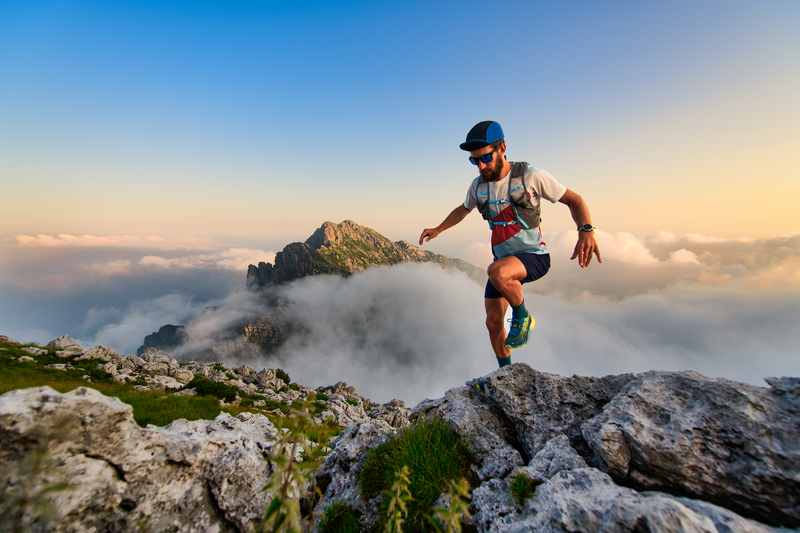
95% of researchers rate our articles as excellent or good
Learn more about the work of our research integrity team to safeguard the quality of each article we publish.
Find out more
REVIEW article
Front. Bioeng. Biotechnol. , 08 December 2022
Sec. Biomaterials
Volume 10 - 2022 | https://doi.org/10.3389/fbioe.2022.1041102
This article is part of the Research Topic Advanced Biomaterials for Drug Delivery in Regenerative Medicine View all 4 articles
Over the past few decades, natural-origin polysaccharides have received increasing attention across different fields of application, including biomedicine and biotechnology, because of their specific physicochemical and biological properties that have afforded the fabrication of a plethora of multifunctional devices for healthcare applications. More recently, marine raw materials from fisheries and aquaculture have emerged as a highly sustainable approach to convert marine biomass into added-value polysaccharides for human benefit. Nowadays, significant efforts have been made to combine such circular bio-based approach with cost-effective and environmentally-friendly technologies that enable the isolation of marine-origin polysaccharides up to the final construction of a biomedical device, thus developing an entirely sustainable pipeline. In this regard, the present review intends to provide an up-to-date outlook on the current green extraction methodologies of marine-origin polysaccharides and their molecular engineering toolbox for designing a multitude of biomaterial platforms for healthcare. Furthermore, we discuss how to foster circular bio-based approaches to pursue the further development of added-value biomedical devices, while preserving the marine ecosystem.
The unparalleled biological diversity of the marine realm is an extraordinary source of a plethora of natural macromolecules with higher bioactivity and chemical novelty when compared with those extracted from terrestrial life forms (Zhang et al., 2016), thus making them particularly attractive to be applied in biomedicine and healthcare (Wan et al., 2021; Iliou et al., 2022). Despite the marine environment reconnaissance as an endless diversity of natural compounds, the exploitation of marine biodiversity as a source of high added-value natural compounds is still at its early-stage, thus deserving further comprehensive attention (Vieira et al., 2020). For instance, amongst the world capture of marine species, more than 35% of the total weight has been discarded as by-products of processed marine organisms (Vázquez et al., 2013). Such biomass constitutes a valuable source of a diverse set of novel macromolecules and bioactive products with key properties and functions that extensively surpass those obtained by synthetic means. Furthermore, the inherent renewable character of marine resources will assure new and promising benefits, including the provision of innovative compounds with a lower carbon footprint, as well as a highly valuable alternative to polymers obtained from fossil fuels. However, one should look at a conscious, prudent and sustainable use and management of marine living resources, which implies the reduction of overfishing, pollution and habitat destruction, in order to protect, valorize and secure their survival and maintain the marine ecosystem (Samalens et al., 2022).
Among the source of natural compounds found in the oceans, polysaccharides obtained from marine organisms (Figure 1) are currently being investigated for different biomedical applications, namely tissue engineering (TE) (Arokiarajan et al., 2022), drug delivery (Sun et al., 2021) and regenerative medicine (RM) (Mahendiran et al., 2021; Rial-Hermida et al., 2021). The requirements for a material to be suitable for such biomedical applications are biocompatibility, biodegradability and non-immunogenic properties. Besides, marine-origin polysaccharides are readily and widely available, thus enhancing their potential as a low-cost and green strategy for the development of new materials for the biomedical field (Chiellini and Morelli, 2011).
FIGURE 1. Marine-origin polysaccharide portfolio: chemical structures and representative marine sources.
With the aim of maximizing the value of marine by-products towards the development of high value-added biomaterials, this review provides an up-to-date outlook on efficient, cost-effective and environmentally-friendly strategies that have been employed from the isolation of marine-origin polysaccharides up to obtaining the envisage biomedical device. Finally, we highlight some guidelines to facilitate the translation of marine-derived biomaterial platforms to the market and their benefits for society, while fulfilling the United Nations Sustainable Development Goals.
Most conventional extraction processes (e.g., soxhlet, solid-liquid extraction, or liquid-liquid extraction) are characterized by long extraction times and comprise hazardous acidic and alkali treatments (Ibañez et al., 2012). These conventional extraction methods are also associated with both low selectivity and extraction yields of bioactive compounds. To tackle these issues, the scientific community has been looking for eco-friendly and efficient approaches following the Green Chemistry principles (Ibañez et al., 2012), aimed at supporting scientists and engineers on the design of new materials (Ibañez et al., 2012). Those techniques are mostly based on compressed fluids, including Supercritical Fluid Extraction, Pressurized Hot Water Extraction, and Pressurized Liquid Extraction, also known as Accelerated Solvent Extraction.
Aside from compressed fluids, extraction processes encompassing Subcritical Water Extraction (SWE), Ultrasound-Assisted Extraction (UAE) and Microwave Assisted Extraction (MAE) have also been used to surpass the bottlenecks of the standard extraction procedures, since they could be applied at pilot scale, thus offering the possibility to be integrated into a process chain within a biorefinery. Table 1 summarizes the main features beyond each green extraction technique, as well its advantages and major drawbacks.
This section provides an overview of the extraction methods applied to date to recover the most studied marine polysaccharides, including chitin, chitosan, hyaluronic acid, chondroitin sulfate, ulvan, alginate, fucoidan, laminarin, carrageenan and agarose (Figure 1).
Chitin is the second most common polysaccharide in nature, after cellulose, being present in the exoskeleton of animals including crustaceans and shrimps. Chitosan (CHT) is a cationic polymer that is obtained by the deacetylation of chitin (Venugopal, 2011).
Standard processes to extract chitin and CHT usually involve the use of high temperatures combined with strong harsh solvents, which consequently contribute to the generation of hazardous effluents and the quality decrease of the extracted polymers (Nouri et al., 2016). In this regard, some researchers have been focused on finding more environmentally friendly alternatives for their extraction. One of the successfully applied techniques is Subcritical Water Extraction (SWE). To recover chitin from the by-products of shrimp cephalothorax (Espíndola-Cortés et al., 2017), in a 82.2% yield (wt; Table 2), one of the highest chitin content already reported in the form of α-chitin. The optimal conditions for the extraction involved a process time of 30 min at 260°C and a by-product:water ratio of 0.17% (w/w). Moreover, SWE has afforded the complete removal of the protein fraction from the polymeric structure, which has been a bottleneck in the use of chitin due to allergic reactions in sensitive customers (Espíndola-Cortés et al., 2017).
In another study, chitin was extracted from the shells of the shrimp Litopenaeus vannamei by employing an enzyme from Streptomyces griseus. After 3 h treatment, the deproteination percentage was 91.1% (Hongkulsup et al., 2016). Additionally, the chitin recovered by enzymatic extraction had a lower degree of crystallinity than the one retrieved from chemical extraction, thus exhibiting higher solubility properties. The authors concluded that the mild conditions associated to the enzymatic extraction process contributed to the maintenance of the chitin properties, avoiding its degradation.
CHT has been mainly extracted by green techniques like MAE, which holds great promise due to the decrease of the extraction time and increased yield (Table 1). CHT extracted from the fishing processing wastes of shrimps by MAE has showcased a significantly enhanced deacetylation degree yield when compared to autoclave (Alishahi et al., 2011) and alkaline methods (Nouri et al., 2016). Such works have still highlighted the potential of MAE technique to achieve these outcomes in very low reaction times, thus reducing the energy consumption.
HA and CS are part of the glycosaminoglycan (GAG) family and can be extracted from the cartilage, bones and fins of several marine organisms (e.g., whales, sharks, rays, salmon, etc.) (Cheng et al., 2013; Sadhasivam et al., 2013; Abdallah et al., 2020). In general, cartilage is the most commonly used source of CS, while the vitreous humor is the main source of HA (Abdallah et al., 2020).
Both HA and CS display interesting physicochemical and biological properties and are widely present in the extracellular matrix (ECM) of connective tissues. Owing to their negative surface charge, they are capable to absorb a large amount of water, thus favouring tissue hydration. In general, HA is known for providing viscoelasticity, lubrification and immune system modulation, while CS displays anti-inflammatory activity, having a central role in the biological processes (Abdallah et al., 2020).
The enzymatic hydrolysis is the most standard applied method for the extraction of GAGs, requiring the use of specific enzymes (e.g., papain, trypsin, pepsin and pronase) to breakdown the protein core and isolate HA and CS macromolecules from other polysaccharide complexes (Sadhasivam et al., 2013; Abdallah et al., 2020). Among the enzymes’ diversity, the most commonly employed to recover CS and HA polysaccharides is papain owing to its highest efficiency. For instance, HA has been extracted from the liver of marine stingray Aetobatus narinari (usually discarded in the sea as a waste by food processing companies) through the use of papain (Sadhasivam et al., 2013). The enzymatic degradation can also be coupled with other extraction methods. In this regard, the CS isolation from thornback skate (Raja clavata) was carried out by combining the enzymatic degradation approach with chemical hydrolysis through the use of papain in the presence of an alkaline hydroalcoholic solution (Murado et al., 2010).
Although less exploited, other enzymes have also been used for enzymatic digestion. For instance, HA was extracted from the vitreous humor of fish eyes using a protease from Streptomyces griseus (Amagai et al., 2009).
Despite being the most standard employed extraction procedure, enzymatic digestion is time-consuming, requiring high energy consumption and several purification steps that lead to low extraction rates. To tackle these issues, the recovery of CS from Tilapia fish species encompassing ultrasonic-microwave synergistic extraction was attempted (Cheng et al., 2013). Several experimental variables were investigated, aiming to establish the optimal extraction conditions. Although being time and energy saving, the extraction yield was low (2.5%). Furthermore, more recently, one attempt to recovery CS from fish bones was carried out by high intensity pulsed electric fields (He et al., 2014). Results have shown that CS content (Table 2) was higher in comparison with the yield values obtained either by enzyme digestion or alkali extraction, holding a great promising strategy to recover bioactive compounds.
Ulvan (UL) is one of the sulfated polysaccharides extracted from the cell wall of green algae (Chlorophyceae). It can be isolated from seaweed from the genera Ulva and Enteromorpha, that usually have a negative connotation because they are associated with the phenomenon of eutrophication, a detrimental process for the aquatic environment (Chiellini and Morelli, 2011). Therefore, the exploitation of UL can be a remediation strategy towards this problem.
Owing to its variable chemical structure, different molecular weights have been reported for UL (Cunha and Grenha, 2016). Also, the extraction temperature has shown a significant impact in molecular weights variations between ULs extracted from the same species, pointing out that high temperatures are required to extract high molecular weights (Lahaye and Robic, 2007). UL extraction is usually performed using warm water solution (80–90°C) and could be enhanced by the presence of chelating agents (e.g., ammonium oxalate or calcium), acidic or alkaline solutions. Afterwards, UL is generally recovered by precipitation with organic solvents.
UL polysaccharide has also shown to possess different biological properties, such antioxidant (Li et al., 2018), antibacterial (Tran et al., 2018), immunomodulating (Tabarsa et al., 2012), antitumor (Lee et al., 2004), antihyperlipidemic (Pengzhan et al., 2003), antiviral (Aguilar-Briseño et al., 2015) and anticoagulant activities (de Carvalho et al., 2018). The sulphate groups in UL structure are known to be responsible for the aforementioned biological features (Chiellini and Morelli, 2011; Tziveleka et al., 2019).
In the literature, there is not much information regarding the use of green techniques in UL extraction. A recent work has reported the microwave-assisted hydrothermal extraction of UL from two different species, namely U. meridionalis and U. ohnoi (Tsubaki et al., 2016), attaining maximum extraction yields of 40.4 ± 3.2% and 36.5 ± 3.1%, respectively. Such values were significantly higher than that achieved with conventional hot water extraction (18.7% U. meridionalis) (Tsubaki et al., 2014). Furthermore, microwave-assisted procedure has also allowed to reduce drastically the extraction time (from 3 h to 14 min), without requiring the use of chelating agents and hazardous catalysts. In addition, UL extraction through microwave afforded the control of molecular weights and viscosity by varying the extraction temperature.
Alginate (ALG), fucoidan (FU) and laminarin (LAM) are extracted from marine brown algae (Phaeophyceae). Additionally, FU can also be extracted from some marine invertebrates (sea urchins and sea cucumbers) (Lee and Mooney, 2012; Quitain et al., 2013; Cunha and Grenha, 2016). Although LAM is still relatively unexplored, it has recently attracted the attention of the scientific community in the biomedical field due to its low viscosity, cytocompatibility and biocompatibility (Zargarzadeh et al., 2022).
Polysaccharides extracted from brown algae display biological properties, including antioxidant, anticoagulant, antiviral, anti-inflammatory, immunomodulation, and antitumor activities, highlighting their importance in the biomedical field (Saravana et al., 2018). These polysaccharides have been usually extracted from brown algae through several time-consuming extraction approaches, namely hot water, dilute alkali or acid, and various purification and fractionation processes.
Therefore, alternative methods encompassing less extraction times and the use of non-harsh solvents have been investigated, including microwave-assisted extraction (MAE) combined with hydrothermal treatment. One work has investigated a wide range of operational conditions (reaction time, pressure and alga/water ratio), aiming at establishing the best ones that could maximize the FU extraction yield (Rodriguez-Jasso et al., 2011; Quitain et al., 2013). The optimal extraction conditions (pressure 120 psi, 1 min extraction and 1/25 (g ml−1) algae/water ratio) have afforded a FU extraction yield of 18.2%. In another work, the microwave–hydrothermal extraction was combined with supercritical carbon dioxide to remove the lipid fraction from the seaweed, prior to FU recovery (Quitain et al., 2013). The hydrothermal process with microwave irradiation has enhanced the degradation of fucoidan into low molecular-weight fractions when compared to conventional heating. Lastly, attempts have been performed to extract fucoidan from Saccharina japonica through PLE, which doesn’t require the use of organic solvent. Although the crude FU yield was the best one achieved (8.2%) comparing to conventional methods (1.8%), microwave-assisted extraction seems to be faster and more efficient than PLE.
Apart from microwave, ultrasound-assisted extraction (UAE) has also been applied as an eco-friendly and time saving technique for the recovery of polysaccharides from brown seaweeds, namely laminarin and alginate (Kadam et al., 2015; Youssouf et al., 2017). In both studies, ultrasound has afforded the reduction of extraction time as well as enhanced the extraction yield over conventional methods.
More recently, one work has reported the synergistic effect of coupling deep eutectic solvent (DES) with subcritical water extraction (SWE) of brown seaweed polysaccharides (FU and ALG) from Saccharina japonica (Saravana et al., 2018). In this regard, the extraction solvent has involved the mixture of different types of DES with water at various concentrations. Alginate and fucoidan yields were different across DES solvents owing to the different electrostatic interaction strength established between these compounds and polysaccharides. Therefore, only one DES solvent (choline chloride:glycerol) has showcased higher extraction efficiency for both polysaccharides. At the optimal experimental conditions, the polysaccharides’ yield obtained in this DES catalyst solvent was at least twice (Table 2) comparing to dilute acid treatment.
Carrageenan (k-CG) and agar (or agar-agar) are marine polysaccharides derived from red seaweed (Rhodophyta) (Cunha and Grenha, 2016).
Owing to their benign nature, ionic liquids (ILs) and deep eutectic solvents (DES) have aroused particular interest as green catalyst in the extraction procedure of carrageenan (k-CG). They can be used either alone or combined with another eco-friendly extraction technique to enhance the polysaccharide yield. For instance, extraction of k-CG from Kappaphycus alvarezii was carried out by using either deep eutectic solvents prepared by the complexation of choline chloride with urea, ethylene glycol and glycerol or by water as standalone extraction media (Das et al., 2016). Interestingly, for the best DES formulation, k-CG yield has achieved the highest value when combined with water (60.3%) due to the higher affinity of DES charges towards k-CG, which are absent in pure water, thus showing lower extraction efficiency (46.9%). In another work, k-CG was extracted from the red marine macroalgae Kappaphycus alvarezii via subcritical water extraction (SWE) using ILs as catalyst solvent (Gereniu et al., 2018). Using aqueous SWE alone, the k-CG yield was slightly lower (71.0%) in comparison to SWE combined with ILs (78.8%) (Table 2). Nevertheless, the extraction efficiency of SWE technique was higher either in aqueous or ILs media when compared to conventional alkaline treatments (55.3%).
Concerning agar polysaccharide, one should be mentioned that its structure comprises agaropectin and agarose (AGR); the latter is a neutral and linear polysaccharide that results from enzymatic depolymerization and desulfation of agar (Tiwari and Troy, 2015; Cardoso et al., 2016). Therefore, agarose is usually retrieved by the purification of agar through chromatographic fractionations (Sharma et al., 2015).
Agar is only soluble in hot water (>85°C) and its cooling leads to the formation of thermoreversible gels (Laurienzo, 2010). Therefore, hot-water extraction with alkali pretreatment has been traditionally employed in agar extraction processes. Non-etheless, agar could be leached in hot alkali solution, negatively affecting the extraction yield. To tackle such concerns, an eco-friendly extraction approach of agar was recently explored by combining ultrasonication with two different strategies: i) a controlled alkali pretreatment followed by enzyme-assisted extraction (EAE) and ii) EAE standalone (Li et al., 2021), aiming at generating a synergistic effect by coupling more than one extraction procedure. In fact, EAE has shown to be relevant in both strategies since it has allowed the increase of agar extraction yield by 2 to 6-fold compared to the yield of a non-enzyme method for a total extraction time of 2–3 h. The ultrasonication technique has enabled the reduction of time extraction in 1h, which could be even shorter, at least 30 min, with an alkali pretreatment.
Novel materials based on polysaccharides are being intensively pursued through bulk and surface modifications. The chemical and structural modification of marine polysaccharides can improve their solubility, chemical and mechanical stability, processing routes, and the properties of the final polysaccharide-based materials (Siddhanta et al., 2015). Their unique functionalities can be adjusted throughout the coupling of specific functional groups, opening new perspectives for the design of advanced biomaterials. Among the functional groups present in polysaccharides, the hydroxyl groups (–OH) are the most widely explored and chemically modified, although other functional groups have also been used for chemical reactions, including amino (–NH2), carboxylic acid groups (–COOH) and aldehydes (–CHO) (BeMiller, 2004).
The modification of the polysaccharides’ structure through the introduction of acidic, basic, hydrophilic, hydrophobic, or other molecules with specific properties will not change fundamentally the polysaccharide backbone. However, it will unlock advanced modifications required for specific applications, and will change the final properties of the developed biomaterials. The development of novel chemical methodologies is an ongoing research area that is expected to become more important over the years, as the importance on using renewable starting materials and sustainable chemical processes are expanding. The methods described in this section (Figure 2) include nucleophilic reactions of the amines or hydroxyl groups, oxidation of polysaccharides, introduction of nucleophiles into their chains, and activation of functional groups, always taking in account green chemistry approaches or mild conditions commonly employed to modify the marine polysaccharides’ backbone.
FIGURE 2. Schematic illustration of major modification strategies applied in polysaccharides based on their functional groups: (A) Conjugation to the polysaccharides structure with EDC/NHS coupling chemistry; (B) Insertion of methacrylated moieties into the polysaccharide backbone and photo-crosslinking reaction to produce hydrogels; (C) Click-chemistry and (D) Oxidative cleavage.
Covalent chemical crosslinking is a widely used technique to enhance physical and chemical stability of the polysaccharide derivatives (Parhi, 2017). In the past decade, 1-ethyl-3-(3-dimethyl-aminopropyl-1-carbodiimide) (EDC) was the most common used chemical agent in carbodiimide crosslinking chemistry and emerged as a popular coupling agent in reactions involving carboxylic acid and amino functional groups, owing to its mild reaction condition, water solubility and self-degradability (Azevedo et al., 2017; Silva et al., 2017; Sousa and Mano, 2017). This graft approach allows to coupling specific molecules with distinct properties, such as methacrylates (Diolosà et al., 2014), catechols (Azevedo et al., 2017; Sousa and Mano, 2017; Costa et al., 2020), among others, into the polysaccharide backbone through a one-step amidation reaction between the amino and carboxylic acid groups. Such coupling reaction is conventionally employed to endow the developing material with application-specific properties (Ye et al., 2017; An et al., 2018; Wang Z. et al., 2018; Sánchez-Téllez et al., 2020). The EDC coupling agent can be used alone or with an additive, usually N-hydroxysuccinimide (NHS), which catalyzes the formation of an amide bond between the carboxyl and the amino groups (Sideris et al., 2016), thus improving the reaction efficiency. Toxicity is absent in the carbodiimide reaction, since EDC is transformed into a non-toxic urea derivative (Park et al., 2002). Other carbodiimide agents like dicyclohexylcarbodiimide (DCC) and N,N′-diisopropylcarbodiimide (DIC) are water-insoluble requiring organic solvents wherein most polysaccharides are non-soluble. Under extreme acid or basic conditions, the EDC/NHS coupling reaction gives rise to the formation of secondary products, thus decreasing the yield efficiency. After the EDC activation of the carboxylic groups, which is more efficient at a pH ∼ 4.5, NHS can be added to the reaction mixture, thus converting the extremely unstable O-acylisourea intermediate into a stable NHS ester. The optimal pH range for the reaction of NHS ester with amine group occurs between 7.2 and 9.0. According to these straightforward reaction conditions, the use of (4-(4,6-dimethoxy-1,3,5-triazin-2-yl)-4-methyl-morpholinium chloride) (DMTMM) showcases major advantages over the conventional EDC/NHS chemistry, since the coupling reaction doesn’t require an accurate pH control for the formation of the amide bonds (D’Este et al., 2014; Borke et al., 2015; Fan et al., 2019) as a result of the coupling reaction between the amino group and the activated ester, produced from the addition of DMTMM to a carboxylate anion (D’Este et al., 2014).
Light-initiated polymerization is an important process for the development of novel biomaterials. The insertion of methacrylate groups (MA) into the polymer backbone to be further photo-crosslinked is a widely applied chemical route in natural polysaccharides and used in the fabrication of several biomedical devices, such as 3D hydrogel structures (Bjørge et al., 2018; Martins et al., 2018), cell encapsulation systems (Khademhosseini et al., 2006), and in drug delivery platforms (Jeon et al., 2009). The introduction of photo-crosslinkable moieties into polysaccharide backbones can occur across two distinct functional groups: i) primary hydroxyl groups (Custódio et al., 2016; Martins et al., 2018; Zargarzadeh et al., 2022) and ii) amine groups (Costa and Mano, 2015; Bjørge et al., 2018). The methacrylation reaction via hydroxyl and amine groups is carried out through substitution reaction, achieving a higher substitution degree for the latter one due to its nucleophilic strength (higher for amines than hydroxyl groups) (Wang H. et al., 2018; Kolawole et al., 2018). Concerning polysaccharides containing carboxylic acid groups, the metacrylation step is carried out through a coupling reaction with amino groups (Jeon et al., 2009). In such case, the methacrylation degree is commonly lower than the substitution reaction owing to the non-controllable side reactions (Costa and Mano, 2015).
Click chemistry (CC) has emerged as a powerful tool for design and synthesis novel polysaccharide derivatives in a more controlled and modular manner (Meng and Edgar, 2016), overcoming major drawbacks from conventional synthetic pathways such as low selectivity, side reactions, low substitution efficiency and low yields. Therefore, click chemistry (CC) is a sophisticated and selective chemical route to covalently link two molecular components via simple and high-yielding chemistry. This reaction still fulfills other requirements accounted in the broader Sharpless’ click definition (Kolb et al., 2001), namely both high chemoselectivity and efficiency, wide in scope, simple reaction condition and product isolation as well as fast reaction rate generating inoffensive and easily removable by-products.
The term “click-chemistry” is especially well-known when related to the copper catalyzed azide-alkyne cycloaddition (AAC). The AAC is still the leading technology among CC reactions due to the easy modification and incorporation of azide and alkyne groups into the polysaccharide structure. The application of AAC chemistry in polysaccharides has contributed to generate complex glycoconjugates that allowed the development of new biomaterials exhibiting improved properties and functions (Elchinger et al., 2011), assuring high yields and highly selective products by carbon-hetero bond formation reactions, which results in the formation of a triazole ring unit (Tan et al., 2018). On the other hand, it is also possible to use the biorthogonal click reaction through the strain-promoted azide-alkyne cycloaddition (SPAAC), thus avoiding the use of the cytotoxic copper catalyst (copper-free chemistry). In this scope, we highlight the recent Nobel Prize in Chemistry in which Carolyn Bertozzi has applied such elegant and efficient chemical reaction inside living organisms, without disrupting the normal cell chemistry. Such development has leveraged click chemistry to a new dimension, affording to map cells and track biological processes as well as to improve the targeting of cancer pharmaceuticals (Cioce et al., 2022; Stanczak et al., 2022).
Several reviews have discussed the multiple applications of the (bio)conjugates arise from click-chemistry (Meng and Edgar, 2016; Fantoni et al., 2021; Solberg et al., 2022). Here, we will not summarize the works-to-date on the application of CC to natural polymers but rather focus more precisely on the description of cycloaddition reactions employed in marine polysaccharides, highlighting this powerful chemical binding strategy. Thus, incorporating “click” moieties (e.g., azide or alkyne) into the polysaccharides structure affords either the creation of new materials by “click” crosslinking (Han et al., 2017; Castanheira et al., 2020; Heida et al., 2020; Moody et al., 2020) or the introduction of several functional active molecules for different purposes (Lee et al., 2014; Breger et al., 2015; Duan et al., 2018; Hui et al., 2021).
Oxidation in polysaccharides can occur at primary and/or secondary hydroxyl groups according to the chemical nature of the mild oxidant agent, thus producing different type of structures (e.g., aldehyde, ketone or carboxylic acid). Typically, the oxidation of primary hydroxyl groups in polysaccharides gives rise to an aldehyde or further to a carboxylic acid group with structural similarity to alginate, while oxidizing secondary alcohols leads to a ring-opening oxidative C–C bond cleavage giving dicarbonyl compounds (Cumpstey, 2013).
Selective oxidation has been attempted using nitrogen dioxide, nitroxyl radical 2,2,6,6-tetramethylpiperidine-1-oxyl (TEMPO) or periodate salts as mild oxidants. Using nitrogen dioxide, primary hydroxyl groups at the C6 position can be oxidized into carboxyl groups. However, oxidative cleavage of polysaccharides may concurrently occur as side reaction, modifying the polysaccharide composition, which is a major drawback (Bragd et al., 2004). In contrast, TEMPO-mediated oxidation features comprise moderate molecular degradation, high selectivity and fast reaction rate (Matsumura and Rajan, 2021). This oxidant agent is suitable for selective oxidation of primary alcohol groups into aldehydes and/or carboxylic acid groups. The selective oxidation of polysaccharide primary alcohols either to the aldehyde or the carboxylic acid levels will rely from the proper selection of oxidizing conditions.
Concerning secondary hydroxyl groups (C-2 and C-3), their oxidation is usually performed by using periodate that causes the oxidative scission of vicinal diols and, ultimately, the formation of dialdehydes or diketones. These groups exhibit high reactivity via Schiff base chemistry with compounds bearing amine moieties, thereby widely used for the fabrication of functional biomaterials as hydrogel crosslinking strategy and drug conjugation (Lavrador et al., 2020; Noser et al., 2021).
The development of polysaccharide-based fibers and particles has gathered considerable attention in the biomedical field due to their high surface-to-volume ratio, enhancing loading of cargo as well as efficient nutrient and metabolite transfer and cell-matrix interactions (Kee Jo et al., 2020; Iliou et al., 2022). Advances in precision processing technologies have triggered the design of engineered polysaccharide-based biomaterials with well-defined shapes and sizes, especially aimed at tuning their physicochemical properties for the targeted biomedical application. One of those highly precise and versatile technologies is electrospinning that allows the fabrication of a plethora of polymeric fibrous mesh architectures with controllable fiber diameters, morphologies and arrangements by tailoring electrospinning parameters. These electrospun fiber mats have been widely used as scaffolds in tissue engineering sector (Table 3) due to their inherent fiber morphology that resemble the morphological structure found in native ECM (Silver et al., 2003; Järvinen et al., 2004; Bürck et al., 2013; Wade et al., 2015).
TABLE 3. Marine-derived polysaccharides and their processing as fiber/particulate systems for biomedical applications.
Despite the attractiveness of marine polysaccharides for producing biocompatible electrospun scaffolds, electrospinning of polysaccharide pristine solutions has proven to be challenging since they have poor solubility in common volatile organic solvents as well as low molecular weight, thus hampering stable fiber formation through conventional electrospinning methods. Likewise, marine polysaccharides are often combined with synthetic water-soluble biopolymers, including polyvinyl alcohol (PVA), polyvinyl pyrrolidone (PVP) and polyethylene oxide (PEO), thus providing the desired electrospinnability for continuous fiber formation (Toskas et al., 2011; Sousa et al., 2015b; Guo et al., 2016; Ahire et al., 2017). In particular, the use of electrospinning aqueous medium instead of the harsh organic standard solvents has rendered this technique more attractive not only to produce fully biocompatible tissue-engineered scaffolds, but also to load hydrophilic bioactive compounds that could be intolerant to organic solvents. Antibiotics and antibacterial agents are the most representative hydrophilic biocides that have been successfully blended with water-soluble marine polysaccharides to produce drug-loaded fibers with controlled release profile, thus enhancing wound healing performance (Guo et al., 2016; Ahire et al., 2017; Kyzioł et al., 2017; Amiri et al., 2020). However, in the blending electrospinning method, the drug is mostly localized on the electrospun fiber surface, giving rise to an obvious initial burst release. Rather than blending electrospinning, another spinning method that enables the development of drug loaded polysaccharide fibers is coaxial electrospinning wherein core-shell fibers are formed. Drugs encapsulated in the core layer have exhibited a gradual drug release, while being protected from direct exposure to the biological environment by the polymer shell (Hu et al., 2014). Drugs can also be loaded within both core and shell layers, endowing the electrospun fibers with dual drug release profile. Electrospun core-shell fibers with Nylon-6 as the inner core and CHT/polyethylene oxide as the outer shell were loaded with different antibacterial drugs, yielding a binary antimicrobial system with spatial control release (Keirouz et al., 2020).
Apart from water-based solvents, other green spinning solvents (Avossa et al., 2022), namely formic acid, acetic acid, dimethyl sulfoxide, or a combination thereof, have also been employed to produce electrospun fibers based on CHT (Li et al., 2015; Amiri et al., 2020; Keirouz et al., 2020; Vargas-Osorio et al., 2022), which is soluble in concentrated organic acids. Despite the acid character of those solvents, resulting fiber mats have showcased no cytotoxic effects on cellular responses, thus being suitable to be applied as tissue engineered scaffolds (Li et al., 2015; Kong et al., 2021; Vargas-Osorio et al., 2022). Non-etheless, marine polysaccharide fiber mats generated in aqueous conditions have limited mechanical stability in water-based media, losing their fibrous morphology over time. Therefore, polysaccharide fiber mats have been crosslinked during (in-situ) or after (post) electrospinning process, thus enabling the formation of stable hydrogel-based nanofibers. Moreover, crosslinking treatments also contribute to a sustained and prolonged drug release, thus preventing an initial burst release.
Although glutaraldehyde has been reported as the most commonly used crosslinker agent (Guo et al., 2016; Zhang et al., 2017), its hazardous nature could impair scaffold transplantation in tissue engineering scenarios. In this regard, research efforts have been focused on the use of less cytotoxic and more environmental friendly crosslinking strategies, encompassing benign crosslinkers (e.g., genipin, enzymes, EDC alone or with NHS) (Gruppuso et al., 2022), polysaccharide complexation (Chen et al., 2021), thermal treatment (C Madruga et al., 2021) or UV-light exposure (Kianfar et al., 2019). While these green methods require the use of catalysts or external energy inputs for post-crosslinking electrospun fibers, a fast and one-step fiber drawing and crosslinking approach was recently proposed in the form of multiple fibrous hydrogel populations (Figure 3A). These fibers comprised hyaluronic acid-based polysaccharide functionalized with complementary chemical moieties (e.g., aldehyde and hydrazide groups) that were able to form covalently hydrazone bonds within minutes, when brought into contact under mechanical load (Davidson et al., 2020). The combination of multifiber electrospinning with complementary dynamic chemistry has enable the development of fibrous hydrogel networks with mechano-sensitive properties, emulating both structural and mechanochemical features of the native ECM.
FIGURE 3. (A) Multifiber electrospinning to fabricate fibrous hydrogel networks with complementary chemical moieties (aldehyde and hydrazide groups) that form covalent bonds under mechanical. Adapted from ref (Davidson et al., 2020). with permission from John Wiley & Sons, Copyright 2019 WILEY-VCH Verlag GmbH & Co. KGaA, Weinheim; (B) Production of spheroid-like microgels by using superhydrophobic surfaces. Adapted from (Bjørge et al., 2018) with permission from American Chemical Society, Copyright 2018.
Polysaccharide-based particulates, namely microparticles, microgels and microcapsules, have also been broadly applied as individual drug carriers and cell culture platforms (Table 3). Different environmentally friendly strategies have been employed to fabricate spherical polysaccharide-based particulates, spanning from conventional emulsion techniques, droplet microfluidics and electrospraying to superhydrophobic surfaces. The innate presence of functional groups on marine polysaccharides affords specific chemical modification that could be synergistically combined with the aforementioned processing technologies to give rise to advanced particulate systems. Recently, biodegradable LAM-based microparticles were produced via triazole crosslinking between alkyne-modified LAM and hydrazine-modified LAM through standard microemulsion (Castanheira et al., 2020). Marine polysaccharide microparticles could also be functionalized with specific antibodies for selective recruitment of different cell types from a mixed cell population (Custódio et al., 2015; Martins et al., 2018). Beyond cell sorting applicability, these platforms could also function as microcarriers for cell expansion to be further applied in TERM either as injectable scaffolds or as modular units for large-scale scaffolding constructs.
Other particulate systems that have also been gained momentum as injectable platforms and building blocks for bottom-up TE approach are microgels, where different populations could be combined into a single granular structure, affording multiple functionalities (Mealy et al., 2018). Micropatterned superhydrophobic surfaces have emerged as one promising eco-friendly and cost-effective approach for high-throughput fabrication of marine polysaccharide-based microgel particles and microcapsules, with multiplex shapes and sizes (Neto et al., 2016; Nadine et al., 2020). Also, standalone superhydrophobic surfaces have afforded the fabrication of core-shell and multicompartmental microgels comprising a photo-crosslinkable CHT outer layer and an ALG core, which could be further liquefied giving rise to a liquid microenvironment that enhanced nutrient up-take and disposal of waste products by encapsulated cells (Costa and Mano, 2017). Non-modified CHT and ALG polysaccharides have also been used as layer-by-layer (LbL) building-blocks in the fabrication of either hollow (Ribeiro et al., 2018) or liquefied multilayered capsules (Nadine et al., 2019, 2022; Bjørge et al., 2022), which could be used for tailoring cellular internalization and bone repair, respectively. Recently, the production of marine polysaccharide-based particulate systems through superhydrophobic surfaces expanded beyond the standard spherical-shaped ones. In this regard, droplets of the precursor microgel were squeezed between two superhydrophobic surfaces separated with spacers with different height (Figure 3B), and then photo-crosslinked to maintain the acquired spheroidal shape after “de-sandwiching” (Bjørge et al., 2018). Those spheroid-like microgels have showcased an improved viability of encapsulated cells due to enhanced nutrient diffusion to the core as well as faster drug release rate from the polymer network.
Marine-origin polysaccharides have also been widely used either alone or in combination with other (bio)polymeric materials to prepare membranes, denoting fine-tuned properties and functions at the nanoscale, by resorting to green processing methodologies. Solvent casting/evaporation, LbL assembly technology, and polyelectrolyte complexation have been among the most commonly used methodologies to process biocompatible marine polysaccharide-derived self-standing membranes (Table 4) of the desired size and geometry and without the need for specialized equipment to be used in a wide range of biomedical applications.
TABLE 4. Membrane-shaped biomaterials based on marine-derived polysaccharides for biomedical applications.
Over the last 2 decades, native and crosslinked wholly marine polysaccharide and blended membranes have been produced at a fast pace by solvent casting followed by solvent evaporation and their physicochemical, structural and biological properties studied (Silva et al., 2005; Wu et al., 2010; Yang et al., 2016), revealing that the crosslinking triggers the preparation of stiffer membranes with enhanced cell functions (Silva et al., 2013; Khaliq et al., 2022). Due to their physicochemical, structural and biological properties, these membranes have shown to be promising biomedical devices for the loading and sustained release of drug/therapeutic agents (da Silva et al., 2008; Murata et al., 2010) and as wound dressings (Silva et al., 2013; Khaliq et al., 2022). Moreover, hot press techniques, namely thermo-compression molding has been used to process marine polysaccharide-based composite biofilms/membranes, denoting good mechanical strength and swelling behavior for sustained drug release (Hashizume et al., 2016), controlled molecular permeability (Sagawa et al., 2022), and cell adhesion and proliferation (Iijima et al., 2017). Furthermore, due to their cost-effective and ease of scalability, they can be easily translated into industrial applications. However, the composite films produced with CHT via either solvent casting or thermo-compression molding resort to common organic acids for enabling its solubilization. As such, the scientific community has been looking for benign solvents to enable the production of biofilms. In particular, deep eutectic solvents have emerged as alternative green plasticizers for the sustainable production of more homogeneous, compact, and environmentally friendly marine polysaccharide derived composite biofilms/membranes, denoting improved mechanical properties and flexibility (Sousa et al., 2014; 2015a; Galvis-Sánchez et al., 2016, 2018; Zdanowicz et al., 2018). Although such biofilms have been mostly processed for food packaging applications, they hold great potential for being applied in biomedical applications, particularly as wound dressings. Still, the fact that high temperatures are employed in the membranes’ production process poses a major challenge to work with sensitive macromolecules susceptible to thermal degradation and that would benefit from alternative processing strategies under physiological temperature. In this regard, compact biofunctional polyelectrolyte complexes membranes encompassing two oppositely charged marine polysaccharides have been produced at physiological temperature by polyelectrolyte complexation, in the presence of salt, followed by sedimentation and temperature-assisted solvent evaporation for compaction. In particular, microsized soft compact saloplastic membranes encompassing CHT/ALG and CHT/CS have been produced and their physicochemical and biological properties studied aiming for being used as drug/therapeutic carriers and adhesives for soft tissue engineering/regeneration (Wang et al., 2002; Costa et al., 2015; Rodrigues et al., 2016; Zhu et al., 2017; Hardy et al., 2018). It was found that their physicochemical and biological properties could be easily tailored to meet the tissues to be regenerated by playing with the polyelectrolyte types, assembly conditions (e.g., pH, ionic strength, temperature, concentration) and compaction strength.
The production methodology can be easily scaled-up and at a faster pace than the classical solvent casting and LbL assembly methodology due to the high concentration of the polyelectrolyte complexes at the beginning of the drying step. Compact membranes denoting improved adhesive strength to soft tissues at physiological conditions have also been produced by polyelectrolyte complexation and compaction by resorting to hybrid synthetic/natural polyelectrolyte complexes, showing great promise as biomimetic sealants for the regeneration of soft biological tissues (Costa et al., 2019).
Over the last 2 decades, we have witnessed to the emergence of the LbL assembly technology as a green bottom-up assembly methodology to process multilayered films/membranes, under mild conditions, in entirely aqueous solutions and on virtually any type of substrate for biomedical applications (Borges and Mano, 2014). The LbL assembly process is a rather time-consuming methodology when compared with the aforementioned methodologies but enables the incorporation of multicomponents and, thus, multifunctionalities in a single membrane and a better control in thickness. Smooth flat hydrophobic and hydrophilic non-patterned or patterned inert templates have been used to produce biocompatible multilayered films, with well-defined structures, compositions, and thicknesses at the nanoscale by repeating their alternate immersion in aqueous solutions of at least two oppositely charged marine polysaccharides via dip-assisted LbL assembly methodology (Larkin et al., 2010; Caridade et al., 2013, 2015; Silva et al., 2014; Martins et al., 2017; Sousa et al., 2017). In the end of the assembly process, the multilayered films are easily detached into robust and easily handled free-standing multilayered membranes whose nanotopography recreates the substrate’s features. For instance, CHT/ALG multilayered films were assembled on hydrophobic poly (propylene) substrates and further detached, without any post-processing step, into free-standing membranes by playing with the solution pH, polyelectrolyte concentration and number of adsorbed layers (Caridade et al., 2013). These membranes demonstrated to be stable in physiological media and enabled a faster permeation of model drugs, thus holding great potential for being used as reservoir of therapeutic agents. Furthermore, C2C12 myoblast cells adhered preferentially onto the ALG-ended membranes, thus being potentially used as wound dressings for muscle tissue engineering strategies. This work launched the foundations of several studies encompassing either solely marine polysaccharides or hybrid multilayered systems and further covalently crosslinked aiming to develop free-standing membranes with increased stiffness and better cell response (Silva et al., 2014; Caridade et al., 2015; Gil et al., 2015). In this regard, CHT/ALG freestanding membranes chemically crosslinked with EDC enabled the proliferation and differentiation of C2C12 myoblasts into myotubes, a process whose extent can be easily modulated by playing with the concentration of EDC. Furthermore, the membranes with the highest concentration of EDC demonstrated to be osteoinductive in vivo, thus holding great promise for bone repair (Caridade et al., 2015). The effects of either hydration or ionic cross-linking have also been demonstrated as contributing to the development of smart self-sustained membranes denoting adhesive properties and shape memory capability (Figure 4A) to be used as implantable biomaterials via minimally invasive surgery (Borges et al., 2015; Silva et al., 2016). In addition, bioinspired free-standing membranes functionalized with catechol moieties have shown enhanced mechanical strength, cellular adhesion and proliferation, and adhesive properties for being used as bio-adhesive patches for wound healing and general surgery applications (Scognamiglio et al., 2016; Sousa et al., 2018; Moreira et al., 2020).
FIGURE 4. (A) Shape memory behavior of (CHT/ALG)100 freestanding multilayered membranes induced by hydration. Reproduced from ref (Borges et al., 2015). with permission from John Wiley & Sons, Copyright 2015 WILEY-VCH Verlag GmbH & Co. KGaA, Weinheim; (B) Freestanding multilayered membranes patterned with micro-wells, wherein cells tended to colonize preferentially. Adapted from (Martins et al., 2017) with permission from Elsevier, Copyright 2017.
Besides, enzymatic-sensitive polysaccharide-based freestanding membranes have been developed as smart carrier vehicles for the controlled release of bioactive agents in tissue engineering and regenerative medicine strategies (Cardoso et al., 2016). Such strategy was also recently applied to produce protein-loaded mucoadhesive buccal patches for sublingual drug delivery (Paris et al., 2021). Those patches consisted on biodegradable freestanding membranes comprising CHT and HA multilayers, in which the CHT outer layers afforded mucoadhesion owing to the electrostatic interaction of CHT positive charges with negative glycoprotein acid residues from the mucosa surface. Through pH changes, protein rich-ovalbumin was efficiently loaded into the freestanding LbL membranes, producing bioactive patches. Upon sublingual administration in a mouse model, the protein delivered by bioactive patches was detected into the sublingual epithelium 10 min after patch administration, while remaining in the mouse mouth for at least 30 min, even after the enzymatic degradation of the membrane. Such delivery system could open a promising alternative to oral administration, affording a sustained drug release profile when compared to sublingual liquid administration wherein the liquid drug formulation was quickly washed-out in the saliva and poorly delivered at mucosal sites.
More recently, flat patterned templates have gained momentum due to the need to produce bioinstructive patterned freestanding membranes that could recreate not only the structural but also the topographical features of highly aligned tissues, such as bone or nervous tissue, enabling a better regulation of cell functions. Micro-patterned polydimethylsiloxane substrates have been used to produce (CHT/ALG)100 free-standing membranes denoting a well-defined array of geometrical features at the microscale in which osteoblast-like cells tended to colonize preferentially (Figure 4B), holding great promise as micro-reservoirs of therapeutic agents or as cell carriers in bone tissue regeneration (Martins et al., 2017). More recently, nanogrooved CHT/CS freestanding membranes assembled onto nanopatterned polycarbonate substrates guided C2C12 myoblast cells’ alignment along the nanopattern direction and triggered their differentiation into myotubes (Sousa et al., 2017). Such an approach is of high value-added for muscle tissue regeneration but could also be extend for the regeneration of other tissues whose cells respond to topographical cues, such as neuronal cells, thus potentially enabling the regeneration of the nervous system.
Hydrogels have been one of the bioengineering platforms highly explored in the biomedical field to mimic and restore the native tissues’ functionalities. They offer similarity to the ECM, high-water content, mechanical support for cellular activities, and the opportunity to incorporate different molecules (e.g., drugs, cytokines or growth factors). Owing to their structural similarity to ECM compounds, marine polysaccharides have also been extensively employed as precursor building blocks in the hydrogels’ design either in the form of injectable systems or as implantable 3D constructs (Table 5).
Although providing an adequate microenvironments to guide cells, conventional polysaccharide hydrogel-networks have been widely applied as passive and static 3D supports for embedded cells, thus lacking the innate ECM viscoelasticity and its highly dynamic behavior that enables matrix remodeling (Neves et al., 2020). Aiming at recapitulating such features, innovative chemistry toolboxes have been actively investigated toward the design of ECM biomimetic networks (Kirschning et al., 2018; Jiang et al., 2019). Among available chemical strategies, incorporating dynamic bonds into marine polysaccharide hydrogels has been emerged as one of the most attractive approach to recapitulate specific ECM functionalities. In this regard, viscoelastic and adaptable hydrogels comprising laminarin chemically modified with pendant aldehyde moieties and gelatin were engineered via Schiff base crosslinking chemistry (Figure 5A) (Lavrador et al., 2020). Adjusting aldehyde-to-amine ratios afforded a fine-tuned control over crosslinking kinetics, viscoelastic properties, and degradability profile. Furthermore, complex topographies were able to be imprinted on post-crosslinked hydrogel through mechanical imprinting process due to the covalently adaptable hydrogel networks that underwent spatially rearrangement during compressive loading.
FIGURE 5. (A) Dynamic covalently adaptable hydrogels imprinted with an anisotropic nanotopographical configuration where cells have aligned along the intercalating nanoridge topography. Adapted from (Lavrador et al., 2020) with permission from John Wiley & Sons, Copyright 2020 WILEY-VCH Verlag GmbH & Co. KGaA, Weinheim; (B) Ascidian-inspired gallol-modified hyaluronic acid hydrogel adhered to various organs through covalent binding of gallol moieties with nucleophilic groups present at tissues interface. Adapted from (Cho et al., 2018) with permission from John Wiley & Sons, Copyright 2017 WILEY-VCH Verlag GmbH & Co. KGaA, Weinheim.
The incorporation of dynamic bonds into hydrogels also leverages on-demand hydrogel functionalities as self-healing and/or shape-memory properties with peculiar interest on injectable tissue engineering strategies (Mehrali et al., 2017). Inspired on the mussel byssus adhesiveness, a robust marine polysaccharide-based hydrogel was fabricated by combining a chemically crosslinked chitosan-based network with dynamic non-covalent bonds through metal coordination of iron (III) cations with pendant catechol moieties present into the CHT backbone (Azevedo et al., 2017). Such physical crosslinking has enabled efficient energy dissipation as sacrificial bonds upon compressive deformation and, ultimately, excellent toughness, fast self-recovery capability, good fatigue resistance, shear-thinning and self-healing properties. Besides coordination bonds capability, catechol and other phenolic groups also endow polysaccharide-based hydrogels with outstanding self-adhesive properties on wet conditions, thus making them suitable as a surgical sealant or glue for sutureless wound closure (Kim et al., 2020; Li et al., 2020; Zhou et al., 2020). The mechanism behind tissue adhesion mainly relies on the strong binding affinity of oxidized catechol moieties towards different nucleophilic groups (e.g., amine, thiol and imidazole) of peptides/proteins found at tissues interface (Kim et al., 2020). So, catechol- and gallol-grafted hyaluronic acid hydrogels have showcased strong adhesion onto the surface of various organs (Shin et al., 2015; Cho et al., 2018), including liver, heart and kidney (Figure 5B), remaining tightly bound for up to 2 weeks. Considering the strong tissue adhesiveness of these mussel-inspired hydrogels, they could be also applied as delivery therapeutic cells without injection or invasive procedures (Cho et al., 2018; Koivusalo et al., 2019). For instance, human adipose-derived stem cells (hASCs) encapsulated in catechol-grafted hyaluronic hydrogel were directly painted onto the infarcted area of rat hearts, providing an effective injection-free cell transplantation into the ischemic myocardium (Shin et al., 2015). Owing to their high affinity to nucleophiles, mussel-inspired polysaccharide-based hydrogels can also immobilize various growth factors and bioactive molecules that would impact cellular behavior and functions. It was found that incorporating basic fibroblast growth factor (bFGF) into catechol-grafted hyaluronic acid hydrogels increased the viability and hepatic function of human hepatocytes without a loss of loaded biomolecules over 2 weeks (Shin et al., 2015), thus indicating that the embedded growth factors could be chemically conjugated to hydrogel catechol moieties rather than being physically entrapped within it (Lee et al., 2013).
Recently, self-healable hydrogels have also been employed as supporting baths for embedding shear-thinning hydrogel inks (Highley et al., 2015; Hoon Song et al., 2018). Both hydrogels were assembled through guest–host interactions by functionalizing HA with guest–host pairs of adamantane (Ad) (Ad-HA) and β-cyclodextrin (β-CD) (CDHA). Additionally, in one of those studies (Hoon Song et al., 2018) the support hydrogel was further reinforced with norbornene (Nor) (AdNor-HA) to be covalently crosslinked through a thiol-ene reaction. Such chemistry facilitated the stabilization of the support hydrogel, while removing the self-healable hydrogel ink by disrupting the guest–host bonds with a solution containing excess β-CD. Such approach has been particularly attractive for printing complex and freestanding 3D constructs with open channels. Aside from providing the stabilization of supporting bath, norbornene pendant moieties have also allowed the further anchorage of arginine–glycine–aspartic acid (RGD) peptides, enabling the endothelial cells’ seeding and their sprouting into the supporting hydrogel, which underwent on-demand protease-sensitive degradation when cells were exposed to angiogenic factors. The formation of confluent cell monolayers within complex and different blood vessel-shaped microchannels hold great potential for engineering vascular grafts.
One should be mentioned that the use of cyclodextrin and adamantane guest–host interactions has also been advantageous to self-assembly hyaluronic-based microgels, affording the formation of granular hydrogel systems (Mealy et al., 2018). In particular, such guest-host interparticle crosslinking have imparted shear-thinning and self-healing properties to the granular hydrogel, rendering it amenable to injection into dynamic environments. The possibility of combining microgels with varied compositions into a single granular hydrogel hold great potential for the therapeutic design of hydrogels with multiplex functionalities.
Other recent strategy to design functional and perfusable vascular networks for embedding into large-scale hydrogel constructs, wherein nutrient/oxygen diffusion limitations compromised cellular viability into the hydrogel core region, involves the synergistic combination of the 3D printing and the layer-by-layer (LbL) assembly technologies (Sousa et al., 2021). In such approach, alginate was employed as ink to produce customizable 3D sacrificial microstructures that were further coated with bioinstructive CHT and RGD-coupled ALG multilayers via LbL assembly. After embedding the LbL-coated ALG structures into a shear-thinning photo-crosslinkable supporting hydrogel, the alginate core template was liquefied giving rise to perfusable microchannels wherein the multilayered membrane shell acts as physical selective barrier, thus mimicking the native basement membranes that surrounded most mammalian tissues. This work was an interesting approach to recapitulate the native 3D microenvironment where cells reside in vivo.
With the significant advances on the hydrogels’ design, new classes of hydrogel-based biomaterials have been emerged such as cryogels (Eggermont et al., 2020) and ionogels (Luo et al., 2022). Cryogels are injectable preformed scaffolds with shape–memory properties, typically formed in water solvent with controlled polymerization subzero temperatures (Jones et al., 2009). Such conditions affords the formation of sponge-like structures with highly interconnected macroporous network that facilitates cellular infiltration, cell organization and proliferation, thus being suitable for TERM applications, drug delivery and immunotherapy (Memic et al., 2019). Recently, covalently crosslinked cryogels made of methacrylated-hyaluronic acid functionalized with RGD domains were engineered to withstand autoclave sterilization, which has substantially improved the cryogel biocompatibility after subcutaneous injection, with minimal induced immune reaction at the implantation site (Villard et al., 2019).
Concerning ionogels, they are polymeric networks that are swollen with ionic liquids (ILs). In particular, the use of ILs with polysaccharides are attractive to endow them with electrical properties towards the development of electroactive tissues (e.g., cardiac, skeletal, muscle, brain, and nerve). For instance, VPImBF4 ionic liquid was grafted onto methacrylated-chitosan upon UV-light exposure, enabling the formation of an electroactive hydrogel, which conductivity matched the one reported for spinal cord tissue (Wang et al., 2022). Therefore, such ECM-like electroactive hydrogel has enhanced the neuronal differentiation response, also triggering the neovascularization. Such ionogel offers a new approach to engineer electroactive implants for spinal cord injury repair.
The bioeconomy paradigm aims to foster a sustainable and circular economy supported by renewable biological resources. However, the growing interest on the use of marine organisms for a multitude of high-end uses may impact involuntarily their natural populations and negatively affect the marine ecosystems. Even marine taxa which have been explored for several decades are now being more closely monitored and their collection from the wild more strictly regulated. This is, for example, the case of Gelidium, the highly prized red seaweed used to produce Agar since the end of the 1800s. When mixed with water, this gelatinous substance forms hard gels and is used around the world by biologists to culture microorganisms. The growing concern with the conservation status of natural populations has led authorities from the main supplying countries (e.g., Morocco and Spain) to restrict the harvest of Gelidium. With no signs for the global demand for Agar to slow down in upcoming years, with no unexplored wild populations of Gelidium available to supply algal biomass and with its production through aquaculture not being technically nor economically feasible, prices for this polysaccharide have skyrocketed.
The scenario described above for Gelidium may eventually also occur for other seaweeds currently harvested from the wild and whose highly prized polysaccharides may also experience an increase in price. From brown seaweeds yielding FU (e.g., Fucus), ALGs and LAM (e.g., Laminaria and Saccharina), to red seaweeds sourced for k-CG (e.g., Chondrus and Eucheuma) and green seaweeds to produce UL (e.g., Ulva), their natural populations may soon be unable to cope with the high demand if aquaculture is unable to boost supply. Seaweeds biodiversity is mirrored by their chemodiversity (Leal et al., 2013), as their life history allowed them to colonise different habitats along the depth gradient. While green seaweeds commonly occur in shallower waters, as their photosynthetic pigments are less efficient harvesting light wave lengths that can penetrate deeper in the water column, brown seaweeds have evolved to colonise deeper waters. Non-etheless, red seaweeds were the ones that have evolved to better harness the light wave lengths that can still penetrate to the lower limit of the photic zone (Figure 6). If one focus in Europe, it is possible to see that over the last two decades the harvesting of seaweed populations from the wild continued to be the primary production source for macroalgal biomass. It is therefore urgent to boost aquaculture production to keep-up with demand (Araújo et al., 2021). While offshore aquaculture is the one that presents a higher potential for scaling up production, land-based aquaculture allows to have a much higher control over the quality of algal biomass being produced, as well as their potential contamination by a multitude of pollutants. While certainly more costly than offshore aquaculture, land-based production when performed under bio-secure protocols can deliver algal biomass that fetches significantly higher values in premium cosmeceutical, nutraceutical, and biomedical markets. Indeed, the higher the position in the value chain of the biomolecules being sourced, the more likely it is that aquaculture of the species yielding them is commercially feasible (Araújo et al., 2021).
Several polysaccharides of commercial relevance are also sourced from marine animals. For instance, CHT is mostly derived from the exoskeletons of decapod crustaceans, namely shrimps. Non-etheless, most shrimp exoskeletons employed for this purpose result from the peeling processing of specimens sourced from fisheries for human consumption (e.g., the northern prawn Pandalus borealis) or farmed in Asian countries (e.g., whiteleg shrimp Penaeus vannamei). This is an excellent example of a circular bio-based approach that aims to add value to a side-stream (the exoskeletons) of a perfectly well-established value chain associated with the global processing of wild and farmed shrimp (De Aguiar et al., 2021). For example, PRIMEX is a recognized Icelandic marine biotechnology company, which is leader in processing shrimp shells raw-materials of Pandalus borealis into high quality CHT powder and based products for use in medical devices and personal care. Currently, PRIMEX product portfolio comprises CHT capsules for effective weight management (LIPOSAN), CHT medical spray and gel for natural healing (ChitoCare), CHT pool clarifier (SeaKlear) and animal CHT gel and spray for damaged skin as well as chronic wounds and ulcers (ChitoClear).
Also, the use of side streams from the processing of farmed fish, such as Atlantic salmon (Salmo salar), is certainly a sustainable way to obtain some polysaccharides of commercial relevance. For instance, while producing hyaluronan from the bacteria Streptococcus zooepidemicus remains as the most economically appealing approach (Chen et al., 2019), marine derived hyaluronan can be sourced from farmed Atlantic salmon (Kakizaki et al., 2017). This polysaccharide can also be yielded from the eye bulbs of several fish species targeted by commercial fisheries (e.g., swordfish, Xiphias gladius). This type of biorefinery approach is paramount to foster circularity and safeguard that non-edible parts (eye bubs, bones, skin, viscera … ) of marine living resources sourced from fisheries are used to its full potential. HA can also be sourced from other marine organisms, but the sustainability of such approach is certainly more questionable, as cnidarians and echinoderms are less likely to endure high fishing pressure. This premise is particularly relevant for sharks and rays, which can also yield marine HA, as well as CS. This last polysaccharide has been signalled from several species whose conservation status are of significant concern. This is the case of the shortfin mako shark (Isurus oxyrinchus) or the blue shark (Prionace glauca), whose populations worldwide are facing an unprecedented level of fishing pressure that is rapidly pushing them towards extinction (Dulvy et al., 2008). While other sharks and rays body parts can also yield CS, this polysaccharide has been often reported from the cartilages of shark fins. Shark fins are often obtained through a dreaded and illegal practice called finning–the dorsal, pectoral, and caudal fins of sharks are cut onboard, and specimens are thrown back to the sea still alive, where they suffer a slow and painful end, either by drowning or bleeding to death. The use of side streams from the processing of sharks sourced from commercial fisheries may be a solution, but its sustainability may only be valid if the fishery itself is sustainable. It is also worth referring that, non-governmental organizations and social media, are particularly active in exposing the cruelty of shark finning and banning the capture of several emblematic shark species already signalled in the Red List of Threatened Species by the International Union for Conservation of Nature (IUCN). Consumers of beauty and health-related products that incorporate marine origin polysaccharides are increasingly aware of sustainability issues, particularly those in the western markets of the northern hemisphere. Consequently, such products incorporating marine polysaccharides derived from sharks, rays and other marine species known to be endangered may rapidly become unpopular and be less appealing for high-end commercial uses.
Overall, while algae-derived marine polysaccharides will likely experience a growing acceptance by consumers that commonly perceive them as sustainable, this may not exactly be the case for those derived from marine animals, namely those originating from flagship species for marine conservation, such as sharks. With the forecasted growth of marine seaweeds and finfish aquaculture, along with increasing concerns to properly manage world fisheries, more sustainably sourced biomass will be available to yield marine polysaccharides of commercial relevance. The sustainable use of marine polysaccharides can contribute to the 2030 United Nations Agenda for Sustainable Development, namely to the Sustainable Development Goal (SDG) 3 (Good Health and Wellbeing), by yielding health promoting biomolecules, as well as to SDG 12 (Responsible Consumption and Production), by fostering biorefinery approaches aligned with a circular bioeconomy, that allow to fully use marine resources from fisheries and aquaculture sourced for human consumption. Ultimately, by adding value to marine chemodiversity, the use of marine polysaccharides can raise awareness towards the need to preserve marine biodiversity and contribute to achieve SDG 14 (Life Bellow Water). Marine biomass produced under high-biosecurity standards, as well as that sourced from side-streams from the transformation industry of seaweeds and marine seafood will likely become the cornerstone of marine polysaccharides value-chains, in line with the Blue Bioeconomy framework currently advocated.
The marine environment has proven to be an extraordinary source of bioactive molecules for a multitude of biomedical and biotechnological applications owing to their renewable character and very appealing physicochemical and biological properties. As such, marine-origin polysaccharides extracted from marine biomass are a highly valuable alternative to polymers obtained from petroleum sources, having a huge impact on the preservation of the environment, wellbeing of society, and the sustainability of our Planet. In fact, to date, marine biopolymers have already ignited a great interest in several commercial applications in different fields such as food, cosmetics, packaging, or biomedicine. However, when massively used, a major burden is imposed in their natural populations and marine ecosystems. Also, the chemical strategies most commonly employed to enable the extraction of marine polysaccharides encompass the use of hazardous chemicals and the generation of hazardous by-products, often being disposed, together with the marine biomass waste, into and contaminating the ocean, which imposes a major burden to the natural ecosystem and human health.
In this regard, the continuous exploitation of efficient, cost-effective, environmentally-friendly and sustainable methodologies for enabling and valorising the efficient extraction of polysaccharide from marine biomass to produce added-value biomaterials for biomedicine is of upmost importance to address the United Nations Sustainable Development Goals (UN SDGs) and enabling a sustainable future globally.
In this context, we would suggest some guidelines for the valorisation of marine biomass as high-added value products for healthcare applications: i) establish efficient, simple and eco-friendly extraction processes that could be applied in pilot plants; ii) unlock multiple functionalities for marine polysaccharides by combining computational and synthetic green chemistry towards the man-designed advanced biomaterials; iii) pursuit advanced research to exploit their full market potential encompassing scientists’ collaboration from interdisciplinary fields; and iv) promote synergistic interactions between academia and industries to foster marine-derived biomaterials into ready-to-use market products.
Conceptualization, JM; Literature review, MS, JB, JR, SP, and RC; Writing–original draft, MS; Infographics, FC; Writing, review and editing, JB, SP, JR, RC, and JM; Funding acquisition, JB, SP, JR, and JM. All authors have read and agreed to the published version of the manuscript.
This work was developed within the scope of the project CICECO-Aveiro Institute of Materials (UIDB/50011/2020, UIDP/50011/2020 & LA/P/0006/2020), financed by national funds (OE) through the FCT/MEC (PIDDAC). This work was also financed by Fundo Azul Call–N.° 5/2017 and Direção-Geral de Política do Mar (DGMP) do Ministério do Mar Português within the scope of project BLUEGLUE (FA_05_2017_031), as well as by OE through FCT in the scope of the following projects: “MIMETIc” (PTDC/BTM-MAT/31210/2017) and “COP2P” (PTDC/QUI-QOR/30771/2017), both also supported Programa Operacional Competitividade e Internacionalização (POCI-01-0145-FEDER-031210 and POCI-01-0145-FEDER-030771), respectively, in its FEDER component; and “SUPRASORT” (PTDC/QUI-OUT/30658/2017), also supported by Programa Operacional Regional do Centro–Centro 2020 (CENTRO-01-0145-FEDER-030658) in the component FEDER.
MS, JB, SP, and JR gratefully acknowledge FCT for the individual PhD grant (2020.07156. BD - MS) and individual researcher contracts (2020.00758. CEECIND - JB; 2020.00366. CEECIND - SP; CEECIND/01363/2018 - JR, respectively).
The authors declare that the research was conducted in the absence of any commercial or financial relationships that could be construed as a potential conflict of interest.
All claims expressed in this article are solely those of the authors and do not necessarily represent those of their affiliated organizations, or those of the publisher, the editors and the reviewers. Any product that may be evaluated in this article, or claim that may be made by its manufacturer, is not guaranteed or endorsed by the publisher.
Abdallah, M. M., Fernández, N., Matias, A. A., and Bronze, M. do R. (2020). Hyaluronic acid and Chondroitin sulfate from marine and terrestrial sources: Extraction and purification methods. Carbohydr. Polym. 243, 116441. doi:10.1016/j.carbpol.2020.116441
Aguilar-Briseño, J., Cruz-Suarez, L., Sassi, J.-F., Ricque-Marie, D., Zapata-Benavides, P., Mendoza-Gamboa, E., et al. (2015). Sulphated polysaccharides from ulva clathrata and cladosiphon okamuranus seaweeds both inhibit viral attachment/entry and cell-cell fusion, in NDV infection. Mar. Drugs 13, 697–712. doi:10.3390/md13020697
Ahearne, M., and Kelly, D. J. (2013). A comparison of fibrin, agarose and gellan gum hydrogels as carriers of stem cells and growth factor delivery microspheres for cartilage regeneration. Biomed. Mater 8, 035004. doi:10.1088/1748-6041/8/3/035004
Ahire, J. J., Robertson, D. D., van Reenen, A. J., and Dicks, L. M. T. (2017). Polyethylene oxide (PEO)-hyaluronic acid (HA) nanofibers with kanamycin inhibits the growth of Listeria monocytogenes. Biomed. Pharmacother. 86, 143–148. doi:10.1016/J.BIOPHA.2016.12.006
Alishahi, A., Mirvaghefi, A., Tehrani, M. R., Farahmand, H., Shojaosadati, S. A., Dorkoosh, F. A., et al. (2011). Enhancement and characterization of chitosan extraction from the wastes of shrimp packaging plants. J. Polym. Environ. 193 19, 776–783. doi:10.1007/S10924-011-0321-5
Amaral, A. J. R., Gaspar, V. M., Lavrador, P., and Mano, J. F. (2021). Double network laminarin-boronic/alginate dynamic bioink for 3D bioprinting cell-laden constructs. Biofabrication 13, 035045. doi:10.1088/1758-5090/ABFD79
Amaral, A. J. R., Gaspar, V. M., and Mano, J. F. (2020). Responsive laminarin-boronic acid self-healing hydrogels for biomedical applications. Polym. J. 52 (8), 997–1006. doi:10.1038/s41428-020-0348-3
Amagai, I., Tashiro, Y., and Ogawa, H. (2009). Improvement of the extraction procedure for hyaluronan from fish eyeball and the molecular characterization. Fish. Sci. 75, 805–810. doi:10.1007/s12562-009-0092-2
Amiri, N., Ajami, S., Shahroodi, A., Jannatabadi, N., Amiri Darban, S., Fazly Bazzaz, B. S., et al. (2020). Teicoplanin-loaded chitosan-PEO nanofibers for local antibiotic delivery and wound healing. Int. J. Biol. Macromol. 162, 645–656. doi:10.1016/J.IJBIOMAC.2020.06.195
An, H., Lee, J. W., Lee, H. J., Seo, Y., Park, H., and Lee, K. Y. (2018). Hyaluronate-alginate hybrid hydrogels modified with biomimetic peptides for controlling the chondrocyte phenotype. Carbohydr. Polym. 197, 422–430. doi:10.1016/J.CARBPOL.2018.06.016
Araújo, R., Vázquez Calderón, F., Sánchez López, J., Azevedo, I. C., Bruhn, A., Fluch, S., et al. (2021). Acidification in the U.S. Southeast: Causes, potential consequences and the role of the southeast ocean and coastal acidification network. Front. Mar. Sci. 7, 1–548. doi:10.3389/fmars.2020.00548
Arokiarajan, M. S., Thirunavukkarasu, R., Joseph, J., Ekaterina, O., and Aruni, W. (2022). Advance research in biomedical applications on marine sulfated polysaccharide. Int. J. Biol. Macromol. 194, 870–881. doi:10.1016/J.IJBIOMAC.2021.11.142
Avossa, J., Herwig, G., Toncelli, C., Itel, F., and Rossi, R. M. (2022). Electrospinning based on benign solvents: Current definitions, implications and strategies. Green Chem. 24, 2347–2375. doi:10.1039/D1GC04252A
Azevedo, S., Costa, A. M. S., Andersen, A., Choi, I. S., Birkedal, H., and Mano, J. F. (2017). Bioinspired ultratough hydrogel with fast recovery, self-healing, injectability and cytocompatibility. Adv. Mat. 29, 1700759–1700766. doi:10.1002/adma.201700759
Azizi, S., Mohamad, R., Abdul Rahim, R., Mohammadinejad, R., and Bin Ariff, A. (2017). Hydrogel beads bio-nanocomposite based on Kappa-Carrageenan and green synthesized silver nanoparticles for biomedical applications. Int. J. Biol. Macromol. 104, 423–431. doi:10.1016/J.IJBIOMAC.2017.06.010
Babo, P. S., Pires, R. L., Santos, L., Franco, A., Rodrigues, F., Leonor, I., et al. (2017). Platelet lysate-loaded photocrosslinkable hyaluronic acid hydrogels for periodontal endogenous regenerative technology. ACS Biomater. Sci. Eng. 3, 1359–1369. doi:10.1021/ACSBIOMATERIALS.6B00508
Bardajee, G. R., Khamooshi, N., Nasri, S., and Vancaeyzeele, C. (2020). Multi-stimuli responsive nanogel/hydrogel nanocomposites based on κ-carrageenan for prolonged release of levodopa as model drug. Int. J. Biol. Macromol. 153, 180–189. doi:10.1016/J.IJBIOMAC.2020.02.329
Bauleth-Ramos, T., Shih, T. Y., Shahbazi, M. A., Najibi, A. J., Mao, A. S., Liu, D., et al. (2019). Acetalated dextran nanoparticles loaded into an injectable alginate cryogel for combined chemotherapy and cancer vaccination. Adv. Funct. Mat. 29, 1903686. doi:10.1002/adfm.201903686
BeMiller, J. N. (2004). “Carbohydrates,” in Kirk-othmer encycl. Chem. Technol. (New Jersey, United States: Wiley Online Library). doi:10.1002/0471238961.0301180202051309.A01
Bjørge, I. M., Costa, A. M. S., Silva, A. S., Vidal, J. P. O., Nóbrega, J. M., and Mano, J. F. (2018). Tuneable spheroidal hydrogel particles for cell and drug encapsulation. Soft Matter 14, 5622–5627. doi:10.1039/C8SM00921J
Bjørge, I. M., De Sousa, B. M., Patrício, S. G., Silva, A. S., Nogueira, L. P., Santos, L. F., et al. (2022). Bioengineered hierarchical bonelike compartmentalized microconstructs using nanogrooved microdiscs. ACS Appl. Mat. Interfaces 14, 19116–19128. doi:10.1021/acsami.2c01161
Borges, J., Caridade, S. G., Silva, J. M., and Mano, J. F. (2015). Unraveling the effect of the hydration level on the molecular mobility of nanolayered polymeric systems. Macromol. Rapid Commun. 36, 405–412. doi:10.1002/MARC.201400568
Borges, J., and Mano, J. F. (2014). Molecular interactions driving the layer-by-layer assembly of multilayers. Chem. Rev. 114, 8883–8942. doi:10.1021/cr400531v
Borke, T., Winnik, F. M., Tenhu, H., and Hietala, S. (2015). Optimized triazine-mediated amidation for efficient and controlled functionalization of hyaluronic acid. Carbohydr. Polym. 116, 42–50. doi:10.1016/J.CARBPOL.2014.04.012
Bragd, P. L., Van Bekkum, H., and Besemer, A. C. (2004). TEMPO-mediated oxidation of polysaccharides: Survey of methods and applications. Top. Catal. 271 27, 49–66. doi:10.1023/B:TOCA.0000013540.69309.46
Breger, J. C., Fisher, B., Samy, R., Pollack, S., Wang, N. S., and Isayeva, I. (2015). Synthesis of “click” alginate hydrogel capsules and comparison of their stability, water swelling, and diffusion properties with that of Ca+2 crosslinked alginate capsules. J. Biomed. Mat. Res. 103, 1120–1132. doi:10.1002/JBM.B.33282
Bürck, J., Heissler, S., Geckle, U., Ardakani, M. F., Schneider, R., Ulrich, A. S., et al. (2013). Resemblance of electrospun collagen nanofibers to their native structure. Langmuir 29, 1562–1572. doi:10.1021/la3033258
Cardoso, M. J., Caridade, S. G., Costa, R. R., and Mano, J. F. (2016). Enzymatic degradation of polysaccharide-based layer-by-layer structures. Biomacromolecules 17, 1347–1357. doi:10.1021/acs.biomac.5b01742
Caridade, S. G., Monge, C., Almodóvar, J., Guillot, R., Lavaud, J., Josserand, V., et al. (2015). Myoconductive and osteoinductive free-standing polysaccharide membranes. Acta Biomater. 15, 139–149. doi:10.1016/J.ACTBIO.2014.12.027
Caridade, S. G., Monge, C., Gilde, F., Boudou, T., Mano, J. F., and Picart, C. (2013). Free-standing polyelectrolyte membranes made of chitosan and alginate. Biomacromolecules 14, 1653–1660. doi:10.1021/bm400314s
Carlos, C. R., Everton, E. L., Araujo, L. F. S., Leonira, L. M., Feitosa, J. P. A., Cunha, A. F., et al. (2021). Synthesis and characterization of scaffolds produced under mild conditions based on oxidized cashew gums and carboxyethyl chitosan. Int. J. Biol. Macromol. 176, 26–36. doi:10.1016/J.IJBIOMAC.2021.01.178
Carvalho, D. N., Williams, D. S., Sotelo, C. G., Pérez-Martín, R. I., Mearns-Spragg, A., Reis, R. L., et al. (2022). Marine origin biomaterials using a compressive and absorption methodology as cell-laden hydrogel envisaging cartilage tissue engineering. Biomater. Adv. 137, 212843. doi:10.1016/J.BIOADV.2022.212843
Castanheira, E. J., Correia, T. R., Rodrigues, J. M. M., and Mano, J. F. (2020). Novel biodegradable laminarin microparticles for biomedical applications. Bull. Chem. Soc. Jpn. 93, 713–719. doi:10.1246/BCSJ.20200034
Chen, F., Yu, S., Liu, B., Ni, Y., Yu, C., Su, Y., et al. (2016). An injectable enzymatically crosslinked carboxymethylated pullulan/chondroitin sulfate hydrogel for cartilage tissue engineering. Sci. Rep. 61 6, 1–12. doi:10.1038/srep20014
Chen, J., Gao, J., Yu, Y., and Yang, S. (2019). A hyaluronan-based polysaccharide peptide generated by a genetically modified Streptococcus zooepidemicus. Carbohydr. Res. 478, 25–32. doi:10.1016/J.CARRES.2019.04.005
Chen, Y., Zhu, H., Hao, Y., Sun, Z., Shen, P., and Zhou, Q. (2021). Preparation of fucoidan-based electrospun nanofibers and their interaction with endothelial cells. Front. Bioeng. Biotechnol. 9, 739209. doi:10.3389/FBIOE.2021.739209
Cheng, C. Y., Duan, W. W., Duan, Z. H., Hai, Y., Lei, X. G., and Chang, H. (2013). Extraction of chondroitin sulfate from Tilapia byproducts with ultrasonic-microwave synergistic. Adv. Mat. Res. 726–731, 4381–4385. doi:10.4028/www.scientific.net/amr.726-731.4381
Chiellini, F., and Morelli, A. (2011). “Ulvan: A versatile platform of biomaterials from renewable resources,” in Biomaterials - physics and chemistry (London, UK: IntechOpen Limited). doi:10.5772/24901
Cho, J. H., Lee, J. S., Shin, J., Jeon, E. J., An, S., Choi, Y. S., et al. (2018). Ascidian-inspired fast-forming hydrogel system for versatile biomedical applications: Pyrogallol chemistry for dual modes of crosslinking mechanism. Adv. Funct. Mat. 28, 1705244. doi:10.1002/ADFM.201705244
Cioce, A., Calle, B., Rizou, T., Lowery, S. C., Bridgeman, V. L., Mahoney, K. E., et al. (2022). Cell-specific bioorthogonal tagging of glycoproteins. Nat. Commun. 131 (13), 6237–6318. doi:10.1038/s41467-022-33854-0
Costa, A. M. S., and Mano, J. F. (2015). Highly robust hydrogels via a fast, simple and cytocompatible dual crosslinking-based process. Chem. Commun. 51, 15673–15676. doi:10.1039/C5CC05564D
Costa, A. M. S., and Mano, J. F. (2017). Solvent-free strategy yields size and shape-uniform capsules. J. Am. Chem. Soc. 139, 1057–1060. doi:10.1021/jacs.6b11925
Costa, A. M. S., Rodrigues, J. M. M., Pérez-Madrigal, M. M., Dove, A. P., and Mano, J. F. (2020). Modular functionalization of laminarin to create value-added naturally derived macromolecules. J. Am. Chem. Soc. 142, 19689–19697. doi:10.1021/jacs.0c09489
Costa, D. C. S., Costa, P. D. C., Gomes, M. C., Chandrakar, A., Wieringa, P. A., Moroni, L., et al. (2022). Universal strategy for designing shape memory hydrogels. ACS Mat. Lett. 4, 701–706. doi:10.1021/ACSMATERIALSLETT.2C00107
Costa, R. R., Costa, A. M. S., Caridade, S. G., and Mano, J. F. (2015). Compact saloplastic membranes of natural polysaccharides for soft tissue engineering. Chem. Mat. 27, 7490–7502. doi:10.1021/acs.chemmater.5b03648
Costa, R. R., Soares da Costa, D., Reis, R. L., and Pashkuleva, I. (2019). Bioinspired baroplastic glycosaminoglycan sealants for soft tissues. Acta Biomater. 87, 108–117. doi:10.1016/J.ACTBIO.2019.01.040
Cumpstey, I. (2013). Chemical modification of polysaccharides. ISRN Org. Chem. 2013, 1–27. doi:10.1155/2013/417672
Cunha, L., and Grenha, A. (2016). Sulfated seaweed polysaccharides as multifunctional materials in drug delivery applications. Mar. Drugs 14, 42. doi:10.3390/md14030042
Custódio, C. A., Cerqueira, M. T., Marques, A. P., Reis, R. L., and Mano, J. F. (2015). Cell selective chitosan microparticles as injectable cell carriers for tissue regeneration. Biomaterials 43, 23–31. doi:10.1016/J.BIOMATERIALS.2014.11.047
Custódio, C. A., Reis, R. L., and Mano, J. F. (2016). Photo-cross-linked laminarin-based hydrogels for biomedical applications. Biomacromolecules 17, 1602–1609. doi:10.1021/acs.biomac.5b01736
da Silva, R. M. P., Caridade, S. G., San Román, J., Mano, J. F., and Reis, R. L. (2008). Transport of small anionic and neutral solutes through Chitosan membranes: Dependence on cross-linking and chelation of divalent cations. Biomacromolecules 9, 2132–2138. doi:10.1021/bm8001649
Das, A. K., Sharma, M., Mondal, D., and Prasad, K. (2016). Deep eutectic solvents as efficient solvent system for the extraction of κ-carrageenan from Kappaphycus alvarezii. Carbohydr. Polym. 136, 930–935. doi:10.1016/J.CARBPOL.2015.09.114
Dash, M., Samal, S. K., Morelli, A., Bartoli, C., Declercq, H. A., Douglas, T. E. L., et al. (2018). Ulvan-chitosan polyelectrolyte complexes as matrices for enzyme induced biomimetic mineralization. Carbohydr. Polym. 182, 254–264. doi:10.1016/J.CARBPOL.2017.11.016
Davidson, M. D., Ban, E., Schoonen, A. C. M., Lee, M. H., D’Este, M., Shenoy, V. B., et al. (2020). Mechanochemical adhesion and plasticity in multifiber hydrogel networks. Adv. Mat. 32, 1905719. doi:10.1002/ADMA.201905719
De Aguiar, A. C., Pinheiro, S., Martí-Quijal, F. J., Barba, F. J., Tappi, S., Rocculi, P., et al. (2021). Innovative non-thermal technologies for recovery and valorization of value-added products from Crustacean processing by-products—an opportunity for a circular economy approach. Foods 10, 2030. doi:10.3390/FOODS10092030
de Carvalho, M. M., de Freitas, R. A., Ducatti, D. R. B., Ferreira, L. G., Gonçalves, A. G., Colodi, F. G., et al. (2018). Modification of ulvans via periodate-chlorite oxidation: Chemical characterization and anticoagulant activity. Carbohydr. Polym. 197, 631–640. doi:10.1016/j.carbpol.2018.06.041
D’Este, M., Eglin, D., and Alini, M. (2014). A systematic analysis of DMTMM vs EDC/NHS for ligation of amines to Hyaluronan in water. Carbohydr. Polym. 108, 239–246. doi:10.1016/J.CARBPOL.2014.02.070
Deng, Y., Sun, A. X., Overholt, K. J., Yu, G. Z., Fritch, M. R., Alexander, P. G., et al. (2019). Enhancing chondrogenesis and mechanical strength retention in physiologically relevant hydrogels with incorporation of hyaluronic acid and direct loading of TGF-β. Acta Biomater. 83, 167–176. doi:10.1016/J.ACTBIO.2018.11.022
Diolosà, M., Donati, I., Turco, G., Cadenaro, M., Di Lenarda, R., Breschi, L., et al. (2014). Use of methacrylate-modified chitosan to increase the durability of dentine bonding systems. Biomacromolecules 15, 4606–4613. doi:10.1021/bm5014124
Duan, H., Donovan, M., Foucher, A., Schultze, X., and Lecommandoux, S. (2018). Multivalent and multifunctional polysaccharide-based particles for controlled receptor recognition. Sci. Rep. 81 (8), 14730–14739. doi:10.1038/s41598-018-32994-y
Dulvy, N. K., Baum, J. K., Clarke, S., Compagno, L. J. V., Cortés, E., Domingo, A., et al. (2008). You can swim but you can’t hide: The global status and conservation of oceanic pelagic sharks and rays. Aquat. Conserv. 18, 459–482. doi:10.1002/AQC.975
Eggermont, L. J., Rogers, Z. J., Colombani, T., Memic, A., and Bencherif, S. A. (2020). Injectable cryogels for biomedical applications. Trends Biotechnol. 38, 418–431. doi:10.1016/J.TIBTECH.2019.09.008
Elchinger, P. H., Faugeras, P. A., Boëns, B., Brouillette, F., Montplaisir, D., Zerrouki, R., et al. (2011). Polysaccharides: The “click” chemistry impact. Polym. (Basel). 3, 1607–1651. doi:10.3390/POLYM3041607
Espíndola-Cortés, A., Moreno-Tovar, R., Bucio, L., Gimeno, M., Ruvalcaba-Sil, J. L., and Shirai, K. (2017). Hydroxyapatite crystallization in shrimp cephalothorax wastes during subcritical water treatment for chitin extraction. Carbohydr. Polym. 172, 332–341. doi:10.1016/J.CARBPOL.2017.05.055
Fan, F., Zhang, P., Wang, L., Sun, T., Cai, C., and Yu, G. (2019). Synthesis and properties of functional glycomimetics through click grafting of fucose onto chondroitin sulfates. Biomacromolecules 20, 3798–3808. doi:10.1021/acs.biomac.9b00878
Fantoni, N. Z., El-Sagheer, A. H., and Brown, T. (2021). A hitchhiker’s guide to click-chemistry with nucleic acids. Chem. Rev. 121, 7122–7154. doi:10.1021/acs.chemrev.0c00928
Freitas, B., Lavrador, P., Almeida, R. J., Gaspar, V. M., and Mano, J. F. (2020). Self-assembled bioactive colloidal gels as injectable multiparticle shedding platforms. ACS Appl. Mat. Interfaces 12, 31282–31291. doi:10.1021/ACSAMI.0C09270
Galvis-Sánchez, A. C., Castro, M. C. R., Biernacki, K., Gonçalves, M. P., and Souza, H. K. S. (2018). Natural deep eutectic solvents as green plasticizers for chitosan thermoplastic production with controlled/desired mechanical and barrier properties. Food Hydrocoll. 82, 478–489. doi:10.1016/J.FOODHYD.2018.04.026
Galvis-Sánchez, A. C., Sousa, A. M. M., Hilliou, L., Gonçalves, M. P., and Souza, H. K. S. (2016). Thermo-compression molding of chitosan with a deep eutectic mixture for biofilms development. Green Chem. 18, 1571–1580. doi:10.1039/C5GC02231B
Gan, D., Han, L., Wang, M., Xing, W., Xu, T., Zhang, H., et al. (2018). Conductive and tough hydrogels based on biopolymer molecular templates for controlling in situ formation of polypyrrole nanorods. ACS Appl. Mat. Interfaces 10, 36218–36228. doi:10.1021/ACSAMI.8B10280
Gereniu, C. R. N., Saravana, P. S., and Chun, B. S. (2018). Recovery of carrageenan from Solomon Islands red seaweed using ionic liquid-assisted subcritical water extraction. Sep. Purif. Technol. 196, 309–317. doi:10.1016/J.SEPPUR.2017.06.055
Gil, S., Silva, J. M., and Mano, J. F. (2015). Magnetically multilayer polysaccharide membranes for biomedical applications. ACS Biomater. Sci. Eng. 1, 1016–1025. doi:10.1021/acsbiomaterials.5b00292
Gruppuso, M., Iorio, F., Turco, G., Marsich, E., and Porrelli, D. (2022). Hyaluronic acid/lactose-modified chitosan electrospun wound dressings – crosslinking and stability criticalities. Carbohydr. Polym. 288, 119375. doi:10.1016/J.CARBPOL.2022.119375
Guo, J., Zhou, H., Akram, M. Y., Mu, X., Nie, J., and Ma, G. (2016). Characterization and application of chondroitin sulfate/polyvinyl alcohol nanofibres prepared by electrospinning. Carbohydr. Polym. 143, 239–245. doi:10.1016/J.CARBPOL.2016.02.013
Han, S. S., Yoon, H. Y., Yhee, J. Y., Cho, M. O., Shim, H. E., Jeong, J. E., et al. (2017). In situ cross-linkable hyaluronic acid hydrogels using copper free click chemistry for cartilage tissue engineering. Polym. Chem. 9, 20–27. doi:10.1039/C7PY01654A
Hao, Y., Zheng, W., Sun, Z., Zhang, D., Sui, K., Shen, P., et al. (2021). Marine polysaccharide-based composite hydrogels containing fucoidan: Preparation, physicochemical characterization, and biocompatible evaluation. Int. J. Biol. Macromol. 183, 1978–1986. doi:10.1016/J.IJBIOMAC.2021.05.190
Hardy, A., Seguin, C., Brion, A., Lavalle, P., Schaaf, P., Fournel, S., et al. (2018). β-Cyclodextrin-Functionalized chitosan/alginate compact polyelectrolyte complexes (CoPECs) as functional biomaterials with anti-inflammatory properties. ACS Appl. Mat. Interfaces 10, 29347–29356. doi:10.1021/acsami.8b09733
Hashizume, M., Murata, Y., Iijima, K., and Shibata, T. (2016). Drug loading and release behaviors of freestanding polysaccharide composite films. Polym. J. 484 48, 545–550. doi:10.1038/pj.2015.126
He, G., Yin, Y., Yan, X., and Yu, Q. (2014). Optimisation extraction of chondroitin sulfate from fish bone by high intensity pulsed electric fields. Food Chem. x. 164, 205–210. doi:10.1016/J.FOODCHEM.2014.05.032
Heida, T., Otto, O., Biedenweg, D., Hauck, N., and Thiele, J. (2020). Microfluidic fabrication of click chemistry-mediated hyaluronic acid microgels: A bottom-up material guide to tailor a microgel’s physicochemical and mechanical properties. Polym. (Basel). 12, 1760. doi:10.3390/POLYM12081760
Highley, C. B., Rodell, C. B., Burdick, J. A., Highley, C. B., Rodell, C. B., and Burdick, J. A. (2015). Direct 3D printing of shear-thinning hydrogels into self-healing hydrogels. Adv. Mat. 27, 5075–5079. doi:10.1002/ADMA.201501234
Hongkulsup, C., Khutoryanskiy, V. V., and Niranjan, K. (2016). Enzyme assisted extraction of chitin from shrimp shells (Litopenaeus vannamei). J. Chem. Technol. Biotechnol. 91, 1250–1256. doi:10.1002/JCTB.4714
Hoon Song, K., Highley, C. B., Rouff, A., Burdick, J. A., Song, K. H., Highley, C. B., et al. (2018). Complex 3D-printed microchannels within cell-degradable hydrogels. Adv. Funct. Mat. 28, 1801331. doi:10.1002/ADFM.201801331
Hu, X., Liu, S., Zhou, G., Huang, Y., Xie, Z., and Jing, X. (2014). Electrospinning of polymeric nanofibers for drug delivery applications. J. Control. Release 185, 12–21. doi:10.1016/J.JCONREL.2014.04.018
Hui, E., Sumey, J. L., and Caliari, S. R. (2021). Click-functionalized hydrogel design for mechanobiology investigations. Mol. Syst. Des. Eng. 6, 670–707. doi:10.1039/D1ME00049G
Ibañez, E., Herrero, M., Mendiola, J. A., and Castro-Puyana, M. (2012). “Chapter 2 - extraction and characterization of bioactive compounds with health benefi ts from marine resources: Macro and micro algae, cyanobacteria, and invertebrates,” in Marine bioactive compounds: Sources, characterization and applications (Berlin, Germany: Springer Science), 55–98. doi:10.1007/978-1-4614-1247-2-2
Iijima, K., Tsuji, Y., Kuriki, I., Kakimoto, A., Nikaido, Y., Ninomiya, R., et al. (2017). Control of cell adhesion and proliferation utilizing polysaccharide composite film scaffolds. Colloids Surfaces B Biointerfaces 160, 228–237. doi:10.1016/J.COLSURFB.2017.09.025
Iliou, K., Kikionis, S., Ioannou, E., and Roussis, V. (2022). Marine biopolymers as bioactive functional ingredients of electrospun nanofibrous scaffolds for biomedical applications. Mar. Drugs 20, 314. doi:10.3390/MD20050314
Järvinen, T. A. H., Järvinen, T. L. N., Kannus, P., Józsa, L., and Järvinen, M. (2004). Collagen fibres of the spontaneously ruptured human tendons display decreased thickness and crimp angle. J. Orthop. Res. 22, 1303–1309. doi:10.1016/J.ORTHRES.2004.04.003
Jeon, O., Bouhadir, K. H., Mansour, J. M., and Alsberg, E. (2009). Photocrosslinked alginate hydrogels with tunable biodegradation rates and mechanical properties. Biomaterials 30, 2724–2734. doi:10.1016/J.BIOMATERIALS.2009.01.034
Jiang, Z., Bhaskaran, A., Aitken, H. M., G Shackleford, I. C., Connal, L. A., Jiang, Z., et al. (2019). Using synergistic multiple dynamic bonds to construct polymers with engineered properties. Macromol. Rapid Commun. 40, 1900038. doi:10.1002/MARC.201900038
Jin, R., Moreira Teixeira, L. S., Dijkstra, P. J., van Blitterswijk, C. A., Karperien, M., and Feijen, J. (2010). Enzymatically-crosslinked injectable hydrogels based on biomimetic dextran–hyaluronic acid conjugates for cartilage tissue engineering. Biomaterials 31, 3103–3113. doi:10.1016/J.BIOMATERIALS.2010.01.013
Jones, R. G., Wilks, E. S., Metanomski, W. V., Kahovec, J., Hess, M., and Stepto, R. (2009). Compendium of polymer terminology and nomenclature. Cambridge, UK: Royal Society of Chemistry. doi:10.1039/9781847559425
Kadam, S. U., O’Donnell, C. P., Rai, D. K., Hossain, M. B., Burgess, C. M., Walsh, D., et al. (2015). Laminarin from Irish Brown seaweeds ascophyllum nodosum and Laminaria hyperborea: Ultrasound assisted extraction, characterization and bioactivity. Mar. Drugs 13, 4270–4280. doi:10.3390/MD13074270
Kakizaki, I., Miura, A., Mineta, T., Hong, J., and Kato, Y. (2017). Characterization of proteoglycan and hyaluronan in hot water extract from salmon cartilage. J. Appl. Glycosci. 64, 83–90. doi:10.5458/JAG.JAG.JAG-2017_005
Kadri, R., Elkhoury, K., Ben Messaoud, G., Kahn, C., Tamayol, A., Mano, J. F., et al. (2021). Physicochemical interactions in nanofunctionalized alginate/GelMA IPN hydrogels. Nanomaterials 11, 2256. doi:10.3390/NANO11092256
Kearney, C. J., Skaat, H., Kennedy, S. M., Hu, J., Darnell, M., Raimondo, T. M., et al. (2015). Switchable release of entrapped nanoparticles from alginate hydrogels. Adv. Healthc. Mat. 4, 1634–1639. doi:10.1002/ADHM.201500254
Kee Jo, Y., Lee, D., Jo, Y. K., and Lee, D. (2020). Biopolymer microparticles prepared by microfluidics for biomedical applications. Small 16, 1903736. doi:10.1002/SMLL.201903736
Keirouz, A., Radacsi, N., Ren, Q., Dommann, A., Beldi, G., Maniura-Weber, K., et al. (2020). Nylon-6/chitosan core/shell antimicrobial nanofibers for the prevention of mesh-associated surgical site infection. J. Nanobiotechnology 18, 51–17. doi:10.1186/S12951-020-00602-9
Kennedy, S., Hu, J., Kearney, C., Skaat, H., Gu, L., Gentili, M., et al. (2016). Sequential release of nanoparticle payloads from ultrasonically burstable capsules. Biomaterials 75, 91–101. doi:10.1016/J.BIOMATERIALS.2015.10.008
Khademhosseini, A., Eng, G., Yeh, J., Fukuda, J., Blumling, J., Langer, R., et al. (2006). Micromolding of photocrosslinkable hyaluronic acid for cell encapsulation and entrapment. J. Biomed. Mat. Res. A 79A, 522–532. doi:10.1002/JBM.A.30821
Khaliq, T., Sohail, M., Minhas, M. U., Ahmed Shah, S., Jabeen, N., Khan, S., et al. (2022). Self-crosslinked chitosan/κ-carrageenan-based biomimetic membranes to combat diabetic burn wound infections. Int. J. Biol. Macromol. 197, 157–168. doi:10.1016/J.IJBIOMAC.2021.12.100
Kianfar, P., Vitale, A., Dalle Vacche, S., and Bongiovanni, R. (2019). Photo-crosslinking of chitosan/poly(ethylene oxide) electrospun nanofibers. Carbohydr. Polym. 217, 144–151. doi:10.1016/J.CARBPOL.2019.04.062
Kikionis, S., Ioannou, E., Aggelidou, E., Tziveleka, L. A., Demiri, E., Bakopoulou, A., et al. (2021). The marine polysaccharide ulvan confers potent osteoinductive capacity to PCL-based scaffolds for bone tissue engineering applications. Int. J. Mol. Sci. 22, 3086. doi:10.3390/IJMS22063086
Kim, H. D., Lee, E. A., An, Y. H., Kim, S. L., Lee, S. S., Yu, S. J., et al. (2017). Chondroitin sulfate-based biomineralizing surface hydrogels for bone tissue engineering. ACS Appl. Mat. Interfaces 9, 21639–21650. doi:10.1021/ACSAMI.7B04114
Kim, S. H., Kim, K., Kim, B. S., An, Y. H., Lee, U. J., Lee, S. H., et al. (2020). Fabrication of polyphenol-incorporated anti-inflammatory hydrogel via high-affinity enzymatic crosslinking for wet tissue adhesion. Biomaterials 242, 119905. doi:10.1016/j.biomaterials.2020.119905
Kirschning, A., Dibbert, N., and Dräger, G. (2018). Chemical functionalization of polysaccharides—towards biocompatible hydrogels for biomedical applications. Chem. Eur. J. 24, 1231–1240. doi:10.1002/CHEM.201701906
Koivusalo, L., Kauppila, M., Samanta, S., Parihar, V. S., Ilmarinen, T., Miettinen, S., et al. (2019). Tissue adhesive hyaluronic acid hydrogels for sutureless stem cell delivery and regeneration of corneal epithelium and stroma. Biomaterials 225, 119516. doi:10.1016/J.BIOMATERIALS.2019.119516
Kolawole, O. M., Lau, W. M., and Khutoryanskiy, V. V. (2018). Methacrylated chitosan as a polymer with enhanced mucoadhesive properties for transmucosal drug delivery. Int. J. Pharm. X. 550, 123–129. doi:10.1016/J.IJPHARM.2018.08.034
Kolb, H. C., Finn, M. G., and Sharpless, K. B. (2001). Click chemistry: Diverse chemical function from a few good reactions. Angew. Chem. Int. Ed. 40, 2004–2021. doi:10.1002/1521-3773(20010601)40:11<2004::aid-anie2004>3.0.co;2-5
Kong, X., He, Y., Zhou, H., Gao, P., Xu, L., Han, Z., et al. (2021). Chondroitin sulfate/polycaprolactone/gelatin electrospun nanofibers with antithrombogenicity and enhanced endothelial cell affinity as a potential scaffold for blood vessel tissue engineering. Nanoscale Res. Lett. 16, 62–11. doi:10.1186/s11671-021-03518-x
Kyzioł, A., Michna, J., Moreno, I., Gamez, E., and Irusta, S. (2017). Preparation and characterization of electrospun alginate nanofibers loaded with ciprofloxacin hydrochloride. Eur. Polym. J. 96, 350–360. doi:10.1016/J.EURPOLYMJ.2017.09.020
Lahaye, M., and Robic, A. (2007). Structure and functional properties of ulvan, a polysaccharide from green seaweeds. Biomacromolecules 8, 1765–1774. doi:10.1021/bm061185q
Larkin, A. L., Davis, R. M., and Rajagopalan, P. (2010). Biocompatible, detachable, and free-standing polyelectrolyte multilayer films. Biomacromolecules 11, 2788–2796. doi:10.1021/bm100867h
Laurienzo, P. (2010). Marine polysaccharides in pharmaceutical applications: An overview. Mar. Drugs 8, 2435–2465. doi:10.3390/md8092435
Lavrador, P., Gaspar, V. M., and Mano, J. F. (2020). Mechanochemical patternable ECM-mimetic hydrogels for programmed cell orientation. Adv. Healthc. Mat. 9, 1901860. doi:10.1002/ADHM.201901860
Leal, M. C., Munro, M. H. G., Blunt, J. W., Puga, J., Jesus, B., Calado, R., et al. (2013). Biogeography and biodiscovery hotspots of macroalgal marine natural products. Nat. Prod. Rep. 30, 1380–1390. doi:10.1039/C3NP70057G
Lee, C., Shin, J., Lee, J. S., Byun, E., Ryu, J. H., Um, S. H., et al. (2013). Bioinspired, calcium-free alginate hydrogels with tunable physical and mechanical properties and improved biocompatibility. Biomacromolecules 14, 2004–2013. doi:10.1021/bm400352d
Lee, D. G., Hyun, J. W., Kang, K. A., Lee, J. O., Lee, S. H., Ha, B. J., et al. (2004). Ulva lactuca: A potential seaweed for tumor treatment and immune stimulation. Biotechnol. Bioprocess Eng. 9, 236–238. doi:10.1007/BF02942299
Lee, K. Y., and Mooney, D. J. (2012). Alginate: Properties and biomedical applications. Prog. Polym. Sci. 37, 106–126. doi:10.1016/j.progpolymsci.2011.06.003
Lee, S., Koo, H., Na, J. H., Han, S. J., Min, H. S., Lee, S. J., et al. (2014). Chemical tumor-targeting of nanoparticles based on metabolic glycoengineering and click chemistry. ACS Nano 8, 2048–2063. doi:10.1021/nn406584y
Li, Q., Wang, X., Lou, X., Yuan, H., Tu, H., Li, B., et al. (2015). Genipin-crosslinked electrospun chitosan nanofibers: Determination of crosslinking conditions and evaluation of cytocompatibility. Carbohydr. Polym. 130, 166–174. doi:10.1016/J.CARBPOL.2015.05.039
Li, S., Zhou, J., Huang, Y. H., Roy, J., Zhou, N., Yum, K., et al. (2020). Injectable click chemistry-based bioadhesives for accelerated wound closure. Acta Biomater. 110, 95–104. doi:10.1016/J.ACTBIO.2020.04.004
Li, W., Jiang, N., Li, B., Wan, M., Chang, X., Liu, H., et al. (2018). Antioxidant activity of purified ulvan in hyperlipidemic mice. Int. J. Biol. Macromol. 113, 971–975. doi:10.1016/j.ijbiomac.2018.02.104
Li, Y., Zhao, M., Gomez, L. P., Senthamaraikannan, R., Padamati, R. B., O’Donnell, C. P., et al. (2021). Investigation of enzyme-assisted methods combined with ultrasonication under a controlled alkali pretreatment for agar extraction from Gelidium sesquipedale. Food Hydrocoll. 120, 106905. doi:10.1016/J.FOODHYD.2021.106905
Liu, Z., Wang, H., Liu, C., Jiang, Y., Yu, G., Mu, X., et al. (2012). Magnetic cellulose–chitosan hydrogels prepared from ionic liquids as reusable adsorbent for removal of heavy metal ions. Chem. Commun. 48, 7350–7352. doi:10.1039/C2CC17795A
Luo, Z., Li, W., Yan, J., Sun, J., Luo, Z., Li, W., et al. (2022). Roles of ionic liquids in adjusting nature of ionogels: A mini review. Adv. Funct. Mat. 32, 2203988. doi:10.1002/ADFM.202203988
Lynam, D., Peterson, C., Maloney, R., Shahriari, D., Garrison, A., Saleh, S., et al. (2014). Augmenting protein release from layer-by-layer functionalized agarose hydrogels. Carbohydr. Polym. 103, 377–384. doi:10.1016/J.CARBPOL.2013.12.069
Madruga, L. Y., Balaban, R. C., Popat, K. C., Kipper, M. J., C Madruga, L. Y., Balaban, R. C., et al. (2021). Biocompatible crosslinked nanofibers of poly(vinyl alcohol)/carboxymethyl-kappa-carrageenan produced by a green process. Macromol. Biosci. 21, 2000292. doi:10.1002/MABI.202000292
Mahendiran, B., Muthusamy, S., Sampath, S., Jaisankar, S. N., Popat, K. C., Selvakumar, R., et al. (2021). Recent trends in natural polysaccharide based bioinks for multiscale 3D printing in tissue regeneration: A review. Int. J. Biol. Macromol. 183, 564–588. doi:10.1016/J.IJBIOMAC.2021.04.179
Marras-Marquez, T., Peña, J., and Veiga-Ochoa, M. D. (2015). Robust and versatile pectin-based drug delivery systems. Int. J. Pharm. X. 479, 265–276. doi:10.1016/J.IJPHARM.2014.12.045
Martins, C. R., Custódio, C. A., and Mano, J. F. (2018). Multifunctional laminarin microparticles for cell adhesion and expansion. Carbohydr. Polym. 202, 91–98. doi:10.1016/j.carbpol.2018.08.029
Martins, N. I., Sousa, M. P., Custódio, C. A., Pinto, V. C., Sousa, P. J., Minas, G., et al. (2017). Multilayered membranes with tuned well arrays to be used as regenerative patches. Acta Biomater. 57, 313–323. doi:10.1016/J.ACTBIO.2017.04.021
Matsumura, K., and Rajan, R. (2021). Oxidized polysaccharides as green and sustainable biomaterials. Curr. Org. Chem. 25, 1483–1496. doi:10.2174/1385272825666210428140052
Mealy, J. E., Chung, J. J., Jeong, H.-H., Issadore, D., Lee, D., Atluri, P., et al. (2018). Injectable granular hydrogels with multifunctional properties for biomedical applications. Adv. Mat. 30, 1705912. doi:10.1002/ADMA.201705912
Mehrali, M., Thakur, A., Pennisi, C. P., Talebian, S., Arpanaei, A., Nikkhah, M., et al. (2017). Nanoreinforced hydrogels for tissue engineering: Biomaterials that are compatible with load-bearing and electroactive tissues. Adv. Mat. 29, 1603612. doi:10.1002/ADMA.201603612
Mehrotra, S., Lynam, D., Maloney, R., Pawelec, K. M., Tuszynski, M. H., Lee, I., et al. (2010). Time controlled protein release from layer-by-layer assembled multilayer functionalized agarose hydrogels. Adv. Funct. Mat. 20, 247–258. doi:10.1002/ADFM.200901172
Memic, A., Colombani, T., Eggermont, L. J., Rezaeeyazdi, M., Steingold, J., Rogers, Z. J., et al. (2019). Latest advances in cryogel technology for biomedical applications. Adv. Ther. (Weinh). 2, 1800114. doi:10.1002/ADTP.201800114
Meng, X., and Edgar, K. J. (2016). Click” reactions in polysaccharide modification. Prog. Polym. Sci. 53, 52–85. doi:10.1016/J.PROGPOLYMSCI.2015.07.006
Moghassemi, S., Hadjizadeh, A., Hakamivala, A., and Omidfar, K. (2017). Growth factor-loaded nano-niosomal gel formulation and characterization. AAPS PharmSciTech 18, 34–41. doi:10.1208/S12249-016-0579-Y
Moody, C. T., Palvai, S., and Brudno, Y. (2020). Click cross-linking improves retention and targeting of refillable alginate depots. Acta Biomater. 112, 112–121. doi:10.1016/J.ACTBIO.2020.05.033
Morelli, A., Betti, M., Puppi, D., and Chiellini, F. (2016). Design, preparation and characterization of ulvan based thermosensitive hydrogels. Carbohydr. Polym. 136, 1108–1117. doi:10.1016/J.CARBPOL.2015.09.068
Moreira, J., Vale, A. C., Pires, R. A., Botelho, G., Reis, R. L., and Alves, N. M. (2020). Spin-coated polysaccharide-based multilayered freestanding films with adhesive and bioactive moieties. Molecules 25, 840. doi:10.3390/MOLECULES25040840
Murado, M. A., Fraguas, J., Montemayor, M. I., Vázquez, J. A., and González, P. (2010). Preparation of highly purified chondroitin sulphate from skate (Raja clavata) cartilage by-products. Process optimization including a new procedure of alkaline hydroalcoholic hydrolysis. Biochem. Eng. J. 49, 126–132. doi:10.1016/J.BEJ.2009.12.006
Murata, Y., Isobe, T., Kofuji, K., Nishida, N., and Kamaguchi, R. (2010). Preparation of fast dissolving films for oral dosage from natural polysaccharides. Materials 3 (3), 4291–4299. doi:10.3390/MA3084291
Nadine, S., Fernandes, I., Patrício, S. G., Correia, C. R., and Mano, J. F. (2022). Liquefied microcapsules compartmentalizing macrophages and umbilical cord-derived cells for bone tissue engineering. Adv. Healthc. Mat. 11, 2200651. doi:10.1002/ADHM.202200651
Nadine, S., Patrício, S. G., Barrias, C. C., Choi, I. S., Matsusaki, M., Correia, C. R., et al. (2020). Geometrically controlled liquefied capsules for modular tissue engineering strategies. Adv. Biosyst. 4, 2000127. doi:10.1002/ADBI.202000127
Nadine, S., Patrício, S. G., Correia, C. R., and Mano, J. F. (2019). Dynamic microfactories co-encapsulating osteoblastic and adipose-derived stromal cells for the biofabrication of bone units. Biofabrication 12, 015005. doi:10.1088/1758-5090/AB3E16
Neto, A. I., Demir, K., Popova, A. A., Oliveira, M. B., Mano, J. F., Levkin, P. A., et al. (2016). Fabrication of hydrogel particles of defined shapes using superhydrophobic-hydrophilic micropatterns. Adv. Mat. 28, 7613–7619. doi:10.1002/ADMA.201602350
Neves, S. C., Moroni, L., Barrias, C. C., and Granja, P. L. (2020). Leveling up hydrogels: Hybrid systems in tissue engineering. Trends Biotechnol. 38, 292–315. doi:10.1016/J.TIBTECH.2019.09.004
Noser, A. A., Abdelmonsef, A. H., El-Naggar, M., and Salem, M. M. (2021). New amino acid Schiff bases as anticancer agents via potential mitochondrial complex I-associated hexokinase inhibition and targeting AMP-protein kinases/mTOR signaling pathway. Molecules 26, 5332. doi:10.3390/MOLECULES26175332
Nouri, M., Khodaiyan, F., Razavi, S. H., and Mousavi, M. (2016). Improvement of chitosan production from Persian Gulf shrimp waste by response surface methodology. Food Hydrocoll. 59, 50–58. doi:10.1016/J.FOODHYD.2015.08.027
Nunes, C. S., Rufato, K. B., Souza, P. R., de Almeida, E. A. M. S., da Silva, M. J. V., Scariot, D. B., et al. (2017). Chitosan/chondroitin sulfate hydrogels prepared in [Hmim] [HSO4] ionic liquid. Carbohydr. Polym. 170, 99–106. doi:10.1016/J.CARBPOL.2017.04.073
Oliveira, M. B., Bastos, H. X. S., and Mano, J. F. (2018). Sequentially moldable and bondable four-dimensional hydrogels compatible with cell encapsulation. Biomacromolecules 19, 2742–2749. doi:10.1021/ACS.BIOMAC.8B00337
Parhi, R. (2017). Cross-linked hydrogel for pharmaceutical applications: A review. Adv. Pharm. Bull. 7, 515–530. doi:10.15171/APB.2017.064
Paris, A. L., Caridade, S., Colomb, E., Bellina, M., Boucard, E., Verrier, B., et al. (2021). Sublingual protein delivery by a mucoadhesive patch made of natural polymers. Acta Biomater. 128, 222–235. doi:10.1016/J.ACTBIO.2021.04.024
Park, S. N., Park, J. C., Kim, H. O., Song, M. J., and Suh, H. (2002). Characterization of porous collagen/hyaluronic acid scaffold modified by 1-ethyl-3-(3-dimethylaminopropyl)carbodiimide cross-linking. Biomaterials 23, 1205–1212. doi:10.1016/S0142-9612(01)00235-6
Pengzhan, Y., Ning, L., Xiguang, L., Gefei, Z., Quanbin, Z., and Pengcheng, L. (2003). Antihyperlipidemic effects of different molecular weight sulfated polysaccharides from Ulva pertusa (Chlorophyta). Pharmacol. Res. 48, 543–549. doi:10.1016/S1043-6618(03)00215-9
Polat, T. G., Duman, O., and Tunç, S. (2020). Agar/κ-carrageenan/montmorillonite nanocomposite hydrogels for wound dressing applications. Int. J. Biol. Macromol. 164, 4591–4602. doi:10.1016/J.IJBIOMAC.2020.09.048
Qi, X., Su, T., Zhang, M., Tong, X., Pan, W., Zeng, Q., et al. (2020). Macroporous hydrogel scaffolds with tunable physicochemical properties for tissue engineering constructed using renewable polysaccharides. ACS Appl. Mat. Interfaces 12, 13256–13264. doi:10.1021/ACSAMI.9B20794
Quitain, A. T., Kai, T., Sasaki, M., and Goto, M. (2013). Microwave–hydrothermal extraction and degradation of fucoidan from supercritical carbon dioxide deoiled undaria pinnatifida. Ind. Eng. Chem. Res. 52, 7940–7946. doi:10.1021/IE400527B
Rial-Hermida, M. I., Rey-Rico, A., Blanco-Fernandez, B., Carballo-Pedrares, N., Byrne, E. M., and Mano, J. F. (2021). Recent progress on polysaccharide-based hydrogels for controlled delivery of therapeutic biomolecules. ACS Biomater. Sci. Eng. 7, 4102–4127. doi:10.1021/acsbiomaterials.0c01784
Ribeiro, C., Borges, J., Costa, A. M. S., Gaspar, V. M., de Zea Bermudez, V., and Mano, J. F. (2018). Preparation of well-dispersed chitosan/alginate hollow multilayered microcapsules for enhanced cellular internalization. Molecules 23, 625. doi:10.3390/MOLECULES23030625
Rodrigues, M. N., Oliveira, M. B., Costa, R. R., and Mano, J. F. (2016). Chitosan/chondroitin sulfate membranes produced by polyelectrolyte complexation for cartilage engineering. Biomacromolecules 17, 2178–2188. doi:10.1021/ACS.BIOMAC.6B00399
Rodriguez-Jasso, R. M., Mussatto, S. I., Pastrana, L., Aguilar, C. N., and Teixeira, J. A. (2011). Microwave-assisted extraction of sulfated polysaccharides (fucoidan) from Brown seaweed. Carbohydr. Polym. 86, 1137–1144. doi:10.1016/J.CARBPOL.2011.06.006
Ren, Y., Ailierken, A., Zhao, L., Lin, Z., Jiang, J., Li, B., et al. (2022). hUC-MSCs lyophilized powder loaded polysaccharide ulvan driven functional hydrogel for chronic diabetic wound healing. Carbohydr. Polym. 288, 119404. doi:10.1016/J.CARBPOL.2022.119404
Sadhasivam, G., Muthuvel, A., Pachaiyappan, A., and Thangavel, B. (2013). Isolation and characterization of hyaluronic acid from the liver of marine stingray Aetobatus narinari. Int. J. Biol. Macromol. 54, 84–89. doi:10.1016/J.IJBIOMAC.2012.11.028
Sagawa, T., Oishi, M., Yataka, Y., Sato, R., Iijima, K., and Hashizume, M. (2022). Control of the molecular permeability of polysaccharide composite films utilizing a molecular imprinting approach. Polym. J. 544 54, 571–579. doi:10.1038/s41428-021-00605-9
Samalens, F., Thomas, M., Claverie, M., Castejon, N., Zhang, Y., Pigot, T., et al. (2022). Progresses and future prospects in biodegradation of marine biopolymers and emerging biopolymer-based materials for sustainable marine ecosystems. Green Chem. 24, 1762–1779. doi:10.1039/D1GC04327G
Sánchez-Téllez, D. A., Rodríguez-Lorenzo, L. M., and Téllez-Jurado, L. (2020). Siloxane-inorganic chemical crosslinking of hyaluronic acid – based hybrid hydrogels: Structural characterization. Carbohydr. Polym. 230, 115590. doi:10.1016/J.CARBPOL.2019.115590
Saravana, P. S., Cho, Y. N., Woo, H. C., and Chun, B. S. (2018). Green and efficient extraction of polysaccharides from Brown seaweed by adding deep eutectic solvent in subcritical water hydrolysis. J. Clean. Prod. 198, 1474–1484. doi:10.1016/J.JCLEPRO.2018.07.151
Scognamiglio, F., Travan, A., Borgogna, M., Donati, I., Marsich, E., Bosmans, J. W. A. M., et al. (2016). Enhanced bioadhesivity of dopamine-functionalized polysaccharidic membranes for general surgery applications. Acta Biomater. 44, 232–242. doi:10.1016/J.ACTBIO.2016.08.017
Serrano, M. C., Nardecchia, S., García-Rama, C., Ferrer, M. L., Collazos-Castro, J. E., Del Monte, F., et al. (2014). Chondroitin sulphate-based 3D scaffolds containing MWCNTs for nervous tissue repair. Biomaterials 35, 1543–1551. doi:10.1016/J.BIOMATERIALS.2013.11.017
Shanmugapriya, K., Kim, H., and Kang, H. W. (2020). Fucoidan-loaded hydrogels facilitates wound healing using photodynamic therapy by in vitro and in vivo evaluation. Carbohydr. Polym. 247, 116624. doi:10.1016/J.CARBPOL.2020.116624
Sharma, M., Chaudhary, J. P., Mondal, D., Meena, R., and Prasad, K. (2015). A green and sustainable approach to utilize bio-ionic liquids for the selective precipitation of high purity agarose from an agarophyte extract. Green Chem. 17, 2867–2873. doi:10.1039/C4GC02498B
Sharma, S., Swetha, K. L., and Roy, A. (2019). Chitosan-Chondroitin sulfate based polyelectrolyte complex for effective management of chronic wounds. Int. J. Biol. Macromol. 132, 97–108. doi:10.1016/J.IJBIOMAC.2019.03.186
Shen, X., Shamshina, J. L., Berton, P., Bandomir, J., Wang, H., Gurau, G., et al. (2016). Comparison of hydrogels prepared with ionic-liquid-isolated vs commercial chitin and cellulose. ACS Sustain. Chem. Eng. 4, 471–480. doi:10.1021/ACSSUSCHEMENG.5B01400
Shin, J., Lee, J. S., Lee, C., Park, H. J., Yang, K., Jin, Y., et al. (2015). Tissue adhesive catechol-modified hyaluronic acid hydrogel for effective, minimally invasive cell therapy. Adv. Funct. Mat. 25, 3814–3824. doi:10.1002/ADFM.201500006
Siddhanta, A. K., Sanandiya, N. D., Chejara, D. R., and Kondaveeti, S. (2015). Functional modification mediated value addition of seaweed polysaccharides – A perspective. RSC Adv. 5, 59226–59239. doi:10.1039/C5RA09027J
Sideris, E., Griffin, D. R., Ding, Y., Li, S., Weaver, W. M., Di Carlo, D., et al. (2016). Particle hydrogels based on hyaluronic acid building blocks. ACS Biomater. Sci. Eng. 2, 2034–2041. doi:10.1021/acsbiomaterials.6b00444
Silva, C. R., Babo, P. S., Gulino, M., Costa, L., Oliveira, J. M., Silva-Correia, J., et al. (2018). Injectable and tunable hyaluronic acid hydrogels releasing chemotactic and angiogenic growth factors for endodontic regeneration. Acta Biomater. 77, 155–171. doi:10.1016/J.ACTBIO.2018.07.035
Silva, J. M., Caridade, S. G., Reis, R. L., and Mano, J. F. (2016). Polysaccharide-based freestanding multilayered membranes exhibiting reversible switchable properties. Soft Matter 12, 1200–1209. doi:10.1039/C5SM02458G
Silva, J. M., Duarte, A. R. C., Caridade, S. G., Picart, C., Reis, R. L., and Mano, J. F. (2014). Tailored freestanding multilayered membranes based on chitosan and alginate. Biomacromolecules 15, 3817–3826. doi:10.1021/bm501156v
Silva, J. M., García, J. R., Reis, R. L., García, A. J., and Mano, J. F. (2017). Tuning cell adhesive properties via layer-by-layer assembly of chitosan and alginate. Acta Biomater. 51, 279–293. doi:10.1016/J.ACTBIO.2017.01.058
Silva, S. S., Caridade, S. G., Mano, J. F., and Reis, R. L. (2013). Effect of crosslinking in chitosan/aloe vera-based membranes for biomedical applications. Carbohydr. Polym. 98, 581–588. doi:10.1016/J.CARBPOL.2013.06.022
Silva, S. S., Santos, M. I., Coutinho, O. P., Mano, J. F., and Reis, R. L. (2005). Physical properties and biocompatibility of chitosan/soy blended membranes. J. Mat. Sci. Mat. Med. 166 16, 575–579. doi:10.1007/S10856-005-0534-Z
Silva, S. S., Santos, T. C., Cerqueira, M. T., Marques, A. P., Reys, L. L., Silva, T. H., et al. (2012). The use of ionic liquids in the processing of chitosan/silk hydrogels for biomedical applications. Green Chem. 14, 1463–1470. doi:10.1039/C2GC16535J
Silver, F. H., Freeman, J. W., and Seehra, G. P. (2003). Collagen self-assembly and the development of tendon mechanical properties. J. Biomech. 36, 1529–1553. doi:10.1016/S0021-9290(03)00135-0
Solberg, A., Mo, I. V., Omtvedt, L. A., Strand, B. L., Aachmann, F. L., Schatz, C., et al. (2022). Click chemistry for block polysaccharides with dihydrazide and dioxyamine linkers - a review. Carbohydr. Polym. 278, 118840. doi:10.1016/J.CARBPOL.2021.118840
Sousa, A. M. M., Souza, H. K. S., Latona, N., Liu, C. K., Gonçalves, M. P., and Liu, L. (2014). Choline chloride based ionic liquid analogues as tool for the fabrication of agar films with improved mechanical properties. Carbohydr. Polym. 111, 206–214. doi:10.1016/J.CARBPOL.2014.04.019
Sousa, A. M. M., Souza, H. K. S., Liu, L. S., and Gonçalves, M. P. (2015a). Alternative plasticizers for the production of thermo-compressed agar films. Int. J. Biol. Macromol. 76, 138–145. doi:10.1016/J.IJBIOMAC.2015.02.030
Sousa, A. M. M., Souza, H. K. S., Uknalis, J., Liu, S. C., Gonçalves, M. P., and Liu, L. (2015b). Electrospinning of agar/PVA aqueous solutions and its relation with rheological properties. Carbohydr. Polym. 115, 348–355. doi:10.1016/J.CARBPOL.2014.08.074
Sousa, C. F. V., Saraiva, C. A., Correia, T. R., Pesqueira, T., Patrício, S. G., Rial-Hermida, M. I., et al. (2021). Bioinstructive layer-by-layer-coated customizable 3D printed perfusable microchannels embedded in photocrosslinkable hydrogels for vascular tissue engineering. Biomolecules 11, 863. doi:10.3390/BIOM11060863
Sousa, M. P., Caridade, S. G., Mano, J. F., Sousa, M. P., Caridade, S. G., and Mano, J. F. (2017). Control of cell alignment and morphology by redesigning ECM-mimetic nanotopography on multilayer membranes. Adv. Healthc. Mat. 6, 1601462. doi:10.1002/ADHM.201601462
Sousa, M. P., and Mano, J. F. (2017). Cell-adhesive bioinspired and catechol-based multilayer freestanding membranes for bone tissue engineering. Biomimetics 2, 19. doi:10.3390/BIOMIMETICS2040019
Sousa, M. P., Neto, A. I., Correia, T. R., Miguel, S. P., Matsusaki, M., Correia, I. J., et al. (2018). Bioinspired multilayer membranes as potential adhesive patches for skin wound healing. Biomater. Sci. 6, 1962–1975. doi:10.1039/C8BM00319J
Stanczak, M. A., Rodrigues Mantuano, N., Kirchhammer, N., Sanin, D. E., Jacob, F., Coelho, R., et al. (2022). Targeting cancer glycosylation repolarizes tumor-associated macrophages allowing effective immune checkpoint blockade. Sci. Transl. Med. 14, eabj1270. doi:10.1126/scitranslmed.abj1270
Sukul, M., Sahariah, P., Lauzon, H. L., Borges, J., Másson, M., Mano, J. F., et al. (2021). In vitro biological response of human osteoblasts in 3D chitosan sponges with controlled degree of deacetylation and molecular weight. Carbohydr. Polym. 254, 117434. doi:10.1016/J.CARBPOL.2020.117434
Sun, Y., Ma, X., Hu, H., Wang, G., Edrada-Ebel, R., and Wang, C.-Y. (2021). Marine polysaccharides as a versatile biomass for the construction of nano drug delivery systems. Mar. Drugs 19, 345. doi:10.3390/MD19060345
Tabarsa, M., Han, J. H., Kim, C. Y., and You, S. G. (2012). Molecular characteristics and immunomodulatory activities of water-soluble sulfated polysaccharides from ulva pertusa. J. Med. Food 15, 135–144. doi:10.1089/jmf.2011.1716
Tan, W., Zhang, J., Zhao, X., Dong, F., Li, Q., and Guo, Z. (2018). Synthesis and antioxidant action of chitosan derivatives with amino-containing groups via azide-alkyne click reaction and N-methylation. Carbohydr. Polym. 199, 583–592. doi:10.1016/J.CARBPOL.2018.07.056
Tokatlian, T., Cam, C., Segura, T., Tokatlian, T., Segura, T., and Cam, C. (2015). Porous hyaluronic acid hydrogels for localized nonviral DNA delivery in a diabetic wound healing model. Adv. Healthc. Mat. 4, 1084–1091. doi:10.1002/ADHM.201400783
Toskas, G., Hund, R. D., Laourine, E., Cherif, C., Smyrniotopoulos, V., and Roussis, V. (2011). Nanofibers based on polysaccharides from the green seaweed Ulva Rigida. Carbohydr. Polym. 84, 1093–1102. doi:10.1016/J.CARBPOL.2010.12.075
Tran, T. T. Van, Truong, H. B., Tran, N. H. V., Quach, T. M. T., Nguyen, T. N., Bui, M. L., et al. (2018). Structure, conformation in aqueous solution and antimicrobial activity of ulvan extracted from green seaweed Ulva reticulata. Nat. Prod. Res. 32, 2291–2296. doi:10.1080/14786419.2017.1408098
Trivedi, T. J., Rao, K. S., and Kumar, A. (2013). Facile preparation of agarose–chitosan hybrid materials and nanocomposite ionogels using an ionic liquid via dissolution, regeneration and sol–gel transition. Green Chem. 16, 320–330. doi:10.1039/C3GC41317A
Trivedi, T. J., Srivastava, D. N., Rogers, R. D., and Kumar, A. (2012). Agarose processing in protic and mixed protic–aprotic ionic liquids: Dissolution, regeneration and high conductivity, high strength ionogels. Green Chem. 14, 2831–2839. doi:10.1039/C2GC35906E
Tsubaki, S., Oono, K., Hiraoka, M., Onda, A., and Mitani, T. (2016). Microwave-assisted hydrothermal extraction of sulfated polysaccharides from Ulva spp. and Monostroma latissimum. Food Chem. x. 210, 311–316. doi:10.1016/J.FOODCHEM.2016.04.121
Tsubaki, S., Oono, K., Hiraoka, M., Ueda, T., Onda, A., Yanagisawa, K., et al. (2014). Hydrolysis of green-tide forming Ulva spp. by microwave irradiation with polyoxometalate clusters. Green Chem. 16, 2227–2233. doi:10.1039/C3GC42027B
Tziveleka, L. A., Ioannou, E., and Roussis, V. (2019). Ulvan, a bioactive marine sulphated polysaccharide as a key constituent of hybrid biomaterials: A review. Carbohydr. Polym. 218, 355–370. doi:10.1016/j.carbpol.2019.04.074
Tziveleka, L. A., Sapalidis, A., Kikionis, S., Aggelidou, E., Demiri, E., Kritis, A., et al. (2020). Hybrid sponge-like scaffolds based on ulvan and gelatin: Design, characterization and evaluation of their potential use in bone tissue engineering. Materials 13, 1763. doi:10.3390/MA13071763
Vargas-Osorio, Z., Ruther, F., Chen, S., Sengupta, S., Liverani, L., Michálek, M., et al. (2022). Environmentally friendly fabrication of electrospun nanofibers made of polycaprolactone, chitosan and κ-carrageenan (PCL/CS/κ-C). Biomed. Mat. 17, 045019. doi:10.1088/1748-605X/AC6EAA
Vázquez, J. A., Rodríguez-Amado, I., Montemayor, M. I., Fraguas, J., Del González, M. P., and Murado, M. A. (2013). Chondroitin sulfate, hyaluronic acid and chitin/chitosan production using marine waste sources: Characteristics, applications and eco-friendly processes: A review. Mar. Drugs 11, 747–774. doi:10.3390/md11030747
Venugopal, V. (2011). Marine polysaccharides - food applications. New York: C. PRESS. doi:10.1201/9780429058653-11
Vieira, H., Leal, M. C., and Calado, R. (2020). Fifty shades of blue: How blue biotechnology is shaping the bioeconomy. Trends Biotechnol. 38, 940–943. doi:10.1016/J.TIBTECH.2020.03.011
Villard, P., Rezaeeyazdi, M., Colombani, T., Joshi-Navare, K., Rana, D., Memic, A., et al. (2019). Autoclavable and injectable cryogels for biomedical applications. Adv. Healthc. Mat. 8, 1900679. doi:10.1002/ADHM.201900679
Villate-Beitia, I., Truong, N. F., Gallego, I., Zárate, J., Puras, G., Pedraz, J. L., et al. (2018). Hyaluronic acid hydrogel scaffolds loaded with cationic niosomes for efficient non-viral gene delivery. RSC Adv. 8, 31934–31942. doi:10.1039/C8RA05125A
Wade, R. J., Bassin, E. J., Rodell, C. B., and Burdick, J. A. (2015). Protease-degradable electrospun fibrous hydrogels. Nat. Commun. 61 6, 6639–6710. doi:10.1038/ncomms7639
Wan, M., Qin, W., Lei, C., Li, Q., Meng, M., Fang, M., et al. (2021). Biomaterials from the sea: Future building blocks for biomedical applications. Bioact. Mat. 6, 4255–4285. doi:10.1016/J.BIOACTMAT.2021.04.028
Wang, H., Xu, Z., Wu, Y., Li, H., and Liu, W. (2018a). A high strength semi-degradable polysaccharide-based hybrid hydrogel for promoting cell adhesion and proliferation. J. Mat. Sci. 53, 6302–6312. doi:10.1007/S10853-018-2019-8
Wang, L., Cao, J., Lei, D. L., Cheng, X. B., Zhou, H. Z., Hou, R., et al. (2010). Application of nerve growth factor by gel increases formation of bone in mandibular distraction osteogenesis in rabbits. Br. J. Oral Maxillofac. Surg. 48, 515–519. doi:10.1016/J.BJOMS.2009.08.042
Wang, L., Khor, E., Wee, A., and Lim, L. Y. (2002). Chitosan-alginate PEC membrane as a wound dressing: Assessment of incisional wound healing. J. Biomed. Mat. Res. 63, 610–618. doi:10.1002/JBM.10382
Wang, W., Chang, L., Shao, Y., Yu, D., Parajuli, J., Xu, C., et al. (2022). Conductive ionic liquid/chitosan hydrogels for neuronal cell differentiation. Eng. Regen. 3, 1–12. doi:10.1016/J.ENGREG.2022.01.007
Wang, Z., Zhang, H., Chu, A. J., Jackson, J., Lin, K., Lim, C. J., et al. (2018b). Mechanically enhanced nested-network hydrogels as a coating material for biomedical devices. Acta Biomater. 70, 98–109. doi:10.1016/J.ACTBIO.2018.02.003
Wu, H., Liu, C., Chen, J., Chen, Y., Anderson, D. P., and Chang, P. R. (2010). Oxidized pea starch/chitosan composite films: Structural characterization and properties. J. Appl. Polym. Sci. 118, 3082–3088. doi:10.1002/APP.32753
Yang, M., Xia, Y., Wang, Y., Zhao, X., Xue, Z., Quan, F., et al. (2016). Preparation and property investigation of crosslinked alginate/silicon dioxide nanocomposite films. J. Appl. Polym. Sci. 133, 43489. doi:10.1002/APP.43489
Yao, Y., Zaw, A. M., Anderson, D. E. J., Hinds, M. T., and Yim, E. K. F. (2020). Fucoidan functionalization on poly(vinyl alcohol) hydrogels for improved endothelialization and hemocompatibility. Biomaterials 249, 120011. doi:10.1016/J.BIOMATERIALS.2020.120011
Ye, X., Li, X., Shen, Y., Chang, G., Yang, J., and Gu, Z. (2017). Self-healing pH-sensitive cytosine- and guanosine-modified hyaluronic acid hydrogels via hydrogen bonding. Polym. Guildf. 108, 348–360. doi:10.1016/J.POLYMER.2016.11.063
Youssouf, L., Lallemand, L., Giraud, P., Soulé, F., Bhaw-Luximon, A., Meilhac, O., et al. (2017). Ultrasound-assisted extraction and structural characterization by NMR of alginates and carrageenans from seaweeds. Carbohydr. Polym. 166, 55–63. doi:10.1016/J.CARBPOL.2017.01.041
Zargarzadeh, M., Silva, A. S., Nunes, C., Coimbra, M. A., Custódio, C. A., and Mano, J. F. (2022). Self-glucose feeding hydrogels by enzyme empowered degradation for 3D cell culture. Mat. Horiz. 9, 694–707. doi:10.1039/D0MH01982H
Zdanowicz, M., Wilpiszewska, K., and Spychaj, T. (2018). Deep eutectic solvents for polysaccharides processing. A review. Carbohydr. Polym. 200, 361–380. doi:10.1016/J.CARBPOL.2018.07.078
Zhang, G., Li, J., Zhu, T., Gu, Q., and Li, D. (2016). Advanced tools in marine natural drug discovery. Curr. Opin. Biotechnol. 42, 13–23. doi:10.1016/J.COPBIO.2016.02.021
Zhang, W., Zhao, L., Ma, J., Wang, X., Wang, Y., Ran, F., et al. (2017). Electrospinning of fucoidan/chitosan/poly(vinyl alcohol) scaffolds for vascular tissue engineering. Fibers Polym. 185 (18), 922–932. doi:10.1007/S12221-017-1197-3
Zheng, W., Hao, Y., Wang, D., Huang, H., Guo, F., Sun, Z., et al. (2021). Preparation of triamcinolone acetonide-loaded chitosan/fucoidan hydrogel and its potential application as an oral mucosa patch. Carbohydr. Polym. 272, 118493. doi:10.1016/J.CARBPOL.2021.118493
Zhou, D., Li, S., Pei, M., Yang, H., Gu, S., Tao, Y., et al. (2020). Dopamine-modified hyaluronic acid hydrogel adhesives with fast-forming and high tissue adhesion. ACS Appl. Mat. Interfaces 12, 18225–18234. doi:10.1021/acsami.9b22120
Zhou, F., Zhang, X., Cai, D., Li, J., Mu, Q., Zhang, W., et al. (2017). Silk fibroin-chondroitin sulfate scaffold with immuno-inhibition property for articular cartilage repair. Acta Biomater. 63, 64–75. doi:10.1016/J.ACTBIO.2017.09.005
Zhu, F., Bo, Y., chao, H., Lei, W., Ren, K., Qian, J., et al. (2017). Tough polyion complex hydrogel films of natural polysaccharides. Chin. J. Polym. Sci. 3510 35, 1276–1285. doi:10.1007/S10118-017-1977-7
Zhu, Y., Yao, Z., Liu, Y., Zhang, W., Geng, L., and Ni, T. (2020). Incorporation of ROS-responsive substance P-loaded zeolite imidazolate framework-8 nanoparticles into a Ca 2+-cross-linked alginate/ pectin hydrogel for wound dressing applications. Int. J. Nanomedicine 15, 333–346. doi:10.2147/IJN.S225197
AAC Azyde-alkyne cycloaddition
AGR Agarose
ALG Alginate
CC Click-chemistry
CHT chitosan
CS chondroitin sulfate
DES Deep eutectic solvent
DIC N,N′-diisopropylcarbodiimide
ECM Extracellular matrix
EE Enzymatic extraction
EDC 1-ethyl-3-(3-dimethyl-aminopropyl-1-carbodiimide)
FU Fucoidan
GAG Glycosaminoglycan
HA Hyaluronic acid
ILs Ionic liquids
k-CG Carrageenan
LAM laminarin
LbL Layer-by-layer
MAE Microwave-assisted extraction
NHS N-hydroxysuccinmide
PLE Pressurized liquid extraction
RGD Arginine-glycine-aspartic acid
RM Regenerative medicine
SDG Sustainable Development Goal
SPAAC Strain-promoted azyde-alkyne cycloaddition
SWE Subcritical water extraction;
TE Tissue engineering
TERM tissue engineering and regenerative medicine
UAE Ultrasound-assisted extraction
UL Ulvan
UN SDGs United Nations Sustainable Development Goals.
Keywords: marine-origin polysaccharides, marine biomass, green chemistry, circular economy, sustainability, biomedical applications, blue biotechnology
Citation: Sacramento MMA, Borges J, Correia FJS, Calado R, Rodrigues JMM, Patrício SG and Mano JF (2022) Green approaches for extraction, chemical modification and processing of marine polysaccharides for biomedical applications. Front. Bioeng. Biotechnol. 10:1041102. doi: 10.3389/fbioe.2022.1041102
Received: 10 September 2022; Accepted: 24 November 2022;
Published: 08 December 2022.
Edited by:
Mehmet Berat Taskin, Leibniz Institute of Polymer Research (LG), GermanyReviewed by:
Jaydee Cabral, University of Otago, New ZealandCopyright © 2022 Sacramento, Borges, Correia, Calado, Rodrigues, Patrício and Mano. This is an open-access article distributed under the terms of the Creative Commons Attribution License (CC BY). The use, distribution or reproduction in other forums is permitted, provided the original author(s) and the copyright owner(s) are credited and that the original publication in this journal is cited, in accordance with accepted academic practice. No use, distribution or reproduction is permitted which does not comply with these terms.
*Correspondence: João M. M. Rodrigues, anJvZHJpZ3Vlc0B1YS5wdA==; Sónia G. Patrício, c2dwYXRyaWNpb0B1YS5wdA==; João F. Mano, am1hbm9AdWEucHQ=
Disclaimer: All claims expressed in this article are solely those of the authors and do not necessarily represent those of their affiliated organizations, or those of the publisher, the editors and the reviewers. Any product that may be evaluated in this article or claim that may be made by its manufacturer is not guaranteed or endorsed by the publisher.
Research integrity at Frontiers
Learn more about the work of our research integrity team to safeguard the quality of each article we publish.