- 1S-Inova Biotech, Programa de Pós-Graduação Em Biotecnologia, Universidade Católica Dom Bosco (UCDB), Campo Grande, Brazil
- 2Centro de Análises Proteômicas e Bioquímica (CAPB), Programa de Pós-Graduação Em Ciências Genômicas e Biotecnologia, Universidade Católica de Brasília (UCB), Brasília, Brazil
- 3Faculdade de Agronomia e Medicina Veterinária (FAV), Universidade de Brasília (UnB), Brasília, Brazil
- 4Programa de Pós-Graduação Em Biologia Animal, Universidade de Brasília (UnB), Brasília, Brazil
- 5Programa de Pós-Graduação Em Patologia Molecular, Universidade de Brasília (UnB), Brasília, Brazil
Antimicrobial peptides (AMPs) have shown cell membrane-directed mechanisms of action. This specificity can be effective against infectious agents that have acquired resistance to conventional drugs. The AMPs’ membrane-specificity and their great potential to combat resistant microbes has brought hope to the medical/therapeutic scene. The high death rate worldwide due to antimicrobial resistance (AMR) has pushed forward the search for new molecules and product developments, mainly antibiotics. In the current scenario, other strategies including the association of two or more drugs have contributed to the treatment of difficult-to-treat infectious diseases, above all, those caused by bacteria. In this context, the synergistic action of AMPs associated with current antibiotic therapy can bring important results for the production of new and effective drugs to overcome AMR. This review presents the advances obtained in the last 5 years in medical/antibiotic therapy, with the use of products based on AMPs, as well as perspectives on the potentialized effects of current drugs combined with AMPs for the treatment of bacterial infectious diseases.
1 Introduction
Over the years, antibiotic therapy has been the main tool for treating infectious diseases. Discoveries revealed that antibiotics have been used since ancient civilizations, and it is believed that antibiotics have been in human treatment since 350–550 CE (Iskandar et al., 2022). Since the first discovered classes, it is possible to affirm that antibiotics have been responsible for saving thousands of lives annually since the early 20th century (Yin et al., 2021). Even so, the emergence of difficult-to-treat infectious disease was inevitable, given the co-evolution of pathogens. Selective pressure by antibiotic use has resulted in infectious diseases that are effectively untreatable with conventional antibiotics due to antimicrobial resistance (AMR). An increasing range of AMR organisms including bacteria, viruses, parasites and fungi have caused increased mortality rates around the world (Asokan and Vanitha, 2018). Because of this, AMR has increased considerably worldwide in the hospital environment and has alarmed world health authorities (World Health Organization, 2019). The selective pressure for antibiotic use can be considered mainly responsible for the emergence and increase in AMR (Larsson and Flach, 2022). The fight against multidrug-resistant (MDR) agents is a complex problem in both developed and developing countries (Majumder et al., 2020; Ting et al., 2020; Murray et al., 2022).
In fact, AMR has primarily been associated with selective pressure due to the increased use of antibiotics, and to their indiscriminate use due to self-medication. In addition, AMR can be related to social problems such as world overpopulation, poor sanitation, and invasion of preserved biomes. The use of antibiotics in the agricultural sector and also in the treatment of animals in veterinary clinics has contributed to AMR, as reviewed in (Rather et al., 2017; Aslam et al., 2018). Annual reports associate AMR with high mortality rates, prolonged hospital admission and, consequently, high costs in the healthcare sector. The increase in AMR limits treatment options, as it reduces the possibility of choosing effective drugs that have been used in clinical practice for years (Dadgostar, 2019; World Health Organization, 2020). It is estimated that by 2050 approximately 10 million deaths will be caused by infections caused by AMR (World Health Organization, 2017; Ting et al., 2020).
In a recent report (Global Antimicrobial Resistance and Use Surveillance System (GLASS) Report: Early implementation 2020) (World Health Organization, 2020), the WHO issued an emergency alert on the need to develop new treatment alternatives. Among clinical isolates, bacteria stand out in antimicrobial resistant groups. It is increasingly common to note the inefficiency of traditional antibiotics due to AMR bacteria. In a systematic review published in The Lancet journal, the authors observed that in 2019 alone, among the reports of deaths, about 1.27 million (95% of analyzed reports) were caused by bacteria infections (Murray et al., 2022). Besides stressing the urgency of developing new treatments, 12 representatives of some bacterial families were added to the priority pathogen list by the WHO (Table 1). According to the WHO, those families pose a great threat to humanity (Asokan and Vanitha, 2018).
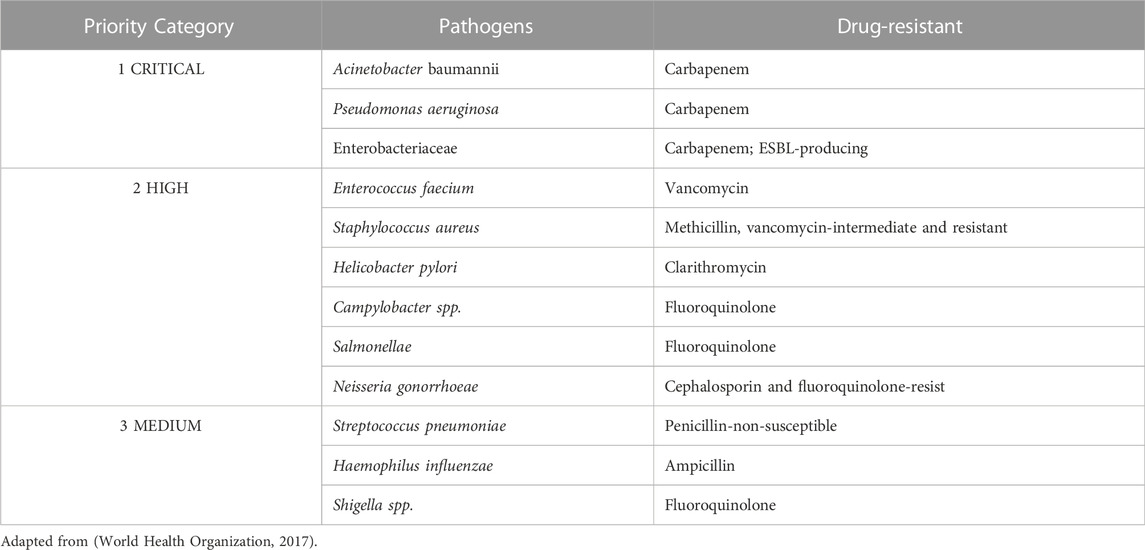
TABLE 1. The bacteria (n = 12) considered the greatest threat to human health that were added to the priority pathogen list by the WHO.
Despite the need for new therapeutic options, and regardless of the increase in antimicrobial resistance, it is possible to observe a decline in the development of new drugs and/or discovery of new molecules. There has been no discovery of a new antibiotic class since the “golden era”, the period between the 1950s and 1970s, when the main known classes were discovered (Hutchings et al., 2019). However, this decline is mainly due to the lack of interest from governments and large industries in investing in research. Certainly, evaluating cost-benefit, heavy investments are needed to prospect, obtain and commercialize new drugs. This results in low financial returns in the face of rapidly increasing microbial resistance, even with the high rate of deaths. In addition to the high costs, long periods are generally required for the production and marketing of a new drug. To put this in perspective, it takes 10–15 years for a new product to be approved and made available to the market, with costs of approximately 2 billion dollars (Wouters et al., 2020).
Thus, it is urgent to search for new alternatives that overcome the therapeutic failures of traditional antibiotics. These alternatives include combined therapy or efficient molecule development that is capable of overcoming the increasing wave of threats from resistant organisms. Following the urgency of developing new antimicrobial drugs, many studies have reported proofs-of-concept, indicating the high therapeutic potential of defense peptides, and some peptides are already in clinical trials (Ting et al., 2020). Peptides are also able to boost the effects of other antibiotics, showing an improved action with these drugs. Among these peptides are known antimicrobial peptides (AMPs). AMPs could also be considered host defense peptides (HDR). HDRs have been involved in the living organisms defense system for millions of years (Mishra et al., 2017).
These peptides besides modulating the immune system, was reported that, on in vivo models, it can also neutralize endotoxins (Mishra et al., 2017; Boto et al., 2018). HDR also act against pathogens invasion (e.g., viruses, fungi, bacteria, and parasites), and can prevent biofilm formation (Mishra et al., 2017; Boto et al., 2018). In addition, it is possible to find AMPs as anticancer agents (Franco, 2011; Mishra et al., 2017). Additionally, AMPs also can be used for medical products development such as, drugs to be applied in wound healing as well as skin care use, due to antioxidant peptides action (Golonka et al., 2021; Moretta et al., 2021). AMPs can also act as inhibitors of ACE1 (angiotensin converting enzyme 1) and pancreatic lipase, being useful to control metabolic syndrome (Moretta et al., 2021).
As mentioned, those peptides have broad activity spectrum, and although it is possible (Maron et al., 2022), AMP resistance is not prevalent. It is an advantage compared to conventional drugs, and AMPs can act synergistically with conventional antibiotics potentializing the effects of them (Duong et al., 2021; Zhu et al., 2022). In this context, the synergistic action of AMPs associated with current antibiotic therapy can bring important results for the production of effective new drugs to overcome AMR. This review presents the advances obtained over the last 5 years in the medical/antibiotic therapy scene, with the use of products based on AMPs, as well as perspectives on the synergic effect of AMPs in association with current drugs for the treatment of bacterial infectious diseases.
2 Synergic effect of drug combination therapy used in clinical practice
For decades, antibiotics have facilitated the treatment of bacterial infectious diseases. However, over the years, selective pressure, by antibiotics use, allowed that bacteria to acquire a random change in their DNA. With those changes, the bacteria can survive in the antibiotic’s presence. This way, bacteria developed resistance mechanisms to conventional antibiotics (Ghosh et al., 2020). Also, those mechanisms can be horizontally transferred. Basic horizontal gene transfer (HGT) mechanisms were discovered more than 50 years ago. In general, HGT is an important form of bacterial survival and evolution (Ting et al., 2020; Power et al., 2021). In this way, horizontal transfer can occur with resistance genes. Resistance gene are also transferred between pathogenic and non-pathogenic bacteria including antibiotic resistance (ABR) ones. HGT afford pathogenic bacteria to develop resistance by obtaining ABR genes by exchange between other pathogenic ones, or commensal bacteria present in surrounding environments (Ting et al., 2020). However, antimicrobial misuse can cause resistance and effect the efficacy of drugs (Boto et al., 2018).
The therapeutic potential of existing antibiotics through collaborations with other biological or chemical molecules represents an approach to enhance antibacterial activity and inhibit the emergence of resistance (Shang et al., 2019). Combined action between two or more drugs that contribute to the final result against a multi-resistant microorganism is known as synergism. Synergistic interaction involves lower doses of the combination constituents, allowing a therapeutic effect (Lloyd et al., 2020). The enhanced effect of therapeutical molecules reflects an efficient means of increasing the amplitude of cellular responses induced by stimulation levels, like AMPs, for example (Sechet et al., 2018; Duong et al., 2021).
Drug–drug interactions (DDIs) happen when one drug modifies another’s pharmaceutical activity, but these require multiple dose levels in the analysis of each drug, either alone or in different combination ratios (Niu et al., 2019). Intuitively, molecule combination may represent higher effectiveness. However, drugs that could be combined, commonly did not improved the collateral effects if it not has dosage reduction of each molecule present in the combination (García-Fuente et al., 2018). DDIs are significant since metabolic biotransformations arise at some point between drug absorption into the circulation and its elimination (Katzung and Trevor, 2012). The biotransformation process can leave this xenobiotic in bioactivation through changes in elimination rates. Therefore, synergistic molecular combinations can overcome toxicity and the effects associated with high doses of drugs, allowing the dosage of each compound to be reduced or accessing a specific target (Roemhild et al., 2022).
Valuable examples of combinatorial effects were described in tests using colistin associated with 19 antibiotics against 20 colistin-resistant and 15 carbapenem-resistant Enterobacteriaceae isolates. The method used in this work was inkjet printer-assisted digital dispensing checkerboard array (Brennan-Krohn et al., 2018). Eighteen of nineteen combinations demonstrated synergy against two or more isolates. In addition, four higher synergic combinations (colistin combined with linezolid, rifampin, azithromycin, and fusidic acid) show potentialized effects against ≥90% of strains. These results suggest that colistin may exert a sub-inhibitory permeabilizing effects on the Gram-negative bacterial outer membrane, even in isolates that are resistant to it (Brennan-Krohn et al., 2018).
Another in vitro study tested the combination of colistin with teicoplanin multidrug-resistant Acinetobacter spp (Rady et al., 2022). This combination was tested against 29 multidrug-resistant Acinetobacter spp isolates. The researchers used 1 mg l−1 colistin and 10 mg l−1 teicoplanin in combination and demonstrated in vitro synergism against all tested Acinetobacter isolates except one (Acinetobacter lowffii). This combination also demonstrated a bactericidal effect at 6 h against 100% of A. baumannii isolates with no bacterial regrowth at 24 h. The same combination was bactericidal against three out of seven non-baumannii Acinetobacter isolates. Colistin can act on Acinetobacter spp. Outer membrane and permit teicoplanin to reach its target in the cell wall (Rady et al., 2022).
As explained, the association of two or more antibiotics demonstrates therapeutic potential through association against microorganisms; the production of antibiotics is not fully species-specific. Antibiotics can be composed of organisms associated with different species, genera, or even orders. An antibiotic’s mechanism of action must begin when it identifies the target macromolecule and its function. For example, the susceptibility of different bacteria to antibiotics relies chiefly on the structure of their cell walls, as it completes the capability of the antibiotic to penetrate the bacterial cell (Long, 1951; Liu et al., 2021). However, pathogen resistance is a result of pathogen selective pressure over many years. The molecular modification of the pathogen to cheat the antibiotic mechanism of action makes the pathogen more efficient, making infections even more difficult to treat (Uddin et al., 2021). However, synergic effects of AMPs with other antimicrobials can reduce the risk of developing bacterial resistance, due to the high lethality level (Duong et al., 2021).
3 Clinically significant advances in synergism between AMP/antibiotic and AMP/AMP
As mentioned, new therapies are urgently needed to tackle the global problem of AMR. To overcome the therapeutic limitations created by bacterial resistance, some strategies have been considered (Vargas-Casanova et al., 2019). In addition to antibiotic combinations, which are a common practice in the hospital environment, antibiotic association with AMPs has been demonstrated as a potential resource against AMR agents (dos Santos et al., 2021; Li et al., 2021). Recent reports can demonstrate the increased activity of the combination (AMP-antibiotic), revealing a potentialized effect. Table 2 summarizes some proof-of-concept works that have reported synergistic AMP-antibiotic combinations in the last 5 years.
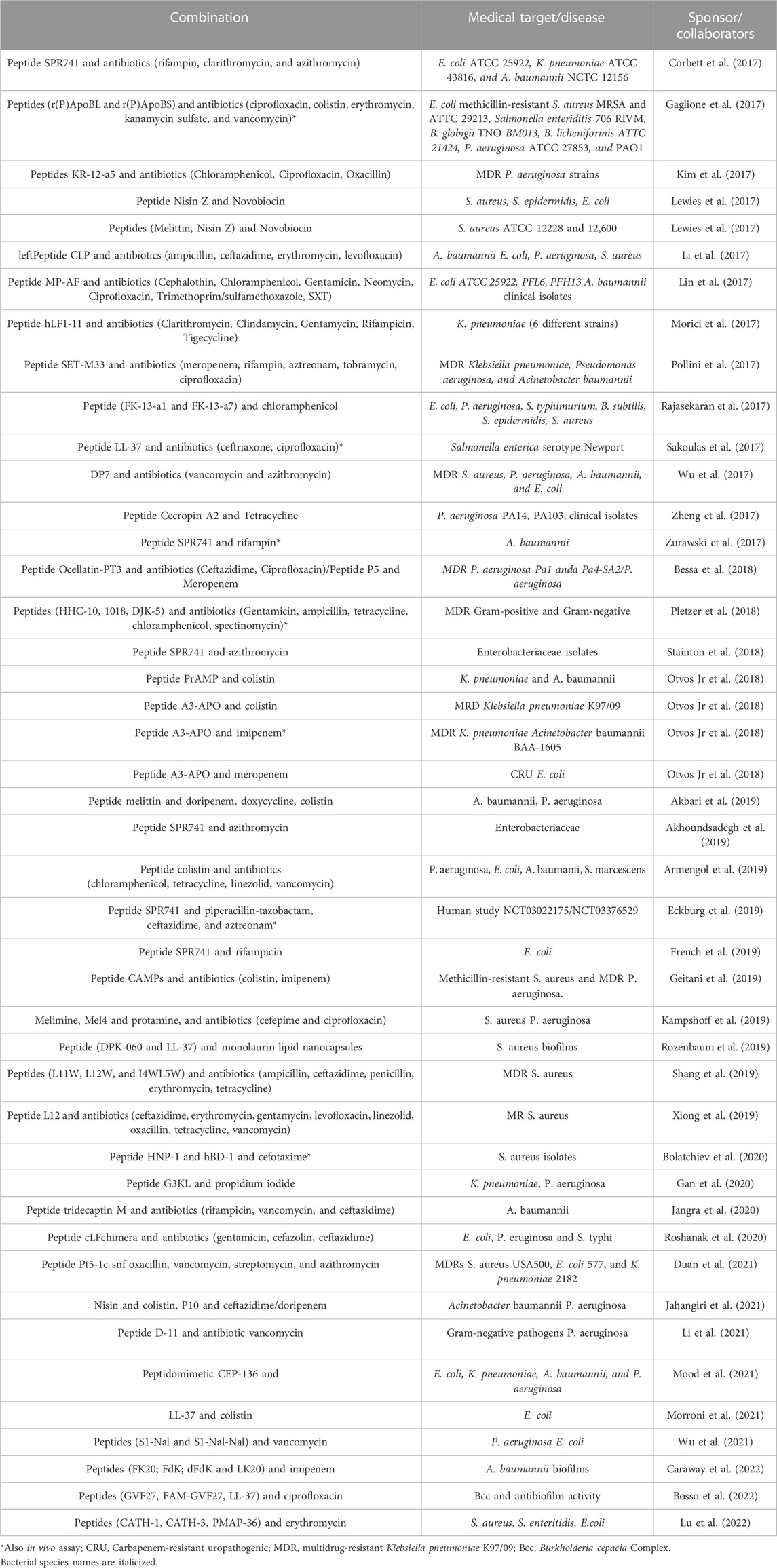
TABLE 2. Some reports of in vitro studies evaluating the synergistic effect of AMP and commercial antibiotic combination, published in the last 5 years.
AMPs can be biological or chemical molecules working with antibiotics. Broadly, two physical features are accepted for AMPs: a cationic charge and a significant measurement of hydrophobic residues. The first property promotes selectivity for negatively charged microbial cytoplasmic membranes, and the second property facilitates interactions with the fatty acyl chains (Shen et al., 2018; Cantor et al., 2019). AMPs interacting with bacterial membranes may cause pores leading to an osmotic imbalance and promoting cell disruption (Hollmann et al., 2018). In this way, it can facilitate the entry of other molecules such as conventional antibiotics and further affect different targets within the cell (Hollmann et al., 2018) such as, cell wall biosynthesis, mechanism of protein synthesis, interference of metabolic activity and synthesis and integrity of nucleic acids (Sarkar et al., 2017; Lin et al., 2018; Stokes et al., 2019; Lade and Kim, 2021).
Combination therapy consist in a strategy to overcome bacterial resistance to conventional antibiotics, as well as, enhance the molecules efficiency (Wang et al., 2022). This is because some AMPs-antibiotics combination can allow bacterial pores to open longer preventing pore repair, and further increasing cellular osmolarity unbalance. In addition, it can complement bacterial killing by imparting mechanisms other including the reduction of bacterial resistance and host cell toxicity (Duong et al., 2021). Bacterial resistance reduction could be considered in AMP-antibiotic combination, once that this synergistic combination involves multiple targets. In general, those targets are in independent bacteria cell pathways. Therefore, to overcome the mechanism of action to both molecules, present in the combination, also would be necessary multiples independents, and simultaneous, set of mutations, in the bacteria for it to become resistant (Duong et al., 2021; Zhu et al., 2022). Thus, the AMP-antibiotic combination can boost antibacterial effects as well as interrupting biofilm formation, and can act with more efficiency than individual drugs (Wu et al., 2017).
Given that AMPs have dominant activities against multidrug-resistant organisms, they can be used to treat the increasing number of antibiotic-resistant infections (Giuliani et al., 2007). It is known that membrane disarray is the principal mechanism of AMPs that can kill or inhibit microorganisms (Atefyekta, 2020), since the notable targets of AMPs are the cell membranes of microbes. The mechanism of action for AMPs has been extensively studied in recent years, and readers are directed to three reviews that detail this mechanism (Moravej et al., 2018; Raheem and Straus, 2019; Benfield and Henriques, 2020).
Other studies demonstrated the action of synergic combinations between AMPs and antibiotics. The combination of colistin sulfate-tobramycin was tested to kill Pseudomonas aeruginosa biofilms. In vivo tests were conducted by groups of 10 female Lewis rats (age, 7 weeks) who were challenged intratracheally with 0.1 ml of a suspension of alginate beads containing 1 x 108 cfu/mL P. aeruginosa PAO1 in the left lung. After one hour, the rats received 0.1 ml of the colistimethate or tobramycin or a simultaneous combination of both drugs or 0.9% saline via intratracheal. After 7 days, the rats who received the antibiotic combination therapy significantly reduced the number of P. aeruginosa cells. In addition, the authors also did a pilot study with 5 patients who presented cystic fibrosis. They inhaled colistin and then tobramycin for 4 weeks, and the results demonstrated a reduction of 2.52 ± 2.5 log10 cfu of P. aeruginosa per milliliter of sputum (p = 0.027) (Herrmann et al., 2010).
The human neutrophil peptide (HNP)-1 was used in combination with isoniazid and rifampicin against Mycobacterium tuberculosis. Results in vitro demonstrated the reduction of >1-log unit of M tuberculosis even when HNP-1 and anti-TB drugs were used at 1/16 MICs. The in vivo analyses demonstrated that the use of HNP-1 in conjunction with anti-TB drugs resulted in a significant decrease in bacterial load in the lungs, liver and spleen of the infected animals, compared with control animals (Kalita et al., 2004).
Another study evaluated the potentialized effect in cryptdin 2 (a Paneth cell antimicrobial peptide) and ampicillin (Amp) combination against Salmonella enterica serovar Typhimurium. For in vivo tests, mice were infected with 107 CFU of S. typhimurium orally. After 7 days the mice were separated into 11 groups of five mice each and treated with cryptdin 2 and ampicillin. Cryptdin 2 was injected subcutaneously (s.c.) at a dose of 5 μg/mouse, while Amp was administered s. c. at 16, 32, and 64 mg/kg of body weight, individually and in combination. Results suggest larger log unit decreases in all target organs of mice treated with the combination than those for the drugs used alone. According to these studies, the synergic effect of AMPs with other antimicrobials are responsible for the efficient control or killing of bacteria. (Duong et al., 2021).
As cited, some works have reported promising results with the antibiotic-AMP or AMP-AMP associations, and these have been proven to be a potent antimicrobial therapy (Grassi et al., 2017; Sierra and Viñas, 2021). Furthermore, the AMP-AMP combination also can display stronger effect. The expectation of potentialized antimicrobial effect in AMPs combination seems likely to master the problem of resistance to conventional antibiotics, being the AMPs potential candidates against multi-drug resistant bacteria (Pirtskhalava et al., 2021). In the last 5 years some studies have explored the synergistic effects of combining two or more AMPs in the control of MDR (Table 3).
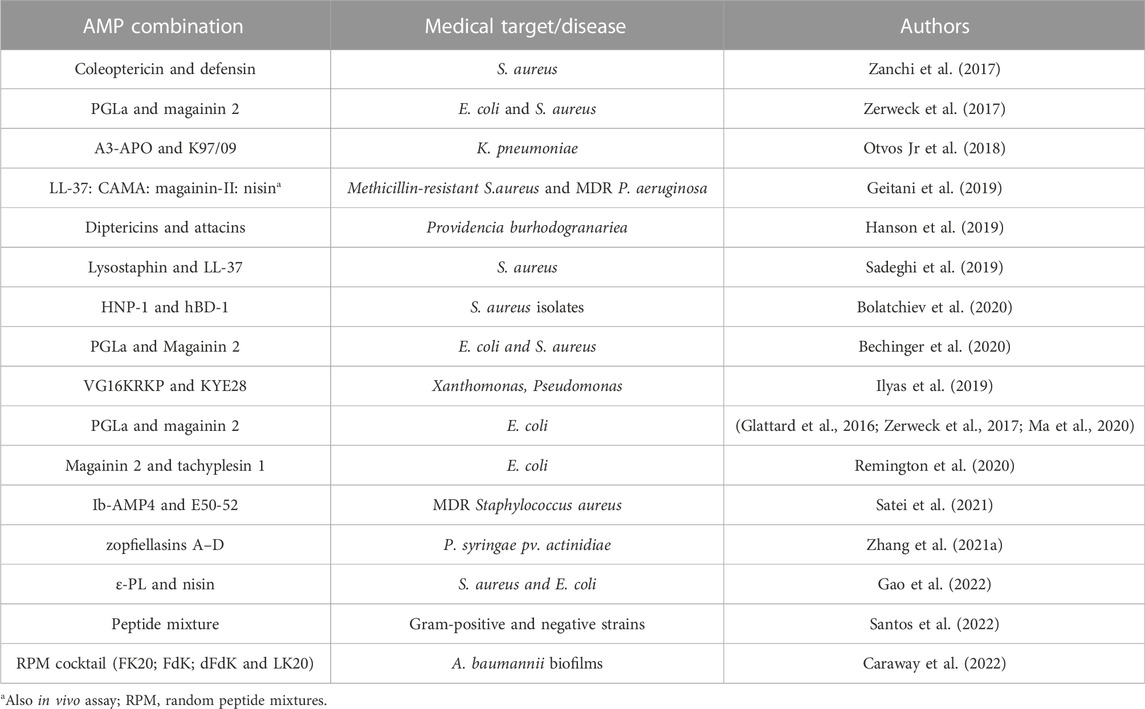
TABLE 3. Some reports evaluating the synergistic effect of AMP-AMP combination, published in the last 5 years.
The combination of two or more AMPs is a strategy that can act on multiple targets, in different pathogens. Also, it looks like the peptide mixture might be safe, as demonstrate a work that evaluated the peptide pool. Peptides mixture can also besides to being considered to improve antimicrobial and antibiofilm activity, in vitro bioassay also was demonstrated that the mixture can be safe to human tissue (Santos et al., 2022). Random peptide mixture (RPM) has been an alternative to overcoming to antimicrobial treatment potentialization. Same RPM can attenuate mouse mortality when submitted to sepsis infection (Caraway et al., 2022). RPM consist in performed synthetic peptide by incorporation of a defined proportion of two or more amino acids, making RPM extreme diversity. So, it tourn improbably the rapid bacterial resistance (Bauer et al., 2020; Bennett et al., 2021). In other study was evaluated the RPM cocktail effect (dFDK, LK20, FK20, and FdK peptides) in vitro, and demonstrated that four RPM (5 µg ml−1 each of RPM) in a cocktail (20 µg ml−1) were more effective against all 3 A. baumannii isolates tested than single RPM (100 µg ml−1), even the single RPM having been the able to capable to eradicate A. baumannii biofilms and inhibited mouse models of infection (Caraway et al., 2022). The results in vitro also However, the single RPM was capable to eradicated A. baumannii biofilms (Bennett et al., 2021; Caraway et al., 2022). In addition to increasing the lethality, this may be more efficient from the point of view of the variety of pathogens that a single combination can control (Geitani et al., 2019; Hanson et al., 2019; Bechinger et al., 2020).
Combination therapy with AMPs can be an alternative to reduce cytotoxicity by synergism. Those strategies not only can surpass the bactericide effect of conventional antibiotics but also can decrease the dose of bactericide necessary, and decrease toxicity rates and side effects of individual or combined antibiotics (Duong et al., 2021; Sierra and Viñas, 2021). AMP-antibiotic and AMP-AMP combinations have the objective of boosting the effects of traditional antibiotics that are still in use. They can also boost the action of antibiotics that have lost their efficacy over the years due to selective pressure from resistant bacteria. The improved effect of the AMP-antibiotic association can mitigate the potential mechanism of bacterial resistance. However, clinical trials involving AMP-antibiotic or AMP-AMP interaction are still scarce. Synergism studies and clinical trials urgently need to get the health agencies’ approval and rapidly obtains products to be marketed. AMP-antibiotic or AMP-AMP combination strategies may bolster the fight against difficult-to-treat infections (Zhang Q.-Y. et al., 2021).
4 Concluding remarks
The increase in the number of deaths worldwide, caused by infections that are difficult to treat, has caused serious concern among world health authorities. The WHO has been warning for some time about the need to develop new drugs. The spread of AMR is not a new event, but even so, there is still a lack of tools for adequate treatment (Luong et al., 2022). Since the golden age of antibiotics, there have been no reports of new molecules, and it is urgently necessary to discover new classes of antimicrobial agents capable of combating infections caused by MDR organisms. Antimicrobial peptides have shown great potential, emerging as a new source of bioactive compounds, capable of combating MDR agents. AMPs have been extensively studied, and over the years some have already been accepted for clinical trials and others have already been used in some therapies. It is known that AMPs promote low toxicity and, due to the high specificity of these molecules, the development of resistance to AMPs is rare, a fact that confirms that AMPs are a powerful weapon in the fight against AMRs. (Luong et al., 2022).
Even so, co-evolution of the pathogen, as observed with conventional antibiotics, can also occur with AMPs, so it is needed to be prepared. The selective pressure that has led many bacteria to resist conventional antibiotics can also be observed using AMP therapies. Although resistance to AMPs is rarer, some isolates have shown such resistance. The combination therapy strategy, with two or more antibiotics, is common in clinical practice, but the risks and benefits have to be weighed carefully (Fatsis-Kavalopoulos et al., 2022). Antibiotic combination has been successful, but there are already clinical isolates that proved to be multidrug resistant. In addition to conventional combination therapy (among antibiotics), positive effects of AMPs-antibiotics combination have been reported. The main advantages of this combination can reduce the annual costs caused by infections and can also prevent emergence of AMR agents (Wang et al., 2022).
This AMP-antibiotic combination has attracted attention due to the benefits it may have. Several studies have been reported in recent years with promising results from AMP-antibiotic combinations. The various proof-of-concept studies with this combination have standardized methods. They have also facilitated the determination of the mechanism of action and synergism mechanisms in AMP combinations. (Pizzolato-Cezar et al., 2019).
However, despite the results demonstrating the positive action of AMP-antibiotic combinations, some challenges are still faced, especially in relation to the permeability/stability of AMPs (Pizzolato-Cezar et al., 2019). Stabilization of AMPs is still the main challenge in clinical therapy. Although there is no direct solution, some work involving nanostructures for the delivery of these molecules has drawn attention in the search to stabilize AMPs and ensure interaction with the target. Nanostructures can be a reinforcement in drug delivery, including for AMPs (Mercer et al., 2020; Yang et al., 2021).
Several AMPs have been discovered with pharmaceutical potential, showing promise for the development of alternative antimicrobial drugs. They have provided support for the management of AMR, including the possibility of AMP-AMP combinations. Studies involving the combination reveal that AMPs act synergistically with other AMPs, with conserved characteristics, due to the high specificity of these molecules. The higher the specificity, the lower the doses needed to obtain results. AMPs can be used in combination with antibiotics and other AMPs and exhibit strong antimicrobial effects even at low concentrations. The dose-effect relation also reflects on the adverse effects caused by the therapy. The fewer doses needed, the more limited the unwanted side effects. Besides the low doses required, another advantage of the increased effect of the AMP-antibiotic or AMP-AMP combination is the reduction of production costs and hospital costs with patient interaction. New drugs will be developed in the future, based on proofs of concept that demonstrate the great potential of AMPs and their combinations (Duong et al., 2021).
Author contributions
SC performed draft preparation and wrote the manuscript. RGR, LLF, RMCG, DSC, CNB, and FLO wrote or contributed to the writing of the manuscript. FLO revised the manuscript.
Acknowledgments
Fundação de Apoio ao Desenvolvimento do Ensino, Ciência e Tecnologia do Estado de Mato Grosso do Sul (FUNDECT) postdoctoral scholarship, the Fundação de Apoio à Pesquisa do Distrito Federal (FAPDF), the Coordenação de Aperfeiçoamento de Pessoal de Nível Superior (CAPES) and the Conselho Nacional de Desenvolvimento Científico e Tecnológico (CNPq).
Conflict of interest
The authors declare that the research was conducted in the absence of any commercial or financial relationships that could be construed as a potential conflict of interest.
The handling editor declared a past co-authorship with the author CS.
Publisher’s note
All claims expressed in this article are solely those of the authors and do not necessarily represent those of their affiliated organizations, or those of the publisher, the editors and the reviewers. Any product that may be evaluated in this article, or claim that may be made by its manufacturer, is not guaranteed or endorsed by the publisher.
References
Akbari, R., Hakemi-Vala, M., Pashaie, F., Bevalian, P., Hashemi, A., and Pooshang Bagheri, K. (2019). Highly synergistic effects of melittin with conventional antibiotics against multidrug-resistant isolates of Acinetobacter baumannii and Pseudomonas aeruginosa. Microb. Drug Resist. 25, 193–202. doi:10.1089/mdr.2018.0016
Akhoundsadegh, N., Belanger, C. R., and Hancock, R. E. W. (2019). Outer membrane interaction kinetics of new polymyxin B analogs in Gram-negative bacilli. Antimicrob. Agents Chemother. 63, e00935-19–e00919. doi:10.1128/aac.00935-19
Armengol, E., Domenech, O., Fusté, E., Pérez-Guillén, I., Borrell, J. H., Sierra, J. M., et al. (2019). <p>Efficacy of combinations of colistin with other antimicrobials involves membrane fluidity and efflux machinery</p>. Infect. Drug Resist 12, 2031–2038. doi:10.2147/idr.s207844
Aslam, B., Wang, W., Arshad, M. I., Khurshid, M., Muzammil, S., Rasool, M. H., et al. (2018). Antibiotic resistance: A rundown of a global crisis. Infect. drug Resist. 11, 1645–1658. doi:10.2147/idr.s173867
Asokan, G. V., and Vanitha, A. (2018). WHO global priority pathogens list on antibiotic resistance: An urgent need for action to integrate one health data. Perspect. Public Health 138, 87–88. doi:10.1177/1757913917743881
Atefyekta, S. (2020). Antibacterial surfaces for biomedical applications. Gothenburg, Sweden: Chalmers University of Technology.
Bauer, S., Mekonnen, D. W., Geist, B., Lange, B., Ghirardo, A., Zhang, W., et al. (2020). The isoleucic acid triad: Distinct impacts on plant defense, root growth, and formation of reactive oxygen species. J. Exp. Bot. 71, 4258–4270. doi:10.1093/jxb/eraa160
Bechinger, B., Juhl, D. W., Glattard, E., and Aisenbrey, C. (2020). Revealing the mechanisms of synergistic action of two magainin antimicrobial peptides. Front. Med. Technol. 2, 615494. doi:10.3389/fmedt.2020.615494
Benfield, A. H., and Henriques, S. T. (2020). Mode-of-action of antimicrobial peptides: Membrane disruption vs. intracellular mechanisms. Front. Med. Technol. 2, 610997. doi:10.3389/fmedt.2020.610997
Bennett, R. C., Oh, M. W., Kuo, S. H., Belo, Y., Maron, B., Malach, E., et al. (2021). Random peptide mixtures as safe and effective antimicrobials against Pseudomonas aeruginosa and MRSA in mouse models of bacteremia and pneumonia. ACS Infect. Dis. 7, 672–680. doi:10.1021/acsinfecdis.0c00871
Bessa, L. J., Eaton, P., Dematei, A., Plácido, A., Vale, N., Gomes, P., et al. (2018). Synergistic and antibiofilm properties of ocellatin peptides against multidrug-resistant Pseudomonas aeruginosa. Future Microbiol. 13, 151–163. doi:10.2217/fmb-2017-0175
Bolatchiev, A., Baturin, V., Bazikov, I., Maltsev, A., and Kunitsina, E. (2020). Effect of antimicrobial peptides HNP-1 and hBD-1 on Staphylococcus aureus strains in vitro and in vivo. Fundam. Clin. Pharmacol. 34, 102–108. doi:10.1111/fcp.12499
Bosso, A., Gaglione, R., Di Girolamo, R., Veldhuizen, E. J. A., García-Vello, P., Fusco, S., et al. (2022). Human cryptic host defence peptide GVF27 exhibits anti-infective properties against biofilm forming members of the Burkholderia cepacia complex. Pharmaceuticals 15, 260. doi:10.3390/ph15020260
Boto, A., Pérez De La Lastra, J. M., and González, C. C. (2018). The road from host-defense peptides to a new generation of antimicrobial drugs. Molecules 23, 311. doi:10.3390/molecules23020311
Brennan-Krohn, T., Pironti, A., and Kirby, J. E. (2018). Synergistic activity of colistin-containing combinations against colistin-resistant Enterobacteriaceae. Antimicrob. Agents Chemother. 62, e00873-18–e00818. doi:10.1128/aac.00873-18
Cantor, S., Vargas, L., Rojas A, O. E., Yarce, C. J., Salamanca, C. H., and Oñate-Garzón, J. (2019). Evaluation of the antimicrobial activity of cationic peptides loaded in surface-modified nanoliposomes against foodborne bacteria. Int. J. Mol. Sci. 20, 680. doi:10.3390/ijms20030680
Caraway, H. E., Lau, J. Z., Maron, B., Oh, M. W., Belo, Y., Brill, A., et al. (2022). Antimicrobial random peptide mixtures eradicate acinetobacter baumannii biofilms and inhibit mouse models of infection. Antibiotics 11, 413. doi:10.3390/antibiotics11030413
Corbett, D., Wise, A., Langley, T., Skinner, K., Trimby, E., Birchall, S., et al. (2017). Potentiation of antibiotic activity by a novel cationic peptide: Potency and spectrum of activity of SPR741. Antimicrob. Agents Chemother. 61, e00200-17–e00217. doi:10.1128/aac.00200-17
Dadgostar, P. (2019). <p>Antimicrobial Resistance: Implications and Costs</p>. Infect. drug Resist. 12, 3903–3910. doi:10.2147/idr.s234610
Dos Santos, C., Dos Santos, L. S., and Franco, O. L. (2021). Fosfomycin and nitrofurantoin: Classic antibiotics and perspectives. J. Antibiot. 74, 547–558. doi:10.1038/s41429-021-00444-z
Duan, H., Zhang, X., Li, Z., Yuan, J., Shen, F., and Zhang, S. (2021). Synergistic effect and antibiofilm activity of an antimicrobial peptide with traditional antibiotics against multi-drug resistant bacteria. Microb. Pathog. 158, 105056. doi:10.1016/j.micpath.2021.105056
Duong, L., Gross, S. P., and Siryaporn, A. (2021). Developing antimicrobial synergy with AMPs. Front. Med. Technol. 3, 640981. doi:10.3389/fmedt.2021.640981
Eckburg, P. B., Lister, T., Walpole, S., Keutzer, T., Utley, L., Tomayko, J., et al. (2019). Safety, tolerability, pharmacokinetics, and drug interaction potential of SPR741, an intravenous potentiator, after single and multiple ascending doses and when combined with β-lactam antibiotics in healthy subjects. Antimicrob. Agents Chemother. 63, e00892-19–e00819. doi:10.1128/aac.00892-19
Fatsis-Kavalopoulos, N., Roelofs, L., and Andersson, D. I. (2022). Potential risks of treating bacterial infections with a combination of β-lactam and aminoglycoside antibiotics: A systematic quantification of antibiotic interactions in E. coli blood stream infection isolates. EBioMedicine 78, 103979. doi:10.1016/j.ebiom.2022.103979
Franco, O. L. (2011). Peptide promiscuity: An evolutionary concept for plant defense. FEBS Lett. 585, 995–1000. doi:10.1016/j.febslet.2011.03.008
French, S., Farha, M., Ellis, M. J., Sameer, Z., CôTé, J.-P., Cotroneo, N., et al. (2019). Potentiation of antibiotics against Gram-negative bacteria by polymyxin B analogue SPR741 from unique perturbation of the outer membrane. ACS Infect. Dis. 6, 1405–1412. doi:10.1021/acsinfecdis.9b00159
Gaglione, R., Dell'olmo, E., Bosso, A., Chino, M., Pane, K., Ascione, F., et al. (2017). Novel human bioactive peptides identified in Apolipoprotein B: Evaluation of their therapeutic potential. Biochem. Pharmacol. 130, 34–50. doi:10.1016/j.bcp.2017.01.009
Gan, B.-H., Cai, X., Javor, S., Köhler, T., and Reymond, J.-L. (2020). Synergistic effect of propidium iodide and small molecule antibiotics with the antimicrobial peptide dendrimer G3KL against gram-negative bacteria. Molecules 25, 5643. doi:10.3390/molecules25235643
Gao, S., Zhai, X., Cheng, Y., Zhang, R., Wang, W., and Hou, H. (2022). Starch/PBAT blown antimicrobial films based on the synergistic effects of two commercial antimicrobial peptides. Int. J. Biol. Macromol. 204, 457–465. doi:10.1016/j.ijbiomac.2022.01.183
García-Fuente, A., Vázquez, F., Viéitez, J. M., Garcia Alonso, F. J., Martín, J. I., and Ferrer, J. (2018). Cisne: An accurate description of dose-effect and synergism in combination therapies. Sci. Rep. 8, 4964–4969. doi:10.1038/s41598-018-23321-6
Geitani, R., Ayoub Moubareck, C., Touqui, L., and Karam Sarkis, D. (2019). Cationic antimicrobial peptides: Alternatives and/or adjuvants to antibiotics active against methicillin-resistant Staphylococcus aureus and multidrug-resistant Pseudomonas aeruginosa. BMC Microbiol. 19, 54–12. doi:10.1186/s12866-019-1416-8
Ghosh, D., Veeraraghavan, B., Elangovan, R., and Vivekanandan, P. (2020). Antibiotic resistance and epigenetics: More to it than meets the eye. Antimicrob. Agents Chemother. 64, e02225-19–e02219. doi:10.1128/aac.02225-19
Giuliani, A., Pirri, G., and Nicoletto, S. (2007). Antimicrobial peptides: An overview of a promising class of therapeutics. Open Life Sci. 2, 1–33. doi:10.2478/s11535-007-0010-5
Glattard, E., Salnikov, E. S., Aisenbrey, C., and Bechinger, B. (2016). Investigations of the synergistic enhancement of antimicrobial activity in mixtures of magainin 2 and PGLa. Biophys. Chem. 210, 35–44. doi:10.1016/j.bpc.2015.06.002
Golonka, I., Greber, K. E., Oleksy-Wawrzyniak, M., Paleczny, J., Dryś, A., Junka, A., et al. (2021). Antimicrobial and antioxidative activity of newly synthesized peptides absorbed into bacterial cellulose carrier against acne vulgaris. Int. J. Mol. Sci. 22, 7466. doi:10.3390/ijms22147466
Grassi, L., Maisetta, G., Esin, S., and Batoni, G. (2017). Combination strategies to enhance the efficacy of antimicrobial peptides against bacterial biofilms. Front. Microbiol. 8, 2409. doi:10.3389/fmicb.2017.02409
Hanson, M. A., Dostálová, A., Ceroni, C., Poidevin, M., Kondo, S., and Lemaitre, B. (2019). Synergy and remarkable specificity of antimicrobial peptides in vivo using a systematic knockout approach. Elife 8, e44341. doi:10.7554/elife.44341
Herrmann, G., Yang, L., Wu, H., Song, Z., Wang, H., Høiby, N., et al. (2010). Colistin-tobramycin combinations are superior to monotherapy concerning the killing of biofilm Pseudomonas aeruginosa. J. Infect. Dis. 202, 1585–1592. doi:10.1086/656788
Hollmann, A., Martinez, M., Maturana, P., Semorile, L. C., and Maffia, P. C. (2018). Antimicrobial peptides: Interaction with model and biological membranes and synergism with chemical antibiotics. Front. Chem. 6, 204. doi:10.3389/fchem.2018.00204
Hutchings, M. I., Truman, A. W., and Wilkinson, B. (2019). Antibiotics: Past, present and future. Curr. Opin. Microbiol. 51, 72–80. doi:10.1016/j.mib.2019.10.008
Ilyas, H., Kim, J., Lee, D., Malmsten, M., and Bhunia, A. (2019). Structural insights into the combinatorial effects of antimicrobial peptides reveal a role of aromatic–aromatic interactions in antibacterial synergism. J. Biol. Chem. 294, 14615–14633. doi:10.1074/jbc.ra119.009955
Iskandar, K., Murugaiyan, J., Halat, H. D., El Hage, S., Chibabhai, V., Adukkadukkam, S., et al. (2022). Antibiotic discovery and resistance: The chase and the race. Antibiotics 11 (2), 182–220. doi:10.3390/antibiotics11020182
Jahangiri, A., Neshani, A., Mirhosseini, S. A., Ghazvini, K., Zare, H., and Sedighian, H. (2021). Synergistic effect of two antimicrobial peptides, Nisin and P10 with conventional antibiotics against extensively drug-resistant Acinetobacter baumannii and colistin-resistant Pseudomonas aeruginosa isolates. Microb. Pathog. 150, 104700. doi:10.1016/j.micpath.2020.104700
Jangra, M., Raka, V., and Nandanwar, H. (2020). Vitro evaluation of antimicrobial peptide tridecaptin M in combination with other antibiotics against multidrug resistant acinetobacter baumannii. Molecules 25, 3255. doi:10.3390/molecules25143255
Kalita, A., Verma, I., and Khuller, G. K. (2004). Role of human neutrophil peptide-1 as a possible adjunct to antituberculosis chemotherapy. J. Infect. Dis. 190, 1476–1480. doi:10.1086/424463
Kampshoff, F., Willcox, M. D. P., and Dutta, D. (2019). A pilot study of the synergy between two antimicrobial peptides and two common antibiotics. Antibiotics 8, 60. doi:10.3390/antibiotics8020060
Kim, E. Y., Rajasekaran, G., and Shin, S. Y. (2017). LL-37-derived short antimicrobial peptide KR-12-a5 and its d-amino acid substituted analogs with cell selectivity, anti-biofilm activity, synergistic effect with conventional antibiotics, and anti-inflammatory activity. Eur. J. Med. Chem. 136, 428–441. doi:10.1016/j.ejmech.2017.05.028
Lade, H., and Kim, J.-S. (2021). Bacterial targets of antibiotics in methicillin-resistant Staphylococcus aureus. Antibiotics 10, 398. doi:10.3390/antibiotics10040398
Larsson, D. G. J., and Flach, C. F. (2022). Antibiotic resistance in the environment. Nat. Rev. Microbiol. 20, 257–269. doi:10.1038/s41579-021-00649-x
Lewies, A., Wentzel, J. F., Jordaan, A., Bezuidenhout, C., and Du Plessis, L. H. (2017). Interactions of the antimicrobial peptide nisin Z with conventional antibiotics and the use of nanostructured lipid carriers to enhance antimicrobial activity. Int. J. Pharm. 526, 244–253. doi:10.1016/j.ijpharm.2017.04.071
Li, D., Yang, Y., Tian, Z., Lv, J., Sun, F., Wang, Q., et al. (2017). Synergistic antibiotic effect of looped antimicrobial peptide CLP-19 with bactericidal and bacteriostatic agents. Oncotarget 8, 55958–55966. doi:10.18632/oncotarget.18124
Li, Q., Cebrián, R., Montalbán-López, M., Ren, H., Wu, W., and Kuipers, O. P. (2021). Outer-membrane-acting peptides and lipid II-targeting antibiotics cooperatively kill Gram-negative pathogens. Commun. Biol. 4, 31–11. doi:10.1038/s42003-020-01511-1
Lin, C.-H., Lee, M.-C., Tzen, J. T. C., Lee, H.-M., Chang, S.-M., Tu, W.-C., et al. (2017). Efficacy of Mastoparan-AF alone and in combination with clinically used antibiotics on nosocomial multidrug-resistant Acinetobacter baumannii. Saudi J. Biol. Sci. 24, 1023–1029. doi:10.1016/j.sjbs.2016.12.013
Lin, J., Zhou, D., Steitz, T. A., Polikanov, Y. S., and Gagnon, M. G. (2018). Ribosome-targeting antibiotics: Modes of action, mechanisms of resistance, and implications for drug design. Annu. Rev. Biochem. 87, 451–478. doi:10.1146/annurev-biochem-062917-011942
Liu, Y., Tong, Z., Shi, J., Li, R., Upton, M., and Wang, Z. (2021). Drug repurposing for next-generation combination therapies against multidrug-resistant bacteria. Theranostics 11, 4910–4928. doi:10.7150/thno.56205
Lloyd, R. L., Wijnhoven, P. W. G., Ramos-Montoya, A., Wilson, Z., Illuzzi, G., Falenta, K., et al. (2020). Combined PARP and ATR inhibition potentiates genome instability and cell death in ATM-deficient cancer cells. Oncogene 39, 4869–4883. doi:10.1038/s41388-020-1328-y
Lu, Y., Tian, H., Chen, R., Liu, Q., Jia, K., Hu, D.-L., et al. (2022). Synergistic antimicrobial effect of antimicrobial peptides CATH-1, CATH-3, and PMAP-36 with erythromycin against bacterial pathogens. Front. Microbiol. 13, 953720. doi:10.3389/fmicb.2022.953720
Luong, H. X., Ngan, H. D., Thi Phuong, H. B., Quoc, T. N., and Tung, T. T. (2022). Multiple roles of ribosomal antimicrobial peptides in tackling global antimicrobial resistance. R. Soc. Open Sci. 9, 211583. doi:10.1098/rsos.211583
Ma, W., Sun, S., Li, W., Zhang, Z., Lin, Z., Xia, Y., et al. (2020). Individual roles of peptides PGLa and magainin 2 in synergistic membrane poration. Langmuir 36, 7190–7199. doi:10.1021/acs.langmuir.0c00194
Majumder, M. a. A., Rahman, S., Cohall, D., Bharatha, A., Singh, K., Haque, M., et al. (2020). Antimicrobial stewardship: Fighting antimicrobial resistance and protecting global public health. Infect. drug Resist. 13, 4713–4738. doi:10.2147/idr.s290835
Maron, B., Rolff, J., Friedman, J., and Hayouka, Z. (2022). Antimicrobial peptide combination can hinder resistance evolution. Microbiol. Spectr. 10, 1–10. doi:10.1128/spectrum.00973-22
Mercer, D. K., Torres, M. D. T., Duay, S. S., Lovie, E., Simpson, L., Von Köckritz-Blickwede, M., et al. (2020). Antimicrobial susceptibility testing of antimicrobial peptides to better predict efficacy. Front. Cell. Infect. Microbiol. 10, 326. doi:10.3389/fcimb.2020.00326
Mishra, B., Reiling, S., Zarena, D., and Wang, G. (2017). Host defense antimicrobial peptides as antibiotics: Design and application strategies. Curr. Opin. Chem. Biol. 38, 87–96. doi:10.1016/j.cbpa.2017.03.014
Mood, E. H., Goltermann, L., Brolin, C., Cavaco, L. M., Nejad, A. J., Yavari, N., et al. (2021). Antibiotic potentiation in multidrug-resistant Gram-negative pathogenic bacteria by a synthetic Peptidomimetic. ACS Infect. Dis. 7, 2152–2163. doi:10.1021/acsinfecdis.1c00147
Moravej, H., Moravej, Z., Yazdanparast, M., Heiat, M., Mirhosseini, A., Moosazadeh Moghaddam, M., et al. (2018). Antimicrobial peptides: Features, action, and their resistance mechanisms in bacteria. Microb. Drug Resist. 24, 747–767. doi:10.1089/mdr.2017.0392
Moretta, A., Scieuzo, C., Petrone, A. M., Salvia, R., Manniello, M. D., Franco, A., et al. (2021). Antimicrobial peptides: A new hope in biomedical and pharmaceutical fields. Front. Cell. Infect. Microbiol. 11, 668632. doi:10.3389/fcimb.2021.668632
Morici, P., Florio, W., Rizzato, C., Ghelardi, E., Tavanti, A., Rossolini, G. M., et al. (2017). Synergistic activity of synthetic N-terminal peptide of human lactoferrin in combination with various antibiotics against carbapenem-resistant Klebsiella pneumoniae strains. Eur. J. Clin. Microbiol. Infect. Dis. 36, 1739–1748. doi:10.1007/s10096-017-2987-7
Morroni, G., Sante, L. D., Simonetti, O., Brescini, L., Kamysz, W., Kamysz, E., et al. (2021). Synergistic effect of antimicrobial peptide LL-37 and colistin combination against multidrug-resistant Escherichia coli isolates. Future Microbiol. 16, 221–227. doi:10.2217/fmb-2020-0204
Murray, C. J. L., Ikuta, K. S., Sharara, F., Swetschinski, L., Aguilar, G. R., Gray, A., et al. (2022). Global burden of bacterial antimicrobial resistance in 2019: A systematic analysis. Lancet 399, 629–655. doi:10.1016/S0140-6736(21)02724-0
Niu, J., Straubinger, R. M., and Mager, D. E. (2019). Pharmacodynamic drug–drug interactions. Clin. Pharmacol. Ther. 105, 1395–1406. doi:10.1002/cpt.1434
Otvos, L., Ostorhazi, E., Szabo, D., Zumbrun, S. D., Miller, L. L., Halasohoris, S. A., et al. (2018). Synergy between proline-rich antimicrobial peptides and small molecule antibiotics against selected gram-negative pathogens in vitro and in vivo. Front. Chem. 6, 309. doi:10.3389/fchem.2018.00309
Pirtskhalava, M., Vishnepolsky, B., Grigolava, M., and Managadze, G. (2021). Physicochemical features and peculiarities of interaction of AMP with the membrane. Pharmaceuticals 14, 471. doi:10.3390/ph14050471
Pizzolato-Cezar, L. R., Okuda-Shinagawa, N. M., and Machini, M. T. (2019). Combinatory therapy antimicrobial peptide-antibiotic to minimize the ongoing rise of resistance. Front. Microbiol. 10, 1703. doi:10.3389/fmicb.2019.01703
Pletzer, D., Mansour, S. C., and Hancock, R. E. W. (2018). Synergy between conventional antibiotics and anti-biofilm peptides in a murine, sub-cutaneous abscess model caused by recalcitrant ESKAPE pathogens. PLoS Pathog. 14, e1007084. doi:10.1371/journal.ppat.1007084
Pollini, S., Brunetti, J., Sennati, S., Rossolini, G. M., Bracci, L., Pini, A., et al. (2017). Synergistic activity profile of an antimicrobial peptide against multidrug-resistant and extensively drug-resistant strains of Gram-negative bacterial pathogens. J. Pept. Sci. 23, 329–333. doi:10.1002/psc.2978
Power, J. J., Pinheiro, F., Pompei, S., Kovacova, V., Yüksel, M., Rathmann, I., et al. (2021). Adaptive evolution of hybrid bacteria by horizontal gene transfer. Proc. Natl. Acad. Sci. U. S. A. 118, e2007873118. doi:10.1073/pnas.2007873118
Rady, O. M. S. M., El-Attar, L., and Amine, A. (2022). In vitro synergistic activity of colistin and teicoplanin combination against multidrug-resistant Acinetobacter spp. J. Antibiot. 75, 181–184. doi:10.1038/s41429-022-00509-7
Raheem, N., and Straus, S. K. (2019). Mechanisms of action for antimicrobial peptides with antibacterial and antibiofilm functions. Front. Microbiol. 10, 2866. doi:10.3389/fmicb.2019.02866
Rajasekaran, G., Kim, E. Y., and Shin, S. Y. (2017). LL-37-derived membrane-active FK-13 analogs possessing cell selectivity, anti-biofilm activity and synergy with chloramphenicol and anti-inflammatory activity. Biochimica Biophysica Acta - Biomembr. 1859, 722–733. doi:10.1016/j.bbamem.2017.01.037
Rather, I. A., Kim, B.-C., Bajpai, V. K., and Park, Y.-H. (2017). Self-medication and antibiotic resistance: Crisis, current challenges, and prevention. Saudi J. Biol. Sci. 24, 808–812. doi:10.1016/j.sjbs.2017.01.004
Remington, J. M., Liao, C., Sharafi, M., SteMarie, E. J., Ferrell, J. B., Hondal, R. J., et al. (2020). Aggregation state of synergistic antimicrobial peptides. J. Phys. Chem. Lett. 11, 9501–9506. doi:10.1021/acs.jpclett.0c02094
Roemhild, R., Bollenbach, T., and Andersson, D. I. (2022). The physiology and genetics of bacterial responses to antibiotic combinations. Nat. Rev. Microbiol. 20, 478–490. doi:10.1038/s41579-022-00700-5
Roshanak, S., Pirkhezranian, Z., Shahidi, F., and Hadi Sekhavati, M. (2020). Antibacterial activity of cLFchimera and its synergistic potential with antibiotics against some foodborne pathogens bacteria. Res. Sq. 1, 1–16. doi:10.21203/rs.3.rs-31015/v1
Rozenbaum, R. T., Su, L., Umerska, A., Eveillard, M., Håkansson, J., Mahlapuu, M., et al. (2019). Antimicrobial synergy of monolaurin lipid nanocapsules with adsorbed antimicrobial peptides against Staphylococcus aureus biofilms in vitro is absent in vivo. J. Control. release 293, 73–83. doi:10.1016/j.jconrel.2018.11.018
Sadeghi, S., Bakhshandeh, H., Cohan, R. A., Peirovi, A., Ehsani, P., and Norouzian, D. (2019). <p>Synergistic anti-staphylococcal activity of niosomal recombinant lysostaphin-LL-37</p>. Int. J. nanomedicine 14, 9777–9792. doi:10.2147/ijn.s230269
Sakoulas, G., Kumaraswamy, M., Kousha, A., and Nizet, V. (2017). Interaction of antibiotics with innate host defense factors against Salmonella enterica serotype newport. MSphere 2, e00410-17–e00417. doi:10.1128/msphere.00410-17
Santos, M. F. D. S., Freitas, C. S., Verissimo Da Costa, G. C., Pereira, P. R., and Paschoalin, V. M. F. (2022). Identification of antibacterial peptide candidates encrypted in stress-related and metabolic Saccharomyces cerevisiae proteins. Pharmaceuticals 15, 163. doi:10.3390/ph15020163
Sarkar, P., Yarlagadda, V., Ghosh, C., and Haldar, J. (2017). A review on cell wall synthesis inhibitors with an emphasis on glycopeptide antibiotics. Med. Chem. Commun. 8, 516–533. doi:10.1039/c6md00585c
Satei, P., Ghaznavi-Rad, E., Fahimirad, S., and Abtahi, H. (2021). Recombinant production of Trx-Ib-AMP4 and Trx-E50-52 antimicrobial peptides and antimicrobial synergistic assessment on the treatment of methicillin-resistant Staphylococcus aureus under in vitro and in vivo situations. Protein Expr. Purif. 188, 105949. doi:10.1016/j.pep.2021.105949
Sechet, E., Telford, E., Bonamy, C., Sansonetti, P. J., and Sperandio, B. (2018). Natural molecules induce and synergize to boost expression of the human antimicrobial peptide β-defensin-3. Proc. Natl. Acad. Sci. U. S. A. 115, E9869–E9878. doi:10.1073/pnas.1805298115
Shang, D., Liu, Y., Jiang, F., Ji, F., Wang, H., and Han, X. (2019). Synergistic antibacterial activity of designed Trp-containing antibacterial peptides in combination with antibiotics against multidrug-resistant Staphylococcus epidermidis. Front. Microbiol. 10, 2719. doi:10.3389/fmicb.2019.02719
Shen, W., He, P., Xiao, C., and Chen, X. (2018). From antimicrobial peptides to antimicrobial poly (α-amino acid) s. Adv. Healthc. Mat. 7, 1800354. doi:10.1002/adhm.201800354
Sierra, J. M., and Viñas, M. (2021). Future prospects for Antimicrobial peptide development: Peptidomimetics and antimicrobial combinations. Expert Opin. Drug Discov. 16, 601–604. doi:10.1080/17460441.2021.1892072
Stainton, S. M., Abdelraouf, K., Utley, L., Pucci, M. J., Lister, T., and Nicolau, D. P. (2018). Assessment of the in vivo activity of SPR741 in combination with azithromycin against multidrug-resistant Enterobacteriaceae isolates in the neutropenic murine thigh infection model. Antimicrob. Agents Chemother. 62, e00239-18–e00218. doi:10.1128/aac.00239-18
Stokes, J. M., Lopatkin, A. J., Lobritz, M. A., and Collins, J. J. (2019). Bacterial metabolism and antibiotic efficacy. Cell metab. 30, 251–259. doi:10.1016/j.cmet.2019.06.009
Ting, D. S. J., Beuerman, R. W., Dua, H. S., Lakshminarayanan, R., and Mohammed, I. (2020). Strategies in translating the therapeutic potentials of host defense peptides. Front. Immunol. 11, 983. doi:10.3389/fimmu.2020.00983
Uddin, T. M., Chakraborty, A. J., Khusro, A., Zidan, B. M. R. M., Mitra, S., Emran, T. B., et al. (2021). Antibiotic resistance in microbes: History, mechanisms, therapeutic strategies and future prospects. J. Infect. Public Health 14, 1750–1766. doi:10.1016/j.jiph.2021.10.020
Vargas-Casanova, Y., Rodríguez-Mayor, A. V., Cardenas, K. J., Leal-Castro, A. L., Muñoz-Molina, L. C., Fierro-Medina, R., et al. (2019). Synergistic bactericide and antibiotic effects of dimeric, tetrameric, or palindromic peptides containing the RWQWR motif against Gram-positive and Gram-negative strains. RSC Adv. 9, 7239–7245. doi:10.1039/c9ra00708c
Wang, N., Luo, J., Deng, F., Huang, Y., and Zhou, H. (2022). Antibiotic combination therapy: A strategy to overcome bacterial resistance to aminoglycoside antibiotics. Front. Pharmacol. 13, 839808. doi:10.3389/fphar.2022.839808
World Health Organization (2020). Global antimicrobial resistance surveillance system (GLASS) report: Early implementation 2020.
World Health Organization (2019). International organizations unite on critical recommendations to combat drug-resistant infections and prevent staggering number of deaths each.
World Health Organization (2017). WHO publishes list of bacteria for which new antibiotics are urgently needed [Online]. Geneva.
Wouters, O. J., Mckee, M., and Luyten, J. (2020). Estimated research and development investment needed to bring a new medicine to market, 2009-2018. Jama 323, 844–853. doi:10.1001/jama.2020.1166
Wu, C.-L., Peng, K.-L., Yip, B.-S., Chih, Y.-H., and Cheng, J.-W. (2021). Boosting synergistic effects of short antimicrobial peptides with conventional antibiotics against resistant bacteria. Front. Microbiol. 12, 747760. doi:10.3389/fmicb.2021.747760
Wu, X., Li, Z., Li, X., Tian, Y., Fan, Y., Yu, C., et al. (2017). Synergistic effects of antimicrobial peptide DP7 combined with antibiotics against multidrug-resistant bacteria. Drug Des. Dev. Ther. 11, 939–946. doi:10.2147/dddt.s107195
Xiong, F., Dai, X., Li, Y. X., Wei, R., An, L., Wang, Y., et al. (2019). Effects of the antimicrobial peptide L12 against multidrug-resistant Staphylococcus aureus. Mol. Med. Rep. 19, 3337–3344. doi:10.3892/mmr.2019.9988
Yang, Z., He, S., Wu, H., Yin, T., Wang, L., and Shan, A. (2021). Nanostructured antimicrobial peptides: Crucial steps of overcoming the bottleneck for clinics. Front. Microbiol. 12, 710199. doi:10.3389/fmicb.2021.710199
Yin, J., Li, H., and Sun, Q. (2021). Analysis of antibiotic consumption by AWaRe classification in shandong province, China, 2012-2019: A panel data analysis. Front. Pharmacol. 12, 790817. doi:10.3389/fphar.2021.790817
Zanchi, C., Johnston, P. R., and Rolff, J. (2017). Evolution of defence cocktails: Antimicrobial peptide combinations reduce mortality and persistent infection. Mol. Ecol. 26, 5334–5343. doi:10.1111/mec.14267
Zerweck, J., Strandberg, E., Kukharenko, O., Reichert, J., Bürck, J., Wadhwani, P., et al. (2017). Molecular mechanism of synergy between the antimicrobial peptides PGLa and magainin 2. Sci. Rep. 7, 1–21. doi:10.1038/s41598-017-12599-7
Zhang, J.-Y., He, J., Li, Z.-H., Feng, T., and Liu, J.-K. (2021a). Zopfiellasins A–D, two pairs of epimeric cytochalasins from kiwi-associated fungus zopfiella sp. and their antibacterial assessment. Molecules 26, 5611. doi:10.3390/molecules26185611
Zhang, Q.-Y., Yan, Z.-B., Meng, Y.-M., Hong, X.-Y., Shao, G., Ma, J.-J., et al. (2021b). Antimicrobial peptides: Mechanism of action, activity and clinical potential. Mil. Med. Res. 8, 48–25. doi:10.1186/s40779-021-00343-2
Zheng, Z., Tharmalingam, N., Liu, Q., Jayamani, E., Kim, W., Fuchs, B. B., et al. (2017). Synergistic efficacy of Aedes aegypti antimicrobial peptide cecropin A2 and tetracycline against Pseudomonas aeruginosa. Antimicrob. Agents Chemother. 61, e00686-17–e00617. doi:10.1128/aac.00686-17
Zhu, Y., Hao, W., Wang, X., Ouyang, J., Deng, X., Yu, H., et al. (2022). Antimicrobial peptides, conventional antibiotics, and their synergistic utility for the treatment of drug-resistant infections. Med. Res. Rev. 42, 1377–1422. doi:10.1002/med.21879
Zurawski, D. V., Reinhart, A. A., Alamneh, Y. A., Pucci, M. J., Si, Y., Abu-Taleb, R., et al. (2017). SPR741, an antibiotic adjuvant, potentiates the in vitro and in vivo activity of rifampin against clinically relevant extensively drug-resistant Acinetobacter baumannii. Antimicrob. Agents Chemother. 61, e01239-17–e01217. doi:10.1128/aac.01239-17
Keywords: infection medication, antibiotic-therapy combination, antimicrobial resistance, synergic effect, infectious diseases
Citation: C S, G. R R, L. F L, M.C.G dR, N.B C, S.C D and O. L F (2022) Advances and perspectives for antimicrobial peptide and combinatory therapies. Front. Bioeng. Biotechnol. 10:1051456. doi: 10.3389/fbioe.2022.1051456
Received: 22 September 2022; Accepted: 28 November 2022;
Published: 12 December 2022.
Edited by:
Marcelo Der Torossian Torres, University of Pennsylvania, United StatesReviewed by:
Rosa Gaglione, University of Naples Federico II, ItalyRobert Bucki, Medical University of Bialystok, Poland
Copyright © 2022 C, G. R, L. F, M.C.G, N.B, S.C and O. L. This is an open-access article distributed under the terms of the Creative Commons Attribution License (CC BY). The use, distribution or reproduction in other forums is permitted, provided the original author(s) and the copyright owner(s) are credited and that the original publication in this journal is cited, in accordance with accepted academic practice. No use, distribution or reproduction is permitted which does not comply with these terms.
*Correspondence: Santos C, Y3Jpc3RpbnliYUBnbWFpbC5jb20=