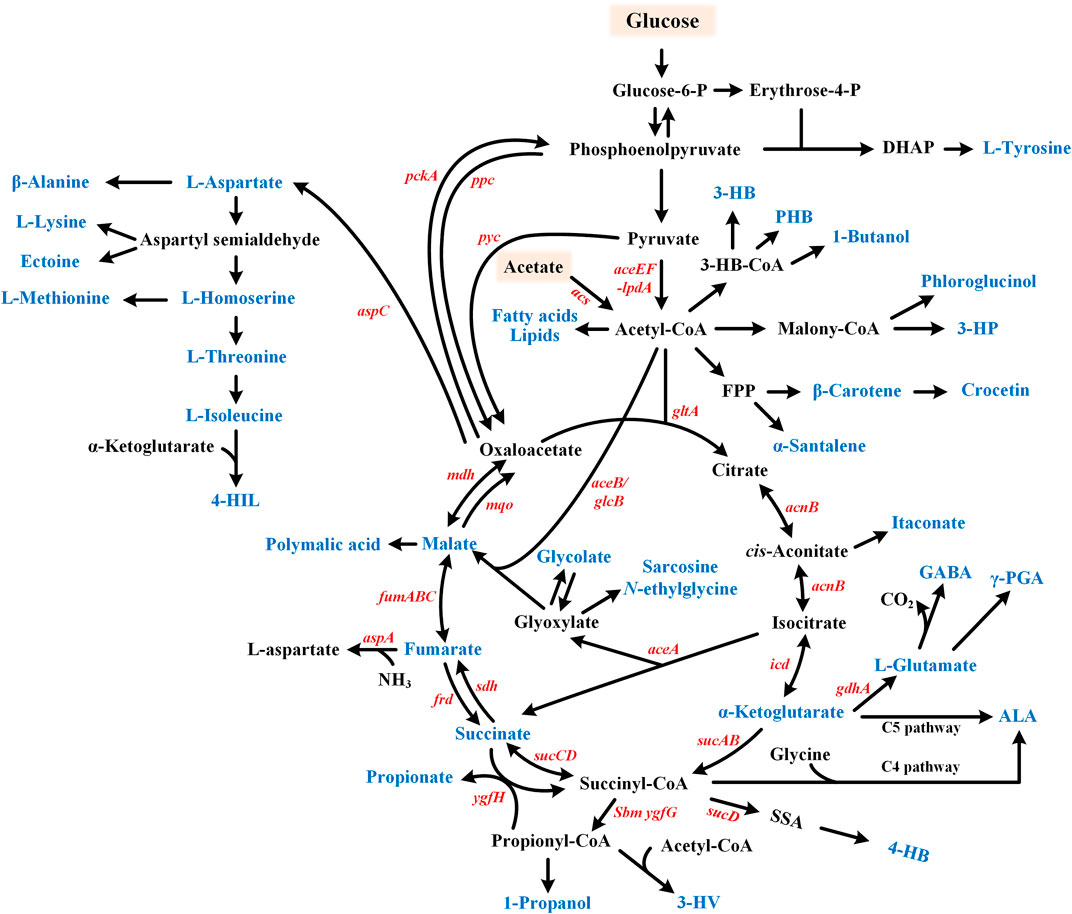
FIGURE 3. Metabolic pathway for the glyoxylate cycle involved chemicals production. Target products include organic acids, amino acids, fatty acid-related chemicals, etc. and are shown in blue. Glucose or acetate can be used as substrates. GABA, γ-aminobutyric acid; 4-HIL, 4-hydroxyisoleucine; ALA, 5-aminolevulinic acid; γ-PGA, Poly-γ-glutamic acid; 3-HB, 3-hydroxybutyrate; PHB, poly β-hydroxybutyric acid; 3-HP, 3-hydroxypropionate; 4-HB, 4-hydroxybutyrate; and 3-HV, 3-hydroxyvalerate.
To achieve the theoretical maximum, the distribution of metabolic flux between carboxylation of PEP to OAA and decarboxylation of PEP to acetyl-CoA should be 5:2 (Vemuri et al., 2002). Skorokhodova et al. constitutively expressed the NAD+-reducing pyruvate dehydrogenase complex (aceEF-lpdA) to enhance the flux toward the glyoxylate cycle while supplying additional NADH at the same time (Skorokhodova et al., 2015). In an E. coli strain with blocked lactate, acetate, and ethonal pathways, they inactivated the pyruvate formate lyase (pflB) to drive acetyl-CoA formation solely by the overexpressed pyruvate dehydrogenase under anaerobic conditions. Further deletion of IclR with a concomitant heterologous pyruvate carboxylase (pyc) overexpression enabled a succinate yield of 1.69 mol/mol at tube-scale experiments with NaHCO3 addition.
Efforts were also put into the aerobic succinate production due to the faster cell growth and higher productivity compared with the anaerobic production. Recruitment of the glyoxylate cycle improved theoretical yield to 1.33 mol/mol from 1.0 mol/mol using the oxidative TCA part solely (Vuoristo et al., 2016). Under anaerobic conditions, ICD is many folds less active than under aerobic conditions (Chao et al., 1997). Also, considering its higher affinity to isocitrate compared with ICL, it is necessary to reduce or eliminate the expression of ICD to strengthen the glyoxylate pathway, and thus to improve the yield under aerobic conditions (Lin et al., 2005; Li et al., 2013; Li Y. et al., 2016). Currently, the titer or yield of succinate using aerobic fermentation is still low (Liu et al., 2022).
The glyoxylate cycle was also engineered in other microbes for succinate production except for E. coli (Zhu et al., 2014a; Cui et al., 2017; Babaei et al., 2019; Durall et al., 2021). Specially, Nguyen et al. integrated the GS enzymes from E. coli into a methanotroph Methylomonas sp. DH-1 (Nguyen et al., 2019). The GS pathway supplied intermediates for biomass synthesis in an sdh mutant and activited the serine cycle to provide more acetyl-CoA, which may have improved the cell growth and succinate production. Finally, 195 mg/L succinate, corresponding to 6.41% of the theoretical yield was produced from methane.
Production of fumarate and malate
Succinate, fumarate, and malate can be interconverted but synthesis of fumarate and malate needs less reducing power compared with succinate under anaerobic conditions. In a study to produce fumarate, IclR was deleted to redirect carbon flux into the glyoxylate cycle (Song et al., 2013) (Figure 3). The phosphoenolpyruvate carboxylase (ppc) was overexpressed, then arcA (encoding a global transcriptional regulator) and ptsG (one of the phosphotransferase system genes) were deleted to reinforce the oxidative TCA cycle. Additionally, fumarate consumption was blocked through fumABC and aspA gene deletion. Finally, glucose uptake was increased by substituting the galP promoter. The engineered E. coli strain produced 28.2 g/L fumarate in 63 h under aerobic conditions (Song et al., 2013).
In another study, the decreased activity of ICD and activated GS was demonstrated to be effective to reduce acetate accumulation and increase the fumarate production. However, aceBA overexpression dramatically slowed cell gowth, which demonstrated the importance of flux balance between the GS and oxiditive TCA cycle (Li et al., 2014). Chen et al. engineered E. coli for fumarate production via the glyoxylate pathway (Chen et al., 2020). They explored the effect of pyruvate carboxylase (pyc), citrate synthase (gltA), aconitase (acnB), isocitrate lyase (aceA), and succinate dehydrogenase (sdh) overexpression on fumarate production, and found that pyruvate carboxylase and isocitrate lyase played crucial roles. The expression level of these two enzymes varied to balance the glyoxylate cycle and oxiditive TCA flux. Through pathway optimization, the fumarate titer was increased from 8.7 g/L to 16.2 g/L.
The glyoxylate pathway was also engineered for malate production in E. coli by the same research group (Gao et al., 2018). First, they constructed an in vitro malate production system containing five enzymes, which were, pyruvate carboxylase (PC), citrate synthase (CS), aconitase (ACN), isocitrate lyase (ICL), and malate synthase (MS), and explored the optimal level for each enzyme. Then, CRISPRi was employed for enzyme modular coordination in vivo. Through the moderate inhibition of PC and CS expression, and fixing the level of ACN: ICL: MS at 4: 5: 4, malate titer was increased by two folds with a yield of 0.85 mol/mol glucose. In addition, iclR and arcA were inactivated to enhance the flux toward glyoxylate cycle and oxiditive TCA cycle.
Trichez et al. engineered an E. coli strain for malate production through deletion of the iclR and arcA genes, block of malate consuming, and overexpression of malate-insensitive PEP carboxylase PpcK620S and NADH-insensitive citrate synthase GltAR164L. A malate yield of 0.82 mol/mol was obtained. The metabolic flux analysis indicated that the engineered strains had a very high flux over the glyoxylate shunt with almost no flux passing through the isocitrate dehydrogenase reaction (Trichez et al., 2018).
Liu et al. engineered L-malate production in Aspergillus oryzae (Liu et al., 2018). First, accumulation of intermediate pyruvate was decreased by overexpressing a pyruvate carboxylase from Rhizopus oryzae in the cytosol and mitochondria. Then, malate synthesis via the glyoxylate cycle in the mitochondria was enhanced by overexpression of isocitrate lyase and malate synthase. Interestingly, strengthening the oxidative TCA route diminished spore formation and malate production, while downregulation of the oxidative TCA cycle enhanced the L-malate titer by 10.7%, indicating that the TCA cycle route was not suitable for the malate production in this case. Finally, through the expression of a dicarboxylate carrier and modulation of the NADH/NAD+ ratio, 117.2 g/L L-malate was produced, with an L-malate yield of 0.9 g/g corn starch and a productivity of 1.17 g/L/h. Polymalic acid (PMA), a high added-value polyester composed of L-malic acid monomers, can be produced via the glyoxylate cycle naturally by Aureobasidium pullulans. A few studies engineered PMA production through enhancement of the glyoxylate cycle (Yang et al., 2018; Zeng et al., 2019).
Production of itaconate
The glyoxylate cycle can also play a positive role in the production of itaconate, an excellent polymeric precursor derived from cis-aconitate (Figure 3). Through overexpression of pyruvate carboxylase, citrate synthase, aconitase, and the cis-aconitate decarboxylase (AT-CAD, cad) from A. terreus, flux toward the itaconate production was enhanced. Together with isocitrate dehydrogenase deletion, the titer of itaconate reached to 43 g/L in 32 h from glycerol under high aeration. Elimination of the glyoxylate shunt demonstrated harmful impact on the itaconate production, although this pathway pulled out the carbon flux via the isocitrate node. This may be due to its positive role in regeneration of oxaloacetate, which was important for itaconate synthesis in turn (Chang et al., 2017).
In another study, an acid-tolerant E. coli strain was engineered for itaconate production from acetate (Noh et al., 2018). By overexpression of the cis-aconitate decarboxylase, only 0.13 g/L itaconic acid was produced because of low acetate uptake. Then, acetate assimilation was enhanced through overexpression of the acetyl-CoA synthetase and activation of the glyoxylate cycle. The high ICL activity was proved to be benificial for the itaconate production. Together with gltA overexpression, 3.57 g/L itaconic acid (16.1% of theoretical maximum yield) was produced from acetate.
Production of glycolate
Glycolate, or glycolic acid (GA) is a small two-carbon α-hydroxy acid used in multiple daily applications (Figure 3). There are no known natural microbial pathways to directly produce GA from relatively cheap feedstock, yet this chemical can be produced via several synthetic pathways including the modified GS pathway (Salusjärvi et al., 2019). In an attempt to produce GA using E. coli, flux toward the glyoxylate cycle was enhanced through the overexpression of ICL and deletion of acetate forming pathways, icd, arcA, and iclR. GA synthesis was further enhanced by glyoxylate reductase (ycdW) overexpression. Consumption of glyoxylate and GA was blocked through inactivation of MS, glyoxylate carboligase (gcl), 2-keto 3-deoxygluconate 6-phosphate aldolase (eda), glycolate oxidase (glcDEFGB) and glycolaldehyde dehydrogenase (aldA). To increase the availability of NADPH, which is needed by YcdW, edd encoding the 6-phosphogluconate dehydratase was deleted. Finally, 52.2 g/L GA was produced from glucose (Dischert and Soucaille, 2015).
Sometimes complete block of the TCA cycle through icd deletion may cause growth retardation. Deng et al. tried to weaken the flux to α-ketoglutarate (α-KG) through overexpression of AceK, which could repress the ICD activity. In this way, the ICD activity was decreased by 83.03%, but poor growth of strain was incurred concomitantly. Then, adaptive evolution was performed to increase the growth rate. Nevertheless, the evolved strain still grew much more slowly than the wild type, which suggested the importance of balance between the TCA cycle and GS reactions. In fed-batch fermentation, the engineered strain produced 56.4 g/L GA (Deng et al., 2015). Subsequently, Deng et al. further improved the producer strain (Deng et al., 2018). Citrate synthase was overexpressed in addition to ICL, YcdW, and AceK. Lactate dehydrogenase (ldhA), which competed for the carbon flux to glycolate and AldA involved in GA consumption were deleted. The following optimization of fermentation, 65.5 g/L GA was produced with a yield of 0.765 g/g glucose (90.0% of the theoretical yield).
Pereira et al. engineered an E. coli strain to produce GA from xylose, the most abundant pentose (Pereira et al., 2016). Through introduction of the exogenetic D-tagatose epimerase, 44.0 g/L GA was produced via the D-ribulose-1-phosphate pathway. Bu combining the D-ribulose-1-phosphate pathway and glyoxylate cycle, the yield was increased from 0.44 to 0.62 g/g xylose (theoretical yield 1 g/g), although the titer did nott improve obviously. Cam et al. engineered GA production in E. coli from xylose via a synthetic xylulose-1 phosphate (X1P) pathway (Cam et al., 2016). In this pathway, D-xylose was converted to glycolaldehyde and DHAP, both of which can be converted to GA with a theoretical yield higher than 20% via the glyoxylate shunt alone. Simultaneous operation of the glyoxylate and X1P pathways enabled a yield of 0.63 g/g, when growing on the glucose/xylose mixture (Alkim et al., 2016).It is worth noting that when produced from xylose via the Dahms pathway, the yield of GA was only 0.5 g/g xylose (Cabulong et al., 2021).
Li et al. engineered GA production in E. coli from acetate via the glyoxylate cycle (Li et al., 2019). The glyoxylate bypass was reinforced by overexpression of ICL and AceK. YcdW was overexpressed to convert glyoxylate to glycolate. MS, glyoxylate carboligase, and glycolate oxidase were inactivated to prevent loss of glyoxylate and glycolate. To strengthen the TCA cycle and acetate utilization, citrate synthase, phosphotransacetylase, and acetate kinase (ackA) were co-overexpressed. As a result, the glycolate titer was improved to 2.75 g/L with the pH control in shake flasks. The GA production pathway can be integrated with other metabolic routes to produce value-added chemicals including 3-hydroxy-γ-butyrolactone (3HBL) and GA-containing polymer directly from glucose or/and xylose (Dhamankar et al., 2014; Choi S. Y. et al., 2016; Li Z.-J. et al., 2016; Li Z.-J. et al., 2017). In addition, the glyoxylate cycle was also engineered to produce GA in microbes other than E. coli, although the titer was relatively low (Koivistoinen et al., 2013; Zahoor et al., 2014; Lee et al., 2020).
Production of amino acids and derivatives
Production of L-aspartate family amino acids
Several amino acids and their derivatives are synthesized from the GS and TCA cycle intermediates, including OAA, α-KG and succinyl-CoA (Figure 3). L-Aspartate family amino acids (AFAAs) refer to amino acids synthesized from L-aspartate such as L-lysine, L-methionine, L-threonine, and L-isoleucine. Because L-aspartate is directly synthesized from OAA, the OAA supply has been considered as a bottleneck for the production of AFAAs (Li Y. et al., 2017). In one study, the OAA pool was increased to improve L-homoserine production in E. coli (Liu et al., 2020). First, IclR was deleted to derepress the glyoxylate cycle. Then, citrate synthase (gltA) was deleted to conserve OAA. Subsequently, pyruvate kinase (pykA and pykF) was deleted to drive more anaplerotic flux into OAA. Together with disrupting the competitive and degradative pathways, 35.8 g/L L-homoserine was produced in the fed-batch fermentation. Conversely, simultaneous activation of the glyoxylate cycle and overexpression of gltA (rather than weakening its expression) improved AFAAs production in some other studies, which suggested the complexity of metabolism (Flores et al., 2005; Zhu et al., 2019). Sometimes, the glyoxylate cycle could be activated through attenuating the isocitrate dehydrogenase activity, which also resulted in replenishment of OAA (Schwentner et al., 2018).
In another study, L-threonine, which can be synthesized from L-homoserine, was produced in E. coli (Zhao et al., 2018). To conserve precursor OAA, the gene iclR was deleted, and the native promoter of the aceBA operon was replaced by the strong trc promoter in the chromosome. Then, the L-threonine biosynthesis pathway was overexpressed via replacing the native promoter of aspC (aspartate aminotransferase) by the trc promoter in the chromosome and plasmid overexpression of thrA* (a mutated thrA), thrB, thrC, and asd (encoding aspartate kinase I, homoserine kinase, threonine synthase and aspartate semialdehyde dehydrogenase, respectively). The final strain TWF006/pFW01-thrA*BC-asd produced 15.85 g/l L-threonine with a yield of 0.53 g/g glucose. In a subsequent study, the same group found that increase of the acetyl-CoA pool positively affected L-threonine production (Yang et al., 2019). The acetyl-CoA pool was increased through: deletion of fatty acid degradation/synthesis regulator FadR and FabR; overexpression of acetyl-CoA synthetase (acs) to convert acetate into acetyl-CoA; and overexpression of fadBA to facilitate fatty acid degradation. The fatty acid degradation and L-threonine biosynthesis pathway were coupled via the glyoxylate shunt, and 103.89 g/l L-threonine was produced after 48-h fed-batch fermentation.
Ectoine is a protective agent and stabilizer which can be synthesized from L-Aspartate. Ning et al. introduced the synthesis pathway of ectoine (encoded by the ectABC gene cluster from Halomonas elongata) into E. coli to produce this valuable chemical (Ning et al., 2016). To increase the OAA pool, iclR was deleted to enhance the glyoxylate shunt and the expression level of ppc (encoding the phosphoenolpyruvate carboxylase) was improved through promoter change. Then, the synthetic pathway of L-lysine and L-threonine was blocked, and a feedback resistant LysC from C. glutamicum (encoding the aspartate kinase) was introduced to enhance the flux to ectoine. Together with the overexpression of ectABC, 25.1 g/L ectoine was produced by fed-batch fermentation. Increase of the OAA pool via deletion of iclR was also proved to be effective in a study to produce β-alanine, another AFAA in E. coli (Wang et al., 2021). However, further enhancement of GS genes through promoter change did not improve β-alanine production, suggesting the importance of flux balance.
Production of α-ketoglutarate-sourced amino acids
α-KG is the precursor of numerous amino acids, such as L-glutamine, L-glutamate, and L-proline (Figure 3). In a C. glutamicum L-glutamate producer strain, glyoxylate pathway was blocked by knocking out aceA to conserve the isocitrate pool, and glutamate synthesis was blocked by knocking out gdh (encoding glutamate dehydrogenase). As a result, α-KG production was increased by 16-fold to 12.4 g/L in flask culture and 47.5 g/L in 5-L fermentor (Jo et al., 2012). In another study to produce Poly-γ-glutamic acid (γ-PGA) using Bacillus licheniformis, the glyoxylate cycle was also reduced to improve the titer (Li et al., 2021). The expression level of aceBA was varied through promoter modulation, and with a weak promoter PbacA, the activity of ICL was decreased by 41.51%, which resulted in 23.24% increase in γ-PGA yield. In addition, pyruvate dehydrogenase and citrate synthase were overexpressed to strengthen the flux into the TCA cycle, and pyruvate formate-lyase was deleted to conserve pyruvate. Finally, γ-PGA titer was enhanced to 12.02 g/L.
In biofermentation, it is essential to address the conflict between cell growth and target chemical production, as the overexpressed production pathways often lead to metabolic burden for the cell. In a study to produce γ-aminobutyric acid (GABA), a genetic switch was designed to balance the GABA production and bacterial cell growth (Soma et al., 2017). The cell growth was controlled by conditional interruption of GS and the TCA cycle. In the cell growth mode, α-ketoglutarate decarboxylase (sucA) of the TCA cycle and ICL (aceA) of the glyoxylate cycle were actively expressed, while turned off in the GABA production mode. On the contrary, pyruvate carboxylase (pyc), glutamate dehydrogenase (gdhA), glutamate decarboxylase (gadB), and the GABA transporter (gadC) involved in GABA synthesis were turned on only during the production mode. This strategy balanced the competition for isocitrate and α-KG between cell growth and GABA production, resulting in a 3-fold improvement in the total GABA production titer and yield.
In another study to produce 4-hydroxyisoleucine (4-HIL), a potential medicine for diabetes, the glyoxylate cycle was blocked (by deleting aceA) to increase one of the substrates α-KG (Shi et al., 2019). 4-HIL was synthesized from L-isoleucine and α-KG under the activity of L-isoleucine dioxygenase (IDO). The deletion of aceA increased the concentration of both α-KG and L-isoleucine, resulting in an 18.9% increase in the titer of 4-HIL. Then, ido3 (another IDO encoding gene) and mqo (encoding malate:quinone oxidoreductase) were coexpressed with ido to draw more flux into the Ile and 4-HIL biosynthetic pathways, resulting in another 31.8% increase of the titer. Further expression of the Vitreoscilla hemoglobin (vgb) and optimization of the fermentation medium led to a final 4-HIL titer of 17.2 g/L.
5-Aminolevulinic acid (ALA) is an α-KG derived non-proteinogenic amino acid with multiple applications in medical, agricultural, and food industries. There are two distinct routes to produce this chemical biologically, the C5 pathway and the C4 pathway. In the C5 pathway, ALA is synthesized from α-KG. To conserve α-KG, the TCA cycle was blocked by deletion of sucA (encoding α-ketoglutarate decarboxylase) (Noh et al., 2017). Although the specific ALA production was increased obviously, both ALA titer and cell biomass were reduced probably due to the insufficient production of energy and building blocks. Overexpression of gltA encoding citrate synthase only marginally improved cell biomass. Next, the glyoxylate cycle was finely tuned through modulating the promoter of aceA. Under the optimal strength, cell biomass and ALA production were increased by 4.45-fold and 2.93-fold, respectively, as compared to the parental strain. After fermentation optimization, high productivity (0.19 g/L/h) and yield (0.28 g/g) for ALA production from glucose as a sole carbon source was achieved.
In the C4 pathway, ALA was synthesized from succinyl-CoA and glycine. To reduce the conversion of ALA to downstream tetrapyrrole/porphyrin, Miscevic et al. applied CRISPRi to repress hemB expression (Miscevic et al., 2021b). Under microaerobic condition, succinyl-CoA is mainly derived from reductive the TCA cycle and the glyoxylate cycle. The authors inactivated iclR to deregulate the glyoxylate shunt while deleting sdhA simultaneously to further redirect the carbon flux toward ALA biosynthesis. Finally, 6.93 g/L ALA was produced from 30 g/L glycerol in E. coli. In another study, an exogenous glyoxylate transaminase from the human, which enabled autogenous synthesis of glycine from glyoxylate, was introduced into E. coli to produce ALA using glucose as the sole carbon source (Ren et al., 2018). The transaminase used alanine as the amino donor, with ATP, PLP, and CoA as cofactors. To increase the supply of glyoxylate, aceA was overexpressed. As a proof-of-concept, 521 mg/L 5-ALA was produced in 18 h of fermentation.
Production of tyrosine and N-alkylated glyoxylate
Tyrosine is an aromatic amino acid, which can be utilized in many aspects. In a study to engineer tyrosine production from acetate in E. coli, precise tuning of the glyoxylate cycle was proved to be vital (Jo et al., 2019). In this case, the two important intermediates for tyrosine synthesis, erythrose-4-phosphate and PEP were both derived from OAA. As acetate was the sole carbon source, it was critical to balance the glyoxylate cycle and TCA cycle, in other words, to balance precursor supply and generation of ATP and NADH. The glyoxylate cycle was precisely controlled by modulating the promoter of aceA. As a result, the best engineered strain produced 0.70 g/L tyrosine.
Glyoxylate can be N-alkylated by monomethylamine or monoethylamine, forming sarcosine and N-ethylglycine, respectively, under the activity of imine reductases DpkA (Mindt et al., 2019b). To increase glyoxylate supply, aceB was deleted and the activity of isocitrate dehydrogenase was reduced by changing the preferred translational start codon ATG to GTG. To further activate the glyoxylate cycle, acetate was added into the culture media. Together with other strategies including optimization of carbon source species, the amount and addition time of monomethylamine and acetate, 8.7 g/L sarcosine was produced from xylose and potassium acetate blends using the engineered C. glutamicum. Subsequently, DpkA was mutated, which accelerated the production of sarcosine (Mindt et al., 2019a). Using this mutant, production of N-ethylglycine from xylose and monoethylamine or from rice straw hydrolysate was demonstrated.
Production of fatty acid-related chemicals and farnesyl diphosphate-derived bioactive compounds
Production of acetyl-CoA derivatives
Synthesis of fatty acids originates from acetyl-CoA in microbial cells. Acetyl-CoA lies at the entrance into the TCA cycle, and is closely related with the glyoxylate cycle. Many polyhydroxyalkanoate monomers and several bioactive compounds can be synthesized from acetyl-CoA, as shown in Figure 3. On the other hand, propionate and 4-hydroxybutyrate (4-HB) can be synthesized from succinyl-CoA, another intermediate strongly linked with GS. Thus, the glyoxylate cycle was engineered to improve production of related chemicals in a few studies.
Nitta et al. engineered an E. coli strain to produce 1-butanol, a bulk chemical and promising biofuel (Nitta et al., 2019). All native fermentation pathways were blocked (through deletion of ldhA, adhE, and frdBC) and the strain relied on 1-butanol formation as the sole electron sink to regenerate NAD+. 1-Butanol was produced using a heterologous Clostridial CoA-dependent pathway. Then, formate dehydrogenase (fdh) from Candida boidinii was introduced to supply more NADH. Also, phosphate acetyltransferase (pta) was deleted to prevent formation of by-product acetate. In addition, the expression of AdhE2, the key enzyme for 1-butanol synthesis was optimized, which resulted in a 1-butanol titer of 18.3 g/L. However, considerable amounts of acetate accumulated (Ohtake et al., 2017). Subsequent metabolome analysis revealed increased accumulation of glyoxylate and acetyl-P with a decrease of α-KG and glutamate in the engineered strain, compared with the base strain. By knocking out aceA, acetate production decreased by 72% and the TCA cycle metabolites (including α-KG and glutamate) increased, which resulted in a 10% increase of the cell growth. Finally, 1-butanol production was improved by 39% (Nitta et al., 2019).
In another study, the expression of the glyoxylate cycle was enhanced by knocking out iclR to overcome acetate overflow and improve the production of two acetyl-CoA derived chemicals, phloroglucinol (PG) and 3-hydroxypropionate (3-HP) in E. coli BL21 (Liu et al., 2017). Acetate accumulation inhibits protein synthesis and depletes the acetyl-CoA pool. Deletion of iclR decreased acetate formation and improved the glucose utilization efficiency, which could reduce the production cost. At the same time, the metabolic flux from acetate and PEP to acetyl-CoA was enhanced, resulting in a more than 2-fold increase in the production of PG or 3HP. In a previous study, deletion of arcA, the redox regulator known to repress the TCA cycle and glyoxylate cycle, demonstrated similar effects on cell physiology and production of acetyl-CoA derived chemicals (Liu et al., 2016). However, the arcA and iclR double mutant showed no better results for PG and the 3HP production than arcA or iclR single mutant and the mechanisms were unclear. In another study, to produce 3-HP in E. coli from acetate, substrate assimilation was enhanced by overexpressing acs and the GS was activated by deleting iclR. As a result, acetate uptake and cell biomass synthesis were enhanced significantly, and 3-HP production was improved by 2.54-fold (Lee et al., 2018).
To balance the cell growth and 3-HP synthesis, a two-stage strategy was adopted by Lama et al., whereby glucose is used for the cell growth and acetate for 3-HP synthesis (Lama et al., 2021). To increase biomass yield on glucose, pathways for synthesis of by-products lactic acid, ethanol, and acetic acid were removed. Then, the effects of GS and the gluconeogenesis pathways on the cell growth and 3-HP formation during the production stage were studied. As a result, block of gluconeogenesis or GS was detrimental for 3-HP production, while theactivation of GS (via deletion of iclR) improved the titer of 3-HP. Using fed-batch fermentation, 7.3 g/L 3-HP was produced with a yield of 0.26 mol/mol acetate. Notably, the yield was still low compared with the theoretical maximum of 0.5 mol/mol acetate, which may reflect the operational cost of GS and subsequent gluconeogenesis.
3-HP biosynthesis was also pursued in non-model microorganisms. Using P. denitrificans as the producer, activation of GS by deleting iclR or enhancement of the oxidative TCA branch by aceK deletion did not improve 3-HP significantly, when a similar two-stage production strategy was adopted (Zhou et al., 2020). Lai et al. deleted the fatty acid degradation repressor FadR to enhance acetic acid utilization, which also activated the GS and improved 3-HP production. Using whole-cell biocatalysis, 15.8 g/L and 11.2 g/L of 3-HP was produced from acetate or syngas-derived acetate, respectively (Lai et al., 2021). Sometimes, when a target chemical not so closely-related was produced, the impact of GS would be complicated (Lin et al., 2013; Zhang et al., 2019).
To enhance production of FPP-derived bioactive compounds, the key point was usually the acetyl-CoA level. Citric acid synthase (CIT2 in peroxidase body) or malic acid synthase (MLS1 in the cytoplasm) was knocked out in a study to enhance the supply of precursor acetyl-CoA for production of crocetin, a potential drug in Saccharomyces cerevisiae (Song et al., 2020). The CIT2 mutant achieved 50% improvement on the total acetyl-CoA content and crocetin production, compared with the parent strain. The MLS1 mutant demonstrated similar effects, but to a lesser degree. Followed by suited fusion expression of key enzymes CrtZ and CCD2 and medium optimization, 12.43 mg/L crocetin was produced in fed-batch fermentation. Deletion of CIT2 and/or MLS1 also proved beneficial to increase the acetyl-CoA level in other studies. In a study to produce α-santalene (an acetyl-CoA derived sesquiterpene) in an engineered yeast strain, deletion of CIT2 or MLS1 improved the titer by 36% and 127%, respectively (Chen et al., 2013). Using CIT2 or MLS1 mutant, the titer of butanol was improved to 14.0 and 16.3 mg/l, respectively in another study (compared with 10.3 mg/L in the parent yeast strain) (Krivoruchko et al., 2013). However, block of the glyoxylate cycle through deletion of CIT2 or MLS1 compromised cell growth of a PHB-producing yeast strain and resulted in accumulation of acetate and a decrease in PHB content (Kocharin et al., 2012).
Production of succinyl-CoA derivatives
Under the anaerobic condition, succinate was mainly derived from the reductive TCA branch. However, the glyoxylate cycle could play an important role under the aerobic/microaerobic condition. Thus, effects of GS on the succinyl-CoA level were quite DO-dependent. In a study to produce propionate using the sleeping beauty mutase (Sbm) pathway in E. coli, the oxidative TCA cycle was blocked via deletion of sdhA and the glyoxylate cycle was deregulated by iclR deletion to improve the succinyl-CoA level. Through this modification, propionate titer was increased to 3.68 g/L from 1.1 g/L. Finally, 30.9 g/L of propionate was produced with an overall yield of 49.7% under optimized aeration condition using fed-batch fermentation (Miscevic et al., 2020b). Subsequently, 3-hydroxyvalerate was produced through condensation of propionyl-CoA and acetyl-CoA via a heterologous pathway using glycerol as the carbon source (Miscevic et al., 2020a; Miscevic et al., 2020c). The effects of aeration condition on activity of GS, and thus, flux distribution into the Sbm pathway were further demonstrated during production of poly (3-hydroxybutyrate-co-3-hydroxyvalerate) (Miscevic et al., 2021a).
4-HB is another chemical derived from succinyl-CoA that can be converted into various industrial chemicals and polymers. Producer strains of E. coli engineered for both aerobic and microaerobic conditions were constructed by Choi et al. based on a genome-scale metabolic model (Choi S. et al., 2016). Under the aerobic condition, the oxidative TCA branch and GS were proved to be essential for the supply of precursor succinate and production of 4-HB. Deletion of sdhAB and iclR was efficient to increase the titer of 4-HB. However, neither enhancement of GS (via deletion of iclR) nor block of GS (via deletion of aceBA) were effective in enhancing 4-HB production under the microaerobic condition, and there seemed to exist an optimal expression level of GS. In addition, glycerol was proved to be effective only for the microaerobic producer. Finally, 103.4 g/L of 4-HB was produced by microaerobic fed-batch fermentation from glycerol.
Santala et al. engineered wax esters production in Acinetobacter baylyi ADP1 from acetate and gluconate (Santala et al., 2021). By deleting aceA, GS was blocked and consumed acetate was dedicated to wax esters biosynthesis. On the other hand, gluconate was used for the synthesis of cofactors and biomass precursors. This design overcame the trade-offs between biomass and product production, an issue frequently encountered in bioengineering. Through optimization of gluconate feed rate and enhancement of the wax esters pathway, wax esters content, titer and productivity were improved significantly and a yield of 18% C/C-total-substrates was achieved.
Concluding remarks and outlook
As outlined in this review, the biochemistry and regulation of glyoxylate cycle was demonstrated in two industrial microorganisms (E. coli and C. glutamicum) and two potential pathogenic microorganisms (M. tuberculosis and P. fluorescens). Although applications in biosynthesis by M. tuberculosis and P. fluorescens are limited due to the pathogenicity, the GS control mechanisms may be instructive. Production of target chemicals, divided into several categories, including organic acids, amino acids and fatty acid-related chemicals were also briefly introduced.
Currently, metabolic engineering studies are focused on a small number of model microorganisms, especially E. coli. Thus, other potential producer strains with better robust traits, production capacity and feedstock compatibility may have been missed (Zeng, 2019). Considering the deep involvement of glyoxylate cycle in the synthesis of so many chemicals, clear interpretation of the glyoxylate metabolism in other industry relevant microorganisms will undoubtedly facilitate chemical bioproduction in the future (such as yeast, cyanobacteria, streptomyces and Clostridia (Chen et al., 2012; Huang et al., 2015; Zhang and Bryant, 2015)). On the other hand, studies on more detailed effects of exogenously introduced the GS pathway on the physiology and production performance of the producers are encouraged (Kabisch et al., 2013; Schada von Borzyskowski et al., 2018; Shimizu et al., 2019). In addition, protein engineering studies of the GS pathway, particularly for biosynthesis purpose are barren to our knowledge.
Metabolism of substrates which could be facilitated by GS are not just limited to acetate, glycerol or fatty acids. Recently, Mainguet et al. constructed a reverse GS which could convert C4 carboxylates into two molecules of acetyl-CoA without loss of CO2 (Mainguet et al., 2013). Heterologous enzymes malate thiokinase, malyl-CoA lyase and ATP-citrate lyase were utilized to drive the thermodynamically unfavorable steps, namely the conversion of malate to glyoxylate and acetyl-CoA, and conversion of citrate to OAA and acetyl-CoA at the expense of ATP. When adapted and integrated with central metabolism, conversion of C1 or CO2 carbon source to acetyl-CoA can be realized (Yu et al., 2018; Yu and Liao, 2018). Although no practical production use of this pathway has been implemented, this non-native route holds the potential for producing various acetyl-CoA derived chemicals, including alcohols, fatty acids, and isoprenoids in the future.
Another challenge is the balance of the cell growth and product biosynthesis. This may be overcome in two steps, that is,, accurate prediction of the appropriate GS strength and fine-tuning of its expression level accordingly. Prediction of the optimized metabolic flux can be promoted by multi-omics and more precise genome-scale metabolic models, such as flux balance analysis (FBA)-based models, elementary mode analysis-based ones and kinetic models (Srirangan et al., 2013; Machado and Herrgård, 2015). Then, fine-tuning of the GS pathway could be realized through CRISPRi, CRISPRa, sRNA, or dynamic control (Choi et al., 2019). The outline for future studies is shown in Figure 4. With the development of new techniques and expansion of the online database, the product portfolio and substrate spectrum can be further expanded in the future.
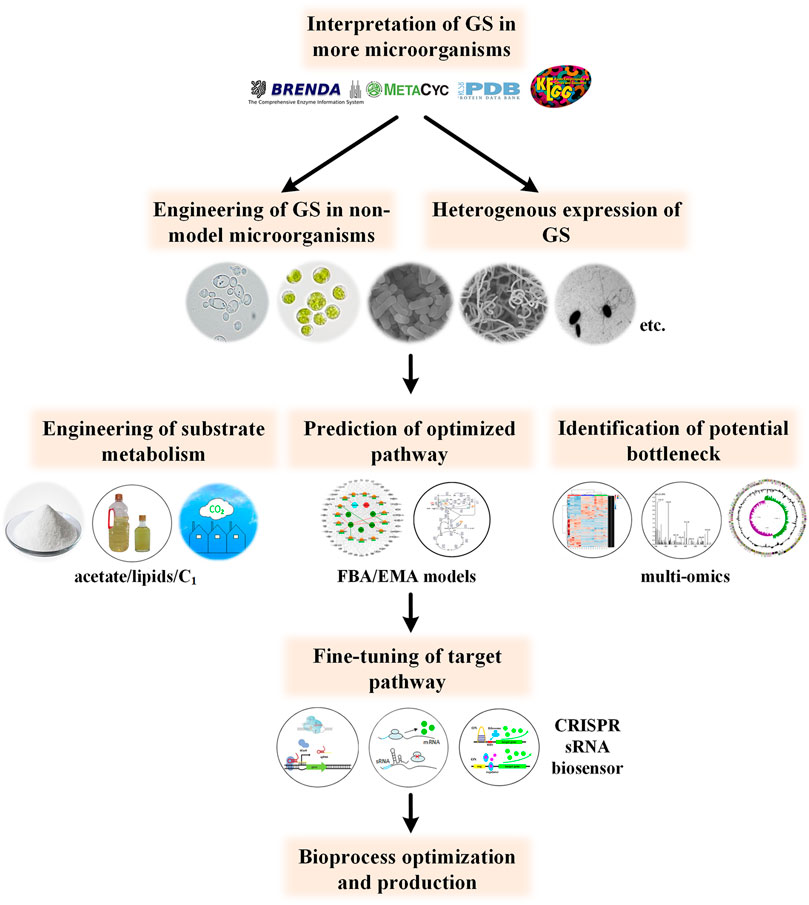
FIGURE 4. Perspective for future studies concerning GS engineering. Further understanding of GS metabolism in more potential producer microorganisms and more precise modulation of related pathways will promote the bioproduction process.
Author contributions
Conceptualization: PY and A-DG; writing—original draft preparation: PY, WL, and YC; writing—review and editing: PY and A-DG; supervision: A-DG; and funding acquisition: PY and A-DG. All authors read and agreed to the published version of the manuscript.
Funding
This work was funded by grants from the National Natural Science Foundation of China (31800074), Nanhu Scholars Program for Young Scholars of XYNU, Scientific and Technological Project of Henan Province (212102110447), and Natural Science Foundation of Henan (222300420519 and 222301420111).
Conflict of interest
The authors declare that the research was conducted in the absence of any commercial or financial relationships that could be construed as a potential conflict of interest.
Publisher’s note
All claims expressed in this article are solely those of the authors and do not necessarily represent those of their affiliated organizations, or those of the publisher, the editors, and the reviewers. Any product that may be evaluated in this article, or claim that may be made by its manufacturer, is not guaranteed or endorsed by the publisher.
Abbreviations
AFAA, L-aspartate family amino acid; ALA, 5-aminolevulinic acid; GS, glyoxylate shunt; GABA, γ-aminobutyric acid; 4-HIL, 4-hydroxyisoleucine; 3-HP, 3-hydroxypropionate; 4-HB, 4-hydroxybutyric acid; ICL, isocitrate lyase; ICD, isocitrate dehydrogenase; IclR, isocitrate lyase regulator; α-KG, α-ketoglutarate; MS, malate synthase; OAA, oxaloacetate; PEP, phosphoenolpyruvate.
References
Ahn, S., Jung, J., Jang, I. A., Madsen, E. L., and Park, W. (2016). Role of glyoxylate shunt in oxidative stress response. J. Biol. Chem. 291 (22), 11928–11938. doi:10.1074/jbc.M115.708149
Alkim, C., Trichez, D., Cam, Y., Spina, L., François, J. M., and Walther, T. (2016). The synthetic xylulose-1 phosphate pathway increases production of glycolic acid from xylose-rich sugar mixtures. Biotechnol. Biofuels 9 (1), 201–211. doi:10.1186/s13068-016-0610-2
Anstrom, D. M., Kallio, K., and Remington, S. J. (2003). Structure of the Escherichia coli malate synthase G: Pyruvate: Acetyl-coenzyme A abortive ternary complex at 1.95 Å resolution. Protein Sci. 12 (9), 1822–1832. doi:10.1110/ps.03174303
Babaei, M., Rueksomtawin Kildegaard, K., Niaei, A., Hosseini, M., Ebrahimi, S., Sudarsan, S., et al. (2019). Engineering oleaginous yeast as the host for fermentative succinic acid production from glucose. Front. Bioeng. Biotechnol. 7, 361. doi:10.3389/fbioe.2019.00361
Bhusal, R. P., Jiao, W., Kwai, B. X., Reynisson, J., Collins, A. J., Sperry, J., et al. (2019). Acetyl-CoA-mediated activation of Mycobacterium tuberculosis isocitrate lyase 2. Nat. Commun. 10 (1), 4639–4647. doi:10.1038/s41467-019-12614-7
Bi, J., Wang, Y., Yu, H., Qian, X., Wang, H., Liu, J., et al. (2017). Modulation of central carbon metabolism by acetylation of isocitrate lyase in Mycobacterium tuberculosis. Sci. Rep. 7 (1), 44826–44911. doi:10.1038/srep44826
Borja, G. M., Rodriguez, A., Campbell, K., Borodina, I., Chen, Y., and Nielsen, J. (2019). Metabolic engineering and transcriptomic analysis of Saccharomyces cerevisiae producing p-coumaric acid from xylose. Microb. Cell Fact. 18 (1), 191. doi:10.1186/s12934-019-1244-4
Cabulong, R. B., Bañares, A. B., Nisola, G. M., Lee, W.-K., and Chung, W.-J. (2021). Enhanced glycolic acid yield through xylose and cellobiose utilization by metabolically engineered Escherichia coli. Bioprocess Biosyst. Eng. 44 (6), 1081–1091. doi:10.1007/s00449-020-02502-6
Cam, Y., Alkim, C., Trichez, D., Trebosc, V., Vax, A., Bartolo, F., et al. (2016). Engineering of a synthetic metabolic pathway for the assimilation of (D)-xylose into value-added chemicals. ACS Synth. Biol. 5 (7), 607–618. doi:10.1021/acssynbio.5b00103
Campilongo, R., Fung, R. K. Y., Little, R. H., Grenga, L., Trampari, E., Pepe, S., et al. (2017). One ligand, two regulators and three binding sites: How KDPG controls primary carbon metabolism in Pseudomonas. PLoS Genet. 13 (6), e1006839. doi:10.1371/journal.pgen.1006839
Castano-Cerezo, S., Bernal, V., Post, H., Fuhrer, T., Cappadona, S., Sanchez-Diaz, N. C., et al. (2014). Protein acetylation affects acetate metabolism, motility and acid stress response in Escherichia coli. Mol. Syst. Biol. 10, 762. doi:10.15252/msb.20145227
Chang, P., Chen, G. S., Chu, H.-Y., Lu, K. W., and Shen, C. R. (2017). Engineering efficient production of itaconic acid from diverse substrates in Escherichia coli. J. Biotechnol. 249, 73–81. doi:10.1016/j.jbiotec.2017.03.026
Chao, G., Shen, J., Tseng, C. P., Park, S. J., and Gunsalus, R. P. (1997). Aerobic regulation of isocitrate dehydrogenase gene (icd) expression in Escherichia coli by the arcA and fnr gene products. J. Bacteriol. 179 (13), 4299–4304. doi:10.1128/jb.179.13.4299-4304.1997
Chen, X., Ma, D., Liu, J., Luo, Q., and Liu, L. (2020). Engineering the transmission efficiency of the noncyclic glyoxylate pathway for fumarate production in Escherichia coli. Biotechnol. Biofuels 13 (1), 132–210. doi:10.1186/s13068-020-01771-3
Chen, Y., Daviet, L., Schalk, M., Siewers, V., and Nielsen, J. (2013). Establishing a platform cell factory through engineering of yeast acetyl-CoA metabolism. Metab. Eng. 15, 48–54. doi:10.1016/j.ymben.2012.11.002
Chen, Y., Siewers, V., and Nielsen, J. (2012). Profiling of cytosolic and peroxisomal acetyl-CoA metabolism in Saccharomyces cerevisiae. PLoS ONE 7 (8), e42475. doi:10.1371/journal.pone.0042475
Choi, K. R., Jang, W. D., Yang, D., Cho, J. S., Park, D., and Lee, S. Y. (2019). Systems metabolic engineering strategies: Integrating systems and synthetic biology with metabolic engineering. Trends Biotechnol. 37 (8), 817–837. doi:10.1016/j.tibtech.2019.01.003
Choi, S., Kim, H. U., Kim, T. Y., and Lee, S. Y. (2016). Systematic engineering of TCA cycle for optimal production of a four-carbon platform chemical 4-hydroxybutyric acid in Escherichia coli. Metab. Eng. 38, 264–273. doi:10.1016/j.ymben.2016.09.004
Choi, S. Y., Park, S. J., Kim, W. J., Yang, J. E., Lee, H., Shin, J., et al. (2016). One-step fermentative production of poly (lactate-co-glycolate) from carbohydrates in Escherichia coli. Nat. Biotechnol. 34 (4), 435–440. doi:10.1038/nbt.3485
Cozzone, A. J. (1998). Regulation of acetate metabolism by protein phosphorylation in enteric bacteria. Annu. Rev. Microbiol. 52 (1), 127–164. doi:10.1146/annurev.micro.52.1.127
Cramer, A., Gerstmeir, R., Schaffer, S., Bott, M., and Eikmanns, B. J. (2006). Identification of RamA, a novel LuxR-type transcriptional regulator of genes involved in acetate metabolism of Corynebacterium glutamicum. J. Bacteriol. 188 (7), 2554–2567. doi:10.1128/JB.188.7.2554-2567.2006
Cui, Z., Gao, C., Li, J., Hou, J., Lin, C. S. K., and Qi, Q. (2017). Engineering of unconventional yeast Yarrowia lipolytica for efficient succinic acid production from glycerol at low pH. Metab. Eng. 42, 126–133. doi:10.1016/j.ymben.2017.06.007
Deng, Y., Ma, N., Zhu, K., Mao, Y., Wei, X., and Zhao, Y. (2018). Balancing the carbon flux distributions between the TCA cycle and glyoxylate shunt to produce glycolate at high yield and titer in Escherichia coli. Metab. Eng. 46, 28–34. doi:10.1016/j.ymben.2018.02.008
Deng, Y., Mao, Y., and Zhang, X. (2015). Metabolic engineering of E. coli for efficient production of glycolic acid from glucose. Biochem. Eng. J. 103, 256–262. doi:10.1016/j.bej.2015.08.008
Dhamankar, H., Tarasova, Y., Martin, C. H., and Prather, K. L. (2014). Engineering E. coli for the biosynthesis of 3-hydroxy-γ-butyrolactone (3HBL) and 3, 4-dihydroxybutyric acid (3, 4-DHBA) as value-added chemicals from glucose as a sole carbon source. Metab. Eng. 25, 72–81. doi:10.1016/j.ymben.2014.06.004
Dischert, W., and Soucaille, P. (2015). Method for producing high amount of glycolic acid by fermentation. U.S. Patent No 8,945,888. Washington, DC: U.S. Patent and Trademark Office.
Dolan, S. K., and Welch, M. (2018). The glyoxylate shunt, 60 years on. Annu. Rev. Microbiol. 72, 309–330. doi:10.1146/annurev-micro-090817-062257
Durall, C., Kukil, K., Hawkes, J. A., Albergati, A., Lindblad, P., and Lindberg, P. (2021). Production of succinate by engineered strains of Synechocystis PCC 6803 overexpressing phosphoenolpyruvate carboxylase and a glyoxylate shunt. Microb. Cell Fact. 20 (1), 39–14. doi:10.1186/s12934-021-01529-y
Flores, N., Flores, S., Escalante, A., de Anda, R., Leal, L., Malpica, R., et al. (2005). Adaptation for fast growth on glucose by differential expression of central carbon metabolism and gal regulon genes in an Escherichia coli strain lacking the phosphoenolpyruvate: Carbohydrate phosphotransferase system. Metab. Eng. 7 (2), 70–87. doi:10.1016/j.ymben.2004.10.002
Gao, C., Wang, S., Hu, G., Guo, L., Chen, X., Xu, P., et al. (2018). Engineering Escherichia coli for malate production by integrating modular pathway characterization with CRISPRi-guided multiplexed metabolic tuning. Biotechnol. Bioeng. 115 (3), 661–672. doi:10.1002/bit.26486
Gerstmeir, R., Cramer, A., Dangel, P., Schaffer, S., and Eikmanns, B. J. (2004). RamB, a novel transcriptional regulator of genes involved in acetate metabolism of Corynebacterium glutamicum. J. Bacteriol. 186 (9), 2798–2809. doi:10.1128/JB.186.9.2798-2809.2004
Gui, L., Sunnarborg, A., and LaPorte, D. C. (1996). Regulated expression of a repressor protein: FadR activates iclR. J. Bacteriol. 178 (15), 4704–4709. doi:10.1128/jb.178.15.4704-4709.1996
Huang, H., Sivapragasam, S., and Grove, A. (2015). The regulatory role of Streptomyces coelicolor TamR in central metabolism. Biochem. J. 466 (2), 347–358. doi:10.1042/BJ20130838
Jo, J.-H., Seol, H.-Y., Lee, Y.-B., Kim, M.-H., Hyun, H.-H., and Lee, H.-H. (2012). Disruption of genes for the enhanced biosynthesis of α-ketoglutarate in Corynebacterium glutamicum. Can. J. Microbiol. 58 (3), 278–286. doi:10.1139/w11-132
Jo, M., Noh, M. H., Lim, H. G., Kang, C. W., Im, D.-K., Oh, M.-K., et al. (2019). Precise tuning of the glyoxylate cycle in Escherichia coli for efficient tyrosine production from acetate. Microb. Cell Fact. 18 (1), 57–59. doi:10.1186/s12934-019-1106-0
Kabisch, J., Pratzka, I., Meyer, H., Albrecht, D., Lalk, M., Ehrenreich, A., et al. (2013). Metabolic engineering of Bacillus subtilis for growth on overflow metabolites. Microb. Cell Fact. 12 (1), 72–10. doi:10.1186/1475-2859-12-72
Kim, H. J., Kim, T. H., Kim, Y., and Lee, H. S. (2004). Identification and characterization of glxR, a gene involved in regulation of glyoxylate bypass in Corynebacterium glutamicum. J. Bacteriol. 186 (11), 3453–3460. doi:10.1128/JB.186.11.3453-3460.2004
Kocharin, K., Chen, Y., Siewers, V., and Nielsen, J. (2012). Engineering of acetyl-CoA metabolism for the improved production of polyhydroxybutyrate in Saccharomyces cerevisiae. Amb. Express 2 (1), 52–11. doi:10.1186/2191-0855-2-52
Koivistoinen, O. M., Kuivanen, J., Barth, D., Turkia, H., Pitkänen, J.-P., Penttilä, M., et al. (2013). Glycolic acid production in the engineered yeasts Saccharomyces cerevisiae and Kluyveromyces lactis. Microb. Cell Fact. 12 (1), 82–16. doi:10.1186/1475-2859-12-82
Kornberg, H. L., and Krebs, H. A. (1957). Synthesis of cell constituents from C2-units by a modified tricarboxylic acid cycle. Nature 179 (4568), 988–991. doi:10.1038/179988a0
Krivoruchko, A., Serrano-Amatriain, C., Chen, Y., Siewers, V., and Nielsen, J. (2013). Improving biobutanol production in engineered Saccharomyces cerevisiae by manipulation of acetyl-CoA metabolism. J. Ind. Microbiol. Biotechnol. 40 (9), 1051–1056. doi:10.1007/s10295-013-1296-0
Kroner, G. M., Wolfe, M. B., and Freddolino, P. L. (2019). Escherichia coli Lrp regulates one-third of the genome via direct, cooperative, and indirect routes. J. Bacteriol. 201 (3), e00411–e00418. doi:10.1128/JB.00411-18
Lai, N., Luo, Y., Fei, P., Hu, P., and Wu, H. (2021). One stone two birds: Biosynthesis of 3-hydroxypropionic acid from CO2 and syngas-derived acetic acid in Escherichia coli. Synthetic Syst. Biotechnol. 6 (3), 144–152. doi:10.1016/j.synbio.2021.06.003
Lama, S., Kim, Y., Nguyen, D. T., Im, C. H., Sankaranarayanan, M., and Park, S. (2021). Production of 3-hydroxypropionic acid from acetate using metabolically-engineered and glucose-grown Escherichia coli. Bioresour. Technol. 320, 124362. doi:10.1016/j.biortech.2020.124362
LaPorte, D. C., Thorsness, P. E., and Koshland, D. E. (1985). Compensatory phosphorylation of isocitrate dehydrogenase. A mechanism for adaptation to the intracellular environment. J. Biol. Chem. 260 (19), 10563–10568. doi:10.1016/S0021-9258(19)85122-0
Lee, J. H., Cha, S., Kang, C. W., Lee, G. M., Lim, H. G., and Jung, G. Y. (2018). Efficient conversion of acetate to 3-hydroxypropionic acid by engineered Escherichia coli. Catalysts 8 (11), 525. doi:10.3390/catal8110525
Lee, S. S., Park, J., Heo, Y. B., and Woo, H. M. (2020). Case study of xylose conversion to glycolate in Corynebacterium glutamicum: Current limitation and future perspective of the CRISPR-Cas systems. Enzyme Microb. Technol. 132, 109395. doi:10.1016/j.enzmictec.2019.109395
Lee, W., VanderVen, B. C., Walker, S., and Russell, D. G. (2017). Novel protein acetyltransferase, Rv2170, modulates carbon and energy metabolism in Mycobacterium tuberculosis. Sci. Rep. 7 (1), 72–11. doi:10.1038/s41598-017-00067-1
Li, B., Cai, D., and Chen, S. (2021). Metabolic engineering of central carbon metabolism of Bacillus licheniformis for enhanced production of poly-γ-glutamic acid. Appl. Biochem. Biotechnol. 193 (11), 3540–3552. doi:10.1007/s12010-021-03619-4
Li, N., Zhang, B., Chen, T., Wang, Z., Tang, Y. J., and Zhao, X. (2013). Directed pathway evolution of the glyoxylate shunt in Escherichia coli for improved aerobic succinate production from glycerol. J. Ind. Microbiol. Biotechnol. 40 (12), 1461–1475. doi:10.1007/s10295-013-1342-y
Li, N., Zhang, B., Wang, Z., Tang, Y. J., Chen, T., and Zhao, X. (2014). Engineering Escherichia coli for fumaric acid production from glycerol. Bioresour. Technol. 174, 81–87. doi:10.1016/j.biortech.2014.09.147
Li, W., Chen, J., Liu, C.-X., Yuan, Q.-P., and Li, Z.-J. (2019). Microbial production of glycolate from acetate by metabolically engineered Escherichia coli. J. Biotechnol. 291, 41–45. doi:10.1016/j.jbiotec.2018.12.012
Li, Y., Huang, B., Wu, H., Li, Z., Ye, Q., and Zhang, Y. P. (2016). Production of succinate from acetate by metabolically engineered Escherichia coli. ACS Synth. Biol. 5 (11), 1299–1307. doi:10.1021/acssynbio.6b00052
Li, Y., Wei, H., Wang, T., Xu, Q., Zhang, C., Fan, X., et al. (2017). Current status on metabolic engineering for the production of l-aspartate family amino acids and derivatives. Bioresour. Technol. 245, 1588–1602. doi:10.1016/j.biortech.2017.05.145
Li, Z.-J., Qiao, K., Che, X.-M., and Stephanopoulos, G. (2017). Metabolic engineering of Escherichia coli for the synthesis of the quadripolymer poly (glycolate-co-lactate-co-3-hydroxybutyrate-co-4-hydroxybutyrate) from glucose. Metab. Eng. 44, 38–44. doi:10.1016/j.ymben.2017.09.003
Li, Z.-J., Qiao, K., Shi, W., Pereira, B., Zhang, H., Olsen, B. D., et al. (2016). Biosynthesis of poly (glycolate-co-lactate-co-3-hydroxybutyrate) from glucose by metabolically engineered Escherichia coli. Metab. Eng. 35, 1–8. doi:10.1016/j.ymben.2016.01.004
Lin, F., Chen, Y., Levine, R., Lee, K., Yuan, Y., and Lin, X. N. (2013). Improving fatty acid availability for bio-hydrocarbon production in Escherichia coli by metabolic engineering. PloS one 8 (10), e78595. doi:10.1371/journal.pone.0078595
Lin, H., Bennett, G. N., and San, K. Y. (2005). Genetic reconstruction of the aerobic central metabolism in Escherichia coli for the absolute aerobic production of succinate. Biotechnol. Bioeng. 89 (2), 148–156. doi:10.1002/bit.20298
Liu, J., Li, J., Liu, Y., Shin, H.-d., Ledesma-Amaro, R., Du, G., et al. (2018). Synergistic rewiring of carbon metabolism and redox metabolism in cytoplasm and mitochondria of Aspergillus oryzae for increased L-malate production. ACS Synth. Biol. 7 (9), 2139–2147. doi:10.1021/acssynbio.8b00130
Liu, M., Ding, Y., Chen, H., Zhao, Z., Liu, H., Xian, M., et al. (2017). Improving the production of acetyl-CoA-derived chemicals in Escherichia coli BL21 (DE3) through iclR and arcA deletion. BMC Microbiol. 17 (1), 10–19. doi:10.1186/s12866-016-0913-2
Liu, M., Lou, J., Gu, J., Lyu, X.-M., Wang, F.-Q., and Wei, D.-Z. (2020). Increasing L-homoserine production in Escherichia coli by engineering the central metabolic pathways. J. Biotechnol. 314, 1–7. doi:10.1016/j.jbiotec.2020.03.010
Liu, M., Yao, L., Xian, M., Ding, Y., Liu, H., and Zhao, G. (2016). Deletion of arcA increased the production of acetyl-CoA-derived chemicals in recombinant Escherichia coli. Biotechnol. Lett. 38 (1), 97–101. doi:10.1007/s10529-015-1953-7
Liu, X., Zhao, G., Sun, S., Fan, C., Feng, X., and Xiong, P. (2022). Biosynthetic pathway and metabolic engineering of succinic acid. Front. Bioeng. Biotechnol. 10, 843887. doi:10.3389/fbioe.2022.843887
Lorca, G. L., Ezersky, A., Lunin, V. V., Walker, J. R., Altamentova, S., Evdokimova, E., et al. (2007). Glyoxylate and pyruvate are antagonistic effectors of the Escherichia coli IclR transcriptional regulator. J. Biol. Chem. 282 (22), 16476–16491. doi:10.1074/jbc.M610838200
Machado, D., and Herrgård, M. J. (2015). Co-evolution of strain design methods based on flux balance and elementary mode analysis. Metab. Eng. Commun. 2, 85–92. doi:10.1016/j.meteno.2015.04.001
Maeda, T., and Wachi, M. (2012). 3' Untranslated region-dependent degradation of the aceA mRNA, encoding the glyoxylate cycle enzyme isocitrate lyase, by RNase E/G in Corynebacterium glutamicum. Appl. Environ. Microbiol. 78 (24), 8753–8761. doi:10.1128/AEM.02304-12
Mainguet, S. E., Gronenberg, L. S., Wong, S. S., and Liao, J. C. (2013). A reverse glyoxylate shunt to build a non-native route from C4 to C2 in Escherichia coli. Metab. Eng. 19, 116–127. doi:10.1016/j.ymben.2013.06.004
Martinez, I., Bennett, G. N., and San, K. Y. (2010). Metabolic impact of the level of aeration during cell growth on anaerobic succinate production by an engineered Escherichia coli strain. Metab. Eng. 12 (6), 499–509. doi:10.1016/j.ymben.2010.09.002
Masiewicz, P., Brzostek, A., Wolański, M., Dziadek, J., and Zakrzewska-Czerwińska, J. (2012). A novel role of the PrpR as a transcription factor involved in the regulation of methylcitrate pathway in Mycobacterium tuberculosis. PloS one 13 (12), e43651. doi:10.1371/journal.pone.0043651
Micklinghoff, J. C., Breitinger, K. J., Schmidt, M., Geffers, R., Eikmanns, B. J., and Bange, F.-C. (2009). Role of the transcriptional regulator RamB (Rv0465c) in the control of the glyoxylate cycle in Mycobacterium tuberculosis. J. Bacteriol. 191 (23), 7260–7269. doi:10.1128/JB.01009-09
Mindt, M., Hannibal, S., Heuser, M., Risse, J. M., Sasikumar, K., Nampoothiri, K. M., et al. (2019a). Fermentative production of N-alkylated glycine derivatives by recombinant Corynebacterium glutamicum using a mutant of imine reductase DpkA from Pseudomonas putida. Front. Bioeng. Biotechnol. 7, 232. doi:10.3389/fbioe.2019.00232
Mindt, M., Heuser, M., and Wendisch, V. F. (2019b). Xylose as preferred substrate for sarcosine production by recombinant Corynebacterium glutamicum. Bioresour. Technol. 281, 135–142. doi:10.1016/j.biortech.2019.02.084
Miscevic, D., Mao, J.-Y., Kefale, T., Abedi, D., Huang, C.-C., Moo-Young, M., et al. (2020a). Integrated strain engineering and bioprocessing strategies for high-level bio-based production of 3-hydroxyvalerate in Escherichia coli. Appl. Microbiol. Biotechnol. 104 (12), 5259–5272. doi:10.1007/s00253-020-10580-5
Miscevic, D., Mao, J.-Y., Mozell, B., Srirangan, K., Abedi, D., Moo-Young, M., et al. (2021a). Bio-based production of poly (3-hydroxybutyrate-co-3-hydroxyvalerate) with modulated monomeric fraction in Escherichia coli. Appl. Microbiol. Biotechnol. 105 (4), 1435–1446. doi:10.1007/s00253-021-11108-1
Miscevic, D., Mao, J. Y., Kefale, T., Abedi, D., Moo-Young, M., and Perry Chou, C. (2021b). Strain engineering for high-level 5-aminolevulinic acid production in Escherichia coli. Biotechnol. Bioeng. 118 (1), 30–42. doi:10.1002/bit.27547
Miscevic, D., Mao, J. Y., Moo-Young, M., and Chou, C. H. P. (2020b). High-level heterologous production of propionate in engineered Escherichia coli. Biotechnol. Bioeng. 117 (5), 1304–1315. doi:10.1002/bit.27276
Miscevic, D., Srirangan, K., Kefale, T., Kilpatrick, S., Chung, D. A., Moo-Young, M., et al. (2020c). Heterologous production of 3-hydroxyvalerate in engineered Escherichia coli. Metab. Eng. 61, 141–151. doi:10.1016/j.ymben.2019.11.005
Munoz-Elias, E. J., and McKinney, J. D. (2005). Mycobacterium tuberculosis isocitrate lyases 1 and 2 are jointly required for in vivo growth and virulence. Nat. Med. 11 (6), 638–644. doi:10.1038/nm1252
Murima, P., Zimmermann, M., Chopra, T., Pojer, F., Fonti, G., Dal Peraro, M., et al. (2016). A rheostat mechanism governs the bifurcation of carbon flux in mycobacteria. Nat. Commun. 7, 12527. doi:10.1038/ncomms12527
Nguyen, D. T. N., Lee, O. K., Hadiyati, S., Affifah, A. N., Kim, M. S., and Lee, E. Y. (2019). Metabolic engineering of the type I methanotroph Methylomonas sp. DH-1 for production of succinate from methane. Metab. Eng. 54, 170–179. doi:10.1016/j.ymben.2019.03.013
Ning, Y., Wu, X., Zhang, C., Xu, Q., Chen, N., and Xie, X. (2016). Pathway construction and metabolic engineering for fermentative production of ectoine in Escherichia coli. Metab. Eng. 36, 10–18. doi:10.1016/j.ymben.2016.02.013
Nitta, K., Laviña, W. A., Pontrelli, S., Liao, J. C., Putri, S. P., and Fukusaki, E. (2019). Metabolome analysis revealed the knockout of glyoxylate shunt as an effective strategy for improvement of 1-butanol production in transgenic Escherichia coli. J. Biosci. Bioeng. 127 (3), 301–308. doi:10.1016/j.jbiosc.2018.08.013
Noh, M. H., Lim, H. G., Park, S., Seo, S. W., and Jung, G. Y. (2017). Precise flux redistribution to glyoxylate cycle for 5-aminolevulinic acid production in Escherichia coli. Metab. Eng. 43, 1–8. doi:10.1016/j.ymben.2017.07.006
Noh, M. H., Lim, H. G., Woo, S. H., Song, J., and Jung, G. Y. (2018). Production of itaconic acid from acetate by engineering acid-tolerant Escherichia coli W. Biotechnol. Bioeng. 115 (3), 729–738. doi:10.1002/bit.26508
Ogawa, T., Murakami, K., Mori, H., Ishii, N., Tomita, M., and Yoshin, M. (2007). Role of phosphoenolpyruvate in the NADP-isocitrate dehydrogenase and isocitrate lyase reaction in Escherichia coli. J. Bacteriol. 189 (3), 1176–1178. doi:10.1128/JB.01628-06
Ohtake, T., Pontrelli, S., Laviña, W. A., Liao, J. C., Putri, S. P., and Fukusaki, E. (2017). Metabolomics-driven approach to solving a CoA imbalance for improved 1-butanol production in Escherichia coli. Metab. Eng. 41, 135–143. doi:10.1016/j.ymben.2017.04.003
Pereira, B., Li, Z.-J., De Mey, M., Lim, C. G., Zhang, H., Hoeltgen, C., et al. (2016). Efficient utilization of pentoses for bioproduction of the renewable two-carbon compounds ethylene glycol and glycolate. Metab. Eng. 34, 80–87. doi:10.1016/j.ymben.2015.12.004
Ren, J., Zhou, L., Wang, C., Lin, C., Li, Z., and Zeng, A.-P. (2018). An unnatural pathway for efficient 5-aminolevulinic acid biosynthesis with glycine from glyoxylate based on retrobiosynthetic design. ACS Synth. Biol. 7 (12), 2750–2757. doi:10.1021/acssynbio.8b00354
Salusjärvi, L., Havukainen, S., Koivistoinen, O., and Toivari, M. (2019). Biotechnological production of glycolic acid and ethylene glycol: Current state and perspectives. Appl. Microbiol. Biotechnol. 103 (6), 2525–2535. doi:10.1007/s00253-019-09640-2
Sanchez, A. M., Bennett, G. N., and San, K. Y. (2005). Novel pathway engineering design of the anaerobic central metabolic pathway in Escherichia coli to increase succinate yield and productivity. Metab. Eng. 7 (3), 229–239. doi:10.1016/j.ymben.2005.03.001
Santala, S., Santala, V., Liu, N., and Stephanopoulos, G. (2021). Partitioning metabolism between growth and product synthesis for coordinated production of wax esters in Acinetobacter baylyi ADP1. Biotechnol. Bioeng. 118 (6), 2283–2292. doi:10.1002/bit.27740
Schada von Borzyskowski, L., Sonntag, F., Pöschel, L., Vorholt, J. A., Schrader, J., Erb, T. J., et al. (2018). Replacing the ethylmalonyl-CoA pathway with the glyoxylate shunt provides metabolic flexibility in the central carbon metabolism of Methylobacterium extorquens AM1. ACS Synth. Biol. 7 (1), 86–97. doi:10.1021/acssynbio.7b00229
Schwentner, A., Feith, A., Münch, E., Busche, T., Rückert, C., Kalinowski, J., et al. (2018). Metabolic engineering to guide evolution–Creating a novel mode for L-valine production with Corynebacterium glutamicum. Metab. Eng. 47, 31–41. doi:10.1016/j.ymben.2018.02.015
Shi, F., Zhang, S., Li, Y., and Lu, Z. (2019). Enhancement of substrate supply and Ido expression to improve 4-hydroxyisoleucine production in recombinant Corynebacterium glutamicum ssp. Appl. Microbiol. Biotechnol. 103 (10), 4113–4124. doi:10.1007/s00253-019-09791-2
Shimizu, T., Teramoto, H., and Inui, M. (2019). Introduction of glyoxylate bypass increases hydrogen gas yield from acetate and L-glutamate in Rhodobacter sphaeroides. Appl. Environ. Microbiol. 85 (2), e01873–e01818. doi:10.1128/AEM.01873-18
Skorokhodova, A. Y., Morzhakova, A. A., Gulevich, A. Y., and Debabov, V. G. (2015). Manipulating pyruvate to acetyl-CoA conversion in Escherichia coli for anaerobic succinate biosynthesis from glucose with the yield close to the stoichiometric maximum. J. Biotechnol. 214, 33–42. doi:10.1016/j.jbiotec.2015.09.003
Soma, Y., Fujiwara, Y., Nakagawa, T., Tsuruno, K., and Hanai, T. (2017). Reconstruction of a metabolic regulatory network in Escherichia coli for purposeful switching from cell growth mode to production mode in direct GABA fermentation from glucose. Metab. Eng. 43, 54–63. doi:10.1016/j.ymben.2017.08.002
Song, C. W., Kim, D. I., Choi, S., Jang, J. W., and Lee, S. Y. (2013). Metabolic engineering of Escherichia coli for the production of fumaric acid. Biotechnol. Bioeng. 110 (7), 2025–2034. doi:10.1002/bit.24868
Song, T., Wu, N., Wang, C., Wang, Y., Chai, F., Ding, M., et al. (2020). Crocetin overproduction in engineered Saccharomyces cerevisiae via tuning key enzymes coupled with precursor engineering. Front. Bioeng. Biotechnol. 8, 578005. doi:10.3389/fbioe.2020.578005
Srirangan, K., Akawi, L., Liu, X., Westbrook, A., Blondeel, E. J., Aucoin, M. G., et al. (2013). Manipulating the sleeping beauty mutase operon for the production of 1-propanol in engineered Escherichia coli. Biotechnol. Biofuels 6 (1), 139–214. doi:10.1186/1754-6834-6-139
Toyoda, K., Teramoto, H., Gunji, W., Inui, M., and Yukawa, H. (2013). Involvement of regulatory interactions among global regulators GlxR, SugR, and RamA in expression of ramA in Corynebacterium glutamicum. J. Bacteriol. 195 (8), 1718–1726. doi:10.1128/JB.00016-13
Trichez, D., Auriol, C., Baylac, A., Irague, R., Dressaire, C., Carnicer-Heras, M., et al. (2018). Engineering of Escherichia coli for Krebs cycle-dependent production of malic acid. Microb. Cell Fact. 17 (1), 113–212. doi:10.1186/s12934-018-0959-y
Vemuri, G. N., Eiteman, M. A., and Altman, E. (2002). Effects of growth mode and pyruvate carboxylase on succinic acid production by metabolically engineered strains of Escherichia coli. Appl. Environ. Microbiol. 68 (4), 1715–1727. doi:10.1128/aem.68.4.1715-1727.2002
Vuoristo, K. S., Mars, A. E., Sanders, J. P. M., Eggink, G., and Weusthuis, R. A. (2016). Metabolic engineering of tca cycle for production of chemicals. Trends Biotechnol. 34 (3), 191–197. doi:10.1016/j.tibtech.2015.11.002
Wang, P., Zhou, H.-Y., Li, B., Ding, W.-Q., Liu, Z.-Q., and Zheng, Y.-G. (2021). Multiplex modification of Escherichia coli for enhanced β-alanine biosynthesis through metabolic engineering. Bioresour. Technol. 342, 126050. doi:10.1016/j.biortech.2021.126050
Wei, L. N., Zhu, L. W., and Tang, Y. J. (2016). Succinate production positively correlates with the affinity of the global transcription factor Cra for its effector FBP in Escherichia coli. Biotechnol. Biofuels 9, 264. doi:10.1186/s13068-016-0679-7
Yang, J., Fang, Y., Wang, J., Wang, C., Zhao, L., and Wang, X. (2019). Deletion of regulator-encoding genes fadR, fabR and iclR to increase l-threonine production in Escherichia coli. Appl. Microbiol. Biotechnol. 103 (11), 4549–4564. doi:10.1007/s00253-019-09818-8
Yang, J., Yang, W., Feng, J., Chen, J., Jiang, M., and Zou, X. (2018). Enhanced polymalic acid production from the glyoxylate shunt pathway under exogenous alcohol stress. J. Biotechnol. 275, 24–30. doi:10.1016/j.jbiotec.2018.04.001
Yates, S. P., Edwards, T. E., Bryan, C. M., Stein, A. J., Van Voorhis, W. C., Myler, P. J., et al. (2011). Structural basis of the substrate specificity of bifunctional isocitrate dehydrogenase kinase/phosphatase. Biochemistry 50 (38), 8103–8106. doi:10.1021/bi200809p
Yu, H., Li, X., Duchoud, F., Chuang, D. S., and Liao, J. C. (2018). Augmenting the Calvin–Benson–Bassham cycle by a synthetic malyl-CoA-glycerate carbon fixation pathway. Nat. Commun. 9 (1), 2008–2010. doi:10.1038/s41467-018-04417-z
Yu, H., and Liao, J. C. (2018). A modified serine cycle in Escherichia coli coverts methanol and CO2 to two-carbon compounds. Nat. Commun. 9 (1), 3992–4010. doi:10.1038/s41467-018-06496-4
Zahoor, A., Otten, A., and Wendisch, V. F. (2014). Metabolic engineering of Corynebacterium glutamicum for glycolate production. J. Biotechnol. 192, 366–375. doi:10.1016/j.jbiotec.2013.12.020
Zeng, A.-P. (2019). New bioproduction systems for chemicals and fuels: Needs and new development. Biotechnol. Adv. 37 (4), 508–518. doi:10.1016/j.biotechadv.2019.01.003
Zeng, W., Zhang, B., Chen, G., Li, M., and Liang, Z. (2019). Efficient production of polymalic acid by a novel isolated Aureobasidium pullulans using metabolic intermediates and inhibitors. Appl. Biochem. Biotechnol. 187 (2), 612–627. doi:10.1007/s12010-018-2825-0
Zhang, K., Mohsin, A., Dai, Y., Chen, Z., Zhuang, Y., Chu, J., et al. (2019). Combinatorial effect of ARTP mutagenesis and ribosome engineering on an industrial strain of Streptomyces albus S12 for enhanced biosynthesis of salinomycin. Front. Bioeng. Biotechnol. 7, 212. doi:10.3389/fbioe.2019.00212
Zhang, S., and Bryant, D. A. (2015). Biochemical validation of the glyoxylate cycle in the cyanobacterium Chlorogloeopsis fritschii strain PCC 9212. J. Biol. Chem. 290 (22), 14019–14030. doi:10.1074/jbc.M115.648170
Zhao, H., Fang, Y., Wang, X., Zhao, L., Wang, J., and Li, Y. (2018). Increasing L-threonine production in Escherichia coli by engineering the glyoxylate shunt and the L-threonine biosynthesis pathway. Appl. Microbiol. Biotechnol. 102 (13), 5505–5518. doi:10.1007/s00253-018-9024-3
Zhou, S., Lama, S., Jiang, J., Sankaranarayanan, M., and Park, S. (2020). Use of acetate for the production of 3-hydroxypropionic acid by metabolically-engineered Pseudomonas denitrificans. Bioresour. Technol. 307, 123194. doi:10.1016/j.biortech.2020.123194
Zhu, L., Fang, Y., Ding, Z., Zhang, S., and Wang, X. (2019). Developing an l-threonine-producing strain from wild-type Escherichia coli by modifying the glucose uptake, glyoxylate shunt, and l-threonine biosynthetic pathway. Biotechnol. Appl. Biochem. 66 (6), 962–976. doi:10.1002/bab.1813
Keywords: glyoxylate cycle, metabolic engineering, biosynthesis, TCA cycle, organic acids, amino acids, fatty acids
Citation: Yang P, Liu W, Chen Y and Gong A-D (2022) Engineering the glyoxylate cycle for chemical bioproduction. Front. Bioeng. Biotechnol. 10:1066651. doi: 10.3389/fbioe.2022.1066651
Received: 11 October 2022; Accepted: 17 November 2022;
Published: 02 December 2022.
Edited by:
Qi Xianghui, Jiangsu University, ChinaReviewed by:
Gao-Qiang Liu, Central South University of Forestry and Technology, ChinaJunping Zhou, Zhejiang University of Technology, China
Copyright © 2022 Yang, Liu, Chen and Gong. This is an open-access article distributed under the terms of the Creative Commons Attribution License (CC BY). The use, distribution or reproduction in other forums is permitted, provided the original author(s) and the copyright owner(s) are credited and that the original publication in this journal is cited, in accordance with accepted academic practice. No use, distribution or reproduction is permitted which does not comply with these terms.
*Correspondence: An-Dong Gong, ZndqdDYzMjk4QDEyNi5jb20=