- Northeast Forestry University, Harbin, China
Nature is the source of human design inspiration. In order to adapt to the environment better, creatures in nature have formed various morphological structures during billions of years of evolution, among which the superhydrophobic characteristics of some animal and plant surface structures have attracted wide attention. At present, the preparation methods of bionic superhydrophobic surface based on the microstructure of animal and plant body surface include vapor deposition, etching modification, sol-gel method, template method, electrostatic spinning method and electrostatic spraying method, etc., which have been used in medical care, military industry, shipping, textile and other fields. Based on nature, this paper expounds the development history of superhydrophobic principle, summarizes the structure and wettability of superhydrophobic surfaces in nature, and introduces the characteristics differences and applications of different superhydrophobic surfaces in detail. Finally, the challenge of bionic superhydrophobic surface is discussed, and the future development direction of this field is prospected.
1 Introduction
During the long evolution of the earth, it is not difficult to find that many unrelated organisms, such as lotus leaves (aquatic plants) (Yu et al., 2007; Bai et al., 2018; Han et al., 2019; Yun et al., 2020; Ghasemlou et al., 2021), roses (terrestrial plants) (Bhushan, 2018; Chen et al., 2019; Dai et al., 2019; Zong et al., 2019; Kang et al., 2021), butterflies (insects) (Qian et al., 1900; Saison et al., 2008; Wang and Guo, 2013; Bixler and Bhushan, 2014; Han et al., 2017), geckos (terrestrial animals) (Li et al., 2011; Darmanin and Guittard, 2015; Stark et al., 2016; Wang et al., 2019a; Weng et al., 2022) and sharks (fish) (Chen et al., 2018; Gose et al., 2018; Jiaqiang et al., 2018; Bilgiç and Bilgiç, 2019; Zhao et al., 2021), have evolved superhydrophobic properties. Researchers determine whether the surface is super-hydrophobic according to the contact angle of water droplets on the solid surface, that is, when the contact angle of water on the solid surface is greater than 150°, the surface is called super-hydrophobic (Huang and Guo, 2018; Shahabadi and Brant, 2019; Hasan and Nosonovsky, 2020; Hu et al., 2022). In fact, due to the difference in microstructure of each organism’s body surface, apart from superhydrophobic properties, different structures also give them different additional properties, such as self-cleaning (Dalawai et al., 2020; Wang et al., 2021a), anti-icing (Lin et al., 2018; Li et al., 2021a; Wu et al., 2021a), anti-fogging (Varshney et al., 2018; Varshney and Mohapatra, 2018; Domke et al., 2019; Fromel et al., 2021), resistance reduction (Li and Guo, 2018; Li et al., 2019) and so on. In the past few decades, superhydrophobic surfaces, as an extreme surface non-wetting state, have attracted great attention in the scientific and technological circles because of their potential applications in many fields, such as self-cleaning, anti-fouling, anti-corrosion, anti-icing and drag reduction. Inspired by these creatures, modern researchers have prepared special superhydrophobic surfaces suitable for different fields by using bionics (Ahmad and Kan, 2016; Shang et al., 2019a; Shang et al., 2019b; Wang et al., 2020a; Lin et al., 2022).
The earliest basic theory to systematically describe the phenomenon of superhydrophobic surface wetting comes from Young’s work (Young, 1805). However, in the real world, few surfaces meet the assumptions of Young’s equation, so Wenzel (uniform wetting) (Wenzel, 1949) and Cassie–Baxter (non-uniform wetting) (Cassie, 1948) respectively established new models to further improve and optimize this problem. In the later period, many scientists also put forward methods to optimize the superhydrophobic model according to different situations (Nosonovsky and Bhushan, 2005; Bhushan et al., 2007; Bittoun and Marmur, 2009; Xie et al., 2018; Jiang et al., 2020). As a hot spot in the field of material research, with the development of bionic superhydrophobic surface theory, the preparation methods of superhydrophobic surface are gradually diversified. Commonly used methods include sol-gel method (Yang et al., 2018; Vidal et al., 2019; Mahadik and Mahadik, 2021), vapor deposition method (Aljumaily et al., 2018; Pour et al., 2019; Mosayebi et al., 2020; Bayram et al., 2021; Zheng et al., 2022), etching modification method (Zhang et al., 2019a; Ma et al., 2020; Wei et al., 2021), electrochemical deposition method (Zhou et al., 2018; Xue et al., 2019a; Xue et al., 2019b; Wang et al., 2020b; Li et al., 2021b) and template pressing method (Xu et al., 2011; Victor et al., 2012). Among them, the template method can completely copy the microstructure of the biological surface, while other methods can imitate the existing structures in nature or create new structures.
In our previous review (Ge-Zhang et al., 2022), various preparation methods of bionic superhydrophobic surfaces, especially etching modification methods, were compared and described in detail. Therefore, in this mini-review, we will follow the course of human development, from using the primitive things of nature to imitating and transforming all things of nature, and then to realizing self-creation. This article focuses on the exploration and discovery of nature by human beings before self-creation. Starting from the essence, it introduces in detail the development process of superhydrophobic principle and superhydrophobic of natural organisms. This review reviews the development of superhydrophobic principle (Part 2), summarizes the structure and wettability of superhydrophobic surfaces of different animals and plants in nature (Part 3), and lists the differences and applications of different superhydrophobic surfaces. Finally, the function and application of bionic superhydrophobic surface are summarized, and the next research direction of bionic superhydrophobic surface is put forward. The current difficulties and future development directions are summarized and prospected (Part 4).
2 Basic principle of superhydrophobic surface
To explore the bionic superhydrophobic surface, we must first have a deep understanding of the principle. This chapter will introduce the concepts and principles of various superhydrophobic surfaces and physical models closely related to superhydrophobic properties.
2.1 Angle
The static wetting performance of droplets on superhydrophobic surface is usually expressed by contact angle (Voronov et al., 2008), while the rolling angle can be used to evaluate the dynamic performance of droplets on superhydrophobic surface (Hao et al., 2010).
2.2 Superhydrophobic model
In order to describe the relationship between the static contact angle of droplets on solid surface and the surface tension of liquid, solid and gas systems, T. Young established Young’s equation of ideal smooth solid surface state, which set a theoretical precedent for studying the wettability of materials. After that, Wensel and Cassie summarized Wensel model (Wenzel, 1949) and Cassie–Baxter model (Cassie, 1948) by studying the relationship between surface roughness and wettability, and pointed out that superhydrophobicity increased with the decrease of surface free energy and the increase of surface roughness. In modern times, more models have been optimized and pointed out (Miljkovic et al., 2013; Jiang et al., 2020; Mohseni et al., 2021; Shen et al., 2021).
2.2.1 Young’s equation
For an ideal solid surface which is uniform, smooth and rigid, Young put forward Young’s equation by means of the thermodynamic equilibrium equation in order to explain the quantitative relationship between contact angle and solid-liquid-gas interface (Figure 1):
Where
However, it is found that even the smooth surface constructed by the lowest surface energy substance (fluoride) has a contact angle of only 119°, which is far lower than the superhydrophobic surface with rough surface microstructure in nature. This is because the surface roughness will also affect the contact angle. In reality, many surfaces often have a certain degree of roughness, which is not completely smooth, undistorted and uniform. Therefore, Young’s equation can only be applied to ideal surfaces, but not to realistic rough solid surfaces (Marmur, 1983). There are many modifications to Young’s equation to deal with the shortcoming that the contact angle cannot be explained and predicted for rough surfaces (White, 1977; Dobbs, 1999; Butt et al., 2007; Alizada and Sofiyev, 2011; Makkonen, 2016; Liu et al., 2020). Starov and Velarde. (2009) considered the influence of absorption liquid layer and liquid vapor, and made the following modifications and improvements to Young’s equation:
They defined the contact angle in this case as an angle between the horizontal axis and the tangent to the droplet cap profile at the point where it touches the absorbed layer of molecules (also called the precursor film). Where
In order to further expand the application range of Young’s equation, Lin and Hong. (2019) further deduced the Young’s equation considering the contact between oil droplets and ideal smooth solid surface:
Among them, the underwater oil contact angle (
2.2.2 Wenzel model
In 1936 (Wenzel, 1949), Wenzel hypothesized that droplets in contact with a rough solid surface would produce a complete wetting phenomenon, that is, filling the grooves of the surface so that the actual contact area of solid-liquid on the rough surface is larger than the apparent contact area. Because the surface energy of rough surface is low, the contact angle of droplets is high, while the surface energy of smooth surface is high and the contact angle of droplets is low, Wenzel introduced the surface roughness (i.e., the ratio of the real surface area of the solid to the apparent geometric area, whose value is usually greater than 1):
where denotes the actual surface area of the solid surface and denotes the apparent surface area of the solid surface. Then the Wenzel model can be expressed as:
Where
Seo and Kim. (2015) derived the modified Wenzel equation by considering the constant volume of droplets as an auxiliary condition and a transverse condition:
where
In order to solve the problem that the Wenzel model is only suitable for ordered arrays or uniform porous media with uniform characteristics, Han et al. (2007) proposed a modified Wenzel model to describe heterogeneous surfaces as follows:
where
2.2.3 Cassie–Baxter model
In 1944, Cassie and Baxter (Cassie, 1948) considered the influence of surface tension, and put forward the concept of compound contact. Because the size of the roughened surface structural unit is smaller than that of the droplet, the droplet on the surface can not completely penetrate into the groove on the surface, which results in air staying in the groove. Therefore, the Cassie–Baxter model of solid-liquid-gas three-phase composite contact is established:
where
From this model, it can be clearly seen that the smaller the solid-liquid contact area ratio, the larger the contact angle of the rough surface and the better the hydrophobicity. This model explains some phenomena, for example, droplets on super-hydrophobic surfaces such as lotus leaves and rice leaves show very small rolling angle and hysteresis angles, which is difficult to be explained by Wenzel model. Figure 2 shows the difference between Wenzel model and Cassie–Baxter model.
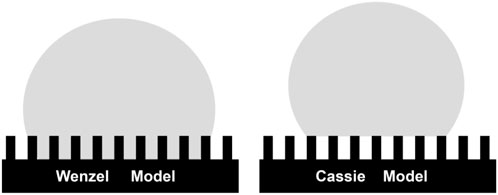
FIGURE 2. Microscopic diagram of droplets on the Wenzel model (left) and Cassie–Baxter model (right).
It is worth noting that Wenzel model and Cassie–Baxter model have their respective applicable scopes. The Cassie–Baxter model is applicable to the highly hydrophobic region where the surface adhesion force is small, while the Wenzel model is applicable to the moderately hydrophobic region where the surface adhesion force is large. As a practical matter, if the droplet overcomes the energy barrier between the two modes and reaches the corresponding energy state under the action of an external force, its wettable viscous state can be transformed between the two models. That is, the wetting state of a droplet on a rough solid surface may then be transformed between both Wenzel and Cassie–Baxter.
In addition, Wang and Jiang. (2007) further refined the existence of five superhydrophobic surfaces based on the previous work (Figure 3): the Wenzel state (droplets are embedded on the surface in a fully wetted state and contact angle hysteresis can be observed), the Cassie state (droplets are independently in contact with the surface in a non-wetted state, with low surface adhesion and easy roll-off), the Lotus state (Cassie state special case, similar to the microscopic raised structure on the surface of lotus leaf, which is important for the design and construction of bionic superhydrophobic surfaces with self-cleaning properties), the Wenzel- Cassie transition state (the state that mainly exists in reality), and Gecko state (the state where droplets on polystyrene nanotube films have extremely high surface adhesion). Ideally, the contact angle of the droplet in Wenzel state is close to 0°, while droplets in the Cassie state would form perfect spheres (ignoring gravity), with the contact angle close to 180°.
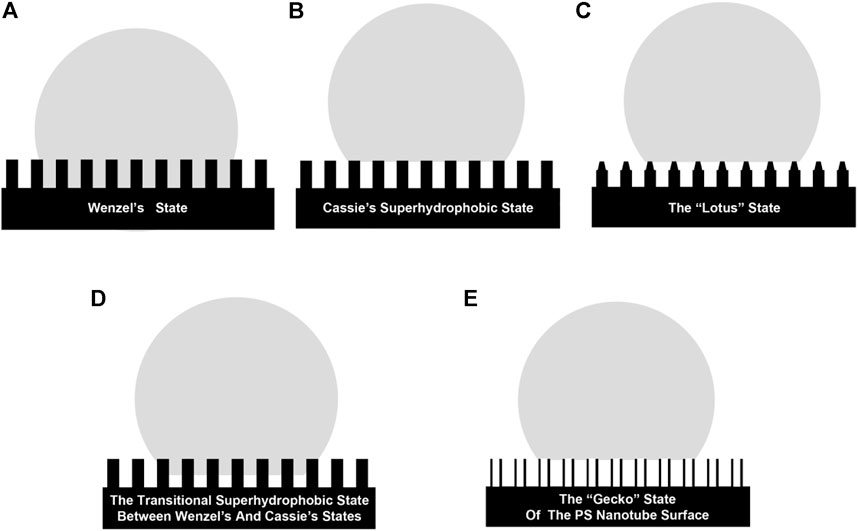
FIGURE 3. Diagram of the existence state of droplets on five superhydrophobic surfaces: Wenzel’s state (A), Cassie state (B), Lotus state (C), Wenzel- Cassie transition state (D), Gecko state (E).
3 Nature's biological superhydrophobic surfaces
Through 3.7 billion years of evolution and species selection, most of the creatures in nature have survived with various unique biological functions and structures, which enable them to quickly adapt to changes in the ecosystem and surrounding environment. According to the order of research objectives in the history of superhydrophobic surface development, this chapter follows the order from plants to animals, and lists many surface structures and multifunctional applications. In addition, according to the relationship between the multifunction of superhydrophobic surfaces from simple to complex, the representative examples of plants and animals are introduced in detail.
3.1 The surface structures of typical plants
Table 1 lists the superhydrophobic phenomena and characteristics of many plants in nature. In fact, the first study of superhydrophobic surface by human beings started with the structure of plant surface. From dust and dirt on lotus leaves easily taken away by dew and rain, to small water drops firmly attached to rose petals on the surface, to water drops on rice leaves easily rolling towards the growth direction of leaves, natural plants have inspired us in many aspects.
3.1.1 Lotus leaves
The lotus leaf was described by the ancient Chinese as “dirt-free plant rising from soil”, which is the most typical super-hydrophobic surface of plants (Latthe et al., 2014), and it is also one of the earliest research goals of human beings, which is why the “lotus leaf effect” is still synonymous with superhydrophobic characteristics. Later, Jiang et al. (Barthlott and Neinhuis, 1997) determined that the surface of lotus leaves is a hierarchical structure formed by micron-sized papillae and nanoscale wax crystals covering the surface, and they also explained the relationship between superhydrophobicity and self-cleaning. It is worth mentioning that in the water condensation experiment, water is hydrophilic on lotus leaves that have experienced water condensation, which shows that lotus leaves can be hydrophobic or hydrophilic, depending on how the water reaches their surface (Cheng and Rodak, 2005). Considering the characteristics of the lotus leaf and the bionic means of scientists, it has a rich and broad application prospect in production and life (Table 2).
One of the more common is the application of lotus leaf in the medical field (Lim et al., 2013; Yang et al., 2014c; Wu et al., 2021b; Huang et al., 2022). Klicova et al., (2022) developed a biocompatible nanofiber pad with anti-adhesion surface by imitating the nanostructure on the lotus leaf by using needle-free electrospraying and polycaprolactone electrospinning technology, which not only shortens the operation time but also greatly reduces the postoperative risk. At the same time, inspired by the self-cleaning characteristics of lotus leaves, Li et al. (2020a) developed a new type of anti-adhesion and antibacterial gauze through three simple dipping steps. With its excellent anti-adhesion and bactericidal activity, it can promote infectious wound regeneration and meet clinical needs. Due to the increasing demand for blood compatibility of biomaterials, Mao et al. (2009) focused on the preparation of an anticoagulant biomaterial-polystyrene nanotube film, which can prevent thrombosis and tissue capsule, and is of great significance in organ transplantation. In addition, the application of super hydrophobicity of biomimetic lotus leaf is also involved in the field of gas sensors (Li et al., 2008) and meteorology (Wang et al., 2021b).
3.1.2 Rose petals
In contrast to the lotus leaf, the rose petal is the canonical example in the Wenzel model. As its petal fibers have a micro-nano double-order structure scale larger than that of the lotus leaf surface, the droplets tend to completely wet the larger scale surface grooves, resulting in increased surface roughness, high surface adhesion, and strong contact angle hysteresis. This shows that even if the petals are inverted, the droplets on the surface will not fall off. Jiang et al. first discovered this phenomenon in 2008 and called it the “petal effect” (Feng et al., 2008). Subsequently, Zheng et al. (2019) studied the dynamic wetting law of viscous superhydrophobic substrates for the first time by comparing and analyzing simple artificial petal-like substrates and superhydrophobic substrates. As shown in Table 3 is bionic product with rose petals as template.
It can be predicted that the self-cleaning functional surface with the “lotus leaf effect” has played an important role in drag reduction, cell culture, dust control (Nosonovsky and Bhushan, 2009; Ueda and Levkin, 2013), while the application prospect of “petal effect” is much broader for non-destructive fluid transfer and biotechnology (Sun et al., 2005; Lai et al., 2013; Yue et al., 2020).
It is worth noting that because the super-hydrophobic rose petals have different surface microstructure and nanostructure, the adhesion of different petals is also different. On the basis of studying two kinds of super-hydrophobic rose petals with high and low adhesion, Bhushan and Her. (2010) prepared artificial super-hydrophobic surfaces with high and low adhesion by wax evaporation, in which the droplets with high adhesion will not fall when the substrate is vertically inclined or inverted.
In addition, since rose petals and lotus leaves are natural examples of the Wenzel-Cassie transition state and the Cassie–Baxter model, respectively, an increasing number of scholars have compared the two with the intention of exploring the relationship and transition between them (Zhang et al., 2012b). The researchers realized the reversible transition between the Cassie–Baxter state and the Cassie impregnation state of the superhydrophobic surface by adjusting the micro/nanostructure of the shape memory polymer SMP. This surface controls the adhesion behavior of liquids and has an important impact on rewritable patterns and the transport and collection of controlled droplets (Shao et al., 2020). In order to apply the superhydrophobic surface to droplet microfluidic chip and microfluidic transmission, Drotlef et al. (2014) Chen et al., 2021b) focused on the magnetic response surface, and proposed a magneto rheological elastomer superhydrophobic surface with magnetic response, which can be quickly and reversibly replaced between “lotus effect” and “rose petal effect”. For large general conductor materials, Liu et al. (2014) developed a one-step electrodeposition method to prepare controllable superhydrophobic surface with excellent stability and corrosion resistance.
3.1.3 Rice leaves
Compared with the former two, rice leaves show another interesting new feature: by macroscopic observation, droplets on rice leaves are easier to slide down in the growth direction of rice leaf (from the stem to the petiole or from the stem to the tip). Microscopically, the surface of rice leaves is also a super hydrophobic surface suitable for the Cassie–Baxter model, but the arrangement of its surface structure is quite different from that of lotus leaves and rose petals. Micro-nano double-stage structures are arranged orderly along the growth direction of rice leaves, but randomly in the vertical direction (Bhushan et al., 2009; Wu et al., 2011), just like the roof tile structure in ancient China. The geometric structure of micro-grooves arranged in order along the same direction makes the energy barrier overcome by liquid droplets rolling along the parallel direction of leaves and stems much smaller than the energy barrier perpendicular to the direction of leaves and stems, resulting in anisotropy of surface adhesion. The rolling angles measured by experiments are 3°–5° along the direction parallel to leaves and stems and 9°–15° in the vertical direction (Feng et al., 2002). As shown in Table 4 is bionic product with rice leaves as template.
With the intensive study of the unique anisotropic (also called liquid-oriented) superhydrophobicity of rice leaves, once again, the field of liquid-oriented drag reduction, water collection and transport has been promoted (Gleiche et al., 2000; Higgins and Jones, 2000; Chen et al., 2005).
Therefore, the researchers are committed to constructing an anisotropic hierarchical structure based on the unidirectional sliding of water droplets in rice leaves (Zhang et al., 2012b; Gao et al., 2018; Xu et al., 2020). Yang et al. (2021) transformed the bionic superhydrophobic surface from isotropic to anisotropic by laser grating scanning, and obtained an anisotropic superhydrophobic aluminum surface with rice leaf shape. Inspired by the microstructure of lotus leaf and rice leaf, Cheng et al. (2018) proposed a new functional material. By repeatedly controlling the surface microstructure shape between lotus leaf structure and rice leaf structure, the reversible transition between isotropic and anisotropic wetting state of superhydrophobic was realized. In addition, the superhydrophobic surface has good stability, even after 1 month, intelligent transformation can be observed, and it is widely used in controlled droplet transportation. In order to highly reproduce the surface structure of rice leaves, Fang et al. Fang et al. (2018) used two-step soft transfer to develop the structure of artificial rice leaves. The structure has the sliding characteristic of anisotropy clearly. The systematic measurement shows that the sliding angles of the structure parallel to the vein direction and perpendicular to the vein direction are 25° and 40° respectively, which can be used for the rapid fabrication of large area artificial rice leaf surface without expensive instruments and complex techniques.
3.1.4 Chapter summary
By comparing the superhydrophobicity of plant surface, it can be easily found that small differences in surface morphology or characteristic size will lead to great differences in surface wetting behavior. For example, the microstructure of rose petals has a larger distance than lotus leaves, which brings a completely different phenomenon, and the micro-morphology of rice leaves arranged regularly will limit the rolling direction of droplets, and so on. Therefore, when constructing and preparing superhydrophobic biomimetic materials, researchers often not only take one organism as a reference, but also combine different structures of various organisms according to the target field to achieve the purpose of meeting the application requirements.
3.2 The surface structures of typical animals
Plants are not the only creatures with superhydrophobic properties. Superhydrophobicity can also be found in different animals, some of which are listed in Table 5, and typical ones will be selected to be elaborated in more detail.
3.2.1 Gecko feet
Gecko has the ability to crawl on smooth vertical walls, which has aroused researchers’ interest. With the strengthening of research in the past century, the description of the gecko crawling instincts has expanded from macroscopic grasping and suction cup to microscopic Van der Waals forces, which is more and more correct and rigorous. As shown in Table 6 is bionic product with gecko feet as template.
Different from the self-cleaning ability of lotus leaf in wet environment, gecko foot has good hydrophobicity, but also has high surface adhesion and self-cleaning performance in dry environment, which provides a direction for the research of dry self-cleaning materials.
Its microscopic state applies to the Gecko state among the five superhydrophobic surface existence states, due to the growth of about half a million micron-level extremely fine bristles on the gecko foot, each bristle end also exists a large number of nanoscale villi branches, which makes the distance between the micro-nano double-order array and the contact surface further reduced and the contact area further increased, so that the sum of the weak Van der Waals forces is sufficient to generate a strong surface adhesion force. The energy barrier for droplet movement increases, so it has the ability to climb walls (Autumn et al., 2000; Autumn et al., 2002; Wang et al., 2012).
As for the mechanism of drying self-cleaning,Xu et al. (2015)showed that geckos used a unique toe-off action in rapid movement, and this dynamic process resulted in a very large instantaneous separation rate of their bristles and contact surfaces. Due to the bristle and shovel-like tentacle system with micro-nano dual-stage structure, the surface adhesion between the foot walls has little to do with the detachment speed, while the detachment force of the microsphere increases with the increase of detachment speed. It is this subtle difference that makes it easy to achieve dry self-cleaning effect during the rapid movement of the gecko. The research results not only provide new design ideas for the long-standing industrial particle manipulation, but also provide a new research direction for the preparation of functional surfaces that can be used repeatedly and have self-cleaning and particle manipulation properties (Kamperman et al., 2010; Liu et al., 2010; Darmanin and Guittard, 2015).
According to the characteristics of geckos, researchers have produced various adhesive materials with high surface adhesion (Li et al., 2011; Liu et al., 2012). In order to design a new type of adhesive film, Zhang et al. (2021) proposed a shape memory film with adhesion to solids and liquids. With high water repellency and low adhesion (about 51 N), this film provides a new idea for the design of different adhesives. Sauer et al. (Tan et al., 2020) prepared nanotube arrays (Eiof∼3 GP) with similar size to gecko bristles from hydrophobic polystyrene, which provided guidance for adhesives designed in wet or underwater environments. In addition, the researchers also used AAO template to prepare multi-scale structure of gecko-like polyimide film. On the basis of stable superhydrophobicity, the film has a high adhesion to water (about 66 μN), and can be used as a manipulator to capture water droplets from a low-adhesion superhydrophobic surface (Liu et al., 2012).
3.2.2 Cicada wings
Compared with the century-old research of gecko, the discovery of super-hydrophobic cicada wings is much later. The Chinese idiom “as thin as a cicada’s wing” is used to describe the extremely small thickness of an object. The scanning electron microscope shows that the thickness of a cicada’s wing is only 8–10 μm, but the self-cleaning and anti-reflection characteristics of cicada’s wings provide another way to discover the superhydrophobic characteristics (Zhang et al., 2006; Dellieu et al., 2014). As shown in Table 7 is bionic product with cicada wings as template.
Similar to the liquid thin layer at the mouth of pitcher plant, the regular hexagonal micro-nano two-level structure on the surface of cicada wings makes cicada wings have better superhydrophobic performance and self-cleaning ability, especially the micro-nano structure composed of three-dimensional waxy structure is easier to adsorb the air thin layer (Lee et al., 2004; Nguyen et al., 2014b).
Because of its different characteristics, cicada wing is widely used in medical treatment, optoelectronic devices and other fields, mainly due to its antibacterial and anti-reflection properties.
First of all, there are some similarities between cicada wings and lotus leaves in antimicrobial activity (Hasan et al., 2013; Kelleher et al., 2016). In order to limit the spread of infection without antibiotics, Ivanova et al. (2012) used anodization, lithography, micellar lithography and self-assembly to simulate the penetration of nanotube arrays on the surface of cicada wings. They solved the huge losses caused by antibiotic resistance and antibiotic action of pathogens by preparing antibacterial surfaces. The researchers prepared a nanostructured ‘hypersurface’ based on the deep reactive ion etching of silicon wafers. The surface is sustainably antibacterial, kills mammalian cells (mouse osteoblasts), and is used in surgical instruments (Hasan et al., 2015).
In addition, Watson and Watson. (2004) found that compared with plants such as lotus leaves, the hexagonal array of cicada wings has a circular tip extending outward about 150–350 nm. To some extent, this unique structure can be regarded as a kind of gradient refractive index material, which leads to the change of photoimpedance, the decrease of light reflection and the enhancement of antireflectivity (Stoddart et al., 2006; Xie et al., 2017). Inspired by the cicada wing structure, the researchers successfully prepared antireflective films with an average transmittance of 98% and nano-solar cells with strong absorptivity in a wide spectral range. Similarly, Liu et al. (2016) used PDMS to replicate the nano-cone structure of cicada wings to prepare the multi-functional surface of artificial cicada wings. Not only the antireflection effect is outstanding, but also the contact angle of the forward PDMS replica can reach 152°. It has a broad application prospect in many optical equipment.
3.2.3 Penguin feathers
Penguins living in the Antarctic often go to sea to feed, but their feathers do not get wet and are extremely difficult to freeze, which has aroused the interest of researchers. Penguin feathers, as a super hydrophobic material with high ice resistance, which has aroused the interest of researchers and become a hot research object in recent years. In view of the waterproof and ice resistance of penguins, Alizadeh-Birjandi explained the main mechanism of delayed solidification of waterproof materials by developing a heat transfer model, which was extended to general superhydrophobic surfaces (Alizadeh-Birjandi et al., 2020). As shown in Table 8 is bionic product with penguin feathers as template.
(Bormashenko et al. (2012) found that hook-like structures with a diameter of about 3 μm and a spacing of about 20 μm are arranged in an orderly manner on the feather branches parallel to penguin micro-scale and sub-micron feathers. The micro-nano double-stage structure has good hydrophobicity and liquid guiding property, so that the droplets falling on it slide down along the growth direction of the feather.
Further research by Wang et al. (2016) found that the surfaces of feather twigs and feather hooks are not smooth, but lined with grooves with a depth of about 100 nm. These grooves can save air, so that droplets cannot be completely wetted, but exist in Cassie state among five super-hydrophobic surface states, that is, droplets can be regarded as spherical on feather surface, which is easier to slide down and slower in heat dissipation. This multi-stage structure reduces the adhesion between ice and makes penguin feathers have excellent anti-icing performance. In addition, the penguin tail evolved a gland that can secrete oil. Penguin use their beaks to spread oil on feathers, which can play a role in waterproof.
According to the excellent anti-icing and anti-condensation properties of penguin feathers, many applications in heavy industries such as aerospace and ships have been derived. Inspired by the three-dimensional microstructure network of penguin body hair, Wang et al. fabricated a novel polyimide nanofiber film on asymmetric electrodes by electrospinning. The film has good mechanical strength at low temperature (no brittle fracture in liquid nitrogen), which prevents the accumulation of pinning droplets and realizes hydrophobicity. It can be used in the aerospace field to avoid the great danger caused by aircraft icing during flight in extreme weather (Wang et al., 2016). Vicente et al. (2021) used electrospinning technology to prepare functional polyvinylidene fluoride (PVDF) fibers for the excellent hydrophobicity and anti-stickiness of penguin feathers. It can not only prevent the aircraft from drift and resistance caused by atmospheric icing caused by supercooled droplets, but also has excellent mechanical strength, thermal stability and very good corrosion resistance. In the field of ship navigation, researchers used a sprayable mixture of hydrophobic silica nanoparticles embedded in a silica gel matrix to create a bionic superhydrophobic surface that can be used for turbulent drag reduction, thus solving the problem that ships consume a lot of energy to overcome underwater resistance (Golovin et al., 2016). In addition, (Li et al. (2021c) using a simple and potentially low-cost method, a flexible hydrophobic surface was prepared by combining a mechanical durable nickel skeleton with an interconnected microwall array filled with hydrophobic polytetrafluoroethylene (PTFE). Even under the pressure of 0.12 MPa, the prepared surface can remain hydrophobic after more than 1,000 times of linear wear. Compared with the inherent hydrophilic metal surface, the good hydrophobicity also enhances the anti-ice function, and can be used as a multi-functional environmental protection coating in navigation engineering.
3.2.5 Chapter summary
Different super-hydrophobic characteristics of animals are closely related to their living environment. For example, the hydrophobic and anti-icing characteristics of warm feathers are of great significance to the survival of animals in cold regions, while underwater fish have evolved to reduce underwater resistance. People use these different properties and structures to design and manufacture many engineering materials, which provide a reliable guarantee for people’s medical health, aerospace and many other fields.
4 Summary and outlook
The hierarchical structure formed by micron-scale papillae and nanoscale wax crystals covering the surface of a lotus leaf, the larger micro-nano double-ordered structure and grooves of rose petal fibres, the geometry of micro-grooves ordered along the same direction in a rice leaf, the bristles and spatula-like tentacle system of the micro-nano double-ordered structure of a gecko foot, the micro-nano structure consisting of regular hexagonal micro-nano two-stage structures and three-dimensional wax structures on the surface of a cicada wing, the micron-scale and The ordered arrangement of the feather branches of sub-micron feathers. All these excellent structures and functions in nature are achieved through multi-level and multi-scale assembly from simple to complex and from disorder to order, which also provides good inspiration for intelligent bionanism in humans. The rich diversity of nature and the adaptive changes of organisms inspire us to think endlessly, and the inventions using the surface hydrophobicity of animals and plants are unique and diverse. From daily necessities to heavy equipment, superhydrophobic materials have attracted people’s unremitting pursuit and exploration for their high performance, low cost and simple preparation process. Based on the principle and concept of superhydrophobic surfaces, this paper mainly introduces the superhydrophobic properties of various animals and plants in nature and their great practical application value, and summarizes the differences and application fields of different superhydrophobic surfaces. Finally, we will put forward a reasonable assumption and plan for the future development prospect of bionic superhydrophobic technology. In view of the achievements and efforts made by our predecessors in constantly exploring the principles and methods of bionic superhydrophobicity, it has laid a solid foundation for us to further develop bionic superhydrophobic materials with simpler, more environmentally friendly materials and lower cost. At present, part of the bionic superhydrophobic technology is gradually changing from the laboratory scale to large-scale industrial production, which has a broad prospect, but the existing problems and shortcomings are also gradually emerging, such as low production efficiency, high production cost, unfriendly to the environment and so on. In this paper, the following ideas are put forward for the future bionic superhydrophobic from natural organism to artificial functional surface:
1) Green, environmentally friendly and sustainable materials make what we are looking for. At present, the Main materials used in the manufacture of superhydrophobic materials are mainly harmful reagents, such as fluorinated superhydrophobic materials, which successfully reduce the surface free energy, but are challenging to the growing environmental and human health problems. We should further develop biodegradable, nontoxic and environmentally friendly new materials into the process of preparing superhydrophobic surfaces, so as to avoid biological pollution and environmental pollution, resulting in irreversible consequences.
2) In light of the fact that the structure and function of these excellent superhydrophobic properties of natural organisms are achieved through multi-level and multi-scale assemblies from simple to complex and from disordered to ordered. Therefore, the development of novel high-performance nanocomposite structures and materials can be achieved by drawing on multiple structures and models.
3) How to make the application materials have sustainable durability has become a big problem. At present, the durability of nanostructure coating on mechanical wear and impact caused by flowing fluid is lower than expected. On the one hand, we need to make some exquisite surface structures, such as micro-nano hierarchical structures or nanostructures, in order to obtain the final superhydrophobic properties. On the other hand, we require the surface to have good surface mechanical properties to meet the requirements of the application. The two are opposing in nature. Therefore, it will be an important research direction in the future that how to achieve a balance or improve its surface mechanical properties on the premise of keeping its surface super-hydrophobic.
4) Compared with the traditional micro-nano processing methods (ion etching, chemical vapor deposition, template method, etc.), femtosecond laser technology has the advantages of high precision, good controllability and applicability to different materials. Therefore, intelligent bionic design with the help of advanced manufacturing technologies and tools such as femtosecond laser machining is also a focus of future research (Yong et al., 2015; Zhang et al., 2020; Fang et al., 2022; Yong et al., 2022; Zhang et al., 2022).
5) The structural and functional design can be coherent and consistent, and the functional design can be considered in conjunction with the natural optical properties of the creature, thus imparting a more aesthetic character.
Author contributions
SG-Z: writing—original draft, writing—review and editing, visualization, methodology; TC: visualization, methodology, investigation, writing—review and editing; HY: writing—review and editing, investigation; YD: writing—review and editing; MS: supervision, writing—review and editing.
Funding
This research was funded by the Project Fund of Resource Value Evaluation of Important Wild Economic Plants in Natural Forests in Prohibited Logging Areas of Northeast China (2019FY100505).
Conflict of interest
The authors declare that the research was conducted in the absence of any commercial or financial relationships that could be construed as a potential conflict of interest.
Publisher’s note
All claims expressed in this article are solely those of the authors and do not necessarily represent those of their affiliated organizations, or those of the publisher, the editors and the reviewers. Any product that may be evaluated in this article, or claim that may be made by its manufacturer, is not guaranteed or endorsed by the publisher.
References
Ahmad, I., and Kan, C. (2016). A review on development and applications of bio-inspired superhydrophobic textiles. Materials 9 (11), 892. doi:10.3390/ma9110892
Alizada, A. N., and Sofiyev, A. H. (2011). Modified Young’s moduli of nano-materials taking into account the scale effects and vacancies. Meccanica 46 (5), 915–920. doi:10.1007/s11012-010-9349-1
Alizadeh-Birjandi, E., Tavakoli-Dastjerdi, F., Leger, J. S., Faull, K. F., Davis, S. H., Rothstein, J. P., et al. (2020). Delay of ice formation on penguin feathers. Eur. Phys. J. Spec. Top. 229 (10), 1881–1896. doi:10.1140/epjst/e2020-900273-x
Aljumaily, M. M., Alsaadi, M. A., and Das, R. (2018). Optimization of the synthesis of superhydrophobic carbon nanomaterials by chemical vapor deposition[J]. Sci. Rep. 8 (1), 1–12.
Autumn, K., Liang, Y. A., Hsieh, S. T., Zesch, W., Chan, W. P., Kenny, T. W., et al. (2000). Adhesive force of a single gecko foot-hair. Nature 405 (6787), 681–685. doi:10.1038/35015073
Autumn, K., Sitti, M., Liang, Y. A., Peattie, A. M., Hansen, W. R., Sponberg, S., et al. (2002). Evidence for van der Waals adhesion in gecko setae. Proc. Natl. Acad. Sci. U. S. A. 99 (19), 12252–12256. doi:10.1073/pnas.192252799
Bahrami, H. R. T., Ahmadi, B., and Saffari, H. (2017). Preparing superhydrophobic copper surfaces with rose petal or lotus leaf property using a simple etching approach[J]. Mater. Res. Express 4 (5), 055014.
Bai, H., Zhang, L., and Gu, D. (2018). Micrometer-sized spherulites as building blocks for lotus leaf-like superhydrophobic coatings. Appl. Surf. Sci. 459, 54–62. doi:10.1016/j.apsusc.2018.07.183
Barraza, B., Olate-Moya, F., Montecinos, G., Ortega, J. H., Rosenkranz, A., Tamburrino, A., et al. (2022). Superhydrophobic SLA 3D printed materials modified with nanoparticles biomimicking the hierarchical structure of a rice leaf. Sci. Technol. Adv. Mater. 23 (1), 300–321. doi:10.1080/14686996.2022.2063035
Barthlott, W., and Neinhuis, C. (1997). Purity of the sacred lotus, or escape from contamination in biological surfaces. Planta 202, 1–8. doi:10.1007/s004250050096
Bayram, F., Mercan, E. S., and Karaman, M. (2021). One-step fabrication of superhydrophobic-superoleophilic membrane by initiated chemical vapor deposition method for oil–water separation. Colloid Polym. Sci. 299 (9), 1469–1477. doi:10.1007/s00396-021-04870-1
Bhushan, B., and Her, E. K. (2010). Fabrication of superhydrophobic surfaces with high and low adhesion inspired from rose petal. Langmuir 26 (11), 8207–8217. doi:10.1021/la904585j
Bhushan, B., Nosonovsky, M., and Chae Jung, Y. (2007). Towards optimization of patterned superhydrophobic surfaces. J. R. Soc. Interface 4 (15), 643–648. doi:10.1098/rsif.2006.0211
Bhushan, B. (2018). Characterization of rose petals and fabrication and characterization of superhydrophobic surfaces with high and low adhesion[M]//Biomimetics. Cham: Springer, 259–287.
Bhushan, B., Jung, Y. C., and Koch, K. (2009). Micro-nano- and hierarchical structures for superhydrophobicity, self-cleaning and low adhesion. Phil. Trans. R. Soc. A 367, 1631–1672. doi:10.1098/rsta.2009.0014
Bilgiç, C., and Bilgiç, Ş. (2019). Innovative superhydrophobic materials designed with inspiration from nature[J]. Theory Res. Eng. II 163.
Bittoun, E., and Marmur, A. (2009). Optimizing super-hydrophobic surfaces: Criteria for comparison of surface topographies. J. Adhesion Sci. Technol. 23 (3), 401–411. doi:10.1163/156856108x369958
Bixler, G. D., and Bhushan, B. (2012). Bioinspired rice leaf and butterfly wing surface structures combining shark skin and lotus effects. Soft matter 8 (44), 11271–11284. doi:10.1039/c2sm26655e
Bixler, G. D., and Bhushan, B. (2014). Rice- and butterfly-wing effect inspired self-cleaning and low drag micro/nanopatterned surfaces in water, oil, and air flow. Nanoscale 6 (1), 76–96. doi:10.1039/c3nr04755e
Bormashenko, E., Gendelman, O., and Whyman, G. (2012). Superhydrophobicity of Lotus leaves versus birds wings: Different physical mechanisms leading to similar phenomena. Langmuir 28 (42), 14992–14997. doi:10.1021/la303340x
Butt, H. J., Golovko, D. S., and Bonaccurso, E. (2007). On the derivation of young's equation for sessile Drops: nonequilibrium effects due to evaporation. J. Phys. Chem. B 111 (19), 5277–5283. doi:10.1021/jp065348g
Cai, Y. (2019). Design and fabrication of superhydrophobic and antimicrobial surfaces on AISI 316L stainless steel[J].
Carmichael, S. W. (2021). Earthworms inspire the creation of fabrics that aggressively repel water. Micros. Today 29 (4), 8–9. doi:10.1017/s1551929521000857
Chang, K. C., Lu, H. I., Peng, C. W., Lai, M. C., Hsu, S. C., Hsu, M. H., et al. (2013). Nanocasting technique to prepare lotus-leaf-like superhydrophobic electroactive polyimide as advanced anticorrosive coatings. ACS Appl. Mat. Interfaces 5 (4), 1460–1467. doi:10.1021/am3029377
Cheeseman, S., Owen, S., Truong, V. K., Meyer, D., Ng, S. H., Vongsvivut, J., et al. (2018). Pillars of life: Is there a relationship between lifestyle factors and the surface characteristics of dragonfly wings? ACS Omega 3, 6039–6046. doi:10.1021/acsomega.8b00776
Chen, C., Liu, M., Zhang, L., Hou, Y., Yu, M., and Fu, S. (2019). Mimicking from rose petal to Lotus leaf: Biomimetic multiscale hierarchical particles with tunable water adhesion. ACS Appl. Mat. Interfaces 11 (7), 7431–7440. doi:10.1021/acsami.8b21494
Chen, C., Pan, L., Li, H., Liu, Q., Li, F., Tu, J., et al. (2021). Conversion of superhydrophilicity to superhydrophobicity by changing the microstructure of carbon-high fly ash. Mater. Lett. 299, 130051. doi:10.1016/j.matlet.2021.130051
Chen, D., Liu, Y., Chen, H., and Zhang, D. (2018). Bio‐inspired drag reduction surface from sharkskin. Biosurface Biotribology 4 (2), 39–45. doi:10.1049/bsbt.2018.0006
Chen, S., Zhu, M., Zhang, Y., Dong, S., and Wang, X. (2021). Magnetic-responsive superhydrophobic surface of magnetorheological elastomers mimicking from Lotus leaves to rose petals. Langmuir 37 (7), 2312–2321. doi:10.1021/acs.langmuir.0c03122
Chen, Y. C., Huang, Z. S., and Yang, H. (2015). Cicada-wing-inspired self-cleaning antireflection coatings on polymer substrates. ACS Appl. Mat. Interfaces 7 (45), 25495–25505. doi:10.1021/acsami.5b08743
Chen, Y., He, B., Lee, J., and Patankar, N. A. (2005). Anisotropy in the wetting of rough surfaces. J. colloid interface Sci. 281 (2), 458–464. doi:10.1016/j.jcis.2004.07.038
Cheng, Y. T., and Rodak, D. E. (2005). Is the lotus leaf superhydrophobic?[J]. Appl. Phys. Lett. 86 (14), 144101. doi:10.1063/1.1895487
Cheng, Z., Zhang, D., Lv, T., Lai, H., Zhang, E., Kang, H., et al. (2018). Superhydrophobic shape memory polymer arrays with switchable isotropic/anisotropic wetting. Adv. Funct. Mat. 28 (7), 1705002. doi:10.1002/adfm.201705002
Dai, S., Zhu, Y., Gu, Y., and Du, Z. (2019). Biomimetic fabrication and photoelectric properties of superhydrophobic ZnO nanostructures on flexible PDMS substrates replicated from rose petal. Appl. Phys. A 125 (2), 138–211. doi:10.1007/s00339-019-2438-7
Dalawai, S. P., Aly, M. A. S., Latthe, S. S., Xing, R., Sutar, R. S., Nagappan, S., et al. (2020). Recent advances in durability of superhydrophobic self-cleaning technology: A critical review. Prog. Org. Coatings 138, 105381. doi:10.1016/j.porgcoat.2019.105381
Darmanin, T., and Guittard, F. (2015). Superhydrophobic and superoleophobic properties in nature. Mater. today 18 (5), 273–285. doi:10.1016/j.mattod.2015.01.001
Dellieu, L., Sarrazin, M., Simonis, P., Deparis, O., and Vigneron, J. P. (2014). A two-in-one superhydrophobic and anti-reflective nanodevice in the grey cicadaCicada orni(Hemiptera). J. Appl. Phys. 116 (2), 024701. doi:10.1063/1.4889849
Dobbs, H. (1999). The modified Young's equation for the contact angle of a small sessile drop from an interface displacement model[J]. Int. J. Mod. Phys. B 13 (27), 3255–3259. doi:10.1142/s0217979299003003
Domke, M., Sonderegger, G., and Kostal, E. (2019). Transparent laser-structured glasses with superhydrophilic properties for anti-fogging applications[J]. Appl. Phys. A 125 (10), 1–10.
Drotlef, D. M., Blümler, P., and del Campo, A. (2014). Magnetically actuated patterns for bioinspired reversible adhesion (dry and wet). Adv. Mat. 26, 775–779. doi:10.1002/adma.201303087
Fang, Y., Sun, G., Cong, Q., Chen, G. h., and Ren, L. q. (2008). Effects of methanol on wettability of the non-smooth surface on butterfly wing. J. Bionic Eng. 5 (2), 127–133. doi:10.1016/s1672-6529(08)60016-5
Fang, Y., Yong, J., Chen, F., Huo, J., Yang, Q., Zhang, J., et al. (2018). Bioinspired fabrication of Bi/tridirectionally anisotropic sliding superhydrophobic PDMS surfaces by femtosecond laser. Adv. Mat. Interfaces 5 (6), 1701245. doi:10.1002/admi.201701245
Fang, Z., Cheng, Y., Yang, Q., Lu, Y., Zhang, C., Li, M., et al. (2022). Design of metal-based slippery liquid-infused porous surfaces (SLIPSs) with effective liquid repellency achieved with a femtosecond laser. Micromachines 13 (8), 1160. doi:10.3390/mi13081160
Feng, L., Li, S., Li, Y., Li, H., Zhang, L., Zhai, J., et al. (2002). Super-hydrophobic surfaces: From natural to artificial. Adv. Mat. 14 (24), 1857–1860. doi:10.1002/adma.200290020
Feng, L., Zhang, Y., Xi, J., Zhu, Y., Wang, N., Xia, F., et al. (2008). Petal Effect: A superhydrophobic state with high adhesive force. Langmuir 24 (8), 4114–4119. doi:10.1021/la703821h
Fromel, M., Sweeder, D. M., Jang, S., Williams, T. A., Kim, S. H., and Pester, C. W. (2021). Superhydrophilic polymer brushes with high durability and anti-fogging activity. ACS Appl. Polym. Mat. 3 (10), 5291–5301. doi:10.1021/acsapm.1c01090
Gao, F., Yao, Y., Wang, W., Wang, X., Li, L., Zhuang, Q., et al. (2018). Light-driven transformation of bio-inspired superhydrophobic structure via reconfigurable PAzoMA microarrays: From Lotus leaf to rice leaf. Macromolecules 51 (7), 2742–2749. doi:10.1021/acs.macromol.8b00059
Gao, X., Su, L., Jiang, G., Pang, J., and Lin, L. (2020). Dimensional stability of lotus leaf-like nanostructure superhydrophobic bamboo by modification using xylan. BioResources 15 (2), 3443–3457. doi:10.15376/biores.15.2.3443-3457
Gao, X., Yan, X., Yao, X., Xu, L., Zhang, K., Zhang, J., et al. (2007). The dry-style antifogging properties of mosquito compound eyes and artificial analogues prepared by soft lithography. Adv. Mat. 19 (17), 2213–2217. doi:10.1002/adma.200601946
Ge-Zhang, S., Yang, H., and Ni, H. (2022). Biomimetic superhydrophobic metal/nonmetal surface manufactured by etching methods: A mini review[J]. Front. Bioeng. Biotechnol., 10. doi:10.3389/fbioe.2022.95809
Ghasemlou, M., Le, P. H., Daver, F., Murdoch, B. J., Ivanova, E. P., and Adhikari, B. (2021). Robust and eco-friendly superhydrophobic starch nanohybrid materials with engineered Lotus leaf mimetic multiscale hierarchical structures. ACS Appl. Mat. Interfaces 13 (30), 36558–36573. doi:10.1021/acsami.1c09959
Gleiche, M., Chi, L. F., and Fuchs, H. (2000). Nanoscopic channel lattices with controlled anisotropic wetting. Nature 403 (6766), 173–175. doi:10.1038/35003149
Golovin, K. B., Gose, J. W., Perlin, M., Ceccio, S. L., and Tuteja, A. (2016). Bioinspired surfaces for turbulent drag reduction. Phil. Trans. R. Soc. A 374 (2073), 20160189. doi:10.1098/rsta.2016.0189
Gose, J. W., Golovin, K., Boban, M., Mabry, J. M., Tuteja, A., Perlin, M., et al. (2018). Characterization of superhydrophobic surfaces for drag reduction in turbulent flow. J. Fluid Mech. 845, 560–580. doi:10.1017/jfm.2018.210
Gou, X., and Guo, Z. (2018). Superhydrophobic plant leaves with micro-line structures: An optimal biomimetic objective in bionic engineering. J. Bionic Eng. 15 (5), 851–858. doi:10.1007/s42235-018-0072-2
Guo, Z., and Liu, W. (2007). Biomimic from the superhydrophobic plant leaves in nature: Binary structure and unitary structure. Plant Sci. 172 (6), 1103–1112. doi:10.1016/j.plantsci.2007.03.005
Gustafsson, L., Jansson, R., Hedhammar, M., and van der Wijngaart, W. (2018). Structuring of functional spider silk wires, coatings, and sheets by self‐assembly on superhydrophobic pillar surfaces. Adv. Mat. 30 (3), 1704325. doi:10.1002/adma.201704325
Han, K., Park, T. Y., Yong, K., and Cha, H. J. (2019). Combinational biomimicking of Lotus leaf, mussel, and sandcastle worm for robust superhydrophobic surfaces with biomedical multifunctionality: Antithrombotic, antibiofouling, and tissue closure capabilities. ACS Appl. Mat. Interfaces 11 (10), 9777–9785. doi:10.1021/acsami.8b21122
Han, T. Y., Shr, J. F., Wu, C. F., and Hsieh, C. T. (2007). A modified Wenzel model for hydrophobic behavior of nanostructured surfaces. Thin Solid Films 515 (11), 4666–4669. doi:10.1016/j.tsf.2006.11.008
Han, Z., Fu, J., Wang, Z., Wang, Y., Li, B., Mu, Z., et al. (2017). Long-term durability of superhydrophobic properties of butterfly wing scales after continuous contact with water. Colloids Surfaces A Physicochem. Eng. Aspects 518, 139–144. doi:10.1016/j.colsurfa.2017.01.030
Hao, P., Lv, C., Yao, Z., and He, F. (2010). Sliding behavior of water droplet on superhydrophobic surface. EPL Europhys. Lett. 90 (6), 66003. doi:10.1209/0295-5075/90/66003
Hasan, J., Raj, S., Yadav, L., and Chatterjee, K. (2015). Engineering a nanostructured “super surface” with superhydrophobic and superkilling properties. RSC Adv. 5 (56), 44953–44959. doi:10.1039/c5ra05206h
Hasan, J., Webb, H. K., Truong, V. K., Pogodin, S., Baulin, V. A., Watson, G. S., et al. (2013). Selective bactericidal activity of nanopatterned superhydrophobic cicada Psaltoda claripennis wing surfaces. Appl. Microbiol. Biotechnol. 97 (20), 9257–9262. doi:10.1007/s00253-012-4628-5
Hasan, M. S., and Nosonovsky, M. (2020). Lotus effect and friction: Does nonsticky mean slippery? Biomimetics (Basel). 5 (2), 28. doi:10.3390/biomimetics5020028
He, J., He, J., Yuan, M., Xue, M., Ma, X., Hou, L., et al. (2018). Facile fabrication of eco-friendly durable superhydrophobic material from eggshell with oil/water separation property. Adv. Eng. Mat. 20 (9), 1701180. doi:10.1002/adem.201701180
Herminghaus, S. (2007). Roughness-induced non-wetting. Europhys. Lett. 79 (5), 59901. doi:10.1209/0295-5075/79/59901
Higgins, A. M., and Jones, R. A. L. (2000). Anisotropic spinodal dewetting as a route to self-assembly of patterned surfaces. Nature 404 (6777), 476–478. doi:10.1038/35006597
Hoefnagels, H. F., Wu, D., De With, G., and Ming, W. (2007). Biomimetic superhydrophobic and highly oleophobic cotton textiles. Langmuir 23 (26), 13158–13163. doi:10.1021/la702174x
Hu, H., Eluchie, C., and Huang, W. (2022). An experimental study to compare water droplet impinging dynamics and wind-driven water runback process over laser treated surfaces with different wettability characteristics[C]. AIAA Aviat. 2022 Forum, 4095.
Huang, C., and Guo, Z. (2018). The wettability of gas bubbles: From macro behavior to nano structures to applications. Nanoscale 10 (42), 19659–19672. doi:10.1039/c8nr07315e
Huang, H., Huang, C., Xu, C., and Liu, R. (2022). Development and characterization of lotus-leaf-inspired bionic antibacterial adhesion film through beeswax. Food Packag. Shelf Life 33, 100906. doi:10.1016/j.fpsl.2022.100906
Ivanova, E. P., Hasan, J., Webb, H. K., Truong, V. K., Watson, G. S., Watson, J. A., et al. (2012). Natural bactericidal surfaces: Mechanical rupture of Pseudomonas aeruginosa cells by cicada wings. Small 8 (16), 2489–2494. doi:10.1002/smll.201200528
Jiang, S., Zhang, H., Jiang, C., and Liu, X. (2020). Antifrosting performance of a superhydrophobic surface by optimizing the surface morphology. Langmuir 36 (34), 10156–10165. doi:10.1021/acs.langmuir.0c01618
Jiaqiang, E., Jin, Y., and Deng, Y. (2018). Wetting models and working mechanisms of typical surfaces existing in nature and their application on superhydrophobic surfaces: A review[J]. Adv. Mat. Interfaces 5, 1701052.
Jin, M., Feng, X., Feng, L., Sun, T., Zhai, J., Li, T., et al. (2005). Superhydrophobic aligned polystyrene nanotube films with high adhesive force. Adv. Mat. 17 (16), 1977–1981. doi:10.1002/adma.200401726
Kamperman, M., Kroner, E., del Campo, A., McMeeking, R. M., and Arzt, E. (2010). Functional adhesive surfaces with “gecko” effect: The concept of contact splitting. Adv. Eng. Mat. 12 (5), 335–348. doi:10.1002/adem.201000104
Kang, F., Yi, Z., Zhao, B., and Qin, Z. (2021). Surface physical structure and durability of superhydrophobic wood surface with epoxy resin. BioResources 16 (2), 3235–3254. doi:10.15376/biores.16.2.3235-3254
Kelleher, S. M., Habimana, O., Lawler, J., O’ Reilly, B., Daniels, S., Casey, E., et al. (2016). Cicada wing surface topography: An investigation into the bactericidal properties of nanostructural features. ACS Appl. Mat. Interfaces 8 (24), 14966–14974. doi:10.1021/acsami.5b08309
Khandavalli, S., Rogers, P., and Rothstein, J. P. (2018). Roll-to-roll fabrication of hierarchical superhydrophobic surfaces. Appl. Phys. Lett. 113 (4), 041601. doi:10.1063/1.5037946
Klicova, M., Oulehlova, Z., Klapstova, A., Hejda, M., Krejcik, M., Novak, O., et al. (2022). Biomimetic hierarchical nanofibrous surfaces inspired by superhydrophobic lotus leaf structure for preventing tissue adhesions. Mater. Des. 217, 110661. doi:10.1016/j.matdes.2022.110661
Kumar, M., and Bhardwaj, R. (2020). Wetting characteristics of Colocasia esculenta (Taro) leaf and a bioinspired surface thereof[J]. Sci. Rep. 10 (1), 1–15.
Lai, D. L., Kong, G., Li, X. C., and Che, C. S. (2019). Corrosion resistance of ZnO nanorod superhydrophobic coatings with rose petal effect or Lotus leaf effect. J. Nanosci. Nanotechnol. 19 (7), 3919–3928. doi:10.1166/jnn.2019.16313
Lai, Y. K., Tang, Y. X., and Huang, J. Y. (2013). Bioinspired TiO2 nanostructure films with special wettability and adhesion for droplets manipulation and patterning[J]. Sci. Rep. 3 (1), 1–8.
Latthe, S. S., Sutar, R. S., Bhosale, A. K., Nagappan, S., Ha, C. S., Sadasivuni, K. K., et al. (2019). Recent developments in air-trapped superhydrophobic and liquid-infused slippery surfaces for anti-icing application. Prog. Org. Coatings 137, 105373. doi:10.1016/j.porgcoat.2019.105373
Latthe, S. S., Terashima, C., Nakata, K., and Fujishima, A. (2014). Superhydrophobic surfaces developed by mimicking hierarchical surface morphology of Lotus leaf. Molecules 19 (4), 4256–4283. doi:10.3390/molecules19044256
Lee, W., Jin, M. K., Yoo, W. C., and Lee, J. K. (2004). Nanostructuring of a polymeric substrate with well-defined nanometer-scale topography and tailored surface wettability. Langmuir 20 (18), 7665–7669. doi:10.1021/la049411+
Letellier, P., Mayaffre, A., and Turmine, M. (2007). Drop size effect on contact angle explained by nonextensive thermodynamics. Young's equation revisited. J. colloid interface Sci. 314 (2), 604–614. doi:10.1016/j.jcis.2007.05.085
Li, B., Ouyang, Y., Haider, Z., Zhu, Y., Qiu, R., Hu, S., et al. (2021). One-step electrochemical deposition leading to superhydrophobic matrix for inhibiting abiotic and microbiologically influenced corrosion of Cu in seawater environment. Colloids Surfaces A Physicochem. Eng. Aspects 616, 126337. doi:10.1016/j.colsurfa.2021.126337
Li, D., and Guo, Z. (2018). Metal-organic framework superhydrophobic coating on Kevlar fabric with efficient drag reduction and wear resistance. Appl. Surf. Sci. 443, 548–557. doi:10.1016/j.apsusc.2018.03.030
Li, J., Liu, X., Ye, Y., Zhou, H., and Chen, J. (2011). Gecko-inspired synthesis of superhydrophobic ZnO surfaces with high water adhesion. Colloids Surfaces A Physicochem. Eng. Aspects 384 (1-3), 109–114. doi:10.1016/j.colsurfa.2011.03.024
Li, M., Chen, Y., Luo, W., and Cheng, X. (2021). Durable and flexible hydrophobic surface with a micropatterned composite metal–polymer structure. Langmuir 37 (19), 5838–5845. doi:10.1021/acs.langmuir.1c00227
Li, R., Gao, Q., Dong, Q., Luo, C., Sheng, L., and Liang, J. (2020). Template-free electrodeposition of ultra-high adhesive superhydrophobic Zn/Zn stearate coating with ordered hierarchical structure from deep eutectic solvent. Surf. Coatings Technol. 403, 126267. doi:10.1016/j.surfcoat.2020.126267
Li, S., Chen, A., Chen, Y., Yang, Y., Zhang, Q., Luo, S., et al. (2020). Lotus leaf inspired antiadhesive and antibacterial gauze for enhanced infected dermal wound regeneration. Chem. Eng. J. 402, 126202. doi:10.1016/j.cej.2020.126202
Li, W., Zhan, Y., and Yu, S. (2021). Applications of superhydrophobic coatings in anti-icing: Theory, mechanisms, impact factors, challenges and perspectives. Prog. Org. Coatings 152, 106117. doi:10.1016/j.porgcoat.2020.106117
Li, Y., Zheng, M., Ma, L., Zhong, M., and Shen, W. (2008). Fabrication of hierarchical ZnO architectures and their superhydrophobic surfaces with strong adhesive force. Inorg. Chem. 47 (8), 3140–3143. doi:10.1021/ic7021598
Li, Z., Marlena, J., Pranantyo, D., Nguyen, B. L., and Yap, C. H. (2019). A porous superhydrophobic surface with active air plastron control for drag reduction and fluid impalement resistance. J. Mat. Chem. A Mat. 7 (27), 16387–16396. doi:10.1039/c9ta02745a
Lian, Z., Xu, J., Yu, Z., Yu, P., and Yu, H. (2019). A simple two-step approach for the fabrication of bio-inspired superhydrophobic and anisotropic wetting surfaces having corrosion resistance. J. Alloys Compd. 793, 326–335. doi:10.1016/j.jallcom.2019.04.169
Liang, L., Liu, P., Su, H., Qian, H., and Ma, H. (2020). One‐step fabrication of superhydrophobic sponge with magnetic controllable and flame‐retardancy for oil removing and collecting. J. Appl. Polym. Sci. 137 (44), 49353. doi:10.1002/app.49353
Liang, Y., Peng, J., Li, X., Huang, J., Qiu, R., Zhang, Z., et al. (2017). Wettability and contact time on a biomimetic superhydrophobic surface. Materials 10 (3), 254. doi:10.3390/ma10030254
Lim, J. I., Kim, S. I., and Jung, Y. (2013). Fabrication and medical applications of lotus-leaf-like structured superhydrophobic surfaces. Polym. Korea 37 (4), 411–419. doi:10.7317/pk.2013.37.4.411
Lin, J., Du, J., Xie, S., Yu, F., Fang, S., Yan, Z., et al. (2022). Durable superhydrophobic polyvinylidene fluoride membranes via facile spray-coating for effective membrane distillation. Desalination 538, 115925. doi:10.1016/j.desal.2022.115925
Lin, X., and Hong, J. (2019). Recent advances in robust superwettable membranes for oil–water separation. Adv. Mat. Interfaces 6 (12), 1900126. doi:10.1002/admi.201900126
Lin, Y., Chen, H., Wang, G., and Liu, A. (2018). Recent progress in preparation and anti-icing applications of superhydrophobic coatings. Coatings (Basel). 8 (6), 208. doi:10.3390/coatings8060208
Liu, J., Zhang, X., Wang, R., Long, F., Zhao, P., and Liu, L. (2021). A mosquito-eye-like superhydrophobic coating with super robustness against abrasion. Mater. Des. 203, 109552. doi:10.1016/j.matdes.2021.109552
Liu, K., Du, J., Wu, J., and Jiang, L. (2012). Superhydrophobic gecko feet with high adhesive forces towards water and their bio-inspired materials. Nanoscale 4 (3), 768–772. doi:10.1039/c1nr11369k
Liu, M., Zheng, Y., Zhai, J., and Jiang, L. (2010). Bioinspired super-antiwetting interfaces with special Liquid−Solid adhesion. Acc. Chem. Res. 43 (3), 368–377. doi:10.1021/ar900205g
Liu, T., Liu, Z., Jagota, A., and Hui, C. Y. (2020). Droplets on an elastic membrane: Configurational energy balance and modified Young equation. J. Mech. Phys. Solids 138, 103902. doi:10.1016/j.jmps.2020.103902
Liu, Y., Gu, H., Jia, Y., Liu, J., Zhang, H., Wang, R., et al. (2019). Design and preparation of biomimetic polydimethylsiloxane (PDMS) films with superhydrophobic, self-healing and drag reduction properties via replication of shark skin and SI-ATRP. Chem. Eng. J. 356, 318–328. doi:10.1016/j.cej.2018.09.022
Liu, Y., Li, S., Zhang, J., Wang, Y., Han, Z., and Ren, L. (2014). Fabrication of biomimetic superhydrophobic surface with controlled adhesion by electrodeposition. Chem. Eng. J. 248, 440–447. doi:10.1016/j.cej.2014.03.046
Liu, Y., Song, Y., Niu, S., Zhang, Y., Han, Z., and Ren, L. (2016). Integrated super-hydrophobic and antireflective PDMS bio-templated from nano-conical structures of cicada wings. RSC Adv. 6 (110), 108974–108980. doi:10.1039/c6ra23811d
Long, J., Fan, P., Gong, D., Jiang, D., Zhang, H., Li, L., et al. (2015). Superhydrophobic surfaces fabricated by femtosecond laser with tunable water adhesion: From Lotus leaf to rose petal. ACS Appl. Mat. Interfaces 7 (18), 9858–9865. doi:10.1021/acsami.5b01870
Ma, L., Li, H., and Hu, H. (2017). An experimental study on the dynamics of water droplets impingement onto a goose feather[C]//55th AIAA aerospace sciences meeting, 0442.
Ma, N., Cheng, D., Zhang, J., Zhao, S., and Lu, Y. (2020). A simple, inexpensive and environmental-friendly electrochemical etching method to fabricate superhydrophobic GH4169 surfaces. Surf. Coatings Technol. 399, 126180. doi:10.1016/j.surfcoat.2020.126180
Mahadik, S. A., and Mahadik, S. S. (2021). Surface morphological and topographical analysis of multifunctional superhydrophobic sol-gel coatings. Ceram. Int. 47 (20), 29475–29482. doi:10.1016/j.ceramint.2021.07.115
Makkonen, L. (2016). Young’s equation revisited. J. Phys. Condens. Matter 28 (13), 135001. doi:10.1088/0953-8984/28/13/135001
Mao, C., Zhao, W. B., and Luo, W. P. (2009). Geometric bionics: Lotus effect helps polystyrene nanotube films get good blood compatibility[J]. Nat. Preced., 1.
Marmur, A. (1983). Equilibrium and spreading of liquids on solid surfaces. Adv. Colloid Interface Sci. 19 (1-2), 75–102. doi:10.1016/0001-8686(83)80004-9
Miljkovic, N., Enright, R., and Wang, E. N. (2013). Modeling and optimization of superhydrophobic condensation. J. Heat Transf. 135 (11). doi:10.1115/1.4024597
Mohseni, M., Far, H. S., Hasanzadeh, M., and Golovin, K. (2021). Non-fluorinated sprayable fabric finish for durable and comfortable superhydrophobic textiles. Prog. Org. Coatings 157, 106319. doi:10.1016/j.porgcoat.2021.106319
Monfared, M., Alidoostan, M. A., and Saranjam, B. (2019). Experimental study on the friction drag reduction of superhydrophobic surfaces in closed channel flow. J. Appl. Fluid Mech. 12 (1), 69–76. doi:10.29252/jafm.75.253.28442
Mosayebi, E., Azizian, S., and Noei, N. (2020). Preparation of robust superhydrophobic sand by chemical vapor deposition of polydimethylsiloxane for oil/water separation. Macromol. Mat. Eng. 305 (12), 2000425. doi:10.1002/mame.202000425
Nagappan, S., Park, J. J., Park, S. S., Lee, W. K., and Ha, C. S. (2013). Bio-inspired, multi-purpose and instant superhydrophobic–superoleophilic lotus leaf powder hybrid micro–nanocomposites for selective oil spill capture. J. Mat. Chem. A Mat. 1 (23), 6761–6769. doi:10.1039/c3ta00001j
Nguyen, S. H., Webb, H. K., Mahon, P. J., Crawford, R., and Ivanova, E. (2014). Natural insect and plant micro-/nanostructsured surfaces: An excellent selection of valuable templates with superhydrophobic and self-cleaning properties. Molecules 19 (9), 13614–13630. doi:10.3390/molecules190913614
Nguyen, S. H. T., Webb, H. K., Hasan, J., Tobin, M. J., Crawford, R. J., and Ivanova, E. P. (2013). Dual role of outer epicuticular lipids in determining the wettability of dragonfly wings. Colloids Surfaces B Biointerfaces 106, 126–134. doi:10.1016/j.colsurfb.2013.01.042
Nguyen, S. H., Webb, H. K., Hasan, J., Tobin, M. J., Mainwaring, D. E., Mahon, P. J., et al. (2014). Wing wettability of Odonata species as a function of quantity of epicuticular waxes. Vib. Spectrosc. 75, 173–177. doi:10.1016/j.vibspec.2014.07.006
Nosonovsky, M., and Bhushan, B. (2005). Roughness optimization for biomimetic superhydrophobic surfaces. Microsyst. Technol. 11 (7), 535–549. doi:10.1007/s00542-005-0602-9
Nosonovsky, M., and Bhushan, B. (2009). Superhydrophobic surfaces and emerging applications: Non-adhesion, energy, green engineering. Curr. Opin. Colloid & Interface Sci. 14 (4), 270–280. doi:10.1016/j.cocis.2009.05.004
Nuraje, N., Khan, W. S., Lei, Y., Ceylan, M., and Asmatulu, R. (2013). Superhydrophobic electrospun nanofibers. J. Mat. Chem. A 1 (6), 1929–1946. doi:10.1039/c2ta00189f
Oh, J., Yin, S., Dana, C. E., Hong, S., Roman, J. K., Jo, K. D., et al. (2019). Cicada-inspired self-cleaning superhydrophobic surfaces. J. Heat Transf. 141 (10). doi:10.1115/1.4044677
Pieniazek, F., Dasgupta, M., and Messina, V. (2021). Differential occurrence of cuticular wax and its role in leaf tissues of three edible aroids of Northeast India[J].
Pour, F. Z., Karimi, H., and Avargani, V. M. (2019). Preparation of a superhydrophobic and superoleophilic polyester textile by chemical vapor deposition of dichlorodimethylsilane for Water–Oil separation[J]. Polyhedron 159, 54–63.
Qian, C., Guang-hua, C., and Yan, F. (1900). Super-hydrophobic characteristics of butterfly wing surface[J]. J. Bionic Eng. 1 (4), 249–255.
Rius-Ayra, O., Castellote-Alvarez, R., and Escobar, A. M. (2018). Superhydrophobic coating bioinspired on rice leaf: A first attempt to enhance erosion resistance properties at environmental conditions with ceramic particles[C]. Mater. Sci. Forum 941, 1874–1879. Trans Tech Publications Ltd. doi:10.4028/www.scientific.net/MSF.941
Román Kustas, J., Hoffman, J. B., Reed, J. H., Gonsalves, A. E., Oh, J., Li, L., et al. (2020). Molecular and topographical organization: Influence on cicada wing wettability and bactericidal properties. Adv. Mat. Interfaces 7 (10), 2000112. doi:10.1002/admi.202000112
Saison, T., Peroz, C., Chauveau, V., Berthier, S., Sondergard, E., and Arribart, H. (2008). Replication of butterfly wing and natural lotus leaf structures by nanoimprint on silica sol–gel films. Bioinspir. Biomim. 3 (4), 046004. doi:10.1088/1748-3182/3/4/046004
Sauer, R. A. (2010). A computational model for nanoscale Adhesion between deformable solids and its application to gecko adhesion. J. adhesion Sci. Technol. 24 (11-12), 1807–1818. doi:10.1163/016942410x507588
Seo, K., and Kim, M. (2015). Re-derivation of Young’s equation, Wenzel equation, and Cassie-Baxter equation based on energy minimization[M]//Surface energy. IntechOpen.
Sethi, S. K., Manik, G., and Sahoo, S. K. (2019). Fundamentals of superhydrophobic surfaces[M]//Superhydrophobic polymer coatings. Elsevier, 3–29.
Shahabadi, S. M. S., and Brant, J. A. (2019). Bio-inspired superhydrophobic and superoleophilic nanofibrous membranes for non-aqueous solvent and oil separation from water[J]. Sep. Purif. Technol., 210: 587–599.
Shang, Q. Q., Chen, J. Q., and Yang, X. H. (2019). Fabrication and oil absorbency of superhydrophobic magnetic cellulose aerogels[J]. J. For. Eng. 4 (6), 105–111.
Shang, Q. Q., Hu, Y., and Liu, C. G. (2019). Fabrication of superhydrophobic cellulose composite aerogels for oil/water separation[J]. J. For. Eng. 4 (3), 86–92.
Shao, Y., Zhao, J., Fan, Y., Wan, Z., Lu, L., Zhang, Z., et al. (2020). Shape memory superhydrophobic surface with switchable transition between “Lotus Effect” to “Rose Petal Effect”. Chem. Eng. J. 382, 122989. doi:10.1016/j.cej.2019.122989
Sharma, S. (2021). Droplet behaviour on metastable hydrophobic and superhydrophobic nonwoven materials[D]. New Delhi: Indian Institute of Technology Delhi (IITD).
Shen, D., Ming, W., Ren, X., Xie, Z., and Liu, X. (2021). Progress in non-traditional processing for fabricating superhydrophobic surfaces. Micromachines 12 (9), 1003. doi:10.3390/mi12091003
Si, Y., Dong, Z., and Jiang, L. (2018). Bioinspired designs of superhydrophobic and superhydrophilic materials. ACS Cent. Sci. 4 (9), 1102–1112. doi:10.1021/acscentsci.8b00504
Stark, A. Y., Subarajan, S., Jain, D., Niewiarowski, P. H., and Dhinojwala, A. (2016). Superhydrophobicity of the gecko toe pad: Biological optimization versus laboratory maximization. Phil. Trans. R. Soc. A 374 (2073), 20160184. doi:10.1098/rsta.2016.0184
Starov, V. M., and Velarde, M. G. (2009). Surface forces and wetting phenomena. J. Phys. Condens. Matter 21, 464121. doi:10.1088/0953-8984/21/46/464121
Stoddart, P. R., Cadusch, P. J., Boyce, T. M., Erasmus, R. M., and Comins, J. D. (2006). Optical properties of chitin: Surface-enhanced Raman scattering substrates based on antireflection structures on cicada wings. Nanotechnology 17 (3), 680–686. doi:10.1088/0957-4484/17/3/011
Sun, T., Feng, L., Gao, X., and Jiang, L. (2005). Bioinspired surfaces with special wettability. Acc. Chem. Res. 38 (8), 644–652. doi:10.1021/ar040224c
Tan, D., Luo, A., Wang, X., Shi, Z., Lei, Y., Steinhart, M., et al. (2020). Humidity-modulated core–shell nanopillars for enhancement of gecko-inspired adhesion. ACS Appl. Nano Mat. 3 (4), 3596–3603. doi:10.1021/acsanm.0c00314
Tan, Y., Hu, B., Chu, Z., and Wu, W. (2019). Bioinspired superhydrophobic papillae with tunable adhesive force and ultralarge liquid capacity for microdroplet manipulation. Adv. Funct. Mat. 29 (15), 1900266. doi:10.1002/adfm.201900266
Teisala, H., and Butt, H. J. (2018). Hierarchical structures for superhydrophobic and superoleophobic surfaces. Langmuir 35 (33), 10689–10703. doi:10.1021/acs.langmuir.8b03088
Teodorescu, M. (2014). Applied biomimetics: A new fresh look of textiles. J. Text. 2014, 1–9. doi:10.1155/2014/154184
Tuo, Y., Zhang, H., Rong, W., Jiang, S., Chen, W., and Liu, X. (2019). Drag reduction of anisotropic superhydrophobic surfaces prepared by laser etching. Langmuir 35 (34), 11016–11022. doi:10.1021/acs.langmuir.9b01040
Ueda, E., and Levkin, P. A. (2013). Emerging applications of superhydrophilic-superhydrophobic micropatterns. Adv. Mat. 25 (9), 1234–1247. doi:10.1002/adma.201204120
Varshney, P., Lomga, J., Gupta, P. K., Mohapatra, S., and Kumar, A. (2018). Durable and regenerable superhydrophobic coatings for aluminium surfaces with excellent self-cleaning and anti-fogging properties. Tribol. Int. 119, 38–44. doi:10.1016/j.triboint.2017.10.033
Varshney, P., and Mohapatra, S. S. (2018). Durable and regenerable superhydrophobic coatings for brass surfaces with excellent self-cleaning and anti-fogging properties prepared by immersion technique. Tribol. Int. 123, 17–25. doi:10.1016/j.triboint.2018.02.036
Verbanic, S., Brady, O., Sanda, A., Gustafson, C., and Donhauser, Z. J. (2014). A novel general chemistry laboratory: Creation of biomimetic superhydrophobic surfaces through replica molding. J. Chem. Educ. 91 (9), 1477–1480. doi:10.1021/ed4007056
Vicente, A., Rivero, P. J., Palacio, J. F., and Rodriguez, R. (2021). The role of the fiber/bead hierarchical microstructure on the properties of PVDF coatings deposited by electrospinning. Polymers 13 (3), 464. doi:10.3390/polym13030464
Victor, J. J., Facchini, D., and Erb, U. (2012). A low-cost method to produce superhydrophobic polymer surfaces. J. Mat. Sci. 47 (8), 3690–3697. doi:10.1007/s10853-011-6217-x
Vidal, K., Gómez, E., Goitandia, A. M., Angulo-Ibanez, A., and Aranzabe, E. (2019). The synthesis of a superhydrophobic and thermal stable silica coating via sol-gel process. Coatings (Basel). 9 (10), 627. doi:10.3390/coatings9100627
Voronov, R. S., Papavassiliou, D. V., and Lee, L. L. (2008). Review of fluid slip over superhydrophobic surfaces and its dependence on the contact angle. Ind. Eng. Chem. Res. 47 (8), 2455–2477. doi:10.1021/ie0712941
Walsh, M. J. (1983). Riblets as a viscous drag reduction technique. AIAA J. 21 (4), 485–486. doi:10.2514/3.60126
Wang, B., and Guo, Z. (2013). Superhydrophobic copper mesh films with rapid oil/water separation properties by electrochemical deposition inspired from butterfly wing. Appl. Phys. Lett. 103 (6), 063704. doi:10.1063/1.4817922
Wang, D., Zhao, A., Jiang, R., Li, D., Zhang, M., Gan, Z., et al. (2012). Surface properties of bionic micro-pillar arrays with various shapes of tips. Appl. Surf. Sci. 259, 93–98. doi:10.1016/j.apsusc.2012.06.106
Wang, L., Huang, X., Wang, D., Zhang, W., Gao, S., Luo, J., et al. (2021). Lotus leaf inspired superhydrophobic rubber composites for temperature stable piezoresistive sensors with ultrahigh compressibility and linear working range. Chem. Eng. J. 405, 127025. doi:10.1016/j.cej.2020.127025
Wang, L., Wang, F., Huang, B., and Tang, Q. (2020). Recent advances in superhydrophobic composites based on clay minerals. Appl. Clay Sci. 198, 105793. doi:10.1016/j.clay.2020.105793
Wang, M., Liu, Q., Zhang, H., Wang, C., Wang, L., Xiang, B., et al. (2017). Laser direct writing of tree-shaped hierarchical cones on a superhydrophobic film for high-efficiency water collection. ACS Appl. Mat. Interfaces 9 (34), 29248–29254. doi:10.1021/acsami.7b08116
Wang, S., and Jiang, L. (2007). Definition of superhydrophobic states. Adv. Mat. 19 (21), 3423–3424. doi:10.1002/adma.200700934
Wang, S., Xue, Y., Ban, C., Taleb, A., and Jin, Y. (2020). Fabrication of robust tungsten carbide particles reinforced Co Ni super-hydrophobic composite coating by electrochemical deposition. Surf. Coatings Technol. 385, 125390. doi:10.1016/j.surfcoat.2020.125390
Wang, S., Yang, Z., Gong, G., Wang, J., Wu, J., et al. (2016). Icephobicity of penguins Spheniscus humboldti and an artificial replica of penguin feather with air-infused hierarchical rough structures. J. Phys. Chem. C 120 (29), 15923–15929. doi:10.1021/acs.jpcc.5b12298
Wang, X., Ding, H., Sun, S., Zhang, H., Zhou, R., Li, Y., et al. (2021). Preparation of a temperature-sensitive superhydrophobic self-cleaning SiO2-TiO2@PDMS coating with photocatalytic activity. Surf. Coatings Technol. 408, 126853. doi:10.1016/j.surfcoat.2021.126853
Wang, Y., Lai, H., Cheng, Z., Zhang, H., Zhang, E., Lv, T., et al. (2019). Gecko toe pads inspired in situ switchable superhydrophobic shape memory adhesive film. Nanoscale 11 (18), 8984–8993. doi:10.1039/c9nr00154a
Wang, Y., Zhang, D., Deng, J., Zhou, F., Duan, Z., Su, Q., et al. (2019). Mosquito’s compound eyes as inspiration for fabrication of conductive superhydrophobic nanocarbon materials from waste wheat straw. ACS Sustain. Chem. Eng. 7 (4), 3883–3894. doi:10.1021/acssuschemeng.8b04906
Watson, G. S., Green, D. W., Schwarzkopf, L., Li, X., Cribb, B. W., Myhra, S., et al. (2015). A gecko skin micro/nano structure – a low adhesion, superhydrophobic, anti-wetting, self-cleaning, biocompatible, antibacterial surface. Acta biomater. 21, 109–122. doi:10.1016/j.actbio.2015.03.007
Watson, G. S., and Watson, J. A. (2004). Natural nano-structures on insects—Possible functions of ordered arrays characterized by atomic force microscopy. Appl. Surf. Sci. 235 (1-2), 139–144. doi:10.1016/j.apsusc.2004.05.129
Wei, D., Wang, J., Liu, Y., Li, S., and Wang, H. (2021). Controllable superhydrophobic surfaces with tunable adhesion on Mg alloys by a simple etching method and its corrosion inhibition performance. Chem. Eng. J. 404, 126444. doi:10.1016/j.cej.2020.126444
Weng, W., Tenjimbayashi, M., Hu, W. H., and Naito, M. (2022). Evolution of and disparity among biomimetic superhydrophobic surfaces with gecko, petal, and Lotus effect. Small 18 (18), 2200349. doi:10.1002/smll.202200349
Wenzel, R. N. (1949). Surface roughness and contact angle. J. Phys. Colloid Chem. 53 (9), 1466–1467. doi:10.1021/j150474a015
White, L. R. (1977). On deviations from Young's equation. J. Chem. Soc. Faraday Trans. 1. 73, 390–398. doi:10.1039/f19777300390
Wolfs, M., Darmanin, T., and Guittard, F. (2013). Superhydrophobic fibrous polymers. Polym. Rev. 53 (3), 460–505. doi:10.1080/15583724.2013.808666
Wong, T. S., Kang, S. H., Tang, S. K. Y., Smythe, E. J., Hatton, B. D., Grinthal, A., et al. (2011). Bioinspired self-repairing slippery surfaces with pressure-stable omniphobicity. Nature 477, 443–447. doi:10.1038/nature10447
Wu, B., Cui, X., Jiang, H., Wu, N., Peng, C., Hu, Z., et al. (2021). A superhydrophobic coating harvesting mechanical robustness, passive anti-icing and active de-icing performances. J. Colloid Interface Sci. 590, 301–310. doi:10.1016/j.jcis.2021.01.054
Wu, D., Wang, J. N., Wu, S. Z., Chen, Q. D., Zhao, S., Zhang, H., et al. (2011). Three-level biomimetic rice-leaf surfaces with controllable anisotropic sliding. Adv. Funct. Mat. 21 (15), 2927–2932. doi:10.1002/adfm.201002733
Wu, W., Liang, R., Lu, L., Wang, W., Ran, X., and Yue, D. (2020). Preparation of superhydrophobic laser-induced graphene using taro leaf structure as templates. Surf. Coatings Technol. 393, 125744. doi:10.1016/j.surfcoat.2020.125744
Wu, X. H., Liew, Y. K., Mai, C. W., and Then, Y. Y. (2021). Potential of superhydrophobic surface for blood-contacting medical devices. Int. J. Mol. Sci. 22 (7), 3341. doi:10.3390/ijms22073341
Wu, Y., Hang, T., Yu, Z., Xu, L., and Li, M. (2014). Lotus leaf-like dual-scale silver film applied as a superhydrophobic and self-cleaning substrate. Chem. Commun. 50 (61), 8405–8407. doi:10.1039/c4cc03878a
Xiang, S., and Liu, W. (2021). Self‐Healing superhydrophobic surfaces: Self‐healing superhydrophobic surfaces: Healing principles and applications (adv. Mater. Interfaces 12/2021). Adv. Mat. Interfaces 8 (12), 2170065. doi:10.1002/admi.202170065
Xie, H., Huang, H. X., and Peng, Y. J. (2017). Rapid fabrication of bio-inspired nanostructure with hydrophobicity and antireflectivity on polystyrene surface replicating from cicada wings. Nanoscale 9 (33), 11951–11958. doi:10.1039/c7nr04176d
Xie, J., Xu, J., Shang, W., and Zhang, K. (2018). Dropwise condensation on superhydrophobic nanostructure surface, part II: Mathematical model. Int. J. Heat Mass Transf. 127, 1170–1187. doi:10.1016/j.ijheatmasstransfer.2018.07.008
Xu, J., Hou, Y., Lian, Z., Yu, Z., Wang, Z., and Yu, H. (2020). Bio-inspired design of bi/tridirectionally anisotropic sliding superhydrophobic titanium alloy surfaces. Nanomater. (Basel). 10 (11), 2140. doi:10.3390/nano10112140
Xu, L., Yang, L., Yang, S., Xu, Z., Lin, G., Shi, J., et al. (2021). Earthworm-inspired ultradurable superhydrophobic fabrics from adaptive wrinkled skin. ACS Appl. Mat. Interfaces 13 (5), 6758–6766. doi:10.1021/acsami.0c18528
Xu, Q. F., Mondal, B., and Lyons, A. M. (2011). Fabricating superhydrophobic polymer surfaces with excellent abrasion resistance by a simple lamination templating method. ACS Appl. Mat. Interfaces 3 (9), 3508–3514. doi:10.1021/am200741f
Xu, Q., Wan, Y., and Hu, T. S. (2015). Robust self-cleaning and micromanipulation capabilities of gecko spatulae and their bio-mimics[J]. Nat. Commun. 6 (1), 1–9.
Xu, S., Wang, Q., and Wang, N. (2021). Chemical fabrication strategies for achieving bioinspired superhydrophobic surfaces with micro and nanostructures: A review. Adv. Eng. Mat. 23 (3), 2001083. doi:10.1002/adem.202001083
Xue, Y., Wang, S., Bi, P., Zhao, G., and Jin, Y. (2019). Super-hydrophobic Co–Ni coating with high abrasion resistance prepared by electrodeposition. Coatings 9 (4), 232. doi:10.3390/coatings9040232
Xue, Y., Wang, S., Zhao, G., Taleb, A., and Jin, Y. (2019). Fabrication of Ni Co coating by electrochemical deposition with high super-hydrophobic properties for corrosion protection. Surf. coatings Technol. 363, 352–361. doi:10.1016/j.surfcoat.2019.02.056
Yang, H., You, W., Shen, Q., Wang, X., Sheng, J., Cheng, D., et al. (2014). Preparation of lotus-leaf-like antibacterial film based on mesoporous silica microcapsule-supported Ag nanoparticles. RSC Adv. 4 (6), 2793–2796. doi:10.1039/c3ra45382k
Yang, L., Shen, X., Yang, Q., Liu, J., Wu, W., Li, D., et al. (2021). Fabrication of biomimetic anisotropic super-hydrophobic surface with rice leaf-like structures by femtosecond laser. Opt. Mater. 112, 110740. doi:10.1016/j.optmat.2020.110740
Yang, M., Liu, W., Jiang, C., He, S., Xie, Y., and Wang, Z. (2018). Fabrication of superhydrophobic cotton fabric with fluorinated TiO2 sol by a green and one-step sol-gel process. Carbohydr. Polym. 197, 75–82. doi:10.1016/j.carbpol.2018.05.075
Yang, Q., Luo, Z., Jiang, F., Luo, Y., Tan, S., Lu, Z., et al. (2016). Air cushion convection inhibiting icing of self-cleaning surfaces. ACS Appl. Mat. Interfaces 8 (42), 29169–29178. doi:10.1021/acsami.6b10165
Yang, S., Ju, J., Qiu, Y., He, Y., Wang, X., Dou, S., et al. (2014). Peanut leaf inspired multifunctional surfaces. Small 10 (2), 294–299. doi:10.1002/smll.201301029
Yang, S., Ju, J., Qiu, Y., He, Y., Wang, X., Dou, S., et al. (2014). Superhydrophobic materials: Peanut leaf inspired multifunctional surfaces (small 2/2014). Small 10 (2), 214. doi:10.1002/smll.201470010
Yang, Y., He, H., Li, Y., and Qiu, J. (2019). Using nanoimprint lithography to create robust, buoyant, superhydrophobic PVB/SiO2 coatings on wood surfaces inspired by red roses petal. Sci. Rep. 9 (1), 9961–9969. doi:10.1038/s41598-019-46337-y
Yong, J., Chen, F., Yang, Q., and Hou, X. (2015). Femtosecond laser controlled wettability of solid surfaces. Soft Matter 11 (46), 8897–8906. doi:10.1039/c5sm02153g
Yong, J., Yang, Q., and Hou, X. (2022). Nature-inspired superwettability achieved by femtosecond lasers[J]. Ultrafast Sci. 2022.
Young, T. (1805). An essay on the cohesion of fluids[J]. Philosophical Trans. R. Soc. Lond. 95, 65–87.
Yu, Y., Zhao, Z. H., and Zheng, Q. S. (2007). Mechanical and superhydrophobic stabilities of two-scale surfacial structure of Lotus leaves. Langmuir 23 (15), 8212–8216. doi:10.1021/la7003485
Yuan, Z., Bin, J., Wang, X., Peng, C., Wang, M., Xing, S., et al. (2014). Fabrication of superhydrophobic surface with hierarchical multi-scale structure on copper foil. Surf. Coatings Technol. 254, 151–156. doi:10.1016/j.surfcoat.2014.06.004
Yue, G., Wang, Y., Li, D., Hou, L., Cui, Z., Li, Q., et al. (2020). Bioinspired surface with special wettability for liquid transportation and separation. Sustain. Mater. Technol. 25, e00175. doi:10.1016/j.susmat.2020.e00175
Yun, X., Xiong, Z., He, Y., and Wang, X. (2020). Superhydrophobic lotus-leaf-like surface made from reduced graphene oxide through soft-lithographic duplication. RSC Adv. 10 (9), 5478–5486. doi:10.1039/c9ra10373b
Zhang, B., and Xu, W. (2021). Superhydrophobic, superamphiphobic and SLIPS materials as anti-corrosion and anti-biofouling barriers[J]. New J. Chem.
Zhang, G., Zhang, J., Xie, G., Liu, Z., and Shao, H. (2006). Cicada wings: A stamp from nature for nanoimprint lithography. Small 2 (12), 1440–1443. doi:10.1002/smll.200600255
Zhang, H., Lai, H., Cheng, Z., Zhang, D., Wang, W., Liu, P., et al. (2021). Superhydrophobic shape memory film with switchable adhesion to both water and solid. Chem. Eng. J. 420, 129862. doi:10.1016/j.cej.2021.129862
Zhang, J., Yang, Q., and Cheng, Y. (2022). Slippery liquid‐infused porous surface on metal material with excellent ice resistance fabricated by femtosecond bessel laser[J]. Adv. Eng. Mater., 2101738. doi:10.1002/adem.202101738
Zhang, K., Li, H., Yin, X., and Wang, Z. (2019). Oil/water separation on structure-controllable Cu mesh: Transition of superhydrophilic-superoleophilic to superhydrophobic-superoleophilic without chemical modification. Surf. Coatings Technol. 358, 416–426. doi:10.1016/j.surfcoat.2018.11.061
Zhang, X., Zhao, J., Mo, J., Sun, R., Li, Z., and Guo, Z. (2019). Fabrication of superhydrophobic aluminum surface by droplet etching and chemical modification. Colloids Surfaces A Physicochem. Eng. Aspects 567, 205–212. doi:10.1016/j.colsurfa.2019.01.046
Zhang, Y., Chen, Y., Shi, L., Li, J., and Guo, Z. (2012). Recent progress of double-structural and functional materials with special wettability. J. Mat. Chem. 22 (3), 799–815. doi:10.1039/c1jm14327a
Zhang, Y., Jiao, Y., Li, C., Chen, C., Li, J., Hu, Y., et al. (2020). Bioinspired micro/nanostructured surfaces prepared by femtosecond laser direct writing for multi-functional applications. Int. J. Extrem. Manuf. 2 (3), 032002. doi:10.1088/2631-7990/ab95f6
Zhang, Y. L., Xia, H., Kim, E., and Sun, H. B. (2012). Recent developments in superhydrophobic surfaces with unique structural and functional properties. Soft Matter 8 (44), 11217–11231. doi:10.1039/c2sm26517f
Zhao, H., Sun, Q., Deng, X., and Cui, J. (2018). Earthworm-inspired rough polymer coatings with self-replenishing lubrication for adaptive friction-reduction and antifouling surfaces. Adv. Mat. 30 (29), 1802141. doi:10.1002/adma.201802141
Zhao, Z., Xiang, J., and Tan, Y. (2021). Preparation of superhydrophobic coating on 5083 aluminum alloy for corrosion protection in simulated marine environment containing SRB[J]. Phys. Metals Metallogr. 122 (14), 1581–1587.
Zheng, J., Yang, J., and Cao, W. (2022). Fabrication of transparent wear-resistant superhydrophobic SiO2 film via phase separation and chemical vapor deposition methods[J]. Ceram. Int. 407. doi:10.1016/j.apsusc.2017.02.207
Zheng, Y., Bai, H., Huang, Z., Tian, X., Nie, F. Q., Zhao, Y., et al. (2010). Directional water collection on wetted spider silk. Nature 463 (7281), 640–643. doi:10.1038/nature08729
Zheng, Y., Gao, X., and Jiang, L. (2007). Directional adhesion of superhydrophobic butterfly wings. Soft Matter 3 (2), 178–182. doi:10.1039/b612667g
Zheng, Y., Zhang, C., Wang, J., Liu, Y., Shen, C., and Yang, J. (2019). Robust adhesion of droplets via heterogeneous dynamic petal effects. J. colloid interface Sci. 557, 737–745. doi:10.1016/j.jcis.2019.09.070
Zhou, S., Zhu, X., and Yan, Q. (2018). One-step electrochemical deposition to achieve superhydrophobic cobalt incorporated amorphous carbon-based film with self-cleaning and anti-corrosion. Surf. Interface Anal. 50 (3), 290–296. doi:10.1002/sia.6367
Zong, C., Hu, M., Azhar, U., Chen, X., Zhang, Y., Zhang, S., et al. (2019). Smart copolymer-functionalized flexible surfaces with photoswitchable wettability: From superhydrophobicity with “rose petal” effect to superhydrophilicity. ACS Appl. Mat. Interfaces 11 (28), 25436–25444. doi:10.1021/acsami.9b07767
Keywords: nature, bionics, superhydrophobic principle, wettability, review
Citation: Ge-Zhang S, Cai T, Yang H, Ding Y and Song M (2022) Biology and nature: Bionic superhydrophobic surface and principle. Front. Bioeng. Biotechnol. 10:1033514. doi: 10.3389/fbioe.2022.1033514
Received: 31 August 2022; Accepted: 27 September 2022;
Published: 17 October 2022.
Edited by:
Hongliang Liu, Yantai University, ChinaReviewed by:
Jiale Yong, University of Science and Technology of China, ChinaCopyright © 2022 Ge-Zhang, Cai, Yang, Ding and Song. This is an open-access article distributed under the terms of the Creative Commons Attribution License (CC BY). The use, distribution or reproduction in other forums is permitted, provided the original author(s) and the copyright owner(s) are credited and that the original publication in this journal is cited, in accordance with accepted academic practice. No use, distribution or reproduction is permitted which does not comply with these terms.
*Correspondence: Mingbo Song, c29uZ21iQDEyNi5jb20=
†These authors have contributed equally to this work