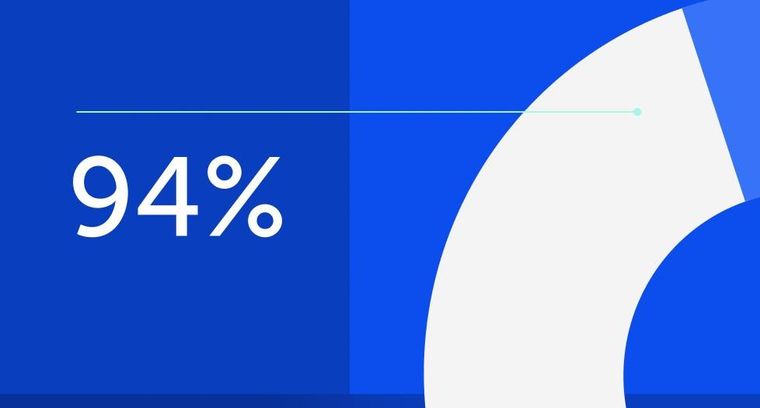
94% of researchers rate our articles as excellent or good
Learn more about the work of our research integrity team to safeguard the quality of each article we publish.
Find out more
MINI REVIEW article
Front. Bioeng. Biotechnol., 18 October 2022
Sec. Biomaterials
Volume 10 - 2022 | https://doi.org/10.3389/fbioe.2022.1039777
This article is part of the Research TopicAdvancement of Stem Cells and Regenerative Agents in Treating Degenerations of Locomotor SystemView all 7 articles
Peripheral nerve injury is a clinically common injury that causes sensory dysfunction and locomotor system degeneration, which seriously affects the quality of the patients’ daily life. Long gapped defects in large nerve are difficult to repair via surgery and limited donor source of autologous nerve greatly challenges the successful nerve repair by transplantation. Significantly, remarkable progress has been made in repairing the peripheral nerve injury using artificial nerve grafts and a variety of products for peripheral nerve repair have emerged been approved globally in recent years. The raw materials of these commercial products includes natural/synthetic polymers, extracellular matrix. Despite a lot of effort, the desirable functional recovery still remains great challenges in long gapped nerve defects. Thus this review discusses the recent development of tissue engineering products for peripheral nerve repair and the design of bionic grafts improving the local microenvironment for accelerating nerve regeneration against locomotor disorder, which may provide potential strategies for the repair of long gaps or thick nerve defects by multifunctional biomaterials.
Peripheral nerve injury mainly occurs in severe accidents such as work-related injuries and traffic accidents often accompanied by symptoms such as sensory and locomotor dysfunction, and it is poor repair would seriously affect the quality of the patients’ daily life (Yi et al., 2019; Vijayavenkataraman, 2020). Peripheral nerve injury can be divided into traction injury, cutting injury, firearm injury, ischemic injury and iatrogenic injury. The phenotypes of peripheral nerve injury include nerve entrapment, nerve hemisection, nerve transection, and nerve defects (Spinner and Kline, 2000; Tezcan, 2017). Significantly, peripheral nerve injury can result in varying degrees of neuronal degeneration, muscle atrophy, and fibrosis (Yang et al., 2022c). Due to the slow rate of regeneration (approximately 1 mm/day), irreversible atrophy usually occurs before skeletal muscle reinnervation. This seriously affects the functional reconstruction of target muscles and the recovery of locomotor function after nerve injury (Sun et al., 2021). Therefore, assessment of functional recovery is critical to determine the degree of regeneration following peripheral nerve injury (Navarro, 2016), which is especially true for studies devoted to developing tissue-engineered products to replace autologous nerve grafts for peripheral nerve injury. (Angius et al., 2012; Heinzel et al., 2020).
Traditionally, autologous nerve repair is the golden line for repairing the injured peripheral nerves. However, it still has many disadvantages: 1) Repairing the defective nerve with normal nerves from other parts may cause secondary surgical damage; 2) cable-like repair is not accurate 3) It is easy to cause mismatched growth of regenerative nerves, resulting in neuroma; 4) Limited source can hardly meet the needs of long gaps or thick nerve defects, and therefore limited its clinical applications (Rutkowski and Health, 1998; Gvans, 2000).
Thanks to the continuous development of biomedical materials for nerve repair, remarkable progress has been made in the application of artificial nerve grafts in repairing the peripheral nerve injury (Gu et al., 2011; Gu et al., 2015a). There have been a variety of products for peripheral nerve repair emerged in recent years. Generally, the ideal tissue-engineered nerve grafts should be compounded with trophic factors and bionic structures within the bioscaffold (Tang et al., 2021b; Zhang Y. et al., 2022; Chen et al., 2022). Furthermore, various kinds of cells can be seeded into bioactive implants to promote the recovery of nerve function by differentiating into locomotor neurons to innervate muscles or secreting neurotrophic factors to repair injury (Du et al., 2018). Therefore, how to choose ideal seed cells and neurotrophic factors with appropriate concentration and combination to further equip tissue engineering products for peripheral nerve repair with better biosafety and bioactivity for functional reconstruction of nerve tissue is a crucial in this fields. Thus, this review discusses the research status and latest progress of tissue engineering products for peripheral nerve repair.
Peripheral nerve repair grafts that have been approved in China belong to Class III medical devices. Until March of 2022, the Chinese Food and Drug Administration has approved a total of five domestic and two imported medical device products (Table 1). The raw materials of these products include collagen, chitosan, polyglycolide lactide (PGLA), DL-lactide-co-ε-caprolactone and polyglycolic acid (PGA), extracellular matrix (ECM).
The decellularized allogeneic nerve repair material (trade name: Shenqiao) is produced by Guangzhou Zhongda Medical Instrument Co., Ltd., which is made from the peripheral nerves collected from the human body (cadavers) followed by decellularization. It is mainly composed of collagen fibers and the extracellular matrix retaining the structure of nerve basement membrane tube, perineurium, epineurium and other supporting structures, which is suitable for repairing 1–5 cm traumatic sensory nerve defects.
Acellular matrix peripheral nerve repair membrane produced by Shandong Junxiu Biotechnology Co., Ltd. Has obtained the National Medical Device Registration Certificate in 2019. It is a transparent or translucent film based on the extracellular matrix of porcine peripheral nerves, which retains the nerve scaffold structure and the active ingredients of the abundant nerve extracellular matrix. This membrane is suitable for auxiliary repair of non-defective or anastomosed peripheral nerve injury.
Artificial nerve sheath tube produced by Beijing Tianxinfu Medical Equipment Co., Ltd. is suitable for repairing peripheral nerve defects those are less than 2 cm obtained the National Medical Device Registration Certificate in 2016. The material of this product is mainly derived from the bovine achilles tendon with high-purity type I collagen and made into a spongy collagen sheath to guide nerve growth. It has good biocompatibility and can be degraded and absorbed within 3–6 months, degradation products of which are amino acids and water.
Peripheral nerve repair graft from Jiangsu Yitong Biotechnology Co., Ltd. is composed of a catheter and a built-in fiber to guide directed nerve growth. The catheter is prepared by freeze-drying chitosan, chitin and medicinal gelatin. The built-in fiber is polyglycolide lactide (PGLA) fiber. The graft is developed for repair of sensory function in the digital nerve, superficial branch of the radial nerve, and median nerve of the forearm with a defective length of less than 30 mm, which can be degraded and absorbed within 3 months.
Neurolac Peripheral Nerve guide and GEM Neurotube are two imported products from Polyganics Innovations B.V. in Netherlands and Synovis Micro Companies Alliance, Inc., which were both awarded the National Imported Medical Device Registration Certificate. Neurolac Peripheral Nerve guide possesses a tubular structure to repair peripheral nerve defects up to 20 mm, prepared from (DL-lactide-co-ε-caprolactone) copolymer. The absorbable GEM Neurotube is an polyglycolic acid mesh tube, of which the pipe wall is corrugated to increase its strength and flexibility. It can be absorbed into the human body through a hydrolysis process and used to repair 8–30 mm digital nerve defects to restore the sensory function.
Although a variety of tissue engineering products for peripheral nerve repair have been approved, several disadvantages still exist, such as the mismatch between material degradation and nerve regeneration, the inability to repair long-distance injuries, poor locomotor functional recovery (Gu et al., 2014; Wu et al., 2017). In recent years, many studies have focused on selection of raw materials, construction of biomimetic structures, improvement of the regenerative microenvironment and implantation with seed cells.
Ideal biomaterials should have good biocompatibility, biodegradability and minimal immunogenicity, be non-toxicity, non-teratogenic and non-carcinogenic, supporting for nerve regeneration and recovery, etc. (Nectow et al., 2012; Fakhri et al., 2020). The chemical and physical properties of biomaterials directly affect their therapeutic effect for nerve regeneration, emphasizing the importance of materials selection for tissue-engineered nerve grafts. Recently, many biodegradable materials have been used in designing scaffolds for neural tissue engineering, including synthetic polymers such as polyglycolic acid (PGA) (Kusuhara et al., 2019; Sayanagi et al., 2020), polylactic acid (PLA) (Jahromi et al., 2020), polyglycolide lactide (PGLA) (Xu et al., 2017; Dos Santos et al., 2019; Lu P. et al., 2021; Manto et al., 2021), poly (ε-caprolactone) (PCL) (Dong et al., 2020; Dursun Usal et al., 2022) and polyurethane (PU) (Yang H. et al., 2022), etc. For example, Reid et al. used a thin film of PCL to prepare nerve catheter for repairing 10 mm sciatic nerve injury in rats, and after 18 weeks, the growth rate of regenerated axons in PCL nerve catheter was comparable to that of autologous nerves (Reid et al., 2013). However, PCL conduit has high rigidity, which is inconvenient for clinical use. However, the degradation products of synthetic polymers such as PGA, PLA, and PGLA are acidic, and may stimulate inflammatory responses to surrounding tissues and hinder peripheral nerve repair and regeneration.
Other natural materials, including native fibrin (Du et al., 2017; Wang et al., 2018; Razavi et al., 2021), collagen (Saeki et al., 2018; Yao et al., 2018; He et al., 2021; Wang et al., 2021; Zheng et al., 2021), keratin (Apel et al., 2008; Lin et al., 2012; Pace et al., 2014), alginate (Lin et al., 2017; Rahmati et al., 2021; Abdelbasset et al., 2022), chitin (Bak et al., 2017; Lu C. F. et al., 2021; Yang et al., 2022b), chitosan (Li et al., 2018; Vishnoi et al., 2019), and silk fibroin (Carvalho et al., 2021; Kim et al., 2021; Zhao et al., 2020), as well as extracellular matrix (ECM) (Li T. et al., 2021; Kong et al., 2021; Kong et al., 2022), have shown great potential in treating long gap nerve defects. For instance, Wang et al. prepared a nerve catheter using chitosan/chitin to achieve excellent angiogenesis in nerve regeneration process for successfully repairing the 10 mm sciatic nerve defect in rats (Wang et al., 2016). These natural biomaterials such as chitosan and collagen have good biocompatibility, but the in vivo degradation time is too long to match the process of peripheral nerve regeneration. In addition, ECM has good biodegradability and biomimetic structure, but its application is mainly limited to the standardization of the decellular method and sterilization methods. To meet the various requirements for nerve regeneration, combination of different raw materials into one graft is reasonable to prepare nerve grafts with excellent chemical and physical performance.
It is well known that the peripheral nerve is morphologically composed of many root nerve fibers. The fibers form the root nerve bundles which then constitute the nerve stems (Tezcan, 2017). Neural fibers are aligned in parallel. Once the peripheral nerve is impaired, the distal nerve axon and myelin undergo fragmentation and collapse, leading to the invasion of a large number of phagocytes to clear axon myelin debris and more importantly, to stimulate the resting Schwann cells to divide and proliferate. After that, the stimulated cells keep growing along each nerve fiber intima and basement membrane into a line, named Buengner cell zone (Mirsky and Jessen, 1999). Eventually, the regenerated axons growing from the proximal end extend along the pipeline to the target organ, thereby restore its function. Therefore, the morphology of biomaterials should be beneficial to induce Schwan cells to arrange into lines and provide a pipeline for the extension of regenerated nerves (Figure 1). Bionic structures can provide a suitable microenvironment for the migration, proliferation and functionalization of nerve cells, and provides contact guidance for the directional growth of axons to improve the accuracy of nerve alignment. However, due to the complexity of natural neural structures, it still remains great challenges to prepare artificial nerve grafts with nerve-like structures in micro-nano scales. Current studies mainly focus on isotropic hydrogel fillers to provide intraluminal support for nerve regeneration (Huang et al., 2018), fibrotic intraluminal topographic guidance for neurites (Zou et al., 2016), and patterned cavity stand to provide three-dimensional (3D) structural support for nerve growth (Qu et al., 2011). For example, Du et al. prepared a 3D hierarchically arranged fibrin nanofiber hydrogel (AFG) to resemble the structure and biological function of natural fibrin cables. The AFG served as filler for tissue-engineered chitosan tubes, bridging a 10-mm-long sciatic nerve defect in rats. The results showed that AFG provided a suitable microenvironment that supported Schwann cell cable formation and axon regeneration within 2 weeks. (Du et al., 2017).
Nerve injury regeneration is a very complex pathophysiological process and the local microenvironment has strong temporal and spatial characteristics, covering different levels such as molecules, cells, and organisms, and involving many fields such as physiology, pathology, biochemistry, biophysics, and bioinformatics (Rajaram et al., 2012; Chen et al., 2015; Radhakrishnan et al., 2015; Li G. et al., 2021). It was found that the use of artificial nerve grafts alone can only repair peripheral nerve defects in short distance. It is difficult to support the regeneration of large gap defects, which may be due to the lack of local support of cells and neurotrophic factors (Li T. et al., 2021; Chen et al., 2022). Nerve regrowth largely depends on the biological function exerted by the neurotrophins secreted by the nerve cells for regulating cell viability, migration, and differentiation in the peripheral and central nervous systems. Therefore, nerve conduits with neurotrophic factors or related components are essential tools for nerve repair (Gordon, 2009). For peripheral nerve defects with large gaps, insufficient fibrin formation between proximal and distal nerves restricts Schwann cell motility and Büngner band formation. Hence, the nutrient support of nerve conduits for cells is indispensable. Similar to skin tissue repair materials by encapsulation, graft copolymerization and so on, adding probiotics (Zhu et al., 2021), growth factors (Hao et al., 2022), etc., to improve the regenerative microenvironment, the functionalization of nerve repair materials can be achieved by similar means. Nerve conduits loaded with factors such as vascular endothelial growth factor (VEGF) (Rao et al., 2020), nerve growth factor (NGF) (Fine et al., 2002), insulin-like growth factor-I (IGF-I) (Zhang J. et al., 2022), fibroblast growth factor (FGF) (Ma et al., 2017), and glial growth factor (GGF) (Alsmadi et al., 2018), have been proven to have a positive role in peripheral nerve regrowth. (Kemp et al., 2008; Catrina et al., 2013). Yao et al. used longitudinally oriented collagen conduitloaded with NGF to repair 35-mm-long sciatic nerve defects in Beagle. After 9 months, the graft effectively achieved the sciatic nerve axonal regeneration and recovery of motor function than LOCC alone (Yao et al., 2018).
In addition, many types of physical or chemical stimulation, such as pH, optical signal, and electric current, can also promote nerve repair by activating the release of bioactive factors in neural cells (Tang et al., 2021a; Tang et al., 2021b; Chen et al., 2022; Tang et al., 2022). A growing amount of studies have found that electric stimulation can regulate Schwann cell proliferation (Gu et al., 2015b), neuronal differentiation (Meng et al., 2020), axon regrowth (Gordon, 2016), and the neurotrophic factors production (English et al., 2007). Some studies suggest that extracellular electrical signals can control gene expression, growth factor release, cell polarization and remodeling, etc. (Xue et al., 2018).
Seed cells are an essential component for tissue engineering, which can proliferate and differentiate to form target tissues to repair defects (Liu et al., 2022). Functional seed cells combined within nerve scaffolds can secrete growth factors, provide a favorable microenvironment for nerve regeneration, and further accelerate the nerve regeneration process. Ideal tissue-engineered neural seed cells require a wide range of sources, good safety, high efficacy without ethics and immune rejection (Lategan et al., 2022; Tang et al., 2022). Peripheral nerve tissue has a particular structure, and its regeneration includes regeneration of non-dead and functional neurons and myelin sheath. Highly differentiated neurons cannot be used as seed cells due to their inability of proliferation and division. On the other hand, glial cells and Schwann cells in peripheral nerve tissue playing important roles in regeneration process can proliferate and differentiate into mature cells to promote peripheral nerve regeneration combing with grafts (Ghane et al., 2021).
Neural stem cells can also differentiate into neurons and glia, the two major cell types of the peripheral and central nervous systems. As seed cells, they can promote the maturation of the nervous system. It has been shown that neural stem cells can promote the recovery of motor function by differentiation into locomotor neurons that innervate muscles. Neural stem cells also secrete a series of neurotrophic factors that promote the repair of nerve function. A novel stem cell-based pulsed electrical stimulation therapy was developed Du et al. for repairing 15 mm sciatic nerve injury of athymic nude mice. The results showed a significant improvement in locomotor function recovery and differentiation of Schwann cell using stem cells therapy, which was comparable to autograft in treatment of long gapped defects (Du et al., 2018). In addition, neural stem cells would promote angiogenesis and immune regulation (Wang et al., 2017). Moreover, BMSCs, olfactory sheath cells, etc., have also been used as seed cells for tissue-engineered nerves (Cooney et al., 2016; Sayad Fathi and Zaminy, 2017; Miah et al., 2021).
Given the inherent drawbacks of autologous transplantation, the development of artificial nerve grafts is considered as a promising strategy in the field of peripheral nerve. Researchers in tissue engineering have focused on the development of biomaterials and tissue processing techniques to create various types of equipment and substrates to support peripheral nerve regeneration. In the past few decades, breakthroughs have been made in materials science and tissue engineering techniques to mimic or protect the neural tissue microenvironment for promoting nerve regrowth. Allogeneic nerve grafts and artificial catheters have been increasingly applied in clinical practice. At present, artificial and non-artificial nerve grafts have been achieved good clinical outcomes in treating short-distance peripheral nerve defects. However, there is still no commercial product that can be comparable to autologous transplantation in treating long gaps defects in large nerve. Effective control of growth factors release in the process of nerve regeneration in real-time still remains a challenge in this field. Notably, numerous studies are devoted to promote the directional growth of cells and remyelination by altering the morphology and structure within the grafts, or involving seed cells/growth factors to create better microenvironments for improving locomotor function recovery after nerve injury. However, most of the established neural stem cell lines come from mice, but there are obvious species differences between mice and humans. Therefore, the source, isolation, culture and identification of neural stem cells should be optimized, and the mechanism of neural stem cell differentiation needs to be further studied. In summary, These ongoing studies have laid a solid foundation for the development of future tissue engineering products for peripheral nerve repair.
All authors contributed to the design of the study and writing of the manuscript. YJ and XT wrote the main manuscript text and prepared figures. TL helped the manuscript editing and discussions. JL and YY revised the article critically. All authors reviewed the manuscript.
This work was financially supported by the National Natural Science Foundation of China (Project No: 32230057), the Jiangsu Provincial Key Research and Development Program (Project No: BE2022766), and Jiangsu Provincial Key Medical Center.
The authors declare that the research was conducted in the absence of any commercial or financial relationships that could be construed as a potential conflict of interest.
All claims expressed in this article are solely those of the authors and do not necessarily represent those of their affiliated organizations, or those of the publisher, the editors and the reviewers. Any product that may be evaluated in this article, or claim that may be made by its manufacturer, is not guaranteed or endorsed by the publisher.
Abdelbasset, W. K., Jasim, S. A., Sharma, S. K., Margiana, R., Bokov, D. O., Obaid, M. A., et al. (2022). Alginate-based hydrogels and tubes, as biological macromolecule-based platforms for peripheral nerve tissue engineering: A review. Ann. Biomed. Eng. 50 (6), 628–653. doi:10.1007/s10439-022-02955-8
Alsmadi, N. Z., Bendale, G. S., Kanneganti, A., Shihabeddin, T., Nguyen, A. H., Hor, E., et al. (2018). Glial-derived growth factor and pleiotrophin synergistically promote axonal regeneration in critical nerve injuries. Acta Biomater. 78, 165–177. doi:10.1016/j.actbio.2018.07.048
Angius, D., Wang, H., Spinner, R. J., Gutierrez-Cotto, Y., Yaszemski, M. J., and Windebank, A. J. (2012). A systematic review of animal models used to study nerve regeneration in tissue-engineered scaffolds. Biomaterials 33 (32), 8034–8039. doi:10.1016/j.biomaterials.2012.07.056
Apel, P. J., Garrett, J. P., Sierpinski, P., Ma, J., Atala, A., Smith, T. L., et al. (2008). Peripheral nerve regeneration using a keratin-based scaffold: Long-term functional and histological outcomes in a mouse model. J. Hand Surg. Br. 33 (9), 1541–1547. doi:10.1016/j.jhsa.2008.05.034
Bak, M., Gutkowska, O. N., Wagner, E., and Gosk, J. (2017). The role of chitin and chitosan in peripheral nerve reconstruction. Polim. Med. 47 (1), 43–47. doi:10.17219/pim/75653
Carvalho, C. R., Chang, W., Silva-Correia, J., Reis, R. L., Oliveira, J. M., and Kohn, J. (2021). Engineering silk fibroin-based nerve conduit with neurotrophic factors for proximal protection after peripheral nerve injury. Adv. Healthc. Mat. 10 (2), e2000753. doi:10.1002/adhm.202000753
Catrina, S., Gander, B., and Madduri, S. (2013). Nerve conduit scaffolds for discrete delivery of two neurotrophic factors. Eur. J. Pharm. Biopharm. 85 (1), 139–142. doi:10.1016/j.ejpb.2013.03.030
Chen, P., Piao, X., and Bonaldo, P. (2015). Role of macrophages in Wallerian degeneration and axonal regeneration after peripheral nerve injury. Acta Neuropathol. 130 (5), 605–618. doi:10.1007/s00401-015-1482-4
Chen, X., Tang, X., Wang, Y., Gu, X., Huang, T., Yang, Y., et al. (2022). Silk-inspired fiber implant with multi-cues enhanced bionic microenvironment for promoting peripheral nerve repair. Biomater. Adv. 135, 112674. doi:10.1016/j.msec.2022.112674
Cooney, D. S., Wimmers, E. G., Ibrahim, Z., Grahammer, J., Christensen, J. M., Brat, G. A., et al. (2016). Mesenchymal stem cells enhance nerve regeneration in a rat sciatic nerve repair and hindlimb transplant model. Sci. Rep. 6, 31306. doi:10.1038/srep31306
Dong, R., Liu, C., Tian, S., Bai, J., Yu, K., Liu, L., et al. (2020). Electrospun polycaprolactone (PCL)-amnion nanofibrous membrane prevents adhesions and promotes nerve repair in a rat model of sciatic nerve compression. PLoS One 15 (12), e0244301. doi:10.1371/journal.pone.0244301
Dos Santos, F. P., Peruch, T., Katami, S. J. V., Martini, A. P. R., Crestani, T. A., Quintiliano, K., et al. (2019). Poly (lactide-co-glycolide) (PLGA) scaffold induces short-term nerve regeneration and functional recovery following sciatic nerve transection in rats. Neuroscience 396, 94–107. doi:10.1016/j.neuroscience.2018.11.007
Du, J., Liu, J., Yao, S., Mao, H., Peng, J., Sun, X., et al. (2017). Prompt peripheral nerve regeneration induced by a hierarchically aligned fibrin nanofiber hydrogel. Acta Biomater. 55, 296–309. doi:10.1016/j.actbio.2017.04.010
Du, J., Zhen, G., Chen, H., Zhang, S., Qing, L., Yang, X., et al. (2018). Optimal electrical stimulation boosts stem cell therapy in nerve regeneration. Biomaterials 181, 347–359. doi:10.1016/j.biomaterials.2018.07.015
Dursun Usal, T., Yesiltepe, M., Yucel, D., Sara, Y., and Hasirci, V. (2022). Fabrication of a 3D printed PCL nerve guide: In vitro and in vivo testing. Macromol. Biosci. 22 (3), 2100389. doi:10.1002/mabi.202100389
English, A. W., Schwartz, G., Meador, W., Sabatier, M. J., and Mulligan, A. (2007). Electrical stimulation promotes peripheral axon regeneration by enhanced neuronal neurotrophin signaling. Dev. Neurobiol. 67 (2), 158–172. doi:10.1002/dneu.20339
Fakhri, E., Eslami, H., Maroufi, P., Pakdel, F., Taghizadeh, S., Ganbarov, K., et al. (2020). Chitosan biomaterials application in dentistry. Int. J. Biol. Macromol. 162, 956–974. doi:10.1016/j.ijbiomac.2020.06.211
Fine, E. G., Decosterd, I., Papaloïzos, M., Zurn, A. D., and Aebischer, P. (2002). GDNF and NGF released by synthetic guidance channels support sciatic nerve regeneration across a long gap. Eur. J. Neurosci. 15, 589–601. doi:10.1046/j.1460-9568.2002.01892.x
Ghane, N., Khalili, S., Nouri Khorasani, S., Esmaeely Neisiany, R., Das, O., and Ramakrishna, S. (2021). Regeneration of the peripheral nerve via multifunctional electrospun scaffolds. J. Biomed. Mat. Res. A 109 (4), 437–452. doi:10.1002/jbm.a.37092
Gordon, T. (2016). Electrical stimulation to enhance axon regeneration after peripheral nerve injuries in animal models and humans. Neurotherapeutics 13 (2), 295–310. doi:10.1007/s13311-015-0415-1
Gordon, T. (2009). The role of neurotrophic factors in nerve regeneration. Neurosurg. Focus 26, E3–E10. doi:10.3171/FOC.2009.26.2.E3
Gu, X., Ding, F., and Williams, D. F. (2014). Neural tissue engineering options for peripheral nerve regeneration. Biomaterials 35 (24), 6143–6156. doi:10.1016/j.biomaterials.2014.04.064
Gu, X., Ding, F., Yang, Y., and Liu, J. (2011). Construction of tissue engineered nerve grafts and their application in peripheral nerve regeneration. Prog. Neurobiol. 93 (2), 204–230. doi:10.1016/j.pneurobio.2010.11.002
Gu, X., Ding, F., Yang, Y., and Liu, J. (2015a). Tissue engineering in peripheral nerve regeneration. Neural Regen., 73–99. doi:10.1016/b978-0-12-801732-6.00005-7
Gu, X., Fu, J., Bai, J., Zhang, C., Wang, J., and Pan, W. (2015b). Low-frequency electrical stimulation induces the proliferation and differentiation of peripheral blood stem cells into Schwann cells. Am. J. Med. Sci. 349 (2), 157–161. doi:10.1097/MAJ.0000000000000385
Gvans, E. (2000). Challenges_to_nerve_regeneration. Seminars Surg. Oncol. 19, 312–318. doi:10.1002/1098-2388(200010/11)19:3<312::AID-SSU13>3.0.CO;2-M
Hao, Y., Zhao, W., Zhang, H., Zheng, W., and Zhou, Q. (2022). Carboxymethyl chitosan-based hydrogels containing fibroblast growth factors for triggering diabetic wound healing. Carbohydr. Polym. 287 (1), 119336. doi:10.1016/j.carbpol.2022.119336
He, J., Zhang, N., Zhu, Y., Jin, R., and Wu, F. (2021). MSC spheroids-loaded collagen hydrogels simultaneously promote neuronal differentiation and suppress inflammatory reaction through PI3K-Akt signaling pathway. Biomaterials 265, 120448. doi:10.1016/j.biomaterials.2020.120448
Heinzel, J., Langle, G., Oberhauser, V., Hausner, T., Kolbenschlag, J., Prahm, C., et al. (2020). Use of the CatWalk gait analysis system to assess functional recovery in rodent models of peripheral nerve injury - a systematic review. J. Neurosci. Methods 345, 108889. doi:10.1016/j.jneumeth.2020.108889
Huang, L., Zhu, L., Shi, X., Xia, B., Liu, Z., Zhu, S., et al. (2018). A compound scaffold with uniform longitudinally oriented guidance cues and a porous sheath promotes peripheral nerve regeneration in vivo. Acta Biomater. 68, 223–236. doi:10.1016/j.actbio.2017.12.010
Jahromi, H. K., Farzin, A., Hasanzadeh, E., Barough, S. E., Mahmoodi, N., Najafabadi, M. R. H., et al. (2020). Enhanced sciatic nerve regeneration by poly-L-lactic acid/multi-wall carbon nanotube neural guidance conduit containing Schwann cells and curcumin encapsulated chitosan nanoparticles in rat. Mater. Sci. Eng. C 109, 110564. doi:10.1016/j.msec.2019.110564
Kemp, S. W., Walsh, S. K., and Midha, R. (2008). Growth factor and stem cell enhanced conduits in peripheral nerve regeneration and repair. Neurological Res. 30 (10), 1030–1038. doi:10.1179/174313208X362505
Kim, S. H., Hong, H., Ajiteru, O., Sultan, M. T., Lee, Y. J., Lee, J. S., et al. (2021). 3D bioprinted silk fibroin hydrogels for tissue engineering. Nat. Protoc. 16 (12), 5484–5532. doi:10.1038/s41596-021-00622-1
Kong, Y., Wang, D., Wei, Q., and Yang, Y. (2021). Nerve decellularized matrix composite scaffold with high antibacterial activity for nerve regeneration. Front. Bioeng. Biotechnol. 9, 840421. doi:10.3389/fbioe.2021.840421
Kong, Y., Xu, J., Han, Q., Zheng, T., Wu, L., Li, G., et al. (2022). Electrospinning porcine decellularized nerve matrix scaffold for peripheral nerve regeneration. Int. J. Biol. Macromol. 209, 1867–1881. doi:10.1016/j.ijbiomac.2022.04.161
Kusuhara, H., Hirase, Y., Isogai, N., and Sueyoshi, Y. (2019). A clinical multi-center registry study on digital nerve repair using a biodegradable nerve conduit of PGA with external and internal collagen scaffolding. Microsurgery 39 (5), 395–399. doi:10.1002/micr.30417
Lategan, M., Kumar, P., and Choonara, Y. E. (2022). Functionalizing nanofibrous platforms for neural tissue engineering applications. Drug Discov. Today 27 (5), 1381–1403. doi:10.1016/j.drudis.2022.01.005
Li, G., Xue, C., Wang, H., Yang, X., Zhao, Y., Zhang, L., et al. (2018). Spatially featured porous chitosan conduits with micropatterned inner wall and seamless sidewall for bridging peripheral nerve regeneration. Carbohydr. Polym. 194, 225–235. doi:10.1016/j.carbpol.2018.04.049
Li, G., Zheng, T., Wu, L., Han, Q., Le, Y., Xue, L., et al. (2021a). Bionic microenvironment-inspired synergistic effect of anisotropic micro-nanocomposite topology and biology cues on peripheral nerve regeneration. Sci. Adv. 7, eabi5812. doi:10.1126/sciadv.abi5812
Li, T., Javed, R., and Ao, Q. (2021b). Xenogeneic decellularized extracellular matrix-based biomaterials for peripheral nerve repair and regeneration. Curr. Neuropharmacol. 19 (12), 2152–2163. doi:10.2174/1570159X18666201111103815
Lin, S. C., Wang, Y., Wertheim, D. F., and Coombes, A. G. A. (2017). Production and in vitro evaluation of macroporous, cell-encapsulating alginate fibres for nerve repair. Mater. Sci. Eng. C 73, 653–664. doi:10.1016/j.msec.2016.12.016
Lin, Y. C., Ramadan, M., Van Dyke, M., Kokai, L. E., Philips, B. J., Rubin, J. P., et al. (2012). Keratin gel filler for peripheral nerve repair in a rodent sciatic nerve injury model. Plastic Reconstr. Surg. 129 (1), 67–78. doi:10.1097/PRS.0b013e3182268ae0
Liu, S., Yang, H., Chen, D., Xie, Y., Tai, C., Wang, L., et al. (2022). Three-dimensional bioprinting sodium alginate/gelatin scaffold combined with neural stem cells and oligodendrocytes markedly promoting nerve regeneration after spinal cord injury. Regen. Biomater. 9, rbac038. rbac038. doi:10.1093/rb/rbac038
Lu, C. F., Wang, B., Zhang, P. X., Han, S., Pi, W., Kou, Y. H., et al. (2021a). Combining chitin biological conduits with small autogenous nerves and platelet-rich plasma for the repair of sciatic nerve defects in rats. CNS Neurosci. Ther. 27 (7), 805–819. doi:10.1111/cns.13640
Lu, P., Wang, G., Qian, T., Cai, X., Zhang, P., Li, M., et al. (2021b). The balanced microenvironment regulated by the degradants of appropriate PLGA scaffolds and chitosan conduit promotes peripheral nerve regeneration. Mat. Today Bio 12, 100158. doi:10.1016/j.mtbio.2021.100158
Ma, F., Zhu, T., Xu, F., Wang, Z., Zheng, Y., Tang, Q., et al. (2017). Neural stem/progenitor cells on collagen with anchored basic fibroblast growth factor as potential natural nerve conduits for facial nerve regeneration. Acta Biomater. 50, 188–197. doi:10.1016/j.actbio.2016.11.064
Manto, K. M., Govindappa, P. K., Parisi, D., Karuman, Z., Martinazzi, B., Hegarty, J. P., et al. (2021). (4-Aminopyridine)-PLGA-PEG as a novel thermosensitive and locally injectable treatment for acute peripheral nerve injury. ACS Appl. Bio Mat. 4 (5), 4140–4151. doi:10.1021/acsabm.0c01566
Meng, X., Du, Y., Dong, Z., Wang, G., Dong, B., Guan, X., et al. (2020). Combination of electrical stimulation and bFGF synergistically promote neuronal differentiation of neural stem cells and neurite extension to construct 3D engineered neural tissue. J. Neural Eng. 17 (5), 056048. doi:10.1088/1741-2552/abaac0
Miah, M., Ferretti, P., and Choi, D. (2021). Considering the cellular composition of olfactory ensheathing cell transplants for spinal cord injury repair: A review of the literature. Front. Cell. Neurosci. 15, 781489. doi:10.3389/fncel.2021.781489
Mirsky, R., and Jessen, K. R. (1999). The neurobiology of Schwann cells. Brain Pathol. (Zurich, Switz) 9 (2), 293–311. doi:10.1111/j.1750-3639.1999.tb00228.x
Navarro, X. (2016). Functional evaluation of peripheral nerve regeneration and target reinnervation in animal models: A critical overview. Eur. J. Neurosci. 43 (3), 271–286. doi:10.1111/ejn.13033
Nectow, A. R., Marra, K. G., and Kaplan, D. L. (2012). Biomaterials for the development of peripheral nerve guidance conduits. Tissue Eng. Part B Rev. 18 (1), 40–50. doi:10.1089/ten.TEB.2011.0240
Pace, L. A., Plate, J. F., Mannava, S., Barnwell, J. C., Koman, L. A., Li, Z., et al. (2014). A human hair keratin hydrogel scaffold enhances median nerve regeneration in nonhuman primates: An electrophysiological and histological study. Tissue Eng. Part A 20 (3-4), 507–517. doi:10.1089/ten.TEA.2013.0084
Qu, D., Li, J., Li, Y., Khadka, A., Zuo, Y., Wang, H., et al. (2011). Ectopic osteochondral formation of biomimetic porous PVA-n-HA/PA6 bilayered scaffold and BMSCs construct in rabbit. J. Biomed. Mat. Res. 96B (1), 9–15. doi:10.1002/jbm.b.31697
Radhakrishnan, J., Kuppuswamy, A. A., Sethuraman, S., and Subramanian, A. (2015). Topographic cue from electrospun scaffolds regulate myelin-related gene expressions in Schwann cells. J. Biomed. Nanotechnol. 11 (3), 512–521. doi:10.1166/jbn.2015.1921
Rahmati, M., Ehterami, A., Saberani, R., Abbaszadeh-Goudarzi, G., Rezaei Kolarijani, N., Khastar, H., et al. (2021). Improving sciatic nerve regeneration by using alginate/chitosan hydrogel containing berberine. Drug Deliv. Transl. Res. 11 (5), 1983–1993. doi:10.1007/s13346-020-00860-y
Rajaram, A., Chen, X. B., and Schreyer, D. J. (2012). Strategic design and recent fabrication techniques for bioengineered tissue scaffolds to improve peripheral nerve regeneration. Tissue Eng. Part B Rev. 18 (6), 454–467. doi:10.1089/ten.TEB.2012.0006
Rao, F., Wang, Y., Zhang, D., Lu, C., Cao, Z., Sui, J., et al. (2020). Aligned chitosan nanofiber hydrogel grafted with peptides mimicking bioactive brain-derived neurotrophic factor and vascular endothelial growth factor repair long-distance sciatic nerve defects in rats. Theranostics 10 (4), 1590–1603. doi:10.7150/thno.36272
Razavi, S., Jahromi, M., Vatankhah, E., and Seyedebrahimi, R. (2021). Differential effects of rat ADSCs encapsulation in fibrin matrix and combination delivery of BDNF and Gold nanoparticles on peripheral nerve regeneration. BMC Neurosci. 22 (1), 50. doi:10.1186/s12868-021-00655-y
Reid, A. J., de Luca, A. C., Faroni, A., Downes, S., Sun, M., Terenghi, G., et al. (2013). Long term peripheral nerve regeneration using a novel PCL nerve conduit. Neurosci. Lett. 544, 125–130. doi:10.1016/j.neulet.2013.04.001
Rutkowski, G. E., and Heath, C. A. (1998). The development of bioartificial nerve grafts for peripheral-nerve regeneration. Trends Biotechnol. 16 (4), 163–168. doi:10.1016/S0167-7799(97)01165-7
Saeki, M., Tanaka, K., Imatani, J., Okamoto, H., Watanabe, K., Nakamura, T., et al. (2018). Efficacy and safety of novel collagen conduits filled with collagen filaments to treat patients with peripheral nerve injury: A multicenter, controlled, open-label clinical trial. Injury 49 (4), 766–774. doi:10.1016/j.injury.2018.03.011
Sayad Fathi, S., and Zaminy, A. (2017). Stem cell therapy for nerve injury. World J. Stem Cells 9 (9), 144–151. doi:10.4252/wjsc.v9.i9.144
Sayanagi, J., Tanaka, H., Ebara, M., Okada, K., Oka, K., Murase, T., et al. (2020). Combination of electrospun nanofiber sheet incorporating methylcobalamin and PGA-collagen tube for treatment of a sciatic nerve defect in a rat model. J. Bone Jt. Surg. 102 (3), 245–253. doi:10.2106/JBJS.19.00254
Spinner, R. J., and Kline, D. G. (2000). Surgery for peripheral nerve and brachial plexus injuries or other nerve lesions. John Wiley Sons, Inc. 23 (5), 680–695. doi:10.1002/(sici)1097-4598(200005)23:5<680::aid-mus4>3.0.co;2-h
Sun, H., Sun, J., Li, M., Qian, L., Zhang, L., Huang, Z., et al. (2021). Transcriptome analysis of immune receptor activation and energy metabolism reduction as the underlying mechanisms in interleukin-6-induced skeletal muscle atrophy. Front. Immunol. 12, 730070. doi:10.3389/fimmu.2021.730070
Tang, X., Chen, X., Zhang, S., Gu, X., Wu, R., Huang, T., et al. (2021a). Silk-Inspired in situ hydrogel with anti‐tumor immunity enhanced photodynamic therapy for melanoma and infected wound healing. Adv. Funct. Mat. 31 (17), 2101320. doi:10.1002/adfm.202101320
Tang, X., Gu, X., Huang, T., Chen, X., Zhou, Z., Yang, Y., et al. (2021b). Anisotropic silk-inspired nerve conduit with peptides improved the microenvironment for long-distance peripheral nerve regeneration. ACS Macro Lett. 10 (12), 1501–1509. doi:10.1021/acsmacrolett.1c00533
Tang, X., Li, Q., Huang, T., Zhang, H., Chen, X., Ling, J., et al. (2022). Regenerative role of T cells in nerve repair and functional recovery. Front. Immunol. 13, 923152. doi:10.3389/fimmu.2022.923152
Tezcan, A. H. (2017). Peripheral nerve injury and current treatment strategies. IntechOpen. doi:10.5772/intechopen.68345
Vijayavenkataraman, S. (2020). Nerve guide conduits for peripheral nerve injury repair: A review on design, materials and fabrication methods. Acta Biomater. 106, 54–69. doi:10.1016/j.actbio.2020.02.003
Vishnoi, T., Singh, A., Teotia, A. K., and Kumar, A. (2019). Chitosan-Gelatin-polypyrrole cryogel matrix for stem cell differentiation into neural lineage and sciatic nerve regeneration in peripheral nerve injury model. ACS Biomater. Sci. Eng. 5 (6), 3007–3021. doi:10.1021/acsbiomaterials.9b00242
Wang, C., Lu, C. F., Peng, J., Hu, C. D., and Wang, Y. (2017). Roles of neural stem cells in the repair of peripheral nerve injury. Neural Regen. Res. 12 (12), 2106–2112. doi:10.4103/1673-5374.221171
Wang, H., Wang, Y., Xue, C., Li, Z., Huang, J., Zhao, Y., et al. (2016). Angiogenesis in tissue-engineered nerves evaluated objectively using MICROFIL perfusion and micro-CT scanning. Neural Regen. Res. 11 (1), 168–173. doi:10.4103/1673-5374.175065
Wang, W., Degrugillier, L., Tremp, M., Prautsch, K., Sottaz, L., Schaefer, D. J., et al. (2018). Nerve repair with fibrin nerve conduit and modified suture placement. Anat. Rec. Hob. 301 (10), 1690–1696. doi:10.1002/ar.23921
Wang, Z., Mei, L., Liu, X., and Zhou, Q. (2021). Hierarchically hybrid biocoatings on Ti implants for enhanced antibacterial activity and osteogenesis. Colloids Surfaces B Biointerfaces 204, 111802. doi:10.1016/j.colsurfb.2021.111802
Wu, X., He, L., Li, W., Li, H., Wong, W. M., Ramakrishna, S., et al. (2017). Functional self-assembling peptide nanofiber hydrogel for peripheral nerve regeneration. Regen. Biomater. 4 (1), 21–30. doi:10.1093/rb/rbw034
Xu, F., Zhang, K., Lv, P., Lu, R., Zheng, L., and Zhao, J. (2017). NECL1 coated PLGA as favorable conduits for repair of injured peripheral nerve. Mater. Sci. Eng. C 70 (2), 1132–1140. doi:10.1016/j.msec.2016.03.043
Xue, J., Zhu, C., Li, J., Li, H., and Xia, Y. (2018). Integration of phase-change materials with electrospun fibers for promoting neurite outgrowth under controlled release. Adv. Funct. Mat. 28 (15), 1705563. doi:10.1002/adfm.201705563
Yang, H., Li, Q., Li, L., Chen, S., Zhao, Y., Hu, Y., et al. (2022a). Gastrodin modified polyurethane conduit promotes nerve repair via optimizing Schwann cells function. Bioact. Mat. 8, 355–367. doi:10.1016/j.bioactmat.2021.06.020
Yang, X., Huang, L., Yi, X., Huang, S., Duan, B., and Yu, A. (2022b). Multifunctional chitin-based hollow nerve conduit for peripheral nerve regeneration and neuroma inhibition. Carbohydr. Polym. 289, 119443. doi:10.1016/j.carbpol.2022.119443
Yang, X., Li, M., Ji, Y., Lin, Y., Xu, L., Gu, X., et al. (2022c). Changes of gene expression patterns of muscle pathophysiology-related transcription factors during denervated muscle atrophy. Front. Physiol. 13, 923190. doi:10.3389/fphys.2022.923190
Yao, Y., Cui, Y., Zhao, Y., Xiao, Z., Li, X., Han, S., et al. (2018). Efect of longitudinally oriented collagen conduit combined with nerve growth factor on nerve regeneration after dog sciatic nerve injury. J. Biomed. Mat. Res. 106 (6), 2131–2139. doi:10.1002/jbm.b.34020
Yi, S., Xu, L., and Gu, X. (2019). Scaffolds for peripheral nerve repair and reconstruction. Exp. Neurol. 319, 112761. doi:10.1016/j.expneurol.2018.05.016
Zhang, J., Zhang, Y., Jiang, Y. K., Li, J. A., Wei, W. F., Shi, M. P., et al. (2022a). The effect of poly(lactic‐co‐glycolic acid) conduit loading insulin‐like growth factor 1 modified by a collagen‐binding domain on peripheral nerve injury in rats. J. Biomed. Mat. Res. 110 (9), 2100–2109. doi:10.1002/jbm.b.35064
Zhang, Y., Wang, X., Li, Y., Liang, J., Jiang, P., Huang, Q., et al. (2022b). Cell osteogenic bioactivity mediated precisely by varying scaled micro-pits on ordered micro/nano hierarchical structures of titanium. Regen. Biomater. 9, rbac046. doi:10.1093/rb/rbac046
Zheng, W., Hao, Y., Wang, D., Huang, H., Guo, F., Sun, Z., et al. (2021). Preparation of triamcinolone acetonide-loaded chitosan/fucoidan hydrogel and its potential application as an oral mucosa patch. Carbohydr. Polym. 272, 118493. doi:10.1016/j.carbpol.2021.118493
Zhu, Y., Wang, Z., Bai, L., Deng, J., and Zhou, Q. (2021). Biomaterial-based encapsulated probiotics for biomedical applications: Current status and future perspectives. Mater. Des. 210 (15), 110018. doi:10.1016/j.matdes.2021.110018
Keywords: nerve regeneration, locomotor disorder, biomaterials, neural stem cells, bionic grafts
Citation: Jiang Y, Tang X, Li T, Ling J and Yang Y (2022) The success of biomaterial-based tissue engineering strategies for peripheral nerve regeneration. Front. Bioeng. Biotechnol. 10:1039777. doi: 10.3389/fbioe.2022.1039777
Received: 08 September 2022; Accepted: 04 October 2022;
Published: 18 October 2022.
Edited by:
Qihui Zhou, Qingdao University, ChinaReviewed by:
Jinfei Yang, University of Health and Rehabilitation Sciences, ChinaCopyright © 2022 Jiang, Tang, Li, Ling and Yang. This is an open-access article distributed under the terms of the Creative Commons Attribution License (CC BY). The use, distribution or reproduction in other forums is permitted, provided the original author(s) and the copyright owner(s) are credited and that the original publication in this journal is cited, in accordance with accepted academic practice. No use, distribution or reproduction is permitted which does not comply with these terms.
*Correspondence: Jue Ling, amwyMDE2QG50dS5lZHUuY24=; Yumin Yang, eWFuZ3ltQG50dS5lZHUuY24=
†These authors have contributed equally to this work
Disclaimer: All claims expressed in this article are solely those of the authors and do not necessarily represent those of their affiliated organizations, or those of the publisher, the editors and the reviewers. Any product that may be evaluated in this article or claim that may be made by its manufacturer is not guaranteed or endorsed by the publisher.
Research integrity at Frontiers
Learn more about the work of our research integrity team to safeguard the quality of each article we publish.