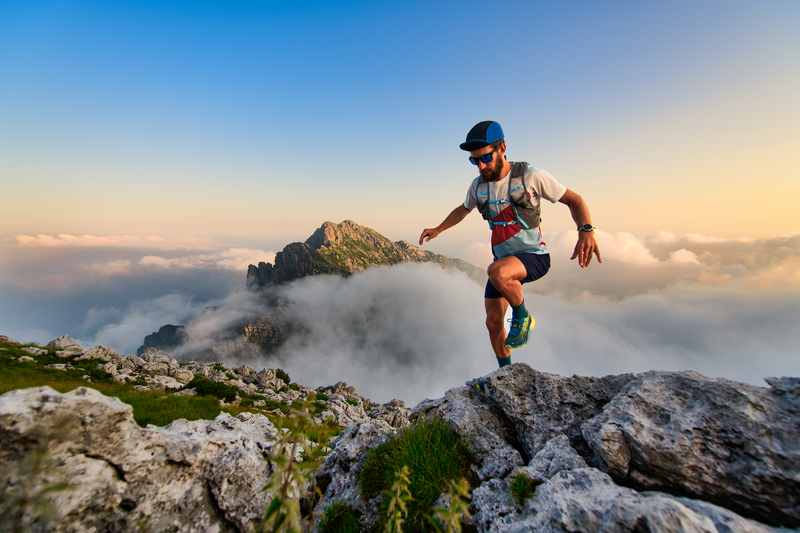
95% of researchers rate our articles as excellent or good
Learn more about the work of our research integrity team to safeguard the quality of each article we publish.
Find out more
ORIGINAL RESEARCH article
Front. Bioeng. Biotechnol. , 17 October 2022
Sec. Nanobiotechnology
Volume 10 - 2022 | https://doi.org/10.3389/fbioe.2022.1013541
This article is part of the Research Topic Nanotechnology and Bioengineering Platforms for Drug and Gene Delivery View all 13 articles
A retraction of this article was approved in:
Retraction: Targeted delivery of 5-fluorouracil, miR-532-3p, and si-KRAS to the colorectal tumor using layer-by-layer liposomes
Citation: Shahidi M, Abazari O, Dayati P, Haghiralsadat BF, Oroojalian F and Tofighi D (2022) Targeted delivery of 5-fluorouracil, miR-532-3p, and si-KRAS to the colorectal tumor using layer-by-layer liposomes. Front. Bioeng. Biotechnol. 10:1013541. doi: 10.3389/fbioe.2022.1013541
Received: 07 August 2022; Accepted: 22 September 2022;
Published: 17 October 2022; Retracted: 22 August 2024.
Edited by:
Gang Chen, University of Health and Rehabilitation Sciences, ChinaDisclaimer: All claims expressed in this article are solely those of the authors and do not necessarily represent those of their affiliated organizations, or those of the publisher, the editors and the reviewers. Any product that may be evaluated in this article or claim that may be made by its manufacturer is not guaranteed or endorsed by the publisher.
Research integrity at Frontiers
Learn more about the work of our research integrity team to safeguard the quality of each article we publish.