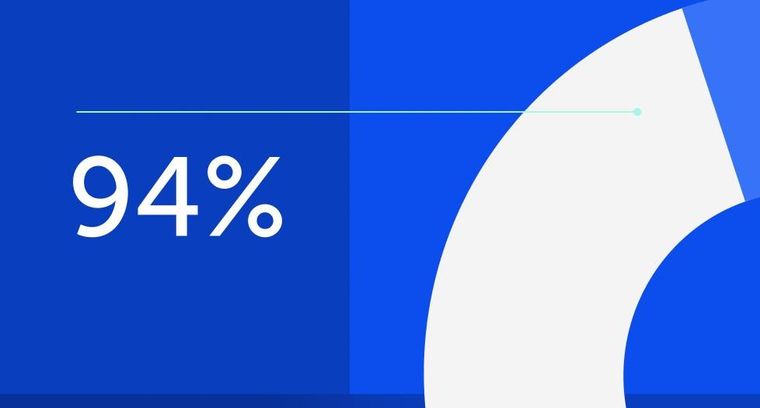
94% of researchers rate our articles as excellent or good
Learn more about the work of our research integrity team to safeguard the quality of each article we publish.
Find out more
REVIEW article
Front. Bioeng. Biotechnol., 15 September 2022
Sec. Biomaterials
Volume 10 - 2022 | https://doi.org/10.3389/fbioe.2022.1020020
This article is part of the Research TopicMicroorganisms and Their Derivatives for Cancer TherapyView all 6 articles
Therapeutic bacteria have shown great potential on anti-tumor therapy. Compared with traditional therapeutic strategy, living bacteria present unique advantages. Bacteria show high targeting and great colonization ability in tumor microenvironment with hypoxic and nutritious conditions. Bacterial-medicated antitumor therapy has been successfully applied on mouse models, but the low therapeutic effect and biosafe limit its application on clinical treatment. With the development of material science, coating living bacteria with suitable materials has received widespread attention to achieve synergetic therapy on tumor. In this review, we summarize various materials for coating living bacteria in cancer therapy and envision the opportunities and challenges of bacteria-medicated antitumor therapy.
With the rapid development of modern medical technology and industry, the average human lifetime is gradually extended (Vaupel et al., 2021). However, the treatment of tumor still is a global problem, despite that some mild tumor could be surgically excised. The improvement of symptoms, survival quality and period are currently the main purpose of tumor therapy (Wyld et al., 2015). Radiotherapy is the common therapy strategy for antitumor, while the insufficient tissue penetration and non-targeting limit its widespread application (Jain, 1998; Minchinton and Tannock, 2006; St-Jean et al., 2008; Grierson et al., 2017). Chemotherapy is an effective way to suppress the growth and spread of tumor with chemical drugs throughout the whole body, but its non-specificity on tumor cells could cause damage to normal tissues (Pérez-Herrero and Fernández-Medarde, 2015). Surgery could not completely clear metastatic cancer cells with the recurrent risk (Wyld et al., 2015). Chimeric antigen receptor T (CAR-T) cell therapy is regarded as an effective solution for relapsed or refractory tumors, due to high tumor targeting (Curran et al., 2012; Sadelain et al., 2013; Mirzaei et al., 2016). Potential side effects restrict the clinical application of CAR-T cell therapy, such as B cell abnormalities (Marofi et al., 2021). New therapy strategy with high tumor targeting, low side effect and good effect is needed for antitumor treatment.
Bacteria therapy could be a promising strategy on tumor treatment (Dang et al., 2001; Silva-Valenzuela et al., 2016). The hypoxic and nutrient-rich tumor microenvironment is uniquely attractive to bacteria (Nguyen and Min, 2017). In the early 19th centuries, Dr. Busch firstly noticed that patients with malignant tumors improved after being infected with Streptococcus pyogenes (S. pyogenes). In the mid-19th century, Coley (1910) found that people with neck cancer recovered from infection of erysipelas. Then Coley tried to treat tumors with inactivated bacteria, such as S. pyogenes and Serrati amarcescens and established the foundation of bacterial therapies on cancer (Richardson et al., 1999). In recent years, people find various bacteria with good tumor-targeting property, including Salmonella (Pawelek et al., 1997), Escherichia, Clostridium (Malmgren and Flanigan, 1955; Minton, 2003), Bifidobacterium (Kohwi et al., 1978), Caulobacter, Listeria (Pan et al., 1999; Kim et al., 2009), Proteus (Arakawa et al., 1968), and Streptococcus (Maletzki et al., 2008). Caulobacter crescentus (C. crescentus) as a Gram-negative non-pathogenic bacterium presented tumor suppressive effects in unmodified form (Bhatnagar et al., 2006). After non-tumorigenic activity in mouse models of transplantable tumors, the prolonged survival and reduced tumor mass of C. crescentus group presented better antitumorigenic activity in mouse models of lung tumor, breast tumor and leukemia tumors than saline controls. These results suggest that C. crescentus may be a safe bacterial immunomodulator for tumor treatment (Bhatnagar et al., 2006).
The ability to induce and amplify antigen-specific immune responses has been considered a potentially valuable tool on the treatment of cancer. Most cancer vaccines induce cytotoxic T lymphocyte (CTL) responses to tumor-associated antigens (TAA). An attenuated vaccine against Listeria monocytogenes (L. monocytogenes) eradicated metastases and the entire primary tumor of breast cancer in mice by TAA-specific CTL-mediated cytolysis to kill tumor cells (Kim et al., 2009). The vaccine mode of action of Listeria provides a new direction in bacterial research in targeting metastatic breast cancer. Further study found that L. monocytogenes could serve as an effective vehicle for tumor-specific antigen targeting (Kim et al., 2009). The engineered L. monocytogenes expressing tumor-specific antigen induced primary tumor regression and identified pulmonary metastases by parenteral immunization in murine model of melanoma B16F10 (Pan et al., 1999). The non-pathogenic parthenogenic anaerobic bacterium Salmonella can specifically target tumor sites to regulate immune response (Pawelek et al., 1997; Lee et al., 2005; Zhao et al., 2005; Forbes, 2010; Chang and Lee, 2014). The modulation of the antitumor effect of Salmonella encapsulated with polyallylamine hydrochloride not only greatly enhanced antitumor activity but also maintained tumor targeting (Lee et al., 2017).
In recent years, biomaterials have been used to decorate bacteria for achieving gastrointestinal protection and synergetic treatment on tumor based on its biodegradability, biocompatibility and immunomodulatory activity (Lee et al., 2013; Zhu et al., 2013). Feng et al. (2020) found that bacteria can be temporarily inactivated by confining them individually in an intact polymer coating. Bacteria are intelligently restored to vital activity after shedding, thus achieving targeted release of bacterial drugs. This approach greatly improves the bioavailability as well as the effectiveness and stability of bacteria during in vivo delivery, providing an important means to prepare bacterial-mediated smart biologics for tumor therapy. This paper reviews biomaterial styles and synergetic strategy for coating bacteria on tumor therapy in recent years. We hope this review could enlighten researcher on bacteria-mediated tumor therapy and guide more biomaterials used on bacterial coating.
Bacteria therapy offers a new perspective on anti-tumor treatment, but the instability and biosafety of living bacteria limit its clinical application (Sarotra and Medhi, 2016). Enteric bacteria can be directly transported to the host via intravenous injection, oral administration, or anal perfusion (Wu et al., 2013; West et al., 2020). Gavage and oral administration are considered as the most convenient and widely applicated method for bacteria delivery compared with anal perfusion considering the low patient compliance and local delivery (Zaloga, 2006).
The low pH environment, proteolytic enzyme, and high concentration of bile salts in gastrointestinal tract (GI tract) could significantly reduce the activity and therapeutic effects of oral bacteria (Evans et al., 1988; Cook et al., 2012; Sohail et al., 2018). The limitation restricts the use of oral bacteria. An effective approach of bacteria therapy is coating bacteria with suitable materials, hence achieving protection and controlled release of living bacteria (Prakash and Jones, 2005). Obviously, these materials should be acid-proof, safe, mild, and automatically degraded under certain conditions. The combination of biomaterials and encapsulation technologies could raise the efficiency of oral delivery and decrease the side effects of bacteria.
Bacteria-medicated cancer therapy has made remarkable progress in recent years. The combination of bacteria with suitable materials could increase bacterial tumor-colonization and offset the shortage of drug supply into intra-tumoral regions, hence reducing side effects and improving antitumor efficacy (Felfoul et al., 2016; Lou et al., 2021). Drug-loaded bacteria could preferentially translocate to tumor stroma after intravenous administration and selectively release the drugs in response to the tumor microenvironment (Li et al., 2021). At present, a variety of biological materials have been used to encapsulate bacteria for tumor treatment, including natural materials, synthetic materials, and cell-based materials (Figure 1).
Up to now, plenty of studies have demonstrated that a variety of natural polymers are suitable for coating bacteria, such as polysaccharides, Eudragit, proteins, poly (amino acids), and lipids (Wee and Gombotz, 1998; Li et al., 2016; Yeung et al., 2016; Dafe et al., 2017; Li et al., 2020).
Alginate comprised with a linear polysaccharide consisting of 1,4’- linked β-D-mannuronic acid and α-L-gluconic acid residues originating from microbes (e.g., Pseudomonas) or brown algae, is particularly suitable to encapsulate bacteria due to its nontoxicity and mild gelling conditions (Zhou et al., 1998; Rinaudo, 2008). Alginate can form an “egg box structure” between four G resides by contacting with divalent metal ions (e.g., Ca2+, Cd2+, Zn2+) (Stokke et al., 1997; Wahab et al., 1997; Cook et al., 2012), because of the carboxylic acid groups on both monomer molecules (Draget et al., 1994). The property of alginate has been exploited to produce microcapsules by an extrusion process. The alginate solution is dropped into cationic propyl gallate (commonly calcium chloride) gelling into a microcapsule. The size of the microcapsules formed by the external gel depends on the droplet size formed in an extrusion process, which is typically between tens of microns and millimetre size (Sun and Griffiths, 2000; Chandramouli et al., 2004; Ding and Shah, 2009).
Therefore, alginate is also a common tool of intestinal delivery vehicles. Numerous studies have shown that coating lactobacillus with alginate gel can help them resist gastric acid and ensure that enough living bacteria into the small intestine (Adhikari et al., 2000; Sultana et al., 2000; Pan et al., 2013). Interestingly, Lee and Heo, 2000) found that the survival rate of living bacteria elevated with increasing the alginate concentration. In addition, alginate could enhance bacterial resistance to antibiotics by fabricating biomimetic biofilm to entrap probiotics crossing-link with calcium ions by electrospray (Li et al., 2018). The application of alginate is restricted due to its instability, uncontrollable swelling, and fragility (Kim et al., 2014). Diffusion-gelled alginate degrades with exposure to biological buffers for a long time both in vivo and in vitro (Peirone et al., 1998; De Vos et al., 1999). The main reason could be the gel dissolution caused by the exchange of calcium and monovalent sodium ions (LeRoux et al., 1999; Van Raamsdonk and Chang, 2001). Therefore, many new strategies are attempted to strengthen the stability of alginate. For example, the co-coating of alginate and chitosan on the surface of bacteria remarkably elevated the stability and survival rate of bacteria (Wee and Gombotz, 1998; Călinoiu et al., 2019). Lin et al. (2008) have found that alginate-chitosan-alginate (ACA) microcapsules could entrap living bacteria to achieve ascendant chemical stability of bacteria in stimulated-gastric fluid in vitro. In-vivo studies proved that ACA microcapsules were more resistant to GI enzyme degradation than alginate-poly-lysine-alginate microcapsules.
Chitosan as a natural cationic polysaccharide is also considered as a coating material for bacteria due to its biodegradability, low toxicity, and biocompatibility (Lai and Lin, 2009; Netsomboon and Bernkop-Schnurch, 2016). Cook et al. (2011) found that Bifidobacterium breve coated with chitosan and alginate showed more tolerant to the GI tract than that by single alginate coating, because that chitosan could stable alginate microcapsules and maintain the stability of probiotics in the stomach. Cationic chitosan and anionic alginate could repeatedly encapsulate bacteria through electrostatic interaction (Anselmo et al., 2016). The layer-by-layer coating technique greatly improved the viability and stability of oral bacteria in the GI tract (Lin et al., 2008; Anselmo et al., 2016). In addition to chitosan and alginate, other materials also are used to encapsulate living bacteria applicated in the biomedical field, such as poly-L-lysine (Krasaekoopt et al., 2004), protamine (Mei et al., 2014), starch (Saberi-Rise and Moradi-Pour, 2020) or gelatin (Tu et al., 2015; Pour et al., 2019).
Poly-L-lysine (PLL), natural cationic polymers, could complex with alginate to form microcapsules via electrostatic attraction. Chen et al. (2005) designed alginate-poly-L-lysine-alginate (APA) microcapsules for oral administration of Lactobacillus plantarum. The APA microcapsules could maintain morphological stability under a simulated stomach condition, but failed to retain structural integrity after long-term exposure in a simulated gastro-intestinal medium. To enhance the stability of coating bacteria in the GI tract, Ouyang et al. (2004) prepared a novel multilayer APPPA (alginate-PLL-pectin-PLL-alginate) system to coat intestinal bacteria and showed better stability than APA microcapsules in simulated gastrointestinal fluid.
Phospholipids, the main components of cell membranes, are often used as delivery carriers for drugs or small molecules because of their biocompatibility, easy modification, low immune response and biodegradability (Matthay et al., 1984). Chowdhuri et al. (2016) used liposomes encapsulating Escherichia coli (E. coli) by the inverse-emulsion way and assessed the effect of liposomes on bacteria activity and viability. The inverse-emulsion method has been reported to coat efficiently biological macromolecules, such as proteins and living cells (Zhu H. et al., 2018). Cao et al. (2019b) utilized lipids to generate a myriad of super gut microbes by bio-interfacial supramolecular self-assembly, which not only improved the bioavailability of oral bacteria but also maintained the bioactivity (Figure 2).
Proteins are another important group of polymers for the encapsulation of bacteria due to their amphiphilic nature (Kim et al., 2016). The most common proteins used for encapsulation of probiotics include gelatin (Paula et al., 2019), whey protein (Yoda et al., 2015), and so on. Silk fibroin from a natural protein of silk-worm cocoon has great biocompatibility, biodegradability, non-immunogenicity, and mechanical robustness (Keten et al., 2010; Rockwood et al., 2011; Zhu Y. et al., 2018; Shi et al., 2019). Silk fibroin nanoparticles could specifically target inflammation sites and damaged intestinal tract, hence assisting with delivering drugs to inflamed tissues (Lamprecht et al., 2001; Gobin et al., 2006; Fathi et al., 2019). Silk fibroin could self-assemble on the surface of bacteria by transforming beta-sheet conformation from a random coil to form the core-shell structure for bacteria delivery (Figure 3) (Hou et al., 2021).
With the development of encapsulation technology and materials science, synthetic materials also are used for coating living bacteria based on their tumor targeting, tumor tissue penetration, and anti-tumor effects. Chen et al. (2019) prepared indocyanine green-loaded nanoparticles and attached them to the surface of a genetically modified Salmonella Typhimurium YB1 through amide bonds to create a biotic/abiotic cross-linked system for large solid tumor precision therapy (Figure 4). This system showed stable and efficient photothermal killing ability after intravenous injection and completely eliminated large solid tumors. Taherkhani et al. (2014) proposed that combining carboxyl-modified drug-carrying nanoliposomes with amino groups on the surface of magnetotactic bacteria MC-1 could deeply penetrate hypoxic tumor sites by the external magnetic field. Doxorubicin (DOX) conjugated to E. coli Nissle 1917 (EcN) by acid-labile linkers of cis-Aconitum anhydride can release under an acidic environment and achieve directly anticancer drug accumulation (Xie et al., 2017).
FIGURE 4. Encapsulation of indocyanine green-loaded nanoparticles on the surface of bacteria for tumor therapy.
Zhang et al. (2018) reported a heat-sensitive drug oral-delivery system in which thermally-sensitive programmable bacteria expressing therapeutic protein TNF-α were decorated with bio-mineralized gold nanoparticles. The engineered bacteria could reach the tumor regions through the GI tract after oral administration. Irradiating tumor sites with near-infrared light, gold nanoparticles could induce the expression of TNF-α from the engineered bacteria, hence inhibiting the growth of tumor cells.
Spore, the dormant life forms of bacteria, could enclose drugs and improve the bioavailability due to the resistance to the acidic enzyme-rich digestive tract. Spore loading with a chemotherapy drug DOX was modified with deoxycholic acid to create anti-tumor nanoparticles. The nanoparticles could effectually protect DOX in the GI tract and enhance the accumulation of DOX in tumor regions (Song et al., 2019).
Except for natural materials and synthetic materials, cell-based materials have been applied in the field of nano and micro motors considering their attractive properties such as biocompatibility and low immunogenicity. Red cell membrane-coated nanoparticles could prolong the circulation time of particles in vivo (Hu et al., 2011), and (Kulkarni et al., 2011) platelet membrane-coated nanoparticles could target specific tumor tissues and enhance the ability of injured sites colonization (Wang et al., 2019). The cell membrane is usually stripped from cells as the materials to decorate bacteria. For red blood cells, cells were first separated from the whole blood and the intracellular components were removed by hypotonic treatment. The hollowed-out red blood cells are then washed and extruded from the porous membrane to form small vesicles derived from the erythrocyte membrane (Luk and Zhang, 2015). Cao et al. (2019a) extruded erythrocyte membrane with EcN to obtain cell membrane-coated bacteria (CMCB). The CMCB achieved lower immunogenicity, inherent bioactivities and blood reservation up to 48 h after injection (Figure 5). Alapan et al. (2018) combined E. coli MG1655 with red blood cells to wrap doxorubicin and superparamagnetic iron oxide nanoparticles via biotin-avidin affinity, hence facilitating the delivery of drugs.
Except for drug delivery, cell membrane coating could also be applied to detoxification of pathogenic virulence factors (Hu et al., 2013a) and anti-virulence vaccination (Hu et al., 2013b) to treat bacterial infections led by Staphylococcus aureus, Enterobacteriaceae, and others. Cell membrane coating shows great potential for targeted delivery of therapeutic agents and bacteria to reduce undesirable off-target effects, since that a variety of targeting ligands and cell membranes can achieve cell-specific binding and uptake. The encapsulation of living bacteria by materials maximizes the survival rate and bioavailability of oral administration in the GI tract, and provides low immunogenicity of bacteria in the blood. However, bacteria-mediated bio-therapy is mainly based on animal models and the potential challenges should be solved before clinical application.
In brief, it is difficult for natural materials to be modified simultaneously while maintaining great bioavailability. Reversely, synthetic materials are very versatile. Synthetic materials can be designed with different functions according to the requirement of drug administration. However, most synthetic materials could be low bioavailability to the delivering bacteria or the delivered animals, which limits their application in clinic (Pan et al., 2021). Cell-based materials present high safety and long systemic circulation in vivo, while the specifical source of each cell membrane could impact on the commonality of cell-based materials. Therefore, natural materials or cell-based materials modified with synthetic materials could endow synergetic therapy with high bioavailability and low immunogenicity, which will accelerate the application of bacteria-mediated therapy in clinic.
Bacterial therapy in oncology could date back at least 150 years, because of the unique ability and easily manipulated genes of bacteria (Kulp and Kuehn, 2010). Some bacteria have well tumor colonization as facultative or strict anaerobes due to the hypoxic microenvironment on tumor, such as E. coli and Salmonella typhimurium. Tumor targeting bacteria have been applied on various tumors for diagnosis, imaging and treatment with or without functional decoration (Figure 6).
Breast cancer is the most common cancer among women worldwide with low survival rate (Alberg and Singh, 2001; Althuis et al., 2005). Current treatment options for metastatic cancer include surgery followed by chemotherapy or radiation therapy and/or adjuvant therapy (Scart et al., 2002). Tumor targeting bacteria could assist in the delivery of chemotherapy or radiation drugs to improve antitumor therapy. Zhang et al. (2018) constructed nanoscale microcells by genetically engineered ECN as a carrier for the targeted delivery of chemotherapeutic drugs to tumor hypoxic zone. The drug-carrying microcells showed significant inhibition on the growth of breast cancer without any significant toxicity. Raman et al. (2021) developed a Salmonella vector with controlled drug synthesis and cellular invasion and achieved effectively reduced tumor growth and metastasis. Salmonella-based protein delivery shows a safe and effective treatment for tumors to provide new therapy for untreatable cancers.
The facultative anaerobe Salmonella strain VNP20009 prefers to colonize hypoxic areas of tumor core (Chen et al., 2017) and necrotic tumor tissue (Chang and Lee, 2014). The attenuated strain VNP20009 can carry exogenous gene expression plasmids to target tumors and express exogenous proteins specifically in tumor tissues. Study showed that VNP20009 combined with photothermal therapy can achieve higher specificity and anti-tumor effects. The photothermal agent converts the incident light into heat in the irradiated target tissue to kill the surrounding tumor cells (Liu et al., 2013; Fan et al., 2018; Jung et al., 2018; Vankayala and Hwang, 2018). Chen et al. (2018) encapsulated polydopamine on the outer layer of VNP20009 to induce apoptosis and necrosis of melanoma cells in mouse model, thereby inhibiting tumor growth (Figure 7). The antitumor of different Salmonella strains was investigated (Avogadri et al., 2008; Crull et al., 2011; Kocijancic et al., 2017). A nutrient-deficient Salmonella enterica with aroA mutant was identified to possess immunostimulatory potential and hence achieved the inhibition of melanoma cells growth and the improved survival rate of mice (Johnson et al., 2021).
Based on the metabolic characteristics of bacteria, Shi et al. (2022) showed that the metabolism of oncolytic bacteria with synthetic photosensitizer-label could specifically clear anaerobic regions of tumors in concert with photodynamic therapy. The new functionalized lysing bacterium combined with the bio-photodynamic-immunotherapy promised minimally invasive removal of malignant melanoma, hence providing a new tool for post-operative recurrence prevention. These studies have shown great potential of bacterial therapy on the treatment of melanoma to provide a more effective and safe treatment strategy.
Colorectal cancer (CRC) is the third most common cancer and the second leading cause of cancer deaths in the United States (Goldberg et al., 2007; Jemal et al., 2008). Except for traditional radiotherapy and chemotherapy, bacterial therapy was used on the CRC treatment long ago and is booming with the development of biomedical technology and nano materials science. Back in the late 1800s, Coley firstly treated sarcomas with a mixture of Serratia marcescens and Streptococcus pyogenes to reduce tumors and prolong survival of CRC patients (Ebrahimzadeh et al., 2021). Chiang and Huang (2021) developed a bacterial treatment for colorectal cancer using ECN to deliver therapeutic proteins and inhibits tumor growth. Kefayat et al. (2018) used alive attenuated Salmonella Typhi Ty21a as a vehicle for smart delivery of gold nanoparticles to the hypoxic regions of tumor and achieved high accumulation of folic acid functionalized gold nanoparticles.
Unique coating strategy of living bacteria could be need for special tumors on anti-tumor therapy. Intravenous injection could be the mainly delivery strategy of living bacteria on therapy of breast cancer. Coating materials should endow bacteria low immunogenicity to escape from immune attack rapidly into tumor sites for killing tumor cells. In-situ injection or subcutaneous injection adjacent to tumor is commonly used on bacteria-mediated anti-tumor on the solid melanoma. Synergistic antitumor materials with chemotherapy drug or photothermal therapy adjuvant could be suitable to coat living bacteria for efficient tumor cytotoxicity. Oral administration is an excellent strategy to deliver bacteria directly reach colorectal tumor through the GI tract. Therefore, the gastrointestinal protection, intestinal adhesion and inflammation targeting are key considerations for choosing coating materials of living bacteria on anti-tumor therapy.
Bacterial therapy is a promising strategy for anti-tumor therapy due to its inherent tumor-targeting properties. Based on the natural tendency of tumor-targeted bacteria, various materials can be grafted onto the surface of bacteria by physical, chemical or biological methods to achieve a highly efficient and stable anti-tumor system by enhancing gastrointestinal stability, tumor tissue targeting, and tumor inhibition response. In this review, we have briefly summarized three kinds of materials used to coat bacteria in cancer therapy. Natural polymers could be easily acquired without a complex synthesis process. Synthetic materials could link with functionalized decoration on the surface of bacteria, hence achieving synergetic therapy on cancer. Cell-based materials show well biocompatibility and low immunogenicity to implement targeted delivery of bacteria on in vivo tumor. Tumor targeting bacteria has been applied on various tumors for diagnosis, imaging and treatment. The effective combination of bacterial therapy by functionalized encapsulation with other approaches is the current research hotspot, particularly immunotherapy and photothermal therapy. The intelligent application of bacterial tumor targeting could facilitate the development of sustainable bacteria-mediated therapies for routine clinical use.
Despite the attractive and promising therapeutic prospects, there are still many limitations and challenges restricting the development and application of bacteria-based delivery system. Safety is a major concern due to the immunogenicity of living bacteria. Higher microbe concentrations potentially could induce systemic toxicity (Din et al., 2016). The trial of Bacillus Calmette-Guérin or modified Salmonella typhimurium as medication in anti-tumor therapy is firstly carried out to validate their safety not therapeutic efficacy (Liang et al., 2019; Mukherjee et al., 2021). Therefore, the appropriate number of bacteria is essential to carry enough drugs ensuring both therapeutic effects and safety.
The manufacturing process of bacteria-based delivery system is more complex than that of small molecule anticancer drugs, which are different from traditional pharmaceutical processes. Therefore, a new methodology is needed for large-scale production, sterilization technology, storage and transportation of bacteria-based delivery products. Developing more effective and rational designs, bacteria-mediated therapies could be one of the most powerful tools against cancer in the future.
HQ and WH designed the study and revised the manuscript, JW and NG wrote the manuscript.
This work was financially supported by the National Natural Science Foundation of China (32100070), the Science and Technology Commission of Shanghai Municipality (YDZX20213100003690).
Authors are thankful to Shanghai Tenth People’s Hospital, Tongji University, Shanghai, China for the quality education and moral support. They would also like to acknowledge the financial support of the Science and Technology Commission of Shanghai Municipality, China.
The authors declare that the research was conducted in the absence of any commercial or financial relationships that could be construed as a potential conflict of interest.
All claims expressed in this article are solely those of the authors and do not necessarily represent those of their affiliated organizations, or those of the publisher, the editors and the reviewers. Any product that may be evaluated in this article, or claim that may be made by its manufacturer, is not guaranteed or endorsed by the publisher.
Adhikari, K., Mustapha, A., Grün, I. U., and Fernando, L. (2000). Viability of microencapsulated bifidobacteria in set yogurt during refrigerated storage. J. Dairy Sci. 83 (9), 1946–1951. doi:10.3168/jds.S0022-0302(00)75070-3
Alapan, Y., Yasa, O., Schauer, O., Giltinan, J., Tabak, A. F., Sourjik, V., et al. (2018). Soft erythrocyte-based bacterial microswimmers for cargo delivery. Sci. Robot. 3 (17), eaar4423. doi:10.1126/scirobotics.aar4423
Alberg, A. J., and Singh, S. (2001). Epidemiology of breast cancer in older women. Drugs & Aging 18 (10), 761–772. doi:10.2165/00002512-200118100-00005
Althuis, M. D., Dozier, J. M., Anderson, W. F., Devesa, S. S., and Brinton, L. A. (2005). Global trends in breast cancer incidence and mortality 1973-1997. Int. J. Epidemiol. 34 (2), 405–412. doi:10.1093/ije/dyh414
Anselmo, A. C., McHugh, K. J., Webster, J., Langer, R., and Jaklenec, A. (2016). Layer-by-layer encapsulation of probiotics for delivery to the microbiome. Adv. Mat. 28 (43), 9486–9490. doi:10.1002/adma.201603270
Arakawa, M., Sugiura, K., Reilly, H. C., and Stock, C. C. (1968). Oncolytic effect of Proteus mirabilis upon tumor-bearing animals. II. Effect on transplantable mouse and rat tumors. Gan 59 (2), 117–122.
Avogadri, F., Mittal, D., Saccheri, F ., Sarrafiore, M., Ciocca, P ., and Larghi, R. (2008). Intra-tumoral Salmonella T yphimurium induces a systemic anti-tumor response that is directed by low-dose radiation to treat distal disease. Eur. J. Immunol. 38 (7), 1937–1947. doi:10.1002/eji.200738035
Bhatnagar, P. K., Awasthi, A., Nomellini, J. F., Smit, J., and Suresh, M. R. (2006). Anti-tumor effects of the bacterium Caulobacter crescentus in murine tumor models. Cancer Biol. Ther. 5 (5), 485–491. doi:10.4161/cbt.5.5.2553
Călinoiu, L. F., Ștefănescu, B. E., Pop, I., Muntean, L. J., and Vodnar, D. C. (2019). Chitosan coating applications in probiotic microencapsulation. Coatings 9, 194. doi:10.3390/coatings9030194
Cao, Z., Cheng, S., Wang, X., Pang, Y., and Liu, J. (2019a). Camouflaging bacteria by wrapping with cell membranes. Nat. Commun. 10 (1), 3452. doi:10.1038/s41467-019-11390-8
Cao, Z., Wang, X., Pang, Y., Cheng, S., and Liu, J. (2019b). Biointerfacial self-assembly generates lipid membrane coated bacteria for enhanced oral delivery and treatment. Nat. Commun. 10 (1), 5783. doi:10.1038/s41467-019-13727-9
Chandramouli, V., Kailasapathy, K., Peiris, P., and Jones, M. (2004). An improved method of microencapsulation and its evaluation to protect Lactobacillus spp. in simulated gastric conditions. J. Microbiol. Methods 56 (1), 27–35. doi:10.1016/j.mimet.2003.09.002
Chang, W. W., and Lee, C. H. (2014). Salmonella as an innovative therapeutic antitumor agent. Int. J. Mol. Sci. 15 (8), 14546–14554. doi:10.3390/ijms150814546
Chen, F., Zang, Z., Chen, Z., Cui, L., Chang, Z., Ma, A., et al. (2019). Nanophotosensitizer-engineered Salmonella bacteria with hypoxia targeting and photothermal-assisted mutual bioaccumulation for solid tumor therapy. Biomaterials 214, 119226. doi:10.1016/j.biomaterials.2019.119226
Chen, H., Ouyang, W., Jones, M., Haque, T., Lawuyi, B., and Prakash, S. (2005). In-vitro analysis of APA microcapsules for oral delivery of live bacterial cells. J. Microencapsul. 22 (5), 539–547. doi:10.1080/02652040500162162
Chen, J., Qiao, Y., Tang, B., Chen, G., Liu, X., Yang, B., et al. (2017). Modulation of Salmonella tumor-colonization and intratumoral anti-angiogenesis by triptolide and its mechanism. Theranostics 7 (8), 2250–2260. doi:10.7150/thno.18816
Chen, W., Wang, Y., Qin, M., Zhang, X., Zhang, Z., Sun, X., et al. (2018). Bacteria-driven hypoxia targeting for combined biotherapy and photothermal therapy. ACS Nano 12 (6), 5995–6005. doi:10.1021/acsnano.8b02235
Chiang, C. J., and Huang, P. H. (2021). Metabolic engineering of probiotic Escherichia coli for cytolytic therapy of tumors. Sci. Rep. 11 (1), 5853. doi:10.1038/s41598-021-85372-6
Chowdhuri, S., Cole, C. M., and Devaraj, N. K. (2016). Encapsulation of living cells within giant phospholipid liposomes formed by the inverse-emulsion technique. Chembiochem 17 (10), 886–889. doi:10.1002/cbic.201500643
Coley, W. B. (1910). The treatment of inoperable sarcoma by bacterial toxins (the mixed toxins of the Streptococcus erysipelas and the Bacillus prodigiosus). Proc. R. Soc. Med. 3, 1–48. doi:10.1177/003591571000301601
Cook, M. T., Tzortzis, G., Charalampopoulos, D., and Khutoryanskiy, V. V. (2012). Microencapsulation of probiotics for gastrointestinal delivery. J. Control Release 162 (1), 56–67. doi:10.1016/j.jconrel.2012.06.003
Cook, M. T., Tzortzis, G., Charalampopoulos, D., and Khutoryanskiy, V. V. (2011). Production and evaluation of dry alginate-chitosan microcapsules as an enteric delivery vehicle for probiotic bacteria. Biomacromolecules 12 (7), 2834–2840. doi:10.1021/bm200576h
Crull, K., Bumann, D., and Weiss, S. (2011). Infuence of infection route and viru-lence factors on colonization of solid tumors by Salmonella enterica serovar T yphimurium. FEMS Immunol. Med. Micl 62 (1), 75–83. doi:10.1111/j.1574-695X.2011.00790.x
Curran, K. J., Pegram, H. J., and Brentjens, R. J. (2012). Chimeric antigen receptors for T cell immunotherapy: Current understanding and future directions. J. Gene Med. 14 (6), 405–415. doi:10.1002/jgm.2604
Dafe, A., Etemadi, H., Dilmaghani, A., and Mahdavinia, G. R. (2017). Investigation of pectin/starch hydrogel as a carrier for oral delivery of probiotic bacteria. Int. J. Biol. Macromol. 97, 536–543. doi:10.1016/j.ijbiomac.2017.01.060
Dang, L. H., Bettegowda, C., Huso, D. L., Kinzler, K. W., and Vogelstein, B. (2001). Combination bacteriolytic therapy for the treatment of experimental tumors. Proc. Natl. Acad. Sci. U. S. A. 98 (26), 15155–15160. doi:10.1073/pnas.251543698
De Vos, P., Van Straaten, J. F., Nieuwenhuizen, A. G., de Groot, M., Ploeg, R. J., De Haan, B. J., et al. (1999). Why do microencapsulated islet grafts fail in the absence of fibrotic overgrowth? Diabetes 48 (7), 1381–1388. doi:10.2337/diabetes.48.7.1381
Din, M. O., Danino, T., Prindle, A., Skalak, M., Selimkhanov, J., Allen, K., et al. (2016). Synchronized cycles of bacterial lysis for in vivo delivery. Nature 536 (7614), 81–85. doi:10.1038/nature18930
Ding, W. K., and Shah, N. P. (2009). An improved method of microencapsulation of probiotic bacteria for their stability in acidic and bile conditions during storage. J. Food Sci. 74 (2), M53–M61. doi:10.1111/j.1750-3841.2008.01030.x
Draget, K. I., Bræk, G. S., and Smidsrod, O. A. (1994). Alginic acid gels: The effect of alginate chemical composition and molecular weight. Carbohydr. Polym. 25, 31–38. doi:10.1016/0144-8617(94)90159-7
Ebrahimzadeh, S., Ahangari, H., Soleimanian, A., Hosseini, K., Ebrahimi, V ., Ghasemnejad, T., et al. (2021). Colorectal cancer treatment using bacteria: Focus on molecular mechanisms. BMC Microbiol. 21 (1), 218. doi:10.1186/s12866-021-02274-3
Evans, D. F., Pye, G., Bramley, R., Clark, A. G., Dyson, T. J., and Hardcastle, J. D. (1988). Measurement of gastrointestinal pH profiles in normal ambulant human subjects. Gut 29 (8), 1035–1041. doi:10.1136/gut.29.8.1035
Fan, J. X., Li, Z. H., Liu, X. H., Zheng, D. W., Chen, Y., and Zhang, X. Z. (2018). Bacteria-mediated tumor therapy utilizing photothermally-controlled TNF-α expression via oral administration. Nano Lett. 18 (4), 2373–2380. doi:10.1021/acs.nanolett.7b05323
Fathi, M., Akbari, B., and Taheriazam, A. (2019). Antibiotics drug release controlling and osteoblast adhesion from Titania nanotubes arrays using silk fibroin coating. Mater Sci. Eng. C Mater Biol. Appl. 103, 109743. doi:10.1016/j.msec.2019.109743
Felfoul, O., Mohammadi, M., Taherkhani, S., de Lanauze, D., Zhong, X. Y., Loghin, D., et al. (2016). Magneto-aerotactic bacteria deliver drug-containing nanoliposomes to tumour hypoxic regions. Nat. Nanotechnol. 11 (11), 941–947. doi:10.1038/nnano.2016.137
Feng, P., Cao, Z., Wang, X., Li, J ., and Liu, J. (2020). On-emand bacterial reactivation by restraining within a triggerable nanocoating. Adv. Mater 32 (34), e2002406. doi:10.1002/adma.202002406
Forbes, N. S. (2010). Engineering the perfect (bacterial) cancer therapy. Nat. Rev. Cancer 10 (11), 785–794. doi:10.1038/nrc2934
Gobin, A. S., Rhea, R., Newman, R. A., and Mathur, A. B. (2006). Silk-fibroin-coated liposomes for long-term and targeted drug delivery. Int. J. Nanomedicine 1 (1), 81–87. doi:10.2147/nano.2006.1.1.81
Goldberg, R. M., Rothenberg, M. L., Van Cutsem, E., Benson, A. B., Blanke, C. D., Diasio, R. B., et al. (2007). The continuum of care: A paradigm for the management of metastatic colorectal cancer. Oncology 12 (1), 38–50. doi:10.1634/theoncologist.12-1-38
Grierson, P., Lim, K. H., and Amin, M. (2017). Immunotherapy in gastrointestinal cancers. J. Gastrointest. Oncol. 8 (3), 474–484. doi:10.21037/jgo.2017.05.01
Hou, W., Li, J., Cao, Z., Lin, S., Pan, C., Pang, Y., et al. (2021). Decorating bacteria with a therapeutic nanocoating for synergistically enhanced biotherapy. Small 17 (37), e2101810. doi:10.1002/smll.202101810
Hu, C. M., Fang, R. H., Copp, J., Luk, B. T., and Zhang, L. (2013a). A biomimetic nanosponge that absorbs pore-forming toxins. Nat. Nanotechnol. 8 (5), 336–340. doi:10.1038/nnano.2013.54
Hu, C. M., Fang, R. H., Luk, B. T., and Zhang, L. (2013b). Nanoparticle-detained toxins for safe and effective vaccination. Nat. Nanotechnol. 8 (12), 933–938. doi:10.1038/nnano.2013.254
Hu, C. M., Zhang, L., Aryal, S., Cheung, C., Fang, R. H., and Zhang, L. (2011). Erythrocyte membrane-camouflaged polymeric nanoparticles as a biomimetic delivery platform. Proc. Natl. Acad. Sci. U. S. A. 108 (27), 10980–10985. doi:10.1073/pnas.1106634108
Jain, R. K. (1998). The next frontier of molecular medicine: Delivery of therapeutics. Nat. Med. 4 (6), 655–657. doi:10.1038/nm0698-655
Jemal, A., Siegel, R., Ward, E., Hao, Y ., Xu, J., Murray, T., et al. (2008). Cancer statistics, 2008. CA Cancer J. Clin. 58 (2), 71–96. doi:10.3322/CA.2007.0010
Johnson, S. A., Ormsby, M. J., Wessel, H. M., Hulme, H. E., Bravo-Blas, A., McIntosh, A., et al. (2021). Monocytes mediate Salmonella Typhimurium-induced tumor growth inhibition in a mouse melanoma model. Eur. J. Immunol. 51 (12), 3228–3238. doi:10.1002/eji.202048913
Jung, H. S., Verwilst, P., Sharma, A., Shin, J., Sessler, J. L., and Kim, J. S. (2018). Organic molecule-based photothermal agents: An expanding photothermal therapy universe. Chem. Soc. Rev. 47 (7), 2280–2297. doi:10.1039/c7cs00522a
Kefayat, A., Ghahremani, F., Motaghi, H., Rostami, S., and Mehrgardi, M. A. (2018). Alive attenuated Salmonella as a cargo shuttle for smart carrying of gold nanoparticles to tumor hypoxic regions. J. Drug Target 27 (3), 315–324. doi:10.1080/1061186X.2018.1523417
Keten, S., Xu, Z., Ihle, B., and Buehler, M. J. (2010). Nanoconfinement controls stiffness, strength and mechanical toughness of beta-sheet crystals in silk. Nat. Mater 9 (4), 359–367. doi:10.1038/nmat2704
Kim, B. J., Park, T., Moon, H. C., Park, S. Y., Hong, D., Ko, E. H., et al. (2014). Cytoprotective alginate/polydopamine core/shell microcapsules in microbial encapsulation. Angew. Chem. Int. Ed. 53, 14443–14446. doi:10.1002/anie.201408454
Kim, J., Muhammad, N., Jhun, B. H., and Yoo, J. W. (2016). Probiotic delivery systems: A brief overview. J. Pharm. Investig. 46 (4), 377–386. doi:10.1007/s40005-016-0259-7
Kim, S. H., Castro, F., Paterson, Y., and Gravekamp, C. (2009). High efficacy of a Listeria-based vaccine against metastatic breast cancer reveals a dual mode of action. Cancer Res. 69 (14), 5860–5866. doi:10.1158/0008-5472.CAN-5808-4855
Kocijancic, D., Leschner, S., Felgner, S., Komoll, R., Frahm, M., Pawar, V., et al. (2017). Therapeutic benefit of Salmonella attributed to LPS and TNF-α is exhaustible and dictated by tumor susceptibility. Oncotarget 8 (22), 36492–36508. doi:10.18632/oncotarget.16906
Kohwi, Y., Imai, K., Tamura, Z., and Hashimoto, Y. (1978). Antitumor effect of Bifidobacterium infantis in mice. Gan 69 (5), 613–618.
Krasaekoopt, W., Bhandari, B., and Deeth, H. (2004). The influence of coating materials on some properties of alginate beads and survivability of microencapsulated probiotic bacteria. Int. Dairy J. 14 (8), 737–743. doi:10.1016/J.IDAIRYJ.2004.01.004
Kulkarni, P. R., Yadav, J. D., and Vaidya, K. A. (2011). Liposomes: A novel drug delivery system. Int. J. Curr. Phar Res. 3 (2), 10–18.
Kulp, A., and Kuehn, M. J. (2010). Biological functions and biogenesis of secreted bacterial outer membrane vesicles. Annu. Rev. Microbiol. 64, 163–184. doi:10.1146/annurev.micro.091208.073413
Lai, W. F., and Lin, M. C. (2009). Nucleic acid delivery with chitosan and its derivatives. J. Control Release 134 (3), 158–168. doi:10.1016/j.jconrel.2008.11.021
Lamprecht, A., Schäfer, U., and Lehr, C. M. (2001). Size-dependent bioadhesion of micro- and nanoparticulate carriers to the inflamed colonic mucosa. Pharm. Res. 18 (6), 788–793. doi:10.1023/a:1011032328064
Lee, C. H., Lin, Y. H., Hsieh, J. L., Chen, M. C., and Kuo, W. L. (2013). A polymer coating applied to Salmonella prevents the binding of Salmonella-specific antibodies. Int. J. Cancer 132 (3), 717–725. doi:10.1002/ijc.277010.1002/ijc.27700
Lee, C. H., Nishikawa, T., and Kaneda, Y. (2017). Salmonella mediated the hemagglutinating virus of Japan-envelope transfer suppresses tumor growth. Oncotarget 8 (21), 35048–35060. doi:10.18632/oncotarget.17037
Lee, C. H., Wu, C. L., Tai, Y. S., and Shiau, A. L. (2005). Systemic administration of attenuated Salmonella choleraesuis in combination with cisplatin for cancer therapy. Mol. Ther. 11 (5), 707–716. doi:10.1016/j.ymthe.2005.01.008
Lee, K. Y., and Heo, T. R. (2000). Survival of Bifidobacterium longum immobilized in calcium alginate beads in simulated gastric juices and bile salt solution. Appl. Environ. Microbiol. 66 (2), 869–873. doi:10.1128/AEM.66.2.869-873.2000
LeRoux, M. A., Guilak, F., and Setton, L. A. (1999). Compressive and shear properties of alginate gel: Effects of sodium ions and alginate concentration. J. Biomed. Mater Res. 47 (1), 46–53. doi:10.1002/(sici)1097-4636(199910)47:1<46:aid-jbm6>3.0.co;2-n
Li, S., Jiang, W., Zheng, C., Shao, D., Liu, Y., Huang, S., et al. (2020). Oral delivery of bacteria: Basic principles and biomedical applications. J. Control Release 327, 801–833. doi:10.1016/j.jconrel.2020.09.011
Li, W., Luo, X., Song, R., Zhu, Y., Li, B., and Liu, S. (2016). Porous cellulose microgel particle: A fascinating host for the encapsulation, protection, and delivery of lactobacillus plantarum. J. Agric. Food Chem. 64 (17), 3430–3436. doi:10.1021/acs.jafc.6b00481
Li, Z., Behrens, A. M., Ginat, N., Tzeng, S. Y., Lu, X., Sivan, S., et al. (2018). Biofilm-inspired encapsulation of probiotics for the treatment of complex infections. Adv. Mater 30 (51), e1803925. doi:10.1002/adma.201803925
Li, Z., Wang, Y., Liu, J., Rawding, P., Bu, J., Hong, S., et al. (2021). Chemically and biologically engineered bacteria-based delivery systems for emerging diagnosis and advanced therapy. Adv. Mater 33 (38), 2102580. doi:10.1002/adma.202102580
Liang, K., Liu, Q., Li, P., Luo, H., Wang, H., and Kong, Q. (2019). Genetically engineered Salmonella Typhimurium: Recent advances in cancer therapy. Cancer Lett. 28 (448), 168–181. doi:10.1016/j.canlet.2019.01.037
Lin, J., Yu, W., Liu, X., Xie, H., Wang, W., and Ma, X. (2008). In vitro and in vivo characterization of alginate-chitosan-alginate artificial microcapsules for therapeutic oral delivery of live bacterial cells. J. Biosci. Bioeng. 105 (6), 660–665. doi:10.1263/jbb.105.660
Liu, Y., Ai, K., Liu, J., Deng, M., He, Y., and Lu, L. (2013). Dopamine-melanin colloidal nanospheres: An efficient near-infrared photothermal therapeutic agent for in vivo cancer therapy. Adv. Mater 25 (9), 1353–1359. doi:10.1002/adma.201204683
Lou, X., Chen, Z., He, Z., Sun, M., and Sun, J. (2021). Bacteria-mediated synergistic Cancer therapy: Small microbiome has a big hope. Nanomicro Lett. 13 (1), 37. doi:10.1007/s40820-020-00560-9
Luk, B. T., and Zhang, L. (2015). Cell membrane-camouflaged nanoparticles for drug delivery. J. Control Release 220, 600–607. doi:10.1016/j.jconrel.2015.07.019
Maletzki, C., Linnebacher, M., Kreikemeyer, B., and Emmrich, J. (2008). Pancreatic cancer regression by intratumoural injection of live Streptococcus pyogenes in a syngeneic mouse model. Gut 57 (4), 483–491. doi:10.1136/gut.2007.125419
Malmgren, R. A., and Flanigan, C. C. (1955). Localization of the vegetative form of Clostridium tetani in mouse tumors following intravenous spore administration. Cancer Res. 15 (7), 473–478. doi:10.1038/bjc.1955.49
Marofi, F., Motavalli, R., Safonov, V. A., Thangavelu, L., Yumashev, A. V., Alexander, M., et al. (2021). CAR T cells in solid tumors: Challenges and opportunities. Stem Cell Res. Ther. 12 (1), 81. doi:10.1186/s13287-13020-02128-1328110.1186/s13287-020-02128-1
Matthay, K. K., Heath, T. D., and Papahadjopoulos, D. (1984). Specific enhancement of drug delivery to AKR lymphoma by antibody-targeted small unilamellar vesicles. Cancer Res. 44 (5), 1880–1886.
Mei, L., He, F., Zhou, R. Q., Wu, C. D., Liang, R., Xie, R., et al. (2014). Novel intestinal-targeted Ca-alginate-based carrier for pH-responsive protection and release of lactic acid bacteria. ACS Appl. Mater Interfaces 6 (8), 5962–5970. doi:10.1021/am501011j
Minchinton, A. I., and Tannock, I. F. (2006). Drug penetration in solid tumours. Nat. Rev. Cancer 6 (8), 583–592. doi:10.1038/nrc1893
Minton, N. P. (2003). Clostridia in cancer therapy. Nat. Rev. Microbiol. 1 (3), 237–242. doi:10.1038/nrmicro777
Mirzaei, H. R., Mirzaei, H., Lee, S. Y., Hadjati, J., and Till, B. G. (2016). Prospects for chimeric antigen receptor (CAR) gammadelta T cells: A potential game changer for adoptive T cell cancer immunotherapy. Cancer Lett. 380 (2), 413–423. doi:10.1016/j.canlet.2016.07.001
Mukherjee, N., Julián, E., Torrelles, J. B., and Svatek, R. S. (2021). Effects of Mycobacterium bovis Calmette et Guérin (BCG) in oncotherapy: Bladder cancer and beyond. Vaccine 39 (50), 7332–7340. doi:10.1016/j.vaccine.2021.09.053
Netsomboon, K., and Bernkop-Schnürch, A. (2016). Mucoadhesive vs. mucopenetrating particulate drug delivery. Eur. J. Pharm. Biopharm. 98, 76–89. doi:10.1016/j.ejpb.2015.11.003
Nguyen, V. H., and Min, J. J. (2017). Salmonella-mediated cancer therapy: Roles and potential. Nucl. Med. Mol. Imaging 51 (2), 118–126. doi:10.1007/s13139-016-0415-z
Ouyang, W., Chen, H., Jones, M. L., Metz, T., Haque, T., Martoni, C., et al. (2004). Artificial cell microcapsule for oral delivery of live bacterial cells for therapy: Design, preparation, and in-vitro characterization. J. Pharm. Pharm. Sci. 7 (3), 315–324.
Pan, C., Li, J. J., Hou, W. L., Lin, S. S., Wang, L., Pang, Y., et al. (2021). Polymerization-mediated multifunctionalization of living cells for enhanced cell-based therapy. Adv. Mater 33 (13), e2007379. doi:10.1002/adma.202007379
Pan, L. X., Fang, X. J., Yu, Z., Xin, Y., Liu, X. Y., Shi, L. E., et al. (2013). Encapsulation in alginate-skim milk microspheres improves viability of Lactobacillus bulgaricus in stimulated gastrointestinal conditions. Int. J. Food Sci. Nutr. 64 (3), 380–384. doi:10.3109/09637486.2012.749841
Pan, Z. K., Weiskirch, L. M., and Paterson, Y. (1999). Regression of established B16F10 melanoma with a recombinant Listeria monocytogenes vaccine. Cancer Res. 59 (20), 5264–5269.
Paula, D. D., Martins, E. M. F., Costa, N. de., de Oliveira, P. M. de., de Oliveira, E. B. de., and Ramos, A. M. (2019). Use of gelatin and gum Arabic for microencapsulation of probiotic cells from Lactobacillus plantarum by a dual process combining double emulsification followed by complex coacervation. Int. J. Biol. Macromol. 133, 722–731. doi:10.1016/j.ijbiomac.2019.04.110
Pawelek, J. M., Low, K. B., and Bermudes, D. (1997). Tumor-targeted Salmonella as a novel anticancer vector. Cancer Res. 57 (20), 4537–4544.
Peirone, M. A., Delaney, K., Kwiecin, J., Fletch, A., and Chang, P. L. (1998). Delivery of recombinant gene product to canines with nonautologous microencapsulated cells. Hum. Gene Ther. 9 (2), 195–206. doi:10.1089/hum.1998.9.2-195
Pérez-Herrero, E., and Fernández-Medarde, A. (2015). Advanced targeted therapies in cancer: Drug nanocarriers, the future of chemotherapy. Eur. J. Pharm. Biopharm. 93, 52–79. doi:10.1016/j.ejpb.2015.03.018
Pour, M. M., Saberi-Riseh, R., Mohammadinejad, R., and Hosseini, A. (2019). Investigating the formulation of alginate- gelatin encapsulated Pseudomonas fluorescens (VUPF5 and T17-4 strains) for controlling Fusarium solani on potato. Int. J. Biol. Macromol. 133, 603–613. doi:10.1016/j.ijbiomac.2019.04.071
Prakash, S., and Jones, M. L. (2005). Artificial cell therapy: New strategies for the therapeutic delivery of live bacteria. J. Biomed. Biotechnol. 2005 (1), 44–56. doi:10.1155/jbb.2005.44
Raman, V., Van Dessel, N., Hall, C. L., Wetherby, V. E., Whitney, S. A., and Kolewe, E. L. (2021). Intracellular delivery of protein drugs with an autonomously lysing bacterial system reduces tumor growth and metastases. Nat. Commun. 12 (1), 6116. doi:10.1038/s41467-021-26367-9
Richardson, M. A., Ramirez, T., Russell, N. C., and Moye, L. A. (1999). Coley toxins immunotherapy: A retrospective review. Altern. Ther. Health Med. 5 (3), 42–47.
Rinaudo, M. (2008). Main properties and current applications of some polysaccharides as biomaterials. Polym. Int. 57 (3), 397–430. doi:10.1002/pi.2378
Rockwood, D. N., Preda, R. C., Yücel, T., Wang, X., Lovett, M. L., and Kaplan, D. L. (2011). Materials fabrication from Bombyx mori silk fibroin. Nat. Protoc. 6 (10), 1612–1631. doi:10.1038/nprot.2011.379
Saberi-Rise, R., and Moradi-Pour, M. (2020). The effect of Bacillus subtilis Vru1 encapsulated in alginate - bentonite coating enriched with titanium nanoparticles against Rhizoctonia solani on bean. Int. J. Biol. Macromol. 152, 1089–1097. doi:10.1016/j.ijbiomac.2019.10.197
Sadelain, M., Brentjens, R., and Rivière, I. (2013). The basic principles of chimeric antigen receptor design. Cancer Discov. 3 (4), 388–398. doi:10.1158/2159-8290.CD-12-0548
Sarotra, P., and Medhi, B. (2016). Use of bacteria in cancer therapy. Recent Results Cancer Res. 209, 111–121. doi:10.1007/978-3-319-42934-2_8
Scart, H., Cantin, J., and Levin, M. (2002). Clinical practice guidelines for the care and treatment of breast cancer: Mastectomy or lumpectomy? The choice of operation for clinicalstages I and II breast cancer (summary of the 2002 update). CMAJ 167 (2), 145–155.
Shi, C., Xing, Y., Patil, A., Meng, Z., Yu, R., Lin, N., et al. (2019). Primary and secondary mesoscopic hybrid materials of Au nanoparticles@Silk fibroin and applications. ACS Appl. Mater Interfaces 11 (33), 30125–30136. doi:10.1021/acsami.9b07846
Shi, L., Liu, X., Li, Y., Li, S., Wu, W., and Gao, X. (2022). Living bacteria-based immuno-photodynamic therapy: Metabolic labeling of Clostridium butyricum for eradicating malignant melanoma. Adv. Sci. 9 (14), e2105807. doi:10.1002/advs.202105807
Silva-Valenzuela, C. A., Desai, P. T., Molina-Quiroz, R. C., Pezoa, D., Zhang, Y., Porwollik, S., et al. (2016). Solid tumors provide niche-specific conditions that lead to preferential growth of Salmonella. Oncotarget 7 (23), 35169–35180. doi:10.18632/oncotarget.9071
Sohail, M. F., Rehman, M., Sarwar, H. S., Naveed, S., Salman, O., Bukhari, N. I., et al. (2018). Advancements in the oral delivery of docetaxel: Challenges, current state-of-the-art and future trends. Int. J. Nanomedicine 13, 3145–3161. doi:10.2147/IJN.S164518
Song, Q., Zheng, C., Jia, J., Zhao, H., Feng, Q., Zhang, H., et al. (2019). A Probiotic spore-based oral autonomous nanoparticles generator for cancer therapy. Adv. Mater 31 (43), e1903793. doi:10.1002/adma.201903793
St-Jean, A. T., Zhang, M., and Forbes, N. S. (2008). Bacterial therapies: Completing the cancer treatment toolbox. Curr. Opin. Biotechnol. 19 (5), 511–517. doi:10.1016/j.copbio.2008.08.004
Stokke, B. T., Drager, K. I., Yuguchi, Y., Urakawa, H., and Kajiwara, K. (1997). Small-angle X-ray scattering and rheological characterization of alginate gels. Macromol. Symp. 120 (1), 91–101. doi:10.1002/masy.19971200111
Sultana, K., Godward, G., Reynolds, N., Arumugaswamy, R., Peiris, P., and Kailasapathy, K. (2000). Encapsulation of probiotic bacteria with alginate-starch and evaluation of survival in simulated gastrointestinal conditions and in yoghurt. Int. J. Food Microbiol. 62 (1-2), 47–55. doi:10.1016/s0168-1605(00)00380-9
Sun, W., and Griffiths, M. W. (2000). Survival of bifidobacteria in yogurt and simulated gastric juice following immobilization in gellan-xanthan beads. Int. J. Food Microbiol. 61 (1), 17–25. doi:10.1016/s0168-1605(00)00327-5
Taherkhani, S., Mohammadi, M., Daoud, J., Martel, S., and Tabrizian, M. (2014). Covalent binding of nanoliposomes to the surface of magnetotactic bacteria for the synthesis of self-propelled therapeutic agents. ACS Nano 8 (5), 5049–5060. doi:10.1021/nn5011304
Tu, L., He, Y., Yang, H., Wu, Z., and Yi, L. (2015). Preparation and characterization of alginate-gelatin microencapsulated Bacillus subtilis SL-13 by emulsification/internal gelation. J. Biomater. Sci. Polym. Ed. 26 (12), 735–749. doi:10.1080/09205063.2015.1056075
Van Raamsdonk, J. M., and Chang, P. L. (2001). Osmotic pressure test: A simple, quantitative method to assess the mechanical stability of alginate microcapsules. J. Biomed. Mater Res. 54 (2), 264–271. doi:10.1002/1097-4636(200102)54:2<264:aid-jbm14>3.0.co;2-7
Vankayala, R., and Hwang, K. C. (2018). Near-infrared-light-activatable nanomaterial-mediated phototheranostic nanomedicines: An emerging paradigm for cancer treatment. Adv. Mater 30 (23), e1706320. doi:10.1002/adma.201706320
Vaupel, J. W., Villavicencio, F., and Bergeron-Boucher, M. P. (2021). Demographic perspectives on the rise of longevity. Pro Natl. Acad. Sci. U. S. A. 118 (9), e2019536118. doi:10.1073/pnas.2019536118
Wahab, S. M. A., Ahmed, M. A., Radwan, F. A., Hassan, R. M., and El-Refae, A. M. (1997). Relative permittivity and electrical conductivity of some divalent metal alginate complexes. Mater. Lett. 30 (2), 183–188. doi:10.1016/S0167-577X(96)00196-6
Wang, H., Wu, J., Williams, G. R., Fan, Q., Niu, S., Wu, J., et al. (2019). Platelet-membrane-biomimetic nanoparticles for targeted antitumor drug delivery. J. Nanobiotechnology 17 (1), 60. doi:10.1186/s12951-019-0494-y
Wee, S., and Gombotz, W. R. (1998). Protein release from alginate matrices. Adv. Drug Deliv. Rev. 31 (3), 267–285. doi:10.1016/s0169-409x(97)00124-5
West, C. L., Stanisz, A. M., Mao, Y. K., Champagne-Jorgensen, K., Bienenstock, J., and Kunze, W. A. (2020). Microvesicles from Lactobacillus reuteri (DSM-17938) completely reproduce modulation of gut motility by bacteria in mice. PLoS One 15 (1), e0225481. doi:10.1371/journal.pone.0225481
Wu, R. Y., Pasyk, M., Wang, B., Forsythe, P., Bienenstock, J., Mao, Y. K., et al. (2013). Spatiotemporal maps reveal regional differences in the effects on gut motility for Lactobacillus reuteri and rhamnosus strains. Neurogastroenterol. Motil. 25 (3), e205–214. doi:10.1111/nmo.12072
Wyld, L., Audisio, R. A., and Poston, G. J. (2015). The evolution of cancer surgery and future perspectives. Nat. Rev. Clin. Oncol. 12 (2), 115–124. doi:10.1038/nrclinonc.2014.191
Xie, S., Zhao, L., Song, X., Tang, M., Mo, C., and Li, X. (2017). Doxorubicin-conjugated Escherichia coli Nissle 1917 swimmers to achieve tumor targeting and responsive drug release. J. Control Release 268, 390–399. doi:10.1016/j.jconrel.2017.10.041
Yeung, T. W., Üçok, E. F., Tiani, K. A., McClements, D. J., and Sela, D. A. (2016). Microencapsulation in alginate and chitosan microgels to enhance viability of Bifidobacterium longum for oral delivery. Front. Microbiol. 7, 494. doi:10.3389/fmicb.2016.00494
Yoda, K., Sun, X., Kawase, M., Kubota, A., Miyazawa, K., Harata, G. M., et al. (2015). A combination of probiotics and whey proteins enhances anti-obesity effects of calcium and dairy products during nutritional energy restriction in aP2-agouti transgenic mice. Br. J. Nutr. 113 (11), 1689–1696. doi:10.1017/S0007114515000914
Zaloga, G. P. (2006). Parenteral nutrition in adult inpatients with functioning gastrointestinal tracts: Assessment of outcomes. Lancet 367 (9516), 1101–1111. doi:10.1016/S0140-6736(06)68307-4
Zhang, Y., Ji, W., He, L., Chen, Y., Ding, X., and Sun, Y. (2018). E. coli Nissle 1917-derived minicells for targeted delivery of chemotherapeutic drug to hypoxic regions for cancer therapy. Theranostics 8 (6), 1690–1705. doi:10.7150/thno.21575
Zhao, M., Yang, M., Li, X. M., Jiang, P., Baranov, E., Li, S., et al. (2005). Tumor-targeting bacterial therapy with amino acid auxotrophs of GFP-expressing Salmonella typhimurium. Proc. Natl. Acad. Sci. U. S. A. 8 (6), 755–760. doi:10.1073/pnas.0408422102
Zhou, L., Srisatjaluk, R., Justus, D. E., and Doyle, R. J. (1998). On the origin of membrane vesicles in gram-negative bacteria. FEMS Microbiol. Lett. 163 (2), 223–228. doi:10.1111/j.1574-6968.1998.tb13049.x
Zhu, C., Yang, Q., Lv, F., Liu, L., and Wang, S. (2013). Conjugated polymer-coated bacteria for multimodal intracellular and extracellular anticancer activity. Adv. Mater 25 (8), 1203–1208. doi:10.1002/adma.2012045501203-1208
Zhu, H., Cheng, P., Chen, P., and Pu, K. (2018a). Recent Progress in the development of near-infrared organic photothermal and photodynamic nanotherapeutics. Biomater. Sci. 6 (4), 746–765. doi:10.1039/c7bm01210a
Keywords: tumor therapy, tumor targeting, coating, bacteria, materials
Citation: Wang J, Guo N, Hou W and Qin H (2022) Coating bacteria for anti-tumor therapy. Front. Bioeng. Biotechnol. 10:1020020. doi: 10.3389/fbioe.2022.1020020
Received: 15 August 2022; Accepted: 01 September 2022;
Published: 15 September 2022.
Edited by:
Yunlei Zhang, Nanjing Medical University, ChinaReviewed by:
Zhongmin Geng,Qingdao Cancer Institute Qingdao University, ChinaCopyright © 2022 Wang, Guo, Hou and Qin. This is an open-access article distributed under the terms of the Creative Commons Attribution License (CC BY). The use, distribution or reproduction in other forums is permitted, provided the original author(s) and the copyright owner(s) are credited and that the original publication in this journal is cited, in accordance with accepted academic practice. No use, distribution or reproduction is permitted which does not comply with these terms.
*Correspondence: Ning Guo, Z3VvbmluZzE2QG1haWxzLnVjYXMuZWR1LmNu; Weiliang Hou, MjQ5MjUwNTA0QHFxLmNvbQ==; Huanlong Qin, aHVhbmxvbmdfcWluQGxpdmUuY24=
Disclaimer: All claims expressed in this article are solely those of the authors and do not necessarily represent those of their affiliated organizations, or those of the publisher, the editors and the reviewers. Any product that may be evaluated in this article or claim that may be made by its manufacturer is not guaranteed or endorsed by the publisher.
Research integrity at Frontiers
Learn more about the work of our research integrity team to safeguard the quality of each article we publish.