- 1Instituto Tecnológico Vale, Belém, Brazil
- 2Facultad de Ingeniería, Arquitectura y Diseño. Universidad San Sebastián, Concepción, Chile
- 3REDEMAT/Universidade Federal de Ouro Preto (UFOP), Ouro Preto, Brazil
- 4Instituto SENAI de Inovação em Tecnologias Minerais, Belém, Brazil
Sulfate-reducing bioreactors are a biotechnological alternative for the treatment of acid mine drainage (AMD). In this study, two separate bioreactors with pH and temperature-controlled (Bio I and II) were operated with two different acidophilic microbial consortia to determine their efficiencies in sulfate removal from a synthetic acidic mine water. The bioreactors were operated for 302 days in continuous flow mode under the same parameters: fed with a sulfate solution of ∼30 mM with a pH of 2.5, the temperature at 30°C, stirred gently at 40 rpm and using a continuous stream of nitrogen to help remove the H2S produced in the bioreactor. The glycerol consumption, acetate production, and sulfate removal were monitored throughout the course of the experiment. The community composition and potential metabolic functional groups were analyzed via 16S rRNA partial gene sequencing. Bio I consortium reduced the sulfate, achieving a range of sulfate concentration from 4.7 to 19 mM in the effluent liquor. The removal of sulfate in Bio II was between 5.6 and 18 mM. Both bioreactors’ communities showed the presence of the genus Desulfosporosinus as the main sulfate-reducing bacteria (SRB). Despite differences in microbial composition, both bioreactors have similar potential metabolism, with a higher percentage of microorganisms that can use sulfate in respiration. Overall, both bioreactors showed similar performance in treating acidic mine water containing mostly sulfate using two different acidophilic sulfidogenic consortia obtained from different global locations.
Introduction
Acid mine drainage (AMD) is one of the major environmental problems caused by the mining industry and can cause local and regional impacts if not properly treated. AMD is wastewater that generally contains an elevated concentration of dissolved metals and metalloids, high sulfate concentration, and a low pH (Nordstrom and Alpers, 1999; Younger et al., 2002; Johnson and Halberg 2005; González et al., 2019). Their inappropriate treatment could lead to severe damage to the health of many organisms and the environment (Simate and Ndlovu, 2014). In order to prevent the damages of AMD and enhance ecological sustainability, a proper treatment and management system is required.
Conventional treatment to treat AMD involves the addition of lime, allowing the increase of pH and leading to a variety of metal ions to precipitate (Johnson and Hallberg, 2005). Despite increasing the effluent pH, this method is disadvantageous due to their high operational costs of lime transport and application. Besides, the metal-rich sludge generated must be removed from landfill areas, and the removal of sulfate sometimes is not enough to meet environmental regulations to permit the discharge of processed water into streams and rivers (Coulton et al., 2003; Johnson and Hallberg, 2005; Nancucheo and Johnson, 2014; Hernández et al., 2022). Moreover, this AMD chemical treatment does not allow the recovery of soluble metals.
In the pursuit of a more sustainable-regarded approach, the use of acidophilic sulfate-reducing bioreactors for AMD treatment has been developed at laboratory scale (Johnson and Sánchez-Andrea, 2019). In this process, sulfate-reducing bacteria (SRB) can be used to promote the recovery of valuable industrial metals, such as copper, and also to remove sulfate. Under acidic conditions, this process is a proton-consuming reaction, enabling to increase the pH of the effluent (Nancucheo et al., 2017). Furthermore, dissimilatory sulfate reduction releases hydrogen sulfide that reacts with dissolved metals, promoting their immobilization via precipitation of insoluble metal sulfides, such as copper sulfides (Colipai et al., 2018).
Most known SRB species are neutrophilic and have a narrow optimum growth pH (between 6 and 8), and therefore these bacteria need to be protected from direct exposure to AMD (Johnson and Santos, 2020). In addition, previous studies have shown that some SRB are acidophiles (Alazard et al., 2010; Sánchez-Andrea et al., 2015), though further research is necessary to evaluate the performance of the treatment of AMD.
This study aims to compare the performance of two acidophilic microbial consortia in bioreactors fed with a synthetic acid mine drainage, containing mostly sulfate and based on the chemical composition of wastewater from a Brazilian acidic mine water. The glycerol consumption, acetate, and sulfate concentration were measured throughout the 302 days of the experiment as well as the community composition and metabolic functional groups of the two microbial consortia were assessed.
Materials and methods
Set up and operation of the sulfidogenic upflow biofilm bioreactors
Two microbial consortia from different locations were used. A novel consortium (Bioreactor I; Bio I) obtained from acidic sediment of a river impacted by an abandoned sulfur mine in the Chilean Altiplano (Guerra et al., 2016; González et al., 2019) was used. A second consortium used (Bioreactor II; Bio II), obtained from mine sites in Spain and Wales (Rowe et al., 2007; Santos and Johnson, 2018), was kindly provided by Dr. Barrie Johnson, from Bangor University, United Kingdom. The set-up for the two bioreactors was the same.
Two continuous flow bench-scale bioreactors populated with each consortium were set up based on the system described previously (Santos and Johnson, 2017). A FerMac 310/60 (Eletrolab, UK) monitored the conditions from each sulfidogenic bioreactor. Each reactor was maintained at 30°C and operated at 40 rpm stirring speed. A continuous sparging (150 mL min-1) of sterile (0.22 μm filter) oxygen-free nitrogen gas was used to provide the anoxic conditions and remove the H2S produced by the SRB.
Each consortium was immobilized on porous sterile glass beads (1–2 mm diameter) that occupied ∼50% of the bioreactor vessels working volumes. The beads were covered with 800 ml of acidophilic SRB medium containing as described (Nancucheo et al., 2016): 1) autotrophic basal salts and trace elements; 2) glycerol (3 mM); 3) 0.01% (w/v) yeast extract; 4) zinc sulfate (0.02 mM); 5) 4 mM of magnesium sulfate. The inoculated medium was maintained in batch mode for 40 days for both sulfidogenic systems recirculating the beads within the bioreactor to encourage attachment and colonization of the beads. After the start-up period of each bioreactor, the continuous operation was initiated with a flow rate of 70 ml.h−1. The bioreactors were fed with synthetic AMD (sAMD) (Santos and Johnson, 2017), with pH adjusted to 2.5 with 5 mM glycerol as carbon and electron donor and 0.01% (w/v) yeast extract. The experiment was carried out for 302 days under continuous flow mode. The sulfate concentration in the feed liquor was maintained at ∼30 mM throughout the experiment. Samples from the effluent were taken periodically and filtered with a 0.22 μm sterile filter (Merck Millipore, United States) and stored at 4°C prior to further analysis.
Physicochemical analysis and bacterial cell counting
Glycerol, acetate, and sulfate concentrations from the effluent liquor were periodically determined by using ion chromatography (Dionex™ ICS-5000, Thermo Fisher Scientific, MA, United States). Glycerol concentration was quantified by using a CarboPac MA1 column coupled to an ED amperometric detector. Sulfate and acetate concentrations were measured using an IonPac AS-11 column equipped with a conductivity detector. Bacteria in the effluent were enumerated using a Neubauer chamber (Brand, Germany) and viewed with a Leica DM 3000 LED optical microscope (Leica Microsystems, Germany).
Microbial community diversity in the bioreactors and metabolic function prediction
Diversity analysis of the microbial community in the bioreactors was performed by high throughput sequencing of the 16S rRNA gene V4 region, followed by bioinformatics analysis using QIIME2 (Bolyen et al., 2019). The samples used in this study were collected every ∼50 days and samples of each inoculum were also sequenced (each bioreactor had different priming phase; therefore, samples were taken at non-identical times). A total of seven samples were sequenced and analyzed for each bioreactor (Table 1).
For genomic DNA extraction, 40 ml from liquid samples was filtered through a 0.22 μm sterile cellulose acetate filter membrane (Merck Millipore, United States). Bacteria attached to the membranes were subjected to DNA extraction using the UltraClean Microbial DNA Isolation Kit (MoBio, CA, United States), according to the manufacturer’s instructions. DNA integrity and quality were checked by gel electrophoresis. DNA was quantified by fluorescence using the Qubit® 2.0 fluorometer with the QubitTM dsDNA HS Assay Kit (Invitrogen ™), following the manufacturer´s instructions.
The primers 515F (5′-GTGCCAGCMGCCGCGGTAA-3′) and 806R (5′-GGACTACHVGGGTWTCTAAT-3′) were used for the amplification of bacterial consortium 16S rRNA gene V4 region (Caporaso et al., 2012; Pylro et al., 2014). Amplicons were sequenced using an Ion Torrent™ PGMTM Hi-Q™ (Thermo Fisher Scientific, MA, United States) at the Genomic Core of the Instituto Tecnológico Vale, Belém, Brazil; following the manufacturer’s instructions for sequencing single-end libraries. After sequencing, all samples were analyzed using quantitative insights into microbial ecology QIIME2 (Bolyen et al., 2019) for Linux. Genome sequences were deposited in the NCBI database, with SRA run accessions SRR21279213 (BIO I) and SRR21279225 (Bio II) in BioProjects PRJNA872571 (BIO I) and PRJNA873019 (Bio II).
FAPROTAX version 1.2.4 (Louca et al., 2016) was used to map the studied bacterial consortia’s main predicted putative metabolic functions. FAPROTAX uses the current literature on cultured strains and converts the microbial sample’s taxonomic profile into putative functional profiles based on taxa. The results were plotted on R version 4.1.1 and RSTUDIO version 1.2.5001 (R Development Core Team, 2019; RStudio Team, 2021) with ggplot 2 package (Wickham et al., 2016).
Statistical analysis
Alfa diversity, Beta diversity, and Principal Component Analysis (PCA) were performed by program R version 4.1.1 and RSTUDIO version 1.2.5001 (R Development Core Team, 2019; RStudio Team, 2021). Alfa diversity was performed with the package Phyloseq, ggplot2, and ape (Paradis et al., 2004; McMurdie and Holmes, 2013; Wickham et al., 2016; Zucchini et al., 2018). Beta diversity was estimated by Weighted UniFrac distance with Phyloseq (McMurdie and Holmes, 2013).
Principal Component Analysis (PCA) was performed with package devtools (Zucchini et al., 2018) and factoextra (Kassambara and Mundt, 2017) and Pearson’s correlation with package Corrplot (Wei and Simko, 2021) using the data obtained from the QIIME2 and the physical-chemical analyzes referring to the days of the samples collected.
Results
Operation of a low sulfidogenic bioreactor
Bacterial numbers taken from the effluent in both bioreactors varied between 1.0 × 104 and 4.4 × 106 mL−1 (Supplementary Figures S1,S2), demonstrating that the bacteria present in both consortia were able to tolerate the acidic solution used as influent sAMD. Cell numbers are planktonic bacteria which derive from the detachment of the biofilm bed (50% of the total volume of the vessel) and the variability can be explained from a random process produced by loss of large pieces of biofilm during the operation.
Glycerol concentrations were below 1 mM in most of the samples (Figure 1), indicating the effective consumption by the SRB as carbon source and electron donor. Acetate concentration was also quantified in the bioreactors, as the main by-product metabolite, maintaining below 5.5 mM in both bioreactors (Figure 1).
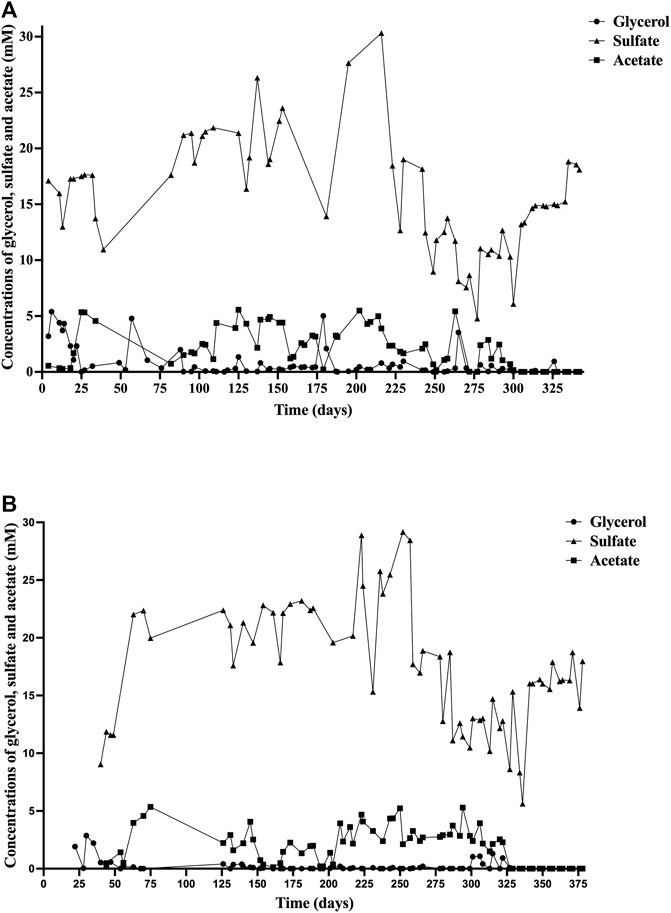
FIGURE 1. Concentrations of glycerol (○), sulfate (▲), and acetate (■) during the experiment in: (A) Bioreactor I (Bio I); (B) Bioreactor II (Bio II).
Sulfate concentration in Bio I (Figure 1A) ranged from 10.9 to 30 mM until day 216. From that day, the concentration decreased, reaching a maximum of 19 mM on day 230 and a minimum of 4.7 mM on day 277. The sulfate concentration in Bio II (Figure 1B) was maintained in a range of 9.03–29.1 mM until day 257. After that day, the concentration reached a maximum of 18.7 mM on day 258 and a minimum of 5.6 mM on day 336.
Microbial communities’ diversity in the bioreactors
The most represented bacterial phylum in Bio I community (Figure 2A) was Firmicutes (>90%), followed by Actinobacteria in the inoculum (T0) and T1 (Figure 2A). The most abundant genus belonging to the phylum Firmicutes was Desulfosporosinus, a well-known anaerobic acidophilic SRB. Their population shifted between T2 and T4, being the most abundant again at T5 to T6. Between T2 and T4, the dominant microorganism was the Firmicutes genus Clostridium (Figure 2A). Microbial diversity showed low diversity in T1-T3, enhancing in T4-T6 (Supplementary Figure S3A).
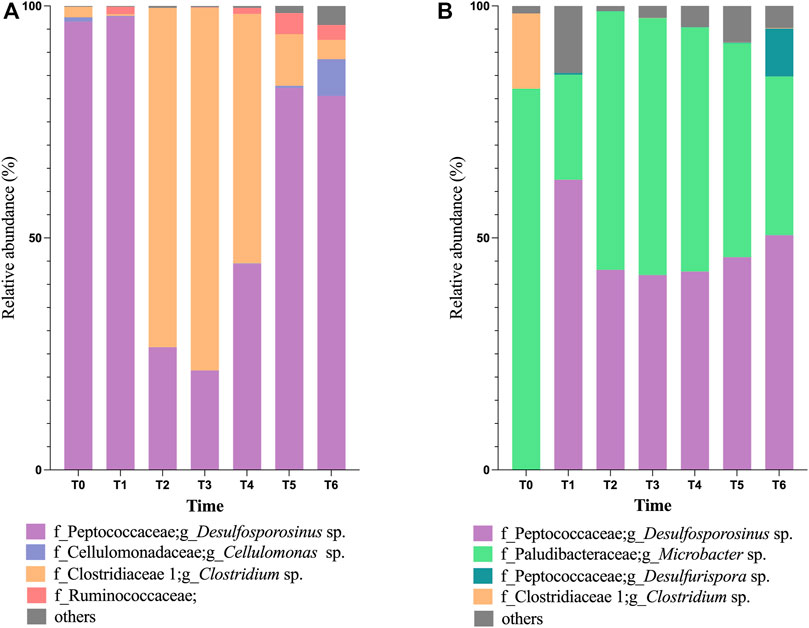
FIGURE 2. Taxonomical classification, at the genus level, of bacterial population in Bioreactor I (A) and Bioreactor II (B), being T0 representing the inoculum population.
The microbial community in Bio II inoculum (T0, in Figure 2B) is dominated by the phylum Bacteroidetes, with most bacteria belonging to the genus Microbacter (79%), followed by the genus Clostridium (19%). Shifts in the microbial community were observed at T1, increasing the phylum Firmicutes (66%) in detriment of Bacteroidetes (18%). Between T2-T4 sampling dates, the genus Microbacter was again the dominant, ranging between 51% and 62%, followed by Desulfosporosinus (range of 34.7%–37%). The presence of bacteria belonging to the genus Desulfurispora was observed at T1 and T6 sampling dates. The microbial diversity on Bio II showed variation in T1-T3, enhanced at the end of the experiment (T4 to T6) (Supplementary Figure S3B).
The PCA first principal coordinate axe (PC1) responded to 54.4% of the variability in bioreactor 1 metadata, whereas the second principal coordinates (PC2) responded to 25.6% (Figure 3). Desulfosporosinus and Clostridium showed a negative association at PCA (Figure 3) and at correlation analysis (Supplementary Figure S4A). Also, a negative correlation between microbial cells (Cells) and glycerol was observed.
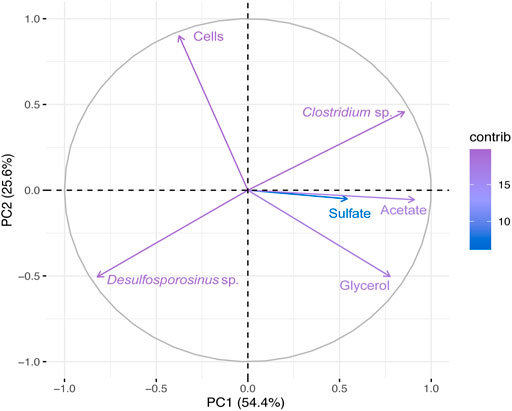
FIGURE 3. Principal Component Analyses (PCA) of the total bacterial community based on 16S rRNA gene sequences and physical-chemical analysis data from Bio I. First principal coordinates (PC1 54.4%) and the second principal coordinates (PC2 25.6%).
In Bio II (Figure 4 and Supplementary Figure S4B), the first principal coordinate axe (PC1) responded to 39.4% of the variability, whereas the second principal coordinates (PC2) responded to 22.5%. PCA shows a negative correlation between Desulfosporosinus and Microbacter, and a positive correlation between Desulfosporosinus and cells. The genus Desulfosporosinus showed a positive correlation with acetate and pH.
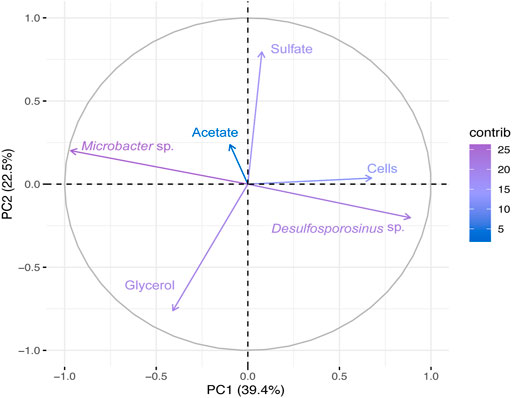
FIGURE 4. Principal Component Analyses (PCA) of the total bacterial community based on 16S rRNA gene sequences and physical-chemical analysis data from Bio II. First principal coordinates (PC1 39.4%) and the second principal coordinates (PC2 22.5%).
Beta diversity obtained by the weighted (quantitative) UniFrac distance (Figure 5) shows two clusters separating the samples by bioreactor. Only the inoculum sample (T0) from Bio II was grouped with the Bio I cluster.
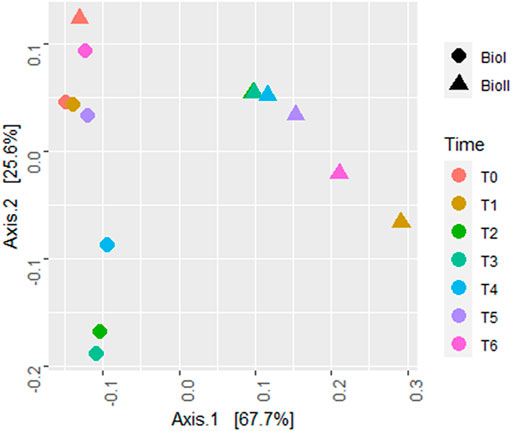
FIGURE 5. Beta diversity plot estimated by UniFrac with weights measure. Bio I (●); Bio II (▲); Each color represents a different sample time.
The mapping of the predictive metabolic functions of Bio I (Figure 6) revealed a prevalence of microorganisms that produce cellular energy using sulfur compounds and sulfate. Furthermore, fermenters, chemoheterotrophs, a small portion of aerobic chemoheterotrophs microorganisms, and a reduction in the identification of predictive metabolic pathways were observed in samples T2, T3, and T4.
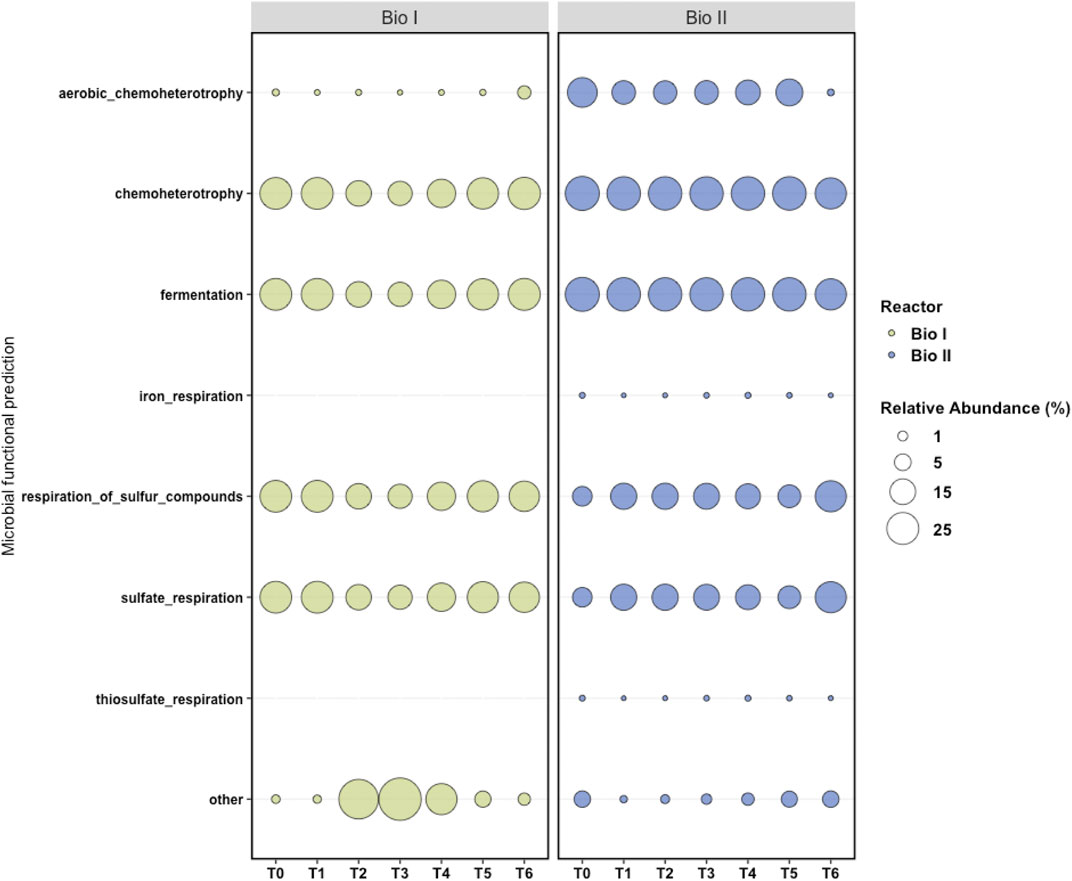
FIGURE 6. Metabolic maps generated by FAPROTAX. FAPROTAX converted taxonomic data at the level of genus and species generated by QIIME2 into functional profiles. Each column represents a sample (T0 to T6) and each line a metabolic function. In the graph, other represents the proportion of microorganisms that did not have the identified physiological functions.
As well as Bio I, a predominance of microorganisms that use sulfur compounds to produce cellular energy, fermenters, and chemoheterotrophs was observed on the map of Bio II (Figure 6). Chemoheterotrophic microorganisms were also represented and other functions were observed in a small proportion, including iron respiration and thiosulfate respiration.
Discussion
The mining industry frequently generates AMD, wastewater characterized by a low pH, a high concentration of dissolved metals, metalloids and sulfate (Sen and Johnson, 1999a; Hallberg, 2010; González et al., 2019). One of the main goals of this study was to compare the ability of two different SRB consortia to operate for a long term for the removal of sulfate using an acidic mine water solution.
During the operation, both bioreactors exhibited an acid-tolerant consortium with the ability to reduce sulfate under acidic conditions. Glycerol was chosen as a carbon source for being an adequate substrate for cultivating and isolating acidophilic SRB (Sen and Johnson, 1999b; Nancucheo et al., 2016; Santos and Johnson, 2017). Both consortia, in Bio I and Bio II, were efficient in consuming glycerol, maintaining below 1 mM throughout the experiment. Similar experiments carried out by Kimura et al. (2006) showed the efficiency in glycerol oxidation at pH ranging from 4.2–3.8 using an acidophilic bacteria belonging to the genus Desulfosporosinus.
The production of acetate concentration in Bio I and II occurs due to the presence of acetogenic SRB as Desulfosporosinus. One of the problems of acetogenic SRB growing at low pH is the production of acetic acid, being toxic to SRB in concentrations higher than 0.9 mM (Reis et al., 1990; Johnson and Hallberg, 2008). In addition, Microbacter detected in Bio II can also produce acetate and has been previously found in anaerobic acidic sediments (Sánchez-Andrea et al., 2014b).
The removal of sulfate in both bioreactors was variable during the experiment. In the first 100 days of the operation in Bio I, sulfate concentration was below 20 mM, and in the last 110 days of the experiment, the concentration ranged from 4.7 to 18 mM (Figure 1A), unlike Bio II, which maintained the sulfate concentration above 20 mM, except in the last 100 days when the concentration decreased, staying in a range of 5.6–18.7 mM (Figure 1B). These variations in sulfate removal might be explained due to that the consortium in Bio I stabilizes its sulfidogenic population in a shorter period than in Bio II. The range of sulfate concentration observed in the last 110 days of Bio I was similar to that described by Santos and Johnson (2017), in which at a pH of 4 (35°C), sulfate concentration was 6.5–20 mM. Since both reactors had a priming phase (up to day 75 for Bio II), the mean and standard deviation for sulfate measured in the effluent from day 75 for Bio I was 17 and 4 mM (51 sampling points for 267 days of operation). Even considering the variability, the data obtained reflects that the maximum amount of sulfate that can be removed based in carbon source added. Note that, 5 mM of glycerol can remove up to ∼9 mM of sulfate using the stoichiometry glycerol:sulfate (4:7) that described biosulfidogenesis. In addition, the yeast extract added can remove ∼3 mM. Therefore, the amount of sulfate removed is 12 mM (fed with 30 mM). Based in this calculation, the amount of sulfate in the reactor expected would be 18 mM which is close to the mean (17 mM). Similar results were obtained for Bio II.
Protons and carbon sources limit the reduction of sulfate in acidophilic sulfidogenic bioreactors. Nancucheo and Johnson (2014) showed removal of 98% of sulfate (fed with 20 mM) when the pH of the feed liquor was decreased from 3 to 1.6 and glycerol was provided as an electron donor (up to 30 mM). Eq. 1 described by Kimura et al. (2006) shows that the reduction of sulfate to H2S is proton-consuming, therefore the partial removal of sulfate achieved in this study suggests a limitation of protons and glycerol supply.
Organisms from the phyla Firmicutes, Bacteroidetes, Proteobacteria, and Actinobacteria are generally found in bioreactors with the same configuration and objective as those studied here (Nancucheo and Johnson, 2012; Sánchez-Andrea et al., 2014a; Sato et al., 2019). Bio I presented as a dominant microorganism in most samples an SRB, Desulfosporosinus which is commonly found in anaerobic acidophilic bioreactors used in AMD treatment (Sánchez-Andrea et al., 2012; Santos and Johnson, 2017; Sato et al., 2019; Hernández et al., 2022). Desulfosporosinus is able to form spores under stress conditions, highly relevant in extreme environments found in mine sites (Sato et al., 2019). Moreover, the genus Desulfosporosinus is the only validated genus of mesophilic SRB with two described species able to grow in acidic environments, D. acidiphilus and D. acididurans (Alazard et al., 2010; Sánchez-Andrea et al., 2015).
Another dominant microorganism in Bio I was Clostridium, an anaerobic fermenting microorganism. Some of its representatives use glycerol in fermentative processes, such as Clostridium pasteurianum (Biebl, 2001), and some of them produce acetic acid, like Clostridium beijerinckii (Chen and Blaschek, 1999). Clostridium spp. has also been found in bioreactors for AMD treatment (Sánchez-Andrea et al., 2012) and recently Santos and Johnson (2022) reported the presence of this genus in a sulfidogenic reactor maintained at moderately low pH. Although Clostridium is not the dominant microorganism in most samples (see Figure 2A), the competition by carbon source (glycerol) and acetic acid production could be problematic to SRBs in the bioreactor. The positive correlation between acetate and Clostridium in PCA and Pearson’s correlation graphs (Figure 3 and Supplementary Figure S4A) showed that this bacterium might ferment glycerol in the bioreactor and generates acetic acid.
In Bio II, besides Desulfosporosinus, the other dominant microorganism was from the genus Microbacter, a microorganism found in acid rock drainage sediments (Sánchez-Andrea et al., 2014b). The observed diversity changes in both bioreactors and changes in predicted microbial function could explain how Bio I showed high acetate concentration. Interestingly, Microbacter had not been previously found in Bio II (Santos and Johnson, 2022), though the specie Microbacter margulisiae was described as strictly anaerobic and able to grow between pH 3-7 using a variety of sugars, producing acetate, lactate and propionate as major products of fermentation.
Bio II presents a slightly more diverse consortium than Bio I (Supplementary Figure S3) and a more stable predicted microbial function condition, which could help in AMD’s treatment. This bioreactor showed more stability with microorganisms that use sulfur respiration (Figure 2), presenting a slighter variation between 38%—62%, than Bio I, which varied in 10%—77% (in T2—T6). However, at the end of the experiment, Bio I showed more stability and percentage of SRBs (>83%) in T5—T6 (Figure 2) than Bio II.
Conclusion
This work has demonstrated how two different acidophilic sulfidogenic consortia obtained from different global locations can be operated under continuous flow mode to treat a synthetic acidic mine water. Both bioreactors showed the presence of the genus Desulfosporosinus, a known acidophilic SRB, able to partially oxidize glycerol to acetate, which is not a desirable by-product, though during the operation both sulfidogenic systems showed the presence of other microorganisms with the ability to produce acetate and highlight the role of acetate degraders in acidophilic sulfate-reducing bioreactor.
Data availability statement
The genomic data presented in the study were deposited in the NCBI, SRA run accessions SRR21279213 (BIO I) and SRR21279225 (Bio II) in BioProjects PRJNA872571 (BIO I) and PRJNA873019 (Bio II).
Author contributions
IN, JA, GO, and JB were involved in the conception of the idea for this research, discussing methodological guidelines, and participating in the study’s design and coordination. TF, WF, DB, PS, and AL developed methodological guidelines and analyses. RN and EP sequenced the samples and achieved molecular studies. TF, IN, and JB wrote this paper.
Funding
This project was supported by the Industry Innovation Call (CNI-Brazil) and Vale. Fellowships were granted by the Coordination for the Improvement of Higher Education Personnel (CAPES-Brazil), FONDECYT-Chile n° 1221604, and FONDEF n° ID21I10111.
Conflict of interest
The authors declare that the research was conducted in the absence of any commercial or financial relationships that could be construed as a potential conflict of interest.
Publisher’s note
All claims expressed in this article are solely those of the authors and do not necessarily represent those of their affiliated organizations, or those of the publisher, the editors and the reviewers. Any product that may be evaluated in this article, or claim that may be made by its manufacturer, is not guaranteed or endorsed by the publisher.
Supplementary material
The Supplementary Material for this article can be found online at: https://www.frontiersin.org/articles/10.3389/fbioe.2022.1048412/full#supplementary-material
References
Alazard, D., Joseph, M., Battaglia-Brunet, F., Cayol, J.-L., and Ollivier, B. (2010). Desulfosporosinus acidiphilus sp. nov.: A moderately acidophilic sulfate-reducing bacterium isolated from acid mining drainage sediments. Extremophiles 14, 305–312. doi:10.1007/s00792-010-0309-4
Biebl, H. (2001). Fermentation of glycerol by Clostridium pasteurianum — Batch and continuous culture studies. J. Ind. Microbiol. Biotechnol. 27, 18–26. doi:10.1038/sj.jim.7000155
Bolyen, E., Rideout, J. R., Dillon, M. R., Bokulich, N. A., Abnet, C. C., Al-Ghalith, G. A., et al. (2019). Reproducible, interactive, scalable and extensible microbiome data science using QIIME 2. Nat. Biotechnol. 37, 852–857. doi:10.1038/s41587-019-0209-9
Caporaso, J. G., Lauber, C. L., Walters, W. A., Berg-Lyons, D., Huntley, J., Fierer, N., et al. (2012). Ultra-high-throughput microbial community analysis on the Illumina HiSeq and MiSeq platforms. ISME J. 6, 1621–1624. doi:10.1038/ismej.2012.8
Chen, C.-K., and Blaschek, H. P. (1999). Effect of acetate on molecular and physiological aspects of Clostridium beijerinckii NCIMB 8052 solvent production and strain degeneration. Appl. Environ. Microbiol. 65, 499–505. doi:10.1128/aem.65.2.499-505.1999
Colipai, C., Southam, G., Oyarzún, P., González, D., Díaz, V., Contreras, B., et al. (2018). Synthesis of copper sulfide nanoparticles using biogenic H2S produced by a low-pH sulfidogenic bioreactor. Minerals 8, 35. doi:10.3390/min8020035
Coulton, R., Bullen, C., and Hallett, C. (2003). The design and optimisation of active mine water treatment plants. Land Contam. Reclam. 11, 273–279. doi:10.2462/09670513.825
González, D., Liu, Y., Gomez, D. V., Southam, G., Hedrich, S., Galleguillos, P., et al. (2019). Performance of a sulfidogenic bioreactor inoculated with indigenous acidic communities for treating an extremely acidic mine water. Min. Eng. 131, 370–375. doi:10.1016/j.mineng.2018.11.011
Guerra, P., Gonzalez, C., Escauriaza, C., Pizarro, G., and Pasten, P. (2016). Incomplete mixing in the fate and transport of arsenic at a river affected by acid drainage. Water Air Soil Pollut. 227, 73. doi:10.1007/s11270-016-2767-5
Hallberg, K. B. (2010). New perspectives in acid mine drainage microbiology. Hydrometallurgy 104, 448–453. doi:10.1016/j.hydromet.2009.12.013
Hernández, P., Recio, G., Canales, C., Schwarz, A., Villa-Gomez, D., Southam, G., et al. (2022). Evaluation of operating conditions on sulfate reduction from acidic wastewater in a fixed-bed bioreactor. Min. Eng. 177, 107370. doi:10.1016/j.mineng.2021.107370
Johnson, D. B., and Hallberg, K. B. (2005). Acid mine drainage remediation options: A review. Sci. total Environ. 338, 3–14. doi:10.1016/j.scitotenv.2004.09.002
Johnson, D. B., and Hallberg, K. B. (2008). Carbon, iron and sulfur metabolism in acidophilic micro-organisms. Adv. Microb. Physiol. 54, 201–255.
Johnson, D. B., and Sánchez-Andrea, I. (2019). “Dissimilatory reduction of sulfate and zero-valent sulfur at low pH and its significance for bioremediation and metal recovery,” in Advances in microbial physiology. Editor R. K. Poole (Academic Press), 205–231. doi:10.1016/bs.ampbs.2019.07.002
Johnson, D. B., and Santos, A. L. (2020). “Biological removal of sulfurous compounds and metals from inorganic wastewaters,” in Environmental technologies to treat sulfur pollution: Principles and engineering. Editor P. N. L. Lens (London: IWA Publishing). doi:10.2166/9781789060966_0215
Kassambara, A., and Mundt, F. (2017). Factoextra: Extract and visualize the results of multivariate data analyses. URL Available at: http://www.sthda.com/english/rpkgs/factoextra BugReports.
Louca, S., Parfrey, L. W., and Doebeli, M. (2016). Decoupling function and taxonomy in the global ocean microbiome. Science 353, 1272–1277. doi:10.1126/science.aaf4507
McMurdie, P. J., and Holmes, S. (2013). phyloseq: an R package for reproducible interactive analysis and graphics of microbiome census data. PLoS One 8, e61217. doi:10.1371/journal.pone.0061217
Nancucheo, I., Bitencourt, J. A. P., Sahoo, P. K., Alves, J. O., Siqueira, J. O., and Oliveira, G. (2017). Recent developments for remediating acidic mine waters using sulfidogenic bacteria. Biomed. Res. Int. 2017, 1–17. doi:10.1155/2017/7256582
Nancucheo, I., and Johnson, D. B. (2014). Removal of sulfate from extremely acidic mine waters using low pH sulfidogenic bioreactors. Hydrometallurgy 150, 222–226. doi:10.1016/j.hydromet.2014.04.025
Nancucheo, I., and Johnson, D. B. (2012). Selective removal of transition metals from acidic mine waters by novel consortia of acidophilic sulfidogenic bacteria. Microb. Biotechnol. 5, 34–44. doi:10.1111/j.1751-7915.2011.00285.x
Nancucheo, I., Rowe, O. F., Hedrich, S., and Johnson, D. B. (2016). Solid and liquid media for isolating and cultivating acidophilic and acid-tolerant sulfate-reducing bacteria. FEMS Microbiol. Lett. 363, fnw083. doi:10.1093/femsle/fnw083
Nordstrom, D. K., and Alpers, C. N. (1999). “The geochemistry of acid mine waters,” in The environmental geochemistry of mineral deposits. Editors Geoffrey S. Plumlee, M. J. Logsdon, and L. F. Filipek (Littleton, CO: Society of Economic Geologists), 133–160.
Paradis, E., Claude, J., and Strimmer, K. (2004). Ape: Analyses of phylogenetics and evolution in R language. Bioinformatics 20, 289–290. doi:10.1093/bioinformatics/btg412
Pylro, V. S., Roesch, L. F. W., Morais, D. K., Clark, I. M., Hirsch, P. R., and Tótola, M. R. (2014). Data analysis for 16S microbial profiling from different benchtop sequencing platforms. J. Microbiol. Methods 107, 30–37. doi:10.1016/j.mimet.2014.08.018
R Development Core Team, (2019). R Core Team (2020). R: A language and environment for statistical computing. Vienna, Austria: R Foundation for Statistical Computing. URL Available at: https://www.R-project.org/.
Reis, M. A. M., Lemos, P. C., Almeida, J. S., and Carrondo, M. J. T. (1990). Influence of produced acetic acid on growth of sulfate reducing bacteria. Biotechnol. Lett. 12, 145–148. doi:10.1007/bf01022432
Rowe, O. F., Sánchez-España, J., Hallberg, K. B., and Johnson, D. B. (2007). Microbial communities and geochemical dynamics in an extremely acidic, metal-rich stream at an abandoned sulfide mine (Huelva, Spain) underpinned by two functional primary production systems. Environ. Microbiol. 9, 1761–1771. doi:10.1111/j.1462-2920.2007.01294.x
Sánchez-Andrea, I., Sanz, J. L., Bijmans, M. F. M., and Stams, A. J. M. (2014a). Sulfate reduction at low pH to remediate acid mine drainage. J. Hazard. Mat. 269, 98–109. doi:10.1016/j.jhazmat.2013.12.032
Sánchez-Andrea, I., Sanz, J. L., and Stams, A. J. M. (2014b). Microbacter margulisiae gen. nov., sp. nov., a propionigenic bacterium isolated from sediments of an acid rock drainage pond. Int. J. Syst. Evol. Microbiol. 64, 3936–3942. doi:10.1099/ijs.0.066241-0
Sánchez-Andrea, I., Stams, A. J. M., Hedrich, S., Nancucheo, I., and Johnson, D. B. (2015). Desulfosporosinus acididurans sp. nov.: An acidophilic sulfate-reducing bacterium isolated from acidic sediments. Extremophiles 19, 39–47. doi:10.1007/s00792-014-0701-6
Sánchez-Andrea, I., Triana, D., and Sanz, J. L. (2012). Bioremediation of acid mine drainage coupled with domestic wastewater treatment. Water Sci. Technol. 66, 2425–2431. doi:10.2166/wst.2012.477
Santos, A. L., and Johnson, D. B. (2022). Comparison of different small molecular weight alcohols for sustaining sulfidogenic bioreactors maintained at moderately low pH. Front. Bioeng. Biotechnol. 10, 937987. doi:10.3389/fbioe.2022.937987
Santos, A. L., and Johnson, D. B. (2018). Design and application of a low pH upflow biofilm sulfidogenic bioreactor for recovering transition metals from synthetic waste water at a Brazilian copper mine. Front. Microbiol. 9, 2051. doi:10.3389/fmicb.2018.02051
Santos, A. L., and Johnson, D. B. (2017). The effects of temperature and pH on the kinetics of an acidophilic sulfidogenic bioreactor and indigenous microbial communities. Hydrometallurgy 168, 116–120. doi:10.1016/j.hydromet.2016.07.018
Sato, Y., Hamai, T., Hori, T., Aoyagi, T., Inaba, T., Kobayashi, M., et al. (2019). Desulfosporosinus spp. were the most predominant sulfate-reducing bacteria in pilot-and laboratory-scale passive bioreactors for acid mine drainage treatment. Appl. Microbiol. Biotechnol. 103, 7783–7793. doi:10.1007/s00253-019-10063-2
Sen, A. M., and Johnson, B. (1999a). Acidophilic sulphate-reducing bacteria: Candidates for bioremediation of acid mine drainage. Process Metall. 9, 709–718. doi:10.1016/S1572-4409(99)80073-X
Sen, A. M., and Johnson, B. (1999b). “Acidophilic sulphate-reducing bacteria: Candidates for bioremediation of acid mine drainage,” in Process metallurgy (Elsevier), 709–718.
Simate, G. S., and Ndlovu, S. (2014). Acid mine drainage: Challenges and opportunities. J. Environ. Chem. Eng. 2, 1785–1803. doi:10.1016/j.jece.2014.07.021
Wei, T., and Simko, V. (2021). R package 'corrplot': Visualization of a correlation matrix. Version 0.92 URL Available at: https://github.com/taiyun/corrplot.
Wickham, H., Chang, W., and Wickham, M. H. (2016). Package ‘ggplot2’. Create elegant data visualisations using the grammar of graphics.
Younger, P. L., Banwart, S. A., and Hedin, R. S. (2002). “Mine water chemistry,” in Mine water: Hydrology, pollution, remediation. Editors P. L. Younger, S. A. Banwart, and R. S. Hedin (Dordrecht: Springer Netherlands), 65–126.
Keywords: acidophilic sulfate-reducing bacteria, bioreactors, acid mine drainage, biological treatments, sulfate treatment
Citation: Frederico TD, Nancucheo I, Santos WCB, Oliveira RRM, Buzzi DC, Pires ES, Silva PMP, Lucheta AR, Alves JO, Oliveira GCd and Bitencourt JAP (2022) Comparison of two acidophilic sulfidogenic consortia for the treatment of acidic mine water. Front. Bioeng. Biotechnol. 10:1048412. doi: 10.3389/fbioe.2022.1048412
Received: 19 September 2022; Accepted: 02 November 2022;
Published: 29 November 2022.
Edited by:
Rajesh K. Sani, South Dakota School of Mines and Technology, United StatesReviewed by:
Pedro Antonio Galleguillos, Centro de Investigación Científico y Tecnológico para la Minería (CICITEM), ChileJavier Sánchez-España, Spanish National Research Council (CSIC), Spain
Copyright © 2022 Frederico, Nancucheo, Santos, Oliveira, Buzzi, Pires, Silva, Lucheta, Alves, Oliveira and Bitencourt. This is an open-access article distributed under the terms of the Creative Commons Attribution License (CC BY). The use, distribution or reproduction in other forums is permitted, provided the original author(s) and the copyright owner(s) are credited and that the original publication in this journal is cited, in accordance with accepted academic practice. No use, distribution or reproduction is permitted which does not comply with these terms.
*Correspondence: Ivan Nancucheo, aXZhbi5uYW5jdWNoZW9AdXNzLmNs; José Augusto Pires Bitencourt, am9zZS5hdWd1c3RvLmJpdGVuY291cnRAaXR2Lm9yZw==